- 1School of Geography and Sustainable Development, University of St. Andrews, St. Andrews, United Kingdom
- 2Marine Scotland Science, Aberdeen, United Kingdom
- 3Scottish Association for Marine Science, Scottish Marine Institute, Oban, United Kingdom
It is estimated that within the UK exclusive economic zone (UK EEZ), 524 Mt of organic carbon (OC) is stored within seabed sediment. However, the stability and potential vulnerability of OC in these sediments under anthropogenic stressors, such as bottom trawling activity, remains poorly quantified. To improve our understanding of the areas where sedimentary OC is likely to be at greatest risk from trawling events, we have developed a carbon vulnerability ranking (CVR) to identify areas of the seabed where preventative protection may be most beneficial to help maintain current OC stocks while further research continues to shed light on the fate of OC after trawling (e.g., remineralization, transport, and consumption). Predictive maps of currently available fishing intensity, OC and sediment distribution, and sediment OC lability have been generated within ArcGIS using fuzzy set theory. Our results show that the west coast of Scotland represents one of the key areas where sedimentary OC is potentially at greatest risk from bottom trawling activity. This is due mainly to the high reactivity of these OC rich sediments combined with the pressures of repetitive trawling activity within inshore waters. Our research shows that these OC hotspots are potentially at risk of disturbance from bottom trawling activity and should be prioritized for the consideration of future safeguarding (management) measures to ensure emissions are minimized and to provide greater protection of this natural carbon capital resource.
1 Introduction
As marine sediments are deposited and accumulate on the seafloor, they act as a trap and long-term store (> 103 years) of large quantities of carbon (C). It is estimated that marine sediments contain 87,000 ± 43,000 Mt of organic carbon (OC) within global surficial sediments (Lee et al., 2019). It has also been predicted that globally, marine sediments may hold up to 3,117,000 Mt OC within the top 1 m (Atwood et al., 2020). These global stores of OC are estimated to increase by 160 Mt annually through accumulation and burial (Hedges and Keil, 1995). Due to the highly heterogeneous nature of sedimentary marine environments, OC is not uniformly stored but instead exhibits a high degree of spatial heterogeneity across the seabed (Hunt et al., 2020; Smeaton et al., 2021a). Under natural hydrodynamic processes, OC can accumulate and add to the long-term store within marine sediments (Berner, 1982; Hedges and Keil, 1995). However, increasing anthropogenic activity which disturbs the seabed is potentially putting these OC stores at risk, notably through elevated rates of OC remineralisation to carbon dioxide (CO2) (Duplisea et al., 2001), which may potentially contribute towards global emissions.
Fishing alongside other anthropogenic activities such as offshore drilling, aggregate dredging, aquaculture farming, and shipping exert significant pressure on benthic marine environments (Kavadas et al., 2015). Out of all these activities, bottom trawling is commonly recognised as the most frequent and widespread cause of anthropogenic disturbance and pressure on the seabed, putting coastal and shelf sea environments at risk (Kaiser et al., 2006; Rijnsdorp et al., 2016; Eigaard et al., 2017; Kenny et al., 2018). During bottom contact fishing disturbance, the resuspension of sediments occurs. This potentially results in the exposure of buried OC to oxygen-rich overlying waters, enhancing organic matter (OM) remineralisation processes (Arndt et al., 2013) and reducing the quantity of OC stored. In some regions where accumulation and deposition of OC is low or where OC is especially reactive (e.g. coastal inlets and fjords) (Smeaton and Austin, 2022), this could result in a more significant overall loss of OC from the sediment when disturbed. Resuspended OC rich sediment may also undergo lateral sediment transport, resulting in a loss of fine flocculant material from the trawl site (Epstein et al., 2022). Additional biological factors such as the reduction in biomass of flora and fauna may also negatively impact OC storage within sediments (Epstein et al., 2022).
However, it is worth noting that the loss of OC due to bottom trawling activity may be offset by a reduction of faunal bioturbation, reduced respiration rates, and an increase in primary production due to increased nutrient availability (Epstein et al., 2022). In addition to this, while sediment may be resuspended and laterally transported during trawling, this material may be deposited and stored elsewhere. Current studies investigating the net impact of bottom trawling have shown mixed results, highlighting the need for further study within this field. It is estimated that 1.3% (4.9 million km2) of the global seabed is trawled annually (Sala et al., 2021). Unlike the environmental threats to sedimentary OC stores, such as changes in ocean temperature and oxygen availability, direct anthropogenic pressures such as bottom trawling can be monitored and controlled to safeguard the most vulnerable OC stores. By managing marine protected areas (MPA’s) and highly protected marine areas (HPMA’s), it may be possible to alleviate some of this fishing pressure and protect marine life and sedimentary environments with a high OC storage capacity. However, globally, only 2.7% of the marine environment is currently under complete or high-level protection from fishing impacts, with the majority (577/1,013) of these protected areas being less than 10 km2 (Marine Conservation Institute, 2021).
It is estimated that 52% (1,606,000 Mt) of the global 1 m OC stock is located within the 200-mile Exclusive Economic Zones (EEZ) (Atwood et al., 2020). In the case of the UK EEZ, it is estimated that these marine sediments store greater quantities of OC (524 Mt) than their terrestrial equivalents (Smeaton et al., 2021a).
The UK EEZ has one of the most comprehensively mapped seabeds in terms of available sedimentary datasets. In recent years, there have been multiple studies looking at the distribution and content of OC within marine sediments in the greater North Sea region (Diesing et al., 2017; Luisetti et al., 2019; Legge et al., 2020; Diesing et al., 2021). However, more recently, fjords, estuaries, and deep-water environments have been included in these efforts, allowing the first complete spatial dataset of OC in the UK EEZ to be developed (Smeaton et al., 2021a). This improved understanding of OC distribution within the UK EEZ allows the development of new tools to assess the vulnerability of these OC stores to anthropogenic disturbances.
In this study, we use a multi-criteria decision analysis approach using open-source data to calculate and spatially define the relative vulnerability of OC in marine sediments within the UK EEZ. We evaluate the impacts of different fishing gear pressures (e.g. otter trawls, beam trawls, and towed dredging) by creating a sedimentary OC vulnerability ranking (CVR) map. This CVR map details areas of the UK EEZ seabed where surficial OC stores are at the highest risk from bottom-contact fishing activity and may highlight areas that would benefit from some form of management intervention to deliver OC safeguarding alongside other criteria for marine protection. The calculations and outputs presented for the UK EEZ represent one of the first detailed mapping efforts to understand the potential vulnerability of sedimentary OC from bottom trawling.
Within this study, vulnerability is taken to be a relative measure of the probability that an aspect (i.e. OC content) of a pre-defined geographical area is likely to be damaged or disrupted by the impact of a particular hazard, in this case, bottom trawling. In addition, we define the “loss” of OC from the seabed as the OC which is disturbed from its original depositional location after a trawling event, with the potential to be either transported and re-deposited, remineralised, or consumed. This model-based approach aims to determine which areas of the seabed contain OC that is most vulnerable to bottom trawling. However, our study does not determine the fate of these sediments after they are disturbed by trawling; neither do we consider ongoing natural processes at the seafloor. Instead, the results from this study are intended to support new decision making related to the monitoring, prioritisation of research, implementation of preventative management, and protection of the seabed within the UK EEZ.
2 Methods
2.1 Study area
The UK EEZ covers an area of 743,470 km2 (including Rockall and the Isle of Man). Within this study, the term EEZ is used to define the entire area under the exclusive jurisdiction of the UK as a coastal state. Throughout the UK EEZ, there is considerable variation in sediments across both water depth and environment type (e.g. fjordic, coastal and inshore, continental shelf etc.) (See Figure 1A), all of which play a critical role in the various biogeochemical processes which occur, most notably in the C cycle (Burrows et al., 2017; Smeaton and Austin, 2017; Smeaton et al., 2021a). The surficial sediments of the UK EEZ are estimated to hold 524.4 ± 68.4 Mt of OC (Smeaton et al., 2021a), much of this is concentrated in distinct accumulation hotspots such as fjords, estuaries, and coastal muds, where OC supply is enhanced by proximity to land and higher marine productivity rates (Figure 1B). The influence of fjordic and sheltered environments is most notable in the estimates for Scottish sedimentary OC stores, which are estimated to contain 356.5 ± 72.2 Mt OC compared to English sedimentary OC stores estimated to contain 142.1 ± 28.5 Mt OC (Smeaton et al., 2021a). There are also muddy sediment areas with higher OC content on the shelf. However, it is unknown whether or not OC is continuously deposited and stored within these sediments as elsewhere, such as in the southern North Sea, sediment accumulation rates are low (De Haas et al., 1997; De Haas et al., 2002; Diesing et al., 2021).
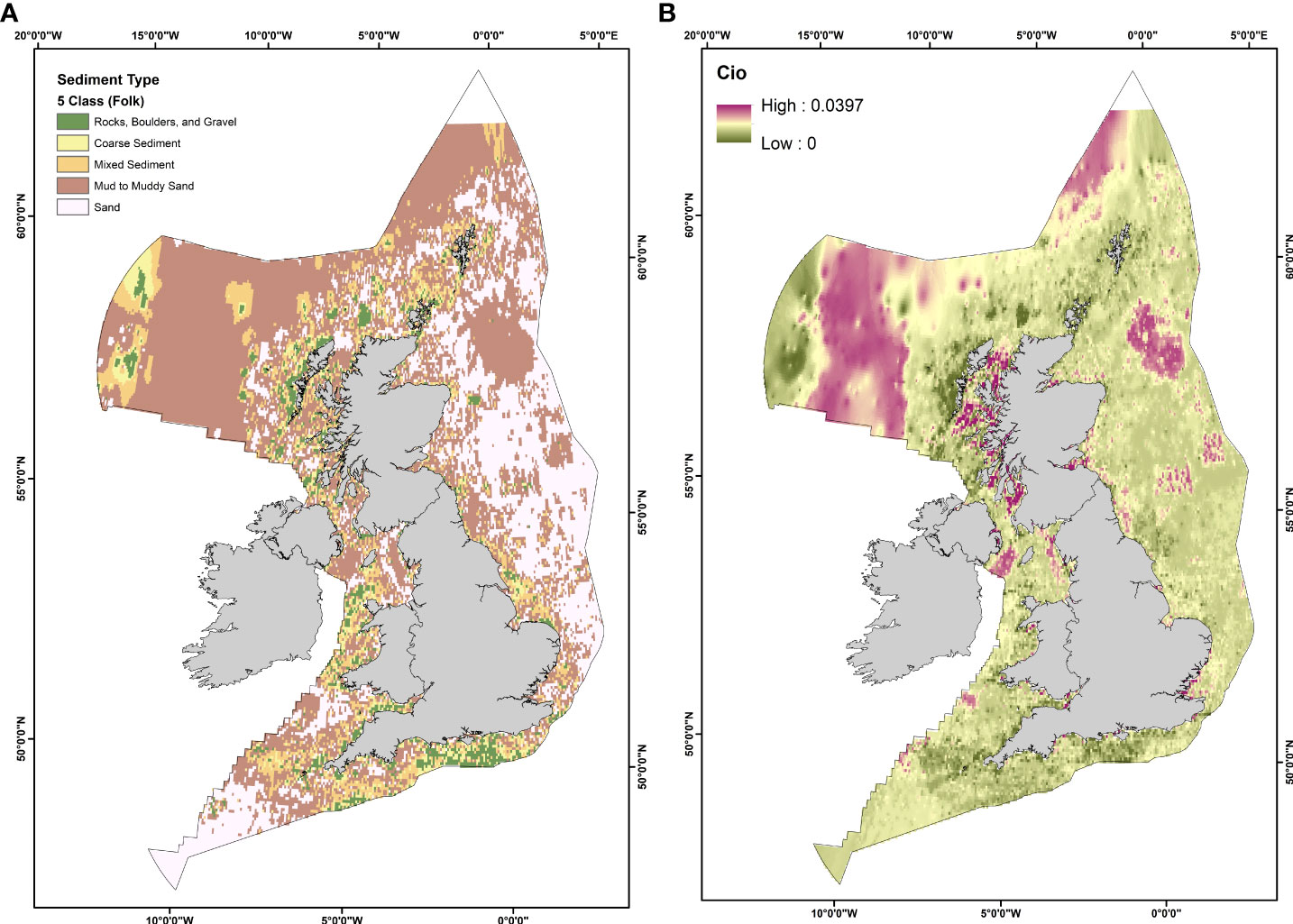
Figure 1 (A) Simplified five classification scheme sediment map of the UK EEZ, (B) Estimated Organic Carbon (OC) content (%) of marine sediments in the UK EEZ. Raster pixel resolution in both figures have been decreased for this study. For higher resolution rasters, see Smeaton et al. (2021a).
2.1.1 Benthic trawling activity
Benthic trawling activity is the most frequent and widespread cause of disturbance to the seabed (Jones, 1992; Amoroso et al., 2018), including within the UK EEZ (de Groot, 1984; Kaiser et al., 1996; Hiddink et al., 2006; Dunkley and Solandt, 2020). As of December 2019, the UK fishing fleet was the seventh-largest fleet within the EU by the number of vessels and the second-largest by gross tonnage (Uberoi et al., 2020). In 2020, the UK fishing fleet consisted of 4,301 active vessels; 61% were 10 metres or under in length and operated predominantly within inshore waters. The types of fishing gear used by British vessels vary with vessel size, power, and target species. Approximately 64% of vessels use static or passive gear types (e.g. pots, traps, hooks, drift and fixed nets etc.) (Quintana et al., 2021). Due to this, vessels under 10 meters in length only account for around 5% of the annual UK catch (Uberoi et al., 2021).
Under European legislation, all vessels greater than 12 meters in length must report their spatial fishing activity via vessel monitoring systems (VMS) to monitor fishing activity spatially and temporally within European waters (Gerritsen and Lordan, 2011; Rouse et al., 2017). By coupling logbook and VMS datasets, the International Council for the Exploration of the Sea (ICES) data centre can analyse this information to estimate the spatial and temporal distribution of bottom trawling activity and its pressure on the seabed within the OSPAR maritime region. Due to the lack of implementation of VMS on vessels under 12 meters in length, there is currently a large knowledge gap surrounding the spatial and temporal fishing patterns of the inshore fishing fleet.
As shown in Figure 2A, fishing activity (e.g., the total time spent fishing) typically occurs within coastal “hotspot” areas primarily on the west coast of Scotland, west of the Isle of Man, and off southwest and southern England. Figure 2B presents the swept area ratio (SAR, see section 2.3.5) for all fishing gear types across the UK EEZ. However, this information can be broken down further to detail the specific gear usage patterns throughout UK waters.
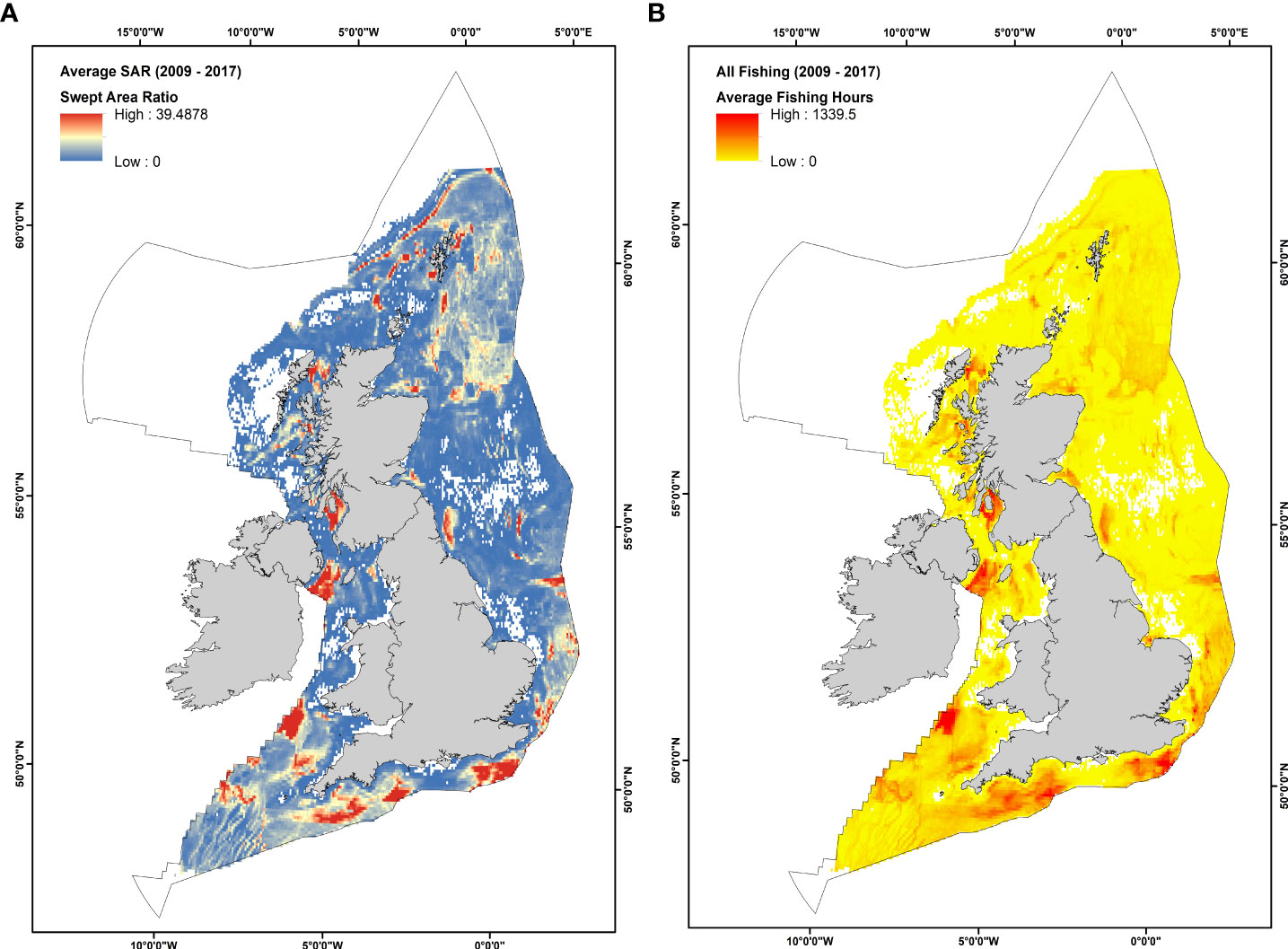
Figure 2 (A) Average fishing effort in hours within the UK EEZ between 2009 – 2017 for all bottom trawling types. (B) Average Swept Area ratio within the UK EEZ between 2009 – 2017 for all bottom trawling types (Data Source: OSPAR, 2016). White patches are areas of no data.
Within the UK EEZ, bottom trawling activity can be grouped into one of the four main gear types: otter trawls (Figure 3A), beam trawls (Figure 3B), dredges (Figure 3C), and seine trawls (Figure 3D). All of these differ in the trawling technique used and the sediment penetration depth of the gears.
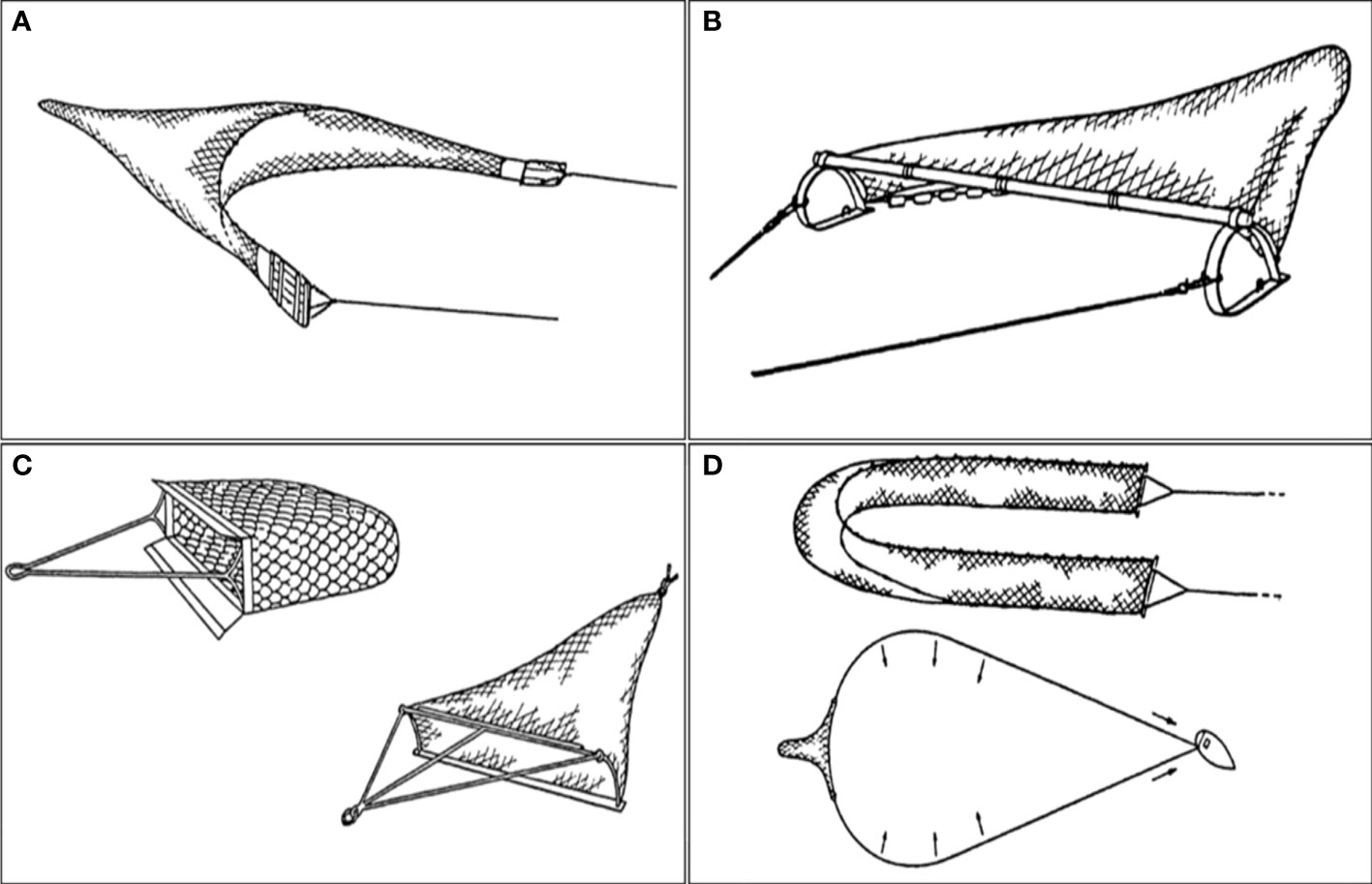
Figure 3 The four main bottom trawling gear types used within the UK EEZ. (A) Otter Trawl, (B) Beam Trawl, (C) Towed Dredge, and (D) Seine Trawl (Source: FAO, Technology Fact Sheets. https://www.fao.org/fishery/en/geartype/104/en). .
The activity of these four gear types for vessels >12 meters is reported by The Convention for the Protection of the Marine Environment of the North-East Atlantic (OSPAR Convention) and ICES as VMS data. As this data is only reported for vessels >12 meters, there is an underestimation of trawling occurring within British waters, particularly within coastal areas where vessels are typically under 12 meters. Otter trawls are the most widely used due to their compact nature, allowing for their use across various vessel sizes. As a result, they have a widely used spatial distribution throughout the entire UK EEZ (Figure 4B). Beam trawling activity occurs primarily within English and Welsh waters, with no records from vessels >12 meters reported within Scottish waters (Figure 4A). Dredging is concentrated mainly within coastal environments across the UK, predominantly around the entire Scottish coast, western Welsh coast, and western and southern English coast (Figure 4C). There is reduced dredging activity reported along the east coast of England. This study did not include seine fishing due to the limited data availability regarding gear penetration depth for Danish and Scottish seining gear (Eigaard et al., 2016).
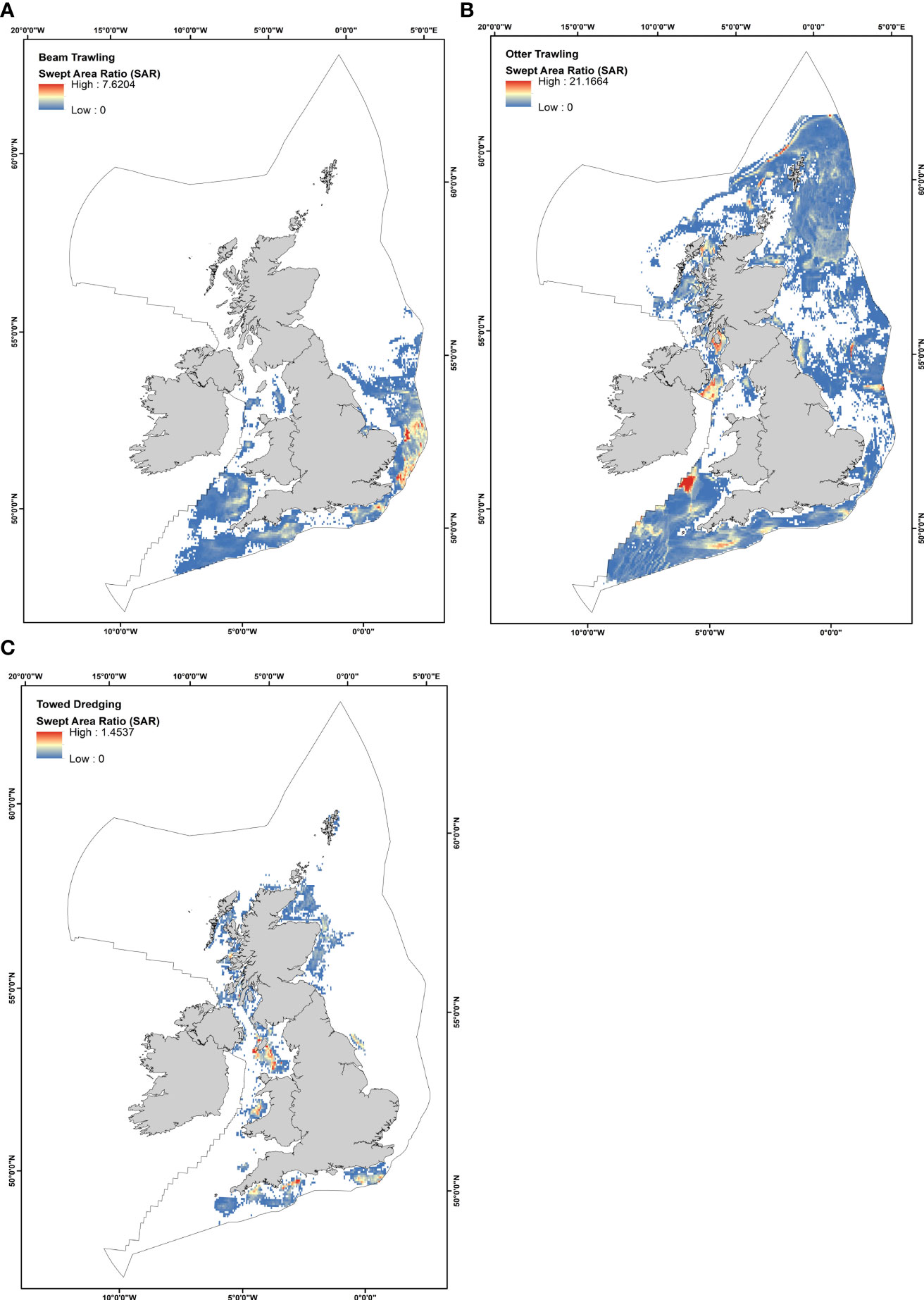
Figure 4 The average swept area ratio (SAR) within the UK EEZ between 2009 2017 for (A) Beam Trawling, (B) Otter Trawling, (C) Towed Dredging, and (D) All Fisheries (Beam, Otter, Dredge, and Seine).
2.2 Selection of criteria and data processing
Previously, attempts at estimating the impact of bottom trawling activity on sedimentary OC stores have been conducted at various scales, from local (Paradis et al., 2019; Tiano et al., 2019; Paradis et al., 2020) to global (Sala et al., 2021). However, due to the nature of the underlying calculations required to estimate these impacts, great care is required before any reliable estimates of risk can be made. Here, we present a revised methodology for generating sedimentary CVR maps to determine areas of seabed where OC is most at risk following disturbance by bottom trawling within the UK EEZ. Our methodology is modified after that of Sala et al. (2021). However, several significant modifications to the data, underlying assumptions, and methods of ranking used in this calculation have been made possible due to the availability of high-quality data sources for the UK EEZ (e.g. Smeaton et al., 2021a), which help overcome some of the limitations of applying the original methodology at the global scale (Epstein et al., 2021).
To estimate the potential vulnerability of OC in marine sediments, we used multiple data sources to generate a sufficient dataset to be implemented within a multi-criteria decision analysis (MCDA). MCDA is a process where conflicting criteria may be evaluated against each other to aid in informed and effective decision-making processes. A complete list of the different types of data, their values, and references to data sources used within this study can be seen in Table 1.
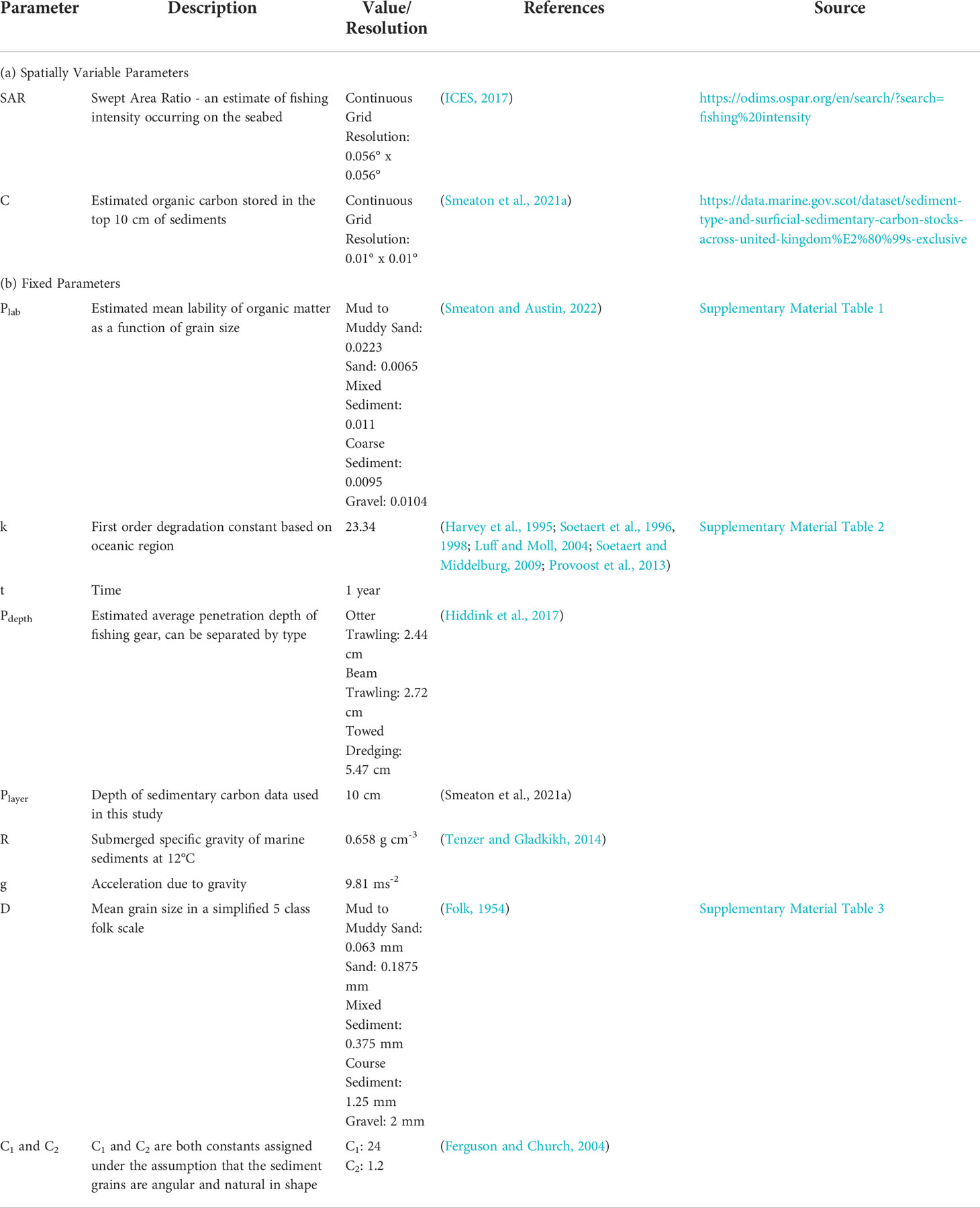
Table 1 Parameters used for modelling the potential vulnerability of UK EEZ marine sediments under different fishing gear types and their sources.
In our mapping analysis, we first conducted a series of smaller calculations and intermediary steps to determine factors such as the spatial impact of fishing gear on the seabed with gear type and penetration depth and the proportion of material which may resettle after bottom trawling disturbance based on sediment type (Figure 5 orange and blue boxes). Combined with data on the estimated reactivity of marine sediments, these factors are then used to estimate the fraction of material that may be “lost” from the seabed during a trawling event (Figure 5. yellow boxes).
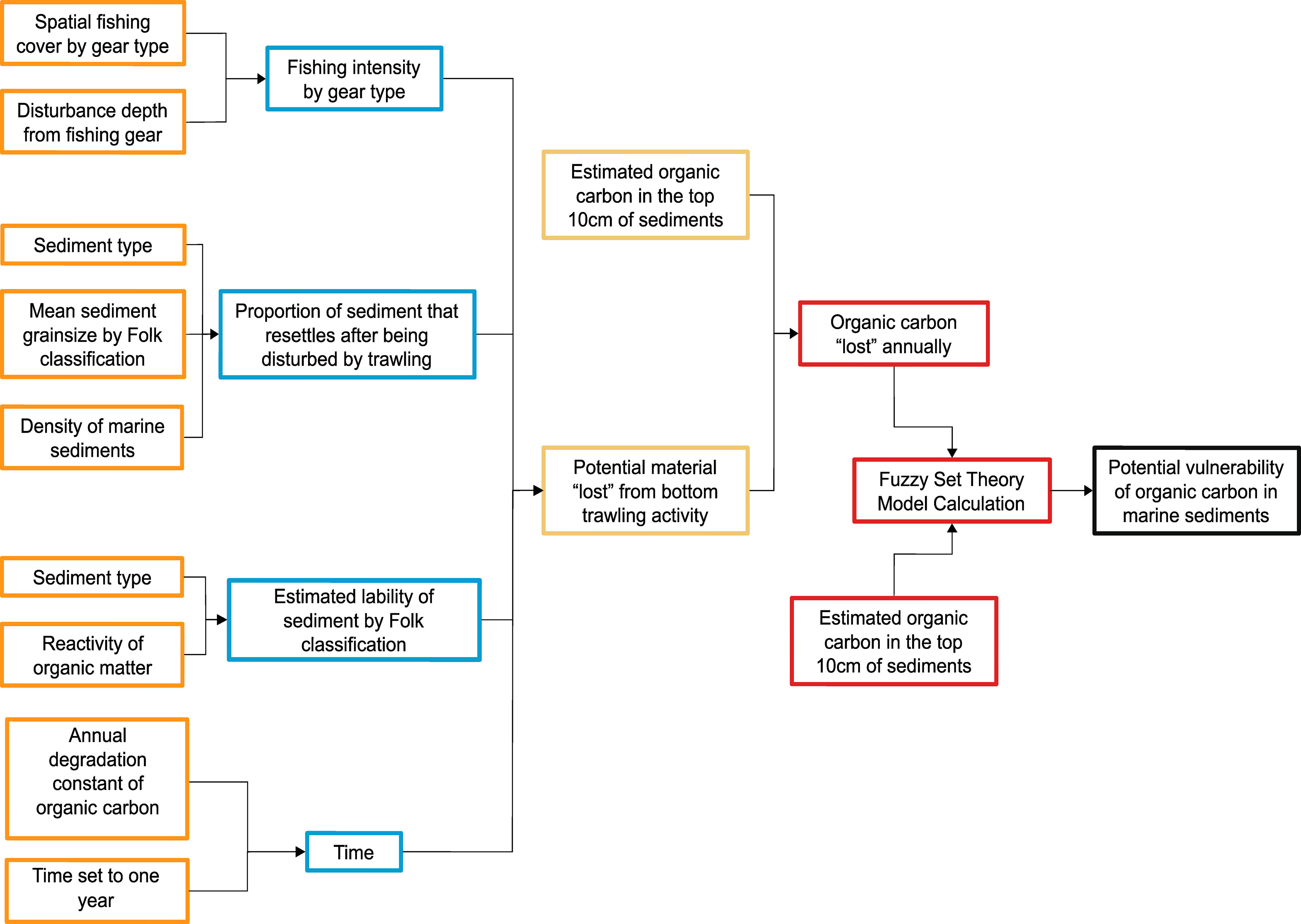
Figure 5 Simplified schematic of the workflow steps taken to generate the organic carbon vulnerability estimates. Coloured boxes represent different stages within the calculation. Preparation of the data used in this study was conducted within RStudio (Ver. 2021.09.1 + 372) and ArcGIS (Ver. 10.7). Rasters were rescaled where necessary to be on the same scale to ensure a single-pixel calculation overlay. Calculations and spatial analysis were conducted within ArcGIS using the raster calculator tool, fuzzy membership tool, and fuzzy overlay tool.
By combining the potential fraction of “lost” material with the amount of OC estimated to be contained within marine sediments, we can improve estimates for the amount of OC that may be “lost” during trawling events (Figure 5 red boxes). The estimated fraction that may be lost can then be combined with the fraction of OC currently estimated to be present in marine sediments across the entire UK EEZ to generate a multi-criteria map which identifies areas where OC is potentially most vulnerable to bottom trawling pressures (Figure 5. black box).
2.2.1 Grain size data
Information on the particle size characteristics of marine sediments is crucial when investigating their role and significance for long term OC storage. Sediment grain size is routinely measured under OSPAR and the ICES commitments within the UK EEZ. As a result, the marine sedimentary environment of the UK EEZ is well mapped and studied, allowing a high-quality dataset of the spatial characteristics of the seabed to be used within our model (BGS, 2019; Smeaton et al., 2021a).
2.2.2 Sedimentary carbon data
Estimates and modelling of OC within the UK EEZ and North-East Atlantic have been steadily growing and improving over the last few years (Diesing et al., 2017; Luisetti et al., 2019; Legge et al., 2020; Smeaton et al., 2020; Diesing et al., 2021; Smeaton et al., 2021a). As a result of these efforts, high-resolution bulk (top 10 cm) OC spatial estimates are now available for the entire UK EEZ (Smeaton et al., 2021a).
2.2.3 Sediment lability data
Just as OC content varies across sediment types, the lability of OM associated with the sediments also varies. Thermal analysis of sediments allows for data regarding the quantity, quality, and reactivity of OM to be obtained. The reactivity of OM can be split into three key components: the labile, the recalcitrant, and the refractory fractions. In simple terms, the labile fraction of OM represents the most biologically available material and is prone to degradation. In contrast, the refractory fraction is the least biologically available and least reactive. Recent analytical developments have allowed the quantification of the different OM pools (labile, recalcitrant, and refractory) within the marine sediment of the UK EEZ (Smeaton and Austin, 2022).
These new results highlight that the quantity of labile OM in the continental shelf sediments are strongly linked to sediment type. The high-resolution spatial data for sediment type within the UK EEZ makes it possible to de-evolve sediment classifications from a 16 Folk classification scheme to the simplified 5 Folk classification scheme (Supplementary Material Figure 1) (Kaskela et al., 2019; Smeaton et al., 2021a). Using this simplified classification scheme, we were able to assign mean sediment OM lability (OMlabile%) for each class within the UK EEZ (sediment samples, n = 375, across a range of environmental types (Supplementary Material: Table 1) (Smeaton and Austin, 2022)).
2.2.4 Disturbed sediment exposure
Once sediment has been disturbed by bottom trawling, it will be resuspended from the seabed into the water column (e.g. Breimann et al., 2022). While in the water column, OC associated with the sediment will be exposed to chemical processes, such as remineralisation, which may lead to its permanent loss from the seabed. Hence, the more time disturbed sediment remains in the water column, the greater the potential for OC to be lost. The length of time the sediment remains above the seabed is determined by the speed it falls out of the water and the height above the seabed to which it is raised by disturbance. However, it is not possible to model in detail the physical disturbance of sediment under all the different physical conditions associated with bottom trawling in UK water or elsewhere. Therefore, a theoretical fall speed can be estimated for any sediment type based on particle size, and this can be used to assess the relative difference in possible exposure during resuspension of disturbed sediment.
Using the universal settling speed calculation proposed by Ferguson and Church (2004), it is possible to calculate a theoretical rate of sediment resettling for each of the five sediment class types used in this study. Settlement speeds are then used to derive a non-dimensional relative estimate of potential exposure. However, it should be noted that this calculation does not consider external factors such as turbulence (Nielsen, 1993), currents and wave movement (Coughlan et al., 2021), which may be critical processes in natural and anthropogenically disturbed shelf systems.
2.2.5 Fishing data
Within the UK EEZ, specific gear types have significant regional patterns related to the fishing intensity (e.g., otter trawling, beam trawling, and towed dredging). VMS can be used to provide high quality temporal and spatial data about the type and intensity of fishing activity occurring across the UK EEZ. Using OSPAR VMS fishing data, we can estimate the CVR of sediments for each of the three main fishing types on a 0.05° x 0.05° cell resolution (C-square geocode system, see Rees, 2003). VMS datasets were chosen for this study instead of the alternative automatic identification systems (AIS) because VMS separates SAR data by gear type, unlike AIS data which reports trawling as a singular activity with no distinction of the gear type being used. Additionally, due to the ability of fishers to turn off AIS tracking systems and known issues with data reporting, AIS data is only accurately reported approximately 26% of the time spent fishing (Shepperson et al., 2018). By EU law, it is required that VMS always remains switched on for vessels >12 meters in length, making the spatial coverage of the fishing data more reliable. However, as previously noted, this data does not include the fishing activity for vessels under 12 meters in length. As a result, there may be some underestimation in fishing intensity, particularly within coastal areas from the inshore fleet, which are typically smaller vessels.
Using the reported VMS hours fished data combined with log-book records, it is possible to calculate the seabed swept area ratio (SAR) (Figure 2B) using Eq. 1.
The SAR indicates the theoretical number of times a grid cell would be “swept” annually if the fishing effort was evenly distributed throughout a set grid cell area (ICES, 2020). Using the OSPAR VMS data, it is possible to establish the annual average SAR of each pixel. To avoid a single year bias, the SAR of each cell has been averaged from 2009 to 2017.
2.3 Calculation method
2.3.1 Model overview
MCDA is a GIS-based spatial analysis method that allows for both spatial and non-spatial data to be brought together to generate outputs that can inform about complex interactions within datasets. A CVR value for each pixel within a raster dataset was estimated using the MCDA method known as fuzzy set theory, which is a widely used modelling approach in complex systems where the information supplied to a model is vague, imprecise, or ambiguous (Kahraman and Kaya, 2010; Balezentiene et al., 2013). Fuzzy set theory is commonly used within spatial planning and evaluation as it allows spatial information to be treated as members of a set. This means that a degree of membership between different types of spatial information can be determined. Unlike other MCDA methodologies, the degree of membership from fuzzy set theory will take on values between 0 and 1, denoting the strength of the membership between the candidate objects being assessed. This allows for a ranking system to be used. For example, the OC ranking of a pixel is a numerical ranking that highlights where sedimentary OC is most vulnerable, where 1 is the most vulnerable location, and 0 is the least vulnerable location. It should be noted that the use of fuzzy set theory estimates the possibility, i.e., the capacity of something being true/occurring, not the probability, which refers to the likelihood of something being true/occurring.
2.3.2 Data processing for model set up
In order to generate a MCDA map of the potential vulnerability of sedimentary C stores we must generate two key layers, the amount of OC currently in sediments before bottom trawling and the amount of OC estimated to remain in sediments after a bottom trawl pass. This was done using the following methodology. Firstly, the total fraction of OC present within each pixel, , is estimated from the UK EEZ sediment OC mapping of Smeaton et al. (2021a), shown here as Ci0
While the fraction of total OC assumed to remain if the sediment in pixel i is exposed to fishing based disturbance using gear g is denoted as .
Where Cio is from Eq. 2. Lai,g is the estimated fraction of labile OM (%) which would be “lost” from the sediment annually in the presence of fishing activity by gear type g in pixel i (Eq. 4). Note that in Eq. 3, if (1-Lai,g) has a value of zero, there is no potential loss of OC from pixel i due to gear g. If it has a value of unity, then there is a theoretical potential for total loss of OC. Lai,g may be calculated via Eq. 4:
Where SVRi,g is the swept volume ratio calculated via Eq. 5 and PERi is the potential exposure ratio calculated via Eq. 6 (See below). Plabi is the estimated mean lability of OM in each pixel as a function of sediment type using the UK EEZ sediment classification from Smeaton et al. (2021a) (Supplementary Table 1). The first-order degradation rate constant, ki, was assigned based on the oceanic region and was estimated for this study to be 23.34 using an average of values for oxic sediment types in the North Sea (Table 1). It should be noted that ki is assigned relative to the oceanic region being studied, meaning that its influence on the rate of loss for labile OM will vary geographically depending where this approach is being applied due to factors such as diffusive oxygen flux, the total OC content of surface sediments, and bottom water oxygen concentration (Seiter et al., 2005; Arndt et al., 2013; Paraska et al., 2014). Time, t, was set to one year.
The swept volume ratio, SVRi,g, from Eq. 4 may be calculated via Eq. 5:
Where SARi,g (Eq. 1.) is the swept area ratio in pixel i by vessels using gear type g, from publicly available OSPAR VMS datasets (ICES, 2016). Pdepth,g is the estimated average penetration depth of gear type g from Hiddink et al., 2017. These depths are 2.44 cm for otter trawling, 2.72 cm for beam trawling, and 5.47 cm for towed dredging. Player is the depth of sediment used in this study for the carbon estimates, in this case, 10 cm.
The potential exposure ratio, PERi, in Eq. 4 was estimated by creating a ratio of the settling of the largest sediment size-class speed (wmax) to the settling speeds (wi) for each of the 5 sediment classes outlined in Table 1 so that:
The settling speed (w) for each sediment grain size class was calculated using the following equation from Ferguson and Church, 2004:
Where R is the submerged specific gravity (0.658 g cm-3) for marine sediments, using the density of marine sediment from Tenzer and Gladkikh (2014) and the density of seawater at 12°C, g is the acceleration due to gravity, and D is the mean diameter of the grain size within each of the sediment classes reported by Smeaton et al. (2021a). C1 is constant with a value of 18, and C2 is constant with a value of 1.0 (Ferguson and Church, 2004), v is the kinematic viscosity of seawater at 12°C (Supplementary Material: Table 3). Several calculation steps were necessary to get the data ready to be applied to the fuzzy overlay model. First, the data fed into the model must undergo fuzzification to allow the source data to be rescaled to fit the 0 to 1 scale (Raines et al., 2010). This was applied using the fuzzy membership tool (membership type: linear) to the estimated OC stored in the top 10 cm of the sediment () and the predicted amount of total OC lost from the sediment due to bottom trawling () in each pixel for each gear type.
To model the CVR for the UK EEZ, fuzzy set theory models were run within ArcMap (ArcGIS 10.7). The final suitability map was generated using the fuzzy overlay tool (overlay function: AND), which allows for a continuous 0 to 1 scale map to be generated (Nyimbili and Erden, 2020). Finally, the model outputs were defuzzied via the reclassification tool, where classes were set for each gear type using the natural breaks (Jenks) classification method to develop a five-class vulnerability ranking of the seabed within the UK EEZ.
While it is possible to calculate the potential OC lost from benthic trawling, we have chosen not to calculate the benthic efflux of CO2 because of the high levels of uncertainty and large-scale assumptions that must be made. Recent studies within this field have attempted to calculate the efflux of CO2 from bottom trawling (Luisetti et al., 2019; Sala et al., 2021). These studies assume that all the labile OC resuspended during a disturbance event is completely remineralised to aqueous CO2. From here, it is also assumed by Luisetti et al., 2019, that all remineralised OC is then released into the atmosphere in a singular process. These gross assumptions are unlikely to be correct and lead to vast overestimates in the amount of CO2 lost from long term sedimentary OC stores upon disturbance. Processes such as elemental stoichiometry, biological turnover, solubility cycling, and carbonate cycling will all influence the fate of remineralised OC and should not be assumed to be negligible. More data on the efflux of OC across the sediment-water interface in natural and post-disturbance environments is needed before this type of calculation can be attempted with any confidence in the results.
3 Results
The spatial distribution of estimated CVR values across the UK EEZ was mapped using the methodology detailed above at a gridded resolution of 0.05° x 0.05°. An area of 743,470 km2 of the UK seabed was mapped; the most northern tip of the UK EEZ (an area of approximately 24,000 km2) remains unmapped due to limitations in data availability (Figure 2). However, there are no reported VMS fishing records for this area, and it can be assumed that the impact of bottom trawling in this area is low. Additionally, OC within this area is also reported to be low (Smeaton et al., 2021a), which would, in turn, reduce the overall CVR of the area.
The lack of VMS data available for inshore fisheries also means that their impact is underestimated and prevents us from reliably modelling the CVR within some inshore areas, particularly within fjords. The CVR of some of these inshore sediments must therefore be considered as conservative estimates at present. Our analysis of the areas of the UK EEZ seabed where OC is estimated to be most vulnerable from bottom trawling activity is calculated as a function of the predicted OC stored within surficial sediments and the relative lability of the OM in these sediments. Therefore, the methodology presented here represents the development of a new tool that can be used to assess the potential vulnerability of sedimentary OC stores from bottom trawling pressures.
Based on previous literature and knowledge, we hypothesised that OC is most at risk from anthropogenic pressure within coastal sediments where there is a constant supply of fresh labile matter and a naturally elevated OC store. Likewise, we can assume that as the distance from shore increases, the vulnerability of OC will decrease due to the decline in lability of overall OM accumulation (Smeaton and Austin, 2022). The CVR risk model estimates a typically lower CVR within sands, coarse sediments, mixed sediments, and gravels/rocks compared to finer-grained sediment types. Our results show that distributions of CVR within the UK EEZ are associated with specific “hot spot” areas for the three major fishing gear types.
3.1 Model validation
Validation of the new model is a fundamental development step and is crucial for determining its predictive capabilities. Our CVR maps have been developed using multiyear spatial monitoring fisheries data (OSPAR, 2016), in addition to previously validated sedimentary OC quantity and sediment type datasets which have been tested via extensive ground-truthing efforts and Markov chain Monte Carlo (MCMC) simulations (Smeaton et al., 2021a). In addition to this, we utilise fuzzy set analysis MCDA techniques in place of traditional weighted overlay methods. Weighted overlay works as a Boolean operator where areas are defined as either being a member (1) or not being a member (0). These results fall within clear cut risk or no risk areas, offering no room for further interpretation. However, fuzzy overlay addresses the possibility of inaccuracies within the attribute data used within the model by assigning a membership ranking between 0 and 1, thus accounting for uncertainties within the calculation where the datasets are more limited (e.g., the lability of sediments assigned as a function of sediment type).
3.2 Carbon vulnerability ranking
Within this model, the CVR is an estimate of those areas of the seabed where sedimentary OC is predicted to be the most susceptible to “loss” due to direct bottom trawling action by specific gears. Our model shows that the most vulnerable areas of the seabed are typically concentrated within inshore muddy sediments and impacted by otter and dredge trawling activity, while beam trawling impacts are spread across muddy, mixed, and coarse sediment types. Despite having the largest spatial coverage overall, otter trawling predominantly results in a low CVR for much of the UK EEZ, with a smaller estimate of areas where vulnerability is high or very high (Table 2 and Figure 6). Otter trawling has the highest proportion of very low, low, medium, and very high CVR areas compared to other fishery types (Table 2).
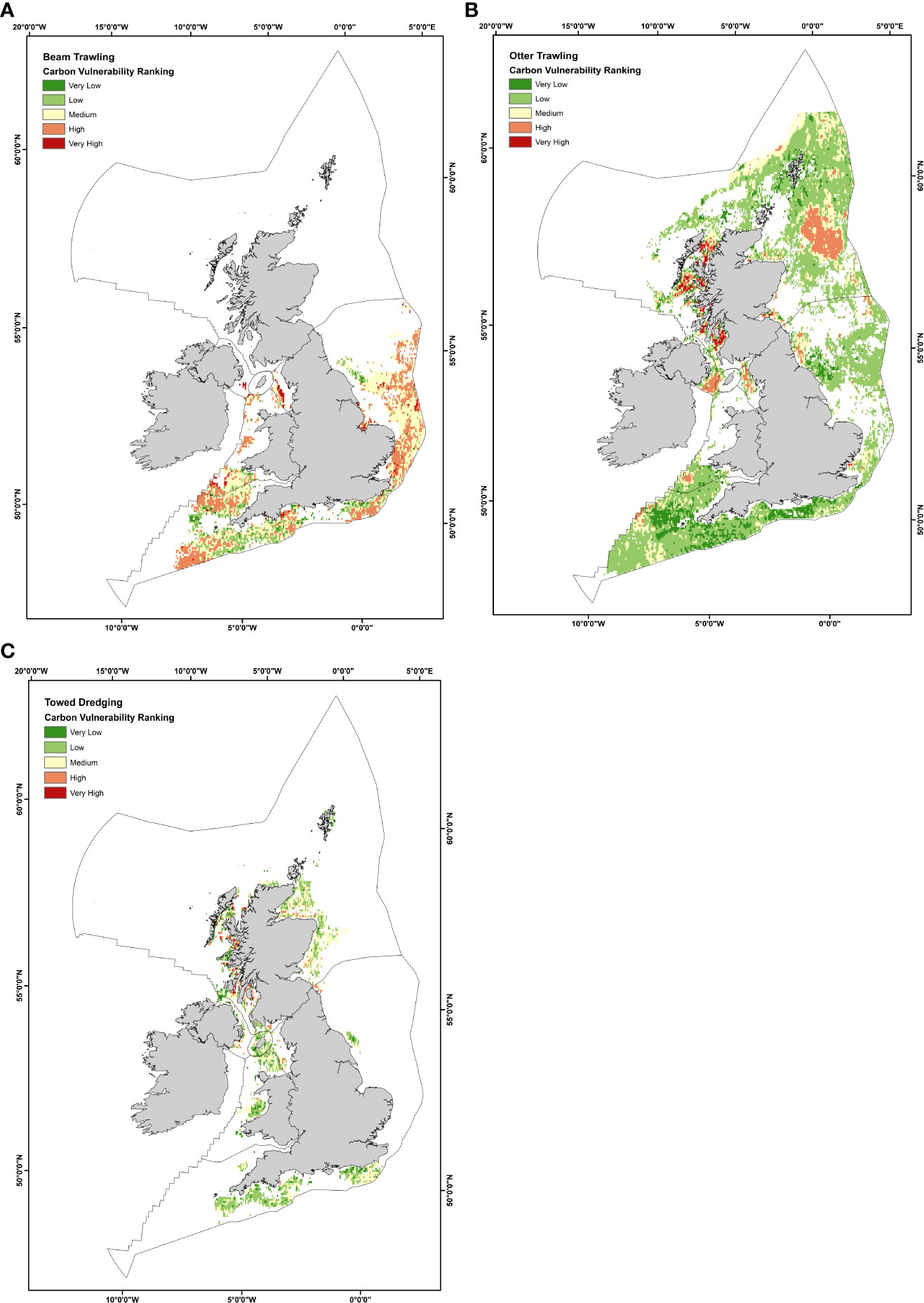
Figure 6 Carbon vulnerability ranking (CVR) by fishery type. All three fishing types are associated with regional hot spots where the CVR is very high. These hotspot areas typically coincide with muddy sediment types where labile organic matter content is high; a high swept area ratio also characterises these inshore fishing areas. (A) Beam Trawling, (B) Otter Trawling, (C) Towed Dredging.
Beam trawling has an estimated total spatial coverage of 53,814 km2, heavily concentrated within the English sector. Within the medium, high, and very high CVR categories, beam trawling has the highest estimated area coverage of 23,079 km2, 19,294 km2, and 1,458 km2, respectively (Table 2 and Figure 6A). Medium CVR areas are scattered for beam fisheries (Figure 6A). However, high CVR areas linked to beam trawling are more closely grouped across England’s south-western, southern, and south-eastern coastline. Areas of very high CVR for beam trawling can be found on the west coast of the UK in the Irish Sea and on the east coast in the Wash.
Overall, otter trawling has the greatest estimated spatial coverage (180,504 km2) out of the three main fishery types considered within this study. However, despite this large footprint, a significant proportion of the actively fished area from otter trawling is classified by our model to be within very low (17,689 km2), low (117,380 km2), and medium (29,376 km2) CVR categories (Table 2). It is estimated that approximately 13,855 km2 of the seabed is categorised as having a high CVR, with a further 2,205 km2 being estimated to have a very high CVR. For otter trawling, these high and very high CVR areas are predominantly located in hot spots across the fjordic west coast of Scotland (Figure 6B), where labile OC muddy sediments dominate, OC content is high, and repetitive trawl passes are common (Figure 2). Regions of high CVR can also be found in the Irish Sea and the Fladen Grounds (Northeast Scotland).
Dredging has the lowest estimated spatial coverage of 30,748 km2 compared to the other two fishing types. Towed dredging across the UK EEZ is concentrated mainly in small areas of activity (Figure 6C), unlike the other two fisheries, which are spread across a much greater area. Our model estimates that approximately 3,320 km2 of actively dredged seabed is categorised as having a very low ranking. A further 12,912 km2 is estimated as being within the low CVR category (Table 2). An estimated 11,993 km2 of the seabed is classified as having a medium CVR, with a further estimated 1,923 km2 and 600 km2 classified as having high to very high CVRs, respectively. Similarly, to otter trawling, hotspots of high to very high CVRs are located on the fjordic west coast of Scotland. Prevailing sediment type, high OC contents and the repetitive nature of fishing disturbances in this region drive high to very high CVRs (Figure 2).
4 Discussion
The main aim of this study was to determine the areas of the seabed where sedimentary OC might be most vulnerable to the impacts of benthic trawling activity within the UK EEZ. We present a new methodological approach to determine the potential vulnerability of the seabed across the UK EEZ using open-source data. The outputs of our model identify the fjords and coastal sediments on the west coast of Scotland as having the highest potential OC vulnerability to disturbances from bottom trawling. Three main determining factors occur in these environments. Firstly, sedimentary OC content is high (Smeaton and Austin, 2019). Secondly, there is a high level of repetitive trawling disturbance within these coastal areas (Figure 4). Thirdly, sediments in this region are largely fine-grained, meaning that they will remain in suspension longer once disturbed. This means that these OC rich sediments have a greater chance of being transported away from their original location and resettling elsewhere.
While we have developed a new OC vulnerability model using fuzzy set theory to identify where OC is potentially most vulnerable due to benthic trawling activity, these models all lack key information that may result in some over- and under-estimations of CVRs. This is most likely the case with the sediment OM lability data (Smeaton and Austin, 2022). It is likely that sediment OM lability is higher within coastal and fjordic areas in comparison to muddy shelf sediments. This is due to differences in productivity between the two environments and the transportation of OM from coastal to shelf environments, and the ongoing degradation of this matter which occurs during this process. There is currently a data gap within UK EEZ sedimentary OM data, which restricts us from applying OM as a function of sediment type rather than as a spatially modelled estimate. Additionally, a lack of data to adequately monitor the impact of the inshore fishing fleet (i.e., vessels <12 meters) implies that the CVR of sediments in coastal waters may be underestimated. We have intentionally used a flexible modelling framework to account for these data limitations that can be adapted as new data sources are available. For example, previous evidence shows that fuzzy logic modelling frameworks have been successful within other data-poor conservation and safeguarding projects involving trawling datasets (Hattab et al., 2013).
It should also be remembered that the UK EEZ is a region with a long and ubiquitous history of fishing activity, implying that the sediments and surficial OC data used in this study may have already been subject to decades of modernised industrial fishing methods. Therefore, this study does not provide an undisturbed natural baseline for sedimentary OC. Instead, this study provides a present-day snapshot of the current state of the potential areas of OC vulnerability within the UK EEZ. Previous research has shown that the first pass of towed benthic gear on the seabed is the most destructive pass to benthic ecosystems, regardless of the hours spent fishing in the area (Lindegarth et al., 2000; Kaiser et al., 2006; Lambert et al., 2014; Rijnsdorp et al., 2018).
While previous attempts at understanding the impact of bottom trawling on sedimentary OC stores have provided an insight into the potential risk to C, they are conducted at too broad a scale and with too many uncertainties and assumptions to be utilised and implemented within policymaking. Instead, our adapted CVR methodology could provide a policy steppingstone, allowing for greater consideration about the sedimentary environment being investigated and the data availability at the regional scale.
Despite our use of improved data sets and the wealth of understanding of the UK EEZ, we have chosen not to estimate the efflux of CO2 from the seabed arising from benthic disturbance because of the assumptions required to make this type of calculation and the misleading outputs that can arise. Cross-comparisons of this type of calculation with in-situ data are difficult due to the lack of studies investigating the post-disturbance effects of trawling on benthic CO2 efflux and nutrient efflux within the UK EEZ, or elsewhere. In addition, we do not include the influence of nutrient release from the sediments during trawling, which can result in increased primary productivity, indirectly resulting in increased OM and OC supply to the sediments. There is currently not enough supporting information about the efflux of nutrients in a post-trawl environment within the UK EEZ to confidently include this information within our calculations. Equally, these models do not account for the removal of benthic fauna during a trawling event.
Similarly, it is also challenging to estimate the accumulation of multiple years of fishing impact on sedimentary OC stores within the UK EEZ due to a lack of information about sediment accumulation rates and OC sequestration rates. Large assumptions would have to be made with this type of calculation. For example, based on current data, we would have to assume that there was a single accumulation rate throughout the UK EEZ and that OC is lost from the sediments at the same rate annually. However, we know this is not the case, especially within coastal fjordic environments where accumulation is reported to be high (Smeaton et al., 2016; Smeaton et al., 2021b) in comparison to the low accumulation rate reported within the North Sea (De Haas et al., 1997; De Haas et al., 2002; Evans et al., 2002; Scourse et al., 2002; Diesing et al., 2021). Recent research has allowed for the sediment accumulation rate to be modelled within the Greater North Sea region (Diesing et al., 2021), highlighting that these types of predictive models are possible. However, for multiyear sedimentary OC losses to be calculated with a high degree of confidence, the sediment accumulation rate across different sedimentary environments and marine environment types (e.g., fjords, inshore, offshore, deep sea) within the UK EEZ need to be spatially resolved.
4.1 Management of marine sedimentary organic carbon
We have shown that marine sediments within the UK EEZ combine with the distinction of fishing activity to produce a spatially variable pattern of OC vulnerability, organic-rich sediments from the fjordic complex of the west coast of Scotland being potentially most vulnerable. Our model can identify at-risk zones where OC is most likely to be vulnerable to bottom trawling activity. While marine sedimentary OC is not currently considered within the frameworks of national OC inventories for greenhouse gas reporting, there is growing interest in the potential and significance of including these stocks (Avelar et al., 2017). Further research into how sedimentary OC can be accounted for within nationally determined contributions is necessary, alongside new accounting guidance and frameworks (Luisetti et al., 2020).
Recent research has shown that marine sediments store significant amounts of OC both globally (Lee et al., 2019; Atwood et al., 2020) and locally within the UK EEZ and greater North Sea region (Diesing et al., 2017; Luisetti et al., 2019; Diesing et al., 2021; Smeaton et al., 2021a). While OC in shelf sea sediments is prone to natural processes of recycling and remineralisation, it is possible that anthropogenic disturbances are driving enhanced OC loss. Our research maps the potential vulnerability of OC to bottom trawling activity. via While this research considers estimated surficial OC stores of the UK EEZ and the anthropogenic impacts of fishing, further detail is needed about the role of and interaction with natural disturbances on these sediments (e.g., current, wave, tidal, and storm activity) to understand the full disturbance pattern. There has been some recent development in this natural disturbance field on sediment mobilisation (Coughlan et al., 2021), but further detail about the impact of natural forcing on OC storage is required. While there are other anthropogenic disturbances that impact the seabed across the UK EEZ, including commercial aggregate dredging, offshore structure construction, and sediment disposal activity, these happen on a localised basis. Thus the impact of these types of activity on sedimentary OC stores is thought to be minimal compared to widespread practices such as trawling (Kenny et al., 2018). Current literature surrounding the fate of OC under bottom trawling activity is conflicting, with some studies estimating that sediment OC may increase (Pusceddu et al., 2005; Martín et al., 2014; Sciberras et al., 2016), while others estimate a decrease (Jennings et al., 2001; Durrieu De Madron et al., 2005; Pusceddu et al., 2014; Oberle et al., 2016). Further research is needed to address some of these uncertainties.
A suggestion for the management of sedimentary OC stores has been to explore the use of MPA’s and HPMA’s as a tool to protect and enhance carbon stores (Roberts et al., 2017), with accumulating evidence in the literature to support the potential of MPA’s to provide an area of enhanced mitigation, adaptation, and resilience towards climate change (Duffy et al., 2016; Roberts et al., 2017; Gaines et al., 2018). Our CVR results for the UK EEZ could be used to help inform potential MPA selection. This type of management approach, where measures limit the activity of bottom trawling may provide scope for the potential limitation of unintentional emissions and possible recovery of benthic communities (Hiddink et al., 2017). The net reaction and relative timescales for the response of benthic ecosystems and sediment OC stores following closure of the seabed to bottom trawling are yet to be determined.
5 Conclusion
We present a new workflow for predicting regions of the seabed where OC is potentially most vulnerable from disturbance. This study has allowed for the first detailed assessment of the relative vulnerability of sedimentary OC towards fishing disturbance. Our model results highlight that coastal muddy sediments dominated by otter and beam trawling have the highest CVRs. We estimate the sedimentary OC stores on the west coast of Scotland to be amongst the most vulnerable areas of the seabed within the UK EEZ.
Data availability statement
Datasets generated/analysed for this study are included in the article/Supplementary Material. The GIS files generated in this study are available at Marine Scotland Data (https://doi.org/10.7489/12426-1).
Author contributions
The study was conceived jointly by KB and WA. KB led the development of the methodology used this study with assistance from CS, WT, and WA. KB undertook the formal analysis and investigation of this study and wrote the first draft of the manuscript as part of her Ph.D. at the University of St Andrews under the supervision of WA. All authors contributed towards manuscript revisions and have approved the submitted version.
Funding
This work received joint funding from the University of St Andrews and Marine Scotland through the Scottish Blue Carbon Forum.
Acknowledgments
We would like to acknowledge the support of The St Andrews Institutional Open Access Fund which supported the cost of Open Access.
Conflict of interest
The authors declare that the research was conducted in the absence of any commercial or financial relationships that could be construed as a potential conflict of interest.
Publisher’s note
All claims expressed in this article are solely those of the authors and do not necessarily represent those of their affiliated organizations, or those of the publisher, the editors and the reviewers. Any product that may be evaluated in this article, or claim that may be made by its manufacturer, is not guaranteed or endorsed by the publisher.
Supplementary material
The Supplementary Material for this article can be found online at: https://www.frontiersin.org/articles/10.3389/fmars.2022.892892/full#supplementary-material
Key data used within this study can be found in the supplementary material. All GIS files will be made available on a data repository
References
Amoroso R. O., Pitcher C. R., Rijnsdorp A. D., McConnaughey R. A., Parma A. M., Suuronen P., et al. (2018). Bottom trawl fishing footprints on the world’s continental shelves. Proc. Natl. Acad. Sci. U. S. A. 115, E10275–E10282. doi: 10.1073/pnas.1802379115
Arndt S., Jørgensen B. B., LaRowe D. E., Middelburg J. J., Pancost R. D., Regnier P. (2013). Quantifying the degradation of organic matter in marine sediments: A review and synthesis. Earth Sci. Rev. 123, 53–86. doi: 10.1016/j.earscirev.2013.02.008
Atwood T. B., Witt A., Mayorga J., Hammill E., Sala E. (2020). Global patterns in marine sediment carbon stocks. Front. Mar. Sci. 7. doi: 10.3389/fmars.2020.00165
Avelar S., van der Voort T. S., Eglinton T. I. (2017). Relevance of carbon stocks of marine sediments for national greenhouse gas inventories of maritime nations. Carbon Balance Manage. 12, 10. doi: 10.1186/s13021-017-0077-x
Balezentiene L., Streimikiene D., Balezentis T. (2013). Fuzzy decision support methodology for sustainable energy crop selection. Renew. Sustain. Energy Rev. 17, 83–93. doi: 10.1016/j.rser.2012.09.016
Berner R. A. (1982). Burial of organic carbon and pyrite sulfur in the modern ocean: Its geochemical and environmental significance. Am. J. Sci. 282, 451–473. doi: 10.2475/ajs.282.4.451
BGS (2019) Offshore sediment index. Available at: http://mapapps2.bgs.ac.uk/geoindex_offshore/home.html (Accessed 2.10.21).
Breimann S. A., O’Neill F. G., Summerbell K., Mayor D. J. (2022). Quantifying the resuspension of nutrients and sediment by demersal trawling. Cont. Shelf Res. 233, 104628. doi: 10.1016/j.csr.2021.104628
Burrows M. T., Hughes D. J., Austin W. E. N., Smeaton C., Hicks N., Howe J. A., et al. (2017). Assessment of blue carbon resources in scotland’s inshore marine protected area network. Scottish Nat. Herit. Commun. Rep. 1–283.
Coughlan M., Guerrini M., Creane S., O’Shea M., Ward S. L., Van Landeghem K. J. J., et al. (2021). A new seabed mobility index for the Irish Sea: Modelling seabed shear stress and classifying sediment mobilisation to help predict erosion, deposition, and sediment distribution. Cont. Shelf Res. 229, 1–17. doi: 10.1016/j.csr.2021.104574
de Groot S. J. (1984). The impact of bottom trawling on benthic fauna of the north Sea. Ocean Manag. 9, 177–190. doi: 10.1016/0302-184X(84)90002-7
De Haas H., Boer W., Van Weering T. C. E. (1997). Recent sedimentation and organic carbon burial in a shelf sea: The north Sea. Mar. Geol. 144, 131–146. doi: 10.1016/S00253227(97)00082-0
De Haas H., Van Weering T. C. E., De Stigter H. (2002). Organic carbon in shelf seas: sinks or sources, processes and products. Cont. Shelf Res. 22, 691–717. doi: 10.1016/S0278-4343(01)00093-0
Diesing M., Kröeger S., Parker R., Jenkins C., Mason C., Weston K. (2017). Predicting the standing stock of organic carbon in surface sediments of the north-west european continental shelf. Biogeochemistry 135, 183–200. doi: 10.1007/s10533-017-0310-4
Diesing M., Thorsnes T., Bjarnadóttir L. R. (2021). Organic carbon densities and accumulation rates in surface sediments of the north sea and skagerrak. Biogeosciences 18, 2139–2160. doi: 10.5194/bg-18-2139-2021
Duffy J. E., Lefcheck J. S., Stuart-Smith R. D., Navarrete S. A., Edgar G. J. (2016). Biodiversity enhances reef fish biomass and resistance to climate change. Proc. Natl. Acad. Sci. U. S. A. 113, 6230–6235. doi: 10.1073/pnas.1524465113
Dunkley F., Solandt J.-L. (2020). A case for a just transition to ban bottom trawl and dredge fishing in offshore marine protected areas. Marine Conservation Society: Ross-on-Wye.
Duplisea D. E., Jennings S., Malcolm S. J., Parker R., Sivyer D. B. (2001). Modelling potential impacts of bottom trawl fisheries on soft sediment biogeochemistry in the north Sea. Geochem. Trans. 2, 112–117. doi: 10.1186/1467-4866-2-112
Durrieu De Madron X., Ferré B., Le Corre G., Grenz C., Conan P., Pujo-Pay M., et al. (2005). Trawling-induced resuspension and dispersal of muddy sediments and dissolved elements in the gulf of lion (NW Mediterranean). Cont. Shelf Res. 25, 2387–2409. doi: 10.1016/j.csr.2005.08.002
Eigaard O. R., Bastardie F., Breen M., Dinesen G. E., Hintzen N. T., Laffargue P, et al. (2016). Estimating seabed pressure from demersal trawls, seines, and dredges based on gear design and dimensions. ICES J. Mar. Sci 73, i27–i43
Eigaard O. R., Bastardie F., Hintzen N. T., Buhl-Mortensen L., Buhl-Mortensen P., Catarino R., et al. (2017). The footprint of bottom trawling in European waters: Distribution, intensity, and seabed integrity. ICES J. Mar. Sci. 74, 847–865. doi: 10.1093/icesjms/fsw194
Epstein G., Hawkins J. P., Norris C. R., Roberts C. M. (2021). The potential for mobile demersal fishing to reduce carbon storage and sequestration in seabed sediments. bioRxiv preprint, 1–29. doi: 10.1101/2021.07.07.450307
Epstein G., Middelburg J. J., Hawkins J. P., Norris C. R., Roberts C. M. (2022). The impact of mobile demersal fishing on carbon storage in seabed sediments. Glob. Change Biol. 28, 2875–2894. doi: 10.1111/gcb.16105
Evans J. R., Austin W. E. N., Brew D. S., Wilkinson I. P., Kennedy H. A. (2002). Holocene Shelf sea evolution offshore northeast England. Mar. Geol. 191, 147–164. doi: 10.1016/S0025-3227(02)00529-7
Ferguson R. I., Church M. (2004). A simple universal equation for grain settling velocity. J. Sediment. Res. 74, 933–937. doi: 10.1306/051204740933
Folk R .L. (1954). The Distinction between Grain Size and Mineral Composition in Sedimentary-Rock. Nomenclature. J. Geol. 62, 344–359.
Gaines S. D., Costello C., Owashi B., Mangin T., Bone J., Molinos J. G., et al. (2018). Improved fisheries management could offset many negative effects of climate change. Sci. Adv. 4, 1–9. doi: 10.1126/sciadv.aao1378
Gerritsen H., Lordan C. (2011). Integrating vessel monitoring systems (VMS) data with daily catch data from logbooks to explore the spatial distribution of catch and effort at high resolution. ICES J. Mar. Sci. 68, 245–252. doi: 10.1093/icesjms/fsq137
Harvey H. R., Tuttle J. H., Bell J. T.. (1995). Kinetics of phytoplankton decay during simulated sedimentation: Changes in biochemical composition and microbial activity under oxic and anoxic conditions. Geochim. Cosmochim. Acta 59, 3367–3377. doi: 10.1371/journal.pone.0076430
Hattab T., Lasram F. B. R., Albouy C., Sammari C., Romdhane M. S., Cury P., et al. (2013). The use of a predictive habitat model and a fuzzy logic approach for marine management and planning. PloS One 8, 1–13. doi: 10.1371/journal.pone.0076430
Hedges J. I., Keil R. G. (1995). Sedimentary organic matter preservation: an assessment and speculative synthesis. Mar. Chem. 49, 81–115. doi: 10.1016/0304-4203(95)00008-F
Hiddink J. G., Jennings S., Kaiser M. J. (2006). Indicators of the ecological impact of bottom-trawl disturbance on seabed communities. Ecosystems 9, 1190–1199. doi: 10.1007/s10021-005-0164-9
Hiddink J. G., Jennings S., Sciberras M., Szostek C. L., Hughes K. M., Ellis N., et al. (2017). Global analysis of depletion and recovery of seabed biota after bottom trawling disturbance. Proc. Natl. Acad. Sci. U. S. A. 114, 8301–8306. doi: 10.1073/pnas.1618858114
Hunt C., Demšar U., Dove D., Smeaton C., Cooper R., Austin W. E. N. (2020). Quantifying marine sedimentary carbon: A new spatial analysis approach using seafloor acoustics, imagery, and ground-truthing data in Scotland. Front. Mar. Sci. 7. doi: 10.3389/fmars.2020.00588
ICES (2016). OSPAR request for further development of fishing intensity and pressure mapping, ICES advice 2016.
ICES (2017). EU request on indicators of the pressure and impact of bottom-contacting fishing gear on the seabed, and of tradeoffs in the catch and the value of landings. ICES Spec. Req. advice ICES:Denmark, 1–29.
ICES (2020). VMS/Log book data for fishing activities in the north East Atlantic and Baltic Sea for the provision of ICES advice on the spatial distribution and impact of fisheries 2009 to 2019 (No. H.4/NH/AB/av) (ICESCopenhagen).
Jennings S., Kaiser M. J., Reynolds J. D. (2001). “Fishing gears and technniques,” in Marine fisheries ecology (Oxford: Blackwell Publishing), 90–111.
Jones J. B. (1992). Environmental impact of trawling on the sea bed: a review. New Zeal. J. Mar. Freshw. Res. 26, 59–67. doi: 10.1080/00288330.1992.9516500
Kahraman C., Kaya I. (2010). Investment analyses using fuzzy probability concept. Technol. Econ. Dev. Econ. 16, 43–57. doi: 10.3846/tede.2010.03
Kaiser M. J., Clarke K. R., Hinz H., Austen M. C. V., Somerfield P. J., Karakassis I. (2006). Global analysis of response and recovery of benthic biota to fishing. Mar. Ecol. Prog. Ser. 311, 1–14. doi: 10.3354/meps311001
Kaiser M. J., Hill A. S., Ramsay K., Spencer B. E., Brand A. R., Veale L., et al. (1996). Benthic disturbance by fishing gear in the Irish Sea: a comparison of beam trawling and scallop dredging. Aquat. Conserv. Mar. Freshw. Ecosyst. 6, 269–285. doi: 10.1002/(SICI)1099-0755(199612)6:4<269::AID-AQC202>3.0.CO;2-C
Kaskela A. M., Kotilainen A. T., Alanen U., Cooper R., Green S., Guinan J., et al. (2019). Picking up the pieces–harmonising and collating seabed substrate data for European maritime areas. Geosciences 9, 1–18. doi: 10.3390/geosciences9020084
Kavadas S., Maina I., Damalas D., Dokos I., Pantazi M., Vassilopoulou V. (2015). Multi-criteria decision analysis as a tool to extract fishing footprints: Application to small scale fisheries and implications for management in the context of the maritime spatial planning directive. Mediterr. Mar. Sci. 16, 294–304. doi: 10.12681/mms.1087
Kenny A. J., Jenkins C., Wood D., Bolam S. G., Mitchell P., Scougal C., et al. (2018). Assessing cumulative human activities, pressures, and impacts on north Sea benthic habitats using a biological traits approach. ICES J. Mar. Sci. 75, 1080–1092. doi: 10.1093/icesjms/fsx205
Lambert G. I., Jennings S., Kaiser M. J., Davies T. W., Hiddink J. G. (2014). Quantifying recovery rates and resilience of seabed habitats impacted by bottom fishing. J. Appl. Ecol. 51, 1326–1336. doi: 10.1111/1365-2664.12277
Lee T. R., Wood W. T., Phrampus B. J. (2019). A machine learning (kNN) approach to predicting global seafloor total organic carbon. Global Biogeochem. Cycles 33, 37–46. doi: 10.1029/2018GB005992
Legge O., Johnson M., Hicks N., Jickells T., Diesing M., Aldridge J., et al. (2020). Carbon on the northwest european shelf: contemporary budget and future influences. Front. Mar. Sci. 7, 143. doi: 10.3389/fmars.2020.00143
Lindegarth M., Valentinsson D., Hansson M., Ulmestrand M. (2000). Effects of trawling disturbances on temporal and spatial structure of benthic soft-sediment assemblages in gullmarsfjorden, Sweden. ICES J. Mar. Sci. 57, 1369–1376. doi: 10.1006/jmsc.2000.0903
Luff R., Moll A.. (2004). Seasonal dynamics of the North Sea sediments using a threedimensional coupled sediment-water model system. Cont. Shelf Res 24, 1099–127
Luisetti T., Ferrini S., Grilli G., Jickells T. D., Kennedy H., Kröger S., et al. (2020). Climate action requires new accounting guidance and governance frameworks to manage carbon in shelf seas. Nat. Commun. 11, 1–10. doi: 10.1038/s41467-020-18242-w
Luisetti T., Turner R. K., Andrews J. E., Jickells T. D., Kröger S., Diesing M., et al. (2019). Quantifying and valuing carbon flows and stores in coastal and shelf ecosystems in the UK. Ecosyst. Serv. 35, 67–76. doi: 10.1016/j.ecoser.2018.10.013
Marine Conservation Institute (2021) Marine protection atlas. Available at: https://mpatlas.org/countries/ (Accessed 8.19.21).
Martín J., Puig P., Masqué P., Palanques A., Sá Nchez-Gó Mez A. (2014). Impact of bottom trawling on deep-Sea sediment properties along the flanks of a submarine canyon. PLoS One 9, 1–11.
Nielsen P. (1993). Turbulence effects on the settling of suspended particles. J. Sediment. Petrol. 63, 835–838. doi: 10.1306/d4267c1c2b26-11d78648000102c1865d
Nyimbili H. P., Erden T. (2020). A combined model of gis and fuzzy logic evaluation for locating emergency facilities: a case study of istanbul. 8th Int. Conf. Cartogr. GIS 1, 191–203.
Oberle F. K. J., Storlazzi C. D., Hanebuth T. J. J. (2016). What a drag: Quantifying the global impact of chronic bottom trawling on continental shelf sediment. J. Mar. Syst. 159, 109–119. doi: 10.1016/j.jmarsys.2015.12.007
OSPAR (2016) Ospar data files including trawler data. Available at: https://odims.ospar.org/odims_data_files/ (Accessed 6.18.19).
Paradis S., Goni M., Masque P., Duran R., Arjona-Camas M., Palanques A., et al. (2020). Persistence of biogeochemical alterations of deep-sea sediments by bottom trawling geophysical research letters. Geophys. Res. Lett. 48, 1–12. doi: 10.1029/2020GL091279
Paradis S., Pusceddu A., Masqué P., Puig P., Moccia D., Russo T., et al. (2019). Organic matter contents and degradation in a highly trawled area during fresh particle inputs (Gulf of castellammare, southwestern Mediterranean). Biogeosciences 16, 4307–4320. doi: 10.5194/bg-16-4307-2019
Paraska D. W., Hipsey M. R., Ursula Salmon S. (2014). Sediment diagenesis models: Review of approaches, challenges and opportunities. Environ. Model. Software 61, 297–325. doi: 10.1016/j.envsoft.2014.05.011
Pusceddu A., Bianchelli S., Martin J., Puig P., Palanques A., Masque P., et al. (2014). Chronic and intensive bottom trawling impairs deep-sea biodiversity and ecosystem functioning. PNAS 111, 8861–8866. doi: 10.1073/pnas.1405454111
Pusceddu A., Grémare A., Escoubeyrou K., Amouroux J. M., Fiordelmondo C., Danovaro R. (2005). Impact of natural (storm) and anthropogenic (trawling) sediment resuspension on particulate organic matter in coastal environments. Cont. Shelf Res. 25, 2506–2520. doi: 10.1016/j.csr.2005.08.012
Provoost P., Braeckman U., Van Gansbeke D., Moodley L., Soetaert K., Middelburg J.J., et al (2013). Modelling benthic oxygen consumption and benthic-pelagic coupling at a shallow station in the southern North Sea. Estuar. Coast. Shelf Sci. 120, 111.
Quintana M. M., Motova A., Wilkie O., Patience N. (2021). Economics of the UK fishing fleet 2020 (Edinburgh:Seafish Industry Authority).
Raines G. L., Sawatzky D. L., Bonham-Carter G. F. (2010). New fuzzy logic tools in ArcGIS. ArcUser 10, 8–13.
Rees T. (2003). C-squares”, a new spatial indexing system and its applicability to the description of oceanographic datasets. Oceanography 16, 11–19. doi: 10.5670/oceanog.2003.52
Rijnsdorp A. D., Bastardie F., Bolam S. G., Buhl-Mortensen L., Eigaard O. R., Hamon K. G., et al. (2016). Towards a framework for the quantitative assessment of trawling impact on the seabed and benthic ecosystem. ICES J. Mar. Sci. 73, i127–i138. doi: 10.1093/icesjms/fsv207
Rijnsdorp A. D., Bolam S. G., Garcia C., Hiddink J. G., Hintzen N. T., Van Denderen P. D., et al. (2018). Estimating sensitivity of seabed habitats to disturbance by bottom trawling based on the longevity of benthic fauna. Ecol. Appl. 28, 1302–1312. doi: 10.1002/eap.1731
Roberts C. M., Leary B. C. O., Mccauley D. J., Maurice P., Duarte C. M., Castilla J. C. (2017). Marine reserves can mitigate and promote adaptation to climate change. PNAS 114, 6167–6175. doi: 10.1073/pnas.1701262114
Rouse S., Kafas A., Hayes P., Wilding T. A. (2017). Development of data layers to show the fishing intensity associated with individual pipeline sections as an aid for decommissioning decision-making. Underw. Technol. 34, 171–178. doi: 10.3723/ut.34.171
Sala E., Mayorga J., Bradley D., Cabral R. B., Atwood T. B., Auber A., et al. (2021). Protecting the global ocean for biodiversity, food and climate. Nature 592, 397–402. doi: 10.1038/s41586-021-03371-z
Sciberras M., Parker R., Powell C., Robertson C., Kroger S., Bolam S., et al. (2016). Impacts of bottom fishing on the sediment infaunal community and biogeochemistry of cohesive and non-cohesive sediments. Limnol. Oceanogr. 61, 2076–2089. doi: 10.1002/lno.10354
Scourse J. D., Austin W. E. N., Long B. T., Assinder D. J., Huws D. (2002). Holocene Evolution of seasonal stratification in the celtic Sea: Refined age model, mixing depths and foraminiferal stratigraphy. Mar. Geol. 191, 119–145. doi: 10.1016/S0025-3227(02)00528-5
Seiter K., Hensen C., Zabel M. (2005). Benthic carbon mineralization on a global scale. Global Biogeochem. Cycles 19, 1–26. doi: 10.1029/2004GB002225
Shepperson J. L., Hintzen N. T., Szostek C. L., Bell E., Murray L. G., Kaiser M. J. (2018). A comparison of VMS and AIS data: The effect of data coverage and vessel position recording frequency on estimates of fishing footprints. ICES J. Mar. Sci. 75, 988–998. doi: 10.1093/icesjms/fsx230
Smeaton C., Austin W. E. N. (2017). Sources, sinks, and subsidies: Terrestrial carbon storage in mid-latitude fjords. J. Geophys. Res. Biogeosci. 122, 2754–2768. doi: 10.1002/2017JG003952
Smeaton C., Austin W. E. N. (2019). Where’s the carbon: exploring the spatial heterogeneity of sedimentary carbon in mid-latitude fjords. Front. Earth Sci. 7, 1–16. doi: 10.3389/feart.2019.00269
Smeaton C., Austin W. E. N. (2022). Quality not quantity: Prioritizing the management of sedimentary organic matter across continental shelf seas. Geophys. Res. Lett. 49, 1–11. doi: 10.1029/2021GL097481
Smeaton C., Austin W. E. N., Davies A. L., Baltzer A., Abell R. E., Howe J. A. (2016). Substantial stores of sedimentary carbon held in mid-latitude fjords. Biogeosciences 13, 5771–5787. doi: 10.5194/bg-13-5771-2016
Smeaton C., Austin W. E. N., Turrell W. R. (2020). Re-evaluating scotland’s sedimentary carbon stocks (Aberdeen:Marine Scotland Science).
Smeaton C., Hunt C. A., Turrell W. R., Austin W. E. N. (2021a). Marine sedimentary carbon stocks of the united kingdom’s exclusive economic zone. Front. Earth Sci. 9, 1–21. doi: 10.3389/feart.2021.593324
Smeaton C., Yang H., Austin W. E. N. (2021b). Carbon burial in the mid-latitude fjords of Scotland. Mar. Geol. 441, 106618. doi: 10.1016/j.margeo.2021.106618
Soetaert K., Herman P. M. J., Middelburg J. J. (1996). A model of early diagenetic processes from the shelf to abyssal depths. Geochim. Cosmochim. Acta 601019–601040.
Soetaert K., Herman P. M. J., Middelburg J. J., Heip C (1998). Assessing organic matter mineralization, degradability and mixing rate in an ocean margin sediment (Northeast Atlantic) by diagenetic modeling. J. Mar. Res. 56, 519–534.
Soetaert K., Middelburg J. J. (2009). Modeling eutrophication and oligotrophication of shallow-water marine systems: The importance of sediments under stratified and wellmixed conditions. Hydrobiologia 629, 239–254.
Tenzer R., Gladkikh V. (2014). Assessment of density variations of marine sediments with ocean and sediment depths. Sci. World J. 2014, 1–9. doi: 10.1155/2014/823296
Tiano J. C., Witbaard R., Bergman M. J. N., van Rijswijk P., Tramper A., van Oevelen D., et al. (2019). Acute impacts of bottom trawl gears on benthic metabolism and nutrient cycling. ICES J. Mar. Sci 76, 19171930. doi: 10.1093/icesjms/fsz060
Uberoi E., Hutton G., Ward M., Ares E. (2020). UK Fisheries statistics 2020. (London: UK parliament house of commons library).
Keywords: organic carbon (OC), sediment, fishing gear, seabed, mapping, disturbance
Citation: Black KE, Smeaton C, Turrell WR and Austin WEN (2022) Assessing the potential vulnerability of sedimentary carbon stores to bottom trawling disturbance within the UK EEZ. Front. Mar. Sci. 9:892892. doi: 10.3389/fmars.2022.892892
Received: 09 March 2022; Accepted: 07 July 2022;
Published: 04 August 2022.
Edited by:
Iris Eline Hendriks, Spanish National Research Council (CSIC), Madrid, SpainReviewed by:
Hilmar Hinz, University of the Balearic Islands, SpainChristian Lønborg, Aarhus University, Denmark
Copyright © 2022 Black, Smeaton, Turrell and Austin. This is an open-access article distributed under the terms of the Creative Commons Attribution License (CC BY). The use, distribution or reproduction in other forums is permitted, provided the original author(s) and the copyright owner(s) are credited and that the original publication in this journal is cited, in accordance with accepted academic practice. No use, distribution or reproduction is permitted which does not comply with these terms.
*Correspondence: Kirsty E. Black, a2I5OUBzdC1hbmRyZXdzLmFjLnVr