- 1Fishery College, Guangdong Ocean University, Zhanjiang, China
- 2Guangdong Provincial Key Laboratory of Pathogenic Biology and Epidemiology for Aquatic Economic Animals, Zhanjiang, China
Combining feeding trials and metabolomics analyses of tissues and biofluids could shed light on nutrient effects and changes in feed intake. In the present study, hybrid grouper (F1 hybrid Epinephelus fuscoguttatus♀×Epinephelus polyphekadion♂) was used as the marine fish model to quantify the impacts of quercetin and sodium quercetin-5′-sulfonates on serum biochemistry and the status of hepatic lipid accumulation and the changes of metabolites in the liver using ultra-performance liquid chromatography-electrospray triple quadrupole mass spectrometry (UPLC-QTRAP/MS/MS). The study shows that total protein, albumin, alkaline phosphatase and glucose were not significantly different among the three groups (P > 0.05). Total cholesterol, triglyceride, and alanine aminotransferase of fish fed quercetin, and sodium quercetin-5′-sulfonates were significantly lower than fish fed without additives (P < 0.05). Low-density lipoprotein cholesterol and aspartate aminotransferase of fish fed quercetin were significantly lower than that of fish fed without additives and sodium quercetin-5′-sulfonates (P < 0.05). The high-density lipoprotein cholesterol of fish fed quercetin, and sodium quercetin-5′-sulfonates were significantly higher than that of fish fed without additives (P < 0.05). Lipid accumulation in the quercetin and sodium quercetin-5′-sulfonates groups decreased significantly. Quercetin and sodium quercetin-5′-sulfonates were effective by increasing hypolipidemic and hepatoprotective compounds that are known for reducing blood lipid levels and liver fat accumulation. As a result of this study, we provide international data for metabolic adaptations during the additives feeding using the fish liver as the study model. By understanding the metabolic effects of these feed additives, this study provides a first step toward understanding the molecular mechanisms of these additives and how they function.
Introduction
Aquaculture nutrition has evolved into a more efficient and sustainable industry with continuous feed formulation innovation over the last two decades. It usually depends on the fish oil (FO) and fish meal (FM) for fish nutrition at higher trophic levels, such as salmonids and marine species, since these nutrition resources provide adequate nutrition for the fish. Natural prey consumed by wild fish has the most similar nutritional composition to FM and FO diets. FM indeed contains highly digestible, high-quality proteins with an appropriate essential amino acid profile, and FO contains a high fatty acid profile suitable for many fish species, especially marine fish (Oliva-Teles et al., 2015; Roques et al., 2020). A shortage of marine resources for aquaculture will result from increased aquaculture production and other markets for pharmaceutics, cosmetics, and human nutrition (FAO, 2016; Roques et al., 2020). As a result, the aquaculture industry’s FM and FO market values will not be sustainable in the future. As a result of these constraints, FM and FO in fish feed need to be replaced by more sustainable raw materials. In the past decade, incorporating plant ingredients to replace marine ingredients has resulted in many enhancements in the formulation of sustainable diets. Since plant resources are now being used as feed for fish, persistent organic pollutants found in feed and fish have decreased (Berntssen et al., 2015). FO substitution is largely dependent on the supply of essential fatty acids. In fact, a number of marine species (Bell and Koppe, 2011; Roques et al., 2020) are unable to produce long-chain PUFAs from the nutritional poly-unsaturated fatty acids (PUFAs) linolenic and linoleic acid because of their enzymatic limitations. Furthermore, a decrease in the docosahexaenoic acid (DHA) and eicosapentaenoic acid (EPA) content in fish flesh results from substituting vegetable oils, while the other qualities of fish flesh remain the same (Oliva-Teles et al., 2015).
FM and FO are only about 10–15% of the total FM, and FO needed for fish feed (Medale et al., 2013; Ytrestøyl et al., 2015) to meet carnivorous and marine species’ nutritional needs. In this regard, replacing fish feed with fish resources is complicated for carnivorous fishes, and earlier researches have resulted in decreased growth rates and metabolic changes which are not compatible with the production of aquaculture (Geay et al., 2011; Collins et al., 2013). Hence, there is a need to include new feedstuffs in fish diets to compensate for these shortcomings, but researchers must first accurately characterise them to fully comprehend their impacts on fish metabolism.
Known as the most abundant flavonoid in foods (Petersen et al., 2016), quercetin (QE) has been linked to healthy and functional diets (Spagnuolo et al., 2012; Bigliardi and Galati, 2013). According to previous studies, QE mostly occurs in its glycosylated form in fruits and vegetables (Wang et al., 2020), exhibiting anti-cancer antiviral and anti-bacterial properties, neuroprotective effects, and anti-inflammatory properties beneficial to human health (Wang et al., 2020). Selective sulfonation of QE has been shown to exert liver-protective effects against heavy metal and carbon tetrachloride-induced liver damage in rodents and has potential as a chemopreventive and chemotherapeutic agent for liver disease (Cui et al., 2014). Notably, compared with the parent compound QE, sodium quercetin-5′-sulfonates (QS) showed stronger biological activity than Czerwonka et al. (2020) reported. Furthermore, QS was shown to have antioxidant (Robak and Kopacz, 1989), anti-bacterial (Woznicka et al., 2013) and antitumor activities (Krol et al., 2002) and exhibited hepatoprotective activity in rodent models stimulated by heavy metals (Szelag et al., 2003; Magdalan et al., 2007; Chlebda et al., 2010; Trocha et al., 2012). However, no studies have examined the impact of QE and QS on hybrid grouper (F1 hybrid Epinephelus fuscoguttatus♀×Epinephelus polyphekadion♂) metabolism, which needs to be studied further.
Typically, new feed formulae are evaluated by determining their analytic composition and digestibility and then evaluating their influences on growth performance, food intake, and further zootechnical indices. In spite of this, these techniques may not be complete enough to understand the effects of fish feed on the metabolism of fish. Chemical analyses of fish diets give a rough idea of the composition; nonetheless, they do not offer data about the profiles of small compounds, like non-essential nutrients such as creatine and taurine and other nutrition-related factors, and several under nutrition-related factors like phytic acid, polyphenols, or mycotoxins found in plant feeds (Glencross et al., 2007; Roques et al., 2020). An extensive and expensive analysis is needed for each of these factors and compounds. In metabolomics, the global set of metabolites in a biological system is investigated in order to gain insight into the system’s metabolic state. Combining feeding trials and metabolomics analyses of tissues and biofluids could shed light on nutrient effects and changes in feed intake.
Hepatic is a multifunctional organ with a high metabolic rate and biotransformation capacity, making it highly susceptible to drugs, feed, pollutants and toxins (Cuykx et al., 2018; Song et al., 2019; Zhou et al., 2019). Interestingly, hepatic is involved in the metabolism of QE and its derivatives and represents one of the essential metabolic organs (Wang et al., 2016b). Hybrid grouper is an essential marine aquaculture fish-breed developed through hybridisation technology and widely cultured in the southeast coast of China and other countries (Amenyogbe et al., 2020; Xie et al., 2021). The in vivo absorption, distribution, metabolism, and bioavailability of QE have been extensively studied in animal models and humans (Wang et al., 2016b). After digestion and absorption by the digestive system in vertebrates, QE forms glucuronic acid or sulfate derivatives in the liver (Wang et al., 2016b). The content and structure type of QE derivatives play a key role in their absorption and distribution (Spencer et al., 2008). Based on high performance liquid chromatography (HPLC) detection method, it was found that QE was mainly deposited in the body in the form of aglycone after being absorbed by tilapia, and the changes of QE concentration were found in plasma, liver and whole-body homogenate (Park et al., 2009). For a better insight into metabolic adjustment, serum biochemical parameters, and liver fat accumulation through feeding additives (QE and QS) to hybrid groupers, the liver hepatic metabolic profile was examined after 56 days of feeding.
Materials and Methods
Chemical Synthesis of QS
All chemicals and reagents are analytically pure and used without further purification. QE (97%, w/w) was purchased from Macklin Biochemical Co., Ltd (Shanghai, China). 1H NMR and 13C NMR spectra were measured at room temperature on a Bruker Avance III 400 MHz NMR spectrometer. Tetramethylsilane (TMS) served as the internal standard.
QS were prepared using the method outlined by Czerwonka et al. (2020). This method includes the following two steps: synthesis of quercetin-5′-sulfonic acid was carried out; the product of sulfonic acid was neutralised with NaOH solution to get the targeted material QS. In brief, the QE powder was added to the appropriate 98% sulfuric acid and put in an 80°C water bath for 2 h to heated with stirring. After the reaction, the mixture was cooled down to room temperature (25°C), and purified water was added while stirring continuously. The quercetin-5′-sulfonic acid precipitate was collected by filtration and then double crystalised from the saturated aqueous solution. The final product of QS was obtained by neutralising the synthesised acid in NaOH aqueous solution and double crystallising the resulting precipitate from the saturated water solution.
1H NMR (400 MHz, DMSO-d6) δ: 12.47 (1H, s, 5-OH), 10.98 (1H, s, 4’-OH), 10.78 (1H, s, 7-OH), 9.46 (1H, s, 3-OH), 9.26 (1H, s, 3’-OH), 7.87(1H, d, H-2’), 7.62(1H, d, H-6’), 6.40 (1H, d, H-8), 6.19(1H, d, H-6).
13C NMR (101 MHz, DMSO-d6) δ: 175.84 (C-4), 163.99 (C-7), 160.76 (C-5), 156.12 (C-9), 145.96 (C-2), 145.46 (C-3’), 144.20 (C-4’), 136.10 (C-3), 131.19 (C-5’), 120.81 (C-1’), 117.76 (C-2’), 115.37 (C-6’), 103.04 (C-10), 98.22 (C-6), 93.26 (C-8).
The molecular structure of the compound is shown in Figure 1.
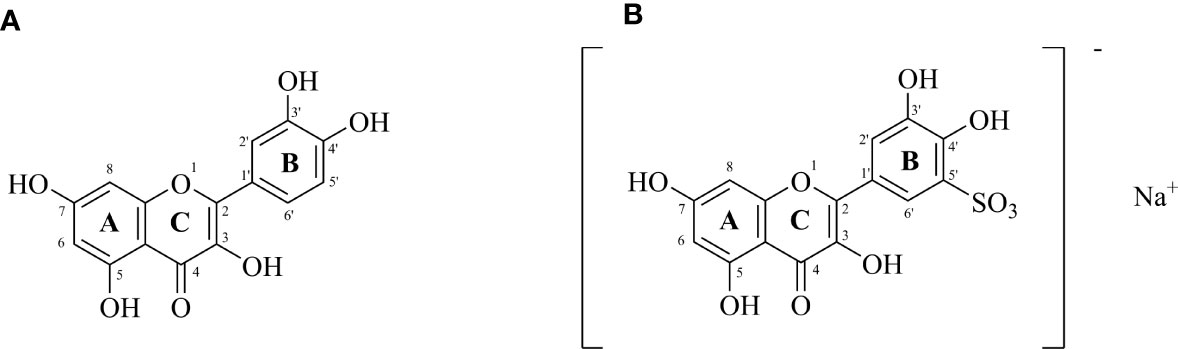
Figure 1 (A) Structure of quercetin (3,3′,4′,5,7-pentahydroxyflavone); (B) Sodium Quercetin -5’-Sulfonates (sodium 3,3’,4’,5,7-pentahydroxyflavon-5’-sulfonate) (Czerwonka et al., 2020).
Experimental Design and Diets
Referring to the study of grouper (Ye et al., 2020; Xie et al., 2021), the ingredients and proximate composition of the primary feed are presented in Tables 1, 2. We opted for QE dosage based on a previous study (Shin et al., 2010; Zhai and Liu, 2013; Xu et al., 2019). Group CT was fed a non-additive (QE or QS) diet; group QE was fed a diet containing 0.8 mmol/kg of QE; group QS was fed a diet containing 0.8 mmol/kg of QS. Before the feed was prepared, all the raw materials had to be crushed, sieved and weighed precisely according to the formula. Mixed all the powder ingredients, and then added oil and water mixed thoroughly with a groove-type mixer (CDH-100, Sichuan Chuanda Drying Technology Engineering Co., Ltd., China). In this study, dough with a diameter of 4 mm was wet extruded with a pelletiser (F-26, South China University of Technology, Guangzhou, China) and dried under air conditioning at 16°C, stored in a sealed bag at - 20°C until feeding.
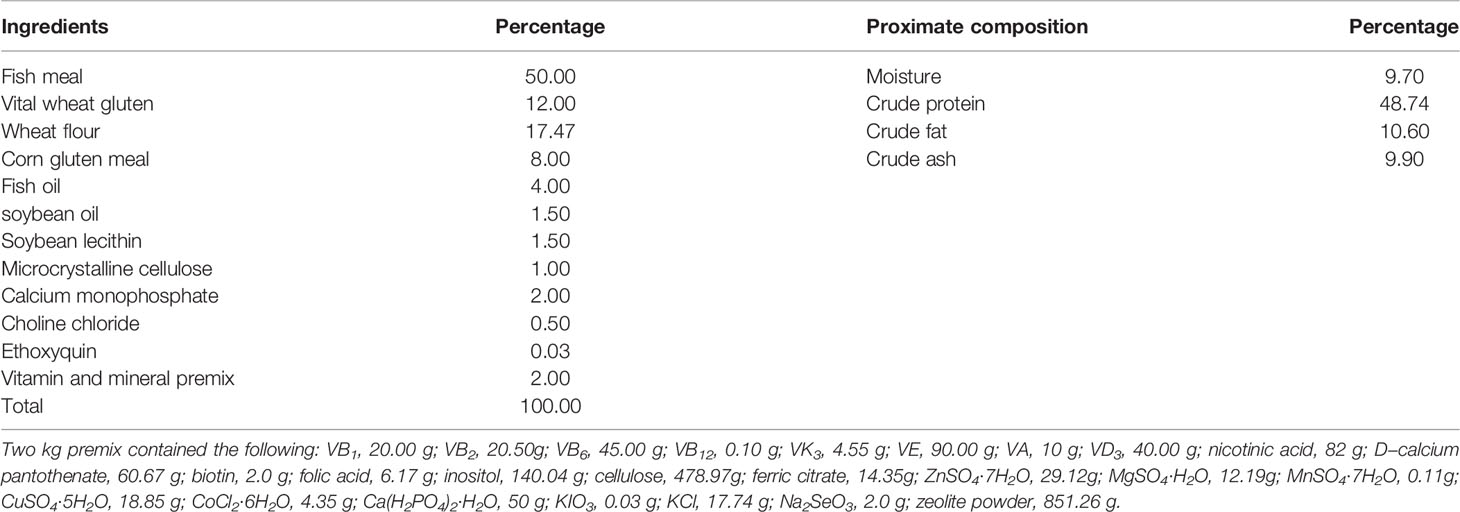
Table 1 Ingredients and proximate composition analysis (on dry weight basis) of the experimental diets.

Table 2 Preparation of quercetin mixture for the different additive levels used in experimental diets.
Experimental Conditions and Fish
Experimental fish were taken from the self-breeding and self-raising seedlings of Zhanjiang Ocean High-tech Park, Guangdong Ocean University. The fish were selected and transported with a special fish transportation vehicle to the breeding base of Guangdong Evergreen Feed Industry Co., Ltd., Zhanjiang, China. They were given the basal diet (crude protein ≥ 40%, crude fibre ≤ 5.0%, crude ash ≤ 16%, crude lipid ≥ 6%, moisture ≤ 12%, total phosphorus 0.90-1.60 and lysine ≥ 2.10) for two weeks to become accustomed to the experimental diets and farming conditions. After 24 hours of fasting, 225 fish (weighed 10.10 ± 0.02 g) were distributed evenly among 9 fibreglass tanks (500L). 25 fish per tank, 3 replicates per treatment. The experiment lasted eight weeks; fish were fed twice a day at 9:00 and 16:00 at 5 - 8% of their body weight. During this experimentation, the rearing water (flow-through natural seawater processed with sand filtration and sedimentation) was constantly aerated and maintained under the following conditions: temperature 25 - 29°C, pH 7.5 - 8.0, salinity 28.0 - 32.0g/L, dissolved oxygen 6 - 8 mg/L, and ammonia stayed 0.03 ± 0.01 mg/L. The fish were reared in a continuous 24-h ventilated flowing water culture system.
Sample Collection
Before sampling, the fish fasted for 24 hours at the end of the feeding trial and were weighed with the average fish size (54.36 ± 3.95 g); then, they were anesthetised with 8 mg/L methane-sulfonate (MS-222, Sigma-Aldrich). Blood samples were collected from the caudal vein of fish (n = 6 per tank) randomly selected from each tank and pooled together. The collected blood (approximately 0.7 mL per fish) was transferred into 1.5 mL anticoagulant-free centrifuge tubes and then stored at 4°C for 12 h. After centrifugation at 3500 rpm and 4°C for 15 min, the separated serum was obtained and immediately stored at -80°C.
Eighteen individuals of hybrid grouper were randomly selected for each treatment to collect livers for metabolomic analysis. Six (n = 6 per tank) liver samples from each replicate tank were pooled together in two tubes each. For histological analysis (Oil red O staining in the liver), the livers of two fishes from each tank were sampled. We immediately froze liver tissues in liquid nitrogen and stored them at -80°C. During sample collection, low temperatures and rapidity were maintained.
Biochemical Parameters in Serum
Cryopreserved serum samples were thawed on ice. The serum total protein (TP, A045-4), albumin (ALB, A028-2-1), total cholesterol (T-CHO, A111-1-1), triglyceride (TG, A110-1-1), alkaline phosphatase (AKP, A059-2), glucose (GLU, A154-1-1), low-density lipoprotein cholesterol (LDL, A113-1-1), high-density lipoprotein cholesterol (HDL, A112-1-1), aspartate aminotransferase (AST, C010-2-1) and alanine aminotransferase (ALT, C009-2-1) levels were measured using the methods described in the detection kits (Nanjing Jiancheng Bioengineering Institute, Jiangsu, China) through the use of a VICTOR Nivo Multimode Microplate Reader (PerkinElmer).
Oil Red O Staining
The trimmed liver tissue was embedded with an embedding agent (OCT) and then placed in a frozen sectioning machine for cryofixation. The sectioning was started after waiting for solidification. Frozen sections were stained in fresh oil red O staining solution for 8-10 min at room temperature, rinsed in tap water, and counterstained with hematoxylin until the desired degree of staining (Servicebio, China). Finally, glycerol jelly mounting medium was added to the sections, and the slides were sealed with cover glass. Lipid droplets were examined with the Nikon Eclipse microscope (Nikon, Japan). Quantification of the relative area of oil red O solution staining was performed using Image-Pro Plus 6.0 software. Six images of each sample were randomly selected, and the average relative area of Oil Red O staining was calculated to determine the relative content of lipid droplets.
Metabolomics Analysis
Extraction and Preparation of Samples
Hepatic samples were thawed on ice. Take 50 ± 2 mg of one sample, add cold steel balls to the mixture, and homogenate at 30 Hz for 3 min. Add 1 mL 70% methanol with internal standard extract to the homogenised centrifuge tube, whirl the mixture for 5 min, and then centrifuge it with 12,000 rpm at 4°C for 10 min. After centrifugation, draw 400 uL of supernatant into the corresponding EP tube and store in a -20°C refrigerator overnight, centrifuge at 12000 r/min at 4°C for 3 min, and take 200 uL of supernatant in the liner of the corresponding injection bottle for on-board analysis.
HPLC Conditions
Liver sample extracts were analyzed using an LC-ESI-MS/MS system (UPLC, ExionLC AD, https://sciex.com.cn/; MS, QTRAP® System, https://sciex.com/).The analytical conditions were as follows (Zhang et al., 2021), UPLC: column, Waters ACQUITY UPLC HSS T3 C18 (1.8 µm, 2.1 mm*100 mm); column temperature, 40°C; flow rate, 0.4 mL/min; injection volume, 2μL; solvent system, water (0.1% formic acid): acetonitrile (0.1% formic acid); gradient program, 95:5 V/V at 0 min, 10:90 V/V at 11.0 min, 10:90 V/V at 12.0 min, 95:5 V/V at 12.1 min, 95:5 V/V at 14.0 min.
ESI-QTRAP-MS/MS
LIT and triple quadrupole (QQQ) scans were acquired on a triple quadrupole-linear ion trap mass spectrometer (QTRAP), QTRAP® LC-MS/MS System, equipped with an ESI Turbo Ion-Spray interface, operating in positive and negative ion mode and controlled by Analyst 1.6.3 software (Sciex). The ESI source operation parameters were as follows (Li et al., 2022): source temperature 500°C; ion spray voltage (IS) 5500 V (positive), -4500 V (negative); ion source gas I (GSI), gas II (GSII), curtain gas (CUR) were set at 55, 60, and 25.0 psi, respectively; the collision gas (CAD) was high. Instrument tuning and mass calibration were performed with 10 and 100 μmol/L polypropylene glycol solutions in QQQ and LIT modes. A specific set of MRM transitions were monitored for each period according to the metabolites eluted within this period.
Data Processing and Statistical Analysis
MS data acquisition and processing were performed previously described (Chen et al., 2013). Metabolites were annotated using the Metware in-house MS2 spectral tag (MS2T) library (Wuhan Metware Biotechnology Co., Ltd.; http://www.metware.cn, Wuhan, China). Differential variables of the metabolites were analysed using orthogonal partial least squares-discriminant analysis (OPLS-DA). VIP ≥ 1 with fold change ≥ 2 or fold change ≤ 0.5 was considered statistically significant. The results of the biochemical indexes were presented as the mean ± standard error (SEM). The experimental data were subjected to one-way ANOVA. Multiple treatment groups were compared by Duncan’s honestly significant difference test at P ≤ 0.05. Statistical analysis of all data was performed using SPSS 22.0 software (SPSS, Inc., Chicago, IL, USA).
Results
Serum Biochemical Indices
Table 3 presents the effects of different diets on serum biochemical parameters in grouper. The result shows that TP, ALB, AKP and GLU were not significantly different among the three groups (P > 0.05). T-CHO, TG and ALT of fish fed QE and QS were significantly lower than those of fish fed CT (P < 0.05). LDL and AST of fish fed QE were significantly lower than that of fish fed CT and QS (P < 0.05). The HDL of fish fed QE and QS was significantly higher than those fish fed CT (P < 0.05).
Lipid Accumulation in the Liver of Fish Fed Different Diets
For the comparison of lipid accumulation in the liver, oil red O staining was performed, shown in Figure 2. Lipid droplets and nuclei were stained red and blue for oil red O staining, respectively. The CT group showed more red spots and lipid droplets than the QE and QS groups in the present study. Compared to the CT group (25.67%), lipid accumulation in the QE and QS groups decreased significantly to 19.55% and 19.32%, respectively (P < 0.05).
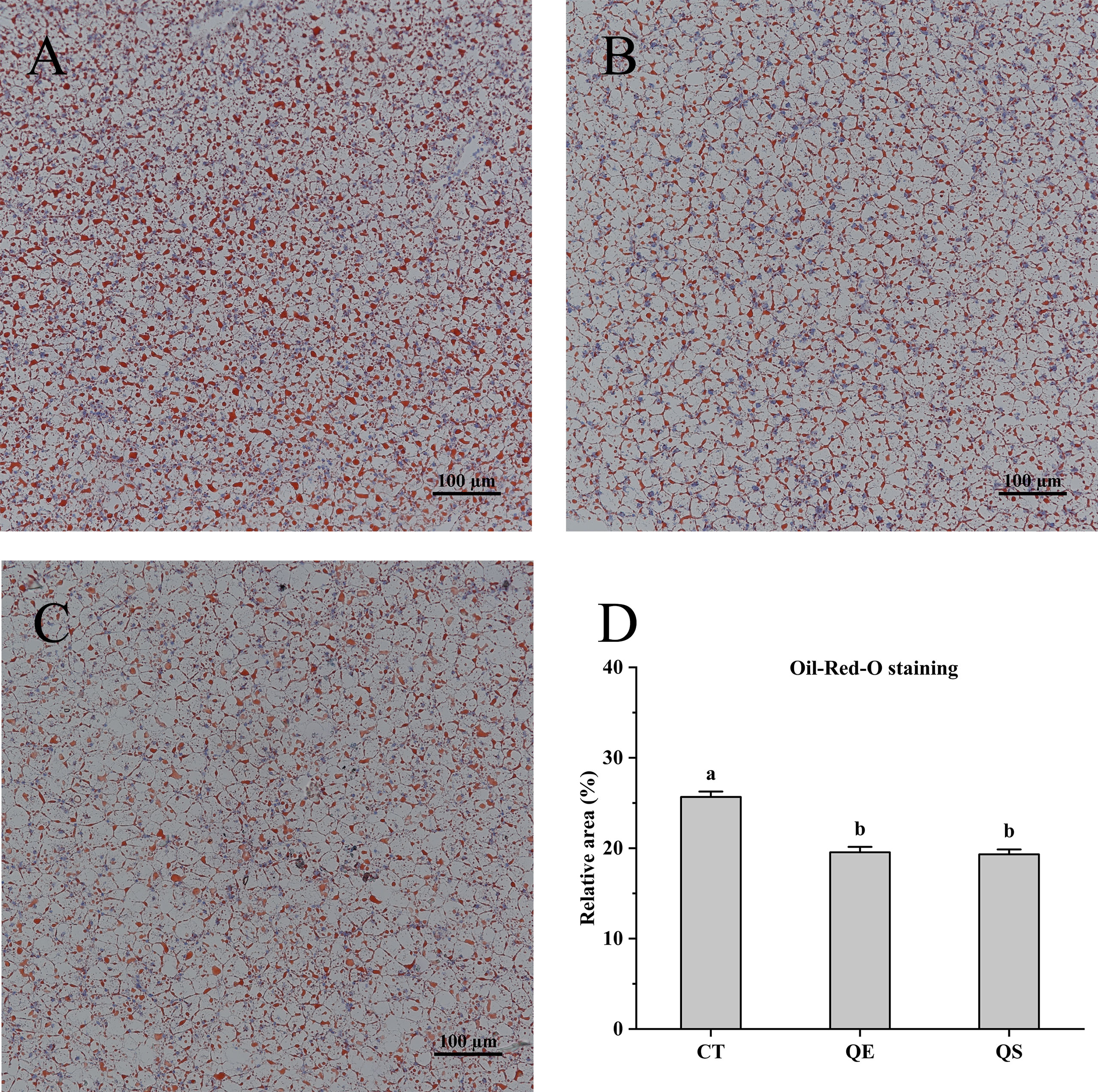
Figure 2 Hepatocyte oil red O (ORO) staining section of hybrid grouper treated with different diets (Magnification × 20). (A) CT; (B) QE; (C) QS; (D) The relative area of red lipid droplets in the hepatic section area (%). Values were presented as means ± SEM, and bars with different letters are significantly different (P < 0.05).
Differential Metabolites in the Liver in Response to QE Treatment
To investigate changes in liver metabolites in QE-treated hybrid grouper, livers from the CT and QE groups were collected for an extensive targeted metabolomic analysis. OPLS-DA model was used to determine metabolite differences between the CT and QE groups. OPLS-DA combines orthogonal signal correction (OSC) and PLS-DA methods to screen for differential variables by removing uncorrelated differences, allowing further indication of differences between groups. OPLS-DA scores clearly classify samples into distinct clusters, indicating significant differences in metabolites between the CT and QE groups. The number of differential metabolites is clearly shown in the OPLS-DA S plot. A total of 91 differential metabolites (Supplementary Table S1) were detected in the CT and QE groups (VIP ≥ 1, fold change ≥ 2 or ≤ 0.5). After qualitative and quantitative analysis of the detected differential metabolites, the results of the metabolites with the top-ranked changes are presented in Figure 3C. Differential metabolites from CT vs. QE could be divided into 11 categories, mainly from fatty acyl groups (FA; 42.86%) and amino acids and their metabolites (21.98%). Oxylipins accounted for 71.05% of FA.
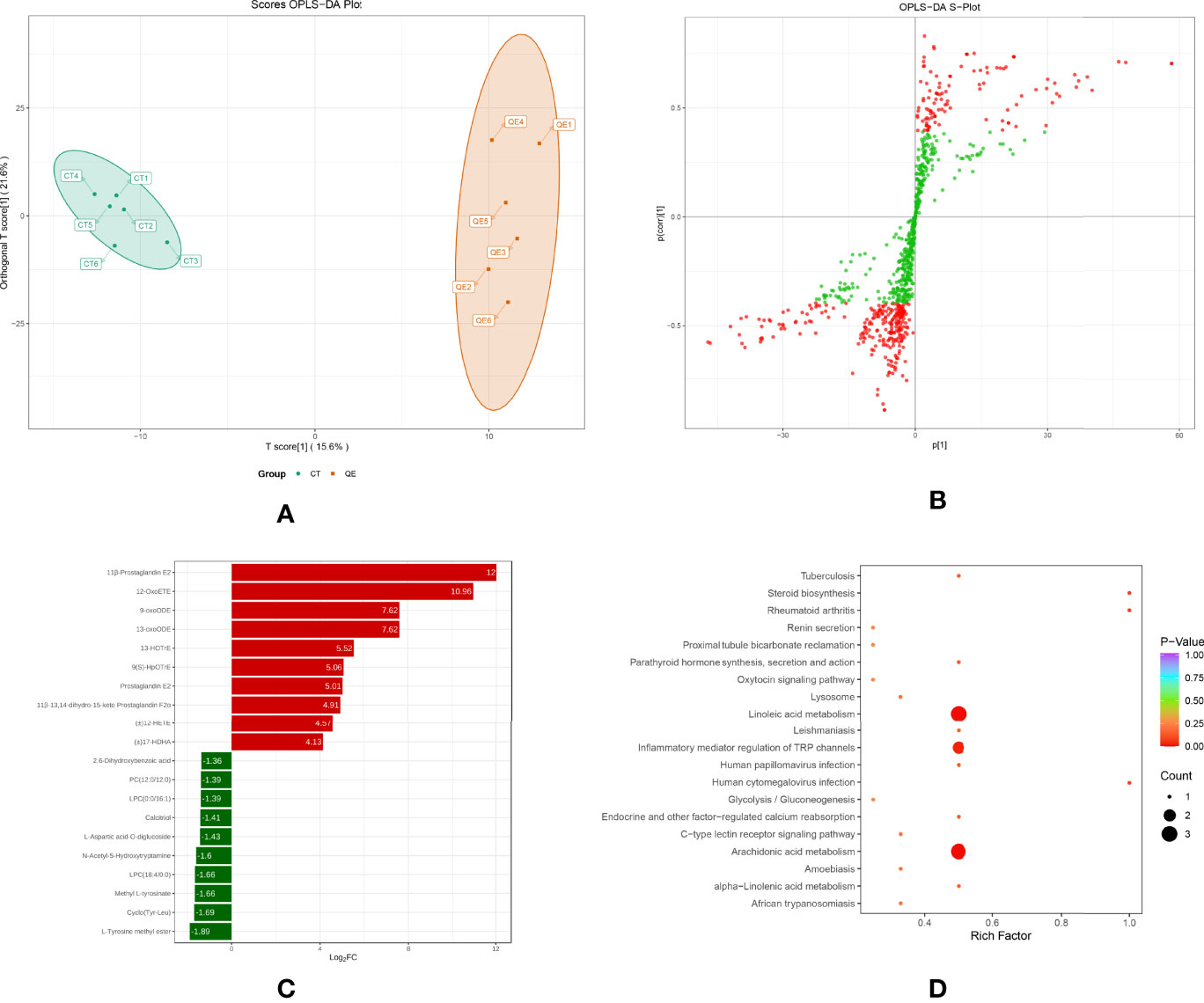
Figure 3 Effect of QE on oxylipins in the liver of hybrid grouper. (A) The OPLS-DA scores of the CT group (green) and QE group (red). (B) The OPLS-DA S-plot. The X-axis indicates the correlation coefficient between the principal components and oxylipins, and the Y-axis indicates the correlation coefficient between the principal components and oxylipins. The red dot represents VIP ≥ 1, and the green dot represents VIP ≤ 1. (C) Bar chart showing the differential oxylipins in the CT group (green) and QE group (red). (D) Differential oxylipins enrich KEGG pathways in the CT group and QE group. (VIP ≥ 1, fold change ≥ 2 or ≤ 0.5, n = 6).
Table 4 classifies 28 different oxylipins, with the most derived from arachidonic acid (ARA, 35.71%), docosahexaenoic acid (DHA, 21.43%) derived oxylipins ranking second, and linoleic acid (LA, 14.29%), alpha-linolenic acid (ALA, 10.71%) and eicosapentaenoic acid (EPA, 10.71%) derived oxylipins as follows. Further KEGG analysis showed that arachidonic acid metabolism, inflammatory mediator regulation of TRP channels and linoleic acid metabolism were significantly enriched after QE supplementation (Figure 3D).
Effect of QS on Metabolites in the Liver of Hybrid Grouper
To further investigate the effect of QS on the liver metabolites of hybrid grouper, liver tissues from the QS group were also analysed. A total of 83 differential metabolites (Supplementary Table S2) were identified (VIP ≥ 1, fold change ≥ 2 or ≤ 0.5), and the results of OPLS-DA and OPLS-DA S plots are shown in Figures 4A, 4B. Among the differential metabolite, 11β-Prostaglandin E2 (11β-PGE2) was the top metabolite that increased the most (Figure 4C). The total differential metabolites CT vs. QS could be divided into 10 main categories, of which 42.17% belonged to FA and 36.14% to amino acids and their metabolites. Among the 35 FA species, 29 belonged to oxylipins.
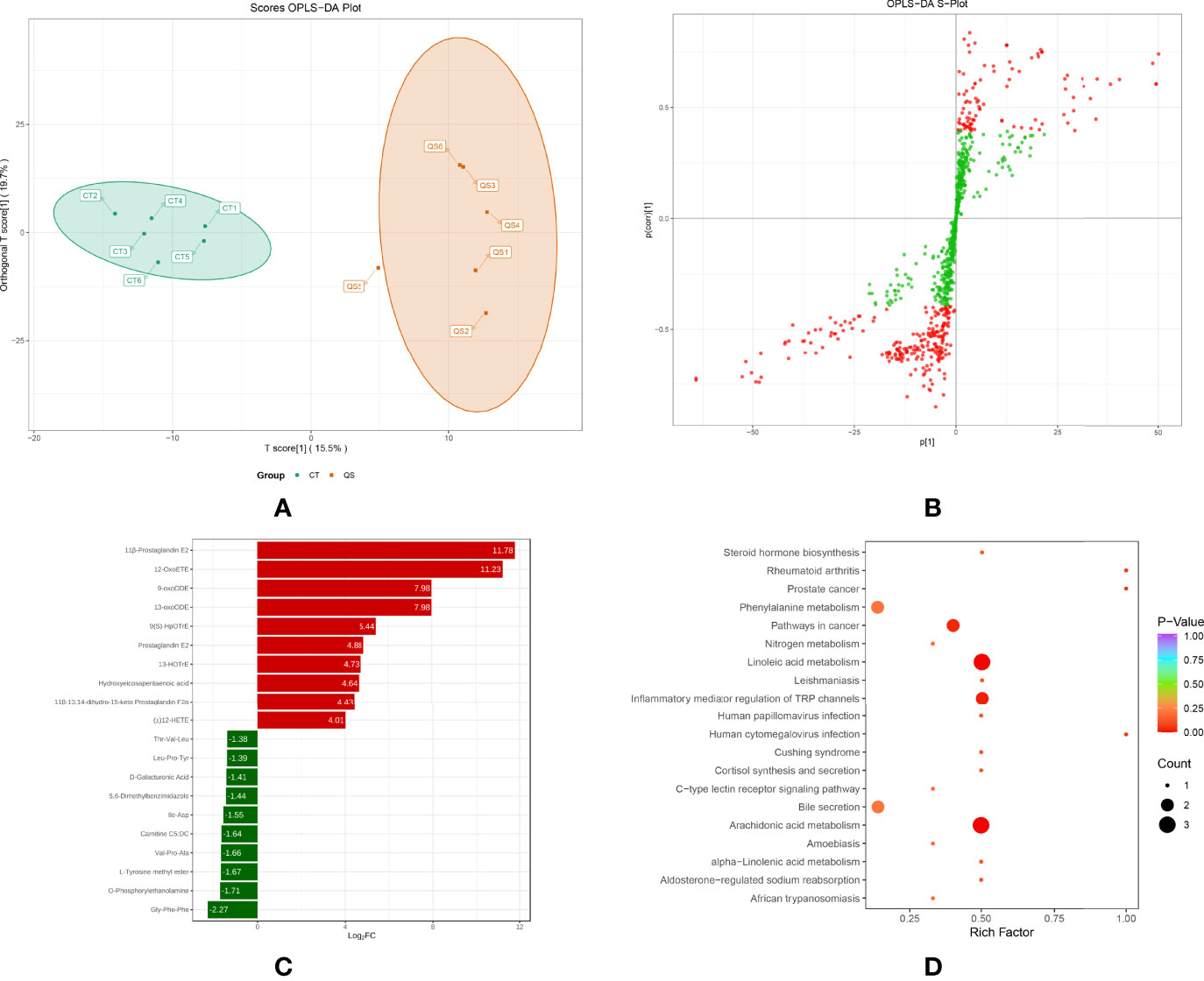
Figure 4 Effect of QS on oxylipins in the liver of hybrid grouper. (A) OPLS-DA scores (B) OPLS-DA S-plot. (C) Bar chart showing the differential oxylipins in the CT group (green) and QS group (red). (D) Differential oxylipins enriched KEGG pathways in the CT group and QS group. (VIP ≥ 1, fold change ≥ 2 or ≤ 0.5, n = 6).
Table 5 classifies 29 different oxylipins, of which the most derived from ARA (31.03%), DHA (27.59%) derived oxylipins ranked second, and LA (13.79%), ALA (10.34%) and EPA (10.34%) derived oxylipins were as follows. Further KEGG analysis showed that arachidonic acid metabolism, inflammatory mediator regulation of TRP channels and linoleic acid metabolism were significantly enriched after QE supplementation (Figure 4D).
Discussion
Changes in serum biochemical indicators reflect the physiological and metabolic status of fish, which is helpful for us to determine the response of fish to dietary supplements (Tan et al., 2018; Xie et al., 2021). In general, if lipid parameters including TG, T-CHO, and LDL increase and HDL in fish decreases, is an indication that fish may have some lipid metabolic disorders and liver damage (Zhai and Liu, 2013). In our results, QE and QS decreased serum TG, T-CHO, LDL levels and increased HDL levels in hybrid grouper. Consistent with our findings, Shin et al. (2010) reported that feeding diets supplemented with 0.25% or 0.50% QE significantly reduced T-CHO levels in flounder, with 60 days of feeding better than 30 days. Repeated use significantly increases the bioavailability of QE (Rangel-Ordonez et al., 2010). Zhai and Liu (2013) indicated that QE could decrease serum TG levels and increase HDL level in tilapia. In addition, QE has a modulating effect on low-density lipoprotein receptor expression (Moon et al., 2012). It reduces blood lipids by increasing the rate of LDL clearance from the blood. Similar results with QE for lowering serum or liver lipids have been reported in rats (Padma et al., 2012), chickens (Qureshi et al., 2011), and rabbits (Kamada et al., 2005), and humans (Egert et al., 2010). In this study, the sulfonated product QS obtained consistent results with QE, indicating that QE and QS showed potential activity in lowering cholesterol and triglycerides and helping to avoid pathological changes in the fatty liver.
QE has preventive and therapeutic effects on hepatic damage, such as fatty liver, cirrhosis and liver fibrosis (Miltonprabu et al., 2017). Cui et al. (2014) revealed that QE significantly reduced the activity of serum AST and ALT in rats. AST and ALT plays an important role in the liver’s metabolic function. Increased ALT enzyme activity is an indicator of the degree of liver cell membrane damage, and increased AST level is another indicator of liver mitochondrial damage (Xu et al., 2010; Yang et al., 2011). In this study, QE and QS significantly reduced the levels of AST and ALT in the serum of hybrid grouper and had the effect on protecting the liver. On this basis, it is suggested that both QE and QS can stabilise the liver cell membrane and have a protective effect on mitochondria. Cui et al. (2014) believe that QE and quercetin-5’,8-disulfonate (QDS) effectively attenuate the increase of AST and ALT in the serum of mice induced by carbon tetrachloride, and the selective sulfonation of QE increases the hepatoprotection effect. Water-soluble QDS was more effective than QE in reducing AST and ALT release.
In contrast, QE was more potent than QS in our results, probably due to the high dose of QS. Remarkably, Significant increases in markers of hepatotoxicity (AST and ALT) were found in mice injected with high doses of QE (1500 and 2000 mg/kg), mediated in part through oxidative stress (Singh et al., 2022). Related findings were found in acute doses of apigenin (Singh et al., 2012) and genistein (flavonoids) (Singh et al., 2014). From a molecular point of view, the toxic effects of QE are likely related to the oxidation of QE to potentially toxic products during ROS scavenging, namely quercetin-quinine, which is highly reactive with thiols, and GSH might be the principal reactant (Galati et al., 2001; Awad et al., 2002; Boots et al., 2007).
In fish, the liver is the primary site for lipid synthesis and storage (Martin et al., 2017; Zhou et al., 2019). Lipid droplets (LDs) are intracellular organelles dedicated to storing energy in the form of neutral lipids and are involved in lipid metabolism (Welte and Gould, 2017). According to previous studies, QE treatment attenuate inflammation and fibrosis in mice nonalcoholic fatty liver disease (NAFLD), alleviate hepatic fat accumulation (Marcolin et al., 2012; Liu et al., 2018). Thus, the current study also observed the lipid accumulation status in hepatic by oil red sections. ORO results show that QE and QS treatments significantly reduced the lipid droplet area in the liver of hybrid grouper, indicating that QE and QS helped to reduce liver fat accumulation. Derivatives of QE have also revealed consistent results in in vitro and in vivo research (Yu et al., 2016; Qin et al., 2018). Amelioration of fat deposition by QE and its derivatives was probably related to the regulation of adipogenic gene expression and antioxidant function (Jung et al., 2013; Wang et al., 2016a; Liu et al., 2018).
This study provides basic metabolic profiling data for studying the marine fish liver response to QE supplementation by ultra-performance liquid chromatography-electrospray ionization triple quadrupole mass spectrometry (UPLC-QTRAP/MS/MS). These data increased our understanding of QE. Indeed, a study has explored the metabolism of QE (Wang et al., 2016b). On this basis, it has been suggested that high phenolic intake increases intestinal hippurate production (Mullen et al., 2008; de Mello et al., 2017; Ulaszewska et al., 2020). However, only dogs produce hippuric acid primarily in the kidneys; in other species, such as mice and rats, hippuric acid production depends on hepatocytes (Toromanovic et al., 2008; Lees et al., 2013). In particular, most QE-derived metabolites are identified as 3-hydroxyphenylacetic acid, benzoic acid and hippuric acid (Mullen et al., 2008). In this experiment, hepatic hippuric acid was significantly increased after dietary QE and QS (Tables S1, S2), indicating that the liver is directly or indirectly involved in the metabolism of QE and QS in fish.
Analysing the metabolomic data, supplementation with QE and QS had the greatest effect on oxylipins metabolites in vivo. Oxylipins are a class of bioactive lipids that play important roles in regulating the intensity and duration of inflammatory responses as well as tissue repair, blood coagulation, vascular permeability and energy regulation (Dennis and Norris, 2015). Therefore, we focused on further analysis of oxylipins in FA. Oxylipins are oxidised PUFAs; depending on the source of their parent PUFAs, oxylipins can be divided into two categories: oxylipins derived from omega-6 PUFAs, such as ARA and LA; and those derived from omega-3 PUFAs, such as α-Linolenic acid (18:3n-3) (ALA), eicosapentaenoic acid (20:5n-3) (EPA) and docosahexaenoic acid (22:6n-3) (DHA) (Gabbs et al., 2015; Picklo and Newman, 2015). Studies showed that oxylipins are rapidly produced and eliminated in the body, and the detectable oxylipins represent their biologically active form (Shaik et al., 2013; Barquissau et al., 2017). A large number of oxylipins were detected in this experiment, indicating that the basic physiological reactions requiring the participation of oxylipins may have been going on in the fish liver all the time.
ARA-derived oxylipins are more diverse and are thought to play important roles in most tissues and organs (Leng et al., 2018; Gao et al., 2021). In the present study, 28 and 29 oxylipins were found in the livers of QE and QS, respectively, most of which were ARA-derived (Tables 4, 5). KEGG annotation also showed that ARA metabolism was mainly enriched (Figures 3, 4), suggesting that QE and QS may be involved in the body’s basal metabolism by regulating ARA levels. Studies on oxylipins in fish are relatively scarce; however, a recent study found 57 targeted lipids of five major n-6 and n-3 PUFAs in the plasma of large yellow croaker and rainbow trout, approximately 42.1% from ARA (Gao et al., 2021). In addition, Leng et al. (2018) identified the 70 oxylipins quantified in the rat liver, about two-thirds of the oxylipins were derived from n-6 PUFAs, and ARA-derived oxylipins accounted for about two-thirds of them. Of the 87 oxylipins present in the rat brain, half (51%) were derived from ARA, accounting for 81-90% of the total oxylipins mass (Ferdouse et al., 2019b). It is speculated that the predominant oxylipins in animals may be roughly similar even with different dietary conditions; that is, ARA-type oxylipins generally have more types and qualities, suggesting that they may play an important role in life activities.
Oxylipins show a wide range of functions, most of which are still being discovered. Interestingly, oxylipins obtained from different PUFA substrates and pathways can have similar or opposite effects so it is important to fully identify the entire oxylipins profile to properly understand their overall biological effects (Deline et al., 2015). In this study, in addition to ARA, EPA and DHA were also significantly increased after QE and QS treatment. Studies have shown that n-3 EPA and DHA have antithrombotic and antiarrhythmic properties (Endo and Arita, 2016; Lee et al., 2016a). In addition, the n-3 fatty acid EPA has a strong anti-inflammatory effect and can affect the T cell response to infection (Wu and Meydani, 1998; Harris et al., 2000). Based on the significant health benefits of EPA and DHA, many studies have begun using EPA and DHA dietary supplements to increase the content of n-3 PUFA oxylipins. Dietary supplements EPA and DHA increased anti-inflammatory n-3 LC-PUFA oxylipins and decreased pro-inflammatory n-6 oxylipins in plasma, liver, kidney, heart, brain, and other tissues (Leng et al., 2018; Ferdouse et al., 2019a; Rey et al., 2019; Kutzner et al., 2020). As a low-toxic natural flavonoid, QE will find more meaningful value and far-reaching effects if further studies on ARA, EPA, and DHA respond to treatments.
Furthermore, prostaglandin E2 (PGE2) and prostaglandin F2α (PGF2α) have inhibitory effects on adipogenesis and can inhibit the early differentiation of adipocytes (Stewart and Fisher, 2015; Barquissau et al., 2017). In the study, PGE2 was significantly increased after QE and QS treatment (Table S1, S2). Increased synthesis and release of PGE2 and PGF2α activate prostaglandin membrane receptors that bind to PGE2 and PGF2α, resulting in sustained calcium oscillations that inhibit the expression of PPARγ target genes, including the uncoupling protein 1 (UCP1) gene, and ultimately inhibit the white adipose tissue browning (Stewart and Fisher, 2015). In this study, 11β-PGE2 was significantly increased after treatment, presumably related to the significant increase in PGE2. Due to its properties, including activation of endogenous stem cells, immunoregulation, and angiogenesis, PGE2 plays a vital role in regenerating various organ systems following injury. As a result, PGE2 can help to reduce inflammation, fibrosis, necrosis, and other adverse effects of liver ischemia or reperfusion injury (Cheng et al., 2021). There is convincing evidence in vitro and in vivo that PGE2 signalling pathways protect various organs from inflammation, oxidative stress, and fibrosis. Hence the present study suggests that QE and QS help to increase the PGE2 production, which could be beneficial to the hybrid grouper, but further studies are needed to elucidate the exact role.
Conclusion
In conclusion, UPLC-QTRAP/MS/MS study of hybrid grouper liver samples brought to light universal information concerning the metabolic metabolism of the liver in response to nutritional additives QE and QS. In addition to providing new ideas for studying feeding additives, these data confirmed the existing research results. This study showed that QE and QS effectively increase hypolipidemic and hepatoprotective compounds that are known for reducing blood lipid levels and liver fat accumulation in groupers. Additionally, we found that QE and QS altered oxylipin levels in the liver of grouper fish, suggesting that the QE and QS are partly mediated through the regulation of oxylipins. There are similarities in the biological effects of quercetin and its sulfonated derivatives. It is believed that sulfation in the structural transformation of flavonoids may provide a novel mechanism to regulate their bioavailability and bioactivity, and further research is needed to evaluate the mechanism underlying the hepatoprotective effects of QE and QS.
Data Availability Statement
The original contributions presented in the study are included in the article/Supplementary Material. Further inquiries can be directed to the corresponding author.
Ethics Statement
This study was conducted following the regulations for the administration of laboratory animals in Guangdong province, China, reviewed and approved by the Guangdong Ocean University Research Council for the care and use of laboratory animals (approval number: GDOU-LAE-2021-021).
Author Contributions
JL was involved in project administration, data collection, formal analysis, processing, and writing of the original draft. EA was involved in writing – original draft and writing – reviewing and editing. J-SH supervision and involvement in resources. GC: conceptualised, involved in methodology, acquired funding, supervision and involved in resources. All authors contributed to the article and approved the submitted version.
Funding
This work was supported by grants from the Development Program of China, Blue Granary Technology Innovation Key Special Project (2020YFD0900200) and Guangdong Provincial Science and Technology program (2016B0201009).
Conflict of Interest
The authors declare that the research was conducted in the absence of any commercial or financial relationships that could be construed as a potential conflict of interest.
Publisher’s Note
All claims expressed in this article are solely those of the authors and do not necessarily represent those of their affiliated organizations, or those of the publisher, the editors and the reviewers. Any product that may be evaluated in this article, or claim that may be made by its manufacturer, is not guaranteed or endorsed by the publisher.
Acknowledgments
Our acknowledgement goes to all the founders of this study.
Supplementary Material
The Supplementary Material for this article can be found online at: https://www.frontiersin.org/articles/10.3389/fmars.2022.891080/full#supplementary-material
References
Amenyogbe E., Chen G., Wang Z. (2020). Identification, Characterisation, and Expressions Profile Analysis of Growth Hormone Receptors (GHR1 and GHR2) in Hybrid Grouper (Epinephelus Fuscoguttatus Female X Epinephelus Polyphekadion Male). Genomics 112 (1), 1–9. doi: 10.1016/j.ygeno.2019.05.012
Awad H. M., Boersma M. G., Boeren S., van Bladeren P. J., Vervoort J., Rietjens I. (2002). The Regioselectivity of Glutathione Adduct Formation With Flavonoid Quinone/Quinone Methides is pH-Dependent. Chem. Res. Toxicol. 15 (3), 343–351. doi: 10.1021/tx010132l
Barquissau V., Ghandour R. A., Ailhaud G., Klingenspor M., Langin D., Amri E.-Z., et al. (2017). Control of Adipogenesis by Oxylipins, GPCRs and PPARs. Biochimie. 136, 3–11. doi: 10.1016/j.biochi.2016.12.012
Bell J., Koppe W. (2011). “Lipids in Aquafeeds,” in Fish Oil Replacement and Alternative Lipid Sources in Aquaculture Feeds. Eds. Turchini G. M., Ng W.-K., Tocher D. R. (Boca Raton Fla: CRC Press), 21–59.
Berntssen M. H. G., Ornsrud R., Hamre K., Lie K. K. (2015). Polyaromatic Hydrocarbons in Aquafeeds, Source, Effects and Potential Implications for Vitamin Status of Farmed Fish Species: A Review. Aquacult Nutr. 21 (3), 257–273. doi: 10.1111/anu.12309
Bigliardi B., Galati F. (2013). Innovation Trends in the Food Industry: The Case of Functional Foods. Trends Food Sci. Technol. 31, 118–129. doi: 10.1016/j.tifs.2013.03.006
Boots A. W., Li H., Schins R. P. F., Duffin R., Heemskerk J. W. M., Bast A., et al. (2007). The Quercetin Paradox. Toxicol. Appl. Pharmacol. 222 (1), 89–96. doi: 10.1016/j.taap.2007.04.004
Cheng H., Huang H., Guo Z., Chang Y., Li Z. (2021). Role of Prostaglandin E2 in Tissue Repair and Regeneration. Theranostics 11 (18), 8836–8854. doi: 10.7150/thno.63396
Chen W., Gong L., Guo Z., Wang W., Zhang H., Liu X., et al. (2013). A Novel Integrated Method for Large-Scale Detection, Identification, and Quantification of Widely Targeted Metabolites: Application in the Study of Rice Metabolomics. Mol. Plant 6 (6), 1769–1780. doi: 10.1093/mp/sst080
Chlebda E., Magdalan J., Merwid-Lad A., Trocha M., Kopacz M., Kuzniar A., et al. (2010). Influence of Water-Soluble Flavonoids, Quercetin-5 ’-Sulfonic Acid Sodium Salt and Morin-5 ’-Sulfonic Acid Sodium Salt, on Antioxidant Parameters in the Subacute Cadmium Intoxication Mouse Model. Exp. Toxicol. Pathol. 62 (2), 105–108. doi: 10.1016/j.etp.2009.02.118
Collins S. A., Øverland M., Skrede A., Drew M. D. (2013). Effect of Plant Protein Sources on Growth Rate in Salmonids: Meta-Analysis of Dietary Inclusion of Soybean, Pea and Canola/Rapeseed Meals and Protein Concentrates. Aquaculture 400–401, 85–100. doi: 10.1016/j.aquaculture.2013.03.006
Cui Y., Han Y., Yang X., Sun Y., Zhao Y. (2014). Protective Effects of Quercetin and Quercetin-5 ’,8-Disulfonate Against Carbon Tetrachloride-Caused Oxidative Liver Injury in Mice. Molecules 19 (1), 291–305. doi: 10.3390/molecules19010291
Cuykx M., Rodrigues R. M., Laukens K., Vanhaecke T., Covaci A. (2018). In Vitro Assessment of Hepatotoxicity by Metabolomics: A Review. Arch. Toxicol. 92 (10), 3007–3029. doi: 10.1007/s00204-018-2286-9
Czerwonka A., Maciolek U., Kalafut J., Mendyk E., Kuzniar A., Rzeski W. (2020). Anti-Cancer Effects of Sodium and Potassium Quercetin-5 ’-Sulfonates Through Inhibition of Proliferation, Induction of Apoptosis, and Cell Cycle Arrest in the HT-29 Human Adenocarcinoma Cell Line. Bioorg. Chem. 94, 103426. doi: 10.1016/j.bioorg.2019.103426
Deline M., Keller J., Rothe M., Schunck W.-H., Menzel R., Watts J. L. (2015). Epoxides Derived From Dietary Dihomo-Gamma-Linolenic Acid Induce Germ Cell Death in C. Elegans. Sci. Rep. 5, 15417. doi: 10.1038/srep15417
de Mello V. D., Lankinen M. A., Lindstrom J., Puupponen-Pimia R., Laaksonen D. E., Pihlajamaki J., et al. (2017). Fasting Serum Hippuric Acid Is Elevated After Bilberry (Vaccinium Myrtillus) Consumption and Associates With Improvement of Fasting Glucose Levels and Insulin Secretion in Persons at High Risk of Developing Type 2 Diabetes. Mol. Nutr. Food Res. 61 (9), 1700019. doi: 10.1002/mnfr.201700019
Dennis E. A., Norris P. C. (2015). Eicosanoid Storm in Infection and Inflammation. Nat. Rev. Immunol. 15 (8), 511–523. doi: 10.1038/nri3859
Egert S., Boesch-Saadatmandi C., Wolffram S., Rimbach G., Mueller M. J. (2010). Serum Lipid and Blood Pressure Responses to Quercetin Vary in Overweight Patients by Apolipoprotein E Genotype. J. Nutr. 140 (2), 278–284. doi: 10.3945/jn.109.117655
Endo J., Arita M. (2016). Cardioprotective Mechanism of Omega-3 Poly-Unsaturated Fatty Acids. J. Cardiol. 67 (1-2), 22–27. doi: 10.1016/j.jjcc.2015.08.002
FAO. (2016). La Situation Mondiale Des Pêches Et De L’aquaculture 2016 (Rome (I: Contribuer à la sécurité alimentaire et à la nutrition de tous FAO). FAO. 2016.
Ferdouse A., Leng S., Winter T., Aukema H. M. (2019a). Dietary N-6 and N-3 PUFA Alter the Free Oxylipin Profile Differently in Male and Female Rat Hearts. Br. J. Nutr. 122 (3), 252–261. doi: 10.1017/s0007114519001211
Ferdouse A., Leng S., Winter T., Aukema H. M. (2019b). The Brain Oxylipin Profile Is Resistant to Modulation by Dietary N-6 and N-3 Polyunsaturated Fatty Acids in Male and Female Rats. Lipids 54 (1), 67–80. doi: 10.1002/lipd.12122
Gabbs M., Leng S., Devassy J. G., Monirujjaman M., Aukema H. M. (2015). Advances in Our Understanding of Oxylipins Derived From Dietary PUFAs. Adv. Nutr. 6 (5), 513–540. doi: 10.3945/an.114.007732
Galati G., Moridani M. Y., Chan T. S., O’Brien P. J. (2001). Peroxidative Metabolism of Apigenin and Naringenin Versus Luteolin and Quercetin: Glutathione Oxidation and Conjugation. Free Radical Biol. Med. 30 (4), 370–382. doi: 10.1016/s0891-5849(00)00481-0
Gao S., Cui K., Li Y., Pang Y., Fang W., Mai K., et al. (2021). Comparation of Oxylipin Profiles as Well as Their Substrates and Synthetic Enzymes Transcriptional Expression Between Marine Fish Larimichthys Crocea and Freshwater Fish Oncorhynchus Mykiss. Aquaculture 539, 736641. doi: 10.1016/j.aquaculture.2021.736641
Geay F., Ferraresso S., Zambonino-Infante J. L., Bargelloni L., Quentel C., Vandeputte M., et al. (2011). Effects of the Total Replacement of Fish-Based Diet With Plant-Based Diet on the Hepatic Transcriptome of Two European Sea Bass (Dicentrarchus Labrax) Half-Sibfamilies Showing Different Growth Rates With the Plant-Based Diet. BMC Genomics 12, 522. doi: 10.1186/1471-2164-12-522
Glencross B. D., Booth M., Allan G. L. (2007). A Feed is Only as Good as its Ingredients - A Review of Ingredient Evaluation Strategies for Aquaculture Feeds. Aquacult. Nutr. 13 (1), 17–34. doi: 10.1111/j.1365-2095.2007.00450.x
Harris H. W., Gosnell J. E., Kumwenda Z. L. (2000). The Lipemia of Sepsis: Triglyceride-Rich Lipoproteins as Agents of Innate Immunity. J. endotoxin Res. 6 (6), 421–430. doi: 10.1179/096805100101532351
Jung C. H., Cho I., Ahn J., Jeon T.-I., Ha T.-Y. (2013). Quercetin Reduces High-Fat Diet-Induced Fat Accumulation in the Liver by Regulating Lipid Metabolism Genes. Phytother Res. 27 (1), 139–143. doi: 10.1002/ptr.4687
Kamada C., Da Silva E. L., Ohnishi-Kameyama M., Moon J. H., Terao J. (2005). Attenuation of Lipid Peroxidation and Hyperlipidemia by Quercetin Glucoside in the Aorta of High Cholesterol-Fed Rabbit. Free Radical Res. 39 (2), 185–194. doi: 10.1080/10715760400019638
Krol W., Dworniczak S., Pietsz G., Czuba Z. P., Kunicka M., Kopacz M., et al. (2002). Synthesis and Tumoricidal Activity Evaluation of New Morin and Quercetin Sulfonic Derivatives. Acta Poloniae pharm. 59 (1), 77–79. doi: 12026118
Kutzner L., Esselun C., Franke N., Schoenfeld K., Eckert G. P., Schebb N. H. (2020). Effect of Dietary EPA and DHA on Murine Blood and Liver Fatty Acid Profile and Liver Oxylipin Pattern Depending on High and Low Dietary N6-PUFA. Food Funct. 11 (10), 9177–9191. doi: 10.1039/d0fo01462a
Lee J. M., Lee H., Kang S., Park W. J. (2016a). Fatty Acid Desaturases, Polyunsaturated Fatty Acid Regulation, and Biotechnological Advances. Nutrients 8 (1), 23. doi: 10.3390/nu8010023
Lees H. J., Swann J. R., Wilson I. D., Nicholson J. K., Holmes E. (2013). Hippurate: The Natural History of a Mammalian-Microbial Cometabolite. J. Proteome Res. 12 (4), 1527–1546. doi: 10.1021/pr300900b
Leng S., Winter T., Aukema H. M. (2018). Dietary ALA, EPA and DHA Have Distinct Effects on Oxylipin Profiles in Female and Male Rat Kidney, Liver and Serum. J. Nutr. Biochem. 57, 228–237. doi: 10.1016/j.jnutbio.2018.04.002
Li H., Lv Q., Liu A., Wang J., Sun X., Deng J., et al. (2022). Comparative Metabolomics Study of Tartary (Fagopyrum Tataricum (L.) Gaertn) and Common (Fagopyrum Esculentum Moench) Buckwheat Seeds. Food Chem. 371, 131125. doi: 10.1016/j.foodchem.2021.131125
Liu P., Lin H., Xu Y., Zhou F., Wang J., Liu J., et al. (2018). Frataxin-Mediated PINK1-Parkin-Dependent Mitophagy in Hepatic Steatosis: The Protective Effects of Quercetin. Mol. Nutr. Food Res. 62 (16), e1800164. doi: 10.1002/mnfr.201800164
Magdalan J., Szeląg A., Chlebda E., Merwid-Ląd A., PR D. (2007). Quercetin-5’-Sulfonic Acid Sodium Salt and Morin-5’-Sulfonic Acid Sodium Salt as Antidotes in the Subacute Cadmium Intoxication in Mice. Pharmacol. Rep. 59, suppl. 1, 210–216. doi: 236844549
Marcolin E., San-Miguel B., Vallejo D., Tieppo J., Marroni N., Gonzalez-Gallego J., et al. (2012). Quercetin Treatment Ameliorates Inflammation and Fibrosis in Mice With Nonalcoholic Steatohepatitis. J. Nutr. 142 (10), 1821–1828. doi: 10.3945/jn.112.165274
Martin B. T., Heintz R., Danner E. M., Nisbet R. M. (2017). Integrating Lipid Storage Into General Representations of Fish Energetics. J. Anim. Ecol. 86 (4), 812–825. doi: 10.1111/1365-2656.12667
Medale F., Le Bouche R., Dupont-Nivet M., Quillet E., Aubin J., Panserat S. (2013). Des Aliments a Base De V_Eg_Etaux Pour Les Poissons D’_Elevage. INRA Productions Animales 26, 303–316. doi: 10.20870/productions-animales.2013.26.4.3159
Miltonprabu S., Tomczyk M., Skalicka-Wozniak K., Rastrelli L., Daglia M., Nabavi S. F., et al. (2017). Hepatoprotective Effect of Quercetin: From Chemistry to Medicine. Food Chem. Toxicol. 108, 365–374. doi: 10.1016/j.fct.2016.08.034
Moon J., Lee S.-M., Do H. J., Cho Y., Chung J. H., Shin M.-J. (2012). Quercetin Up-Regulates LDL Receptor Expression in HepG2 Cells. Phytother Res. 26 (11), 1688–1694. doi: 10.1002/ptr.4646
Mullen W., Rouanet J.-M., Auger C., Teissedre P.-L., Caldwell S. T., Hartley R. C., et al. (2008). Bioavailability of 2-C-14 Quercetin-4 ’-Glucoside in Rats. J. Agric. Food Chem. 56 (24), 12127–12137. doi: 10.1021/jf802754s
Oliva-Teles A., Enes P., Peres H. (2015). Replacing Fishmeal and Fish Oil in Industrial Aquafeeds for Carnivorous Fish. Feed Feeding Practices Aquacult, 203–233. doi: 10.1016/B978-0-08-100506-4.00008-8
Padma V. V., Lalitha G., Shirony N. P., Baskaran R. (2012). Effect of Quercetin Against Lindane Induced Alterations in the Serum and Hepatic Tissue Lipids in Wistar Rats. Asian Pacific J. Trop. Biomed. 2 (11), 910–915. doi: 10.1016/s2221-1691(12)60252-4
Park K. H., Rodriguez-Montes de Oca G. A., Bonello P., Lee K.-J., Dabrowski K. (2009). Determination of Quercetin Concentrations in Fish Tissues After Feeding Quercetin-Containing Diets. Aquacult. Int. 17 (6), 537–544. doi: 10.1007/s10499-008-9222-6
Petersen B., Egert S., Bosywestphal A., Müller M. J., Wolffram S., Hubbermann E. M., et al. (2016). Bioavailability of Quercetin in Humans and the Influence of Food Matrix Comparing Quercetin Capsules and Different Apple Sources. Food Res. Int. 88, 159–165. doi: 10.1016/j.foodres.2016.02.013
Picklo M. J., Newman J. W. (2015). Antioxidant Supplementation and Obesity Have Independent Effects on Hepatic Oxylipin Profiles in Insulin-Resistant, Obesity-Prone Rats. Free Radical Biol. Med. 89, 182–191. doi: 10.1016/j.freeradbiomed.2015.07.152
Qin G., Ma J., Huang Q., Yin H., Han J., Li M., et al. (2018). Isoquercetin Improves Hepatic Lipid Accumulation by Activating AMPK Pathway and Suppressing TGF-β Signaling on an HFD-Induced Nonalcoholic Fatty Liver Disease Rat Model. Int. J. Mol. Sci. 19 (12), 4126. doi: 10.3390/ijms19124126
Qureshi A. A., Reis J. C., Qureshi N., Papasian C. J., Schaefer D. M. (2011). δ-Tocotrienol and Quercetin Reduce Serum Levels of Nitric Oxide and Lipid Parameters in Female Chickens. Lipids Health Dis. 10 (1), 39. doi: 10.1186/1476-511X-10-39
Rangel-Ordonez L., Noeldner M., Schubert-Zsilavecz M., Wurglics M. (2010). Plasma Levels and Distribution of Flavonoids in Rat Brain After Single and Repeated Doses of Standardised Ginkgo Biloba Extract EGb 761 (R). Planta Med. 76 (15), 1683–1690. doi: 10.1055/s-0030-1249962
Rey C., Delpech J. C., Madore C., Nadjar A., Greenhalgh A. D., Amadieu C., et al. (2019). Dietary N-3 Long Chain PUFA Supplementation Promotes a Pro-Resolving Oxylipin Profile in the Brain. Brain Behav. Immun. 76, 17–27. doi: 10.1016/j.bbi.2018.07.025
Robak J., Kopacz M. (1989). The Influence of Sulfonated Bioflavonoids on Enzymatic Oxidation of Arachidonic Acid and on Non-Enzymatic Lipid Oxidation. Polish J. Pharmacol. Pharm. 41 (5), 469–473. doi: 2518221
Roques S., Deborde C., Richard N., Skiba-Cassy S., Moing A., Fauconneau B. (2020). Metabolomics and Fish Nutrition: A Review in the Context of Sustainable Feed Development. Rev. Aquacult. 12 (1), 261–282. doi: 10.1111/raq.12316
Shaik J. S. B., Ahmad M., Li W., Rose M. E., Foley L. M., Hitchens T. K., et al. (2013). Soluble Epoxide Hydrolase Inhibitor Trans-4- 4-(3-Adamantan-1-Yl-Ureido)-Cyclohexyloxy -Benzoic Acid is Neuroprotective in Rat Model of Ischemic Stroke. Am. J. Physiol-Heart Circulatory Physiol. 305 (11), H1605–H1613. doi: 10.1152/ajpheart.00471.2013
Shin H. S., Yoo J. H., Min T. S., Lee K. Y., Choi C. Y. (2010). The Effects of Quercetin on Physiological Characteristics and Oxidative Stress Resistance in Olive Flounder, Paralichthys Olivaceus. Asian-Australasian J. Anim. Sci. 23 (5), 588–597. doi: 10.5713/ajas.2010.90624
Singh P., Mishra S. K., Noel S., Sharma S., Rath S. K. (2012). Acute Exposure of Apigenin Induces Hepatotoxicity in Swiss Mice. PloS One 7 (2), e31964. doi: 10.1371/journal.pone.0031964
Singh P., Sharma S., Rath S. K. (2014). Genistein Induces Deleterious Effects During Its Acute Exposure in Swiss Mice. BioMed. Res. Int. 2014, 1–14. doi: 10.1155/2014/619617
Singh P., Sharma S., Rath S. K. (2022). A Versatile Flavonoid Quercetin: Study of its Toxicity and Differential Gene Expression in the Liver of Mice. Phytomed Plus. 2 (1), 100148. doi: 10.1016/j.phyplu.2021.100148
Song H., Xu D., Tian L., Chen R., Wang L., Tan P., et al. (2019). Overwinter Mortality in Yellow Drum (Nibea Albiflora): Insights From Growth and Immune Responses to Cold and Starvation Stress. Fish Shellfish Immunol. 92, 341–347. doi: 10.1016/j.fsi.2019.06.030
Spagnuolo C., Russo M., Bilotto S., Tedesco I., Laratta B., Russo G. L. (2012). Dietary Polyphenols in Cancer Prevention: The Example of the Flavonoid Quercetin in Leukemia. Ann. N. Y. Acad. 1259, 95–103. doi: 10.1111/j.1749-6632.2012.06599.x
Spencer J. P. E., El Mohsen M. M. A., Minihane A.-M., Mathers J. C. (2008). Biomarkers of the Intake of Dietary Polyphenols: Strengths, Limitations and Application in Nutrition Research. Br. J. Nutr. 99 (1), 12–22. doi: 10.1017/s0007114507798938
Stewart A., Fisher R. A. (2015). “Introduction: G Protein-Coupled Receptors and RGS Proteins,” in Rgs Protein Physiology and Pathophysiology. Ed. Fisher R. A., 1–11. doi: 10.1016/bs.pmbts.2015.03.002
Szelag A., Magdalan J., Kopacz M., Kuzniar A., Kowalski P., Piesniewska M. (2003). Assessment of Efficacy of Quercetin-5 ’-Sulfonic Acid Sodium Salt in the Treatment of Acute Chromium Poisoning: Experimental Studies. Polish J. Pharmacol. 55 (6), 1097–1103.
Tan X., Sun Z., Liu Q., Ye H., Zou C., Ye C., et al. (2018). Effects of Dietary Ginkgo Biloba Leaf Extract on Growth Performance, Plasma Biochemical Parameters, Fish Composition, Immune Responses, Liver Histology, and Immune and Apoptosis-Related Genes Expression of Hybrid Grouper (Epinephelus Lanceolatus Male X Epinephelus Fuscoguttatus Female) Fed High Lipid Diets. Fish Shellfish Immunol. 72, 399–409. doi: 10.1016/j.fsi.2017.10.022
Toromanovic J., Kovac-Besovic E., Sapcanin A., Tahirovic I., Rimpapa Z., Kroyer G., et al. (2008). Urinary Hippuric Acid After Ingestion of Edible Fruits. Bosnian J. Basic Med. Sci. 8 (1), 38–43. doi: 10.17305/bjbms.2008.2994
Trocha M., Merwid-Lad A., Szuba A., Sozanski T., Magdalan J., Szelag A., et al. (2012). Effect of Quercetin-5 ’-Sulfonic Acid Sodium Salt on SOD Activity and ADMA/DDAH Pathway in Extracorporeal Liver Perfusion in Rats. Adv. Clin. Exp. Med. 21 (4), 423–431. doi: 23240447
Ulaszewska M., Garcia-Aloy M., Vazquez-Manjarrez N., Soria-Florido M. T., Llorach R., Mattivi F., et al. (2020). Food Intake Biomarkers for Berries and Grapes. Genes Nutr. 15 (1), 17. doi: 10.1186/s12263-020-00675-z
Wang W., Sun C., Mao L., Ma P., Liu F., Yang J., et al. (2016b). The Biological Activities, Chemical Stability, Metabolism and Delivery Systems of Quercetin: A Review. Trends Food Sci. Technol. 56, 21–38. doi: 10.1016/j.tifs.2016.07.004
Wang L. L., Zhang Z. C., Hassan W., Li Y., Liu J., Shang J. (2016a). Amelioration of Free Fatty Acid-Induced Fatty Liver by Quercetin-3-O-β-D-Glucuronide Through Modulation of Peroxisome Proliferator-Activated Receptor-Alpha/Sterol Regulatory Element-Binding Protein-1c Signaling. Hepatol. Res. 46 (2), 225–238. doi: 10.1111/hepr.12557
Wang J., Zhang C., Zhang J., Xie J., Yang L., Xing Y., et al. (2020). The Effects of Quercetin on Immunity, Antioxidant Indices, and Disease Resistance in Zebrafish (Danio Rerio). Fish Physiol. Biochem. 46, 759–770. doi: 10.1007/s10695-019-00750-2
Welte M. A., Gould A. P. (2017). Lipid Droplet Functions Beyond Energy Storage. Biochim. Et Biophys. Acta-Molecular Cell Biol. Lipids 1862 (10), 1260–1272. doi: 10.1016/j.bbalip.2017.07.006
Woznicka E., Kuzniar A., Nowak D., Nykiel E., Kopacz M., Gruszecka J., et al. (2013). Comparative Study on the Anti-Bacterial Activity of Some Flavonoids and Their Sulfonic Derivatives. Acta Poloniae Pharm. 70 (3), 567–571. doi: 23757948
Wu D., Meydani S. N. (1998). N-3 Poly-Unsaturated Fatty Acids and Immune Function. Proc. Nutr. Soc. 57 (4), 503–509. doi: 10.1079/pns19980074
Xie R.-T., Amenyogbe E., Chen G., Huang J.-s. (2021). Effects of Feed Fat Level on Growth Performance, Body Composition and Serum Biochemical Indices of Hybrid Grouper (Epinephelus Fuscoguttatus X Epinephelus Polyphekadion). Aquaculture 530, 735813. doi: 10.1016/j.aquaculture.2020.735813
Xu Z., Li X., Yang H., Liang G., Gao B., Leng X. (2019). Dietary Quercetin Improved the Growth, Antioxidation, and Flesh Quality of Grass Carp (Ctenopharyngodon Idella). J. World Aquacult. Soc. 50 (6), 1182–1195. doi: 10.1111/jwas.12663
Xu J.-Y., Su Y.-Y., Cheng J.-S., Li S.-X., Liu R., Li W.-X., et al. (2010). Protective Effects of Fullerenol on Carbon Tetrachloride-Induced Acute Hepatotoxicity and Nephrotoxicity in Rats. Carbon 48 (5), 1388–1396. doi: 10.1016/j.carbon.2009.12.029
Yang X., Dong C., Ren G. (2011). Effect of Soyasaponins-Rich Extract From Soybean on Acute Alcohol-Induced Hepatotoxicity in Mice. J. Agric. Food Chem. 59 (4), 1138–1144. doi: 10.1021/jf103749r
Ye G., Dong X., Yang Q., Chi S., Liu H., Zhang H., et al. (2020). Low-Gossypol Cottonseed Protein Concentrate Used as a Replacement of Fish Meal for Juvenile Hybrid Grouper (Epinephelus Fuscoguttatus Female X Epinephelus Lanceolatus Male) Effects on Growth Performance, Immune Responses and Intestinal Microbiota. Aquaculture 524, 735309. doi: 10.1016/j.aquaculture.2020.735309
Ytrestøyl T., Aas T. S., Asgard T. (2015). Utilisation of Feed Resources in Production of Atlantic Salmon (Salmo Salar) in Norway. Aquaculture 448, 365–374. doi: 10.1016/j.aquaculture.2015.06.023
Yu Z., Ming G., Cai W., Yu L., Cheng H. (2016). Dietary Component Isorhamnetin Is a Pparγ Antagonist and Ameliorates Metabolic Disorders Induced by Diet or Leptin Deficiency. Sci. Rep. 6, 19288. doi: 10.1038/srep19288
Zhai S.-W., Liu S.-L. (2013). Effects of Dietary Quercetin on Growth Performance, Serum Lipids Level and Body Composition of Tilapia. Ital. J. Anim. Sci. 12 (4), e85. doi: 10.4081/ijas.2013.e85
Zhang Z., Zhou C., Fan K., Zhang L., Liu Y., Liu P. (2021). Metabolomics Analysis of the Effects of Temperature on the Growth and Development of Juvenile European Seabass (Dicentrarchus Labrax). Sci. Total Environment. 769, 145155. doi: 10.1016/j.scitotenv.2021.145155
Keywords: Quercetin, Sodium quercetin-5′-sulfonates, oxylipins, metabolomics, marine fish
Citation: Luo J, Amenyogbe E, Huang J-s and Chen G (2022) Hepatic Metabolomics Analysis of Hybrid Grouper (Epinephelus fuscoguttatus♀×Epinephelus polyphekadion♂) Fed With Quercetin and Sodium Quercetin-5′-Sulfonates. Front. Mar. Sci. 9:891080. doi: 10.3389/fmars.2022.891080
Received: 07 March 2022; Accepted: 21 April 2022;
Published: 16 May 2022.
Edited by:
Brett Glencross, University of Stirling, United KingdomCopyright © 2022 Luo, Amenyogbe, Huang and Chen. This is an open-access article distributed under the terms of the Creative Commons Attribution License (CC BY). The use, distribution or reproduction in other forums is permitted, provided the original author(s) and the copyright owner(s) are credited and that the original publication in this journal is cited, in accordance with accepted academic practice. No use, distribution or reproduction is permitted which does not comply with these terms.
*Correspondence: Gang Chen, Y2hlbmdAZ2RvdS5lZHUuY24=