- 1National Centre for Marine Sciences, National Council for Scientific Research in Lebanon (CNRS-L), Beirut, Lebanon
- 2Marine Biogeochemistry, GEOMAR Helmholtz Centre for Ocean Research Kiel, Kiel, Germany
- 3IAEA International Atomic Energy Agency, Monaco, Monaco
- 4National Institute of Oceanography and Applied Geophysics-OGS, National Institute of Oceanography and Applied Geophysics-OGS, Trieste, Italy
- 5Laboratoire d'Océanographie de Villefranche, CNRS and Sorbonne Université, Villefranche sur Mer Msida, France
- 6Institute of Earth Systems, University of Malta, Msida, Malta
- 7Institute for Sustainable Development and International Relations, Sciences Po, Paris, France
- 8Institute of Environmental Science and Technology (ICTA-UAB), Universitat Autònoma de Barcelona, Barcelona, Spain
- 9Mediterranean Institute for Advanced Studies (IMEDEA - CSIC-UIB), Esporles, Spain
- 10Middle East Technical University, Institute of Marine Sciences (METU-IMS), Mersin, Turkey
- 11Oceanography Department, Institut National de Recherche Halieutique (INRH), Casablanca, Morocco
- 12University of the Aegean, Department of Marine Sciences, University Hill, Mytilene, Greece
- 13Marine Chemistry Lab, Marine Environment Division, National Institute of Oceanography and Fisheries, Cairo, Egypt
- 14ICTP, International Centre for Theoretical Physic, Trieste, Italy
- 15Catalan Institution for Research and Advanced Studies (ICREA), Barcelona, Spain
Ocean acidification (OA) is a serious consequence of climate change with complex organism-to-ecosystem effects that have been observed through field observations but are mainly derived from experimental studies. Although OA trends and the resulting biological impacts are likely exacerbated in the semi-enclosed and highly populated Mediterranean Sea, some fundamental knowledge gaps still exist. These gaps are at tributed to both the uneven capacity for OA research that exists between Mediterranean countries, as well as to the subtle and long-term biological, physical and chemical interactions that define OA impacts. In this paper, we systematically analyzed the different aspects of OA research in the Mediterranean region based on two sources: the United Nation’s International Atomic Energy Agency’s (IAEA) Ocean Acidification International Coordination Center (OA-ICC) database, and an extensive survey. Our analysis shows that 1) there is an uneven geographic capacity in OA research, and illustrates that both the Algero-Provencal and Ionian sub-basins are currently the least studied Mediterranean areas, 2) the carbonate system is still poorly quantified in coastal zones, and long-term time-series are still sparse across the Mediterranean Sea, which is a challenge for studying its variability and assessing coastal OA trends, 3) the most studied groups of organisms are autotrophs (algae, phanerogams, phytoplankton), mollusks, and corals, while microbes, small mollusks (mainly pteropods), and sponges are among the least studied, 4) there is an overall paucity in socio-economic, paleontological, and modeling studies in the Mediterranean Sea, and 5) in spite of general resource availability and the agreement for improved and coordinated OA governance, there is a lack of consistent OA policies in the Mediterranean Sea. In addition to highlighting the current status, trends and gaps of OA research, this work also provides recommendations, based on both our literature assessment and a survey that targeted the Mediterranean OA scientific community. In light of the ongoing 2021-2030 United Nations Decade of Ocean Science for Sustainable Development, this work might provide a guideline to close gaps of knowledge in the Mediterranean OA research.
Systematic Review Registration: https://www.oceandecade.org/
1 Introduction
Carbon dioxide (CO2) levels in the atmosphere have increased from 280 ppm to more than 420 ppm as reported in May 2022, an increase that is almost 50% higher than at the beginning of the industrial era (https://www.co2.earth/). This sustained CO2 rise is caused by enhanced anthropogenic activities, such as the burning of fossil fuels, deforestation, cement production, and large-scale land-use changes, emitting 43 billion tons of CO2 to the atmosphere in 2019 (Friedlingstein et al., 2020). Only half of the CO2 released by human activities has remained in the atmosphere. Between 1800 and 1994, the ocean absorbed about 9.5 billion tons of CO2 per year (Gruber et al., 2019; Watson et al., 2020; Friedlingstein et al., 2020). This demonstrates that the ocean has been an effective atmospheric CO2 sink (Le Quéré et al., 2015); however, this ecosystem service comes at a very steep price. Today’s ocean is undergoing a fundamental shift in its chemistry, commonly referred to as “ocean acidification” (OA). OA is attributed to a series of chemical reactions that ultimately leads to a reduction of seawater pH and carbonate ion concentrations [CO32–] and an increase in dissolved CO2, dissolved inorganic carbon and bicarbonate ions (Orr et al., 2005). OA affects not only the carbonate system of seawater but also marine life (Gattuso and Hansson, 2011; Riebesell et al., 2013; IPCC, 2021), which depends on some key carbonate chemistry parameters for their fitness. These changes are occurring globally and therefore are also observed in regional seas, such as the Mediterranean Sea.
The Mediterranean Sea is surrounded by 23 countries that are located on three continents. These countries have very diverse economies and rates of development, but all have deep ties to the Mediterranean Sea for trade, food, tourism and overall well-being. This iconic sea is one of the world's busiest, harboring 20% of all seaborne trade, 10% of the world’s shipping container throughput, and over 200 million tourists per year, thus adding additional environmental pressures (Med QSR, 2017). Also, the Mediterranean Sea is the world’s leading tourism destination (Med QSR, 2017; Tovar-Sánchez et al., 2019). At the same time, the Mediterranean Sea combines a vast range of marine biological diversity and productivity, progressive environmental changes, and densely-settled human communities (MedECC, 2020). All those factors make the Mediterranean Sea an ideal basin-study to evaluate the complex linkages between OA, heightened human pressures, and marine physico-chemical and ecological systems.
A surprisingly large number of studies are devoted to OA in the Mediterranean Sea, mostly addressing its chemical and biological aspects (MedECC, 2020, and references therein). The diversity of these studies, the various methodologies that have been adopted, and the importance of quantifying different aspects of OA requires standardization of best practices, and an effective dissemination of knowledge and investments in capacity building. All these key activities are addressed by the IAEA’s Ocean Acidification International Coordination Centre (OA-ICC; Home: Ocean Acidification International Coordination Centre (OA-ICC) | IAEA) that promotes comprehensive OA data portals with publicly available data (https://www.iaea.org/services/oa-icc), and supports global and regional ocean acidification networks, including the Ocean Acidification Mediterranean-Hub (OA Med Hub), a Mediterranean network affiliated to the Global Ocean Acidification Observing Network (GOA-ON).
It is currently well recognized that the ocean holds the key to an equitable and sustainable planet and communities (UN Ocean Decade; https://www.oceandecade.org/). Nevertheless, this depends on the data produced by the scientific community. Consequently, producing reliable OA biogeochemical and biological data is crucial for the much needed ocean governance and mitigation of OA. In order to systematically evaluate the OA research status and gaps, and to give recommendations on the best ways to proceed in this field of research, bibliometric studies have proved to be insightful (Nisumaa et al., 2010; Yang et al., 2016). The UN Ocean Decade (The Ocean Decade - The Science we need for the Ocean we want) provides a great opportunity to upgrade marine research to review, develop, and report on the best OA information needed by policymakers, so that the best science-based decisions can be made to protect our ocean and the communities that rely on a healthy and vibrant ocean. This is indeed challenging for the Mediterranean Sea, where results of comprehensive OA studies are still sparse and not easily accessible and/or scalable. In addition to the remarkable discrepancy of some OA research within the different Mediterranean countries, a clear and straightforward identification of gaps in the various OA research aspects has not been previously addressed.
This paper focuses on the much needed assessment of the current state of OA research progress, geographic discrepancies and gaps in this regional sea, based on the OA-ICC bibliometric data and on the responses of researchers from the Mediterranean OA sphere collected via a survey. Overall, this paper i) summarizes the state of art of OA research in the Mediterranean Sea, ii) identifies various aspects of ongoing OA research in the Mediterranean, iii) discusses main knowledge gaps and, iv) highlights agreed-upon recommendations that might serve as a roadmap for the community in the context of the UN Ocean Decade.
The paper is structured into 9 sections. Section 2 describes the database and the survey used in this study. Section 3 provides an overview of the Mediterranean OA research, including themes and geographic distribution of OA studies. Sections 4, 5, 6 and 7 highlight the status quo of OA in terms of chemistry, modeling and predictions, effects of OA on key species, and on the connections between organisms-ecosystems and ecosystem services, respectively. Section 8 discusses the socio-economical consequences of OA. In section 9, specific aspects related to OA policy and legislation are analyzed. In section 10, specific recommendations are identified for every OA theme in response to the identified gaps of knowledge. Finally, section 11 provides a long-term perspective of the needed actions for the understanding and management of OA among the Mediterranean countries.
2 Methodology
Using the OA-ICC database (Bibliographic databases|Ocean Acidification (news-ocean acidification-icc.org)/ https://www.iaea.org/services/oa-icc) via Zotero (Zotero | Groups > OA-ICC), 534 articles (Tables S1, S2), compiled by December 2021, on ocean acidification in the Mediterranean Sea were collected (out of a total of 8955 articles worldwide). The OA-ICC database keywords were used to filter the geographic location, date and type of the publication, studied organisms, process and parameters, and the applied methodology (see Microsoft Word - UserInstructions for the OA-ICC Bibliographic Database 2021 final.docx (news-oceanacidification-icc.org)).
Afterwards, the selected 534 articles were carefully reviewed to confirm the OA relevance of the study, the specific region of interest, including the type of basin and sub-basin (i.e., whether Eastern, Western, or entire Mediterranean Sea), the country of affiliation of the first and/or corresponding author(s), including the region (i.e. whether North or South of the Mediterranean Sea), and the type of study (e.g. chemistry, biology, etc.) and the studied organisms, if any (Figure 1). All the studies that did not meet such criteria were removed from the current review (Table S2).
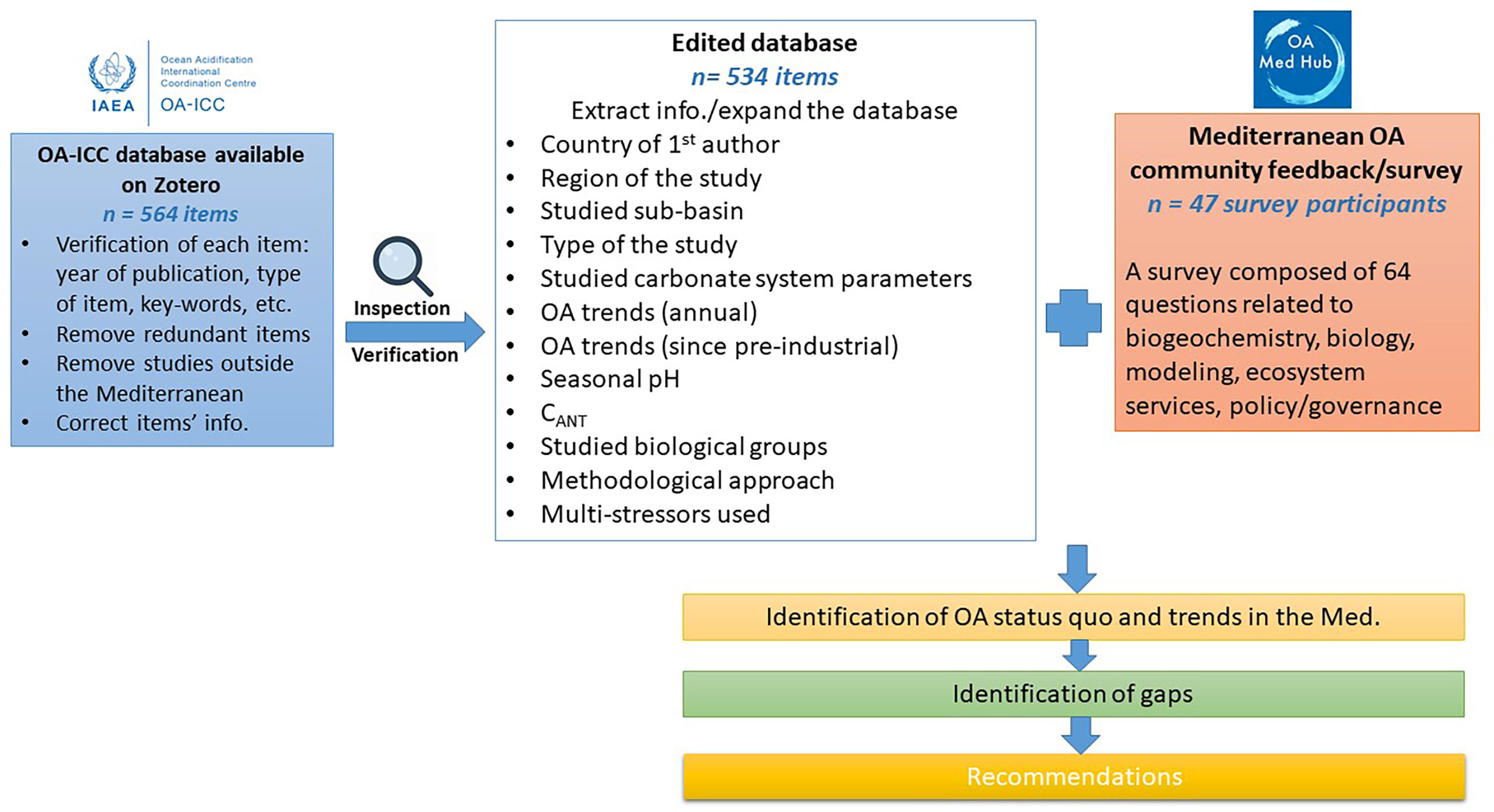
Figure 1 The methodology adopted in this study to derive the status quo, trends, gaps of OA in the Mediterranean and to provide appropriate recommendations.
Furthermore, a targeted survey that consisted of 64 OA-related questions was launched between 1 December 2021 and mid-January 2022 to assess current views of the Mediterranean Sea OA research community (questions and answers can be solicited in Table S6). These included technical capacities related to chemical and biological research, the North-South knowledge gaps, governance issues related to OA, etc. The survey was disseminated via the OA Med-Hub regular newsletter, the mailing list which contained 74 researchers working on OA in the Mediterranean, and via the OA Med-Hub social media accounts (Facebook and Twitter). The number of survey respondents reached a total of 47, more than 63% of the OA Med-Hub members (Figure 1).
3 The current OA research landscape in the Mediterranean
To assess the status of the Mediterranean OA research, we determined in the selected documents the type of OA publications, type of studies, areas of studies, type of organisms studied, and the geographical distribution.
3.1 Trends in OA-related publications
This paper is based on the analysis of a total of 534 entries (i.e., ‘items’, see below) retrieved from the OA-ICC database. These included: 86% peer-reviewed journal articles, 4.5% PhD theses, 4.5% book chapters, 3% conference papers, and 2% other documents (i.e., gray-literature reports, etc.) (Fig. 2A). This reflects the fact that the scientific community is more interested in producing scientific material (peer-reviewed articles), while communication products dedicated to disseminate OA results to a broader audience, such as conference papers, books, reports and targeted policy-briefs, are not a priority.
On average, around 1 to 4 OA items were published annually before 2008. After this year, the number increased to 20 OA items per year, mainly consisting of book chapters, in parallel with the launching of the European Project on Ocean Acidification (EPOCA project - 2008-2012) and the publication of the CIESM report containing multiple book chapters tackling the topic of OA (CIESM, 2008). The number of items continued to increase over the years during the MedSeA project (2011-2014), eventually leading to a peak of 60 OA items in 2017 alone. This was followed by a remarkable decrease in OA-related items up till present (Figure 2A).
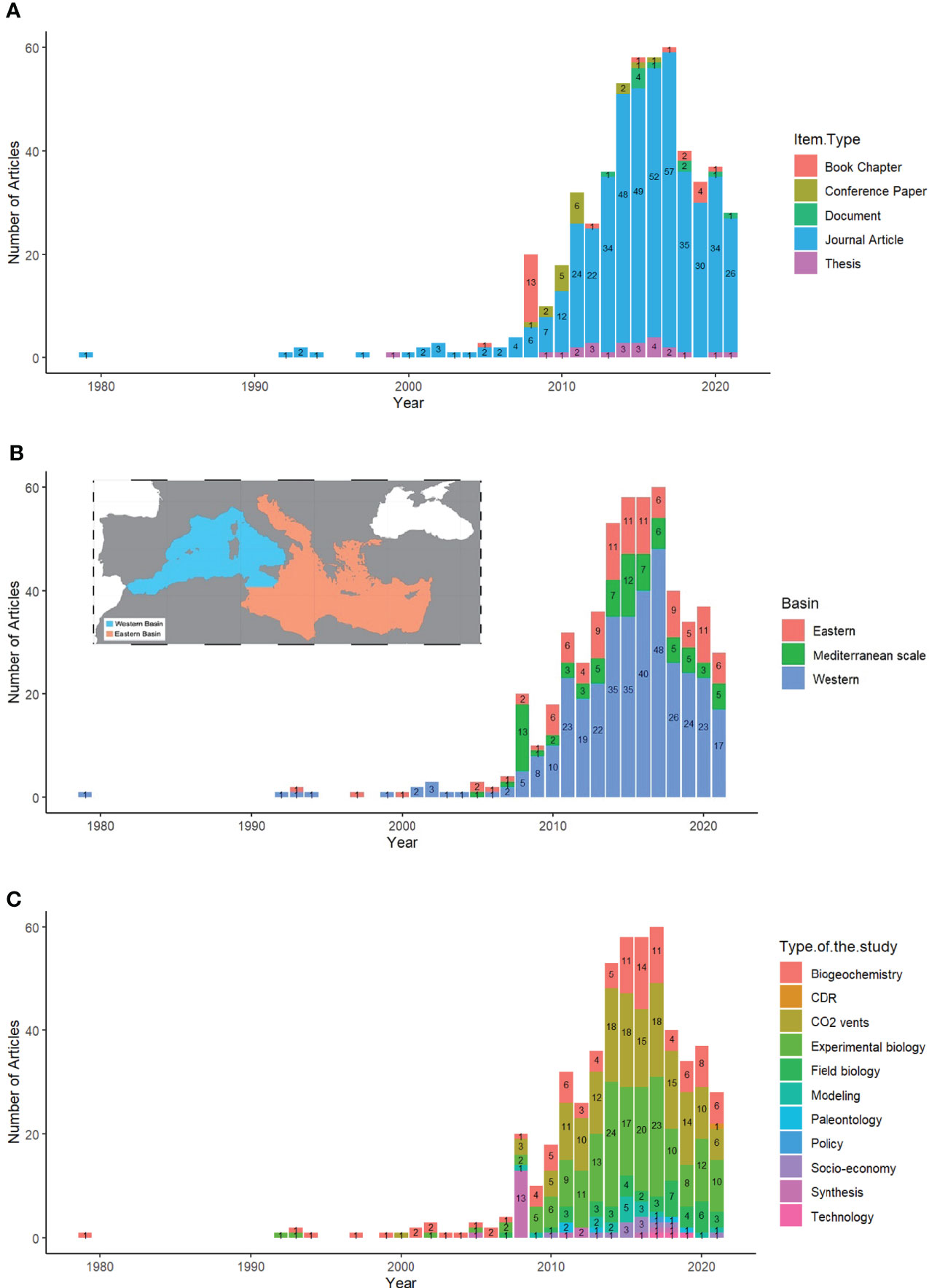
Figure 2 The evolution of OA-related publications through years in the Mediterranean: (A) showing the various types of OA-related items, (B) studies conducted in the main units of the Mediterranean basin, (C) the different types of OA studies.
3.2 Thematics of the OA research
Data show that most of the OA publications are dedicated towards the understanding of the biological response to OA through experimental studies (33%) and research conducted in natural CO2 gradients, particularly in CO2 vent sites (29%), followed by biogeochemical studies intended to quantify the carbonate system and OA trends (19%). This is followed by studies in the field of biological observations (7.5%) studying the in-situ responses of various biological groups, followed by reviews (4%), modeling (3%) and socio-economic studies, as well as paleontological, technological, policy-aspect studies, and carbon dioxide removal known as CDR (2%). There is only one recent article in 2021 tackling the carbon dioxide removal, reflecting that the Mediterranean OA community is still far behind in evaluating and assessing CDR techniques that might be applied in this sea (Figure 2C).
3.3 Distribution of OA studies in the Mediterranean
The interest of Mediterranean countries in the topic of OA has clearly blossomed thanks to large, trans-boundary projects that integrated multiple Mediterranean countries, starting in 2008 and revamped in 2010 (Figure 2B). This study shows that over the years OA studies were mainly implemented in the Western basin of the Mediterranean with more than 65% of the total studies, while only 20% were conducted in the Eastern basin, followed by 15% at the Mediterranean scale (Figure 2B).
Specifically, Italy, France, and Spain are the countries with the highest number of OA studies in the region, with 143, 91, and 67 publications, respectively (Figure 3A). This explains the large number of OA studies in the Western basin, predominantly conducted by these countries (Figure 3D), mainly in the Tyrrhenian (32%) sub-basin where CO2 vents are well studied, followed by the Liguro-Provencal (~ 26%) sub-basin where mesocosm studies have been implemented. These two sub-basins are then followed by studies conducted in the Adriatic (8%), Aegean (7%), Levantine (4%), Alboran (3%), Ionian (1.5%), and the Algero-Provencal (1%) sub-basins. The reason why the Algero-Provencal Sub-basin is the least studied can be explained by the almost non-existing OA items produced in the neighboring Mediterranean countries with a total of three in Tunisia, two in Algeria, and zero OA items from Morocco (Figure 3).
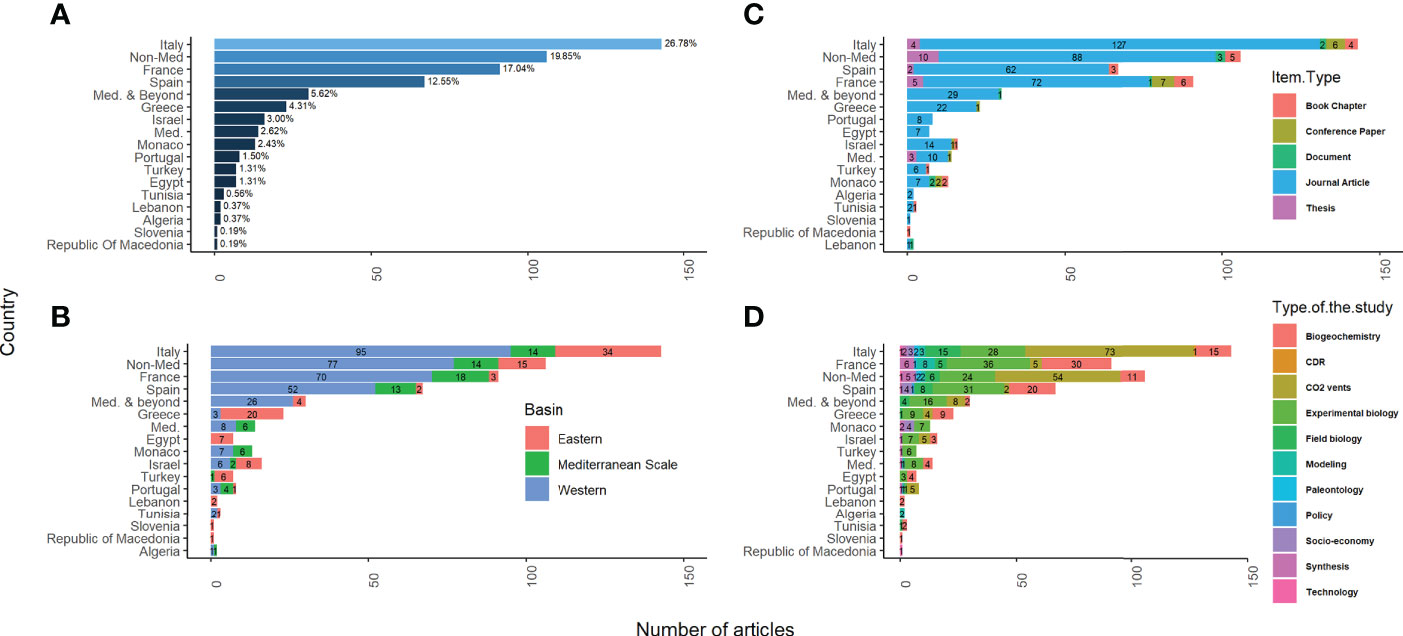
Figure 3 Productivity of OA-related studies: (A) per country* and groups, (B) per type of the OA-related items, (C) per the geographic location of the study, (D) per type of the tackled OA-related topic. Articles published by multiple Mediterranean countries are referred to as “Med.”, by Mediterranean and non-Mediterranean countries as “Med. & Beyond”, and by non-Mediterranean countries as “Non-Med.”. *Per country corresponds to the number of OA items published by the 1st/corresponding author(s) affiliated to institutions in a specific country or countries.
More than 90% of all OA-related studies were performed by scientists that come from the Northern region of the Mediterranean (Figure 4). Thus, current OA conclusions are predominantly derived from European institutions. In fact, there were only a total of seven joint OA studies between North and South (N-S) Mediterranean countries (~ 1%) found in the database, reflecting a significant lack of collaboration. However, OA items published between Northern Mediterranean institutions and research centers outside the Mediterranean were 2.5-fold higher than the ones conducted between N-S Mediterranean institutions (Med. & beyond in Figures 3, 4). Similar to studies originated in the South, these few collaborative N-S studies were focusing on chemical, biological and modeling OA aspects and are sparse in time with a better productivity after 2010. Studies originating in the North were covering most aspects of OA (Figure 3D).
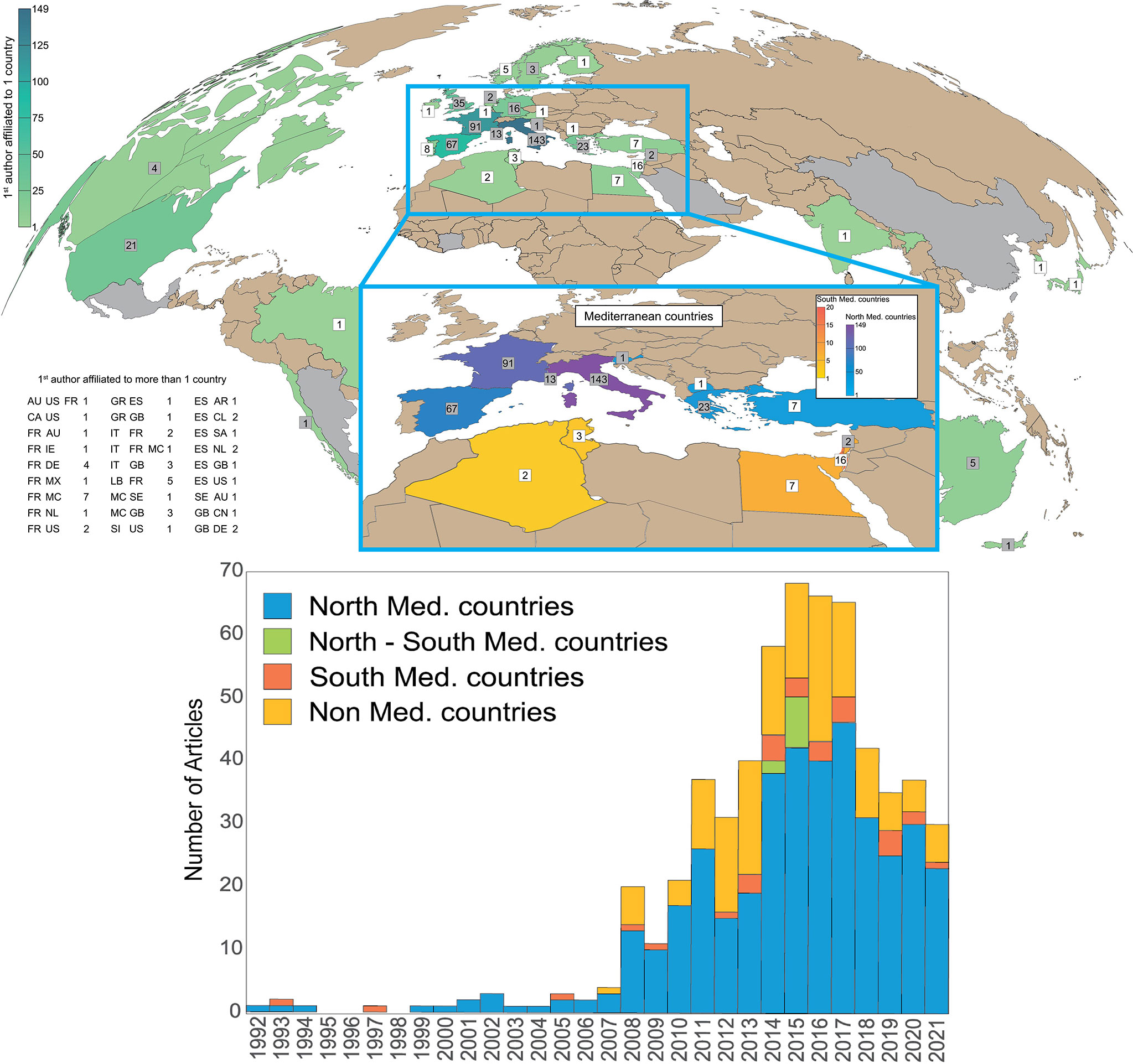
Figure 4 Top) Number of OA-related items per country of the 1st/Corresponding author(s). Gray-background numbers are referring to countries having a 1st/Corresponding author affiliated to more than one country. In the latter case, countries’ abbreviations are shown in details on the bottom left panel according to the UN/LOCODE Code List for every country and territory (UN/LOCODE Code List by Country and TerritoryUNECE). Bottom) The OA-related productivity in function of years for the North, South, North-South Mediterranean, and the non-Mediterranean regions.
These observations are supported by the results of the survey made for this study, where participants (n = 47) expressed the lower availability of financial and technical resources for institutions located in the Southern shores of the Mediterranean, as well as the lack of cooperation with institutions from the Northern shores as the main reasons behind this difference. In contrast, the lack of expertise in the South, the poor research infrastructure in the South, and the low consideration of proposals from the South are considered somehow relevant reasons (Table S6).
Surprisingly, OA items from authors not affiliated to Mediterranean countries are the second most productive group, with about 20% of all OA-related studies (Figure 4). This is likely due to the classification of the Mediterranean Sea as a climate-change impacts’ hotspot (Giorgi, 2006), the uniqueness and vulnerability of its marine ecosystems (Balzan et al., 2020), and the presence of natural CO2 vents and seeps used as proxies to evaluate the resilience of marine species towards OA. All of these factors have attracted the attention of scientists from outside the Mediterranean region, such as from the UK, USA, and Germany, as well as Argentina, Chile, Brazil, and China (Figure 4). This may explain why CO2 vents and experimental biology studies are the main type of studies tackled by non-Mediterranean countries (Figure 3D).
4 Ocean acidification trends: OA biogeochemistry
4.1 A glimpse into of OA-related chemical studies
The bibliometric assessment clearly shows that a considerable effort has been made over the last decade to characterize the carbonate system in order to estimate the concentration of anthropogenic CO2 that has already been absorbed, and to evaluate the corresponding pH trend in the Mediterranean. Most of the studies (51%) were conducted in the Western Mediterranean basin, followed by 36% in the Eastern basin, and 13% at trans-Mediterranean level. The Liguro-Provencal sub-basin is the most studied compartment of the Western basin, while the Adriatic sub-basin is the most studied in the Eastern basin (Figure 5). Articles dealing with water exchanges between the Mediterranean Sea and the adjacent oceanic compartments (Atlantic Ocean, Marmara Sea and Red Sea), as well as between the Mediterranean sub-basins, correspond to 15% of the working dataset, with a special focus on the Strait of Gibraltar (12%).
To constrain the carbonate chemistry in seawater, two out of four carbonate system parameters should be measured: total alkalinity (AT), pH, partial pressure of CO2 (pCO2) and/or total dissolved inorganic carbon (CT) (Touratier and Goyet, 2011; Hassoun et al., 2015; Krasakopoulou et al., 2017). The vast majority of research dealing with OA chemistry includes two different combinations of the seawater carbonate system measurable parameters (pH and AT: 34%, CT and AT: 26%).
In 16% of the scrutinized studies, three out of the four widely-measured carbonate system parameters were measured to quantify the CO2-carbonate system (Figure 6). In three studies, in addition to the pair pH-AT, the individual concentrations of Ca2+, CO32- and the stable carbon isotope ratio of dissolved inorganic carbon (δ13CDIC) were determined. A single parameter was measured in 17% of the studies, thus providing limited information about the carbonate chemistry. Studies based on continuous measurements of surface water pCO2 were less common (10%). Moreover, almost one fourth (24%) of the considered articles provided estimations of anthropogenic CO2 penetration in the Mediterranean Sea mainly using the TrOCA approach (Touratier and Goyet, 2004; Touratier et al., 2007). Interestingly, in some of the studies more than one of the methods calculating anthropogenic CO2 have been applied [e.g. Flecha et al., 2012, TrOCA, back-calculation technique (ΔC*) and φCT° method; Keraghel et al., 2020, TrOCA and back-calculation technique of Chen and Millero (1979)].
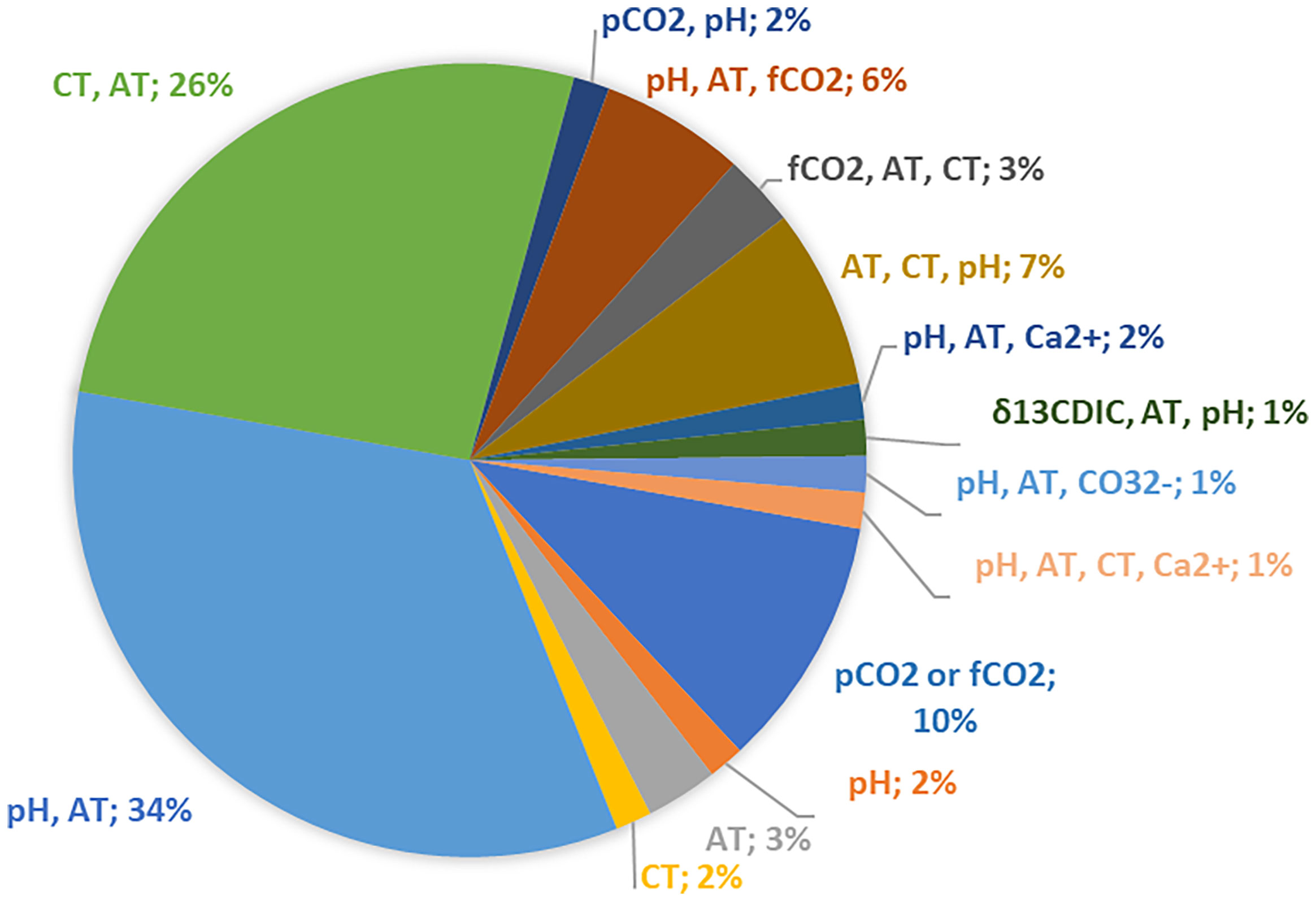
Figure 6 The measured parameters used to constrain the carbonate system in OA biogeochemical studies.
The overall analysis revealed a paucity and sparseness of measurements of the carbonate system parameters, since most studies rely on discrete water samples acquired across different periods of time, covering particular regions of the Mediterranean (Table S4). This spatio-temporal scatter of the marine carbonate system observations is mainly attributed to resources’ availability (both EU and national). The present study reveals a gap in research conducted in the Aegean (4 articles) compared to the Adriatic (10 articles), although in both areas Eastern Mediterranean deep water mass formations take place, playing thus an important role in the sequestration of anthropogenic CO2. Important studies on the Rhodes cyclonic gyre (Northern Levantine), where open-sea convection occurs in Winter, leading mostly to the formation of the LIW (Levantine Intermediate Water) but also episodically to deep waters (Nittis and Lascaratos, 1998), are completely missing. This is a critical gap since LIW is the most voluminous water type of the Mediterranean that spreads westwards, outflows into the Atlantic through the Strait of Gibraltar forming the warm and salty tongue of the Mediterranean waters that contribute to the global ocean circulation.
There are relatively few stations with time-series to monitor the carbonate system in the Mediterranean (GOA-ON Portal : Home), with the vast majority located in the Northern sector of the Mediterranean: DYFAMED and W1-M3A stations (offshore), SOLEMIO (Wimart-Rousseau et al., 2020) and Point B (Kapsenberg et al., 2017) stations (coastal) in the NW Mediterranean Sea, E2-M3A (offshore; E2-M3A Southern Adriatic Observatory - OGS), C1-Miramare (coastal; http://nettuno.ogs.trieste.it/ilter/GoTTs/en_index.html, Ingrosso et al., 2016a) and PALOMA (coastal; Cantoni et al., 2012) stations in the Adriatic, B1 (coastal) and B2 and A3 (offshore) stations in front of Lebanon-Levantine (Hassoun et al., 2019; Hassoun et al., 2022), G1, G2 and G3 (GIFT) stations in the Gibraltar Strait (Flecha et al., 2015; Flecha et al., 2019)]. However, datasets originating from these time-series stations have fueled only one-fourth of the published articles. High-frequency measurements of the seawater carbon chemistry provided by sensors on moored observatories, volunteer observing ships, floats and gliders seem to be relatively scarce in the Mediterranean contributing to around 10% of journal articles in the database, which possibly reveal two major challenges: 1- the high cost, and 2- the complexity of use, thus preventing the widespread utilization of in situ instrumentations (Byrne et al., 2010). In most of these studies, only pCO2 in surface waters was measured (Hood and Merlivat, 2001; Turk et al., 2010; Turk et al., 2013; Merlivat et al., 2018), whilst in two studies the pH variability was recorded with a SENSORLAB-pH system located at a fixed mooring (González-Dávila et al., 2016) and with an ISFET sensor on an underwater glider (Hemming et al., 2017). In another study, both SAMI-pH and SAMI-CO2 Submersible Autonomous Moored Instruments were used (Flecha et al., 2015).
The survey results validated the database analysis. Around 70% of respondents measure the carbonate system parameters in the following order: pH (86%), AT (77%), CT (49%), pCO2 (43%), followed by carbonate ions (23%). Moreover, more than 75% of the respondents encounter problems in: (1) securing Certified Reference Materials (CRMs) mostly from the USA Dickson’s lab (> 85%), (2) assessing the quality of the carbonate system measurements mainly due to limited or no funds (64%), (3) shipment (18%), and other reasons such as limited CRMs production, difficulty in paying in foreign currency, and institutional administrative issues. Although 78% of survey respondents are able to share their OA chemistry data, only 30% are submitting it to the IOC-UNESCO for the reporting of the SDG indicator 14.3.1., while only few respondents are uploading their data in different databases such as PANGAEA (via OA-ICC), GOA-ON data portal, SOCAT and GLODAP. This study therefore reveals a serious issue related to data access and availability within the OA research community.
4.2 Overview of the Mediterranean carbonate system
4.2.1 The carbonate system variability
The Mediterranean Sea is a semi-enclosed basin where Atlantic Ocean water is exchanged through the Strait of Gibraltar. The salinity of the Mediterranean Sea gradually increases due to evaporation processes during basin-wide circulation. The total alkalinity increases in eastward direction (Schneider et al., 2007; Rivaro et al., 2010; Touratier and Goyet, 2011; Álvarez et al., 2014; Hassoun et al., 2015). Freshwater inputs from rivers and the Black Sea also contribute to increases in AT (Copin-Montégut, 1993), especially in the marginal sub-basins, such as the Adriatic (Tamše et al., 2015; Urbini et al., 2020; Brush et al., 2021) and the Aegean (Cossarini et al., 2015a; Krasakopoulou et al., 2017). The Atlantic water inflow is compensated by a deep outflow of saltier and denser Mediterranean water which is, in the long term, slightly smaller than the former in order to balance the freshwater deficit of the Mediterranean Sea (Huertas et al., 2009). The North Atlantic Surface Water is characterized by low AT and CT concentrations, with AT ranging from 2381±5 to 2427±9 μmol kg−1 and CT varying from 2100±6 to 2176±10 μmol kg−1, and a mean pHT of 7.950±0.043. In contrast, the Mediterranean Outflowing Water (MOW) is characterized by high AT and CT concentrations, with AT and CT concentrations of 2569±1.5 μmol kg−1 and 2314±2 μmol kg−1, respectively, and a mean pHT of 7.877±0.015 in the Levantine Intermediate Water, one of the main recognized water masses of the MOW, detected in the Strait of Gibraltar (Huertas et al., 2009; Flecha et al., 2019; Table S5).
The Mediterranean Sea is characterized by impressive deep-water formations that take place where winter strong influxes of CO2 are observed, such as in the Gulf of Lion (Touratier and Goyet, 2009), the Northern Adriatic (Cantoni et al., 2012; Querin et al., 2013; Ingrosso et al., 2016a; Ingrosso et al., 2016b), the Southern Adriatic (Cantoni et al., 2016; Ingrosso et al., 2017), and the Aegean sub-basins (Krasakopoulou et al., 2009). This reflects how seasonality affects the biogeochemical features of the deep Mediterranean waters. The variability of both AT and CT is triggered by seasonal cycles of summer concentration due to evaporation and winter vertical mixing in the Eastern sub-basins, by intense biological processes in the North-Western Mediterranean, and by the horizontal transport in the South-Western Mediterranean. Although the physical contribution to the AT variability is a factor of 5–8 times greater than the biological one, biological processes can have an important contribution in surface layers to the annual variability through increasing AT during the winter-spring period (Cossarini et al., 2015b). The different intensities of the biological and physical processes in the two main Mediterranean basins also shape the surface seasonal patterns of both AT and CT. In the Eastern basin, characterized by weak biological processes and poor vertical mixing, the range of CT variation for example is quite limited (~20 µmol kg-1), while in the Western basin where winter mixing, primary production, and remineralization processes are more intense, the fluctuations are greater (up to -80 µmol kg-1 in the Alboran sub-basin; Gemayel et al., 2015). For analogous reasons, the CT pattern of the intermediate layer increases westward, just in the opposite way compared to the surface trend. Indeed, the higher productivity of the Western basin triggers a larger sinking and degradation of organic matter from the surface to the intermediate layer, which in turn generates a remarkable rise of CT (Álvarez et al., 2014; Gemayel et al., 2015). Total alkalinity, on the other hand, slightly increases in the intermediate layer all over the basin, mirroring the salinity feature (Álvarez et al., 2014). Contrariwise, the progressive westward decrease of AT, in the subsurface, reflects both the salinity gradient and the mineralization processes (more intense in the West) acting to decrease AT concentration.
4.2.2 CO2 air-sea fluxes: Source or sink?
The CO2 winter fluxes can range from -3.8 mmol m-2 d-1 in the North-Western Mediterranean (Copin-Montégut and Bégovic, 2002) to -6.2/-11.8 mmol m-2 d-1 in the Aegean sub-basin (Krasakopoulou et al., 2009), and up to -11.9/-24.2 mmol m-2 d-1 in the Gulf of Trieste (Cantoni et al., 2012; Turk et al., 2013; Ingrosso et al., 2016a) under strong Bora wind events (a typical cold and dry wind in the Northern Adriatic). Otherwise, summer outgassing of CO2 towards the atmosphere has been estimated in the North Adriatic (Turk et al., 2010; Cossarini et al., 2015a; Urbini et al., 2020), and is primarily attributed to an increase of pCO2 caused by warming of surface waters and to degradation of organic matter. Generally, at an annual scale, the Adriatic shelf acts as a net sink of 0.46 TgC yr-1 (0.04 Tmol yr-1; 1 Tg = 1012 g) for the CO2 (Cossarini et al., 2015b). The ultra-oligotrophic and warm South-Eastern Levantine sub-basin behaves differently in function of seasons, by acting as a CO2 source to the atmosphere during most of the year (May to December) with outfluxes ranging between 0.9±0.3 and 6.2±1.4 mmol m-2 d-1, whereas it acts as a sink of atmospheric CO2 in Winter (January to April) with influxes varying between -0.3±0.04 and -0.9±0.15 mmol m-2 d-1 (Sisma-Ventura et al., 2017). The disproportionality in flux direction and magnitude leads to an annual air-sea CO2 outgassing of 845±270 mmol C m−2 y−1 (0.98 Tg C y−1), indicating that the South-Eastern Levantine is a significant net source of CO2 to the atmosphere in contrast to other studied sub-basins in the Mediterranean that predominantly act as net sinks (Sisma-Ventura et al., 2017). The temporal succession of the sink/source status for atmospheric CO2 of the adjacent North-Western Levantine sub-basin is ascribed to AT variations that regulate the seawater pCO2 and the air-sea CO2 exchanges, in addition to the thermodynamic and biological drivers (Wimart-Rousseau et al., 2021). Further, Canu et al. (2015) indicates that CO2 fluxes vary from year to year as a function of both the input from the Strait of Gibraltar and meteorological forcings, quantified fluxes at the country level, and proved that plankton biological activity is a key factor in increasing the dissolution fluxes (sink) that can switch a sub-basin’s role from source to sink.
4.3 Anthropogenic CO2 and acidification trends
Ocean acidification trends also vary spatially across the Mediterranean Sea. The pH trends calculated since the pre-industrial era are mainly derived from two combinations of the carbonate system parameters (AT-CT) and (AT-pH) based on three main approaches used to estimate the amount of anthropogenic carbon (CANT), a variable that cannot be measured directly (Álvarez et al., 2009; Vázquez‐Rodriguez et al., 2009; Álvarez et al., 2014; Hassoun et al., 2015; Touratier et al., 2016; Krasakopoulou et al., 2017). These approaches were applied in various parts of the Mediterranean, and are: TrOCA (Touratier and Goyet, 2004; Touratier et al., 2007), Transit Time Distribution (Waugh et al., 2006), and the Back-calculation (Vázquez-Rodríguez et al., 2009). Since the pre-industrial era, the acidification trend has varied between -0.055 and -0.157 pH units in both basins with slight differences between intermediate and deep water masses (Table S3).
For the yearly pH trends, the Eastern basin presented homogenous trends varying from -0.0021 to -0.0024 pH units y-1 in the upper 80 m, while high annual acidification rate was reported in shallow waters (< 5m) of the South-Eastern coastal area, namely offshore Lebanon (-0.009±0.004 pH unit y-1; Table S3). The Western basin, however, showed wider annual pH ranges, varying between -0.0013 and -0.0015 pH.y-1 in the upper 75 m, and from -0.0017 to -0.003 pH unit y-1 in surface waters (Table S3). The pH trends of coastal and offshore time-series do not appear to be significantly different, so far.
When compared to the Atlantic and the global ocean (-0.001 to -0.0026 pH units y-1; Takahashi et al., 2014 and references therein, Bates et al., 2014), the Mediterranean Sea displays a wider range of acidification rates (-0.001 to -0.009 pH units y-1; Table S3). The relatively higher estimates have been associated to a specific capability of this sea to absorb larger amount of CANT that is efficiently transferred to deep layers, compared to the global ocean (Schneider et al., 2010; Hassoun et al., 2015). In fact, CANT has already penetrated all water masses of the Mediterranean Sea (Touratier and Goyet, 2011; Hassoun et al., 2015); in its various sub-basins: North-Western Mediterranean (Touratier and Goyet, 2009), Algerian (Keraghel et al., 2020), Adriatic (Krasakopolou et al., 2011; Ingrosso et al., 2017), Aegean (Krasakopoulou et al., 2017), and the Levantine (Schneider et al., 2010; Touratier and Goyet, 2011; Sisma-Ventura et al., 2016; Hassoun et al., 2019). This is due predominantly to the following reasons: i) high AT favoring the dissolution of atmospheric CO2 (Álvarez et al., 2014), ii) low Revelle factor ranging between 9.4-11.2 (Álvarez et al., 2014), iii) deep water formation processes and the relatively short ventilation time scale of deep waters (Schneider et al., 2014; Stöven and Tanhua, 2014) and, iv) constant inputs of anthropogenic CO2 at the Strait of Gibraltar (Schneider et al., 2010; Flecha et al., 2012). Similarly, AT in the Mediterranean Sea (>2500 µmol kg-1) is higher than the global ocean due to the high evaporation rates, and the inputs from rivers and the Black Sea (Copin-Montégut, 1993; Schneider et al., 2007). This peculiarity leads to an increase of the buffering capacity of the carbonate system, allowing to uptake a larger amount of CO2 from the atmosphere (Álvarez et al., 2014; Hassoun et al., 2015), as discussed also by Palmiéri et al. (2015) who, in a modeling experiment, showed a net increase of CO2 uptake (10%) in response to an increase of 10% of AT. In addition, the overturning time of the Mediterranean waters is very fast and allows a complete renewal of its water in a short range of time (60 to 220 years; Stöven and Tanhua, 2014) in comparison to the global ocean (more than 1000 years; Khatiwala et al., 2012). As a consequence, surface waters affected by the increase of anthropogenic carbon in the atmosphere can transfer this signature to deep layers in a shorter time frame, making the whole sea quickly invaded by CANT.
The absorbed CANT is estimated to range between 29 and 137 μmol kg−1, depending on the depth and type of the water mass (Table S3). Moreover, the Mediterranean Sea imports from the Atlantic Ocean a large amount of CANT (3.5 Tg yr-1, Schneider et al., 2010) that is dissolved in the surface waters entering the Strait of Gibraltar (Flecha et al., 2012). The intensity of the pH decreasing trend has been estimated by a few observational studies conducted on multi-annual and decadal scales (Yao et al., 2016; Flecha et al., 2019; Hassoun et al., 2019; Coppola et al., 2020 and references therein; Table S3). Along the water column, the pH decrease varies differently, with maximum and minimum decreasing trends in the surface and the intermediate layers, respectively (Table S3). Contrary to what is reported for the global ocean (Santana-Casiano et al., 2007; Dore et al., 2009), deep layers of the Mediterranean also display a noticeable decrease of pH, slightly lower than the surface waters’ values. This vertical pattern is attributed to water column processes and dynamics. Surface water, being in direct contact with the atmosphere, is more affected by an increase in atmospheric CO2. Deep waters also display an evident pH decreasing trend due to dense water formation processes that transfer surface water, enriched in anthropogenic CO2 to intermediate and deep layers. In the global ocean, as the intermediate and deep waters are much older than the surface water, the anthropogenic carbon signal is not particularly evident. However, in the Mediterranean Sea, the intermediate and deep waters are much younger, therefore showing anthropogenic CO2 invasion and consequently clearer acidification signals.
Acidification trends have been assessed also through model re-analysis. Cossarini et al. (2021) focused on the reconstruction of changes of pH and spatio-temporal dynamics of the major carbonate system parameters in the Mediterranean Sea during the last 2 decades, as a part of the Copernicus model-based reanalysis of biogeochemical parameters in the Mediterranean, now available as Copernicus Marine Service product (Data | Copernicus Marine). In this study, model simulations are constrained by qualified available information through data-assimilation techniques, and the final result -named reanalysis- can be considered as the best possible spatio-temporal interpolation of available information, since it is performed by using all the physical and ecological knowledge on ecosystem dynamic that is embedded in the model. The reanalysis suggests that, in the last 30 years, there were significant trends in all carbonate variables, with an increase of AT (1.7 and 0.7 μmol kg-1 y-1 in the Western and Eastern Mediterranean basins, respectively) as well as in CT (2 and 0.6 μmol kg-1 y-1), and a related decrease in pH of approximately -0.0006 to -0.0012 units y–1 (higher in the Eastern sub-basins). Since those trends are computed over the last 30 years period, they cannot be linearly extrapolated over the last two centuries, which have been characterized by a constant increase in atmospheric CO2. In fact, such an exercise would return higher pH changes than those computed in Palmiéri et al. (2015), which is coherent with the different assumption in the two studies.
5 Projections of future ocean acidification
During the last decades, several 3D coupled transport-biogeochemical models of different complexities have been developed to describe the biogeochemical cycling of carbon and major nutrients throughout the Mediterranean Sea. But only a few of these models have the capability to simulate carbonate system dynamics. In fact, while several studies discuss in details recent trends, spatio-temporal dynamics, and future projections of temperature, salinity, nutrients and plankton concentrations, the dynamic of carbonate system and acidification processes in the Mediterranean Sea have been explicitly discussed using a 3D coupled transport-biogeochemical model only in two recent studies (Cossarini et al., 2021; Solidoro et al., 2022). Previously, Palmiéri et al. (2015) attempted to assess changes in the pH of the Mediterranean Sea by relating them to the assimilation of anthropogenic carbon storage, which was estimated by treating it as a single passive tracer constrained by atmospheric boundary condition. That approach, therefore, did not consider the relationship between the carbonate system and biogeochemical processes, and assessed only the changes in pH with respect to a non-quantified pre-industrial state. Otherwise, relying on thermodynamic equations of the carbonate system equilibrium in the seawater, Goyet et al. (2016) calculated future variations of pH as a function of theoretical anthropogenic CO2 concentrations. Their results show that, since the pre-industrial era to the end of this century, pH may decrease down to -0.245 and -0.242 units in the Western and Eastern Mediterranean basins respectively, under the most optimistic SRES scenario, while it can reach -0.462 and -0.457 pH units in the Western and Eastern Mediterranean basins respectively, under the most pessimistic SRES scenario (Goyet et al., 2016). Nevertheless, these estimations do not take into consideration the warming effects on the CO2 sea-atmosphere fluxes and the penetration of anthropogenic CO2 to the Mediterranean Sea’s interior, which may be the reason behind these overestimated projections (MedECC, 2020).
In contrast, both Reale et al. (2022) and Solidoro et al. (2022) consider carbonate dynamic transformations as one of the components of the carbon biogeochemical cycles and therefore explicitly take into account the major interactions among acidification and biogeochemical cycling of nitrogen, phosphorus and carbon, including the impact of plankton photosynthesis and ecosystem respiration, as well as the effect of transport processes. In particular, Solidoro et al. (2022) focus on potential future evolutions and project that, under a high CO2 emission scenario (SRES-A2), the Mediterranean Sea pH will decrease by the end of this century of about -0.28 units in epipelagic waters (0-250 m) (WMed.= -0.29, EMed.= -0.28), of about -0.2 units (WMed.= -0.18, EMed.= -0.21) in mesopelagic waters (500-1000 m), and -0.1 units (WMed.= -0.09, EMed.= -0.14) in bathypelagic waters (1000-5000 m). Changes are therefore expected to be higher in the Eastern part of the basin, and the difference between changes expected in Eastern and Western Mediterranean basins increases with depth, possibly because of changes in the circulation as well as in water temperature, expected to be different in both basins. Reale et al. (2022) confirmed those projections by using a higher spatial resolution model to compute the expected changes in the Mediterranean pH under intermediate (RCP4.5) and high (RCP8.5) CO2 emission scenarios. Also in this case, model projections forecast for the high CO2 emission scenario a pH decrease at the end of the century of about -0.25 units in the upper waters and -0.2 units in the intermediate waters, with stronger changes in the Eastern Mediterranean compared to the Western Mediterranean. Interestingly, this modeling work also provides projections for an intermediate CO2 emission level, showing that under a more conservative scenario the decrease of pH significantly slows down in the second half of the century, yielding a change of -0.08 pH units at the end of century, one third of the pH decrease expected under the extreme scenario (RCP8.5).
Models have also been used to assess the impact of ocean acidification on selected marine organisms and habitat-forming species, with consequent impacts on ecosystem functioning and related ecosystem services. In particular, model projection suggests that the pH reduction expected for the end of the century might have very significant effects on seagrass meadows and coralligenous reefs (Zunino et al., 2020). Also, model results forecast that a healthy ecosystem might be able to buffer, at least partially, the OA impacts by reorganizing their structure, but with some consequences in terms of the capability to provide services. More work needs to be done on these aspects, taking into consideration the cumulative effects of multiple stressors on organisms, food webs, and ecosystems (Zunino et al., 2021).
Only 35% of the survey participants are modeling the carbonate system parameters with the following order: pH (69%), CT (50%), pCO2 (50%), AT (44%), and carbonate ions (19%). Their models are predominantly with yearly time scale resolution (>80%), mainly one or three dimensional (40% each), with a resolution in the order of 5 km (50%) and rarely better than 1/16° (< 20%). To compare their model outputs, participants use mostly PANGAEA via OA-ICC (56%) and GOA-ON data portal (22%). Remarkably, only 15% are satisfied with the skill of their OA model outputs, while 54% of participants confirm that their models need to be improved.
6 Key species and ecosystems threatened
The increasing proton concentrations [H+] and the decreasing concentration of CO32- and saturation states of calcite (ΩCa) and aragonite (ΩAr) (e.g., Feely et al., 2004) can be a major threat for marine organisms, particularly shell-forming and calcifying organisms (Kleypas et al., 1999; Riebesell et al., 2000). A large part of the scientific efforts to determine the effect of OA on marine organisms has focused on calcifying organisms (~ 37%, Figure 7) such as mollusks, corals, echinoderms, crustaceans, etc. However, many of these organisms live in the coastal benthos, where they are often subject to large fluctuations of the carbonate system caused by hydrodynamics and/or biological activities, especially in shallow and sheltered ecosystems (Duarte et al., 2013; Waldbusser and Salisbury, 2014). Long-term measurements at various sites show that pH fluctuations in coastal waters can exceed those in the open ocean by an order of one magnitude or more (e.g., Hofmann et al., 2011). Substantial diurnal oscillations of pH and pCO2 in productive coastal communities are driven by metabolism, i.e. biological photosynthesis/respiration cycles. Ecosystems consisting predominantly of macrophytes like seagrass meadows (Invers et al., 1997; Semesi et al., 2009; Unsworth et al., 2012; Buapet et al., 2013; Hendriks et al., 2014) or macroalgal habitats (e.g. Middelboe and Hansen, 2007; Krause-Jensen et al., 2015; Krause-Jensen et al., 2016; Wahl et al., 2018) can raise the pH considerably during daytime in habitats that can be important for calcifiers. The amplitude of the changes in pH relative to the bulk water are dependent on the spatial scale under consideration, i.e., small scale pH fluctuations can be quite large (> 1 pH unit) in the diffusive boundary layer over macrophyte blades and depend on light intensity and blade anatomy, which is species-specific (Hendriks et al., 2017a), whereas large-scale seasonal pH fluctuations in coastal waters are less pronounced (< 1 pH unit) (Hendriks et al., 2015; Wahl et al., 2016). Organisms living on blade surfaces, like epiphytes (i.e. bryozoa, and benthic foraminifera, 1.3% and 0.3% of studies respectively), are therefore likely exposed to more extreme conditions in pH/pCO2 than organisms living in open water like pteropods (0.3% of studies). By shifting acidification-sensitive processes toward time windows of high pH, calcifiers living within macrophyte canopies (i.e. echinoderms, mollusks) could benefit from pH fluctuations (Thomsen et al., 2015) generated through photosynthesis, with temporarily raised ΩCa and ΩAr, thereby creating a refuge for calcifiers during daylight hours, as demonstrated in several habitats (e.g. Manzello et al., 2012; Duarte et al., 2013; Hendriks et al., 2014; Wahl et al., 2018; Ramajo et al., 2019). Nevertheless, low pH at night associated with respiration could in turn have deleterious effects on some organisms (Rivest et al., 2017). However, calcifiers that live in conditions where there is no such temporary refuge, or no possibility of compensation of the high costs of calcification; through energy gained via symbionts, such as in the case of coral under bleaching conditions or animals with limited food availability (Ramajo et al., 2016), would be most at risk from OA.
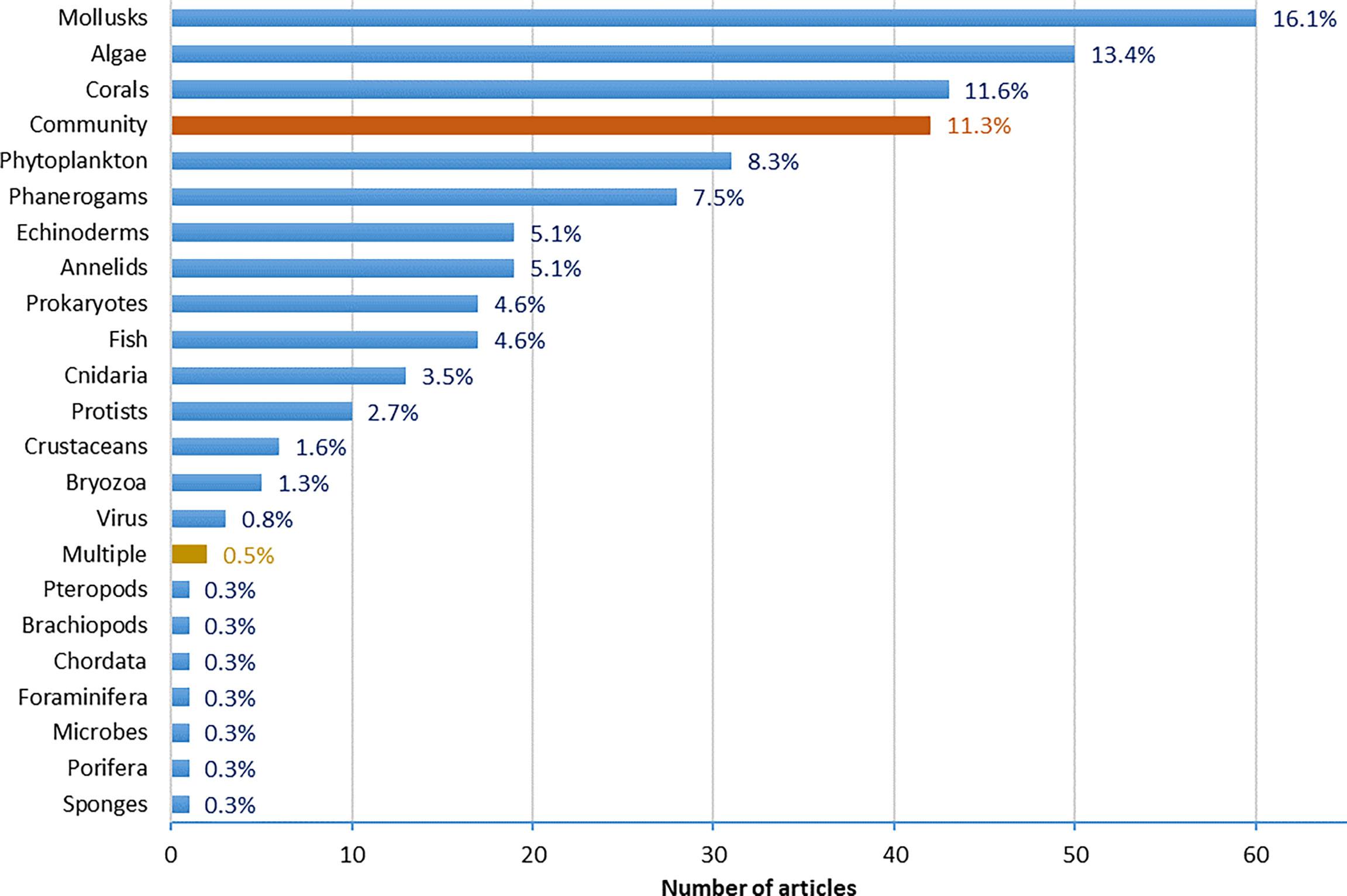
Figure 7 Groups of organisms assessed in Mediterranean OA studies. Organisms’ classification is based on the OA-ICC instructions.
6.1 Insight into OA biological studies
Even though they are arguably not directly at risk from OA, ~ 29% of the studies evaluating the effects of OA on marine organisms focus on autotrophs (algae, phanerogams, phytoplankton; Figure 7). This is due to their presence at most investigated field sites, like CO₂ vent sites that represent 38.5% of biological study approaches and 71% of all field studies (Figure 8). The vast majority of field studies using the natural pH gradients provided by CO₂ vents have been conducted in the Tyrrhenian sub-basin (90%), specifically in the volcanic Island Ischia, and to a lesser extent in Vulcano and Panarea islands (all in Italy). A smaller part (8%) has been performed in the Aegean sub-basin, namely in Milos and Methana islands (Greece). Proper site selection or experiments at a wide range of CO₂ vent sites would be necessary to assure the comparability of results with future conditions. This is notable because site-specific trace metals accumulation that can affect seagrass performance are often associated with CO₂ seeps. Currently, it is robustly clear that phanerogams (seagrasses) will be able to actually benefit from future elevated CO2 levels, although many recent studies are showing positive to no effects in specific species (Koch et al., 2013; Hendriks et al., 2017b; Guerrero-Meseguer et al., 2020). Meta-analyses show neutral effects of OA (Kroeker et al., 2013) or slightly positive effects (Hendriks et al., 2010; Guilini et al., 2017) on macrophytes. The effects of increased CO₂ on autotrophs are difficult to predict because some species utilize carbon concentrating mechanisms that buffer their sensitivity to ambient CO₂ levels and require variable energy investments. However, it seems that photosynthetic responses to OA are highly variable throughout taxa and might be relatively small for most species. The photosynthetic benefits of high CO₂ could be minor relative to the cell's overall energy and material balances, or other negative effects, such as possible respiratory costs from low pH outweigh the benefits of photosynthesis at high pCO2 levels (Mackey et al., 2015).
A major advantage of using pH gradients in CO₂ vent sites is the possibility of studying effects at a community level, under natural light, nutrients’ concentrations, and current conditions, which explains why 38% of OA biological studies adopted the field vent approach (Figure 8). In many laboratory studies (>40% of biological study approaches, Figure 8), stable pH conditions are used to infer effects of OA, which might have limited comparability to realistic conditions in natural environments. Due to the complexity of studying whole communities, only ~11% of the studies (42 in total) have tackled the community-level (Figure 7), with more than half (57%) of community studies implemented in field settings and the majority (45% of community level studies) in field vents. Laboratory settings were used in 14% of the studies, while 26% of the studies used a review of existing literature to unravel the effects of OA on communities.
The results based on the OA-ICC database are in consonance with the survey outcomes, showing that 40% of participants are doing only field biological observations, 20% are doing only experimental biological studies, and 40% are using both biological approaches. Although field biological observations can realistically reflect the feedback of organisms and ecosystems towards OA, there are significant obstacles related to limited-funding needed to sustain long-term observations (81%), the necessity of long-term monitoring to derive conclusions (69%), and the difficulty in distinguishing the main drivers of change (41%). Whereas for experimental studies, difficulties are mainly attributed to sustaining long-term experiments (66%), the complexity of factors that should be taken into consideration (47%), and the absence of unified methodologies (25%), according to the Mediterranean OA community (survey; Table S6).
The main studied biological groups are algae (36%), phytoplankton (36%), mollusks (36%), zooplankton (32%) and corals (27%) with lesser to non-existent interest in other groups, in harmony with the results deduced from the database analysis. Moreover, the main studied processes are the abundance (73%) of organisms, followed by community composition (40%), primary production (37%), calcification and growth (37%), then mortality (33%), morphology (33%) and photosynthesis (30%), with less interest in other biological processes. These results reflect the gap in assessing the potential effects of OA on some biological groups (i.e., nematodes, vertebrate organisms, worms, etc.), and key processes (i.e., performance, die ecology, cellular and sub-cellular responses, production of biotoxins, bioaccumulation of chemicals, etc.).
6.2 Multi-stressors experiments
Of all studies looking at the effects of OA on marine organisms, ~78% (337 studies, Figure 9) looked at the conventional OA single driver (pH or CO₂). Nevertheless, as future scenarios not only predict decreasing pH (increasing CO₂), but also forecast changes in other key drivers, it is becoming crucial to include all relevant drivers when evaluating organismal responses. Temperature is especially relevant as the Mediterranean Sea ranks among the ocean regions warming up the fastest (Marbà et al., 2015) and, together with habitat degradation, eutrophication, hypoxia, ocean acidification and biological invasions, warming has been identified as a major driver of change for the Mediterranean biodiversity since the last century (Coll et al., 2010; Marbà et al., 2014; Marbà et al., 2015; MedECC, 2020; Hassoun et al., 2021).
Coupled atmosphere-ocean general and regional circulation models project rapid warming in the Mediterranean region and an increase of the frequency and intensity of heat waves for the 21st century that could affect coastal marine ecosystems (Jordà et al., 2012). Because of the semi-enclosed nature of the Mediterranean Sea, marine species, in particular benthic organisms, have limited scope to avoid new thermal conditions resulting from global warming by keeping pace with poleward migration of isotherms as occurs elsewhere (Poloczanska et al., 2016). Temperature is also the most used parameter in studies using more than one driver, with 14% of all experimental studies on OA (Figure 9) including temperature, which represents 58% of the studies taking into consideration multiple drivers. Other drivers are also included in multiple-stressor studies, such as nutrients (27% of studies using multiple drivers), light (11% of studies looking at multiple drivers), salinity, and UV (2% each of studies looking at multiple drivers). Manipulation of multiple drivers is even more logistically challenging in field conditions, as reflected by the high percentage of single driver studies (92, 80 and 93% respectively for field, field mesocosm and field vent studies) compared to 56 and 38% of single driver studies in laboratory and laboratory mesocosm settings, respectively. Only 3% of studies looked at more than two drivers, and only one laboratory study included four drivers (0.2%), OA, light, nutrients and UV when evaluating phytoplankton responses.
These results also reflect the community’s perception (Table S6), since in addition to controlling pH/CO2, the main factors manipulated during multi-stressors experiments are temperature (81%), oxygen (42%), salinity (38.5%) and light (35%), while pathogens and bioturbation are almost not considered.
6.3 Evidence of variability in organismal responses: Examples of calcifiers
6.3.1 Corals
OA Effects on Mediterranean corals have received a lot of attention from the scientific community (~ 12% of published articles). This interest was motivated by numerous studies on tropical corals that demonstrated a reduction of calcification with decreasing pH (Kornder et al., 2018; Cornwall et al., 2021). Most of the attention has been on studying the effect of OA in isolation (84% of studies), which is slightly more elevated than the average number across studies on all organisms combined (78%). Cladocora caespitosa was the most studied shallow water species (9 studies). Overall, it was found that this species is particularly resistant to OA when exposed to pCO2 levels expected by the end of the century (Rodolpho-Metalpa et al., 2010; Rodolpho-Metalpa et al., 2011; Carbonne et al., 2021). This was the case during both laboratory studies on corals from control sites and CO2 vent sites (Rodolpho-Metalpa, 2010; Carbonne et al., 2021) and during in situ field studies at CO2 vents (Rodolpho-Metalpa et al., 2011). Negative effects of OA on the calcification of this species were reported once in laboratory (Movilla et al., 2012) and in the field when exposed to very high pCO2 (Rodolpho-Metalpa et al., 2011). Similarly, OA impacts on the calcification rate of Balanophyllia europea have been found to be mostly neutral (Rodolpho-Metalpa et al., 2011; Prada et al., 2017). This was attributed to its capacity to maintain a constant chemistry at its site of calcification along a gradient of pH found at the seeps of Panarea Island, Italy (Wall et al., 2019).
While the data are sparser for other species, they are rather contradictory and range from negative OA effects on the calcification of Astrangia paculata (Holcomb et al., 2010), Astroides calicularis (Prada et al., 2017) and Leptopsammia pruvoti (Prada et al., 2017) to neutral effects on the same species Astroides calicularis (Movilla et al., 2016; Teixido et al., 2020; Carbonne et al., 2021) and Leptopsammia pruvoti (Movilla et al., 2015).
6.3.2 Calcifying phytoplankton: Coccolithophores
On planktonic species, ~ 8% of the total number of biological studies addressed the effects of OA on phytoplankton (Figure 7). Within the phytoplankton group, coccolithophores are major calcifiers and several studies have addressed their vulnerability to OA (3% of the OA biological studies). These unicellular taxa are also dominating the Mediterranean oligotrophic phytoplankton communities and are the main contributors to the CaCO3 production and pump (Knappertsbusch, 1993; Ziveri et al., 2000; Malinverno et al., 2003). They also provide the possibility to address different temporal scale responses to environmental change from culture and mesocosm experiments to geological evolutionary records, being a main builder of CaCO3 marine sediments since the Mesozoic (Ridgwell and Zeebe, 2005; Suchéras-Marx et al., 2019). At a global scale, numerous culture studies addressing OA demonstrated a reduction of calcification and particulate inorganic and organic carbon with decreasing pH (Meyer and Riebesell, 2015 and references herein). The Mediterranean strains are not spared, as culture experiments focusing on OA only, or along with other expected changes (warming and nutrient limitation) show similar impacts (Fiorini et al., 2011; Milner et al., 2016).
However, OA field mesocosm experiments show that for CO2 conditions projected at the end of this century, the pelagic communities in both oligo- to meso-trophic Northwestern Mediterranean Sea system are generally resilient to OA, with coccolithophores presenting minor or undetectable impacts compared to the effects of nutrients’ depletion (Oviedo et al., 2017). Detrimental effects are caused by the rapid and extreme sea surface warming and the synergy with OA (Milner et al., 2016; D'Amario et al., 2017; D’Amario et al., 2020). Laboratory experiments (Fiorini et al., 2011; Milner et al., 2016; Johnson et al., 2022), long-term sediment trap series and observations in naturally high CO2 concentration sites (CO2 vents, off Vulcano island, Italy, Tyrrhenian sub-basin) show negative impacts of OA on coccolith weight and biodiversity (Triantaphyllou et al., 2010; Meier et al., 2014; Ziveri et al., 2014). Another study in natural CO2 gradient sites (CO2 vents, Aegean sub-basin) had reported increased biodiversity with high CO2 conditions (Triantaphyllou et al., 2018). Emiliania huxleyi is the dominant coccolithophore species and the most tested in OA laboratory experiments. It is found that changes in seawater carbonate chemistry can modulate their morphology and that OA has a negative effect on their calcification when exposed to pCO2 levels expected by the end of the century (Oviedo et al., 2014; D’Amario et al., 2018).
Despite the importance of seawater nutrients and temperatures for coccolithophores’ ecology in the Mediterranean, field observations from oceanographic cruise transects that had included seawater carbonate chemistry demonstrated its ecological and physiological importance for understanding their distribution as well.
6.3.3 Pteropods
Thecosome pteropods are specialized free-swimming pelagic sea snails and an important component of the marine food web. They are known for their sensitivity to OA, as their thin shells are made of aragonite (e.g. Comeau et al., 2012; Manno et al., 2012; Bednaršek et al., 2016; Bednaršek et al., 2019). In the Mediterranean Sea, only very few studies (n = 2, < 1% of OA biological studies; Figure 7) addressed this group in very restricted geographical and/or coastal regions. For this reason, conclusions cannot be derived at the basin level. As there are difficulties associated with maintaining a full life cycle of pteropods in laboratory studies, field time-series and observations are fundamental to improve the current knowledge on their ecology and vulnerability to climate change impacts. Interestingly, time-series analysis in a coastal setting in the Northwestern Mediterranean shows no effect on pteropods when pH is declining during the past decades (e.g., Howes et al., 2015). Decreased pteropod shell biomass combined with increased shell fractures is recorded at a CO2 vent site in the Tyrrhenian sub-basin, South-West Mediterranean Sea (Manno et al., 2019).
7 Studies connecting organisms-ecosystems and ecosystem services
Several studies elaborating experimental data and information, using meta-analysis and models, indicated that OA is already occurring and impacting several ecosystems’ structures, functions and services at the global scale (Kroeker et al., 2013; Barange et al., 2014), as well as in the Mediterranean. Studies highlighted that OA is expected to induce adverse effects on ecosystem services provided by pristine coralligenous and vermetid reefs (Milazzo et al., 2014; Zunino et al., 2017). Such effects are ranging from adverse impacts on coastal protection, carbon sequestration and habitat provision, to direct and indirect impacts on relevant coastal socio-economic systems, such as recreational scuba diving, fisheries, aquaculture and different coastal uses (Rodrigues et al., 2013; Hilmi et al., 2014; Zunino et al., 2019; Zunino et al, 2020; Zunino et al., 2021). However, as many of those ecosystem services are deeply linked to coastal processes, their assessment presents a bigger challenge. Assessing and valuing the potential changes and impacts induced by OA at the coastal scale require a deep understanding of the interactions among drivers and processes occurring at different spatial scales- from global to local- and responding at different time scales –from days to centuries (Duarte et al., 2013). Thus, there is a need for tools able to describe and assess these systems at a very high spatial resolution. However, coastal modeling systems are still poorly represented. In the last 30 years, CMEMS (Copernicus Marine Environment Monitoring Service) and other observational forecasting integrated systems have been established in the Mediterranean Sea to provide updates on the state of the basin. These products, however, are still limited to physics and to few main biogeochemical variables, and their resolution is not suitable to properly address the ecological features characterizing coastal areas (Cappelletto et al., 2021).
The public understanding of OA is hampered by several limitations, such as: 1) the cross-scale dimension of OA, both in time and space, 2) the uncertainty related to the interaction of several confounding processes, in particular in coastal areas, 3) the fact that OA effects are less visible and perceptible compared to other pressures such as marine litter, warming, nutrients’ loading, among others (Tiller et al., 2015). In a recent survey conducted in the frame of SHAREMED project (https://sharemed.interreg-med.eu/) addressing multiple pressures and threats in the Mediterranean, institutional stakeholders ranked marine litter much higher compared to OA, which received very little attention. This shows that even among the environmental management-sphere, the perception of OA threats is weak compared to other pressures.
Our survey (Table S6) indicates that most of the socio-economic research on OA in the Mediterranean area addresses provisioning and regulating ecosystem services (ES) (67% of them addresses both), while only 24% addresses cultural ecosystem services. Most of the participants (82%) focus on coastal habitats (such as coralligenous ecosystems, seagrass meadows, rocky habitats including caves, gulfs and lagoons, etc.), or at the Mediterranean scale (39%), while only 30% address ecosystem services at sub-basin levels. Among the ecosystem functions targeted by the community, the list comprises the productivity, trophic relationship, food provision, carbon sequestration and climate regulation, aggregation, refuge, nursery, structuring, biodiversity, sediment trapping, adaptation and physico-biogeochemical processes and interactions. To assess OA effects on ecosystems’ functions, the community uses various types of data, including chlorophyll a concentration, phytoplankton abundance and structure, occurrence of Harmful Algal Blooms (HABs), concentrations of biotoxins, stock growth, mortality/growth/metabolism, aquaculture and fisheries. Data are either produced through research or collected from public databases (PANGAEA via OA-ICC and GOA-ON data portal, 37.5% each, and EMODnet portal, 12.5%). Just a minority of researchers (26%) provides an economic valuation for the ES through cost-benefit, transfer-value and contingent analysis, and sometimes via establishing collaborative works between ecologists and socio-economists.
8 Socio-economic consequences of OA
The potential socio-economic impacts of OA in the Mediterranean Sea can affect different sectors such as fisheries, aquaculture, recreational tourism, jobs, ecosystem services, food security, trades and profits, having thus social and culture impacts on wellbeing, poverty alleviation, conflicts and population migration (Cooley et al., 2012; Hilmi et al., 2014). Consequently, the OA problem is now one of the latest from a list of anthropogenic stressors that are putting at risk both marine and coastal ecosystems of the Mediterranean. For example, it is very likely that in combination with other stressors, such as ocean warming, OA will increasingly impact the fisheries sector during the coming decades. Mediterranean countries are already importing more fish products than they export, as a result of the increasing demand for seafood. Despite being major exporters, countries like France, Spain and Italy suffer the highest trade deficits for seafood. Climate model projections show that by 2060 more than 20% of exploited fishes and invertebrates currently found in the Eastern Mediterranean could become locally extinct due to climate change (Jones and Cheung, 2015; Cheung et al., 2016). By 2100, more than half of all endemic marine species in the Mediterranean are expected to make it on the IUCN Red List of Threatened Species (Ben Rais Lasram et al., 2010). This will partly be brought about by the expected migration of marine species to cooler areas within an otherwise highly restricted Mediterranean basin (Poloczanska et al., 2016).
OA negatively affects both the society and the natural environment (Galdies et al., 2021). Rodrigues et al. (2013) and Zunino et al. (2020) described how OA can lead to significant direct and indirect socio-economic impacts in the Mediterranean on the detriment of an important number of ecosystems. The provision of food through fisheries (Zunino et al., 2021), carbon sequestration (Canu et al., 2015), coastal protection, support of recreational activities and cultural services (Zunino et al., 2020) are some examples of important services that are expected to be negatively affected. This will have negative consequences, especially in the less developed Mediterranean countries where direct income from fishing and related industries support high levels of employment and greatly contribute to the countries’ GDP (Hilmi et al., 2014). Fish consumption in developing countries, like Tunisia and Morocco, constitute some of the highest in the region (Malvarosa and De Young, 2010), and goes in line with the observed 216% increase in fish consumption observed between 1961-2005 by Southern and Eastern developing Mediterranean countries.
The assessment of socio-economic impacts of OA can indeed be quite complex, since it would require the quantification of several direct and indirect indicators such as productivity, employment, income and trade. While the latter are considered to be direct socio-economic impacts, the reduction in the Mediterranean’s ability to continue sequestering carbon, coupled with losses of non-market values associated with the exploitation of particular species and habitats are seen as indirect impacts that can arise from OA.
The survey results (Table S6) show that scientists working on socio-economic impacts related to OA are interested mostly in associated impacts to fisheries (62.5%), followed by aquaculture (58%), and tourism (42%). Most of the OA-related potential direct economic losses/impacts are believed to be influencing the following sectors differently: fisheries (75%), aquaculture (58%), tourism and recreation (50%), and coral/red coral extraction (25%). To assess the OA socio-economic effects, respondents use multiple sources of data such as experiments, modeling, literature and also coverage maps from targeted sectors (such as fisheries’ income, etc.). Only 33% of the respondents provide an economic valuation for some socio-economic sectors that is based on various methods such as numeric approach, cost-benefit, and transfer-value. Most of the research addresses the coastal areas (92%), at the sub-basins and Mediterranean scales (32% each).
9 OA policies and governance
Global strategies to mitigate OA contain few provisions to directly address this problem despite a growing body of knowledge on the subject matter. Some have argued that current global strategies to reduce CO2 emissions through for example, the UNFCCC and its various instruments, are not yet realistic solutions. This stems from the fact that OA requires a collective global action – something that is still mostly elusive, and as a result leads to suboptimal outcomes (Jagers et al., 2019). For example, both the G7 and subsequently the COP26 summits have demonstrated how difficult it is to commit the actors’ willingness to concretely limit CO2 emissions (and thus indirectly help address OA) and to pay for offsets to remedy the effects of global warming in the shortest time possible (i.e., by 2050). This lack of global commitment by the major emitters implies that only through voluntary multilateral cooperation and hard work in the form of mutual agreements and treaties, that a mitigation plan can be successful. Unfortunately, this approach is still elusive and ineffective when it comes to immediate reductions in CO2 emissions that would address OA.
9.1 Knowledge gaps lead to ineffective policies
Heightened anthropogenic pressures that affect both coastal and marine ecosystems of the Mediterranean Sea basin were recognized by all surrounding countries as they adopted the first-ever Regional Seas Programme under the auspices of UNEP in 1975. Through this Programme, several basin-wide monitoring initiatives and programmes were subsequently implemented to provide baseline information on the state of the health of marine ecosystems as well as to establish monitoring guidelines to address marine pollution in the Mediterranean Sea (Galdies, 1999; Galdies, 2008).
The Mediterranean Sea’s unique resilience with respect to a continuous increase in OA deserved special attention (see section 3), as this will in turn help inform and direct decision- and policy-makers to focus on targeted, realistic measures. Unfortunately, there is still a general lack of understanding at the scientific and public level regarding best policy options, which can be attributed to the complex management of both direct and indirect impacts (Riebesell and Gattuso, 2015; Osborne et al., 2017). These barriers are leading to inadequate access and sharing of data and information, and are pushing back the much-needed progress towards effective decision-making at the regional level (Cramer et al., 2018).
9.2 Framing OA governance for the Mediterranean
National policies could provide incentives to penalize the use of fossil fuels and at the same time encourage a move towards a greener economy. However, despite increasing knowledge of OA, the global community does not have any legal provisions that specifically address OA mitigation strategies. According to Harrould-Kolieb and Hoegh-Guldberg, 2019, this has led to “significant gap in the global governance of this issue with no multilateral agreement understood as having jurisdiction over the mitigation of rising ocean acidity”. One possible reaction to this was the suggestion to formulate a number of proposals ranging from the elaboration of a specific international agreement focusing on combating OA (Kim, 2012), to the inclusion of the mitigation of OA within the core obligations of UNFCCC (Harrould-Kolieb and Herr, 2012), or even proposing a new treaty on OA (Lamirande, 2011). Currently, the only comprehensive solution that the global community has to address OA is to drastically reduce, and as soon as possible, global anthropogenic CO2 emissions and the resulting uptake of this gas by the ocean. At the Mediterranean Sea scale, multi-level policy tools targeting CO2 emissions and adaptation strategies do exist in Europe, such as the EU Climate Action and the European Green Deal. However, these are not designed to address OA specifically, but can only be marginally adapted to do so (Billé et al., 2013; Jagers et al., 2019).
Until this is achieved, Mediterranean Sea countries need to continue to refer to currently fragmented legislations which can mitigate, albeit indirectly, OA. Galdies et al. (2020) showed how within the Mediterranean Sea basin, the OA problem is at best uncoordinated even at the European Union level, which spans much of the northern waters of the Mediterranean Sea. Although OA is acknowledged at the higher levels of governance, its status as an environmental challenge is greatly diluted at the European Union Member State level. All major EU measures in these areas take the form of directives rather than directly applicable regulations, and they are transposed into binding measures at the national level. However, a quick review of reports submitted by EU states as part of the MSFD to achieve Good Environmental Status (GES) in European waters (Dupont et al., 2014) shows that consistent EU-wide monitoring of the degree of OA and its impacts in European waters is still not in place. For example, a quick look at the monitoring activities conducted by EU member states for the descriptor D7 (Hydrographical changes) as part of the EU’s Marine Strategy Framework Directive to achieve Good Environmental Status (GES) shows that only Italy has marine acidification as part of its monitoring programme within its territorial waters (MSFD, 2014). Other EU Mediterranean countries are either not participating in such monitoring exercises or opting out from measuring marine acidity in their monitoring programmes. This reflects how even at the EU level, with its available funding and advanced environmental legislation, European OA-related policy and legislation must urgently close this gap by improving their strategies to support OA mitigation, primarily through continuous and systematic monitoring. It is interesting to note that the measurement of pH constitutes perhaps the easiest metric to use for the determination of the success of mitigation measures (Bernie et al., 2010). This metric should be adopted by UNEP/MAP and introduced as part of marine pollution monitoring that should be conducted by all Mediterranean countries in order to ensure a Mediterranean wide action to assess and monitor OA. Indeed, OA is recognized among the list of pressures threatening the coralligenous reefs in the Mediterranean Action Plan on climate change impact on marine biodiversity in the marine protected areas. However, direct measures of OA are not included among the list of monitoring indicators. Two of the four Regional Seas Programs in Europe have set specific assessing and monitoring strategies for OA, such as setting the OSPAR acidification study working group (ICES, 2014), and defining the acidification indicators, as in the Baltic program in 2018.
Many studies show how the impacts of OA can have serious social and environmental consequences that are in turn rooted in a highly fragmented and complex institutional setting (Jagers et al., 2019). This complex nature is even more pronounced in the Mediterranean because a good number of national institutions are faced by poor political, administrative and technical constraints, resulting in lengthy socio-economic and environmental progress. Within all of this, OA is indeed an important thematic, the management of which can become exacerbated within such a multi-faceted governance structure characterized by painfully slow progress. It must be made clear that this sluggishness of the political and administrative structures in the Mediterranean cannot be solely attributed to types of politics such as in those where democracy is still a contested process. Indeed, Jagers et al. (2019) explains how even democratic institutions can fail to provide public goods such as the case for Good Environmental Status of European and Mediterranean waters, as discussed in the previous section. Gorjanc et al. (2020), for example, showed how the ambitions of the Programmes of Measures within the MSFD framework are deemed to be low, characterized by non-existent coordination between EU-member states at sub-regional and regional levels. One can argue that the EU leaves it up to its individual States to choose their own instruments, methods and political preferences in order to reach the final common goal. At the same time, one should be aware of the fact that EU-Member states bordering the Mediterranean Sea are amongst the most advanced and rich Mediterranean nations with adequate finances to support such a public good (e.g. GES).
9.3 The Mediterranean perception
Taking into consideration the current gap in OA legislations and policies in the Mediterranean Sea, the organizations that can best address this issue and make it more mainstream at the Mediterranean political level were classified by the survey participants (n=47; Table S6), from the most relevant as follows: the United Nations Environment Programme (UNEP), Mediterranean Action Plan-Barcelona Convention and IOC-UNESCO (Intergovernmental Oceanographic Commission-UNESCO), followed by CIESM (The Mediterranean Science Commission), Governments, national, regional and local entities, then the IAEA (The International Atomic Energy Agency), UfM (Union for the Mediterranean), Plan Bleu, and non-governmental organizations (e.g., WWF, Greenpeace, etc.). The level of OA-related discussion by the most relevant parties, favorably perceived to address OA by the Mediterranean community, is mostly acceptable (60%), while 17.5% refer to it as an avenue for scientific collaboration and exchange of management practices, and 15% see that they are not interested so much in OA issue, whereas only 7.5% of participants mention that this discussion is highly mature and ambitious. These various perceptions reflect the different influence of the listed parties in the Mediterranean countries, and the need to enforce the bridges of communication to better address OA within these local, regional, and international organizations.
At a local level, more than 60% of participants stated that OA is irrelevant or neutrally perceived by their country’s environmental authorities/government, while only 36% mention that these local authorities are perceiving OA as an important environmental issue. This supports their negative replies (>52% said no) when asked if they are aware of any project or initiative in their countries to mitigate OA. And when such projects are taking place, they are predominantly targeting mitigation of anthropogenic CO2 emissions (67%), and equally (9.5% each) for mitigation of other greenhouse gas emissions, adaptation of ecosystems and human activities that build resilience to OA, restoration activity, lesser (5%) for mitigation of other local/regional factors that contribute to (or compound the effect of) OA (such as pollution, eutrophication, etc.), and none for establishing OA refugia. Besides, for non-EU member states, participants are not aware of any national legislation that is relevant to address OA.
Moreover, survey respondents identified the following activities that are taking place in their respective countries: vulnerability assessments of the resource(s) as a function of sensitivity, exposure and adaptive capacity (39%), clear management goals, including selection of the organism(s) and biological processes of interest for conservation (31%), potential refugia are being identified and/or determined (25%), whereas the majority (42%) confirmed that none of the above activities are taking place in their respective countries.
Upon closer inspection of the research and knowledge equity in the region, the majority of the survey respondents from non-EU Mediterranean countries sense a big difference when it comes to the number of OA-related studies, which they consider to be a relevant matter. Equally relevant (and concerning) is their perception of the current state of funding to manage OA, of the current lack of expertise in the South and to the poor research infrastructure. If true, such a situation is able to manifest itself in lack of equity when it comes to the level of participation and cooperation from the North; such a scenario was in fact rated by the respondents as very relevant.
10 Recommendations
In light of our current understanding of chemical trends and organism-to-ecosystem impacts of OA in the Mediterranean Sea based on our literature assessment, and taking into consideration the gaps and recommendations collected from this study’s survey (referred below as the ‘community’), the following research recommendations were selected. In line with the United Nations Decade of Ocean Science for Sustainable Development, which commenced on January 1, 2021, and as many of our deduced recommendations are in harmony with other OA plans adopted globally (e.g., NOAA’s plan for Ocean, Coastal and Great Lakes Acidification Research for 2020-2029), the ones provided hereafter might be useful as a roadplan to improve the OA research landscape specifically in the Mediterranean region:
10.1 To improve OA chemistry studies in the Mediterranean
10.1.1 Data quality
To overcome the universal challenge in affordable and readily accessible Certified Reference Materials (CRMs) that are essential to high quality carbonate system measurements, the OA community strongly supports the development and distribution of Mediterranean seawater specific CRMs that are calibrated against the UCSD Scripps Institute of Oceanography standards and produced in a suitable, accredited European/Mediterranean laboratory. Moreover, the community overwhelmingly (82%) recognizes the importance of regular inter-calibration proficiency exercises (i.e., WEPAL/QUASIMEME) to make sure all interested laboratories are generating the most accurate and reliable data possible. It is also recognized that essential investments in data quality will require additional and sustained funding.
10.1.2 Data coverage
Taking into consideration the importance of covering under-studied sub-basins to better understand its biogeochemical changes, more oceanographic cruises and regular monitoring efforts should be conducted in order to quantify their carbonate system (such as the Algero-Provencal and Ionian sub-basins), and to complete the missing puzzles of the Mediterranean biogeochemistry. Also, there are very few studies in straits and channels (i.e., Bosphorus, Dardanelles, Sicily, Otranto) which inhibits the understanding of the connectivity between sub-basins on one hand and the Mediterranean with its surrounding areas in another hand. More studies are also needed in deep and intermediate water formation areas (i.e., Rhodes gyre, South Adriatic gyre, North-Eastern Levantine sub-basin) which would help to better estimate the annual sequestration rate of anthropogenic CO2, and the role played by these areas (as sink or source). Furthermore, as freshwater inputs (i.e., river and groundwater discharge) play an important role in modifying the chemistry of seawater, the community needs to systematically constrain their contribution in the seawater carbonate system (Ingrosso et al., 2016b ; Hassoun et al., 2019).
10.1.3 Data availability
To date, too few scientists consistently share their OA-related data on open data portals (section 4). Thus, significant efforts should be channeled towards pushing institutions to produce FAIR data (which meet principles of Findability, Accessibility, Interoperability, and Reusability) at national and regional levels. To quantify and monitor OA trends and variability, the community needs high quality, consistent ocean biogeochemical data that can be archived in a state-of-the-art data resource. This will make data submission and retrieval machine-compatible and efficient (Tanhua et al., 2021). Similarly to the IAEA’s OA-ICC biological database, a biogeochemical database would be helpful to gather all OA-related data in one platform that can be used by biogeochemists and modelers for comparisons and future projections (e.g., The Global Ocean Data Analysis Project Version 2 (glodap.info), with specific data for the Mediterranean Sea [CARIMED]).
Time-series data are more readily available from the northern realm of the Mediterranean Sea while they are too scarce in its southern part. Carbonate system parameters together with some physico-chemical parameters (e.g., T, S, O2, nutrients, etc.) are recommended to be measured on a monthly basis in the context of a national monitoring network. New monitoring programmes need therefore to be established to systematically quantify the OA chemistry in vulnerable coastal and open-sea ecosystems. More than one carbonate system parameter is recommended to be analyzed to better constrain the carbonate system variability and determine the seasonal and interannual drivers controlling it.
10.2 To improve OA modeling studies in the Mediterranean
There is an urgent need for higher resolution, integrated (physical-biogeochemical-ecological) and spatially-nested models which would provide new perspectives on where OA impacts could be more pronounced. Models should be able to address the cumulative effect of OA and other stressors, also considering synergistic effects and non-linear interactions. This is crucial to better understand the multiple effects of changes in stressors and to explore adaptation/mitigation measures that can be applied at different spatial and temporal scales (Duarte et al., 2013; Zunino et al., 2021; Cappelletto et al., 2021). Furthermore, it would be important to at least try to incorporate organism plasticity and long-term adaptation in modeling ecosystem response, possibly also considering different life time-span of different organisms and therefore turnover rates (Solidoro et al., 2010).
Focus should be given to forecast direct consequences of OA on habitat structuring species and other ecologically relevant species, as well as on cascading consequences on the ecosystem network, its functioning and related services. Moreover, a quantitative assessment of uncertainties in model projection would be needed, in order to properly inform research priorities and management choices.
Models should be improved to provide answers to fundamental questions, such as: what are the impact of OA on marine functional biodiversity and possible consequences of OA-induced biodiversity loss on ecosystem functioning and resilience? and more applied questions, including: What are the local time-response of mitigation measures applied to global emissions? Can a model assess the effects of alternative mitigation solutions?
10.3 To improve the OA biological and ecological studies in the Mediterranean
Biological aspects of OA research should address:
(1) multiple environmental stressors. It is more realistic to study OA with different combinations of abiotic and biotic parameters (e.g., temperature, light, salinity, nutrients, grazing, respiration, hypoxia, etc.) in both in situ and experimental studies (Riebesell and Gattuso, 2015). Organismal response to OA is not homogeneous nor easily predictable and appears to depend on biological coping mechanisms to help offset high ‘costs’ of physiological processes (i.e., calcification) under reduced pH conditions. It is thus important to assess multiple stressors under future scenarios, such as natural diurnal pH fluctuations, food availability, including additional potential drivers [e.g., deoxygenation, warming, salinification, etc.] (Boyd et al., 2018). Also, as multiple-drivers studies are relatively scarce, especially on large scales or using larger organisms, cooperation between Mediterranean countries in sharing and enabling larger/more developed mesocosm facilities would be highly beneficial and cost effective.
(2) biotic response at different organizational levels from genes to food webs and ecosystems to assess its potential adaptation strategies in specific ecosystems, and the genes responsible for such plasticity (Riebesell and Gattuso, 2015). This work should take into account the different life stages of species through ecophysiological studies that might yield further insight into detailed physiological processes that are impacted by OA (not only calcification and respiration processes).
(3) key and most vulnerable groups/ecosystems. For example, calcifiers that live in conditions where there is no temporary refuge for calcification (do not live within canopies), or do not have any possibility of compensation for the high costs of physiological processes (i.e., calcification) like in conditions with limited food availability, would be most at risk from OA. The limitations to biological compensation of the negative effects of OA would be a necessary future direction for research, and could elucidate when organisms would be most vulnerable. An interesting case is the group of coralline algae, able to use CO2 as a substrate but also potentially affected negatively through calcification processes. Also, future OA studies should pay more attention to habitat-forming species, various larval-stages of commercial species, and potential OA effects on harmful/toxic and invasive species as well.
(4) not only short-time scale physiological responses but also generational population responses over years and even longer time spans. OA requires a high resolution and long-term monitoring strategy to detect the variability at short-term and -spatial scales and to enable disentangling long-term (multidecadal) anthropogenic signals from short- and medium-term spatial and temporal variability (Doney et al., 2020). There is a clear need to implement long-term monitoring of biological ecosystems to track any potential responses based on near-real-time observations. This is feasible through merging the chemical and biological monitoring in key areas (e.g., highly urbanized coastal areas, MPAs, seagrass meadows, etc.), while focusing on specific biological indicators (i.e., coccolithophore, pteropods, coralligenous, etc.) to simplify the long-term monitoring. Long-term OA observations are crucial to understand the plasticity, micro-evolution and adaptation of key species.
10.4 To improve OA socio-economic studies in the Mediterranean
Robust socio-economic studies should be developed to better assess impacts of the prolonged OA impacts in the Mediterranean Sea. In order to do so, there is a need to invest in research that can address the interactions among drivers and processes at different spatial and temporal scales. Such research should focus on species/habitats/ecosystems of commercial and cultural values and possibly be supported by modeling and assessment performed at the relevant (high) spatial and temporal scales. Quantification of uncertainties across broad scales would be very important (Solidoro, 2021).
Moreover, there is a necessity to assess the loss of ecosystem services due to OA, and to value the costs/benefits of adaptation/mitigation measures using projected future scenarios based on global SSP, and IPCC AR6, and local downscaling scenarios. Such studies will help to bridge the knowledge gaps between policymakers and the OA community by reflecting on the importance of supporting OA research and implementing OA mitigation strategies to sustain the resources and avoid projected losses.
10.5 To bridge the gap of OA research discrepancy in the Mediterranean
Mediterranean Sea countries are particularly vulnerable to OA, specially those in the Middle East and North Africa (MENA) (Hilmi et al., 2017), thus regional budget and governmental resources should be directed to capacity building of these countries’ institutions to ensure quality and sustainability of the obtained data. Moreover, OA research, particularly on regional key economic species, in the Southern Mediterranean countries should be encouraged and systematically supported in order to conserve their economic resources and save threatened habitats, and therefore the livelihoods of coastal vulnerable communities.
Furthermore, it is important to encourage research to fill gaps in understanding chemical, biological, ecological and socio-economic impacts of OA at regional and interregional scales (see Tilbrook et al., 2019), especially in the MENA region. This can be widely recognized and adopted in national monitoring programs of the Mediterranean countries in support of legislations aimed at mitigating OA (similar to Barcelona convention).
According to the survey’s respondents, to reduce the discrepancy related to OA-related research, knowledge and governance within the Mediterranean the following additional measures should be implemented (from the most relevant to the least): 1) Promote more scientific exchanges (training, capacity building efforts, etc.), 2) Involve researchers from the South in Mediterranean oceanographic cruises and experimental studies, 3) Increase the regional and North-South projects, 4) Support more PhD students from South to North Mediterranean countries, 5) Fix a quota (minimum number of partners) from the Southern countries for the project to be eligible. More North-South and East-West projects and programmes can be supported through including the diversity of involved countries and researchers as a basic criteria in projects’ selection procedures to encourage researchers to cooperate with peers from under-represented areas and to fortify scientific exchanges, inter-comparison exercises and experimental collaborations. This should not only be conducted through short-term capacity-building efforts, but also via common trans-Mediterranean oceanographic cruises, and by supporting associated graduate and postgraduate projects.
10.6 To improve OA policies and governance in the Mediterranean
In parallel with the need to further fine-tune research related to the understanding of OA trends and consequences in the Mediterranean Sea (see the above recommendations), the available governance and legal opportunities remain the most relevant tools to address OA. It is time to re-enforce coordination and harmony of global, regional and national governance bodies so that they are tailored for effective governance of OA in the Mediterranean Sea (see Mossler et al., 2017 for more information about the effects of OA and other carbon emissions frames). Such structures would include existing EU and regional multilateral environmental laws, conventions and protocols that are pertinent to the protection of the Mediterranean Sea. For example, Mediterranean countries can use Marine Spatial Planning (MSP) techniques to improve their location of mariculture activities on the basis of OA impacts and survival thresholds of economically important species (Meaden et al., 2016).
10.7 To improve OA literacy and capacity building in the Mediterranean
Tools to monitor, model, analyze and make informed decisions should be developed together with innovative ocean literacy approaches, such as direct discussions with marine scientists (Fauville, 2017) and Virtual Reality (VR) techniques (Fauville, 2016; Fauville et al., 2021), to disseminate concomitantly key knowledge that might help in creating a public momentum about OA. Ocean literacy is needed to improve the awareness among practitioners, institutions and the Mediterranean wide and diverse public. This is feasible through re-elaborating the results of key trans-Mediterranean OA-related projects (such as EPOCA and MedSeA), and some key findings published in peer-reviewed articles and OA-related networks (OA Med-Hub and GOA-ON) using public-friendly terminologies. Although such literacy-awareness efforts already took place in some countries, the public is still only vaguely aware of OA and its threats in the Mediterranean Sea. OA literacy should not be exclusively restricted to posters, flyers, and a few videos on social media platforms, but also dovetailed with national ministries (ministries of education/research/environment), municipalities and NGOs to publicize this knowledge efficiently at a national-level, in educational curriculums and via national platforms (TVs, journals, radio stations, etc.). Only through this suggested approach, can OA become an environmental public cause that may trigger national representatives into adopting actions and long-term adaptation/mitigation strategies.
11 Time to act now on the science we need for the Mediterranean Sea we want
Just as our understanding of the science and management of global OA are still very much under development, so it is also for the Mediterranean Sea. This has been indirectly communicated in the final press briefing following the recent G7 meeting. In their press briefing, the wealthiest countries (which are also the main emitters of GHGs), only gave a single, generic reference to OA: “Increased carbon dioxide absorption is also leading to increased ocean acidification”, and promised to “continue our efforts to strengthen the conservation, protection and restoration of coral reefs, mangroves, seagrass beds, salt marshes, polar regions and other ecosystems and we recognise the value of blue carbon ecosystems, which can provide climate resilience benefits while also sequestering carbon. We recognise the importance of sustainable resilience for coastal communities and marine ecosystems and will strengthen our support for the Ocean Risk and Resilience Action Alliance (ORRAA)” (G7, 2021).
To address OA in the Mediterranean Sea, there is a strong need to adopt and implement firm statements in parallel with systematic and clear actions, particularly by the most industrialized countries. Knowing that our Mediterranean Sea is facing a combination of climate change-related consequences, the OA community, and the Mediterranean cities and nations surrounding the Mediterranean Sea basin should declare a de facto OA emergency: Only through systemic cooperation, innovative collaborations and solutions, and by conducting careful OA research that includes biological impacts, realistic modeling and socio-economic forecasts, can innovative governance and policies produce viable solutions to the effects of OA in the Mediterranean Sea. In direct support of the UN Ocean Decade, it is time to collectively support the science we need to protect the Mediterranean Sea we want.
Data availability statement
The original contributions presented in the study are included in the article/Supplementary Material, Further inquiries can be directed to the corresponding author.
Author contributions
AH, PS, and J-PG participated in the conceptualization and outlines' drafting of the paper, and also in refining and fine-tuning the text. AH participated in developing the sub-outlines of the paper, forming the team of authors, drawing the main figures, coordinating between the different authors, and reviewing the different elements of the work. DC, SC, CG, MG, MGr, IH, VI, MI, EK, NS, CS, and PZ, have all participated in writing different sections and sub-sections of the paper, in building the supplementary tables, and in drawing some figures. PS and AB supported the establishement of this paper and its funding. All authors contributed to the article and approved the submitted version.
Acknowledgments
The IAEA is grateful for the support provided to its Marine Environment Laboratories by the Government of the Principality of Monaco. This is a contribution of the IAEA’s Ocean Acidification International Coordination Centre (OA-ICC), a project generously funded through the Peaceful Uses Initiative of the IAEA. The authors would like to thank the OA-ICC for supporting the OA Med-Hub in expanding its activities and visibility. MG and PZ acknowledge funding from MINECO (BIOCAL Project - PID2020-113526RB-I00.
Conflict of interest
The authors declare that the research was conducted in the absence of any commercial or financial relationships that could be construed as a potential conflict of interest.
Publisher’s note
All claims expressed in this article are solely those of the authors and do not necessarily represent those of their affiliated organizations, or those of the publisher, the editors and the reviewers. Any product that may be evaluated in this article, or claim that may be made by its manufacturer, is not guaranteed or endorsed by the publisher.
Supplementary material
The Supplementary Material for this article can be found online at: https://www.frontiersin.org/articles/10.3389/fmars.2022.892670/full#supplementary-material
References
Álvarez M., Monaco C. L., Tanhua T., Yool A., Oschlies A., Bullister J. L., et al. (2009). Estimating the storage of anthropogenic carbon in the subtropical Indian ocean: a comparison of five different approaches. Biogeosciences 6 (4), 681–703. doi: 10.5194/bg-6-681-2009
Álvarez M., Sanleón-Bartolomé H., Tanhua T., Mintrop L., Luchetta A., Cantoni C., et al. (2014). The CO2 system in the Mediterranean Sea: A basin wide perspective. Ocean Sci. 10, 69–92. doi: 10.5194/os-10-69-2014
Balzan M. V., Hassoun A. E. R., Aroua N., Baldy V., Bou Dagher M., Branquinho C., et al. (2020). “Ecosystems,” in Climate and environmental change in the Mediterranean basin – current situation and risks for the future. first Mediterranean assessment report. Eds. Cramer W., Guiot J., Marini K. (Marseille, France: Union for the Mediterranean, Plan Bleu, UNEP/MAP), 323–468.
Barange M., Merino G., Blanchard J. L., Scholtens J., Harle J., Allison E. H., et al. (2014). Impacts of climate change on marine ecosystem production in societies dependent on fisheries. Nat. Climate Change 4 (3), 211–216. doi: 10.1038/nclimate2119
Bates N. R., Astor Y. M., Church M. J., Currie K., Dore J. E., González- Dávila M. (2014). A time-series view of changing ocean chemistry due to ocean uptake of anthropogenic CO2 and ocean acidification. Oceanogr. 27, 126–141. doi: 10.5670/oceanog.2014.16
Bednaršek N., Feely R. A., Howes E. L., Hunt B. P., Kessouri F., León P., et al. (2019). Systematic review and meta-analysis toward synthesis of thresholds of ocean acidification impacts on calcifying pteropods and interactions with warming. Front. Mar. Sci. 6 227. doi: 10.3389/fmars.2019.00227
Bednaršek N., Harvey C. J., Kaplan I. C., Feely R. A., Možina J. (2016). Pteropods on the edge: Cumulative effects of ocean acidification, warming, and deoxygenation. Prog. Oceanography. 145, 1–24. doi: 10.1016/j.pocean.2016.04.002
Ben Rais Lasram F., Guilhaumon F., Albouy C., Somot S., Thuiller W., Mouillot D. (2010). The Mediterranean Sea as a ‘cul-de-sac’for endemic fishes facing climate change. Global Change Biol. 16 (12), 3233–3245. doi: 10.1111/j.1365-2486.2010.02224.x
Bernie D., Lowe J., Tyrrell T., Legge O. (2010). Influence of mitigation policy on ocean acidification. Geophysical. Res. Lett. 37 (15). doi: 10.1029/2010GL043181
Billé R., Kelly R., Biastoch A., Harrould-Kolieb E., Herr D., Joos F., et al. (2013). Taking action against ocean acidification: a review of management and policy options. Environ. Manage. 52 (4), 761–779. doi: 10.1007/s00267-013-0132-7
Boyd P. W., Collins S., Dupont S., Fabricius K., Gattuso J. P., Havenhand J., et al. (2018). Experimental strategies to assess the biological ramifications of multiple drivers of global ocean change–a review. Global Change Biol. 24 (6), 2239–2261. doi: 10.1111/gcb.14102
Brush M., Giani M., Totti C., Testa J., Faganeli J., Ogrinc N., et al. (2021). “Eutrophication: toxic algal events, oxygen depletion, and acidification,” in Coastal ecosystems in transition: A comparative analysis of the northern Adriatic and Chesapeake bay, geophysical monograph, 1st Edition, vol. 256 . Eds. Malone T., Malej A., Faganeli J. (Hoboken, NJ 07030, USA: Wiley & Sons Ltd , ISBN), 75–104.
Buapet P., Gullström M., Björk M. (2013). Photosynthetic activity of seagrasses and macroalgae in temperate shallow waters can alter seawater pH and total inorganic carbon content at the scale of a coastal embayment. Mar. Freshw. Res. 64, 1040–1048. doi: 10.1071/MF12124
Byrne R. H., DeGrandpre M. D., Short R. T., Martz T. R., Merlivat L., McNeil C., et al. (2010). “Sensors and systems for in situ observations of marine carbon dioxide system variables,” in Proceedings of OceanObs’09: Sustained ocean observations and information for society . ; 2. Eds. Hall J., Harrison D. E., Stammer D. (Venice, Italy: ESA Publication), 8. WPP-306 . OceanObs'09. doi: 10.5270/OceanObs09.cwp.13
Cantoni C., Luchetta A., Celio M., Cozzi S., Raicich F., Catalano G. (2012). Carbonate system variability in the gulf of Trieste (North Adriatic Sea). Estuarine Coast. Shelf Sci. 115, 51–62. doi: 10.1016/j.ecss.2012.07.006
Cantoni C., Luchetta A., Chiggiato J., Cozzi S., Schroeder K., Langone L. (2016). Dense water flow and carbonate system in the southern Adriatic: A focus on the 2012 event. Mar. Geology 375, 15–27. doi: 10.1016/j.margeo.2015.08.013
Canu D. M., Ghermandi A., Nunes P. A., Lazzari P., Cossarini G., Solidoro C. (2015). Estimating the value of carbon sequestration ecosystem services in the Mediterranean Sea: An ecological economics approach. Global Environ. Change 32, 87–95. doi: 10.1016/j.gloenvcha.2015.02.008
Cappelletto M., Santoleri R., Evangelista L., Galgani F., Garcés E., Giorgetti A., et al. (2021). The Mediterranean Sea we want. Ocean Coast. Res. 69 (suppl 1). doi: 10.1590/2675-2824069.21019mc
Carbonne C., Teixidó N., Moore B., Mirasole A., Guttierez T., Gattuso J. P., et al. (2021). Two temperate corals are tolerant to low pH regardless of previous exposure to natural CO2 vents. Limnology Oceanography. 66 (11), 4046–4061. doi: 10.1002/lno.11942
Chen G. T., Millero F. J. (1979). Gradual increase of oceanic CO2. Nature 277 (5693), 205–206. doi: 10.1038/277205a0
Cheung W. W., Jones M. C., Reygondeau G., Stock C. A., Lam V. W., Frölicher T. L. (2016). Structural uncertainty in projecting global fisheries catches under climate change. Ecol. Model. 325, 57–66. doi: 10.1016/j.ecolmodel.2015.12.018
Coll M., Piroddi C., Steenbeek J., Kaschner K., Ben Rais Lasram F., Aguzzi J., et al. (2010). The biodiversity of the Mediterranean Sea: estimates, patterns, and threats. PLoS One 5 (8), e11842. doi: 10.1371/journal.pone.0011842
Comeau S., Alliouane S., Gattuso J. P. (2012). Effects of ocean acidification on overwintering juvenile Arctic pteropods limacina helicina. Mar. Ecol. Prog. Ser. 456, 279–284. doi: 10.3354/meps09696
Cooley S. R., Lucey N., Kite-Powell H., Doney S. C. (2012). Nutrition and income from molluscs today imply vulnerability to ocean acidification tomorrow. Fish Fisheries 13 (2), 182–215. doi: 10.1111/j.1467-2979.2011.00424.x
Copin-Montégut C. (1993). Alkalinity and carbon budgets in the Mediterranean Sea. Glob. Biogeochem. Cycles 7 (4), 915–925. doi: 10.1029/93GB01826
Copin-Montégut C., Bégovic M. (2002). Distributions of carbonate properties and oxygen along the water column (0–2000 m) in the central part of the NWMediterranean Sea (DYFAMED site): influence of winter vertical mixing on air–sea CO2 and O2 exchanges. Deep-Sea Res. II 49, 2049–2066. doi: 10.1016/S0967-0645(02)00027-9
Coppola L., Boutin J., Gattuso J. P., Lefèvre D., Metzl N. (2020). “The carbonate system in the ligurian Sea,” in The Mediterranean Sea in the era of global change 1: 30 years of multidisciplinary study of the ligurian Sea. Migon C., and Nival P., Sciandra A. Eds. (ISTE Ltd and John Wiley & Sons, Inc), 79–103. doi: 10.1002/9781119706960.ch4
Cornwall C. E., Comeau S., Kornder N. A., Perry C. T., van Hooidonk R., DeCarlo T. M., et al. (2021). Global declines in coral reef calcium carbonate production under ocean acidification and warming. Proc. Natl. Acad. Sci. 118 (21), e2015265118. doi: 10.1073/pnas.2015265118
Cossarini G., Feudale L., Teruzzi A., Bolzon G., Coidessa G., Solidoro C., et al. (2021). High-resolution reanalysis of the Mediterranean Sea biogeochemistry (1999–2019). Front. Mar. Sci. 8, 741486. doi: 10.25423/CMCC/MEDSEA_MULTIYEAR_BGC_006_008_MEDBFM3I
Cossarini G., Lazzari P., Solidoro C. (2015b). Spatiotemporal variability of alkalinity in the Mediterranean Sea. Biogeosciences 12, 1647–1658. doi: 10.5194/bg-12-1647-2015
Cossarini G., Querin S., Solidoro C. (2015a). The continental shelf carbon pump in the northern Adriatic Sea (Mediterranean sea): Influence of wintertime variability. Ecol. Model. 314, 118–134. doi: 10.1016/j.ecolmodel.2015.07.024
Cramer W., Guiot J., Fader M., Garrabou J., Gattuso J. P., Iglesias A., et al. (2018). Climate change and interconnected risks to sustainable development in the Mediterranean. Nat. Climate Change 8 (11), 972–980. doi: 10.1038/s41558-018-0299-2
D'Amario B., Ziveri P., Grelaud M., Oviedo A., Kralj M. (2017). Coccolithophore haploid and diploid distribution patterns in the Mediterranean Sea: can a haplo-diploid life cycle be advantageous under climate change? J. Plankton Res. 39 (5), 781–794. doi: 10.1093/plankt/fbx044
D’Amario B., Pérez C., Grelaud M., Pitta P., Krasakopoulou E., Ziveri P. (2020). Coccolithophore community response to ocean acidification and warming in the Eastern Mediterranean Sea: results from a mesocosm experiment. Sci. Rep. 10 (1), 1–14. doi: 10.1038/s41598-020-69519-5
D'Amario B., Ziveri P., Grelaud M., Oviedo A. (2018). Emiliania huxleyi coccolith calcite mass modulation by morphological changes and ecology in the Mediterranean Sea. PLoS ONE 137 (7), e0201161. doi: 10.1371/journal.pone.0201161
Doney S. C., Shallin Busch D., Sarah R., Cooley K., Kroeker J. (2020). The impacts of ocean acidification on marine ecosystems and reliant human communities. Annu. Rev. Environ. Resour. 45, 1, 83–1,112. doi: 10.1146/annurev-environ-012320-083019
Dore J. E., Lukas R., Sadler D. W., Church M. J., Karl D. M. (2009). Physical and biogeochemical modulation of ocean acidification in the central north pacific. Proc. Natl. Acad. Sci. 106 (30), 12235–12240. doi: 10.1073/pnas.0906044106
Duarte C. M., Hendriks I. E., Moore T. S., Olsen Y. S., Steckbauer A., Ramajo L., et al. (2013). Is ocean acidification an open-ocean syndrome? understanding anthropogenic impacts on seawater pH. Estuaries Coasts 36 (2), 221–236. doi: 10.1007/s12237-013-9594-3
Dupont C., Belin A., Vermonden B., Moreira G., Cochrane S., Wilson L., et al. (2014). Article 12 technical assessment of the MSFD 2012 obligations, milieu Ltd, Brussels 2014, 67.
Fauville G., Lantz-Andersson A., Mäkitalo Å., Dupont S., Säljö R. (2016). The carbon footprint as a mediating tool in students’ online reasoning about climate change. In Learning across contexts in the knowledge society, 179–201. Available at: https://brill.com/view/book/edcoll/9789463004145/BP000011.xml
Fauville G. (2017). Questions as indicators of ocean literacy: students’ online asynchronous discussion with a marine scientist. Int. J. Sci. Educ. 39:16, 2151–2170. doi: 10.1080/09500693.2017.1365184
Fauville G., Queiroz A. C.M., Brown L. H. B. A., Bailenson J. N. (2021). Participatory research on using virtual reality to teach ocean acidification: a study in the marine education community. Environ. Educ. Res. 27:2, 254–278. doi: 10.1080/13504622.2020.1803797
Feely R. A., Sabine C. L., Lee K., Berelson W., Kleypas J., Fabry V. J., et al. (2004). Impact of anthropogenic CO2 on the CaCO3 system in the oceans. Science 305, 362–366. doi: 10.1126/science.1097329
Fiorini S., Middelburg J. J., Gattuso J. P. (2011). Testing the effects of elevated Pco2 on coccolithophores (Prymnesiophyceae): Comparison between haploid and diploid life stages 1. J. Phycology 47 (6), 1281–1291. doi: 10.1111/j.1529-8817.2011.01080.x
Flecha S., Pérez F. F., García-Lafuente J., Sammartino S., Ríos A. F., Huertas I. E. (2015). Trends of pH decrease in the Mediterranean Sea through high frequency observational data: indication of ocean acidification in the basin. Sci. Rep. 5, 16770. doi: 10.1038/srep16770
Flecha S., Pérez F. F., Murata A., Makaoui A., Huertas I. E. (2019). Decadal acidification in Atlantic and Mediterranean water masses exchanging at the strait of Gibraltar. Sci. Rep. 9, 15533. doi: 10.1038/s41598-019-52084-x
Flecha S., Pérez F. F., Navarro G., Ruiz J., Olivé I., Rodríguez-Gálvez S., et al. (2012). Anthropogenic carbon inventory in the gulf of cádiz. J. Mar. Syst. 92 (1), 67–75. doi: 10.1016/j.jmarsys.2011.10.010
Friedlingstein P., O'sullivan M., Jones M. W., Andrew R. M., Hauck J., Olsen A., et al. (2020). Global carbon budget 2020. Earth System Sci. Data 12 (4), 3269–3340. doi: 10.5194/essd-12-3269-2020
Galdies C. (1999). Manual on the biomarkers recommended for the MED POL biomonitoring programme (Athens: UNITED NATIONS ENVIRONMENT PROGRAMME, MEDITERRANEAN ACTION PLAN), 49pp.
Galdies C. (2008). “Maritime traffic effects on biodiversity in the Mediterranean Sea,” in Review of impacts, priority areas and mitigation measures. Ed. Abdulla A. (Malaga, ES: IUCN Centre for Mediterranean Cooperation), 182.
Galdies C., Bellerby R., Canu D., Chen W., Garcia-Luque E., Gašparović B., et al. (2020). European Policies and legislation targeting ocean acidification in european waters - current state. Mar. Policy Volume 118, 103947. doi: 10.1016/j.marpol.2020.103947
Galdies C., Melaku Canu D., Guerra R., Martinez Romera B., Gjelsvik Tiller R. (2021). Policy brief: global and regional management of ocean acidification. OceanGov.
Gemayel E., Hassoun A. E. R., Benallal M. A., Goyet C., Rivaro P., Abboud-Abi Saab M., et al. (2015). Climatological variations of total alkalinity and total dissolved inorganic carbon in the Mediterranean Sea surface waters. Earth Syst. Dynam. 6, 789–800. doi: 10.5194/esd-6-789-2015,2015
Giorgi F. (2006). Climate change hot-spots. Geophys. Res. Lett. 33, L08707. doi: 10.1029/2006GL025734
González-Dávila M., Santana-Casiano J. M., Petihakis G., Ntoumas M., Suárez de Tangil M., Krasakopoulou E. (2016). Seasonal pH variability in the saronikos gulf: A year-study using a new photometric pH sensor. J. Mar. Syst. 162, 37–46. doi: 10.1016/j.jmarsys.2016.03.007
Gorjanc S., Klančnik K., Murillas-Maza A., Uyarra M. C., Papadopoulou N. K., Th Paramana C., et al. (2020). Coordination of pollution-related MSFD measures in the Mediterranean - where we stand now and insights for the future. Mar. pollut. Bull. 159, 111476. doi: 10.1016/j.marpolbul.2020.111476
Goyet C., Hassoun A., Gemayel E., Touratier F., Abboud-Abi Saab M., Guglielmi V. (2016). Thermodynamic forecasts of the Mediterranean Sea acidification. Mediterr. Mar. Sci. 17 (2), 508–518. doi: 10.12681/mms.1487
Gruber N., Clement D., Carter B. R., Feely R. A., Van Heuven S., Hoppema M., et al. (2019). The oceanic sink for anthropogenic CO2 from 1994 to 2007. Science 363 (6432), 1193–1199. doi: 10.1126/science.aau5153
Guerrero-Meseguer L., Cox T. E., Sanz-Lázaro C., Schmid S., Enzor L. A., Major K., et al. (2020). Mesocosm experiment in the northern gulf of Mexico. Estuaries Coasts 43 (6), 1377–1393. doi: 10.1007/s12237-020-00720-5
Guilini K., Weber M., de Beer D., Schneider M., Molari M., Lott C., et al. (2017). Response of posidonia oceanica seagrass and its epibiont communities to ocean acidification. PLoS One 12 (8), e0181531. doi: 10.1371/journal.pone.0181531
G7. (2021). Climate and environment: Ministers’ communiqué. (London), 21. – GOV.UK www.gov.uk.
Harrould-Kolieb E. R., Herr D. (2012). Ocean acidification and climate change: synergies and challenges of addressing both under the UNFCCC. Climate Policy 12 (3), 378–389. doi: 10.1080/14693062.2012.620788
Harrould-Kolieb E. R., Hoegh-Guldberg O. (2019). A governing framework for international ocean acidification policy. Mar. Policy 102, 10–20. doi: 10.1016/j.marpol.2019.02.004
Hassoun A. E. R., Fakhri M., Raad N., Abboud-Abi Saab M., Gemayel E., De Carlo Heinen E. (2019). The carbonate system of the easternmost Mediterranean Sea, levantine sub-basin: Variations and drivers. Deep-Sea Res. Part II 102, 1–15. doi: 10.1016/j.dsr2.2019.03.008
Hassoun A. E. R., Gemayel E., Krasakopoulou E., Goyet C., Abboud-Abi Saab M., Guglielmi V., et al. (2015). Acidification of the Mediterranean Sea from anthropogenic carbon penetration. Deep Sea research part I: Oceanographic research papers 102, 1–15. doi: 10.1016/j.marenvres.2016.02.016
Hassoun A. E. R., Guiot J., Marini K., Cramer W. (2021). The changing Mediterranean basin through the lens of Mediterranean experts. Int. J. Euro-Mediterranean Stud. 13 (2), 117–137.
Hassoun A. E. R., Hernández-Moresino R., Barbieri E. S., Carbajal J. C., Crespi-Abril A., De Cian A., et al. (2022). “Coastal monitoring in the context of climate change: Time-series efforts in Lebanon and Argentina,” in Frontiers in ocean observing: Documenting ecosystems, UnderstandingEnvironmental changes, forecasting hazards, vol. 34 . Eds. Kappel E. S., Juniper S. K., Seeyave S., Smith E., Visbeck M. (USA: TOS), 12–Pp. 13. doi: 10.5670/oceanog.2021.supplement.02-05. A Supplement to Oceanography.
Hemming M. P., Kaiser J., Heywood K. J., Bakker D. C. E., Boutin J., Shitashima K., et al. (2017). Measuring pH variability using an experimental sensor on an underwater glider. Ocean Sci. 13, 427–442. doi: 10.5194/os-13-427-2017
Hendriks I. E., Duarte C. M., Álvarez M. (2010). Vulnerability of marine biodiversity to ocean acidification: a meta-analysis. Estuarine Coast. Shelf Sci. 86 (2), 157–164. doi: 10.1016/j.ecss.2009.11.022
Hendriks I. E., Duarte C. M., Marbà N., Krause-Jensen D. (2017a). pH gradients in the diffusive boundary layer of subarctic macrophytes. Polar Biol. 40 (12), 2343–2348. doi: 10.1007/s00300-017-2143-y
Hendriks I. E., Duarte C. M., Olsen Y. S., Steckbauer A., Ramajo L., Moore T. S., et al. (2015). Biological mechanisms supporting adaptation to ocean acidification in coastal ecosystems. Estuarine Coast. Shelf Sci. 152, A1–A8. doi: 10.1016/j.ecss.2014.07.019
Hendriks I. E., Olsen Y. S., Duarte C. M. (2017b). Light availability and temperature, not increased CO2, will structure future meadows of posidonia oceanica. Aquat. Bot. 139, 32–36. doi: 10.1016/j.aquabot.2017.02.004
Hendriks I. E., Olsen Y. S., Ramajo L., Basso L., Steckbauer A., Moore T. S., et al. (2014). Photosynthetic activity buffers ocean acidification in seagrass meadows. Biogeosciences 11, 333–346. doi: 10.5194/bg-11-333-2014
Hilmi N., Allemand D., Cinar M., Cooley S., Hall-Spencer J. M., Haraldsson G., et al. (2014). Exposure of Mediterranean countries to ocean acidification. Water 6, 1719–1744. doi: 10.3390/w6061719. no. 6.
Hilmi N., Safa A., Planas-Bielsa V., Kadmiri Y., Cinar M. (2017). Ocean acidification in the middle East and north African region. Reg. Dev. 46, 43–57.
Hofmann G. E., Smith J. E., Johnson K. S., Send U., Levin L. A., Micheli F., et al. (2011). High-frequency dynamics of ocean pH: a multi-ecosystem comparison. PLoS One 6 (12), e28983. doi: 10.1371/journal.pone.0028983
Holcomb M., McCorkle D. C., Cohen A. L. (2010). Long-term effects of nutrient and CO2 enrichment on the temperate coral astrangia poculata (Ellis and solander 1786). J. Exp. Mar. Biol. Ecol. 386 (1-2), 27–33. doi: 10.1016/j.jembe.2010.02.007
Hood E. M., Merlivat L. (2001). Annual to interannual variations of fCO2 in the northwestern Mediterranean Sea: Results from hourly measurements made by CARIOCA buoys 1995-1997. J. Mar. Res. 59 (1), 113–131. doi: 10.1357/002224001321237399
Howes E. L., Stemmann L., Assailly C., Irisson J. O., Dima M., Bijma J., et al. (2015). Pteropod time series from the north Western mediterranean (1967-2003): impacts of pH and climate variability. Mar. Ecol. Prog. Ser. 531, 193–206. doi: 10.3354/meps11322
Huertas I. E., Ríos A. F., García-Lafuente J., Makaoui A., Rodríguez-Gálvez S., Sánchez-Román A., et al. (2009). Anthropogenic and natural CO2 exchange through the strait of Gibraltar. Biogeosciences 6, 647–662. doi: 10.5194/bg-6-647-2009
ICES (2014). Final report to OSPAR of the joint OSPAR/ICES ocean acidification study group (SGOA). ICES 67, 141.
Ingrosso G., Bensi M., Cardin V., Giani M. (2017). Anthropogenic CO2 in a dense water formation area of the Mediterranean Sea. Deep-Sea Res. Part I 123, 118–128. doi: 10.1016/j.dsr.2017.04.004
Ingrosso G., Giani M., Cibic T., Karuza A., Kralj M., Del Negro P. (2016a). Carbonate dynamics and biological processes along a river-sea gradient (Gulf of Trieste, northern Adriatic Sea). J. Mar. Syst. 155, 35–49. doi: 10.1016/j.jmarsys.2015.10.013
Ingrosso G., Giani M., Comici C., Kralj M., Piacentino S., De Vittor C., et al. (2016b). Drivers of the carbonate system seasonal variations in a Mediterranean gulf. Estuarine Coast. Shelf Sci. 168, 58–70. doi: 10.1016/j.ecss.2015.11.001
Invers O., Romero J., Perez M. (1997). Effects of pH on seagrass photosynthesis: A laboratory and field assessment. Aquat. Bot. 59, 185–194. doi: 10.1016/S0304-3770(97)00072-7
IPCC (2021). Climate change 2021: The physical science basis. contribution of working group I to the sixth assessment report of the intergovernmental panel on climate change. Eds. Masson-Delmotte V., Zhai P., Pirani A., Connors S. L., Péan C., Berger S., Caud N., Chen Y., Goldfarb L., Gomis M. I., Huang M., Leitzell K., Lonnoy E., Matthews J. B. R., Maycock T. K., Waterfield T., Yelekçi O., Yu R., Zhou B. (Cambridge University Press).
Jagers S. C., Martinsson J., Matti S. (2019). The impact of compensatory measures on public support for carbon taxation: An experimental study in Sweden. Climate Policy 19 (2), 147–160. doi: 10.1080/14693062.2018.1470963
Jones M. C., Cheung W. W. (2015). Multi-model ensemble projections of climate change effects on global marine biodiversity. ICES J. Mar. Sci. 72 (3), 741–752. doi: 10.1093/icesjms/fsu172
Johnson R., Langer G., Rossi S., Probert I., Mammone M., Ziveri P. (2022). Nutritional response of a coccolithophore to changing pH and temperature. Limnol Oceanogr.. doi: 10.1002/lno.12204
Jordà G., Marbà N., Duarte. C. M. (2012). Mediterranean Seagrass vulnerable to regional climate warming. Nat. Climate Change 2, 821–824. doi: 10.1038/nclimate1533
Kapsenberg L., Alliouane S., Gazeau F., Mousseau L., Gattuso J. P. (2017). Coastal ocean acidification and increasing total alkalinity in the northwestern Mediterranean Sea. Ocean Sci. 13 (3), 411–426. doi: 10.5194/os-13-411-2017
Keraghel M., Louanchi F., Zerrouki M., Ait Kaci M., Aït-Ameur N., Labaste M., et al. (2020). Carbonate system properties and anthropogenic carbon inventory in the Algerian basin during SOMBA cruiss (2014): acidification rate estimate. Mar. Chem. 221, 103783. doi: 10.1016/j.marchem.2020.103783
Khatiwala S., Primeau F., Holzer M. (2012). Ventilation of the deep ocean constrained with tracer observations and implications for radiocarbon estimates of ideal mean age. Earth Planetary Sci. Lett. 325, 116–125. doi: 10.1016/j.epsl.2012.01.038
Kim R. E. (2012). Is a new multilateral environmental agreement on ocean acidification necessary? Rev. Eur. Community Int. Environ. Law 21 (3), 243–258. doi: 10.1111/reel.12000.x
Kleypas J. A., Buddemeier R. W., Archer D., Gattuso J. P., Langdon C., Opdyke B. N. (1999). Geochemical consequences of increased atmospheric carbon dioxide on coral reefs. science 284 (5411), 118–120. doi: 10.1126/science.284.5411.118
Knappertsbusch M. (1993). Geographic distribution of living and Holocene coccolithophores in the Mediterranean Sea. Mar. Micropaleontology 21 (1-3), 219–247. doi: 10.1016/0377-8398(93)90016-Q
Koch M., Bowes G., Ross C., Zhang X.-H. (2013). Climate change and ocean acidification effects on seagrasses and marine macroalgae. Glob Change Biol. 19, 103–132. doi: 10.1111/j.1365-2486.2012.02791.x
Kornder N. A., Riegl B. M., Figueiredo J. (2018). Thresholds and drivers of coral calcification responses to climate change. Global Change Biol. 24 (11), 5084–5095. doi: 10.1111/gcb.14431
Krasakopoulou E., Rapsomanikis S., Papadopoulos A., Papathanassiou E. (2009). Partial pressure and air-sea CO2 flux in the Aegean Sea during February 2006. Continental Shelf Res. 29, 1477–1488. doi: 10.1016/j.csr.2009.03.015
Krasakopoulou E., Souvermezoglou E., Goyet C. (2011). Anthropogenic CO2 fluxes in the otranto strait (E. Mediterranean) February 1995. Deep-Sea Res. Part I 58, 1103–1114. doi: 10.1016/j.dsr.2011.08.008
Krasakopoulou E., Souvermezoglou E., Goyet C. (2017). Carbonate system parameters and anthropogenic CO2 in the north Aegean Sea during October 2013. Continental Shelf Res. 149, 69–81. doi: 10.1016/j.csr.2017.04.002
Krause-Jensen D., Duarte C. M., Hendriks I. E., Meire L., Blicher M. E., Marba N., et al. (2015). Macroalgae contribute to nested mosaics of pH variability in a subarctic fjord. Biogeosciences 12, 4895–4911. doi: 10.5194/bg-12-4895-2015
Krause-Jensen D., Marb a N., Sanz-Martin M., Hendriks I. E., Thyrring J., Carstensen J., et al. (2016). Long photoperiods sustain high pH in arctic kelp forests. Sci. Adv. 2, e1501938. doi: 10.1126/sciadv.1501938
Kroeker K. J., Kordas R. L., Crim R., Hendriks I. E., Ramajo L., Singh G. S., et al. (2013). Impacts of ocean acidification on marine organisms: quantifying sensitivities and interaction with warming. Global Change Biol. 19 (6), 1884–1896. doi: 10.1111/gcb.12179
Lamirande H. R. (2011). From sea to carbon cesspool: preventing the world's marine ecosystems from falling victim to ocean acidification. Suffolk Transnat'l L. Rev. 34, 183.
Le Quéré C., Moriarty R., Andrew R. M., Canadell J. G., Sitch S., Korsbakken J. I., et al. (2015). Global carbon budget 2015. Earth System Sci. Data 7 (2), 349–396. doi: 10.5194/essd-7-349-2015
Mackey K. R. M., Morris J. J., Morel F. M. M., Kranz S. A. (2015). Response of photosynthesis to ocean acidification. Oceanography 28 (2), 74–91. doi: 10.5670/oceanog.2015.33
Malinverno E., Ziveri P., Corselli C. (2003). Coccolithophorid distribution in the Ionian Sea and its relationship to eastern Mediterranean circulation during late fall to early winter 1997. J. Geophys Res. 108, 2156–2202. doi: 10.1029/2002JC001346
Malvarosa L., De Young C. (2010). Fish trade among Mediterranean countries: Intraregional trade and import-export with the European union.
Manno C., Morata N., Primicerio R. (2012). Limacina retroversa's response to combined effects of ocean acidification and sea water freshening. Estuarine Coast. Shelf Sci. 113, 163–171. doi: 10.1016/j.ecss.2012.07.019
Manno C., Rumolo P., Barra M., d’Albero S., Basilone G., Genovese S., et al. (2019). Condition of pteropod shells near a volcanic CO2 vent region. Mar. Environ. Res. 143, 39–48. doi: 10.1016/j.marenvres.2018.11.003
Manzello D. P., Enochs I. C., Melo N., Gledhill D. K., Johns E. M. (2012). Ocean acidification refugia of the Florida reef tract. PLoS One 7, e41715. doi: 10.1371/journal.pone.0041715
Marbà N., Díaz-Almela E., Duarte C. M. (2014). Mediterranean Seagrass (Posidonia oceanica) loss between 1842 and 2009. Biol. Conserv. 176, 183–190. doi: 10.1016/j.biocon.2014.05.024
Marbà N., Jordà G., Agusti S., Girard C., Duarte C. M. (2015). Footprints of climate change on Mediterranean Sea biota. Front. Mar. Sci. 2, 00056. doi: 10.3389/fmars.2015.00056
Meaden G. J., Aguilar-Manjarrez J., Corner R. A., O'Hagan A. M., Cardia F. (2016). Marine spatial planning for enhanced fisheries and aquaculture sustainability: Its application in the near East. FAO fisheries aquaculture Tech. paper, I.
MedECC (2020). Climate and environmental change in the Mediterranean basin – current situation and risks for the future. first Mediterranean assessment report. Eds. Cramer W., Guiot J., Marini K. (Marseille, France: Union for the Mediterranean, Plan Bleu, UNEP/MAP), 632pp. doi: 10.5281/zenodo.4768833
Med QSR (2017). Mediterranean 2017 quality status report. Mediterranean 2017 quality status report | UNEPMAP QSR.
Meier K. J. S., Beaufort L., Heussner S., Ziveri P. (2014). The role of ocean acidification in emiliania huxleyi coccolith thinning in the Mediterranean Sea. Biogeosciences 11 (10), 2857–2869. doi: 10.5194/bg-11-2857-2014
Merlivat L., Boutin J., Antoine D., Beaumont L., Melek Golbol M., Vellucci V. (2018). Increase of dissolved inorganic carbon and decrease in pH in near-surface waters in the Mediterranean Sea during the past two decades. Biogeosciences 15, 5653–5662. doi: 10.5194/bg-15-5653-201
Meyer J., Riebesell U. (2015). Reviews and syntheses: Responses of coccolithophores to ocean acidification: a meta-analysis. Biogeosciences 12 (6), 1671–1682. doi: 10.5194/bg-12-1671-2015
Middelboe A. L., Hansen P. J. (2007). High pH in shallow-water macroalgal habitats. Mar. Ecol. Prog. Ser. 338, 107–117. doi: 10.3354/meps338107
Milazzo M., Rodolfo-Metalpa R., Chan V. B. S., Fine M., Alessi C., Thiyagarajan V., et al. (2014). Ocean acidification impairs vermetid reef recruitment. Sci. Rep. 4 (1), 1–7. doi: 10.1038/srep04189
Milner S., Langer G., Grelaud M., Ziveri P. (2016). Ocean warming modulates the effects of acidification on emiliania huxleyi calcification and sinking. Limnology Oceanography. 61 (4), 1322–1336. doi: 10.1002/lno.10292
Mossler M. V., Bostrom A., Kelly R. P., Crosman K. M., Moy P. (2017). How does framing affect policy support for emissions mitigation? testing the effects of ocean acidification and other carbon emissions frames. Global Environ. Change 45, 63–78. doi: 10.1016/j.gloenvcha.2017.04.002
Movilla J., Calvo E., Coma R., López-Sanz A., Serrano E., Pelejero C. (2015). Long-term response of two Mediterranean azooxanthellate temperate corals to low-pH and high-temperature conditions. Effects ocean acidification Mediterr. coral, 93.
Movilla J., Calvo E., Coma R., Serrano E., Lopez-Sanz A., Pelejero C. (2016). Annual response of two Mediterranean azooxanthellate temperate corals to low-pH and high-temperature conditions. Mar. Biol. 163 (6), 1–14. doi: 10.1007/s00227-016-2908-9
Movilla J., Calvo E., Pelejero C., Coma R., Serrano E., Fernández-Vallejo P., et al. (2012). Calcification reduction and recovery in native and non-native Mediterranean corals in response to ocean acidification. J. Exp. Mar. Biol. Ecol. 438, 144–153. doi: 10.1016/j.jembe.2012.09.014
MSFD (2014). Article 12 technical assessment of the MSFD 2014 reporting on monitoring programmes Mediterranean regional report; coherence assessment by descriptor 2015, 36.
Nisumaa A.-M., Pesant S., Bellerby R. G. J., Delille B., Middelburg J. J., Orr J. C., et al. (2010). EPOCA/EUR-OCEANS data compilation on the biological and biogeochemical responses to ocean acidification, earth syst. Sci. Data 2, 167–175. doi: 10.5194/essd-2-167-2010
Nittis K., Lascaratos A. (1998). Diagnostic and prognostic numerical studies of LIW formation. J. Mar.Syst. 18, 179–195. doi: 10.1016/S0924-7963(98)00011-6
Orr J. C., Fabry V. J., Aumont O., Bopp L., Doney S. C., Feely R. A., et al. (2005). Anthropogenic ocean acidification over the twenty-first century and its impact on calcifying organisms. Nature 437 (7059), 681–686. doi: 10.1038/nature04095
Osborne K., Thompson A. A., Cheal A. J., Emslie M. J., Johns K. A., Jonker M. J., et al. (2017). Delayed coral recovery in a warming ocean. Global Change Biol. 23 (9), 3869–3881. doi: 10.1111/gcb.13707
Oviedo A. M., Ziveri P., Álvarez M., Tanhua T. (2014). Is coccolithophore distribution in the Mediterranean Sea related to seawater carbonate chemistry. Ocean Sci. Discuss 11, 613–653. doi: 10.5194/osd-11-613-2014
Oviedo A. M., Ziveri P., Gazeau F. (2017). Coccolithophore community response to increasing pCO2 in Mediterranean oligotrophic waters. Estuarine, coastal and shelf science 186, 58–71. doi: 10.1016/j.ecss.2015.12.007
Palmiéri J., Orr J. C., Dutay J. C., Béranger K., Schneider A., Beuvier J., et al. (2015). Simulated anthropogenic CO 2 storage and acidification of the Mediterranean Sea. Biogeosciences 12 (3), 781–802. doi: 10.5194/bg-12-781-2015
Poloczanska E. S., Burrows M. T., Brown C. J., García Molinos J., Halpern B. S., Hoegh-Guldberg O., et al. (2016). Responses of marine organisms to climate change across oceans. Front. Mar. Sci. 3, 62. doi: 10.3389/fmars.2016.00062
Prada F., Caroselli E., Mengoli S., Brizi L., Fantazzini P., Capaccioni B., et al. (2017). Ocean warming and acidification synergistically increase coral mortality. Sci. Rep. 7 (1), 1–10. doi: 10.1038/srep40842
Querin S., Cossarini G., Solidoro C. (2013). Simulating the formation and fate of dense water in a midlatitude marginal sea during normal and warm winter conditions. J. Geophysical. Research: Oceans 118 (2), 885–900. doi: 10.1002/jgrc.20092
Ramajo L., Lagos N. A., Duarte C. M. (2019). Seagrass posidonia oceanica diel pH fluctuations reduce the mortality of epiphytic forams under experimental ocean acidification. Mar. pollut. Bull. 146, 247–254. doi: 10.1016/j.marpolbul.2019.06.011
Ramajo L., Pérez-León E., Hendriks I. E., Marbà N., Krause-Jensen D., Sejr M. K., et al. (2016). Food supply confers calcifiers resistance to ocean acidification. Sci. Rep. 6 (1), 1–6. doi: 10.1038/srep19374
Reale M., Cossarini G., Lazzari P., Lovato T., Bolzon G., Masina S., et al. (2022). Acidification, deoxygenation, nutrient and biomasses decline in a warming Mediterranean Sea. Biogeosciences Discuss. doi: 10.5194/bg-2021-301
Ridgwell A., Zeebe R. E. (2005). The role of the global carbonate cycle in the regulation and evolution of the earth system. Earth Planetary Sci. Lett. 234 (3-4), 299–315. doi: 10.1016/j.epsl.2005.03.006
Riebesell U., Czerny J., von Bröckel K., Boxhammer T., Büdenbender J., Deckelnick M., et al. (2013). A mobile sea-going mesocosm system–new opportunities for ocean change research. Biogeosciences 10 (3), 1835–1847. doi: 10.5194/bg-10-1835-2013
Riebesell U., Gattuso J. P. (2015). Lessons learned from ocean acidification research. Nat. Climate Change 5 (1), 12–14. doi: 10.1038/nclimate2456
Riebesell U., Zondervan I., Rost B., Tortell P. D., Zeebe R. E., Morel F. M. (2000). Reduced calcification of marine plankton in response to increased atmospheric CO2. Nature 407 (6802), 364–367. doi: 10.1038/35030078
Rivaro P., Messa R., Massolo S., Frache R. (2010). Distributions of carbonate properties along the water column in the Mediterranean Sea: spatial and temporal variations. Mar. Chem. 121, 236–254. doi: 10.1016/j.marchem.2010.05.003
Rivest E. B., Comeau S., Cornwall C. E. (2017). The role of natural variability in shaping the response of coral reef organisms to climate change. Curr. Climate Change Rep. 3, 271–281. doi: 10.1007/s40641-017-0082-x
Rodolfo-Metalpa R., Houlbrèque F., Tambutté É., Boisson F., Baggini C., Patti F. P., et al. (2011). Coral and mollusc resistance to ocean acidification adversely affected by warming. Nat. Climate Change 1 (6), 308–312. doi: 10.1038/nclimate1200
Rodolfo-Metalpa R., Martin S., Ferrier-Pagès C., Gattuso J. P. (2010). Response of the temperate coral cladocora caespitosa to mid-and long-term exposure to pCO 2 and temperature levels projected for the year 2100 AD. Biogeosciences 7 (1), 289–300. doi: 10.5194/bg-7-289-2010
Rodrigues L. C., van den Bergh J. C., Ghermandi A. (2013). Socio-economic impacts of ocean acidification in the Mediterranean Sea. Mar. Policy 38, 447–456. doi: 10.1016/j.marpol.2012.07.005
Santana-Casiano J. M., Gonzalez-Davila M., Rueda M. J., Llinas O., Gonzalez-Davila E. F. (2007). The interannual variability of oceanic CO2 parameters in the northeast Atlantic subtropical gyre at the ESTOC site. Glob. Biogeochem. Cycle 21, GB1015. doi: 10.1029/2006GB002788
Schneider A., Tanhua T., Körtzinger A., Wallace D. W. (2010). High anthropogenic carbon content in the eastern Mediterranean. J. Geophysical. Research: Oceans 115 (C12), C12050. doi: 10.1029/2010JC006171
Schneider A., Tanhua T., Roether W., Steinfeldt R. (2014). Changes in ventilation of the Mediterranean Sea during the past 25 year. Ocean Sci. 10, 1–16. doi: 10.5194/os-10-1-2014
Schneider A., Wallace D. W. R., Körtzinger A. (2007). Alkalinity of the Mediterranean Sea: alkalinity of the Mediterranean Sea. Geophys. Res. Lett 34, L15608. doi: 10.1029/2006GL028842
Semesi I. S., Beer S., Björk M. (2009). Seagrass photosynthesis controls rates of calcification and photosynthesis of calcareous macroalgae in a tropical seagrass meadow. Mar. Ecol. Prog. Ser. 382, 41–47. doi: 10.3354/meps07973
Sisma-Ventura G., Yam R., Kress N., Shemesh A. (2016). Water column distribution of stable isotopes and carbonate properties in the South-eastern Levantine basin (Eastern Mediterranean): Vertical and temporal change. Journal of Marine Systems 158, 13–25. doi: 10.1016/j.jmarsys.2016.01.012
Sisma-Ventura G., Or B. M., Yam R., Herut B., Silverman J. (2017). pCO2 variability in the surface waters of the ultra-oligotrophic levantine Sea: exploring the air-sea CO2 fluxes in a fast warming region. Mar. Chem. 196, 13–23. doi: 10.1016/j.marchem.2017.06.006
Solidoro C. (2021). Observing ocean state and marine life. Bull. Geophysics Oceanography. 62 (3), 79–85.
Solidoro C., Cossarini G., Lazzari P., Galli G., Bolzon G., Somot S., et al. (2022). Modeling carbon budgets and acidification in the Mediterranean Sea ecosystem under contemporary and future climate. Front. Mar. Sci. 8. doi: 10.3389/fmars.2021.781522
Solidoro C., Cossarini G., Libralato S., Salon S. (2010). Remarks on the redefinition of system boundaries and model parameterization for downscaling experiments. Prog. Oceanography. 84 (1-2), 134–137. doi: 10.1016/j.pocean.2009.09.017
Stöven T., Tanhua T. (2014). Ventilation of the Mediterranean Sea constrained by multiple transient tracer measurements. Ocean Sci. 10, 439–457. doi: 10.5194/os-10-439-2014
Suchéras-Marx B., Mattioli E., Allemand P., Giraud F., Pittet B., Plancq J., et al. (2019). The colonization of the oceans by calcifying pelagic algae. Biogeosciences 16 (12), 2501–2510. doi: 10.5194/bg-16-2501-2019
Takahashi T., Sutherland S. C., Chipman D. W., Goddard J. G., Ho C., Newberger T., et al. (2014). Climatological distributions of pH, pCO2, total CO2, alkalinity, and CaCO3 saturation in the global surface ocean, and temporal changes at selected locations. Mar. Chem. 164, 95–125. doi: 10.1016/j.marchem.2014.06.004
Tamše S., Ogrinc N., Walter L. M., Turk D., Faganeli J. (2015). River sources of dissolved inorganic carbon in the gulf of Trieste (N adriatic): Stable carbon isotope evidence. Estuaries Coasts 38, 151–164. doi: 10.1007/s12237-014-9812-7
Tanhua T., Lauvset S. K., Lange N., Olsen A., Álvarez M., Diggs S., et al. (2021). A vision for FAIR ocean data products. Commun. Earth Environ. 2 (1), 1–4. doi: 10.1038/s43247-021-00209-4
Teixidó N., Caroselli E., Alliouane S., Ceccarelli C., Comeau S., Gattuso J. P., et al. (2020). Ocean acidification causes variable trait-shifts in a coral species. Global Change Biol. 26 (12), 6813–6830. doi: 10.1111/gcb.15372
Thomsen J., Haynert K., Wegner K. M., Melzner F. (2015). Impact of seawater carbonate chemistry on the calcification of marine bivalves. Biogeosciences 12, 4209–4220. doi: 10.5194/bg-12-4209-2015
Tilbrook B., Jewett E. B., DeGrandpre M. D., Hernandez-Ayon J. M., Feely R. A., Gledhill D. K., et al. (2019). An enhanced ocean acidification observing network: From people to technology to data synthesis and information exchange. Front. Mar. Sci. 6. doi: 10.3389/fmars.2019.00337
Tiller R., Arenas F., Galdies C., Leitão F., Malej A., Romera R. M., et al. (2015). Who cares about ocean acidification in the plasticene? Ocean Coast. Manage. 174, 170–180. doi: 10.1016/j.ocecoaman.2019.03.020
Touratier F., Azouzi L., Goyet C. (2007). CFC-11, Δ14C and 3H tracers as a means to assess anthropogenic CO2 concentrations in the ocean. Tellus B: Chem. Phys. Meteorology 59 (2), 318–325. doi: 10.1111/j.1600-0889.2006.00247.x
Touratier F., Goyet C. (2004). Applying the new TrOCA approach to assess the distribution of anthropogenic CO2 in the Atlantic ocean. J. Mar. Syst. 46 (1-4), 181–197. doi: 10.1016/j.jmarsys.2003.11.020
Touratier F., Goyet C. (2009). Decadal evolution of anthropogenic CO2 in the northwestern Mediterranean Sea from the mid-1990s to the mid-2000s. Deep-Sea Res. I 56, 1708–1716. doi: 10.1016/j.dsr.2009.05.015
Touratier F., Goyet C. (2011). Impact of the Eastern Mediterranean transient on the distribution of anthropogenic CO2 and first estimate of acidification for the Mediterranean Sea. Deep-Sea Res. I 58, 1–15. doi: 10.1016/j.dsr.2010.10.002
Touratier F., Goyet C., Houpert L., De Madron X. D., Lefevre D., Stabholz M., et al. (2016). Role of deep convection on anthropogenic CO2 sequestration in the gulf of lions (northwestern Mediterranean Sea). Deep Sea Res. Part I: Oceanographic 113, 33–48. doi: 10.1016/j.dsr.2016.04.003
Tovar-Sánchez A., Sánchez-Quiles D., Rodríguez-Romero A. (2019). Massive coastal tourism influx to the Mediterranean Sea: The environmental risk of sunscreens. Sci. Total Environ. 656, 316–321. doi: 10.1016/j.scitotenv.2018.11.399
Triantaphyllou M. V., Baumann K. H., Karatsolis B. T., Dimiza M. D., Psarra S., Skampa E., et al. (2018). Coccolithophore community response along a natural CO2 gradient off methana (SW saronikos gulf, Greece, NE Mediterranean). PLoS One 13 (7), e0200012. doi: 10.1371/journal.pone.0200012
Triantaphyllou M., Dimiza M., Krasakopoulou E., Malinverno E., Lianou V., Souvermezoglou E. (2010). Seasonal variation in emiliania huxleyi coccolith morphology and calcification in the Aegean Sea (Eastern Mediterranean). Geobios 43 (1), 99–110. doi: 10.1016/j.geobios.2009.09.002
Turk D., Book J. W., McGillis W. R. (2013). pCO2 and CO2 exchange during high bora winds in the northern Adriatic. J. Mar. Syst. 117-118, 65–71. doi: 10.1016/j.jmarsys.2013.02.010
Turk D., Malačič V., DeGrandpre M. D., McGillis W. R. (2010). Carbon dioxide variability and air-sea fluxes in the northern Adriatic Sea. J. Geophysical. Res. 115, C10043. doi: 10.1029/2009JC006034
Unsworth R. K. F., Collier C. J., Henderson G. M., Mckenzie L. J. (2012). Tropical seagrass meadows modify seawater carbon chemistry: Implications for coral reefs impacted by ocean acidification. Environ. Res. Lett. 7, 24026–24034. doi: 10.1088/1748-9326/7/2/024026
Urbini L., Ingrosso G., Djakovac T., Piacentino S., Giani M. (2020). Temporal and spatial variability of the CO2 system in a riverine influenced area of the Mediterranean Sea, the northern Adriatic. Front. Mar. Sci., 679. doi: 10.3389/fmars.2020.00679
Vazquez-Rodriguez M., Touratier F., Monaco C. L., Waugh D. W., Padin X. A., Bellerby R. G., et al. (2009). Anthropogenic carbon distributions in the Atlantic ocean: data-based estimates from the Arctic to the Antarctic. Biogeosciences 6 (3), 439–451. doi: 10.5194/bg-6-439-2009
Wahl M., Saderne V., Sawall Y. (2016). How good are we at assessing the impact of ocean acidification in coastal systems? limitations, omissions and strengths of commonly used experimental approaches with special emphasis on the neglected role of fluctuations. Mar. Freshw. Res. 67, 25–36. doi: 10.1071/MF14154
Wahl M., Schneider Covachã S., Saderne V., Hiebenthal C., Müller J. D., Pansch C., et al. (2018). Macroalgae may mitigate ocean acidification effects on mussel calcification by increasing pH and its fluctuations. Limnol. Oceanogr. 63, 3–21. doi: 10.1002/lno.10608
Waldbusser G. G., Salisbury J. E. (2014). Ocean acidification in the coastal zone from an organism’s perspective: Multiple system parameters, frequency domains, and habitats. Annu. Rev. Mar. Sci. 6, 221–247. doi: 10.1146/annurev-marine-121211-172238
Wall C. B., Ritson-Williams R., Popp B. N., Gates R. D. (2019). Spatial variation in the biochemical and isotopic composition of corals during bleaching and recovery. Limnology Oceanography. 64 (5), 2011–2028. doi: 10.1002/lno.11166
Watson A. J., Schuster U., Shutler J. D., Holding T., Ashton I. G., Landschützer P., et al. (2020). Revised estimates of ocean-atmosphere CO2 flux are consistent with ocean carbon inventory. Nat. Commun. 11 (1), 1–6. doi: 10.1038/s41467-020-18203-3
Waugh D. W., Hall T. M., McNeil B. I., Key R., Matear R. J. (2006). Anthropogenic CO2 in the oceans estimated using transit time distributions. Tellus B: Chem. Phys. Meteorology 58 (5), 376–389. doi: 10.1111/j.1600-0889.2006.00222.x
Wimart-Rousseau C., Lajaunie-Salla K., Marrec P., Armengaud A., Lefèvre D. (2020). Temporal variability of the carbonate system and air-sea CO2 exchanges in a Mediterranean human-impacted coastal site. Estuarine Coast. Shelf Sci. 236, 106641. doi: 10.1016/j.ecss.2020.106641
Wimart-Rousseau C., Wagener T., Álvarez M., Moutin T., Fourrier M., Coppola L., et al. (2021). Seasonal and interannual variability of the CO2 system in the Eastern Mediterranean Sea: A case study in the north Western levantine basin. Front. Mar. Sci. 8. doi: 10.3389/fmars.2021.649246
Yang Y., Hansson L., Gattuso J.-P. (2016). Data compilation on the biological response to ocean acidification: an update. Earth Syst. Sci. Data 8, 79–87. doi: 10.5194/essd-8-79-2016
Yao K. M., Marcou O., Goyet C., Savy J.-P. (2016). Time variability of the north-western Mediterranean Sea pH over 1995–2011. Mar. Environ. Res. 116, 51–60. doi: 10.1016/j.marenvres.2016.02.016
Ziveri P., Passaro M., Incarbona A., Milazzo M., Rodolfo-Metalpa R., Hall-Spencer J. M. (2014). Decline in coccolithophore diversity and impact on coccolith morphogenesis along a natural CO2 gradient. Biol. Bull. 226 (3), 282–290. doi: 10.1086/BBLv226n3p282
Ziveri P., Rutten A., de Lange G., Thomson J., Corselli C. (2000). Present-day coccolith fluxes recorded in central eastern Mediterranean sediment traps and surface sediments. Palaeogeography, Paleoclimatology and Palaeoecology 158 (3-4), 175–195.
Zunino S., Canu D. M., Zupo V., Solidoro C. (2019). Direct and indirect impacts of marine acidification on the ecosystem services provided by coralligenous reefs and seagrass systems. Global Ecol. Conserv. Vol. 18, 00625. doi: 10.1016/j.gecco.2019.e00625
Zunino S., Libralato S., Melaku Canu D., Solidoro C. (2021). Impact of ocean acidification on ecosystem functioning and services in habitat-forming species and marine ecosystems. Ecosystems. doi: 10.1007/s10021-021-00601-3
Zunino S., Melaku Canu D., Bandelj V., Solidoro C. (2017). Effects of ocean acidification on benthic organisms in the Mediterranean Sea under realistic climatic scenarios: A meta-analysis. Regional Stud. Mar. Sci. 10, 86–89. doi: 10.1016/j.rsma.2016.12.011
Keywords: ocean acidification, Mediterranean Sea, socio-economy, policies, climate change, UN ocean decade
Citation: Hassoun AER, Bantelman A, Canu D, Comeau S, Galdies C, Gattuso J-P, Giani M, Grelaud M, Hendriks IE, Ibello V, Idrissi M, Krasakopoulou E, Shaltout N, Solidoro C, Swarzenski PW and Ziveri P (2022) Ocean acidification research in the Mediterranean Sea: Status, trends and next steps. Front. Mar. Sci. 9:892670. doi: 10.3389/fmars.2022.892670
Received: 09 March 2022; Accepted: 31 August 2022;
Published: 27 September 2022.
Edited by:
Marta Álvarez, Spanish Institute of Oceanography (IEO), SpainReviewed by:
Chen-Feng You, National Cheng Kung University, TaiwanElizabeth B. Jewett, National Oceanic and Atmospheric Administration (NOAA), United States
Copyright © 2022 Hassoun, Bantelman, Canu, Comeau, Galdies, Gattuso, Giani, Grelaud, Hendriks, Ibello, Idrissi, Krasakopoulou, Shaltout, Solidoro, Swarzenski and Ziveri. This is an open-access article distributed under the terms of the Creative Commons Attribution License (CC BY). The use, distribution or reproduction in other forums is permitted, provided the original author(s) and the copyright owner(s) are credited and that the original publication in this journal is cited, in accordance with accepted academic practice. No use, distribution or reproduction is permitted which does not comply with these terms.
*Correspondence: Abed El Rahman Hassoun, YWJlZGhhc3NvdW5AY25ycy5lZHUubGI=; YWhhc3NvdW5AZ2VvbWFyLmRl