- 1Smithsonian Marine Station (SMS), Fort Pierce, FL, United States
- 2SECORE International, Miami, FL, United States
- 3Georgia Institute of Technology, Atlanta, GA, United States
- 4Scripps Institution of Oceanography, University of California, San Diego, La Jolla, CA, United States
Tetrabromopyrrole (TBP) is a readily biosynthesized marine proteobacterial compound that induces coral settlement (attachment and metamorphosis) at concentrations ranging from 50 – 250 ng ml-1 (0.13 – 0.65 µM). This suggests a great potential for the use of this compound as a settlement inducer for restoration purposes. However, the applicability and optimal concentration of TBP for many coral species is not yet known. Furthermore, TBP is an unstable compound, which may present both challenges and benefits to its potential use for restoration purposes. In order to assess the utility of this compound for restoration, settlement induction by TBP was assessed among a wide range of Caribbean coral species. Additionally, a suite of halogenated compounds (tribromopyrrole, pentabromopseudolin, dibromophenol, tribromophenol, bromophene, n-methyl tetrabromopyrrole, tetrachloropyrrole, dibromoindole, n-methyl tetrachloropyrrole and dibromopyridine) that are related to TBP, some of which have similar antibiotic and antialgal properties, were also tested for settlement induction activity. These compounds were chosen based on their structural similarity to TBP or their identity as a product within the bacterial TBP biosynthetic pathway. TBP induced settlement in nine of ten coral species tested including seven not previously reported (Dendrogyra cylindrus, Orbicella faveolata, Colpophyllia natans, Diploria labyrinthiformis, Pseudodiploria clivosa, Acropora cervicornis, Montastraea cavernosa) at concentrations ranging from 0.375 – 1.5 µM. No other compound tested induced settlement, demonstrating a high degree of specificity for TBP.
1 Introduction
Coral reefs are undergoing rapid degradation globally (Gardner et al., 2003; Pandolfi et al., 2003; Hughes et al., 2017). In many parts of the world, reefs have reached a level of degradation that requires a shift in efforts from conservation to restoration (Boström-Einarsson et al., 2020; Knowlton et al., 2021; Suggett and Van Oppen, 2022). Coral restoration is typically approached using one of two propagation methods: 1) asexual fragmentation of adult corals or 2) production of sexually propagated juvenile corals (Omori, 2019; Boström-Einarsson et al., 2020; Suggett and Van Oppen, 2022). The latter has the benefit of producing higher genetic diversity and the potential to greatly increase the scale of restoration efforts (Baums et al., 2019). However, the use of sexually propagated juveniles has a variety of challenges. The success of restoration using sexually propagated corals is dependent on the settlement (attachment and metamorphosis) of swimming planula larvae onto appropriate substrates (Randall et al., 2020; Banaszak et al., 2023). For restoration purposes, settlement substrates are generally placed in a reef or oceanic environment for weeks to months to obtain the necessary microbial biofilms and other settlement inducing organisms (Suzuki et al., 2020; Miller et al., 2022). Alternatively, settlement inducing crustose coralline algae (CCA) are harvested and placed in containers where settlement is staged (Henry et al., 2019). Neither approach is scalable (i.e., labor costs are high and CCA supply is limiting) and both can have inconsistent outcomes (i.e., settlement is not even across substrates). Therefore, the development of a consistent, low-cost settlement cue that could be applied to substrates or to the water column to enhance settlement would greatly increase the effectiveness of restoration via sexual propagation (Randall et al., 2020). The identification of biochemical cues that induce settlement in a wide range of coral species would be invaluable, filling a high-priority knowledge gap for successful coral restoration efforts (Randall et al., 2020).
The use of chemical cues to enhance coral settlement for restoration purposes was first proposed in the 1990’s (Morse et al., 1994). There have been several attempts to identify chemical cues responsible for the natural induction of coral settlement as well as compounds that could be used to induce settlement artificially. Although not all fully characterized, several compounds extracted from crustose coralline algae and their associated bacteria have been discovered that induce settlement in some corals (Morse and Morse, 1991; Harrington et al., 2004; Kitamura et al., 2007; Tebben et al., 2011; Sneed et al., 2014; Tebben et al., 2015; Petersen et al., 2021). These include two fully characterized, bacterially produced settlement cues, tetrabromopyrrole (Tebben et al., 2011; Sneed et al., 2014) and cycloprodigiosin (Petersen et al., 2021; Petersen et al., 2023). Several cnidarian neurotransmitters (Hym-248, dopamine, glutamic acid, epinephrine) also induce coral settlement, presumably by hijacking part of the signaling cascade responsible for attachment and metamorphosis (Iwao et al., 2002; Erwin and Szmant, 2010; Moeller et al., 2019). These cues have not yet been successfully applied to restoration purposes. Some are likely too complex to make them feasible for cost-effective large-scale synthesis. None have been tested on more than a few coral species.
Tetrabromopyrrole (TBP) 1 (Figure 1) is a polyhalogenated compound produced by marine bacteria that was first noted for its antibiotic properties against both Gram-positive and Gram-negative bacteria (Andersen et al., 1974). In addition, TBP exhibits antialgal, anti-protozoan and antifungal activity (Tebben et al., 2014; Whalen et al., 2018). The biosynthetic genes for TBP production were first characterized from Pseudoalteromonas Gammaproteobacteria (El Gamal et al., 2016; Busch et al., 2019), and gene homologs have been found globally, largely associated with coastal environments (within 10 km of shorelines) (Whalen et al., 2018). More recently TBP has gained attention for its ability to induce metamorphosis in corals. In some cases, this metamorphosis occurs without attachment (Tebben et al., 2011; Tebben et al., 2015), interrupting the full settlement process, while in others, exposure to TBP results in complete settlement (attachment and metamorphosis) (Sneed et al., 2014; Nietzer et al., 2018; Alker et al., 2023). The latter was demonstrated with the Caribbean spawning corals Acropora palmata and Orbicella franski and the brooding coral Porites astreoides (Sneed et al., 2014) as well as the Pacific brooding coral Leptastrea purpurea (Nietzer et al., 2018). The response of corals to TBP is concentration-dependent. If concentrations are too low there is no change in coral settlement behavior, while concentrations that are too high result in death of the larvae (Sneed et al., 2014; Nietzer et al., 2018). TBP is unstable and light-sensitive (Andersen et al., 1974) and it degrades easily in aqueous solutions (Agarwal et al., 2014). These qualities present challenges to its application in restoration practice. However, it is a simple molecule that is readily synthesized (Palmer, 1967; Tebben et al., 2011; El Gamal et al., 2016). Several related brominated pyrroles have demonstrated antibiotic and antialgal activity similar to TBP but have not been tested for coral settlement activity (Burkholder et al., 1966; Whalen et al., 2018). In order to evaluate the potential for chemical induction of coral settlement for restoration, we tested TBP and a suite of related compounds against a broad range of Caribbean corals, including ten species, eight genera, seven families and two reproductive strategies. Compounds were tested at a range of concentrations to test for dose dependent activity.
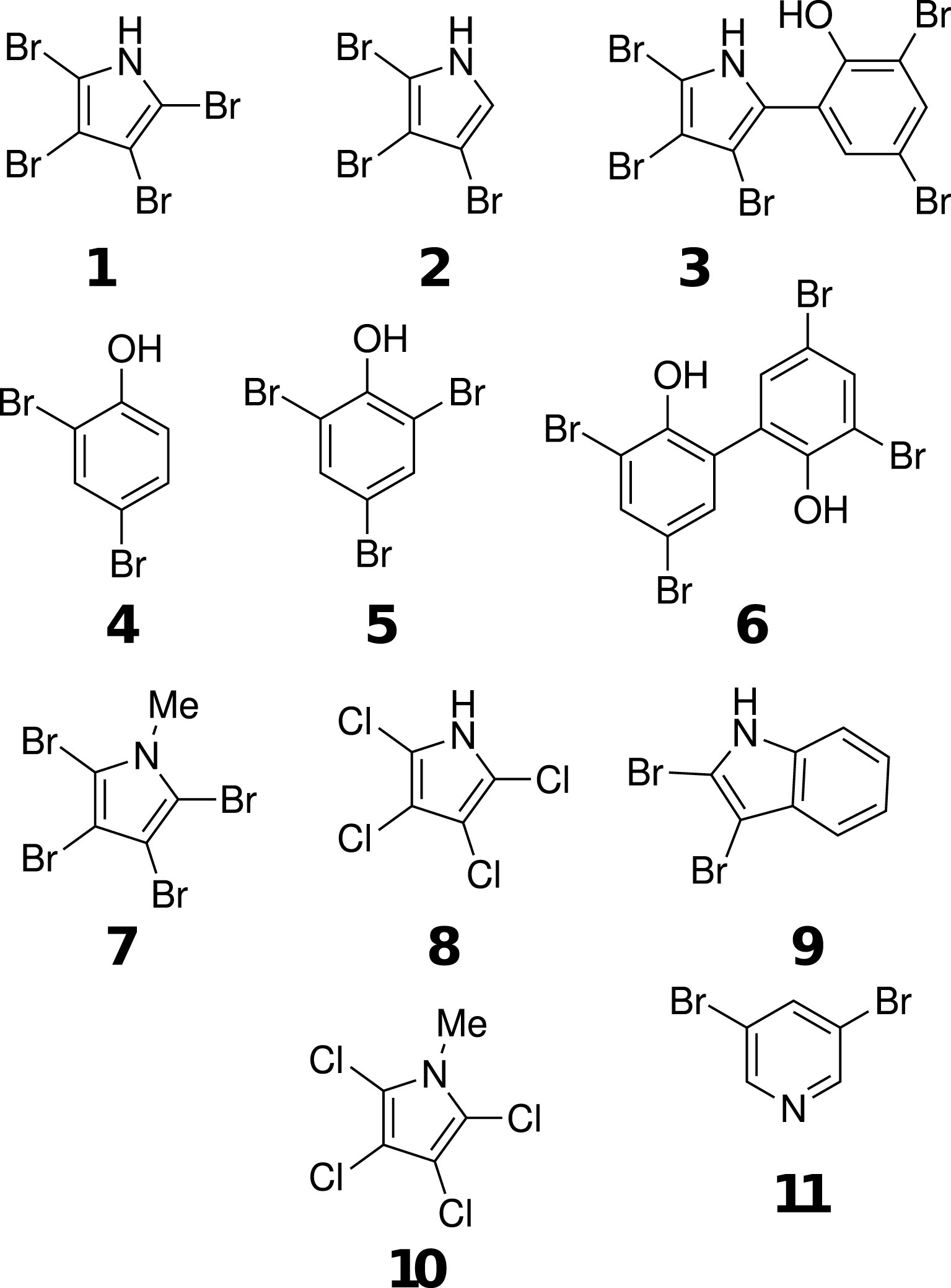
Figure 1 Structures of compounds tested in settlement assays. Tetrabromopyrrole (TBP) 1, tribromopyrrole (Br3Py) 2, pentabromopseudolin (Penta) 3, dibromophenol (DiBrPh) 4, tribromophenol (TrBrPh) 5, bromophene (Bromo) 6, n-methyl tetrabromopyrrole (Me-TBP) 7, tetrachloropyrrole (TCP) 8, dibromoindole (DBI) 9, n-methyl tetrachloropyrrole (Me-TCP) 10 and dibromopyridine (DiBPy) 11.
2 Methods
Two sets of compounds were tested for settlement induction activity. In the first set, five products of the Pseudoalteromonas luteoviolacea polybrominated marine pyrrole/phenol biosynthetic pathway (tribromopyrrole 2, pentabromopseudolin 3, dibromophenol 4, tribromophenol 5 and bromophene 6, Figure 1) (El Gamal et al., 2016) and TBP 1 itself were tested for settlement induction at a range of concentrations (0.375 μM, 0.75 μM, and 1.5 μM) in the brooding coral Porites astreoides and the spawning coral Acropora palmata. TBP alone was also tested in the spawning coral A. cervicornis at these concentrations. In the second set, five compounds structurally similar to TBP (n-methyl tetrabromopyrrole 7, tetrachloropyrrole 8, dibromoindole 9, n-methyl tetrachloropyrrole 10, dibromopyridine 11, Figure 1) and TBP were tested at 0.25 μM and 0.5 μM with Colpophyllia natans, Dendrogyra cylindrus, Diploria labyrinthiformis, Orbicella faveolata, Montastraea cavernosa, Porites astreoides and Pseudodiploria strigosa. TBP alone was also tested with Pseudodiploria clivosa at 0.25 μM and 0.5 μM concentrations. TBP, n-methyl tetrabromopyrrole 7, tetrachloropyrrole 8, and n-methyl tetrachloropyrrole 10 were also tested at higher concentrations (up to 2 μM) with O. faveolata larvae. All compounds were tested within the range of concentrations previously determined to be optimal for TBP induced settlement (Sneed et al., 2014). Details on compound synthesis for TBP 1, n-methyl tetrabromopyrrole 7, tetrachloropyrrole 8, n-methyl tetrachloropyrrole 10, and dibromopyridine 11 used in this study are provided in the Supplementary Information (Supplementary Figures 1–5). Synthesis of tribromopyrrole 2 used in this study is described in El Gamal et al. (2016). Pentabromopseudolin 3 and bromophene 6 were isolated from Pseudoalteromonas sp. cultures (Agarwal et al., 2014). Dibromophenol 4, tribromophenol 5 and dibromoindole 9 were purchased commercially from Sigma.
2.1 Larval collection and maintenance
Acropora cervicornis and A. palmata were collected during the annual spawning event in August of 2016 at Carrie Bow Cay, Belize following methods described in Ritson-Williams et al. (2014). Porites astreoides larvae were collected in the Florida Keys in 2016 and 2021 as described in Kuffner et al. (2006). All other larvae were provided by coral restoration and research partners (Table 1). All larvae provided by external partners were transported to the Smithsonian Marine Station (SMS) in 1 L Nalgene bottles of seawater. At SMS, larvae were stored in polystyrene take-out containers in 0.2 µm filter-sterilized seawater (FSW) at room temperature (~ 25-28°C) until use in assays. Larvae were transferred to clean containers with FSW as needed. Larvae of the brooding coral P. astreoides are competent for metamorphosis when they are released and were used within 3 days after release. Larval development and competency occur 3-4 days post-fertilization in the spawning species tested here. Larvae were used in assays upon demonstrating swimming behavior and morphology consistent with competency, 3-9 days post-fertilization (Table 1).
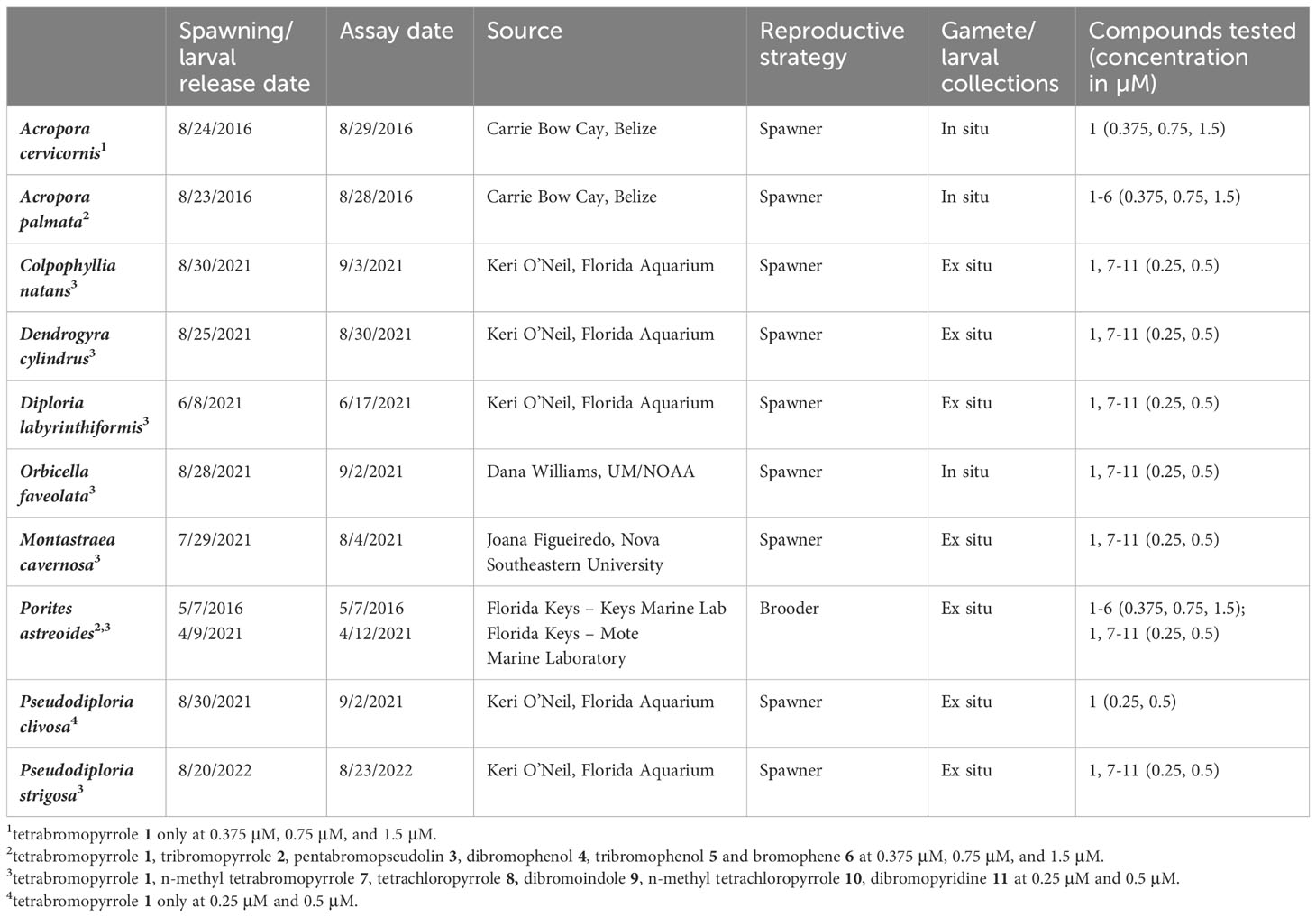
Table 1 Spawn dates, assay dates and sources of larvae from different coral species used in this study.
2.2 Larval assays
Stock solutions of all compounds were prepared in dimethyl sulfoxide (DMSO) and stored at -20 °C until use. Settlement assays were performed in sterile, polystyrene 6-well plates with 10 ml FSW. Compounds were diluted in DMSO to 1000x the final concentration and 10 µl was added to each assay well. In addition to compound treatments, each experiment included a solvent control (1‰ DMSO) as well as a FSW control with nothing added. The first set of assays (compounds 1-6) also included a small piece of Hydrolithon boergesenii or unidentified crustose coralline algae (CCA) as a positive control while the second set (compounds 1, 7-11) did not because of a lack of availability of CCA. Except for the wells with CCA, no other substrate was added to the wells. Immediately after treatments were added, 10 larvae were counted into each well in a minimal amount of seawater to minimize effects on compound concentration. Spawning or larval release dates and assay dates are given in Table 1. Five replicate wells were used per treatment and were randomized among the well plates using a random number generator. Settlement was scored as the total number metamorphosed and attached to the plastic well or CCA after 24 hours. Attachment was confirmed by gentle shaking of the plate and/or gentle water flow from a glass pipet. Larvae that remained swimming and those that metamorphosed but did not attach were also recorded. Survival was scored as the total number of live corals (swimming larvae and metamorphosed combined). Mean proportion settlement, unattached metamorphosed, and survival were calculated based on the starting number of larvae (10).
2.3 Statistics
Proportion data were tested for normality and homogeneity of variance using the Shapiro-Wilk and Bartlett tests and failed to meet these assumptions. Arcsine square root transformation did not change the normality or homogeneity of variance; therefore, the data were subjected to non-parametric statistical analysis using the Kruskal-Wallis Rank Sum Test followed by a Dunn’s posthoc test using the Benjamini-Hochberg correction for multiple comparisons to identify differences between treatments. Alpha was set at p = 0.05 and the null hypothesis rejected when p < α/2 according to the Benjamini-Hochberg method. Statistical analyses were run in R version 4.2.0 (R Core Team, 2023) using the package dunn.test (Dinno, 2017). All results are reported as mean proportion ± standard error. Significance of settlement induction was determined relative to DMSO controls since all compounds were dissolved in DMSO for the assays.
3 Results and discussion
Tetrabromopyrrole (TBP) 1 induced significant attachment and metamorphosis (referred to as settlement hereafter) in nine of ten coral species (Figures 2A, 3) at concentrations similar to the range (125 – 250 ng ml-1 = 0.375 - 0.65 µM) that induced the most settlement in P. astreoides and O. franksi in previous work (Sneed et al., 2014). The tenth species, Pseudodiploria strigosa, settled at higher numbers when exposed to TBP (36 ± 18% and 42 ± 17% at 0.25 µM and 0.5 µM TBP) compared to DMSO controls (4 ± 2%, Figure 2A), but this difference was not statistically significant according to the non-parametric Dunn’s Test (p = 0.077 and 0.091 respectively).
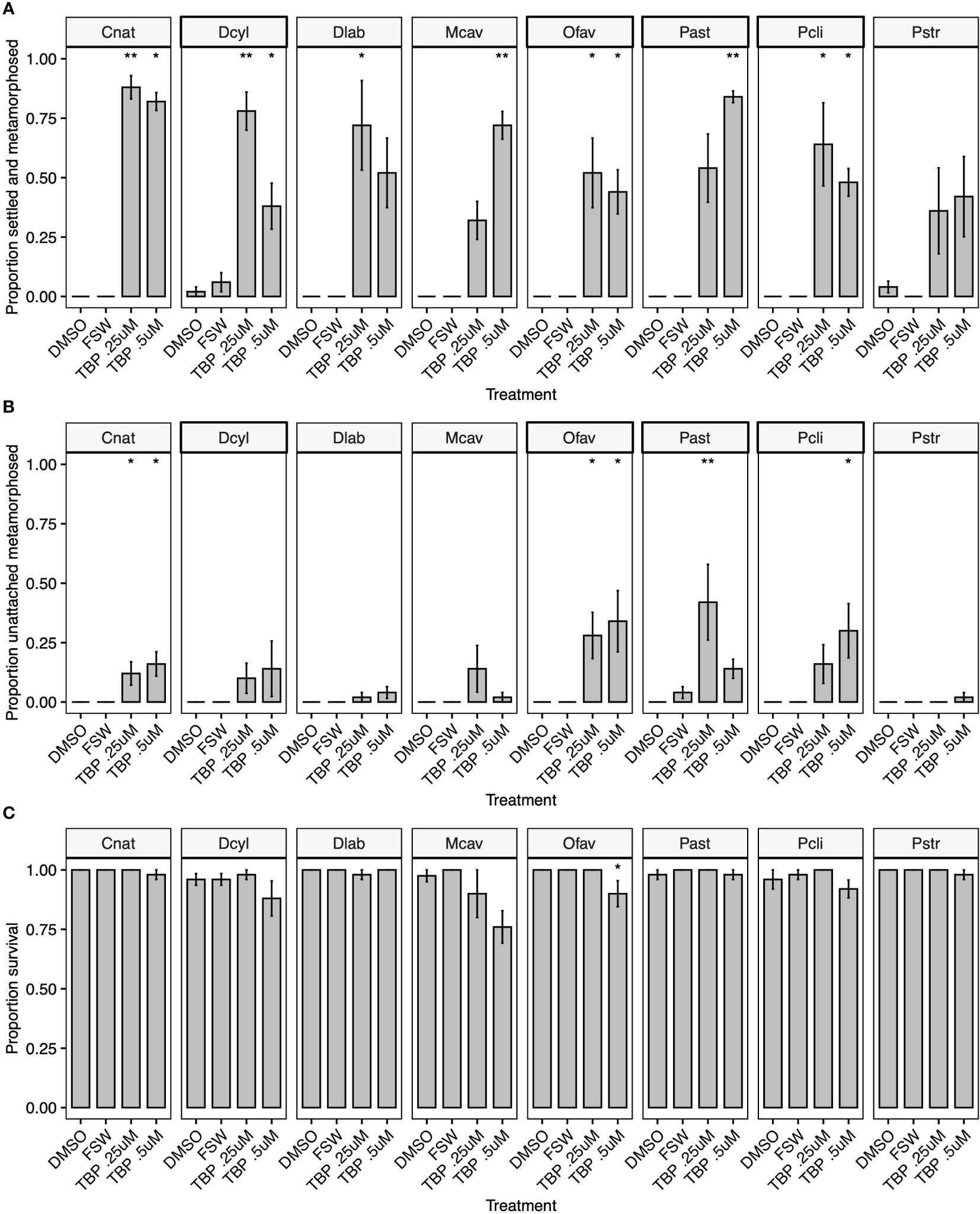
Figure 2 (A) Settlement response (attachment and metamorphosis), (B) metamorphosis without attachment and (C) total survival of eight coral species (Colpophyllia natans – Cnat, Dendrogyra cylindrus – Dcyl, Diploria labyrinthiformis – Dlab, Montastraea cavernosa – Mcav, Orbicella faveolata – Ofav, Porites astreoides – Past, Pseudodiploria clivosa – Pcli, Pseudodiploria strigosa – Pstr) 24 hours after treatment with 0.25 µM or 0.5 µM TBP delivered in 1‰ DMSO, solvent alone (1‰ DMSO) and filter-sterilized seawater controls (FSW). Bars indicate mean proportion settled and metamorphosed ± SE, n = 5. Asterisks indicate significant difference from DMSO controls determined by a Dunn’s test using a Benjamini-Hochberg correction for multiple comparisons (*p<0.05, **p<0.01).
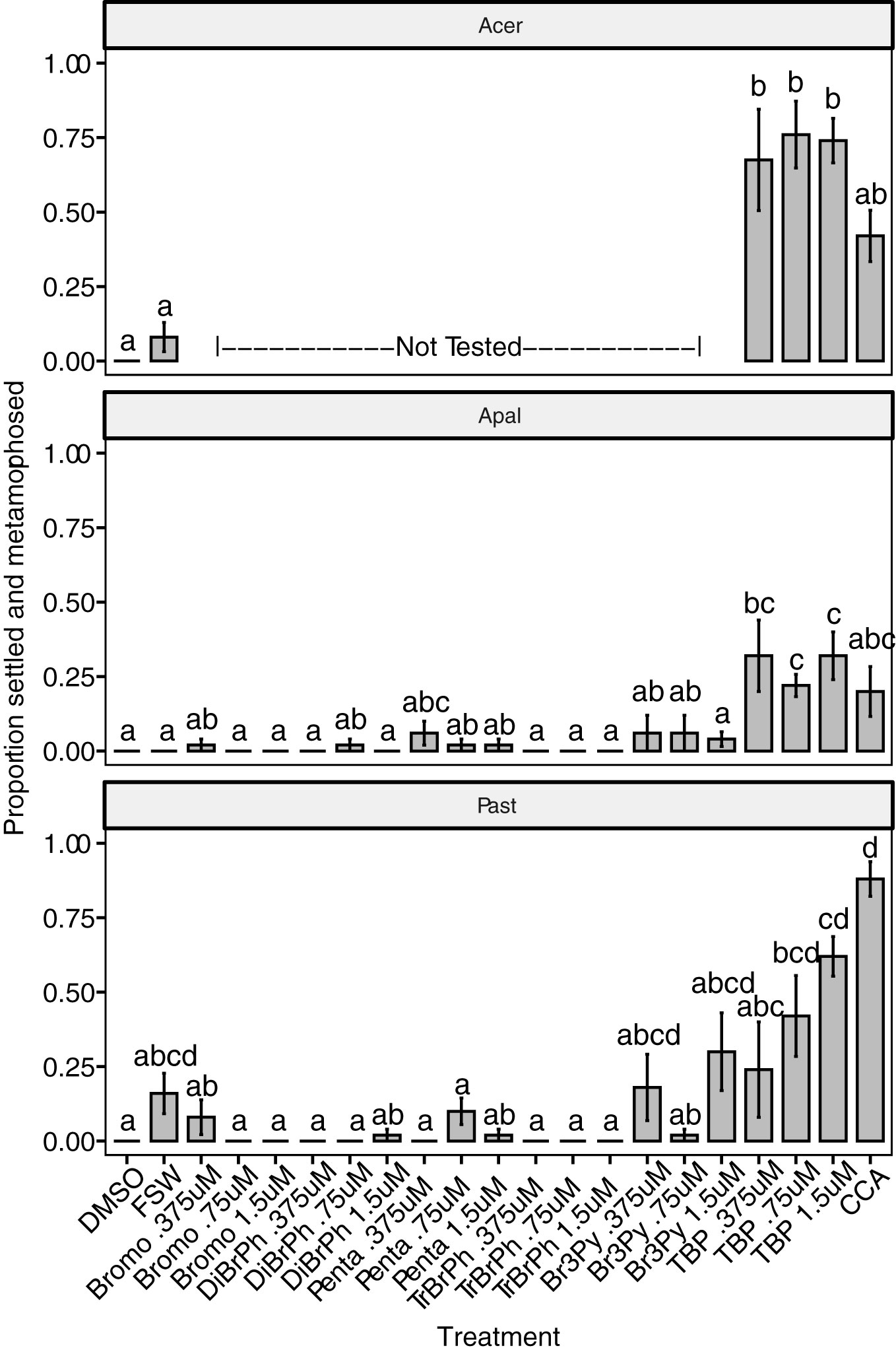
Figure 3 Settlement response (attachment and metamorphosis) of three coral species (Acropora cervicornis – Acer, A. palmata – Apal, Porites astreoides – Past) after treatment with six different compounds (bromophene – Bromo 6, dibromophenol – DiBrPh 4, pentabromopseudolin – Penta 3, tribromophenol – TrBrPh 5, tribromopyrrole – Br3Py 2, tetrabromopyrrole – TBP 1) at three concentrations (0.375 µM, 0.75 µM, 1.5 µM) delivered in 1‰ DMSO, solvent alone (1‰ DMSO), the CCA Hydrolithon boergesenii, and filter-sterilized seawater controls (FSW). Bars indicate mean proportion settled and metamorphosed ± SE, n = 5. Different letters above bars indicate significant differences among treatments as determined by a Dunn’s test using a Benjamini-Hochberg correction for multiple comparisons.
This is the first reported assay to test TBP settlement induction for eight of the coral species tested (Acropora cervicornis, Colpophyllia natans, Dendrogyra cylindrus, Diploria labyrinthiformis, Montastraea cavernosa, Orbicella faveolata, Pseudodiploria clivosa and P. strigosa). Excluding P. strigosa, each species settled significantly in response to at least one of the TBP concentrations tested, while some settled at multiple concentrations (Figures 2A, 3). M. cavernosa and P. astreoides had significant settlement at higher TBP concentrations (0.5 µM and 0.5 µM - 1.5 µM, respectively), but not lower (Figures 2A, 3). Conversely, D. labyrinthiformis had significantly higher settlement in 0.25 µM TBP compared to DMSO controls, but not at 0.5 µM (Figure 2A), demonstrating the difference in sensitivity to this compound among coral species. For some coral species, TBP induced not only significant settlement, but also significant amounts of metamorphosis without attachment (Figure 2B). The proportion unattached and metamorphosed appears to be inversely related to the proportion attached and metamorphosed at the concentrations tested here (Figure 2B). The loss of activity at higher concentrations was previously observed in other species and is likely caused by an overdose effect in these larvae, although there is no significant decrease in survival in most of these species at 0.5 µM (Figure 2C, Sneed et al., 2014). TBP did significantly reduce survival in O. faveolata at 0.5 µM but did not affect survival in any other coral species at any concentration tested (Figures 2C, 4). In a subsequent experiment with O. faveolata at higher concentrations of TBP, n-methyl tetrabromopyrrole, tetrachloropyrrole and n-methyl tetrachloropyrrole, survival was not affected at 0.5 µM TBP but was significantly reduced at 1.0 µM TBP (Figure 5). All other species had significant settlement in response to all TBP concentrations tested (Figures 2A, 3, 0.25 µM and 0.5 µM for C. natans, D. cylindrus, O. faveolata, P. clivosa and 0.375, 0.75 and 1.5 µM for A. palmata, A. cervicornis).
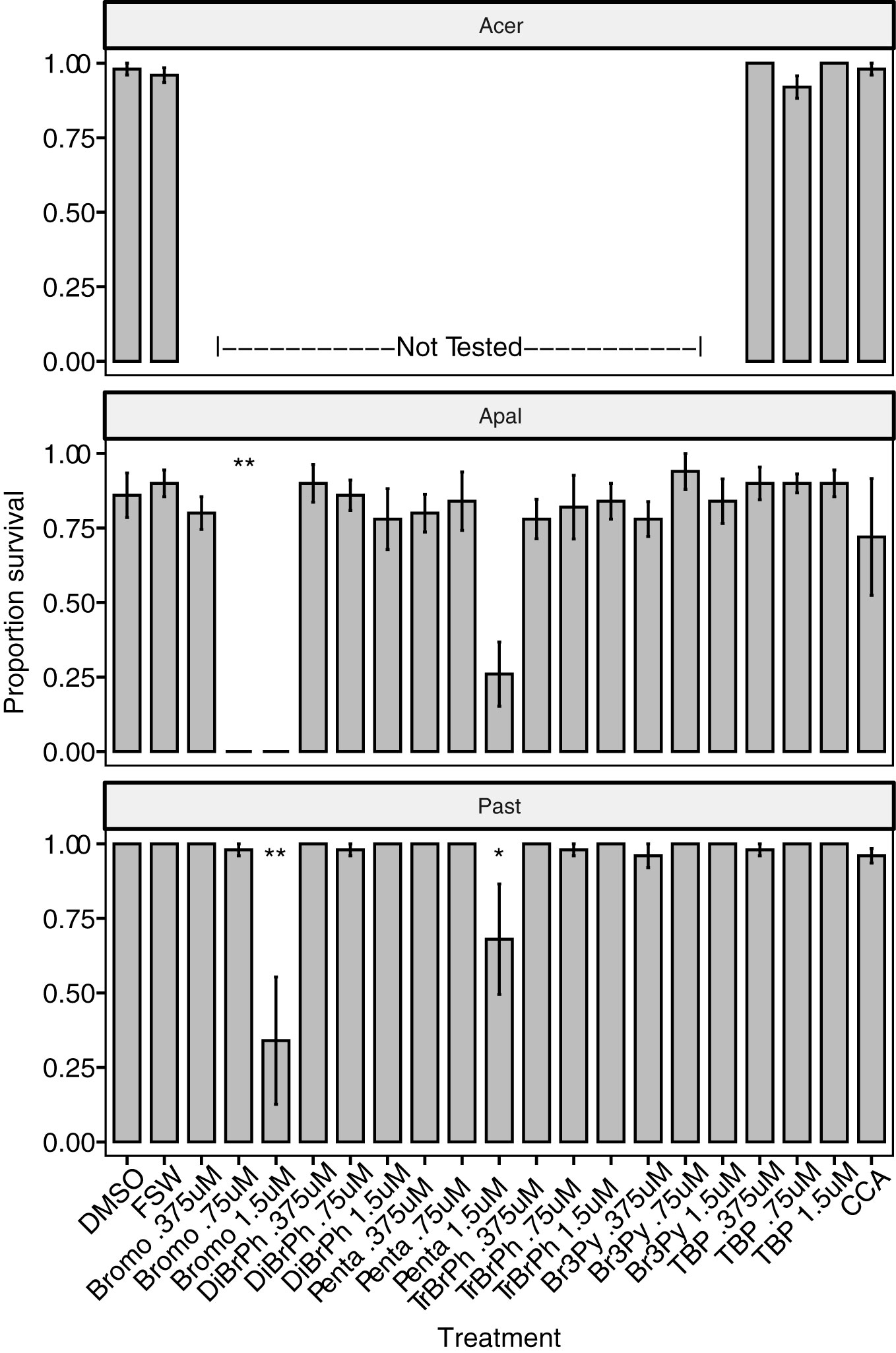
Figure 4 Survival of three coral species (Acropora cervicornis – Acer, A. palmata – Apal, Porites astreoides – Past) 24 hours after treatment with six different compounds (bromophene – Bromo 6, dibromophenol – DiBrPh 4, pentabromopseudolin – Penta 3, tribromophenol – TrBrPh 5, tribromopyrrole – Br3Py 2, tetrabromopyrrole – TBP 1) at three concentrations (0.375 µM, 0.75 µM, 1.5 µM) delivered in 1‰ DMSO, solvent alone (1‰ DMSO), the CCA Hydrolithon boergesenii and filter-sterilized seawater (FSW) controls. Bars indicate mean proportion survival ± SE, n = 5. Asterisks indicate significant difference from DMSO controls determined by a Dunn’s test using a Benjamini-Hochberg correction for multiple comparisons (*p<0.05, **p<0.01).
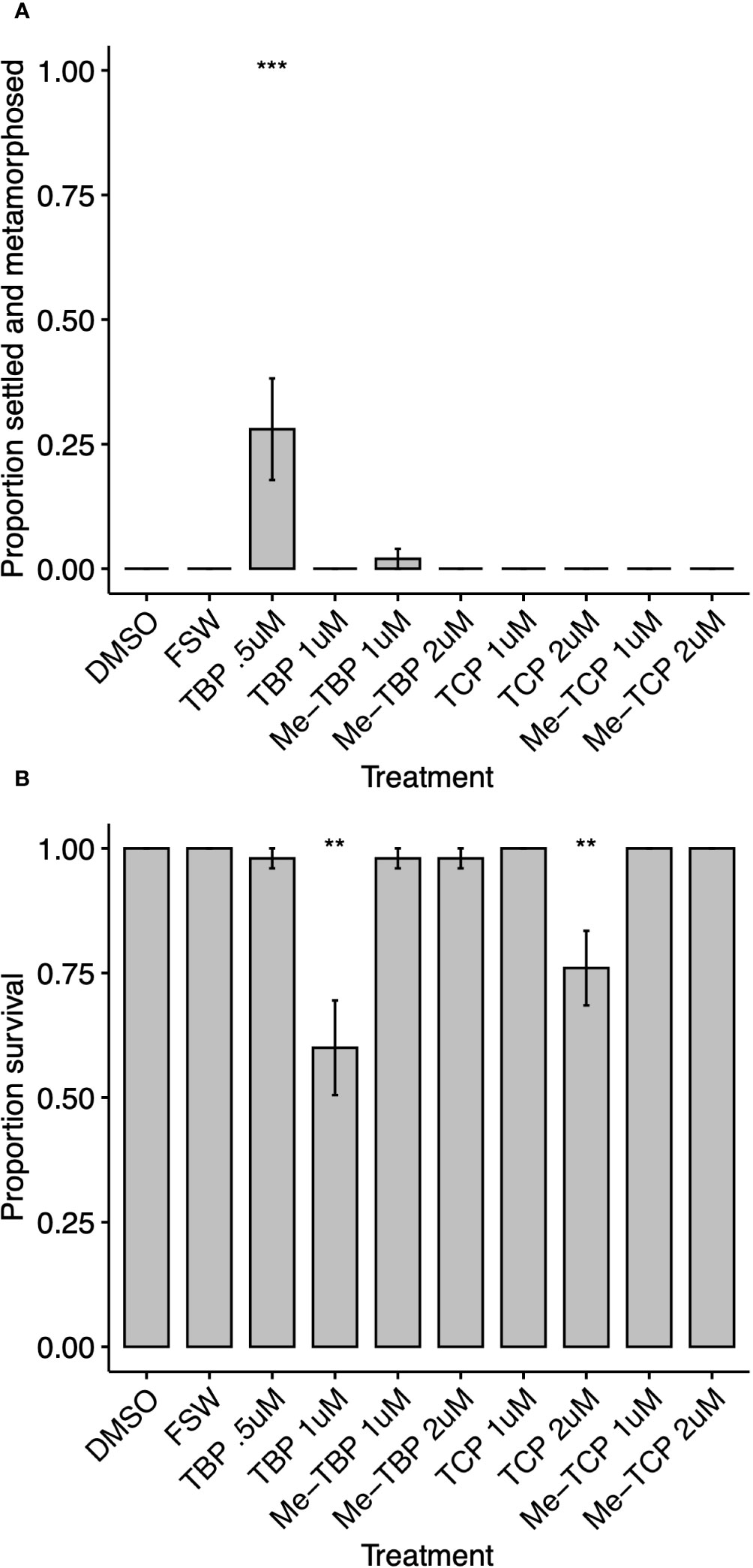
Figure 5 (A) Settlement response (attachment and metamorphosis) and (B) survival of Orbicella faveolata 24 hours after treatment with TBP 1 (0.5 and 1 µM), n-methyl tetrabromopyrrole – Me-TBP 7, tetrachloropyrrole - TCP 8, n-methyl tetrachloropyrrole – Me-TCP 10 (1 and 2 µM) delivered in 1‰ DMSO, solvent alone (1‰ DMSO) and filter-sterilized seawater controls (FSW). Bars indicate mean proportion settled and metamorphosed ± SE, n = 5. Asterisks indicate significant difference from DMSO controls determined by a Dunn’s test using a Benjamini-Hochberg correction for multiple comparisons (**p<0.01, ***p<0.001).
Two of the species tested (A. palmata and P. astreoides) had previously been reported to settle in response to TBP (Sneed et al., 2014). Our results confirm the settlement of A. palmata and P. astreoides in response to TBP although the active concentration ranges varied slightly from previous reports. A. palmata settled in response to 0.375 and 0.75 µM TBP, which are within the range previously reported (12.5 - 250 ng ml-1; 0.037 - 0.65 µM) (Sneed et al., 2014), but also to a higher concentration (1.5 µM) of TBP which is close to the concentration (500 ng ml-1; 1.3 µM) that caused a decline in settlement in previous work (Figure 3). P. astreoides settled at 0.75 - 1.5 µM TBP concentrations, which corresponds to the upper end of the range previously reported (50 – 500 ng ml-1; 0.13 - 1.3 µM), but did not have significant settlement relative to DMSO controls at lower concentrations (0.25 - 0.375 µM, Figures 2A, 3). It should be noted that TBP is unstable after isolation, and therefore concentrations may be slightly skewed from one experiment to another depending on the amount of compound degradation that has occurred. Further work is necessary to determine the rate of degradation under varying storage and use conditions.
Coral larvae demonstrated a high degree of specificity in their settlement response to TBP. There was no significant settlement after exposure to any of the ten other compounds tested at a range of concentrations from 0.25 – 2 µM (Table 2, Figures 3, 5) despite structural and/or biosynthetic similarities to TBP (Figure 1). It is interesting to note that studies of the antibiotic and antialgal effects of TBP find that similar brominated, pyrrole-containing compounds retain activity, typically associated with the degree of bromination (Tebben et al., 2015; Whalen et al., 2018). Pentabromopseudilin 3, another marine bacterial antibiotic compound that contains a TBP-derived polybrominated pyrrole ring (El Gamal et al., 2016) caused inhibition of growth in the coccolithophore Emiliana huxleyi with an IC50 (1.3 nM) that was almost two orders of magnitude less than TBP (99 nM) (Whalen et al., 2018). TBP is produced as an intermediate in the biosynthetic pathway for pentabromopseudilin (El Gamal et al., 2016). Despite its similar molecular characteristics, biosynthetic relationship and similar antialgal (Whalen et al., 2018) and antibacterial activity (Burkholder et al., 1966) to TBP, pentabromopseudilin does not induce settlement in coral larvae (Figure 3). Tribromopyrrole 2, which differs from TBP only by the loss of one bromine atom, also inhibited E. huxleyi growth at concentrations within the range tested here (0.106 µM and 0.476 µM) (Whalen et al., 2018), but did not induce settlement of any coral species tested (Figure 3).
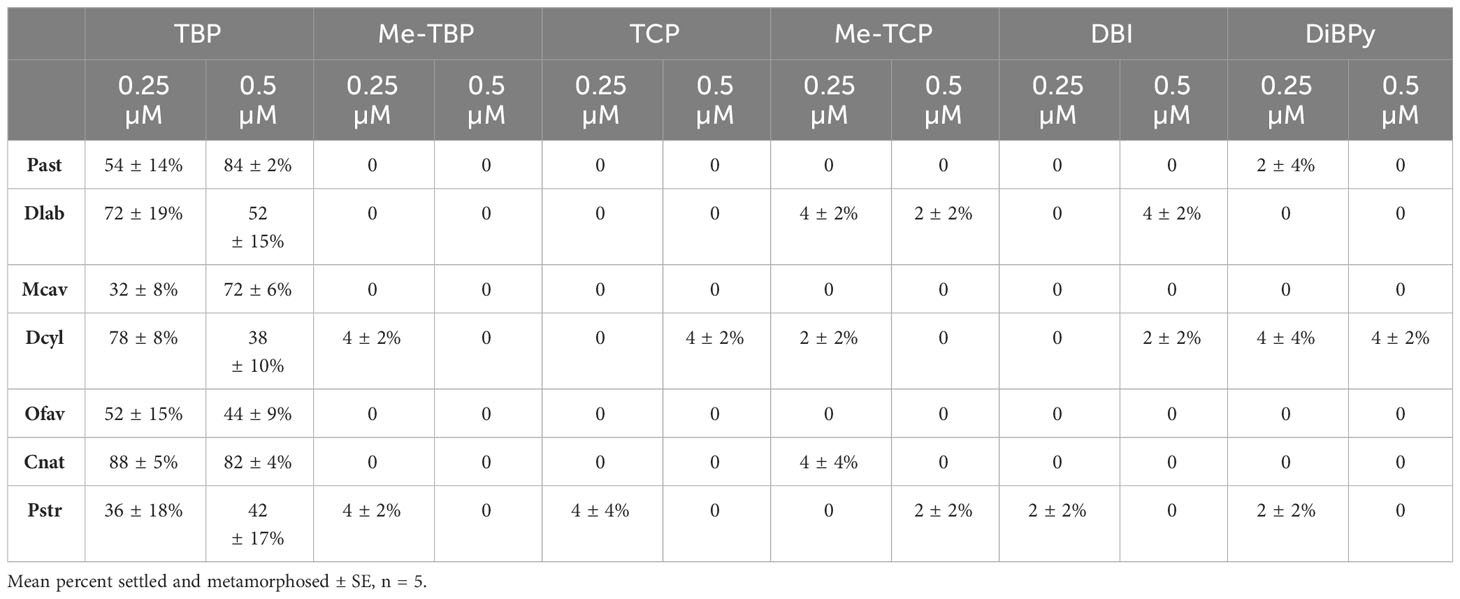
Table 2 Settlement response (attachment and metamorphosis) of coral species (Colpophyllia natans – Cnat, Dendrogyra cylindrus – Dcyl, Diploria labyrinthiformis – Dlab, Montastraea cavernosa – Mcav, Orbicella faveolata – Ofav, Porites astreoides – Past, Pseudodiploria strigosa - Pstr) after treatment with TBP and five other compounds (n-methyl tetrabromopyrrole – Me-TBP 7, tetrachloropyrrole – TCP 8, n-methyl tetrachloropyrrole – Me-TCP 10, dibromoindole – DBI 9, dibromopyridine – DiBPy 11) at 0.25 µM or 0.5 µM TBP delivered in 1‰ DMSO.
While the mode of action for TBP settlement induction is still unknown, TBP causes increases in intracellular reactive oxygen species (ROS) and Ca2+ ions in E. huxleyi cells at very low concentrations (10 and 100 nM TBP) (Whalen et al., 2018). Petersen et al. (2023) found that the bacterial pigment cycloprodigiosin induces settlement (attachment and metamorphosis) in Leptastrea purpurea and suggest that production of the ROS H2O2 resulting from the light-dependent degradation of cycloprodigisin is responsible for the metamorphosis that occurs during settlement. Exogenous application of H2O2 also induces settlement in the brooding corals Favia fragum and P. astreoides but not the spawning corals A. palmata and O. franksi (Ross et al., 2017). It may be that TBP causes increases in intracellular ROS in coral larvae similar to those in E. huxleyi that plays a role in coral metamorphosis. However, settlement induction by H2O2 has only been demonstrated in larvae of brooding corals that contain algal symbionts while TBP induces settlement in brooding and spawning corals and specifically A. palmata and O. franksi, which did not respond to H2O2 in previous studies (Sneed et al., 2014; Ross et al., 2017). Zheng et al. (2018) also identified a potential role for TBP in cellular Ca2+ dynamics through interactions with mammalian ryanodine receptors, and direct application of Ca2+ promotes settlement in the brooding coral Pocillopora damicornis (Yang et al., 2022). It is possible that TBP is triggering Ca2+ dependent signaling cascades resulting in coral settlement by increasing intracellular Ca2+.
TBP has demonstrated antibacterial, antifungal, antialgal activities and has been shown to inhibit embryo development in sea urchins (Tebben et al., 2014; Whalen et al., 2018; Akkipeddi et al., 2021). In at least some cases, the negative impacts of TBP reported are at higher concentrations than those needed to induce settlement (Akkipeddi et al., 2021). While the antibacterial activity could be beneficial to coral settled with TBP, the toxicity of TBP to other marine organisms is a concern that needs to be accounted for in the use of this compound in situ for restoration. The rapid degradation of TBP presents a challenge in terms of application of precise concentrations especially in coral restoration contexts remote from laboratory facilities. However, its instability could be beneficial in that it will break down quickly in the environment, limiting any unintended effects of its application (Zheng et al., 2018).
4 Conclusions
TBP induces settlement in a broad range of Caribbean corals spanning ten species, nine genera and seven families. The species tested represent members of both the spawning and brooding reproductive strategies. The optimal concentration differs slightly among coral species, but a concentration near 0.25 µM induced settlement in all coral species tested, while 0.5 µM was too high for some species and may become toxic. The broad-spectrum activity of TBP among corals makes it a good candidate for applications to enhance settlement in restoration pipelines; however, further research on its stability, application methods and broader impacts on marine organisms is needed. Methods of transportation and compound handling need to be optimized to ensure its effectiveness. Application methods to the larvae during the settlement process need to be explored to determine the feasibility of using TBP to induce settlement on a large scale. Innovation in the stabilization and time release of TBP could prove invaluable.
Data availability statement
The datasets presented in this study can be found in online repositories. The names of the repository/repositories and accession number(s) can be found below: https://figshare.com/s/ffd00e932fa6ed29b67d.
Ethics statement
The manuscript presents research on animals that do not require ethical approval for their study.
Author contributions
JS: Conceptualization, Data curation, Formal analysis, Funding acquisition, Investigation, Methodology, Project administration, Validation, Visualization, Writing – original draft, Writing – review & editing. AD: Investigation, Writing – review & editing. MM: Conceptualization, Funding acquisition, Writing – review & editing. DY: Investigation, Methodology, Resources, Writing – review & editing. BM: Investigation, Resources, Writing – review & editing. VA: Conceptualization, Investigation, Resources, Writing – review & editing. VP: Conceptualization, Funding acquisition, Investigation, Supervision, Writing – review & editing.
Funding
The author(s) declare financial support was received for the research, authorship, and/or publication of this article. Funding was provided by Oceankind Labs Initiative (MM, JS, VP), Florida Department of Environmental Protection (JS, VP), NIH (NIGMS) grant R35GM142882 (VA) and NSF grant OCE-1837116 (BM).
Acknowledgments
Porites astreoides larvae were collected under permits FKNMS-2015-11 and FKNMS-2019-024. We thank Belize Fisheries Department for supporting research performed in Belize. We thank Joana Figueiredo and her lab at Nova Southeastern University, Keri O’Neil and the Florida Aquarium Coral Conservation Program and Dana Williams with University of Miami Cooperative Institute for Marine and Atmospheric Studies for providing larvae. We thank Aurora Giorgi, Tommy DeMarco and Amanda Alker for assistance setting up and scoring experiments. We would like to thank Erich Bartels and Cory Walter for field assistance in coral collection and return. Orbicella faveolata collections used for this work were funded by NOAA Coral Reef Conservation Program and NOAA Southeast Fisheries Science Center. Gametes were collected under FKNMS permit number FKNMS-2018-163 by NOAA SEFSC’s Coral Team. This is Smithsonian Marine Station contribution no. 1209 and CCRE contribution no. 1075.
Conflict of interest
The authors declare that the research was conducted in the absence of any commercial or financial relationships that could be construed as a potential conflict of interest.
Publisher’s note
All claims expressed in this article are solely those of the authors and do not necessarily represent those of their affiliated organizations, or those of the publisher, the editors and the reviewers. Any product that may be evaluated in this article, or claim that may be made by its manufacturer, is not guaranteed or endorsed by the publisher.
Supplementary material
The Supplementary Material for this article can be found online at: https://www.frontiersin.org/articles/10.3389/fmars.2023.1298518/full#supplementary-material
References
Agarwal V., El Gamal A. A., Yamanaka K., Poth D., Kersten R. D., Schorn M., et al. (2014). Biosynthesis of polybrominated aromatic organic compounds by marine bacteria. Nat. Chem. Biol. 10, 640–647. doi: 10.1038/nchembio.1564
Akkipeddi S. M. K., Xu M., Chan K. Y. K. (2021). Halogenated compound secreted by marine bacteria halts larval urchin development. J. Exp. Mar. Biol. Ecol. 538, 151540. doi: 10.1016/j.jembe.2021.151540
Alker A. T., Farrell M. V., Demko A. M., Purdy T. N., Adak S., Moore B. S., et al. (2023). Linking bacterial tetrabromopyrrole biosynthesis to coral metamorphosis. ISME Commun. 3, 98. doi: 10.1038/s43705-023-00309-6
Andersen R. J., Wolfe M. S., Faulkner D. J. (1974). Autotoxic antibiotic production by a marine chromobacterium. Mar. Biol. 27, 281–285. doi: 10.1007/BF00394363
Banaszak A. T., Marhaver K. L., Miller M. W., Hartmann A. C., Albright R., Hagedorn M., et al. (2023). Applying coral breeding to reef restoration: best practices, knowledge gaps, and priority actions in a rapidly-evolving field. Restor. Ecol. 31, e13913. doi: 10.1111/rec.13913
Baums I. B., Baker A. C., Davies S. W., Grottoli A. G., Kenkel C. D., Kitchen S. A., et al. (2019). Considerations for maximizing the adaptive potential of restored coral populations in the western Atlantic. Ecol. Appl. 29, e01978. doi: 10.1002/eap.1978
Boström-Einarsson L., Babcock R. C., Bayraktarov E., Ceccarelli D., Cook N., Ferse S. C. A., et al. (2020). Coral restoration – A systematic review of current methods, successes, failures and future directions. PloS One 15, e0226631. doi: 10.1371/journal.pone.0226631
Burkholder P. R., Pfister R. M., Leitz F. H. (1966). Production of a pyrrole antibiotic by a marine bacterium. Appl. Microbiol. 14, 649–653. doi: 10.1128/am.14.4.649-653.1966
Busch J., Agarwal V., Schorn M., MaChado H., Moore B. S., Rouse G. W., et al. (2019). Diversity and distribution of the bmp gene cluster and its polybrominated products in the genus Pseudoalteromonas. Environ. Microbiol. 21, 1575–1585. doi: 10.1111/1462-2920.14532
Dinno A. (2017). dunn.test: dunn’s test of multiple comparisons using rank sums. R package version 1.3.5. Available at: https://CRAN.R-project.org/package=dunn.test.
El Gamal A., Agarwal V., Diethelm S., Rahman I., Schorn M. A., Sneed J. M., et al. (2016). Biosynthesis of coral settlement cue tetrabromopyrrole in marine bacteria by a uniquely adapted brominase–thioesterase enzyme pair. Proc. Natl. Acad. Sci. 113, 3797–3802. doi: 10.1073/pnas.1519695113
Erwin P. M., Szmant A. M. (2010). Settlement induction of Acropora palmata planulae by a GLW-amide neuropeptide. Coral Reefs 29, 929–939. doi: 10.1007/s00338-010-0634-1
Gardner T. A., Cote I. M., Gill J. A., Grant A., Watkinson A. R. (2003). Long-term region-wide declines in Caribbean corals. Science 301, 958–960. doi: 10.1126/science.1086050
Harrington L., Fabricius K., De'ath G., Negri A. (2004). Recognition and selection of settlement substrata determine post-settlement survival in corals. Ecology 85, 3428–3437. doi: 10.1890/04-0298
Henry J. A., O’neil K. L., Patterson J. T. (2019). Native herbivores improve sexual propagation of threatened staghorn coral Acropora cervicornis. Front. Mar. Sci., 6. doi: 10.3389/fmars.2019.00713
Hughes T. P., Kerry J. T., Alvarez-Noriega M., Alvarez-Romero J. G., Anderson K. D., Baird A. H., et al. (2017). Global warming and recurrent mass bleaching of corals. Nature 543, 373–377. doi: 10.1038/nature21707
Iwao K., Fujisawa T., Hatta M. (2002). A cnidarian neuropeptide of the GLWamide family induces metamorphosis of reef-building corals in the genus Acropora. Coral Reefs 21, 127–129. doi: 10.1007/s00338-002-0219-8
Kitamura M., Koyama T., Nakano Y., Uemura D. (2007). Characterization of a natural inducer of coral larval metamorphosis. J. Exp. Mar. Biol. Ecol. 340, 96–102. doi: 10.1016/j.jembe.2006.08.012
Knowlton N., Grottoli A. G., Kleypas J., Obura D., Corcoran E., De Goeij J., et al. (2021). Rebuilding coral reefs: a decadal grand challenge. Int. Coral Reef Soc. Future Earth Coasts, 56. doi: 10.53642/NRKY9386
Kuffner I., Walters L., Becerro M. A., Paul V. J., Ritson-Williams R., Beach K. (2006). Inhibition of coral recruitment by macrolagae and cyanobacteria. Mar. Ecol. Prog. Ser. 323, 107–117. doi: 10.3354/meps323107
Miller M. W., Latijnhouwers K. R. W., Bickel A., Mendoza-Quiroz S., Schick M., Burton K., et al. (2022). Settlement yields in large-scale in situ culture of Caribbean coral larvae for restoration. Restor. Ecol. 30, e13512. doi: 10.1111/rec.13512
Moeller M., Nietzer S., Schupp P. J. (2019). Neuroactive compounds induce larval settlement in the scleractinian coral Leptastrea purpurea. Sci. Rep. 9, 2291. doi: 10.1038/s41598-019-38794-2
Morse D. E., Morse A. N. C. (1991). Enzymatic characterization of the morphogen recognized by Agaricia humilis (Scleractinian Coral) larvae. Biol. Bull. 181, 104–122. doi: 10.2307/1542493
Morse D. E., Morse A. N. C., Raimondi P. T., Hooker N. (1994). Morphogen-based chemical flypaper for Agaricia humilis coral larvae. Biol. Bull. 186, 172–181. doi: 10.2307/1542051
Nietzer S., Moeller M., Kitamura M., Schupp P. J. (2018). Coral larvae every day: Leptastrea purpurea, a brooding species that could accelerate coral research. Front. Mar. Sci., 5. doi: 10.3389/fmars.2018.00466
Omori M. (2019). Coral restoration research and technical developments: what we have learned so far. Mar. Biol. Res. 15, 377–409. doi: 10.1080/17451000.2019.1662050
Pandolfi J. M., Bradbury R. H., Sala E., Hughes T. P., Bjorndal K. A., Cooke R. G., et al. (2003). Global trajectories of the long-term decline of coral reef ecosystems. Science 301, 955–958. doi: 10.1126/science.1085706
Petersen L.-E., Kellermann M. Y., Fiegel L. J., Nietzer S., Bickmeyer U., Abele D., et al. (2023). Photodegradation of a bacterial pigment and resulting hydrogen peroxide release enable coral settlement. Sci. Rep. 13, 3562. doi: 10.1038/s41598-023-30470-w
Petersen L.-E., Kellermann M. Y., Nietzer S., Schupp P. J. (2021). Photosensitivity of the bacterial pigment cycloprodigiosin enables settlement in coral larvae—Light as an understudied environmental factor. Front. Mar. Sci. 8. doi: 10.3389/fmars.2021.749070
Randall C. J., Negri A. P., Quigley K. M., Foster T., Ricardo G. F., Webster N. S., et al. (2020). Sexual production of corals for reef restoration in the Anthropocene. Mar. Ecol. Prog. Ser. 635, 203–232. doi: 10.3354/meps13206
R Core Team (2023). R: A Language and Environment for Statistical Computing (Vienna, Austria: R Foundation for Statistical Computing).
Ritson-Williams R., Arnold S. N., Paul V. J., Steneck R. S. (2014). Larval settlement preferences of Acropora palmata and Montastraea faveolata in response to diverse red algae. Coral Reefs 33, 59–66. doi: 10.1007/s00338-013-1113-2
Ross C., Fogarty N. D., Ritson-Williams R., Paul V. J. (2017). Interspecific variation in coral settlement and fertilization success in response to hydrogen peroxide exposure. Biol. Bull. 233, 206–218. doi: 10.1086/696215
Sneed J. M., Sharp K. H., Ritchie K. B., Paul V. J. (2014). The chemical cue tetrabromopyrrole from a biofilm bacterium induces settlement of multiple Caribbean corals. Proc. R. Soc. B-Biol. Sci. 281, 20133086. doi: 10.1098/rspb.2013.3086
Suggett D. J., Van Oppen M. J. H. (2022). Horizon scan of rapidly advancing coral restoration approaches for 21st century reef management. Emerg. Topics Life Sci. 6, 125–136. doi: 10.1042/ETLS20210240
Suzuki G., Okada W., Yasutake Y., Yamamoto H., Tanita I., Yamashita H., et al. (2020). Enhancing coral larval supply and seedling production using a special bundle collection system “coral larval cradle” for large-scale coral restoration. Restor. Ecol. 28, 1172–1182. doi: 10.1111/rec.13178
Tebben J., Motti C. A., Siboni N., Tapiolas D. M., Negri A. P., Schupp P. J., et al. (2015). Chemical mediation of coral larval settlement by crustose coralline algae. Sci. Rep. 5, 10803. doi: 10.1038/srep10803
Tebben J., Motti C., Tapiolas D., Thomas-Hall P., Harder T. (2014). A coralline algal-associated bacterium, Pseudoalteromonas strain J010, yields five new korormicins and a bromopyrrole. Mar. Drugs 12, 2802–2815. doi: 10.3390/md12052802
Tebben J., Tapiolas D. M., Motti C. A., Abrego D., Negri A. P., Blackall L. L., et al. (2011). Induction of larval metamorphosis of the coral Acropora millepora by tetrabromopyrrole isolated from a Pseudoalteromonas bacterium. PloS One 6 (4), e19082. doi: 10.1371/journal.pone.0019082
Whalen K. E., Kirby C., Nicholson R. M., O’reilly M., Moore B. S., Harvey E. L. (2018). The chemical cue tetrabromopyrrole induces rapid cellular stress and mortality in phytoplankton. Sci. Rep. 8, 15498. doi: 10.1038/s41598-018-33945-3
Yang Q., Zhang W., Zhang Y., Tang X., Ling J., Zhang Y., et al. (2022). Promoting larval settlement of coral Pocillopora damicornis by calcium. Coral Reefs 41, 223–235. doi: 10.1007/s00338-021-02216-5
Zheng J., Mckinnie S. M. K., El Gamal A., Feng W., Dong Y., Agarwal V., et al. (2018). Organohalogens naturally biosynthesized in marine environments and produced as disinfection byproducts alter sarco/endoplasmic reticulum Ca2+ dynamics. Environ. Sci. Technol. 52, 5469–5478. doi: 10.1021/acs.est.8b00512
Keywords: coral restoration, coral reef, chemical ecology, larval settlement, coral reproduction, marine microbiology
Citation: Sneed JM, Demko AM, Miller MW, Yi D, Moore BS, Agarwal V and Paul VJ (2024) Coral settlement induction by tetrabromopyrrole is widespread among Caribbean corals and compound specific. Front. Mar. Sci. 10:1298518. doi: 10.3389/fmars.2023.1298518
Received: 21 September 2023; Accepted: 26 December 2023;
Published: 12 January 2024.
Edited by:
Cliff Ross, University of North Florida, United StatesReviewed by:
Esti Kramarsky-Winter, Ben-Gurion University of the Negev, IsraelMatthias Y. Kellermann, University of Oldenburg, Germany
Copyright © 2024 Sneed, Demko, Miller, Yi, Moore, Agarwal and Paul. This is an open-access article distributed under the terms of the Creative Commons Attribution License (CC BY). The use, distribution or reproduction in other forums is permitted, provided the original author(s) and the copyright owner(s) are credited and that the original publication in this journal is cited, in accordance with accepted academic practice. No use, distribution or reproduction is permitted which does not comply with these terms.
*Correspondence: Jennifer M. Sneed, c25lZWRqQHNpLmVkdQ==