- 1Marine Systems Modelling, National Oceanography Centre, Southampton, United Kingdom
- 2Centre of Biogeochemistry in the Anthropocene and Department of Bioscience, University of Oslo, Oslo, Norway
- 3Department of Engineering Mathematics, Dalhousie University, Halifax, NS, Canada
- 4Ocean Biogeosciences, National Oceanography Centre, Southampton, United Kingdom
Copepods are a critical component of ocean ecosystems, providing an important link between phytoplankton and higher trophic levels as well as regulating biogeochemical cycles of carbon (C) and nutrients. Lipid-rich animals overwinter in deep waters where their respiration may sequester a similar quantity of C as that due to sinking detritus. This ‘seasonal lipid pump’ nevertheless remains absent from global biogeochemical models that are used to project future ocean-climate interactions. Here, we make an important step to resolving this omission by investigating the biogeochemical cycling of C and nitrogen (N) by high-latitude copepods using a new individual-based stoichiometric model that includes explicit representation of lipid reserves. Simulations are presented for Calanus finmarchicus throughout its life cycle at Station Mike (66°N, 2°E) in the Norwegian Sea, although the model is applicable to any suitable location and species with a similar life history. Results indicate that growth, development and egg production in surface waters are driven primarily by food intake (quantity) which provides a good stoichiometric match to metabolic requirements. In contrast, the main function of stored lipid is to support overwintering respiration and gonad development with these two processes respectively accounting for 19 and 55% of the lipid accumulated during the previous spring/summer. The animals also catabolise 41% of body protein in order to provide N for the maintenance of structural biomass. In total, each individual copepod sequesters 9.6 μmol C in deep water. If the areal density of animals is 15,000–40,000 m-2, these losses correspond to a sequestration of 1.7–4.6 g C m-2 yr-1. Lipids contribute only 1% of the C used in egg production in the following year. Accumulating extra lipid in spring would potentially increase egg production but our analysis suggests that any such benefit is outweighed by a higher risk of predator mortality. Our work indicates that the seasonal lipid pump may be of similar magnitude to C sequestration via sinking particles in the North Atlantic and highlights the need for improved physiological understanding of lipid use by high-latitude copepods in order to better constrain C fluxes in ocean food-webs and biogeochemical models.
1 Introduction
Calanoid copepods lie at the heart of high-latitude marine ecosystems, acting as key grazers on phytoplankton and as a major source of food for higher trophic levels including fish and whales (Bachiller et al., 2016; Plourde et al., 2019). They also play important biogeochemical roles including export of carbon (C) and nitrogen (N) to the ocean interior. Together with other epipelagic consumers, their faecal pellets contribute substantially to the classic biological gravitational pump that promotes ocean C sequestration and storage via organic particles that sink vertically within the water column (Boyd et al., 2019).
A key aspect of these animals is their complex life cycle. In the case of Calanus finmarchicus, which is the main focus of our study, adult females typically spawn immediately prior to, and during, the spring phytoplankton bloom (Niehoff et al., 1999; Mayor et al., 2009a) after which their offspring develop from egg to adult via 6 naupliar and 6 copepodite stages. Towards the end of summer, copepodites, typically stage 5 (CV), interrupt their development and enter into a dormant phase, diapause, which may last for 6-9 months (Hirche, 1991; Halvorsen et al., 2003; Heath et al., 2004; Melle et al., 2014). Copepodites descend into deep waters at the onset of diapause where they persist with reduced metabolic rates and minimise visual predation in the dark, cold environment, before ascending as adults between late winter and early spring to spawn. To successfully diapause and emerge as adults, C. finmarchicus and many other polar copepods accumulate and store substantial lipid reserves (Figure 1) during spring and summer when food is abundant (Kattner and Krause, 1987; Sargent and Falk-Petersen, 1988; Hygum et al., 2000). This storage may account for >60% of their body weight (Sargent and Henderson, 1986). Lipid reserves have been suggested to play multiple roles throughout the copepod life cycle, supplying energy for metabolism during diapause (Baumgartner and Tarrant, 2017), supporting gonad development (Sargent and Falk-Petersen, 1988; Rey-Rassat et al., 2002) and egg production (Niehoff et al., 2002), as well as in buoyancy control (Visser and Jónasdóttir, 1999, Pond, 2012).
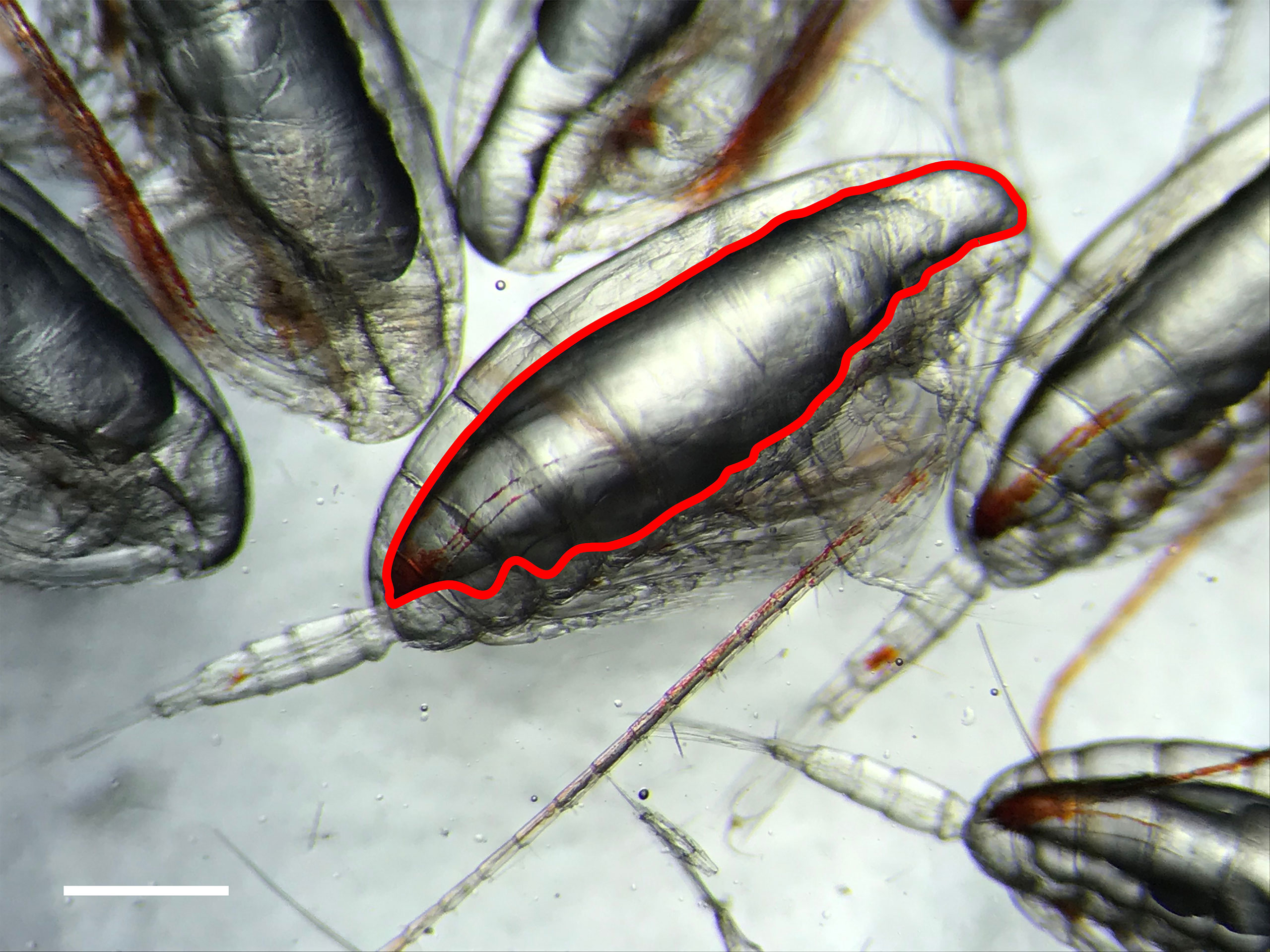
Figure 1 Fat-laden lipid sac (outlined in red) within Calanus finmarchicus; scale bar is 1 mm. (image from Mayor et al., 2020; © D.J. Mayor).
The use of stored lipids to support metabolism in deep waters over winter contributes to C sequestration via the “seasonal lipid pump” which may be similar in magnitude to the gravitational pump at high latitudes (Jónasdóttir et al., 2015; Jónasdóttir et al., 2019). Nevertheless, this process has not been incorporated into the global ocean biogeochemical models that are used to project the future change of marine ecosystems due to natural and anthropogenic factors, along with associated biogeochemical cycling and ocean C sequestration. Our aim here is to pave the way for this incorporation by presenting a new model, LILICOP_1.0 (model of LIpids in the LIfe cycle of a high latitude COPepod, version 1.0), that explicitly represents structural biomass and storage lipids as separate entities, simulating the life cycle of an individual animal throughout its life cycle. The cycling of C is inextricably linked to nutrient elements, notably N, via growth and metabolic processes. Protein (N) intake is essential for zooplankton growth and egg production, while metabolism involves not only energy but also protein costs associated with biomass turnover (Mayor et al., 2022; Lahtvee et al., 2014). Under severe starvation, protein may be catabolised as an additional source of energy (Lemcke and Lampert, 1975). Our model therefore includes both C and N as currencies, with metabolism formulated using the latest state-of-the-art stoichiometric equations (Anderson et al., 2020; Anderson et al., 2021). We use it to quantitatively investigate the different roles of lipid reserves by generating metabolic budgets for C and N throughout the life cycle of an individual high-latitude copepod, C. finmarchicus, from which C sequestration via the seasonal lipid pump is estimated. The model is applicable to any suitable location in the ocean; we use input data for Station Mike (66°N, 2°E) in the Norwegian Sea, which exhibits the characteristic seasonality in primary production at high latitude sites with a spring phytoplankton bloom in May, and which has been well studied in terms of the life cycle of C. finmarchicus and associated egg production (Hirche, 1991; Irigoien et al., 1998; Niehoff et al., 1999).
2 Methods
2.1 Model outline
A new model of high-latitude copepods is developed that incorporates the stoichiometry of lipid storage and use, in conjunction with food protein and carbohydrate, with C and N as currencies. The zooplankton model is forced with food fields and environmental temperature to simulate the life cycle of a calanoid copepod at Station Mike (66°N, 2°E) in the Norwegian Sea. Modelling these animals is challenging because their life history comprises 13 stages, which are simplified to 6 Phases in the model (Figure 2). The non-feeding stages, which include eggs and nauplii (NI and NII), are collectively represented in Phase 1. Feeding and rapid growth takes place from NIII to copepodite stage CII (Phase 2), followed by the continued development to CV during which lipid reserves are laid down (Phase 3). Animals then enter diapause (Phase 4), descending into cool deep waters to minimise metabolic losses and reduce predation. Gonad development takes place in Phase 5, after which animals re-emerge as adults (CVI) in surface waters to spawn eggs in the second year of their life cycle (Phase 6). Each simulation follows the development of a single individual, spawned as an egg on a chosen day of year and thereafter transiting through the different Phases in sequence. The model has the potential to be extended to population level, although population dynamics are not the focus of our study here.
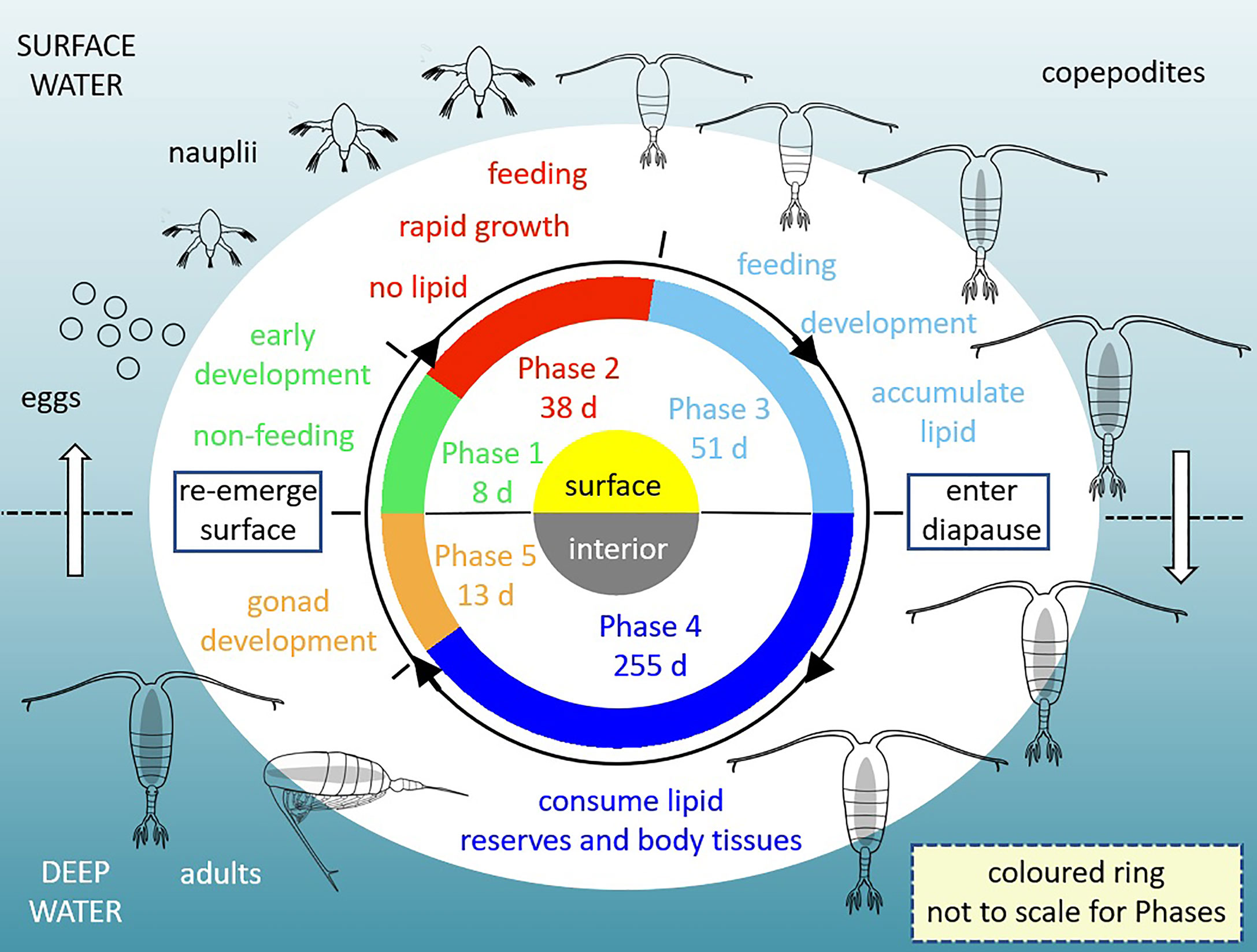
Figure 2 Diagram showing model Phases 1-5 representing the first year in the life history of a Calanoid copepod (outer images; redrawn from Mayor et al., 2020; grey interior represents lipid sac). Phase durations shown are for the standard model run with default parameter values. Egg production in spring and summer of the second year (Phase 6) is not shown.
Copepod biomass is represented by two state variables, structural biomass, ZS, with a fixed C:N ratio of 4.9 mol C mol N-1 (parameter θZS; Tande, 1982; Durbin et al., 1995; Mayor et al., 2009a; Swalethorp et al., 2011) and storage lipids, ZL, that contain only C, with C as the base model currency. For the purpose of calculating biochemical transformations, structural C is subdivided into protein, with a fixed C:N ratio, θV = 3.7, and non-protein C (nominally carbohydrate, although it may also include lipid; Anderson et al., 2020). Growth (increase in structural biomass), development (progression through the Phases) and reproduction are governed by differential equations that explicitly represent the physiology and associated stoichiometry of these processes.
Metabolism, growth and egg production are calculated using the latest developments in metabolic stoichiometry, here including lipid storage as an additional source of C and energy. We provide a brief summary of this approach (Figure 3); the reader is referred to Supplementary Appendix 2 and Anderson et al. (2020), Anderson et al. (2021) for a complete description, including a comprehensive list of equations. Ingested substrates are subject to absorption efficiencies βV = 0.62 and βH = 0.53 for protein and non-protein (carbohydrate and lipid), respectively (Anderson et al., 2021), with the remainder lost as faecal material. Absorbed substrates are prioritised for metabolism which is quantified using explicit terms for biomass turnover, other basal metabolism and specific dynamic action, SDA (Anderson et al., 2017; Anderson et al., 2020; Anderson et al., 2021). Biomass turnover (parameter τT, d-1) is akin to protein turnover and represents tissue breakdown and replacement, requiring C and N in ratio θZS. Other basal metabolism (ξT, d-1) includes basic cellular processes such as production of adenosine triphosphate (ATP) and maintenance of molecular and ionic gradients (Karr et al., 2012). It is an energetic cost that is preferentially met using non-nitrogenous compounds (lipid and carbohydrate), thereby sparing protein for growth. Both τT and ξT are temperature-dependent, calculated using a Q10 relationship (parameter QM = 2.0) where τTref and ξTref are model parameters for rates at the reference temperature, Tref of 10°C. SDA is an additional energetic expenditure that accounts for the costs of feeding, absorption and assimilation (Secor, 2009) and is expressed as a fixed fraction, η, of total C intake. Calculation of growth and egg production is on the same basis as that of biomass turnover, except that new biomass is produced, rather than replacement biomass. Despite the preferential use of non-protein substrates, it is assumed that protein sparing is not 100% efficient such that a fraction of protein, 1- (where is the maximum N net synthesis efficiency), is always lost as excretion. Use of protein in metabolism gives rise to losses of both CO2 via respiration and excretion of N, while use of carbohydrate or lipid results only in release of CO2. If intake is insufficient to meet the costs of metabolism, lipid reserve and structural biomass are used instead. This is necessarily so during Phases 4 and 5 (diapause and gonad maturation) when the modelled copepod is away from the ocean surface and without access to food.
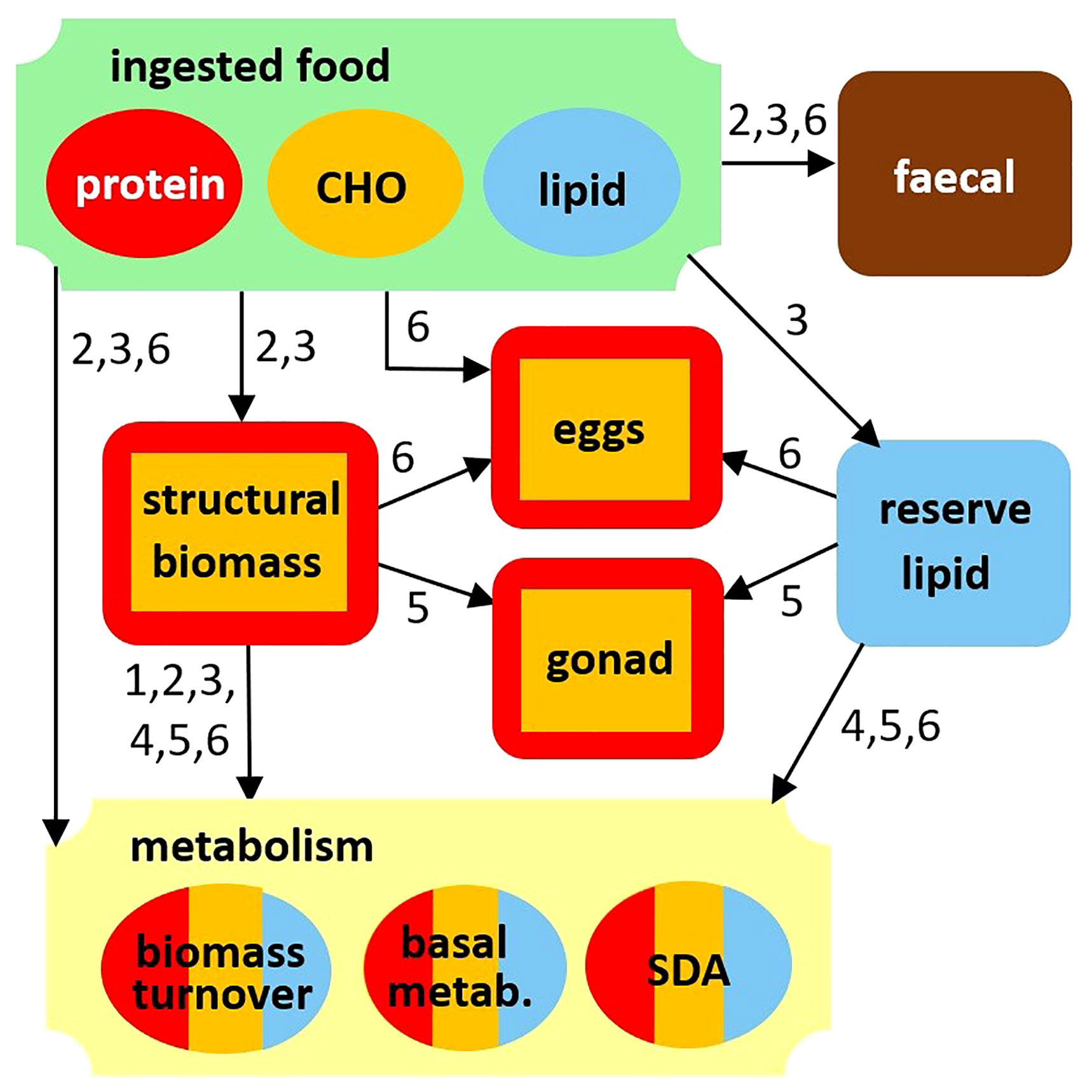
Figure 3 Model diagram showing use of protein (red), carbohydrate (CHO, orange) and lipid (blue) in food, as well as storage lipid and structural biomass, for growth, metabolism and egg production. Numbers refer to the Phases in which particular flows take place.
Limitation of growth and egg production is by either C or N, depending on C:N ratios in zooplankton tissues and food, and utilisation efficiencies for each element which vary with food quantity and temperature. Metabolism is relatively more expensive in terms of C relative to N (has a higher C:N ratio) and so C requirements are relatively higher when food is scarce and metabolism dominates over growth. A threshold elemental ratio (TER) can be calculated which represents the optimal C:N in food in order to meet the combined requirements of growth and metabolism (Anderson and Hessen, 1995; Anderson and Hessen, 2005; Anderson et al., 2021). C limits growth when food C:N < TER, with limitation by N when food C:N > TER. The latter occurs when prey items contain levels of non-protein C that give rise to excess C that is assumed to be respired in order to maintain homeostasis. Protein can never be in excess because it contains both C and N and can always be profitably used for either growth or metabolism. Protein is used as a source of energy when zooplankton are C-limited, in which case the associated N is deaminated and excreted, potentially incurring a metabolic penalty (Anderson et al., 2020).
Stages CIII to CV copepodites of Calanus primarily accumulate storage lipids in the form of wax esters (Kattner and Krause, 1987; Sargent and Falk-Petersen, 1988; Hygum et al., 2000). These lipids create a new dimension to the stoichiometric model, providing an extra source of C to meet energetic costs in metabolism and for growth but require rules for prioritisation throughout the life cycle of the animals (Figure 3). Reserve lipid is accumulated in Phase 3 and is subsequently available in Phases 4, 5 and 6 to support metabolism in diapause, gonad development and egg production. Food C takes priority, followed by lipid, while structural tissues in biomass are only used as a last resort if minimum costs in maintenance cannot otherwise be met.
Given that we are modelling a single individual throughout its life cycle, there is no representation of competition for food, nor do we represent top-down processes, i.e., mortality due to higher predators (except for an ancillary analysis in Section 3.2). The modelled individual can nevertheless die from starvation. Structural biomass is consumed to meet the costs of metabolism when food intake is insufficient and we assume that death occurs if it declines below a critical threshold that we call carcass weight, ZC (μmol C). This is calculated as a fraction, ψ, of the maximum structural biomass achieved by an individual. Chossat’s rule (Chossat, 1843, in Kleiber, 1961) posits that animals die when starvation reduces their body mass by half, based on experiments on a range of vertebrates. Greater losses have been recorded in zooplankton (Threlkeld, 1976; Kirk et al., 1999) and so we set ψ = 0.3. For example, if ZS reaches 5 μmol C, the animal dies if this subsequently drops back to 1.5 μmol C.
The representation of environment in the model is simple, based on Station Mike in the Norwegian Sea. The seasonal cycles of surface food fields (diatoms, non-diatoms, microzooplankton and detritus), together with surface temperature, are taken from an existing high-resolution simulation of the NEMO-MEDUSA model (Yool et al., 2015). Output was extracted for years 2000-2009, a representative 10-year “present-day” period, and was averaged to a climatological year to decrease the importance of interannual variability. Surface food concentration and temperature were calculated as mixed layer depth averages. More details of this simulation can be found in Yool et al. (2015), and a full description of the MEDUSA model is provided in Yool et al. (2013). Deep interior temperature was set to a value of 4°C (Gammelsrød et al., 1992). Note that the model can be used at any suitable ocean location; we show results for Ocean Weather Station India (60°N, 20° W) in Supplementary Appendix 5. The following sections describe the different model Phases in detail noting that the parameterisation is primarily based on C. finmarchicus. Model parameters are presented in Table 1, along with differential equations and associated description in Supplementary Appendix 1. The model code and supporting files are available via an online repository (see Data availability statement).
2.2 Development to copepodite stage CV (Phases 1 to 3)
Each model simulation starts by spawning a single copepod egg in surface waters on a specified day of the year. Prior to diapause, the egg develops through model Phases 1 (non-feeding: eggs and naupliar stages NI and NII), 2 (feeding but without lipid deposition; stages NIII to CII) and 3 (feeding with lipid deposition: stages CIII to mature active CV). Development is driven by temperature and food. Phase 1 depends solely on the former and is modelled using a specified duration of 7 days at a reference temperature of 10°C (parameter L1,Tref; Hirche et al., 2001), adjusted for ambient temperature using a Q10 of 2 (parameter QD). Biomass necessarily decreases during Phase 1 due to the costs of metabolism in the absence of feeding. For all subsequent Phases, the animal can gain weight if there is sufficient food available (ingestion is in excess of metabolic demands). The transitions between Phases 2 and 3, and 3 and 4, are assumed to occur at critical moulting biomasses (Hirche et al., 2001), in common with other stage-structured models (Carlotti and Sciandra, 1989; Carlotti and Radach, 1996; Moll and Stegert, 2007; Eisenhaur et al., 2009; Maps et al., 2010).
Parameters that define the critical masses for the transition between Phases were assigned as follows. Initial egg biomass, Begg, is 0.025 μmol C, derived as the average of 0.026 (average in Mayor et al., 2009b), 0.019 (Hirche, 1996; Hirche et al., 2001) and 0.03 (Harris et al., 2000). Egg C:N is set to θegg = 5.8, which is within the typical range seen in high-latitude copepods of ~5.5–6.5 (Ohman and Runge, 1994; Mayor, 2005; Pond et al., 1996; Rey et al., 2001; Mayor et al., 2009b; Mayor et al., 2022). The end-point values assigned to Phases 1 (equivalent to end of naupliar stage NII) and 2 (copepodite stage CII) are Z1 = 0.021 and Z2 = 0.7 μmol C, respectively (Hirche et al., 2001). The transition from active growth and lipid accumulation (Phase 3) to diapause (Phase 4) is triggered by the attainment of maximum biomass (structure plus lipid; Irigoien, 2004; Pond et al., 2012; Häfker et al., 2018). Measured values for this biomass for C. finmarchicus CV vary widely between ~100-250 μg C (Harris et al., 2000; Campbell et al., 2001; Hirche et al., 2001; Gislason, 2005; Mayor et al., 2006; Häfker et al., 2018). We use a value of 16 μmol C (192 μg C). Using this value in combination with a C:N ratio immediately prior to diapause of 12 (parameter θZ3; Tande, 1982), total biomass comprises 6.5 μmol C as structure (parameter Z3S; calculated using θZS = 4.9) and 9.4 μmol C as lipid.
Feeding is quantified using a multiple-prey Sigmoidal (Holling III) functional response (Gentleman et al., 2003) that is defined by a maximum grazing rate, gT, half saturation constant, kg, and grazing preference parameters ωPn, ωPd, ωZmi and ωD for non-diatoms, diatoms, microzooplankton and detritus, respectively. The maximum grazing rate is temperature-dependent, again calculated using a Q10 of 2 (parameter QI) and a reference value at 10°C, parameter gTref. The use of ingested food for growth and metabolism is calculated using metabolic stoichiometry, as described above, noting that metabolic rates are also temperature-dependent (Q10 function; parameter QM).
Parameters for the multiple-prey functional response for grazing were derived as follows. Maximum specific ingestion rates decrease with increasing body size in C. finmarchicus, from ~0.5 d-1 in nauplii and early copepodite stages, to ~0.3 d-1 for late copepodite stages and adults (Gamble, 1978; Harris et al., 2000; Irigoien et al., 2003; Castellani et al., 2008). The latter value is normalised against total biomass, i.e., structure plus lipid. Our rate in the model is specified with respect to structural biomass only and is as a consequence higher because structure is only a fraction of the total. We therefore use a maximum grazing rate, parameter gTref, of 0.5 d-1 for all stages, modulated by a Q10 of 2 for temperature-dependence (Gamble, 1978; Carlotti and Radach, 1996). This value of gTref also corresponds to the maximum specific grazing rate used in the MEDUSA marine ecosystem model (Yool et al., 2013). Assigning a value for the so-called half-saturation constant of a multiple-prey functional response is complicated because it relates to a measure of total food that is preferentially weighted for the different food types. We set kg = 1 mmol C m-3; for details of the derivation of this parameter value see Supplementary Appendix 3. A sensitivity analysis on functional response parameters is presented in Supplementary Appendix 4. Remaining grazing parameters are losses to “messy feeding”, ϕ = 0.2, and grazing preference parameters ωPn, ωPd, ωZmi, ωD = 0.15, 0.35, 0.35, 0.15 (Yool et al., 2013). These preference parameters dictate the actual density-dependent prey preferences (i.e. proportion in the diet vs. environment) and derive from differences among Holling’s attack rate for each single prey type (Gentleman et al., 2003).
An advantage of using metabolic stoichiometry to calculate copepod growth and metabolism is that values for the associated model parameters can be assigned directly from observation and experiment. We use temperature-dependent rates of biomass turnover and basal metabolism (for a reference temperature of 10°C) of τTref = 0.032 d-1 and ξTref = 0.015 d-1, respectively. These values are based on a survey of turnover rates in starved animals for various Calanus species in which average rates for C and N turnover were 0.047 and 0.032 d-1 for C and N, respectively (Mayor et al., 2022). Biomass turnover equals the N rate and other basal metabolism is the difference between the C and N rates. The associated Q10 for temperature-dependence is QM = 2.0 (Anderson et al., 2017). SDA, parameter η, is assigned a value of 0.12 (Thor et al., 2002; Anderson et al., 2020). Finally, maximum N utilisation efficiency, = 0.9 (Anderson et al., 2020; Anderson et al., 2021).
Accumulation of reserve lipids takes place in Phase 3 and is configured in the model to account for lipids obtained both directly in the diet and those synthesised de novo (Graeve et al., 2020). Fractions of protein, carbohydrate and lipid in food are calculated for each food type based on C:N ratios. The protein fraction is calculated from protein C:N ratio, θV = 3.7, along with C:N ratios of θP = 6.625 for phytoplankton (the Redfield ratio) and θZmi = 5.5 for microzooplankton (Verity, 1985), with a variable ratio in detritus. Remaining non-protein C is subdivided into carbohydrate and lipid. Data indicate that the lipid fraction of total C in food is ~0.25 from data (Opute, 1974; Grosse et al., 2017; Jónasdóttir, 2019). If the total non-protein C is ~0.5 (based on the C:N ratios above), the lipid fraction of non-protein C is then 0.5 (parameter ν). This lipid fraction is prioritised for synthesis of storage lipids throughout Phase 3. Protein and carbohydrate in food are allocated to structural growth until the maximum structural biomass (Z3S) is reached and thereafter, beyond the requirements of maintenance, are used for de novo lipid synthesis. The synthesis efficiency of storage lipids, parameter γL, is assumed to be high, corresponding to a theoretical maximum of 0.75 (Calow, 1977).
2.3 Diapause and reproduction (Phases 4 to 6)
The modelled copepod enters diapause (Phase 4) when it reaches lipid-replete CV status. At this point, it is assumed to descend into deep waters although there is no explicit representation of the vertical water column in our model. The simulated animal has no access to food during diapause and so metabolic costs are preferentially met using lipid reserves, and if these run out then by consuming structural biomass. Respiring lipid generates CO2 while utilisation of biomass also gives rise to excretion of N. Metabolic rates in diapause are significantly lower than in surface waters, even when adjusted for the lower temperature in the deep water column. Other basal metabolism, parameter ξdiaTref, is assigned a value of 0.0016 d-1 based on the rate of wax ester turnover in diapausing C. finmarchicus in Loch Etive, Scotland (Mayor et al., 2022) and is consistent with respiration rates decreasing by ~20-fold over winter in Calanus CV (Ingvarsdöttir et al., 1999). Copepod C:N ratio declines over winter (Tande, 1982) indicating that metabolic losses during diapause are dominated by basal metabolism which is solely an energetic cost that can be met using lipid, in contrast to biomass turnover which requires both C and N. Likewise, Freese et al. (2016) noted that the protein content of Calanus glacialis did not change significantly over winter in an Arctic fjord. We therefore set the rate of biomass turnover during diapause, τdiaTref, to a nominal value of 0.0003 d-1, 1% of the non-diapausing value. Parameters τdiaTref and ξdiaTref are temperature-dependent, as per their surface water equivalents. Diapausing animals do not feed such that there are no associated energetic costs and SDA is zero.
In preparation for reproduction, gonad development takes place during the transition from stage CV copepodite to adult (Phase 5). The precise timing of advanced gonad development is not well known. It may occur entirely prior to arrival back in surface waters in which case internal lipid reserves are used for metabolism or, alternatively, may be fueled, at least in part, by food after animals return to the ocean surface (Niehoff, 2007). We adopt the former scenario and assume that the main costs of gonad maturation are incurred while copepods remain deep in the water column and during ascent to the surface, over a specified period, Lgonad = 14 days (e.g., Plourde and Runge, 1993). Although no feeding takes place during this period, we assume that Phase 5 animals return to an active state and that their metabolic rates revert to non-diapause values (τTref = 0.032 d-1 and ξTref = 0.015 d-1). For simplicity, we assume that the C:N ratio of gonad tissue is the same as that of structural biomass. This being so, the process of gonad maturation can be modelled as equivalent to biomass turnover where structural biomass is replaced with gonad tissue. Gonad biomass synthesis and associated energetic costs are represented by parameters τgonadTref and ξgonadTref, respectively, where the latter is supported by the use of storage lipid to meet energetic costs. Providing values for these parameters is not straightforward due to lack of empirical studies, although gonad development is known to be energetically expensive (Rey-Rassat et al., 2002). We base our parameterisation on the observation that copepod C:N is ~8 when animals reappear in surface waters (Tande, 1982), i.e., at the completion of gonad development in the model (end of Phase 5). The maturation parameters were tuned to achieve this C:N, keeping them in the same proportion as for surface metabolism, resulting in τgonadTref = 0.11 d-1 and ξgonadTref = 0.055 d-1. Re-emergence in surface waters takes place on a specified day in spring of the following year, Demerge.
The modelled copepod is assumed to arrive back in surface waters as an adult on completion of gonad development. Feeding resumes and the resulting intake is used to fuel egg production throughout the year (Phase 6), continuing until food density decreases to a level that is insufficient to meet the costs of maintenance. At this point, the animal has to start using structural biomass to meet these costs. Body weight decreases and death by starvation occurs when it reaches carcass weight. The calculation of egg production as a function of ingested food is the same as for structural growth in Phases 2 and 3, using metabolic stoichiometry, with two additional considerations. First, egg C:N (θegg = 5.8) is greater than that of structural biomass (θZS = 4.9). Second, lipid reserves are used to supplement ingested food if needed to offset C limitation (these reserves were accumulated, rather than utilised, during development in Phase 3). Animals focus solely on egg production in Phase 6, with no growth in terms of structural biomass or accumulation of lipid reserves.
3 Results
3.1 Simulated life cycle
The simulated development of a single C. finmarchicus individual, spawned as an egg on day 120 at Station Mike, is shown in Figure 4. This represents an ideal start date as the spring phytoplankton bloom was taking off at this time, peaking about 30 days later at a biomass of 19.4 mmol C m-3. Food is dominated by phytoplankton which account for 77% of the total (annual average), with contributions of 17% and 6% by microzooplankton and detritus, respectively (note that the food field is supplied as weekly averages, leading to the somewhat jagged appearance in the Figure). Development through Phases 1, 2 and 3 took 8, 38 and 51 days, respectively (Figure 4A), noting that surface temperature varied between 6 and 11°C. Total development time to lipid-replete CV was thus 97 days. Predicted intake and growth rates (structure + lipid) increased steadily to 2.4 and 0.56 μmol C d-1, respectively, during this period as the animal gained weight, reaching peak structural biomass of 6.5 μmol C on day 206 and total CV biomass (structure plus lipid) of 16 μmol C on day 217 (Figure 4B). At this point, it entered diapause.
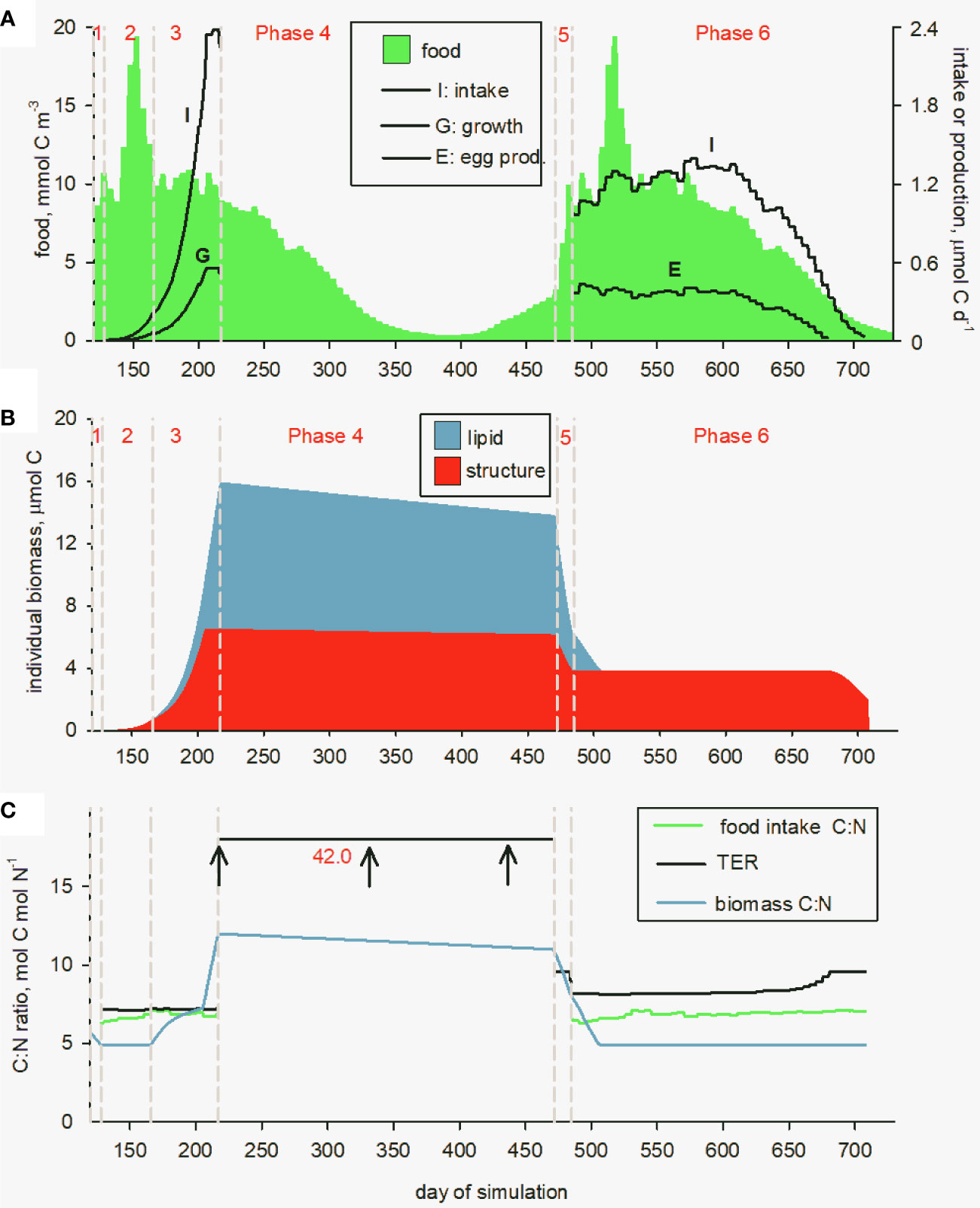
Figure 4 Predicted development of a C. finmarchicus individual throughout its life cycle at Station Mike. (A) Intake (I), growth (G) and egg production (E), along with the concentration of available food from the MEDUSA model output (green); (B) structural biomass (red) and lipid reserves (blue); (C) comparison of C:N ratios for food intake (green), copepod biomass (structure + lipid; blue) and the Threshold Elemental Ratio (TER; black).
Based on metabolic rates in diapause of 0.0011 and 0.0002 d-1 for basal metabolism and biomass turnover at 4°C (standard rates of 0.0016 and 0.0003 d-1 adjusted for temperature), predicted lipid and structural biomass decreased by 1.8 and 0.35 μmol C during diapause (Phase 4), respectively. This loss of structural biomass, which necessarily occurs because protein turnover involves both C and N and cannot therefore be met using lipid, is relatively low but nevertheless accounts for 16% of the total C consumed. Gonad development was in comparison expensive, with predicted release of CO2 due to respiration of 5.1 and 2.3 μmol C via lipid and structural biomass, respectively. The copepod thus lost 6.9 from the available 9.4 μmol C lipid during diapause and gonad development (19 and 55% during Phases 4 and 5, respectively), as well as 2.7 from 6.5 μmol C structural biomass (41%).
Returning to surface waters on day 120 of the second year of the simulation as a mature female, the modelled copepod produced a total of 2513 eggs over the next 195 days, at an average of 12.9 eggs d-1 during the spawning period. A total of 2.5 μmol C of storage lipid was consumed in the process (Figure 4B). The animal finally ran out of sufficient food to meet the costs of metabolism and died of starvation on day 711 of the simulation (day 346 of the second year) after decreasing in size below carcass weight.
The predicted stoichiometry of the C. finmarchicus individual throughout its life cycle is shown in Figure 4C. As an egg, it starts out with a C:N ratio of 5.8 which declines to 4.9 as energy is consumed during non-feeding development (Phase 1). The ratio then increases to 12 as lipid reserve is laid down in Phase 3, followed by a decline to 8.1 as the lipid is subsequently respired during diapause and gonad development. Remaining lipid is used in egg production after which C:N ratio returns to the baseline value of 4.9 for structural biomass. The C:N of food intake averaged 6.8. The calculated TER was only slightly above this intake ratio during Phases 2 and 3, averaging 7.5, indicating that copepods are limited by C, i.e., food quantity, but only marginally so meaning that food was a good stoichiometric match to requirements (Mayor et al., 2009b; Anderson et al., 2021). The strength of C limitation increased for egg production because egg C:N > structural biomass C:N (5.8 and 4.9, respectively), resulting in rapid exhaustion of the remaining post-diapause lipid in Phase 6. The predicted TER increased from 8.5 to 10.9 during this Phase because, with decreasing food availability, the energetic demand for C becomes an increasingly large proportion of an animal’s metabolic budget (Anderson et al., 2017; Anderson et al., 2020). The concept of TER usually represents an ideal food C:N ratio. This is evidently not so for the non-feeding animals during diapause and gonad development. We nevertheless show the TER of 42.0 which in this case represents the high C:N demands in maintenance.
Budgets for C and N throughout the different Phases of the copepod’s life cycle are shown in Figure 5. All fluxes ultimately originate from food intake in Phases 2, 3 and 6 and Phases 3 and 6 therefore dominate the overall C budget in terms of quantity. Predicted C GGE is 0.3, 0.25 and 0.28 for these three Phases (combined height of the orange, pale blue and pink bars), consistent with typical values for copepods (Straile, 1997). GGE for N is 0.41 and 0.33 in Phases 2 and 6, higher than that for C because the absorption efficiency for protein is greater than that of carbohydrate (βV = 0.62, βH = 0.53) and due to the relatively greater costs of C in metabolism. These values of N GGE are consistent with previous empirical and modelling studies (Kiørboe, 1989; Anderson et al., 2021). The growth efficiency for N is however only 0.13 in Phase 3 because animals are accumulating lipid at this time in which case food N is in excess and excreted. In contrast to Phases 3 and 6, the total C fluxes in Phases 4 and 5 are small, 2.1 and 12.8 μmol respectively, noting these fluxes are nevertheless important because C losses via respiration have the potential to be sequestered in the ocean interior. The contribution of lipid C to egg production (Phase 6) was minor (about 1%), with most C supplied via food intake.
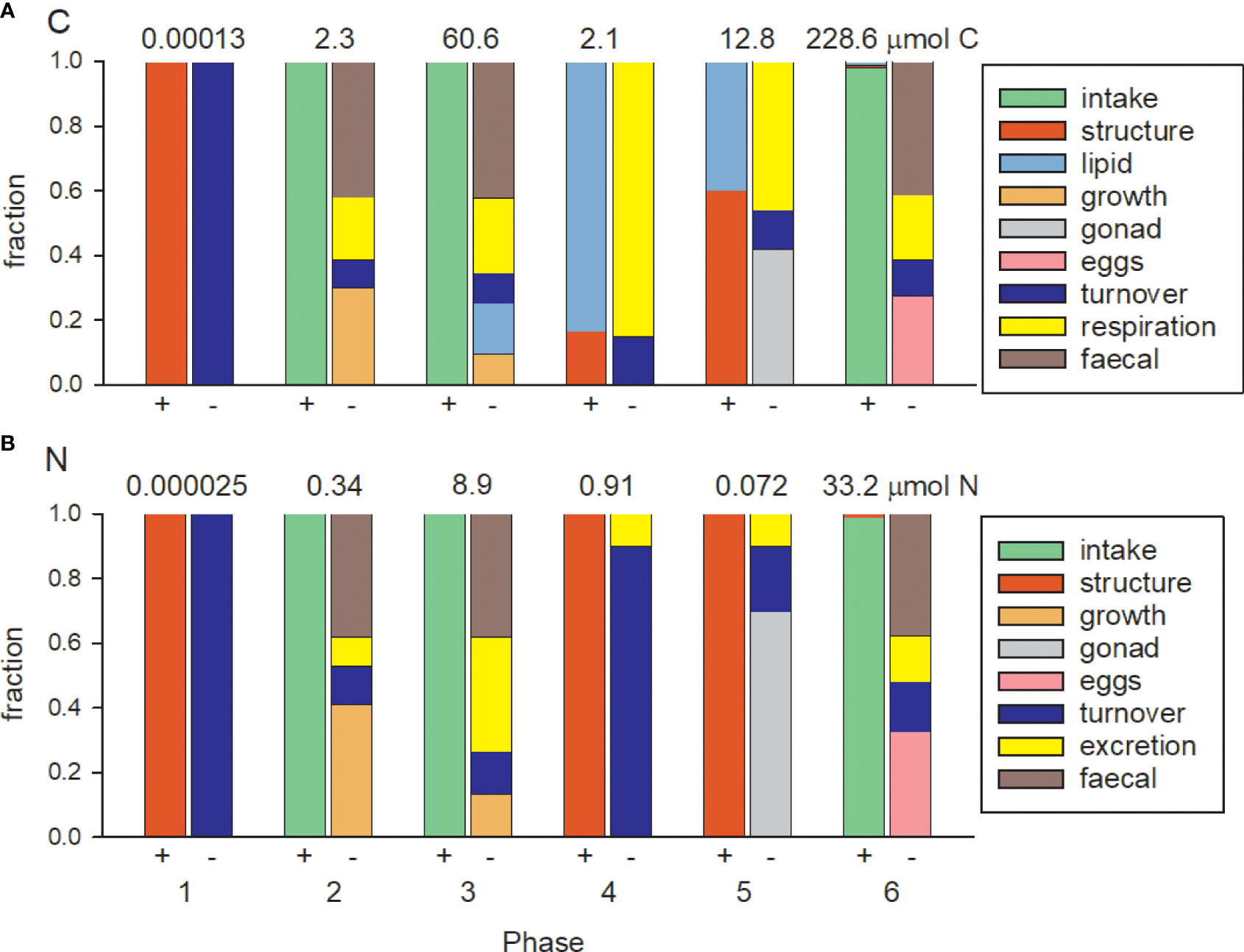
Figure 5 C and N budgets by Phase. The bars are normalised to total C and N fluxes showing source (-) and sink (+) terms. Values for the total fluxes, which balance for each Phase, are shown above each pair of bars. (A) carbon: intake, structural biomass, lipid, structural growth, biomass turnover (contributes to respiration), direct respiration of CO2, faecal, gonad and eggs; (B) nitrogen: intake, structural biomass, structural growth, biomass turnover (contributes to excretion), direct excretion, faecal, gonad and eggs.
3.2 Lipid and egg production: Further analysis
The results presented above indicate the importance of reserve lipids in diapause and gonad development, whereas their role in egg production is less clear. The associated stoichiometric analysis suggests that limitation of egg production is by C in which case reserve lipid ought to be of benefit, complementing food resources. However, only 2.5 from the accumulated 9.4 μmol C lipid (replete CV) remained after diapause and gonad development and so the overall contribution to predicted egg production was small. Would copepods therefore benefit from laying down greater lipid reserves in spring in order to maximise egg production in the following year? We investigate this question here. As a prelude, two initial simulations were carried out as sensitivity tests. In the first, the modelled copepod was denied access to stored lipid in Phase 6. Total predicted egg production was 2426, a decrease of only 3.5% from the 2513 eggs predicted in our standard simulation. On the other hand, if the copepod is supplied with unlimited lipid during this Phase, in which case egg production is limited by food N, total predicted egg production reached 3070, an increase of 22%.
Accumulating additional lipid reserves means feeding for longer in spring and thereby entails risk from exposure to mortality from predators. As such, we propose that there is a tradeoff between the benefit of increased lipid for egg production and the risk of predation. We investigate this tradeoff by introducing a mortality term, mZ, which is the daily probability of an individual copepod dying due to predation during development (Phases 1 to 3), prior to diapause. Thus, the probability of survival on any one day is 1-mZ and over a period of, for example, 80 days is (1-mZ)80. Model simulations were carried out in which the maximum reserve lipid at full-size copepodite stage CV (Z3L; completion of Phase 3, immediately prior to diapause) varied between 5 and 25 μmol C (by adjusting the corresponding C:N ratio, parameter θZ3). For comparison, Z3L with default parameters is 9.4 μmol C. Model solutions are shown in Figure 6 for mZ = 0, 0.01, 0.02 d-1, which are conservative rates for copepods (Hirst and Kiørboe, 2002; Cruz et al., 2021). Increasing the size of the lipid pool means that more time is spent feeding and so predicted development times (Phases 1 to 3) are markedly longer, from 89 days for Z3L = 5 μmol C, to 128 days for Z3L = 25 μmol C (Figure 6A). If Z3L is decreased below the default setting, e.g., to 5 μmol C, there is not enough lipid to support metabolism through diapause and gonad development (Phases 4 and 5) and the animal arrives back in surface waters with no lipid reserve and in poor condition because structural biomass is used to meet the metabolic demands over winter (Figure 6B). In contrast, if Z3L is increased to 25 μmol C, then 19.3 μmol C are predicted to remain, in which case copepod C:N ratio is 29.5. If mortality is zero, then predicted egg production increases with increasing lipid availability, up to 3106 eggs for Z3L = 25 μmol C (Figure 6D). Imposing even low mortality rates drastically reduces survival probability during the spring period, with calculated rates decreasing markedly with increasing development time (Figure 6C). Average fecundity, calculated by multiplying egg production of a surviving individual by survival probability, then decreases with increasing lipid storage for m = 0.01, 0.02 d-1 (Figure 6D). In other words, the analysis indicates that the potential benefit of extra lipid for fueling egg production on emergence from diapause in early winter is outweighed by the predation risk when accumulating that lipid in surface waters during spring.
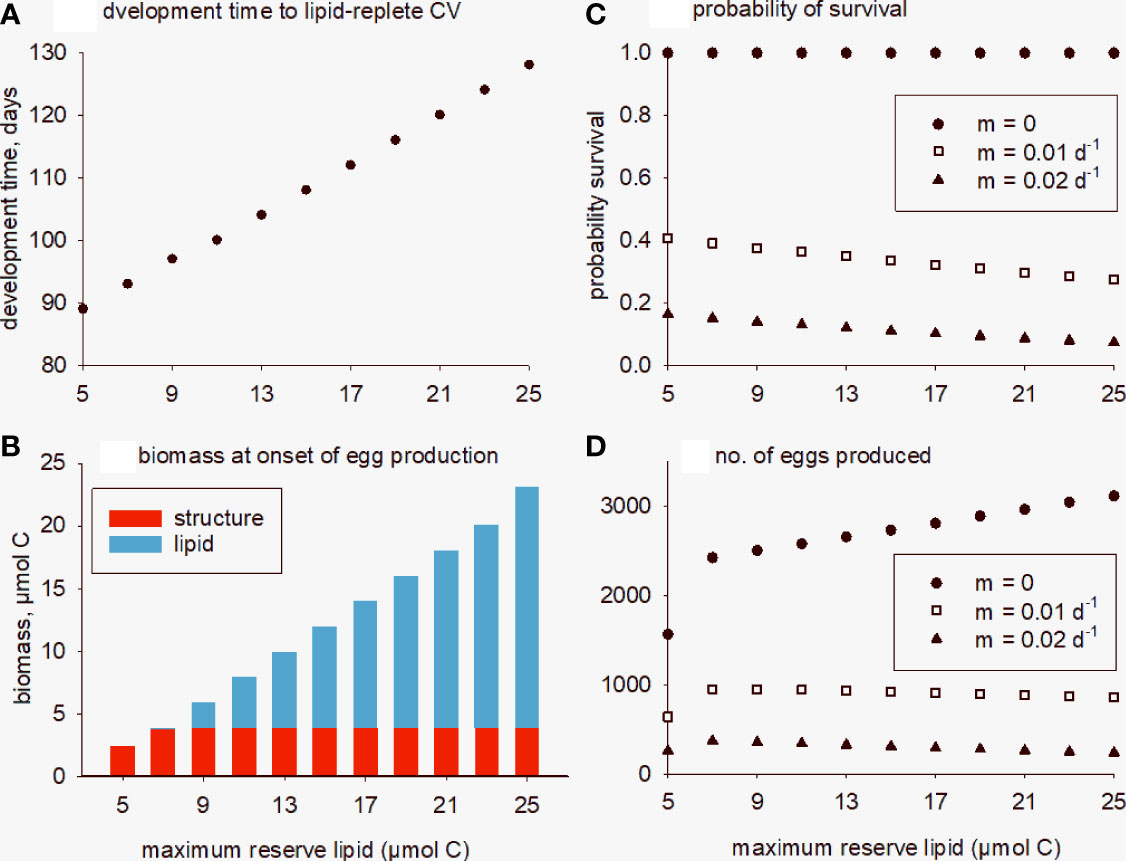
Figure 6 Model simulations in which lipid biomass at full-size copepodite stage CV (Z3L) was varied between 5 and 25 μmol C, noting that the default value is 9.4 (close to the third point/bar). (A) development time through Phases 1-3; (B) biomass (structure + lipid) remaining at the onset of egg production (Phase 6); (C) probability of individual survival based on development time and mortality rates of mZ = 0, 0.01, 0.02 per day; (D) average fecundity taking into account survival probability.
3.3 Development in spring
Thus far, we have examined the metabolic and stoichiometric requirements throughout the life history of an individual that was spawned as an egg on day 120 and established the significance of development time in spring as a driver for survival and reproductive success in C. finmarchicus. We now examine how egg spawning date, in conjunction with food density and temperature, influence development time and the ability of an animal to successfully build up sufficient biomass, including lipid, to enter diapause. Model simulations for copepods spawned on days 60, 90, 120 (default), …, 240 are shown in Figure 7. All except the last two successfully reached lipid-replete stage CV (completion of Phase 3; biomass of 15.9 μmol C), at which point they entered diapause. Predicted development times were 146, 112, 97, 95 and 101 days for the animals spawned on days 60, 90, 120, 150 and 180, respectively. Insufficient food, combined with declining temperature, meant that the animals spawned on days 210 and 240 failed to mature and died of starvation after being overwhelmed by metabolic costs.
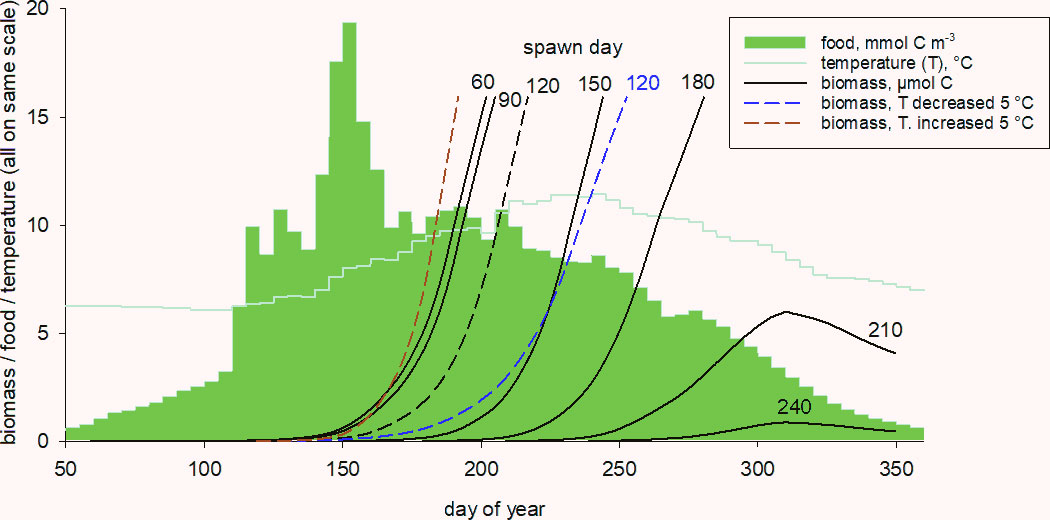
Figure 7 Predicted biomass (structure + lipid) development in spring to full-size CV (end of Phase 3) for animals spawned on days 60, 90, 120, …, 240. The default setting (day 120) is subjected to a sensitivity analysis where temperature is decreased/increased by 5°C (colour dashed lines). Seasonal food fields and temperature also shown (all plots are on the numerical same scale, although with different units).
Sensitivity experiments were carried out for the animal spawned on day 120, subjecting it to temperatures of ± 5°C relative to the standard seasonal pattern. Predicted development time was markedly affected, increasing from 97 to 133 days when temperature was decreased by 5°C, while decreasing to 72 days for plus 5°C (Figure 7). The predicted metabolic budgets for C and N at the three temperatures were near identical when summed over the development period. Metabolism and intake respond to increasing or decreasing temperature in equal proportion if the two processes have the same Q10, in which case growth, which is the difference between intake and metabolism, proceeds faster or slower (Anderson et al., 2017). Although development time decreases or increases accordingly, the total amount of food required to complete development remains the same noting that small differences exist between the simulations at different temperatures because animals developing more slowly are exposed to diminishing food quantity.
The relationship between egg spawning date and development time is shown in Figure 8, for standard seasonal temperature and ± 5°C, along with a model simulation in which food density was doubled. The ranges in spawn date represent the bounds for successful completion of development and were 39-191, 30-153 and 47-216 for standard temperature, decrease and increase of 5°C, respectively, and 21-229 when food was doubled. Eggs spawned before day 100 have a longer development period because of relatively low temperature and food scarcity. The earlier minimum start day when temperature was decreased by 5°C is because metabolic rate is less at low temperature in which case copepods can get by on a smaller food ration. Decreasing food availability at the end of the year likewise increases development time. Doubling food increases the window at either end of the year for spawning eggs that will successfully mature to full-size CV. We also carried out simulations in which food density was halved. In that case, all individuals, regardless of spawn date, failed to mature. Overall, the results highlight the importance of both food availability and temperature in the timing of copepod development.
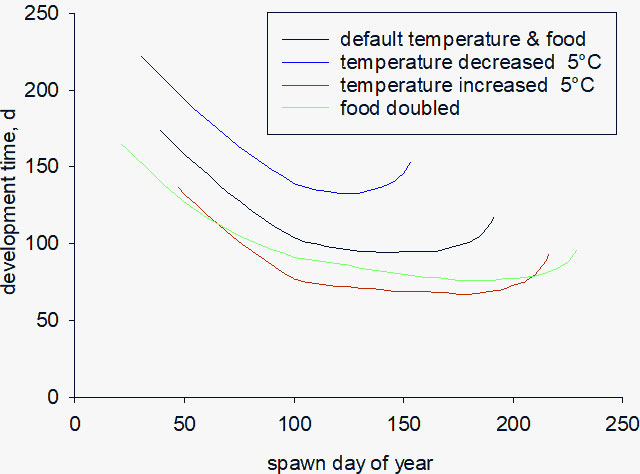
Figure 8 Relationship between development time and day eggs are spawned, showing simulations with default forcing for temperature and food (black line), temperature decreased or increased by 5°C (blue, red), and doubling of food density (green).
4 Discussion
Polar copepods play a critical role in driving the dynamics and biogeochemistry of high-latitude ecosystems. Their current representation in global biogeochemical models is nevertheless simplistic, typically as a single “mesozooplankton” variable without explicit representation of multiple life history stages or the dynamics of stored lipid. A fixed C:N ratio in biomass, i.e., homeostasis, is usually assumed (e.g., Yool et al., 2013; Alver et al., 2016) based on experimental budgets that have been constructed for non-lipid-storing copepods (Kuijper et al., 2004; Anderson et al., 2017) or copepods with exhausted lipid reserves (Mayor et al., 2009a; Mayor et al., 2009b). The resulting equations used for trophic transfer and export flux are inconsistent and simplified, compromising our ability to quantify C export and sequestration in the deep ocean and how they may respond to climate change driven by natural and anthropogenic factors (Anderson et al., 2013). Lipid storage by zooplankton fundamentally alters the C and N cycling landscape (Tande, 1982; Aubert et al., 2014), noting that the “seasonal lipid pump” may sequester similar amounts of C in deep water as the flux of sinking particles (Jónasdóttir et al., 2015; Jónasdóttir et al., 2019; Boyd et al., 2019). Nevertheless, this fundamental process remains absent from contemporary marine biogeochemical models. Here, we developed a new model of high-latitude copepods, focusing on Calanus finmarchicus as a representative species, which includes explicit representation of lipid storage and multiple life history stages, paving the way for incorporation of the seasonal lipid pump into global biogeochemical models. The model formulations are robust, employing a wealth of observed estimates for rate parameters including the latest values for metabolism of C and N (Mayor et al., 2022) in conjunction with state-of-the-art stoichiometric equations for zooplankton growth, respiration and excretion (Anderson et al., 2020; Anderson et al., 2021). The model was successfully run to simulate the entire life-cycle of an individual high-latitude copepod, including lipid dynamics and rates of development, using key physiological parameters derived from observation and experiment. This achievement is by no means a given outcome and the exercise in doing so both endorses the chosen parameter values and associated observations, such as measurements of copepod metabolic rates, and highlights key areas of uncertainty that warrant future research.
4.1 Growth and development to stage CV
Predicted development time from egg to lipid-replete CV, for an animal spawned on day 120 and driven by food and temperature, was 97 days, consistent with the field observations for C. finmarchicus (Tande, 1982; Hirche et al., 2001). The lipid reserves accumulated during this period were sufficient to meet the costs of metabolism during diapause, as well as requirements for gonad development that occurs prior to arrival back in surface waters. In contrast, the animal is predicted to die of starvation if lipid reserves are artificially removed at the onset of diapause, leaving gonad development incomplete and with zero egg production. Predicted growth and development to lipid-replete stage CV (biomass of 16 μmol C that includes 9.4 μmol C reserve lipid) are driven by food availability, mediated by the effect of temperature on intake and metabolism. Food, being rich in protein, is shown to provide a good stoichiometric match to metabolic requirements. The calculated Threshold Elemental Ratio, which represents the optimal food C:N ratio at a given point in time, averaged 7.5 in spring and early summer (Phases 2 and 3), slightly higher than food C:N which remained close to the canonical Redfield ratio of 6.625 throughout the year. This result indicates that the growth of C. finmarchicus is limited by C, although only marginally so, as we have previously shown for the non-lipid storing copepod, Acartia tonsa (Anderson et al., 2021). Limitation by C is more straightforwardly thought of as limitation by food quantity because growth increases with total intake of organic matter, irrespective of its C:N ratio, because all organic matter contains C by definition (Anderson et al., 2021). The accumulation of lipid reserves imposes an additional constraint on development in spring. If zooplankton are limited by N, lipid could be generated from the stoichiometric excess of C intake (Hessen and Anderson, 2008). In the case of limitation by C, however, lipid accumulation imposes an extra demand for food, increasing development time to mature CV from 88 days (the outcome of an ancillary simulation in which the model was run without lipid reserve) to 97 days (the result of our standard simulation).
Animals that inhabit highly seasonal environments need to ensure that the timing of reproduction coincides with available food resources (Varpe et al., 2007; Seebens et al., 2009). Our model results indicate a “window of opportunity” of 152 days (day of year 39-191) at Station Mike that allows C. finmarchicus individuals to develop and successfully acquire sufficient lipid reserves before entering diapause. Spawning either too early or too late leads to food scarcity, incomplete development and starvation (Figure 7; Varpe et al., 2007; Tarling et al., 2022). Our simulations showed that even lipid-replete CVs were unable to sustain metabolism over winter when prevented from entering diapause, experiencing negative metabolic balance and death.
Future climate change will likely alter the seasonal timing of food resources and, without rapid acclimation to changed conditions, will lead to trophic mismatch, reduced fecundity and a decline in the population sizes of animals (Edwards and Richardson, 2004). This may be particularly so for copepods, given that the progression of phytoplankton blooms is closely coupled to ocean physics and its meteorological forcing (Henson et al., 2010; Lewandowska et al., 2014). We used a Q10 of 2.0 to model the temperature-dependence of copepod development rate (Gamble, 1978; Carlotti and Radach, 1996). The predicted window of opportunity for individual survival decreased and increased in breadth to 123 and 169 days, respectively (day of year 30-153 and 47-216) when model simulations were carried out with a cooling and warming of 5°C. Likewise, doubling food availability promotes faster development, giving rise to a window of 208 days (day of year 21-229). Our results highlight the importance of understanding the phenology of zooplankton life history and reproduction, and their coupling to environmental forcing, if the impact of climate warming on marine ecosystems, and associated biogeochemistry, are to be successfully represented in ocean models.
4.2 Diapause and gonad development
Model results indicate that the main function of stored lipids in copepods is to support copepod metabolism and development during diapause and gonad maturation, with 1.8 and 5.1 μmol C consumed during these two phases, respectively (corresponding to 19 and 55% of the 9.4 μmol C lipid accumulated in spring). Stored lipids are ideal for meeting the energetic costs of metabolism but are unlikely to offset protein (N) requirements arising from biomass turnover, the magnitude of which is poorly known. A further 0.35 μmol C originating from maternal biomass was therefore released as CO2 during diapause, with an associated 0.07 μmol excretion of N. Accurately quantifying metabolic rates is essential for understanding lipid use by high-latitude copepods and the associated biogeochemical cycling and sequestration of C. Our default values for basal metabolism and biomass turnover for non-diapausing C. finmarchicus (in surface waters) are 0.032 d-1 and 0.015 d-1 at a reference temperature of 10°C, based on data from incubation experiments involving starved copepods (Mayor et al., 2022). Metabolic rates in diapause may be ~20 fold lower (Ingvarsdöttir et al., 1999) as a consequence of mechanisms of metabolic downregulation (Podrabsky and Hand, 2015) including suppression of mitochondrial function and metabolism (Hand et al., 2018). We set basal metabolism during diapause to 0.0016 d-1, 20 times less than that for non-diapausing animals, while biomass turnover was assigned a value of 0.0003 d-1, 100 times less. These values must be considered tentative noting that other studies, with equally uncertain parameter estimates, have suggested that metabolic activities during diapause may be reduced by only 50-70%: Maps et al., 2014; Freese et al., 2016).
Gonad development begins several months before copepods ascend back to the ocean surface (Hirche, 1996; Niehoff et al., 2002), although a large part takes place in the final weeks (Tande, 1982; Rey-Rassat et al., 2002). The last moult occurs at depth or during ascent (Ingsvardöttir et al., 1999). In the model, we assumed that gonad development follows after diapause and takes place prior to re-emergence in surface waters and thereby in the absence of food. The process is energetically expensive (Rey-Rassat et al., 2002) and so diapause was terminated and pre-diapause metabolic rates reinstated during this period of development. The modelled costs of gonad development and metabolism were substantial, consuming 5.1 μmol C reserved lipid (55% of the 9.4 μmol C accumulated in spring) and net 2.3 μmol C maternal biomass (35% of the 6.5 μmol C at mature CV). It is essential to know where, i.e., at what depth, this energy-expensive process takes place because it will only contribute to C sequestration via the seasonal lipid pump if it occurs at depths where mixing does not return it to surface waters.
Many studies of high latitude copepods, including the present one, principally view lipids as an energy store for overwintering. While this is undoubtably the case, such an energy-centric approach may obscure our appreciation of their wider role in facilitating other important aspects of their life histories. There is growing awareness that copepods carefully regulate and reconfigure the composition of their lipids to confer changes in their density, potentially generating periods of negative, neutral and positive buoyancy (Pond, 2012). In the case of gonad development, achieving this at depth by catabolizing >50% of their lipid reserve seems unlikely if the same lipid is also required for the maintenance of neutral buoyancy and/or the provision of positive buoyancy to help the animal re-ascend from diapause. Better understanding how, why, when and where high latitude copepods use their prodigious lipid reserves is essential for improving their representation in mechanistic models of the seasonal lipid pump and increasing confidence in projections of how it will respond to environmental perturbation.
4.3 Carbon sequestration
Assuming that both diapause and gonad development take place in the deep ocean interior, our model predictions for respiration of storage lipids can be used to estimate C sequestration via the seasonal lipid pump at Station Mike. The predicted total CO2 generated via respiration during these two phases is 1.8 + 5.1 = 6.9 μmol C per individual. If copepod densities are 15,000-40,000 individuals m-2 (Jonasdottir et al., 2015), this translates as a C sequestration via the seasonal lipid pump of 1.2 – 3.3 g C m-2 yr-1. Despite using completely different approaches to estimate the seasonal lipid pump, our values are remarkably similar to those of Jonasdottir et al., 2015. Our estimate of C sequestration increases to 1.7 – 4.6 g C m-2 yr-1, if CO2 produced via respiration of maternal biomass, 2.7 μmol C per individual, is included in the calculation. On the other hand, it may be that, at least in some animals, gonad development is completed during ascent or with access to food after re-emergence in surface waters (Tande and Hopkins, 1981; Niehoff and Hirche, 1996; Harris et al., 2000), in which case the associated release of C does not contribute to the seasonal lipid pump. The calculated C sequestration in this scenario, accruing solely from respiration of lipid during diapause, is only 0.3 – 0.9 g C m-2 yr-1. All these estimates are strongly dependent on model rate parameters for metabolism during diapause and gonad development which, as noted above, are highly uncertain.
The parameterisation of metabolism in the model involves not only C, but also N. This led to a predicted net consumption of 0.55 μmol N in maternal biomass over winter which, in the absence of food, is the only source of available N in the modelled copepod. The estimated excretion of N by the population as a whole is then 0.12–0.31 g N m-2 yr-1, calculated as for C above, and represents a “lipid shunt” where C and nutrient cycling are decoupled (Jonasdottir et al., 2015). What we call “structural biomass” in the model is in reality a multitude of different tissues including exoskeleton, gut, reproductive organs, etc. While it is well known that animals can store energy in the form of fat, it is a common misconception that they are unable to store N because there are no specific tissues or organs for this purpose. Reserves could be mobilised from the haemolymph (Carlotti and Hirche, 1997; Hirche et al., 1997; Niehoff, 2000) and it may also be the case that some lipids contain N (Mayor et al., 2015). N may also be recovered from other tissues. Copepods have no need for their gut during diapause and so, in order to save energy because of high tissue turnover rates, it could be resorbed thereby freeing up N (Hallberg and Hirche, 1980).
4.4 Egg production
A total of 2513 eggs were produced by the modelled copepod, at an average of 12.9 eggs d-1, slightly below values of 14-44 d-1 observed during the bloom period at Station Mike (Irigoien et al., 1998; Niehoff et al., 1999). Observed egg production rates for individual C. finmarchicus vary widely between 0-90 d-1 (Helland et al., 2003; Jonasdottir et al., 2005; Runge et al., 2006; Koski, 2007; Gislason et al., 2008; Head et al., 2013; Pasternak et al., 2013). The predicted contribution of the lipid reserve to egg production was small, 1% of the C requirement, noting that our model calculates egg production on an income, rather than capital basis, i.e., recently ingested food provides the main source of nutrition. Capital reproduction, whereby offspring are produced from stored reserves and which is not included in our model, may also be important in high latitude copepods, particularly prior to the feeding season (Varpe et al., 2009), because eggs produced at this time have a disproportionally high chance of recruiting into the population (Varpe et al., 2007). Eggs have a greater C:N ratio than structural biomass, 5.8 vs 4.9, and so the predicted TER for egg production was slightly higher than that of growth, between 8.5 and 10.9 (varying with food quantity). Thus, production was once again limited by food quantity (C), indicating that that availability of reserve lipid could be beneficial in this regard. We used the model to test this theory by conducting a simulation in which lipid storage at mature CV was increased from 9.4 to 25 μmol C ind-1. The number of eggs produced was 3245, a significant increase of 24%. However, accruing extra lipid in spring entails a greater demand for food resources and therefore more time feeding in surface waters and a longer and potentially higher exposure to predators. There is thus a tradeoff between accruing reserve lipids for egg production and the potential for increased mortality. We investigated this tradeoff by introducing a copepod mortality term in spring, using conservative rates of 0.01-0.02 d-1 (Hirst and Kiørboe, 2002; Cruz et al., 2021). The predicted average fecundity of individuals within a population as a whole was negatively impacted with increasing mortality as the adverse impact of predation due to extended surface feeding outweighed the potential increase in egg production from the extra accrued lipid. Our results indicate that the best strategy for copepods is to minimise time in surface waters in spring, building up lipid reserves for essential use in diapause and gonad development but that they should not accumulate extra lipid for subsequent use in egg production. Predation is a strong driver of life history strategies in copepods (Kvile et al., 2021) and future modelling work, focusing on population dynamics, could investigate the tradeoff between lipid gain and mortality in greater detail.
5 Conclusion
In conclusion, we have developed a new stoichiometric model that, for the first time, explicitly includes copepod lipid reserves and thereby improves upon the usual assumption of homeostasis (fixed C:N) in zooplankton. The model allowed us to quantitatively explore the interactions between lipid-storing copepods and their environment and how these influence ocean biogeochemistry in both the surface and deep ocean. Results indicate that the primary function of reserve lipid is to support metabolism in diapause and subsequent gonad development during the period when copepods are without access to food. The modelled respiration during this period leads to an estimated C sequestration via the seasonal lipid pump that is remarkably similar to previous estimates (Jonasdottir et al., 2015), indicating that this flux is comparable in magnitude to the gravitational sinking pump. Our work highlights that, in order to accurately quantify the seasonal lipid pump in the ocean, there is a need for improved mechanistic understanding of the ecology and physiology of lipid use by high-latitude copepods, including as a supply of energy, for gonad development, egg production, and in the regulation of buoyancy. Better resolving these knowledge gaps will facilitate the incorporation of the seasonal lipid pump into the global ocean biogeochemical models used to investigate carbon sequestration in the ocean and its response to climate change.
Data availability statement
The LILICOP_1.0 model is coded in R as stand-alone files (main R file, “LILICOP_1.0.R”, along with input files for model parameters, settings and station forcing), with no dependency on R libraries. The model files are freely available for online download at the Zenodo repository; the citation is: Anderson, T.R. (2022). LILICOP_1.0: model of LIpids in the LIfe cycle of a high latitude COPepod, version 1.0. Zenodo, doi: 10.5281/zenodo.6615006.
Author contributions
TA and DM conceived the study. TA led the work, developed and coded the model and carried out the simulation runs. He was extensively supported by DH, WG, and DM with developing the model assumptions and parameterisation, as well as with interpretation of results. AY provided the input data from the global NEMO-MEDUSA ocean biogeochemical model that was used as forcing input. All authors provided intellectual input and contributed significantly to the writing of the manuscript.
Funding
TA, AY and DM received funding from the Natural Environment Research Council, UK, programs DIAPOD (award reference NE/P006353/1) and BIOPOLE (NE/W004933/1). WG was funded the by Natural Sciences and Engineering Research Council of Canada.
Conflict of interest
The authors declare that the research was conducted in the absence of any commercial or financial relationships that could be construed as a potential conflict of interest.
The handling editor AP declared a past co-authorship with the author DM.
Publisher’s note
All claims expressed in this article are solely those of the authors and do not necessarily represent those of their affiliated organizations, or those of the publisher, the editors and the reviewers. Any product that may be evaluated in this article, or claim that may be made by its manufacturer, is not guaranteed or endorsed by the publisher.
Supplementary material
The Supplementary Material for this article can be found online at: https://www.frontiersin.org/articles/10.3389/fmars.2022.928209/full#supplementary-material
References
Alver M. O., Broch O. J., Melle W., Bagøien E., Slagstad D. (2016). Validation of an eulerian population model for the marine copepod Calanus finmarchicus in the Norwegian Sea. J. Mar. Syst. 160, 81–93. doi: 10.1016/j.jmarsys.2016.04.004
Anderson T. R., Hessen D. O. (1995). Carbon or nitrogen limitation in marine copepods? J. Plankton. Res. 17, 317–331. doi: 10.1093/plankt/17.2.317
Anderson T. R., Hessen D. O. (2005). Threshold elemental ratios for carbon versus phosphorus limitation in Daphnia. Freshw. Biol. 50, 2063–2075. doi: 10.1111/j.1365-2427.2005.01450.x
Anderson T. R., Hessen D. O., Boersma M., Urabe J., Mayor D. J. (2017). Will invertebrates require increasingly carbon-rich food in a warming world? Amer. Nat. 190, 725–742. doi: 10.1086/694122
Anderson T. R., Hessen D. O., Mayor D. J. (2021). Is the growth of marine copepods limited by food quantity or quality? Limnol. Oceanogr. Lett. 6, 127–133. doi: 10.1002/lol2.10184
Anderson T. R., Hessen D. O., Mitra A., Mayor D. J., Yool A. (2013). Sensitivity of secondary production and export flux to choice of trophic transfer formulation in marine ecosystem models. J. Mar. Syst. 125, 41–53. doi: 10.1016/j.jmarsys.2012.09.008
Anderson T. R., Raubenheimer D., Hessen D. O., Jensen K., Gentleman W. C., Mayor D. J. (2020). Geometric stoichiometry: unifying concepts of animal nutrition to understand how protein-rich diets can be “too much of a good thing”. Front. Ecol. Evol. 8. doi: 10.3389/fevo.2020.00196
Aubert A. B., Svensen C., Hessen D. O., Tamelander T. (2013). CNP stoichiometry of a lipid-synthesising zooplankton, Calanus finmarchicus, from winter to spring bloom in a sub-Arctic sound. J. Mar. Syst. 111–112, 19–28. doi: 10.1016/j.jmarsys.2012.09.004
Bachiller E., Skaret G., Nøttestad L., Slotte A. (2016). Feeding ecology of northeast Atlantic mackerel, Norwegian spring-spawning herring and blue whiting in the Norwegian Sea. PLoS One 11 (2), e0149238. doi: 10.1371/journal.pone.0149238
Baumgartner M. F., Tarrant A. M. (2017). The physiology and ecology of diapause in marine copepods. Annu. Rev. Mar. Sci. 9, 387–411. doi: 10.1146/annurev-marine-010816-060505
Boyd P. W., Claustre H., Levy M., Siegel D. A., Weber T. (2019). Multi-faceted particle pumps drive carbon sequestration in the ocean. Nature 568, 327–335. doi: 10.1038/s41586-019-1098-2
Calow P. (1977). Conversion efficiencies in heterotrophic organisms. Biol. Rev. 52, 385–409. doi: 10.1111/j.1469-185X.1977.tb00840.x
Campbell R. G., Wagner M. W., Teegarden G. J., Boudreau C. A., Durbin E. G. (2001). Growth and development rates of the copepod Calanus finmarchicus reared in the laboratory. Mar. Ecol. Prog. Ser. 221, 161–183. doi: 10.3354/meps221161
Carlotti F., Hirche H. J. (1997). Growth and egg production of female Calanus finmarchicus: an individual-based physiological model and experimental validation. Mar. Ecol. Prog. Ser. 149, 91–104. doi: 10.3354/meps149091
Carlotti F., Radach G. (1996). Seasonal dynamics of phytoplankton and Calanus finmarchicus in the North Sea as revealed by a coupled one-dimensional model. Limnol. Oceanogr. 41, 522–539. doi: 10.4319/lo.1996.41.3.0522
Carlotti F., Sciandra A. (1989). Population dynamics model of Euterpina acutifrons (Copepoda: Harpacticoida) coupling individual growth and larval development. Mar. Ecol. Prog. Ser. 56, 225–242. doi: 10.3354/meps056225
Castellani C., Irigoien X., Mayor D. J., Harris R. P., Wilson D. (2008). Feeding of Calanus finmarchicus and Oithona similis on the microplankton assemblage in the Irminger Sea, North Atlantic. J. Plankton. Res. 30, 1095–1116. doi: 10.1093/plankt/fbn074
Chossat C. (1843). Reserches experimentales sur l’inanition Vol. 8 (Paris: Mem. L’acad. Sci. Paris Inst. France).
Cruz M. H., Kriest I., José Y. S., Kiko R., Hauss H., Oschlies A. (2021). Zooplankton mortality effects on the plankton community of the northern Humboldt Current system: sensitivity of a regional biogeochemical model. Biogeosciences 18, 2891–2916. doi: 10.5194/bg-18-2891-2021
Durbin E. G., Gilman S. L., Campbell R. G., Durbin A. G. (1995). Abundance, biomass, vertical migration and estimated development rate of the copepod Calanus finmarchicus in the southern Gulf of Maine during late spring. Cont. Shelf Res. 15, 571–591. doi: 10.1016/0278-4343(94)00060-Z
Edwards M., Richardson A. J. (2004). Impact of climate change on marine pelagic phonology and trophic mismatch. Nature 430, 881–884. doi: 10.1038/nature02808
Eisenhauer L., Carlotti F., Baklouti M., Diaz F. (2009). Zooplankton population model coupled to a biogeochemical model of the NorthWestern Mediterranean Sea ecosystem. Ecol. Mod. 220, 2865–2876. doi: 10.1016/j.ecolmodel.2009.06.024
Freese D., Søreide J. E., Graeve M., Niehoff B. (2016). A year−round study on metabolic enzymes and body composition of the Arctic copepod Calanus glacialis: implications for the timing and intensity of diapause. Mar. Biol. 164, 3. doi: 10.1007/s00227-016-3036-2
Gamble J. C. (1978). Copepod grazing during a declining spring phytoplankton bloom in the northern North Sea. Mar. Biol. 49, 303–315. doi: 10.1007/BF00455025
Gammelsrød T., Østerhus S., Godøy Ø. (1992). Decadal variations of ocean climate in the Norwegian Sea observed at ocean station “Mike”. ICES Mar. Sci. Symp. 195, 68–75.
Gentleman W., Leising A., Frost B., Strom S., Murray J. (2003). Functional responses for zooplankton feeding on multiple resources: a review of assumptions and biological dynamics. Deep-Sea. Res. II. 50, 2847–2875. doi: 10.1016/j.dsr2.2003.07.001
Gislason A. (2005). Seasonal and spatial variability in egg production and biomass of Calanus finmarchicus around Iceland. Mar. Ecol. Prog. Ser. 286, 177–192. doi: 10.3354/meps286177
Gislason A., Gaard E., Debes H., Falkenhaug T. (2008). Abundance, feeding and reproduction of Calanus finmarchicus in the Irminger Sea and on the northern Mid-Atlantic Ridge in June. Deep-Sea. Res. II. 55, 72–82. doi: 10.1016/j.dsr2.2007.09.008
Graeve M., Boissonnot L., Niehoff B., Hagen W., Kattner G. (2020). Assimilation and turnover rates of lipid compounds in dominant Antarctic copepods fed with 13C-enriched diatoms. Phil. Trans. R. Soc B 375, 20190647. doi: 10.1098/rstb.2019.0647
Grosse J., Burson A., Stomp M., Huisman J., Boschker H. T. S. (2017). From ecological stoichiometry to biochemical composition: variation in N and P supply alters key biosynthetic rates in marine phytoplankton. Front. Microbiol. 8. doi: 10.3389/fmicb.2017.01299
Häfker N. S., Teschke M., Last K. S., Pond D. W., Hüppe L., Meyer B. (2018). Calanus finmarchicus seasonal cycle and diapause in relation to gene expression, physiology, and endogenous clocks. Limnol. Oceanogr. 63, 2815–2838. doi: 10.1002/lno.11011
Hallberg E., Hirche H. J. (1980). Differentiation of mid-gut in adults and overwintering copepodids of Calanus finmarchicus (Gunnerus) and C. helgolandicus Claus. J. Exp. Mar. Biol. Ecol. 48, 283–295. doi: 10.1016/0022-0981(80)90083-0
Halvorsen E., Tande K. S., Edvardsen A., Slagstad D., Pedersen O. P. (2003). Habitat selection of overwintering Calanus finmarchicus in the NE Norwegian Sea and shelf waters off northern Norway in 2000–02. Fish. Oceanogr. 12 (4-5), 339–351. doi: 10.1046/j.1365-2419.2003.00255.x
Hand S. C., Moore D. S., Patil Y. (2018). Challenges during diapause and anhydrobiosis: Mitochondrial bioenergetics and desiccation tolerance. IUBMB Life 70, 1251–1259. doi: 10.1002/iub.1953
Harris R. P., Irigoien X., Head R. N., Rey C., Hygum B. H., Hansen B. W., et al. (2000). Feeding, growth, and reproduction in the genus Calanus. ICES J. Mar. Sci. 57, 1708–1726. doi: 10.1006/jmsc.2000.0959
Head E. J. H., Harris L. R., Ringuette M., Campbel R. W. (2013). Characteristics of egg production of the planktonic copepod, Calanus finmarchicus, in the Labrador Sea: 1997–2010. J. Plank. Res. 35, 281–298. doi: 10.1093/plankt/fbs097
Heath M. R., Boyle P. R., Gislason A., Gurney W. S. C., Hay S. J., Head E. J. H., et al. (2004). Comparative ecology of over-wintering Calanus finmarchicus in the northern North Atlantic, and implications for life-cycle patterns. ICES J. Mar. Sci. 61, 698–708. doi: 10.1016/j.icesjms.2004.03.013
Helland S., Nejstgaard J. C., Humlen R., Fyhn H. J., Båmstedt U. (2003). Effects of season and maternal food on Calanus finmarchicus reproduction, with emphasis on free amino acids. Mar. Biol. 142, 1141–1151. doi: 10.1007/s00227-003-1045-4
Henson S. A., Sarmiento J. L., Dunne J. P., Bopp L., Lima I., Doney S. C., et al. (2010). Detection of anthropogenic climate change in satellite records of ocean chlorophyll and productivity. Biogeosciences 7, 621–640. doi: 10.5194/bg-7-621-2010
Hessen D. O., Anderson T. R. (2008). Excess carbon in aquatic organisms and ecosystems: Physiological, ecological, and evolutionary implications. Limnol. Oceanogr. 53, 1685–1696. doi: 10.4319/lo.2008.53.4.1685
Hirche H.-J. (1991). Distribution of dominant calanoid copepod species in the Greenland Sea during late fall. Polar Biol. 11, 351–362. doi: 10.1007/BF00239687
Hirche H.-J. (1996). The reproductive biology of the marine copepod, Calanus finmarchicus – a review. Ophelia 44, 111–128. doi: 10.1080/00785326.1995.10429842
Hirche H.-J., Brey T., Niehoff B. (2001). A high-frequency time series at Ocean Weather Ship Station M: population dynamics of Calanus finmarchicus. Mar. Ecol. Prog. Ser. 219, 205–219. doi: 10.3354/meps219205
Hirche H.-J., Meyer U., Niehoff B. (1997). Egg production of Calanus finmarchicus: effect of temperature, food and season. Mar. Biol. 127, 609–620. doi: 10.1007/s002270050051
Hirst A. G., Kiørboe T. (2002). Mortality of marine planktonic copepods: global rates and patterns. Mar. Ecol. Prog. Ser. 230, 195–209. doi: 10.3354/meps230195
Hygum B. H., Rey C., Hansen B. W. (2000). Growth and development rates of Calanus finmarchicus nauplii during a diatom spring bloom. Mar. Biol. 136, 1075–1085. doi: 10.1007/s002270000313
Ingvarsdöttir A., Houlihan D. F., Heath M. R., Hay S. J. (1999). Seasonal changes in respiration rates of copepodite stage V Calanus finmarchicus (Gunnerus). Fish. Oceanogr. 8 (Suppl. 1), 73–83. doi: 10.1046/j.1365-2419.1999.00002.x
Irigoien X. (2004). Some ideas about the role of lipids in the life cycle of Calanus finmarchicus. J. Plankton. Res. 26, 259–263. doi: 10.1093/plankt/fbh030
Irigoien X., Head R., Klenke U., Meyer-Harms B., Harbour D., Niehoff B., et al. (1998). A high frequency time series at weathership M, Norwegian Sea, during the 1997 spring bloom: feeding of adult female Calanus finmarchicus. Mar. Ecol. Prog. Ser. 172, 127–137. doi: 10.3354/meps172127
Irigoien X., Titelman J., Harris R. P., Harbour D., Castellani C. (2003). Feeding of Calanus finmarchicus in the Irminger Sea. Mar. Ecol. Prog. Ser. 262, 193–200. doi: 10.3354/meps262193
Jónasdóttir S. H. (2019). Fatty acid profiles and production in marine phytoplankton. Mar. Drugs 17, 151. doi: 10.3390/md17030151
Jónasdottir S. H., Trung N. H., Hansen F., Gärtner S. (2005). Egg production and hatching success in the calanoid copepods Calanus helgolandicus and Calanus finmarchicus in the North Sea from march to September 2001. J. Plankton. Res. 27, 1239–1259. doi: 10.1093/plankt/fbi091
Jónasdóttir S. H., Visser A. W., Richardson K., Heath M. R. (2015). Seasonal copepod lipid pump promotes carbon sequestration in the deep North Atlantic. Proc. Nat. Acad. Sci. U.S.A. 112, 12122–12126. doi: 10.1073/pnas.1512110112
Jónasdóttir S. H., Wilson R. J., Gislason A., Heath M. R. (2019). Lipid content in overwintering Calanus finmarchicus across the subpolar eastern North Atlantic ocean. Limnol. Oceanogr. 64, 2029–2043. doi: 10.1002/lno.11167
Karr J. R., Sanghvi J. C., Macklin D. N., Gutschow M. V., Jacobs J. M., Bolival B. Jr., et al. (2012). A whole-cell computational model predicts phenotype from genotype. Cell 150, 389–401. doi: 10.1016/j.cell.2012.05.044
Kattner G., Krause M. (1987). Changes in lipids during the development of Calanus finmarchicus s.l. from copepodid I to adult. Mar. Biol. 96, 511–518. doi: 10.1007/BF00397968
Kiørboe T. (1989). Phytoplankton growth rate and nitrogen content: implications for feeding and fecundity in a herbivorous copepod. Mar. Ecol. Prog. Ser. 55, 229–234. doi: 10.3354/meps055229
Kirk K. L., Ellis J., Taylor J. (1999). Physiological responses to variable environments: storage and respiration in starving rotifers. Freshw. Biol. 42, 637–644. doi: 10.1046/j.1365-2427.1999.00502.x
Koski M. (2007). High reproduction of Calanus finmarchicus during a diatom-dominated spring bloom. Mar. Biol. 151, 1785–1798. doi: 10.1007/s00227-007-0615-2
Kuijper L. D. J., Anderson T. R., Kooijman S. A. L. M. (2004). C and N gross growth efficiencies of copepod egg production studied using a dynamic energy budget model. J. Plankton. Res. 26, 213–226. doi: 10.1093/plankt/fbh020
Kvile K.Ä., Altin D., Thommesen L., Titelman J. (2021). Predation risk alters life history strategies in an oceanic copepod. Ecology 102, e03214. doi: 10.1002/ecy.3214
Lahtvee P.-J., Seiman A., Arike L., Adamberg K., Vilu R. (2014). Protein turnover forms one of the highest maintenance costs in Lactococcus lactis. Microbiology 160, 1501–1512. doi: 10.1099/mic.0.078089-0
Lemcke H. W., Lampert W. (1975). Veränderungen im gewicht und der chemischen zusammensetzung von Daphnia pulex im hunger. Arch. Hydrobiol. 48, 108–137.
Lewandowska A. M., Boyce D. G., Hofmann M., Matthiessen B., Sommer U., Worm B. (2014). Effects of sea surface warming on marine plankton. Ecol. Lett. 17, 614–623. doi: 10.1111/ele.12265
Maps F., Plourde S., Zakardjian B. (2010). Control of dormancy by lipid metabolism in Calanus finmarchicus: a population model test. Mar. Ecol. Prog. Ser. 403, 165–180. doi: 10.3354/meps08525
Maps F., Record N. R., Pershing A. J. (2014). A metabolic approach to dormancy in pelagic copepods helps explaining inter- and intra-specific variability in life-history strategies. J. Plankton. Res. 36, 18–30. doi: 10.1007/s00227-016-3036-2
Mayor D. J. (2005). Nutritional regulation of egg production of Calanus finmarchicus in the North Atlantic (UK: Univ. Southampton). Available at: http://eprints.soton.ac.uk/id/eprint/17240.
Mayor D. J., Anderson T. R., Irigoien X., Harris R. (2006). Feeding and reproduction of Calanus finmarchicus during non-bloom conditions in the Irminger Sea. J. Plankton. Res. 28, 1167–1179. doi: 10.1093/plankt/fbl047
Mayor D. J., Anderson T. R., Pond D. W., Irigoien X. (2009a). Egg production and associated losses of carbon, nitrogen and fatty acids from maternal biomass in Calanus finmarchicus before the spring bloom. J. Mar. Syst. 78, 505–510. doi: 10.1016/j.jmarsys.2008.12.019
Mayor D. J., Anderson T. R., Pond D. W., Irigoien X. (2009b). Limitation of egg production in Calanus finmarchicus in the field: A stoichiometric analysis. J. Mar. Syst. 78, 511–517. doi: 10.1016/j.jmarsys.2008.12.020
Mayor D. J., Cook K. B., Anderson T. R., Belcher A., Jenkins H., Lindeque P., et al. (2020). Marine copepods, the wildebeest of the ocean. front. Young. Minds. 8. doi: 10.3389/frym.2020.00018
Mayor D. J., Cook K. B., Atherden F., Tarling G. A., Thornton B., Anderson T. R. (2022). Biomass turnover rates in metabolically active and inactive marine calanoid copepods. Front. Mar. Sci. doi: 10.3389/fmars.2022.907290
Mayor D. J., Sommer U., Cook K. B., Viant M. R. (2015). The metabolic response of marine copepods to environmental warming and ocean acidification in the absence of food. Sci. Rep. 5, 13690. doi: 10.1038/srep13690
Melle W., Runge J., Head E., Plourde S., Castellani C., Licandro P., et al. (2014). The North Atlantic Ocean as habitat for Calanus finmarchicus: Environmental factors and life history traits. Prog. Oceanogr. 129, 244–284. doi: 10.1016/j.pocean.2014.04.026
Moll A., Stegert C. (2007). Modelling Pseudocalanus elongatus stage-structured population dynamics embedded in a water column ecosystem model for the northern North Sea. J. Mar. Syst. 64, 35–46. doi: 10.1016/j.jmarsys.2006.03.015
Niehoff B. (2000). The effect of starvation on the reproductive potential of Calanus finmarchicus. ICES J. Mar. Sci. 57, 1764–1772. doi: 10.1006/jmsc.2000.0971
Niehoff B. (2007). Life history strategies in zooplankton communities: The significance of female gonad morphology and maturation types for the reproductive biology of marine calanoid copepods. Prog. Oceanogr. 74, 1–47. doi: 10.1016/j.pocean.2006.05.005
Niehoff B., Hirche H.-J. (1996). Oogenesis and gonad maturation in the copepod Calanus finmarchicus and the prediction of egg production from preserved samples. Polar Biol. 16, 601–612. doi: 10.1007/BF02329058
Niehoff B., Klenke U., Hirche H.-J., Irigoien X., Head R., Harris R. (1999). A high frequency time series at Weathersip M, Norwegian Sea, during the 1997 spring bloom: the reproductive biology of Calanus finmarchicus. Mar. Ecol. Prog. Ser. 176, 81–92. doi: 10.3354/meps172127
Niehoff B., Madsen S., Hansen B., Nielsen T. (2002). Reproductive cycles of three dominant Calanus species in Disko bay, West Greenland. Mar. Biol. 140, 567–576. doi: 10.1007/s00227-001-0731-3
Ohman M. D., Runge J. A. (1994). Sustained fecundity when phytoplankton resources are in short supply: Omnivory by Calanus finmarchicus in the Gulf of St. Lawrence. Limnol. Oceanogr. 39, 21–36. doi: 10.4319/lo.1994.39.1.0021
Opute F. I. (1974). Studies on fat accumulation in nitzschia palea kütz. Annal. Bot. 38, 889–902. doi: 10.1093/oxfordjournals.aob.a084883
Pasternak A. F., Arashkevich E. G., Grothe U., Nikishina A. B., Solovyev K. A. (2013). Different effects of increased water temperature on egg production of Calanus finmarchicus and C. glacialis. Oceanology 53, 547–553. doi: 10.1134/S0001437013040085
Plourde S., Lehoux C., Johnson C. L., Perrin G., Lesage V. (2019). North Atlantic right whale (Eubalaena glacialis) and its food: (I) a spatial climatology of Calanus biomass and potential foraging habitats in Canadian waters. J. Plankton. Res. 41, 667–685. doi: 10.1093/plankt/fbz024
Plourde S., Runge J. A. (1993). Reproduction of the planktonic copepod Calanus finmarchicus in the Lower St. Lawrence Estuary: relation to the cycle of phytoplankton production and evidence for a Calanus pump. Mar. Ecol. Prog. Ser. 102, 217–227. doi: 10.3354/meps095217
Podrabsky J. E., Hand S. C. (2015). Physiological strategies during animal diapause: lessons from brine shrimp and annual killifish. J. Exp. Biol. 218, 1897–1906. doi: 10.1242/jeb.116194
Pond D. W. (2012). The physical properties of lipids and their role in controlling the distribution of zooplankton in the ocean. J. Plankton. Res. 34, 443–453. doi: 10.1093/plankt/fbs027
Pond D., Harris R., Head R., Harbour D. (1996). Environmental and nutritional factors determining seasonal variability in the fecundity and egg viability of Calanus helgolandicus in coastal waters off Plymouth, UK. Mar. Ecol. Prog. Ser. 143, 45–63. doi: 10.3354/meps143045
Pond D. W., Tarling G. A., Ward P., Mayor D. J. (2012). Wax ester composition influences the diapause patterns in the copepod Calanoides acutus. Deep-Sea. Res. II. 59, 93–104. doi: 10.1016/j.dsr2.2011.05.009
Rey C., Harris R., Irigoien X., Head R., Carlotti F. (2001). Influence of algal diet on growth and ingestion of Calanus helgolandicus nauplii. Mar. Ecol. Prog. Ser. 216, 151–165. doi: 10.3354/meps216151
Rey-Rassat C., Irigoien X., Harris R., Carlotti F. (2002). Energetic cost of gonad development in Calanus finmarchicus and C. helgolandicus. Mar. Ecol. Prog. Ser. 238, 301–306. doi: 10.3354/meps238301
Runge J. A., Plourde S., Joly P., Niehoff B., Durbin E. (2006). Characteristics of egg production of the planktonic copepod, Calanus finmarchicus, on Georges Bank: 1994–1999. Deep-Sea. Res. II. 53, 2618–2631. doi: 10.1016/j.dsr2.2006.08.010
Sargent J. R., Falk-Petersen S. (1988). The lipid biochemistry of calanoid copepods. Hydrobiologia, 101–114. doi: 10.1007/BF00026297
Sargent J. R., Henderson R. J. (1986). “Lipids,” in The biological chemistry of marine copepods. Eds. Corner E. D. S., O'Hara S. C. M. (Oxford: Clarendon Press), 59–108.
Secor S. M. (2009). Specific dynamic action: a review of the postprandial metabolic response. J. Comp. Physiol. B 179, 1–56. doi: 10.1007/s00360-008-0283-7
Seebens H., Einsle U., Straile D. (2009). Copepod life cycle adaptations and success in response to phytoplankton spring bloom phenology. Global Change Biol. 15, 1394–1404. doi: 10.1111/j.1365-2486.2008.01806.x
Straile D. (1997). Gross growth efficiencies of protozoan and metazoan zooplankton and their dependence on food concentration, predator-prey weight ratio, and taxonomic group. Limnol. Oceanogr. 42, 1375–1385. doi: 10.4319/lo.1997.42.6.1375
Swalethorp R., Kjellerup S., Dünweber M., Nielsen T. G., Møller E. F., Rysgaard S., et al. (2011). Grazing, egg production, and biochemical evidence of differences in the life strategies of Calanus finmarchicus, C. glacialis and C. hyperboreus in Disko bay, western Greenland. Mar. Ecol. Prog. Ser. 429, 125–144. doi: 10.3354/meps09065
Tande K. S. (1982). Ecological investigations on the zooplankton community of Balsfjorden, northern Norway: generation cycles and variations in weight and body content of carbon and nitrogen related to overwintering and reproduction in the copepod Calanus finmarchicus (Gunnerus). J. Exp. Mar. Biol. Ecol. 62, 129–142. doi: 10.1016/0022-0981(82)90087-9
Tande K. S., Hopkins C. C. E. (1981). Ecological investigations of the zooplankton community of Balsfjorden, northern Norway: the genital system in Calanus finmarchicus and the role of gonad development in overwintering strategy. Mar. Biol. 63, 159–164. doi: 10.1007/BF00406824
Tarling G. A., Freer J. J., Banas N. S., Belcher A., Blackwell M., Castellani C., et al. (2022). Can a key boreal Calanus copepod species now complete its lifecycle in the Arctic? evidence and implications for Arctic foodwebs. Ambio 51, 333–344. doi: 10.1007/s13280-021-01667-y
Thor P., Cervetto G., Besiktepe S., Ribera-Maycas E., Tang K. W., Dam H. G. (2002). Influence of two different green algal diets on specific dynamic action and incorporation of carbon into biochemical fractions in the copepod Acartia tonsa. J. Plankton. Res. 24, 293–300. doi: 10.1093/plankt/24.4.293
Threlkeld S. T. (1976). Starvation and the size structure of zooplankton communities. Freshw. Biol. 6, 489–496. doi: 10.1111/j.1365-2427.1976.tb01640.x
Varpe Ø., Jørgensen C., Tarling G. A., Fiksen Ø. (2007). Early is better: seasonal egg fitness and timing of reproduction in a zooplankton life-history model. Oikos 116, 1131–1142. doi: 10.1111/j.0030-1299.2007.15893.x
Varpe Ø., Jørgensen C., Tarling G. A., Fiksen Ø. (2009). The adaptive value of energy storage and capital breeding in seasonal environments. Oikos 118, 363–370. doi: 10.1111/j.1600-0706.2008.17036.x
Verity P. G. (1985). Grazing, respiration, excretion, and growth rates of tintinnids. Limnol. Oceanogr. 30, 1268–1282. doi: 10.4319/lo.1985.30.6.1268
Visser A. W., Jónasdóttir S. H. (1999). Lipids, buoyancy and the seasonal vertical migration of Calanus finmarchicus. Fish. Oceanogr. 8 (Suppl. 1), 100–06. doi: 10.1046/j.1365-2419.1999.00001.x
Yool A., Popova E. E., Anderson T. R. (2013). MEDUSA-2.0: an intermediate complexity biogeochemical model of the marine carbon cycle for climate change and ocean acidification studies. Geosci. Mod. Dev. 6, 1767–1811. doi: 10.5194/gmd-6-1767-2013
Keywords: zooplankton, diapause, gonad development, seasonal lipid pump, egg production
Citation: Anderson TR, Hessen DO, Gentleman WC, Yool A and Mayor DJ (2022) Quantifying the roles of food intake and stored lipid for growth and development throughout the life cycle of a high-latitude copepod, and consequences for ocean carbon sequestration. Front. Mar. Sci. 9:928209. doi: 10.3389/fmars.2022.928209
Received: 25 April 2022; Accepted: 29 June 2022;
Published: 26 July 2022.
Edited by:
Alex J. Poulton, Heriot-Watt University, United KingdomReviewed by:
Jessica Garzke, University of British Columbia, CanadaTaketoshi Kodama, Japan Fisheries Research and Education Agency (FRA), Japan
Copyright © 2022 Anderson, Hessen, Gentleman, Yool and Mayor. This is an open-access article distributed under the terms of the Creative Commons Attribution License (CC BY). The use, distribution or reproduction in other forums is permitted, provided the original author(s) and the copyright owner(s) are credited and that the original publication in this journal is cited, in accordance with accepted academic practice. No use, distribution or reproduction is permitted which does not comply with these terms.
*Correspondence: Thomas R. Anderson, dHJhQG5vYy5hYy51aw==; Daniel J. Mayor, ZGFuLm1heW9yQG5vYy5hYy51aw==