Dinoflagellate cysts production, excystment and transport in the upwelling off Cape Blanc (NW Africa)
- 1MARUM, Center for Marine Environmental Sciences, University of Bremen, Bremen, Germany
- 2Geosciences Department, University of Bremen, Bremen, Germany
- 3Marine Geochemistry, Alfred-Wegener-Institut, Helmholtz Zentrum für Polar- und Meeresforschung, Bremerhaven, Germany
- 4Department of Physics and Earth Sciences, Jacobs University Bremen, Bremen, Germany
To extend the understanding of dinoflagellate cysts production, excystment and vertical/lateral transport in the water column, we compared upper water cyst export production with cysts associations and concentrations in the subsurface nepheloid layer, bottom nepheloid layer and deeper water column during active upwelling off Cape Blanc (NW Africa) in August 2020. Export production was collected by two drifting trap surveys; DTS1 in an active upwelling cell for 4 days and DTS2 in an offshore drifting upwelling filament for 2 days. Subsurface, bottom nepheloid layers and deeper waters were sampled by in-situ pumps along two transects perpendicular to the shelf break. During DTS1, light limitation hampered phytoplankton production which might have influenced cyst production negatively due to up- and downward movement of water masses. Cyst export production increased at the rim of the upwelling cell. For DTS2, upwelling filament cyst export production was up to 3 times lower than that of DTS1. Echinidinium delicatum had highest relative and absolute abundances in the active upwelling, Echinidinium zonneveldiae and Bitectatodinium spongium in the upwelling filament, and Impagidinium spp. and cysts of Gymnodinium microreticulatum/nolleri at the most distal stations. Comparison of concentrations of cysts with and without cell contents showed that the majority of cysts hatched before reaching deeper waters and displayed a dormancy period of less than 6 days. About 5% of the living cysts reached deeper waters and/or the ocean floor. Living cysts were transported offshore in the upwelling filament. In case ships exchange ballast waters in the studied region, they will take up laterally transported living cysts. Upon release of the ballast waters in the port of arrival, these cysts have the potential to become “invader species” that can threaten economy and/or health. Lateral transport of cysts was observed in the bottom nepheloid layer and in deeper waters (800 - 1200m depth) with a maximal extension of about 130km off the shelf break. Therefore, sediments in the region will contain a mixture of regionally and locally produced dinoflagellate cysts. This insight contributes to the improvement of environmental reconstructions of the Cape blanc upwelling system based on downcore cyst associations.
Introduction
Dinoflagellates form a diverse group of unicellular eukaryotes that constitute an important part of the marine upper water planktic biomass (e.g. Ellegaard et al., 2017). Many dinoflagellate species can be associated with harmful algal blooms (HAB) and their dispersal forms a major economic and health threat. More than 10% of the modern dinoflagellate species produce cysts as part of their complex life cycle (Bravo and Figueroa, 2014). Embedded in the sediments some species/specimens can remain viable for more than a century (Lundholm et al., 2011; Ellegaard and Ribeiro, 2018; Delebecq et al., 2020). Especially sedimentary “seed banks” of cysts of HAB species form a potential risk for future hazards (Figueroa et al., 2018). Therefore, it is important to obtain detailed insight into the dispersal of dinoflagellate cysts from their production to their embedding in the sediment. Apart from being of major importance for HAB studies, information about production and vertical/lateral transport of dinoflagellate cysts in the water column is of major importance for dinoflagellate-cyst reconstructions of palaeoclimate, -oceanography and -environment (e.g. García-Moreiras et al., 2018; de Vernal et al., 2020 and references therein). Here, cyst associations are studied in sedimentary archives to reconstruct the past oceanographic and environmental conditions above the archive sites at times of deposition. For an adequate interpretation of the signal it is of major importance to determine if the deposited cysts are formed by an autochthonous or allochthonous association or a combination of both.
Although over the last years, information increased about cyst dispersal due to human activities (e.g. Hallegraeff and Bolch, 1992; Casas-Monroy et al., 2013; Rodrígez-Villegas et al., 2020) as well as natural dispersal in coastal settings (e.g. Brosnahan et al., 2017 and references therein) extremely limited information exists on cyst dispersal in the open ocean (e.g. Dale and Dale, 1992; Zonneveld and Brummer, 2000; Aretxabaleta et al., 2014; Butman et al., 2014; Zonneveld et al., 2018). Recently, Zonneveld et al. (2018) observed that dinoflagellate cysts could be laterally transported offshore for about 130 km within intermediate and bottom-water nepheloid layers in the active upwelling area off Cape Blanc. It is however not clear if this lateral transport in nepheloid layers is a permanent feature in the studied region or corresponds to sporadic or seasonal events. Furthermore, it is not known if 130 km is the maximal extension of lateral transport of dinoflagellate cysts in the studied region.
The upwelling system off Cape Blanc is part of the Canary Current Eastern Boundary Upwelling Environment (CC-EBUE). Surface waters are characterized by high nutrient concentrations as a result of year-round upwelling of intermediate waters into the photic zone, as well as frequent input of Sahara dust (e.g. Mittelstaedt, 1991; Kolber et al., 1994; Friese et al., 2017). Both upwelling and dust fertilize the upper ocean resulting in the Cape Blanc upwelling region to be one of the most productive regions in the world. The active upwelling cells are concentrated in a small band along the shelf edge about 20-30 km offshore (Figure 1). The upwelled water can be transported further offshore along the ocean surface in the form of eddies and filaments (Mittelstaedt, 1991). In nepheloid layers, particle densities are higher than in the surrounding waters. They are frequently observed in the subsurface waters in this region (Nowald et al., 2006; Fischer et al., 2009; Basse et al., 2014; Nowald et al., 2014). Generally, three types of nepheloid layers can be distinguished: sub-surface nepheloid layers (SNL) that are mainly associated with biologically active surface mixed layers; intermediate nepheloid layers (INL) at different levels in the water column below the subsurface and a bottom nepheloid layer (BNL) in the lowermost water column just above the ocean floor (Inthorn et al., 2006). Zonneveld et al. (2018) showed that particles of the BNL and one INL observed at deeper water depths had a similar source, most probably in the region of the active upwelling cells at the shelf break whereas a second intermediate layer showed an association of a different origin. However, until now it is not clear if the nepheloid layer particles originate in active upwelling cells/filaments or represent resuspended shelf material.
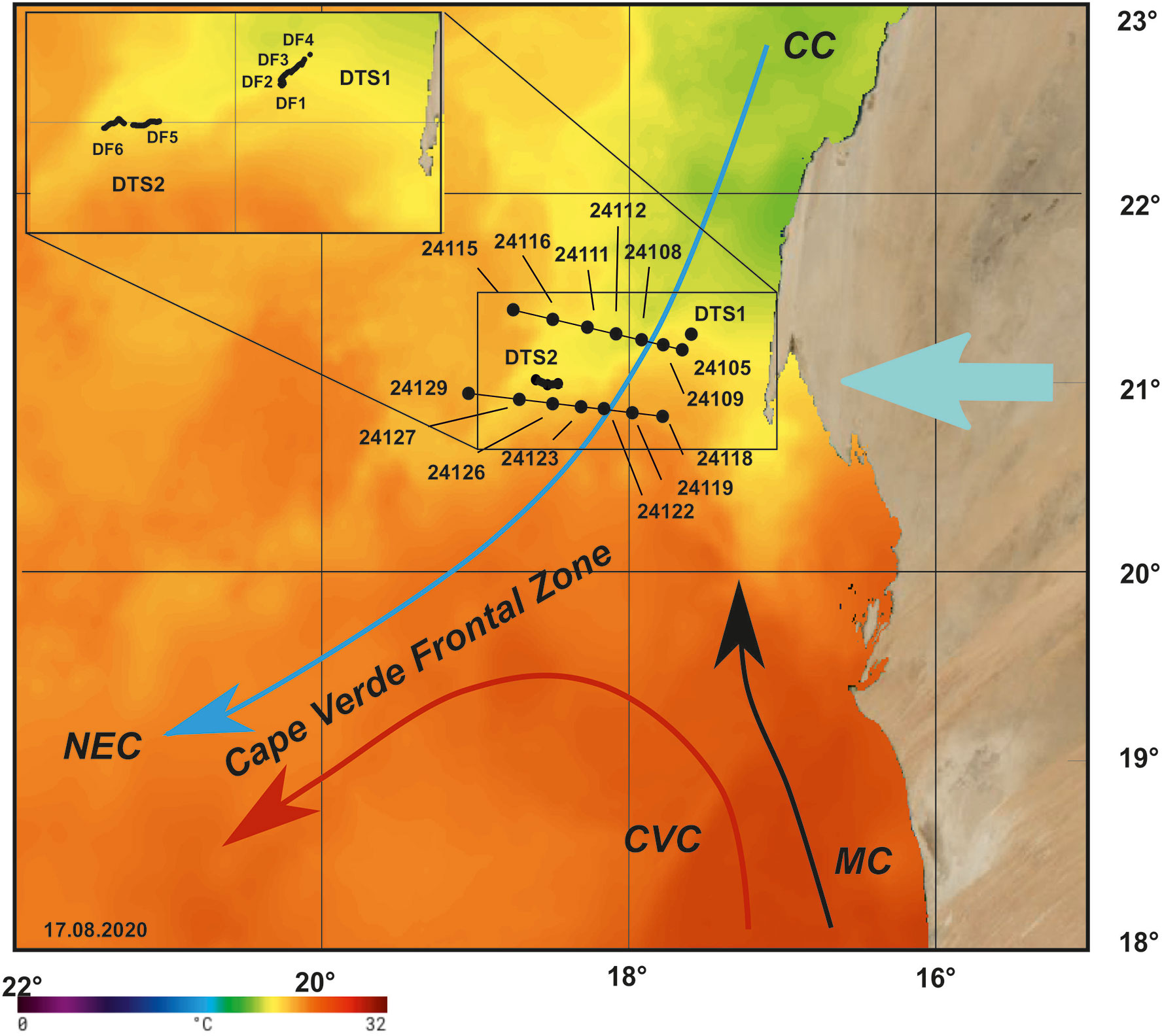
Figure 1 Satellite derived surface water temperature in the research area with major upper water current systems and station positions (satellite image with courtesy of State of the Ocean: https://podaac-tools.jpl.nasa.gov/soto/). DTS1= Drifting trap survey 1, DF1-4 = Drifting trap array tracks during DTS1 on day 1, 2, 3, 4, DTS2 = Drifting trap survey 2, DF5,6 = Drifting trap array track on day 1 and 2 during DTS2, numbers 5 and 6 refer to the deployment numbers as given in the cruise report (Zonneveld et al., 2021a), CC, Canary Current, NEC, North Equatorial Current, CVC, Cape Verde Current, MC, Mauritania Current. Blue arrow represents the direction of the Sahara air layer. Redrawn from Zonneveld et al., 2021b.
A drifting trap study executed at maximal upwelling in November 2018 (Zonneveld et al., 2021a) indicated that resuspension of shelf material can occur in the Cape Blanc region. By following the export production of cysts in an active upwelling cell during a 7-day survey, Zonneveld et al. (2021b) revealed that particles resuspended from shelf sediment formed a considerable part of the cyst association collected at 400m water depth in a water column that was characterized by active upwelling. However, resuspended cysts disappeared from the association when the trap had drifted to more offshore waters where the water column was more stratified. This latter study does not provide information about whether such resuspension of shelf material is momentum- or season-bound, or occurs throughout the year.
To investigate if lateral transport of cysts within nepheloid layers and resuspension of cysts from the shelf is momentum- or season-bound, we executed a 4-day drifting trap survey (DTS1) in an active upwelling cell located at the shelf break and a 2-day drifting trap survey (DTS2) in an offshore moving upwelling filament (Figure 1) in the Cape Blanc region during the season with low upwelling activity in August 2020. We compared the cysts associations (recording empty cysts and cysts with cell content) of the trap samples with cysts associations collected by in-situ pumps from the SNL in the subsurface water column, deeper water layers and BNL along two onshore - offshore transects (Figure 1). This allowed us to obtain insight in the possible source areas and potential lateral transport of cysts recovered from the water column.
Material and methods
Study area
The region of active upwelling off Cape Blanc (NW Africa) is characterized by permanent upwelling of which the intensity depends on the seasonal variation in wind direction and strength, ocean currents as well as the shelf width and the seafloor topography along northwestern Africa (e.g. Mittelstaedt, 1991). Apart from upwelled water, surface waters are additionally fertilized by dust input bringing nutrients and trace elements such as iron and phosphorus into the photic zone (e.g. Kolber et al., 1994).
The surface currents are the southward flowing Canary Current (CC) and the combined northward flowing Mauritania Current (MC) and Poleward Undercurrent (PUC, Figure 1, e.g. Mittelstaedt, 1991; Alves et al., 2002). The Canary Current flows along the northwest African coast as far as Cape Blanc where it detaches from the shelf between 7° and 20°N, forming the westward-flowing North Equatorial Current (NEC). The MC/PUC gradually flow northwards up to 20° N. Towards late autumn, the MC is gradually replaced by a southward flow associated with upwelling water due to the increasing influence of trade winds south of 20° N (Zenk et al., 1991). During summer the MC is enhanced and is pushed onto the shelf by the relatively strong North Equatorial Countercurrent. The presence of strong coastal currents in the region leads to substantial horizontal shear within the surface layer forming a convergence zone: the Cape Verde Frontal Zone (CVFZ, Figure 1; Zenk et al., 1991). Ekman transport induced by the prevailing north-easterly winds in the region result in coastal upwelling, typically along a band off the continental shelf. The source of upwelling waters is either North Atlantic Central Water (NACW), or the South Atlantic Central Water (SACW, Meunier et al., 2012). The NACW has higher salinity and temperature but less nutrients than the SACW. It has its source in the North Atlantic and reaches subsurface waters at lower latitudes through the thermohaline circulation. The SACW originates in the Subantarctic Zone of the Southern Ocean. South of the CVFZ upwelled waters are mainly formed by SACW whereas the more northern upwelled waters are a mixture of NACW and SACW (see references in Olivar et al., 2016).
The position and strength of the surface currents are strongly coupled to the atmospheric circulation, which in turn is mainly controlled by the seasonal migration of the intertropical convergence zone (e.g. Mittelstaedt, 1991). Upwelling intensity is modified by short-term intensification and relaxation of the wind stress on a scale of days to weeks. Most intense upwelling is observed during late winter and spring (Fischer et al., 2016). Off Cape Blanc, coastal waters and upwelling filaments can reach to >280 km offshore (e.g. Meunier et al., 2012; Ohde et al., 2015; Lovecchio et al., 2017).
Material
Material was collected during R.V. METEOR cruise M165, 18 - 25 August 2020 (Zonneveld et al., 2021a) during a drifting trap survey of 4-days (DTS1) and one of 2-days (DTS2) as well as by deploying in-situ pump systems (ISP, Zonneveld et al., 2021b). ISPs were deployed along two transects perpendicular to the coast from the shelf break (ca 500m water depth) towards the deep ocean (> 3000m water depth). Prior to deployment upwelling cells in the region were monitored on a daily basis using the NOAA satellite sea surface temperature record available at the “State of the Ocean” website: https://podaac-tools.jpl.nasa.gov/soto/. For this the characteristic of freshly upwelled waters having a lower temperature than surrounding ocean waters was used. Water column characteristics were determined with a CTD system (Seabird 9+) with additional oxygen, chlorophyll-a and turbidity sensors.
The first trap array was deployed in the center of a newly formed active upwelling cell (Figure 1). Trap arrays were exchanged every day (Supplementary Table 1). Traps collected material at 150 m, 250m and 450m water depths. During the third day, the array drifted towards shallower waters. Therefore, the deepest trap was dismantled and replaced by a trap at 350m water depth during the fourth day of the survey. During the second survey the drifting trap was released in an offshore moving upwelling filament. The arrays collected material at 150m, 250m and 450m water depth. Each particle trap consisted of four collection cylinders that were arranged in a free-swinging mounting bracket. Two alternating sets of tubes with a diameter of 10 cm and a volume of 8 L were used. Prior to deployments, the tubes were filled with brine water that consisted in filtered seawater (0.75 µm pore size, GFF) with NaCl added to reach salinities between 40 to 44. This brine water prevented wash out of settled material during trap recovery. The complete drifting array consisted of a surface buoy with an Iridium satellite unit and an AIS transmitter sending four signals every hour. Two surface floats and 26 small floats served as wave breakers. During deployment, the array drifted freely with the surface currents. Upon recovery, tubes were kept upright and steady until further treatment. Immediately after recovery, the contents of two tubes were sieved with filtered seawater through a metal sieve with pore size of 150 µm. The fraction < 150 µm was ultrasonically treated and sieved with tap water over a 20 µm metal sieve (Storck-Veco) to remove the salt. Although this method caused lysis of thecae and naked dinoflagellates it did not the morphology or lysis of cysts. The material was successively transferred to centrifuge tubes to let the material settle for 24 h. Subsequently, the upper part of the water was carefully removed. The settled material and remaining water were transferred to Eppendorff tubes that were centrifuged at 3000 rpm for 1 min and concentrated to 1 ml of suspension. After homogenization of the material a known aliquot was transferred onto a glass slide where it was embedded in glycerin-gelatin, covered with a cover slip and sealed with wax to prevent oxidation of the organic material. Cysts were counted by light microscopy at 400x magnification. Cyst fluxes were calculated by multiplying the cyst counts with the aliquot fraction and dividing by the tube opening surface and hours of sampling.
Suspended matter of the SNL, deeper water column and BNL was collected at 10 stations with ISP systems. The ISP pumped water volumes of 434 to 965 L though polycarbonate filters with pore size of 10 μm and diameter of 142mm (Zonneveld et al., 2020). Immediately after the pumps were recovered from the water column, filters were carefully removed from the pumps and washed in tap water to collect all the filtered particles. Successively, the material was ultrasonically treated and sieved with tap water through a high precision metal sieve (Storck-Veco) with pore size of 20 μm to remove the salt. The residue was transferred to Eppendorff cups and concentrated to 1 ml. After homogenization of the material a known aliquot was transferred onto a glass slide where it was embedded in glycerin-gelatin, covered with a cover slip and sealed with wax to prevent oxidation of the organic material. Cysts were counted by light microscopy at 400x magnification. Cyst concentrations were calculated by multiplying the cyst counts with the aliquot fraction and dividing through the volume pumped (L) by the ISP.
Taxonomy of organic dinoflagellates was according to Zonneveld and Pospelova (2015); Mertens et al. (2020) and van Nieuwenhoven et al. (2020). The count data were deposited in the PANGAEA database.
An as yet undescribed cyst morphotype was observed (Plate 1). The morphotype is marked throughout the manuscript as “brown cruciform”. These cysts had a cruciform cavate morphology. Both inner wall and outer wall had a brown color. The average diameter of the outer wall of the girdle was 28 µm, that of the apex-antapex 25 µm (n=10). Cysts contained an intercalary archeopyle (Plate 1). 41 specimens in 16 samples were recorded in this study.
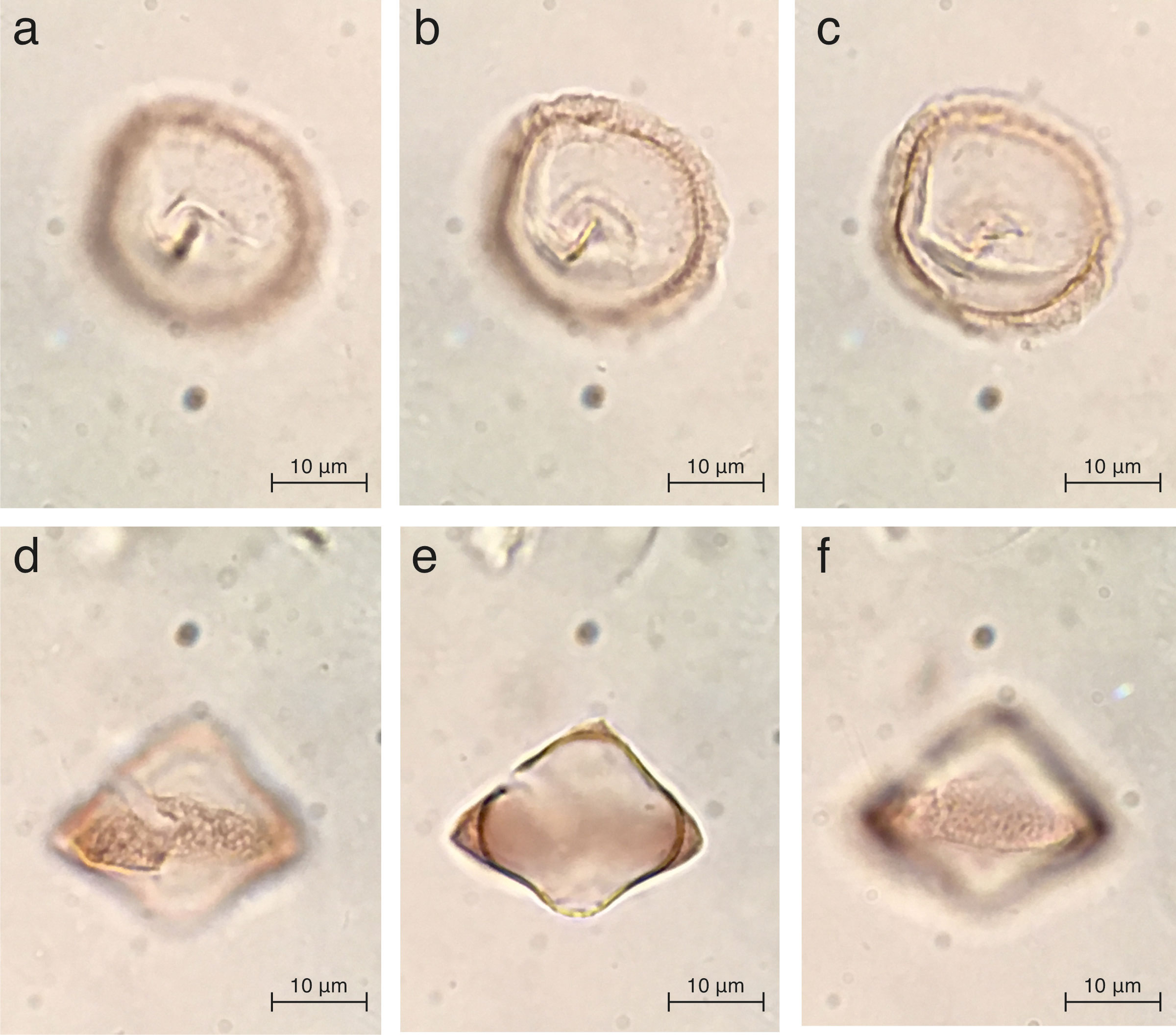
PLATE 1 Cruciform brown cysts. (A) Apical Vew; Epicyst with Split Archeopyle, (B) Apical View Cross Section, (C)Apical View; Hypocyst, (D) Ventral View, (E) Vross Section,(F) Dorsal View.
Species of which less than 3 cysts were recorded in the samples or occurred in a single sample only, were combined in species groups. The following groups were established:
Brigantedinium spp.: all round brown cysts without processes and without signs of tabulation other than the archeopyle.
Echinidinium spp.: cysts of Archaeperidinium constrictum, unidentified cysts of the genus Echinidinium, cysts of Polykrikos hartmannii.Echinidinium zonneveldiae: Echinidinium zonneveldiae, Echinidinium transparantum.
“Other peridiniacean”: Lejeunecysta invisitatum, Lejeunecysta paratenella, Trinovantedinium applanatum, Quinquecuspis concreta, Votadinium spinosum, Xandarodinium xanthum.
Impagidinium spp.: Impagidinium aculeatum, Impagidinium patulum, Impagidinium paradoxum, Impagidinium sphaericum, Impagidinium strialatum, unidentified cysts of the genus Impagidinium.
Other phototrophic dinoflagellate cysts: Ataxiodinium choanum, Dalella chathamensis, Nematosphaeropsis labyrinthus, Operculodinium centrocarpum, Pyxidinopsis reticulatum, Spiniferites mirabilis, unindentified cysts of the genus Spiniferites and Tectatodinium psilatum.
Microreticulate cysts: cysts of Gymnodinium microreticulatum, cysts of Gymnodinium nolleri. Note, none of the microreticulate cysts observed displayed more than 4 paravesicle rows in the cingulum. Since in the majority of the microreticulate specimens observed in this study did range in size between 25 and 30 µm, and not show a clear archeopyle, no separation was made between G. microreticulatum and G. nolleri.
Statistical analyses
Samples were analyzed by Hierarchic Cluster Analysis (HCA) based on euclidian distances using PAST 4.08 (Hammer et al., 2001) as well as Principal Component Analyses (PCA) and Detrended Correspondence Analysis (DCA) using the software package Canoco-5 (Smilauer and Leps, 2014). Multivariate analyses were performed on relative abundance data by exclusion of samples that contained less than 70 dinoflagellate cysts. DCA has been carried out previous to further analyses to determine the length of the gradient to verify if the species response curves in the dataset indeed had a linear character.
Results
Oceanographic conditions during sampling
During day 1 and 2 of DTS1 (DF-1, DF-2), upper water conditions were relatively similar, with temperature (∼ 21°C), salinity (∼36), oxygen (∼ 4.5 ml/l), chlorophyll-a ∼ 1.8 mg/m3) and turbidity (NTU ∼ 0.25) in the upper 50 m of the water column (Figure 3). An oxygen minimum was observed between 100 m and 200 m water depth (< 1 ml/l). Below 200 m depth water masses had, relative to the upper waters, low temperatures (14-16°C), low salinity (∼ 35.7), low turbidity (NTU ∼ 0.1) and intermediate oxygen concentration (suboxic conditions ∼ 1-2 ml/l). Chlorophyll-a concentrations were below the detection limit (Figure 2).
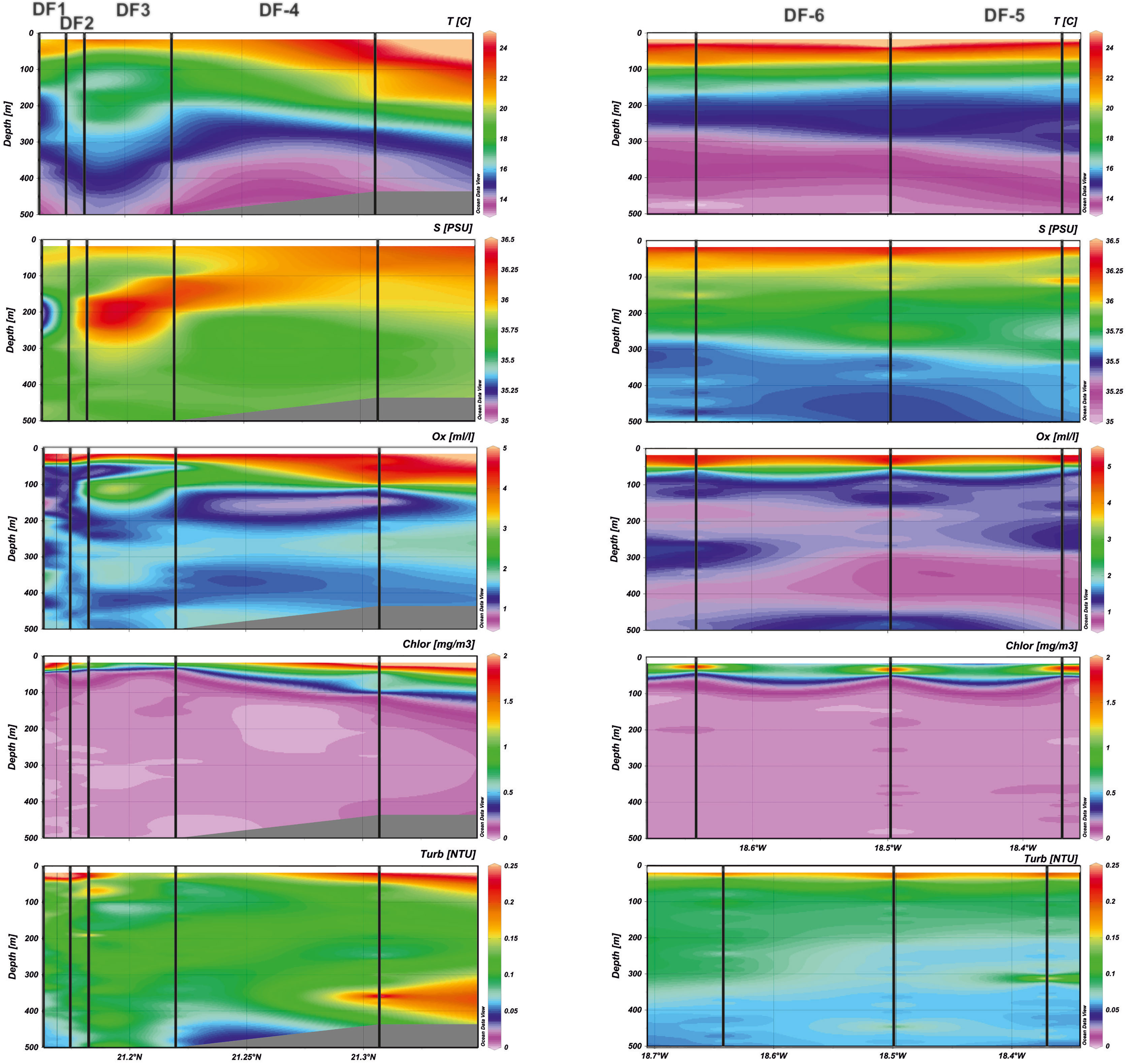
Figure 2 Cross section of the upper 500 m of the water column depicting CTD derived temperature, salinity, oxygen concentrations, chlorophyll-a concentrations and turbidity along the drifting trap tracks. Thick black lines represent the position of the CTD casts along the drifting trap track. CTD casts are deployed at the start and the end of the individual trap array deployments.
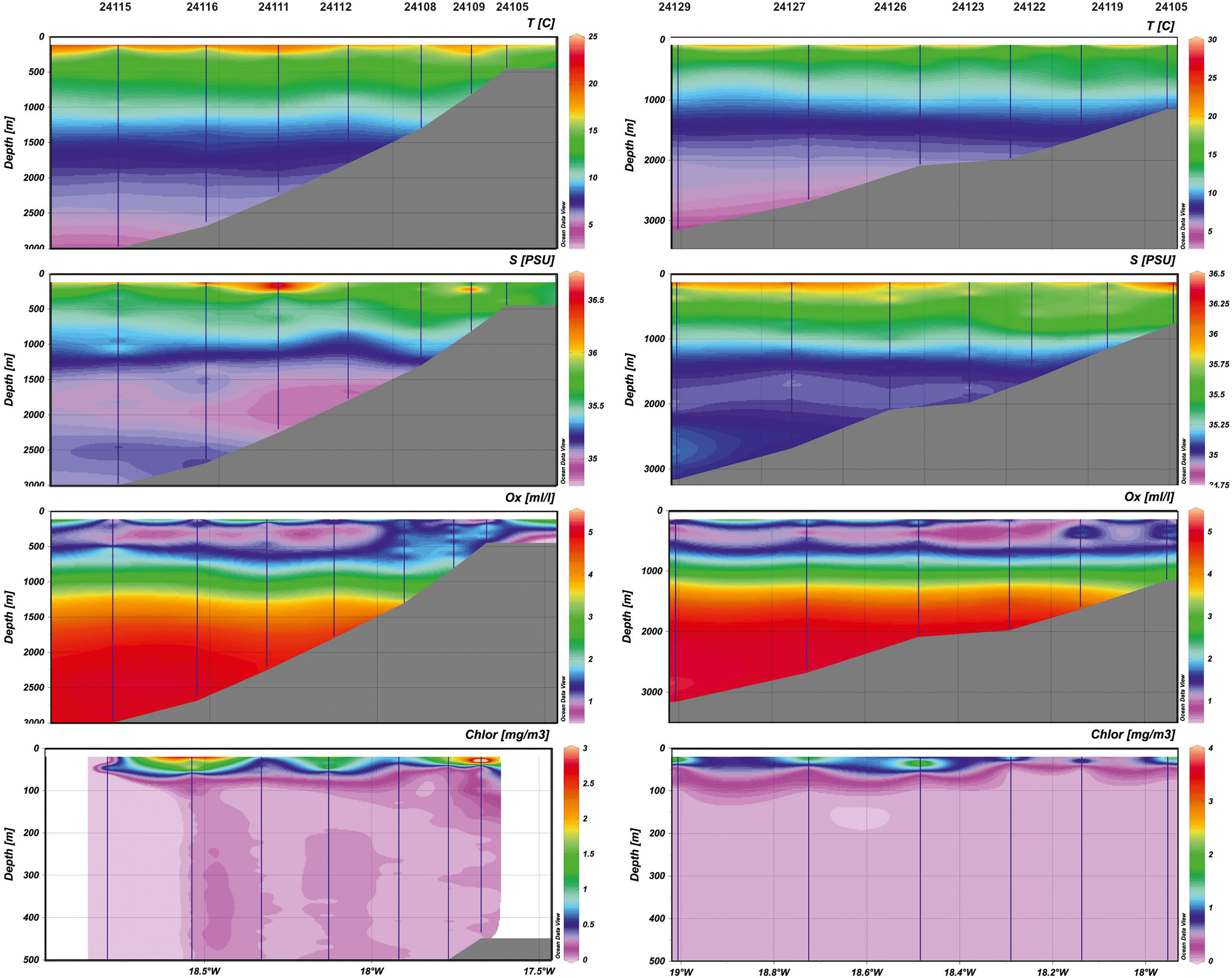
Figure 3 Cross section of the upper 500 m of the water column depicting CTD derived temperature, salinity oxygen and chlorophyll-a concentrations along the northern and southern transects. Blue lines represent the positions of the CTD casts at the studied stations.
At day 3 (DF-3), a waterbody with relatively higher temperatures, salinities, oxygen concentrations and low particle concentrations was present between 150 m and 250 m depth. During day 4 (DF-4) temperature and salinity increased to about 25°C and 36.5 respectively in the water column above 200 m. The water column was characterized by a subsurface oxygen minimum zone were oxygen concentrations were less than 1 ml/l. Again, chlorophyll-a concentrations were below detection limit below 200 m water depth. The water column contained high particle concentrations (NTU > 0.2) notably above 50 m water depth and between 325 and 425m water depth.
Oceanographic conditions were similar at both days of DTS2. Compared to the first survey, we observed higher surface water temperatures (∼ 24°C), salinity (∼ 36.5), oxygen (∼ > 5 ml/l), chlorophyll-a (> 1.5 mg/m3) and turbidity (NTU > 0.2) in the upper 100 m of the water column. Temperature and salinity increased gradually with depth. Below 100 m water depth, oxygen concentrations were low (< 1.5 ml/l). Chlorophyll-a could not be detected below 50 m water depth. Intermediate turbidity values were observed between 50 m and 300 m water depth (NTU ∼ 0.1 - 0.15). Below 300 m water depth turbidity was very low (NTU < 0.05). Comparison of oceanographic conditions in the subsurface water column and satellite imaging reveals that surface and subsurface waters belong to an offshore drifting upwelling filament.
Except for chlorophyll-a and turbidity, the oceanographic conditions at the northern and southern transect were similar (Figures 4, 7). Highest temperatures (∼ 24°C) and salinity (> 36) were observed in the upper 100 m of the water column. A clear oxygen minimum zone with oxygen concentrations < 1 ml/l was present between ∼150 and 800 m water depth. Below 800 m water depth oxygen concentrations increased rapidly and reached < 5 ml/l below 1200 m water depth.
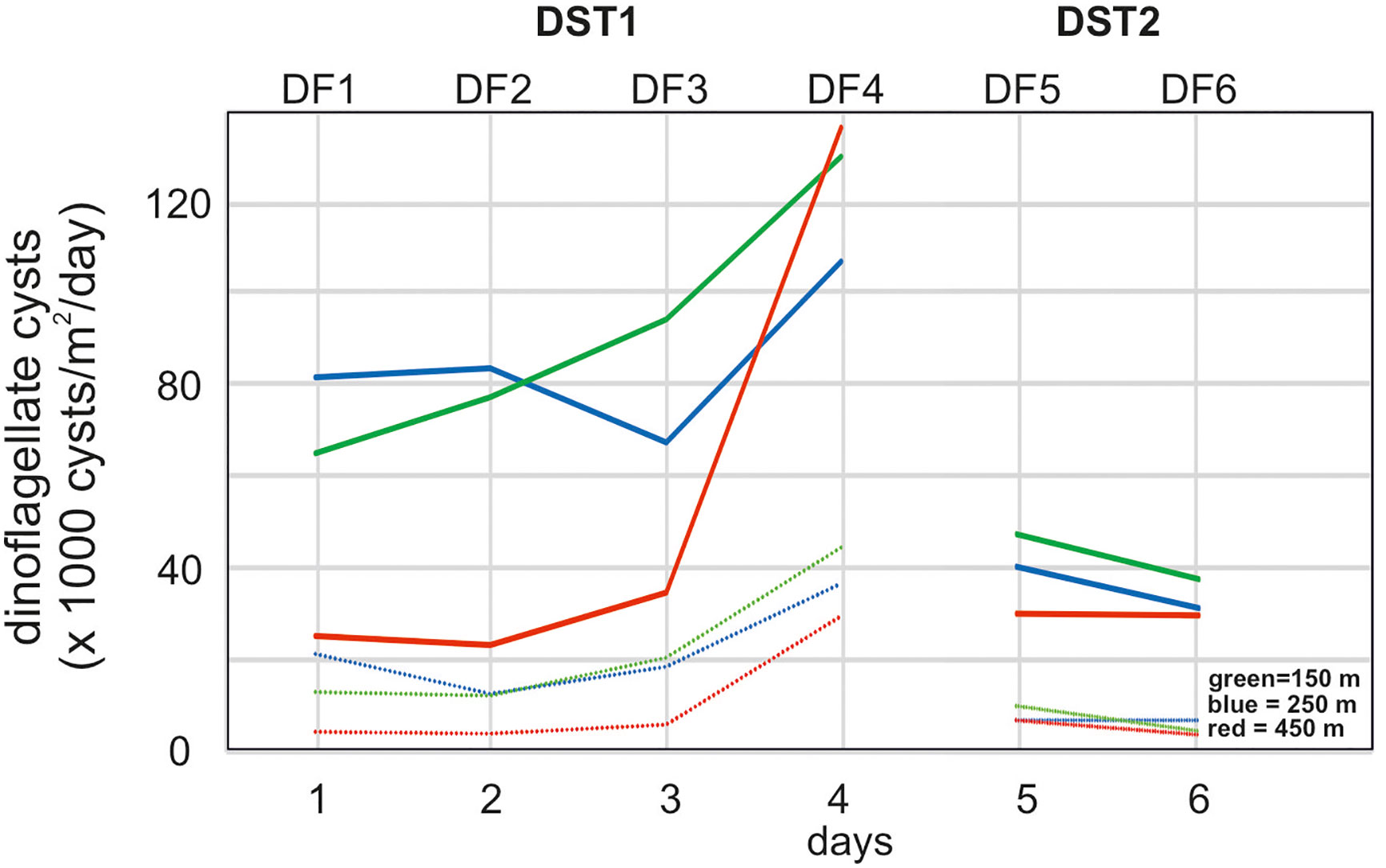
Figure 4 Total flux of cysts with and without cell content during drifting trap surveys 1 and 2. Solid lines = cysts with and without cell content, dotted lines = cysts with cell content.
At both the northern and southern transect chlorophyll-a concentrations could not be detected below 50 m water depth. Chlorophyll-a concentrations at the northern transect were higher and patchier than at the southern transect (Figure 3). Comparison with satellite images revealed that these patches of high chlorophyll-a concentration corresponded to active upwelling cells and offshore drifting upwelling filaments. Upwelling intensity was more pronounced at the northern transect compared to the southern transect at times of sampling.At both transects, turbidity in the upper water column decreased with distance to the shelf edge. Higher turbidity was observed close to the ocean floor forming a bottom nepheloid layer (BNL; Figure 3). In contrast to the northern transect slightly higher particle concentrations were present between about 800 -1200 m water depth at the southern transect.
Drifting trap surveys
Accumulation rates of total dinoflagellate cysts (with and without cell contents) were 2 to 3 times higher in the active upwelling cell than in the more offshore upwelling filament (Figure 4). During DTS1, cyst accumulation rates at day 4 were considerably higher than at the previous three days. Accumulation rates remained constant during DTS2.
The dinoflagellate cyst association in both the upwelling cell and upwelling filament were dominated by cysts of dinoflagellates with a heterotrophic life strategy (Figure 5).
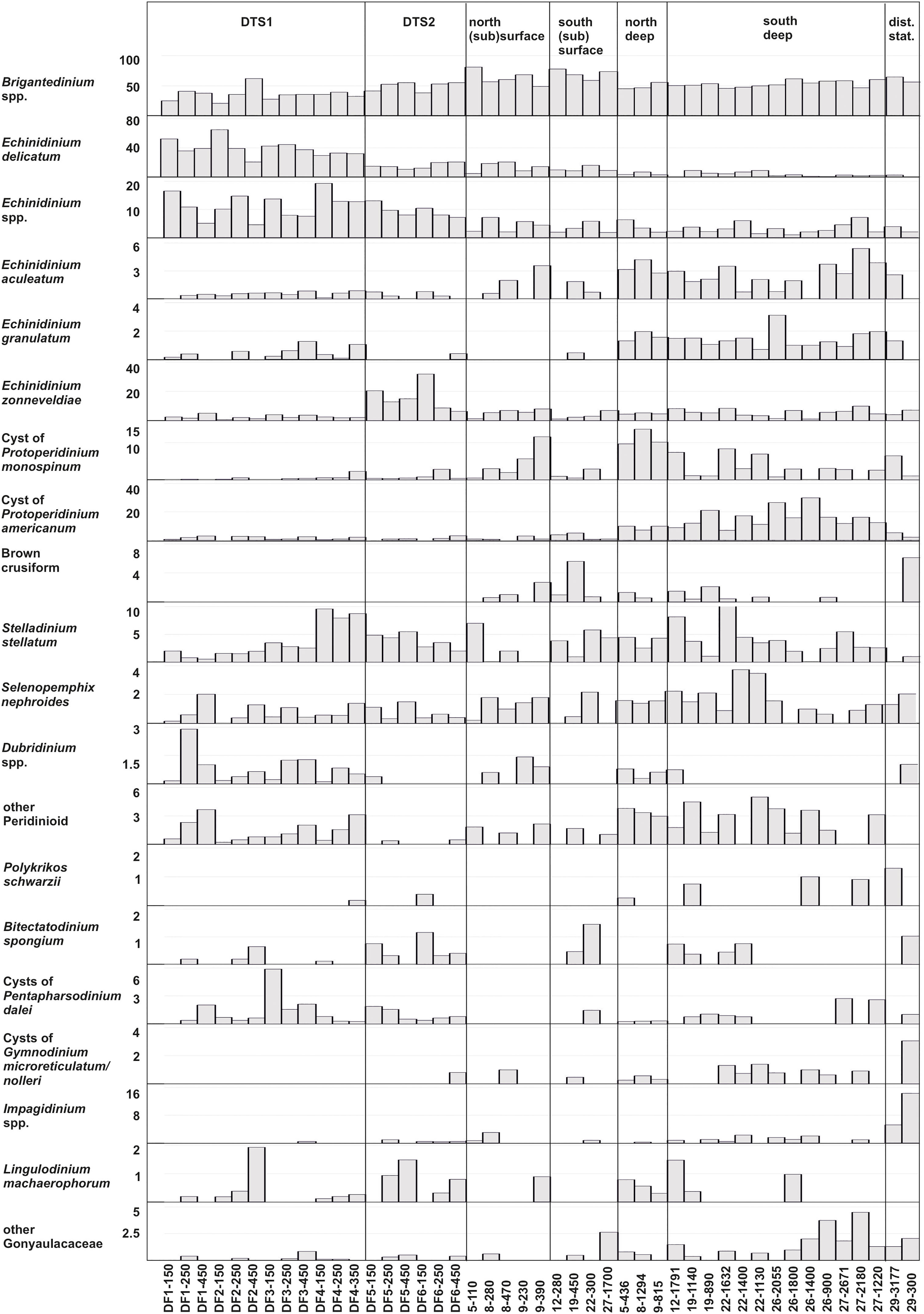
Figure 5 Relative abundances of dinoflagellate cysts. DF-survey 1 and 2: Sample numbers xx-xxx = sample number - sampling depth, north (sub)surface = samples collected in the upper water column at the northern transect, south (sub)surface = samples collected in the upper water column at the southern transect, north deep = samples collected in the deeper water column and bottom nepheloid layer at the northern transect, south deep = samples collected in the deeper water column and bottom nepheloid layer at the southern transect, dist. stat. = most distal station at the southern transect. Sample numbers: xx - xxxx = discriminating station number - sampling depth.
In the upwelling cell (DTS1), the cyst association was dominated by Echinidinium delicatum (ca. 39%) followed by Brigantedinium spp. (ca. 36%) and Echinidinium spp. (ca. 11%). Cysts of the heterotrophic species Stelladinium stellatum (ca. 3.6%), Echinidinium zonneveldiae (ca. 2.5%), and Protoperidinium mericanum (ca. 1.5%) formed a minor part of the association. All other species formed less than 1% of the association. At day 4, a strong increase in export flux and relative abundance of Stelladinium stellatum (ca. 8.7%) was observed. Cysts of phototrophic species formed about 2% of the association and were mainly formed by Pentapharsodinium dalei (ca 1.3%). Both empty and living cysts of Pentapharsodinium dalei increased over time during the first three days of the survey but disappeared almost from the association at day 4.
The cyst association in DTS2 (upwelling filament) was also dominated by Brigantedinium spp. (ca. 49%) followed by Echinidinium zonneveldiae (ca. 16%) and Echinidinium delicatum (ca. 15%). Relative abundances of Echinidinium zonneveldiae were about twice that of DTS1. Echinidinium spp. (9.4%), Stelladinium stellatum (3.8%) and cysts of Protoperidinium mericanum (1.1%) formed a minor part of the association. All other cyst species formed less than 1% of the association. Cysts of phototrophic species formed about 2.7% of the association. Living cysts of Impagidinium spp. And a few empty microreticulate cysts were collected in the upwelling filament during DTS2.
The percentage of cysts with cell content compared to the total cysts was generally higher in the upper traps compared to the deepest trap. Exceptions were DF-2 and DF-5 where there was no change with depth (Figure 6).
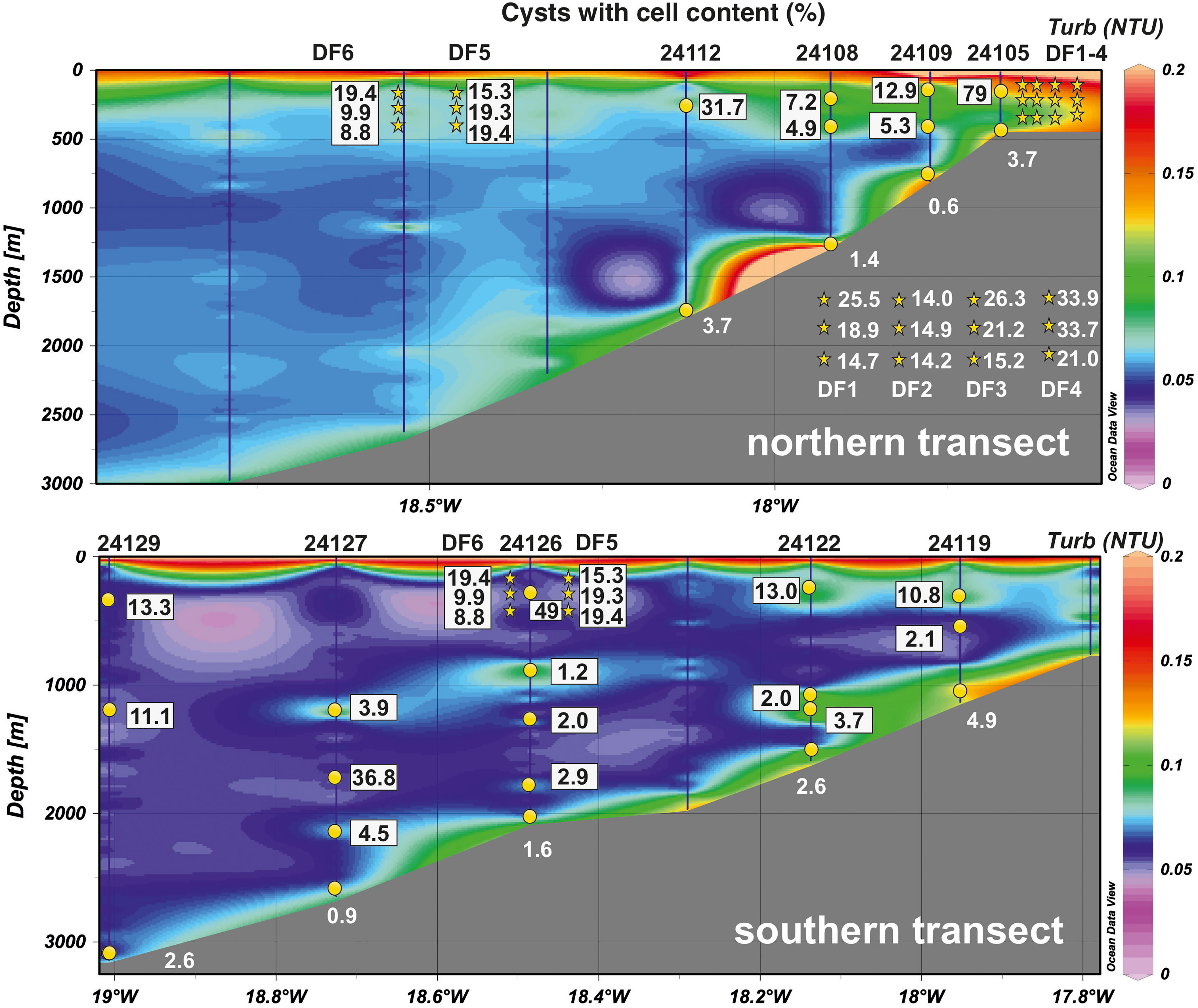
Figure 6 Cross section of the water column of the northern and southern transect depicting turbidity in the water column with relative amount of cysts of the total cyst association that contained cell content. Yellow stars present drifting trap samples, yellow dots in-situ pump samples and vertical dark blue lines CTD-casts.
Cyst concentration and association composition along the northern and southern transects
Cyst concentrations in the water column of both the northern and southern transect were highest on the upper shelf and decreased in the more offshore samples (Figure 7). East of about 18.1° W cyst concentrations in the bottom nepheloid layer were remarkably higher than in the SNL and intermediate waters. West of this point, cyst concentrations were slightly enhanced in intermediate water layers compared to the subsurface and bottom water layers.
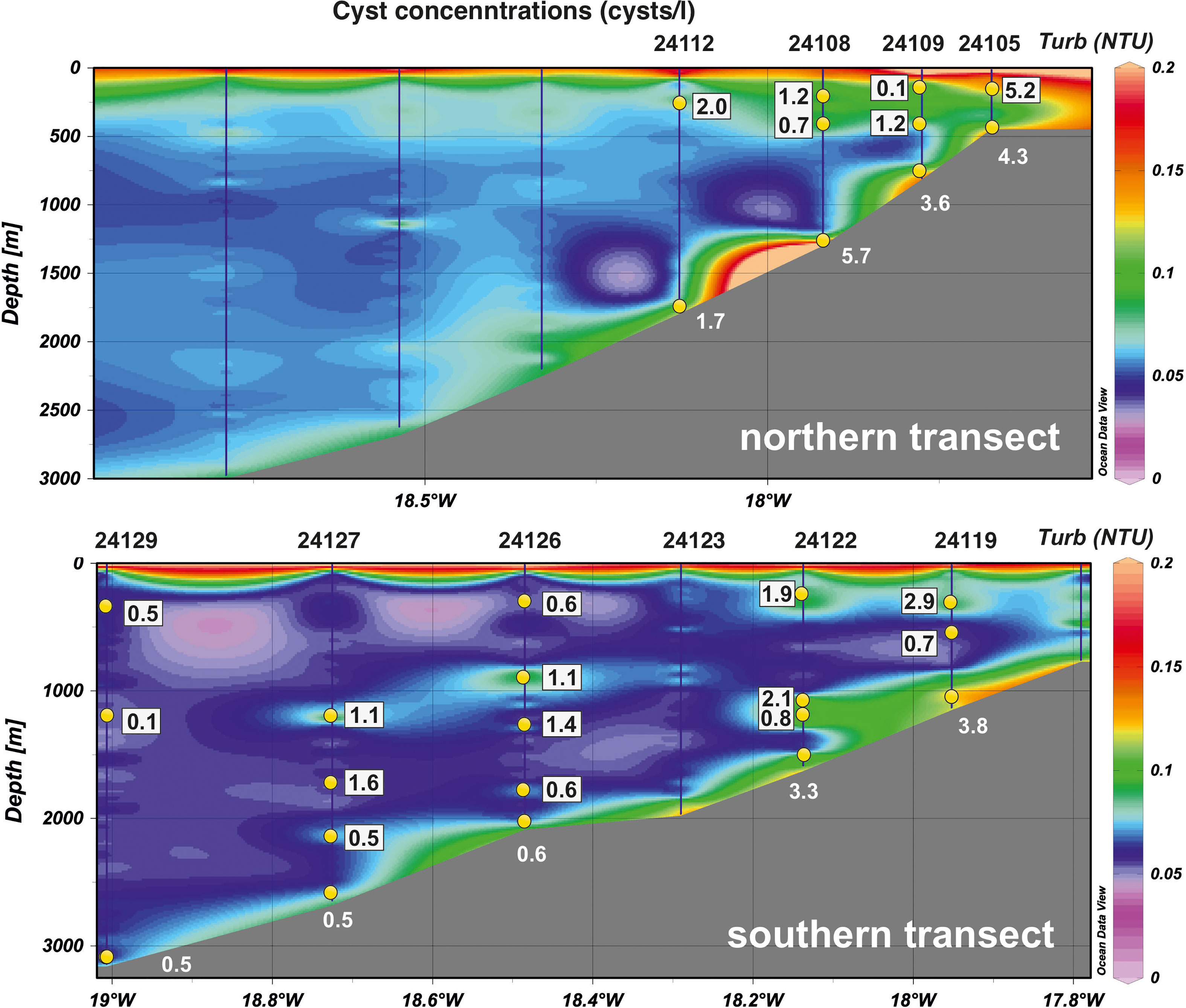
Figure 7 Turbidity map of the northern and southern transects with cyst concentrations (cysts/l). Yellow dots represent in-situ pump samples and vertical dark blue lines represent CTD-casts.
Cyst associations of the water column at the northern and southern transects were similar (Figures 5, 8). They were dominated by Brigantedinium spp. (ca. 58%) followed by cysts of Protoperidinium americanum (9.5%), Echinidinium delicatum (6.4%), Echinidinium zonneveldiae (5%) and cysts of Protoperidinium monospinum (4%). Cysts of phototrophic dinoflagellates formed about 3.5% of the association and were dominated by Impagidinium aculeatum (ca. 1.3%).
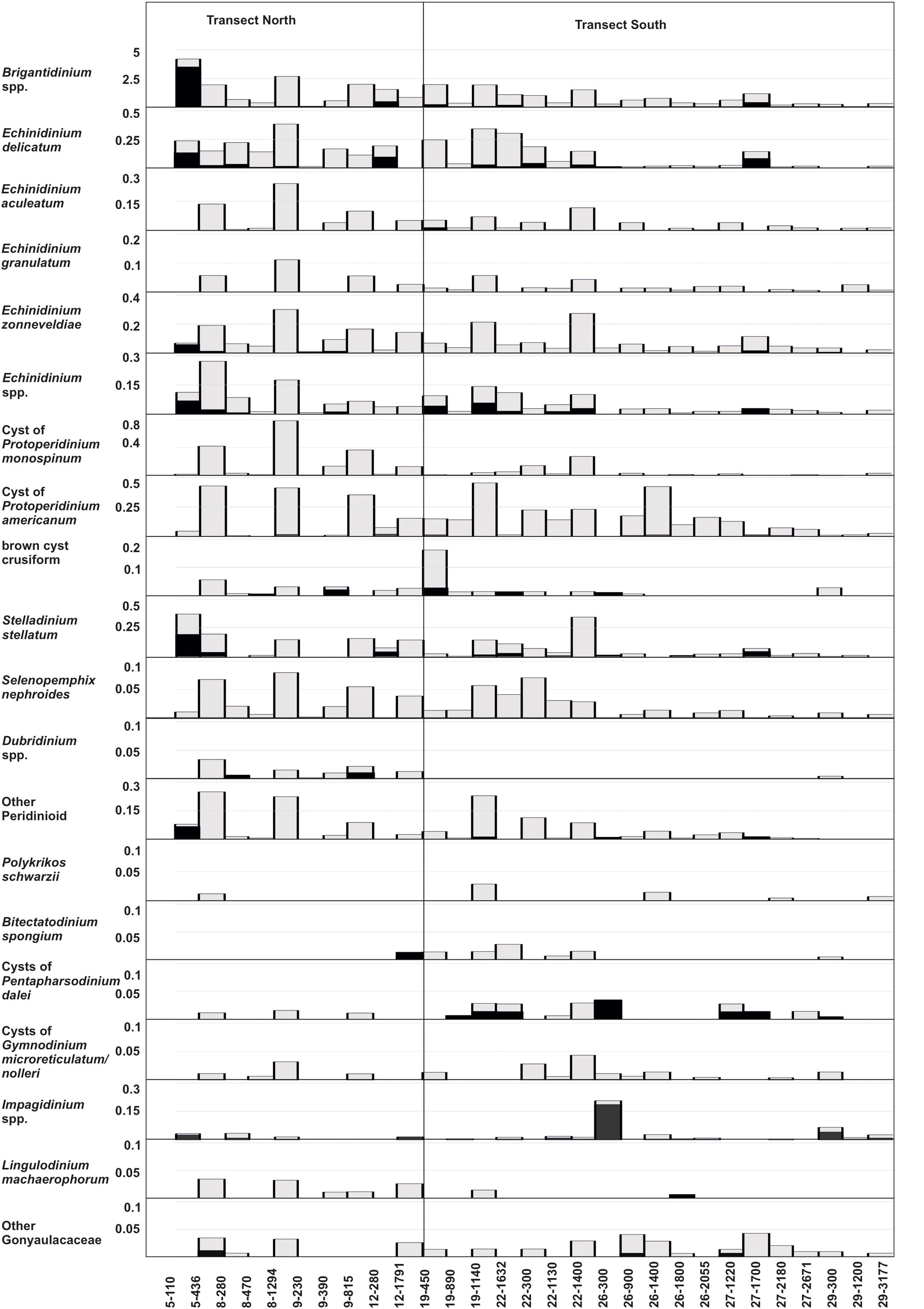
Figure 8 Cyst concentrations in the in-situ pump samples. Black: cysts with cell contents, grey: empty cysts. Sample numbers: xx - xxxx = discriminating station number - sampling depth.
Samples collected in the upper 300 m of the water column contained higher relative amounts of cysts with cell content compared to below 300 m (Figure 6) except at station GeoB 24127 where highest relative abundances of cysts with cell content were observed at 1700 m water depth (Figure 6).
Comparison of drifting trap associations with northern and southern transects
Combined results from the HCA and PCA showed roughly 5 groups of samples and two outliers (samples DF-2_150 and 24129-300; Figures 9, 10). Samples from group 1 were ordinated at the positive side of PCA axis 1 (Figure 9). This group combined all samples from the first drifting trap survey except for the upper and deepest samples of DF-2. Samples of group 1 had high relative abundances of Echinidinium delicatum (38%), and Echinidinium spp. (12%) and comparably low relative abundances of Brigantedinium spp. (34.6%). Cysts from phototrophic dinoflagellates formed less than 2% of the association (1.8%).
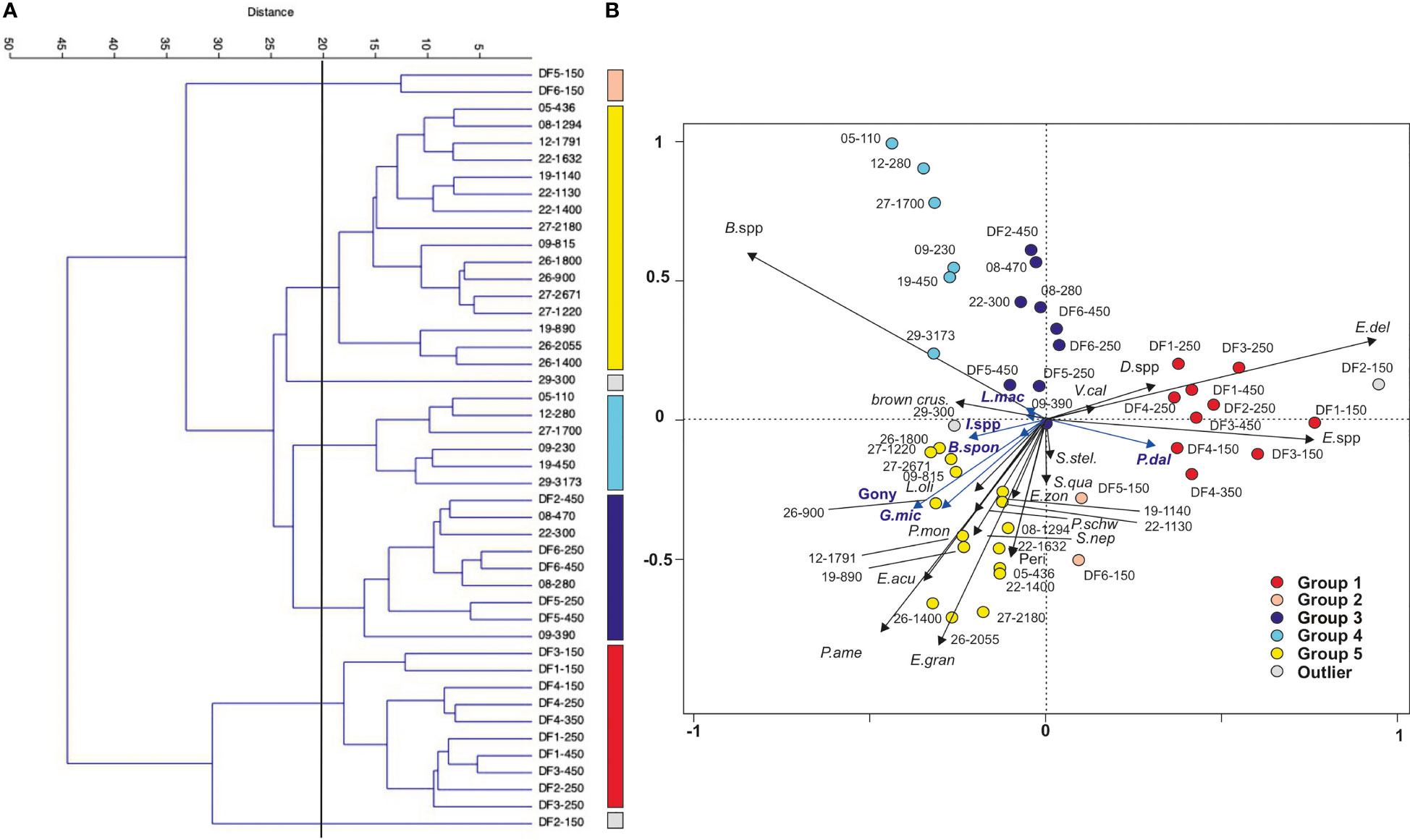
Figure 9 Multivariate analyses of combined drifting trap and in-situ pump samples. (A) Cluster analysis, (B) principal component analysis with samples represented by dots and species by arrows. Bold species abbreviations: B. spon, Bitectatodinium spongium; B.spp, Brigantedinium spp.; brown crus., brown cruciform; D.spp, Dubridinium spp.; E.acu, Echinidinium aculeatum; E. del, Echinidinium delicatum; E. gran, Echinidinium granulatum; E.zon, Echinidinium zonneveldiae; E.spp, Echinidinium spp.; Gony, other Gonyaulacoid; G.mic, Gymnodinium microreticulatum/nolleri; I.spp, Impagidinium spp.; L.mac, Lingulodinium machaerophorum; L.oli, Lejeunecysta oliva; P.ame, Protoperidinium americanum; P.dal, Pentapharsodinium dalei; Peri, other Peridinioid; P.mon, Protoperidinium monospinum; P.sch, Polykrikos schwartzii; S.nep, Selenopemphix nephroides; S.stel, Stelladinium stellatum; S.qua, Selenopemphix quanta; V.cal, Votadinium calvum; Blue arrows, phototrophic species; Black arrows, heterotrophic species.
The upper sample of DF-2 (DF-2_150) was clustered as outlier, apart from samples of group 1 but ordinated at the most positive side of PCA axis 1. Similar to the species composition of group 1 its assemblage was dominated by Echinidinium delicatum (65%) followed by Brigantedinium spp. (21.1%) and Echinidinium spp. (10%). Cysts from phototrophic dinoflagellates formed less than 1% of the association (1.8%).
Samples from group 2 consist of the two upper samples of DTS2 (DF-5_150, DF-6_150) and were ordinated at the positive side of PCA axis 1 and negative side of PCA axis 2. These samples had high relative abundances of Echinidinium zonneveldiae (26%) and Echinidinium spp. (11.7%). Compared to group 1 these samples contained higher relative abundances of Brigantedinium spp. (40%) whereas Echinidinium delicatum (13.4%), formed a much lower part of the association. Relative abundances of cysts of phototrophic species were also higher in group 2 than in group 1 (2.3%) and were mainly formed by Bitectatodinium spongium (1%) and cysts of Pentapharsodinium dalei (1.1%).
Samples from group 3 were ordinated at the positive side of PCA axis 2 and consisted of samples collected in subsurface waters by in-situ pumps at stations 24108, 24109, 24122 and deeper samples collected during DTS2 (DF5-250, DF5-450, DF6-250, DF6-450). These samples were dominated by Brigantedinium spp. (56%) followed by Echinidinium delicatum (17.2%). Echinidinium zonneveldiae (7.4%) and Echinidinium spp. (6.3%) formed a notable part of the association although not as pronounced as in samples from group 2. Cysts of photosynthetic species formed 2.7% of the association with, compared to group 1 and 2, higher relative abundances of Impagidinium spp. (0.6%) and Lingulodinium machaerophorum (0.7%) and lower relative abundances of cysts of Pentapharsodinium dalei (0.6%).
Group 4 includes all other subsurface samples as well as sample 24127-1700 m depth and 24129-3173 m depth. The composition of the species association is quite similar to that of group 3 although relative abundances differ. These samples were dominated by Brigantedinium spp. (73%) followed by Echinidinium delicatum (6.3%), Echinidinium zonneveldiae (3.8%) and Echinidinium spp. (3.1%). Cysts of photosynthetic species formed 2% of the association notably formed by Impagidinium spp. (0.7%).
Group 5 consisted of all samples from the bottom- and deeper intermediate nepheloid layers with exception of the sample of the most offshore station. Group 5 was ordinated at the negative sides of PCA axes 1 and 2. The cyst association of these samples was dominated by Brigantedinium spp. (53.4%) followed by cysts of Protoperidinium americanum (14.6%), Echinidinium zonneveldiae (5.1%) and cysts of Protoperidinium monospinum (4.4%). Cysts of phototrophic species formed 3.2% of the association and were mainly formed by “other Gonyaulacoid (1.2%), Impagidinium spp. (0.6), cysts of Pentapharsodinium dalei (0.6%) and cysts of Gymnodinium microreticulatum/nolleri (0.5%).
Sample 24129-300 is clustered separate from group 5 but ordinated in the vicinity of this group. Similar to group 5 its association was dominated by Brigantedinium spp. (56%) and relatively high abundances of Echinidinium zonneveldiae (7.2%). However, in contrast to the other samples it contained high relative abundances of brown cruciform cysts (6%) and of phototrophic cysts, notably Impagidinium spp. (14%) and cysts of Gymnodinium microreticulatum/nolleri (3%).
Discussion
Drifting trap surveys
Satellite imaging of surface water temperatures indicated that during DTS1, the drifting trap array collected particles in an active upwelling cell on 4 successive days. During days 2 and 3 a subsurface body of water with higher temperature, salinity, oxygen and low turbidity was observed (Figure 2). The T/S characteristics of this water body were different from NACW or SACW but similar to surface waters. In combination with the high oxygen concentrations of this water body, this suggests that this water mass was formed by downward movement of surface water, indicating active vertical movement and instability of water masses within the upwelling cell. Intense vertical movement of waters during the first three days of the survey was also reflected by relatively low temperatures in the upper 50 m indicating that upwelled colder deeper waters reached the ocean surface. Therefore, we can assume that during the first three days of the survey both upwelling and downwelling of waters occurred in the upper 500 m of the water column. During day 4, the upper waters had higher temperatures and salinities and became more stratified indicating that the trap had drifted out of the zone of active upwelling towards the rim of the upwelling cell (Figure 2). Both chlorophyll-a and turbidity increased in the upper water column during day 4 as well as turbidity at 350 m depth. In the research area, the waters of the photic zone can be fertilized by upwelling of nutrient-rich subsurface waters and by mineral input through Sahara dust. During the research cruise M165 no dust was recorded in the atmosphere so that fertilization of the upper waters solely resulted from upwelling.
At day 4, the export flux of cysts with and without cell content increased with the increasing chlorophyll-a concentrations in the upper 100 m of the water column (Figure 2). Vertical movement of water masses in upwelling cells might limit phytoplankton production due to light limitation as plankton being trapped in these masses is present consecutively within the photic zone and below (e.g. Smayda and Trainer, 2010; Trainer et al., 2010; Armengol et al., 2019; Villemaña et al., 2019; Burger et al., 2020 and references therein). Based on laboratory experiments that showed that extremely strong turbulence decreased or inhibited phototrophic dinoflagellate growth, it was assumed that turbulence itself had a negative effect on dinoflagellate growth and production (e.g. Smayda, 2000 and references therein). However, later experiments showed that these early experiments investigated turbulence levels several factors higher than those occurring in nature (e.g. Sullivan and Swift, 2003). Sullivan and Swift (2003) showed that enhanced turbulence, comparable to naturally occurring levels, known from upwelling areas did not decrease or inhibited growth in 9 of the 10-studied species, but could enhance growth. rates. Smayda (2000; 2010) showed that swimming speed plays an important role with respect to tolerance of species towards turbulence, with faster swimming species having a larger tolerance. He concluded that mobility rates of upwelling dinoflagellates generally exceed upwelling rates. Protoperidinians appear to be among the fastest swimming dinoflagellates. Since the association recovered in our study is largely dominated by protoperidinians we, therefore, assume that turbulence itself did not affect growth rate and cyst export production.
Our association is largely dominated by heterotrophic dinoflagellates of which production and cyst formation is largely dependent on the availability of their food source. The observation of lower chlorophyll-a concentrations during the first 3 days with active vertical movement of upper waters might indicate that plankton growth was light limited. As such, light limitation might have indirectly influenced cyst production as well.
The increase in cyst flux of both living and empty cysts at day 4 was most pronounced for Stelladinium stellatum. This species was observed typically in sediment trap time series of the Arabian Sea, Cariaco Basin and off NW Africa during active upwelling (Zonneveld and Brummer, 2000; Zonneveld et al., 2010; Bringué et al., 2019). Bringué et al. (2019) could clearly link high abundances of this species to large abundances of haptophytes (primarily coccolithophorids), their potential food source, in a 12.5-year sediment trap time series of the Cariaco Basin. Unfortunately, we did not have information about the haptophyte production during our trap survey and therefore cannot confirm this link. Nevertheless, our observation support the characterization of Stelladinium stellatum as a species that can increase its cyst production in freshly upwelled waters.
On first sight, our results seemed in contrast to observations from November 2018 in the same area (Zonneveld et al., 2021a). In November 2018, a drifting trap survey was executed at the rim of an active upwelling cell where the cyst export flux succession was followed over a period of 7 days. In 2018, cyst export fluxes decreased over time when upper waters became more stratified, whereas in this study we observed an increase in cyst export flux when waters become more stratified. However, in November 2018, the first trap array was placed at the rim of an upwelling cell and not in the center. In contrast to the present study, no vertical movement of water masses was observed at that location in 2018. We therefore assume that light limitation due to active vertical water movement did not limit cyst production during the survey in 2018.
Satellite imaging indicated that the sediment traps drifted in an upwelling filament during DTS2 (Figure 1). CTD profiles of the water column support this by showing highly stratified upper waters (Figure 2). Compared to the active upwelling cell, cyst export fluxes were strongly reduced (Figure 2). This agreed with the survey of November 2018 (Zonneveld et al., 2021b) where the first trap drifted from the rim of an active upwelling cell slowly offshore with surface waters becoming increasingly stratified. Also in that case, cyst export flux decreased in the more stratified surface waters.
Compared to the 2018 survey, cyst concentrations in the traps of both DTS1 and DTS2 were higher (Figure 4). In the research area, upwelled waters are formed by a mixture of North Atlantic Central Water (NACW) and South Atlantic Central Water (SACW) with NACW forming a larger part of the upwelled waters in boreal winter, whereas SACW contributes more upwelled water in boreal summer. Compared to NACW, SACW contains more nutrients. Many sediment trap studies showed that cyst production is enhanced with enhanced nutrient availability in surface waters (e.g. Sin et al., 2018; Bringué et al., 2019). The observation of higher cyst export fluxes in summer (this study) compared to the winter situation (2018) might, therefore, be a consequence of different source waters of the waters of the upwelling cell sampled during boreal summer compared to boreal winter.
Resuspension of cysts
During the survey in 2018 the lowermost traps contained large amounts of empty cysts of species that were not recorded in the upper traps (Zonneveld et al., 2021a). This suggested that the deepest trap captured a considerable number of cysts, that had been resuspended from the nearby shelf. In the present drifting trap survey, percentage of cysts with cell contents on the total cysts remained constant in traps at all depths (Figure 6). We also did not observe a difference in species association in the deeper traps compared to the waters above (Figure 5). We therefore had no indications that reworked sediments formed part of the material collected with the drifting traps in summer 2020.
Cysts with cell content
We observed a considerable number of cysts with cell content in our drifting trap and water column samples (Figures 6, 8). We assumed that these cysts represented living cysts since micro-organisms rapidly degrade the cell contents upon death (e.g. Price and Pospelova, 2011; Bravo and Figueroa, 2014; Heikkilä et al., 2016). Encystment experiments have shown that cysts that finished their dormancy period, quickly hatched when exposed to oxic conditions (e.g. Bravo and Figueroa, 2014; Ellegaard and Ribeiro, 2018). We did not observe higher concentrations or relative amounts of cysts with cell content in the oxygen minimum zone and since the majority of sediments in the research area were covered by oxic waters, it could be assumed that cysts that became resuspended, would have hatched quickly. We therefore assumed that the cysts with cell content represented recently produced living cells.
We observed that both the drifting trap and in-situ pump samples collected in the uppermost waters, had higher concentrations of cysts with cell content compared to the samples collected in deeper waters (Figure 6). Since we had no indication of resuspended material in the drifting traps we assumed that in our two surveys, the majority of the empty cysts in the deepest traps have hatched before reaching deeper waters. This agrees with our findings in 2018 and confirms many suggestions made in the literature (e.g. Dale, 1986; Dale and Dale, 1992; Price and Pospelova, 2011). Based on in-situ high resolution camera observations and drifting trap studies in the research area, it has been estimated that material collected at 400 m water depth is about 3 to 6 days older than material collected at 100 m water depth (Iversen et al., 2010; Thiele et al., 2015). This suggests that the dormancy period of the species recovered here is less than 6 days. However, we did observe that cysts with cell content formed up to 5% of the cyst association in the samples collected from the intermediate waters and the bottom nepheloid layer. This indicated that a considerable part of the living population of cysts produced in the upper waters did not hatch before reaching deep waters. A first explanation could be that the length of the dormancy period varies within the species. Differences in dormancy periods between individuals of a cyst population have been documented in several studies (e.g. Bravo and Figueroa, 2014 and references therein). As an example, the dormancy of Alexandrium catenella cysts varied between 69 and 113 +/- 13.5 days in a culture experiment with cysts from Patagonian fjords (Chile). To what extent this applies to the species observed here requires further investigation. A second explanation might be that encystment was inhibited by ambient environmental conditions for a part of the cyst population. Oxygen depletion is a major factor that can inhibit excystment (e.g. Bravo and Figueroa, 2014). Within the research area, a major part of the particulate organic matter produced in the upper water column is thought to be transported towards the deeper ocean by aggregates (e.g. Iversen and Ploug, 2013). Depending on the size and texture, the oxygen concentration of the inner parts of marine aggregates can be strongly reduced (e.g. Iversen and Ploug, 2010; Zetsche et al., 2020). As a consequence, when living cysts are being incorporated within such aggregates, the low oxygen concentrations might inhibit cyst hatching. Again, further investigations are required to see to what extend this is the case for cysts being produced in the upper waters in the upwelling area off Cape Blanc.
On first sight, it might appear that the part of the cyst population that reaches waters below the photic zone is lost for further reproduction. However, it is likely that these cysts remain vital for a longer time. Remaining vital for a longer time period is shown to be extremely efficient in more shallow waters where cysts can form a so called “seed bank” from which upper water can be (re-)colonized (Lundholm et al., 2011; Bravo and Figueroa, 2014; Ellegaard and Ribeiro, 2018; Rodrígez-Villegas et al., 2020). For deep sea environments, remaining vital for a longer period might also be an efficient strategy for dispersal. Many studies showed that cyst forming species can colonize new regions through cysts dispersal by ocean currents (e.g. Anderson, 1997; Ribeiro et al., 2012; Genovesi et al., 2013; Mardones et al., 2016; Murray and Hallegraeff, 2018). We therefore assumed that the part of the cyst population with cell content reaching deeper water was not simply “lost” but formed a part of the life strategy of the species.
We did observe one outlier. At station 24127, 33% of the cyst collected at 1700 m water depth contained cell content (Figure 6). Sediment trap studies from the North Atlantic and Indian Ocean have shown that short pulses of particle transport containing high amounts of cysts can sporadically occur (Dale and Dale, 1992; Dale and Dale, 1992; Zonneveld and Brummer, 2000). Although we observed these high concentrations of cysts with cell content in one sample only, we might have collected material of such a short pulse of downward particle transport here as well.
Cyst associations in relation to surface water conditions
Statistical analyses of the drifting trap and water column samples revealed five sample groups (Figures 9, 10). Four of these groups included surface and subsurface samples along the onshore-offshore gradient whereas group 5 included samples from deeper water masses and the bottom nepheloid layer.
Along the surface/subsurface onshore-offshore gradient environment changes from active upwelling along the shelf break at about 17.3°W, via upwelling further offshore to open oceanic and warm oligotrophic conditions at the most distal station at 19°W. Satellite imaging showed that upwelling filaments increased in temperature on their way west indicating that the upwelled surface waters slowly became stratified and progressively mixed with warm oligotrophic oceanic waters on their way offshore (Figure 1). Visual examination and statistical analyses revealed that the cyst associations of the region with active upwelling were dominated by Echinidinium species and Brigantedinium spp. (Figure 5). Further offshore the relative abundances of Echinidinium species gradually decreased in favor of Brigantedinium spp. Both groups showed a strong decrease in cyst export flux (drifting traps) or concentrations of cysts/l (in-situ pumps) with distance to the active upwelling cells at the shelf break (Figures 5, 8). High concentrations and relative abundances of Echinidinium and Brigantedinium species have also been observed in surface sediments below other areas of active upwelling in the (sub)tropics (e.g. Verleye and Louwye, 2010; Limoges et al., 2010; Zonneveld et al., 2013; Ribeiro et al., 2016; Garcia-Moreiras et al., 2021).
In this study, we observed that Echinidinium delicatum is the most abundant Echinidinium species in the active upwelling whereas it is replaced by Echinidinium zonneveldiae in the upwelling filaments. Echinidinium delicatum as the most dominant Echinidinium species was regularly observed in high relative and absolute abundances in sediment traps recording intensive upwelling (e.g. in the Arabian Sea (Zonneveld and Brummer, 2000), the Cape Blanc region (Zonneveld et al., 2010) and Cariaco Basin (Bringué et al., 2019). In the Santa Barbara Basin, E. delicatum was typically recorded at the onset of upwelling relaxation (Bringué et al., 2013). However, in November 2018, E. zonneveldiae was the dominant species of the cyst export flux at the rim of an active upwelling cell whereas only a few specimens of E. delicatum were observed. This suggests that the cyst composition in individual upwelling cells in the Cape Blanc region may be very different. Since upwelled waters drift offshore in filaments, the higher abundance of E. zonneveldiae might reflect its dominance in the source upwelling cell. Long term sediment trap studies from the Cape Blanc region show that the cyst associations may vary between different events of increased upwelling (see data stored in PANGAEA.de; Romero et al., 2020). Also in Cariaco Basin large variability in associations was observed during the individual upwelling stages over a 12.5-year period. This suggests that although a set of species are typically associated to active upwelling, not all these species occur in every upwelling event.
A clear onshore-offshore change is also documented in the cyst association of phototrophic species. Like other upwelling areas, the active upwelling samples have low relative abundances of cysts of phototrophic species whereas concentrations increased with distance to the shelf. On the species level, a clear trend was seen in the distribution of Bitectatodinium spongium (Figures 5, 8). This species was almost absent in the active upwelling but formed a more prominent part of the association in the upwelling filament area and decreased in relative abundance to the most offshore samples. In other upwelling areas fluxes of this species were enhanced during the southeast monsoon upwelling phase (Zonneveld and Brummer, 2000; Susek et al., 2005; Zonneveld et al., 2010; Bringué et al., 2019). This suggests that this species was formed in a previous upwelling cell and drifted offshore with the filament or was formed in the more stratified waters of the filament itself.
At the most offshore sites, highest relative abundances are observed for the phototrophic Impagidinium spp. (10%) and cysts of Gymnodinium microreticulatum/nolleri (1.6%, Figures 5, 9). Whereas Impagidinium spp. was also present in the active upwelling and in the upwelling filaments, cysts of Gymnodinium microreticulatum/nolleri were restricted to the most offshore stations. Also in other upwelling areas, this species is typically observed in stratified upper waters later in the upwelling succession, when active upwelling has terminated (e.g. Ribeiro et al., 2016; Band-Schmidt et al., 2019; Garcia-Moreiras et al., 2021).
Lateral and vertical transport
Along the studied transects large environmental gradients were present ranging from active upwelling at the shelf break, via offshore drifting upwelling filaments at the more offshore locations towards a stratified open ocean environment at the distal end of the transect. The following hypotheses could be formulated with respect to horizontal and vertical transport of cysts in the water column. In case vertical cyst transport would dominate, cyst association composition would be station specific, independent of the depth the associations were sampled. In case lateral transport would dominate, cyst associations would be related to the water layer and independent of the station position along the gradient. Both visual and statistical analyses showed a clear difference in cyst associations between surface/subsurface and deeper water masses (Figures 5, 8, 9). Along the onshore-offshore transects the subsurface associations collected by in-situ pumps and during drifting trap DTS2 showed large similarity (groups 2, 3, 4; Figure 9, 10). Furthermore, the bottom-, deeper waters contained a similar cyst association as well. Consequently, it is likely that lateral cyst displacement of cysts from the shelf break towards the open ocean has occurred both in (sub)surface water layers through offshore drifting upwelling filaments and in deeper water layers. Lateral transport in subsurface waters has been observed up to the most offshore station about 140 km off the shelf break (= 75 nm; 100 nm/200 km from the coast). This agrees with float and satellite studies that showed that filaments can reach up to >280 km offshore (e.g. Meunier et al., 2012; Ohde et al., 2015; Lovecchio et al., 2017). However, our study shows that although the cyst association in these filaments was similar to that in the subsurface waters, a gradual change in association in the surface waters occurred.
Lateral transport in deeper waters has been observed before in the research area, both in intermediate nepheloid layers (INL) and the bottom nepheloid layers (BNL; Zonneveld et al., 2018). Although in this study particle concentrations in the bottom nepheloid layer above the ocean floor were clearly enhanced, in the deeper water column turbidity was near the detection limit and it is not clear if the layers with slightly enhanced turbidity values represent intermediate INL. We observed however clear lateral transport within the BNL and waters at about 800-1200 m water depth.
The principal component analyses indicated that for the most distal station the subsurface waters and bottom waters had a similar association (Figures 9, 10). However, the association of the subsurface sample showed similarity to more coastal subsurface samples as well. This suggests that apart from the lateral transport discussed above, at this station vertical transport was prominent. At station 24127 the cyst association at 1700 m depth was similar to that at the subsurface. As discussed above, the large abundance of cysts with cell content in this sample suggests that we collected a short pulse of downward particle transport. The water layers above this samples and the BNL at this station had a cyst association similar to the more onshore BNL and the deep-water samples. This suggests that in addition to vertical transport lateral transport of cysts occurred at this station. The occurrence of a combination of lateral and vertical transport of particles in this area is in line with previous studies on sediment trap studies (e.g. Fischer et al., 2016; Romero and Fischer, 2017; Fischer et al., 2019; Romero et al., 2021).
Both in November 2015 during high upwelling intensity and in the present study during low upwelling intensity, we observed that the most distal traces of lateral transport of cysts in deeper water layers can be found at about 18.7° W (=about 130 km off the shelf break). On first sight, this suggests that the maximal distance of lateral transport of particles within (sub)surface waters, deeper waters and BNL does not extend much further offshore. This agrees with the observation that INL’s in the region do not extent further west than about 19-20°W (e.g. (Helmke et al., 2005; Nowald et al., 2006; Basse et al., 2014, Helmke et al., 2005; Fischer et al., 2009; Basse et al., 2014). However, a long year sediment trap study at 20.52°W (CBmeso, about 250 km from the shelf break) reveals that about half of the total organic carbon collected at 3600 m depth originated from the coastal area (Fischer et al., 2019 and references therein). Unfortunately, information is absent on what part of this carbon accounts for particles in the size range of dinoflagellate cysts. However, at the same location and depth, a considerable part of the diatom association consisted of benthic species that likely had a coastal origin (Romero et al., 2021). Although benthic diatoms often colonize pelagic species in this region it is not automatically implied that benthic diatoms observed in sediment trap samples originate from the shelf (pers. observation 2018, 2020, 2021) we cannot exclude that maximal lateral transport of particles including dinoflagellate cysts might reach more distal regions as documented until now.
Implications for (harmful) algal bloom studies and environmental reconstructions
We documented lateral transport of cysts in surface/subsurface water filaments to about 100 nm (140 km) west of the Cape Blanc peninsula. According to the International Convention for the Control and Management of Ships’ Ballast Water and Sediments (BWM) ships carrying ballast waters are obliged to replace 95% of their ballast waters at least 50 nm from the shore. Although we observed only a few specimens of potentially toxic species (Lingulodinium machaerophorum and microreticulate cysts), several toxic species have been reported from the region (e.g. Holzwarth et al., 2010; Zonneveld et al., 2010; Zonneveld et al., 2021a). Ballast waters can contain viable populations of cysts of toxic and non-toxic dinoflagellate species (Lin et al., 2021). Within the region the main ship route along southern Africa towards Europe crosses the research area and generally the shortest coastal route is followed, less than 100 nm offshore (e.g. https://www.canadiangeographic.ca/article/mapping-cargo-ship-routes-around-world). This implies that ships replacing their ballast waters in the study region might take in potential harmful or toxic species that are either produced in the upwelling filaments or drifted offshore. Many species observed in this study that have their main distribution world-wide in coastal waters (Zonneveld et al., 2013). This means that taking in ballast waters in the Cape Blanc region may increase the risk of transporting these cyst species to regions that were previously not inhabited by them which could result in the introduction of exotic species in the target harbors.
Our observation that considerable lateral transport occurs in deeper and bottom water layers implies that sediments in the region contain a mixture of regional and a local signal in agreement with Zonneveld et al. (2021b). As such our observation, will lead to an improvement of reconstructions of past upper water bio-production and environmental conditions based on cyst associations in sediments from the region.
Conclusions
The dinoflagellate cyst export fluxes were investigated by means of drifting traps sampling at 150 m, 250 m and 450 m depth in an active upwelling cell and in an upwelling filament. In the center of the active upwelling cell, active vertical movement of water masses of the upper water may have limited cyst production. At the rim of the upwelling cell, cyst export production increased, notably of Stelladinium stellatum. Cyst export production in the upwelling filament was up to a factor 3 lower than in the active upwelling. No resuspension of cysts into the upper water column was observed within the drifting traps.
Higher concentrations of cysts with cell content in the uppermost drifting trap and in-situ pump samples showed that a major part of the cysts hatched before reaching deeper waters. Taking into account regional sinking velocities, the cyst dormancy period of the hatched specimens was less than 6 days. A considerable part of the living cysts reached deeper waters and the ocean floor and we ascribed this to variation in dormancy period or to hatching inhibition by unfavorable conditions during sinking, for instance as a result of encapsulating in e.g. aggregates. These unhatched cysts have the potential to support the wider dispersal of species. Along the studied transects environmental conditions ranged from active upwelling at the shelf break, via offshore drifting upwelling filaments at the more offshore locations to a stratified open ocean environment at the distal end of the transect. Cyst associations in the (sub)surface showed higher relative abundances of Echinidinium delicatum in the active upwelling, Echinidinium zonneveldiae and Bitectatodinium spongium in the upwelling filament and Impagidinium spp. and cysts of Gymnodinium microreticulatum/nolleri at the most distal stations.The cyst assemblages indicated lateral transport from the shelf break towards the open ocean in (sub)surface waters and intermediate/bottom nepheloid layers. Within the deeper water column, the extension of lateral transport of cysts was up to 130 km from the shelf break. At the distal part of the transect both lateral transport and vertical transport of cysts could be observed.
Our study revealed that downcore sediment in the region will contain a mixed regional and local dinoflagellate cyst deposition signal. In case ships replace ballast waters in the studied region there is a risk they take in potential harmful or toxic species that are either produced in the upwelling filaments or drifted offshore. This furthermore increases the risk of introducing exotic species into their destination harbor environments.
Data availability statement
The original contributions presented in the study are included in the article/Supplementary Material. Count data are stored in PANGAEA. Further inquiries can be directed to the corresponding author.
Author contributions
KZ analysed the samples, performed the statistical analyses together with author GV and wrote the manuscript. HG collected the samples and was involved in the interpretation of the results. GV performed statistical analyses, was involved in the interpretation of the results and in writing the manuscript. All authors contributed to the article and approved the submitted version.
Funding
The expedition was funded by the Deutsche Forschungsgemeinschaft GPF 18-1_18. The financial support in the form of scholarships and positions of participants of the cruise was provided by the Deutsche Forschungsgemeinschaft GPF 18-1_18 and the MARUM Cluster of Excellence “The Ocean Floor – Earth’s Uncharted Interface”.
Acknowledgments
We thank captain Detlef Korte and the crew of the R.V. METEOR for the professional support and excellent cooperation during cruise M165. We especially thank Götz Ruhland, Marco Klann, and Kristina Kotzem for their support during sampling. We greatly appreciate the support, excellent cooperation and flexibility of the head office Gutachterpanel Forschungsschiffe (GPF), the German Research Fleet Control Centre (LDF) and Briese Research Forschungsschifffahrt that provided us this unique possibility to execute the research cruise M165 in Corona Pandemic times.
Conflict of interest
The authors declare that the research was conducted in the absence of any commercial or financial relationships that could be construed as a potential conflict of interest.
Publisher’s note
All claims expressed in this article are solely those of the authors and do not necessarily represent those of their affiliated organizations, or those of the publisher, the editors and the reviewers. Any product that may be evaluated in this article, or claim that may be made by its manufacturer, is not guaranteed or endorsed by the publisher.
Supplementary material
The Supplementary Material for this article can be found online at: https://www.frontiersin.org/articles/10.3389/fmars.2022.915755/full#supplementary-material
References
Alves M., Gaillard F., Sparrow M., Knoll M., Giraud S. (2002). Circulation patterns and transport of the Azores front-current system. Deep. Sea. Res. Part II: Top. Stud. Ocean.49, 3983–4002. doi: 10.1016/S0967-0645(02)00138-8
Anderson D. M. (1997). Bloom dynamics of toxic alexandrium species in the northeastern US. Limn. Ocean.42, 1009–1022. doi: 10.4319/lo.1997.42.5_part_2.1009
Aretxabaleta A. L., Butman B., Signell R. P., Dalyander P. S., Sherwood C. R., Sheremet V. A., et al. (2014). Near-bottom circulation and dispersion of sediment containing Alexandrium fundyense cysts in the gulf of Maine during 2010–2011. Deep. Sea. Res. Part. II: Top. Stud. Ocean103, 96–111. doi: 10.1016/j.dsr2.2013.11.003
Armengol L., Calbet A., Franchy G., Rodríguez-Santos A., Hernández-León S. (2019). Planktonic food web structure and trophic transfer efficiency along a productivity gradient in the tropical and subtropical Atlantic ocean. Sci. Rep.9, 1–19. doi: 10.1038/s41598-019-38507-9
Band-Schmidt C., Durán-Riveroll L., Bustillos-Guzmán J., Leyva-Valencia I., López-Cortés D., Núnez-Vázquez E., et al. (2019). Paralytic toxin producing dinoflagellates in Latin America: Ecology and physiology. Front. Mar. Sci.21, 1–39. doi: 10.3389/fmars.2019.00042
Basse A., Zhu C., Versteegh G. J., Fischer G., Hinrichs K.-U., Mollenhauer G. (2014). Distribution of intact and core tetraether lipids in water column profiles of suspended particulate matter off cape blanc. NW Africa. Org. Geochem.72, 1–13. doi: 10.1016/j.orggeochem.2014.04.007
Bravo I., Figueroa R. I. (2014). Towards an ecological understanding of dinoflagellate cyst functions. Microorg.2, 11–32. doi: 10.3390/microorganisms2010011
Bringué M., Pospelova V., Pak D. (2013). Seasonal production of organic-walled dinoflagellate cysts in an upwelling system: A sediment trap study from the Santa Barbara basin, California. Mar. Mic.100, 34–51. doi: 10.1016/j.marmicro.2013.03.007
Bringué M., Pospelova V., Tappa Eric J., Thunell R. C. (2019). Dinoflagellate cyst production in the cariaco basin: A 12.5 year-long sediment trap study. Progr. Ocean.171, 175–211. doi: 10.1016/j.pocean.2018.12.007
Brosnahan M. L., Ralston D. K., Fischer A. D., Solow A. R., Anderson D. M. (2017). Bloom termination of the toxic dinoflagellate Alexandrium catenella: Vertical migration behavior, sediment infiltration, and benthic cyst yield. Limn. Ocean.62, 2829–2849. doi: 10.1002/lno.10664
Burger J., Moloney C., Walker D., Parrot R., Fawset S. (2020). Drivers of short-term variability in phytoplankton production in an embayment of the southern benguela upwelling system. J. Mar. Sys.208, 103341. doi: 10.1016/j.jmarsys.2020.103341
Butman B., Aretxabaleta A. L., Dickhudt P. J., Dalyander P. S., Sherwood C. R., Anderson D. M., et al. (2014). Investigating the importance of sediment resuspension in Alexandrium fundyense cyst population dynamics in the gulf of Maine. Deep. Sea. Res. Part. II: Top. Stud. Ocean.103, 79–95. doi: 10.1016/j.dsr2.2013.10.011
Casas-Monroy O., Roy S., Rochon A. (2013). Dinoflagellate cysts in ballast sediments: differences between canada’s east coast, west coast and the great lakes. Aq. Cons. Mar. Freshw. Ecosys.23, 254–276. doi: 10.1002/aqc.2310
Dale B. (1986). Life cycle strategies of oceanic dinoflagellates. UNESCO. Techn. Pap. Mar. Sci.49, 65–72.
Dale B., Dale A. L. (1992). Dinoflagellate contributions to the deep sea, woods hole oceanographic institution, woods hole, ocean biocoe. Ser.5, 1–73.
Delebecq G., Schmidt S., Ehrhold A., Latimier M., Siano R. (2020). Revival of ancient marine dinoflagellates using molecular biostimulation. J. Phycol.56, 1077–1089. doi: 10.1111/jpy.13010
de Vernal A., Hillaire-Marcel C., Duc C. L., Roberge P., Brice C., Matthiessen J., et al. (2020). Natural variability of the Arctic ocean sea ice during the present interglacial. Proc. Natl. Acad. Sci. U.S.A.117, 26069–26075. doi: 10.1073/pnas.2008996117
Ellegaard M., Dale B., Mertens K., et al. (2017). “Dinoflagellate cysts as proxies for Holocene environmental change in estuaries: diversity, abundance and morphology,” in Applications of paleoenvironmental techniques in estuarine studies (The Netherlands: Springer, Dordrecht), 295–312.
Ellegaard M., Ribeiro S. (2018). The long-term persistence of phytoplankton resting stages in aquatic ‘seed banks’. Biol. Rev. Camb. Philos. Soc93, 166–183. doi: 10.1111/brv.12338
Figueroa R. I., Estrada M., Garcés E. (2018). Life histories of microalgal species causing harmful blooms: Haploids, diploids and the relevance of benthic stages. Harm. Alg.73, 44–57. doi: 10.1016/j.hal.2018.01.006
Fischer G., Reuter C., Karakas G., Nowald N., Wefer G. (2009). Offshore advection of particles within the cape blanc filament, Mauritania: Results from observational and modelling studies. Prog. Ocean.83, 322–330. doi: 10.1016/j.pocean.2009.07.023
Fischer G., Romero O., Merkel U., Donner B., Iversen M., Nowald N., et al. (2016). Deep ocean mass fluxes in the coastal upwelling off Mauritania from 1988 to 2012: variability on seasonal to decadal timescales. Biogeosc.13, 3071–3090. doi: 10.5194/bg-13-3071-2016
Fischer G., Romero O., Toby E., Iversen M., Donner B., Mollenhauer G., et al. (2019). Changes in the dust-influenced biological carbon pump in the canary current system: Implications from a coastal and an offshore sediment trap record off cape blanc, Mauritania. Glob. Biogeochem. Cycl.33, 1100–1128. doi: 10.1029/2019GB006194
Friese C. A., Hateren J. A. V., Vogt C., Fischer G., Stuut J.-B. W. (2017). Seasonal provenance changes in present-day Saharan dust collected in and off Mauritania. Atmos. Chem. Phys.17, 10163–10193. doi: 10.5194/acp-17-10163-2017
Garcia-Moreiras I., Oliveira A., Santos A. I., Oliveira P. B., Amorim A. (2021). Environmental factors affecting spatial dinoflagellate cyst distribution in surface sediments off aveiro-figueira de foz (Atlantic Iberian margin). Front. Mar. Sci.8, 21. doi: 10.3389/fmars.2021.699483
García-Moreiras I., Pospelova V., García-Gil S., Sobrino C. M. (2018). Climatic and anthropogenic impacts on the ría de vigo (NW Iberia) over the last two centuries: A high-resolution dinoflagellate cyst sedimentary record. Palaeogeogr. Palaeoclim. Palaeoecol.504, 201–218. doi: 10.1016/j.palaeo.2018.05.032
Genovesi B., Mouillot D., Laugier T., Fiandrino A., Laabir M., Vaquer A., et al. (2013). Influences of sedimentation and hydrodynamics on the spatial distribution of alexandrium catenella/tamarense resting cysts in a shellfish farming lagoon impacted by toxic blooms. Harm. Alg.25, 15–25. doi: 10.1016/j.hal.2013.02.002
Hallegraeff G. M., Bolch C. J. (1992). Transport of diatom and dinoflagellate resting spores in ships’ ballast water: implications for plankton biogeography and aquaculture. J. Plankt. Res.14, 1067–1084. doi: 10.1093/plankt/14.8.1067
Hammer O., Harper D.A.T., Ryan P.D. (2001). Past: Paleontological statistics software package for education and data analysis. Palaeontologia Electronicca4, 1−9.
Heikkilä M., Pospelova V., Forest A., Stern G. A., Fortier L., Macdonald R. W. (2016). Dinoflagellate cyst production over an annual cycle in seasonally ice-covered Hudson bay. Mar. Mic.125, 1–24. doi: 10.1016/j.marmicro.2016.02.005
Helmke P., Romero O., Fischer G. (2005). Northwest African Upwelling and its effect on offshore organic carbon export to the deep sea. Glob. Biogeochem. Cyc.19. doi: 10.1029/2004GB002265
Holzwarth U., Esper O., Zonneveld K. A. F. (2010). Organic-walled dinoflagellate cysts as indicators of oceanographic conditions and terrigenous input in the NW African upwelling region. Rev. Palaeobot. Palynol.159, 35–55. doi: 10.1016/j.revpalbo.2009.10.005
Inthorn M., Mohrholz V., Zabel M. (2006). Nepheloid layer distribution in the benguela upwelling area offshore Namibia. Deep. Sea. Res. Part. I: Oceanogr. Res. Pap.53, 1423–1438. doi: 10.1016/j.dsr.2006.06.004
Iversen M. H., Nowald N., Plough H., Jackson G. A., Fischer G. (2010). High resolution profiles of vertical particle organic matter export off cape blanc, Mauritania: degradation processes and ballasting effects. Deep. Sea. Res. I57, 771–784. doi: 10.1016/j.dsr.2010.03.007
Iversen M. H., Ploug H. (2010). Ballast minerals and the sinking carbon flux in the ocean: carbon-specific respiration rates and sinking velocity of marine snow aggregates. Biogeosc.7, 2613–2624. doi: 10.5194/bg-7-2613-2010
Iversen M. H., Ploug H. (2013). Temperature effects on carbon-specific respiration rate and sinking velocity of diatom aggregates; potential implications for deep ocean export processes. Biogeosc.10, 4073–4085. doi: 10.5194/bg-10-4073-2013
Kolber Z. S., Barber R. T., Coale K. H., Fitzwater S. E., Greene R. M., Johnson K. S., et al. (1994). Iron limitation of phytoplankton photosynthesis in the equatoral pacific ocean. Nat.371, 145–148. doi: 10.1038/371145a0
Limoges A., Kielt J. F., Radi T., Ruiz-Fernandez A. C., de Vernal A. (2010). Dinoflagellate cyst distribution in surface sediments along the south-western Mexican coast (14.76 degrees n to 24.75 degrees n). Mar. Mic.76, 104–123. doi: 10.1016/j.marmicro.2010.06.003
Lin L., Wang Q., Wi H. (2021). Study on the dinoflagellate cysts in ballast tank sediments of international vessels in Chinese shipyards. Mar. Environ. Res.169, 105348. doi: 10.1016/j.marenvres.2021.105348
Lovecchio E., Gruber N., Münnich M., Lachkar Z. (2017). On the long-range offshore transport of organic carbon from the canary upwelling system to the open north Atlantic. Biogeosc.14, 3337. doi: 10.5194/bg-14-3337-2017
Lundholm N., Ribeiro S., Andersen T. J., Koch T., Godhe A., Ekelund F., et al. (2011). Buried alive - germination of up to a century-old marine protist resting stages. Phycol.50, 629–640. doi: 10.2216/11-16.1
Mardones J. I., Bolch C., Guzmán L., Paredes J., Varela D., Hallegraeff G. M. (2016). Role of resting cysts in Chilean Alexandrium catenella dinoflagellate blooms revisited. Harm. Alg.55, 238–249. doi: 10.1016/j.hal.2016.03.020
Mertens K. N., Gu H., Gurdebeke P., Takano Y., Clark D., Aidin H., et al. (2020). A review of rare, poorly known, and morphologically problematic extant marine organic-walled dinoflagellate cyst taxa of the orders gymnodiniales and peridiniales from the northern hemisphere. Mar. Mic.159, 1–35. doi: 10.1016/j.marmicro.2019.101773
Meunier T., Barton E. D., Barreiro B., Torres R. (2012). Upwelling filaments off cap blanc: Interaction of the NW African upwelling current and the cape Verde frontal zone eddy field? J. Geophys. Res.: Oceans.117, 1–18. doi: 10.1029/2012JC007905
Mittelstaedt E. (1991). The ocean boundary along the northwest African coast: circulation and oceanographic properties at the sea surface. Progr. Ocean.28, 167–188. doi: 10.1016/0079-6611(91)90011-A
Murray S., Hallegraeff G. (2018). “Harmful Algae Introductions: Vectors of Transfer, Mitigation and Management,” in Harmfull Algal Blooms, A Compendium Desk Reference, WileyBlackwell Publishing Inc., United States493–505.
Nowald N., Iversen M. H., Fischer G., Ratmeyer V., Wefer G. (2014). Time series of in-situ particle properties and sediment trap fluxes in the coastal upwelling filament off cape blanc, Mauritania. Prog. Ocean.137, 1–11. doi: 10.1016/j.pocean.2014.12.015
Nowald N., Karakas G., Ratmeyer V., Fischer G., Schlitzer R., Davenport R. A., et al. (2006). Distribution and transport processes of marine particulate matter off cape blanc (NW-africa): results from vertical camera profiles. Ocean Sci. Disc.3, 903–938. doi: 10.5194/osd-3-903-2006
Ohde T., Fiedler B., Körtzinger A. (2015). Spatio-temporal distribution and transport of particulate matter in the eastern tropical north Atlantic observed by argo floats. Deep. Sea. Res. Part I: Ocean. Res. Pap.102, 26–42. doi: 10.1016/j.dsr.2015.04.007
Olivar M., Sabantés A., Pastor M. V., Pelegrí J. L. (2016). Water masses and mesoscale control on latitudinal and cross-shelf variations in larval fish assemblages off NW Africa. Deep. Sea. Res. Part I: Ocean. Res. Pap.117, 120–137. doi: 10.1016/j.dsr.2016.10.003
Price A. M., Pospelova V. (2011). High-resolution sediment trap study of organic-walled dinoflagellate cyst production and biogenic silica flux in saanich inlet (BC, Canada). Mar. Mic.80, 18–43. doi: 10.1016/j.marmicro.2011.03.003
Ribeiro S., Amorim A., Abrantes F., Ellegaard M. (2016). Environmental change in the Western Iberia upwelling ecosystem since the preindustrial period revealed by dinoflagellate cyst records. Holoc.26, 874–889. doi: 10.1177/0959683615622548
Ribeiro S., Amorim A., Andersen T. J., Abrantes F., Ellegaard M. (2012). Reconstructing the history of an invasion: the toxic phytoplankton species Gymnodinium catenatum in the northeast Atlantic. Biol. Inv.5, 969–985. doi: 10.1007/s10530-011-0132-6
Rodrígez-Villegas C., Díaz P., Pizarro G., Saögado P., Pérez-Santos I., Díaz M., et al. (2020). Alexandrium catenella cyst accumulation by passive and active dispersal agents: Implications for the potential spreading risk in Chilean Patagonian fjords. Harm. Alg.96, 101832. doi: 10.1016/j.hal.2020.101832
Romero O. E., Baumann K.-H., Zonneveld K. A. F., Donner B., Hefter J., Hamady B., et al. (2020). Flux variability of phyto- and zooplankton communities in the Mauritanian coastal upwelling between 2003 and 2008. Biogeosc.17, 187–214. doi: 10.5194/bg-17-187-2020
Romero O. E., Fischer G. (2017). Shift in the species composition of the diatom community in the eutrophic Mauritanian coastal upwelling: Results from a multi-year sediment trap experiment, (2003–2010). Prog. Ocean.159, 31–44. doi: 10.1016/j.pocean.2017.09.010
Romero O. E., Ramondec S., Fischer G. (2021). A 2-decade, (1988–2009) record of diatom fluxes in the Mauritanian coastal upwelling: impact of low-frequency forcing and a two-step shift in the species composition. Biogeosc.18, 1873–1891. doi: 10.5194/bg-18-1873-2021
Sin H. H., Li Z., Lin D., Lee K., Seo M. H., Lim W. A. (2018). Seasonal production of dinoflagellate cysts in relation to environmental characteristics in jinhae-masan bay, Korea: One-year sediment trap observation. Est. Coast. Shelf Sci.215, 83–93. doi: 10.1016/j.ecss.2018.09.031
Smayda T. J. (2000). Ecological features of harmful algal blooms in coastal upwelling ecosystems. S. Afr. J. Mar. Sci.22, 219–253. doi: 10.2989/025776100784125816
Smayda T. J. (2010). Adaptations and selection of harmful and other dinoflagellate species in upwelling systems. 2. motility and migratory behavior. Progr. Ocean.85, 71–91. doi: 10.1016/j.pocean.2010.02.005
Smayda T. J., Trainer V. L. (2010). Dinoflagellate blooms in upwelling systems: Seeding, variability, and contrasts with diatom bloom behavior. Progr. Ocean.85, 92–107. doi: 10.1016/j.pocean.2010.02.006
Smilauer P., Leps J. & , (2014). Multivariate analysis of ecological data using Canoco5. 1–362Cambridge University Press, Cambridge.
Sullivan J. M., Swift E. (2003). Effects of small-scale turbulence on net growth rate and size of ten species of marine dinoflagellates. J. Phycol.39, 83–94. doi: 10.1046/j.1529-8817.2003.02094.x
Susek E., Zonneveld K. A. F., Fischer G., Versteegh G. J. M., Willems H. (2005). Organic-walled dinoflagellate cyst production in relation to upwelling intensity and lithogenic influx in the cape blanc region (off north-west Africa).Phyc. Res.53, 97–112. doi: 10.1111/j.1440-1835.2005.tb00362.x
Thiele S., Fuchs B. M., Amann R., Iversen M. H. (2015). Colonization in the photic zone and subsequent changes during sinking determine bacterial community composition in marine snow. Appl. Env. Microbiol.81, 1463–1471. doi: 10.1128/AEM.02570-14
Trainer V. L., Pitcher G. C., Reguera B., Smayda T. J. (2010). The distribution and impacts of harmful algal bloom species in eastern boundary upwelling systems. Prog. Ocean.85, 33–52. doi: 10.1016/j.pocean.2010.02.003
van Nieuwenhoven N., Head M. J., Limoges A., Pospelova V., Mertens K., Matthiessen J., et al. (2020). An overview and brief description of common marine organic-walled dinoflagellate cyst taxa occurring in surface sediments of the northern hemisphere -Mar. Mic.159, 1–46. doi: 10.1016/j.marmicro.2019.101814
Verleye T. J., Louwye S. (2010). Recent geographical distribution of organic-walled dinoflagellate cysts in the southeast pacific (25-53 degrees s) and their relation to the prevailing hydrographical conditions. Palaeogeogr. Palaeoclim. Palaeoecol.298, 319–340. doi: 10.1016/j.palaeo.2010.10.006
Villemaña M., Marañon E., Cermeño P., Estrada M., Fernández-Castro B., Figueiras F.G., latas M., Otero-Ferrer J.L., Reguera B., Mouriño-Carballido B. (2019). The role of mixing in controlling resource availability and phytoplankton community composition. Prog. in Ocean. 178, 1−15. doi: 10.1016/j.pocean.2019.102181
Zetsche E.-M., Larsson A. I., Iversen M. H., Ploug H. (2020). Flow and diffusion around and within diatom aggregates: Effects of aggregate composition and shape. Limn. Oceanogr.65, 1818–1833. doi: 10.1002/lno.11420
Zonneveld K. A. F., Brummer G. J. A. (2000). (Palaeo-)ecological significance, transport and preservation of organic-walled dinoflagellate cysts in the Somali basin, NW Arabian Sea. Deep. Sea. Res. Part II: Top. Stud. Oceanogr.47, 2229–2256. doi: 10.1016/S0967-0645(00)00023-0
Zonneveld K. A. F., Coulibaly O., Flintrop C., Grotheer H., Klann M., Knoke M., et al. (2021a). Particles from source to sink off northwest Africa (PASTOSI). Meteor-Berichte.M165, 1–49. doi: 10.48433/cr_m165
Zonneveld K. A. F., Ebersbach F., Maeke M., Versteegh G. J. M. (2018). Transport of organic-walled dinoflagellate cysts in nepheloid layers off cape blanc(N-W Africa). Deep. Sea. Res. Part. II: Top. St. Oceanogr.139, 55–67. doi: 10.1016/j.dsr.2018.06.003
Zonneveld K. A., Marret F., Versteegh G. J., Bogus K., Bonnet S., Bouimetarhan I., et al. (2013). Atlas of modern dinoflagellate cyst distribution based on 2405 datapoints. Rev. Palaeobot. Palynol.191, 1–197. doi: 10.1016/j.revpalbo.2012.08.003
Zonneveld K. A., Meilland J., Donner B., Versteegh G. J. (2021b). Export flux succession of dinoflagellate cysts and planktonic foraminifera in an active upwelling cell off cape blanc (NW Africa). Eur. J. Phycol., 571–18. doi: 10.1080/09670262.2021.1885066
Zonneveld K. A. F., Pospelova V. (2015). A determination key for modern dinoflagellate cysts. Palynology.39, 387–409. doi: 10.1080/01916122.2014.990115
Zonneveld K. A. F., Susek E., Fischer G. (2010). Seasonal variability of the organic-walled dinoflagellate cyst production in the coastal upwelling region off cape blanc (Mauritania): a five-year survey.J. Phyc.46, 202–215. doi: 10.1111/j.1529-8817.2009.00799.x
Keywords: dinoflagellate cysts, fluxes, succession, excystment, dispersal, ecology, upwelling
Citation: Zonneveld KAF, Grotheer H and Versteegh GJM (2022) Dinoflagellate cysts production, excystment and transport in the upwelling off Cape Blanc (NW Africa). Front. Mar. Sci. 9:915755. doi: 10.3389/fmars.2022.915755
Received: 08 April 2022; Accepted: 28 June 2022;
Published: 26 July 2022.
Edited by:
Jose Luis Iriarte, Austral University of Chile, ChileReviewed by:
Manuel Bringué, Department of Natural Resources, CanadaSofia Ribeiro, Geological Survey of Denmark and Greenland, Denmark
Copyright © 2022 Zonneveld, Grotheer and Versteegh. This is an open-access article distributed under the terms of the Creative Commons Attribution License (CC BY). The use, distribution or reproduction in other forums is permitted, provided the original author(s) and the copyright owner(s) are credited and that the original publication in this journal is cited, in accordance with accepted academic practice. No use, distribution or reproduction is permitted which does not comply with these terms.
*Correspondence: Karin A. F. Zonneveld, kzonneveld@marum.de