Theory, practice, and design criteria for utilizing artificial reefs to increase production of marine fishes
- 1Department of Biology, Vantuna Research Group, Occidental College, Los Angeles, CA, United States
- 2Department of Biological Sciences, California State Polytechnic University, Pomona, Pomona, CA, United States
Increasing the production of marine fishes is a tractable goal with wide socioeconomic and ecological appeal. Ecosystem restoration projects that increase the amount of suitable habitat in an area and/or habitat quality enhance both fishery production and ecosystem services. Fortuitously, there are a wealth of studies documenting the specific examples of restoring these services from successful artificial reef deployments. Considering the need to create future structures in a variety of scenarios and locations, it is salient to summarize the mechanisms through which increased secondary production occurs and the design considerations. To achieve this objective maximizing ecological processes including the provisioning of planktonic and epibenthic food resources and related trophic pathways, and those associated with life-stage specific habitat use (e.g., recruitment, juvenile survival, reproductive output) are critical mechanisms of productive reefs. We synthesized this information by addressing the structural and ecological theory of artificial reef design based upon physical attributes such as complexity, vertical relief, habitat heterogeneity, and spatial scale. Within this framework we summarized the mechanisms that may be used to increase secondary fish production and propose a general theory for optimization of these variables.
Introduction
There is no debate that marine resources are crucial for current and future socioeconomic processes. Unfortunately, these resources have declined over time due to a variety of stressors including the depletion of species at higher trophic levels, habitat loss, pollution, and climate change, dramatically impacting marine ecosystems worldwide (Jackson et al., 2001; Worm et al., 2006). Reduced fishery stocks and deterioration or loss of habitat, compounded by the increasing socioeconomic need to access marine resources highlights the theory that current secondary (fish) production and services were below the potential, and certainly historical potential, for marine ecosystems. While this theory is generally not tested directly (i.e., manipulation and/or calculation of a maximum versus current production potential), it has been indirectly demonstrated and is at the core of a variety of fishery management actions to improve stock sizes and sustainability (Knowlton, 2021). Well-designed ecosystem restoration projects increase the amount of suitable habitat area and/or quality, enhancing both fishery production and ecological services. Similarly, marine protected areas increase fishery stock biomass within their boundaries (Claudet et al., 2008; Edgar and Stuart-Smith, 2009), supporting this fundamental concept: current production is less than potential production in marine ecosystems.
Ongoing macroscale alterations of marine habitats and the implementation of infrastructure projects challenge us from a variety of perspectives. For thousands of years ‘human-made’, or as the literature primarily refers to them ‘artificial’ reefs, were installed to enhance marine habitats and there are continuous proposals for future implementation (Whitmarsh et al., 2008; Seaman, 2019; Tickell et al., 2019; Bugnot et al., 2020). Optimal artificial reef design is salient for deploying these structures to adequately restore lost ecosystem services. Herein, we delineate the theory and applicability of reversing or offsetting these losses, synthesizing the current empirical evidence for contributions of artificial reef habitats to local and regional ecosystems worldwide and the mechanisms supporting this goal. These theoretical constructs are essential for establishing design and evaluation criteria for utilizing reefing technology to improve ecosystem health and associated services.
Artificial reefs deployments have resulted in substantial increases of local and regional fish and invertebrate production. Johnson et al. (1994) found the rate of secondary production for a reef fish assemblage on a quarry rock artificial reef in southern California, USA was nine times greater than the sand-bottom fish assemblage the artificial reef replaced (i.e., was constructed on top of). Similarly, artificial reef habitat in Delaware Bay, USA supported up to two orders of magnitude higher secondary production (benthic macrofauna) per unit area than the comparable soft bottom habitat it replaced (Steimle et al., 2002). Large-scale artificial reef deployment assessments provide evidence for regional increases of production in fishes and invertebrates. In Japan, analyses of catch data revealed regional increases in octopus production in response to approximately 50,000 m3 of artificial reef habitat construction (Polovina and Sakai, 1989). Following the deployment of an artificial reef complex in Algarve, southern Portugal, Roa-Ureta et al. (2019) analyzed 27 years of landings data to estimate the regional carrying capacity (K) of two-banded seabream, Diplodus vulgaris, increased by 35% (an additional 895 tons). This large-scale reefing effort comprised over 21,500 concrete modules (small 3 m3 or large 174 m3) covering an area of 43 km2 (Santos et al., 2011). The Algarve artificial reef design focused on adding habitat for high-value (€12–18/kg in 2011) juvenile and adult fishes, which were targets of an artisanal fishery, to a soft bottom area where this habitat was previously rare (Santos et al., 2011). An economic analysis found revenues from fishing the areas around this artificial reef complex was greater than at control sites and catch rates and associated revenues had risen consistently over a 15 year period following deployment of the reef (Whitmarsh et al., 2008). Additionally, the artificial reef in Algarve relieved pressure from natural reefs in the region by redistributing fishing effort across a larger area (Leitao et al., 2009). These researchers also emphasized the need for additional fishery management regulations to prevent overfishing (Santos et al., 2011; Roa-Ureta et al., 2019). Thus, increased regional secondary production is achievable, but necessitates holistic design strategies that incorporate local and regional ecological processes, proper spatial scale and location, and socioeconomic factors such as management.
Impacts of a given artificial reef project were typically species- or taxa-specific (or even specific to a life-stage within a species), because responses to artificial reef habitats depend on species-specific functional attributes (e.g., diet, habitat use, life history) (Leitão, 2013; Smith et al., 2015; Cresson et al., 2019). Impacts were so diverse across taxa that predicting potential effects for entire assemblages was difficult and therefore a ‘case-by-case’ approach was generally more accurate (Brickhill et al., 2005). In paired comparisons between natural rocky or coral reefs and nearby artificial habitats (e.g., artificial reefs, shipwrecks, energy infrastructure), fish density, biomass and richness were often similar, demonstrating that artificial reefs have the potential to mimic natural reefs, if that is the objective (Paxton et al., 2020b). Most assessments of artificial reef designs examining specific objectives concentrated on important focal species (e.g., fishery or keystone species) and/or functional groups (e.g., those with specific trophic roles or habitat use patterns). Similarly, comparing reef success across ecosystems was highly context-specific and necessitates an understanding of regional goals as well as fine-scale biological and physical processes. The goal of increasing local and/or regional production is feasible, but a “one size fits all” approach is not advised (Komyakova et al., 2019; Paxton et al., 2020b; Blount et al., 2021).
Thus, a substantial amount of literature documented specific effects of individual artificial reef deployments, the mechanisms through which increased production occurred, and design considerations for achieving these objectives. Recently there has been more focus in the literature on the specific mechanisms, sometimes referred to as “enhanced ecological functions” (Glarou et al., 2020), that lead to increased secondary production of fish on artificial reefs, even above rates observed on natural reefs (e.g., Cresson et al., 2014a; Glarou et al., 2020; Rouse et al., 2020; Puckeridge et al., 2021). These mechanisms provide benefits to individual species, typically through provision of shelter and/or food supporting survival or growth. While in some instances data were not available to directly quantify regional or local secondary production of fishes, evidence of these mechanisms, often in the context of a focal species’ life history and behavioral ecology, support an assumption that reefs were contributing to secondary production. Yet, across ecosystems, there were consistent elements in artificial reef design that support increased secondary production and these criteria are useful for planning, evaluation, and design of current and future projects and have the potential to increase production on a regional scale. We posit that the most tractable metric to evaluate ecological services across habitats is secondary production.
Ecological process considerations of reef design
Planktonic and epibenthic food resources
A sizable proportion of the secondary production of fishes and invertebrates in artificial reef systems is fueled through planktonic resources, with a lesser proportion being supported by macroalgae or detritus. In a stable isotope and diet study of the largest artificial reef complex in the Mediterranean Sea (RECIFS PRADO program), organic matter of pelagic origin (i.e., phytoplankton and zooplankton) was the primary source supporting the system through artificial reef resident fishes feeding on sessile filter feeding invertebrates or directly on zooplankton (Cresson et al., 2014a; Cresson et al., 2014b). Similarly, a study of trophic pathways of fishes on a natural reef system in Australia observed that planktonic resources supported 60% of fishes, compared to approximately 30% by macroalgae and 10% by detritus (Truong et al., 2017). Current flow that delivers the associated flux of plankton and nutrients are critical factors supporting secondary production and thus are essential for overall performance. Therefore, understanding trophic bottlenecks and maximizing planktonic flux is necessary for increasing secondary production.
Zooplanktivorous fishes on artificial reefs are often the key trophic link between zooplankton and the larger reef-associated community (Bray et al., 1981; Cresson et al., 2014a; Holland et al., 2021; Puckeridge et al., 2021). Schooling water column and zooplanktivorous fishes were in greater abundance above higher relief purpose-built artificial reefs in temperate Australia (Puckeridge et al., 2021) and wrecks off of North Carolina, USA (Lemoine et al., 2019), when compared to lower relief natural reefs within each region. An Australian study directly documented the trophic link between Bluespotted Flathead (Platycephalus caeruleopunctatus), a resident benthic ambush predator, feeding on pelagic forage fish above the high relief artificial reef modules (Holland et al., 2021). This mechanism has also involved nutrient transfer, such as the case with diurnal planktivorous pomacentrids in California that increase overall reef production with their metabolic byproducts being used by algae (Bray et al., 1981). Many artificial reef systems are therefore more productive than natural reefs because they provide sufficient habitat for zooplanktivorous fishes (Champion et al., 2015; Pondella et al., 2018; Holland et al., 2021).
With this in mind, flux of planktonic resources and even inducing upwelling to increase primary productivity (e.g., Itosu et al., 1995; Otake et al., 1995) are important considerations. Further, for reefs that receive higher current flow, such has offshore oil and gas platforms in California, this translated to increased growth rates for mussels (Mytilus spp.), a dominant filter feeding invertebrate, and the planktivorous Blue Rockfish (Sebastes mystinus) than individuals in nearby natural nearshore habitats (Page and Hubbard, 1987; Blanchette et al., 2007; Love et al., 2007). Similarly, a study of an experimental artificial reef off of Scotland demonstrated the importance of water flow for the secondary production of a suspension feeding bryozoan both on the outer-facing reef surfaces and into internal reefs spaces, with higher secondary invertebrate production found on reefs with more complex designs and larger internal reef spaces (Rouse et al., 2020). Clearly in order to maximize secondary production, optimizing both benthic and pelagic food resources is essential.
Shelter
Proper shelter allows fishes to maximize the acquisition of planktonic and epibenthic food resources while minimizing the amount of energy needed to maintain themselves. In a system with underutilized food resources, the increase of shelter and associated use by various life history stages of fishes theoretically results in greater production. Beyond increasing appropriate habitat where it is limited or lost, properly designed habitats provide a direct survival and metabolic benefit to the fish sheltering on them. In Atlantic Cod, metabolic rates associated with reef habitat (as compared to sand) were lower, suggesting that the energy saved by sheltering contributed to increased somatic growth and thus production rates (Schwartzbach et al., 2020). Reef holes and cavities (i.e., void spaces) provide refuges, and hole size was often positively associated with the body sizes of fishes found sheltering in them (Hixon and Beets, 1993; Beets and Hixon, 1994; Friedlander and Parrish, 1998). For example, smaller reef fishes had higher survival rates on reefs with smaller hole sizes compared to similar sized fish on reefs with larger holes (Hixon and Beets, 1993). Some reef designs utilized models to predict hole/crevice size and number based on the rock size (Barry and Wickins, 1992), suggesting the possibility of designing reefs with appropriate hole sizes for a specific taxa or life history stages (e.g., Glarou et al., 2020). Survival and growth of fishes is a function of both predator avoidance and the reduced energy associated with sheltering from current flow in appropriately sized holes. Productive reefs have shelter characteristics enabling fishes to minimize their energy expenditures from flow regimes and predator avoidance while allowing them to maximize the flux of energy from the plankton and epibenthic prey. Theoretically increasing shelter increases the secondary production a reef supports. This is ulitmately limited by the carrying capacity of the system (e.g, food availability, species and life-stage specific survival rates) in a density dependent manner resulting in an asymptotic relationship between shelter and production (Figure 1).
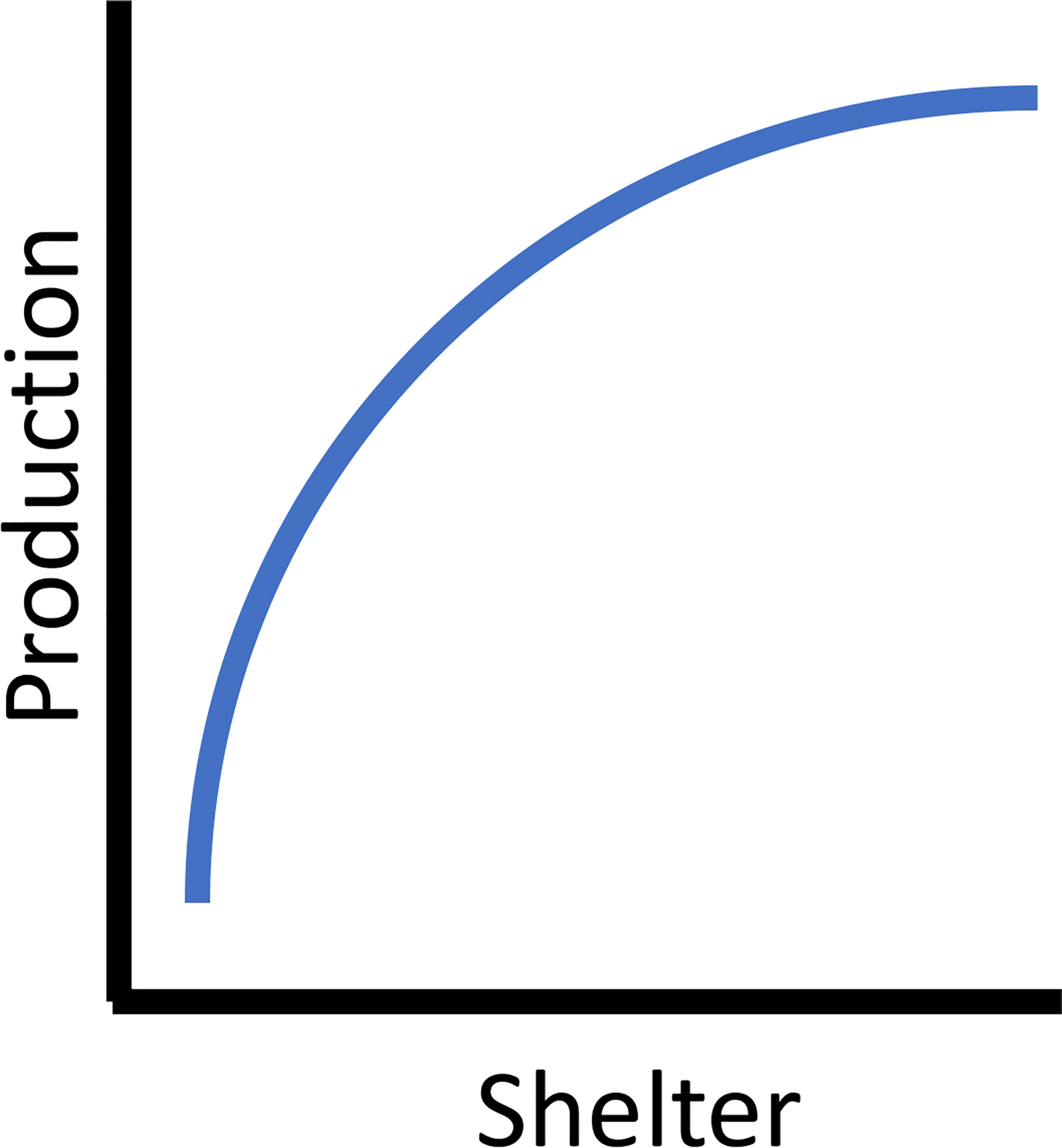
Figure 1 The asymptotic theoretical relationship between available shelter and secondary (fish) production.
Recruitment and juvenile survivorship
The function of artificial reefs as shelter for recruitment, survivorship, and growth of young-of-year and juvenile reef-associated fishes is another essential component for increasing local and regional production. Recruitment and juvenile success, the hallmarks of fishery models (Ricker, 1975), are a critical factor for stock management. There are multiple examples of artificial reefs providing habitat for newly settled and/or juvenile fish, which increased recruitment rates and cohort success, which in turn increased ecosystem-wide services. High prevalence of newly recruited and/or juvenile fishes on artificial reefs was common (e.g., Santos et al., 2011; Reubens et al., 2014; Granneman and Steele, 2015; Krone et al., 2017). Fish assemblages on more complex, larger-scale artificial reefs supported a higher proportion of smaller juvenile fishes (e.g., Santos et al., 2011; Granneman and Steele, 2015; Love et al., 2019) suggesting these patterns documented on small experimental reefs (cm2 to m2) are scalable to larger systems (10’s to 100’s of m2). Additionally, Komyakova et al. (2019) found in temperate southern Australia that the location relative to larger reefs, either natural rocky reefs or large breakwaters, had a greater influence on fish densities at smaller artificial reef modules. This was attributed to increased recruitment of young-of-the-year fishes to these larger reefs and subsequent movement of those fishes to the artificial reef modules. Undoubtedly, incorporation of a nursery design is a key component for successful reefing programs.
In a variety of ecosystems, increasing habitat for juvenile fish resulted in corresponding production increases. Windmill artificial reefs (WARs), consisting of monopile offshore wind turbines surrounded by quarry rock scour protection and deployed in largely soft bottom regions of the North Sea, supported high abundances of juvenile fishes. Specifically, they were suitable feeding grounds for juvenile Atlantic Cod (Gadus morhua) and Pouting (Trisopterus luscus), which fed upon epifaunal invertebrate prey and showed high seasonal site fidelity (De Troch et al., 2013; Reubens et al., 2014). Juvenile Atlantic Cod sheltered on WARs between dawn and dusk (crepuscular) feeding periods, suggesting sheltering from predators provides a survival advantage (Reubens et al., 2014), in addition to metabolic energy savings (Schwartzbach et al., 2020). In passive acoustic tracking of Atlantic Cod, these patterns were consistent over 2-5 month periods, with fish sheltering on the same WAR reef daily (Reubens et al., 2014). While these increases were in local production and regional-scale increases had not yet been observed, these juvenile fish likely emigrated as they matured, benefitting commercial fisheries. Similarly, in a mark-recapture study, juvenile Gag Grouper (Mycteroperca microlepis) had high site fidelity (average 300 days) on an experimental concrete artificial reef off Florida. Adults then moved further offshore where they became part of the broader fished stock, with two fish being recaptured on other side of the Gulf of Mexico in Texas and Mexico (Lindberg et al., 2006). In the Algarve artificial reef complex, which was designed to specifically provide shelter for juvenile fishes of fishery importance (Santos et al., 2011), this juvenile sheltering effect made an important contribution to the increases in regional carrying capacity (K) and production of Two-Banded Seabream (Roa-Ureta et al., 2019). Up to 88% of the fish assemblage associated with the Algarve reef modules were juveniles, with consistent recruitment of young-of-the-year fishes observed for some species. Juveniles were also documented as sheltering in the structure and feeding directly on the benthic macrofauna or in the water column near the structures (Leitao et al., 2009; Santos et al., 2011). These examples illustrate the progression necessary for increasing production: creating habitat where appropriate, documenting the density of critical life stages and their associated habitat use, then linking this to regional production. Clearly, reducing habitat and recruitment limitations by increasing nursery areas has significant benefits.
Reproductive output
In addition to providing a nursery function and sheltering juvenile fishes with high rates of somatic production, a frequently overlooked component in artificial reef studies is reproductive output, or gonadal production. Reproductive output is a function of the number of larger adult fishes residing on a reef, and is a “major component of energy flow through the fishes in [a] reef system” (Demartini et al., 1994). In this study, fish gonadal production on artificial reefs was equivalent to, or a large multiple of, the somatic production estimates. For territorial species, reefs with more suitable habitat have a corresponding greater number of potential feeding and mating territories (Hixon, 1980), which can lead to increased secondary production compared to less complex natural reefs (Pondella et al., 2002). These concepts are frequently incorporated in fisheries management and conservation network designs, such as MPA connectivity, with the overall goal of increasing localized spawning stock biomass and exporting it throughout the ecosystem (Russ et al., 2004; Pérez-Ruzafa et al., 2008; Almany et al., 2009; Botsford et al., 2009; Cudney-Bueno et al., 2009; Foley et al., 2010; Berumen et al., 2012; Schmiing et al., 2017; Baetscher et al., 2019; Marshall et al., 2019). Further, since most fishes have a pelagic larval stage, understanding gonadal production was a critical feature in modeling connectivity and the link between biomass density and recruitment (Pondella et al., 2015). Particularly well-studied for fishes, gonadal production and egg quality is a function of female size and age. Larger females have higher fecundity and allocate more energy for egg production, and in some instances, produce more fit larvae than smaller females (Berkeley et al., 2004). Importantly, this relationship was not linear, instead being hyperallometric with size (Barneche et al., 2018), and supported the idea that the protection and production of larger females disproportionately and positively affects stock structure. Larger adults have lower annual somatic production but theoretically are a major link to regional increases, especially if habitats are not limiting recruitment. Thus, habitat design and/or management actions taking this into account (e.g., the implementation of MPAs or fishery management practices such as slot limits and protection of spawning aggregations) have a greater impact on recovery due to increased gonadal production and potentially more fit larvae (Claudet et al., 2008; Edgar and Stuart-Smith, 2009; Marshall et al., 2019). Habitat characteristics that optimize spawning aggregations, generating larval sources throughout an ecosystem were also important considerations (Schmiing et al., 2017). Some studies identified larval production as a key management action for fishery replenishment (Kough et al., 2019). Higher quality habitats also support greater densities of large individuals, magnifying this effect.
Physical considerations of reef design
Structural complexity
Increasing reef complexity, both externally (rugosity) and internally (void space), improves secondary production (Blount et al., 2021). Simply increasing the complexity of reef modules in the French Mediterranean doubled overall fish species richness, increased density by ten times, and increased biomass by forty times (Charbonnel et al., 2002). Experimental artificial reefs across a broad geographic range consistently found increased fish density, biomass, and/or species diversity associated with more complex structures (North Carolina, USA : Potts and Hulbert, 1994, South Carolina, USA: Kellison and Sedberry, 1998, Gulf of Mexico, Florida, USA: Sherman et al., 2002, Mediterranean, Italy: Relini et al., 2007, Scotland, UK: Hunter and Sayer, 2009, temperate southern Australia: Komyakova et al., 2019). Often fish density and size structure have a greater impact on rates of secondary production than relative differences in individual growth rates. For example, Granneman and Steele (2014) compared somatic growth rates, estimated from otolith back-calculation, on paired artificial and adjacent natural reefs in southern California, USA. When combined with observed density and size structure of the fish assemblage, they found secondary production rates on quarry rock artificial reefs were similar to or greater than natural reefs, and positively correlated with habitat characteristics (i.e., the abundance of larger boulders on artificial reefs). The authors also found that size-specific differences in fish density made a large contribution to the differences in production, while growth rates among sites were similar. The general mechanism discussed for this phenomenon relates direct sheltering opportunities for a variety of sizes of fishes provided by the increased number and diversity of sizes of holes and crevices in the reefs. Further, more rugose reefs are more suitable for attachment of both algae and sessile invertebrates (Harlin and Lindbergh, 1977; Walters and Wethey, 1996). As such, there is a positive relationship between complexity and production. In addition, three-dimensional complexity adds increased surface area, elevating the overall abundance of benthic forage resources, which results in increased fish density, an integral factor in secondary production models. Theoretically, this relationship asymptotes with increasing complexity (Figure 2). While increasing complexity adds structural elements that correspond to species-specific functional attributes (e.g., diet, habitat use, life history) of the fish assemblage, similar to within reef habitat heterogeneity, this is ultimately limited by the surface area to volume structural relationship and the availability of internal spaces of a reef of a given size (Kim et al., 1994; Lemoine et al., 2019; Rouse et al., 2020). Thus, increasing complexity to maximize production is a theoretical and practical design consideration.
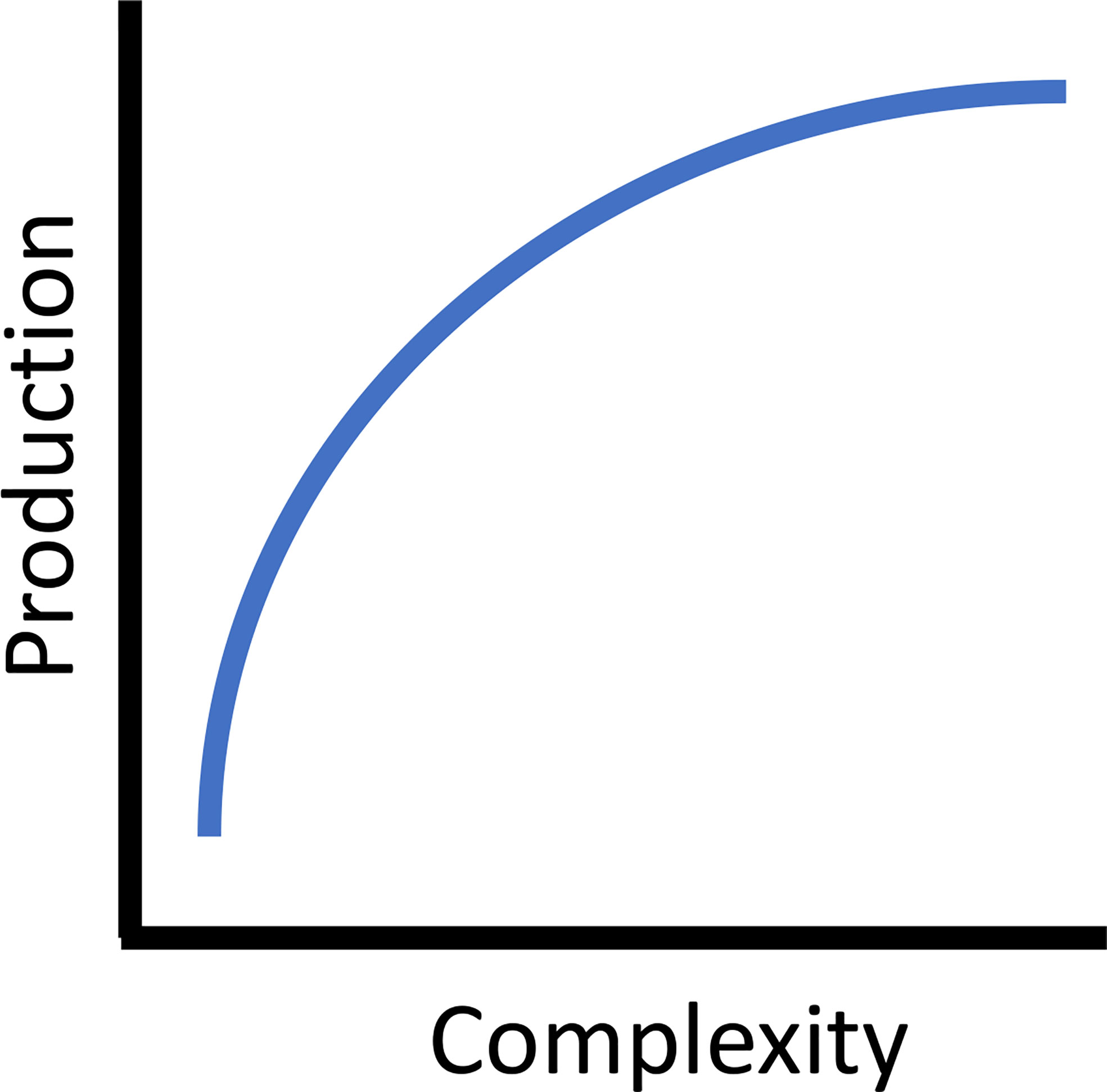
Figure 2 The asymptotic theoretical relationship between reef complexity and secondary (fish) production.
Vertical relief
Vertical relief (i.e., height above the seafloor) is another commonly investigated factor in reef design and artificial reefs that provide sufficient vertical relief support greater taxonomic diversity (Ogawa, 1967; Molles and Manuel, 1978; Bohnsack et al., 1994). Positive relationships between vertical relief and fish density and/or biomass were documented at quarry rock artificial reefs in California, USA (e.g., Ambrose and Swarbrick, 1989; Pondella et al., 2006; Granneman and Steele, 2015). Studies comparing fish assemblages on shipwrecks (i.e., high-relief artificial reefs) with natural reefs and/or concrete artificial reefs found higher-relief wrecks have higher mean densities of fishes (northeast Pacific: Bulger et al., 2019, North Carolina, USA: Lemoine et al., 2019; Paxton et al., 2020a), as well as differences in trophic guilds. Similarly, Paxton et al. (2020a) observed wrecks had higher densities of transient predators (e.g., jacks, mackerel, barracuda, sharks) than concrete and natural reefs, and their review of the artificial reef literature suggested this pattern of more high trophic level (i.e., predatory, typically transient) fishes on artificial reefs than natural reefs was a global pattern. Yet, shipwrecks were not necessarily adequate surrogates for natural reefs (Medeiros et al., 2021). This is representative of a general pattern of predators being more common on artificial reefs globally, with transient predators being observed in higher densities on taller structures (Paxton et al., 2020a). While there were numerous objective and anecdotal observations of this phenomenon, salient are the mechanisms and their potential utility in reef design.
Reefs with greater relief perform better than low relief reefs. At the spatial scale of typical reefs (meters), theoretically this will asymptote (Figure 3). While many species of fish orient to high relief structures for feeding purposes (as described previously) they also utilize them for spawning sites. Such spawning aggregations were often associated with the highest relief components within a reef and in some taxa optimal spawning sites were favored. Additionally, adults return to traditional spawning sites based upon a learned response, yet in the absence of this learning, they chose new sites based upon reef features implying there was an optimization of assessed reef features to maximize spawning success (Warner, 1988). Interestingly, on newly deployed reefs adult fishes sometimes within hours, but certainly within days orient to these structures, which, since they were just placed in the water, do not yet have epibenthic resources (Turner et al., 1969). Over the long term, large predatory fishes migrate and orient to these vertical features (Barilotti et al., 2020; Burns et al., 2020; Paxton et al., 2020a). However, very high-relief reefs, particularly those with an intertidal component (i.e., breakwaters, some shipwrecks, and oil platforms), had increased ecosystem heterogeneity and associated production (e.g., Stephens et al., 1994; Claisse et al., 2014). For example, breakwaters incorporate the intertidal zone, increasing habitat heterogeneity and have high rates of secondary production (Pondella et al., 2002). These processes are consistent with the inclusion of kelp, whereby a three-dimensional relief component is extended to the surface by a canopy providing habitat heterogeneity and trophic resources that support an increased suite of life stages and species (Graham, 2004). Structures with these high-relief components also act as fish aggregating devices (FADs) for more transient pelagic fishes. This attraction can occur on a non-random consistent basis, resulting in increased overall production through the inclusion of planktivores into the system (Smith et al., 2016). Relief is an attraction mechanism of adults and larval fishes, potential spawning sites, and food resources. On a typical reef module without an intertidal component, production as a function of relief asymptotes (especially if resource dependent), then adding an additional three-dimensional FAD/kelp/intertidal component increases production to a theoretical maximum (Figure 3).
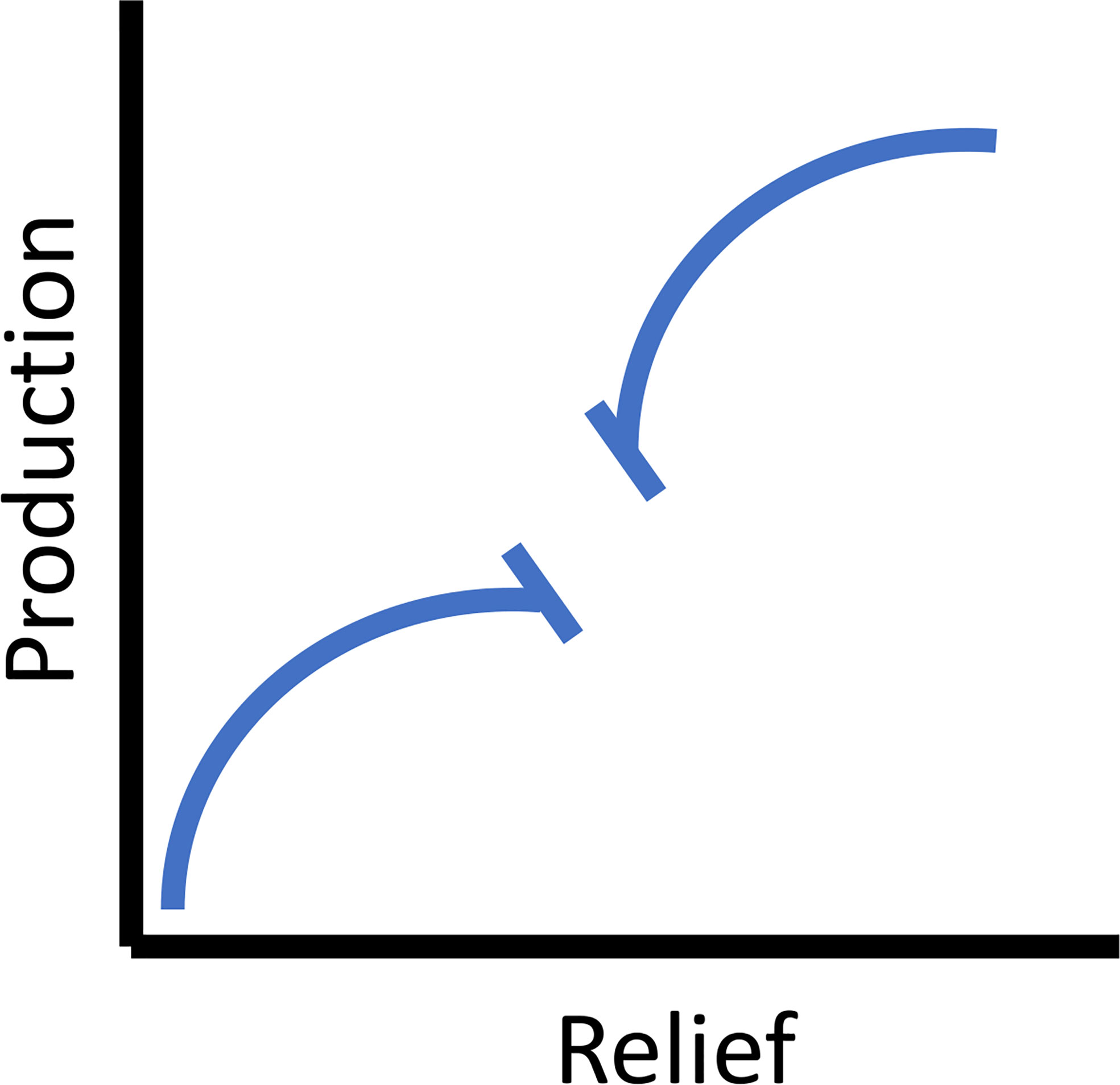
Figure 3 The theoretical relationship between vertical relief and secondary (fish) production. For the scale of typical reefs (meters) the relationship asymptotes (left side on x-axis), and then for very high relief reefs (10’s of meters) and those that include an intertidal component (e.g., breakwaters, some shipwrecks, and oil platforms) the relationship is extended until it asymptotes again (right side on x-axis).
Habitat heterogeneity, reef size, and spacing
In many systems, nursery areas are often decoupled from adult habitats. Thus, increasing habitat heterogeneity (i.e., variability of habitat types across a given landscape area) (Wedding and Yoklavich, 2015), is a critical step towards optimizing habitat for multiple life stages. Fishes, either through ontogenetic changes or during specific life history stages, often use various habitats within an ecosystem. For many reef associated species this includes foraging in surrounding soft-bottom areas. A “halo effect” documented around artificial and natural reef structures that were surrounded by soft-bottom habitat largely refers to direct and secondary effects of the organic enrichment of these sediments. These halo effects were due to complex processes. Hydrodynamic changes from the increased vertical relief of an artificial reef directly affected the composition and size of sediments, and created a plume of detritus and waste generated by reef inhabitants (e.g., filter feeding invertebrates, planktivorous fishes, macroalgae) organically enriching sediments at scales of meters to tens of meters around an artificial reef (Heery et al., 2017; Bugnot et al., 2020). Changes in the composition and densities of sediment infauna (e.g., polychaetes and amphipods) at these scales [e.g., out to 30 m in Reeds et al. (2018) and out to 80 m in Bortone et al. (1998)], were a result of being fed by the additional organic material and increased predation from reef-associated fishes and crabs. These soft-bottom foraging grounds were important in supporting the diets (i.e., production) of many species that shelter in reefs. Many taxa utilize these reef/soft bottom ecotones, emphasizing the importance of the halo effects. Multiple studies have focused on assessing the foraging distance of fishes from artificial reefs (Bortone et al., 1998; Brandt and Jackson, 2013) to minimize the overlap of foraging areas in reef design (Champion et al., 2015; Rosemond et al., 2018), which has the potential to limit growth and reef productivity (e.g., Lindberg et al., 2006). Johnson et al. (1994) found 20-25% of reef associated fish diets on a California artificial quarry rock reef derived from sand-rock ecotone habitats. These ecotones in southern California support increased densities of a commercial serranid, Barred Sandbass (Paralabrax nebulifer) (Anderson et al., 1989). Pondella et al. (2018) increased the amount of ecotone habitat by designing a reef with offset modules to increase the reef perimeter. Rosemond et al. (2018) found that reef-associated fish species were mostly foraging in the soft-bottom habitat up to 30 m away from a variety of artificial reef types (e.g., concrete pipes, metal ships) off North Carolina, USA. Reeds et al. (2018) documented reef fish foraging at elevated densities only out to 15 m from a steel fishery enhancement, but because the reef had a relatively small footprint (12 m x 16 m), they calculated that reef associated fishes were using an area 15 times greater that the reef footprint itself, demonstrating that for some species, a relatively large area of these surrounding sediments play an important ecological role. In the Adriatic Sea, Tyrrhenian Sea, and southern California, physical factors associated with artificial reef proximity outweighed the influence of predators (Ambrose and Anderson, 1990; Danovaro et al., 2002; Fabi et al., 2002) illustrating the importance of these ‘halos’.
Extending the ‘halo effect’ by incorporating the utilization of planktonic resources is conceptually a ‘dome effect’, as reefs extend three-dimensionally up into the water column. Multiple studies have taken a mechanistic approach in examining how the amount of shelter a reef provides interacts with the trophic processes of artificial reef residents who feed in adjacent habitats (e.g., water column, soft bottom, or a mix of soft bottom and natural reef). These studies suggested there is a trade-off (and potentially thresholds) between providing shelter for more individuals within the artificial reef and competition among those individuals for planktonic (Champion et al., 2015) or benthic food resources in the surrounding habitats (Lindberg et al., 2006; Brandt and Jackson, 2013), or both (Lindquist et al., 1994). Champion et al. (2015) empirically derived the amount of zooplankton that flowed across an artificial reef in Australia and the amount consumed per day by individuals of a resident fish species (Atypichthys strigatus, a smaller Kyphosidae). They modeled thresholds at which reefs were able to shelter more fish than the zooplankton flow could support, suggesting an optimal reef size exists to balance this tradeoff. They also noted that reef shape was important. Lindberg et al. (2006) found that growth was density-dependent in larger juvenile and young adult Gag Grouper (Mycteroperca microlepis) sheltering in concrete artificial reefs off Florida, USA and attributed it to competition for food in the surrounding feeding grounds. When also factoring in the potential for increased fishing mortality, if fish were in higher densities, the authors argued that most positive effect on stocks was achieved through widely scattered patch reefs with appropriately sized cavities, as that should increase growth rates of individual fish while they were residing in the reefs. It is also important to note that this species used these reefs during an older juvenile/younger adult stage prior to moving further offshore, again emphasizing that habitat use patterns associated with artificial reefs are species and life stage dependent. For example, adult wrasses (Labridae) shelter nocturnally in sand proximate to reefs. Some wrasses on coral reefs have a post-settlement stage where they shelter in the sand prior to recruitment to the reef (Hamilton et al., 2008). For overall fish productivity, there are tradeoffs between distance between reef modules, size, spacing, and maximization of the halo and ecotone effects, as these effects are synergistic to overall performance (Figure 4). But adding more structure without optimizing spacing criteria may have diminishing returns. As such, in a particular reef system, the heterogeneity of subhabitats has a maximum (Figure 4). Theoretically, each increase in subhabitat type theoretically increases the production to a maximum.
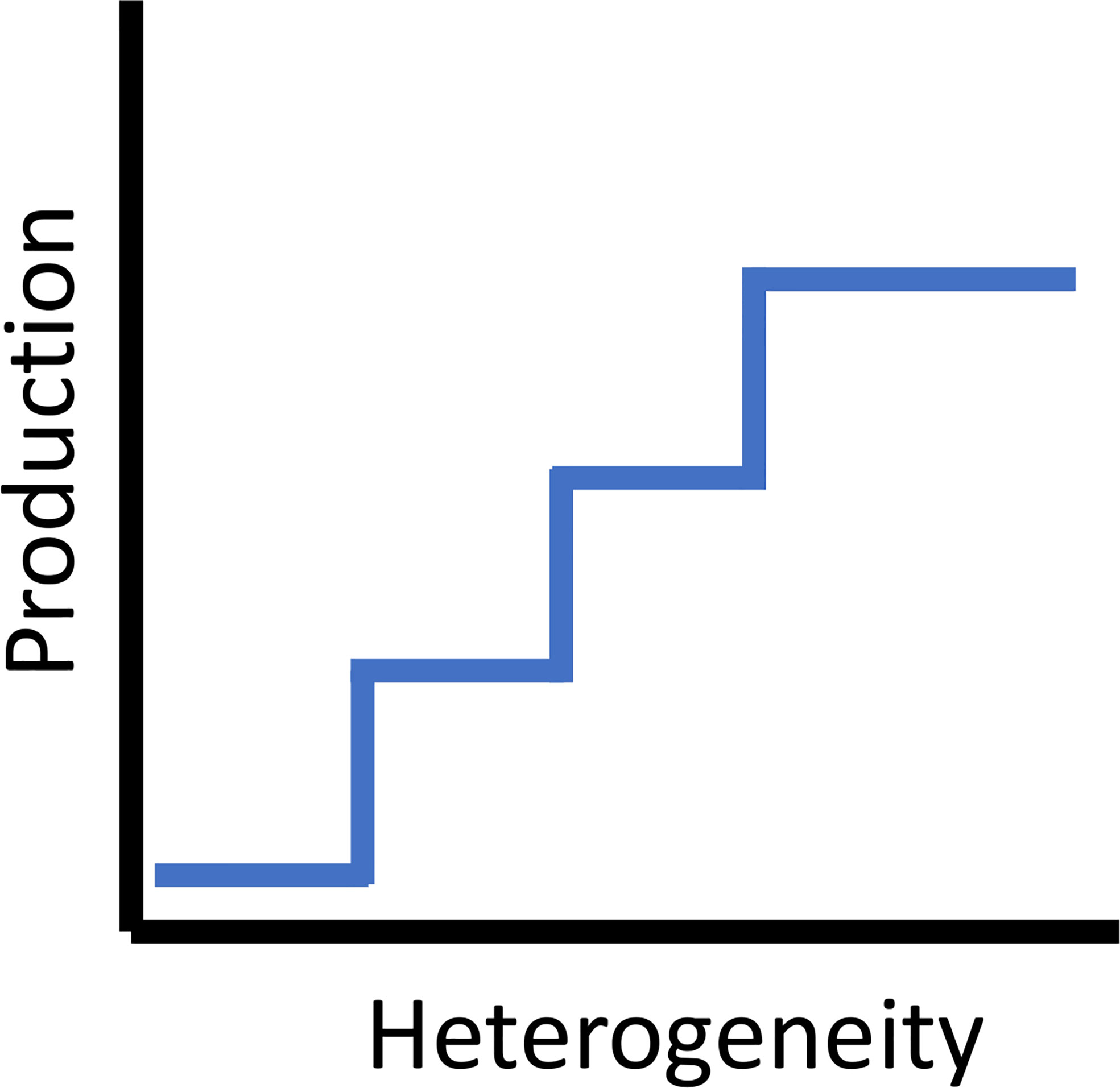
Figure 4 The theoretical step relationship between habitat heterogeneity and secondary (fish) production.
Construction logistics, available funding, engineering, regulation restrictions or boundaries, and costs constrain reef size and associated local and regional effects (Lan and Hsui, 2006). Often it is necessary to weigh the benefits of one or a few larger reefs versus many smaller reefs optimizing the use of limited resources (e.g., Pondella et al., 2018). Some studies that have directly addressed reef size suggested intermediate sizes were most productive (reef volumes from 400-4000 cubic meters, (Ogawa et al., 1977), as cited and discussed in Glarou (et al. 2020). For example, on reefs constructed with concrete cubes with holes in Taiwan, the biomass of resident fish species increased sigmoidally when compared to reef size, indicating that intermediate sizes produced the best ratio of standing stock biomass to construction cost (Jan et al., 2003). Further, reefs that are too large will reduce habitat heterogeneity and ultimately reduce production for the suite of species that depend on these varied resources (Figure 5) The trade-offs between costs and design criteria are important considerations for maximizing fish production with available resources.
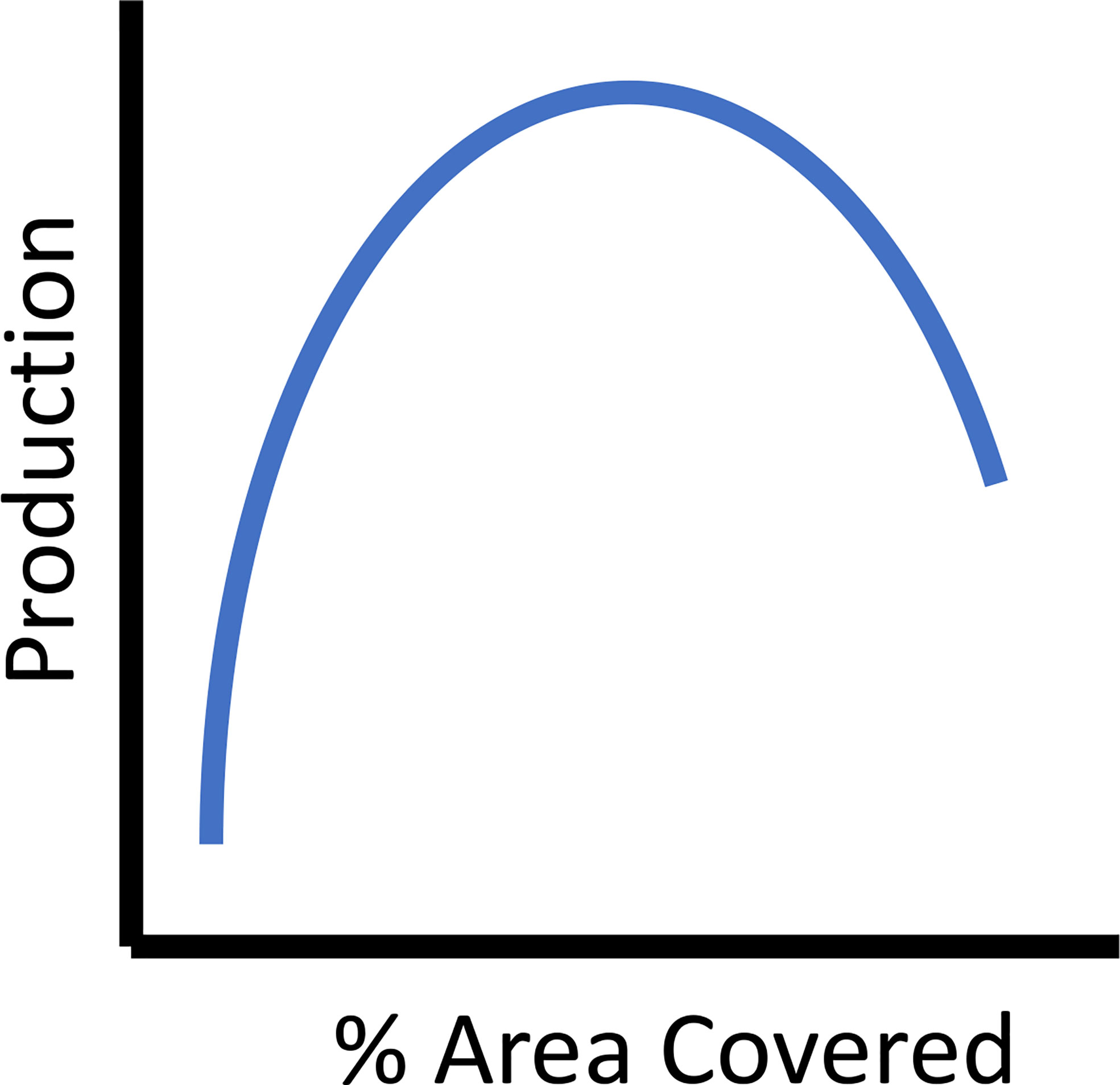
Figure 5 The theoretical relationship between percent of area covered, as a metric of reef spacing criteria and secondary (fish) production.
Additional considerations for realizing benefits
There are species-specific timescales for expected fishery benefits, based on life history and behavioral ecological traits. These benefits follow periods of good larval survival and recruitment. In Algarve, the Seabream fishery regional carrying capacity increased by 35% eight years post construction (construction took five years) as new production attributed to the artificial reef spilled over to the surrounding continental shelf. It followed multiple strong recruitment years, and then a four year lag for the species to enter the fishery (Roa-Ureta et al., 2019). An earlier economic analysis for the initial pilot-scale deployments of artificial reefs in this region also demonstrated fishery value (i.e., revenue) per unit effort slowly increased over a 15 year period following reef deployment and was higher near artificial reef modules when compared to fishing near control areas (Whitmarsh et al., 2008). These are consistent with timelines reported for maturity in Marine Protected Areas (MPA). Typically, post-closure fish densities and biomass asymptote at an interval of at least 15-20 years with older reserves outperforming younger reserves (Abesamis and Russ, 2005; Claudet et al., 2006). These time frames for maximizing reef contribution on both local and regional scales parallel typical age at maturity, the significantly greater gonadal output for larger females, and overall longevity for many focal species.
The installation of an artificial reef complex took pressure off natural reefs in the region by redistributing fishing effort across a larger area (Leitao et al., 2009). As such, spacing and connectivity to other reefs are important design criteria. In some instances, reef development and spacing protect soft-bottom species by acting as barriers to bottom trawling (Guillen et al., 1994). Shallow anthropogenic seafloor structures influence the foraging of marine mammals (Arnould et al., 2015; Todd et al., 2020). The interplay among the potential to redistribute and/or enhance mammal populations as well as attracting these predators to artificial reefs (Fernandez-Betelu et al., 2022) is an open question necessitating further investigation as these predators could increase the natural mortality of fish and invertebrate populations. Determining mechanisms for (or direct evidence of) increased survival or growth of fishes also provided evidence that artificial reef systems are not functioning as ecological traps (Reubens et al., 2014). In such traps, an organism is attracted to and settles preferably in a habitat with suboptimal conditions relative to other available and more suitable habitats (Robertson and Hutto, 2006). If an ecological trap occurs, growth, recruitment and survival rates are lower than at reference habitats, as was recently described for Reef Ball reefs in Australia (Komyakova et al., 2021). Although better alternative habitats are available, the suboptimal habitat is unfortunately chosen, resulting in reduced production. While there are few examples of this in the literature, demonstrating a net benefit to a species with certain characteristics (e.g., life history, habitat use or movement patterns, trophic traits/groups) is critical. There is also the potential for artificial reefs to support or expand the range of invasive species (Dahl and Patterson, 2014; Dafforn, 2017; Schulze et al., 2020). In heavily overfished populations (i.e., the current standing stock is not producing enough reproductive output to begin with), adding additional, even higher quality, habitat will not realize increased production if recruitment levels are low (Powers et al., 2003). In these cases, considering larger location-specific integrative coastal management approaches (Komyakova et al., 2019; Paxton et al., 2020b) and additional fishery management is necessary to achieve or sustain positive outcomes (Powers et al., 2003; Whitmarsh et al., 2008; Roa-Ureta et al., 2019).
Artificial reefs have a long history of use in fishery management (Bortone et al., 2011). An emerging concern in the literature was the consideration of artificial reefs as part of larger location-specific integrative coastal management approaches (Komyakova et al., 2019; Paxton et al., 2020b) and the potential necessity of additional fishery management for achieving or sustaining positive outcomes (Powers et al., 2003; Whitmarsh et al., 2008; Santos et al., 2011; Roa-Ureta et al., 2019). While not a concern when artificial reefs are primarily providing a nursery function to life stages with sizes below those targeted by fisheries (e.g., oyster reef restoration in estuaries in southeastern USA: Peterson et al., 2003, WARs in the North Sea: Reubens et al., 2014), this was a consideration when artificial reefs are being used by adult stages of species targeted heavily in local fisheries. On one hand, if an artificial reef or complex is relatively large (with respect to natural reefs in the area), then it may take pressure off natural reefs in the region by redistributing fishing effort across a larger total area reef habitat (Leitao et al., 2009). There are complex interactions within habitats, for instance reviews cite many studies where artificial reefs essentially stop bottom trawling in the area and may have positive impacts for soft bottom species (Relini et al., 2007; Brandini, 2014; Heery et al., 2017; Glarou et al., 2020). However, if the artificial reefs instead aggregated existing biomass in the region making target species easier to exploit (the goal of Fish Aggregating Devices [FADs]), and if this fishing mortality was not counter-balanced with a proportional increase in localized production (Lindberg et al., 2006; Smith et al., 2015), essentially the crux of the ‘attraction vs production’ debate (Osenberg et al., 2002), then consideration of additional fisheries management actions is prudent. In the case where an artificial reef serves as a spawning aggregation site (Schmiing et al., 2017), this necessitates potential permanent or reproductive season fishing closures. Balancing this against the potential secondary production increases due to increasing gonadal production are the challenges managers face. More broadly though, if objectives include amplifying regional impacts of deploying an artificial habitat (e.g., population recovery or increasing regional carrying capacity), then considerations including additional management actions focused on spawning stock biomass recovery, and particularly the protection of larger/older individuals, like in the implementation of MPAs and size limits, were practical (Claudet et al., 2008; Edgar and Stuart-Smith, 2009; Barneche et al., 2018; Marshall et al., 2019). Thus, incorporating an ecosystem-wide approach in reef design is necessary.
Overall successful reefing projects optimize all these discussed criteria (shelter, complexity, heterogeneity, relief and spacing) to maximize reproductive output and recruitment success. These parameters are ecosystem- and taxa- specific; thus, wherever possible a thorough and understanding of the geographical, ecological, and socioeconomic context is necessary.
Author contributions
All authors listed have made a substantial, direct, and intellectual contribution to the work and approved it for publication.
Funding
Funding for this study was provided by Chevron Technical Center, a division of Chevron USA Inc., under Service Order No. 0015115104.
Acknowledgments
We would like to thank Travis Elsdon, Gary Wolinsky, and William Gala for their feedback during initial project development and manuscript preparation.
Conflict of interest
This paper is the result of a study funded by Chevron Technical Center, a division of Chevron USA Inc., which supported the core project team to conduct a literature review on factors influencing secondary production on artificial reef structures. The funder was not involved in the study design, literature review, interpretation of data, the writing of this article or the decision to submit it for publication. This funding source has not influenced the authors’ objectivity. The authors have no further potential conflicts of interest to disclose.
Publisher’s note
All claims expressed in this article are solely those of the authors and do not necessarily represent those of their affiliated organizations, or those of the publisher, the editors and the reviewers. Any product that may be evaluated in this article, or claim that may be made by its manufacturer, is not guaranteed or endorsed by the publisher.
References
Abesamis R. A., Russ G. R. (2005). Density-dependent spillover from a marine reserve: long-term evidence. Ecol. Appl. 15, 1798–1812. doi: 10.1890/05-0174
Almany G., Connolly S., Heath D., Hogan J., Jones G., McCook L., et al. (2009). Connectivity, biodiversity conservation and the design of marine reserve networks for coral reefs. Coral reefs 28, 339–351. doi: 10.1007/s00338-009-0484-x
Ambrose R. F., Anderson T. W. (1990). Influence of an artifical reef on the surrounding infaunal community. Mar. Biol. 107, 41–52. doi: 10.1007/BF01313240
Ambrose R. F., Swarbrick S. L. (1989). Comparison of fish assemblages on artificial and natural reefs off the coast of southern California. Bull. Mar. Sci. 44, 718–733.
Anderson T. W., DeMartini E. E., Roberts D. A. (1989). The relationship between habitat structure, body size and distribution of fishes at a temperate artificial reef. Bull. Mar. Sci. 44, 681–697.
Arnould J. P. Y., Monk J., Ierodiaconou D., Hiindell M. A., Semmens J., Hoskins A. J., et al. (2015). Use of anthropogenic sea floor structures by Australian fur seals: potential positive ecological impacts of marine industrial development? PloS One 107, e0130581. doi: 10.1371/journal.pone.0130581
Baetscher D. S., Anderson E. C., Gilbert-Horvath E. A., Malone D. P., Saarman E. T., Carr M. H., et al. (2019). Dispersal of a nearshore marine fish connects marine reserves and adjacent fished areas along an open coast. Mol. Ecol. 28, 1611–1623. doi: 10.1111/mec.15044
Barilotti A. A., White C. F., Lowe C. G. (2020). Are Fishes Attracted to Piers? Movements and Association of Marine Fishes to a Public Fishing Pier within a Commercial Harbor. Bulletin, Southern California Academy of Sciences 119(1):18–34
Barneche D. R., Robertson D. R., White C. R., Marshall D. J. (2018). Fish reproductive-energy output increases disproportionately with body size. Science 360, 642–645. doi: 10.1126/science.aao6868
Barry J., Wickins J. F. (1992). A model for the number and sizes of crevices that can be seen on the exposed surface of submerged rock reefs. Environmetrics 3, 55–69. doi: 10.1002/env.3170030104
Beets J., Hixon M. A. (1994). Distribution, persistence, and growth of groupers (Pisces: Serranidae) on artificial and natural patch reefs in the virgin islands. Bull. Mar. Sci. 55, 470–483.
Berkeley S. A., Hixon M. A., Larson R. J., Love M. S. (2004). Fisheries sustainability via protection of age structure and spatial distribution of fish populations. Fisheries 29, 23–32. doi: 10.1577/1548-8446(2004)29[23:FSVPOA]2.0.CO;2
Berumen M. L., Almany G. R., Planes S., Jones G. P., Saenz-Agudelo P., Thorrold S. R. (2012). Persistence of self-recruitment and patterns of larval connectivity in a marine protected area network. Ecol. Evol. 2, 444–452. doi: 10.1002/ece3.208
Blanchette C. A., Helmuth B., Gaines S. D. (2007). Spatial patterns of growth in the mussel, Mytilus californianus, across a major oceanographic and biogeographic boundary at point conception, California, USA. J. Exp. Mar. Biol. Ecol. 340, 126–148. doi: 10.1016/j.jembe.2006.09.022
Blount C., Komyakova V., Barnes L., Smith M. L., Zhang D., Reeds K., et al. (2021). Using ecological evidence to refine approaches to deploying offshore artificial reefs for recreational fisheries. Bull. Mar. Sci. 97, 665–698. doi: 10.5343/bms.2020.0059
Bohnsack J. A., Harper D. E., McClellan D. B., Hulsbeck M. (1994). Effects of reef size on colonization and assemblage structure of fishes at artificial reefs off southeastern Florida, USA. Bull. Mar. Sci. 55, 796–823.
Bortone S. A., Brandini F. P., Fabi G., Otake S. (Eds.) (2011). Artificial reefs in fishery management (Boca Raton, Florida: CRC Press, Taylor & Francis Group).
Bortone S. A., Cody R. P., Turpin R. K., Bundrick C. M. (1998). The impact of artificial-reef fish assemblages on their potential forage area. Ital. J. Zool. 65, 265–267. doi: 10.1080/11250009809386830
Botsford L., White J. W., Coffroth M.-A., Paris C., Planes S., Shearer T., et al. (2009). Connectivity and resilience of coral reef metapopulations in marine protected areas: matching empirical efforts to predictive needs. Coral reefs 28, 327–337. doi: 10.1007/s00338-009-0466-z
Brandini F. (2014). Marine biodiversity and sustainability of fishing resources in Brazil: a case study of the coast of paraná state. Regional Environ. Change 14, 2127–2137. doi: 10.1007/s10113-013-0458-y
Brandt J. R., Jackson D. C. (2013). Influences of artificial reefs on juvenile red snapper along the Mississippi gulf coast. Mar. Coast. Fisheries 5, 1–10. doi: 10.1080/19425120.2012.736445
Bray R. N., Miller A. C., Geesey G. G. (1981). The fish connection: A trophic link between planktonic and rocky reef communities? Science 214, 204–205. doi: 10.1126/science.214.4517.204
Brickhill M. J., Lee S. Y., Connolly R. M. (2005). Fishes associated with artificial reefs: attributing changes to attraction or production using novel approaches. J. Fish Biol. 67, 53–71. doi: 10.1111/j.0022-1112.2005.00915.x
Bugnot A. B., Mayer-Pinto M., Airoldi L., Heery E. C., Johnston E. L., Critchley L. P., et al. (2020). Current and projected global extent of marine built structures. Nat. Sustain, 33–41. doi: 10.1038/s41893-020-00595-1
Bulger D. S., Volpe J. P., Fisher J. T. (2019). Differences in fish communities on natural versus artificial temperate reefs, groundfish conservation applications in British Columbia. Mar. Environ. Res. 152, 104788. doi: 10.1016/j.marenvres.2019.104788
Burns E. S., Clevenstine A. J., Logan R. K., Lowe C. G. (2020). Evidence of artificial habitat use by a recovering marine predator in southern California. J. Fish Biol 97, 1857–1860. doi: 10.1111/jfb.14539
Champion C., Suthers I. M., Smith J. A. (2015). Zooplanktivory is a key process for fish production on a coastal artificial reef. Mar. Ecol. Prog. Ser. 541, 1–14. doi: 10.3354/meps11529
Charbonnel E., Serre C., Ruitton S., Harmelin J.-G., Jensen A. (2002). Effects of increased habitat complexity on fish assemblages associated with large artificial reef units (French Mediterranean coast). ICES J. Mar. Sci. 59, S208–S213. doi: 10.1006/jmsc.2002.1263
Claisse J. T., Pondella D. J. II, Love M., Zahn L. A., Williams C. M., Williams J. P., et al. (2014). Oil platforms off California are among the most productive marine fish habitats globally. Proc. Natl. Acad. Sci. U.S.A. 111, 15462–15467. doi: 10.1073/pnas.1411477111
Claudet J., Osenberg C. W., Benedetti-Cecchi L., Domenici P., García-Charton J.-A., Pérez-Ruzafa Á., et al. (2008). Marine reserves: size and age do matter. Ecol. Lett. 11, 481–489. doi: 10.1111/j.1461-0248.2008.01166.x
Claudet J., Pelletier D., Jouvenel J., Bachet F., Galzin R. (2006). Assessing the effects of marine protected area (MPA) on a reef fish assemblage in a northwestern Mediterranean marine reserve: identifiying community-based indicators. Biol. Conserv. 130, 349–369. doi: 10.1016/j.biocon.2005.12.030
Cresson P., Le Direach L., Rouanet E., Goberville E., Astruch P., Ourgaud M., et al. (2019). Functional traits unravel temporal changes in fish biomass production on artificial reefs. Mar. Environ. Res. 145, 137–146. doi: 10.1016/j.marenvres.2019.02.018
Cresson P., Ruitton S., Harmelin-Vivien M. (2014a). Artificial reefs do increase secondary biomass production: mechanisms evidenced by stable isotopes. Mar. Ecol. Prog. Ser. 509, 15–26. doi: 10.3354/meps10866
Cresson P., Ruitton S., Ourgaud M., Harmelin-Viivien M. (2014b). Contrasting perception of fish trophic level from stomach content and stable isotope analyses: A Mediterranean artificial reef experience. J. Exp. Mar. Biol. Ecol. 452, 54–62. doi: 10.1016/j.jembe.2013.11.014
Cudney-Bueno R., Lavín M. F., Marinone S. G., Raimondi P. T., Shaw W. W. (2009). Rapid effects of marine reserves via larval dispersal. PloS One 4, e4140. doi: 10.1371/journal.pone.0004140
Dafforn K. A. (2017). Eco-engineering and management strategies for marine infrastructure to reduce establishment and dispersal of non-indigenous species. Manage. Biol. Invasions 8, 153–161. doi: 10.3391/mbi.2017.8.2.03
Dahl K. A., Patterson W. F. III (2014). Habitat-specific density and diet of rapidly expanding invasive red lionfish, Pterois volitans, populations in the northern gulf of Mexico. PloS One 9, e105852. doi: 10.1371/journal.pone.0105852
Danovaro R., Gambi C., Mazzola A., Mirto S. (2002). Influence of artificial reefs on the surrounding infauna: analysis of meiofauna. ICES J. Mar. Sci. 59, S356–S362. doi: 10.1006/jmsc.2002.1223
Demartini E. E., Barnett A. M., Johnson T. D., Ambrose R. F. (1994). Growth and production estimates for biomass-dominant fishes on a southern California artificial reef. Bull. Mar. Sci. 55, 484–500.
De Troch M., Reubens J. T., Heirman E., Degraer S., Vincx M. (2013). Energy profiling of demersal fish: A case-study in wind farm artificial reefs. Mar. Environ. Res. 92, 224–233. doi: 10.1016/j.marenvres.2013.10.001
Edgar G. J., Stuart-Smith R. D. (2009). Ecological effects of marine protected areas on rocky reef communities–a continental-scale analysis. Mar. Ecol. Prog. Ser. 388, 51–62. doi: 10.3354/meps08149
Fabi G., Luccarini F., Panfili M., Solustri C., Spagnolo A. (2002). Effects of an artificial reef on the surrounding soft-bottom community (central Adriatic Sea). ICES J. Mar. Sci. 59, S343–S349. doi: 10.1006/jmsc.2002.1308
Fernandez-Betelu O., Graham I. M., Thompson P. M. (2022). Reef effect of offshore structures on the occurrence and foraging activity of harbour porpoises. Front. Mar. Sci. 9. doi: 10.3389/fmars.2022.980388
Foley M. M., Halpern B. S., Micheli F., Armsby M. H., Caldwell M. R., Crain C. M., et al. (2010). Guiding ecological principles for marine spatial planning. Mar. Policy 34, 955–966. doi: 10.1016/j.marpol.2010.02.001
Friedlander A. M., Parrish J. D. (1998). Temporal dynamics of fish communities on an exposed shoreline in Hawaii. Environ. Biol. Fishes 53, 1–18. doi: 10.1023/A:1007497210998
Glarou M., Zrust M., Svendsen J. C. (2020). Using artificial-reef knowledge to enhance the ecological function of offshore wind turbine foundations: Implications for fish abundance and diversity. J. Mar. Sci. Eng. 8:1–25. doi: 10.3390/jmse8050332
Graham M. H. (2004). Effects of local deforestation on the diversity and structure of southern California giant kelp forest food webs. Ecosystems 7, 341–357. doi: 10.1007/s10021-003-0245-6
Granneman J. E., Steele M. A. (2014). Fish growth, reproduction, and tissue production on artificial reefs relative to natural reefs. ICES J. Mar. Sci. 71, 2494–2504. doi: 10.1093/icesjms/fsu082
Granneman J. E., Steele M. A. (2015). Effects of reef attributes on fish assemblage similarity between artificial and natural reefs. ICES J. Mar. Sci. 72, 2385–2397. doi: 10.1093/icesjms/fsv094
Guillen J. E., Ramos A. A., Martinez L., Sanchez Lizaso J. L. (1994). Antitrawling reefs and the protection of Posidonia oceanica (L.) delile meadows in the Western Mediterranean Sea: demand and aims. Bull. Mar. Sci. 55, 645–650.
Hamilton S. L., Regetz J., Warner R. R. (2008). Postsettlement survival linked to larval life in a marine fish. Proc. Natl. Acad. Sci. 105, 1561–1566. doi: 10.1073/pnas.0707676105
Harlin M. M., Lindbergh J. M. (1977). Selection of substrata by seaweeds: Optimal surface relief. Mar. Biol. 40, 33–40. doi: 10.1007/BF00390625
Heery E. C., Bishop M. J., Critchley L. P., Bugnot A. B., Airoldi L., Mayer-Pinto M., et al. (2017). Identifying the consequences of ocean sprawl for sedimentary habitats. J. Exp. Mar. Biol. Ecol. 492, 31–48. doi: 10.1016/j.jembe.2017.01.020
Hixon M. A. (1980). Comparative interactions between California reef fishes of the genus Embiotoca. Ecology 61, 918–931. doi: 10.2307/1936761
Hixon M. A., Beets J. P. (1993). Predation, prey refuges, and the structure of coral-reef fish assemblages. Ecol. Monogr. 63, 77–101. doi: 10.2307/2937124
Holland M. M., Becker A., Smith J. A., Everett J. D., Suthers I. M. (2021). Fine-scale spatial and diel dynamics of zooplanktivorous fish on temperate rocky and artificial reefs. Mar. Ecol. Prog. Ser. 674, 221–239. doi: 10.3354/meps13831
Hunter W. R., Sayer M. D. J. (2009). The comparative effects of habitat complexity on faunal assemblages of northern temperate artificial and natural reefs. Ices J. Mar. Sci. 66, 691–698. doi: 10.1093/icesjms/fsp058
Itosu C., Otake S., Nakamura M. (1995). “Excess energy of separated eddies due to its spin-down and upwelling,” in ECOSET ‘95 international conference on ecological system enhancement technology for aquatic environments (Tokyo, Japan: Japan Internationa Marine Science and Technology Federation), 251–256.
Jackson J. B. C., Kirby M. X., Berger W. H., Bjorndal K. A., Botsford L. W., Bourque B. J., et al. (2001). Historical overfishing and the recent collapse of coastal ecosystems. Science 293, 629–638. doi: 10.1126/science.1059199
Jan R.-Q., Liu Y.-H., Chen C.-Y., Wang M.-C., Song G.-S., Lin H.-C., et al. (2003). Effects of pile size of artificial reefs on the standing stocks of fishes. Fisheries Res. 63, 327–337. doi: 10.1016/S0165-7836(03)00081-X
Johnson T. D., Barnett A. M., DeMartini E. E., Craft L. L., Ambrose R. F., Purcell L. J. (1994). Fish production and habitat utilization on a southern California artificial reef. Bull. Mar. Sci. 55, 709–723.
Kellison T. G., Sedberry G. R. (1998). The effects of artificial reef vertical profile and hole diameter on fishes off south Carolina. Bull. Mar. Sci. 62, 763–780.
Kim C. G., Lee J. W, Park J. S (1994). Artificial reef designs for Korean coastal waters. Bulletin Mar. Sci., 55(2–3), 858–866.
Knowlton N. (2021). Ocean optimism: moving beyond the obituaries in marine conservation. Annu. Rev. 13, 2.1–2.21. doi: 10.1146/annurev-marine-040220-101608
Komyakova V., Chamberlain D., Jones G. P., Swearer S. E. (2019). Assessing the performance of artificial reefs as substitute habitat for temperate reef fishes: Implications for reef design and placement. Sci. Total Environ. 668, 139–152. doi: 10.1016/j.scitotenv.2019.02.357
Komyakova V., Chamberlain D., Swearer S. E. (2021). A multi-species assessment of artificial reefs as ecological traps. Ecol. Eng. 171, 106394. doi: 10.1016/j.ecoleng.2021.106394
Kough A. S., Belak C. A., Paris C. B., Lundy A., Cronin H., Gnanalingam G., et al. (2019). Ecological spillover from a marine protected area replenishes an over-exploited population across an island chain. Conserv. Sci. Pract. 1, e17. doi: 10.1111/csp2.17
Krone R., Dederer G., Kanstinger P., Kramer P., Schneider C., Schmalenbach I. (2017). Mobile demersal megafauna at common offshore wind turbine foundations in the German bight (North Sea) two years after deployment - increased production rate of cancer pagurus. Mar. Environ. Res. 123, 53–61. doi: 10.1016/j.marenvres.2016.11.011
Lan C.-H., Hsui C.-y. (2006). Insight from complexity: a new approach to designing the deplyment of artificial reef communities. Bull. Mar. Sci. 78, 21–28.
Leitão F. (2013). Artificial reefs: from ecological processes to fishing enhancement tools. Braz. J. Oceanogr. 61, 77–81. doi: 10.1590/S1679-87592013000100009
Leitao F., Santos M. N., Erzini K., Monteiro C. C. (2009). Diplodus spp. assemblages on artificial reefs: importance for near shore fisheries. Fisheries Manage. Ecol. 16, 88–99. doi: 10.1111/j.1365-2400.2008.00646.x
Lemoine H. R., Paxton A. B., Anisfeld S. C., Rosemond R. C., Peterson C. H. (2019). Selecting the optimal artificial reefs to achieve fish habitat enhancement goals. Biol. Conserv. 238:1–9. doi: 10.1016/j.biocon.2019.108200
Lindberg W. J., Frazer T. K., Portier K. M., Vose F., Loftin J., Murie D. J., et al. (2006). Density-dependent habitat selection and performance by a large mobile reef fish. Ecol. Appl. 16, 731–746. doi: 10.1890/1051-0761(2006)016[0731:DHSAPB]2.0.CO;2
Lindquist D. G., Cahoon L. B., Clavijo I. E., Posey M. H., Bolden S. K., Pike L. A., et al. (1994). Reef fish stomach contents and prey abundance on reef and sand substrata associated with adjacent artificial and natural reefs in onslow bay, North Carolina. Bull. Mar. Sci. 55, 308–318.
Love M. S., Brothers E., Schroeder D. M., Lenarz W. H. (2007). Ecological performance of young-of-the-year blue rockfish (Sebastes mystinus) associated with oil platforms and natural reefs in California as measured by daily growth rates. Bull. Mar. Sci. 80, 147–157.
Love M. S., Claisse J. T., Roeper A. (2019). An analysis of the fish assemblages around 23 oil and gas platforms off California with comparisons with natural habitats. Bull. Mar. Sci. 95, 477–514. doi: 10.5343/bms.2018.0061
Marshall D. J., Gaines S., Warner R., Barneche D. R., Bode M. (2019). Underestimating the benefits of marine protected areas for the replenishment of fished populations. Front. Ecol. Environ. 17, 407–413. doi: 10.1002/fee.2075
Medeiros A. P., Ferreira B. P., Betancur-R R., Cardoso A. P., Matos M. R., Santos B. A. (2021). Centenary shipwrecks reveal the limits of artificial habitats in protecting regional reef fish diversity. J. Appl. Ecol. 59, 286–299. doi: 10.1111/1365-2664.14053
Molles J., Manuel C. (1978). Fish species diversity on model and natural reef patches: experimental insular biogeography. Ecol. Monogr. 48, 289–305. doi: 10.2307/2937232
Ogawa Y. (1967). Experiments on the attractiveness of artificial reefs for marine fishes. VII. Attraction Fishes to Various Sizes Model. Reefs. Bull. Jap. Soc Sci. Fish 33, 801–811. doi: 10.2331/suisan.33.801
Ogawa Y., Takeuchi S., Hattori A. (1977). An estimate for the optimum size of artificial reef. Bull. Japanese Soc. Fisheries Oceanogr. (Japan) 30, 39–45.
Osenberg C. W., St C. M., Wilson J. A., Lindberg W. J. (2002). A quantitative framework to evaluate the attraction-production controversy. ICES J. Mar. Sci. 598, S214–S221. doi: 10.1006/jmsc.2002.1222
Otake S., Itosu C., Nakamura M. (1995). “Artificial upwelling due to parallel walls,” in ECOSET ‘95 international conference on ecological system enhancement technology for aquatic environments (Tokyo, Japan: Japan Internationa Marine Science and Technology Federation), 245–250.
Page H. M., Hubbard D. M. (1987). Temporal and spatial patterns of growth in mussels Mytilus edulis on an offshore platform: relationships to water temperature and food availability. J. Exp. Mar. Biol. Ecol. 111, 159–179. doi: 10.1016/0022-0981(87)90053-0
Paxton A. B., Newton E. A., Adler A. M., Van Hoeck R. V., Iversen E. S. Jr., Taylor J. C., et al. (2020a). Artificial habitats host elevated densities of large reef-associated predators. PloS One 15, e0237374. doi: 10.1371/journal.pone.0237374
Paxton A. B., Shertzer K. W., Bacheler N. M., Kellison G. T., Riley K. L., Taylor J. C. (2020b). Meta-analysis reveals artificial reefs can be effective tools for fish community enhancement but are not one-Size-Fits-All. Front. Mar. Sci. 7. doi: 10.3389/fmars.2020.00282
Pérez-Ruzafa A., Martín E., Marcos C., Zamarro J. M., Stobart B., Harmelin-Vivien M., et al. (2008). Modelling spatial and temporal scales for spill-over and biomass exportation from MPAs and their potential for fisheries enhancement. J. Nat. Conserv. 16, 234–255. doi: 10.1016/j.jnc.2008.09.003
Peterson C. H., Grabowski J. H., Powers S. P. (2003). Estimated enhancement of fish production resulting from restoring oyster reef habitat: quantitative valuation. Mar. Ecol. Prog. Ser. 264, 249–264. doi: 10.3354/meps264249
Polovina J. J., Sakai I. (1989). Impacts of artificial reefs on fishery production in Shimamaki, Japan. Bull. Mar. Sci. 44, 997–1003.
Pondella D. J. II, Allen L. G., Craig M. T., Gintert B. (2006). Evaluation of eelgrass mitigation and fishery enhancement structures in San Diego bay, California. Bull. Mar. Sci. 78, 115–131.
Pondella D. J. II, Stephens J. S., Craig M. T. (2002). Fish production of a temperate artificial reef based on the density of embiotocids (Teleostei: Perciformes). ICES J. Mar. Sci. 59, S88–S93. doi: 10.1006/jmsc.2002.1219
Pondella D. J. II, Williams J. P., Williams C. M., Claisse J. T., Witting D. (2018). “Restoring a nearshore rocky reef ecosystem in the challenge of an urban setting,” in Marine artificial reef research and development: Integrating fisheries management objectives (Bethesda, Maryland, USA: American Fisheries Society), 165–186.
Pondella D. J. II, Zahn L. A., Love M. S., Siegel D., Bernstein B. B. (2015). Modeling fish production for southern California’s petroleum platforms. Integrated Environ. Assess. Manage. 11, 584–593. doi: 10.1002/ieam.1689
Potts T. A., Hulbert A. W. (1994). Structural influences of artificial and natural habitats on fish aggregations in onslow bay, North Carolina. Bull. Mar. Sci. 55, 609–622.
Powers S. P., Grabowski J. H., Peterson C. H., Lindberg W. J. (2003). Estimating enhancement of fish production by offshore artificial reefs: uncertainty exhibited by divergent scenarios. Mar. Ecol. Prog. Ser. 264, 265–277. doi: 10.3354/meps264265
Puckeridge A. C., Becker A., Taylor M. D., Lowry M. B., McLeod J., Schilling H. T., et al. (2021). Foraging behaviour and movements of an ambush predator reveal benthopelagic coupling on artificial reefs. Mar. Ecol. Prog. Ser. 666, 171–182. doi: 10.3354/meps13691
Reeds K. A., Smith J. A., Suthers I. M., Johnston E. L. (2018). An ecological halo surrounding a large offshore artificial reef: Sediments, infauna, and fish foraging. Mar. Environ. Res. 141, 30–38. doi: 10.1016/j.marenvres.2018.07.011
Relini G., Relini M., Palandri G., Merello S., Beccornia E. (2007). History, ecology and trends for artificial reefs of the Ligurian sea, Italy. Hydrobiologia 580, 193–217. doi: 10.1007/s10750-006-0453-0
Reubens J. T., Degraer S., Vincx M. (2014). The ecology of benthopelagic fishes at offshore wind farms: a synthesis of 4 years of research. Hydrobiologia 727, 121–136. doi: 10.1007/s10750-013-1793-1
Ricker W. E. (1975). Computation and interpretation of biological statistics of fish populations. Bull. Fisheries Res. Board Canada 191, 382.
Roa-Ureta R. H., Santos M. N., Leitão F. (2019). Modelling long-term fisheries data to resolve the attraction versus production dilemma of artificial reefs. Ecol. Model. 407:1–12. doi: 10.1016/j.ecolmodel.2019.108727
Robertson B. A., Hutto R. L. (2006). A framework for understanding ecological traps and an evaluationn of existing evidence. Ecology 87, 1075–1085. doi: 10.1890/0012-9658(2006)87[1075:AFFUET]2.0.CO;2
Rosemond R. C., Paxton A. B., Lemoine H. R., Fegley S. R., Peterson C. H. (2018). Fish use of reef structures and adjacent sand flats: implications for selecting minimum buffer zones between new artificial reefs and existing reefs. Mar. Ecol. Prog. Ser. 587, 187–199. doi: 10.3354/meps12428
Rouse S., Porter J. S., Wilding T. A. (2020). Artificial reef design affects benthic secondary productivity and provision of functional habitat. Ecol. Evol. 10(4), 2122–2130. doi: 10.1002/ece3.6047
Russ G. R., Alcala A. C., Maypa A. P., Calumpong H. P., White A. T. (2004). Marine reserve benefits local fisheries. Ecol. Appl. 14, 597–606. doi: 10.1890/03-5076
Santos M. N., Monteiro C. C., Leitão F. (2011). “The role of artificial reefs in the sustainability of artisanal fisheries,” in Artificial reefs in fisheries management (New York: Taylor and Francis Group), 221–237.
Schmiing M., Fontes J., Afonso P. (2017). Predictive mapping of reproductive fish habitats to aid marine conservation planning. Can. J. Fisheries Aquat. Sci. 74, 1016–1027. doi: 10.1139/cjfas-2015-0538
Schulze A., Erdner D. L., Grimes C. J., Holstein D. M., Miglietta M. P. (2020). Artificial reefs in the northern gulf of Mexico: Community ecology amid the “Ocean sprawl”. Front. Mar. Sci. 7, 447. doi: 10.3389/fmars.2020.00447
Schwartzbach A., Behrens J. W., Svendsen J. C. (2020). Atlantic Cod gadus morhua save energy on stone reefs: implications for the attraction versus production debate in relation to reefs. Mar. Ecol. Prog. Ser. 635, 81–87. doi: 10.3354/meps13192
Seaman W. (2019). “Artificial reefs,” in Encyclopedia of ocean sciences, 3rd edition, vol. 1 . Eds. Cochran J.K, Bokuniewicz H., Yager L. P., Elsevier: Amsterdam, Netherlands 662–670.
Sherman R. L., Gilliam D. S., Spieler R. E. (2002). Artificial reef design: void space, complexity, and attractants. ICES J. Mar. Sci. 59, S196–S200. doi: 10.1006/jmsc.2001.1163
Smith J. A., Lowry M. B., Champion C., Suthers I. M. (2016). A designed artificial reef is among the most productive marine fish habitats: new metrics to address ‘production versus attraction’. Mar. Biol. 163, 1–8. doi: 10.1007/s00227-016-2967-y
Smith J. A., Lowry M. B., Suthers I. M. (2015). Fish attraction to artificial reefs not always harmful: a simulation study. Ecol. Evol. 5, 4590–4602. doi: 10.1002/ece3.1730
Steimle F., Foster K., Kropp R., Conlin B. (2002). Benthic macrofauna productivity enhancement by and artificial reef in Delaware Bay, USA. ICES J. Mar. Sci. 59, S100–S105. doi: 10.1006/jmsc.2002.1268
Stephens J. S., Morris P. A., Pondella D. J. II, Koonce T. A., Jordan G. A. (1994). Overview of the dynamics of an urban artificial reef fish assemblage at king Harbor, California, USA, 1974-1991: A recruitment driven system. Bull. Mar. Sci. 55, 1224–1239.
Tickell S. C., Sáenz-Arroyo A., Milner-Gulland E. J. (2019). Sunken worlds: The past and future of human-made reefs in marine conservation. BioScience 69, 725–735. doi: 10.1093/biosci/biz079
Todd V. L. G., Lazar L., Williamson L. D., Peters I. T., Hoover A. L., Cox S. E., et al. (2020). Underwater visual records of marine megafauna around offshore anthropogenic structures. Front. Mar. Sci. 7, 230. doi: 10.3389/fmars.2020.00230
Truong L., Suthers I. M., Cruz D. O., Smith J. A. (2017). Plankton supports the majority of fish biomass on temperate rocky reefs. Mar. Biol. 164:1–12. doi: 10.1007/s00227-017-3101-5
Turner C. H., Ebert E. E., Given R. R. (1969). Fish Bulletin 146. Man-Made Reef Ecology. (UC San Diego: Library – Scripps Digital Collection). Retrieved from https://escholarship.org/uc/item/2s55m47r
Walters L. J., Wethey D. S. (1996). Settlement and early post-settlement survival of sessile marine invertebrates on topographically complex surfaces: the importance of refuge dimensions and adult morphology. Mar. Ecol. Prog. Ser. 137, 161–171. doi: 10.3354/meps137161
Warner R. R. (1988). Traditionality of mating-site preferences in a coral reef fish. Nature 335, 719–721. doi: 10.1038/335719a0
Wedding L., Yoklavich M. M. (2015). Habitat-based predictive mapping of rockfish density and biomass off the central California coast. Mar. Ecol. Prog. Ser. 540, 235–250. doi: 10.3354/meps11442
Whitmarsh D., Santos M. N., Ramos J., Monteiro C. C. (2008). Marine habitat modification through artificial reefs off the Algarve (southern Portugal): An economic analysis of the fisheries and the prospects for management. Ocean Coast. Manage. 51, 463–468. doi: 10.1016/j.ocecoaman.2008.04.004
Keywords: secondary fish production, habitat restoration, shelter, habitat complexity, habitat heterogeneity, trophic pathways, ecosystem services
Citation: Pondella DJ II, Claisse JT and Williams CM (2022) Theory, practice, and design criteria for utilizing artificial reefs to increase production of marine fishes. Front. Mar. Sci. 9:983253. doi: 10.3389/fmars.2022.983253
Received: 30 June 2022; Accepted: 29 September 2022;
Published: 20 October 2022.
Edited by:
Ibon Galparsoro, Technological Center Expert in Marine and Food Innovation (AZTI), SpainReviewed by:
Daniel Ierodiaconou, Deakin University, AustraliaGladwin Asir, Suganthi Devadason Marine Research Institute (SDMRI), India
Copyright © 2022 Pondella, Claisse and Williams. This is an open-access article distributed under the terms of the Creative Commons Attribution License (CC BY). The use, distribution or reproduction in other forums is permitted, provided the original author(s) and the copyright owner(s) are credited and that the original publication in this journal is cited, in accordance with accepted academic practice. No use, distribution or reproduction is permitted which does not comply with these terms.
*Correspondence: Daniel J. Pondella II, pondella@oxy.edu