Revisiting the footprints of climate change in Arctic marine food webs: An assessment of knowledge gained since 2010
- 1Institute for Ecosystem Research, Kiel University, Kiel, Germany
- 2Department of Arctic and Marine Biology, UiT - The Arctic University of Norway, Tromsø, Norway
- 3Functional Ecology, Alfred Wegener Institute, Helmholtz Centre for Polar and Marine Research, Bremerhaven, Germany
- 4Functional Ecology, Helmholtz Institute for Functional Marine Biodiversity, Oldenburg, Germany
In 2011, a first comprehensive assessment of the footprints of climate change on Arctic marine ecosystems (such as altered distribution ranges, abundances, growth and body conditions, behaviours and phenologies, as well as community and regime shifts) was published. Here, we re-assess the climate-driven impacts reported since then, to elucidate to which extent and how observed ecological footprints have changed in the following decade (2011 to 2021). In total, 98 footprints have been described and analysed. Most of those impacts reported in the 2011 assessment are reconfirmed and can, hence, be assumed as continuing trends. In addition, novel footprints (behavioural changes, diet changes, altered competition and pathogen load) are described. As in 2011, most reported footprints are related to changes in distribution ranges, abundances, biomass and production. Range shifts have mostly been observed for fish species, while behavioural changes have mainly been reported for mammals. Primary production has been observed to further increase in Arctic seas. The footprints on pelagic herbivores, particularly the key species Calanus spp., are less clear. In comparison to 2011, more complex, cascading effects of climate change, such as increased bowhead whale body conditions due to increased primary production, have been reported. The observed footprints, and the trends that they indicate, strongly suggest that due to further northward range shifts of sub-Arctic and boreal species Arctic seas are likely to experience increasing species richness in the future. However, a tipping point may be reached, characterized by subsequent biodiversity decline, when Arctic-endemic species will go extinct as ocean warming and/or acidification will exceed their physiological adaptation capacity. Furthermore, as invading boreal species have a competitive advantage due to their wider physiological and trophic range, Arctic species abundances are predicted to decrease. Overall, the future Arctic Ocean will very likely experience increasing numbers and intensities of climate-change footprints.
1 Introduction
Climate change is particularly rapid, pronounced, and momentous in polar regions (Fox-Kemper et al., 2021; Gutiérrez et al., 2021; Ranasinghe et al., 2021), and its environmental and ecological impacts and risks are particularly severe (Meredith et al., 2019; Constable et al., 2022). The impacts in polar regions have become commonly acknowledged as both important drivers and indicators of general climate change, with manifold effects on natural and human systems on both regional and global scales (Meredith et al., 2019; Constable et al., 2022). For instance, the Arctic atmosphere plays a major role in the global climate system, and to understand how the future climate – and its impacts - will possibly look like, understanding current changes is key (Cohen et al., 2020 and references therein; Wang, 2021). Therefore, public interest in polar regions has steadily increased in the past two decades and, hence, research efforts in Arctic and Antarctic regions have also risen (Figure 1). Since 2011, the number of publications for the Arctic and Antarctic has nearly doubled, continuing a trend that is visible since the 1990s (Figure 1).
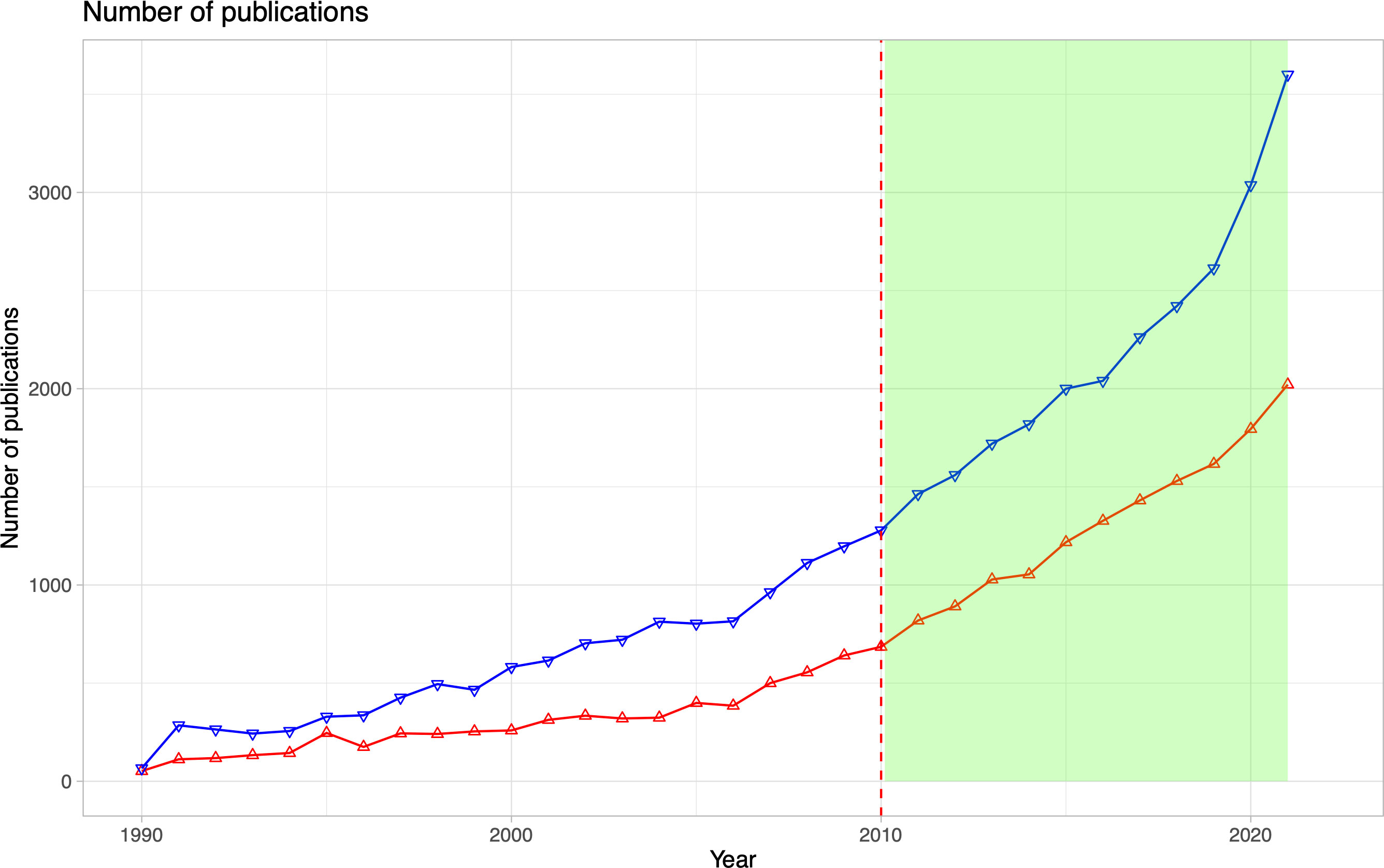
Figure 1 The number of publications published after 1990 on Arctic (red crosses) and Antarctic (blue triangles) marine biological and ecological topics. The Web of Science ™ research has been used with the Web of Science Core Collection Database on 23 September 2022. For the Arctic, the following search string was used: “Arctic AND (Bio* OR Eco*) AND (Marine OR Ocean*)” and for the Antarctic: “(Antarctica* OR ‘Southern Ocean’) AND (Bio* OR Eco*) AND (Marine OR Ocean*)”. The green highlight shows the time frame (2011-2021) assessed in this paper. The red dashed line symbolises the year of the last publication reported in Wassmann et al. (2011).
Warming, the most prominent effect of climate change, is particularly pronounced in the Arctic (Benton, 1970; Stocker et al., 2013), with near-surface air temperatures rising 2-3 times more rapidly compared to the global average (Screen and Simmonds, 2010; Cohen et al., 2014). Some investigations even indicate a six times higher increase (Huang et al., 2017). For instance, Svalbard experienced atmospheric warming rates of 3-5°C from 1971 to 2017 (equivalent to 0.81°C per decade), particularly during winter (Hanssen-Bauer et al., 2019). The higher-than-average warming exemplifies the Arctic amplification (Manabe and Stouffer, 1980) that has been widely addressed in climate change research (Serreze et al., 2009; Screen and Simmonds, 2010; Lang et al., 2017). Sea-ice decline, in combination with reduced snow cover on land, leads to an albedo decrease, mainly caused by larger open-water areas, which adsorb more solar radiation and thus cause further warming, which in turn leads to further inhibited sea-ice growth in winter and accelerated melt of sea ice in spring and summer (Perovich et al., 2007; Arrigo et al., 2008). This positive feedback results in a further reduction of ice extent and increase of open-water areas, resulting in additional increased atmospheric heat input. In addition, changes in ocean dynamics have also contributed to the warming of the Arctic (Blunden and Arndt, 2016). Waters inflowing from the North Atlantic and Pacific Oceans have warmed the Barents and Chukchi Seas, respectively (Blunden and Arndt, 2016). The inflow of Atlantic water into the Barents Sea has doubled in the last 30 years (Oziel et al., 2016; Oziel et al., 2020), and the warming of Arctic surface waters is generally more than double than the global average (Blunden and Arndt, 2016; Neukermans et al., 2018).
The sweeping and fast changes in climate-driven environmental conditions resulted in profound and far-reaching ecological impacts, as reported by Wassmann et al. (2011) in a pan-Arctic review of observed footprints of climate change on Arctic marine food webs. A total of 51 footprints reported in the scientific literature published between 1995 and 2010 were assessed (Wassmann et al., 2011 and references therein). Note that these footprint records are solely based on observations, and experimental and modelling evidence published in the literature was not considered in this study. Most pronounced impacts were found for fish and marine mammals, especially polar bears. For phytoplankton, an increase in primary production and biomass was observed in response to decreasing ice cover. For small zooplankton, such as amphipods and copepods, changes in community structure and biomass were reported. Observed impacts on benthic biotas were diverse. From macroalgae to snow crabs and clams, a variety of footprints were reported, mostly regarding declines in abundance, northward distribution shifts of some species (e.g., for the blue mussel Mytilus edulis) and subsequent changes in community composition. Most studies of fish communities did also find a northward shift in species distributions, increasing spawning biomass, and increased recruitment and replacement of cod populations by krill. For Arctic seabirds, warming and change in sea-ice extent resulted in declining colony sizes, increased mortalities, and diet changes. For mammals, only one footprint for whales and two footprints for seals [harp seal (Pagophilus groenlandicus) and ringed seal (Pusa hispida)] were reported, while nine footprints were described for polar bears, such as decreasing population sizes, landward shift of denning sites, as well as increased drowning and cannibalism, and declining cub survival.
Based on their assessment, Wassmann et al. (2011) suggested four foci of future research efforts: 1) time series, 2) adequate seasonal coverage in key regions, 3) new technology and 4) making existing Soviet/Russian literature available. Research into these directions should regionally emphasize the central AO, Fram Strait and Siberian shelves, due to their particular sensitivity to climate impacts. More in-depth studies were also regarded as necessary, to provide a sound baseline needed to detect further impacts of climate change (Wassmann et al., 2011). Moreover, there was a need for new tools, such as molecular methods, to distinguish between different populations and taxa, while the use of more remote-sensing technology was assumed to likely not contribute much additional information due to the extensive cloud and ice cover of Arctic seas (Wassmann et al., 2011). After a decade of accelerating climate warming since 2010, worldwide and particularly in the Arctic, it seems timely and topical to repeat the assessment that Wassmann et al. (2011) made at the end of the first decade of the second millennium
2 Approach, material and methods
To update the work of Wassmann et al. (2011) and assess the progress in knowledge on the impacts of climate change in Arctic marine ecosystems in the past decade, we reviewed all reports on ecological footprints published in the scientific peer-reviewed literature since 2011. We elucidated whether previous trends have been continuing or stopped or whether even new footprints were detected as compared to the findings summarized in Wassmann et al. (2011). Our assessment was based on extensive queries in both Web of Science™ and Google Scholar™, as well as references in the retrieved articles themselves. To ensure comparability, we used a similar definition of a “footprint of climate change” as Wassmann et al. (2011). Again, only observational studies that covered a time span of at least 10 years and reported climate-driven changes/trends have been considered in the repeated assessment. Impacts caused by multiple factors or with more than one potential explanation will be mentioned or discussed. Model results were only taken into account in hindcast mode. Experimental evidence was not regarded as indicating a ‘footprint’ in a strict sense and was, hence, used only as supplementary information (underpinning knowledge gained on physiological footprints). Those papers that report footprints as defined, plus those showing clear trends, are presented in Supplementary Tables 1–8. Please note that a single paper can report multiple footprints. Moreover, we differentiated between primary (direct) footprints, which are based on changing environmental factors, and secondary (indirect) footprints, which are cascading effects of primary ones, i.e., mostly biotic changes, such as new species interactions or increased exposure to pathogens (Table 1). Figures 2–4 were plotted using R package “ggOceanMaps” (Vihtakari, 2022).
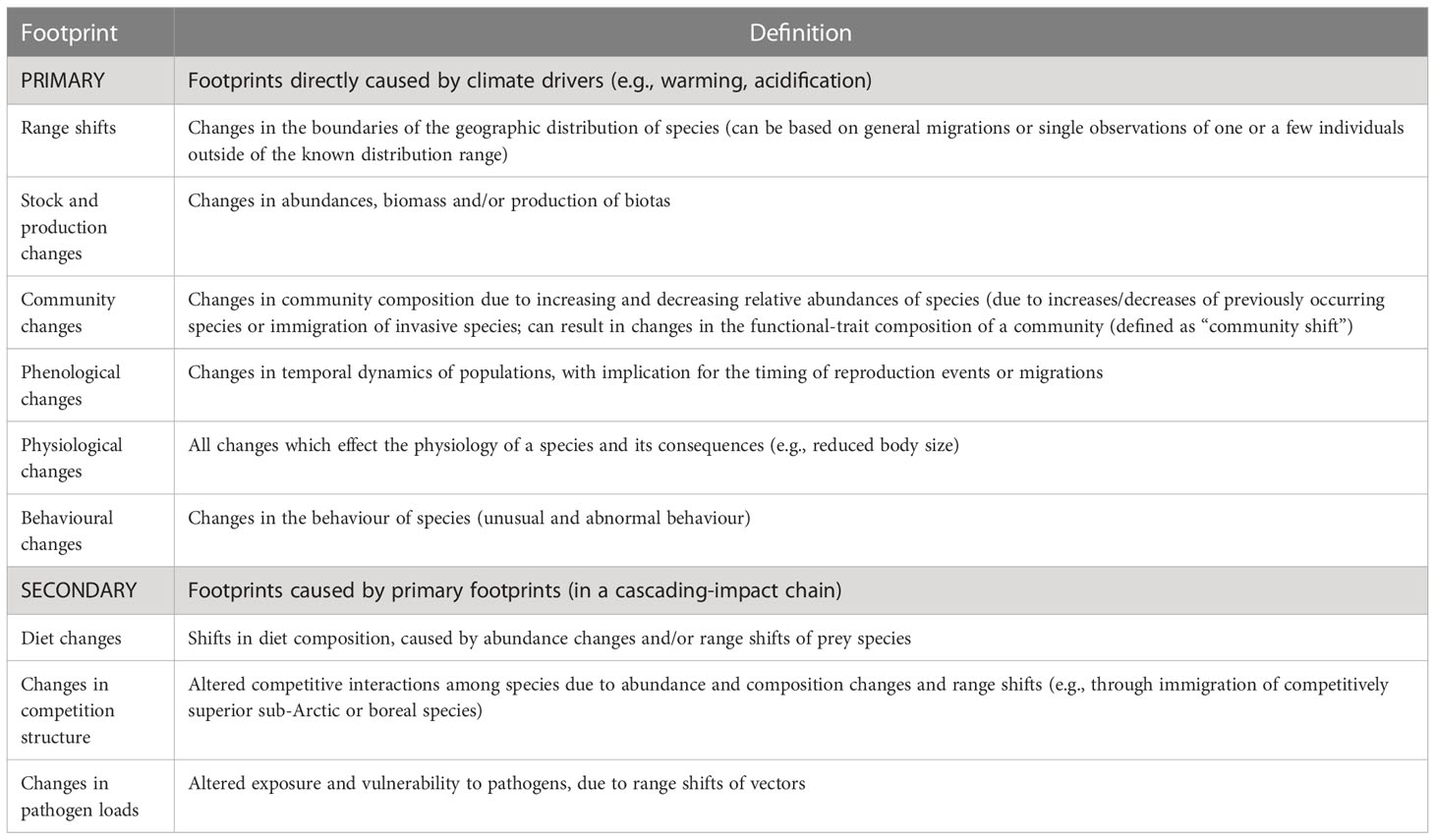
Table 1 Definition of primary and secondary footprints of climate change in Arctic marine ecosystems.
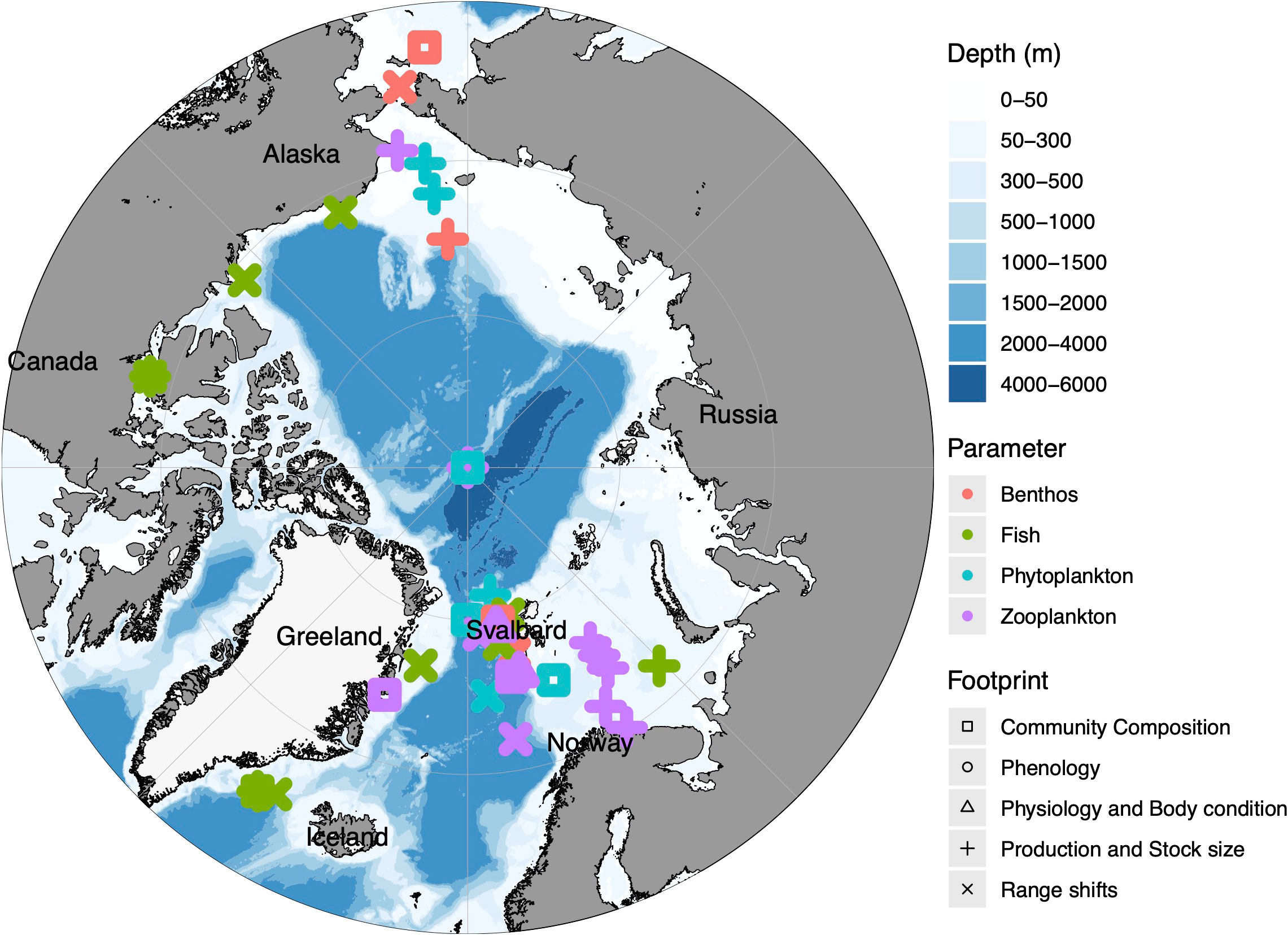
Figure 2 Map of the geographical distribution of primary footprints on a pan-Arctic scale Part 1 (Primary production, zooplankton, benthos, fish). The colours represent the different parameters, while the shapes represent the type of footprint. Please note that the geographical North Pole was chosen as a representative point for all pan-Arctic studies, therefore overlapping can occur. A summary of all relevant papers sorted by footprint types and parameters in Supplementary Tables 1-6.
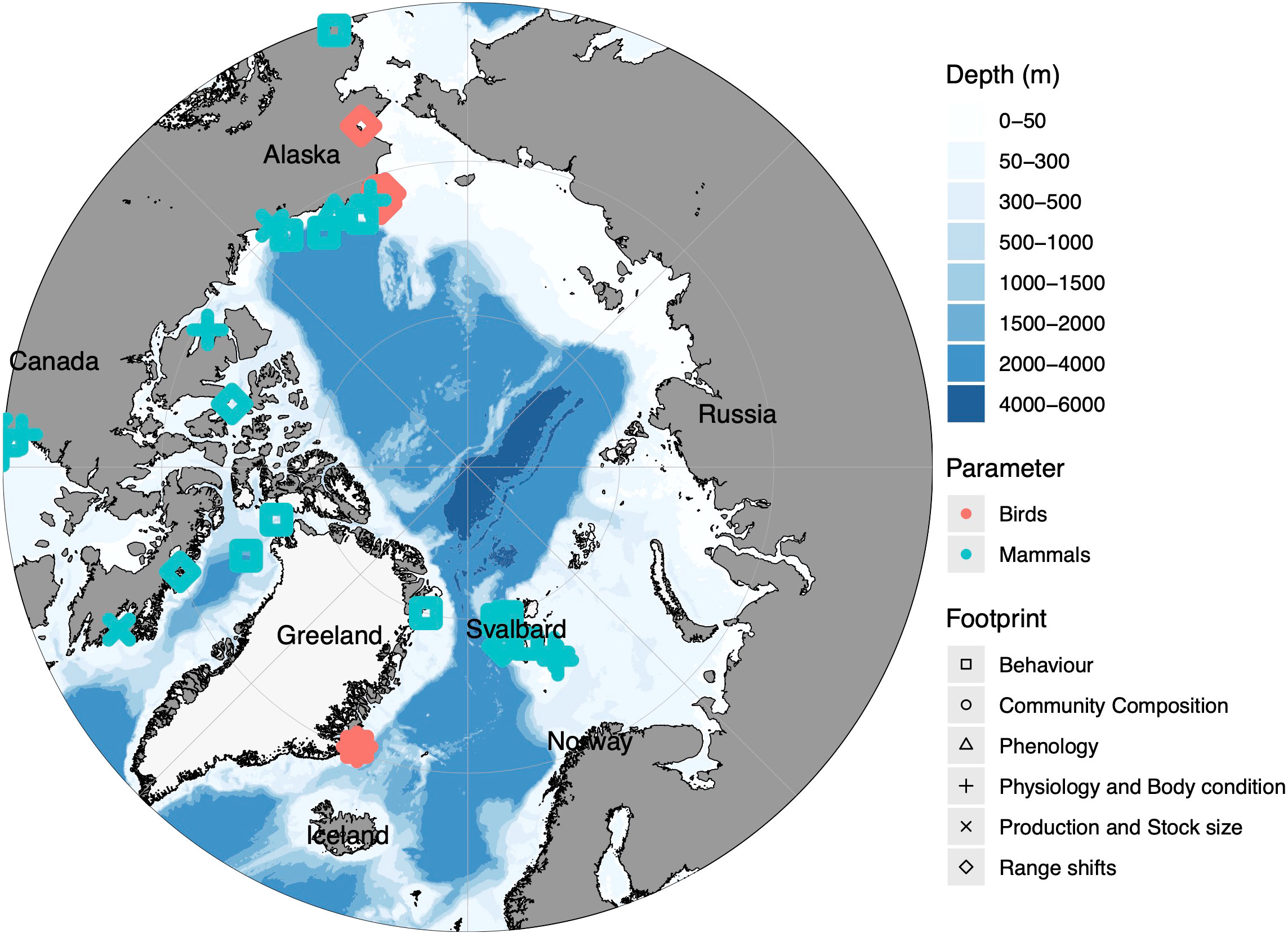
Figure 3 Map of the geographical distribution of primary footprints on a pan-Arctic scale Part 2 (Birds and Mammals). The colours present the different parameters, while the shape represent the type of footprint. Please note that the geographical North Pole was chosen as a representative point for all pan-arctic studies, therefore overlapping can occur. Further, not all footprints are represented in this plot, due to their geographical location. A summary of all relevant papers sorted by footprint types and parameters in Supplementary Tables 1-6.
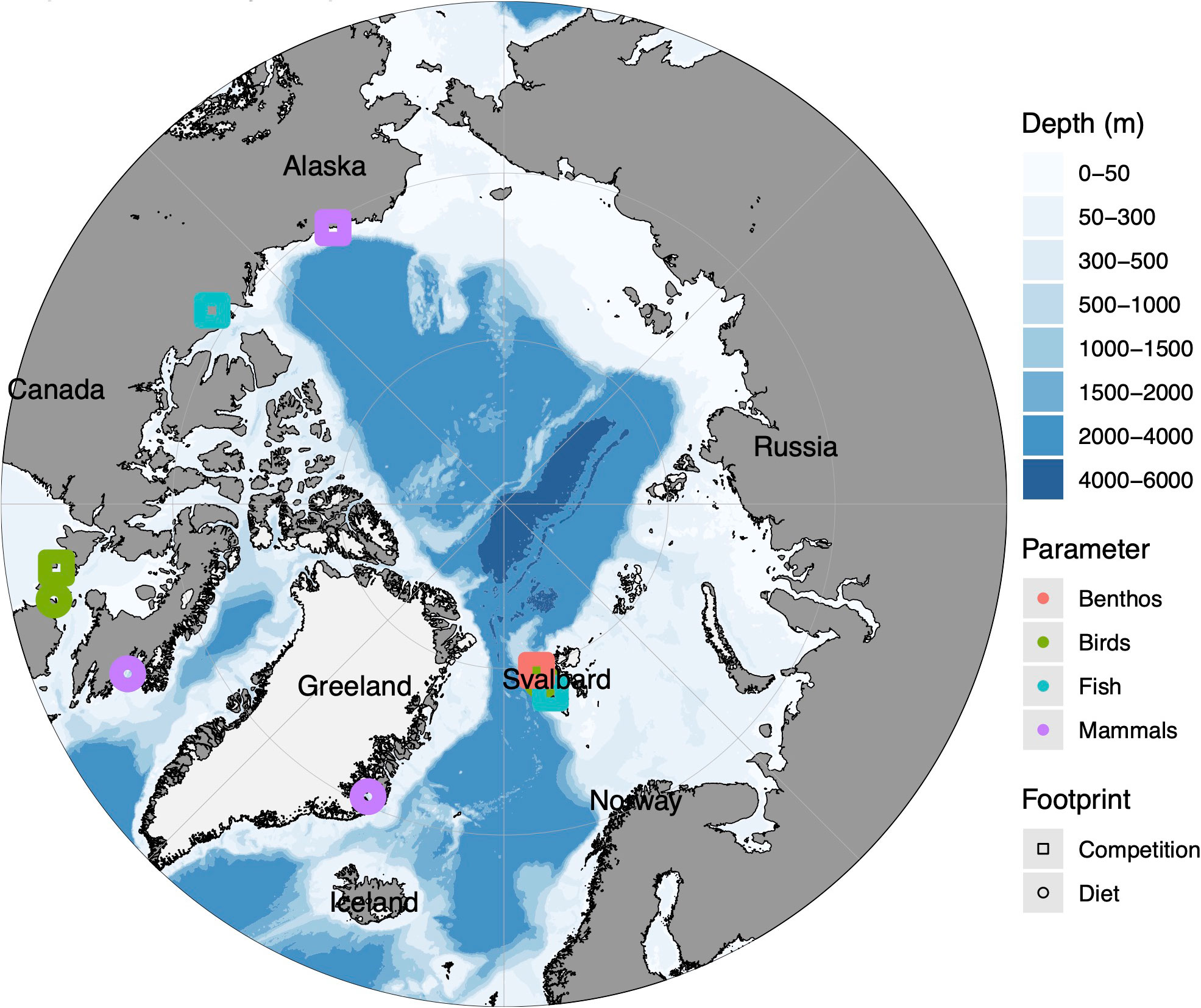
Figure 4 Map of the geographical distribution of all recorded secondary footprints on a pan-Arctic scale. The colours present the different animal parameters, while the shape represents the type of footprint. A summary of all relevant papers sorted by footprint types and parameters in Supplementary Tables 7, 8.
3 Observed ecological footprints and their implications
3.1 Primary footprints
Primary footprint (range shifts, production and stock size, community changes, phenological changes, physiological changes, behavioural changes) have been recorded from various Arctic regions for primary production, zooplankton, benthos, fish (Figure 2) and birds and mammals (Figure 3).
3.1.1 Range shifts
Phytoplankton studies suggest ongoing northward range shifts, as reflected in the geographic position of spring blooms, as well as by the occurrence of the coccolithophorid Emiliania huxleyi, a temperate species, in Arctic waters (Oziel et al., 2017; Neukermans et al., 2018; Renaut et al., 2018; Oziel et al., 2020). The northward shift in the Barents and Kara Seas is most likely caused by reduced sea-ice cover, thinner ice and an earlier retreat of sea ice, which is the result of an increased inflow of warm Atlantic waters (Renaut et al., 2018), or stronger and accelerated surface currents, irrespective of higher water temperatures (Oziel et al., 2020).
The Atlantic hyperiid amphipod Themisto compressa showed a northward shift in range and abundance (Kraft et al., 2013). The shrimp species Pandalus borealis was found for the first time on the Northeast Greenland shelf (Andrews et al., 2019). In Kongsfjorden (Svalbard), the boreal krill species Thysanoessa longicaudata and Meganyctiphanes norvegica, as well as the sub-tropical-boreal species Nematoscelis megalops, are now occurring occasionally in Arctic seas, due to increased inflow of Atlantic Water (Buchholz et al., 2010; Huenerlage et al., 2016). Note that the investigation by Buchholz et al. (2010) on euphausiids cannot be defined as a climate-change footprint according to the strict definition given above, since the first report was published before 2011. The very first observation of Thysanoessa raschii spawning in the Arctic was reported in 2011, suggested as evidence for a changing environment concerning temperature and food-web composition (Buchholz et al., 2012). The northward range shift is ongoing, as indicated by the most recent observation of T. raschii in Svalbard waters (Kongsfjorden) in August 2018 (Brandt, pers. obs.). Moreover, there is a well-documented advance of the boreal amphipod species Gammarus oceanicus on the expense of its Arctic twin species G. setosus off Svalbard (Węsławski et al., 2018; Węsławski et al., 2020). The crown jellyfish Periphylla periphylla was reported for the very first time in a high-Arctic fjord (>78° N) in January 2017 (Geoffroy et al., 2018). Abundances of P. periphylla have been increasing in the northern Barents Sea since 2014 when it has been found there for the first time, likely because of the increasing warm Atlantic water inflow, which combined with the low light conditions in winter makes it a more suitable condition for jellyfish (Geoffroy et al., 2018).
The northward extension of the distribution of the benthic blue mussel Mytilus edulis as reported in Wassmann et al. (2011), continues (Leopold et al., 2019). Another yet mostly overseen migratory factor for Mytilus spp. is plastic debris, which combined with the increasing favourable environmental factors in the Arctic enhances the northward expansion of Mytilus spp. (Kotwicki et al., 2021). Furthermore, data from Wiktor et al. (2022) suggests changes in the macroalgae composition in the Arctic with ongoing climate change (Wiktor et al., 2022). Three new starfish species were reported off the western coasts of Svalbard the first time: Doplopteraster multipes, Marthasterias glacialis and Asterias rubens, which had previously been reported only further south at the Norwegian coast (Deja et al., 2016).
Fish communities also showed a shift in their northward boundaries, with Arctic species being displaced by a boreal fish species immigrating from the south (Fossheim et al., 2015). The distribution centre of the Arctic fish community moved 159 km northward between 2004 and 2012 and was dominated by the bigeye sculpin (Triglops nybelini), Greenland halibut (Reinhardtius hippoglossoides), and snailfishes (Liparis spp.) (Fossheim et al., 2015). Furthermore, Arctic fish species were found north and east of their common distribution areas. This northward shift is reported on a community level. Because of the northward distribution the Arctic fish community can be found in deeper waters (Fossheim et al., 2015). Some areas have switched from an Arctic fish-dominated to boreal fish-dominated community (Fossheim et al., 2015). Furthermore, it was shown that the in situ range shifts are much faster than models predicted (Fossheim et al., 2015). In the Beaufort Sea, the northward extension of the known distribution ranges of walleye pollock (Theragra chalogramma), Pacific cod (Gadus macrocephalus), festive snailfish (Liparis marmoratus), eyeshade sculpin (Nautichthys pribiloviu) and Bering flounder (Hippoglossoides robustus) was documented (Rand and Logerwell, 2011). Atlantic mackerels (Scomber scomber) were observed at the west coast of Svalbard during a short period of time in late summer 2015 (Berge et al., 2015b), two years after it had first been reported in 2013 by a local newspaper (Aaserud, 2013). Since the 2000s, adult Atlantic cod (Gadus morhua) has been caught regularly in bottom trawls around Svalbard, and in 2008 also juveniles for the first time (Berge et al., 2015b). The spawning grounds and migrations of the most northern populations of Atlantic cod and haddock (Melanogrammus aeglefinus) have generally shifted northward (Drinkwater, 2009). The first reports of Atlantic cod, beaked redfish (Sebastes mentella) and capelin (Mallotus villosus) off Northeast Greenland are from 2015 (Andrews et al., 2019). Pacific sand lances (Ammodytes hexapterus) have regularly been caught, in increasing numbers, in the Beaufort Sea since 2010 (Falardeau et al., 2014; Suzuki et al., 2015). Their successful reproduction in the region is assumed (Suzuki et al., 2015), and further evidence for an ongoing eastward range shift since 2017 is reported (Falardeau et al., 2017). Bluefin tuna (Thunnus thynnus) have been reported off the eastern coast of Greenland (65°N) in Denmark Strait for the first time in 342 years, likely indicating the influence of increasing water temperatures (MacKenzie et al., 2014).
The ranges of seabirds did also shift, in response to the northward relocation of their zooplankton prey caused by sea-ice retreat (Hunt et al., 2018). Three boreal seabird species - short-tailed albatross (Phoebastria albatrus), northern gannet (Morus bassanus) and rhinoceros auklet (Cerorhinca monocerata) - were reported for the first time in the high-Arctic eastern Chukchi and Beaufort Seas (Day et al., 2013). Furthermore, ancient murrelets (Synthliboramphus antiquus) had expanded their range towards the eastern Chukchi Seas before 2006 and have now reached the Beaufort Sea (Day et al., 2013). A similar range shift was found for black-legged kittiwakes (Rissa tridsctyla), common murres (Uria aalge) and thick-billed murres (U. lomvia) over 12 years, in addition to oscillating relocations of feeding grounds between cold and warm years: during the former, seabirds used preferentially oceanic prey, while during the latter, they mostly fed on shelf organisms (Will and Kitaysky, 2018).
Whales also shifted their distribution ranges. For instance, due to reduced sea ice extent, two bowhead whale populations (Balaena mysticetus) had gotten into contact via the Northwest Passage, which is the very first report of its kind (Heide-Jørgensen et al., 2012). First evidence for a northward range expansion of the Atlantic blue whale population (Balaenoptera musculus) to the waters off Svalbard was reported, accompanied by similar shifts for minke whales (Balaenoptera acutorostrata), fin whales (Balaenoptera physalus), and humpback whales (Megaptera novaeangliae) (Storrie et al., 2018). Off Iceland, climate change also led to changes in the cetacean community, one of which is the northward range shift of blue whales (Víkingsson et al., 2015). In addition, there is increasing evidence for the occurrence of killer whales (Orcinus orca) in the high Arctic (Higdon and Ferguson, 2009; Higdon et al., 2012), despite the risk of being trapped by sea ice and dying during winter, as reported from the southern Hudson Bay (Matthews et al., 2019).
A polar bear subpopulation in Baffin Bay has become increasingly isolated, due to several reasons including a general northward range shift of the entire population, a reduction in overall population range extent, and less migration between the subpopulations in Hudson Bay, Kane Basin and Davis Strait (Scientific Working Group to the Canada-Greenland Joint Commission on Polar Bear, 2016; Laidre et al., 2018b).
3.1.2 Changes in production and stock size
The general finding of an increase in annual primary production in Arctic seas in response to spatially and temporally increasing open-water areas and a longer growing season (Arrigo et al., 2008) has been corroborated in further studies (e.g., Arrigo and van Dijken, 2011; Bélanger et al., 2013; Hill et al., 2018; Dalpadado et al., 2020). However, the increase differs among regions, being most pronounced in the Barents and Kara Seas (Renaut et al., 2018). The general trend apparently continues unabated with a rate of 30% per decade, based on large-scale remote-sensing studies of surface-water Chl a dynamics (Arrigo and van Dijken, 2015). This general trend is dampened by 3.5% per decade, despite a significant decrease in photosynthetic active radiation over the sea surface (-8% per decade) due to increasing cloudiness in summer (Bélanger et al., 2013). The general increase in marine Arctic primary production has been attributed to a number of reasons, including ocean warming and more open-water areas, leading to more mixing and a higher nutrient supply to the euphotic zone (Hill et al., 2018; Randelhoff and Sundfjord, 2018).
In addition to increasing primary production, two further phenomena have been reported. First, a huge under-ice phytoplankton bloom was first observed in 2011 as a result of reduced sea-ice thickness (Arrigo et al., 2012). This bloom, observed over 100 km under the sea surface, was estimated to be 4-times higher than the open-water bloom (Arrigo et al., 2012). Previous studies had already reported some rather anecdotal evidence of such under-ice phytoplankton blooms but did not investigate this phenomenon in detail (Strass and Nöthig, 1996; Fortier et al., 2002; Mundy et al., 2009). Other small-scale studies related under-ice phytoplankton blooms to melt-water ponds (Arrigo et al., 2014; Horvat et al., 2017). The second phenomenon discovered in ice-covered Arctic seas for the first time was a red tide caused by the phototrophic ciliate Mesodinium rubrum, which is correlated to the thinning of sea ice (Olsen et al., 2019).
Zooplankton production and biomass have increased in the Chukchi Sea, which has been correlated to the Arctic Oscillation and Pacific Decadal Oscillation resulting in an increased advection of species-rich Pacific waters through Bering Strait (Ershova et al., 2015). Also, the abundance of the copepod species Calanus glacialis has risen in the White Sea, most likely due to increased water temperatures and changes in salinity (Persson et al., 2012). Climate-driven biomass variations of the two copepod species C. glacialis and C. hyperboreus are closely correlated (Carstensen et al., 2012). Despite warming, no increase in the biomass of C. finmarchicus in the Barents Sea was observed, while there was a significant decline of the Arctic key amphipod species Themisto libellula due to decreased Arctic water masses in this region (Dalpadado et al., 2012). Euphausiid abundances and biomass increased, possibly due to increased inflow of relatively warm Atlantic waters (Eriksen and Dalpadado, 2011; Johannesen et al., 2012; Eriksen et al., 2016). Furthermore, the abundances of under-ice- amphipods, such as Gammarus wilkitzkii and Onisimus spp., declined, in response to the reduction of multi-year sea ice (Hop et al., 2021).
Benthic biotas did also show changes in stock size. For instance, in the southern Chukchi Sea echinoderm biomass decreased from 2004 to 2012, mainly due to the declines of the brittle star Ophiura sarsi and the sea cucumber Myriotrochus rinkii, while the biomass of benthic arthropods and molluscs peaked in 2009, mainly due to the higher abundances of snow crabs (Chionoecetes opilio) and moon snails (Cryptonatica affinis) (Grebmeier et al., 2015). These changes were most likely caused by multiple drivers, such as sea-ice retreat, altered ocean dynamics and a changing phenology of seasonal production events (Grebmeier et al., 2015). While in the Bering Strait region benthic macrofaunal biomass decreased, there was an increase at time-series stations (1998-2015) dominated by bivalves in the southeastern Chukchi Sea, caused by changes in ocean currents, which affected sediment grain-size composition and benthic food supply (Grebmeier et al., 2018).
Arctic fish abundances and biomass were also impacted by climate change. For instance, in the Barents Sea reduced abundances were reported for Greenland halibut, sculpins (Icelus spp.), and snailfishes (Liparis spp.) (Fossheim et al., 2015). In contrast, increasing numbers of Pacific sand lances were found in the Beaufort Sea, in response to warming and ice loss (Falardeau et al., 2017). The findings of Falardeau et al. (2017) are supported by the niche-based models run under climate change scenarios (Wisz et al., 2015). At two stations off eastern Greenland, a loss of deep-water fish abundance, related to warming, changed species interactions and habitats, has been reported (Emblemsvåg et al., 2020). More specifically, the Greenland halibut, different wolffish species (Anarhichas minor, A. lupus and A. denticulatus) as well as the Kaup´s arrowtooth eel (Synaphobranchus kaupii) decreased at the northern station, while Greenland halibut, Günther´s grenadier (Coryphae noides guentheri), blue antimoral (Antimora rostrata), Murray´s longsnout grenadier (Trachyrhynchus murrayi), roundnouse grenadier (Coryphaenoides rupestris) and Agassiz´s slickhead (Alepochephalus agassizii) decreased at the southern station (Emblemsvåg et al., 2020).
Seabird populations are still in a steady state in Hornsund, although increased glacier melt has been reported over the last 15 years (Stempniewicz et al., 2017). Glacier fronts are generally known as attractive foraging grounds of seabirds for a number of reasons (Apollonio, 1973; Węsławski and Legezytńska, 1998; Lydersen et al., 2014), and glacier melt due to warming will most likely cause a shrinking of this important feeding places (Kohler et al., 2007), in particular if the glacier withdraws from the fjord. Due to climate change, the phenology of flora and fauna is changing (Brown and Arrigo, 2013; Cherry et al., 2013; Kahru et al., 2016; Hauser et al., 2017). Phenological changes in lower trophic levels have effects on higher trophic levels. The prolonged growth season and the subsequent increase of primary and secondary production have had positive effects on 9 of 15 seabird species investigated near the sub-Arctic Alaska Gyre, while only one species declined in abundance (Thompson et al., 2012).
Harp seal populations declined in the White Sea and the Northwest Atlantic, due to climate-driven sea-ice decline leading to a reduction of reproduction rates and increased pup mortalities (Johnston et al., 2012; Stenson et al., 2016). The Hudson Bay ringed seal population also showed a decline due to multiple factors, such as sea-ice loss (Ferguson et al., 2017).
Evidence of the development of polar bear (Ursus maritimus) populations varied regionally. In Greenland, polar bear abundances rose (Peacock et al., 2013), due to a significant correlation between seal abundances and polar bear survival rates (Peacock et al., 2013). In contrast, declining polar bear populations have been reported from the Hudson Bay and Beaufort Sea, due to changing sea-ice conditions (Bromaghin et al., 2015; Lunn et al., 2016). The above-mentioned finding of Lunn et al. (2016) might be substantially influenced by the hunt of polar bears by humans, as the authors discussed.
3.1.3 Changes in community composition
For phytoplankton, data about community composition is limited. Nevertheless, an increasing trend for Phaeocystis pouchetii has been reported. This led to a shift from diatom-dominated communities towards communities dominated by P. pouchetii and other pico- and nanoplankton in Fram Strait (Nöthig et al., 2015). This increasing trend was once more confirmed in bio-optical investigations in the Barents Sea between 2002 to 2018 (Orkney et al., 2020).
Over the last three decades the diversity for sea-ice protists declined in the AO due to reduced multi-year sea-ice extent and concentration (Hop et al., 2020).
There have been multiple reports of changes in Arctic zooplankton community composition since 2011. In general, there is ample evidence that the Arctic zooplankton is changing from the dominance of the Arctic species C. glacialis towards that of the boreal C. finmarchicus (Weydmann et al., 2014; Gluchowska et al., 2017). A reduction in the relative abundances of Pseudocalanus spp. in northeast Greenland waters have also been reported (Middelbo et al., 2019).
Littoral seabed communities in Kongsfjorden and Smeerenburgfjorden (Svalbard) changed in composition to a brown algae-dominated biota, in response to increasing water temperatures, reduced control mechanisms and reduced competition among algal species (Węsławski et al., 2010; Kortsch et al., 2012). In the Bering Sea south of St. Lawrence Island, the zoobenthos has become dominated by maldanid polychaetes instead of bivalves since 2009, driven by changes in bottom-water temperatures and the silt and clay content of the sediments (Grebmeier and Cooper, 2016; Grebmeier et al., 2018). The echinoderm assemblages along the western coast of Svalbard are becoming more homogenous, most likely due to the dominance of subpolar species (Deja et al., 2016).
Emblemsvåg et al. (2020) described species-specific deep-water fish stock size changes off East Greenland (see above), leading to a “borealisation” of the fish community composition.
In the eastern Chukchi Sea, populations of piscivorous and planktivorous surface-feeding seabirds, e.g., black-legged kittiwakes (Rissa tridactyla) and murres (Uria spp.), declined during three decades from 1975-1981 to 2008-2012, while populations of diving planktivorous birds, e.g., crested auklets (Aethia cristatella) and short-tailed shearwaters (Puffinus tenuirostris), increased. This is very likely a response to declining sea ice and increasing availability of large zooplankton prey, which in turn were caused by increased primary production and increased advection of phyto- and zooplankton (Gall et al., 2017).
3.1.4 Changes in phenology
Spring phytoplankton blooms have been shown to start earlier in some Arctic regions (about a tenth of the overall spatial extent) (Kahru et al., 2011; Kahru et al., 2016), most pronounced in areas with significantly reduced sea ice, such as Hudson Bay, Foxe Basin, Baffin Sea, Greenland coasts, the waters off Novaya Zemlya, and the Kara Sea (Kahru et al., 2011). In the northern Barents Sea, the onset of the blooms occurred on average three days earlier each year since 1998 (Kahru et al., 2016), resulting in the overall prolongation of the productive season by 47 days (from 15 days in 1998 to 62 days in 2015). However, this trend will only be able to prolong the growth season until the astronomical light limits phytoplankton growth. Interestingly, the ending does not occur later, which was explained by the effects of nutrient limitation after the primary bloom. The changed phenology led to an increase in total primary production (Kahru et al., 2016). In addition, first evidence for a fall bloom in Arctic seas have been found over a 14-year study period, which is also attributed to reduced sea-ice extent (Ardyna et al., 2014) that together with strong wind events can induce secondary blooms through vertical mixing and the provision of regenerated nutrients (Fujiwara et al., 2018). Similar findings are reported by Oziel et al. (2017) and by Waga and Hirawake (2020).
A delayed autumn migration of beluga whales (Delphinapterus leucas) to the eastern Chukchi and Beaufort Seas by two to four weeks has been recorded, significantly correlated to the later sea-ice freeze-up (Hauser et al., 2017).
3.1.5 Changes in physiology and body condition
There is evidence that the growth season of C. glacialis has become longer and its critical developmental times shorter, attributed to warming and increased radiation (Feng et al., 2018). A faster development and earlier spawning have also been found for C. finmarchicus (Weydmann et al., 2018). Similar findings were reported for the jellyfish Aglantha digitale in the West Spitsbergen current (Mańko et al., 2022).
Polar cod (Boreogadus saida) and saffron cod (Eleginus gracilis) have become smaller in the northern Bering and Chukchi Sea. Increasing temperature and reduced sea ice extent have been discussed as potential reasons, but none of these factors could actually explain the reduction of body size (Helser et al., 2017).
Little auks (Alle alle) off East Greenland have likely benefited from reducing sea-ice extent (Amélineau et al., 2019), as there is evidence that in years with a low sea-ice concentrations their fitness was higher, while in years with much sea ice the adult body size and chick growth rate was reduced (Fort et al., 2009; Amélineau et al., 2019). A similar trend has been observed for red-legged kittiwakes (Rissa brevirostris), with lower nutritional stress in years with reduced sea-ice extent (Will et al., 2018).
The reproductive success as well as body condition of polar bears are clearly negatively impacted by sea-ice decline (Derocher et al., 2011; Obbard et al., 2016). The total population storage energy for polar bears in western Hudson Bay decreased by 56% and a decline of 53% in the total population energy density over a time period of about three decades has been reported (Johnson et al., 2020). Those declines are related to earlier ice break-up and longer open-water periods. Ringed seals showed a significant decline in body condition due to an earlier sea-ice breakup in spring, resulting in changed prey availability and increased competition between ringed seals and more temperate species (Harwood et al., 2012). Increased predation pressure by newly arrived predators, as well as warmer temperatures, have also been discussed as potential drivers of this development, which also led to lower pregnancy rates and a lower pup harvests by Inuits (Chambellant et al., 2012; Ferguson et al., 2017). Similarly, the body condition of harp seals in the Barents Sea also declined, likely as a result of climate-driven changes in food availability (Øigård et al., 2013).
In contrast to the negative climate-change effects discussed above, a two-decade study by George et al. (2015) showed a positive response of bowhead whales, including a positive trend in body condition in response to reduced sea-ice extent, prolonged open-water season, higher primary production in the Pacific Arctic and higher upwelling potentials due to more wind stress. The study of Mazurkiewicz et al. (2020) do also not support negative climate effects. Their findings indicate that body size of polychaetes and generally soft infauna is an inherited factor rather than being affected by ongoing warming, suggesting a certain degree of resilience of the benthic community.
3.1.6 Behavioural changes of upper trophic-level organisms
Several studies have shown that polar bears stay closer to land in response to changing environmental factors, primarily related to sea-ice retreat (Laidre et al., 2015; Laidre et al., 2018a; Laidre et al., 2018b). Increasing land use has also been reported (Cherry et al., 2013; Rode et al., 2015; Atwood et al., 2016). A statistically significant change in the phenology, elevation, slope and aspect of polar bear dens in the Baffin Bay region has been reported to be most likely the result of changed snow conditions and sea-ice dynamics (Escajeda et al., 2018). In Svalbard, polar bears spent less time at tide glacier fronts in summer and autumn than before the strong sea-ice decline in 2006 (Hamilton et al., 2017). Polar bears move longer distances and stay longer near ground-nesting bird areas (Hamilton et al., 2017). In search for alternative prey, polar bears have been reported of feeding on whale carcasses and foraging eggs of ground-nesting birds, which is expected to have a negative impact on the breeding success of bird populations, particularly of barnacle geese (Branta leucopsis), common eiders (Somateria mollissima) and glaucous gulls (Larus hyperboreus) (Prop et al., 2015). The finding that lipid and protein contents in the blood of land-using bears are higher than in sea-ice-faring individuals suggest that feeding on bird eggs can be beneficial for polar bear body conditions (Whiteman et al., 2018). In addition, adaptations in hunting behaviour have been reported for polar bears (Stempniewicz et al., 2014; Stempniewicz et al., 2021). While polar bears have typically hunted seals by stalking their breathing holes or stalking them over longer distances, with sea-ice retreat they apparently “adapt” new hunting techniques (e.g., ambushing resting and sleeping reindeer (Rangifer tarandus) as well as longer chases on land and in water) and new food sources (e.g., adult black guillemonts (Cepphus grylle) and Arctic tern chicks (Sterna paradisea)) (Stempniewicz et al., 2014). Polar bears were observed to successfully hunt fully grown, adult Spitsbergen Reindeer (R. tarandus platyrhynchus) (Stempniewicz et al., 2021). Moreover, polar bears face higher energy demands by up to 4% per year because of increased activities or faster migrations in response to changed sea-ice drift patterns (Durner et al., 2017).
Foraging behavioural changes were also reported for the little auk, more specifically their modified diving behaviour in response to increased ocean temperatures and reduced sea ice (Amélineau et al., 2019).
Sub-adult ringed seals have been shown to swim greater distances, are less area-restricted in their search for food, spend less time on the sea ice, and dive longer with shorter surface intervals in response to changing sea-ice dynamics (Hamilton et al., 2015). Moreover, driven by the increasing Atlantic water influx, ringed seals at Svalbard spent significantly more time at tide glacier fronts (Hamilton et al., 2019).
Similarly, beluga whales stayed shorter near glacier fronts in Svalbard waters but more time in the open fjord waters, indicating a behavioural adaptation to climate-driven environmental changes (Vacquié-Garcia et al., 2018). The authors further suggested that their results indicate a resilience of the beluga whales to changes caused by global climate change, at least in the short run (Vacquié-Garcia et al., 2018). These findings are once more supported by a more recent publication of Hamilton et al. (2019), who found that beluga whales in Svalbard waters spend significantly less time at tide water glacier fronts since 1995 as response to declining sea ice extent and increased Atlantic water influx in the Arctic. Bowhead whales in the western Arctic spend more time in open waters further away from the coasts, which are likely characterized by better prey availability due to reduced sea-ice extent (Druckenmiller et al., 2018).
3.2 Secondary footprints
For the secondary footprints diet changes, changes in competition structure and changes in pathogene load reports can be found for numbers of regions of the Arctic (Figure 4), here for fish, benthos birds and mammals,
3.2.1 Diet changes
In relation to changing biotic and abiotic factors, including decreasing sea-ice concentrations, increasing summer sea-surface temperatures and a changed fish composition in Cumberland Sound, Canada, the diets of Greenland halibuts shifted over the past 22 years towards higher shares of forage fish (Yurkowski et al., 2018). This trend to an increased non-endemic fish consumption has also been found in the diet of black-legged kittiwakes. From 1982 to 1987 and 1997 to 2016, the diets of black-legged kittiwakes in Kongsfjorden changed from a dominance of Arctic fish towards higher shares of Atlantic fish, reflecting the “Atlantification” of the fjord fish fauna driven due to the immigration of boreal species (Vihtakari et al., 2018).
Other studies, however, for instance on the thick-billed murres and black-legged kittiwakes on two Pribilof Islands (Alaska), did not show clear trends or changes in diets (Renner et al., 2012).
The trends in the Arctic top-predator diets are more diverse. Polar bears in eastern Greenland fed less on ringed seals and more on hooded seals (Cystophora cristata), likely reflecting the influence of the Northern Atlantic Oscillation rather than changing sea-ice conditions (McKinney et al., 2013). Bearded seals (Erignathus barbatus) and ringed seals living at the west coast of Spitsbergen changed their diets. Matching the changed diving behaviour, the diet of the bearded seal showed increasing pelagic share and a lower benthic share. The diet of the ringed seals shows an increasing share of boreal species (Lowther et al., 2017; Hamilton et al., 2018).
For cetaceans, diet changes are also reported. Increasing shares of non-endemic fish were reported for beluga whales in Cumberland Sound, Canada (Yurkowski et al., 2018). Fatty acid analysis showed that more capelin and less polar cod has been eaten by beluga whales in the Beaufort Sea, reflecting the retreat of sea ice (Watt et al., 2016). Similarly, the diets of narwhals (Monodon monoceros) in the northern Hudson Bay and in Svalbard waters were also characterized by increasing shares of capelin or Atlantic fish (Watt and Ferguson, 2015).
3.2.2 Altered competition structure and pathogen load
Since the Arctic is subjected to particularly rapid and profound climate change, it is a suitable place to study the effect of changing competition dynamics caused by the invasion of non-native species. It is well known that any increase in competition causes stress for affected species (Fossheim et al., 2015), and that closely related species have similar diets (Horstkotte and Strecker, 2005; Olsen et al., 2009). Due to climate change, gadid fish species such as polar cod, Atlantic cod and haddock do co-occur now in Svalbard waters, but their dietary overlap is less than 40%, indicating little food competition between the three species (Renaud et al., 2012). Opposing results were found in the western Canadian Arctic, where dietary overlaps and niche widths indicated a relatively high level of food competition between co-occurring immature capelin and polar cod (McNicholl et al., 2018).
However, not all new non-endemic species in the Arctic seem to interact with endemic species. The boreal G. oceanicus and the endemic G. setosus are currently co-occuring in the waters off western Svalbard (Węsławski et al., 2018; Węsławski et al., 2021), at least on a macrohabitat scale. Current evidence suggest that they do not on a microhabitat scale, though, which makes a direct competition unlikely (Węsławski et al., 2021), but further studies are needed to fully understand this pattern (Węsławski et al., 2021).
Due to significantly increased mosquito parasitism and polar bear predation at Cape Pembroke (Hudson Bay), adult thick-billed murres showed unusual high mortality, resulting bird carcasses are leading to increased scavenging and abandoned egg predation by foxes and gulls, and an increase in overall mortality by 20% per year and a decrease in colony productivity by 20% (Gaston and Elliott, 2013).
Polar bears from the Southern Beaufort Sea showed a potential increase of antibodies for the pathogens Brucella spp. and Toxoplasma gondii, as well as for the first time for the terrestrial microbial pathogens Francisella tularensis, Neospora caninum and Coxiella burnetti (Atwood et al., 2017). Individuals on land showed highest exposure to T. gondii (Atwood et al., 2017). The exposure of an Arctic marine mammal to C. burnetti is the very first report of this type. Therefore, this study represents the first report for a northward expansion of a sub-Arctic pathogen.
3.2.3 Projections and cascading impacts based on primary or secondary footprints
Ecological footprints of climate change are related to each other in manifold ways, for instance by sharing the same or similar drivers (single or multiple) or resulting in (or from) other footprints in a cascading-impact chain. For example, the increase in marine primary production has had – and very likely will have - cascading positive effects on the higher trophic levels of marine food webs, from zooplankton to seabirds and whales (Thompson et al., 2012; George et al., 2015; Waga et al., 2019).
Model results indicate that increased phytoplankton densities can potentially amplify ocean warming in the Arctic through increased heat absorption (Lengaigne et al., 2009; Park et al., 2015). An earlier onset of the bloom does not consequently mean a later ending of the bloom due to nutrient limitation (Kahru et al., 2016). However, increased river discharge can sustain and prolong primary production by increasing nutrient availability (McClelland, 2004; McClelland et al., 2006; Terhaar et al., 2019; Terhaar et al., 2021). Moreover, increased atmosphere-ocean interactions (wind events/strong wind events) have been shown to boost primary production by increased vertical mixing (Nishion et al., 2015; Uchimiya et al., 2016).
Conversely, climate-driven changes in the distribution and composition of Arctic zooplankton communities had some potential negative impacts on little auks (Kwasniewski et al., 2010), including longer foraging trips or increased foraging time, leading to increasing energy demand for feeding activities (Jakubas et al., 2016), which in turn results in less food and higher mortality of chicks that increasingly fed on C. finmarchicus (Kwasniewski et al., 2010). However, there was also evidence for an adaptive flexibility in little auks’ behaviour that may lessen or even reverse the consequences of prey community shifts (Kwasniewski et al., 2012; Amélineau et al., 2019).
Surprisingly limited observational data are available for zooplankton community shifts in response to the changing production regime. Model results suggest an increasing energy content through a more efficient energy transfer from primary production to higher trophic levels (Renaud et al., 2018) because of the observed and projected shift to a C. finmarchicus-dominated zooplankton community (Feng et al., 2018; Weydmann et al., 2018). These model results support of the findings of Thompson et al. (2012) and George et al. (2015), who described increasing body conditions for bowhead whales and seabirds resulting from improved prey availability due to increased production of lower trophic levels, which is in turn in line with the finding of decreased developmental times of Calanus spp. due to ocean warming and a prolonged growth season (Renaud et al., 2018).
The climate-driven shifts in the marine Arctic production regime, such as an earlier phytoplankton bloom and an increasing gap between the ice-algal and phytoplankton blooms have negative impacts on secondary producers (Søreide et al., 2010) and the entire marine food web (Dezutter et al., 2019). The recently observed secondary phytoplankton blooms in early fall in Arctic seas has yet unknown implications for the function of the marine ecosystems (Kahru et al., 2016; Uchimiya et al., 2016; Chen et al., 2017). Based on a similar development observed in the temperate North Atlantic where a changed zooplankton community is now dominated by smaller herbivorous Calanus species (e.g. C. helgolandicus), the smaller copepod has a higher grazing rate, two abundance peaks and utilises the fall phytoplankton bloom better (Martinez et al., 2011). C. finmarchicus in the Arctic may also be able to take advantage of the new fall bloom in Arctic waters, especially since there is evidence that this species can reproduce twice during the growing season (Häfker et al., 2018). C. glacialis may be able to develop under favourable conditions to an overwintering stage from a second spawning stage (Daase et al., 2013).
Polar bears have shown more variability and flexibility in their diet than expected, by switching from seals to birds, eggs, and carcasses (Stempniewicz et al., 2014; Prop et al., 2015; Galicia et al., 2016). Utilizing increasing numbers of walrus and bowhead whale carcasses, due to increased killer whale predation, positively contributes to the physiological condition of polar bears (Fischbach and Monson, 2009; Galicia et al., 2016), as well as the use of further potential prey, such as geese or reindeers (Stempniewicz et al., 2014; Heldbjerg et al., 2019).
Further cascading negative impacts for marine wildlife result from an indirect effect of climate change in the Arctic, the rising levels of human activities in this region, especially in terms of increased ship traffic leading to higher stress and risk levels for Arctic biotas (Reeves et al., 2014; Hauser et al., 2018). These risks include the introduction of new species. By now, a total of 54 introduction events led to the introduction of 34 non-indigenous species to Arctic waters (Chan et al., 2019). For about half of the single-pathway introductions of new species, the vectors were vessels, strongly suggesting that increasing vessel traffic will lead to even more introduced species which in turn will result in increased competition for endemic species (Chan et al., 2019). An additional source of new organisms introduced into the Arctic Ocean is plastic debris (Csapó et al., 2021; Kotwicki et al., 2021). Animals attached to plastic debris have more time to acclimate to the new environment, due to the slow travel speed of plastic debris, and thus are more likely to arrive vital in the Arctic (Csapó et al., 2021).
4 Conclusions
4.1 How did the footprints evolve since 2011?
By 2022, the numbers of published research papers, which address the impacts of climate change in Arctic seas or provide large-scale biodiversity assessments, e.g. (Piepenburg et al. (2011); Goldsmit et al. (2014); Logerwell et al. (2015)), has more than doubled since the publication of Wassmann et al. (2011) (Figure 1). The number of observed footprints increased from 51 reported in Wassmann et al. (2011) to 98 reported here. However, the footprints reported in this paper are more diverse and complex than those described in 2011, as slightly different assessment approaches have been applied. While the footprints in Wassmann et al. (2011) were exclusively driven by abiotic factors, such as ocean warming and changes in the sea-ice regime, we also report biologically driven (“secondary”) footprints, representing cascading effects of the physical “primary” footprints, e.g., the sea-ice loss induced declining seal populations that in turn caused a decline of polar bear populations (Bromaghin et al., 2015). Overall, we conclude, however, that most footprints and trends reported in Wassmann et al. (2011) were largely confirmed, indicating continuing trends in the increase of climate-change impacts and risks.
4.2 How did the perspectives and outlooks develop since 2011?
Wassmann et al. (2011) suggested in their outlook and future perspectives to particularly strengthen the research efforts on four main aspects: 1) new technology, 2) accessibility of old Russian data, 3) long-term monitoring programmes and 4) seasonal research.
In three of these aspects, we detected progress during the last decade. Since 2010, various new research technologies have been successfully applied in Arctic marine research, such as tissue-specific stable isotope analysis (Barton et al., 2019), use of molecular markers for studies on distribution ranges (Hardy et al., 2011), and analysis of environmental DNA (eDNA) samples (Lacoursière-Roussel et al., 2018). In contrast to the prognoses of Wassmann et al. (2011), remote-sensing research is still carried out and likely to continue, despite the predicted increase in cloud cover (Bélanger et al., 2013).
There are some notable long-term monitoring efforts in Arctic seas, such as the Long-Term Ecological Research (LTER) observatory HAUSGARTEN in Fram Strait (Soltwedel et al., 2005), the Distributed Biological Observatory (DBO) program in the western Arctic (Grebmeier et al., 2019) or the annual ecosystem cruises (https://www.hi.no/en/hi/cruises-and-field-work [latest access: 17.04.2021). The Nansen Legacy is probably the newest multidisciplinary, long-term research project, which has been implemented in 2018 (https://arvenetternansen.com/mission/ [17.04.2021]). However, there is still a need for more sustained long-term monitoring. The Russian Arctic remains particularly under-studied in that regard.
Although the ‘polar night’ (the time period of late fall, winter, early spring), as well as the central Arctic Ocean, remains under-studied, there have been efforts since 2011 that specifically targeted these important research challenges, such as the dedicated 3-year ‘polar night’ field study in Kongsfjorden (Berge et al., 2015a) or the one-year drift study across the central Arctic Ocean in 2019/20 (MOSAiC: Multidisciplinary drifting Observatory for the Study of Arctic Climate) (Kintisch, 2019). Additionally, studies on marine species during polar night are available (e.g., Grigor et al. (2015); Lischka and Riebesell (2017)). Most of those papers are one-time observations and do not have a time-series character. Nevertheless, they do provide (or are going to provide) valuable–and partly surprising–new insights about the processes in these scarcely known time periods and regions. For the central Arctic Ocean, a handful of publications is also available (e.g., Fernández-Méndez et al., 2014; David et al., 2016; Schanke et al., 2021). Like the polar night data, these publications have a low temporal resolution and are diverse in their research interest. Our research let to the conclusion that data for the central Arctic Ocean and the polar night are increasingly available. However, due to the low temporal and spatial resolution, adequate coverage is not given yet. Moreover, there are plans to continue with further international and multidisciplinary efforts to gradually close the still existing knowledge gaps in the central Arctic Ocean (van Pelt et al., 2017), preferably in the framework of a coordinated pan-Arctic Synoptic Arctic Survey programme (SAS; Paasche et al., 2019).
Russian research data gathered during Soviet times, the problematic availability of which was discussed in Wassmann et al. (2011), are still difficult to access for international research, despite many efforts to address this issue. However, some data on snow and ice conditions are available from the soviet drifting ice stations (Kahl, 1993; NASA National Snow and Ice Data Center Distributed Active Archive Center, 1993).
5 Outlook
In the last few years, significant progress in polar marine research has been made. However, climate change is accelerating, particularly in the Arctic (Fox-Kemper et al., 2021; Gutiérrez et al., 2021), and its impacts are becoming more profound (Meredith et al., 2019; Ranasinghe et al., 2021) due to feedback loops (Perovich et al., 2007; Arrigo et al., 2008). Therefore, one of the major recommendations of Wassmann et al. (2011) – more collaboration on an international level to fund and coordinate large-scale and multidisciplinary research – has remained valid, as shown for instance by the Third International Conference on Arctic Research Planning (ICARP III) (Werner et al., 2016), and it continues to be, as highlighted for instance in the context of ICARP IV 2022–2026 (Ruck et al., 2022; https://icarp.iasc.info [accessed 3 January 2023]), the 2018–2028 strategic plan of SAON (Sustaining Arctic Observing Networks) to develop a Roadmap for Arctic Observing and Data Systems (ROADS; Starkweather et al., 2021) and the regional ‘Arctic Action Plan’ that has been produced for the United Nations Decade for Ocean Science for Sustainable Development (2021–2030) (‘Ocean Decade’) (https://www.oceandecade.dk/decade-actions/arctic-process; accessed 3 January 2023).
The assessment of the impacts of climate change on Arctic marine food webs shows that these have reached new levels. The research published since 2011 provided growing evidence that not only environmental “primary” climate drivers but also cascading (biologically driven and direct anthropogenic) “secondary” impacts lead to important ecological footprints. Therefore, not only continuing and further long-term, year-round monitoring programs and observational field studies, using traditional and molecular methods, are still necessary but also experimental studies that enable us to understand how physiological constraints and resource competition will affect Arctic endemic species.
Microbial food webs have long been identified as particularly under-studied components of Arctic marine ecosystems, including their interaction with higher trophic levels and how climate change affects them (Vincent, 2010; this paper). This assessment is still valid, as only relatively small numbers of research papers on Arctic marine microbial food webs have been published since then (e.g. Iversen and Seuthe, 2011; Seuthe et al., 2011). This remaining knowledge gap does also mean that there is no valid baseline that potential ecological footprints can be gauged against. This lack is particularly concerning, since potential impacts are likely to be significant, given the importance of microbes for biodiversity, biomass, nutrient cycling and energy flow of any ecosystem (Vincent, 2010). Experimental evidence for the microbial activity from Isfjorden (Svalbard) suggests that phytoplankton production will decrease and bacterial production increase with ocean acidification, leading to a changed carbon flux pattern and a decreased net production of the Arctic fjord community (Vaqué et al., 2019). Furthermore, results from Kongsfjorden (Svalbard) suggest that ciliates and dinoflagellates heavily influence the phytoplankton community (Seuthe et al., 2011).
Since acidification rates are particularly high at the low ambient temperatures in polar waters, they potentially have significant implications for Arctic marine food webs (AMAP, 2018). Accordingly, there are a number of reports about how acidification will impact Arctic marine biotas in the future (Fabry et al., 2009; Bates et al., 2012; Lischka and Riebesell, 2012; Bates et al., 2013; Lewis et al., 2013) but no studies have reported footprints caused by ocean acidification yet.
Ocean freshening is a further physical effect of climate change, due to increased Arctic precipitation and river discharge (Vihma et al., 2016; Box et al., 2019) and glacial meltwater input, from both terrestrial glaciers and the Greenland Ice Sheet (Fox-Kemper et al., 2021), with potentially far-reaching and profound ecological ramifications for Arctic marine food webs. However, there are not yet observed ecological footprints documented in the scientific literature.
Secondary climate-change footprints, such as increased pathogen loads, rising competition levels, and altered diets, can have severe further cascading effects, e.g., on animal fitness, as for instance the diet directly affects body conditions and reproductive success of a species (Harding et al., 2011; Divoky et al., 2015; Will and Kitaysky, 2018). Therefore, these changes will likely have negative but hard-to-predict impacts, particularly on endemic Arctic species (Divoky et al., 2015; Nakano et al., 2016).
Irrespective of the mostly negative impacts of climate change, there is also some evidence of resilience through adaptation in several species, such as little auk (Kwasniewski et al., 2010; Grémillet et al., 2012) and polar cod (Drost et al., 2016), which have shown propensity for plasticity and acclimatisation to climate change. Polar bears increasingly display new hunting behaviours (Stempniewicz et al., 2014) and spend more time on land instead on sea ice (Cherry et al., 2013; Laidre et al., 2015; Laidre et al., 2018b), which have been suggested as adaptations towards altering environmental conditions that can compensate for negative climate-change impacts.
Here, we show that the impacts of climate change on Arctic marine food webs have continued and become more profound since the assessment of Wassmann et al. (2011). Based on the trends that are evident for several impacts, ecosystems are heading towards tipping points and irreversible regime shifts. Our assessment also shows that footprints of climate change are not static, but evolve and change in their extent, scale, amplitude and complexity, particularly with regard to the “secondary” impacts deriving from cascading feedback loops. Therefore, we propose further repeated assessments of climate-change impacts on Arctic marine food webs about every ten years, using a comparable approach as Wassmann et al. (2011) and us a decade later. Finally, assessments like those by Wassmann et al. (2011) and us do provide further evidence for the need of coordinated synoptic research, as for instance Paasche et al. (2019) proposed with a synoptic SAS framework, to document and analyse the development in Arctic waters, including possible surpassings of tipping points in response to the predicted accelerating climate change for the decades to come (Fox-Kemper et al., 2021; Gutiérrez et al., 2021).
Author contributions
SB and DP conceived and designed the study. SB analysed the data. SB, DP and PW wrote the manuscript. All authors contributed to the article and approved the submitted version.
Funding
We acknowledge support by the Open Access Publication Funds of Alfred-Wegener-Institut Helmholtz-Zentrum für Polar- und Meeresforschung.
Acknowledgments
This paper is based on SB’s MSc thesis (University of Kiel, Germany). We would like to thank Allison Baily, Lena Seuthe, Rolf Gradinger, Maline Daasen, Paul Renaud and Philipp Assmy for their advice. PW acknowledges the support of the research group Arctic SIZE (http://site.uit.no/arcticsize/) through UiT – The Arctic University of Norway. Last but not least we thank two peer reviewers for their valuable and constructive comments that greatly contributed to improving the manuscript.
Conflict of interest
The authors declare that the research was conducted in the absence of any commercial or financial relationships that could be construed as a potential conflict of interest.
Publisher’s note
All claims expressed in this article are solely those of the authors and do not necessarily represent those of their affiliated organizations, or those of the publisher, the editors and the reviewers. Any product that may be evaluated in this article, or claim that may be made by its manufacturer, is not guaranteed or endorsed by the publisher.
Supplementary material
The Supplementary Material for this article can be found online at: https://www.frontiersin.org/articles/10.3389/fmars.2023.1096222/full#supplementary-material
References
Aaserud M. (2013). Pionermakrellen har kommet til Longyearbyen [The pioneer mackerel has arrived in Longyearbyen]. Svalbardposten 2013, 4–5.
Amélineau F., Grémillet D., Harding A. M. A., Walkusz W., Choquet R., Fort J. (2019). Arctic Climate change and pollution impact little auk foraging and fitness across a decade. Sci. Rep. 9, 1014. doi: 10.1038/s41598-018-38042-z
AMAP (2018). AMAP assessment 2018: Arctic ocean acidification (Tromsø, Norway: Arctic Monitoring and Assessment Programme (AMAP), vi+187.
Andrews A. J., Christiansen J. S., Bhat S., Lynghammar A., Westgaard J.-I., Pampoulie C., et al. (2019). Boreal Marine fauna from the Barents Sea disperse to Arctic northeast Greenland. Sci. Rep. 9, 5799. doi: 10.1038/s41598-019-42097-x
Apollonio S. (1973). Glaciers and nutrients in arctic seas. Science 180, 491–493. doi: 10.1126/science.180.4085.491
Arrigo K. R., Perovich D. K., Pickart R. S., Brown Z. W., van Dijken G. L., Lowry K. E., et al. (2012). Massive phytoplankton blooms under Arctic sea ice. Science 336, 1408. doi: 10.1126/science.1215065
Arrigo K. R., Perovich D. K., Pickart R. S., Brown Z. W., van Dijken G. L., Lowry K. E., et al. (2014). Phytoplankton blooms beneath the sea ice in the chukchi Sea. Deep Sea Res. II 105, 1–16. doi: 10.1016/j.dsr2.2014.03.018
Arrigo K. R., van Dijken G. L. (2011). Secular trends in Arctic ocean net primary production. J. Geophys. Res. 116, L19603. doi: 10.1029/2011JC007151
Arrigo K. R., van Dijken G. L. (2015). Continued increases in Arctic ocean primary production. Prog. Oceanogr. 136, 60–70. doi: 10.1016/j.pocean.2015.05.002
Arrigo K. R., van Dijken G., Pabi S. (2008). Impact of a shrinking Arctic ice cover on marine primary production. Geophys. Res. Lett. 35, 529. doi: 10.1029/2008GL035028
Ardyna M., Babin M., Gosselin M., Devred E., Rainville L., Tremblay J.-É. (2014). Recent Arctic ccean sea ice loss triggers novel fall phytoplankton blooms. Geophys Res. Lett. 41 (17), 6207–6212. doi: 10.1002/2014GL061047
Atwood T. C., Duncan C., Patyk K. A., Nol P., Rhyan J., McCollum M., et al. (2017). Environmental and behavioral changes may influence the exposure of an Arctic apex predator to pathogens and contaminants. Sci. Rep. 7, 13193. doi: 10.1038/s41598-017-13496-9
Atwood T. C., Peacock E., McKinney M. A., Lillie K., Wilson R., Douglas D. C., et al. (2016). Rapid environmental change drives increased land ice by an Arctic marine predator. PloS One 11, e0155932. doi: 10.1371/journal.pone.0155932
Barton M. B., Litvin S. Y., Vollenweider J. J., Heintz R. A., Norcross B. L., Boswell K. M. (2019). Experimental determination of tissue turnover rates and trophic discrimination factors for stable carbon and nitrogen isotopes of Arctic sculpin (Myoxocephalus scorpioides): A common Arctic nearshore fish. J. Exp. Mar. Biol. Ecol. 511, 60–67. doi: 10.1016/j.jembe.2018.11.005
Bates N. R., Orchowska M. I., Garley R., Mathis J. T. (2012). Seasonal calcium carbonate undersaturation in shelf waters of the western Arctic ocean; how biological processes exacerbate the impact of ocean acidification. Biogeosci. Disc. 9, 14255–14290. doi: 10.5194/bgd-9-14255-2012
Bates N. R., Orchowska M. I., Garley R., Mathis J. T. (2013). Summertime calcium carbonate undersaturation in shelf waters of the western Arctic ocean - how biological processes exacerbate the impact of ocean acidification. Biogeosciences 10, 5281–5309. doi: 10.5194/bg-10-5281-2013
Bélanger S., Babin M., Tremblay J.-É. (2013). Increasing cloudiness in Arctic damps the increase in phytoplankton primary production due to sea ice receding. Biogeosciences 10, 4087–4101. doi: 10.5194/bg-10-4087-2013
Benton G. S. (1970). Carbon dioxide and its role in climate change. Proc. Natl. Acad. Sci. U.S.A. 67, 898–899. doi: 10.1073/pnas.67.2.898
Berge J., Daase M., Renaud P. E., Ambrose W. G., Darnis G., Last K. S., et al. (2015a). Unexpected levels of biological activity during the polar night offer new perspectives on a warming arctic. Curr. Biol. 25, 2555–2561. doi: 10.1016/J.CUB.2015.08.024
Berge J., Heggland K., Lønne O. J., Cottier F., Hop H., Gabrielsen G. W., et al. (2015b). First records of Atlantic mackerel (Scomber scombrus) from the Svalbard archipelago, Norway, with possible explanations for the extension of its distribution. Arctic 68, 54. doi: 10.14430/arctic4455
Blunden J., Arndt D. S. (2016). State of the climate in 2015. Bull. Am. Meteorol. Soc. 97, Si–S275. doi: 10.1175/2016BAMSStateoftheClimate.1
Bromaghin J. F., McDonald T. L., Stirling I., Derocher A. E., Richardson E. S., Regehr E., et al. (2015). Polar bear population dynamics in the southern Beaufort Sea during a period of sea ice decline. Ecol. Appl. 25, 634–651. doi: 10.1890/14-1129.1
Brown Z. W., Arrigo K. R. (2013). Sea Ice impacts on spring bloom dynamics and net primary production in the Eastern Bering Sea. J. Geophys. Res. Oceans 118, 43–62. doi: 10.1029/2012JC008034
Box J. E., Colgan W. T., Christensen T. R., Schmidt N. M., Lund M., Parmentier F.-J. W., et al. (2019). Key indicators of Arctic climate change: 1971–2017. Environ. Res. Lett. 14 (4), 45010. doi: 10.1088/1748-9326/aafc1b
Buchholz F., Buchholz C., Węsławski J. M. (2010). Ten years after: Krill as indicator of changes in the macro-zooplankton communities of two Arctic fjords. Polar Biol. 33, 101–113. doi: 10.1007/s00300-009-0688-0
Buchholz F., Werner T., Buchholz C. (2012). First observation of krill spawning in the high Arctic kongsfjorden, west spitsbergen. Polar Biol. 35, 1273–1279. doi: 10.1007/s00300-012-1186-3
Carstensen J., Weydmann A., Olszewska A., Kwasniewski S. (2012). Effects of environmental conditions on the biomass of Calanus spp. in the Nordic seas. J. Plankton Res. 34, 951–966. doi: 10.1093/plankt/fbs059
Chambellant M., Stirling I., Gough W. A., Ferguson S. H. (2012). Temporal variations in Hudson bay ringed seal (Phoca hispida) life-history parameters in relation to environment. J. Mamm 93, 267–281. doi: 10.1644/10-MAMM-A-253.1
Chan F. T., Stanislawczyk K., Sneekes A. C., Dvoretsky A., Gollasch S., Minchin D., et al. (2019). Climate change opens new frontiers for marine species in the Arctic: Current trends and future invasion risks. Glob. Chang. Biol. 25, 25–38. doi: 10.1111/gcb.14469
Chen M., Nam S.-I., Kim J.-H., Kwon Y.-J., Hong S., Jung J., et al. (2017). High abundance of protein-like fluorescence in the amerasian basin of Arctic ocean: Potential implication of a fall phytoplankton bloom. Sci. Total Environ. 599–600, 355–363. doi: 10.1016/j.scitotenv.2017.04.233
Cherry S. G., Derocher A. E., Thiemann G. W., Lunn N. J. (2013). Migration phenology and seasonal fidelity of an Arctic marine predator in relation to sea ice dynamics. J. Anim. Ecol. 82, 912–921. doi: 10.1111/1365-2656.12050
Cohen J., Screen J. A., Furtado J. C., Barlow M., Whittleston D., Coumou D., et al. (2014). Recent Arctic amplification and extreme mid-latitude weather. Nat. Geosci. 7, 627–637. doi: 10.1038/ngeo2234
Cohen J., Zhang X., Francis J., Jung T., Kwok R., Overland J., et al. (2020). Divergent consensuses on Arctic amplification influence on midlatitude severe winter weather. Nat. Clim. Chang. 10, 20–29. doi: 10.1038/s41558-019-0662-y
Constable A.J., Harper J., Dawson K., Holsman T., Mustonen D., Piepenburg D., et al. (2022). “Cross-Chapter Paper 6: Polar Regions”. In Climate Change 2022: Impacts, Adaptation, and Vulnerability. Contribution of Working Group II to the Sixth Assessment Report of the Intergovernmental Panel on Climate Change. Eds. Pörtner H.-O., Roberts D.C., Tignor M., Poloczanska E.S., Mintenbeck K., Alegría A., Craig M., Langsdorf S., Löschke S., Möller V., Okem A., Rama B.. (Cambridge:Cambridge University Press), 2319–2368. doi: 10.1017/9781009325844.023
Csapó H. K., Grabowski M., Węsławski J. M. (2021). Coming home - Boreal ecosystem claims Atlantic sector of the Arctic. Sci. Total Environ. 771, 144817. doi: 10.1016/j.scitotenv.2020.144817
Daase M., Falk-Petersen S., Varpe Ø., Darnis G., Søreide J. E., Wold A., et al. (2013). Timing of reproductive events in the marine copepod Calanus glacialis: A pan-Arctic perspective. Can. J. Fish. Aquat. Sci. 70, 871–884. doi: 10.1139/cjfas-2012-0401
Dalpadado P., Arrigo K. R., Dijken G. L., Rune H., Bagøien E., Dolgov A., et al. (2020). Climate effects on temporal and spatial dynamics of phytoplankton and zooplankton in the Barents Sea. Prog. Oceanogr. 185, 102320. doi: 10.1016/j.pocean.2020.102320
Dalpadado P., Ingvaldsen R. B., Stige L. C., Bogstad B., Knutsen T., Ottersen G., et al. (2012). Climate effects on Barents Sea ecosystem dynamics. ICES J. Mar. Sci. 69, 1303–1316. doi: 10.1093/icesjms/fss063
David C., Lange B., Krumpen T., Schaafsma F., van Franeker J. A., Flores H. (2016). Under-ice distribution of polar cod Boreogadus saida in the central Arctic ocean and their association with sea-ice habitat properties. Polar Biol. 39, 981–994. doi: 10.1007/s00300-015-1774-0
Day R. H., Gall A. E., Morgan T. C., Rose J. R., Sanzenbacher P. M., Fenneman J. D., et al. (2013). Seabirds new to the eastern chukchi and Beaufort seas, Alaska: Response to a changing climate? West Birds 44, 174–182.
Deja K., Węsławski J. M., Borszcz T., Włodarska-Kowalczuk M., Kukliński P., Bałazy P., et al. (2016). Recent distribution of Echinodermata species in spitsbergen coastal waters. Pol. Polar Res. 37, 511–526. doi: 10.1515/popore-2016-0027
Derocher A. E., Andersen M., Wiig Ø., Aars J., Hansen E., Biuw M. (2011). Sea Ice and polar bear den ecology at hopen island, Svalbard. Mar. Ecol. Prog. Ser. 441, 273–279. doi: 10.3354/meps09406
Dezutter T., Lalande C., Dufresne C., Darnis G., Fortier L. (2019). Mismatch between microalgae and herbivorous copepods due to the record sea ice minimum extent of 2012 and the late sea ice break-up of 2013 in the Beaufort Sea. Prog. Oceanogr. 173, 66–77. doi: 10.1016/j.pocean.2019.02.008
Divoky G. J., Lukacs P. M., Druckenmiller M. L. (2015). Effects of recent decreases in Arctic sea ice on an ice-associated marine bird. Prog. Oceanogr. 136, 151–161. doi: 10.1016/j.pocean.2015.05.010
Drinkwater K. (2009). Comparison of the response of Atlantic cod (Gadus morhua) in the high-latitude regions of the north Atlantic during the warm periods of the 1920s–1960s and the 1990s–2000s. Deep Sea Res. Part II: Topic. Stud. Oceanogr. 56, 2087–2096. doi: 10.1016/j.dsr2.2008.12.001
Drost H. E., Lo M., Carmack E. C., Farrell A. P. (2016). Acclimation potential of Arctic cod (Boreogadus saida) from the rapidly warming Arctic ocean. J. Exp. Biol. 219, 3114–3125. doi: 10.1242/jeb.140194
Druckenmiller M. L., Citta J. J., Ferguson M. C., Clarke J. T., George J. C., Quakenbush L. (2018). Trends in sea-ice cover within bowhead whale habitats in the pacific Arctic. Deep Sea Res. Part II: Topic. Stud. Oceanogr. 152, 95–107. doi: 10.1016/j.dsr2.2017.10.017
Durner G. M., Douglas D. C., Albeke S. E., Whiteman J. P., Amstrup S. C., Richardson E., et al. (2017). Increased Arctic sea ice drift alters adult female polar bear movements and energetics. Glob. Chang. Biol. 23, 3460–3473. doi: 10.1111/gcb.13746
Emblemsvåg M., Núñez-riboni I., Christensen H. T., Nogueira A., Gundersen A., Primicerio R. (2020). Increasing temperatures, diversity loss and reorganization of deep-sea fish communities east of Greenland. Mar. Ecol. Prog. Ser. 654, 127–141. doi: 10.3354/meps13495
Eriksen E., Dalpadado P. (2011). Long-term changes in krill biomass and distribution in the Barents Sea: Are the changes mainly related to capelin stock size and temperature conditions? Polar Biol. 34, 1399–1409. doi: 10.1007/s00300-011-0995-0
Eriksen E., Skjoldal H. R., Dolgov A., Dalpadado P., Orlova E. L., Prozorkevich D. V. (2016). The Barents Sea euphausiids: Methodological aspects of monitoring and estimation of abundance and biomass. ICES J. Mar. Sci. 73, 1533–1544. doi: 10.1093/icesjms/fsw022
Ershova E., Hopcroft R., Kosobokova K., Matsuno K., Nelson R. J., Yamaguchi A., et al. (2015). Long-term changes in summer zooplankton communities of the western chukchi Sea 1945–2012. Oceanography 28, 100–115. doi: 10.5670/oceanog.2015.60
Escajeda E., Laidre K. L., Born E. W., Wiig Ø., Atkinson S., Dyck M., et al. (2018). Identifying shifts in maternity den phenology and habitat characteristics of polar bears (Ursus maritimus) in Baffin bay and Kane basin. Polar Biol. 41, 87–100. doi: 10.1007/s00300-017-2172-6
Fabry V., McClintock J., Mathis J., Grebmeier J. (2009). Ocean acidification at high latitudes: The bellwether. Oceanography 22, 160–171. doi: 10.5670/oceanog.2009.105
Falardeau M., Bouchard C., Robert D., Fortier L. (2017). First records of pacific sand lance (Ammodytes hexapterus) in the Canadian Arctic archipelago. Polar Biol. 40, 2291–2296. doi: 10.1007/s00300-017-2141-0
Falardeau M., Robert D., Fortier L. (2014). Could the planktonic stages of polar cod and pacific sand lance compete for food in the warming Beaufort Sea? ICES J. Mar. Sci. 71, 1956–1965. doi: 10.1093/icesjms/fst221
Feng Z., Ji R., Ashjian C., Campbell R., Zhang J. (2018). Biogeographic responses of the copepod Calanus glacialis to a changing Arctic marine environment. Glob. Chang. Biol. 24, e159–e170. doi: 10.1111/gcb.13890
Ferguson S. H., Young B. G., Yurkowski D. J., Anderson R., Willing C., Nielsen O. (2017). Demographic, ecological, and physiological responses of ringed seals to an abrupt decline in sea ice availability. PeerJ 5, e2957. doi: 10.7717/peerj.2957
Fernández-Méndez M., Wenzhöfer F., Peeken I., Sørensen H. L., Glud R. N., Boetius A. (2014). Composition, buoyancy regulation and fate of ice algal aggregates in the central Arctic ocean. PloS One 9, e107452. doi: 10.1371/journal.pone.0107452
Fischbach A. S., Monson D. H. (2009). Enumeration of pacific walrus carcasses on beaches of the chukchi Sea in Alaska following a mortality event, September 2009. U.S. Geol. Surv. Open-File Rep. 4, 1–11. doi: 10.3133/ofr20091291
Fortier M., Fortier L., Michel C., Legendre L. (2002). Climatic and biological forcing of the vertical flux of biogenic particles under seasonal Arctic sea ice. Mar. Ecol. Prog. Ser. 225, 1–16. doi: 10.3354/meps225001
Fort J., Porter W. P., Gremillet D. (2009). Thermodynamic modelling predicts energetic bottleneck for seabirds wintering in the northwest Atlantic. J. Exp. Biol. 212, 2483–2490. doi: 10.1242/jeb.032300
Fossheim M., Primicerio R., Johannesen E., Ingvaldsen R. B., Aschan M. M., Dolgov A. V. (2015). Recent warming leads to a rapid borealization of fish communities in the Arctic. Nat. Clim. Chang. 5, 673–677. doi: 10.1038/NCLIMATE2647
Fox-Kemper B., Hewitt H. T., Xiao C., Aðalgeirsdóttir G., Drijfhout S. S., Edwards T. L., et al. (2021). “Ocean, cryosphere and Sea level change,” in Climate change 2021: The physical science basis. contribution of working group I to the sixth assessment report of the intergovernmental panel on climate change. Eds. Masson-Delmotte V., Zhai P., Pirani A., Connors S. L., Péan C., Berger S., et al (Cambridge: Cambridge University Press). doi: 10.1017/9781009157896.011
Fujiwara A., Nishino S., Matsuno K., Onodera J., Kawaguchi Y., Hirawake T., et al. (2018). Changes in phytoplankton community structure during wind-induced fall bloom on the central chukchi shelf. Polar Biol. 41, 1279–1295. doi: 10.1007/s00300-018-2284-7
Galicia M. P., Thiemann G. W., Dyck M. G., Ferguson S. H., Higdon J. W. (2016). Dietary habits of polar bears in foxe basin, Canada: Possible evidence of a trophic regime shift mediated by a new top predator. Ecol. Evol. 6, 6005–6018. doi: 10.1002/ece3.2173
Gall A. E., Morgan T. C., Day R. H., Kuletz K. J. (2017). Ecological shift from piscivorous to planktivorous seabirds in the chukchi sea 1975–2012. Polar Biol. 40, 61–78. doi: 10.1007/s00300-016-1924-z
Gaston A. J., Elliott K. H. (2013). Effects of climate-induced changes in parasitism, predation and predator-predator interactions on reproduction and survival of an Arctic marine bird. Arctic 66, 43–52. doi: 10.14430/arctic4265
Geoffroy M., Berge J., Majaneva S., Johnsen G., Langbehn T. J., Cottier F., et al. (2018). Increased occurrence of the jellyfish Periphylla periphylla in the European high Arctic. Polar Biol. 41, 2615–2619. doi: 10.1007/s00300-018-2368-4
George J. C., Druckenmiller M. L., Laidre K. L., Suydam R., Person B. (2015). Bowhead whale body condition and links to summer sea ice and upwelling in the Beaufort Sea. Prog. Oceanogr. 136, 250–262. doi: 10.1016/j.pocean.2015.05.001
Gluchowska M., Dalpadado P., Beszczynska-Möller A., Olszewska A., Ingvaldsen R. B., Kwasniewski S., et al. (2017). Interannual zooplankton variability in the main pathways of the Atlantic water flow into the Arctic ocean (Fram strait and Barents Sea branches). ICES J. Mar. Sci. 74, 1921–1936. doi: 10.1093/icesjms/fsx033
Goldsmit J., Howland K., Archambault P. (2014). Establishing a baseline for early detection of non-indigenous species in ports of the Canadian Arctic. Aquat. Invasion. 9, 327–342. doi: 10.3391/ai.2014.9.3.08
Grebmeier J., Bluhm B., Cooper L., Denisenko S., Iken K., Kedra M., et al. (2015). Time-series benthic community composition and biomass and associated environmental characteristics in the chukchi Sea during the RUSALCA 2004–2012 program. Oceanography 28, 116–133. doi: 10.5670/oceanog.2015.61
Grebmeier J. M., Cooper L. W. (2016). “The Saint Lawrence island polynya: A 25-year evaluation of an analogue for climate change in polar regions,” in Aquatic microbial ecology and biogeochemistry. Eds. Glibert P. M., Kana T. M. (Cham: Springer).
Grebmeier J., Frey K., Cooper L., Kedra M. (2018). Trends in benthic macrofaunal populations, seasonal sea ice persistence, and bottom water temperatures in the Bering strait region. Oceanography 31, 136–151. doi: 10.5670/oceanog.2018.224
Grebmeier J. M., Moore S. E., Cooper L. W., Frey K. E. (2019). The distributed biological observatory: A change detection array in the pacific Arctic – an introduction. Deep Sea Res. II 162, 1–7. doi: 10.1016/J.DSR2.2019.05.005
Grémillet D., Welcker J., Karnovsky N. J., Walkusz W., Hall M. E., Fort J., et al. (2012). Little auks buffer the impact of current Arctic climate change. Mar. Ecol. Prog. Ser. 454, 197–206. doi: 10.3354/meps09590
Grigor J. J., Marais A. E., Falk-Petersen S., Varpe Ø. (2015). Polar night ecology of a pelagic predator, the chaetognath Parasagitta elegans. Polar Biol. 38, 87–98. doi: 10.1007/s00300-014-1577-8
Gutiérrez J. M., Jones R. G., Narisma G. T., Alves L. M., Amjad M., Gorodetskaya I. V., et al. (2021). “Atlas,” in Climate change 2021: The physical science basis. contribution of working group I to the sixth assessment report of the intergovernmental panel on climate change. Eds. Masson-Delmotte V., Zhai P., Pirani A., Connors S. L., Péan C., Berger S., et al (Cambridge:Cambridge University Press). 1927–2058. doi: 10.1017/9781009157896.021
Häfker N. S., Teschke M., Last K. S., Pond D. W., Hüppe L., Meyer B. (2018). Calanus finmarchicus seasonal cycle and diapause in relation to gene expression, physiology, and endogenous clocks. Limnol. Oceanogr. 63, 2815–2838. doi: 10.1002/lno.11011
Hamilton C. D., Kovacs K. M., Ims R. A., Aars J., Lydersen C. (2017). An Arctic predator-prey system in flux: Climate change impacts on coastal space use by polar bears and ringed seals. J. Anim. Ecol. 86, 1054–1064. doi: 10.1111/1365-2656.12685
Hamilton C. D., Kovacs K. M., Lydersen C. (2018). Individual variability in diving, movement and activity patterns of adult bearded seals in Svalbard, Norway. Sci. Rep. 8, 16988. doi: 10.1038/s41598-018-35306-6
Hamilton C. D., Lydersen C., Ims R. A., Kovacs K. M. (2015). Predictions replaced by facts: A keystone species’ behavioural responses to declining Arctic sea-ice. Biol. Lett. 11, 20150803. doi: 10.1098/rsbl.2015.0803
Hamilton C. D., Vacquié-Garcia J., Kovacs K. M., Ims R. A., Kohler J., Lydersen C. (2019). Contrasting changes in space use induced by climate change in two Arctic marine mammal species. Biol. Lett. 15, 20180834. doi: 10.1098/rsbl.2018.0834
Hanssen-Bauer I., Førland E. J., Hisdal H., Mayer S., Sandø A. B., Sorteberg A., et al. (2019). Climate in Svalbard 2100 - a knowledge base for climate adaptation. NCCS Rep. 1/2019, 1–207. doi: 10.13140/RG.2.2.10183.75687
Harding A. M. A., Welcker J., Steen H., Hamer K. C., Kitaysky A. S., Fort J., et al. (2011). Adverse foraging conditions may impact body mass and survival of a high Arctic seabird. Oecologia 167, 49–59. doi: 10.1007/s00442-011-1971-7
Hardy S. M., Carr C. M., Hardman M., Steinke D., Corstorphine E., Mah C. (2011). Biodiversity and phylogeography of Arctic marine fauna: Insights from molecular tools. Mar. Biodiv. 41, 195–210. doi: 10.1007/s12526-010-0056-x
Harwood L. A., Smith T. G., Melling H., Alikamik J., Kingsley M. C. S. (2012). Ringed seals and sea ice in canada’s Western Arctic: Harvest-based monitoring 1992–2011. Arctic 65, 377–390. doi: 10.14430/arctic4236
Hauser D. D. W., Laidre K. L., Stafford K. M., Stern H. L., Suydam R. S., Richard P. R. (2017). Decadal shifts in autumn migration timing by pacific Arctic beluga whales are related to delayed annual sea ice formation. Glob. Chang. Biol. 23, 2206–2217. doi: 10.1111/gcb.13564
Hauser D. D. W., Laidre K. L., Stern H. L. (2018). Vulnerability of Arctic marine mammals to vessel traffic in the increasingly ice-free Northwest passage and northern Sea route. Proc. Natl. Acad. Sci. U.S.A. 115, 7617–7622. doi: 10.1073/pnas.1803543115
Heide-Jørgensen M. P., Laidre K. L., Quakenbush L. T., Citta J. J. (2012). The Northwest passage opens for bowhead whales. Biol. Lett. 8, 270–273. doi: 10.1098/rsbl.2011.0731
Heldbjerg H., Madsen J., Amstrup J., Bakken J., Balsby T. T. J., Christensen T. K., et al. (2019). Pink-footed goose Svalbard population status report 2018-2019. AEWA Eur. Goose Manage. Platform S.l., 1–13.
Helser T. E., Colman J. R., Anderl D. M., Kastelle C. R. (2017). Growth dynamics of saffron cod (Eleginus gracilis) and Arctic cod (Boreogadus saida) in the northern Bering and chukchi seas. Deep Sea Res. II 135, 66–77. doi: 10.1016/j.dsr2.2015.12.009
Higdon J. W., Ferguson S. H. (2009). Loss of Arctic sea ice causing punctuated change in sightings of killer whales (Orcinus orca) over the past century. Ecol. Appl. 19, 1365–1375. doi: 10.1890/07-1941.1
Higdon J. W., Hauser D. D. W., Ferguson S. H. (2012). Killer whales (Orcinus orca) in the Canadian Arctic: Distribution, prey items, group sizes, and seasonality. Mar. Mamm. Sci. 28, E93–E109. doi: 10.1111/j.1748-7692.2011.00489.x
Hill V., Ardyna M., Lee S. H., Varela D. E. (2018). Decadal trends in phytoplankton production in the pacific Arctic region from 1950 to 2012. Deep Sea Res. II 152, 82–94. doi: 10.1016/j.dsr2.2016.12.015
Hop H., Vihtakari M., Bluhm B. A., Assmy P., Poulin M., Gradinger R., et al. (2020). Changes in sea-ice protist diversity with declining sea ice in the Arctic ocean from the 1980s to 2010s. Front. Mar. Sci. 7. doi: 10.3389/fmars.2020.00243
Hop H., Vihtakari M., Bluhm B. A., Daase M., Gradinger R., Melnikov I. A. (2021). Ice-associated amphipods in a pan-Arctic scenario of declining sea ice. Front. Mar. Sci. 8. doi: 10.3389/fmars.2021.743152
Horstkotte J., Strecker U. (2005). Trophic differentiation in the phylogenetically young Cyprinodon species flock (Cyprinodontidae, teleostei) from Laguna chichancanab (Mexico). Biol. J. Linn. Soc. 85, 125–134. doi: 10.1111/j.1095-8312.2005.00476.x
Horvat C., Jones D. R., Iams S., Schroeder D., Flocco D., Feltham D. (2017). The frequency and extent of sub-ice phytoplankton blooms in the Arctic ocean. Sci. Adv. 3, e1601191. doi: 10.1126/sciadv.1601191
Huang J., Zhang X., Zhang Q., Lin Y., Hao M., Luo Y., et al. (2017). Recently amplified arctic warming has contributed to a continual global warming trend. Nat. Clim. Chang. 7, 875–879. doi: 10.1038/s41558-017-0009-5
Huenerlage K., Graeve M., Buchholz F. (2016). Lipid composition and trophic relationships of krill species in a high Arctic fjord. Polar Biol. 39, 1803–1817. doi: 10.1007/s00300-014-1607-6
Hunt G. L., Renner M., Kuletz K. J., Salo S., Eisner L., Ressler P. H., et al. (2018). Timing of sea-ice retreat affects the distribution of seabirds and their prey in the southeastern Bering Sea. Mar. Ecol. Prog. Ser. 593, 209–230. doi: 10.3354/meps12383
Iversen K. R., Seuthe L. (2011). Seasonal microbial processes in a high-latitude fjord (Kongsfjorden , Svalbard ): I . heterotrophic bacteria, picoplankton and nanoflagellates. Polar Biol. 34, 731–749. doi: 10.1007/s00300-010-0929-2
Jakubas D., Iliszko L. M., Strøm H., Darecki M., Jerstad K., Stempniewicz L. (2016). Foraging behavior of a high-Arctic zooplanktivorous alcid, the little auk, at the southern edge of its breeding range. J. Exp. Mar. Biol. Ecol. 475, 89–99. doi: 10.1016/j.jembe.2015.11.010
Johannesen E., Ingvaldsen R. B., Bogstad B., Dalpadado P., Eriksen E., Gjøsæter H., et al. (2012). Changes in Barents Sea ecosystem state 1970–2009: Climate fluctuations, human impact, and trophic interactions. ICES J. Mar. Sci. 69, 880–889. doi: 10.1093/icesjms/fss046
Johnson A. C., Reimer J. R., Lunn N. J., Stirling I., Mcgeachy D., Derocher A. E. (2020). Influence of sea ice dynamics on population energetics of western Hudson bay polar bears. Conserv. Physiol. 8, 1–16. doi: 10.1093/conphys/coaa132
Johnston D. W., Bowers M. T., Friedlaender A. S., Lavigne D. M. (2012). The effects of climate change on harp seals (Pagophilus groenlandicus). PloS One 7, e29158. doi: 10.1371/journal.pone.0029158
Kahl J. (1997). Daily Arctic Ocean rawinsonde data from Soviet drifting ice stations, version 1 (Boulder: NASA National Snow and Ice Data Center Distributed Active Archive Center). doi: 10.5067/AH8D9CN70VN6
Kahru M., Brotas V., Manzano-Sarabia M., Mitchell B. G. (2011). Are phytoplankton blooms occurring earlier in the Arctic? Glob. Chang. Biol. 17, 1733–1739. doi: 10.1111/j.1365-2486.2010.02312.x
Kahru M., Lee Z., Mitchell B. G., Nevison C. D. (2016). Effects of sea ice cover on satellite-detected primary production in the Arctic ocean. Biol. Lett. 12, 20160223. doi: 10.1098/rsbl.2016.0223
Kintisch E. (2019). Arctic Researchers prepare to go with the floes. Science 365, 728–729. doi: 10.1126/science.365.6455.728
Kohler J., James T. D., Murray T., Nuth C., Brandt O., Barrand N. E., et al. (2007). Acceleration in thinning rate on western Svalbard glaciers. Geophys. Res. Lett. 34, F04007. doi: 10.1029/2007GL030681
Kortsch S., Primicerio R., Beuchel F., Renaud P. E., Rodrigues J., Lønne O. J., et al. (2012). Climate-driven regime shifts in Arctic marine benthos. Proc. Natl. Acad. Sci. U.S.A. 109, 14052–14057. doi: 10.1073/pnas.1207509109
Kotwicki L., Węsławski J. M., Włodarska-Kowalczuk M., Mazurkiewicz M., Wenne R., Zbawicka M., et al. (2021). The re-appearance of the mytilus spp. complex in Svalbard, Arctic, during the Holocene: The case for an arrival by anthropogenic flotsam. Glob. Planet. Change 202, 103502. doi: 10.1016/j.gloplacha.2021.103502
Kraft A., Nöthig E. M., Bauerfeind E., Wildish D. J., Pohle G. W., Bathmann U., et al. (2013). First evidence of reproductive success in a southern invader indicates possible community shifts among Arctic zooplankton. Mar. Ecol. Prog. Ser. 493, 291–296. doi: 10.3354/meps10507
Kwasniewski S., Gluchowska M., Jakubas D., Wojczulanis-Jakubas K., Walkusz W., Karnovsky N., et al. (2010). The impact of different hydrographic conditions and zooplankton communities on provisioning little auks along the West coast of spitsbergen. Prog. Oceanogr. 87, 72–82. doi: 10.1016/j.pocean.2010.06.004
Kwasniewski S., Gluchowska M., Walkusz W., Karnovsky N. J., Jakubas D., Wojczulanis-Jakubas K., et al. (2012). Interannual changes in zooplankton on the West spitsbergen shelf in relation to hydrography and their consequences for the diet of planktivorous seabirds. ICES J. Mar. Sci. 69, 890–901. doi: 10.1093/icesjms/fss076
Lacoursière-Roussel A., Howland K., Normandeau E., Grey E. K., Archambault P., Deiner K., et al. (2018). eDNA metabarcoding as a new surveillance approach for coastal Arctic biodiversity. Ecol. Evol. 8, 7763–7777. doi: 10.1002/ece3.4213
Laidre K. L., Born E. W., Atkinson S. N., Wiig Ø., Andersen L. W., Lunn N. J., et al. (2018a). Range contraction and increasing isolation of a polar bear subpopulation in an era of sea-ice loss. Ecol. Evol. 8, 2062–2075. doi: 10.1002/ece3.3809
Laidre K. L., Born E. W., Heagerty P., Wiig Ø., Stern H., Dietz R., et al. (2015). Shifts in female polar bear (Ursus maritimus) habitat use in East Greenland. Polar Biol. 38, 879–893. doi: 10.1007/s00300-015-1648-5
Laidre K. L., Stern H., Born E. W., Heagerty P., Atkinson S., Wiig Ø., et al. (2018b). Changes in winter and spring resource selection by polar bears Ursus maritimus in Baffin bay over two decades of sea-ice loss. Endang. Spec. Res. 36, 1–14. doi: 10.3354/esr00886
Lang A., Yang S., Kaas E. (2017). Sea Ice thickness and recent Arctic warming. Geophys. Res. Lett. 44, 409–418. doi: 10.1002/2016GL071274
Lengaigne M., Madec G., Bopp L., Menkes C., Aumont O., Cadule P. (2009). Bio-physical feedbacks in the Arctic ocean using an earth system model. Geophys. Res. Lett. 36, 401. doi: 10.1029/2009GL040145
Leopold P., Renaud P. E., Ambrose W. G. A. JR., Berge J. (2019). High Arctic Mytilus spp.: occurrence , distribution and history of dispersal. Polar Biol. 42, 237–244. doi: 10.1007/s00300-018-2415-1
Lewis C. N., Brown K. A., Edwards L. A., Cooper G., Findlay H. S. (2013). Sensitivity to ocean acidification parallels natural pCO2 gradients experienced by Arctic copepods under winter sea ice. Proc. Natl. Acad. Sci. U.S.A. 110, E4960–E4967. doi: 10.1073/pnas.1315162110
Lischka S., Riebesell U. (2012). Synergistic effects of ocean acidification and warming on overwintering pteropods in the Arctic. Glob. Chang. Biol. 18, 3517–3528. doi: 10.1111/gcb.12020
Lischka S., Riebesell U. (2017). Metabolic response of Arctic pteropods to ocean acidification and warming during the polar night / twilight phase in kongsfjord (Spitsbergen). Polar Biol. 40, 1211–1227. doi: 10.1007/s00300-016-2044-5
Logerwell E., Busby M., Carothers C., Cotton S., Duffy-Anderson J., Farley E., et al. (2015). Fish communities across a spectrum of habitats in the western Beaufort Sea and chukchi Sea. Prog. Oceanogr. 136, 115–132. doi: 10.1016/j.pocean.2015.05.013
Lowther A. D., Fisk A., Kovacs K. M., Lydersen C. (2017). Interdecadal changes in the marine food web along the west spitsbergen coast detected in the stable isotope composition of ringed seal (Pusa hispida) whiskers. Polar Biol. 40, 2027–2033. doi: 10.1007/s00300-017-2122-3
Lunn N. J., Servanty S., Regehr E., Converse S. J., Richardson E., Stirling I. (2016). Demography of an apex predator at the edge of its range: Impacts of changing sea ice on polar bears in Hudson bay. Ecol. Appl. 26, 1302–1320. doi: 10.1890/15-1256
Lydersen C., Assmy P., Falk-Petersen S., Kohler J., Kovacs K. M., Reigstad M., et al. (2014). The importance of tidewater glaciers for marine mammals and seabirds in Svalbard, Norway. J. Mar. Syst. 129, 452–471. doi: 10.1016/j.jmarsys.2013.09.006
MacKenzie B. R., Payne M. R., Boje J., Høyer J. L., Siegstad H. (2014). A cascade of warming impacts brings bluefin tuna to Greenland waters. Glob. Chang. Biol. 20, 2484–2491. doi: 10.1111/gcb.12597
Manabe S., Stouffer R. J. (1980). Sensitivity of a global climate model to an increase of CO2 concentration in the atmosphere. J. Geophys. Res. 85, 5529. doi: 10.1029/JC085iC10p05529
Mańko M. K., Merchel M., Kwaśniewski S., Weydmann-Zwolicka A. (2022). Atlantification alters the reproduction of jellyfish Aglantha digitale in the European Arctic. Limnol. Oceanog. 67, 1836–1849. doi: 10.1002/lno.12170
Martinez E., Antoine D., D’Ortenzio F., de Boyer Montégut C. (2011). Phytoplankton spring and fall blooms in the north Atlantic in the 1980s and 2000s. J. Geophys. Res. 116, 43. doi: 10.1029/2010JC006836
Matthews C. J. D., Raverty S. A., Noren D. P., Arragutainaq L., Ferguson S. H. (2019). Ice entrapment mortality may slow expanding presence of Arctic killer whales. Polar Biol. 42, 639–644. doi: 10.1007/s00300-018-02447-3
Mazurkiewicz M., Górska B., Renaud P. E., Włodarska-Kowalczuk M. (2020). Latitudinal consistency of biomass size spectra - benthic resilience despite environmental, taxonomic and functional trait variability. Sci. Rep. 10, 4164. doi: 10.1038/s41598-020-60889-4
McClelland J. W. (2004). Increasing river discharge in the Eurasian Arctic: Consideration of dams, permafrost thaw, and fires as potential agents of change. J. Geophys. Res. 109, 291. doi: 10.1029/2004JD004583
McClelland J. W., Déry S. J., Peterson B. J., Holmes R. M., Wood E. F. (2006). A pan-arctic evaluation of changes in river discharge during the latter half of the 20th century. Geophys. Res. Lett. 33, 863. doi: 10.1029/2006GL025753
McKinney M. A., Iverson S. J., Fisk A. T., Sonne C., Rigét F. F., Letcher R. J., et al. (2013). Global change effects on the long-term feeding ecology and contaminant exposures of East Greenland polar bears. Glob. Chang. Biol. 19, 2360–2372. doi: 10.1111/gcb.12241
McNicholl D. G., Davoren G. K., Majewski A. R., Reist J. D. (2018). Isotopic niche overlap between co-occurring capelin (Mallotus villosus) and polar cod (Boreogadus saida) and the effect of lipid extraction on stable isotope ratios. Polar Biol. 41, 423–432. doi: 10.1007/s00300-017-2199-8
Meredith M., Sommerkorn M., Cassotta S., Derksen C., Ekaykin A., Hollowed A., et al. (2019). “Polar regions,” in IPCC special report on the ocean and cryosphere in a changing climate. Eds. Pörtner H.-O., Roberts D. C., Masson-Delmotte V., Zhai P., Tignor M., Poloczanska E., et al (Cambridge: Cambridge University Press), 203–320. doi: 10.1017/9781009157964.00
Middelbo A. B., Møller E. F., Arendt K. E., Thyrring J., Sejr M. K. (2019). Spatial, seasonal and inter-annual variation in abundance and carbon turnover of small copepods in young sound, northeast Greenland. Polar Biol. 42, 179–193. doi: 10.1007/s00300-018-2416-0
Mundy C. J., Gosselin M., Ehn J., Gratton Y., Rossnagel A., Barber D. G., et al. (2009). Contribution of under-ice primary production to an ice-edge upwelling phytoplankton bloom in the Canadian Beaufort Sea. Geophys. Res. Lett. 36, 1111. doi: 10.1029/2009GL038837
Nakano T., Matsuno K., Nishizawa B., Iwahara Y., Mitani Y., Yamamoto J., et al. (2016). Diets and body condition of polar cod (Boreogadus saida) in the northern Bering Sea and chukchi Sea. Polar Biol. 39, 1081–1086. doi: 10.1007/s00300-015-1769-x
NASA National Snow and Ice Data Center Distributed Active Archive Center and R (1993). doi: 10.5065/V47S-KW40. CISL RDA: AARI Russian North Polar Drifting Station Data, from NSIDC.
Neukermans G., Oziel L., Babin M. (2018). Increased intrusion of warming Atlantic water leads to rapid expansion of temperate phytoplankton in the Arctic. Glob. Chang. Biol. 24, 2545–2553. doi: 10.1111/gcb.14075
Nishion S., Kawaguchi Y., Inoue J., Hirawake T., Fujiwara A., Futsuki R., et al. (2015). Nutrient supply and biological response to wind-induced mixing, inertial motion, internal waves, and currents in the northern chukchi Sea. J. Geophys. Res. Oceans 120, 1975–1992. doi: 10.1002/2014JC010407
Nöthig E.-M., Bracher A., Engel A., Metfies K., Niehoff B., Peeken I., et al. (2015). Summertime plankton ecology in fram strait – a compilation of long- and short-term observations. Polar Res. 8369, 1751–8369. doi: 10.3402/polar.v34.23349
Obbard M. E., Cattet M. R. L., Howe E. J., Middel K. R., Newton E. J., Kolenosky G. B., et al. (2016). Trends in body condition in polar bears (Ursus maritimus) from the southern Hudson bay subpopulation in relation to changes in sea ice. Arct. Sci. 2, 15–32. doi: 10.1139/as-2015-0027
Øigård T. A., Lindstrøm U., Haug T., Nilssen K. T., Smout S. (2013). Functional relationship between harp seal body condition and available prey in the Barents Sea. Mar. Ecol. Prog. Ser. 484, 287–301. doi: 10.3354/meps10272
Olsen E., Aanes S., Mehl S., Holst J. C., Aglen A., Gjosaeter H. (2009). Cod, haddock, saithe, herring, and capelin in the Barents Sea and adjacent waters: A review of the biological value of the area. ICES J. Mar. Sci. 67, 87–101. doi: 10.1093/icesjms/fsp229
Olsen L. M., Duarte P., Peralta-Ferriz C., Kauko H. M., Johansson M., Peeken I., et al. (2019). A red tide in the pack ice of the Arctic ocean. Sci. Rep. 9, 9536. doi: 10.1038/s41598-019-45935-0
Orkney A., Platt T., Narayanaswamy B. E., Kostakis I., Bouman H. A. (2020). Bio-optical evidence for increasing Phaeocystis dominance in the barents. Philos. Trans. R Soc. A 378, 20190357. doi: 10.1098/rsta.2019.0357
Oziel L., Baudena A., Ardyna M., Massicotte P., Randelhoff A., Sallée J.-B., et al. (2020). Faster Atlantic currents drive poleward expansion of temperate phytoplankton in the Arctic ocean. Nat. Commun. 11, 1705. doi: 10.1038/s41467-020-15485-5
Oziel L., Neukermans G., Ardyna M., Lancelot C., Tison J.-L., Wassmann P. F., et al. (2017). Role for Atlantic inflows and sea ice loss on shifting phytoplankton blooms in the Barents Sea. J. Geophys. Res. Oceans 120, 5121–5139. doi: 10.1002/2016JC012582
Oziel L., Sirven J., Gascard J.-C. (2016). The Barents Sea frontal zones and water masses variability, (1980–2011). Ocean Sci. 12, 169–184. doi: 10.5194/os-12-169-2016
Paasche Ø., Olsen A., Årthun M., Anderson L. G., Wängberg S-Å., Ashjian C. J., et al. (2019). Addressing Arctic challenges requires a synoptic ocean survey. Eos 100, 52688. doi: 10.1029/2019EO136200
Park J.-Y., Kug J.-S., Bader J., Rolph R., Kwon M. (2015). Amplified Arctic warming by phytoplankton under greenhouse warming. Proc. Natl. Acad. Sci. U.S.A. 112, 5921–5926. doi: 10.1073/pnas.1416884112
Peacock E., Taylor M. K., Laake J., Stirling I. (2013). Population ecology of polar bears in Davis strait, Canada and Greenland. J. Wildl. Manage 77, 463–476. doi: 10.1002/jwmg.489
Perovich D. K., Light B., Eicken H., Jones K. F., Runciman K., Nghiem S. V. (2007). Increasing solar heating of the Arctic ocean and adjacent seas 1979–2005: Attribution and role in the ice-albedo feedback. Geophys. Res. Lett. 34, 509. doi: 10.1029/2007GL031480
Persson J., Stige L. C., Stenseth N. C., Usov N., Martynova D. (2012). Scale-dependent effects of climate on two copepod species, Calanus glacialis and Pseudocalanus minutus, in an Arctic-boreal sea. Mar. Ecol. Prog. Ser. 468, 71–83. doi: 10.3354/meps09944
Piepenburg D., Archambault P., Ambrose W. G., Blanchard A. L., Bluhm B. A., Carroll M. L., et al. (2011). Towards a pan-Arctic inventory of the species diversity of the macro- and megabenthic fauna of the Arctic shelf seas. Mar. Biodivers. 41, 51–70. doi: 10.1007/s12526-010-0059-7
Prop J., Aars J., Bårdsen B.-J., Hanssen S. A., Bech C., Bourgeon S., et al. (2015). Climate change and the increasing impact of polar bears on bird populations. Front. Ecol. Evol. 3. doi: 10.3389/fevo.2015.00033
Ranasinghe R., Ruane A. C., Vautard R., Arnell N., Coppola E., Cruz F. A., et al. (2021). “Climate change information for regional impact and for risk assessment,” in In climate change 2021: The physical science basis. contribution of working group I to the sixth assessment report of the intergovernmental panel on climate change. Eds. Masson-Delmotte, Zhai P., Pirani A., Connors S. L., Péan C., Berger S., et al (Cambridge: Cambridge University Press), 1767–1926. doi: 10.1017/9781009157896.014
Randelhoff A., Sundfjord A. (2018). Short commentary on marine productivity at Arctic shelf breaks: Upwelling, advection and vertical mixing. Ocean Sci. 14, 293–300. doi: 10.5194/os-14-293-2018
Rand K. M., Logerwell E. A. (2011). The first demersal trawl survey of benthic fish and invertebrates in the Beaufort Sea since the late 1970s. Polar Biol. 34, 475–488. doi: 10.1007/s00300-010-0900-2
Reeves R. R., Ewins P. J., Agbayani S., Heide-Jørgensen M. P., Kovacs K. M., Lydersen C., et al. (2014). Distribution of endemic cetaceans in relation to hydrocarbon development and commercial shipping in a warming Arctic. Mar. Policy 44, 375–389. doi: 10.1016/j.marpol.2013.10.005
Renaud P. E., Berge J., Varpe Ø., Lønne O. J., Nahrgang J., Ottesen C., et al. (2012). Is the poleward expansion by Atlantic cod and haddock threatening native polar cod, Boreogadus saida? Polar Biol. 35, 401–412. doi: 10.1007/s00300-011-1085-z
Renaud P. E., Daase M., Banas N. S., Gabrielsen T. M., Søreide J. E., Varpe Ø., et al. (2018). Pelagic food-webs in a changing Arctic: A trait-based perspective suggests a mode of resilience. ICES J. Mar. Sci. 75, 1871–1881. doi: 10.1093/icesjms/fsy063
Renaut S., Devred E., Babin M. (2018). Northward expansion and intensification of phytoplankton growth during the early ice–free season in Arctic. Geophys. Res. Lett. 45, 10,510–590,598. doi: 10.1029/2018GL078995
Renner H. M., Mueter F., Drummond B. A., Warzybok J. A., Sinclair E. H. (2012). Patterns of change in diets of two piscivorous seabird species during 35 years in the pribilof islands. Deep Sea Res. II 65–70, 273–291. doi: 10.1016/j.dsr2.2012.02.014
Rode K. D., Wilson R. R., Regehr E., St Martin M., Douglas D. C., Olson J. (2015). Increased land use by chukchi Sea polar bears in relation to changing sea ice conditions. PloS One 10, e0142213. doi: 10.1371/journal.pone.0142213
Ruck K., Arndal M. F., Biebow N., Dahl J., Flått S., Granskog M., et al. (2022). International access to research infrastructure in the Arctic. Polar Rec. 58, 1–5. doi: 10.1017/S0032247422000249
Søreide J. E., Leu E. V. A., Berge J., Graeve M., Falk-Petersen S. (2010). Timing of blooms, algal food quality and Calanus glacialis reproduction and growth in a changing Arctic. Glob. Chang. Biol. 12, no–no. doi: 10.1111/j.1365-2486.2010.02175.x
Schanke N. L., Bolinesi F., Mangoni O., Katlein C., Anhaus P., Hoppmann M., et al. (2021). Biogeochemical and ecological variability during the late summer – early autumn transition at an ice-floe drift station in the central Arctic ocean. Limnol. Oceanogr. 66, 363–382. doi: 10.1002/lno.11676
Scientific Working Group to the Canada-Greenland Joint Commission on Polar Bear (2016) Re-assessment of the Baffin bay and Kane basin polar bear subpopulations: Final report to the Canada-Greenland joint commission on polar bear from the scientific working group (SWG) of the Canada-Greenland joint commission on polar bear. Available at: https://www.gov.nu.ca/sites/default/files/re-assessment_of_the_baffin_bay_and_kane_basin_polar_bear_subpopulations_ch_1-2.pdf.
Screen J. A., Simmonds I. (2010). The central role of diminishing sea ice in recent Arctic temperature amplification. Nature 464, 1334–1337. doi: 10.1038/nature09051
Serreze M. C., Barrett A. P., Stroeve J. C., Kindig D. N., Holland M. M. (2009). The emergence of surface-based Arctic amplification. Cryosphere 3, 11–19. doi: 10.5194/tc-3-11-2009
Seuthe L., Rokkan Iversen K., Narcy F. (2011). Microbial processes in a high-latitude fjord (Kongsfjorden, svalbard): II. ciliates and dinoflagellates. Polar Biol. 34, 751–766. doi: 10.1007/s00300-010-0930-9
Soltwedel T., Bauerfeind E., Bergmann M., Budaeva N., Hoste E., Jaeckisch N., et al. (2005). HAUSGARTEN: Multidisciplinary investigations at a deep-Sea, long-term observatory in the Arctic ocean. Oceanography 18, 46–61. doi: 10.5670/oceanog.2005.24
Starkweather S., Larsen J. R., Kruemmel E., Eicken H., Arthurs D., Bradley A. C., et al. (2021). Sustaining Arctic observing networks’ (SAON) roadmap for Arctic observing and data systems (ROADS). Arctic 74, 56–68. doi: 10.14430/arctic74330
Stempniewicz L., Goc M., Kidawa D., Urbański J., Hadwiczak M., Zwolicki A. (2017). Marine birds and mammals foraging in the rapidly deglaciating Arctic fjord - numbers, distribution and habitat preferences. Clim. Change 140, 533–548. doi: 10.1007/s10584-016-1853-4
Stempniewicz L., Kidawa D., Barcikowski M., Iliszko L. (2014). Unusual hunting and feeding behaviour of polar bears on spitsbergen. Polar Rec. 50, 216–219. doi: 10.1017/S0032247413000053
Stempniewicz L., Kulaszewicz I., Aars J. (2021). Yes, they can: polar bears Ursus maritimus successfully hunt Svalbard reindeer Rangifer tarandus platyrhynchus. Polar Biol. 44, 2199–2206. doi: 10.1007/s00300-021-02954-w
Stenson G. B., Buren A. D., Koen-Alonso M. (2016). The impact of changing climate and abundance on reproduction in an ice-dependent species, the Northwest Atlantic harp seal, Pagophilus groenlandicus. ICES J. Mar. Sci. 73, 250–262. doi: 10.1093/icesjms/fsv202
Stocker T. F., Qin D., Plattner G.-K., Tignor M., Allen S. K., Boschung J., et al (Eds.) (2013). “Climate change 2013: The physical science basis,” in Contribution of working group I to the fifth assessment report of the intergovernmental panel on climate change (Cambridge: Cambridge University Press).
Storrie L., Lydersen C., Andersen M., Wynn R. B., Kovacs K. M. (2018). Determining the species assemblage and habitat use of cetaceans in the Svalbard archipelago, based on observations from 2002 to 2014. Polar Res. 37, 1463065. doi: 10.1080/17518369.2018.1463065
Strass V. H., Nöthig E.-M. (1996). Seasonal shifts in ice edge phytoplankton blooms in the Barents Sea related to the water column stability. Polar Biol. 16, 409–422. doi: 10.1007/BF02390423
Suzuki K. W., Bouchard C., Robert D., Fortier L. (2015). Spatiotemporal occurrence of summer ichthyoplankton in the southeast Beaufort Sea. Polar Biol. 38, 1379–1389. doi: 10.1007/s00300-015-1701-4
Terhaar J., Lauerwald R., Regnier P., Gruber N., Bopp L. (2021). Around one third of current Arctic ocean primary production sustained by rivers and coastal erosion. Nat. Commun. 12, 1–10. doi: 10.1038/s41467-020-20470-z
Terhaar J., Orr J. C., Ethé C., Regnier P., Bopp L. (2019). Simulated Arctic ocean response to doubling of riverine carbon and nutrient delivery. Glob. Biogeochem. Cycl. 33, 1048–1070. doi: 10.1029/2019GB006200
Thompson S. A., Sydeman W. J., Santora J. A., Morgan K. H., Crawford W., Burrows M. T. (2012). Phenology of pelagic seabird abundance relative to marine climate change in the Alaska gyre. Mar. Ecol. Prog. Ser. 454, 159–170. doi: 10.3354/meps09598
Uchimiya M., Motegi C., Nishino S., Kawaguchi Y., Inoue J., Ogawa H., et al. (2016). Coupled response of bacterial production to a wind-induced fall phytoplankton bloom and sediment resuspension in the chukchi Sea shelf, Western Arctic ocean. Front. Mar. Sci. 3. doi: 10.3389/fmars.2016.00231
Vacquié-Garcia J., Lydersen C., Ims R. A., Kovacs K. M. (2018). Habitats and movement patterns of white whales Delphinapterus leucas in Svalbard, Norway in a changing climate. Mov. Ecol. 6, 21. doi: 10.1186/s40462-018-0139-z
van Pelt T. I., Huntington H. P., Romanenko O. V., Mueter F. J. (2017). The missing middle: Central Arctic ocean gaps in fishery research and science coordination. Mar. Policy 85, 79–86. doi: 10.1016/j.marpol.2017.08.008
Vaqué D., Lara E., Arrieta J. M., Holding J., Sà E. L., Hendriks I. E., et al. (2019). Warming and CO2 enhance arctic heterotrophic microbial activity. Front. Microbiol. 10. doi: 10.3389/fmicb.2019.00494
Vihtakari M. (2022). ggOceanMaps: Plot data on oceanographic maps using ‘ggplot2’. R package version 2.1.4. Available at: https://mikkovihtakari.github.io/ggOceanMaps/ [accessed September 16, 2022]
Vihtakari M., Welcker J., Moe B., Chastel O., Tartu S., Hop H., et al. (2018). Black-legged kittiwakes as messengers of atlantification in the Arctic. Sci. Rep. 8, 1178. doi: 10.1038/s41598-017-19118-8
Vihma T., Screen J., Tjernström M., Newton B., Zhang X., Popova V., et al. (2016). The atmospheric role in the Arctic water cycle: A review on processes, past and future changes, and their impacts. J. Geophy Res: Biogeosci 121 (3), 586–620. doi: 10.1002/2015JG003132
Víkingsson G. A., Pike D. G., Valdimarsson H., Schleimer A., Gunnlaugsson T., Silva T., et al. (2015). Distribution, abundance, and feeding ecology of baleen whales in icelandic waters: Have recent environmental changes had an effect? Front. Ecol. Evol. 3. doi: 10.3389/fevo.2015.00006
Vincent W. F. (2010). Microbial ecosystem responses to rapid climate change in the Arctic. ISME J. 4, 1087–1090. doi: 10.1038/ismej.2010.108
Węsławski J. M., Dragańska-Deja K., Legeżyńska J., Walczowski W. (2018). Range extension of a boreal amphipod Gammarus oceanicus in the warming Arctic. Ecol. Evol. 8, 7624–7632. doi: 10.1002/ece3.4281
Węsławski J. M., Legeżyńska J., Kotwicki L., Mazurkiewicz M., Olenin S. (2021). Gammarus (Amphipoda) species competitive exclusion or coexistence as a result of climate change in the Arctic? Pol. Polar Res. 42 (4), 287–302. doi: 10.24425/ppr.2021.138586
Węsławski J. M., Legeżyńska J., Włodarska-Kowalczuk M. (2020). Will shrinking body size and increasing species diversity of crustaceans follow the warming of the Arctic littoral? Ecol. Evol. 10, 10305–10313. doi: 10.1002/ece3.6780
Węsławski J. M., Legezytńska J. (1998). Glaciers caused zooplankton mortality? J. Plankton Res. 20, 1233–1240. doi: 10.1093/plankt/20.7.1233
Węsławski J. M., Wiktor J., Kotwicki L. (2010). Increase in biodiversity in the arctic rocky littoral, sorkappland, Svalbard, after 20 years of climate warming. Mar. Biodivers. 40, 123–130. doi: 10.1007/s12526-010-0038-z
Waga H., Hirawake T. (2020). Changing occurrences of fall blooms associated with variations in phytoplankton size structure in the pacific Arctic. Front. Mar. Sci. 7. doi: 10.3389/fmars.2020.00209
Waga H., Hirawake T., Fujiwara A., Grebmeier J. M., Saitoh S.-I. (2019). Impact of spatiotemporal variability in phytoplankton size structure on benthic macrofaunal distribution in the pacific Arctic. Deep Sea Res. Part II: Topic. Stud. Oceanogr. 162, 114–126. doi: 10.1016/j.dsr2.2018.10.008
Wang Q. (2021). Stronger variability in the Arctic ocean induced by sea ice decline in a warming climate: Freshwater storage, dynamic sea level and surface circulation. J. Geophys. Res. Oceans 126, e2020JC016886. doi: 10.1029/2020JC016886
Wassmann P., Duarte C. M., Agustí S., Sejr M. K. (2011). Footprints of climate change in the Arctic marine ecosystem. Glob. Chang. Biol. 17, 1235–1249. doi: 10.1111/j.1365-2486.2010.02311.x
Watt C. A., Ferguson S. H. (2015). Fatty acids and stable isotopes (δ13 c and δ15 n) reveal temporal changes in narwhal (Monodon monoceros) diet linked to migration patterns. Mar. Mamm. Sci. 31, 21–44. doi: 10.1111/mms.12131
Watt C. A., Orr J., Ferguson S. H. (2016). A shift in foraging behaviour of beluga whales Delphinapterus leucas from the threatened Cumberland sound population may reflect a changing Arctic food web. Endang. Spec. Res. 31, 259–270. doi: 10.3354/esr00768
Werner K., Fritz M., Morata N., Keil K., Pavlov A., Peeken I., et al. (2016). Arctic In rapid transition: Priorities for the future of marine and coastal research in the Arctic. Polar Sci. 10, 364–373. doi: 10.1016/j.polar.2016.04.005
Weydmann A., Carstensen J., Goszczko I., Dmoch K., Olszewska A., Kwasniewski S. (2014). Shift towards the dominance of boreal species in the Arctic: Inter-annual and spatial zooplankton variability in the West spitsbergen current. Mar. Ecol. Prog. Ser. 501, 41–52. doi: 10.3354/meps10694
Weydmann A., Walczowski W., Carstensen J., Kwaśniewski S. (2018). Warming of subarctic waters accelerates development of a key marine zooplankton Calanus finmarchicus. Glob. Chang. Biol. 24, 172–183. doi: 10.1111/gcb.13864
Whiteman J. P., Harlow H. J., Durner G. M., Regehr E., Amstrup S. C., Ben-David M. (2018). Phenotypic plasticity and climate change: Can polar bears respond to longer Arctic summers with an adaptive fast? Oecologia 186, 369–381. doi: 10.1007/s00442-017-4023-0
Wiktor J. M., Tatarek A., Kruss A., Singh R. K., Wiktor J. M., Søreide J. E. (2022). Comparison of macroalgae meadows in warm Atlantic versus cold Arctic regimes in the high-Arctic Svalbard. Front. Mar. Sci. 9. doi: 10.3389/fmars.2022.1021675
Will A. P., Kitaiskaia E. V., Kitaysky A. S. (2018). Red-legged kittiwake feathers link food availability to environmental changes in the Bering Sea. Mar. Ecol. Prog. Ser. 593, 261–274. doi: 10.3354/meps12509
Will A. P., Kitaysky A. S. (2018). Variability in trophic level and habitat use in response to environmental forcing: Isotopic niche dynamics of breeding seabirds in the southeastern Bering Sea. Mar. Ecol. Prog. Ser. 593, 247–260. doi: 10.3354/meps12471
Wisz M. S., Broennimann O., Grønkjær P., Møller P. R., Olsen S. M., Swingedouw D., et al. (2015). Arctic Warming will promote Atlantic–pacific fish interchange. Nat. Clim. Chang. 5, 261–265. doi: 10.1038/NCLIMATE2500
Keywords: impacts, Arctic, climate change, marine ecosystems, food webs, footprints
Citation: Brandt S, Wassmann P and Piepenburg D (2023) Revisiting the footprints of climate change in Arctic marine food webs: An assessment of knowledge gained since 2010. Front. Mar. Sci. 10:1096222. doi: 10.3389/fmars.2023.1096222
Received: 11 November 2022; Accepted: 09 January 2023;
Published: 30 January 2023.
Edited by:
Kui Zhang, South China Sea Fisheries Research Institute (CAFS), ChinaReviewed by:
Jan Marcin Weslawski, Polish Academy of Sciences, PolandJacqueline Mary Grebmeier, University of Maryland, College Park, United States
Copyright © 2023 Brandt, Wassmann and Piepenburg. This is an open-access article distributed under the terms of the Creative Commons Attribution License (CC BY). The use, distribution or reproduction in other forums is permitted, provided the original author(s) and the copyright owner(s) are credited and that the original publication in this journal is cited, in accordance with accepted academic practice. No use, distribution or reproduction is permitted which does not comply with these terms.
*Correspondence: Dieter Piepenburg, Dieter.Piepenburg@awi.de