The combined effects of acidification and acute warming on the embryos of Pacific herring (Clupea pallasii)
- 1Department of Environmental Sciences, College of the Environment, Western Washington University, Bellingham, WA, United States
- 2Marine and Coastal Science, Western Washington University, Bellingham, WA, United States
- 3School of Marine and Environmental Affairs and Washington Ocean Acidification Center, University of Washington, Seattle, WA, United States
- 4Biology Department, Woods Hole Oceanographic Institution, Woods Hole, MA, United States
- 5Department of Biology, College of Science and Engineering, Western Washington University, Bellingham, WA, United States
Anthropogenic climate change is projected to affect marine ecosystems by challenging the environmental tolerance of individuals. Marine fishes may be particularly vulnerable to emergent climate stressors during early life stages. Here we focus on embryos of Pacific herring (Clupea pallasii), an important forage fish species widely distributed across the North Pacific. Embryos were reared under a range of temperatures (10-16°C) crossed with two pCO2 levels (600 and 2000 μatm) to investigate effects on metabolism and survival. We further tested how elevated pCO2 affects critical thermal tolerance (CTmax) by challenging embryos to short-term temperature fluctuations. Experiments were repeated on embryos collected from winter and spring spawning populations to determine if spawning phenology corresponds with different limits of environmental tolerance in offspring. We found that embryos could withstand acute exposure to 20°C regardless of spawning population or incubation treatment, but that survival was greatly reduced after 2-3 hours at 25°C. We found that pCO2 had limited effects on CTmax. The survival of embryos reared under chronically warm conditions (12°, 14°, or 16°C) was significantly lower relative to 10°C treatments in both populations. Oxygen consumption rates (MO2) were also higher at elevated temperatures and pCO2 levels. However, heart contraction measurements made 48 hours after CTmax exposure revealed a greater increase in heart rate in embryos reared at 10°C compared to 16°C, suggesting acclimation at higher incubation temperatures. Our results indicate that Pacific herring are generally tolerant of pCO2 but are vulnerable to acute temperature stress. Importantly, spring-spawning embryos did not clearly exhibit a higher tolerance to heat stress compared to winter offspring.
1 Introduction
Coastal ecosystems are vulnerable to the effects of climate change which include increased mean temperatures, a higher frequency of marine heat waves, and ocean acidification (Bindoff et al., 2019). Changes to abiotic factors such as marine carbonate chemistry and sea surface temperature have affected the physiological performance of marine ectotherms resulting in distributional shifts for many marine species that are projected to become more pronounced throughout the 21st century (Arias et al., 2021). The Salish Sea, a large estuarine ecosystem off the coast of Washington, USA and British Columbia, Canada, supports wildlife habitat that is important to the economic and cultural livelihood of the local people (Sobocinski et al., 2022). Projections indicate that this region is expected to experience higher air and water temperatures in combination with shifts in seawater carbonate chemistry (e.g. acidification) as global climate change progresses (Khangaonkar et al., 2019). The development, survival, and hatching success of multiple marine fishes are known to be adversely affected by both abnormally high temperatures and increases in the partial pressure of CO2 (pCO2). These effects are most prevalent in the early life stages when many fishes exhibit a narrower range of environmental tolerance relative to older conspecifics (Fabry et al., 2008; Ishimatsu et al., 2008; Pörtner, 2008; Peck et al., 2012; Clements and Hunt, 2015; Cattano et al., 2018).
Anthropogenic increases in atmospheric CO2 contribute to both global climate change and ocean acidification (Arias et al., 2021). Elevated pCO2 may adversely affect fishes through several mechanisms, including altered acid-base homeostasis, behavioral impairments, skeletal abnormalities, reduced larval growth, and increased organ damage (Fabry et al., 2008; Frommel et al., 2012; Frommel et al., 2014; Clements and Hunt, 2015). Early life stages that lack developed acid-base regulatory competency and key regulatory organs (e.g., gills) are particularly vulnerable to simultaneous increases in pCO2 and temperature, which have been shown to increase embryonic mortality (Ishimatsu et al., 2008; Pörtner, 2008; Dahlke et al., 2017; Cattano et al., 2018; Sswat et al., 2018; Dahlke et al., 2020; Villalobos et al., 2020). In ectotherms, elevated temperatures will increase oxygen demand to support higher metabolic rates, while simultaneously lowering oxygen availability by reducing the solubility of O2 in seawater (Pörtner and Knust, 2007; Oschlies et al., 2018; Kwiatkowski et al., 2020). This can produce a lethal oxygen deficiency or impair fitness by reducing the energy available for growth and reproduction (Pörtner, 2008; Dahlke et al., 2020). Other sublethal effects observed in fish embryos include increased frequency of developmental malformations, elevated heart rates, and reduced metabolic efficiency (Dahlke et al., 2017; Villalobos et al., 2020; Murray and Klinger, 2022).
This study focused on the effects of elevated pCO2 and temperature on the embryonic development of Pacific herring (Clupea pallasii; Cuvier and Valenciennes, 1847). The Pacific herring is an ecologically critical forage fish species of high commercial value that is widely distributed along the Pacific Coast of the United States and Canada (Dinnel et al., 2011). Forage fishes are vital components of marine ecosystems that serve as energy conduits from the lower trophic levels to higher order predators such as marine mammals, piscivorous fishes, and seabirds (Cury et al., 2000; Pikitch et al., 2014). The cultural importance of Pacific herring to the indigenous Coast Salish peoples (McKechnie et al., 2014) and the economic value of their fisheries further support the need for understanding factors that affect their population dynamics (Schweigert et al., 2010; DFO, 2019).
There are 21 defined Pacific herring spawning populations (stocks) in the Southern Salish Sea, some of which are genetically distinct (Stick et al., 2014). Salish Sea Pacific herring reproduce predominantly from January through April in shallow nearshore waters where they attach adhesive eggs to submerged vegetation (Penttila, 2007). Distinct reproductive stocks show considerable variation in spawning phenology and the offspring of different populations can therefore experience quite different thermal conditions during development (Sandell et al., 2016). Furthermore, the Salish Sea is considered to be a naturally acidified system that is currently experiencing further acidification from anthropogenic causes (Cai et al., 2021). In nearshore systems, pCO2 conditions reach seasonal extremes during winter so that herring embryos are routinely exposed to relatively high levels of pCO2 during this time (Lowe et al., 2019).
Pacific herring populations in the Salish Sea have undergone severe declines during the last 50 years (Sandell et al., 2016; Sandell In prep). Stock biomass reductions have been attributed to habitat loss (Gaeckle et al., 2011), pollutants (West et al., 2008), and other potential causes such as climate change (Landis and Bryant, 2010; Sandell In prep), but the extent to which any of these factors have contributed to their decline is not yet clear. The Cherry Point spring spawning stock, once the largest in Washington state, has declined by at least 97% since monitoring began in 1973 (Stick et al., 2014), with spawning failure reported for the first time in 2023 (P. Doinne, Washington Department of Fish and Wildlife, personal communication). This stock is unique in its spawning phenology in that they reproduce substantially later than most other populations (April–June), so that their developing embryos are already subjected to warmer water temperatures that may be close to the upper thermal limits for viable embryogenesis in this species (Alderdice and Velsen, 1971). Thus, the Cherry Point stock may be particularly vulnerable to marine heat stress and other effects of climate change that are likely to affect the region (Landis and Bryant, 2010; Frölicher et al., 2018).
Salish Sea herring present an opportunity to study how differences in spawning phenology can alter the environmental tolerance of offspring and their sensitivity to climate change. In this study, we assessed the combined impacts of ocean warming and elevated pCO2 on Pacific herring offspring sourced from winter-spawning (Port Gamble Stock) and spring-spawning (Cherry Point Stock) herring populations. In addition to static rearing experiments, we tested embryos in critical thermal tolerance assays that simulated extreme temperature fluctuations consistent with marine heatwave events. We hypothesize that chronic exposure to elevated pCO2 and warm conditions will reduce embryo survival to hatch, increase metabolic rates, promote developmental malformations, and increase mortality after exposure to acute temperature stress. Furthermore, we hypothesize that Cherry Point offspring will show higher tolerance to chronic and acute warming indicative of adaptation to a later spawning phenology when temperatures are both warmer and more variable.
2 Methods
2.1 Collection of animals and fertilization
The experiments were conducted in March (winter) and May (spring) 2021 at Western Washington University’s Shannon Point Marine Center (SPMC) in Anacortes, WA, USA. For the winter experiment, mature Pacific herring were collected at Port Gamble (47.8543°N, 122.5838°W) as part of the annual Washington Department of Fish and Wildlife (WDFW) spawning survey. Sampled fish were stored on ice and transported in a cooler to SPMC where dissection and fertilization were performed immediately. In the spring experiment, naturally spawned eggs deposited on vegetation were collected by WDFW at the Cherry Point spawning site (48.8618°N, 122.7482°W) and transferred to SPMC. Eggs were immediately aged under a stereomicroscope (SZ40 stereomicroscope, Olympus®) and the embryos chosen for experimentation were at approximately 90% epiboly (~54 hours post fertilization; Kawakami et al., 2011). The discrepancy in collection methods was due to a reluctance to collect fish from the diminished Cherry Point population (collection of eggs has a much smaller impact than whole animal harvesting).
2.2 Fertilization protocol
A gamete mixture from 10 males and 10 females was used for the winter experiment. Fertilization methods followed established protocols (Dinnel et al., 2011; Murray and Klinger, 2022) with small modifications. Ovaries were carefully dissected and placed in individual petri dishes. Using a single-edged razor blade, a 2 cm × 2 cm piece of testis from each male was macerated and gametes from the 10 males were combined in 100 ml of filtered seawater at 10°C and a salinity of 32 practical salinity units (psu). 25 ml of sperm suspension was added to 4 individual 9 × 13 cm polyethylene containers that were lined with nylon mesh screening and filled with 2 L of filtered and sterilized seawater. Using a silicone spatula, roughly 200 eggs at a time were selected from one female and were haphazardly spread onto the mesh screening of each spawning container. This process was repeated for each female, such that each container received an equal proportion of eggs from all 10 females. Eggs adhere to the mesh screening upon contact with seawater, which facilitated their enumeration and distribution to the experiment. The fertilization process occurred in an enclosed, temperature-controlled room maintained at 10°C (ambient sea temperature) to optimize fertilization success. After 30 minutes, fertilization success was quantified for each container by sampling three randomly selected sections of mesh screen containing ~30 eggs each. These eggs were imaged using a digital camera (MC170 HD, Leica®) attached to a dissection microscope (SZ40 stereomicroscope, Olympus®) and fertilization success (indicated by the presence of a raised fertilization membrane) was calculated as the average number of fertilized eggs over the total number of imaged embryos. Fertilization success ranged from 68% to 75% in the four containers (overall mean: 72.7%).
2.3 Experimental treatment conditions
Experimental treatments were selected based on current conditions and near-future predictions for both temperature and pCO2 in the Salish Sea. Current temperature and pCO2 treatment conditions (10°C, ~600 μatm) were chosen based on records of Salish Sea surface seawater in late winter, which we refer to here as ambient conditions (Fassbender et al., 2018; Pacella et al., 2018). The elevated pCO2 treatment of ~2000 μatm is on par with predicted business-as-usual global change values for the coast of Washington state (Pacella et al., 2018; Evans et al., 2019). An elevated temperature of 16°C was chosen as the upper temperature limit for late-spawning herring populations. This temperature is regularly reported from Salish Sea nearshore waters in late spring and early summer and has been shown to be a survivable temperature for Pacific herring development (Dinnel et al., 2008; Villalobos et al., 2020). Mean temperatures in the Salish Sea are projected to increase by an average of 3°C by 2095 in comparison to the year 2000, with some areas experiencing an average of 5°C of warming (Khangaonkar et al., 2019). Intermediate temperatures of 12° and 14°C were used in the winter experiment to calculate response curves of the temperature effects between 10° and 16°C.
2.3.1 Experimental system
Seawater was drawn from the Guemes Channel into the laboratory at SPMC where pCO2 was adjusted in three replicate header tanks per pCO2 treatment (Figure S1). Individual header tanks received filtered seawater (filtered to 5 µm) at a rate of ~1 L min-1. Salinity averaged ~30 psu as determined via refractometer. Low pCO2 header tanks were aerated with CO2-free air (CO2 adsorber, Twin Tower Engineering) to reduce pCO2 to ~600 μatm. An 8 channel Masterflex L/S® Digital Drive (model UX-77921-75) peristaltic pump was attached to a 20 lb. food grade CO2 gas tank to inject a stream of CO2 bubbles into individual powerhead pumps (Marineland Maxi-jet 900) that quickly dissolved CO2 into the high pCO2 header tanks (Figure S1). The rate of CO2 delivery and water flow through the tank were tuned to produce the desired pCO2 similar to flowthrough systems described in Jokiel et al. (2014) and Villalobos et al. (2020). Continuous bubbling with room air in addition to the CO2 injection system ensured dissolved oxygen saturation remained near 100% (verified by a YSI Model 55).
Four main temperature baths (40 L) were positioned below the header tanks, outfitted with independent temperature controls, and were maintained at 10°, 12°, 14°, and 16°C, respectively. Water temperature was adjusted with two Aqueon® Submersible Aquarium heaters controlled by Elitech® STC-1000 temperature controllers that sustained targets within ± 0.3°C. Submersible pumps (Hydor Koralia Nano 240) circulated water within temperature baths to prevent hot-spots and promote temperature equilibration within cups and tubing (see below).
Embryos were reared in customized polyethylene cups (0.5 L) that were fitted with nylon mesh bottoms (300 µm) and were floated within the temperature baths (Figure S2). pCO2-adjusted seawater was gravity fed into individual cups using 8 feet (2.4 m) of thin plastic tubing that was coiled and submerged within the temperature baths to equalize pCO2-adjusted seawater with the treatment temperature before entering rearing cups (Figure S2). Flow rate into rearing cups was set at ~30-40 ml per minute by adjusting a stopcock at the end of each tube. Daily temperature and pH values were measured and recorded using an Orion Star™ pHNBS conductivity meter calibrated daily with NBS-buffers (Fisher Chemical®) at 10°C. Seawater samples were collected and preserved regularly for analysis of carbonate chemistry parameters (see below).
2.3.2 Experimental design
In winter we applied four temperature treatments (10°, 12°, 14°, and 16°C) crossed with two pCO2 levels (600 and 2000 μatm) for a total of eight treatment combinations. Each treatment was allotted six rearing cups; three cups received 50 embryos each for quantification of developmental and survival traits while the other three cups received approximately 250 embryos to be tested in the CTmax and respirometry trials. All embryos were dispersed to rearing cups within two hours of fertilization. Initial temperatures were set to 10°C for all water baths and then gradually increased to the set treatment temperatures treatments over four hours.
In spring the 12° and 14°C temperature treatments were removed to focus on the two extremes (10° and 16°C) that were crossed with two pCO2 levels (600 and 2000 μatm) for a total of four treatment combinations. A total of eight rearing cups were allotted to each treatment combination; four cups contained 50 embryos for quantification of survival and developmental traits and the remaining four cups received approximately 250 embryos for CTmax trials. Selected embryos were immediately introduced to rearing cups after determination of developmental stage. The 16°C bath was warmed to the target level from an initial temperature of 10°C over four hours.
2.4 Carbonate chemistry
Seawater samples were drawn from all header tanks every other day and immediately measured for spectrophotometric pH (total scale, pHT) and dissolved inorganic carbon (DIC) following Love et al. (2017). Seawater was sampled from individual rearing cups every other day during the experiments and preserved in 20 ml scintillation vials (winter) or 40 ml volatile organic chemical (VOC) vials (spring) to which we added 10-μL of a saturated solution of mercuric-chloride (HgCl2), stored at 2°C, and analyzed within 3 weeks post-experiment for pHT and DIC. A diode array spectrophotometer (Ocean Optics S-UV-VIS Flame) was used to assess duplicate measurements of pHT. Seawater samples were brought to 25°C in a water bath and systematically transferred into a jacketed 5 cm cuvette. The samples received two 20-μL aliquots of m-cresol indicator dye to determine absorbance at three wavelengths (730 nm, 578 nm, 434 nm; Dickson et al., 2007). Dye impurities were corrected according to Douglas and Byrne (2017). DIC was determined by acidification of duplicate aliquots of seawater samples, followed by nitrogen extraction and gas phase analysis (Apollo SciTech AS-C3). Room temperature and salinity measurements were used to calculate density. The carbonate chemistry program CO2 SYS (Lewis et al., 1998) used direct measurements of pHT, DIC, typical nutrient concentrations of the seawater source, and the treatment temperature at time of sampling to calculate in situ pCO2 (μatm), total alkalinity (AT, kg−1), and carbonate and bicarbonate ion concentrations (CO3−2, HCO3−; μmol kg−1. See Tables S1, S2 for a complete summary of carbonate chemistry parameters.
2.5 Critical thermal maximum protocol
2.5.1 Winter experiment
CTmax trials were performed on embryos reared under the two temperature extremes of 10° and 16°C. Embryos were sampled for the trials during the early stages of segmentation at 43- and 69-hours post-fertilization (hpf) at 16° and 10°C, respectively (~25% of full development, Stages F and G in Kawakami et al., 2011). A total of 210 normally developing embryos were drawn from each of the three replicate cups per treatment group and divided into seven 10 ml vials (30 embryos per vial) that were filled with seawater adjusted to source treatment pCO2 and temperature conditions with 100% dissolved oxygen saturation. Of these seven vials, one was held at treatment conditions as a control and the remaining vials were exposed to one of the six CTmax temperature × duration combinations (20°C for 1, 2, or 3 hours; 25°C for 1, 2, or 3 hours). The replicate and treatment combinations resulted in a total of 84 vials (7 vials × 3 replicate rearing cups × 2 temperatures × 2 pCO2 treatments). However, since the trials for each temperature were held on different days, only 42 vials were used for each temperature trial.
The CTmax trials were conducted using a high precision water bath (Versa Model V-HC) and target temperatures were verified using a thermistor (Fluke 1523 Thermometer). Embryos from 10°C treatments were warmed to 16°C by increasing the bath temperature by 1°C every 5 minutes. After reaching 16°C the temperature of all vials was increased by 1°C every 15 minutes. Upon reaching 20°C, three vials per replicate were removed and placed in a separate water bath set to 20°C. Three vials per replicate were removed after 1, 2, and 3 hours, respectively, and placed in a separate water bath for re-acclimation to the original incubation temperature at a rate of -1°C every 15 minutes. The remaining three vials per treatment underwent further heating to 25°C (by 1°C every 15 minutes) and again, 1 vial per replicate was removed after 1, 2, and 3 hours, respectively, and re-acclimated to the original rearing temperature as noted above. After trials were completed, embryos were transferred to petri dishes filled with pCO2 adjusted seawater and placed within large temperature-controlled units set to the original incubation temperatures. Seawater was exchanged regularly to maintain high oxygen and treatment pCO2 levels. Embryo survival was assessed 37 hours post-exposure (hpe) for 16°C treatments and 68 hpe for 10°C treatments when embryos were predicted to have reached 50% of full development (3.5 and 5.5-days post-fertilization at 16°C and 10°C, respectively). Eggs were inspected using a SZ40 stereomicroscope (Olympus®) and normally developing embryos with heartbeats were considered alive, while developmentally arrested, coagulated, or embryos with obvious developmental anomalies (e.g., shortened body length, twisted notochord, missing eyes) were designated as dead.
2.5.2 Spring experiment
CTmax trials for the spring experiment were conducted using specimens at a later developmental stage (~60% of full development with appearance of eye pigmentation; stage I-J in Kawakami et al., 2011) to allow the wild-collected embryos to develop under experimental treatments for at least two days. The methodology for the CTmax trials was kept constant between the winter and spring experiments. Due to the addition of one replicate cup per treatment, the spring experiment contained 24 more vials than winter (7 vials × 4 replicates × 2 temperatures × 2 pCO2 treatments) for a total of 112 vials, 56 vials for each temperature trial. In contrast with the winter trials, after the CTmax trial embryos were held in 20 ml vials and submerged in the original experimental temperature tanks until assessment in order to reduce the need for additional temperature control units. Due to the spring CTmax assessment taking place at a later developmental stage, mortality was assessed ~24 hours post-exposure and prior to hatching. The methods for the assessment of mortality were the same in the winter and spring studies.
2.6 Respirometry
A subset of living embryos from each temperature × pCO2 treatment were used to estimate oxygen consumption rates (MO2) during the winter experiment. MO2 was not evaluated in spring due to logistical constraints. Trials were conducted separately for each temperature treatment when embryos reached 50% epiboly (Stage C in Kawakami et al., 2011; 24, 26, 31, and 46 hpf at 16°, 14°, 12°, and 10°C, respectively). An early developmental stage was chosen because previous work with this species showed limited effects of high pCO2 on the MO2 of older eyed-staged embryos (Murray and Klinger, 2022), and because evidence from work with other temperate fish species indicated that earlier embryonic stages may be more sensitive to elevated pCO2 levels (Dahlke et al., 2020).
Respirometry methods followed Murray and Klinger (2022). Ten embryos were collected from three replicate cups per treatment and placed in customized 2-ml glass respirometry vials, prefilled with autoclaved seawater (readjusted to 32 psu) that was adjusted to treatment temperature and pCO2 conditions and 100% oxygen saturation. Three blank vials were filled with the same autoclaved water and a single glass mixing bead (2.4 mm diameter) and were measured alongside the 6 embryo vials for a total of 9 vials per trial (3 replicate cups × 2 treatments + 3 blanks). Sample vials did not receive a mixing bead because the embryos fulfilled this purpose.
Respirometry vials were equipped with a single planar trace oxygen sensor spot (PreSens®) that allows for optical measurements of dissolved oxygen through the glass. Trials were performed in a temperature-controlled walk-in chamber to maintain experimental temperature during sampling. Vials were submerged in a temperature-controlled water bath adjusted to the appropriate treatment temperature. Vials were then measured for oxygen concentrations (μmol/L) every 10 minutes using a Fibox 4 oxygen meter with a PSt3 fiber optic sensor (PreSens®) running the PreSens® Measurement Studio 2 Microsoft program. All vials were inverted every 5 minutes to avoid oxygen stagnation. The trials were terminated after 10 measurements had been taken. After the trials, the exact mass of water in a vial was estimated using a precision micro balance (Mettler Toledo AT21 Comparator) and converted into volume using the known density of water within each vial. Post-trial, embryos were inspected and then preserved in a 4% formalin/freshwater solution for storage.
Oxygen consumption data were calculated following Murray and Klinger (2022). Linear regressions between oxygen consumption and time were fit for each respirometry vial. Bonferroni outlier tests were used to identify outlying oxygen measurements that deviated significantly from the linear fit. All outliers were removed from the oxygen time series and the regression was refit. Resulting slopes were used to calculate MO2 for an individual embryo (nmol O2h-1) after controlling for background oxygen change (calculated as the mean ΔO2 of all blank vials), the precise seawater volume of the vial, and the number of embryos per vial.
2.7 Heart contractions
Heart contraction measurements were used to assess cardiac stress in embryos from the spring experiment CTmax trials. Surviving embryos were held in temperature-controlled baths at treatment pCO2 conditions for 3 days post CTmax trial (2 days post mortality assessment) and then imaged. Embryos from the control and 25°C exposure treatments (1, 2, and 3 hours) were selected for measurements. Individual embryos were recorded for 60 seconds using a digital camera that captured up to 30 frames per second (MC170 HD, Leica®) attached to a SZ40 stereomicroscope (Olympus®). All surviving embryos were tested, up to a maximum of 10 per replicate. Videos were then used to quantify embryonic heart rates (beats per minute, BPM), which were then compared between treatments.
2.8 Embryo survival and hatching morphometrics
Starting at 6 dpf, all survival cups were checked daily for newly hatched larvae starting at 6 dpf. Hatchlings were counted, euthanized with an overdose of MS-222 (1000 mg/L, Dinnel et al., 2008), and immediately photographed individually at 8× magnification to produce calibrated digital images for the identification of developmental malformations (SZ40 stereomicroscope, Olympus®). For the spring experiment, hatchlings were first preserved in 5% buffered formalin, then stored and imaged later. Daily hatch, cumulative hatch, time to first hatch (TFH), time to peak hatch (TPH), and hatching success were analyzed for each survival replicate cup. Daily hatch was calculated as the ratio of larvae (healthy + malformed) that hatched each day post fertilization (dpf) over the total starting embryos (N = 50). Cumulative hatch was calculated as the number of larvae (healthy + malformed) that hatched across time over the total starting embryos. TFH was calculated as the number of days post fertilization where hatching first occurred. TPH was calculated by determining the day on which the highest quantity of eggs hatched. Percent hatching success was calculated as the ratio of healthy hatched larvae (cumulative hatch minus malformed larvae) to the number of starting embryos. Identification of larval malformations included developmental anomalies such as shortening, curvatures in the body that surpassed 90°, swelling or distortions of the yolk sac, underdeveloped jaws, and spinal deviation (Murray and Klinger, 2022; Figure S3). Larval malformation rate was defined two ways: 1) the number of malformed larvae relative to the number of starting embryos, and 2) the number of malformed larvae relative to the total number of hatchlings. Differences in survival and hatching were only examined within experiments.
2.9 Statistical analysis
All statistical analyses were performed in R using RStudio version 1.3.1073 (R Core Team, 2022). Ratio data (hatching success and malformation rates) were logit transformed prior to analysis (Warton and Hui, 2011). Linear mixed-effects models (nlme package) were constructed to test for temperature × CO2 on hatching success, malformation rates, time to first hatch, and MO2 with “header tank” assigned as a random factor and fit by the restricted maximum likelihood method (REML). However, the header tank only accounted for a small amount of total variance in each trait (<1%). Hatching success, malformation rates, and MO2 were therefore assessed using two-way analysis of variance (ANOVA) with temperature and pCO2 set as fixed factors. Tukey’s HSD (multicomp package) was used for post hoc multiple comparison tests.
Statistical assessments of CTmax survival were performed separately for embryos incubated at 10° and 16°C. In a separate analysis incubation temperatures were tested for significant differences in survival. Survival (%) was logit transformed and tested in a three-factor ANOVA, with pCO2 and CTmax temperature set as fixed factors and duration set as a numeric factor. The multcomp package was used for post hoc comparisons (Tukey’s HSD), which assessed significance values within treatments.
A linear mixed-effects model (LME) was used to analyze the treatment effects on heart contraction data using “replicate cup” as a random factor. A fully factorial model was used with incubation temperature, pCO2 and CTmax temperature as factors and duration as a numeric predictor. pCO2 and all pCO2 interactions were non-significant, and therefore a second LME was constructed using data from combined pCO2 treatments.
3 Results
3.1 Critical thermal maximum
3.1.1 Winter experiment
We found that embryos incubated at 10° and 16°C responded similarly to the CTmax trials with respect to survival (Figure 1). In both groups, post CTmax survival was significantly influenced by an interactive effect between CTmax temperature and duration (ANOVA, df = 1, p < 0.04, Table 1). Acute exposure to 20°C for one, two, and three hours did not reduce survival relative to control groups. However, for clutches tested at a CTmax of 25°C, survival after one hour was similar to that seen in control embryos but decreased after two hours of exposure (by 21% for 10°C embryos and 22% for 16°C embryos) and decreased further after three hours (by 41% for 10°C embryos and 34% for 16°C embryos). We found that pCO2 treatment had small but opposing effects on CTmax survival between 10° and 16°C embryo groups (Figure 1). For embryos reared under 10°C there was a significant interaction effect between pCO2 and CTmax temperature (ANOVA, df = 2, p = 0.007, Table 1), as high pCO2 increased the survival of embryos exposed to 25°C by 11% relative to low pCO2 embryos when averaged across all durations. For the 16°C treatment we found an overall negative effect of pCO2 (ANOVA, df = 1, p = 0.008, Table 1) where high pCO2 exposure reduced survival by 10% in comparison to low pCO2 conspecifics when averaged across all combinations of CTmax temperature and duration (Figure 1).
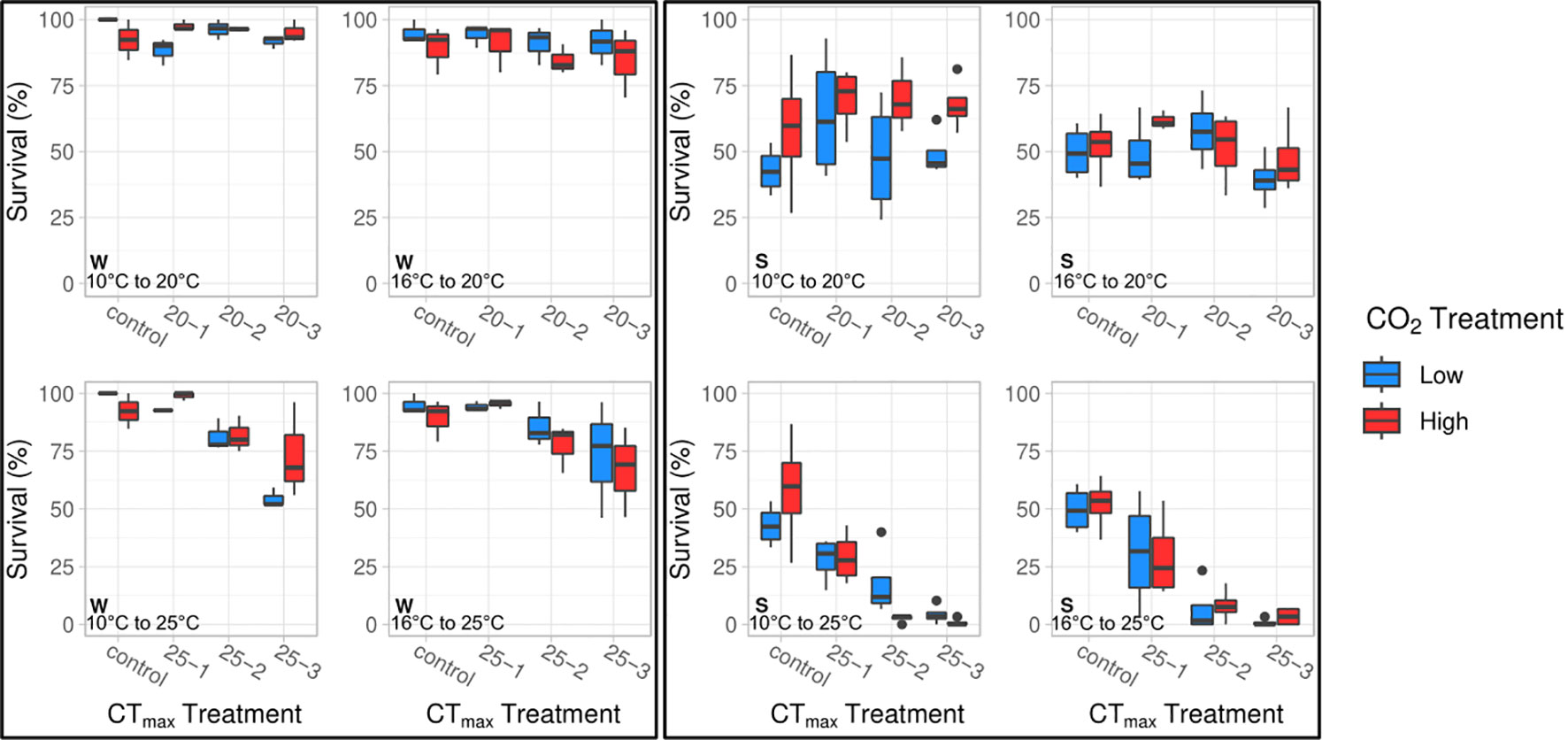
Figure 1 The interactive effects of CTmax temperature (20°, 25°C) and exposure duration (control, 1, 2, 3 hrs.) on embryo survival (%) in winter (left) and spring (right) experiments at two incubation temperatures (10°, 16°C). CTmax, duration, and CTmax × duration significantly impacted survival in winter and spring embryos. pCO2 was significant in winter at 16°C (ANOVA, df = 1, p = 0.008). CTmax × pCO2 significantly affected survival in 10°C embryos in winter and spring (ANOVA, df = 2, p ≤ 0.008).
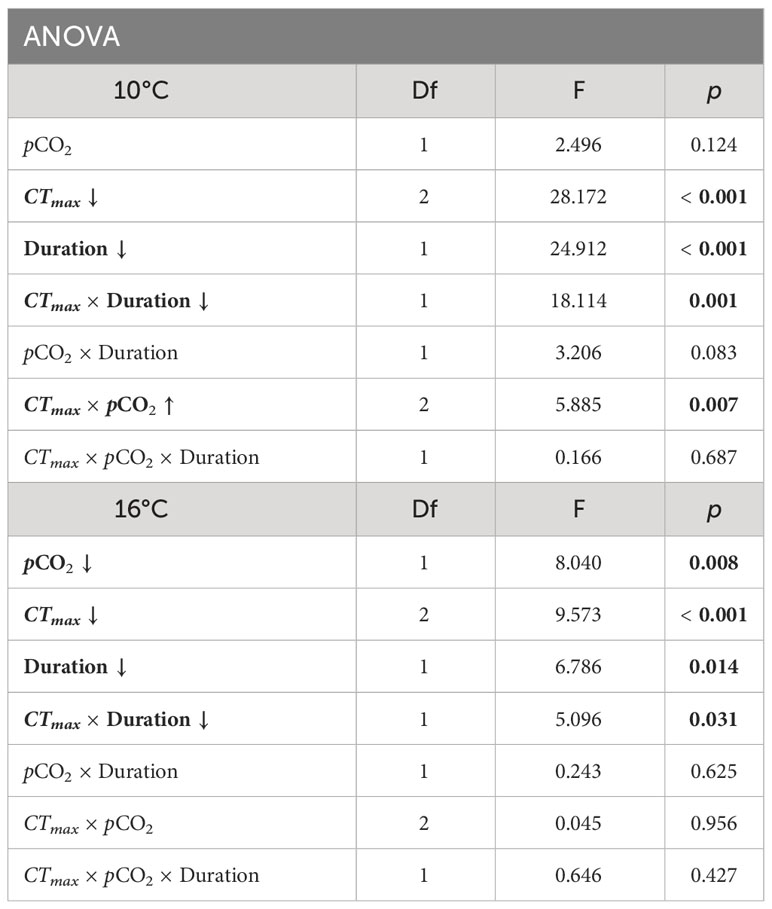
Table 1 Winter ANOVA results for embryo survival in Critical Thermal Maximum (CTmax) experiments at incubation temperatures of 10° and 16°C. Parameters include pCO2 (600 and 2000 μatm), CTmax temperature (20 or 25°C), duration of exposure to CTmax (1, 2, or 3 hours), and the interaction effects of these parameters. Significant factors are indicated in bold. Arrow indicates the direction of trait effect trend with increasing factor.
3.1.2 Spring experiment
Overall, results from the springtime CTmax trial were similar to those seen in the winter trial (Figure 1), as survival was primarily affected by an interaction between CTmax temperature and the duration of exposure (ANOVA, df = 1, p ≤ 0.001, Table 2). Embryos from 10° and 16°C rearing treatments showed no reduction in survival under a CTmax of 20°C across durations. However, exposure to a CTmax of 25°C progressively reduced survival with duration, resulting in near complete mortality after 2 and 3 hours (Figure 1). However, overall survival during the spring CTmax trial was considerably lower than in the winter trial, as the survival of even the control groups was reduced by a factor of 50% (Figure 1). The only significant effect of pCO2 during spring was an interaction effect between CTmax and pCO2 for embryos reared under 10°C (ANOVA, df = 2, p = 0.008, Table 2). High pCO2 slightly increased survival of embryos exposed to a CTmax of 20°C across all durations, whereas high pCO2 reduced CTmax survival for embryos exposed to 25°C, particularly after 2 hours (Figure 1).
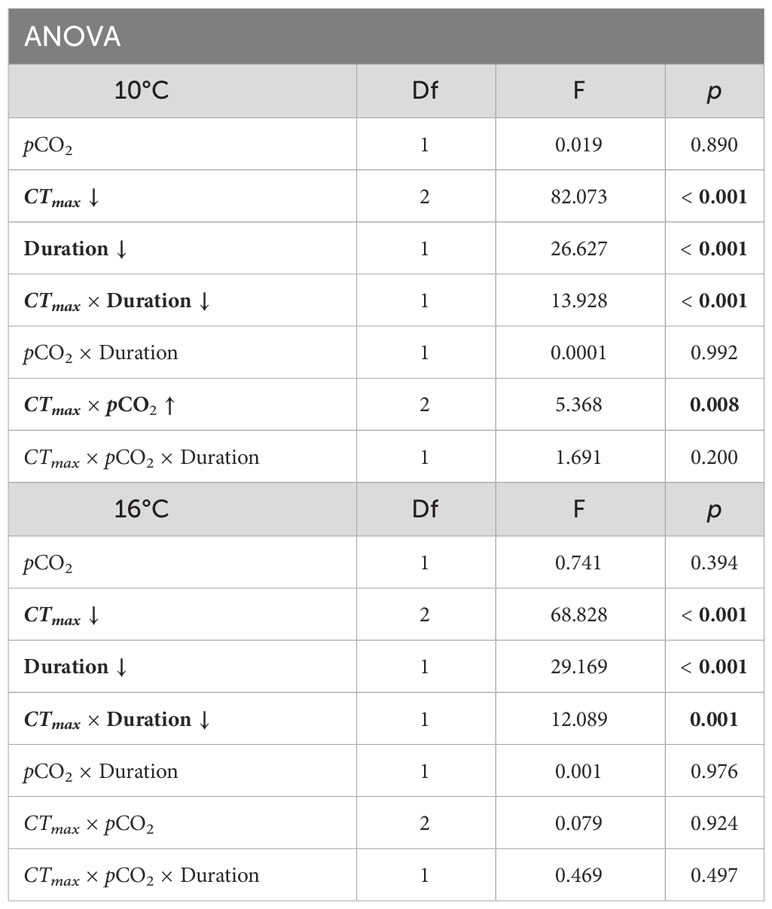
Table 2 Spring ANOVA results for embryo survival in Critical Thermal Maximum (CTmax) experiments at incubation temperatures of 10° and 16°C. Parameters include pCO2 (600 and 2000 μatm), CTmax temperature (20 or 25°C), duration of exposure to CTmax (1, 2, or 3 hours), and the interaction effects of these parameters. Significant factors are indicated in bold. Arrow indicates the direction of trait effect trend with increasing factor.
3.2 Oxygen consumption rate
During the winter experiment we found that both temperature and pCO2 significantly affected the MO2 of early developing embryos (ANOVA, p < 0.01, Table S3; Figure 2). Post hoc testing indicated that embryos reared at 10°C showed significantly lower MO2 values by an average of 41.97% in comparison to those held at 12° and 16°C (Tukey’s HSD, p < 0.03). No significant difference was found between the 10° and 14°C treatments. Embryos in the high pCO2 treatment displayed significantly higher oxygen consumption rates in comparison to those exposed to ambient pCO2 conditions by 24.5% when averaged across temperature regimes (ANOVA, df = 1, p = 0.012, Figure 2; Table S3).
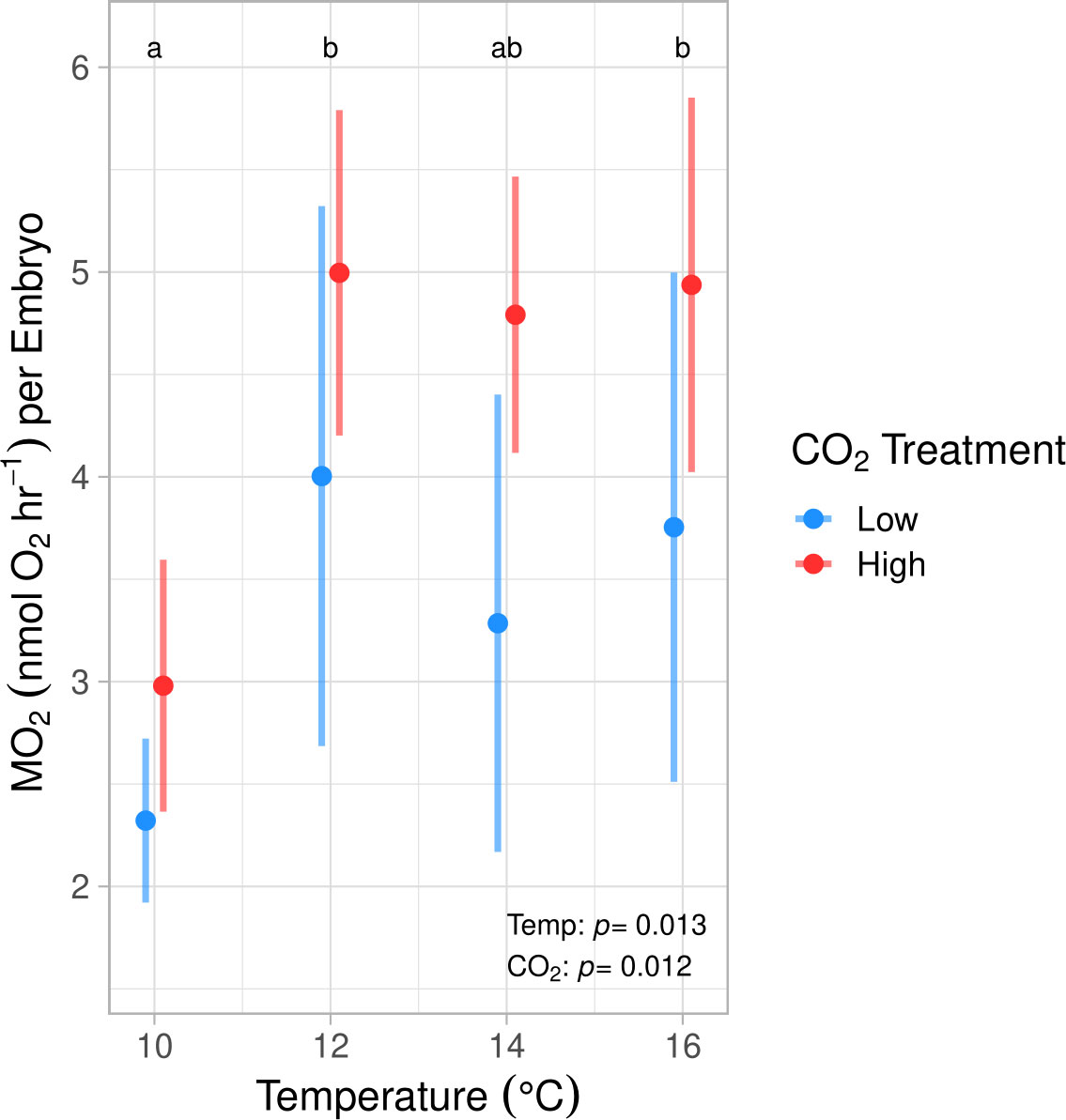
Figure 2 Embryonic oxygen consumption rates (MO2) at 50% epiboly development (stage C in Kawakami et al., 2011) at 4 discrete temperatures and 2 discrete pCO2 treatments in the winter experiment (means ± std. dev). MO2 was significantly impacted by temperature and pCO2, yet no pCO2 effect was found within any temperature group. MO2 was significantly lower at 10°C (a) compared to 12° and 16°C (b) and was not significantly different from 14°C (ab) (Tukey’s HSD, p < 0.03). Across temperature treatments, MO2 was significantly higher at high pCO2 compared to ambient (ANOVA, df = 1, p = 0.012).
3.3 Heart contractions
Heart contractions were not affected by pCO2 or any pCO2 interaction (ANOVA, p > 0.05) and analyses were therefore completed on combined pCO2 data (high pCO2 + low pCO2) within each treatment category. The average heart contraction rate (BPM) for control samples kept at their original incubation temperatures were similar between the 10°C (87.95 ± 14.57) and 16°C (92.25 ± 14.75, Figure 3) treatments. Heart contraction rates increased linearly for embryos held at a CTmax of 25°C for 1, 2, and 3 hours (LMM, df = 195, p = 0.001, Table S4; Figure 3). However, a significant interaction effect of incubation temperature and duration of CTmax exposure indicated that the slope of heart rate increase was nearly significantly greater for embryos reared at 10°C (LMM, df = 198, p = 0.053, Table S4). At 3 hours of exposure the average heart rate was 119 ± 7.63 BPM (10°C) and 103.85 ± 15.25 (16°C) (Figure 3).
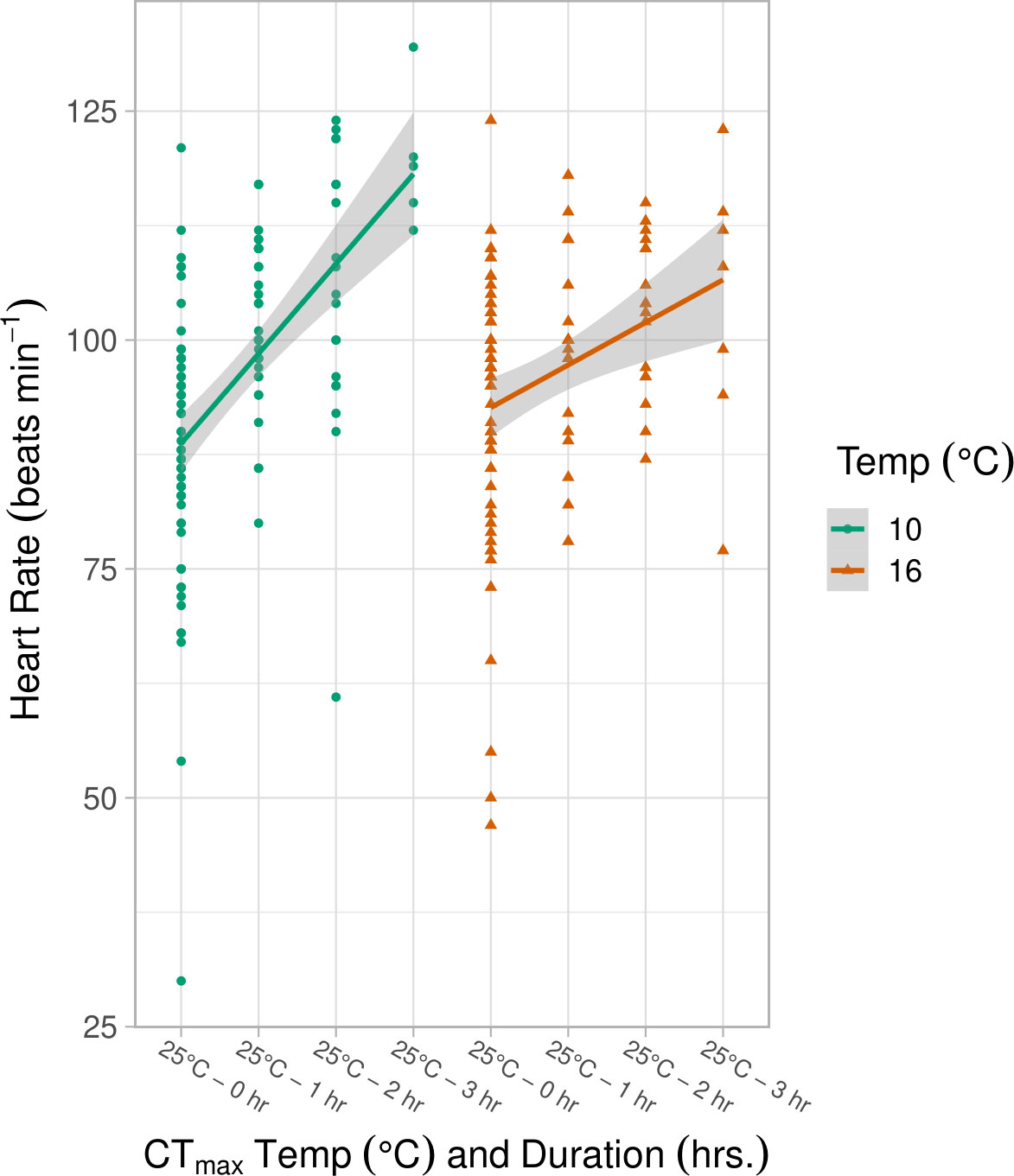
Figure 3 Heart rate (beats min-1) at incubation temperature (10°, 16°C) measured 3 days post CTmax exposure at 25°C with duration (control, 1, 2, 3 hrs.) in spring. Duration of CTmax exposure at 25°C and the interaction of duration and incubation temperature significantly impacted heart rate (LMM, p ≤ 0.05, Table S4), as seen in the differing slopes of the best fit lines for each temperature treatment. The shaded area indicates the 95% confidence interval.
3.4 Hatching success
3.4.1 Winter experiment
Time to first hatch was influenced by temperature and the temperature × pCO2 interaction. Larvae emerged first at 7 days post fertilization (dpf) when kept at 16°C and at 11 dpf at 10°C (ANOVA, df = 3, p < 0.001, Figure 4; Table S5). Time to first hatch was significantly longer under elevated pCO2 at 12°C, although no pCO2 effects were found at other temperatures (Tukey’s HSD, p < 0.001, Figure 4). Time to peak hatch decreased significantly with elevated temperature (ANOVA, df = 3, p < 0.001, Figure 4; Table S5) and was significantly faster in the ambient pCO2 treatment (ANOVA, df = 1, p = 0.039, Figure 4). Cumulative hatch was not significantly impacted by temperature, pCO2, or their interaction (ANOVA, p > 0.05, Table S5).
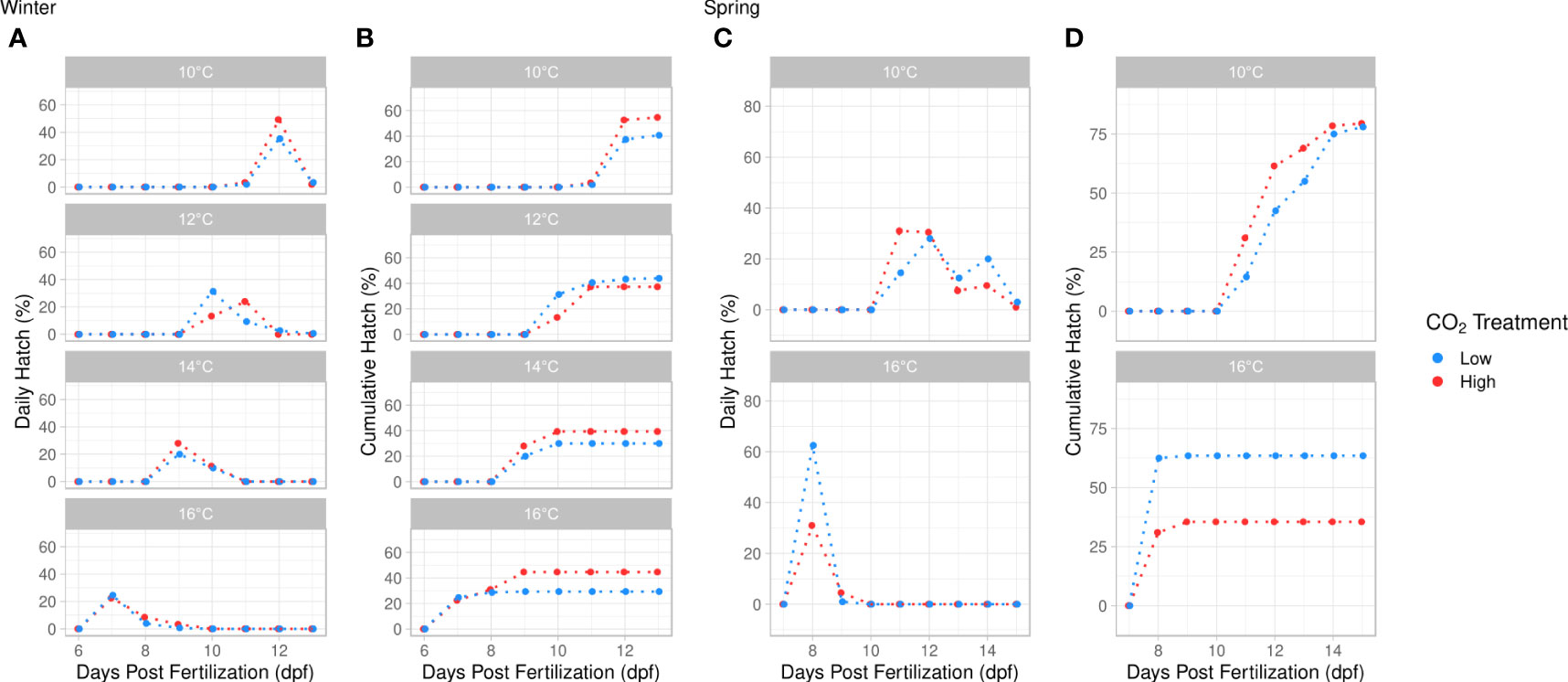
Figure 4 Daily hatch (%) and cumulative hatch (%) versus days post fertilization (dpf) within each incubation temperature treatment. (A, B) represent the daily hatch (%) and cumulative hatch (%) in the winter experiment at 4 discrete temperatures (10°, 12°, 14°, 16°C). (C, D) represent the daily hatch (%) and cumulative hatch (%) in the spring experiment at 2 discrete temperatures (10°, 16°C). pCO2 treatment is indicated by color (blue = ambient, red = high). Temperature significantly reduced time to first hatch (TFH) and time to peak hatch (TPH) in both winter and spring (ANOVA, p < 0.001). TFH was significantly reduced by the temperature × pCO2 interaction at 12°C in winter (Tukey’s HSD, p < 0.001). TPH was significantly faster at low pCO2 in winter (ANOVA, df = 1, p = 0.039). Cumulative hatch was significantly lower at 16°C in spring (ANOVA, df = 1, p = 0.003).
Larval malformation rates (compared to total embryos per replicate cup) were significantly affected by temperature, pCO2, and their interaction (ANOVA, p < 0.04, Table S5). Malformation rates were significantly lower at 10°C than all other temperature treatments (Tukey’s HSD, p ≤ 0.02). As temperature increased, malformation rates remained low under low pCO2 (0–8%) but increased significantly to ~12% for embryos exposed to high pCO2 at 12-16°C (Tukey’s HSD, p < 0.03, Figure 5). When malformation rates were analyzed relative to the number of total hatched larvae and excluded embryos that did not hatch, malformations were seen to increase with temperature only (ANOVA, df = 3, p = 0.003, Table S5 and Figure 6). After accounting for malformation rates, we found that temperature had a near significant effect, with generally lower hatching success under warmer treatments (ANOVA, df = 3, p = 0.054, Table S5), yet no significant pCO2 effect was found (Figure 4; Table 3). Embryos reared at 14°C had a significantly lower hatching success than those reared at 10°C (Tukey’s HSD, p = 0.048, Figure 7) and no other pairwise comparison between temperature treatments were significant (Tukey’s HSD, p > 0.10).
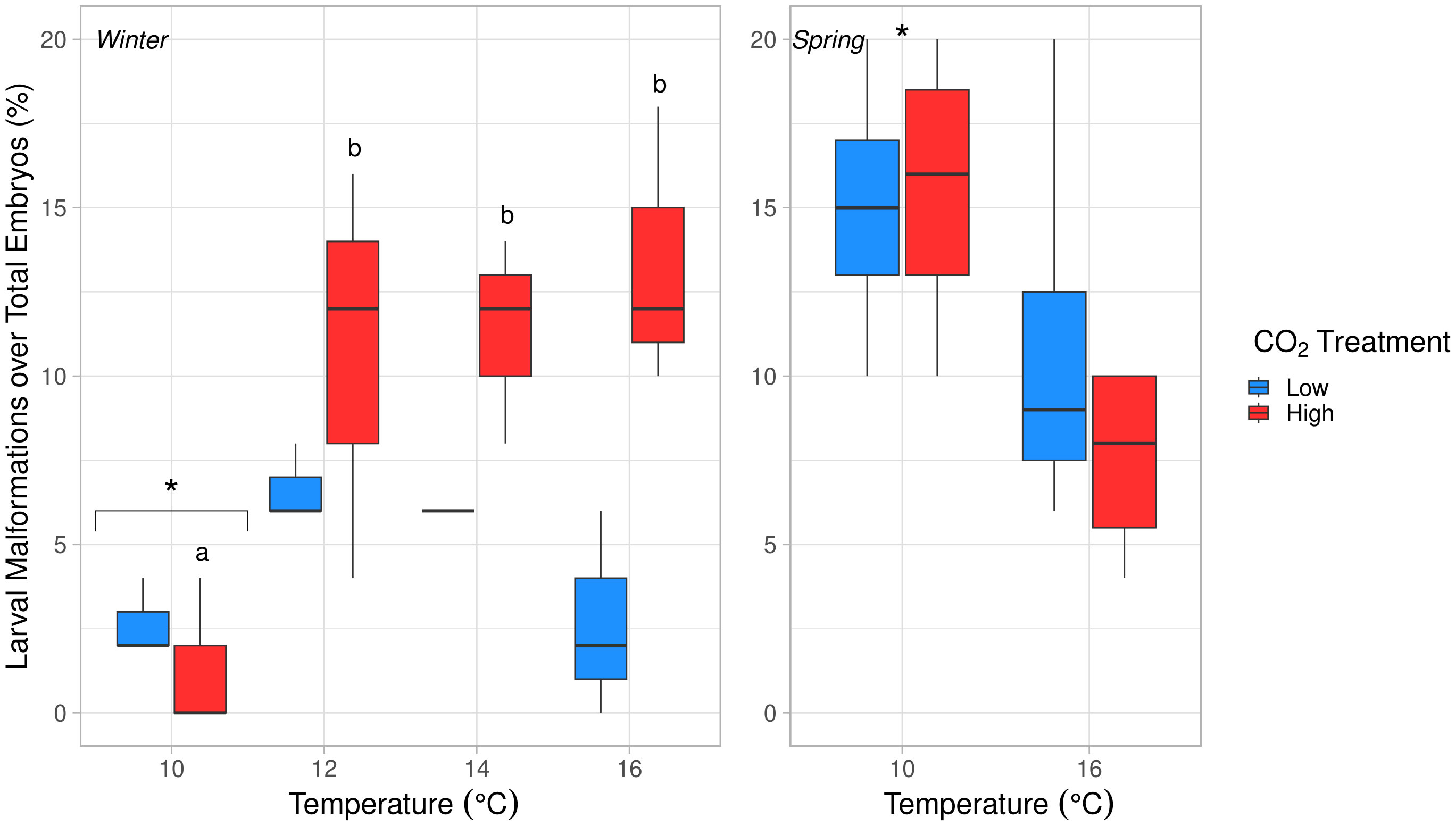
Figure 5 Effects of temperature on larval malformations over total embryos per replicate (%) in winter (left) and spring (right) experiments. Temperature, pCO2, and their interaction had significant effects in winter (ANOVA, p < 0.04). Malformations were significantly less common at 10°C in comparison to all other temperatures in winter (Tukey’s HSD, p < 0.03). Letters (a, b) denote significant difference between temperatures in the high pCO2 condition (Tukey’s HSD, p < 0.04). Temperature had a significant, negative effect in spring (ANOVA, df = 1, p = 0.023). Significantly different temperature treatments are marked with an asterisk (*).
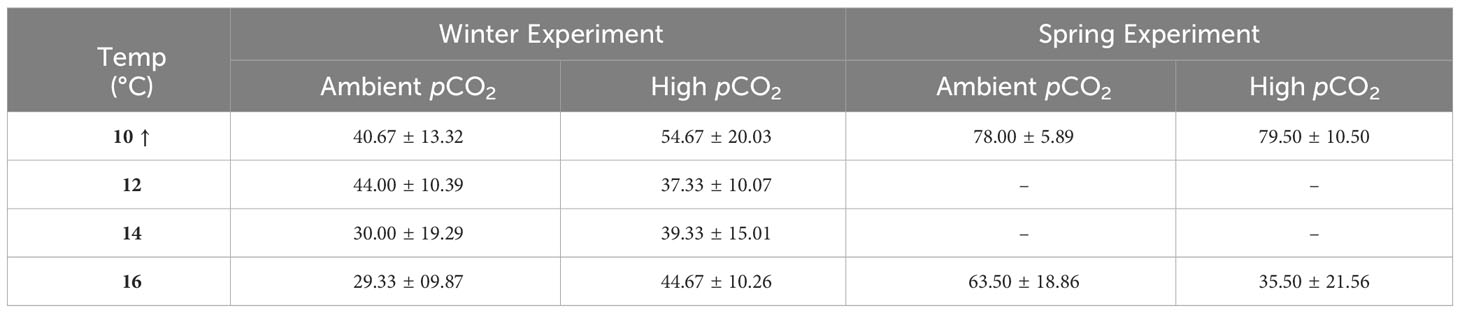
Table 3 Average hatching success (%, ± 1 SD) at different incubation conditions in winter and spring experiments. Treatment combinations of pCO2 + temperature are presented at ambient and high pCO2 values and 4 discrete temperatures. Percent hatching is negatively impacted by temperature treatment with no significant pCO2 effect for both winter and spring. The arrow indicates that hatching success was significantly higher in the 10°C treatment compared to all other temperatures.
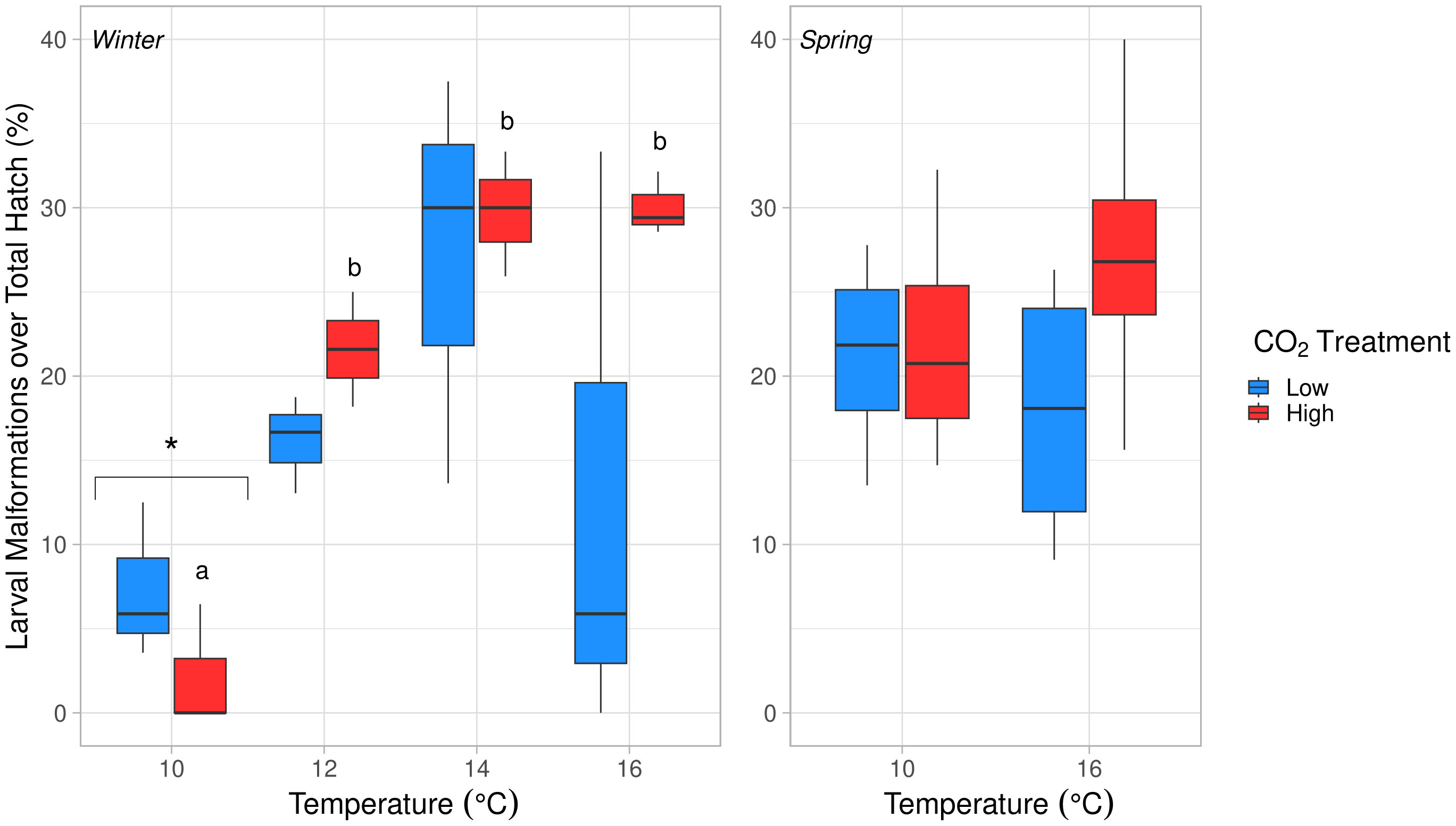
Figure 6 Effects of temperature on larval malformations over total hatch count (%) in winter (left) and spring (right). Temperature had a significant, positive effect in winter (ANOVA, df = 3, p = 0.003). Malformations were significantly lower at 10°C compared to all other temperatures in winter (Tukey’s HSD, p < 0.03). Letters (a, b) denote significant differences between temperatures in the high pCO2 condition (Tukey’s HSD, p < 0.05). No significant differences were found in spring (ANOVA, p > 0.05). The significantly different temperature treatment in winter is marked with an asterisk (*).
3.4.2 Spring experiment
Embryos reared at 16°C showed a significantly reduced time to first hatch and peak hatch (ANOVA, df = 1, p < 0.001, Table S6) and no pCO2 effect was found (Figure 4). Cumulative hatch and hatching success for embryos held at 10°C were substantially higher than those for embryos held at 16°C (ANOVA, df = 1, p < 0.04, Figures 4, 7; Table S6). Within each temperature treatment, TFH was observed in a single day, resulting in standard deviations of zero and preventing the calculation of F-values (Table S6). Overall, pCO2 and the temperature × pCO2 interaction did not significantly affect any of the hatching parameters examined in the spring experiment (ANOVA, df = 1, p > 0.05, Table S6).
Springtime larvae exhibited inverse effects of temperature on total embryo malformation rates in comparison to winter specimens, with significantly higher proportions of malformations occurring in larvae reared at 10°C in comparison to those reared at 16°C (ANOVA, df = 1, p = 0.023, Figure 5; Table S6). In contrast to the winter experiment, no significant temperature × pCO2 interaction effects were found. When malformation rates were measured using only hatched larvae, we saw no significant difference between temperatures in spring (Figure 7; Table S6).
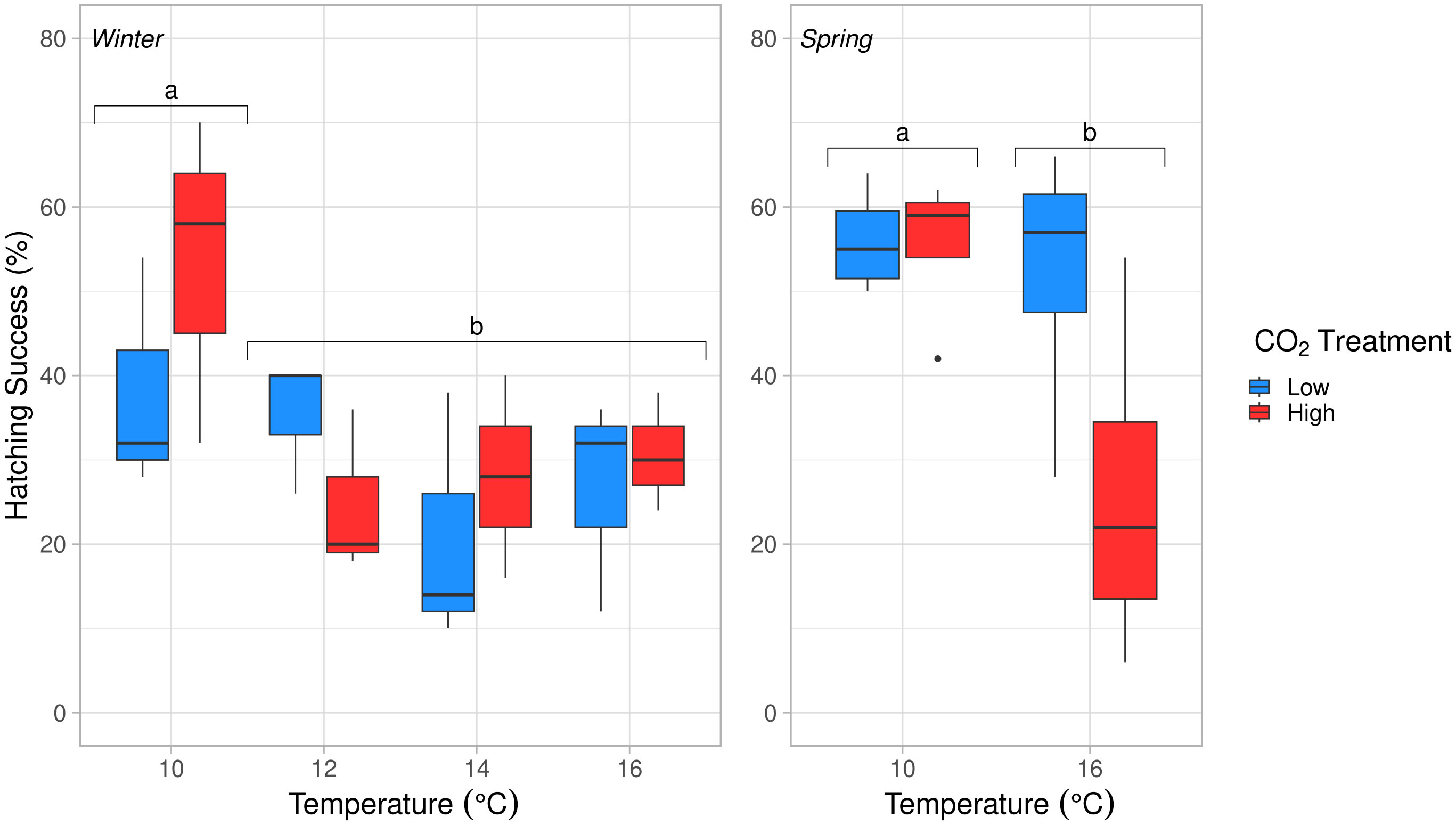
Figure 7 Hatching success (%) at 4 discrete temperature treatments for winter (10°, 12°, 14°, 16°C; left) and two discrete temperatures for spring (10°, 16°C; right) experiments. Temperature had a significant effect on hatching success in winter (ANOVA, df = 3, p = 0.054) and spring (ANOVA, df = 1, p = 0.039). The pCO2 and temperature × pCO2 treatments were not significant in either experiment. Embryos at 10°C had significantly higher hatching success than all other temperature treatments, indicated with letters (a, b) (Tukey’s HSD, p < 0.05).
4 Discussion
The purpose of this study was to determine the effects of ocean acidification, temperature rise, and extreme heating events on the physiology and survival of embryonic Pacific herring. Early life stages of fishes tend to have narrower thermal tolerances and lower resistance to pCO2 fluctuations relative to juveniles and adults because their regulatory pathways and central organ systems are not fully developed (Pörtner et al., 2006). Even small changes in growth and mortality during early fish development are known to impact the recruitment success of marine fishes at the population level (Pörtner and Peck, 2010).
4.1 Critical thermal maximum
The significant decline in survival at 25°C and the downward trend in survival that accompanied increasing hours of exposure to high temperatures indicates that extended hours/days of exposure at or around 25°C could have severe impacts on embryo survival that would reduce recruitment success and herring population biomass. These conditions are more likely to be encountered by late spawning populations as the potential for marine heat waves increases (Frölicher et al., 2018). We found no significant effect of incubation temperature on survival rates during the CTmax trials, which indicates that changes in embryonic rearing temperature up to 16°C do not extend the upper limits of thermal tolerance. In contrast to our findings, Moyano et al. (2017) found that CTmax was significantly higher in Atlantic herring larvae acclimated to elevated incubation temperatures throughout their development (~60 days). This suggests that longer developmental periods under warmer conditions may have a greater effect on the ability of herring offspring to withstand extreme temperature fluctuations. Extending the current study to include more larval stages would allow for a useful comparison between these two species.
While Pacific herring embryos from late spawning populations are more likely to encounter unfavorable temperatures, our data do not clearly resolve the question of whether fish from different stocks are likely to exhibit differences in their physiological responses to these conditions. Although we cannot statistically compare the winter (Port Gamble) embryos with the spring (Cherry Point) embryos, the patterns in the survival data for the CTmax trials are similar for the two populations. This suggests that they may exhibit similar responses to short term temperature excursions. Survival was higher for all tested CTmax parameters in winter spawners in comparison to spring spawners, including the controls. It is unclear as to why spring control embryos exhibited lower survival rates in comparison to their winter counterparts, but differences in methodology (lab fertilization versus field collection of embryos) may have contributed to the variation in survival between experiments, as wild spawned embryos could have lower tolerance to laboratory manipulations. Moreover, spring embryos underwent the CTmax trials at a later developmental stage in comparison with winter embryos, which could be associated with lower thermal plasticity and diminished survival (Eme et al., 2015). There is some evidence of temperature acclimation and higher survival in the Cherry Point stock in comparison to two other Salish Sea herring stocks that spawn in the Puget Sound (Marshall, 2012), but further work is necessary to identify population-level differences in the susceptibility of Pacific herring to global climate change.
Acidification seems to have limited effects on the thermal window of Pacific herring. Among the 16°C winter embryos, those maintained at ambient pCO2 exhibited significantly higher survival than the high pCO2 treatment. This may predict a lethal physiological effect of combined increases in pCO2 and incubation temperature on wild herring embryos, especially during marine heat stress events. However, for embryos incubated at 10°C, exposure to high pCO2 resulted in higher survival after the CTmax trial. This finding could indicate that ocean acidification conditions can activate protective mechanisms that reduce mortality under moderate heat stress. Similar effects have been observed in studies where a moderate heat shock or contaminant resulted in a protective effect against subsequent physiological challenges (Brown et al., 1992; Renfro et al., 1993; Iwama et al., 1998).
4.2 Oxygen consumption rates
We found significantly higher embryonic MO2 values at high pCO2 levels in comparison to ambient pCO2 at all temperatures. These findings contrast with prior studies of Atlantic cod, Atlantic herring, and other recent work with Pacific herring, where pCO2 was not found to influence embryonic oxygen consumption (Dahlke et al., 2017; Leo et al., 2018; Murray and Klinger, 2022). However, in this study we analyzed the effects of pCO2 at an earlier developmental stage in comparison to previous work on Pacific herring (Murray and Klinger, 2022) by taking MO2 measurements from embryos during gastrulation; a developmental stage that has been identified as a critical window of sensitivity to elevated pCO2 in Atlantic cod (Dahlke et al., 2020). Our results indicate a similar pattern may exist in Pacific herring embryos. Acclimation to elevated pCO2 prior to organogenesis requires a substantial increase in metabolic rate, likely due to the upregulation and maintenance of metabolically costly acid/base regulatory proteins to buffer against intracellular acidosis (Dahlke et al., 2020). Given that embryonic development operates on a tight metabolic budget, such a shift in energetic allocation may have contributed to the increased rates of developmental malformations observed in embryos exposed to elevated pCO2 and warmer temperatures. By contrast, prior work on Atlantic herring found a direct effect of temperature on MO2 with no pCO2 effect in measurements performed at similar development stages (Leo et al., 2018). The impact of acidification on MO2 should be studied further to determine how energetic restraints imposed by global change may affect fish metabolism during the early stages of embryonic development.
Many temperate fishes show a parabolic relationship between MO2 and temperature (Lefevre, 2016). In our study we saw significantly lower MO2 values at 10°C, and similar values at 12–16°C, with a plateau which may indicate that this is the peak of a parabolic relationship between temperature and MO2. The oxygen consumption rates of Atlantic cod (Gadus morhua) were influenced by an interaction effect of pCO2 and temperature, where embryos exposed to elevated pCO2 at cold and intermediate temperatures (0-9°C) exhibited an increase in MO2 relative to embryos at low pCO2 levels, yet MO2 significantly declined in fish exposed to both the highest temperature (12°C) and highest pCO2 combination (Dahlke et al., 2017).
Although we did not identify an interaction effect of pCO2 and temperature, we did not assess the effects on MO2 at elevated pCO2 or temperatures nearing the upper thermal limit of this species. Doing so might conceivably produce a response pattern similar to those reported for Atlantic cod by Dahlke et al. (2017). In that study, metabolic activity under extreme warm conditions was compromised by functional defects in the mitochondrial electron transport chain and a reduction in ATP production (Dahlke et al., 2017). A parabolic relationship between temperature and MO2 implies the existence of oxygen supply limits at the plateau and subsequent drops in oxygen demand during prolonged exposure above optimal temperatures, possibly because of cardiac disfunction and physiological damage (Pörtner and Knust, 2007).
4.3 Heart contractions
Heart rate was significantly higher in post-exposure measurements of CTmax embryos in comparison to control embryos kept at a baseline incubation temperature, which indicates that acute exposure to critical temperatures can affect cardiac function, regardless of initial incubation temperature. This suggests that heat stress events could have long-term impacts on the cardiac function of surviving embryos that could reduce the health and fitness of later life stages. For each hour of CTmax exposure, heart rate increased linearly when measured two – three days post-exposure, which suggests that this is not a threshold effect, but the result of damage accumulated during longer exposures. Embryos do not depend on blood circulation for oxygen delivery since they do not yet have functioning gills, but chronically elevated heart rates may indicate the presence of long-term, negative impacts on cardiac function (Steinhausen et al., 2008).
Differences in embryonic heart rate are tied to how their systems respond to environmental cues and to their overall stress response but does not reflect differences in oxygen demand (Barrionuevo and Burggren, 1999; Burggren, 2004). We found that embryos incubated under the lower temperature regime of 10°C showed a larger persistent increase in heart rate after acute exposure to 25°C compared to embryos incubated at 16°C. This indicates a more effective recovery or perhaps a reduced maximum heart rate for embryos acclimated to elevated basal temperature. It also suggests that embryos incubated at 10°C suffered an enhanced stress response that prohibited a return to normal function. Alfonso et al. (2020) found that fish acclimated to elevated basal temperatures had lower cortisol levels than those reared at ambient temperatures, indicating that temperature exposure history can alter the thermal stress response. Although rearing temperature did not have a strong independent effect on increasing heart rates as it did in Villalobos et al. (2020), this dataset was not designed to detect differences between basal conditions, but to examine the responses to simulated acute heat waves.
While embryonic mortality was not impacted by original incubation temperature, deleterious sub-lethal effects on hatched larvae, such as chronically elevated heart rates, could result in diminished recruitment due to reduced allocation of energy to feeding, predator avoidance, and other behaviors critical to survival (Leo et al., 2018). This finding is consistent with recent work on Pacific herring, which showed that chronic exposure to heat wave conditions significantly reduced metabolic efficiency of developing embryos (Murray and Klinger, 2022). On the molecular scale, a review on the expression of heat shock proteins in fish found that increased expression of heat shock proteins from environmental conditions led to a decrease in metabolic capacity, which indicates that stress responses may impair other biological pathways (Iwama et al., 1999). Future research examining heart rates at the time of acute temperature exposure could assess the relationship between basal temperature acclimation and heart rate, which may supply additional evidence of short and long-term cardiac effects.
4.4 Hatching parameters
We found lower hatching success at higher rearing temperatures in both winter and spring spawning stocks, which is consistent with the findings of a previous Pacific herring study (Villalobos et al., 2020). An interesting feature of our data is the lower mean hatching success seen in winter embryos (40%) in comparison with those from the spring (64%). Although we did not determine why hatching success was lower in winter spawners, there may be variation in the viability of offspring from different parents. Egg viability also differed between artificially fertilized (winter) versus field collected embryos (spring), which was likely in part due to the different methods used. Hatching success in other Pacific herring studies ranged from 61% to 78% at ~10°C (Villalobos et al., 2020; Murray and Klinger, 2022, respectively). The decrease in hatching success seen at elevated temperatures aligns with our understanding of temperature-dependent hatching dynamics in herring (Alderdice and Velsen, 1971; Leo et al., 2018; Villalobos et al., 2020; Murray and Klinger, 2022; Toomey et al., 2023).
Our results show that pCO2 and its interaction with temperature did not significantly impact hatching success or cumulative hatch, which is broadly consistent with previous Pacific herring studies (Villalobos et al., 2020; Frommel et al., 2022; Murray and Klinger, 2022). However, our data suggest there may be a negative trend of reduced hatching success with elevated pCO2 in the spring experiment that was non-significant due to high variability. Similarly, Murray and Klinger (2022) found a trend towards lower hatching success in Pacific herring in embryos exposed to a heat wave × high pCO2 in comparison to heat wave × low pCO2, though this trend was not statistically significant. Both studies suggest a potential small pCO2 effect on embryo viability, perhaps through an increased frequency of developmental malformations.
4.5 Malformation rates
Malformations in winter larvae became more frequent at increased temperatures, as expected based on the general literature, regardless of count methodology. The low malformation rates at both pCO2 levels at ambient temperature, and sharp increase in the combined effects of elevated temperature and high pCO2 treatments suggest that the effect of pCO2 on malformation rates in herring could be associated with increased energy allocation to pH buffering, and reduced levels of ATP available for growth, development, and homeostasis (Pörtner, 2008; Dahlke et al., 2017). Although temperature is considered a main driver of developmental defects, acidification can impair fish development by causing them to expend energy on regulating their internal pH at the expense of other processes (Pörtner, 2008; Dahlke et al., 2017). Developmental malformations can also result from ATP shortages at critically high or low temperatures (Leo et al., 2018; Dahlke et al., 2020) and from thermal damage to proteins at extreme temperatures (Somero, 2010), which may cause more damage in development compared to adult fish (Finn and Kapoor, 2008). Our winter malformation results align with those of previous studies on herring (Franke and Clemmesen, 2011; Murray and Klinger, 2022) yet contrast with a study on Atlantic herring in which malformations were higher in groups exposed to elevated pCO2, but where no differences in the prevalence of malformation was seen among temperature treatments (Leo et al., 2018).
Spring larvae exhibited a decrease in malformations at elevated temperatures when malformation rates were calculated as a percentage of total starting embryos. This appears to be atypical, since previous studies saw either an increase in malformations with elevated temperature (Dahlke et al., 2017; Murray and Klinger, 2022) or no effect (Leo et al., 2018; Villalobos et al., 2020). We postulate that elevated temperatures may lead to increased embryo mortality, but since we did not assess embryo malformations of unhatched eggs, we may have underestimated true malformation rates in offspring that survived to hatch. It is likely that heightened temperatures prevent some damaged larvae from hatching, resulting in lower hatching success and, as a result, reduced malformation rates. Malformation counts over total hatching did not exhibit the decrease in malformation rate at high temperature, which supplies evidence for an embryo mortality effect related to malformation before hatching. Thus, it is probable that both populations exhibit a higher frequency of developmental malformations under a combined exposure to warm temperatures and elevated pCO2.
4.6 Summary
Our findings indicate that critical thermal tolerance of embryos will be influenced by duration of extreme heat exposure, which are likely to be variable in nature. We found that Pacific herring embryos from both winter and spring spawning populations can withstand temperatures of ~20°C for 3+ hours regardless of incubation temperature and pCO2 level. Resilience to acute heat stress diminished at 25°C, where survival decreased by ~36% in winter and ~95% in spring when exposed for 3+ hours. Nearshore temperatures in the Puget Sound frequently exceed 20°C in late-spring and summer and can reach temperatures above 25°C (NOAA, 2023; Figure 8). High temperature exposures typically last for 1-3 hours during low spring tides at Cherry Point and sometimes are associated with air exposure of similar duration (Love et al., Unpubl. data), which may have some beneficial effects on hatching success and embryonic survival at ambient pCO2 levels (Frommel et al., 2022).
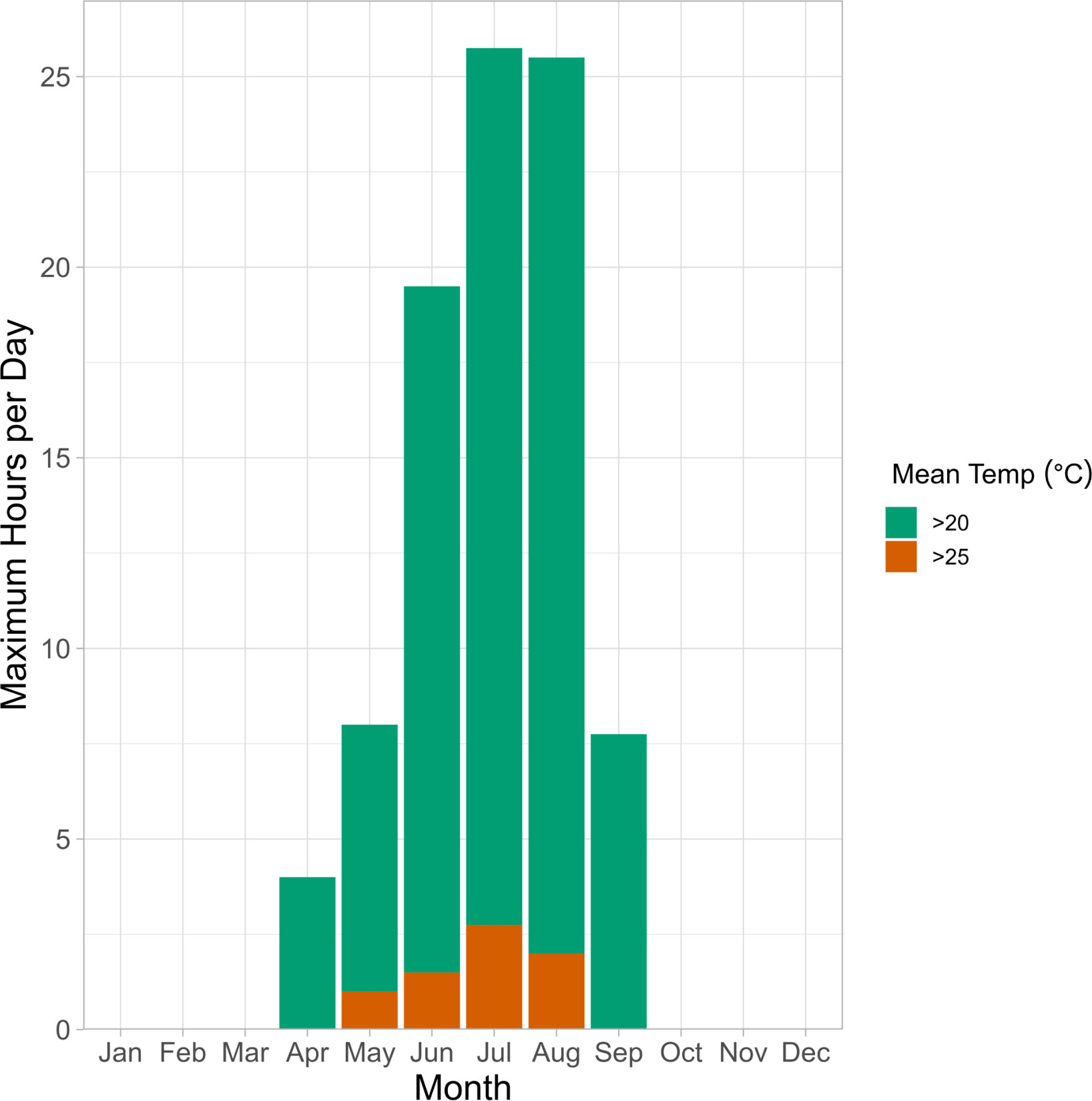
Figure 8 Vertical bars represent the maximum duration of hours per day that seawater temperatures were above 20°C (green) and 25°C (orange) recorded monthly at the NERRS Padilla Bay Ploeg Channel Station between 2001 and 2022. This station records environmental conditions in large seagrass ecosystem that is characteristic of Pacific herring spawning habitat. Temperatures exceeded 20°C between April and September and exceeded 25°C between May and August.
Marine heat waves are 9× as prevalent today as they were in preindustrial times (Frölicher et al., 2018) and their frequency is projected to increase because of anthropogenic climate change. The combination of marine and atmospheric heat waves will increase the potential for acute temperature rise in shallow, tidal habitats, particularly during low tide when atmospheric heat waves can rapidly warm shallow water (Schlegel et al., 2017). Our results suggest that embryonic survival during acute temperature change is both duration and temperature sensitive. Therefore, if heat wave duration and intensity (maximum temperature) are increasing, then their impacts on early life stage mortality, annual stock recruitment, and the overall abundance of Pacific herring may also increase in the coming years. Although variation in temperature and pCO2 is common in nearshore spawning habitats, heat waves nearing 25°C will expose embryos to temperatures at the edge of their thermal window for hours and/or days. This is especially alarming for herring stocks that spawn in the spring, such as the Cherry Point population, because they experience greater maximum temperatures. However, the observed interactive effects of incubation temperature and duration of exposure to heat stress on embryo heart rates suggest that acclimation to warmer temperatures during development could help herring withstand acute temperature fluctuations.
Increased oxygen consumption at elevated temperatures and pCO2 levels indicates that oxygen supply could become limiting much faster under these conditions in comparison to ambient conditions. Although there has been some research into the effects of temperature on MO2 in Pacific herring, the pCO2 effect found in this study contributes to our understanding of the physiological impacts of acidification during the early life stages in this species. Although the mechanism that produces increased MO2 at elevated temperatures is well understood, the heightened MO2 response to elevated pCO2 should be investigated further.
Adult Pacific herring and their eggs and larvae are available in the Salish Sea throughout most of the winter and spring, which provides an important food resource for a wide variety of organisms over an extended time period. This contributes to their importance as a forage fish species and reinforces interest in the effects of global change on Pacific herring populations (Stick et al., 2014; Sandell In prep). The results presented here can inform practices and policy related to the conservation of this species.
Data availability statement
The datasets presented in this study can be found in online repositories. The names of the repository/repositories and accession number(s) can be found below: All data have been published with SEANOE. https://doi.org/10.17882/96693 https://doi.org/10.17882/96694 https://doi.org/10.17882/96694 https://doi.org/10.17882/96696 https://doi.org/10.17882/96697 https://doi.org/10.17882/96698.
Ethics statement
The animal study was approved by Animal Care and Use Committee, Western Washington University. The study was conducted in accordance with the local legislation and institutional requirements.
Author contributions
NS: Conceptualization, Data curation, Formal analysis, Investigation, Methodology, Project administration, Writing – original draft, Writing – review & editing. BL: Conceptualization, Funding acquisition, Investigation, Methodology, Resources, Supervision, Writing – review & editing. CM: Conceptualization, Data curation, Formal analysis, Investigation, Methodology, Supervision, Writing – review & editing. KS: Supervision, Validation, Writing – review & editing. WC: Supervision, Validation, Writing – review & editing.
Funding
The author(s) declare financial support was received for the research, authorship, and/or publication of this article. This work was funded by the Washington Ocean Acidification Center Agreement BPO54883, Western Washington University College of the Environment small grant, Shannon Point Marine Center - Marine and Coastal Science summer funding, and Sulkin Graduate Research Fund.
Acknowledgments
We thank the Washington Department of Fish and Wildlife for providing the whole-animal fish and egg specimens that made this research possible. We also thank Ariel Shiley and Abi Lee for their research support. Thank you to the faculty and staff at SPMC for providing the facilities, equipment, and assistance during the experimental process.
Conflict of interest
The authors declare that the research was conducted in the absence of any commercial or financial relationships that could be construed as a potential conflict of interest.
Publisher’s note
All claims expressed in this article are solely those of the authors and do not necessarily represent those of their affiliated organizations, or those of the publisher, the editors and the reviewers. Any product that may be evaluated in this article, or claim that may be made by its manufacturer, is not guaranteed or endorsed by the publisher.
Supplementary material
The Supplementary Material for this article can be found online at: https://www.frontiersin.org/articles/10.3389/fmars.2023.1307617/full#supplementary-material
References
Alderdice D., Velsen F. (1971). Some effects of salinity and temperature on early development of Pacific herring (Clupea pallasi). J. Fish. Res. Board Can. 28, 1545–1562. doi: 10.1139/f71-234
Alfonso S., Gesto M., Sadoul B. (2020). Temperature increase and its effects on fish stress physiology in the context of global warming. J. Fish Biol. 98 (6), 1496–1508. doi: 10.1111/jfb.14599
Arias P. A., Bellouin N., Coppola E., Jones R. G., Krinner G., Marotzke J., IPCC, et al (2021). Climate Change 2021: The Physical Science Basis: Working Group I Contribution to the Sixth Assessment Report of the Intergovernmental Panel on Climate Change. Eds. Masson-Delmotte V., Zhai P., Pirani A., Connors S. L., Péan C., Berger S., et al (Cambridge and New York: Cambridge University Press). doi: 10.1017/9781009157896
Barrionuevo W. R., Burggren W. W. (1999). O2 consumption and heart rate in developing zebrafish (Danio rerio): influence of temperature and ambient O2. Am. J. Physiol. 276 (2), R505–R513. doi: 10.1152/ajpregu.1999.276.2.R505
Bindoff N. L., Cheung W. W. L., Kairo J. G., Arístegui J., Guinder V. A., Hallberg R., et al. (2019). “Changing ocean, marine ecosystems, and dependent communities,” in IPCC special report on the ocean and cryosphere in a changing climate. Eds. Pörtner H. O., Roberts D. C., Masson-Delmotte V., Zhai P., Tignor M., Poloczanska E., et al (Cambridge and New York: Cambridge University Press), 447–587. doi: 10.1017/9781009157964.007
Brown T. J., Jardine J., Ison C. A. (1992). Antibodies against Haemophilus ducreyi heat shock proteins. Microb. Pathog. 15 (2), 131–139. doi: 10.1006/mpat.1993.1063
Burggren W. W. (2004). What is the purpose of the embryonic heart beat? Or how facts can ultimately prevail over physiological dogma. Physiol. Biochem. Zool 77 (3), 333–345. doi: 10.1086/422230
Cai W.-J., Feely R. A., Testa J. M., Li M., Evans W., Alin S. R., et al. (2021). Natural and anthropogenic drivers of acidification in large estuaries. Annu. Rev. Mar. Sci. 13, 23–55. doi: 10.1146/annurev-marine-010419-011004
Cattano C., Claudet J., Domenici P., Milazzo M. (2018). Living in a high CO2 world: A global meta-analysis shows multiple trait-mediated fish responses to ocean acidification. Ecol. Monogr. 88 (3), 320–335. doi: 10.1002/ecm.1297
Clements J., Hunt H. (2015). Marine animal behavior in a high CO2 ocean. Mar. Ecol. Prog. Ser. 536, 259–279. doi: 10.3354/meps11426
Cury P., Bakun A., Crawford R. J. M., Jarre A., Quiñones R. A., Shannon L. J., et al. (2000). Small pelagics in upwelling systems: patterns of interaction and structural changes in ‘‘wasp-waist’’ ecosystems. ICES J. Mar. Sci. 57, 603–618. doi: 10.1006/jmsc.2000.0712
Cuvier G., Valenciennes A. (1847). Histoire naturelle des poissons. Tome vingtième. Livre vingt et unième. de la famille des Clupéoïdes. Hist. Nat. Poiss 20i-xviii + 1, 1–472, Pls. 591-606.
Dahlke F. T., Leo E., Mark F. C., Pörtner H. O., Bickmeyer U., Frickenhaus S., et al. (2017). Effects of ocean acidification increase embryonic sensitivity to thermal extremes in Atlantic cod, Gadus morhua. Glob. Change Biol. 23 (4), 1499–1510. doi: 10.1111/gcb.13527
Dahlke F., Lucassen M., Bickmeyer U., Wohlrab S., Puvanendran V., Mortensen A., et al. (2020). Fish embryo vulnerability to combined acidification and warming coincides with a low capacity for homeostatic regulation. J. Exp. Biol. 223 (11), jeb212589. doi: 10.1242/jeb.212589
DFO (2019). Status of Pacific Herring (Clupea pallasii) in 2018 and forecast for 2019 (Center for Science Advice, Pacific Region, Fisheries and Oceans Canada, Nanaimo, BC: DFO Can. Sci. Advis. Sec. Sci. Resp). Available at: https://waves-vagues.dfo-mpo.gc.ca/Library/40884909.pdf.
Dickson A. G., Sabine C. L., Christian J. R. (2007). “Guide to best practices for ocean CO2 measurements,” in PICES Spec. Pu. Report No. 8, vol. 3. , 1–191. Available at: https://cdiac.ess-dive.lbl.gov/ftp/oceans/Handbook_2007/Guide_all_in_one.pdf.
Dinnel P. A., Hoover R., Lechuga L. (2008). Development of larval Pacific herring, Clupea Pallasii, bioassay protocols: Refinement, validation, refinery effluent and Cherry Point ambient water testing during 2007 (Western Washington University, Anacortes: Washington Department of Ecology by Shannon Point Marine Center).
Dinnel P. A., Middaugh D. P., Schwarck N. T., Farren H. M., Haley R. K., Hoover R. A., et al. (2011). Methods for conducting bioassays using embryos and larvae of Pacific herring, Clupea pallasi. Arc. Environ. Contam. Toxicol. 60 (2), 290–308. doi: 10.1007/s00244-010-9600-8
Douglas N. K., Byrne R. H. (2017). Achieving accurate spectrophotometric pH measurements using unpurified meta-cresol purple. Mar. Chem. 190, 66–72. doi: 10.1016/j.marchem.2017.02.004
Eme J., Mueller C. A., Manzon R. G., Somers C. M., Boreham D. R., Wilson J. Y. (2015). Critical windows in embryonic development: Shifting incubation temperatures alter heart rate and oxygen consumption of Lake Whitefish (Coregonus clupeaformis) embryos and hatchlings. Comp. Biochem. Physiol. A. Mol. Integr. Physiol. 179, 71–80. doi: 10.1016/j.cbpa.2014.09.005
Evans W., Pocock K., Hare A., Weekes C., Hales B., Jackson J., et al. (2019). Marine CO2 patterns in the Northern Salish Sea. Front. Mar. Sci. 5. doi: 10.3389/fmars.2018.00536
Fabry V. J., Seibel B. A., Feely R. A., Orr J. C. (2008). Impacts of ocean acidification on marine fauna and ecosystem processes. ICES J. Mar. Sci. 65 (3), 414–432. doi: 10.1093/icesjms/fsn048
Fassbender A. J., Alin S. R., Feely R. A., Sutton A. J., Newton J. A., Krembs C., et al. (2018). Seasonal carbonate chemistry variability in marine surface waters of the US Pacific Northwest. Earth Syst. Sci. Data. 10 (3), 1367–1401. doi: 10.5194/essd-10-1367-2018
Franke A., Clemmesen C. (2011). Effect of ocean acidification on early life stages of Atlantic herring (Clupea harengus L.). Biogeosciences 8 (12), 3697–3707. doi: 10.5194/bg-8-3697-2011
Frölicher T. L., Fischer E. M., Gruber N. (2018). Marine heatwaves under global warming. Nature 560 (7718), 360–364. doi: 10.1038/s41586-018-0383-9
Frommel A. Y., Lye S. L. R., Brauner C. J., Hunt B. P. V. (2022). Air exposure moderates ocean acidification effects during embryonic development of intertidally spawning fish. Sci. Rep. 12 (1), 12270. doi: 10.1038/s41598-022-16399-6
Frommel A. Y., Maneja R., Lowe D., Malzahn A. M., Geffen A. J., Folkvord A., et al. (2012). Severe tissue damage in Atlantic cod larvae under increasing ocean acidification. Nat. Climate Change 2 (1), 42–46. doi: 10.1038/nclimate1324
Frommel A. Y., Maneja R., Lowe D., Pascoe C. K., Geffen A. J., Folkvord A., et al. (2014). Organ damage in Atlantic herring larvae as a result of ocean acidification. Ecol. Appl. 2 (5), 1131–1143. doi: 10.1890/13-0297.1
Gaeckle J., Dowty P., Berry H., Ferrier L. (2011). Puget Sound submerged vegetation Monitoring project: 2009 report (Olympia, WA: Nearshore Habitat Program, Aquatic Resources Division, Washington State Department of Natural Resources). Available at: https://www.dnr.wa.gov/publications/aqr_eelgrass_svmp_report.pdf.
Ishimatsu A., Hayashi M., Kikkawa T. (2008). Fishes in high- CO2, acidified oceans. Mar. Ecol. Prog. Ser. 373, 295–302. doi: 10.3354/meps07823
Iwama G. K., Thomas P. T., Forsyth R. B., Vijayan M. M. (1998). Heat shock protein expression in fish. Rev. Fish Biol. Fish. 8, 35–56. doi: 10.1023/A:1008812500650
Iwama G. K., Vijayan M. M., Forsyth R. B., Ackerman P. A. (1999). Heat shock proteins and physiological stress in fish. Amer. Zool. 39 (6), 901–909. doi: 10.1093/icb/39.6.901
Jokiel P. L., Bahr K. D., Rodgers K. S. (2014). Low-cost, high-flow mesocosm system for simulation ocean acidification with CO2 gas. Limnol. Oceanogr. Method 12 (5), 313–322. doi: 10.4319/lom.2014.12.313
Kawakami T., Okouchi H., Aritaki M., Aoyama J., Tsukamoto K. (2011). Embryonic development and morphology of eggs and newly hatched larvae of Pacific herring (Clupea pallasii). Fish. Sci. 77, 183–190. doi: 10.1007/s12562-010-0317-4
Khangaonkar T., Nugraha A., Xu W., Balaguru K. (2019). Salish Sea response to global climate change, sea level rise, and future nutrient loads. J. Geophys. Res. Oceans 124 (6), 3876–3904. doi: 10.1029/2018JC014670
Kwiatkowski L., Torres O., Bopp L., Aumont O., Chamberlain M., Christian J. R., et al. (2020). Twenty-first century ocean warming, acidification, deoxygenation, and upper-ocean nutrient and primary production decline from CMIP6 model projections. Biogeosciences 1 (13), 3439–3470. doi: 10.5194/bg-17-3439-2020
Landis W. G., Bryant P. T. (2010). Using weight of evidence characterization and modeling to investigate the cause of the changes in Pacific Herring (Clupea pallasi) population dynamics in Puget Sound and at Cherry Point, Washington. Risk. Anal. 30 (2), 183–202. doi: 10.1111/j.1539-6924.2009.01288.x
Lefevre S. (2016). Are global warming and ocean acidification conspiring against marine ectotherms? A meta-analysis of the respiratory effects of elevated temperature, high CO2 and their interaction. Conserv. Physiol. 4 (1), cow009. doi: 10.1093/conphys/cow009
Leo E., Dahlke F. T., Storch D., Pörtner H. O., Mark F. C. (2018). Impact of ocean acidification and warming on the bioenergetics of developing eggs of Atlantic herring Clupea harengus. Conserv. Physiol. 6 (1), coy050. doi: 10.1093/conphys/coy050
Lewis E., Wallace D., Allison L. J. (1998). Program developed for CO2 system calculations (United States: N.p). doi: 10.2172/639712
Love B. A., Olson M. B., Wuori T. (2017). Technical Note: A minimally invasive experimental system for pCO2 manipulation in plankton cultures using passive gas exchange (atmospheric carbon control simulator). Biogeosciences 14 (10), 2675–2684. doi: 10.5194/bg-14-2675-2017
Lowe A. T., Bos J., Ruesink J. (2019). Ecosystem metabolism drives pH variability and modulates long-term ocean acidification in the Northeast Pacific coastal ocean. Sci. Rep. 9, 963. doi: 10.1038/s41598-018-37764-4
Marshall R. (2012). Final report on Pacific herring (Clupea pallasii) test development and Validation. Pub No. 11-10-086 (Olympia, WA: Washington State Department of Ecology, Water Quality Program). Available at: https://apps.ecology.wa.gov/publications/documents/1110086.pdf.
McKechnie I., Lepofsky D., Moss M. L., Butler V. L., Orchard T. J., Coupland G. (2014). Archaeological data provide alternative hypotheses on Pacific herring (Clupea pallasii) distribution, abundance, and variability. PNAS 111 (9), E807–E816. doi: 10.1073/pnas.1316072111
Moyano M., Candebat C., Ruhbaum Y., Álvarez-Fernández S., Claireaux G., Zambonino Infante J.-L., et al. (2017). Effects of warming rate, acclimation temperature and ontogeny on the critical thermal maximum of temperate marine fish larvae. PloS One 12 (7), e0179928. doi: 10.1371/journal.pone.0179928
Murray C. S., Klinger T. (2022). High pCO2 does not alter the thermal plasticity of developing Pacific herring embryos during a marine heatwave. J. Exp. Biol. 225, jeb.243501. doi: 10.1242/jeb.243501
NOAA (2023). Data from: Ploeg Channel, Padilla Bay Reserve, WA (PBLW1) (National Data Buoy Center, Stennis Space Center, MS).
Oschlies A., Brandt P., Stramma L., Schmidtko S. (2018). Drivers and mechanisms of Ocean deoxygenation. Nat. Geosci. 11, 467–473. doi: 10.1038/s41561-018-0152-2
Pacella S. R., Brown C. A., Waldbusser G. G., Labiosa R. G., Hales B. (2018). Seagrass habitat metabolism increases short-term extremes and long-term offset of CO2 under future ocean acidification. Proc. Natl. Acad. Sci. 115 (15), 3870–3875. doi: 10.1073/pnas.1703445115
Peck M. A., Kanstinger P., Holste L., Martin M. (2012). Thermal windows supporting survival of the earliest life stages of Baltic herring (Clupea harengus). ICES J. Mar. Sci. 69 (4), 529–536. doi: 10.1093/icesjms/fss038
Penttila D. (2007). Marine Forage Fishes in Puget Sound (Olympia, WA: Technical Report 2007-03, Washington Department of Fish and Wildlife), 23. Available at: https://wdfw.wa.gov/sites/default/files/publications/02193/wdfw02193.pdf.
Pikitch E. K., Rountos K. J., Essington T. E., Santora C., Pauly D., Watson R., et al. (2014). The global contribution of forage fish to marine fisheries and ecosystems. Fish Fish. 15 (1), 43–64. doi: 10.1111/faf.12004
Pörtner H. O. (2008). Ecosystem effects of ocean acidification in times of ocean warming: A physiologist’s view. Mar. Ecol. Prog. Ser. 373, 203–217. doi: 10.3354/meps07768
Pörtner H. O., Knust R. (2007). Climate change affects marine fishes through the oxygen limitation of thermal tolerance. Science 315 (5808), 95–97. doi: 10.1126/science.1135471
Pörtner H. O., Peck M. A. (2010). Climate change effects on fishes and fisheries: Towards a cause-and-effect understanding. J. Fish Biol. 77 (8), 1745–1779. doi: 10.1111/j.1095-8649.2010.02783.x
Pörtner H. O., Peck L. S., Hirse T. (2006). Hyperoxia alleviates thermal stress in the Antarctic bivalve, Laternula elliptica: Evidence for oxygen limited thermal tolerance. Polar. Biol. 29 (9), 688–693. doi: 10.1007/s00300-005-0106-1
R Core Team (2022). R: A language and environment for statistical computing (Vienna, Austria: R Foundation for Statistical Computing).
Renfro J. L., Brown M. A., Parker S. L., Hightower L. E. (1993). Relationship of thermal and Chemical tolerance to transepithelial transport by cultured flounder renal epithelium. J. Pharmacol. Exp. Ther. 265, 992–1000.
Sandell T., Lindquist A., Biondo P., Bruestle E., Dionne P. 2020 Washington State Herring Stock Status Report (Olympia, WA: Washington Department of Fish and Wildlife). (In Prep).
Sandell T., Lindquist A., Dionne P., Lowry D. (2016). 2016 Washington State Herring Stock (Olympia, WA: Fish Program Technical Report No. FPT 19-07, Washington Department of Fish and Wildlife). Available at: https://wdfw.wa.gov/sites/default/files/publications/02105/wdfw02105.pdf.
Schlegel R. W., Oliver E. C. J., Perkins-Kirkpatrick S., Kruger A., Smit A. J. (2017). Predominant atmospheric and oceanic patterns during coastal marine heatwaves. Front. Mar. Sci. 4. doi: 10.3389/fmars.2017.00323
Schweigert J. F., Boldt J. L., Flostrand L., Cleary J. S. (2010). A review of factors limiting recovery of Pacific herring stocks in Canada. ICES J. Mar. Sci. 67 (9), 1903–1913. doi: 10.1093/icesjms/fsq134
Sobocinski K. L., Harvell C. D., Baloy N. J. K., Broadhurst G., Dethier M. N., Flower A., et al. (2022). Urban seas are hotspots of stress in the Anthropocene ocean: The Salish Sea example. Elementa: Sci. Anthro 10 (1), 55. doi: 10.1525/elementa.2022.00055
Somero G. N. (2010). The physiology of climate change: how potentials for acclimatization and genetic adaptation will determine ‘winners’ and ‘losers’. J. Exp. Biol. 213, 912–920. doi: 10.1242/jeb.037473
Sswat M., Stiasny M. H., Taucher J., Algueró-Muñiz M., Bach L. T., Jutfelt F., et al. (2018). Food web changes under ocean acidification promote herring larvae survival. Nat. Ecol. Evol. 2, 836–840. doi: 10.1038/s41559-018-0514-6
Steinhausen M. F., Sandblom E., Eliason E. J., Verhille C., Farrell A. P. (2008). The effect of acute temperature increases on the cardiorespiratory performance of resting and swimming sockeye salmon (Oncorhynchus nerka). J. Exp. Biol. 211 (24), 3915–3926. doi: 10.1242/jeb.019281
Stick K. C., Lindquist A., Lowry D. (2014). “2012 Washington State herring stock status report,” in Fish Program Technical Report No. FPA 14-09 (Olympia, WA: Washington Department of Fish and Wildlife). Available at: https://wdfw.wa.gov/sites/default/files/publications/01628/wdfw01628.pdf.
Toomey L., Giraldo C., Loots C., Mahé K., Marchal P., MacKenzie K. (2023). Impact of temperature on Downs herring (Clupea harengus) embryonic stages: First insights from an experimental approach. PloS One 18 (4), e0284125. doi: 10.1371/journal.pone.0284125
Villalobos C., Love B. A., Olson M. B. (2020). Ocean acidification and ocean warming effects on Pacific herring (Clupea pallasi) early life stages. Front. Mar. Sci. 7. doi: 10.3389/fmars.2020.597899
Warton D. I., Hui F. K. (2011). The arcsine is asinine: the analysis of proportions in ecology. Ecol. 92 (1), 3–10. doi: 10.1890/10-0340.1
West J. E., O’Neill S. M., Ylitalo G. M. (2008). Spatial extent, magnitude, and patterns of persistent organochlorine pollutants in Pacific herring (Clupea pallasi) populations in the Puget Sound (USA) and Strait of Georgia (Canada). Sci. Total Environ. 394 (2-3), 369–378. doi: 10.1016/j.scitotenv.2007.12.027
Keywords: Pacific herring, ocean acidification, temperature rise, climate change, critical thermal limits, oxygen consumption rates, heart rate, forage fishes
Citation: Singh NR, Love B, Murray CS, Sobocinski KL and Cooper WJ (2023) The combined effects of acidification and acute warming on the embryos of Pacific herring (Clupea pallasii). Front. Mar. Sci. 10:1307617. doi: 10.3389/fmars.2023.1307617
Received: 04 October 2023; Accepted: 27 November 2023;
Published: 18 December 2023.
Edited by:
Gretchen E. Hofmann, University of California, Santa Barbara, United StatesReviewed by:
Andrea Y. Frommel, University of British Columbia, CanadaLiqiang Zhao, Guangdong Ocean University, China
Copyright © 2023 Singh, Love, Murray, Sobocinski and Cooper. This is an open-access article distributed under the terms of the Creative Commons Attribution License (CC BY). The use, distribution or reproduction in other forums is permitted, provided the original author(s) and the copyright owner(s) are credited and that the original publication in this journal is cited, in accordance with accepted academic practice. No use, distribution or reproduction is permitted which does not comply with these terms.
*Correspondence: Nicole R. Singh, singh.nicole13@gmail.com