- 1Laboratorio de Investigación Acuático Ambiental (LACER), HUB AMBIENTAL UPLA, Universidad de Playa Ancha, Valparaíso, Chile
- 2Departamento de Ciencias y Geografía, Facultad de Ciencias Naturales y Exactas, Universidad de Playa Ancha, Valparaíso, Chile
- 3Departamento de Ciencias del Mar y Biología Aplicada, Facultad de Ciencias, Universidad de Alicante, Alicante, Spain
- 4Doctorado Interdisciplinario en Ciencias Ambientales, Facultad de Ciencias Naturales y Exactas, Universidad de Playa Ancha, Valparaíso, Chile
- 5Departamento de Ciencias y Recursos Naturales, Facultad de Ciencias, Universidad de Magallanes, Punta Arenas, Chile
- 6Centro de Investigación Dinámica de Ecosistemas Marinos de Altas Latitudes (IDEAL), Valdivia-Punta Arenas, Chile
- 7School of Biological and Marine Sciences, University of Plymouth, Plymouth, United Kingdom
- 8Instituto de Ciencias Marinas y Limnológicas, Facultad de Ciencias, Universidad Austral de Chile, Valdivia, Chile
- 9Instituto de biotecnología y Desarrollo Azul (IBYDA), Centro Experimental Grice Hutchinson, Universidad de Málaga, Málaga, Spain
The Antarctic Peninsula is experiencing one of the highest warming rates globally. In polar regions, macroalgae thrive under extreme environmental conditions, which could worsen because of future climate change scenarios, including increased ultraviolet exposure, extremely low light availability, and fluctuating temperatures, particularly in the intertidal zones. To investigate the potential role of photoprotective and antioxidant mechanisms in response to future increases in sea surface temperatures caused by climate change, we conducted laboratory experiments using three intertidal macroalgae model species: Adenocystis utricularis (Ochrophyta, Phaeophyceae), Pyropia endiviifolia (Rhodophyta, Bangiophyceae), and Monostroma hariotii (Chlorophyta, Ulvophyceae). These algae were collected in Punta Artigas (King George Island, Antarctica) and acclimated at 2°C for 48 h. They were then assessed in laboratory experiments for up to 5 days under two treatments: (1) control conditions at 2°C and (2) elevated tem.perature conditions at 8°C, representing the most negative increment in SSTs estimated by the end of the 21st century. Carbon, nitrogen, pigments (chlorophylls and carotenoids), mycosporine-like amino acids (MAAs), and phenolic compounds were quantified after 3 and 5 days of exposure. For M. hariotii, elevated temperatures led to an increase in the C/N ratio, total antioxidant capacity, and levels of nitrogen, total carotenoids, chlorophyll-a, pigments (chlorophyll-b and violaxanthin), and phenolic compounds. For A. utricularis, elevated temperatures led to elevated C/N ratio and levels of chlorophyll-a and carotenoids (fucoxanthin and β-carotene). For P. endiviifolia, elevated temperatures resulted in elevated levels of carotenoids (lutein and β-carotene), phenolic compounds, and MAAs (porphyra-334, shinorine, and palythine). Thus, our study suggests that increasing water temperatures due to global warming can enhance the photoprotective abilities of three Antarctic intertidal macroalgae (M. hariotii, A. utricularis, and P. endiviifolia), with each species showing specific responses.
1 Introduction
Since the pre-industrial revolution era, anthropogenic greenhouse gas emissions have led to significant increases in atmospheric concentrations of carbon dioxide (CO2), methane (CH4), and nitrous oxide (N2O), among other gases. Consequently, average global temperatures have increased, resulting in climate change. According to the Intergovernmental Panel on Climate Change (IPCC, 2023), atmospheric CO2 concentrations are expected to reach 800–1,000 ppm by the end of the 21st century. During the past 50 years, average sea surface temperatures (SSTs) have increased by 0.13°C per decade, with predictions varying from 2°C to 6°C by the end of the century (Solomon et al., 2007). The Antarctic Peninsula’s warming rate has been identified as one of the fastest events globally (Turner et al., 2016; Cárdenas et al., 2018).
Such anticipated environmental changes are thought to be key aspects that determine the ecological responses of intertidal Antarctic seaweeds. This includes investigating their tolerance to desiccation under higher temperatures and understanding the consequences at higher levels of biological organization (Gómez, 2015; Navarro et al., 2016; Celis-Plá et al., 2020; Gómez and Huovinen, 2020). Therefore, it is essential to deepen our understanding of the ecophysiological responses of intertidal macroalgae species under simulated (as close as possible) conditions encountered in the future to assess their maximum tolerance thresholds to upcoming environmental changes and their potential effect on ecosystems (Gómez, 2015; Celis-Plá et al., 2020). Additionally, increasing temperatures may influence future levels of sea-ice cover levels, which play a role in attenuating incident light and protecting subtidal (and maybe lesser in intertidal) primary producers from excessive exposure to ultraviolet (UV) irradiance (UV radiation, UVR: UVB, λ = 280–315 nm and UVA, λ = 315–400 nm) and high photosynthetically active radiation (PAR, λ = 400–700 nm). The extent of underwater variations in UV/PAR penetration will depend on changes in water turbidity related to phytoplankton production and terrestrial particles (Gómez and Huovinen, 2020). Therefore, the predicted future increase in SSTs, combined with increased UVR exposure, is expected to exacerbate the existing environmental challenges experienced by polar macroalgae (Rautenberger et al., 2015; Celis-Plá et al., 2020)—for example, during spring and summer, macroalgae must endure high levels (540 ± 84 µmol photons m−2 s−1) of PAR and short-wavelength radiation (10.7 ± 3.8 Wm−2), particularly UVR (Huovinen and Gómez, 2013).
Exposure to increasing temperatures and dosage of UVR can induce excess reactive oxygen species (ROS) and lead to the overproduction of photoprotective compounds (Celis-Plá et al., 2020; Gómez and Huovinen, 2020). ROS overproduction associated with excess UVR is primarily due to the disruption of electron transport chains and excess energy transfer to oxygen in the chloroplasts, while the effects of temperature-mediated oxidative stress can occur through different processes such as photodamage associated with the excess accumulation of ROS (Kim and Portis, 2004).
The main photoprotective compounds produced by macroalgae under environmental stress are chlorophylls, carotenoids, phycobiliproteins, mycosporine-like amino acids (MAAs), and phenolic compounds (PCs) (Celis-Plá et al., 2017; Gómez and Huovinen, 2020). These compounds can harvest excess light and act as efficient ROS scavengers under oxidative stress conditions (Stahl and Sies, 2007; Celis-Plá et al., 2020). The xanthophyll cycle, mediated by zeaxanthin and catalyzed by epoximerase, is recognized as the most important photoprotective mechanism in several algal divisions (Niyogi et al., 2001; Rodrigues et al., 2002), and their importance in macroalgae underlies their ability to dissipate excess thermal energy (Adams et al., 2008; Esteban et al., 2008; Goss and Jakob, 2010). In brown algae, the xanthophyll cycle involves a rapid reversible de-epoxidation of violaxanthin through antheraxanthin to zeaxanthin (Marquardt and Hanelt, 2004).
While the specific response of polar seaweeds to global temperature increases is not yet fully established, their survival will be associated with metabolic adjustments, including the synthesis of photoprotective and antioxidant compounds (Kremb et al., 2012; Heinrich et al., 2015; Gómez and Huovinen, 2020). MAAs have been extensively studied because of their photoprotective ability as UV-screening compounds and antioxidant properties, effectively absorbing UVR. In red algae, MAA induction is dependent on radiation (Karsten et al., 2003; Figueroa et al., 2014; Álvarez-Gómez et al., 2017), and they exhibit a high antioxidant potential (De la Coba et al., 2009; Carreto and Carignan, 2011). Similarly, phlorotannins, a heterogeneous group of phenolics compounds (PCs) present primarily in brown macroalgae (Pavia and Brock, 2000; Celis-Plá et al., 2022) and some red and green algae (Álvarez-Gómez et al., 2017; Figueroa et al., 2021), have various potential biological activities, including ROS-scavenging potential (Sáez et al., 2015; Celis-Plá et al., 2020).
In this study, we focused on three intertidal macroalgae species that are abundant in Fildes Bay, Antarctic Peninsula—Pyropia endiviifolia (red algae), Adenocystis utricularis (brown algae), and Monostroma hariotii (green algae) —with relevant ecological roles (Gómez, 2015). In this sense, A. utricularis covers 80% of the substrate in King George Island and is associated with larger algae such as Desmarestia spp. and fauna such as amphipods, bivalves, and gastropods (Martín et al., 2016). The endemic Antarctic algae, M. hariotii and P. endiviifolia, are among the most abundant species as well, providing added niches where fungal taxa can survive and coexist with their host in extreme conditions (Furbino et al., 2014). However, M. hariotii has a great richness of the fauna associated as mollusks, copepods, and polychaetas, which would be related to its morphology as well as oceanic circulation patterns (Elias-Piera et al., 2020). We aimed to evaluate their ecophysiological and photoprotective responses by subjecting them to environmentally controlled experiments at 6°C above the current average SSTs for up to 5 days. We hypothesized that seaweed responses to elevated SST conditions would result in an exacerbated ecophysiological response, leading to increased production of light-harvesting and photoprotective metabolites, such as chlorophylls, carotenoids, PCs, and MAAs.
2 Materials and methods
2.1 Macroalgae collection and sampling site
The thalli of M. hariotii (Bory; intertidal pools), A. utricularis (Gain; present in the mid-intertidal), and P. endiviifolia (A. Gepp and E. Gepp; present in the top intertidal zones) (Gómez, 2015) were sampled from Fildes Bay, King George Island, Antarctic Peninsula (62°02′00″ S; 58°21′00″ W), on January 4, 2019 (Figure 1). The algal samples were transported to the research laboratory at “Base Professor Julio Escudero,” operated by the Chilean Antarctic Institute (INACH; 62°12′01″ S to 58°57′34″ W). The samples were washed with seawater (filtered with 1-µm membrane filter) and acclimated to laboratory conditions in plastic containers for 48 h, wherein seawater was under constant aeration at 2°C at a photoperiod of 20:4 (day/night) and daylight conditions of 140 μmol m−2 s−1 (fluorescent light TL12; Phillips, Amsterdam, the Netherlands) of PAR (Celis-Plá et al., 2020).
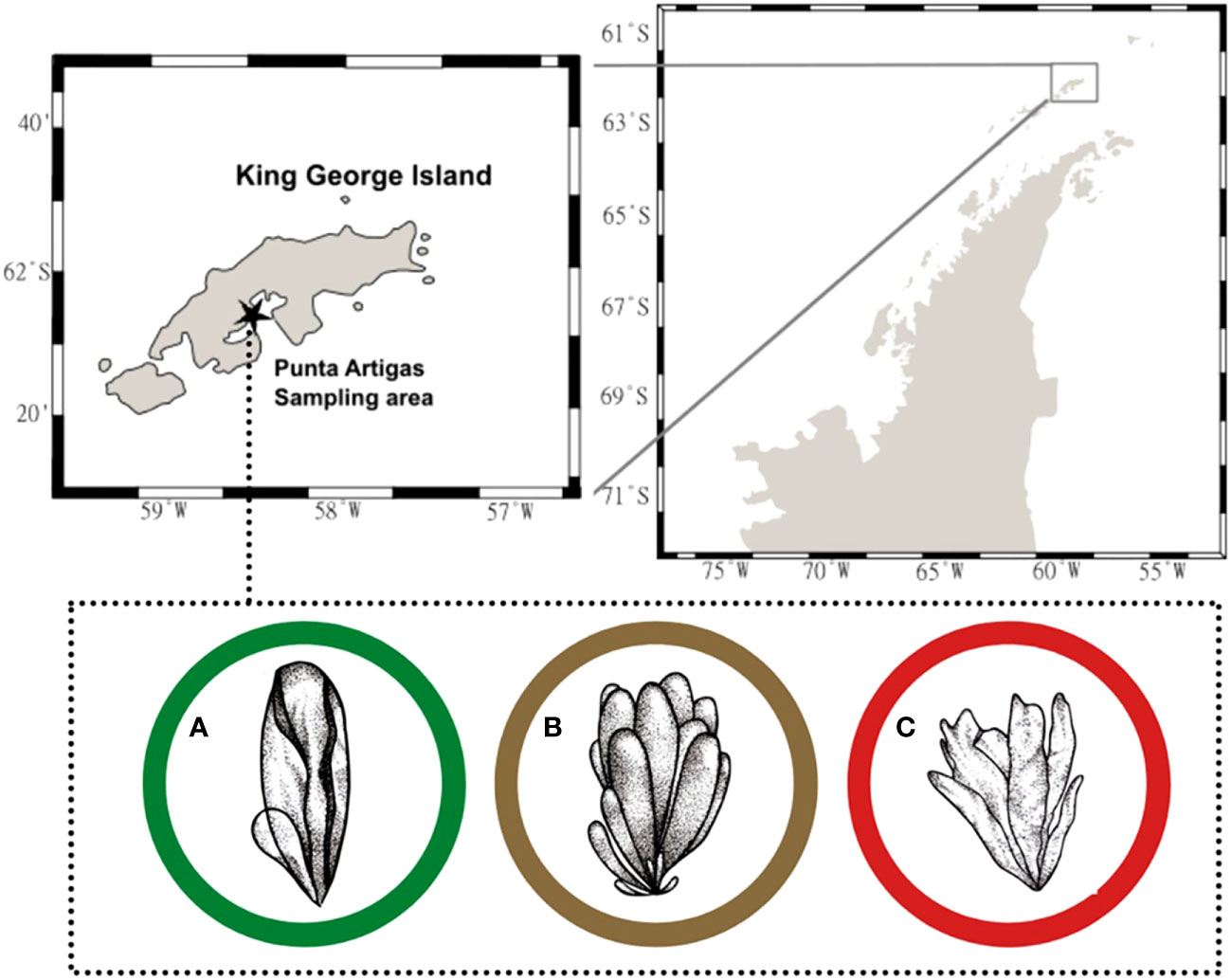
Figure 1 Sampling area in Punta Artigas, King George Island, Antarctic Peninsula (62°02′ S, 58°21′ W) on January 4, 2019 (black star). Macroalga species were collected: (A) Monostroma hariotii, (B) Adenocystis utricularis, and (C) Pyropia endiviifolia.
2.2 Experimental design
After the acclimation period, different individuals of each species (30 g of fresh biomass per vessel) were transferred to 1-L containers (30 × 20 × 15) in triplicates for two treatments: control environmental temperature at 2°C and control temperature + 6°C (i.e., 8°C; upon worst IPCC predictions by the end of the 21st century). The temperature maintenance and increase were achieved using LAUDA Compact Low-Temperature Thermostat (RC 6 CP, LAUDA, Germany). The remaining culture conditions were the same as those described for the acclimation process.
The experiment was conducted for up to 5 days; in this regard, investigations related to the exposure of macroalgae (also Antarctic) to environmental stress (including elevated temperatures) suggest that, when the macroalgae were exposed to environmental pressure for more than 2 to 3 days, they demonstrated potential responses in the long term (Cruces et al., 2012; Flores-Molina et al., 2016; Moenne et al., 2016; Celis-Plá et al., 2019; Rodríguez-Rojas et al., 2019; Celis-Plá et al., 2020).
The samples were collected during the early days of the experiment and initial time (on days 3 and 5 of the experiment), immediately frozen in liquid nitrogen, and transported in a dry shipper device (CXR500-0 Cryogenic Shippers; Worthington Industries, Columbus, OH, USA) to the Laboratory of Aquatic Environmental Research (LACER) at the HUB AMBIENTAL UPLA Research Centre, in the University of Playa Ancha, where the samples were maintained at −80°C until further analyses.
2.3 Quantification of total carbon and nitrogen
Total carbon (C) and nitrogen (N) concentrations were determined on dry weight (DW) basis using the element analyzer CNHS-932 (LECO) (Celis-Plá et al., 2017). All biochemical variables were expressed as mg g−1 DW after determining the fresh-weight-to-DW ratio, estimated as 4.79 for A. utricularis, 5.93 for M. hariotii, and 3.75 for P. endiviifolia.
2.4 Quantification of pigments
The concentrations of chlorophyll a (Chla) and pigments were quantified using high-performance liquid chromatography (HPLC), with modifications to the methods reported by Celis-Plá et al. (2017). Chla and pigments were extracted overnight at 4°C from 20 mg (fresh weight) of samples using 500 µL of dimethylformamide, and then the extract was filtered (using 0.22-µM nylon filters). The concentrations were determined using HPLC–mass spectrometry (MS; Ultimate 3000, Thermo Fisher Scientific, Waltham, MA, USA).
The concentrations of carotenoids were determined using commercial standards [chlorophyll b (Chlb), chlorophyll c (Chlc), fucoxanthin (Fuco), lutein (Lut), violaxanthin (viola), zeaxanthin (Zea), and β-carotene (β-Car)] of DHI LAB Products (Hørsholm, Denmark).
2.5 Quantification of photoprotective compounds
PCs were extracted from 0.25 g of frozen fresh tissue with 2.5 mL of a mixture solution containing 80% methanol and 20% water and incubated overnight at 4°C in the dark under agitation (Abdala-Díaz et al., 2006; Cérantola et al., 2006; Figueroa et al., 2014; Celis-Plá et al., 2016). After extraction, the samples were centrifuged at 2,253 ×g for 30 min at 4°C. The supernatant was collected to measure the PC content colorimetrically using the Folin–Ciocalteu reagent (Sigma-Aldrich, St. Louis, MO, USA), with phloroglucinol (1,3,5-trihydroxybenzene; P-3502; Sigma St. Louis, MO, USA) as the standard. Finally, the absorbance was determined at 760 nm using a microplate reader. The concentration of PCs was expressed as mg g−1 DW. Using the same extract, phenolic single compounds were determined in the spectral sweep from 250 to 500 nm using commercial standards.
The composition of PCs (single phenolics, SP) was determined using HPLC, according to the method described by Celis-Plá et al. (2018). SP was extracted from 0.25 g of frozen fresh tissue at 4°C in the dark under agitation. The extract was mixed with 2.5 mL of 80% methanol and then filtered (using a 0.22-µM nylon filter). The components were analyzed using HPLC (Shimadzu, Kyoto, Japan), equipped with a UV detector set at 260 nm (254–340 nm; SPD M20A; Shimadzu). SP composition was determined using commercial standards (p-coumaric acid, rutin, 3,4-dihydroxy benzaldehyde, 4-hydroxybenzoic acid, esculetin, trans-cinnamic acid, protocatechuic acid, and phloroglucinol; Sigma-Aldrich, St. Louis, MO, USA).
The PCs were determined in the spectral sweeps for the three species and for all treatments using a microplate spectrophotometer, and the results were compared with commercial standards of phenols (p-coumaric acid, rutin, 3,4-dihydroxy benzaldehyde, 4-hydroxybenzoic acid, esculetin, trans-cinnamic acid, protocatechuic acid, and phloroglucinol; Sigma-Aldrich, St. Louis, MO, USA).
The concentrations of total and individual MAAs were determined from the extract prepared using 20 mg of DW material mixed with 1 mL of 20% methanol (Korbee-Peinado et al., 2004). MAAs present in methanolic extracts were quantified using HPLC-MS (Ultimate 3000; Thermo Fisher Scientific, Waltham, MA, USA) according to the protocols described by Korbee-Peinado et al. (2004) and Álvarez-Gómez et al. (2017) in the extracts of the red algae P. endiviifolia. The HPLC system was coupled to an MS (Orbitrap Q Exactive) with an atmospheric pressure ionization system and heated electrospray H‐ESI (Thermo Fisher Scientific, Waltham, MA, USA).
The HPLC was equipped with a thermostat autosampler (operating at 5°C) and a 4 μM, 250 mm × 4.6 mm C8 column (Luna Phenomenex, Aschaffenburg, Germany). The fluxes of the mobile phases (A: H2O + 5 mM of NH4 at pH 4.5; B: acetonitrile) and the MS parameters were adjusted as follows: resolution = 70.000 full width at half-maximum (FWHM), sheath gas = 40, spray voltage = 4 kV, capillary temperature = 320°C, and S‐Lens level = 55. Mass spectra were acquired at an interval of 100–1,200 m/z in positive and negative ion modes. Quantification calculations were performed using previously reported extinction coefficients (Korbee-Peinado et al., 2004), and the results were expressed as mg g−1 DW.
2.6 Total antioxidant capacity
The 2,2-diphenyl-1-picrylhydrazyl (DPPH) method was used as a proxy for determining total antioxidant capacity; the same extracts used for PC determination were used for the total antioxidant capacity assessment (Celis-Plá et al., 2022). Each extract (150 μL) was added to a stock solution of DPPH (1.27 mM concentration; 90 MeOH:10 H2O), and the reaction was completed after 30 min in the dark at an ambient temperature (~20°C); the absorbance was read at 517 nm using a microplate reader. The calibration curve constructed based on the DPPH assay was used to calculate the remaining concentration of DPPH in the reaction mixture after incubation. Finally, Trolox (6-hydroxy-2,5,7,8-tetramethylchromane-2-carboxylic acid; 0–50 µM) was used as the reference antioxidant. The results obtained were expressed as micromole of Trolox equivalent antioxidant capacity per gram of DW (Álvarez-Gómez et al., 2017).
2.7 Statistical analyses
The effects of treatments on the photoprotective responses of M. hariotii, A. utricularis, and P. endiviifolia were assessed using two-way analysis of variance (ANOVA) (Underwood, 1997). Two fixed factors were considered: time (third and fifth days) and temperature (2°C—control and 8°C—elevated). This design allowed us to test the interactive and additive effects of the variables on photoprotective responses after the 5-day experimental period (Underwood, 1997). Student–Newman–Keuls tests were performed on significant interactions in ANOVA. Homogeneity of variance was tested using Cochran’s C test statistic and by visual inspection of the residuals. All data conformed to the homogeneity of variance (n = 3). Analyses were performed using RStudio (R Core Team, 2023, Vienna, Austria). The general variation patterns between biochemical and photoprotective variables measured in M. hariotii, A. utricularis, and P. endiviifolia were explored using a multivariate approach. Principal component analysis (PCA) was performed based on Euclidean distance, using the PERMANOVA+ for PRIMER 6 package (Anderson et al., 2008). This multivariate ordination was applied because it reveals the variation in the concentration of biochemical and photoprotective compounds concurrently from observing the ordination plot. Each variable was represented by an arrow in the ordination plot to point the samples with the highest concentration of that compound. Each replicate represented the concentration of all compounds calculated from the replicate thalli (n = 3) collected at any time during the sampling period for each time point or temperature, and grouping was performed per seaweed species.
3 Results
3.1 Carbon and nitrogen concentrations and C/N ratio
In the initial samples (48 h after acclimatization), C concentration was the highest in P. endiviifolia (350.99 ± 1.78 mg g−1 DW), followed by M. hariotii (285.26 ± 12.28 mg g−1 DW) and then A. utricularis (225.15 ± 28.25 mg g−1 DW). A significant difference in the total C concentration was observed in M. hariotii for both factors, with high values under control temperature at 3 days (Figure 2A; Supplementary Table S1). In A. utricularis, the total C concentration was significantly lower at 8°C at both sampling time points (Figure 2B; Supplementary Table S1). By contrast, no significant changes in the C concentration were observed in P. endiviifolia during the experiment (Figure 2C; Supplementary Table S1).
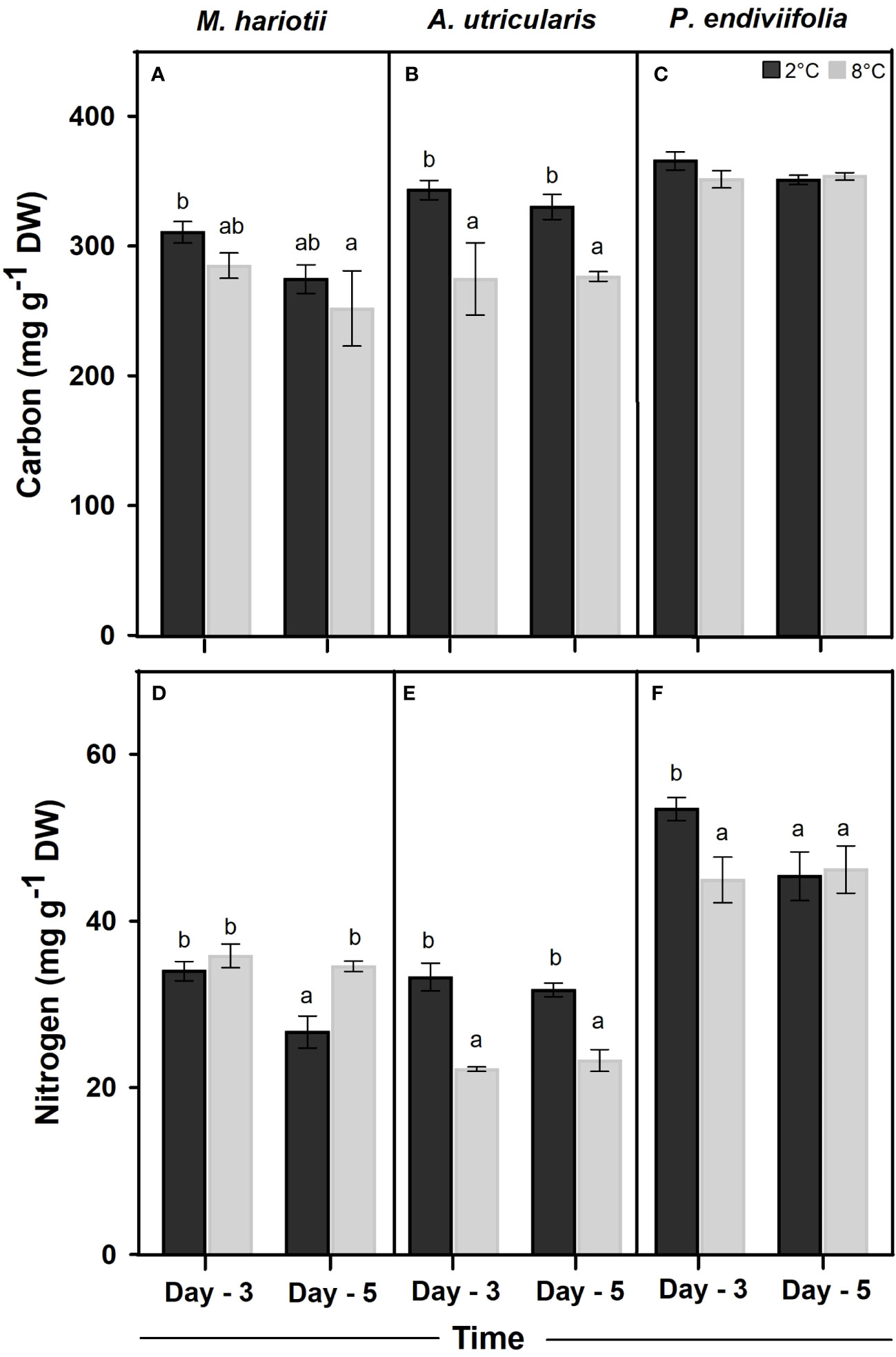
Figure 2 Total Carbon concentrations in (A) Monostroma hariotii, (B) Adenocystis utricularis, (C) Pyropia endiviifolia, and total nitrogen content in (D) Monostroma hariotii, (E) Adenocystis utricularis, (F) Pyropia endiviifolia, exposed to 2°C (dark bars) and 8°C (gray bars) on days 3 and 5 of the experiment. Different letters indicate significant differences obtained using the Student Newman-Keuls test (lowercase letters), within 95% confidence intervals (p < 0.05). Error bars show the standard error of the mean of three independent replicates.
The internal N concentration in P. endiviifolia, M. hariotii, and A. utricularis in the initial samples was 45.34 ± 2.40, 36.93 ± 2.75, and 19.75 ± 2.86 mg g−1 DW, respectively. Significant differences in the internal N concentration existed for both factors in M. hariotii and P. endiviifolia. By contrast, the N concentration in A. utricularis was affected only by temperature (Figure 2; Supplementary Table S2). Finally, the C/N ratio was the highest in the thallus of A. utricularis at elevated temperatures (Table 1), whereas the C/N ratio was significantly lower at the same temperature in M. hariotii (Table 1). No significant differences were observed in P. endiviifolia between both factors (Table 1 and Supplementary Table S3).
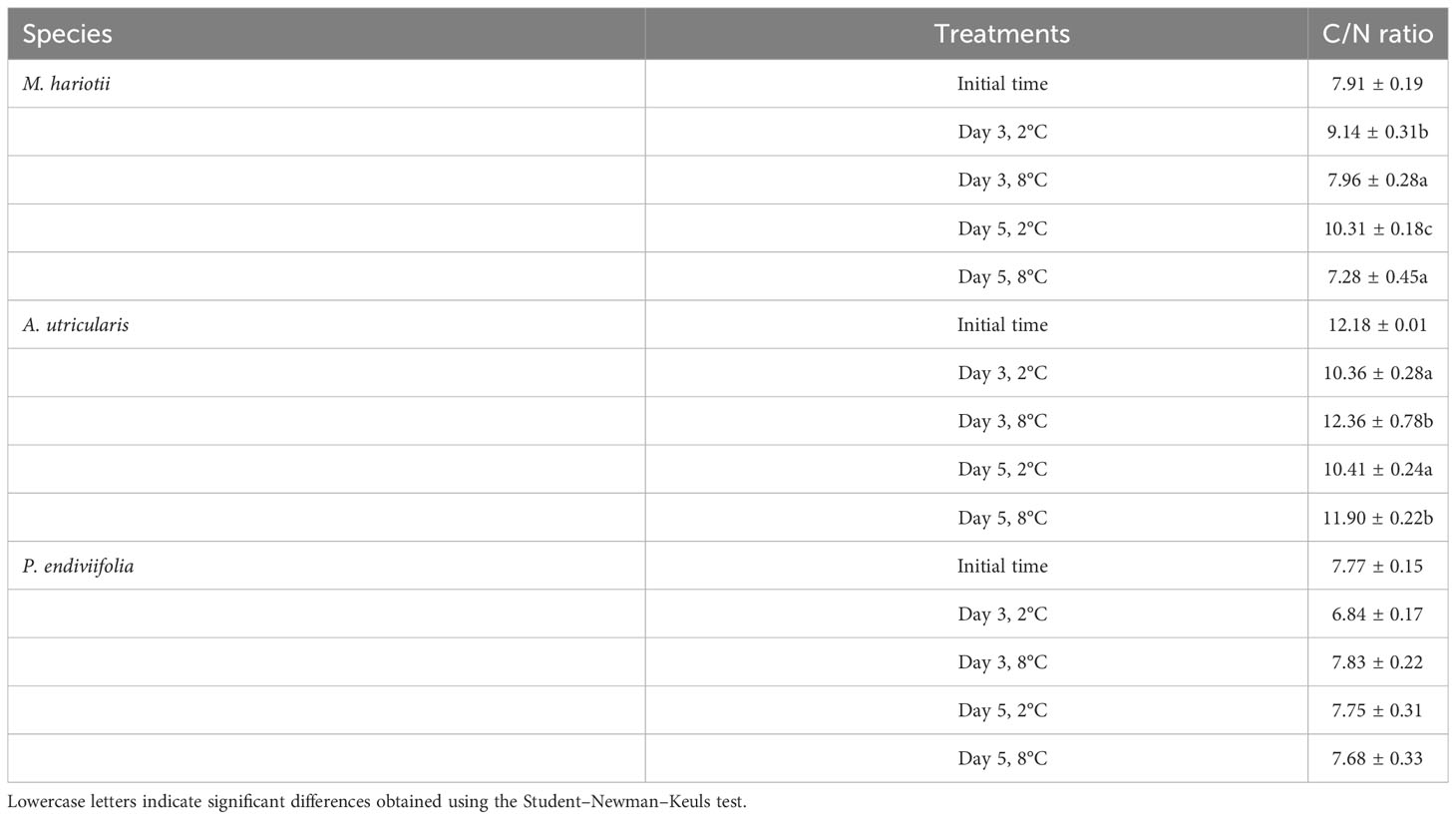
Table 1 C/N ratio (mean ± standard error, n = 3) in Monostroma hariotii, Adenocystis utricularis, and Pyropia endiviifolia in the initial samples.
3.2 Pigment concentration
In the initial samples, the Chla in relative contents was 39.49% ± 3.17%, 62.56% ± 1.76%, and 27.59% ± 0.91% for M. hariotii, A. utricularis, and P. endiviifolia, respectively (Table 2). The Chla relative contents showed significant differences between both factors in M. hariotii and A. utricularis, and the Chla relative contents increased at elevated temperatures (Figures 3A, B; Supplementary Table S4). By contrast, in P. endiviifolia, the Chla relative contents decreased significantly at elevated temperatures (Figure 3C; Supplementary Table S4).
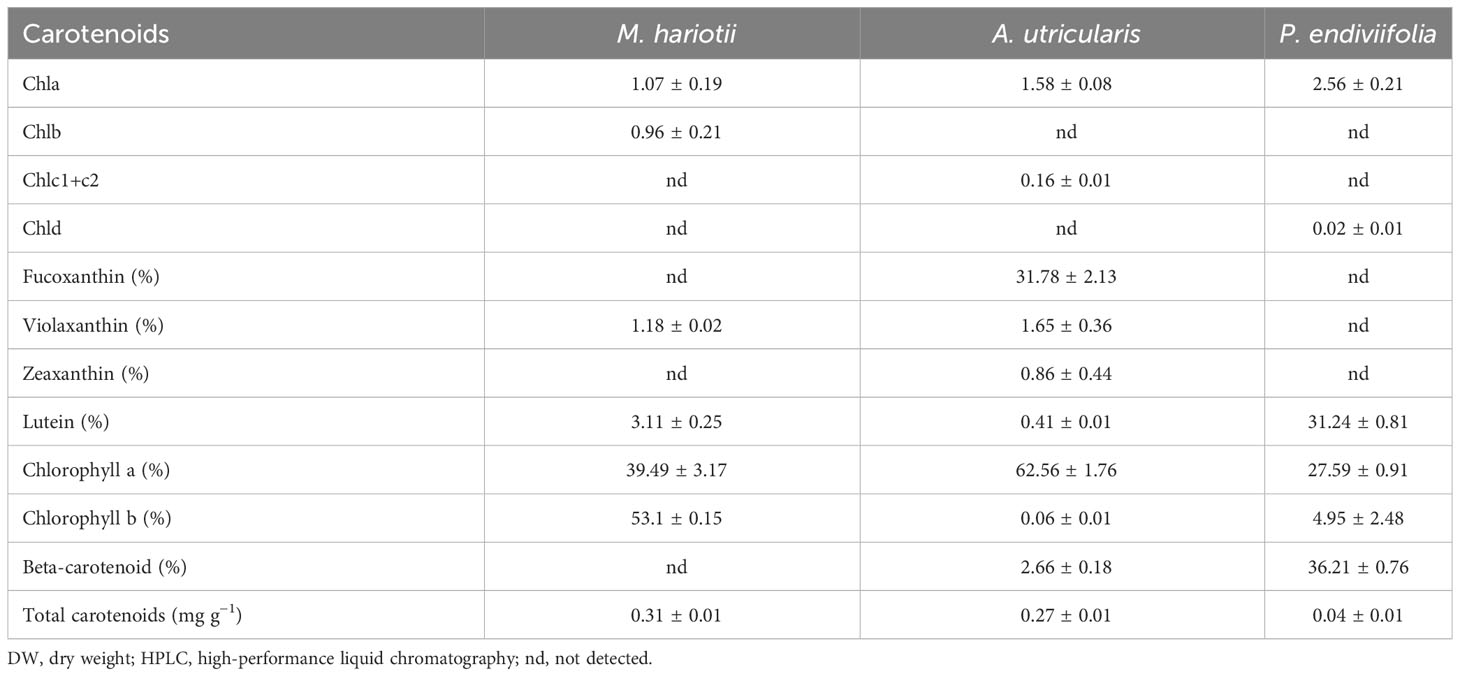
Table 2 Concentrations of pigments (mg g−1 DW), carotenoids (%), and total carotenoids (mg g−1 DW) (mean ± standard error, n = 3) detected by using HPLC in Monostroma hariotii, Adenocystis utricularis, and Pyropia endiviifolia in the initial samples.
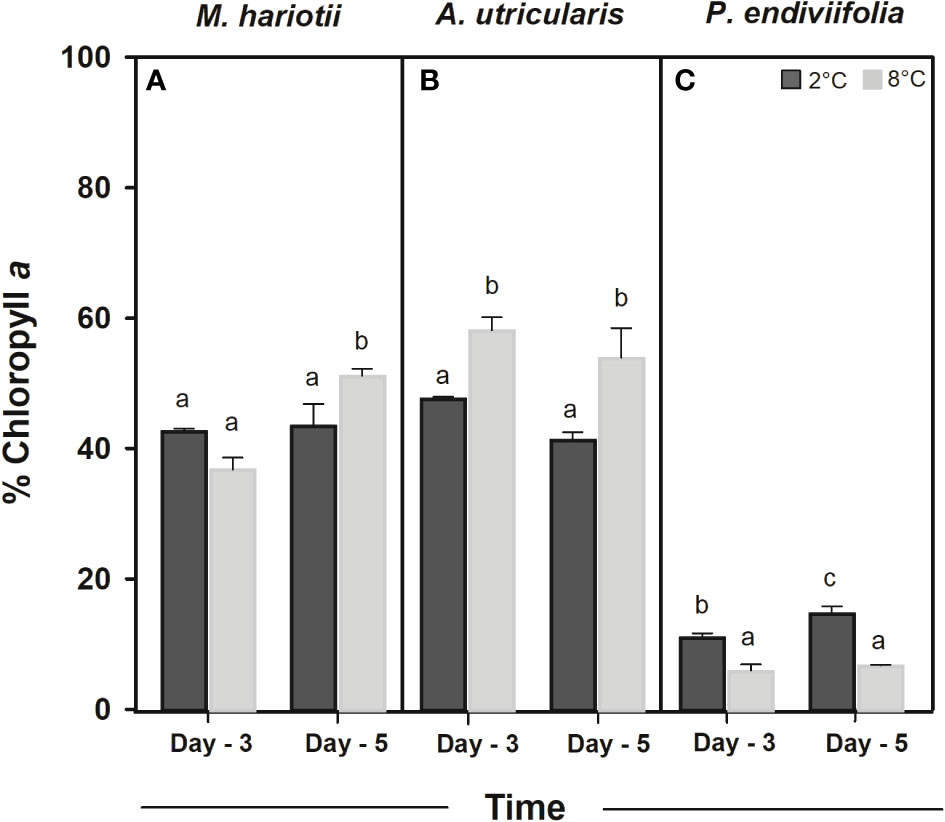
Figure 3 Chlorophyll a relative content (%) in (A) Monostroma hariotii, (B) Adenocystis utricularis, and (C) Pyropia endiviifolia, exposed to 2°C (dark bars) and 8°C (gray bars) on days 3 and 5 of the experiments. Different letters indicate significant differences obtained using the Student Newman-Keuls results (lowercase letters), within 95% confidence interval (p < 0.05). Error bars show the standard error of the mean of three independent replicates.
The concentration of total carotenoids (TC) was 0.31 ± 0.01, 0.27 ± 0.01, and 0.04 ± 0.01 mg g−1 DW for M. hariotii, A. utricularis, and P. endiviifolia, respectively (Table 2). The concentration of TC showed significant differences between the factors in all three species (Table 3). In M. hariotii and A. utricularis, the TC concentration increased at elevated temperatures, whereas in P. endiviifolia the TC concentration decreased in smaller proportions (Table 3). Similarly, the proportion of carotenoids showed differences in M. hariotii, with the highest levels for viola and β-carotene at day 3 under elevated temperature levels, and the proportion of Chlb was lower at an elevated temperature for both days 3 and 5 (Figure 4A). In A. utricularis, the proportion of Fuco was approximately 80%–90% of TC at elevated temperatures on days 3 and 5; smaller proportions of β-carotene, violaxanthin, and zeaxanthin were also found in A. utricularis (Figure 4B). In P. endiviifolia, the proportions of β-carotene increase under elevated temperatures on day 3 but decrease at 5 days. In contrast, the reverse behavior occurs for lutein, with smaller proportions of Chlb (Figure 4C).
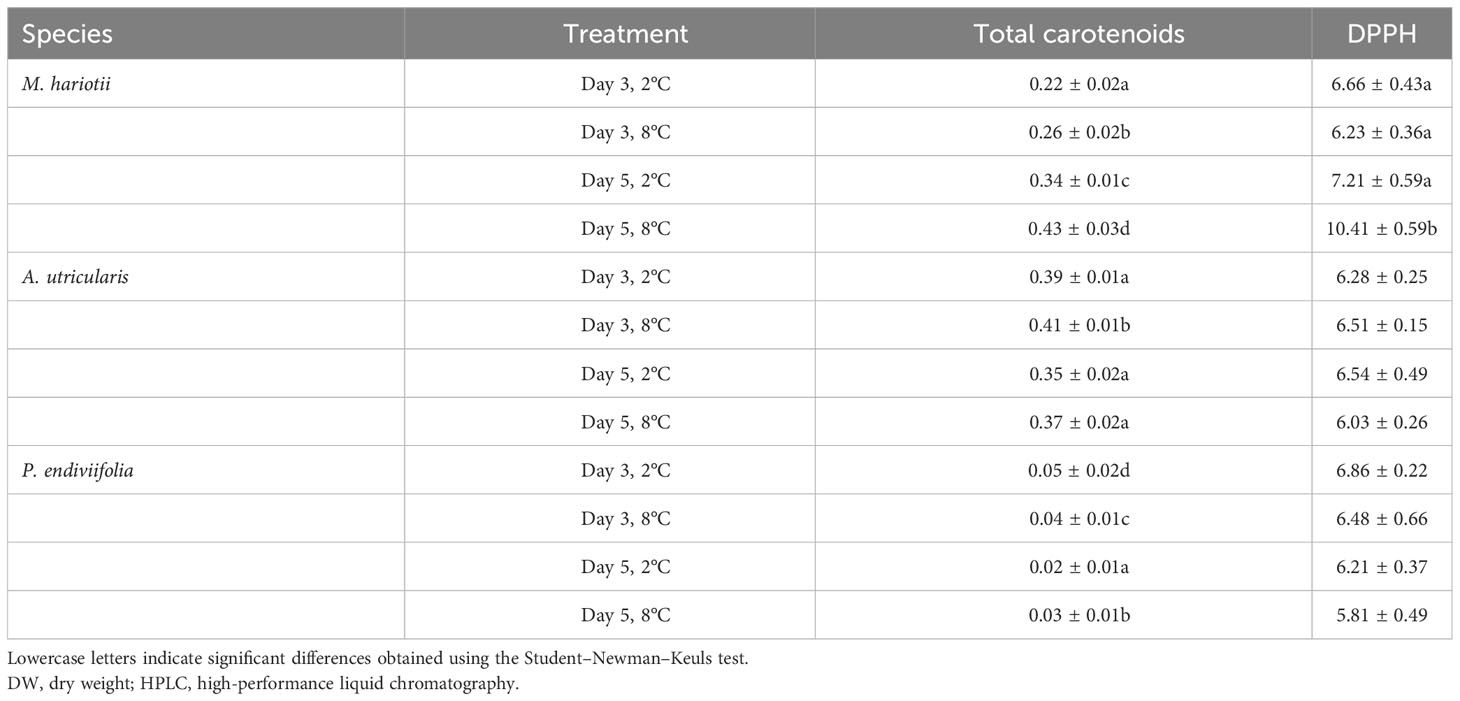
Table 3 Total carotenoids (expressed as mg g−1 DW) (mean ± standard error, n = 3) detected using HPLC and DPPH (µmol TEAC g-1 DW) in Monostroma hariotii, Adenocystis utricularis, and Pyropia endiviifolia on days 3 and 5.
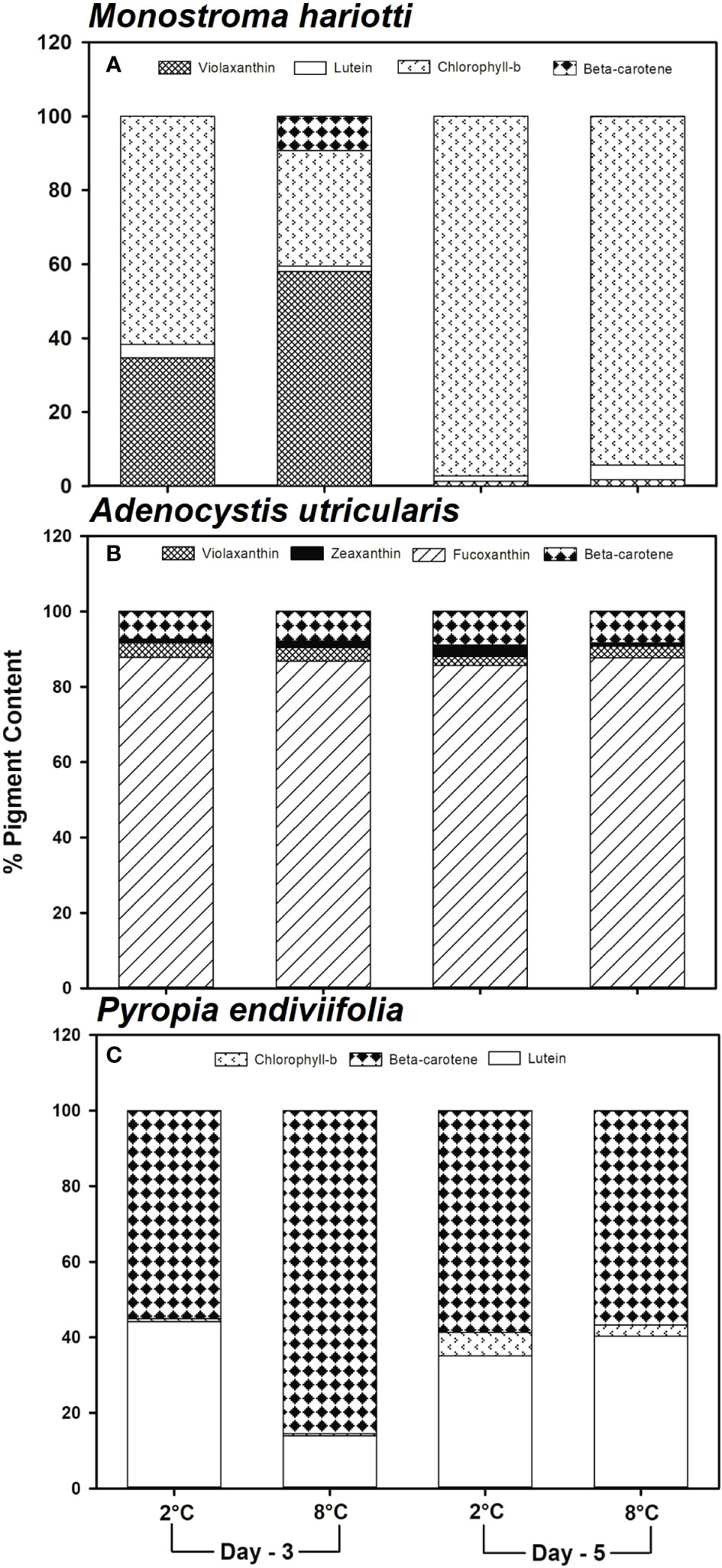
Figure 4 Determination of the carotenoid pigment (%) using high-performance liquid chromatography in (A) Monostroma hariotii, (B) Adenocystis utricularis, and (C) Pyropia endiviifolia, exposed to 2°C (dark bars) and 8°C (gray bars), on days 3 and 5 of the experiment.
3.3 Photoprotective responses (PCs and MAAs)
The PC concentrations at day 0 for M. hariotii, A. utricularis, and P. endiviifolia were 8.32 ± 0.70, 25.2 ± 3.08, and 8.80 ± 0.13 mg g−1 DW, respectively. The PCs showed significant differences in all species (Figure 5; Supplementary Table S5). In M. hariotii (Figure 5A), the PC concentrations were higher at elevated temperatures in time factor; in A. utricularis (Figure 5B), the PC concentration decreased at higher temperatures independent of the time factor; and in P. endiviifolia, the PC concentration increased under elevated temperatures (Figure 5C).
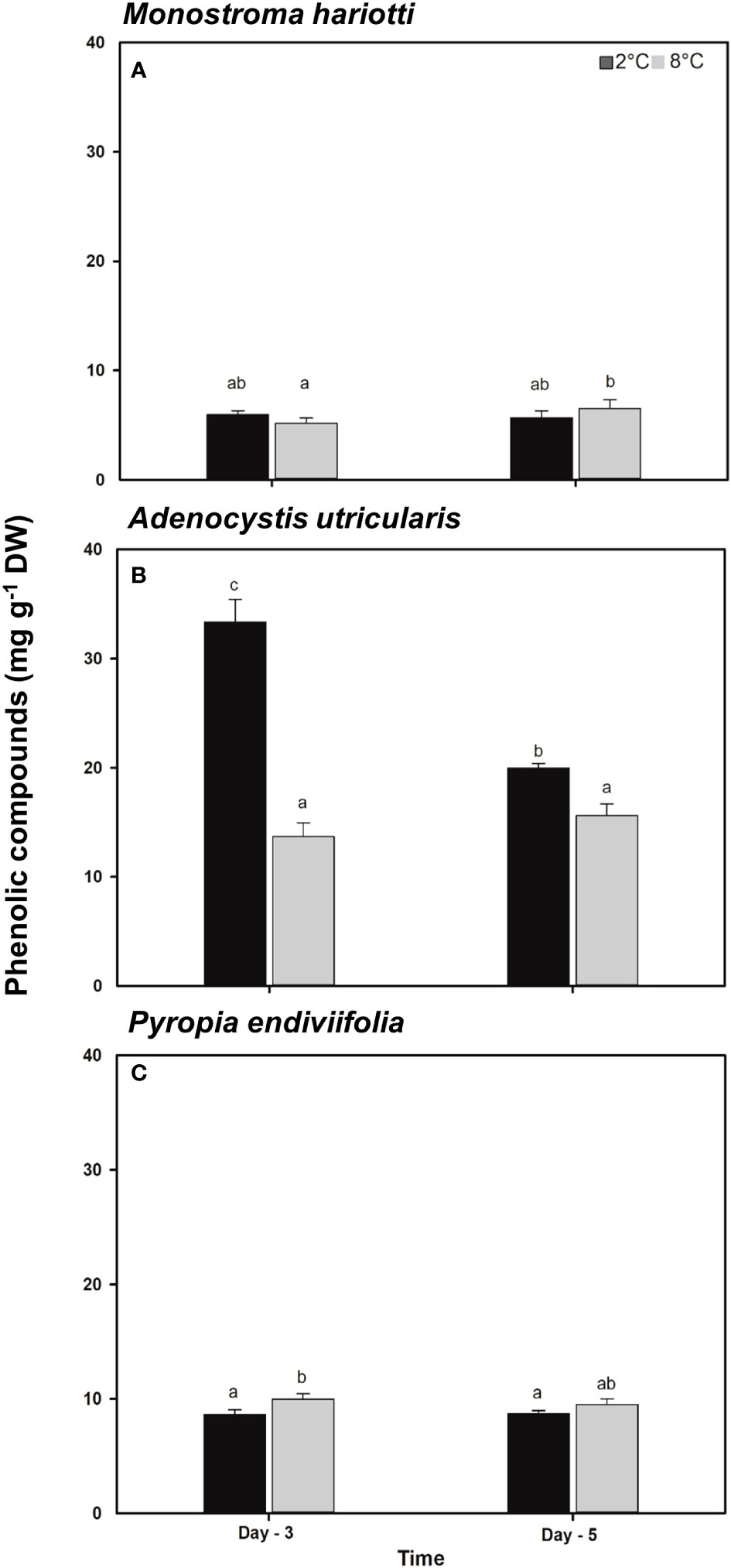
Figure 5 Total phenolic compounds concentrations in (A) Monostroma hariotii, (B) Adenocystis utricularis, and (C) Pyropia endiviifolia exposed to 2°C (dark bars) and 8°C (gray bars) on days 3 and 5 of the experiments. Different letters indicate significant differences obtained with the Student Newman-Keuls results (lowercase letters), within 95% confidence interval (p < 0.05). Error bars show the standard error of the mean of three independent replicates.
SP in A. utricularis showed significant differences among all factors (Table 4). Indeed in A. utricularis, phloroglucinol was detected in both treatments, whereas gallic acid was not detected at the end of the experimental period at elevated temperatures. The concentration of total PCs was higher on day 3 at the control temperature (2°C). However, in M. hariotii, phloroglucinol was present only on day 3 at 8°C, and kaempferol was detected on day 3 at 2°C (Table 4), whereas in P. endiviifolia, these PCs were not detected (Table 4).
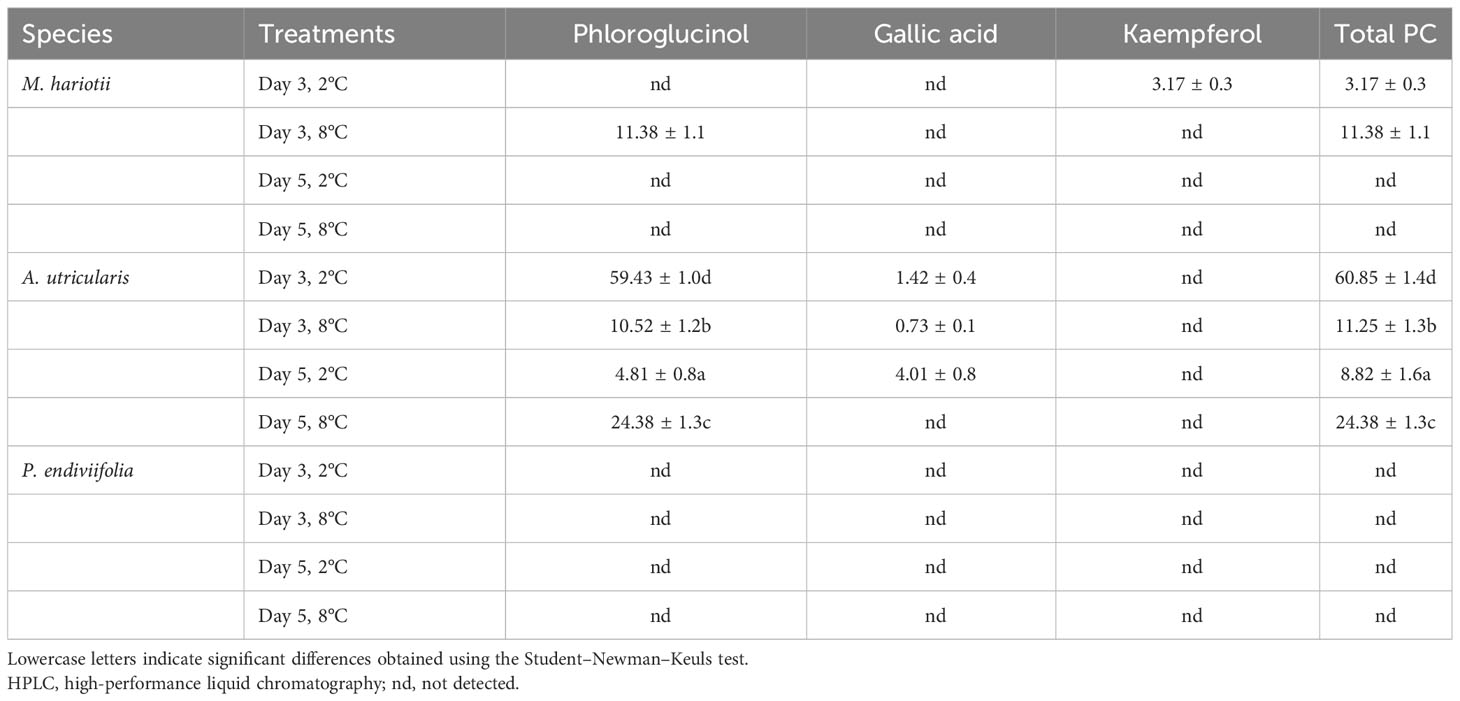
Table 4 Phenolic composition and total phenolic compounds (mg g−1 DW) detected using HPLC in Monostroma hariotii, Adenocystis utricularis, and Pyropia endiviifolia on days 3 and 5.
In the spectral sweeps for phenolic single compounds (Supplementary Figure S1), the presence of phloroglucinol was observed in M. hariotii and A. utricularis but not in P. endiviifolia.
The total MAA concentration in P. endiviifolia showed significant differences for both factors (Figure 6A; Supplementary Table S7). As a general pattern, a significant decrease existed in the total MAA concentration for both time and temperature factors (Figure 6A). As for the identified single MAAs, four compounds were identified: porphyra-334, shinorine, palythine, and palythinol. Porphyra-334 constituted the highest proportion (83.28% on day 3 and 82.81% on day 5) at 8°C. By contrast, palythine and palythinol (detected only on day 3) constituted the least abundance under all treatments (Figure 6B).
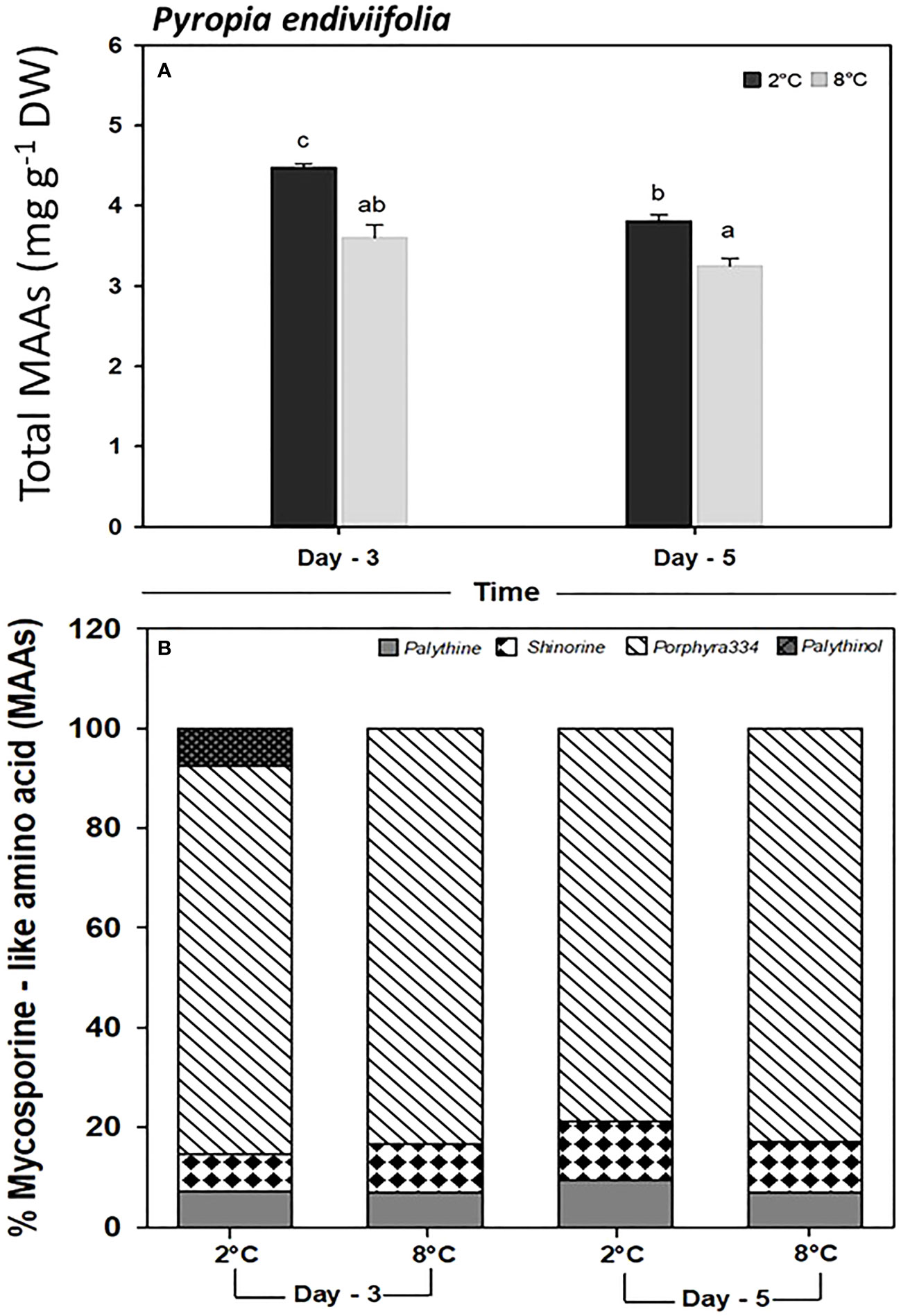
Figure 6 Determination of (A) total mycosporine-like amino acid content, and (B) single mycosporine-like amino acid (%), in Pyropia endiviifolia exposed to 2°C (dark bars) and 8°C (gray bars) on days 3 and 5 of the experiment. Different letters indicate significant differences obtained with the Student Newman-Keuls results (lowercase letters), within 95% confidence interval (p < 0.05). Error bars show the standard error of the mean of three independent replicates.
The DPPH concentration was significantly higher in M. hariotii (p < 0.05, Supplementary Table S6) on day 5 at 8°C.
3.4 Multivariable analyses
The principal component analysis of the experimental results demonstrated a positive correlation, suggesting 50.1% of total variation in the first axis and 79.5% of the total variation in both axes. The first dimension had correlations with higher values of Car, β-Car, PC, and Lut in A. utricularis (Figure 7); similarly, the second dimension had a positive correlation with the variables carbon, Fuco, Zea, nitrogen, and Chla (%), with higher concentrations in P. endiviifolia than in the other alga. Finally, the PCA results showed that M. hariotii had higher concentrations of Chlb, viola, and DPPH (Figure 7).
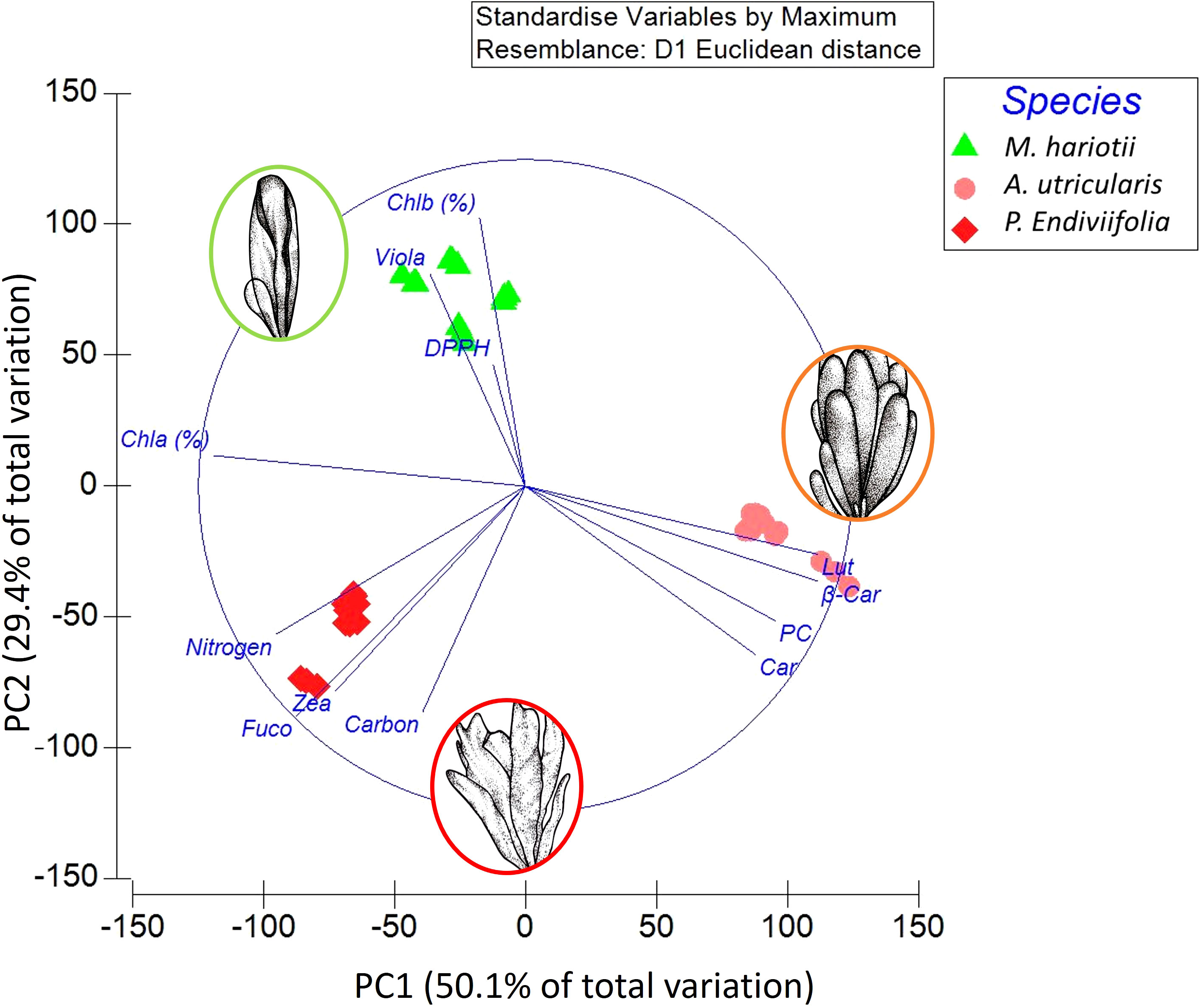
Figure 7 Principal component analysis based on 13 variables and data of all treatments from the green, brown, and red macroalga Monostroma hariotii, Adenocystis utricularis, and Pyropia endiviifolia, respectively. All antioxidant and photoprotective variables were considered, as well as carbon, nitrogen, Car, PC, DPPH, Fuco, viola, Zea, Lut, Chla (%), Chlb (%), and β-Car.
4 Discussion
In this study, we investigated the ecophysiological responses of three intertidal Antarctic macroalga and evaluated their different bioactive compounds and photoprotective responses to understand their behavior under temperature stress and its potential positive or negative effects. Recent research indicates that climate change is gradually leading to species-specific responses in macroalgae, especially in Antarctica. Understanding these responses is crucial for assessing worst-case scenarios for species and ecosystems by the end of the 21st century (Turner et al., 2016; Celis-Plá et al., 2020; Gómez and Huovinen, 2020). Temperature increase can affect the concentration of photoprotective compounds and antioxidant capacity in macroalgae, particularly those from temperate latitudes, such as carotenoids, xanthophyll cycle components, polyphenols, and MAAs (Demmig-Adams and Adams, 2006; Figueroa et al., 2021).
The ecophysiological status of seaweeds can be estimated through their stoichiometric ratios (Figueroa et al., 2021; Celis-Plá et al., 2022). In our study, we found that M. hariotii exhibited a significant decrease in the C/N ratio under elevated temperatures over time. By contrast, A. utricularis showed a significantly higher C/N ratio under elevated temperatures over time. However, no significant differences were observed in P. endiviifolia among treatments. The C/N ratio provides information about the nutritional status of the macroalgae and can indicate any possible damage to internal compounds caused by environmental stressors (Korbee et al., 2005; Sterner et al., 2008; Celis-Plá et al., 2016). Additionally, we observed that the N concentration in M. hariotii was significantly higher at elevated temperatures over time (30–35 mg g−1), while in A. utricularis the N concentration was significantly lower at elevated temperatures (30–35 mg g−1). In P. endiviifolia, the N concentration was lower over time in all treatments (45–50 mg g−1). Similar findings were reported by Gordillo et al. (2022) for arctic seaweeds: the N concentration in Saccharina latissimi and Alaria esculenta (brown macroalga) as well as Phycodrys rubens and Ptilota gunneri (red macroalgae) was approximately 32–35 mg g−1 at a low temperature (3°C). Piquet et al. (2014) and van De Poll et al. (2016) reported that, in Arctic waters, N exists mostly as nitrate during winter and early spring, being completely depleted in summer, thus favoring the production of secondary metabolites. This indicates that these algae have a higher reservoir of N at a low temperature, for example, in winter, than at a high temperature (Cárdenas et al., 2018), probably because the decrease in nitrate in the water column under Antarctic summer conditions increased—primarily as a result of global change conditions and secondly because of a possible use of N source at high temperatures to maintain metabolic fitness (Oren and Gunde-Cimerman, 2007).
Our study found that the responses to carotenoid and other pigment contents were higher in the thalli of M. hariotii, A. utricularis, and P. endiviifolia collected from waters with elevated temperature, indicating that these algae invest in accumulating bioactive compounds or developing photoprotection systems. This observation is in line with the findings of Celis-Plá et al. (2017), who reported higher concentrations of carotenoids and Chla in Ericaria selaginoides (formerly Cystoseira tamariscifolia) in waters at elevated temperatures with nutrient enrichment. Significant differences were observed in terms of the quantities of violaxanthin (V-) in M. hariotii, relative to control and elevated temperature treatments on day 3; however, this carotenoid decreased significantly on day 5. By contrast, A. utricularis showed a smaller proportion of violaxanthin (V-) and zeaxanthin (Z). Indeed the violaxanthin level (-V) decreased under elevated temperature, while the zeaxanthin (Z) level increased at 3 days, with the reverse patterns on the 5 days, suggesting more activation of the xanthophyll cycle. These carotenoids were used in the xanthophyll cycle; under elevated temperature conditions, β-carotene and fucoxanthin decreased their proportions as well. In this sense, the increased violaxanthin (V-) levels have been associated with enhanced photoprotection through the xanthophyll cycle (Demmig-Adams and Adams, 2006; García-Mendoza and Colombo-Pallotta, 2007). In P. endiviifolia, β-carotene and lutein levels increased at higher temperatures. Responses of the xanthophyll cycle could reflect a regulatory and photoprotective mechanism that downregulates the delivery of excitation energy into the electron transport chain to match the rates at which products of electron transport can be consumed in these algae (Demmig-Adams and Adams, 2006). In our study, significant quantities of violaxanthin (V-) were found in M. hariotii and A. utricularis, with only traces of zeaxanthin detected in A. utricularis, indicating their involvement in the xanthophyll cycle. The activity of the xanthophyll or violaxanthin (V-) cycle with the corresponding formation of zeaxanthin (Z) has been described by Demmig-Adams and Adams (2006).
Macroalgae can also display phenotypic plasticity, which includes the presence of PCs in brown macroalgae (Gómez and Huovinen, 2020; Celis-Plá et al., 2022) and MAAs in red seaweeds (Oren and Gunde-Cimerman, 2007; De la Coba et al., 2009). Our findings showed that, in M. hariotii and P. endiviifolia, the concentration of PCs increased at elevated temperatures over time. By contrast, A. utricularis exhibited decreased PC levels at higher temperatures independent of the time factor, possibly compensated by other photoprotectors such as carotenoids. These observations highlight the importance of biological mechanisms that allow for metabolic adaptations, including the development of photoprotective compounds (Kremb et al., 2012; Heinrich et al., 2015; Rangel et al., 2020). Regarding the concentration of phenols, Celis-Plá et al. (2017) reported the decreased concentration of PCs in the brown macroalgae E. selaginoides after a +4°C increase in control temperature conditions in a mesocosm system. On the contrary, Flores-Molina et al. (2016) reported an increase in the concentration of PCs in Desmarestia anceps, a brown Antarctic alga, at elevated temperatures, with values reaching 50 mg g−1 DW at 7°C and approximately 80–100 mg g−1 DW at 12°C. These polyphenolic compounds not only act as UV screen photoprotectors but also have a higher antioxidant potential, which makes them effective photoprotectors in waters with high UV penetration (Abdala-Díaz et al., 2006; Celis-Plá et al., 2016). The increased production of photoprotective compounds may help counteract the effects of the depleted ozone layer in the Antarctic region, leading to increased UV exposure (Jofré et al., 2020).
MAAs are one of the most crucial photoprotective compounds present in red macroalgae, with their synthesis being a light-dependent process (Karsten et al., 2003). Previous studies on Antarctic macroalgae have shown that the concentration and composition of MAAs can be modified under elevated temperatures and increased PAR and UVR (Hoyer et al., 2001; Karsten et al., 2003). In our study, the total MAAs in P. endiviifolia significantly decreased under elevated temperature (8°C). Moreover, specific MAA compounds exhibited variations in their proportions after the experimental period, with changes from 77% for porphyra-334, 8% for palythine, 8% for shinorine, and 6.5% for palythinol at 2°C to 85% for porphyra-334, 10% for shinorine, and 5% for palythine at 8°C. Compounds such as shinorine and porphyra-334 have been extensively studied due to their photochemical properties (Tedetti and Sempéré, 2006). Therefore, the study of red algal extracts from P. endiviifolia could be intriguing, as they contain palythine and asterina-330 and have a high content of MAAs. Jofré et al. (2020) also demonstrated similar patterns in three red macroalgae collected from the Magellan Strait in the Sub-Antarctic region under elevated temperatures during summer. The proportions of MAAs were as follows: 75% for porphyra-334, 20% for palythinol, and 5% for shinorine in Nothogenia fastigiata (Rhodophyta) 60% for palythine, 20% for palythinol, 17.5% for shinorine, and 2.5% for asterina-330 in Iridaea tuberculosa (Rhodophyta); and 40% for palythine, 30% for shinorine, 10% for porphyra-334, 10% for palythinol, and 10% for asterina-330 in Corallina officinalis (Rhodophyta). However, our results are in contrast with those of Hoyer et al. (2001), who identified shinorine and palythine as the most abundant components, followed by porphyra-334 and asterina-330, in eight distinct species of Rhodophyta at King George Island. In the Antarctic area, high levels of MAAs are expected during summer due to the increase of solar irradiance. However, elevated temperatures may reduce the level of MAAs, possibly associated with an increase in stress conditions and higher ROS production (Celis-Plá et al., 2020). In our study, the MAAs decreased in P. endiviifolia at elevated temperatures, suggesting that future climate scenarios with elevated temperatures could lead to a reduction in photoprotective capacity (Navarro et al., 2016; Celis-Plá et al., 2020).
5 Conclusions
Elevated temperatures allowed three intertidal macroalga—M. hariotii, A. utricularis, and P. endiviifolia—to have an upregulated production of responses to photoprotective compounds. Our study findings showed that, with increased SSTs, beneficial effects on the accumulation of photoprotective carotenoids and other compounds of the Antarctic three intertidal macroalgae (M. hariotii, A. utricularis, and P. endiviifolia) can be expected, with each species showing specific responses.
Data availability statement
The original contributions presented in the study are publicly available. This data can be found here: https://data.mendeley.com/datasets/3cxt36v48p/1.
Author contributions
PC-P and CS: investigation, conceptualization, formal analysis, writing—original draft and review and editing. NN, MB, IG, and FF: conceptualization, formal analysis, statistical analysis, and writing—review and editing. MT, CN, FR-R, CL, AT, and DP: experimental research, conceptualization, formal analysis, and statistical analysis. All authors contributed to the article and approved the submitted version.
Funding
Financial and logistical support were granted by the Projects INACH N° RG_10_18 and RT_09_16 granted to PC-P and CS, respectively.
Acknowledgments
The authors would like to thank the Mass Spectrometry Unit and the Photobiology Units, the Central Research Support Services (SCAI), Málaga University, Spain, the Project InES I+D 2021 (INID210013), and the General Direction of Research of the University of Playa Ancha.
Conflict of interest
The authors declare that the research was conducted in the absence of any commercial or financial relationships that could be construed as a potential conflict of interest.
Publisher’s note
All claims expressed in this article are solely those of the authors and do not necessarily represent those of their affiliated organizations, or those of the publisher, the editors and the reviewers. Any product that may be evaluated in this article, or claim that may be made by its manufacturer, is not guaranteed or endorsed by the publisher.
Supplementary material
The Supplementary Material for this article can be found online at: https://www.frontiersin.org/articles/10.3389/fmars.2023.1223853/full#supplementary-material
References
Abdala-Díaz R. T., Cabello-Pasini A., Pérez-Rodríguez E., Conde-Álvarez R. M., Figueroa F. L. (2006). Daily and seasonal variations of optimum quantum yield and phenolic compounds in Cystoseira tamariscifolia (Phaeophyta). Mar. Biol. 148, 459–465. doi: 10.1007/s00227-005-0102-6
Adams W. W. III, Zarter C. R., Mueh K. E., Amiard V. S. E., Demmig-Adams B. (2008). ““Energy dissipation and photoinhibition: A continuum of photoprotection,” in Photoprotection, Photoinhibition, Gene Regulation, and Environment. Eds. Demmig-Adams B., Adams. W. W. III., Mattoo A. (Dordrecht: Springer), 49–64.
Álvarez-Gómez F., Bouzon Z. L., Korbee N., Celis-Plá P. S. M., Schmidt É.C., Figueroa F. L. (2017). Combined effects of UVR and nutrients on cell ultrastructure, photosynthesis, and biochemistry in Gracilariopsis longissima (Gracilariales, Rhodophyta). Algal Res. 26, 190–202. doi: 10.1016/j.algal.2017.07.022
Anderson M., Gorley R. N., Clarke R. K. (2008). Permanova+ for Primer: Guide to Software and Statistical Methods (Plymouth, England: PRIMER-E).
Cárdenas C. A., González-Aravena M., Santibañez P. A. (2018). The importance of local settings: Within-year variability in seawater temperature at South Bay, Western Antarctic Peninsula. PeerJ 6, e4289. doi: 10.7717/peerj.4289
Carreto J. I., Carignan M. O. (2011). Mycosporine-like amino acids: Relevant secondary metabolites. Chemical and ecological aspects. Mar. Drugs 9, 387–446. doi: 10.3390/md9030387
Celis-Plá P. S. M., Bouzon Z. L., Hall-Spencer J. M., Schmidt E. C., Korbee N., Figueroa F. L. (2016). Seasonal biochemical and photophysiological responses in the intertidal macroalga Cystoseira tamariscifolia (Ochrophyta). Mar. Environ. Res. 115, 89–97. doi: 10.1016/j.marenvres.2015.11.014
Celis-Plá P. S. M., Brown M. T., Santillán-Sarmiento A., Korbee N., Sáez C. A., Figueroa F. L. (2018). Ecophysiological and metabolic responses to interactive exposure to nutrients and copper excess in the brown macroalga Cystoseira tamariscifolia. Mar. Pollut. Bull. 128, 214–222. doi: 10.1016/j.marpolbul.2018.01.005
Celis-Plá P. S. M., Martínez B., Korbee N., Hall-Spencer J. M., Figueroa F. L. (2017). Photoprotective responses in a brown macroalgae Cystoseira tamariscifolia to increases in CO2 and temperature. Mar. Environ. Res. 130, 157–165. doi: 10.1016/j.marenvres.2017.07.015
Celis-Plá P. S. M., Moenne F., Rodríguez-Rojas F., Pardo D., Lavergne C., Moenne A., et al. (2020). Antarctic intertidal macroalgae under predicted increased temperatures mediated by global climate change: Would they cope? Sci. Total Environ. 740, 140379. doi: 10.1016/j.scitotenv.2020.140379
Celis-Plá P. S. M., Rodríguez-Rojas F., Méndez L., Moenne F., Muñoz P. T., Lobos M. G., et al. (2019). MAPK pathway under chronic copper excess in green macroalgae (Chlorophyta): Influence on metal exclusion/extrusion mechanisms and photosynthesis. Int. J. Mol. Sci. 20, 4547. doi: 10.3390/ijms20184547
Celis-Plá P. S. M., Trabal A., Navarrete C., Troncoso M., Moenne F., Zúñiga A., et al. (2022). Daily changes on seasonal ecophysiological responses of the intertidal brown macroalga Lessonia spicata: Implications of climate change. Front. Plant Sci. 13, 941061. doi: 10.3389/fpls.2022.941061
Cérantola S., Breton F., Ar Gall E., Deslandes E. (2006). Co-occurrence and antioxidant activities of fucol and fucophlorethol classes of polymeric phenols in Fucus spiralis. Bot. Mar. 49, 347–351. doi: 10.1515/BOT.2006.042
Cruces E., Huovinen P., Gómez I. (2012). Phlorotannin and antioxidant responses upon short-term exposure to UV radiation and elevated temperature in three South Pacific kelps. Photochem. Photobiol. 88, 58–66. doi: 10.1111/j.1751-1097.2011.01013.x
De la Coba F., Aguilera J., Figueroa F. L., De Gálvez M. V., Herrera E. (2009). Antioxidant activity of mycosporine-like amino acids isolated from three red macroalgae and one marine lichen. J. Appl. Phycol. 21, 161–169. doi: 10.1007/s10811-008-9345-1
Demmig-Adams B., Adams W. W. III (2006). Photoprotection in an ecological context: The remarkable complexity of thermal dissipation. New Phytol. 172, 11–21. doi: 10.1111/j.1469-8137.2006.01835.x
Elias-Piera F., Rossi S., Petti M. A. V., Campos L. S., Valério-Berardo M. T., Corbisier T. N. (2020). Fauna associated with morphologically distinct macroalgae from Admiralty Bay, King George Island (Antarctica). Polar Biol. 43, 1535–1547. doi: 10.1007/s00300-020-02726-y
Esteban R., Martínez B., Fernández-Martín B., Becerril J. M., García-Plazaola I. (2008). Carotenoid composition in Rhodophyta: Insights into xanthophyll regulation in Corallina elongata. Eur. J. Phycol. 44, 221–230. doi: 10.1080/09670260802439109
Figueroa F. L., Bonomi-Barufi J., Celis-Plá P. S. M., Nitschke U., Arenas F., Connan S., et al. (2021). Short-term effects of increased CO2, nitrate and temperature on photosynthetic activity in Ulva rigida (Chlorophyta) estimated by different pulse amplitude modulated fluorometers and oxygen evolution. J. Exp. Bot. 72, 491–509. doi: 10.1093/jxb/eraa473
Figueroa F. L., Domínguez-González B., Korbee N. (2014). Vulnerability and acclimation to increased UVB in the three intertidal macroalgae of different morpho-functional groups. Mar. Environ. Res. 101, 8–21. doi: 10.1016/j.marenvres.2014.01.009
Flores-Molina M. R., Rautenberger R., Muñoz P., Huovinen P., Gómez I. (2016). Stress tolerance of the endemic Antarctic brown alga Desmarestia anceps to UV radiation and temperature is mediated by high concentrations of phlorotannins. Photochem. Photobiol. 92, 455–466. doi: 10.1111/php.12580
Furbino L. E., Godinho V. M., Santiago I. F., Pellizari F. M., Alves T. M., Zani C. L., et al. (2014). Diversity patterns, ecology and biological activities of fungal communities associated with the endemic macroalgae across the Antarctic Peninsula. Microb. Ecol. 67, 775–787. doi: 10.1007/s00248-014-0374-9
García-Mendoza E., Colombo-Pallotta M. F. (2007). The giant kelp Macrocystis pyrifera presents a different nonphotochemical quenching control than higher plants. New Phytol. 173, 526–536. doi: 10.1111/j.1469-8137.2006.01951.x
Gómez I., Huovinen P. (2020). Antarctic Seaweeds: Diversity, Adaptation and Ecosystem Services (Cham: Springer), 397.
Gordillo F. J. L., Carmona R., Jiménez C. (2022). A warmer Arctic compromises winter survival of habitat-forming seaweeds. Front. Mar. Sci. 8. doi: 10.3389/fmars.2021.750209
Goss R., Jakob T. (2010). Regulation and function of xanthophyll cycle-dependent photoprotection in algae. Photosynth. Res. 106, 103–122. doi: 10.1007/s11120-010-9536-x
Heinrich S., Valentin K., Frickenhaus S., Wiencke C. (2015). Temperature and light interactively modulate gene expression in Saccharina latissima (Phaeophyceae). J. Phycol. 51, 93–108. doi: 10.1111/jpy.12255
Hoyer K., Karsten U., Sawall T., Wiencke C. (2001). Photoprotective substances in Antarctic macroalgae and their variation with respect to depth distribution, different tissues and developmental stages. Mar. Ecol. Prog. Ser. 211, 117–129. doi: 10.3354/meps211117
Huovinen P., Gómez I. (2013). Photosynthetic characteristics and UV stress tolerance of Antarctic seaweeds along the depth gradient. Polar Biol. 36, 1319–1332. doi: 10.1007/s00300-013-1351-3
IPCC. (2023). “Climate change 2023: synthesis report,” in A report of the Intergovernmental Panel on Climate Change. Contribution of Working Groups I, II and III to the Sixth Assessment Report of the Intergovernmental Panel on Climate Change. Eds. Lee H., Romero J. (Geneva, Switzerland: In Press). Core Writing Team.
Jofré J., Celis-Plá P. S. M., Figueroa F. L., Navarro N. P. (2020). Seasonal variation of mycosporine-like amino acids in three subantarctic red seaweeds. Mar. Drugs 18, 75. doi: 10.3390/md18020075
Karsten U., Dummermuth A., Hoyer K., Wiencke C. (2003). Interactive effects of ultraviolet radiation and salinity on the ecophysiology of two Arctic red algae from shallow waters. Polar Biol. 26, 249–258. doi: 10.1007/s00300-002-0462-z
Kim K., Portis J. A. R. (2004). Oxygen-dependent H2O2 production by Rubisco. FEBS Lett. 571, 124–128. doi: 10.1016/j.febslet.2004.06.064
Korbee N., Huovinen P., Figueroa F. L., Aguilera J., Karsten U. (2005). Availability of ammonium influences photosynthesis and the accumulation of mycosporine-like amino acids in two Porphyra species (Bangiales, Rhodophyta). Mar. Biol. 146, 645–654. doi: 10.1007/s00227-004-1484-6
Korbee-Peinado N., Abdala-Díaz R. T., Figueroa F. L., Helbling E. W. (2004). Ammonium and UV radiation stimulate the accumulation of mycosporine-like amino acids in Porphyra columbina (Rhodophyta) from Patagonia. Argentina. J. Phycol. 40, 248–259. doi: 10.1046/j.1529-8817.2004.03013.x
Kremb S., Ernst D., Heller W., Wiencke C. (2012). Gene expression analysis in the green macroalga Acrosiphonia arcta (Dillw.) J. Ag.: Method optimization and influence of ultraviolet radiation. Phycol. Res. 60, 151–160. doi: 10.1111/j.1440-1835.2012.00647.x
Marquardt J., Hanelt D. (2004). Carotenoid composition of Delesseria lancifolia and other marine red algae from polar and temperate habitats. Eur. J. Phycol. 39, 285–292. doi: 10.1080/09670260410001712572
Martín A., Miloslavich P., Díaz Y., Ortega I., Klein E., Troncoso J., et al. (2016). Intertidal benthic communities associated with the macroalgae Iridaea cordata and Adenocystis utricularis in King George Island, Antarctica. Polar Biol. 39, 207–220. doi: 10.1007/s00300-015-1773-1
Moenne A., González A., Sáez C. A. (2016). Mechanisms of metal tolerance in marine macroalgae, with emphasis on copper tolerance in Chlorophyta and Rhodophyta. Aquat. Toxicol. 176, 30–37. doi: 10.1016/j.aquatox.2016.04.015
Navarro N. P., Huovinen P., Gómez I. (2016). Stress tolerance of Antarctic macroalgae in the early life stages. Rev. Chil. Hist. Nat. 89, 1–9. doi: 10.1186/s40693-016-0051-0
Niyogi K. K., Shih C., Chow W. S., Pogson B. J., DellaPenna D., Björkman O. (2001). Photoprotection in a zeaxanthin- and lutein-deficient double mutant of Arabidopsis. Photosynth. Res. 67, 139–145. doi: 10.1023/A:1010661102365
Oren A., Gunde-Cimerman N. (2007). Mycosporines and mycosporine-like amino acids: UV protectants or multipurpose secondary metabolites? FEMS Microbiol. Lett. 269, 1–10. doi: 10.1111/j.1574-6968.2007.00650.x
Pavia H., Brock E. (2000). Extrinsic factors influencing phlorotannin production in the brown alga Ascophyllum nodosum. Mar. Ecol. Prog. Ser. 193, 285–294. doi: 10.3354/meps193285
Piquet A. M.-T., van de Poll W. H., Visser R. J. W., Wiencke C., Bolhuis H., Buma A. G. J. (2014). Springtime phytoplankton dynamics in Arctic Krossfjorden and Kongsfjorden (Spitsbergen) as a function of glacier proximity. Biogeosciences 11, 2263–2279. doi: 10.5194/bg-11-2263-2014
Rangel K. C., Villela L. Z., de Castro Pereira K., Colepicolo P., Debonsi H. M., Gaspar L. R. (2020). Assessment of the photoprotective potential and toxicity of Antarctic red macroalgae extracts from Curdiea racovitzae and Iridaea cordata for cosmetic use. Algal Res. 50, 101984. doi: 10.1016/j.algal.2020.101984
Rautenberger R., Huovinen P., Gómez I. (2015). Effects of increased seawater temperature on UV-tolerance of Antarctic marine macroalgae. Mar. Biol. 162, 1087–1097. doi: 10.1007/s00227-015-2651-7
R Core Team. (2023). R: A language and environment for statistical computing (Vienna, Austria: R Foundation for Statistical Computing). Available at: https://www.R-project.org/.
Rodrigues M. A., Dos Santos C. P., Young A. J., Strbac D., Hall D. O. (2002). A smaller and impaired xanthophyll cycle makes the deep sea macroalgae Laminaria abyssalis (Phaeophyceae) highly sensitive to daylight when compared with shallow water Laminaria digitata. J. Phycol. 38, 939–947. doi: 10.1046/j.1529-8817.2002.t01-1-01231.x
Rodríguez-Rojas F., Celis-Plá P. S. M., Méndez L., Moenne F., Muñoz P. T., Lobos M. G., et al. (2019). MAPK pathway under chronic copper excess in green macroalgae (Chlorophyta): Involvement in the regulation of detoxification mechanisms. Int. J. Mol. Sci. 20, 4546. doi: 10.3390/ijms20184546
Sáez C. A., Roncarati F., Moenne A., Moody A. J., Brown M. T. (2015). Copper-induced intra-specific oxidative damage and antioxidant responses in strains of the brown alga Ectocarpus siliculosus with different pollution histories. Aquat. Toxicol. 159, 81–89. doi: 10.1016/j.aquatox.2014.11.019
Solomon S., Qin D., Manning M., Chen Z., Marquis M., Averyt K. B., et al. (2007). Climate Change 2007: The Physical Science Basis (Cambridge, UK, and New York, USA: Cambridge University Press), 996.
Stahl W., Sies H. (2007). Carotenoids and flavonoids contribute to nutritional protection against skin damage from sunlight. Mol. Biotechnol. 37, 26–30. doi: 10.1007/s12033-007-0051-z
Sterner R. W., Anderson T. R., Elser J. J., Hessen D. O., Hood J. M., McCauley E., et al. (2008). Scale-dependent carbon: Nitrogen: Phosphorus seston stoichiometry in marine and freshwaters. Limnol. Oceanogr. 53, 1169–1180. doi: 10.4319/lo.2008.53.3.1169
Tedetti M., Sempéré R. (2006). Penetration of ultraviolet radiation in the marine environment. A review. Photochem. Photobiol. 82, 389–397. doi: 10.1562/2005-11-09-IR-733
Turner J., Lu H., White I., King J. C., Phillips T., Hosking J. S., et al. (2016). Absence of 21st century warming on Antarctic Peninsula consistent with natural variability. Nature 535, 411–415. doi: 10.1038/nature18645
Underwood T. (1997). “Experiments in ecology,” in Their Logical Design and Interpretation Using Analysis of Variance (Cambridge UK: Cambridge University Press), 504.
van De Poll W. H., Maat D. S., Fischer P., Rozema P. D., Daly O. B., Koppelle S., et al. (2016). Atlantic advection driven changes in glacial melt- water: Effects on phytoplankton chlorophyll a and taxonomic composition in Kongsfjorden, Spitsbergen. Front. Mar. Sci. 3, 200. doi: 10.3389/fmars.2016.00200
Keywords: Antarctic seaweeds, photoprotective responses, carotenoids, phenolic compounds, mycosporine-like amino acids
Citation: Sáez CA, Troncoso M, Navarrete C, Rodríguez-Rojas F, Navarro N, Trabal A, Lavergne C, Pardo D, Brown MT, Gómez I, Figueroa FL and Celis-Plá PSM (2023) Photoprotective responses of three intertidal Antarctic macroalgae to short-term temperature stress. Front. Mar. Sci. 10:1223853. doi: 10.3389/fmars.2023.1223853
Received: 16 May 2023; Accepted: 23 August 2023;
Published: 25 October 2023.
Edited by:
Patrick J. Neale, Smithsonian Environmental Research Center (SI), United StatesReviewed by:
Ralf Rautenberger, Norwegian Institute of Bioeconomy Research (NIBIO), NorwayLeonel Pereira, University of Coimbra, Portugal
Copyright © 2023 Sáez, Troncoso, Navarrete, Rodríguez-Rojas, Navarro, Trabal, Lavergne, Pardo, Brown, Gómez, Figueroa and Celis-Plá. This is an open-access article distributed under the terms of the Creative Commons Attribution License (CC BY). The use, distribution or reproduction in other forums is permitted, provided the original author(s) and the copyright owner(s) are credited and that the original publication in this journal is cited, in accordance with accepted academic practice. No use, distribution or reproduction is permitted which does not comply with these terms.
*Correspondence: Paula S. M. Celis-Plá, cGF1bGFjZWxpc3BsYUB1cGxhLmNs