- 1Instituto de Ciencias del Mar-CSIC, Barcelona, Spain
- 2Instituto de Investigaciones Marinas-CSIC, Vigo, Spain
Plastic debris reaching the ocean is exponentially increasing in parallel with plastic production. Once into seawater, plastic starts to leach organic compounds that are presumably additives and plastic oligomers, and that process is enhanced by solar radiation. From previous studies with virgin plastic, it has been estimated that up to 23,600 metric tons of dissolved organic carbon (DOC) can be released into seawater every year from all the plastic entering the ocean. However, most plastic found in the ocean has been aged through weathering and sunlight radiation, which could result in enhanced leaching. Despite this, dissolved organic matter (DOM) leaching from aged plastic and the effect on microbial communities and their activity has never been explored. Here we studied DOM leaching by aged plastic collected from a sand beach and how it compares with that from virgin plastic. After characterizing the DOM leached from plastic through fluorescence techniques, we also explored the bioavailability of the DOM leached and its fluorescent fraction through biodegradation experiments. Finally, we determined single cell activities of the prokaryotic community growing on the plastic leachates. We found that the release of DOC by aged plastic was two order of magnitude higher than that leached by virgin new plastic. Extrapolating that into the ocean and assuming that most of the plastic arriving there is not new, we estimated that up to 57,000 metric tons of DOC can be released by plastic debris every year. A fraction of the DOM released by plastic was fluorescent (FDOM), especially in the protein-like region, and bioavailable to microbial uptake, as it is also shown by the increase in the single cell activities of the bacteria growing on the leachates. Since most plastics in the ocean have been exposed to sunlight radiation provoking aging, our results unveil that the amount of carbon released by plastics is much higher than hitherto recognized, and thus will have a stronger impact in the oceanic carbon cycle and in marine ecosystems.
Introduction
In 2020, 367 million metric tons of plastic were produced worldwide (Plastic Europe, 2021). From that, about 4% ended in the ocean and the predictions are that this amount will increase twice by 2025, in parallel with the increasing plastic production (Jambeck et al., 2015). Marine plastic debris is known to negatively affect marine fauna through entangling and affecting their health after ingestion (Wesch et al., 2016). When plastics enter in contact with seawater where organic pollutants are present, they may adsorb and absorb these pollutants, which can be posteriorly released into the surrounding environment (Chen et al., 2018). Also, during plastic manufacture, different types of additives are added to the polymer to provide it with the properties required for its use, such as color, durability, and elasticity, among others. Up to 60% of plastic weight can be due to additives (Hermabessiere et al., 2017), which may be released into seawater (Suhrhoff and Scholz-Böttcher, 2016), but also inside the organisms once the plastic is ingested (Hermabessiere et al., 2017). The impact of these organic compounds in the carbon cycle has recently started to be studied. A previous work found that plastic entering the ocean can release up to 23,600 metric tons of dissolved organic carbon every year (Romera-Castillo et al., 2018), but this value was estimated using new commercial plastic. Plastic found in the ocean has been exposed to environmental factors such as UV radiation, oxidation, and erosion which contribute to its aging (Andrady, 2011). This causes degradation in form of scratches and fragmentation of the plastic into smaller pieces. Therefore, it is crucial to study aged plastic leaching into seawater and how it compares with new plastic. Only one work studied the leaching from aged plastic collected in the North Pacific but it did not determine the optical characterization of the leachates nor the biodegradation of the material released (Zhu et al., 2020). Fluorescence spectroscopy has been widely applied to the study of natural dissolved organic matter in aquatic systems (Stedmon and Nelson, 2015). Its application to the DOM leached from plastic gives information about the optical and chemical characteristics of the material released into seawater in a fast and non-expensive way. Also, this technique could show if the fluorescent signal associated to the DOM leached from plastic is distinguishable from that naturally occurring in aquatic systems.
Only a few studies have focused on the impact of plastic leaching on the microbial food web. For instance, dissolved organic compounds leached by plastic can enhance heterotrophic microbial growth (Romera-Castillo et al., 2018) but they have also been found to inhibit Procchlorochoccus photosynthesis and growth (Tetu et al., 2019). However, the effect of aged plastic leachates on marine prokaryotes is mostly unknown. Also, among the few works studying the microbial bioavailability of DOM leached from plastic (Romera-Castillo et al., 2018; Zhu et al., 2020), no one has studied the activity of prokaryotes growing in the leachates and how the leachates from aged plastic compare with new virgin plastic.
The aim of this work is to compare the amount of DOC and fluorescent characteristics of the DOM leached from a mix of naturally aged plastics with that from virgin low density polyethylene (LDPE) as well as other plastic types from the literature. It also investigates the response of a natural bacterial community to these different leachates. To this end, we measured the single-cell protein synthesis capacity of a natural bacterial community growing in plastic leachates applying Bioorthogonal non-canonical amino acid tagging (BONCAT, Dieterich et al., 2006; Hatzenpichler et al., 2014; Leizeaga et al., 2017). We hypothesize that aged plastics leach more DOC in contact with water due to the degradation of their surface and that their leachates will have a stronger impact on the marine bacterial communities than those from virgin commercial plastic.
Materials and Methods
Plastic Leaching Photodegradation (Abiotic Production)
The plastic types used in this study were low density polyethylene (LDPE) film (0.5 mm thickness, Goodfellow), cut in 5 × 5 mm side squares, and a mix of aged plastics collected in Famara Beach, Lanzarote (Canary Islands, Spain). This beach is affected by predominant N-NE winds and swells which bring large amounts of plastic debris into its sand. This plastic is probably allochthonous, reaching Famara Beach with the Canary Current, which is fed by the Azores Current, coming from the western North Atlantic, and the Portugal Current, coming from the Iberian basin (Pérez-Hernández et al., 2013). The mix of aged plastics was composed by irregular flat pieces between 0.4 and 1 cm size. The total weight of plastic added to each flask was of 2.48 g for the new LDPE and ∼7.5 g for aged plastic. The amount of LDPE added was chosen following the methodology in Romera-Castillo et al. (2018), who determined the optimal plastic surface values, for a better comparison of the results. Adding the same surface of the aged plastic would have resulted in too few pieces with the risk of not having enough signal. Therefore, for aged plastic, we added ∼180 items L–1 which is a concentration within the range commonly found in very polluted coastal areas such as Jade Bay in the Sourthern North Sea (64 items L–1 on average, Dubaish and Liebezeit, 2013) or Mumbai coast (372 items L–1 on average, Gurjar et al., 2022).
Aged plastic was characterized by FTIR using a Nicolet iS10 coupled with a ATR Smart iTR-Diamond. The mix was composed of 65% of polyethylene, 30% polypropylene, and 5% polyvinyl chloride. We decided to use new LDPE for comparison since polyethylene is the most abundant plastic found in the ocean (e.g., Cózar et al., 2014) and it represented 65% of the aged sample. A few plastic pieces were observed on the scanning electronic microscope (Hitachi S-3500N) and no bacteria or microorganisms were observed on their surface. The pictures also show that the plastic presented signs of aging with multiple fractures and scaly surface (Supplementary Figure 1). Before the experiment, the aged plastics were sonicated (Cole Parmer 8890, 42 KHz, 80 W) three times with MQ water for 10 min. Then, they were soaked in 1% oxygen peroxide for 1 h, to remove any organic debris and any potential bacterial contamination (e.g., Zhu et al., 2020). After that, the plastics were sonicated three times in MQ water for 5 min.
For the photodegradation experiment, plastic pieces were placed in 500 mL quartz tubes filled with 400 mL of 0.2 μm filtered and autoclaved aged seawater (AAS). This AAS had been collected at 2000 m depth in the NW Mediterranean Sea and aged in the dark for 2 years to ensure a low concentration of DOC (down to 52.0 μmol L–1 at the beginning of our experiment). Non-irradiated treatments were placed in 500 mL borosilicate bottles wrapped with aluminum foil. Controls of AAS without plastic were also incubated. All the treatments were performed in triplicates. The flasks were incubated for 6 days in a solar simulator placed in a flow-through water bath maintaining the temperature of the incubation at 28°C, similar to the water temperature in the tropics. The solar simulator provided artificial photosynthetic active radiation (PAR) by a HQI-T Powerstar lamp (250 W, Osram), UV-A radiation by 2 Philips TL100W/10 R fluorescent tubes and, and UV-B radiation by 2 UVA-340 fluorescent lamps (Q-Panel Company, United Kingdom). The radiation intensity for each wavelength or wavelength range was as follows: PAR (400–700 nm), 700 μmol m–2 s–1; 380 nm, 28.47 μW cm–2 nm–1; 340 nm, 16.31 μW cm–2 nm–1; 320 nm, 7.95 μW cm–2 nm–1; 305 nm, 1.09 μW cm–2 nm–1. The radiation dose rate represents the solar radiation in the subtropical North Atlantic Gyre measured at noon at 15 m depth. Artificial solar radiation was measured at 305, 320, 340, 380 nm, and PAR with a Biospherical PUV-510 radiometer. The light treatments received continuous artificial solar radiation.
Dissolved organic carbon and fluorescent dissolved organic matter (FDOM) were measured before and after the incubation period from the irradiated and non-irradiated treatments to estimate the DOM leaching from the plastic material and the effect of the radiation on it. The treatments with aged plastic were filtered through a pre-combusted (4 h, 450°C) Whatman GF/F filter at the end of the incubation since some plastic particles were observed in the water. In the controls of AAS without plastic, DOC variation during the leaching time was not significant (p > 0.05), being 1.16 ± 0.86 μmol L–1 for irradiated and 1.57 ± 1.09 for non-irradiated treatments. DOC release was normalized to the surface area of the plastic used in every flask (8,000 mm2 for LDPE and 15,000 mm2 for aged plastic). The total surface area for each treatment was calculated from pictures using the software ImageJ. Bacterial abundance was measured at the end of the experiment to check that microbial growth did not take place during this photodegradation phase.
Prokaryotic Degradation of Plastic Leachates
After exposure to irradiation, plastic pieces were removed and the leachates obtained in the photodegradation experiment were inoculated with fresh surface seawater from the Blanes Bay Microbial Observatory, in the coastal NW Mediterranean Sea (41°40′N, 2°48′E), at a ratio of 9:1 (leachate: inoculum). The inoculum was collected the same day of the experiment and was filtered by 0.8 μm to remove grazers and other eukaryotic cells, since they could mask the prokaryotic response to leachates. The water for the inoculum was collected on January 30, 2020. The treatments were amended with NH4Cl and NaH2PO4 to a final concentration of 10 and 2 μmol mL–1, respectively, to avoid growth limitation by either nitrogen or phosphorus availability. The flasks were incubated in the dark at 23°C until the prokaryotic community reached the stationary phase. Samples for prokaryotic abundance were collected daily. DOC and FDOM samples were collected at the beginning of the incubation and at the end of the exponential growth phase. At the end of the experiment, samples for DOC and FDOM were filtered through pre-combusted Whatman GF/F filters to remove cells. Samples for BONCAT analyses were collected at 24 h and 96 h after the inoculation.
Analyses
Dissolved organic carbon samples were collected in pre-combusted 20 mL glass vials, acidified with concentrated HCl to pH < 2 and stored at 4°C until analysis. DOC was measured on a Shimatzu TOC-V organic carbon analyzer (Álvarez-Salgado and Miller, 1998) after the removal of CO2 by vigorous sparging with high purity N2. Therefore, only the non-volatile fraction of DOC was measured. The accuracy was tested daily with the DOC reference materials provided by D.A. Hansell (University of Miami). We obtained average concentrations of 44.3 ± 0.8 μmol L–1 for the deep ocean reference (Batch 18–Lot 08–18) minus blank reference materials. The nominal range of DOC values provided by the reference laboratory was 43.2–45.2 μmol L–1.
Plastic carbon loss in the form of DOC was calculated as the mg of DOC leached per kg of plastic carbon (ppm). The carbon content for the plastics used in this work was determined on an Elemental Analyzer Perkin Elmer 2400. We obtained that carbon represented 85.3% for LDPE, and an average of 82.7% for aged beach plastic.
Excitation Emission matrices (EEMs) were performed with a LS 55 PerkinElmer Luminescence spectrometer, equipped with a xenon discharge lamp, equivalent to 20 kW for 8-ms duration. The detector was a red-sensitive R928 photomultiplier, and a photodiode worked as a reference detector. Measurements were performed at a constant room temperature of 20°C in a 1-cm quartz fluorescence cell. The excitation wavelength ranged from 240 to 440 nm at 10 nm steps increments and emissions wavelength from 300 to 560 nm at 0.5 nm increments, with a bandwidth of 5 nm, and the integration time was 0.1 s (scan speed was 250 nm min). Data was standardized and normalized to the Raman area. Raman area and its baseline correction was performed with the emission scan at 350 nm of the MQ blanks and the area was calculated based on measurements of Raman peak at 350 nm (Lawaetz and Stedmon, 2009). Samples were not diluted since the absorbance was always lower than 10 m–1 both at 240 nm and 254 nm (Stedmon and Bro, 2008). Raman-normalized MQ blanks were subtracted to remove the Raman scattering signal (e.g., Stedmon et al., 2003). The drEEM 0.2.0 toolbox was used to standardize the EEMs (Murphy et al., 2013).
Integrated fluorescence was calculated as the sum of the fluorescence intensity values inside the range Ex: 300–400 and Em: 370–500, for the humic-like region, and Ex: 240–300 and Em: 300–370, for the protein-like region. Although the fluorescence observed due to the plastic leaching is not due to the humic- or protein-like substances present in natural DOM, we will keep the terms humic- and protein-like regions for an easier comparison with the Ex:Em regions where the natural dissolved organic matter fluoresces.
Bacterial abundances were determined daily by flow cytometry as described in Gasol and Del Giorgio (2000). Bacterial carbon biomass was calculated from bacterial abundance using the conversion factor 20 fg C cell–1 (Lee and Fuhrman, 1987). Bacterial growth efficiency (BGE, as%) was calculated as the increase in bacterial carbon biomass and the decrease in DOC during the incubation time.
Single-cell activity was estimated using BONCAT (Bioorthogonal non-canonical amino acid tagging, Dieterich et al., 2006) following the protocol detailed in Leizeaga et al. (2017). This protocol consists on the incubation of a natural sample with an artificial amino acid (L-homopropargylglycine, HPG) that is structurally analogue to L-methionine, which is incorporated into the newly synthesized proteins. The HPG-containing cells can get later fluorescently labeled via copper-catalyzed azide-alkyne click chemistry, and visualized and quantified with epifluorescence microscopy.
Samples for BONCAT were collected 24 h and 96 h after inoculation. Nine mL samples were incubated with L-homopropargylglycine (HPG) (1 μM final concentration) during 2 h. The incubation was stopped by fixing the samples with 0.2 μm-filtered formaldehyde (final concentration 4% [v/v]) overnight at 4°C. Samples were gently filtered through a 0.2 μm pore size polycarbonate filter placed on top of a 0.8 μm cellulose acetate membrane filter, washed three times with sterile milliQ water, and kept frozen at −80°C until further processing. Samples were processed following Leizeaga et al. (2017) with small modifications (see Supplementary Material for details).
Images were acquired in black and white using an Axio Imager.Z2m epifluorescence microscope connected to a Zeiss camera (AxioCam MRm, Carl Zeiss MicroImaging, S.L., Barcelona, Spain) at 630 × magnification through the Axiovision software, and analyzed using ACMEtool. A Colibri LED light source was used (Carl Zeiss) with two light-emitting diodes (UV-emitting LED 365 for DAPI; red-emitting LED 590 for the tyramide Alexa Fluor 594), combined with the HE-62 multifilter module (Carl Zeiss). 100 ms exposure time was used for DAPI, and 120 ms for the BONCAT images. On average, 5,000 cells were counted per portion of filter. Image analyses were performed with ACMEtool over two black and white pictures of the same visual field: one for the DAPI stain and one for the BONCAT+ cells. The percentage of BONCAT+ cells was calculated in relation to the DAPI counts.
The intensity of the BONCAT+ cells was assessed using the mean gray value (MGV), which is the sum of the gray values of all the pixels in the cell divided by the number of pixels. Cells were categorized as displaying high, medium or low activity based on this signal intensity. To this end, all the BONCAT+ labeled cells were first rank-ordered by intensity. Then the maximum intensity and the minimum intensity were obtained. This intensity range (between the maximum and minimum intensity) was equally divided in three groups: high intensity (top third), medium intensity (middle third), and low intensity cells (bottom third). Finally, the percentage of each category within the BONCAT+ cells was calculated at each time point.
Statistical Tests
We used ANOVA followed by Tukey’s Honestly Significant Difference (HSD) test to explore differences in cell activity between the different treatments.
Results
Photodegradation of Plastic Leachates (Abiotic Production)
All the plastic treatments showed leaching of DOC ranging from 0.09 ± 0.09 μg cm–2 of plastic surface, for non-irradiated LDPE, to 21.50 ± 0.78 μg cm–2 of plastic surface, for irradiated aged plastic (Figure 1A and Table 1). The aged plastic collected on the beach released between 30 and 94 times more DOC than the commercial LDPE for irradiated and non-irradiated treatments, respectively. Irradiation significantly enhanced the release of DOC in both plastic types, particularly in the LDPE that released 8 times more DOC after irradiation than its dark counterpart. After 6 days, all plastic types lost weight in form of DOC. The highest loss corresponded to the aged plastic with 542 ± 20 mg of DOC per kg of plastic carbon (ppm), for the irradiated, and 225 ± 2 ppm, for the dark treatments. After irradiation, LDPE lost only 29 ± 7 ppm while this plastic kept in the dark lost 3.9 ± 4 ppm.
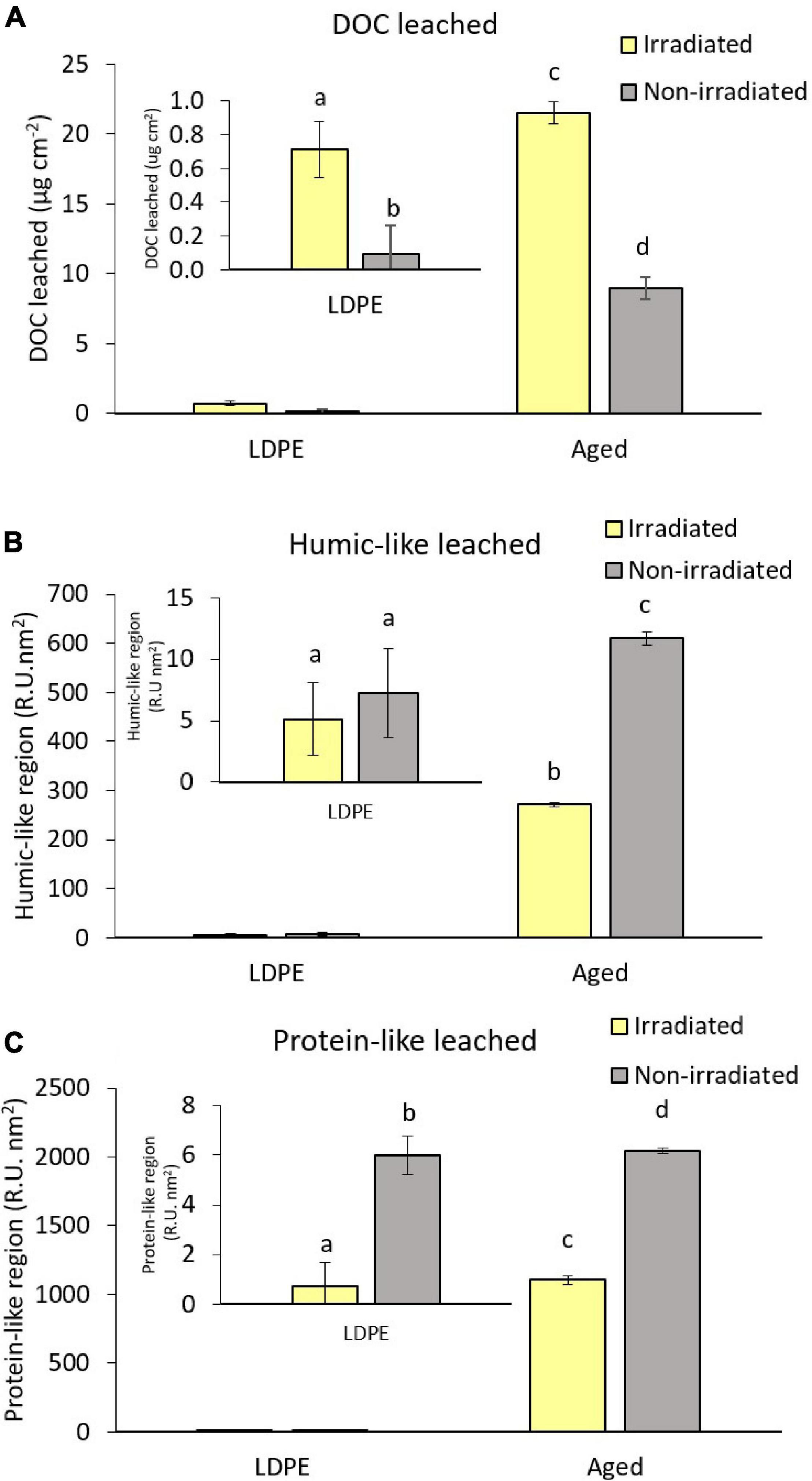
Figure 1. Dissolved organic matter leached from LDPE and aged plastics in the irradiated and non-irradiated treatments during the photodegradation experiment (A) dissolved organic carbon; (B) fluorescent dissolved organic matter integrated in the humic-like region; (C) fluorescent dissolved organic matter integrated in the protein-like region. An enlargement of the LDPE values during the leaching incubation is included in all panels. The same letter over different bars indicate non-significant differences between them. Error bars represent the standard deviation of the mean of the triplicate cultures.
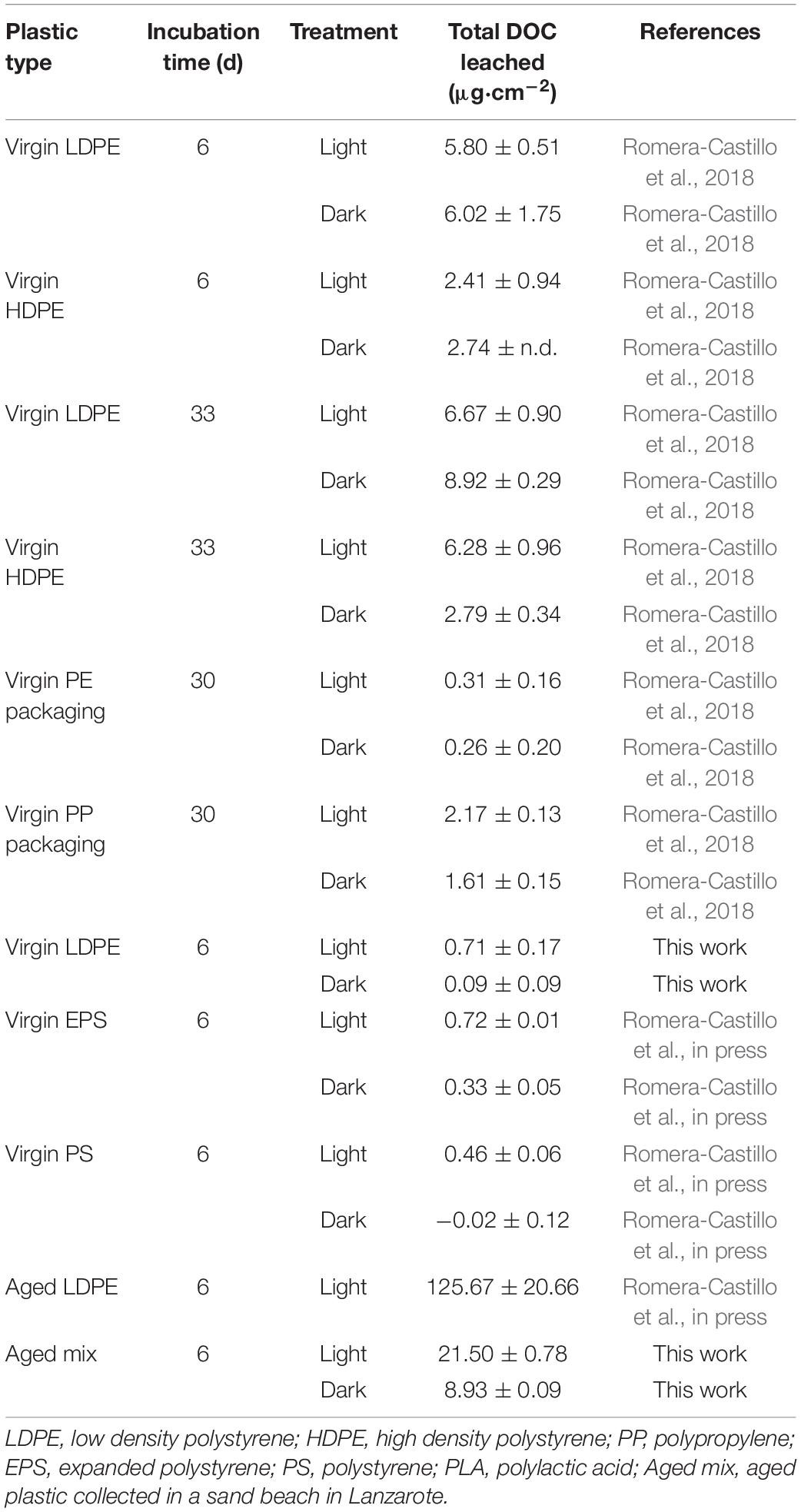
Table 1. Total dissolved organic carbon (μg⋅cm–2) leached by different types of virgin plastic and aged plastics.
Both new LDPE and aged plastics released fluorescent dissolved organic matter (FDOM) in the humic-like region (Figure 1B). The highest release was observed in the aged plastic treatments with 271.1 ± 4.2 R.U.⋅ nm2, for the irradiated treatments, and 609.8 ± 13.4 R.U. ⋅ nm2, for the non-irradiated ones. The non-irradiated treatments presented higher FDOM in the humic-like region than irradiated ones, being not significant for LDPE but more than double in the aged plastic. Regarding FDOM in the protein-like region, only irradiated LDPE did not show a net release (Figure 1C). Aged plastic leached the highest amount being 1105.4 ± 31.9 R.U. ⋅ nm2, for the irradiated treatments, and almost twice that amount (2042.3 ± 20.8 R.U. ⋅ nm2) for the non-irradiated ones.
The leached material of the aged plastic showed Excitation Emission Matrices (EEMs) characterized by two well-defined peaks: one in the protein-like region at Ex:Em around 268 nm:352 nm and a smaller one, around 3 times lower than the former, at Ex:Em around 312 nm:362 nm (Figures 2A,B). Both peaks were absent in the LDPE and control treatments. The intensity of these peaks was higher in the non-irradiated treatments (about 0.61 and 0.2 R.U., respectively) than in the irradiated ones (about 0.10 and 0.03 R.U., respectively) indicating that irradiation could have photodegraded both peaks. In the humic-like region it is also observable a fluorescent signal in the non-irradiated treatments that almost disappeared in the irradiated ones, showing that it was also photodegraded.
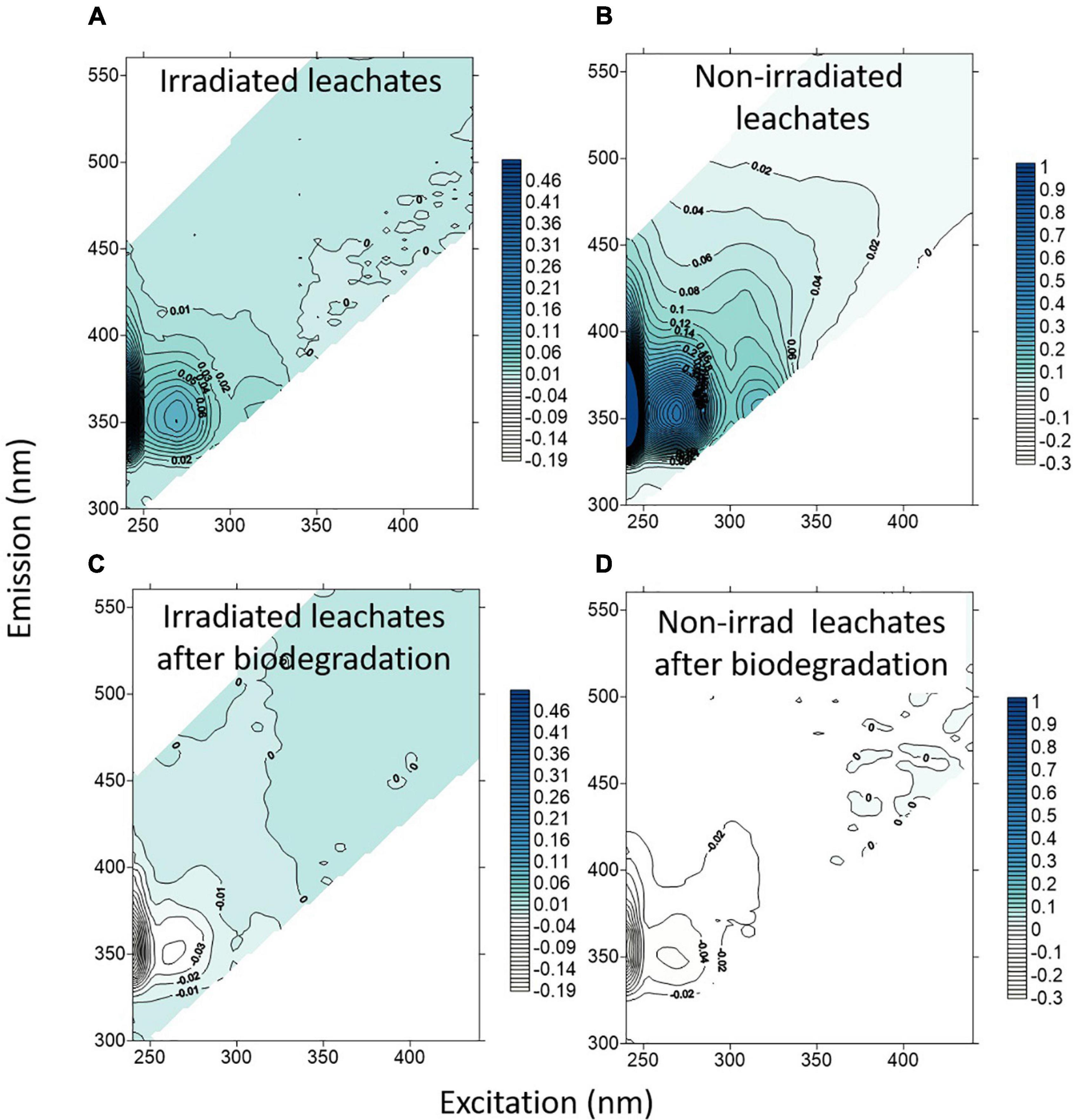
Figure 2. Net Excitation Emission matrices (EEMs) variation in the leachates from aged plastic, as the difference between final and initial time points after (A) 6 days of abiotic irradiation; (B) 6 days of abiotic incubation with no irradiation; (C) 4 days of biodegradation by a marine natural community of the irradiated leachates; and (D) 4 days of biodegradation by a marine natural community of the non-irradiated leachates. Note the difference in the color scale for the irradiated and non-irradiated treatments.
Biodegradation of Plastic Leachates
All treatments showed an increase in the bacterial abundance that reached between 2.71 × 106 ± 2.32 × 105 cell mL–1 in the non-irradiated control, and 1.34 × 107 ± 2.75 × 106 cell mL–1 in the irradiated aged plastic leachates (Figure 3). The exponential phase started after 20 h of incubation. We stopped the incubations at day 4 when the communities reached the stationary phase. Bacterial growth on aged plastic leachates was one order of magnitude higher than in the control without plastic and in the LDPE leachates, for both, irradiated and non-irradiated treatments. The bacterial abundance reached in the LDPE leachates did not show a significant difference compared to the control without plastic for both irradiated (about 3.10 × 106 cell mL–1) and non-irradiated treatments (about 2.75 × 106 cell mL–1). At the end of the biodegradation experiment, all the previously irradiated treatments presented higher bacterial abundance compared to their non-irradiated counterparts, although the difference was not significant for the treatments with aged plastic leachates.
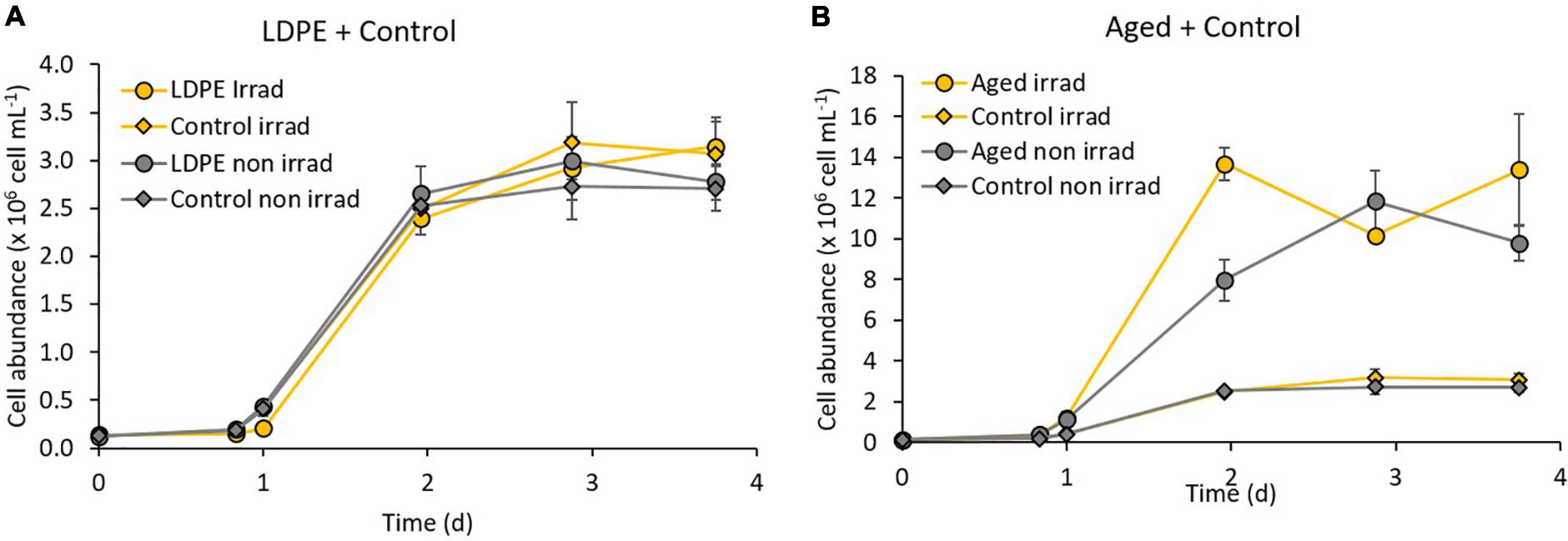
Figure 3. Growth of natural bacterial communities on (A) LDPE leachates and (B) aged plastic leachates produced during the abiotic degradation experiment (irradiated and non-irradiated). The controls with no plastics are also shown. Error bars represent the standard deviation of the mean of the triplicate cultures. Note the different scales of cell abundance in panels (A,B).
Dissolved organic carbon consumption during the biodegradation experiments ranged between 15.4 ± 5.4 μmol L–1 in the non-irradiated control, and 194.9 ± 12.8 μmol L–1 in the irradiated aged plastic leachates (Figure 4A). The consumption of DOC in the aged plastic leachates was significantly higher than in the LDPE and control, which showed similar values between them for both the irradiated and non-irradiated treatments. All the irradiated treatments presented a significantly (p < 0.05) higher DOC consumption than their non-irradiated counterparts. Since the DOC concentration at the beginning of the biodegradation experiment was different in each treatment, we also calculated the fraction of DOC consumed in relation to the initial concentration. In the irradiated treatments, between 35.7 and 30.0% of the available DOC was utilized with no significant differences between treatments. The fraction of DOC consumed in the non-irradiated treatments (between 25.7 and 20.7%) was significantly lower (p < 0.05) than in the irradiated ones but with no significant differences between plastic types or controls.
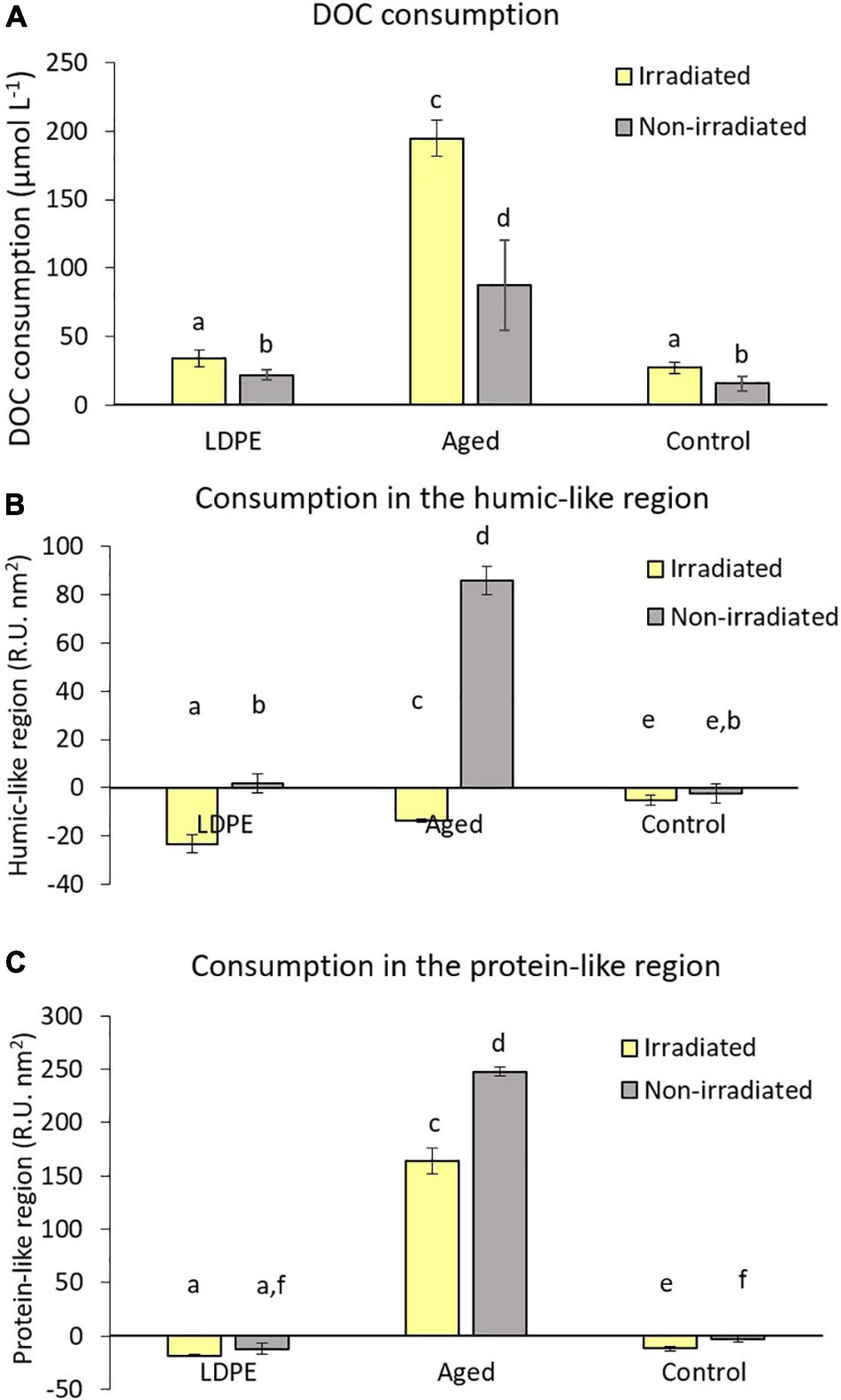
Figure 4. Net variation of dissolved organic matter leached by LDPE and aged plastic after 4 days of bacterial biodegradation, calculated as the difference between the initial and final time points for (A) dissolved organic carbon consumption; Integrated fluorescence of dissolved organic matter in the (B) humic-like region and (C) protein-like region. Positive values indicate consumption while negatives indicate production. The same letter over different bars indicate non-significant differences between them. Error bars represent the standard deviation of the mean of the triplicate cultures.
For the treatments with plastic, average bacterial growth efficiency (BGE) was 19 ± 10% while for the controls without plastic, it was 25 ± 8%. No significant differences were observed between both. Our BGE values are within the range of those found in coastal waters (Del Giorgio and Cole, 1998), but slightly higher than those found in similar experiments using a natural bacterial inoculum from the Adriatic Sea (Romera-Castillo et al., 2018). In the irradiated treatments, the average BGE was 15 ± 4%, not significantly different from the one observed in the non-irradiated treatments (27 ± 14%).
During the biodegradation phase, both irradiated plastic treatments showed a significantly (p < 0.05) higher production of FDOM in the humic-like region than the irradiated control without plastic with the highest value observed in the LDPE leachates (23.2 ± 3.7 R.U. ⋅ nm2) followed by the aged plastic leachates (13.6 ± 0.6 R.U. ⋅ nm2) (Figure 4B). The non-irradiated LDPE and control treatments did not show a significant change in the humic-like material over the biodegradation experiment. However, the non-irradiated aged plastic leachates experienced a notable decrease of 86.0 ± 5.8 R.U. ⋅ nm2, showing the consumption of compounds fluorescing in the humic-like region by the marine prokaryote community. We also normalized the FDOM values to the DOC concentration at the beginning of the biodegradation experiment and the consumption/production trends were the same than those before the normalization.
A production of FDOM in the protein-like region was observed in all the treatments except in those with the aged plastic leachates, where consumption took place instead (Figure 4C). The production of these compounds in the irradiated LDPE treatments (18.5 ± 1.0 R.U. ⋅ nm2) was significantly (p < 0.05) higher than in the irradiated control (11.5 ± 2.1 R.U. ⋅ nm2). However, although the non-irradiated LDPE leachates showed higher production (12.2 ± 5.3 2.45 ± 0.10 R.U. ⋅ nm2) than the non-irradiated control (2.7 ± 2.7 R.U. ⋅ nm2), the differences between them were not significant. The production of protein-like material was similar in both irradiated and non-irradiated LDPE plastic leachates while in the control without plastic, the production was significantly higher in the irradiated than in its non-irradiated counterpart. In the aged plastic leachates, the consumption of protein-like FDOM was higher (p < 0.05) in the non-irradiated treatments (248.0 ± 4.4 R.U. ⋅ nm2) than in the irradiated ones (164.0 ± 12.5 R.U. ⋅ nm2).
During the biodegradation experiment, the peak observed in the protein-like region (Ex:Em around 268 nm:352 nm) of the aged plastic leachates decreased in intensity (Figures 2C,D) by 0.042 R.U. in the irradiated treatment and by 0.060 R.U. in the non-irradiated one. For the peak centered at Ex:Em around 312 nm:362 nm, the consumption was 0.012 R.U. in the irradiated and 0.020 R.U. in the non-irradiated treatments. Consumption at the boundary between the protein- and humic-like regions was also observed centered at 305nm:395 nm with higher variation in the dark.
To further investigate the response of the bacterial community to the plastic leachates we used Bioorthogonal non-canonical amino acid tagging (BONCAT, Dieterich et al., 2006; Hatzenpichler et al., 2014; Leizeaga et al., 2017), which achieves the fluorescent labeling of proteins that have been synthesized during the incubation with a methionine surrogate, allowing the quantification of translationally active cells. The aged plastic leachates resulted in a 4–5-fold-increase in the number of active cells compared to the LDPE leachates and the control treatment at the end of the experiment (Figure 5A). However, no significant differences were found in the number of active cells between the irradiated and the non-irradiated treatments in the control or any of the plastics leachates (ANOVA test, p > 0.05). The number of active cells in the LDPE leachates was not significantly different to that in the control treatment at any of the sampled time-points.
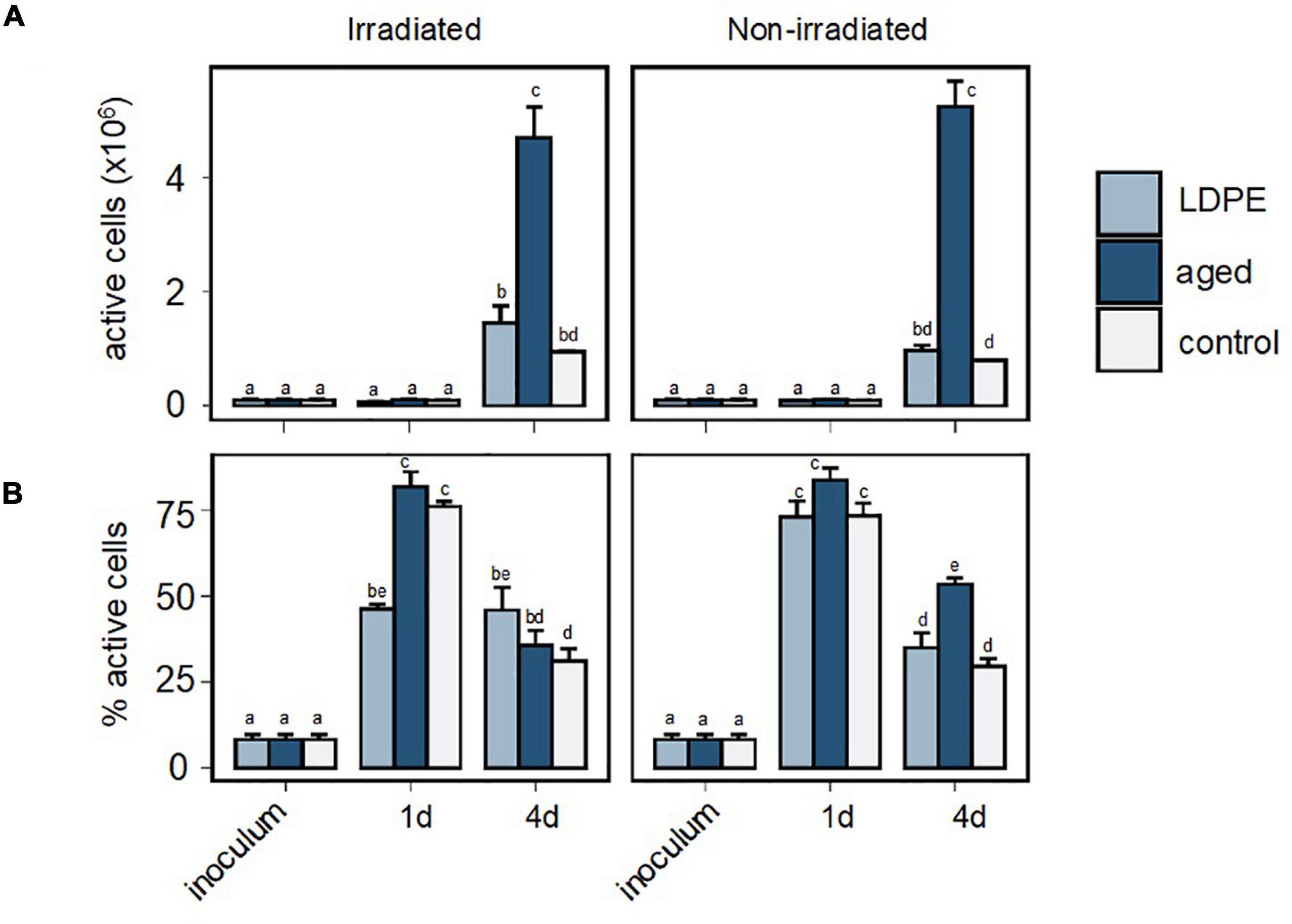
Figure 5. Bacterial single-cell activity estimated with BONCAT in the inoculum and during the incubation with plastic leachates on day 1 and 4. (A) Number of active cells in the incubations. (B) Proportion of active cells. Different letters indicate significant differences among treatments. Error bars represent the standard deviation of the mean of the triplicate cultures.
Although the number of active cells was low one day after bacterial inoculation, the increase in the proportion of active cells observed from the inoculum to the control and plastic treatments (Figure 5B) indicated that the prokaryotic cells were rapidly stimulated by the 1:9 dilution. Nonetheless, this stimulation was significantly lower in the irradiated LDPE leachates, where 50% of the cells were active, than in the control and aged plastic leachates, where ∼75% of the cells were active (Tukey’s post hoc test, p < 0.001). On day 4, the proportion of active cells significantly decreased in all treatments except in the irradiated LDPE leachates, where it barely changed. Furthermore, the proportion of active cells was lower in the irradiated aged plastic leachates than in the non-irradiated counterpart (p = 0.00006), whereas the opposite trend was observed with the LDPE leachates (p = 0.04).
Besides the decrease in the proportion of active cells, we also observed a drop in the single-cell translational activity of the individual cells in the aged plastic leachates on day 4, as evidenced by the fact that low activity cells accounted for most of the active pool of cells in both, irradiated and non-irradiated treatments (>75% of the cells, Figure 6). However, the proportion of low activity cells was significantly higher in the irradiated treatments than in the non-irradiated ones (p = 0.01). In contrast, medium and high activity cells represented roughly half of the active cells in the LDPE leachates and the control. These findings may indicate that in the aged-plastic leachates bacteria used all the biodegradable DOC faster, and the remaining DOC on day 4 (particularly in the irradiated treatment) was more resistant to utilization than the one in the LDPE leachates or the control treatment.
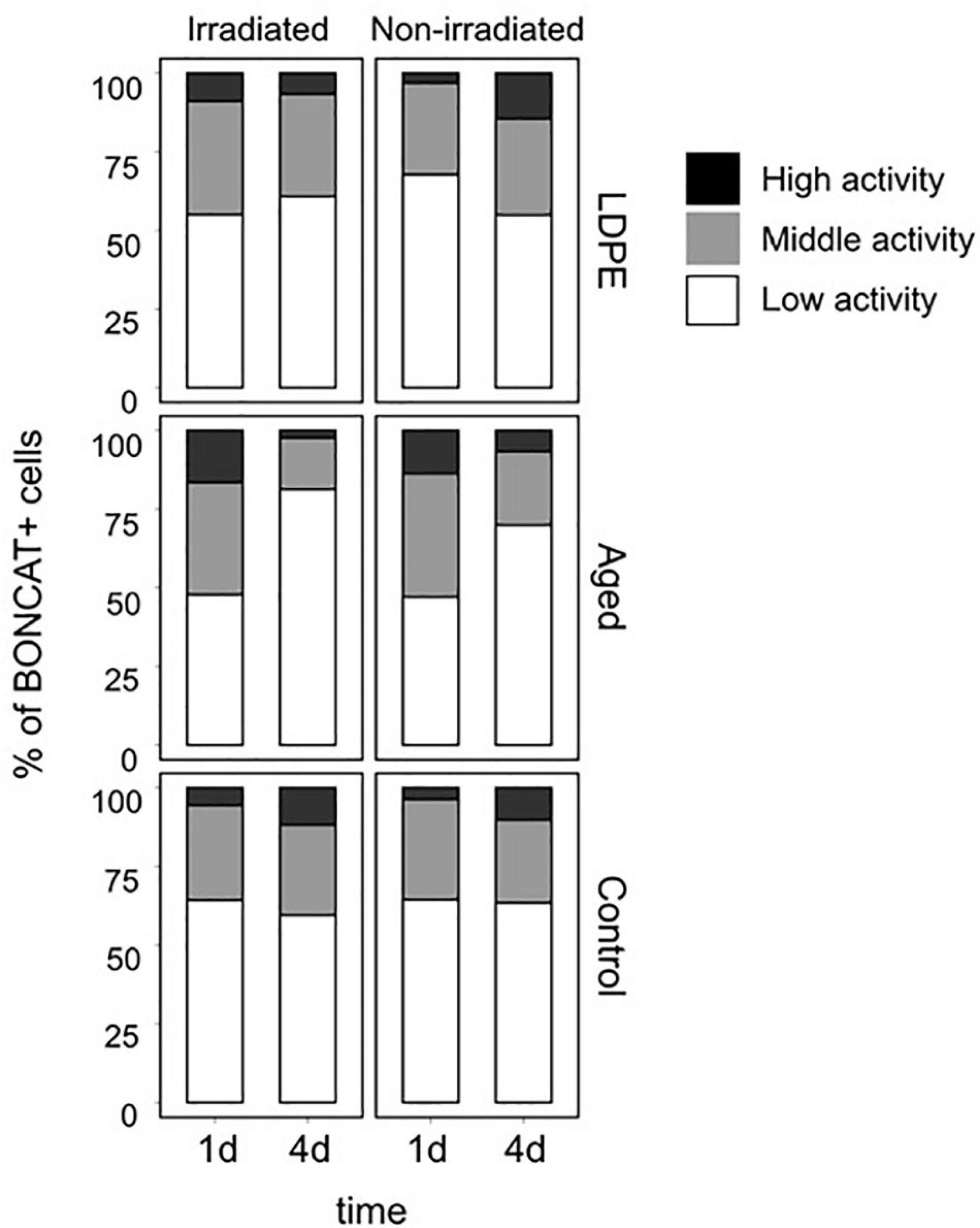
Figure 6. Distribution of activity in the pool of active cells. Active cells were categorized as having low, medium, or high activity based on their BONCAT signal intensity, as in Sebastián et al. (2019) (see Section “Materials and Methods”).
Discussion
Plastic Leaching of Dissolved Organic Matter
The amounts of DOC leached from LDPE were within the low range of those previously reported for the same plastic type (Romera-Castillo et al., 2018; Table 1). Similar experiments were performed in our lab, as part of another work, with virgin expanded polystyrene (EPS), virgin polystyrene (PS), and artificially aged LDPE (exactly the same used as virgin in this experiment, Table 1). The aged plastic collected on the sand beach leached an amount of DOC up to two order of magnitude higher than those virgin plastic types, including PE and PP which made the 95% of the aged mix composition. Finally, the DOC leaching from the artificially aged LDPE was three order of magnitude higher than the virgin LDPE. This indicates that aged plastic releases much more DOM than virgin plastic.
In our study, between 3.9 and 542 ppm of carbon was lost from plastic in form of DOC after 6 days of abiotic incubation. The highest loss corresponded to the irradiated aged plastic. The amounts of plastic carbon lost in form of DOC (225–542 ppm) in the aged plastic treatments were one order of magnitude higher than the previously reported for new plastic after 6 days of irradiation which showed a loss of 86.7 ppm for expanded polystyrene and 28 ppm for LDPE (Romera-Castillo et al., in press). However, these values were lower than those reported by Zhu et al. (2020) of 4.8 g kg–1 (ppt) for standard polyethylene and 68 ppt for EPS after 54 days of irradiation. This could be because the amount of DOC leached from plastic was found to increase exponentially with incubation time (Zhu et al., 2020). New plastic has been shown to leach out the highest DOC amount right after the plastic get in touch with the water (Romera-Castillo et al., 2018). However, in longer incubation experiments using post-consumed plastic, the leaching increased exponentially at different rates depending on the plastic type, but accelerating after 54 days of light exposure (Zhu et al., 2020). The chemical composition of the commercial plastic includes antioxidants and other additives to prevent or retard degradation. Most of those additives are not chemically bound and can easily migrate to the fluid (Hermabessiere et al., 2017). Cracks and fragmentation make accessible light and oxygen to internal layers accelerating the aging process (Luo et al., 2020). Therefore, after a long time exposure to UV radiation, the additives can be lost making plastic even more vulnerable to degradation and leaching. After certain point, the plastic could be constantly leaking chemical compounds. This agrees with a previous experiment using a mix of plastic collected in the North Pacific Subtropical Gyre (NPSG), presumably old, which showed a lineal increase of DOC leached with time of exposure to irradiation (Zhu et al., 2020). All this explains why our new plastic released much less amount of DOC than the aged plastic which had been exposed to radiation for longer than 54 days. The previous estimate of 23,600 metric tons of DOC released by plastic in the ocean every year (Romera-Castillo et al., 2018) could be markedly underestimated, as it was derived from experiments with virgin plastic. Considering that aged plastic leached 21.50 μg cm–2, which is 2.4 times the maximum leaching previously obtained with virgin plastics (Romera-Castillo et al., 2018), the amount of DOC released by plastic entering the ocean would increase up to 56,882 metric tons of DOC every year. The amount of plastic debris floating in the NPSG, one of the most polluted areas of the open ocean, is meant to be 96,400 metric tons (Eriksen et al., 2014). That plastic is known to be aged, since it takes about 1 year to arrive there from the closest coast (Lebreton et al., 2018) but they can be retained in the gyre for more than 3 years (Ebbesmeyer et al., 2007). Along that journey, it has been exposed to weathering factors such as sunlight radiation, oxidation and erosion. Therefore, the amount of DOC released per year from the plastic accumulated in that “garbage patch” is estimated to be, at least, 432 metric tons.
Here, the aged plastic collected on the beach released between 30 times more DOC, for irradiated, and 94 times more DOC, for non-irradiated treatments, than the commercial LDPE showing that sunlight radiation had more effect on the release of DOC from new than from aged plastic. The higher release of DOC after irradiation of plastic found here has been previously observed (Lee et al., 2020a). However, other studies reported contrasting results on plastic leaching (Suhrhoff and Scholz-Böttcher, 2016; Romera-Castillo et al., 2018). This difference could be due to the chemical composition of the material used, its degree of degradation and, maybe, the matrix where the plastic is releasing its compounds (fresh, brackish, or marine waters).
Possible compounds within plastic leachates could be the same oligomers from the polymer or additives (e.g., plasticizers or antioxidants). Some of these additives are known to present aromatic moieties that emit fluorescence, such as bisphenol A (BPA, Em/Em 220/309) and diethylhexyl phthalate (Em/Em 220/340, Lee et al., 2020b). Fluorescent additives would explain the FDOM observed in this work in both LDPE and aged plastics leachates, which was especially higher in the latter. Irradiated treatments showed lower values of leached FDOM than their non-irradiated counterparts both in the humic- and protein-like regions. This indicates that the FDOM leached from plastics was partially photodegraded during irradiation. The effect of irradiation on the FDOM was the opposite than on DOC which presented higher release in irradiated treatments. Therefore, DOM released by plastic under sunlight will have a higher proportion of less fluorescent material than that released in the dark. In the aged plastic leachates, the main fluorescing area was the protein-like region while in the humic-like region the intensity was lower. The ratio between the integrated fluorescence in the humic-like region of the irradiated aged plastic leachates vs. those from the non-irradiated aged plastic leachates was lower (0.34 ± 0.09) than the same ratio for the protein-like region (0.54 ± 0.09). This suggests that the compounds fluorescing in the humic-like region were more photo-labile than those in the protein-like area. This agrees with the fact that the UV radiation reaching the Earth surface starts at wavelengths > 295 nm. Since the protein-like fluorophores are usually excited bellow that value, they do not undergo a direct photochemical decomposition and the effect of radiation on them is lower.
Aged plastic leachates EEMs showed two characteristic peaks that were not present in the LDPE leachates. Those peaks presented an intensity about six times higher in the non-irradiated than in the irradiated treatments, indicating, again, that irradiation photodegraded them. There are two typical protein-like peaks commonly found in natural organic matter: peak-B (Ex:Em 275 nm:310 nm) and peak-T (275 nm:340 nm, Coble, 1996). The highest peak observed in the aged plastic leachates (Ex:Em about 268 nm:352 nm) is close to the peak-T, but shifted to longer emission wavelengths. In a previous experiment with expanded polyestyrene (EPS) leachates, a predominant peak was also found in the protein-like region but shifted to shorter excitation and emission wavelengths (Ex:Em ∼250 nm:306 nm, Romera-Castillo et al., in press). This peak was attributed to polycyclic aromatic hydrocarbons (PAHs) from plastic impurities or additives, since those compounds fluoresce in that region (Ferretto et al., 2014). A recent study using new grinded virgin polyvinyl chloride (PVC) and polystyrene plastics, but in artificial freshwater, observed two peaks also in the protein-like region but their Ex:Em maxima did not coincide with ours (Lee et al., 2020b; Lee et al., 2021), being ours shifted to longer emission wavelengths. Agostino et al. (2021) found two peaks in the protein-like region coinciding with peak-T and peak-B in leachates from PP in MQ water. However, the peak found here at Ex:Em 312nm:362 nm seems to not coincide with any other peak previously found in natural organic matter nor in leachates from new plastic. This indicates that the mix of aged plastics, which is the most representative plastic debris found in the ocean (e.g., Cózar et al., 2014), releases much more FDOM than virgin plastic and presents a unique fluorescence fingerprint. However, more research needs to be done to see if that signal is observed with other plastic samples.
In any case, according to this work and to similar studies mentioned above, it seems that plastic releases FDOM with a characteristic signal in the protein-like region. So, special care should be taken when sampling for DOM, and particularly for FDOM, in very polluted areas such as some regions of the Mediterranean Sea or some very polluted coastal areas, since plastic signal could mask natural FDOM.
Biodegradation of Plastic Leachates and Bacterial Activity
Bacterial abundance and single-cell activity in the LDPE treatments were similar to those in the control without plastic, with no significant differences among them. This contrasts with previous studies, which found a significantly higher bacterial abundance in LDPE treatments than in control without plastics (Romera-Castillo et al., 2018). On the other hand, DOC consumption was higher in those treatments where the plastic leaching was higher, which agrees with previous studies (Romera-Castillo et al., 2018), indicating that DOC leachates were labile to microbial uptake. This is supported by the observation of a significantly positive correlation between the bacterial abundance (p < 0.05; R2 = 0.97) and single-cell activity (p < 0.05; R2 = 0.76) at the end of the experiment with the amount of DOC leached, indicating that the prokaryotic response depended on the amount of DOC released. Since LDPE released a low amount of DOC in this experiment, it seems it was not high enough to provoke a big change in the bacterial abundance and single-cell activity regarding the control without plastic. The fluorescent fraction of the material leached by the aged plastic was also found to be bioavailable, as evidenced by a decrease of the DOM fluorescing in the protein-like region and in the humic-like region. This is also supported by the decrease of the two peaks observed in the EEMs of the aged plastic leachates, indicating a consumption during the bacterial incubation. All this shows that the fluorescent material released by aged plastic was labile to microbial uptake. The aged plastic was a mix of plastic types with a 65% of them being polyethylene, but remarkably, we did not observe those protein-like peaks in the LDPE treatment. Thus, probably, it was not the plastic type but the aging condition that made plastic release that labile fluorescent material.
Compounds fluorescing in the protein-like region were preferentially consumed by bacteria compared to those in the humic-like region. Coincidently, in natural DOM, protein-like compounds are also usually more labile than humic-like compounds (e.g., Lønborg et al., 2010; Cory and Kaplan, 2012). FDOM consumption was higher in the non-irradiated than in the irradiated treatments, but the opposite occurred for the DOC. This indicates that the DOM consumed by prokaryotes in the non-irradiated treatments was richer in fluorescent compounds than the DOM in the irradiated leachates. This could be due to the fact that fluorescent compounds were previously photodegraded in the irradiated treatments, so less amounts of FDOM were left there and prokaryotes used different compounds than in the treatments non-exposed to radiation. This shows that photodegradation makes the FDOM leached from aged plastic less labile to microbial uptake. For general DOC instead, photo-reactions could be rendering the non-fluorescent fraction of the DOM more labile to microbial uptake that would explain the higher consumption in the irradiated treatments. This agrees with the observation that sunlight can change the lability of natural DOM, turning the labile DOM into recalcitrant and making the recalcitrant DOM into labile (Obernosterer et al., 1999).
The presence of microplastics has been shown to stimulate marine microbial production of chromophoric DOM (CDOM) during a microcosm experiments containing microplastics and bacteria simultaneously (Galgani et al., 2018). In our study, in which marine bacteria were never in contact with plastic but only with the plastic leachates, we only observed a higher production of FDOM by bacteria, both in humic- and protein-like regions, in plastic leachates of the virgin LDPE treatments, especially in the irradiated samples. However, in the aged plastic leachates, only production of FDOM in the humic-like region of the irradiated treatments was observed. This could indicate that marine bacteria produce FDOM during the reworking of new virgin plastic leachates. It is likely that they also produce it during the biodegradation of the aged plastic leachates, but the consumption is probably masking the production.
Despite the amount of DOC released in the irradiated aged plastic was higher than in its non-irradiated counterpart, no significant differences were found in the number of active cells between both treatments. Looking at the quality of DOM, the protein-like FDOM to DOC ratio was higher in the non-irradiated aged plastic treatment than in the irradiated one, both at the beginning and at the end of the biodegradation experiments. The lack of differences in the number of active cells between both treatments may indicate that the response of the bacterial community is driven by the interplay between both quantity and quality of DOM. The drop in the percentage of active cells and their corresponding single cell-activity observed in all treatments on day 4 of the experiment suggests that a large fraction of the DOM amenable to utilization had been consumed by then (Figures 5B, 6) or, alternatively, that the bacterial community became limited by some micronutrient (i.e., vitamins or cofactors), as inorganic nutrients were added to prevent nutrient limitation. This drop in single-cell activity was more remarkable with the aged plastic leachates (and particularly in the irradiated treatment), where DOC consumption had been the highest compared to the other treatments. At the end of the biodegradation experiment, the remaining DOM in the irradiated aged plastic leachates presented a protein-like to DOC ratio significantly lower than the non-irradiated aged plastic leachates (0.38 ± 0.14 vs. 0.99 ± 0.13, respectively). This suggests that the remaining DOM could have a higher quality in the non-irradiated aged plastic treatment than in the irradiated one, which explains why bacterial cells in the non-irradiated aged plastic treatment displayed comparatively higher per cell activity. In any case, bacterial cells were remarkably stimulated by the aged plastic leachates, with the number of active cells increasing 5-fold relative to the control (Figure 5). This stimulation is similar in magnitude to the one observed with a long-term carbon starved dark ocean prokaryotic community upon fresh organic carbon inputs (Sebastián et al., 2019). Thus, these findings suggest that leachates of ocean plastic debris may have a strong impact on microbial metabolism.
Conclusion
Here we have seen that aged plastic releases much more DOC than virgin plastic into seawater. Such DOM presents a characteristic fluorescent signal in the protein-like region, which is photodegradable under the sunlight. The fluorescent material released by aged plastic was labile to microbial uptake, specially that fluorescing in the protein-like region. The aged plastic leachates showed a characteristic fluorescent signal at Ex:Em 312 nm:362 nm, which could potentially be used to track unseen plastic pollution in areas of the oceans that are heavily polluted. However, this signal could be masked by photodegradation and microbial processes. The single cell activity of marine bacteria was notably enhanced by the DOM released by aged plastic. Since plastic can last in the ocean for many years becoming old and smaller in size, most of the plastic that is found in the ocean is presumably aged and it is releasing more DOC into the ocean than previously thought, stimulating prokaryote activity. This means that DOM released by plastic has the capacity to alter the biogeochemistry of the carbon cycle in the ocean.
Data Availability Statement
The original contributions presented in the study are included in the article/Supplementary Material, further inquiries can be directed to the corresponding author.
Author Contributions
CR-C, MS, and XAÁ-S designed the research. CR-C and SB performed the experimental work. CR-C run the FDOM and bacterial abundance analyses and wrote the first draft of the manuscript. SB and MS performed the BONCAT analyses. XAÁ-S run the DOC samples. All authors made comments and amendments, and approved the final version.
Funding
This work and CR-C involvement was supported by ComFuturo Program from Fundación General CSIC and a JIN-2019 project (PID2019-109889RJ-I00) from the Spanish Ministry of Science and Innovation and the Agencia Estatal de Investigación. CR-C also thanks the support by the Spanish Ministry of Science and the Agencia Estatal de Investigación through the Thematic Network of Micro- and Nanoplastics in the Environment (RED2018-102345-T, EnviroPlaNet) as well as the support provided by ISDIN. MS was supported by MIAU (RTI2018-101025-B-I00) from the Spanish Ministry of Science and Innovation. SB was funded by the Erasmus+ Program.
Conflict of Interest
The authors declare that the research was conducted in the absence of any commercial or financial relationships that could be construed as a potential conflict of interest.
Publisher’s Note
All claims expressed in this article are solely those of the authors and do not necessarily represent those of their affiliated organizations, or those of the publisher, the editors and the reviewers. Any product that may be evaluated in this article, or claim that may be made by its manufacturer, is not guaranteed or endorsed by the publisher.
Acknowledgments
We acknowledges the “Severo Ochoa Centre of Excellence” accreditation (CEX2019-000928-S). We also thank Roberto Rosal García and Carlos Edo for the FTIR measurements of the sand beach plastic mix, Ariadna Martínez-Dios for provide the plastics from the sand beach in Lanzarote and Marola Lourdes Saá Yanez for lab assistance.
Supplementary Material
The Supplementary Material for this article can be found online at: https://www.frontiersin.org/articles/10.3389/fmars.2022.861557/full#supplementary-material
References
Agostino, A., Rao, N. R. H., Paul, S., Zhang, Z., Leslie, G., Le-Clech, P., et al. (2021). Polymer leachates emulate naturally derived fluorescent dissolved organic matter: understanding and managing sample container interferences. Water Res. 204:117614. doi: 10.1016/j.watres.2021.117614
Álvarez-Salgado, X. A., and Miller, A. E. J. (1998). Simultaneous determination of dissolved organic carbon and total dissolved nitrogen in seawater by high temperature catalytic oxidation: conditions for precise shipboard measurements. Mar. Chem. 62, 325–333. doi: 10.1016/s0304-4203(98)00037-1
Chen, Q., Reisser, J., Cunsolo, S., Kwadijk, C., Kotterman, M., Proietti, M., et al. (2018). Pollutants in plastics within the North Pacific subtropical gyre. Environ. Sci. Technol. 52, 446–456. doi: 10.1021/acs.est.7b04682
Coble, P. G. (1996). Characterization of marine and terrestrial DOM in seawater using excitation-emission matrix spectroscopy. Mar. Chem. 51, 325–346. doi: 10.1016/0304-4203(95)00062-3
Cory, R. M., and Kaplan, L. A. (2012). Biological lability of streamwater fluorescent dissolved organic matter. Limnol. Oceanogr. 57, 1347–1360. doi: 10.1038/nature08580
Cózar, A., Echevarría, F., González-Gordillo, J. I., Irigoien, X., Úbeda, B., Hernández-León, S., et al. (2014). Plastic debris in the open ocean. Proc. Natl. Acad. Sci. U.S.A. 111, 10239–10244. doi: 10.1073/pnas.1314705111
Del Giorgio, P. A., and Cole, J. J. (1998). Bacterial growth efficiency in natural aquatic systems. Annu. Rev. Ecol. Syst. 29, 503–541.
Dieterich, D. C., Link, A. J., Graumann, J., Tirrell, D. A., and Schuman, E. M. (2006). Selective identification of newly synthesized proteins in mammalian cells using bioorthogonal noncanonical amino acid tagging (BONCAT). Proc. Natl. Acad. Sci. U.S.A. 103, 9482–9487. doi: 10.1073/pnas.0601637103
Dubaish, F., and Liebezeit, G. (2013). Suspended microplastics and black carbon particles in the jade system, Southern North Sea. Water Air Soil Pollut. 224:1352.
Ebbesmeyer, C. C., Ingraham, W. J., Royer, T. C., and Grosch, C. E. (2007). Tub toys orbit the Pacific subarctic gyre. EOS 88, 1–4.
Eriksen, M., Lebreton, L. C. M., Carson, H. S., Thiel, M., Moore, C. J., Borerro, J. C., et al. (2014). Plastic pollution in the world’s oceans: more than 5 trillion plastic pieces weighing over 250,000 tons Afloat at Sea. PLoS One 9:e111913. doi: 10.1371/journal.pone.0111913
Ferretto, N., Tedetti, M., Guigue, C., Mounier, S., Redon, R., and Goutx, M. (2014). Identification and quantification of known polycyclic aromatic hydrocarbons and pesticides in complex mixtures using fluorescence excitation–emission matrices and parallel factor analysis. Chemosphere 107, 344–353. doi: 10.1016/j.chemosphere.2013.12.087
Galgani, L., Engel, A., Rossi, C., Donati, A., and Loiselle, S. A. (2018). Polystyrene microplastics increase microbial release of marine chromophoric dissolved organic matter in microcosm experiments. Sci. Rep. 8:14635. doi: 10.1038/s41598-018-32805-4
Gasol, J. M., and Del Giorgio, P. A. (2000). Using flow cytometry for counting natural planktonic bacteria and understanding the structure of planktonic bacterial communities. Sci. Mar. 64, 197–224. doi: 10.3989/scimar.2000.64n2197
Gurjar, U. R., Xavier, K. A. M., Shukla, S. P., Jaiswar, A. K., Deshmukhe, G., and Nayak, B. B. (2022). Microplastic pollution in coastal ecosystem off Mumbai coast, India. Chemosphere 288:132484. doi: 10.1016/j.chemosphere.2021.132484
Hatzenpichler, R., Scheller, S., Tavormina, P. L., Babin, B. M., Tirrell, D. A., and Orphan, V. J. (2014). In situ visualization of newly synthesized proteins in environmental microbes using amino acid tagging and click chemistry. Environ. Microbiol. 16, 2568–2590. doi: 10.1111/1462-2920.12436
Hermabessiere, L., Dehaut, A., Paul-Pont, I., Lacroix, C., Jezequel, R., Soudant, P., et al. (2017). Occurrence and effects of plastic additives on marine environments and organisms: a review. Chemosphere 182, 781–793. doi: 10.1016/j.chemosphere.2017.05.096
Jambeck, J. R., Geyer, R., Wilcox, C., Siegler, T. R., Perryman, M., Andrady, A., et al. (2015). Plastic waste inputs from land into the ocean. Science 347, 768–771. doi: 10.1126/science.1260352
Lawaetz, A. J., and Stedmon, C. A. (2009). Fluorescence intensity calibration using the Raman scatter peak of water. Appl. Spectrosc. 63, 936–940. doi: 10.1366/000370209788964548
Lebreton, L., Slat, B., Ferrari, F., Sainte-Rose, B., Aitken, J., Marthouse, R., et al. (2018). Evidence that the Great Pacific Garbage Patch is rapidly accumulating plastic. Sci. Rep. 8:4666. doi: 10.1038/s41598-018-22939-w
Lee, S., and Fuhrman, J. A. (1987). Relationships between biovolume and biomass of naturally derived marine bacterioplankton. Appl. Environ. Microbiol. 53, 1298–1303. doi: 10.1128/aem.53.6.1298-1303.1987
Lee, Y. K., Hong, S., and Hur, J. (2021). A fluorescence indicator for source discrimination between microplastic-derived dissolved organic matter and aquatic natural organic matter. Water Res. 207:117833. doi: 10.1016/j.watres.2021.117833
Lee, Y. K., Romera-Castillo, C., Hong, S., and Hur, J. (2020a). Characteristics of microplastic polymer-derived dissolved organic matter and its potential as a disinfection byproduct precursor. Water Res. 175:115678. doi: 10.1016/j.watres.2020.115678
Lee, Y. K., Murphy, K. R., and Hur, J. (2020b). Fluorescence signatures of dissolved organic matter leached from microplastics: polymers and additives. Environ. Sci. Technol. 54, 11905–11914. doi: 10.1021/acs.est.0c00942
Leizeaga, A., Estrany, M., Forn, I., and Sebastián, M. (2017). Using click-chemistry for visualizing in situ changes of translational activity in planktonic marine bacteria. Front. Microbiol. 8:2360. doi: 10.3389/fmicb.2017.02360
Lønborg, C., Álvarez-Salgado, X. A., Davidson, K., Martínez-García, S., and Teira, E. (2010). Assessing the microbial bioavailability and degradation rate constants of dissolved organic matter by fluorescence spectroscopy in the coastal upwelling system of the Ría de Vigo. Mar. Chem. 119, 121–129.
Luo, H., Zhao, Y., Li, Y., Xiang, Y., He, D., and Pan, X. (2020). Aging of microplastics affects their surface properties, thermal decomposition, additives leaching and interactions in simulated fluids. Sci. Total Environ. 714:136862. doi: 10.1016/j.scitotenv.2020.136862
Murphy, K. R., Stedmon, C. A., Graeber, D., and Bro, R. (2013). Fluorescence spectroscopy and multi-way techniques. PARAFAC. Anal. Methods 5, 6557–6566.
Obernosterer, I., Reitner, B., and Herndl, G. J. (1999). Contrasting effects of solar radiation on dissolved organic matter and its bioavailability to marine bacterioplankton. Limnol. Oceanogr. 44, 1645–1654.
Pérez-Hernández, D. M., Hernández-Guerra, A., Fraile-Nuez, E., Comas-Rodríguez, I., Benítez-Barrios, V. M., Domínguez-Yanes, J. F., et al. (2013). The source of the canary current in fall 2009. J. Geophys. Res. Oceans 118, 2874–2891. doi: 10.1002/jgrc.20227
Plastic Europe (2021). Plastic Europe. Available Online at: https://plasticseurope.org/wp-content/uploads/2021/12/Plastics-the-Facts-2021-web-final.pdf
Romera-Castillo, C., Mallenco-Fornies, R., Saá-Yánez, M., and Alvarez-Salgado, X. A. (in press). Leaching and bioavailability of dissolved organic matter from petrol-based and biodegradable plastics. Mar. Environ. Res. doi: 10.1016/j.marenvres.2022.105607
Romera-Castillo, C., Pinto, M., Langer, T. M., Álvarez-Salgado, X. A., and Herndl, G. J. (2018). Dissolved organic carbon leaching from plastics stimulates microbial activity in the ocean. Nat. Commun. 9:1430. doi: 10.1038/s41467-018-03798-5
Sebastián, M., Estrany, M., Ruiz-González, C., Forn, I., Sala, M. M., Gasol, J. M., et al. (2019). High growth potential of long-term starved deep ocean opportunistic heterotrophic bacteria. Front. Microbiol. 10:760. doi: 10.3389/fmicb.2019.00760
Stedmon, C., and Nelson, N. B. (2015). “The optical properties of DOM in the ocean,” in Biogeochemistry of Marine Dissolved Organic Matter, 2nd Edn, eds D. A. Hansell and C. A. Carlson (Amsterdam: Elsevier), 481–508. doi: 10.1016/b978-0-12-405940-5.00010-8
Stedmon, C. A., and Bro, R. (2008). Characterizing dissolved organic matter fluorescence with parallel factor analysis: a tutorial. Limnol. Oceanogr. Methods 6, 572–579. doi: 10.4319/lom.2008.6.572b
Stedmon, C. A., Markager, S., and Bro, R. (2003). Tracing dissolved organic matter in aquatic environments using a new approach to fluorescence spectroscopy. Mar. Chem. 82, 239–254. doi: 10.1021/acs.est.8b02648
Suhrhoff, T. J., and Scholz-Böttcher, B. M. (2016). Qualitative impact of salinity, UV radiation and turbulence on leaching of organic plastic additives from four common plastics — a lab experiment. Mar. Pollut. Bull. 102, 84–94. doi: 10.1016/j.marpolbul.2015.11.054
Tetu, S. G., Sarker, I., Schrameyer, V., Pickford, R., Elbourne, L. D. H., Moore, L. R., et al. (2019). Plastic leachates impair growth and oxygen production in Prochlorococcus, the ocean’s most abundant photosynthetic bacteria. Commun. Biol. 2:184. doi: 10.1038/s42003-019-0410-x
Wesch, C., Bredimus, K., Paulus, M., and Klein, R. (2016). Towards the suitable monitoring of ingestion of microplastics by marine biota: a review. Environ. Pollut. 218, 1200–1208. doi: 10.1016/j.envpol.2016.08.076
Keywords: plastic leaching, FDOM, DOM, aged microplastics, BONCAT
Citation: Romera-Castillo C, Birnstiel S, Álvarez-Salgado XA and Sebastián M (2022) Aged Plastic Leaching of Dissolved Organic Matter Is Two Orders of Magnitude Higher Than Virgin Plastic Leading to a Strong Uplift in Marine Microbial Activity. Front. Mar. Sci. 9:861557. doi: 10.3389/fmars.2022.861557
Received: 24 January 2022; Accepted: 21 February 2022;
Published: 31 March 2022.
Edited by:
Meilian Chen, Guangdong Technion-Israel Institute of Technology, ChinaCopyright © 2022 Romera-Castillo, Birnstiel, Álvarez-Salgado and Sebastián. This is an open-access article distributed under the terms of the Creative Commons Attribution License (CC BY). The use, distribution or reproduction in other forums is permitted, provided the original author(s) and the copyright owner(s) are credited and that the original publication in this journal is cited, in accordance with accepted academic practice. No use, distribution or reproduction is permitted which does not comply with these terms.
*Correspondence: Cristina Romera-Castillo, Y3Jpc3JjQGljbS5jc2ljLmVz