Thiomicrorhabdus marina sp.nov., an obligate chemolithoautotroph isolated from tidal zone sediment, and genome insight into the genus Thiomicrorhabdus
- 1Marine College, Shandong University, Weihai, Shandong, China
- 2College of Bioengineering and Biotechnology, Tianshui Normal University, Tianshui, Gansu, China
- 3Weihai Research Institute of Industrial Technology, Shandong University, Weihai, Shandong, China
- 4Shenzhen Research Institute of Shandong University, Shenzhen, Guangdong, China
The contribution of microbes to the marine sulfur cycle has received considerable attention in recent years. In this study, a new Gram-stain-negative, aerobic sulfur-oxidizing bacterium, designated strain 6S2-11T, was isolated from tidal zone sediment of the coast of Weihai, China. Strain 6S2-11T was an obligate chemolithoautotroph utilizing thiosulfate as the energy source. Physiological and biochemical experiments, phylogenetic analysis, and comparative genomic analysis were done with strain 6S2-11T. According to genomic analysis, strain 6S2-11T owned a complete thiosulfate oxidation pathway and an untypical nitrogen metabolism pathway. Its relatively small genome also has multiple environmental adaptation mechanisms. The DNA G+C content of strain 6S2-11T was 44.1%. Strain 6S2-11T was observed to grow at 20-37°C (optimum, 35°C), pH 6.0-9.5 (optimum, pH 7.5), and 0.5-5% (w/v) NaCl (optimum, 2.5%). The major cellular fatty acids (>10%) of strain 6S2-11T were Summed Feature 8 (C18:1ω7c/C18:1ω6c), C16:0 and Summed Feature 3 (C16:1ω7c/C16:1ω6c). The comparison of 16S rRNA gene sequences indicated that strain 6S2-11T was most closely to Thiomicrorhabdus xiamenensis G2T (96.8%). Based on the results of phylogenetic analysis, the strain 6S2-11T is a novel specie of the genus Thiomicrorhabdus, for which name Thiomicrorhabdus marina sp.nov. is proposed with the type strain 6S2-11T (=MCCC 1H00523T=KCTC 82994T).
Introduction
The genus Thiomicrorhabdus now has 11 species, four of which were reclassified from the genus Thiomicrospira in 2017 (Boden et al., 2017). Members of the genus Thiomicrorhabdus are capable of inorganic autotrophic growth using thiosulfates, sulfides, and monosulfides (Boden et al., 2017). They are all obligately chemolithoautotrophic sulfur-oxidizing bacteria (SOB) (Brinkhoff et al., 1999a; Boden et al., 2017). All members of genus Thiomicrorhabdus have been found in diverse environments, from deep-sea hydrothermal vents where SOB dominates to continental shelf sediments and intertidal mud flats (Brinkhoff et al., 1999a; Brinkhoff et al., 1999b; Liu et al., 2020). In this study, the strain 6S2-11T was isolated from coastal sediments.
A critical step in the biogeochemical sulfur cycle is the sulfur redox reactions. (Jørgensen, 1990; Jørgensen and Bak, 1991). About 11.3 teramoles of sulfate are reduced to hydrogen sulfide in seafloor sediments each year, which in turn is oxidized by biotic or abiotic means (Bowles et al., 2014). One study showed that sulfate-reducing bacteria (SRB) drive the remineralization of up to 29% of seafloor organic matter globally, reducing it to form hydrogen sulfide and other sulfides, which are then oxidized by SOB (Bowles et al., 2014). Therefore, the role of SOB and SRB in biogeochemical sulfur cycling is significant.
In addition to playing an important role in the global biogeochemical sulfur cycle, microorganisms are also extensively involved in the nitrogen cycle, including nitrification, denitrification, assimilatory nitrate reduction (ANR), and dissimilatory nitrate reduction to ammonia (DNRA), etc (Hutchins and Capone, 2022). In the context of human activities that have increased the flux of reactive nitrogen (Nr, mainly nitrate) from land to aquatic ecosystems and the eutrophication of many lakes and coastal waters, many studies are exploring the contribution of microbial activities in these aquatic ecosystems to nitrate reduction (Galloway et al., 2004, 2008).
Therefore, this study focused on the metabolic pathways of sulfur and nitrogen in a novel strain 6S2-11T isolated from coastal sediments, which can help to understand the microbiome-mediated sulfur and nitrogen transformation pathways in coastal sediments.
Experimental procedure
Bacteria isolation and cultivation
The strain 6S2-11T was isolated from tidal zone sediment of the coast of Weihai, China (36°58′37″ N, 122°2′37″ E). After sample enrichment, 100 μL of samples with dilution gradients of 10-3 and 10-4 were spread on SOB medium (Peptone 5 g/L, yeast powder 1 g/L, sodium pyruvate 0.3 g/L, NH4Cl 0.26 g/L, Na2S2O3 4.96 g/L) and incubated at 30°C. The isolated strain 6S2-11T was cultured on SOB medium at 30°C, stored in a sterile mixture of 1% (w/v) saline and 15% glycerol at -80°C. The type strain Thiomicrorhabdus xiamenensis G2T and. Thiomicrorhabdus sediminis G1T were used as reference strains which are also cultivated on SOB medium at 30°C.
Gene extraction and analysis
Gene sequence acquisition
The 16S rRNA gene sequence of strain 6S2-11T was obtained by PCR with universal primers 27F and 1492R (Lane, 1991). The sequence was ligated to the pMD18-T vector (Takara) and cloned to obtain a complete 16S rRNA gene sequence. The genome was extracted using a DNA kit (Takara) and then sequenced on the Hiseq X Ten platform (Illumina Inc., San Diego, USA) at Beijing Novogene Bioinformatics Technology, using the pair-end 350-bp sequencing protocol. All sequenced reads were assembled by SOAPdenovo software v2.04. The 16S rRNA gene sequences and whole genome sequences of other strains were downloaded from the NCBI database.
Genetic analysis
The obtained 16S rRNA gene sequences were compared in the EzBioCloud database (https://www.ezbiocloud.net/) and the NCBI database (https://www.ncbi.nlm.nih.gov/) for preliminary confirmation of taxonomic status. The ANI values (https://www.ezbiocloud.net/tools/ani) (Yoon et al., 2017) and DDH values (https://ggdc.dsmz.de/ggdc.php) (Meier-Kolthoff et al., 2013) betwee the strain 6S2-11T and other Thiomicrorhabdus strains were calculated based on genome sequences to confirm their taxonomic status further. Phylogenetic trees were built using neighbor-joining (NJ), maximum-likelihood (ML), and maximum-parsimony (MP) algorithms in MEGA version 7.0 with the bootstrap value being set to 1000 (Kumar et al., 2016). The genome phylogenetic trees were built using IQ-Tree (Nguyen et al., 2015). The genome was annotated using the KEGG database to study the metabolic pathways of the strains (Kanehisa et al., 2016). The antiSMASH database (https://antismash.secondarymetabolites.org/#!/start) was used to predict the secondary metabolites of the strain (Blin et al., 2021). Prediction of antibiotic resistance of strains was performed using the CARD database (https://card.mcmaster.ca/) (Alcock et al., 2020). The analysis of metabolic pathways and insight into environmental adaptation mechanisms are based on the classification results of KEGG annotations. Strains for genome analysis were all Thiomicrorhabdus strains for which the genomes were available from NCBI. Details of the strains and their genome sequence accession numbers are shown in Table 1.
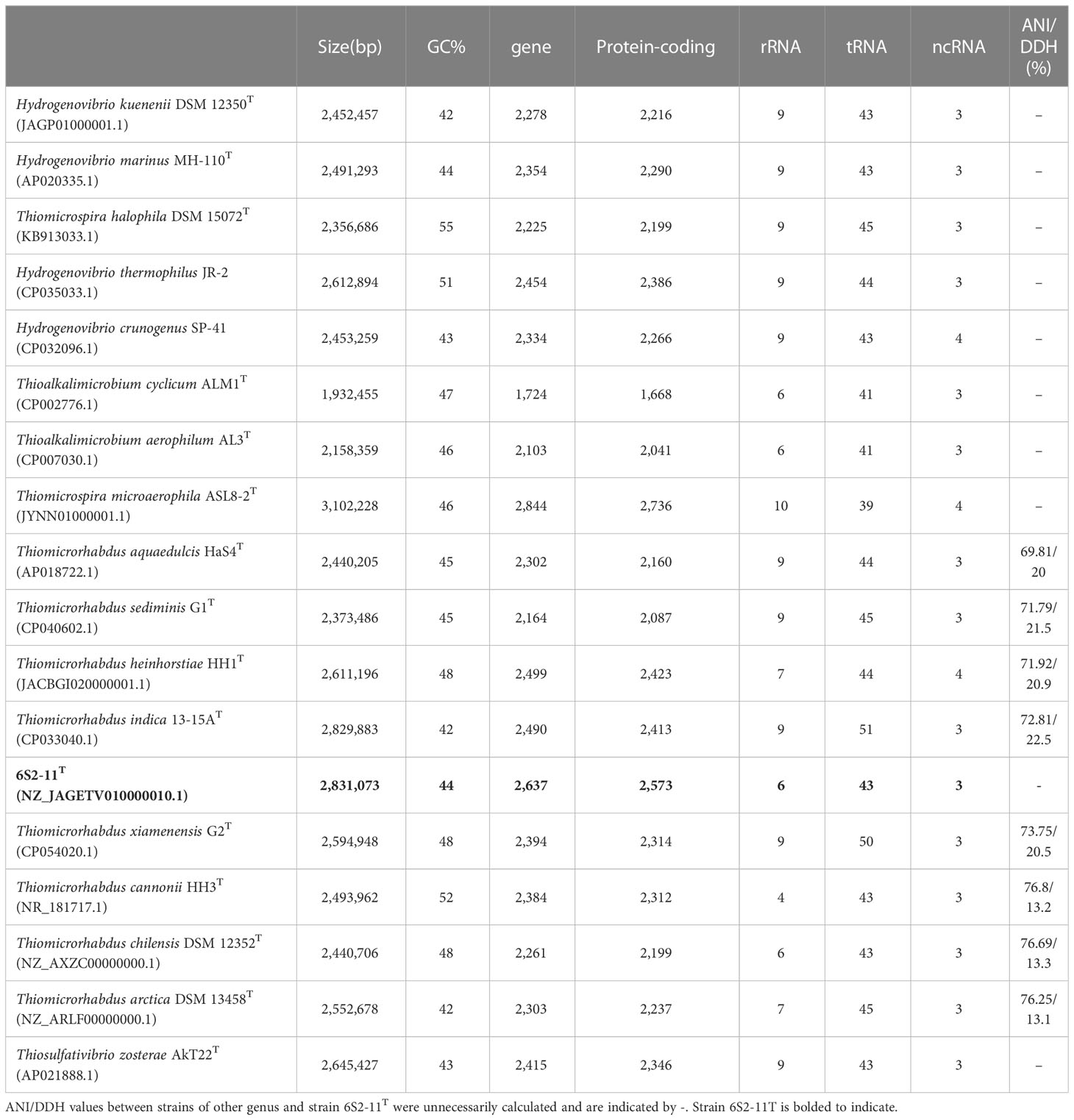
Table 1 Genomic characteristics of all strains and ANI/DDH values between strains of genus Thiomicrorhabdus and strain 6S2-11T.
Physiology, biochemical and chemotaxonomic analyses
The experiments to investigate the morphological and physiological characteristics of the strains were performed using the microorganisms that had been cultured on SOB medium for three days. Among them, the size and morphology of the cells were observed by light microscopy (E600; Nikon) and transmission electron microscope (JEM-1200, JEOL). Gram-staining experiments were performed by the method described by Smibert (Smibert, 1994). The motility experiments were then performed on SOB medium with 0.3% agar, and the results were obtained by direct observation. The temperature range for growth and the optimum growth temperature were tested at different temperatures of 0, 4, 10, 15, 20, 25, 28, 30, 33, 35, 37, 40, and 43°C. The suitable salinity range and optimum salinity for growth were tested in SOB medium with different NaCl concentrations (0, 0.5, 1, 1.5, 2, 2.5, 3, 4, 5, 6, 7, 8, 9, 10%, w/v) and the aged seawater was replaced with artificial seawater (MgSO4 1.62g/L, MgCl2 4.83 g/L, CaCl2 1.15 g/L, KCl 0.72 g/L). The appropriate pH range for growth was tested by adjusting pH to 5.5-9.5 at the interval of 0.5 with appropriate amounts of HCl or NaOH as well as the different buffers before sterilization (including MES-pH 5.5 and 6.0, PIPES-pH 6.5 and 7.0, HEPES-pH 7.5 and 8.0, Tricine-pH 8.5, CAPSO-9.0 and 9.5). The OD600 values were determined in the stationary phase of the strain growth curve. Three experimental groups and one control group were set up for SOX validation experiments with strains 6S2-11T, G1Tand G2T. To exclude interference, the medium was prepared using artificial seawater without sulfate (MgCl2 4.83 g/L, CaCl2 1.15 g/L, KCl 0.72 g/L, NaCl 30 g/L). After 5 days of incubation, 200 µL of culture solution was taken in a ninety-six-well plate, 50 µL of ferric chloride and barium chloride were added respectively to observe whether precipitation was produced (Zhu et al., 2021). The nitrate utilization validation experiment was designed with three parallel experimental groups (2 g/L potassium nitrate and 2% bacterial inoculum) and three parallel control groups (2 g/L potassium nitrate and 2% sterilized medium). In addition, blank groups (without potassium nitrate, 2% bacterial inoculum or 2% sterilized medium) was set up separately. These were all performed in liquid medium. After 0h, 12h, 24h and 36h of incubation respectively, 5mL of liquid medium was taken as samples for detection. After centrifugation, filtration, and dilution (with pure water in a 1:50 ratio), the absorbance of the samples was measured at 220 nm using a UV spectrophotometer. Catalase activity was tested by direct observation of bubble production in a 3% hydrogen peroxide solution. The strains were tested for hydrolytic activity against agar, sodium alginate, cellulose, starch, DNA, casein, and Tween 20, 40, 60, and 80 according to the method of Smibert (Smibert, 1994). The antibiotic susceptibility of strains was tested using the disc diffusion method described by Du (Du et al., 2014), and strain susceptibility was described according to the guidelines of the Clinical and Laboratory Standards Institute (CLSI). Other physiological and biochemical characteristics of the strains were determined using API 20E and Biolog GENIII, referring to their instructions for specific steps (except for salinity, which was adjusted to 3%).
Polar lipids were extracted using a chloroform/methanol system according to the method described by Komagata and Suzuki (Komagata and Suzuki, 1988) and measured by two-dimensional thin-layer assay chromatography (TLC) (Minnikin et al., 1984). Fatty acids were extracted according to the standard protocol of MIDI (Sherlock Microbial Identification System, version 6.1). Analysis was performed with a gas chromatograph. Cellular fatty acids were identified using the TSBA40 database of the Microbial Identification System to determine fatty acid names and percentages (Sasser, 1990).
Results and discussion
Phylogenetic analysis and genome comparison
The almost complete 16S rRNA gene sequence of strain 6S2-11T was amplified (MW712743) and the genomic sequence of the strain were extracted (NZ_JAGETV010000010). The preliminary identification of the 16S rRNA gene sequence was performed by the EzBioCloud database. The 16S rRNA gene sequence of Thiomicrorhabdus xiamenensis G2T was found to be the closest to that of strain 6S2-11T with 96.81% similarity. To further confirm the relationship between strain 6S2-11T and related taxa, their ANI and DDH values were calculated (Table 1). The results showed that the ANI values were all less than the classification threshold for ANI (95-96%) (Goris et al., 2007). The DDH values are also below the species identification boundary for DDH (70%) (Meier-Kolthoff et al., 2013). Phylogenetic trees were built using NJ, ML, and MP methods for 16S rRNA genes (Figure 1) and IQ-Tree for genomes (Figure 2). The results all indicated that strain 6S2-11T belongs to the genus Thiomicrorhabdus. The genome of the strain 6S2-11T was annotated by NCBI PGAP and compared with the genomic data of several other strains of the genus Thiomicrorhabdus, Thiomicrospira, Hydrogenovibrio, and Thiosulfativibrio (Figure 2).
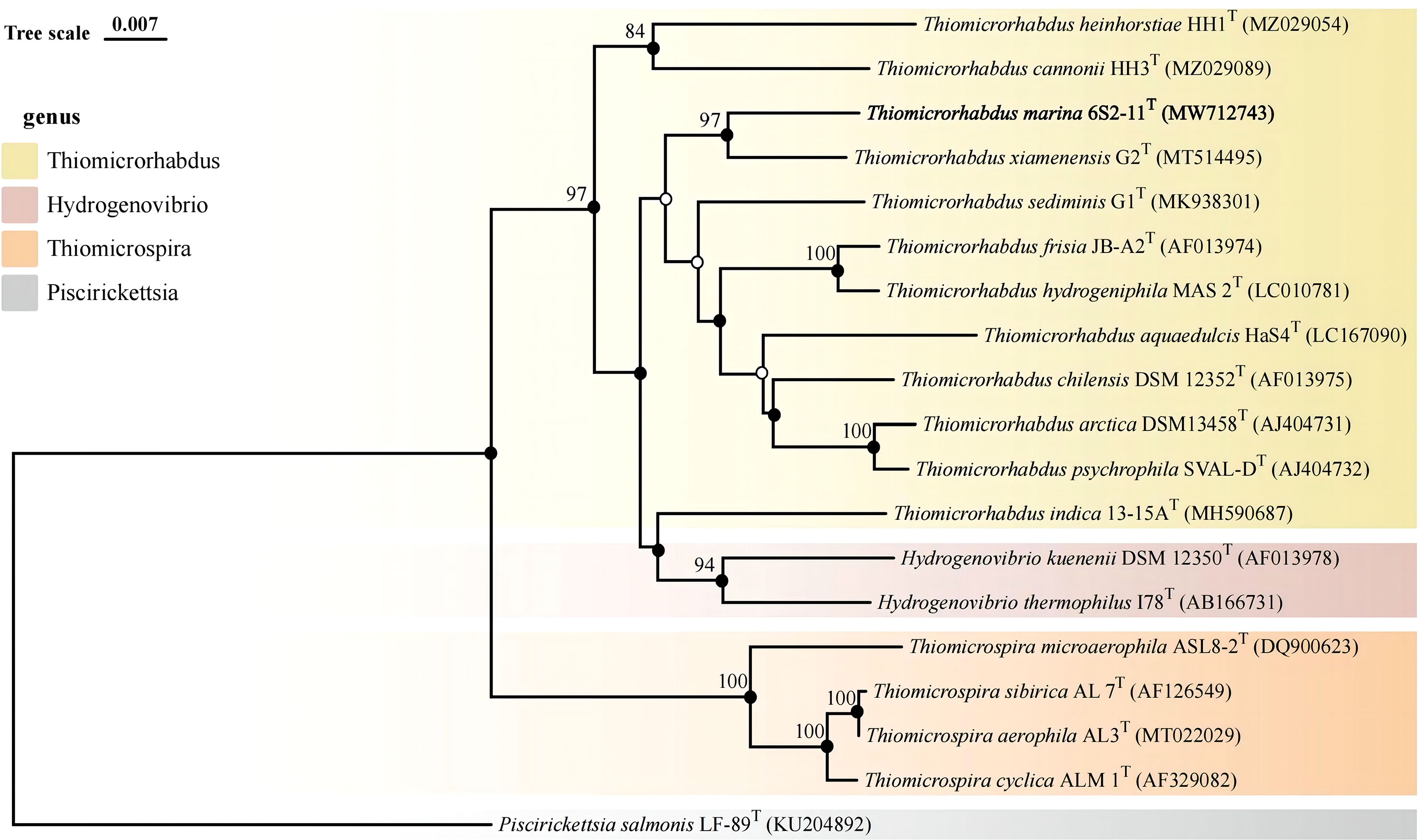
Figure 1 Phylogenetic tree of 16S rDNA based on NJ algorithm. Bootstrap values are shown on the branch nodes (only bootstrap values higher than 70% are marked on the branches). Solid circles represent consistent results with ML and MP algorithms.
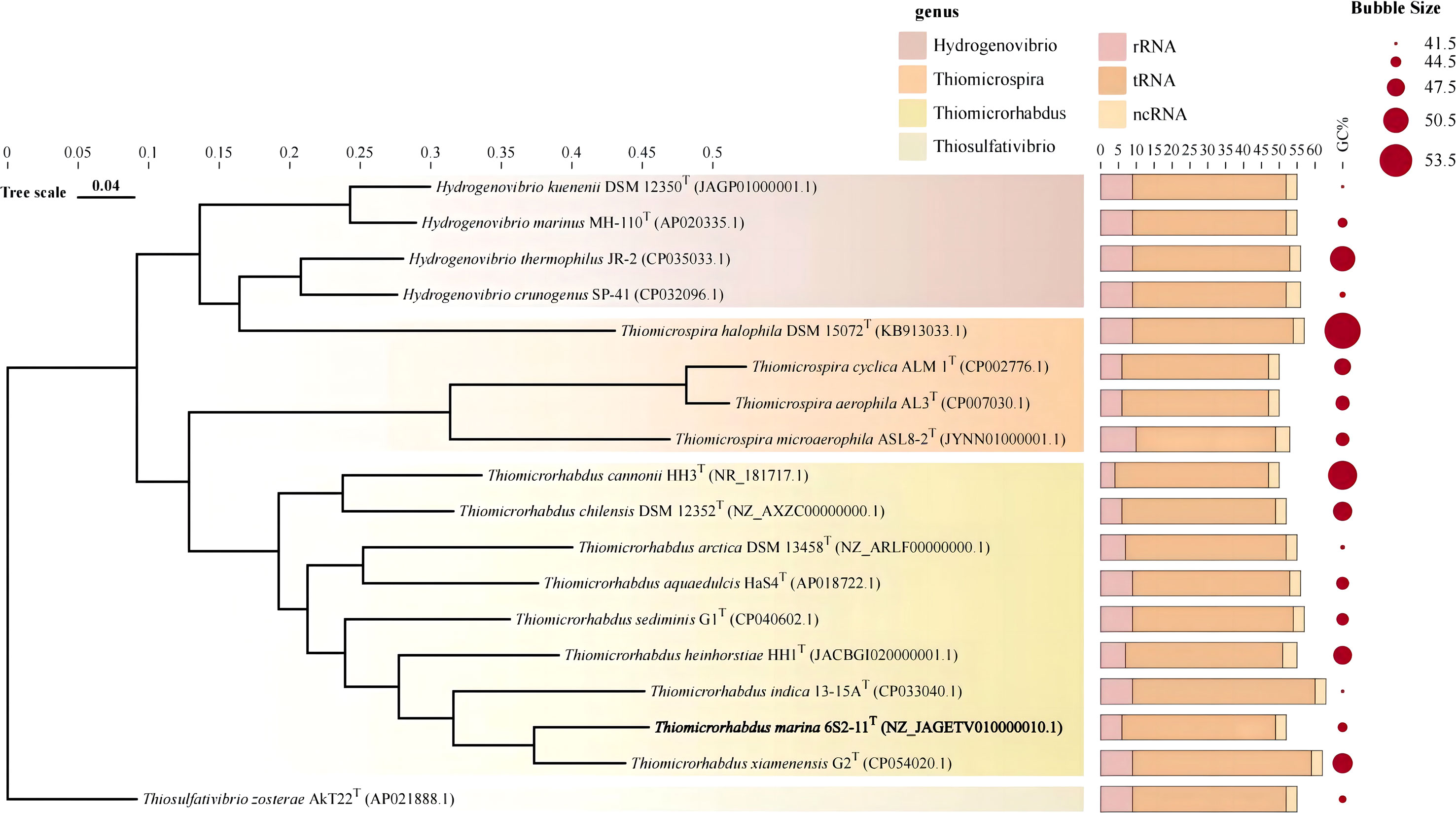
Figure 2 Genome phylogenetic tree. Stacked bar charts represent the number of different RNAs. Circle plots indicate GC content.
Genomic features of different species of strains were collected and compared in Table 1. Strain 6S2-11T contained 2637 genes, including 2573 protein-coding genes, and 52 RNAs genes (6 rRNA genes, 43 tRNA genes, and 3 ncRNA). The G+C content was 44.1%. The strain 6S2-11T has 6 rRNA genes, and in comparison to other strains (except the strain HH3T) it has the least amount. This may indicate that strain 6S2-11T is not outstanding in its ability to respond to complex environmental resources compared with other strains in this genus. It has been shown that the number of rRNA genes correlates with the rate of bacterial response to resource availability(Klappenbach et al., 2000). The bacteria exposed to nutrient-complex media that rapidly form colonies contain an average of 5.5 small-subunit rRNA genes (Klappenbach et al., 2000). Thus, the number of rRNA genes in the strains of genus Thiomicrorhabdus may help improve their ability to respond to complex environmental resource.
Metabolic pathway analysis
The genomes of nine strains of the genus Thiomicrorhabdus, including strain 6S2-11T, were annotated (These nine strains are all the strains of genus Thiomicrorhabdus for which the genomes were vailable from NCBI). The pathway types and gene count of strain 6S2-11T (Supplementary Figure 1) and the metabolic modules integrity of Thiomicrorhabdus strains (Figure 3) were obtained from the annotation. Metabolic module integrity was obtained from the annotation results by calculating the proportion of pathways that the strain has to the total pathways required for this module to proceed. All pathways required for the corresponding metabolic module to proceed are obtained from KEGG.
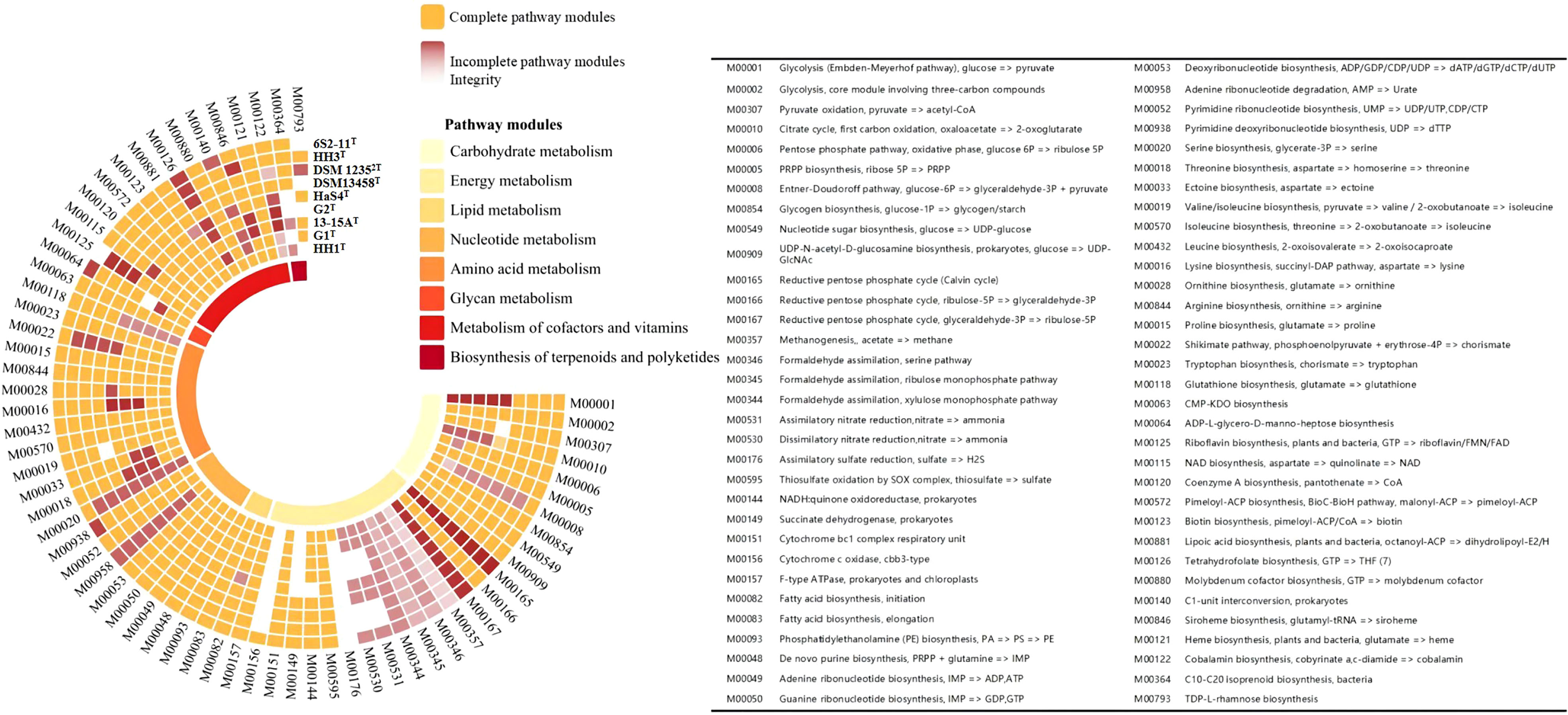
Figure 3 The metabolic modules integrity of Thiomicrorhabdus strains. The orange color blocks represent intact metabolic modules, and the red-brown color blocks represent incomplete metabolic modules. The integrity of incomplete metabolic modules is indicated by color shades. The lowest integrity is 0 for white.
Nitrogen metabolism
Of all the strains analyzed, strain 6S2-11T, DSM 13458T, HaS4T, G1Tand HH1T have pathway modules related to nitrogen metabolism. These strains have half of DNRA (M00530, NirBD, nitrite reductase, EC 1.7.1.15) and ANR (M00531, NasA, assimilatory nitrate reductase, EC 1.7.99.-), without Nitrogen fixation, Denitrification, and Nitrification.
The integrity of both DNRA and ANR metabolic modules is only 50%, but this does not mean that the strains are incapable of nitrogen metabolism. In a previous study, it was found that nirBD-encoded nitrite reductase can not only catalyze fermentation-mode-dependent DNRA but also regulate ANR (Luque-Almagro et al., 2011). Nitrite reductase (NirBD) and assimilatory nitrate reductase (NasA) can work together to enable ANR to proceed properly (Hu et al., 2022). In the microbial nitrogen cycle, ANR is a form of anabolic metabolism in which nitrate is first reduced to nitrite by NasA, then nitrite is converted to ammonia (Reitzer, 2003). Ammonia is synthesized into glutamate by glutamine synthetase (glnA, EC 6.3.1.2) and glutamate synthase (gltBD, EC 1.4.1.13) (These two enzymes were also annotated in the genome of strain 6S2-11T) (Reitzer, 2003). Eventually, the nitrogen in nitrate is incorporated into the organism (Reitzer, 2003). DNRA is a catabolic pathway that does not incorporate nitrogen into the organism (Sparacino-Watkins et al., 2014). Instead, after reduction to obtain nitrite, the end product ammonia obtained by NirBD is secreted from the cells (Reitzer, 2003; Sparacino-Watkins et al., 2014). We also realized that this ANR process works only if the strain has a system capable of transporting nitrate to the cytoplasm. Through genomic analysis, we found the existence of nasF (nitrate/nitrite transport system substrate-binding protein), nasE (nitrate/nitrite transport system permease protein), and nasD (nitrate/nitrite transport system ATP-binding protein), which form an ATP dependent nitrate transport system. Due to the combination of proteins, ANR allows these strains to assimilate nitrate and use NirBD to remove nitrogen pollutants from aquatic ecosystems under low ammonium conditions (Jack and Joop, 2000). The reactive nitrogen transformation pathway via ANR sometimes occurs at more than the DNRA and denitrification (Hu et al., 2022).
To verify whether the strain is nitrate-reducing, we used API 20E and UV spectrophotometric method to detect. The results of 20E showed that the strain was negative for nitrate reduction (Supplementary Table 1). This may be because the experiment was conducted to determine whether the strains had nitrate-reducing ability by the nitrite content in the environment. But the ANR process mentioned above always occurs in the cytoplasm and no nitrite is released into the environment and detected. The result of UV spectrophotometric detection at 220 nm was shown in the Supplementary Figure 2. The measured data were subjected to one-way ANOVA test using SPSS software, and it was concluded that the nitrate content in the environmental samples with the strain 6S2-11T growth was significantly reduced in 0-12 hours (p< 0.01). While the change of nitrate content in 12-36 hours was not significant. The difference in nitrate concentration between the experimental and control groups at 0 h was not significant. Compared to the control group (without bacteria), the differences in nitrate content during 36 hours were at highly significant levels. Therefore, the utilization of nitrate by strain 6S2-11T can be observed using UV spectrophotometry.
The confirmation that strain 6S2-11T has such a nitrogen metabolism pathway on the one hand corroborates previous findings that chemoautotrophic sulfur-oxidizing bacterium is the dominant nitrogen-assimilating bacteria in hydrothermal vent (Jiang et al., 2015). On the other hand, it further illustrates the importance of understanding its nitrogen metabolism for understanding the nitrogen cycle in its habitat.
Sulfur metabolism
All nine strains of the genus Thiomicrorhabdus have the complete thiosulfate oxidation pathway-SOX system (M00595), and HH1T also has the complete assimilatory sulfate reduction pathway (M00176). However, no strain was found to have the Dissimilatory sulfate reduction pathway. The sulfur metabolic pathway diagram of strain 6S2-11T was compiled from the annotated results (Figure 4).
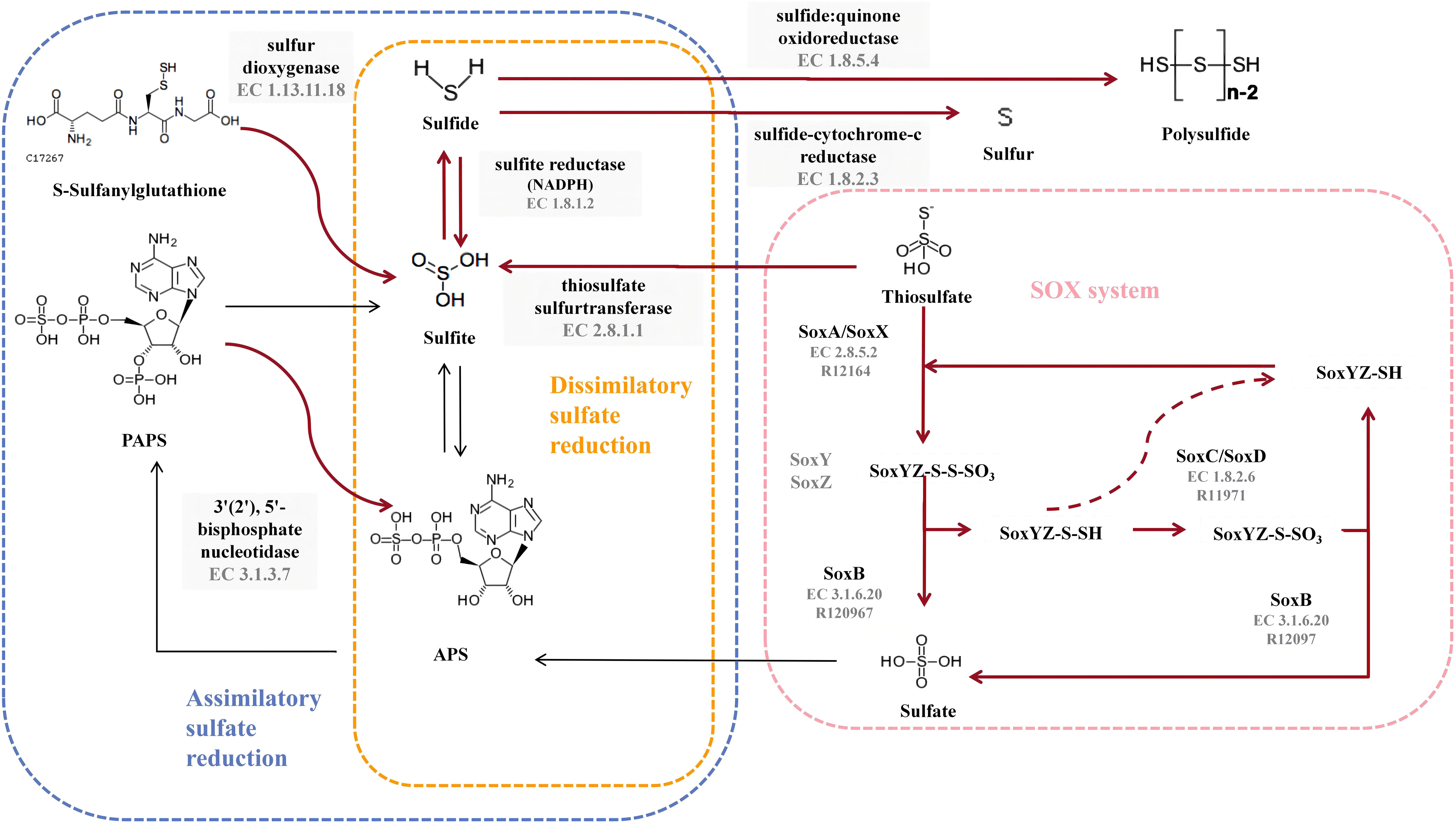
Figure 4 Diagram of the sulfur metabolic pathway. The pink blocks indicate SOX pathway-related enzymes, and the gray blocks indicate enzymes owned by strain 6S2-11T that are not related to SOX but are related to sulfur metabolism. The red bolded arrows indicate pathways available to strain 6S2-11T.
To validate the results obtained from genome annotation, we designed experiments to verify whether strain 6S2-11T can oxidize thiosulfate to sulfate via SOX. It was found that after incubation, there were significant precipitates in the bacterial suspension of all three strains with barium chloride and no precipitates in the bacterial suspension of all three strains with iron chloride compared to the bacterial suspension of day 0 (Supplementary Table 2). This indicates that after incubation, all three strains produced that could be precipitated by Ba2+. It was verified that these three strains do have the ability to oxidize thiosulfate to sulfate. In combination with the genomic analysis, only the SOX system was annotated in all pathways of sulfur metabolism to produce sulfate. Also, the annotated SOX system was complete. Therefore, it is presumed that these strains possess the complete SOX system.
In strain 6S2-11T, besides annotating the complete SOX system, it also annotates thiosulfate sulfurtransferase (EC 2.8.1.1), sulfite reductase (NADPH) (EC 1.8.1.2), sulfide-cytochrome -c reductase (FCC, EC 1.8.2.3), sulfide: quinone oxidoreductase (SQR, EC 1.8.5.4), 3’(2’), 5’-bisphosphate nucleotidase (EC 3.1.3.7), and sulfur dioxygenase (EC 1.13.11.18). Among them, FCC is a periplasmic enzyme, together with thiosulfate sulfurtransferase (EC 2.8.1.1) and sulfite reductase (NADPH) (EC 1.8.1.2), mediating the reduction of thiosulfate to monosulfide (Nguyen et al., 2022). Meanwhile, sulfide: quinone oxidoreductase (EC 1.8.5.4) acts as a membrane-bound enzyme to mediate the formation of the inorganic polysulfide H2Sn from H2S, which in turn spontaneously forms S8 in the cytoplasm and aggregates into spheres (Wang et al., 2022). S8 is the major component of elemental sulfur (Wang et al., 2022). Therefore, it can be assumed that strain 6S2-11T has two different pathways for the formation of elemental sulfur. However, the importance of these two elemental sulfur production pathways varies for different species. For example, in Allochromatium vinosum, mutational inactivation of FCC did not affect its sulfide oxidation, whereas in the genus Thioalkalivibrio, FCC plays a crucial role in sulfide oxidatio (Griesbeck et al., 2000). Both FCC and SQR were present in strain 6S2-11T, and the effect of FCC and SQR on their specific sulfide oxidation still needs further investigation.In general, these sulfur metabolic processes are not only a metabolic and detoxification mechanism for Chemoenergetic autotrophic bacteria but also an essential microbial involvement in the biogeochemical sulfur cycle.
Carbon metabolism
In the analysis of the pathway modules, both strains 6S2-11T and HH1T have an intact ED pathway (M00008), while the remaining four strains have a low ED pathway integrity (Figure 3). Compared to the intact EMP, the ED pathway is expected to require much fewer enzymatic proteins to achieve the same rate of glucose conversion as the EMP (Flamholz et al., 2013). It reflects a trade-off between energy (ATP) production and the amount of enzymatic protein required, which is a metabolic strategy of the strain to adapt to its environment (Flamholz et al., 2013). Furthermore, all nine strains were annotated to have an almost complete Calvin cycle, indicating that all nine strains of the genus Thiomicrorhabdus are likely to be able to obtain energy through carbon fixation via the CBB cycle, which is also the main mode of carbon fixation by autotrophic Gammaproteobacteria (Hügler and Sievert, 2011).
Other metabolic modules
Strains 6S2-11T, HaS4T, G1T, and HH1T all have complete ectoine synthesis pathways, while the integrity of the ectoine synthesis pathway in G2T and 13-15AT is also high. It is also the case that four strains 6S2-11T, HaS4T, G1T, and HH1T have complete siroheme synthesis pathways. Siroheme is a heme-like prosthetic group in microorganisms and some plants, which is involved in the assimilation of nitrate as a cofactor for nitrite reductase (Murphy et al., 1974; Dietl et al., 2018).
Environmental adaptation mechanisms
The role of secondary metabolites in adverse environments
Nine strains of the genus Thiomicrorhabdus were annotated using the antiSMASH database to predict secondary metabolites. The results obtained revealed that all strains produce ectoine and TypeIII polyketides synthase (T3PKS). In addition, G1T, 13-15AT, DSM 12352T, DSM 13458T and HH3T produce RiPP-like (other unspecified ribosomally synthesised and post-translationally modified peptide product); DSM 13458T and HH3T produce arylpolyene; HH1T produce N-acetylglutaminylglutamine amide (NAGGN) (Figure 5).
Ectoine is a compatible solute that can be found in a wide range of different salt-loving and salt-tolerant microorganisms (Reed, 1990). It protects the organism from hyperosmotic stress by regulating the intracellular concentration of low molecular weight solutes (Reed, 1990; Roesser and Müller, 2001). It can prevent cell leakage, irreversible plasmolysis, and dehydration. It also has the ability to generate swelling pressure within the range required for growth, all of which are survival strategies for a bacterial adaptation to the environment (Reed, 1990; Roesser and Müller, 2001). Other recent studies have revealed that ectoine production is related to the mechanism of thermal adaptation of bacteria (Salvador et al., 2018).Ectoine can be seen as a heterocyclic amino acid (Galinski et al., 1985). Moreover, HH1T can produce NAGGN, which is also a compatible solute that protects the cell by regulating the concentration of small molecular weight solutes. However, the mechanism of its biosynthesis is not clear (Csonka and Hanson, 1991). The phenomenon that different cells select different solutes is mainly due to different growth conditions (Ventosa et al., 1998). In general, the secondary metabolites of all nine strains of the genus Thiomicrorhabdus are functioning mostly to adapt to a hyperosmotic environment or high-temperature environment. It suggests that their survival strategies are not only the production of secondary metabolites that help them survive in extreme environments. They also choose to ensure their survival by reducing unnecessary energy expenditure in metabolic processes.
Heavy metal resistance and environmental adaptation mechanisms
Combined with the above analysis in this study, it can be found that nine strains of the genus Thiomicrorhabdus, including strain 6S2-11T, have multiple mechanisms of adaptation to the environment. For example, more rRNA genes, ED pathway, and production of secondary metabolites Ectoine and NAGGN. It can also be seen in Supplementary Figure 1 that there are some genes of the environmental adaptation pathway present in the genome of strain 6S2-11T.Therefore, the genes related to environmental adaptation were predicted and analyzed by KEGG. The czc-A protein, czc-B protein, and czc-C protein of the CzcCBA-mediated heavy metal efflux system were predicted in several strains of the genus Thiomicrorhabdus (Supplementary Table 3) (Nies, 2003). CzcCBA mainly mediates resistance to Co2+, Zn2+, and Cd2+ (Mergeay et al., 1985). This resistance is based on the reduced cellular accumulation of the three cations due to proton-driven cation efflux (Nies and Silver, 1989; Nies, 1995).
Analysis and validation of antibiotic resistance
The antibiotic resistance of nine strains of the genus Thiomicrorhabdus, including strain 6S2-11T, was predicted using the CARD database to analyze their potential antibiotic resistance properties. The results are displayed in Figure 6. And the results of the antibiotic resistance properties of strain 6S2-11T using drug-sensitive paper are shown in Supplementary Table 4.
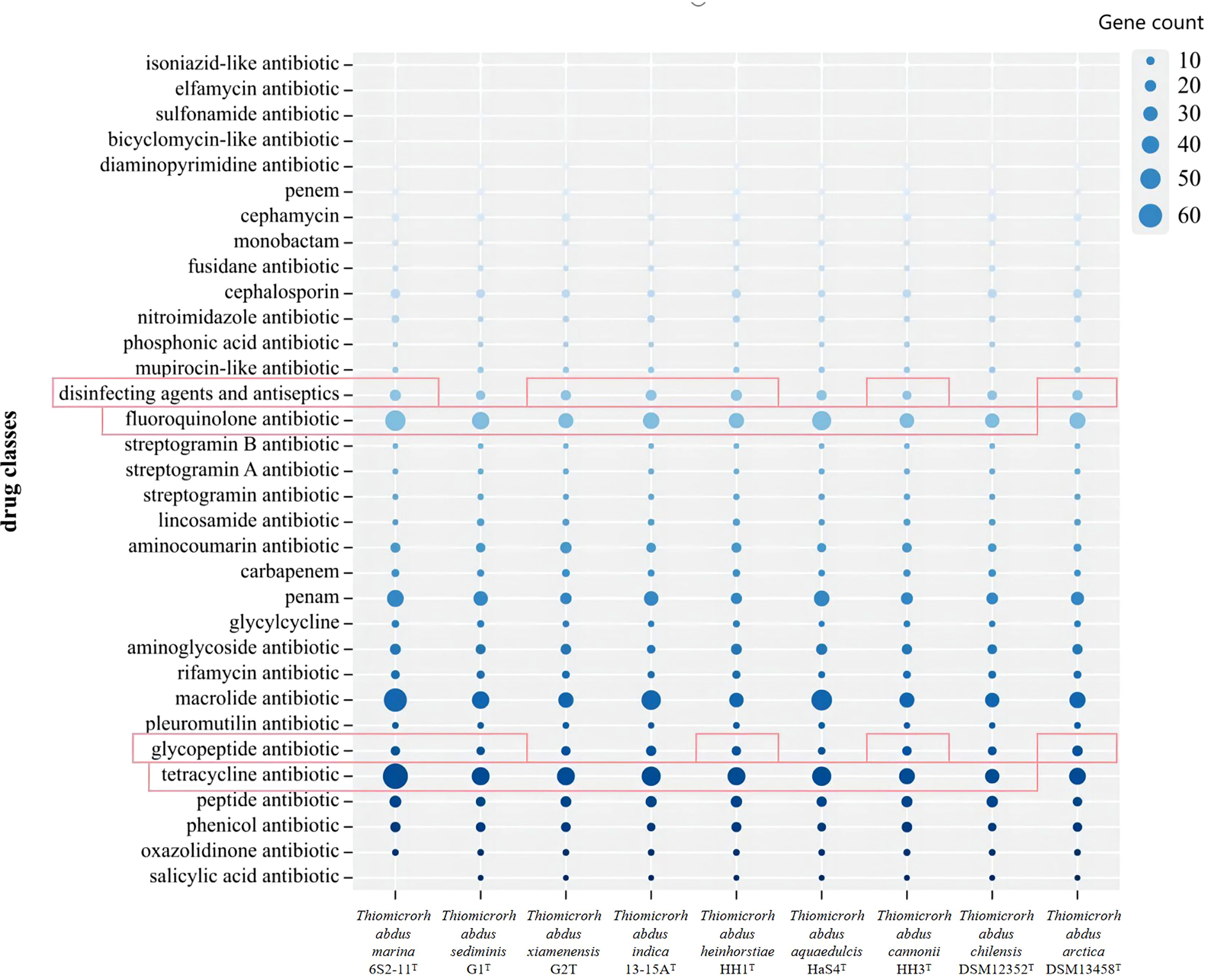
Figure 6 The gene count of different antibiotics resistance genes annotated for Thiomicrorhabdus strains. Red highlighting indicates that it has genes that are annotated by a rigorous algorithm.
Thiomicrorhabdus strains behaved similarly in gene count of different antibiotics resistance. In the CARD database, antibiotics resistance is annotated by both rigorous and loose algorithms. All the Thiomicrorhabdus strains (except DSM 13458T) annotated with tetracycline antibiotic and fluoroquinolone antibiotic resistance by rigorous algorithm, and the gene count was significantly greater than the other antibiotics resistant genes. In addition, strain 6S2-11T was also annotated with glycopeptide antibiotics, disinfecting agents, and antiseptics resistant genes by rigorous algorithm. The experimental results supported part of the analysis, strain 6S2-11T was indeed highly resistant to tetracycline, Polymyxin B, and Vancomycin (Polymyxin B and Vancomycin are both glycopeptide antibiotics). 30μg of tetracycline, 30μg of vancomycin, and 300μg of Polymyxin B all did not affect strain 6S2-11T. The results also showed that strain 6S2-11T was highly resistant to lincomycin. 2μg of lincomycin did not affect its growth. However, all nine strains in the biographical analysis were not significantly resistant to lincomycin, strain 6S2-11T had the lowest number of genes (2).
These antibiotic resistances possessed by strain 6S2-11T may be related to the composition of the microbial community in its habitat as a way of adapting to unfavorable environments. It fights for survival by resisting the antibiotics released by microorganisms in its habitat.
Physiological and biochemical characteristics
Strain 6S2-11T is an oval-shape, aerobic, Gram-negative bacterium with a single polar flagellum that grows at 20-37°C (optimum, 35°C), pH 6.0-9.5 (optimum, pH 7.5-8.0), and 0.5-5% (w/v) NaCl (optimum, 2.5%) (Supplementary Figure 3). It was positive for oxidase activity and peroxidase activity and was unable to hydrolyze agar, sodium alginate, CM-cellulose, starch, Tween20, Tween40, Tween60, Tween80, DNA, and casein. In addition, the carbon sources that both strain 6S2-11T and reference strains G1T and G2T could oxidize d-Galacturonic Acid, d-Fucose, d-Glucuronic Acid, Glucuronamide, l-Fucose, d-Fructose-6-PO4, l-Rhamnose (Supplementary Table 5). And in API 20E, both strain 6S2-11T and reference strain G1T and G2T were positive for TDA and GEL only (Supplementary Table 1).
Chemotaxonomic analyses
The dominant fatty acids (>10%) of strain 6S2-11T were Summed Feature 8 (C18:1ω7c/C18:1ω6c) (46.58%), C16:0 (21.69%), Summed Feature 3 (C16:1ω7c/C16:1ω6c) (14.57%). The fatty acid composition of strain 6S2-11T and the reference strains G1T, G2T are shown in Supplementary Table 6.
The major polar lipids of strain 6S2-11T were phosphatidylglycerol (PG), phosphatidylethanolamine (PE), aminophospholipids (APL) and three unidentifified lipids (L) (Supplementary Figure 4).
Description of Thiomicrorhabdus marina sp. nov.
Thiomicrorhabdus marina (ma.ri’na. L. fem. adj. marina, of the sea, marine)
Cells of Thiomicrorhabdus marina are oval-shaped with a single polar flagellum. It is an aerobic, Gram-negative bacterium that grows at 20-37°C (optimum, 35°C), pH 6.0-9.5 (optimum, pH 7.5-8.0), and 0.5-5% (w/v) NaCl (optimum, 2.5%). It was positive for oxidase activity and peroxidase activity and was unable to hydrolyze agar, sodium alginate, CM-cellulose, starch, Tween20, Tween40, Tween60, Tween80, DNA, and casein. The dominant fatty acids (>10%) of Thiomicrorhabdus marina were Summed Feature 8 (C18:1ω7c/C18:1ω6c), C16:0, Summed Feature 3 (C16:1ω7c/C16:1ω6c).
The type strain, 6S2-11T (=MCCC 1H00523T=KCTC 82994T), was isolated from marine sediment from the coast of Weihai, China (36°58′37″ N, 122°2′37″ E). The Genebank accession number for the 16S rRNA gene sequence of strain 6S2-11T was MW712743 and the draft genome has been deposited in GenBank under the accession number NZ_JAGETV010000010.
Data availability statement
The datasets presented in this study can be found in online repositories. The names of the repository/repositories and accession number(s) can be found in the article/Supplementary Material.
Author contributions
X-JL isolated the strain strains 6S2-11T. X-YT, X-JL, FY, HY performed material preparation, experimental operation, data collection and analysis. X-JL and X-YT finished the experiment and manuscript. ZL performed genomic analysis. Z-JD and M-QY offered experiment guidance and critical revision of manuscripts. All authors contributed to the article and approved the submitted version.
Funding
This work was funded by National Science and Technology Fundamental Resources Investigation Program of China (2019FY100700), National Natural Science Foundation of China (32200003), Natural Science Foundation of Shandong Province (ZR2022QC106), Guangdong Basic and Applied Basic Research Foundation (2022A1515110773), Youth Science and Technology Fund Program of Gansu Province (20JR10RA803), Science and Technology Support Program of Qinzhou District Tianshui City (2021-JHGLG-4062) and Innovation Fund of Tianshui Normal University (CXJ2020-01).
Conflict of interest
The authors declare that the research was conducted in the absence of any commercial or financial relationships that could be construed as a potential conflict of interest.
Publisher’s note
All claims expressed in this article are solely those of the authors and do not necessarily represent those of their affiliated organizations, or those of the publisher, the editors and the reviewers. Any product that may be evaluated in this article, or claim that may be made by its manufacturer, is not guaranteed or endorsed by the publisher.
Supplementary material
The Supplementary Material for this article can be found online at: https://www.frontiersin.org/articles/10.3389/fmars.2023.1144912/full#supplementary-material
Abbreviations
KCTC, Korean Collection for Type Cultures; MCCC, Marine Culture Collection of China; DDH, Digital DNA–DNA hybridization; ANI, Average nucleotide identity; TLC, Thin-layer chromatography; MEGA, Molecular evolutionary genetics analysis.
References
Alcock B. P., Raphenya A. R., Lau T. T. Y., Tsang K. K., Bouchard M., Edalatmand A., et al. (2020). CARD 2020: antibiotic resistome surveillance with the comprehensive antibiotic resistance database. Nucleic Acids Res. 48, D517–D525. doi: 10.1093/nar/gkz935
Blin K., Shaw S., Kloosterman A. M., Charlop-Powers Z., van Wezel G. P., Medema M. H., et al. (2021). antiSMASH 6.0: improving cluster detection and comparison capabilities. Nucleic Acids Res. 49, W29–W35. doi: 10.1093/nar/gkab335
Boden R., Scott K. M., Williams J., Russel S., Antonen K., Rae A. W., et al. (2017). An evaluation of thiomicrospira, hydrogenovibrio and thioalkalimicrobium: reclassification of four species of thiomicrospira to each thiomicrorhabdus gen. nov. and hydrogenovibrio, and reclassification of all four species of thioalkalimicrobium to thiomi. Int. J. Syst. Evol. Microbiol. 67, 1140–1151. doi: 10.1099/ijsem.0.001855
Bowles M. W., Mogollón J. M., Kasten S., Zabel M., Hinrichs K.-U. (2014). Global rates of marine sulfate reduction and implications for sub-sea-floor metabolic activities. Science 344, 889–891. doi: 10.1126/science.1249213
Brinkhoff T., Muyzer G., Wirsen C. O., Kuever J. (1999a). Thiomicrospira chilensis sp. nov., a mesophilic obligately chemolithoautotrophic sulfuroxidizing bacterium isolated from a thioploca mat. Int. J. Syst. Bacteriol. 49 (Pt 2), 875–879. doi: 10.1099/00207713-49-2-875
Brinkhoff T., Muyzer G., Wirsen C. O., Kuever J. (1999b). Thiomicrospira kuenenii sp. nov. and thiomicrospira frisia sp. nov., two mesophilic obligately chemolithoautotrophic sulfur-oxidizing bacteria isolated from an intertidal mud flat. Int. J. Syst. Evol. Microbiol. 49, 385–392. doi: 10.1099/00207713-49-2-385
Csonka L. N., Hanson A. D. (1991). Prokaryotic osmoregulation: genetics and physiology. Annu. Rev. Microbiol. 45, 569–606. doi: 10.1146/annurev.mi.45.100191.003033
Dietl A.-M., Binder U., Shadkchan Y., Osherov N., Haas H. (2018). Siroheme is essential for assimilation of nitrate and sulfate as well as detoxification of nitric oxide but dispensable for murine virulence of aspergillus fumigatus. Front. Microbiol. 9. doi: 10.3389/fmicb.2018.02615
Du Z.-J., Wang Y., Dunlap C., Rooney A. P., Chen G.-J. (2014). Draconibacterium orientale gen. nov., sp. nov., isolated from two distinct marine environments, and proposal of draconibacteriaceae fam. nov. Int. J. Syst. Evol. Microbiol. 64, 1690–1696. doi: 10.1099/ijs.0.056812-0
Flamholz A., Noor E., Bar-Even A., Liebermeister W., Milo R. (2013). Glycolytic strategy as a tradeoff between energy yield and protein cost. Proc. Natl. Acad. Sci. U. S. A. 110, 10039–10044. doi: 10.1073/pnas.1215283110
Galloway J. N., Dentener F. J., Capone D. G., Boyer E. W., Howarth R. W., Seitzinger S. P., et al. (2004). Nitrogen cycles: past, present, and future. Biogeochemistry 70, 153–226. doi: 10.1007/s10533-004-0370-0
Galloway J. N., Townsend A. R., Erisman J. W., Bekunda M., Cai Z., Freney J. R. (2008). Transformation of the nitrogen cycle: recent trends, questions, and potential solutions. Science 320, 889–892. doi: 10.1126/science.1136674
Goris J., Konstantinidis K. T., Klappenbach J. A., Coenye T., Vandamme P., Tiedje J. M. (2007). DNA–DNA Hybridization values and their relationship to whole-genome sequence similarities. Int. J. Syst. Evol. Microbiol. 57, 81–91. doi: 10.1099/ijs.0.64483-0
Griesbeck C., Hauska G., Schütz M. (2000). Biological sulfide oxidation: Sulfide-quinone reductase (SQR), the primary reaction. Recent Res. Dev. Microbiol. 4, 179–203.
Hu R., Liu S., Huang W., Nan Q., Strong P. J., Saleem M., et al. (2022). Evidence for assimilatory nitrate reduction as a previously overlooked pathway of reactive nitrogen transformation in estuarine suspended particulate matter. Environ. Sci. Technol. 56, 14852–14866. doi: 10.1021/acs.est.2c04390
Hügler M., Sievert S. M. (2011). Beyond the Calvin cycle: autotrophic carbon fixation in the ocean. Ann. Rev. Mar. Sci. 3, 261–289. doi: 10.1146/annurev-marine-120709-142712
Hutchins D. A., Capone D. G. (2022). The marine nitrogen cycle: new developments and global change. Nat. Rev. Microbiol. 20, 401–414. doi: 10.1038/s41579-022-00687-z
Jørgensen B. B. (1990). A thiosulfate shunt in the sulfur cycle of marine sediments. Science 249, 152–154. doi: 10.1126/science.249.4965.152
Jørgensen B. B., Bak F. (1991). Pathways and microbiology of thiosulfate transformations and sulfate reduction in a marine sediment (kattegat, denmark). Appl. Environ. Microbiol. 57, 847–856. doi: 10.1128/aem.57.3.847-856.1991
Jack J. M., Joop N. (2000). Nitrogen uptake by heterotrophic bacteria and phytoplankton in the nitrate-rich Thames estuary. Mar. Ecol. Prog. Ser. 203, 13–21. doi: 10.3354/meps203013
Jiang X., Dang H., Jiao N. (2015). Ubiquity and diversity of heterotrophic bacterial nasA genes in diverse marine environments. PloS One 10, e0117473. doi: 10.1371/journal.pone.0117473
Kanehisa M., Sato Y., Kawashima M., Furumichi M., Tanabe M. (2016). KEGG as a reference resource for gene and protein annotation. Nucleic Acids Res. 44, D457–D462. doi: 10.1093/nar/gkv1070
Klappenbach J. A., Dunbar J. M., Schmidt T. M. (2000). rRNA operon copy number reflects ecological strategies of bacteria. Appl. Environ. Microbiol. 66, 1328–1333. doi: 10.1128/AEM.66.4.1328-1333.2000
Komagata K., Suzuki K.-I. (1988). “4 lipid and cell-wall analysis in bacterial systematics,” in Methods in microbiology (Elsevier) 19, 161–207. doi: 10.1016/S0580-9517(08)70410-0
Kumar S., Stecher G., Tamura K. (2016). MEGA7: molecular evolutionary genetics analysis version 7.0 for bigger datasets. Mol. Biol. Evol. 33, 1870–1874. doi: 10.1093/molbev/msw054
Liu X., Jiang L., Hu Q., Lyu J., Shao Z. (2020). Thiomicrorhabdus indica sp. nov., an obligately chemolithoautotrophic, sulfur-oxidizing bacterium isolated from a deep-sea hydrothermal vent environment. Int. J. Syst. Evol. Microbiol. 70, 234–239. doi: 10.1099/ijsem.0.003744
Luque-Almagro V. M., Gates A. J., Moreno-Vivián C., Ferguson S. J., Richardson D. J., Roldán M. D. (2011). Bacterial nitrate assimilation: gene distribution and regulation. Biochem. Soc Trans. 39, 1838–1843. doi: 10.1042/BST20110688
Meier-Kolthoff J. P., Auch A. F., Klenk H.-P., Göker M. (2013). Genome sequence-based species delimitation with confidence intervals and improved distance functions. BMC Bioinf. 14, 1–14. doi: 10.1186/1471-2105-14-60
Mergeay M., Nies D., Schlegel H. G., Gerits J., Charles P., Van Gijsegem F. (1985). Alcaligenes eutrophus CH34 is a facultative chemolithotroph with plasmid-bound resistance to heavy metals. J. Bacteriol. 162, 328–334. doi: 10.1128/jb.162.1.328-334.1985
Minnikin D. E., O’donnell A. G., Goodfellow M., Alderson G., Athalye M., Schaal A., et al. (1984). An integrated procedure for the extraction of bacterial isoprenoid quinones and polar lipids. J. Microbiol. Methods 2, 233–241. doi: 10.1016/0167-7012(84)90018-6
Murphy M. J., Siegel L. M., Tove S. R., Kamin H. (1974). Siroheme: a new prosthetic group participating in six-electron reduction reactions catalyzed by both sulfite and nitrite reductases. Proc. Natl. Acad. Sci. U. S. A. 71, 612–616. doi: 10.1073/pnas.71.3.612
Nguyen P. M., Do P. T., Pham Y. B., Doan T. O., Nguyen X. C., Lee W. K., et al. (2022). Roles, mechanism of action, and potential applications of sulfur-oxidizing bacteria for environmental bioremediation. Sci. Total Environ. 852, 158203. doi: 10.1016/j.scitotenv.2022.158203
Nguyen L.-T., Schmidt H. A., Von Haeseler A., Minh B. Q. (2015). IQ-TREE: a fast and effective stochastic algorithm for estimating maximum-likelihood phylogenies. Mol. Biol. Evol. 32, 268–274. doi: 10.1093/molbev/msu300
Nies D. H. (1995). The cobalt, zinc, and cadmium efflux system CzcABC from alcaligenes eutrophus functions as a cation-proton antiporter in escherichia coli. J. Bacteriol. 177, 2707–2712. doi: 10.1128/jb.177.10.2707-2712.1995
Nies D. H. (2003). Efflux-mediated heavy metal resistance in prokaryotes. FEMS Microbiol. Rev. 27, 313–339. doi: 10.1016/S0168-6445(03)00048-2
Nies D. H., Silver S. (1989). Plasmid-determined inducible efflux is responsible for resistance to cadmium, zinc, and cobalt in alcaligenes eutrophus. J. Bacteriol. 171, 896–900. doi: 10.1128/jb.171.2.896-900.1989
Reed R. H. (1990). Microbial water stress physiology: principles and perspectives. Trends Biotechnol. 8, 365.
Reitzer L. (2003). Nitrogen assimilation and global regulation in escherichia coli. Annu. Rev. Microbiol. 57, 155–176. doi: 10.1146/annurev.micro.57.030502.090820
Roesser M., Müller V. (2001). Osmoadaptation in bacteria and archaea: common principles and differences. Environ. Microbiol. 3, 743–754. doi: 10.1046/j.1462-2920.2001.00252.x
Salvador M., Argandoña M., Naranjo E., Piubeli F., Nieto J. J., Csonka L. N., et al. (2018). Quantitative RNA-seq analysis unveils osmotic and thermal adaptation mechanisms relevant for ectoine production in chromohalobacter salexigens. Front. Microbiol. 9. doi: 10.3389/fmicb.2018.01845
Sasser M. (1990). Identification of bacteria by gas chromatography of cellular fatty acids. Usfcc Newsl. 20, 1–7.
Sparacino-Watkins C., Stolz J. F., Basu P. (2014). Nitrate and periplasmic nitrate reductases. Chem. Soc Rev. 43, 676–706. doi: 10.1039/c3cs60249d
Ventosa A., Nieto J. J., Oren A. (1998). Biology of moderately halophilic aerobic bacteria. Microbiol. Mol. Biol. Rev. 62, 504–544. doi: 10.1128/MMBR.62.2.504-544.1998
Wang T., Ran M., Li X., Liu Y., Xin Y., Liu H., et al. (2022). The pathway of sulfide oxidation to octasulfur globules in the cytoplasm of aerobic bacteria. Appl. Environ. Microbiol. 88, e0194121. doi: 10.1128/AEM.01941-21
Yoon S.-H., Ha S.-M., Kwon S., Lim J., Kim Y., Seo H., et al. (2017). Introducing EzBioCloud: a taxonomically united database of 16S rRNA gene sequences and whole-genome assemblies. Int. J. Syst. Evol. Microbiol. 67, 1613–1617. doi: 10.1099/ijsem.0.001755
Keywords: Thiomicrorhabdus, comparative genomic analysis, obligate chemolithoautotroph, carbon, nitrogen and sulfur metabolism, environmental adaptation
Citation: Tan X-Y, Liu X-J, Li Z, Yu F, Yang H, Du Z-J and Ye M-Q (2023) Thiomicrorhabdus marina sp.nov., an obligate chemolithoautotroph isolated from tidal zone sediment, and genome insight into the genus Thiomicrorhabdus. Front. Mar. Sci. 10:1144912. doi: 10.3389/fmars.2023.1144912
Received: 15 January 2023; Accepted: 29 March 2023;
Published: 12 April 2023.
Edited by:
Xue-Wei Xu, Ministry of Natural Resources, ChinaReviewed by:
Rosa León-Zayas, Willamette University, United StatesHeng-Lin Cui, Jiangsu University, China
Copyright © 2023 Tan, Liu, Li, Yu, Yang, Du and Ye. This is an open-access article distributed under the terms of the Creative Commons Attribution License (CC BY). The use, distribution or reproduction in other forums is permitted, provided the original author(s) and the copyright owner(s) are credited and that the original publication in this journal is cited, in accordance with accepted academic practice. No use, distribution or reproduction is permitted which does not comply with these terms.
*Correspondence: Zong-Jun Du, duzongjun@sdu.edu.cn; Meng-Qi Ye, yemengqi@126.com
†These authors have contributed equally to this work and share first authorship