- 1Institut des Sciences de la Mer, Université du Québec à Rimouski, Rimouski, QC, Canada
- 2BOREA, UMR-MNHN, CNRS, UPMC, IRD, UCN, UA, Muséum National d’Histoire Naturelle, Paris, France
- 3CNRS, LEMAR, UMR 6539, Institut Universitaire Européen de la Mer, Plouzané, France
- 4TBM environnement et SOMME, Plouzané, France
- 5Department of Biology, University of Victoria, Victoria, BC, Canada
The uncontrolled colonization of benthic organisms on submerged surfaces, also called biofouling, causes severe damage in the shipping and aquaculture industries. Biofouling starts with a benthic biofilm composed of a complex assemblage of microbes, bacteria and benthic diatoms, called microfouling, on which macrofouling invertebrate species settle and grow. Invertebrate larvae may use natural soundscapes to orientate inshore and choose their optimal habitat. Recent studies have demonstrated that ship sounds enhance the larval settlement and growth of several invertebrate species, such as mussels, associated with biofouling. Among invertebrates, effects of sound generated by offshore human activities are still poorly studied. This study aims to assess the effect of pile driving, drilling and vessel sounds on model species associated with micro and macrofouling. First, the biofilm development of Navicula pelliculosa and Amphora coffeaeformis was assessed, then, the larval development of the blue mussel (Mytilus edulis) was evaluated from the D-veliger to the postlarval stage. Mussel larvae and microalgae were exposed 12 h each day in tanks (Larvosonic) adapted to sound experiments under controlled conditions. All anthropogenic sounds induced a thinner N. pelliculosa biofilm coupled with a lower microalgae concentration. The drilling sound had a stronger effect on the biofilm thickness. The drilling sound significantly reduced the pediveliger settlement and the postlarvae clearance rate by 70.4% and tended to diminish settler sizes compared to control sound. Contrary to our expectation, pile driving tended to enhance larval recruitment by 22% (P=0.077) and the boat sound did not stimulate larval settlements or recruitment. Drilling sound generated a stressful acoustic environment for pediveliger settlements and postlarvae seem to maintain their shell valves closed to preserve energy. We identify potential causes and mechanisms involved in these impacts of anthropophony on larval ecology and microfouling dynamics.
1 Introduction
Consideration of ambient underwater sound as an important process of recruitment is growing in marine benthic ecology. Natural ambient underwater sounds act as pelagic cues to orientate fish (Montgomery et al., 2006; Simpson et al., 2016), crustaceans (Radford et al., 2007) and coral (Vermeij et al., 2010) larvae. Sounds emitted by reefs and other natural soundscapes, like waves on rocks seem to indicate beneficial conditions for larval settlement (Montgomery et al., 2006) increasing recruitment success and thus affecting local benthic population dynamics. However, the rapid colonization of macro invertebrates on oceanographic equipment, aquaculture systems, water pumps and particularly on vessel hulls is a big concern for the industry as it generates substantial costs for the cleaning of impacted infrastructure (Schultz et al., 2011). For example, mussels, Mytilus galloprovincialis biofouling in New Zealand creates around $16 million yr−1 economic loss in Perna canaliculus aquaculture production (Forrest and Atalah, 2017).
Biofouling starts with a benthic biofilm composed of a complex assemblage of microbes, bacteria and benthic diatoms, called microfouling, on which macrofouling invertebrate species settle and grow. Briefly, organic compounds and microbes are deposited on a clean surface to form an organic “conditioning layer”. This layer acts as a stimulus to bacterial settlement (Dobretsov et al., 2009) and the micro communities develop a quorum sensing communication mechanism (Beitelshees et al., 2018). Bacteria exude a matrix of extracellular polymeric substances (EPS) (Flemming and Wingender, 2010) which facilitate microalgae colonization, usually dominated by diatoms (Bao et al., 2007), followed by fungal and protozoan spores (Callow and Callow, 2011). Mature, thicker and heterogenous biofilm will signal and increase the adhesion abilities of invertebrate larvae or their attachment strength to the substrate (Hadfield, 2011) as shown for the mussel Mytilus edulis (Toupoint et al., 2012b). As other marine benthic bivalves, mussels produce pelagic planktotrophic larvae that develop through several veliger stages until the pediveliger, the competent stage to settlement (Bayne, 1965). Pediveliger larvae use environmental stimuli to settle in an optimal habitat and undertake their metamorphosis (Hadfield and Paul, 2001). If settlement conditions are unsuitable, pediveliger larvae can prolong their pelagic dispersal life and delay their metamorphosis for several weeks (Pechenik, 1990; Martel et al., 2014). These larvae can also settle, metamorphosis and carry out a secondary migration process to find a more suitable environment (Günther, 1992; Forêt et al., 2018).
Some anthropogenic noise can mimic natural sounds, like waves on rocks and thereby stimulate the settlement of benthic invertebrates (McDonald et al., 2014). For example, vessels sounds emitted in the laboratory increased by an order of 4 the larval settlement of mussels, M. edulis, when combined with a trophic cue (Jolivet et al., 2016). Wilkens et al. (2012) found that loud sounds emitted by a freight ferry reduced the median time to attachment by 40% for the mussel, Perna canaliculus. However, the impact seems related to the nature of the anthropogenic sound where louder sounds, like turbine or seismic pulses could interfere with the capacity of larvae to detect trigger settlement cues delaying the metamorphosis of crab megalopae (Pine et al., 2012) or cause direct detrimental effects to the development of scallop veligers (de Soto et al., 2013). Each human marine activity produces its own acoustic signature depending on the gear used and the nature of the bedrock (Hawkins et al., 2015; Carroll et al., 2017; Chauvaud et al., 2018; Solé et al., 2023). Marine shipping constitutes > 90% of the acoustic energy emitted into the sea (Green et al., 1994; McDonald et al., 2014). Vessel and ferry sounds are produced by propellers, motor engines, diesel generators and other equipment involved in the boat machinery producing sound intensities between 140 and 190 dB re 1 μPa m-1 depending on vessel size, speed and power engines (Mitson, 1995; Gervaise et al., 2012; Chauvaud et al., 2018). Oil and gas exploration and exploitation, port area maintenance and expansion, or the development of offshore wind farms require construction phases that produce high levels of sound emission (Chauvaud et al., 2018). Pile driving and drilling are commonly used in marine shipyards and belong to the most powerful activities, after seismic surveys (Chauvaud et al., 2018). Modern pile driving consists of striking large diameter piles with a hydraulic hammer into the seabed. The contact between the hammer and piles produce short (~ 0.1s) and loud pulses (Tougaard et al., 2008) ranging from 192 to 270 dB re 1 μPa m-1 (Bailey et al., 2010). Drilling sound is generated by the drill bit’s high-speed rotation crushing the seabed sediment/rocks. It generates a continuous sound with a relatively loud intensity ranging from 145 to 190 dB re 1 μPa m-1 (Chauvaud et al., 2018).
Documentation of the effects of pile driving and drilling sounds on micro and macrofouling development are lacking in the literature. It is important to understand anthropogenic sound effects on the microorganisms that shape and modulate biofilm dynamics and which have a critical role in the recruitment of species from higher benthic trophic levels (Antunes et al., 2019). The main goal of this study is to understand micro- and macrofouling development exposed to different anthropogenic sound sources. Biofilm development is assessed, including benthic bacterial and algae density during the establishment of two benthic diatoms under pile driving, drilling and boat sounds emission. M. edulis was used as a macrofouling model species to measure the impact of the same anthropogenic sound emissions on mussel planktonic development and recruitment success on artificial collectors without biofilm. We expect that boat sound will stimulate the development of the diatom biofilm and the recruitment success of the mussel. However, we suggest that louder sounds, particularly pile driving, could have a detrimental effect on micro and macrofouling development.
2 Materials and methods
2.1 Experimental emission system
Experiments were carried out at the ISMER-UQAR wet laboratory facilities (Rimouski, Qc, Canada). To limit sound reverberation generated in a small tank (Jézéquel et al., 2018) and to obtain sound treatments as similar as possible to the original sound recorded in situ, we used Larvosonicmesocosms (Figure 1), which included acoustic panels on the internal tank walls, developed and described by Olivier et al. (2023). Multifuser DC2 panels set at the center of each tank wall provided multi-reflection on both vertical and horizontal planes with maximum efficiency in mid and high frequencies (maximum absorption in air between 0.8-2.5 kHz). Trap Fuser set at each corner allowed the sound energy to be trapped in the cavities and/or scattered by the plain surface. The main tank was fully filled with fresh water until the level reached the lid that supported 6 semi-submerged experimental cylinders (5 L) and 6 multiwell plates (6 x 15 mL) (Figure 1). The main structure has the same dimensions as the Larvosonicdescribed by Olivier et al. (2023), but was made of plywood coated with epoxy and rested on 4 steel adjustable feet, compared to the Larvosonicin plexiglass set on an aluminum frame. Three Larvosonictanks were used for the three sound treatments and another without sound (room ambient sound only) considered to the control treatment. Clark synthesis AQ339 Diluvio™ underwater loudspeakers (8Ohms/20-17000Hz, Littleton, CO, USA) set on the bottom center played the sound treatments (Figure 1). As discussed in Olivier et al. (2023), one tank was not an exposure condition, but a sonorous environment where cylinders are isolated from the main tank as they are also and completely isolated and independent from each other, representing a replication level of 6. During each experiment, abiotic conditions (temperature, salinity, etc.) were monitored in each cylinder to ensure that all cylinders displayed similar conditions.
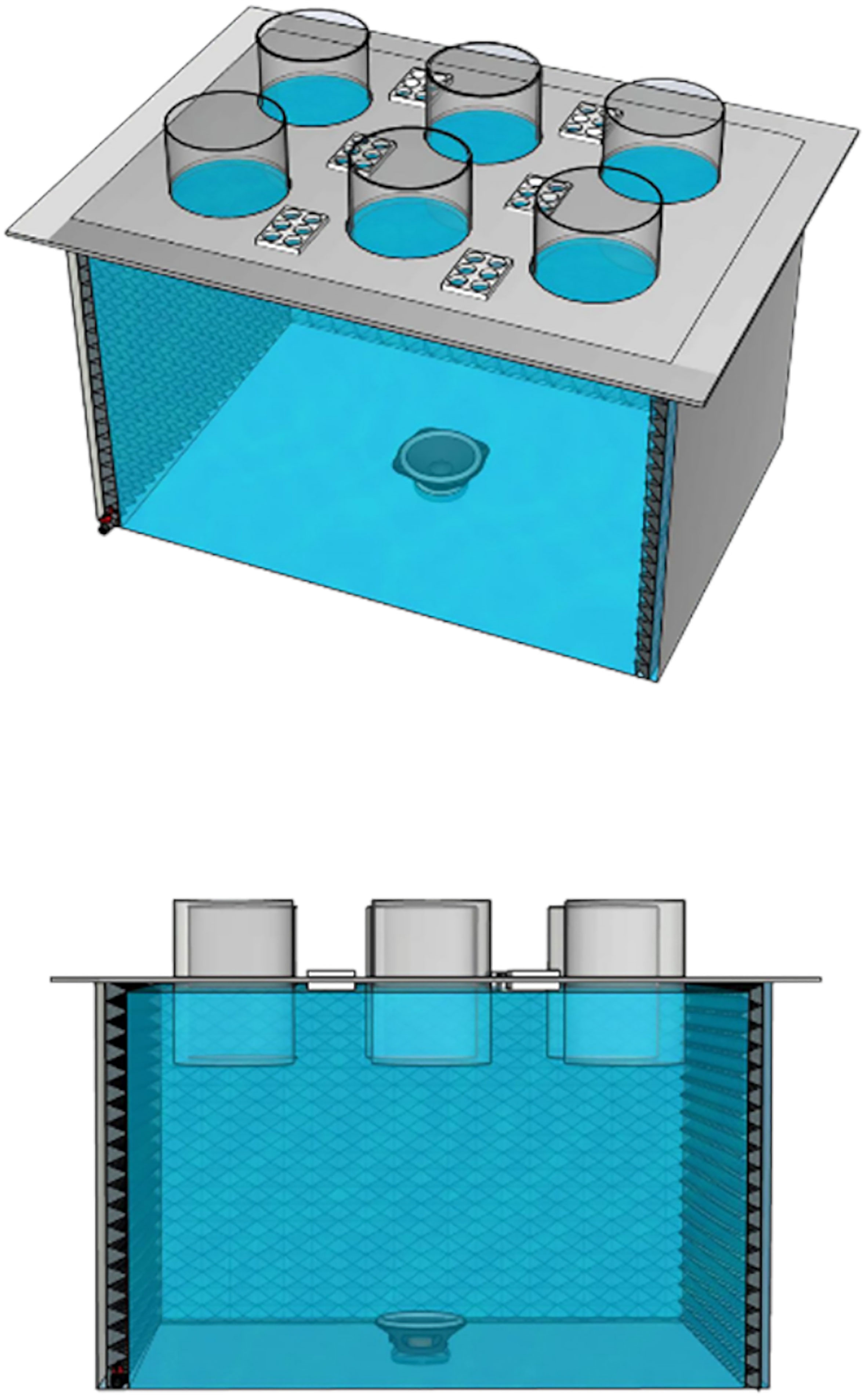
Figure 1 Larvosonic tank developed by Olivier et al. (2023) which includes acoustic Multifuser DC2 and Trap Fuser panels on the vertical walls, 6 semi-submerged cylinders (5L) and 6 multiwell plates (6x15mL).
Speakers were connected to an amplifier (DENON/DN-300Z/16–bit/20-20000Hz/44.1KHz, Cumberland, RI, USA), then to a matrix mixer with a signal processor (Yamaha 26x8 MTX3, Buena Park, CA, USA). Pile driving sound was played via an SD card, directly inserted into the amplifier set in repeat mode. Drilling and boat sounds were played independently with 2 computers connected to the amplifier using VLC media player software set in repeat mode, with both volumes adjusted to 100%. Sound treatments were recorded for 30s in each 5L experimental cylinders (10 cm above the bottom) with an underwater acoustic recorder (Loggerhead LS1, HTI 96-MIN/3V/LED/-170 dB/44.1 KHz, Sarasota, FL, USA) and calibrated to obtain a similar level to that measured in the field. The sound pressure level (dB re 1µPa) - peak to peak - was calculated using the following equation:
where t is the length of the sound and p the pressure units after correcting from volts to μPa. Fourier transformation was applied to each recording to analyze the power spectral density (PSD) using a custom Matlab script (The MathWorks Inc.). Sound treatments were emitted 12 h each day with an alternating sound exposure period of 6 hours followed by 6 hours of silence. A 30 s sequence was looped during experiments. The boat sound used was from a 11 m long D & H Boatbuilding hull equipped with a diesel motor (Cummins 300 hp C series) and was the same originally recorded and used by Jolivet et al. (2016). Drilling and pile driving sounds were recorded during an offshore wind farm installation in the Bay of Saint-Brieuc (France) with a calibrated hydrophone (High Tech, Inc., Mississippi, USA, HTI-99-HF: sensitivity −169.7 dB re 1 V/μ Pa; frequency range 2 Hz to 125 kHz flat response) and the output captured with a calibrated underwater acoustic recorder (RTSYS-Marine Technologies, France, EA-SDA14, 156 kHz, 24-bit resolution). Pile driving is an impulsive sound (one 200 ms impulse every 3 s) dominated by low-frequencies (40 - 800 Hz). The pile driving playback level corresponds to a distance to the source of approximatively 300-400 meters, depending on the project and environmental conditions. Drilling is continuous and its spectrum is characterized by high levels in the 150 - 600 Hz and 4000 - 7000 Hz frequencies range. It corresponds to a distance to the source of approximatively 500 meters.
2.2 Microfouling experiment
Benthic pennate diatoms strains (Amphora coffeaeformis CCMP 127 and Navicula pelliculosa CCMP 543) were obtained from the Center for Culture of Marine Phytoplankton (CCMP), Bigelow Laboratory for Ocean Sciences (West Boothbay Harbor, ME, USA) and cultivated with an autoclaved medium F/2 with silica (Guillard, 1975). Microalgae were cultured under an LED system (72 mixed blue (24) and white (48) LEDs, 14 W, 6500 Ka) at an intensity corresponding to a photon flux of 200 μmol photons m−2 s−1 and ultrafiltered (0.02 µm) and UVs treated seawater with a salinity of 27.1, at 20°C. For each diatom species, 20L of culture was prepared until concentrations over 300 000 cell mL-1 were obtained. For the sound emission experiments, 2 cylinders tank-1 by species illuminated by one LED system (intensity 200 μmol photons m−2 s−1; 14h day : 8h night cycle) were inoculated with 5 million cells of A. coffeaeformis or N. pelliculosa. The volume of the cylinders was completed with ultrafiltered-UVs treated seawater added with F/2 silicate autoclave medium culture added. Into each cylinders, 2 x 3.5 cm-diameter discs roughened by carborundum paper were inserted. Before immersion into the cylinders, discs were washed in a neutral detergent, submerged in 70% aqueous ethanol for 5 min, and then irradiated under UV for 2-h to eliminate any microbial contamination (Leyton and Riquelme, 2008). The use of roughened plexiglass discs promoted benthic biofilm development and facilitated harvesting during sampling. Sounds were emitted for 8 days for N. pelliculosa and 9 days for A. coffeaeformis, and each of the biofilms on the discs was developed enough to be examined without loss. The first disc in each experimental cylinder was used to measure the biofilm thickness by confocal microscopy (Zeiss inverted microscope Axio observer Z1, Oberkochen, Germany, Figure 2 for example). Discs were stored in individual Petri dishes with the upper face upwards and 5 mL utltrafitered-UVs seawater was added to keep biofilms moist until confocal analysis. The biofilm thickness was measured at 5 random spots on the upper face at a magnification of 10x. On each spot, 3-D images of the biofilm were obtained by mosaic of stitching images at each 10 µm using a laser scanning microscope LSM 700 and analyzed by ZEN 2009 software. The second disc in each experimental cylinder was used to estimate the microalgae and bacteria cell abundance (concentration) in the biofilm with the use of CytoFLEX flow cytometry (Beckman Coulter, IN, USA). Briefly, the biofilms were collected by scraping all the upper face with a razor blade. The samples were placed in a 4.5 mL of 25% glutaraldehyde solution and deionized water for 15 min before storing at -80°C. After thawing, samples were ultrasonicated for 10 min to break down cell agglomerations. For each sample, 500 µl was sieved over a 35 µm filter and heterotrophic bacteria were quantified following staining with SYBR Green I nucleic acid bounder (Molecular Probes Inc., OR, USA). Pigmented microalgae cells were quantified by their natural fluorescence (Belzile et al., 2008; Tremblay et al., 2009).
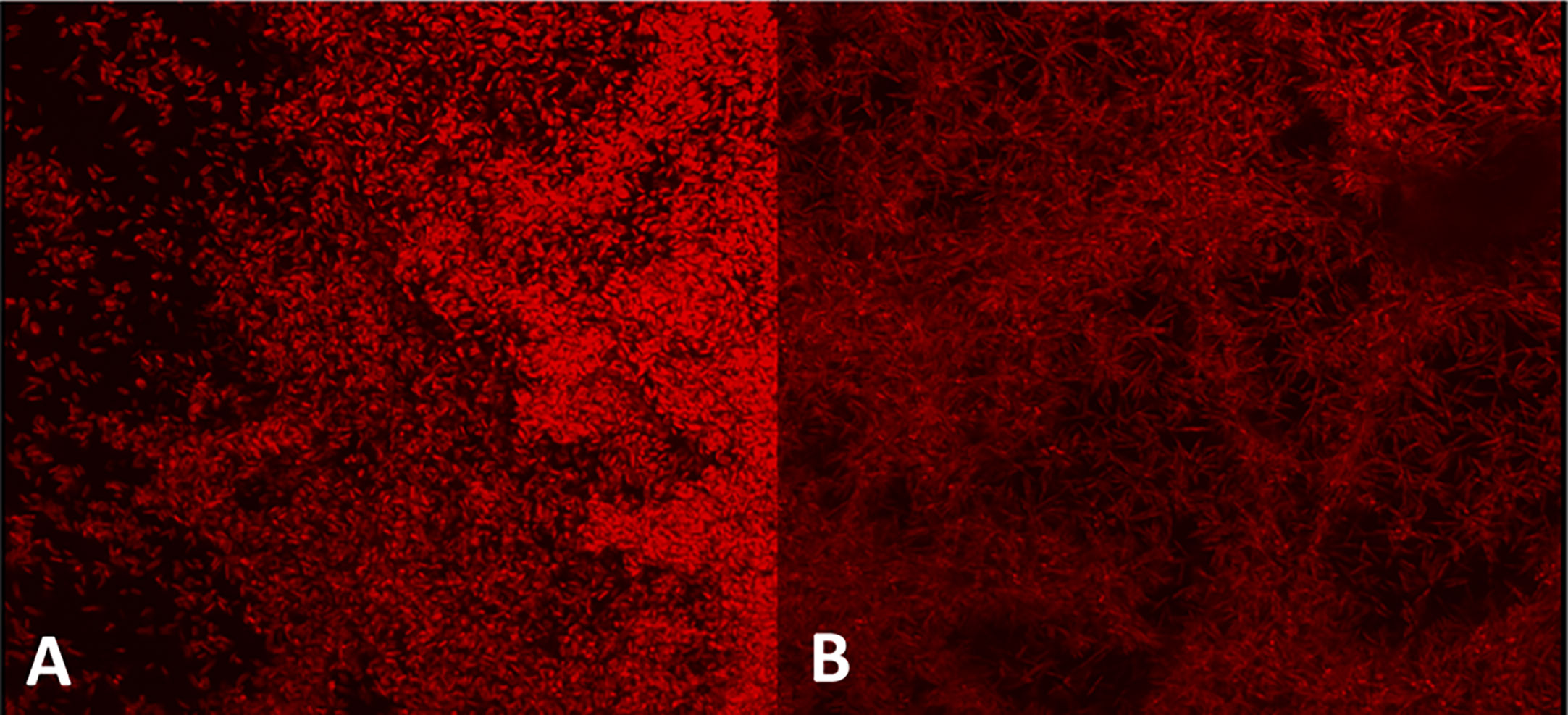
Figure 2 3-D images of the biofilm of Amphora coffeaeformis (A) and Navicula pelliculosa (B) obtained by confocal microscopy (Zeiss inverted microscope Axio observer Z1, Oberkochen, Germany). Images were obtained by mosaic of stitching images at each 10µm using a laser scanning microscope LSM 700 and analyzed by ZEN 2009 software.
2.3 Macrofouling experiments
Mussels, M. edulis, from St. Peters Bay, Prince Edward Island (Canada) were transferred to ISMER-UQAR wet laboratory facilities for larval rearing as described in Rayssac et al. (2010). Spawning was induced on 30 individuals by thermal shock and gametes from different parents were used in a pool-cross design to produce one random larval family. Post-fertilized eggs (66.4 ± 5.3 µm) were transferred to a 100 L bottom flat tank filled with filtered (1 µm) and ultraviolet (UVs) treated seawater at 18-20°C. After 72 h, 25000 D-larvae (113.1 ± 6.5 µm) were transferred into each 5 L experimental cylinder (5 larvae mL-1). During all sound emission experiments, larvae were fed with a mixture of Pavlova lutheri, Tisochrysis lutea, Chaetoceros muelleri, Tetraselmis suecica and Nannochloropsis oculata (1:1:1:1:1, 60000 cell mL-1). Low intensity cool white lights (2.5 ± 0.4 μmol photon m−2 s−1) were aligned and adjusted above each tank with a natural light period of 14h day : 8h night. The temperature during all larvae and postlarvae rearing was maintained between 20 and 22°C.
At 48 h intervals, larvae from each cylinderwere collected on a 35 μm nylon mesh screens and resuspended with 300 mL of 1 μm ultrafiltered and UVs treated seawater to sample 1 mL of larvae for survival and growth estimation. For the growth rates, 30 larvae were measured with the image analysis software Image-Pro Plus coupled to the Evolution VF camera (Media Cybernetics, Silver Spring, MD, USA) and an Olympus BX41 microscope. Survival rates were expressed as the total number of individuals minus the cumulative number of empty shells and based on the first sampling time point. After cleaning the cylinders with Virkon VKS10 disinfectant (LANXESS Deutschland GmbH, Cologne, Germany), the larvae were put back into the growing cylinders with 5 L of 1 µm filtered-UVs treated seawater, with the addition of the microalgae mixture (1:1:1:1:1, 60 000 cell mL-1). When more than 50% of larvae were pediveligers at 14 days post-fertilization (dpf), two collectors consisting of 30 cm polypropylene rope were added to each cylinder. For each following 48 h cleaning session (until the end of the experiment at 24 dpf), the collectors were carefully removed and hung up in the air to avoid juvenile detachment. In parallel, the pelagic larvae were collected on 53 µm nylon mesh screens to estimate survival and growth as described above. At 17 dpf, pelagic larvae were subsampled from each cylinder of the control tank and redistributed randomly into 3 (6 x 20 mL) multiwell plates of all the tanks. In each plate, 3 wells were filled with 10 larvae and 15 mL ultra-filtered-UVs treated seawater with the addition of 60 000 cell mL-1 of microalgae mixture as already described. After 72 hours of sound treatments (until 20 dpf), 1 mL of 4% formaldehyde solution was added to each well and the proportion of settled pediveliger larvae (larvae attached to the well surface) and unattached larvae were counted under a binocular microscope. The ratio between settled and the sum of all larvae was considered as the settlement rate (%).
At 24 dpf, the collectors were carefully removed and gently rinsed with a sprayer over a 100 µm nylon mesh screens to collect the settled postlarvae. The cylinders were sieved on 100 µm nylon mesh screens to collect all the pelagic larvae. 10 mL of the water sieved was sampled to estimate the clearance rate (21 to 24 dpf) with a M4e multisizer coulter counter fitted with a 50 µm aperture tube (Beckman, Mississauga, ON, Canada) using a modified formula described in Comeau et al. (2008):
where Ci is the initial microalgal concentration at T0 (cell mL-1), Cf is the final concentration, V is the volume of seawater (mL), T is the duration (days) of the experiment and N is the number of postlarvae in each well.
Settled postlarvae on the growing cylinder walls were carefully brushed and pooled with the postlarvae settled on the collectors to estimate the total wet biomass of recruits. Around 50 pelagic larvae and 50 settled postlarvae were collected and kept at -80°C for measurements of the prodissochonch (PII) and larval total length (TL) (Martel et al., 2014) with the use of a Keyence VHX-2000 Series digital microscope with VH-Z100UR objectives (Osaka, Japan, 1µm and HDR resolution). A postlarval growth index (PL) was calculated using the following formula:
The remaining postlarvae were weighed and stored in 2 mL of dichloromethane (CH2CL2) in amber glass vials with Teflon-lined caps at -80°C. Lipid extraction was carried out with dichloromethane and methanol following the method described by Parrish (1999), adapted from Folch et al. (1957). Lipid extracts were separated into neutral and polar fractions using a 6% hydrated silica gel column (Marty et al., 1992). The neutral fraction of each sample was eluted with 10 mL of dichloromethane:methanol (98:2) and the polar fraction with 20 mL of methanol, then the neutral fraction was purified on an activated silica gel with 1 mL of hexane:ethyl acetate (v/v) to eliminate free sterols. Fatty acid methyl esters (FAME) were prepared according to the method described in Lepage and Roy (1984) and analyzed using a multichannel Trace GC ultra (Thermo Scientific) gas chromatograph equipped with a Triplus autosampler, a PTV injector, and a ITQ900 (Thermo Scientific) mass detector, and analyzed with Xcalibur v.2.1 software (ThermoScientific, Mississauga, ON, CA). Methyl nonadecanoate (19:0) was used as an internal standard and FAME were identified and quantified using known standards (Supelco 37 Component FAME Mix and menhaden oil; Supleco) and were further confirmed by mass spectrometry.
2.4 Data analysis
For micro and macrofouling experiments, means (± se) of each variable are presented by sound treatment (tank) and defined as the fixed factor to be tested (4 levels corresponding to control, pile driving, drilling and boat noises). PRIMER (version 7.0.13) was used to perform univariate PERMANOVA (based on Euclidean dissimilarities) analyses to compare differences among sound treatments. Homoscedasticity was previously evaluated with PERMDISP tests. When significant differences were obtained (α ≤ 0.05), pairwise multiple comparison tests were used to identify differences among sound treatments. For the mussel experiment, neutral and polar fatty acid composition was tested with a multivariate PERMANOVA with the use of sound treatments as fixed factors.
3 Results
3.1 Acoustic
The sound pressure level recorded in all cylinders of each tank is summarized in Table 1. We observed similar measures among cylinders in the same tank with less than 1% variability. The control sound treatment was subjected to 8% contamination from emission from other tanks with a mean control sound pressure enhanced by 9 dB re 1 µPa compared to the room ambient sound.
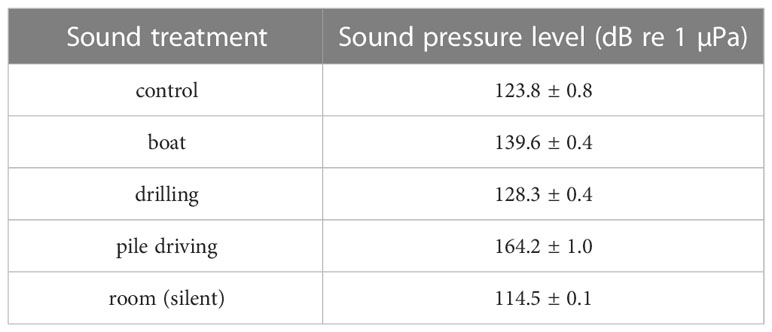
Table 1 Mean sound pressure level (dB re 1 µPa, pk to pk) of pile driving, drilling, boat and control sound emission recorded in LARVOSONIC cylinders (N = 6) during the sound emissions and before the experiments (room).
Pile driving sound recorded in the cylinders reached its maximum power in the 100-500 Hz bandwidth with a maximum peak (200 Hz) around 125 dB re 1 µPa2/Hz. The 150-800Hz frequencies were amplified by 20-30 dB re 1 µPa2/Hz versus their open water values. In the highest frequencies [1000-2000 Hz], the spectrum recorded corroborated with the in situ spectrum varying from 60 to 80 dB re 1 µPa2/Hz, characterized by a series of alternating minima and maxima peaks. Sound power at > 5000 Hz decreased smoothly to 50-60 dB re 1 µPa2/Hz and was slightly amplified by 10 dB compared to in situ spectrum. The drilling sound emitted by the source (Figure 3) had a low energy content in the 30-80 Hz range, matching with the sound recorded during in-situ experiments. Furthermore, the drilling sound emitted by the source displays a slight lower energy content in the 40-60 Hz that could be attributed to temporal variations in the electrical use and pump activities of the experimental wet laboratory. The powerful pile driving sound contaminated the other tank spectra recorded amplifying slightly the 200-800 Hz bandwidth. The nearest tank (drilling) from the pile driving source was the most impacted and exposed from +20 dB to +30 dB re 1 µPa2/Hz in the 250-800 Hz bandwidth versus its in situ intensity. Sound distortion also occurred in the 1000-2000 Hz bandwidth inducing a reduction of about -30 dB (except a peak around 1700 Hz) of the drilling cylinders spectrum. In the 3000-8000 Hz bandwidth, j cylinders ar spectrum power was higher (+5 to + 20dB re 1 µPa2/Hz) than the in situ spectrum. Less distortion occurred due to the boat sound power which maintained its open water soundscape (Figure 3). Frequencies in the 100-1000 Hz were slightly amplified by 5-10 dB re 1 µPa2/Hz. For frequencies > 1000 Hz, cylinders sound power was reduced from -5 dB to -20 dB re 1 µPa2/Hz depending on frequency. Maximum mean boat sound power reached 80 dB re 1 µPa2/Hz< 20Hz. The control sound power was maximum under 50 Hz reaching 60-65 dB re 1 µPa2/Hz. Increase of power occurred in the 200-850Hz bandwidth with maximum values around 200 and 800 Hz, and smoothly decreasing in higher frequencies around 32 dB re 1 µPa2/Hz.
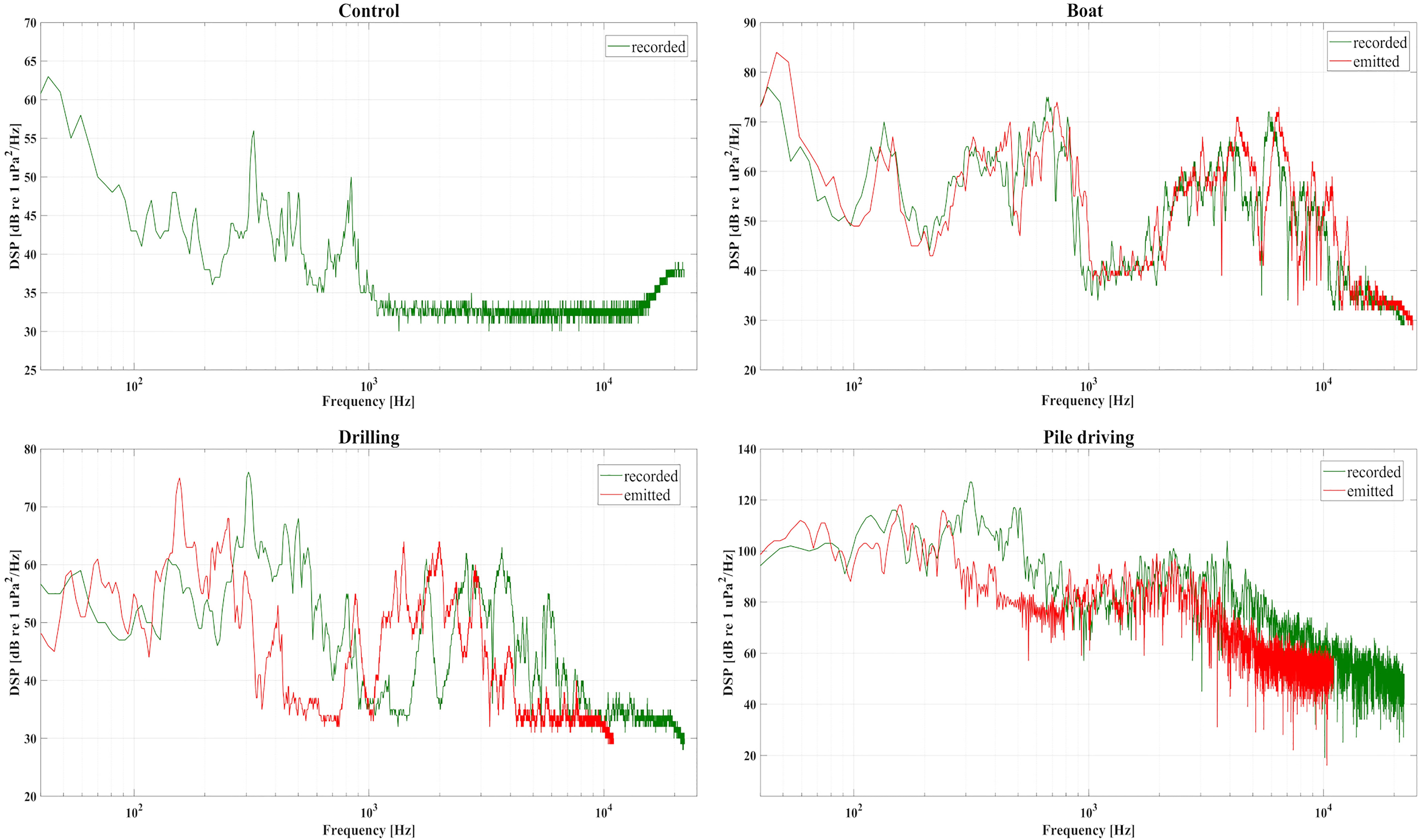
Figure 3 Sound power spectra (dB re 1 µPa/Hz) of the control, boat, drilling and pile driving sound emitted (red) into each tank and recorded (green) in each tank’s central cylinder.
3.2 Microfouling experiments
After 8 days of sound treatment, the N. pelliculosa biofilm (Figures 4A–C) was thinner when exposed to anthropogenic sounds. Drilling and pile driving had a stronger effect and reduced by 47% and 32% respectively the biofilm thickness (Figure 4A). These reductions could be explained by a lower mean concentration of N. pelliculosa cells structuring the biofouling in all anthropogenic sound treatments with a stronger effect when pile driving (-73%) and drilling (-45%) sounds were emitted (Figure 4B). Mean bacteria cell concentration did not differ significantly showing large variations, particularly when boats sound was emitted, as 123% higher bacteria concentrations were measured in the biofilm compared to the control (Figure 4C). The A. coffeaeformis biofilm showed strong variation so that no differences between treatments (sound emissions and control) for each variable measured (thickness, microalgae and bacteria cells concentration) in relation to emission of the different anthropogenic sound (Figures 4D–F) were found.
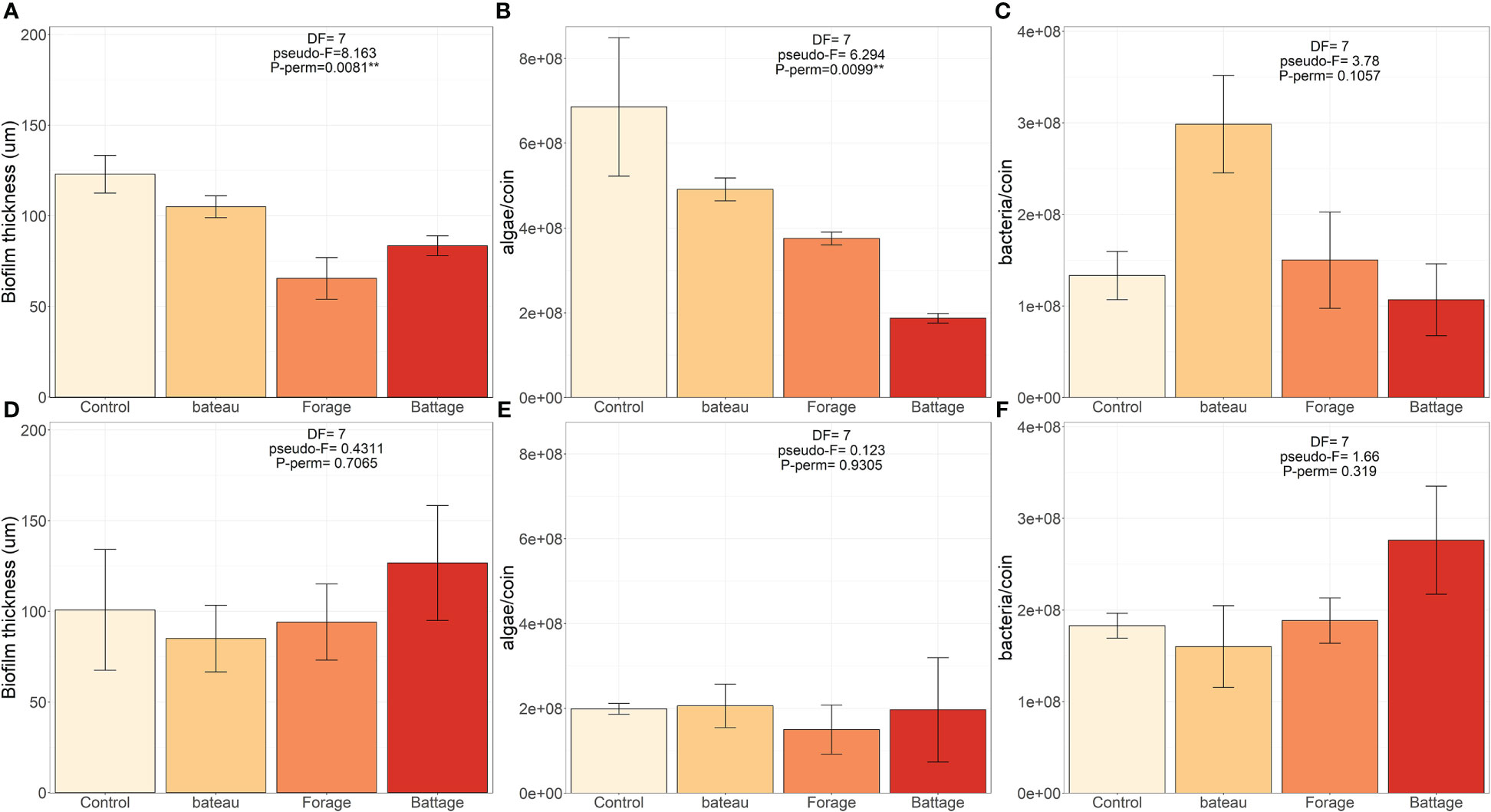
Figure 4 Effect of pile driving, drilling and boat sound treatment on the thickness (µm), total microalgae and bacteria cell count of Navicula pelliculosa (A-C) and Amphora coffeaeformis (D-F) biofilms.
3.3 Macrofouling experiments
Pile driving and drilling sounds tended to reduce by 10% and 11%, respectively, the mean larval survival compared to the control tank, but without a significant effect (Figure 5A). No effect of sound on the larval daily growth was observed (Figure 5B) with mean values > 15 µm day-1 until the appearance of the pediveliger stage (14 dpf).
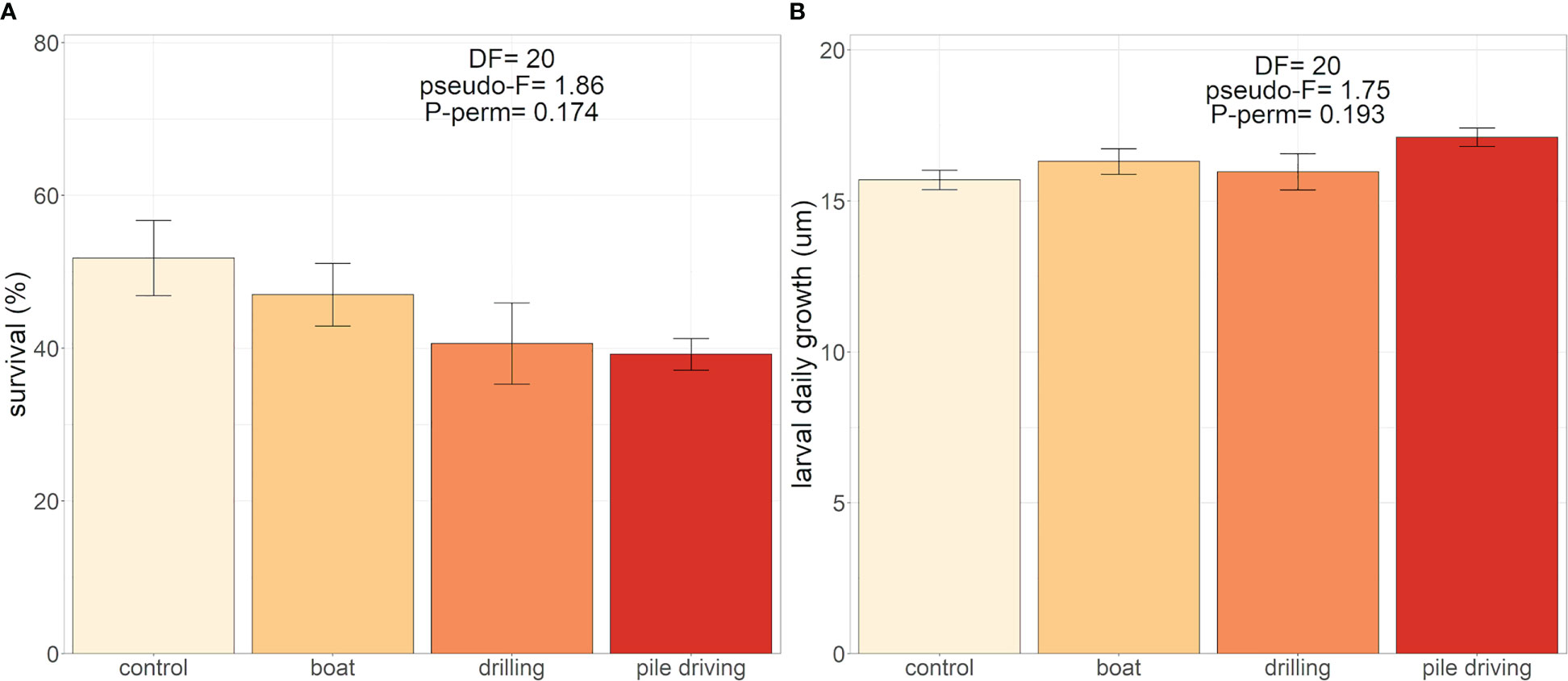
Figure 5 Effect of boat, drilling and pile driving sound treatments on (A) larval survival (%) (N = 6) and (B) the larval daily growth (µm), of Mytilus edulis after 12 days of sound treatments (N = 6).
The settlement rate was significantly different among sound treatments (Figure 6A) and was 36.7% lower (PMC = 0.044) than the control treatment for the pediveliger larvae exposed to drilling sound. Pile driving sound also reduced the settlement rate by 20% but the difference with control treatment was not significant as shown by the pairwise test (PMC = 0.123). Boat and drilling sounds also reduced drastically the clearance rate in postlarvae (Figure 6B) with values 70% lower in the drilling sound treatment (Pperm = 0.037). However, the 57% reduction observed in the boat sound treatment was not significant (Pperm = 0.074). After 21 days of sound treatments, pile-driving sound tended to increase by 21.9% of the total wet mass of spats recruited, a result close to the significant threshold with a p-value of 0.077 (Figure 6C). Sizes at metamorphosis (PII) for settled and swimming postlarvae were similar for all sound treatments (Table 2) with no differences in total length (TL) detected for settled and swimming postlarvae. However, drilling sound tended to reduce the TL of both settled (-7.8%) and swimming (-5.9%) postlarvae. These decreases triggered a lower postlarval growth index (PL< 0.4) but was not significant (Table 2). Drilling sound tended to enhance the total neutral (+59.2%) and polar (+63.8%) fatty acid concentrations in settled recruits (Table 3), but due to large variability among the 6 replicates, differences were not significant. The fatty acid composition (Annex 1) of recruits exposed to different sound treatments was similar for each lipid fraction (neutral: pseudo-F = 1.07, Pperm = 0.38 and polar: pseudo-F = 0.54, P-perm = 0.72).
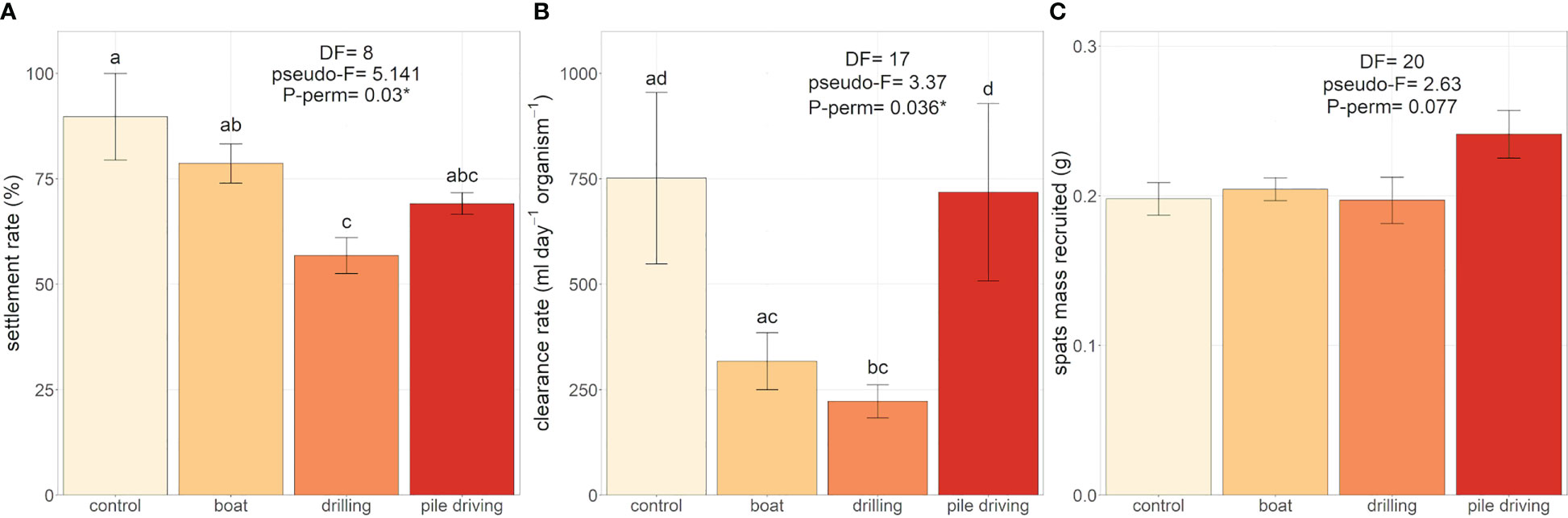
Figure 6 Effect of boat, drilling and pile driving sounds on: (A) pediveliger (17 to 21 dpf) settlement rate and (B) postlarvae (21 to 24 dpf) clearance rate (mL day-1 organism-1) after 72 h of sound exposure in the multiwell plates (N = 3), (C) total mass of spats (g) recruited on collectors and cylinder walls after 21 days of sound treatment (N = 6). “*” means a significant difference detected by the PERMANOVA post-hoc pairwise comparison (α< 0.05).
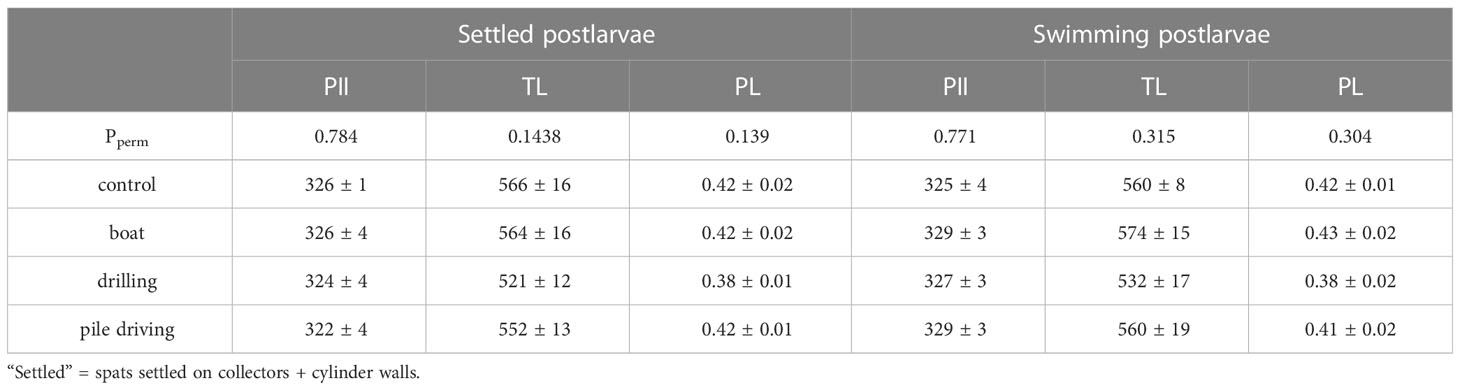
Table 2 Effect of different anthropogenic sound on the sizes (µm) at metamorphosis PII (N = 6), total length TL (µm) (N = 6) and postlarval growth index PL (N = 6) of Mytilus edulis larvae after 21 days of sound treatments.
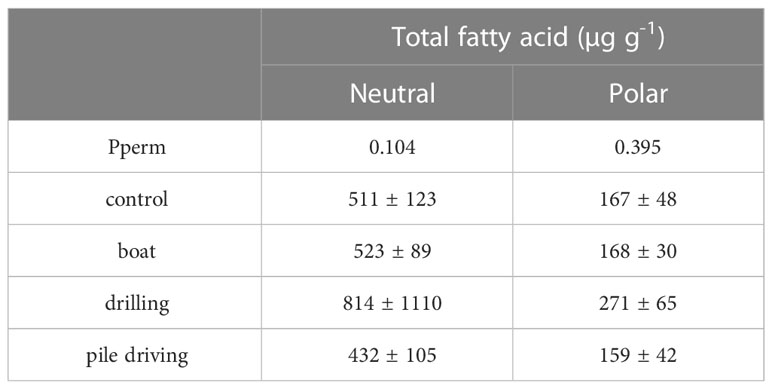
Table 3 Total fatty acid amount of Mytilus edulis spats recruited after 21 days of boat, drilling and pile driving sound treatments (N = 6).
4 Discussion
The experimental platform developed to study the impact of anthropogenic sound on model species structuring biofouling showed high acoustic quality with minimal variability among the sound intensity of the 6 cylinders units in each tank. Thus, the tanks were sonorous environment where cylinders were isolated from the main tank, completely isolated and independent from each other and thus be considered as true replicate as described by Hurlbert (1984). With the use of trap diffusers on the wall of the tanks, reverberation phenomena still occurred in the pile-driving tank amplifying the 200-800 Hz bandwidth frequency and were slightly different than sounds measured in the field. The drilling sound power spectrum was also weakly affected by the powerful sound of pile driving in the same frequencies maybe due to its low intensity. All sound treatments induced a thinner N. pelliculosa biofilm related to a lower development of these microalgae on the discs, particularly when drilling and pile driving sounds were emitted. In these treatments, biofilm thickness was less than 50% compared to the control. However, this impact of anthropogenic sounds on microfouling development seems species specific, as no impact was measured on the development of A. coffeaeformis. Furthermore, we observed that some anthropogenic sounds could also influence the development of the macrofouling. Our study showed for the first time that drilling sound effect (128 dBpk to pk re 1 µPa) the ontogeny of M. edulis and confirmed our hypothesis of a reduction of the settlement rate of the pediveliger larvae (-36.7%) and the clearance rate of post larvae (-70.4%). After 21 days of sound treatments, pile-driving sound (164 dBpk to pk re 1 µPa) showed an intriguing 21.9% increase in the mass of recruited spats, a tendency close to significance (p-value = 0.077). However, this increasing trend in recruitment was not observed for the boat sound treatment, in contrast to our expectations (Figure 7).
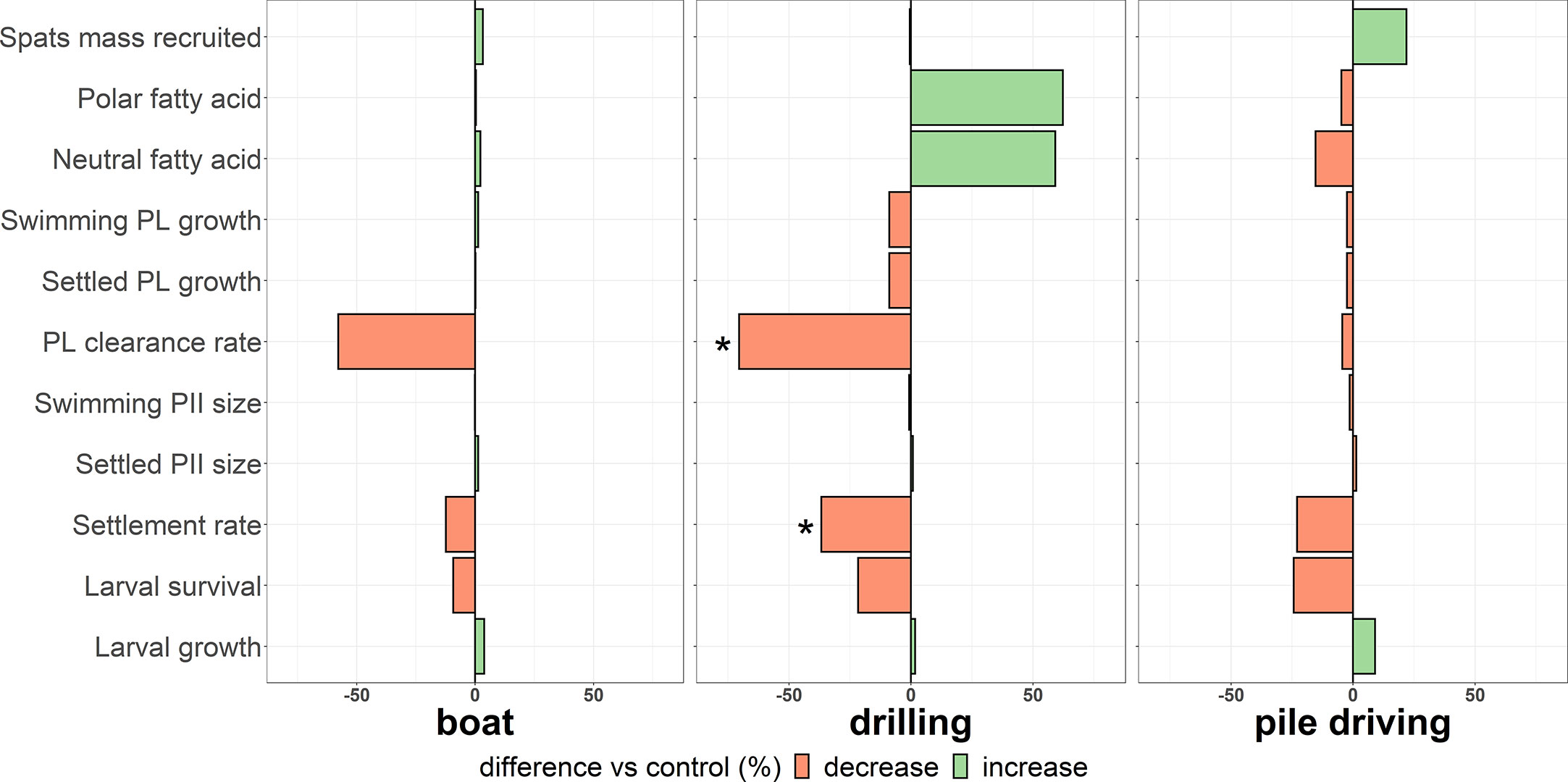
Figure 7 Differences (%) between control and anthropogenic sound treatments for all variables measured on the larval development of Mytilus edulis. “*” means a significant difference detected by the PERMANOVA post-hoc pairwise comparison (α<0.05), “PL” = post larval growth, “PII” = prodissochonch II (pelagic larval shell).
4.1 Drilling sound
All sound treatments tended to induce a thinner N. pelliculosa biofilm, but the drilling treatment had the strongest effect inducing a 47% reduction associated with 45% less cell concentration. Diatoms are a major component of microbial slime and of the global primary production of coastal systems (Smetacek, 1999), and dominate the microphytobenthic community in intertidal mudflats (Doghri et al., 2017). They are the prime colonizer with bacterial communities and largely involved in ship hull fouling (Schultz, 2004; Schultz, 2007; Hakim et al., 2019). The presence of a 1 mm thick slime layer increases significantly the hull drag, reducing ship speed by 15% (Lewthwaite et al., 1985). Diatoms are characterized by a unique silicified cell called a frustule, which is a kind of box composed of 2 halves (Wang et al., 2013; Chen et al., 2019). The epitheca (the lid) closes the hypotheca (the box) connected by one or more girdles that facilitate cell expansion and growth (Molino and Wetherbee, 2008; Stefano et al., 2009). A. coffeaeformis and N. pelliculosa dominate microfouling communities and are pennate diatoms (Mitbavkar and Anil, 2006; Mitbavkar and Anil, 2007; Khandeparker et al., 2014). This group is characterized by the presence of a raphe on both cell valves which is an elongated slit system found on the frustule (Molino and Wetherbee, 2008). This structure allows diatom cells to move or “glide” over a surface to avoid being buried under soft sediments but also to migrate to sufficient light reception and higher nutrient concentrations (Molino and Wetherbee, 2008; Wang et al., 2013; Lachnit et al., 2019). While gliding, diatoms secrete an exopolysaccharide (EPS) mucilage, composed of proteins and carbohydrates with bio-adhesive properties that allow cells to slide but also increase the adhesion of other cells (Higgins et al., 2002; Molino and Wetherbee, 2008; Chen et al., 2019). Diatom adhesion is intimately related to the physico-chemical properties of the submerged surface. It is known that surface roughness, temperature, pH, ionic strength, surface charge, chemical compounds, cell exopolymer, contact time and the nature of the cell are factors involved in the adhesion strength of diatom cells (Klein et al., 2014). The concentration and nature of the bacterial communities are also related to the diatoms and plays a major role in the complex interaction developed by all these microorganisms through EPS, also called “Quorum sensing” (Beitelshees et al., 2018). The diatom-bacteria interactions are species-specific, depend on biofilm maturity, diatom community composition and structure, and environmental conditions (Doghri et al., 2017; Koedooder et al., 2019). Bacterial communities can inhibit or accelerate diatom growth. Moreover, bacterial influence differs whether the biofilm is composed of one strain or several diatoms species and can induce a change in the community and diatom-diatom relationships (Koedooder et al., 2019).
Drilling sound strongly inhibited the development of N. pelliculosa biofilm but did not affect A. coffeaformis. The pressure variation, vibration or particle motion (Popper and Hawkins, 2018) through the viscous-sublayer could generate physical, hydrodynamic conditions that may disturb the ability of N. pelliculosa to adhere onto the discs. The pressure variation or vibration of the cylinders and discs could have induced unfavourable surface physico-chemical properties for N. pelliculosa development (Ozkan and Berberoglu, 2013). Moreover, the sound wave disturbances could induce negative bacterial-diatom interactions resulting in a negative dynamic for N. pelliculosa growth (Koedooder et al., 2019). However, we observed no interaction of drilling sound on bacterial concentration in the biofilm collected. The medium for biofilm growth was Plexiglas discs and only two discs per basin were used for each variable. Therefore, post-hoc tests could not be performed. This lack of statistical power makes it impossible to discriminate whether a single or multiple treatments induced a significant reduction effect on microalgal thickness and concentration in N. pelliculosa. The slowing effect induced by drilling should therefore be interpreted with caution. The biofilm structure and adhesion strength vary according to hydrodynamic conditions (Zargiel and Swain, 2014) and the nature of the submerged surface. A biofilm developing on a ship’s hull will not have the same characteristics as a biofilm developing on a rocky or soft substrate (Klein et al., 2014). In our study, we did not quantify the EPS production accumulated on each disc, nor determine the more precise assembly of the bacterial communities. Furthermore, the biofilms were developed in a static environment without turbulence. Further investigations using different natural surfaces, diatom species, as well as a larger number of replicates are needed to understand if the drilling sound effects the adhesion process of pennate diatoms, their cell physiology or the relationship with bacteria.
According to Rittschof et al. (1998), environmental cues determine the larval settlement process of macrofouling species such as ascidians, barnacles, bryozoans and oysters rather than larval choice (Rittschof and Costlow, 1989; Rittschof et al., 1998). Larval settlement responses differ among species according to surface energy (dispersive polar forces as measured by wettability), light and vibration (Rittschof et al., 1998). Pine et al. (2012) found that sound from both wind and tidal turbines (145 dB re 1 µPa) delay the median time (about 18 hours vs silent treatment) to metamorphosis of crab megalopae. The authors argued that this delay is generated by unfavourable conditions generated by anthropogenic sounds or by the “absence of habitat-specific acoustic settlement cues” (Pine et al., 2012; Stanley et al., 2012). Here, we highlight for the first time that drilling sound affects larvae of aquatic invertebrates. In our experiment, the pediveliger settlement rate was reduced but no effect was detected for the size at metamorphosis compared to the control treatment (Table 2). This reduced settlement rate might be related to shell valve closure, which is usually a response of bivalve species under suboptimal or stressful conditions (Riisgard, 1991; Roberts et al., 2015; Durier et al., 2021). The drilling sound could generate stressful “suboptimal acoustic conditions”, potential vibration or particle motion (Popper and Hawkins, 2018) that increase the closure periods of the larval shell valve, decreasing foot activity (Bayne, 1965; Bayne, 1971) and the capacity of competent larvae to explore the substrate and to settle. The decreasing clearance rates observed in postlarvae exposed to drilling sound seem to be in accordance with this hypothesis, as does the 8% decreasing tendency of the postlarval growth (Table 2). Direct physical effects on the mussel epidermal sensory cells or the adductor muscle might also occur and shell valve closure could be a response to stressful neurophysiological stimulation generated by the drilling sound (Lacourse and Northshop, 1978; Roberts et al., 2015). Negative sound effects could also occur in the larval attachment process. Several exogenous factors can affect the byssal attachment of mussel juveniles such as temperature (Lachance et al., 2008), air bubbles or water motion (Alfaro, 2006). Moreover, to reach the substratum, larvae need to cross a potential thin viscous boundary layer present on the substratum surface (Crimaldi et al., 2002; Hendriks et al., 2006; Koehl, 2007). A dysfunction in the byssal thread secretion or complex interactions between the sound wave propagation and vibration with the substratum boundary layer (McDonald et al., 2014) could affect the capacity of mussel larvae to attach. The settlement reduction observed in the 15 mL multiwell plates was not detected in the long-term recruitment process, as the total mass of spat recruited onto the 5 L cylinder collectors and walls was similar to the control. Drilling sound tended to enhance by 60% the total fatty acid amount (neutral and polar) in the juvenile tissue, but these differences were not significant. Fatty acids reflect the physiological condition of an organism (Toupoint, 2012) and correspond to a major source of metabolic energy used for growth, energy storage and other essential physiological maintenance functions (Tremblay et al., 2007; Parrish, 2009; Lee et al., 2018). It remains difficult to understand why these different effects were not detected with the other anthropogenic sounds. Drilling sound was the weakest sound treatment (128 dBpk to pk re 1 µPa) and no such effect was recorded in the loud pile driving tank (164 dBpk to pk re 1 µPa). Pile driving sound is associated with powerful but short (0.1s) pulses (Chauvaud et al., 2018) compared to drilling sound which is characterized by continuous sound wave emission. A continuous and constant sound emission, with a lower sound pressure level such as our drilling sound treatment, versus powerful pile driving pulses, can still have detrimental effects on benthic bivalves.
4.2 Pile-driving sound
In contrast to the drilling treatment, pile driving had less effect on the N. pelliculosa biofilm thickness (-32%) but reduced drastically (-73%) the N. pelliculosa concentration. Similar to the other anthropogenic sound treatments, bacteria concentration in the N. pelliculosa biofilm was not impacted, along with all the indicators of biofilm development of A. coffeaeformis. It is therefore difficult to stipulate that pile driving sound impacts the overall dynamics of the biofilm. Clearly, N. pelliculosa biofilm development was largely sensitive to high energy, particle motion, pressure variation and/or vibration generated by pile driving sound emitted in our experimental conditions.
The non-significant increase of 21.9% mass of mussel recruits in the pile driving sound treatment is still intriguing. In particular, some cylinders in the pile-driving sound exhibited an increase higher than 40% of mass recruited compared to the control. The pile driving treatment is characterized by a higher power emission in the 100-1000 Hz frequency range compared to the other treatments (Figure 3). Animal activities in coastal habitats produce a wide spectrum of sounds but mostly concentrated in the 100-1000 Hz frequency range. These frequencies are known to attract and indicate favorable conditions (Montgomery et al., 2006) for the settlement of coral (Vermeij et al., 2010), fish (Simpson et al., 2016) and crab (Radford et al., 2007) larvae. The pile driving sound could stimulate in a certain way (physical component) or indicate appropriate acoustic conditions for the settlement of mussels which prefer to settle into noisy habitats such as rocky shores (Wilkens et al., 2012). However, this tendency to stimulate mussel recruitment was not found in the multiwell plates. The variation in settlement response observed between the sound treatment and within the tank could also be explained due to resonance phenomena under specific frequencies (Jézéquel et al., 2018). Further experiments testing the effect of different frequencies from powerful sound could potentially demonstrate the implications of certain frequency ranges on the settlement process of invertebrates.
In our experiments, particle motion and vibration were not measured. Sound waves can be transmitted across the substrate and can also generate waves at the interface of the water and the substrate. Interface waves are characterized by low frequencies (> 30 Hz) associated with large particle motion amplitude (Popper and Hawkins, 2018). Energy from these waves are maximum close to the substrate, which could be of major significance and provide “key information” about the environment to the organisms living close to or in the substrate (Popper and Hawkins, 2018). Potential vibration of the adhesion surfaces (cylinders and collectors) could promote mussel larval recruitment. This hypothesis was also mentioned by McDonald et al. (2014) who suggested that boat hull vibration could influence the settlement behavior or stimulate the adhesive release of fouling species such as the ascidian C. intestinalis. These explanations remain hypothetical and raise the complex nature of sound waves propagating into the substrate but also at the interface between the substrate and the water, across the bottom sublayer (Koehl, 2007). Further investigations into the potential effect of particle motion and vibration are required to better understand their implication in the larval settlement process of benthic invertebrates.
De Soto et al. (2013) studied the effect of playback seismic pulses (131rms dB ref 1 µPa) on the New Zealand scallop (Pecten novaezelandiae) larvae for 90 hours, immediately after fertilization. D-veliger showed significant developmental delays and 46% of larvae exposed showed body malformations suggesting that physiological stress was induced by this cumulative sound exposure (De Soto et al., 2013). No such effect was observed on M. edulis larval development with the pile driving sound emitted, as no differences with the control treatment were observed in larval survival, larval growth (Figure 5) or mean size at metamorphosis (Table 2). Similar results were obtained with flatfish Solea solea larvae after 7 days of pile driving (210 dB re 1 µPa2, peak pressure level, 50-1000Hz) (Bolle et al., 2012). Roberts et al. (2015) observed an increase in shell valve closure in M. edulis adults, as a response to sinusoidal vibratory signals in the frequency range of 5 to 410 Hz. This sensitivity increased with lower frequencies (except a response at 410 Hz) leading to potential negative effects on mussel fitness (Roberts et al., 2015). Higher sound wave transmission could be more important in adults due to their bigger size, through external (shell) or internal structures (mantle, foot, statocyst, etc.). Conversely, unsettled larvae devoid of a solid shell (dissochonch) potentially do not interact with these different waveforms. The absence of a short-term effect does not mean that any chronic or sub-lethal effects would not occur over a longer time period. Loud anthropogenic sound exposure during a complete life cycle could potentially highlight chronic or physiological effects on M. edulis fitness (Roberts et al., 2015).
4.3 Boat sound
Boat sound showed the least acoustic distortion and was well preserved the in situ acoustic signature (Figure 3). Boat sound pressure levels emitted in our experimental system were higher (139 dB re 1 µPa) than that of the drilling treatment (128 dB re 1 µPa). This greater intensity could facilitate a better preservation of the acoustic signature. Wilkens et al. (2012) studied the effect of two sound intensities (high and low) of a ferry sound, on the “time to attachment” of Perna canaliculus over 8 hours in 50 mL plastic vials (placed in water baths). Overall, high intensity vessel noise (126 dBRMS re 1 µPa) induced a 40% shorter time for larvae to settle compared to the silent treatment. Larvae exposed to the high intensity noise were attached during the first 72h (Wilkens et al., 2012). Similar results have been observed by Jolivet and colleagues (2016) with the presence of Nannocloropsis occulata, a species with a high level of polyunsaturated fatty acids acting as a settlement cues in mussels (Toupoint et al., 2012a). Other biological and chemical cues have been also determined for the settlement of pediveliger mussel larvae, like mature biofilms (Bao et al., 2007; Toupoint et al., 2012b) and neuroactive compounds (Satuito et al., 1999). However, in our study no enhancement of the settlement was observed as reflected by the absence of increased biomass of recruits at 24 dpf. Without biofilms, trophic triggers and water motion, the sterile substrate of the 15 mL wells was probably not a suitable habitat to stimulate pediveliger settlement. Jolivet et al. (2016) performed settlement experiments in 240 mL cylinders whereas Wilkens et al. (2012) used 50 mL vials. Furthermore, food condition of mussels was not modified during settlement experiments strengthening the results of Jolivet et al. (2016) demonstrating that the boat sound increases settlement of pediveliger mussel larvae when combined to trophic settlement triggers. Each well unit was previously sanded to enhance substrate roughness and facilitate larval adhesion abilities (Abelson and Denny, 1997). Nevertheless, the bottom microstructures apparently did not promote larval attachment in addition to the expected positive boat sound effect. The substratum surface microstructures were smaller than larvae and possibly did not disrupt the potential thin viscous boundary layer (Koehl, 2007). On the other hand, anthropogenic sound waves might also maintain certain hydrodynamic forces in the low water volume that prevent larvae from attaching. Additional water flow analyses at a finer scale in experimental units could provide information on the hydrodynamic properties (Koehl, 2007) and eventual disruption generated by soundwave propagation in the substrate/sublayer interface.
Hydrodynamic forces influence fluid motion and are an essential physical component that determines the larval recruitment success of biofouler larvae (Crimaldi et al., 2002; Koehl, 2007). The larval development of mussels occurs naturally in turbulent shallow waters and pediveliger larvae have strong adhesive abilities (byssus threads) that allow larval settlement under high velocities on hard substrata (Koehl, 2007). Eyster and Pechenik (1987) recorded that water agitation enhanced M. edulis larval attachment onto filaments two to eight fold in 2 L beakers. Carrying out settlement assays in a relatively small water volume probably underestimated the number of settled larvae (Pechenik, 1990). The lack of water motion likely generated a mismatch to the larval response under natural physical processes that naturally drive mussel settlement. The use of flowing water and higher-volume experimental units could be a better choice with which to perform future larval settlement experiments. Such an experimental setup would offer potentially more favorable physical conditions for mussel settlements and the implementation of essential small-scale hydrodynamic analyses (Koehl, 2007). Studying the development of micro and macrofouling during the same experiment under different anthropogenic sounds is necessary to assess the effect of anthropophony on the complete establishment of biofouling.
5 Conclusion
We observed that anthropogenic sound induced different effects on the biofilm development dependent on species involved and specific sounds. With respect to microfouling, all types of sounds tested showed no impact on bacteria concentration constituting the biofilm and on the development of A. coffeaeformis, but drilling and pile driving sounds impacted negatively the development of N. pelliculosa biofilm. These results suggest that these sound emissions were characterized by intensity and/or power spectrum generating substrate interference and unfavourable conditions for the establishment of the N. pelliculosa diatom slime layer. More research is needed to understand the sensitivity mechanisms of diatoms species in relation to substrate interference related to sound emission. Evaluating the sound effect on macrofouling development, we selected the blue mussel as a model species for this study. It was negatively impacted by the drilling sound characterized by emission of 128 dB re 1 µPa, particularly at the competent stage to settle and after metamorphosis and settlement on the substrate. Thus, negative impacts have been measured only when mussels were in contact with the substrate. We suggest that the sound treatment could induce a stressful acoustic environment for the development of postlarvae which prefer to reduce their metabolism and conserve their energy. The variation in settlement response between the experimental units raises some questions about resonance and distortion of sound spectra in the tanks and might explain why a 21.9% increase in recruitment success in the pile-driving treatment was non-significant. Bivalves start their life in the water column, then swim and crawl at the interface of the substrate and the bottom layer to finally attach and connect to the seabed. This transition from pelagic life to a benthic environment should be considered a sensitive stage for anthropogenic activities interacting with the seabed. However, in accordance to Slabbekoorn and Bouton (2008), the responses could be certainly best tested, not only by using replicate set of individuals, but also as well as a replicate set of call recordings. Also, further studies on the potential effect of noise on the complex interactions between substrate sound propagation/vibration and particle motion with the viscous sublayer and particularly the larval perception of sound propagation or substrate borne vibration are required to better understand the larval settlement process on a finer scale.
Data availability statement
The raw data supporting the conclusions of this article will be made available by the authors, without undue reservation.
Author contributions
GC carried out the experiments, performed statistical analysis and has written the manuscript. RT supervised all the study, established the methodology and provided technical support. FO and LC developed the LARVOSONIC basins, provided the underwater acoustic recorder, acoustic support and participate to data analyses. DM performed the acoustic analysis. FJ and GW participated in data analyses. All authors contributed to the article and approved the submitted version.
Funding
We are grateful for the financial support provided for two years by the Natural Sciences and Engineering Research Council of Canada’s (NSERC) Strategic Network, Canadian Healthy Oceans Network (CHONe) (Grant ID: 468437), and its partners: Fisheries and Oceans Canada and the Northern Institute for Research in Environment and Occupational Health and Safety (INREST), representing the Port de Sept-Îles and Ville de Sept-Îles. This work was also supported by complementary funding provided by Ressources Aquatiques Québec (RAQ), a strategic research network funded by the Fonds de recherche du Québec – Nature et technologies (FRQNT, #2014-RS-171172).
Acknowledgments
Our sincere thanks to Nathalie Gauthier and Élodie Bouchard for their help at the Aquaculture Station of UQAR-ISMER. Marine Sciences Institute The study is a contribution to the international laboratory BeBEST.
Conflict of interest
The authors declare that the research was conducted in the absence of any commercial or financial relationships that could be construed as a potential conflict of interest.
Publisher’s note
All claims expressed in this article are solely those of the authors and do not necessarily represent those of their affiliated organizations, or those of the publisher, the editors and the reviewers. Any product that may be evaluated in this article, or claim that may be made by its manufacturer, is not guaranteed or endorsed by the publisher.
Supplementary material
The Supplementary Material for this article can be found online at: https://www.frontiersin.org/articles/10.3389/fmars.2023.1111505/full#supplementary-material
References
Abelson A., Denny M. (1997). Settlement of marine organisms in flow. Annu. Rev. Ecol. System. 28 (1), 317–339. doi: 10.1146/annurev.ecolsys.28.1.317
Alfaro A. C. (2006). Byssal attachment of juvenile mussels, Perna Canaliculus, affected by water motion and air bubbles. Aquaculture 255 (1), 357–361. doi: 10.1016/j.aquaculture.2005.11.059
Antunes J., Leão P., Vasconcelos V. (2019). Marine biofilms: diversity of communities and of chemical cues. Environ. Microbiol. Rep. 11 (3), 287–305. doi: 10.1111/1758-2229.12694
Bailey H., Senior B., Simmons D., Rusin J., Picken G., Thompson P. M. (2010). Assessing underwater noise levels during pile-driving at an offshore windfarm and its potential effects on marine mammals. Mar. Pollut. Bull. 60, 888–897. doi: 10.1016/j.marpolbul.2010.01.003
Bao W. Y., Satuito C. G., Yang J. L., Kitamura H. (2007). Larval settlement and metamorphosis of the mussel Mytilus galloprovincialis in response to biofilms. Mar. Biol. 150, 565–574. doi: 10.1007/s00227-006-0383-4
Bayne B. L. (1965). Growth and the delay of metamorphosis of the larvae of Mytilus edulis (L.). Ophelia 2 (1), 1–47. doi: 10.1080/00785326.1965.10409596
Bayne B. L. (1971). “Some morphological changes that occur at the metamorphosis of the larvae of mytilus edulis,” in Fourth European marine biology symposium. Ed. Crisp D. J. (Cambridge, U.K.: Cambridge University Press), 259–280.
Beitelshees M., Hill A., Jones C. H., Pfeifer B. A. (2018). Phenotypic variation during biofilm formation: implications for anti-biofilm therapeutic design. Materials 11(7), 1086. doi: 10.3390/ma11071086
Belzile C., Brugel S., Nozais C., Gratton Y., Demers S. (2008). Variations of the abundance and nucleic acid content of heterotrophic bacteria in Beaufort shelf waters during winter and spring. J. Mar. Syst. 74 (3), 946–956. doi: 10.1016/j.jmarsys.2007.12.010
Bolle L. J., de Jong C. A. F., Bierman S. M., van Beek P. J. G., van Keeken O. A., Wessels P. W., et al. (2012). Common sole larvae survive high levels of pile-driving sound in controlled exposure experiments. PloS One 7 (3), e33052. doi: 10.1371/journal.pone.0033052
Callow J. A., Callow M. E. (2011). Friendly fouling-resistant marine coatings. Nat. Commun. 2, 210–244. doi: 10.1038/ncomms1251
Carroll A. G., Przeslawski R., Duncan A., Gunning M., Bruce B. (2017). A critical review of the potential impacts of marine seismic surveys on fish & invertebrates. Mar. pollut. Bull. 114, 9–24. doi: 10.1016/j.marpolbul.2016.11.038
Chauvaud S., Chauvaud L., Jolivet A. (2018). Impacts des sons anthropiques sur la faune marine (Versailles Cedex, France: Quae).
Chen L., Weng D., Du C., Wang J., Cao S. (2019). Contribution of frustules and mucilage trails to the mobility of diatom Navicula sp. Sci. Rep. 9 (1), 7342. doi: 10.1038/s41598-019-43663-z
Comeau L. A., Pernet F., Tremblay R., Bates S. S., Leblanc A. (2008). Comparison of Eastern oyster (Crassostrea virginica) and blue mussel (Mytilus edulis) filtration rates at low temperatures. Can. Tech. Rep. Fish. Aquat. Sci., 2810.
Crimaldi J. P., Thompson J. K., Rosman J. H., Lowe R. J., Koseff J. R. (2002). Hydrodynamics of larval settlement: the influence of turbulent stress events at potential recruitment sites. Limnol. Oceanogr. 47 (4), 1137–1151. doi: 10.4319/lo.2002.47.4.1137
Dobretsov S., Teplitski M., Paul V. (2009). Mini-review: quorum sensing in the marine environment and its relationship to biofouling. Biofouling 25 (5), 413–427. doi: 10.1080/08927010902853516
Doghri I., Lavaud J., Dufour A., Bazire A., Lanneluc I., Sablé S. (2017). Cell-bound exopolysaccharides from an axenic culture of the intertidal mudflat Navicula phyllepta diatom affect biofilm formation by benthic bacteria. J. Appl. Phycol. 29 (1), 165–177. doi: 10.1007/s10811-016-0943-z
Durier G., Nadalini J. B., Saint-Louis R., Genard B., Comeau L., Tremblay R. (2021). Sensitivity to oil dispersants: effects on the valve movements of the blue mussel Mytilus edulis and the giant scallop Placopecten magellanicus, in Sub-Arctic conditions. Aquat. Toxicol. 234, 105797. doi: 10.1016/j.aquatox.2021.105797
Eyster L. S., Pechenik J. A. (1987). Attachment of Mytilus edulis l. larvae on algal and byssal filaments is enhanced by water agitation. J. Exp. Mar. Biol. Ecol. 114 (2), 99–110. doi: 10.1016/0022-0981(88)90131-1
Flemming H. C., Wingender J. (2010). The biofilm matrix. Nat. Rev. Microbiol. 8 (9), 623–633. doi: 10.1038/nrmicro2415
Folch J., Lees M., Sloane Stanley G. H. (1957). A smple method for the isolation and purification of total lipides from animal tissues. J. Biol. Chem. 226 (1), 497–509. doi: 10.1016/S0021-9258(18)64849-5
Forêt M., Barbier P., Tremblay R., Meziane T., Neumeier U., Duvieilbourg E., et al. (2018). Trophic cues promote secondary migrations of bivalve recruits in a highly dynamic temperate intertidal system. Ecosphere 9 (12), e02510. doi: 10.1002/ecs2.2510
Forrest B. M., Atalah J. (2017). Significant impact from blue mussel Mytilus galloprovincialis biofouling on aquaculture production of green-lipped mussels in new zealand. Aquacult. Environ. Interact. 9, 115–126. doi: 10.3354/aei00220
Gervaise C., Simard Y., Roy N., Kinda B., Me N. (2012). Shipping noise in whale Habitat : characteristics, sources, budget, and impact on belugas in saguenay – st. Lawrence marine park hub. J. Acoustical Soc. Am. 132 (1), 76–89. doi: 10.1121/1.4728190
Green D. M., DeFerrari H. A., McFadden D., Pearse J. S., Popper A. N., Richardson W. J., et al. (1994). Low-frequency sound and marine mammals: current knowledge and research needs (Washington (DC: National Academy Press).
Guillard R. R.L. (1975). Culture of phytoplankton for feeding marine invertebrates BT - culture of marine invertebrate animals: proceedings — 1st conference on culture of marine invertebrate animals greenport. Eds. Smith W. L., Chanley M. H. (Boston, MA: Springer US), 29–60. doi: 10.1007/978-1-4615-8714-9_3
Günther C. P. (1992). Dispersal of intertidal invertebrates: a strategy to react to disturbance of different scales? Netherlands J. Sea Res. 30, 45–56. doi: 10.1016/0077-7579(92)90044-F
Hadfield M. G. (2011). Biofilms and marine invertebrate larvae: what bacteria produce that larvae use to choose settlement sites. Annu. Rev. Mar. Sci. 3 (1), 453–470. doi: 10.1146/annurev-marine-120709-142753
Hadfield M. G., Paul V. J. (2001). “Natural chemical cues for settlement and metamorphosis of marine- invertebrate larvae,” in Marine chemical ecology. Eds. McClintock J. B., Baker B. J. (Boca Raton, USA: CRC. Press), 431–461. doi: 10.1201/9781420036602.ch13
Hakim M. L., Nugroho B., Nurrohman M. N., Suastika I. K., Utama I. K. A. P. (2019). Investigation of fuel consumption on an operating ship due to biofouling growth and quality of anti-fouling coating. IOP Conf. Series: Earth Environ. Sci. 339, 12037. doi: 10.1088/1755-1315/339/1/012037
Hawkins A. D., Pembroke A. E., Popper A. N. (2015). Information gaps in understanding the effects of noise on fishes and invertebrates. Rev. Fish Biol. Fish. 25 (1), 39–64. doi: 10.1007/s11160-014-9369-3
Hendriks I. E., van Duren L. A., Herman P. M. J. (2006). Turbulence levels in a flume compared to the field: implications for larval settlement studies. J. Sea Res. 55, 15–29. doi: 10.1016/j.seares.2005.09.005
Higgins M. J., Crawford S. A., Mulvaney P., Wetherbee R. (2002). Characterization of the adhesive mucilages secreted by live diatom cells using atomic force microscopy. Protist 153 (1), 25–38. doi: 10.1078/1434-4610-00080
Hurlbert S. H. (1984). Pseudoreplication and the design of ecological field experiments. Ecol. Monogr. 54 (2), 187–211. doi: 10.2307/1942661
Jézéquel Y., Bonnel J., Coston-Guarini J., Guarini J. M., Chauvaud L. (2018). Sound characterization of the European lobster Homarus gammarus in tanks. Aquat. Biol. 27, 13–23. doi: 10.3354/ab00692
Jolivet A., Tremblay R., Olivier F., Gervaise C., Sonier R., Genard B., et al. (2016). Validation of trophic and anthropic underwater noise as settlement trigger in blue mussels. Sci. Rep. 6 (September), 33829. doi: 10.1038/srep33829
Khandeparker L., D’Costa P. M., Anil A. C., Sawant S. S. (2014). Interactions of bacteria with diatoms: influence on natural marine biofilms. Mar. Ecol. 35 (2), 233–248. doi: 10.1111/maec.12077
Klein G. L., Pierre G., Bellon-Fontaine M. N., Zhao J. M., Breret M., Maugard T., et al. (2014). Marine diatom Navicula jeffreyi from biochemical composition and physico-chemical surface properties to understanding the first step of benthic biofilm formation. J. Adhesion Sci. Technol. 28 (17), 1739–1753. doi: 10.1080/01694243.2014.920461
Koedooder C., Stock W., Willems A., Mangelinckx S., De Troch M., Vyverman W., et al. (2019). Diatom-bacteria interactions modulate the composition and productivity of benthic diatom biofilms. Front. Microbiol. 10. doi: 10.3389/fmicb.2019.01255
Koehl M. R. A. (2007). Mini review: hydrodynamics of larval settlement into fouling communities. Biofouling 23 (5), 357–368. doi: 10.1080/08927010701492250
Lachance A. A., Myrand B., Tremblay R., Koutitonsky V., Carrington E. (2008). Biotic and abiotic factors influencing attachment strength of blue mussels Mytilus edulis in suspended culture. Aquat. Biol. 2, 119–129. doi: 10.3354/ab00041
Lachnit M., Buhmann T., Klemm J., Kröger N., Poulsen N. (2019). Identification of proteins in the adhesive trails of the diatom Amphora coffeaeformis. Philos. Trans. R. Soc. B: Biol. Sci. 374, 20190196. doi: 10.1098/rstb.2019.0196
Lacourse J. R., Northshop R. B. (1978). A preliminary study of mechanoreceptors within the anterior byssus retractor muscle of Mytilus edulis l. Biol. Bull. 155 (1), 161–168. doi: 10.2307/1540873
Lee M. C., Park J. C., Lee J. S. (2018). Effects of environmental stressors on lipid metabolism in aquatic invertebrates. Aquat. Toxicol. 200, 83–92. doi: 10.1016/j.aquatox.2018.04.016
Lepage G., Roy C. C. (1984). Improved recovery of fatty acid through direct transesterification without prior extraction or purification. J. Lipid Res. 25 (12), 1391–1396. doi: 10.1016/S0022-2275(20)34457-6
Lewthwaite J. C., Molland A. F., Thomas K. W. (1985). An investigation into the variation of ship skin frictional resistance with fouling. R. Institution Naval Architects Trans. 127, 16.
Leyton Y. E., Riquelme C. E. (2008). Use of specific bacterial-microalgal biofilms for improving the larval settlement of Argopecten purpuratus (Lamarck 1819) on three types of artificial spat-collecting materials. Aquaculture 276 (1), 78–82. doi: 10.1016/j.aquaculture.2008.01.037
Martel A. L., Tremblay R., Toupoint N., Olivier F., Myrand B. (2014). Veliger size at metamorphosis and temporal variability in prodissoconch II morphometry in the blue mussel (Mytilus edulis): potential impact on recruitment. J. Shellfish Res. 33 (2), 443–456. doi: 10.2983/035.033.0213
Marty Y., Delaunay F., Moal J., Samain J.-F. (1992). Changes in the fatty acid composition of Pecten maximus (L.) during larval development. J. Exp. Mar. Biol. Ecol. 163 (2), 221–234. doi: 10.1016/0022-0981(92)90051-B
McDonald J. I., Wilkens S. L., Stanley J. A., Jeffs A. G. (2014). Vessel generator noise as a settlement cue for marine biofouling species. Biofouling 30 (6), 741–749. doi: 10.1080/08927014.2014.919630
Mitbavkar S., Anil A. C. (2006). Diatoms of the microphytobenthic community in a tropical intertidal sand flat influenced by monsoons: spatial and temporal variations. Mar. Biol. 148 (4), 693–709. doi: 10.1007/s00227-005-0112-4
Mitbavkar S., Anil A. C. (2007). Species interactions within a fouling diatom community: roles of nutrients, initial inoculum and competitive strategies. Biofouling 23 (2), 99–112. doi: 10.1080/08927010701191753
Mitson R. B. (1995). Underwater noise of research vessels: review and recommendations: ICES cooperative research report 209: 1-61 (Copenhagen: ICES).
Molino P. J., Wetherbee R. (2008). The biology of biofouling diatoms and their role in the development of microbial slimes. Biofouling 24 (5), 365–379. doi: 10.1080/08927010802254583
Montgomery J. C., Jeffs A., Simpson S. D., Meekan M., Tindle C. B. T. (2006). Sound as an orientation cue for the pelagic larvae of reef fishes and decapod crustaceans. Adv. Mar. Biol. 51, 143–196. doi: 10.1016/S0065-2881(06)51003-X
Olivier F., Gigot M., Mathias D., Bonnel J., Jezequel Y., Meziane T., et al. (2023). Impacts of anthropogenic sounds on early stages of benthic invertebrates: the ‘Larvosonic system’. Limnol. Oceanogr. Methods 21, 53–68. doi: 10.1002/lom3.10527
Ozkan A., Berberoglu H. (2013). Physico-chemical surface properties of microalgae. Colloids Surfaces B: Biointerf. 112, 287–293. doi: 10.1016/j.colsurfb.2013.08.001
Parrish C. C. (1999). “Determination of total lipid, lipid classes, and fatty acids in aquatic samples,” in Lipids in freshwater ecosystems. Eds. Arts M. T., Wainman B. C. (New York, NY: Springer New York), 4–20. doi: 10.1007/978-1-4612-0547-0_2
Parrish C. C. (2009). “Essential fatty acids in aquatic food webs,” in Lipids in aquatic ecosystems. Eds. Kainz M., Brett M. T., Arts M. T. (New York, NY: Springer New York), 309–326. doi: 10.1007/978-0-387-89366-2_13
Pechenik J. A. (1990). Delayed metamorphosis by larvae of benthic marine invertebrates: does it occur? is there a price to pay? Ophelia 32 (1–2), 63–94. doi: 10.1080/00785236.1990.10422025
Pine M. K., Jeffs A. G., Radford C. A. (2012). Turbine sound may influence the metamorphosis behaviour of estuarine crab megalopae. PloS One 7 (12), e51790. doi: 10.1371/journal.pone.0051790
Popper A. N., Hastings M. C. (2009). The effects of anthropogenic sources of sound on fishes. J. Fish Biol. 75 (3), 455–489. doi: 10.1111/j.1095-8649.2009.02319.x
Popper A. N., Hawkins A. D. (2018). The importance of particle motion to fishes and invertebrates. J. Acoustical Soc. America 143 (1), 470–488. doi: 10.1121/1.5021594
Radford C. A., Jeffs A. G., Montgomery J. C. (2007). Directional swimming behavior by five species of crab postlarvae in response to reef sound. Bull. Mar. Sci. 80 (2), 369–378. doi: 10.1080/09524622.2008.9753776
Rayssac N., Pernet F., Lacasse O., Tremblay R. (2010). Temperature effect on survival, growth, and triacylglycerol content during the early ontogeny of Mytilus edulis and M. Trossulus. Mar. Ecol. Prog. Ser. 417, 183–191. doi: 10.3354/meps08774
Riisgard H. U. (1991). Filtration rate and growth in the blue mussel, Mytilus edulis linneaus. 1758: dependance on algal concentration. J. Shellfish Res. 10 (1), 29–35.
Rittschof D., Costlow J. D. (1989). Bryozoan and barnacle settlement in relation to initial surface wettability: a comparison of laboratory and field studies. Scientia Marina 53 (2–3), 145–754.
Rittschof D., Forward R. B., Cannon G., Welch J. M., McClary M., Holm E. R., et al. (1998). Cues and context: larval responses to physical and chemical cues. Biofouling 12 (1–3), 31–44. doi: 10.1080/08927019809378344
Roberts L., Cheesman S., Breithaupt T., Elliott M. (2015). Sensitivity of the mussel Mytilus edulis to Substrate-borne vibration in relation to anthropogenically generated noise. Mar. Ecol. Prog. Ser. 538, 185–195. doi: 10.3354/meps11468
Satuito C. G., Natoyama K., Yamazaki M., Shimizu K., Fusetani N. (1999). Induction of metamorphosis in the pediveliger larvae of the mussel Mytilus galloprovincialis by neuroactive compounds. Fish. Sci. 65 (3), 384–389. doi: 10.2331/fishsci.65.384
Schultz M. P. (2004). Frictional resistance of antifouling coating systems. J. Fluids Eng. 126 (6), 1039–1047. doi: 10.1115/1.1845552
Schultz M. P. (2007). Effects of coating roughness and biofouling on ship resistance and powering. Biofouling 23 (5), 331–341. doi: 10.1080/08927010701461974
Schultz M. P., Bendick J. A., Holm E. R., Hertel W. M. (2011). Economic impact of biofouling on a naval surface ship. Biofouling 27 (1), 87–98. doi: 10.1080/08927014.2010.542809
Simpson S. D., Radford A. N., Holles S., Ferarri M. C. O., Chivers D. P., McCormick M. I., et al. (2016). “Small-boat noise impacts natural settlement behavior of coral reef fish larvae,” in The effects of noise on aquatic life II. Eds. Popper A. N., Hawkins A. (New York, NY: Springer New York), 1041–1048.
Slabbekoorn H., Bouton N. (2008). Soundscape orientation: a new field in need of sound investigation. Anim. Behav. 76, e5–8. doi: 10.1016/j.anbehav.2008.06.010
Smetacek V. (1999). Diatoms and the ocean carbon cycle. Protist 150 (1), 25–32. doi: 10.1016/S1434-4610(99)70006-4
Solé M., Kaifu K., Mooney T. A., Nedelec S. L., Olivier F., Radford A. N., et al. (2023). Marine invertebrates and noise. Front. Mar. Sci. 10. doi: 10.3389/fmars.2023.1129057
Soto N.A. de, Delorme N., Atkins J., Howard S., Williams J., Johnson M. (2013). Anthropogenic noise causes body malformations and delays development in marine larvae. Sci. Rep. 3, 2831. doi: 10.1038/srep02831
Stanley J. A., Radford C. A., Jeffs A. G. (2012). Location, location, location: finding a suitable home among the noise. Proc. R. Soc. B: Biol. Sci. 279 (1742), 3622–3631. doi: 10.1098/rspb.2012.0697
Stefano M., De Stefano L., Congestri R. (2009). Functional morphology of micro- and nanostructures in two distinct diatom frustules. Superlattices Microstructures 46 (1), 64–685. doi: 10.1016/j.spmi.2008.12.007
Tougaard J., Madsen P. T., Wahlberg M. (2008). Underwater noise from construction and operation of offshore wind farms. Bioacoustics 17 (1–3), 143–146. doi: 10.1080/09524622.2008.9753795
Toupoint N. (2012). Le succès de recrutement de la moule bleue: influence de la qualité de la ressource trophique. [Thèse de doctorat] (Rimouski, Canada: Université du Québec à Rimouski).
Toupoint N., Gilmore-Solomon L., Bourque F., Myrand B., Pernet F., Olivier F., et al. (2012a). Match/Mismatch between the Mytilus edulis larval supply and seston quality: effect on recruitment. Ecology 93 (8), 1922–1934. doi: 10.1890/11-1292.1
Toupoint N., Mohit V., Linossier I., Bourgougnon N., Myrand B., Olivier F., et al. (2012b). Effect of biofilm age on settlement of Mytilus edulis. Biofouling 28 (9), 985–1001. doi: 10.1080/08927014.2012.725202
Tremblay G., Belzile C., Gosselin M., Poulin M., Roy S. (2009). Late summer phytoplankton distribution along a 3500 km transect in Canadian Arctic waters: strong numerical dominance by picoeukaryotes. Aquat. Microb. Ecol. 54 (1), 55–70. doi: 10.3354/ame01257
Tremblay R., Cartier S., Miner P., Pernet F., Quéré C., Moal J., et al. (2007). Effect of Rhodomonas salina addition to a standard hatchery diet during the early ontogeny of the scallop Pecten maximus. Aquaculture 262 (2), 410–418. doi: 10.1016/j.aquaculture.2006.10.009
Vermeij M. J. A., Marhaver K. L., Huijbers C. M., Nagelkerken I., Simpson S. D. (2010). Coral larvae move toward reef sounds. PloS One 5 (5), e10660. doi: 10.1371/journal.pone.0010660
Wang J., Shan C., Chuan D., Chen D. (2013). Underwater locomotion strategy by a benthic pennate diatom Navicula sp. Protoplasma 250, 1203–1212. doi: 10.1007/s00709-013-0502-2
Wilkens S. L., Stanley J. A., Jeffs A. G. (2012). Induction of settlement in mussel (Perna canaliculus) larvae by vessel noise. Biofouling 28 (1), 65–72. doi: 10.1080/08927014.2011.651717
Keywords: bioacoustics, biofouling, anthropogenic sounds, benthic diatoms, larval development, settlement
Citation: Cervello G, Olivier F, Chauvaud L, Winkler G, Mathias D, Juanes F and Tremblay R (2023) Impact of anthropogenic sounds (pile driving, drilling and vessels) on the development of model species involved in marine biofouling. Front. Mar. Sci. 10:1111505. doi: 10.3389/fmars.2023.1111505
Received: 29 November 2022; Accepted: 17 April 2023;
Published: 05 May 2023.
Edited by:
Marta Solé, BarcelonaTech (UPC), SpainReviewed by:
Satheesh Sathianeson, King Abdulaziz University, Saudi ArabiaJenni Stanley, University of Waikato, New Zealand
Copyright © 2023 Cervello, Olivier, Chauvaud, Winkler, Mathias, Juanes and Tremblay. This is an open-access article distributed under the terms of the Creative Commons Attribution License (CC BY). The use, distribution or reproduction in other forums is permitted, provided the original author(s) and the copyright owner(s) are credited and that the original publication in this journal is cited, in accordance with accepted academic practice. No use, distribution or reproduction is permitted which does not comply with these terms.
*Correspondence: Réjean Tremblay, UmVqZWFuX1RyZW1ibGF5QHVxYXIuY2E=