Dietary Use of the Microalga Chlorella fusca Improves Growth, Metabolism, and Digestive Functionality in Thick-Lipped Grey Mullet (Chelon labrosus, Risso 1827) Juveniles
- 1Departamento de Microbiología, Facultad de Ciencias, Instituto Andaluz de Biotecnología y Desarrollo Azul (IBYDA), Universidad de Málaga, Ceimar-Universidad de Málaga, Málaga, Spain
- 2Departamento de Biología y Geología, Universidad de Almería, Ceimar-Universidad de Almería, Almería, Spain
- 3Departamento de Biología, Facultad de Ciencias del Mar y Ambientales, Instituto Universitario de Investigación Marina (INMAR), Universidad de Cádiz, Ceimar-Universidad de Cádiz, Cádiz, Spain
- 4Departamento de Ingeniería Química, Universidad de Almería, Ceimar-Universidad de Almería, Almería, Spain
- 5Departamento de Ecología y Geología, Facultad de Ciencias, Instituto Andaluz de Biotecnología y Desarrollo Azul (IBYDA), Universidad de Málaga, Ceimar-Universidad de Málaga, Málaga, Spain
In recent years, a clear emphasis has been placed on replacing fishmeal and fish oil in aquafeeds with other alternative ingredients, including algae, particularly in low trophic omnivorous fish species. This work aimed at evaluating the effects of moderate dietary supplementation with the green microalga Chlorella fusca on growth, metabolism, and digestive functionality in juvenile thick-lipped grey mullet (Chelon labrosus). Fish were fed a control diet (CT) or a diet containing 15% C. fusca (C-15) biomass during 90 days. C. labrosus fed with the C-15 diet showed higher growth performance (in terms of final weight and length, weight gain, and specific growth rate) than the control group. Somatic indices and muscle proximate composition were similar at the end of the feeding trial. Regarding fatty acids profile, C. fusca-fed fish showed a selective retention of docosahexaenoic acid (DHA) in the liver, and arachidonic acid (ARA), eicosapentaenoic acid (EPA), and DHA in the muscle. Dietary inclusion of this microalga significantly increased intestinal total alkaline protease, leucine aminopeptidase, and alkaline phosphatase activities in specimens fed with C-15 diet. Furthermore, intestine histological analysis revealed the absence of damage signs on gut morphology in fish fed the microalgae supplemented diet. Thick-lipped grey mullets fed the C-15 diet increased plasma glucose and decreased plasma lactate. Overall, the effects observed on liver (lipid metabolism, glycolysis and glycogenolysis) enzyme activities, together with adequate fatty acid profile, metabolic response, and gut morphology, and a significant increase in the intestinal mucosa’s digestive and absorptive capacity, could explain the positive effects on growth performance obtained in fish fed the microalgae-supplemented diet. In conclusion, the results obtained showed that C. fusca is suitable as dietary ingredient for feeding thick-lipped grey mullet juveniles.
Introduction
Fishmeal represents a vital protein source for cultured fish species in aquafeeds (Jannathulla et al., 2019; Oliva-Teles et al., 2020). However, constraints related to high prices, limited availability, and environmental concerns have promoted extensive research efforts focused on assessing lower-cost alternative protein sources (Tibaldi et al., 2015; Minjarez-Osorio et al., 2016).
Microalgae are a promising alternative to fishmeal and crop-based ingredients since they have several features that make them attractive for the aquafeed industry. From an economical point of view, a model facility for microalgae production of 111 ha would produce 2,750 tonnes yr−1 of protein and 2,330 tonnes yr−1 of algal oil, at a capital cost of $29.3 M, such a facility would generate $5.5 M in average annual net income over its 30-year lifetime (Beal et al., 2018). From a nutritional perspective, microalgae have high levels of protein (30 to 60%, dry matter basis), relatively well-balanced amino acid profile, essential fatty acid (specifically eicosapentaenoic and docosahexaenoic acids, EPA and DHA, respectively) (Shah et al., 2018; Tibbetts, 2018) and bioactive compounds, including minerals, vitamins, and pigments (Camacho et al., 2019).
In this sense, the suitability of microalgae in practical diets for different fish species has been previously studied (Vizcaíno et al., 2014; Tibaldi et al., 2015; Yeganeh et al., 2015; Sørensen et al., 2016; Perera et al., 2020). Although depending on species tested and levels of inclusion (Shah et al., 2018), low, moderate, and even total substitution of fishmeal by microalgae in aquafeeds evidenced beneficial effects on growth, nutrient utilization, digestibility, metabolism, or survival rate (Rahimnejad et al., 2017; Vizcaíno et al., 2018; García-Márquez et al., 2020; Perera et al., 2020). Microalgae also have health-promoting effects in fish, modulate the fatty acid profile and the quality of the fish flesh and also improve the stress resistance, which is also of great interest today for both producers and consumers (Sarker et al., 2016; Gong et al., 2019; Silveira Júnior et al., 2019).
Overall, herbivorous and omnivorous fish tolerate higher inclusion levels of algae than carnivorous species. However, results reported in the literature indicate that the optimum dietary algae inclusion level should vary depending on the algae and the farmed fish species (Shah et al., 2018). Therefore, specific research should be carried out on each particular algae strain and fish species. Owing to the high nutritional value, species of the genus Chlorella have been used as dietary protein source, and abundant literature assessing their effects on a wide range of farmed fish species is available (Atlantic salmon, Salmo salar: Tibbetts et al., 2017; common carp, Cyprinus carpio: Abdulrahman et al., 2018; European sea bass, Dicentrarchus labrax: Hasanein et al., 2018; grey mullet, Mugil cephalus: Akbary and Aminikhoei, 2019). The microalga Chlorella fusca shows a high content of various high-value compounds, including carotenoids, specifically lutein (Becker, 2013). Moreover, this species can accumulate a high content of essential fatty acids such as linoleic, and α-linolenic acids (Pratoomyot et al., 2005). However, it is well-known that chlorophyte microalgae of the Chlorella genus possess recalcitrant cell walls (Domozych et al., 2012; Liu and Hu, 2013), and in fact, itself may act as protective barrier, diminishing the digestibility and assimilation of intracellular nutrients (Lavecchia et al., 2016). Moreover, the nutritional effects of Chlorella species depend on its inclusion levels in aquaculture feeds. In this sense, Ahmad et al. (2020) reported controversial effects on growth performance and feed utilization in different species of fish, which may be attributed to the levels of inclusion of the microalga, as levels above 20% compromises growth performance (Hasan and Chakrabarti, 2009; Lupatsch and Blake, 2013).
The thick-lipped grey mullet (Chelon labrosus, Risso 1827) has been described as an easily cultivable species and could constitute a low-trophic level new candidate for aquaculture diversification (Zouiten et al., 2008; García‐Márquez et al., 2021). Recent studies have been focused on practical aspects of its culture, like its sensitivity to stress or its digestive physiology (de las Heras et al., 2015; Pujante et al., 2015; Pujante et al., 2017; Pujante et al., 2018). Nevertheless, there is a lack of information regarding feed utilization or growth performance in C. labrosus fed compound diets.
Thus, the aim of this study is to assess the potential of the microalga C. fusca as dietary ingredient for feeding C. labrosus juveniles. We hypothesise that C. fusca might improve growth performance, nutrient utilization, and several parameters related to physiological metabolism, and digestive functionality in juvenile C. labrosus when tested at moderate dietary inclusion level (15%) through a 90-day feeding trial.
Materials and Methods
Microalgae
The microalga Chlorella fusca was produced in pilot-scale photobioreactors (PBR) at the SABANA facilities of the University of Almeria (Spain). The inoculum was produced in a bubble column photobioreactor (100-L water capacity, 0.20 m diameter, 2.0 m height) with automatic temperature and pH control, and air bubbling of 0.2 vol vol-1 min−1 (volume of air per volume of reactor per time). After that, 3.0 m3 tubular photobioreactors (0.10 m tube diameter) were used to produce the final amount of biomass required. The pH, temperature, and dissolved oxygen were continuously monitored on these reactors using specific probes (Crison Instruments, Spain). The pH was controlled automatically by the on-demand injection of CO2. The temperature was kept within the range required for optimal growth of C. fusca (20-25°C) by controlling the greenhouse’s temperature on which the reactors were located. The culture medium used was the one described by Sorokin and Krauss (1958), which was prepared by dissolving fertilizers in tap water and then sterilized by filtration (0.02 µm) and ozone (1 mg L−1). Microalgal biomass was harvested by centrifugation (SSD6 GEA Westfalia, Germany). Cell disruption was performed using a high-pressure homogenizer (GEA Ariete NS3015H) at 600 bars in a single pass, these operational conditions being previously optimized. For drying, a spray-dryer was utilized (GEA Mobile MinorTM Spray dryer), performed at an inlet temperature of 160 °C, while the outlet temperature was kept below 80 °C. The final powder was stored in the dark at −20°C until further preparation of the experimental diet.
Experimental Diets and Feeding Trial
Two iso-nitrogenous and isolipidic (40% and 7%, respectively, on a dry weight basis) experimental diets were formulated and elaborated by the Service of Experimental Diets (CEIMAR-University of Almeria, Spain, grant EQC2019-006380-P) using standard aquafeed processing procedures to obtain 3 mm floating pellets. The diet designed as C-15 included 15% (w/w) dry C. fusca biomass, and an algae-free diet was used as control (CT). The ingredient composition and fatty acid profile of the experimental diets and C. fusca are shown in Tables 1, 2, respectively. Feed ingredients were finely ground and mixed in a vertical helix ribbon mixer (Sammic BM-10, 10-L capacity, Sammic, Azpeitia, Spain) before fish oil and diluted choline chloride were added. All the ingredients were mixed together for 15 min, and then water (350 mL kg-1) was added to the mixture to obtain a homogeneous dough. The dough was passed through a single screw laboratory extruder (Miltenz 51SP, JSConwell Ltd, New Zealand). The extruder barrel consisted of four sections, and the temperature profile in each section (from inlet to outlet) was 95°C, 98°C, 100°C, and 110°C, respectively. Finally, pellets were dried at 27°C in a drying chamber (Airfrio, Almeria, Spain) for 24 h, and kept in sealed plastic bags at −20°C until use.
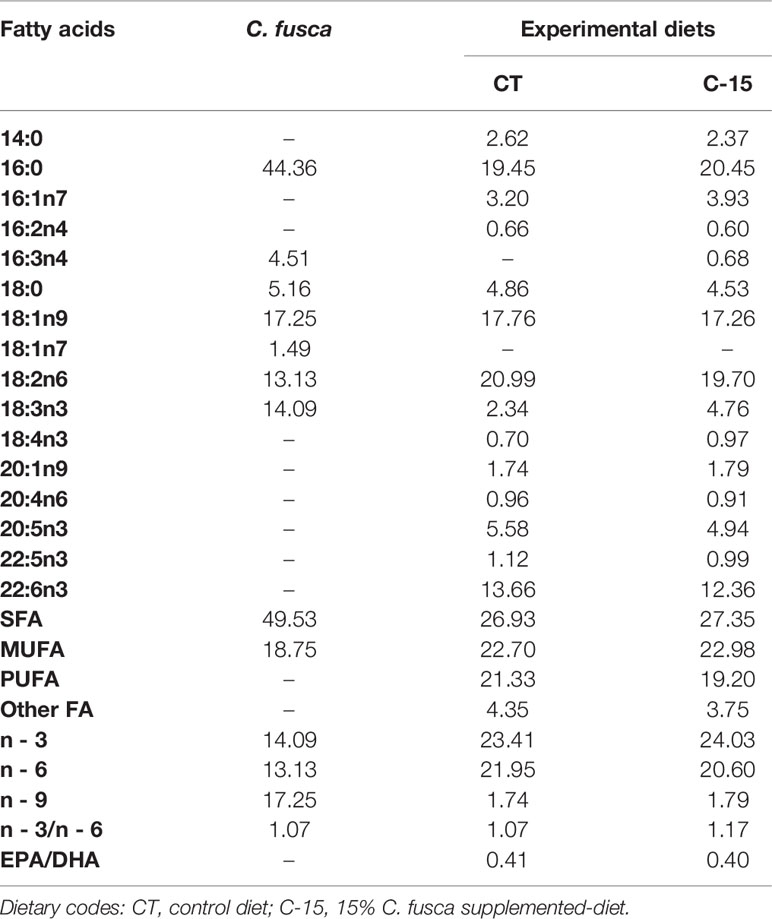
Table 2 Fatty acid composition (% of total fatty acids) of microalga and experimental diets used in the feeding trial.
Thick-lipped grey mullet (Chelon labrosus) specimens (n = 180) were provided by the Centro Integrado de Formación Profesional C.I.F.P. Marítimo Zaporito (San Fernando, Cadiz, Spain), and transferred to the Centro de Experimentación de Ecología y Microbiología de Sistemas Acuáticos Controlados Grice-Hutchinson (CEMSAC) of the University of Malaga (Malaga, Spain; Spanish Operational Code REGA ES290670002043). The fish were acclimated to experimental conditions and fed with a commercial diet (32% protein, 6% fat, TI-3 Tilapia, Skretting, Spain) for 30 days before starting the feeding trial. Six homogeneous groups of 30 fish (84.7 ± 0.3 g) were randomly distributed in 1000 L tanks coupled to a recirculation aquaculture system (RAS), equipped with physical and biological filters, and maintained under natural photoperiod (November 2019 – February 2020), in the range of 17.9–23.8°C, and salinity 1.0–1.2 ‰. Supplemental aeration was provided to maintain dissolved oxygen at 6.8 ± 0.4 mg L-1. Ammonia (<0.1 mg L-1), nitrite (<0.2 mg L-1), and nitrate (<50 mg L-1) were determined weekly at 9:00 AM. The two experimental dietary groups (CT and C-15) were then established in triplicates. Fish were hand-fed twice per day (9:00 AM and 5:00 PM) at a rate of 1.5% of their body weight for 90 days. The uneaten pellets were collected after 1 h and then dried and weighed.
Fish Sampling
Fish were counted and group-weighed every 3 weeks, and feed intake was recorded for each experimental replicate to calculate growth performance parameters. At the end of the trial (day 90), overnight fasted fish (3 fish per replicate, 9 per experimental diet) were randomly selected, deeply anesthetized with 2-phenoxyethanol (1 mL L−1, Sigma-Aldrich 77699), and then sampled for blood and tissue collection. Blood was drawn from caudal vessels with heparinized syringes, centrifuged at 3000 × g for 5 min at 4°C, and plasma samples were snap-frozen in liquid nitrogen. Immediately, whole viscera were obtained, the intestines were separated from the other organs, and all visible perivisceral fat was removed. Liver, total viscera, and perivisceral fat were weighed for hepatosomatic, viscerosomatic, and perivisceral indices, respectively. Plasma, liver, and white muscle samples were stored at −80 °C until biochemical analysis.
Moreover, for the enzymatic analysis intestines from four fish per each experimental tank were randomly grouped, which allowed obtaining four different enzymatic extracts per experimental tank (12 enzymatic extracts per dietary treatment). Intestinal samples were homogenized in distilled water at 4°C (w/v 1:2). Supernatants were obtained after centrifugation (13,000 × g, 12 min, 4°C) and stored at −20°C until further analysis. In parallel, 1 cm length portions of the proximal intestine of three specimens from each tank (9 fish per dietary treatment) were collected for further examination under light (LM), transmission (TEM), and scanning (SEM) electron microscopy.
Growth Performance and Biometric Parameters
The following growth parameters were evaluated: (1) weight gain (WG) = ((final fish weight – initial fish weight) x 100); (2) specific growth ratio (SGR) = (100 x [(ln final fish weight) – (ln initial fish weight)]/experimental days); (3) feed conversion ratio (FCR) = dry feed intake (g)/weight gain (g); (4) protein efficiency ratio (PER) = weight gain/intake of particular protein; (5) condition factor (K, %) = ((fish weight/fish length3) x 100); (6) hepatosomatic index (HSI, %) = ((liver weight/body weight) x 100); (7) viscerosomatic index (VSI, %) = ((total viscera weight/body weight) x 100); (8) perivisceral index (PVI, %) = ((total fat viscera weight/total viscera weight) x 100).
Proximate Composition, Fatty Acid Profile, and Indices of Lipid Metabolism and Quality
Proximate analyses of feeds and fish muscle samples were carried out according to AOAC (2000) for dry matter and ash. Crude protein content (N × 6.25) was determined using elemental analysis (C:H:N) (Fisons EA 1108 analyzer, Fisons Instruments, USA), and total lipid content was quantified according to the methods described by Folch et al. (1957). Fatty acid composition of feeds, liver, and muscle samples was determined by gas chromatograph following the procedure described by Rodríguez-Ruiz et al. (1998).
From the fatty acid profile of fish muscle, different indices were calculated (Arakawa and Sagai, 1986; Senso et al., 2007): (1) peroxidability index (PI)= (% monoenoic × 0.025) + (% dienoic × 1) + (% trienoic × 2) + (% tetraenoic × 4) + (% pentaenoic × 6) + (% hexaenoic × 8); (2) index of thrombogenicity (IT) = (14:0 + 16:0 + 18:0)/[(0.5 × 18:1) + (0.5 × ΣMUFAs) + (0.5 × n-6 PUFAs) + (3 × n-3 PUFAs) + (n-3/n6)]; (3) index of atherogenicity (IA) = (12:0 + 4 × 14:0 + 16:0)/[(n-6 + n-3) PUFAs + 18:1 + other MUFAs]; (4) fish lipid quality (FLQ, %) = [(20:5n-3 + 22:6n-3)/total lipid] × 100. MUFAs and PUFAs stand for monounsaturated fatty acids and polyunsaturated fatty acids, respectively.
Determination of Digestive Enzyme Activities
Total alkaline protease activity was measured using buffered 5 g L−1 casein (50 mM Tris-HCl, pH 9.0) as substrate following the method described by Alarcón et al. (1998). One unit of activity (UA) was defined as the amount of enzyme releasing 1 µg tyrosine per minute measured spectrophotometrically at 280 nm (extinction coefficient for tyrosine of 0.008 μg−1 cm−1 mL−1). Trypsin and chymotrypsin activities were determined using 0.5 mM BAPNA (N-α-benzoyl-DL-arginine-4-nitroanilide) as substrate according to Erlanger et al. (1961) and 0.2 mM SAPNA (N-succinyl-(Ala)2-Pro-Phe-P-nitroanilide) according to DelMar et al. (1979), respectively, in 50 mM Tris–HCl buffer, pH 8.5, containing 10 mM CaCl2. Leucine aminopeptidase activity was assayed using buffered 2 mM L-leucine -p-nitroanilide (LpNa) (100 mM Tris-HCl, pH 8.8) as substrate according to Pfleiderer (1970), and alkaline phosphatase activity was determined using buffered p-nitrophenyl phosphate (pH 9.5) as substrate, according to the methodology described by Bergmeyer (1974). For trypsin, chymotrypsin, and leucine aminopeptidase activities, one UA was defined as the amount of enzyme that released 1 µmol of p-nitroanilide (pNA) per minute (extinction coefficient 8,800 M cm−1 at 405 nm). For alkaline phosphatase, one UA was defined as the amount of enzyme that released 1 µg of nitrophenyl per min (extinction coefficient 17,800 M cm−1 at 405 nm). All assays were performed in triplicate in each one of the 12 enzymatic extracts obtained per dietary treatment, and specific enzyme activities were expressed as U g tissue−1 (Galafat et al., 2020).
In addition, a substrate-SDS-PAGE (sodium dodecyl sulfate polyacrylamide gel electrophoresis) electrophoresis gel was carried out to visualize the active proteases present in intestinal extracts of fish. Intestinal extracts were mixed with SDS sample buffer (1:1), and SDS-PAGE was performed according to Laemmli (1970) using 11% polyacrylamide (100 V per gel, 60 min, 4°C). Zymograms revealing protease active bands were made according to Alarcón et al. (1998). After electrophoresis, gels were washed with distilled water and incubated for 30 min at 4°C in buffer 0.75% (w/v) casein solution (50 mM Tris–HCl buffer, pH 9). Then, gels were incubated in the same solution for 90 min at 37°C. After the incubation, gels were washed and fixed in 12% trichloroacetic acid solution (TCA) for 105 min to stop the reaction prior to staining with Coomassie Brilliant Blue R-250 in a solution of methanol-acetic acid-water overnight. Finally, gels were destained using a methanol–acetic acid–water solution. Clear gel zones revealed the presence of active proteases with caseinolytic activity.
Histological and Ultrastructural Study of the Intestinal Mucosa
Anterior intestinal samples from three fish per tank (9 per dietary treatment) were collected for examination by light and electron microscopy. For light microscopy examination, intestine samples were fixed for 24 h in phosphate-buffered formalin (4% v/v, pH 7.2), dehydrated, and embedded in paraffin according to standard histological techniques described in Vizcaíno et al. (2014). Briefly, samples were cut in 5 μm transversal sections, and the slides were stained with hematoxylin-eosin (H&E). The stained preparations were examined under a light microscope (Olympus ix51, Olympus, Barcelona, Spain) equipped with a digital camera (CC12, Olympus Soft Imaging Solutions GmbH, Muenster, Germany). Images were analyzed with specific software (Image J, National Institutes of Health, USA). The length and diameter of mucosal folds, total enterocyte height, as well as the thickness of the lamina propria of the submucosa, muscular and serous layer were analyzed (50 independent measurements per treatment) in intestinal samples.
Samples for transmission (TEM) and scanning (SEM) electron microscopy were processed as described in Vizcaíno et al. (2014). For TEM, intestine samples were fixed with glutaraldehyde 25 g L−1 and formaldehyde 40 g L−1 in phosphate buffer saline (PBS) pH 7.5 for 4 h at 4°C. Then, they were washed three times with PBS, and a 2 h post-fixation with 20 g L−1 osmium tetroxide was carried out. After that, samples were dehydrated by consecutive immersions in gradient ethanol solutions (from 50% to 100%; v/v). The dehydrated tissue was embedded in a mixture of 1:1 ethanol 100% (v/v) and EPON resin for 2 h with stirring. Then, it was included in pure EPON resin for 24 h and polymerized at 60°C. The ultra-fine cuts were placed on 700 copper mesh and stained with uranyl acetate and lead citrate. The observation was performed with a transmission electron microscope Zeiss 10C at 100 kV (Carl Zeiss, Barcelona, Spain). For SEM, samples were fixed for 24 h in phosphate-buffered formalin (4% v/v, pH 7.2). Then, they were washed and progressively dehydrated in graded ethanol. Then samples were dried by critical point (CDP 030 Critical point dryer, Leica Microsystems, Madrid, Spain) with absolute ethanol as the intermediate fluid and CO2 as the transition fluid. Dried samples were mounted on supports, fixed with graphite (PELCO® Colloidal Graphite, Ted Pella Inc., Ca, USA), and gold sputter-coated (SCD 005 Sputter Coater, Leica Microsystems). Finally, all samples were screened with a scanning electron microscopy (HITACHI model S-3500, Hitachi High-Technologies Corporation, Japan). All digital images were analyzed with UTHSCA ImageTool software and morphometric analysis to determine the microvilli length (ML), the microvilli diameter (MD), the number of microvilli over 1 μm distance, and the enterocyte apical area (EA) was carried out. Finally, the total absorption surface per enterocyte (TAS) was estimated following the procedure described byVizcaíno et al. (2014).
Tissue Metabolites
For assessing tissue metabolite levels, samples from liver and muscle (three fish per tank, 9 per dietary treatment) were individually minced on an ice-cold Petri dish and subsequently homogenized by mechanical disruption (Ultra-Turrax®, T25basic with an S25N-8G dispersing tool, IKA®-Werke) with 7.5 vol. (w/v) of ice-cold 0.6 N perchloric acid and neutralized after adding the same volume of 1 M KHCO3. Subsequently, the homogenates were centrifuged (3500 × g, 30 min, 4°C), and the supernatants were recovered in different aliquots. The aliquots were then stored at −80°C until used in metabolite assays. Metabolite concentrations in plasma (glucose, lactate, and triglycerides) and liver (glucose and triglycerides) were determined using commercial kits from Spinreact (Barcelona, Spain) (Glucose-HK Ref. 1001200; Lactate Ref. 1001330; Triglycerides ref. 1001311) with reactions adapted to 96-well microplates. Liver glycogen levels were assessed using the method from Keppler and Decker (1974). After subtracting free glucose levels, the glucose obtained after glycogen was determined using the commercial kit described above for glucose. Plasma total protein concentration was determined using bovine serum albumin (BSA) as the standard with BCA Protein AssayKit (PIERCE, Thermo Fisher Scientific, USA, #23225). Total α-amino acid levels were assessed colorimetrically using the ninhydrin method from Moore (1968) adapted to 96-well microplates. All standards and samples were measured in duplicate. All the assays were run on an Automated Microplate Reader (PowerWave 340, BioTek Instrument Inc., Winooski, VT, USA) using KCjunior™ software.
Activity of Metabolic Enzymes in the Liver
Frozen liver tissues (three fish per tank, 9 per dietary treatment) for enzyme activity assays were homogenized by mechanical disruption (Ultra-Turrax®) with 10 vol. (w/v) of ice-cold homogenization buffer (in mM: 50 imidazole, 1 2-mercaptoethanol, 50 NaF, 4 EDTA, 0.5 phenylmethylsulfonyl fluoride (PMSF), and 250 sucrose; pH 7.5). Homogenates were centrifuged for 30 min at 3220 × g and 4°C, and supernatants were stored at −80°C for further analysis. The assays of several enzymes involved in glycogenolysis (GPase [active]: glycogen phosphorylase, EC 2.4.1.1), glycolysis (HK: hexokinase, EC 2.7.1.1; PK: pyruvate kinase, EC 2.7.1.40), gluconeogenesis (LDH: lactate dehydrogenase, EC 1.1.1.27; FBP: fructose 1,6-bisphosphatase, EC 3.1.3.11) and lipid metabolism (HOAD: 3-hydroxyacyl-CoA dehydrogenase, EC 1.1.1.35) were performed as previously described for gilthead seabream (Sparus aurata) tissues (Perera et al., 2020). All the assays were run on an Automated Microplate Reader (PowerWave 340, BioTek Instrument Inc., Winooski, VT, USA) using KCjunior™ software. Activities were expressed as specific activities per mg of protein in the homogenate (U mg prot−1). Proteins were assayed in duplicate, as described above for plasma samples.
Statistical Analysis
Results are reported as means ± SEM (n = 9). Normal distribution was checked for all data with the Shapiro–Wilk test, while the homogeneity of the variances was obtained using the Levene test. When necessary, an arcsin transformation was performed. Differences between the two experimental diets (CT and C-15) were tested using Student’s t-test. In all statistical tests performed, p < 0.05 was considered significantly different. All analyses were performed with SPSS Statistics 25 software (SPSS Inc, IBM Company, NY, USA).
Results
Growth Performance, Nutrient Utilization, and Proximate Composition
No mortality occurred during the experimental period. The inclusion of C. fusca statistically stimulated growth performance of C. labrosus juveniles (Table 3). After 90 days, C-15 group animals enhanced significantly body weight and length as well as weight gain and specific growth ratio respect to fish fed CT diet. Fish fed with Chlorella increased protein efficiency and decreased feed conversion ratio compared to the control group; however, no statistical differences were found between experimental groups. Furthermore, no significant differences were found in Fulton’s Condition Factor (K) and somatic indices (HSI, VSI, and PVI).
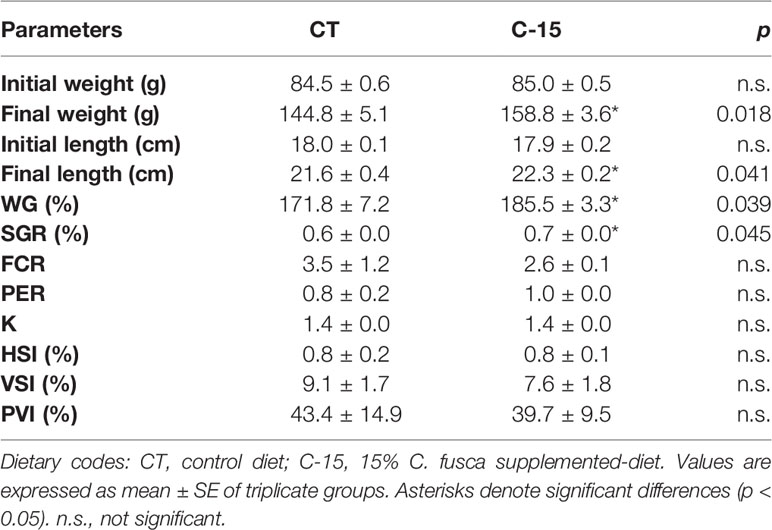
Table 3 Growth performance and somatic indices of juvenile C. labrosus fed control (CT) and C-15 diets during 90 days.
The proximate composition of C. labrosus specimens is presented in Table 4. Body composition of fish did not show any significant variation between fish fed CT and C-15 diets.
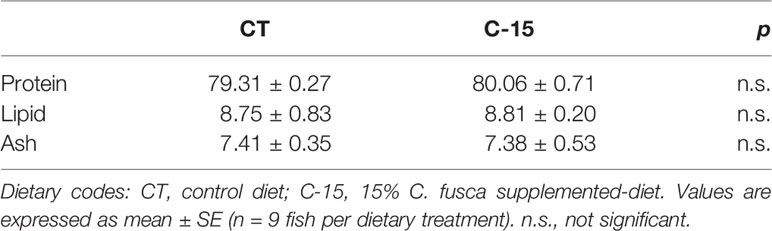
Table 4 Muscle proximate composition (% dry weight) of juvenile C. labrosus fed control (CT) and C-15 diets during 90 days.
Tissue Fatty Acids
Fatty acid profiles results are shown in Table 5. The fatty acid profiles of liver and muscle differed between experimental groups. Palmitic acid (16:0) and oleic acid (18:1n-9) were both tissues’ most abundant fatty acids. Liver from the CT group had significantly higher proportions of oleic acid, linolenic acid (18:3n-3), and arachidonic acid (ARA, 20:4n-6). However, liver from the C-15 group showed significantly higher proportions of vaccenic acid (18:1n-7), eicosenoic acid (20:1n-9), and docosahexaenoic acid (DHA, 22:6n-3). In muscle, C-15 specimens presented statistically higher proportions of palmitic acid, stearic acid (18:0), ARA, eicosapentaenoic acid (EPA, 20:5n-3), docosapentaenoic acid (22:5n-3), and DHA. On the other hand, muscle from the control group had higher oleic acid, vaccenic acid, linoleic acid (18:2n6), linolenic acid, and eicosenoic acid. Hepatic percentage of total saturated, monounsaturated and polyunsaturated fatty acids (SFA, MUFA, and PUFA, respectively) was not modified by the inclusion of C. fusca. In contrast, while a significantly higher proportion of SFA, PUFA, and n-3 content was found in muscle of the C-15 diet, higher content of MUFA, n-6, and n-9 were detected in muscle of control specimens. Altogether, the n-3/n-6 ratio enhanced significantly in both tissues from C-15-fed fish, while EPA/DHA ratio decreased significantly. As a result of these differences due to the inclusion of C. fusca, C-15 specimens showed a significantly higher peroxidability index (PI), flesh lipid quality (FLQ), and atherogenicity index (IA), as well as a lower index of thrombogenicity (IT).
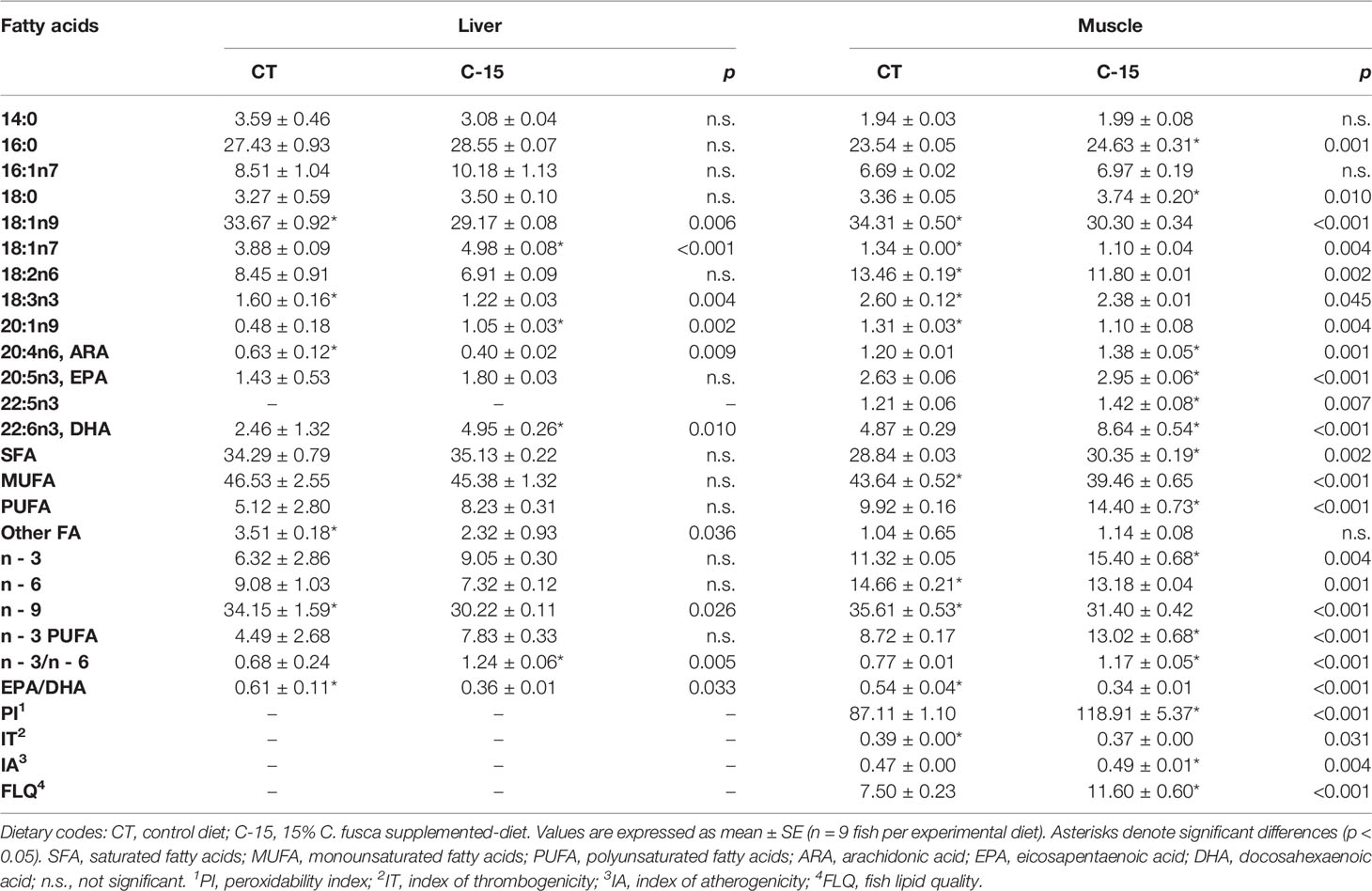
Table 5 Liver and muscle fatty acid profile (% of total fatty acids) of juvenile C. labrosus fed control (CT) and C-15 diets during 90 days.
Digestive Functionality
Enzyme activities measured in the intestinal extracts of C. labrosus specimens fed with the experimental diets are shown in Table 6. Total alkaline protease activity was significantly higher in the C-15 group than fish fed the control diet, whilst trypsin and chymotrypsin activity levels were similar between both groups. Regarding brush border enzymes, dietary inclusion of C. fusca significantly increased both leucine aminopeptidase and alkaline phosphatase activity levels compared to CT group. In addition, the zymogram of the intestinal proteases revealed the same profile of active fractions in both CT and C-15 fed fish (Figure 1).
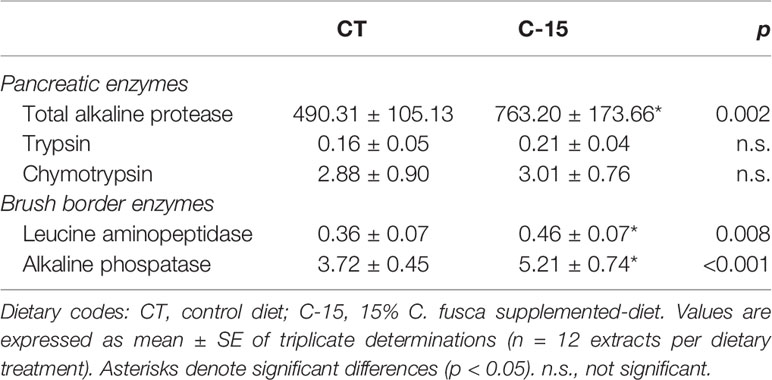
Table 6 Enzyme activities (U g tissue−1) measured in the whole intestinal extracts of juvenile C. labrosus fed control (CT) and C-15 diets during 90 days.
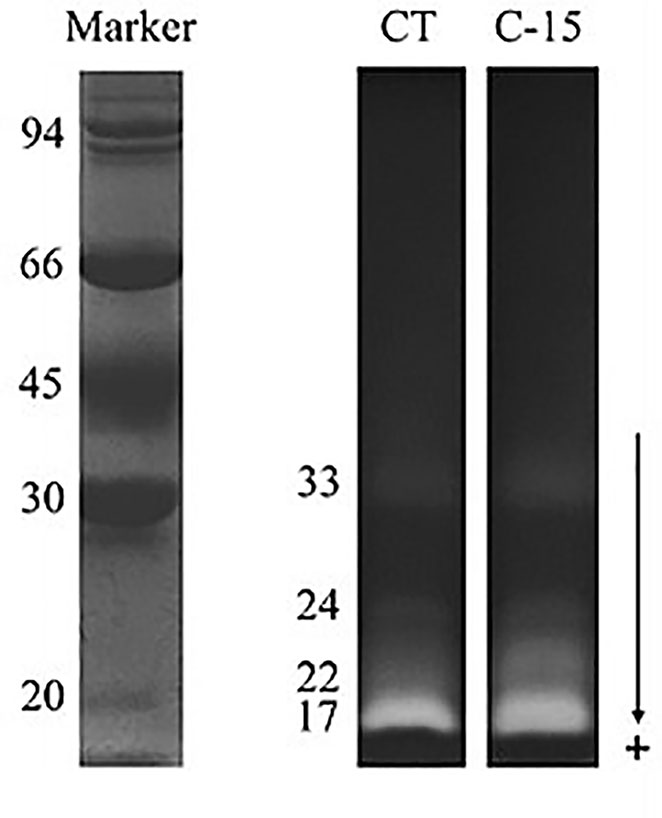
Figure 1 Zymogram showing total proteolytic activity from pooled intestinal extracts of fish. Dietary codes: CT, control diet; C-15, 15% C. fusca supplemented-diet. Protein standards employed were phosphorylase b (94), bovine serum albumin (66), ovalbumin (45), carbonic anhydrase (30), soybean trypsin inhibitor (20). The molecular mass (in kDa) of proteins was measured using a linear plot of log Mr. of protein standards (M) vs. relative mobility (Rf). Five microliters of molecular weight marker (M) were loaded.
The histological characteristics of intestinal sections from fish receiving the two dietary treatments are shown in Figure 2. Overall, no signs of intestinal damage were found as all specimens presented intestinal mucosa without evidence of abnormality. The morphometric analysis (Table 7) revealed a significant increase of fold length in fish fed the C-15 diet, while fold diameter and enterocyte height were similar in both dietary groups. Additionally, thickness of the serosa and muscular layers, as well as of the lamina propria was significantly reduced in specimens fed with C-15 diet.
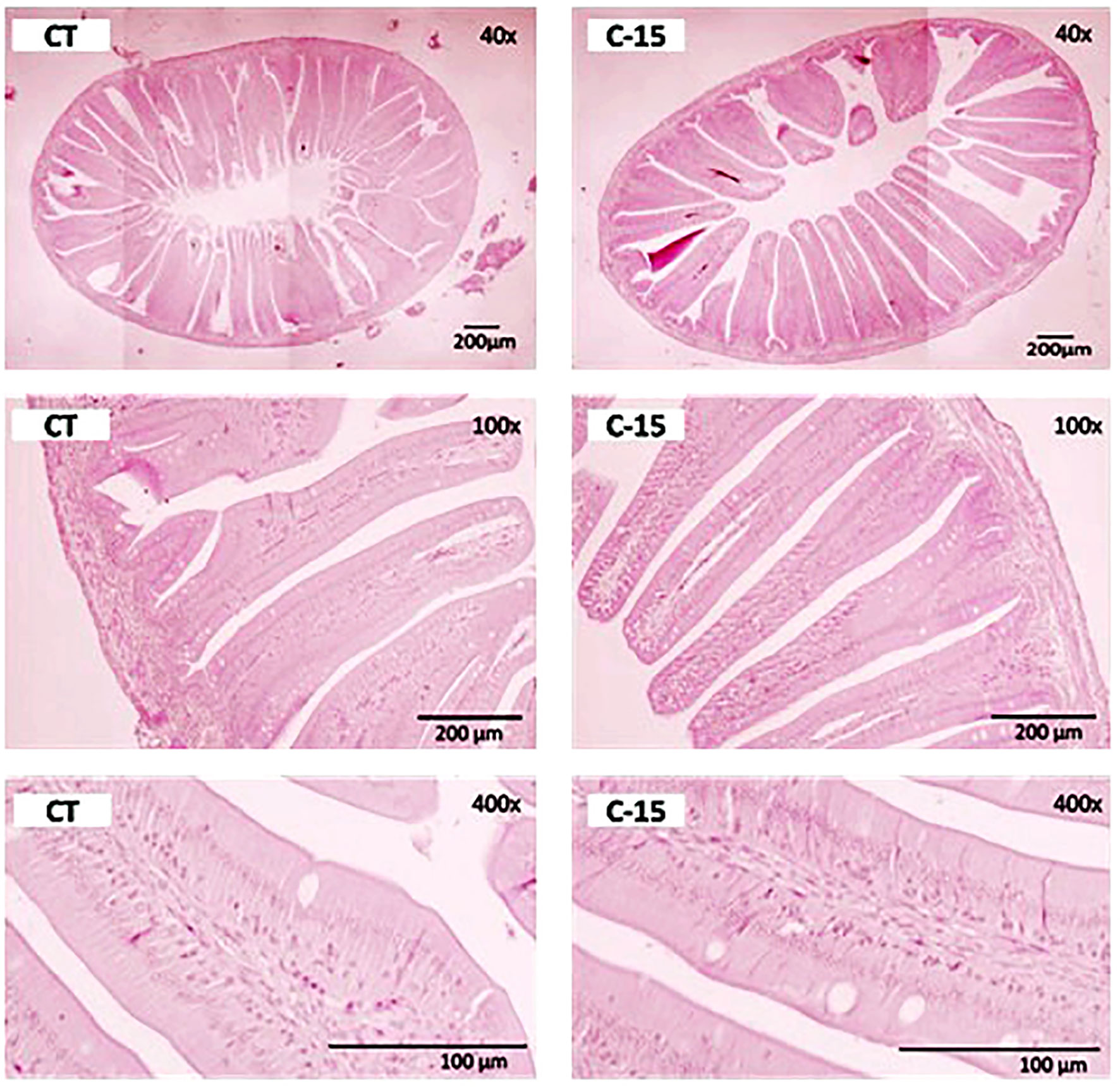
Figure 2 Transversal sections from the proximal intestine of C. labrosus juveniles fed control (CT) or experimental (C-15) diets during 90 days. H&E stain, scale bar 200 μm, and 100 μm. Dietary codes: CT, control diet; C-15, 15% C. fusca supplemented-diet.
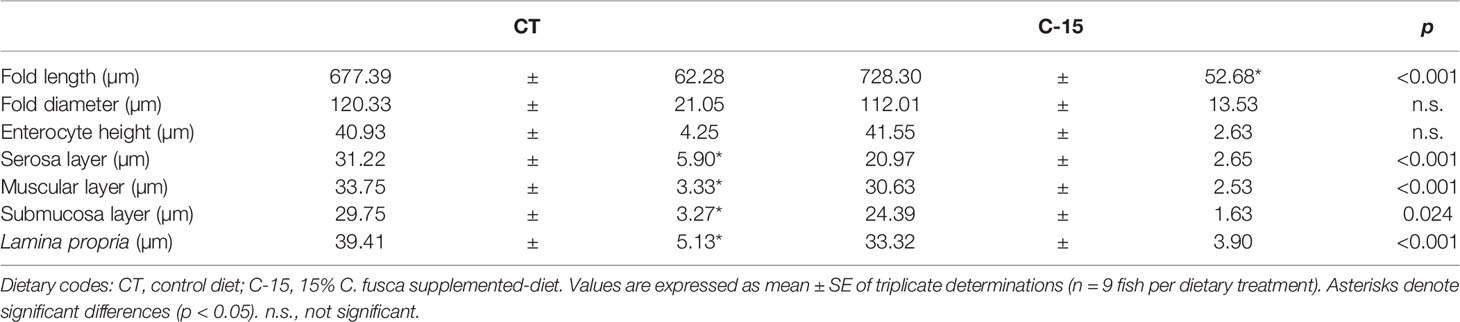
Table 7 Quantification of the histological parameters assessed in the intestine of juvenile C. labrosus fed control (CT) and C-15 diets during 90 days.
TEM and SEM observations confirmed a well-defined and organized intestinal brush border membrane in both dietary groups (Figure 3). Morphometric analysis of TEM and SEM images revealed that enterocytes from proximal intestine of fish fed with C-15 diet increased significantly microvilli length, apical area, as well as total absorption surface compared to the CT group (Table 8).
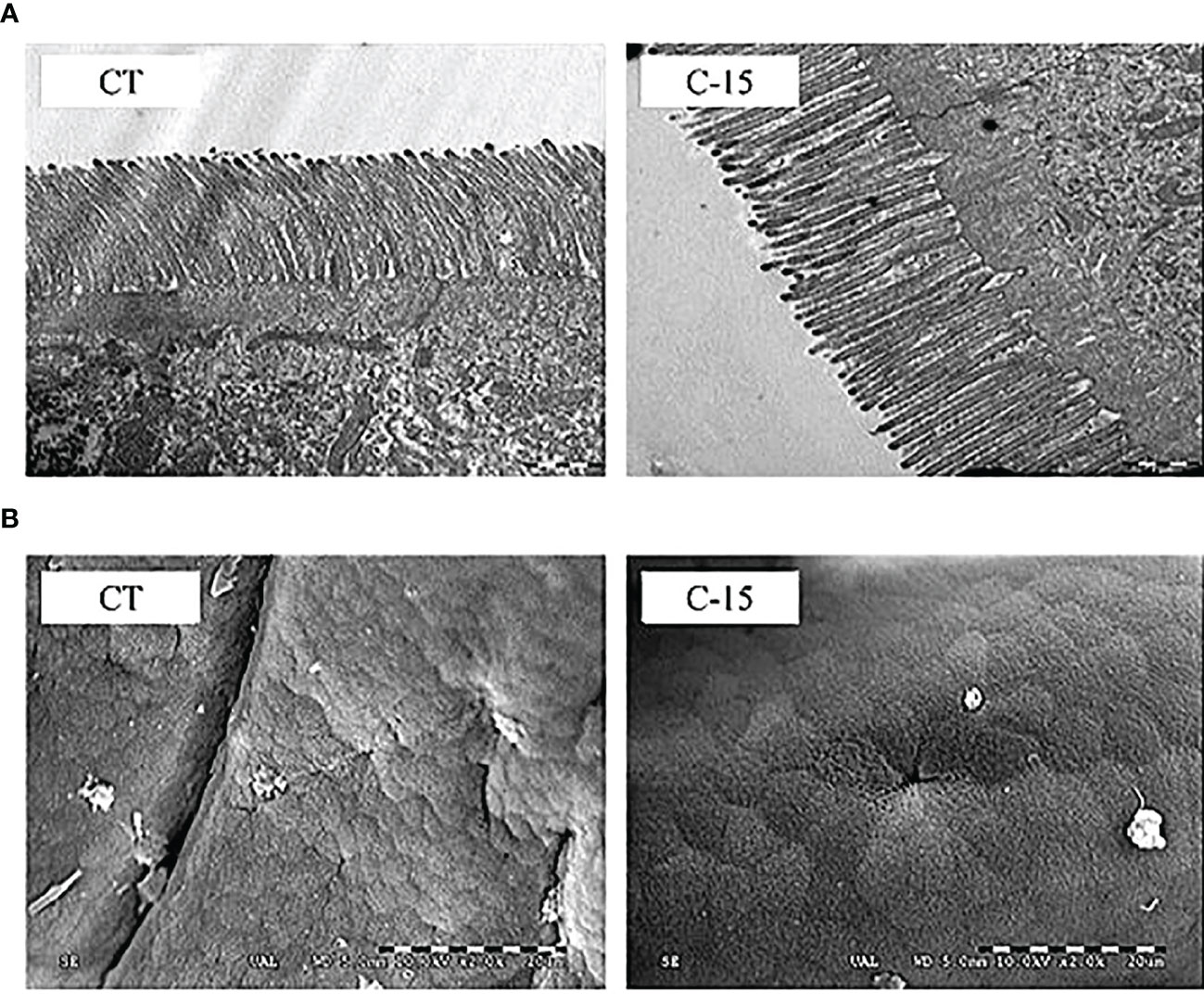
Figure 3 Comparative TEM (A) and SEM (B) micrographs from the anterior intestine of C. labrosus juveniles at the end of the feeding trial. Dietary codes: CT, control diet; C-15: 15% C. fusca supplemented-diet. (TEM bar: 1 mm, SEM bar: 20 mm).

Table 8 Microvillar morphology of juvenile C. labrosus fed control (CT) and C-15 diets during 90 days.
Plasma, Liver, and Muscle Metabolites and Enzyme Metabolic Activities
Plasma, liver, and muscle metabolites are shown in Table 9. The experimental diets did not cause significant plasma triglycerides and protein changes. A statistical increase in plasma glucose and a reduction in lactate values were observed in C-15 group compared to the CT group. In the liver, triglycerides levels decreased statistically in C-15 group respect to CT group, while amino acid levels enhanced significantly. No significant differences were detected among experimental groups in hepatic free glucose, glycogen, or lactate values. In muscle, no significant differences were detected among experimental groups for any metabolite assessed.
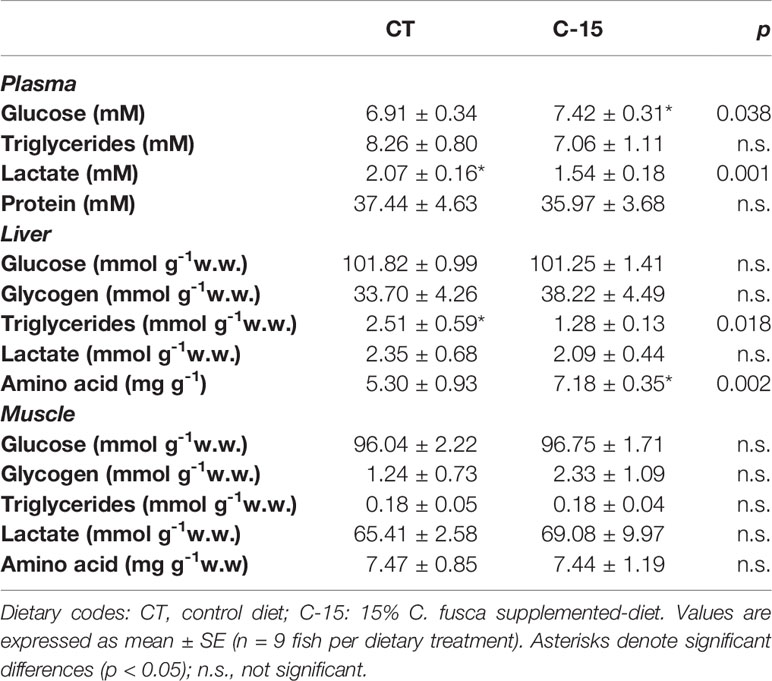
Table 9 Tissue metabolites content of juvenile C. labrosus fed control (CT) and C-15 diets during 90 days.
The effect of the dietary inclusion of C. fusca was also evaluated in several metabolic enzymes related to glycogenolysis, glycolysis, gluconeogenesis, and lipid metabolism in the liver (Figure 4). HOAD (lipid metabolism), HK (glycolysis), and GPact (glycogenolysis) enzymes displayed a significant increase in C-15 group after 90 days of feeding. In contrast, hepatic activity of the FBP (gluconeogenesis) enzyme decreased in this group. No effects were found between experimental groups on the hepatic activity of LDH (gluconeogenesis) and PK (glycolysis) enzymes.
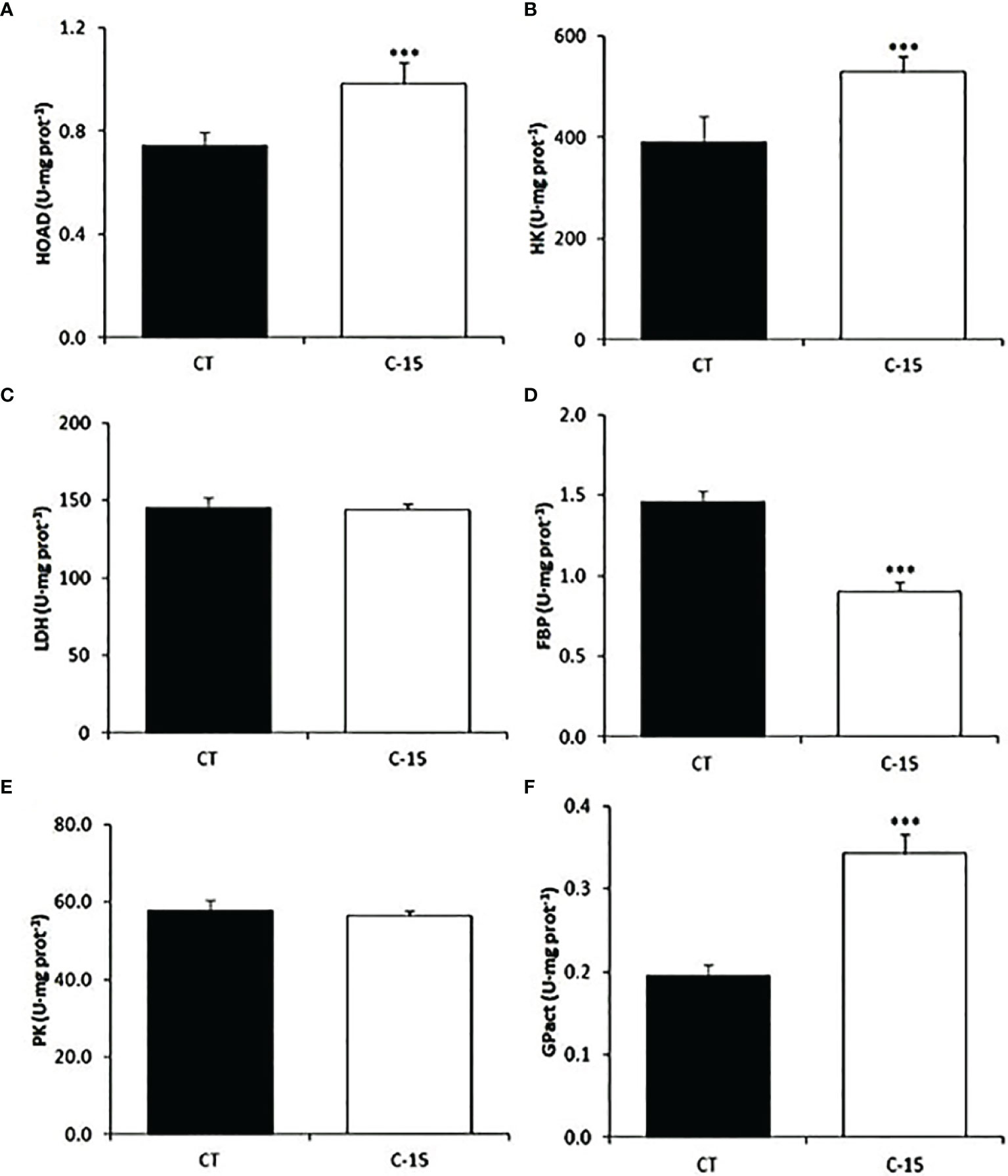
Figure 4 Specific activity (U mg protein−1 as mean ± SEM) of metabolic enzymes in the liver of juvenile (C) labrosus fed control (CT) and C-15 diets during 90 days. Asterisks denote significant differences at p < 0.001 (***). (A) HOAD, 3-hydroxyacyl-CoA dehydrogenase; (B) HK, hexokinase; (C) LDH, lactate dehydrogenase; (D) FBP, fructose 1,6-bisphosphatase; (E) PK, pyruvate kinase; (F) GPase, glycogen phosphorylase (active).
Discussion
Microalgae have been evaluated as functional dietary ingredients or for replacement of fishmeal in different aquaculture species, generally with positive effects on growth and fatty acid profile of fish fillet (Vizcaíno et al., 2014; Pakravan et al., 2017; Sarker et al., 2018; García-Márquez et al., 2020), thereby not only improving fish health but also increasing productivity and the value of the commercial product obtained. The result of this work revealed that the inclusion of 15% of C. fusca stimulated specific growth rate and body weight, which is in broad agreement with that previously published for other fish species fed with different microalgae (Vizcaíno et al., 2018; Gong et al., 2019; Sarker et al., 2020). Furthermore, the feed efficiency (PER and FCR) was not negatively affected by the inclusion of C. fusca. Different studies have reported both favourable and unfavourable effects of Chlorella species at varying supplementation levels in aquaculture feeds on growth performance and feed utilization in different fish species. For instance, Rahimnejad et al. (2017) reported that dietary inclusion of 10–15% of Chlorella vulgaris enhanced significantly growth performance and specific growth rate in Olive flounder (Paralichthys olivaceus). Similarly, Teuling et al. (2017) reported the overall performance in both Nile tilapia (Oreochromis niloticus) and African catfish (Clarus gariepinus) were similar or superior compared to fish fed the reference diet when C. vulgaris meal was included at 30% in the aquafeeds. In contrast, when Chlorella sp. meal was supplemented at 1.6% and 2% in diets for gibel carp (Carassius auratus gibelio) feed conversion ratio was significantly lower than the control group (Xu et al., 2014). To resume, the varied effects on fish performance reported at higher and lower dietary Chlorella inclusion levels could be attributed to the different inclusion levels, the specific microalgae strain and fish species, and/or the duration of the experiment. Even so, the positive effects observed in the present study strongly suggest its use as suitable ingredient in herbivorous/omnivorous marine species, at least at 15% of biomass. However, it has been reported that C. labrosus changes its digestive enzyme profile according to the size/age of specimens (Pujante et al., 2017). For this reason, the beneficial effects observed of dietary inclusion of C. fusca (i.e. 15%) could be confirmed using specimens with different stages of development.
Similar to that reported previously in feeding trials using aquafeed with inclusion of other microalgae, no differences in proximate muscle composition attributable to the dietary inclusion of C. fusca were observed (Vizcaíno et al., 2014; Vizcaíno et al., 2018; Sales et al., 2021). However, disparate effects of microalgae inclusion on muscle proximate composition can be described. Thus, some authors described that microalgal biomass inclusion may increase protein and decrease lipid content in different farmed fish species owing to the presence of several bioactive compounds in microalgae biomass that may activate fish metabolism, and particularly the use of lipid as energy source (Roohani et al., 2019; Galafat et al., 2020).
Regarding fatty acids profile, the inclusion of C. fusca induced selective retention of DHA in the liver, and ARA, EPA, and DHA in the muscle. This fact has been previously pointed out in other studies, which evidenced a relationship between microalgae inclusion and higher efficiency of mobilizing lipids from liver (He et al., 2018; Vizcaíno et al., 2018; García-Márquez et al., 2020). Besides this, the relative increase of structural fatty acids in muscle (ARA, EPA, and DHA) is also reflected in the significant fish lipid quality index (FLQ), n3/n6 ratio, and index of atherogenicity (IA) enhancement in fish fed on C-15 diet, which could be beneficial from a human nutrition standpoint (Abdelhamid et al., 2018; Román et al., 2019).
According to Furné et al. (2008), adequate feed efficiency is highly related to the physiological capacity of fish to digest and transform the ingested nutrients, and therefore to the presence of an appropriate set of digestive enzymes. Indeed, any change in their activity levels could reveal a significant impact on fish growth and proper nutrient utilization. Previous works evidenced that dietary inclusion of different microalgal biomasses may exert substantial changes in the activity of several enzymes involved in the digestive and absorptive processes at intestinal level (Vizcaíno et al., 2014; Vizcaíno et al., 2018; Gong et al., 2019; Galafat et al., 2020; Galafat et al., 2022). In agreement with these studies, the results obtained in this piece of research evidenced noticeable differences in enzyme activity levels between fish fed C-15 diet and those fed on the control diet. Dietary inclusion of 15% C. fusca did not cause any adverse effects on enzymatic activities from the pancreatic secretion, as it can be observed in the zymogram, which evidenced that microalgae-fed fish showed the same pattern of intestinal proteases that control (CT) specimens. However, protease activity was significantly higher in fish fed C-15 diet than the CT group. Similar results were shown by Akbary and Raeisi (2020), who found an increase of total alkaline protease activity in grey mullet (M. cephalus) specimens fed on diets supplemented with up to 15% C. vulgaris. This increase in proteolytic activity seems to be related to an improvement in digestive functionality that could lead to better absorption and utilization of nutrients (Engrola et al., 2007), in line with the higher growth performance observed.
On the other hand, there are previous works focused on evaluating the changes in the digestive enzyme profile in fish that modify their feeding habits during the ontogeny such as mullets, which showed an omnivorous feeding habits during their early stages with a trend to become herbivorous with age (Wassef et al., 2001). In this regard, Pujante et al. (2017), found a noticeable increase in the protease activity levels in C. labrosus as consequence of the changes in the fish-feeding habits. These modifications might reflect a possible compensation mechanism (i.e. by increasing enzyme secretion) that ensure efficient digestive processes, as it has been described in other herbivorous fish species such as carp or tilapia (Uscanga-Martínez et al., 2011; Hernández-Sámano et al., 2017). Regarding brush border enzymes, a significant increase in leucine aminopeptidase and alkaline phosphatase activity levels was observed in fish fed the diets supplemented with 15% C. fusca. Both enzymes play a key role in the final stages of protein digestion, hydrolysing the oligopeptides released by pancreatic enzymes into free amino acids, dipeptides or tripeptides (Gisbert et al., 2018) and allowing absorption or transport of amino acids through the enterocytes (Cahu and Zambonino Infante, 2001). These enzymes, especially alkaline phosphatase, can be used as indicator of intestinal integrity and nutrient absorption, so increased activity levels seem to be related to an improvement in digestive process efficiency and absorptive capability of the intestinal mucosa (Vizcaíno et al., 2014).
In addition, it was observed that these positive changes in enzymatic intestinal activities concur with the histological and ultrastructural measurements carried out on the intestinal mucosa. In herbivorous fish, intestines are generally larger than in carnivorous ones, which is thought to allow for additional processing of relatively difficult-to-digest items (Wilson and Castro, 2010). The structural condition of the intestine is considered a reliable nutritional and physiological biomarker since it reflects any physiological disorder caused by unbalanced diets or inadequate feeding conditions (Gisbert et al., 2008). Similar to other previous studies (Vizcaíno et al., 2014; Galafat et al., 2022), histological observations indicated that microalgae inclusion did not cause adverse effects on gut morphology. Besides the increase in mucosal fold length observed in fish fed on C. fusca-supplemented diet, the histological analysis revealed the absence of inflammatory processes in the lamina propria or accumulation of lipid vacuoles inside the enterocytes that could evidence intestinal enteritis and/or steatosis (Uran et al., 2008). Indeed, the serosa, muscular, and submucosa layers, as well as the lamina propria, were significantly thinner in fish fed with C. fusca than those fed with the microalgae-free diet. Those results pointed out that dietary microalgae inclusion might be useful to prevent intestinal pro-inflammatory processes, but further research is required to elucidate if longer feeding period or if higher microalgae dietary inclusion level can produce morphological alteration on the intestinal morphology in this species.
Electron microscopy analysis did not evidence structural alterations or signs of damage on intestinal brush border attributable to dietary inclusion of C. fusca biomass. It was observed that all the specimens showed a particular morphology and disposition of their intestinal folds, which was different from that observed in other fish species such as the carnivorous seabream (S. aurata), seabass (D. labrax), or Senegalese sole (Solea senegalensis) (Vizcaíno et al., 2014; Vizcaíno et al., 2018). C. labrosus folds were characterized by the presence of numerous laminar ridges with a flattened apex and a random distribution similar to that observed in other Mugilidae species such as gold grey mullet (Liza aurata) (Ferrando et al., 2006) (see Supplementary Material 1). Regarding the morphometric analysis of TEM and SEM images, microvilli length, enterocyte apical area, and enterocyte absorption surface significantly increased in fish fed C. fusca-supplemented diet. These results are consistent with those observed in previous studies performed in S. aurata (Vizcaíno et al., 2014; Galafat et al., 2020; Galafat et al., 2022), so it seems that the use of this microalga in aquafeeds enhanced absorption capacity, reinforcing the idea of the function of the intestinal mucosa as a physical barrier.
The response of the metabolism concerning the experimental diets was also characterized. The C-15 diet significantly mobilized carbohydrate metabolism by increasing plasma glucose and concomitantly decreasing plasma lactate. Thus, we suggest that C. fusca inclusion in the aquafeed may promote depletion of plasma lactate, which is previously originated in white muscle due to anaerobic metabolism, for later be partially incorporated into the liver according to what Perera et al. (2020) observed. However, the conversion of lactate to pyruvate by LDH in the liver (i.e. Cori cycle), and its further conversion to glycogen, are not supported neither by LDH activity nor glucose-glycogen trends in the present study, which remained unchanged regardless of the experimental diet. In this regard, high plasma glucose levels might indicate a proficient digestive breakdown of carbohydrates from the diet in this species and a potential source for glycogen reservoirs (Omlin et al., 2014). It has also been found that C. fusca inclusion increased hepatic HK activity, whereas PK activity was unaltered. HK is the first step in glycolysis, phosphorylating glucose to be used by cells, while PK catalyzes the last step of glycolysis, producing pyruvate and ATP. Even though hepatic glycogen content was not significantly higher, its increase supports that the C-15 diet enhanced hepatic glucose uptake capacity to be stored as glycogen instead of oxidized for energy, as previously observed in gilthead seabream (Perera et al., 2020).
The above-mentioned metabolic stage agrees with the observed reduction of hepatic gluconeogenic enzyme (FBP) activity in C-15 group, further supporting subsequent non-significant increases in muscle and hepatic glycogen within this same group. Although the metabolic significance of higher glycogen phosphorylase activity remains unknown in fish, it could be related to the turnover of liver glycogen for glucose in other metabolic pathways, such as synthesizing specific fatty acids as occurs in humans (Adeva-Andany et al., 2016). In this regard, the significant decrease in liver triglycerides values (the fundamental unit of lipid metabolism) in the C-15 group supports this hypothesis.
Increased glucose uptake by the liver, or production of glucose from glycogen, is known to have a stimulatory effect on the lipogenic enzymes, glucose-6-phosphate dehydrogenase (G6PDH), and malate dehydrogenase (MDH) (Perera et al., 2020), which provide NADPH for the biosynthesis of fatty acids, and subsequently lead to higher lipid storage or export from the liver (Alvarez et al., 2000; Laliotis et al., 2010). However, we cannot rule out the possibility that some of the changes in specific fatty acids depositions are due to the existence of other nutrients in this microalga. The higher muscular HOAD activity (the third step of beta-oxidation) observed in specimens of C-15 group is remarkable. In higher vertebrates, lipid availability increases mitochondrial fatty acid oxidative capacity in muscle (Turner et al., 2007). Thus, we suggest that HOAD activity in the present study may be a compensatory mechanism to control excessive fat accumulation in fish muscles supplemented with microalgae or might be involved in lipid muscle deposition remodeling. Additionally, we also observed a significant increase in hepatic α-amino acids. The liver is an important organ for protein synthesis, degradation, and detoxification as well as amino acid metabolism. Overall hepatic amino acids are involved in various cellular metabolisms, the synthesis of lipids and nucleotides as well as detoxification reactions (Lee and Kim, 2019).
In conclusion, the results obtained in this work are in accordance with previous studies using microalgae-based aquafeeds and confirmed that C. fusca biomass is suitable for using as dietary ingredient in C. labrosus juveniles. The effects observed on digestive and metabolic enzyme activities, together with adequate metabolic response and gut morphology, as well as a significant increase in intestinal mucosa’s digestive and absorptive capacity, could explain the positive effects on growth performance obtained in fish fed the microalgae. However, given the scarce information related to the optimization of specific aquafeeds for this species and the changes in intestinal digestive capacity associated with developmental stages (Pujante et al., 2017), further studies to determine optimal inclusion levels of C. fusca in a long-term feeding trial and/or different developmental stages in this fish species are needed.
Data Availability Statement
The raw data supporting the conclusions of this article will be made available by the authors, without undue reservation.
Ethics Statement
The animal study was reviewed and approved by Ethical Committee from the Autonomous Andalusian Government (Junta de Andalucía reference number 11/07/2020/082).
Author Contributions
Conceptualization: JG-M, SA, and RA-D; Methodology: JG-M, JM-S, FA, and GA; Validation: JG-M, JM-S, FA, JM, SA, and RA-D; Formal analysis: JG-M, AG, and AV; Resources: JM-S, FA, JM, and FF; Data curation: JG-M, AG, AV, AB, and JM-S; Visualization: JG-M, AG, AV, AB, and JM-S; Supervision: FA, JM, SA, and RA-D; Project administration: SA and RA-D; Funding acquisition: FA, JM, GA, and FF; Writing-original draft: JG-M; Writing-review and editing: all authors. All authors have read and agreed to the published version of the manuscript.
Funding
This research was funded by the Project ‘Design and evaluation of probiotics and feed in uncoupled aquaponic system of fishes, plants and microalgae’ (AGL‐2017‐83260R) granted by Agencia Estatal de Investigación (MINECO, Spanish Government), by AquaTech4Feed (grant # PCI2020-112204) granted by MCIN/AEI/10.13039/501100011033, the EU “NextGenerationEU”/PRTR within the ERA-NET BioBlue COFUND, the project PROBIVAC (Plan Nacional de Investigación, AGL2010-17789), and co-financed by the spin-off from the University of Almería LifeBioencapsulation S.L. and the European Union under the 2014-2020 ERDF Operational Programme and by the Department of Economic Transformation, Industry, Knowledge, and Universities of the Regional Government of Andalusia (Project reference: FEDER-UCA18-107182). This research was performed in collaboration with the EU-H2020 SABANA project (grant # 727874). AB is supported by a Postdoctoral Research Fellow “Margarita Salas” (University of Cádiz).
Conflict of Interest
The authors declare that the research was conducted in the absence of any commercial or financial relationships that could be construed as a potential conflict of interest.
The handling editor BC declared a past co-authorship with the authors AB and JM.
Publisher’s Note
All claims expressed in this article are solely those of the authors and do not necessarily represent those of their affiliated organizations, or those of the publisher, the editors and the reviewers. Any product that may be evaluated in this article, or claim that may be made by its manufacturer, is not guaranteed or endorsed by the publisher.
Acknowledgments
The authors want to thank David López Paniagua and Fabian López Palica for the use of the facilities of the Centro de Experimentación de Ecología y Microbiología de Sistemas Acuáticos Controlados Grice-Hutchinson (CEMSAC), University of Málaga, Spain, to carry out the experiment. Besides, we acknowledge the support of the University of Almería (Experimental feeds Service, https://www.ual.es/universidad/serviciosgenerales/stecnicos/perifericos-convenio/piensos-experimentales) for aquafeed elaboration.
Supplementary Material
The Supplementary Material for this article can be found online at: https://www.frontiersin.org/articles/10.3389/fmars.2022.902203/full#supplementary-material
References
Abdelhamid A. S., Brown T. J., Brainard J. S., Biswas P., Thorpe G. C., Moore H. J., et al. (2018). Omega-3 Fatty Acids for the Primary and Secondary Prevention of Cardiovascular Disease. Cochrane Database Syst. Rev. 11, CD003177. doi: 10.1002/14651858.cd003177.pub4
Abdulrahman N., Hoshyar Abid S., Aziz Khidir A., Bakhtyar Omer B., Bahman Hama Rasheed D., Hıwa Baha Alddin L. (2018). Effect of Adding Microalgae Chlorella Sp. On Some Biological Parameters and Proximate Analysis of Common Carp Cyprinus Carpio L. Iran. J. Vet. Med. 12 (3), 199–206. doi: 10.22059/IJVM.2018.244747.1004856
Adeva-Andany M. M., Pérez-Felpete N., Fernández-Fernández C., Donapetry-García C., Pazos-García C. (2016). Liver Glucose Metabolism in Humans. Biosci. Rep. 36 (6), 416. doi: 10.1042/BSR20160385/56473
Ahmad M. T., Shariff M., Md. Yusoff F., Goh Y. M., Banerjee S. (2020). Applications of Microalga Chlorella Vulgaris in Aquaculture. Rev. Aquac. 12 (1), 328–346. doi: 10.1111/RAQ.12320
Akbary P., Aminikhoei Z. (2019). Impact of Dietary Supplementation of Chlorella Vulgaris (Beijerinck 1890) on the Growth, Antioxidant Defense and Immune Status of the Grey Mullet, Mugil Cephalus (Linnaeus 1758). Iran. J. Aquat. Anim. Heal. 5 (1), 57–70. doi: 10.29252/IJAAH.5.1.57
Akbary P., Raeisi E. (2020). Optimum Dietary Level of Chlorella Vulgaris Powder as a Feed Additive for Some Blood Parameters and Digestive Enzymatic Activities of Grey Mullet, Mugil Cephalus. Iran. J. Fish. Sci. 19 (3), 1130–1139. doi: 10.22092/IJFS.2019.119600.0
Alarcón F. J., Díaz M., Moyano F. J., Abellán E. (1998). Characterization and Functional Properties of Digestive Proteases in Two Sparids; Gilthead Seabream (Sparus Aurata) and Common Dentex (Dentex Dentex). Fish Physiol. Biochem. 19 (3), 257–267. doi: 10.1023/A:1007717708491
Alvarez M. J., Díez A., López-Bote C., Gallego M., Bautista J. M. (2000). Short-Term Modulation of Lipogenesis by Macronutrients in Rainbow Trout (Oncorhynchus Mykiss) Hepatocytes. Br. J. Nutr. 84 (5), 619–628. doi: 10.1017/S0007114500001951
AOAC. (2000). Official Methods of Analysis. 17th Edition (Washington: Association of Official Analytical Chemists).
Arakawa K., Sagai M. (1986). Species Differences in Lipid Peroxide Levels in Lung Tissue and Investigation of Their Determining Factors. Lipids 21, 769–775. doi: 10.1007/BF02535410
Beal C. M., Gerber L. N., Thongrod S., Phromkunthong W., Kiron V., Granados J., et al. (2018). Marine Microalgae Commercial Production Improves Sustainability of Global Fisheries and Aquaculture. Sci. Rep. 8 (1), 15064. doi: 10.1038/s41598-018-33504-w
Becker E. W. (2013). Microalgae for Aquaculture: Nutritional Aspects. Handbook of Microalgal Culture: Applied Phycology and Biotechnology, 2nd ed. UK: John Wiley & Sons, Ltd. 671–691. doi: 10.1002/9781118567166.CH36
Cahu C., Zambonino Infante J. (2001). Substitution of Live Food by Formulated Diets in Marine Fish Larvae. Aquaculture 200 (1–2), 161–180. doi: 10.1016/S0044-8486(01)00699-8
Camacho F., Macedo A., Malcata F. (2019). Potential Industrial Applications and Commercialization of Microalgae in the Functional Food and Feed Industries: A Short Review. Mar. Drugs 17 (6), 312. doi: 10.3390/MD17060312
de las Heras V., Martos-Sitcha J. A., Yúfera M., Mancera J. M., Martínez-Rodríguez G. (2015). Influence of Stocking Density on Growth, Metabolism and Stress of Thick- Lipped Grey Mullet (Chelon Labrosus) Juveniles. Aquaculture 448, 29–37. doi: 10.1016/J.AQUACULTURE.2015.05.033
DelMar E. G., Largman C., Brodrick J. W., Geokas M. C. (1979). A Sensitive New Substrate for Chymotrypsin. Anal. Biochem. 99 (2), 316–320. doi: 10.1016/S0003-2697(79)80013-5
Domozych D. S., Ciancia M., Fangel J. U., Mikkelsen M. D., Ulvskov P., Willats W. G. T. (2012). The Cell Walls of Green Algae: A Journey Through Evolution and Diversity. Front. Plant Sci. 3. doi: 10.3389/FPLS.2012.00082/BIBTEX
Engrola S., Conceição L. E. C., Dias L., Pereira R., Ribeiro L., Dinis M. T. (2007). Improving Weaning Strategies for Senegalese Sole: Effects of Body Weight and Digestive Capacity. Aquac. Res. 38 (7), 696–707. doi: 10.1111/J.1365-2109.2007.01701.X
Erlanger B. F., Kokowsky N., Cohen W. (1961). The Preparation and Properties of Two New Chromogenic Substrates of Trypsin. Arch. Biochem. Biophys. 95 (2), 271–278. doi: 10.1016/0003-9861(61)90145-X
Ferrando S., Maisano M., Parrino V., Ferrando T., Girosi L., Tagliafierro G. (2006). Gut Morphology and Metallothionein Immunoreactivity in Liza Aurata From Different Heavy Metal Polluted Environments. Ital. J. Zool. 73 (1), 7–14. doi: 10.1080/11250000500502228
Folch J., Lees M., Sloane Stanley G. H. (1957). A Simple Method for the Isolation and Purification of Total Lipides From Animal Tissues. J. Biol. Chem. 226 (1), 497–509. doi: 10.3989/scimar.2005.69n187
Furné M., García-Gallego M., Hidalgo M. C., Morales A. E., Domezain A., Domezain J., et al. (2008). Effect of Starvation and Refeeding on Digestive Enzyme Activities in Sturgeon (Acipenser Naccarii) and Trout (Oncorhynchus Mykiss). Comp. Biochem. Physiol. -Part A Mol. Integr. Physiol. 149 (4), 420–425. doi: 10.1016/J.CBPA.2008.02.002
Galafat A., Vizcaíno A. J., Sáez M. I., Martínez T. F., Arizcun M., Chaves-Pozo E., et al. (2022). Assessment of Dietary Inclusion of Crude or Hydrolysed Arthrospira Platensis Biomass in Starter Diets for Gilthead Seabream (Sparus Aurata). Aquaculture 548, 737680. doi: 10.1016/J.AQUACULTURE.2021.737680
Galafat A., Vizcaíno A. J., Sáez M. I., Martínez T. F., Jérez-Cepa I., Mancera J. M., et al. (2020). Evaluation of Arthrospira Sp. Enzyme Hydrolysate as Dietary Additive in Gilthead Seabream (Sparus Aurata) Juveniles. J. Appl. Phycol. 32 (5), 3089–3100. doi: 10.1007/S10811-020-02141-0/TABLES/6
García‐Márquez J., Galafat A., Alarcón F. J., Figueroa F. L., Martínez‐Manzanares E., Arijo S., et al. (2021). Cultivated and Wild Juvenile Thick-Lipped Grey Mullet, Chelon Labrosus: A Comparison From a Nutritional Point of View. Animals 11 (7), 2112. doi: 10.3390/ANI11072112
García-Márquez J., Rico R. M., del Pilar Sánchez-Saavedra M., Gómez-Pinchetti J. L., Acién F. G., Figueroa F. L., et al. (2020). A Short Pulse of Dietary Algae Boosts Immune Response and Modulates Fatty Acid Composition in Juvenile Oreochromis Niloticus. Aquac. Res. 51, 4397–4409. doi: 10.1111/are.14781
Gisbert E., Fournier V., Solovyev M., Skalli A., Andree K. B. (2018). Diets Containing Shrimp Protein Hydrolysates Provided Protection to European Sea Bass (Dicentrarchus Labrax) Affected by a Vibrio Pelagius Natural Infection Outbreak. Aquaculture 495, 136–143. doi: 10.1016/J.AQUACULTURE.2018.04.051
Gisbert E., Ortiz-Delgado J. B., Sarasquete C. (2008). Nutritional Cellular Biomarkers in Early Life Stages of Fish. Histol. Histopathol. 23 (12), 1525–1539. doi: 10.14670/HH-23.1525
Gong Y., Bandara T., Huntley M., Johnson Z. I., Dias J., Dahle D., et al. (2019). Microalgae Scenedesmus Sp. As a Potential Ingredient in Low Fishmeal Diets for Atlantic Salmon (Salmo Salar L.). Aquaculture 501, 455–464. doi: 10.1016/j.aquaculture.2018.11.049
Hasan M. R., Chakrabarti R. (2009). Use of Algae and Aquatic Macrophytes as Feed in Small-Scale Aquaculture. A Review. Fisheries and Aquaculture Technical Paper 531 (Rome: FAO), 123.
Hasanein S. S., Saleh N. E., El-Sayed H. S., Helal A. M. (2018). The Effect of Dietary Supplementation of Spirulina Platensis and Chlorella Vulgaris Algae on the Growth and Disease Resistance of the Sea Bass (Dicentrarchus Labrax). Egypt. J. Aquat. Biol. Fish. 22 (4), 249–262. doi: 10.21608/ejabf.2018.17160
He Y., Lin G., Rao X., Chen L., Jian H., Wang M., et al. (2018). Microalga Isochrysis Galbana in Feed for Trachinotus Ovatus: Effect on Growth Performance and Fatty Acid Composition of Fish Fillet and Liver. Aquac. Int. 26 (5), 1261–1280. doi: 10.1007/S10499-018-0282-Y
Hernández-Sámano A. C., Guzmán-García X., García-Barrientos R., Guerrero-Legarreta I. (2017). Actividad Enzimática De Proteasas De Cyprinus Carpio (Cypriniformes: Cyprinidae) Extraídas De Una Laguna Contaminada En México. Rev. Biol. Trop. 65 (2), 589–597. doi: 10.15517/RBT.V65I2.24486
Jannathulla R., Rajaram V., Kalanjiam R., Ambasankar K., Muralidhar M., Dayal J. S. (2019). Fishmeal Availability in the Scenarios of Climate Change: Inevitability of Fishmeal Replacement in Aquafeeds and Approaches for the Utilization of Plant Protein Sources. Aquac. Res. 50 (12), 3493–3506. doi: 10.1111/ARE.14324
Keppler D., Decker K. (1974). “Glycogen Determination With Amyloglucosidase,” in Methods of Enzymatic Analysis. Ed. Bergmeyer H. U. (New York: Academic Press), 1127–1131.
Laemmli U. K. (1970). Cleavage of Structural Proteins During the Assembly of the Head of Bacteriophage T4. Nature 227 (5259), 680–685. doi: 10.1038/227680A0
Laliotis G. P., Bizelis I., Rogdakis E. (2010). Comparative Approach of the De Novo Fatty Acid Synthesis (Lipogenesis) Between Ruminant and Non Ruminant Mammalian Species: From Biochemical Level to the Main Regulatory Lipogenic Genes. Curr. Genomics 11 (3), 168–183. doi: 10.2174/138920210791110960
Lavecchia R., Maffei G., Zuorro A. (2016). Evaluation of Dissolved Organic Carbon (DOC) as a Measure of Cell Wall Degradation During Enzymatic Treatment of Microalgae. Chem. Eng. Trans. 49, 373–378. doi: 10.3303/CET1649063
Lee D.-Y., Kim E.-H. (2019). Therapeutic Effects of Amino Acids in Liver Diseases: Current Studies and Future Perspectives. J. Cancer Prev. 24 (2), 72. doi: 10.15430/JCP.2019.24.2.72
Liu J., Hu Q. (2013). Chlorella: Industrial Production of Cell Mass and Chemicals. Handbook of Microalgal Culture: Applied Phycology and Biotechnology, 2nd ed. UK: John Wiley & Sons, Ltd. 327–338. doi: 10.1002/9781118567166.CH16
Lupatsch I., Blake C. (2013). Algae Alternative: Chlorella Studied as Protein Source in Tilapia Feeds. Global Aquacul. Advocate 16, 78–79.
Minjarez-Osorio C., Castillo-Alvarado S., Gatlin D. M., González-Félix M. L., Perez-Velazquez M., Rossi W. (2016). Plant Protein Sources in the Diets of the Sciaenids Red Drum (Sciaenops Ocellatus) and Shortfin Corvina (Cynoscion Parvipinnis): A Comparative Study. Aquaculture 453, 122–129. doi: 10.1016/J.AQUACULTURE.2015.11.042
Moore S. (1968). Amino Acid Analysis: Aqueous Dimethyl Sulfoxide as Solvent for the Ninhydrin Reaction. J. Biol. Chem. 243 (23), 6281–6283. doi: 10.1016/S0021-9258(18)94488-1
Oliva-Teles A., Couto A., Enes P., Peres H. (2020). Dietary Protein Requirements of Fish – A Meta-Analysis. Rev. Aquac. 12 (3), 1445–1477. doi: 10.1111/RAQ.12391
Omlin T., Langevin K., Weber J. M. (2014). Exogenous Lactate Supply Affects Lactate Kinetics of Rainbow Trout, Not Swimming Performance. Am. J. Physiol. - Regul. Integr. Comp. Physiol. 307 (8), R1018–R1024. doi: 10.1152/AJPREGU.00200.2014
Pakravan S., Akbarzadeh A., Sajjadi M. M., Hajimoradloo A., Noori F. (2017). Partial and Total Replacement of Fish Meal by Marine Microalga Spirulina Platensis in the Diet of Pacific White Shrimp Litopenaeus Vannamei: Growth, Digestive Enzyme Activities, Fatty Acid Composition and Responses to Ammonia and Hypoxia Stress. Aquac. Res. 48 (11), 5576–5586. doi: 10.1111/are.13379
Perera E., Sánchez-Ruiz D., Sáez M. I., Galafat A., Barany A., Fernández-Castro M., et al. (2020). Low Dietary Inclusion of Nutraceuticals From Microalgae Improves Feed Efficiency and Modifies Intermediary Metabolisms in Gilthead Sea Bream (Sparus Aurata). Sci. Rep. 10 (1), 1–14. doi: 10.1038/s41598-020-75693-3
Pfleiderer G. (1970). “Particle-Bound Aminopeptidase From Pig Kidney,” in Methods in Enzymology, 19th edn. Eds. Penman G. E., Lowland L. (London: Academic Press), 514–521. doi: 10.1016/0076-6879(70)19038-0
Pratoomyot J., Srivilas P., Noiraksar T. (2005). Fatty Acids Composition of 10 Microalgal Species. Songklanakarin J. Sci. Technol. 27, 1179–1187.
Pujante I. M., Díaz-López M., Mancera J. M., Moyano F. J. (2017). Characterization of Digestive Enzymes Protease and Alpha-Amylase Activities in the Thick-Lipped Grey Mullet (Chelon Labrosus, Risso 1827). Aquac. Res. 48 (2), 367–376. doi: 10.1111/ARE.13038
Pujante I. M., Martos-Sitcha J. A., Moyano F. J., Ruiz-Jarabo I., Martínez-Rodríguez G., Mancera J. M. (2015). Starving/re-Feeding Processes Induce Metabolic Modifications in Thick-Lipped Grey Mullet (Chelon Labrosus, Risso 1827). Comp. Biochem. Physiol. Part - B Biochem. Mol. Biol. 180, 57–67. doi: 10.1016/J.CBPB.2014.10.005
Pujante I. M., Moyano F. J., Martos-Sitcha J. A., Mancera J. M., Martínez-Rodríguez G. (2018). Effect of Different Salinities on Gene Expression and Activity of Digestive Enzymes in the Thick-Lipped Grey Mullet (Chelon Labrosus). Fish Physiol. Biochem. 44, 349–373. doi: 10.1007/s10695-017-0440-6
Rahimnejad S., Park H.-G., Lee S.-M., Choi J. (2017). Effects of Dietary Inclusion of Chlorella Vulgaris on Growth, Blood Biochemical Parameters and Antioxidant Enzymes Activity in Olive Flounder, Paralichthys Olivaceus. Fish Shellfish Immunol. 53, 103–112. doi: 10.1016/j.fsi.2016.04.067
Rodríguez-Ruiz J., Belarbi E. H., Sanchez J. L. G., Alonso D. L. (1998). Rapid Simultaneous Lipid Extraction and Transesterification for Fatty Acid Analyses. Biotechnol. Tech. 12 (9), 689–691. doi: 10.1023/A:1008812904017
Román G. C., Jackson R. E., Gadhia R., Román A. N., Reis J. (2019). Mediterranean Diet: The Role of Long-Chain ω-3 Fatty Acids in Fish; Polyphenols in Fruits, Vegetables, Cereals, Coffee, Tea, Cacao and Wine; Probiotics and Vitamins in Prevention of Stroke, Age-Related Cognitive Decline, and Alzheimer Disease. Rev. Neurol. 175, 724–741. doi: 10.1016/j.neurol.2019.08.005
Roohani A. M., Abedian Kenari A., Fallahi Kapoorchali M., Borani M. S., Zoriezahra S. J., Smiley A. H., et al. (2019). Effect of Spirulina Spirulina Platensis as a Complementary Ingredient to Reduce Dietary Fish Meal on the Growth Performance, Whole-Body Composition, Fatty Acid and Amino Acid Profiles, and Pigmentation of Caspian Brown Trout (Salmo Trutta Caspius) Juveniles. Aquac. Nutr. 25 (3), 633–645. doi: 10.1111/ANU.12885
Sørensen M., Berge G. M., Reitan K. I., Ruyter B. (2016). Microalga Phaeodactylum Tricornutum in Feed for Atlantic Salmon (Salmo Salar) —Effect on Nutrient Digestibility, Growth and Utilization of Feed. Aquaculture 460, 116–123. doi: 10.1016/J.AQUACULTURE.2016.04.010
Sales R., Galafat A., Vizcaíno A. J., Sáez M. I., Martínez T. F., Cerón-García M. C., et al. (2021). Effects of Dietary Use of Two Lipid Extracts From the Microalga Nannochloropsis Gaditana (Lubián 1982) Alone and in Combination on Growth and Muscle Composition in Juvenile Gilthead Seabream, Sparus Aurata. Algal Res. 53, 102162. doi: 10.1016/J.ALGAL.2020.102162
Sarker P. K., Kapuscinski A. R., Bae A. Y., Donaldson E., Sitek A. J., Fitzgerald D. S., et al. (2018). Towards Sustainable Aquafeeds: Evaluating Substitution of Fishmeal With Lipid-Extracted Microalgal Co-Product (Nannochloropsis Oculata) in Diets of Juvenile Nile Tilapia (Oreochromis Niloticus). PloS One 13 (7), e0201315. doi: 10.1371/journal.pone.0201315
Sarker P. K., Kapuscinski A. R., Lanois A. J., Livesey E. D., Bernhard K. P., Coley M. L. (2016). Towards Sustainable Aquafeeds: Complete Substitution of Fish Oil With Marine Microalga Schizochytrium Sp. Improves Growth and Fatty Acid Deposition in Juvenile Nile Tilapia (Oreochromis Niloticus). PloS One 11 (6), e0156684. doi: 10.1371/JOURNAL.PONE.0156684
Sarker P. K., Kapuscinski A. R., McKuin B., Fitzgerald D. S., Nash H. M., Greenwood C. (2020). Microalgae-Blend Tilapia Feed Eliminates Fishmeal and Fish Oil, Improves Growth, and is Cost Viable. Sci. Rep. 10 (1), 1–14. doi: 10.1038/s41598-020-75289-x
Senso L., Suarez M. D., Ruiz-Cara T., García-Gallego M. (2007). On the Possible Effects of Harvesting Season and Chilled Storage on the Fatty Acid Profile of the Fillet of Farmed Gilthead Sea Bream (Sparus Aurata). Food Chem. 101 (1), 298–307. doi: 10.1016/j.foodchem.2006.01.036
Shah M. R., Lutzu G. A., Alam A., Sarker P., Kabir Chowdhury M. A., Parsaeimehr A., et al. (2018). Microalgae in Aquafeeds for a Sustainable Aquaculture Industry. J. Appl. Phycol. 30 (1), 197–213. doi: 10.1007/s10811-017-1234-z
Silveira Júnior A. M., Faustino S. M. M., Cunha A. C. (2019). Bioprospection of Biocompounds and Dietary Supplements of Microalgae With Immunostimulating Activity: A Comprehensive Review. PeerJ 2019 (10), e7685. doi: 10.7717/PEERJ.7685/TABLE-4
Sorokin C., Krauss R. W. (1958). The Effects of Light Intensity on the Growth Rates of Green Algae. Plant Physiol. 33 (2), 109–113. doi: 10.1104/PP.33.2.109
Teuling E., Schrama J. W., Gruppen H., Wierenga P. A. (2017). Effect of Cell Wall Characteristics on Algae Nutrient Digestibility in Nile Tilapia (Oreochromis Niloticus) and African Catfish (Clarus Gariepinus). Aquaculture 479, 490–500. doi: 10.1016/J.AQUACULTURE.2017.06.025
Tibaldi E., Chini Zittelli G., Parisi G., Bruno M., Giorgi G., Tulli F., et al. (2015). Growth Performance and Quality Traits of European Sea Bass (D. Labrax) Fed Diets Including Increasing Levels of Freeze-Dried Isochrysis Sp. (T-ISO) Biomass as a Source of Protein and N-3 Long Chain PUFA in Partial Substitution of Fish Derivatives. Aquaculture 440, 60–68. doi: 10.1016/j.aquaculture.2015.02.002
Tibbetts S. M. (2018). The Potential for ‘Next-Generation’, Microalgae-Based Feed Ingredients for Salmonid Aquaculture in Context of the Blue Revolution. Microalgal Biotechnol. 151–175. doi: 10.5772/INTECHOPEN.73551
Tibbetts S. M., Mann J., Dumas A. (2017). Apparent Digestibility of Nutrients, Energy, Essential Amino Acids and Fatty Acids of Juvenile Atlantic Salmon (Salmo Salar L.) Diets Containing Whole-Cell or Cell-Ruptured Chlorella Vulgaris Meals at Five Dietary Inclusion Levels. Aquaculture 481, 25–39. doi: 10.1016/j.aquaculture.2017.08.018
Turner N., Bruce C. R., Beale S. M., Hoehn K. L., So T., Rolph M. S., et al. (2007). Excess Lipid Availability Increases Mitochondrial Fatty Acid Oxidative Capacity in Muscle Evidence Against a Role for Reduced Fatty Acid Oxidation in Lipid-Induced Insulin Resistance in Rodents. Diabetes 56 (8), 2085–2092. doi: 10.2337/DB07-0093
Uran P. A., Schrama J. W., Rombout J. H. W. M., Obach A., Jensen L., Koppe W., et al. (2008). Soybean Meal-Induced Enteritis in Atlantic Salmon (Salmo Salar L.) at Different Temperatures. Aquac. Nutr. 14 (4), 324–330. doi: 10.1111/J.1365-2095.2007.00534.X
Uscanga-Martínez A., Perales-García N., Álvarez-González C. A., Moyano F. J., Tovar-Ramírez D., Gisbert G. E., et al. (2011). Changes in Digestive Enzyme Activity During Initial Ontogeny of Bay Snook Petenia Splendida. Fish Physiol. Biochem. 37 (3), 667–680. doi: 10.1007/S10695-011-9467-2
Vizcaíno A. J., López G., Sáez M. I., Jiménez J. A., Barros A., Hidalgo L., et al. (2014). Effects of the Microalga Scenedesmus Almeriensis as Fishmeal Alternative in Diets for Gilthead Sea Bream, Sparus Aurata, Juveniles. Aquaculture 431, 34–43. doi: 10.1016/j.aquaculture.2014.05.010
Vizcaíno A. J., Rodiles A., López G., Sáez M. I., Herrera M., Hachero I., et al. (2018). Growth Performance, Body Composition and Digestive Functionality of Senegalese Sole (Solea Senegalensis Kaup 1858) Juveniles Fed Diets Including Microalgae Freeze-Dried Biomass. Fish Physiol. Biochem. 44, 661–677. doi: 10.1007/s10695-018-0462-8
Wassef E. A., El Masry M. H., Mikhail F. R. (2001). Growth Enhancement and Muscle Structure of Striped Mullet, Mugil Cephalus, L., Fingerlings by Feeding Algal Mealbased Diets. Aquac. Res. 32, 315–322. doi: 10.1046/j.1355-557x.2001.00043.x
Wilson J. M., Castro L. F. C. (2010). Morphological Diversity of the Gastrointestinal Tract in Fishes. Fish Physiol. 30 (C), 1–55. doi: 10.1016/S1546-5098(10)03001-3
Xu W., Gao Z., Qi Z., Qiu M., Peng J., Shao R. (2014). Effect of Dietary Chlorella on the Growth Performance and Physiological Parameters of Gibel Carp, Carassius Auratus Gibelio. Turkish J. Fish. Aquat. Sci. 14, 53–57. doi: 10.4194/1303-2712-v14_1_07
Yeganeh S., Teimouri M., Amirkolaie A. K. (2015). Dietary Effects of Spirulina Platensis on Hematological and Serum Biochemical Parameters of Rainbow Trout (Oncorhynchus Mykiss). Res. Vet. Sci. 101, 84–88. doi: 10.1016/J.RVSC.2015.06.002
Keywords: absorptive capacity, aquafeed, Chlorophyta, enzymatic activity, fatty acids, fish quality, low-trophic species, Mugilidae
Citation: García-Márquez J, Galafat A, Vizcaíno AJ, Barany A, Martos-Sitcha JA, Mancera JM, Acién G, Figueroa FL, Alarcón FJ, Arijo S and Abdala-Díaz RT (2022) Dietary Use of the Microalga Chlorella fusca Improves Growth, Metabolism, and Digestive Functionality in Thick-Lipped Grey Mullet (Chelon labrosus, Risso 1827) Juveniles. Front. Mar. Sci. 9:902203. doi: 10.3389/fmars.2022.902203
Received: 22 March 2022; Accepted: 29 April 2022;
Published: 26 May 2022.
Edited by:
Benjamin Costas, University of Porto, PortugalReviewed by:
Mahmoud A.O. Dawood, Kafrelsheikh University, EgyptAna Couto, University of Porto, Portugal
Inês Guerreiro, University of Porto, Portugal
Copyright © 2022 García-Márquez, Galafat, Vizcaíno, Barany, Martos-Sitcha, Mancera, Acién, Figueroa, Alarcón, Arijo and Abdala-Díaz. This is an open-access article distributed under the terms of the Creative Commons Attribution License (CC BY). The use, distribution or reproduction in other forums is permitted, provided the original author(s) and the copyright owner(s) are credited and that the original publication in this journal is cited, in accordance with accepted academic practice. No use, distribution or reproduction is permitted which does not comply with these terms.
*Correspondence: Jorge García-Márquez, j.garcia@uma.es