Understanding nitrogen dynamics in coral holobionts: comprehensive review of processes, advancements, gaps, and future directions
- 1State Key Laboratory of Marine Environmental Sciences, College of Ocean and Earth Science, Xiamen University, Xiamen, China
- 2State Key Laboratory of Pollution Control and Resource Reuse, School of the Environment, Nanjing University, Nanjing, China
- 3State Key Laboratory of Marine Resource Utilization in South China Sea, Hainan University, Haikou, China
Coral reefs are known for being highly productive ecosystems in oligotrophic oceans, which is commonly referred to as the Darwin’s Paradox. Nitrogen is an essential component of organisms, but it limits primary productivity in most euphotic ocean, including the coral reef system. Therefore, understanding nitrogen’s transfer and transformation within the coral holobiont is essential to comprehend the holobiont homeostasis and functioning mechanisms, which may help to explain the Darwin’s Paradox. Previous studies have pointed out the fundamental importance of nitrogen cycling between coral host and symbiotic algae. Recently, increasing researches, particularly in quantitative aspect, have significantly improved our understandings of the various roles of nitrogen pathways in regulating the inter-relationship among coral host and symbiotic algae and the associated microbiome. In this paper, we synthesized knowledge advances of different nitrogen processes in coral holobionts standing on the nitrogen cycle perspective. We extracted consensus and contradictions from published research results regarding nitrogen flows of coral holobiont. This review presented the temporal and spatial variation of nitrogen fixation and analyzed the global nitrogen processes rates in coral holobionts. We also summarized projections of specific nitrogen processes of coral holobionts facing climate change from limited reports. We realized that there are significant gaps in our understanding of nitrogen processes in coral holobionts, which hindering our comprehension of nitrogen balance in coral holobionts and, therefore, the coral reef systems. These gaps include the roles and relative importance of nitrification, denitrification, and DNRA in coral holobionts, as well as the self-regulation mechanisms to maintain nitrogen-homeostasis in short-term and long-term, particularly in the context of environmental changes. At the end, we provide our opinions on research methods regarding quantitative coral research in the future.
1 Introduction
Coral reefs are thriving ecosystems in oligotrophic seas, similar to an oasis in the desert. This phenomenon was first noticed by Darwin (Kleypas et al., 1999; O'neil and Capone, 2008; Radecker et al., 2015). There are two main hypotheses for explaining this paradox (O'neil and Capone, 2008; Brandl et al., 2019). Firstly, external input of ‘new nutrients’, such as the supply from nitrogen fixation (Cardini et al., 2015; Roth et al., 2020), upwelling (Andrews and Gentien, 1982; Rougerie et al., 1992), groundwater (Umezawa et al., 2002; Wang et al., 2018; Oehler et al., 2019; Silbiger et al., 2020), and seabird-producing nutrients (Smith and Johnson, 1995; Savage, 2019), can facilitate the growth of corals and the expansion of coral reefs. Secondly, the efficient internal energy utilization and nutrient cycling, e.g., recycling and utilization by different communities tightly connected in coral holobionts (Muscatine and Porter, 1977) and coral reef ecosystems (de Goeij et al., 2013; Brandl et al., 2019), promote self-sustainability of the system. Since nitrogen is the critical limiting nutrient in oligotrophic sunlit ocean (Falkowski, 1997; Moore et al., 2013), understanding the supply, availability, and cycling of nitrogen in coral holobionts and coral reefs is essential to resolve the Darwin’s paradox and to predict the future of coral reef systems under global change.
Reef-building coral is an animal that scarcely survive on its own. The coral holobiont, a meta-organism consisting of coral host, symbiotic algae, fungi, bacteria, archaea, and viruses (Wegley et al., 2007), forms a complex system with intricate interactions. The symbiotic relationship between the coral host and symbiotic algae is fundamental for the holobiont, and the nitrogen-limited microenvironment created by coral holobiont is crucial for maintaining this relationship (Muscatine et al., 1997; Burkepile et al., 2020; Roberty et al., 2020; Rivera and Davies, 2021). In addition, there are abundant nitrogen-associated functional microorganisms living in coral holobionts (Kimes et al., 2010; Bourne et al., 2016), which create a network to maintain the internal nitrogen balance (Tilstra et al., 2019a) and productivity (Bednarz et al., 2018) of coral holobionts. Studies have shown that nitrogen is one of the key elements for the regulation among components within the coral holobiont (Cui et al., 2019; Ferrier-Pagès et al., 2021; Rivera and Davies, 2021). Therefore, it is necessary to elucidate and discuss from a system perspective the nitrogen transfer and transformation occurring among these components in coral holobionts.
In 2015, Rädecker et al. summarized the contemporary knowledge of the nitrogen cycle in coral holobionts (Radecker et al., 2015). However, there are amounts of significant progresses about nitrogen cycle in coral holobionts, especially in the quantitative aspect and the responses to environmental change after 2015, greatly promoting our understanding in this field. Given the advances after their review, this review compiles quantitative data of coral holobionts’ nitrogen processes globally and examines the consistent and inconsistent research results for different nitrogen processes. Additionally, we discuss the nitrogen flow through coral holobionts and the response of nitrogen pathways in coral holobionts to global change. Here we review and reexamine current knowledges and try to expand our understanding about the nitrogen dynamics within coral holobiont from a system perspective. Finally, we suggested specific areas for future research to fill the knowledge gaps in coral ecosystems and propose opinions on research methods for quantitative coral studies.
2 Dynamic environmental gradients in the coral ecosystem and holobiont
Despite the serene appearance of the transparent ocean, the chemical and physical properties of coral habitat are highly dynamic. Corals are subject to a range of physical and hydrodynamic forces, including light, waves, tides, storms, and riverine discharges, which combined with biological feedbacks create strong and constantly shifting environmental gradients for chemicals, such as dissolved oxygen (DO), partial pressure of carbon dioxide (pCO2), and pH both inside and outside the coral. These gradients provide distinctive conditions for microorganisms associated with various nitrogen pathways, meanwhile, the dynamic change in gradients regulate the pathways and intensity of nitrogen cycling in coral holobionts.
Diel light cycles are the most prominent forcing in coral habitats, with the light intensity changing significantly as it passes through the coral tissue. As much as 90% - 99% of photosynthetically active radiation (PAR) is absorbed or scattered before it reaches the coral skeleton (Schlichter et al., 1997; Magnusson et al., 2007), which not only affects the assimilation of nutrients (Domotor and Delia, 1984; Falkowski et al., 1984; Almoghrabi et al., 1993; Grover et al., 2002) and carbon transfer efficiency between the coral symbiont and coral host (Tremblay et al., 2013; Ezzat et al., 2017; Wall et al., 2020), but also regulates many light-sensitive nitrogen processes such as ammonia oxidation and nitrite oxidation (Horrigan et al., 1981; Olson, 1981; Horrigan and Springer, 1990; Guerrero and Jones, 1996). Hydrodynamic force also plays a crucial role in shaping the coral reef environment. While extreme weather events can physically damage coral reefs, regular hydrodynamics, such as waves and tides, which corals encountered every day can shape the reefs’ environmental properties, and associated with biogeochemical processes closely, for example, by imperceptible diffusion and sensible advection of nutrients.
The chemical property of coral ecosystems is largely controlled by benthos. Diel light cycles primarily control the photosynthesis and respiration of benthos, which in turn modulates DO and pCO2 cycles (Jiang et al., 2011). Meanwhile, tidal cycles and episodic storm events tune the degree of water exchange between coral habitat and surrounding surface and subsurface waters with varying chemical and physical property, such as DO, pCO2, pH, nutrients, and temperature. Accordingly, coral habitats experience vigorous oxygen fluctuations over diel and tidal cycles, with diurnal variations in oxygen saturation from 200% at dusk to 50% at dawn (Sournia, 1976; Guadayol et al., 2014; Nelson and Altieri, 2019). These fluctuations are particularly pronounced in atolls and lagoons, where oxygen exchange is weak due to the temporal decoupling of oxygen consumption by abundant organisms’ respiration and diel photosynthesis. Reasonably, the range of DO variation in coral is much larger, particularly in its diffusive boundary layer, than that in the ambient seawater (Shashar et al., 1993; Wangpraseurt et al., 2012). The main cause is the temporal decoupling between the photosynthetic oxygen production by symbiont algae in the day and the overall oxygen consumption of the holobiont throughout the day (Shashar et al., 1993; O'neil and Capone, 2008). In addition, the production of organic-rich mucus by corals on a daily basis (Wild et al., 2004) obstructs the diffusion of oxygen and accelerates microbial respiration on the coral surface, creating an low oxygen microenvironment (Ritchie and Smith, 2004). Meanwhile, hypoxia is also frequently observed in the coral gastric cavity where water exchange is limited (Agostini et al., 2011). Oxygen not only affects the oxygen consumption pattern of coral in turn (Hughes et al., 2022), but also regulates the path and intensity of nitrogen processes sensitive to redox.
Similar to oxygen, coral reefs are also subject to temporally decoupled diel and tidal cycles on pCO2 and pH, which is opposite to oxygen due to photosynthesis and respiration of multiple communities (Jiang et al., 2011; Jiang et al., 2018; George and Lugendo, 2023). Furthermore, microenvironments with unique pH have been detected in coral holobionts, such as the calcifying fluid under calicoblastic epithelium, where the pH is 0.5-1.9 units higher than the surrounding water due to the active proton pump (Ries, 2011; Venn et al., 2011). Conversely, the pH in the semi-enclosed gastric cavity is lower than the ambient condition (Agostini et al., 2011). As pH can regulate the availability and enzymes activity (Stein et al., 1997; Park and Bae, 2009; Beman et al., 2011), it plays a direct role in driving -related nitrogen reaction chains.
Significant nutrient gradients have been reported between inside and outside corals (Risk and Muller, 1983; Schiller and Herndl, 1989; Agostini et al., 2011). For example, nutrient concentrations are even hundreds of times higher in corals’ gastric cavity and porewater than that in ambient seawater (Agostini et al., 2011). Regardless the mechanisms, the availability of nitrogen directly affects the activities of nitrogen-related microorganisms and thus the symbiotic relationship between the coral host and its symbiotic microorganisms.
Overall, coral reef environmental parameters change strongly due to tidal-induced exchange or storm-induced riverine influence superimposed onto the diurnal variation of light-induced DO, pCO2 and pH fluctuations. Such fluctuations of environmental may become more intense in the future due to the intensification of global warming, acidification, eutrophication, and hypoxia. These changes may promote coral adaptability (Oliver and Palumbi, 2011; Comeau et al., 2014; Palumbi et al., 2014; Schoepf et al., 2015; Safaie et al., 2018; Lima et al., 2022), sellect corals with stronger adaptability (Grottoli et al., 2014), and/or affect the health of coral host and symbionts, shaking the stability and development of coral reef ecosystems. However, answering this question is still challenging due to the intricate interaction of climate change and adaptive capacity (Logan et al., 2021).
3 Fundamental nutrition supplies for coral holobiont via hosts’ heterotrophy and symbiotic algae’ mixotrophy
Hosts’ heterotrophy and symbiotic algae’ mixotrophy are two essential pathways for coral holobiont to acquire nutrients. The proportion of the two pathways varies depending on nutrient regimes, environmental conditions and symbiotic algae type (Muscatine et al., 1997; Grover et al., 2002; Baker et al., 2013; Leal et al., 2015; Ezzat et al., 2017; Fox et al., 2018; Ferrier-Pagès et al., 2021), highlighting the trophic flexibility of coral holobionts under varying or stressful conditions.
In oligotrophic seas, dissolved inorganic nitrogen (DIN) is limited, making heterotrophy a vital pathway for many corals (Martinez et al., 2020; Pupier et al., 2021). Heterotrophic path can satisfy 15% - 50% of corals’ daily metabolism, and this proportion can further increase up to 100% for bleaching corals (Houlbreque and Ferrier-Pages, 2009; Meunier et al., 2019; Price et al., 2021). Actually, the plasticity and resilience of heterotrophy for corals are crucial in stress resistance, bleaching, and recovery (Grottoli et al., 2006; Tremblay et al., 2016; Meunier et al., 2022; Radice et al., 2022), whether the stressor is thermal (Lyndby et al., 2020) or high-CO2 (Schoepf et al., 2013). Meanwhile, through heterotrophic feeding, coral hosts can acquire nitrogen, which is deficient in organic substances provided by symbiotic algae (Falkowski et al., 1993; Burriesci et al., 2012).
Coral hosts have the ability to ingest different types of organic matter, including live particulate organic matter (POM) (Ribes et al., 2003; Tremblay et al., 2011), detrital particulate organic matter (Anthony, 1999; Anthony, 2000), and dissolved organic matter (DOM) (Levas et al., 2013; Levas et al., 2015). Among these, the ingestion of POM, such as zooplankton (Palardy et al., 2008), picoplankton, and nanoplankton (Hoadley et al., 2021; Meunier et al., 2021b), has been widely studied. The contribution of heterotrophic feeding on zooplankton to the corals’ total fixed carbon budget appears to be highly species-specific, ranging from less than 4% (Grottoli et al., 2014) to as much as 46% (Palardy et al., 2008). While the relationship between bleaching events and heterotrophic feeding on zooplankton in corals is complex and not yet fully understood. Some cases show an increase in heterotrophic feeding on zooplankton during bleaching, while others do not (Grottoli et al., 2006; Palardy et al., 2008; Grottoli et al., 2014). Additionally, some studies suggest that differences in heterotrophic plasticity between single and repeat bleaching corals may explain the observed differences (Grottoli et al., 2014; Levas et al., 2015). As for pico- and nanoplankton, a major source of nitrogen for coral reef communities, there has been increased attention on coral’s selective ingestion of pico- and nanoplankton in recent years, especially regarding coral acclimation under stress. For example, Meunier (Meunier et al., 2019; Meunier et al., 2021b) found that some corals feed more on Synechococcus than Prochlorococcus and picoeukaryotes in bleaching or in high pCO2 conditions. They attributed such selective ingestion to higher nitrogen demand for physiological stress reliefs, as Synechococcus contains higher nitrogen content (Meunier et al., 2019; Meunier et al., 2021b). Corals can also selectively ingest diazotrophs to relieve stress (Meunier et al., 2019), which are abundant in coral reefs (Foster et al., 2009; Turk-Kubo et al., 2015; Messer et al., 2016; Bonnet et al., 2017; Messer et al., 2017). Actually, nitrogen sourced from heterotrophic feeding on diazotrophs (the host’s heterotrophy) can be even up to a thousand times higher than that from symbiotic N2 fixation (N2 fixation process), regardless of stresses, for some corals (Meunier et al., 2021a). N2 fixation will be discussed further in section 4. Coral hosts’ assimilation of DIN (coral hosts’ autotrophy) will not be discussed since the uptake rate by coral hosts is negligible (Pernice et al., 2012) and is often thought to be assimilated by symbiotic algae only (Kopp et al., 2013).
Besides the heterotrophy, symbiotic algae’ mixotrophy is another vital pathway for coral holobionts to acquire nitrogen (Grover et al., 2008; Krueger et al., 2020; Diroberts et al., 2021), in the forms of DIN and partly micromolecular DON (e. g. urea and some amino acids) (Wilkerson and Trench, 1986; Grover et al., 2006; Grover et al., 2008; Pernice et al., 2012; Martinez et al., 2022). Although symbiotic algae preferred DIN obviously (Grover et al., 2008), DON uptake cannot be ignored, as Renaud Grover found that the uptake rate of DON accounts for 1/4 of total nitrogen demand in the scleractinian coral Stylophora pistillata (Grover et al., 2006; Grover et al., 2008). Symbiotic algae showed different affinities for DIN species (Domotor and Delia, 1984; Grover et al., 2003; Diroberts et al., 2021; Li et al., 2021), among which is highly preferred due to energy-saving. Moreover, the uptake of DIN by symbiotic algae is a quick process (within an hour), as evidenced by using nanoscale secondary ion mass spectrometry (NanoSIMS) (Pernice et al., 2012; Kopp et al., 2013; Kopp et al., 2015; Lema et al., 2016), a powerful tool to visualize and quantify simultaneously the (sub) cellular-level nitrogen dynamic processes.
Coral habitats face various environmental stressors such as warming, acidification, and eutrophication, which have been extensively studied for their impacts on coral physiology, particularly photosynthesis and calcification (Towle et al., 2015; Simancas-Giraldo et al., 2021). However, the impact of these stressors on nitrogen assimilation has often been overlooked. Emerging evidences show that environmental stressors may modulate the pathway allocation of nitrogen sourced from the hosts’ heterotrophy and symbiotic algae’ mixotrophy, thus altering the symbiotic relationship between the symbiotic algae and coral host. Coral holobionts can adapt to moderately high level of nutrient enrichment (Krueger et al., 2020; Dobson et al., 2021), but nutrient levels exceeding the threshold can cause a drop in coral physiological functions, leading to bleaching or even mortality (Nalley et al., 2023). Different forms of excess nitrogen have differential impacts on coral physiology (Shantz and Burkepile, 2014; Burkepile et al., 2020; Fernandes De Barros Marangoni et al., 2020; Diroberts et al., 2021; Zhao et al., 2021). For example, makes coral bleach more frequently relative to and urea, likely due to the induced oxidative stress (Burkepile et al., 2020; Fernandes De Barros Marangoni et al., 2020; Blanckaert et al., 2021).
It is believed that food supply helped in preventing damage to the coral physiology performance. In response to deviations in temperature or pH levels, corals can adjust their heterotrophic feeding and inorganic nutrient acquisition to help counteract these adverse effects (Ferrier-Pages et al., 2010; Houlbreque et al., 2015; Towle et al., 2015). However, the extent to which these responses occur may vary depending on factors such as coral species (Ferrier-Pages et al., 2010; Ezzat et al., 2016), symbiotic algae clade (Baker et al., 2013), stress history (Towle et al., 2016; Gibbin et al., 2018), and synergistic control of multiple environmental factors (Godinot et al., 2011). For example, high pCO2 can significantly reduce the acquisition of organic nutrients such as dissolved free amino acids and zooplankton (Houlbreque et al., 2015). While Towle et al. show corals experienced ocean acidification have a higher feeding rate. Nowadays, more experiments were conducted to study the combined effects of environmental stressors (Donovan et al., 2020; Blanckaert et al., 2021; Logan et al., 2021). For example, excess nitrogen will increase the susceptibility of bleaching under thermal stress (Burkepile et al., 2020; Donovan et al., 2020), while additional application of low levels of ultra-violet radiation may relax the deleterious effects of heat and nutrients (Blanckaert et al., 2021).
Overall, the drastic changes of hosts’ heterotrophic and symbiotic algae’ mixotrophic pathways will shake the foundation of coral holobionts. However, coral holobionts have demonstrated remarkable trophic flexibility and adaptability, suggesting that management and restoration of coral reefs are still feasible. A deeper understanding of the nutritional requirements of the coral holobiont is essential for developing more effective management strategies for coral reefs.
4 Nitrogen introduced from nitrogen fixation to coral holobionts
Nitrogen fixation is an important pathway for supplying supplementary nitrogen to coral systems, with cyanobacteria and heterotrophic bacteria being the key player (Sohm et al., 2011). If coral-associated diazotrophs conduct N2 fixation, it is a symbiotic process that can satisfy even up to 10% of symbiotic algae’s nitrogen requirements (Cardini et al., 2015; Bednarz et al., 2017). Such additional nitrogen is crucial for the survival and growth of autotrophic corals (Pogoreutz et al., 2017b).
4.1 Measurements of nitrogen fixation for coral holobionts
Over the years, the acetylene reduction assay (ARA) has been widely used to quantify N2 fixation of coral holobiont due to its easy operation and low cost (Crossland and Barnes, 1976; Williams et al., 1987; Shashar et al., 1994a; Shashar et al., 1994b; Davey et al., 2007; Lesser et al., 2007; Rädecker et al., 2014; Bednarz et al., 2015; Cardini et al., 2015; Cardini et al., 2016a; Cardini et al., 2016b; Bednarz et al., 2018; Tilstra et al., 2019a; El-Khaled et al., 2020b). However, ARA cannot provide DDN (Diazotroph-Derived Nitrogen) measurement because its measurement object is C2H4 gas derived from the whole coral holobiont in the incubator. Grover et al. were the first to use the 15N tracer method (the bubble method (Montoya et al., 1996)) to measure the symbiotic N2 fixation rates of coral holobionts (Grover et al., 2014). The 15N tracer method not only provides net N2 fixation rates but also enables to trace the fate and assimilation of DDN within different coral compartments. Currently, there are three protocols to measure N2 fixation using 15N2 tracer: the bubble method, dissolution method, and the modified bubble method (reviewed in (Wilson et al., 2012; White et al., 2020)). However, the bubble method underestimates the N2 fixation rates seriously due to the slow N2 dissolution. While the modified bubble method is less preferred since it requires shaking incubator, which may interfere coral activity. Therefore, the most popular method is the dissolution method. Inevitably, the dissolution method has the potential to introduce trace metal contamination and alter pH and alkalinity, which can bias the results (White et al., 2020).
4.2 Temporal and spatial variability of nitrogen fixation in coral holobionts
N2 fixation of coral holobionts have temporal and spatial variation. From temporal aspect, diazotrophs (Lema et al., 2014; Zhang et al., 2016; Cai et al., 2018) and N2 fixation rates (Bednarz et al., 2015; Cardini et al., 2015; Cardini et al., 2016a; Bednarz et al., 2018) in coral holobiont show significant seasonal variation regulated by environmental factors. For example, in summer season with lower DIN supply and stronger light relative to winter, the rates of N2 fixation in coral holobionts are higher correspondingly (Bednarz et al., 2015; Cardini et al., 2015; Cardini et al., 2016a; Bednarz et al., 2018; Cai et al., 2018; Tong et al., 2020). An opposite case presented by Bednarz (Bednarz et al., 2021) showed that N2 fixation is detectable in winter under opposite environmental conditions, but not detected in summer, suggesting extra factors, such as food availability may also influence coral holobionts’ N2 fixation.
Besides seasonality, diel light cycle also modulates N2 fixation of coral holobionts. Most research results show higher N2 fixation rates in the light compared with the dark (Crossland and Barnes, 1976; Williams et al., 1987; Shashar et al., 1994a; Rädecker et al., 2014; Cardini et al., 2016b; Zhang et al., 2016), yet, the light stimulation is likely indirect. More specifically, light provide energy for photosynthesis in algae, and the supply of photosynthate promotes N2 fixation of heterotrophic bacterial diazotrophs (HBDs) subsequently (Shashar et al., 1994a). This statement is supported by molecular biology showing HBDs such as α and γ-proteobacteria are the main diazotrophs in many coral holobionts (Olson et al., 2009; Lema et al., 2014; Santos et al., 2014; Bednarz et al., 2019; Bednarz et al., 2021; Glaze et al., 2022; Sheng et al., 2023) and by manipulation experiments, which showed N2 fixation activity was enhanced after adding DOC (Pogoreutz et al., 2017a). Couple researches showed that there was no significant difference of N2 fixation rates between the day and night (Cardini et al., 2016b; Lesser et al., 2018; Glaze et al., 2022), such phenomenon can be explained by sufficient carbon provision to HBDs in coral holobionts in the night. Interestingly, Lesser’s experiments not only show a higher rate at night but also the second peak of N2 fixation between 6 a.m. and 8 a.m. (Lesser et al., 2007). That may be explained by the effect of oxygen, an important factor controlling N2 fixation, although it has become clear that different diazotrophs could adopt different strategies to combat oxygen damage (Gallon, 1981; Zehr and Capone, 2020). For oceanic N2 fixation, there are still amounts of unknowns about HBDs themselves’ physiological information and their relative roles and contribution (Zehr and Capone, 2020), however, their wide-distributed and high-diverse characteristics suggest HBDs definitely play an important role (Delmont et al., 2018; Salazar et al., 2019; Zehr and Capone, 2020). The existing limited evidences show that HBDs are largely associated with organic-rich and low-DO environment (Pedersen et al., 2018; Farnelid et al., 2019). Actually, that is exactly consistent with the microenvironment that coral holobionts provide. The specific mechanism of the diel variation of N2 fixation in coral holobiont is still not clear, but the inconsistencies suggests that the mechanism is far more complex than we imagined. In deeper waters where environment is relatively stable, N2 fixation showed less seasonality (Bednarz et al., 2018), and reasonably less diel variation.
Here, we compiled data of global N2 fixation rates in coral holobionts (Figure 1). It is worth mentioning that the N2 fixation rates reported in “15N based studies” only reflect the rates in bulk tissue, which includes both algal symbiont and coral host tissue. However, N2 fixation in coral skeleton may also play a non-negligible role for the coral holobiont (Bednarz et al., 2019; Bednarz et al., 2021; Moynihan et al., 2022). Some studies have found diazotrophs in coral skeletons (Yang et al., 2016; Yang et al., 2019) and measured nitrogen fixation rates (Shashar et al., 1994a; Bednarz et al., 2019; Bednarz et al., 2021; Moynihan et al., 2022). Existing literature indicates that the nitrogen fixation rate in coral skeletons can be 20% to 300% of the rate in bulk tissues (Bednarz et al., 2019; Bednarz et al., 2021; Moynihan et al., 2022). However, due to the limited research on this topic, we will not delve into it further here. Nonetheless, we call on coral scientists to pay attention to the role of coral skeletons. Additionally, while it is unclear whether coral-released mucus benefits the coral holobiont itself, it does stimulate N2 fixation process in the mucus and water column (Rädecker et al., 2014).
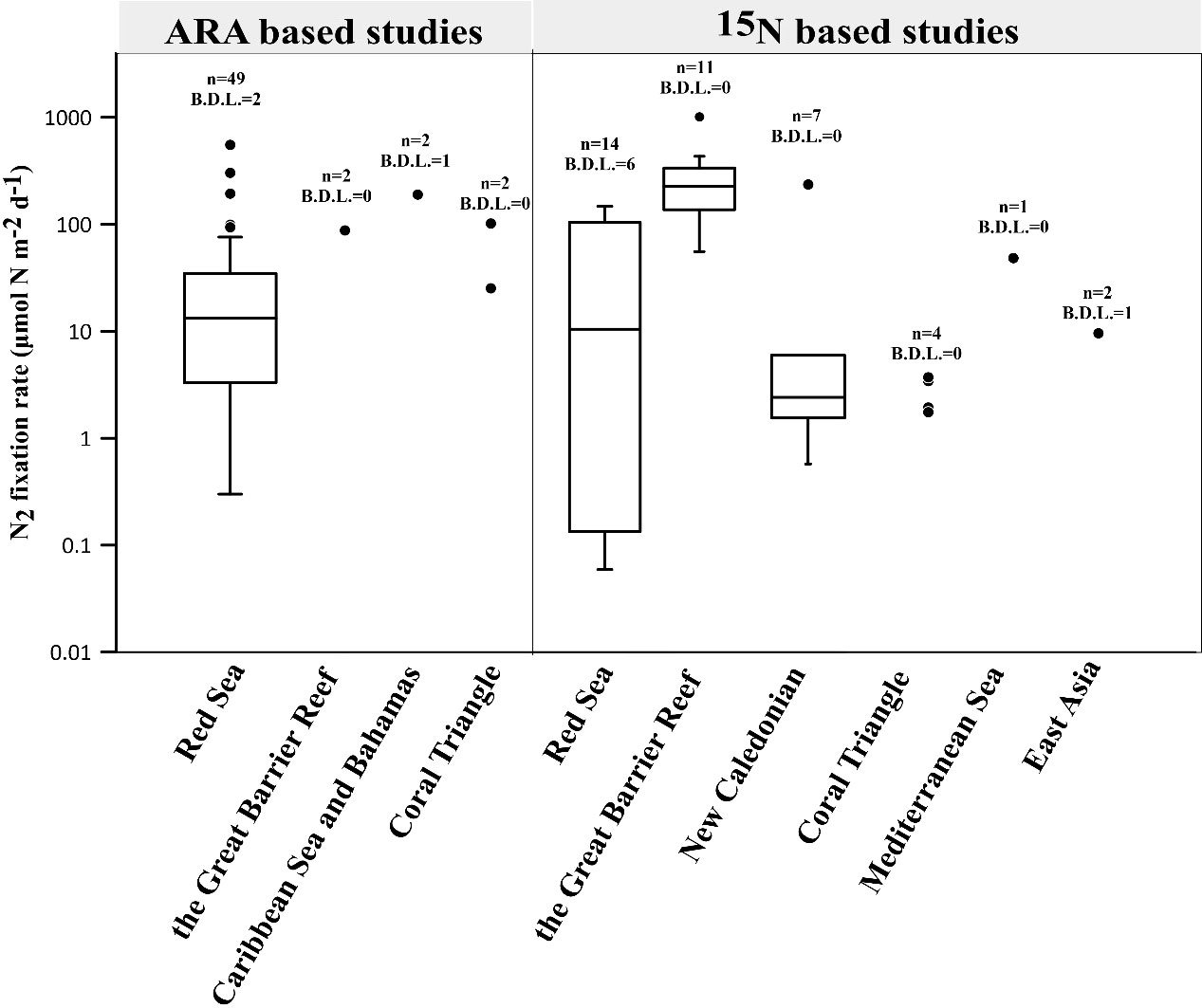
Figure 1 Summary of global N2 fixation rates in coral holobionts (Shashar et al., 1994a; Davey et al., 2007; Lesser et al., 2007; Grover et al., 2014; Rädecker et al., 2014; Cardini et al., 2015; Benavides et al., 2016; Cardini et al., 2016a; Cardini et al., 2016b; Bednarz et al., 2017; Pogoreutz et al., 2017b; Radecker et al., 2017; Sangsawang et al., 2017; Bednarz et al., 2018; Lesser et al., 2018; Bednarz et al., 2019; Lesser et al., 2019; Meunier et al., 2019; Pupier et al., 2019; Tilstra et al., 2019a; El-Khaled et al., 2020b; Bednarz et al., 2021; El-Khaled et al., 2021b; Meunier et al., 2021a; Meunier et al., 2021b; Glaze et al., 2022; Moynihan et al., 2022; Radecker et al., 2022; Sheng et al., 2023) (see Table S1 for reported rates). Scattered points of a non-box diagram represent data with less than five points, while points with a value of 0 are not shown. The number indicates the total number of data points and the number of data points below the detection limit (B.D.L.). C2H4 fluxes were converted into corresponding N2 fluxes assuming a theoretical molar ratio of C2H4:N2 = 4 and normalized to the surface area of coral fragments.
Spatially, there are significant differences in N2 fixation rates in coral holobionts within and between regions (Figure 1), which can be attributed to coral heterogeneity (Pogoreutz et al., 2017b), environmental condition such as light (Lesser et al., 2018), and food availability (Benavides et al., 2016; Pupier et al., 2019; El-Khaled et al., 2020b). In Figure 1, the median of 4 boxes (ARA based studies: Red sea; 15N based studies: Red sea, the Great Barrier Reef and New Caledonian) analyzed are 13.2, 10.5, 225.6, 2.4 μmol N m-2d-1 respectively. For 15N based studies, N2 fixation rates in the Great Barrier Reef were relatively higher than those in Red Sea and New Caledonian (P< 0.05), probably indicating that the corals in the Great Barrier Reef are more autotrophic and require more nitrogen compensation. There is also a significant difference in the amount of data available for N2 fixation rates between different regions (Figure 1), which is inseparable from the convenience of research in certain areas and the level of attention paid to coral-related research. The Coral Triangle and the coastal areas of East Asia have high coral abundance and diversity (Veron et al., 2015), but there is a lack of sufficient research, especially the Coral Triangle, which is significantly impacted by human activities (Andrello et al., 2022). Therefore, in addition to emphasizing conservation efforts, it is important to increase research in these regions.
On the vertical scale, the research results show consistency basically. The relative nifH abundance associated with coral holobionts decreased with the water depth (Tilstra et al., 2019b; Bednarz et al., 2021), but the assimilation of DDN increased (Grover et al., 2014; Bednarz et al., 2017; Bednarz et al., 2021), especially in winter (Bednarz et al., 2018). That is explained by the shift of trophic strategy for coral holobionts as depth increases (Bednarz et al., 2017; Tilstra et al., 2019b). Mesophotic coral holobionts generally rely on heterotrophy, which may result more DDN assimilation from heterotrophic path. However, the profile of N2 fixation in coral holobionts is affected by multiple factors, thus, in some cases N2 fixation rates show no significant effect of depth (Lesser et al., 2018) even may decrease with depth in summer due to the enhancement of N2 fixation of coral holobionts in the upper layer (Bednarz et al., 2018).
4.3 Nitrogen fixation of coral holobionts under stress
Recently, the impact of environmental stresses on the N2 fixation of coral holobionts has garnered significant attention. For the heat stress, studies have shown an increase in the abundances and diversities of diazotrophs and the total N2 fixation activity (from ARA) in coral holobiont, especially during the daytime (Santos et al., 2014; Cardini et al., 2016b; Radecker et al., 2022). However, the net N2 fixation rates were found to decrease in parallel experiments using 15N2, indicating reduced utilization efficiency of DDN by coral holobionts (Radecker et al., 2022). For acidification, which mainly focused on pCO2 elevation, inconsistent results were documented. Some studies demonstrate a decrease in N2 fixation rates as pCO2 increased, explained by the reallocation of energy for calcification (Rädecker et al., 2014; Zheng et al., 2021). However, some field studies showed that N2 fixation rates and diazotrophs in coral holobionts were higher in high pCO2 sites than low pCO2 sites (Biagi et al., 2020; Meunier et al., 2021b; Prada et al., 2023), such enhancement of N2 fixation in space was attributable to the higher N requirements induced by the enhanced photosynthesis under high pCO2 condition (Biagi et al., 2020). These contrasting findings were not surprising, since the effects of acidification, including pCO2 and pH effect, on N2 fixation is a complicated issue (Hutchins et al., 2015; Hong et al., 2017). For nutrient stress, elevated nutrient levels may stimulate N2 fixation (Bednarz et al., 2017; El-Khaled et al., 2020b) after coral holobionts’ reorganizing in-and-out nitrogen pathways. The above results indicate that diazotrophs in coral holobionts could take different strategies to counter various adverse circumstances.
Oceanic environment changes, such as warming (Schoepf et al., 2015), acidification (Anthony et al., 2008), eutrophication (Hall et al., 2018) and deoxygenation (Johnson et al., 2021; Alderdice et al., 2022), all may lead to coral bleaching after transgressing a certain threshold eventually. Researches have shown that after bleaching coral holobionts’ N2 fixation increases dozens of times due to occupation of some pioneer communities on coral substrate (Larkum, 1988; Davey et al., 2007; Bednarz et al., 2019; Meunier et al., 2019; Roth et al., 2020), and such enhancement of N2 fixation is crucial for coral recovery and coral reef succession. Obviously, the sustainability and resilience of coral system depends not only to understand and predict the persistence of coral reefs. Further studies are necessary to subdivide the effects of different nutrients, such as and phosphorus, to avoid coordinated effect. Furthermore, investigating the relationship between diazotrophs and other coral compartments’ response to environmental change is essential.
5 The nitrogen exchange inside the coral holobiont
The nitrogen transfer in coral holobionts among coral hosts, symbiotic algae and microbes is a crucial aspect for their survival, in addition to the acquisition of nitrogen sources (Falkowski et al., 1993; Kopp et al., 2015). The symbiotic relationship between the coral host and symbiotic algae is the foundation of a coral holobiont. Coral hosts provide symbiotic algae with a habitat that can withstand damage from the outside world and nutrient-rich metabolites, such as (Wilkerson and Trench, 1986; Falkowski et al., 1993). They also exert control over symbiotic algae through some mechanisms (Burris, 1983; Sutton and Hoegh-Guldberg, 1990; Radecker et al., 2015; Cui et al., 2019) that allow for a degree of ‘fault tolerance’ (Krueger et al., 2020). Meanwhile, symbiotic algae handed over most (>95%) of photosynthate to the coral host in the form of glucose mainly (Muscatine et al., 1984; Falkowski et al., 1993; Davy and Turner, 1996; Burriesci et al., 2012), being further proved in the sea anemone, another symbiotic cnidarian recently (Cui et al., 2023). While nitrogen restriction as the key for the symbiotic relationship (Tremblay et al., 2013; Ezzat et al., 2015), environmental stress such as heat (Radecker et al., 2021) and nutrient stress (Ezzat et al., 2015) can disrupt the nutrient cycling and transform the symbiotic relationship from nitrogen restriction to carbon restriction (Radecker et al., 2021), leading to the symbiotic breakdown (Petrou et al., 2021; Radecker et al., 2021).
Until recently, the transfer of nitrogen in coral holobionts remained unclear. However, by using the 15N tracer method combining centrifugation separation or in situ imaging technique, we can now identify the reallocations of autotrophic, heterotrophic nitrogen and N2 transfer from ambient environment into different coral compartments and subsequent translocation (Kopp et al., 2013; Kopp et al., 2015; Martinez et al., 2022). For example, 15N-enriched DFAAs and plankton were used to trace their pathways within the coral holobiont even down to cell level by using NanoSIMS (Kopp et al., 2013; Martinez et al., 2022).
For autotrophy, the translocation and utilization of nitrogen in coral holobionts are rapid (Kopp et al., 2013; Kopp et al., 2015) and efficient (Muscatine and Porter, 1977). After assimilation, DIN can be incorporated immediately into uric acid crystals, proved to be mobilized rapidly in algal symbionts (Clode et al., 2009), forming a transient nitrogen pool in the symbiotic algae (after ~45 min) and then it is translocated to the coral host (starting after 6 hours), though the translocation of carbon-containing photosynthates is even faster (just in 15 min) (Kopp et al., 2015). And the transfer efficiency can be affected by the symbiotic algae clade (Sproles et al., 2020; Wall et al., 2020), light (Tremblay et al., 2013; Ezzat et al., 2017; Wall et al., 2020), feeding regimes (Tremblay et al., 2013) and other factors. Moreover, Chiles et al. found that dipeptide pools have a high turnover rate and that separate biosynthetic mechanisms mediate the production of dipeptides and their precursor amino acids in coral holobionts, as indicated by a 15 addition experiment (Chiles et al., 2022).
For heterotrophic nitrogen, the efficiencies of heterotrophic assimilation of DFAAs (here, aspartic acid) is similar with autotrophic (Kopp et al., 2013). Furthermore, research has confirmed that symbiotic algae absolutely benefits from heterotrophic amino acids or recycled from its associated coral hosts (Martinez et al., 2022). This presents a reverse translocation about amino acids, which used to be described as a unidirectional supply from symbiotic algae (Martinez et al., 2022).
For DDN, it is introduced from functional microorganisms in coral holobionts and then transfer to both coral host and symbiotic algae. At present, most researches show the symbiotic algae has higher signal within the incubation time from 4h to 48h (Benavides et al., 2016; Meunier et al., 2019; Meunier et al., 2021a; Meunier et al., 2021b; Moynihan et al., 2022). Although it is uncertain whether there is the influence of diazotrophs’ signal in symbiotic algae’s 15N signal by centrifugation separation, the conclusion in in situ imaging technique is clear (Radecker et al., 2022). We cannot yet determine the specific sequential transfer order due to lack of higher resolution of time series pulse-chase experiments and more sophisticated instrument, but we confirm that the process of the transfer to coral hosts and symbiotic algae from diazotrophs will proceed in a short time.
6 The other nitrogen pathways associated with coral holobionts
6.1 Nitrification
Nitrification is crucial process for coral holobionts, involving two steps of ammonia oxidation and nitrite oxidation. Ammonia oxidation converts NH3/ to by either ammonia oxidation archaea (AOA) or ammonia oxidation bacteria (AOB). Nitrite oxidation then further converts to by nitrite oxidation bacteria (NOB). This process helps to reduce their internal levels to maintain the growth of symbiotic algae stable, meanwhile, retain the dissolved inorganic nitrogen in oxidized state (Radecker et al., 2015).
Actually, molecular biological evidences shows that ammonia oxidation occurs in coral tissues (Wafar et al., 1990; Yang et al., 2013), mucus (Siboni et al., 2008; Kimes et al., 2010) and skeletons (Risk and Muller, 1983). Furthermore, different coral holobionts in various areas may contain varying species and abundance of ammonia-oxidizing organisms, e.g., solely AOA (Beman et al., 2007; Siboni et al., 2008; Siboni et al., 2012), solely AOB (Yang et al., 2013), AOA coexisted with AOB (Zhang et al., 2015; Zhang et al., 2021) and none (Beman et al., 2007), although the survival strategies of AOA and AOB are completely different. It remains unclear what determines the habitat to host AOA or/and AOB in coral holobionts, which requires further studied.
Molecular biology evidences suggest that coral holobionts have the potential to conduct nitrification, but it remains uncertain whether they actually do so. However, there have been limited quantitative studies on the topic. In 1990, Wafar et al. were the first to quantify the gross nitrification rate in coral holobionts after holding in the dark in running seawater for 1 or 2 d to minimize dark uptake of by the symbiotic algae, which was found to be on average of 9. 4 ± 6. 0 nmol (mg coral tissue N) -1 h-1, accounting for even up to 17% of the total consumption (Wafar et al., 1990). This suggests a potential competitive relationship between nitrification and the uptake process of symbiotic algae. However, until 2021, there have been other quantitative studies on the nitrification rate in coral holobionts. Babbin and Glaze measured the net nitrification in coral holobiont using 15N tracer method and their results were less than 2 μmol N m-2 d-1 and an average 2. 70 µmol N m-2 d-1 respectively (Babbin et al., 2021; Glaze et al., 2022). However, their studies did not report the uptake rate, so the percentage of nitrification to total consumption remains unknown.
Furthermore, Glaze’s experiment demonstrated that the rate of dark incubation (4. 75 µmol N m-2 d-1) was significantly greater than the rate observed in light (0. 65 µmol N m-2 d-1) (Glaze et al., 2022). This finding is in line with our current understanding of the photoinhibition on the ammonia oxidation process (Schoen and Engel, 1962; Merbt et al., 2012; Horak et al., 2018; Glaze et al., 2022). However, in comparison to other processes such as the well-studied N2 fixation, these rates are relatively low. Thus, upcoming research efforts should concentrate on examining the allocation and condition of the consumption pathway to verify the role of nitrification.
6.2 Denitrification
Denitrification is a complex process that involves a sequence of four steps, whereby the is reduced to , NO, N2O and finally N2, with each step corresponding to different reductase genes (nas/narG/napA/euk-nr, nir, norB, nosZ). The significance of denitrification for coral holobionts may lie in the fact that, during the night, when the nitrification is more intense (Glaze et al., 2022), corals convert excess to and through nitrification, while anoxic microenvironment causes denitrifying bacteria to expel nitrogen from the coral holobiont. By coupling of nitrification and denitrification, corals can not only survive when the nutrient level increases, but also avoid the excessive growth of symbiotic algae in coral holobionts (Siboni et al., 2008; Radecker et al., 2015). Research on denitrification in coral holobionts began relatively late compared to nitrification. With the advent of molecular biology, it was gradually discovered that there are denitrifying microorganisms present in coral holobionts (Siboni et al., 2008), including in tissues and mucus (Kimes et al., 2010). Further studies have shown that different corals or even different color morphs of the same coral, have different types and numbers of denitrifying-related genes (Yang et al., 2013; Lesser et al., 2019; Tilstra et al., 2019a). It is now widely accepted that coral holobionts have the potential to perform denitrification.
As for quantitative studies, currently, there are the two methods used to measure denitrification in coral holobionts, 15N tracer method and COBRA (a combination of acetylene blockage analysis and acetylene reduction assay) (El-Khaled et al., 2020a). Acetylene blockage analysis uses the property of acetylene to inhibit the reduction of N2O, which leads to the accumulation of N2O in the incubation system. The denitrification rate can be calculated by measuring the change in N2O content using gas chromatography (Balderston et al., 1976; Yoshinari and Knowles, 1976). Recent studies have measured the denitrification rate of coral holobionts (Middelburg et al., 2015; El-Khaled et al., 2020a; El-Khaled et al., 2021a; El-Khaled et al., 2021b; Zhang et al., 2021; Glaze et al., 2022), and almost all samples have detected denitrification, indicating that this process is widespread in coral holobionts (Tilstra et al., 2019a; El-Khaled et al., 2020b; Babbin et al., 2021; El-Khaled et al., 2021b; Glaze et al., 2022). The results showed higher rates of denitrification at night, which may be closely related to lower oxygen concentrations, although not all corals exhibit this characteristic (Babbin et al., 2021; Glaze et al., 2022). Interestingly, Glaze’s results indicate stimulates the production of the combined gases of NO, N2O and N2 less than (Glaze et al., 2022). From a symbiotic perspective, can stimulate nitrogen-related gas production to reduce the nitrogen content in the holobiont, which may be an important part of coral internal regulation to maintain the density of symbiotic algae and homeostasis.
Figure 2 presents the denitrification and nitrification rates of coral holobionts. The median rates for the 4 boxes (Denitrification: Red sea, the Great Barrier Reef and Caribbean Sea and Bahamas; Nitrification: the Great Barrier Reef) are 1.8, 13.3, 0.4 and 3.4 μmol N m-2 d-1 respectively. Although the range of these rates is smaller than that of N2 fixation, they still show differ by orders of magnitude, possibly due to coral heterogeneity, regional differences and variations in denitrification rate measurement protocols (Table S2).
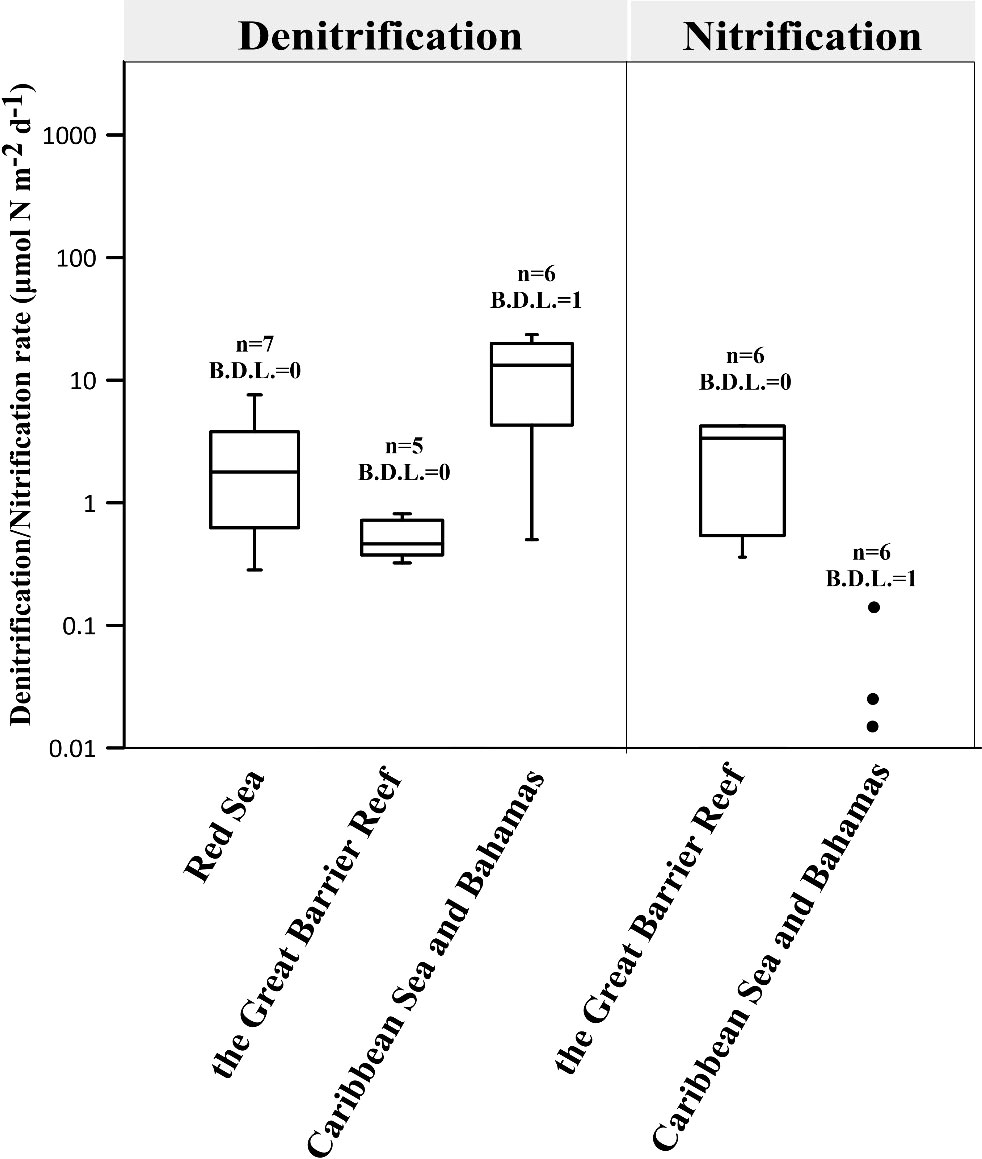
Figure 2 Summary of global denitrification and nitrification rates in coral holobionts (Tilstra et al., 2019a; El-Khaled et al., 2020b; Babbin et al., 2021; El-Khaled et al., 2021b; Glaze et al., 2022) (see Tables S2, S3 for reported rates). Scattered points of a non-box diagram represent data with less than five points, while points with a value of 0 are not shown. The number indicates the total number of data points and the number of data points below the detection limit (B.D.L.).
The relationship of denitrification and N2 fixation is also discussed these years. Previous studies have shown a positive correlation between denitrification and N2 fixation rates in coral holobiont, suggesting the balance between denitrification and N2 fixation process in coral holobionts (Tilstra et al., 2019a). Furthermore, Tilstra et al. (El-Khaled et al., 2021b) found that nirS/nifH of different coral species was positively correlated with the concentration of in the environment, suggesting the availability of environmental could drive the changes of denitrification and N2 fixation in coral holobionts. Further experiment has confirmed that denitrification of coral holobionts is enhanced with the increase of nutrient concentration (El-Khaled et al., 2020b). Additionally, Redfield ratio of coral holobionts may be an important index presenting the metabolic balance of coral, which is also used to explain the balance of denitrification and N2 fixation (Tilstra et al., 2019a; El-Khaled et al., 2021b).
Furthermore, Xiang et al. found when the external environment had an increased DOC concentration, the C/N ratio of corals increased, while the abundance of denitrifying organisms in coral holobionts decreased by an order of magnitude (Xiang et al., 2022). Hypotheses for this phenomenon were presented by Xiang et al.: (1) The abundance of denitrifying organisms is mainly controlled by the availability of nitrogen, so they grow normally under nitrogen restriction even if DOC content is increased. (2) Different species of denitrifying organisms prefer different carbon sources, and using glucose as the only carbon source in the experiment may affect the experimental results (Xiang et al., 2022). Together, these funding suggested that changes in the external environment may cause Redfield ratio changes in coral holobionts, thereby promoting changes in the denitrification and N2 fixation to maintain the original ratio.
It is possible that denitrifying organisms within coral holobiont may be opportunistic rather than dominant. As ocean nutrient levels continue to rise, denitrification is likely to play a more significant role in regulating the carbon and nitrogen balance of coral holobionts. It is important to consider whether corals can actively promote changes in denitrification and N2 fixation to maintain homeostasis under varying environmental conditions, and this should be discussed in future studies.
6.3 DNRA
DNRA (dissimilatory nitrate reduction to ammonium) is a process reducing to (Burgin and Hamilton, 2007). This pathway has the potential to enhance the efficiency of internal nitrogen circulation within coral holobionts, resulting in the retention of dissolved inorganic nitrogen in the form of . When coupled with nitrification, this bidirectional process helps to maintain a stable level, promoting healthy coral growth. However, direct measurement of DNRA in coral holobionts using 15N tracer method is challenging, and therefore, there are limited studies on this process. Molecular evidences showed that DNRA-associated organisms such as fungi and vibrios, are common in coral holobionts, but their prevalence varies with coral species, regions and depths (Wegley et al., 2007; Zhang et al., 2015; Babbin et al., 2021; Bednarz et al., 2021; Zhang et al., 2021; Glaze et al., 2022). Some researches showed that the DNRA-associated genes can account for up to ~12% of the nitrogen-associated genes (Glaze et al., 2022), underscoring the importance of DNRA. Indirect calculation using mass balance models has shown that the DNRA rates obtained at night could reach 460–600 µmol N m-2 d-1, assuming nitrification is less than 1% of ammonia assimilation (Glaze et al., 2022). However, due to the considerable variation of nitrogen processes rates within coral holobionts, the indirect rate obtained was with great uncertainty.
6.4 ANAMMOX
ANAMMOX (anaerobic ammonium oxidation) is a reaction between and that produces N2, which is currently thought to occur only in a strictly anoxic environment (Dalsgaard et al., 2005). ANAMMOX has been hypothesized as a possible means of nitrogen removal for coral holobionts (Radecker et al., 2015). However, only a few studies have been conducted on this topic, and they have found that ANAMMOX-associated genes exist in small amounts in coral holobionts in South China Sea. These studies also found a weak positive correlation between ANAMMOX-associated genes and concentration (Zhang et al., 2015; Zhang et al., 2021). In contrast, other studies have not found any evidences of ANAMMOX in coral holobionts (Babbin et al., 2021; Glaze et al., 2022). These findings suggest that ANAMMOX may not play an important role in coral holobionts.
7 Current situation and future perspectives
Coral holobiont, a classic symbiotic system, exhibit high plasticty, which may explain their long survival on earth. The intricate nitrogen cycling network within coral holobionts is regulated not only by external factors but also by the coordination of members to maintain nitrogen balance, enabling them to resist harsh environments.
7.1 Differential advancements according to technical difficulties
Mixotrophy, the primary mechanism by which coral holobionts acquire energy, has been extensively studied for its role in coral physiology. However, there is still much to be learned about nitrogen fixation, which has only recently begun to be explored in depth. Current investigations have been limited to different regions, seasons, and responses to environmental stress. Quantitative research on nitrification and denitrification processes has just begun, while DNRA and other processes have yet to receive sufficient attention. Based on the number of studies published on various nitrogen processes related to corals (as shown in Figure 3), we can gain some insight into the above-mentioned issue. The amount of researches on assimilation and N2 fixation related to coral is the largest, which is closely related to their long research history and their important role in balance nutrition, while the studies about nitrification and other processes is less significantly due to technical difficulties. We still need to strengthen further nitrogen research of coral holobionts in the future. So that we can know more about the influence of the different processes on coral health, which is crucial for understanding how coral holobionts build and collapse.
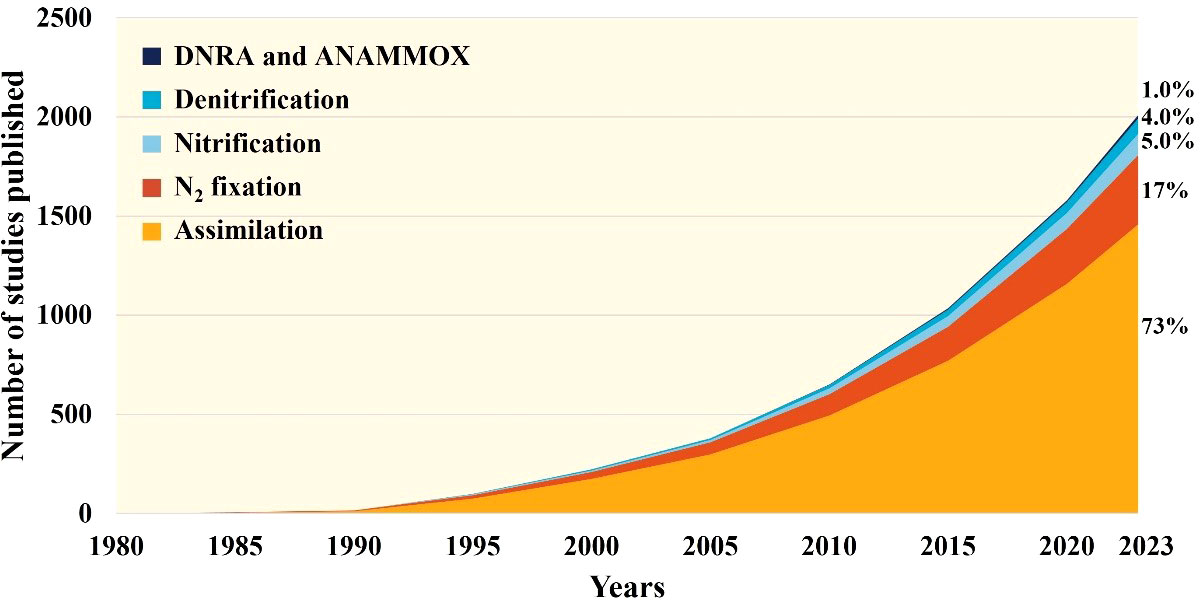
Figure 3 Number of scientific papers about nitrogen processes associated to coral (1980-2023). This diagram was obtained using Web of Science Core Collection. More details about the retrieval strategy displayed in supplementary information.
7.2 Considerations for the experiment in coral holobiont studies
Coral holobionts are complex ecosystems consisting of the coral host and a diverse range of microorganisms. Below we listed some of the critical factors to consider when designing and conducting experiments in order to obtain accurate and reliable data for studying this ecosystem.
Recent experiment designs have been closer to real in situ conditions, such as pulsed nutrient addition (Van Der Zande et al., 2021), fluctuation of pCO2 control (Jiang et al., 2018), and short-term acute heat stress (Guan et al., 2020). These experiments have produced results significantly different from those maintaining constant external conditions, providing a more accurate assessment of the impact of the environment on corals. Hence, researchers should focus more on these types of experiments.
Nitrogen processes in coral holobiont are dynamic, and different phenomena may be observed in a longer incubation time, just like sheng et al.’s (Sheng et al., 2023) experiment results show. Therefore, new ideas and methods need to be developed to explore more detailed processes in the future. For example, future experiments exploring nitrogen processes in coral holobionts should set more time points in the sequential experiment to estimate nitrogen migration and transformation rates. And as various processes occur simultaneously in coral holobiont, this brings some difficulties in analyzing a single path. Researches can also use isotope matrix methods, which proposed by Xu et al., in 2017 for the quantification of simultaneous multi-path nitrogen flow (Xu et al., 2017), to study coral holobionts and clarify the respective contributions of each path under different environmental stresses.
Maintaining the appropriate balance of environmental factors in incubation experiments is crucial. Some experiments require long incubation periods in a gas-tight incubator, which can cause a sharp decrease in dissolved oxygen levels. This low level of dissolved oxygen can affect the redox-sensitive processes like nitrification (Bristow et al., 2016) and denitrification. Therefore, it is important to pay attention to the synergistic control of environmental factors in the incubation experiments, for example, maintaining a suitable balance between oxygen consumption rate and incubator volume.
Enhancing comparability across experiments and data is a critical challenge in coral research, given that the coral holobiont is a meta-organism with multiple dimensions. This complexity creates numerous design and operational choices for coral experiments compared to other marine investigations. For instance, heat-stress experiments require careful consideration of several factors, such as exposure duration, light conditions, feeding regimes, collection, preservation, and laboratory operation as highlighted by existing reviews (Mclachlan et al., 2020; Grottoli et al., 2021; Mclachlan et al., 2021; Vega Thurber et al., 2022). Furthermore, quantitative normalization is fundamental for data analysis. Different studies have used various normalization units, like cross-section area, dry weight, nitrogen content of coral tissue, and coral surface area. The mainstream method is to normalize tothe surface area, representing the habitat of coral microbes. At present, several methods are available for measuring surface area, including simple geometry, advanced geometry, aluminum foil, methylene blue dye, secondary wax drops, primary wax drops, CT, and laser scanning. Therefore, it is recommended to measure the coral surface area at least as a constant quantitative indicator. This approach would help standardize the results across different studies and avoid the confusion that may arise from using different normalization units.
7.3 Future direction
Research on nitrogen processes, such as nitrification, denitrification, and DNRA, in coral holobionts is still limited and requires futher efforts. In addition, there is a lack of research that measures all nitrogen processes simultaneously in coral holobionts, even for model species. The heterogeneity of coral holobionts creates great uncertainty in what we have learned about nitrogen fluxes. Therefore, future work on fundamental nitrogen flux of coral holobiont still need to be strengthened.
Understanding the short-term responses of corals to turbulent environments on diurnal and tidal timescales, as well as regulation of internal nitrogen cycle, is essential to explain the inconsistencies in previous studies. Invitigations into the effects of long-term environmental changes, such as warming (Radecker et al., 2021), acidification (Hoegh-Guldberg et al., 2017), eutrophication (Zhao et al., 2021), hypoxia (Altieri et al., 2021; Hughes et al., 2022), and synergistic effects of those factors (Donovan et al., 2020; Zhang et al., 2023), are also critical for predicting future of coral reefs. However, these investigations should be treated differently and their differences and connections should be clarifed by purposeful experiments in future.
Given the dynamic nature of coral holobionts including symbiont transfer and symbiosis evolution, it is currently challenging to quantify relative contributions of different factors to coral holobiont function, making it difficult to predict future impacts accurately. Thus, researchers must remain attentive to new technologies, and the development and utilization of new tools and methods may represent important breakthroughs in furthering our understanding of coral holobionts.
Author contributions
LMY: Conceptualization; Writing - original draft; Writing - review and editing; H-XS: Conceptualization; Writing - review and editing; DMY: Data curation; Formal analysis; Visualization; review and editing; S-JK: Conceptualization; Funding acquisition; Resources; Supervision; Writing - review and editing. All authors contributed to the article and approved the submitted version.
Funding
This research was supported by the National Natural Science Foundation of China (41721005, 92058204, 92251306, 41890802) and the Ph.D. Fellowship of Xiamen University.
Acknowledgments
The authors would like to express their gratitude to Li-Li Han, Moge Du, Xiaoyu Guo, Jin-Ming Tang and Zhibo Shao for their valuable discussions and assistance during the writing process. Additionally, the authors would like to thank the State Key Laboratory of Marine Environmental Science, Xiamen University, for providing a platform that allows us to freely explore scientific ideas. We thank the editor and two reviewers for their dedication to the peer review process to make this review better.
Conflict of interest
The authors declare that the research was conducted in the absence of any commercial or financial relationships that could be construed as a potential conflict of interest.
Publisher’s note
All claims expressed in this article are solely those of the authors and do not necessarily represent those of their affiliated organizations, or those of the publisher, the editors and the reviewers. Any product that may be evaluated in this article, or claim that may be made by its manufacturer, is not guaranteed or endorsed by the publisher.
Supplementary material
The Supplementary Material for this article can be found online at: https://www.frontiersin.org/articles/10.3389/fmars.2023.1203399/full#supplementary-material
References
Agostini S., Suzuki Y., Higuchi T., Casareto B. E., Yoshinaga K., Nakano Y., et al. (2011). Biological and chemical characteristics of the coral gastric cavity. Coral Reefs 31 (1), 147–156.
Alderdice R., Perna G., Cardenas A., Hume B. C. C., Wolf M., Kuhl M., et al. (2022). Deoxygenation lowers the thermal threshold of coral bleaching. Sci. Rep. 12 (1), 18273.
Almoghrabi S., Allemand D., Jaubert J. (1993). Valine uptake by the scleractinian coral galaxea-fascicularis - characterization and effect of light and nutritional-status. J. Comp. Physiol. B-Biochem. Syst. Environ. Physiol. 163 (5), 355–362.
Altieri A. H., Johnson M. D., Swaminathan S. D., Nelson H. R., Gedan K. B. (2021). Resilience of tropical ecosystems to ocean deoxygenation. Trends Ecol. Evol. 36 (3), 227–238. doi: 10.1016/j.tree.2020.11.003
Andrello M., Darling E. S., Wenger A., Suarez-Castro A. F., Gelfand S., Ahmadia G. N. (2022). A global map of human pressures on tropical coral reefs. Conserv. Lett. 15 (1), e12858. doi: 10.1111/conl.12858
Andrews J. C., Gentien P. (1982). Upwelling as a source of nutrients for the great barrier-reef ecosystems - a solution to Darwin question. Mar. Ecol. Prog. Ser. 8 (3), 257–269. doi: 10.3354/meps008257
Anthony K. R. N. (1999). Coral suspension feeding on fine particulate matter. J. Exp. Mar. Biol. Ecol. 232 (1), 85–106. doi: 10.1016/S0022-0981(98)00099-9
Anthony K. R. N. (2000). Enhanced particle-feeding capacity of corals on turbid reefs (Great barrier reef, Australia). Coral Reefs 19 (1), 59–67. doi: 10.1007/s003380050227
Anthony K. R., Kline D. I., Diaz-Pulido G., Dove S., Hoegh-Guldberg O. (2008). Ocean acidification causes bleaching and productivity loss in coral reef builders. Proc. Natl. Acad. Sci. U. S. A. 105 (45), 17442–17446. doi: 10.1073/pnas.0804478105
Babbin A. R., Tamasi T., Dumit D., Weber L., Rodriguez M. V. I., Schwartz S. L., et al. (2021). Discovery and quantification of anaerobic nitrogen metabolisms among oxygenated tropical Cuban stony corals. ISME J. 15 (4), 1222–1235. doi: 10.1038/s41396-020-00845-2
Baker D. M., Andras J. P., Jordan-Garza A. G., Fogel M. L. (2013). Nitrate competition in a coral symbiosis varies with temperature among symbiodinium clades. ISME J. 7 (6), 1248–1251. doi: 10.1038/ismej.2013.12
Balderston W. L., Sherr B., Payne W. J. (1976). Blockage by acetylene of nitrous oxide reduction in pseudomonas perfectomarinus. Appl. Environ. Microbiol. 31 (4), 504–508. doi: 10.1128/aem.31.4.504-508.1976
Bednarz V. N., Cardini U., Van Hoytema N., Al-Rshaidat M. M. D., Wild C. (2015). Seasonal variation in dinitrogen fixation and oxygen fluxes associated with two dominant zooxanthellate soft corals from the northern red Sea. Mar. Ecol. Prog. Ser. 519, 141–152. doi: 10.3354/meps11091
Bednarz V. N., Grover R., Maguer J. F., Fine M., Ferrier-Pages C. (2017). The assimilation of diazotroph-derived nitrogen by scleractinian corals depends on their metabolic status. mBio 8 (1), e02058–16. doi: 10.1128/mBio.02058-16
Bednarz V. N., Naumann M. S., Cardini U., Van Hoytema N., Rix L., Al-Rshaidat M. M. D., et al. (2018). Contrasting seasonal responses in dinitrogen fixation between shallow and deep-water colonies of the model coral stylophora pistillata in the northern red Sea. PloS One 13 (6), e0199022. doi: 10.1371/journal.pone.0199022
Bednarz V. N., Van De Water J., Grover R., Maguer J. F., Fine M., Ferrier-Pages C. (2021). Unravelling the importance of diazotrophy in corals - combined assessment of nitrogen assimilation, diazotrophic community and natural stable isotope signatures. Front. Microbiol. 12, 631244. doi: 10.3389/fmicb.2021.631244
Bednarz V. N., Van De Water J., Rabouille S., Maguer J. F., Grover R., Ferrier-Pages C. (2019). Diazotrophic community and associated dinitrogen fixation within the temperate coral oculina patagonica. Environ. Microbiol. 21 (1), 480–495. doi: 10.1111/1462-2920.14480
Beman J. M., Chow C. E., King A. L., Feng Y., Fuhrman J. A., Andersson A., et al. (2011). Global declines in oceanic nitrification rates as a consequence of ocean acidification. Proc. Natl. Acad. Sci. U. S. A. 108 (1), 208–213. doi: 10.1073/pnas.1011053108
Beman J. M., Roberts K. J., Wegley L., Rohwer F., Francis C. A. (2007). Distribution and diversity of archaeal ammonia monooxygenase genes associated with corals. Appl. Environ. Microbiol. 73 (17), 5642–5647. doi: 10.1128/AEM.00461-07
Benavides M., Houlbreque F., Camps M., Lorrain A., Grosso O., Bonnet S. (2016). Diazotrophs: a non-negligible source of nitrogen for the tropical coral stylophora pistillata. J. Exp. Biol. 219 (Pt 17), 2608–2612. doi: 10.1242/jeb.139451
Biagi E., Caroselli E., Barone M., Pezzimenti M., Teixido N., Soverini M., et al. (2020). Patterns in microbiome composition differ with ocean acidification in anatomic compartments of the Mediterranean coral astroides calycularis living at CO(2) vents. Sci. Total Environ. 724, 138048. doi: 10.1016/j.scitotenv.2020.138048
Blanckaert A. C. A., De Barros Marangoni L. F., Rottier C., Grover R., Ferrier-Pages C. (2021). Low levels of ultra-violet radiation mitigate the deleterious effects of nitrate and thermal stress on coral photosynthesis. Mar. Pollut. Bull. 167, 112257. doi: 10.1016/j.marpolbul.2021.112257
Bonnet S., Caffin M., Berthelot H., Moutin T. (2017). Hot spot of N(2) fixation in the western tropical south pacific pleads for a spatial decoupling between N(2) fixation and denitrification. Proc. Natl. Acad. Sci. U. S. A. 114 (14), E2800–E2801. doi: 10.1073/pnas.1619514114
Bourne D. G., Morrow K. M., Webster N. S. (2016). Insights into the coral microbiome: underpinning the health and resilience of reef ecosystems. Annu. Rev. Microbiol. 70, 317–340. doi: 10.1146/annurev-micro-102215-095440
Brandl S. J., Tornabene L., Goatley C. H. R., Casey J. M., Morais R. A., Côté I. M., et al. (2019). Demographic dynamics of the smallest marine vertebrates fuel coral reef ecosystem functioning. Science 364 (6446), 1189–1192.
Bristow L. A., Dalsgaard T., Tiano L., Mills D. B., Bertagnolli A. D., Wright J. J., et al. (2016). Ammonium and nitrite oxidation at nanomolar oxygen concentrations in oxygen minimum zone waters. Proc. Natl. Acad. Sci. U. S. A. 113 (38), 10601–10606. doi: 10.1073/pnas.1600359113
Burgin A. J., Hamilton S. K. (2007). Have we overemphasized the role of denitrification in aquatic ecosystems? a review of nitrate removal pathways. Front. Ecol. Environ. 5 (2), 89–96. doi: 10.1890/1540-9295(2007)5[89:HWOTRO]2.0.CO;2
Burkepile D. E., Shantz A. A., Adam T. C., Munsterman K. S., Speare K. E., Ladd M. C., et al. (2020). Nitrogen identity drives differential impacts of nutrients on coral bleaching and mortality. Ecosystems 23 (4), 798–811. doi: 10.1007/s10021-019-00433-2
Burriesci M. S., Raab T. K., Pringle J. R. (2012). Evidence that glucose is the major transferred metabolite in dinoflagellate-cnidarian symbiosis. J. Exp. Biol. 215 (Pt 19), 3467–3477. doi: 10.1242/jeb.070946
Burris R. H. (1983). Uptake and assimilation of (Nh4+)-N-15 by a variety of corals. Mar. Biol. 75 (2-3), 151–155. doi: 10.1007/BF00405997
Cai L., Zhou G., Tong H., Tian R. M., Zhang W., Ding W., et al. (2018). Season structures prokaryotic partners but not algal symbionts in subtropical hard corals. Appl. Microbiol. Biotechnol. 102 (11), 4963–4973. doi: 10.1007/s00253-018-8909-5
Cardini U., Bednarz V. N., Naumann M. S., Van Hoytema N., Rix L., Foster R. A., et al. (2015). Functional significance of dinitrogen fixation in sustaining coral productivity under oligotrophic conditions. Proc. Biol. Sci. 282 (1818), 20152257. doi: 10.1098/rspb.2015.2257
Cardini U., Bednarz V. N., Van Hoytema N., Rovere A., Naumann M. S., Al-Rshaidat M. M. D., et al. (2016a). Budget of primary production and dinitrogen fixation in a highly seasonal red Sea coral reef. Ecosystems 19 (5), 771–785. doi: 10.1007/s10021-016-9966-1
Cardini U., Van Hoytema N., Bednarz V. N., Rix L., Foster R. A., Al-Rshaidat M. M., et al. (2016b). Microbial dinitrogen fixation in coral holobionts exposed to thermal stress and bleaching. Environ. Microbiol. 18 (8), 2620–2633. doi: 10.1111/1462-2920.13385
Chiles E. N., Huffmyer A. S., Drury C., Putnam H. M., Bhattacharya D., Su X. Y. (2022). Stable isotope tracing reveals compartmentalized nitrogen assimilation in scleractinian corals. Front. Mar. Sci. 9. doi: 10.3389/fmars.2022.1035523
Clode P. L., Saunders M., Maker G., Ludwig M., Atkins C. A. (2009). Uric acid deposits in symbiotic marine algae. Plant Cell Environ. 32 (2), 170–177. doi: 10.1111/j.1365-3040.2008.01909.x
Comeau S., Edmunds P. J., Spindel N. B., Carpenter R. C. (2014). Diel pCO2 oscillations modulate the response of the coral acropora hyacinthus to ocean acidification. Mar. Ecol. Prog. Ser. 501, 99–111. doi: 10.3354/meps10690
Crossland C. J., Barnes D. J. (1976). Acetylene reduction by coral skeletons. Limnol. Oceanography 21 (1), 153–156. doi: 10.4319/lo.1976.21.1.0153
Cui G., Konciute M. K., Ling L., Esau L., Raina J. B., Han B., et al. (2023). Molecular insights into the Darwin paradox of coral reefs from the sea anemone aiptasia. Sci. Adv. 9 (11), eadf7108.
Cui G., Liew Y. J., Li Y., Kharbatia N., Zahran N. I., Emwas A. H., et al. (2019). Host-dependent nitrogen recycling as a mechanism of symbiont control in aiptasia. PloS Genet. 15 (6), e1008189. doi: 10.1371/journal.pgen.1008189
Dalsgaard T., Thamdrup B., Canfield D. E. (2005). Anaerobic ammonium oxidation (anammox) in the marine environment. Res. Microbiol. 156 (4), 457–464. doi: 10.1016/j.resmic.2005.01.011
Davey M., Holmes G., Johnstone R. (2007). High rates of nitrogen fixation (acetylene reduction) on coral skeletons following bleaching mortality. Coral Reefs 27 (1), 227–236.
Davy S. K., Turner J. R. (1996). Carbon budgets in temperate anthozoan-dinoflagellate symbioses. Mar. Biol. 126 (4), 773–783. doi: 10.1007/BF00351344
de Goeij J. M. D., Oevelen D. V., Vermeij M. J. A., Osinga R., Middelburg J. J., Anton F. P. M., et al. (2013). Surviving in a marine desert: the sponge loop retains resources within coral reefs. Science 342 (6154), 108–110.
Delmont T. O., Quince C., Shaiber A., Esen O. C., Lee S. T., Rappe M. S., et al. (2018). Nitrogen-fixing populations of planctomycetes and proteobacteria are abundant in surface ocean metagenomes. Nat. Microbiol. 3 (7), 804–813. doi: 10.1038/s41564-018-0176-9
Diroberts L. E., Dudek A., Ray N. E., Fulweiler R. W., Rotjan R. D. (2021). Testing assumptions of nitrogen cycling between a temperate, model coral host and its facultative symbiont: symbiotic contributions to dissolved inorganic nitrogen assimilation. Mar. Ecol. Prog. Ser. 670, 61–74. doi: 10.3354/meps13731
Dobson K. L., Levas S., Schoepf V., Warner M. E., Cai W.-J., Hoadley K. D., et al. (2021). Moderate nutrient concentrations are not detrimental to corals under future ocean conditions. Mar. Biol. 168 (7), 98. doi: 10.1007/s00227-021-03901-3
Domotor S. L., Delia C. F. (1984). Nutrient-uptake kinetics and growth of zooxanthellae maintained in laboratory culture. Mar. Biol. 80 (1), 93–101. doi: 10.1007/BF00393132
Donovan M. K., Adam T. C., Shantz A. A., Speare K. E., Munsterman K. S., Rice M. M., et al. (2020). Nitrogen pollution interacts with heat stress to increase coral bleaching across the seascape. Proc. Natl. Acad. Sci. U. S. A. 117 (10), 5351–5357. doi: 10.1073/pnas.1915395117
El-Khaled Y. C., Nafeh R., Roth F., Radecker N., Karcher D. B., Jones B. H., et al. (2021a). High plasticity of nitrogen fixation and denitrification of common coral reef substrates in response to nitrate availability. Mar. Pollut. Bull. 168, 112430. doi: 10.1016/j.marpolbul.2021.112430
El-Khaled Y. C., Roth F., Rädecker N., Kharbatia N., Jones B. H., Voolstra C. R., et al. (2020a). Simultaneous measurements of dinitrogen fixation and denitrification associated with coral reef substrates: advantages and limitations of a combined acetylene assay. Front. Mar. Sci. 7. doi: 10.3389/fmars.2020.00411
El-Khaled Y. C., Roth F., Radecker N., Tilstra A., Karcher D. B., Kurten B., et al. (2021b). Nitrogen fixation and denitrification activity differ between coral- and algae-dominated red Sea reefs. Sci. Rep. 11 (1), 11820. doi: 10.1038/s41598-021-90204-8
El-Khaled Y. C., Roth F., Tilstra A., Radecker N., Karcher D. B., Kurten B., et al. (2020b). In situ eutrophication stimulates dinitrogen fixation, denitrification, and productivity in red Sea coral reefs. Mar. Ecol. Prog. Ser. 645, 55–66. doi: 10.3354/meps13352
Ezzat L., Fine M., Maguer J. F., Grover R., Ferrier-Pages C. (2017). Carbon and nitrogen acquisition in shallow and deep holobionts of the scleractinian coral s. pistillata. Front. Mar. Sci. 4. doi: 10.3389/fmars.2017.00102
Ezzat L., Maguer J. F., Grover R., Ferrier-Pages C. (2015). New insights into carbon acquisition and exchanges within the coral-dinoflagellate symbiosis under NH4+ and NO3- supply. Proc. Biol. Sci. 282 (1812), 20150610.
Ezzat L., Maguer J. F., Grover R., Ferrier-Pages C. (2016). Limited phosphorus availability is the Achilles heel of tropical reef corals in a warming ocean. Sci. Rep. 6, 31768. doi: 10.1038/srep31768
Falkowski P. G. (1997). Evolution of the nitrogen cycle and its influence on the biological sequestration of CO2 in the ocean. Nature 387 (6630), 272–275.
Falkowski P. G., Dubinsky Z., Muscatine L., Mccloskey L. (1993). Population-control in symbiotic corals. Bioscience 43 (9), 606–611. doi: 10.2307/1312147
Falkowski P. G., Dubinsky Z., Muscatine L., Porter J. W. (1984). Light and the bioenergetics of a symbiotic coral. Bioscience 34 (11), 705–709. doi: 10.2307/1309663
Farnelid H., Turk-Kubo K., Ploug H., Ossolinski J. E., Collins J. R., Van Mooy B., et al. (2019). Diverse diazotrophs are present on sinking particles in the north pacific subtropical gyre. ISME J. 13 (1), 170–182. doi: 10.1038/s41396-018-0259-x
Fernandes De Barros Marangoni L., Ferrier-Pages C., Rottier C., Bianchini A., Grover R. (2020). Unravelling the different causes of nitrate and ammonium effects on coral bleaching. Sci. Rep. 10 (1), 11975.
Ferrier-Pagès C., Bednarz V., Grover R., Benayahu Y., Maguer J. F., Rottier C., et al. (2021). Symbiotic stony and soft corals: is their host-algae relationship really mutualistic at lower mesophotic reefs? Limnol. Oceanography 67 (1), 261–271.
Ferrier-Pages C., Rottier C., Beraud E., Levy O. (2010). Experimental assessment of the feeding effort of three scleractinian coral species during a thermal stress: effect on the rates of photosynthesis. J. Exp. Mar. Biol. Ecol. 390 (2), 118–124. doi: 10.1016/j.jembe.2010.05.007
Foster R. A., Paytan A., Zehr J. P. (2009). Seasonality of N2 fixation and nifH gene diversity in the gulf of aqaba (Red Sea). Limnol. Oceanography 54 (1), 219–233. doi: 10.4319/lo.2009.54.1.0219
Fox M. D., Williams G. J., Johnson M. D., Radice V. Z., Zgliczynski B. J., Kelly E. L. A., et al. (2018). Gradients in primary production predict trophic strategies of mixotrophic corals across spatial scales. Curr. Biol. 28 (21), 3355–3363 e3354. doi: 10.1016/j.cub.2018.08.057
Gallon J. R. (1981). The oxygen sensitivity of nitrogenase - a problem for biochemists and microorganisms. Trends Biochem. Sci. 6 (1), 19–23. doi: 10.1016/0968-0004(81)90008-6
George R., Lugendo B. R. (2023). Tidal cycle and time of day control pH levels in coastal habitats of the western Indian ocean: the case of mnazi and chwaka bays in Tanzania. Western Indian Ocean J. Mar. Sci. 21 (2), 141–150. doi: 10.4314/wiojms.v21i2.12
Gibbin E. M., Krueger T., Putnam H. M., Barott K. L., Bodin J., Gates R. D., et al. (2018). Short-term thermal acclimation modifies the metabolic condition o the coral holobiont. Front. Mar. Sci. 5. doi: 10.3389/fmars.2018.00010
Glaze T. D., Erler D. V., Siljanen H. M. P. (2022). Microbially facilitated nitrogen cycling in tropical corals. ISME J. 16 (1), 68–77. doi: 10.1038/s41396-021-01038-1
Godinot C., Houlbreque F., Grover R., Ferrier-Pages C. (2011). Coral uptake of inorganic phosphorus and nitrogen negatively affected by simultaneous changes in temperature and pH. PloS One 6 (9), e25024. doi: 10.1371/journal.pone.0025024
Grottoli A. G., Rodrigues L. J., Palardy J. E. (2006). Heterotrophic plasticity and resilience in bleached corals. Nature 440 (7088), 1186–1189.
Grottoli A. G., Toonen R. J., Van Woesik R., Vega Thurber R., Warner M. E., Mclachlan R. H., et al. (2021). Increasing comparability among coral bleaching experiments. Ecol. Appl. 31 (4), e02262. doi: 10.1002/eap.2262
Grottoli A. G., Warner M. E., Levas S. J., Aschaffenburg M. D., Schoepf V., Mcginley M., et al. (2014). The cumulative impact of annual coral bleaching can turn some coral species winners into losers. Global Change Biol. 20 (12), 3823–3833. doi: 10.1111/gcb.12658
Grover R., Ferrier-Pages C., Maguer J. F., Ezzat L., Fine M. (2014). Nitrogen fixation in the mucus of red Sea corals. J. Exp. Biol. 217 (Pt 22), 3962–3963. doi: 10.1242/jeb.111591
Grover R., Maguer J. F., Allemand D., Ferrier-Pages C. (2003). Nitrate uptake in the scleractinian coral stylophora pistillata. Limnol. Oceanography 48 (6), 2266–2274. doi: 10.4319/lo.2003.48.6.2266
Grover R., Maguer J. F., Allemand D., Ferrier-Pages C. (2006). Urea uptake by the scleractinian coral stylophora pistillata. J. Exp. Mar. Biol. Ecol. 332 (2), 216–225. doi: 10.1016/j.jembe.2005.11.020
Grover R., Maguer J. F., Allemand D., Ferrier-Pages C. (2008). Uptake of dissolved free amino acids by the scleractinian coral stylophora pistillata. J. Exp. Biol. 211 (Pt 6), 860–865. doi: 10.1242/jeb.012807
Grover R., Maguer J. F., Reynaud-Vaganay S., Ferrier-Pages C. (2002). Uptake of ammonium by the scleractinian coral stylophora pistillata: effect of feeding, light, and ammonium concentrations. Limnol. Oceanography 47 (3), 782–790. doi: 10.4319/lo.2002.47.3.0782
Guadayol O., Silbiger N. J., Donahue M. J., Thomas F. I. (2014). Patterns in temporal variability of temperature, oxygen and pH along an environmental gradient in a coral reef. PloS One 9 (1), e85213. doi: 10.1371/journal.pone.0085213
Guan Y., Hohn S., Wild C., Merico A. (2020). Vulnerability of global coral reef habitat suitability to ocean warming, acidification and eutrophication. Global Change Biol. 26 (10), 5646–5660. doi: 10.1111/gcb.15293
Guerrero M. A., Jones R. D. (1996). Photoinhibition of marine nitrifying bacteria .2. dark recovery after monochromatic or polychromatic irradiation. Mar. Ecol. Prog. Ser. 141 (1-3), 193–198.
Hall E. R., Muller E. M., Goulet T., Bellworthy J., Ritchie K. B., Fine M. (2018). Eutrophication may compromise the resilience of the red Sea coral stylophora pistillata to global change. Mar. Pollut. Bull. 131 (Pt A), 701–711. doi: 10.1016/j.marpolbul.2018.04.067
Hoadley K. D., Hamilton M., Poirier C. L., Choi C. J., Yung C. M., Worden A. Z. (2021). Selective uptake of pelagic microbial community members by Caribbean reef corals. Appl. Environ. Microbiol. 87 (9), e03175–20. doi: 10.1128/AEM.03175-20
Hoegh-Guldberg O., Poloczanska E. S., Skirving W., Dove S. (2017). Coral reef ecosystems under climate change and ocean acidification. Front. Mar. Sci. 4. doi: 10.3389/fmars.2017.00158
Hong H., Shen R., Zhang F., Wen Z., Chang S., Lin W., et al. (2017). The complex effects of ocean acidification on the prominent N(2)-fixing cyanobacterium trichodesmium. Science 356 (6337), 527–531.
Horak R. E. A., Qin W., Bertagnolli A. D., Nelson A., Heal K. R., Han H., et al. (2018). Relative impacts of light, temperature, and reactive oxygen on thaumarchaeal ammonia oxidation in the north pacific ocean. Limnol. Oceanography 63 (2), 741–757. doi: 10.1002/lno.10665
Horrigan S. G., Carlucci A. F., Williams P. M. (1981). Light inhibition of nitrification in Sea-surface films. J. Mar. Res. 39 (3), 557–565.
Horrigan S. G., Springer A. L. (1990). Oceanic and estuarine ammonium oxidation - effects of light. Limnol. Oceanography 35 (2), 479–482. doi: 10.4319/lo.1990.35.2.0479
Houlbreque F., Ferrier-Pages C. (2009). Heterotrophy in tropical scleractinian corals. Biol. Rev. Camb Philos. Soc. 84 (1), 1–17.
Houlbreque F., Reynaud S., Godinot C., Oberhansli F., Rodolfo-Metalpa R., Ferrier-Pages C. (2015). Ocean acidification reduces feeding rates in the scleractinian coral stylophora pistillata. Limnol. Oceanography 60 (1), 89–99. doi: 10.1002/lno.10003
Hughes D. J., Alexander J., Cobbs G., Kuhl M., Cooney C., Pernice M., et al. (2022). Widespread oxyregulation in tropical corals under hypoxia. Mar. Pollut. Bull. 179, 113722. doi: 10.1016/j.marpolbul.2022.113722
Hutchins D. A., Walworth N. G., Webb E. A., Saito M. A., Moran D., Mcilvin M. R., et al. (2015). Irreversibly increased nitrogen fixation in trichodesmium experimentally adapted to elevated carbon dioxide. Nat. Commun. 6 (1), 8155. doi: 10.1038/ncomms9155
Jiang L., Guo Y. J., Zhang F., Zhang Y. Y., Mccook L. J., Yuan X. C., et al. (2018). Diurnally fluctuating pCO(2) modifies the physiological responses of coral recruits under ocean acidification. Front. Physiol. 9, 1952. doi: 10.3389/fphys.2018.01952
Jiang Z. P., Huang J. C., Dai M. H., Kao S. J., Hydes D. J., Chou W. C., et al. (2011). Short-term dynamics of oxygen and carbon in productive nearshore shallow seawater systems off Taiwan: observations and modeling. Limnol. Oceanography 56 (5), 1832–1849. doi: 10.4319/lo.2011.56.5.1832
Johnson M. D., Swaminathan S. D., Nixon E. N., Paul V. J., Altieri A. H. (2021). Differential susceptibility of reef-building corals to deoxygenation reveals remarkable hypoxia tolerance. Sci. Rep. 11 (1), 23168.
Kimes N. E., Van Nostrand J. D., Weil E., Zhou J., Morris P. J. (2010). Microbial functional structure of montastraea faveolata, an important Caribbean reef-building coral, differs between healthy and yellow-band diseased colonies. Environ. Microbiol. 12 (2), 541–556. doi: 10.1111/j.1462-2920.2009.02113.x
Kleypas J. A., Mcmanus J. W., Meñez L. (1999). Environmental limits to coral reef development: where do we draw the line? Am. Zool. 39 (1), 146–159. doi: 10.1093/icb/39.1.146
Kopp C., Domart-Coulon I., Escrig S., Humbel B. M., Hignette M., Meibom A. (2015). Subcellular investigation of photosynthesis-driven carbon assimilation in the symbiotic reef coral pocillopora damicornis. mBio 6 (1), e02299–14. doi: 10.1128/mBio.02299-14
Kopp C., Pernice M., Domart-Coulon I., Djediat C., Spangenberg J. E., Alexander D. T., et al. (2013). Highly dynamic cellular-level response of symbiotic coral to a sudden increase in environmental nitrogen. mBio 4 (3), e00052–e00013. doi: 10.1128/mBio.00052-13
Krueger T., Horwitz N., Bodin J., Giovani M. E., Escrig S., Fine M., et al. (2020). Intracellular competition for nitrogen controls dinoflagellate population density in corals. Proc. Biol. Sci. 287 (1922), 20200049. doi: 10.1098/rspb.2020.0049
Larkum A. W. D. (1988). High-rates of nitrogen-fixation on coral skeletons after predation by the crown of thorns starfish acanthaster-planci. Mar. Biol. 97 (4), 503–506. doi: 10.1007/BF00391046
Leal M. C., Hoadley K., Pettay D. T., Grajales A., Calado R., Warner M. E. (2015). Symbiont type influences trophic plasticity of a model cnidarian-dinoflagellate symbiosis. J. Exp. Biol. 218 (Pt 6), 858–863. doi: 10.1242/jeb.115519
Lema K. A., Clode P. L., Kilburn M. R., Thornton R., Willis B. L., Bourne D. G. (2016). Imaging the uptake of nitrogen-fixing bacteria into larvae of the coral acropora millepora. ISME J. 10 (7), 1804–1808. doi: 10.1038/ismej.2015.229
Lema K. A., Willis B. L., Bourne D. G. (2014). Amplicon pyrosequencing reveals spatial and temporal consistency in diazotroph assemblages of the acropora millepora microbiome. Environ. Microbiol. 16 (10), 3345–3359.
Lesser M. P., Falcon L. I., Rodriguez-Roman A., Enriquez S., Hoegh-Guldberg O., Iglesias-Prieto R. (2007). Nitrogen fixation by symbiotic cyanobacteria provides a source of nitrogen for the scleractinian coral montastraea cavernosa. Mar. Ecol. Prog. Ser. 346, 143–152. doi: 10.3354/meps07008
Lesser M. P., Morrow K. M., Pankey M. S. (2019). N2 fixation, and the relative contribution of fixed n, in corals from curaçao and Hawaii. Coral Reefs 38 (6), 1145–1158. doi: 10.1007/s00338-019-01863-z
Lesser M. P., Morrow K. M., Pankey S. M., Noonan S. H. C. (2018). Diazotroph diversity and nitrogen fixation in the coral stylophora pistillata from the great barrier reef. ISME J. 12 (3), 813–824. doi: 10.1038/s41396-017-0008-6
Levas S. J., Grottoli A. G., Hughes A., Osburn C. L., Matsui Y. (2013). Physiological and biogeochemical traits of bleaching and recovery in the mounding species of coral porites lobata: implications for resilience in mounding corals. PloS One 8 (5), e63267. doi: 10.1371/journal.pone.0063267
Levas S., Grottoli A. G., Schoepf V., Aschaffenburg M., Baumann J., Bauer J. E., et al. (2015). Can heterotrophic uptake of dissolved organic carbon and zooplankton mitigate carbon budget deficits in annually bleached corals? Coral Reefs 35 (2), 495–506. doi: 10.1007/s00338-015-1390-z
Li T., Chen X., Lin S. (2021). Physiological and transcriptomic responses to n-deficiency and ammonium: nitrate shift in fugacium kawagutii (Symbiodiniaceae). Sci. Total Environ. 753, 141906. doi: 10.1016/j.scitotenv.2020.141906
Lima L. F., Alker A. T., Papudeshi B., Morris M. M., Edwards R. A., De Putron S. J., et al. (2022). Coral and seawater metagenomes reveal key microbial functions to coral health and ecosystem functioning shaped at reef scale. Microbial. Ecol, 1–16. doi: 10.1007/s00248-022-02094-6
Logan C. A., Dunne J. P., Ryan J. S., Baskett M. L., Donner S. D. (2021). Quantifying global potential for coral evolutionary response to climate change. Nat. Climate Change 11 (6), 537. doi: 10.1038/s41558-021-01037-2
Lyndby N. H., Holm J. B., Wangpraseurt D., Grover R., Rottier C., Kuhl M., et al. (2020). Effect of temperature and feeding on carbon budgets and O-2 dynamics in pocillopora damicornis. Mar. Ecol. Prog. Ser. 652, 49–62. doi: 10.3354/meps13474
Magnusson S. H., Fine M., Kuhl M. (2007). Light microclimate of endolithic phototrophs in the scleractinian corals montipora monasteriata and porites cylindrica. Mar. Ecol. Prog. Ser. 332, 119–128. doi: 10.3354/meps332119
Martinez S., Grover R., Baker D. M., Ferrier-Pages C. (2022). Symbiodiniaceae are the first site of heterotrophic nitrogen assimilation in reef-building corals. mBio 13 (5), e0160122. doi: 10.1128/mbio.01601-22
Martinez S., Kolodny Y., Shemesh E., Scucchia F., Nevo R., Levin-Zaidman S., et al. (2020). Energy sources of the depth-generalist mixotrophic coral stylophora pistillata. Front. Mar. Sci. 7, 988. doi: 10.3389/fmars.2020.566663
Mclachlan R. H., Dobson K. L., Schmeltzer E. R., Vega Thurber R., Grottoli A. G. (2021). A review of coral bleaching specimen collection, preservation, and laboratory processing methods. PeerJ 9, e11763. doi: 10.7717/peerj.11763
Mclachlan R. H., Price J. T., Solomon S. L., Grottoli A. G. (2020). Thirty years of coral heat-stress experiments: a review of methods. Coral Reefs 39 (4), 885–902. doi: 10.1007/s00338-020-01931-9
Merbt S. N., Stahl D. A., Casamayor E. O., Marti E., Nicol G. W., Prosser J. I. (2012). Differential photoinhibition of bacterial and archaeal ammonia oxidation. FEMS Microbiol. Lett. 327 (1), 41–46. doi: 10.1111/j.1574-6968.2011.02457.x
Messer L. F., Brown M. V., Furnas M. J., Carney R. L., Mckinnon A. D., Seymour J. R. (2017). Diversity and activity of diazotrophs in great barrier reef surface waters. Front. Microbiol. 8, 967. doi: 10.3389/fmicb.2017.00967
Messer L. F., Mahaffey C C. M. R., Jeffries T. C., Baker K. G., Bibiloni Isaksson J., Ostrowski M., et al. (2016). High levels of heterogeneity in diazotroph diversity and activity within a putative hotspot for marine nitrogen fixation. ISME J. 10 (6), 1499–1513. doi: 10.1038/ismej.2015.205
Meunier V., Bonnet S., Benavides M., Ravache A., Grosso O., Lambert C., et al. (2021a). Diazotroph-derived nitrogen assimilation strategies differ by scleractinian coral species. Front. Mar. Sci. 8. doi: 10.3389/fmars.2021.692248
Meunier V., Bonnet S., Camps M., Benavides M., Dubosc J., Rodolfo-Metalpa R., et al. (2022). Ingestion of diazotrophs makes corals more resistant to heat stress. Biomolecules 12 (4), 537. doi: 10.3390/biom12040537
Meunier V., Bonnet S., Pernice M., Benavides M., Lorrain A., Grosso O., et al. (2019). Bleaching forces coral's heterotrophy on diazotrophs and synechococcus. ISME J. 13 (11), 2882–2886. doi: 10.1038/s41396-019-0456-2
Meunier V., Geissler L., Bonnet S., Radecker N., Perna G., Grosso O., et al. (2021b). Microbes support enhanced nitrogen requirements of coral holobionts in a high CO(2) environment. Mol. Ecol. 30 (22), 5888–5899. doi: 10.1111/mec.16163
Middelburg J. J., Mueller C. E., Veuger B., Larsson A. I., Form A., Van Oevelen D. (2015). Discovery of symbiotic nitrogen fixation and chemoautotrophy in cold-water corals. Sci. Rep. 5, 17962. doi: 10.1038/srep17962
Montoya J. P., Voss M., Kahler P., Capone D. G. (1996). A simple, high-precision, high-sensitivity tracer assay for N(inf2) fixation. Appl. Environ. Microbiol. 62 (3), 986–993. doi: 10.1128/aem.62.3.986-993.1996
Moore C. M., Mills M. M., Arrigo K. R., Berman-Frank I., Bopp L., Boyd P. W., et al. (2013). Processes and patterns of oceanic nutrient limitation. Nat. Geosci. 6 (9), 701–710. doi: 10.1038/ngeo1765
Moynihan M. A., Goodkin N. F., Morgan K. M., Kho P. Y. Y., Lopes Dos Santos A., Lauro F. M., et al. (2022). Coral-associated nitrogen fixation rates and diazotrophic diversity on a nutrient-replete equatorial reef. ISME J. 16 (1), 233–246. doi: 10.1038/s41396-021-01054-1
Muscatine L., Falkowski P. G., Dubinsky Z., Cook P. A., Mccloskey L. R. (1997). The effect of external nutrient resources on the population dynamics of zooxanthellae in a reef coral. proceedings of the royal society of London. B. Biol. Sci. 236 (1284), 311–324.
Muscatine L., Falkowski P. G., Porter J. W., Dubinsky Z. (1984). Fate of photosynthetic fixed carbon in light-adapted and shade-adapted colonies of the symbiotic coral stylophora-pistillata. Proc. R. Soc. Ser. B-Biol. Sci. 222 (1227), 181–202.
Muscatine L., Porter J. W. (1977). Reef corals: mutualistic symbioses adapted to nutrient-poor environments. Bioscience 27 (7), 454–460. doi: 10.2307/1297526
Nalley E. M., Tuttle L. J., Conklin E. E., Barkman A. L., Wulstein D. M., Schmidbauer M. C., et al. (2023). A systematic review and meta-analysis of the direct effects of nutrients on corals. Sci. Total Environ. 856 (Pt 1), 159093.
Nelson H. R., Altieri A. H. (2019). Oxygen: the universal currency on coral reefs. Coral Reefs 38 (2), 177–198. doi: 10.1007/s00338-019-01765-0
O'neil J. M., Capone D. G. (2008). “Nitrogen cycling in coral reef environments.” in Nitrogen in the marine environment. Eds. Capone D. G., Bronk D. A., Mulholland M. R., Carpenter E. J. (San Diego: Academic Press), 949–989.
Oehler T., Bakti H., Lubis R. F., Purwoarminta A., Delinom R., Moosdorf N. (2019). Nutrient dynamics in submarine groundwater discharge through a coral reef (western lombok, Indonesia). Limnol. Oceanography 64 (6), 2646–2661. doi: 10.1002/lno.11240
Oliver T. A., Palumbi S. R. (2011). Do fluctuating temperature environments elevate coral thermal tolerance? Coral Reefs 30 (2), 429–440. doi: 10.1007/s00338-011-0721-y
Olson R. J. (1981). Differential photoinhibition of marine nitrifying bacteria - a possible mechanism for the formation of the primary nitrite maximum. J. Mar. Res. 39 (2), 227–238.
Olson N. D., Ainsworth T. D., Gates R. D., Takabayashi M. (2009). Diazotrophic bacteria associated with Hawaiian montipora corals: diversity and abundance in correlation with symbiotic dinoflagellates. J. Exp. Mar. Biol. Ecol. 371 (2), 140–146. doi: 10.1016/j.jembe.2009.01.012
Palardy J. E., Rodrigues L. J., Grottoli A. G. (2008). The importance of zooplankton to the daily metabolic carbon requirements of healthy and bleached corals at two depths. J. Exp. Mar. Biol. Ecol. 367 (2), 180–188. doi: 10.1016/j.jembe.2008.09.015
Palumbi S. R., Barshis D. J., Traylor-Knowles N., Bay R. A. (2014). Mechanisms of reef coral resistance to future climate change. Science 344 (6186), 895–898.
Park S., Bae W. (2009). Modeling kinetics of ammonium oxidation and nitrite oxidation under simultaneous inhibition by free ammonia and free nitrous acid. Process Biochem. 44 (6), 631–640. doi: 10.1016/j.procbio.2009.02.002
Pedersen J. N., Bombar D., Paerl R. W., Riemann L. (2018). Diazotrophs and N(2)-fixation associated with particles in coastal estuarine waters. Front. Microbiol. 9, 2759. doi: 10.3389/fmicb.2018.02759
Pernice M., Meibom A., Van Den Heuvel A., Kopp C., Domart-Coulon I., Hoegh-Guldberg O., et al. (2012). A single-cell view of ammonium assimilation in coral-dinoflagellate symbiosis. ISME J. 6 (7), 1314–1324. doi: 10.1038/ismej.2011.196
Petrou K., Nunn B. L., Padula M. P., Miller D. J., Nielsen D. A. (2021). Broad scale proteomic analysis of heat-destabilised symbiosis in the hard coral acropora millepora. Sci. Rep. 11 (1), 19061.
Pogoreutz C., Radecker N., Cardenas A., Gardes A., Voolstra C. R., Wild C. (2017a). Sugar enrichment provides evidence for a role of nitrogen fixation in coral bleaching. Global Change Biol. 23 (9), 3838–3848. doi: 10.1111/gcb.13695
Pogoreutz C., Radecker N., Cardenas A., Gardes A., Wild C., Voolstra C. R. (2017b). Nitrogen fixation aligns with nifH abundance and expression in two coral trophic functional groups. Front. Microbiol. 8, 1187. doi: 10.3389/fmicb.2017.01187
Prada F., Franzellitti S., Caroselli E., Cohen I., Marini M., Campanelli A., et al. (2023). Acclimatization of a coral-dinoflagellate mutualism at a CO(2) vent. Commun. Biol. 6 (1), 66.
Price J. T., Mclachlan R. H., Jury C. P., Toonen R. J., Grottoli A. G. (2021). Isotopic approaches to estimating the contribution of heterotrophic sources to Hawaiian corals. Limnol. Oceanography 66 (6), 2393–2407. doi: 10.1002/lno.11760
Pupier C. A., Bednarz V. N., Grover R., Fine M., Maguer J. F., Ferrier-Pages C. (2019). Divergent capacity of scleractinian and soft corals to assimilate and transfer diazotrophically derived nitrogen to the reef environment. Front. Microbiol. 10, 1860. doi: 10.3389/fmicb.2019.01860
Pupier C. A., Mies M., Fine M., Francini R. B., Brandini F. P., Zambotti-Villela L., et al. (2021). Lipid biomarkers reveal the trophic plasticity of octocorals along a depth gradient. Limnol. Oceanography 66 (5), 2078–2087. doi: 10.1002/lno.11746
Rädecker N., Meyer F. W., Bednarz V. N., Cardini U., Wild C. (2014). Ocean acidification rapidly reduces dinitrogen fixation associated with the hermatypic coral seriatopora hystrix. Mar. Ecol. Prog. Ser. 511, 297–302. doi: 10.3354/meps10912
Radecker N., Pogoreutz C., Gegner H. M., Cardenas A., Perna G., Geissler L., et al. (2022). Heat stress reduces the contribution of diazotrophs to coral holobiont nitrogen cycling. ISME J. 16 (4), 1110–1118. doi: 10.1038/s41396-021-01158-8
Radecker N., Pogoreutz C., Gegner H. M., Cardenas A., Roth F., Bougoure J., et al. (2021). Heat stress destabilizes symbiotic nutrient cycling in corals. Proc. Natl. Acad. Sci. U. S. A. 118 (5), e2022653118. doi: 10.1073/pnas.2022653118
Radecker N., Pogoreutz C., Voolstra C. R., Wiedenmann J., Wild C. (2015). Nitrogen cycling in corals: the key to understanding holobiont functioning? Trends Microbiol. 23 (8), 490–497.
Radecker N., Pogoreutz C., Ziegler M., Ashok A., Barreto M. M., Chaidez V., et al. (2017). Assessing the effects of iron enrichment across holobiont compartments reveals reduced microbial nitrogen fixation in the red Sea coral pocillopora verrucosa. Ecol. Evol. 7 (16), 6614–6621. doi: 10.1002/ece3.3293
Radice V. Z., Fry B., Brown K. T., Dove S., Hoegh-Guldberg O. (2022). Biogeochemical niches and trophic plasticity of shallow and mesophotic corals recovering from mass bleaching. Limnol. Oceanography 67 (7), 1617–1630. doi: 10.1002/lno.12157
Ribes M., Coma R., Atkinson M. J., Kinzie R. A. (2003). Particle removal by coral reef communities: picoplankton is a major source of nitrogen. Mar. Ecol. Prog. Ser. 257, 13–23. doi: 10.3354/meps257013
Ries J. B. (2011). A physicochemical framework for interpreting the biological calcification response to CO2-induced ocean acidification. Geochim. Et Cosmochimica Acta 75 (14), 4053–4064. doi: 10.1016/j.gca.2011.04.025
Risk M. J., Muller H. R. (1983). Porewater in coral heads - evidence for nutrient regeneration. Limnol. Oceanography 28 (5), 1004–1008. doi: 10.4319/lo.1983.28.5.1004
Ritchie K. B., Smith G. W. (2004). “Microbial communities of coral surface mucopolysaccharide layers.” In Coral Health and Disease. Rosenberg E., Loya Y. eds. Coral Health and Disease (Berlin: Springer-Verlag), 259–264.
Rivera H. E., Davies S. W. (2021). Symbiosis maintenance in the facultative coral, oculina arbuscula, relies on nitrogen cycling, cell cycle modulation, and immunity. Sci. Rep. 11 (1), 21226.
Roberty S., Beraud E., Grover R., Ferrier-Pages C. (2020). Coral productivity is Co-limited by bicarbonate and ammonium availability. Microorganisms 8 (5), 640. doi: 10.3390/microorganisms8050640
Roth F., Karcher D. B., Radecker N., Hohn S., Carvalho S., Thomson T., et al. (2020). High rates of carbon and dinitrogen fixation suggest a critical role of benthic pioneer communities in the energy and nutrient dynamics of coral reefs. Funct. Ecol. 34 (9), 1991–2004. doi: 10.1111/1365-2435.13625
Rougerie F., Fagerstrom J. A., Andrie C. (1992). Geothermal endoupwelling - a solution to the reef nutrient paradox. Continental Shelf Res. 12 (7-8), 785–798. doi: 10.1016/0278-4343(92)90044-K
Safaie A., Silbiger N. J., Mcclanahan T. R., Pawlak G., Barshis D. J., Hench J. L., et al. (2018). High frequency temperature variability reduces the risk of coral bleaching. Nat. Commun. 9 (1), 1671. doi: 10.1038/s41467-018-04074-2
Salazar G., Paoli L., Alberti A., Huerta-Cepas J., Ruscheweyh H. J., Cuenca M., et al. (2019). Gene expression changes and community turnover differentially shape the global ocean metatranscriptome. Cell 179 (5), 1068–1083 e1021. doi: 10.1016/j.cell.2019.10.014
Sangsawang L., Casareto B. E., Ohba H., Vu H. M., Meekaew A., Suzuki T., et al. (2017). (13)C and (15)N assimilation and organic matter translocation by the endolithic community in the massive coral porites lutea. R. Soc. Open Sci. 4 (12), 171201. doi: 10.1098/rsos.171201
Santos H. F., Carmo F. L., Duarte G., Dini-Andreote F., Castro C. B., Rosado A. S., et al. (2014). Climate change affects key nitrogen-fixing bacterial populations on coral reefs. ISME J. 8 (11), 2272–2279. doi: 10.1038/ismej.2014.70
Savage C. (2019). Seabird nutrients are assimilated by corals and enhance coral growth rates. Sci. Rep. 9 (1), 4284.
Schiller C., Herndl G. J. (1989). Evidence of enhanced microbial activity in the interstitial space of branched corals - possible implications for coral metabolism. Coral Reefs 7 (4), 179–184. doi: 10.1007/BF00301596
Schlichter D., Kampmann H., Conrady S. (1997). Trophic potential and photoecology of endolithic algae living within coral skeletons. Mar. Ecol.-Pubblicazioni Della Stazione Zool. Di Napoli I 18 (4), 299–317. doi: 10.1111/j.1439-0485.1997.tb00444.x
Schoen G. H., Engel H. (1962). [The effect of light on nitrosomonas europaea win]. Arch. Mikrobiol. 42, 415–428. doi: 10.1007/BF00409076
Schoepf V., Grottoli A. G., Warner M. E., Cai W. J., Melman T. F., Hoadley K. D., et al. (2013). Coral energy reserves and calcification in a high-CO2 world at two temperatures. PloS One 8 (10), e75049. doi: 10.1371/journal.pone.0075049
Schoepf V., Stat M., Falter J. L., Mcculloch M. T. (2015). Limits to the thermal tolerance of corals adapted to a highly fluctuating, naturally extreme temperature environment. Sci. Rep. 5 (1), 17639.
Shantz A. A., Burkepile D. E. (2014). Context-dependent effects of nutrient loading on the coral-algal mutualism. ECOLOGY 95 (7), 1995–2005. doi: 10.1890/13-1407.1
Shashar N., Cohen Y., Loya Y. (1993). Extreme diel fluctuations of oxygen in diffusive boundary layers surrounding stony corals. Biol. Bull. 185 (3), 455–461. doi: 10.2307/1542485
Shashar N., Cohen Y., Loya Y., Sar N. (1994a). Nitrogen-fixation (Acetylene-reduction) in stony corals - evidence for coral-bacteria interactions. Mar. Ecol. Prog. Ser. 111 (3), 259–264. doi: 10.3354/meps111259
Shashar N., Feldstein T., Cohen Y., Loya Y. (1994b). Nitrogen-fixation (Acetylene-reduction) on a coral-reef. Coral Reefs 13 (3), 171–174. doi: 10.1007/BF00301195
Sheng H. X., Wan X. H. S., Zou B. B., Sun Y. F., Hanoch B., Zou W. B., et al. (2023). An efficient diazotroph-derived nitrogen transfer pathway in coral reef system. Limnol. Oceanography 68 (4), 963–981. doi: 10.1002/lno.12324
Siboni N., Ben-Dov E., Sivan A., Kushmaro A. (2008). Global distribution and diversity of coral-associated archaea and their possible role in the coral holobiont nitrogen cycle. Environ. Microbiol. 10 (11), 2979–2990. doi: 10.1111/j.1462-2920.2008.01718.x
Siboni N., Ben-Dov E., Sivan A., Kushmaro A. (2012). Geographic specific coral-associated ammonia-oxidizing archaea in the northern gulf of eilat (Red Sea). Microbial. Ecol. 64 (1), 18–24. doi: 10.1007/s00248-011-0006-6
Silbiger N. J., Donahue M. J., Lubarsky K. (2020). Submarine groundwater discharge alters coral reef ecosystem metabolism. Proc. Biol. Sci. 287 (1941), 20202743. doi: 10.1098/rspb.2020.2743
Simancas-Giraldo S. M., Xiang N., Kennedy M. M., Nafeh R., Zelli E., Wild C. (2021). Photosynthesis and respiration of the soft coral Xenia umbellata respond to warming but not to organic carbon eutrophication. PeerJ 9, e11663. doi: 10.7717/peerj.11663
Smith J. S., Johnson C. R. (1995). Nutrient inputs from seabirds and humans on a populated coral cay. Mar. Ecol. Prog. Ser. 124 (1-3), 189–200. doi: 10.3354/meps124189
Sohm J. A., Webb E. A., Capone D. G. (2011). Emerging patterns of marine nitrogen fixation. Nat. Rev. Microbiol. 9 (7), 499–508. doi: 10.1038/nrmicro2594
Sournia A. (1976). Oxygen metabolism of a fringing reef in French Polynesia. Helgol. Mar. Res. 28 (3), 401–410. doi: 10.1007/BF01610589
Sproles A. E., Oakley C. A., Krueger T., Grossman A. R., Weis V. M., Meibom A., et al. (2020). Sub-Cellular imaging shows reduced photosynthetic carbon and increased nitrogen assimilation by the non-native endosymbiont durusdinium trenchii in the model cnidarian aiptasia. Environ. Microbiol. 22 (9), 3741–3753. doi: 10.1111/1462-2920.15142
Stein L. Y., Arp D. J., Hyman M. R. (1997). Regulation of the synthesis and activity of ammonia monooxygenase in nitrosomonas europaea by altering pH to affect NH(inf3) availability. Appl. Environ. Microbiol. 63 (11), 4588–4592. doi: 10.1128/aem.63.11.4588-4592.1997
Sutton D. C., Hoegh-Guldberg O. (1990). Host-zooxanthella interactions in four temperate marine invertebrate symbioses: assessment of effect of host extracts on symbionts. Biol. Bull. 178 (2), 175–186. doi: 10.2307/1541975
Tilstra A., El-Khaled Y. C., Roth F., Radecker N., Pogoreutz C., Voolstra C. R., et al. (2019a). Denitrification aligns with N(2) fixation in red Sea corals. Sci. Rep. 9 (1), 19460. doi: 10.1038/s41598-019-55408-z
Tilstra A., Pogoreutz C., Radecker N., Ziegler M., Wild C., Voolstra C. R. (2019b). Relative diazotroph abundance in symbiotic red Sea corals decreases with water depth. Front. Mar. Sci. 6. doi: 10.3389/fmars.2019.00372
Tong H., Cai L., Zhou G., Zhang W., Huang H., Qian P. Y. (2020). Correlations between prokaryotic microbes and stress-resistant algae in different corals subjected to environmental stress in Hong Kong. Front. Microbiol. 11, 686. doi: 10.3389/fmicb.2020.00686
Towle E. K., Baker A. C., Langdon C. (2016). Preconditioning to high CO2 exacerbates the response of the Caribbean branching coral porites porites to high temperature stress. Mar. Ecol. Prog. Ser. 546, 75–84. doi: 10.3354/meps11655
Towle E. K., Enochs I. C., Langdon C. (2015). Threatened Caribbean coral is able to mitigate the adverse effects of ocean acidification on calcification by increasing feeding rate. PloS One 10 (4), e0123394.
Tremblay P., Gori A., Maguer J. F., Hoogenboom M., Ferrier-Pages C. (2016). Heterotrophy promotes the re-establishment of photosynthate translocation in a symbiotic coral after heat stress. Sci. Rep. 6, 38112. doi: 10.1038/srep38112
Tremblay P., Grover R., Maguer J. F., Hoogenboom M., Ferrier-Pagès C. (2013). Carbon translocation from symbiont to host depends on irradiance and food availability in the tropical coral stylophora pistillata. Coral Reefs 33 (1), 1–13.
Tremblay P., Peirano A., Ferrier-Pages C. (2011). Heterotrophy in the Mediterranean symbiotic coral cladocora caespitosa: comparison with two other scleractinian species. Mar. Ecol. Prog. Ser. 422, 165–177. doi: 10.3354/meps08902
Turk-Kubo K. A., Frank I. E., Hogan M. E., Desnues A., Bonnet S., Zehr J. P. (2015). Diazotroph community succession during the VAHINE mesocosm experiment (New Caledonia lagoon). Biogeosciences 12 (24), 7435–7452. doi: 10.5194/bg-12-7435-2015
Umezawa Y., Miyajima T., Kayanne H., Koike I. (2002). Significance of groundwater nitrogen discharge into coral reefs at ishigaki island, southwest of Japan. Coral Reefs 21 (4), 346–356. doi: 10.1007/s00338-002-0254-5
Van Der Zande R. M., Mulders Y. R., Bender-Champ D., Hoegh-Guldberg O., Dove S. (2021). Asymmetric physiological response of a reef-building coral to pulsed versus continuous addition of inorganic nutrients. Sci. Rep. 11 (1), 13165.
Vega Thurber R., Schmeltzer E. R., Grottoli A. G., Van Woesik R., Toonen R. J., Warner M., et al. (2022). Unified methods in collecting, preserving, and archiving coral bleaching and restoration specimens to increase sample utility and interdisciplinary collaboration. PeerJ 10, e14176. doi: 10.7717/peerj.14176
Venn A., Tambutte E., Holcomb M., Allemand D., Tambutte S. (2011). Live tissue imaging shows reef corals elevate pH under their calcifying tissue relative to seawater. PloS One 6 (5), e20013. doi: 10.1371/journal.pone.0020013
Veron J., Stafford-Smith M., Devantier L., Turak E. (2015). Overview of distribution patterns of zooxanthellate scleractinia. Front. Mar. Sci. 2. doi: 10.3389/fmars.2014.00081
Wafar M., Wafar S., David J. J. (1990). Nitrification in reef corals. Limnol. Oceanography 35 (3), 725–730. doi: 10.4319/lo.1990.35.3.0725
Wall C. B., Kaluhiokalani M., Popp B. N., Donahue M. J., Gates R. D. (2020). Divergent symbiont communities determine the physiology and nutrition of a reef coral across a light-availability gradient. ISME J. 14 (4), 945–958. doi: 10.1038/s41396-019-0570-1
Wang G. Z., Wang S. L., Wang Z. Y., Jing W. P., Xu Y., Zhang Z. L., et al. (2018). Tidal variability of nutrients in a coastal coral reef system influenced by groundwater. Biogeosciences 15 (4), 997–1009. doi: 10.5194/bg-15-997-2018
Wangpraseurt D., Weber M., Roy H., Polerecky L., De Beer D., Suharsono, et al. (2012). In situ oxygen dynamics in coral-algal interactions. PloS One 7 (2), e31192. doi: 10.1371/journal.pone.0031192
Wegley L., Edwards R., Rodriguez-Brito B., Liu H., Rohwer F. (2007). Metagenomic analysis of the microbial community associated with the coral porites astreoides. Environ. Microbiol. 9 (11), 2707–2719. doi: 10.1111/j.1462-2920.2007.01383.x
White A. E., Granger J., Selden C., Gradoville M. R., Potts L., Bourbonnais A., et al. (2020). A critical review of the n-15(2) tracer method to measure diazotrophic production in pelagic ecosystems. Limnol. Oceanography-Methods 18 (4), 129–147. doi: 10.1002/lom3.10353
Wild C., Huettel M., Klueter A., Kremb S. G., Rasheed M. Y., Jorgensen B. B. (2004). Coral mucus functions as an energy carrier and particle trap in the reef ecosystem. Nature 428 (6978), 66–70.
Wilkerson F. P., Trench R. K. (1986). Uptake of dissolved inorganic nitrogen by the symbiotic clam tridacna-gigas and the coral acropora sp. Mar. Biol. 93 (2), 237–246. doi: 10.1007/BF00508261
Williams W. M., Viner A. B., Broughton W. J. (1987). Nitrogen-fixation (Acetylene-reduction) associated with the living coral acropora-variabilis. Mar. Biol. 94 (4), 531–535. doi: 10.1007/BF00431399
Wilson S. T., Bottjer D., Church M. J., Karl D. M. (2012). Comparative assessment of nitrogen fixation methodologies, conducted in the oligotrophic north pacific ocean. Appl. Environ. Microbiol. 78 (18), 6516–6523. doi: 10.1128/AEM.01146-12
Xiang N., Hassenruck C., Pogoreutz C., Radecker N., Simancas-Giraldo S. M., Voolstra C. R., et al. (2022). Contrasting microbiome dynamics of putative denitrifying bacteria in two octocoral species exposed to dissolved organic carbon (DOC) and warming. Appl. Environ. Microbiol. 88 (2), e0188621. doi: 10.1128/AEM.01886-21
Xu M. N. N., Wu Y. H., Zheng L. W., Zheng Z. Z., Zhao H. D., Laws E. A., et al. (2017). Quantification of multiple simultaneously occurring nitrogen flows in the euphotic ocean. Biogeosciences 14 (4), 1021–1038. doi: 10.5194/bg-14-1021-2017
Yang S. H., Lee S. T. M., Huang C. R., Tseng C. H., Chiang P. W., Chen C. P., et al. (2016). Prevalence of potential nitrogen-fixing, green sulfur bacteria in the skeleton of reef-building coral isopora palifera. Limnol. Oceanography 61 (3), 1078–1086. doi: 10.1002/lno.10277
Yang S., Sun W., Zhang F., Li Z. (2013). Phylogenetically diverse denitrifying and ammonia-oxidizing bacteria in corals alcyonium gracillimum and tubastraea coccinea. Mar. Biotechnol. (NY) 15 (5), 540–551. doi: 10.1007/s10126-013-9503-6
Yang S. H., Tandon K., Lu C. Y., Wada N., Shih C. J., Hsiao S. S., et al. (2019). Metagenomic, phylogenetic, and functional characterization of predominant endolithic green sulfur bacteria in the coral isopora palifera. Microbiome 7 (1), 3. doi: 10.1186/s40168-018-0616-z
Yoshinari T., Knowles R. (1976). Acetylene inhibition of nitrous oxide reduction by denitrifying bacteria. Biochem. Biophys. Res. Commun. 69 (3), 705–710. doi: 10.1016/0006-291X(76)90932-3
Zehr J. P., Capone D. G. (2020). Changing perspectives in marine nitrogen fixation. Science 368 (6492), eaay9514. doi: 10.1126/science.aay9514
Zhang J., Huang Z., Li Y., Fu D., Li Q., Pei L., et al. (2023). Synergistic/antagonistic effects of nitrate/ammonium enrichment on fatty acid biosynthesis and translocation in coral under heat stress. Sci. Total Environ. 876, 162834. doi: 10.1016/j.scitotenv.2023.162834
Zhang Y., Ling J., Yang Q., Wen C., Yan Q., Sun H., et al. (2015). The functional gene composition and metabolic potential of coral-associated microbial communities. Sci. Rep. 5, 16191. doi: 10.1038/srep16191
Zhang Y., Yang Q., Ling J., Van Nostrand J. D., Shi Z., Zhou J., et al. (2016). The shifts of diazotrophic communities in spring and summer associated with coral galaxea astreata, pavona decussata, and porites lutea. Front. Microbiol. 7, 1870. doi: 10.3389/fmicb.2016.01870
Zhang Y., Yang Q., Zhang Y., Ahmad M., Ling J., Dong J., et al. (2021). The diversity and metabolic potential of the microbial functional gene associated with porites pukoensis. Ecotoxicology 30 (5), 986–995. doi: 10.1007/s10646-021-02419-0
Zhao H. W., Yuan M. L., Strokal M., Wu H. C., Liu X. H., Murk A., et al. (2021). Impacts of nitrogen pollution on corals in the context of global climate change and potential strategies to conserve coral reefs. Sci. Total Environ. 774, 145017. doi: 10.1016/j.scitotenv.2021.145017
Keywords: coral holobiont, nitrogen, quantification, symbiosis, diazotroph
Citation: Li M, Sheng H-X, Dai M and Kao S-J (2023) Understanding nitrogen dynamics in coral holobionts: comprehensive review of processes, advancements, gaps, and future directions. Front. Mar. Sci. 10:1203399. doi: 10.3389/fmars.2023.1203399
Received: 10 April 2023; Accepted: 08 May 2023;
Published: 26 May 2023.
Edited by:
Wei Jiang, Guangxi University, ChinaReviewed by:
Christine Ferrier-Pagès, Centre Scientifique de Monaco, MonacoHao Jiang, Chinese Academy of Sciences (CAS), China
Copyright © 2023 Li, Sheng, Dai and Kao. This is an open-access article distributed under the terms of the Creative Commons Attribution License (CC BY). The use, distribution or reproduction in other forums is permitted, provided the original author(s) and the copyright owner(s) are credited and that the original publication in this journal is cited, in accordance with accepted academic practice. No use, distribution or reproduction is permitted which does not comply with these terms.
*Correspondence: Shuh-Ji Kao, sjkao@xmu.edu.cn