Size matters: Physiological sensitivity of the scallop Argopecten purpuratus to seasonal cooling and deoxygenation upwelling-driven events
- 1Centro de Estudios Avanzados en Zonas Áridas (CEAZA), Coquimbo, Chile
- 2Departamento de Biología Marina, Facultad de Ciencias del Mar, Universidad Católica del Norte (UCN), Coquimbo, Chile
- 3Center for Climate and Resilience Research (CR2), Santiago, Chile
- 4ONG Jáukén, Santiago, Chile
Environment imposes physiological constraints which are life-stage specific as growth-maintenance and/or growth-reproduction energetic requirements are size and volume-dependent. The scallop Argopecten purpuratus, one of the most important bivalve species subjected to fishery and aquaculture along the Humboldt Current System, inhabits spaces affected by continuous changes in temperature, pH, oxygen, and food availability driven by remote and local oceanographic processes. Specifically, in Chile, this species is mainly cultured in central-north Chile where is permanently affected by upwelling events of dissimilar intensity and duration which generate local conditions of acidification, deoxygenation, and cooling with different magnitudes. However, to date, it remains unknown how this economic valuable resource is physiologically affected throughout its life cycle by the continuous environmental changes driven by upwelling events of different intensities and duration along the year. Here, for the first time, A. purpuratus life-stage physiological sensitivity was assessed at a seasonal scale through a year-field experiment where growth, calcification, and survivorship were evaluated. Our study shows how seasonal differences in the upwelling phenology (here measured as changes in temperature, dissolved oxygen, pH, and primary productivity, but also as the number, duration, and intensity of cooling and de-oxygenation events) notably impacted the A. purpuratus physiological performance from juvenile to adult life-stages. This was especially noticeable during the spring season which showed the most intense cooling and deoxygenation events driven by stronger favorable-upwelling winds and the lowest growth and gross calcification rates (the highest decalcification rates) where adult stages showed the lowest performance. On the other hand, A. purpuratus survivorship was not significantly affected by upwelling intensity which would be providing evidence of the high physiological flexibility and well-locally adapted is this species to fluctuating and occasional stressful environmental conditions. Our results are significantly relevant in the climate change context as some upwelling systems are at risk to change shortly (i.e., an upwelling intensification in frequency and intensity) as a consequence of changes in the atmospheric pressures that modulate favourable-upwelling winds. These changes may certainly increase the climate related-risks of the entire socio-ecological systems related to the fishery and aquaculture of A. purpuratus along the Humboldt Current System.
1. Introduction
Physiological performance is highly dependent on environmental conditions (Thomsen et al., 2015; Byrne, 2012). Elevated temperatures increase organism’s metabolic rates accelerating growth and sexual maturity, while when habitat temperatures exceed the organism tolerance thresholds can promote the rapid deterioration of cellular processes (Pörtner, 2008). Also, low seawater pH values can cause hypercapnia with cascading effects on multiple physiological responses of diverse taxa, being specifically detrimental in calcifiers as shell precipitation and shell integrity are highly dependent on pH and carbonate concentration conditions (e.g., Kroeker et al., 2013; Ramajo et al., 2016; Ramajo et al., 2020). Indeed, lower pH levels result in lower calcification rates, increasing shell malformations (Waldbusser et al., 2015) and shell mechanical changes (e.g., weaker shells) (Zhao et al., 2020; Lagos et al., 2021) which also may increase the vulnerability to predation, parasitism, and/or stress by wave action (Gazeau et al., 2013; MacLeod and Poulin, 2015; Gaylord et al., 2015). Likewise, critical physiological processes such as respiration are dependent on the flux of oxygen (Seibel, 2011) where low oxygen availability is usually related to reduced growth and low reproduction success, altered behavior patterns, and increased risk of predation with resulting lower survivorship for many marine species (Breitburg et al., 2018).
Moreover, physiological sensitivity to environmental changes is life-stage specific (Talmage and Gobler, 2010; Baumann et al., 2012). Early life-stages such as larvae or juveniles usually show lower energy reserves and smaller tolerance limits making them more sensitive than adults to higher temperatures (e.g., Truebano et al., 2018), decreasing pH levels (e.g., Kroeker et al., 2013; Wessel et al., 2018), and hypoxic conditions (e.g., Clark and Gobler, 2016). Also, growth-maintenance and/or growth-reproduction trade-offs are more evident when organisms get older as maintenance, growth, and reproduction related-costs are size and volume-dependent (Bayne and Newell, 1983; Kooijman, 2000; Thompson and MacDonald, 2006; Chauvaud et al., 2012). In particular, for calcifying organisms, some physiological trade-offs emerge as a consequence of the higher energetic requirements needed for biomineralization and calcification processes including carbonate precipitation and shell organic and periostracum production (Palmer, 1981; Trussell and Smith, 2000; Clark et al., 2020; Ramajo et al., 2019; Ramajo et al., 2020) which could represent up to 75-410% of the energy invested for growth and reproduction (Palmer, 1981).
The scallop Argopecten purpuratus is one of the most important bivalve species subjected to fishery and aquaculture along the Peruvian and Chilean Humboldt Current System (Yáñez et al., 2017). Historical A. purpuratus landings show an important variability as a consequence of changes in environmental conditions (Lagos et al., 2016; Yáñez et al., 2017) with significant impacts on Chilean and Peruvian markets (von Brand et al., 2016). Previous experimental and field studies on this resource show significant sensitivity to changes in temperature, pH, oxygen, and food availability (Lagos et al., 2016; Ramajo et al., 2016; Lardies et al., 2017; Ramajo et al., 2019; Ramajo et al., 2020; Avendaño and Cantillánez, 2022) which is particularly important in Chile as this scallop species is mostly cultured (i.e., Tongoy Bay) under the effects of one of the most active upwelling centers on the Chilean coast (Figueroa and Moffat, 2000; Rutllant and Montecino, 2002; Aravena et al., 2014; Rahn and Garreaud, 2014). Environmental conditions at Tongoy Bay have important physiological impacts on A. purpuratus juveniles (Ramajo et al., 2020), especially during the upwelling season (spring-summer) when the intensity and duration of upwelling events are higher (Moraga-Opazo et al., 2011; Bravo et al., 2016). However, to date, remains unknown which is the life-stage A. purpuratus sensitivity to changes in the environmental conditions driven by the seasonal upwelling cycle. This missing information is significantly relevant in the climate change context as some Eastern Boundary Upwelling Systems (EBUS) are at risk to change shortly (i.e., an upwelling intensification as a consequence of changes in the frequency and intensity) which could also favor local ocean acidification and deoxygenation (see Garreaud and Muñoz, 2005; Aravena et al., 2014; Sydeman et al., 2014; Rykaczewski et al., 2015; IPCC, 2021). Certainly, potential changes in the upwelling phenology may threaten the fishery and aquaculture scallop industry and the related-socioecological system along the Humboldt Current System (Lluch-Cota et al., 2014; Bakun et al., 2015; Ramajo et al., 2020; IPCC, 2021).
Based on that, here was assessed, for the first time, the level of physiological sensitivity of different life-stages of A. purpuratus at a seasonal scale in the context of upwelling. To do that, a year-field experiment was performed in Tongoy Bay where physiological responses (growth, calcification, and survivorship) were evaluated in a large range of scallop sizes (from the juvenile to adult stages) aiming to capture how seasonal changes in the upwelling phenology (number of events, duration, and intensity) could be modulating the physiological performance and fitness of this economical valuable resource for the fishery and shellfish aquaculture along the Humboldt Current System.
2. Material and methods
2.1. Study site
Tongoy Bay, in north-central Chile, is a key place for the culture of the scallop Argopecten purpuratus. This bay is located just north of Punta Lengua de Vaca (PLV), one of the most important and active upwelling centers that modulate environmental conditions on the central-north Chilean coast (Figueroa and Moffat, 2000; Rutllant and Montecino, 2002; Rahn and Garreaud, 2014) (Figure 1A). Previous studies have determined that Tongoy Bay shows a semi-permanent upwelling regime with higher upwelling intensities during the austral spring-summer seasons (Moraga-Opazo et al., 2011; Bravo et al., 2016). By the year, seasonal differences in the upwelling phenology provoke important oceanographic, biogeochemical, and environmental heterogeneity in the nearby coastal areas (Torres et al., 1999; Torres and Ampuero, 2009; Ramajo et al., 2020). As a consequence of this seasonal pattern and upwelling, Tongoy Bay shows along the year high fluctuations in terms of temperature (10.6°C - 19.7°C), pH (7.6 - 8.1), oxygen concentrations (1.45 - 4.17 ml L-1) (Ramajo et al., 2019; Ramajo et al., 2020), and primary productivity (ranging from 2.40 to 10.45 mg m-3) (Rutllant and Montecino, 2002; Ramajo et al., 2020).
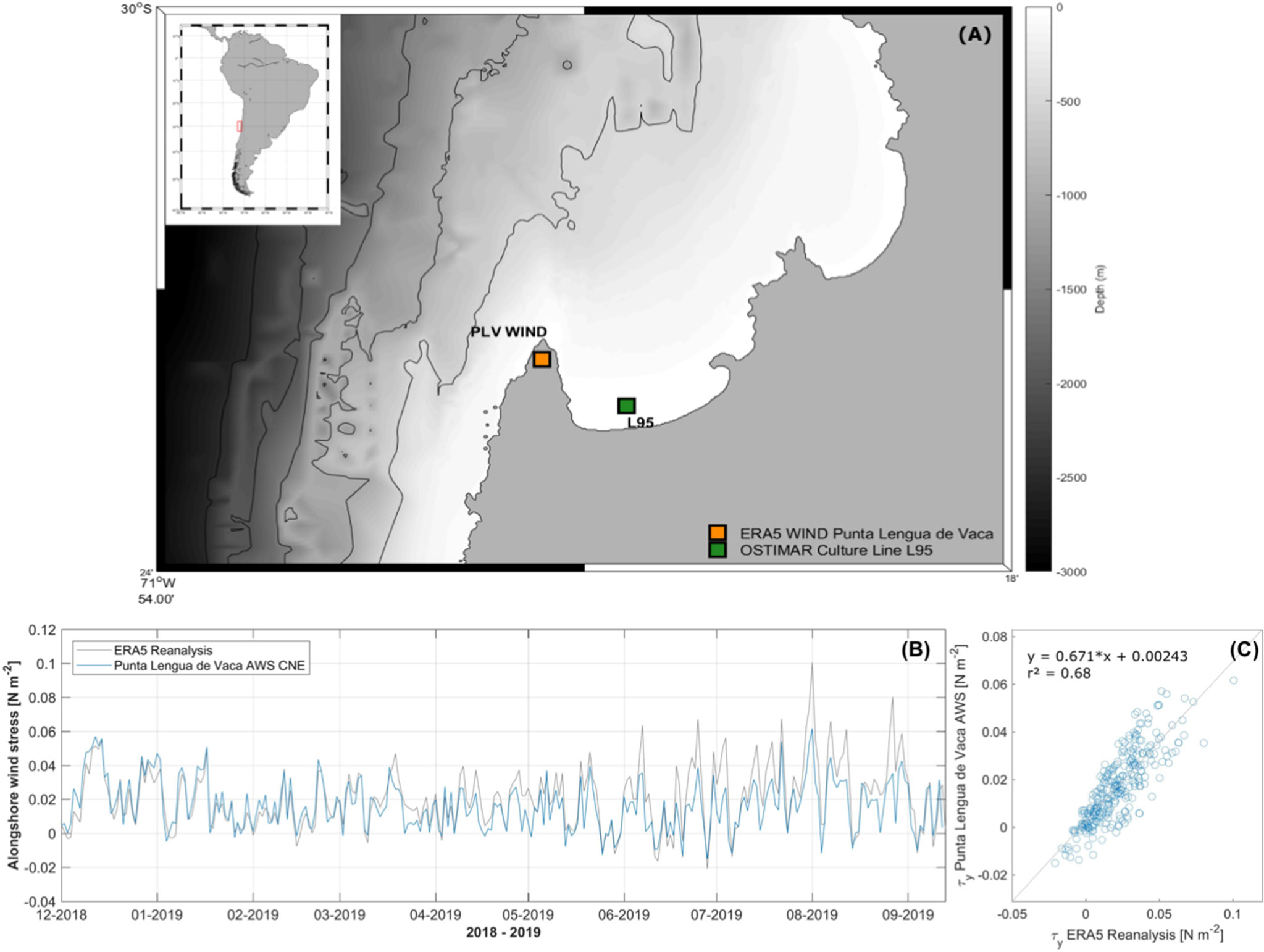
Figure 1 Study site and wind conditions. (A) Tongoy Bay coastal area with positions of the experiment (green) (culture line L95) and the location of the automatic weather station (AWS) placed close to the upwelling centre Punta Lengua de Vaca (PLV) (orange). (B) Validation of ERA5 reanalysis alongshore wind component with the in situ PLV-AWS data from 2018 to 2019. (C) Least-square linear regression between ERA5 reanalysis and AWS alongshore wind data.
2.2. Organismal collection and experimental design
Wild scallops from Tongoy Bay (30°16’S; 71°35’W), with no shell damage and parasite presence (Basilio et al., 1995), were provided by the scallop aquaculture company OSTIMAR S.A. The experimental design consisted of four analogous field experiments (hereafter, seasonal experiments) that aimed to determine how seasonal environmental changes are modulated by upwelling phenological changes (i.e. a semi-permanent upwelling regime with lower intensity during the autumn and winter months, and higher intensity during spring-summer seasons, Thiel et al., 2007; Torres and Ampuero, 2009; Rahn and Garreaud, 2014) and may be affecting different life-stages (from juveniles to adults) of the scallop A. purpuratus at Tongoy Bay. To do that, around 180 scallops were used in each seasonal experiment with a body-size range between 10 to 90 mm (Figure S1). After collection, experimental scallops were transported under-insulated conditions to the laboratory and labeled with numbered tags glued onto the shells allowing their identification during the experiment. After that, between 100 - 120 scallops (hereafter, alive scallops) were maintained in aquaria under controlled conditions (14°C, pHNBS near 8.0, and >90% of oxygen saturation). In addition, ~ 60 scallops were sacrificed and their soft parts were removed, and shells were kept for the experiment (hereafter, empty shells). For both, alive scallops and empty shells, the maximum shell size (length and width), and the shell buoyant weight were recorded at the initial time (Figure S1). After that, alive scallops and empty shells were randomly distributed in 4 pearl nets (i.e. common culture structures used by the aquaculture industry to avoid denso-dependency issues). Each pearl net was composed of 2 different nets separated by less than 25 cm between them. Each pearl net contained 30 alive scallops and 30 empty shells (see Figure 2). The four experimental pearl nets were located in the culture line number L95 belonging to OSTIMAR S.A (30°16’49.4′′S; 71°34’03.7′′W) (Figure 1A) at 9 m depth. Each alive scallop and each empty shell were considered independent replicates. The four seasonal experiments followed similar experimental protocols. The Summer experiment was carried out between January to March 2019, the Autumn experiment between March to July 2019, Winter from July to October 2019, and the Spring experiment from October 2019 to January 2020 (see Figure 2). At the end of each seasonal experiment, pearl nets with alive scallops and empty shells were removed from the L95 and transported to the laboratory where shell size (maximum length and width) and buoyant weight were measured. At the end of each seasonal experiment, all alive scallops were sacrificed (not returned to the field).
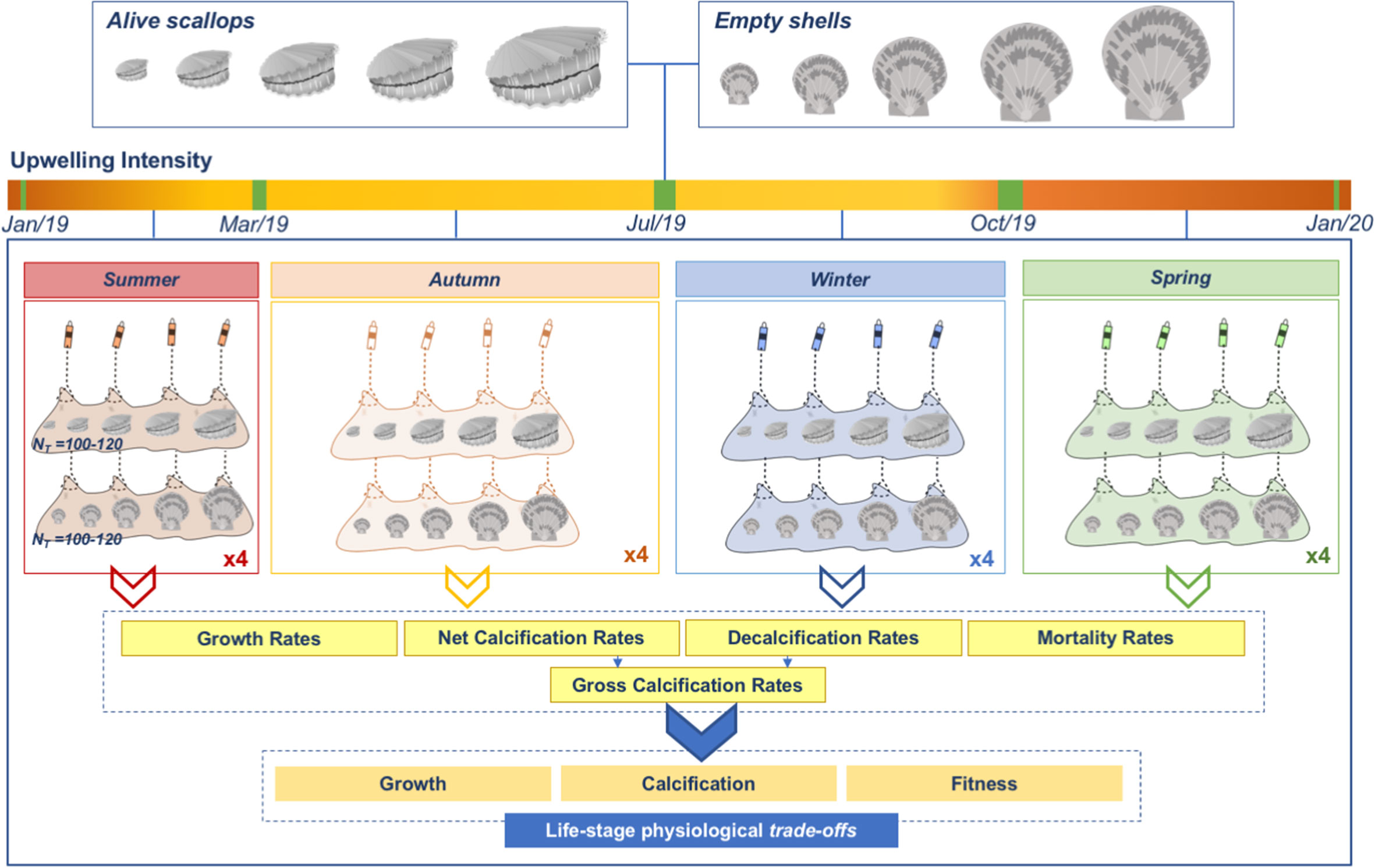
Figure 2 Experimental design. General scheme of the experimental design used where alive scallops and empty shells were exposed to four different seasonal experiments (Summer, Autumn, Winter, and Spring) from January 2019 to January 2020. Four pearl nets composed of 2 nets containing between 100-120 alive scallops and empty shells were used by seasonal experiment. At the end of each experiment, growth, net calcification, decalcification, and gross calcification rates were determined, and mortality was recorded. The intensity of the upwelling is based on the environmental data monitored during the experiments (i.e. higher intensity is shown as orange).
2.3. Environmental conditions at Tongoy Bay
Tongoy Bay environmental conditions, at high and low frequencies, were monitored during the four seasonal experiments. High-frequency (each 15 min) temperature and dissolved oxygen (DO) conditions were registered by using HOBO U26 Dissolved Oxygen Data Loggers (ONSET®) which were attached to the experimental pearl nets. According to the manufacturer’s instructions, every six months the oxygen logger membranes were replaced with new ones. Anti-fouling sensor maintenance, as well as, data downloading from each sensor were performed at the end of each seasonal experiment. At low frequency (weekly), pHNBS conditions were determined by collecting water samples through a NISKIN bottle (5L vol). Three water samples per depth were analyzed within 60 min after water collection using a Metrohm 780 Meter (Metrohm®) connected to a combined electrode (Aquatrode Plus with Pt1000, Metrohm®). The electrode was calibrated using three NBS buffers (Metrohm®) at 25°C before each measurement. In addition, surface chlorophyll-a (Chl-a) by each seasonal experiment was obtained through 8-day composites of Moderate Resolution Imaging Spectroradiometer (MODIS-Aqua) 4km products retrieved from NASA (http://oceancolor.gsfc.nasa.gov) comprising an area limited by 30.6° - 30°S and 71.9°W to the coast. MODIS Chl-a data were averaged at a monthly scale and then averaged for each seasonal experiment. Finally, the intensity and variability of wind-driven coastal upwelling were obtained from ERA5 reanalysis (https://rda.ucar.edu/datasets/ds633.0/) at PLV during the experiment. PLV is representative of the environmental conditions occurring at Tongoy Bay as it was recently observed by Ramajo et al. (2020) (see also Figure 1A). Reanalysis dataset was validated against available continuous 10m wind data from an Automatic Weather Station (AWS) at the same geographic location between December 2018 to September 2019 (see Figure 1B). Daily alongshore wind magnitude measured by the AWS at PLV and ERA5 reanalysis showed a high and significant correlation (Pearson correlation: R2 = 0.68, P-value = 0.000) (see Figure 1C).
2.4. Growth, calcification, and mortality
Growth rates (GR), net calcification rates (NCRs), decalcification rates (DCRs), and gross calcification rates (GCR) were estimated for all seasonal experiments. GRs estimations correspond to the difference in the total area of alive scallops (estimated as an ellipsoid from shell maximum length and width measurements) divided by the number of days that the experiment lasted. Empty shells were used to estimate size differences in DCRs for each seasonal experiment (see Figure S2). Previous studies have found that low pH conditions generate corrosive and undersaturated carbonate conditions which are responsible for shell layers dissolution and higher decalcification rates (i.e., Waldbusser et al., 2011; Langer et al., 2014). Both NCRs and DCRs were estimated by using the buoyant weight technique (Davies, 1989). Buoyant weight data was converted into shell dry weight (DW) using the seawater density (average conditions at Tongoy Bay, salinity = 34‰, temperature = 14°C), and the density of calcite (2.71 g cm-3) (see Ramajo et al., 2019). Finally, NCRs and DCRs were computed as the change in the shell dry (DW) weight goes by between two different periods (i.e. duration of each seasonal experiment). Lastly, GCRs for each alive scallop and seasonal experiment were determined by subtracting DCRs from the NCRs. All physiological rates (i.e. GRs, NCR, DCRs, and GCRs) are shown as non-normalized and body-size normalized. Dead organisms were counted at the end of each seasonal experiment, and mortality rates were estimated as the percentage of dead organisms per day (% d-1).
2.5. Data analyses
Environmental and biogeochemical seawater properties (i.e. pHNBS, temperature and DO), based on the mean state (± standard error, SE) and their variability (coefficient of variation, CV) were established for the four seasonal experiments.
Upwelling was characterized by calculating alongshore wind stress (τalongshore) after rotating ERA5 daily wind vectors to align them approximately with the direction of the coast (Equation 1).
where ρair is air density (assumed constant at 1.22 kg m-3), Cd is the drag coefficient varying with ν, and ν is the meridional alongshore wind component.
Further, across-shelf Ekman transport (M, units = m2 s-1 per meter of the coast) was computed following:
where ρw is the water density (assumed constant at 1024 kg m-3), f is the Coriolis parameter (s-1), and τ is the alongshore wind stress. Positive values of M indicate onshore transport due to downwelling favorable winds, while negative values imply offshore transport caused by upwelling favorable winds (Bakun, 1975). Posteriorly, following Tapia et al. (2009) and Ramajo et al. (2020) methodologies, the impact of upwelling on temperature changes (i.e. cooling) and DO conditions (i.e. deoxygenation) at Tongoy Bay were estimated. The present study spans one entire year in a semi-permanent upwelling region encompassing upwelling, downwelling favorable winds, and relaxation periods (Rahn and Garreaud, 2014), some extra methodological modifications to Ramajo et al. (2020) were implemented to properly attribute cooling and de-oxygenating events to upwelling. To do that, first, we defined a threshold of alongshore wind stress of 5m s-1 in the equatorward direction (see Cury and Roy, 1989; García-Reyes et al., 2014). Secondly, to highlight the synoptic variability dominance in upwelling systems (Renault et al., 2009), a 30-day running mean was subtracted from the daily average time series of seawater temperature and DO. Daily anomalies in temperature and DO time series were used to quantify the number, duration, and intensity of the different cooling and de-oxygenations episodes recorded during each seasonal experiment (Figure 5A) (for further details, see Ramajo et al., 2020).
Average specific differences in GRs, NCRs, DRs, and GCRs (non- and body-size normalized), as well as, the size distribution of alive and dead scallops after each seasonal experiment were evaluated by using Kruskal Wallis and Dunn tests. The role of the tested factors (Size and Season) for all physiological rates was addressed by using hierarchical linear mixed-effects models (e.g., Ramajo et al., 2020). Size and Season and the two-way interaction between these were modeled as fixed effects. Random effects at the individual level were employed to control for potential organismal effects. Restricted maximum likelihood (REML) was used to fit the model for each physiological rate (GRs, NCRs, DRs, and GCRs), as well as, to calculate unbiased estimates of parameter variance and standard error according to the general model:
where y is the vector of the observations (i.e., physiological rates), b and 0 is the vector of fixed effects (i.e., Size and Season), a is the vector of random effects (i.e., individuals), e represents the residual effects, and X and Z are the corresponding incidence matrices. Linear mixed-effects models were estimated by using the lme4 package. The AFEX package was used to obtain parameter P-values for the linear mixed-effects models using the Kenward-Roger approximation for degrees of freedom. Optimal models were identified as those yielding the greatest number of significant (P< 0.05) fixed effects. Variance and standard deviation of the random effect (individual) are also reported. Differences in mortality (i.e. number of dead scallops) were determined by using Poisson regressions. Finally, to identify potential associations between environmental variables and physiological rates measured, Pearson correlation analyses were carried out, and multivariate comparisons between all physiological rates and environmental variables were assessed using redundancy analysis (RDA). Before RDA analyses, environmental variables and physiological rates were standardized (from zero to one). RDA analysis was carried out using the vegan package. All these analyses were carried out on R software (version 3.5.0).
3. Results
3.1. Seasonal environmental variability at Tongoy Bay
During 2019 at Tongoy Bay, coastal upwelling conditions (i.e. negative Ekman transport, M) dominated the year-round experimental study showing an increasing intensity from summer towards the spring season (observed in mean and minimum values) exhibiting a maximum mean of -0.43 m2 s-1 in the winter season. On the other hand, downwelling favorable conditions (i.e., positive Ekman transport, M) were mostly observed during the autumn season. Regarding upwelling relaxation events (i.e., Ekman transport close to zero), these were observed throughout the year on a synoptic time scale (~1 to 16 days) (Figure 3A; Table 1).
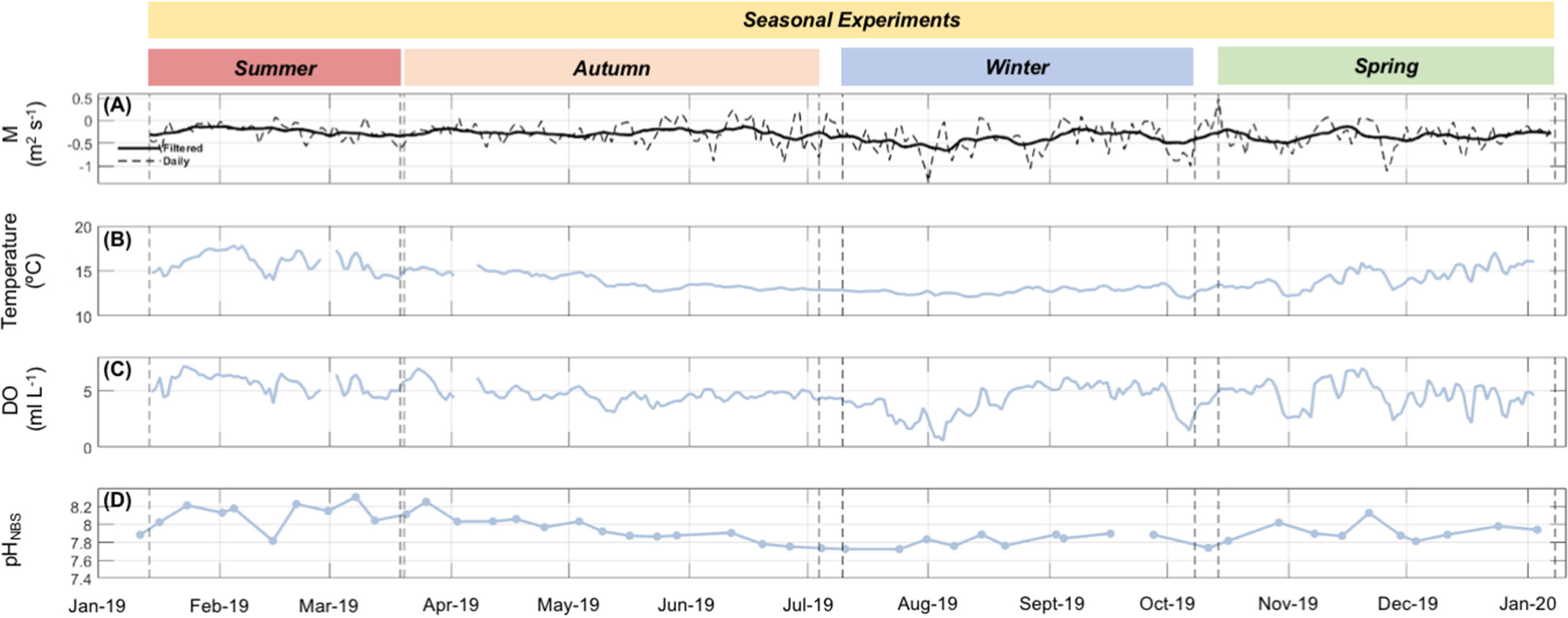
Figure 3 Field Conditions. (A) Daily averaged and high-pass filtered time series of Ekman transport at PLV, in segmented and continuous lines, respectively. Daily and weekly time series of (B) seawater temperature, (C) dissolved oxygen (DO), and (D) pHNBS at Tongoy Bay (9 m depth). Vertical segmented lines separate the four seasonal experiments.
Surface Chl-a at Tongoy Bay presented maximum values during the spring and the lowest concentrations during the autumn and winter months (Figure 4; Figure S3).
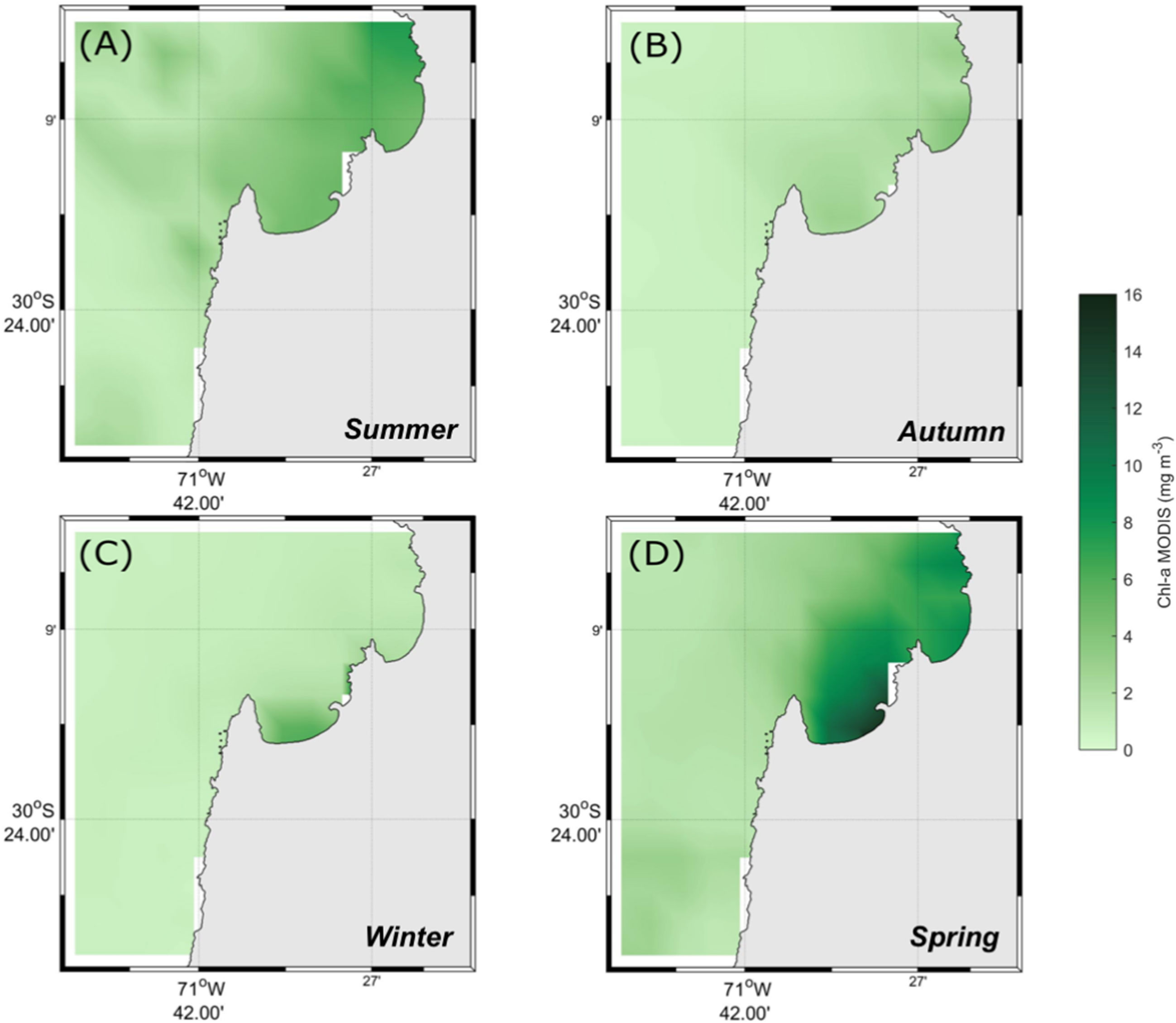
Figure 4 Chlorophyll-a variability. Seasonally-averaged Chl-a fields derived from MODIS-Aqua 8-day composites for each seasonal experiment.. (A) Summer, (B) Autumn, (C) Winter, and (D) Spring season.
In terms of variability (here measured as CV, %), temperature and pH exhibited a decline towards winter with a subsequent rise in spring. Maximum variability in temperature and pH was found during spring and summer, with temperature CV peaking in spring and pH CV in summer (Figures 3B, D; Table 1). DO variability increased from summer to spring, with a maximum in winter (Figure 3C; Table 1).
The number and duration of cooling and de-oxygenation events showed differences among seasonal experiments and were highly correlated with Ekman transport magnitude ((Figures 5B–D)) revealing a negative relationship between M and temperature and DO conditions (Figures 3A–D). A slight increase in the number and duration of cooling and de-oxygenation events was observed from summer to winter then showing a little reduction during the spring season. Winter presented the highest number of cooling and de-oxygenation events, as well as, the longest duration compared with the rest of the seasons. However, spring and summer exhibited the highest intensity and cooling and de-oxygenation rates (Figures 5E–H).
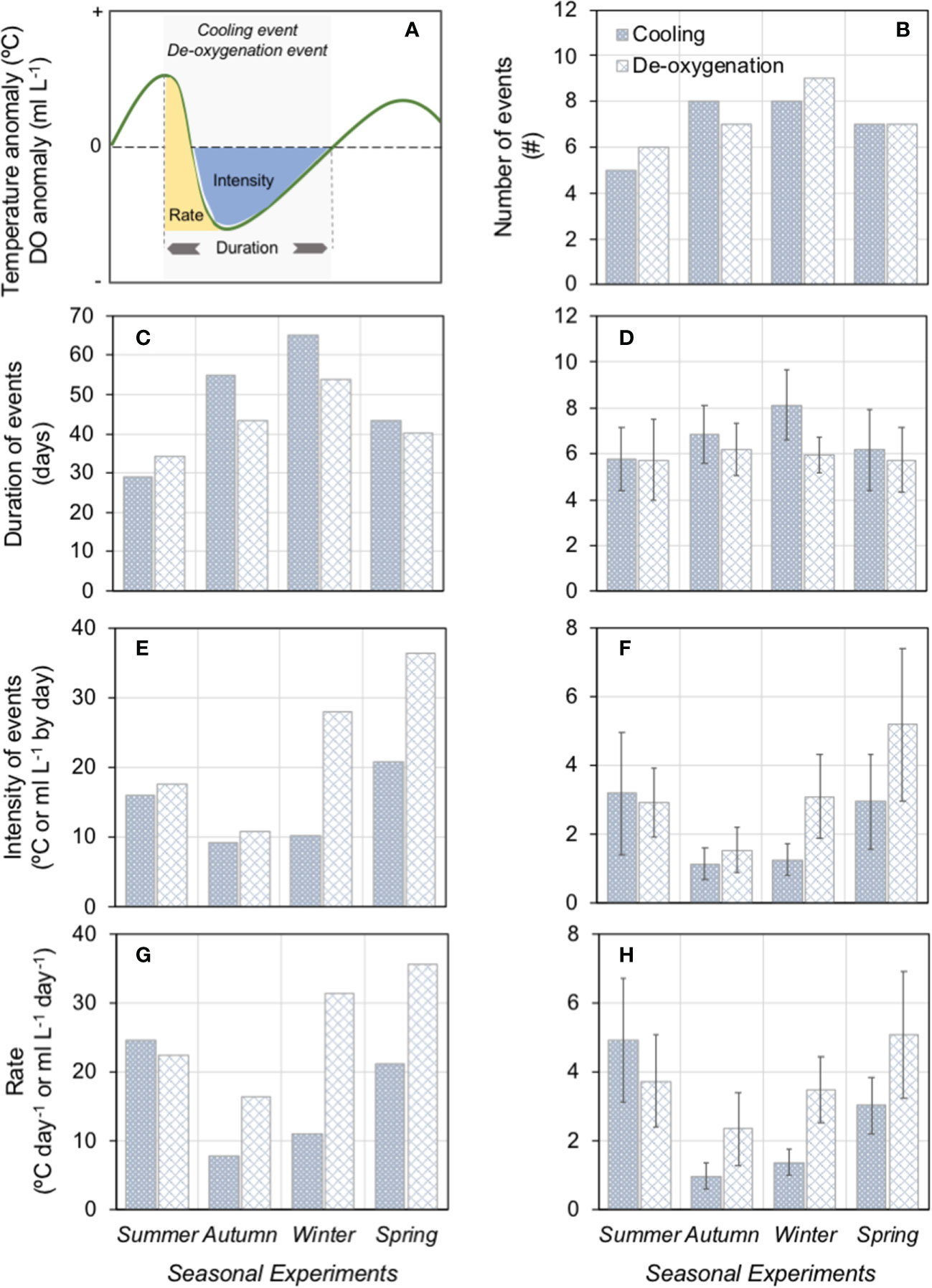
Figure 5 Cooling and de-oxygenation at Tongoy Bay. (A) Scheme of the methodology used to determine the upwelling impact on cooling and de-oxygenation events based on thermal and dissolved oxygen anomalies (see section 2.1 for further details). Number (B); accumulated (C) and mean (D) duration; accumulated (E) and mean (F) intensity (as an integrated anomaly); accumulated (G) and mean (H) rate (as integrated cooling/deoxygenation) of events registered each seasonal experiment. Data in D, F, and H are means ± SE.
3.2. Growth and calcification
Both, average non-body-size normalized and body-size normalized GRs showed significant differences among the different seasonal experiments (Kruskal-Wallis test: χ2 = 24.86, P-value< 0.001, and χ2 = 50.58, P-value = 0.000, respectively). Average GRs (non-body-size normalized) showed similar values between the summer, autumn, and winter seasons (Dunn’s test: P-value = 1.000), however a significant reduction in the GRs was detected during the spring in comparison with summer (Dunn’s test: P-value = 0.000), autumn (Dunn’s test: P-value = 0.000) and winter (Dunn’s test: P-value< 0.001) (see Figure 6A). On the other hand, average normalized body-size GRs showed a different trend with a visible decreasing trend from the summer to the spring. Normalized body-size GRs were significantly higher during the summer in comparison with the winter (Dunn’s test: P-value = 0.001) and spring seasons (P-value = 0.000). No significant differences were found between summer and autumn (P-value = 0.080), as well as between autumn and winter (Dunn’s test: P-value = 0.844) (see Figure 6B). Linear mixed models controlled for the random effects of individuals confirmed that the scallops’ size (P-value< 0.001), season (i.e., the environmental conditions occurring during each seasonal experiment) (P-value< 0.037), and the interaction between size and season (P-value = 0.030) predicted significantly the GRs observed (Figure 7A; Table 2).
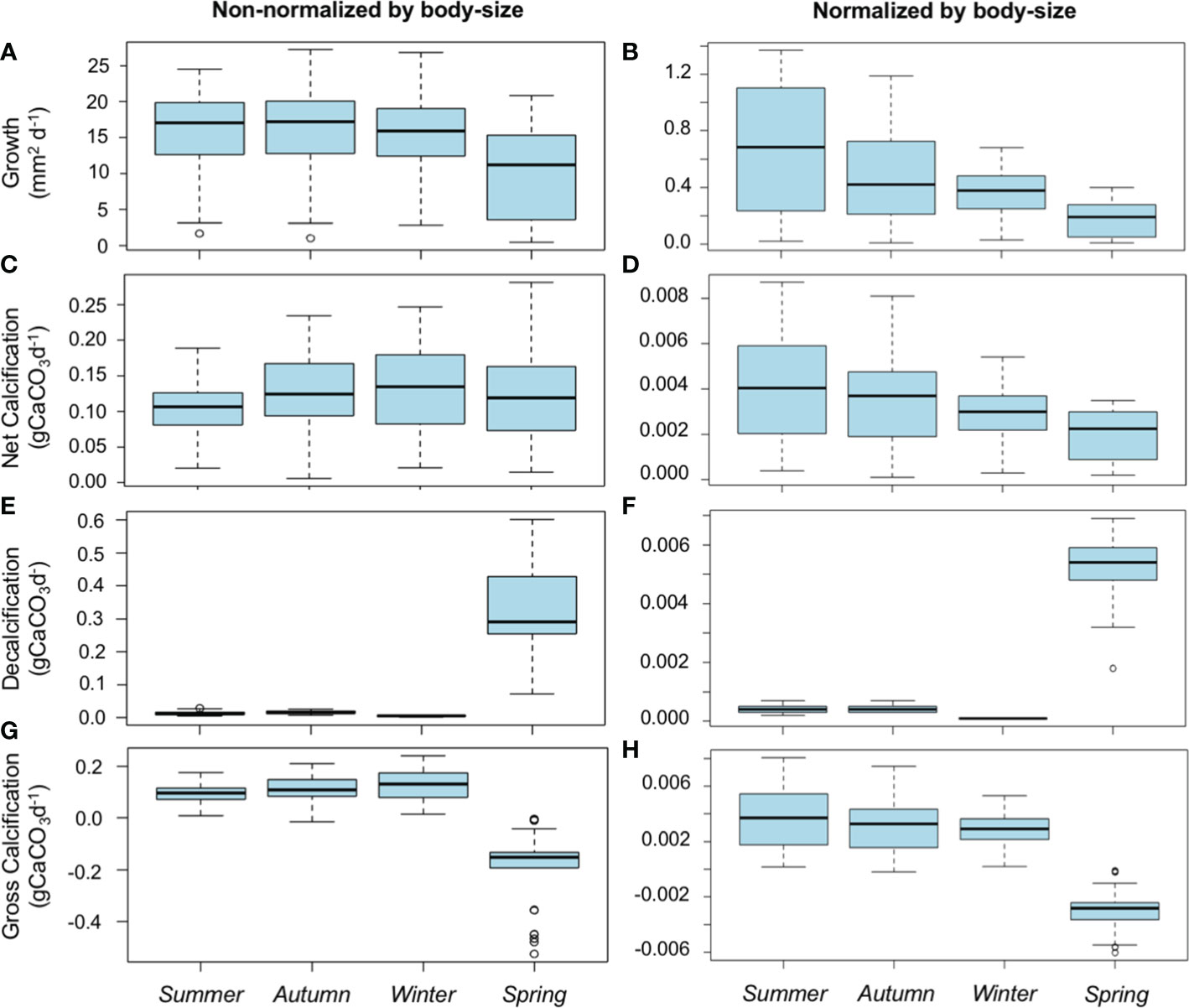
Figure 6 A. purpuratus physiological rates. Average non-normalized and normalized body-size growth (A, B), net calcification (C, D), decalcification (E, F), and gross calcification rates (G, H) were recorded during the four seasonal experiments. Data shown are mean ± SE.
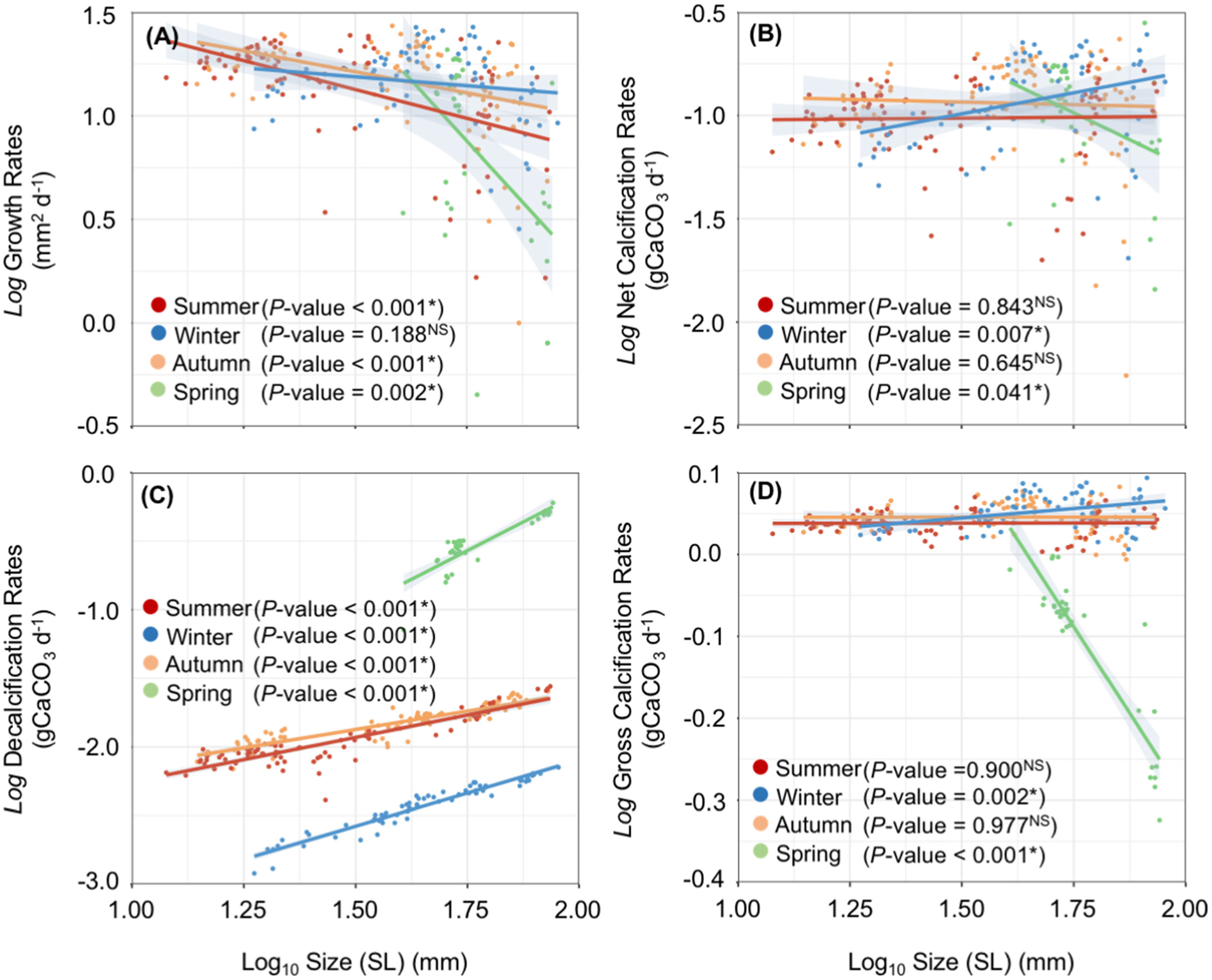
Figure 7 Size-related physiological performance. Growth (A), net calcification(B), decalcification (C), and gross calcification rates (D) rates by shell maximum large (SL) were recorded during the four seasonal experiments. Regression P-values are shown for each season and physiological trait. * = P-value< 0.05; NS = Not significant.
Both average NCR (non-normalized and normalized body-size) showed significant differences among the different seasonal experiments (Kruskal-Wallis’s test: χ2 = 13.17, P-value = 0.004, and χ2 = 29.77, P-value = 0.000, respectively). Non-normalized body-size NCRs did not show a clear pattern along the year-round experiment showing similar values during the autumn (Dunn test: P-value = 1.000), winter (Dunn’s test: P-value = 1.000), and spring (Dunn’s test: P-value = 1.000) However, non-normalized body-size NCRs were significantly lower in summer in comparison with autumn (Dunn’s test: P-value = 0.011), and winter (Dunn’s test: P-value = 0.0120) (see Figure 6C). On the other hand, normalized body-size NCRs showed a clear pattern with decreasing values from summer to spring (Figure 6D), presenting significant lower values during spring in comparison with autumn (Dunn’s test: P-value< 0.001) and winter (Dunn’s test: P-value< 0.001). Normalized body-size NCRs were also higher during summer in comparison with winter (Dunn’s test: P-value = 0.016). No significant differences were found between autumn and summer (Dunn test: P-value = 1.000) and winter (Dunn’s test: P-value = 0.330), as well as between winter and spring (Dunn’s test: P-value = 0.054) (see Figure 6D).
Linear mixed models controlled for the random effects of individuals confirmed that both seasons (i.e., environmental conditions occurring at each seasonal experiment) and size factors predicted significantly the NCRs observed (Size x Season; P-value= 0.002) (Figure 7; Table 2).
DRs in alive scallops (non-body-size and normalized body-size) showed significant differences among the seasonal experiments (Kruskal-Wallis’s test: χ2 = 211.58, P-value = 0.000, and χ2 = 211.11, P-value = 0.000, respectively), being significantly higher during the spring (see Figures 6E, F). Linear mixed models controlled for the random effects of individuals confirmed that the interaction between size and season factors predicted significantly the DRs observed (P-value< 0.011) (see Figure 7; Table 2).
Both, non- and normalized body-size GCRs were significantly different among the different seasonal experiments (Kruskal-Wallis’s test: χ2 = 102.07, P-value = 0.000, and χ2 = 93.96, P-value = 0.000, respectively). Spring season showed the lowest GCRs in comparison with summer, autumn, and winter seasons in both non-normalized (Dunn test: P-value = 0.000) and normalized body-size GCRs (Dunn`s test: P-value = 0.000) (Figures 6G, H). GCRs (non-normalized and normalized body-size) did not show significant differences between the autumn, winter, and summer seasons (Dunn`s tests: P-value< 0.05 for all combinations). Linear mixed models controlled for the random effects of individuals confirmed that size (P-value< 0.001), season (i.e., the environmental conditions occurring during each seasonal experiment) (P-value = 0.023), and the interaction between size and season factors (P-value = 0.001) predicted significantly the GCRs observed (Figure 7; Table 2).
3.3. Mortality
Higher mortality rates were found during the spring and summer seasons which corresponded to the 57% and 35% of initial experimental scallops used for each seasonal experiment, respectively. On average, dead scallops in summer and spring seasons mortalities occurred in those organisms with larger sizes (88.65 and 92.97 mm maximum shell length, respectively) (see Figure 8A). However, mortality rates (Poisson regression: χ2 = 4.3825, P-value = 0.223) and size of the dead scallops (Kruskal-Wallis test: χ2 = 7.6931, P-value = 0.053) did not show any significant difference among the seasonal experiments (Figure 8B).
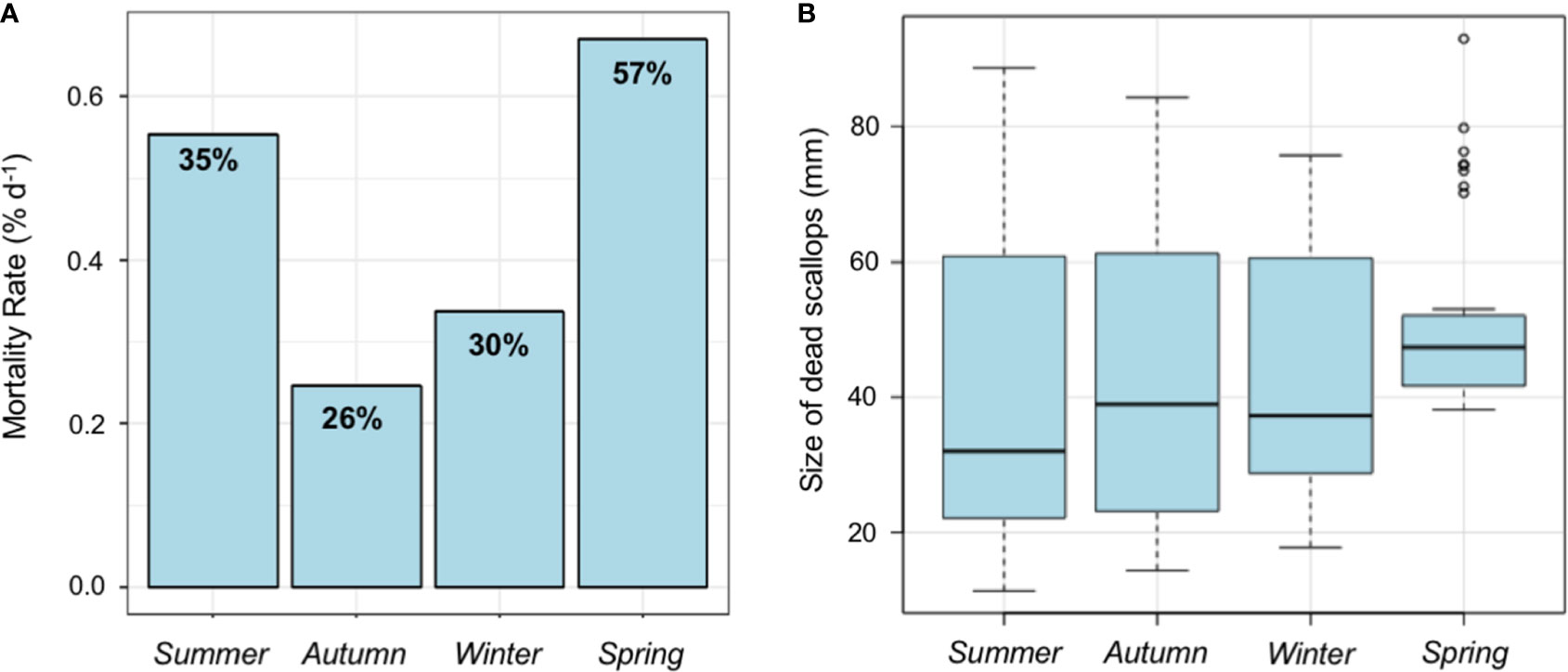
Figure 8 Mortality. (A) Mortality rates (% d−1) and percentage of dead scallops recorded, and (B) size of dead scallop A. purpuratus recorded at the end of each seasonal experiment.
3.4. Interaction between environmental conditions and scallop physiology
High collinearity between environmental conditions recorded at each seasonal experiment and physiological A. purpuratus performance was corroborated by the RDA analyses (Figure 9A). The two main components explained, in total, more than 99% of the observed variance (89.8% of Component RDA1 and 9.96% of Component RDA2). Ekman transport, pH, DO and temperature were all highly correlated (RDA2), while Chl-a showed a higher load along the RDA1 axis, and thus, a lower correlation with the variables previously mentioned (Figure 9A).
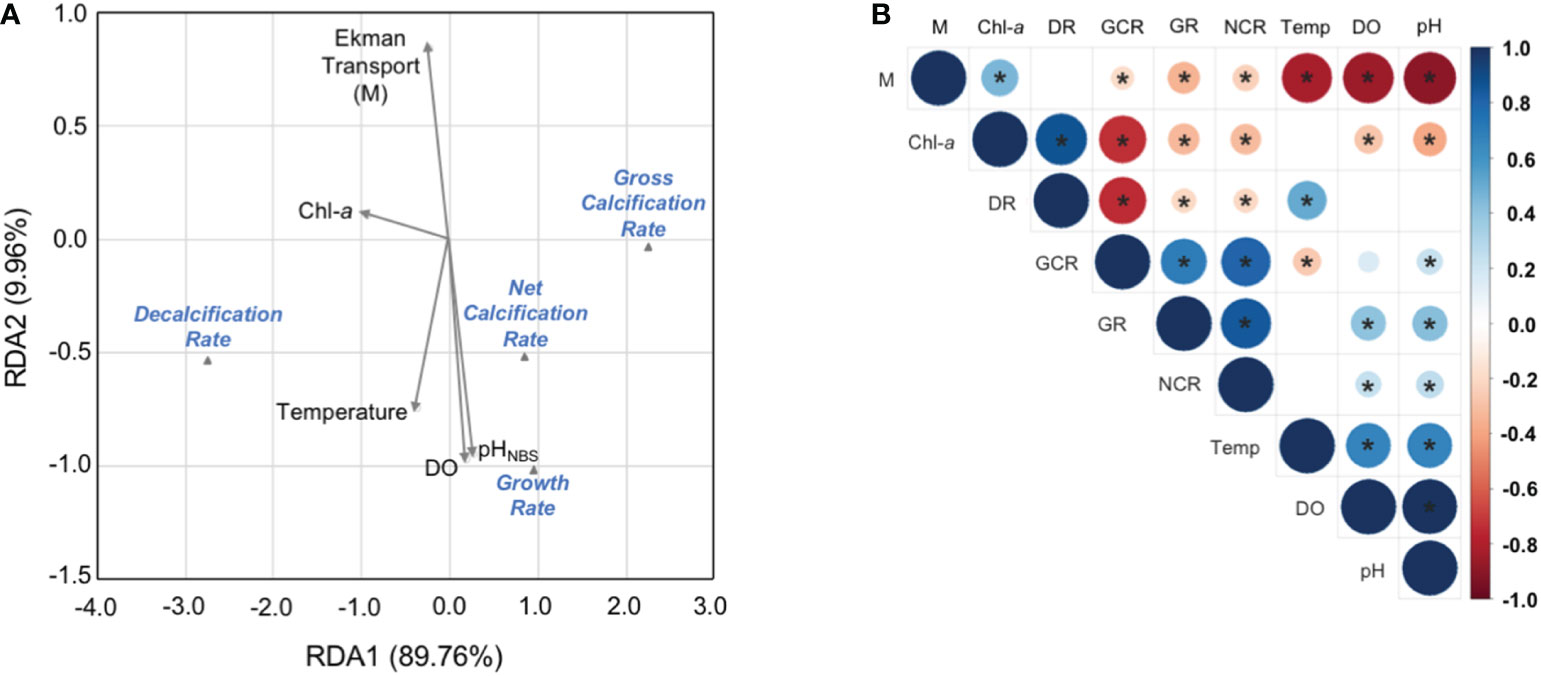
Figure 9 Environment and physiology. (A) Correlation matrix of environmental variables of Tongoy Bay with A. purpuratus physiological rates. Significance values at a = 0.05 are shown by asterisks (P-value< 0.05). (B) Redundancy analysis (RDA) for environmental variables (i.e. Ekman transport, temperature, DO, and pHNBS) with A. purpuratus physiological rates (i.e. GRs, NCRs, DCRs, and GCRs).
In particular, strong negative and significant correlations were found between Ekman transport and the temperature, DO, and pH levels of Tongoy Bay. On the other hand, pH and DO showed stronger positive and significant correlations. Weak correlation values were observed (positive and significant) between temperature and pH and DO. Chl-a showed a positive and significant correlation with Ekman transport, as well as a negative and significant correlation with DO and pH values.
Related to physiological responses, GRs showed a positive and significant correlation with NCR and GCRs, and a negative and significant correlation with DRs. NCRs showed a negative and significant correlation with DRs, and a positive and significant correlation with GCRs. GCRs and DCs showed a significant and strong negative correlation
Finally, strong correlations were found between the environmental variables (Ekman transport, temperature, DO, pH, and Chl-a) and A. purpuratus physiological responses (GRs, NCRs, DCs, and GCRs) (Figure 9B). GRs and NCRs showed significant positive correlations with DO and pH, while this correlation was negative with Ekman transport and Chl-a, and null with temperature. DRs showed significant and positive correlations with temperature and Chl-a values, however, no significant correlations were observed between DRs with DO, pH, and Ekman transport. GCRs showed a negative significant correlation with Ekman transport, temperature, and Chl-a values, and positive with pH values.
4. Discussion
Marine environmental conditions are modulated by remote and local drivers that alter, at multiple spatiotemporal scales, several physical, chemical, biological and socio-ecological processes (e.g., Bakun et al., 2015; Sprogis et al., 2018). Environmental fluctuations have important effects on the physiological performance of marine organisms with significant consequences on the ecosystem structure and functioning, as well as, on the state of the socio-ecological systems (SES) related to fisheries or aquaculture (Sprogis et al., 2018; Bertrand et al., 2020). Our study shows the major role of upwelling shaping the annual physiological performance and fitness of the scallop A. purpuratus, one of the most important resources subjected to fishery and aquaculture along the Humboldt Current System.
Tongoy Bay, in central-north Chile, is the main location where the scallop A. purpuratus is cultured in Chile. This area is permanently affected by changes in seawater temperature, dissolved oxygen, pH, and primary productivity as a consequence of the upwelling transport forced by coastal wind patterns (e.g., Ramajo et al., 2020). The magnitude of environmental changes is modulated by the seasonal and intra-seasonal variability of the local atmospheric forcing controlling the phenological upwelling pattern (i.e., number, intensity, and duration of upwelling, relaxation, and downwelling events). Seasonal differences in upwelling phenology (here measured as changes in temperature, oxygen pH, and primary productivity, but also as the number, duration, and intensity of cooling and de-oxygenation events) affected the physiological performance and fitness of the different A. purpuratus life-stages tested. Spring and summer experimental periods showed higher intensities of cooling and deoxygenation events which, on average, produced lower growth and gross calcification rates (higher decalcification rates), especially in A. purpuratus adults. On the other hand, although higher mortalities were recorded under more intense upwelling periods (spring and summer), environmental conditions driven by upwelling did not significantly affect the A. purpuratus survivorship confirming the high physiological flexibility and well-locally-adaptation of this species to the fluctuating and stressful environmental conditions imposed by upwelling (Lagos et al., 2016; Ramajo et al., 2016; Ramajo et al., 2020).
It is known that upwelling, forced by the alongshore southerly favourable-winds, provokes a replacement of surface seawater by deeper water masses (Chapman, 1996; Bravo et al., 2016) which are colder, richer in nutrients and pCO2 (low pH), and poor in oxygen concentrations (Huyer, 1983; Garcia-Reyes et al., 2015, Ramajo et al., 2020; Kämpf and Chapman, 2016). Alongshore upwelling-favourable winds at PLV (nearest upwelling center to Tongoy Bay) had significant effects on Tongoy Bay’s environmental and biogeochemical conditions during the experiment, where the highest strength of upwelling (i.e., more negative Ekman transport values) occurred during the winter and spring months. Environmental conditions recorded during 2019-2020 partially agrees with previous studies that show year-round upwelling activity, peaking during the spring season at 30°S (Strub et al., 1998; Torres et al., 1999; Rutllant and Montecino, 2002; Torres and Ampuero, 2009; Aravena et al., 2014; Rahn and Garreaud, 2014). However, climate change is forcing shifts in major atmospheric high-pressure Hadley cells that trigger an intensification and a change of the intraseasonal variability of the alongshore favourable-upwelling winds (Lu et al., 2007; Rykaczewski et al., 2015; Schneider et al., 2017) particularly, in the poleward part of the Humbolt Current System (e.g., Aguirre et al., 2018; Aguirre et al., 2021; Belmadani et al., 2014) which may be explaining why intense upwelling events occurred during the winter season.
Major Ekman transport values (M) were significantly correlated with lower seawater temperatures, DO and pH values, and higher Chl-a concentrations similar to those recently observed at Tongoy Bay by Ramajo et al. (2020). Annual seawater temperature pattern followed the solar radiation annual cycle with higher mean values recorded in summer and spring, and lower in winter months. However, the summer and spring months presented higher temperature variability with values ranging between 12-13°C to 18°C. Higher temperature values can be explained by the higher solar radiation occurring during the summer and spring months, while lower temperatures can be attributed to the higher cooling rates associated with upwelling events of higher intensity observed at Tongoy Bay. The lowest dissolved oxygen and pH average values were recorded in the winter months when Ekman transport values were higher. Winter and spring months showed a high number of de-oxygenation events with higher duration and intensity. Moreover, the higher speed of loss of dissolved oxygen in the water column (i.e., upwelling rate) recorded in the winter and spring seasons may be explaining the higher variability in oxygen conditions. On average, seasonal pH values were also highly correlated with higher Ekman transport values. This agrees with previous studies that determine that multiple processes such as organic matter remineralization, high oxygen consumption, and dissolved inorganic carbon (DIC) production occur at deeper depths making upwelled waters poor in oxygen and rich pCO2 concentrations (low pH) (Umasangaji et al., 2021). Average seasonal surface Chl-a at Tongoy Bay also responded to the radiation and temperature annual cycle (highest values recorded in summer and spring seasons). However, during the winter months, when stronger upwelling-favourable winds were recorded at PLV, a peak of Chl-a (with values ranging between 0.6 and 18.6 mg m-3) was registered. This agrees with several studies across different upwelling systems showing how nearshore productivity is coupled to local upwelling conditions (e.g., Daneri et al., 2012; Du and Peterson, 2014; Aguirre et al., 2021).
Upwelling generates important coastal environmental fluctuations at local and larger scales as a consequence of deep upwelled waters properties (i.e., colder, hypoxic-anoxic, acidic) which, in many cases, can cause physiological constraints for marine biota. Paradoxically, these areas highlight by their high productivity, high biodiversity, and significant socio-economical contributions to humans (Carr et al., 2002; Chavez and Messié, 2009; Yáñez et al., 2017). In particular, the Humboldt Current System is recognized as one of the most productive areas on a global scale (300 gC/m2 per year, Kämpf and Chapman, 2016) holding one of the highest fisheries worldwide (Chavez et al., 2008). Only in Chile in 2018, 3.7 million tons of fish (including mollusks and crustaceans) were captured with a value of USD 11,544.1 million being aquaculture responsible for 91% of this value (OECD, 2021). In particular, scallop aquaculture in Chile is an important socioeconomic activity whose production reached 7,600 tones in 2018 (SERNAPESCA, 2019) being exported to multiple international target markets (e.g. Spain, France, Singapore, and Brazil), and contributing to more than US$5.5 million to the Chilean’s export basket (Yáñez et al., 2017). However, to date, the culture of A. purpuratus in Chile is completely carried out in the natural environment being highly vulnerable to environmental fluctuations and climate change impact-drivers.
Our study show how A. purpuratus physiology was significantly altered by seasonal environmental conditions which were modulated by the presence and intensity of favourable-upwelling winds. The lowest A. purpuratus performance (lowest GRs and GCRs) occurred during the spring season when upwelling generated an elevated number and intense cooling and de-oxygenation events resulting in rapid losses of temperature and oxygen in the water column. Environment imposes biological constraints (e.g., major energy requirement) which are especially notorious when habitat conditions reach values near (or out) of the physiological tolerance ranges of species (Pörtner, 2008). In particular, cold stress has significant consequences from molecular to major physiological scales affecting cellular processes, development, metabolism, growth, and swimming capacities, among others (Pernet et al., 2007; Boroda et al., 2020). In addition, oxygen levels below physiological thresholds impact the performance, fitness, and behavior of the majority of marine taxa (e.g., Vaquer-Sunyer and Duarte, 2008). Previous studies on A. purpuratus show higher survivorship and growth under higher temperatures (Arntz et al., 2006) in agreement with Waller (1969) who described this species as warmed-adapted due to its tropical/sub-tropical origin. Also, more recent studies show how A. purpuratus is also seriously affected by hypoxia (e.g., lower growth and net calcification rates), especially when low oxygen conditions are also accompanied by changes in temperature or pH conditions (i.e., see Aguirre-Velarde et al., 2016; Ramajo et al., 2019; Ramajo et al., 2020). Indeed, multiple studies warn that low pH values are physiologically stressful for the majority of calcifying biota (Kroeker et al., 2013) affecting particularly calcification and metabolic rates, shell growth, and larval development among others (Kroeker et al., 2013). However, A. purpuratus living at Tongoy Bay seems to be highly adapted to acidified conditions (i.e., higher growth and calcification rates, see Ramajo et al., 2016) which have been attributed to acclimatization and local adaptation processes (Ramajo et al., 2016; Ramajo et al., 2019). Still, the magnitude and direction of changes in physiological responses to pH fluctuations depend greatly on temperature, oxygen, or food availability conditions (Ramajo et al., 2016; Lagos et al., 2016; Ramajo et al., 2019; Ramajo et al., 2020) which could explain the lowest GCRs observed during spring months when pH did not show the lowest values of the year, but oxygen and temperature showed important reductions.
Nevertheless, A. purpuratus was not only exposed to the most intense cooling and de-oxygenation events during the spring season but also to the most rapid reductions in temperature (cooling rate) and oxygen concentrations (de-oxygenation rate). Certainly, this had serious consequences on the physiological performance of this species (lowest GR and GCRs, and higher DRCs). Coping responses to physiological stress in marine organisms can take many shapes as they depend on the direction, duration, magnitude, and velocity of the environmental changes (Kristensen et al., 2020). In particular, the velocity of environmental changes is extremely relevant from the point of view of evolutionary processes and the expression of biological mechanisms, but also in the current context of climate change where unprecedented rapid changes are occurring in all world oceans (i.e., climate velocities, e.g., Brito-Morales et al., 2018).
Lower physiological performance during spring can be also explained by the annual environmental cycle as a continuous decrease in temperature, oxygen, and pH occurring from summer to winter, where winter also showed the lowest average temperatures, and oxygen and pH values. Continuous environmental changes and previous stressful conditions may have affected the total A. purpuratus energetic budget, and thus the ability to express physiological mechanisms to cope with rapid changes in temperature and oxygen during spring. Fluctuating and stressful environmental conditions foster the expression of biological mechanisms that require high consumption of energy (Hendriks et al., 2015; Ramajo et al., 2020) which also promote the energetic requirements re-allocation and the apparition of physiological trade-offs, lower physiological performance, and decreased fitness (e.g., Pan et al., 2015). This may be explaining the steady decay in GRs, NCRs and GCRs observed during the experiment. On the other hand, food supply could also explain why A. purpuratus fitness was not affected during the summer and spring months, as both seasons also presented the highest food availability conditions (i.e., higher Chl-a concentrations). Previous studies have observed and suggested that A. purpuratus can increase its feeding rates under low pH (Ramajo et al., 2016) and after intense upwelling events to recover from physiological stressful conditions (see Ramajo et al., 2020).
Energetic demands for maintenance and reproduction change across sizes and ages (Kooijman, 2000). Thus, it can be expected that not all life-stages cope equally with environmental fluctuations as different life-stages present different necessities in terms of food, oxygen availability, and calcium carbonate supply (Baumann et al., 2012). Our study shows how seasonal physiological responses of A. purpuratus were significantly modulated by the size (age) (Table 1), especially when environmental conditions pose a physiologically stressful scenario with rapid cooling and deoxygenation/corrosive rates (i.e., Spring season, see Figure 7). Size seems to be a key factor explaining the thermal tolerances of A. purpuratus which may explain different results among previous studies. Meanwhile, Arntz et al. (2006) and Lagos et al. (2016) studies detected a higher tolerance of A. purpuratus under higher than lower temperatures, Ramajo et al. (2019) concluded that A. purpuratus seems to be a species better adapted to cold than warmer conditions. These differences among studies may be attributed to differences in the life-stages tested as Arntz et al. (2006) and Lagos et al. (2016) studies were performed on larvae or adult organisms, while Ramajo et al. (2019) experiment was accomplished on juveniles. A similar physiological pattern has been observed to compare the physiological performance of juveniles and adults to changes in pH (see Ramajo et al., 2016; Lagos et al., 2016). Unfortunately, to date, no studies addressing the oxygen availability impact among different A. purpuratus life-stages have been yet performed.
The lower physiological performance observed in A. purpuratus adult stages as a consequence of more intense upwelling conditions occurring during the spring season may have additional impacts on aquaculture production as the scallop industry in Tongoy Bay mainly relies on natural larvae captured from the natural environment. Although A. purpuratus gametes release can occur throughout the year, this is more active in the austral spring and summer seasons when higher food availability (Chl-a concentrations) is present due to nutrients supplied by upwelling (Avendaño et al., 2008). However, unusual intense upwelling events and related stressful conditions could have important impacts on the reproductive A. purpuratus conditions (i.e., gamete release, spawning time, as well as egg production and larval survivorship). Multiple studies in bivalve species show how temperature, pH, and oxygen conditions modulate multiple reproductive adult traits including larvae availability and their survivorship (i.e., Kroeker et al., 2013; Breitburg et al., 2018). In particular, for A. purpuratus, studies performed by Cantillánez et al. (2005, 2006, 2008) and Avendaño et al. (2005) show how dates of spawning events occur under higher water temperatures and food availability conditions. Here, although A. purpuratus reproductive-related traits were not addressed, our results (i.e., lower performance in older scallops) suggest that intense cooling and deoxygenation/corrosive conditions driven by intense upwelling events may constrain reproductive A. purpuratus cycle due to growth-maintenance-reproduction trade-offs which are more evident in older stages as energetic costs are size and volume-dependent (see the introduction for references).
In brief, our study concludes that physiological sensitivity to isolated environmental variables is not able to entirely explain the seasonal physiological performance of A. purpuratus. Upwelling imposes differential physiological pressures across A. purpuratus life-stages, where the combination of intensity and the velocity of the changes, as well as the food availability, modulated the performance and the energy available to cope with uninterrupted (and sometimes stressful) environmental conditions. This highlight the necessity of considering marine upwelling habitats as highly complex and heterogenous environmental entities where fluctuations ranges (and not only average values), the speed of changes, and the nature of the interaction (antagonist, synergistic, additive) among environmental variables need to be considered to make better projections about the future of fisheries and shell marine aquaculture (SMA) developed in EBUS (Henson et al., 2017; Kristensen et al., 2020). In particular, this is highly relevant along the Humboldt Current System, as the coastal invertebrates’ catches have shown sustained growth since 1950, and specifically, SMA is a growing productive activity with an elevated socio-economic impact on the region and worldwide (FAO 2005-2021; Gutiérrez et al., 2017). However, to date, understanding how SMA in this region will cope with future climate conditions driven by changes in upwelling, ocean acidification, or ocean deoxygenation as a consequence of climate change is not completely understood as it requires an adequate comprehension of the environmental dynamics of marine systems, as well as, better knowledge about the physiological tolerance of the species and their different life-stages to heterogeneous conditions.
Data availability statement
The raw data supporting the conclusions of this article will be made available by the authors, without undue reservation.
Author contributions
LR designed and conceived the study. CS-H, MV, JI, and LR conducted the experiment. LR, MV, and JI registered and measured the environmental data. MV and OA provided wind data and upwelling index estimations. LR, CS-H, and MV analyzed the data. LR, CS-H, and MV prepared the manuscript. All authors contributed to the article and approved the submitted version.
Funding
This study was funded by FONDECYT Project #3170156 to LR. LR and CS-H acknowledge the support from the “Center for the study of multiple drivers on marine socio-ecological systems (MUSELS) from the Ministerio de Economía, Fomento y Turismo. OA acknowledges the support from FONDECYT project #11190999. LR, MV, OA, and JI acknowledge the support from ANID-CENTROS REGIONALES R20F0008 (CEAZA). LR acknowledges the support from FONDAP/ANID 15110009, and grants PID2020-116660GB-I00 (Ministerio de Ciencia e Innovación, Spain), grupo RNM-938, B-RNM-265-UGR18 and P20_00207 (Junta de Andalucía, Spain).
Acknowledgments
We are very grateful to Julia Godoy, Pamela Tapia, Christian Tapia from OSTIMAR S.A., and Tongoy local school (Liceo Carmen Rodríguez Henriquez) for their support during the experiments. We thank Carolina Fernández, Paul Watt-Arévalo, Manuel Núñez, Marco A. Lardies, Bernardo Broitman, William Farias, and Fernanda Oyarzún for their support and help during the study. Finally, we want to acknowledge Cristian Duarte and Loretto Contreras for their valuable comments and suggestions while the research was conducted. Scientific illustrations in the figures are courtesy of the Integration and Application Network (http://ian.umces.edu/symbols), University of Maryland Center for Environmental Science.
Conflict of interest
The authors declare that the research was conducted in the absence of any commercial or financial relationships that could be construed as a potential conflict of interest.
Publisher’s note
All claims expressed in this article are solely those of the authors and do not necessarily represent those of their affiliated organizations, or those of the publisher, the editors and the reviewers. Any product that may be evaluated in this article, or claim that may be made by its manufacturer, is not guaranteed or endorsed by the publisher.
Supplementary material
The Supplementary Material for this article can be found online at: https://www.frontiersin.org/articles/10.3389/fmars.2022.992319/full#supplementary-material
References
Aguirre C., García-Loyola S., Testa G., Silva D., Farias L. (2018). Insight into anthropogenic forcing on coastal upwelling off south-central Chile. Elem. Sci. Anth. 6 (1), 59. doi: 10.1525/elementa.314
Aguirre-Velarde A., Jean F., Thouzeau G., Flye-Sainte-Marie J. (2016). Effects of progressive hypoxia on oxygen uptake in juveniles of the Peruvian scallop, Argopecten purpuratus (Lamarck 1819). Aquaculture 451, 385–389. doi: 10.1016/j.aquaculture.2015.07.030
Aguirre C., Garreaud R., Belmar L., Farías L., Ramajo L., Barrera F. (2021). High-Frequency Variability of the Surface Ocean Properties Off Central Chile During the Upwelling Season. Front. Mar. Sci. doi: 10.3389/fmars.2021.702051
Aravena G., Broitman B., Stenseth N. C. (2014). Twelve years of change in coastal upwelling along the central-northern coast of Chile: spatially heterogeneous responses to climatic variability. PloS One 9 (2), e90276. doi: 10.1371/journal.pone.0090276
Arntz W. E., Gallardo V. A., Gutiérrez D., Isla E., Levin L. A., Mendo J., et al (2006). El Niño and similar perturbation effects on the benthos of the Humboldt, California, and Benguela Current upwelling ecosystems. Adv. Geosci. 6, 243–265. doi: 10.5194/adgeo-6-243-2006
Avendaño M., Cantillánez M. (2022). Argopecten purpuratus (Mollusca, pectinidae) post-El niño 1997-98 response in la rinconada marine reserve (Antofagasta, Chile). Lat. Am. J. Aquat. Res. 50 (2), 168–180. doi: 10.3856/vol50-issue2-fulltext-2776
Avendaño M., Cantillánez M., Le Pennec M., Thouzeau G. (2008). Reproductive and larval cycle of the scallop argopecten purpuratus (Ostreoida: Pectinidae), during El niño-la niña events and normal weather conditions in antofagasta, Chile. Rev. Biología Trop. 56 (1), 121–132.
Avendaño M., Cantillánez M., Peña J. (2006). Effect of immersion time of culthch on spatfall of the scallop argopecten purpuratus (Lamarck 1819) in the marine reserve at la rinconada, antofagasta, Chile. Aquacul. Int. 14, 267–283. doi: 10.1007/s10499-005-9033-y
Avendaño M., Cantillánez M., Rodríguez L., Zúñiga O., Escribano R., Oliva M. (2005). Crecimiento y estructura demográfica de Argopecten purpuratus en la reserva marina La Rinconada (Antofagasta, Chile: Ciencias marina). 31 (3), 491–503.
Bakun A., Black B. A., Bograd S. J., García-Reyes M., Miller A. J., Rykaczewki R. R., et al (2015). Anticipated effects of climate change on coastal upwelling ecosystems. Curr. Clim. Change Rep. 1, 85–93. doi: 10.1007/s40641-015-0008-4
Bakun A. (1975). Daily and weekly upwelling indices, west coast of North Americ-73 (U.S: Department of Commerce), 114. Available at: https://repository.library.noaa.gov/view/noaa/15387.
Basilio C. D., Cañete J. I., Rozbaczylo N. (1995). Polydora sp. (Spionidae), un poliqueto perforador de las valvas del ostión argopecten purpuratus (Bivalvia: Pectinidae) en bahía tongoy, Chile. Rev. Biología Marina 30, 71–77. doi: 10.4067/S0718-19572001000100009
Baumann H., Talmage S. C., Gobler J. (2012). Reduced early life growth and survival in a fish in direct response to increased carbon dioxide. Nat. Clim. Change 2, 38–41. doi: 10.1038/nclimate1291
Bayne B. L., Newell R. C. (1983). Physiological Energetics of Marine Molluscs. Eds Saleuddin A. S. M, Wilbur K. M. B. T.-T. M. (Academic press), 407–515. doi: 10.1016/B978-0-12-751404-8.50017-7
Belmadani A., Echevin V., Codron F., Takahashi K., Junquas C. (2014). What dynamics drive future wind scenarios for coastal upwelling off Peru and Chile? Clim. Dyn. 43, 1893–1914. doi: 10.1007/s00382-013-2015-2
Bertrand A., Lengaigne M., Takahashi K., Avadi A., Poulain F., Harrod C (2020). El Niño southern oscillation (ENSO) effects on fisheries and aquaculture. FAO fisheries and aquaculture technical paper no. 660 (Rome: FAO). doi: 10.4060/ca8348en
Boroda A. V., Kipryushina Y. O., Odintsova N. A. (2020). The effects of cold stress on mytilus species in the natural environment. Cell Stress Chaperones 25 (6), 821–832. doi: 10.1007/s12192-020-01109-w
Bravo L., Ramos M., Astudillo O., Dewitte B., Goubanova K. (2016). Seasonal variability of the ekman transport and pumping in the upwelling system off central-northern Chile (∼ 30° s) based on a high-resolution atmospheric regional model (WRF). Ocean Sci. 12 (5), 1049–1065. doi: 10.5194/os-12-1049-2016
Breitburg D., Levin L. A., Oschlies A., Grégoire M., Chaves F. P., Conley D. J., et al (2018). Declining oxygen in the global ocean and coastal waters. Science 359 (6371), eaam7240. doi: 10.1126/science.aam7240
Brito-Morales I., García-Molinos J., Schoeman D. S., Burrows M. T., Poloczanska E. S., Brown C. J., et al (2018). Climate Velocity Can Inform Conservation in a Warming World. Trends Ecol. Evol. 33 (6), 441–457. doi: 10.1016/j.tree.2018.03.009
Byrne M. (2012). Global change ecotoxicology: Identification of early life history bottlenecks in marine invertebrates, variable species responses and variable experimental approaches. Mar. Environ. Res 76, 3–15. doi: 10.1016/j.marenvres.2011.10.004
Cantillánez M., Avendaño M., Thouzeau G., Le Pennec M. (2005). Reproductive cycle of argopecten purpuratus (Bivalvia: Pectinidae) in la rinconada marine reserve, (Antofagasta, chile): response to environmental effects of El niño and la niña. Aquaculture 246, 181–195. doi: 10.1016/j.aquaculture.2004.12.031
Carr M.-E., Strub P. T., Thomas A. C., Blanco J. L. (2002). Evolutions of the 1996–1999 la niña and El niño conditions off the western coast of south America: a remote sensing perspective. J. Geophys. Res. 108 (C12), 3236. doi: 10.1029/2001JC001183
Chapman P. (1996). Upwelling in the ocean: Modern processes and ancient records. Limnology Oceanography 41. doi: 10.4319/lo.1996.41.7.1585
Chauvaud L., Patry Y., Jolivet A., Cam E., Le Goff C., Strand Ø., et al (2012). Variation in size and growth of the great scallop pecten maximus along a latitudinal gradient. PloS One 7 (5), e37717. doi: 10.1371/journal.pone.0037717
Chavez F. P., Messié M. (2009). A comparison of eastern boundary upwelling ecosystems. Prog. Oceanogr. 83, 80–96. doi: 10.1016/j.pocean.2009.07.032
Chavez F. P., Bertrand A., Guevara-Carrasco R., Soler P., Csirke J. (2008). The Northern Humboldt Current System: ocean dynamics, ecosystem processes, and fisheries. Prog. Oceanogr 79, 95–105. doi: 10.1016/j.pocean.2008.10.012
Clark M. S., Peck L. S., Arivalagan J., Backeljau T., Berland S., Cardoso J. C., et al (2020). Deciphering mollusc shell production: the roles of genetic mechanisms through to ecology, aquaculture and biomimetics. Biol. Rev. 95 (6), 1812–1837. doi: 10.1111/brv.12640
Clark H. R., Gobler C. J. (2016). Diurnal fluctuations in CO2 and dissolved oxygen concentrations do not provide a refuge from hypoxia and acidification for early-life-stage bivalves. Mar. Ecol. Prog. Ser. 558, 1–14. doi: 10.3354/meps11852
Cury P., Roy C. (1989). Optimal environmental window and pelagic fish recruitment success in upwelling areas. Can. J. Fish. Aquat. Sci. 46 (4), 670–680. doi: 10.1139/f89-086
Daneri G., Lizárraga L., Montero P., González H. E., Tapia ,. F. J. (2012). Wind forcing and short-term variability of phytoplankton and heterotrophic bacterioplankton in the coastal zone of the Concepción upwelling system (Central Chile). Prog. Oceanography 92–95, 92–96. doi: 10.1016/j.pocean.2011.07.013
Davies P. S. (1989). Short-term growth measurements of corals using an accurate buoyant weighing technique. Mar. Biol. 101, 389–395. doi: 10.1007/BF00428135
Du X., Peterson W. T. (2014). Seasonal Cycle of Phytoplankton Community Composition in the Coastal Upwelling System Off Central Oregon in 2009. Estuaries Coasts 37, 299–311. doi: 10.1007/s12237-013-9679-z
Falvey M., Garreaud R. D. (2009). Regional cooling in a warming world: Recent temperature trends in the southeast pacific and along the west coast of subtropical south america, (1979–2006). J. Geophys. Res.: Atmospheres 114 (D4), D04102. doi: 10.1029/2008JD010519
FAO (2005-2021). “World inventory of fisheries. Subsidies and trade distortion. Issues Fact Sheets,” in Text by Audun Lem (Rome: FAO Fisheries Division [online]).
Figueroa D., Moffat C. (2000). On the influence of topography in the induction of coastal upwelling along the Chilean coast. Geophys. Res. Lett. 27 (23), 3905–3908. doi: 10.1029/1999GL011302
García-Reyes M., Largier J. L., Sydeman W. J. (2014). Synoptic-scale upwelling indices and predictions of phyto- and zooplankton populations. Prog. Oceanogr. 120, 177–188. doi: 10.1016/j.pocean.2013.08.004
García-Reyes M., Sydeman W. J., Schoeman D. S., Rykaczewski R. R., Black B. A., Smit A. J., et al (2015). Under Pressure: Climate Change, Upwelling, and Eastern Boundary Upwelling Ecosystems. Front. Mar. Sci. doi: 10.3389/fmars.2015.00109
Garreaud R., Muñoz R. C. (2005). The low-level jet off the west coast of subtropical south America: Structure and variability. Monthly Weather Rev. 133 (8), 2246–2261. doi: 10.1175/MWR2972.1
Gaylord B., Kroeker K. J., Sunday J. M., Anderson K. M., Barry J. P., Brown N. E., et al (2015). Ocean acidification through the lens of ecological theory. Ecology 96 (1), 3–15. doi: 10.1890/14-0802.1
Gazeau F., Parker L. M., Comeau S., Gattuso J. P., O’Connor W. A., Martin S., et al (2013). Impacts of ocean acidification on marine shelled molluscs. Mar. Biol. 160, 2207–2245. doi: 10.1007/s00227-013-2219-3
Gutiérrez D., Akester M., Naranjo L. (2017). Productivity and Sustainable Management of the Humboldt Current Large Marine Ecosystem under climate change. Environ. Dev. 17, 126–144. doi: 10.1016/j.envdev.2015.11.004
Hendriks I. E., Duarte C. M., Olsen Y. S., Steckbauer A., Ramajo L., Moore T. S., et al (2015). Biological mechanisms supporting adaptation to ocean acidification in coastal ecosystems. Estuarine Coast. Shelf Sci. 152, A1–A8. doi: 10.1016/j.ecss.2014.07.019
Henson S., Beaulieu C., Ilyina T., John J. G., Long M., Séférian R., et al (2017). Rapid emergence of climate change in environmental drivers of marine ecosystems. Nat. Commun. 8, 14682. doi: 10.1038/ncomms14682
Huyer A. (1983). Coastal upwelling in the California current system. Prog. Oceanogr. 12, 259–284. doi: 10.1016/0079-6611(83)90010-1
IPCC (2021). “Climate Change 2021: The Physical Science Basis. Contribution of Working Group I to the Sixth Assessment Report of the Intergovernmental Panel on Climate Change,”. Eds. Masson-Delmotte V., Zhai P., Pirani A., Connors S. L., Péan C., Berger S., et al (Cambridge, United Kingdom and New York, NY, USA: Cambridge University Press). doi: 10.1017/9781009157896
Kämpf J., Chapman P. (2016). Upwelling systems of the world. A scientific journey to the most productive marine ecosystems (Switzerland: Springer International Publishing). doi: 10.1007/978-3-319-42524-5
Kooijman S (2000). Dynamic Energy and Mass Budgets in Biological Systems (2nd ed.). Cambridge: Cambridge University Press. doi: 10.1017/CBO9780511565403
Kristensen T. N., Ketola T., Kronholm I. (2020). Adaptation to environmental stress at different timescales. Ann. N.Y. Acad. Sci. 1476, 5–22. doi: 10.1111/nyas.13974
Kroeker K. J., Kordas R. L., Crim R., Hendriks I. E., Ramajo L., Singh G. S., et al (2013). Impacts of ocean acidification on marine organisms: quantifying sensitivities and interaction with warming. Glob Change Biol 19, 1884–1896. doi: 10.1111/gcb.12179
Lagos N. A., Benítez S., Duarte C., Lardies M. A., Broitman B. R., Tapia C., et al (2016). Effects of temperature and ocean acidification on shell characteristics of argopecten purpuratus: implications for scallop aquaculture in an upwelling-influenced area. Aquacult. Environ. Interact. 8, 357–370. doi: 10.3354/aei00183
Lagos N. A., Benítez S., Grenier C., Rodriguez-Navarro A. B., García-Herrera C., Abarca-Ortega A., et al (2021). Plasticity in organic composition maintains biomechanical performance in shells of juvenile scallops exposed to altered temperature and pH conditions. Sci. Rep. 11, 24201. doi: 10.1038/s41598-021-03532-0
Langer G., Nehrke G., Baggini C., Rodolfo-Metalpa R., Hall-Spencer J. M., Bijma J. (2014). Limpets counteract ocean acidification induced shell corrosion by thickening of aragonitic shell layers. Biogeosciences 11, 7363–7368. doi: 10.5194/bg-11-7363-2014
Lardies M. A., Benitez S., Osores S., Vargas C. A., Duarte C., Lohrmann K. B., et al (2017). Physiological and histopathological impacts of increased carbon dioxide and temperature on the scallops argopecten purpuratus cultured under upwelling influences in northern Chile. Aquaculture 479, 455–466. doi: 10.1016/j.aquaculture.2017.06.008
Lluch-Cota S. E., Hoegh-Guldberg O., Karl D., Pörtner H. O., Sundby S., Gattuso J. P. (2014). “Cross-chapter box on uncertain trends in major upwelling ecosystems,” in Climate change 2014: Impacts, adaptation, and vulnerability. part a: Global and sectoral aspects. contribution of working group II to the fifth assessment report of the intergovernmental panel of climate change. Eds. Field C. B., Barros V. R., Dokken D. J., Mach K. J., Mastrandrea M. D. (Cambridge, Uk: New York, NY: Cambridge University Press), 149–151.
Lu J., Vecchi G. A., Reichler T. (2007). Expansion of the Hadley cell under global warming. Geophys. Res. Lett. 34, L06805. doi: 10.1029/2006GL028443
MacLeod C. D., Poulin R. (2015). Interactive effects of parasitic infection and ocean acidification on the calcification of a marine gastropod. Marine Ecology Progress Series. 537, 137–150. doi: 10.1016/j.ijpara.2015.02.007
Moraga-Opazo J., Valle-Levinson A., Ramos M., Pizarro-Koch M. (2011). Upwelling-triggered near-geostrophic recirculation in an equatorward facing embayment. Continental Shelf Res. 31 (19-20), 1991–1999. doi: 10.1016/j.csr.2011.10.002
OECD (2021). “The Organization for Economic Cooperation and Developmen),” in Fisheries and Aquaculture in Chile January 2021 (Paris (France: . OECD Review of Fisheries Country Notes).
Palmer A. R. (1981). Do carbonate skeletons limit the rate of body growth? Nature 292 (5819), 150–152. doi: 10.1038/292150a0
Pan T. C. F., Applebaum S. L., Manahan ,. D. T. (2015). Experimental ocean acidification alters the allocation of metabolic energy. Proc. Natl. Acad. Sci. 112 (15), 4696–4701. doi: 10.1073/pnas.1416967112
Pernet F., Tremblay R., Comeau L., Guderley H. (2007). Temperature adaptation in two bivalve species from different thermal habitats: energetics and remodelling of membrane lipids. J. Exp. Biol. 210 (17), 2999–3014. doi: 10.1242/jeb.006007
Pörtner H. (2008). Ecosystem effects of ocean acidification in times of ocean warming: a physiologist’s view. Mar. Ecol. Prog. Ser. 373, 203–217. doi: 10.3354/meps07768
Rahn D. A., Garreaud R. D. (2014). A synoptic climatology of the near-surface wind along the west coast of south America. Int. J. Climatol. 34 (3), 780–792. doi: 10.1002/joc.3724
Ramajo L., Prado L., Rodriguez-Navarro A. B., Lardies M. A., Duarte C. M., Lagos N. A. (2016). Plasticity and trade-offs in physiological traits of intertidal mussels subjected to freshwater-induced environmental variation. Mar. Ecol. Prog. Ser. 553, 93–109. doi: 10.3354/meps11764
Ramajo L., Fernández C., Núñez Y., Caballero P., Lardies M. A., Poupin M. J. (2019). Physiological responses of juvenile Chilean scallops (Argopecten purpuratus) to isolated and combined environmental drivers of coastal upwelling. ICES J. Mar. Sci. 76 (6), 1836–1849. doi: 10.1093/icesjms/fsz080
Ramajo L., Valladares M., Astudillo O., Fernández C., Rodríguez-Navarro B. A., Watt-Arévalo P., et al (2020). Upwelling intensity modulates the fitness and physiological performance of coastal species: Implications for the aquaculture of the scallop argopecten purpuratus in the Humboldt current system. Sci. Total Environ 745, 140949. doi: 10.1016/j.scitotenv.2020.140949
Renault L., Dewitte B., Falvey M., Garreaud R., Echevin V., Bonjean F. (2009). Impact of atmospheric coastal jet off central Chile on sea surface temperature from satellite observations, (2000–2007). J. Geophys. Res. 114, C08006. doi: 10.1029/2008JC005083
Rutllant J., Montecino V. (2002). Multiscale upwelling forcing cycles and biological response off north-central Chile. Rev. Chil. Hist. Natural 75 (217), e231. doi: 10.4067/S0716-078X2002000100020
Rykaczewski R. R., Dunne J. P., Sydeman W. J., García-Reyes M., Black B. A., Bograd S. J. (2015). Poleward displacement of coastal upwelling-favorable winds in the ocean’s eastern boundary currents through the 21st century. Geophys. Res. Lett. 42, 6424–6431. doi: 10.1002/2015GL064694
Schneider W., Donoso D., Garcés-Vargas J., Escribano R. (2017). Water-column cooling and sea surface salinity increase in the upwelling region off central Chile driven by a pole-ward displacement of the south pacific high. Prog. Oceanogr. 151, 38–48. doi: 10.1016/j.pocean.2016.11.004
Seibel B. A. (2011). Critical oxygen levels and metabolic suppression in oceanic oxygen minimum zones. J. Exp. Biol. 214 (2), 326–336. doi: 10.1242/jeb.049171
SERNAPESCA (2019) Desembarque total. Available at: http://www.sernapesca.cl/sites/default/files/2019_0301_desembarque_total.pdf (Accessed December 9, 2022).
Sprogis K. R., Christiansen F., Wandres M., Bejder L. (2018). El Niño southern oscillation influences the abundance and movements of a marine top predator in coastal waters. Glob Change Biol. 24, 1085–1096. doi: 10.1111/gcb.13892
Strub P. T., Shillington F. A., James C, Weeks S. J. (1998). Satellite comparison of the seasonal circulation in the Benguela and California current systems. South African Journal of Marine Science, 19 (1), 99–112. doi: 10.2989/025776198784126836
Sydeman W. J., García-Reyes M., Schoeman D. S., Rykaczewski R. R., Thompson S. A., Black B. A., et al (2014). Climate change and wind intensification in coastal upwelling ecosystems. Science 345 (6192), 77–80. doi: 10.1126/science.1251635
Talmage S. C., Gobler ,. C. J. (2010). Effects of past, present, and future ocean carbon dioxide concentrations on the growth and survival of larval shellfish. Proc. Natl. Acad. Sci. 107 (40), 17246–17251. doi: 10.1073/pnas.0913804107
Tapia F. J., Navarrete S. A., Castillo M., Menge B. A., Castilla J. C., Largier J., et al (2009). Thermal indices of upwelling effects on inner-shelf habitats. Prog. Oceanography 83, 278–287. doi: 10.1016/j.pocean.2009.07.035
Thiel M., et al (2007) The Humboldt current system of northern and central Chile. Available at: https://www.webofscience.com/wos/WOSCC/full-record/000252476600006.
Thompson R. J., MacDonald B. A. (2006). “Chapter 8 Physiological integrations and energy partitioning,” in Shumway S. E., Parsons F. S. Eds. Scallops: Biology, Ecology and Aquaculture. (Elsevier), 35, 493–520. doi: 10.1016/S0167-9309(06)80035-9
Thomsen J., Haynert K., Wegner K.M., Melzner F. (2015). Impact of seawater carbonate chemistry on the calcification of marine bivalves. Biogeosciences 12, 4209–4220. doi: 10.5194/bg-12-4209-2015
Torres R., Ampuero P. (2009). Strong CO2 outgassing from high nutrient low chlorophyll coastal waters off central Chile (30 s): The role of dissolved iron. Estuarine Coast. Shelf Sci. 83 (2), 126–132. doi: 10.1016/j.ecss.2009.02.030
Torres R., Turner D. R., Silva N., Rutllant J. (1999). High short-term variability of CO2 fluxes during an upwelling event off the Chilean coast at 30 s. Deep Sea Res. Part I: Oceanogr. Res. Papers 46 (7), 1161–1179. doi: 10.1016/S0967-0637(99)00003-5
Truebano M., Fenner P., Tills O., Rundle S. D., Rezende E. L. (2018). Thermal strategies vary with life history stage. J. Exp. Biol. 221 (8), jeb171629. doi: 10.1242/jeb.171629
Trussell G. C., Smith L. D. (2000). Induced defenses in response to an invading crab predator: an explanation of historical and geographic phenotypic change. Proc. Natl. Acad. Sci. 97 (5), 2123–2127. doi: 10.1073/pnas.040423397
Umasangaji H., Ramili Y. (2021). “Mini review: Characteristics of upwelling in several coastal areas in the world,” in IOP Conference Series: Earth and Environmental Science, Vol. 890. 012004 (IOP Publishing). doi: 10.1088/1755-1315/890/1/012004
Vaquer-Sunyer R., Duarte C. M. (2008). Thresholds of hypoxia for marine biodiversity. Proc Natl Acad Sci U S A. doi: 10.1073/pnas.0803833105
von Brand E., Abarca A., Merino G. E., Stotz W. (2016). “Scallop fishery and aquaculture in Chile: A history of developments and declines,” in Scallop, biology, ecology, aquaculture and fisheries. Eds. Shumway S. E., G. J. (Oxford: Elsevier), 1047–1072. doi: 10.1016/B978-0-444-62710-0.00026-2
Waldbusser G. G., Hales B., Langdon C. J., Haley B. A., Schrader P., Brunner E. L., et al (2015). Ocean acidification has multiple modes of action on bivalve larvae. PloS One 10 (6), e0128376. doi: 10.1371/journal.pone.0128376
Waldbusser G. G., Voigt E. P., Bergschneider H., Green M. A., Newell R. I. E. (2011). Biocalcification in the Eastern oyster (Crassostrea virginica) in relation to long-term trends in Chesapeake bay pH. Estuaries Coasts 34, 221–231. doi: 10.1007/s12237-010-9307-0
Waller T. R. (1969). The evolution of the Argopecten gibbus stock (Mollusca: Bivalvia), with emphasis on the Tertiary and Quaternary species of eastern North America. Memoir (The Paleontological Society) 3, i–125.
Wessel N., Martin S., Badou A., Dubois P., Huchette S., Julia V., et al (2018). Effect of CO2–induced ocean acidification on the early development and shell mineralization of the European abalone (Haliotis tuberculata). J. Exp. Mar. Biol. Ecol 508, 52–63. doi: 10.1016/j.jembe.2018.08.005
Yáñez E., Lagos N.A., Norambuena R., Silva C., Letelier J., Muck K.-P., et al (2017). Impacts of climate change on marine fisheries and aquaculture in Chile. Climate Change Impacts Fish. Aquacult.: A Global Anal. 1 (1), 239–342. doi: 10.1002/9781119154051.ch10
Keywords: shellfish aquaculture, Humboldt Current System, climate change, upwelling intensification, physiological impacts, ocean deoxygenation, ocean acidification, cooling
Citation: Ramajo L, Sola-Hidalgo C, Valladares M, Astudillo O and Inostroza J (2022) Size matters: Physiological sensitivity of the scallop Argopecten purpuratus to seasonal cooling and deoxygenation upwelling-driven events. Front. Mar. Sci. 9:992319. doi: 10.3389/fmars.2022.992319
Received: 12 July 2022; Accepted: 01 December 2022;
Published: 16 December 2022.
Edited by:
Hongsheng Yang, Chinese Academy of Sciences (CAS), ChinaReviewed by:
Burke Hales, Oregon State University, United StatesLiqiang Zhao, Guangdong Ocean University, China
Friedrich Buchholz, University of Hamburg, Germany
Copyright © 2022 Ramajo, Sola-Hidalgo, Valladares, Astudillo and Inostroza. This is an open-access article distributed under the terms of the Creative Commons Attribution License (CC BY). The use, distribution or reproduction in other forums is permitted, provided the original author(s) and the copyright owner(s) are credited and that the original publication in this journal is cited, in accordance with accepted academic practice. No use, distribution or reproduction is permitted which does not comply with these terms.
*Correspondence: Laura Ramajo, laura.ramajo@ceaza.cl