- 1National Marine Science Centre, Southern Cross University, Coffs Harbour, NSW, Australia
- 2School of Geosciences, University of Sydney, Sydney, NSW, Australia
The health of coral reefs is declining from the effects of human activity and climate change. Mass coral bleaching is often triggered by elevated water temperature and excessive solar irradiance. Shading can reduce coral bleaching risk. Shading-based management interventions, such as whole-of-reef marine fogging, have been proposed as a conservation tool for periods when coral undergoes excessive thermal stress. This study examined the effect of intermittent shade (30% for 0, 4, or 24 h) on two coral species, Duncanopsammia axifuga and Turbinaria reniformis, held at either 26.4°C or 32.4°C for 18 days. Coral fragments were assessed for bleaching (relative mean intensity of grey, chlorophyll a, and symbiont density), photochemistry (PAM fluorometry), and antioxidant biomarkers (SOD and CAT). Shading responses were species-specific, with T. reniformis more responsive to shading than D. axifuga. Thirty per cent shading delayed bleaching up to three-degree heat weeks (DHW), and 24 h shade was more protective than 4 h shade. Shading suppressed catalase activity in T. reniformis. Overall, our results suggest that intermittently shading corals for 4 h can moderate light stress and slow bleaching in some corals and could improve the efficiency of active solar radiation management in marine ecosystems.
1 Introduction
The unprecedented decline of coral reefs globally has been robustly documented over the last two decades (De'ath et al., 2012; Hughes et al., 2018a). Coral cover in some iconic ecosystems, such as the Great Barrier Reef, has been reduced by up to 70% (Bell et al., 2014), while at other places, 100% mortality has been reported (Guzman, 1991). Crown-of-thorns predation (De'ath et al., 2012), the severe effects of climate change and ocean acidification, and the increasing frequency and intensity of tropical storms and bleaching events are considered the main drivers of the current coral crisis (Hughes et al., 2003; Grottoli et al., 2014; Hughes et al., 2018b). Recently, extreme weather events triggered some of the worst mass bleaching events on the Great Barrier Reef during 2016–2017 (Hughes et al., 2017). The aftermath of this third global-scale coral bleaching event showed that the accumulated heat exposure exceeding a critical threshold of 3–4 degree heating weeks (DHW) contributed to bleaching on 91% of reefs, coral cover loss up to 75–100% in some reefs, and an 80% decline in specific species (e.g. staghorn Acropora, Seriatopora hystrix, Styllophora pistillata) (Hughes et al., 2018a; Hughes et al., 2018b).
The most recent Intergovernmental Panel on Climate Change report indicated that global sea surface temperatures would continue to rise even under the lowest emission scenario presented (Cooley et al., 2022). Thus, the frequency and severity of coral bleaching events are expected to increase in the coming decades (Donner, 2009; Jackson et al., 2022). This presents an urgent challenge to develop and implement management interventions that reduce the impacts of environmental stressors on corals and facilitate their recovery following bleaching events (Bay et al., 2019).
It is well known that high irradiance levels coupled with high temperatures can trigger bleaching events (Brown, 1997; Berkelmans, 2002; Courtial et al., 2017; Cropp et al., 2018; Jackson et al., 2018). The possible benefits of coral shading have been demonstrated over several decades of coral research (Wethey and Porter, 1976; Shick et al., 1996; Baker et al., 2008). Previous attempts to shade reefs have been limited and do not operate at a reef scale, i.e., 10’s to 100’s km2. In their review of coral shading, Tagliafico et al. (2022) showed several artificial coverings have been used to reduce light intensity and assist coral rehabilitation such as screens (Coles and Jokiel, 1978), aeration and sprinklers (Kramer et al., 2016), awnings (Simpson et al., 2008), shade cloths (Coelho et al., 2017), fabric (Thinesh et al., 2017), and plastic sheeting (Muller and van Woesik, 2009; Muller and van Woesik, 2011). Consequently, shading reefs is a potential solution to reduce irradiance levels reaching corals and may mitigate bleaching, although implementing reef scale solutions remain elusive. Recent projections suggest that by 2040, coral cover on shaded reefs could double that found on unshaded reefs (Condie et al., 2021). Current research attempts to scale shading to the scale of individual reefs or even the entire Great Barrier Reef (e.g. fogging and cloud and sky brightening) (Tollefson, 2021). However, this research is in an early phase, and up-scaling equipment may present a technical and financial challenge (Baker et al., 2021), and the required light attenuation levels and implementation times to reduce the risk of coral bleaching are still unclear (Coelho et al., 2017). Nonetheless, shading the entire Great Barrier Reef is predicted to reduce degree heating weeks (DHW) and could limit coral mortality during severe bleaching events (Harrison et al., 2019).
Some of the shading technologies being trialled on the Great Barrier Reef (i.e., fogging and cloud brightening) require energy (e.g. diesel generator, vessel generator), and improvements in energy consumption and cost-effectiveness are desirable. Satellite imaging and weather models can help to predict when shading technologies may be necessary (Coelho et al., 2017), but variable weather conditions can impact deployment. To assess the effectiveness of intermittent sub-daily shading targeted around peak sunlight, we tested the hypothesis that different levels of shading (4 h and 24 h per day) at two different temperatures [collection site temperature: 26.4°C and four degrees above the maximum monthly mean (28.6°C), reaching 32.6°C] would significantly affect the bleaching response of corals during a high-temperature episode of up to 5 DHW. Coral bleaching is a general stress response of symbiotic corals to a wide variety of environmental stressors that results in the loss of unicellular symbiotic algae in corals (Fitt et al., 2001; Lesser and Farrell, 2004; Ainsworth et al., 2016). Elevated sea temperature and its interaction with excess solar radiation are the key environmental triggers of coral bleaching (Brown, 1997; Fitt et al., 2001; Ainsworth et al., 2016). The interaction between excessive light and water temperature can result in oxidative stress, where excessive generation of reactive oxygen species is the primary cause of coral bleaching (Smith et al., 2005; Suggett et al., 2008; Baird et al., 2009). Superoxide dismutase and catalase are important coral antioxidant defences addressing the products of oxidative stress (Weydert and Cullen, 2010).
We used chlorophyll a concentration, symbiont density, and relative mean intensity of grey as bleaching proxies. Pulse amplitude modulation (PAM) fluorometry was used to evaluate symbiont photochemistry. In addition, we used the activity of superoxide dismutase and catalase to assess the antioxidant response to oxidative stress.
2 Materials and methods
2.1 Coral species selection, collection, and preparation
The branching coral, Duncanopsammia axifuga was selected due to a demonstrated quick recovery and high survival rate after the fragmentation process, as well as the large coral tissue yield that can be obtained per small fragments of around 3 cm (mean ± SD = 1.01 ± 0.52 g), which allows laboratory analyses of different variables response (Tagliafico et al., 2017; Tagliafico et al., 2018). The plate coral, Turbinaria reniformis, was selected as it has been previously used in experiments looking at the effects of different levels of irradiance and other stressors (Courtial et al., 2017; Jones et al., 2020; Dobson et al., 2021).
Colonies of Duncanopsammia axifuga and Turbinaria reniformis were collected at 10 m depth from Arlington Reef (-16.724°, 146.063°) and Vlasoff Reef (-16.655°, 145.992°), respectively. The water temperature at each collection site was 26.4°C (November 2021), and the maximum monthly mean (MMM) at each collection site was estimated at 28.6°C using eReefs data from the 1 km resolution hydrodynamic model (Steven et al., 2019).
The collected corals were transported to the National Marine Science Centre, Coffs Harbour, New South Wales, Australia (-30.268°, 153.138°), and acclimatised in a 1200 L flow-through filtered seawater tank for one week at 26 ± 0.5°C. Corals were fed twice with Artemia salina (1d old). Afterwards, colonies were fragmented into similar sizes using a Dremel rotary tool with a diamond wheel. No significant differences were found for buoyant weight among fragments of the same species (p > 0.05).
A total of 152 fragments (76 per species, 12 replicates per treatment) were acclimatised for an additional week in conditions similar to those described above. Post-acclimation, no supplemental feeding was provided. Each fragment was randomly allocated to one of six treatments (see below). In-situ and destructive sampling of coral occurred on four occasions: (i) before starting the experiment [baseline], (ii) after 3.1 DHW [day 13], at 5 DHW [day 19], and (iv) after seven days of recovery [day 26].
2.2 Experimental setup
In the experimental system, 144 x 600 mL experimental tanks were supplied with 100 mL min-1 of flow-through, sand-filtered seawater from Charlesworth Bay (-30.267°, 153.141°). The experimental tanks were arranged in orthogonal combinations of temperature (two levels): 1) collection site temperature: 26.4°C, hereafter referred to as lower temperature (LT) and 2) MMM + 4°C: 32.6°C, henceforth higher temperature (HT); and two levels of 30% shade (4h around solar noon and 24 h). Shade cloth that reduced PAR by 30.1 ± 0.85% (mean ± SE, n = 3340) was used for the shaded treatments. For the 4 h shade treatment, the shade cloth was placed over each tank for two hours on either side of solar noon (approximately 10 am to 2 pm), and for the 24 h shade treatment, the shade cloth cover remained in place throughout the experiment. Controls with no shade were established at 26.4°C and 32.6°C. Temperature was maintained at 26 ± 0.5°C and 32.6 ± 0.5°C using heat pumps (2100 Lh-1, EVO-F5, Australia). The higher temperature treatment was increased over a week by 1.1°C per day to reach the experimental temperature of 32.6°C. To minimise possible tank position effects, the experimental tanks were randomly relocated every four days.
2.3 Environmental parameters
Visible light was measured thrice daily (11:00; 13:00 and 15:00) with a LI-250A meter with an attached LI-192 underwater quantum sensor (LI-COR, Nebraska) and over 24 h, with photosynthetic irradiance loggers (Odyssey) placed under direct sunlight and underwater with and without shade, respectively (Supplementary Figure 1). The PAR loggers were calibrated under solar radiation against a recently calibrated LI-250A. A multiplier of –143.68 and –189.66 was applied to calibrate the PAR loggers deployed in air and water, respectively.
Nitrite (0.012 ± 0.008 mg L-1 NO2), phosphate (0.23 ± 0.33 mg L-1 PO4) and alkalinity (163 ± 36 mg L-1 CaCO3) were measured from incoming water twice a week with a photometer system (Palintest® model 7100, UK). Dissolved oxygen (HT: 7.78 ± 0.29 mg L-1, LT: 8.03 ± 0.25 mg L-1) and conductivity (HT: 57.61 ± 5.26 mS cm-1, LT: 52.55 ± 5.95 mS cm-1) were recorded thrice daily (11:00, 13:00 and 15:00) with a handheld probe meter (Hach HQ40d).
2.4 In situ sampling
Maximum quantum yield (Fv/Fm) was measured using a diving pulse amplitude modulation (DIVING-PAM©) fluorometer (Heinz Walz GmbH, Germany) from dark-adapted corals after 1 h dark acclimation. Each coral fragment was measured once per sample period. PAM settings were set to avoid low signal or overflow readings (measuring light intensity 8, damping 2, gain 12, actinic light intensity 5, saturation pulse intensity 8, and saturation pulse width 0.8). PAM readings were made with the probe 1 cm from the coral. At the start of the experiment, no significant differences were found in maximum quantum yield among coral fragments within D. axifuga (df = 2, 66; pseudo-F = 0.28; p = 0.76) and T. reniformis (df = 2, 66; pseudo-F = 1.75; p = 0.17).
Each coral was photographed while underwater in their experimental tank from above using a Canon EOS 5D Mark II DSLR camera fitted with a Canon Macro EF 100mm f/2.8L IS USM lens. Camera settings were slightly modified from those suggested by Winters et al. (2009), ISO-100, F = 3.5, 1/60 and white balance manually set to 6000 K. Each image contained a white tile as a true colour reference. The camera was mounted on a video tripod with a centred mid-level spreader (Cayer BV30L, China). A Neewer T120 18W filming light (WB 5600 K, brightness 100%) was mounted on a different tripod (Evo-2 mini tripod, Manfrotto, Italy) 15 cm from the coral angled 30° off-nadir. The camera was manually focused using the two-step expanded focus (10X) function, accounting for differences in fragment shape. The tripod position (camera and light) and distance between the camera lens and coral were consistent for all pictures.
Each photograph was analysed using ImageJ (ver. 1.53k) following Amid et al. (2018) and Mclachlan and Grottoli (2021). Images were converted to 8-bit greyscale and the mean grey value for twenty 60-pixel diameter (~1 mm) areas in each image was collected. These values were normalised to a white reference and then averaged to obtain the mean intensity of grey (MIG). The relative MIG was calculated using the initial and final MIG values (Amid et al., 2018). At the start of the experiment, there was no significant difference in MIG among fragments of D. axifuga (df = 2, 66; pseudo-F = 0.45; p = 0.64) and T. reniformis (df = 2, 66; pseudo-F = 0.1; p = 0.90).
2.5 Tissue collection
Tissue from four coral fragments from each of the six treatments (n = 24 per species) was extracted using an air pistol connected to an electric 1 HP air compressor delivering air at 40 L min-1 at 7 bar (Black Ridge, Australia) by adding 0.6 mL or 1.2 mL of 0.1 M phosphate-buffered saline (PBS, Sigma Aldrich: P3813) for D. axifuga and T. reniformis, respectively. Individual plastic bags were used to maximise tissue recovery which was subsampled into vials for chlorophyll a and symbiont density and for enzymatic analysis and snap frozen (Rangel et al., 2019).
2.6 Laboratory analyses
Tissues for chlorophyll a and symbiont density were lysed in 0.8 mL of ice-cold 0.1 M PBS using a TissueLyser LT (Qiagen) at 40 Hz for 120 s. The resulting lysate was centrifuged at 3500 ×g (4°C) for 5 min (Beckman Coulter, USA), and the supernatant was discarded. The pellet was resuspended in 0.8 mL PBS and centrifuged with the same settings, and the supernatant was discarded. The pellet was then resuspended in 1.6 mL of PBS, and 0.8 mL was aliquoted for chlorophyll a analysis. The remainder of the sample was used to determine symbiont density using a Leica DM750 compound microscope and a Neubauer haemocytometer.
The aliquot for chlorophyll analysis was centrifuged with the same settings as above and the supernatant discarded. Chlorophyll a was extracted using 0.8 mL of 100% undenatured ethanol and incubated without light at 4°C overnight (Ritchie, 2006). Chlorophyll a concentration was determined by absorbance at 629 and 665 nm following Warren (2008) and quantified using Ritchie (2006). Chlorophyll and symbiont density data were normalised against surface area, obtained using the wax dipping method (Veal et al., 2010) for D. axifuga and the aluminium foil technique (Marsh, 1970) for T. reniformis.
Tissues for enzyme analysis were homogenised in cold 0.1 M Trizma-HCl (pH 7.4) with 0.5% Triton X-100, 5 nM β-mercaptoethanol and protease inhibitor cocktail (Sigma Aldrich: P2714) for 180s at 50 Hz. The homogenate was centrifuged at 14000 ×g at 4°C for 10 min and the supernatant collected for analysis of SOD and CAT activity. Superoxide dismutase (SOD) activity was estimated using the SOD Activity kit (Sigma Aldrich: CS0009). SOD activity was estimated by comparison to an inhibition curve, normalised to protein content, and reported as U mg-1 protein, where 1 unit of SOD inhibits the rate of reduction of cytochrome c by 50% in a coupled xanthine and xanthine oxidase system.
Catalase (CAT) activity was estimated following Aebi (1984) and Li and Schellhorn (2007) using 0.01 M H2O2 as a substrate in a UV transparent microplate (Merck: CLS3635) at 240 nm. CAT activity was calculated using an extinction coefficient of 43.6 M-1 cm-1 and expressed as nmol min-1 mg-1 protein. Soluble protein content was determined at 595 nm following Bradford (1976) and used to normalise enzyme activity. All assays were completed on a BMG FLUOstar® Omega microplate reader (BMG Labtech, Australia) and corrected for path length.
2.7 Statistical analysis
Permutational multivariate analysis of variance (PERMANOVA, Anderson, 2017) was used to test for significant differences in SOD and CAT activity, growth, chlorophyll a, and symbiont concentration for each species using PRIMER + PERMANOVA v7 (PRIMER-e). A two-factor PERMANOVA model tested for significant differences within each sample event according to temperature (fixed, 2-levels: 26.4°C and 32.6°C) and shading (fixed, 3-levels: control (no shade), 4 h, and 24 h shade) and their interactions. Univariate PERMANOVA using the same model tested for significant differences in relative mean intensity of grey and maximum quantum yield within each sample event. Pairwise comparisons were run when the main factor or their interaction terms were significant. All analyses were based on a Euclidean distance dissimilarity matrix.
The potential effect of coral genotype was included in tests at each sample event, and no significant difference was found among genotypes within each treatment for any of the tests. Therefore, the coral genotype was not a significant factor in the bleaching responses detected.
3 Results
There was a significant temperature and shade effect, but no interaction for relative MIG in D. axifuga and T. reniformis on day 13 (Table 1). After 13 days, shading for either 4 or 24 h significantly reduced bleaching compared to the unshaded corals in each species (Figures 1A, D), and less bleaching occurred in the 24 h than the 4 h shaded corals. The colouration of D. axifuga shaded for 24 h improved compared to the initial measurement (Figure 1A). A significant temperature effect was found at 19 and 26 days for D. axifuga (Table 1). The higher temperature increased bleaching in D. axifuga compared to the lower temperature (Figures 1A–C). A significant shade × temperature interaction was detected for relative MIG for T. reniformis after 19 and 26 days (Table 1). A similar bleaching trend was found for each day. After 19 and 26 days, less bleaching occurred in T. reniformis at the lower compared to the higher temperature (Figures 1E, F). At the lower temperature, less bleaching occurred as shade duration increased in T. reniformis. At the higher temperature, T. reniformis bleaching was unaffected by shading. Bleaching of unshaded T. reniformis was similar at either temperature after 19 days (Figures 1E, F).
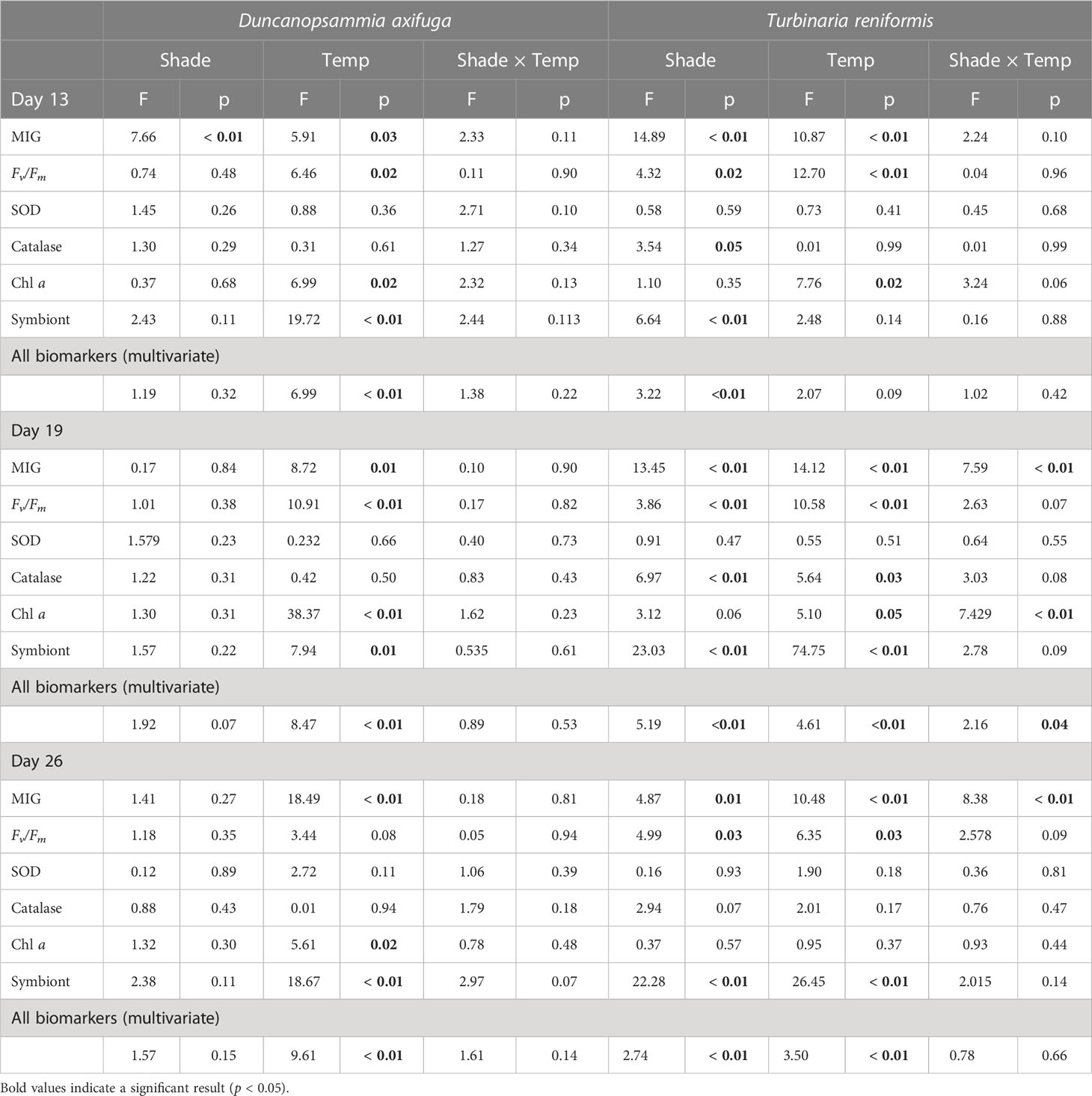
Table 1 PERMANOVA summaries using a 2-factor model (shading: 3 levels and temperature: 2 levels) for Duncanopsammia axifuga and Turbinaria reniformis exposed to either a higher (32.6°C) or lower (26.4°C) water temperature and unshaded or shaded for 4 or 24 h per day after 13 and 19 days and after a 7d recovery (Day 26).
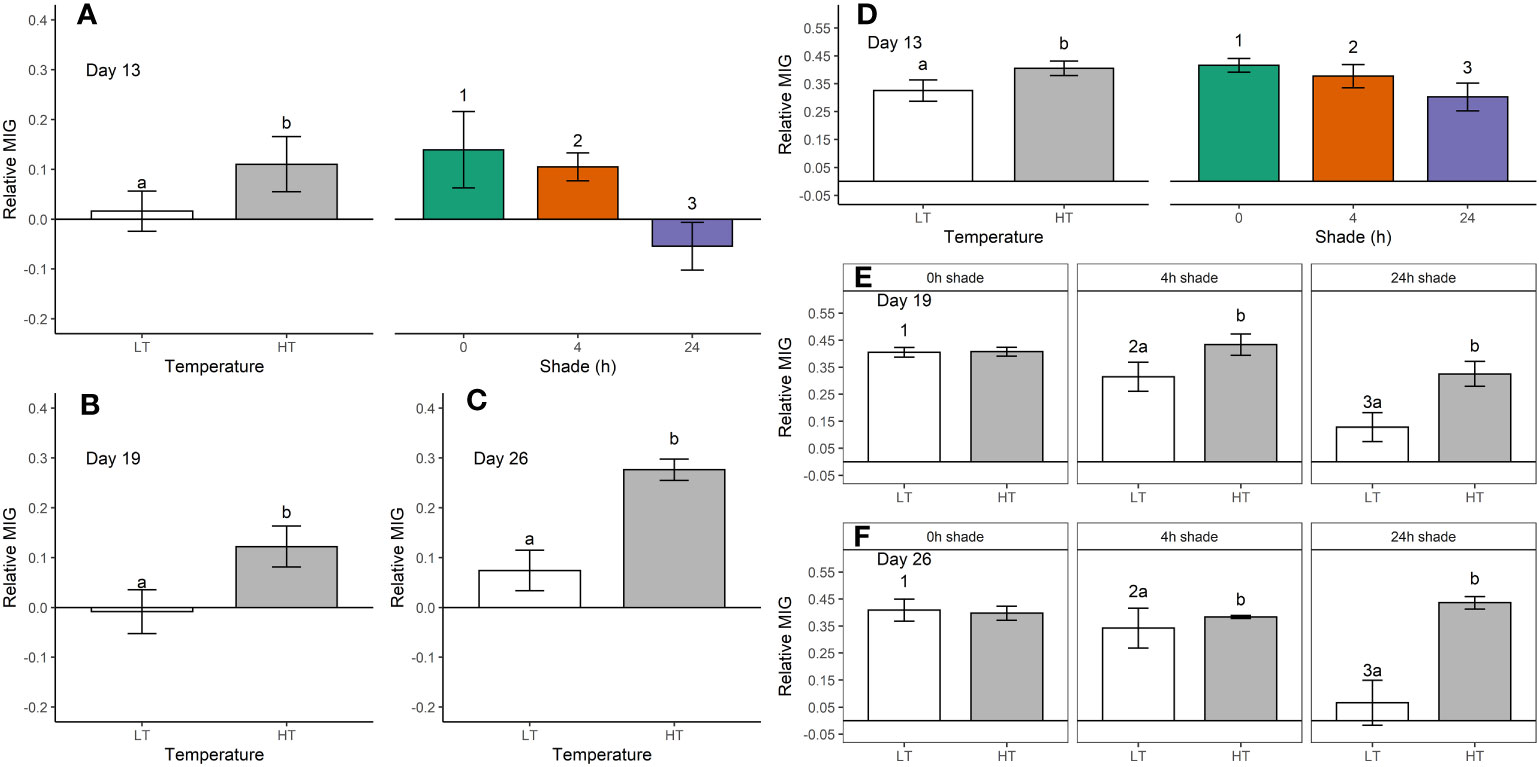
Figure 1 Relative mean intensity of grey (MIG, mean ± SE) in Duncanopsammia axifuga (A-C) and Turbinaria reniformis (D–F) shaded for 0, 4, or 24 h daily at a higher temperature (HT, 32.6°C) or a lower temperature (LT, 26.4°C) after day 13 (A, D), day 19 (B, E), and day 26 (C, F). Only the significant results are shown where letters indicate a significant difference between temperatures, and numbers indicate significant differences among shade treatments within each day.
Higher temperature significantly reduced the maximum quantum yield (Fv/Fm) of D. axifuga and T. reniformis at day 13 and day 19 compared to the lower temperature (Table 1; Figure 2). Fv/Fm of T. reniformis remained lower in the higher temperature at day 26 (Figure 2E). A significant shading effect was found for Fv/Fm in T. reniformis at days 13, 19, and 26 (Table 1). On day 13, Fv/Fm in T. reniformis was significantly higher with 24 h shade than with 4 h or no shade (Figure 2C). By day 19, the Fv/Fm of the 4 h shaded T. reniformis had recovered slightly to be similar to the 24 h shade treatment, while Fv/Fm of the unshaded coral was significantly lower than the 24 h shaded coral (Figure 2D). By day 26, Fv/Fm in the 24 h shaded T. reniformis was significantly higher than the 4 h shaded and unshaded coral (Figure 2E). The maximum quantum yield of D. axifuga was unaffected by shading.
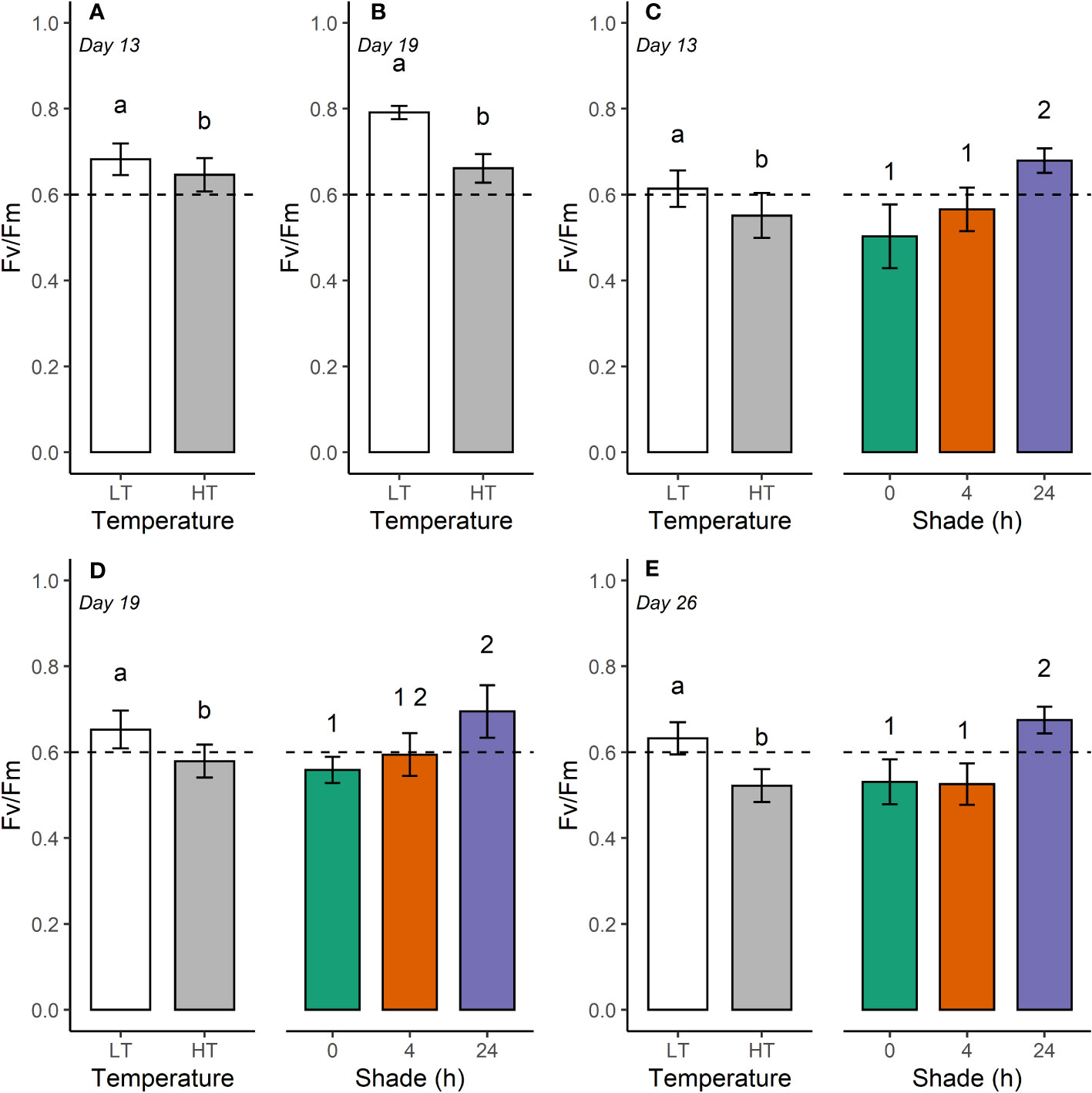
Figure 2 Maximum quantum yield (Fv/Fm, mean ± SE) for Duncanopsammia axifuga (A, B) and Turbinaria reniformis (C–E) held at 26.4°C (LT) and 32.6°C (HT) at day 13, 19, and 26. The dashed horizontal line indicates a lower threshold for Fv/Fm for healthy coral of 0.6. Letters indicate a significant difference between temperatures, and numbers denote a significant difference among shade treatments.
Chlorophyll a was significantly reduced by higher temperature in D. axifuga and T. reniformis on days 13 and 19, and in D. axifuga on day 26 (Table 1). A significant shade × temperature interaction was detected for chlorophyll a in T. reniformis on day 19 (Figure 3A). Higher temperature significantly reduced chlorophyll a in unshaded T. reniformis, and shading reduced chlorophyll a in the 24 h shade treatment compared to the 4 h shaded and unshaded coral (Figure 3A). Higher temperature significantly reduced symbiont density for D. axifuga on days 13, 19, and 26, and for T. reniformis on days 19 and 26 (Table 1). A significant shading effect was found for symbiont density in T. reniformis on days 13, 19, and 26 (Table 1). Symbiont density was significantly higher in the 24 h shaded T. reniformis than the 4 h or unshaded coral (Figure 3B). On day 19, symbiont density was significantly greater in the shaded compared to the unshaded T. reniformis. By day 26, symbiont density increased significantly as the shade duration increased (Figure 3B). Shading or temperature did not affect symbiont density in D. axifuga (Table 1).
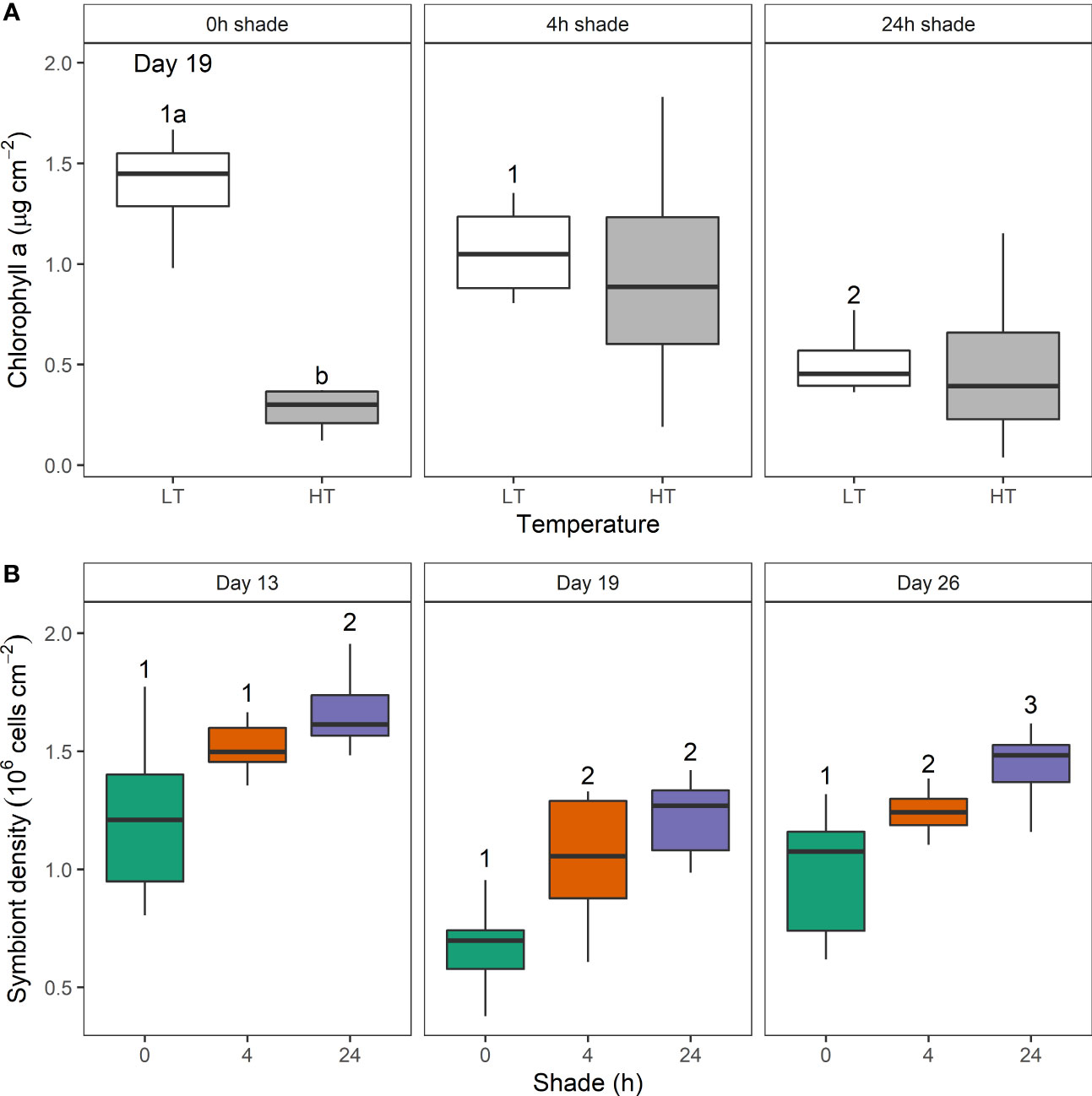
Figure 3 The effect of shade duration (0, 4, or 24 h) and higher (HT) and lower temperature (LT) on (A) chlorophyll a concentration (µg cm-2) from Turbinaria reniformis on day 19 and (B) symbiont density (106 cells cm-2) on day 13, 19, and 26. Box plots show the percentiles (25, 50, and 75%) and range. Letters show a significant temperature difference within shade treatment, and numbers indicate a significant difference among shade treatments each day.
Catalase activity was significantly greater in the unshaded than the shaded T. reniformis at days 13 and 19 (Table 1; Figure 4). Higher temperature increased catalase activity in T. reniformis on day 19 (Table 1). Neither shade nor temperature affected catalase in D. axifuga on days 13, 19, or 26. Superoxide dismutase activity was not significantly affected by temperature or shading in D. axifuga or T. reniformis (Table 1).
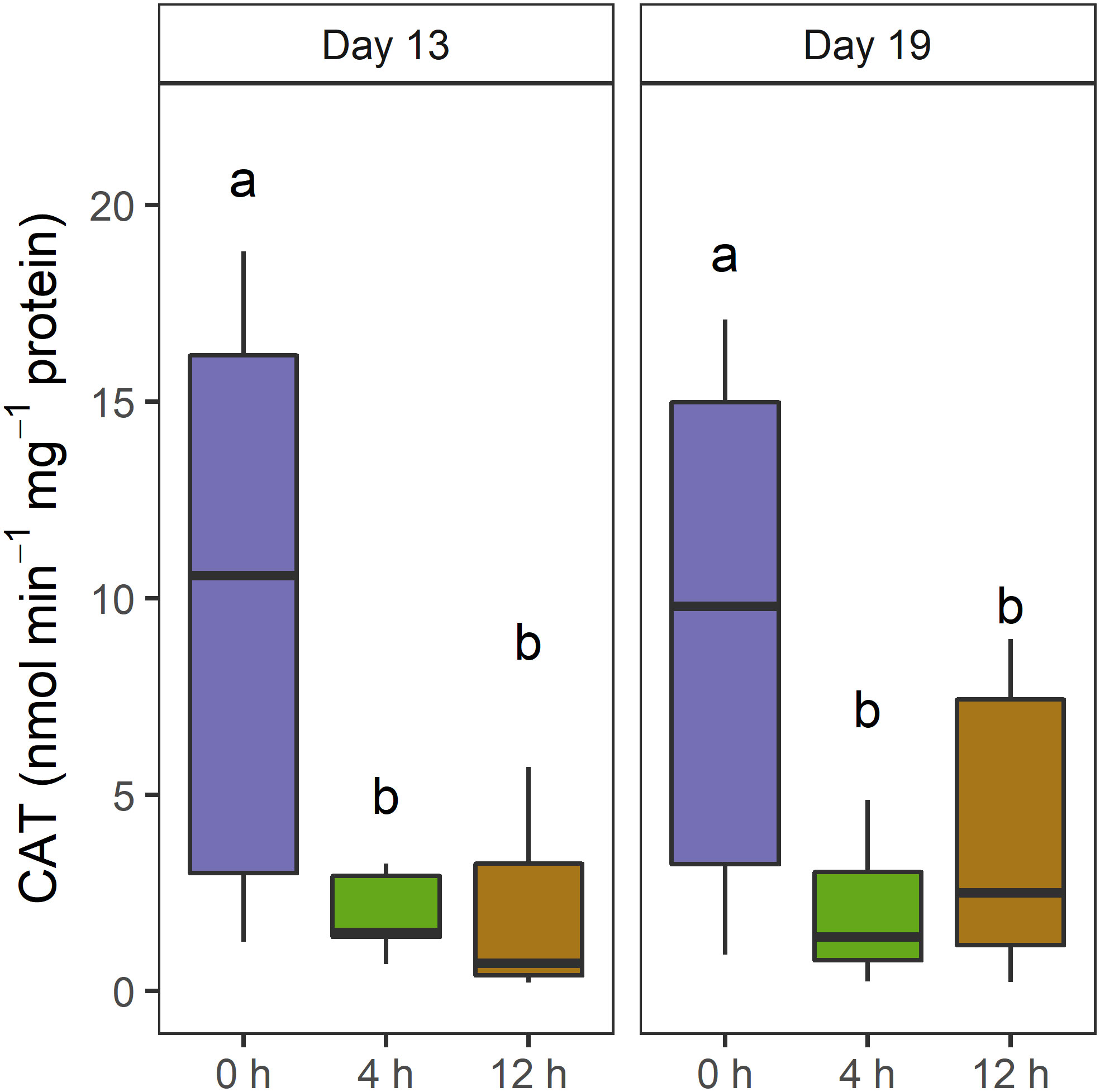
Figure 4 Catalase activity (nmol min-1 mg-1 protein) in Turbinaria reniformis tissue at day 13 and 19 when shaded for 0, 4, and 24 h. The letters indicate significant differences within each day.
4 Discussion
Intermittently shading corals by 30% for 4 h per day can delay bleaching in a thermally stressed shallow coral species, T. reniformis. Conversely, D. axifuga responded to 24 h shading until 3 DHW, but was unresponsive to shading as the degree heat weeks increased. Thermal stress had the most significant effect on bleaching in these two species, and as the thermal stress increased, the beneficial effect of intermittent shading declined. The novel shading regime, of 30% shading for 4 h around solar noon, slowed bleaching, and the decline of chlorophyll a and symbiont density and reduced antioxidant activity. Intermittent shading has the potential to moderate the projected impacts of warming oceans on at-risk corals during bleaching events.
Thermal stress had the greatest effect on bleaching of D. axifuga and T. reniformis. Elevated water temperature is the primary stressor of many bleaching events (Coles et al., 1976; Berkelmans, 2002; Ainsworth et al., 2016; Sully et al., 2019), where heat stress leads to a breakdown of biochemical and physiological pathways (Fitt et al., 2001). Bleaching reduces coral colouration either by ejection of symbionts, shuffling or loss of chlorophyll or symbionts or both (Brown and Dunne, 2015). Turbinaria reniformis and D. axifuga showed a decrease in chlorophyll a and symbiont density at higher temperatures, resulting in an elevated relative MIG and decreased maximum quantum yield. Coral responses to thermal stress are well known; subsequently, the response to the novel shading regime will be the focus of the remainder of this discussion.
Shading reduced relative MIG and catalase activity, moderating the bleaching of T. reniformis, with relative MIG lower when shading corals continuously. Reduced antioxidant activity suggests reactive oxygen species production decreased, limiting a key mechanism of the bleaching response in thermal and/or light-stressed corals (Downs et al., 2002; Higuchi et al., 2008; Krueger et al., 2015; Wietheger et al., 2018). Continual shading of thermally stressed Acropora muricata, Pocillopora damicormis, and Porites cylindrica by either 50 or 75% was very effective at reducing bleaching until 4 DHW and offered some protection up to 8 DHW, and the heavier shading was more effective (Coelho et al., 2017). Here, 30% shade for 4 h reduced bleaching of T. reniformis up to 3 DHW, after which the protection decreased up to 5 DHW. Although the protection offered by the 30% shading was less than that shown by Coelho et al. (2017) using higher levels of shade. This is a particularly relevant result as there is a 40% probability of severe mass bleaching on the Great Barrier Reef bleaching at 3 DHW (Hughes et al., 2017), and 30% shade may be more achievable over reef scale areas. However, further work is needed to establish the shading level required for other coral species and the physiological and biological responses to intermittent shading.
Shading delayed the decline of maximum quantum yield indicating reduced physiological stress. The decline in PSII efficiency during bleaching has been well described (Jones et al., 1998; Hill et al., 2012; Roth, 2014) and is likely caused by damage to the D1 protein (Warner et al., 1999). Declining PSII efficiency can lead to the accumulation of reactive oxygen species, damaging cellular membranes and triggering bleaching (Downs et al., 2002). Furthermore, loss of PSII efficiency can significantly impact coral nutritional status where symbiote-derived products can account for up to 30% of total nitrogen (Bythell, 1988) and 95% of carbon for growth, reproduction, and maintenance (Muscatine and Porter, 1977; West and Salm, 2003). Slowing the decline in maximum quantum yield by shading corals, can reduce reactive oxygen species production and maintain coral energy reserves for recovery when stressors decrease. Energy reserves are particularly important in oligotrophic waters where coral reefs abound.
Interspecific differences existed in the bleaching response between D. axifuga and T. reniformis. T. reniformis responded to shading, whereas D. axifuga was less responsive to shading. The interaction of light with temperature is subtle and is more pronounced at the thermal limits of a coral (Coles and Jokiel, 1978). Pratchett et al. (2020) showed that a significant bleaching response in D. axifuga in warming waters was exacerbated by high light intensity. The limited response of D. axifuga to shading in the present study suggests that the coral may not be at its thermal limit, although it is unclear if it is above or below the thermal limit. Furthermore, bleaching susceptibility can vary due to nutritional status (Hoogenboom et al., 2012; Beraud et al., 2013), morphological (Brown and Dunne, 2015), physiological (Dias et al., 2019), or symbiont variation (Hoadley et al., 2016), and acclimation to differing thermal or light conditions (Fitt et al., 2001). Regardless of the cause, a range of responses to shading can be expected and understanding the interactions between environmental conditions and the long-term health of corals is critical for managing potential shading interventions (Bay et al., 2019). Further studies are needed to assess coral responses to short-term localised shading and should include a multifactorial approach with a range of environmental variables, such as ocean acidification and nutrient loading.
Intermittent shading could reduce the costs associated with active marine ecosystem management. Shading corals is an unconventional local strategy to minimise the impacts of temperature and carbon dioxide as global stressors of marine ecosystems (Rau et al., 2012; Tagliafico et al., 2022). The success of many of these shading interventions depends on water and atmospheric conditions (Bay et al., 2019). For example, in-water interventions such as shade cloth deployment (Coelho et al., 2017) depend on wave conditions and currents, while atmospheric conditions affect interventions such as smoke generation (Russell et al., 2013) or marine cloud brightening or fogging (Harrison et al., 2019). Interventions could be targeted in response to bleaching forecasts or, more broadly, over the high-risk summer months (Berkelmans, 2002; Coelho et al., 2017) as weather conditions permit. Targeted intermittent deployment of energy-intensive interventions, such as marine fogging or cloud brightening, shorten operating time, reduce power consumption, and could lower operational and maintenance expenses. The intermittent deployment of in-water shading, such as shade cloths, reduces the risks of invasive species, algal overgrowth, and the potential to shelter more sensitive coral species or symbionts less adapted to the prevailing conditions that may be impacted when shading is removed (Donner, 2009, Hughes 2018a). However, further research is necessary to investigate the indirect effects of these novel shading techniques. The bleaching protection shown here indicates that 30% shading for 4 h is sufficient to delay bleaching and improve the viability of active interventions that depend on variable climatic conditions.
Temperature is the predominant stressor during bleaching events; however, solar radiation management can delay bleaching responses up to 3 DHW, beyond which the protective benefits of intermittent shading diminish. Intermittent shading delayed the decline in relative bleaching and maximum quantum yield, symbiont density, and chlorophyll a concentration. Shading of T. reniformis reduced catalase activity, indicating decreased reactive oxygen species production, a key driver of coral bleaching. Shading responses were species-specific, with T. reniformis more responsive to shading than D. axifuga, possibly due to thermal stress susceptibility. Overall, practical management interventions at appropriate scales (e.g. reef scale marine fogging) could be targeted to periods of maximum coral vulnerability and suitable environmental conditions for deployment, lowering costs and reducing the risk of invasive species while protecting marine ecosystems.
Data availability statement
The raw data supporting the conclusions of this article will be made available by the authors, without undue reservation.
Ethics statement
Ethical approval was not required for the study involving animals in accordance with the local legislation and institutional requirements because it is not required for research involving corals.
Author contributions
PB: Data curation, Formal analysis, Investigation, Visualisation, Writing – original draft. AT: Conceptualisation, Investigation, Methodology, Writing – original draft. SE: Investigation, Writing – review and editing. BK: Methodology, Writing – review and editing. CH: Investigation, Writing – review and editing. DH: Conceptualisation, Methodology, Supervision, Funding acquisition, Writing – review and editing. All authors contributed to the article and approved the submitted version.
Funding
This work was undertaken for the Reef Restoration and Adaptation Program (Cooling and shading sub-program), funded by the partnership between the Australian Government’s Reef Trust and the Great Barrier Reef Foundation.
Acknowledgments
We extend our deepest respect and recognition to all Traditional Owners of the Gumbaynggirr country where this study was conducted and to all Traditional Owners of the Great Barrier Reef and its Catchments as First Nations Peoples holding the hopes, dreams, traditions and cultures of the Reef.
Conflict of interest
The authors declare that the research was conducted in the absence of any commercial or financial relationships that could be construed as a potential conflict of interest.
Publisher’s note
All claims expressed in this article are solely those of the authors and do not necessarily represent those of their affiliated organizations, or those of the publisher, the editors and the reviewers. Any product that may be evaluated in this article, or claim that may be made by its manufacturer, is not guaranteed or endorsed by the publisher.
Supplementary material
The Supplementary Material for this article can be found online at: https://www.frontiersin.org/articles/10.3389/fmars.2023.1162896/full#supplementary-material
References
Aebi H. (1984). Catalase in vitro. Methods Enzymol. 105, 121–126. doi: 10.1016/s0076-6879(84)05016-3
Ainsworth T. D., Heron S. F., Ortiz J. C., Mumby P. J., Grech A., Ogawa D., et al. (2016). Climate change disables coral bleaching protection on the Great Barrier Reef. Science 352 (6283), 338–342. doi: 10.1126/science.aac7125
Amid C., Olstedt M., Gunnarsson J. S., Le Lan H., Tran Thi Minh H., Van den Brink P. J., et al. (2018). Additive effects of the herbicide glyphosate and elevated temperature on the branched coral Acropora formosa in Nha Trang, Vietnam. Environ. Sci. pollut. Res. Int. 25 (14), 13360–13372. doi: 10.1007/s11356-016-8320-7
Anderson M. J. (2017). “Permutational multivariate analysis of variance (PERMANOVA),” in Wiley statsRef: statistics reference online (eds. Balakrishnan N., Colton T., Everitt B., Piegorsch W., Ruggeri F., Teugels J. L.), 1–15. doi: 10.1002/9781118445112.stat07841
Baird A. H., Bhagooli R., Ralph P. J., Takahashi S. (2009). Coral bleaching: the role of the host. Trends Ecol. Evol. 24 (1), 16–20. doi: 10.1016/j.tree.2008.09.005
Baker P., Alroe J., Tagliafico A., Ellis S., Harrison L., Hernandez D., et al. (2021). Fogging feasibility stage 1 report, In A Report Provided to the Australian Government by the Reef Restoration and Adaptation Program (Townsville: Reef Restoration and Adaptation Program).
Baker A. C., Glynn P. W., Riegl B. (2008). Climate change and coral reef bleaching: An ecological assessment of long-term impacts, recovery trends and future outlook. Estuar. Coast. Shelf Sci. 80 (4), 435–471. doi: 10.1016/j.ecss.2008.09.003
Bay L. K., Rocker M., Bostrom-Einarsson L., Babcock R. C., Buerger P., Cleves P., et al. (2019). Reef restoration and adaptation program: intervention technical summary. A report provided to the Australian Government by the Reef Restoration and Adaptation Program. (89pp).
Bell P. R., Elmetri I., Lapointe B. E. (2014). Evidence of large-scale chronic eutrophication in the Great Barrier Reef: Quantification of chlorophyll a thresholds for sustaining coral reef communities. AMBIO 43 (3), 361–376. doi: 10.1007/s13280-013-0443-1
Beraud E., Gevaert F., Rottier C., Ferrier-Pages C. (2013). The response of the scleractinian coral Turbinaria reniformis to thermal stress depends on the nitrogen status of the coral holobiont. J. Exp. Biol. 216 (Pt 14), 2665–2674. doi: 10.1242/jeb.085183
Berkelmans R. (2002). Time-integrated thermal bleaching thresholds of reefs and their variation on the Great Barrier Reef. Mar. Ecol. Prog. Ser. 229, 73–82. doi: 10.3354/meps229073
Bradford M. M. (1976). A rapid and sensitive method for the quantitiation of microgram quantities of protein utilizing the principle of protein-dye binding. Anal. Biochem. 72), 248–254. doi: 10.1016/0003-2697(76)90527-3
Brown B. E. (1997). Coral bleaching: Causes and consequences. Coral Reefs 16 (0), S129–S138. doi: 10.1007/s003380050249
Brown B. E., Dunne R. P. (2015). Coral Bleaching: The roles of sea temperature and solar radiation. Dis. Coral. 18, 266–283. doi: 10.1002/9781118828502.ch18
Bythell J. C. (1988). “A total nitrogen and carbon budget for the elkhorn coral Acropora palmata (Lamark),” in Proceedings of the 6th International Coral Reef Symposium, Townsville, Australia.
Coelho V., Fenner D., Caruso C., Bayles B. R., Huang Y., Birkeland C. (2017). Shading as a mitigation tool for coral bleaching in three common Indo-Pacific species. J. Exp. Mar. Biol. Ecol. 497, 152–163. doi: 10.1016/j.jembe.2017.09.016
Coles S. L., Jokiel P. L. (1978). Synergistic effects of temperature, salinity and light on the hermatypic coral Montipora verrucosa. Mar. Biol. 49 (3), 187–195. doi: 10.1007/BF00391130
Coles S. L., Jokiel P. L., Lewis C. R. (1976). Thermal tolerance in tropical versus subtropical Pacific reef corals. Pacific Science. 30 (2), 159–166.
Condie S. A., Anthony K. R. N., Babcock R. C., Baird M. E., Beeden R., Fletcher C. S., et al. (2021). Large-scale interventions may delay decline of the Great Barrier Reef. R. Soc. Open Sci. 8 (4), 201296. doi: 10.1098/rsos.201296
Cooley S., Schoeman D., Bopp L., Boyd P., Donner S., Ghebrehiwet D. Y., et al. (2022). “Oceans and coastal ecosystems and their services,” in Climate change 2022: impacts, adaptation and vulnerability. Eds. Pörtner H.-O., Roberts D. C., Tignor M., Poloczanska E. S., Mintenbeck K., Alegría A., Craig M., Langsdorf S., Löschke S., Möller V., Okem A., Rama andB. (UK and New York: Cambridge University Press) pp. 379–550. doi: 10.1017/9781009325844.005
Courtial L., Roberty S., Shick J. M., Houlbrèque F., Ferrier-Pagès C. (2017). Interactive effects of ultraviolet radiation and thermal stress on two reef-building corals. Limnol. Oceanog. 62 (3), 1000–1013. doi: 10.1002/lno.10481
Cropp R., Gabric A., van Tran D., Jones G., Swan H., Butler H. (2018). Coral reef aerosol emissions in response to irradiance stress in the Great Barrier Reef, Australia. AMBIO 47 (6), 671–681. doi: 10.1007/s13280-018-1018-y
De'ath G., Fabricius K. E., Sweatman H., Puotinen M. (2012). The 27-year decline of coral cover on the Great Barrier Reef and its causes. Proc. Natl. Acad. Sci. U.S.A. 109 (44), 17995–17999. doi: 10.1073/pnas.1208909109
Dias M., Ferreira A., Gouveia R., Madeira C., Jogee N., Cabral H., et al. (2019). Long-term exposure to increasing temperatures on scleractinian coral fragments reveals oxidative stress. Mar. Environ. Res. 150, 104758. doi: 10.1016/j.marenvres.2019.104758
Dobson K. L., Ferrier-Pagès C., Saup C. M., Grottoli A. G. (2021). The effects of temperature, light, and feeding on the physiology of Pocillopora damicornis, Stylophora pistillata, and Turbinaria reniformis corals. Water 13 (15), 1–20. doi: 10.3390/w13152048
Donner S. D. (2009). Coping with commitment: Projected thermal stress on coral reefs under different future scenarios. PloS One 4 (6), e5712. doi: 10.1371/journal.pone.0005712
Downs C. A., Fauth J. E., Halas J. C., Dustan P., Bemiss J., Woodley C. M. (2002). Oxidative stress and seasonal coral bleaching. Free Radical Biol. Med. 33 (4), 533–543. doi: 10.1016/S0891-5849(02)00907-3
Fitt W. K., Brown B. E., Warner M. E., Dunne R. P. (2001). Coral bleaching: Interpretation of thermal tolerance limits and thermal thresholds in tropical corals. Coral Reefs 20 (1), 51–65. doi: 10.1007/s003380100146
Grottoli A. G., Warner M. E., Levas S. J., Aschaffenburg M. D., Schoepf V., McGinley M., et al. (2014). The cumulative impact of annual coral bleaching can turn some coral species winners into losers. Glob Chang Biol. 20 (12), 3823–3833. doi: 10.1111/gcb.12658
Guzman H. M. (1991). Restoration of coral reefs in Pacific Costa Rica. Conserv. Biol. 5 (2), 189–194. doi: 10.1111/j.1523-1739.1991.tb00123.x
Harrison D. P, Baird M., Harrison L., Utembe S., Schofield R., Escobar Correa R., et al. (2019). Reef restoration and adaptation program: Environmental modelling of large scale solar radiation management. A report provided to the Australian Government by the Reef Restoration and Adaptation Program. (83pp).
Higuchi T., Fujimura H., Arakaki T., Oomori T. (2008). “Activities of antioxidant enzymes (SOD and CAT) in the coral Galaxea fascicularis against increased hydrogen peroxide concentrations in seawater,” in 11th International Coral Reef Symposium, Ft. Lauderdale, Florida.
Hill R., Larkum A. W. D., Prášil O., Kramer D. M., Szabó M., Kumar V., et al. (2012). Light-induced dissociation of antenna complexes in the symbionts of scleractinian corals correlates with sensitivity to coral bleaching. Coral Reefs 31 (4), 963–975. doi: 10.1007/s00338-012-0914-z
Hoadley K. D., Pettay D. T., Grottoli A. G., Cai W.-J., Melman T. F., Levas S., et al. (2016). High-temperature acclimation strategies within the thermally tolerant endosymbiont Symbiodinium trenchii and its coral host, Turbinaria reniformis, differ with changing pCO2 and nutrients. Mar. Biol. 163 (6), 1–13. doi: 10.1007/s00227-016-2909-8
Hoogenboom M. O., Campbell D. A., Beraud E., Dezeeuw K., Ferrier-Pages C. (2012). Effects of light, food availability and temperature stress on the function of photosystem II and photosystem I of coral symbionts. PloS One 7 (1), e30167. doi: 10.1371/journal.pone.0030167
Hughes T. P., Anderson K. D., Connolly S. R., Heron S. F., Kerry J. T., Lough J. M., et al. (2018a). Spatial and temporal patterns of mass bleaching of corals in the Anthropocene. Science 359 (6371), 80–83. doi: 10.1126/science.aan8048
Hughes T. P., Baird A. H., Bellwood D. R., Card M., Connolly S. R., Folke C., et al. (2003). Climate change, human impacts, and the resilience of coral reefs. Science 301 (5635), 929–933. doi: 10.1126/science.1085046
Hughes T. P., Kerry J. T., Alvarez-Noriega M., Alvarez-Romero J. G., Anderson K. D., Baird A. H., et al. (2017). Global warming and recurrent mass bleaching of corals. Nature 543 (7645), 373–377. doi: 10.1038/nature21707
Hughes T. P., Kerry J. T., Baird A. H., Connolly S. R., Dietzel A., Eakin C. M., et al. (2018b). Global warming transforms coral reef assemblages. Nature 556 (7702), 492–496. doi: 10.1038/s41586-018-0041-2
Jackson R., Gabric A., Cropp R. (2018). Effects of ocean warming and coral bleaching on aerosol emissions in the Great Barrier Reef, Australia. Sci. Rep. 8 (1), 14048. doi: 10.1038/s41598-018-32470-7
Jackson R. L., Woodhouse M. T., Gabric A. J., Cropp R. A. (2022). CMIP6 projections of ocean warming and the impact on dimethylsulfide emissions from the Great Barrier Reef, Australia. Front. Mar. Sci. 9. doi: 10.3389/fmars.2022.910420
Jones R., Giofre N., Luter H. M., Neoh T. L., Fisher R., Duckworth A. (2020). Responses of corals to chronic turbidity. Sci. Rep. 10 (1), 4762. doi: 10.1038/s41598-020-61712-w
Jones R. J., Hoegh-Guldberg O., Larkum A. W. D., Schreiber U. (1998). Temperature-induced bleaching of corals begins with impairment of the CO2 fixation mechanism in zooxanthellae. Plant Cell Environ. 21 (12), 1219–1230. doi: 10.1046/j.1365-3040.1998.00345.x
Kramer J., Ripkey N., Segard L., Troisi M., Kramer P. R., Coraspe P., et al. (2016). Turn down the heat: An innovative citizen science pilot project to reduce impacts of coral bleaching. Proc. Gulf Caribb. Fish. Inst. 69, 231–242.
Krueger T., Hawkins T. D., Becker S., Pontasch S., Dove S., Hoegh-Guldberg O., et al. (2015). Differential coral bleaching - Contrasting the activity and response of enzymatic antioxidants in symbiotic partners under thermal stress. Comp. Biochem. Physiol. A Mol. Integr. Physiol. 190, 15–25. doi: 10.1016/j.cbpa.2015.08.012
Lesser M. P., Farrell J. H. (2004). Exposure to solar radiation increases damage to both host tissues and algal symbionts of corals during thermal stress. Coral Reefs 23 (3), 367–377. doi: 10.1007/s00338-004-0392-z
Li Y., Schellhorn H. E. (2007). Rapid kinetic microassay for catalase activity. J. biomol. techniques 18 (4), 185–187.
Marsh J. A. (1970). Primary productivity of reef-building calcareous red algae. Ecology 51 (2), 255–263. doi: 10.2307/1933661
Mclachlan R., Grottoli A. G. (2021). Image analysis to quantify coral bleaching using greyscale model. doi: 10.17504/protocols.io.bx8wprxe
Muller E. M., van Woesik R. (2009). Shading reduces coral-disease progression. Coral Reefs 28 (3), 757–760. doi: 10.1007/s00338-009-0504-x
Muller E. M., van Woesik R. (2011). Black-band disease dynamics: Prevalence, incidence, and acclimatization to light. J. Exp. Mar. Biol. Ecol. 397 (1), 52–57. doi: 10.1016/j.jembe.2010.11.002
Muscatine L., Porter J. W. (1977). Reef corals: Mutualistic symbioses adapted to nutrient-poor environments. BioScience 27 (7), 454–460. doi: 10.2307/1297526
Pratchett M. S., Caballes C. F., Newman S. J., Wilson S. K., Messmer V., Pratchett D. J. (2020). Bleaching susceptibility of aquarium corals collected across northern Australia. Coral Reefs 39 (3), 663–673. doi: 10.1007/s00338-020-01939-1
Rangel M. S., Erler D., Tagliafico A., Cowden K., Scheffers S., Christidis L. (2019). Quantifying the transfer of prey δ15N signatures into coral holobiont nitrogen pools. Mar. Ecol. Prog. Ser. 610, 33–49. doi: 10.3354/meps12847
Rau G. H., McLeod E. L., Hoegh-Guldberg O. (2012). The need for new ocean conservation strategies in a high-carbon dioxide world. Nat. Climate Change 2 (10), 720–724. doi: 10.1038/nclimate1555
Ritchie R. J. (2006). Consistent sets of spectrophotometric chlorophyll equations for acetone, methanol and ethanol solvents. Photosynthesis Res. 89 (1), 27–41. doi: 10.1007/s11120-006-9065-9
Roth M. S. (2014). The engine of the reef: photobiology of the coral-algal symbiosis. Front. Microbiol. 5. doi: 10.3389/fmicb.2014.00422
Russell L. M., Sorooshian A., Seinfeld J. H., Albrecht B. A., Nenes A., Ahlm L., et al. (2013). Eastern Pacific emitted aerosol cloud experiment. Bull. Am. Meteorol. Soc. 94 (5), 709–729. doi: 10.1175/BAMS-D-12-00015.1
Shick J. M., Lesser M. P., Jokiel P. L. (1996). Effects of ultraviolet radiation on corals and other coral reef organisms. Global Change Biol. 2 (6), 527–545. doi: 10.1111/j.1365-2486.1996.tb00065.x
Simpson M. C., Gossling S., Scott D., Hall C. M., Gladin E. (2008). “Climate change adaptation and mitigation in the tourism sector: Frameworks, tools and practices,” in Climate change adaptation and mitigation in the tourism sector: Frameworks, tools and practices (CABI) [(Paris, France: United Nations Environmental Programme (UNEP)].
Smith D. J., Suggett D. J., Baker N. R. (2005). Is photoinhibition of zooxanthellae photosynthesis the primary cause of thermal bleaching in corals? Global Change Biol. 11 (1), 1–11. doi: 10.1111/j.1529-8817.2003.00895.x
Steven A. D. L., Baird M. E., Brinkman R., Car N. J., Cox S. J., Herzfeld M., et al. (2019). eReefs: An operational information system for managing the Great Barrier Reef. J. Operat. Oceanog. 12 (sup2), S12–S28. doi: 10.1080/1755876x.2019.1650589
Suggett D. J., Warner M. E., Smith D. J., Davey P., Hennige S., Baker N. R. (2008). Photosynthesis and production of hydrogen peroxide by Symbiodinium (Pyrrhophyta) phylotypes with different thermal tolerances. J. Phycol. 44 (4), 948–956. doi: 10.1111/j.1529-8817.2008.00537.x
Sully S., Burkepile D. E., Donovan M. K., Hodgson G., van Woesik R. (2019). A global analysis of coral bleaching over the past two decades. Nat. Commun. 10 (1), 1264. doi: 10.1038/s41467-019-09238-2
Tagliafico A., Baker P., Kelaher B., Ellis S., Harrison D. (2022). The effects of shade and light on corals in the context of coral bleaching and shading technologies. Front. Mar. Sci. 9. doi: 10.3389/fmars.2022.919382
Tagliafico A., Rangel S., Kelaher B., Christidis L. (2018). Optimizing heterotrophic feeding rates of three commercially important scleractinian corals. Aquaculture 483, 96–101. doi: 10.1016/j.aquaculture.2017.10.013
Tagliafico A., Rudd D., Rangel M. S., Kelaher B. P., Christidis L., Cowden K., et al. (2017). Lipid-enriched diets reduce the impacts of thermal stress in corals. Mar. Ecol. Prog. Ser. 573, 129–141. doi: 10.3354/meps12177
Thinesh T., Meenatchi R., Pasiyappazham R., Jose P. A., Selvan M., KIran G. S., et al. (2017). Short-term in situ shading effectively mitigates linear progression of coral-killing sponge Terpios hoshinota. PloS One 12 (8), e0182365. doi: 10.1371/journal.pone.0182365
Tollefson J. (2021). Can clouds save the Great Barrier Reef? Nature 596, 476–478. doi: 10.1038/d41586-021-02290-3
Veal C. J., Carmi M., Fine M., Hoegh-Guldberg O. (2010). Increasing the accuracy of surface area estimation using single wax dipping of coral fragments. Coral Reefs 29 (4), 893–897. doi: 10.1007/s00338-010-0647-9
Warner M. E., Fitt W. K., Schmidt G. W. (1999). Damage to photosystem II in symbiotic dinoflagellates: A determinant of coral bleaching. Proc. Natl. Acad. Sci. 96 (14), 8007–8012. doi: 10.1073/pnas.96.14.8007
Warren C. R. (2008). Rapid measurement of chlorophylls with a microplate reader. J. Plant Nutr. 31 (7), 1321–1332. doi: 10.1080/01904160802135092
West J. M., Salm R. V. (2003). Resistance and resilience to coral bleaching: Implications for coral reef conservation and management. Conserv. Biol. 17 (4), 956–967. doi: 10.1046/j.1523-1739.2003.02055.x
Wethey D. S., Porter J. W. (1976). Sun and shade differences in productivity of reef corals. Nature 262 (5566), 281–282. doi: 10.1038/262281a0
Weydert C. J., Cullen J. J. (2010). Measurement of superoxide dismutase, catalase and glutathione peroxidase in cultured cells and tissue. Nat. Protoc. 5 (1), 51–66. doi: 10.1038/nprot.2009.197
Wietheger A., Starzak D. E., Gould K. S., Davy S. K. (2018). Differential ROS Generation in response to stress in Symbiodinium spp. Biol. Bull. 234 (1), 11–21. doi: 10.1086/696977
Keywords: irradiance, bleaching, photochemistry, climate change, oxidative stress, solar-radiation management, shading
Citation: Butcherine P, Tagliafico A, Ellis SL, Kelaher BP, Hendrickson C and Harrison D (2023) Intermittent shading can moderate coral bleaching on shallow reefs. Front. Mar. Sci. 10:1162896. doi: 10.3389/fmars.2023.1162896
Received: 10 February 2023; Accepted: 07 August 2023;
Published: 20 September 2023.
Edited by:
Charles Alan Jacoby, St. Johns River Water Management District, United StatesReviewed by:
Albert Gabric, Griffith University, AustraliaKeisha D. Bahr, Texas AandM University Corpus Christi, United States
Copyright © 2023 Butcherine, Tagliafico, Ellis, Kelaher, Hendrickson and Harrison. This is an open-access article distributed under the terms of the Creative Commons Attribution License (CC BY). The use, distribution or reproduction in other forums is permitted, provided the original author(s) and the copyright owner(s) are credited and that the original publication in this journal is cited, in accordance with accepted academic practice. No use, distribution or reproduction is permitted which does not comply with these terms.
*Correspondence: Peter Butcherine, cGV0ZXIuYnV0Y2hlcmluZUBzY3UuZWR1LmF1