- School of Marine Science, Ningbo University, Ningbo, China
Phosphorus (P) accumulation in aquaculture systems is damaging our environment beyond acceptable levels. Devising strategies to potentially recover P from aquaculture systems in a reusable bioresource form is paramount and aligns with circular economy policies. In this study, we constructed two culture models, monoculture (Mon) and tandem culture (Tan), using Exopalaemon carinicauda and Mercenaria mercenaria. By monitoring the performance of rearing organisms, P dynamic patterns, and pollutant emissions, we found that: i) Compared to the Mon system, the Tan system demonstrated no differences in the performance of E. carinicauda and M. mercenaria, suggesting that the Tan model was viable in terms of fishery yield; ii) P in the Tan system could be efficiently recovered and removed from water and sediment, as indicated by the lower phosphate concentration in water (0.01 mg L−1), and the decrease in labile P in surface sediment (from 0.04 to 0.02 mg L−1). A combination of assimilatory and dissimilatory processes, mediated by phototrophic (bait-microalgae) and heterotrophic organisms (bivalves), appeared to be the primary mechanism for P utilization and removal; iii) The Tan system reduced pollutant emissions four times lower than the Mon system due to its minimal tailwater discharge (10%, 230 L). The emissions of total P, phosphate, total organic carbon, ammonium, and chemical oxygen demand from the Tan systems were 19 mg m−2 d−1, 2 mg m−2 d−1, 2 g m−2 d−1, 38 mg m−2 d−1, and 11 g m−2 d−1, respectively, 1.3, 1.7, 1.4, 1.3, and 1.2 times lower than those from the Mon systems. The eco-friendly Tan culture model fully exploited the resources of pond culture, a solution with environmental and health co-benefits for P recovery and emission reduction.
1 Introduction
The increasing global demand for aquatic animal proteins has led to a rapid expansion of aquaculture, with production growing from 59.0 million tons in 2010 to 122.6 million tons in 2020 (FAO, 2022). Although beneficial from a production point of view, this expansion is inevitably coupled with a myriad of negative environmental implications, especially concerning nitrogen (N) and phosphorus (P) emissions. Due to low assimilation efficiency, only 10%–33% of the P added as fertilizer, feed, and food additives was assimilated by organisms in mariculture (Bouwman et al., 2013). The remaining portion was accumulated in sediments or discharged to neighboring areas, accelerating water degradation and restricting the sustainable development of aquaculture (Hicks et al., 2019). Therefore, reconciling the exploitation of fishery resources with water protection is one of the major challenges that we must address judiciously and cautiously.
The expansion of aquaculture toward sustainability necessitates technologies that focus on the recycling of matter and energy (Jegatheesan et al., 2011). Numerous technological approaches are in practice, such as microalgal/macroalgal biofiltration, recirculating aquaculture systems (RAS), and integrated multi-trophic aquaculture (IMTA) (Dalsgaard et al., 2013; Paolacci et al., 2022; Mishra et al., 2023; Nissar et al., 2023). Microalgal biofiltration is important for wastewater remediation by removing carbon, N, and P from the aquaculture system (El-Maghrabi et al., 2022; Mishra et al., 2023). For example, Andreotti et al. (2017) reported that 90% of N and 79% of P were removed from fishery wastewater by using Isochrysis galbana, Tetraselmis suecica, and Dunaliella tertiolecta. RAS, typically used for intensive shrimp and fish production, have the merits of high-density culture without being limited by season and water availability (Dalsgaard et al., 2013; Xiao et al., 2019). IMTA has been most popular in recent decades because it supports the farming of aquatic species belonging to different trophic levels in the same space in such a manner that the waste, by-products, or uneaten feed of one species is reutilized by another crop (as energy, fertilizer, or feed), thereby addressing the main plights of aquaculture pollution, feed inputs, and paucity of space (Omont et al., 2020; Nissar et al., 2023). For example, the co-culture of Gracilaria lemaneiformis and Chlamys farreri can remove 83.75% of ammonium and 70.4% of phosphorus (Mao et al., 2009). The extraction rates of N and P were 1112.45 and 134.69 mg thallus-1 in the co-culture system of Sargassum hemiphyllum and oyster (Yu et al., 2016). Although the above approaches are promising for improving nutrient utilization efficiency, the inability to maintain the desired algal species during microalgal biofiltration, the high cost of RAS operation, and the rapid disease transmission in IMTA systems are recognized as non-negligible drawbacks (Dalsgaard et al., 2013; Dong et al., 2022; Mishra et al., 2023). Therefore, new avenues in aquaculture are urgently needed to recover nutrients from aquaculture systems into reusable bioresources to help close the nutrient cycle and make fishery production more sustainable.
We propose an advanced solution for the microalgae-mediated tandem culture of shrimp and bivalve shellfish (hereafter referred to as shellfish) based on a combination of assimilatory and dissimilatory processes, in which the excess nutrients from the shrimp feed are used to produce a crop of microalgae, the microalgae are fed to the shellfish by passing the algae-laden water through the shellfish pond, and the water is then returned to the shrimp pond, allowing for efficient nutrient recovery and utilization. Although a similar conceptual design has been proposed in previous pioneering studies (Wang, 2003), no other relevant work has comprehensively reported the nutrient turnover of such a design. Thus, we experimented with comparing tandem and traditional monoculture shrimp and shellfish cultures. Exopalaemon carinicauda and Mercenaria mercenaria species, which are widely cultured in China, were selected as the cultured organisms (Lin et al., 2008; Zhang et al., 2014). This study aims to guide pond aquaculture engineering and achieve environmental and economic sustainability by assessing nutrient recovery and pollutant emissions from the tandem culture system.
2 Materials and methods
2.1 Experimental system setup
The feeding trial (from 10 November to 4 December 2021) with tandem culture and monoculture models was conducted in 16 cement ponds (1.6 m×1.8 m×1.0 m) at the Chunlin Aquaculture Farm, Ningbo, China (29°70′71” N, 121°84′61” E). We introduced sediment into the cement ponds to make the culture conditions more similar to those in the natural earthen ponds surrounding Ningbo. Sediment (total nitrogen: 0.7 mg g-1 dw; total phosphorus: 0.5 mg g-1 dw; organic matter: 27.3 mg g-1 dw) was collected from Xiangshan Bay (29°41′18″ N, 121°50′30″ E) and subsequently passed through a sieve (0.5 cm mesh size), mixed, and added to each pond to obtain a sediment layer of 5 cm. Afterward, these cement ponds were filled with 2300 L of brackish water (salinity: 14.23 ppt) from an adjacent estuary using a submerged pump, and the water depth was maintained at 80 cm. Meanwhile, sufficient seawater was stored in a pond (1000 m2) covered with a thermally insulated shed for water replenishment and exchange during farming. Daily water exchange was applied by regulating individual valves in each cement pond, and the water level was maintained at 80 cm above the sediment surface during the experiment.
2.2 Experimental design
Two treatments with four replicates were established: shrimp–shellfish tandem culture (coded as Tan) and shrimp–shellfish monoculture (Mon;Figure 1). In the Tan model (Figure 1A), the shrimp pond (coded as ShrimpTan) and the shellfish pond (ShellfishTan) were connected once a day using a water pump (running for 2 hours) during daily water changes. The bait-microalgae, which provides food for the shellfish, was added to the ShrimpTan ponds first, which were able to use the residual bait and nutrients in the ShrimpTan ponds to maintain a stable community. Then, 20% (460 L) of the ShrimpTan pond water containing abundant microalgae was pumped into the ShellfishTan pond and 10% (230 L) was reflowed after filter-feeding by the shellfish. To maintain a stable water level of 0.8 m, 10% of the water loss in the ShrimpTan pond was replenished using the stored water. The extra 10% of water in the ShellfishTan pond was discharged directly. In contrast, in the Mon model (Figure 1B), the shrimp pond (coded as ShrimpMon) and the shellfish pond (ShellfishMon) were independent of each other, and there was no water exchange between them during the farming period. Bait-microalgae was added directly to the ShellfishMon ponds. Approximately 20% (460 L) of the wastewater was discharged daily from the ShrimpMon and ShellfishMon ponds. The water loss was compensated using stored seawater. The mass of daily water inflow and discharge is shown in Table 1.
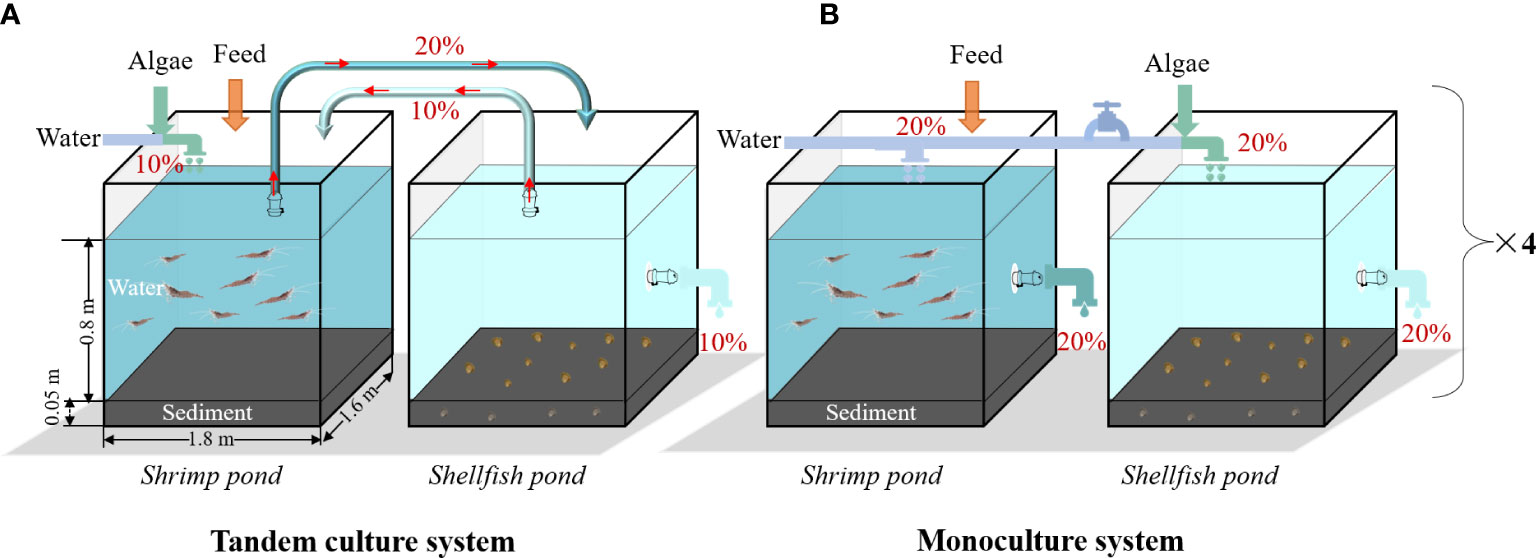
Figure 1 Experimental system. (A) Tandem culture system, (B) Monoculture system. The volume of the culture tank: 2300 L for each pond. The cultured organisms were Exopalaemon carinicauda and Mercenaria. Totals of 10% (230 L) or 20% (460 L) represent the proportion of water inflow or outflow to the total volume of pond water.
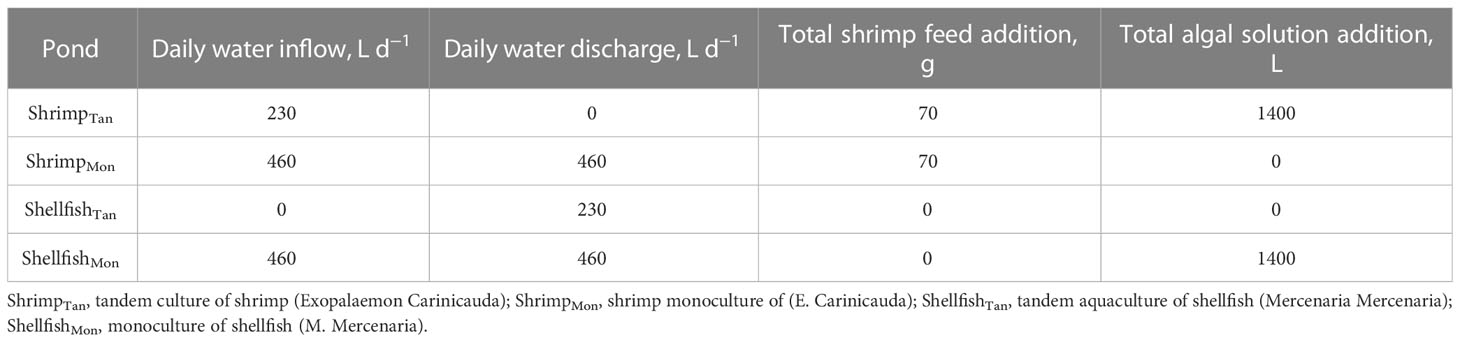
Table 1 Information on daily water inflow, daily water discharge, shrimp feed addition, and algal solution addition in monoculture (ShrimpMon and ShellfishMon) and tandem culture (ShrimpTan and ShellfishTan) ponds.
In this study, E. carinicauda and M. mercenaria were selected as the cultivated species. On 5 November 2021, E. carinicauda was collected from the nearby earthen ponds. Similar-sized shrimp (body length: 4.05 ± 0.2 cm; body weight: 2.82 ± 0.18 g) with better vitality were selected and stocked in the shrimp ponds at a density of 122 ind·m−2 (220 ind·pond−1). M. Mercenaria (body length: 2.2 ± 0.1 cm; body weight: 94.7 ± 9.6 g) purchased from Xianglian Aodalai Technology Co. (Ningde, China) was placed in the shellfish ponds at a density of 90 ind·m−2.
The bait-microalgae providing food availability to the shellfish were a mixture of Nannochloropsis oceanica (density: 2×106 cell mL−1) and Thalassiosira weissflogii (density: 4×104 cell mL−1) in a volume ratio of 1:1. Nearly 40 L of the algal solution was pumped into each ShrimpTan and ShellfishMon pond every two to three days, for a total of 720 L in both Tan and Mon ponds (Table 1). The bait-microalgae were cultivated and expanded in the following steps: in step 1, Nannochloropsis sp. and Thalassiosira sp. were purely cultured in a 3 L conical flask with NMB3 medium containing KNO3 (100 g), KH2PO4 (10 g), FeSO4·7H2O (2.5 g), MnSO4·H2O (0.25 g), EDTA·Na2 (10 g), vitamin B1 (6 mg L−1), and vitamin B12 (0.05 mg L−1). The culture conditions were light intensity of 100 mmol photon m−2 s−1 under 23°C (light: dark=12:12 h; Cao et al., 2021). In step 2, 0.5 L of the algal solution from step 1 was inoculated into a 5 L conical flask for activation and expansion. The culture conditions were the same as in step 1, and the final densities of Nannochloropsis sp. and Thalassiosira sp. were 2×107 cell mL−1 and 4×105 cell mL−1, respectively. In step 3, 1 L of the algal solution from step 2 was inoculated into a 50 L white plastic barrel filled with seawater (disinfected with sodium hypochlorite and dechlorinated with sodium thiosulfate before use). The final densities of Nannochloropsis sp. and Thalassiosira sp. were 3×106 cell mL−1 and 5×104 cell mL−1, respectively. In step 4, 10 L of the algal solution from step 3 was inoculated into a 500 L white plastic barrel for further spread cultivation. The culture process was in line with step 3; the final densities of Nannochloropsis sp. and Thalassiosira sp. were 2×106 cell mL−1 and 4×104 cell mL−1, respectively.
2.3 Aquaculture system operation and daily management
Throughout the farming period, the aquaculture systems were inspected in the morning and evening to ensure that the production facilities and breeding animals were in good condition. Shrimp were fed twice a day with commercial feed pellets containing 42% crude protein (Yuehai™, Guangzhou, China), once in the morning (7: 00 am) and once in the afternoon (4:00 pm). The feeding rate was maintained at 1%–4% of shrimp weight (Salame, 1993), and all shrimp ponds received the same amount of feed (7−8 g per day, 224 g in total) during the farming period (Table 1). On 10 November and 25 November, five shrimp were collected from each pond to measure shrimp weight for feeding rate determination. In each pond, two air pumps were operated daily to facilitate aeration. No drugs (e.g., probiotics and antibiotics) were used during the breeding process.
2.4 Sampling and analysis
Water temperature (WT), salinity (SAL), dissolved oxygen (DO), and pH were recorded in situ at four-day intervals (on 10, 15, 20, 25, 30 November, and on 4 December, respectively) with a YSI ProPlus (Yellow Spring Inc., USA). Water samples (2 L) were taken at three randomly chosen locations at mid-water depth within each cement pond using a water collector (5 L in volume). One liter of well-mixed water was immediately fixed with Lugol’s solution (3%–5% final concentration) for phytoplankton analysis. Phytoplankton taxa were counted in sedimentation chambers (Hydro-Bios Apparatebau GmbH, Kiel, Germany) using an inverted microscope (CK2, Olympus Corporation, Tokyo, Japan), and the biomass was calculated using geometric approximations using the computerized counting program (Yang et al., 2020). A total of 0.2 L was filtered on 0.45µm cellulose acetate membrane filters for analysis of ammonium (NH4+-N), nitrate (NO3−-N), nitrite (NO2−-N), and phosphate (PO43−-P). NH4+-N, NO3−-N, and NO2−-N were measured by the hypobromite oxidation method, the zinc-cadmium reduction method, and the on-line flow injection method, respectively, using an automated spectrophotometer (Smart-Chem 400 Discrete Analyzer, Westco Scientific Instruments, Brookfield, USA) (AQSIQ, 2007). PO43−-P was determined using an ammonium molybdate ultraviolet spectrophotometric method (Ma et al., 2018). The remaining unfiltered water was used for the determination of total phosphorus (TP), total organic carbon (TOC), phytoplankton chlorophyll a (Chl a), and chemical oxygen demand (COD). TP and COD were determined following the standard methods (AQSIQ, 2007). TOC was determined with a total organic carbon analyzer (model Aurora 1030, OI Analytical, USA) (Zeng et al., 2021). Chl a was extracted with 90% acetone (at 4°C for 24 h) after filtration through GF/C filters (Whatman, GE Healthcare UK Limited, Buckinghamshire, UK). Absorbance was then read at 665 and 750 nm before and after acidification with 10% HCl using a spectrophotometer (Ma et al., 2021).
The distribution of labile P (referred to as easily changeable or mobile P fractions) at the sediment–water interface profiles was determined using Zr-oxide diffusive gradients in thin films (DGT). In total, 32 Zr-oxide DGT probes (EasySensor Co. Ltd., Nanjing, China) assembled with standard DGT holders were inserted across the sediment–water interface on 8 November (at the start of cultivation) and 4 December (after 25 days of cultivation) using a release device (Ma et al., 2021). The probes were forced 2 cm into the sediment and kept 4 cm above the water surface. After 48 h, the probes were retrieved and brought to the laboratory for analysis. The binding gels were removed from the DGT probes and cut into 2 mm strips using a ceramic blade. The accumulated masses of DRP in the Zr-oxide binding gels were extracted with NaOH (1 M). The concentration of labile P measured by DGT was calculated as follows (Ma et al., 2018):
where M is the accumulated mass of P on the Zr-oxide gel (µg), Ceis the labile P concentration in the alkaline eluate (mg L-1), Ve is the volume of extraction solvent (mL), Vg is the volume of the gel (mL), fe is the elution efficiency, Δg is the thickness of the diffusive layer (cm), D is the diffusion coefficient of the phosphate in the diffusive layer (cm2 s-1), A is the exposed area of the gel (cm2), and t is the deployment time (s).
At the end of the experiment, we harvested all shrimp and shellfish and recorded their length and weight. Their growth performance was evaluated in terms of survival rate (SR), daily growth rate (DGR), and specific growth rate (SGR), according to Turkmen (2007).
2.5 Calculation of pollutants in aquaculture tailwater discharge
During water discharge, 0.5 L of tailwater was collected at a five-day interval from the outflow tube for measurement of TP, PO43−-P, TOC, NH4+-N, NO3−-N, and COD. The pollutants in the tailwater discharge were calculated according to Cai et al. (2013):
where daily emission M (mg m-2 d-1) was calculated as the mean for each of the five days of water quality sampling and then summed for the entire month of cultivation. C is the concentration of the pollutant (mg L−1) taken from the outflow tube at a five-day interval, and V is the volume of water discharged as tailwater (L), which was determined by the water level scale on the wall of each pond. T is the cultivation time (d).
2.6 Statistical analyses
Origin 9.0 and SPSS 2.5 software were used for basic drawing and data processing. Results were expressed as means and standard errors. Following Bin Othman and Heng (2014) and Park et al. (2009), we used repeated measures ANOVA (RM-ANOVA) and the Mann–Whitney U test (MWU) to compare the means between the groups of interest (statistical significance was accepted at p< 0.05). RM-ANOVA was applied to the repeated measures data, such as TP, TOC, NH4+-N, NO3−-N, NO2−-N, PO43−-P, and Chl a (monitored at five-day intervals). MWU was applied to the independent data, such as the growth data of the rearing organisms (monitored only once at the end of the experiment).
3 Results
3.1 Composition, biomass, and abundance of phytoplankton in the Tan and Mon systems
According to the data monitored on 20 November, there were 11 phytoplankton species belonging to Cyanophyta, Chlorophyta, Bacillariophyta, and Euglenophyta. In ShrimpTan ponds, Nannochloropsis sp. and Thalassiosira sp. were the dominant species as added bait-microalgae, accounting for 37.4% and 37.0% of total algal biomass and abundance, respectively (Figure 2). Similar patterns were found in ShellfishTan ponds. In ShrimpMon ponds, Lygbya sp. and Coelosphaerium dubium, belonging to the Chlorophyta phylum, were the top two dominant species, contributing 35.2% of the total algal biomass and 31.0% of the total algal abundance. In ShellfishMon ponds, the most dominant algal species were Cosmarium sp., Lygbya sp., and C. dubium, accounting for 40.4% of the total algal biomass and 35.0% of the algal abundance (Figure 2).
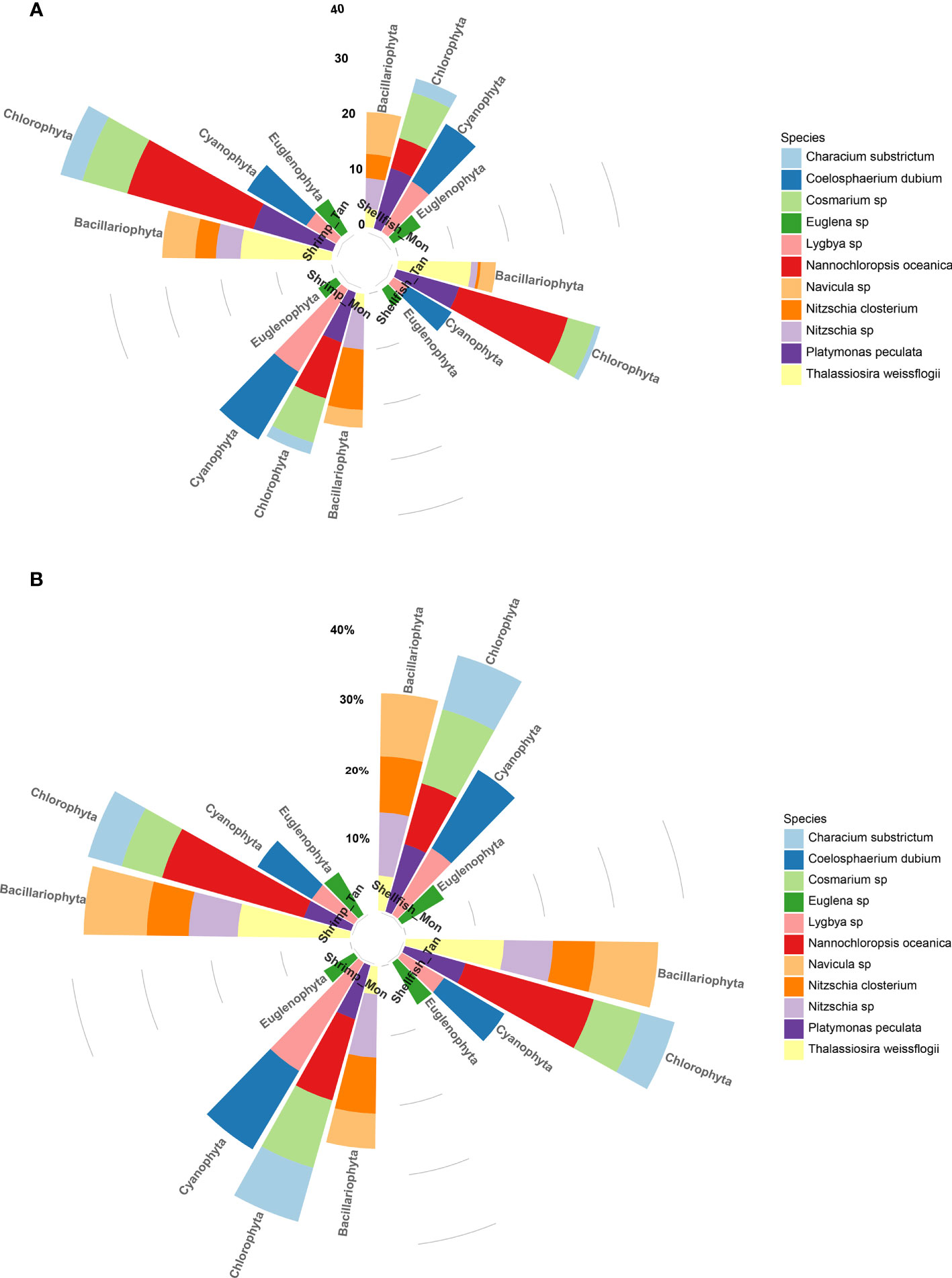
Figure 2 Circular stacked bar plots demonstrating the biomass (10−3 mg L−1) (A) and percent abundance (B) of phytoplankton species monitored on 20 November in monoculture (ShrimpMon and ShellfishMon) or tandem culture (ShrimpTan and ShellfishTan) experiments. Different species are displayed in different colors. The total number of species abundances was 16.51, 27.63, 20.07, and 44.24 in ShrimpMon, ShellfishMon, ShrimpTan, and ShellfishTan ponds, respectively.
During the experiment, Chl a concentration, an indicator of algal density, was significantly higher in ShrimpTan or ShellfishTan than in ShrimpMon or ShellfishMon (p = 0.001; 0.03). The mean values of Chl a were 0.98, 0.49, 0.34, and 0.11 mg L−1 in ShrimpTan, ShellfishTan, ShrimpMon, and ShellfishMon, respectively (Figure 3A).
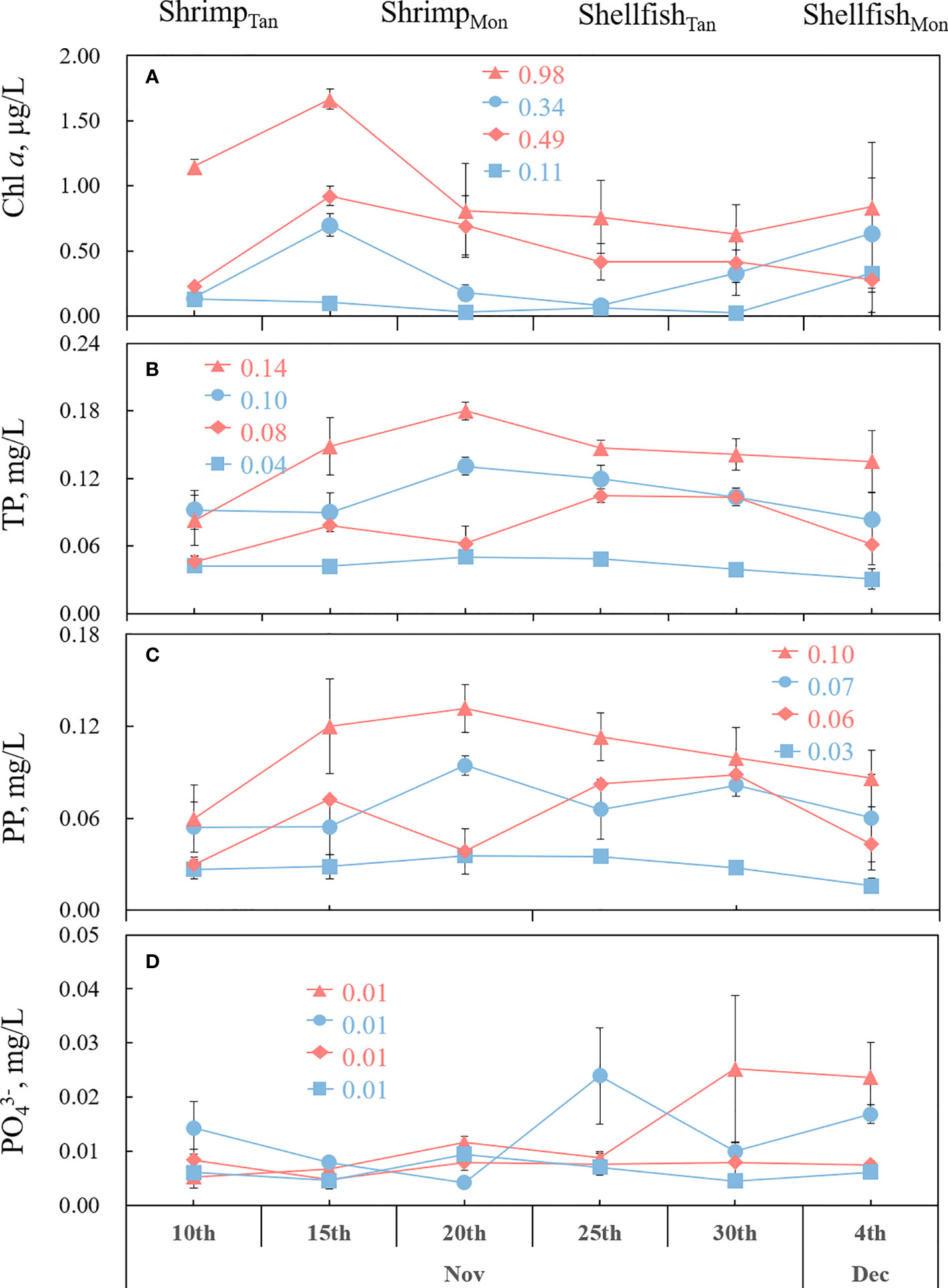
Figure 3 Mean values ( ± SE) of phytoplankton chlorophyll a (Chl a) (A), total phosphorus (TP) (B), particulate phosphorus (PP) (C) and phosphate (PO43−-P) (D) for 25 days of growth of shrimp and shellfish in monoculture (ShrimpMon and ShellfishMon) or tandem culture (ShrimpTan and ShellfishTan) experiments. The data above the lines indicate the average value of the different treatments.
3.2 Growth performance of reared organisms in the Tan and Mon systems
We selected SR, DGR, and SGR to measure the growth performance of reared organisms since they are highly recommended in the majority of previous studies (Turkmen, 2007). No statistical differences were observed between the Tan and Mon models for SR, DGR, and SGR of shrimp (Table 2). There were no significant differences between Tan and Mon systems for shellfish’s SR, although it tended to be lower in ShellfishTan ponds (82%), than in ShellfishMon ponds (87%; Table 2). In contrast, shellfish DGR was higher in ShellfishTan ponds (6‰ d−1) than in ShellfishMon ponds (2‰ d−1). SGR displayed similar patterns as DGR.
3.3 Variations in water quality variables and their associated relationships
As for TP (Figure 3B), ShrimpTan was significantly higher than ShrimpMon (p< 0.001), and ShellfishTan was significantly higher than ShellfishMon (p< 0.001). Particulate phosphorus (PP) exhibited a similar pattern to TP, being significantly higher in ShrimpTan or ShellfishTan than in ShrimpMon or ShellfishMon (p = 0.001, 0.02; Figure 3C). No significant differences were observed for PO43−-P between ShrimpTan and ShrimpMon (p = 0.82) and between ShellfishTan and ShellfishMon (p = 0.72, 0.70; Figure 3D).
In terms of labile P at the sediment–water interface (Figure 4), both ShrimpTan and ShrimpMon ponds were higher at the end of the experiment (4 December) compared to the initial stage of the culture (10 November). In the ShellfishTan ponds, the labile P in the sediment (0 to −20 mm) monitored on 4 December decreased compared to the initial condition, together with the occurrence of a static layer (sediment or water layer with an extremely low concentration of labile P). On the other hand, the labile P in the water (0 to 30 mm) monitored on 4 December increased with respect to the initial value. During the experiment, there was no noticeable trend change in labile P for ShellfishMon ponds.
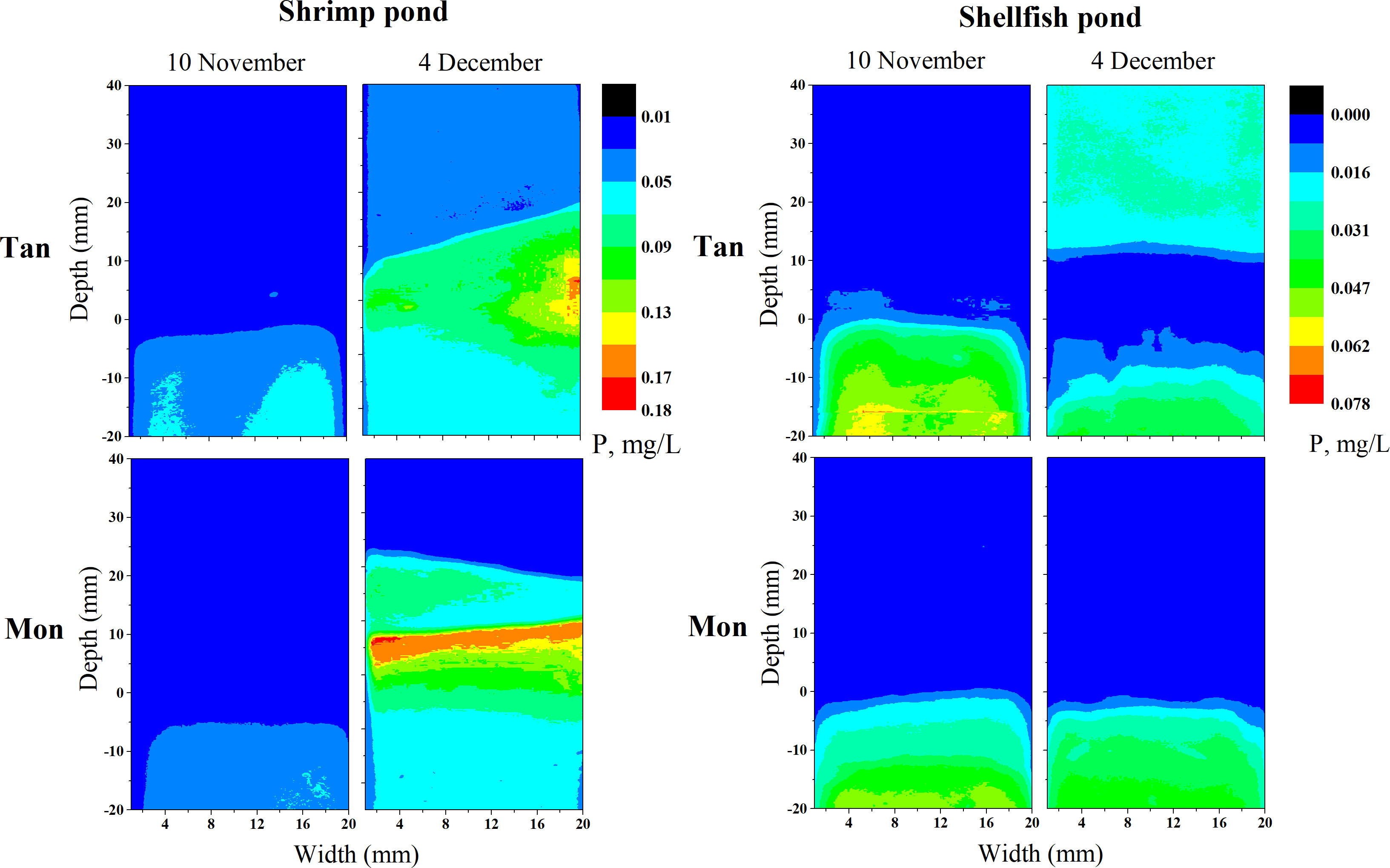
Figure 4 Two-dimensional distribution images of labile phosphorus concentration (labile P) at a spatial resolution of 0.45 mm at sediment-water interface profiles at the beginning (10 November) and end (4 December) of the culture experiment. The location of the sediment-water interface is represented by zero. Tan and Mon represent tandem and monoculture modes, respectively.
No significant differences were observed for TOC between ShrimpTan and ShrimpMon ponds (p = 0.40) and between ShellfishTan and ShellfishMon ponds (p = 0.25) (Figure 5A). NH4+-N was significantly lower in ShrimpTan than in ShrimpMon (p = 0.02), while it was significantly higher in ShellfishTan than in ShellfishMon (p = 0.002; Figure 5B). NO3−-N was notably higher in ShrimpTan or ShellfishTan than in ShrimpMon or ShellfishMon (p = 0.01, 0.006; Figure 5C). For NO2−-N (Figure 5D), no difference was found between ShrimpTan and ShrimpMon (p = 0.88), while ShellfishTan was significantly higher than ShellfishMon (p = 0.02).
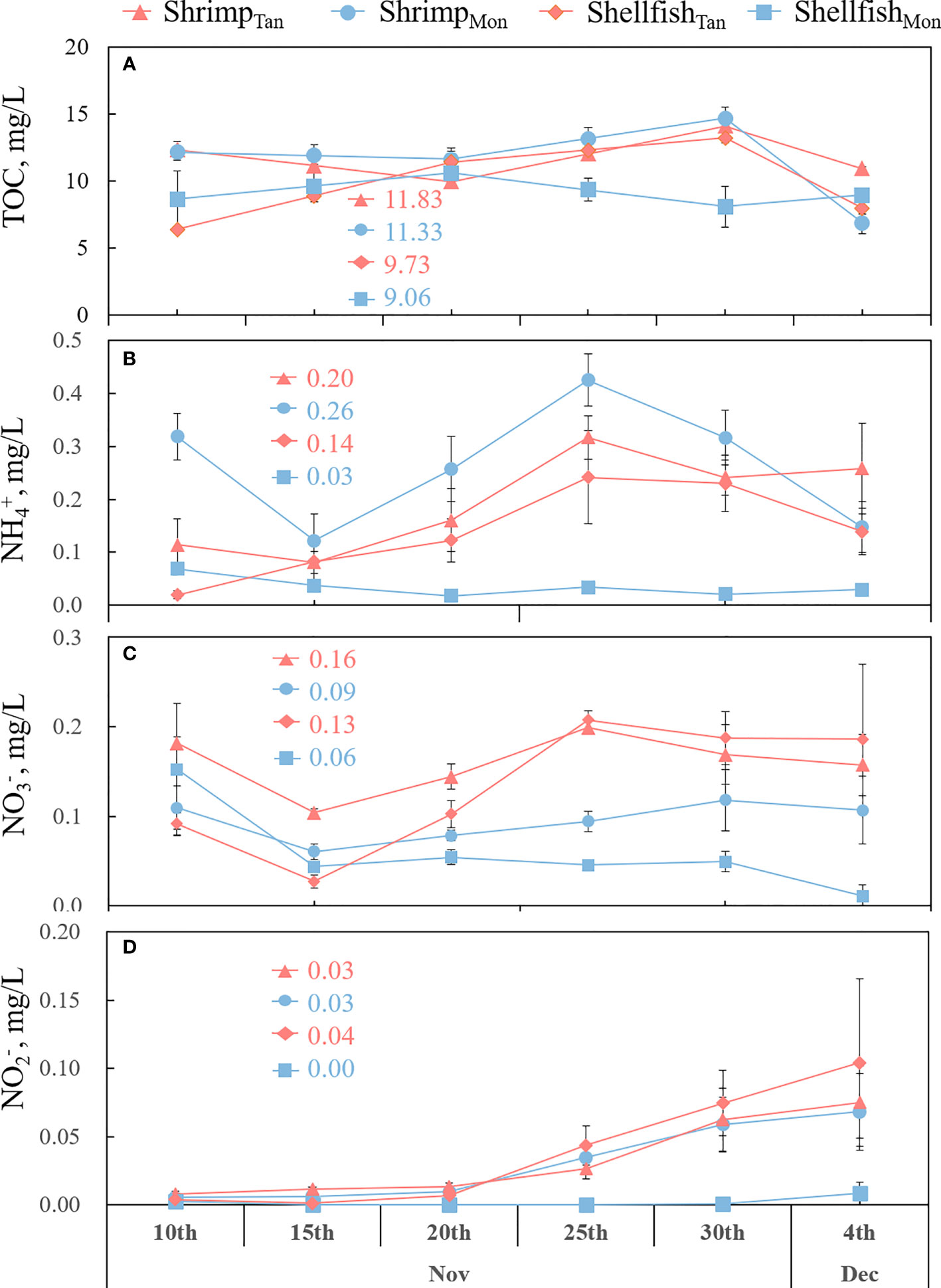
Figure 5 Mean values ( ± SE) of total organic carbon (TOC) (A), ammonium (NH4+-N) (B), nitrate (NO3−-N) (C), and nitrite (NO2−-N) (D) during 25 days of growth of shrimp and shellfish in monoculture (ShrimpMon and ShellfishMon) or tandem culture (ShrimpTan and ShellfishTan) experiments. The data above the lines indicate the average value of the different treatments.
As for the relationships between water quality variables (Table 3), TP correlated positively with Chl a, NO2−-N, NO3−-N, NH4+-N, PP, and PO43−-P and correlated negatively with pH. NO2−-N and NO3−-N correlated positively with Chl a, NH4+-N, PP, and PO43−-P and negatively with pH and WT. NH4+-N showed significant positive correlations with TP, PP, and PO43−-P and negative correlations with pH and COD.
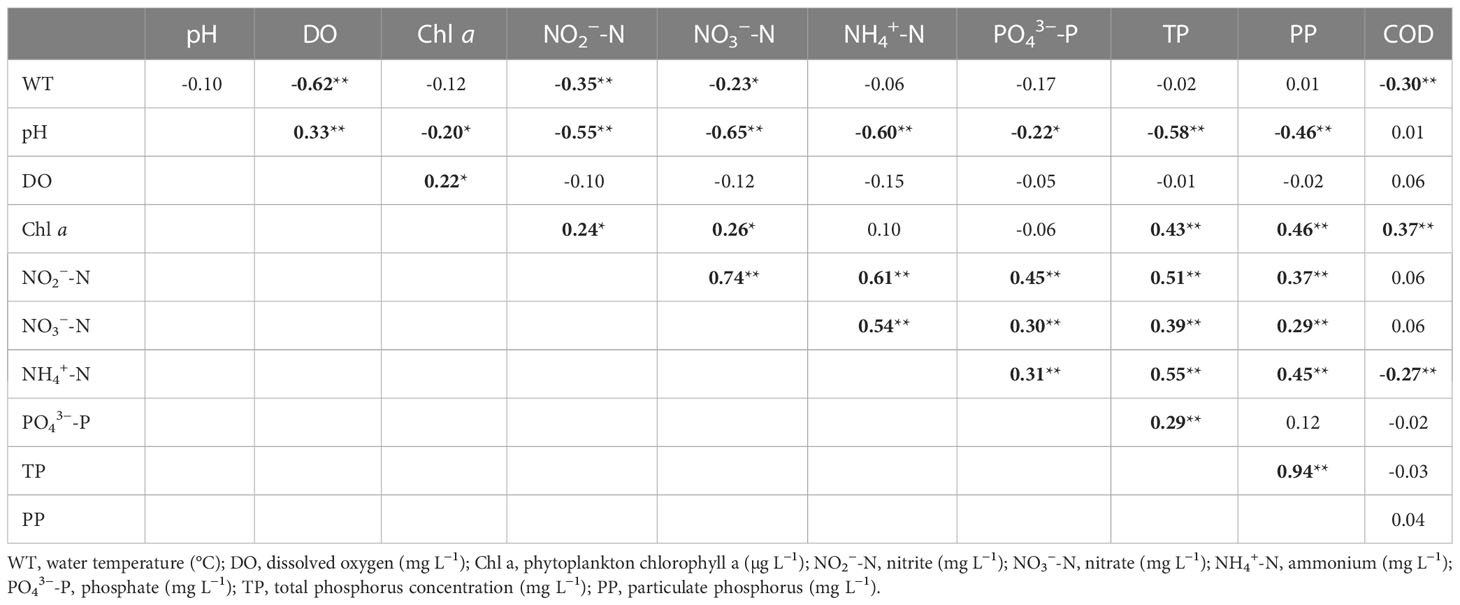
Table 3 Spearman’s rank correlations (r value) between environmental variables (significant correlations in bold, *p<0.05, **p<0.01).
3.4 Tailwater discharge in the Tan and Mon systems
The pollutants discharged from mariculture are depicted in Figure 6. Except for NO3−-N, all pollutant emissions, including PO43−, TP, TOC, NH4+-N, and COD, were significantly lower in Tan than in the Mon system. The Tan system discharged 19 mg TP m−2 d−1, 2 mg PO43−-P m−2 d−1, and 2 g TOC m−2 d−1 to the surrounding environment, which was 1.3, 1.7, and 1.4 times lower than those observed in the Mon systems, respectively. Approximately 38 mg NH4+-N m−2 d−1 and 11 g COD m−2 d−1 were discharged from the Tan system, 1.3 and 1.2 times lower than from the Mon system, respectively. In contrast, NO3−-N discharge was slightly higher in the Tan system (up to 30 mg m−2 d−1) than in the Mon system (27 mg m−2 d−1), although we found no statistical difference between them.
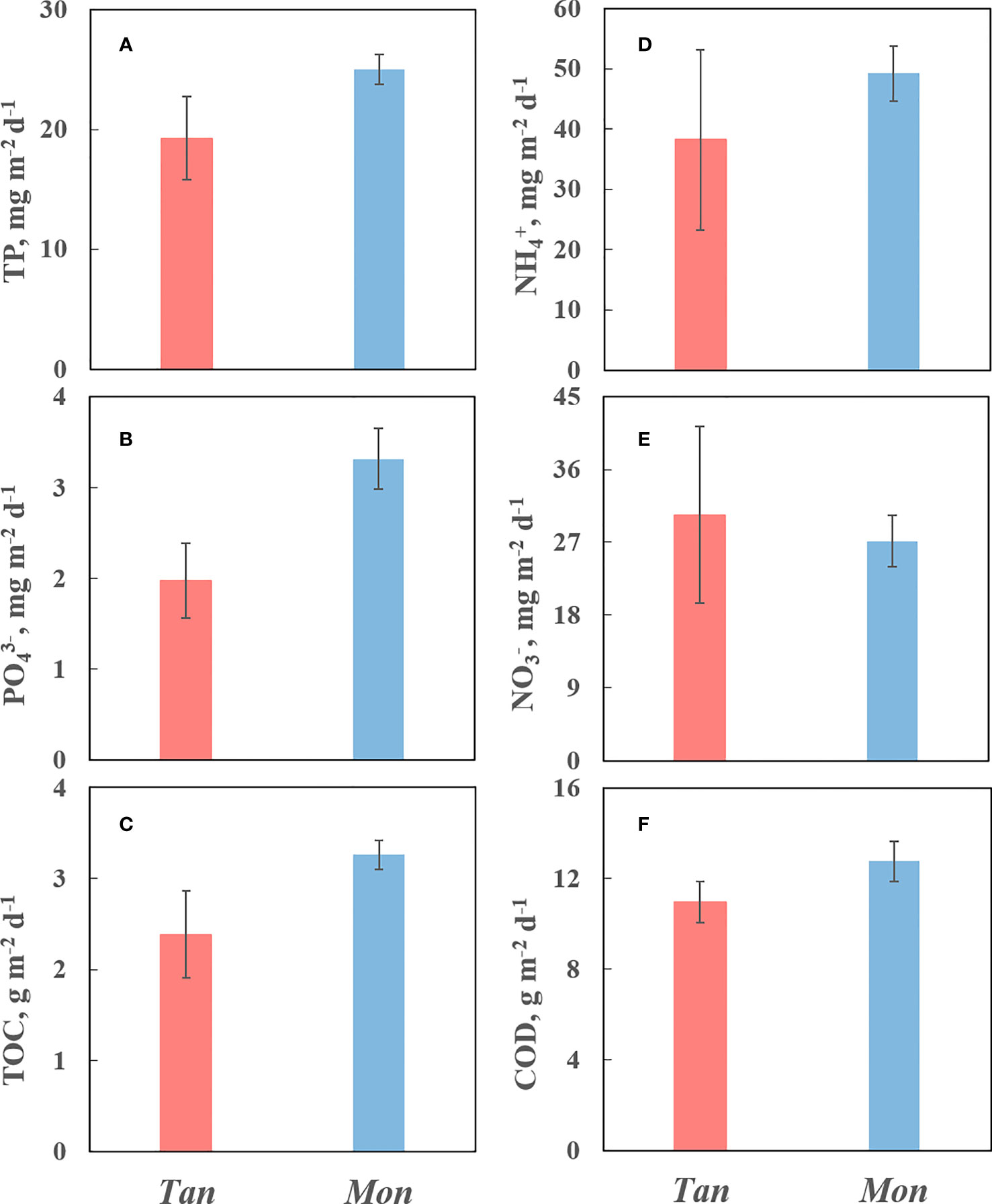
Figure 6 Mean values (± SE) of total phosphorus (TP) (A), phosphate (PO43−-P) (B), (TOC) (C), ammonium (NH4+-N) (D), nitrate (NO3−-N) (E) and chemical oxygen demand (COD) (F) discharged from mariculture. Tan and Mon represent tandem and monoculture modes, respectively.
4 Discussion
4.1 Performance of reared organisms in Tan and Mon systems
Within the culture period (10 November– 4 December 2021), we found no statistical differences in the performance of shrimp and shellfish between the Tan and Mon systems, which suggested that the tandem culture model was viable in terms of animal harvesting compared to the monoculture model. However, the survival rate of shrimp in all ponds was relatively low (50%–51%) compared with the data (67%–80%) reported in previous studies (Abdelrahman et al., 2018; Yang et al., 2021). The following three reasons may explain the low survival rate of shrimp: i) the temperature dropped with the season (from 17°C to 4°C), which was regarded as a major factor in reduced shrimp survival (Perez-Velazquez et al., 2012). The optimum temperature for shrimp is 23°C–30°C; above and below this temperature, their survival is considerably lower (Perez-Velazquez et al., 2012; Abdelrahman et al., 2018). ii) No protective drugs such as probiotics and antibiotics were used during the farming period, which could reduce shrimp resistance to various pathogens (Butt et al., 2021). iii) Generally, the safety threshold of NO2−-N concentrations in the overlying water of shrimp aquaculture ponds is 0.01 mg L−1 (Lai, 2014). However, we observed mean concentrations of NO2−-N (0.03–0.04 mg L−1) in all shrimp ponds that were considerably higher than the safety threshold, indicating that the water quality could be harmful to the shrimp and reduce their survival and growth through a variety of physiological dysfunctions (Hu et al., 2012; Yang et al., 2021). It is worth noting that, except for the ShellfinsMon ponds, the NO2−-N levels gradually increased in the middle of the experiment. This can be attributed to the organic loading (indicated by TOC increase) and its related decomposition processes: high levels of organic matter occurred together with high levels of NO2−-N, particularly in the later stages of cultivation (Milstein et al., 2001). However, the relatively short duration of this experiment, covering only part of the organism’s growth cycle, may underestimate some of the problems typically occurring in the longer term (e.g., organic matter accumulation and disease outbreaks) (Dong et al., 2022). Shellfish survival in this study remained at 82%–87%, which is close to the survival rate of 90% reported by Washitani et al. (2017). Notably, the daily growth rate of shellfish tended to be higher in ShellfishTan ponds (6‰ d−1) than in ShellfishMon ponds (2‰ d−1), which may be due to the adequate food (bait-microalgae) in Tan systems, as indicated by the more stable and higher algal biomass (as indicated by Chl a).
Algae, as primary producers of aquaculture ecosystems, differed in biomass and composition between the Tan and Mon systems, with higher biomass of Nannochloropsis sp. and Thalassiosira sp. in the Tan than in the Mon systems. This suggested that the Tan model facilitated the colonization and stabilization of the added bait-microalgae. An increase in nutrient availability was expected to cause dynamics in the phytoplankton community and an increase in biomass, as indicated by the positive correlations between Chl a and the nutrients N and P. This was in line with previous studies (Laiolo et al., 2014).
4.2 Tandem culture model in support of P utilization and P loop closure
P use efficiency differed between the Tan and Mon systems, likely due to differences in algal uptake efficiency and recirculating water. Microalgae had a higher potential for P utilization in the Tan systems. On the one hand, as an intermediary medium to remove the excess nutrients from the shrimp ponds and subsequently, as feed for the shellfish, microalgae absorbed and removed large portions of water P, reducing PO43−-P to low levels (≤ 0.01 mg L−1). In this way, P was removed by combining assimilatory and dissimilatory processes, which can fully exploit the P resources of the pond culture and help close the P cycle (Van Rijn, 2013). The efficiency of algae in nutrient uptake and removal has been widely recognized (Xu et al., 2017; Paw et al., 2019; Abdelfattah et al., 2023). For instance, Chlorella minutissima removed 88% of N and 99% of P from the aquaculture wastewater (Paw et al., 2019). On the other hand, microalgae can also utilize sediment P resources by increasing the upward diffusion of sediment P (referred to as the “pumping” effect of algae in the previous study by Xie et al., 2003), as indicated by the decreased labile P in the surficial 2 cm sediment (decreased from 0.04 to 0.02 mg L−1 in ShellfishTan ponds). In contrast, P use efficiency in Mon systems was less successful. This was likely because the bait-microalgae in the Mon system were added directly to the ShellfishMon ponds and immediately filtered by the shellfish, and thus did not have time to regrow and reproduce.
Furthermore, the recirculating water in the Tan systems allowed for accelerated P turnover and utilization, which explains well why nutrients (NH4+-N, TP, and PP) were higher in the Tan than in the Mon systems. In contrast, shrimp and shellfish ponds in Mon systems were independent of each other, which hindered the P flow between them and, in turn, reduced the efficiency of P utilization.
4.3 Pollutant emissions from the Tan and Mon systems
The Tan system with recirculating water makes it possible to reduce water consumption and pollutant emissions. Indeed, the shrimp (algae)−shellfish tandem culture operation can be achieved with a minimum daily water exchange rate of only 10% (230 L). In contrast, traditional pond aquaculture consumes a large amount of water. For example, each kg of aquatic product from pond aquaculture in China requires 3−13.4 m3 of water (Liu et al., 2021), which is 4−19 times higher than our Tan system. Such minimal tailwater discharge maintained system stability and reduced pollutant emissions (Wang, 2003). For example, the daily emissions of N and P from the Tan system were 78 mg m−2 d−1 and 2 mg m−2 d−1, which were 1.2−1.5 and 3 times lower than the intensive shrimp ponds in Australia (de Lacerda et al., 2006). Similarly, TP, PO43−-P, TOC, NH4+-N, NO3−-N, and COD emissions from Tan systems were 1.3, 1.7, 1.4, 1.3, 1.1, and 1.2 times lower than those observed in Mon systems, indicating that tandem systems had clear advantages in terms of pollution reduction. Furthermore, the growth and reproduction of bait-microalgae were more stable in Tan systems than in Mon systems, which may increase P uptake by microalgae. Overall, the Tan model was eco-friendly and preferred because it demonstrated high stability, high nutrient utilization efficiency, minimal water input, and low wastewater discharge while allowing full control of the culture environment.
5 Conclusions
Overall, the microalgae-mediated tandem culture of shrimp and shellfish made the use of P resources more efficient and sustainable through the recirculation of the water and the high uptake efficiency of algae. As an intermediate medium to remove the excess nutrients from the shrimp ponds and then as feed for the bivalve, the microalgae not only absorbed water P but also utilized sediment P by stimulating the upward diffusion of sediment P. Furthermore, the tandem culture system can reduce pollutant emissions by minimizing water discharge. In addition, TP, PO43−-P, TOC, NH4+-N, and COD emissions were 1.3, 1.7, 1.4, 1.3, and 1.2 times lower than those observed in monoculture systems, respectively. The environmental and health co-benefits of such a tandem culture are an effective approach to recovering and removing P and therefore deserve to be prioritized.
Data availability statement
The original contributions presented in the study are included in the article/Supplementary Material. Further inquiries can be directed to the corresponding author.
Ethics statement
The studies involving animals were reviewed and approved by the Ningbo University Laboratory Animal Center under permit number no. SYXK (ZHE2008-0110).
Author contributions
SM and XD conceived and designed the study. SM, XD, and CL collected the samples.XD, and CZ raised and managed the experimental shellfish and shrimp. XD and CL performed the biochemical. SM and JX wrote the manuscript. All authors read and approved the final manuscript for submission.
Funding
The research was supported by the Ningbo Public Welfare Science and Technology Program (Grant No. 2021S060), the Zhejiang Basic Public Welfare Research Program (Grant No. LQ21C030005), the National Natural Science Foundation of China (Grant No. 42107399), and the earmarked fund for CARS-49.
Acknowledgments
We thank Anne Mette Poulsen and Freescience Information Technology Ltd. for their valuable editorial assistance.
Conflict of interest
The authors declare that the research was conducted in the absence of any commercial or financial relationships that could be construed as a potential conflict of interest.
Publisher’s note
All claims expressed in this article are solely those of the authors and do not necessarily represent those of their affiliated organizations, or those of the publisher, the editors and the reviewers. Any product that may be evaluated in this article, or claim that may be made by its manufacturer, is not guaranteed or endorsed by the publisher.
References
Abdelfattah A., Ali S. S., Ramadan H., El-Aswar E. I., Eltawab R., Ho S. H., et al. (2023). Microalgae-based wastewater treatment: mechanisms, challenges, recent advances, and future prospects. environ. Sci. Ecotech. 13, 100205. doi: 10.1016/j.ese.2022.1002052666-4984
Abdelrahman H. A., Abebe A., Boyd C. E. (2018). Influence of variation in water temperature on survival, growth and yield of pacific white shrimp Litopenaeus vannamei in inland ponds for low-salinity culture. Aquac. Res. 50 (2), 658–672. doi: 10.1111/are.13943
Andreotti V., Chindris A., Brundu G., Vallainc D., Francavilla M., Garcia J. (2017). Bioremediation of aquaculture wastewater from Mugilcephalus (Linnaeus 1758) with different microalgae species. Chem. Ecol. 33 (8), 750–761. doi: 10.1080/02757540.2017.1378351
AQSIQ P. R. C. (2007) The specification for marine monitoring of China-part 4: seawater analysis (GB 17378.4–2007) (General administration of quality supervision, inspection and quarantine (Aqsiq) of the people’s republic of China (in Chinese). Available at: http://openstd.samr.gov.cn/bzgk/gb/newGbInfo?hcno=9FB14D0EE23D77A96D54A9BDAAF6EA07.
Bin Othman A. R., Heng L. C. (2014). Sensitivity analysis of the refinement to the Mann-Whitney test. Sains Malays. 43 (7), 1095–1100.
Bouwman L., Beusen A., Glibert P. M., Overbeek C., Pawlowski M., Herrera J., et al. (2013). Mariculture: significant and expanding cause of coastal nutrient enrichment. Environ. Res. Lett. 8 (4), 44026. doi: 10.1088/1748-9326/8/4/044026
Butt U. D., Lin N., Akhter N., Siddiqui T., Li S. H., Wu B. (2021). Overview of the latest developments in the role of probiotics, prebiotics and synbiotics in shrimp aquaculture. Fish Shellfish Immun. 114, 263–281. doi: 10.1016/j.fsi.2021.05.003
Cai C. F., Gu X. H., Ye Y. T., Yang C. G., Dai X. Y., Chen D. X., et al. (2013). Assessment of pollutant loads discharged from aquaculture ponds around taihu lake, China. Aquac. Res. 44 (5), 795–806. doi: 10.1111/j.1365-2109.2011.03088.x
Cao J. Y., Wang Y. Y., Wu M. N., Kong Z. Y., Lin J. H., Ling T., et al. (2021). RNA-Seq insights into the impact of alteromonas macleodii on isochrysis galbana. Front. Microbiol. 12. doi: 10.3389/fmicb.2021.711998
Dalsgaard J., Lund I., Thorarinsdottir R., Drengstig A., Arvonen K., Pedersen P. B. (2013). Farming different species in RAS in Nordic countries: current status and future perspectives. Aquacult. Eng. 53, 2–13. doi: 10.1016/j.aquaeng.2012.11.008
de Lacerda L. D., Vaisman A. G., Maia L. P., Silva C. A. R. E., Cunha E. M. S. (2006). Relative importance of nitrogen and phosphorus emissions from shrimp farming and other anthropogenic sources for six estuaries along the NE Brazilian coast. Aquaculture 253, 433–446. doi: 10.1016/j.aquaculture.2005.09.005
Dong S. P., Shan H. W., Yu L. Y., Liu X. B., Ren Z. W., Wang F. (2022). An ecosystem approach for integrated pond aquaculture practice: application of food web models and ecosystem indices. Ecol. Indic. 141, 109154. doi: 10.1016/j.ecolind.2022.109154
El-Maghrabi N., Fawzy M., Mahmoud A. E. (2022). Efficient removal of phosphate from wastewater by a novel phyto-graphene composite derived from palm byproducts. ACS Omenga. 7 (49), 45386–45402. doi: 10.1021/acsomega.2c05985
FAO (2022). The state of world fisheries and aquaculture 2022 (Rome: Towards Blue Transformation). doi: 10.4060/cc0461en
Hicks C. C., Cohen P. J., Graham N. A. J., Nash K. L., Allison E. H., D’Lima C., et al. (2019). Harnessing global fisheries to tackle micronutrient deficiencies. Nature 574, 95–98. doi: 10.1038/s41586-019-1592-6
Hu Z., Lee J. W., Chandran K., Kim S., Khanal S. K. (2012). Nitrous oxide (N2O) emission from aquaculture: a review. Environ. Sci. Technol. 46, 6470–6480. doi: 10.1021/es300110x
Jegatheesan V., Shu L., Visvanathan C. (2011). Aquaculture effluent: impacts and remedies for protecting the environment and human health. Encycl. Environ. Health, 123–135. doi: 10.1016/B978-0-444-52272-6.00340-8
Laiolo L., Barausse A., Dubinsky Z., Palmeri L., Goffredo S., Kamenir Y., et al. (2014). Phytoplankton dynamics in the gulf of aqaba (Eilat, red sea): a simulation study of mariculture effects. Mar. pollut. Bull. 86, 481–493. doi: 10.1016/j.marpolbul.2014.06.026
Lin Z. H., Lu Z. M., Chai X. L., Fang J., Zhang J. M. (2008). Karyotypes of diploid and triploid Mercenaria (Linnaeus). J. Shellfish Res. 27 (2), 297–300. doi: 10.2983/0730-8000(2008)27[297:KODATM]2.0.CO;2
Liu X. G., Shao Z. Y., Cheng G. F., Lu S. M., Gu Z. J., Zhu H., et al. (2021). Ecological engineering in pond aquaculture: a review from the whole-process perspective in China. Rev. Aquacult. 13, 1060–1076. doi: 10.1111/raq.12512
Ma S. N., Wang H. J., Wang H. Z., Li Y., Liu M., Liang X. M. (2018). High ammonium loading can increase alkaline phosphatase activity and promote sediment phosphorus release: a two-month mesocosm experiment. Water Res. 145, 388–397. doi: 10.1016/j.watres.2018.08.043
Ma S. N., Wang H. J., Wang H. Z., Zhang M., Li Y., Shi J. B. (2021). Effects of nitrate on phosphorus release from lake sediments. Water Res. 194, 116894. doi: 10.1016/j.watres.2021.116894
Mao Y., Yang H., Zhou Y., Ye N., Fang J. (2009). Potential of the seaweed (Gracilaria lemaneiformis) for integrated multi-trophic aquaculture with scallop (Chlamys farreri) in north China. J. Appl. Phycol. 21 (6), 649–656. doi: 10.1007/s10811-008-9398-1
Milstein A., Zoran M., Kochba M., Avnimelech Y. (2001). Effect of different management practices on water quality of intensive tilapia culture systems in Israel. Aquacult. Int. 9, 133–152. doi: 10.1023/A:1014209124855
Mishra B., Tiwari A., Mahmoud A. E. (2023). Microalgal potential for sustainable aquaculture applications: bioremediation, biocontrol, aquafeed. Clean Technol. Envir. 25, 675–687. doi: 10.1007/s10098-021-02254-1
Nissar S., Bakhtiyar Y., Arafat M. Y., Andrabi S., Mir Z. A., Khan N. A., et al. (2023). The evolution of integrated multi-trophic aquaculture in context of its design and components paving way to valorization via optimization and diversification. Aquaculture 565, 739074. doi: 10.1016/j.aquaculture.2022.739074
Omont A., Elizondo-Gonzalez R., Quiroz-Guzman E., Escobedo-Fregoso C., Hernandez-Herrera R., Pena-Rodriguez A. (2020). Digestive microbiota of shrimp Penaeus vannamei and oyster Crassostrea gigas co-cultured in integrated multi-trophic aquaculture system. Aquaculture 521, 735059. doi: 10.1016/j.aquaculture.2020.735059
Paolacci S., Stejskal V., Toner D., Jansen M. A. K. (2022). Wastewater valorisation in an integrated multitrophic aquaculture system; assessing nutrient removal and biomass production by duckweed species. Environ. pollut. 302, 119059. doi: 10.1016/j.envpol.2022.119059
Park E., Cho M., Ki C. S. (2009). Correct use of repeated measures analysis of variance. Korean J. Lab. Med. 29 (1), 1–9. doi: 10.3343/kjlm.2009.29.1.1
Paw M. H., Koniuszy A., Galczynska M., Zajac G., Barglowicz J. S. (2019). Production of microalgal biomass using aquaculture wastewater as growth medium. Water 12 (1), 106. doi: 10.3390/w12010106
Perez-Velazquez M., Davis D. A., Roy L. A., Gonzalez-Felix M. L. (2012). Effects of water temperature and na+: k+ ratio on physiological and production parameters of Litopenaeus vannamei reared in low salinity water. Aquaculture 342, 13–17. doi: 10.1016/j.aquaculture.2012.02.008
Turkmen G. (2007). Experimental commercial growout of penaeus semisulcatus (Decapoda: penaeidae). Isr. J. Aquacult-Bamid. 59 (1), 52–57. doi: 10.46989/001c.20502
Van Rijn J. (2013). Waste treatment in recirculating aquaculture systems. Aquacult. Eng. 53, 49–56. doi: 10.1016/j.aquaeng.2012.11.010
Wang J. K. (2003). Conceptual design of a microalgae-based recirculating oyster and shrimp system. Aquacult. Eng. 28, 37–46. doi: 10.1016/S0144-8609(03)00020-7
Washitani Y., Hayakawa R., Li M., Shibata S. (2017). Comparing the survival rate of mangrove clam, polymesoda (Geloina) spp. (Solander 1876) through field experiments in mangrove forests of iriomote island. Zool. Sci. 34 (3), 223–228. doi: 10.2108/zs160021
Xiao R. C., Wei Y. G., An D., Li D. L., Ta X. X., Wu Y. H., et al. (2019). A review on the research status and development trend of equipment in water treatment processes of recirculating aquaculture systems. Rev. Aquacult. 11, 863–895. doi: 10.1111/raq.12270
Xie L. Q., Xie P., Li S. X., Tang H. J., Liu H. (2003). The low TN: TP ratio, a cause or a result of microcystis blooms? Water Res. 37 (9), 2073–2080. doi: 10.1016/S0043-1354(02)00532-8
Xu W. Q., Li R. H., Liu S. M., Ning Z. M., Jiang Z. J. (2017). The phosphorus cycle in the sanggou bay. Acta Oceanol. Sin. 36 (1), 90–100. doi: 10.1007/s13131-017-0997-z
Yang P., Zhao G. H., Tong C., Tang K. W., Lai D. Y. F., Li L., et al. (2021). Assessing nutrient budgets and environmental impacts of coastal land-based aquaculture system in southeastern China. Agr. Ecosyst. Environ. 322, 107662. doi: 10.1016/j.agee.2021.107662
Yang W., Zheng Z. M., Lu K. H., Zheng C., Du Y., Wang J. P., et al. (2020). Manipulating the phytoplankton community has the potential to create a stable bacterioplankton community in a shrimp rearing environment. Aquaculture 520, 734789. doi: 10.1016/j.aquaculture.2019.734789
Yu Z., Robinson S. M. C., Xia J., Sun H., Hu C. (2016). Growth, bioaccumulation and fodder potentials of the seaweed (Sargassum hemiphyllum) grown in oyster and fish farms of south China. Aquaculture 464, 459–468. doi: 10.1016/j.aquaculture.2016.07.031
Zeng S. Z., Wei D. D., Hou D. W., Wang H. J., Liu J., Weng S. P., et al. (2021). Sediment microbiota in polyculture of shrimp and fish pattern is distinctive from those in monoculture intensive shrimp or fish ponds. Sci. Total Environ. 787, 147594. doi: 10.1016/j.scitotenv.2021.147594
Keywords: phosphorus recovery, emission reduction, culture model, microalgae, circular economy
Citation: Ma S, Dong X, Luo C, Zhao C and Xu J (2023) Microalgae-mediated tandem culture of shrimp and bivalve: an environmental and health co-benefits solution for phosphorus recovery and emission reduction. Front. Mar. Sci. 10:1163640. doi: 10.3389/fmars.2023.1163640
Received: 11 February 2023; Accepted: 26 June 2023;
Published: 18 July 2023.
Edited by:
Jose Luis Iriarte, Austral University of Chile, ChileReviewed by:
Alaa El Din Mahmoud, Alexandria University, EgyptWenguang Zhou, Nanchang University, China
Copyright © 2023 Ma, Dong, Luo, Zhao and Xu. This is an open-access article distributed under the terms of the Creative Commons Attribution License (CC BY). The use, distribution or reproduction in other forums is permitted, provided the original author(s) and the copyright owner(s) are credited and that the original publication in this journal is cited, in accordance with accepted academic practice. No use, distribution or reproduction is permitted which does not comply with these terms.
*Correspondence: Jilin Xu, eHVqaWxpbkBuYnUuZWR1LmNu