- 1Department of Arctic Biology, University Centre in Svalbard, Longyearbyen, Norway
- 2Faculty of Biosciences and Aquaculture, Nord University, Bodø, Norway
- 3Shirshov Institute of Oceanology, Department of Marine Ecology, Russian Academy of Sciences, Moscow, Russia
- 4Department of Arctic and Marine Biology, Faculty for Bioscience, Fisheries and Economy, UiT The Arctic University of Norway, Tromsø, Norway
The calanoid copepod Calanus glacialis dominates the mesozooplankton biomass in the Arctic shelf seas, but its smaller North Atlantic sibling Calanus finmarchicus is expanding northwards and may potentially replace it if the climate continues to warm. Here we studied the population structure, overwintering strategies, gonad maturation and egg production of C. glacialis and C. finmarchicus over a period of 15 consecutive months in a high-Arctic fjord with sub-Arctic ocean climate and no sea ice formation in winter. The relative proportions of C. glacialis and C. finmarchicus varied throughout the study period, but with an overall dominance of C. glacialis. The overwintering population of C. glacialis was dominated by copepodite stage CIV (74%) while C. finmarchicus overwintered mainly as CV (65%), reflecting a primarily two- and one-year life cycle, respectively. Adult males and females of C. glacialis appeared as early as October with a peak during December-January, two months earlier than in C. finmarchicus, with a corresponding one-month earlier peak in recruitment for C. glacialis. While C. glacialis reproduced prior to the bloom with egg production peaking during the bloom, C. finmarchicus started egg laying during the bloom and continued to reproduce throughout the summer. Seasonal changes in the population structure suggest that C. finmarchicus born early in spring are able to develop to CV during summer and overwinter successfully, while offspring born later in the season do most likely not reach the CV overwintering stage. The ability to reproduce early and the flexibility to alter between 1- and 2-year life cycles give C. glacialis an advantage over C. finmarchicus in high-Arctic unpredictable environments with short-pulsed primary production regimes. Our data indicate that C. glacialis and C. finmarchicus occupy similar environmental niches, but different timing in reproduction reduces the competition. If sea temperatures remain within their temperature-tolerance ranges, both C. glacialis and C. finmarchicus seem to benefit from warming due to accelerating growth and higher survival of the recruits as long as C. glacialis has access to a colder refuge by descending to deeper depths.
1 Introduction
Calanoid copepods of the genus Calanus dominate (>60%) the mesozooplankton biomass in Arctic and sub-Arctic seas (Jaschnov, 1970; Kosobokova et al., 1998 ; Blachowiak-Samolyk et al., 2008; Kosobokova and Hirche, 2009; Kosobokova et al., 2011; Kosobokova and Pertsova, 2018) and constitute the key link between primary producers and higher trophic levels (Falk-Petersen et al., 2007). Calanus are filter feeders, primarily feeding on microscopic algae, but do also consume alternative prey such as eggs, fungi and heterotrophic microorganisms outside the peak productive spring and summer season (Cleary et al., 2017; Frank-Gopolos et al., 2017). At high latitudes, the spring phytoplankton bloom lasts from two to eight weeks (reviewed by Leu et al., 2015). During this short period, these relatively large copepods convert their food to energy-rich lipids which may comprise up to 70% of their dry weight (Sargent and Falk-Petersen, 1988; Lee et al., 2006). Thus, Calanus is a major contributor to the rapid and efficient transfer of energy and essential fatty acids from primary producers to higher trophic levels in the lipid driven arctic marine food web (Falk-Petersen et al., 1990; Dahl et al., 2003).
Three species of the genus Calanus co-occur in the European Arctic: the two arctic species Calanus hyperboreus and C. glacialis, and the boreal C. finmarchicus (Conover, 1988; Choquet et al., 2017). These three species are all morphologically similar but differ in body sizes (Kwasniewski et al., 2003; Choquet et al., 2018). All three perform seasonal migrations, descending to deeper depths towards the end of the summer to spend the unproductive winter in a state of dormancy (diapause) (Falk-Petersen et al., 2009). The three species have different core distribution areas and have evolved different life strategies in response to the physical and biological environment they are exposed to. The smallest of the three species, the boreal C. finmarchicus, has a 1-year life cycle in the northernmost part of its distribution range and is considered to be primarily an income breeder, i.e. fueling egg production by ingested food (Plourde and Runge, 1993; Madsen et al., 2001; Kjellerup et al., 2012). The mid-sized species, the arctic C. glacialis, inhabits the Arctic Ocean with highest abundance confined at present to the outer shelf and slope regions (Ershova et al., 2021). Calanus glacialis has a 1–2-year life cycle and performs both income breeding and capital breeding, i.e. it fuels egg production using both ingested food and internal lipid resources (Hirche and Kattner, 1993; Kosobokova, 1999; Madsen et al., 2001). The largest arctic oceanic species C. hyperboreus has adapted to the extreme conditions of the oligotrophic Arctic Ocean with a life strategy where reproduction is not as tightly coupled with the spring bloom as in the two smaller ones. Calanus hyperboreus starts reproduction at depth in winter purely fueled by internal resources (Hirche, 1997), and the life cycle can be as long as 4 years depending on the extremeness of the environment (Hirche and Niehoff, 1996; Ashjian et al., 2003; Varpe et al., 2009; Ejsmond et al., 2018). All the three species develop through six naupliar (NI-NVI) stages, followed by six copepodite stages (CI-CVI), of which stage CV develops into either adult male (CVI, AM) or adult female (CVI, AF). Adult males of Calanus have a short life span compared to females (Kosobokova, 1999; Hatlebakk et al., 2019), thus the timing of male presence, and consequent mating, is an important and sensitive aspect in the Calanus life cycle (Kosobokova, 1998; Kosobokova, 1999; Daase et al., 2018). Females on the other hand are long-lived and females of C. hyperboreus and C. glacialis may even survive one more winter and reproduce again (Hirche and Niehoff, 1996; Kosobokova, 1999). To develop female gonads is energy demanding. Jónasdóttir (1999) estimated that C. finmarchicus required 50% of its stored lipids (wax ester and triacylglycerol) to ripen the gonads. To be ready for descent and overwintering, Calanus need to reach a copepodite stage capable of storing a sufficient amount of lipids that allows them to successfully overwinter and fuel molting and/or maturation at the end of winter (Falk-Petersen et al., 2009; Bailey, 2010). Calanus hyperboreus can overwinter already as CIII, C. glacialis primarily as CIV, and C. finmarchicus primarily as CV (Hirche, 1997; Kosobokova, 1999; Falk-Petersen et al., 2009; Daase et al., 2013; Kosobokova and Pertsova, 2018).
Calanus finmarchicus and C. glacialis are regarded as important climate beacons in the Svalbard-Barents Sea region (Wassmann et al., 2015). In regions of sympatry, a high proportion of C. finmarchicus over C. glacialis indicates a strong influence of Atlantic water and a relatively warm climate, often with sea temperatures above zero year-round (Conover, 1988). The predominance of C. glacialis, on the other hand, is usually found in regions characterized by a colder arctic regime encompassing a seasonal ice cover and sub-zero water temperatures (Arnkværn et al., 2005). In regions where C. glacialis and C. finmarchicus co-exist, it has been suggested that extensive hybridization occurs (Parent et al., 2012). However, recent studies show that this is very unlikely (Choquet et al., 2017), even when adult males and females of both species are present at the same time (Choquet et al., 2021). Calanus finmarchicus is considered to be a boreal expatriate in the Arctic (Hirche and Kosobokova, 2007; Kosobokova, 2012; Wassmann et al., 2015). It is regularly transported in high numbers into the Arctic with the Atlantic currents, but the late onset of the phytoplankton spring bloom is assumed to limit its ability to mature and reproduce in the Arctic Ocean proper, which prevents it from establishing its own local arctic population (Hirche and Kosobokova, 2007).
Typically, the onset of the productive season is delayed with increasing latitude due to the more extreme seasonality of sunlight and more persistent ice and snow cover preventing light from reaching the water column (Sakshaug, 2004; Falk-Petersen et al., 2007; Leu et al., 2011; Leu et al., 2015). In the seasonally ice-covered waters, the productive season starts with a bloom of ice algae which are adapted to utilize low light levels early in the season (Hancke et al., 2018; Kvernvik et al., 2020). As the ice melts, stratification and increasing light availability in the water column triggers the main pelagic bloom. Warming of the Arctic and the consequential decrease in ice cover is changing the arctic bloom phenology (Carmack and Wassmann, 2006; Leu et al., 2015; Tremblay et al., 2015). This has potentially cascading effects on the distribution of Calanus copepods, as their reproduction and life cycles are closely linked to the timing of the algal bloom and the length of the productive season (Søreide et al., 2010; Wold et al., 2011; Daase et al., 2013; Dezutter et al., 2019).
Recently, a substantial borealization of the arctic zooplankton community has been predicted with global warming (Hays et al., 2005; Polyakov et al., 2020). Higher sea water temperatures, reduction in sea ice and a prolonged algal growth season will make the environment more favorable for C. finmarchicus in the high Arctic (Freer et al., 2021). While there is currently no conclusive evidence that C. finmarchicus successfully recruit new generations locally in the high Arctic (Hirche and Kosobokova, 2007; Dvoretsky, 2011; Wassmann et al., 2015; Choquet et al., 2017), there are indications of accelerated development at the northern edge of its current distributional range in the Fram Strait (Tarling et al., 2021) and north of Svalbard (Hop et al., 2021), potentially leading to the production of a second generation in warmer years (Weydmann et al., 2018). In some Arctic regions, such as the areas and fjords surrounding the path of the North Atlantic Current into the Fram Strait and the Barents Sea, an increase in abundances of C. finmarchicus has recently been observed (Wassmann et al., 2006; Chust et al., 2014; Weydmann et al., 2014; Aarflot et al., 2018; Hop et al., 2019; Møller and Nielsen, 2020). The latter indicates not only increased advection but also suggests better survival and successful recruitment of C. finmarchicus in areas where C. glacialis and/or C. hyperboreus normally prevail. Subsequently, one may expect that C. finmarchicus could become the more dominant species if warming continues. A change from a dominance of larger arctic zooplankton species to smaller boreal ones may have cascading effects on the higher trophic levels, especially those species (e.g. little auks) preying on one item at the time (Kitaysky and Golubova, 2000; Hirche and Kosobokova, 2007; Falk-Petersen et al., 2007; Vihtakari et al., 2018).
For most parts of the Arctic, seasonal zooplankton studies are still rare due to logistical challenges of accessing remote areas regularly over an entire annual cycle, and previous studies describing the seasonal cycle in the Calanus community in Svalbard fjords largely lack data from the polar night (Arnkværn et al., 2005; Søreide et al., 2010; Lischka and Hagen, 2016). Here, we investigated the population development of two sympatric Calanus species, C. glacialis and C. finmarchicus, over a period of 15 consecutive months, including the polar night, to study similarities and dissimilarities in their life histories in a high-Arctic fjord (78°N) characterized by sub-Arctic water temperatures (~1-6°C) with no sea ice formation in winter. To gain a better understanding of these two species life histories, we also studied the gonad maturation in C. glacialis and C. finmarchicus. Specifically, we compared the following between the two species (1) population size and structure, (2) timing of the seasonal vertical migration and (3) timing of the reproductive events in terms of sexual maturation and egg production.
2 Materials and Methods
2.1 Sampling Area and Sample Processing
Calanus glacialis and C. finmarchicus were collected once a month from June 2015 to August 2016 at Karlskronadjupet (78°19’N; 015°10’E) (IsK, Figure 1), a 274 m deep basin in the central part of the Isfjorden system, Svalbard. The mouth of Isfjorden is open towards the shelf and slope area facing west Spitsbergen, with no sill limiting the inflow of Atlantic and Arctic water reaching this area via the West Spitsbergen Current (WSC) and the East Spitsbergen Current (ESC), respectively. Both these currents are flowing from south to north along the shelf break and on the shelf, respectively (Nilsen et al., 2008).
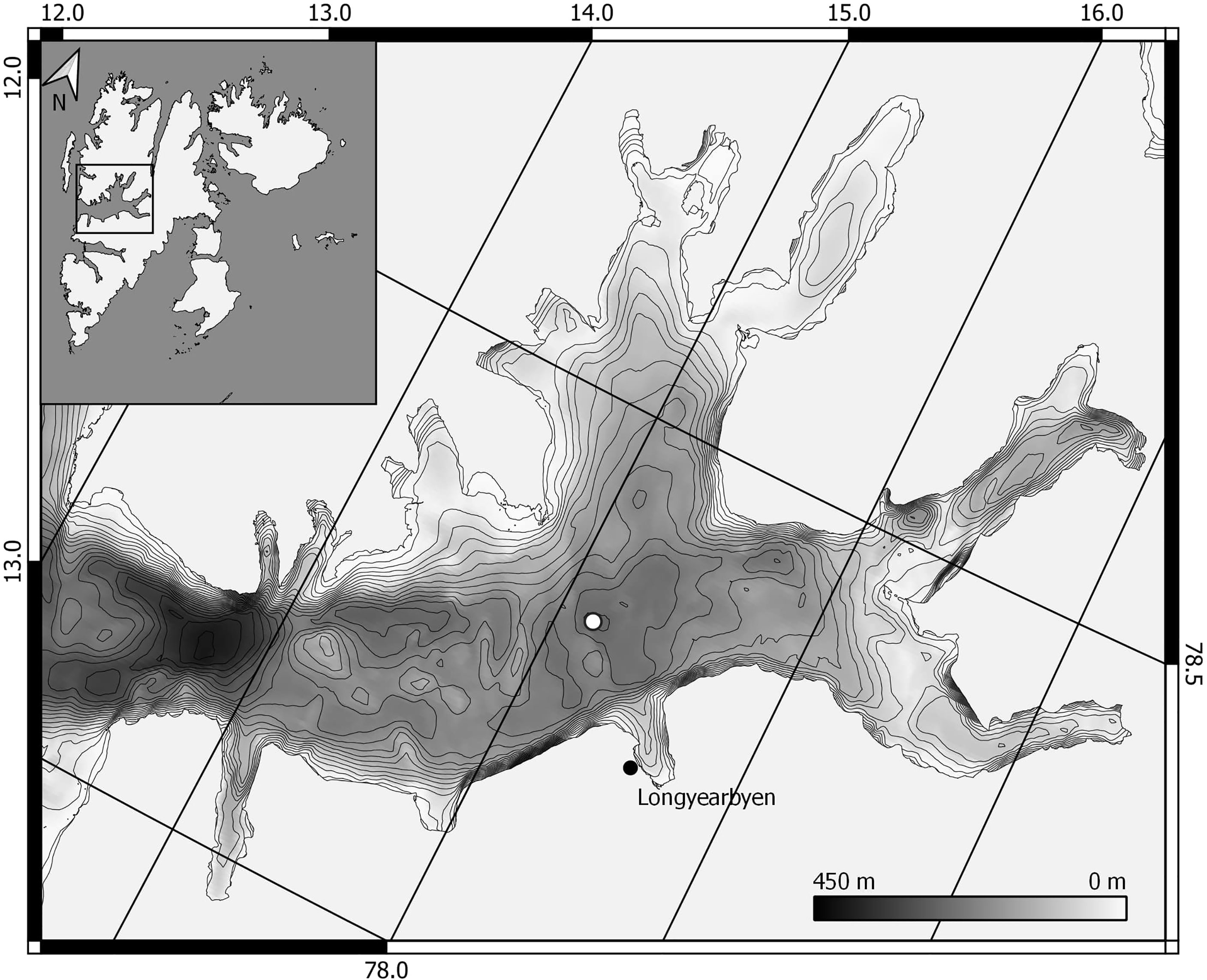
Figure 1 Map of the study area. Sampling station at Karlskronadjupet Isfjorden, Svalbard marked with white marker. (Map data source: NPI, GEBCO).
Sampling was conducted from either larger vessels (R/V Helmer Hanssen, K/V Svalbard, R/V Dalnie Zelentsy) or smaller boats (R/V Viking Explorer and UNIS Polaris). Data on the properties of the water column were collected using a SAIV SD204 CTD or a SBE Seabird Electronics CTD. The water mass definition by Skogseth et al. (2020) was used to identify the different water masses throughout the study period. Isfjorden is influenced by both Arctic and Atlantic water coming into the fjord, as well as local hydro-physical processes. In summer, the water column is typically stratified, with fresher surface water overlaying a layer of Atlantic-influenced water, and often a layer of colder local or winter cooled water at the bottom. In the fall and winter, vertical mixing takes place, leading to a homogenous water column gradually cooling off throughout the winter and spring (Nilsen et al., 2008; Skogseth et al., 2020). Fluorescence data from fluorometers attached to the CTDs were used to estimate the average Chlorophyll a (Chl a) concentration in the depth intervals where zooplankton was sampled (see below). Fluorescence data from the sensors were verified with Chl a concentrations measured fluorometrically. Water was collected for Chl a at standard depths (1 m, 5 m, 15 m, 50 m, bottom) and the depth of Chl a max. The water was filtered on Whatman grade GF/F glass fiber filters and extracted in methanol for 24 hours at 4°C before the Chl a concentration was measured with an AU10 Turner Fluorometer (Turner Design, Inc.).
Stratified zooplankton samples for Calanus community composition were collected with a MultiNet “Midi” (0.25 m2 mouth opening, mesh size 200 µm, Hydro-Bios, Kiel) or a WP2 closing net (0.25 m2 mouth opening, mesh size 200 µm, Hydro-Bios, Kiel) and fixed in 4% buffered formaldehyde-seawater solution. Samples were collected at the following depth intervals bottom-200-100-50-20-0 m when using the MultiNet, and bottom-100-50-20-0 when using the WP2 closing net (Supplementary, Table S1). In June 2015, the deepest stratum was not collected due to technical problems. Females for egg production incubations were collected from 50-0 m using a WP2 or WP3 net (1 m2 mouth opening, mesh size 1000 µm, Hydro-Bios, Kiel).
2.2 Morphological Identification of Calanus spp.
Due to the close morphological similarity of the Calanus species, they are commonly identified to species using size classes (for prosome length) derived from length frequency analyses. Recent studies using molecular tools have shown, however, a high but regionally variable overlap in prosome length between C. finmarchicus and C. glacialis (e.g., Gabrielsen et al., 2012; Choquet et al., 2018). In the present study, species of Calanus were distinguished based on size classes established earlier by Daase and Eiane (2007) for the study area with adjustments for CV and adult females after Choquet et al. (2018) and Daase et al. (2018) (Table 1). For live specimens, pigmentation can also be used as an additional species indicator, with C. glacialis having more red pigmentation on the antennules and genital segment (females only) compared to C. finmarchicus (Nielsen et al., 2014; Choquet et al., 2018). The latter characteristic was additionally used to identify adult females for egg production incubations (see below).

Table 1 Size ranges (prosome length, mm) used to differentiate between copepodite stages CI-CV and adult females and males (AF/AM) of C. finmarchicus and C. glacialis based on Daase and Eiane (2007) with adjustments for CV and adults after Choquet et al. (2018) and Daase et al. (2018).
It is difficult to distinguish nauplii to species level morphologically (e.g., Daase et al., 2011), therefore they were not included in our analysis.
2.3 Calanus Population Structure
Seasonal changes in the Calanus spp. abundance and stage structure were estimated from the formalin preserved samples. The samples were drained, rinsed and then soaked in filtered sea water to wash out the formalin. Large plankters such as chaetognaths, amphipods and gelatinous taxa were removed from the entire sample using forceps to not interfere with the subsequent subsampling. Subsamples of 2-5 ml were taken from a known total volume of the remaining sample using a micropipette with enlarged opening and analyzed under a stereo microscope. All Calanus spp. copepodites in the subsample were enumerated and identified morphologically to species and development stage. Subsamples were taken until a minimum of 100 Calanus individuals had been counted.
Abundance was estimated from the subsamples following equation 1:
Where ai is the number of individuals per m3 of species a, si is the total number of individuals in the subsamples, Vsi is the subsample volume, Vti is total volume of the sample, and di is the sampled distance in depth stratum i in meters. Because abundance in m3 is influenced by the depth at a given station, the integrated abundance (Ai) was calculated for better comparison to other locations, following equation 2:
Where n is the number of depth strata.
Weighted mean depth (WMD) was calculated for the total population of C. glacialis and C. finmarchicus as well as copepodite stages within the species according to equation 3:
Where Di is average depth in depth stratum i. Further the standard deviation (SD) of WMD was calculated following equation 4:
Results are reported as mean ± SD.
2.4 Gonad Maturation
The gonad maturation of Calanus CVs and adults (CVIs) was examined using formalin-preserved zooplankton samples. Up to thirty specimens of adult females, males and CVs were randomly picked from samples for each date of collection between September 2015 and July 2016. They were stained with 2% borax carmine solution (Tande and Hopkins, 1981), dehydrated and stored in glycerine for further gonad examination. The gonadal stages (GS) of females were assessed under a stereomicroscope (Olympus SZX-10) according to the classification scheme suggested by Kosobokova (1999). Six stages of gonad maturation (GS1 – GS6, Table 2) were distinguished. The gonads of CVs were also examined to discriminate between sexually undifferentiated specimens and potential females/males, according to Kosobokova (1998; 1999). In total, gonad maturation was examined in ca. 3000 specimens of Calanus spp. The prosome length (PL) of each examined individual was measured to nearest 0.1 mm.
2.5 Egg Production
Monthly from February to August, up to 30 adult females of C. glacialis and 30 C. finmarchicus were sorted from WP2 and/or WP3 net samples collected from the upper 50 m. Females were incubated individually in 125 ml incubation chambers equipped with a false bottom of mesh size 300 to 500 µm that allowed eggs to sink through, separating them from the female to avoid predation (Basedow and Tande, 2006). Incubations started within four hours of sampling and lasted for 24 hours in darkness at in situ temperatures specified in Table 3. After 24 hours of the female incubation, the eggs were removed, transferred to a petri dish and counted under a stereo microscope in a cold lab (4-6°C). The eggs were cultivated in petri dishes in darkness at in situ temperatures for eight more days. After eight days, the number of nauplii and unhatched eggs were counted to determine the egg hatching success. Eggs which had not hatched after eight days were considered to be non-viable (Daase et al., 2011).
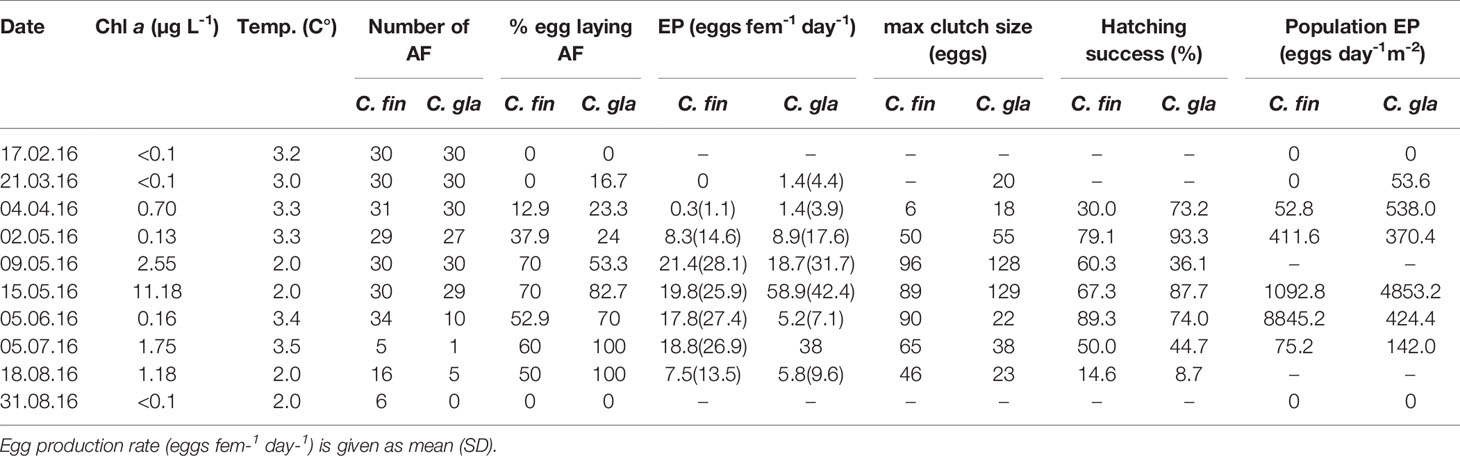
Table 3 Egg production and hatching success for C. glacialis (C. gla) and C. finmarchicus (C. fin) in Isfjorden from February to August 2016.
3 Results
3.1 Environmental Conditions
3.1.1 Hydrography
At the sampling site in Isfjorden proper (Station IsK, 78°N, 015°E, Figure 1), positive water temperatures were measured year-round, except in June and July 2015 at depths below 160 m. From August 2015, the intermediate layers were increasingly influenced by Transformed Atlantic Water (TAW) throughout the fall as evident by the prevailing positive temperatures and relatively high salinity (Figures 2 and S1). A pronounced influx of Atlantic Water was observed in December 2015, detectable as a distinct layer of + 5°C and increased salinity at around 100 m depth (Figures 2A, B). The warm (1-3°C) TAW prevailed in the entire water column until the end of the sampling campaign in August 2016. Water temperature did, however, gradually decrease during winter and spring due to atmospheric cooling and winter convection. Highest water temperatures were measured in the upper 10-20 m in summer, with monthly maximum between 5.1 and 10.6°C in June-August 2015 and between 4.5 and 6.3°C in June-August 2016 (Figure 2A). This is typical for surface water in summer, together with low salinity during the same period with minimum of 29.7 to 33.91 in June-August 2015 and 31.6 to 33.9 in June-August 2016 (Figure 2B, Figure S1).
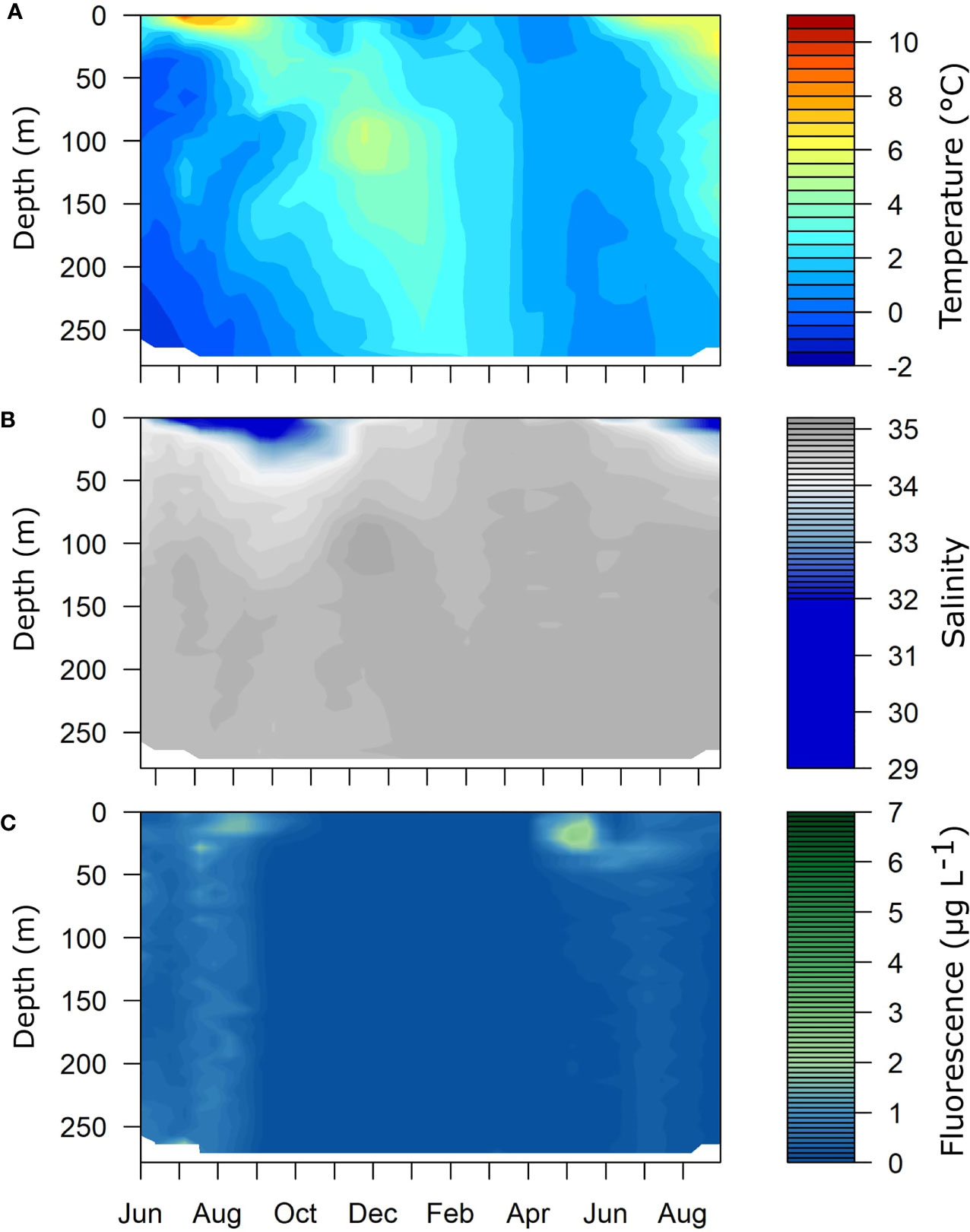
Figure 2 Seasonal changes in (A) water temperature (°C), (B) salinity and (C) fluorescence (µg L-1) in Isfjorden, station IsK, from June 2015 to August 2016.
3.1.2 Chlorophyll a
In Isfjorden, the spring bloom had subsided at the start of the sampling campaign in June 2015 with Chl a concentration of 1.5 µg Chl a L-1. Relatively high Chl a concentrations persisted throughout July and August with 2.2 and 2.4 µg Chl a L-1, respectively (Figure 2C). From October 2015 to April 2016 typically low winter Chl a concentrations of < 0.01 µg Chl a L-1 prevailed with an increase first recorded in early May to 2.1 µg Chl a L-1, and a peak between mid-May and early June when Chl a concentrations were >7.0 µg Chl a L-1. In July, values of 2.1 µg Chl a L-1 were similar to observations from the previous summer, dropping to 0.73 µg Chl a L-1 in late August (Figure 2C).
3.2 Calanus Population Dynamics
We observed large seasonal variability in Calanus abundances. For both species, abundance was particularly low during the winter-spring transition from March to May, followed by peaks in summer and autumn (Figure 3). The relative proportions of C. glacialis and C. finmarchicus varied throughout the study period, but with an overall dominance of C. glacialis, which was 0.8 to 9.0 times the abundance of C. finmarchicus (Figure 3). The strong increase in abundance of both species in September likely resulted from sampling bias, as strong winds and currents caused the nets to go oblique rather than vertical.
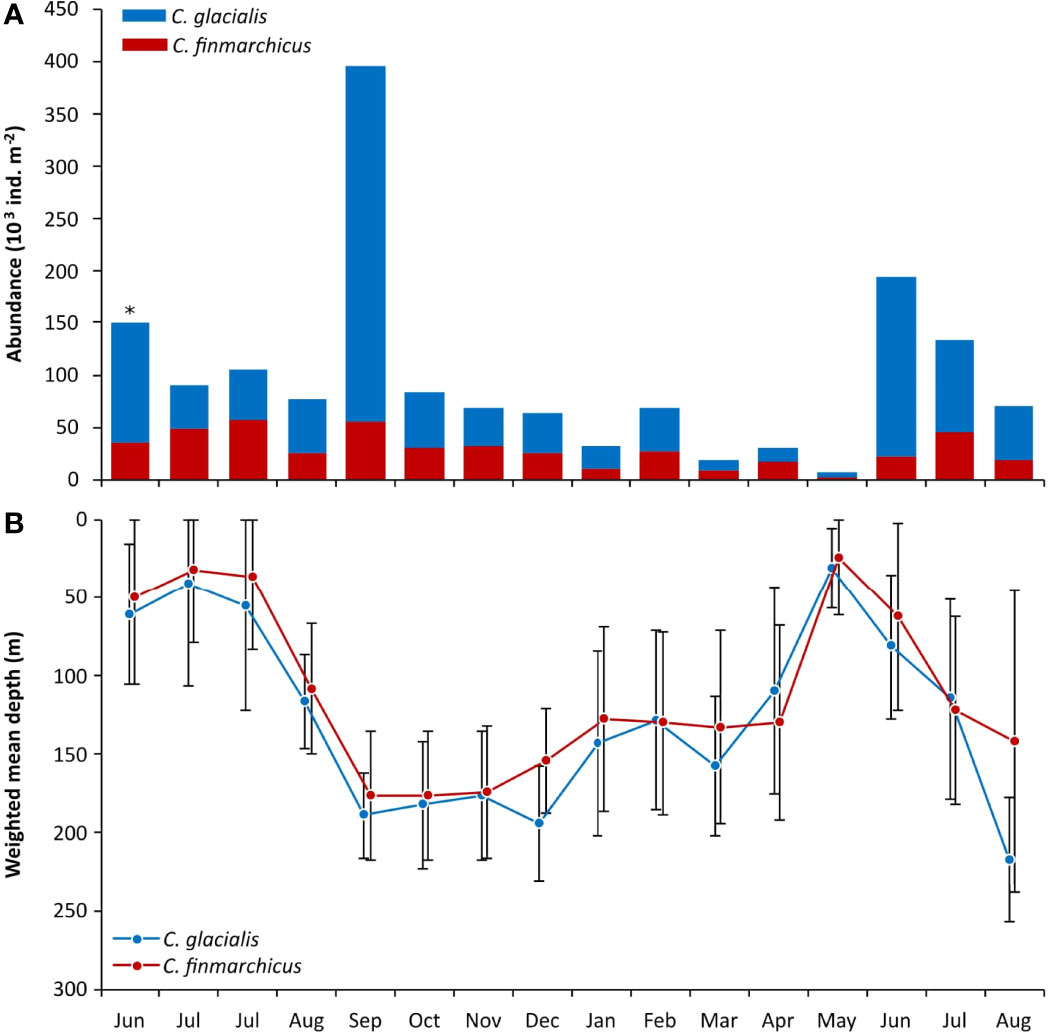
Figure 3 Monthly changes in the (A) total abundance (ind. m-2) and (B) weighted mean depth of C finmarchicus (red) and C glacialis (blue) at Karlskronadjupet, Isfjorden from June 2015 to August 2016. * In June 2015 sampling below 180 meters was not possible due to technical issues.
Both species performed pronounced seasonal vertical migration with comparable monthly weighted mean depth (WMD) for most months except August 2016 (Figure 3), when C. glacialis was found deeper in the water column than C. finmarchicus. From August to January, both species remained deep (WMD > 175 m) until a gradual ascent was observed from January to March (WMD ~125 m). By the onset of the spring bloom in May, both populations were concentrated in the upper 50 m (Figure 3).
From August to April, the C. glacialis population was dominated by CIVs (74%), followed by CVs (17%). Adult females were always present but became more abundant from December. Adult males were observed in low numbers (12 ind. m-2) from October and increased to 812 ind. m-2 in December (Figure 4 and Table 4).
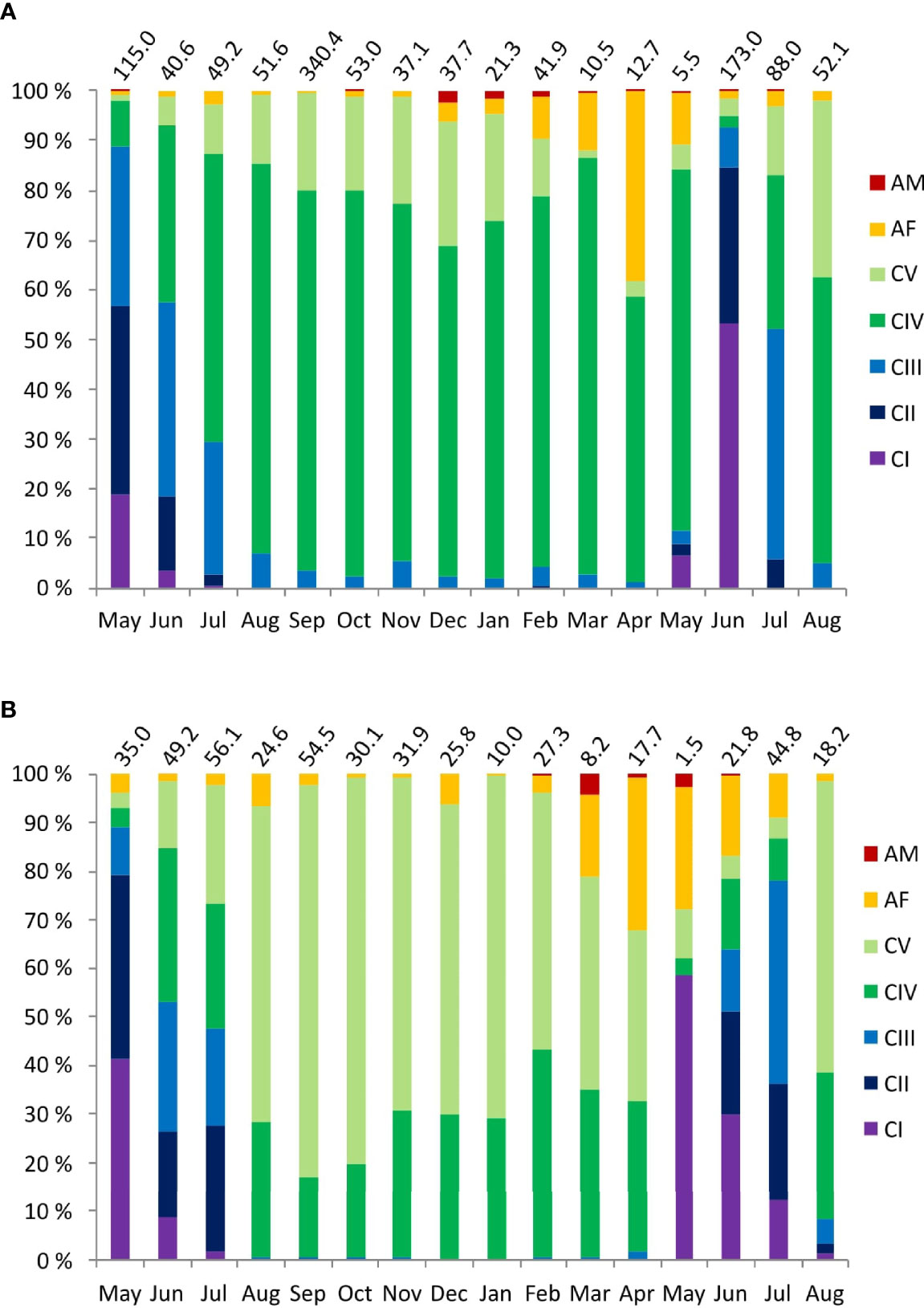
Figure 4 Monthly changes in the relative copepodite stage composition of (A) C glacialis and (B) C finmarchicus at Karlskronadjupet, Isfjorden from June 2015 to August 2016. Total abundance (ind. m2) each month is noted on top of each bar.
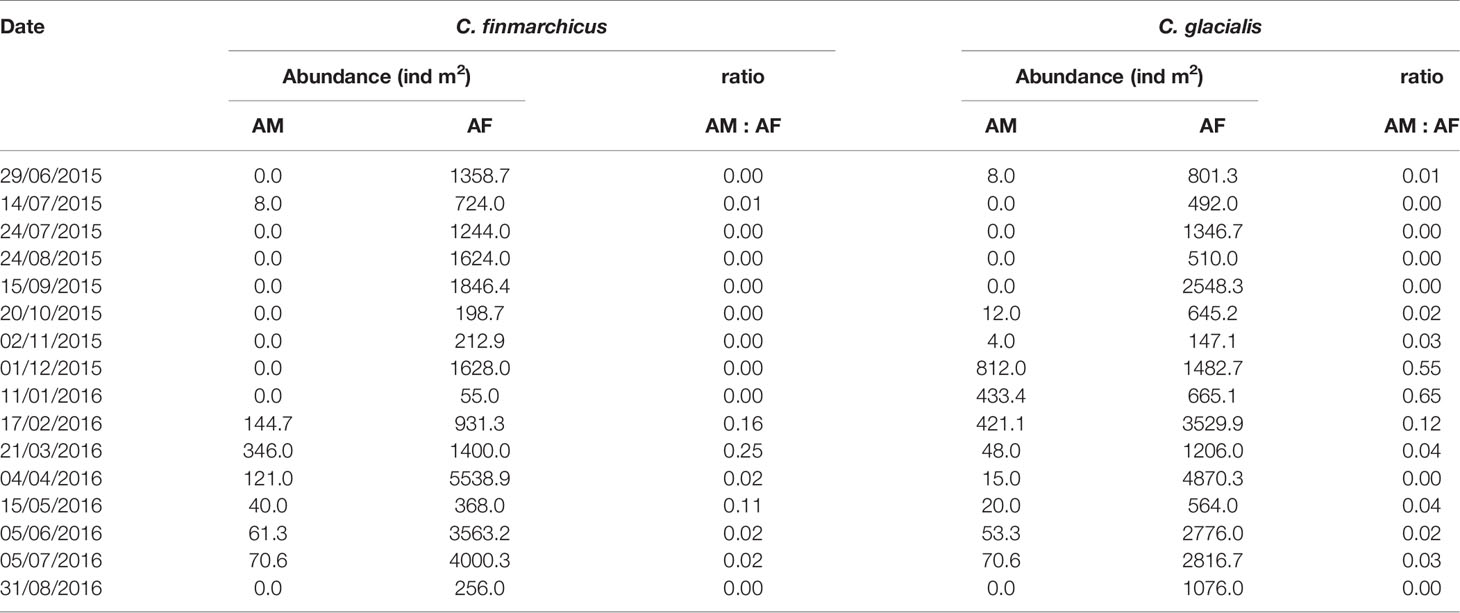
Table 4 Seasonal variations in abundance of adult males (AM) and adult females (AF) and sex ratio (males:females) of C. finmarchicus and C. glacialis adults.
The overwintering population of C. finmarchicus was dominated by CV (65%), followed by CIV (29%). For C. finmarchicus, numbers of adult females and males started to increase in February, two months later than for C. glacialis (Table 4). Females peaked in abundance in May. The C. finmarchicus population was dominated by young copepodite stages CI-CIII in May to July, and from August overwintering stages CIV (29%) and CV (68%) prevailed (Figure 5B).
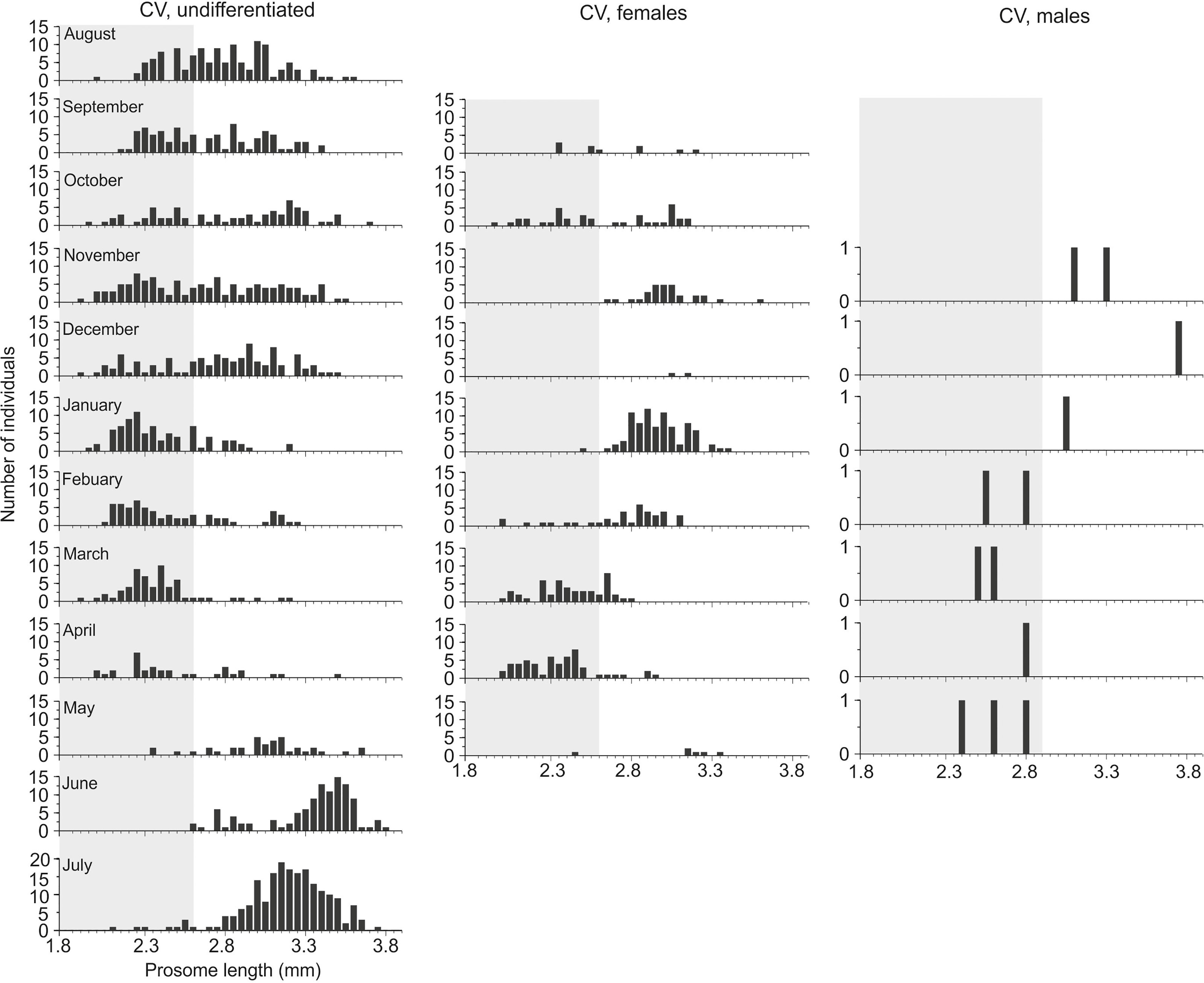
Figure 5 Size structure (prosome length, mm) and maturity stages of Calanus copepodite stage V in Isfjorden from August 2015 to August 2016. The size range of C. finmarchicus CV is highlighted in gray.
The sex ratio (males:females) in the adult Calanus population varied strongly throughout the year. For C. glacialis, the maximum sex ratio of 0.12-0.65 was observed between December and February, for C. finmarchicus it was at maximum of 0.11-0.25 between February and May (Table 4). For the rest of the year the proportion of males was low or males were completely absent.
Ontogenetic differences in WMD of the different developmental stages were observed. In January, females and males of C. glacialis were primarily found in the upper 100 m (WMD = 51 ± 48 m and 107 ± 65 m, respectively) while CIVs were concentrated below 100 m (WMD = 148 ± 51 m) (Supplementary Figure S1C). The abundance of young copepodite stages CI-CIII of C. glacialis was highest in June and July and they were concentrated in the upper 50 m (Supplementary Figure S2). By August the population was composed mainly by the overwintering stages CIV and CV concentrated at depth (WMD = 217 ± 40 m) (Figure 4A).
3.3 Egg Production
Females of C. glacialis commenced egg production in March at a rate of 1.4 ± 4.4 eggs female-1 day-1 and reached peak egg production during the spring bloom in mid-May with individual rate of 58.9 ± 42.4 eggs female-1 day-1, and total egg production of the surface (50-0 m) population of 4853.6 eggs day-1 m-2 (Table 3). By June, the egg production had declined to 5.8 ± 9.6 eggs female-1 day-1, along with a distinct decrease of the number of females in the population (Table 3).
Calanus finmarchicus commenced egg production in early April at a rate of 0.3 ± 1.1 eggs female-1 day-1, with a clear increase in early May to 8.3 ± 14.6 eggs female-1 day-1. From May to July, the egg production rate was stable at around 20 eggs female-1 day-1, then decreased to 7.5 ± 13.5 eggs female-1 day-1 in mid-August and ceased by the end of August. Because of the high female abundance in June, the total egg production of the C. finmarchicus female population peaked in June at 8845.2 eggs day-1 m-2 (Table 3).
3.4 Gonad Maturation
3.4.1 Gonad Development in Calanus CVs
The Calanus CV specimens with sexually undifferentiated gonads were present in Isfjorden all year round, while CVs with sexually differentiated gonads, i.e. potential females (PF) or males (PM) (Kosobokova, 1998), occurred only during particular parts of the year (Figure 5). A bimodal distribution of the CV size frequency was observed and revealed that individuals ≥2.6 mm developed to PF between November and January, while those <2.6 mm developed to PF first in February-March when CVs >2.6 mm had disappeared (Figure 5). Only few CVs developing into PM were observed, with the largest ones ≥2.9 mm appearing first (between November and January), and the smaller ones <2.9 mm appearing later, from February to May (Figure 5).
The pattern above suggests that the small-sized CVs most likely represent C. finmarchicus while the largest CVs represent C. glacialis, but the size delimitation for PF and PM is different, 2.6 and 2.9 mm, respectively. If we apply this revised size delimitation to discriminate between C. finmarchicus and C. glacialis, one can see that C. glacialis CVs with sexually undifferentiated gonads were present in relatively high numbers from May to December (Figure 5), and in very low numbers from February to April. The gonad transition to PF and PM in CVs started in September. C. glacialis CVs with PF gonads peaked between November and February, at the same time as C. glacialis CVs with PM gonads (PL 2.9-3.3 mm) appeared (Figure 5).
The C. finmarchicus CVs with undifferentiated gonads were present in Isfjorden from August to May, but, in contrast to C. glacialis, they were almost absent during June and July 2015 (Figure 5). A few CVs with PF gonads were observed in September-October 2015 and reappeared in February-April 2016. PM of C. finmarchicus CV (PL <2.9 mm) were observed after PM of C. glacialis CV from February to May 2016. The overall abundance of Calanus CV males was, unfortunately, too low to reliably establish size range of potential CV males in any of the two species.
3.4.2 Gonad Development in Calanus Adults
With the new size categories applied above to differentiate between two Calanus species, we analyzed the gonad maturation data for adult females (AF). The female specimens with PL ≥ 2.6 mm (assumed to be C. glacialis) were present in Isfjorden year-round (Figure 6). From June to February, their gonads were in an immature state (GS1). The development of oocytes in the ovaries (GS2) began in February, and by April, almost all AFs were in advanced maturation state ready to spawn (GS3). From June to August, gradual gonad degradation took place in C. glacialis AF indicating termination of spawning. The GS4 females were followed by completely spent GS5 females present between June and January (Figure 6).
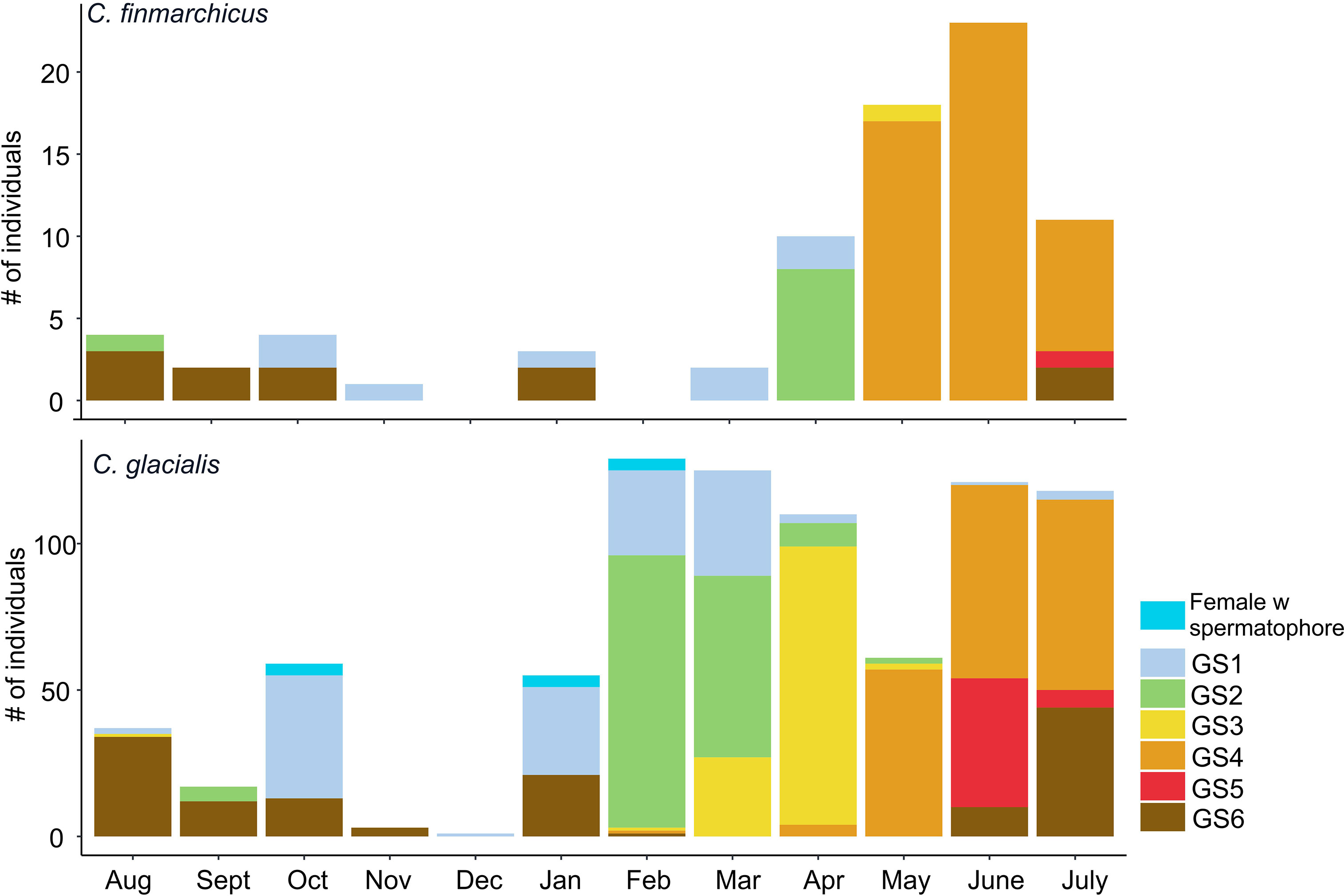
Figure 6 Seasonal changes in gonad maturation of adult females of Calanus in Isfjorden from August 2015 to August 2016. For description of gonadal stages (GS1-GS6) see Table 2.
The GS1 adult females in the size range of C. finmarchicus (<2.6 mm) first appeared in March, in a good agreement with dynamics of the gonad development in C. finmarchicus CVs (see above). In April, the gonads of approximately half of C. finmarchicus AFs passed to the next stage (GS2), and through May, June and July most AFs had mature gonads of GS3. This period corresponds well to the main reproductive season of C. finmarchicus revealed from the egg production experiments. Between August and October abundance of AFs gradually decreased, and all C. finmarchicus AFs had spent gonads (GS5). Only very few C. finmarchicus adult females were observed in the population between October and March.
Calanus adult males were present already from October (Figure 4) which was also confirmed by the observation of females with spermatophores (Figure 6). Largest adult males (PL 2.9-3.85 mm) peaked in January and February in continuation of the presence of the largest CV males (Figure 5). Later, in April and June, the smaller adult males were observed (PL 2.65-3.3 mm, presumably, C. finmarchicus) following the cohort of the smaller CV males.
4 Discussion
4.1 Seasonal Migration and Overwintering
The stage composition of the overwintering Calanus populations, mainly CV for C. finmarchicus and CIV for C. glacialis, suggests 1 and 2-year life cycles in these species, respectively, which is in agreement with observations from other Svalbard fjords and elsewhere in the Arctic (e.g., Conover, 1988; Kosobokova, 1999; Madsen et al., 2001; Ashjian et al., 2003; Arnkværn et al., 2005; Hirche and Kosobokova, 2007; Pertsova and Kosobokova, 2010; Kosobokova, 2012; Kosobokova and Pertsova, 2018). A sharp decrease in Calanus abundance in late winter/early spring indicates high winter mortality, likely due to depletion of lipid reserves at the end of winter when energy storages are used up for metabolism, molting and maturation while food is not yet available (Daase et al., 2014; Berge et al., 2020; Daase and Søreide, 2021). In C. glacialis, the proportion of CVs diminishes over winter, as they develop to adults, which is in accordance with the observed changes in the gonad development of CVs (Figure 5) and the increase in the proportion of AFs and AMs in the population in December and January (Figure 6). Calanus glacialis adult males were only present in high numbers for a short period. They appeared in low numbers in October, peaked in abundance in December to February (Table 4), but disappeared soon, presumably, after mating (Daase et al., 2018; Hatlebakk et al., 2019). In contrast, adult C. glacialis females were present in the population throughout the entire year (Figure 4); their overall abundance was highest between February and July. They were less abundant between July to January suggesting senescence of some spent females after termination of spawning (Kosobokova, 1999).
While the overwintering population of C. finmarchicus was dominated by CVs, almost 30% were comprised by CIVs. The presence of the overwintering CIVs has also been observed in the C. finmarchicus populations in the NE Norwegian Sea (e.g., Arashkevich et al., 2004) where CIVs were suggested to be either late-born Generation 1 (G1) of C. finmarchicus, or potentially Generation 2 (G2). Arashkevich et al. (2004) reported that in the Norwegian Sea the overwintering population (G0) can reproduce in March (G1), complete development and reproduce by the end of summer (G2). The offspring of G2 only manage to develop to CIV before overwintering (Arashkevich et al., 2004). While it is relatively easy to identify the G0 and G1 generations from the population structure data, it is challenging to identify a possible G2 generation (Aksnes and Blindheim, 1996). Advection in particular complicates the identification of the new generations. Calanus finmarchicus CIVs could potentially have been transported to Isfjorden with the influx of Transformed Atlantic Water in December 2015. If these CIVs remained in the upper 600 m in the Norwegian Sea in autumn, as observed by Basedow et al. (2018) in 2014, they were exposed to the Atlantic currents continuously moving northwards (Basedow et al., 2018). However, no significant increase in C. finmarchicus population numbers appeared in conjunction with the advective event in December. Recent molecular studies have highlighted the difficulties to distinguish between C. finmarchicus and C. glacialis which challenge our traditional view of how these two species are distributed geographically (Choquet et al., 2017; Choquet et al., 2018). It is therefore likely that some of the CIVs in our study were misidentified C. glacialis CIVs (e.g., Gabrielsen et al., 2012; Choquet et al., 2017) masking the species-specific differences in the population structure and the life cycle duration of these two species in Isfjorden.
The seasonal vertical migration patterns were remarkably similar for the two species. For the Calanus population as a whole, the deepest WMD was registered from September through November. At this time of the year also the lowest metabolic enzyme activity has been observed (Freese et al., 2017; Hatlebakk et al., this issue), indicating this to be the period when diapause is reaching its deepest phase. By January, CVs and adult specimens of the overwintering stock seem to become more active, migrating further up in the water column (Supplementary Figure S3). Such a seasonal ascent of adult females and males in January (Supplementary Figure S4) was likely related to mating at this time of the year which is in accordance with earlier observation in Svalbard fjords (Freese et al., 2015; Daase et al., 2018). In early May, during the spring bloom both Calanus populations were concentrated in the upper 50 m where the algal food was plentiful (Figures 3 and S2–S4).
In August 2016, at the end of our study period, the WMD of C. glacialis was deeper than that of C. finmarchicus, indicating that the former descends earlier to the overwintering depth compared to the latter. C. glacialis reduces its metabolism in the absence of food (Morata and Søreide, 2015; Freese et al., 2017), and older copepodite stages CIV-CVI leave the surface layer if temperatures become too high seeking refuge in the colder, deeper waters (Niehoff and Hirche, 2005; Pertsova and Kosobokova, 2010). The earlier descent of C. glacialis observed during the present study is most likely also a response to reduced food availability and/or high temperature (Figure 2). Our temporal sampling resolution was too coarse to pinpoint the exact difference in the timing of the two species seasonal descent. In future studies, higher sampling frequency around the time of seasonal migration, particularly the descent, which seems to be more synchronized than the seasonal ascent (Kosobokova and Pertsova, 2005; Bailey, 2010), are recommended. Changes in the population structures can happen rapidly (< 1 month) during times with high biological activities and thus species-specific differences could easily have been missed in our study with monthly sampling resolution.
4.2 Gonad Maturation, Reproduction, and Recruitment
The simultaneous presence of late copepodites CIV-V of both Calanus species during winter and the presence of different generations of C. glacialis during summer months (Kosobokova, 1999) makes it difficult to reliably distinguish the two species and their new and overwintered generations based on seasonal changes of the population age structure alone. The experimental evidence that females of at least C. glacialis are long living and can lay eggs for up to 10 months when fed (Hirche, 1989; Kosobokova, 1999) further complicates our ability to determine life cycle length without additional tools. The examination of the gonad maturation state in copepods is a tool that provides better insights into copepods life history and reproductive biology as well as an assessment of the life spans in the late phases of their life cycles (Tande and Hopkins, 1981; Tande et al., 1985; Norrbin, 1991; Kosobokova, 1998; Kosobokova, 1999).
Our observations of gonad maturation patterns in C. glacialis and C. finmarchicus indicate that these two species utilize different reproductive strategies, differing in timing of: 1) sexual gonad differentiation in pre- adult stage CV, 2) molting of the overwintering CVs to adults, 3) gonad maturation in adults, and 4) mating and spawning. Sex differentiation in C. glacialis CVs, revealed from the gonad morphology, took place predominantly during fall and winter and terminated by the onset of the primary production period. Most C. glacialis CVs developed to potential females during winter, between November and February (Figure 5), as indicated by the almost complete disappearance of CVs with sexually undifferentiated gonads in January-February and simultaneous appearance of potential CV females (Figure 5). A very rare occurrence of potential CV females during later period, from March onward (Figure 5), confirmed that the majority of the overwintering C. glacialis CVs have molted to adult females (CVI) by that time. These observations are in a good agreement with the dynamics revealed by the seasonal variation of the stage composition of C. glacialis (Figure 4A).
The re-appearance of C. glacialis CVs with undifferentiated gonads in May and their presence throughout June and July (Figure 5) reflects the transition of the overwintering C. glacialis CIVs (one-year old generation) into the CVs during the primary production season. All these “young” CVs have undifferentiated gonads; they prioritize to accumulate lipids during the summer season and delay development and sexual differentiation of their gonads to the coming winter (January-February).
The seasonal pattern of gonad maturation in C. glacialis adult females (Figure 6) corresponds well to variation in reproductive activity of this species revealed from the egg production incubations, suggesting the onset of the reproductive season in March, with May being the month of peak production, fueled by the spring bloom. Similar seasonal variations in gonad maturity of C. glacialis have been observed in the White Sea (Kosobokova, 1999) and Disko Bay (Niehoff et al., 2002).
Sex differentiation in C. finmarchicus takes place later in the season compared to C. glacialis, from March onwards (Figure 5). Relatively high proportions of C. finmarchicus PF CVs were found in March and April, and all CVs completed development and molted to adults between March and May. During the same months, adult females with maturing gonads of stages GS1-GS2 appeared (Figure 6). Females in egg-laying condition (GS3-GS4) within the C. finmarchicus size range become most numerous from May to July in a good accordance with results of the egg production incubations, and in August their gonads turn to GS6 stage indicating termination of spawning. Niehoff et al. (2002) followed the gonad maturation of both C. finmarchicus and C. glacialis females in Disko Bay, and though they used a slightly different gonad classification system, they also found an earlier presence of egg laying C. glacialis compared to C. finmarchicus. Differences in the timing of appearance of adults between C. glacialis and C. finmarchicus similar to that observed in our study have been documented previously in other cold-water regions (Kjellerup et al., 2012). The early January appearance of C. glacialis males and females in Isfjorden is consistent with Daase et al. (2018), and appearance of C. finmarchicus adults in February-March corresponds well with observations further south (Arashkevich et al., 2004; Choquet et al., 2021).
Seasonal changes in sex ratio based on the community data confirm the later appearance of C. finmarchicus compared to C. glacialis. However, size measurements of Calanus males are still rare (Daase et al., 2018). Confirmation of size ranges and verification of males’ identification by genetic methods is urgently needed in order to prove the actual differences in the timing of appearance of males of these two species in the study area.
Previous studies have indicated the potential of C. glacialis and C. finmarchicus to interbreed and produce fertile offspring in the North Atlantic and Canadian Arctic (Parent et al., 2012). However, newer studies using nuclear InDel markers, which are regarded as more reliable tools for detecting potential hybrids (Nielsen et al., 2014; Smolina et al., 2014), show that hybridization is very unlikely (Choquet et al., 2017). One additional reason for absence of hybridization could be the difference in timing of appearance of adults as observed in this study which would provide an efficient temporal barrier for inter-breeding. However, hybridization has also not been detected in nauplii or older copepodite stages in fjords located further south (66°N) where adult males and females of C. glacialis and C. finmarchicus co-occur, suggesting that hybridization is not occurring between these two Calanus species (Choquet et al., 2021).
4.3 Reproductive Strategies and Timing of Reproduction
Both C. glacialis and C. finmarchicus reproduced over an extended period in Isfjorden, but with different timing in relation to the spring bloom. Calanus glacialis produced eggs in low numbers already in March, six weeks prior to the spring bloom, indicating a capital breeding strategy early in the season which has been observed across the Arctic and is regarded as one of the reasons why C. glacialis is successful in the Arctic shelf regions (Kosobokova and Hirche, 2001; Swalethorp et al., 2011; Daase et al., 2013; Kwasniewski et al., 2013). However, peak spawning in C. glacialis often coincides with the phytoplankton bloom (Kosobokova and Hirche, 2001; Hirche and Kosobokova, 2003; Niehoff and Hirche, 2005; Daase et al., 2013). A six-fold increase in egg production rates from pre-bloom to peak-bloom conditions in our study indicates that while C. glacialis is capable of capital breeding, the switch to income breeding when food is available yields a higher reproductive output that is in a good agreement with earlier studies (Hirche and Kattner, 1993; Kosobokova and Hirche, 2001; Madsen et al., 2001; Hirche and Kosobokova, 2003; Madsen et al., 2008; Søreide et al., 2010; Swalethorp et al., 2011). In Disko Bay, Swalethorp et al. (2011) observed an increase from an average egg production of 0.6 eggs fem-1 day-1 pre-bloom to 26.4 during the peak of the bloom. Another study from Disko Bay by Madsen et al. (2008) also showed an increase of egg production as the spring bloom peaked. Although Chl a had already started to build up in the water column at the start of their study, a doubling in egg production (to 48.3 eggs fem-1 day-1) was observed from the start of the study to the peak of the bloom.
Another factor determining the overall population recruitment is the female stock abundance. Calanus glacialis had highest numbers of females in April suggesting that an earlier onset of the bloom will be advantageous in ice free fjords to secure early and high egg production. Ice algae was absent at the study location, but fresh algal food is available in low concentrations in ice free parts of Isfjorden/Svalbard fjords from late winter-early spring (Kubiszyn et al., 2017; Kvernvik et al., 2018).)
Calanus finmarchicus does not have the same potential for capital breeding as C. glacialis and is considered to be a pure income breeder. It needs the algae bloom to fuel the final maturation of gonads. Subsequently, it can first start egg production when food has become available (Hirche, 1996; Niehoff and Hirche, 1996; Niehoff, 2004). In accordance with observed patterns of gonad maturation, with later molting of CVs to adult females and later gonad ripening (Figure 6), C. finmarchicus started egg production at the onset of the spring bloom approximately one month later than C. glacialis. A later onset of egg production was also observed in Disko Bay by Madsen et al. (2008), where C. finmarchicus increased from 20% spawning females pre-bloom in April to >90% at the peak of the spring bloom in early May, while C. glacialis was already at 80% spawning females in April. Egg production rates of C. finmarchicus peaked first in June after the peak spring bloom, thus later than in C. glacialis. These differences in timing of reproduction suggest a mismatch between the offspring of C. finmarchicus and the peak spring bloom which provides the optimal feeding conditions. However, C. finmarchicus was able to maintain high egg production rates for a longer period compared to C. glacialis, which also has been observed in Disko Bay (Swalethorp et al., 2011). In Disko Bay, an average of 46% of the C. finmarchicus females were spawning in the post-boom period with an egg production rate of 7 eggs fem-1 day-1, which is somewhat lower than we observed in our study with 50-60% spawning females and 8-19 eggs fem-1 day-1. The longer spawning season may be explained by C. finmarchicus feeding more efficiently on smaller sized algae than C. glacialis (Hansen et al., 1994; Levinsen et al., 2000). Such a late summer reproduction increases the predation pressure on eggs and nauplii (Varpe et al., 2009), and may thus have a negative effect on the population growth of C. finmarchicus compared to C. glacialis which this study suggests with the lower population increase in C. finmarchicus compared to C. glacialis over the summer.
The differences in the timing of reproduction between the two species were also well reflected in the population composition and gonad maturation patterns. The short period of egg production in C. glacialis was followed by a relatively short period when CIs of a new generation were present in the fjord (mainly in June). This suggests that reproduction mainly took place during the spring bloom, leaving time for the offspring to grow and develop to the overwintering stages over summer. For C. finmarchicus, reproduction seems to continue throughout the entire summer, as suggested not only by the high egg production rates in June but also the continuous presence of CIs from May to August. The later presence of young stages in C. finmarchicus compared to C. glacialis was also observed in Disko Bay (Madsen et al., 2001). It is, however, unlikely that the young stages found in late summer will make it to overwintering stage and accumulate enough reserves to survive the winter, but this needs to be addressed in more details in future studies. Furthermore, it should be noted that morphological identification of C. finmarchicus vs. C. glacialis is particularly difficult for the younger stages where the sizes significantly overlap, so we cannot exclude misidentification of the species that might bias our conclusions. Molecular identification of young life stages in particular is needed to gain more insights into differences in the population development and reproductive strategies between these two Calanus species.
4.4 Fate of C. finmarchicus and C. glacialis in a Warming Arctic
The winter of 2015-2016 has been described as the warmest winter on record in the Arctic with the largest positive temperature anomalies observed over the region of the Barents and Kara Seas (Cullather et al., 2016; Overland and Wang, 2016). Locally in Isfjorden, the winter 2015-2016 was characterized by relatively strong inflow of Atlantic water and little sea ice formation, which is anyway restricted to side-fjords in the inner part of the Isfjorden system (Muckenhuber et al., 2016). Such conditions have been defined as a ‘winter open situation’ (Tverberg et al., 2019; Skogseth et al., 2020). The spring and summer following a ‘winter open situation’ are typically relatively warm (Tverberg et al., 2019), which was the case in 2016 in Isfjorden (Skogseth et al., 2020; this study).
Advection of the North Atlantic populations with Atlantic water is considered the main contributor to the C. finmarchicus population in the Arctic (Speirs et al., 2006; Hirche and Kosobokova, 2007; Kosobokova and Hirche, 2009; Wassmann et al., 2015; Choquet et al., 2017). Kwasniewski et al. (2003) argued for two populations of C. finmarchicus in Kongsfjorden, Svalbard: a local population in the inner basin and an advected population in the outer basin. Our study site is comparable to the outer basin of Kongsfjorden, with no sill mitigating the influx of water masses from outside the fjord. Thus, advection likely is contributing to the C. finmarchicus population in Isfjorden, particularly during periods of high Atlantic inflow. Nevertheless, despite the high influx of Transformed Atlantic Water and relatively warm conditions we found a pronounced dominance of the arctic C. glacialis over the boreal C. finmarchicus during most part of our 15 months study period. Particularly in summer, the population was strongly skewed towards C. glacialis, which contributed up to 90% in June 2016. Throughout summer, young copepodite stages with a succession from CI to CIII dominated in the C. glacialis population, indicating successful reproduction and development of a new generation. The abundance of C. glacialis was not only higher compared to C. finmarchicus, but also compared to maximum abundances reported from other fjords in Svalbard of more Arctic character with a seasonal ice cover providing access to early growing ice algae (Arnkværn et al., 2005; Leu et al., 2011; Lischka and Hagen, 2016; Hop et al., 2019). Early access to ice algae provides favorable feeding conditions for C. glacialis at the beginning of the spawning season, speeding up gonad maturation and support early egg production which allows early offspring to be ready to feed when the spring bloom starts (Niehoff et al., 2002; Søreide et al., 2010; Daase et al., 2013). This likely would improve the reproductive output and increase survival, and we would therefore expect higher population size in seasonally ice-covered locations (i.e. Arnkværn et al., 2005, and Lischka and Hagen, 2016) compared to our study site. However, the abundance of both C. glacialis and C. finmarchicus observed in our study was consistently higher year-round compared to other, seasonally ice-covered, study sites (Arnkværn et al., 2005; Leu et al., 2011; Lischka and Hagen, 2016). The high abundance, successful reproduction and dominance of C. glacialis in Isfjorden indicate that this species is thriving also under warmer, more Atlantic-influenced conditions and likely even benefits from these warmer conditions, presumably, due to increased growth rates and a better match between the spring bloom and presence of females. Increased growth and developmental rates at higher temperatures allow a higher portion of offspring to reach the overwintering stage and accumulate sufficient lipids for successful overwintering (Coyle and Gibson, 2017) as long as these temperatures remain within the species temperature tolerance range (Pertsova and Kosobokova, 2010).
While Carstensen et al. (2012) identified a threshold of ~6°C as the temperature range above which C. glacialis abundance and biomass decreases in the Fram Strait, observations of isolated local populations of C. glacialis in the Sub-Arctic White Sea indicate that different life stages of this species are adapted to survive different temperature ranges, and for some of them upper temperature limits are significantly higher than prevailing in situ temperature in the high Arctic (Kosobokova, 1999; Pertsova and Kosobokova, 2010; Kosobokova and Pertsova, 2018). Thus, its older stenothermal stages (CV – CVI) do prefer water temperatures close to and below 0°C and stay in surface waters only as long as temperatures correspond to their preferences (< +6°C). When annual surface temperatures reaches the limits of the comfortable temperature regime and passes over + 6°C, CV-CVI copepodites descend into deeper colder layers. In contrast, the younger copepodite stages I-III of the new generation are resistant to a wider temperature range; they develop successfully near the surface even after the temperature has passed to positive and reach up to 15°C (Pertsova and Kosobokova, 2010).
Freer et al. (2021) recently demonstrated that habitat suitability for C. finmarchicus has increased in areas that experienced warming and a reduction in sea ice. Furthermore, an increase in abundances of the boreal C. finmarchicus has recently been observed in some Arctic regions that experience distinct warming and sea ice loss (Chust et al., 2014; Weydmann et al., 2014; Aarflot et al., 2018; Hop et al., 2019; Møller and Nielsen, 2020), indicating not only increased advection but also more successful recruitment. Moreover, recent studies have shown that autumn blooms will become more common in the Arctic (Ardyna et al., 2014). At temperate latitudes, autumn blooms are triggered by wind driven mixing breaking down stratification that result in an upward supply of nutrients (Chiswell et al., 2013). Loss of sea ice and increased frequency and intensity of fall storms have led to an increase in the average number of stormy days over open waters in the Arctic during September and October (Ardyna et al., 2014). The occurrence of a second peak of algal biomass in autumn and an earlier onset of the spring bloom, in addition to the ability of C. finmarchicus to feed more efficiently than C. glacialis on small algae typically dominating the summer production (Hansen et al., 1994), may benefit C. finmarchicus in the Arctic. Combined with increased developmental rates, this could provide the necessary time and resources for late C. finmarchicus recruits to reach overwintering stage CV and accumulate enough lipids for overwintering, and thus allowing C. finmarchicus to fulfill its life cycle further north than today (Tarling et al., 2021).
So far, there is little evidence that an increase in habitat suitability for C. finmarchicus comes to the detriment of C. glacialis, as time series data show that an increase in C. finmarchicus is not accompanied by a decrease in C. glacialis (Hop et al., 2019; Møller and Nielsen, 2020). The high flexibility in the life history strategies of C. glacialis is regarded as key to explain its success across Arctic shelf seas and the Arctic Ocean (Ershova et al., 2021). The existence of relict populations in Sub-Arctic waters in more southern latitudes demonstrates that this species is adapted to a relatively wide range of environmental conditions (Kosobokova, 1999; Niehoff and Hirche, 2005; Choquet et al., 2017; Kosobokova and Pertsova, 2018). A northward extension of its distribution range into the central Arctic Ocean with retreating sea ice has recently been demonstrated (Ershova et al., 2021), suggesting that C. glacialis needs a certain period of open water to complete its life cycle in the ice-covered regions. Based on our study and earlier data from the White Sea, we conclude that C. glacialis may be more resistant to predicted climate warming than believed (Hirche and Kosobokova, 2007; Pertsova and Kosobokova, 2010; Carstensen et al., 2012). We observed here that C. glacialis is able to thrive in relatively warm waters in the absence of sea ice, but there is likely an upper limit of warming that it will be able to withstand. Apparently, it is able to cope with warming in different parts of its range, as long as cold waters lying under the warmed surface waters will serve as a refuge (Pertsova and Kosobokova, 2010). However, if temperature continues to increase over the entire water column, a tipping point could be reached, and the negative effects may outweigh the positive. In particular, higher winter temperatures at depth can potentially lead to increased metabolic costs during overwintering, risking premature depletion of stored lipids (Coyle and Gibson, 2017) and increased winter mortality (Daase and Søreide, 2021).
Within the Calanus complex, a size shift towards smaller individuals may not necessarily be caused by a shift in species composition, but a change in growth rates of the arctic species under warmer conditions may also lead to overall smaller body sizes of these (Ejsmond et al., 2018; Renaud et al., 2018). Using a life history model, Renaud et al. (2018) suggest that a shift to smaller body size in Calanus populations may be accompanied by shorter life span and higher population turnover rate which actually will lead to a higher total lipid production rate by Calanus spp. However, a shift to smaller-sized Calanus spp. can impact predators who select for larger-sized prey (Martens et al., 2015), such as the little auk (Alle alle). This may result in change of the species composition and impact timing when the lipids in form of Calanus spp. are available for higher trophic levels (Hedeholm et al., 2010; Martens et al., 2015; Blanchard et al., 2017; Møller and Nielsen, 2020).
4.5 Conclusions
Arctic warming and loss of sea ice may facilitate a northward expansion and successful recruitment of new generations of C. finmarchicus locally in Arctic waters. However, due to the extreme seasonality in the light regime, there is a limit in the potential prolongation of the productive season, and the bloom phenology will likely remain short-pulsed and unpredictable due to high inter-annual variability in sea ice coverage. Overall, arctic Calanus species are still better adapted to these conditions than C. finmarchicus (Falk-Petersen et al., 2009; Daase et al., 2013; Ershova et al., 2021). Although the co-existence of different Calanus species is still not completely understood, our data indicate that even though both C. glacialis and C. finmarchicus occupy very similar niches, they don’t necessarily compete for the same resources during recruitment. Calanus glacialis utilizes the early spring bloom, while C. finmarchicus can utilize the post-bloom and autumn bloom to accumulate lipids at a time when the majority of the C. glacialis specimens have migrated to deeper waters for overwintering. As long as sea temperatures remain within their respective temperature-tolerance ranges, both C. glacialis and C. finmarchicus seem to benefit from warming due to accelerating growth and higher survival of the recruits.
Data Availability Statement
The raw data supporting the conclusions of this article will be made available by the authors, without undue reservation.
Author Contributions
MH has contributed to sampling, sample and data analysis, compiling first draft and edited the manuscript based on feedback from co-authors. KK has contributed to sample and data analysis, provided extensive feedback in the editing process. MD has contributed to sampling, sample and data analysis, provided extensive feedback in the editing process. JS has contributed to sampling, data analysis, compiling first draft and extensive feedback in the editing process. All authors contributed to the article and approved the submitted version.
Conflict of Interest
The authors declare that the research was conducted in the absence of any commercial or financial relationships that could be construed as a potential conflict of interest.
Publisher’s Note
All claims expressed in this article are solely those of the authors and do not necessarily represent those of their affiliated organizations, or those of the publisher, the editors and the reviewers. Any product that may be evaluated in this article, or claim that may be made by its manufacturer, is not guaranteed or endorsed by the publisher.
Funding
Funding was provided by the Norwegian ResearchCouncil (NRC) through the project COPPY (227139) and the Fram Centre Flagship Arctic Ocean (project FADE, 2019–2021). KK conducted research within the framework of the state assignment of IO RAS (theme No. FMWE–2021–0007) and was partially supported by the Russian Science Foundation (grant No. 19-17- 00058). MD received additional funding through the NRC project Deep Impact (300333).
Acknowledgments
We thank the captains and crew of RV Helmer Hanssen, RV Dalnie Zelentsy, RV Viking Explorer and UNIS logistics for making it possible to conduct the year-round sampling for this study. We are very grateful to Kasia Dmoch, Helene Eide and Runa Solvang for their contribution to analysis of sample material. This study i s a contribution to the ARCTOS research network (arctos.uit.no).
Supplementary Material
The Supplementary Material for this article can be found online at: https://www.frontiersin.org/articles/10.3389/fmars.2022.877910/full#supplementary-material
Supplementary Figure S1 | TS-diagram of water masses recorded each month at Karlskronadjupet in Isfjorden, split into panels according to season: (a) summer 2015, (b) autumn 2015, (c) winter 2015-2016, (d) spring 2016 and (e) summer 2016. External water masses (Grey shade): AtW = Atlantic water, ArW= Arctic water; Local water masses (135° lines): SW = surface water, LW = Local water, WCW=winter cooled water; mixed water masses (45° lines) TAW = Transformed Atlantic water, IW = intermediate water. Dashed grey line indicates freezing point. Solid grey lines show isopycnals at intervals of 1. Water mass definitions based on Skogseth et al. (2020).
Supplementary Figure S2 | Seasonal changes in vertical distribution of copepodite stages and adults of C. finmarchicus in Isfjorden between June 2015 and August 2016. Note differences in scale of x-axis.
Supplementary Figure S3 | Weighted mean depth for (a) Adult females, (b) CV and (c) CIV of C. finmarchicus (red) and C. glacialis (blue) at Karlskronadjupet, Isfjorden from June 2015 to August 2016.
Supplementary Figure S4 | Seasonal changes in vertical distribution of copepodite stages and adults of C. glacialis in Isfjorden between June 2015 and August 2016. Note differences in scale of x-axis.
References
Aarflot J. M., Skjoldal H. R., Dalpadado P., Skern-Mauritzen M. (2018). Contribution of Calanus Species to the Mesozooplankton Biomass in the Barents Sea. ICES. Mar. Sci. 75 (7), 2342–2354. doi: 10.1093/icesjms/fsx221
Aksnes D., Blindheim J. (1996). Circulation Patterns in the North Atlantic and Possible Impact on Population Dynamics of Calanus Finmarchicus. Ophelia 44, 7–28. doi: 10.1080/00785326.1995.10429836
Arashkevich E., Tande K., Pasternak A., Ellertsen B. (2004). Seasonal Moulting Patterns and the Generation Cycle of Calanus Finmarchicus in the NE Norwegian Sea, as Inferred From Gnathobase Structures, and the Size of Gonads and Oil Sacs. Mar. Biol. 146, 119–132. doi: 10.1007/s00227-004-1416-5
Ardyna M., Babin M., Gosselin M., Devred E., Rainville L., Tremblay J.É. (2014). Recent Arctic Ocean Sea Ice Loss Triggers Novel Fall Phytoplankton Blooms. Geophys. Res. Lett. 41 (17), 6207–6212. doi: 10.1002/2014GL061047
Arnkværn G., Daase M., Eiane K. (2005). Dynamics of Coexisting Calanus Finmarchicus, Calanus Glacialis and Calanus Hyperboreus Populations in a High-Arctic Fjord. Pol. Biol. 28, 528–538. doi: 10.1007/s00300-005-0715-8
Ashjian C. J., Campbell R. G., Welch H. E., Butler M., Van Keuren D. (2003). Annual Cycle in Abundance, Distribution, and Size in Relation to Hydrography of Important Copepod Species in the Western Arctic Ocean. Deep-Sea. Res. Part I-Oceanogr. Res. Pap. 50 (10-11), 1235–1261. doi: 10.1016/S0967-0637(03)00129-8
Bailey A. (2010). Lipids and Diapause in Calanus Spp. In a High-Arctic Fjord: State-Dependent Strategies? Tracking Lipids Through the Polar Night. [Master's thesis] (Tromsø. Norway: University of Tromsø and University Centre in Svalbard).
Basedow S. L., Sundfjord A., von Appen W. J., Halvorsen E., Kwasniewski S., Reigstad M. (2018). Seasonal Variation in Transport of Zooplankton Into the Arctic Basin Through the Atlantic Gateway, Fram Strait. Front. Mar. Sci. 5, 194. doi: 10.3389/fmars.2018.00194
Basedow S. L., Tande K. S. (2006). Cannibalism by Female Calanus Finmarchicus on Naupliar Stages. Mar. Ecol. Prog. Ser. 327, 247–255. doi: 10.3354/meps327247
Berge J., Daase M., Hobbs L., Falk-Petersen S., Darnis G., Søreide J. E. (2020). “Zooplankton in the Polar Night,” in Polar Night Marine Ecology. Eds. Berge J., Johnsen G., Cohen J. H. (Cham: Springer), 113–159.
Blachowiak-Samolyk K., Søreide J. E., Kwasniewski S., Sundfjord A., Hop H., Falk-Petersen S., et al. (2008). Hydrodynamic Control of Mesozooplankton Abundance and Biomass in Northern Svalbard Waters (79–81 N). Deep. Sea. Res. II. 55, 2210–2224. doi: 10.1016/j.dsr2.2008.05.018
Blanchard J. L., Heneghan R. F., Everett J. D., Trebilco R., Richardson A. J. (2017). From Bacteria to Whales: Using Functional Size Spectra to Model Marine Ecosystems. Trends Ecol. Evol. 32, 174–186. doi: 10.1016/j.tree.2016.12.003
Carmack E., Wassmann P. (2006). Food Webs and Physical–Biological Coupling on Pan-Arctic Shelves: Unifying Concepts and Comprehensive Perspectives. Prog. Oceanogr. 71, 446–477. doi: 10.1016/j.pocean.2006.10.004
Carstensen J., Weydmann A., Olszewska A., Kwasniewski S. (2012). Effects of Environmental Conditions on the Biomass of Calanus Spp. In the Nordic Seas. J. Plankt. Res. 34, 951–966. doi: 10.1093/plankt/fbs059
Chiswell S. M., Bradford-Grieve J., Hadfield M. G., Kennan S. C. (2013). Climatology of Surface Chlorophyll a, Autumn-Winter and Spring Blooms in the Southwest Pacific Ocean. J. Geophys. Res.-Ocean. 118 (2), 1003–1018. doi: 10.1002/jgrc.20088
Choquet M., Burckard G., Skreslet S., Hoarau G., Søreide J. E. (2021). No Evidence for Hybridization Between Calanus Finmarchicus and Calanus Glacialis in a Subarctic Area of Sympatry. Limnol. Oceanogr. 66, S314–S325. doi: 10.1002/lno.11583
Choquet M., Hatlebakk M., Dhanasiri A. K. S., Kosobokova K., Smolina I., Søreide J. E., et al. (2017). Genetics Redraws Pelagic Biogeography of Calanus. Biol. Lett. 13 (12), 20170588. doi: 10.1098/rsbl.2017.0588
Choquet M., Kosobokova K., Kwaśniewski S., Hatlebakk M., Dhanasiri A. K., Melle W., et al. (2018). Can Morphology Reliably Distinguish Between the Copepods Calanus Finmarchicus and C. Glacialis, or is DNA the Only Way? Limnol. Oceanogr-Meth. 16, 237–252. doi: 10.1002/lom3.10240
Chust G., Castellani C., Licandro P., Ibaibarriaga L., Sagarminaga Y., Irigoien X. (2014). Are Calanus Spp. Shifting Poleward in the North Atlantic? A Habitat Modelling Approach. ICES. J. Mar. Sci. 71 (2), 241–253. doi: 10.1093/icesjms/fst147
Cleary A. C., Søreide J. E., Freese D., Niehoff B., Gabrielsen T. M., Fields H. E. D. (2017). Feeding by Calanus Glacialis in a High Arctic Fjord: Potential Seasonal Importance of Alternative Prey. ICES. J. Mar. Sci. 74, 1937–1946. doi: 10.1093/icesjms/fsx106
Conover R. J. (1988). Comparative Life Histories in the Genera Calanus and Neocalanus in High Latitudes of the Northern Hemisphere. Hydrobiologia 167, 127–142. doi: 10.1007/BF00026299
Coyle K. O., Gibson G. A. (2017). Calanus on the Bering Sea Shelf: Probable Cause for Population Declines During Warm Years. J. Plankt. Res. 39 (2), 257–270. doi: 10.1093/plankt/fbx005
Cullather R. I., Lim Y. K., Boisvert L. N., Brucker L., Lee J. N., Nowicki S. M. (2016). Analysis of the Warmest Arctic Winter 2015–2016. Geophys. Res. Lett. 43 (20), 10–808. doi: 10.1002/2016GL071228
Daase M., Eiane K. (2007). Mesozooplankton Distribution in Northern Svalbard Waters in Relation to Hydrography. Pol. Biol. 30, 969–981. doi: 10.1007/s00300-007-0255-5
Daase M., Falk-Petersen S., Varpe Ø., Darnis G., Søreide J. E., Wold A., et al. (2013). Timing of Reproductive Events in the Marine Copepod Calanus Glacialis: A Pan-Arctic Perspective. Can. J. Fish. Aquat. Sci. 70 (6), 871–884. doi: 10.1139/cjfas-2012-0401
Daase M., Kosobokova K., Last K. S., Cohen J. H., Choquet M., Hatlebakk M., et al. (2018). New Insights Into the Biology of Calanus Spp.(Copepoda) Males in the Arctic. Mar. Ecol. Prog. Ser. 607, 53–69. doi: 10.3354/meps12788
Daase M., Søreide J. E. (2021). Seasonal Variability in non-Consumptive Mortality of Arctic Zooplankton. J. Plankt. Res. 43, 565–585. doi: 10.1093/plankt/fbab042
Daase M., Søreide J. E., Martynova D. (2011). Effects of Food Quality on Naupliar Development in Calanus Glacialis at Subzero Temperatures. Mar. Ecol. Prog. Ser. 429, 111–124. doi: 10.3354/meps09075
Daase M., Varpe Ø., Falk-Petersen S. (2014). Non-Consumptive Mortality in Copepods: Occurrence of Calanus Spp. Carcasses in the Arctic Ocean During Winter. J. Plankt. Res. 36 (1), 129–144. doi: 10.1093/plankt/fbt079
Dahl T. M., Falk-Petersen S., Gabrielsen G. W., Sargent J. R., Hop H., Millar R.-M. (2003). Lipids and Stable Isotopes in Common Eider, Black-Legged Kittiwake and Northern Fulmar: A Trophic Study From an Arctic Fjord. Mar. Ecol. Prog. Ser. 256, 257–269. doi: 10.3354/meps256257
Dezutter T., Lalande C., Dufresne C., Darnis G., Fortier L. (2019). Mismatch Between Microalgae and Herbivorous Copepods Due to the Record Sea Ice Minimum Extent of 2012 and the Late Sea Ice Break-Up of 2013 in the Beaufort Sea. Prog. Oceanogr. 173, 66–77. doi: 10.1016/j.pocean.2019.02.008
Dvoretsky V. G. (2011). Distribution of Calanus Species Off Franz Josef Land (Arctic Barents Sea). Pol. Sci. 5, 361–373. doi: 10.1016/j.polar.2011.06.004
Ejsmond M. J., McNamara J. M., Soreide J., Varpe Ø. (2018). Gradients of Season Length and Mortality Risk Cause Shifts in Body Size, Reserves and Reproductive Strategies of Determinate Growers. Funct. Ecol. 32, 2395–2406. doi: 10.1111/1365-2435.13191
Ershova E. A., Kosobokova K. N., Banas N. S., Ellingsen I., Niehoff B., Hildebrandt N., et al. (2021). Sea Ice Decline Drives Biogeographical Shifts of Key Calanus Species in the Central Arctic Ocean. Glob. Change Biol. 27 (10), 2128–2143. doi: 10.1111/gcb.15562
Falk-Petersen S., Hopkins C. C. E., Sargent J. R. (1990). “Trophic Relationships in the Pelagic, Arctic Food Web,” in Trophic Relationships in the Marine Environment. Eds. Barnes M., Gibson R. N. (Aberdeen: Aberdeen University Press), 315–333.
Falk-Petersen S., Mayzaud P., Kattner G., Sargent J. R. (2009). Lipids and Life Strategy of Arctic Calanus. Mar. Biol. Res. 5, 18–39. doi: 10.1080/17451000802512267
Falk-Petersen S., Pavlov V., Timofeev S., Sargent J. R. (2007). “Climate Variability and Possible Effects on Arctic Food Chains: The Role of Calanus,” in Arctic Alpine Ecosystems and People in a Changing Environment (Heidelberg: Springer).
Frank-Gopolos T., Friis Møller E., Gissel Nielsen T. (2017). The Role of Egg Cannibalism for the Calanus Succession in the Disko Bay, Western Greenland. Limnol. Oceanogr. 62, 865–883. doi: 10.1002/lno.10472
Freer J. J., Daase M., Tarling G. A. (2021). Modelling the Biogeographic Boundary Shift of Calanus Finmarchicus Reveals Drivers of Arctic Atlantification by Subarctic Zooplankton. Glob. Change Biol. 28, 429–440. doi: 10.1111/gcb.15937
Freese D., Niehoff B., Søreide J. E., Sartoris F. J. (2015). Seasonal Patterns in Extracellular Ion Concentrations and pH of the Arctic Copepod Calanus Glacialis. Limnol. Oceanogr. 60 (6), 2121–2129. doi: 10.1002/lno.10158
Freese D., Søreide J. E., Graeve M., Niehoff B. (2017). A Year-Round Study on Metabolic Enzymes and Body Composition of the Arctic Copepod Calanus glacialis: Implications for the Timing and Intensity of Diapause. Mar. Biol. 164, 3. doi: 10.1007/s00227-016-3036-2
Gabrielsen T. M., Merkel B., Søreide J., Johansson-Karlsson E., Bailey A., Vogedes D., et al. (2012). Potential Misidentifications of Two Climate Indicator Species of the Marine Arctic Ecosystem: Calanus Glacialis and C. Finmarchicus. Pol. Biol. 35, 1621–1628. doi: 10.1007/s00300-012-1202-7
Hancke K., Lund-Hansen L. C., Lamare M. L., Højlund Pedersen S., King M. D., Andersen P., et al. (2018). Extreme Low Light Requirement for Algae Growth Underneath Sea Ice: A Case Study From Station Nord, NE Greenland. J. Geophys. Res-Ocean. 123, 985–1000. doi: 10.1002/2017JC013263
Hansen B., Verity P., Falkenhaug T., Tande K. S., Norrbin F. (1994). On the Trophic Fate of Phaeocystis Pouchetti (Harriot). 5. Trophic Relationships Between Phaeocystis and Zooplankton—an Assessment of Methods and Size Dependence. J. Plankt. Res. 16 (5), 487–511. doi: 10.1093/plankt/16.5.487
Hatlebakk M., Graeve M., Boissonnot L., Søreide J. E. (2019). Lipid Storage Consumption and Feeding Ability of Calanus Glacialis Jaschnov 1955 Males. J. Exp. Mar. Biol. Ecol. 521, 151226. doi: 10.1016/j.jembe.2019.151226
Hatlebakk M., Niehoff B., Choquet M., Hop H., Wold A., Hoarau G., et al. (this issue). Seasonal Enzyme Activities of Sympatric Calanus Glacialis and C. Finmarchicus in the High-Arctic. Fron. Mar. Sci. doi: 10.3389/fmars.2022.877904
Hays G. C., Richardson A. J., Robinson C. (2005). Climate Change and Marine Plankton. Trends Ecol. Evol. 20, 337–344. doi: 10.1016/j.tree.2005.03.004
Hedeholm R., Grønkjær P., Rosing-Asvid A., Rysgaard S. (2010). Variation in Size and Growth of West Greenland Capelin (Mallotus Villosus) Along Latitudinal Gradients. ICES. J. Mar. Sci. 67, 1128–1137. doi: 10.1093/icesjms/fsq024
Hirche H. J. (1989). Egg Production of the Arctic Copepod Calanus Glacialis—Laboratory Experiments. Mar. Biol. 103, 311–318. doi: 10.1007/BF00397264
Hirche H. J. (1996). The Reproductive Biology of the Marine Copepod, Calanus Finmarchicus — A Review. Ophelia 44 (1-3), 111–128. doi: 10.1080/00785326.1995.10429842
Hirche H. J. (1997). Life Cycle of the Copepod Calanus Hyperboreus in the Greenland Sea. Mar. Biol. 128, 607–618. doi: 10.1007/s002270050127
Hirche H. J., Kattner G. (1993). Egg Production and Lipid Content of Calanus Glacialis in Spring: Indication of a Food-Dependent and Food-Independent Reproductive Mode. Mar. Biol. 117, 615–622. doi: 10.1007/BF00349773
Hirche H. J., Kosobokova K. N. (2003). Early Reproduction and Development of Dominant Calanoid Copepods in the Ice Zone of the Barents Sea – Need for a Change of Paradigms? Mar. Biol. 143, 769–781. doi: 10.1007/s00227-003-1122-8
Hirche H. J., Kosobokova K. (2007). Distribution of Calanus Finmarchicus in the Northern North Atlantic and Arctic Ocean—expatriation and Potential Colonization. Deep-Sea. Res.II 54, 2729–2747. doi: 10.1016/j.dsr2.2007.08.006
Hirche H. J., Niehoff B. (1996). Reproduction of the Arctic Copepod Calanus Hyperboreus in the Greenland Sea-Field and Laboratory Observations. Pol. Biol. 16, 209–219. doi: 10.1007/BF02329209
Hop H., Wold A., Meyer A., Bailey A., Hatlebakk M., Kwasniewski S., et al. (2021). Winter-Spring Development of the Zooplankton Community Below Sea Ice in the Arctic Ocean. Front. Mar. Sci. 8. doi: 10.3389/fmars.2021.609480
Hop H., Wold A., Vihtakari M., Daase M., Kwasniewski S., Gluchowska M., et al. (2019). “Zooplankton in Kongsfjorden, (1996–2016) in Relation to Climate Change,” in The Ecosystem of Kongsfjorden. Eds. Hop H., Weincke C. (Svalbard: Springer), P.229–P.300.
Jaschnov W. (1970). Distribution of Calanus Species in the Seas of the Northern Hemisphere. International. Rev. Der. Gesamten. Hydrobiol. Und. Hydrograph. 55, 197–212. doi: 10.1002/iroh.19700550203
Jónasdóttir S. H. (1999). Lipid Content of Calanus Finmarchicus During Overwintering in the Faroe–Shetland Channel. Fish. Oceanogr. 8, 61–72. doi: 10.1046/j.1365-2419.1999.00003.x
Kitaysky A. S., Golubova E. G. (2000). Climate Change Causes Contrasting Trends in Reproductive Performance of Planktivorous and Piscivorous Alcids. J. Anim. Ecol. 69 (2), 248–262. doi: 10.1046/j.1365-2656.2000.00392.x
Kjellerup S., Dunweber M., Swalethorp R., Nielsen T. G., Møller E. F., Markager S., et al. (2012). Effects of a Future Warmer Ocean on the Coexisting Copepods Calanus Finmarchicus and C. Glacialis in Disko Bay, Western Greenland. Mar. Ecol. Prog. Ser. 447, 87–108. doi: 10.3354/meps09551
Kosobokova K. N. (1998). New Data on the Life Cycle of Calanus Glacialis in the White Sea (Based on Seasonal Observations of its Genital System Development). Oceanology 38 (3), 347–355.
Kosobokova K. N. (1999). The Reproductive Cycle and Life History of the Arctic Copepod Calanus Glacialis in the White Sea. Pol. Biol. 22, 254–263. doi: 10.1007/s003000050418
Kosobokova K. N. (2012). “Zooplankton of the Arctic Ocean,” in Community Structure, Ecology, Spatial Distribution (Moscow, Russia: GEOS), 272.
Kosobokova K. N., Hanssen N., Hirche H. J., Knickmeier K. (1998). Composition and Distribution of Zooplankton in the Laptev Sea and Adjacent Nansen Basin in the Summer 1993. Pol. Biol. 19, 63–76. doi: 10.1007/s003000050216
Kosobokova K. N., Hirche H. J. (2001). Reproduction of Calanus Glacialis in the Laptev Sea, Arctic Ocean. Pol. Biol. 24, 33–43. doi: 10.1007/s003000000171
Kosobokova K. N., Hirche H. J. (2009). Biomass of Zooplankton in the Eastern Arctic Ocean – a Base Line Study. Prog. Oceanogr. 82, 265–280. doi: 10.1016/j.pocean.2009.07.006
Kosobokova K. N., Hopcroft R. R., Hirche H. J. (2011). Patterns of Zooplankton Diversity Through the Depths of the Arctic’s Central Basins. Mar. Biodiver. 41, 29–50. doi: 10.1007/s12526-010-0057-9
Kosobokova K. N., Pertsova N. M. (2005). Zooplankton of the Deep Basin of the White Sea at the End of Hydrological Winter. Oceanology 45 (6), 866–878.
Kosobokova K. N., Pertsova N. M. (2018). “Zooplankton of the White Sea: Communities’ Structure, Seasonal Dynamics, Spatial Distribution, and Ecology,” in Biogeochemistry of Atmosphere, Ice and Water of the White Sea: The White Sea Environment. Part I, Hdb Env. Chem. Eds. Lisitzin A. P., Gordeev V. (Cham, Switzerland: Springer), 223–266. doi: 10.1007/698_2018_347
Kubiszyn A. M., Wiktor J. M., Wiktor J. M. Jr., Griffiths C., Kristiansen S., Gabrielsen T. M. (2017). The Annual Planktonic Protist Community Structure in an Ice-Free High Arctic Fjord (Adventfjorden, West Spitsbergen). J. Mar. SSys. 169, 61–72. doi: 10.1016/j.jmarsys.2017.01.013
Kvernvik A. C., Hoppe C. J. M., Lawrenz E., Prášil O., Greenacre M., Wiktor J. M., et al. (2018). Fast Reactivation of Photosynthesis in Arctic Phytoplankton During the Polar Night. J. Phycol. 54, 461–470. doi: 10.1111/jpy.12750
Kvernvik A. C., Rokitta S. D., Leu E., Harms L., Gabrielsen T. M., Rost B., et al. (2020). Higher Sensitivity Towards Light Stress and Ocean Acidification in an Arctic Sea-Ice-Associated Diatom Compared to a Pelagic Diatom. New Phytol. 226 (6), 1708–1724. doi: 10.1111/nph.16501
Kwasniewski S., Hop H., Falk-Petersen S., Pedersen G. (2003). Distribution of Calanus Species in Kongsfjorden, a Glacial Fjord in Svalbard. J. Plankt. Res. 25, 1–20. doi: 10.1093/plankt/25.1.1
Kwasniewski S., Walkusz W., Cottier F. R., Leu E. (2013). Mesozooplankton Dynamics in Relation to Food Availability During Spring and Early Summer in a High Latitude Glaciated Fjord (Kongsfjorden), With Focus on Calanus. J. Mar. Syst. 111-112, 83–96. doi: 10.1016/j.jmarsys.2012.09.012
Lee R. F., Hagen W., Kattner G. (2006). Lipid Storage in Marine Zooplankton. Mar. Ecol. Prog. Ser. 307, 273–306. doi: 10.3354/meps307273
Leu E., Mundy C., Assmy P., Campbell K., Gabrielsen T., Gosselin M., et al. (2015). Arctic Spring Awakening–Steering Principles Behind the Phenology of Vernal Ice Algal Blooms. Prog. Oceanogr. 139, 151–170. doi: 10.1016/j.pocean.2015.07.012
Leu E., Søreide J., Hessen D., Falk-Petersen S., Berge J. (2011). Consequences of Changing Sea-Ice Cover for Primary and Secondary Producers in the European Arctic Shelf Seas: Timing, Quantity, and Quality. Prog. Oceanogr. 90, 18–32. doi: 10.1016/j.pocean.2011.02.004
Leu E., Søreide J., Hessen D., Falk-Petersen S., Berge J. (2011). Consequences of Changing Sea-Ice Cover for Primary and Secondary Producers in the European Arctic Shelf Seas: Timing, Quantity, and Quality. Prog. Oceanogr. 90, 18–32. doi: 10.1016/j.pocean.2011.02.004
Levinsen H., Turner J. T., Nielsen T. G., Hansen B. W. (2000). On the Trophic Coupling Between Protists and Copepods in Arctic Marine Ecosystems. Mar. Ecol. Prog. Ser. 204, 65–77. doi: 10.3354/meps204065
Lischka S., Hagen W. (2016). Seasonal Dynamics of Mesozooplankton in the Arctic Kongsfjord (Svalbard) During Year-Round Observations From August 1998 to July 1999. Pol. Biol. 39, 1859–78. doi: 10.1007/s00300-016-2005-z
Møller E. F., Nielsen T. G. (2020). Borealization of Arctic Zooplankton—Smaller and Less Fat Zooplankton Species in Disko Bay, Western Greenland. Limnol. Oceanogr. 65, 1175–1188. doi: 10.1002/lno.11380
Madsen S. D., Nielsen T. G., Hansen B. W. (2001). Annual Population Development and Production by Calanus Finmarchicus, C. Glacialis and C. Hyperboreus in Disko Bay, Western Greenland. Mar. Biol. 139, 75–93. doi: 10.1007/s002270100552
Madsen S. J., Nielsen T. G., Tervo O. M., Söderkvist J. (2008). Importance of Feeding for Egg Production in Calanus Finmarchicus and C. Glacialis During the Arctic Spring. Mar. Ecol. Prog. Ser. 353, 177–190. doi: 10.3354/meps07129
Martens E. A., Wadhwa N., Jacobsen N. S., Lindemann C., Andersen K. H., Visser A. (2015). Size Structures Sensory Hierarchy in Ocean Life. P. R. Soc B-Biol. Sci. 282 (1815), 20151346. doi: 10.1098/rspb.2015.1346
Morata N., Søreide J. E. (2015). Effect of Light and Food on the Metabolism of the Arctic Copepod Calanus Glacialis. Pol. Biol. 38, 67–73. doi: 10.1007/s00300-013-1417-2
Muckenhuber S., Nilsen F., Korosov A., Sandven S. (2016). Sea Ice Cover in Isfjorden and Hornsund, Svalbard, (2000–2014) From Remote Sensing Data. Cryosphere. 10, 149–158. doi: 10.5194/tc-10-149-2016
Niehoff B. (2004). The Effect of Food Limitation on Gonad Development and Egg Production of the Planktonic Copepod Calanus Finmarchicus. J. Exp. Mar. Biol. Ecol. 307, 237–259. doi: 10.1016/j.jembe.2004.02.006
Niehoff B., Hirche H. J. (1996). Oogenesis and Gonad Maturation in the Copepod Calanus Finmarchicus and the Prediction of Egg Production From Preserved Samples. Pol. Biol. 16 (8), 601–612. doi: 10.1007/BF02329058
Niehoff B., Hirche H. J. (2005). Reproduction of Calanus Glacialis in the Lurefjord (Western Norway): Indication for Temperature-Induced Female Dormancy. Mar. Ecol. Prog. Ser. 285, 107–115. doi: 10.3354/meps285107
Niehoff B., Madsen S. D., Hansen B., Nielsen T. (2002). Reproductive Cycles of Three Dominant Calanus Species in Disko Bay, West Greenland. Mar. Biol. 140, 567–576. doi: 10.1007/s00227-001-0731-3
Nielsen T. G., Kjellerup S., Smolina I., Hoarau G., Lindeque P. (2014). Live Discrimination of Calanus Glacialis and C. Finmarchicus Females: Can We Trust Phenological Differences? Mar. Biol. 161, 1299–1306. doi: 10.1007/s00227-014-2419-5
Nilsen F., Cottier F., Skogseth R., Mattsson S. (2008). Fjord–shelf Exchanges Controlled by Ice and Brine Production: The Interannual Variation of Atlantic Water in Isfjorden, Svalbard. Cont. Shelf. Res. 28, 1838–1853. doi: 10.1016/j.csr.2008.04.015
Norrbin M. F. (1991). Gonad Maturation as an Indication of Seasonal Cycles for Several Species of Small Copepods in the Barents Sea. Pol. Res. 10, 421–432. doi: 10.1111/j.1751-8369.1991.tb00663.x
Overland J. E., Wang M. (2016). Recent Extreme Arctic Temperatures are Due to a Split Polar Vortex. J. Climate 29 (15), 5609–5616. doi: 10.1175/JCLI-D-16-0320.1
Parent G. J., Plourde S., Turgeon J. (2012). Natural Hybridization between Calanus finmarchicus and C. glacialis (Copepoda) in the Arctic and Northwest Atlantic. Limnol. Oceanogr. 57, 1057–1066. doi: 10.4319/lo.2012.57.4.1057
Pertsova N. M., Kosobokova K. N. (2010). Interannual and Seasonal Variation of the Population Structure, Abundance, and Biomass of the Arctic Copepod Calanus Glacialis in the White Sea. Oceanology 50, 531–541. doi: 10.1134/S0001437010040090
Plourde S., Runge J. A. (1993). Reproduction of the Planktonic Copepod Calanus Finmarchicus in the Lower St. Lawrence Estuary: Relation to the Cycle of Phytoplankton Production and Evidence for a Calanus Pump. Mar. Ecol. Prog. Ser. 102, 217–227. doi: 10.3354/meps102217
Polyakov I. V., Alkire M. B., Bluhm B. A., Brown K. A., Carmack E. C., Chierici M., et al. (2020). Borealization of the Arctic Ocean in Response to Anomalous Advection From Sub-Arctic Seas. Front. Mar. Sci. 7, 491. doi: 10.3389/fmars.2020.00491
Renaud P. E., Daase M., Banas N. S., Gabrielsen T. M., Søreide J. E., Varpe Ø., et al. (2018). Pelagic Food-Webs in a Changing Arctic: A Trait-Based Perspective Suggests a Mode of Resilience. ICES. J. Mar. Sci. 75, 1871–1881. doi: 10.1093/icesjms/fsy063
Søreide J. E., Leu E. V., Berge J., Graeve M., Falk-Petersen S. (2010). Timing of Blooms, Algal Food Quality and Calanus Glacialis Reproduction and Growth in a Changing Arctic. Glob. Change Biol. 16 (11), 3154–3163. doi: 10.1111/j.1365-2486.2010.02175.x
Sakshaug E. (2004). “Primary and Secondary Production in the Arctic Seas,” in The Organic Carbon Cycle in the Arctic Ocean. Eds. Stein R., Macdonald R. W. (Berlin, Heidelberg: Springer), 57–81.
Sargent J., Falk-Petersen S. (1988). “The Lipid Biochemistry of Calanoid Copepods,” in Biology of Copepods (Dordrecht, Netherlands: Springer).
Skogseth R., Olivier L. L. A., Nilsen F., Falck E., Fraser N., Tverberg V., et al. (2020). Variability and Decadal Trends in the Isfjorden (Svalbard) Ocean Climate and Circulation - a Thermometer for Climate Change in the Arctic Ocean. Prog. Oceanogr. 187, 102394. doi: 10.1016/j.pocean.2020.102394
Smolina I., Kollias S., Poortvliet M., Nielsen T., Lindeque P., Castellani C., et al. (2014). Genome-and Transcriptome-assisted Development of Nuclear Insertion/deletion Markers for Calanus species (Copepoda: Calanoida) Identification. Molec. Ecol. Res. 14, 1072–1079. doi: 10.1111/1755-0998.12241
Speirs D. C., Gurney W. S., Heath M. R., Horbelt W., Wood S. N., De Cuevas B. A. (2006). Ocean-Scale Modelling of the Distribution, Abundance, and Seasonal Dynamics of the Copepod Calanus Finmarchicus. Mar. Ecol. Prog. Ser. 313, 173–192. doi: 10.3354/meps313173
Swalethorp R., Kjellerup S., Dünweber M., Nielsen T. G., Møller E. F., Rysgaard S., et al. (2011). Grazing, Egg Production, and Biochemical Evidence of Differences in the Life Strategies of Calanus Finmarchicus, C. Glacialis and C. Hyperboreus in Disko Bay, Western Greenland. Mar. Ecol. Prog. Ser. 429, 125–144. doi: 10.3354/meps09065
Tande K. S., Hassel A., Slagstad D. (1985). “Gonad Maturation and Possible Life Cycle Strategies in Calanus Finmarchicus and Calanus Glacialis in the Northwestern Part of the Barents Sea,” in Marine Biology of Polar Regions and Effects of Stress on Marine Organisms. Eds. Gray J. S., Christiansen M. E. (New York: Chichester: Wiley and Sons), P. 141–P. 155.
Tande K. S., Hopkins C. C. E. (1981). Ecological Investigations of the Zooplankton Community of Balsfjorden, Northern Norway: Genital System in Calanus Finmarchicus and the Role of Gonad Development in the Overwintering Strategy. Mar. Biol. 63, 159–164. doi: 10.1007/BF00406824
Tarling G. A., Freer J. J., Banas N. S., Belcher A., Blackwell M., Castellani C., et al. (2021). Can a Key Boreal Calanus Copepod Species Now Complete its Life-Cycle in the Arctic? Evidence and Implications for Arctic Food-Webs. Ambio, 51, 333–344. doi: 10.1007/s13280-021-01667-y
Tremblay J.É., Anderson L. G., Matrai P., Couple P., Bélanger S., Michel C., et al. (2015). Global and Regional Drivers of Nutrient Supply, Primary Production and CO2 Drawdown in the Changing Arctic Ocean. Prog. Oceanogr. 139, 171–196. doi: 10.1016/j.pocean.2015.08.009
Tverberg V., Skogseth R., Cottier F., Sundfjord A., Walczowski W., Inall M. E., et al. (2019). “The Kongsfjorden Transect: Seasonal and Inter-Annual Variability in Hydrography,” in The Ecosystem of Kongsfjorden. Eds. Hop H., Weincke C. (Svalbard: Springer), P. 49–P.104.
Varpe Ø., Jorgensen C., Tarling G. A., Fiksen Ø. (2009). The Adaptive Value of Energy Storage and Capital Breeding in Seasonal Environments. Oikos 118, 363–370. doi: 10.1111/j.1600-0706.2008.17036.x
Vihtakari M., Welcker J., Moe B., Chastel O., Tartu S., Hop H., et al. (2018). Black-Legged Kittiwakes as Messengers of Atlantification in the Arctic. Sci. Rep. 8 (1), 1–11. doi: 10.1038/s41598-017-19118-8
Wassmann P., Kosobokova K. N., Slagstad D., Drinkwater K. F., Hopcroft R. R., Moore S. E., et al. (2015). The Contiguous Domains of Arctic Ocean Advection: Trails of Life and Death. Prog. Oceanogr. 139, 42–65. doi: 10.1016/j.pocean.2015.06.011
Wassmann P., Reigstad M., Haug T., Rudels B., Carroll M. L., Hop H., et al. (2006). Food Webs and Carbon Flux in the Barents Sea. Prog. Oceanogr. 71, 232–287. doi: 10.1016/j.pocean.2006.10.003
Weydmann A., Carstensen J., Goszczko I., Dmoch K., Olszewska A., Kwasniewski S. (2014). Shift Towards the Dominance of Boreal Species in the Arctic: Inter-Annual and Spatial Zooplankton Variability in the West Spitsbergen Current. Mar. Ecol. Prog. Ser. 501, 41–52. doi: 10.3354/meps10694
Weydmann A., Walczowski W., Carstensen J., Kwaśniewski S. (2018). Warming of Subarctic Waters Accelerates Development of a Key Marine Zooplankton Calanus Finmarchicus. Glob. Change Biol. 24 (1), 172–183. doi: 10.1111/gcb.13864
Keywords: calanoid copepods, Svalbard fjords, reproduction, gonad maturation, population structure, climate
Citation: Hatlebakk M, Kosobokova K, Daase M and Søreide JE (2022) Contrasting Life Traits of Sympatric Calanus glacialis and C. finmarchicus in a Warming Arctic Revealed by a Year-Round Study in Isfjorden, Svalbard. Front. Mar. Sci. 9:877910. doi: 10.3389/fmars.2022.877910
Received: 17 February 2022; Accepted: 11 April 2022;
Published: 12 May 2022.
Edited by:
Martin Edwards, Plymouth Marine Laboratory, United KingdomReviewed by:
Vladimir G. Dvoretsky, Murmansk Marine Biological Institute, RussiaTerry Whitledge, Retired, Fairbanks, AK, United States
Copyright © 2022 Hatlebakk, Kosobokova, Daase and Søreide. This is an open-access article distributed under the terms of the Creative Commons Attribution License (CC BY). The use, distribution or reproduction in other forums is permitted, provided the original author(s) and the copyright owner(s) are credited and that the original publication in this journal is cited, in accordance with accepted academic practice. No use, distribution or reproduction is permitted which does not comply with these terms.
*Correspondence: Maja Hatlebakk, bWFqYS5rLnYuaGF0bGViYWtrQG50bnUubm8=
†Present address: Maja Hatlebakk, Institute for Biology, Norwegian University of Science and Technology, Trondheim, Norway