- 1Colegio de Ciencias Biológicas y Ambientales, Universidad San Francisco de Quito USFQ, Quito, Ecuador
- 2Galapagos Science Center GSC, Universidad San Francisco de Quito USFQ & University of North Carolina at Chapel Hill UNC, Puerto Baquerizo Moreno, Galápagos, Ecuador
- 3The Department of Biology, University of North Carolina at Chapel Hill, Chapel Hill, NC, United States
- 4The Department of Biology, Boston University, Boston, MA, United States
Increased standing macroalgal biomass in upwelling zones is generally assumed to be the result of higher nutrient flux due to upwelled waters. However, other factors can strongly impact macroalgal communities. For example, herbivory and temperature, via their effects on primary producers and the metabolic demands of consumers, can also influence macroalgal biomass and productivity, respectively. We assessed the effects of nutrient availability, temperature, and herbivory on macroalgal biomass on a subtidal nearshore rocky reef in the Galápagos Islands. We manipulated nutrient availability and herbivory in field experiments performed in two seasons: the first during a cool, upwelling season, and the second during a warm, non-upwelling season. Excluding macro-herbivores had a clear effect on standing macroalgal biomass, independent of season or nutrient availability. However, we found different interactive effects of nutrients and macro-herbivores between the two seasons. During the cool season, macroalgal biomass was significantly higher in herbivore exclusions than in open areas under ambient nutrient conditions. However, when nutrients were added, macroalgal biomass was not significantly different across all herbivore treatments, which suggests reduced top-down control of herbivores (hence a greater standing algal biomass) in open areas. In the warm season, macroalgal biomass was significantly higher in herbivore exclusions compared to open treatments, both with and without nutrient addition. Furthermore, biomass reached 11X in herbivore exclusions with nutrient additions, which hints nutrient limitation only during warm, low-upwelling conditions. Overall, our results support the hypothesis that macro-herbivores reduce macroalgal biomass in this system and suggest that nutrient availability, but not temperature, modulate herbivory.
Introduction
Shallow subtidal communities in coastal upwelling zones are believed to be largely bottom-up controlled via nutrient fluxes (Bustamante et al., 1995; Broitman et al., 2001; Menge and Branch, 2001; Menge et al., 2002; Nielsen, 2003; Nielsen and Navarrete, 2004; Vinueza et al., 2006; Vinueza et al., 2014). However, predation (top-down control) is also important in marine communities (Menge, 1991; Paine, 1992; Trussell et al., 2002; Harley, 2011), including in upwelling zones. For example, Witman et al. (2010) found that predation intensity was higher at strong upwelling sites compared to weak upwelling sites in the Galápagos. The authors suggested that predators (mostly whelks) track their prey resources (barnacles), which were more abundant in strong upwelling sites due to enhanced productivity (Witman et al., 2010).
Nutrient fluxes linked to upwelling can influence algal biomass in two ways. First, by enhancing algal photosynthesis and growth due to increased nitrogen and other limiting nutrients in the ocean (Figure 1, Bustamante et al., 1995; Broitman et al., 2001; Menge and Branch, 2001; Menge et al., 2002; Nielsen, 2003; Nielsen and Navarrete, 2004; Vinueza et al., 2006; Vinueza et al., 2014). And secondly, by reducing herbivory due to higher nutrient load in algal tissues, which may decrease per capita consumption rates (Figure 1). This happens because herbivores sometimes alter their feeding rates relative to the nutrient content of their food source (Burnell et al., 2013) or in response to decreased macroalgal palatability (Tomas et al., 2011). The opposite (i.e., increased consumption rates of enriched algae) has been found in oligotrophic conditions (Boyer et al., 2004; Russell and Connell, 2007).
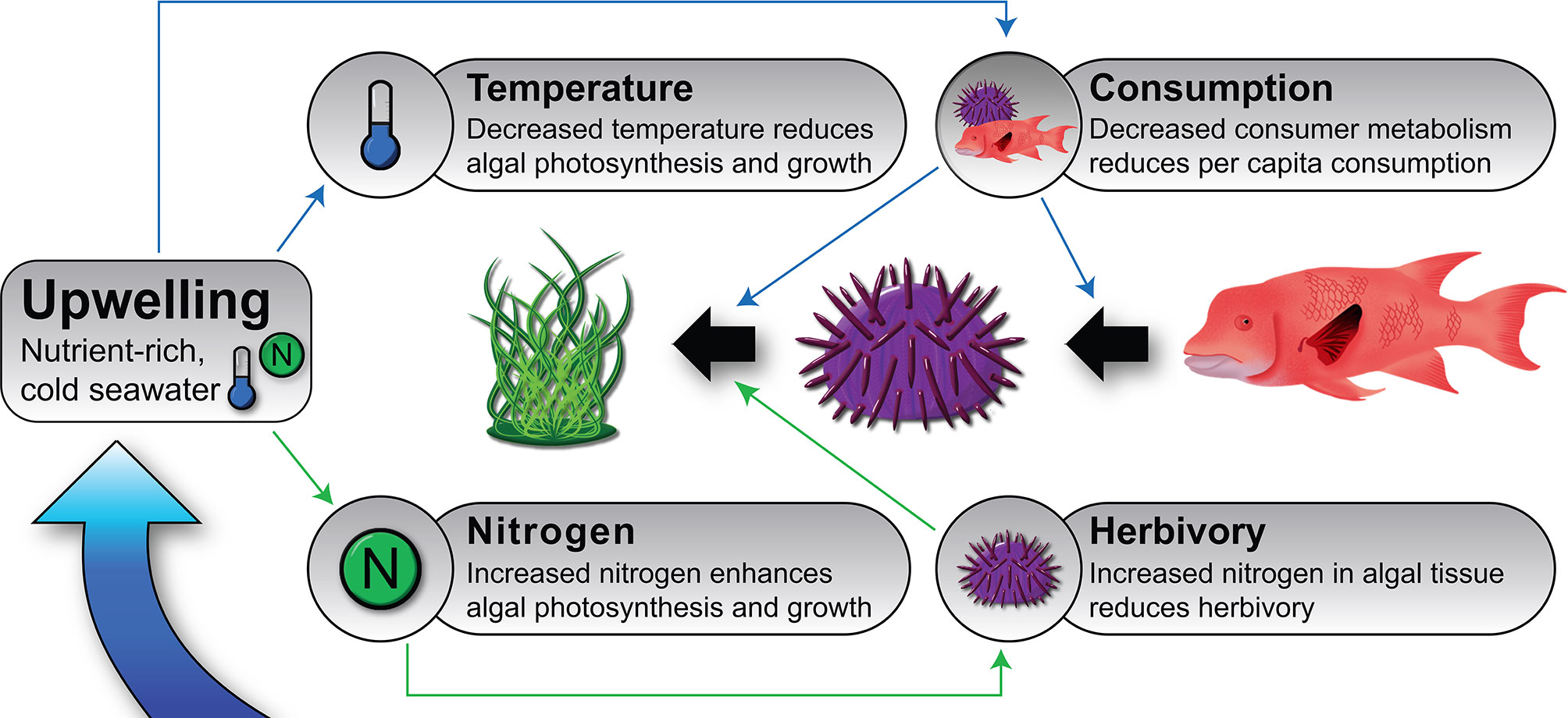
Figure 1 Conceptual model depicting the effects of upwelling on ecological processes and on macroalgal biomass in benthic upwelling communities such as nearshore rocky reefs of the Galápagos Islands. Black arrows represent trophic interactions, and the narrow, colored arrows represent hypothesized effects of upwelling on some important food web dynamics via enhanced nutrient flux (green lines) and lower temperatures (blue lines). Upwelling typically reduces water temperature and increases nutrient flux (particularly of nitrate). These environmental modifications cause numerous changes in organismal rates (respiration, photosynthesis, growth, movement, consumption, etc.) and to the per capita strength of consumer–prey interactions. The relative importance and net balance of the influence of upwelling on these and other processes is largely unknown; for example, upwelling should have both negative (via cooling) and positive (via nutrient delivery) effects on primary productivity; the general observation is that prey populations tend to be larger when and where upwelling is strong (Witman et al., 2010). Not every possible effect is included in this model; for instance, enhanced prey growth and density caused by greater resource flux could increase the fitness and abundance of consumers through donor control.
Although its role is largely unexplored, temperature may also be an important factor in upwelling systems by influencing the strength of bottom-up and top-down effects (Bruno et al., 2015). For example, due to the temperature-dependence of metabolism (Brown et al., 2004), cooler temperatures associated with upwelling should reduce algal photosynthesis and consumer pressure (Figure 1). Reduced consumer pressure during upwelling has been documented in the field (Sanford, 1999; Sanford, 2002; Carr et al., 2018) and is an alternative explanation for the observed association of high algal biomass when or where upwelling is intense; in other words it is a consequence of reduced top-down control for what is assumed to be a bottom-up phenomenon.
Although there is a fair number of studies looking at the interactive effects of herbivores and nutrients in both tropical and temperate regions, there is a lack of studies looking at these effects in tropical or subtropical upwelling regions (Burkepile and Hay, 2006). The purpose of this study was to measure the effects that herbivores, temperature, and nutrient availability have on standing macroalgal biomass. We manipulated nutrient availability and herbivory in two field experiments — one during the cool, upwelling season, and the other during the warm non-upwelling season — on a subtidal nearshore rocky reef in the Galápagos Islands.
Methods
Study Site
We performed the two experiments at 8-10 m depth at Cerro Mundo (0°52’06.0”S; 89°35’04.0”W), a typical Galápagos subtidal reef, located in San Cristóbal Island. The biological communities in the waters surrounding most of the Galápagos Islands are more temperate than tropical and are dominated by green algae (Ulva is typically the foremost benthic Genus), brown foliose algae, and grazing sea urchins. The pencil sea urchin Eucidaris galapagensis was the only sea urchin species present at Cerro Mundo during our experiments (Supplementary Table 1); they are known to have important effects on benthic algae in this system (Ruttenberg, 2001; Irving and Witman, 2009; Edgar et al., 2010; Brandt et al., 2012). Other important grazers include the razor surgeonfish (Prionurus laticlavius), the blue chin parrotfish (Scarus ghobban), sea turtles (Chelonia mydas), and marine iguanas (Amblyrhyncus cristatus).
Experimental Design
We ran the first experiment during the 2019 cool season (22 July – 25 November 2019, 18 weeks), and the second during the 2020 warm season (9 January – 24 February 2020, ~ 6 weeks). The durations differ because the warm season experiment had to be ended abruptly due to COVID-19. Both experiments included two factors in a 3 x 2 factorial design (n=8), with slightly different herbivore treatments. The experiments were based on circular concrete platforms “pizzas” (Witman et al., 2017), 0.5 m in diameter, into which Aquamesh™ (plastic-coated, galvanized with a 5 cm mesh size) was embedded for most treatments. Two substantial advantages of the experimental pizzas are that the substrate qualities are largely held constant among replicates and treatments (which would be impossible using natural substrate), and that they can be moved to other sites for site-level replication or related experiments.
We used four types of experimental pizzas to achieve the herbivore treatments (Figure 2). The 2019 cool season experiment included three herbivore treatments: 1) Open pizzas in which all herbivores could access the experimental substrate (Figure 2A), 2) Fish exclusions in which only sea urchins and meso-herbivores, such as amphipods, small blennies or damselfish had access (Figure 2B), and 3) Full herbivore exclusions (Figure 2C), which prevented access to all macro-herbivores such as sea urchins, fishes (including adult damselfishes and blennies), iguanas, and turtles, but not to meso-herbivores. Given that sea urchins did not access the fish exclusions (Brandt et al. personal obs. and see Results), we removed this treatment and added a procedural control in the 2020 warm season, which consisted of a cage with partial sides and no mesh top (Figure 2D). In both experiments, the herbivore factor was crossed with nutrients (two treatment levels: ambient and nutrient addition). For the cool season experiment, nutrients were added in the 24 nutrient addition plots by attaching two diffusers made from plastic 15 ml falcon tubes onto each addition unit (as in Zaneveld et al., 2016, Figure 2A). The tubes had 25 holes drilled into the sides to facilitate the slow liberation of time-release fertilizer (25 g of Osmocote NPK 19-6-12 without micronutrients). Due to concerns that the nutrients were not leaching out of the plastic tubes, for the warm season experiment we switched to using sheer stockings as diffusers (as in Bruno et al., 2003) and increased the fertilizer volume to 50 g. Nutrient diffusers were replaced bimonthly during the 2019 cool season experiment and weekly during the 2020 warm season experiment. We, and many others, have successfully used these techniques to manipulate nutrient availability (Bruno et al., 2003; Burkepile and Hay, 2009; Zaneveld et al., 2016).
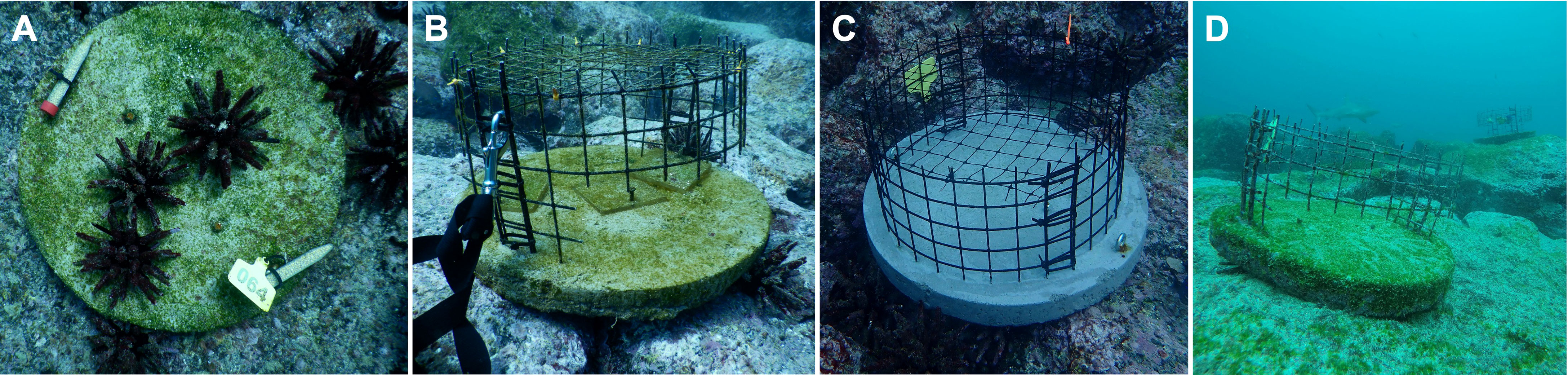
Figure 2 Examples of the four types of experimental pizzas used. (A) Open pizzas with full access to all grazers. (B) Fish exclusions, which allowed access only to sea urchins (shown acrylic plates were used only during the pilot phase of this study). (C) Full exclusions, preventing access to all macro-herbivores such as sea urchins, fishes, iguanas, and turtles, but not to meso-herbivores such as amphipods. Note the clean, uncolonized experimental surface immediately after deployment. Both cages (fish and full exclusions) are covered with a fine, 1cm monofilament mesh top. (D) Procedural control that included partial sides, designed to affect flow and light to a similar degree as other cages to test for experimental artifacts of the herbivore manipulation. Pizzas on (A–C) were used in the cool season experiment, pizzas on (A, C, D) in the warm season experiment. Results of (D) are shown in Supplementary Figure 2. The pizza design is based on Witman et al. (2017). Note the huge Galápagos shark cruising across the reef in (D).
We recorded treatment effects on macroalgal cover and biomass. To track the cover of algal taxa and for the 2019 cool season experiment, we took weekly images of each experimental unit, starting on week 6 and for the next six weeks. For the rest of the experiment (last six weeks) we took pictures every two weeks. As for the 2020 cool season experiment, we took images every two weeks, starting from the first week. We analyzed the images in Adobe Photoshop™ (version 2017.1.6), by superimposing a transparent layer with 100 random points over each image and identifying the substrate category underneath each point. Categories included: Ulva sp., sand, red filamentous algae, brown foliose algae, coarsely branched red algae, Asparagopsis sp., fish, sea urchin and snail. Given that we were interested in the effect of macro-herbivores in general on macroalgal cover, we added the % cover of all algal functional groups/species. To quantify final macroalgal biomass, algae were scraped, vacuumed into 200 µm mesh bags (Supplementary Figure 1A), and returned to the nearby marine lab of the Galapagos Science Center (GSC). For the 2019 cool season experiment this happened at the end of the experiment, i.e., on week 18; for the 2020 warm season experiment we vacuumed the algae on week 6, when we had to unexpectedly and immediately end the experiment due to imminent COVID-19 restrictions. We determined the macroalgae Ash Free Dry Weight (AFDW) by drying each sample in an oven for 24 hrs at 60°C and then burning it in a muffle furnace for 4 hrs at 500°C.
We quantified the effectiveness of the treatments in controlling herbivore access by deploying GoPro (v8) cameras focused on a subset of the pizzas (see Supplementary Figure 1B). This also enabled us determining the composition of the local marine community, and which species and functional groups were grazing or interacting on/with the experimental units. The GoPro set-up video-recorded continuously for ~90 min during each deployment. Finally, we monitored temperature with HOBO Water Pro v2 Temperature Loggers (Onset Computer Corporation, Pocasset, MA) programmed to record water temperatures at 15 min intervals.
Data Analyses
To compare treatment effects within both seasons, we calculated parametric bootstrap confidence intervals (1500 iterations) based on the best-fit generalized linear model with a Gamma distribution and a log-link (AFDW ~ herbivores * nutrients) in R (R Core Team, 2020). This model structure is common for positive-only ecological data (Schmettow, 2021; Anderson, 2022). We also assessed the effects of treatment and time on percent algal cover during the study (see Supplementary Figure 3 for results). Algal cover was treated as count data and the models were fit with a quasipoisson distribution and a square root link (Zeileis et al., 2008). We interpreted non-overlapping bars (i.e., 95% confidence intervals) as “statistically significant” treatment effects at an alpha of 0.05 (Wilcox, 2010; Ranstam, 2012). Code and data are available at: github.com/eagudoadriani/PizzaProject_2019-2020.
Results
The average benthic temperature at Cerro Mundo was 19.5°C during the 2019 cool season experiment (range 15.0 - 22.9°C) and 23.4°C during the 2020 warm season (range 18.0 - 26.6°C, Figure 3). The experimental pizzas remained in place for both experiments, even during storms and rough periods. In addition, the final macroalgal biomass in the open pizzas and our procedural controls in the warm season (Supplementary Figure 2) was not significantly different, suggesting no artifacts of flow or light availability in our exclusion cages. Algae quickly colonized the concrete substrate (Supplementary Figure 3) and within 3-6 weeks the algal community was qualitatively indistinguishable from adjacent natural substrate (Brandt et al. personal obs.). In addition, typical algal succession patterns were evidenced within the cages (Supplementary Figure 4). Forty-seven mobile species were observed at the site in the vicinity of the experiment, nine of which were seen grazing on the pizzas (Supplementary Table 1). Common herbivorous fishes on the open pizzas included blue-chin and bumphead parrotfishes (Scarus ghobban and Scarus perrico, respectively) and razor surgeonfish (Prionurus laticlavius, Supplementary Figure 1B). Pencil sea urchins were also frequently observed grazing on the open pizzas (Figure 2A).
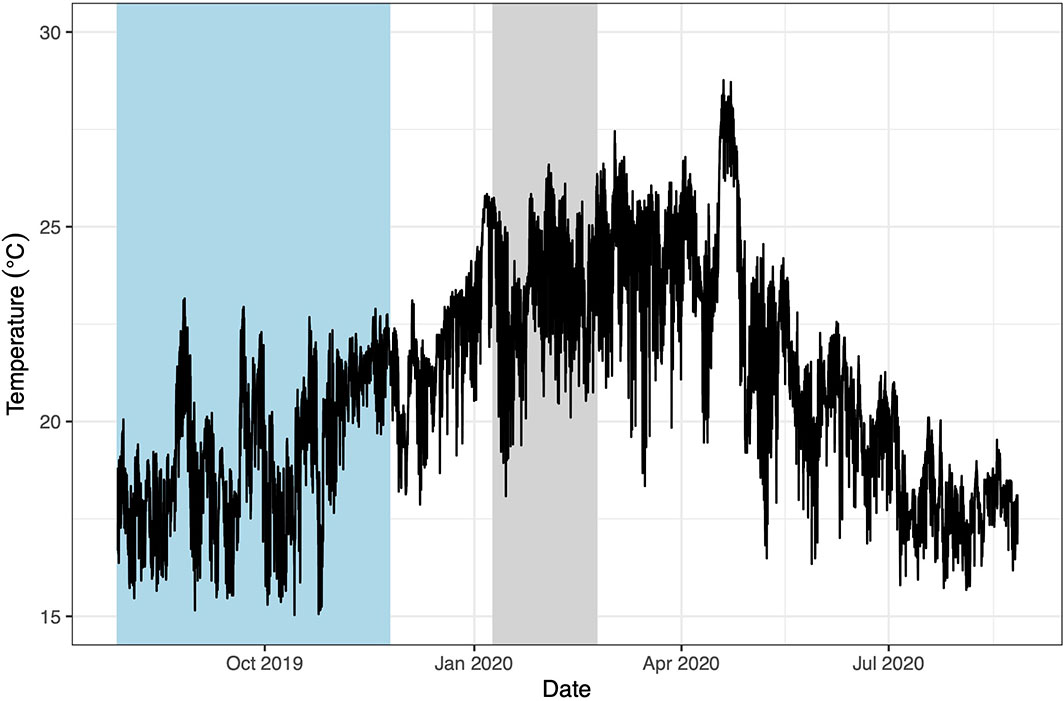
Figure 3 Temporal variation on temperature on the surface of a nearshore rocky reef where our experiments were performed (“Cerro Mundo”, 10 m depth at high tide) off the southeastern coast of San Cristóbal Island, Galápagos. Data were recorded with HOBO Water Pro v2 Temperature Loggers (Onset Computer Corporation, Pocasset, MA). Measurements were taken every 15 min. Min: 15.0°C, Max: 28.7°C, Mean: 21.0°C. The blue bar highlights the temperatures during the cool season experiment and the gray bar during the warm season experiment.
In both the cool and warm season experiments and when all herbivores were allowed access to experimental substrates (open pizzas or gray bars in Figure 4), final algal biomass was relatively low, with mean values ranging from 0.93 to 7.62 g. On the other hand, excluding all macro-herbivores in general produced higher accumulation of final macroalgal biomass with mean values that ranged from 2.85 to 29.56 g (blue bars in Figure 4). The combined effects of nutrients and herbivore exclusions varied however in the cool and the warm season experiments. Excluding macro-herbivores (both fish and all herbivores) during the cool season significantly increased final macroalgal biomass relative to the open pizzas, only with ambient nutrients; there were not significant differences among open pizzas and herbivore exclusions when nutrients were added (Figure 4A). This contrasted with the results of the warm season experiment: final macroalgal biomass significantly increased in herbivore exclusions compared to open pizzas only with nutrient additions, and it reached, ~11X more biomass (Figure 4B). In other words, adding nutrients had contrasting effects in the two experiments: during the cool season experiment the significant differences among herbivore treatments disappeared when nutrients were added (due to a higher macroalgal biomass in open pizzas), while the addition of nutrients during the warm season experiment promoted the significant differences among herbivore treatments (Figure 4).
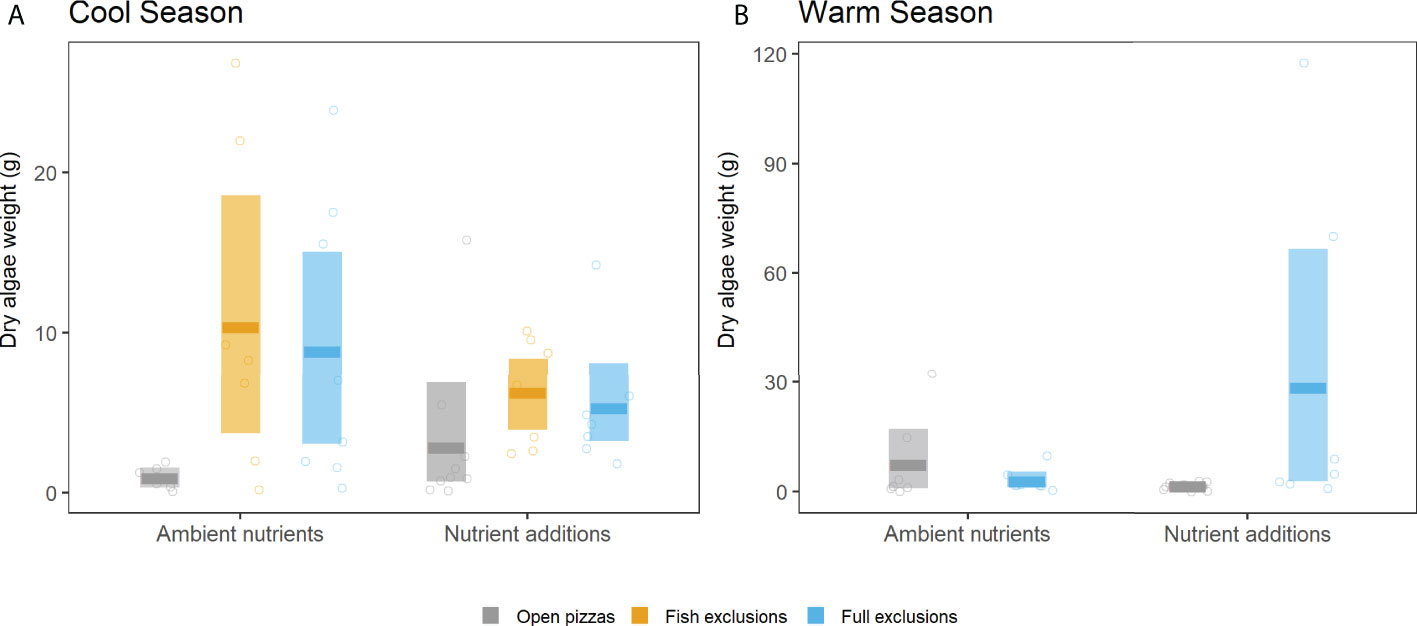
Figure 4 Herbivore treatment effects on final macroalgal biomass (AFDW of all algal taxa, which was largely Ulva) in the 2019 cool season (A) and the 2020 warm season experiments (B). Bars represent bootstrap estimated means (darker, thick horizontal lines) and modelled 95% confidence intervals (entire shaded bar). Circles are the individual data raw points (n = 8).
Discussion
Overall, our results are in agreement with previous findings that macro-herbivores have a strong top-down effect on macroalgal biomass in the Galápagos, even during intense upwelling when nutrient flux was presumably high (Vinueza et al., 2006; Vinueza et al., 2014; Carr et al., 2018). On the other hand, in our study, excluding only fish or all macro-herbivores had the same effect on final macroalgal biomass during the cool season (the only season during which the fish exclusion treatment was included, Figure 4A). This contrasts with Carr et al. (2018), who found that both sea urchins and other herbivores including fishes, had strong effects on standing macroalgal biomass. Our results indicate that the effect of sea urchins alone was negligible, as final macroalgal biomass in the fish exclusions (i.e., open to sea urchins) was not significantly different than in the full exclusions (Figure 4A). A possible explanation for this is that sea urchins may not have accessed and grazed the fish exclusions for a substantial time, limiting their effects on macroalgal biomass. In fact, we recorded sea urchins in only ~11% of the fish exclusions throughout the duration of the experiment. The discrepancies between our results and those of Carr et al. (2018) could also be due to differences in the local sea urchin species composition during the two studies. In our experiments, the pencil sea urchin (E. galapagensis) was the only sea urchin species present, and our cage design allowed sea urchins to enter/exit at their will. In contrast, Carr et al. (2018) used the green sea urchin Lytechinus semituberculatus (the second most common sea urchin species in the Galápagos, Brandt and Guarderas, 2002) in inclusion cages. It thus seems reasonable that the impact of the green sea urchins might have been stronger (and detectable) in Carr et al. (2018). This agrees with other studies that have found a strong top-down effect of both pencil and green sea urchins in inclusion cages in the Galápagos subtidal (Brandt et al., 2012). In addition, it is known that pencil sea urchins have slower algal grazing rates than green sea urchins (Witman et al., 2017). Thus the absence of the faster-grazing green sea urchins in the present experiment could have reduced the total sea urchin grazing effect.
There was no evidence that nutrient addition affected primary productivity in the full exclusions (no herbivory) during the cool, upwelling season experiment, potentially because enhanced nutrient flux via upwelling alleviated nutrient limitation. This period is also when mean water temperature was the lowest (19.5°C), likely reducing algal productivity and nutrient demand. Although final algal biomass was not significantly different in the cool season experiment across open pizzas (accessible to herbivores) and regardless nutrient additions, there was a positive, non-significant trend towards higher biomass when nutrients were added (Figure 4A). This may hint at reduced herbivory when algal tissue nutrient concentration is high, as predicted for non-oligotrophic conditions (Tomas et al., 2011; Burnell et al., 2013).
Compared to open pizzas, excluding all macro-herbivores had a significant effect on algal biomass both in the cool and in the warm season experiments, but with different nutrient treatments: in the cool season experiment significant differences of final macroalgal biomass among herbivore treatments occurred with ambient nutrients (Figure 4A), while it did with nutrient additions in the warm season (Figure 4B). These differences were maximized in the warm season experiment (~20X versus ~10X more biomass comparing full exclusions and open pizzas among herbivore treatments with significant differences, Figure 4). These results suggest that nutrient addition enhances standing macroalgal biomass in the absence of macro-herbivores only during the warm season (Figure 4B). A finding consistent with the ideas that 1) nutrients were limiting only in the warm season due to the relative weakness of upwelling, and 2) that higher temperatures (23.4°C, average temperature during the warm season) caused greater macroalgal biomass accumulation when nutrients were not limiting (as seen in O’Connor et al., 2009). However, given that algal biomass in the open pizzas with ambient nutrients was not significantly lower in the warm season compared to the cool season experiment (which would hint that nutrients were limiting during the warm season) it is also possible that the cool season nutrient additions were ineffective and that the observed increased algal biomass in the herbivore exclusions in the warm season was a result of changing the design (drilled falcon tubes vs. sheer stockings), the frequency (bimonthly vs. weekly), and the amount (25 vs. 50 g) of nutrient delivery between the cool and warm season experiments, respectively (see Methods). It is still interesting however that the increased algal biomass was restricted to the herbivore exclusions when nutrients were added. This could suggest that top-down control in warmer conditions is strong enough to counter the effects of nutrient availability.
In terms of the influence of temperature, we expected to see a greater top-down control of grazers on macroalgal biomass during the warm season (i.e., lower macroalgal biomass in the warm season and higher in the cool season in the open pizzas), due to temperature-dependence of consumer metabolism (Brown et al., 2004). However, grazing intensity in open pizzas was not significantly different across seasons (Figure 4). Carr and Bruno (2013) found that the grazing of the green sea urchin Lytechinus semituberculatus increased at higher temperatures; their temperature treatments differed by 14°C. On average, our temperature “treatment” (which was dependent of in situ temperature fluctuations) only varied 3.9°C across seasons, which was likely insufficient to produce an increase (or to detect a difference) in macro-herbivores’ metabolism and hence in their grazing rates.
As mentioned before, few studies on the interactive effects of herbivores and nutrients have been performed in tropical or subtropical upwelling regions (Burkepile and Hay, 2006). One example is the one by Cordeiro et al. (2020), which found that in shallow depths (1-2 m) sea urchin herbivory strongly influenced macroalgal cover on marginal coral reefs off southern Brazil. Surprisingly, fishes had no effect on macroalgal cover. However, at greater depths (5-6 m), the dynamics of benthic macroalgae were largely determined by abiotic factors related to localized upwelling. However, the question remains: when nutrient fluxes are greater (as in upwelling regions like the Galápagos), do bottom-up forces overwhelm top-down control? Our results illustrate an approach that could be used to untangle these factors in productive and dynamic systems, while also considering the confounding role of temperature. However, an important caveat is that because several aspects of our experimental design differed between the cool and warm season experiments, the results are not directly comparable. For example, final algal biomass could have been distinct between our experiments due to differences in their duration (18 weeks: cold season experiment, 6 weeks: warm season experiment) and have affected our results. We do not think this is the case, as in general, biomass accumulation in full exclusions was surprisingly very similar between experiments (Figure 4), and more importantly, when analyzing our data normalized by the number of days of each experiment (126 days: cold season experiment, 46 days: warm season experiment), the results yielded the exact same patterns as observed in Figure 4 and Supplementary Figure 5. Having said that, there should be replication during warm and cool seasons so that any observed differences could be attributed to environmental characteristics like temperature and nutrient fluxes. Moreover, the concentration of important nutrients (e.g., Nitrogen and Phosphorus) should be tracked in different treatments (remarkably, there is little information on benthic nutrient concentrations in this system). We are actually implementing these and other changes to the study design and repeating the experiment in replicate warm and cool seasons to begin exploring the role of these environmental factors in mediating primary productivity and consumption patterns.
In conclusion, our results suggest that both top-down and bottom-up forcing influence benthic macroalgal communities in this dynamic upwelling system (Figure 1). Additionally, the response of macroalgae to temperature, nutrients, and herbivory hints at an interactive effect among these three factors. Studying top-down and bottom-up controls in complex, highly variable systems like the Galápagos is important, because direct and indirect interactions among species may be altered by anthropogenic ocean heating and other aspects of global change including altered upwelling dynamics and reduced ocean productivity.
Data Availability Statement
Code and data are available at: github.com/eagudoadriani/PizzaProject_2019-2020.
Author Contributions
MB, IS-R and JFB conceived and designed the experiments; MB, IS-R, DFG, EA-A and JFB performed the field experiments; IS-R and DF-G analysed the data; EA-A and CBB ran the statistical analyses and made the graphs; MB and JFB wrote the paper; all authors read, edited, and agreed on the final version of the paper.
Funding
The project was funded by the National Science Foundation (grant OCE #1737071 to JFB).
Conflict of Interest
The authors declare that the research was conducted in the absence of any commercial or financial relationships that could be construed as a potential conflict of interest.
Publisher’s Note
All claims expressed in this article are solely those of the authors and do not necessarily represent those of their affiliated organizations, or those of the publisher, the editors and the reviewers. Any product that may be evaluated in this article, or claim that may be made by its manufacturer, is not guaranteed or endorsed by the publisher.
Acknowledgments
We thank the Galápagos National Park Directorate for granting the permit PC-26-19 to perform the research, the GSC for logistics and facilities support (special thanks to S. Sotamba, J. Sotamba, D. Alarcón, C. Vintimilla, A. Carrión, and S. Sarzosa), the Universidad San Francisco de Quito and The University of North Carolina at Chapel Hill, the divers and field assistants S. Ryburn, J.M. Álava, S. Medor, L. Nelson, L. Turner, J. Hlavin, M. Mejía, and L. Reascos. We are greatful with Captains Manuel Yepez and Yuri Revelo for the long hours at sea. We thank Jamie Kerlin and Emy Miyazawa for preparing the templates of Figure 1. We thank the reviewers for their valuable remarks and suggestions that contributed to improve this manuscript.
Supplementary Material
The Supplementary Material for this article can be found online at: https://www.frontiersin.org/articles/10.3389/fmars.2022.845635/full#supplementary-material
References
Anderson S. (2022). Generalized Linear Mixed-Effects Modeling in R. Available at: https://github.com/seananderson/glmm-course.
Boyer K. E., Fong P., Armitage A. R., Cohen R. A. (2004). Elevated Nutrient Content of Tropical Macroalgae Increases Rates of Herbivory in Coral, Seagrass, and Mangrove Habitats. Coral Reefs 23, 530–538. doi: 10.1007/s00338-004-0421-y
Brandt M., Guarderas P. (2002). “Erizos De Mar,” in Reserva Marina De Galápagos. Línea Base De La Biodiversidad. Fundación Charles Darwin/Servicio Parque Nacional Galápagos, Santa Cruz, Galápagos, Ecuador (Santa Cruz, Galápagos, Ecuador: Fundación Charles Darwin/ Servicio Parque Nacional Galápagos), 396–418.
Brandt M., Witman J. D., Chiriboga A. I. (2012). Influence of a Dominant Consumer Species Reverses at Increased Diversity. Ecology 93, 868–878. doi: 10.1890/10-1785.1
Broitman B. R., Navarrete S. A., Smith F. (2001). Geographic Variation of Southeastern Pacific Intertidal Communities. Marine Ecol. Prog. Ser. 224, 21–34. doi: 10.3354/meps224021
Brown J. H., Gillooly J. F., Allen A. P., Savage V. M., West G. B. (2004). Toward a Metabolic Theory of Ecology. Ecology 85, 1771–1789. doi: 10.1890/03-9000
Bruno J. F., Carr L. A., O’Connor M. I. (2015). Exploring the Role of Temperature in the Ocean Through Metabolic Scaling. Ecology 96, 3126–3140. doi: 10.1890/14-1954.1
Bruno J. F., Petes L. E., Drew Harvell C., Hettinger A. (2003). Nutrient Enrichment Can Increase the Severity of Coral Diseases. Ecol. Lett. 6, 1056–1061. doi: 10.1046/j.1461-0248.2003.00544.x
Burkepile D. E., Hay M. E. (2006). Herbivore vs. Nutrient Control of Marine Primary Producers: Context-Dependent Effects. Ecology 87, 3128–3139. doi: 10.1890/0012-9658(2006)87[3128:HVNCOM]2.0.CO;2
Burkepile D., Hay M. (2009). Nutrient Versus Herbivore Control of Macroalgal Community Development and Coral Growth on a Caribbean Reef. Marine Ecol. Prog. Ser. 389, 71–84. doi: 10.3354/meps08142
Burnell O. W., Russell B. D., Irving A. D., Connell S. D. (2013). Eutrophication Offsets Increased Sea Urchin Grazing on Seagrass Caused by Ocean Warming and Acidification. Mar. Ecol. Prog. Ser. 485, 37–46. doi: 10.3354/meps10323
Bustamante R. H., Branch G. M., Eekhout S., Robertson B., Zoutendyk P., Schleyer M., et al. (1995). Gradients of Intertidal Primary Productivity Around the Coast of South Africa and Their Relationships With Consumer Biomass. Oecologia 102, 189–201. doi: 10.1007/BF00333251
Carr L., Bruno J. F. (2013). Warming Increases the Top-Down Effects and Metabolism of a Subtidal Herbivore. PeerJ 1, e109. doi: 10.7717/peerj.109
Carr L. A., Gittman R. K., Bruno J. F. (2018). Temperature Influences Herbivory and Algal Biomass in the Galápagos Islands. Front. Mar. Sci. 5. doi: 10.3389/fmars.2018.00279
Cordeiro C. A. M. M., Harborne A. R., Ferreira C. E. L. (2020). The Biophysical Controls of Macroalgal Growth on Subtropical Reefs. Front. Mar. Sci. 7. doi: 10.3389/fmars.2020.00488
Edgar G. J., Banks S. A., Brandt M., Bustamante R. H., Chiriboga A., Earle S. A., et al. (2010). El Niño, Grazers and Fisheries Interact to Greatly Elevate Extinction Risk for Galápagos Marine Species. Global Change Biol. 16, 2876–2890. doi: 10.1111/j.1365-2486.2009.02117.x
Harley C. D. G. (2011). Climate Change, Keystone Predation, and Biodiversity Loss. Science 334, 1124–1127. doi: 10.1126/science.1210199
Irving A. D., Witman J. D. (2009). Positive Effects of Damselfish Override Negative Effects of Urchins to Prevent an Algal Habitat Switch. J. Ecol. 97, 337–347. doi: 10.1111/j.1365-2745.2008.01467.x
Menge B. A. (1991). Generalizing From Experiments: Is Predation Strong or Weak in the New England Rocky Intertidal? Oecologia 88, 1–8. doi: 10.1007/BF00328396
Menge B. A., Branch G. (2001). “Rocky Intertidal Communities,” in Marine Community Ecology. Eds. Bertness M. D., Hay M. E., Gaines S. D. (Sunderland, MA: Sinauer Associates), 221–251.
Menge B. A., Sanford E., Daley B., Freidenburg T. L., Hudson G., Lubchenco J. (2002). Inter-Hemispheric Comparison of Bottom-Up Effects on Community Structure: Insights Revealed Using the Comparative-Experimental Approach. Ecol. Res. 17, 1–16. doi: 10.1046/j.1440-1703.2002.00458.x
Nielsen K. J. (2003). Nutrient Loading and Consumers: Agents of Change in Open-Coast Macrophyte Assemblages. Proc. Natl. Acad. Sci. 100, 7660–7665. doi: 10.1073/pnas.0932534100
Nielsen K. J., Navarrete S. A. (2004). Mesoscale Regulation Comes From the Bottom-Up: Intertidal Interactions Between Consumers and Upwelling. Ecol. Lett. 7, 31–41. doi: 10.1046/j.1461-0248.2003.00542.x
O’Connor M. I., Piehler M. F., Leech D. M., Anton A., Bruno J. F. (2009). Warming and Resource Availability Shift Food Web Structure and Metabolism. PloS Biol. 7, 1–6. doi: 10.1371/journal.pbio.1000178
Paine R. T. (1992). Food-Web Analysis Through Field Measurement of Per Capita Interaction Strength. Nature 355, 73–75. doi: 10.1038/355073a0
Ranstam J. (2012). Why the P-Value Culture Is Bad and Confidence Intervals a Better Alternative. Osteoarthr. Cartil. 20, 805–808. doi: 10.1016/j.joca.2012.04.001
R Core Team. (2020). R: A Language and Environment for Statistical Computing (Vienna, Austria: R Foundation for Statistical Computing). Available at: https://www.R-project.org/.
Russell B. D., Connell S. D. (2007). Response of Grazers to Sudden Nutrient Pulses in Oligotrophic Versus Eutrophic Conditions. Mar. Ecol. Prog. Ser. 349, 73–80. doi: 10.3354/meps07097
Ruttenberg B. (2001). Effects of Artisanal Fishing on Marine Communities in the Galápagos Islands. Conserv. Biol. 15 (6), 1691–1699. doi: 10.1046/j.1523-1739.2001.99556.x
Sanford E. (1999). Regulation of Keystone Predation by Small Changes in Ocean Temperature. Science 283, 2095–2097. doi: 10.1126/science.283.5410.2095
Sanford E. (2002). The Feeding, Growth, and Energetics of Two Rocky Intertidal Predators (\Emphpisaster Ochraceus and \Emphnucella Canaliculata) Under Water Temperatures Simulating Episodic Upwelling. J. Exp. Marine Biol. Ecol. 273, 199–218. doi: 10.1016/S0022-0981(02)00164-8
Schmettow M. (2021) 7 Generalized Linear Models | New Statistics for Design Researchers. Available at: https://schmettow.github.io/New_Stats/glm.html.
Tomas F., Abbott J. M., Steinberg C., Balk M. A., Williams S., Stachowicz J. J. (2011). Plant Genotype and Nitrogen Loading Influence Seagrass Productivity, Biochemistry, and Plant–Herbivore Interactions. Ecology 92, 1807–1817. doi: 10.1890/10-2095.1
Trussell G. C., Ewanchuk P. J., Bertness M. D. (2002). Field Evidence of Trait-Mediated Indirect Interactions in a Rocky Intertidal Food Web. Ecol. Lett. 5, 241–245. doi: 10.1046/j.1461-0248.2002.00304.x
Vinueza L. R., Branch G. M., Branch M. L., Bustamante A. R. H. (2006). Top-Down Herbivory and Bottom-Up El Niño Effects on Galápagos Rocky-Shore Ccommunities. Ecol. Monogr. 76, 111–131. doi: 10.1890/04-1957
Vinueza L. R., Menge B. A., Ruiz D., Palacios D. M. (2014). Oceanographic and Climatic Variation Drive Top-Down / Bottom-Up Coupling in the Galápagos Intertidal Meta-Ecosystem. Ecol. Monogr. 84, 411–434. doi: 10.1890/13-0169.1
Wilcox R. R. (2010). Fundamentals of Modern Statistical Methods: Substantially Improving Power and Accuracy. 2nd ed (New York, NY: Springer).
Witman J. D., Brandt M., Smith F. (2010). Coupling Between Subtidal Prey and Consumers Along a Mesoscale Upwelling Gradient in the Galapagos Islands. Ecol. Monogr. 80, 153–177. doi: 10.1890/08-1922.1
Witman J. D., Smith F., Novak M. (2017). Experimental Demonstration of a Trophic Cascade in the Galápagos Rocky Subtidal: Effects of Consumer Identity and Behavior. PloS One 12, e0175705. doi: 10.1371/journal.pone.0175705
Zaneveld J. R., Burkepile D. E., Shantz A. A., Pritchard C. E., Mcminds R., Welsh R., et al. (2016). Overfishing and Nutrient Pollution Interact With Temperature to Disrupt Coral Reefs Down to Microbial Scales. Nat. Commun. 7, 1–12. doi: 10.1038/ncomms11833
Keywords: macroalgae, subtidal, upwelling, nutrients, temperature, bottom-up/top-down control, Galápagos
Citation: Brandt M, Silva-Romero I, Fernández-Garnica D, Agudo-Adriani E, Bove CB and Bruno JF (2022) Top-Down and Bottom-Up Control in the Galápagos Upwelling System. Front. Mar. Sci. 9:845635. doi: 10.3389/fmars.2022.845635
Received: 30 December 2021; Accepted: 13 April 2022;
Published: 12 May 2022.
Edited by:
Rachel Collin, Smithsonian Tropical Research Institute (SI), United StatesReviewed by:
Andrew Sellers, Smithsonian Tropical Research Institute, PanamaCarlos Eduardo Leite Ferreira, Fluminense Federal University, Brazil
Copyright © 2022 Brandt, Silva-Romero, Fernández-Garnica, Agudo-Adriani, Bove and Bruno. This is an open-access article distributed under the terms of the Creative Commons Attribution License (CC BY). The use, distribution or reproduction in other forums is permitted, provided the original author(s) and the copyright owner(s) are credited and that the original publication in this journal is cited, in accordance with accepted academic practice. No use, distribution or reproduction is permitted which does not comply with these terms.
*Correspondence: Margarita Brandt, bWJyYW5kdEB1c2ZxLmVkdS5lYw==