- 1MARBEC (Marine Biodiversity, Exploitation and Conservation), Univ Montpellier, CNRS, Ifremer, IRD, Montpellier, France
- 2Sorbonne Université, CNRS UMR7621 (Laboratoire d’Océanographie Microbienne), Observatoire Océanographique, Banyuls-sur-Mer, France
- 3MARBEC (Marine Biodiversity, Exploitation and Conservation), Univ Montpellier, CNRS, Ifremer, IRD, Sète, France
Temperature and light mainly drive seasonal dynamics of microbial planktonic diversity in coastal ecosystems; however, disentangling their effects is challenging because they are always tightly coupled. Shallow coastal lagoons exhibit intense temperature changes throughout the year and high interannual temperature fluctuations, offering the opportunity to study temperature effects on microbial community diversity and succession. Weekly sampling at 16s and 18s rRNA gene OTU diversity associated with high-frequency meteorological and hydrological monitoring was conducted in the northwestern Mediterranean Thau Lagoon (South of France) from winter to spring in 2015 and 2016. While 2015 was a normal climatic year, 2016 had the warmest winter ever recorded in southern France. Water temperature was found to be the main driver of community diversity and succession from winter to spring. During the normal temperature year of 2015, bacterial communities were dominated by Proteobacteria and Bacteroidetes, archaeal community by Thaumarachaeota, and unicellular eukaryotes mainly by picochlorophytes (Bathycoccus prasinos, Micromonas bravo, and Ostreococcus spp.) in winter and diatoms (Chaetoceros spp.) in spring. The unusually warm year 2016 benefited Actinobacteria (ML602J-51), Cyanobacteria (Synechococcus), the picoeukaryote Ostreococcus spp., and several dinoflagellates. Our results suggest that in a warmer ocean, smaller organisms will dominate microbial communities in shallow coastal waters, potentially affecting ecosystem services.
Introduction
Microbial planktonic diversity, including unicellular eukaryotes and bacteria, plays a critical role in marine ecosystems as these organisms are involved in primary production, matter recycling, and energy transfer to higher trophic levels (Field et al., 1998; Danovaro and Pusceddu, 2007; Buchan et al., 2014; Dolan, 2018). As microbial diversity is strongly influenced by environmental factors, it is constantly reshaped throughout the year, with episodic periods of intense cell proliferation during seasonal blooms (Needham and Fuhrman, 2016; Lambert et al., 2018).
Microbial diversity and succession have been extensively described in coastal zones (Nogales et al., 2007; Gilbert et al., 2012; Ward et al., 2017; Lambert et al., 2018; Tragin and Vaulot, 2019). These zones, at the interface between land and sea, are influenced by various environmental factors such as riverine runoff, tides, and upwelling, which modulate microbial diversity and affect ecosystem functioning and productivity (Boaden and Seed, 1985; Pecqueur et al., 2011; Deininger et al., 2016). In coastal areas, seasonal variations in temperature and day length shape the communities throughout the year (Ward et al., 2017; Lambert et al., 2018; Trombetta et al., 2019; Trombetta et al., 2021). In addition, inter-annual temperature variations and anomalies arising from meteorological events such as wind or floods strongly influenced the microbial composition from one year to another, thereby modifying microbial community diversity (Trombetta et al., 2019; Lambert et al., 2021; Trombetta et al., 2021).
Among coastal ecosystems, coastal lagoons, at the interface between land and open sea, play a major role in nutrient transition, carbon cycling and biodiversity hosting (Kjerfve, 1994; Schubert and Telesh, 2017). In these zones, microbial communities play an important role in the ecosystem functioning and productivity, as notably they mineralize organic matter, are principal primary producers and provide a consequent part of the energy transfer to higher trophic levels. They participate to the sustainability of the biodiversity and are also crucial for local populations, as coastal lagoons also provide food, raw material and recreational activities (Costanza et al., 1997). Coastal lagoons are sensitive to environmental forces such as wind, rain, droughts, currents, small tides, seawater, or riverine inputs (Kjerfve, 1994; Schubert and Telesh, 2017).
The Mediterranean Sea is characterised by a large number of lagoons (Pérez-Ruzafa et al., 2011; Cataudella et al., 2015). In Mediterranean coastal lagoons, light penetrates the whole water column throughout the year and is often non-limiting for organisms such as planktonic primary producers (Trombetta et al., 2019). By contrast, due to the shallowness and inertia of the water body, atmospheric temperature strongly influences the whole water column temperature (Trombetta et al., 2021), which often exhibits a significant seasonal amplitude of more than 25°C difference between winter and summer (Pernet et al., 2012; Cataudella et al., 2015; Trombetta et al., 2019). Consequently, in coastal lagoons, water temperature is suspected to be the predominant factor driving microbial diversity and succession (Trombetta et al., 2021). Therefore, Mediterranean shallow coastal lagoons provide a unique opportunity to study how intra- and inter-annual temperature variations affect microbial diversity and succession.
The Thau Lagoon is a typical Northwestern Mediterranean shallow coastal system where microorganisms have been studied for decades. However, the diversity and succession of planktonic bacterial and unicellular eukaryotes have not been examined at a fine taxonomic level using metabarcoding of 16s and 18s ribotypes. Thus, in the Thau Lagoon, the planktonic bacterial and small eukaryotic communities are still a ‘black box’. This lack of knowledge makes it difficult to assess how actual and future warmer climatic conditions may affect the microbial diversity and functioning of these ecosystems. The study objective was to characterise the taxonomic diversity of microbial communities from winter to late spring in the Thau Lagoon over two consecutive years. A comparison of two contrasting climatic years, a ‘normal’ year (2015) versus an exceptional warmer year (2016), offered the opportunity to assess how temperature influences communities’ microbial diversity and succession. In the Thau Lagoon, we considered as ‘normal’ a year with a significant water cooling (around 4°C) and a constant temperature increase throughout the spring, as occurred in 2015. The 2016 year was an exceptional warm year (https://www.ncdc.noaa.gov/sotc/global/202113#ref), inducing no water cooling and high water temperatures during the winter (Trombetta et al., 2019).
Material and Methods
Study Site and Sampling
The study was carried out in the Thau Lagoon (Supplementary Figure 1), located on the northwestern Mediterranean French coast (43°24’00” N, 3°36’00” E). The Thau Lagoon is a characteristic shallow coastal lagoon with a mean depth of 4 m (max 10 m) and an area of 75 km². Although sporadically limited by phosphorus and nitrogen, it is a mesotrophic lagoon and is predominantly marine with a salinity ranging from 34 to 38 (Souchu et al., 2010). The Thau Lagoon is connected to the sea by two main channels and has a turnover rate of 2% (50 days). It is characterised by large water temperature amplitude throughout the year, ranging from 4°C in winter to 30°C in summer, and is often exposed to high wind speeds (Millet and Cecchi, 1992; Pernet et al., 2012; Trombetta et al., 2019). In addition, the Thau Lagoon is economically important for local populations, mainly due to its oyster farms, representing 10% of French production.
To investigate bacteria, archaea and unicellular eukaryota diversity, the water column was sampled at mid-depth (approximately 1.5 m below the surface) at the fixed meteorological and hydrological monitoring station of ‘Coastal Mediterranean Thau Lagoon Observatory’ (43°24’53” N, 3°41’16” E) (Mostajir et al., 2018) close to the pontoon of the Mediterranean platform for Marine Ecosystem Experimental Research (MEDIMEER). The sampling station is located less than 50 m from the mouth of the main channel connecting the lagoon to the sea; therefore, the water residence at the station is at its lowest (20 days) (Fiandrino et al., 2012). The depth at the station was 2.5–3 m. The water was sampled weekly from winter to spring, encompassing the main annual spring bloom in two consecutive years (2015 and 2016), i.e. from 8 January to 12 May 2015 and from 12 January to 14 June 2016. Samples were taken between 9 and 10 am Local Time, using a Niskin bottle. Subsamples of 1 to 2.3 L were collected, and microbial biomass was firstly collected on 3 µm pore-size polycarbonate filters with pore sizes of 3 µm (Nucleopore, Whatman). Then, the 3 µm screened water helped to collect microbial biomass on 0.22 µm pore-size GV Sterivex cartridges (Millipore). Both 3.0 µm and 0.22 µm filters were stored at -80°C until analysis. Only the 0.22–3.0 µm fraction was analysed for microbial diversity.
In addition, as part of the weekly sampling for measuring nutrient concentrations at the Coastal Mediterranean Thau Lagoon Observatory, a high-frequency recording (every 15 min) of hydrological and meteorological parameters, using automated sensors, was also carried out at this station. Hydrological and meteorological data are available in the Seanoe database (DOI 10.17882/58280 Mostajir et al., 2018); they were also previously published (Trombetta et al., 2019; Trombetta et al., 2021) and are shown in Supplementary Figure 2. Nutrients were nitrite (NO2-), nitrate (NO3-), phosphate (PO4 3-), and silicate (SiO2). Hydrological and meteorological parameters were Chlorophyll-a (Chl-a) fluorescence, air and water temperature, wind speed and direction, photosynthetically active radiation (PAR) (400–700 nm), short wave ultraviolet B radiation (UVBR) (280–320 nm), salinity, turbidity, depth, air pressure, humidity, and precipitation.
DNA Extraction, Amplification and Sequencing
Nucleic acid extraction was adapted from previously published protocols (Hugoni et al., 2013; Lambert et al., 2018), using the Nucleospin Plant II kit (MN, 740770). Briefly, the Sterivex and the 3 µm pore-size filters were thawed on ice; then lysis buffer PL1 supplied in the kit supplemented with lysozyme (1.2 mg ml-) was added. The filters were then incubated on a rotary mixer at 55°C and 900 rpm for 1 h. Total DNA was extracted and purified according to the manufacturer’s instructions. Specific primer pairs were used to target V4-V5 of the bacterial and achaeal16S rRNA gene, 515F-Y (5’-GTGYCAGCMGCCGCGGTAA-3’) and 926-R (5’-CCGYCAATTYMTTTRAGTTT-3’) (Parada et al., 2016) and the V4 region of the 18S rRNA eukaryotic gene, TAReukF1 (5-’CCAGCASCYGCGGTAATTCC-3’, and Euklebon-R (5’-ACTTTCGTTCTTGATYRATGA-3’) (Stoeck et al., 2010). Polymerase chain reaction (PCR) was performed in triplicate and then pooled before metabarcoding. Sequencing was carried out with an Illumina MiSeq 2 × 300bp, V3 kit by GeT-PlaGe (Castanet-Tolosan, France). Sequences were deposited in the DATAREF-Ifremer database.
Sequence Analysis
Raw sequence analysis was performed using FROGS (Find Rapidly OTU with Galaxy Solution) software (https://galaxy-datarmor.ifremer.fr/) used in Galaxy (Escudié et al., 2018). This software analyses large sets of DNA amplicon sequences with precision and speed. It produces an abundance table of operational taxonomic units (OTUs) based on their taxonomic affiliations and is the only tool capable of handling non-overlapping pairs of reads. It was designed to support multiplexed or demultiplexed sequences. The preprocessing step involves matching the reads, cleaning up, and dereplicating the sequences. The sequence clustering step uses Swarm, which works with a local clustering threshold (not global) (often 97% by default), similar to other clustering software. The chimaeric removal step used VSEARCH combined with cross-validation of the original chimaeras. The filtration step removed most of the remaining artefactual sequences. This last step makes it possible to affiliate each OTU to a taxon (up to the species level) with the possibility of a “multi-affiliation” annotation, which can generate a list of all the taxa with the same sequence. The scripts are available at https://github.com/geraldinepascal/FROGS. We analysed 88 samples for the eukaryotic phytoplankton and bacteria data sets and obtained an average of ca. 6023 and 18030 reads per sample, respectively. Taxonomic assignments were made using the Silva 132_16S database for prokaryotic analysis and PR2 4.11.0 for eukaryotic analysis.
As with all primers, there can be biases introduced during the amplification because of the amplified preference or the uneven number of rRNA copies. In our case, primers for 18s rRNA covers only 54.2% of the eukaryota and 2% of the hapthophyta.
Data and Statistical Analysis
As the present study aimed to establish the diversity of planktonic microorganisms, the 18s rRNA genes OTU database was filtered to retain only unicellular organism sequences. Further statistical analyses were performed on the 16s and 18s rRNA genes of unicellular OTU database. All analyses described hereafter were performed using R software (R version 4.0.2).
The temporal dynamics of alpha diversity, from winter to spring, were assessed by computing the Shannon index (Shannon, 1948) at each date for both 16s and 18s rRNA genes in 2015 and 2016. The number of OTUs per date was computed, and an UpSet diagram (Lex et al., 2014) was produced to identify the number of common OTUs between months or periods of consecutive months. UpSet diagrams are a visualisation tool allowing the identification of a number of elements (here OTUs) in intersections between groups (here month), but with more flexibility than classic Venn diagrams. The temporal dynamics of beta diversity, from winter to spring, were assessed by computing the Bray-Curtis dissimilarity index on consecutive sampling dates for both 16s and 18s rRNA genes data in 2015 and 2016. Non-metric multidimensional scaling (nMDS) ordinations were also applied to OTU Bray-Curtis dissimilarity to classify 16s and 18s rRNA genes OTU community diversity from winter to spring. To identify the environmental factors driving the planktonic assembly, hydrological, meteorological, and nutrient variables were ordinated, and their correlation scores with non-metric multidimensional scaling (nMDS) axes were calculated using the envfit function in R (vegan package, version 2.4-2). Phylum composition from winter to spring was assessed by computing the relative OTU abundance clustered by Phylum at each date for both 16s and 18s rRNA genes in 2015 and 2016. The temporal dynamics from winter to spring of the dominant OTU were assessed by computing the relative abundance of dominant OTUs at each date for the three domains (i.e., bacteria, archaea and eukaryote) in 2015 and 2016. Dominant OTUs per domain were those with a maximum relative abundance of > 5%. Furthermore, the temporal associations between all three domains dominant OTUs were studied using Spearman’s rank correlation heatmaps, associated with a hierarchical clustering analysis to identify OTUs with common temporal patterns.
Results
Environmental Parameters and Chlorophyll-a Dynamics
The dynamics of the environmental parameters from winter to spring in 2015 and 2016 are presented in Supplementary Figure 2. Chl-a fluorescence showed different patterns between 2015 and 2016. During 2015, three main blooms were observed, in winter, early spring, and late spring, and in 2016, a low Chl-a level was recorded during the winter and early spring and a slow biomass accumulation bloom was observed during spring (Trombetta et al., 2019). Water temperature in 2015 increased from 4°C in February to 20°C in mid-May. In 2016, the water temperature remained exceptionally high during winter (around 10°C) from January to mid-March, and then slowly increased until June. Air temperature followed the same daily pattern as water temperature for both years but with a higher amplitude. Salinity in 2015 slowly increased from 34 in winter to 35.5 in spring. In 2016, it was higher, with 38 in winter, a substantial decrease in May, reaching 34, and an increase to 38 in early June. Depth, an indicator of a lagoon-sea exchange inflow or outflow, varied between 1.3 and 1.8, with frequent brief increases and decreases in both years. Generally, the depth decreased slightly from winter to spring in 2015 but increased in 2016. Incident PAR and UVBR regularly increased from winter to spring. In both years, regular wind events reaching 8 to 10 m s-1 were observed, generally blowing from the north or northwest (Mistral and Tramontane winds). Air pressure varied between 99.0 to 103.5 kPa and humidity between 40% to 80% in both years, but no particular increase or decrease was observed from winter to spring. Precipitation was generally low from winter to spring in both years. However, 2015 was characterised by longer periods of drought interspersed with short and significant rain events, while 2016 exhibited more regular and less intense rain events. Finally, turbidity peaks were observed in both 2015 and 2016, mainly in winter and early spring. Detailed information about environmental parameters during these periods can also be found in Trombetta et al. (2019; 2021).
Microbial Community OTU Alpha Diversity
The total number of 16s OTUs recovered was 437 (430 in 2015 and 434 in 2016). The archaeal domain was represented by 7 OTUs, and the bacterial by 430. The majority of OTUs (427 OTUs) were common between 2015 and 2016, three specifics were common up to 2015, and seven were common up to 2016. UpSet diagrams of 16s diversity (Figures 1A, B) showed that a large majority of bacterial OTUs were present over the whole period (from January to May or June), in both 2015 (Figure 1A: 259 OTU) and 2016 (Figure 1B: 255 OTU). The Shannon index (alpha diversity) for 16s (Figures 1C, D) revealed differences between years. In 2015, the alpha diversity, as inferred from the Shannon index, was stable from January to mid-February and then slowly increased until the end of the period. Furthermore, the variation in diversity between the two consecutive sampling dates was low. In 2016, the alpha diversity was fairly stable from January to mid-February and then increased until late March, showing low variation between consecutive sampling dates. However, in 2016 from April until the end of the monitoring period, contrary to 2015, the Shannon index was highly variable, and amplitude variations between two consecutive sampling dates were high.
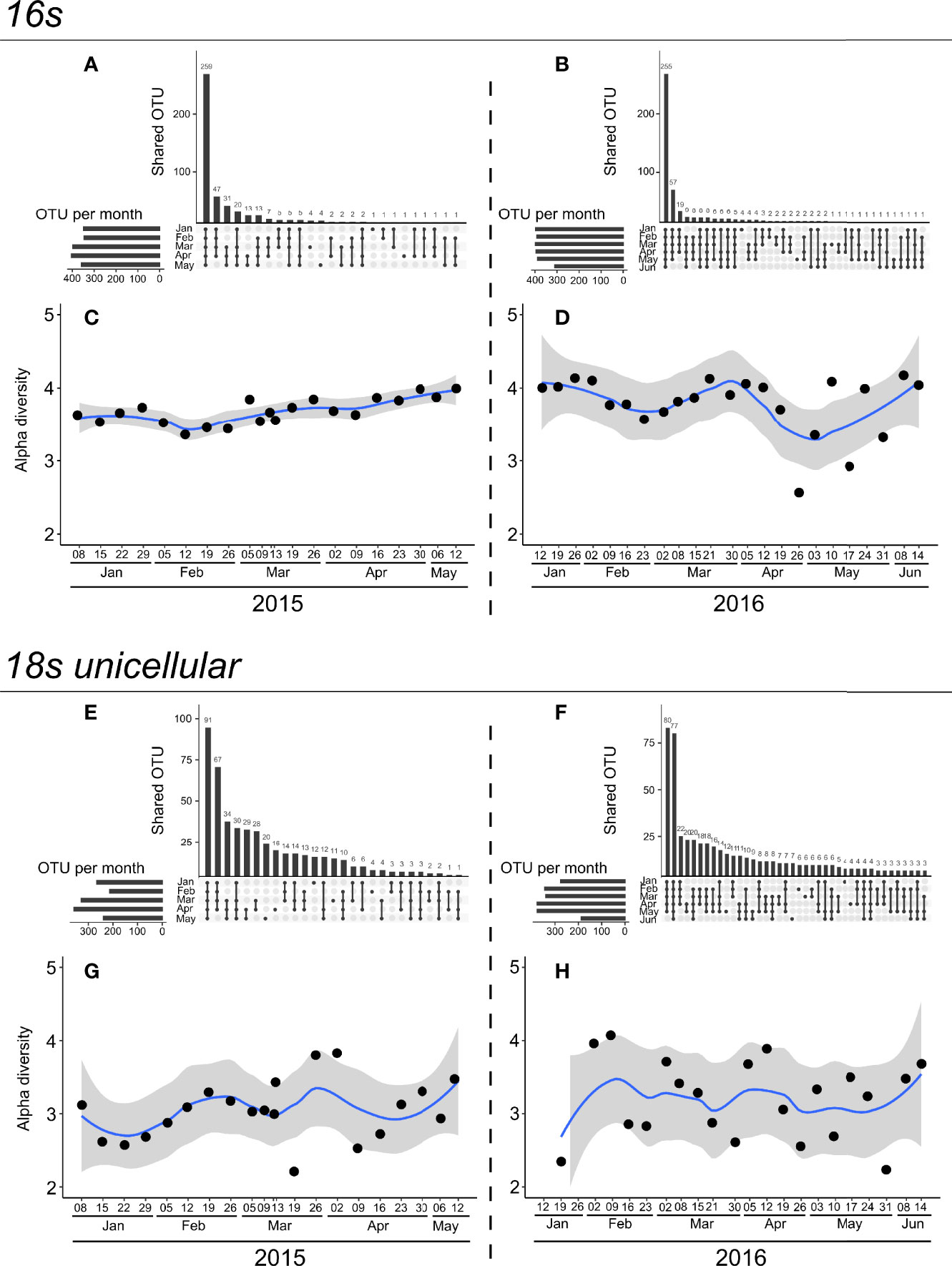
Figure 1 UpSet diagrams (A, B, E, F) of number of OTUs shared between periods and alpha diversity of Shannon index (C, D, G, H) for 16s (upper panel) and 18s rRNA gene unicellular OTUs (lower panel) in 2015 (left) and 2016 (right) years. UpSet diagrams represent the number of common and unique OTUs between months. For example, for 16s in 2015 (A), 13 OTUs are common and represented only in April and May (5th bar). The total numbers of OTUs identified per month are represented as horizontal bars on the left side of each UpSet diagram. Temporal alpha diversity was smoothed using a LOESS function, represented by a blue line. Grey background is the 95% confidence interval of LOESS smoothing function.
The total number of 18s rRNA genes for unicellular OTU found was 508 (494 in 2015 and 439 in 2016). Again, the majority (425 OTUs) were common between 2015 and 2016, 14 being specific to 2015 and 69 to 2016. The UpSet diagram (Figures 1E, F) revealed that the majority of OTUs were common from January to May or June in both 2015 (Figure 1E: 91 OTU) and 2016 (Figure 1F: 80 OTU). The alpha diversity (Shannon index) for 2015 (Figure 1G) revealed an increase during winter (from January to late February), with low variations between consecutive sampling dates. From March to May 2015, the alpha diversity was very erratic, and its amplitude from one week to the other was important. The alpha diversity for 2016 (Figure 1H) showed no particular increase or decrease trends following the periods, and its variation between consecutive sampling dates was significant, with erratic changes.
Microbial Community OTU Beta Diversity
The non-metric multidimensional scaling (nMDS) analysis of 16s (Figures 2A, B) and 18s rRNA genes OTU diversities (Figures 2C, D) revealed similar seasonal patterns for 2015 and 2016. For both years and 16s and 18s rRNA genes OTUs, winter communities (from January to March) were close together and then deviated from April until the end of the study period. Fitting environmental variables on nMDS showed that spring communities in both the 16s and 18s rRNA genes OTUs communities cases occurred with high water and air temperature conditions in 2015 and 2016. Furthermore, the water temperature was correlated with the nMDS axis in the four panels (p < 0.001). Winter community sampling dates were characterised by different environmental conditions in 2015 and 2016. On the one hand, in 2015, winter sampling dates (from January to late March) were characterised by significant high SiO2 concentrations and low salinity. On the other hand, in 2016, winter sampling dates were considered to have significant high salinity. In both years, winter sampling dates were also characterised by significant low water temperatures and light conditions.
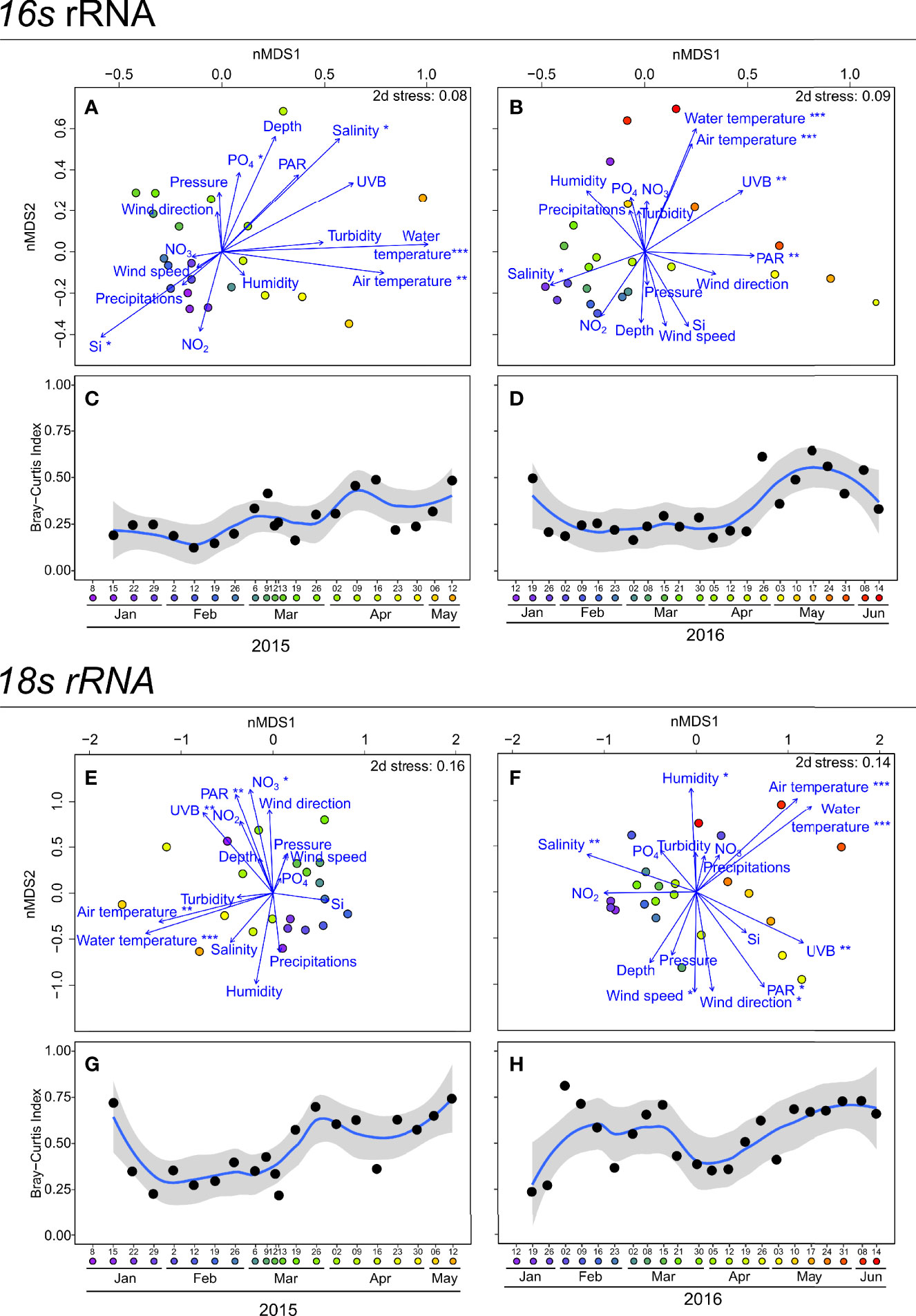
Figure 2 Non-metric multidimensional scaling (nMDS; (A, B, E, F) and Bray-Curtis dissimilarity index (C, D, G, F), of 16s (A, C, E, G) and 18s rRNA gene unicellular OTUs (B, D, F, H) community, for 2015 (right) and 2016 (left) years. Coloured circles represent sampling dates: purple for January, blue for March, green for April, orange for May and red for June. Blue arrows represent the projection of environmental parameters. Proximity between sampling dates indicated proximity in diversity. The more sampling dates are distant, the more different their communities are. Proximity between the environmental parameters and sampling dates indicates the environmental factors characteristic of these sampling dates. Asterisks represent significance levels of correlation between fitted environmental parameters and nMDS axis, calculated using a linear model including both axes. * represents p-value < 0.05; ** represents p-value < 0.01; and *** represents p-value < 0.001. Environmental parameters significantly correlated to axes best explained the community diversity. The Bray-Curtis dissimilarity index point represents the similarity value with the previous date for each sampling date. As an example, in 2015, the 15 January index value is the similarity index between 15 January and 8 January. The temporal Bray-Curtis dissimilarity index dynamic was smoothed using a LOESS function, represented as a blue curve. Grey background is the 95% confidence interval of the LOESS smoothing function.
Seasonal community differences between consecutive sampling dates were highlighted in the Bray-Curtis dissimilarity index dynamic for 16s (Figures 2C, D). In 2015, this index remained low and stable during winter from January to late February (mean of 0.19 ± 0.04). From March, it increased with high variations between successive sampling dates to a maximum of 0.50 on 16 April. In contrast, in 2016, the Bray-Curtis dissimilarity index was high and stable until mid-April (mean of 0.25 ± 0.08), before increasing sharply to reach a maximum of 0.65 on 17 May. The dynamic Bray-Curtis dissimilarity index for 18s rRNA genes OTUs also highlighted seasonal community differences (Figures 2G, H). In 2015, the Bray-Curtis dissimilarity index for 18s rRNA genes OTUs was low and stable from January to mid-March (mean of 0.36 ± 0.14), suggesting low community diversity changes from one date to the other. From late March until the end of the study period, the Bray-Curtis dissimilarity index increased to a maximum of 0.74 on 12 May. In contrast, in 2016, the Bray-Curtis dissimilarity index exhibited a significant amplitude between sampling dates from the beginning of the study period (from January to late March, min = 0.23; max = 0.82), suggesting substantial community changes during this period. This index slowly increased from April to June from 0.35 on 05 April to 0.73 on 08 June, with low variation amplitude between sampling dates.
Phylum Composition of Bacteria, Archaea and Unicellular Eukaryota
For the bacterial domain, Actinobacteria, Bacteroidetes, Cyanobacteria, Proteobacteria, and Verrucomicrobia dominated the bacteria phyla in both years, but differences in their dynamics were observed between 2015 and 2016. In 2015 (Figure 3A), Proteobacteria were dominant in winter from January to late February, preceding Bacteroidetes, which were abundant in spring between March and May. The relative abundance of Actinobacteria was low in winter but increased from April. In contrast to 2015 (Figure 3B), Proteobacteria dominated the winter communities in 2016 (from January to April), and Actinobacteria dominated until the end of the sampling period. Sporadic blooms of cyanobacteria and Verrucomicrobia were observed in late April and early May 2016, respectively. Archaea phyla were represented by Euryarchaeota and Thaumarchaeota. They were low in relative abundance and never exceed 0.01% of the total 16s rRNA genes OTUs abundance.
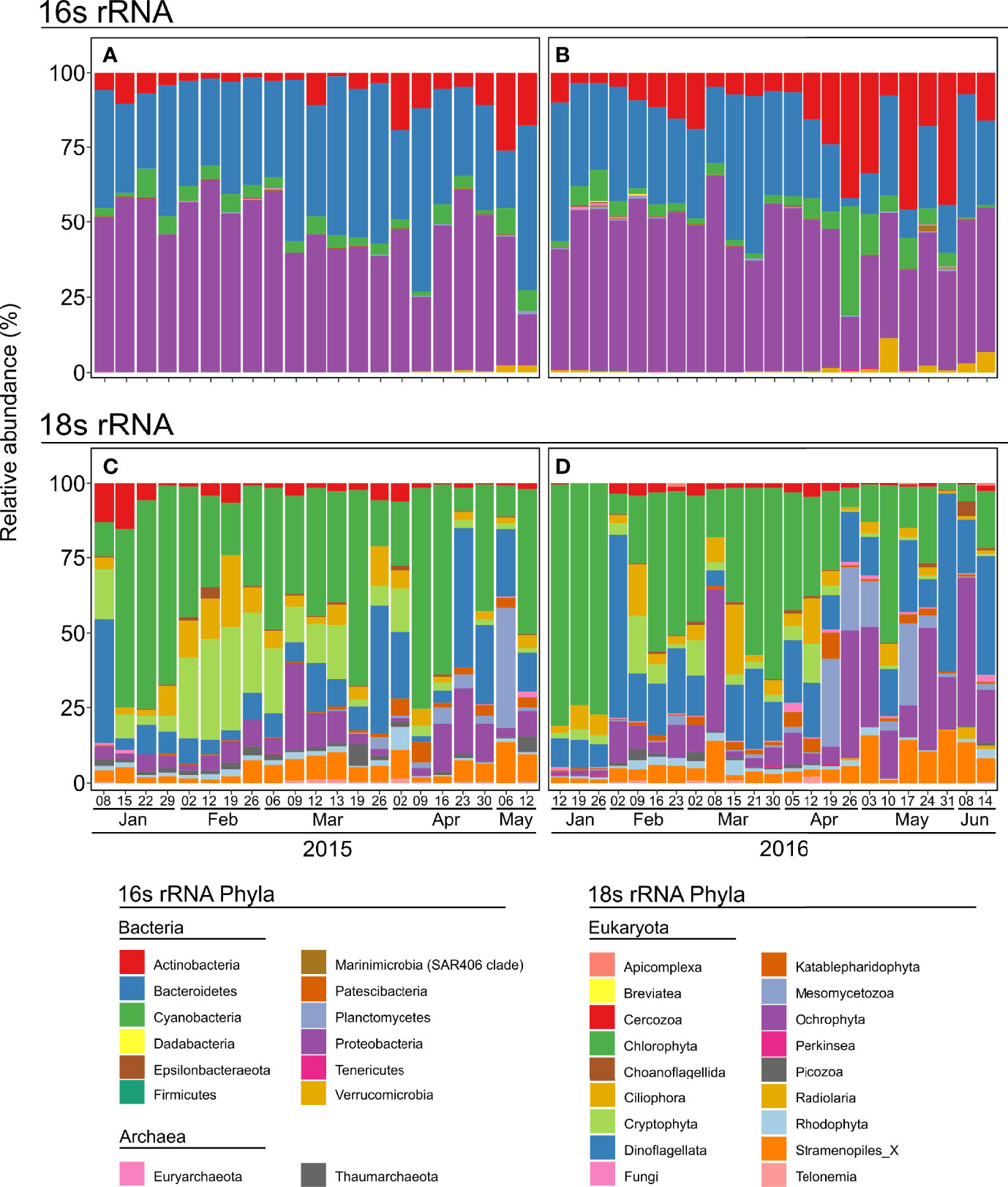
Figure 3 Phylum composition in relative abundance of 16s (A, B) and 18s rRNA gene unicellular OTUs (C, D) for 2015 (left panels) and 2016 (right panels).
Chlorophyta, Dinoflagellata, Ochrophyta, and Cryptophyta dominated the eukaryote community in both years, but with striking differences between 2015 and 2016. In 2015, chlorophytes were abundant over the entire sampling period (Figure 3C). However, in February, the relative abundance of Chlorophyta decreased, which benefited Cryptophyta. The relative abundance of dinoflagellates was low during the winter but increased from mid-March to become dominant at specific sampling dates (e.g. 26 March, 26 April). There were significant changes in the community composition between 2015 and 2016 (Figure 3D). While Cryptophytes were among the dominant groups from January to mid-April in 2015, they did not increase and remained at low levels in 2016. Furthermore, in 2016, Dinoflagellata and Ochrophyta became more significant, especially towards the end of the sampling period.
Bacteria, Archaea and Eukaryota Dominant OTU
Several OTUs dominated the bacterial diversity in each phylum (Figure 4, purple OTU). Bacteroidetes and Actinobacteria were represented by eight and six main OTUs, while Cyanobacteria, Proteobacteria, and Verrucomicrobia were represented by three, two, and one main OTU, respectively. Relative abundance of bacteroides OTUs dominated in 2015, while their relative abundance declined in 2016 to the benefit of the Actinobacteria ML602J-51 sp., which was largely dominant. The cyanobacteria bloom in April 2016 was due to two main Synechococcus OTUs, Synechococcus CC9902 Multi-affiliations and Synechococcus CC9902 sp. (Figure 4). Verrucomicrobia were represented by DEV007 on only one date (12 May) in 2015, while they were represented in 2016 on 11 sampling dates (12 January and 12 April until the end of the sampling period).
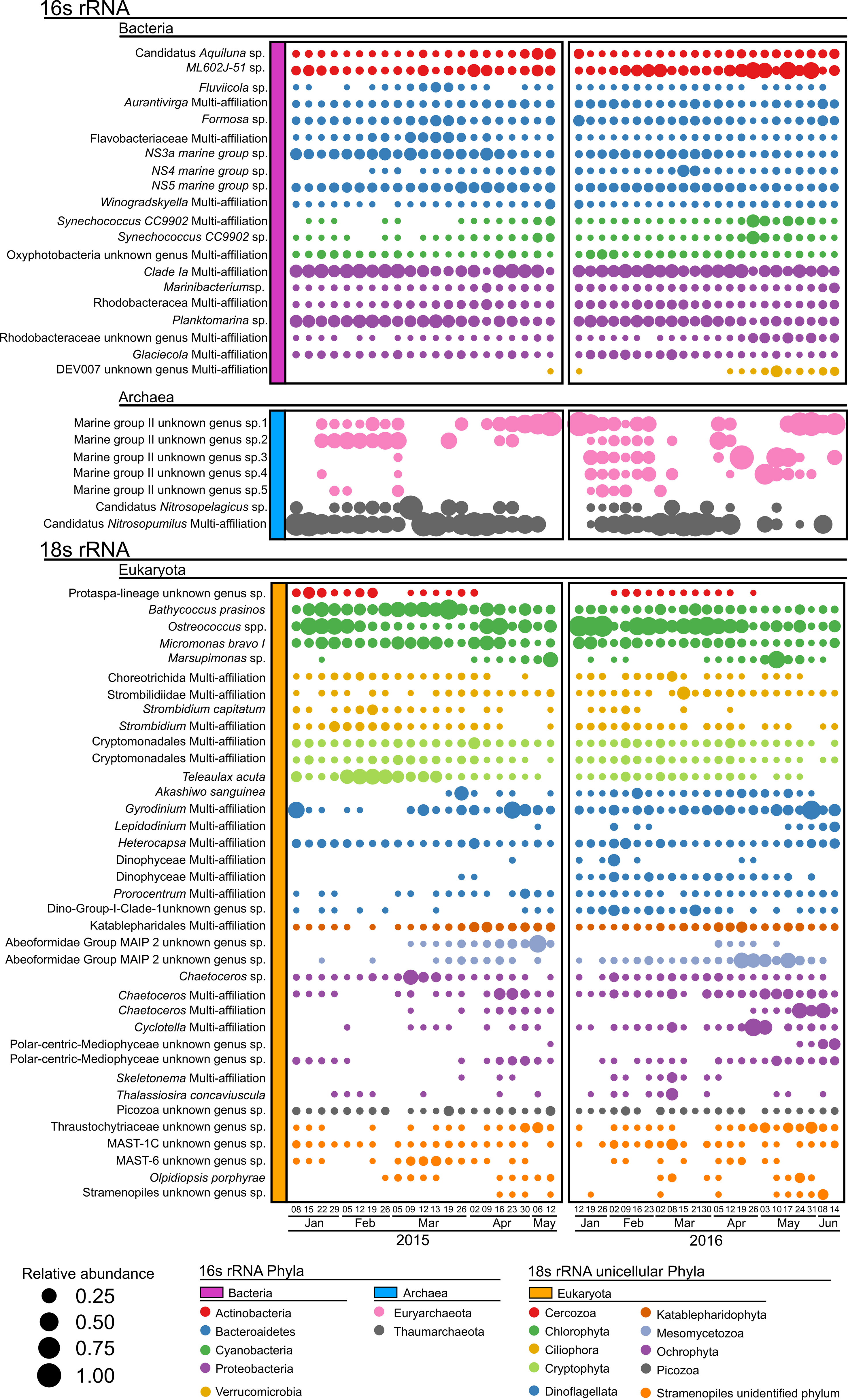
Figure 4 Relative abundances’ dot plot of the dominant OTUs for 2015 (left) and 2016 (right). Dots are proportional to the relative abundance of OTUs. Dominant OTUs are those exhibiting a maximum relative abundance > 5% in 2015 or 2016.
Archaeal diversity (Figure 4, blue OTU) was represented by 5 OTUs of Euryarchaeota and 2 OTUs of Thaumarchaeota. In 2015, Thaumarchaeota OTUs dominated (especially Candidatus Nitrosopumilus Multi-affiliation), from winter to spring. It declines in late spring, benefiting the Euryarchaeota Marine group II unknown genus sp.1. In 2016, Thaumarchaeota OTUs were less dominant, benefiting the 5 OTUs of Marine group II unknown genus.
Several OTUs dominated the eukaryota diversity in each phylum (Figure 4, orange OTU). Chlorophyta, Ciliophora, Cryptophyta, Dinoflagellata, Ochrophyta, and unidentified Stramenopiles were represented by three to eight main OTUs, while only one or two OTUs were identified for Cercozoa, Mesomycetozoa, and Picozoa. Bathycoccus prasinos, Ostreococcus spp., Micromonas bravo I, and Marsupimonas sp. were the main OTUs belonging to the Chlorophyta. The dominant ochrophyte OTUs were all diatoms (8 OTUs), especially Chaetoceros (3 OTUs). Marked differences were observed between 2015 and 2016, with an increased contribution of Ostreococcus spp. at the expense of Bathycoccus prasinos in 2016. The decrease in the relative abundance of Cryptophytes in 2016 was mainly attributed to Teleaulax acuta. While Gyrodinium, Heterocapsa, and Prorocentrum dominated the Dynophyceae in 2015, more diversified OTUs, such as Akashiwo sanguinera, the Dinophyceae Multi-affiliation, and Dino-Group-I-Clade-1 sp. were observed in 2016. The Mesomycetozoa Abeoforminade Group MAIP2 sp. also increased in 2016 compared to 2015. In 2016, most Ochrophyte OTUs were more abundant in the winter to spring transition than in the other sampling periods.
Bacteria, Archaea and Eukaryota Correlations and Clustering Analysis
Analysis of the bacteria, archaea and eukaryota OTU identified in 2015 and 2016 revealed common correlations between them (Figure 5). In both years, the Chlorophyta Bathycoccus prasinos and Micromonas bravos I were positively correlated with Bacteroidetes Fluviicola sp. and Formosa sp. and with the Thaumarchaeota Nitrosopelagicus sp. and Nitrosopumilus Multi-affiliation. In both years, the two diatoms Chaetoceros OTU (Chaetoceros Multi-affiliation 1 and 2) and Cyclotella sp. were positively correlated and clustered together, while these three OTUs were negatively correlated with Chaetoceros sp. and Thalassiossira concaviscula OTUs. A larger cluster in both 2015 and 2016 encompassed the Cryptophyta Teleaulax acuta and Cryptomonadales Multi-affiliation, Ciliophora Strombidium capitatum, Strombidium Multi-affiliation, and Choreotrichida Multi-affiliation, the Bacteroidetes NS3a marine group sp., and the Proteobacteria Planktomarina sp. In addition to these positive associations present in both years, most associations differed between 2015 and 2016. For instance, Ostreococcus spp. was positively correlated with Bathycoccus prasinos, Micromonas bravo and Formosa sp. in 2016, but not in 2015. Both Synechococcus OTUs (CC9902 sp. and CC9902 multi-affiliation), ML602J-51 and DEV007 exhibited strong positive correlations and close association clusters in 2016, but not in 2015. inoflagellates, Dinophyceae Multi-affiliation (2 OTUs), and Akashiwo sanguinea were positively correlated and clustered only in 2016. On the opposite, the Euryarchaeota Marine group II unknown genus sp.2, 3, 4 and 5 are clustered together in 2015 while in 2015, only sp.3 and 4 are clustered together.
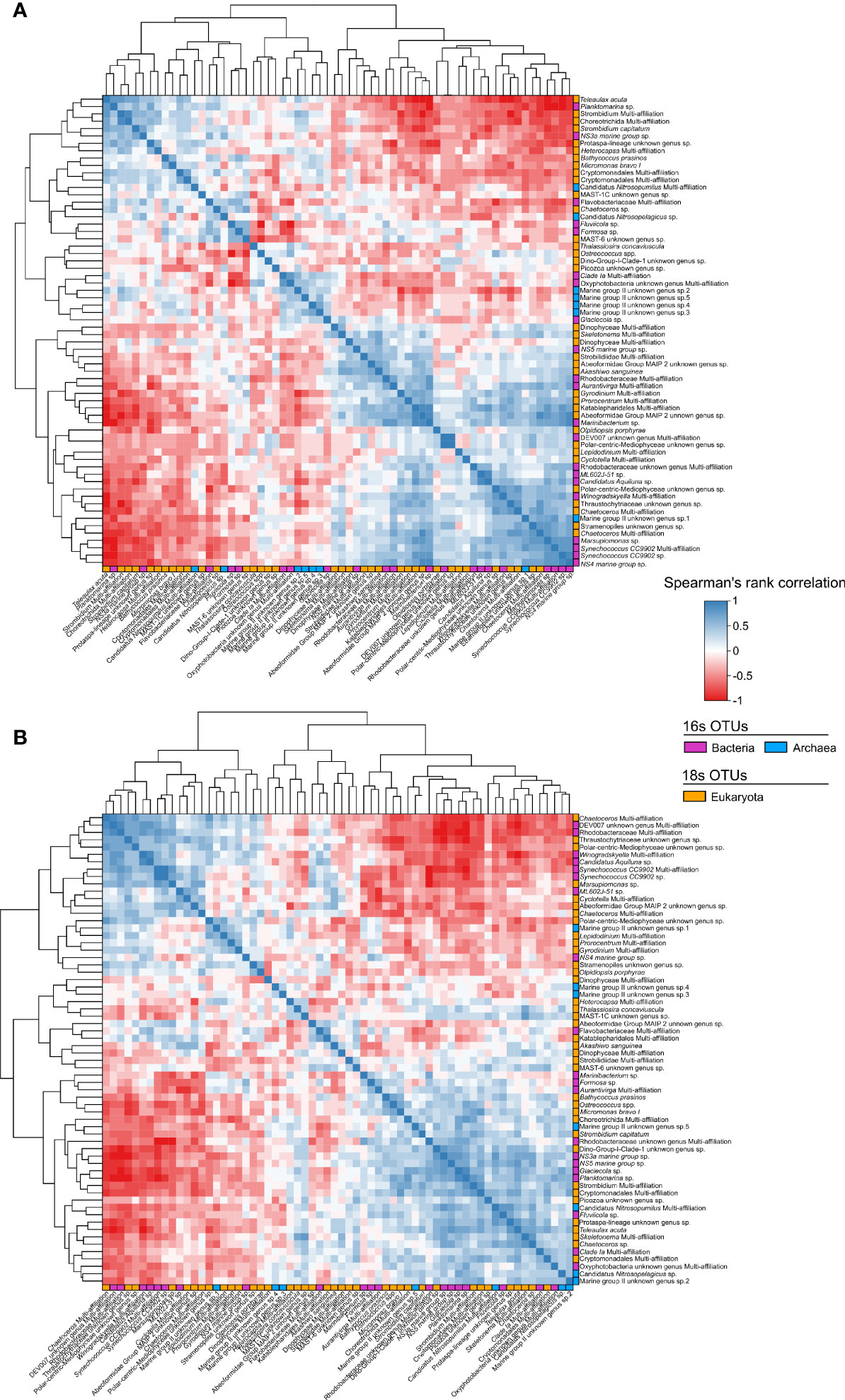
Figure 5 Spearman’s rank correlation heatmap of dominant OTUs for 2015 (A) and 2016 (B). The colour gradient represents the strength of correlations. Blue and red colours represent positive and negative correlations, respectively. Dendrograms are based on hierarchical clustering analysis highlighting dynamic similarities and correlations of taxa that are close to each other.
Discussion
Bacterial and Archaeal Diversity and Succession in Thau Lagoon
Many studies have reported planktonic dynamics using cytometric measurements in the Thau Lagoon, but no in situ studies have used molecular techniques to determine the high-resolution taxonomic diversity of microorganisms over several months. In this study, we revealed that the Thau Lagoon supported characteristic marine bacterial and archaeal communities, with some freshwater signatures following salinity variations. Indeed, the Proteobacteria and Bacteroidetes dominated the bacterial communities, as reported in other coastal lagoons of the Mediterranean Sea, such as in the Adriatic Venice Lagoon (Simonato et al., 2010). The eight dominant OTUs belonged to the Flavobacteriaceae (Bacteroidetes), a group typically existing in marine and coastal waters, suggesting a strong marine influence at the Thau Lagoon study site (Barberán and Casamayor, 2010; Simonato et al., 2010; Ghai et al., 2012; Mohit et al., 2014). In other coastal lagoons, the Flavobacteriaceae are usually associated with Sphingobacteriales, which are more common in freshwater habitats (Alonso et al., 2007; Barberán and Casamayor, 2010; Simonato et al., 2010) but, based on our results, this was not the case for the dominant OTUs in the Thau Lagoon. Proteobacteria are also dominant in marine and coastal ecosystems (Simonato et al., 2010; Ghai et al., 2012; Mohit et al., 2014; Lambert et al., 2018), and the abundance of Proteobacteria OTUs in the Thau Lagoon confirms a strong marine influence (Fiandrino et al., 2012; Trombetta et al., 2019).
The presence of Actinobacteria, known to be typically occurring in freshwater systems (Barberán and Casamayor, 2010; Ghai et al., 2012), demonstrated the interface characteristics of the Thau Lagoon, which is influenced by sporadic riverine inputs (Pecqueur et al., 2011) that could inoculate freshwater bacterial strains. In addition, the relative abundance of Actinobacteria was often low in this study; however, it strongly increased from early April to late May 2016. During this period, Actinobacteria sometimes dominated the bacterial community, and this dominance was mainly due to ML602J-51 strain, often isolated in lakes or coastal zones, coinciding with a substantial freshwater input causing the salinity drop (Supplementary Figure 2). Our results suggest that, despite the marine influence, salinity decreases can induce significant changes in bacterial community composition, notably by promoting the increase of some freshwater signatures and even shifting the OTU dominance.
Arachaea diversity confirms that communities are characteristic from marine and coastal environments. Indeed, Euryarchaeota Marine group II are widely known to be typical components of shallow coastal waters, as their abundance decreasing with depth (Massana et al., 1997; Murray et al., 1999; Santoro et al., 2019). Despite their low relative abundance in the 16s rDNA genes, archaea fill important function in the microbial food web. Indeed, Thaumarchaeota Candidatus Nitrosopumilus and Candidatus Nitrosopelagicus are known to be ammonia-oxydizing (Walker et al., 2010; Santoro et al., 2015) taxa and may contribute to an important part of the nitrification process in the Thau Lagoon benefiting primary production.
Picoeukaryotes Diversity and Succession in Thau Lagoon
In Thau Lagoon, studies on smaller protists (< 5 µm) were limited to cytometric analysis, while larger organisms were studied through microscopy (Dupuy et al., 2000; Bec et al., 2005; Trombetta et al., 2019). To our knowledge, only one study using experimental microcosms carried out in the Thau Lagoon applied techniques to detect high-resolution taxonomic diversity (Domaizon et al., 2012), and studies were mostly dedicated to in-situ monitoring observations. The present study shows that the taxonomic diversity of the picoeukaryote black box was dominated by a succession of four Chlorophyte OTUs from winter to spring in both years. Species corresponding to these OTUs were Bathycoccus prasinos, Ostreococcus spp., and Micromonas bravo I, and, to a lesser extent, Marsupimonas sp. The genera Bathycoccus, Ostreococcus, and Micromonas are commonly found in the oceans (Tragin and Vaulot, 2019). Interestingly, Bathycoccus prasinos and Micromonas bravo I had similar temporal dynamics, which were highlighted in association heatmaps. The Ostreococcus spp. OTU was also abundant, especially in 2016, where its dynamics were clearly different from those of B. prasinos and M. bravo I. Similarly, in Banyuls Bay (north-western Mediterranean Sea), Bathycoccus and Micromonas were the two main eukaryotagenera in the cold winter months, while Ostreoccoccus OTUs were observed only under warmer temperatures (Lambert et al., 2018). On the opposite, in the Venice Lagoon, Mamiellophyceae were not found as a dominant class in any season (Armeli Minicante et al., 2019; Armeli Minicante et al., 2020), maybe due to the lower and stable salinty levels (Tragin and Vaulot, 2019). Ostreoccoccus spp. was the most abundant OTU in the present study, although it has not been recorded as a dominant species in earlier studies of the Mediterranean coastal zone (Tragin and Vaulot, 2019). It has been described as a high-light-adapted strain, characteristic of mesotrophic coastal zones (Rodríguez et al., 2005; Demir-Hilton et al., 2011). The dominance of Ostreococcus spp. in the present study in winter as well as in spring suggests that the species is well adapted to shallow marine coastal lagoons, where the light penetrates the whole water column throughout the year. In previous analyses, Ostreococcus tauri was found to be the dominant Mamiellophyceae in the Thau Lagoon (Courties et al., 1994; Domaizon et al., 2012). However, the present sequencing method used could not allow to discriminate it from other Ostreococcus species. Thus, the Ostreococcus spp. OTU may be a mix between Ostreococcus tauri and Ostreococcus lucimarinus, as the latter is commonly found in other coastal sites (Demir-Hilton et al., 2011).
The dynamics of dominant OTUs such as Chaetoceros spp., Skeletonema sp., Thalassiosira concaviscula, Cyclotella sp., Teleaulax sp., Gyrodinium sp., Heterocapsa sp., and Strombidium spp. in the Thau Lagoon, were consistent with those described in earlier studies based on microscopic observations (Bec et al., 2005; Trombetta et al., 2019; Trombetta et al., 2021). The succession of larger plankton (> 5 µm) throughout the year was apparent. Winter and early spring communities were mostly dominated by Cryptophyceae (3 OTUs: 2 OTU of Cryptomonadales Multi-affiliation and Teleaulax acuta), widely known to dominate in various coastal systems of the Mediterranean Sea, including lagoons and estuaries, and considered to be the major primary producers in these areas (Novarino, 2005; Šupraha et al., 2014). In the Venice Lagoon, the Cryptophyceae Teleaulax acuta was also found to be a major component of the community (Armeli Minicante et al., 2020). Their ubiquity in interface systems, subjected to several stressors, can be attributed to their high tolerance to environmental changes (Klaveness, 1988) and their mixotrophy (Jones, 1997; Roberts and Laybourn‐Parry, 1999), which explains their dominance from winter to early spring across wide environmental gradients in the present study. The spring is accompanied by increased diversity, mainly due to diatom blooms, including Chaetoceros spp., Cyclotella sp., or the Polar-centric Mediophycea OTU. Diatoms are known to be characteristic from spring conditions in various systems such as the Venice Lagoon (Armeli Minicante et al., 2019; Armeli Minicante et al., 2020), as triggered by favourable temperature, nutrient and light conditions. In particular, the Chaetoceros genus is known to cause major blooms in diverse systems, including shallow lagoons (Carstensen et al., 2015). In the Thau Lagoon, the bloom of Chaetoceros spp. in spring, along with other larger diatoms, can diversify the prey for higher trophic levels, potentially diversifying the food web architecture. Ciliophora generally dominated from winter to early spring (four OTUs, including Strombidium spp.). These protozoans are known to be grazers of small plankton (Jonsson, 1986; Bernard and Rassoulzadegan, 1990), and their co-occurrence and association with pico- and nanophytoplankton, along with bacterial OTUs observed during the present study, suggest a link with prey-predator dynamics.
Succession and Increase of Microbial Diversity From winter to Spring Driven by Seasonal Water Temperature Increase
Bacterial and unicellular eukaryotic alpha diversity was generally stable and slightly diversified during the winter, and then from early spring, the microorganism diversity progressively increased (Figures 1, 2). When the temperature increased from winter to spring (Supplementary Figure 2), several prokaryotic phyla and OTUs, as well as eukaryotes, gained prominence, and their relative abundances increased (Figures 3, 4). Among the different studied environmental forcing factors, established correlations highlighted that the seasonal temperature rose, generally starting in late winter and early spring. This seasonal temperature rise was the main driver of the bacteria, archaea and eukaryota community composition, triggering community changes and increasing diversity (Figure 2 and Supplementary Figure 2). This result corroborates previous studies suggesting that temperature is the leading environmental factor triggering phytoplankton blooms and driving planktonic communities and plankton networks in the Thau Lagoon (Trombetta et al., 2019; Trombetta et al., 2021). The diversity increase observed in spring might be due to several species reaching their thermal optimum, being more competitive, and gaining relevance with a temperature rise. Indeed, the relative abundance of an important number of dominant OTUs of various phyla increased in spring, including Cyanobacteria, Ochrophytes, Dinophyceae, and Mesomycetozoa (Figure 4). Among them, a large majority are clustered together (Figure 5) and are known to benefit from high temperatures. Some examples include Synechococcus spp. (Pittera et al., 2014; Mackey et al., 2017), Chaetoceros spp. (Halac et al., 1997; Trombetta et al., 2019; Nagao et al., 2020) or Gyrodinium spp. (Lee et al., 2021; Manning et al., 2021). On the other hand, the water temperature triggered succession shifts through the OTU thermal optimum. For example, among the Mamiellophyceae (Chlorophytes), Bathycoccus prasinos, and Micromonas bravos I dominated when the temperature was low and were replaced by Ostreococcus spp. when the temperature was higher (Figure 4), as already observed in another coastal Mediterranean system, Banyuls Bay (Lambert et al., 2018).
Despite this change in diversity and community composition from early spring, a large number of OTUs were, in fact, present throughout the study period for bacteria and archaea (59.5 ± 1.0% for 2015 and 2016), and to a lesser extent, eukaryota (18.3 ± 0.1% for 2015 and 2016) (Figures 1A, B, E, F). This finding suggests that the ‘microbial core’ (OTUs present throughout the year), especially the bacteria and small protists, is a critical element in the community of microorganisms in the Thau Lagoon. The increase in diversity and composition of microorganisms in spring is mainly due to a change in the relative abundance of these ‘core OTUs’ rather than the inoculation of new ones. However, this is less true for eukaryota, especially larger organisms (diatoms, dinoflagellates, and Ciliophora), with a substantial number of ‘occasional OTUs’ appearing at particular sampling dates, mostly in spring (Figure 4). The appearance of these OTUs could be due to the inoculation and persistence of strains through horizontal (sea or freshwater input) or vertical (sediment resuspension) advection. However, this could only be due to the abundance of these OTUs, which reached the detection threshold.
Light conditions (UVBR and PAR) may also have been an important driver for unicellular eukaryote communities, but not for bacteria and archaea (Figure 2). This was because a substantial number of unicellular eukaryote OTU were photosynthetic (phytoeukaryotes), while only a few bacteria were photosynthetic (cyanobacteria OTU). However, in this shallow lagoon with an average depth of 4 m, the light penetrates the whole water column with the seasonal incident light increase, and influence of light on the unicellular eukaryota community seems lesser than that of seasonal temperature variations.
Microorganism Diversity in a Warmer Year: Comparison Between Two Distinctly Different Climatic Years
The year 2015 was a classic or normal climatic year characterised by winter water-cooling and a substantial water temperature amplitude from winter to spring (Supplementary Figure 2, Trombetta et al., 2019). In contrast, 2016 saw the warmest winter ever recorded in southern France (http://www.meteofrance.fr/climat-passe-et-futur/bilansclimatiques/bilan-2016/hiver), leading to the absence of winter water cooling, which is an exception in this lagoon (Trombetta et al., 2019). This thermal difference between the two consecutive years could explain the striking differences observed in the bacteria, archaea and unicellular eukaryota diversity.
The alpha diversity of the 16s community was erratic in 2016, exhibiting significant variations across consecutive sampling dates from early spring (Figure 1D) due to rapid changes in the community composition (Figure 3B) of particular blooming OTUs in this warmer year, such as Synechococcus CC9902 (Cyanobacteria, 2 OTUs), ML602J-51 (Actinobacteria), and DEV007 (Verrucomicrobia) (Figure 4). Trombetta et al. (2019) used cytometry analysis on the same sampling dates of the same study site in the Thau Lagoon and found significantly more cyanobacterial abundance in 2016 than in 2015. Decadal time-series analyses revealed an increased relevance of picocyanobacteria in Thau Lagoon, probably due to oligotrophication and climatic changes (Collos et al., 2009; Derolez et al., 2020). The present study confirms that cyanobacterial blooms can serve as thermal signatures, while further studies are needed to determine whether Actinobacteria and Verrucomicrobia are thermal sentinels in the Thau Lagoon.
Dinophyceae benefited from the warmer year (Figures 3, 4). It has already been suggested that Akasiwo sanguinea may benefit from future climate change, especially due to acidification, high irradiance, and warming (Ou et al., 2017), and the present results tend to confirm this hypothesis, at least with respect to temperature increase. In general, the Dinophyceae are expected to dominate ecosystems with higher water temperatures due to global warming (Kibler et al., 2015; Xiao et al., 2018; Brandenburg et al., 2019), as they already dominated in warmer Mediterranean lagoons such as the Bizerte lagoon (Pringault et al., 2020). Similarly to cyanobacteria, decadal time-series analyses revealed the prominence of the Dinophyceae in Thau Lagoon, which may be due to oligotrophication and climatic changes (Collos et al., 2009; Derolez et al., 2020). If future warmer conditions benefit the Dinophyceae, then harmful bloom frequency may increase, as in the case of A. sanguinea, which is known to produce powerful surfactant proteins (Jones et al., 2017).
In 2016, with an extremely warm winter, Ostreococcus spp. dominated Chlorophytes throughout the study period (especially during the winter), while B. prasinos and M. bravos I never attained dominance. These results suggest that, under warm conditions, Ostreococcus spp. outcompetes other small chlorophytes and replaces them, as already observed in other coastal systems (Lambert et al., 2018).
In conclusion, the present study revealed that the Thau Lagoon supported bacterial and unicellular eukaryotic plankton communities characteristic of marine coastal zones. Its community composition, succession, and association were mainly driven by increasing water temperatures from winter to spring. In addition, the water temperature increase associated with an exceptionally warm year strongly modified the composition, succession, and association of microbial communities, suggesting that future global warming could strongly shift the diversity of microbial communities in thermal-driven shallow coastal zones such as the Thau Lagoon.
Data Availability Statement
The datasets presented in this study can be found in online repositories. The names of the repository/repositories and accession number(s) can be found below: https://doi.org/10.12770/21dd441d-dfe3-4385-bd70-85f891d8dff8.
Author Contributions
FV and BM designed the experiment. FV and BM performed the experiment. CF performed DNA extraction, amplification and sequencing. FV, F-YB, CF, BM and TT performed the analysis. TT wrote the original draft. All authors contributed to the manuscript revision as well as reading and approval of the final version.
Funding
This study was part of the Photo-Phyto project funded by the French National Research Agency (ANR-14-CE02-0018).
Conflict of Interest
The authors declare that the research was conducted in the absence of any commercial or financial relationships that could be constructed as potential conflict of interest.
Publisher’s Note
All claims expressed in this article are solely those of the authors and do not necessarily represent those of their affiliated organizations, or those of the publisher, the editors and the reviewers. Any product that may be evaluated in this article, or claim that may be made by its manufacturer, is not guaranteed or endorsed by the publisher.
Acknowledgments
We could like to thank Cécile Roques for sampling and filtration of DNA samples as well as Benjamin Sembeil, Oceane Schenkels and Ludovic Pancin for helping during the sampling. We would also like to acknowledge Sébastien Mas and David Parin, who helped during 2 years of the monitoring performed using facilites of the Marine Station of the “Observatoire de Recherche Mediterraneen de l’Environnement” (OSU OREME) in Sete. We would like to thank Editage (www.editage.com) for English language editing.
Supplementary Material
The Supplementary Material for this article can be found online at: https://www.frontiersin.org/articles/10.3389/fmars.2022.858744/full#supplementary-material
References
Alonso C., Warnecke F., Amann R., Pernthaler J. (2007). High Local and Global Diversity of Flavobacteria in Marine Plankton. Environ. Microbiol. 9, 1253–1266. doi: 10.1111/j.1462-2920.2007.01244.x
Armeli Minicante S., Piredda R., Quero G. M., Finotto S., Bernardi Aubry F., Bastianini M., et al. (2019). Habitat Heterogeneity and Connectivity: Effects on the Planktonic Protist Community Structure at Two Adjacent Coastal Sites (the Lagoon and the Gulf of Venice, Northern Adriatic Sea, Italy) Revealed by Meta Barcoding (Accessed March 8, 2022).
Armeli Minicante S., Piredda R., Finotto S., Aubry F. B., Acri F., Pugnetti A., et al. (2020). Spatial Diversity of Planktonic Protists in the Lagoon of Venice (LTER-Italy) Based on 18S rDNA. Adv. Oceanogr. Limnol. 11, 35–44. doi: 10.4081/aiol.2020.8961
Barberán A., Casamayor E. O. (2010). Global Phylogenetic Community Structure and β-Diversity Patterns in Surface Bacterioplankton Metacommunities. Aquat. Microb. Ecol. 59, 1–10. doi: 10.3354/ame01389
Bec B., Husseini-Ratrema J., Collos Y., Souchu P., Vaquer A. (2005). Phytoplankton Seasonal Dynamics in a Mediterranean Coastal Lagoon: Emphasis on the Picoeukaryote Community. J. Plankton Res. 27, 881–894. doi: 10.1093/plankt/fbi061
Bernard C., Rassoulzadegan F. (1990). Bacteria or Microflagellates as a Major Food Source for Marine Ciliates: Possible Implications for the Microzooplankton. Mar Ecol. Prog. Ser. 64, 147–155. doi: 10.3354/meps064147
Boaden P. J., Seed R. (1985). An Introduction to Coastal Ecology (Netherlands: Springer). doi: 10.1007/978-94-011-7100-7
Brandenburg K. M., Velthuis M., de Waal D. B. V. (2019). Meta-Analysis Reveals Enhanced Growth of Marine Harmful Algae From Temperate Regions With Warming and Elevated CO2 Levels. Global Change Biol. 25, 2607–2618. doi: 10.1111/gcb.14678
Buchan A., LeCleir G. R., Gulvik C. A., González J. M. (2014). Master Recyclers: Features and Functions of Bacteria Associated With Phytoplankton Blooms. Nat. Rev. Microbiol. 12, 686–698. doi: 10.1038/nrmicro3326
Carstensen J., Klais R., Cloern J. E. (2015). Phytoplankton Blooms in Estuarine and Coastal Waters: Seasonal Patterns and Key Species. Estuarine Coastal Shelf Sci. 162, 98–109. doi: 10.1016/j.ecss.2015.05.005
(2015). Mediterranean Coastal Lagoons: Sustainable Management and Interactions Among Aquaculture, Capture Fisheries and the Environment. Available at: https://agris.fao.org/agris-search/search.do?recordID=XF2016025618 (Accessed July 7, 2021). F. and A. P. and E. D. eng.
Collos Y., Bec B., Jauzein C., Abadie E., Laugier T., Lautier J., et al. (2009). Oligotrophication and Emergence of Picocyanobacteria and a Toxic Dinoflagellate in Thau Lagoon, Southern France. J. Sea Res. 61, 68–75. doi: 10.1016/j.seares.2008.05.008
Costanza R., d’Arge R., de Groot R., Farber S., Grasso M., Hannon B., et al. (1997). The Value of the World’s Ecosystem Services and Natural Capital. Nature 387, 253. doi: 10.1038/387253a0
Courties C., Vaquer A., Troussellier M., Lautier J., Chrétiennot-Dinet M. J., Neveux J., et al. (1994). Smallest Eukaryotic Organism. Nature 370, 255. doi: 10.1038/370255a0
Danovaro R., Pusceddu A. (2007). Biodiversity and Ecosystem Functioning in Coastal Lagoons: Does Microbial Diversity Play Any Role? Estuarine Coastal Shelf Sci. 75, 4–12. doi: 10.1016/j.ecss.2007.02.030
Deininger A., Faithfull C. L., Lange K., Bayer T., Vidussi F., Liess A. (2016). Simulated Terrestrial Runoff Triggered a Phytoplankton Succession and Changed Seston Stoichiometry in Coastal Lagoon Mesocosms. Mar Environ. Res. 119, 40–50. doi: 10.1016/j.marenvres.2016.05.001
Demir-Hilton E., Sudek S., Cuvelier M. L., Gentemann C. L., Zehr J. P., Worden A. Z. (2011). Global Distribution Patterns of Distinct Clades of the Photosynthetic Picoeukaryote Ostreococcus. Isme J. 5, 1095–1107. doi: 10.1038/ismej.2010.209
Derolez V., Soudant D., Malet N., Chiantella C., Richard M., Abadie E., et al. (2020). Two Decades of Oligotrophication: Evidence for a Phytoplankton Community Shift in the Coastal Lagoon of Thau (Mediterranean Sea, France). Estuarine Coastal Shelf Sci. 241, 106810. doi: 10.1016/j.ecss.2020.106810
Dolan J. R. (2018). Microbial Ecology of the Oceans. J. Plankton Res. 40, 500–502. doi: 10.1093/plankt/fby022
Domaizon I., Lepère C., Debroas D., Bouvy M., Ghiglione J. F., Jacquet S., et al. (2012). Short-Term Responses of Unicellular Planktonic Eukaryotes to Increases in Temperature and UVB Radiation. BMC Microbiol. 12, 202. doi: 10.1186/1471-2180-12-202
Dupuy C., Vaquer A., Lam-Höai T., Rougier C., Mazouni N., Lautier J., et al. (2000). Feeding Rate of the Oyster Crassostrea Gigas in a Natural Planktonic Community of the Mediterranean Thau Lagoon. Mar Ecol. Prog. Ser. 205, 171–184. doi: 10.3354/meps205171
Escudié F., Auer L., Bernard M., Mariadassou M., Cauquil L., Vidal K., et al. (2018). FROGS: Find, Rapidly, OTUs With Galaxy Solution. Bioinformatics 34, 1287–1294. doi: 10.1093/bioinformatics/btx791
Fiandrino A., Giraud A., Robin S., Pinatel C. (2012) Validation D’une Méthode D’estimation Des Volumes D’eau Echangés Entre La Mer Et Les Lagunes Et Définition D’indicateurs Hydrodynamiques Associés. Available at: https://archimer.ifremer.fr/doc/00274/38544/ (Accessed November 15, 2018).
Field C. B., Behrenfeld M. J., Randerson J. T., Falkowski P. (1998). Primary Production of the Biosphere: Integrating Terrestrial and Oceanic Components. Science 281, 237–240. doi: 10.1126/science.281.5374.237
Ghai R., Hernandez C. M., Picazo A., Mizuno C. M., Ininbergs K., Díez B., et al. (2012). Metagenomes of Mediterranean Coastal Lagoons. Sci. Rep. 2, 490. doi: 10.1038/srep00490
Gilbert J. A., Steele J. A., Caporaso J. G., Steinbrück L., Reeder J., Temperton B., et al. (2012). Defining Seasonal Marine Microbial Community Dynamics. ISME J. 6, 298–308. doi: 10.1038/ismej.2011.107
Halac S., Felip M., Camarero L., Sommaruga-Wograth S., Psenner R., Jordi C., et al. (1997). An in Situ Enclosure Experiment to Test the Solar UVB Impact on Plankton in a High-Altitude Mountain Lake. I. Lack of Effect on Phytoplankton Species Composition and Growth. J. Plankton Res. 19, 1671–1686. doi: 10.1093/plankt/19.11.1671
Hugoni M., Taib N., Debroas D., Domaizon I., Dufournel I. J., Bronner G., et al. (2013). Structure of the Rare Archaeal Biosphere and Seasonal Dynamics of Active Ecotypes in Surface Coastal Waters. PNAS 110, 6004–6009. doi: 10.1073/pnas.1216863110
Jones H. (1997). A Classification of Mixotrophic Protists Based on Their Behaviour. Freshwater Biol. 37, 35–43. doi: 10.1046/j.1365-2427.1997.00138.x
Jones T., Parrish J. K., Punt A. E., Trainer V. L., Kudela R., Lang J., et al. (2017). Mass Mortality of Marine Birds in the Northeast Pacific Caused by Akashiwo Sanguinea. Mar Ecol. Prog. Ser. 579, 111–127. doi: 10.3354/meps12253
Jonsson P. R. (1986). Particle Size Selection, Feeding Rates and Growth Dynamics of Marine Planktonic Oligotrichous Ciliates (Ciliophora: Oligotrichina). Mar Ecol. Prog. Ser. 33, 265–277. doi: 10.3354/meps033265
Kibler S. R., Tester P. A., Kunkel K. E., Moore S. K., Litaker R. W. (2015). Effects of Ocean Warming on Growth and Distribution of Dinoflagellates Associated With Ciguatera Fish Poisoning in the Caribbean. Ecol. Modelling 316, 194–210. doi: 10.1016/j.ecolmodel.2015.08.020
Kjerfve B. (1994). “Chapter 1 Coastal Lagoons,” in Elsevier Oceanography Series Coastal Lagoon Processes. Ed. Kjerfve B. (Amsterdam: Elsevier), 1–8. doi: 10.1016/S0422-9894(08)70006-0
Klaveness D. (1988). “Ecology of the Cryptomonadida: A First Review,” in Growth and Reproductive Strategies of Freshwater Phytoplankton (Cambridge: Cambridge University Press), 105–133.
Lambert S., Lozano J.-C., Bouget F.-Y., Galand P. E. (2021). Seasonal Marine Microorganisms Change Neighbours Under Contrasting Environmental Conditions. Environ. Microbiol. 23, 2592–2604. doi: 10.1111/1462-2920.15482
Lambert S., Tragin M., Lozano J.-C., Ghiglione J.-F., Vaulot D., Bouget F.-Y., et al. (2018). Rhythmicity of Coastal Marine Picoeukaryotes, Bacteria and Archaea Despite Irregular Environmental Perturbations. ISME J. 13, 388–401. doi: 10.1038/s41396-018-0281-z
Lee S. Y., Jeong H. J., Kang H. C., Ok J. H., You J. H., Park S. A., et al. (2021). Comparison of the Spatial-Temporal Distributions of the Heterotrophic Dinoflagellates Gyrodinium Dominans, G. Jinhaense and G. Moestrupii in Korean Coastal Waters. Algae 36, 37–50. doi: 10.4490/algae.2021.36.3.4
Lex A., Gehlenborg N., Strobelt H., Vuillemot R., Pfister H. (2014). UpSet: Visualization of Intersecting Sets. IEEE Trans. Vis. Comput. Graph 20, 1983–1992. doi: 10.1109/TVCG.2014.2346248
Mackey K. R. M., Hunter-Cevera K., Britten G. L., Murphy L. G., Sogin M. L., Huber J. A. (2017). Seasonal Succession and Spatial Patterns of Synechococcus Microdiversity in a Salt Marsh Estuary Revealed Through 16S rRNA Gene Oligotyping. Front. Microbiol. 8. doi: 10.3389/fmicb.2017.01496
Manning T., Thilagaraj A. V., Mouradov D., Piola R., Grandison C., Gordon M., et al. (2021). Diversity of Dinoflagellate Assemblages in Coastal Temperate and Offshore Tropical Waters of Australia. BMC Ecol. Evo 21, 27. doi: 10.1186/s12862-021-01745-5
Massana R., Murray A. E., Preston C. M., DeLong E. F. (1997). Vertical Distribution and Phylogenetic Characterization of Marine Planktonic Archaea in the Santa Barbara Channel. Appl. Environ. Microbiol. 63, 50–56. doi: 10.1128/aem.63.1.50-56.1997
Millet B., Cecchi P. (1992). Wind-Induced Hydrodynamic Control of the Phytoplankton Biomass in a Lagoon Ecosystem. Limnol. Oceanogr. 37, 140–146. doi: 10.4319/lo.1992.37.1.0140
Mohit V., Archambault P., Toupoint N., Lovejoy C. (2014). Phylogenetic Differences in Attached and Free-Living Bacterial Communities in a Temperate Coastal Lagoon During Summer, Revealed via High-Throughput 16S rRNA Gene Sequencing. Appl. Environ. Microbiol. 80, 2071–2083. doi: 10.1128/AEM.02916-13
Mostajir B., Mas S., Parin D., Vidussi F. (2018). High-Frequency Physical, Biogeochemical and Meteorological Data of Coastal Mediterranean Thau Lagoon Observatory. SEANOE. doi: 10.17882/58280
Murray A. E., Blakis A., Massana R., Strawzewski S., Passow U., Alldredge A., et al. (1999). A Time Series Assessment of Planktonic Archaeal Variability in the Santa Barbara Channel. Aquat. Microb. Ecol. 20, 129–145. doi: 10.3354/ame020129
Nagao R., Ueno Y., Akimoto S., Shen J.-R. (2020). Effects of CO2 and Temperature on Photosynthetic Performance in the Diatom Chaetoceros Gracilis. Photosynth. Res. 146, 189–195. doi: 10.1007/s11120-020-00729-8
Needham D. M., Fuhrman J. A. (2016). Pronounced Daily Succession of Phytoplankton, Archaea and Bacteria Following a Spring Bloom. Nat. Microbiol. 1, 16005. doi: 10.1038/nmicrobiol.2016.5
Nogales B., Aguiló-Ferretjans M. M., Martín-Cardona C., Lalucat J., Bosch R. (2007). Bacterial Diversity, Composition and Dynamics in and Around Recreational Coastal Areas. Environ. Microbiol. 9, 1913–1929. doi: 10.1111/j.1462-2920.2007.01308.x
Novarino G. (2005). Nanoplankton Protists From the Western Mediterranean Sea. II. Cryptomonads (Cryptophyceae = Crptomonadea). Scientia Marina 69, 47–74. doi: 10.3989/scimar.2005.69n147
Ou G., Wang H., Si R., Guan W. (2017). The Dinoflagellate Akashiwo Sanguinea Will Benefit From Future Climate Change: The Interactive Effects of Ocean Acidification, Warming and High Irradiance on Photophysiology and Hemolytic Activity. Harmful Algae 68, 118–127. doi: 10.1016/j.hal.2017.08.003
Parada A. E., Needham D. M., Fuhrman J. A. (2016). Every Base Matters: Assessing Small Subunit rRNA Primers for Marine Microbiomes With Mock Communities, Time Series and Global Field Samples. Environ. Microbiol. 18, 1403–1414. doi: 10.1111/1462-2920.13023
Pecqueur D., Vidussi F., Fouilland E., Floc’h E. L., Mas S., Roques C., et al. (2011). Dynamics of Microbial Planktonic Food Web Components During a River Flash Flood in a Mediterranean Coastal Lagoon. Hydrobiologia 673, 13–27. doi: 10.1007/s10750-011-0745-x
Pérez-Ruzafa A., Marcos C., Pérez-Ruzafa I. M. (2011). Mediterranean Coastal Lagoons in an Ecosystem and Aquatic Resources Management Context. Phys. Chem. Earth Parts A/B/C 36, 160–166. doi: 10.1016/j.pce.2010.04.013
Pernet F., Malet N., Pastoureaud A., Vaquer A., Quéré C., Dubroca L. (2012). Marine Diatoms Sustain Growth of Bivalves in a Mediterranean Lagoon. J. Sea Res. 68, 20–32. doi: 10.1016/j.seares.2011.11.004
Pittera J., Humily F., Thorel M., Grulois D., Garczarek L., Six C. (2014). Connecting Thermal Physiology and Latitudinal Niche Partitioning in Marine Synechococcus. ISME J. 8, 1221–1236. doi: 10.1038/ismej.2013.228
Pringault O., Bouvy M., Carre C., Fouilland E., Meddeb M., Mejri K., et al. (2020). Impacts of Chemical Contamination on Bacterio-Phytoplankton Coupling. Chemosphere 257, 127165. doi: 10.1016/j.chemosphere.2020.127165
Roberts E. C., Laybourn-Parry J. (1999). Mixotrophic Cryptophytes and Their Predators in the Dry Valley Lakes of Antarctica. Freshwater Biol. 41, 737–746. doi: 10.1046/j.1365-2427.1999.00401.x
Rodríguez F., Derelle E., Guillou L., Le Gall F., Vaulot D., Moreau H. (2005). Ecotype Diversity in the Marine Picoeukaryote Ostreococcus (Chlorophyta, Prasinophyceae). Environ. Microbiol. 7, 853–859. doi: 10.1111/j.1462-2920.2005.00758.x
Santoro A. E., Dupont C. L., Richter R. A., Craig M. T., Carini P., McIlvin M. R., et al. (2015). Genomic and Proteomic Characterization of “Candidatus Nitrosopelagicus Brevis”: An Ammonia-Oxidizing Archaeon From the Open Ocean. Proc. Natl. Acad. Sci. 112, 1173–1178. doi: 10.1073/pnas.1416223112
Santoro A. E., Richter R. A., Dupont C. L. (2019). Planktonic Marine Archaea. Annu. Rev. Mar. Sci. 11, 131–158. doi: 10.1146/annurev-marine-121916-063141
Schubert H., Telesh I. (2017). “Estuaries and Coastal Lagoons”. In: Biological Oceanography of the Baltic Sea (Dordrecht: Springer Netherlands) (Accessed October 12, 2020).
Shannon C. E. (1948). A Mathematical Theory of Communication. Bell Syst Tech. J. 27, 379–423. doi: 10.1002/j.1538-7305.1948.tb01338.x
Simonato F., Gómez-Pereira P. R., Fuchs B. M., Amann R. (2010). Bacterioplankton Diversity and Community Composition in the Southern Lagoon of Venice. Syst. Appl. Microbiol. 33, 128–138. doi: 10.1016/j.syapm.2009.12.006
Souchu P., Bec B., Smith V. H., Laugier T., Fiandrino A., Benau L., et al. (2010). Patterns in Nutrient Limitation and Chlorophyll a Along an Anthropogenic Eutrophication Gradient in French Mediterranean Coastal Lagoons. Can. J. Fish Aquat. Sci. 67, 743–753. doi: 10.1139/F10-018
Stoeck T., Bass D., Nebel M., Christen R., Jones M. D. M., Breiner H.-W., et al. (2010). Multiple Marker Parallel Tag Environmental DNA Sequencing Reveals a Highly Complex Eukaryotic Community in Marine Anoxic Water. Mol. Ecol. 19, 21–31. doi: 10.1111/j.1365-294X.2009.04480.x
Šupraha L., Bosak S., Ljubešić Z., Mihanović H., Olujić G., Mikac I., et al. (2014). Cryptophyte Bloom in a Mediterranean Estuary: High Abundance of Plagioselmis Cf. Prolonga in the Krka River Estuary (Eastern Adriatic Sea). Scientia Marina 78, 329–338. doi: 10.3989/scimar.03998.28C
Tragin M., Vaulot D. (2019). Novel Diversity Within Marine Mamiellophyceae (Chlorophyta) Unveiled by Metabarcoding. Sci. Rep. 9, 5190. doi: 10.1038/s41598-019-41680-6
Trombetta T., Vidussi F., Mas S., Parin D., Simier M., Mostajir B. (2019). Water Temperature Drives Phytoplankton Blooms in Coastal Waters. PloS One 14, e0214933. doi: 10.1371/journal.pone.0214933
Trombetta T., Vidussi F., Roques C., Mas S., Scotti M., Mostajir B. (2021). Co-Occurrence Networks Reveal the Central Role of Temperature in Structuring the Plankton Community of the Thau Lagoon. Sci. Rep. 11, 17675. doi: 10.1038/s41598-021-97173-y
Walker C. B., de la Torre J. R., Klotz M. G., Urakawa H., Pinel N., Arp D. J., et al. (2010). Nitrosopumilus Maritimus Genome Reveals Unique Mechanisms for Nitrification and Autotrophy in Globally Distributed Marine Crenarchaea. Proc. Natl. Acad. Sci. 107, 8818–8823. doi: 10.1073/pnas.0913533107
Ward C. S., Yung C.-M., Davis K. M., Blinebry S. K., Williams T. C., Johnson Z. I., et al. (2017). Annual Community Patterns Are Driven by Seasonal Switching Between Closely Related Marine Bacteria. Isme J. 11, 1412–1422. doi: 10.1038/ismej.2017.4
Keywords: metabarcoding, plankton, water temperature, shallow coastal lagoon, microbial diversity, eukaryota, bacteria, archaea
Citation: Trombetta T, Bouget F-Y, Félix C, Mostajir B and Vidussi F (2022) Microbial Diversity in a North Western Mediterranean Sea Shallow Coastal Lagoon Under Contrasting Water Temperature Conditions. Front. Mar. Sci. 9:858744. doi: 10.3389/fmars.2022.858744
Received: 20 January 2022; Accepted: 21 March 2022;
Published: 20 April 2022.
Edited by:
Jun Sun, China University of Geosciences, ChinaReviewed by:
Sandi Orlic, Rudjer Boskovic Institute, CroatiaKasia Piwosz, National Marine Fisheries Research Institute, Poland
Copyright © 2022 Trombetta, Bouget, Félix, Mostajir and Vidussi. This is an open-access article distributed under the terms of the Creative Commons Attribution License (CC BY). The use, distribution or reproduction in other forums is permitted, provided the original author(s) and the copyright owner(s) are credited and that the original publication in this journal is cited, in accordance with accepted academic practice. No use, distribution or reproduction is permitted which does not comply with these terms.
*Correspondence: Thomas Trombetta, dGhvbWFzLnRyb21iZXR0YUBnbWFpbC5jb20=; Francesca Vidussi, ZnJhbmNlc2NhLnZpZHVzc2lAY25ycy5mcg==