- 1University of Coimbra, Centre for Functional Ecology, Department of Life Sciences, Coimbra 3000-456, Portugal
- 2INRAE, Université de Pau et des Pays de l’Adour, E2S UPPA, NuMéA, Saint-Pée-sur-Nivelle, France
- 3Center for Neuroscience and Cell Biology, University of Coimbra, Coimbra, Portugal
- 4CIVG – Vasco da Gama Research Center, University School Vasco da Gama – EUVG, Coimbra, Portugal
- 5Interdisciplinary Centre of Marine and Environmental Research (CIIMAR), University of Porto, Matosinhos, Portugal
Glycerol is the by-product of biodiesel production and its utilisation in fish feed has recently gained relevance. As an important metabolic intermediate and structural component of triacylglycerol (TAG), it is still unclear whether its supplementation affects lipid utilisation and/or deposition in different tissues. Accordingly, a set of studies was aimed to evaluate how increasing levels of dietary glycerol (0, 2.5 and 5%) affect lipid synthesis in the liver, muscle and adipose tissue. After a growth trial with rainbow trout (8 weeks) and European seabass (6 weeks) fish were sampled at 6 and 24 h to assess mRNA levels of lipid metabolism-related enzymes. The remaining fish were subjected to a metabolic trial consisting of a 6-day residence in deuterated water (2H2O) for metabolic flux calculations. This study stands as the second part of a broader experiment that also aimed at evaluating the carbohydrate metabolism (Viegas et al., 2022). Dietary supplementation at 5% glycerol significantly increased plasma TAG levels in both species and liver TAG levels in seabass, with no repercussions on the perivisceral fat index. Despite responding as expected in a postprandial setting, only fas and Δ6-fad in trout and Δ6-fad in seabass responded significantly by increasing with the dietary supplementation of glycerol. In trout, the observed differences in the regulation of these enzymes were not reflected in the de novo lipogenic fluxes. The fractional synthetic rates (FSR) were overall low in all tissues with an average of 0.04, 0.02 and 0.01% d–1, for liver, muscle and perivisceral fat, respectively. In seabass on the other hand, and despite increased mRNA levels in Δ6-fad, the overall lipid profile in the liver muscle and perivisceral fat was higher in MUFA at the expense of lower PUFA. Moreover, glycerol supplementation altered the lipogenic capacity of seabass with hepatic fractional synthetic rates for TAG-bound FA increasing with increasing glycerol levels (0.32 ± 0.18, 0.57 ± 0.18, and 0.82 ± 0.24 for 0%, 2.5% and 5% glycerol supplementation, respectively). The findings of the present study suggest that supplementation up to 2.5% of glycerol did not severely impact the lipid metabolism nor increased lipogenic potential in liver, muscle and perivisceral fat accumulation.
Introduction
Glycerol (1,2,3-propanetriol or glycerine; ChEBI: 17754) is a water-soluble, almost colorless, odorless, viscous, hygroscopic 3-carbon sugar alcohol. Glycerol is an important structural component of triglycerides and phospholipids, as well as an important metabolic intermediate at the crossroad of several pathways. Its sites of activation to glycerol-3-phosphate, transportation between cells, as well as regulatory roles of related enzymes or genes on lipid metabolism have been extensively studied and reviewed (Xue et al., 2017). However, several aspects of the complex interplay between glycerol and lipid metabolism are yet to be unraveled. Early on, studies in mice hypothesised that dietary glycerol supplementation could cause problems associated with hepatic lipid mobilisation by increasing the liver ability to sequester and re-esterify fatty acids (FA) from tissue (Lin et al., 1976). Glycerol could also stimulate hepatic lipogenesis and speed up the induction of fatty acid desaturases resulting in changes in liver FA composition and in altered membrane permeability (Narayan and Mcmullen, 1979). The metabolisation of glycerol in liver has been clearly associated with the pentose phosphate pathway, from which NADPH, the reducing agent for lipogenesis, is produced (Jin et al., 2014). Besides, the versatility of this 3-carbon substrate puts it at the centre of lipid metabolism also in extrahepatic tissues (Coppack et al., 1999; Nye et al., 2008). This is particularly evident in scenarios of energy storage in adipose tissue (Rotondo et al., 2017; Ho-Palma et al., 2019) and energy mobilisation (e.g., exercise) in muscle tissue (Montell et al., 2002; van Hall et al., 2002).
With the rampant increase in biodiesel production in the early 2000s, where 1 kg of crude glycerol can be generated for every 10 kg of biodiesel (Ayoub and Abdullah, 2012), soon the potential of glycerol as ingredient for livestock feed was identified and evaluated (Food and Agriculture Organization [FAO], 2012). In beef cattle, diets at levels up to 15% glycerol did not impair performance or carcass characteristics (Del Bianco Benedeti et al., 2016). In feedlot young bulls, the fat content in the longissimus dorsi muscle, particularly oleic acid (18:1n-9) increased, while the saturated fatty acids (SFA) content decreased linearly as function of glycerol addition (Carvalho et al., 2014). In dairy cows glycerol supplementation did not affect overall intake but impacted feeding patterns including particle sorting (Carvalho E.R. et al., 2012). Glycerol utilisation in swine was even more appealing due to the metabolisable-to-digestible energy ratio similar to corn or soybean oil (Lammers et al., 2008b). Supplementations up to ∼10% did not impair performance, carcass characteristics, and meat quality (Lammers et al., 2008a; Schieck et al., 2010; Ordoñez-Gomez et al., 2017) as well as plasmatic parameters such as glucose, triglycerides, cholesterol, and urea nitrogen (Carvalho P.L.D.O. et al., 2012). Similar to what has been observed in cattle, adding 5% glycerol also increased significantly the proportion of oleic acid in pig backfat but at the expense of linoleic (18:2n-6) and linolenic acids (18:3n-3; 18:3n-6), consequently decreasing the unsaturation index of fat (Mourot et al., 1994). In broilers diets containing 10% glycerol influenced performance thus 5% is considered the appropriate supplementation level (Cerrate et al., 2006; Freitas et al., 2017). However, while these studies reported no alterations in abdominal fat content, others reported increased abdominal fat pad weight when compared to control diets (Lessard et al., 1993).
The use of glycerol in fish feed was recently reviewed (Mauerwerk et al., 2021a) but studies and insight into lipid metabolism are scarce and its putative lipogenic potential is yet to be evaluated thoroughly. Early studies with rainbow trout (Oncorhynchus mykiss) reported that glycerol was not an effective precursor for lipogenesis (Menton et al., 1986) but since then evidence has been presented that this may not be the case in other species and/or dosages. In juvenile Mozambique tilapia (Oreochromis mossambicus), the incorporation of dietary glycerol into liver and muscle lipids was demonstrated using pellets labeled with 14C-glycerol. This incorporation into muscle was statistically lower in glycerol-supplemented fish while no difference in incorporation was observed into hepatic lipids (Costa et al., 2017). In another study with the same species, glycerol supplementation > 10% increased triacylglycerol (TAG) levels in liver and white muscle (Costa et al., 2015). On the contrary, in channel catfish (Ictalurus punctatus), glycerol supplementation > 15% decreased liver lipids (Li et al., 2010). Fish plasma TAG seems to be irresponsive to glycerol supplementation (Neu et al., 2013; Costa et al., 2015; Mauerwerk et al., 2021b) with the exception of curimbatá (Prochilodus lineatus) (Balen et al., 2017) which presented significantly increased TAG levels at 12% supplementation. Although these studies provide valuable insight, and in the absence of enzymatic studies, plasma TAG or liver lipids are merely indicative of the actual status of lipid metabolism. In order to evaluate how dietary stimuli affects lipid utilisation and deposition in several tissues, namely liver, muscle and adipose tissue, deuterated water (2H2O) has been employed successfully in fish (Viegas et al., 2016, 2019). Deuterium (2H) is differentially incorporated into different positions of the molecules and, if using 2H NMR, it is possible to discriminate such positional 2H-enrichment, an advantage over other types of instrumentation able to detect overall 2H-enrichments. This means that in this case, within a TAG molecule, 2H NMR is able to separately quantify the enrichment in the glycerol backbone and on its three esterified FA. Differential positional labeling should stem, amongst others, from pathways where unlabeled glycerol (in this case, dietary glycerol) is involved. Accordingly, the present study aimed to describe how dietary glycerol affects lipid synthesis and accumulation in different tissues. 2H2O-based metabolic flux calculations were paired with the mRNA levels of important lipid metabolism-related enzymes using as model two representative carnivorous species of European aquaculture: freshwater rainbow trout (Oncorhynchus mykiss) and marine European seabass (Dicentrarchus labrax).
Materials and Methods
This work is the second of two parts focussing on the utilisation of dietary glycerol in carnivorous fish. The first part, providing insights into hepatic carbohydrate metabolism (Viegas et al., 2022), and the present manuscript are based on the same set of experiments. Viegas et al.(2022) will be often referred to as “Part I” whenever necessary and describes in detail all the experimental procedures that led to the production of the data presented hereafter. The “Ethics statement,” “Diet Formulation,” and “Growth trials – fish handling and sampling” sections of Part I cover in its entirety the information needed for replicating the experiment. Further details about diet formulations can be found in Part I as Supplementary Information (Viegas et al., 2022 – Supplementary Table 1). The formulation was the same for both species (D0, D2.5 and D5), but for the sake of clarity and context they will hereafter be referred to T0, T2.5, T5 or S0, S2.5, S5, if when addressing the rainbow trout or European seabass experiment, respectively. All remaining details specific to replicating the present experiment are provided in the following sections. Briefly, the experiment consisted of a 6- to 8-week feeding trial (Viegas et al., 2022 – Table 1), after which samples taken 6 h and 24 h after last meal were used to assess the molecular regulation of hepatic enzymes (two to three fish per tank for a total n = 8 for trout and n = 7 for seabass, per diet, per sampling time). The remaining fish were transferred to 2H2O (4%) for 6 days for a metabolic trial (total n = 24 per diet for both species). Here, 2H gets incorporated into metabolites and molecules following well-defined metabolic steps with consequent analysis of the positional 2H-enrichment by 2H NMR. Further details on the growth trials can be found in Palma et al.(2019) and Magnoni et al.(2021) for rainbow trout and in Palma et al.(2019) for European seabass.
Molecular Analysis of Intermediary Metabolism
Sampling, sample treatment and consequent relative gene mRNA level determination by real-time quantitative polymerase chain reaction (qPCR) are extensively described in Part I, including the PCR protocol and amplification cycles. Plasma and liver TAG were quantified using a commercial kit (TRG050; Sobioda). The primer sequences used in real-time quantitative qPCR assays for lipid metabolism gene and other information for the analysis are presented in Supplementary Table 1 for trout and Supplementary Table 2 for seabass. mRNA level for key enzymes of lipogenesis (fas, fatty acid synthase EC 2.3.1.85); dgat (diglyceride acyltransferase EC 2.3.1.20), lipolysis (hoad, 3-hydroxyacyl-CoA dehydrogenase EC 1.1.1.35; cpt1a and cpt1b carnitine palmitoyltransferase I isoforms a and b EC: 2.3.1.21) and enzymes involved in long chain polyunsaturated fatty acids (PUFA) biosynthesis (Δ6-fad, acyl-CoA 6-desaturase EC:1.14.19.3; elovl2 and elovl5, elongation of very long-chain fatty acid protein 2 and 5 EC 2.3.1.199). Elongation factor-1 alpha (ef1α) was used as reference gene.
Metabolic Trial – Labeling of Triacylglycerol With 2H2O
As described in Part I, the fish remaining from the growth trials were transferred to a closed recirculated system consisting of 3 × 150 L tanks for the metabolic trials. Tank water (TW) contained ∼4% 2H-enriched water, achieved by the addition of 99%-enriched 2H2O (CortecNet, France) to water from the system where the respective growth trial took place as previously described (Viegas et al., 2011). Fish remained in 2H-enriched water for 6 days, being fed once a day (ad libitum) and euthanized 24 h after last meal on day 5. Fish were anesthetised (MS-222; 3-aminobenzoic acid ethyl ester, 0.1 g L–1), euthanized and sampled for blood, liver, muscle and perivisceral fat as described in Part I. Plasma TAG was quantified with commercial kit (I.S.E. S.r.l.; A-R0100000901). In order to ensure samples that yielded 2H NMR spectra with high signal-to-noise ratios, tissue from 2 to 4 fish were pooled (for a final n = 6 per diet).
TAG were extracted from N2-pulverised (liver and muscle) or recently thawed (perivisceral fat) tissue according to Matyash et al.(2008) using methyl tert-butyl ether, methanol and water mixture (MTBE:MeOH:H2O, 10:3:2.5; 20 mL g–1 of tissue), after 1 h in agitation at room temperature. The organic phase was then transferred to glass amber vials, dried under N2 stream and stored at −20 °C. TAG were purified by solid phase extraction with pre-packed 2 g cartridges (Discovery® DSC-NH2 52.641-U, Supelco) and TAG identity was confirmed by spotting onto thin by thin-layer chromatography (TLC) plates alongside TAG standards. The plate was developed with petroleum ether/diethyl ether/acetic acid (7.0/1.0/0.1; v/v/v). After drying, lipid spots were visualised under iodine vapour and correspondent fractions dried under N2 stream and stored at −20 °C until NMR analysis (Viegas et al., 2016).
2H NMR Acquisition and Metabolic Flux Calculations
Proton-decoupled 2H NMR spectra were obtained with a Bruker Avance III HD 500 spectrometer using a 2H-selective 5 mm probe incorporating a 19F-lock channel. Body water (BW) and tank water (TW) 2H-enrichments were quantified as previously described in Part I according to Jones et al.(2001). Triacylglycerol samples were reconstituted in 400 μL of chloroform (Sigma-Aldrich 528730; HPLC grade, ≥99.9%) and transferred to a 5-mm NMR-grade tube with a pyrazine standard and 50 μL of hexafluorobenzene. TAG spectra were obtained at 25 °C: 1H NMR spectra were acquired with a 90 ° pulse, 3 s of acquisition time and 5 s of pulse delay, for 16 scans; 2H NMR spectra were acquired with a 90 ° pulse, 0.37 s of acquisition time and 0.1 s of pulse delay, for 10,000 to 20,000 scans. As control for the TAG extraction, a FA/glycerol ratio (should be ∼3) was calculated from the area of all α protons times 2, divided by TAG-bound glycerol sn1,3 protons (Duarte et al., 2014; Viegas et al., 2016). The FA profile (in percentage) for saturated (SFA) and unsaturated fatty acids (UFA), both poly- (PUFA) and monounsaturated fatty acids (MUFA) were estimated from 1H NMR spectra according to Viegas et al.(2016). 2H-enrichments were quantified from the 1H and 2H NMR spectra by measuring the 1H and 2H intensities of selected signals relative to the 1H and 2H intensities of a pyrazine standard, as described previously (Duarte et al., 2014; Viegas et al., 2016). Briefly, TAG-bound FA synthetised de novo were estimated by determining the 2H-enrichment in the FA terminal methyl site and newly synthesized and/or turned-over TAG-bound glycerol was estimated by determining the 2H-enrichment in the sn-1,3 glycerol site. Estimates on desaturation rates were made by determining the 2H-enrichment in the MUFAs’ allylic protons. Estimating elongation rates are based on the principle that during the residence in 2H2O, the terminal methyl site is enriched during the first round of FA synthesis, while the α protons incorporate 2H in the last round of elongation. Therefore, if elongation occurs on pre-existing (unlabeled) FA, the α- and methyl-protons will be differentially labeled and will inform of the fractional contribution of elongation to lipid synthesis. Excess TAG positional 2H-enrichments were calculated after systematic subtraction of the values with 0.0156%, taken as natural abundance 2H-enrichment. Fractional synthetic rates (FSR; in% day–1) were estimated by dividing these above described positional TAG enrichments by that of body water. Spectra were processed by applying exponential multiplication to the free-induction decay (1H: 0.1 Hz; 2H: 1.0 Hz), and analyzed using the curve-fitting routine supplied with ACD/NMR Processor Academic Edition from ACD/Labs 12.0 software (Advanced Chemistry Development, Inc.).
Statistics
Data are presented as mean ± SD. Normality (Shapiro-Wilk’s test) and homogeneity of variance (Bartlett’s test) were verified prior to the analysis. For the plasma and hepatic parameters and mRNA levels of lipid-related enzymes, the effects of sampling time and diet were analyzed by two-way ANOVA, with time (6 h and 24 h) and glycerol level (D0, D2.5 and D5) as variables. In the case of significant interactions, one-way ANOVA was performed followed by Tukey’s HSD for multiple comparison. For the parameters evaluated following the metabolic trial, such as FSR for de novo lipogenesis, glycerol turnover, elongation and desaturation rates, were analyzed by one-way ANOVA. In the case of detecting significant differences through the one-way ANOVA, a Tukey multiple range test was performed to discriminate differences between diets. Statistical analysis was performed in GraphPad Prism software (GraphPad Software, La Jolla, CA, United States) and differences were considered statistically significant at p < 0.05.
Results
Tank water (TW) 2H-enrichment was maintained between 3.5 and 4.0%. Body water 2H-enrichment ranged from 2.7 to 4.1% (average of 3.6 ± 0.3%) with no statistical differences between dietary treatments for both species. After the growth trial no differences in the final body weight for both diet (D0, D2.5, D5) and sampling time (6 h vs. 24 h after last meal) were observed for trout or seabass (Viegas et al., 2022).
Rainbow Trout
Plasma and liver TAG levels were not significantly affected by diet and sampling time. However, it is worth noting that for TAG levels in plasma p-value for diet was 0.065, which may indicate that increased glycerol dietary levels may lead to hypertriglyceridaemia. This was corroborated by the plasma TAG levels from the fish at the end of the metabolic trial, which were sampled only 24 h after last meal but that were significantly increased in T5. As explained in Part I for liver glycogen analysis the liver tissue assigned for the TAG assay was poorly maintained and this parameter was not measured (Viegas et al., 2022). The perivisceral fat index did not reveal any statistical differences. The results from both trials in trout are summarised in Table 1.
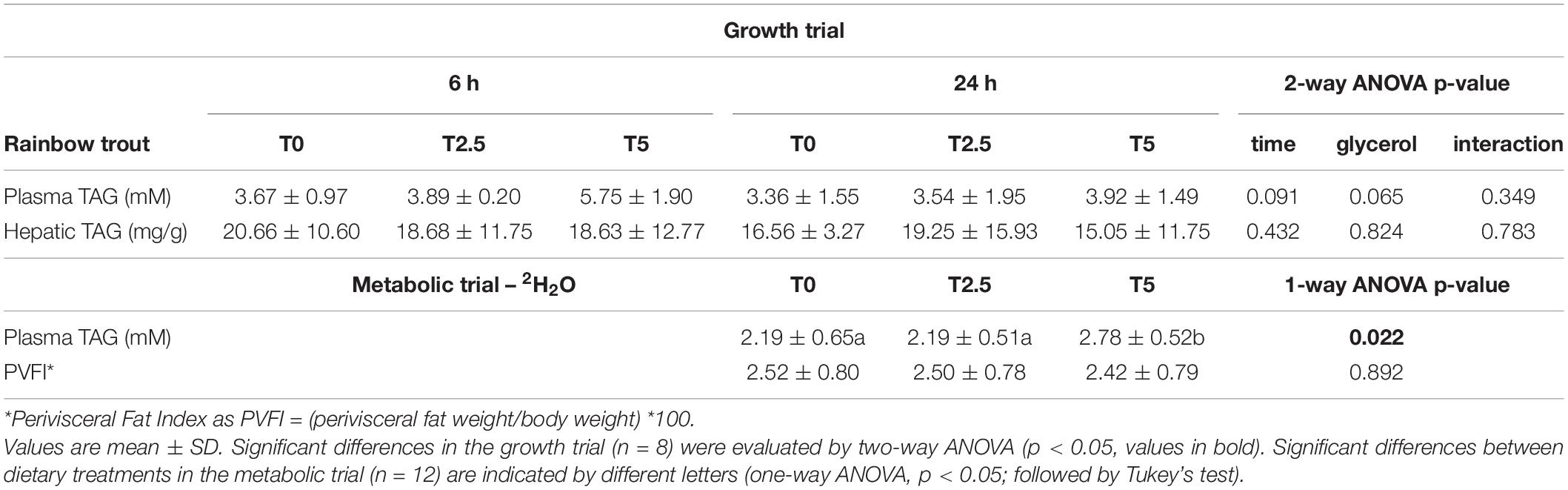
Table 1. Plasma and liver parameters for rainbow trout (Oncorhynchus mykiss) fed with different levels of glycerol (0, 2.5, and 5%) for 8 weeks sampled at the end of the growth trial at 6 h and 24 h after last meal and the metabolic trial (6-day residence in 2H-enriched water) 24 h after last meal.
In order to assess the impact of glycerol-supplemented diets on hepatic metabolism, mRNA levels of key enzymes involved in the regulation of lipid metabolism were assessed after the growth trial at two different sampling times after last meal (6 and 24 h) by real-time qPCR (Figure 1). At 6 h after last meal, as expected in a postprandial setting, and as already observed in Part I with the enzymes related to the carbohydrate metabolism (Viegas et al., 2022), most enzymes related with lipid metabolism significantly differed between sampling times. In addition, as expected in a postprandial setting, the mRNA levels of lipogenic enzymes were up-regulated and the lipolytic enzymes were down-regulated at 6 h after last meal. But contrary to that observed in the enzymes related to carbohydrate metabolism where none of them responded to dietary treatment, fas and Δ6-fad (involved in the de novo synthesis of FA and long chain PUFA biosynthesis, respectively), involved in the de novo synthesis of FA and long chain PUFA biosynthesis, respectively, were significantly increased with up-regulated levels of dietary glycerol. The differences observed in the regulation of enzymes involved in lipid metabolism were not reflected in the overall lipid profile of TAG-bound FA in liver (Table 2). However, in muscle and perivisceral TAG there seemed to be an increase in MUFA at the highest glycerol inclusion (T5) when compared with T2.5 but not when compared with T0.
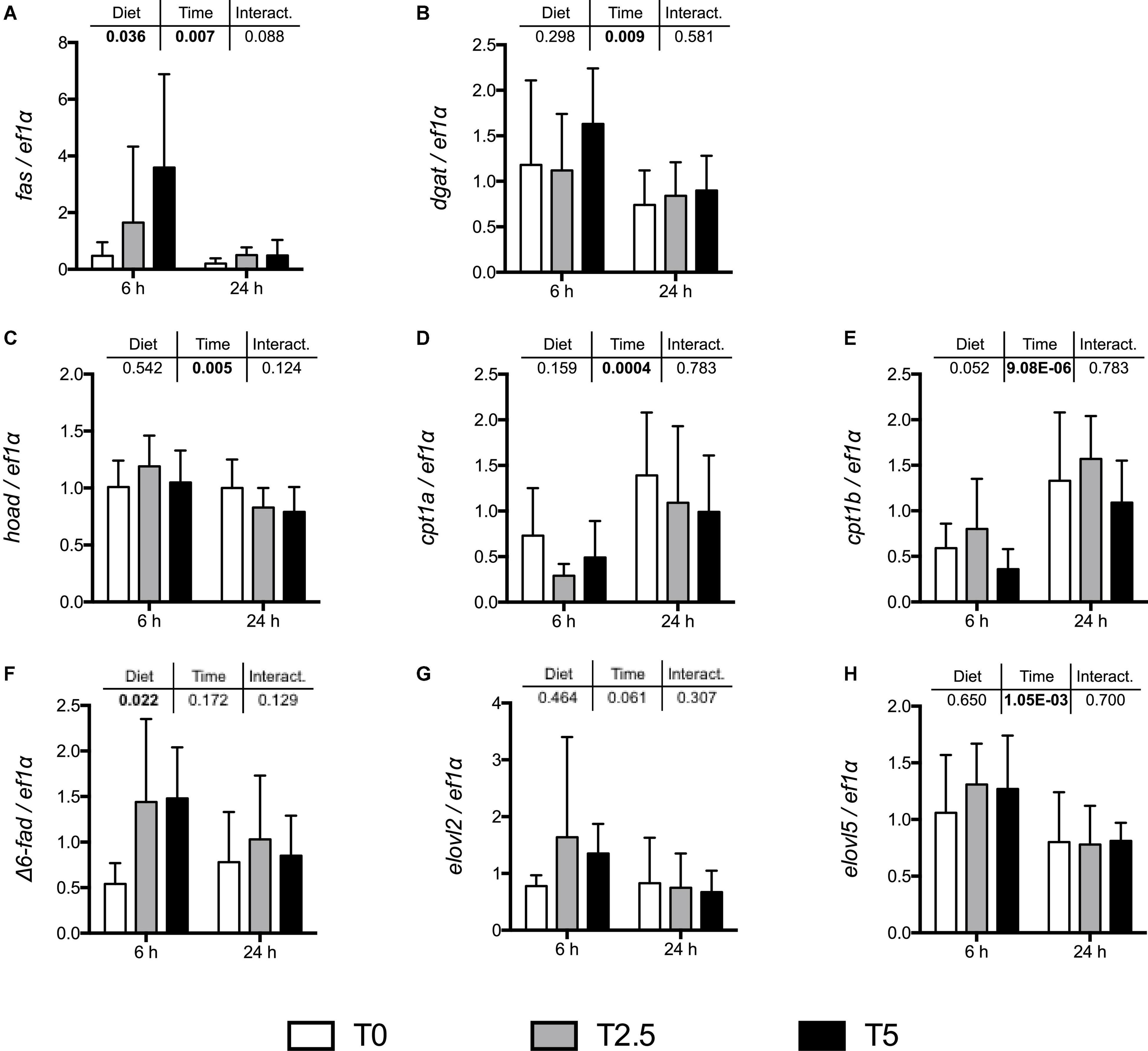
Figure 1. mRNA levels of selected lipogenic enzymes (A) fas (fatty acid synthase), (B) dgat (diglyceride acyltransferase); lipolytic enzymes (C) hoad (3-hydroxyacyl-CoA dehydrogenase), (D) cpt1a and (E) cpt1b (carnitine palmitoyltransferase I a and b, respectively); and enzymes involved in long chain PUFA biosynthesis: (F) Δ6-fad (acyl-CoA 6-desaturase), (G) elovl2 and (H) elovl5 (elongation of very long-chain fatty acid protein 2 and 5, respectively), in the liver of rainbow trout (Oncorhynchus mykiss) fed with different levels of glycerol (0, 2.5, and 5%) for 8 weeks. Values are mean ± SD (n = 8); nd: not detected. Significant differences were evaluated by two-way ANOVA (p < 0.05, values in bold).
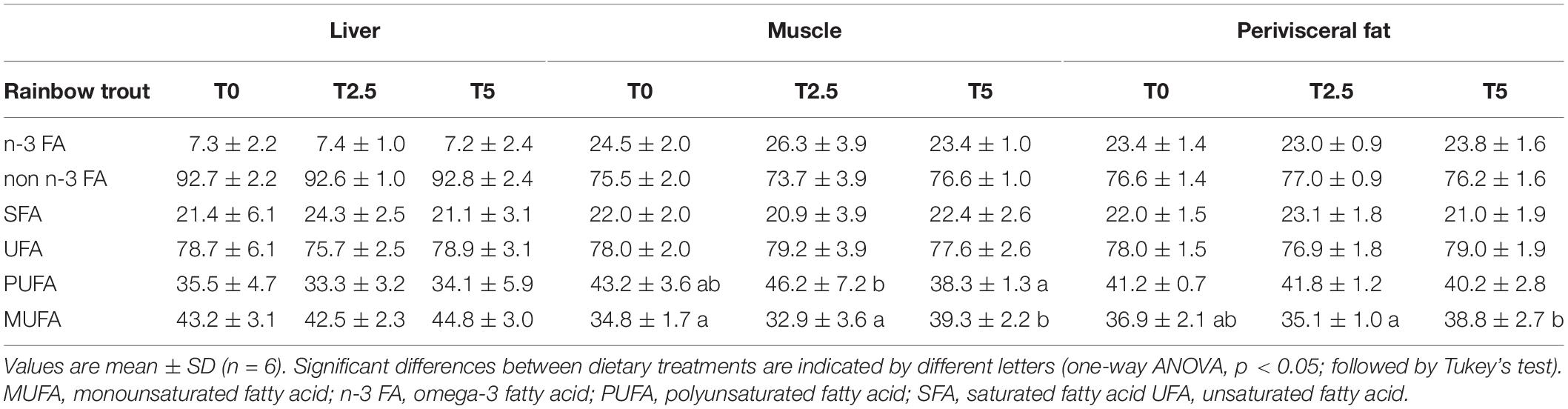
Table 2. Percentage of fatty acid classes as determined from 1H NMR spectra of TAG from liver, muscle and perivisceral fat of rainbow trout (Oncorhynchus mykiss) fed with different levels of glycerol (0, 2.5, and 5%).
A deeper insight into the impact of glycerol-supplemented diets on lipid metabolism was gained through the analysis of the positional 2H-labeling of TAG with 2H2O (Figure 2). Glycerol supplementation did not alter the lipogenic capacity of rainbow trout as evaluated by the 2H-enrichment of TAG-bound FA (Figure 2). These were overall low in all tissues with average fractional synthetic rates at 0.04, 0.02 and 0.01% d–1, for liver, muscle and perivisceral fat, respectively. It is worth noting that the variability was very high amongst fish from the same dietary treatment. TAG-bound glycerol revealed much higher 2H-enrichment relative to TAG-bound FA without significant differences between levels of glycerol supplementation for all tissues. The hepatic glycerol pool was completely turned over with fractional synthetic rates similar to TW 2H-enrichment. Glycerol fractional synthetic rates for muscle and perivisceral fat (0.38 and 0.09% d–1, respectively) were lower compared to the ones observed in the liver.
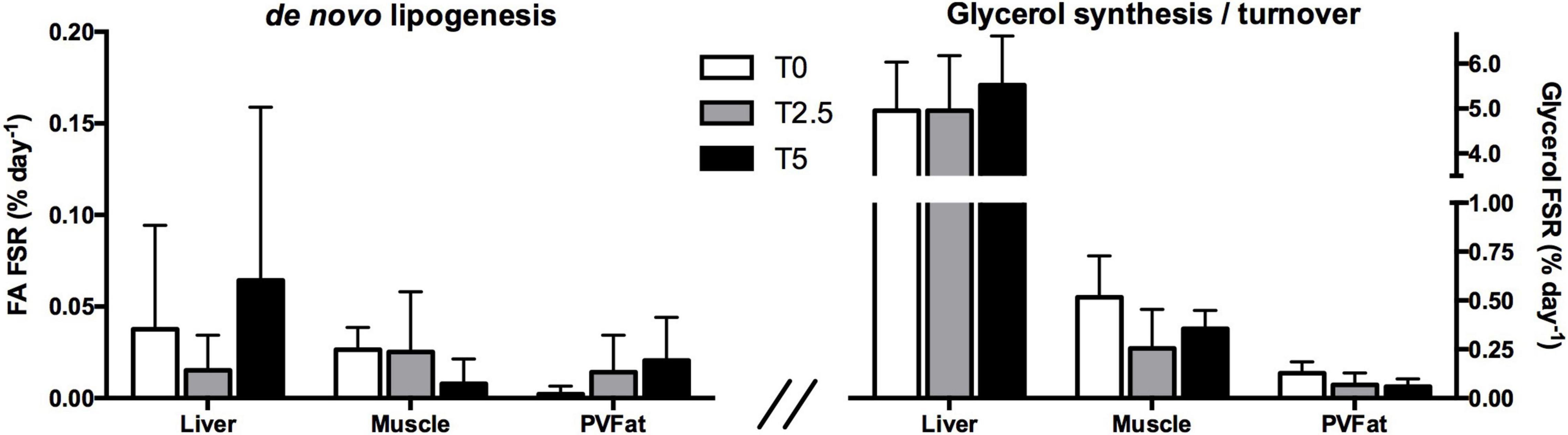
Figure 2. TAG-bound fatty acid fractional synthetic rate (FA FSR; de novo lipogenesis) expressed as percent of newly synthesized fatty acids per day (left) and TAG-bound glycerol fractional synthetic rate (Glycerol FSR; glycerol synthesis/turnover) expressed as percent of newly synthesized/turned over glycerol per day in hepatic, muscular and perivisceral TAG of rainbow trout (Oncorhynchus mykiss) fed with different levels of glycerol (0, 2.5, and 5%) after a 6-day residence in a tank with ∼4% 2H-enriched water. Mean values ± SD are presented (n = 6). Significant differences between dietary treatments are indicated by different letters (one-way ANOVA, p < 0.05; followed by Tukey’s test).
European Seabass
After the growth trial significant differences were found in plasma TAG levels between diets (S0, S2.5, S5) and sampling time (6 h, 24 h after last meal). Significant differences were also found in hepatic TAG but only between sampling time. In the metabolic trial, no alterations were found in plasma TAG levels or in the perivisceral fat index. The results from both trials in seabass are summarised in Table 3.
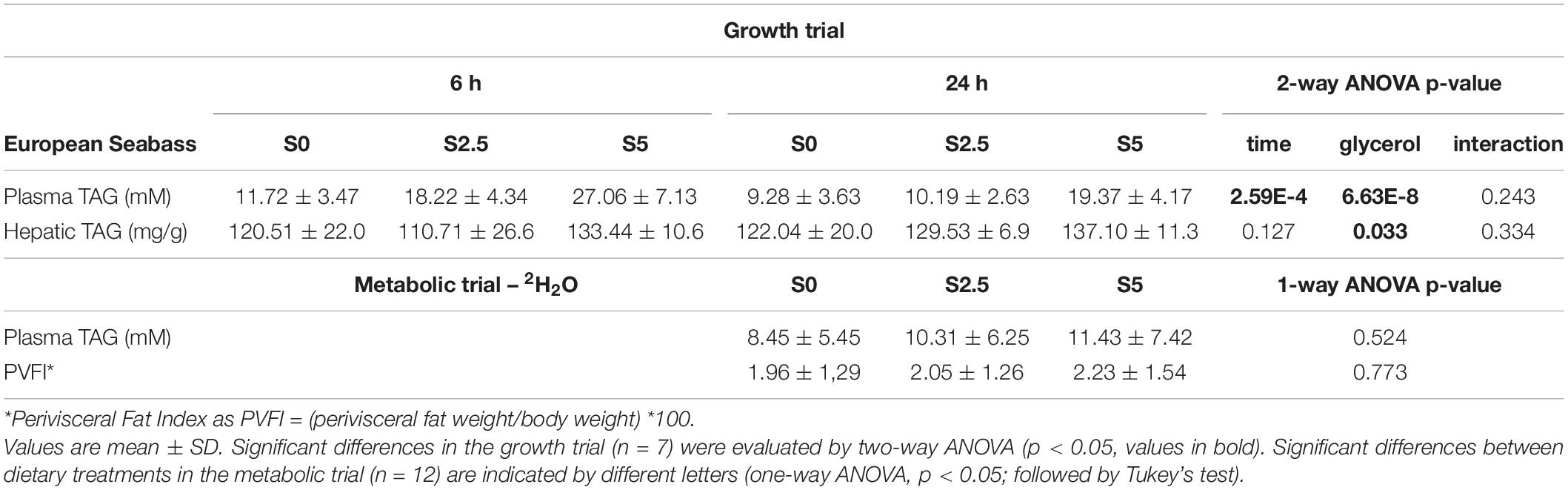
Table 3. Plasma and liver parameters for European seabass (Dicentrarchus labrax) fed with different levels of glycerol (0, 2.5, and 5%) for 6 weeks sampled at the end of the growth trial at 6 h and 24 h after last meal and the metabolic trial (6-day residence in 2H-enriched water) 24 h after last meal.
Similar to that observed in trout, most mRNA levels of key enzymes involved in the regulation of lipid metabolism did not respond significantly to the different levels of dietary glycerol with the exception was Δ6-fad (Figure 3). However, the differences observed in the regulation of this enzyme were not reflected in the overall lipid profile of TAG-bound FA in liver muscle and perivisceral fat TAG. In fact, an increase in MUFA was observed in every tissue while PUFA decrease regardless of increased Δ6-fad expression observed in the liver (Table 4).
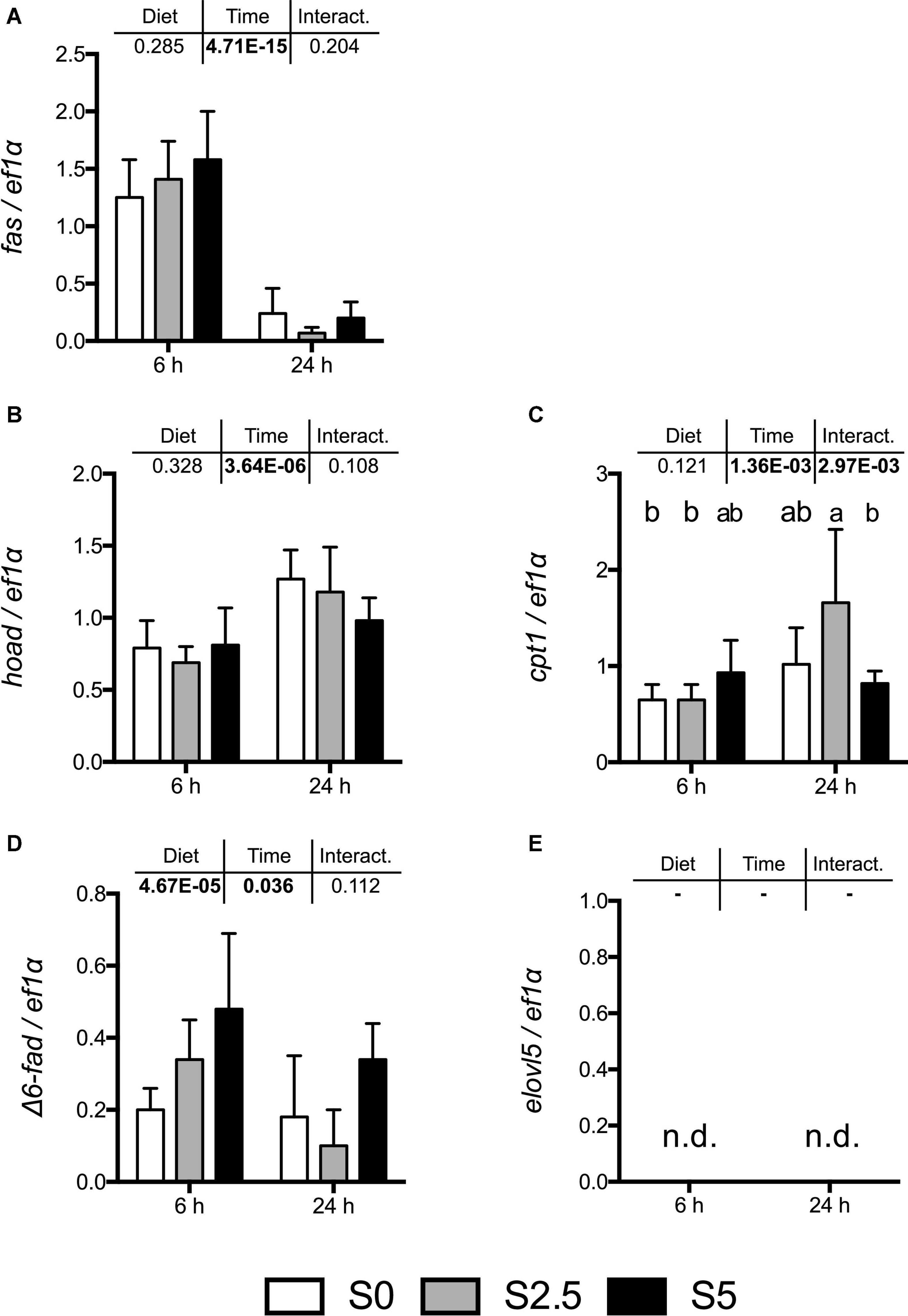
Figure 3. mRNA levels of selected lipogenic enzymes (A) fas (fatty acid synthase); lipolytic enzymes (B) hoad (3-hydroxyacyl-CoA dehydrogenase) and (C) cpt1 (carnitine palmitoyltransferase I); and enzymes involved in long chain PUFA biosynthesis: (D) Δ6-fad (acyl-CoA 6-desaturase) and (E) elovl5 (elongation of very long-chain fatty acid protein 5) in the liver of European seabass (Dicentrarchus labrax) fed with different levels of glycerol (0, 2.5, and 5%) for 8 weeks. Values are mean ± SD (n = 7); n.d.: not detected. Significant differences were evaluated by two-way ANOVA (p < 0.05, values in bold). In the case of significant interactions, significant differences are indicated by different letters (one-way ANOVA, p < 0.05; followed by Tukey’s test).
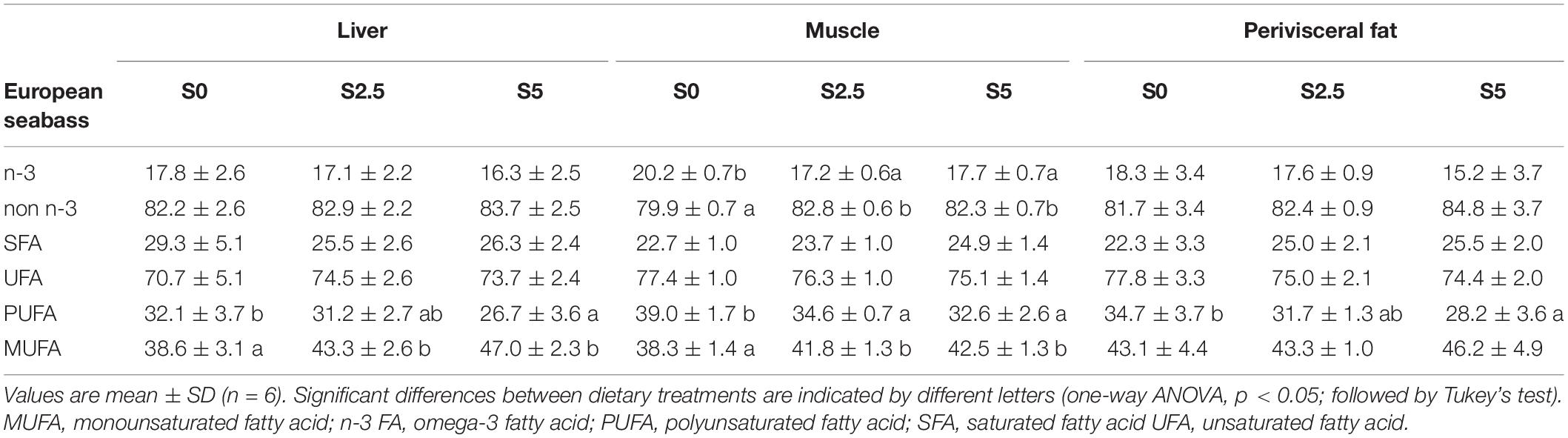
Table 4. Percentage of fatty acid classes as determined from 1H NMR spectra of TAG from liver, muscle and perivisceral fat of European seabass (Dicentrarchus labrax) fed with different levels of glycerol (0, 2.5, and 5%).
Contrary to observed in rainbow trout, glycerol supplementation altered the lipogenic capacity of seabass with hepatic fractional synthetic rates for TAG-bound FA increasing with increasing glycerol levels (Figure 4) (0.32 ± 0.18, 0.57 ± 0.18, and 0.82 ± 0.24 for 0%, 2.5% and 5% glycerol supplementation, respectively). No 2H-enrichment was detected in muscle TAG while the enrichments found in perivisceral fat were extremely low (0.01% d–1) and not influenced by dietary treatment. TAG-bound glycerol revealed much higher 2H-enrichment relative to TAG-bound FA. Also as observed in rainbow trout, the hepatic glycerol pool was completely turned over with fractional synthetic rates similar to TW 2H-enrichment, without significant differences between levels of glycerol supplementation for all tissues.
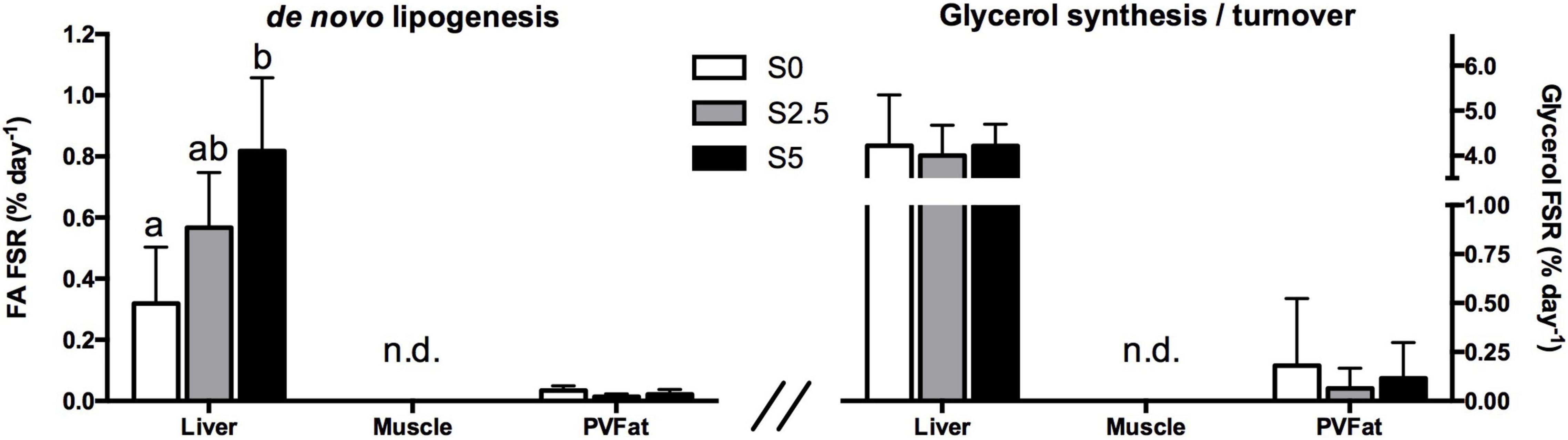
Figure 4. Triacylglycerol-bound fatty acid fractional synthetic rate (FA FSR; de novo lipogenesis) expressed as percent of newly synthesized fatty acids per day (left) and triacylglycerol-bound glycerol fractional synthetic rate (Glycerol FSR; glycerol synthesis/turnover) expressed as percent of newly synthesized/turned over glycerol per day in hepatic, muscular and perivisceral TAG of European seabass (Dicentrarchus labrax) fed with different levels of glycerol (0, 2.5, and 5%) after a 6-day residence in a tank with ∼4% 2H-enriched water. Mean values ± SD are presented (n = 6). Significant differences between dietary treatments are indicated by different letters (one-way ANOVA, p < 0.05; followed by Tukey’s test). n.d.: not detected.
Discussion
Our understanding of fish nutrition and metabolism has advanced substantially and the use of isotopes has greatly assisted to follow the metabolic fate of various metabolites, glycerol included. Delivery strategies may be methodologically challenging in aquatic organisms but are valid both in vitro, by using labeling incubation medium for fish hepatocytes (Lech, 1970) or muscle slices (Kam and Milligan, 2006), and in vivo. Amongst the latter, the feeding of labeling pellets (Costa et al., 2017) raises the issue of leaching of costly compounds into the water. This can be circumvented by forced feeding or gavage which then require handling, anaesthesia and more invasive protocols. The same applies to intraperitoneal (IP) injection (Savina and Wojtczak, 1977; Rito et al., 2019). This methodology assures the delivery of a controlled amount of label, in spite of doing so in a somehow unrealistic transfer (in terms of rate and dosage) of the labeling metabolite into circulation. On the other hand, dorsal aorta cannulation in combination with a continuous infusion of a radiolabeled metabolite requires a surgical procedure, which is highly dependent on fish size for access through the mouth and on the skills of highly trained personnel (Haman and Weber, 1996). This is an invasive method that, if properly setup, can allow for accurate estimation of substrate fluxes (Weber et al., 2016), including glycerol (Bernard et al., 1999; Magnoni et al., 2008), in fully recovered fish under minimal stress. If using radioactive isotopes like [3H] or [14C] glycerol, additional laboratory security procedures and safe discard of leftover solutions and biomass must be in place, amounting to experimental costs. Despite all the technicalities behind these approaches, the aforementioned studies were able to clearly demonstrate that glycerol is incorporated into lipids. Li et al.(2016) using [14C]glycerol on Atlantic cod (Gadus morhua) larvae, were even able to determine that the predominant destination lipid classes were TAG and phosphatidyl-choline (PC), followed by phosphatidylethanolamine (PE). A significant increase in [14C]PC and a decreasing percentage of [14C]TAG over time were also reported. On the other hand, deuterium 2H is stable (non-radioactive) isotope, and if delivered on the form of 2H2O is ideally suited for metabolic studies in fish. It can be incorporated into tank water safely for an indefinite period, if periodically adjusted for evaporation, 2H-enrichment and water quality. 2H-enrichment incorporation allows for a non-invasive measurement of glycerol utilisation on naturally feeding fish, and in the case of TAG, separately quantify the enrichment of the glycerol backbone and its esterified FA.
Small 3-carbon glucose precursors like lactate, pyruvate, alanine and glycerol are metabolically versatile and display inter-organ moiety with several described metabolic shuttles. However, if considered as aquafeed ingredients, glycerol has the advantage of being a gluconeogenic substrate that does not generate nitrogenous disposal. This is particularly relevant in fish farmed at high densities or using a recirculated aquatic system (RAS), and even more relevant to carnivorous fish species that do not tolerate high levels of dietary carbohydrate and depend highly on gluconeogenesis to regulate glycaemia and glycogen reserves (Viegas et al., 2013, 2015, 2019). This was confirmed and thoroughly discussed in Part I of the present study for hepatic glycogen synthesis in rainbow trout and blood glucose regulation in European seabass (Viegas et al., 2022). Moreover, high glycerol turnover allows for incredibly high rate of re-esterification (or TAG/FA cycling) in rainbow trout at rest (Magnoni et al., 2008; Turenne and Weber, 2018) which surprisingly remains unaltered when in exercise. This is a very different paradigm when compared with mammals that need to boost their lipolytic rates (measured as Ra glycerol) by 2–5 fold (Turenne and Weber, 2018). This mechanism in fish has also been suggested as a means to achieve rapid membrane remodeling in variable environments.
Liver
Alteration to lipid metabolism may be revealed by a spectrum of parameters amongst which the most common are plasma and liver TAG/lipid levels. In the present study both species provided evidence that dietary glycerol may in fact increase plasma TAG levels. In Nile tilapia and silver catfish (Rhamdia quelen) dietary glycerol did not influence plasma TAG (Costa et al., 2015; Mauerwerk et al., 2021b) even with diets with up to 15% and 7.5% glycerol, respectively. Moreover, dietary glycerol appeared to result in an increase of liver TAG levels of seabass, as previously observed in Nile tilapia but at supplementation levels of 15% glycerol (Santos et al., 2019). However, in tilapia, indirect measurements of adiposity in liver through the assessment of histological parameters, suggested that there was no strong relationship between diet and the accumulation of lipids in the hepatocytes (Mewes et al., 2016) or alteration in hepatocyte area (Santos et al., 2019). In channel catfish, diets containing 5 and 10% glycerol provoked no significant differences in liver lipid level while fish fed diets containing 15 and 20% glycerol reduced liver lipid content (Li et al., 2010). In seabass, the increase in liver TAG levels was accompanied by a change in its composition in S5 provoking a significant decrease in PUFA at the expense of an increase in MUFA. This pattern in FA composition was observed also in muscle and perivisceral fat. No such alterations in lipid composition were observed in trout liver that was apparently able to compensate by significantly up-regulating Δ6-fad. Despite not displaying any significant alterations to liver TAG and its composition, trout liver responded to dietary glycerol with a significant up-regulation of fas. Excess FA may have been exported to the muscle where the same pattern of decrease in PUFA at the expense of an increase in MUFA verified in seabass tissue was observed. As mentioned before, glycerol supplementation may alter the unsaturation index of fat in cattle and pigs (Mourot et al., 1994; Carvalho et al., 2014) and the mechanisms behind this pattern should be addressed in future studies. Nonetheless, the up-regulation of fas was not corroborated by the metabolic flux data, where no significant increase in de novo lipogenesis was observed in trout. In seabass, the increase in liver TAG levels was corroborated not by not by changes in fas mRNA levels but by a sustained increased in de novo lipogenesis as revealed by 2H-enriched TAG-bound FA terminal CH3. In fact, the FA fractional synthetic rates in seabass were almost 10-fold of those found in trout. This is revealing of the capacity of trout to efficiently retain dietary lipid as previously described in gilthead seabream fed with 5% glycerol (Silva et al., 2012), or in trout where fat is being replaced by starch (Figueiredo-Silva et al., 2012). During the 6-day residence in 2H2O the glycerol turnover in liver was almost complete and not influenced by dietary glycerol. This is asserted by the fact that TAG-bound glycerol 2H-enrichment equalled that of the tank water (average 5.1 ± 0.3 vs. 3.9% TW in trout; average 4.1 ± 0.1 vs. 3.5% TW in seabass). Other than fas in trout and Δ6-fad in trout and seabass, the mRNA levels of the other lipid metabolism-related enzymes did not respond to the dietary treatment. This is in agreement with the results obtained from the glycerol metabolism-related enzymes evaluated in trout from the present study (Panserat et al., 2020). Even if poorly regulated by glycerol, the mRNA levels of these enzymes in liver were overall responsive to time after last meal (6 vs. 24 h), as previously described for both species (Panserat et al., 2009; Kamalam et al., 2013; Castro et al., 2015a,b).
Muscle and Perivisceral Fat
One of the first reports on the effects of dietary glycerol in fish (rainbow trout) revealed that the addition of 1% free glycerol (approximately the amount of bound glycerol found in a 10% addition of lipid to the control diets) resulted in no effect on the final carcass lipid levels (either whole or eviscerated fish) (Menton et al., 1986). This supplementation, even if at a relatively low dosage, was made to low and high-energy diets, and the lack of significant alteration appeared to indicate that dietary free glycerol had no significant effect on lipogenic activity in trout. Other studies in other fish species reported that, in general, fillet protein and fat generally decrease, while moisture increases as dietary glycerol level increase (Li et al., 2010; Moesch et al., 2016; Santos et al., 2019). In humans, glycerol has been associated with increased fluid retention via osmotic effect on the kidneys, being independent of hormonal response (Goulet et al., 2007; Nelson and Robergs, 2007). It is, however, speculative to assert the same mechanisms for aquatic organisms, especially marine species that have to finely osmoregulate, mechanisms to which glycerol has not been clearly associated yet. In spite of the potential impact of glycerol on water retention, whole body moisture in trout reared in the present study was not affected by diet as reported elsewhere (Magnoni et al., 2021).
The association between increasing levels of glycerol and muscle lipids are complex to interpret since most studies test a wide range of supplementations. In Nile tilapia, carcass lipids showed the highest values at 5% and the lowest at 10% (Neu et al., 2013) which may represent not a linear metabolic response but a severe metabolic deregulation at higher dosages. In gilthead seabream, the individual muscle FA analyzed did not differ between diets (control vs. 5% glycerol), and there were no significant differences between major lipid classes (Silva et al., 2012). In the present study there was a decrease in muscle PUFA at the expense of an increase in MUFA in both species, even if apparent digestibility coefficient for crude lipid was not impacted by diet in trout as previously reported elsewhere for the same fish from the present study (Magnoni et al., 2021). Choline is an essential component of cell membranes in the form of PC preventing excessive lipid accumulation. In trout, muscle choline was decreased in the T5 group compared to the T0 group as previously reported for in a metabolomic analysis performed in the same fish from this study (Palma et al., 2019). As stated before, PC is the major fate lipid class to glycerol after TAG (Li et al., 2016), and as most diets shift toward plant-based ingredients an exogenous supply of choline may be essential for normal metabolism. This is particularly true since choline is essential for transport and export of lipids across the mucosa of the pyloric caeca and for prevention of lipid malabsorption syndrome (LMS) (Hansen et al., 2020). At the time of death, gilthead seabream fed with glycerol had a significantly higher ATP content in the muscle (Silva et al., 2012); however, fish from the present study (both species) revealed no effects on the overall energetic state of the muscle as calculated by adenylate energy charge (Palma et al., 2019). From a consumer’s perspective, glycerol increased the hardness of raw gilthead seabream fillets, but this effect dissipated in the cooked fillets (Silva et al., 2012).
From a metabolic point of view, TAG in extrahepatic tissues TAG presented much lower enrichments compared to the liver, since in the muscle of seabass no 2H-enrichment was detected (neither in the TAG-bound FA nor glycerol) as previously described (Viegas et al., 2019). Relatively lower TAG-bound glycerol 2H-enrichments were also observed in extrahepatic tissues of trout (muscle: 0.38 ± 0.13% d–1; perivisceral fat; 0.09 ± 0.04% d–1) and seabass (muscle: not detected; perivisceral fat; 0.12 ± 0.03% d–1). As the liver is the main metabolically active organ, this may stem from the limited capacity of the liver to export labeled metabolites, or of the peripheral tissues to incorporate circulating dietary (unlabeled) glycerol. The latter seems to be the case for seabass since Viegas et al.(2019) reported glycerol 2H-enrichments in muscle between 1 and 2.5% for the same period of residence in 2H2O. Overall, no differences were found due to the dietary treatment in extrahepatic tissues for TAG-bound FA and glycerol. As in the present study, liposomatic index, visceral fat weight or perivisceral fat/viscerosomatic index were not correlated (Silva et al., 2012; Neu et al., 2013; Gonçalves et al., 2015) or were even negatively correlated with glycerol supplementation (Santos et al., 2019) in several other species. Visceral fat composition mimicked what was in generally observed in liver and muscle with a significant decrease in PUFA in seabass and a significant increase in MUFA in trout.
Conclusion
In conclusion, we present evidence that dietary glycerol supplementation at 5% significantly increased plasma TAG levels in both species and liver TAG levels in seabass. A supplementation of 2.5% glycerol is recommended since it did not severely impact the lipid metabolism nor increased lipogenic potential in liver, muscle and perivisceral fat accumulation in both species. Moreover, the TAG-bound FA composition was in general higher in MUFA at the expense of lower PUFA in all tissues. This means that increasing the PUFA profile in the diet formulation should be considered to compensate this effect of glycerol supplementation. Overall, endogenous turnover rates of TAG-bound glycerol were not impacted by dietary glycerol but extrapolations to other species should be cautious. Specific differences were evident since seabass synthesized FA de novo at much higher rates than trout and in proportion to increasing dietary glycerol.
Data Availability Statement
The original contributions presented in the study are included in the article/Supplementary Material, further inquiries can be directed to the corresponding author.
Ethics Statement
The animal study was reviewed and approved by Portuguese Veterinary Authority (1005/92), DGAV-Portugal, according to the guidelines on the protection of animals used for scientific purposes from the European directive 2010/63/UE.
Author Contributions
IV, LJM, and SP: conceptualization, supervision, data treatment, and writing of the original draft. LJM, RO, ES, JR, LFH, LCT, MP, and IV: growth and metabolic trials. SP and EP-J: molecular analysis of enzymes. JR, ES, LFH, LCT, MP, and IV: NMR spectral acquisition and processing. IV and LJM: funding acquisition, project administration, and resources. All authors reviewed, edited, and approved the submitted version.
Funding
This work was supported by Fundação para a Ciência e Tecnologia (FCT; Portugal) through national funds with co-funding from ERDF/FEDER, within the PT2020 Partnership Agreement, and COMPETE 2020: research grant to IV (PTDC/CVT-NUT/2851/2014, PTDC/BAA-AGR/3550/2020); individual grant to MP through Centro2020 (ReNATURE; Centro-01-0145-FEDER-000007); and structural funds to Center for Neuroscience and Cell Biology (UID/NEU/04539/2013) and Centre for Functional Ecology (UID/BIA/04004/2019) and Interdisciplinary Centre of Marine and Environmental Research (UID/Multi/04423/2019). UC-NMR facilities (REEQ/481/QUI/2006, RECI/QEQ-QFI/0168/2012, Centro-07-CT62-FEDER-002012) and Rede Nacional de Ressonância Magnética Nuclear (RNRMN).
Conflict of Interest
The authors declare that the research was conducted in the absence of any commercial or financial relationships that could be construed as a potential conflict of interest.
The handling editor declared past co-authorship with IV, MP, and LCT.
Publisher’s Note
All claims expressed in this article are solely those of the authors and do not necessarily represent those of their affiliated organizations, or those of the publisher, the editors and the reviewers. Any product that may be evaluated in this article, or claim that may be made by its manufacturer, is not guaranteed or endorsed by the publisher.
Acknowledgments
We acknowledge John Jones for the expertise and technical support, and are very grateful for the technical support provided by Paulo Rema, Sara Basto, and Sara Jorge (UTAD) and Francisca Silva-Brito and Thais Oliveira (CIIMAR).
Supplementary Material
The Supplementary Material for this article can be found online at: https://www.frontiersin.org/articles/10.3389/fmars.2022.836612/full#supplementary-material
Abbreviations
2H, deuterium; 2H2O, deuterated water; BW, body water; cpt1, carnitine palmitoyltransferase I (EC: 2.3.1.21); DNL, de novo lipogenesis; elovl, elongation of very long-chain fatty acid protein (EC 2.3.1.199); FA, fatty acids; fas, fatty acid synthase (EC 2.3.1.85); FSR, fractional synthetic rate; dgat, diglyceride acyltransferase (EC 2.3.1.20); hoad, 3-hydroxyacyl-CoA dehydrogenase EC 1.1.1.35; HSI, hepatosomatic index; MUFA, monounsaturated fatty acid; n-3, omega-3; NMR, nuclear magnetic resonance; PUFA, polyunsaturated fatty acid; RAS, recirculated aquatic system; SFA, saturated fatty acid; TAG, triacylglycerol; TW, tank water; UFA, unsaturated fatty acid; Δ6-fad, acyl-CoA 6-desaturase (EC:1.14.19.3).
References
Ayoub, M., and Abdullah, A. Z. (2012). Critical review on the current scenario and significance of crude glycerol resulting from biodiesel industry towards more sustainable renewable energy industry. Renew. Sustain. Energy Rev. 16, 2671–2686. doi: 10.1016/j.rser.2012.01.054
Balen, R. E., Junior, G. B., Colpini, L. M. S., Bombardelli, R. A., Silva, L. C. R., and Meurer, F. (2017). Energia digestível e inclusão da glicerina bruta em dietas para juvenis de curimbatá concentrations of ammonia. Bol. Inst. Pesca 43, 347–357. doi: 10.20950/1678-2305.2017v43n3p347
Bernard, S. F., Reidy, S. P., Zwingelstein, G., and Weber, J. (1999). Glycerol and fatty acid kinetics in rainbow trout: effects of endurance swimming. J. Exp. Biol. 202, 279–288. doi: 10.1242/jeb.202.3.279
Carvalho, E. R., Schmelz-Roberts, N. S., White, H. M., Wilcox, C. S., Eicher, S. D., and Donkin, S. S. (2012). Feeding behaviors of transition dairy cows fed glycerol as a replacement for corn. J. Dairy Sci. 95, 7214–7224. doi: 10.3168/jds.2010-3584
Carvalho, P. L. D. O., Moreira, I., Martins, E. N., Piano, L. M., Toledo, J. B., and Costa Filho, C. D. L. (2012). Crude glycerine in diets for piglets. Rev. Bras. Zootec. 41, 1654–1661. doi: 10.1590/S1516-35982012000700014
Carvalho, J. R. R., Chizzotti, M. L., Ramos, E. M., Machado Neto, O. R., Lanna, D. P. D., Lopes, L. S., et al. (2014). Qualitative characteristics of meat from young bulls fed different levels of crude glycerin. Meat Sci. 96, 977–983. doi: 10.1016/j.meatsci.2013.10.020
Castro, C., Corraze, G., Panserat, S., and Oliva-Teles, A. (2015a). Effects of fish oil replacement by a vegetable oil blend on digestibility, postprandial serum metabolite profile, lipid and glucose metabolism of European sea bass (Dicentrarchus labrax) juveniles. Aquac. Nutr. 21, 592–603. doi: 10.1111/anu.12184
Castro, C., Corraze, G., Pérez-Jiménez, A., Larroquet, L., Cluzeaud, M., Panserat, S., et al. (2015b). Dietary carbohydrate and lipid source affect cholesterol metabolism of European sea bass (Dicentrarchus labrax) juveniles. Br. J. Nutr. 114, 1143–1156. doi: 10.1017/S0007114515002731
Cerrate, S., Yan, F., Wang, Z., Coto, C., Sacakli, P., and Waldroup, P. W. (2006). Evaluation of Glycerine from Biodiesel Production as a Feed Ingredient for Broilers. Int. J. Poult. Sci. 5, 1001–1007. doi: 10.3923/ijps.2006.1001.1007
Coppack, S. W., Persson, M., Judd, R. L., and Miles, J. M. (1999). Glycerol and nonesterified fatty acid metabolism in human muscle and adipose tissue in vivo. Am. J. Physiol. Endocrinol. Metab. 276, E233–E240. doi: 10.1152/ajpendo.1999.276.2.E233
Costa, D. V. D., Dias, J., Colen, R., Rosa, P. V., and Engrola, S. (2017). Partition and metabolic fate of dietary glycerol in muscles and liver of juvenile tilapia. Arch. Anim. Nutr. 71, 165–174. doi: 10.1080/1745039X.2017.1281579
Costa, D. V. D., Paulino, R. R., Okamura, D., Oliveira, M. M. D., and Rosa, P. V. E. (2015). Growth and energy metabolism of Nile tilapia juveniles fed glycerol. Pesqui. Agropecu. Bras. 50, 347–354. doi: 10.1590/S0100-204X2015000500001
Del Bianco Benedeti, P., Paulino, P. V. R., Marcondes, M. I., Maciel, I. F. S., Da Silva, M. C., and Faciola, A. P. (2016). Partial Replacement of Ground Corn with Glycerol in Beef Cattle Diets: intake, Digestibility, Performance, and Carcass Characteristics. PLoS One 11:e0148224. doi: 10.1371/journal.pone.0148224
Duarte, J. A. G., Carvalho, F., Pearson, M., Horton, J. D., Browning, J. D., Jones, J. G., et al. (2014). A high-fat diet suppresses de novo lipogenesis and desaturation but not elongation and triglyceride synthesis in mice. J. Lipid Res. 55, 2541–2553. doi: 10.1194/jlr.M052308
Figueiredo-Silva, A. C., Saravanan, S., Schrama, J. W., Panserat, S., Kaushik, S., and Geurden, I. (2012). A comparative study of the metabolic response in rainbow trout and Nile tilapia to changes in dietary macronutrient composition. Br. J. Nutr. 109, 816–826. doi: 10.1017/S000711451200205X
Food and Agriculture Organization [FAO] (2012). Biofuel co-products as livestock feed – Opportunities and challenges. Rome: FAO. Available online at: http://www.fao.org/3/i3009e/i3009e.pdf
Freitas, L., Menten, J., Zavarize, K., Pereira, R., Romano, G., Lima, M., et al. (2017). Evaluation of Dietary Glycerin Inclusion During Different Broiler Rearing Phases. Braz. J. Poult. Sci. 19, 91–96. doi: 10.1590/1806-9061-2016-0226
Gonçalves, L. U., Cerozi, B. D. S., Silva, T. S. C., Zanon, R. B., and Cyrino, J. E. P. (2015). Crude glycerin as dietary energy source for Nile tilapia. Aquaculture 437, 230–234. doi: 10.1016/j.aquaculture.2014.12.004
Goulet, E. D. B., Aubertin-Leheudre, M., Plante, G. E., and Dionne, I. J. (2007). A Meta-Analysis of the Effects of Glycerol-Induced Hyperhydration on Fluid Retention and Endurance Performance. Int. J. Sport Nutr. Exerc. Metab. 17, 391–410. doi: 10.1123/ijsnem.17.4.391
Haman, F., and Weber, J. M. (1996). Continuous tracer infusion to measure in vivo metabolite turnover rates in trout. J. Exp. Biol. 199, 1157–1162. doi: 10.1242/jeb.199.5.1157
Hansen, A. K. G., Kortner, T. M., Denstadli, V., Måsøval, K., Björkhem, I., Grav, H. J., et al. (2020). Dose–response relationship between dietary choline and lipid accumulation in pyloric enterocytes of Atlantic salmon (Salmo salar L.) in seawater. Br. J. Nutr. 123, 1081–1093. doi: 10.1017/S0007114520000434
Ho-Palma, A. C., Toro, P., Rotondo, F., Romero, M. D. M., Alemany, M., Remesar, X., et al. (2019). Insulin Controls Triacylglycerol Synthesis through Control of Glycerol Metabolism and Despite Increased Lipogenesis. Nutrients 11:513. doi: 10.3390/nu11030513
Jin, E. S., Sherry, A. D., and Malloy, C. R. (2014). Interaction between the Pentose Phosphate Pathway and Gluconeogenesis from Glycerol in the Liver. J. Biol. Chem. 289, 32593–32603. doi: 10.1074/jbc.M114.577692
Jones, J. G., Merritt, M., and Malloy, C. (2001). Quantifying tracer levels of 2H2O enrichment from microliter amounts of plasma and urine by 2H NMR. Magn. Reson. Med. 45, 156–158. doi: 10.1002/1522-2594(200101)45:1<156::aid-mrm1020>3.0.co;2-z
Kam, J. C., and Milligan, C. L. (2006). Fuel use during glycogenesis in rainbow trout (Oncorhynchus mykiss Walbaum) white muscle studied in vitro. J. Exp. Biol. 209, 871–880. doi: 10.1242/jeb.02071
Kamalam, B. S., Médale, F., Larroquet, L., Corraze, G., and Panserat, S. (2013). Metabolism and Fatty Acid Profile in Fat and Lean Rainbow Trout Lines Fed with Vegetable Oil: effect of Carbohydrates. PLoS One 8:e76570. doi: 10.1371/journal.pone.0076570
Lammers, P. J., Kerr, B. J., Weber, T. E., Dozier, W. A. III, Kidd, M. T., Bregendahl, K., et al. (2008b). Digestible and metabolizable energy of crude glycerol for growing pigs. J. Anim. Sci. 86, 602–608. doi: 10.2527/jas.2007-0453
Lammers, P. J., Kerr, B. J., Weber, T. E., Bregendahl, K., Lonergan, S. M., Prusa, K. J., et al. (2008a). Growth performance, carcass characteristics, meat quality, and tissue histology of growing pigs fed crude glycerin-supplemented diets1. J. Anim. Sci. 86, 2962–2970. doi: 10.2527/jas.2008-0972
Lech, J. J. (1970). Glycerol kinase and glycerol utilization in trout (Salmo gairdneri) liver. Comp. Biochem. Physiol. 34, 117–124. doi: 10.1016/0010-406X(70)90058-7
Lessard, P., Lefrançois, M. R., and Bernier, J. F. (1993). Dietary Addition of Cellular Metabolic Intermediates and Carcass Fat Deposition in Broilers. Poult. Sci. 72, 535–545. doi: 10.3382/ps.0720535
Li, K., Olsen, R. E., Østensen, M.-A., Altin, D., Kjørsvik, E., and Olsen, Y. (2016). Atlantic cod (Gadus morhua) larvae can biosynthesis phospholipid de novo from 2-oleoyl-glycerol and glycerol precursors. Fish Physiol. Biochem. 42, 137–147. doi: 10.1007/s10695-015-0125-y
Li, M. H., Minchew, C. D., Oberle, D. F., and Robinson, E. H. (2010). Evaluation of Glycerol from Biodiesel Production as a Feed Ingredient for Channel Catfish, Ictalurus punctatus. J. World Aquac. Soc. 41, 130–136. doi: 10.1111/j.1749-7345.2009.00320.x
Lin, M. H., Romsos, D. R., and Leveille, G. A. (1976). Effect of Glycerol on Lipogenic Enzyme Activities and on Fatty Acid Synthesis in the Rat and Chicken. J. Nutr. 106, 1668–1677. doi: 10.1093/jn/106.11.1668
Magnoni, L., Rema, P., Silva-Brito, F., Rito, J., Palma, M., Ozorio, R., et al. (2021). Dietary glycerol inclusion decreases growth performance and nitrogen retention efficiency in rainbow trout (Oncorhynchus mykiss). Aquaculture 535:736383. doi: 10.1016/j.aquaculture.2021.736383
Magnoni, L., Vaillancourt, E., and Weber, J.-M. (2008). In vivo regulation of rainbow trout lipolysis by catecholamines. J. Exp. Biol. 211, 2460–2466. doi: 10.1242/jeb.018143
Matyash, V., Liebisch, G., Kurzchalia, T. V., Shevchenko, A., and Schwudke, D. (2008). Lipid extraction by methyl-tert-butyl ether for high-throughput lipidomics. J. Lipid Res. 49, 1137–1146. doi: 10.1194/jlr.D700041-JLR200
Mauerwerk, M. T., Zadinelo, I. V., and Meurer, F. (2021a). Use of glycerol in fish nutrition: a review. Rev. Aquac. 13, 853–861. doi: 10.1111/raq.12502
Mauerwerk, M. T., Zadinelo, I. W., Zanella Júnior, M. C., Balen, R. E., Bombardelli, R. A., Da Silva, L. C. R., et al. (2021b). Glycerol effects on silver catfish (Rhamdia quelen) fingerling feeding: morphometric, zootechnical and blood parameters. Aquaculture 535:736361. doi: 10.1016/j.aquaculture.2021.736361
Menton, D. J., Slinger, S. J., and Hilton, J. W. (1986). Utilization of free glycerol as a source of dietary energy in rainbow trout (Salmo gairdneri). Aquaculture 56, 215–227. doi: 10.1016/0044-8486(86)90337-6
Mewes, J. K., Meurer, F., Tessaro, L., Buzzi, A. H., Syperreck, M. A., and Bombardelli, R. A. (2016). Diets containing crude glycerin damage the sperm characteristics and modify the testis histology of Nile tilapia broodstock. Aquaculture 465, 164–171. doi: 10.1016/j.aquaculture.2016.08.035
Moesch, A., Meurer, F., Zadinelo, I. V., Carneiro, W. F., Da Silva, L. C. R., and Dos Santos, L. D. (2016). Growth, body composition and hepatopancreas morphology of Nile tilapia fingerlings fed crude glycerol as a replacement for maize in diets. Anim. Feed Sci. Technol. 219, 122–131. doi: 10.1016/j.anifeedsci.2016.05.009
Montell, E., LeríN, C., Newgard, C. B., and Gómez-Foix, A. M. (2002). Effects of Modulation of Glycerol Kinase Expression on Lipid and Carbohydrate Metabolism in Human Muscle Cells. J. Biol. Chem. 277, 2682–2686. doi: 10.1074/jbc.M107227200
Mourot, J., Aumaitre, A., Mounier, A., Peiniau, P., and François, A. C. (1994). Nutritional and physiological effects of dietary glycerol in the growing pig. Consequences on fatty tissues and post mortem muscular parameters. Livest. Prod. Sci. 38, 237–244. doi: 10.1016/0301-6226(94)90175-9
Narayan, K. A., and Mcmullen, J. J. (1979). The Interactive Effect of Dietary Glycerol and Corn Oil on Rat Liver Lipids, Serum Lipids and Serum Lipoproteins. J. Nutr. 109, 1836–1846. doi: 10.1093/jn/109.11.1836
Nelson, J. L., and Robergs, R. A. (2007). Exploring the Potential Ergogenic Effects of Glycerol Hyperhydration. Sports Med. 37, 981–1000. doi: 10.2165/00007256-200737110-00005
Neu, D. H., Furuya, W. M., Boscolo, W. R., Potrich, F. R., Lui, T. A., and Feiden, A. (2013). Glycerol inclusion in the diet of Nile tilapia (Oreochromis niloticus) juveniles. Aquac. Nutr. 19, 211–217. doi: 10.1111/j.1365-2095.2012.00968.x
Nye, C. K., Hanson, R. W., and Kalhan, S. C. (2008). Glyceroneogenesis is the dominant pathway for triglyceride glycerol synthesis in vivo in the rat. J. Biol. Chem. 283, 27565–27574. doi: 10.1074/jbc.M804393200
Ordoñez-Gomez, C., Afanador-Tellez, G., Castañeda, S., Florez, H., and Ariza-Nieto, C. (2017). Growth performance, carcass characteristics, meat quality of growing pigs fed diets supplemented with crude glycerin derived from palm oil. Ciênc. Anim. Bras. 18:e40769. doi: 10.1590/1089-6891v18e-40769
Palma, M., Tavares, L. C., Rito, J., Henriques, L. F., Silva, J. G., Ozório, R., et al. (2019). Metabolic Effects of Dietary Glycerol Supplementation in Muscle and Liver of European Seabass and Rainbow Trout by 1H NMR Metabolomics. Metabolites 9:202. doi: 10.3390/metabo9100202
Panserat, S., Plagnes-Juan, E., Gazzola, E., Palma, M., Magnoni, L. J., Marandel, L., et al. (2020). Hepatic Glycerol Metabolism-Related Genes in Carnivorous Rainbow Trout (Oncorhynchus mykiss): insights Into Molecular Characteristics, Ontogenesis, and Nutritional Regulation. Front. Physiol. 11:882. doi: 10.3389/fphys.2020.00882
Panserat, S., Skiba-Cassy, S., Seiliez, I., Lansard, M., Plagnes-Juan, E., Vachot, C., et al. (2009). Metformin improves postprandial glucose homeostasis in rainbow trout fed dietary carbohydrates: a link with the induction of hepatic lipogenic capacities? Am. J. Physiol. Regul. Integr. Comp. Physiol. 297, R707–R715. doi: 10.1152/ajpregu.00120.2009
Rito, J., Viegas, I., Pardal, M. Â, Metón, I., Baanante, I. V., and Jones, J. G. (2019). Utilization of glycerol for endogenous glucose and glycogen synthesis in seabass (Dicentrarchus labrax): a potential mechanism for sparing amino acid catabolism in carnivorous fish. Aquaculture 498, 488–495. doi: 10.1016/j.aquaculture.2018.08.066
Rotondo, F., Ho-Palma, A. C., Remesar, X., Fernández-López, J. A., Romero, M. D. M., and Alemany, M. (2017). Glycerol is synthesized and secreted by adipocytes to dispose of excess glucose, via glycerogenesis and increased acyl-glycerol turnover. Sci. Rep. 7:8983. doi: 10.1038/s41598-017-09450-4
Santos, L. D. D., Zadinelo, I. V., Moesch, A., Bombardelli, R. A., and Meurer, F. (2019). Crude glycerol in diets for Nile tilapia in the fattening stage. Pesqui. Agropecu. Bras. 54:e00460. doi: 10.1590/S1678-3921.pab2019.v54.00460
Savina, M. V., and Wojtczak, A. B. (1977). Enzymes of gluconeogenesis and the synthesis of glycogen from glycerol in various organs of the lamprey (Lampetra fluviatilis). Comp. Biochem. Physiol. B Comp. Biochem. 57, 185–190. doi: 10.1016/0305-0491(77)90141-9
Schieck, S. J., Shurson, G. C., Kerr, B. J., and Johnston, L. J. (2010). Evaluation of glycerol, a biodiesel coproduct, in grow-finish pig diets to support growth and pork quality. J. Anim. Sci. 88, 3927–3935. doi: 10.2527/jas.2010-2858
Silva, T. S., Matos, E., Cordeiro, O. D., Colen, R., Wulff, T., Sampaio, E., et al. (2012). Dietary Tools To Modulate Glycogen Storage in Gilthead Seabream Muscle: glycerol Supplementation. J. Agric. Food Chem. 60, 10613–10624. doi: 10.1021/jf3023244
Turenne, E. D., and Weber, J.-M. (2018). Lean, mean, lipolytic machines: lipid mobilization in rainbow trout during graded swimming. J. Exp. Biol. 221:jeb171553. doi: 10.1242/jeb.171553
van Hall, G., Sacchetti, M., Rådegran, G., and Saltin, B. (2002). Human Skeletal Muscle Fatty Acid and Glycerol Metabolism During Rest, Exercise and Recovery. J. Physiol. 543, 1047–1058. doi: 10.1113/jphysiol.2002.023796
Viegas, I., Jarak, I., Rito, J., Carvalho, R. A., Metón, I., Pardal, M. A., et al. (2016). Effects of dietary carbohydrate on hepatic de novo lipogenesis in European seabass (Dicentrarchus labrax L.). J. Lipid Res. 57, 1264–1272. doi: 10.1194/jlr.M067850
Viegas, I., Mendes, V. M., Leston, S., Jarak, I., Carvalho, R. A., Pardal, M. Â, et al. (2011). Analysis of glucose metabolism in farmed European sea bass (Dicentrarchus labrax L.) using deuterated water. Comp. Biochem. Physiol. A Mol. Integr. Physiol. 160, 341–347. doi: 10.1016/j.cbpa.2011.06.029
Viegas, I., Rito, J., González, J. D., Jarak, I., Carvalho, R. A., Metón, I., et al. (2013). Effects of food-deprivation and refeeding on the regulation and sources of blood glucose appearance in European seabass (Dicentrarchus labrax L.). Comp. Biochem. Physiol. A Mol. Integr. Physiol. 166, 399–405. doi: 10.1016/j.cbpa.2013.07.013
Viegas, I., Rito, J., Jarak, I., Leston, S., Caballero-Solares, A., Metón, I., et al. (2015). Contribution of dietary starch to hepatic and systemic carbohydrate fluxes in European seabass (Dicentrarchus labrax L.). Br. J. Nutr. 113, 1345–1354. doi: 10.1017/S0007114515000574
Viegas, I., Trenkner, L. H., Rito, J., Palma, M., Tavares, L. C., Jones, J. G., et al. (2019). Impact of dietary starch on extrahepatic tissue lipid metabolism in farmed European (Dicentrarchus labrax) and Asian seabass (Lates calcarifer). Comp. Biochem. Physiol. A Mol. Integr. Physiol. 231, 170–176. doi: 10.1016/j.cbpa.2019.02.025
Viegas, I., Tavares, L. C., Plagnes-Juan, E., Silva, E., Rito, J., Marandel, L., et al. (2022). On the utilization of dietary glycerol in carnivorous fish – Part I: insights into hepatic carbohydrate metabolism of juvenile rainbow trout (Oncorhynchus mykiss) and European seabass (Dicentrarchus labrax). Front. Mar. Sci. 9:836610. doi: 10.3389/fmars.2022.836610
Weber, J.-M., Choi, K., Gonzalez, A., and Omlin, T. (2016). Metabolic fuel kinetics in fish: swimming, hypoxia and muscle membranes. J. Exp. Biol. 219, 250–258. doi: 10.1242/jeb.125294
Keywords: 2H NMR, aquaculture, circular economy, deuterated water, glycerol, muscle, perivisceral fat, triacylglycerol (TAG)
Citation: Viegas I, Palma M, Plagnes-Juan E, Silva E, Rito J, Henriques LF, Tavares LC, Ozório ROA, Panserat S and Magnoni LJ (2022) On the Utilization of Dietary Glycerol in Carnivorous Fish—Part II: Insights Into Lipid Metabolism of Rainbow Trout (Oncorhynchus mykiss) and European Seabass (Dicentrarchus labrax). Front. Mar. Sci. 9:836612. doi: 10.3389/fmars.2022.836612
Received: 15 December 2021; Accepted: 09 February 2022;
Published: 19 April 2022.
Edited by:
Brett Glencross, University of Stirling, United KingdomReviewed by:
Mansour Torfi Mozanzadeh, South Iran Aquaculture Research Center, IranJesús M. Míguez, University of Vigo, Spain
Copyright © 2022 Viegas, Palma, Plagnes-Juan, Silva, Rito, Henriques, Tavares, Ozório, Panserat and Magnoni. This is an open-access article distributed under the terms of the Creative Commons Attribution License (CC BY). The use, distribution or reproduction in other forums is permitted, provided the original author(s) and the copyright owner(s) are credited and that the original publication in this journal is cited, in accordance with accepted academic practice. No use, distribution or reproduction is permitted which does not comply with these terms.
*Correspondence: Ivan Viegas, aXZpZWdhc0B1Yy5wdA==
†ORCID: Ivan Viegas, orcid.org/0000-0003-2589-2212; Mariana Palma, orcid.org/0000-0002-0564-8442; Rodrigo O. A. Ozório, orcid.org/0000-0002-3533-3126; Stéphane Panserat, orcid.org/0000-0002-4479-9868; Leonardo J. Magnoni, orcid.org/0000-0001-8449-6071
‡Present address: Leonardo J. Magnoni, Plant & Food Research, Nelson, New Zealand