- 1Department of Biotechnology, Faculty of Technology, Khon Kaen University, Khon Kaen, Thailand
- 2Research Group for Development of Microbial Hydrogen Production Process from Biomass, Khon Kaen University, Khon Kaen, Thailand
- 3Academy of Science, Royal Society of Thailand, Bangkok, Thailand
Cost of nutrients is one of the major contributors to the production cost of docosahexaenoic acid (DHA) by thraustochytrids, and this remains the main challenge for economical and sustainable production of DHA. In the present study, cassava pulp (CP) was investigated as an alternative low-cost carbon source for DHA production by Aurantiochytrium limacinum SR21. Cultivation conditions, i.e., salinity level, type of nitrogen source, and concentrations of glucose and nitrogen sources, as well as pH, were optimized for cell growth. CP was enzymatically hydrolyzed, and used as the base medium, supplemented with artificial seawater salts, for DHA production under fed-batch cultivation. A. limacinum grew well at 18 g/L of NaCl (equivalent to 50% salinity level), and initial glucose concentration of 64 g/L, initial yeast extract concentration of 5 g/L, and pH 7.5 were optimum for cell growth. Fed-batch cultivation, with dissolved oxygen (DO) controlled at 10%, using CP-based medium as the carbon source gave a final cell dry mass of 26.3 ± 2.0 g/L, with 15.5 ± 0.1 g/L of lipid. DHA content in the lipid was 37.4 ± 1.5%, equivalent to 5.8 ± 0.3 g/L, and DHA productivity was 960 mg/(L·d). Medium-based economic analysis showed that an economic yield (EY) of this process was 0.86, which was higher than the 0.81 obtained using glucose as the substrate, assuming the same process and conditions. Overall, the results reveal that CP is a highly feasible feedstock for DHA production by A. limacinum SR21.
Introduction
Docosahexaenoic acid (DHA, C22:6n-3) is an omega-3 polyunsaturated fatty acid (PUFA) that has been recognized as a valuable additive in food and feed industries. Fortification of DHA in infant formulas can promote development of the brain, retina, and nervous system. In adult, DHA helps maintain brain functions and it has positive and protective effects against several diseases, including arthritis, atherosclerosis, depression, diabetes, myocardial infarction, thrombosis, and cardiovascular diseases (Innis, 2008; Xu et al., 2020; Chi et al., 2022). DHA is also nutritionally and physiologically essential in animals. It can, for instance, reduce skeletal malformation in larval Trachinotus ovatus and enhances immune function in juvenile grouper (Zhang et al., 2019). DHA can also improve various economic features of aquatic, cattle, and poultry animals, e.g., growth, development, and fecundity (Xu et al., 2020). Presently, most DHA in the market is derived from marine fish, in the form of fish oil or fish flesh, and seafood. These products, however, are relatively expensive due to the costly production process, which was estimated to be at least 10.9 USD/kg-DHA, excluding the cost of DHA recovery and other contributing factors (Chi et al., 2022). DHA production from marine fish can also lead to overfishing, and fish farming seems not a sustainable solution as this can pollute the local environment due to the accumulation of farm waste products (Tičina et al., 2020).
The above-mentioned drawbacks and limitations can be avoided by producing DHA using marine protists (heterotrophic thraustochytrids), which are the primary DHA producer in the food chain (Gladyshev et al., 2013). Among others, Aurantiochytrium limacinum (formerly known as Schizochytrium limacinum) is known to have excellent ability to synthesize and accumulate DHA at high level (Chi et al., 2022). It has been reported to accumulate lipid up to 73% of its dry weight (Liang et al., 2010; Patil and Gogate, 2015), with DHA content up to 35–47% of the total lipid (Wang et al., 2018). This marine protist can be cultivated under controlled conditions to utilize glucose, fructose, glycerol (Patil and Gogate, 2015), as well as volatile fatty acids (Patel et al., 2020b), as the carbon source for cell growth and DHA production. However, the use of pure carbon source, e.g., refined sugar, is actually not economically viable since it is costly. In some cases, cost of sugar could be almost 80% of the total medium cost (Patel et al., 2020a), making DHA production through Aurantiochytrium cultivation more expensive than the traditional fish oil (Xu et al., 2020; Chi et al., 2022). To lower the production cost, there have been attempts to substitute refined sugar with sugars derived from lignocellulosic biomass, e.g., Jurusalem artichoke (Yu et al., 2016), birch wood chips (Patel et al., 2019), and sugarcane bagasse (Nguyen et al., 2018). However, the use of these biomasses often encounters a problem of low saccharification yield. This is due to their complex structure and the presence of lignin at high level, which underlie the need for pretreatment to facilitate the release of sugars from the biomasses. Pretreatment not only complicates the process and is expensive, but also produces wastewater that needs to be treated before discharging into the environment. Another potential source of sugar is cassava (Manihot esculenta Crantz) pulp (CP), which is a by-product of cassava starch production process. CP is abundantly available in many countries, including Thailand. It also has low cost (e.g., 170 USD/ton), high carbohydrate (up to 80%) and relatively low lignin contents, which is beneficial for enzymatic saccharification (Khanpanuek et al., 2022). A previous study showed that enzymatic saccharification of CP at low temperature (up to 50°C) using α-amylase and glucoamylase yielded as high as 0.72 g/g of reducing sugar on CP, with a hydrolysis efficiency of 77.8% (Siriwong et al., 2019). This strongly suggests that CP could be used as a low-cost substrate for Aurantiochytrium cultivation. However, to the best of our knowledge, CP has never been investigated as DHA feedstock. Therefore, the present study was carried out to investigate the feasibility of CP as a substrate for DHA production by A. limacinum SR21. Attempts have also been made to further reduce DHA production cost by investigating the possibility to reduce salt concentration, referred to as salinity level in the present study, in the medium, as well as reducing the number of nutrients (nitrogen source) used in the medium preparation. Cultivation conditions, i.e., initial glucose and yeast extract concentrations, and initial pH, were also optimized statistically to achieved good cell growth. Based on the results, kinetic parameters for cell growth and lipid production were estimated, and a medium-based economic analysis was performed to assess the feasibility of CP as DHA feedstock.
Materials and methods
Microorganism
A. limacinum SR21 (ATCC® MYA-1381™) was used in the present study. It was purchased from the American Type Culture Collection (ATCC, USA). The freeze-dried culture was reactivated in 790 By+ medium at 25°C for 7 days. The medium was prepared by dissolving 5 g/L of glucose, 1 g/L of yeast extract, and 1 g/L of peptone in half-strength artificial seawater (ASW), which consisted of 18 g/L of NaCl, 2.6 g/L of MgSO4.7H2O, 0.6 g/L of KCl, 1 g/L of NaNO3, 0.3 g/L of CaCl2.2H2O, 0.05 g/L of KH2PO4, 0.027 g/L of NH4Cl, 1 g/L of Tris, 1.35×10−4 g/L of vitamin B12, 10 mL/L of P-II metal solution, and 1 mL/L of chelated iron solution (https://utex.org/products/artificial-seawater-medium). After that, the culture was acclimatized to grow at 30 g/L of glucose.
Acclimatization was performed by cultivating the cells in the 790 By+ medium containing 5 g/L of glucose at 25°C, 150 rpm, for 60 h. Then, the culture (10%, v/v) was transferred into the medium containing 10 g/L of glucose and incubated under the same conditions. The cultivation on 10 g/L of glucose was performed twice. After that, the cells were grown in the medium containing 20 g/L of glucose for three times, followed by the sub-cultivation in the medium containing 30 g/L of glucose for three times under the same conditions as above. The acclimatized cells were stored at −80°C in a medium (half-strength ASW containing 30 g/L glucose and 2 g/L of yeast extract) containing 20% (v/v) pure glycerol.
Inoculum preparation
Inoculum was prepared by growing the cells on an agar medium (half-strength ASW containing 30 g/L glucose, 2 g/L of yeast extract, and 15 g/L of agar) at 25°C until single colonies with diameter of 3–5 mm were observed, which took around 4–5 days. Then, one single colony was transferred into 150 mL of half-strength ASW containing 30 g/L glucose, and 2 g/L of yeast extract, and incubated at 25°C, 150 rpm, for 4 days. After that, the culture was transferred into a fresh medium at 10% (v/v) and further incubated at 25°C, 150 rpm, for 48 h in order for the cells to reach the mid-exponential phase before use.
Cassava pulp and enzymes
Cassava pulp (CP) was purchased from a local vendor in Khon Kaen, Thailand. It is commercially available as animal feed. CP was sieved to obtain particles of 0.5–1.0 mm, then stored in an air-tight plastic container until use. It contained 10.3% moisture, 3.4% protein, 1.2% fat, 13.7% fiber, 8.1% ash, 47.7% starch, 20.3% cellulose, 18.3% hemicellulose, and 3.4% lignin by weight.
Three enzymes were used to hydrolyze CP, i.e., iKnowzyme HTAA 40 L (α-amylase), iKnowzyme GA 200 L (glucoamylase), and Cellic® CTec3 HS (cellulase). The iKnowzymes were purchased from Reach Biotechnology Co. Ltd., Bangkok, Thailand. Cellic® CTec3 HS was purchased from Novozyme, Denmark. iKnowzyme HTAA 40 L had an amylase activity of 135 U/mL, iKnowzyme GA 200 L had a glucoamylase activity of 1,300 U/mL, and Cellic® CTec3 HS had a cellulase activity of 240 filter paper unit (FPU) per mL. All the enzymes were stored in screwcap plastic bottles at 4°C until use.
Preparation of cassava pulp hydrolysate
Hydrolysis of CP was conducted using a CP concentration of 150 g/L, α-amylase loading of 29 U/g-CP, glucoamylase loading of 47 U/g-CP, and cellulase loading of 60 FPU/g-CP, at 50°C, for 24 h, on a magnetic stirrer set at 400 rpm. These conditions were pre-optimized in our previous study (unpublished data). After the hydrolysis reaction, the suspension was filtered through a filter cloth to remove the residual solids, and the liquid fraction was incubated in a boiling water bath for 20 min to inactivate the enzymes. After that, the suspension was centrifuged at 10,000 rpm for 10 min, and the supernatant was collected for the subsequent experiments. The hydrolysate thus obtained was designated as cassava pulp hydrolysate (CP-HL) and contained 101.8 ± 0.9 g/L of reducing sugar (glucose equivalent). Glucose was the major component in CP-HL, accounting for 81.3 ± 1.9% of the total reducing sugar, followed by xylose (7.6 ± 0.3%), maltose (7.1 ± 1.9%), and fructose (4.0 ± 0.4%). Yield of reducing sugar on CP was 0.68 g/g.
Effects of salinity levels on growth of A. limacinum
Investigation of the effects of salinity levels on growth of A. limacinum SR21 was conducted using ASW with 25%, 50% (half-strength), and 75% salinity of natural sea water, which contains ca. 36 g/L of sea salts (Dobrowolski et al., 2019). The media were prepared by dissolving 5 g/L glucose, 1 g/L yeast extract and 1 g/L peptone in ASW containing 9, 18, or 27 g/L of NaCl, equivalent to 25%, 50% and 75% salinity levels, respectively. The media were autoclaved at 110°C for 28 min, cooled to room temperature, and inoculated with 10% (v/v) of A. limacinum inoculum. Cultivation was conducted in 500-mL Erlenmeyer flask, with a working volume of 150 mL, at 25°C, 150 rpm, for 72 h. Samples were taken at the beginning and the end of the cultivation for measurement of optical density at 660 nm (OD660), which was converted into cell dry mass (CDM) using Eq. (1), with a coefficient of determination (R2) of 0.9887. The suitable salinity level was selected based on the biomass production and was used in subsequent experiments.
Effects of nitrogen source on growth of A. limacinum
The effects of nitrogen sources on growth of A. limacinum SR21 was investigated by growing the cells in half-strength ASW containing 30 g/L glucose and four different nitrogen sources, i.e., 1 g/L yeast extract and 1 g/L of meat peptone (nitrogen sources in the 790 By+ medium); 2 g/L meat peptone; 2 g/L yeast extract; and 0.97 g/L of sodium nitrate (NaNO3). All the media were prepared to contain ca. 0.3 g/L of total nitrogen (TN) as present in the 790 By+ medium. Cultivation was conducted in 500-mL Erlenmeyer flask, with a working volume of 150 mL, at 25°C, 150 rpm, for 7 days. The growth of the cells was followed periodically in terms of CDM, and the effects of nitrogen sources on the growth were assessed based on the growth characteristics of the cells.
Optimization of cultivation conditions for growth of A. limacinum.
Using the selected salinity level and suitable nitrogen source, initial pH (6.5–7.5), initial glucose concentration (30–70 g/L), and initial yeast extract concentration (5–8 g/L), were optimized using response surface methodology (RSM) with central composite design (CCD). Each independent variable was varied in 5 levels, i.e., −α, −1, 0, + 1, +α, and cell growth in terms of CDM was used as the response. The design matrix consisting of 20 experimental runs are shown in Table 1. The cultivations were performed in 500-mL Erlenmeyer flasks, with a working volume of 150 mL. Half-strength ASW was used as the base medium, added with designated amounts of glucose and yeast extract. pH of the media was adjusted to the designated level using 2 M NaOH and 1 M HCl. A. limacinum inoculum was transferred to the medium at 10% (v/v) to start the cultivation, which was carried out at 25°C, 150 rpm, for 7 days. Samples were taken at the beginning and the end of the cultivation for determination cell growth (in terms of CDM). A confirmation experiment was conducted under the predicted optimum conditions in triplicate. Samples were taken periodically for determinations of cell growth, reducing sugar, and free amino nitrogen (FAN). Noted that FAN was used as an indicator to follow the consumption of assimilable nitrogenous substances (Salakkam et al., 2017).
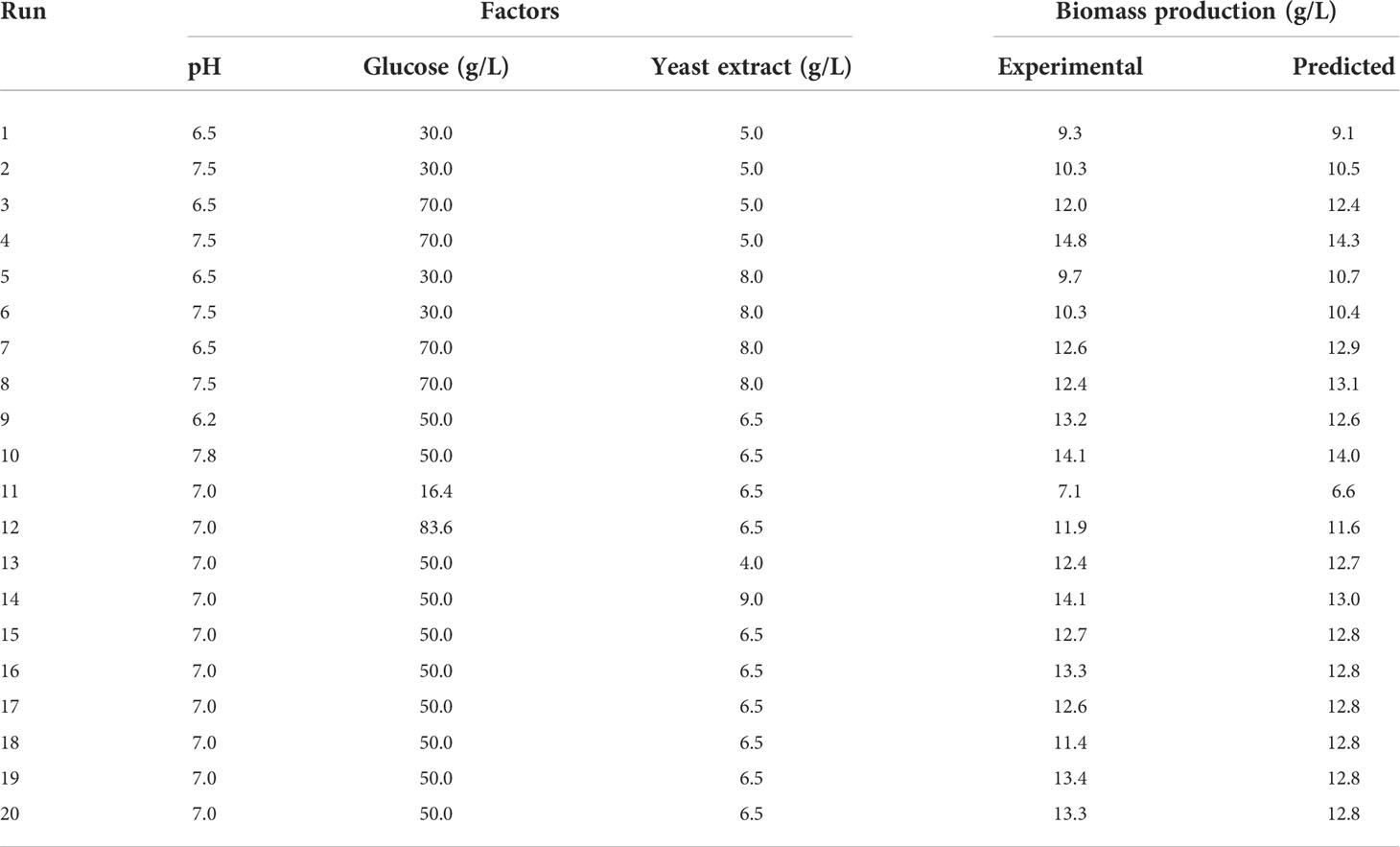
Table 1 Optimization of initial pH, initial glucose and yeast extract concentrations using RSM with CCD.
Fed-batch cultivation using cassava pulp hydrolysate as carbon source
This experiment was conducted in a 2-L bioreactor (Bioneer-neo-2L, B.E. Marubishi, Thailand), with an initial working volume of 0.6 L. CP-HL was diluted with distilled water to contain ca. 64 g/L of reducing sugar and added with ASW components to attain 50% salinity level. Then, the medium was transferred into the bioreactor and autoclaved at 110°C for 40 min. After cooling to room temperature, calculated volume of sterile yeast extract solution was added into the bioreactor to reach a concentration of 5 g/L, and A. limacinum inoculum was transferred into the bioreactor at 10% (v/v). Temperature and pH were controlled at 25°C and pH 7.5, respectively. Aeration rate and dissolved oxygen (DO) level were controlled at 2 vvm, and 10% of oxygen saturation in the medium, respectively. Control of DO level was achieved by automatic adjustment of agitation rate in the range 100 to 500 rpm. Silicone-based antifoam was used to reduce foam formation when necessary. When the concentration of reducing sugar decreased below 20 g/L, 0.2 L of feed (CP-HL supplemented with ASW components to attain 50% salinity level) was transferred into the bioreactor, and the process was allowed to proceed under the same conditions. The feeding was conducted three times. Samples were taken periodically for CDM, reducing sugar, FAN, and lipid determination. This experiment was conducted in duplicate.
Medium-based economic analysis
Economic yield (EY), defined as the monetary unit (USD) of lipid containing around 40% of DHA per monetary unit (USD) spent in nutrients, was calculated using Eq. (2), where Y is the concentration of lipid (kg/L), C is the cost of each nutrient used in the medium preparation (USD/kg), N is the concentration of each nutrient (kg/L), and I is the selling price of the lipid (USD/kg) (Bustos et al., 2004). Price of lipid containing 40% DHA (69 USD/kg) was taken from www.bulknaturaloils.com, and prices of other nutrients were from www.alfa.com (Supplementary Table S1). For the calculation of EY using glucose as the carbon source, the price of food-grade glucose (0.904 USD/kg) was used (Chi et al., 2022).
Analytical methods
OD660 was measured using a UV-VIS spectrophotometer (UVmini-1240, Shimadzu, Japan). CDM was determined gravimetrically after centrifuging 5 mL of samples, washing the cells twice with normal saline, and drying at 80°C for 24 h. Reducing sugar was determined using the 3,5-dinitrosalisylic acid (DNS) method (Miller, 1959), using glucose as the standard. The composition of sugars in CP-HL was analyzed using high performance liquid chromatography (Waters 2414, USA), equipped with Aminex HPX-87H column (Bio-Rad, USA) and a refractive index (RI) detector, following the method of Khamtib and Reungsang (2012). FAN was determined using the ninhydrin method (Abernathy et al., 2009), using glycine as the standard. Activity of cellulase was assayed using the method of the National Renewable Energy Laboratory (NREL) (Adney and Baker, 2008), and is reported as FPU/mL. Activities of α-amylase and glucoamylase were assayed using the method of de Moraes & Ulhoa (1999). One unit of amylase was defined as the amount of enzyme needed to release 1 µmol of reducing sugar (maltose equivalent) per min under the assay conditions. One unit of glucoamylase was defined as the amount of enzyme needed to release 1 µmol of glucose per min under the assay conditions. Lipid concentration was determined using the method of Byreddy et al. (2015). Residual CDM (RCDM) was determined as the difference between CDM and lipid concentration. Lipid composition was analyzed by converting the lipids into fatty acid methyl esters (FAMEs) following the method of Huang et al. (2012), with a slight modification, i.e., NaOH was used in place of KOH in the preparation of 0.5 N KOH in methanol. Analysis of the FAMEs composition was performed using gas chromatography (GC7890, Agilent, USA), equipped with a capillary column (Stabilwax®-MS, Restek, USA) and flame ionization detector (FID). The oven temperature was set at 150°C, with 10°C/s increment to 180°C, followed by 3°C/s to 240°C, and hold for 5 min. Injection volume was 1 µL, and detector and injector temperatures were set at 250°C and 240°C, respectively. Helium was used as the carrier gas at a flow rate of 1 mL/min. Concentration of each FAME was calculated from its corresponding peak area, relative to the peak of respective standards (Marine Oil FAME Mix, catalog no. 35066, Restek, USA). Statistical analysis was performed using IBM® SPSS® Statistics (version 28.0.1.0). One-way analysis of variance (ANOVA) was conducted at 95% confidence level, followed by Tukey’s multiple comparison test.
Mathematical modeling of cell growth and lipid production
A logistic model (Eq. (3)) was used to fit the profiles of CDM and RCDM, where X is the concentration of CDM or RCDM (g/L), X0 is the initial concentration of CDM or RCDM (g/L), Xm is the maximum concentration of CDM or RCDM (g/L), μm is the maximum specific growth rate of CDM or RCDM (1/h), and t is the cultivation time (h). The modified Gompertz model (Eq. (4)) was used to fit lipid production profile, where P is the concentration of lipid (g/L), Pm is the maximum lipid concentration (g/L), Rm is the maximum rate of lipid production (g/(L·h)), e is the Euler’s number (2.71828), and λ is the lag time (h) (Phukoetphim et al., 2017).
Results and discussion
Effects of salinity level on growth of A. limacinum
Previous studies showed that thraustochytrids do not necessarily required full-strength seawater solution (100% salinity level) for optimal growth (Shabala et al., 2009), and these microorganisms can tolerate as low as 0% up to 200% salinity (Raghukumar, 2008), but growth would vary depending upon the salt concentration (Shabala et al., 2009). Based on these reports, the present study investigated effects of salinity level of the medium in the range 9 to 27 g/L of NaCl, equivalent to 25% to 75% salinity, on growth of A. limacinum SR21 and the possibility to reduce the concentration of salts, as components of ASW, to lower the cost of medium. Figure 1 shows that the growth at 9 g/L of NaCl (25% salinity) was statistically lower than that at higher salinity levels. The cells grew to only 2.5 ± 0.1 g/L at the end of the cultivation (72 h). On the other hand, using the salinity level of 18 g/L (50% salinity) resulted in improved growth, and the final CDM concentration was enhanced to 3.4 ± 0.4 g/L. Further increasing the salinity level to 27 g/L (75% salinity) did not result in significant increase in CDM concentration, suggesting that 50% salinity level was adequate to support growth of the microalga.
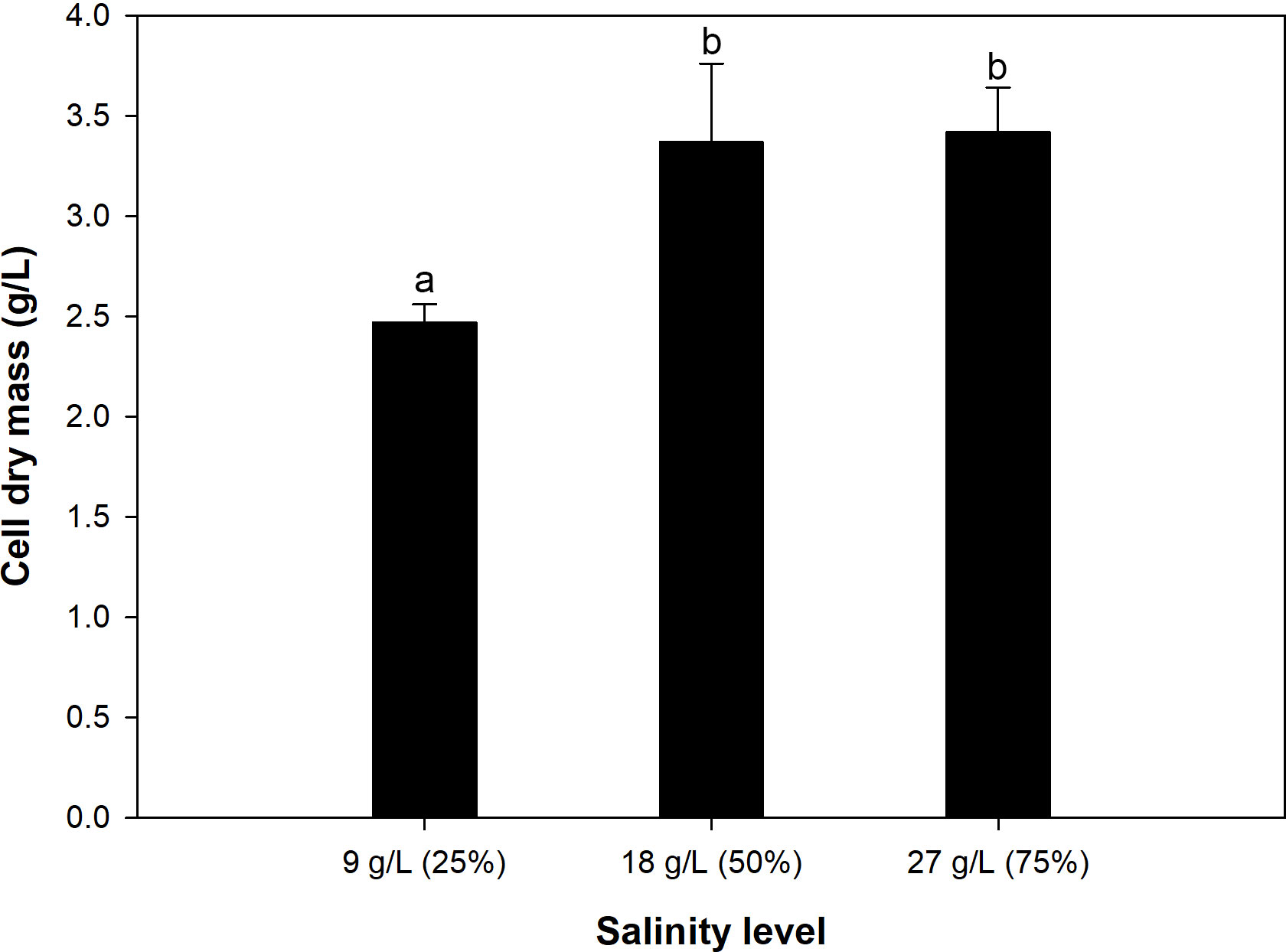
Figure 1 Growth of A. limacinum SR21 in 790 By+ media with 25%, 50% and 75% salinity levels. Different alphabets indicate statistically difference among the results at 95% confidence level.
The low growth at 9 g/L of NaCl was thought to be due to low sodium ion (Na+) concentration in the medium. Since thraustochytrids have an absolute requirement of sodium for osmotic adjustment and cell growth (Sohedein et al., 2020), and this element cannot be replaced by potassium (Fossier Marchan et al., 2018), the low Na+ concentration, and hence hypo-osmotic pressure, could result in cell swelling with changes in cytoplasm composition (Chen and Jiang, 2009) and other undesirable effects that could lead to low growth. Sodium ion also plays a role in transports of some essential elements, e.g., phosphorus (Fan and Chen, 2007), that influence activities of some enzymes, e.g., malic enzyme, glucose-6-phosphate dehydrogenase, and NAD+-isocitrate dehydrogenase (Ren et al., 2013), as well as nucleic acid formation (Fan and Chen, 2007). Similar effects of hypo-osmotic pressure have previously been reported on Aurantiochytrium sp. KRS101 (Kim et al., 2013) and Traustochytrium sp. (Shabala et al., 2009). As for the growths at 18 and 27 g/L of NaCl, the final CDM concentration were more or less the same, suggesting that NaCl at 18 g/L (50% salinity level) was adequate to achieve good growth. This complied well with the reported optimum range of 50–100% salinity (Raghukumar, 2008), and agreed well with a study of Yokochi et al. (1998) that A. limacinum SR21 grew best in media containing at least 50% seawater concentration. Similar results were reported by Kim et al. (2013) that Aurantiochytrium sp. KRS101 grew best at 20 g/L of sea salt, which was equivalent to ca. 56% salinity level. Hong et al. (2011) also reported the optimum concentration of artificial sea salt of at least 15 g/L, which was only slightly lower than that observed in this study. Based on results shown in Figure 1, 50% salinity level was selected for the subsequent experiments. The use of 50% salinity level was considered to have two benefits, i.e., the cost of the cultivation medium could be reduced, and the concentration of chloride ion (Cl−) in the medium would be low, which could help prolong the lifespan of the bioreactor (Sohedein et al., 2020).
Effects of nitrogen source on growth of A. limacinum
Figure 2 shows the growth of A. limacinum in media containing different nitrogen sources. The microalga grew well in media containing yeast extract, and peptone and yeast extract, and lower growths were observed for media containing peptone, and NaNO3, respectively. The final CDM concentration obtained from the media containing yeast extract, peptone and yeast extract, peptone, and NaNO3 were 9.8 ± 0.5, 9.8 ± 0.0, 8.7 ± 0.0, and 7.4 ± 0.2 g/L, and μm, estimated by Eq. (3), were 0.057, 0.053, 0.030, and 0.029 1/h, respectively (Table 2). From the results, it is obvious that the use of yeast extract as the sole nitrogen source resulted in much higher growth as compared with the use of peptone alone. Although both of these nitrogen sources are extensively used in media for thraustochytrids cultivation (Sohedein et al., 2020), yeast extract was considered a richer nitrogen source than peptone, and therefore supported a better growth. Yeast extract contains not only nitrogenous substances, but also several vitamins and minerals that are essentials for cell growth (Jacob et al., 2019; Proust et al., 2019; Yin et al., 2019). On the other hand, peptone, which is typically produced by digesting meat or other proteins, contains primarily amino acids and short peptides with less proportions of vitamin and minerals (Krahulec and Šafránek, 2021). This observation agreed well with a report of Gao et al. (2013) that yeast extract supported better growth than peptone, but contradict to a study of Hong et al. (2011), where peptone was shown to give slightly higher CDM concentration than yeast extract. This discrepancy might be arisen from the differences in the cultivaton conditions, microbial strain, as well as the composition of the media used in the process. Comparing the growth on yeast extract as the sole nitrogen source with that on yeast extract in a combination with peptone, it was found that the growths on both media were very similar, indicating that yeast extract could substitute peptone for the cultivation of A. limacinum without any observable adverse effects. This was considered beneficial as the process could be simplified by reducing the number of nutrients used in medium preparation. Besides, yeast extract is cheaper than peptone (Xu et al., 2020), and so the economics of the process could be improved. The use of NaNO3 as the nitrogen source was also investigated, and it was found that this nitrogen source gave the lowest growth. Despite NaNO3 was shown to support better growth of A. limacinum KRS101 as compared with NH4Cl (Park et al., 2018), due to the lack of other essential compounds (Sohedein et al., 2020), it gave much lower growth than the organic nitrogen sources tested in the present study. This drawback cancels out the low price advantage of inorganic nitrogen source (Raghukumar, 2008), and NaNO3 was considered not suitable for economical production of DHA. Based on these results, yeast extract was used as the sole nitrogen source in the medium for the subsequent experiments.
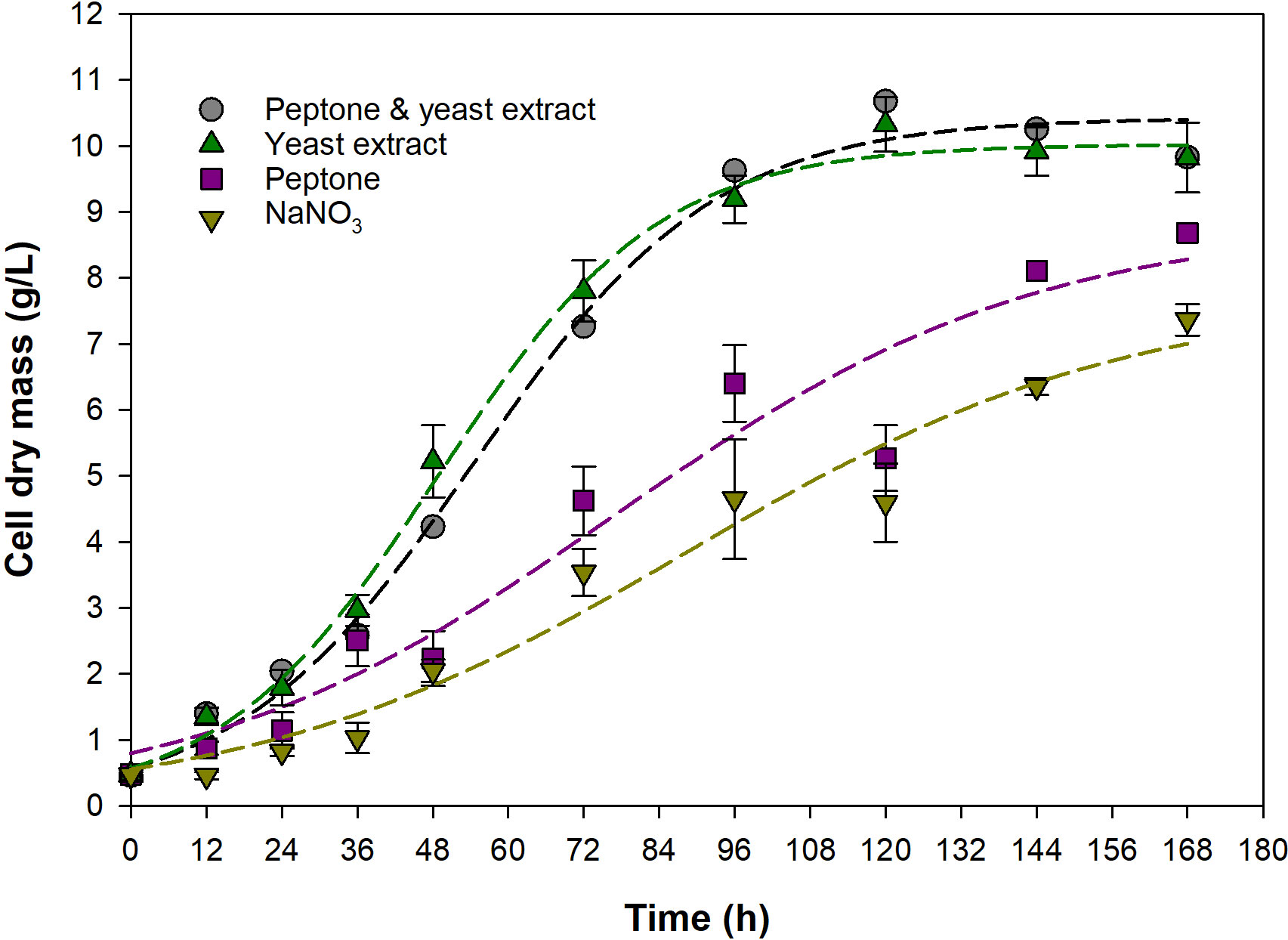
Figure 2 Growth of A. limacinum SR21 in media containing different nitrogen sources. Dashed lines represent data predicted by Eq. (3).
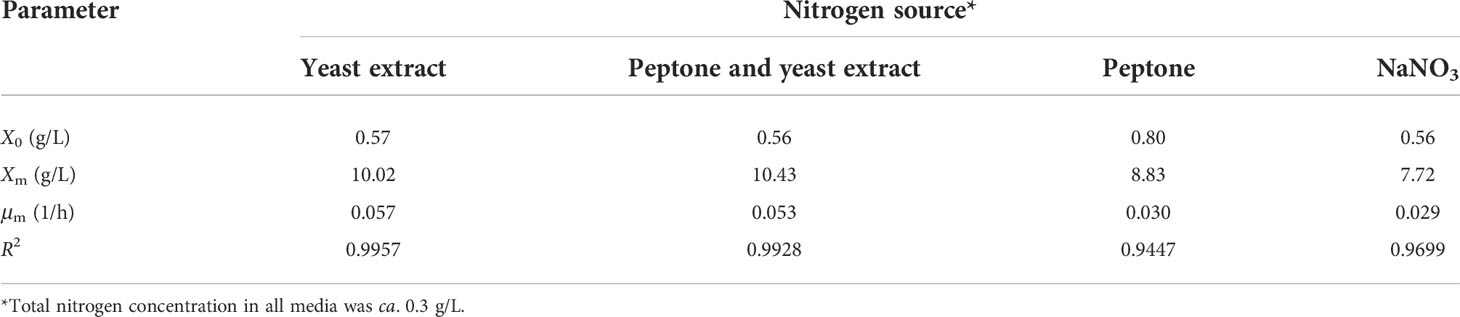
Table 2 Kinetic parameters for growth of A. limacinum SR21 in half-strength ASW-based media containing 30 g/L glucose and four different nitrogen sources.
Optimization of cultivation conditions for growth of A. limacinum
Optimization of the cultivation conditions was carried out using RSM with CCD. Table 1 shows that varying the concentrations of initial glucose and yeast extract, as well as initial pH, led to different growths of the microorganism. From these results, a quadratic model was generated to describe the change in CDM production as functions of the tested factors (Eq. (5)), where Glu is the initial glucose concentration (g/L), and YE is the initial yeast extract concentration (g/L). Results showed that the model fitted the data well with R2 of 0.8964. One-way analysis of variance (ANOVA) was used to examine the effects of each factor, as well as their interactions, on CDM production. It was found that the model was significant (p-value < 0.05) and the lack of fit was not significant (p-value > 0.05) at 95% confidence level (Table 3), indicating that the model was adequate for predicting the biomass growth of A. limacinum SR21 under the ranges of conditions tested.
Table 3 also revealed that only initial glucose concentration in the range tested significantly affected the biomass growth (p-value < 0.05), while pH, initial yeast extract concentration, and interaction between the factors did not. The contour plot between pH and initial glucose concentration (Figure 3A) shows that CDM increased with increasing pH and initial glucose concentration, and CDM reached the highest level at pH 7.50 and 64.2 g/L of glucose. However, increasing the initial concentration of yeast extract simultaneously with pH did not positively affect biomass production, and CDM was highest at pH 7.50 and 5 g/L of yeast extract, which was the lowest level tested (Figure 3B). As for the interaction effect between initial glucose concentration and initial yeast extract concentration (Figure 3C), increasing yeast extract concentration did not improve growth, and the highest level of growth was observed at 64.2 g/L of glucose and 5 g/L of yeast extract. Based on these results, it could be concluded that the optimum conditions for CDM production were pH 7.5, initial glucose concentration of 64.2 g/L, and initial yeast extract concentration of 5 g/L. Using Eq. (5), the predicted CDM production was 14.4 g/L.
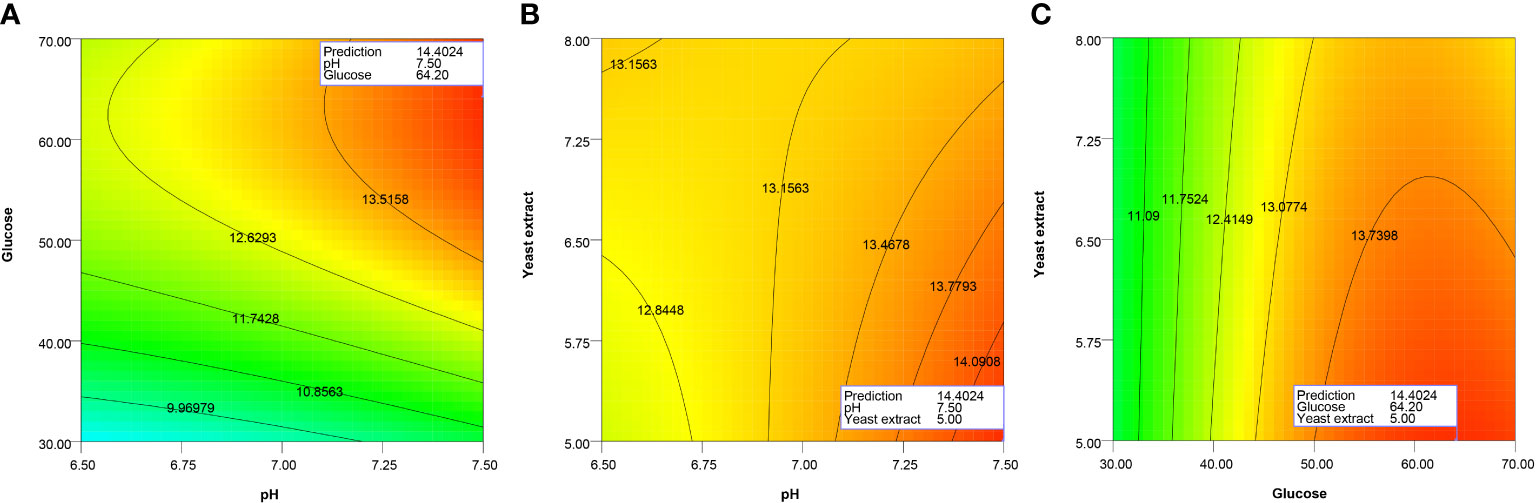
Figure 3 Contour plots showing interactions effects between initial pH and initial glucose concentration (A), initial pH and initial yeast extract concentration (B), and initial glucose and yeast extract concentration (C) on growth of A. limacinum SR21.
A confirmation experiment was conducted under the optimum conditions, yielding 14.3 ± 0.5 g/L of CDM after 7 days of cultivation (Figure 4). This value was very close to the predicted value and fell within the 95% prediction interval (12.1–16.7 g/L), indicating that the predicted optimum conditions were valid. Analysis of samples taken over the course of the cultivation revealed that the cells grew rapidly during the first 72 h, with the CDM productivity of 0.13 ± 0.01 g/(L·h), after which time growth rate decreased, and CDM concentration reached 14.3 g/L at 168 h. Estimation of the growth kinetic parameters using Eq. (3) revealed that the initial and maximum CDM concentrations were 1.8 and 13.1 g/L, respectively, and μm was 0.042 1/h (R2 = 0.9767). FAN concentration decreased rapidly from 191 ± 9 to 31 ± 4 mg/L during the first 24 h, and the concentration stayed relatively constant in a range 18–28 mg/L until the end of cultivation. The presence of the residual FAN in the medium indicated that there was inassimilable FAN in yeast extract, which was around 9.3% of total FAN. Rapid consumption of nitrogen source during the early stage of cultivation was also reported in studies of Jiang et al. (2017) and Kim et al. (2013), suggesting that nitrogen source was used primarily for the biomass production. Despite the relatively constant concentration of FAN after 24 h, CDM concentration continued to increase until the end of cultivation. Previously, Taoka et al. (2009) and Xu et al. (2020) reported that Aurantiochytrium sp. is able to produce protease during its growth. Therefore, it was possible that the cells utilized proteins or large peptides in the medium as additional nitrogen sources for growth. Kim et al. (2013) also suggested that the CDM concentration could increase, simultaneously with cell swelling or enlargement, after the depletion of nitrogen source due to the present of carbon source in the medium that leads to lipid accumulation under nitrogen-deficient condition (Ratledge, 2004; Morita et al., 2006; Nazir et al., 2018). This assumption was supported by the continued consumption of glucose after 24 h. However, this was inconclusive since lipid content in the cells was not determined. The consumption rate of reducing sugar was relatively constant over the course of the process. The concentration decreased from 58.9 ± 0.8 to 11.6 ± 4.2 g/L at 168 h, at a rate of 0.28 ± 0.03 g/(L·h) and reducing sugar consumption efficiency was 80.3 ± 7.4%. It was interesting that the cells did not completely consume reducing sugar, and there was 11.6 ± 4.2 g/L reducing sugar remained at the end of cultivation. This was similar to a report of Chi et al. (2007) that A. limacinum SR21 did not completely consume glucose in the medium. Nazir et al. (2018) also reported similar results that Schizochytrium sp. S31 consumed only ca. 70% of total fructose in the cultivation medium. This was possibly because there was inadequate nitrogen source to support further reducing sugar consumption as suggested by Chi et al. (2007).
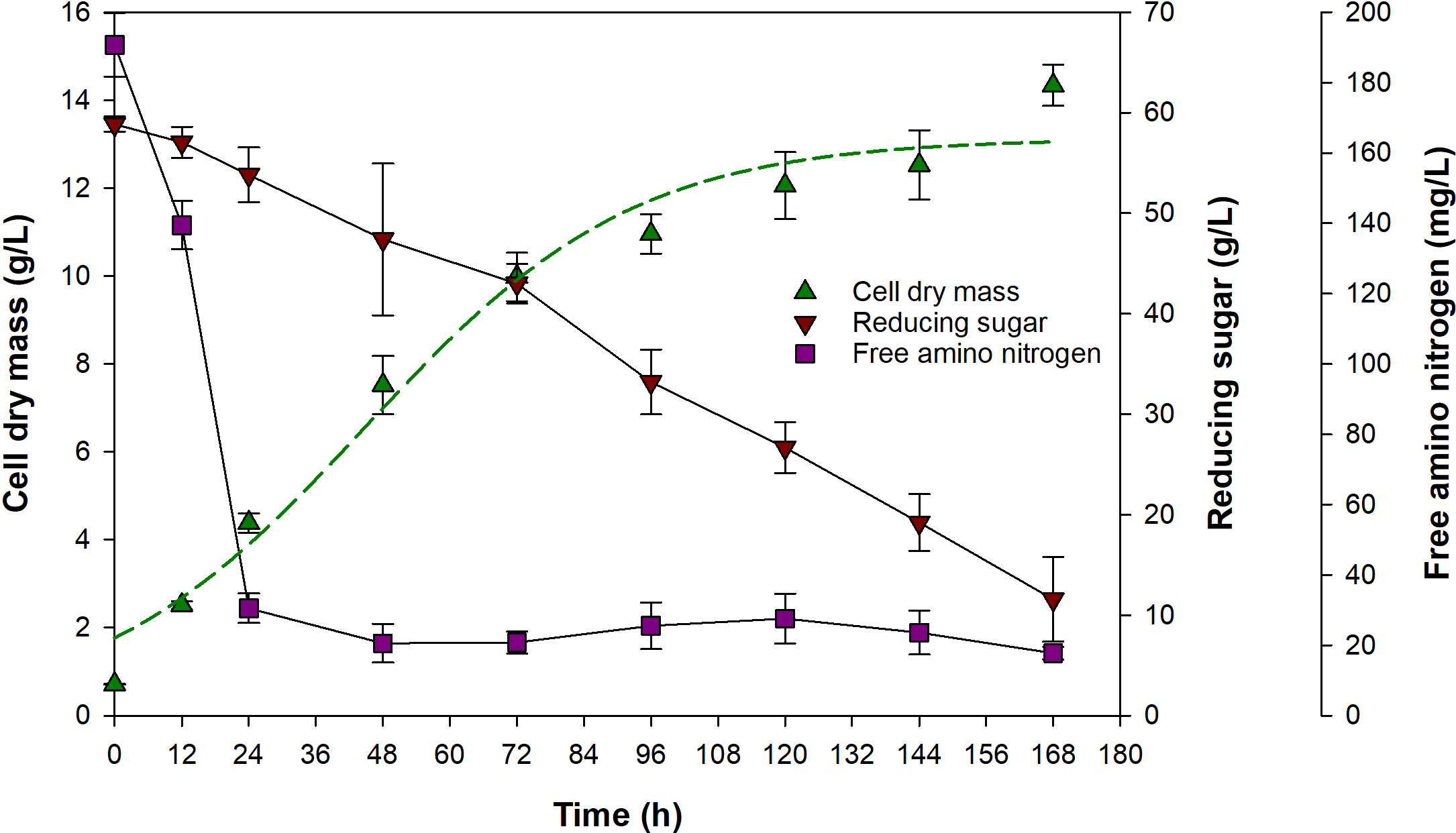
Figure 4 Growth and substrates consumption of A. limacinum SR21 during a cultivation under the optimum conditions. Dashed line represents data predicted by Eq. (3).
Fed-batch cultivation with cassava pulp hydrolysate
Using the optimum initial concentration of glucose (64 g/L), yeast extract (5 g/L), and pH 7.5, fed-batch cultivation was conducted in a 2-L bioreactor using CP-HL with 50% salinity level as the carbon source. The cultivation was started as batch cultivation, and it was fed with 0.2 L of CP-HL when the reducing sugar concentration decreased below 20 g/L. The feeding was performed three times. It is worth mentioning that DO level was controlled at 10% throughout the process. This was because provision of oxygen is vital to cell growth, but high level of oxygen tension might not be required for DHA synthesis (Sohedein et al., 2020) since the polyketide synthase (PKS)-like pathway, which is believed to be the main generator of DHA (Aasen et al., 2016), does not required oxygen-dependent desaturations to introduce double bonds (Morabito et al., 2019). Therefore, the present study controlled DO set point at 10% to maintain cell viability.
Figure 5A shows that CDM and RCDM increased rapidly during the first 24 h, reaching 7.1 ± 0.7 and 5.8 ± 0.9 g/L, respectively, corresponding to a large drop of FAN concentration from 230 ± 12 to 31 ± 5 mg/L, and reducing sugar concentration from 58.4 ± 0.2 to 48.6 ± 2.4 g/L (Figure 5B). Lipid was detected in the biomass at as early as 24 h, indicating the commencement of lipid accumulation phase. Lipid production increased rapidly during 24 to 48 h due to the excess carbon and limited nitrogen conditions (high carbon to nitrogen ratio), which is favorable for lipid synthesis by thraustochytrids (Sohedein et al., 2020). At 48 h, first feeding of CP-HL was performed, increasing the concentration of reducing sugar and FAN to 34.8 ± 0.3 g/L, and 29 ± 5 mg/L, respectively. This further supported lipid production to 12.9 ± 0.1 g/L at 72 h, while the concentration of RCDM was relatively constant, suggesting that the increase in CDM concentration was due primarily to the accumulation of lipid inside the cells. At 72 h, CP-HL was fed for the second time, raising the concentration of reducing sugar to 28.7 ± 4.3 g/L. At 84 h, third feeding was performed and reducing sugar concentration increased from 16.6 ± 1.3 to 28.6 ± 0.8 g/L. It is noteworthy that the concentration of CDM did not increase much after 72 h because feeding of CP-HL into the bioreactor (total volume of feed was 0.6 L) increased the total volume of the medium, thus diluting the biomass concentration. It was also noticeable that FAN concentration remained at very low level after 24 h. Furthermore, it was observed that the production of lipid increased only slightly after the second feeding at 72 h. At the end of the cultivation, CDM, RCDM, and lipid concentration were 26.3 ± 2.0, 10.8 ± 2.0, and 15.5 ± 0.1 g/L, respectively. Determination of lipid content in the biomass showed that it increased rapidly from 18.0% to 51.5% during 24 to 48 h, and further increased to 59.3% at the end of the cultivation. This confirmed that active lipid accumulation phase started at 24 h. DHA content in the total lipid at the end of the cultivation was 37.4 ± 1.5%, equivalent to 5.8 ± 0.3 g/L. Based on these results, lipid and DHA productivity were calculated to be 0.11 g/(L·h) and 40 mg/(L·h), equivalent to 2.6 g/(L·d) and 960 mg/(L·d), respectively. Eicosapentaenoic acid (EPA) was not detected in the samples, implying that the PKS-like pathway was used for PUFAs synthesis (Monroig et al., 2013; Chi et al., 2022).
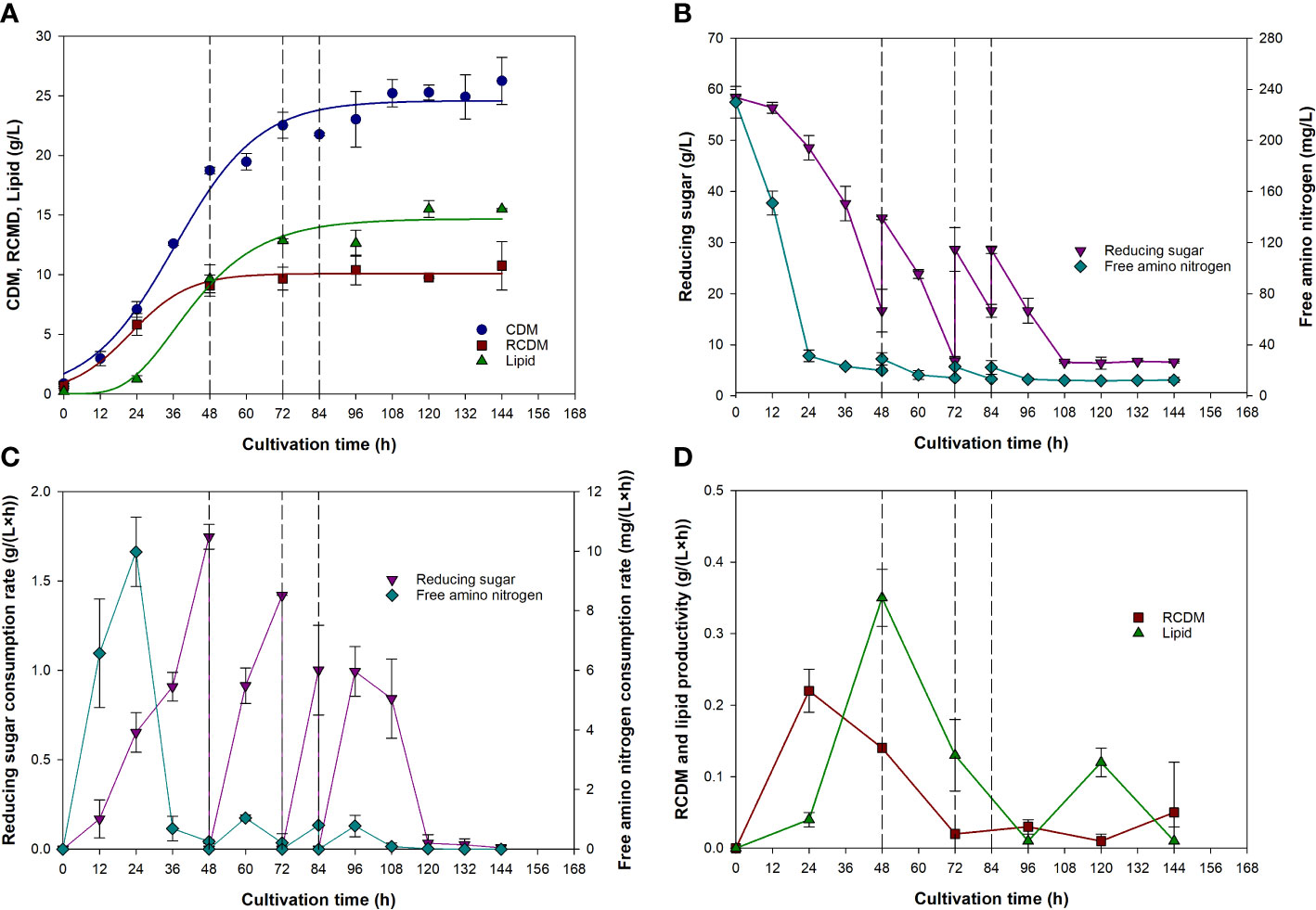
Figure 5 Changes in cell dry mass, residual cell dry mass, and lipid concentrations (A), reducing sugar and free amino nitrogen (B), consumption rate of reducing sugar and free amino nitrogen (C), and residual cell dry mass and lipid productivity (D) during fed-batch cultivation of A. limacinum SR21 in cassava pulp hydrolysate. Blue and red solid lines in (A) represent data predicted by Eq. (3), green solid line represent data predicted by Eq. (4). Dashed lines in (A–D) represent the time at which fresh medium was fed into the bioreactor.
Profiles of CDM and RCDM were fitted with the logistic model (Eq. (3)), and that of lipid was fitted with the modified Gompertz model (Eq. (4)), which are highly applicable for estimating the growth and product formation of microorganisms, respectively (Phukoetphim et al., 2017; Moungprayoon et al., 2022). The maximum specific growth rate estimated for CDM and RCDM (non-lipid biomass) were 0.072 and 0.103 1/h, respectively. These were higher than the 0.042 1/h observed in the previous experiment, suggesting that the control of DO level could have enhanced the growth of the cells. Other kinetic parameters are given in Table 4. Based on curve fitting (Figure 5A), it can be seen that RCDM concentration increased rapidly during the first 24 h, coinciding with the rapid consumption of FAN (Figure 5B). The profile of FAN consumption rate (Figure 5C) and RCDM productivity (Figure 5D) confirmed that FAN was mainly consumed for cell growth. After 24 h, rate of change of RCDM concentration decreased and its concentration was relatively constant after 48 h, corresponding to the low level of FAN in the medium. On the other hand, after 24 h, lipid production rate increased (Figure 5D) with increasing consumption rate of reducing sugar (Figure 5C). This indicated that sugar was mainly utilized for lipid synthesis. The maximum lipid production rate (Rm), estimated using Eq. (4), was 0.34 g/(L·h) (Table 4).
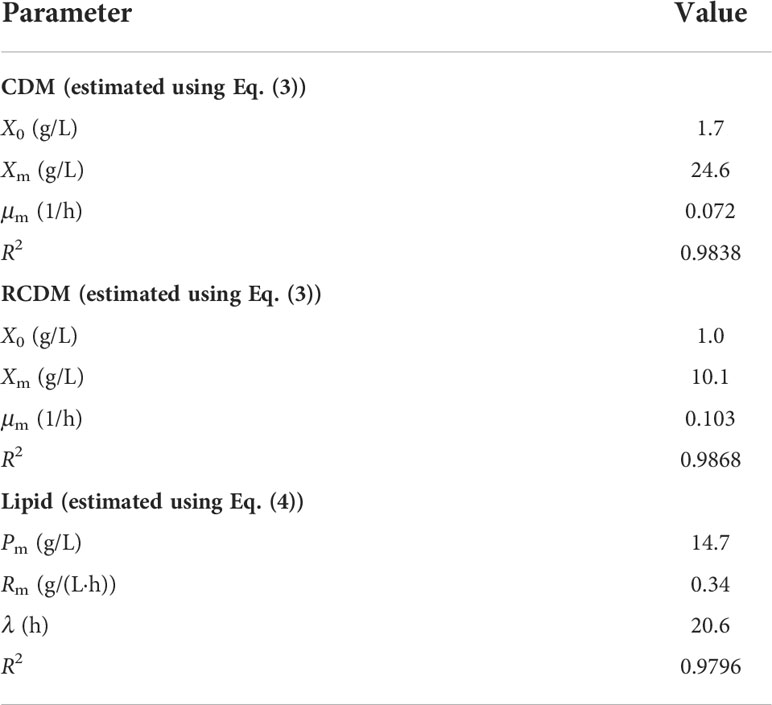
Table 4 Kinetic parameters for growth and lipid production of A. limacinum SR21 in fed-batch cultivation using CP-HL based medium.
Further inspection of the results revealed that there was only little lipid production after the second feeding (72 h), but reducing sugar was continually consumed. This was possibly due to the utilization of sugar for cell maintenance and syntheses of other products. Squalene, for example, could be produced simultaneously with DHA by Aurantiochytrium sp. According to Patel et al. (2020a) and Patel et al. (2019), 16.34 and 88.47 mg/g-cell-dry-weight of squalene were produced when Schizochytrium limacinum SR21 and Aurantiochytrium sp. T66 were grown on spruce and birch hydrolysates, respectively. Additionally, other carotenoids, e.g., astaxanthin, canthaxanthin, β-carotene, could also be produced by Schizochytrium (Aurantiochytrium) as reported by Raghukumar (2008). Since the production of lipid was negligible and the lipid content in CDM was more or less constant after 72 h, it was considered that the cultivation could be terminated at this time, which would improve the lipid productivity to 0.18 g/(L·h). Additionally, assuming that DHA content in total lipid was 37.4%, shortening the cultivation time to 72 h would result in DHA productivity of 67 mg/(L·h), equivalent to 1,600 mg/(L·d).
Feasibility of cassava pulp hydrolysate as carbon source for DHA production
Key results for lipid and DHA productions from the present study along with those reported in the literature using Aurantiochytrium spp. (Schizochytrium spp.) are given in Table 5. It can be seen that lipid and DHA productions vary depending on the strain of the microorganism, cultivation mode and conditions, as well as carbon source, which could be simple sugar (e.g., glucose), sugar alcohol (e.g., glycerol), and sugar-containing hydrolysate derived from biomasses. Among these, highest CDM concentration of 59.3 g/L was attained using Jerusalem artichoke hydrolysate as carbon source, while the lowest of 4.4 g/L was attained using orange peel extract. Lipid content in CDM ranged from 45.2% to 73.6%, and DHA content in total lipid was 14.3% to 41.3%. Comparing the results of this study with the others, it was found that using CP-HL as the carbon source gave relatively high CDM concentration, as well as lipid and DHA contents. Furthermore, DHA productivity observed in the present study (0.96 g/(L·h)) and DHA yield on sugar (0.06 g/g) were among the highest values reported in the literature. These indicated that CP-HL is a feasible substrate for DHA production.
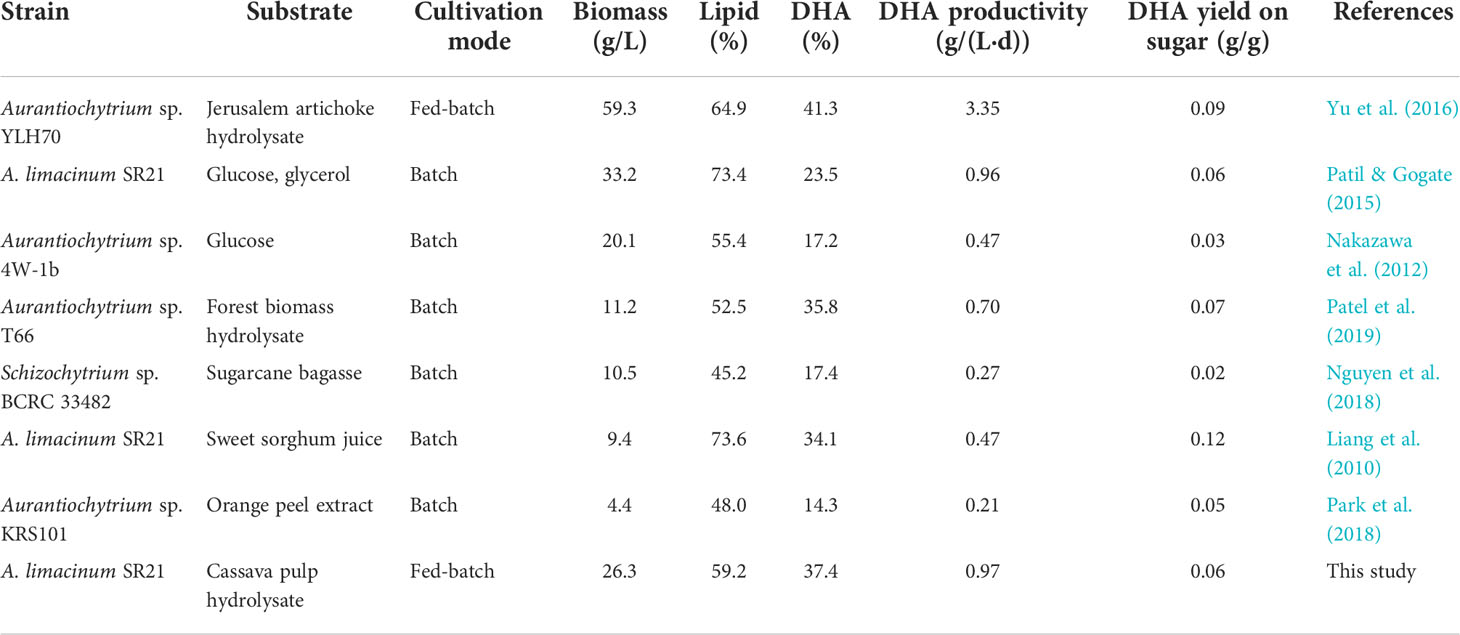
Table 5 Lipid and DHA productions by Aurantiochytrium spp. (Schizochytrium spp.) growing on various substrates.
To further demonstrate the feasibility of CP as DHA feedstock, the cost of carbon source per kg of DHA was calculated. The biomass yield obtained in the present study was 0.24 g-CDM/g-reducing-sugar, and the lipid and DHA contents were 59.3% and 37.4%, respectively. With the reducing sugar yield on CP of 0.68 g/g, and CP price of 170 USD/ton, the cost of CP per kg of DHA was 4.71 USD/kg. This was 63% lower than the estimated value of 12.56 USD/kg-DHA using glucose as the carbon source, assuming the biomass yield on glucose of 0.36 g/g, price of food-grade glucose of 903.9 USD/ton, lipid content in biomass of 50%, and DHA content in total lipid of 40%. It was also lower than the production cost of 10.90 USD/kg for DHA production from fish oil (Chi et al., 2022). Medium-based economic analysis was also performed, considering the cost of other nutrients, e.g., nitrogen source and salts. Results showed that EY obtained using CP as carbon source was 0.86, while that obtained using glucose, assuming the same process and conditions, was 0.81. The better results obtained using CP were considered due to the use of low salinity level (50%) in the medium, which could lower the cost of medium, as well as the low price of CP. These results further confirmed the feasibility of CP as DHA feedstock. It should, however, be noted that other cost factors, e.g., cost of CP-HL preparation and downstream processing, were not considered in the calculation, and so detailed analysis of cost and benefit should be conducted to fully assess the feasibility of the process. Furthermore, EY calculation revealed that the cost of yeast extract was ca. 60% of the total nutrient cost. Consequently, it was considered that yeast extract should be replaced by low-cost nitrogen source to further improve the EY. Among others, soybean residue is a potential substitute for yeast extract. It is a protein-rich waste from soy milk and tofu production processes that is readily available at low cost and was previously shown to be a good source of FAN. Protein in soybean residue could be transformed into FAN by solid-state fermentation followed by hydrolysis of the fermented solids to produce FAN-containing hydrolysate. According to Salakkam et al. (2017), a soybean residue hydrolysate containing as high as 1.8 g-FAN/L was produced through the above-mentioned process. However, the expenditure of the process should be taken into consideration.
Conclusions
Conditions for growth of A. limacinum SR21, i.e, salinity level of medium, nitrogen source, initial glucose and yeast extract concentrations, and initial pH, were optimized; and cassava pulp hydrolysate (CP-HL) was used as the sole carbon source for DHA production. Results showed that the cells grew satisfactorily at 50% salinity level, and yeast extract could be used as the sole nitrogen source in the medium to support cell growth. Optimum initial glucose and yeast extract concentrations, as well as pH, were 64 g/L, 5 g/L, and pH 7.5, respectively. The use of CP-HL as the carbon source in fed-batch cultivation gave final CDM, RCDM, and lipid concentrations of 26.3 ± 2.0, 10.8 ± 2.0, and 15.5 ± 0.1 g/L, respectively. DHA content in the total lipid was 37.4 ± 1.5%, equivalent to 5.8 ± 0.3 g/L, and DHA productivity was 40 mg/(L·h), equivalent to 960 mg/(L·d). The present study shows promising results for DHA production using CP-HL as a low-cost substrate that can reduce the cost of medium preparation, thus enhancing EY of the process.
Data availability statement
The original contributions presented in the study are included in the article/Supplementary Material. Further inquiries can be directed to the corresponding author.
Author contributions
UA: Investigation, Writing – original draft. SL: Methodology, Writing – review and editing. AR: Resources, Funding acquisition. AS: Conceptualization, Methodology, Writing – review and editing, Supervision, Funding acquisition. All authors discussed the results and contributed to the final manuscript.
Funding
This work was supported by the Faculty of Technology Scholarship of Academic Year 2017 and the internal research funding by the Department of Biotechnology; and the ThailandResearch Fund (TRF) Senior Research Scholar (Grant no.RTA6280001).
Acknowledgments
The authors also thank the Fermentation Research Center for Value Added Agricultural Products (FerVAAP), Faculty of Technology, Khon Kaen University, Thailand, for the provision of equipment and chemicals.
Conflict of interest
The authors declare that the research was conducted in the absence of any commercial or financial relationships that could be construed as a potential conflict of interest.
Publisher’s note
All claims expressed in this article are solely those of the authors and do not necessarily represent those of their affiliated organizations, or those of the publisher, the editors and the reviewers. Any product that may be evaluated in this article, or claim that may be made by its manufacturer, is not guaranteed or endorsed by the publisher.
Supplementary material
The Supplementary Material for this article can be found online at: https://www.frontiersin.org/articles/10.3389/fmars.2022.985119/full#supplementary-material
References
Aasen I. M., Ertesvåg H., Heggeset T. M. B., Liu B., Brautaset T., Vadstein O., et al. (2016). Thraustochytrids as production organisms for docosahexaenoic acid (DHA), squalene, and carotenoids. Appl. Microbiol. Biotechnol. 100 (10), 4309–4321. doi: 10.1007/s00253-016-7498-4
Abernathy D. G., Spedding G., Starcher B. (2009). Analysis of protein and total usable nitrogen in beer and wine using a microwell ninhydrin assay. J. Instit. Brewing. 115 (2), 122–127. doi: 10.1002/j.2050-0416.2009.tb00356.x
Adney B., Baker J. (2008). "Measurement of cellulase activities" (Colorado: National Renewable Energy Laboratory). Available at: https://www.nrel.gov/docs/gen/fy08/42628.pdf.
Bustos G., Moldes A. B., Alonso J. L., Vázquez M. (2004). Optimization of d-lactic acid production by Lactobacillus coryniformis using response surface methodology. Food Microbiol. 21 (2), 143–148. doi: 10.1016/S0740-0020(03)00061-3
Byreddy A. R., Gupta A., Barrow C. J., Puri M. (2015). Comparison of cell disruption methods for improving lipid extraction from thraustochytrid strains. Mar. Drugs 13 (8), 5111–5127. doi: 10.3390/md13085111
Chen H., Jiang J. G. (2009). Osmotic responses of Dunaliella to the changes of salinity. J. Cell. Physiol. 219 (2), 251–258. doi: 10.1002/jcp.21715
Chi Z., Pyle D., Wen Z., Frear C., Chen S. (2007). A laboratory study of producing docosahexaenoic acid from biodiesel-waste glycerol by microalgal fermentation. Proc. Biochem. 42 (11), 1537–1545. doi: 10.1016/j.procbio.2007.08.008
Chi G., Xu Y., Cao X., Li Z., Cao M., Chisti Y., et al. (2022). Production of polyunsaturated fatty acids by Schizochytrium (Aurantiochytrium) spp. Biotechnol. Adv. 55, 107897. doi: 10.1016/j.biotechadv.2021.107897
de Moraes L. M., Ulhoa C. J. (1999). Purification and some properties of an α-amylase glucoamylase fusion protein from Saccharomyces cerevisiae. World J. Microbiol. Biotechnol. 15 (5), 561–564. doi: 10.1023/A:1008961015119
Dobrowolski A., Drzymała K., Rzechonek D. A., Mituła P., Mirończuk A. M. (2019). Lipid production from waste materials in seawater-based medium by the yeast Yarrowia lipolytica. Front. Microbiol. 10. doi: 10.3389/fmicb.2019.00547
Fan K. W., Chen F. (2007). “"Production of high-value products by marine microalgae thraustocytrids,",” in Bioprocessing of value-added products from renewable resources. Ed. Yang S.-T. (Amsterdam: Elsevier), 293–323.
Fossier Marchan L., Lee Chang K. J., Nichols P. D., Mitchell W. J., Polglase J. L., Gutierrez T. (2018). Taxonomy, ecology and biotechnological applications of thraustochytrids: A review. Biotechnol. Adv. 36 (1), 26–46. doi: 10.1016/j.biotechadv.2017.09.003
Gao M., Song X., Feng Y., Li W., Cui Q. (2013). Isolation and characterization of Aurantiochytrium species: high docosahexaenoic acid (DHA) production by the newly isolated microalga, Aurantiochytrium sp. SD116. J. Oleo. Sci. 62 (3), 143–151. doi: 10.5650/jos.62.143
Gladyshev M. I., Sushchik N. N., Makhutova O. N. (2013). Production of EPA and DHA in aquatic ecosystems and their transfer to the land. Prostaglandins Other. Lipid Mediators 107, 117–126. doi: 10.1016/j.prostaglandins.2013.03.002
Hong W.-K., Rairakhwada D., Seo P.-S., Park S.-Y., Hur B.-K., Kim C. H., et al. (2011). Production of lipids containing high levels of docosahexaenoic acid by a newly isolated microalga, aurantiochytrium sp. KRS101. Appl. Biochem. Biotechnol. 164 (8), 1468–1480. doi: 10.1007/s12010-011-9227-x
Huang T. Y., Lu W. C., Chu I. M. (2012). A fermentation strategy for producing docosahexaenoic acid in Aurantiochytrium limacinum SR21 and increasing C22: 6 proportions in total fatty acid. Biores. Technol. 123, 8–14. doi: 10.1016/j.biortech.2012.07.068
Innis S. M. (2008). Dietary omega 3 fatty acids and the developing brain. Brain Res. 1237, 35–43. doi: 10.1016/j.brainres.2008.08.078
Jacob F. F., Striegel L., Rychlik M., Hutzler M., Methner F.-J. (2019). Yeast extract production using spent yeast from beer manufacture: influence of industrially applicable disruption methods on selected substance groups with biotechnological relevance. Eur. Food Res. Technol. 245 (6), 1169–1182. doi: 10.1007/s00217-019-03237-9
Jiang X., Zhang J., Zhao J., Gao Z., Zhang C., Chen M. (2017). Regulation of lipid accumulation in Schizochytrium sp. ATCC 20888 in response to different nitrogen sources: Regulation of lipid accumulation in Schizochytrium sp. Eur. J. Lipid Sci. Technol. 119, e201700025. doi: 10.1002/ejlt.201700025
Khamtib S., Reungsang A. (2012). Biohydrogen production from xylose by Thermoanaerobacterium thermosaccharolyticum KKU19 isolated from hot spring sediment. Int. J. Hydrogen. Energy 37 (17), 12219–12228. doi: 10.1016/j.ijhydene.2012.06.038
Khanpanuek S., Lunprom S., Reungsang A., Salakkam A. (2022). Repeated-batch simultaneous saccharification and fermentation of cassava pulp for ethanol production using amylases and Saccharomyces cerevisiae immobilized on bacterial cellulose. Biochem. Eng. J. 177, 108258. doi: 10.1016/j.bej.2021.108258
Kim K., Jung Kim E., Ryu B.-G., Park S., Choi Y.-E., Yang J.-W. (2013). A novel fed-batch process based on the biology of Aurantiochytrium sp. KRS101 for the production of biodiesel and docosahexaenoic acid. Biores. Technol. 135, 269–274. doi: 10.1016/j.biortech.2012.10.139
Krahulec J., Šafránek M. (2021). Impact of media components from different suppliers on enterokinase productivity in Pichia pastoris. BMC Biotechnol. 21 (1), 19–19. doi: 10.1186/s12896-021-00681-y
Liang Y., Sarkany N., Cui Y., Yesuf J., Trushenski J., Blackburn J. W. (2010). Use of sweet sorghum juice for lipid production by Schizochytrium limacinum SR21. Biores. Technol. 101 (10), 3623–3627. doi: 10.1016/j.biortech.2009.12.087
Monroig Ó., Tocher D. R., Navarro J. C. (2013). Biosynthesis of polyunsaturated fatty acids in marine invertebrates: Recent advances in molecular mechanisms. Mar. Drugs 11 (10), 3998–4018. doi: 10.3390/md11103998
Morabito C., Bournaud C., Maës C., Schuler M., Aiese Cigliano R., Dellero Y., et al. (2019). The lipid metabolism in thraustochytrids. Prog. Lipid Res. 76, 101007. doi: 10.1016/j.plipres.2019.101007
Morita E., Kumon Y., Nakahara T., Kagiwada S., Noguchi T. (2006). Docosahexaenoic acid production and lipid-body formation in Schizochytrium limacinum SR21. Mar. Biotechnol. 8 (3), 319–327. doi: 10.1007/s10126-005-5060-y
Moungprayoon A., Lunprom S., Reungsang A., Salakkam A. (2022). High cell density cultivation of Paracoccus sp. on sugarcane juice for poly(3-hydroxybutyrate) production. Front. Bioengin. Biotechnol. 10. doi: 10.3389/fbioe.2022.878688
Nakazawa A., Matsuura H., Kose R., Ito K., Ueda M., Honda D., et al. (2012). Optimization of biomass and fatty acid production by aurantiochytrium sp. strain 4W-1b. Procedia Environmental Sciences 15, 27–33. doi: 10.1016/j.proenv.2012.05.006
Nazir Y., Shuib S., Kalil M. S., Song Y., Hamid A. A. (2018). Optimization of culture conditions for enhanced growth, lipid and docosahexaenoic acid (DHA) production of Aurantiochytrium sw1 by response surface methodology. Sci. Rep. 8 (1), 8909. doi: 10.1038/s41598-018-27309-0
Nguyen H. C., Su C.-H., Yu Y.-K., Huong D. T. M. (2018). Sugarcane bagasse as a novel carbon source for heterotrophic cultivation of oleaginous microalga Schizochytrium sp. Ind. Crops Prod. 121, 99–105. doi: 10.1016/j.indcrop.2018.05.005
Park W.-K., Moon M., Shin S.-E., Cho J. M., Suh W. I., Chang Y. K., et al. (2018). Economical DHA (Docosahexaenoic acid) production from aurantiochytrium sp. KRS101 using orange peel extract and low cost nitrogen sources. Algal. Res. 29, 71–79. doi: 10.1016/j.algal.2017.11.017
Patel A., Liefeldt S., Rova U., Christakopoulos P., Matsakas L. (2020a). Co-Production of DHA and squalene by thraustochytrid from forest biomass. Sci. Rep. 10 (1), 1992. doi: 10.1038/s41598-020-58728-7
Patel A., Rova U., Christakopoulos P., Matsakas L. (2019). Simultaneous production of DHA and squalene from Aurantiochytrium sp. grown on forest biomass hydrolysates. Biotechnol. Biofuels 12 (1), 255. doi: 10.1186/s13068-019-1593-6
Patel A., Rova U., Christakopoulos P., Matsakas L. (2020b). Assessment of fatty acids profile and omega-3 polyunsaturated fatty acid production by the oleaginous marine thraustochytrid Aurantiochytrium sp. T66 cultivated on volatile fatty acids. Biomolecules 10 (5), 694. doi: 10.3390/biom10050694
Patil K. P., Gogate P. R. (2015). Improved synthesis of docosahexaenoic acid (DHA) using Schizochytrium limacinum SR21 and sustainable media. Chem. Eng. J. 268, 187–196. doi: 10.1016/j.cej.2015.01.050
Phukoetphim N., Salakkam A., Laopaiboon P., Laopaiboon L. (2017). Kinetic models for batch ethanol production from sweet sorghum juice under normal and high gravity fermentations: Logistic and modified gompertz models. J. Biotechnol. 243, 69–75. doi: 10.1016/j.jbiotec.2016.12.012
Proust L., Sourabié A., Pedersen M., Besançon I., Haudebourg E., Monnet V., et al. (2019). Insights into the complexity of yeast extract peptides and their utilization by Streptococcus thermophilus. Front. Microbiol. 10, 906. doi: 10.3389/fmicb.2019.00906
Raghukumar S. (2008). Thraustochytrid marine protists: production of PUFAs and other emerging technologies. Mar. Biotechnol. 10 (6), 631–640. doi: 10.1007/s10126-008-9135-4
Ratledge C. (2004). Fatty acid biosynthesis in microorganisms being used for single cell oil production. Biochimie 86 (11), 807–815. doi: 10.1016/j.biochi.2004.09.017
Ren L.-J., Feng Y., Li J., Qu L., Huang H. (2013). Impact of phosphate concentration on docosahexaenoic acid production and related enzyme activities in fermentation of Schizochytrium sp. Biopro. Biosyst. Eng. 36 (9), 1177–1183. doi: 10.1007/s00449-012-0844-8
Salakkam A., Kingpho Y., Najunhom S., Aiamsonthi K., Kaewlao S., Reungsang A. (2017). Bioconversion of soybean residue for use as alternative nutrient source for ethanol fermentation. Biochem. Eng. J. 125, 65–72. doi: 10.1016/j.bej.2017.05.020
Shabala L., McMeekin T., Shabala S. (2009). Osmotic adjustment and requirement for sodium in marine protist thraustochytrid. Environ. Microbiol. 11 (7), 1835–1843. doi: 10.1111/j.1462-2920.2009.01908.x
Siriwong T., Laimeheriwa B., Aini U. N., Cahyanto M. N., Reungsang A., Salakkam A. (2019). Cold hydrolysis of cassava pulp and its use in simultaneous saccharification and fermentation (SSF) process for ethanol fermentation. J. Biotechnol. 292, 57–63. doi: 10.1016/j.jbiotec.2019.01.003
Sohedein M. N. A., Wan-Mohtar W.A.A.Q.I., Ilham Z., Babadi A. A., Hui-Yin Y., Siew-Moi P. (2020). Vital parameters for biomass, lipid, and carotenoid production of thraustochytrids. J. Appl. Phycol. 32 (2), 1003–1016. doi: 10.1007/s10811-019-01970-y
Taoka Y., Nagano N., Okita Y., Izumida H., Sugimoto S., Hayashi M. (2009). Extracellular enzymes produced by marine eukaryotes, thraustochytrids. Biosci. Biotechnol. Biochem. 73 (1), 180–182. doi: 10.1271/bbb.80416
Tičina V., Katavić I., Grubišić L. (2020). Marine aquaculture impacts on marine biota in oligotrophic environments of the mediterranean sea – a review. Front. Mar. Sci. 7. doi: 10.3389/fmars.2020.00217
Wang K., Sun T., Cui J., Liu L., Bi Y., Pei G., et al. (2018). Screening of chemical modulators for lipid accumulation in schizochytrium sp. S31. Biores. Technol. 260, 124–129. doi: 10.1016/j.biortech.2018.03.104
Xu X., Huang C., Xu Z., Xu H., Wang Z., Yu X. (2020). The strategies to reduce cost and improve productivity in DHA production by Aurantiochytrium sp.: from biochemical to genetic respects. Appl. Microbiol. Biotechnol. 104 (22), 9433–9447. doi: 10.1007/s00253-020-10927-y
Yin F.-W., Zhu S.-Y., Guo D.-S., Ren L.-J., Ji X.-J., Huang H., et al. (2019). Development of a strategy for the production of docosahexaenoic acid by schizochytrium sp. from cane molasses and algae-residue. Biores. Technol. 271, 118–124. doi: 10.1016/j.biortech.2018.09.114
Yokochi T., Honda D., Higashihara T., Nakahara T. (1998). Optimization of docosahexaenoic acid production by Schizochytrium limacinum SR21. Appl. Microbiol. Biotechnol. 49 (1), 72–76. doi: 10.1007/s002530051139
Yu X.-J., Liu J.-H., Sun J., Zheng J.-Y., Zhang Y.-J., Wang Z. (2016). Docosahexaenoic acid production from the acidic hydrolysate of Jerusalem artichoke by an efficient sugar-utilizing Aurantiochytrium sp. YLH70. Ind. Crops Prod. 83, 372–378. doi: 10.1016/j.indcrop.2016.01.013
Zhang M., Chen C., You C., Chen B., Wang S., Li Y. (2019). Effects of different dietary ratios of docosahexaenoic to eicosapentaenoic acid (DHA/EPA) on the growth, non-specific immune indices, tissue fatty acid compositions and expression of genes related to LC-PUFA biosynthesis in juvenile golden pompano Trachinotus ovatus. Aquaculture 505, 488–495. doi: 10.1016/j.aquaculture.2019.01.061
Keywords: cassava pulp, docosahexaenoic acid (DHA), thraustochytrids, waste utilization, bioeconomy
Citation: Aini UN, Lunprom S, Reungsang A and Salakkam A (2022) Docosahexaenoic acid (DHA) production by Aurantiochytrium limacinum using cassava pulp hydrolysate as an alternative low-cost carbon source. Front. Mar. Sci. 9:985119. doi: 10.3389/fmars.2022.985119
Received: 03 July 2022; Accepted: 21 July 2022;
Published: 08 August 2022.
Edited by:
Wangbiao Guo, Yale University, United StatesReviewed by:
Tsunehiro Aki, Hiroshima University, JapanXiaojin Song, Qingdao Institute of Bioenergy and Bioprocess Technology, Chinese Academy of Sciences (CAS), China
Chiara Ceccotti, University of Insubria, Italy
Copyright © 2022 Aini, Lunprom, Reungsang and Salakkam. This is an open-access article distributed under the terms of the Creative Commons Attribution License (CC BY). The use, distribution or reproduction in other forums is permitted, provided the original author(s) and the copyright owner(s) are credited and that the original publication in this journal is cited, in accordance with accepted academic practice. No use, distribution or reproduction is permitted which does not comply with these terms.
*Correspondence: Apilak Salakkam, YXBpbHNhQGtrdS5hYy50aA==