- 1Department of Marine, Earth, and Atmospheric Sciences, North Carolina State University, Raleigh, NC, United States
- 2College of Earth, Ocean, and Atmospheric Sciences, Oregon State University, Corvallis, OR, United States
- 3Graduate School of Oceanography, University of Rhode Island, Narragansett, RI, United States
Oxygen limited marine environments, such as oxygen minimum zones, are of profound importance for global nutrient cycling and vertical habitat availability. While it is understood that the extent and intensity of oxygen minimum zones are responsive to climate, the limited suite of viable proxies for low oxygen pelagic environments continues to pose a real barrier for paleoclimate interpretations. Here we investigate the proxy potential of an array of trace element (Mg, Mn, Zn, and Sr) to Ca ratios from the shells of Globorotaloides hexagonus, a planktic foraminifer endemic to tropical through temperate oxygen minimum zones. A species-specific relationship between Mg/Ca and temperature is proposed for quantitative reconstruction of oxygen minimum zone paleotemperatures. Both Mn/Ca and Zn/Ca ratios vary with oxygen concentration and could be useful for reconstructing G. hexagonus habitat where the primary signal can be d\istinguished from diagenetic overprinting. Finally, a robust correlation between Sr/Ca ratios and dissolved oxygen demonstrates a role for Sr as an indicator of oxygen minimum zone intensity, potentially via foraminiferal growth rate. The analysis of these relatively conventional trace element ratios in the shells of an oxygen minimum zone species has tremendous potential to facilitate multiproxy reconstructions from this enigmatic environment.
Introduction
Marine deoxygenation is one consequence of ongoing global change and is likely to be felt most acutely in the expansion of already low oxygen environments in the coastal and open ocean (Keeling et al., 2009; Levin, 2018; Schmidtko et al., 2017; Breitburg et al., 2018). Expansion of marine hypoxia and anoxia alter the global cycling of key nutrients and redox sensitive metals (Gruber, 2008; DeVries et al., 2012), and drive ecological shifts in both benthic and pelagic communities (Levin, 2003; Stramma et al., 2010; Stramma et al., 2012; Horak et al., 2016). Despite growing recognition of the importance of low oxygen environments and the ramifications of their expansion, the short duration (decades at most) of ocean oxygen timeseries fundamentally limits the timescales at which oxygen and associated environmental variability can be studied.
Oxygen minimum zones (OMZs) are subsurface (~100-2000 m deep), generally open ocean features, where dissolved oxygen is low enough to impact biological and chemical cycles. OMZs have changed in step with global climate in the past, especially during periods of rapid warming such as deglaciations (i.e., van Geen et al., 2003; Nameroff et al., 2004; Martinez and Robinson, 2010; Moffitt et al., 2015). However, models still fall short of reconstructing recent deoxygenation, and a greater understanding of long-term drivers of deoxygenation is required to improve future projections (Oschlies et al., 2018). Moreover, while marine deoxygenation is ongoing in the modern ocean (Schmidtko et al., 2017), some oxygen variability may be attributable to decadal scale variability rather than long-term climate change (Deutsch et al., 2014). With a better pre-modern baseline, such cyclical versus secular trends would be easier to tease apart. Thus, there is a particular need to develop and apply additional environmental and oxygenation proxies within the OMZ.
Great strides have been made toward developing new paleo-oxygenation proxies to constrain past OMZ dynamics. One notable example is the refinement and application of the I/Ca proxy for dissolved oxygen using shells of planktic foraminifera, a group of calcifying protists (Zhou et al., 2014; Lu et al., 2016; Hoogakker et al., 2018; Lu et al., 2020). Other trace element to calcium (TE/Ca) ratios in the shells of benthic and planktic foraminifera also have tremendous potential for recording the physical and chemical environment of the OMZ. For example, Mn/Ca ratios in benthic foraminifera are a promising proxy for bottom water hypoxia (Groeneveld and Filipsson, 2013; McKay et al., 2015; Brinkmann et al., 2021).
We explore the proxy potential of several conventional trace elements within the shells of the planktic foraminifer Globorotaloides hexagonus. This species has been widely associated with low oxygen waters (Fairbanks et al., 1982; Ortiz et al., 1995; Birch et al., 2013; Rippert et al., 2016), and was recently recovered live from discrete depth plankton tows through the Eastern Tropical North Pacific (ETNP) OMZ (Davis et al., 2021). Furthermore, G. hexagonus has a rich fossil history, with shells occurring in sediments dating back 14 Mya (Kennett and Srinivasan, 1983), making it an ideal candidate for reconstructing paleo-OMZs. We explore potential controls on Mg/Ca, Mn/Ca, Zn/Ca, and Sr/Ca ratios, which are routinely measured in calcite and are resolvable in foraminifera shells with the use of standard Inductively Couple Plasma Mass Spectrometry (ICP-MS), and in some cases even Inductively Coupled Plasma Optical Emission Spectrometry, after the use of defined cleaning protocols (Barker et al., 2003; Marr et al., 2013a; Fritz-Endres and Fehrenbacher, 2021). As a result, these analyses are accessible to a wide range of researchers and could prove especially valuable for reconstructing past OMZ environments. Moreover, as TE/Ca in calcite may reflect diverse drivers ranging from temperature to oxygenation to source water (see Katz et al., 2010 for a review) and can be analyzed simultaneously, investigating multiple TE/Ca ratios could make multiproxy and multivariate records more accessible. Here we use Laser Ablation ICP-MS for two reasons. The first is that G. hexagonus shells are both rare and light in many sediments (Davis et al., 2021, Davis et al., 2023), such that obtaining a sufficient sample for solution measurements may not always be possible. The second is anticipation of the potential need to use high-resolution intra-shell analyses to analytically distinguish between primary and altered calcite.
Methods
Plankton tows and hydrography
All foraminiferal shells used in this study were collected by an opening-closing zooplankton net system, the MOCNESS (Multiple Opening/Closing Net and Environmental Sensing System; Wiebe et al., 1985), taken onboard the R/V Sikuliaq in January-February of 2017 in the ETNP (21° N, 117° W). Net tows consisted of both depth-stratified vertical profiles from the surface to 1000 m depth in 25-100 m intervals, as well as horizontal sequences of tows through low oxygen features (8 or 9 nets per tow, 222µm mesh) (Wishner et al., 2018, Wishner et al., 2020, Wishner et al., 2021). A suite of environmental data was collected by MOCNESS sensors during each tow, including depth, temperature, salinity, dissolved oxygen, in situ fluorescence, and volume filtered through each net (Figure 1). Each net encompassed a range of environmental data, since an individual net was open for about 10 – 20 minutes and sampled a depth stratum (in the case of vertical tows) from 25 to 100 m thick. Since it is unknown exactly where within the sampled stratum a foraminifer was collected, the environmental data range for the specific net from which an individual was analyzed is shown by the horizontal lines in the following figures. Samples from each net were preserved shipboard in 4% sodium-borate buffered formalin and seawater and then stored in the lab until foraminifera were removed in 2017-2018. All foraminifera were picked from tow material as described by Davis et al. (2021). The shells of live collected (with cytoplasm present) G. hexagonus were isolated from tow material. Because planktic foraminifera are not believed to be highly mobile, it was assumed that much or all of the adult whorl, calcified within the depth and environmental range sampled by the net in which it was captured. We stress that the use of the final or “F” chamber here is not completely analogous to its use in fossil material. In living foraminifera, the final chamber refers to the most recently calcified chamber at the time of capture, and not necessarily the final chamber to calcify prior to reproduction and death. As the relatively large mesh size (222 μm) used excludes juveniles (measured foraminifera ranged from 297 to 631 μm in length), all individuals are inferred to be adults.
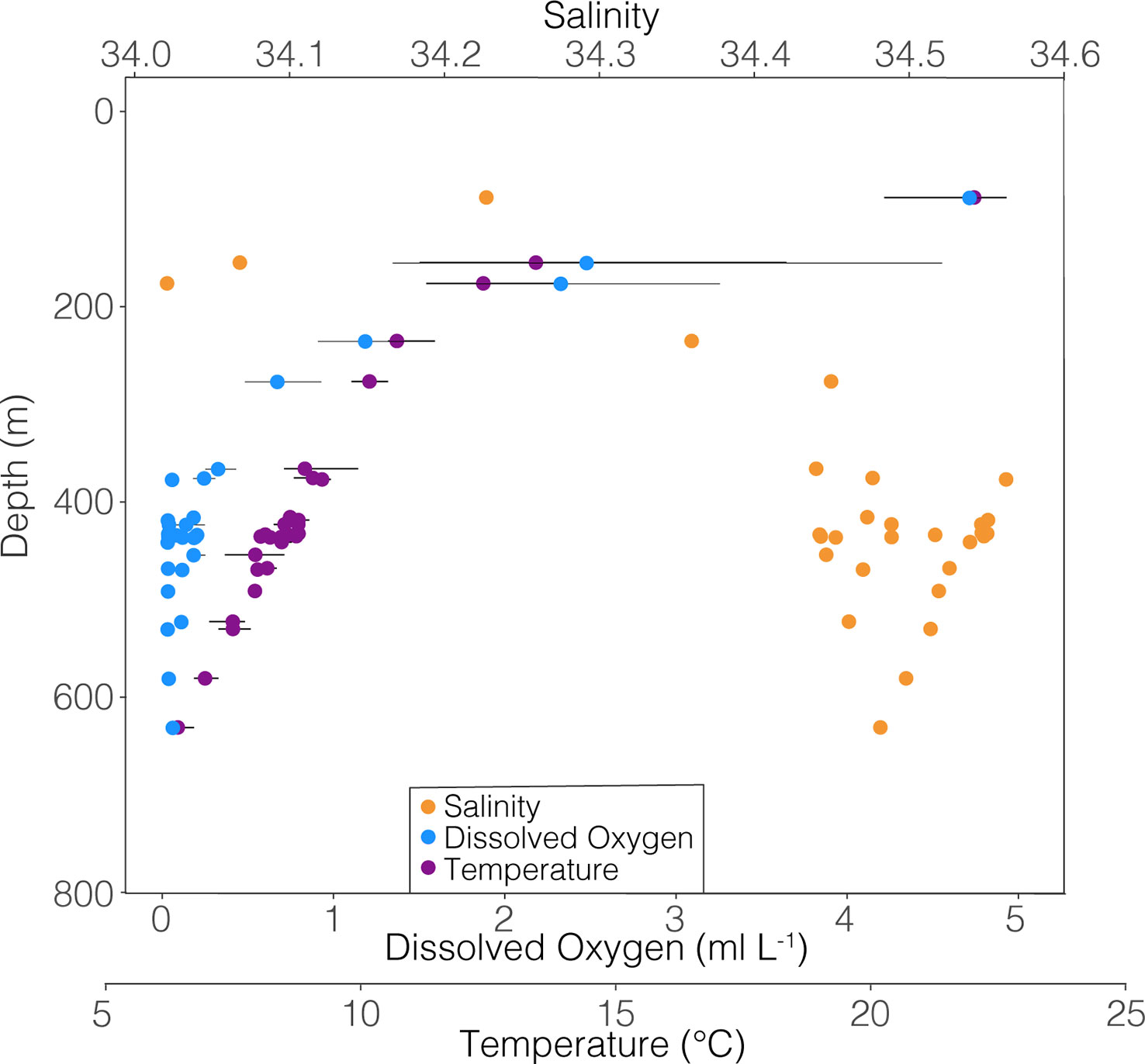
Figure 1 Temperature, salinity, and dissolved oxygen relative to depth of all samples from which G. hexagonus was picked. Points represent the mean, and horizontal bars extend to the minimum and maximum value measured in each net since it is not known where within a particular net an individual foraminiferan was collected. Data represent results from nine tows. Thus hydrographic variables taken together represent an aggregate rather than an instantaneous profile of conditions within the water column.
Trace metal analyses
Preparation for trace elemental analyses involved an oxidative cleaning step to remove residual organic matter from shells, following that described by (Barker et al., 2003) with some modification. Briefly, shells were bathed individually in a 1:1 mixture of NaOH and H2O2 for 10 minutes at 60°C and then triple rinsed in deionized water to remove reagent. While foraminiferal trace element cleaning frequently includes sonication, this step was excluded due to the fragility of G. hexagonus shells and because removal of clays and other infilling from tow collected specimens is unnecessary. Shells were kept whole to facilitate laser ablation ICP-MS and because recent findings demonstrate that fragmentation may artificially decrease some trace element ratios in shells (Fritz-Endres and Fehrenbacher, 2021). Shells were mounted on carbon conductive tape on a glass slide.
Shells were analyzed by a laser ablation system (Photon Machines 193 nm ArF laser with an ANU HelEx dual-volume laser ablation cell) coupled to an iCAP quadrupole ICP-MS in the College of Earth, Ocean, and Atmospheric Sciences at Oregon State University following previously established protocols (Fehrenbacher et al., 2015). Shells were ablated using a 65 μm spot size, a 4 Hz rep rate, and a fluence of 0.85 J cm-2. Analytes presented here include 25Mg, 44Ca, 55Mn, 66Zn, and 88Sr, with ablations of NIST 610 and NIST 612 run between every ~10 samples. Profiles include the data from 1 s after the start of ablation to when the laser broke through the chamber wall. Data from laser ablation analyses were processed using the LATools Software (Branson et al., 2019), and are presented as mean TE/Ca ratios either for a chamber (averaged if more than one ablation was possible within a chamber), or for a shell (presented as an average of every chamber ablated) (Supplementary Data). Ablation times ranged from 3 to 79 seconds, and ablation profiles less than 5 seconds (8 profiles) were excluded from these analyses. Reproducibility was assessed by the mean difference in duplicate ablations through the same chambers. The mean difference between ablations was 0.27 mmol/mol for Mg/Ca, 0.03 mmol/mol for Sr/Ca, 5.15 μmol/mol for Mn/Ca, and 90.86 μmol/mol for Zn/Ca.
Statistics
All statistics were carried out in R. In the case of regression analyses, non-linear least squares regressions were used and are reported here along with standard error. All correlations were carried out using a Pearson method, with a Holm’s correction for multiple hypothesis testing and both correlation coefficient and p-value reported here.
Results
Laser ablation ICP-MS profiles were resolved from 184 individual foraminifera shells spanning 20 different nets over a range of depth, oxygen, and temperature conditions within the ETNP OMZ (Figure 1).
Mg/Ca vs. oxygen and temperature
The average Mg/Ca in individual G. hexagonus shells ranged from 1.02 to 5.85 mmol/mol. Mg/Ca ratios are positively correlated with both the average values of dissolved oxygen (corr = 0.31, p< 0.001) and temperature (corr = 0.31, p< 0.001) recorded in the corresponding nets (Figure S1). The latter ranged from 6.1 to 22.0°C. Due to the bias in vertical distribution of G. hexagonus, with most individuals living well below the thermocline (Davis et al., 2021), only three shells were analyzed from the warmest temperature. Moreover, because this net integrated across the thermocline, it is possible that individuals collected were living preferentially at the low end of that temperature range (deeper), rather than the mean. Despite this potential bias, the mean temperature, is < 2°C greater than the minimum in this sample, and thus is unlikely to be a major source of uncertainty in developing a quantitative relationship.
Regressing individual G. hexagonus shell data against the mean temperature associated with each net and adopting an exponential fit, as has been found most suitable in other species (Lea et al., 1999; Mashiotta et al., 1999; Elderfield and Ganssen, 2000; Dekens et al., 2002; Anand et al., 2003; McConnell and Thunell, 2005; Cléroux et al., 2008; Sadekov et al., 2009; Livsey et al., 2020), results in a relationship between temperature and Mg/Ca that can be described as:
(Equation 1.; Figure 2)
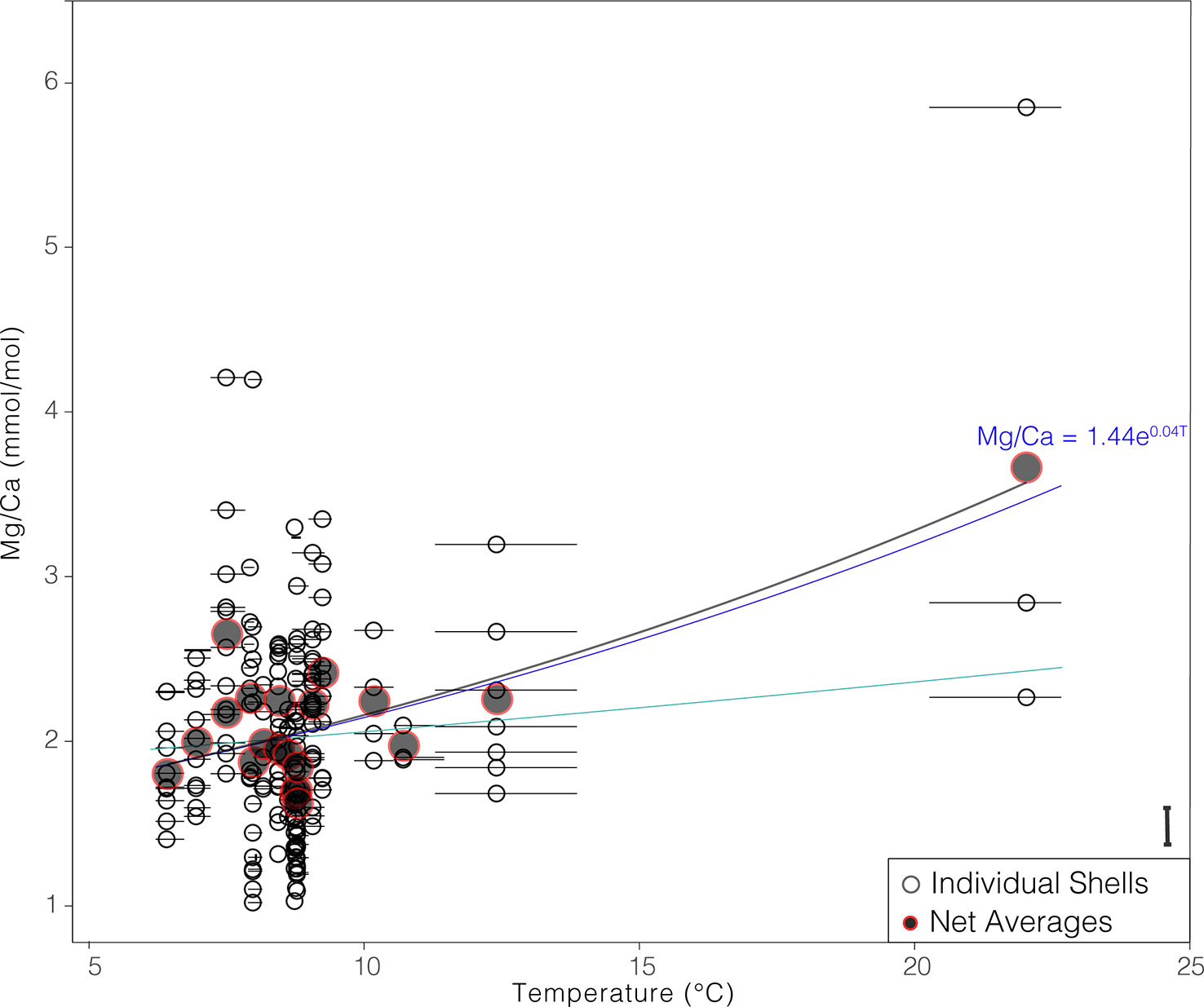
Figure 2 Mg/Ca values of individual shells (open black points), and net means (closed gray/red points) relative to in situ temperature at collection. Points are plotted against the mean temperature from the net in which the foraminifers were collected, and horizontal bars show the minimum to maximum temperature measured in that net. Exponential fit based on individual shell data is shown as a bright blue line, and in black for net means; exponential fit exclusive of the highest temperature is shown in turquise. The mean difference between duplicate ablations of the same chamber is show as a bar in the bottom right.
with T as temperature in degrees Celsius, reported with standard error.
The same analysis can be run using Mg/Ca averages from each net, producing a regression within error of Equation 1 (Mg/Ca = 1.40 (+- 0.1) * e(0.04 +- 0.01)(T)). Given the paucity of datapoints at the warmest temperature, we also regressed individual shell Mg/Ca against temperature with results from the warmest net removed, resulting in an equation again within error of Equation 1 ((Mg/Ca = 1.78 (+- 0.3) * e(0.02 +- 0.02)(T))) and virtually indistinguishable at lower temperatures (Figure 2). We note that over a small temperature range the relationship could be equally well described by a linear relationship. Given the similarity between the three approaches, we selected the first, which is significant despite very high inter-individual variability, considers all available data, and retains an exponential relationship as has been found for other species.
Mn/Ca, Zn/Ca, and Sr/Ca
Ratios of Mn/Ca ranged between 3.79 and 125.75 μmol/mol and Zn/Ca ranged between 32 and 1708.32 μmol/mol across dissolved oxygen values of 0.03 to 4.71 ml/L. The range of Sr/Ca values in individual shells was 1.04-1.47 mmol/mol. All three TE/Ca ratios were found to have highly significant correlations with in situ temperature and the dissolved oxygen levels at which they were recovered despite a large degree of inter-individual variability. Negative correlations are present for Mn/Ca (-0.33, p-value< 0.001) and Zn/Ca (-0.21, p-value = 0.005) (Figures 3, S2, S3). A correlation coefficient of 0.50 was found for individual shell Sr/Ca values and dissolved oxygen (p-value< 0.001). Removal of the highest oxygen value does not meaningfully impact these correlations (-0.31, -0.13, and 0.47, respectively) suggesting that these trends are robust to potential bias in the shallowest sample (Figure S2).
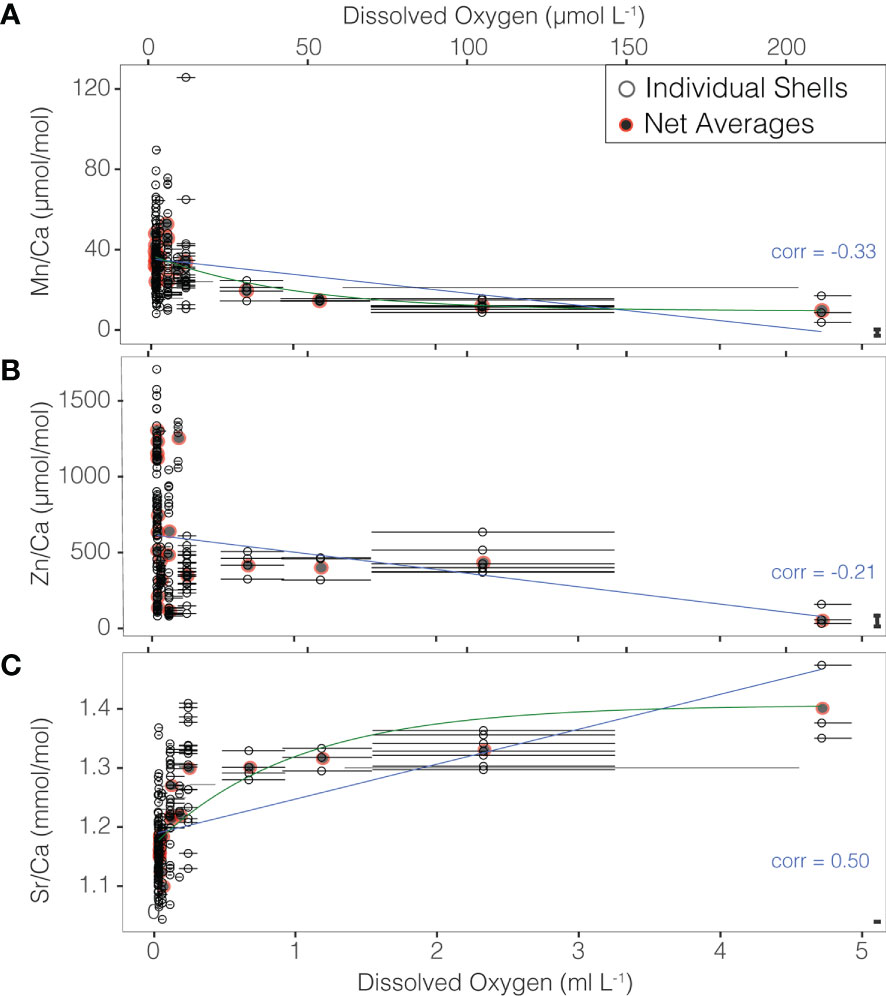
Figure 3 (A) Mn/Ca, (B) Zn/Ca, and (C) Sr/Ca values of individual shells (open black points), and net means (closed gray/red points) relative to in situ oxygen at collection. Points are plotted against the mean oxygen within the net from which the foraminifers were collected, and horizontal bars show the minimum to maximum values of oxygen measured within each net. Significant linear correlations are shown as blue lines. Exponential fits are shown in green in panels (A, C). The mean difference between duplicate ablations of the same chamber is show as a bar in the bottom right of each panel.
Despite, high interindividual variability, the relationship between Mn/Ca and O2 can be described by either a linear regression (r2 = 0.10, p-value< 0.001) or an exponential curve (r2 = 0.12, p-value< 0.001). Similar regressions for Zn/Ca are significant (p-values 0f 0.004 and 0.002 respectively), but with very low r2 values (0.04 and 0.05). In contrast the relationship between Sr/Ca and O2 can be described by either a linear regression (r2 = 0.24, p-value< 0.001) or a logarithmic curve (r2 = 0.46, p-value< 0.001) (Figure 3).
Ontogenetic trends
The use of laser ablation to analyze individual chambers allows for a comparison of chamber-to-chamber differences in TE/Ca, or trends in trace element incorporation through ontogeny. A Kruskal-Wallis test demonstrates that each of the targeted elemental ratios change through ontogeny (p<0.001 in all cases). Significantly lower Mn/Ca, Zn/Ca, and Mg/Ca ratios are observed in younger relative to older chambers. By contrast, higher Sr/Ca and ratios are observed in younger chambers (Figure 4).
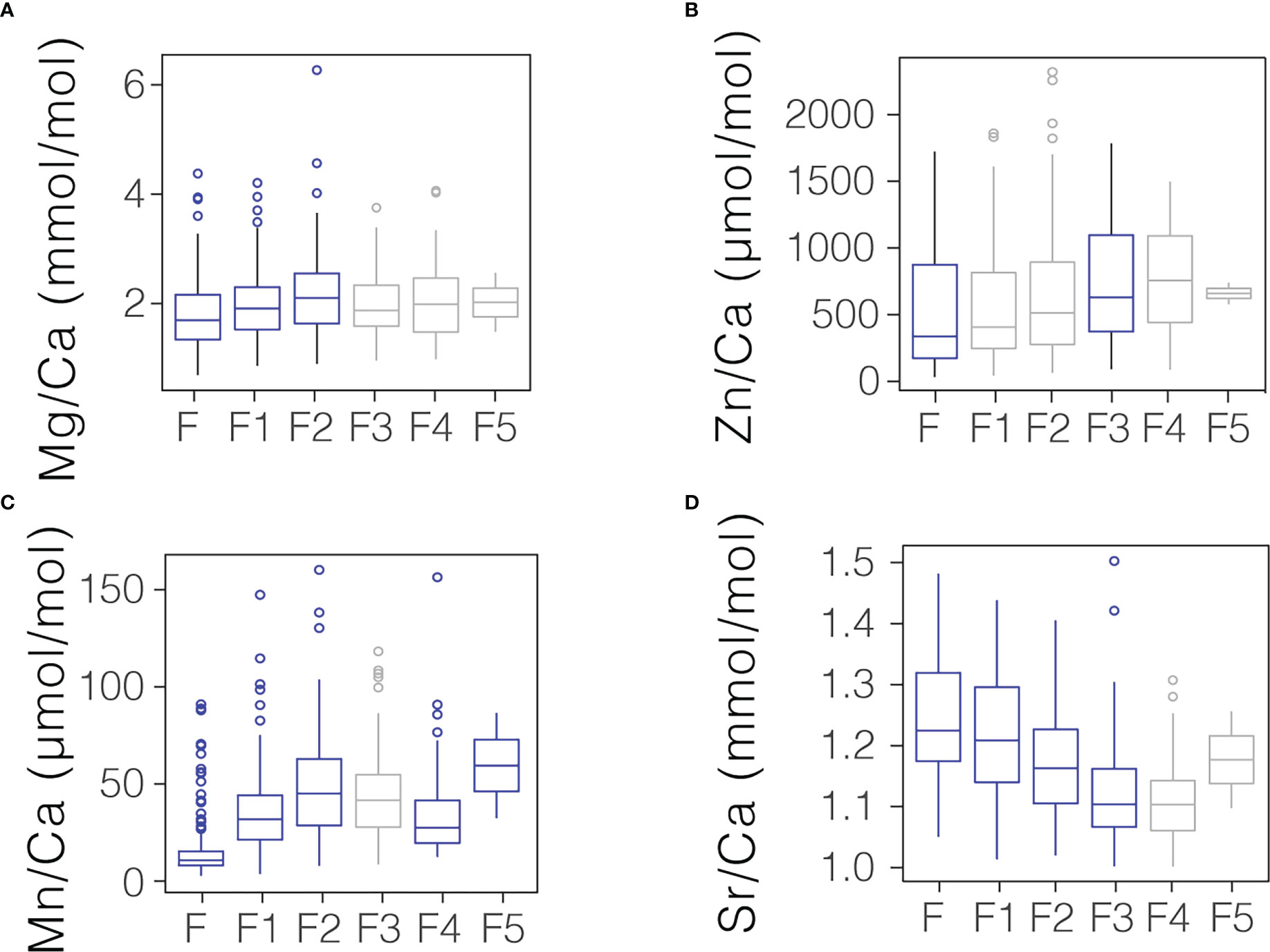
Figure 4 Comparison of (A) Mg/Ca, (B) Zn/Ca, (C) Mn/Ca, and (D) Sr/Ca found in the youngest 6 chambers of G. hexagonus. In each plot, F refers to the youngest or “final” chamber, with older chambers progressing sequentially from this point. Chambers values which were significantly different (p< 0.05) from the subsequent chamber are shown in blue; those that were not significantly different are shown in grey. The boxes denote the 1st and 3rd quartiles and the center bar the median.
Discussion
Calibration of the Mg/Ca paleothermometer for G. hexagonus
The variables of interest (temperature, oxygenation, depth) are highly colinear in this dataset, with all TE/Ca displaying robust correlations with multiple environmental drivers (Figure S1). Therefore, the following discussion will center around connections between environmental parameters and elemental ratios as supported by previous work rather than attempt to statistically deconvolve potential drivers. Given the rich literature on species-specific sensitivity of foraminiferal shells to calcification temperature (e.g., Nürnberg et al., 1996; Lea et al., 1999; Mashiotta et al., 1999; Elderfield and Ganssen, 2000; Lea et al., 2002; Anand et al., 2003), we present Mg/Ca results in the context of calcification temperature. However, in at least some species, salinity (Lea et al., 1999; Kısakürek et al., 2008; Dueñas-Bohórquez et al., 2009; Mathien-Blard and Bassinot, 2009; Hönisch et al., 2013; Gray et al., 2018), and carbonate chemistry (Lea et al., 1999; Russell et al., 2004; Gray et al., 2018; Gray and Evans, 2019) have secondary influences on shell Mg/Ca. Here, salinity can be disregarded as a driver due to the narrow range of salinity across these samples (34.0-34.6), but the same assumption cannot be made about carbonate chemistry. While direct measurements of carbonate chemistry are not available, we would expect pH to be highly correlated with dissolved oxygen (Paulmier et al., 2011; Figure S2) and therefore with temperature (Figure 1). Based on work in other planktic species, Mg/Ca would be expected to increase as pH or carbonate ion ([CO32-]) availability decreases (Russell et al., 2004; Allen et al., 2016; Evans et al., 2016; Gray and Evans, 2019). A potential pH/[CO32-] effect would then act counter to temperature, increasing Mg incorporation at depth. Thus, it is possible that the sensitivity of Mg/Ca to temperature alone in G. hexagonus is higher than predicted by the empirical relationship derived here. This would need to be tested through future work in additional locations or laboratory culture. One further caveat is the degree of interindividual variability observed. Interindividual variability has been observed in culture (Davis et al., 2017), natural living populations (Davis et al., 2020; Livsey et al., 2020; Jonkers et al., 2022), and fossil assemblages (e.g., Groeneveld et al., 2019; Schmitt et al., 2019) of other species of planktic foraminifera. Thus, temperature is an important but not sole driver of Mg/Ca, and single shell Mg/Ca may not produce reliable temperature reconstructions. This is not an issue confined to G. hexagonus. While population-level Mg/Ca bears a clear relationship with temperature, the data presented here highlights uncertainties around the use of individual foraminiferal analysis Mg/Ca as a direct corollary to calcification temperature.
The temperature predicted by a given Mg/Ca value in Equation 1 is within the range of what would be predicted by other species-specific equations. (Supplemental Table 1). The high preexponential constant (1.44 ± 0.1) and low exponential constant (0.04 ± 0.01) indicate a more linear sensitivity to temperature than most other equations. Importantly, the relationship of G. hexagonus shell Mg/Ca to temperature is significantly different (outside standard error) from previously published equations, implying a species-specific calibration is necessary.
Equation 1 should be suitable for deriving calcification temperature from G. hexagonus shells from marine sediments and quantitatively reconstructing temperatures 6-22°C within the overlying OMZ with two caveats. First, temperatures should be interpreted only from populations of fossil G. hexagonus shells rather than individuals. Second, [CO32-] may have a contributing influence. Neither is a limitation specific to G. hexagonus, but likely applies more broadly to other species and settings. Despite these caveats, the relationship presented here raises several possibilities for paleoceanography. For example, G. hexagonus shells could be used to constrain temperature of pelagic intermediate water masses and enable quantitative assessments of oxygen solubility (largely a function of temperature) as a long-term driver of sub-surface oxygenation.
Oxygen and carbonate system controls on Mn and Zn
The robust relationships found between Mn/Ca and Zn/Ca in the shells of G. hexagonus and in situ dissolved oxygen point to a potential environmental control. There is an existing theoretical framework for such a relationship in Mn/Ca. As Mn is readily oxidized to MnO2, Mn2+, the cation assumed to substitute for Ca2+ in the calcite lattice, should be more available in low oxygen water masses. Thus, Mn2+ substitutions for Ca2+ should occur more frequently and Mn/Ca should increase in shells formed at lower oxygen conditions (Barras et al., 2018; van Dijk et al., 2020). While there are other controls on Mn and Mn2+ availability in natural systems, in the ETNP dissolved Mn shows a distinct peak specifically associated with low dissolved oxygen (Bolster et al., 2022; Figure 5). There is also evidence for an oxygenation control on the incorporation of Mn/Ca into benthic foraminiferal calcite (Munsel et al., 2010; Groeneveld and Filipsson, 2013; Koho et al., 2015; McKay et al., 2015; Ní Fhlaithearta et al., 2018; van Dijk et al., 2020). However, increased shell Mn/Ca has also been related to oxygenation or advection of water across the oxycline in live-caught planktic foraminfiera (Steinhardt et al., 2014; Davis et al., 2020). The directionality of the relationship found here is consistent with a dissolved oxygen control on Mn/Ca incorporation into G. hexagonus calcite (Figure 3A).
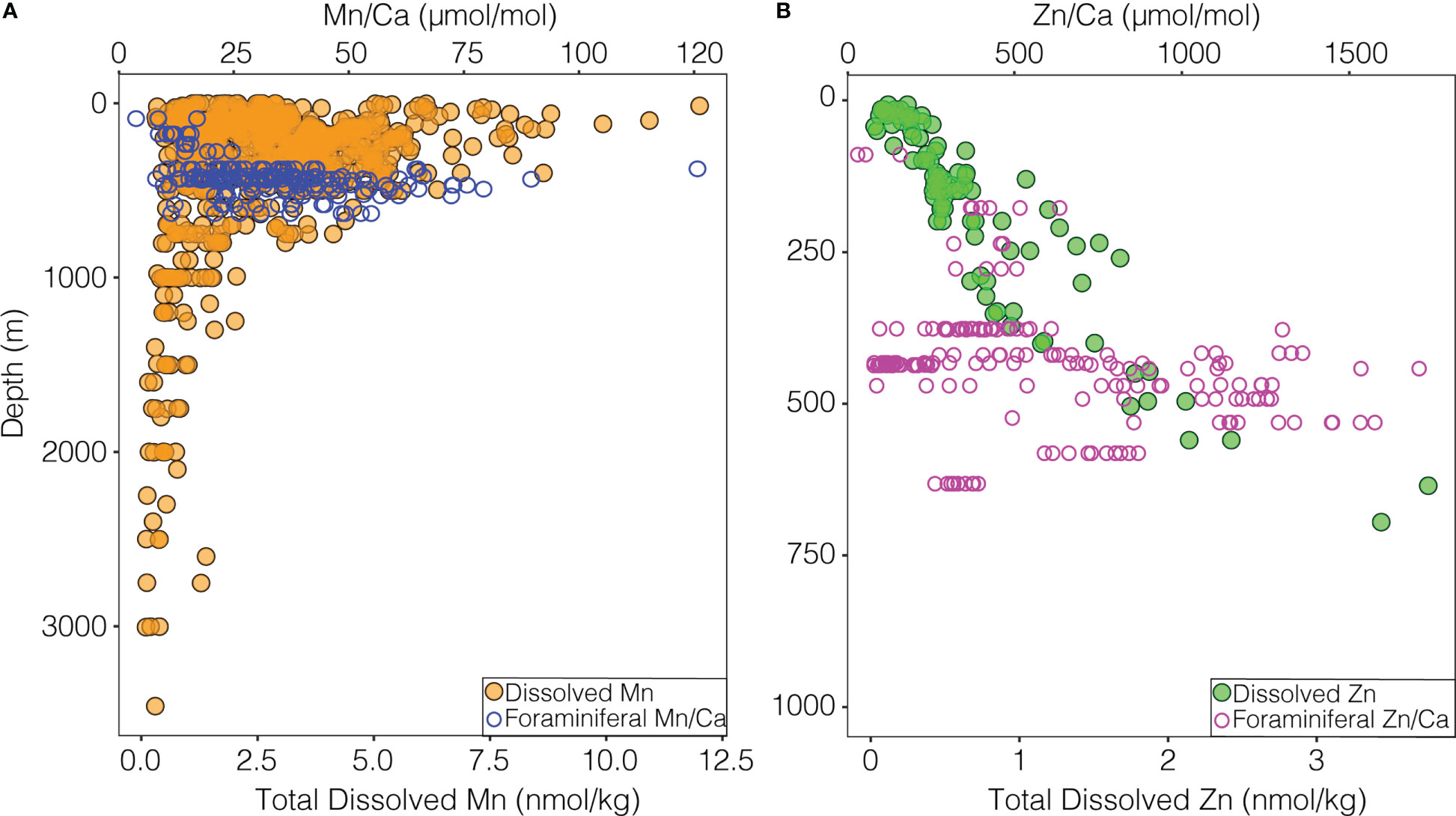
Figure 5 (A) Dissolved Mn with depth in the ETNP (solid orange points) from Moffett (2020) and John et al. (2022). Individual foraminiferal shell Mn/Ca (open blue points) at the mean depth from the net in which they were collected. (B) Dissolved Zn with depth in the ETNP (solid green points) from John and Moffett (2021). Individual foraminiferal shell Zn/Ca (open pink points) at the mean depth from the net in which they were collected.
As multiple parameters covary with depth in this dataset, it is necessary to consider alternate drivers responsible for the higher Mn/Ca ratios, especially the carbonate system (Figures 1, S1, S2). Laboratory culture of the planktic species Orbulina universa demonstrated a negative correlation between shell Mn/Ca and [CO32-] (Allen et al., 2016) and a positive correlation with DIC (Holland et al., 2017). Similarly, cultures of the benthic hyaline species Amphistigina gibbosa as well as the phylogenetically distant porcelaneous Sorites marginalis show increasing shell Mn/Ca values with increasing DIC (van Dijk et al., 2020). These results are consistent with the expectation that increasing DIC (decreasing [CO32-]) would co-occur with decreasing oxygenation in the OMZ (Paulmier et al., 2011). Thus, the carbonate system and dissolved oxygen could act independently or in concert to drive higher Mn/Ca values in nets collected closer to the core of the OMZ. While more work in modern samples will be required to tease apart these drivers, results indicate that shell Mn/Ca may record the intensity of the OMZ and the co-occurring carbon maximum within the habitat of G. hexagonus.
We find lower Zn/Ca ratios are in shells from nets with higher oxygen (Figure 3B) and higher temperature. No previous work has directly compared Zn/Ca in foraminiferal shells to oxygenation, while temperature has a negligible effect on the Zn/Ca of benthic foraminifera (Marchitto et al., 2000; Titelboim et al., 2021). The carbonate system, however, has been widely implicated as a driver of Zn/Ca ratios (Marchitto et al., 2000; van Dijk et al., 2017; van Dijk et al., 2017), and Zn/Ca increases with increasing DIC (decreasing [CO32-]) in multiple species of cultured benthic foraminifera (van Dijk et al., 2016; van Dijk et al., 2017). This has been attributed to pH dependent speciation of Zn, and incorporation into foraminiferal calcite of Zn2+ (van Dijk et al., 2017). Zn also displays a nutrient-like profile, increasing with depth through the OMZ (Conway and John, 2015; Janssen and Cullen, 2015) (Figure 5). Thus, both increased availability of Zn and Zn2+ may act in conjunction to increase Zn/Ca ratios with increasing depth and OMZ intensity.
Results suggest that Zn/Ca and Mn/Ca in G. hexagonus can be related to macroenvironmental drivers, via concentrations of dissolved ions in ambient seawater. This is further supported by observations of an increase in dissolved Mn and Zn at OMZ depths in the ETNP, where Mn/Ca and Zn/Ca also increase in foraminiferal calcite (Bolster et al., 2022; Figure 5). The variability in Zn/Ca and Mn/Ca ratios of G. hexagonus shells and dissolved Mn and Zn are also all higher at OMZ depths (Figure 5; Supplemental Table 2).
Other non-spinose foraminifera such as Neogloboquadrina dutertrei and Globorotalia truncatulanoides, may calcify within an organic aggregate microhabitat, as identified in part by high Ba/Ca values (Fehrenbacher et al., 2018; Richey et al., 2022). Shell Ba/Ca is also high in G. hexagonus (mean 39 μmol/mol across all ablation profiles) and therefore, G. hexagonus may be additionally influenced by the redox conditions inside particle microenvironments. Particle microenvironments can support anaerobic respiration in a low oxygen water column when oxygen is further drawn down by respiration inside the particle (Alldredge and Cohen, 1987; Alldredge and Silver, 1988; Shanks and Reeder, 1993; Bianchi et al., 2018). This oxygen depletion should result in higher Mn2+. These same particulate microenvironments might have a complex and non-linear influence on Zn. When reduced sulfate is available in particulate microenvironments, Zn2+ precipitates into ZnS which can have implications for Zn cycling in the OMZ (Janssen and Cullen, 2015). While respiration within a particulate microenvironment could further decrease pH, increasing Zn2+, sulfate reduction at sufficiently low oxygen levels could decrease the availability of [Zn2+]. Such sulfate-reducing metabolisms may occur within particles in the ETNP OMZ (Carolan et al., 2015), and could be partly responsible for the large range and variance of Zn/Ca ratios found at the lowest oxygen levels (Figure 5; Table S2).
Related controls on Sr/Ca
The strongest correlation of any analyte with oxygen was found for Sr/Ca (Figure 3C). As foraminiferal Sr/Ca ratios have not yet been evaluated with respect to oxygenation, we will first consider alternative controls. From relatively early in the history of foraminiferal trace element analyses, the Sr/Ca of planktic foraminifera shells has been linked to salinity and temperature (Lea et al., 1999; Elderfield et al., 2002; Cléroux et al., 2008; Kısakürek et al., 2008). Salinity differences are minimal in this dataset and therefore an unlikely driver of shell Sr/Ca. Previous studies demonstrate only a weak (Lea et al., 1999) or insignificant (Russell et al., 2004; Kısakürek et al., 2008) relationship between Sr/Ca and temperature in planktic foraminifera. However, a strong correlation between temperature and dissolved oxygen (corr = 0.92; Figure S1) make the two parameters nearly impossible to disentangle, and temperature cannot be discounted as an important influence on G. hexagonus Sr/Ca ratios. Given this uncertainly, other drivers need be considered.
The Sr/Ca ratios of calcareous foraminifera have also been linked to carbonate chemistry, similar to Mn/Ca and Zn/Ca ratios. However, findings are inconsistent. Multiple species of planktic foraminifera show an increase in shell Sr/Ca ratios with an increase in pH and/or [CO32-] (decreasing DIC) (Lea et al., 1999; Russell et al., 2004; Dueñas-Bohórquez et al., 2009; Holland et al., 2017). By contrast, some benthic foraminifera show a decrease in Sr/Ca with decreasing DIC (increasing pH and/or [CO32-]) (Keul et al., 2017; van Dijk et al., 2017). Still other benthic (Dissard et al., 2010; Raitzsch et al., 2010) and planktic species (Lea et al., 1999; Russell et al., 2004; Kısakürek et al., 2008) are apparently insensitive to the carbonate system as a Sr/Ca driver.
One related consideration for the incorporation of Sr into foraminiferal calcite is growth rate. Higher growth rate has been widely associated with increased foraminiferal Sr/Ca ratios especially in planktic species (Elderfield et al., 2002; Kısakürek et al., 2008; Holland et al., 2017; Geerken et al., 2022), and explicitly linked with an apparent influence of temperature and carbonate chemistry (Lea et al., 1999; Russell et al., 2004). The shell mass of cultured planktic foraminifera tends to increase with temperature (Lombard et al., 2009), [CO32-],pH (Spero et al., 1997; Lea et al., 1999; Bijma et al., 2002; Russell et al., 2004; Lombard et al., 2010; Manno et al., 2012), and O2 (Kuroyanagi et al., 2013), which suggests all three parameters could increase growth. Further support for growth rate as a driver of Sr/Ca incorporation in G. hexagonus comes from individual shells. Assuming initial calcification of each chamber takes a similar amount of time, a larger chamber would calcify faster than a smaller chamber and therefore might have higher Sr/Ca. This is supported by chamber-specific ablations which demonstrate that younger, larger chambers have higher Sr/Ca compared to older, smaller chambers (Figure 4). We note that significant decreases in Sr/Ca in younger chambers are not confined to the final chamber alone, indicating that the presence of this trend is unlikely to be driven entirely by incomplete calcification of the youngest chamber.
While we cannot disentangle the potential impacts of temperature, pH, and oxygen on Sr/Ca from this dataset, all three variables would be expected to result in slower shell growth rates within the low-oxygen, low-pH, and low-temperature core of the OMZ. As a result, Sr/Ca presents itself as a potentially useful proxy; a decrease in growth rate, and therefore lower Sr/Ca, may be associated with more intensive OMZ conditions rather than an isolated environmental driver. The use of Sr/Ca as a proxy for OMZ intensity would allow for an assessment of habitat within the OMZ, and therefore the depth habitat of an individual; a shell with higher Sr/Ca likely calcified farther from the core of the OMZ than one with lower Sr/Ca. At the population level, changes in Sr/Ca could point to changing strength of the OMZ, with lower Sr/Ca values indicative of more intense OMZ habitat being available to G. hexagonus.
Diagenesis as a potential complication
One undeniable challenge in the reconstruction of OMZ environments from foraminiferal shell TE/Ca is preservation. It is well known that trace elemental ratios such as Mg/Ca can be altered by either calcite overgrowths and recrystallization (Boyle, 1983; Pena et al., 2005; Pena et al., 2008) or dissolution (Lorens et al., 1977; Dekens et al., 2002; Fehrenbacher et al., 2006; Johnstone et al., 2011; Regenberg et al., 2014; Branson et al., 2015). The use of elements such as Mn and Zn as proxies is especially complicated by postmortem modification of shell chemistry, with Mn alteration frequently noted (Boyle, 1983; Pena et al., 2005; Pena et al., 2008). A combination of chemical and mechanical cleaning may remove postmortem contaminants but can also remove the primary signal of elements including Mn and Zn (Fritz-Endres and Fehrenbacher, 2021). Distinguishing between primary and diagenetic signals via high resolution analytical technics, such as Secondary Ion Mass Spectrometry (SIMS) or Laser Ablation ICP-MS is one possibility (Bice et al., 2005; Marr et al., 2013b). In fact, our rationale for employing Laser Ablation ICP-MS here was partly in recognition that future analyses may need to employ this high-resolution technique to exclude altered shell regions. However, the delicacy of thin and porous G. hexagonus shells present distinct challenges for differentiation of primary and diagenetic signals by microanalytical techniques. For example, altered calcite might be more commonly found on exposed surfaces like the inner and outer shell surface and within pores. This means that mixing of altered and relatively pristine regions would occur in any ablation pit that included a pore. To some extent, this would always be an issue in using LA-ICP-MS to identify altered zones, but would be amplified in a species with relatively large pores and thin walls. These challenges may be partially overcome by future characterization of the natural TE/Ca heterogeneity within G. hexagonus shells in addition to continued evaluation of cleaning protocols and careful site selection.
Although diagenesis may complicate some applications of Mn/Ca and Zn/Ca as proxies in G. hexagonus shells, the immediate outlook for other proxies is brighter. While Mg/Ca diagenesis requires careful consideration, a substantial body of research has already been amassed on cleaning and sample selection with an eye specifically to Mg/Ca preservation in foraminifera (e.g., Barker et al., 2003; Marr et al., 2013a; Fritz-Endres and Fehrenbacher, 2021). The upside is that temperature interpretations from Mg/Ca should be feasible at all but the most altered sites. Moreover, Sr/Ca is not highly susceptible to post-depositional alteration (Lorens et al., 1977), although ratios may decrease if shells experience dissolution (McCorkle et al., 1995; Edgar et al., 2015). Thus, Mg/Ca and Sr/Ca ratios of G. hexagonus shells from sediments show particular promise for reconstructing past OMZs. It is our hope that these conventional trace elements in planktic foraminifera, along with other archives of pelagic calcite such as fish otoliths (Limburg et al., 2015; Limburg and Casini, 2018; Altenritter and Walther, 2019; Cavole et al., 2023) may be used to improve the spatial and temporal resolution of records from low oxygen pelagic environments.
Conclusions
Trace metal analysis of individual G. hexagonus shells demonstrates several ways in which the species could be useful for reconstructing OMZ environments beyond the observational record. As in other species of planktic foraminifera, Mg/Ca ratios of G. hexagonus shells can serve as a proxy for temperature once a species-specific equation is applied. Ratios of Mn/Ca and Zn/Ca both increase with decreasing dissolved oxygen, as would be expected if carbonate chemistry and/or oxygen were dominant controls. An especially strong correlation is observed between low Sr/Ca ratios and more intense OMZ environments. We propose that this effect could be linked to growth rate and that Sr/Ca is a potential proxy for OMZ intensity, especially given relative robustness of Sr/Ca ratios to diagenesis. Thus Sr/Ca, and more tentatively Mn/Ca and Zn/Ca, could be useful in reconstructing OMZ intensity.
Data availability statement
The original contributions presented in the study are included in the article/Supplementary Material. Further inquiries can be directed to the corresponding author.
Author contributions
CD conceived of and designed the study. CD and JF performed analyses, CD and SD analyzed data. KW supplied materials. CD, SD, JF, and KW all contributed to the writing and editing of the manuscript. All authors contributed to the article and approved the submitted version.
Funding
This research was supported by NSF OCE 1851589 to CD, NSF OCE 1459243 to KW.
Acknowledgments
Many thanks to D. Outram and C. Russo for laboratory assistance.
Conflict of interest
The authors declare that the research was conducted in the absence of any commercial or financial relationships that could be construed as a potential conflict of interest.
Publisher’s note
All claims expressed in this article are solely those of the authors and do not necessarily represent those of their affiliated organizations, or those of the publisher, the editors and the reviewers. Any product that may be evaluated in this article, or claim that may be made by its manufacturer, is not guaranteed or endorsed by the publisher.
Supplementary material
The Supplementary Material for this article can be found online at: https://www.frontiersin.org/articles/10.3389/fmars.2023.1145756/full#supplementary-material
References
Alldredge A. L., Cohen Y. (1987). Can microscale chemical patches persist in the sea? microelectrode study of marine snow, fecal pellets. Science 235 (4789), 689–691. doi: 10.1126/science.235.4789.689
Alldredge A. L., Silver M. W. (1988). Characteristics, dynamics and significance of marine snow. Prog. oceanography 20 (1), 41–82. doi: 10.1016/0079-6611(88)90053-5
Allen K. A., Hönisch B., Eggins S. M., Haynes L. L., Rosenthal Y., Yu J. (2016). Trace element proxies for surface ocean conditions: A synthesis of culture calibrations with planktic foraminifera. Geochimica Cosmochimica Acta 193, 197–221. doi: 10.1016/j.gca.2016.08.015
Altenritter M. E., Walther B. D. (2019). The legacy of hypoxia: Tracking carryover effects of low oxygen exposure in a demersal fish using geochemical tracers. Trans. Am. Fisheries Soc. 148 (3), 569–583. doi: 10.1002/tafs.10159
Anand P., Elderfield H., Conte M. H. (2003). Calibration of Mg/Ca thermometry in planktonic foraminifera from a sediment trap time series. Paleoceanography 18 (2), 1050. doi: 10.1029/2002PA000846
Barker S., Greaves M., Elderfield H. (2003). A study of cleaning procedures used for foraminiferal Mg/Ca paleothermometry. Geochemistry Geophysics Geosystems 4 (9), 8407. doi: 10.1029/2003GC000559
Barras C., Mouret A., Nardelli M. P., Metzger E., Petersen J., La C., et al. (2018). Experimental calibration of manganese incorporation in foraminiferal calcite. Geochimica Cosmochimica Acta 237, 49–64. doi: 10.1016/j.gca.2018.06.009
Bianchi D., Weber T. S., Kiko R., Deutsch C. (2018). Global niche of marine anaerobic metabolisms expanded by particle microenvironments. Nat. Geosci. 11 (4), 263–268. doi: 10.1038/s41561-018-0081-0
Bice K. L., Layne G. D., Dahl K. (2005). Application of secondary ion mass spectrometry to the determination of Mg/Ca in rare, delicate, or altered planktonic foraminifera: Examples from the Holocene, paleogene, and Cretaceous. Geochemistry Geophysics Geosystems 6 (12), Q12P07. doi: 10.1029/2005GC000974
Bijma J., Hönisch B., Zeebe R. E. (2002). Impact of the ocean carbonate chemistry on living foraminiferal shell weight: Comment on “Carbonate ion concentration in glacial-age deep waters of the Caribbean sea” by w. s. broecker and e. Clark. Geochemistry Geophysics Geosystems 3 (11), 1064. doi: 10.1029/2002GC000388
Birch H., Coxall H. K., Pearson P. N., Kroon D., O'Regan M. (2013). Planktonic foraminifera stable isotopes and water column structure: Disentangling ecological signals. Mar. Micropaleontology 101, 127–145. doi: 10.1016/j.marmicro.2013.02.002
Boyle E. A. (1983). Manganese carbonate overgrowths on foraminifera tests. Geochimica Cosmochimica Acta 47 (10), 1815–1819. doi: 10.1016/0016-7037(83)90029-7
Branson O., Read E., Redfern S. A., Rau C., Elderfield H. (2015). Revisiting diagenesis on the ontong Java plateau: Evidence for authigenic crust precipitation in globorotalia tumida. Paleoceanography 30 (11), 1490–1502. doi: 10.1002/2014PA002759
Branson O., Fehrenbacher J. S., Vetter L., Sadekov A. Y., Eggins S. M., Spero H. J. (2019). LAtools: A data analysis package for the reproducible reduction of LA-ICPMS data. Chem. Geology 504, 83–95. doi: 10.1016/j.chemgeo.2018.10.029
Breitburg D., Levin L. A., Oschlies A., Grégoire M., Chavez F. P., Conley D. J., et al. (2018). Declining oxygen in the global ocean and coastal waters. Science 359 (6371), eaam7240. doi: 10.1126/science.aam7240
Brinkmann S. N., Schweizer M., Oldham V. E., Quintana Krupinski N. B., et al. (2021). Foraminiferal Mn/Ca as bottom-water hypoxia proxy: An assessment of nonionella stella in the santa barbara basin, USA. Paleoceanography and Paleoclimatology 36 (11), e2020PA004167.
Bolster K. M., Heller M. I., Mulholland M. R., Moffett J. W. (2022). Iron and manganese accumulation within the eastern tropical north pacific oxygen deficient zone. Geochimica et Cosmochimica Acta 334, 259–272.
Carolan M. T., Smith J. M., Beman J. M. (2015). Transcriptomic evidence for microbial sulfur cycling in the eastern tropical north pacific oxygen minimum zone. Front. Microbiol. 6. doi: 10.3389/fmicb.2015.00334
Cavole L. M., Limburg K. E., Gallo N. D., Vea Salvanes A. G., Ramírez-Valdez A., Levin L. A., et al. (2023). Otoliths of marine fishes record evidence of low oxygen, temperature and pH conditions of deep oxygen minimum zones. Deep Sea Res. Part I: Oceanographic Res. Papers 191, 103941. doi: 10.1016/j.dsr.2022.103941
Cléroux C., Cortijo E., Anand P., Labeyrie L., Bassinot F., Caillon N., et al. (2008). Mg/Ca and Sr/Ca ratios in planktonic foraminifera: Proxies for upper water column temperature reconstruction. Paleoceanography 23 (3), n/a–n/a. doi: 10.1029/2007PA001505
Conway T. M., John S. G. (2015). The cycling of iron, zinc and cadmium in the north East pacific ocean – insights from stable isotopes. Geochimica Cosmochimica Acta 164, 262–283. doi: 10.1016/j.gca.2015.05.023
Davis C. V., Fehrenbacher J. S., Hill T. M., Russell A. D., Spero H. J. (2017). Relationships between temperature, pH, and crusting on Mg/Ca ratios in laboratory-grown neogloboquadrina foraminifera. Paleoceanography 32 (11), 1137–1152. doi: 10.1002/2017PA003111
Davis C. V., Fehrenbacher J. S., Benitez-Nelson C., Thunell R. C. (2020). Trace element heterogeneity across individual planktic foraminifera from the modern cariaco basin. J. Foraminiferal Res. 50 (2), 204–218. doi: 10.2113/gsjfr.50.2.204
Davis C. V., Sibert E. C., Jacobs P. H., Burls N., Hull P. M. (2023). Intermediate water circulation drives distribution of pliocene oxygen minimum zones. Nature Communications 14 (1), 40.
Davis C. V., Wishner K., Renema W., Hull P. M. (2021). Vertical distribution of planktic foraminifera through an oxygen minimum zone: how assemblages and test morphology reflect oxygen concentrations. Biogeosciences 18 (3), 977–992. doi: 10.5194/bg-18-977-2021
Dekens P. S., Lea D. W., Pak D. K., Spero H. J. (2002). Core top calibration of Mg/Ca in tropical foraminifera: Refining paleotemperature estimation. Geochemistry Geophysics Geosystems 3 (4), 1–29. doi: 10.1029/2001GC000200
Deutsch C., Berelson W., Thunell R., Weber T., Tems C., McManus J., et al. (2014). Centennial changes in north pacific anoxia linked to tropical trade winds. Science 345 (6197), 665–668. doi: 10.1126/science.1252332
DeVries T., Deutsch C., Primeau F., Chang B., Devol A. (2012). Global rates of water-column denitrification derived from nitrogen gas measurements. Nat. Geosci. 5 (8), 547. doi: 10.1038/ngeo1515
Dissard D., Nehrke G., Reichart G. J., Bijma J. (2010). Impact of seawater pCO2 on calcification and Mg/Ca and Sr/Ca ratios in benthic foraminifera calcite: results from culturing experiments with Ammonia tepida. Biogeosciences 7 (1), 81–93. doi: 10.5194/bg-7-81-2010
Dueñas-Bohórquez A., da Rocha R. E., Kuroyanagi A., Bijma J., Reichart G.-J. (2009). Effect of salinity and seawater calcite saturation state on mg and Sr incorporation in cultured planktonic foraminifera. Mar. Micropaleontology 73 (3), 178–189. doi: 10.1016/j.marmicro.2009.09.002
Edgar K. M., Anagnostou E., Pearson P. N., Foster G. L. (2015). Assessing the impact of diagenesis on δ11B, δ13C, δ18O, Sr/Ca and B/Ca values in fossil planktic foraminiferal calcite. Geochimica Cosmochimica Acta 166, 189–209. doi: 10.1016/j.gca.2015.06.018
Elderfield H., Ganssen G. (2000). Past temperature and δ18O of surface ocean waters inferred from foraminiferal Mg/Ca ratios. Nature 405 (6785), 442–445. doi: 10.1038/35013033
Elderfield H., Vautravers M., Cooper M. (2002). The relationship between shell size and Mg/Ca, Sr/Ca, δ18O, and δ13C of species of planktonic foraminifera. Geochemistry Geophysics Geosystems 3 (8), 1–13. doi: 10.1029/2001GC000194
Evans D., Wade B. S., Henehan M., Erez J., Müller W. (2016). Revisiting carbonate chemistry controls on planktic foraminifera Mg/Ca: implications for sea surface temperature and hydrology shifts over the Paleocene–Eocene thermal maximum and Eocene–oligocene transition. Climate Past 12 (4), 819–835. doi: 10.5194/cp-12-819-2016
Fairbanks R. G., Sverdlove M., Free R., Wiebe P. H., Bé A. W. (1982). Vertical distribution and isotopic fractionation of living planktonic foraminifera from the Panama basin. Nature 298 (5877), 841–844. doi: 10.1038/298841a0
Fehrenbacher J., Martin P. A., Eshel G. (2006). Glacial deep water carbonate chemistry inferred from foraminiferal Mg/Ca: A case study from the western tropical Atlantic. Geochemistry Geophysics Geosystems 7 (9), Q09P16. doi: 10.1029/2005GC001156
Fehrenbacher J. S., Spero H. J., Russell A. D., Vetter L., Eggins S. (2015). Optimizing LA-ICP-MS analytical procedures for elemental depth profiling of foraminifera shells. Chem. Geology 407, 2–9. doi: 10.1016/j.chemgeo.2015.04.007
Fehrenbacher J. S., Russell A. D., Davis C. V., Spero H. J., Chu E., Hönisch B. (2018). Ba/Ca ratios in the non-spinose planktic foraminifer Neogloboquadrina dutertrei: Evidence for an organic aggregate microhabitat. Geochimica Cosmochimica Acta 236, 361–372. doi: 10.1016/j.gca.2018.03.008
Fritz-Endres T., Fehrenbacher J. (2021). Preferential loss of high trace element bearing inner calcite in foraminifera during physical and chemical cleaning. Geochemistry Geophysics Geosystems 22 (1), e2020GC009419. doi: 10.1029/2020GC009419
Geerken E., de Nooijer L., Toyofuku T., Roepert A., Middelburg J. J., Kienhuis M. V., et al. (2022). High precipitation rates characterize biomineralization in the benthic foraminifer Ammonia beccarii. Geochimica Cosmochimica Acta 318, 70–82. doi: 10.1016/j.gca.2021.11.026
Gray W. R., Evans D. (2019). Nonthermal influences on Mg/Ca in planktonic foraminifera: A review of culture studies and application to the last glacial maximum. Paleoceanography Paleoclimatology 34 (3), 306–315. doi: 10.1029/2018PA003517
Gray W. R., Weldeab S., Lea D. W., Rosenthal Y., Gruber N., Donner B., et al. (2018). The effects of temperature, salinity, and the carbonate system on Mg/Ca in Globigerinoides ruber (white): A global sediment trap calibration. Earth Planetary Sci. Lett. 482, 607–620. doi: 10.1016/j.epsl.2017.11.026
Groeneveld J., Filipsson H. L. (2013). Mg/Ca and Mn/Ca ratios in benthic foraminifera: the potential to reconstruct past variations in temperature and hypoxia in shelf regions. Biogeosciences 10 (7), 5125–5138. doi: 10.5194/bg-10-5125-2013
Groeneveld J., Ho S. L., Mackensen A., Mohtadi M., Laepple T. (2019). Deciphering the variability in Mg/Ca and stable oxygen isotopes of individual foraminifera. Paleoceanography Paleoclimatology 34 (5), 755–773. doi: 10.1029/2018PA003533
Gruber N. (2008). The marine nitrogen cycle: overview and challenges. Nitrogen Mar. Environ. 2, 1–50.
Holland K., Eggins S. M., Hönisch B., Haynes L. L., Branson O. (2017). Calcification rate and shell chemistry response of the planktic foraminifer Orbulina universa to changes in microenvironment seawater carbonate chemistry. Earth Planetary Sci. Lett. 464, 124–134. doi: 10.1016/j.epsl.2017.02.018
Hönisch B., Allen K. A., Lea D. W., Spero H. J., Eggins S. M., Arbuszewski J., et al. (2013). The influence of salinity on Mg/Ca in planktic foraminifers–evidence from cultures, core-top sediments and complementary δ18O. Geochimica Cosmochimica Acta 121, 196–213. doi: 10.1016/j.gca.2013.07.028
Hoogakker B. A., Lu Z., Umling N., Jones L., Zhou X., Rickaby R. E., et al. (2018). Glacial expansion of oxygen-depleted seawater in the eastern tropical pacific. Nature 562 (7727), 410–413. doi: 10.1038/s41586-018-0589-x
Horak R. E. A., Ruef W., Ward Bess B., Devol Allan H. (2016). Expansion of denitrification and anoxia in the eastern tropical north pacific from 1972 to 2012. Geophysical Res. Lett. 43 (10), 5252–5260. doi: 10.1002/2016GL068871
Janssen D. J., Cullen J. T. (2015). Decoupling of zinc and silicic acid in the subarctic northeast pacific interior. Mar. Chem. 177, 124–133. doi: 10.1016/j.marchem.2015.03.014
John S. G., Bian X., Moffett J. W. (2022). Concentrations of dissolved cadmium, nickel, manganese, lanthanum, cerium, praseodymium, and neodymium from the Eastern tropical north pacific ocean on R/V revelle cruise RR1804-1805 (OMZ nutrient cycling project). Biol. Chem. Oceanography Data Manage. Office (BCO-DMO).
John S. G., Moffett J. W. (2021). Isotopic composition and concentrations of dissolved and particulate nickel, cadmium, iron, zinc, and copper from the Eastern tropical north pacific ocean on R/V revelle cruise RR1804 and on R/V sikuliaq cruise SKQ201617S. Biol. Chem. Oceanography Data Manage. Office (BCO-DMO). doi: 10.26008/1912/bco-dmo.842086.1
Johnstone H. J. H., Yu J., Elderfield H., Schulz M. (2011). Improving temperature estimates derived from Mg/Ca of planktonic foraminifera using X-ray computed tomography–based dissolution index, XDX. Paleoceanography 26 (1), PA1215. doi: 10.1029/2009PA001902
Jonkers L., Brummer G.-J. A., Meilland J., Groeneveld J., Kucera M. (2022). Variability in Neogloboquadrina pachyderma stable isotope ratios from isothermal conditions: implications for individual foraminifera analysis. Climate Past 18 (1), 89–101. doi: 10.5194/cp-18-89-2022
Katz M. E., Cramer B. S., Franzese A., Hönisch B., Miller K. G., Rosenthal Y., et al. (2010). Traditional and emergin geochemical proxies in foraminifera. J. Foraminiferal Res. 40 (2), 165–192. doi: 10.2113/gsjfr.40.2.165
Keeling R. F., Körtzinger A., Gruber N. (2009). Ocean deoxygenation in a warming world. Annu. Rev. Mar. Sci. 2 (1), 199–229. doi: 10.1146/annurev.marine.010908.163855
Kennett J. P., Srinivasan M. S. (1983). Neogene planktonic foraminifera: a phylogenetic atlas (Hutchinson Ross) 265, 546–548.
Keul N., Langer G., Thoms S., de Nooijer L. J., Reichart G.-J., Bijma J. (2017). Exploring foraminiferal Sr/Ca as a new carbonate system proxy. Geochimica Cosmochimica Acta 202, 374–386. doi: 10.1016/j.gca.2016.11.022
Kısakürek B., Eisenhauer A., Böhm F., Garbe-Schönberg D., Erez J. (2008). Controls on shell Mg/Ca and Sr/Ca in cultured planktonic foraminiferan, Globigerinoides ruber (white). Earth Planetary Sci. Lett. 273 (3-4), 260–269. doi: 10.1016/j.epsl.2008.06.026
Koho K., de Nooijer L., Reichart G. (2015). Combining benthic foraminiferal ecology and shell Mn/Ca to deconvolve past bottom water oxygenation and paleoproductivity. Geochimica Cosmochimica Acta 165, 294–306. doi: 10.1016/j.gca.2015.06.003
Kuroyanagi A., da Rocha R. E., Bijma J., Spero H. J., Russell A. D., Eggins S. M., et al. (2013). Effect of dissolved oxygen concentration on planktonic foraminifera through laboratory culture experiments and implications for oceanic anoxic events. Mar. Micropaleontology 101, 28–32. doi: 10.1016/j.marmicro.2013.04.005
Lea D. W., Martin P. A., Pak D. K., Spero H. J. (2002). Reconstructing a 350 ky history of sea level using planktonic Mg/Ca and oxygen isotope records from a Cocos ridge core. Quaternary Sci. Rev. 21 (1-3), 283–293. doi: 10.1016/S0277-3791(01)00081-6
Lea D. W., Mashiotta T. A., Spero H. J. (1999). Controls on magnesium and strontium uptake in planktonic foraminifera determined by live culturing. Geochimica Cosmochimica Acta 63 (16), 2369–2379. doi: 10.1016/S0016-7037(99)00197-0
Levin L. A. (2003). Oxygen minimum zone benthos: Adaptation and community response to hypoxia. Oceanography Mar. Biol. 41, 1–45.
Levin L. (2018). Manifestation, drivers, and emergence of open ocean deoxygenation. Annu. Rev. Mar. science. 10, 229–260.
Limburg K. E., Casini M. (2018). Effect of marine hypoxia on Baltic Sea cod Gadus morhua: Evidence from otolith chemical proxies. Front. Mar. Sci. 5. doi: 10.3389/fmars.2018.00482
Limburg K. E., Walther B. D., Lu Z., Jackman G., Mohan J., Walther Y., et al. (2015). In search of the dead zone: Use of otoliths for tracking fish exposure to hypoxia. J. Mar. Syst. 141, 167–178. doi: 10.1016/j.jmarsys.2014.02.014
Livsey C. M., Kozdon R., Bauch D., Brummer G.-J. A., Jonkers L., Orland I., et al. (2020). High-resolution Mg/Ca and δ18O patterns in modern Neogloboquadrina pachyderma from the fram strait and irminger Sea. Paleoceanography Paleoclimatology 35 (9), e2020PA003969. doi: 10.1029/2020PA003969
Lombard F., Labeyrie L., Michel E., Spero H. J., Lea D. W. (2009). Modelling the temperature dependent growth rates of planktic foraminifera. Mar. Micropaleontology 70 (1–2), 1–7. doi: 10.1016/j.marmicro.2008.09.004
Lombard F., da Rocha R. E., Bijma J., Gattuso J. P. (2010). Effect of carbonate ion concentration and irradiance on calcification in planktonic foraminifera. Biogeosciences 7 (1), 247–255. doi: 10.5194/bg-7-247-2010
Lorens R. B., Williams D. F., Bender M. L. (1977). The early nonstructural chemical diagenesis of foraminiferal calcite. J. Sedimentary Res. 47 (4), 1602–1609.
Lu W., Dickson A. J., Thomas E., Rickaby R. E., Chapman P., Lu Z. (2020). Refining the planktic foraminiferal I/Ca proxy: Results from the southeast Atlantic ocean. Geochimica Cosmochimica Acta 287, 318–327. doi: 10.1016/j.gca.2019.10.025
Lu Z., Hoogakker B. A., Hillenbrand C.-D., Zhou X., Thomas E., Gutchess K. M., et al. (2016). Oxygen depletion recorded in upper waters of the glacial southern ocean. Nat. Commun. 7 (1), 1–9. doi: 10.1038/ncomms11146
Manno C., Morata N., Bellerby R. (2012). Effect of ocean acidification and temperature increase on the planktonic foraminifer Neogloboquadrina pachyderma (sinistral). Polar Biol. 35 (9), 1311–1319. doi: 10.1007/s00300-012-1174-7
Marchitto T. M., Curry W. B., Oppo D. W. (2000). Zinc concentrations in benthic foraminifera reflect seawater chemistry. Paleoceanography 15 (3), 299–306. doi: 10.1029/1999PA000420
Marr J. P., Bostock H. C., Carter L., Bolton A., Smith E. (2013a). Differential effects of cleaning procedures on the trace element chemistry of planktonic foraminifera. Chem. Geology 351, 310–323. doi: 10.1016/j.chemgeo.2013.05.019
Marr J. P., Carter L., Bostock H. C., Bolton A., Smith E. (2013b). Southwest pacific ocean response to a warming world: Using Mg/Ca, Zn/Ca, and Mn/Ca in foraminifera to track surface ocean water masses during the last deglaciation. Paleoceanography 28 (2), 347–362. doi: 10.1002/palo.20032
Martinez P., Robinson R. S. (2010). Increase in water column denitrification during the last deglaciation: the influence of oxygen demand in the eastern equatorial pacific. Biogeosciences 7 (1), 1–9. doi: 10.5194/bg-7-1-2010
Mashiotta T. A., Lea D. W., Spero H. J. (1999). Glacial–interglacial changes in subantarctic sea surface temperature and δ18O-water using foraminiferal mg. Earth Planetary Sci. Lett. 170 (4), 417–432. doi: 10.1016/S0012-821X(99)00116-8
Mathien-Blard E., Bassinot F. (2009). Salinity bias on the foraminifera Mg/Ca thermometry: Correction procedure and implications for past ocean hydrographic reconstructions. Geochemistry Geophysics Geosystems 10 (12), Q12011. doi: 10.1029/2008GC002353
McConnell M. C., Thunell R. C. (2005). Calibration of the planktonic foraminiferal Mg/Ca paleothermometer: Sediment trap results from the guaymas basin, gulf of California. Paleoceanography 20 (2), PA2016. doi: 10.1029/2004PA001077
McCorkle D. C., Martin P. A., Lea D. W., Klinkhammer G. P. (1995). Evidence of a dissolution effect on benthic foraminiferal shell chemistry: δ13C, Cd/Ca, Ba/Ca, and Sr/Ca results from the ontong Java plateau. Paleoceanography 10 (4), 699–714. doi: 10.1029/95PA01427
McKay C. L., Groeneveld J., Filipsson H. L., Gallego-Torres D., Whitehouse M. J., Toyofuku T., et al. (2015). A comparison of benthic foraminiferal Mn/Ca and sedimentary Mn/Al as proxies of relative bottom-water oxygenation in the low-latitude NE Atlantic upwelling system. Biogeosciences 12 (18), 5415–5428. doi: 10.5194/bg-12-5415-2015
Moffett J. W. (2020). Iron, manganese and nutrient data from four cruises in the eastern tropical north pacific 2012 to 2018. biological and chemical oceanography data management office (BCO-DMO). doi: 10.26008/1912/bco-dmo.828183.1
Moffitt S. E., Moffitt R. A., Sauthoff W., Davis C. V., Hewett K., Hill T. M. (2015). Paleoceanographic insights on recent oxygen minimum zone expansion: Lessons for modern oceanography. PLoS One 10 (1), e0115246. doi: 10.1371/journal.pone.0115246
Munsel D., Kramar U., Dissard D., Nehrke G., Berner Z., Bijma J., et al. (2010). Heavy metal incorporation in foraminiferal calcite: results from multi-element enrichment culture experiments with Ammonia tepida. Biogeosciences 7 (8), 2339–2350. doi: 10.5194/bg-7-2339-2010
Nameroff T. J., Calvert S. E., Murray J. W. (2004). Glacial-interglacial variability in the eastern tropical north pacific oxygen minimum zone recorded by redox-sensitive trace metals. Paleoceanography 19 (1), PA1010. doi: 10.1029/2003PA000912
Ní Fhlaithearta S., Fontanier C., Jorissen F., Mouret A., Dueñas-Bohórquez A., Anschutz P., et al. (2018). Manganese incorporation in living (stained) benthic foraminiferal shells: a bathymetric and in-sediment study in the gulf of lions (NW Mediterranean). Biogeosciences 15 (20), 6315–6328. doi: 10.5194/bg-15-6315-2018
Nürnberg D., Bijma J., Hemleben C. (1996). Assessing the reliability of magnesium in foraminiferal calcite as a proxy for water mass temperatures. Geochimica Cosmochimica Acta 60 (5), 803–814. doi: 10.1016/0016-7037(95)00446-7
Ortiz J. D., Mix A. C., Collier R. W. (1995). Environmental control of living symbiotic and asymbiotic foraminifera of the California current. Paleoceanography 10 (6), 987–1009. doi: 10.1029/95PA02088
Oschlies A., Brandt P., Stramma L., Schmidtko S. (2018). Drivers and mechanisms of ocean deoxygenation. Nat. Geosci. 11 (7), 467–473. doi: 10.1038/s41561-018-0152-2
Paulmier A., Ruiz-Pino D., Garçon V. (2011). CO2 maximum in the oxygen minimum zone (OMZ). Biogeosciences 8 (2), 239–252. doi: 10.5194/bg-8-239-2011
Pena L., Cacho I., Calvo E., Pelejero C., Eggins S., Sadekov A. (2008). Characterization of contaminant phases in foraminifera carbonates by electron microprobe mapping. Geochemistry Geophysics Geosystems 9 (7), Q07012. doi: 10.1029/2008GC002018
Pena L. D., Calvo E., Cacho I., Eggins S., Pelejero C. (2005). Identification and removal of Mn-mg-rich contaminant phases on foraminiferal tests: Implications for Mg/Ca past temperature reconstructions. Geochemistry Geophysics Geosystems 6 (9), Q09P02. doi: 10.1029/2005GC000930
Raitzsch M., Dueñas-Bohórquez A., Reichart G.-J., de Nooijer L. J., Bickert T. (2010). Incorporation of mg and Sr in calcite of cultured benthic foraminifera: impact of calcium concentration and associated calcite saturation state. Biogeosciences 7 (3), 869–881. doi: 10.5194/bg-7-869-2010
Regenberg M., Regenberg A., Garbe-Schönberg D., Lea D. W. (2014). Global dissolution effects on planktonic foraminiferal Mg/Ca ratios controlled by the calcite-saturation state of bottom waters. Paleoceanography 29 (3), 127–142. doi: 10.1002/2013PA002492
Richey J. N., Fehrenbacher J. S., Reynolds C. E., Davis C. V., Spero H. J. (2022). Barium enrichment in the non-spinose planktic foraminifer, globorotalia truncatulinoides. Geochimica Cosmochimica Acta 333, 184–199. doi: 10.1016/j.gca.2022.07.006
Rippert N., Nürnberg D., Raddatz J., Maier E., Hathorne E., Bijma J., et al. (2016). Constraining foraminiferal calcification depths in the western pacific warm pool. Mar. Micropaleontology 128, 14–27. doi: 10.1016/j.marmicro.2016.08.004
Russell A. D., Hönisch B., Spero H. J., Lea D. W. (2004). Effects of seawater carbonate ion concentration and temperature on shell U, mg, and Sr in cultured planktonic foraminifera. Geochimica Cosmochimica Acta 68 (21), 4347–4361. doi: 10.1016/j.gca.2004.03.013
Sadekov A., Eggins S. M., De Deckker P., Ninnemann U., Kuhnt W., Bassinot F. (2009). Surface and subsurface seawater temperature reconstruction using Mg/Ca microanalysis of planktonic foraminifera Globigerinoides ruber, globigerinoides sacculifer, and pulleniatina obliquiloculata. Paleoceanography 24 (3), PA3201. doi: 10.1029/2008PA001664
Schmidtko S., Stramma L., Visbeck M. (2017). Decline in global oceanic oxygen content during the past five decades. Nature 542 (7641), 335–339. doi: 10.1038/nature21399
Schmitt A., Elliot M., Thirumalai K., La C., Bassinot F., Petersen J., et al. (2019). Single foraminifera Mg/Ca analyses of past glacial-interglacial temperatures derived from g. ruber sensu stricto and sensu lato morphotypes. Chem. Geology 511, 510–520. doi: 10.1016/j.chemgeo.2018.11.007
Shanks A. L., Reeder M. L. (1993). Reducing microzones and sulfide production in marine snow. Mar. Ecol. Prog. Ser. 96 (1), 43–47. doi: 10.3354/meps096043
Spero H. J., Bijma J., Lea D. W., Bemis B. E. (1997). Effect of seawater carbonate concentration on foraminiferal carbon and oxygen isotopes. Nature 390 (6659), 497–500.
Steinhardt J., Cléroux C., Ullgren J., de Nooijer L., Durgadoo J. V., Brummer G. J., et al. (2014). Anti-cyclonic eddy imprint on calcite geochemistry of several planktonic foraminiferal species in the Mozambique channel. Mar. Micropaleontology 113, 20–33. doi: 10.1016/j.marmicro.2014.09.001
Stramma L., Schmidtko S., Levin L. A., Johnson G. C. (2010). Ocean oxygen minima expansions and their biological impacts. Deep Sea Res. Part I: Oceanographic Res. Papers 57 (4), 587–595. doi: 10.1016/j.dsr.2010.01.005
Stramma L., Prince E. D., Schmidtko S., Luo J., Hoolihan J. P., Visbeck M., et al. (2012). Expansion of oxygen minimum zones may reduce available habitat for tropical pelagic fishes. Nat. Climate Change 2 (1), 33–37. doi: 10.1038/nclimate1304
Titelboim D., Sadekov A., Blumenfeld M., Almogi-Labin A., Herut B., Halicz L., et al. (2021). Monitoring of heavy metals in seawater using single chamber foraminiferal sclerochronology. Ecological Indicators 120, 106931.
van Dijk I., De Nooijer L., Wolthers M., Reichart G.-J. (2017). Impacts of pH and [CO32-] on the incorporation of zn in foraminiferal calcite. Geochimica Cosmochimica Acta 197. doi: 10.1016/j.gca.2016.10.031
van Dijk I., de Nooijer L. J., Reichart G. J. (2017). Trends in element incorporation in hyaline and porcelaneous foraminifera as a function of pCO2. Biogeosciences 14 (3), 497–510. doi: 10.5194/bg-14-497-2017
van Dijk I., de Nooijer L. J., Barras C., Reichart G.-J. (2020). Mn Incorporation in large benthic foraminifera: Differences between species and the impact of pCO2. Front. Earth Sci. 8. doi: 10.3389/feart.2020.567701
van Geen A., Zheng Y., Bernhard J., Cannariato K., Carriquiry J., Dean W., et al. (2003). On the preservation of laminated sediments along the western margin of north America. Paleoceanography 18 (4), 1098.
Wiebe P., Morton A., Bradley A., Backus R., Craddock J., Barber V., et al. (1985). New development in the MOCNESS, an apparatus for sampling zooplankton and micronekton. Mar. Biol. 87 (3), 313–323. doi: 10.1007/BF00397811
Wishner K. F., Seibel B., Roman C., Deutsch C., Outram D., Shaw C., et al. (2018). Ocean deoxygenation and zooplankton: Very small oxygen differences matter. Sci. Adv. 4 (12), eaau5180. doi: 10.1126/sciadv.aau5180
Wishner K. F., Seibel B., Outram D. (2020). Ocean deoxygenation and copepods: coping with oxygen minimum zone variability. Biogeosciences 17 (8), 2315–2339.
Wishner K., Roman C., Seibel B. (2021). Event log from R/V sikuliaq SKQ201701S from January to February 2017. biological and chemical oceanography data management office (BCO-DMO). doi: 10.26008/1912/bco-dmo.755088.1
Keywords: foraminifera, oxygen minimum zone (OMZ), oxygen proxy, trace element, calcite
Citation: Davis CV, Doherty S, Fehrenbacher J and Wishner K (2023) Trace element composition of modern planktic foraminifera from an oxygen minimum zone: Potential proxies for an enigmatic environment. Front. Mar. Sci. 10:1145756. doi: 10.3389/fmars.2023.1145756
Received: 16 January 2023; Accepted: 15 March 2023;
Published: 29 March 2023.
Edited by:
Ed Hathorne, Helmholtz Association of German Research Centres (HZ), GermanyReviewed by:
Jeroen Groeneveld, National Taiwan University, TaiwanInda Brinkmann, Geological Survey of Denmark and Greenland, Denmark
Copyright © 2023 Davis, Doherty, Fehrenbacher and Wishner. This is an open-access article distributed under the terms of the Creative Commons Attribution License (CC BY). The use, distribution or reproduction in other forums is permitted, provided the original author(s) and the copyright owner(s) are credited and that the original publication in this journal is cited, in accordance with accepted academic practice. No use, distribution or reproduction is permitted which does not comply with these terms.
*Correspondence: Catherine V. Davis, Y2RhdmlzMjRAbmNzdS5lZHU=