- 1Centro de Estudios Avanzados en Zonas Áridas (CEAZA), Universidad Católica del Norte, Coquimbo, Chile
- 2Facultad de Ciencias del Mar, Depto. Biología Marina, Universidad Católica del Norte, Coquimbo, Chile
- 3Laboratorio de Eco-Parasitología y Epidemiología Marina (LEPyEM), Instituto de Ciencias Naturales Alexander von Humboldt, Universidad de Antofagasta, Antofagasta, Chile
- 4Instituto Milenio de Oceanografía, Universidad de Concepción, Concepción, Chile
- 5Departamento de Ecología, Facultad de Ciencias Biológicas, Pontificia Universidad Católica de Chile, Santiago, Chile
- 6School of Biological Sciences and the SWIRE Institute of Marine Sciences, The University of Hong Kong, Hong Kong, Hong Kong SAR, China
- 7Institute for Climate and Carbon Neutrality, The University of Hong Kong, Hong Kong, Hong Kong SAR, China
- 8Coastal Ecosystems & Global Environmental Change Lab (ECCALab), Department of Aquatic System, Faculty of Environmental Sciences, Universidad de Concepción, Concepción, Chile
- 9Instituto Milenio de Socio-Ecología Costera (SECOS), Universidad de Concepción & P. Universidad Católica de Chile, Santiago, Chile
Extreme low pH events in estuaries and upwelling areas can modulate the phenotypic and genetic diversity of natural populations. To test this hypothesis, we explored the linkage between local scale extreme low pH events, genetic diversity, and variation in fecundity-related traits (body size, egg size, and egg production rate) in the broad-dispersal copepod Acartia tonsa. We assessed genetic and phenotypic characteristics of populations by contrasting extreme low pH environments (upwelling and temperate estuary) in the coastal Southeast Pacific, under natural and experimental conditions. These populations showed significant genetic differentiation with higher diversity in mitochondrial and nuclear loci (encoding mtCOI and 18S rRNA) in the estuarine population. Copepods from this population are exposed to more frequent extreme low pH events (< 7.7), and the adult females exhibit consistent phenotypic variation in body size, egg size, and egg production rate across different cohorts. Experimental acclimation to extreme low pH conditions revealed no significant differences in fecundity-related traits between A. tonsa populations. Although these results partially support our hypothesis, the experimental findings suggest other drivers might also influence phenotypic differences in the local environments.
Introduction
Compared to open ocean in which surface pH is progressively lowered solely due to ocean acidification (Gruber et al., 2021), coastal environments are characterized by complex and dynamic pH conditions that vary across time and space (Carstensen and Duarte, 2019). This environmental variability (in terms of predictability, range, and extremes) can promote inter-population differences in phenotypic plasticity and genetic diversity in natural populations (Gaitán-Espitia et al., 2017; Kapsenberg and Cyronak, 2019; Sasaki and Dam, 2019; Sasaki and Dam, 2020). Extreme low pH events in coastal habitats (e.g., freshwater-influenced areas, coastal upwelling areas, and oxygen minimum zones) occur episodically usually as short term intervals (days) (Spisla et al., 2021), and have been associated with greater phenotypic and genetic variations in inhabiting populations compared to those in less variable and extreme habitats (Calosi et al., 2013; Kelly and Hofmann, 2013; Aguilera et al., 2016; Riquelme-Bugueño et al., 2020).
Extreme low pH events (i.e., pH-threshold) affecting marine invertebrate physiology, mainly crustacean, were recently defined as pH values < 7.7 (Bednaršek et al., 2021). These extreme low pH events can vary across biogeographic regions depending on the interactions between the seascape/landscape, natural dynamics of physical-chemical conditions and the specific level of influence of climate and global change (Gruber et al., 2021; Burger and Frölicher, 2023). For temperate estuarine ecosystems, temperature, salinity, and pH conditions vary mostly due to the influence of cold, low alkalinity and high pCO2 freshwater (Waldbusser and Salisbury, 2014; Vargas et al., 2016; Carstensen and Duarte, 2019). This influence is expected to change due to changing hydrological cycles and river runoff driven by climate change, and/or changing land uses by global change, exposing these habitats, for example, to anomalous extreme low pH events (Diffenbaugh et al., 2005; Curra-Sánchez et al., 2022). Conversely, in coastal upwelling regions, temperature and pH conditions can vary dramatically due to wind-driven upwelling, which uplift deep, cold, and low pH water to the ocean surface (Feely et al., 2008; Vargas et al., 2017; Vargas et al., 2022). The projections of increased frequency and intensity of extreme low pH events are related to either more intense upwelling favorable winds (Sydeman et al., 2014) and/or the expansion and shoaling of CO2-rich oxygen minimum zones (Cabré et al., 2015). The specific environmental variability, including frequency and magnitude of extreme pH events that these habitats provide to local populations, can promote specific patterns of physiological plasticity and genetic differentiation (Burger et al., 2020; Gruber et al., 2021).
The global cosmopolitan and broad dispersal copepod species Acartia tonsa (Copepoda, Calanoida) (Chaalali et al., 2013), is distributed in the southeastern Pacific from tropical (5°S) upwelling areas (Aronés et al., 2009), temperate estuaries (Aguilera et al., 2013) and subantarctic channels (∼54°S) (Aguirre et al., 2012). In between, there are contrasting climate-geographic provinces (Camus, 2001; Escribano et al., 2003), each exhibiting specific patterns of local scale environmental variability (Vargas et al., 2017; Vargas et al., 2022). A. tonsa populations inhabiting these specific geographic provinces might present different patterns of genetic and phenotypic variation. In this study, we assessed genetic and phenotypic variation in fecundity related-related traits of two distant (>15° latitude separation) A. tonsa populations inhabiting a temperate-seasonal estuary and sub-tropical year-round upwelling system in the southeastern Pacific. Although contradictory results have been observed with regards to pH effects on copepod traits, fecundity related-traits, such as egg production rate and egg size, tend to be highly sensitive to low pH conditions (Wang et al., 2018). These traits can determine the fitness of the offspring through maternal effects (Vehmaa et al., 2012), while accounting for demographic and biogeochemical processes like secondary production (Poulet et al., 1995). Our results indicate the estuarine A. tonsa population was exposed to more frequent extreme low pH events (< 7.7), and the adult females exhibit consistent phenotypic variation in fecundity-related traits across different cohorts. Although copepod populations were genetically structured, acclimation experiments revealed no significant differences in fecundity-related traits between A. tonsa populations, suggesting other drivers might also influence phenotypic differences in the local environments.
Materials and methods
Study areas
Local scale pH conditions, genetic diversity and copepod fecundity-related traits were characterized in a temperate-estuarine system in Southern Chile (Valdivia River Estuary, 39.8°S 73.2°W) and a coastal upwelling system off northern Chile (Antofagasta, -23.4°S -70.6°W). Strong riverine discharges characterize the estuarine system, with maximum runoff during the austral autumn-winter period (130 ± 93 mm y-1 of precipitation), and occasional rainy events occurring throughout the year (Pérez et al., 2016). The influence of freshwater runoff in this estuarine system is widely affected by tidal cycles that determine regular events of low temperature (< 14°C), low pH (pHT < 7.7) and low salinity conditions (< 33 psu) in the adjacent coastal zone (Aguilera et al., 2013; Garcés-Vargas et al., 2020; Osma et al., 2020). In contrast, the Antofagasta upwelling system is located off the most arid global desert, the Atacama Desert, which lacks seasonality and freshwater discharges (Hartley et al., 2005). Permanent equatorward winds promote year-round uplifts of subsurface cold and low pH (pHT < 7.7) water (Torres et al., 2002; Aguilera et al., 2020a) over an extremely narrow (< 5 km) continental shelf. The estuarine and upwelling habitats are embedded in the Humboldt Current System and located in distant coastal provinces (> 1700 km, > 15° latitude distant), separated by oceanographic clines efficiently structuring the latitudinal distribution and genetic diversity of benthic invertebrates (Haye et al., 2014).
Characterization of environmental variability in each habitat
Environmental characterization in the estuarine system was assessed during the seasonal (austral spring-summer) occurrence and reproduction of the local A. tonsa population, between the years 2010 and 2012, under the influence of flood tides (Aguilera et al., 2013). The environmental variability was characterized at 7 m, likely reflecting the most prevalent environmental niche of adult females in which fecundity-related traits were assessed. Indeed, the spatial niche of A. tonsa might be smaller in the estuarine system due to hydrographic conditions potentially reducing the habitat suitability on the surface (less dense and low pH freshwater lens) and near the bottom (i.e., turbulent and sediment enriched tidal currents) (Pino et al., 1994; Vargas et al., 2003; Osma et al., 2020). Environmental drivers such as temperature, salinity, pHT, and food concentration were characterized on an inter-daily scale (i.e., each 4 – 6 days) by means of 18 oceanographic surveys (Table 1). A small CTD (Ocean Seven 305 Plus, www.idronaut.it) deployed from above the bottom to the surface provided continuous temperature (°C) and salinity (psu) measurements. In addition, water samples were collected at 7 m depth with a 10-L Niskin bottle for determinations of pHT, total alkalinity (AT), and copepod food. Within 2 h of collection, pHT was measured in a closed 25-mL cell thermostated at 25.0°C using a Metrohm 713 pH meter and a glass combined double junction Ag/AgCl electrode (Metrohm model 6.0219.100) calibrated with Tris buffer at 25°C. Samples for AT were collected in 250 mL high-density polypropylene bottles, poisoned with 50 µL of a saturated HgCl2 solution and then stored in darkness at room temperature until analysis no longer than 1 month. AT was analyzed using the automated potentiometric titration method (Haraldsson et al., 1997) controlling the accuracy against certified reference material (CRM, Batch #101, Scripps Institution of Oceanography, San Diego, USA). With pH, AT, temperature, and salinity data, pHT (total scale) and other carbonate system parameters were estimated using the CO2sys_v3.0 software (Pierrot et al., 2021), set with Mehrbach solubility constants (Mehrbach et al., 1973) and refitted after Dickson and Millero (1987). Food concentration was determined as the biomass of nanoflagellates and phytoplankton cells in carbon units (μg C L-1) (e.g., Vargas and González, 2004).
Environmental characterization in the upwelling system was carried out at variable intervals (4 ± 3 d) during 2015 (Table 1). In this system, A. tonsa reproduces throughout the year (Ruz et al., 2015) and its vertical niche seems to be constrained to the upper 20 m of the water column due to a shallow oxygen minimum zone (Escribano et al., 2009). Thus, environmental characterization in the upwelling system was accomplished at 10 m depth by means of 28 oceanographic surveys, all during 2015 (Table 1). Temperature and salinity vertical profiles were recorded from above the bottom (∼ 30 m) to the surface, through vertical deployments of a calibrated SeaBird SBE19 Plus CTD. Seawater was collected through oceanographic sample collections at 10 m depth for determinations of pHT, AT and copepod food. Seawater pHT was measured in triplicate as described above, within 1 h from the time of collection in a closed 25 mL cell thermostated (25°C). AT samples were handled and determined as described above by means of an automated Alkalinity Titrator AS-ALK2 Apollo SciTech. The accuracy for AT determinations was controlled in combination with certified reference material (A. Dickson, Batch #140). The pHT was calculated as described above. Food concentration was determined as chlorophyll-a (Chl, µg Chl L-1) concentration, and then Chl concentration was converted to carbon units by using a Chl:C ratio=120, according to Vargas and González (2004), for the same study area. Triplicate samples (200 mL) of sieved (200 μm mesh) seawater were filtered onto a GF/F filter (nominal pore size=0.7 µM). Chl was extracted for 24 h in 90% acetone v/v and measured in a TD Turner fluorometer (Strickland and Parsons, 1972).
Copepod sampling
Three different cohorts of both the estuarine and upwelling A. tonsa populations were assessed. Three different cohorts were assessed in the estuarine habitat over the period of 2010–2012, while in the northern-upwelling habitat, copepods were sampled throughout 2015. Cohorts were identified because they were either sampled in subsequent seasons (estuary) or by accumulated changes in the body size of adult females across an annual period (upwelling) (Aguilera and Bednaršek, 2022). Copepod samples were collected by gentle oblique hauls with a 200-µm mesh size WP2 net equipped with a non-filtering 1 L cod-end, from 12 to 7 m depth (estuary) and 15 to 10 m depth (upwelling) strata. Samples were gently transferred to a temperature-controlled and well oxygenated container over the duration of the transport to the laboratory.
Genetic diversity
Genetic diversity was assessed using females randomly sorted from samples collected during surveys encompassing months (upwelling, n=29) to years (estuary, n=32) and analyzed individually. For molecular analyses, partial sequences of the mitochondrial gene cytochrome c oxidase subunit I (mtCOI, c. 700bp; Forward LCO1490: 5’-GGTCAACAAATCATAAAGATATTGG-3’ and Reverse HCO2198: 5’-TAAACTTCAGGGTGACCAAAAAATCA-3’; Folmer et al., 1994) and nuclear gene for 18S ribosomal RNA (c.1600 bp; Forward 18A1mod: 5’-CTGGTTGATCCTGCCAGTCATATGC-3’) and Reverse 1800mod: 5’-GATCCTTCCGCAGGTTCACCTACG-3’; Raupach et al., 2009) were amplified through the polymerase chain reaction (PCR). The mtCOI gene is related to the maternal mode of inheritance with sharp intraspecific (up to 4%) and interspecific (∼9 to >25%) genetic divergence (Bucklin et al., 1999; Bucklin et al., 2003; Hebert et al., 2003b; Bucklin et al., 2010). The nuclear 18S gene evolves at a lower rate than mitochondrial genes (Moriyama and Powell, 1997), which has been a valuable complementary tool to disentangle complex phylogenetic relationships and reconstruction in copepod species (Blanco-Bercial et al., 2011; Cornils and Blanco-Bercial, 2013). All PCR amplifications were performed in 30 µl volume reaction with 3µl PCR buffer (Promega), 3µl MgCl2 (Promega), 0.5 µl dNTPs (10 mmol µl-1), 0.5 µl of each primer (100 pmol µl-1), 0.2 µl Taq polymerase (Promega), 17.3 µl of sterile water and 5 µl of DNA template. For mtCOI amplification, a temperature profile with an initial denaturation at 94°C for 1 min was applied, followed by 32 cycles at 94°C for 40 s, annealing at 48°C for 40 s, extension at 72°C for 1 min, and finishing with another extension at 72°C for 1 min. Amplification of the 18S fragment consisted in an initial denaturation at 94°C for 1 min, then 37 cycles at 94°C for 40 s, annealing at 54°C for 1 min, extension at 72°C for 1 min and a final extension at 72°C for 90 s. Successful amplification of fragments was confirmed by 1.2% agarose gel electrophoresis and staining with GelRedTM fluorescent DNA dye. Both DNA strands were directly sequenced (Macrogen, Seoul, Korea; http://www.macrogen.com). Mitochondrial and nuclear sequences were edited and aligned in Geneious R11.1.5 (2018) using a MUSCLE alignment algorithm (Edgar, 2004) with default settings. Population diversity indexes, as the number of polymorphic sites (p), number of mutations (nm), nucleotide diversity (π), mean number of nucleotide differences (k), haplotype diversity(h), number of haplotypes (nh) and number of private haplotypes (np), were estimated in DnaSp software V.6. (Rozas et al., 2017). Genetic divergences between populations (Φst) were estimated in the software Arlequin V.3.5 with 10000 permutations (Excoffier and Lischer, 2010). Finally, genealogical relations between haplotypes were represented with a minimum spanning haplotype network (Bandelt et al., 1999) in PopART software (Leigh and Bryant, 2015).
Fecundity related traits
Assessed fecundity-related traits were female body size, egg production rate and egg size. Within 2 h of collection, only mature and visibly healthy A. tonsa females were sorted out under a stereomicroscope (Supplementary Figure 1). Up to 40 copepod females were immediately preserved in 90% ethanol for further measurements of copepod body length (mm) within two weeks of preservation to diminish ethanol effects on body size (Moksness and Fossum, 1992). Additional copepods were incubated in seawater collected during sampling with the aim of estimating phenotypic plasticity in fecundity-related traits as related to the habitat-specific variations in environmental conditions. Mean incubation temperature was 14 ± 1°C, which corresponded to the mean temperature conditions experienced by the various cohorts in both habitats. Mean egg production rates (EPR) were estimated based on batches of 30–40 copepod females, individually incubated for 17–20 h in 200 mL clean crystallizing dishes filled with sieved (<200 μm) natural seawater. The concave walls of the dishes that converge towards the floor, allow the settlement and grouping of the relatively dense copepod eggs (Tang et al., 1998) onto the floors of the dishes (although the walls of the dishes were surveyed as well). Eggs produced over this period by each group were counted under a stereomicroscope, standardized to daily duration (24 h), with the mean EPR expressed as the egg fem-1 d-1 (± SD) (Aguilera et al., 2011). After being counted, some of the produced eggs (20–30) were preserved (90% ethanol) and their size (i.e., diameter in µm) measured under an inverted microscope within two weeks of preservation to diminish ethanol effects on egg size (Moksness and Fossum, 1992).
Acclimation experiments
Environmental conditions in acclimation experiments should be consistent with the habitat conditions of the respective populations (Stillman, 2003), providing the opportunity of examining phenotypic outcomes in response to specific attributes of environmental variability, such as extreme conditions. This contrasts with common garden experiments in which the mean habitat conditions of one (or more) population is displaced to a common nominal level to allow comparison of phenotypic responses among different populations (Thorpe et al., 2005). Three acclimation experiments with three different cohorts were carried out within each population immediately after estimating phenotypic responses to field conditions. Females were acclimated during 96-h under mean field temperature and salinity, controlled food supply, and high (i.e., control) and extreme low pH levels (Supplementary Figure 3). Events with pH < 7.7 were considered extreme low pH events (Bednaršek et al., 2021). This threshold of pH 7.7 corresponds to the 5th percentile of measurements at the estuarine and upwelling habitats. These conditions are associated either to the predominance of freshwater in the estuary (Aguilera et al., 2013; Osma et al., 2020) or very recently upwelled waters (Torres et al., 2002). In the estuarine system, seawater was collected 8 km northward from the estuarine system and transferred into a micro-mesocosm laboratory at the Calfuco Marine Station, Austral University of Chile. In the upwelling system, seawater was collected during three different opportunities from the Antofagasta Bay and transferred to laboratory facilities at the Marine Sciences Faculty of the Antofagasta University. Mean seawater temperature was maintained relatively constant (± 0.2°C) in a free circulating water system (estuary) or cold room (upwelling) during the incubations. In both systems, the target pHT levels were achieved by mixing seawater with air containing different pCO2 concentrations (Vargas et al., 2017). Mean control and extreme pH values reproduced during acclimation experiments were 7.73 ± 0.05 and 8.0 ± 0.06 in both the estuarine and upwelling system. Copepod females (4 – 6) were pipetted into three 660-mL borosilicate acid-washed bottles filled with pH equilibrated water, with daily exchange of incubation water and food, the latter composed of Isochrysis sp. (estuary) and Isochrysis sp. + Tetraselmis suecica (upwelling) in concentrations above the saturation level for this species (>350 µg C L-1 and > 4 µg Chl L-1; Thompson et al., 1994). After the last 24 h incubation within the 96 h of acclimation, fecundity-related traits (i.e., EPR) were estimated as described above.
Data analysis
To test differences in environmental conditions at the inter-population level (i.e., between A. tonsa populations) we used a one-way ANOVA, while body-size dependent fecundity was compared with an ANCOVA analysis. Parametric tests were carried out after successfully fulfilling requirements of normal distribution (Lilliefors test, p<0.01) and homogeneity of variance (Levene’s tests). Inter-population comparisons with significant differences (p-value< 0.005) were a posteriori compared with Tukey’s HSD test (Sokal and Rohlf, 1995). The numerical relationships of fecundity with environmental pH conditions were explored with Pearson’s correlation tests, such as plastic responses which were expressed as a mean reaction norm that had a significantly non-zero slope (Stearns, 1992). Such a mechanistic approach to detect the relationship between phenotypic plasticity and specific environmental features can yield insights into ecological speciation among populations (Chen and Hare, 2008). Acclimation experiments were carried out with three different cohorts of each population. Although these cohorts were sampled in subsequent chronologies, such sampling was not continuous and thus, assessed cohorts correspond to discrete subgroups within each population (group). To evaluate inter-population differences in the EPR during acclimation experiments, we conducted a linear mixed effects model. Extensions of simple linear models and mixed effects models allow the simultaneous assessment of fixed and random effects on non-independent data sets, such as phenotypic/genetic variations across cohorts of a given population (i.e., hierarchical structure). Experimental conditions (temperature, salinity, and body size) and populations (estuarine and upwelling) were considered as fixed effects, while experiments (I, II, III) as a random effect. Differences in fecundity plasticity were indicated by a significant effect of population. Statistical analyses were performed in PRIMER6+ and STATISTICA package10.
Results
Genetic diversity
A total of 61 mtCOI sequences, 29 from the upwelling and 32 from the estuarine population were aligned in a region of 508 bp (Genbank access code OQ877133-OQ877193). COI sequences showed a moderate to high diversity in both localities with 13 haplotypes in total. Particularly, a relatively higher diversity was revealed in the estuarine with respect to the upwelling population (details in Table 2), with the presence of 6 private haplotypes. In the upwelling population, we found 3 private haplotypes (Figure 1). The most abundant haplotypes were present in both populations, while the private haplotypes were less frequent (Figure 1A). However, the pairwise Φst comparison showed a considerable and significant genetic divergence between populations (Φst=0,414; p-value < 0.001). An alignment of 1460 bp to nuclear 18S was constructed with 19 sequences, 12 from the estuarine and 7 from the upwelling population (Genbank access code OQ875872-OQ875890). These sequences showed a high diversity in both populations with the presence of 12 haplotypes (Figure 1B). As with mtCOI, estuarine individuals showed a greater diversity than upwelling copepods (Table 2), with the presence of 8 haplotypes. No shared haplotypes were found, all being private haplotypes. However, a low and not significant genetic divergence was found between populations (Φst =-0.008; p-value= 0.374), likely related to high nucleotide differentiation (diversity) among estuarine individuals (π=0.133 and see detailed pairwise comparisons in Supplementary Table 1.
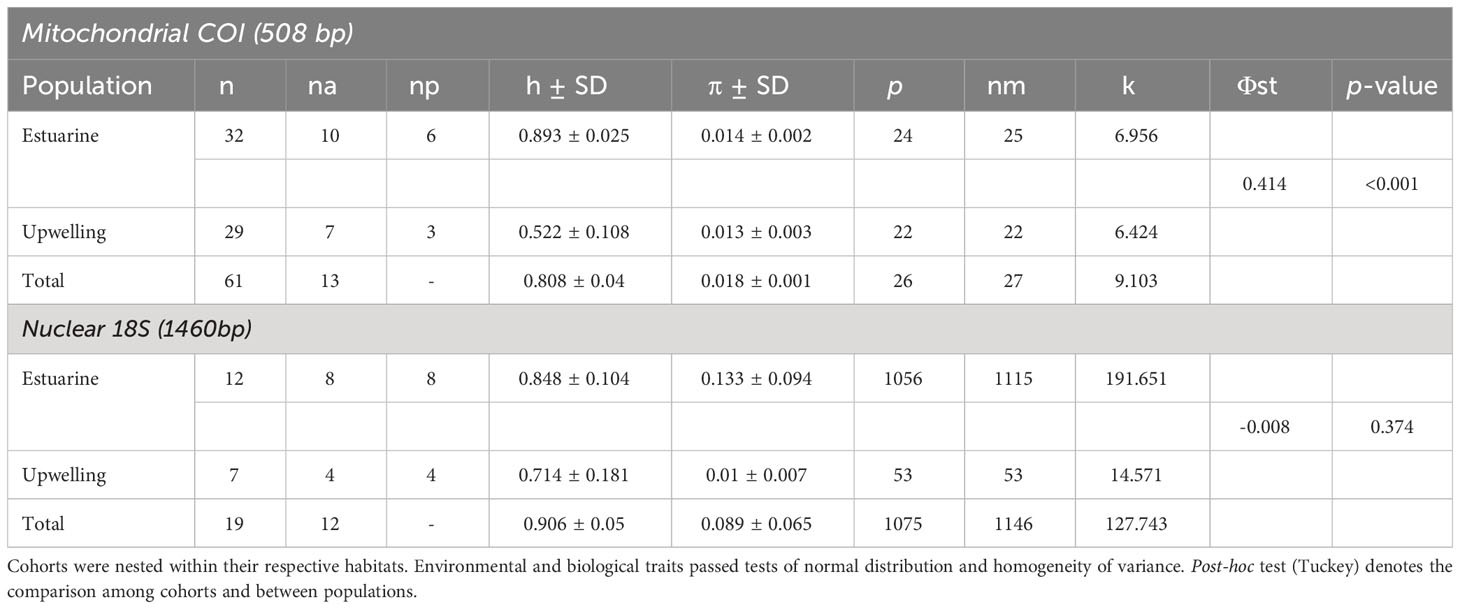
Table 2 Comparison of environmental drivers and copepod traits regarding two variability factors: cohorts (3 levels) and habitats (2 levels).
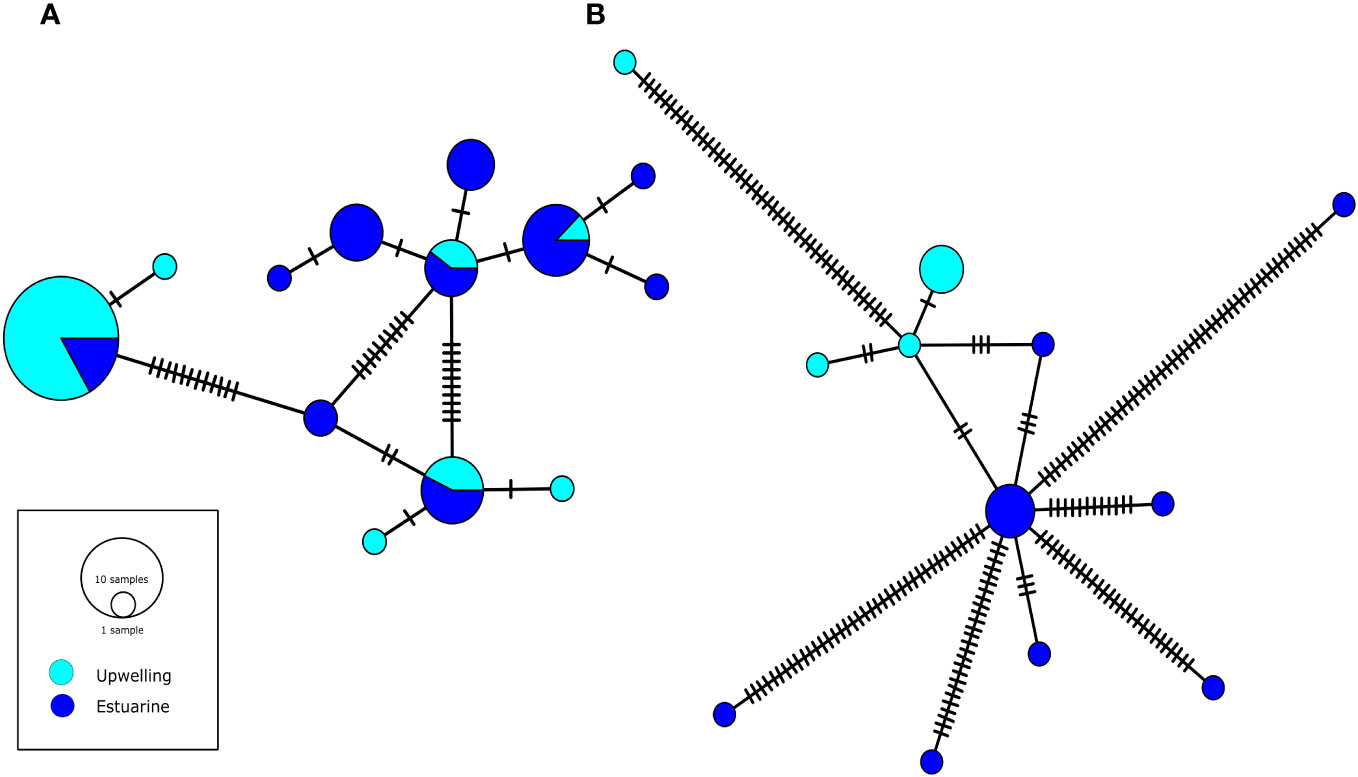
Figure 1 Minimum spanning network to A. tonsa populations from estuary (blue) and upwelling (cyan) populations with mtCOI (A) and nuclear 18S (B). Short lines between haplotypes represent mutational steps among them.
Distribution of drivers and copepod traits
There was a marked divergence between inter-daily temperature variation assessed for the estuarine (11.9–16.9°C) and upwelling (14.0–17.3°C) populations (Figures 2A, B). Temperatures below 13°C were significantly more prevalent (>70%) in the estuarine system (Table 3). Unimodal (13.1°C) distribution of temperature at the estuarine system contrasted with warmer (>15°C), unimodal distribution observed at the upwelling system. A clear divergence in salinity ranges and variability was evidenced between populations (Figures 2C, D). The highest salinity values (33.7 psu) experienced by copepods in the estuarine system were below the lower threshold (34.7 psu) of salinity variations experienced by upwelling copepods. Mean ( ± SD) pHT values, 7.94 ± 0.14 (estuary) and 7.92 ± 0.12 (upwelling), overlap between both coastal habitats (Figures 2E, F). Differences in mean pH conditions were not significant between A. tonsa populations (Table 3). The magnitude of extreme low pH events was similar for both populations (pHT <7.7), although the frequency of such events was three times higher (9.4%) for the estuarine than upwelling (3.6%) population. Food in the estuarine system was higher and less variable (190 ± 76 µg C L-1) than in the upwelling (175 ± 200 µg C L-1) site (Figures 2G, H), such that, there was a significant difference in the food conditions between systems. Mean (± SD) values of environmental conditions experienced by each cohort of both populations are in Supplementary Figure 2.
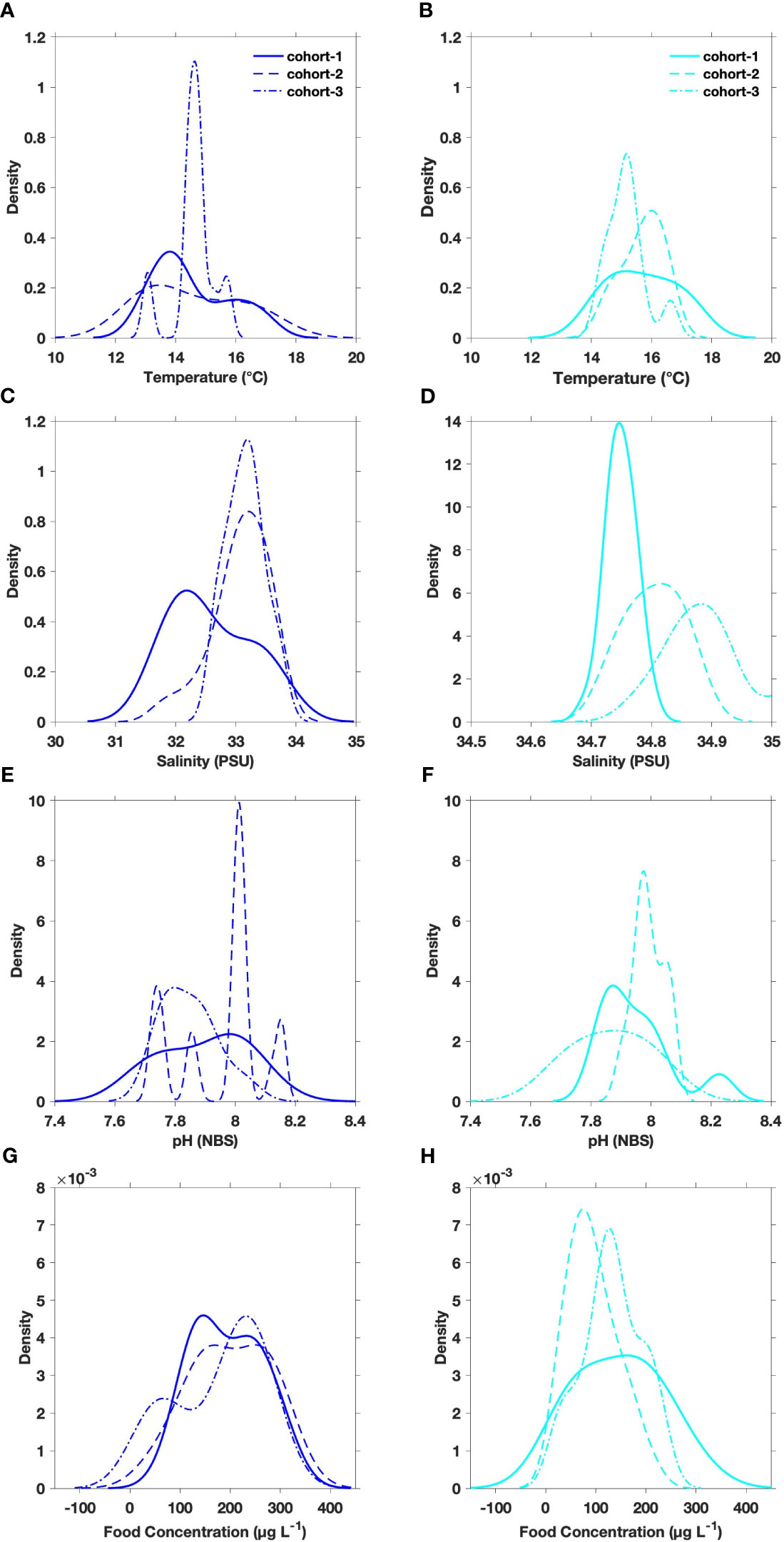
Figure 2 Density plots of (A, B) temperature, (C, D) salinity, (E, F) pHT and (G, H) food concentration assessed for three cohorts of the estuarine (blue) and upwelling (cyan) A. tonsa populations.
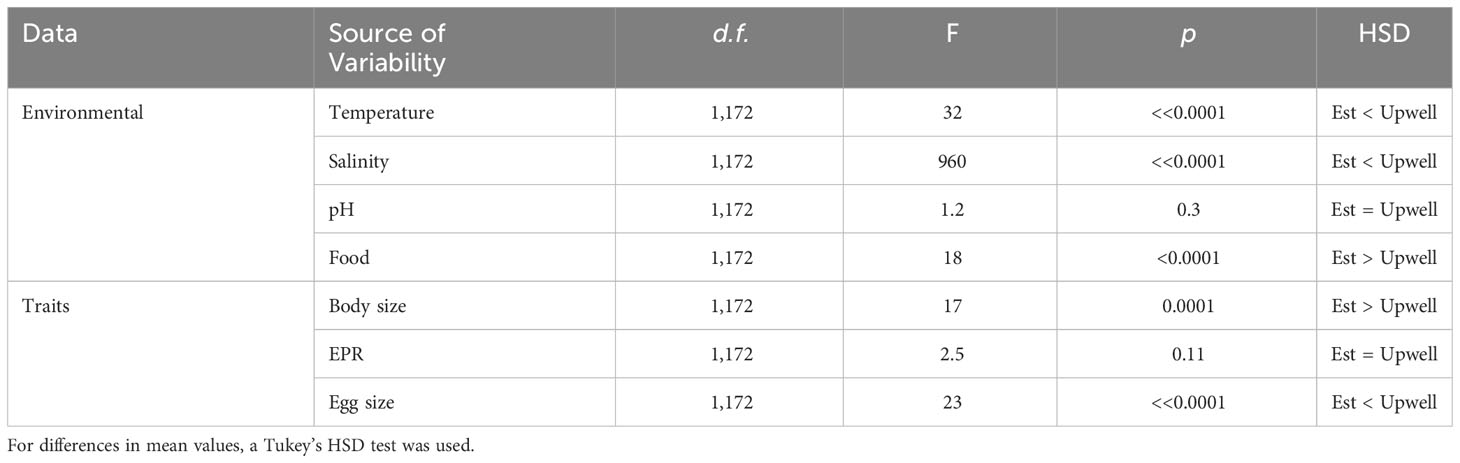
Table 3 Results of ANCOVA comparison of environmental drivers and copepod fecundity-related traits between examined estuarine and upwelling A. tonsa populations.
The distribution of female body size tended to overlap to a certain extent between populations (Figures 3A, B). The prevalence (>20%) of individuals larger than the mode (>1.1 mm) in estuarine females were significantly higher than upwelling congeners (p-value; Table 3). Within EPR ranges extended up to 70 egg ind-1 d-1, the mode in both systems were below 20 egg ind-1 d-1. Relatively smaller copepod females were found in the upwelling habitat, which produced larger brood-sizes (13 egg fem-1 d-1) than those produced by the largest females collected in the estuarine system (11 egg fem-1 d-1) (p-value; Figures 3C, D). There was a relationship between body size and fecundity (ANCOVA, p<0.05) in the estuarine population. Similar ranges of egg size (13–15 µm) were found in both populations (Figures 3E, F), though lower egg size values (<82 mm) were significantly more prevalent (>70%) in the estuarine population (Table 3).
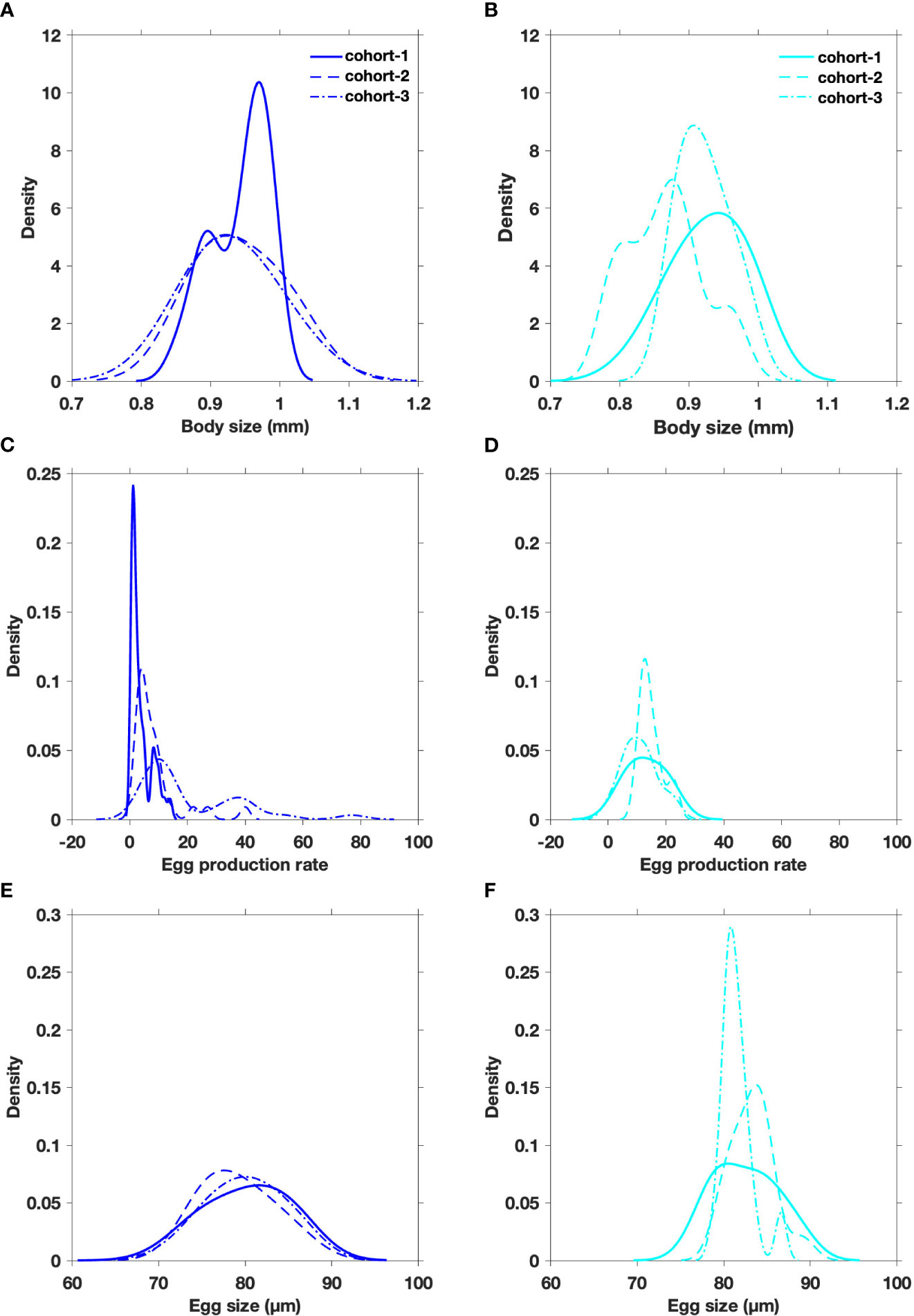
Figure 3 Density plots of body size (A, B), egg production rate (C, D), egg size (E, F) assessed for three cohorts of the estuarine (blue) and upwelling (cyan) A. tonsa populations.
There were population-specific patterns of phenotypic plasticity in fecundity to field pH conditions (Figure 4). Such pH variations explained 58% (estuary, y =-543 + 70*x; p<0.0001; r2 = 0.58) and 47% (upwelling, y=-259 + 34*x; p<0.0001; r2 = 0.47) of the EPR variability according to Pearson’s correlation coefficient, while student’s t-test0.05 indicate significant differences (p=0.002) between population EPR slopes. Accordingly, a positive non-zero EPR slope was observed in both populations with pH increasing (Figure 4), although the sensitivity (i.e., EPR change rate) of estuarine copepods was twice as high as that of the upwelling counterparts.
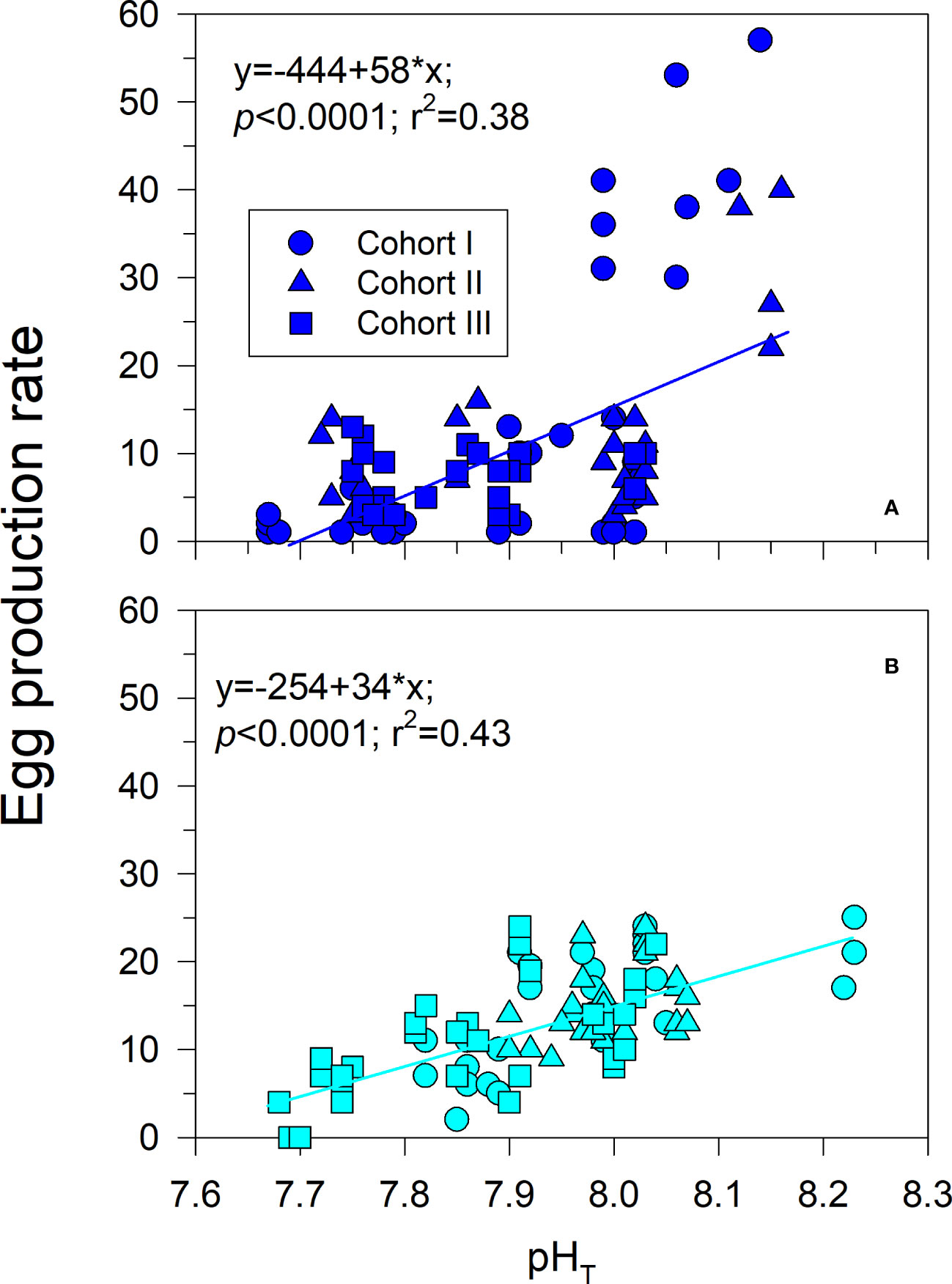
Figure 4 Phenotypic variations in EPR to field pH conditions manifested by three different cohorts of the estuarine (A) and upwelling (B) A. tonsa populations. Plastic responses were expressed as a mean reaction norm that had a significantly non-zero slope. The slope might indicate the relative EPR tolerance/sensitivity to low pH conditions.
Acclimation experiments
Relatively similar in magnitude, extreme low pH levels were three times more frequent in the estuarine than in the upwelling system. Natural extreme (pHT 7.68–7.78) and high (pHT 7.94–8.06) pH levels were replicated in three 96-h acclimation experiments involving females from both populations. There were significant differences in temperature (F1,24 = 1488, p=0.0001) and salinity (F1,24 = 249, p=0.0001) levels between experiments conducted with both populations (Supplementary Table 2). In addition, the temperature (F2, 24 = 11, p=0.0004) of experiment I and salinity (F2, 24 = 6, p=0.006) of experiment III with the estuarine population were significantly lower than other treatments. Both control and extreme pH scenarios were similar among the experiments for both populations (F1,24 = 1.1, p=0.3). The phenotypic plasticity in EPR to extreme pH conditions varied among the three different cohorts assessed for each A. tonsa population (Figure 5). The full linear mixed effects model indicates reproductive outcomes were significantly influenced by salinity, body size, pH-condition, population, and the interaction experiment*pH-condition*population (adj. R2 = 0.78) (Table 4). The effect of body size did not appear in a best-fitting model, while its adjusted R2 was 0.68.
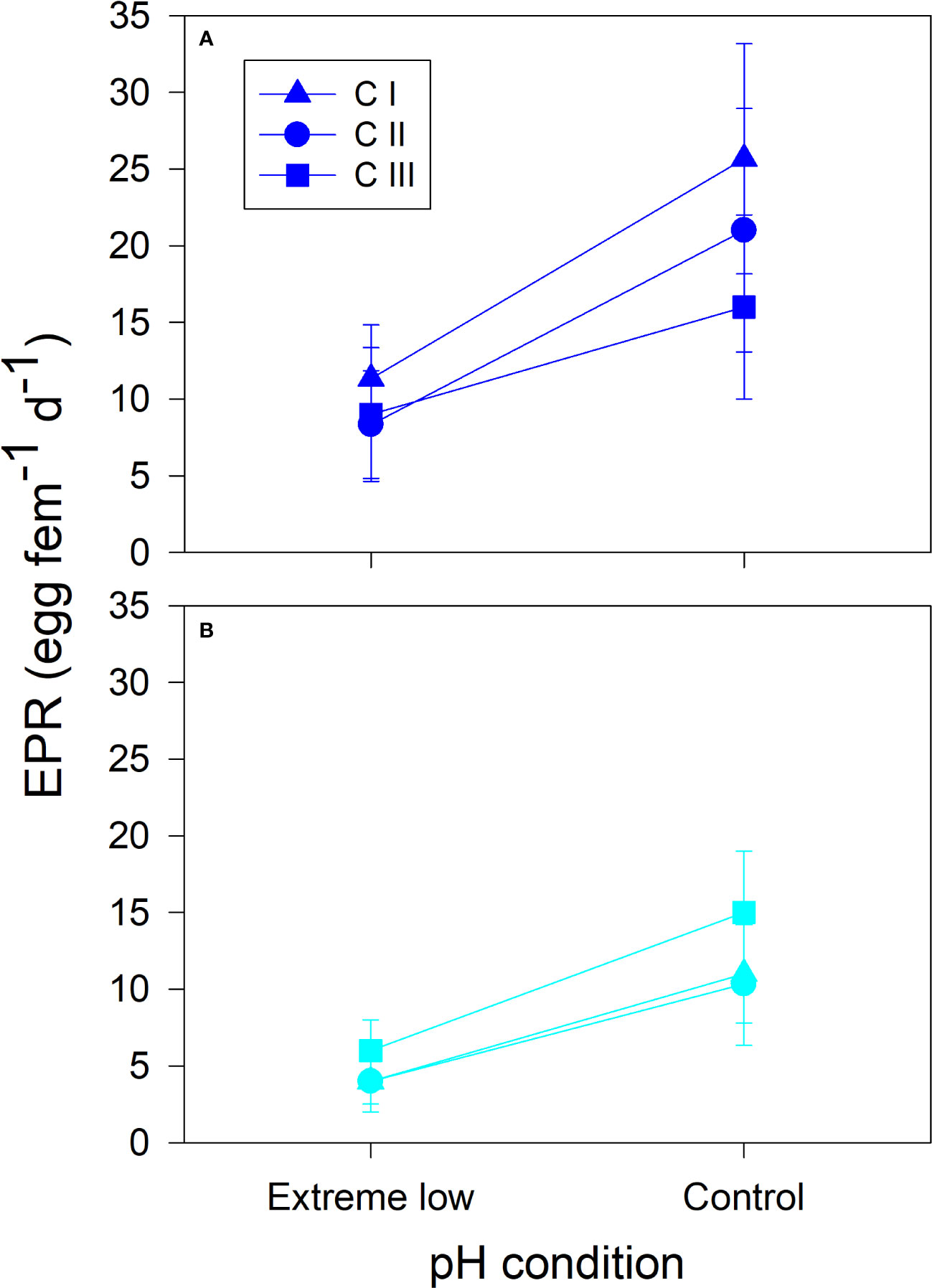
Figure 5 Phenotypic variation in egg production rate (EPR) as a function of mean ( ± SD) extreme low (7.66 ± 0.2) and Control (8.00 ± 0.02) pHT levels. Acclimation experiments were conducted with females belonging to three different cohorts (C I, C II and C III) of the estuarine (A) and upwelling (B) A. tonsa populations.
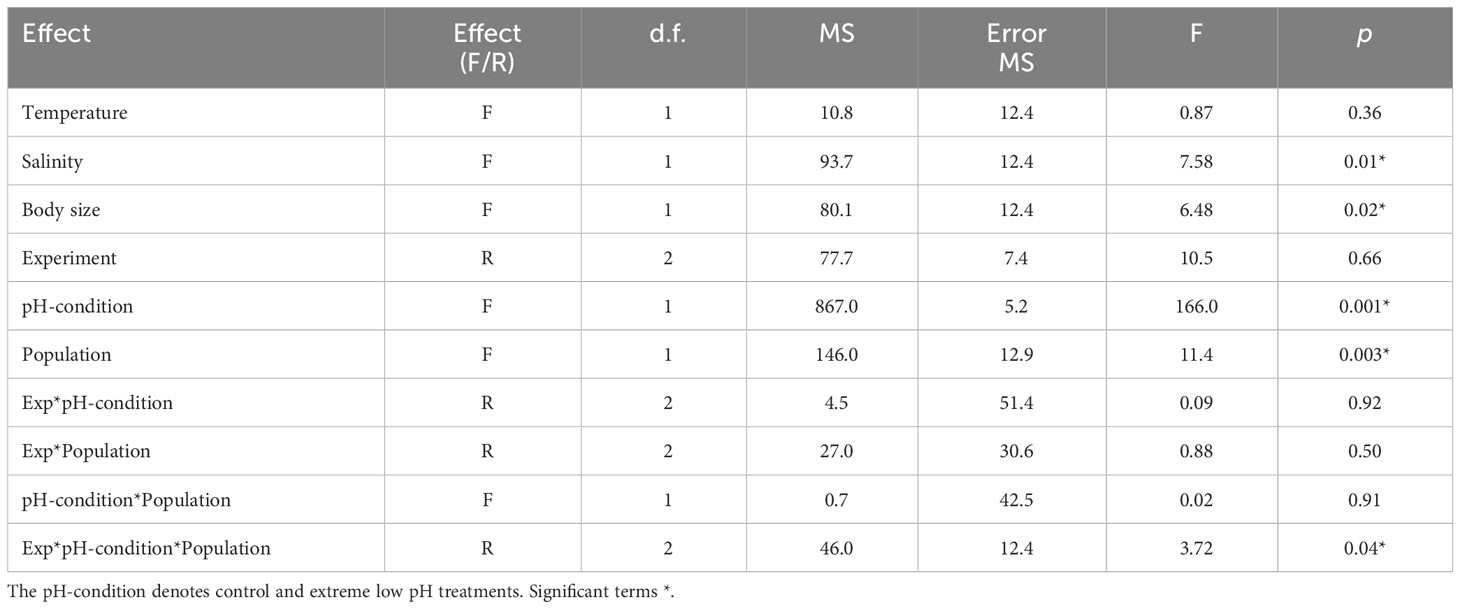
Table 4 Effect of fixed (F) and random (R) factors on EPR plasticity during acclimation experiments according to the linear mixed effects model (full model adj. R2 = 0.78).
Discussion
Extreme climate and biogeochemical events might represent a negative deviation from optimum environmental conditions for natural populations. Thus, such events are expected to influence microevolutionary processes, modulating phenotypic and genetic plasticity among populations (Porlier et al., 2012; Thor et al., 2018; Sasaki and Dam, 2020; Barley et al., 2021). Species distributed over a wide spatial range including highly heterogeneous habitats can be exposed to these microevolutionary processes. Interestingly, marine copepod populations can maintain gene flow despite distance and habitat heterogeneity, for example, through coastal currents (Chen and Hare, 2008). Therefore, in addition to being ecologically important in their own right, copepod species constitute valuable study models to explore mechanisms underpinning intra-specific differences in plasticity in response to local scale conditions. Our environmental characterization of contrasting coastal habitats indicates extreme low pH events were similar in magnitude though three times more frequent in the estuarine habitat. The local population was genetically more diverse and exhibited higher phenotypic variation across cohorts than the upwelling population.
Genetic diversity
The analysis of molecular diversity based on the mtCOI and nuclear 18S genes including the number of haplotypes and polymorphic sites, haplotype, and nucleotide diversity (Table 2), indicates the estuarine population was genetically more diverse and possibly older than that present in the upwelling habitat. The considerable level of genetic variation also suggests that both populations have not faced recent events of drastic population reductions (bottlenecks), which could be caused by strong selection pressure (Lloyd et al., 2016; Gurgel et al., 2020). Furthermore, the observed molecular divergence based on the mtCOI gene, and the absence of shared haplotypes to 18S between estuarine and upwelling populations, suggest genetic structuration and limited genetic connectivity between examined A. tonsa populations. Cryptic lineages have been observed in A. tonsa across latitudinal and environmental gradients (Caudill and Bucklin, 2004; Chen and Hare, 2008), sometimes coexisting in sympatry and manifesting variable phenotypic responses (i.e., reaction norms) to specific habitat drivers (Chen and Hare, 2011).
Distribution of drivers and copepod traits
Environmental data (Figure 2) well represented those environmental drivers, for example, temperature, salinity, and pH conditions, prevailing either seasonally during the reproductive period of the estuarine population (Aguilera et al., 2013; Garcés-Vargas et al., 2020; Osma et al., 2020) or daily and synoptic variations in the upwelling system due to a combination of solar heating and surface mixing (Kaplan et al., 2003; Piñones et al., 2007). Fluctuations in pH conditions, including extreme low events, in the assessed coastal habitats are related to river discharges (Aguilera et al., 2013; Pérez et al., 2016) or wind-driven upwelling (Torres et al., 2002; Vargas et al., 2017; Aguilera et al., 2020a), both characterized by cold water. The significant temperature-pHT relationship (Supplementary Figure 3) provides confidence that these events were well represented in the current study. However, we also acknowledge some potential caveats. At a mean temperature between 15–16°C, observed in both examined systems, A. tonsa individuals can complete its ontogenetic development within 17–18 days (Miller et al., 1977), with a mean life span around 35 days (Ceballos and Kiørboe, 2011; Kiørboe et al., 2015). This suggests our sampling approach might have largely underestimated high frequency (hours-day) variability affecting environmental pH in both coastal systems (see for example Hofmann et al., 2011; Carstensen and Duarte, 2019; Kapsenberg and Cyronak, 2019). Such environmental variability might be critical in shaping the phenotypic plasticity of short life cycle copepods (Gaitán-Espitia et al., 2017; Vargas et al., 2017). For example, day-night pH cycles have been observed in estuaries (Baumann and Smith, 2018) and upwelling (Saderne et al., 2013) ecosystems associated with photosynthesis/respiration balance. In this sense, assessed ranges of pHT in both coastal systems overlapped to a certain extent despite contrasting oceanographic, climatic, and geographic forcing operating in distant eco-geographic provinces (Vargas et al., 2017; Vargas et al., 2022). However, Pearson’s analysis indicated population-specific phenotypic responses to local pH variations (Figure 4) that might reflect physiological adequations to local scale pH conditions (Fitzer et al., 2012; Aguilera and Bednaršek, 2022). Contradictory results have been found with regards to pH effects (null, positive, and negative) on Acartia species through in situ (Hansen et al., 2019; Aguilera et al., 2020b) or laboratory observations by means of short (day-week) to long term (transgenerational) experiments (Langer et al., 2019; Dam et al., 2021). This emphasized that both approaches are needed to draw more comprehensive patterns in the response of copepod species and related biological communities to climate change (Reum et al., 2016). In the case of copepod physiological responses to low pH, such interaction can be modulated if ample and nutritious food is available (Aguilera et al., 2020b; Cominassi et al., 2020), such as that observed in both coastal habitats. Significant correlations between EPR and field pH conditions might also correspond to the effect of other simultaneous environmental drivers affecting copepods physiology, including changes in temperature (Sasaki and Dam, 2019; Dam et al., 2021) and food spectra (Kleppel and Burkart, 1995; Jónasdóttir et al., 2009). For example, river-discharges can introduce organic carbon and nutrients from different land use and the resultant physical-chemical conditions might influence the tolerance of marine organisms to environmental perturbations associated with climate change (Pérez et al., 2016). However, the concentration of nutrients and carbon in the Valdivia estuary are relatively low due to its low human intervention and greater vegetation coverage (Pérez et al., 2015).
Acclimation experiments
An additional characterization of EPR phenotypic variations was accomplished in this study through sequential acclimation experiments (e.g., Stillman, 2003), in which mean habitat conditions (temperature, salinity, pH and food condition) of each population were replicated. Temperature and salinity levels varied among experiments conducted with the estuarine population (Supplementary Table 2), likely affecting phenotypic outcomes of the local population. Since experimental temperature deviations (0.5°C) were still within the most prevalent thermal conditions in that habitat (Figure 2A), and salinity differences (0.2 psu) were well below changes able to impact the metabolic scope of these copepods (Calliari et al., 2008), observed experimental variations might have a relatively low influence on observed phenotypic responses. This is supported by results of the linear mixed effects model that indicated no significant effect of temperature on EPR variation (Table 4). However, body size, which is linked to habitat temperature (Miller et al., 1977), did influence EPR during acclimation experiments according to the full linear mixed effect model (Table 4). Multiple discrete cohorts inherently contain genetic information and manifest phenotypic plasticity in response to specific local environmental conditions (Sasaki and Dam, 2020). Differences in female age and related effects on fecundity (Rodríguez-Graña et al., 2010), not visually detectable during 96-h acclimation experiments, could have reduced the influence of body size on experimental EPR since this effect did not appear on a second best-fitting model.
During acclimation experiments, copepods from both populations were fed at similar food concentration (400 µg C L-1) but different composition. Estuarine A. tonsa females were fed unialgal Isochrysis sp. Diet, whereas upwelling copepods a mixed diet composed by Isochrysis sp. And Tetraselmis suecica (3:1 proportion). Subtle (< 30%) chemical (lipids, carbohydrates, and proteins) differences have been found between different Isochrysis and Tetraselmis strains (da Silva Gorgonio et al., 2013; Custódio et al., 2014). However, such differences seem to not lead to relevant changes in copepods reproduction (Lee et al., 2006), likely due to Isochrysis being the main source of nutritional compounds (Knuckey et al., 2005), which are highly required during invertebrates’ reproduction (Müller-Navarra et al., 2000). Acclimation experiments considered 96-h to acclimate copepod EPR to experimental conditions. Copepod ingestion might require at least 48 h to acclimate to laboratory non-limiting food conditions while digestive enzymes dampened short-term food variations (Mayzaud et al., 1992). Thus, acclimation to food (ingestion, digestion) might have occurred within the first 48–72 h of our experiment. Considering A. tonsa can convert ingested food into egg production within less than 10 h (Tester and Turner, 1990), it is highly possible our acclimation period might have been enough to evaluate copepods physiological outcomes (acclimation) during our experiment.
According to our GLM analysis, the population*pH-condition did not significantly affect EPR during acclimation experiments (Figure 5). Although observed phenotypic variation tended to be higher in the estuarine population in agreement with genetic diversity and plastic variations to field pH conditions, only a single cohort (CIII) of this population exhibited a significantly different performance during acclimation experiments. Environmental conditions observed during the sampling of the CIII in the estuarine habitat were significantly less variable than that affecting other cohorts of the local population (Supplementary Figure 2). The environmental conditions, involving mean values, fluctuations and extreme events, experienced seasonally across the development of the cohort can induce changes in the phenotypic plasticity and tolerance to environmental perturbations in Acartia species (Sasaki and Dam, 2019). Such a plasticity does not necessarily imply adaptive plasticity nor local adaptation. In both cases, transgenerational experiments are required to remove environmental influence on and elucidate adaptive plasticity and genetic components of phenotypic plasticity (Brennan et al., 2022). In order to assess the extent to which this phenotypic variation and plasticity are influenced by genetic components, potentially linked to local adaptation, a different experimental approach will be required using common garden conditions.
Conclusions
Distant and genetically structured A. tonsa populations are exposed to specific natural variability regimes, which also implies different extreme low pH conditions (high pCO2 conditions). Coincident with more frequent extreme low pH events in the estuarine system, the local population showed higher genetic and phenotypic variation. This was not consistent with acclimation experiments in which both populations showed similar phenotypic variation. It is still unclear if the observed pattern in phenotypic plasticity is determined by the level of genetic variation or the local environmental variability. Further long-term studies are needed to understand the effect of local extreme environmental variation (frequency, intensity and duration) modulating the phenotypic variation in natural populations.
Data availability statement
The datasets presented in this study can be found in online repositories. The names of the repository/repositories and accession number(s) can be found below: https://www.ncbi.nlm.nih.gov/genbank/, OQ875872-OQ8758, https://www.ncbi.nlm.nih.gov/genbank/, OQ877133-OQ877193. The datasets presented in this study can be found in PANGAEA: https://doi.pangaea.de/10.1594/PANGAEA.962723.
Ethics statement
The manuscript presents research on animals that do not require ethical approval for their study.
Author contributions
VA performed field campaigns and experiments; VA, FS, CV and AM analyzed and interpreted the data; all authors contributed to writing of the manuscript, contributed critically to the drafts and gave final approval for publication.
Funding
The author(s) declare financial support was received for the research, authorship, and/or publication of this article. VA was supported by the Chilean Scientific and Technologic Agency (ANID) through the Millennium Science Initiative Program–Millennium Institute of Oceanography (IMO) ICN12_019 and Proyecto ANILLOS ACT210071. CV was partially supported by FONDECYT, Chile grant N° 1210171 and from the Agencia Nacional de Investigación y Desarrollo (ANID)–Millennium Science Initiative Program–Millennium Institute of Oceanography (IMO) ICN12_019, the Coastal Socio-Ecological Millennium Institute (SECOS) ICN2019_015.
Acknowledgments
We would like to thank Mr. Mauricio Vegas, Mr. Miguel Barrientos, Mr. Jose Martel, and Mr. Boris Aqueveque for their help during field surveys and experiments. We would also like to thank Paulo and Borja Aguilera for their support.
Conflict of interest
The authors declare that the research was conducted in the absence of any commercial or financial relationships that could be construed as a potential conflict of interest.
Publisher’s note
All claims expressed in this article are solely those of the authors and do not necessarily represent those of their affiliated organizations, or those of the publisher, the editors and the reviewers. Any product that may be evaluated in this article, or claim that may be made by its manufacturer, is not guaranteed or endorsed by the publisher.
Supplementary material
The Supplementary Material for this article can be found online at: https://www.frontiersin.org/articles/10.3389/fmars.2023.1221132/full#supplementary-material
References
Aguilera V. M., Bednaršek N. (2022). Variations in phenotypic plasticity in a cosmopolitan copepod species across latitudinally contrasting climate and eco-geographic coastal provinces. Front. Ecol. Evol. 10. doi: 10.3389/fevo.2022.92564810
Aguilera V. M., Donoso K., Escribano R. (2011). Reproductive performance of small-sized dominant copepods with a highly variable food resource in the coastal upwelling system off the Chilean Humboldt Current. Mar. Biol. Res. 7 (3), 235–249. doi: 10.1080/17451000.2010.499437
Aguilera V. M., Vargas C. A., Dam H. G. (2020b). Antagonistic interplay between pH and food resources affects copepod traits and performance in a year-round upwelling system. Sci. Rep. 10 (1), 62. doi: 10.1038/s41598-019-56621-6
Aguilera V. M., Vargas C. A., Dewitte B. (2020a). Intraseasonal hydrographic variations and nearshore carbonates system off northern Chile during the 2015 El Niño event. J. Geophys. Res.: Biogeosci. 125 (11), e2020JG005704. doi: 10.1029/2020JG005704
Aguilera V. M., Vargas C. A., Lardies M. A., Poupin M. J. (2016). Adaptive variability to low-pH river discharges in Acartia tonsa and stress responses to high pCO2 conditions. Mar. Ecol. 37 (1), 215–226. doi: 10.1111/maec.12282
Aguilera V. M., Vargas C. A., Manríquez P. H., Navarro J. M., Duarte C. (2013). Low-pH freshwater discharges drive Spatial and Temporal Variations in Life History Traits of Neritic Copepod Acartia tonsa. Estuaries. Coasts. 36 (5), 1084–1092. doi: 10.1007/s12237-013-9615-2
Aguirre G. E., Capitanio F. L., Lovrich G. A., Esnal G. B. (2012). Seasonal variability of metazooplankton in coastal sub-Antarctic waters (Beagle Channel). Mar. Biol. Res. 8 (4), 341–353. doi: 10.1080/17451000.2011.627922
Aronés K., Ayón P., Hirche H. J., Schwamborn R. (2009). Hydrographic structure and zooplankton abundance and diversity off Paita, northern Peru (1994 to 2004)—ENSO effects, trends and changes. J. Mar. Syst. 78 (4), 582–598. doi: 10.1016/j.jmarsys.2009.01.002
Bandelt H., Forster P., Röhl A. (1999). Median-joining networks for inferring intraspecific phylogenies. Mol. Biol. Evol. 16 (1), 37–48. doi: 10.1093/oxfordjournals.molbev.a026036
Barley J. M., Cheng B. S., Sasaki M., Gignoux-Wolfsohn S., Hays C. G., Putnam A. B., et al. (2021). Limited plasticity in thermally tolerant ectotherm populations: evidence for a trade-off. Proc. R. Soc. B. 288 (1958), 20210765. doi: 10.1098/rspb.2021.0765
Baumann H., Smith E. M. (2018). Quantifying metabolically driven pH and oxygen fluctuations in US nearshore habitats at diel to interannual time scales. Estuaries. Coasts. 41 (4), 1102–1117. doi: 10.1007/s12237-017-0321-3
Bednaršek N., Ambrose R., Calosi P., Childers R. K., Feely R. A., Litvin S. Y., et al. (2021). Synthesis of thresholds of ocean acidification impacts on decapods. Front. Mar. Sci. 8, 651102. doi: 10.3389/fmars.2021.651102
Blanco-Bercial L., Bradford-Grieve J., Bucklin A. (2011). Molecular phylogeny of the calanoida (Crustacea: Copepoda). Mol. Phylogenet. Evol. 59 (1), 103–113. doi: 10.1016/j.ympev.2011.01.008
Brennan R. S., DeMayo J. A., Dam H. G., Finiguerra M. B., Baumann H., Pespeni M. H. (2022). Loss of transcriptional plasticity but sustained adaptive capacity after adaptation to global change conditions in a marine copepod. Nat. Commun. 13 (1), 1147. doi: 10.1038/s41467-022-28742-6
Bucklin A., Frost B., Bradford-Grieve J., Allen L., Copley N. (2003). Molecular systematic and phylogenetic assessment of 34 calanoid copepod species of the Calanidae and Clausocalanidae. Mar. Biol. 142 (2), 333–343. doi: 10.1007/s00227-002-0943-1
Bucklin A., Guarnieri M., Hill R. S., Bentley A. M., Kaartvedt S. (1999). “Taxonomic and systematic assessment of planktonic copepods using mitochondrial COI sequence variation and competitive, species-specific PCR. In Molecular Ecology of Aquatic Communities,” in Developments in Hydrobiology. Eds. Zehr J. P., Voytek M. A. (Dordrecht: Springer Netherlands), 239–254. doi: 10.1007/978-94-011-4201-4_18
Bucklin A., Ortman B. D., Jennings R. M., Nigro L. M., Sweetman C. J., Copley N. J., et al. (2010). A ‘Rosetta Stone’ for metazoan zooplankton: DNA barcode analysis of species diversity of the Sargasso Sea (Northwest Atlantic Ocean). Deep. Sea. Res. Part II.: Topical. Stud. Oceanogr. Species. Diversity Mar. Zooplankton. 57 (24), 2234–2247. doi: 10.1016/j.dsr2.2010.09.025
Burger F. A., Frölicher T. L. (2023). Drivers of surface ocean acidity extremes in an Earth1system model. Global Biogeochem. Cycles 37 (9). doi: 10.1029/2023GB007785
Burger F. A., John J. G., Frölicher T. L. (2020). Increase in ocean acidity variability and extremes under increasing atmospheric CO 2. Biogeosciences 17 (18), 4633–4662. doi: 10.5194/bg-17-4633-2020
Cabré A., Marinov I., Bernardello R., Bianchi D. (2015). Oxygen minimum zones in the tropical Pacific across CMIP5 models: mean state differences and climate change trends. Biogeosciences 12 (18), 5429–5454. doi: 10.5194/bg-12-5429-2015
Calliari D., Andersen-Borg M., Thor P., Gorokhova E., Tiselius P. (2008). Instantaneous salinity reductions affect the survival and feeding rates of the co-occurring copepods Acartia tonsa Dana and A. clausi Giesbrecht differently. J. Exp. Mar. Biol. Ecol. 362, 18–25. doi: 10.1016/j.jembe.2008.05.005
Calosi P., Rastrick S. P. S., Graziano M., Thomas S. C., Baggini C., Carter H. A., et al. (2013). Distribution of sea urchins living near shallow water CO2 vents is dependent upon species acid–base and ion-regulatory abilities. Mar. pollut. Bull. 73 (2), 470–484. doi: 10.1016/j.marpolbul.2012.11.040
Camus P. A. (2001). Biogeografía marina de Chile continental. Rev. Chil. Hist. Natural 74 (3), 587–617. doi: 10.4067/S0716-078X2001000300008
Carstensen J., Duarte C. M. (2019). Drivers of pH variability in coastal ecosystems. Environ. Sci. Technol. 53 (8), 4020–4029. doi: 10.1021/acs.est.8b03655
Caudill C. C., Bucklin A. (2004). Molecular phylogeography and evolutionary history of the estuarine copepod, Acartia tonsa, on the Northwest Atlantic Coast. Hydrobiologia 511 (1), 91–102. doi: 10.1023/B:HYDR.0000014032.05680.9d
Ceballos S., Kiørboe T. (2011). Senescence and sexual selection in a pelagic copepod. PloS One 6 (4), e18870. doi: 10.1371/journal.pone.0018870
Chaalali A., Beaugrand G., Raybaud V., Goberville E., David V., Boët P., et al. (2013). Climatic facilitation of the colonization of an estuary by Acartia tonsa. PloS One 8 (9), e74531. doi: 10.1371/journal.pone.0074531
Chen G., Hare M. P. (2008). Cryptic ecological diversification of a planktonic estuarine copepod, Acartia tonsa. Mol. Ecol. 17 (6), 1451–1468. doi: 10.1111/j.1365-294X.2007.03657.x
Chen G., Hare M. P. (2011). Cryptic diversity and comparative phylogeography of the estuarine copepod Acartia tonsa on the US Atlantic coast. Mol. Ecol. 20 (11), 2425–2441. doi: 10.1111/j.1365-294X.2011.05079.x
Cominassi L., Moyano M., Claireaux G., Howald S., Mark F. C., Zambonino-Infante J. L., et al. (2020). Food availability modulates the combined effects of ocean acidification and warming on fish growth. Sci. Rep. 10 (1), 1–12. doi: 10.1038/s41598-020-58846-2
Cornils A., Blanco-Bercial L. (2013). Phylogeny of the paracalanid15iesbrechtcht 1888 (Crustacea: copepoda: calanoida). Mol. Phylogenet. Evol. 69 (3), 861–872. doi: 10.1016/j.ympev.2013.06.018
Curra-Sánchez E. D., Lara C., Cornej’-D’Ottone M., Nimptsch J., Aguayo M., Broitman B. R., et al. (2022). Contrasting land-uses in two small river basins impact the colored dissolved organic matter concentration and carbonate system along a river-coastal ocean continuum. Sci. Total. Environ. 806, 150435. doi: 10.1016/j.scitotenv.2021.150435
Custódio L., Soares F., Pereira H., Barreira L., Vietto-Duarte C., João M., et al. (2014). Fatty Acid Composition and Biological Activities of Isochrysis galbana T-ISO, Tetraselmis sp. and Scenedesmus sp.: Possible Application in the pharmaceutical and functional food industries. J. Appl. Phycol. 26 (1), 151–161. doi: 10.1007/s10811-013-0098-0
Dam H. G., deMayo J. A., Park G., Norton L., He X., Finiguerra M. B., et al. (2021). Rapid, but limited, zooplankton adaptation to simultaneous warming and acidification. Nat. Climate Change 11 (9), 780–786. doi: 10.1101/2021.04.07.438881
da Silva Gorgonio C. M., Aranda D. A. G., Couri S. (2013). Morphological and chemical aspects of Chlorella pyrenoidosa, Dunaliella tertiolecta, Isochrysis galbana and Tetraselmis gracilis microalgae. Natural Sci. 5, 783–791. doi: 10.4236/ns.2013.57094
Dickson A. G., Millero F. J. (1987). A comparison of the equilibrium constants for the dissociation of carbonic acid in seawater media. Deep. Sea. Res. Part A. Oceanographic. Res. Papers. 34 (10), 1733–1743. doi: 10.1016/0198-0149(87)90021-5
Diffenbaugh N. S., Pal J. S., Trapp R. J., Giorgi F. (2005). Fine-scale processes regulate the response of extreme events to global climate change. Proc. Natl. Acad. Sci. 102 (44), 15774–15778. doi: 10.1073/pnas.0506042102
Edgar R. C. (2004). MUSCLE: a multiple sequence alignment method with reduced time and space complexity. BMC Bioinf. 5, 113. doi: 10.1186/1471-2105-5-113
Escribano R., Fernández M., Aranís A. (2003). Physical-chemical processes and patterns of diversity of the Chilean eastern boundary pelagic and benthic marine ecosystems: an overview. Gayana 67 (2), 190–205. doi: 10.4067/S0717-65382003000200008
Escribano R., Hidalgo P., Krautz C. (2009). Zooplankton associated with the oxygen minimum zone system in the northern upwelling region of Chile during March 2000. Deep. Sea. Res. Part II.: Topical. Stud. Oceanogr. 56 (16), 1083–1094.
Excoffier L., Lischer H. E. (2010). Arlequin suite ver 3.5: a new series of programs to perform population genetics analyses under Linux and Windows. Mol. Ecol. Resour. 10 (3), 564–567. doi: 10.1111/j.1755-0998.2010.02847.x
Feely R. A., Sabine C. L., Hernandez-Ayon J. M., Ianson D., Hales B. (2008). Evidence for upwelling of corrosive acidified water onto the continental shelf. Science 320 (5882), 1490–1492. doi: 10.1126/science.1155676
Fitzer S. C., Caldwell G. S., Close A. J., Clare A. S., Upstill-Goddard R. C., Bentley M. G. (2012). Ocean acidification induces multi-generational decline in copepod naupliar production with possible conflict for reproductive resource allocation. J. Exp. Mar. Biol. Ecol. 418, 30–36. doi: 10.1016/j.jembe.2012.03.009
Folmer O., Black M., Hoeh W., Lutz R., Vrijenhoek R. (1994). DNA primers for amplification of mitochondrial cytochrome c oxidase subunit I from diverse metazoan invertebrates. Mol. Mar. Biol. Biotechnol. 3 (5), 294–299.
Gaitán-Espitia J. D., Marshall D., Dupont S., Bacigalupe L. D., Bodrossy L., Hobday A. J. (2017). Geographical gradients in selection can reveal genetic constraints for evolutionary responses to ocean acidification. Biol. Lett. 13 (2), 20160784. doi: 10.1098/rsbl.2016.0784
Garcés-Vargas J., Schneider W., Pinochet A., Piñones A., Olguin F., Brieva D., et al. (2020). Tidally forced saltwater intrusions might impact the quality of drinking water, the Valdivia River (40° S), Chile Estuary Case. Water 12 (9), 2387. doi: 10.3390/w12092387
Gruber N., Boyd P. W., Frölicher T. L., Vogt M. (2021). Biogeochemical extremes and compound events in the ocean. Nature 600 (7889), 395–407. doi: 10.3929/ethz-b-000521508
Gurgel C. F. D., Camacho O., Minne A. J., Wernberg T., Coleman M. A. (2020). Marine heatwave drives cryptic loss of genetic diversity in underwater forests. Curr. Biol. 30 (7), 1199–1206. doi: 10.1016/j.cub.2020.01.051
Hansen B. W., Andersen C. M., Hansen P. J., Nielsen T. G., Vismann B., Tiselius P. (2019). In situ and experimental evidence for effects of elevated pH on protistan and metazoan grazers. J. Plankton. Res. 41 (3), 257–271. doi: 10.1093/plankt/fbz020
Haraldsson C., Anderson L. G., Hassellöv M., Hulth S., Olsson K. (1997). Rapid, high-precision potentiometric titration of alkalinity in ocean and sediment pore waters. Deep. Sea. Res. Part I.: Oceanographic. Res. Papers. 44 (12), 2031–2044. doi: 10.1016/S0967-0637(97)00088-5
Hartley A. J., Chong G., Houston J., Mather A. E. (2005). 150 million years of climatic stability: evidence from the Atacama Desert, northern Chile. J. Geological. Soc. 162 (3), 421–424. doi: 10.1144/0016-764904-07
Haye P. A., Segovia N. I., Muñoz-Herrera N. C., Gálvez F. E., Martínez A., Meynard A., et al. (2014). Phylogeographic structure in benthic marine invertebrates of the southeast Pacific coast of Chile with differing dispersal potential. PloS One 9 (2), e88613. doi: 10.1371/journal.pone.0088613
Hebert P. D. N., Ratnasingham S., deWaard J. R. (2003b). Barcoding animal life: cytochrome c oxidase subunit 1 divergences among closely related species. Proc. R. Soc Lond. B. 270, S96–S99. doi: 10.1098/rsbl.2003.0025
Hofmann G. E., Smith J. E., Johnson K. S., Send U., Levin L. A., Micheli F., et al. (2011). High-frequency dynamics of ocean pH: a multi-ecosystem comparison. PloS One 6 (12), e28983. doi: 10.1371/journal.pone.0028983
Jónasdóttir S. H., Visser A. W., Jespersen C. (2009). Assessing the role of food quality in the production and hatching of Temora longicornis eggs. Mar. Ecol. Prog. Ser. 382, 139–150. doi: 10.3354/meps07985
Kaplan D. M., Largier J. L., Navarrete S., Guiñez R., Castilla J. C. (2003). Large diurnal temperature fluctuations in the nearshore water column. Estuarine. Coast. Shelf. Sci. 57 (3), 385–398. doi: 10.1016/S0272-7714(02)00363-3
Kapsenberg L., Cyronak T. (2019). Ocean acidification refugia in variable environments. Global Change Biol. 25 (10), 3201–3214. doi: 10.1111/gcb.14730
Kelly M. W., Hofmann G. E. (2013). Adaptation and the physiology of ocean acidification. Funct. Ecol. 27 (4), 980–990. doi: 10.1111/j.1365-2435.2012.02061.x
Kiørboe T., Ceballos S., Thygesen U. H. (2015). Interrelations between senescence, life-history traits, and behavior in planktonic copepods. Ecology 96 (8), 2225–2235. doi: 10.1890/14-2205.1
Kleppel G. S., Burkart C. A. (1995). Egg production and the nutritional environment of Acartia tonsa: the role of food quality in copepod nutrition. ICES. J. Mar. Sci. 52 (3-4), 297–304. doi: 10.1016/1054-3139(95)80045-X
Knuckey R. M., Semmens G. L., Mayer R. J., Rimmer M. A. (2005). Development of an optimal microalgal diet for the culture of the calanoid copepod Acartia sinjiensis: effect of algal species and feed concentration on copepod development. Aquaculture 249 (1-4), 339–351. doi: 10.1016/j.aquaculture.2005.02.053
Langer J. A., Meunier C. L., Ecker U., Horn H. G., Schwenk K., Boersma M. (2019). Acclimation and adaptation of the coastal calanoid copepod Acartia tonsa to ocean acidification: a long-term laboratory investigation. Mar. Ecol. Prog. Ser. 619, 35–51. doi: 10.3354/meps12950
Lee K. W., Park H. G., Lee S. M., Kang H. K. (2006). Effects of diets on the growth of the brackish water cyclopoid copepod Paracyclopina nana Smirnov. Aquaculture 256 (1-4), 346–353. doi: 10.1016/j.aquaculture.2006.01.015
Leigh J. W., Bryant D. (2015). PopART: full-feature software for haplotype network construction. Methods Ecol. Evol. 6, 1110–1116. doi: 10.1111/2041-210X.12410
Lloyd M. M., Makukhov A. D., Pespeni M. H. (2016). Loss of genetic diversity as a consequence of selection in response to high pCO2. Evol. Appl. 9 (9), 1124–1132. doi: 10.1111/eva.12404
Mayzaud P., Roche-Mayzaud O., Razouls S. (1992). Medium term time acclimation of feeding and digestive enzyme activity in marine copepods: influence of food concentration and copepod species. Mar. Ecol. Prog. Ser. 89, 197–212. doi: 10.3354/meps089197
Mehrbach C., Culberson C. H., Hawley J. E., Pytkowicx R. M. (1973). Measurement of the apparent dissociation constants of carbonic acid in seawater at atmospheric pressure 1. Limnol. Oceanogr. 18 (6), 897–907. doi: 10.4319/lo.1973.18.6.0897
Miller C. B., Johnson J. K., Heinle D. R. (1977). Growth rules in the marine copepod genus Acartia. Limnol. Oceanogr. 22 (2), 326–335. doi: 10.4319/lo.1977.22.2.0326
Moksness E., Fossum P. (1992). Daily growth rate and hatching-date distribution of Norwegian spring-spawning herring (Clupea harengus L.). ICES. J. Mar. Sci. 49 (2), 217–221. doi: 10.1093/icesjms/49.2.217
Moriyama E. N., Powell J. R. (1997). Codon usage bias and tRNA abundance in Drosophila. J. Mol. Evol. 45 (5), 514–523. doi: 10.1007/PL00006256
Müller-Navarra D. C., Brett M. T., Liston A. M., Goldman C. R. (2000). A highly unsaturated fatty acid predicts carbon transfer between primary producers and consumers. Nature 403 (6765), 74–77. doi: 10.1038/47469
Osma N., Latorre-Melín L., Jacob B., Contreras P. Y., von Dassow P., Vargas C. A. (2020). Response of phytoplankton assemblages from naturally acidic coastal ecosystems to elevated pCO2. Front. Mar. Sci. 323. doi: 10.3389/fmars.2020.00323
Pérez C. A., DeGrandpre M. D., Lagos N. A., Saldías G. S., Cascales E. K., Vargas C. A. (2015). Influence of climate and land use in carbon biogeochemistry in lower reaches of rivers in central southern Chile: Implications for the carbonate system in river-influenced rocky shore environments. J. Geophys. Res.: Biogeosci. 120 (4), 673–692. doi: 10.1002/2014JG002699
Pérez C. A., Lagos N. A., Saldías G. S., Waldbusser G., Vargas C. A. (2016). Riverine discharges impact physiological traits and carbon sources for shell carbonate in the marine intertidal mussel Perumytilus purpuratus. Limnol. Oceanogr. 61 (3), 969–983. doi: 10.1002/lno.10265
Pierrot D., Epitalon J.-M., Orr J. C., Lewis E., Wallace D. W. R. (2021) MS Excel program developed for CO2 system calculations – version 3.0, GitHub repository. Available at: https://github.com/dpierrot/co2sys_xl.
Pino M. Q., Perillo G. M. E., Santamarina P. (1994). Residual fluxes in a cross-section of the Valdivia River estuary, Chile. Estuarine. Coast. Shelf. Sci. 38 (5), 491–505. doi: 10.1006/ecss.1994.1034
Piñones A., Castilla J. C., Guiñez R., Largier J. L. (2007). Nearshore surface temperatures in Antofagasta Bay (Chile) and adjacent upwelling centers. Cienc. Marinas. 33 (1), 37–48. doi: 10.7773/cm.v33i1.1226
Porlier M., Charmantier A., Bourgault P., Perret P., Blondel J., Garant D. (2012). Variation in phenotypic plasticity and selection patterns in blue tit breeding time: between-and within-population comparisons. J. Anim. Ecol. 81 (5), 1041–1051. doi: 10.1111/j.1365-2656.2012.01996.x
Poulet S. A., Ianora A., Laabir M., Breteler W. K. (1995). Towards the measurement of secondary production and recruitment in copepods. ICES. J. Mar. Sci. 52 (3-4), 359–368. doi: 10.1016/1054-3139(95)80051-4
Raupach M. J., Mayer C., Malyutina M., Wägele J. W. (2009). Multiple origins of deep-sea Asellota (Crustacea: Isopoda) from shallow waters revealed by molecular data. Proceedings. Biol. Sci. 276 (1658), 799–808. doi: 10.1098/rspb.2008.1063
Reum J. C., Alin S. R., Harvey C. J., Bednaršek N., Evans W., Feely R. A., et al. (2016). Interpretation and design of ocean acidification experiments in upwelling systems in the context of carbonate chemistry co-variation wiemperaturaure and oxygen. ICES. J. Mar. Sci. 73 (3), 582–595. doi: 10.1093/icesjms/fsu231
Riquelme-Bugueño R., Pérez-Santos I., Alegría N., Vargas C. A., Urbina M. A., Escribano R. (2020). Diel vertical migration into anoxic and high-pCO2 waters: acoustic and net-based krill observations in the Humboldt Current. Sci. Rep. 10 (1), 1–11. doi: 10.1038/s41598-020-73702-z
Rodríguez-Graña L., Calliari D., Tiselius P., Hansen B. W., Sköld H. N. (2010). Gender-specific ageing and non-Mendelian inheritance of oxidative damage in marine copepods. Mar. Ecol. Prog. Ser. 401, 1–13. doi: 10.3354/meps08459
Rozas J., Ferrer-Mata A., Sánchez-DelBarrio J. C., Guirao-Rico S., Librado P., Ramos-Onsins S. E., et al. (2017). DnaSP 6: DNA sequence polymorphism analysis of large data sets. Mol. Biol. Evol. 34 (12), 3299–3302. doi: 10.1093/molbev/msx248
Ruz P. M., Hidalgo P., Yáñez S., Escribano R., Keister J. E. (2015). Egg production and hatching success of Calanus Chilensis and Acartia tonsa in the northern Chile upwelling zone (23° S), Humboldt Current System. J. Mar. Syst. 148, 200–212. doi: 10.1016/j.jmarsys.2015.03.007
Saderne V., Fietzek P., Herman P. M. J. (2013). Extreme variations of pCO2 and pH in a macrophyte meadow of the Baltic Sea in summer: evidence of the effect of photosynthesis and local upwelling. PloS One 8 (4), e62689. doi: 10.1371/journal.pone.0062689
Sasaki M. C., Dam H. G. (2019). Integrating patterns of thermal tolerance and phenotypic plasticity with population genetics to improve understanding of vulnerability to warming in a widespread copepod. Global Change Biol. 25 (12), 4147–4164. doi: 10.1111/gcb.14811
Sasaki M. C., Dam H. G. (2020). Genetic differentiation underlies seasonal variation in thermal tolerance, body size, and plasticity in a short-lived copepod. Ecol. Evol. 10 (21), 12200–12210. doi: 10.1002/ece3.6851
Sokal R. R., Rohlf F. J. (1995). Biometry. The principles and practice of statistics in biological research. 3rd edn (New York: W. H. Freeman).
Spisla C., Taucher J., Bach L. T., Haunost M., Boxhammer T., King A. L., et al. (2021). Extreme levels of ocean acidification restructure the plankton community and biogeochemistry of a temperate coastal ecosystem: a mesocosm study. Front. Mar. Sci. 7, 611157. doi: 10.3389/fmars.2020.611157
Stearns S. C. (1992). The evolution of life histories (No. 575 S81) (Oxford: Oxford university press).
Stillman J. H. (2003). Acclimation capacity underlies susceptibility to climate change. Science 301 (5629), 65–65. doi: 10.1126/science.1083073
Strickland J. D. H., Parsons T. R. (1972). A Practical Handbook of Seawater Analysis (Ottawa: Fisheries Research Board of Canada).
Sydeman W. J., García-Reyes M., Schoeman D. S., Rykaczewski R. R., Thompson S. A., Black B. A., et al. (2014). Climate change and wind intensification in coastal upwelling ecosystems. Science 345 (6192), 77–80. doi: 10.1126/science.1251635
Tang K. W., Dam H. G., Feinberg L. R. (1998). The relative importance of egg production rate, hatching success, hatching duration and egg sinking in population recruitment of two species of marine copepods. J. Plankton. Res. 20 (10), 1971–1987. doi: 10.1093/plankt/20.10.1971
Tester P. A., Turner J. T. (1990). How long does it take copepods to make eggs? J. Exp. Mar. Biol. Ecol. 141 (2-3), 169–182. doi: 10.1016/0022-0981(90)90222-X
Thompson A. M., Durbin E. G., Durbin A. G. (1994). Seasonal changes in maximum ingestion rate of Acartia tonsa in Narragansett Bay, Rhode Island, USA. Mar. Ecol. Prog. Ser. 108, 91–105. doi: 10.3354/meps108091
Thor P., Bailey A., Dupont S., Calosi P., Søreide J. E., De Wit P., et al. (2018). Contrasting physiological responses to future ocean acidification among Arctic copepod populations. Global Change Biol. 24 (1), e365–e377. doi: 10.1111/gcb.13870
Thorpe R. S., Reardon J. T., Malhotra A. (2005). Common garden and natural selection experiments support ecotypic differentiation in the Dominican anole (Anolis oculatus). Am. Nat. 165 (4), 495–504. doi: 10.1086/428408
Torres R., Turner D., Rutllant J., Sobarzo M., Antezana T., Gonzalez H. E. (2002). CO2 outgassing off central Chile (31–30 S) and northern Chile (24–23 S) during austral summer 1997: The effect of wind intensity on the upwelling and ventilation of CO2-rich waters. Deep. Sea. Res. Part I.: Oceanographic. Res. Papers. 49 (8), 1413–1429. doi: 10.1016/S0967-0637(02)00034-1
Vargas C. A., Araneda S. E., Valenzuela G. (2003). Influence of tidal phase and circulation on larval fish distribution in a partially mixed estuary, Corral Bay, Chile. J. Mar. Biol. Assoc. United. Kingdom. 83 (1), 217–222. doi: 10.1017/S0025315403006994h
Vargas C. A., Contreras P. Y., Pérez C. A., Sobarzo M., Saldías G. S., Salisbury J. (2016). Influences of riverine and upwelling waters on the coastal carbonate system off Central Chile and their ocean acidification implications. J. Geophys. Res.: Biogeosci. 121 (6), 1468–1483. doi: 10.1002/2015JG003213
Vargas C. A., Cuevas L. A., Broitman B. R., San Martin V. A., Lagos N. A., Gaitán-Espitia J. D., et al. (2022). Upper environmental pCO2 drives sensitivity to ocean acidification in marine invertebrates. Nat. Climate Change 12 (2), 200–207. doi: 10.1038/s41558-021-01269-2
Vargas C. A., González H. E. (2004). Plankton community structure and carbon cycling in a coastal upwelling system. I. Bacteria, microprotozoans and phytoplankton in the diet of copepods and appendicularians. Aquat. Microbial. Ecol. 34 (2), 151–164. doi: 10.3354/ame034151
Vargas C. A., Lagos N. A., Lardies M. A., Duarte C., Manríquez P. H., Aguilera V. M., et al. (2017). Species-specific responses to ocean acidification should account for local adaptation and adaptive plasticity. Nat. Ecol. Evol. 1 (4), 1–7. doi: 10.1038/s41559-017-0084
Vehmaa A., Brutemark A., Engström-Öst J. (2012). Maternal effects may act as an adaptation mechanism for copepods facing pH and temperature changes. PloS One 7 (10), e48538. doi: 10.1371/journal.pone.0048538
Waldbusser G. G., Salisbury J. E. (2014). Ocean acidification in the coastal zone from an organism’s perspective: multiple system parameters, frequency domains, and habitats. Annu. Rev. Mar. Sci. 6, 221–247. doi: 10.1146/annurev-marine-121211-172238
Keywords: coastal variability, carbon chemistry, extreme events, temperate and subtropical systems, gene flow, phenotypic plasticity, copepods
Citation: Aguilera VM, Sepulveda F, von Dassow P, Gaitán-Espitia JD, Mesas A and Vargas CA (2023) Local scale extreme low pH conditions and genetic differences shape phenotypic variation in a broad dispersal copepod species. Front. Mar. Sci. 10:1221132. doi: 10.3389/fmars.2023.1221132
Received: 11 May 2023; Accepted: 08 November 2023;
Published: 07 December 2023.
Edited by:
Katja U. Heubel, University of Kiel, GermanyReviewed by:
Jan Heuschele, University of Oslo, NorwayReid Brennan, Helmholtz Association of German Research Centers (HZ), Germany
Copyright © 2023 Aguilera, Sepulveda, von Dassow, Gaitán-Espitia, Mesas and Vargas. This is an open-access article distributed under the terms of the Creative Commons Attribution License (CC BY). The use, distribution or reproduction in other forums is permitted, provided the original author(s) and the copyright owner(s) are credited and that the original publication in this journal is cited, in accordance with accepted academic practice. No use, distribution or reproduction is permitted which does not comply with these terms.
*Correspondence: Victor M. Aguilera, dmljdG9yLmFndWlsZXJhQGNlYXphLmNs
†ORCID: Victor M. Aguilera, orcid.org/0000-0001-5791-5250