Coastal landforms and fetch influence shoreline restoration effectiveness
- 1School of Aquatic and Fishery Sciences, University of Washington, Seattle, WA, United States
- 2Habitat Program, Washington Department of Fish and Wildlife, Olympia, WA, United States
- 3Northwest Straits Foundation, Bellingham, WA, United States
- 4Vashon Nature Center, Vashon, WA, United States
- 5Friday Harbor Laboratories, University of Washington, Friday Harbor, WA, United States
Coastal shorelines are a key interface between terrestrial and aquatic ecosystems and are vital for human livelihood. As a result, shorelines have experienced substantial human modifications worldwide. Shoreline “hardening” – the construction of armor including seawalls, bulkheads, or rip-rap – is a common modification that has substantial negative ecological effects. Currently, restoration involving the removal of armor and replacement with “living” shorelines is becoming an established practice. Still, the ecological response to armor removal is oftentimes unpredictable and site-specific. We hypothesized that the confluence of larger-scale geophysical features might strongly influence ecological restoration outcomes at particular locations. To measure the effectiveness of armor removal in the context of broad-scale geophysical features across the Salish Sea, WA, USA, we studied 26 paired restored and natural reference beaches of the same shoretype (feeder bluff, accretion shoreform, or pocket beach), as well as corresponding fetch, sub-basin, and percent of shoreline sediment drift cell armored. Sites were restored for an average of six years. We gauged restoration effectiveness based on levels of five ecological response variables: beach wrack (percent, depth), logs (count, width), sediments (percent sand), vegetation (percent overhanging, count of fallen trees), and insects (density, taxa richness). We found that armor removal often restored these variables to natural levels, but that restoration response was dependent on geophysical features such as shoretype and fetch. Natural beaches did have higher measurements of overhanging vegetation, fallen trees, and insect taxa richness, as these features likely take time to mature at restored beaches. Feeder bluffs had a higher proportion of surface sand and number of fallen trees than other shoretypes, coinciding with the erosion of bluff material, whereas natural pocket beaches within bordering rocky headlands had higher insect densities. Sites with a large fetch had higher input of deposited wrack and logs, whereas sites with a small fetch had higher input from localized terrestrial sources – fallen trees and eroding sand. By incorporating the effectiveness of restoration with landscape features such as shoretype and fetch, we can more effectively plan for future restoration actions and better predict their outcomes.
1 Introduction
Geophysical features can govern habitat function on shorelines (Roff and Taylor, 2000) and therefore potentially influence effectiveness of restoration efforts. Coastal landforms such as bluffs, dunes, saltmarshes, and rocky headlands are globally common features that can provide important functions for shore-dwelling biota (Boström et al., 2011; Morris et al., 2022). Restoring functioning shoreline habitat is vital for the recovery of species, such as juvenile fishes and invertebrates that are threatened by a mosaic of stressors including habitat loss and sea level rise (Toft et al., 2018; Lefcheck et al., 2019; Duarte et al., 2020). Providing linkages across coastal seascape habitats can provide scientists and managers a foundation for building effective, data-based recovery plans (Boström et al., 2011; Rogers et al., 2014), and can lead to informed implementation of restoration actions. Our objectives are to build upon these topics by examining the restoration effectiveness of shoreline habitats in the context of their larger geophysical features.
Worldwide, intertidal shorelines have experienced a host of human modifications such as land reclamation and placement of shoreline armor (i.e., seawalls, bulkheads, rip-rap) to protect property and infrastructure from erosion (Ma et al., 2014; Morris et al., 2019a). More than 14% of the total US coastline is armored (Gittman et al., 2015), and a global increase is expected (Bugnot et al., 2021). In the Salish Sea, WA, USA, 29% of shorelines are armored (MacLennan et al., 2017). Armor placement can have negative impacts on biodiversity and organism abundance both regionally (Dethier et al., 2016) and worldwide (Gittman et al., 2016b), and as a result, efforts to remove armor and restore natural processes are increasing. Studies in the Salish Sea have focused on measuring restoration effectiveness in upper intertidal-supratidal areas where impacts of armor (Dethier et al., 2016) and therefore restoration actions (Toft et al., 2021) have been most pronounced on beach characteristics such as wrack, logs, sediments, vegetation, and invertebrates. Armoring and restoration can have important food web implications, as the forage fish surf smelt (Hypomesus pretiosus) and Pacific sand lance (Ammodytes hexapterus) lay their eggs on beach sediments (Rice, 2006), and endangered populations of Chinook salmon (Oncorhynchus tshawytscha) feed on insects along the shore as outmigrating juveniles (Toft et al., 2007). Armor removal is often combined with sediment nourishment, placement of logs, and planting of vegetation (Johannessen et al., 2014), similar to the implementation of living shoreline techniques worldwide that incorporate a number of complementary techniques such as inclusion of marsh sills and oyster beds (Gittman et al., 2016a; Morris et al., 2019b), along with armor removal (Bilkovic et al., 2017; Smith et al., 2020).
In the Salish Sea, our knowledge of nearshore restoration effectiveness has increased as studies are published over multiple scales, as is true elsewhere. For example, in Florida, seagrasses showed a greater response at seven years post-restoration than at three years (Bell et al., 2014). In Chesapeake Bay, nekton use of living shorelines was found to be equivalent to natural marshes at thirteen sites (Guthrie et al., 2022), whereas an initial study of just two sites showed a positive response for some but not all fish species (Davis et al., 2006). Previous studies of restoration effectiveness in our region have assessed site-to-site and annual variability of wrack, logs, and invertebrates in 18 locations (Des Roches et al., 2022), the effectiveness of living shorelines in 10 locations (Toft et al., 2021), a meta-analysis of six case studies (Lee et al., 2018), and invertebrates at one site of armor removal (Toft et al., 2014). Our project builds on this previous research by studying 26 restored beaches, encompassing a broader spatial area and allowing us to determine the effects of multiple large-scale predictor variables, including coastal landforms. To accomplish this effort, the Shoreline Monitoring Database (https://www.shoremonitoring.org) has been instrumental in providing a framework for our collaborative team of academic, agency, and community scientist groups to work together, broadening what we can achieve.
Shoreline ecosystems are shaped by their surrounding coastal landforms. Different categories of coastal landforms can be characterized by processes of sediment erosion and deposition, and the type of coastal landform is likely to influence armor removal and shoreline restoration. In the Salish Sea, shorelines are segmented into “drift cells”, each with sediment sources, transport paths, and deposition areas (Coastal Geologic Services (CGS), 2020). There are three main shoretypes mapped in regional geodatabases at which restoration occurred (Figure 1). First, feeder bluffs (FB) are high-elevation coastal bluffs that erode through time and supply sediment to the nearshore (Coastal Geologic Services (CGS), 2020). Sediment is transported down-drift, maintaining beach structure and providing habitat value. Second, accretion shoreforms (AS) are low-elevation beaches where sediment is deposited from eroding updrift bluffs, leading to landform maintenance and development over time as long as sediment processes in the drift cell are intact (i.e., not interrupted by artificial armor and other infrastructure) (Dawson et al., 2009). Finally, pocket beaches (PB) are closed systems that occur between rocky headlands, and therefore are not formed by littoral drift.
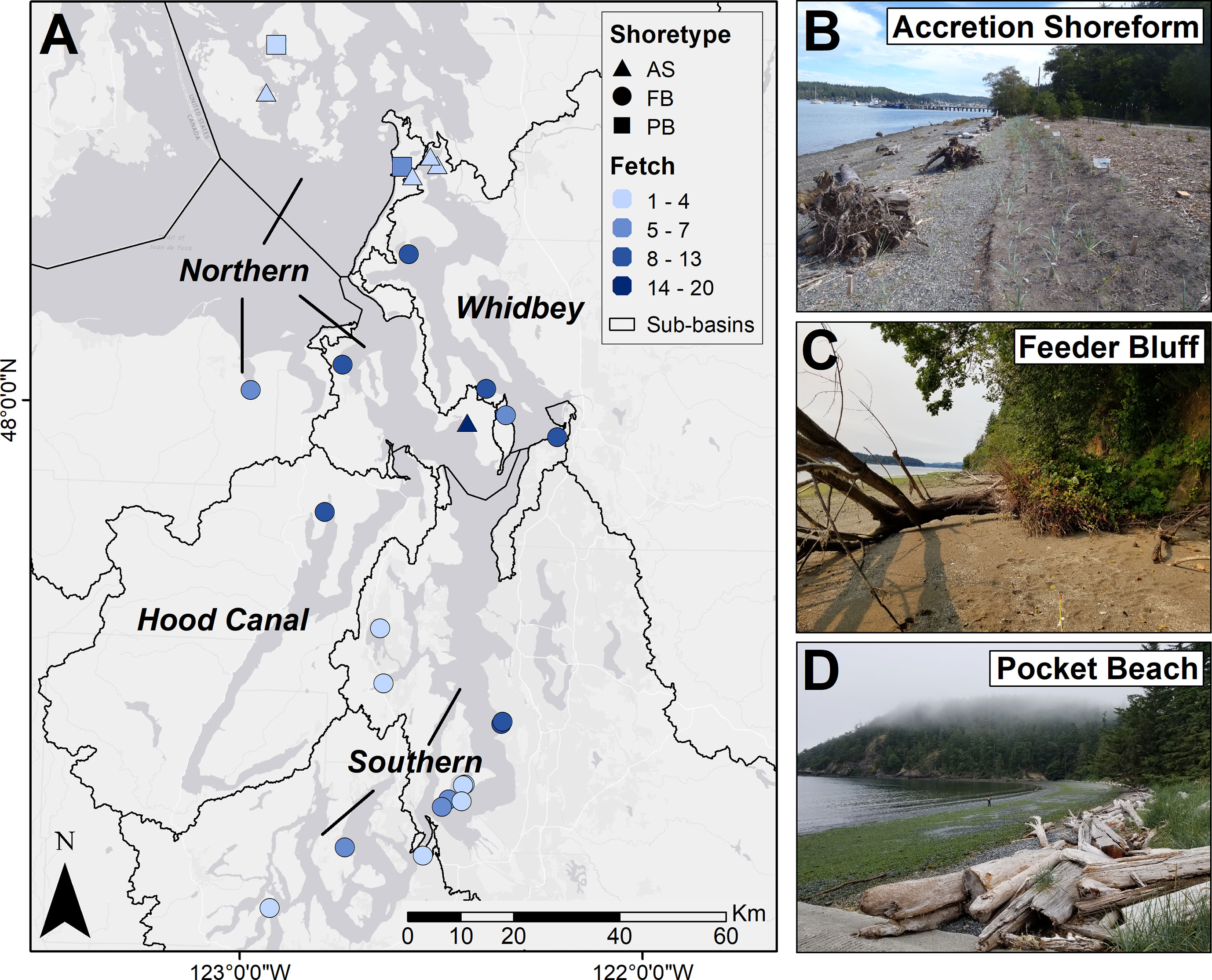
Figure 1 Map of twenty-six restored sites in the Salish Sea, WA, USA (A), each with a paired natural reference beach. Shapes indicate the shoretype: AS = Accretion Shoreform (B), FB = Feeder Bluff (C), and PB = Pocket Beach (D). Colors indicate the fetch distance (miles, binned for display). Text labels and lines show the four regions included in the analysis, based on sub-basins: Northern (Strait of Juan de Fuca, San Juan Islands and Georgia Strait, and north central Puget Sound); Southern (south central and south Puget Sound); Whidbey; and Hood Canal. Map base map source: Esri, HERE, Garmin, (C) OpenStreetMap contributors, and the GIS user community.
In addition to the impact of coastal landforms on the outcome of restoration actions, we predict that several additional landscape-level parameters will be important in shoreline habitat function, including fetch, sub-basin location, and percent of the drift cell that is armored. Fetch is the distance that waves are generated by wind, and may be a governing force in the supply and movement of sediments, wrack, and logs (Nordstrom and Jackson, 2012). Within the protected waters of the Salish Sea, fetch is limited, as opposed to the open coast where ocean swells and storms can generate much larger forces, and along with rising seas are leading to management actions such as managed retreat (Hino et al., 2017). Sub-basins incorporate larger geological features such as tidal flux and position in the landscape, which along the sinuous shorelines of the Salish Sea are influential in some impacts of armor (Dethier et al., 2016). Additionally, the percent of drift cell armored can account for the scale of human impacts along the entire drift cell, as opposed to that at singular sites. Although this parameter can lead to the coarsening of sediments due to the legacy of armor placement (Dethier et al., 2016), the clearest outcomes of restoration actions have been documented at the site scale (Toft et al., 2021).
In this study, we evaluate the effectiveness of removing armor across different shoretypes – feeder bluffs, accretion shoreforms, and pocket beaches. We compare restored beaches (restored for an average of six years) to natural never armored beaches, which serve as a reference point for successful restoration actions because they should have largely intact localized processes and functions (e.g., sediment movement, invertebrate diversity). A broad spatial sampling scale enables us to include effects of shoretype (feeder bluff, pocket beach, accretion shoreform), fetch (distance), sub-basin, and relative amount of armored shoreline in the surrounding drift cell. Due to the underlying factors that may affect habitat response on the landscape level, we hypothesize that these geophysical features will influence restoration effectiveness at the site level. Our sampling regime measures structural habitat components of beach wrack, logs, sediments, and vegetation, as well as insects among shoreline vegetation, at upper intertidal and supratidal elevations (as per Dethier et al., 2016; Toft et al., 2021; Des Roches et al., 2022). Our goal is to assess restoration effectiveness across shoretypes and landscape features in order to adaptively plan for future restoration actions along developed coasts.
2 Materials and methods
2.1 Study area
We surveyed 26 sites within the Salish Sea (Washington State, USA) which is an estuarine fjord with mixed semidiurnal tides (Sobocinski, 2021). Each site included paired transects at beaches of restored and natural status that were of the same shoretype (accretion shoreform, feeder bluff, pocket beach) (Figure 1). Sites were restored for an average of six years, ranging from 1 to 17 years (Supplemental Material). Accretion shoreforms were the shoretype at four sites, Cornet Bay (Deception Pass), Kukutali Preserve (Similk Bay), Similk Bay (Similk Bay), and Sunlight Shores (Whidbey Island). Feeder bluffs were the prevalent shoretype, consisting of 20 sites at Anna Smith Park (Dyes Inlet), Brown Island (San Juan Islands), Dabob Bay (Hood Canal), Dockton Park I and II (Maury Island), Dawley (Sequim Bay), Edgewater Beach (Eld Inlet), Forest Glen (Vashon Island), Fort Townsend (Port Townsend), Howarth Park (Everett), Maylor Point (Whidbey Island), Penrose Point (Carr Inlet), Piner Point (Maury Island), Ross Point (Sinclair Inlet), Seahorse Siesta (Whidbey Island), Seahurst Park I and II (Burien), Tahlequah (Vashon Island), Titlow Park (Tacoma), and Waterman (Whidbey Island). Pocket beaches were the shoretype at two sites, Bowman Bay (Deception Pass) and Family Tides (San Juan Islands).
2.2 Data collection
We collected data during the spring and summer months (May-September) spanning the years 2009 to 2022. Data collections were not consistent across this timespan, as they varied by the year of restoration at any given site. All methods used standardized protocols as described in our previous publications (Dethier et al., 2016; Toft et al., 2021; Des Roches et al., 2022) and are available online at the Shoreline Monitoring Database (https://shoremonitoring.org). Data were collected along 50-meter transects parallel to shore during low tides when the upper beach was exposed. At the most recently deposited beach wrack line, we measured the proportion of wrack cover and wrack depth at ten random points along the transect, using a 0.1 m2 quadrat sub-divided into 25 squares for ease of estimating percentages. We counted the number of logs greater than 1 m in length and 0.1 m in diameter (driftwood) and the width of the log line perpendicular to shore at five random points on the transect. Along the 50 m transect, we measured the linear percent overhanging vegetation on the upper beach and counted the total number of fallen trees. We sampled sediment size using either visual estimates (0.1 m2 quadrat with visual sediment size indicators at five random points) or collection of sediment (sieving and weighing) consistent within a restored-natural pairing, to calculate the proportion of sand (< 2 mm diameter) versus gravel (2 mm - 6 cm) in surface and sub-surface (5 cm) layers (dug with a hand trowel to a 5 cm measurement). We sampled insects and other terrestrial arthropods (e.g., arachnids, collembola), all together shortened to “insects” hereafter, with fallout traps (40 × 25 cm plastic bins with a small amount of soapy water) deployed for 24 hours at five random points along the transect. Samples were sieved at 106 μm, preserved in 70% isopropanol, and returned to the laboratory for identification and enumeration under a dissecting microscope.
2.3 Statistical analysis
To generate a dataset for our study sites that included shoretype, percent of drift cell armored, fetch, and sub-basin, we used the Spatial Join function in ArcMap (ArcGIS Desktop 10.8.2) from the Nearshore Geospatial Framework (Coastal Geologic Services (CGS), 2017) and the Beach Strategies Phase 1 Database (MacLennan et al., 2017). All data were transformed to the same Geographic Coordinate System (GCS_WGS_1984) for analysis and the spatial joins were manually checked for accuracy. Percent of drift cell armored was updated with the length of armor that was removed at our restored beaches. Shoretype was coded as feeder bluff, accretion shoreform, or pocket beach. For feeder bluffs, we included the following bluff-backed sub-categories in our feeder bluff grouping (Coastal Geologic Services (CGS), 2020) – feeder bluff exceptional, feeder bluff, and transport zones that are generally more stable. To increase site replication within spatially adjacent sub-basins, we combined three northern sub-basins into one northern grouping (Strait of Juan de Fuca, San Juan Islands and Georgia Strait, and north central Puget Sound), and two southern sub-basins into one grouping (south central and south Puget Sound), with Whidbey and Hood Canal sub-basins separate.
We conducted statistical analyses using analyses of variance (ANOVA) on generalized linear mixed models. Final models were chosen by comparing potential models using the corrected Akaike Information Criterion (AICc) (Hurvich and Tsai, 1989). Post-hoc analyses included estimating marginal means of significant responses (p < 0.05) across factors, conducting pairwise comparisons of marginal means (with Tukey or multivariate t corrections for multiple post-hoc testing), and calculating the effect size of the comparisons, that is, the magnitude of difference between groups (Cohen, 1988). Potential fixed effects were based on study questions and included the status of the beach (restored or natural), shoretype (feeder bluff, accretion shoreform, pocket beach), fetch (miles), sub-basin (southern, northern, Whidbey, and Hood Canal), and percent armoring of the site’s drift cell. Interactive effects of beach status and shoretype were included, and if no interactive effects were present based on ANOVA results, the main effects were studied. Random effects included year (to account for interannual sampling variation) and a spatial effect to account for variation due to site location and among transects within sites (Bolker et al., 2009). The spatial effect was coded as a unique transect identifier nested within its site. To account for time since restoration for restored sites, years since restoration was tested as a fixed effect, but this did not improve model fits and was subsequently removed from analyses.
Response variables included beach wrack (proportion total cover, wrack depth), logs (total counts, width of log line), vegetation (fallen trees, percent overhanging vegetation), insects (density, taxa richness), and sediments (proportion of sand at surface and subsurface depths). Response variables measured as proportions (e.g., wrack cover and sand) followed a beta distribution. Continuous responses followed a Gaussian distribution, while count data followed a Poisson or negative binomial distribution (Supplemental Material). All statistical analyses were performed using R Statistical Software (v4.2.2; R Core Team, 2022). Data manipulation was performed using “dplyr” (v2.2.1; Wickham et al., 2022). We developed GLMMs using R package “glmmTMB” (v1.1.5; Brooks et al., 2017), designed post-hoc comparisons for beach status and shoretype using the package “emmeans” (v1.8.3; Lenth, 2022), and used “ggplot2” (v3.4.0 Wickham, 2016) for data visualization.
3 Results
Shoretype, fetch, sub-basin, and restored or natural status of beaches accounted for significant differences in at least one of our measured response variables; however, the percent of drift cell armored did not explain significant variation and therefore was not included in our final models (Table 1; Supplemental Material). Final models for vegetation data analyzed the effects of beach status (restored and natural), shoretype (FB, AS, PB), and fetch. Natural beaches had more overhanging vegetation than restored beaches (Figure 2). Feeder bluffs had more fallen trees than the other shoretypes (marginal mean comparisons = -3.44 AS-FB, 5.27 FB-PB), and natural beaches had more fallen trees than restored (Figures 2, 3; Table 1). Fallen trees increased as fetch decreased (Figure 4).
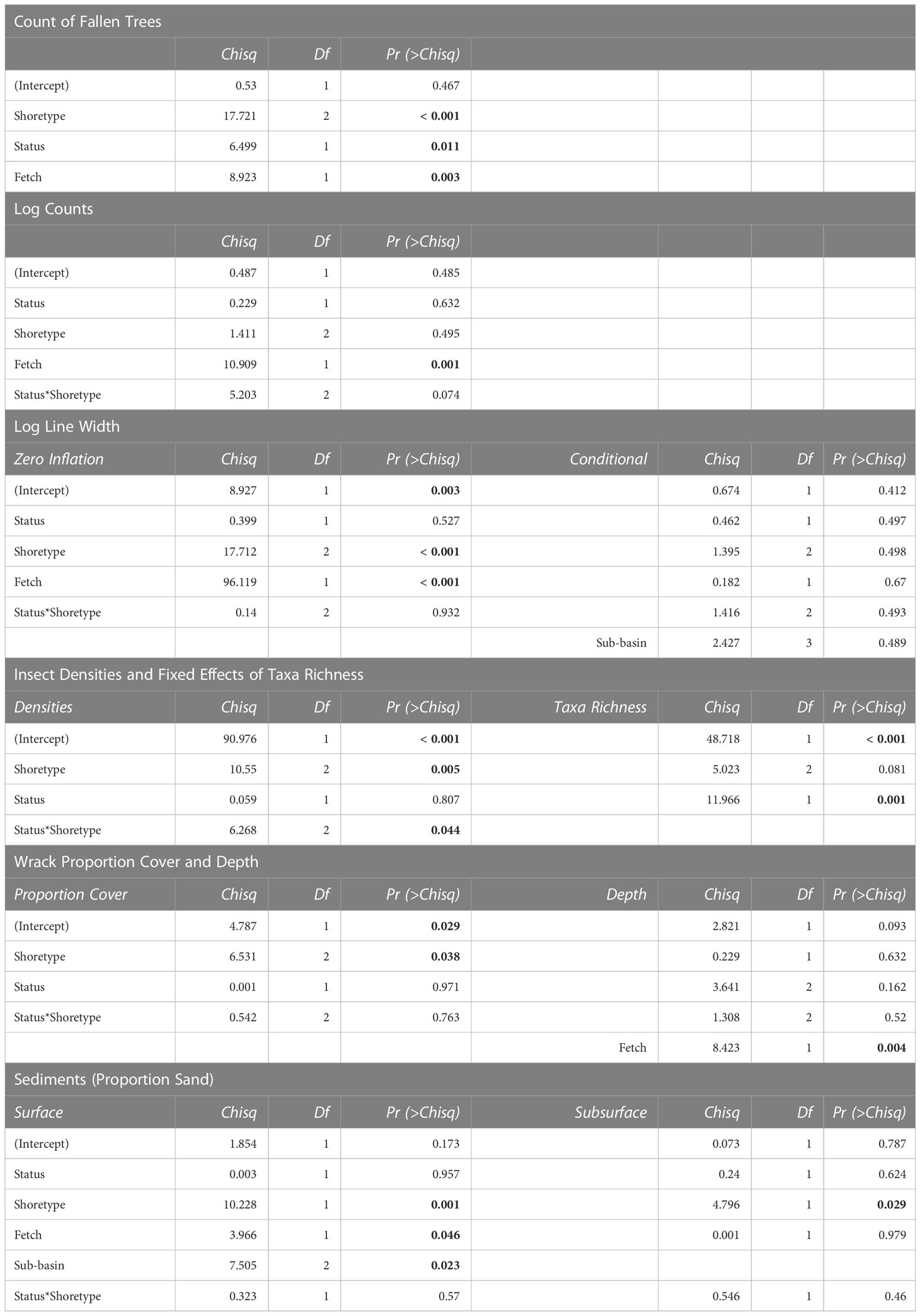
Table 1 ANOVA summaries of response variables (vegetation, logs, insects, wrack, sediments) for generalized linear mixed-effects models with shoretype (accretion shoreform, feeder bluff, pocket beach), beach status (natural or restored), fetch, and sub-basin, including Chi-square values, degrees of freedom, and p-values (highlighted in bold when significant; “*” indicates interactions).
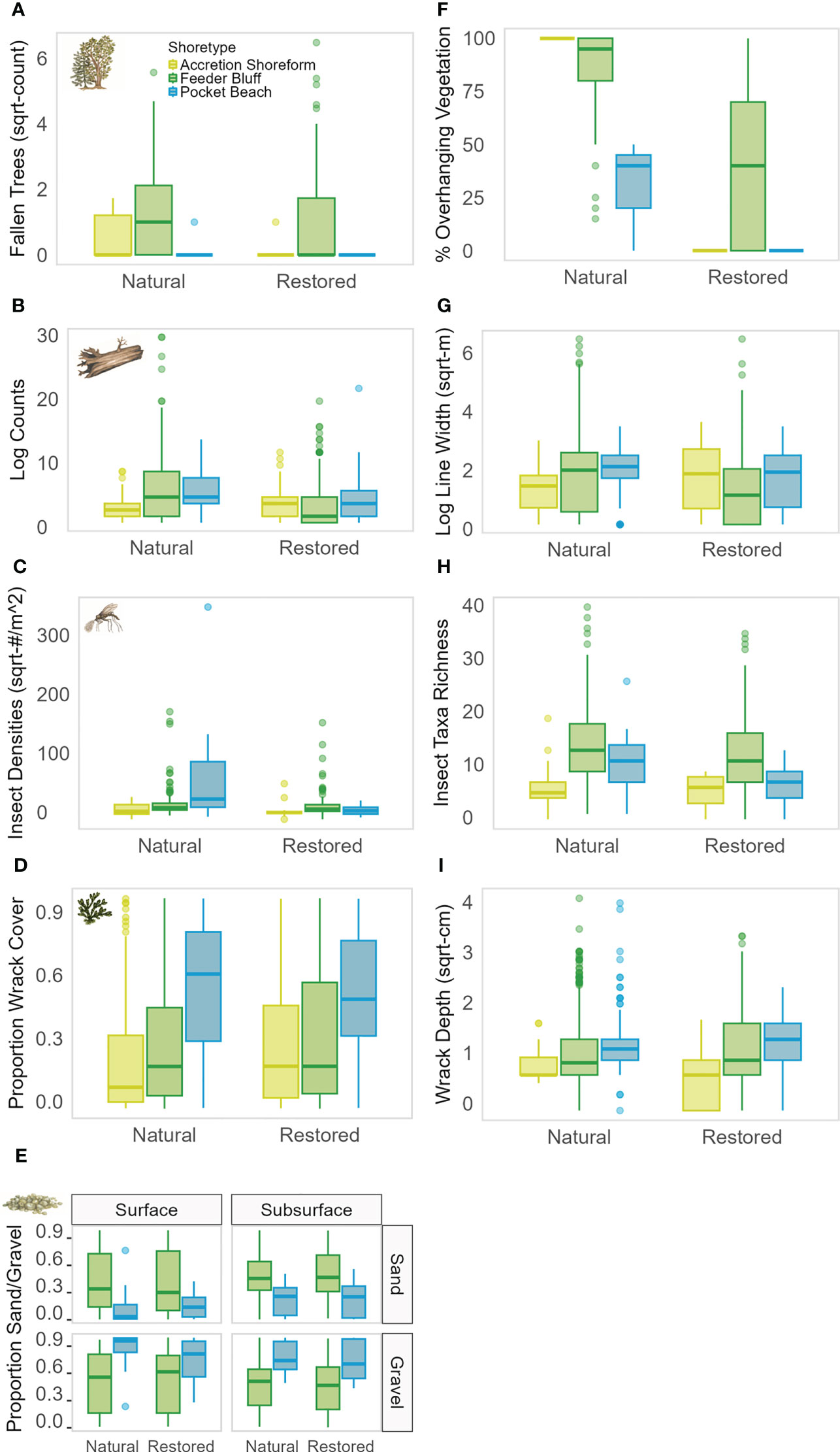
Figure 2 Boxplots depicting response variables by beach status (natural or restored) among shoretypes (AS = yellow, FB = green, PB = blue), including (A) count of fallen trees, (B) log counts, (C) insect densities, (D) proportion wrack cover, (E) proportion sand and gravel, (F) percent overhanging vegetation, (G) log line width, (H) insect taxa richness, and (I) wrack depth. #, indicates number.
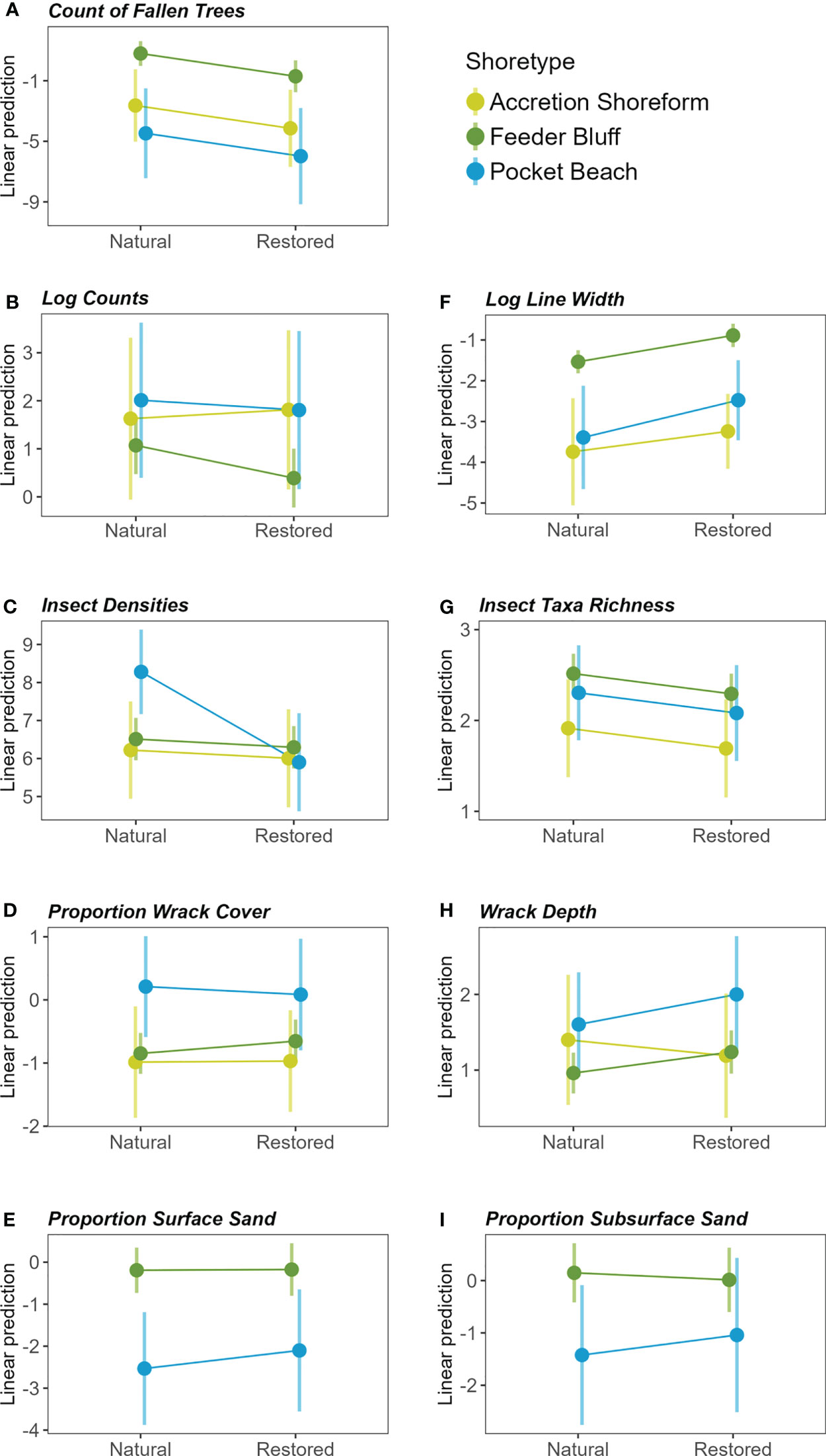
Figure 3 Marginal means plots depicting response variables by beach status (natural or restored) among shoretypes (AS = yellow, FB = green, PB = blue), including (A) count of fallen trees, (B) log counts, (C) insect densities, (D) proportion wrack cover, (E) proportion surface sand, (F) log line width, (G) insect taxa richness, (H) wrack depth, and (I) proportion subsurface sand.
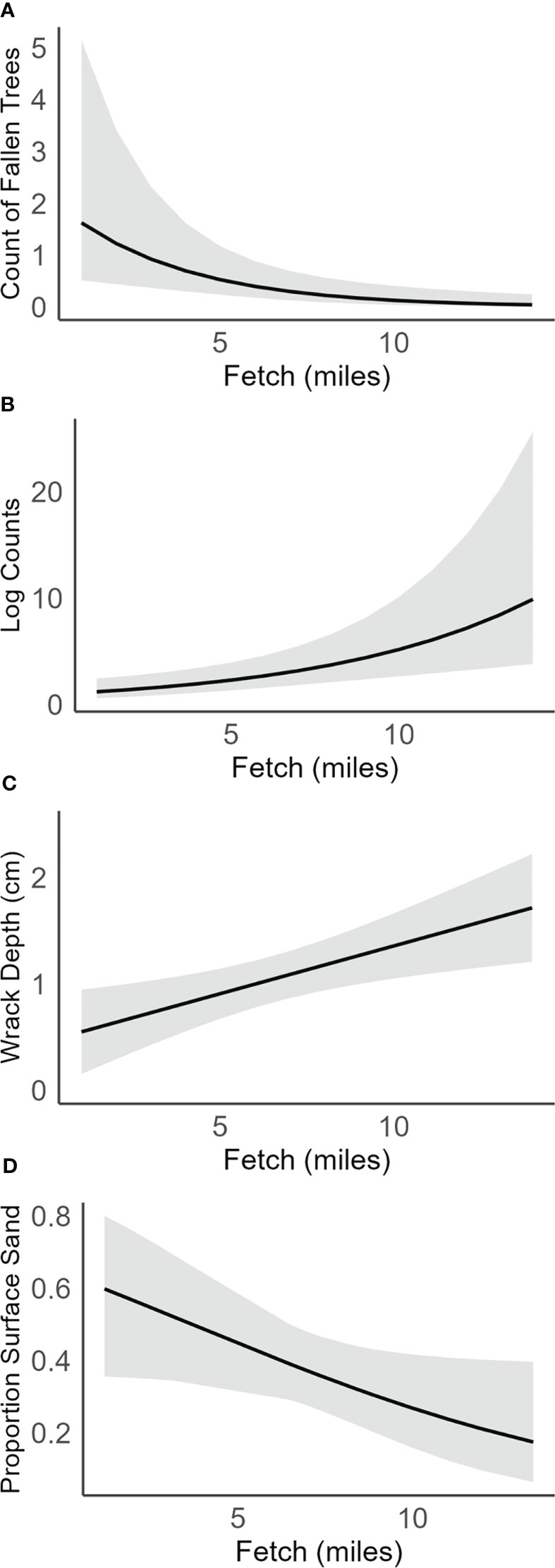
Figure 4 Marginal effects plots of significant responses for fetch, including (A) count of fallen trees, (B) log counts, (C) wrack depth, and (D) proportion surface sand. Error bars are 95% confidence intervals.
The interaction of beach status and shoretype was significant for log counts (Table 1). Pairwise comparisons of marginal means within each shoretype showed that log counts at natural feeder bluffs were higher than those at restored feeder bluffs (marginal mean comparison = 0.70, and a small effect size of 0.14) (Figures 2, 3). There were no pairwise differences in log counts at other shoretypes. However, the width of the log line was significantly more likely to be zero at feeder bluffs than pocket beaches and accretion shoreforms, regardless of beach status (Figure 2; Supplemental Material). Log counts increased along with fetch distance (incidence rate ratio = 1.18, SE = 0.001) (Figure 4), and width of the log line had more occurrences of zeros with lower fetch (estimate = -0.28, SE = 0.03).
The interaction of beach status and shoretype was significant for insect density (i.e., inclusive of insects and other terrestrial arthropods), with pairwise comparisons showing that natural pocket beaches had higher insect densities than natural accretional shoreforms and feeder bluffs (marginal mean comparisons = -2.06 natural AS-PB, -1.77 natural FB-PB) (Figure 2, Table 1; Supplemental Material). Natural beaches had higher insect taxa richness than those that were restored, and there were no interactions with shoretype (Figures 2, 3, Table 1). Final models for insect data did not include fetch or sub-basin.
Restored and natural beach status was not significant in wrack proportion cover or wrack depth. Shoretype was significant for wrack proportion cover, but post-hoc analyses showed no differences, and shoretype was not significant for wrack depth. Due to model selection, fetch and sub-basin were not included in final models for wrack proportion cover (Figures 2, 3, Table 1). Fetch was included in final models for wrack depth, and showed significant increases in wrack depth as fetch increased (estimate = 0.09, SE = 0.03) (Figure 4).
Final models for sediment size included fetch and sub-basin, and feeder bluff and pocket beach shoretypes, as sample sizes at accretion shoreforms were too few for analyses. Sand and gravel sediment sizes were inversely proportional (Figure 2), and proportion sand was the focus of analyses. Feeder bluffs had a higher proportion of surface sand than pocket beaches at both natural and restored beaches (Figures 2, 3, Table 1). Proportion of sand in surface sediments also increased as fetch decreased (Figure 4), and Whidbey Basin had a higher proportion of surface sand than the southern sub-basin (Table 1; Supplemental Material). Effects were not as strong in sub-surface sediments (5 cm depths), yet natural feeder bluffs had a higher proportion of sand than natural pocket beaches (Figures 2, 3, Table 1).
4 Discussion
Our study harnesses data collected from 26 pairs of natural and restored (armor removal) sites to show how geophysical features can influence key indicators of restoration effectiveness including beach wrack cover, the number of beached logs, sediment sand composition, amount of vegetation and fallen trees, and insect density and richness. Shoreline restoration in the Salish Sea is often, but not always, effective at approaching natural conditions, and geophysical features, mainly shoretype and fetch, are associated with variations in restoration responses. One of the aims of shoreline restoration has been to re-establish natural ecological processes, structure, and function to conditions prior to armor construction. Yet, the extent to which ecosystems return to these former conditions is variable and can be unpredictable. Even across natural, never-armored beaches, there is appreciable variation in the deposition of beach wrack and logs and the composition of invertebrate communities (Des Roches et al., 2022). Geophysical features, including coastal landforms, fetch, sub-basin, and drift cell characteristics are likely to affect restoration effectiveness, yet are difficult to study in a controlled manner.
The proportion of wrack cover and depth, the width of the log line, and the proportion of sand were comparable between natural and restored beaches, regardless of shoretype. Similar shoreline restoration benefits have been found regionally (Toft et al., 2021) and internationally (Strain et al., 2018). However, natural beaches had higher percentages of overhanging vegetation, more fallen trees, and higher insect taxa richness (i.e., inclusive of insects and other terrestrial arthropods). These results suggest that more time is necessary for growth and maturation of vegetation at restored sites, relative to rapid responses like wrack and log deposition, and longer term monitoring is needed to track these conditions beyond our average of six years post-restoration. This temporal component is noteworthy given the influence that shoreline vegetation can have on fish and their invertebrate prey (Romanuk and Levings, 2003; Romanuk and Levings, 2006; Toft et al., 2007). Protection of existing riparian vegetation, especially large trees, is an important practice to partner with restoration actions.
We found various parameters of coastal landforms to cause significant variation in the response to shoreline restoration. Bluffs with active sediment input (“feeder bluffs”) are important for providing sediments that create and maintain beaches, and also for supporting diverse biota that are dependent on these habitats. In our study, feeder bluffs were the prevalent shoretype and had a higher proportion of surface sand than pocket beaches at both natural and restored beaches, which is understandable given the localized source of eroding sand at feeder bluffs, in contrast with the closed system of pocket beaches bordered by rocky headlands. Feeder bluffs also had more fallen trees than the other shoretypes, a habitat feature that coincides with the erosion of bluff material which includes riparian vegetation as well as sediments. These autochthonous sources may be important to nearshore food webs, as has been found in restored marshes where estuarine consumers are dependent on localized subsidies (Howe and Simenstad, 2011). Allochthonous (marine) sources of algae and eelgrass in wrack are also important to beach-dwelling talitrid amphipods and other consumers (Toft et al., 2021; Hyndes et al., 2022). Beach wrack abundance was similar across all shoretypes and beach status, pointing to its rapid accumulation at restored beaches and its prevalence across diverse coastal landforms.
Natural pocket beaches had the highest insect densities of all shoretypes. Since pocket beaches are closed systems rather than a portion of a larger drift cell, insects and other terrestrial arthropods may be confined within the rocky headland boundaries more than along open shoreline stretches. Similarly, insect taxa richness increased at a created pocket beach along an urban shoreline bordered by artificial armor (Toft et al., 2013), likely dependent on the amount of supralittoral vegetation that is present along the shoreline (Romanuk and Levings, 2003). Invertebrate community recovery at pocket beaches is a resource for juvenile salmonids, as these are important habitats for foraging (Beamer et al., 2006). Pocket beaches have also proved to be valuable habitat elsewhere, including for horseshoe crab spawning along the US Atlantic Coast (Landi et al., 2015). Although accretion shoreforms were not found to be higher in any of our analyzed metrics compared to the other shoretypes, these depositional beaches are still prevalent features of the landscape with associated habitat functions typical of shallow water systems (Toft et al., 2007; Defeo et al., 2009). Since accretion shoreform and pocket beach shoretypes were less represented in our study sites than feeder bluffs, future studies should seek to more broadly incorporate a diversity of shoretypes.
In addition to beach status and shoretype, fetch was a significant predictor of restoration effectiveness. Because the fetch-limited beaches characteristic of much of the Salish Sea have low-energy waves, less wrack and fewer logs are carried to these sites as compared to exposed coasts (Nordstrom and Jackson, 2012). Our analysis shows that within the Salish Sea, increased fetch leads to an increase in log counts and wrack depth. Conversely, as fetch decreases, fallen trees and surface sand increase, as low local wave energies allow fallen trees to remain in place and function as traps for sediment movement alongshore (Nordstrom and Jackson, 2012). Of our predictor variables, percent armoring of the drift cell was not a significant factor in our analyses. The larger scale of armoring along a drift cell versus at a single site has been shown to matter for overall armor impacts, specifically by coarsening sediment sizes (Dethier et al., 2016), but not for restoration effectiveness (Toft et al., 2021), at least on the more limited spatial and temporal scales that restoration has occurred. As restoration programs expand, shoretype and fetch should be considered influential factors that can mediate the restoration response to deleterious impacts of armor, such as decreased beach logs and wrack (Dethier et al., 2016) and reduction of the variation in ecological responses (Des Roches et al., 2022).
Our results show that landscape context interacts with restoration practices to produce shoreline habitats that vary in the type of restoration benefits that they provide. This variation in restoration response can help to maintain a mosaic of habitat benefits across a large scale, similar to what is found along natural shorelines (Des Roches et al., 2022). The knowledge that recovery of natural functions at restoration sites varies with their larger landscape context can help shoreline managers envision outcomes based on local characteristics. For example, restoring a site with a large fetch may lead to higher input from outside sources – floating logs and wrack that drift into the site – whereas a small fetch may lead to higher input from localized sources – fallen trees, and eroding sand. The degree of wave exposure and habitat type have also been found to be influential in other systems, such as on fish composition along Australian coasts (Valesini et al., 2004; Borland et al., 2017). Log-line width may also be dependent on shoreline conditions, as it was more likely to be zero (i.e., no logs) at high-bank feeder bluffs than low-bank pocket beaches and accretion shoreforms. The subtle differences that we found across sub-basins may reflect the specific sites sampled rather than overall sub-basin characteristics (Dethier et al., 2016). The Whidbey sub-basin did have a higher proportion of sand in surface sediments than the southern sub-basin, likely because Whidbey and Camano Islands have the greatest linear extent of intact unarmored feeder bluffs of any county in Puget Sound (Coastal Geologic Services (CGS), 2020), and therefore may have the greatest potential for eroding sand accumulation on upper beaches.
Our study focused on the upper beach where direct shoreline restoration actions most commonly occur. Further connections across the land-sea interface will help identify how functions operate at variable tidal elevations and distances from shore. For example, previous work with community scientist groups has shown that the taxa richness of lower intertidal biota was higher at feeder bluffs than at accretional and modified shorelines (Toft et al., 2017). Above the beach on the bluff face, piscivorous birds such as Pigeon Guillemots nest and can influence food web dynamics (Bishop et al., 2016). Further, sediment supply to tidal flats are often the main reason that feeder bluffs are prioritized for restoration and protection (Dawson et al., 2009; Coastal Geologic Services (CGS), 2020). Along with forage fish spawning on beaches (Rice, 2006), there are wider benefits of restoration and protection efforts to the fish, birds, and invertebrates that rely on coastal habitats for nursery functions (Lefcheck et al., 2019). These benefits can connect to subtidal zones as well, in the context of shoreline restoration associations for offshore subtidal fish (Francis et al., 2022), as well as subtidal restoration actions for eelgrass (Zostera marina) (Thom et al., 2018) and bull kelp (Nereocystis luetkeana) (Carney et al., 2005).
Geophysical features can be used in the planning of restoration site feasibility, as has been done regionally (Johannessen et al., 2014; Coastal Geologic Services (CGS), 2020) and in Chesapeake Bay, USA, using a suite of fine-scale coastal conditions to provide management recommendations on where “living shorelines” are suitable (Nunez et al., 2022). By incorporating the effectiveness of restoration with landscape features such as shoretype and fetch, we can further expand prioritization guidelines to include likely functions of restoration. Physical and biological factors are tightly linked in coastal systems (Dethier and Schoch, 2005; Defeo et al., 2009), and continued examination of how these relate to aspects of restoration effectiveness and global change should be prioritized by shoreline scientists and managers, both along soft sediment shores as in our study, as well as rocky shores such as at the base of cliffs in the United Kingdom (Jackson and McIlvenny, 2011). Analyzing this context-dependence will allow us to more effectively link our knowledge of coastal seascape ecology (Boström et al., 2011) with planning for the future restoration of our coasts (Morris et al., 2022). By incorporating larger-scale geophysical features, we can guide restoration effectiveness across diverse landscape topographies, beyond the variability that is often noted at the single-site scale within multi-scale analyses (Simenstad et al., 2000; Valesini et al., 2014), and as interpretation of variability in terms of restoration effectiveness (Des Roches et al., 2022; Morris et al., 2022). These elements can improve our collective understanding of not only shoreline restoration, but on a larger scale, of the landscape features that govern shoreline functions.
Data availability statement
The datasets presented in this study can be found in online repositories. The names of the repository/repositories and accession number(s) can be found below: All datasets generated for this study are uploaded to the Shoreline Monitoring Database: https://www.shoremonitoring.org.
Author contributions
JT, HF, JM, BP, and MD contributed to conception and design of the study. JT, SR, KA, HF, JM, BP, MM and JK organized the database. KA and SR performed statistical analysis. All authors contributed to manuscript writing, reading, and approval of the submitted version.
Funding
This project has been funded wholly or in part by the United States Environmental Protection Agency under assistance agreement PC-01 J22301 through the Washington Department of Fish and Wildlife. Additional community science coordination through the Vashon Nature Center was funded by a King County Flood Control District Stewardship Engagement and Learning grant and Rose Foundation for Communities and Environment. The contents of this document do not necessarily reflect the views and policies of the Environmental Protection Agency or the Washington Department of Fish and Wildlife, or any other funders, nor does mention of trade names or commercial products constitute endorsement or recommendation for use.
Acknowledgments
We thank the community scientists and coordinators who have helped collect data over the years through the Northwest Straits Foundation, the Vashon Nature Center, the University of Washington, the Snohomish County WSU Beach Watchers, and the Washington Department of Fish and Wildlife. We thank W Raymond and S Fishman for their helpful reviews, and K Litle for her support throughout this project.
Conflict of interest
The authors declare that the research was conducted in the absence of any commercial or financial relationships that could be construed as a potential conflict of interest.
Publisher’s note
All claims expressed in this article are solely those of the authors and do not necessarily represent those of their affiliated organizations, or those of the publisher, the editors and the reviewers. Any product that may be evaluated in this article, or claim that may be made by its manufacturer, is not guaranteed or endorsed by the publisher.
Supplementary material
The Supplementary Material for this article can be found online at: https://www.frontiersin.org/articles/10.3389/fmars.2023.1199749/full#supplementary-material
References
Beamer E. M., McBride A., Henderson R., Griffith J., Fresh K., Zackey T., et al. (2006). Habitats and fish use of pocket estuaries in the whidbey basin and north skagit county bays 2004 and 2005 (Technical report by The Skagit River System Cooperative).
Bell S. S., Middlebrooks M. L., Hall M. O. (2014). The value of long-term assessment of restoration: support from a seagrass investigation. Restor. Ecol. 22, 304–310. doi: 10.1111/rec.12087
Bilkovic D. M., Mitchell M. M., La Peyre M. K., Toft J. D. (2017). Living shorelines: the science and management of nature-based coastal protection. 1st ed (Boca Raton, FL: CRC Press).
Bishop E., Rosling G., Kind P., Wood F. (2016). Pigeon guillemots on whidbey island, Washington: a six-year monitoring study. Northwest. Nat. 97, 237–245. doi: 10.1898/NWN15-31.1
Bolker B., Brooks M., Clark C., Geange S., Poulsen J., Stevens H., et al. (2009). Generalized linear mixed models: a practical guide for ecology and evolution. Trends Ecol. Evol. 24, 127–135. doi: 10.1016/j.tree.2008.10.008
Borland H. P., Schlacher T. A., Gilby B. L., Connolly R. M., Yabsley N. A., Olds A. D. (2017). Habitat type and beach exposure shape fish assemblages in the surf zones of ocean beaches. Mar. Ecol. Prog. Ser. 570, 203–211. doi: 10.3354/meps12115
Boström C., Pittman S. J., Simenstad C., Kneib R. T. (2011). Seascape ecology of coastal biogenic habitats: advances, gaps, and challenges. Mar. Ecol. Prog. Ser. 427, 191–217. doi: 10.3354/meps09051
Brooks M., Kristensen K., van Benthem K., Magnusson A., Berg C. W., Nielsen A., et al. (2017). GlmmTMB balances speed and flexibility among packages for zero-inflated generalized linear mixed modeling. R J. 9, 378–400. doi: 10.32614/RJ-2017-066
Bugnot A. B., Mayer-Pinto M., Airoldi L., Heery E. C., Johnston E. L., Critchley L. P., et al. (2021). Current and projected global extent of marine built structures. Nat. Sustain. 4, 33–41. doi: 10.1038/s41893-020-00595-1
Carney L. T., Waaland J. R., Klinger R., Ewing K. (2005). Restoration of the bull kelp nereocystis luetkeana in nearshore rocky habitats. Mar. Ecol. Prog. Ser. 302, 49–61. doi: 10.3354/meps302049
Coastal Geologic Services (CGS) (2017). Nearshore geospatial framework final version (ArcGIS map package prepared for the puget sound partnership) (Bellingham, WA).
Coastal Geologic Services (CGS) (2020). Beach strategies for puget sound, phase 2 summary report (Bellingham, WA: Prepared for the Estuary and Salmon Restoration Program of the Washington Department of Fish and Wildlife).
Cohen J. (1988). Statistical power analysis for the behavioral sciences. 2nd ed (Hillsdale, NJ: Lawrence Erlbaum Associates, Publishers).
Davis J. L. D., Takacs R. L., Schnabel R. (2006). “Evaluating ecological impacts of living shorelines and shoreline habitat elements: an example from the upper western Chesapeake bay,” in Management, policy, science, and engineering of nonstructural erosion control in the Chesapeake bay, vol. 08-164 . Eds. Erdle S. Y., Davis J. L. D., Sellner K. G. (Chesapeake Bay: CRC Publ), 55–61.
Dawson R. J., Dickson M., Nicholls R. J., Hall J. W., Walkden M. J. A., Stansby P. K., et al. (2009). Integrated analysis of risks of coastal flooding and cliff erosion under scenarios of log term change. Climatic Change 95, 249–288. doi: 10.1007/s10584-008-9532-8
Defeo O., McLachlan A., Schoeman D. S., Schlacher T. A., Dugan J., Jones A., et al. (2009). Threats to sandy beach ecosystems: a review. Estuar. Coast. Shelf. S. 81, 1–12. doi: 10.1016/j.ecss.2008.09.022
Des Roches S., LaFuente J. R., Faulkner H. S., Morgan J. R., Perla B. S., Metler M., et al. (2022). Shoreline armor removal can restore variability in intertidal ecosystems. Ecol. Indic. 140, 109056. doi: 10.1016/j.ecolind.2022.109056
Dethier M. N., Raymond W. W., McBride A. N., Toft J. D., Cordell J. R., Ogston A. S., et al. (2016). Multiscale impacts of armoring on salish Sea shorelines: evidence for cumulative and threshold effects. Estuar. Coast. Shelf. S. 175, 106–117. doi: 10.1016/j.ecss.2016.03.033
Dethier M. N., Schoch G. C. (2005). The consequences of scale: assessing the distribution of benthic populations in a complex estuarine fjord. Estuar. Coast. Shelf. S. 62, 253–270. doi: 10.1016/j.ecss.2004.08.021
Duarte C. M., Agusti S., Barbier E., Britten G. L., Castilla J. C., Gattuso J.-P., et al. (2020). Rebuilding marine life. Nature 580, 39–51. doi: 10.1038/s41586-020-2146-7
Francis T. B., Sullaway G. H., Feist B. E., Shelton A. O., Chui E., Daley C., et al. (2022). Equivocal associations between small-scale shoreline restoration and subtidal fishes in an urban estuary. Restor. Ecol. 30, e13652. doi: 10.1111/rec.13652
Gittman R. K., Fodrie F. J., Popowich A. M., Keller D. A., Bruno J. F., Currin C. A., et al. (2015). Engineering away our natural defenses: an analysis of shoreline hardening in the US. Front. Ecol. Environ. 13, 301–307. doi: 10.1890/150065
Gittman R. K., Peterson C. H., Currin C. A., Fodrie F. J., Piehler M. F., Bruno J. F. (2016a). Living shorelines can enhance the nursery role of threatened estuarine habitats. Ecol. Appl. 26, 249–263. doi: 10.1890/14-0716
Gittman R. K., Scyphers S. B., Smith C. S., Neylan I. P., Grabowski J. H. (2016b). Ecological consequences of shoreline hardening: a meta-analysis. BioScience 66, 763–773. doi: 10.1093/biosci/biw091
Guthrie A., Bikovic D. M., Mitchell M. M., Chambers R. M., Jessica T., Isdell R. E. (2022). Ecological equivalency of living shorelines and natural marshes for fish and crustacean communities. Ecol. Eng. 176, 106511. doi: 10.1016/j.ecoleng.2021.106511
Hino M., Field C., Mach K. (2017). Managed retreat as a response to natural hazard risk. Nat. Clim. Change 7, 364–370. doi: 10.1038/nclimate3252
Howe E. R., Simenstad C. A. (2011). Isotopic determination of food web origins in restoring and ancient estuarine wetlands of the San Francisco bay and delta. Estuar. Coasts 34, 597–617. doi: 10.1007/s12237-011-9376-8
Hurvich C. M., Tsai C.-L. (1989). Regression and time series model selection in small samples. Biometrika 76, 297–307. doi: 10.1093/biomet/76.2.297
Hyndes G. A., Berdan E., Duarte C., Dugan J. E., Emery K. A., Hambäck P. A., et al. (2022). The role of inputs of marine wrack and carrion in sandy-beach ecosystems: a global review. Biol. Rev., 97, 2127–2161. doi: 10.1111/brv.12886
Jackson A. C., McIlvenny J. (2011). Coastal squeeze on rocky shores in northern Scotland and some possible ecological impacts. J. Exp. Mar. Biol. Ecol. 400, 314–321. doi: 10.1016/j.jembe.2011.02.012
Johannessen J., MacLennan A., Blue A., Waggoner J., Williams S., Gerstel W., et al. (2014). Marine shoreline design guidelines (Washington: Washington Department of Fish and Wildlife. Olympia).
Landi A. A., Vokoun J. C., Howell P., Auster P. (2015). Predicting use of habitat patches by spawning horseshoe crabs (Limulus polyphemus) along a complex coastline with field surveys and geospatial analyses. Aquat. Conserv. 25, 380–395. doi: 10.1002/aqc.2440
Lee T. S., Toft J. D., Cordell J. R., Dethier M. N., Adams J. W., Kelly R. P. (2018). Quantifying the effectiveness of shoreline armoring removal on coastal biota of puget sound. PeerJ 6, e4275. doi: 10.7717/peerj.4275
Lefcheck J. S., Hughes B. B., Johnson A. J., Pfirrmann B. W., Rasher D. B., Smyth A. R., et al. (2019). Are coastal habitats important nurseries? A Meta Analysis. Conserv. Lett. 12, e12645. doi: 10.1111/conl.12645
Lenth R. V. (2022). “Emmeans: estimated marginal means, aka least-squares means,” in R package version 1.7.5. Available at: https://CRAN.R-project.org/package=emmeans.
Ma Z., Melville D. S., Liu J., Chen Y., Yang H., Ren W., et al. (2014). Rethinking china’s new great wall. Science 346, 912–914. doi: 10.1126/science.1257258
MacLennan A., Rishel B., Johannessen J., Lubeck A., Ode L. (2017). Beach strategies phase 1 summary report - identifying target beaches to restore and protect. Prepared Estuary Salmon Restor. Program.
Morris R. L., Bilkovic D. M., Boswell M. K., Bushek D., Cebrian J., Goff J., et al. (2019b). The application of oyster reefs in shoreline protection: are we over-engineering for an ecosystem engineer? J. Appl. Ecol. 56, 1703–1711. doi: 10.1111/1365-2664.13390
Morris R. L., Bilkovic D. M., Walles B., Strain E. M. A. (2022). Nature-based coastal defence: developing the knowledge needed for wider implementation of living shorelines. Ecol. Eng. 185, 106798. doi: 10.1016/j.ecoleng.2022.106798
Morris R. L., Heery E. C., Loke L. H. L., Lau E., Strain E. M. A., Airoldi L., et al. (2019a). Design options, implementation issues and evaluating success of ecologically engineered shorelines. Oceanogr. Mar.Biol. 57, 169–228. doi: 10.1201/9780429026379-4
Nordstrom K. F., Jackson N. L. (2012). Physical processes and landforms on beaches in short fetch environments in estuaries, small lakes and reservoirs: a review. Earth-Sci. Rev. 111, 232e247. doi: 10.1016/j.earscirev.2011.12.004
Nunez K., Rudnicky T., Mason P., Tombleson C., Berman M. (2022). A geospatial modeling approach to assess site suitability of living shorelines and emphasize best shoreline management practices. Ecol. Eng. 179, 106617. doi: 10.1016/j.ecoleng.2022.106617
R Core Team (2022). R: a language and environment for statistical computing (Vienna, Austria: R Foundation for Statistical Computing). Available at: http://www.R-project.org/.
Rice C. A. (2006). Effects of shoreline modification on a northern puget sound beach: microclimate and embryo mortality in surf smelt (Hypomesus pretiosus). Estuar. Coasts 29, 63–71. doi: 10.1007/BF02784699
Roff J. C., Taylor M. E. (2000). National frameworks for marine conservation – a hierarchical geophysical approach. Aquat. Cons. Mar. Fresh. Eco. 10, 209–223. doi: 10.1002/1099-0755(200005/06)10:3%3C209::AID-AQC408%3E3.0.CO;2-J
Rogers L. A., Olsen E. M., Knutsen H., Stenseth N. C. (2014). Habitat effects on population connectivity in a coastal seascape. Mar. Ecol. Prog. Ser. 511, 153–163. doi: 10.3354/meps10944
Romanuk T. N., Levings C. D. (2003). Associations between arthropods and the supralittoral ecotone: dependence of aquatic and terrestrial taxa on riparian vegetation. Environ. Entomol. 32, 1343–1353. doi: 10.1603/0046-225X-32.6.1343
Romanuk T. N., Levings C. D. (2006). Relationships between fish and supralittoral vegetation in nearshore marine habitats. Aquat. Cons. Mar. Fresh. Eco. 16, 115–132. doi: 10.1002/aqc.704
Simenstad C. A., Hood W. G., Thom R. M., Levy D. A., Bottom D. L. (2000). “Landscape structure and scale constraints on restoring estuarine wetlands for pacific coast juvenile fishes,” in Concepts and controversies in tidal marsh ecology. Eds. Weinstein M. P., Kreeger D. A. (Dordrecht: Kluwer Academic Publisher), 597–630.
Smith C. S., Rudd M. E., Gittman R. K., Melvin E. C., Patterson V. S., Renzi J. J., et al. (2020). Coming to terms with living shorelines: a scoping review of novel restoration strategies for shoreline protection. Front. Mar. Sci. 7. doi: 10.3389/fmars.2020.00434
Sobocinski K. L. (2021). State of the salish Sea. Eds. Broadhurst G., Baloy N. J. K. (Salish Sea Institute, Western Washington University). doi: 10.25710/vfhb-3a69
Strain E. M. A., Heath T., Steinberg P. D., Bishop M. J. (2018). Eco-engineering of modified shorelines recovers wrack subsidies. Ecol. Eng. 112, 26–33. doi: 10.1016/j.ecoleng.2017.12.009
Thom R., Gaeckle J., Buenau K., Borde A., Vavrinec J., Aston L., et al. (2018). Eelgrass (Zostera marina l.) restoration in puget sound: development of a site suitability assessment process. Restor. Ecol. 26, 1066–1074. doi: 10.1111/rec.12702
Toft J.D., Ogston A.S., Heerhartz S.M., Cordell J.R., Flemer E.E. (2013). Ecological response and physical stability of habitat enhancements along an urban armored shoreline. Ecol. Eng. 57, 97–108. doi: 110.1016/j.ecoleng.2013.04.022
Toft J. D., Cordell J. R., and Armbrust E. A. (2014). Shoreline armoring impacts and beach restoration effectiveness vary with elevation. Northwest Sci. 88, 367–375. doi: 10.3955/046.088.0410
Toft J. D., Cordell J. R., Simenstad C. A., Stamatiou L. A. (2007). Fish distribution, abundance, and behavior along city shoreline types in puget sound. N. Am. J. Fish. Manage. 27, 465–480. doi: 10.1577/M05-158.1
Toft J. D., Dethier M. N., Howe E. R., Buckner E. V., Cordell J. R. (2021). Effectiveness of living shorelines in the salish Sea. Ecol. Eng. 167, 106255. doi: 10.1016/j.ecoleng.2021.106255
Toft J., Fore L., Hass T., Bennett B., Brubaker L., Brubaker D., et al. (2017). A framework to analyze citizen science data for volunteers, managers, and scientists. Cit. Sci. Theory Pract. 2, 9. doi: 10.5334/cstp.100
Toft J. D., Munsch S. H., Cordell J. R., Siitari K., Hare V. C., Holycross B., et al. (2018). Impact of multiple stressors on juvenile fish in estuaries of the northeast pacific. Glob. Change Biol. 24, 2008–2020. doi: 10.1111/gcb.14055
Valesini F. J., Potter I. C., Clarke K. R. (2004). To what extent are the fish compositions at nearshore sites along a heterogeneous coast related to habitat type? Estuar. Coast. Shelf. S. 60, 737–754. doi: 10.1016/j.ecss.2004.03.012
Valesini F. J., Teedley J. R., Clarke K. R., Potter I. C. (2014). The importance of regional, system-wide and local spatial scales in structuring temperate estuarine fish communities. Estuar. Coasts 37, 525–547. doi: 10.1007/s12237-013-9720-2
Wickham H. (2016). Ggplot2: elegant graphics for data analysis (Springer-Verlag New York). Available at: https://ggplot2.tidyverse.org.
Wickham H., Francois R., Henry L., Muller K. (2022). “Dplyr: a grammar of data manipulation,” in R package version 1.0.9. Available at: https://CRAN.R-project.org/package=dplyr.
Keywords: restore, living shorelines, habitat, armor, coastal protection
Citation: Toft JD, Accola KL, Des Roches S, Kobelt JN, Faulkner HS, Morgan JR, Perla BS, Metler M and Dethier MN (2023) Coastal landforms and fetch influence shoreline restoration effectiveness. Front. Mar. Sci. 10:1199749. doi: 10.3389/fmars.2023.1199749
Received: 03 April 2023; Accepted: 16 June 2023;
Published: 29 June 2023.
Edited by:
Correigh Greene, National Oceanic and Atmospheric Administration (NOAA), United StatesReviewed by:
Jenny R. Hillman, The University of Auckland, New ZealandRochelle Diane Seitz, College of William & Mary, United States
Copyright © 2023 Toft, Accola, Des Roches, Kobelt, Faulkner, Morgan, Perla, Metler and Dethier. This is an open-access article distributed under the terms of the Creative Commons Attribution License (CC BY). The use, distribution or reproduction in other forums is permitted, provided the original author(s) and the copyright owner(s) are credited and that the original publication in this journal is cited, in accordance with accepted academic practice. No use, distribution or reproduction is permitted which does not comply with these terms.
*Correspondence: Jason D. Toft, tofty@uw.edu