- 1Key Laboratory for Earth System Modeling, Ministry of Education, Department of Earth System Science, Tsinghua University, Beijing, China
- 2Key Laboratory of Soil Ecology and Health in Universities of Yunnan Province, School of Ecology and Environmental Sciences, Yunnan University, Kunming, China
- 3Institute of Ocean Engineering, Tsinghua’s Shenzhen International Graduate School, Shenzhen, China
- 4School of Ecology and Environment, Hainan University, Haikou, China
The fast-growing exotic mangrove species (Laguncularia racemosa) has been widely introduced in new territories such as China to restore mangrove ecosystems. However, the invasiveness, as well as the mechanisms for the rapid expansion after the introduction are still not well studied. Here, we try to reveal possible micro-mechanisms for the fast expansion of L. racemosa, using the data on leaf stomata straits, gas-exchange parameters, stable isotope ratios, carbon-nitrogen allocation from L. racemosa and the adjacent native mangroves (Avicennia marina, Aegiceras corniculatum, Bruguiera gymnorhiza, Kandelia obovata) in Hainan Island, China. We found that the higher density but smaller size stoma of L. racemosa enhanced stomatal conductance and shorten the diffusion path of carbon dioxide, thereby increasing the photosynthetic rate. Moreover, the higher stomatal density of L. racemosa exerts a significant positive effect on transpiration, which thus accelerated the water transport and nutrient uptake to meet the advanced need for nutrients and water for fast-growing. The evidence from leaf δ13C and carbon-nitrogen allocation further proved that L. racemosa has a lower intrinsic water use efficiency but a higher rate of photosynthesis than native mangrove species. Our results suggest that stomatal morphological and physiological traits could strongly influence the growth of L. racemosa compared to the adjacent native mangroves, which provides a new perspective for the fast expansion of exotic mangrove species in China. These findings also suggest that L. racemosa has an invasive potential in native mangrove habitats, thereby the mangrove reforestation projects by introducing L. racemosa should be treated with caution.
Introduction
Mangrove forests, one of the most carbon-rich ecosystems, provide numerous ecological functions such as breeding habitats, purifying water quality, protecting coastlines, and sequestrating CO2 (Duke et al., 2007; Lee et al., 2014; Lovelock and Duarte, 2019). However, one-third of the total world mangrove area has been lost during the last half-century due to human activity or climate change (Kuenzer et al., 2011; Hamilton and Casey, 2016). The mangrove in Hainan Island is considered a hotspot for mangrove research and conservation due to the highest mangrove diversity and the highest carbon stock of all mangrove forests in China (Li and Lee, 1997; Lin, 1999). From 1950 to 2010, the mangrove area of Hainan sharply reduced by 60% (Liao and Zhang, 2014). In order to increase mangrove habitats, reforestation projects have been conducted on Hainan Island. However, in these restoration projects, exotic mangroves, especially fast-growing species were introduced into new territories. Furthermore, the invasiveness risk and the potential expansion mechanism of exotic mangroves were neglected and still need to be systematically studied.
Laguncularia racemosa (white mangrove) is one mangrove species, natively distributed on the coasts of western Africa and eastern America, which was introduced to Hainan, China from the coast of LaPaz, Mexico in 1999 (Liao et al., 2006). L. racemosa was the most widely used exotic mangrove species for reforestation due to its high environmental adaptability and fast-growing character (Wang et al., 2018). Previous studies have indicated that L. racemosa is a quality afforestation species that could be widely introduced to mangrove habitats (Zhong et al., 2011; Chen et al., 2013). However, more field observations have shown that L. racemosa grew faster than native mangrove species and was even more aggressive than Sonneratia apetala (another fast-growing mangrove species) (Li et al., 2020). Previous studies of L. racemosa mainly focused on the reforestation effect and planting technology (Zhong et al., 2011; Chen et al., 2014), physiological character (Han et al., 2010), salt tolerance (Sobrado, 2005; Rodríguez et al., 2017). Few studies estimated the invasiveness and invasive mechanism of L. racemosa. The study by Gu et al. (2019) has reported that the combined effects of salinity and light exerted a significant influence on the growth of L. racemosa. Li et al. (2020) believed that L. racemosa was growing faster because of the lower leaf construction cost. Although those studies suggested that L. racemosa has invasive potential, there was no proposed physiological mechanism to explain why L. racemosa grows faster and has a higher salt tolerance and higher photosynthesis rate.
Exotic fast-growing species often have advantages over native species on growth-related traits, such as photosynthetic rate (A) and resource use efficiency (Funk and Vitousek, 2007; Matzek, 2011). Stomata, the pores on the leaf surface, exert a major influence on plant growth and productivity through regulating stomatal conductance (gs) and transpiration (E) by stoma opening or closure, which determine the carbon, water, nutrient cycling between plant and ambient environment (Lin et al., 2015; Henry et al., 2019). The gs to CO2 and water are ultimately driven by the combined effect of size (taken here as guard cell length, S) and density (number per unit area, D) of stomata in the epidermis (Franks et al., 2009; Doheny-Adams et al., 2012). Generally, the relationship between S and D is well described by a negative power function but the slope is different across species (Hetherington and Woodward, 2003). Previous studies have indicated that stomatal density imposed a positive effect on the conductance therefore enhance leaf photosynthesis capacity (Tanaka et al., 2013; Pathare et al., 2020). Furthermore, plants increase water-use efficiency (WUE) by reducing stomatal conductance (gs) via altered stomatal density (D) and size (S) (Franks et al., 2015; Dunn et al., 2019), which is in parallel with the evidence from stable isotope studies (Guerrieri et al., 2019). Meanwhile, in terms of resource use efficiency, plants can adjust the water transport and nutrient uptake by altering transpiration and thereby maintain foliar nutrient supply and water balance (Lu et al., 2018; Lanning et al., 2019). Although the stomatal effect has been intensively investigated in many terrestrial plant species, the stomatal traits and effect of mangrove species have remained unclear, especially in exotic mangrove species. It is therefore essential to elucidate the relationship between stomatal traits and gas exchange in native and exotic mangrove species.
This study aims to unveil the physiological mechanisms by comparing the stomatal effect and other physiological characteristics between native and exotic mangrove species. We collected data on mangrove stomata traits (guard cell length, stomatal density), gas exchange parameters, stable isotope fractionation, carbon and nitrogen contents to examine the linkages between stomatal traits and plant growth features of L. racemosa. Our study was designed to address two questions: (a) what are the differences in stomatal traits and gas-exchange characters between exotic and the adjacent native mangrove species? (b) how do the stomatal traits affect the physiological process of exotic mangroves thereby resulting in faster growth than native mangroves? Those results will strengthen the understanding of the potential expansion risk of exotic mangroves and have important implications for mangrove restoration and conservation.
Materials and methods
Study sites
Field measurements were conducted in two study sites on Hainan Island in the south of China, which was considered a hotspot for mangrove research and conservation due to the highest mangrove diversity and the highest carbon stock of all mangrove forests in China (Li and Lee, 1997; Lin, 1999). Haikou (HK) and Sanya (SY), located in the Northern and Southern of Hainan Island, were selected as two main sites for this study. Haikou Dongzhaigang Mangrove Nature Reserve (113°57’E, 22°35’N) was established as the first National Mangrove Nature Reserve of China in 1986 and was then listed as one of the International Important Wetlands in 1992 (Qiu et al., 2011). Dongzhaigang Mangrove Nature Reserve is the first place where two exotic mangrove species (Sonneratia apetala, Laguncularia racemosa) were introduced and planted. The Laguncularia racemosa was introduced from Mexico in 1999 (Liao et al., 2006). Sanya Qingmeigang Mangrove Nature Reserve (109°36’E, 18°13’N) has a mangrove area of 64 ha-1, which is the largest and best-preserved mangrove region in Sanya City. With a tropical monsoon climate, the mean annual temperature, mean annual precipitation and salinity were 23.8°C, 1685 mm, and 27‰ for the Haikou site, and 25.5°C, 1280 mm, 33‰ for the Sanya site, respectively.
Leaf gas exchange measurements
Gas exchange parameters of leaves were measured using an LI-6400 portable photosynthesis system (Li-COR Inc., Lincoln, NE, USA). The exotic mangrove Laguncularia racemosa and the adjacent native mangroves (Avicennia marina, Aegiceras corniculatum, Bruguiera gymnorhiza, Kandelia obovata) with the same tree age (≈ two years) were chosen to measure the gas exchange parameters. All measurements were conducted between 09:00 and 15:00, with the ambient temperature ranging from 26 to 35°C in Summer. Conditions in the cuvette were maintained around 400 ppm CO2 concentration, approximate local ambient CO2 concentration, and photosynthetic active radiation was set at 1000 μmol photons m-2 s-1, with an airflow rate of 500 μmol s-1. The assimilation rate (A), stomatal conductance (gs), transpiration rate (E), and CO2 concentration (Ci) were recorded after stabilizing control conditions (≈ 30 mins) with a leaf inside the leaf cuvette. Instantaneous water use efficiency (WUEi) was calculated according to the ratio A/E. For each leaf, five consecutive measurements were averaged as one replicate value, and three to five replicates of individual plants were gathered for each mangrove species.
Stomatal traits
The mature and intact leaves of different species under the same environments were chosen to measure stomatal traits. For each leaf, a razor blade was used to scrape the leaf vein and mesophyll, leaving the leaf epidermis, which was then rinsed clean with deionized water. Small sections of the epidermis were cut, and drip stained on glass microscope slides. Under a fluorescence microscope (Nikon Eclipse Ci-L, Tokyo, Japan) at ×400 magnification, guard cell lengths were measured, and stomatal density was calculated as the number of stomata per field of view. Three fields were sampled for each leaf, and three to five leaves were measured for each species. Both sides of the leaf epidermis were imaged, and the stomatal density of both sides was calculated.
Stable isotope analyses
After measuring leaf gas exchange, we collected the plant samples from the exotic and the adjacent native mangroves. The leaf, stem, and root of each plant were separated and dried by oven at 70°C for 48 h until constant weight, then ground to powder using a grinding mill (Jingxin, JXFSTPRP-32, China), and passed through a 60-mesh sieve. The δ13C and δ15N were measured with an isotope ratio mass spectrometer (IRMS) (Delta V Advantage, Thermo Fisher Scientific, Inc., USA), the C and N content were measured by the elemental analyzer (Flash 2000 EA-HT, Thermo Finnigan, Bremen, Germany). The isotope composition of plant tissue (‰, in parts per thousand) was calculated as:
Where Rsample and Rstandard are the isotope ratios of the plant tissue relative to PDB standard for δ13C and N2-atm standard for δ15N, respectively. The δ13C and WUE were demonstrated as (Farquhar and Richards, 1984; Farquhar et al., 1989).
Where A is the assimilation rate of the plant, E is the transpiration rate. ca and ci are the partial pressures of CO2 in the atmosphere and intercellular, respectively. v is the vapor pressure difference between the intercellular and the atmosphere.
where a is the fractionation caused by CO2 diffusion in the air (4.4‰), b is the fractionation due to carboxylation (27‰), and δ13Cplant and δ13Cairare the carbon isotope composition of the plant and CO2 in the atmosphere, respectively (Farquhar et al., 1982).
Statistical analysis
We applied linear and non-linear regressions to test the significance of correlations (p<0.05) between stomatal density and stomatal size. One-way analysis of variance (ANOVA) was used to evaluate the differences in gas exchange parameters among different mangrove species means. A Tukey’s HSD multiple comparison was used to test significance at α=0.05 level. Mean and standard error (S.E.) values of replicates were calculated for each variable. A multi-linear regression model and spearman correlation heat-matrix were created to explore the relationships among stomatal traits, photosynthesis characters by using the “ggplot2” and “ggcor” package in R 4.0.5. The conceptual diagram was made to summarize the physiological link of mangrove stomatal traits and other factors by using Adobe illustrator CC2018 (Adobe Systems Inc., San Jose, CA, USA). The statistical analyses and figures were carried out using Origin software 2019b. (OriginLab Corp., Northampton, MA, USA).
Results
Relationships between stomatal density and stomatal size in mangroves
For the native mangrove K. obovata, the stomata were only found on the lower epidermis (Figures 1A, C). However, stomata are distributed on both the lower and upper epidermis of leaves in L. racemosa (Figures 1B, D). Furthermore, the stomatal density of the upper epidermis was higher than those of the lower epidermis in L. racemosa. Stomatal density tended to increase with the stomatal guard cell length decline, the negative relationship was best estimated (R2 = 0.66, p<0.001) by a power function (Figure 1E). The stomatal density of exotic mangrove species is significantly higher than that of native mangrove species. However, the stomatal guard cell length of exotic mangroves is lower than that native mangrove species (Figure 1E).
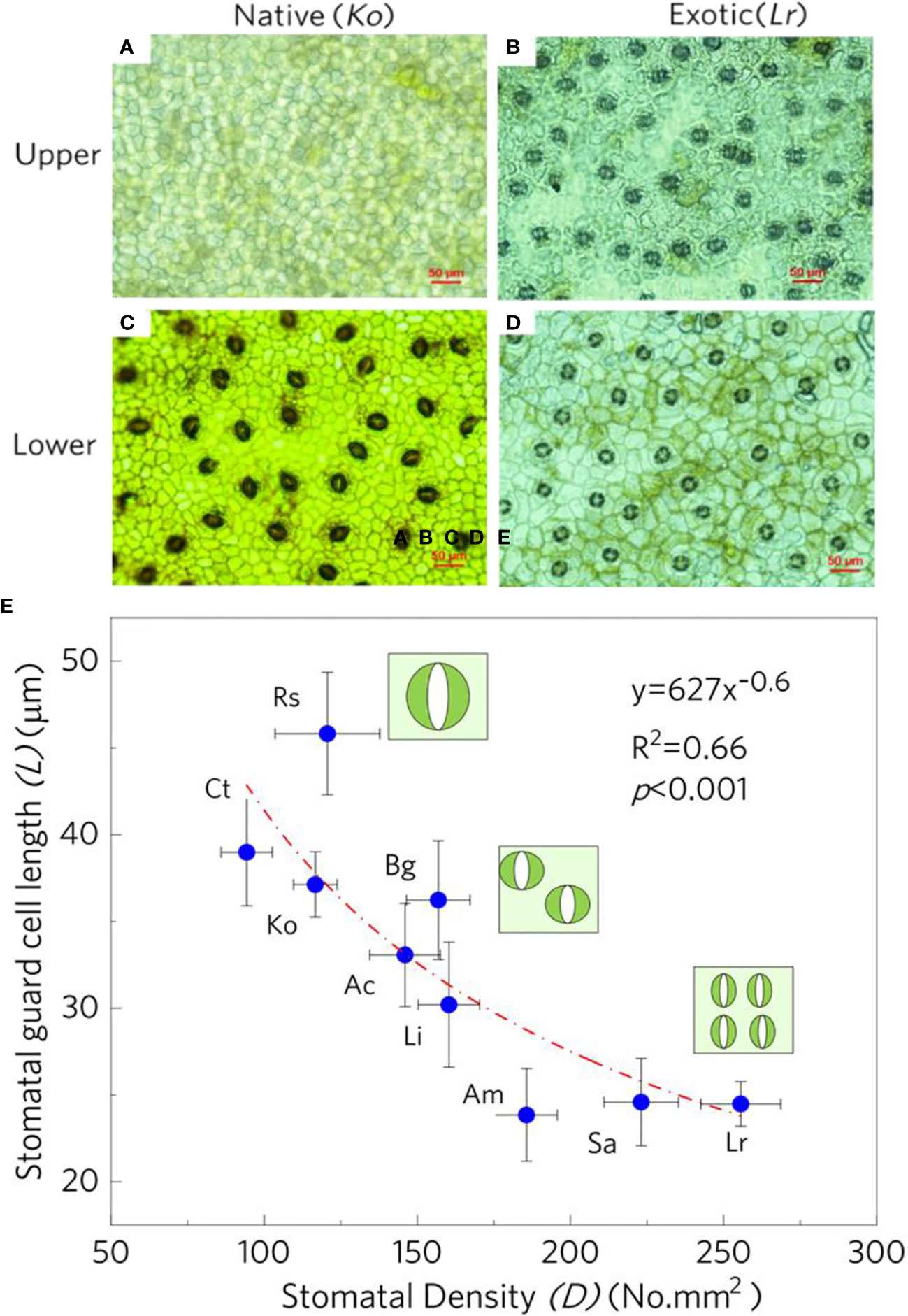
Figure 1 Comparisons in leaf stoma distribution and density between a native mangrove K. obovate and an exotic mangrove L. racemosa, the upper epidermis of K. obovate (A) and L. racemosa (B), the lower epidermis of K. obovate (C) and L. racemosa (D), the relationship between stomatal density and stomatal length pooled for different mangrove species (E).The error bars represent Mean±SE (n=5), and the species name abbreviations are as follows: Rs, Rhizophora stylosa; Ct, Ceriops tagal; Ko, Kandelia obovata; Bg, Bruguiera gymnorhiza; Ac, Aegiceras corniculatum; Ll, Lumnitzera racemosa; Am, Avicennia marina; Sa, Sonneratia apetala; Lr, Laguncularia racemosa.
Photosynthetic gas exchange parameters
Gas exchange parameters varied significantly between species. Specifically, the leaf CO2 assimilation rate was significantly higher in exotic mangroves (Lr) compared with other native mangrove species (Figure 2A). Consistently, the stomatal conductance and transpiration of L. racemosa were significantly higher than those of native species (Figures 2B, C). Instantaneous water use efficiency in L. racemosa showed no significant difference when compared with B. gymnorhiza but was lower than other native mangrove species (Figure 2D). Remarkably, L. racemosa has the largest stomata density but the smallest stomata size (Figures 2E, F).
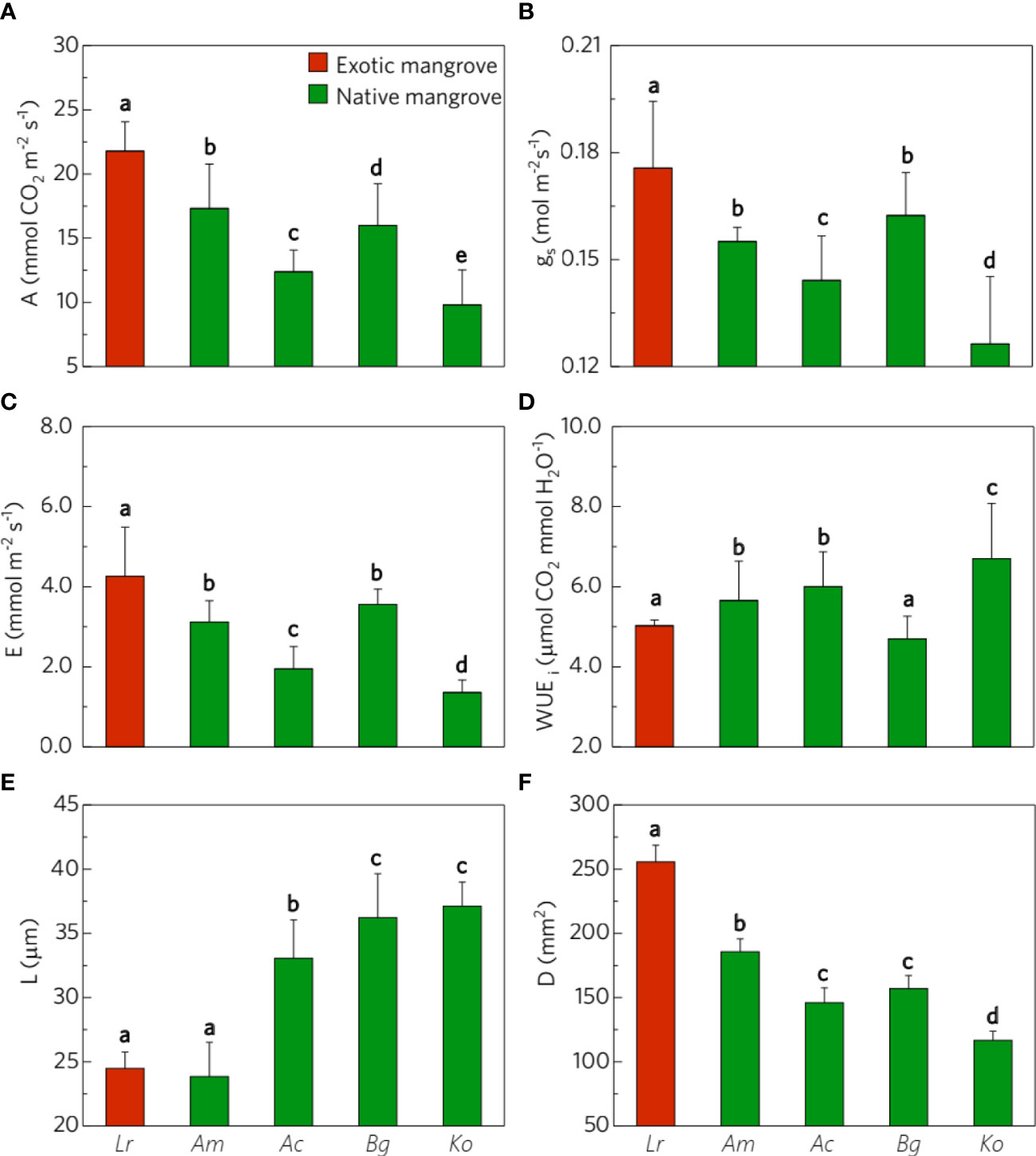
Figure 2 Comparison of gas exchange parameters and stomata traits. (A) photosynthesis (A), (B) transpiration (E), (C) conductance (gs), (D) Instantaneous water use efficiency (WUEi), (E) stomata guard cell length (L), and (F) stomata density (D) between exotic mangrove species (red bar) and native mangrove species (green bar), the error bars represent Mean ± SE(n>10), the letters above the bar indicate different significantly (p<0.05). See Figure 1 for species abbreviations explanations.
Stable isotope compositions
The δ13C of leaf, stem, root in L. racemosa were all significantly lower than native mangrove both in the Haikou site and Sanya site (Figures 3A, B). In addition, the ⊿leaf of L. racemosa was significantly higher than those of native mangroves across two sites (Figures 3C, D). For the Haikou site, the value of δ13C in L.racemosa increased in the order of root>stem>leaf, whereas the value of δ13C in K. obovata was leaf >stem>root. the largest difference (≈3 ‰) in the carbon isotope discrimination between L. racemosa and K. obovata was observed in roots. The δ15N of L. racemosa was not significantly different from those of native mangroves (Figures 3A, B). For Sanya site, the δ13C of L. racemosa were more negative than the natives species, but had a different pattern compared with the δ13C of L. racemosa in the Haikou site, the value of δ13C increased in the order of root >stem>leaf (Figure 3B). Furthermore, the range of δ15N (root-stem-leaf) discrimination in L. racemosa (4.2‰-6.7‰) was wider than those in C. tagal (4.5‰-5.5‰) but lower than those of L. racemosa in Haikou site (6 ‰-10 ‰). And the leaf δ15N of L. racemosa was lower than those of all native mangrove species. Generally, the δ13C of exotic mangrove (L. racemosa) was more negative than native mangrove. Meanwhile, the δ13C discrimination of mangroves in Haikou was lower than mangroves in Sanya, whereas the δ15N discrimination of mangroves in Haikou was higher than mangroves in Sanya.
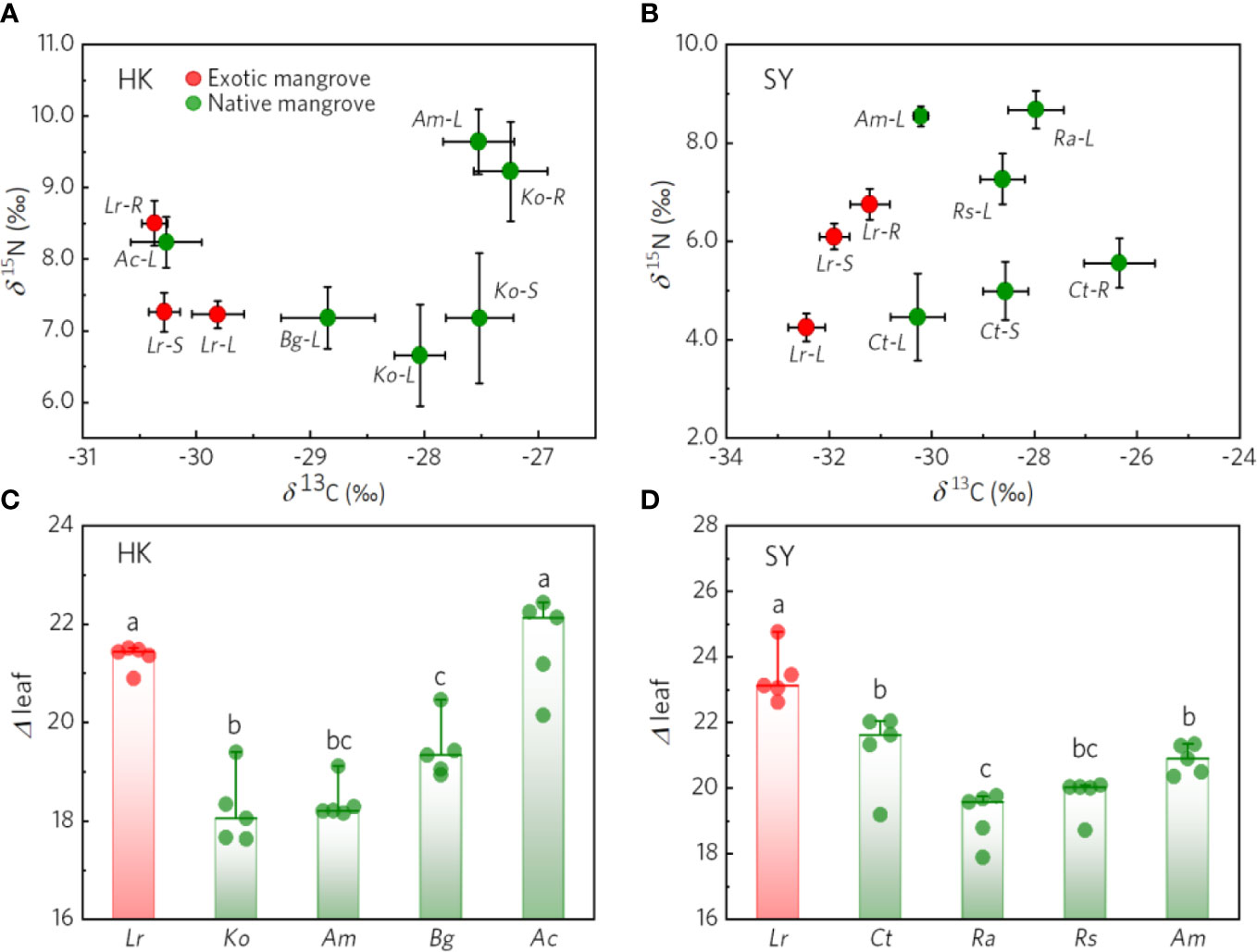
Figure 3 The value of δ13C, δ15N, and ⊿leaf in native and exotic mangroves. Comparing mangrove δ13C and δ15N values of mangrove in HK(Haikou) site (A) and SY(Sanya) site (B). The⊿ leaf of mangrove in HK(Haikou) site (C) and SY(Sanya) site (D). Exotic mangrove (Lr-Laguncularia racemosa) and native mangrove (Ko-Kandelia obovata, Am-Avicennia marina, Rs-Rhizophora stylosa, Ct-Ceriops tagal, Bg-Bruguiera gymnorhiza, Ac-Aegiceras corniculatum,Ra- Rhizophora apiculata), the error bars represent Mean ± SE (n=5). Red dots represent exotic mangroves, green dots represent native mangroves. The letters above the bar indicate different significantly (p<0.05).L, Leaf; S, Stem; R, Root.
Relationship between stomatal traits and gas exchange parameters
The spearman correlation analyses show that stomata size negatively related to stomata density (R=-0.78). This pattern was similarly observed in WUE (R=-0.56) (Figure 4A). Generally, the stomata density was significantly positively correlated with A, E, Gs, whereas the negative coefficients were observed between stomata size and A, E, Gs (Figure 4A). However, the relationships among stomata size, stomata density and A, E, Gs were nonlinear (Figure 4B, Figure S1). For instance, the WUE decreased slowly with low density but sharply declined with high density, which differed from the trend of stomata size (Figure 4B). With the increase of stomata density, conductance increased rapidly with low density and then stably rose with high density, thus leading to an increase in photosynthesis rate and transpiration (Figure 4B). However, the transpiration increased more in high-density samples compared to the trend of stomatal conductance and photosynthesis rate (Figure 4B).
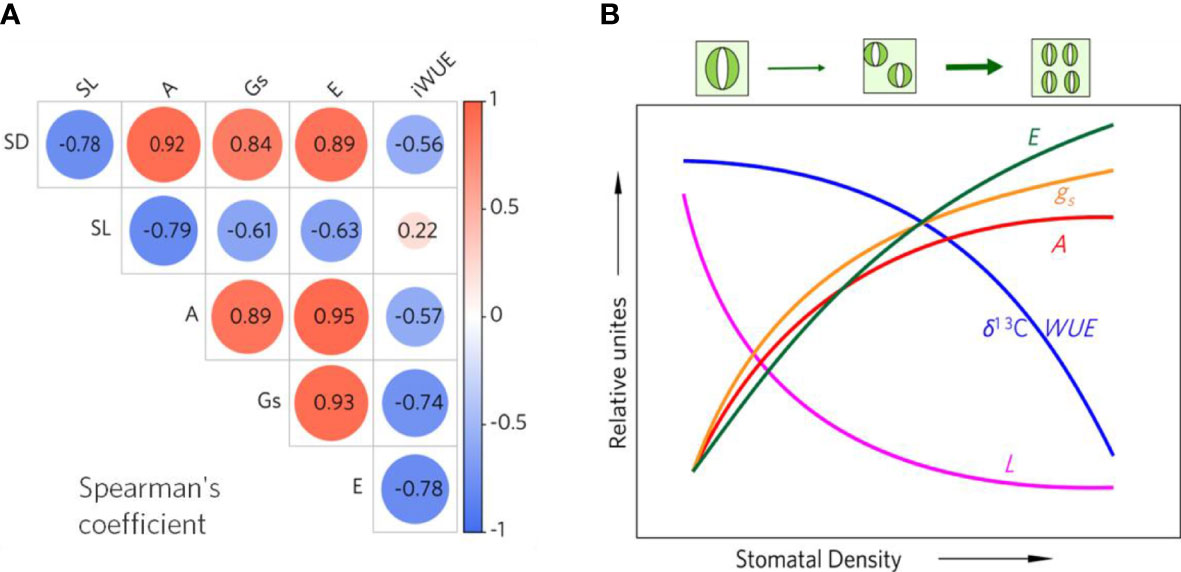
Figure 4 Spearman’s correlation coefficient heat map (A), and a conceptual figure (B) showing the general relationships among stomatal size (L), water use efficiency inferred from carbon isotope ratio (δ13C WUE), photosynthesis (A), transpiration (E), stomatal conductance (gs) to stomatal density (D) in mangroves.
Discussion
Stomatal traits of native and exotic mangrove species
The relationships between stomata size and stomata density exert a significant influence on plant growth and productivity through regulating stomatal conductance and transpiration, which control the carbon, water, nutrient cycling between plant and environment. We found that the relationship between stomata size and stomata density in mangrove was best described by a negative power function (Figure 1E), which was consistent with the previous studies (Franks and Beerling, 2009). Besides, we observed that stomata can be found on both the lower and upper epidermis of the leaves of L. racemosa, whereas stomata exist only on the lower epidermis in most native mangrove species (Figure 1). Also, the exotic mangrove species tend to have higher stomata density and smaller stomata size than the native mangrove species. The negative correlation between stomata size and stomata density of mangrove may be explained by the physical constraints and energy strategy (Franks et al., 2009). On one hand, the limited space of leaves requires the allocation of stomata with the sufficient density and size to achieve the optimized conductance for photosynthesis. On the other hand, the plants balance photosynthetic productivity (energy gain) and respiration (energy loss) through the evolution of stomatal properties.
Contrasting gas exchange parameters between native and exotic mangroves
The higher stomata density corresponding to a higher photosynthetic rate, and stomatal conductance was observed in the L. racemosa (Figures 2A, B, F). The same trend can be found in other mangrove species, namely, that higher stomata density tends to increase stomatal conductance and photosynthetic rate, and that lower stomata density corresponds with a down-regulation of conductance (Figure 4). This trend is consistent with the previous study by (Franks et al., 2009), which showed an enhancement of photosynthetic rate and conductance limited by the relationship between stomatal size and density. This can be explained by the negative relationship between stomata size and density, the allocation traits of stoma constrained by the space-optimized rule. In order to enhance stomatal conductance, plants tend to allocate the small size but high density stoma to optimize the space (Dow et al., 2014). With the higher conductance and photosynthesis of L. racemosa, the transpiration increase results in a decline in instantaneous water use efficiency (WUEi) compared with native mangroves. Although increasing conductance supplies additional CO2 and water for carboxylation, more water is lost through the more numerous stomata. Several previous studies have verified that conductance plays an important role in regulating the WUE, leading to a negative relationship between conductance and WUE (Figure 4) (Franks et al., 2015; Dunn et al., 2019).
Environmental factors affected stomatal traits and gas exchange of mangroves
However, it should be pointed out that mangroves are restricted by salinity due to the saline environment imposing a negative effect on photosynthesis and conductance through regulating the opening and closure of stoma (Ball and Farquhar, 1984; Parida et al., 2004). This suggests that stomata traits and gas exchange parameters of mangrove will shift with environmental changes (Sperry et al., 2016; Dong et al., 2020). Mangroves, therefore, have evolved salt-tolerant function such as salt exclusion in the root, salt secretion by salt glands, osmolyte accumulation to adapt to the high salinity of seawater (Parida and Jha, 2010; Jiang et al., 2017; Cheng et al., 2020). Even so, only a few mangrove species, such as Aegiceras corniculatum, Avicennia marina, have specialized salt glands. Interestingly, we found that L. racemosa has a good salt secretion function owing to abundant salt glands and aqueous tissues distributed in the leaves (Chen et al., 2018; Li and Lin, 2006). Thus, we concluded that despite the lower WUE, there is no strong limit on water supply for L. racemosa compared to native mangroves. These results may explain why L. racemosa possesses a high salt tolerance.
The evidence from the isotope also confirmed that L. racemosa has a lower WUE (more negative δ13C) but higher photosynthetic capacity compared with other native mangrove species (Figure 3). The higher nitrogen (N) content and lower carbon-nitrogen ratio (C:N) of L. racemosa suggest a higher nitrogen uptake efficiency and photosynthetic nitrogen use efficiency (NUE) compared to native species (Figure S2). Moreover, we found that a spatial pattern exists in the δ13C and δ15N values, namely, the δ13C discrimination of mangrove in Haikou site (low salinity) were lower than mangroves in Sanya site (high salinity), whereas the δ15N discrimination of mangroves in Haikou were higher than mangroves in Sanya (Figure 3). The lower foliar δ13C of mangrove reflect a lower WUE in Haikou sites due to the lower salt stress. Foliar δ15N values serve as an indicator of the supply of N relative to plant N demand, and the lower δ15N values represents a greater N limitation (Craine et al., 2018). The results of δ15N shows that the mangroves in Haikou exhibited a lower N limitation compared to the mangroves in Sanya, which was caused by lower salt stress and higher N availability in Haikou mangrove habitats (Bai et al., 2021).
The potential mechanism for rapid expansion of exotic mangrove in new territory
Previous studies have reported that L. racemosa was more invasive than other mangrove species due to fast growth and high salinity tolerance (Gu et al., 2019). However, the potential mechanism of fast growth is still unclear. As Figure 5 shows, the foliar stomata density of L. racemosa is higher than that of native mangrove species, particularly on the upper epidermis, which yields two important physiological functions for its fast growth. On one hand, high stomata density enhances the conductance thus supplies more CO2 and H2O to support the carboxylation. On the other hand, having numerous stomata on the upper epidermis (chloroplasts distribution) shortens the diffusion path of CO2 and H2O. Consequently, more CO2 diffuses into the chloroplasts thus enhancing the rate of photosynthesis. Meanwhile, the transpiration increases with the conductance increasing, which results in more water loss thus decline of WUE (Figures 2D, 3). This is consistent with the study by Tian et al. (2016). The study shows that stomatal density was positively correlated with vein density thereby balancing the leaf-level water transpiration and supply. As mentioned above, however, the lower WUE has no strong restriction on water supply due to the efficient salt secretion function and aqueous tissues of L. racemosa. Furthermore, the increasing transpiration alters the water potential through the plant and accelerates the water transport and nutrient uptake in L. racemosa. It thereby maintains foliar nutrient supply and water balance (Cernusak et al., 2011; Ocheltree et al., 2016; Lu et al., 2018). As a result, the N content of the L. racemosa is higher than those of native mangroves but the C:N ratio is significantly lower than native species, especially for foliar N content (Figure S2), which provides further evidence to verify the advantages of water and nutrient transport and allocation in L. racemosa.
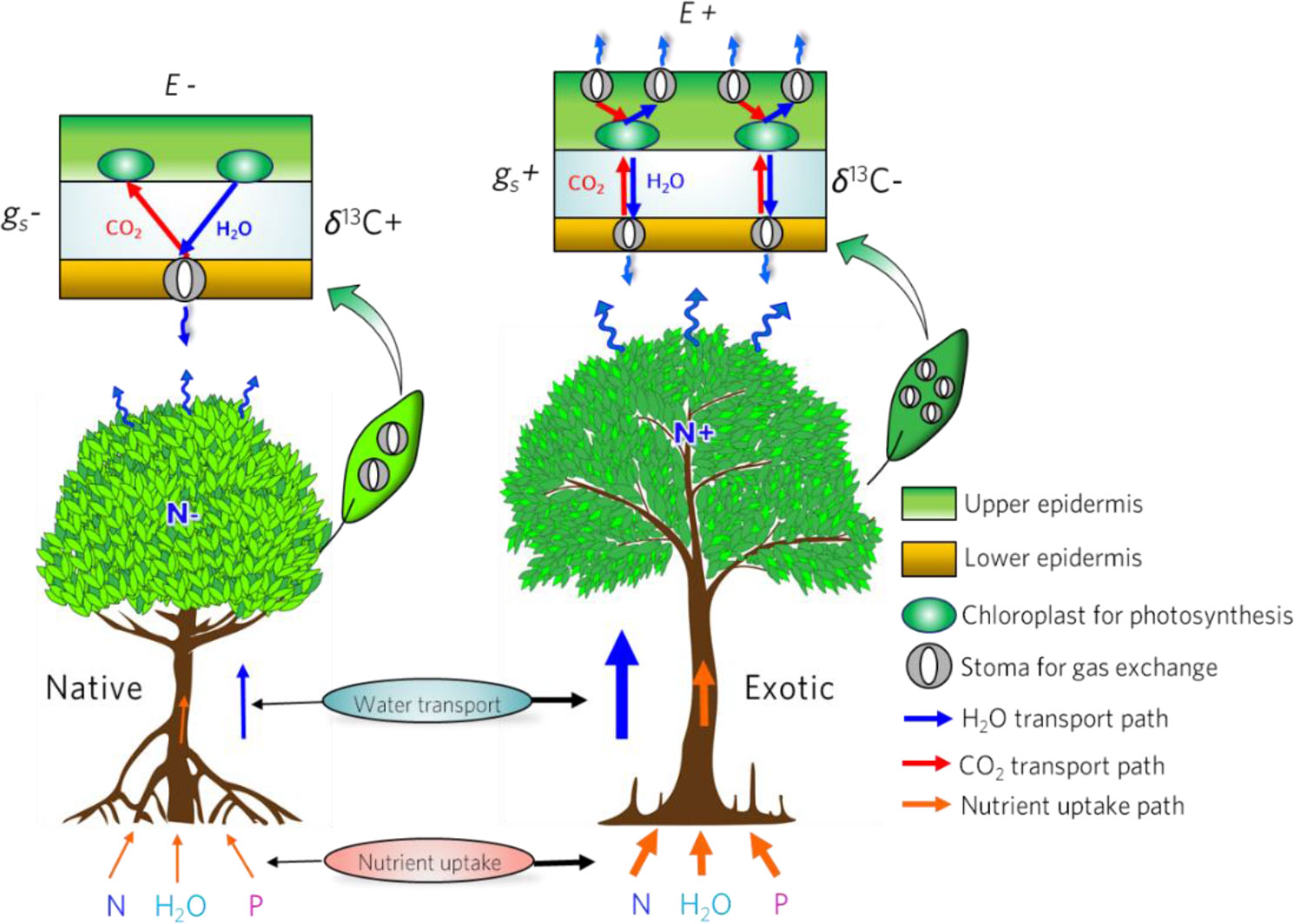
Figure 5 Conceptional diagram for linking stomatal traits to foliar gas exchange performance in native mangroves (right) and exotic mangrove (left) N-nitrogen ability, E-transpiration, gs- conductance, δ13C-the stable carbon isotope of mangrove leaf.
Implications for mangrove conservation under environment change
The ongoing rising atmospheric CO2 (Ca), is expected to affect the stomatal traits of plants, and thus regulate the gas-exchange and energy balance, such as carbon, water, and nutrient cycling of plants (Voelker et al., 2016). Studies from experiments have reported that the water use efficiency tends to increase with a rise in atmospheric CO2 by decreasing the stomatal conductance, thus enhancing assimilation rates (Keenan et al., 2013; Franks et al., 2015). The trend was further corroborated by the increase of 13C/12C isotopic discrimination of plant photosynthesis (Keeling et al., 2017). Whether this trend exists for mangrove plants is not clear. Several studies indicated that elevated CO2 imposes a positive effect on mangrove productivity (Farnsworth et al., 1996; Ball et al., 2010; Reef and Lovelock, 2015). Furthermore, the above- and belowground production of mangroves have different responses to elevated CO2 in combination with other environmental factors (e.g. N availability, salinity, temperature) (McKee and Rooth, 2008; Reef et al., 2016). Normally, the optimized assimilation rate is constrained by the balance of maximum C gain and minimum water loss. However, L. racemosa shows higher photosynthesis but lower WUE due to higher conductance. To some degree, there is minimal water limitation of the L. racemosa. Hence, we hypothesize that the rising atmospheric CO2 is expected to significantly enhance the assimilation rate of L. racemosa and thus facilitate the expansion of L. racemosa into native mangrove habitats. Certainly, more evidence is needed to further test this hypothesis.
Furthermore, more nutrient input could enhance the mangrove productivity in a short term. Aquaculture is a major threat to mangrove forest degradation (Rahman et al., 2013; Richards and Friess, 2016). Aquaculture not only directly reduces the mangrove cover but also increases nutrient input into the neighboring mangroves. Especially in China, the pollution and deforestation caused by aquaculture are the main threats leading to over 50% of mangroves loss (Dan et al., 2016). The increased nutrient loading might also strengthen the CO2–nutrient fertilized effect of exotic mangroves because of the higher assimilation rate and nutrient use efficiency compared to the native mangroves. Those results suggest that climate change and human activity also impose widespread effects and synergistic interactions to accelerate the expansion of exotic mangroves.
Conclusions
This study provides a new perspective showing the linkages between stomatal physiological traits and the rapid growth of the exotic mangrove L. racemosa in new territories. Stomata density and stomata size were major traits that enhance mangrove photosynthetic capacity by increasing conductance, transpiration, and decreasing water use efficiency. Additionally, the evidences from isotopes shows that the stomata traits and gas exchange of mangroves change with ambient environment change. Our results indicate that leaf stomatal and physiological traits strongly affect the rapid expansion of exotic mangroves (L. racemosa) by modulating the gas exchange process. These findings also imply that L. racemosa exhibited an invasive potential in native mangrove habitats and should be cautiously introduced into native mangroves habitats for reforestation.
Data availability statement
The raw data supporting the conclusions of this article will be made available by the authors, without undue reservation.
Author contributions
JB, GL, and XZ designed the research. JB, YM, RG, and ZD performed the field survey, and JB analyzed the data and wrote the manuscript. All authors especially GL and XZ either supported the field surveys and/or contributed to the revisions of manuscript. All authors contributed to the article and approved the submitted version.
Funding
This study was supported by the Ministry of Science and Technology of China (2017FY100703; 2019YEA0606604), the National Natural Science Foundation of China (41877352), and the Guangdong MEPP fund (GDOE [2019] A06).
Acknowledgments
We thank the staff of Hainan University for help during our field plot surveys. We would also like to thank the staff at the Dongzhaigang National Mangrove Nature Reserve, the Qinglangang Provincial Mangrove Nature Reserve, and other county mangrove nature reserves in Hainan for their logistical support during our fieldwork.
Conflict of interest
The authors declare that the research was conducted in the absence of any commercial or financial relationships that could be construed as a potential conflict of interest.
Publisher’s note
All claims expressed in this article are solely those of the authors and do not necessarily represent those of their affiliated organizations, or those of the publisher, the editors and the reviewers. Any product that may be evaluated in this article, or claim that may be made by its manufacturer, is not guaranteed or endorsed by the publisher.
Supplementary material
The Supplementary Material for this article can be found online at: https://www.frontiersin.org/articles/10.3389/fmars.2023.1136443/full#supplementary-material
References
Bai J., Meng Y., Gou R., Lyu J., Dai Z., Diao X., et al. (2021). Mangrove diversity enhances plant biomass production and carbon storage in hainan island, China. Funct. Ecology. 35 (3), 774–786. doi: 10.1111/1365-2435.13753
Ball M. C., Cochrane M. J., Rawson H. M. (2010). Growth and water use of the mangroves rhizophora apiculata and r. stylosa in response to salinity and humidity under ambient and elevated concentrations of atmospheric CO2. Plant Cell Environment. 20 (9), 1158–1166. doi: 10.1046/j.1365-3040.1997.d01-144.x
Ball M. C., Farquhar G. D. (1984). Photosynthetic and stomatal responses of two mangrove species, aegiceras corniculatum and avicennia marina, to long term salinity and humidity conditions. Plant Physiol. 74 (1), 1–6. doi: 10.1104/pp.74.1.1
Cernusak L. A., Klaus W., Turner B. L. (2011). Transpiration modulates phosphorus acquisition in tropical tree seedlings. Tree Physiol. 31 (8), 878–885. doi: 10.1093/treephys/tpr077
Chen J., Li N. Y., Liu Q., Zhong C. R., Huang M. (2013). Antioxidant defense and photosynthesis for non-indigenous mangrove species sonneratia apetala and laguncularia racemosa under NaCl stress. Chin. J. Plant Ecology. 37 (5), 443–453. doi: 10.3724/SP.J.1258.2013.00443
Chen Y., Liao B., Li M., Deng Z., Wei J., Guan W. (2014). Field planting experiments of mangrove on high-salinity beaches. J. South China Agric. University. 56 (2), 78–85. doi: 10.7671/j.issn.1001-411X.2014.02.015
Chen J. H., Miao S. Y., Huang H. F. (2018). Comparative study on leaf structures of five mangrove plants. Guihaia 38 (5), 655–664. doi: 10.11931/guihaia.gxzw201705036
Cheng H., Inyang A., Li C. D., Fei J., Wang Y. S. (2020). Salt tolerance and exclusion in the mangrove plant avicennia marina in relation to root apoplastic barriers. Ecotoxicology 29 (4), 676–683. doi: 10.1007/s10646-020-02203-6
Craine J. M., Elmore A. J., Wang L., Aranibar J., Bauters M., Boeckx P., et al. (2018). Isotopic evidence for oligotrophication of terrestrial ecosystems. Nat. Ecol. Evolution. 2 (11), 1735–1744. doi: 10.1038/s41559-018-0694-0
Dan X., Liao B., Wu Z., Wu H., Bao D., Dan W., et al. (2016). Resources, conservation status and main threats of mangrove wetlands in China. Ecol. Environ. Sci. 25 (007), 1237–1243. doi: 10.16258/j.cnki.1674-5906.2016.07.021
Doheny-Adams T., Hunt L., Franks P. J., Beerling D. J., Gray J. E. (2012). Genetic manipulation of stomatal density influences stomatal size, plant growth and tolerance to restricted water supply across a growth carbon dioxide gradient. Philos. Trans. R. Soc. B: Biol. Sci. 367 (1588), 547–555. doi: 10.1098/rstb.2011.0272
Dong N., Prentice I. C., Wright I. J., Evans B. J., Togashi H. F., Caddy-Retalic S., et al. (2020). Components of leaf-trait variation along environmental gradients. New Phytologist. 228 (3), 82–94. doi: 10.1111/nph.16558
Dow G. J., Berry J. A., Bergmann D. C. (2014). The physiological importance of developmental mechanisms that enforce proper stomatal spacing in arabidopsis thaliana. New Phytologist. 201 (4), 1205–1217. doi: 10.1111/nph.12586
Duke N. C., Meynecke J.-O., Dittmann S., Ellison A. M., Anger K., Berger U., et al. (2007). A world without mangroves? Science 317 (5834), 41–42. doi: 10.1126/science.317.5834.41b
Dunn J., Hunt L., Afsharinafar M., Meselmani M. A., Mitchell A., Howells R., et al. (2019). Reduced stomatal density in bread wheat leads to increased water-use efficiency. J. Exp. botany. 70 (18), 4737–4748. doi: 10.1093/jxb/erz248
Farnsworth E. ,. J., Ellison A. ,. M., Gong W. ,. K. (1996). Elevated CO2 alters anatomy, physiology, growth, and reproduction of red mangrove (Rhizophora mangle l.). Oecologia 108 (4), 599–609. doi: 10.1007/BF00329032
Farquhar G. D., Hubick K. T., Condon A. G., Richards R. A. (1989). “Carbon isotope fractionation and plant water-use efficiency,” in Stable isotopes in ecological research (New York, NY: Springer), 21–40.
Farquhar G. D., O'Leary M. H., Berry J. A. (1982). On the relationship between carbon isotope discrimination and the intercellular carbon dioxide concentration in leaves. Funct. Plant Biol. 9 (2), 121–137. doi: 10.1071/PP9820121
Farquhar G. D., Richards R. A. (1984). Isotopic composition of plant carbon correlates with water-use efficiency of wheat genotypes. Funct. Plant Biol. 11 (6), 539–552. doi: 10.1071/PP9840539
Franks P. J., Beerling D. J. (2009). Maximum leaf conductance driven by CO2 effects on stomatal size and density over geologic time. Proc. Natl. Acad. Sci. 106 (25), 10343–10347. doi: 10.1073/pnas.0904209106
Franks P. J. ,. W., Doheny-Adams T., Britton-Harper Z. J., Gray J. E. (2015). Increasing water-use efficiency directly through genetic manipulation of stomatal density. New Phytologist. 207 (1), 188–195. doi: 10.1111/nph.13347
Franks P. J., Drake P. L., Beerling D. J. (2009). Plasticity in maximum stomatal conductance constrained by negative correlation between stomatal size and density: an analysis using eucalyptus globulus. Plant Cell Environment. 32 (12), 1737–1748. doi: 10.1111/j.1365-3040.2009.002031.x
Funk J. L., Vitousek P. M. (2007). Resource-use efficiency and plant invasion in low-resource systems. Nature 446 (4), 1079–1081. doi: 10.1038/nature05719
Gu X., Feng H., Tang T., Fung N., Chen L. (2019). Predicting the invasive potential of a non-native mangrove reforested plant (Laguncularia racemosa) in China. Ecol. Eng. 139. doi: 10.1016/j.ecoleng.2019.105591
Guerrieri R., Belmecheri S., Ollinger S. V., Asbjornsen H., Jennings K., Xiao J., et al. (2019). Disentangling the role of photosynthesis and stomatal conductance on rising forest water-use efficiency. Proc. Natl. Acad. Sci. 116 (34), 16909–16914. doi: 10.1073/pnas.1905912116
Hamilton S. E., Casey D. (2016). Creation of a high spatiotemporal resolution global database of continuous mangrove forest cover for the 21st century (CGMFC-21). Global Ecol. Biogeography. 25 (6), 729–738. doi: 10.1111/geb.12449
Han S., Li N., He P., Zhong C., Yang Y. (2010). Photosynthetic characteristics of introduced and indigenous mangrove seedlings in China. Acta Botanica Boreali-Occidentalia Sinica. 30 (8), 1667–1674. doi: 000-4025/abos2010/08-1667-08
Henry C., John G. P., Pan R., Bartlett M. K., Fletcher L. R., Scoffoni C., et al. (2019). A stomatal safety-efficiency trade-off constrains responses to leaf dehydration. Nat. Commun. 10 (1), 1–9. doi: 10.1038/s41467-019-11006-1
Hetherington A. M., Woodward F. I. (2003). The role of stomata in sensing and driving environmental change. Nature 424 (6951), 901–908. doi: 10.1038/nature01843
Jiang G.-F., Goodale U. M., Liu Y.-Y., Hao G.-Y., Cao K.-F. (2017). Salt management strategy defines the stem and leaf hydraulic characteristics of six mangrove tree species. Tree Physiol. 37 (3), 389–401. doi: 10.1093/treephys/tpw131
Keeling R. F., Graven H. D., Welp L. R., Resplandy L., Bi J., Piper S. C., et al. (2017). Atmospheric evidence for a global secular increase in carbon isotopic discrimination of land photosynthesis. Proc. Natl. Acad. Sci. 114 (39), 10361–10366. doi: 10.1073/pnas.1619240114
Keenan T. F., Hollinger D. Y., Bohrer G., Dragoni D., Munger J. W., Schmid H. P., et al. (2013). Increase in forest water-use efficiency as atmospheric carbon dioxide concentrations rise. Nature 499 (7458), 324–327. doi: 10.1038/nature12291
Kuenzer C., Bluemel A., Gebhardt S., Quoc T. V., Dech S. (2011). Remote sensing of mangrove ecosystems: a review. Remote Sens 62 (3), 878–928. doi: 10.3390/rs3050878
Lanning M., Wang L., Scanlon T. M., Vadeboncoeur M. A., Adams M. B., Epstein H. E., et al. (2019). Intensified vegetation water use under acid deposition. Sci. Advances. 5 (7), 5168–5177. doi: 10.1126/sciadv.aav5168
Lee S. Y., Primavera J. H., Dahdouh-Guebas F., McKee K., Bosire J. O., Cannicci S., et al. (2014). Ecological role and services of tropical mangrove ecosystems: a reassessment. Global Ecol. Biogeography. 23 (7), 726–743. doi: 10.1111/geb.12155
Li M. S., Lee S. Y. (1997). Mangroves of China: a brief review. For. Ecol. Management. 96 (3), 241–259. doi: 10.1016/S0378-1127(97)00054-6
Li Y., Lin P. (2006). Anatomical characteristics of leaves in three mangrove species. J. Trop. Subtrop Bot. 14 (4), 301–306. doi: 10.3969/j.issn.1005-3395.2006.4.006
Li F. L., Zhong L., Cheung S. G., Wong Y. S., Tam N. F. Y. (2020). Is laguncularia racemosa more invasive than sonneratia apetala in northern fujian, China in terms of leaf energetic cost? Mar. pollut. Bulletin. 152 (11), 897–906. doi: 10.1016/j.marpolbul.2020.110897
Liao B., Zhang Q. (2014). Area,Distribution and species composition of mangroves in China. wetland science. 12 (4), 435–440. doi: 10.14067/j.cnki.1673-923x.2006.03.015
Liao B. W., Zheng S. F., Chen Y. J., Li M., Zheng D. Z. (2006). Preliminary report on introduction of several alien mangrove plants in dongzhai harbor of hainan province. J. Cent. S. For. Univ. 26 (6), 63–67.
Lin Y. S., Medlyn B. E., Duursma R. A. (2015). Optimal stomatal behaviour around the world. Nat. Climate Change. 5 (11), 459–464. doi: 10.1038/nclimate2550
Lovelock C. E., Duarte C. M. (2019). Dimensions of blue carbon and emerging perspectives. Biol. Letters. 15 (3), 781–790. doi: 10.1098/rsbl.2018.0781
Lu X., Vitousek P. M., Mao Q., Gilliam F. S., Luo Y., Zhou G., et al. (2018). Plant acclimation to long-term high nitrogen deposition in an n-rich tropical forest. Proc. Natl. Acad. Sci. 115 (20), 5187–5196. doi: 10.1073/pnas.1720777115
Matzek V. (2011). Superior performance and nutrient-use efficiency of invasive plants over non-invasive congeners in a resource-limited environment. Biol. Invasions. 13 (12), 3005–3014. doi: 10.1007/s10530-011-9985-y
McKee K. L., Rooth J. E. (2008). Where temperate meets tropical: multi-factorial effects of elevated CO2, nitrogen enrichment, and competition on a mangrove-salt marsh community. Global Change Biol. 14 (5), 971–984. doi: 10.1111/j.1365-2486.2008.01547.x
Ocheltree T. W., Nippert J. B., Prasad P. V. V. (2016). A safety vs efficiency trade-off identified in the hydraulic pathway of grass leaves is decoupled from photosynthesis, stomatal conductance and precipitation. New Phytologist. 210 (1), 97–107. doi: 10.1111/nph.13781
Parida A. K., Das A. B., Mittra B. (2004). Effects of salt on growth, ion accumulation, photosynthesis and leaf anatomy of the mangrove, bruguiera parviflora. Trees 18 (2), 167–174. doi: 10.1007/s00468-003-0293-8
Parida A. K., Jha B. (2010). Salt tolerance mechanisms in mangroves: a review. Trees 24 (2), 199–217. doi: 10.1007/s00468-010-0417-x
Pathare V. S., Koteyeva N., Cousins A. B. (2020). Increased adaxial stomatal density is associated with greater mesophyll surface area exposed to intercellular air spaces and mesophyll conductance in diverse C4 grasses. New Phytologist. 225 (1), 169–182. doi: 10.1111/nph.16106
Qiu Y.-W., Yu K.-F., Zhang G., Wang W.-X. (2011). Accumulation and partitioning of seven trace metals in mangroves and sediment cores from three estuarine wetlands of hainan island, China. J. Hazardous Materials. 190 (3), 631–638. doi: 10.1016/j.jhazmat.2011.03.091
Rahman A. F., Dragoni D., Didan K., Barreto-Munoz A., Hutabarat J. A. (2013). Detecting large scale conversion of mangroves to aquaculture with change point and mixed-pixel analyses of high-fidelity MODIS data. Remote Sens. Environment. 130 (8), 96–107. doi: 10.1016/j.rse.2012.11.014
Reef R., Lovelock C. E. (2015). Historical analysis of mangrove leaf traits throughout the 19th and 20th centuries reveals differential responses to increases in atmospheric CO2. Global Ecol. Biogeography. 23 (11), 1209–1214. doi: 10.1111/geb.12211
Reef R., Slot M., Motro U., Motro M., Motro Y., Adame M.F., et al. (2016). The effects of CO2 and nutrient fertilisation on the growth and temperature response of the mangrove avicennia germinans. Photosynthesis Res. 129 (2), 159–170. doi: 10.1007/s11120-016-0278-2
Richards D. R., Friess D. A. (2016). Rates and drivers of mangrove de- forestation in southeast asia 2000-2012. Proc. Natl. Acad. Sci. United States America. 113 (9), 344–349. doi: 10.1073/pnas.1510272113
Rodríguez J. A. R., Pineda J. E. M., Melgarejo L. M., Calderón J. H. M. (2017). Functional traits of leaves and forest structure of Neotropical mangroves under different salinity and nitrogen regimes. Flora 239 (5), 52–61. doi: 10.1016/j.flora.2017.11.004
Sobrado M. A. (2005). Leaf characteristics and gas exchange of the mangrove laguncularia racemosa as affected by salinity. Photosynthetica 43 (2), 217–221. doi: 10.1007/s11099-005-0036-8
Sperry J. S., Venturas M. D., Anderegg W. R. L., Mencuccini M., Mackay D. S., Wang Y., et al. (2016). Predicting stomatal responses to the environment from the optimization of photosynthetic gain and hydraulic cost. Plant Cell Environment. 40 (6), 816–830. doi: 10.1111/pce.12852
Tanaka Y., Sugano S. S., Shimada T., Hara-Nishimura I. (2013). Enhancement of leaf photosynthetic capacity through increased stomatal density in arabidopsis. New Phytologist. 198 (3), 757–764. doi: 10.1111/nph.12186
Tian S. ,. Q., Zhu S.D., Zhu J. ,. J., Shen Z. ,. H., Cao K. ,. F. (2016). Impact of leaf morphological and anatomical traits on mesophyll conductance and leaf hydraulic conductance in mangrove plants. Plant Sci. J. 34 (6), 909–919. doi: 10.11913/PSJ.2095-0837.2016.60909
Voelker S. L., Brooks J. R., Meinzer F. C., Anderson R., Bader M. K. F., Battipaglia G., et al. (2016). A dynamic leaf gas-exchange strategy is conserved in woody plants under changing ambient CO2: evidence from carbon isotope discrimination in paleo and CO2 enrichment studies. Global Change Biol. 22 (2), 889–902. doi: 10.1111/gcb.13102
Wang X., Zhou L., Lu C. (2018). Do environmental factors affect the male frequency of exotic mangrove species laguncularia racemosa (Combretaceae) along the southeast coast of China? Aquat. Ecology. 52 (7), 235–244. doi: 10.1007/s10452-018-9658-3
Keywords: exotic mangrove species, stable isotope, gas exchange, Invasive mechanism, Laguncularia racemosa
Citation: Bai J, Meng Y, Gou R, Dai Z, Zhu X and Lin G (2023) The linkages between stomatal physiological traits and rapid expansion of exotic mangrove species (Laguncularia racemosa) in new territories. Front. Mar. Sci. 10:1136443. doi: 10.3389/fmars.2023.1136443
Received: 03 January 2023; Accepted: 19 April 2023;
Published: 27 April 2023.
Edited by:
Stelios Katsanevakis, University of the Aegean, GreeceReviewed by:
Yunchao Wu, (CAS), ChinaJun-Jian Wang, Southern University of Science and Technology, China
Copyright © 2023 Bai, Meng, Gou, Dai, Zhu and Lin. This is an open-access article distributed under the terms of the Creative Commons Attribution License (CC BY). The use, distribution or reproduction in other forums is permitted, provided the original author(s) and the copyright owner(s) are credited and that the original publication in this journal is cited, in accordance with accepted academic practice. No use, distribution or reproduction is permitted which does not comply with these terms.
*Correspondence: Guanghui Lin, bGluZ2hAdHNpbmdodWEuZWR1LmNu