- 1School of Ocean, Yantai University, Yantai, China
- 2Frontiers Science Center for Deep Ocean Multispheres and Earth System, and Key Laboratory of Marine Chemistry Theory and Technology, Ministry of Education, Ocean University of China, Qingdao, China
- 3College of Chemistry and Chemical Engineering, Ocean University of China, Qingdao, China
Removing fluoroquinolones (FQs) in marine culture tailwater is crucial for the coastal marine environment. The application of a bacteria-based microrobot for FQ removal was discussed. Norfloxacin (NOR) and levofloxacin (LEV) had static maximum adsorption capacities of 114.8 and 49.4 mg/g, respectively, by a magnetic microrobot. The experimental results of NOR adsorption by a magnetic microrobot were well supported by the Langmuir isotherm and Elovich kinetic models. Both the Langmuir isotherm model and the pseudo-second-order kinetic model may be able to accurately represent the LEV adsorption process. The mass transfer mechanism of the NOR and LEV adsorptions was divided into two steps and described better using the intraparticle diffusion (IPD) model. The exothermic and spontaneity of the sorption process were demonstrated through the study of thermodynamics. The magnetic microrobot’s heterogeneous surface was validated by the examination of site energy distribution. Additionally, this study demonstrated that the majority of the NOR and LEV sorption took place at sites with energies over 4.25 and 17.36 kJ/mol, respectively, supporting the notion that NOR and LEV adsorption constitute physical–chemical processes. Based on the above results, a magnetic microrobot, as a new-style green bio-adsorbent, can potentially be used to remove NOR and LEV from the mariculture in an inexpensive and effective manner.
1 Introduction
Fluoroquinolones (FQs) are extensively consumed for disease prevention and growth promotion in animal breeding and aquaculture (Coffie et al., 2021). Mariculture tailwater has drawn much attention, as it brings serious eutrophication offshore and damage to the surrounding estuary ecosystems (Gao et al., 2022). Also, some of the FQs were officially detected in the mariculture system around the world (Wang et al., 2022). Most of the FQs cannot be metabolized or absorbed by the body, and so enter into the environment instead (Oberoi et al., 2019). They have also been recognized as new toxic organic pollutants in the aquatic environment (Saya et al., 2022). Antibiotic residues in water may endanger the ecosystem and human health because they can cause central nervous system stimulation, convulsions, ocular problems, inhibition of the neurotransmitter gamma-aminobutyric acid, reproductive dysfunction, and gastrointestinal disturbances (Saya et al., 2022). It is imperative to develop a green and effective technology to remove antibiotic residues from the aquatic environment.
When FQs enter the environment, they undergo migration and transformation processes such as adsorption, hydrolysis, photolysis, and biodegradation (Sarmah et al., 2006; Patel et al., 2019; Yang et al., 2021). While FQs are relatively stable in water they are not particularly suitable for hydrolysis (Van Doorslaer et al., 2014; Patel et al., 2019). The photolysis process is much easier to carry out (Wammer et al., 2013; Lakshmi Prabavathi and Muthuraj, 2019), but will produce active free radicals and cause secondary pollution. Therefore, adsorption is the primary way of removing FQs in land-based mariculture tailwater since the biodegradation rate of FQs is very low (Oberoi et al., 2019; Saya et al., 2022). Many absorbents have been confirmed to remove FQs from aqueous media, such as clay minerals (Hacıosmanoglu ˘ et al., 2022), biochar (Cheng et al., 2021), activated carbon (Kah et al., 2017) and other carbonaceous materials (Scaria et al., 2022), plastic polymers (Rai et al., 2022), graphene-based nanomaterials (Wang et al., 2019a), and Fe-oxide-based composites (Chu et al., 2022). Some green materials, such as plant leaves, fruit, algae, fungi, and bacteria, were employed to achieve energy-efficient, cost-effective, and environmentally friendly removal of organic pollutants (Altaf et al., 2021). Moreover, the favored adsorption has some strengths, such as a simple operation process, high treatment efficiency, rich adsorbent materials, and no highly toxic by-products (Fang et al., 2021b; Hacıosmanoglu ˘ et al., 2022).
Magnetic substrate-loaded adsorbent has been employed in the removal of FQs with easy regeneration (Fang et al., 2021a). Magnetic micro/nanorobots have piqued the interest of researchers because of their enormous potential for use in biomedicine and environmental remediation (Zhou et al., 2021). In addition to their bio-friendliness, recoverability, and toxin-free nature due to magnetic manipulation, they can actively swim around aquatic contaminants and remove them via capture (adsorption/absorption) or degradation (Zhang et al., 2018). Owing to the strong dynamic intermixing, also known as the magnetic stirring function, the magnetic fields can also be used to speed up reaction kinetics or increase recognition efficiency. They can also be used to retrieve nano/microrobots after the cleaning process is finished (Ji et al., 2020). The magnetic micro/nanorobots will eventually be able to be recycled or reused without their parts being altered. To deal with oil spills, researchers designed a micromotor that moved like a walnut and was made of polycaprolactone, Fe3O4 nanoparticles, and catalase in a solution with H2O2. The micromotor could be navigated and recycled using an external magnetic field (Wang et al., 2019b). Porous biohybrid microrobots made of fungal spores and Fe3O4 nanoparticles were found to be very effective at removing heavy metal ions. The group behaviors and magnetically driven movement of the microrobots may enhance pollutant adsorption more than static microrobots (Zhang et al., 2018). Carbon soot-based micromotors were simultaneously activated by a magnetic field and oxygen microbubbles, allowing for efficient on-the-spot degradation of methyl blue (MB) dye contamination (Singh et al., 2020). Additionally, it has been reported that functional magnetic micromotors were powerful enough to absorb or remove antibiotics from contaminated water (Li et al., 2019b; Liu et al., 2019).
In a previous study, we made magnetic microrobots with iron (II, III) oxide nanoparticles (Fe3O4 NPs) based on hydrocarbon-degrading bacteria (Pi et al., 2022). The magnetic microrobot performed excellently for Congo red adsorption from the aquatic phase (Pi et al., 2022). Owing to FQs having a similar benzene ring structure to that of organic dye, the removal of FQs with the help of a magnetic microrobot was the goal of this work. Norfloxacin (NOR) and levofloxacin (LEV) are FQs that are used widely, and the concentration of ng/L to μg/L has been detected in different aqueous environments (Fang et al., 2021a, b; Yan et al., 2017a; Yan et al., 2017b). They were used as typical FQs in this work. Adsorption capacity, kinetics, isotherm equilibrium, site energy distribution analysis, and mechanism were used to investigate NOR’s and LEV’s removal potential. Adsorption parameters, which corresponded to a specific site energy distribution, were also determined using a variety of isotherm models. Owing to their magnetic characteristics, microrobots were easy to navigate and were recycled under a magnetic field.
2 Materials and methods
2.1 Materials
Without further purification, all chemicals are at an analytical grade. The bacteria were isolated from an antibiotic-producing pharmaceutical factory’s sewage outlet. Our previous research serves as a reference for the isolation procedure (Pi et al., 2015).
2.2 Synthesis of microrobots
The microrobot can be simply defined as a nanocluster magnetite (Fe3O4) attached to bacteria. The Fe3O4 was prepared in accordance with previous studies (Schwertmann and Cornell, 2000; Jiang et al., 2018; Jiang et al., 2020). The preparation of magnetic microrobots is referred to in the literature (Pi et al., 2022; Vaghasiya et al., 2022). The details of the characterization of bio-based materials were also demonstrated according to the field emission scanning electron microscopy (FESEM), transmission electron microscopy (TEM), fourier transform infrared (FTIR), and X-ray diffraction (XRD), nitrogen adsorption-desorption isotherms, magnetic behaviors, and point of zero charges (Pi et al., 2022).
2.3 Adsorption experiments
All adsorption experiments were conducted using 50-mL glass tubes in accordance with our previous work (Wang et al., 2021; Pi et al., 2022). To investigate the adsorption isotherm equilibrium, 50.0 mL of a NOR/LEV solution was mixed with 5 mg Fe3O4 or a magnetic microrobot, with initial NOR/LEV concentrations ranging from 10 to 50 mg/L. In addition, 0.1 mol/L NaOH and/or 0.1 mol/L HCl solutions were used to adjust the NOR/LEV solutions’ pH. The tubes were shaken at 150 rpm at a controlled temperature using a shaker in the dark. Then, the pH value (4–10), the adsorbent dose, and the adsorption temperature were changed to obtain the adsorption capacity.
In the kinetics experiments, a 500-mL NOR/LEV solution was mixed with moderate adsorbents. The NOR/LEV solution was measured using high-performance liquid chromatography (HPLC) after being adsorbed for various amounts of time. To avoid light, all samples were shaken in the dark. Each experiment was replicated three times.
Ultraviolet-(UV-)HPLC (1260, Agilent Technologies) was utilized for the detection of NOR and LEV. With a mobile phase of 60:40 (v/v) acetonitrile anhydrous and formic acid at 1% in deionized water and a flow rate of 0.75 mL/min, the HPLC was equipped with a C18 column (2.7 m, 4.6 mm × 100 mm). The wavelengths used for UV detection were 278 nm (NOR) and 293 nm (LEV).
2.4 Methods of data analysis
2.4.1 Equilibrium adsorption capacity
The following formulae (1–3) were used to calculate the NOR/LEV removal efficiency (adsorption percentage), adsorption capacities at time t, and equilibrium:
where R is the NOR/LEV’s removal efficiency; C0 (mg/L), Ce (mg/L), and Ct (mg/L) are the concentrations of the initial, equilibrium, and time t of the NOR/LEV, respectively; the NOR/LEV’s adsorption capacities at time t and equilibrium are qt (mg/g) and qe (mg/g); and the adsorbent’s mass is w (g) and V (L) is the volume of the solution (Pi et al., 2022).
2.4.2 Fitting of adsorption isotherms
The empirical relationships between adsorption capacities of NOR/LEV on Fe3O4 and the magnetic microrobots with the equilibrium concentration in the liquid phase were fitted with Langmuir, Freundlich, Temkin (Pi et al., 2022), and Langmuir–Freundlich (Yan et al., 2017a; Yan et al., 2017b) isotherm (Carter et al., 1995) equations (4–7):
where KL (L/mg), KF [(mg/g) (L/mg)1/n], and KT (L/mg) are the adsorption coefficients for the Langmuir, Freundlich, and Temkin models, respectively. qm (mg/g) was the calculated maximum value of the adsorbents’ NOR/LEV adsorption capacity. The 1/nF indicates the Freundlich model parameter, while bT (L/mg) is the equilibrium constant for the adsorption of the Temkin model (Yan et al., 2017a; Yan et al., 2017b). b (L/mg) is the equilibrium constant for the adsorption of the Langmuir–Freundlich model.
2.4.3 Fitting of adsorption kinetics
The pseudo-first-order, pseudo-second-order, and Elovich kinetic models were used to establish the rate-governing adsorption process, as shown below (8–10) (Sun et al., 2022):
where k1 (h–1) and k2 (g/mg·h) are the pseudo-first-order and pseudo-second-order rate constants, respectively. In the Elovich model, α and β are constants.
2.4.4 Mass transfer mechanisms
The adsorption process’s speed-limiting steps were investigated using intraparticle diffusion (IPD) (Wang et al., 2010) and liquid film diffusion (LFD) models.
The LFD and IPD models were expressed as:
The constant for the diffusion rate of a liquid film is k3 (min−1), and F is the qt/qe. The IPD diffusion rate constant is kIPD [mg/(g·min1/2)], and the linear intercept c (mg/g) provides information about the thickness of the boundary layer (Foroutan et al., 2021).
2.4.5 Thermodynamic calculation
The following equations were used to calculate the thermodynamic parameters to ascertain the nature of the adsorption process (13–15):
where the coefficient of the adsorption distribution is Kc (L/g). Entropy, enthalpy, and Gibb’s free energy are represented by ΔS (J/mol), ΔH (J/mol), and ΔG (J/mol·K), respectively. The universal gas constant is R (8.314 J/mol K) and the temperature is T (K).
2.4.6 Approximate site energy distribution
The adsorbent’s adsorption site energy distribution was linked to the equilibrium adsorption capacity (Carter et al., 1995). Using the parameters of the Langmuir–Freundlich model, the following equations (16,17) were used to determine the magnetic microrobot’s site energy distribution:
where Ce = Cs, Es is the adsorption energy, E* is the difference in adsorption energies between the adsorbate and solvent on the adsorbent surfaces, and F(E*) is an approximate site energy distribution function. The adsorbate’s maximum solubility is Cs (mg/L). The Cs value of NOR in water is 303.5mg/L, 400 mg/L, 522.1 mg/L, 678.2 mg/L, and 872.5 mg/L at 293.15 K, 298.15 K, 303.15 K, 308.15 K, and 313.15 K (pH=7), respectively (Ross and Riley, 1990; Blokhina et al., 2016). The Cs value of LEV in water is 46, 50, 54, 58, and 62 mg/mL at 293.15, 298.15, 303.15, 308.15, and 313.15 K, respectively (Ross and Riley, 1990; Blokhina et al., 2016).
The adsorption site energy distribution, which can be calculated using the following equation, was used to determine the average site energy:
Incorporating the above equation with Eq. (16) and Eq. (17), the average site energy could be determined as follows:
3 Results and discussion
3.1 Adsorption property for norfloxacin and levofloxacin
3.1.1 Effect of initial norfloxacin and levofloxacin concentration
The impact of the initial concentrations of NOR and LEV on the relative adsorption capacity and removal efficiency on the magnetic microrobot is illustrated in Figures 1A, B. The relative adsorption capacity increased with the initial concentration until 40 and 50 mg/L for NOR and LEV, respectively. The concentration gradient is a vital driving force between the liquid adsorbate and solid adsorbent. When it overwhelmed the resistance to bulk transfer between the adsorbate and adsorbent, the adsorption was enhanced as if the initial concentration of the adsorbate had increased (Altaf et al., 2021). Also, the limited adsorption site of the adsorbent reached a saturation state with the increase of the initial concentration. Thereafter at this point, the absorbent achieved the equilibrium, with the adsorption capacity up to 63.8 and 49.4 mg/g for NOR and LEV, respectively. The removal efficiency of NOR and LEV decreased gradually with the increase of the initial concentration. This can be attributed to the fixed amount of adsorbent dosage in this experiment.
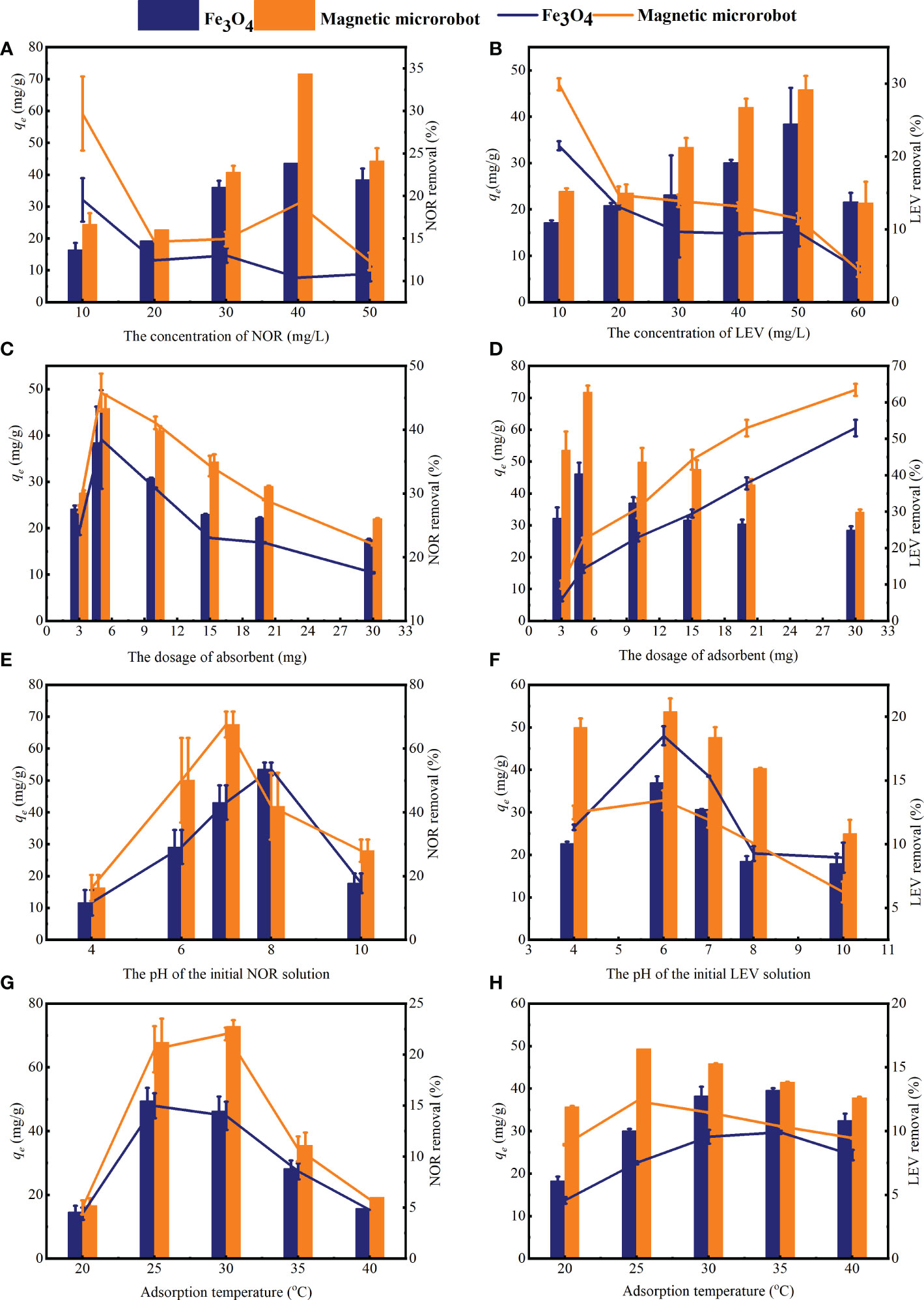
Figure 1 The adsorption properties and removal percentage of norfloxacin (NOR) and levofloxacin (LEV) on Fe3O4 and the magnetic microrobot. (A, B) The initial concentrations of NOR and LEV, (C, D) the adsorbent dosages, (E, F) the initial pH of NOR and LEV, and (G, H) the adsorption temperature. Note: the columns represent the adsorption capacity and the lines represent the removal efficiency.
3.1.2 Effects of adsorbent dosage
The effect of the adsorbent dose on the adsorption capacity and removal efficiency is presented in Figures 1C, D. When the dosage increased from 3 to 30 mg, the removal efficiency increased significantly due to the increase in the number and surface area of the active adsorption sites (Altaf et al., 2021; Fang et al., 2021). Also, the aggregation of the adsorbents resulting from a high dose of nanoparticles enhanced the removal efficiency (Altaf et al., 2021), while aggregation led to a decrease in the total surface area of the adsorbent (Fang et al., 2021b). The adsorption capacity increased with the increasing dosage, from 3 to 5 mg, peaking at 5 mg. The high adsorption capacity at 5 mg could be attributed to the fulfillment of most binding sites and an increase in total surface area (Zhou et al., 2018). Then, there was a decline when the adsorbent dosage was larger than 5 mg, indicating that the absorbent achieved equilibrium. While the capacity was calculated using the relative adsorption dose, the value declined according to the increased dose of the adsorbent.
3.1.3 Effect of pH
The pH of the solution, which plays a crucial role in the adsorption process, can alter the adsorbents’ surface charges and adsorbate ionization, affecting the adsorption capacity even more (Fang et al., 2021a). Because they have two distinct acid dissociation (pKa1 and pKa2) from the carboxyl group and the amino group,respectively, the form and adsorption effects of NOR and LEV were determined by the pH value of the solution, as shown in Figure 2. If the solution pH was< pKa1, -COOH and -NH2+- existed in the molecular of the cationic form. When the solution pH was > pKa2, -COO- and -NH- existed in the molecular in anionic form. When pKa1< pH< pKa2, then NOR and LEV were in the zwitterionic form with -COO- and -NH2+- existed in the molecular form.
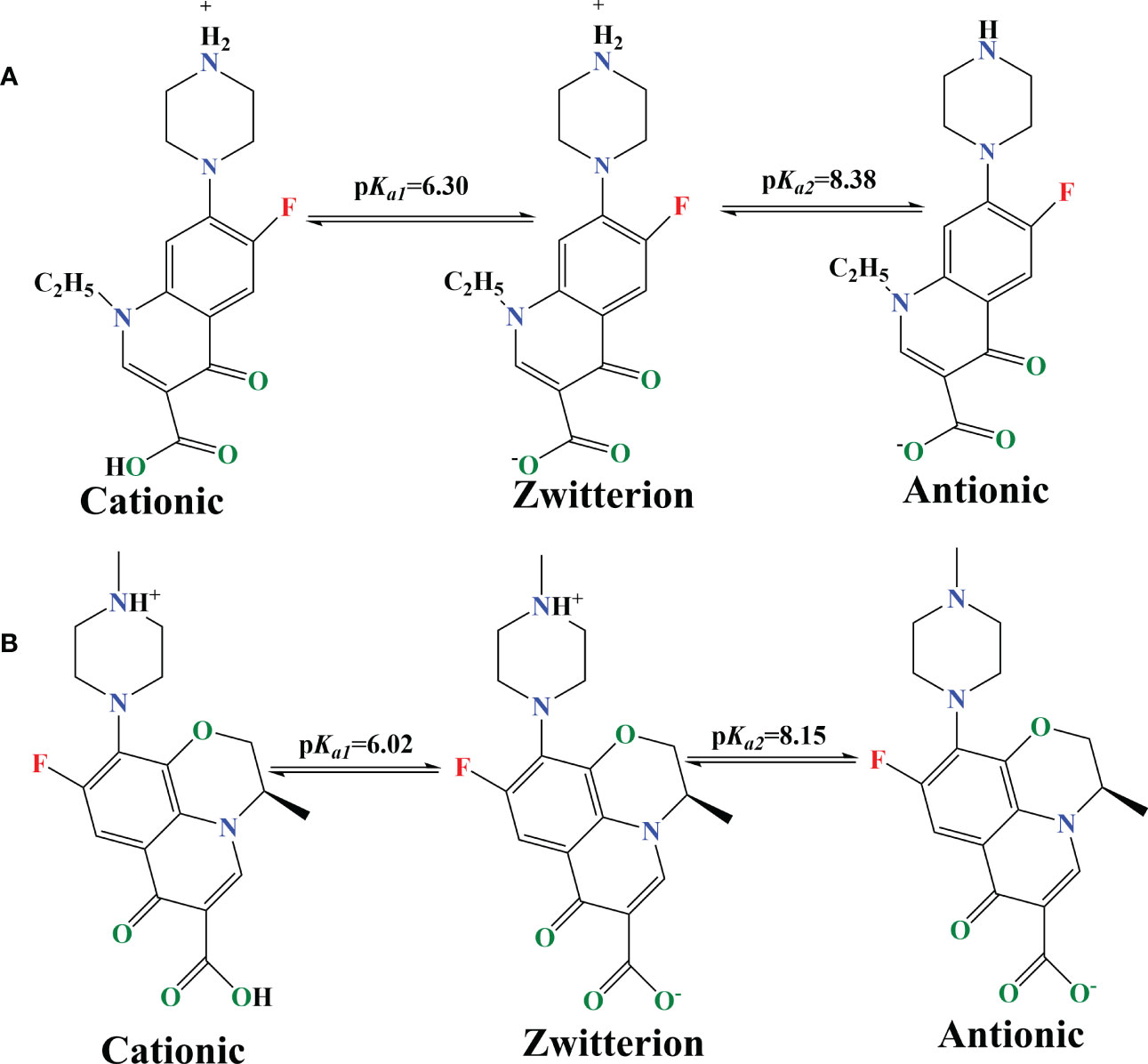
Figure 2 Molecular structure and pH-dependent speciation of (A) NOR (norfloxacin) and (B) LEV (levofloxacin).
Figures 1E, F depict the adsorption capacity and removal efficiency onto a magnetic microrobot at various initial pH levels, from 4 to 10. It can be seen that the adsorption limit and expulsion proficiency under similar circumstances were NOR > LEV. The adsorption capacity and removal efficiency of the capacity increased with the pH ranging from 4 to 7 for NOR, peaking at pH = 7. The LEV adsorption had a similar trend to that of NOR, with the highest adsorption capacity at pH = 6. The removal capacities were 63.8 and 49.2 mg/g, respectively. The adsorption capacity and removal efficiency significantly declined as the pH increased from 7 to 10. In general, the adsorbents’ uptake of FQs was higher under pKa1< pH< pKa2 than acid or alkaline conditions, which was related to the properties of the FQs in zwitterionic form. The results are consistent with the studies conducted by Altaf et al. (2021). The adsorption capacity reached its maximum value at a pH of approximately 7, which is consistent with the structural characteristics of zwitterionic FQs (Yan and Niu, 2017; Yan and Niu, 2018), and the quantity of zwitterionic FQs gradually increased with pH, implying that FQ adsorption results from complementary ion–pair interactions (Jiang et al., 2021). According to the research, Fe3O4 makes a passive oxide layer by coating on the surface when the pH is > 7 and reduces the FQ adsorption from the magnetic microrobot (Rezaei and Vione, 2018).
3.1.4 Effect of temperature
The adsorption performances of NOR and LEV by magnetic microrobot at various temperatures are presented in Figures 1G, H, respectively. The highest adsorption capacity and removal efficiency were achieved at 30°C for NOR and at 25°C for LEV. The interaction between FQs and absorbents was enhanced due to the active site expansion, when the adsorption temperature increased from 20°C to 30°C for NOR and 25°C for LEV (Altaf et al., 2021). While the adsorption temperature continued rising, the updraft movement of FQs was enhanced, which weakened the attraction between active adsorption sites and themselves, leading to the decline of the adsorption capacity and removing efficiency (Chen et al., 2011).
3.2 Adsorption isotherms
The experimental data and model-fitted findings for NOR and LEV adsorption from a magnetic microrobot are shown in Table 1. The non-linearity of the NOR and LEV adsorption isotherms on the absorbents suggests that the NOR and LEV concentrations and distributional effects were related to the magnetic microrobot’s adsorption capacity (Sun et al., 2022). At various temperatures, the R2 values of the Langmuir isotherm model were greater than those of the Freundlich, Temkin, and Langmuir–Freundlich isotherm models, indicating that NOR and LEV mostly adsorb onto the absorbents in monolayers. In the Freundlich model, nF is the parameter relating to the mean energy of adsorption. If nF> 1, it is preferred that adsorption is chemisorption in nature, and a strong adsorbent/adsorbate interaction occurs (Lv et al., 2020; Gaho et al., 2022). The calculated values of nF were all larger than 1, indicating the chemisorption of NOR and LEV on the absorbents.
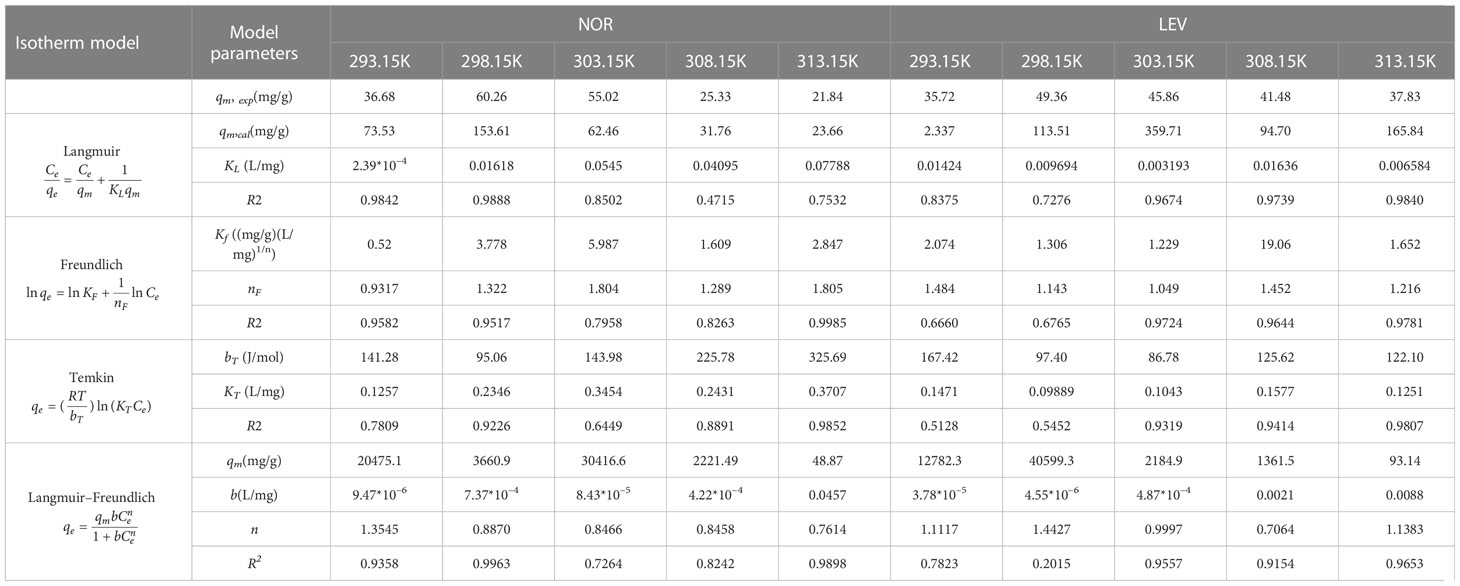
Table 1 Adsorption equilibrium isotherm models on norfloxacin (NOR) and levofloxacin (LEV) using a magnetic microrobot.
Many different adsorbents were listed in the comparison of the adsorption capacity with magnetic microrobots. As shown in Table 2, the Qm for NOR was 698.6 mg/g by FRMB consisting of SDS (Li et al., 2019a). The Qmof Fe3O4-based adsorbents (5-IOW and 14-IOW) for NOR (8.64 and 6.48 mg/g) were significantly lower than those of the magnetic microrobot. The Qm,exp of the magnetic microrobot for NOR was much larger than for most other adsorbents (Table 2). The Qm for LEV was 298.43 mg/g by PCS-KOH (Yang et al., 2020), which was much higher than for the other absorbents. The Qmof the Fe3O4-based adsorbent (Fe3O4-gINPs) for LEV was 22.47 mg/g, which was much lower than that of the magnetic microrobot. Compared with other adsorbents, a magnetic microrobot can be used as one of the markedly superior adsorbent materials in wastewater treatment containing NOR or LEV.
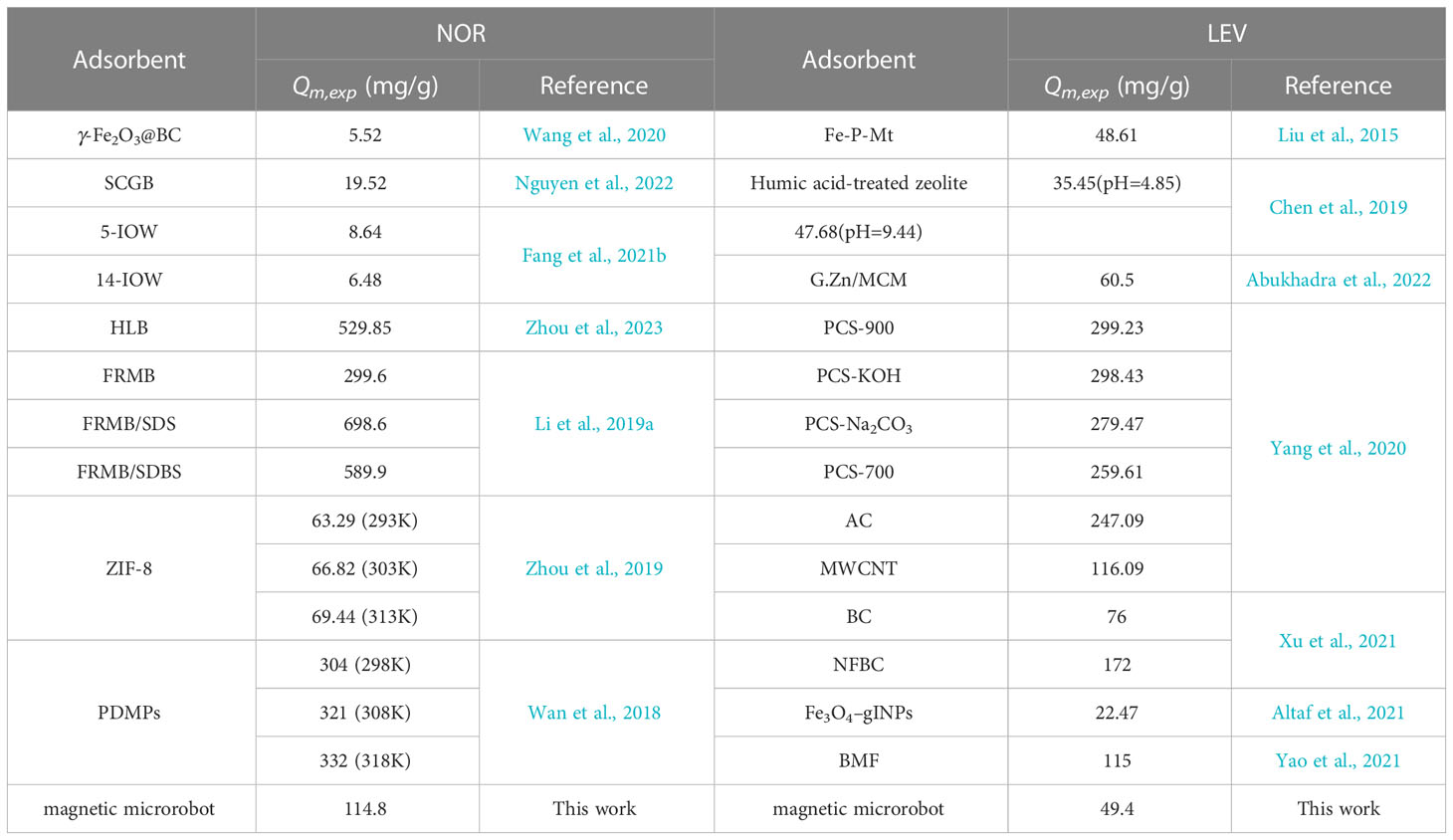
Table 2 Comparison of the adsorption capacities of different adsorbents for the removal of norfloxacin (NOR) and levofloxacin (LEV) at ambient temperatures (25°C).
3.3 Adsorption kinetics
The kinetic data of NOR and LEV fitted with three kinetic models are presented in Figure 3 and Table 3. Based on Figure 3 and the high R2 values in Table 3, the adsorption of NOR fitted the Elovich kinetic model well, while the adsorption of LEV fitted the pseudo-second-order model well. The Elovich model was suitable for simulating the adsorption of NOR, showing that it is the valence electron, rather than just the electrostatic attraction, that causes the adsorption of NOR on to magnetic microrobots (Sun et al., 2022). The main process involved in LEV kinetic adsorption was chemisorption due to the pseudo-second-order model (Sun et al., 2022).
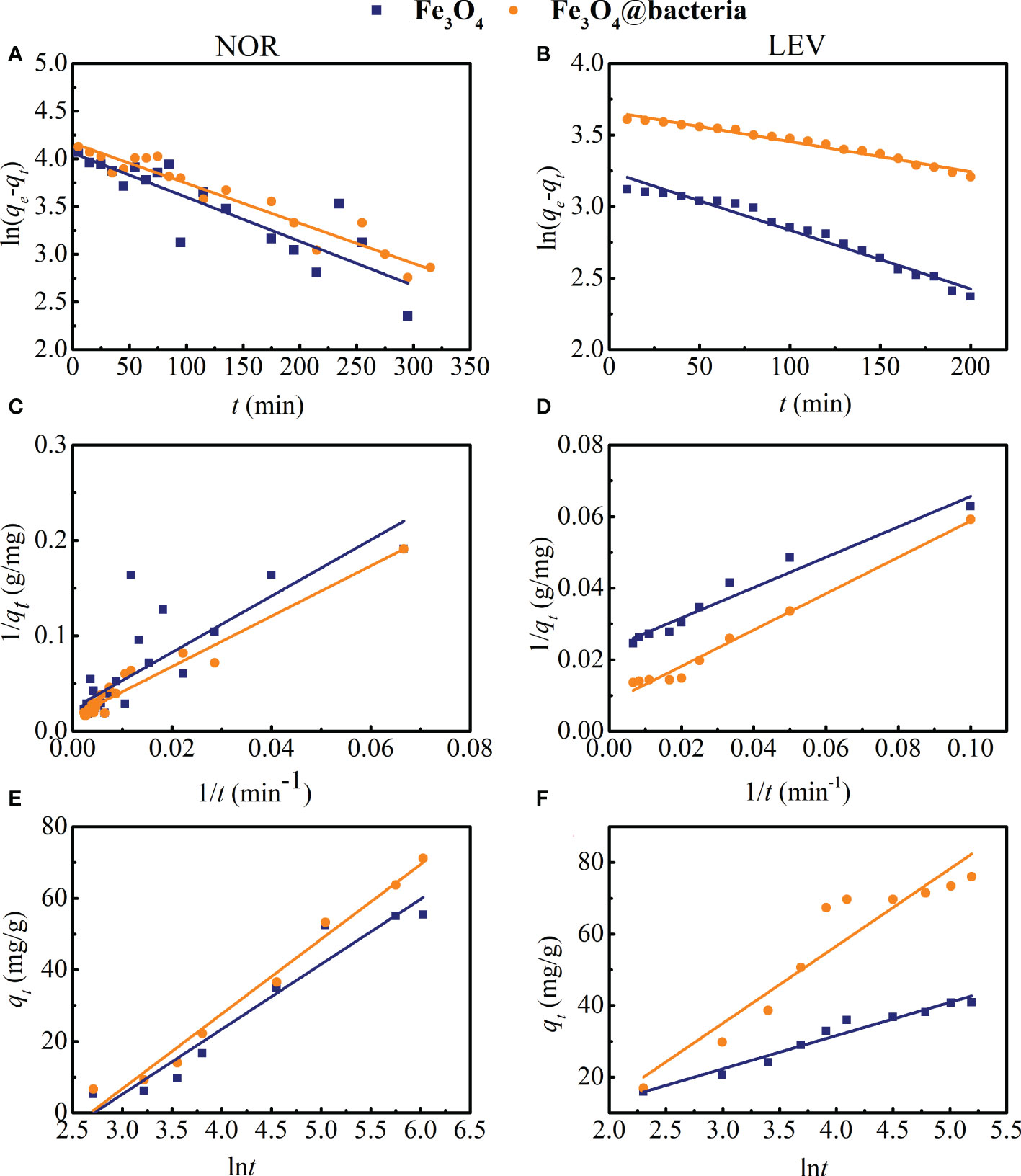
Figure 3 (A, B) Pseudo-first-order kinetic model, (C ,D) Pseudo-second-order kinetic model, and (E, F) Elovich kinetic model for norfloxacin (NOR) and levofloxacin (LEV) adsorption.
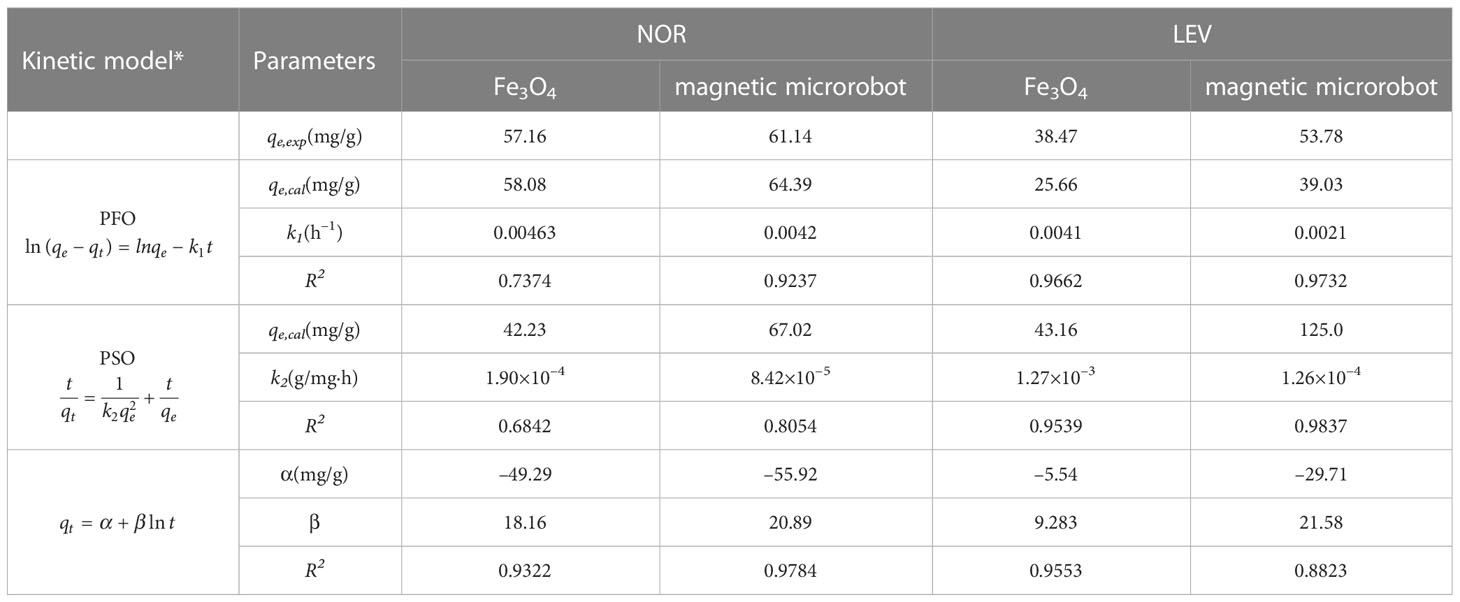
Table 3 Adsorption kinetic models on norfloxacin (NOR) and levofloxacin (LEV) using Fe3O4 and a magnetic microrobot.
3.4 Mass transfer mechanisms
According to temperature, pressure, and the types of adsorbent and adsorbate, varied mass transfer resistances may be able to limit the adsorption rate, which was evaluated using a kinetic analysis. The solid material was characterized by two primary resistances: protection from outer dissemination (interparticle), connected with mass exchange from mass liquid to the outside surface, and intraparticle dispersion, connected with mass exchange from an outside surface to an internal permeable construction. To investigate mechanisms and the potential rate-determining steps, several models have been proposed. Piecewise and linear regressions were used to fit the adsorption data using the IPD and LFD models to investigate the specific adsorption processes of NOR and LEV. The results are shown in Figure 4 and Table 4. The IPD model was better suited to represent the mass transfer process of NOR and LEV adsorption by Fe3O4 and a magnetic microrobot based on the statistical parameter (R2 > 0.90). From Figures 4A, B, the fact that the connection between qt and t1/2 is non-linear and does not pass through the origin point indicates that there are other rate-limiting steps besides IPD and that the rate of adsorption may potentially be controlled by an external diffusion mechanism. IPD and equilibrium are the two steps of the adsorption process. The adsorption rate of NOR and LEV was kIPD1 > kIPD2. The first stage was relatively slow, and the second stage represented the equilibrium condition for the adsorption–desorption process. The internal mass transfer restriction of the magnetic microrobot was stronger than that of Fe3O4 based on the fact that the R2 of the magnetic microrobot for NOR adsorption was higher than that of Fe3O4 (Yu et al., 2021). In conclusion, NOR and LEV were adsorbed on magnetic microrobots by a chemical process that was regulated simultaneously by internal and exterior diffusion processes.
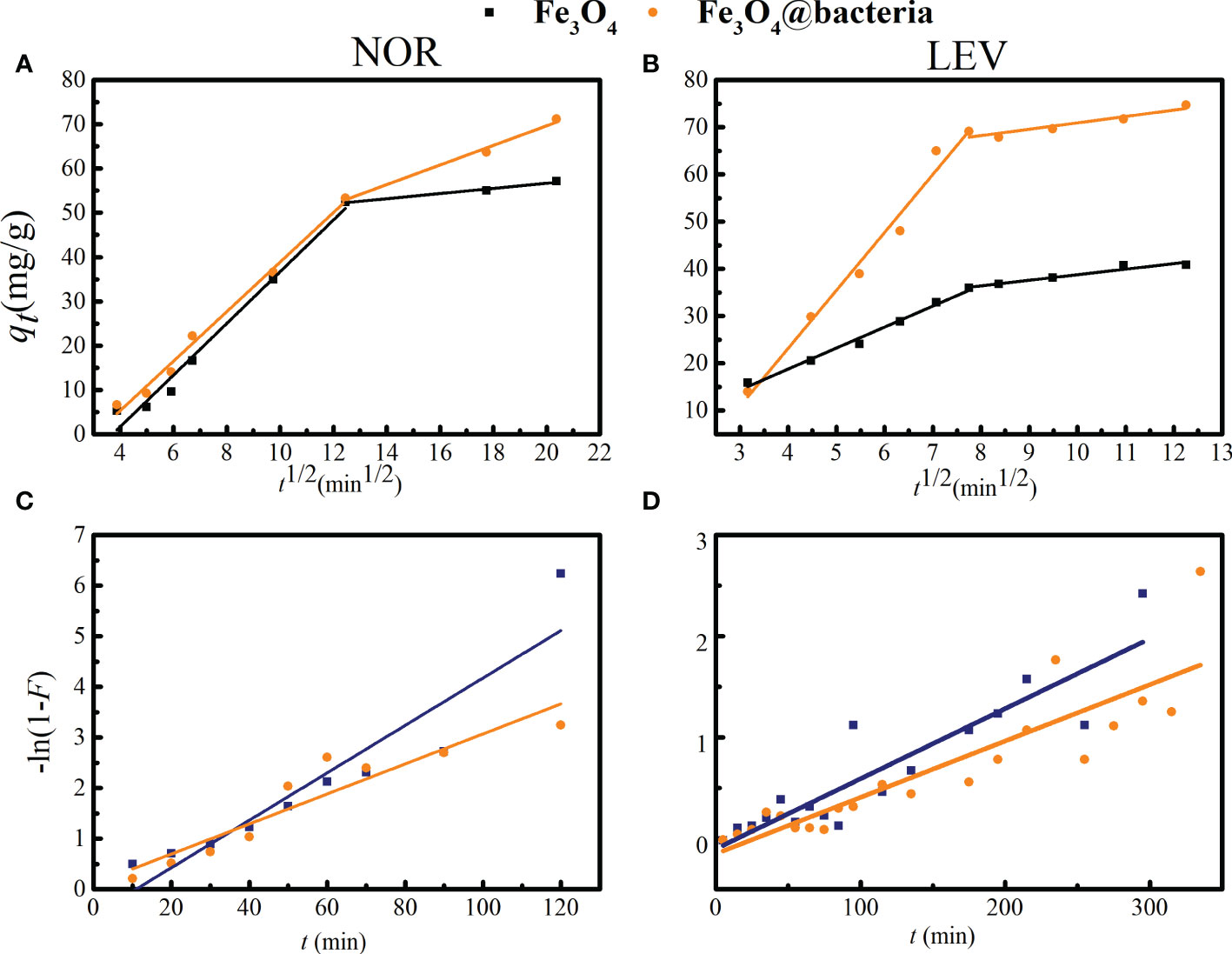
Figure 4 The particle diffusion model (A ,B) and LFD model (C, D) of norfloxacin (NOR) and levofloxacin (LEV) adsorption by Fe3O4 and a magnetic microrobot.
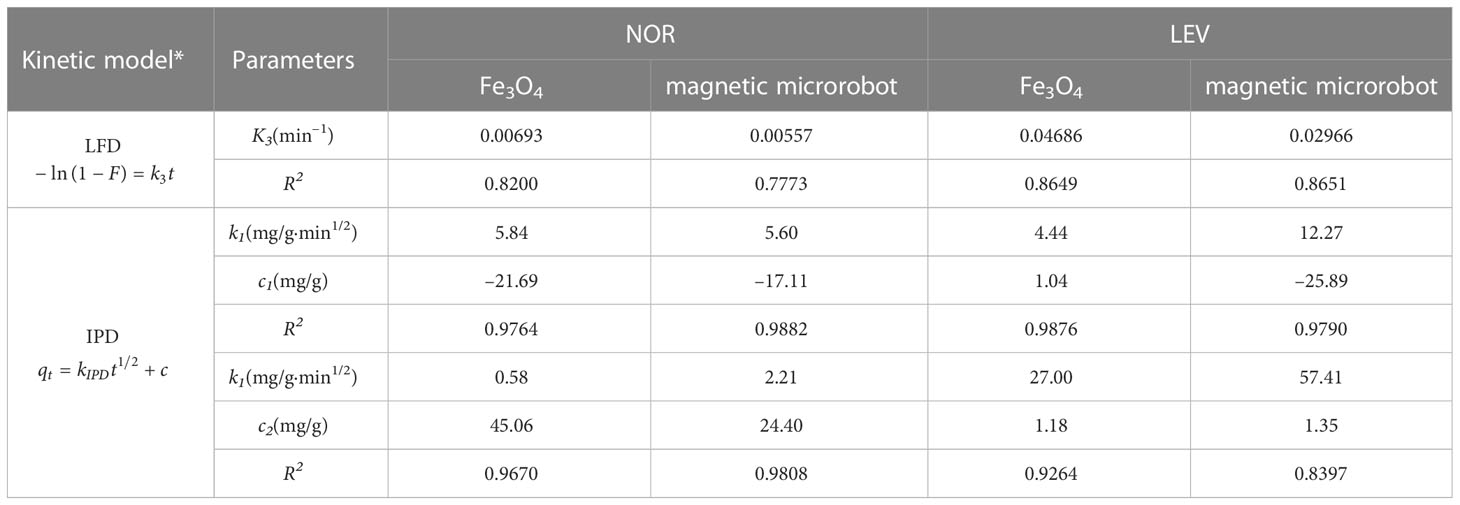
Table 4 Adsorption kinetic models on norfloxacin (NOR) and levofloxacin (LEV) using Fe3O4 and a magnetic microrobot.
3.5 Adsorption thermodynamics
To examine the adsorption thermodynamics of NOR and LEV on a magnetic microrobot, a range of temperatures, including 298, 303, 308, 313, and 318 K were chosen. It is clear from Table 5 that the adsorption of NOR had a negative ΔH value, which indicates that the process was exothermic and involved the adsorption of both chemical and physical substances. LEV adsorption by the magnetic microrobot was an endothermic process (ΔH>0), which shows that chemisorption may have occurred. ΔS was positive, demonstrating that the solid/solution interface contains additional unpredictability. It might be connected to the fact that the molecules of the displaced solvent (like water) gained more translational energy than the molecules of the adsorbate lost (de Andrade et al., 2018).
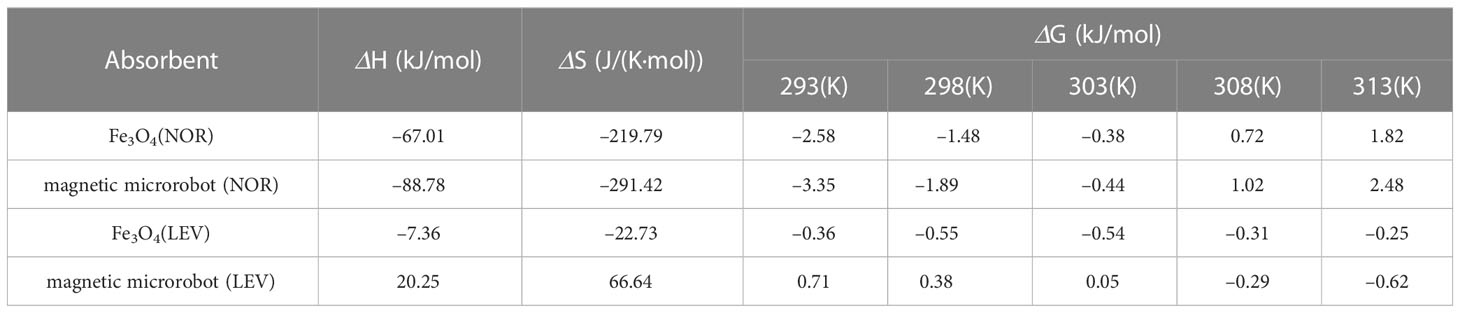
Table 5 Adsorption thermodynamic parameters of norfloxacin (NOR) and levofloxacin (LEV) adsorption by a magnetic microrobot.
To investigate the adsorption thermodynamics of NOR and LEV on magnetic microrobots, a range of temperatures, including 298, 303, 308, 313, and 318 K, were chosen. It can be seen in Table 5 that the ΔH of the adsorption of NOR was negative, demonstrating that both physical and chemical adsorption was involved in the exothermic process. For the LEV adsorption by a magnetic microrobot, it is an endothermic process (ΔH), which suggests that chemisorption may have occurred. Since ΔS was positive, the solid/solution interface must have had additional randomness. It could be related to the fact that the adsorbate molecules lost less translational energy than the displaced solvent molecules (like water) (de Andrade et al., 2018). ΔG< 0, which means that adsorption was advantageous and spontaneous at any temperature.
3.6 Adsorption site energy analysis
Based on Equation (17), the equilibrium NOR and LEV adsorption capacities qe are plotted as a function of the magnetic microrobot’s site energy E* values in Figure 5. The E* values decreased dramatically as the amount of NOR and LEV adsorbed on adsorbents increased. These findings demonstrated that NOR and LEV molecules occupied the magnetic microrobot’s high-energy adsorption sites first, and then the low-energy adsorption sites. After the adsorbate occupied the high-energy sites, low-energy sorption sites interacted with the NOR and LEV molecules as the solution concentration rose. This was in line with the earlier NOR adsorption on carbon nanotubes (Wang et al., 2010). On a magnetic microrobot, the values of NOR’s site energy E* were significantly lower than those of LEV’s. The E* values of NOR adsorbed on the magnetic microrobot fell in the range of 4.25 to 10.73 kJ/mol, and the E* values of LEV ranged from 17.36 to 21.22 kJ/mol. Additionally, the plots of E* showed an increase with temperature, indicating that the NOR and LEV adsorption on the magnetic microrobot benefited from higher temperatures. Temperature increased the rate of molecular diffusion, making it easier for NOR and LEV molecules to cross the magnetic microrobot’s external boundary layer and enter its internal pores.
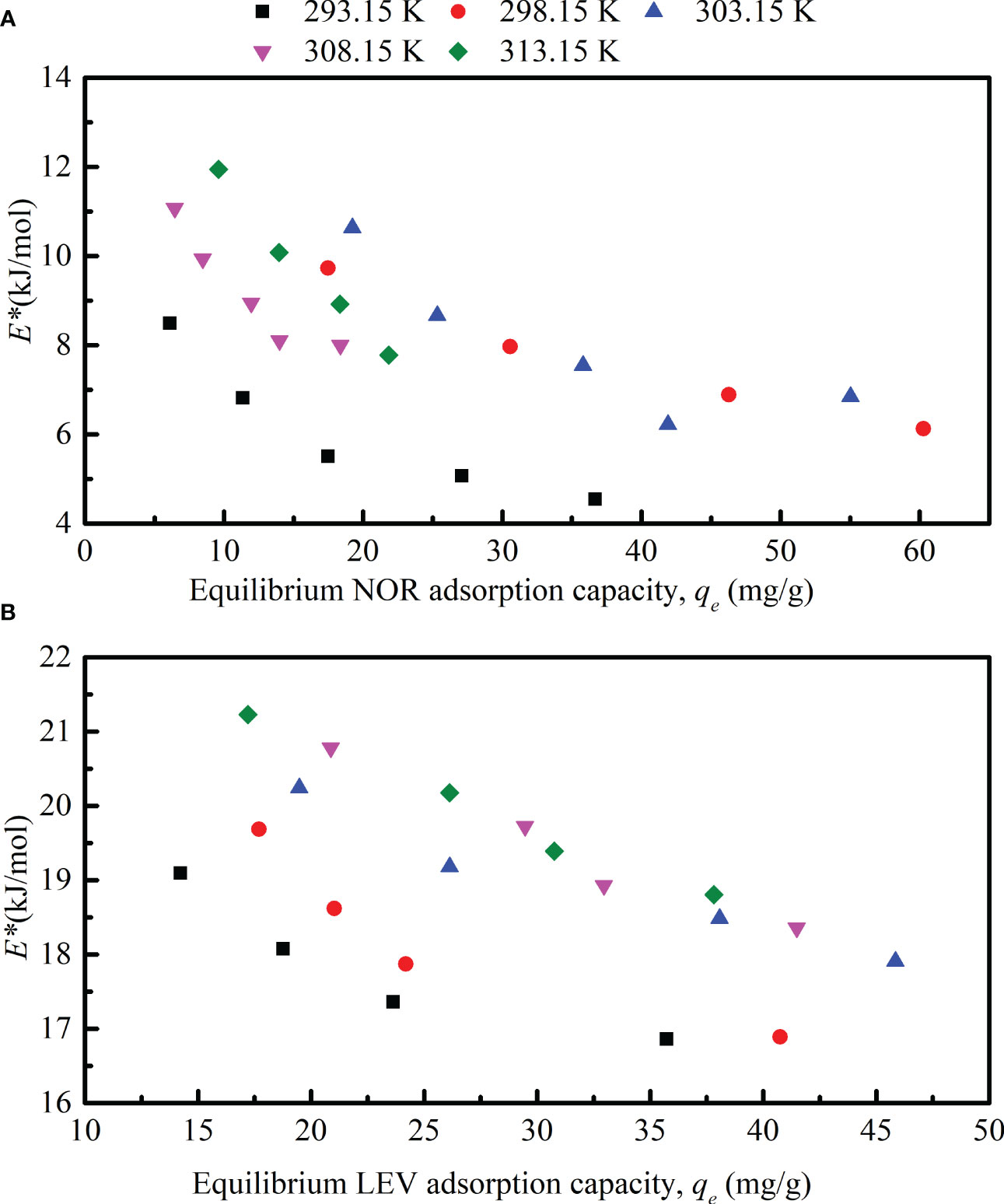
Figure 5 Site energy of norfloxacin (NOR) and levofloxacin (LEV) adsorption. (A) site energy versus equilibrium NOR adsorption capacity; (B) site energy versus equilibrium LEV adsorption capacity.
Based on the Langmuir–Freundlich isotherm model, the approximate site energy distribution (F(E*)) for the adsorption of NOR and LEV to the magnetic microrobot at various temperatures is depicted in Figure 6. For NOR and LEV, the site energy had unimodal distributions. Most of the NOR and LEV sorption occurred on the sites with energy over 4.0 kJ/mol and lower than 16.0 kJ/mol, indicating that NOR and LEV sorption on the biosorbent was a physical-chemical process. First, as the site energy (E*) increased, the frequency function F(E*) decreased until it was close to zero. The upward trend suggests that, at high concentrations, some NOR and LEV molecules were forced to occupy lower-energy sites. Conversely, at low-solution concentrations, NOR and LEV molecules were preferentially captured at the high-energy site (Zhang et al., 2021). Owing to the lower F(E*), however, the extremely high- or low- energy sites contributed very little to the maximum amount of adsorption (Liu et al., 2016).
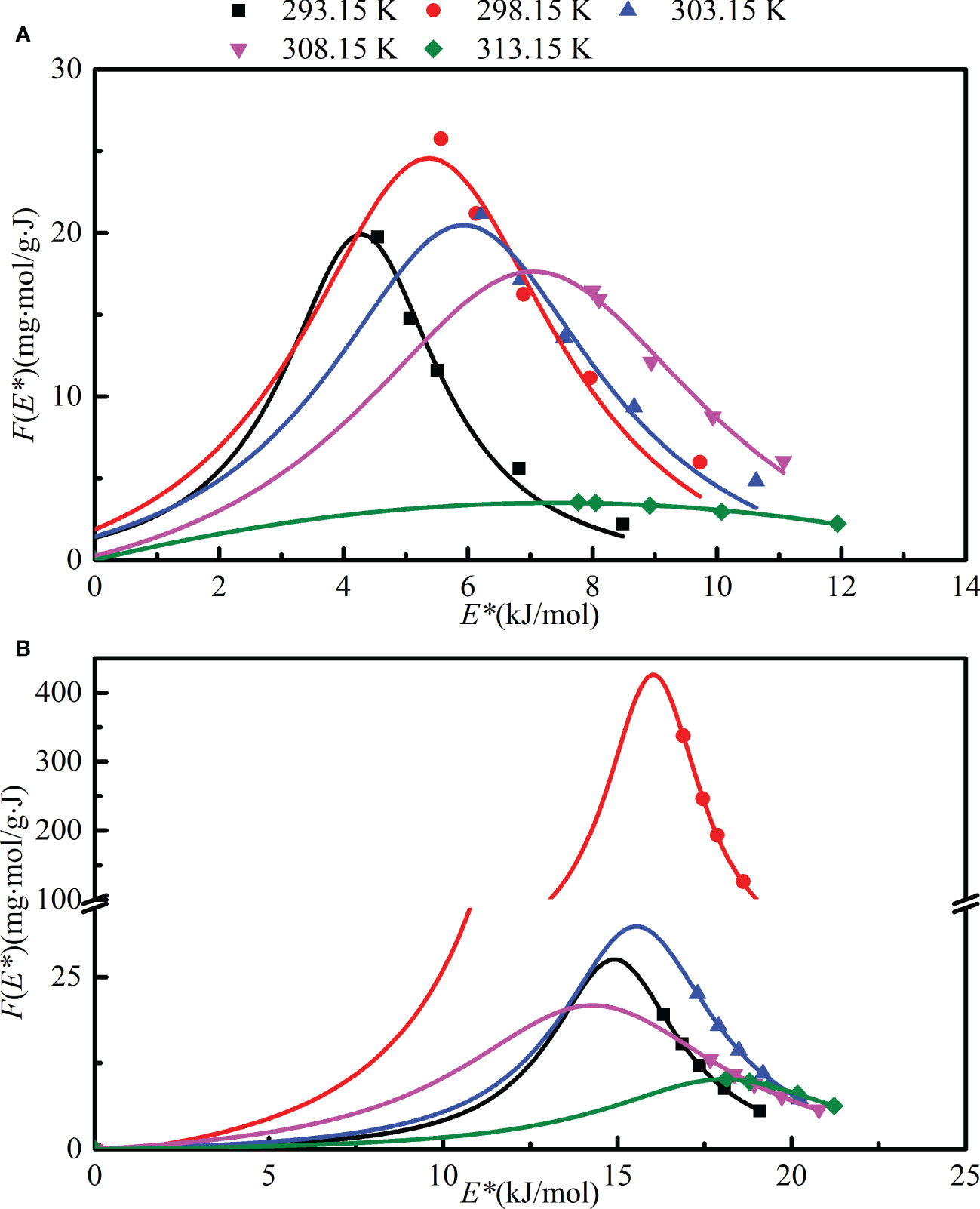
Figure 6 Site energy distribution of norfloxacin (NOR) and levofloxacin (LEV) adsorption on a magnetic microrobot at various temperatures. (A) Site energy distribution of NOR; (B) site energy distribution of LEV.
4 Conclusion
The magnetic microrobot demonstrated outstanding NOR and LEV adsorption capacities. The static maximum adsorption capacities of NOR and LEV on the magnetic microrobot were 114.8 and 49.4 mg/g, respectively. The Langmuir isotherm model and Elovich kinetic models agreed well with the experimental data of NOR adsorption by a magnetic microrobot. The Langmuir isotherm model and the pseudo-second-order kinetic model might be able to adequately describe the LEV adsorption procedure. The IPD was not the only mechanism regulating the adsorption process, and the IPD model was better suited to describe the mass transfer process of the NOR and LEV adsorption by magnetic microrobot. Exothermic and spontaneous characteristics were verified through thermodynamics. The biosorbents’ heterogeneous surface was verified by the analysis of site energy distribution. Additionally, Figure 6 demonstrated that the majority of the NOR and LEV adsorption took place on sites with greater than 4.25 kJ/mol and less than 17.36 kJ/mol, supporting the notion that this adsorption was physical–chemical in nature. Based on the findings, the magnetic microrobot can potentially be employed to inexpensively and efficiently remove NOR and LEV from aqueous wastewater as a new-style green bio-adsorbent.
Data availability statement
The original contributions presented in the study are included in the article/Supplementary Material. Further inquiries can be directed to the corresponding author.
Author contributions
YT: resources, funding acquisition, writing—reviewing and editing. WJ: investigation, methodology, and data handling. MB: writing—reviewing and editing. SQ: funding acquisition, writing—reviewing and editing. YP: conceptualization, resources, writing—reviewing and editing, supervision, funding acquisition. CL: investigation, methodology. JZ: methodology, resources, data handling. All authors contributed to the article and approved the submitted version.
Funding
This research was financially supported by the Shandong Provincial Natural Science Foundation (ZR2022QD018); the National Key R&D Program of China (2018YFD0900802); the National Innovation and Entrepreneurship Training Program for College Students (202111066013); the Yantai University Doctoral Start-up Foundation (HX2018B32); and the Shandong Key Laboratory of Marine Ecological Restoration (201919).
Conflict of interest
The authors declare that the research was conducted in the absence of any commercial or financial relationships that could be construed as a potential conflict of interest.
Publisher’s note
All claims expressed in this article are solely those of the authors and do not necessarily represent those of their affiliated organizations, or those of the publisher, the editors and the reviewers. Any product that may be evaluated in this article, or claim that may be made by its manufacturer, is not guaranteed or endorsed by the publisher.
Supplementary material
The Supplementary Material for this article can be found online at: https://www.frontiersin.org/articles/10.3389/fmars.2023.1169883/full#supplementary-material
References
Abukhadra M. R., AlHammadi A. A., Khim J. S., Ajarem J. S., Allam A. A. (2022). Enhanced decontamination of levofloxacin residuals from water using recycled glass based a green zinc oxide/mesoporous silica nanocomposite; adsorption and advanced oxidation studies. J. Clean. Prod. 356, 131836. doi: 10.1016/j.jclepro.2022.131836
Altaf S., Zafar R., Zaman W. Q., Ahmad S., Yaqoob K., Syed A., et al. (2021). Removal of levofloxacin from aqueous solution by green synthesized magnetite (Fe3O4) nanoparticles using Moringa olifera: kinetics and reaction mechanism analysis. Ecotox. Environ. Safe. 226, 112826. doi: 10.1016/j.ecoenv.2021.112826
Blokhina S. V., Sharapova A. V., Ol’khovich M. V., Volkova T. V., Perlovich G. L. (2016). Solubility, lipophilicity, and membrane permeability of some fluoroquinolone antimicrobials. Eur. J. Pharm. Sci. 93, 29–37. doi: 10.1016/j.ejps.2016.07.016
Carter M. C., Kilduff J. E., Weber W. J. (1995). Site energy distribution analysis of preloaded adsorbents. Environ. Sci. Technol. 29 (7), 1773–1780. doi: 10.1021/es00007a013
Chen Z. X., Jin X. Y., Chen Z., Megharaj M., Naidu R. (2011). Removal of methyl orange from aqueous solution using bentonite-supported nanoscale zero-valent iron. J. Colloid. Interface Sci. 363 (2), 601–607. doi: 10.1016/j.jcis.2011.07.057
Chen Z., Ma W., Lu G., Meng F., Duan S., Zhang Z., et al. (2019). Adsorption of levofloxacin onto mechanochemistry treated zeolite: modeling and site energy distribution analysis. Sep. Purif. Technol. 222, 30–34. doi: 10.1016/j.seppur.2019.04.010
Cheng N., Wang B., Wu P., Lee X., Xing Y., Chen M., et al. (2021). Adsorption of emerging contaminants from water and wastewater by modified biochar: a review. Environ. pollut. 273, 116448. doi: 10.1016/j.envpol.2021.116448
Chu D., Dong H., Li Y., Xiao J., Xiang S., Dong Q., et al. (2022). Insights into the correlation between different adsorption/oxidation/catalytic performance and physiochemical characteristics of fe-Mn oxide-based composites. J. Hazard. Mater. 439, 129631. doi: 10.1016/j.jhazmat.2022.129631
Coffie E. S., Xie L., Hassan M., Shi B., Ashraf G. A., Zhang B. (2021). Energy-positive removal of norfloxacin in the bioelectro fenton system with nanoferrite-based composite electrodes. Energ. Fuel. 35, 4502–4511. doi: 10.1021/acs.energyfuels.0c04170
de Andrade J. R., Oliveira M. F., da Silva M. G. C., Vieira M. G. A. (2018). Adsorption of pharmaceuticals from water and wastewater using nonconventional low-cost materials: a review. Ind. Eng. Chem. Res. 57, 3103–3127. doi: 10.1021/acs.iecr.7b05137
Fang N., He Q., Sheng L., Xi Y., Zhang L., Liu H., et al. (2021b). Toward broader applications of iron ore waste in pollution control: adsorption of norfloxacin. J. Hazard. Mater. 418, 126273. doi: 10.1016/j.jhazmat.2021.126273
Fang L., Miao Y. X., Wei D., Zhang Y., Zhou Y. C. (2021a). Efficient removal of norfloxacin in water using magnetic molecularly imprinted polymer. Chemosphere 262, 128032. doi: 10.1016/j.chemosphere.2020.128032
Foroutan R., Peighambardoust S. J., Peighambardoust S. H., Pateiro M., Lorenzo J. M. (2021). Adsorption of crystal violet dye using activated carbon of lemon wood and activated carbon/Fe3O4 magnetic nanocomposite from aqueous solutions: a kinetic, equilibrium and thermodynamic study. Molecules 26, 2241. doi: 10.3390/molecules26082241
Gaho M. M., Zuhra Memon G., Rehman Memon J., Arain J. B., Arain A. J., Shah A., et al. (2022). Synthesis of novel magnetic molecularly imprinted polymers by solid-phase extraction method for removal of norfloxacin. Chin. J. Anal. Chem. 50 (6), 100079. doi: 10.1016/j.cjac.2022.100079
Gao Y., Guo L., Jin C., Zhao Y., Gao M., She Z., et al. (2022). Metagenomics and network analysis elucidating the coordination between fermentative bacteria and microalgae in a novel bacterial-algal coupling reactor (BACR) for mariculture wastewater treatment. Water Res. 215, 118256. doi: 10.1016/j.watres.2022.118256
Hacıosmanoglu ˘ G. G., Mejías C., Martín J., Santos J. L., Aparicio I., Alonso E. (2022). Antibiotic adsorption by natural and modified clay minerals as designer adsorbents for wastewater treatment: a comprehensive review. J. Environ. Manage. 317, 115397. doi: 10.1016/j.jenvman.2022.115397
Ji F., Wang B., Zhang L. (2020). Light-triggered catalytic performance enhancement using magnetic nanomotor ensembles. Research 2020, 6380794. doi: 10.34133/2020/6380794
Jiang W., Cui W. R., Liang R. P., Qiu J. D. (2021). Zwitterionic surface charge regulation in ionic covalent organic nanosheets: synergistic adsorption of fluoroquinolone antibiotics. Chem. Eng. J. 417, 128034. doi: 10.1016/j.cej.2020.128034
Jiang F., Du R., Yan X., Zhang M., Han Q., Sun X., et al. (2020). Ferroferric oxide nanoclusters decorated Ti3C2Tx nanosheets as high performance anode materials for lithium ion batteries. Electrochim. Acta 329, 135146. doi: 10.1016/j.electacta.2019.135146
Jiang F., Liu Y., Wang Q., Zhou Y. (2018). Hierarchical Fe3O4@NC composites: ultralong cycle life anode materials for lithium-ion batteries. J. Mater. Sci. 53, 2127–2136. doi: 10.1007/s10853-017-1651-z
Kah M., Sigmund G., Xiao F., Hofmann T. (2017). Sorption of ionizable and ionic organic compounds to biochar, activated carbon, and other carbonaceous materials. Water Res. 124, 673–692. doi: 10.1016/j.watres.2017.07.070
Lakshmi Prabavathi S., Muthuraj V. (2019). Superior visible light driven photocatalytic degradation of fluoroquinolone drug norfloxacin over novel NiWO4 nanorods anchored on g-C3N4 nanosheets. Colloid. Surface. A. Physicochem. Eng. Asp. 567, 43–54. doi: 10.1016/j.colsurfa.2019.01.040
Li C., Gao Y., Li A., Zhang L., Ji G., Zhu K., et al. (2019a). Synergistic effects of anionic surfactants on adsorption of norfloxacin by magnetic biochar derived from furfural residue. Environ. Pollut. 254, 113005. doi: 10.1016/j.envpol.2019.113005
Li J., Ji F., Ng D. H. L., Liu J., Bing X., Wang P. (2019b). Bioinspired pt-free molecularly imprinted hydrogel-based magnetic janus micromotors for temperature-responsive recognition and adsorption of erythromycin in water. Chem. Eng. J. 369, 611–620. doi: 10.1016/j.cej.2019.03.101
Liu Y., Dong C., Wei H., Yuan W., Li K. (2015). Adsorption of levofloxacin onto an iron-pillared montmorillonite (clay mineral): kinetics, equilibrium and mechanism. Appl. Clay. Sci. 118, 301–307. doi: 10.1016/j.clay.2015.10.010
Liu J., Li J., Wang G., Yang W., Yang J., Liu Y. (2019). Bioinspired zeolitic imidazolate framework (ZIF-8) magnetic micromotors for highly efficient removal of organic pollutants from water. J. Colloid. Interface Sci. 555, 234–244. doi: 10.1016/j.jcis.2019.07.059
Liu F. F., Zhao J., Wang S., Xing B. (2016). Adsorption of sulfonamides on reduced graphene oxides as affected by pH and dissolved organic matter. Environ. pollut. 210, 85–93. doi: 10.1016/j.envpol.2015.11.053
Lv X., Yan D. Y. S., Lam F. L. Y., Ng Y. H., Yin S., An A. K. (2020). Solvothermal synthesis of copper-doped BiOBr microflowers with enhanced adsorption and visible-light driven photocatalytic degradation of norfloxacin. Chem. Eng. J. 401, 126012. doi: 10.1016/j.cej.2020.126012
Nguyen V. T., Vo T. D. H., Nguyen T. B., Dat N. D., Huu B. T., Nguyen X. C., et al. (2022). Adsorption of norfloxacin from aqueous solution on biochar derived from spent coffee ground: master variables and response surface method optimized adsorption process. Chemosphere 288, 132577. doi: 10.1016/j.chemosphere.2021.132577
Oberoi A. S., Jia Y., Zhang H., Khanal S. K., Lu H. (2019). Insights into the fate and removal of antibiotics in engineered biological treatment systems: a critical review. Environ. Sci. Technol. 53, 7234–7264. doi: 10.1021/acs.est.9b01131
Patel M., Kumar R., Kishor K., Mlsna T., Pittman C. U., Mohan D. (2019). Pharmaceuticals of emerging concern in aquatic systems: chemistry, occurrence, effects, and removal methods. Chem. Rev. 119, 3510–3673. doi: 10.1021/acs.chemrev.8b00299
Pi Y. R., Bao M. T., Li Y. M., Li G. M., Lu J. R., Sun P. Y. (2015). Characterization of crude oil degrading microbial cultures isolated in qingdao China. RSC. Adv. 5 (118), 97665–97674. doi: 10.1039/C5RA16628D
Pi Y., Duan C., Zhou Y., Sun S., Yin Z., Zhang H., et al. (2022). The effective removal of Congo red using a bio-nanocluster: Fe3O4 nanoclusters modified bacteria. J. Hazard. Mater. 424, 127577. doi: 10.1016/j.jhazmat.2021.127577
Rai P. K., Sonne C., Brown R. J. C., Younis S. A., Kim K. (2022). Adsorption of environmental contaminants on micro- and nano-scale plastic polymers and the influence of weathering processes on their adsorptive attributes. J. Hazard. Mater. 427, 127903. doi: 10.1016/j.jhazmat.2021.127903
Rezaei F., Vione D. (2018). Effect of pH on zero valent iron performance in heterogeneous fenton and fenton-like processes: a review. Molecules 23, 3127. doi: 10.3390/molecules23123127
Ross D. L., Riley C. M. (1990). Aqueous solubilities of some variously substituted quinolone antimicrobials. Int. J. Pharm. 63 (3), 237–250. doi: 10.1016/0378-5173(90)90130-V
Sarmah A. K., Meyer M. T., Boxall A. B. (2006). A global perspective on the use, sales, exposure pathways, occurrence, fate and effects of veterinary antibiotics (VAs) in the environment. Chemosphere 65, 725–759. doi: 10.1016/j.chemosphere.2006.03.026
Saya L., Malik V., Gautam D., Gambhir G., Balendra, Singh W. R., et al. (2022). A comprehensive review on recent advances toward sequestration of levofloxacin antibiotic from wastewater. Sci. Total. Environ. 813 (2022), 152529. doi: 10.1016/j.scitotenv.2021.152529
Scaria J., Gopinath A., Ranjith N., Ravindran V., Ummar S., Nidheesh P. V., et al. (2022). Carbonaceous materials as effective adsorbents and catalysts for the removal of emerging contaminants from water. J. Clean. Prod. 350, 131319. doi: 10.1016/j.jclepro.2022.131319
Schwertmann U., Cornell R. M. (2000). Iron oxides in the laboratory: preparation and characterization. 2nd ed. (New York, USA: Wiley-VCH).
Singh A. K., Bhuyan T., Maity S., Mandal T. K., Bandyopadhyay D. (2020). Magnetically actuated carbon soot nano-particle-based catalytic CARBOts coated with Ni/Pt nanofilms for water detoxification and oil-spill recovery. ACS Appl. Nano. Mater. 3, 3459–3470. doi: 10.1021/acsanm.0c00199
Sun M., Yang Y., Huang M., Fu S., Hao Y., Hu S., et al. (2022). Adsorption behaviors and mechanisms of antibiotic norfloxacin on degradable and nondegradable microplastics. Sci. Total. Environ. 807, 151042. doi: 10.1016/j.scitotenv.2021.151042
Vaghasiya J. V., Mayorga-Martinez C. C., Matějková S., Pumera M. (2022). Pick up and dispose of pollutants from water via temperature-responsive micellar copolymers on magnetite nanorobots. Nat. Commun. 13, 1026. doi: 10.1038/s41467-022-28406-5
Van Doorslaer X., Dewulf J., Van Langenhove H., Demeestere K. (2014). Fluoroquinolone antibiotics: an emerging class of environmental micropollutants. Sci. Total. Environ. 500, 250–269. doi: 10.1016/j.scitotenv.2014.08.075
Wammer K. H., Korte A. R., Lundeen R. A., Sundberg J. E., McNeill K., Arnold W. A. (2013). Direct photochemistry of three fluoroquinolone antibacterials norfloxacin, ofloxacin, and enrofloxacin. Water Res. 47, 439–448. doi: 10.1016/j.watres.2012.10.025
Wan Y., Liu X., Liu P., Zhao L., Zou W. (2018). Optimization adsorption of norfloxacin onto polydopamine microspheres from aqueous solution: kinetic, equilibrium and adsorption mechanism studies. Sci. Total. Environ. 639, 428–437. doi: 10.1016/j.scitotenv.2018.05.171
Wang X., Lin Y., Zheng Y., Meng F. (2022). Antibiotics in mariculture systems: a review of occurrence, environmental behavior, and ecological effects. Environ. pollut. 293, 118541. doi: 10.1016/j.envpol.2021.118541
Wang A., Liu C., Ge X., Meng W., Pi Y., Liu C. (2021). Enhanced removal of Congo red dye from aqueous solution by surface modified activated carbon with bacteria. J. Appl. Microbiol. 131, 2270–2279. doi: 10.1111/jam.15100
Wang X., Yin R., Zeng L., Zhu M. (2019a). A review of graphene-based nanomaterials for removal of antibiotics from aqueous environments. Environ. Pollut. 253, 100–110. doi: 10.1016/j.envpol.2019.06.067
Wang J., Zhang M., Zhou R., Li J., Zhao W., Zhou J. (2020). Adsorption characteristics and mechanism of norfloxacin in water by γ-Fe2O3@BC. Water Sci. Technol. 82, 242–254. doi: 10.2166/wst.2020.078
Wang D., Zhao G., Chen C., Zhang H., Duan R., Zhang D., et al. (2019b). One-step fabrication of dual optically/magnetically modulated walnut-like micromotor. Langmuir 35, 2801–2807. doi: 10.1021/acs.langmuir.8b02904
Wang Z. Y., Yu X. D., Pan B., Xing B. S. (2010). Norfloxacin sorption and its thermodynamics on surface-modified carbon nanotubes. Environ. Sci. Technol. 44 (3), 978–984. doi: 10.1021/es902775u
Xu Z., Xiang Y., Zhou H., Yang J., He Y., Zhu Z., et al. (2021). Manganese ferrite modified biochar from vinasse for enhanced adsorption of levofloxacin: effects and mechanisms. Environ. pollut. 272, 115968. doi: 10.1016/j.envpol.2020.115968
Yan B., Niu C. H. (2017). Modeling and site energy distribution analysis of levofloxacin sorption by biosorbents. Chem. Eng. J. 307, 631–642. doi: 10.1016/j.cej.2016.08.065
Yan B., Niu C. H. (2018). Adsorption behavior of norfloxacin and site energy distribution based on the dubinin - astakhov isotherm. Sci. Total. Environ. 631-632, 1525–1533. doi: 10.1016/j.scitotenv.2018.03.119
Yan B., Niu C. H., Wang J. (2017a). Kinetics, electron-donor-acceptor interactions, and site energy distribution analyses of norfloxacin adsorption on pretreated barley straw. Chem. Eng. J. 330, 1211–1221. doi: 10.1016/j.cej.2017.08.056
Yan B., Niu C. H., Wang J. (2017b). Analyses of levofloxacin adsorption on pretreated barley straw with respect to temperature: kinetics, π-π electron-donor-acceptor interaction and site energy distribution. Environ. Sci. Technol. 51, 8048–8056. doi: 10.1021/acs.est.7b00327
Yang Q., Gao Y., Ke J., Show P. L., Ge Y., Liu Y., et al. (2021). Antibiotics: an overview on the environmental occurrence, toxicity, degradation, and removal methods. Bioengineered 12 (1), 7376–7416. doi: 10.1080/21655979.2021.1974657
Yang D., Li J., Luo L., Deng R., He Q., Chen Y. (2020). Exceptional levofloxacin removal using biochar-derived porous carbon sheets: mechanisms and density-functional-theory calculation. Chem. Eng. J. 387, 124103. doi: 10.1016/j.cej.2020.124103
Yao B., Luo Z., Du S., Yang J., Zhi D., Zhou Y. (2021). Sustainable biochar/MgFe2O4 adsorbent for levofloxacin removal: adsorption performances and mechanisms. Bioresour. Technol. 340, 125698. doi: 10.1016/j.biortech.2021.125698
Yu J., Zhang X., Jin B., Chen J., Huang Y., Wang Z. (2021). Silica aluminum xerogel-based sorbent for removal of volatilized PbCl2 during the incineration: improvement on mass-transfer limitations via high porosity. Sci. Total. Environ. 782, 146925. doi: 10.1016/j.scitotenv.2021.146925
Zhang X., Chu Y., Zhang H., Hu J., Wu F., Wu X., et al. (2021). A mechanistic study on removal efficiency of four antibiotics by animal and plant origin precursors-derived biochars. Sci. Total. Environ. 772, 145468. doi: 10.1016/j.scitotenv.2021.145468
Zhang Y., Yan K., Ji F., Zhang L. (2018). Enhanced removal of toxic heavy metals using swarming biohybrid adsorbents. Adv. Funct. Mater. 28, 1806340. doi: 10.1002/adfm.201806340
Zhou L., Li N., Owens G., Chen Z. (2019). Simultaneous removal of mixed contaminants, copper and norfloxacin, from aqueous solution by ZIF-8. Chem. Eng. J. 362, 628–637. doi: 10.1016/j.cej.2019.01.068
Zhou H., Mayorga-Martinez C. C., Pané S., Zhang L., Pumera M. (2021). Magnetically driven micro and nanorobots. Chem. Rev. 121, 4999–5041. doi: 10.1021/acs.chemrev.0c01234
Zhou H., Wang Z., Gao C., Sun Q., Liu J., She D. (2023). Synthesis of honeycomb lignin-based biochar and its high-efficiency adsorption of norfloxacin. Bioresour. Technol. 369, 128402. doi: 10.1016/j.biortech.2022.128402
Zhou A., Zhu C., Chen W., Wan J., Tao T., Zhang T. C., et al. (2018). Phosphorus recovery from water by lanthanum hydroxide embedded interpenetrating network poly (vinyl alcohol)/sodium alginate hydrogel beads. Colloids. Surf. A. Physicochem. Eng. Asp. 554, 237–244. doi: 10.1016/j.colsurfa.2018.05.086
Keywords: magnetic microrobot, fluoroquinolones, kinetics, adsorption mechanisms, site energy distribution
Citation: Tang Y, Jia W, Bao M, Qiu S, Pi YR, Liu C and Zhao J (2023) Pick-up of fluoroquinolones from the aqueous phase via magnetically propeled microrobots: kinetics, thermodynamics, and site energy distribution analysis. Front. Mar. Sci. 10:1169883. doi: 10.3389/fmars.2023.1169883
Received: 20 February 2023; Accepted: 05 May 2023;
Published: 26 May 2023.
Edited by:
Kyu-Jung Chae, Korea Maritime and Ocean University, Republic of KoreaReviewed by:
Sudipta Rakshit, Tennessee State University, United StatesHai-Lei Cao, Fujian Agriculture and Forestry University, China
Copyright © 2023 Tang, Jia, Bao, Qiu, Pi, Liu and Zhao. This is an open-access article distributed under the terms of the Creative Commons Attribution License (CC BY). The use, distribution or reproduction in other forums is permitted, provided the original author(s) and the copyright owner(s) are credited and that the original publication in this journal is cited, in accordance with accepted academic practice. No use, distribution or reproduction is permitted which does not comply with these terms.
*Correspondence: YongRui Pi, eXJwaUBvdXRsb29rLmNvbQ==