- 1Department of Cell Biology, Physiology, and Immunology, Faculty of Biosciences, Universitat Autonoma de Barcelona, Barcelona, Spain
- 2Centro de Biotecnología Acuícola, Facultad de Química y Biología, Universidad de Santiago de Chile, Santiago, Chile
- 3Facultad de Medicina Veterinaria y Agronomía, Universidad de Las Américas, Santiago, Chile
The stress response in fish is characterized by the activation of the HPI axis resulting in the release of cortisol. Previous studies in rainbow trout (Oncorhynchus mykiss) and gilthead sea bream (Sparus aurata) have shown that an acute stressor modulates the expression of immune- and stress-related genes in mucosal-associated lymphoid tissues (MALTs), particularly in the skin (SALT), gills (GIALT), and gut (GALT). However, there are no antecedents on whether the modulation on the mucosal transcriptomic profile is coordinated through the local presence of cortisol in the mucosal tissue surface. Thus, the aim of this study was to evaluate the effect of cortisol upon the modulation of a set of immune- (il-1β, il-6, tnf-α, and cox-2) and stress-related (hsp70, gr1) genes. For this purpose, tissue explants cultures were incubated with cortisol (100 ng/100 mg tissue) for 2-, 4-, and 24 h and the gene expression profile was evaluated at each time-point by real-time PCR. No differences were found in the gene expression between cortisol-incubated tissue explants and mock-incubated tissues in any of the time-points tested for both species. These results suggest that the quick modulation of the gene expression during the first 24 h after the exposure to stressor challenge reported in previous studies, is probably coordinated and mediated through a systemic-dependent mechanism but not through a peripheral/local response on mucosal tissue surfaces.
Introduction
The stress response is a physiological complex mechanism activated by a stimulus that is perceived by the organism as a potential threat (Tort, 2011). It involves a sum of sequential and coordinated activation mechanisms at different functional levels including molecular processes with direct repercussions on gene expression and protein synthesis that, at the end, will activate the responses from cellular to systemic and performance levels (Parra et al., 2015).
Once an external (i.e., fluctuation on water parameters, contaminants, xenobiotics, endocrine disruptors, crowding, and predator attack) or internal stimuli (i.e., homeostasis imbalance, an opportunistic pathogen, increases on the virus/bacterial load) is perceived as a stressor, this information is immediately transmitted to the central nervous system (CNS) for its processing. In case the stimulus is greater than the minimum threshold required, the activation of the Hypothalamus-Pituitary-Interrenal (HPI) and Sympathetic Adreno-Medullar (SAM) axes occurs. The SAM axis is characterized by the neuronal signaling to secrete catecholamines (adrenaline and noradrenaline) by chromaffin cells. This response is also known as the fight-or-flight reaction and it takes place in a matter of seconds to a few minutes (Schreck and Tort, 2016). On the other hand, the HPI axis activation is responsible for the synthesis of hypothalamic corticotrophin-releasing hormone (CRH) from the hypothalamic paraventricular nucleus (PVN), which in turn promotes the secretion of adrenocorticotropic hormone (ACTH) by the pituitary gland (Khansari et al., 2017a). The ACTH is then recognized by the melanocortin receptor 2 (MC2R) of the interrenal cells, thus activating the steroidogenic signaling pathway responsible for cortisol synthesis.
The fate of these two main stress hormones is diametrically different in terms of average lifetime in response to a stressor challenge. While catecholamines are rapidly cleared from circulation, cortisol level remains elevated within minutes to hours in the bloodstream (Vijayan et al., 2010), thus allowing interaction with cortisol receptors in most tissues and also an easier analytical assessment. For this reason, cortisol has been used as the most common physiological indicator of stress in fish (Schreck and Tort, 2016; Reyes-López et al., 2018). The main functions of cortisol include energy homeostasis, osmotic balance maintenance, and metabolic reorganization. In this way, cortisol modulates the normal function of other biological processes including the host defense through a delay or reduction of the immune response (Tort, 2011). There is a generalized consensus that immune suppressive mechanisms in response to stressors in teleost species is more typical of a chronic than an acute stress response (Sunyer and Tort, 1995; Vazzana et al., 2002; Verburg-van Kemenade et al., 2009; Tort, 2011; Schreck and Tort, 2016). This pattern of response is associated to continuous and long-time energy and biological requirements to cope with the stressor challenge (Tort, 2011). By contrast, when fish face up an acute short-term stressor it may happen that the pattern of response is preferentially stimulatory, activating particularly the innate humoral immunity (Sunyer and Tort, 1995; Demers and Bayne, 1997). However, other immune-related processes may not be significantly affected (Tort, 2011). Despite these antecedents, less consensus exists in regard to the effects of the acute stress response in fish. In fact, it has been shown that the magnitude and dynamics of cortisol secretion and the modulation of immune- and stress-related genes against the same stressor are different between different teleost species (Khansari et al., 2019).
In the last years, special attention has focused on the mucosal-associated lymphoid tissues (MALTs) because: (a) their role as a physical barrier in intimate contact with the aquatic environment (Parra et al., 2015); (b) their wide range of biological functions including immune response (Bakke et al., 2010; Nelson and Dehn, 2010; Zhang et al., 2011; Xu et al., 2013; Salinas, 2015); (c) their potential utilization as target tissues for the development of new vaccine administration strategies (Salinas et al., 2015); and (d) their use as an interesting alternative for the less-invasive sampling collection for the evaluation of fish health indicators (Rajan et al., 2013; Cordero et al., 2015; Sanahuja and Ibarz, 2015). In teleost species, four MALTs have been described: nose-associated lymphoid tissue (NALT), skin-associated lymphoid tissue (SALT), gill-associated lymphoid tissue (GIALT), and gut-associated lymphoid tissue (GALT) (Parra et al., 2015). These tissues have attracted increasing interest for the evaluation of different stress indicators, including cortisol, particularly skin mucus (Guardiola et al., 2016; Fernández-Alacid et al., 2018). Previous studies in rainbow trout (Oncorhynchus mykiss) and gilthead sea bream (Sparus aurata) have shown that an acute stressor is able to increase the cortisol in skin mucus (Khansari et al., 2018). Moreover, there is a correlation between plasmatic and skin mucus cortisol levels in the first 24 h after an intense activation of the HPI axis (Carbajal et al., 2019). Importantly, this acute response modulates the expression of MALT immune- and stress-related genes in time-, and species-specific manner (Khansari et al., 2018). Several studies have reported the local effect of cortisol on the modulation of immune- and stress-related genes in the head kidney because of its neuroendocrine function, as an effector organ in the HPI axis activation cascade, as well as for its role as lymphohematopoietic tissue (Castillo et al., 2009; Khansari et al., 2017b,c). Nonetheless, there is no antecedents on whether the modulation of the mucosal transcriptomic profile is coordinated through the local presence of cortisol on the mucosal tissue surface. Thus, the aim of this study was to evaluate the effect of cortisol upon the modulation of a set of immune- and stress-related genes in MALT, including skin (SALT), gills (GIALT), and gut (GALT). For this purpose, tissue explants cultures were incubated with cortisol for 2-, 4-, and 24 h and the gene expression profile was evaluated at each time-point. For this purpose, the study included two commercially relevant species that inhabit two distinct milieus: rainbow trout (a freshwater teleost) and gilthead sea bream (marine teleost), and that have been model species for the study of stress (chronic and acute) and the immune response (Tort, 2011; Balasch and Tort, 2019).
Materials and Methods
Fish
Juvenile rainbow trout (Oncorhynchus mykiss) (130 ± 10 g; ntotal = 36) and gilthead sea bream (Sparus aurata) (65 ± 5.0 g; ntotal = 36) were purchased from commercial hatcheries (TROUTFACTORY, Lleida, Spain; Aquicultura els ALFACS, S.L., Tarragona, Spain) and maintained at the AQUAB-Fish facility (Universitat Autónoma de Barcelona, Cerdanyola del Vallès, Spain). Fish were acclimatized to laboratory conditions for 21 days before the start of the experiment in conic tanks (2.0 m3 total volume capacity) with water pump, recirculating chiller cooling system, sand filter, and biofilter. Fish were maintained at a photoperiod of 12L:12D and at their respective environmental temperature (15°C for trout; 20°C for sea bream). Fish were fed a commercial pellet (Skretting) at 1.5% of total body weight/day. Water quality indicators (dissolved oxygen, ammonia, nitrite, and pH for both species; salinity in the case of sea bream) were analyzed and controlled periodically.
Ethics Statement
The experiment complied with the Guiding Principles for Biomedical Research Involving Animals (EU2010/63), the guidelines of the Spanish laws (law 32/2007 and RD 53/2013) and authorized by the Ethical Committee of the UAB CEEAH (Spain) for the use of laboratory animals (Ref. OH4218_4219).
Tissue Sampling
Rainbow trout (n = 6) and gilthead sea bream (n = 6) were euthanized by MS-222 (Sigma) overdose (200 mg/l). Before tissue sampling collection, blood was harvested in order to avoid the systemic influence of cortisol on mucosal tissues. After blood sampling, the same piece of skin, gills, and gut were taken from each fish according to Khansari et al. (2018) with minor modifications. Skin tissue samples were taken from the upper lateral line area behind the dorsal fin, left side, and roughly the same size. The skin samples were carefully taken to avoid muscle contamination. Thereafter, the body cavity was opened laterally and the gills were perfused with 1X Phosphate-buffered saline (PBS) (Sigma) according to Xu et al. (2016) in order to avoid blood contamination, thus discarding any systemic response influence on data. Then, gills (first lamella from the left side) were also sampled. For gut analysis, the body cavity was opened laterally, and the midgut and hindgut were sampled using a sterile scalpel and forceps. These harvested gut sections were open longitudinally and feces and mucus were carefully removed with forceps. Then, all the tissue sections were immediately transferred to 50-ml tubes and maintained with 20 ml DMEM – high glucose (Sigma; product num. 08168), and kept on ice.
Tissue Explants Culture and Incubation With Cortisol
The mucosal tissues (gills, skin, and gut) from each fish were divided into six sections of 100 mg each [one sample per experimental group (negative control and cortisol-incubated) and time-point evaluated (2-, 4-, and 24 h of incubation); ntotal = 6 fish for each fish species)]. Each piece of tissue was disposed individually in 24-well culture plate (Jet Biofil). All the samples were submerged in 70% ethanol for 20 sec to eliminate any potential bacteria present on the tissue surface, according to Xu et al. (2016). Then, the tissue was rinsed twice in 1X PBS for 2 min, and immediately placed in a new 24-well culture plate (Jet Biofil) and kept on ice with 1 ml DMEM - high glucose supplemented with 10% heat inactivated fetal bovine serum (Gibco) and penicillin (100 IU/l) per well until the beginning of the incubation with cortisol.
Cortisol (Hydrocortisone; Sigma cat #H2882-1G) was dissolved in appropriate solvent (absolute ethanol; Sigma-Aldrich cat #E7023) according to manufacturer’s instructions. Each mucosal tissue sample (gills, skin, and gut) was incubated with cortisol (100 ng/100 mg tissue/1 ml DMEM – high glucose supplemented) and incubated for 2-, 4-, and 24 h under optimal temperature culture conditions (16°C for rainbow trout; 18°C for sea bream; 5% CO2), according to Khansari et al., 2017b,c). The concentration of cortisol used in this current study was chosen because it corresponds to the plasma concentration level of acute stress-subjected fish (for rainbow trout and sea bream) (Castillo et al., 2008; Khansari et al., 2019). This concentration of cortisol has been previously used and its effect has been evaluated in similar approaches (Castillo et al., 2009; Kelly and Chasiotis, 2011; Khansari et al., 2017b,c). The time-points analyzed in this current study were chosen based on the highest cortisol effect on in vitro cell cultures (2 h post-incubation) (Castillo et al., 2009), the recovery limit time period for exogenously elevated cortisol levels (4 h post-incubation) (Algera et al., 2017), and the time established as accepted limit for the recovery of a situation of acute stress of high intensity and short duration (Khansari et al., 2018, 2019; Carbajal et al., 2019). As negative control, the mucosal tissue was mock-incubated under the same conditions. After treatment, the whole tissue was immediately frozen to −80°C until the RNA extraction.
Total RNA Extraction and Complementary DNA Synthesis
Total RNA extraction was carried out from the whole frozen MALT using 1 ml of TriReagent (Sigma, cat#T9424) following manufacturer’s instructions. Total RNA concentration was determined by NanoDrop-1000 spectrophotometer (Thermo Scientific, Waltham, MA, United States) and the integrity was measured by Agilent 2100 Bioanalyzer (Agilent Technologies, Santa Clara, CA, United states). Only those samples with RIN values > 7.5 were chosen for gene expression analysis. One μg of total RNA from all samples were used to synthesize cDNA with High-Capacity cDNA Reverse Transcription Kit (Applied Biosystems) according to the manufacturer’s instructions. The cDNA was used as template for absolute quantification by real-time PCR (qRT-PCR) expression analysis.
Gene Expression Analysis
Real-time PCR (qRT-PCR) was carried out in rainbow trout and sea bream MALTs to analyze the expression pattern of genes associated to pro- (il-1β, il-6, tnf-α, cox-2) and anti-inflammatory response (il-10, tgf-β), and stress response [gr isoform 1 (gr1), hsp-70]. The details about specific primers used for all genes tested including thermal conditions and reagent concentrations, are indicated in Khansari et al. (2017b,2018). Real-time PCR was carried out using 1:20 as optimum cDNA dilution for each tissue based on the set of serial dilutions contained in the primer efficiency curve. All reactions were performed using Bio-Rad CFX384 Real-Time PCR Detection System (Bio-Rad Laboratories, Hercules, CA, United States) and data output was obtained from Bio-Rad CFX Manager software (version 3.1). We tested several candidates for reference genes in rainbow trout (ef1α and β-actin) and sea bream (18s, ef1α, and rpl27) to elucidate which one had less variation. β-Actin (for rainbow trout) and 18s (for sea bream) was included on gene expression analysis. Quantification was done according to Pfaffl (2001) corrected by each primer efficiency using a specific reference gene for each species (β-actin for rainbow trout; 18S for sea bream) and normalized to the control group.
Statistical Analysis
Assumptions were tested for normality (D’Agostino-Pearson normality test), and homogeneity of variance (Spearman test for homoscedasticity). Two-way ANOVA and Sidak’s post-test were used to explore differences in each time-point between the control group and cortisol treatment for each MALT tissue assessed. GraphPad software v6.0 was used to calculate the mean and standard error (SEM), and to perform statistical tests. In the analyses, a p-value < 0.05 was considered statistically significant.
Results
In order to evaluate the cortisol local effect on gills-, skin-, and gut-associated lymphoid tissues, the tissue explants were incubated with the hormone. No differences were found between cortisol-incubated explants and mock-incubated tissues for the expression of genes associated to immune (pro- [il-1β, il-6, tnf-α, cox-2] and anti-inflammatory cytokines [il-10, tgf-β]) or stress response [gr isoform 1 (gr1), hsp-70] for any of the time-points tested (2-, 4-, 24 h of incubation with cortisol) in rainbow trout gills (Figure 1A), skin (Figure 1B), nor gut (Figure 1C). No differences were either registered for gilthead sea bream gills (Figure 1D), skin (Figure 1E), nor gut (Figure 1F). The p-values obtained from post-hoc Sidak’s test for comparison of NRE values between control and cortisol treatment is listed in Supplementary Table 1. These results suggest that exogenous cortisol has no local effect upon the modulation of the genes tested in gills, skin, nor gut mucosal tissues.
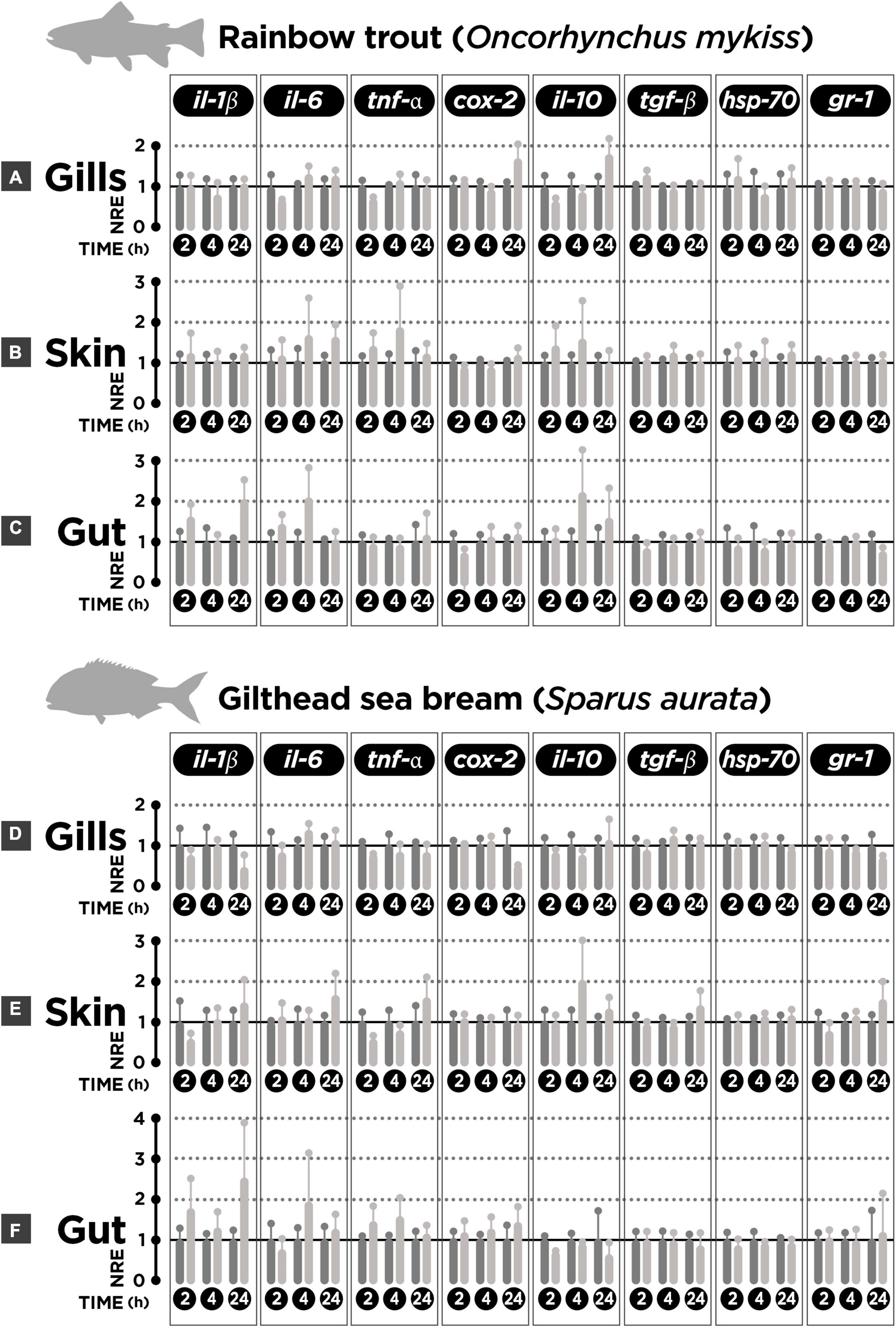
Figure 1. Normalized relative expression (NRE) in rainbow trout (n = 6) and gilthead sea bream (n = 6) skin-(SALT), gills-(GIALT), and gut-associated lymphoid tissue (GALT) explants incubated with cortisol. (A) Rainbow trout gills. (B) Rainbow trout skin. (C) Rainbow trout gut. (D) Sea bream gills. (E) Sea bream skin. (F) Sea bream gut. The gene expression pattern for pro- (il-1β, il-6, tnf-α, and cox-2) and anti-inflammatory cytokines (il-10, tgf-β), and stress-related genes (hsp70, gr1) after 2, 4, and 24 h of incubation with cortisol. Bars represent mean ± SEM (n = 6 fish per condition and time-point assessed). For each time-point, the left bars represents the mock-incubated tissue explant (control); the right bar represents the tissue explant incubated with cortisol. Two-way ANOVA and Sidak’s post-test was used to explore differences (p-value < 0.05) in each time-point between mock-incubated (control) and cortisol treatment groups for each MALT tissue assessed. No significant differences were obtained (p-value > 0.05).
Discussion
In this study, the local effect of cortisol upon the modulation of immune- and stress-related genes in gills (GIALT), skin (SALT), and gut (GALT) was evaluated. Our results clearly show that cortisol by itself does not significantly modulate the expression of the genes evaluated in mucosal surfaces of either rainbow trout and gilthead sea bream at any of the time-points tested (2-, 4-, and 24 h post-incubation) compared to the mock-incubated group. These results suggest that the individual, isolated, and local action of cortisol on MALTs is not sufficient to promote the modulation of the genes tested in this study, and therefore that the relevant physiological modulation would be probably performed through the HPI systemic axis.
It has been well documented that in most fishes, a plasmatic concentration of 100 ng/ml of cortisol is associated with an acute stress response event (Castillo et al., 2008; Khansari et al., 2018, 2019). This concentration is currently measured in the first hour after subjecting rainbow trout to stressors of different nature, or a few hours post-stress (6 h in the case of sea bream) (Khansari et al., 2018, 2019). For this reason, it is not surprising that in several studies the concentration of 100 ng/ml of cortisol was used to evaluate local effects on the modulation of genes associated with immune and stress response (Castillo et al., 2009; Khansari et al., 2017b,c). The local effect of cortisol has been evaluated in the head kidney because (1) its endocrine function associated to the secretion of cortisol and catecholamines; (2) its role in immunity as lymphopoietic tissue; and (3) its role in preserving the physiological and energetic status due to its responsibility as hematopoietic tissue. Previous work showed that the incubation of sea bream head kidney primary cell culture (HKPCC) with 100 ng/ml cortisol induced a down-regulatory effect on the expression of pro- (il-1β, il-6, tnfα) and anti-inflammatory cytokines (il-10, tgfβ) after 2 h (Castillo et al., 2009; Khansari et al., 2017b). These antecedents indicate a high influence of cortisol on the expression of pro- and anti-inflammatory cytokines. However, no regulatory effects upon the expression of those genes were observed in rainbow trout HKPCC incubated with cortisol (100 ng/ml) (Khansari et al., 2017b), suggesting differential sensitivity to cortisol at local level between rainbow trout and sea bream.
It is widely accepted that the perception of a stressor is a centralized mechanism that generates a coordinated response. The stimulus is perceived by sensory elements of the CNS that, once processed and depending on its magnitude and intensity, may conduct to the activation of the HPI axis and the subsequent release of corticosteroids to the bloodstream. These stress hormones, in turn, will be responsible for the effector response at the periphery. At the systemic level, the increase of plasma cortisol has been associated to the in vivo modulation of some genes involved in stress and immunity. In comparative analyses between rainbow trout and sea bream subjected to acute stressors, a differential expression pattern was observed both in magnitude and also response kinetics in the liver and spleen (Khansari et al., 2019). These antecedents suggest that the differential stress reactions among fishes may depend not only on the stressor intensity but on the environmental factors (i.e., salinity and thermopreferendum) and species specificities. In addition, sensitivity to stressors may also depend on the individual response or coping strategy, i. e., a proactive versus reactive response (Vargas et al., 2018). This suggests that more comparative approaches are necessary among teleost species in order to assess the short-term responsiveness to stressors and their eventual common characteristics associated to the acute stress response.
Because of the potential stressor effect of blood sampling, the interest in applying less-invasive sampling techniques associated to welfare issues, and the specific time presence (minutes to hours) of cortisol in the bloodstream (Vijayan et al., 2010), new strategies have been explored to identify cortisol in other biological matrices than in plasma. In this way, cortisol has been measured in the skin mucus of trout and sea bream (Guardiola et al., 2016; Fernández-Alacid et al., 2018; Khansari et al., 2018; Carbajal et al., 2019). Along with the cortisol increased levels both in plasma and skin mucus after acute stress, differences between rainbow trout and sea bream were observed on the expression pattern of genes associated to immune and stress response in gills (il-6, il-10, tgf-β, hsp70), skin (il-6, tnf-α, il-10, tgf-β, hsp70), and gut mucosal tissues (il-6, il-10, hsp70). By contrast, only the upregulation of il-1β and cox-2 showed the same expression pattern in all these mucosal surfaces (Khansari et al., 2018). In gills the upregulation of tnf-α was also registered, while in the gut the upregulation of il-1β, cox-2, and tnf-α was accompanied with the upregulation of tgf-β (Khansari et al., 2018). This differential modulation of genes in mucosal tissues could be directly associated with the systemic (bloodstream) cortisol increase and, in consequence, with the concomitant modulation of the physiological response on the whole organism to recover homeostasis. On the other hand, the presence of cortisol in the mucus matrix could influence the subsequent immediate modulation of the transcriptional machinery. However, in our study, no modulatory local effect of cortisol was observed on a set of immune (il-1β, il-6, tnf-α, cox-2, il-10, tgf-β) nor stress genes (hsp70, gr-1) after incubating mucosal tissue explants with cortisol (100 ng). The absence of the direct effect of cortisol on the transcriptomic tissue explant profile suggests that cortisol is not able to carry out a relevant direct and local influence on mucosal tissues. This antecedent, together with the previous report (Khansari et al., 2018) indicating a modulatory gene expression effect after acute stress in rainbow trout and gilthead sea bream mucosal tissues, suggest that the expression profile associated to stress response in mucosal surfaces would be more influenced by the sum of systemic mechanisms activated in response to a stressor challenge rather than the direct exposure to the exogenous cortisol (Figure 2).
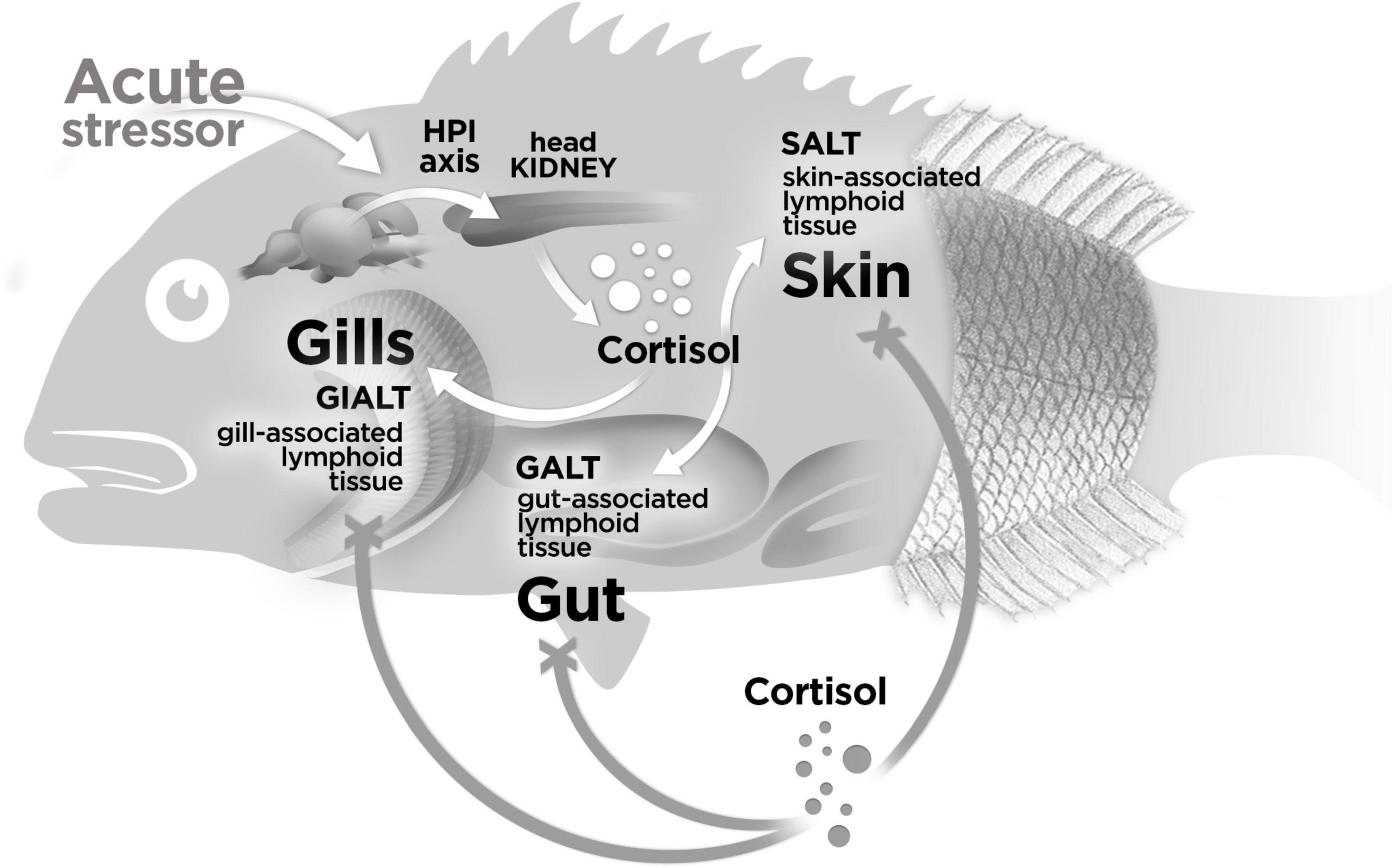
Figure 2. Model of response for the modulation of genes in MALT in response to cortisol. In our study, no effect was registered on gills-(GIALT), skin-(SALT), and gut-associated lymphoid tissue (GALT) explants incubated with exogenous cortisol (represented with gray spheres) upon the modulation of a set of immune- and stress-related genes (represented with a “X” instead an arrow head). In a previous study (23) has been reported that the endogenous cortisol released (represented with white spheres) at the first 24 h in response to a stressor challenge modulates the expression of genes associated to immune and stress response in the same MALTs evaluated in this current study. These antecedents suggest that the modulation of genes on mucosal surfaces associated to a stress response is mainly influenced by the sum of systemic mechanisms activated in response to a stressor challenge but not by the direct exposure of the exogenous cortisol. The fish representation (gilthead sea bream) is merely explicative and also applicable for rainbow trout.
In summary, our results suggest that the quick modulation of the gene expression in the first 24 h after the exposure to stressor challenge reported in previous studies (Khansari et al., 2018) is probably coordinated and mediated through a systemic dependent mechanism but not so through a peripheral local response on mucosal tissues surfaces.
Data Availability Statement
The original contributions presented in the study are included in the article/Supplementary Material, further inquiries can be directed to the corresponding author.
Ethics Statement
The animal study was reviewed and approved by the UAB CEEAH (Spain) for the use of laboratory animals (Reference number OH4218_4219).
Author Contributions
EV-V, AK, LT, and FER-L performed the conceptualization of the experiment. EV-V, AK, LS-D, and LT carried out the experiments. EV-V and FER-L performed the data analysis. EV-V, JCB, LT, and FER-L conceptualized the figures. EV-V, LT, and FER-L wrote the original draft. All authors participated in the interpretation of the results and corrected, read, and approved the final manuscript.
Funding
This study was funded by the AGL2016-76069-C2-2-R and PID2020-117557RB-C21, AEI-MINECO (Spain) grants. EV-V thanks the support of Fondecyt iniciación grant (project number 11221308; Agencia Nacional de Investigación y Desarrollo de Chile, Government of Chile). AK was recipient of a Ministry of Science, Research, and Technology (Iran) fellowship. FER-L thanks the support of Fondecyt regular grant (project number: 1211841; Agencia Nacional de Investigación y Desarrollo de Chile, Government of Chile).
Conflict of Interest
The authors declare that the research was conducted in the absence of any commercial or financial relationships that could be construed as a potential conflict of interest.
Publisher’s Note
All claims expressed in this article are solely those of the authors and do not necessarily represent those of their affiliated organizations, or those of the publisher, the editors and the reviewers. Any product that may be evaluated in this article, or claim that may be made by its manufacturer, is not guaranteed or endorsed by the publisher.
Acknowledgments
We thank Joan Baró (Trout Factory) for the trout supply.
Supplementary Material
The Supplementary Material for this article can be found online at: https://www.frontiersin.org/articles/10.3389/fmars.2022.828050/full#supplementary-material
References
Algera, D. A., Brownscombe, J. W., Gilmour, K. M., Lawrence, M. J., Zolderdo, A. J., and Cooke, S. J. (2017). Cortisol treatment affects locomotor activity and swimming behaviour of male smallmouth bass engaged in paternal care: a field study using acceleration biologgers. Physiol. Behav. 181, 59–68. doi: 10.1016/j.physbeh.2017.08.026
Bakke, A. M., Glover, C., and Krogdahl, Å (2010). Feeding, digestion and absorption of nutrients. Fish Physiol. 30, 57–110. doi: 10.1016/S1546-5098(10)03002-5
Balasch, J. C., and Tort, L. (2019). Netting the Stress Responses in Fish. Front. Endocrinol. 10:62. doi: 10.3389/fendo.2019.00062
Carbajal, A., Reyes-López, F. E., Tallo-Parra, O., Lopez-Bejar, M., and Tort, L. (2019). Comparative assessment of cortisol in plasma, skin mucus and scales as a measure of the hypothalamic-pituitary-interrenal axis activity in fish. Aquaculture 506, 410–416. doi: 10.1016/j.aquaculture.2019.04.005
Castillo, J., Castellana, B., Acerete, L., Planas, J. V., Goetz, F. W., Mackenzie, S., et al. (2008). Stress-induced regulation of steroidogenic acute regulatory protein expression in head kidney of Gilthead seabream (Sparus aurata). J. Endocrinol. 196, 313–322. doi: 10.1677/JOE-07-0440
Castillo, J., Teles, M., Mackenzie, S., and Tort, L. (2009). Stress-related hormones modulate cytokine expression in the head kidney of gilthead seabream (Sparus aurata). Fish Shellfish Immunol. 27, 493–499. doi: 10.1016/j.fsi.2009.06.021
Cordero, H., Brinchmann, M. F., Cuesta, A., Meseguer, J., and Esteban, M. A. (2015). Skin mucus proteome map of European sea bass (Dicentrarchus labrax). Proteomics 15, 4007–4020. doi: 10.1002/pmic.201500120
Demers, N. E., and Bayne, C. J. (1997). The immediate effects of stress on hormones and plasma lysozyme in rainbow trout. Dev. Comp. Immunol. 21, 363–373. [pii], doi: 10.1016/s0145-305x(97)00009-8
Fernández-Alacid, L., Sanahuja, I., Ordóñez-Grande, B., Sánchez-Nuño, S., Viscor, G., Gisbert, E., et al. (2018). Skin mucus metabolites in response to physiological challenges: a valuable non-invasive method to study teleost marine species. Sci. Total Environ. 644, 1323–1335. doi: 10.1016/j.scitotenv.2018.07.083
Guardiola, F. A., Cuesta, A., and Esteban, M. Á (2016). Using skin mucus to evaluate stress in gilthead seabream (Sparus aurata L.). Fish Shellfish Immunol. 59, 323–330. doi: 10.1016/j.fsi.2016.11.005
Kelly, S. P., and Chasiotis, H. (2011). Glucocorticoid and mineralocorticoid receptors regulate paracellular permeability in a primary cultured gill epithelium. J. Exp. Biol. 214, 2308–2318. doi: 10.1242/jeb.055962
Khansari, A. R., Balasch, J. C., Reyes-lópez, F. E., and Tort, L. (2017a). Stressing the Inflammatory Network?: immuno-endocrine Responses to Allostatic Load in Fish. J. Mar. Sci. Res. Technol. 1:2.
Khansari, A. R., Parra, D., Reyes-López, F. E., and Tort, L. (2017b). Cytokine modulation by stress hormones and antagonist specific hormonal inhibition in rainbow trout (Oncorhynchus mykiss) and gilthead sea bream (Sparus aurata) head kidney primary cell culture. Gen. Comp. Endocrinol. 250, 122–135. doi: 10.1016/j.ygcen.2017.06.005
Khansari, A. R., Parra, D., Reyes-López, F. E., and Tort, L. (2017c). Modulatory in vitro effect of stress hormones on the cytokine response of rainbow trout and gilthead sea bream head kidney stimulated with Vibrio anguillarum bacterin. Fish Shellfish Immunol. 70, 736–749. doi: 10.1016/j.fsi.2017.09.009
Khansari, A. R., Balasch, J. C., Vallejos-Vidal, E., Parra, D., Reyes-López, F. E., and Tort, L. (2018). Comparative Immune- and Stress-Related Transcript Response Induced by Air Exposure and Vibrio anguillarum Bacterin in Rainbow Trout (Oncorhynchus mykiss) and Gilthead Seabream (Sparus aurata) Mucosal Surfaces. Front. Immunol. 9:856. doi: 10.3389/fimmu.2018.00856
Khansari, A. R., Balasch, J. C., Vallejos-Vidal, E., Teles, M., Fierro-Castro, C., Tort, L., et al. (2019). Comparative study of stress and immune-related transcript outcomes triggered by Vibrio anguillarum bacterin and air exposure stress in liver and spleen of gilthead seabream (Sparus aurata), zebrafish (Danio rerio) and rainbow trout (Oncorhynchus mykiss). Fish Shellfish Immunol. 86, 436–448. doi: 10.1016/j.fsi.2018.11.063
Nelson, J. A., and Dehn, A. M. (2010). The GI tract in air breathing. First Edit. Elsevier Inc. 30, 395–433. doi: 10.1088/0031-8949/2005/T121/007
Parra, D., Reyes-Lopez, F. E., and Tort, L. (2015). Mucosal Immunity and B Cells in Teleosts: effect of Vaccination and Stress. Front. Immunol. 6:354. doi: 10.3389/fimmu.2015.00354
Pfaffl, M. W. (2001). A new mathematical model for relative quantification in real-time RT-PCR. Nucleic Acids Res. 29:e45. doi: 10.1093/nar/29.9.e45
Rajan, B., Lokesh, J., Kiron, V., and Brinchmann, M. F. (2013). Differentially expressed proteins in the skin mucus of Atlantic cod (Gadus morhua) upon natural infection with Vibrio anguillarum. BMC Vet. Res. 9:103. doi: 10.1186/1746-6148-9-103
Reyes-López, F. E., Aerts, J., Vallejos-Vidal, E., Ampe, B., Dierckens, K., Tort, L., et al. (2018). Modulation of Innate Immune- Related Genes and Glucocorticoid Synthesis in Gnotobiotic Full-Sibling European Sea Bass (Dicentrarchus labrax) Larvae Challenged With Vibrio anguillarum. Front. Immunol. 9:914. doi: 10.3389/fimmu.2018.00914
Salinas, I. (2015). The Mucosal Immune System of Teleost Fish. Biology 4, 525–539. doi: 10.3390/biology4030525
Salinas, I., LaPatra, S. E., and Erhardt, E. B. (2015). Nasal vaccination of young rainbow trout (Oncorhynchus mykiss) against infectious hematopoietic necrosis and enteric red mouth disease. Dev. Comp. Immunol. 53, 105–111. doi: 10.1016/j.dci.2015.05.015
Sanahuja, I., and Ibarz, A. (2015). Skin mucus proteome of gilthead sea bream: a non-invasive method to screen for welfare indicators. Fish Shellfish Immunol. 46, 426–435. doi: 10.1016/j.fsi.2015.05.056
Schreck, C. B., and Tort, L. (2016). “The concept of stress in fish”, in Biology of Stress in Fish. First edit, eds A. P. Farrell and C. J. Brauner (London: Academic Press).
Sunyer, J. O., and Tort, L. (1995). Natural hemolytic and bactericidal activities of sea bream Sparus aurata serum are effected by the alternative complement pathway. Vet. Immunol. Immunopathol. 45, 333–345.
Tort, L. (2011). Stress and immune modulation in fish. Dev. Comp. Immunol. 35, 1366–1375. doi: 10.1016/j.dci.2011.07.002
Vargas, R., Balasch, J. C., Brandts, I., Reyes-López, F., Tort, L., and Teles, M. (2018). Variations in the immune and metabolic response of proactive and reactive Sparus aurata under stimulation with Vibrio anguillarum vaccine. Sci. Rep. 8:17352. doi: 10.1038/s41598-018-35863-w
Vazzana, M., Cammarata, M., Cooper, E. L., and Parrinello, N. (2002). Confinement stress in sea bass (Dicentrarchus labrax) depresses peritoneal leukocyte cytotoxicity. Aquaculture 210, 231–243. doi: 10.1016/S0044-8486(01)00818-3
Verburg-van Kemenade, B. M. L., Stolte, H. H., Metz, J. R., and Chadzinska, M. K. (2009). “Neuroendocrine-immune interactions in teleost fish,” in Fish Neuroendocrinology Fish Physiology?: 28, eds N. J. Bernier, G. Van der Kraak, A. P. Farrell, and C. J. Brauner (London: Academic Press), 313–364. doi: 10.1016/s1546-5098(09)28007-1
Vijayan, M. M., Aluru, N., and Leatherland, J. F. (2010). “Stress response and the role of cortisol. Vol 2: non-Infectious Disorders,” in Fish Diseases and Disorders, eds J. F. Leatherland and P. Woo (Oxfordshire: CAB), 182–201. doi: 10.1079/9781845935535.0182
Xu, Z., Parra, D., Gómez, D., Salinas, I., Zhang, Y.-A., Jørgensen, L. V. G., et al. (2013). Teleost skin, an ancient mucosal surface that elicits gut-like immune responses. Proc. Natl. Acad. Sci. U.S.A. 110, 13097–13102. doi: 10.1073/pnas.1304319110
Xu, Z., Takizawa, F., Parra, D., Gómez, D., Jørgensen, L. V. G., LaPatra, S. E., et al. (2016). Mucosal immunoglobulins at respiratory surfaces mark an ancient association that predates the emergence of tetrapods. Nat. Commun. 7:10728. doi: 10.1038/ncomms10728
Keywords: glucocorticoids, fish stress, immune response, gene expression, mucosal tissues, fish physiology, teleosts
Citation: Vallejos-Vidal E, Khansari AR, Soliva-Dueso L, Balasch JC, Tort L and Reyes-López FE (2022) The Direct Exposure of Cortisol Does Not Modulate the Expression of Immune-Related Genes on Tissue Explants of Mucosal Surfaces in Rainbow Trout (Oncorhynchus mykiss) Nor in Gilthead Sea Bream (Sparus aurata). Front. Mar. Sci. 9:828050. doi: 10.3389/fmars.2022.828050
Received: 02 December 2021; Accepted: 31 January 2022;
Published: 08 March 2022.
Edited by:
Benjamin Costas, University of Porto, PortugalReviewed by:
Mahmoud A. O. Dawood, Kafrelsheikh University, EgyptLuis Vargas-Chacoff, Austral University of Chile, Chile
Copyright © 2022 Vallejos-Vidal, Khansari, Soliva-Dueso, Balasch, Tort and Reyes-López. This is an open-access article distributed under the terms of the Creative Commons Attribution License (CC BY). The use, distribution or reproduction in other forums is permitted, provided the original author(s) and the copyright owner(s) are credited and that the original publication in this journal is cited, in accordance with accepted academic practice. No use, distribution or reproduction is permitted which does not comply with these terms.
*Correspondence: Felipe E. Reyes-López, RmVsaXBlLlJleWVzQHVhYi5jYXQ=; ZmVsaXBlLnJleWVzLmxAdXNhY2guY2w=
†These authors have contributed equally to this work and share first authorship