- 1Zoological Department, SeaWorld San Diego, San Diego, CA, United States
- 2Zoological Department, SeaWorld San Antonio, San Antonio, TX, United States
- 3Zoological Department, SeaWorld Orlando, Orlando, FL, United States
- 4Seaworld and Busch Gardens Species Preservation Laboratory, San Diego, CA, United States
Killer whales are an important sentinel species and developing non-invasive methods of health assessments might provide insight for understanding how wildlife health is influenced by ecosystem change. Rectal temperature (RT) is a proxy for core body temperature in managed-care cetaceans, however, this measurement is impractical for free-ranging cetaceans and infrared imaging has been suggested as an alternative. The aim of the current study was to prospectively compare infrared thermography of the blowhole to rectal temperatures in killer whales, as well as establish a healthy range for rectal temperature using retrospective data. Infrared video was recorded from the blowhole of thirteen healthy killer whales in managed care, immediately followed by rectal temperature measurement. Repeated measures Bland-Altman analysis revealed blowhole temperature (BHT) had a bias of -1.28°C from RT. Considerable proportional bias was observed with agreement between measurements improving as mean temperature increased. RT positively associated with air temperature, and inversely associated with body mass. BHT was not significantly affected by sex or body mass but was significantly affected by water temperature and air temperature. Retrospective analysis from eighteen killer whales (n = 3591 observations) was performed to generate expected RT ranges, partitioning out for sex and body mass. Given the proportional bias observed with Bland Altman analysis, BHT cannot currently be recommended as a measurement for absolute core body temperature, however infrared thermography of the blowhole remains a promising tool for health assessment of free-ranging killer whale populations, as it may serve as a non-contact screening tool to detect pyrexic animals within a group.
1 Introduction
Killer whales (Orcinus orca) are considered a sentinel species for marine ecosystem health (Bossart, 2011; Andvik et al., 2021) and while populations are distributed worldwide, the “southern resident” killer whale population (SRKW) inhabiting the Salish Sea currently has 75 individuals (Center for Whale Research, 2023) and is listed as endangered by the Canadian Commission for the Status on Endangered Wildlife in Canada and the United States under the Endangered Species Act (National Marine Fisheries Service, 2005). Health surveillance of these individuals is paramount for their conservation, and investigating minimally or non-invasive methods for health assessment are important due to the logistic difficulty of collecting direct physiologic samples or measurements from these large animals (Hunt et al., 2013; Rhodes et al., 2022). Non-invasive techniques can reduce stress due to handling by minimizing or preventing physiological responses resulting from functional changes in the hypothalamus-pituitary-adrenal axis and the sympathetic-adrenal-medullary axis. Activation of these systems during handling of cetaceans can potentially result in stress-induced hyperthermia, electrolyte derangements, and changes in circulating thyroid hormones (Hao et al., 2009; Karaer et al., 2023). In addition, non-invasive methods avoid the potentially serious complications associated with the use of sedatives for chemical restraint (Dold and Ridgway, 2014).
Core body temperature is a physiological parameter that provides insight into thermoregulation, physiology, clinical health status, activity level, and response to environment (McCafferty, 2007; McCafferty et al., 2015). Rectal temperature (RT) is a proxy used to estimate core body temperature in marine mammals as part of a clinical health assessment, and is typically employed in animals conditioned to accept a rectal thermometer without the use of restraint (Sweeney and Ridgway, 1975; Katsumata et al., 2006). There are no reported reference intervals for rectal temperature in killer whales, and previous reported ranges of 35.5-35.9°C, and 37.1-38.5°C, are each based off of individual cases (Whittow et al., 1974; Kusuda et al., 2011). Rectal temperature is not practical to obtain in managed-care animals that are not conditioned for the behavior, in animals choosing not to participate due to illness or other factors, or in free-ranging animals (McCafferty et al., 2015). Therefore, a non-invasive measurement method may be more suitable for these individuals.
Infrared thermography allows for identification of thermal windows (i.e., anatomical regions characterized by poor insulation, glabrous skin, and dense vascularization (Andrade, 2015)) and can detect changes in thermal patterns that may be associated with vasoconstriction or vasodilation of peripheral vasculature, that can occur secondary to environmental stimuli, internal metabolic processes, or pathology. It has the potential to screen for any disease process that induces inflammation or pyrexia (Rekant et al., 2016; Mota-Rojas et al., 2022). Use of infrared thermography in wildlife and marine mammal medicine is well established and in managed-care animals is used to evaluate wounds, musculoskeletal injury, skin conditions, or diseases of the oral cavity (Sweeney and Ridgway, 1975; Walsh and Gaynor, 2001; McCafferty, 2007; Cilulko et al., 2013; Melero et al., 2015). These types of conditions are particularly amenable to infrared imaging, as inflammatory processes cause vasodilation and a subsequent increase in surface temperature, or in the case of cetaceans, injury may cause detectable breaches of the temperature-insulating blubber layer (Barbieri et al., 2010; Rekant et al., 2016; Mota-Rojas et al., 2022; Verdegaal et al., 2024).
Under normal resting conditions, the body generates and dissipates heat as a mechanism to control deep body temperature. This is regulated primarily by neurological positive and negative feedback circuits. Peripheral vasodilation is induced when the central nervous system receives thermal cutaneous signals (e.g., external temperature fluctuations, hyperthermia, pyrexia), which allows for the dissipation of heat at the skin surface, and likewise vasoconstriction reduces the amount of heat lost from peripheral circulation (Morrison and Nakamura, 2011; Romanovsky, 2014; Mota-Rojas et al., 2021). In marine mammals, several thermal adaptations are utilized, such as the specialized blubber layer instead of the subcutaneous fat employed by terrestrial mammals. A complex network of arteriovenous anastomoses perfuses the skin and blubber layers, and since they cannot respond to heat stress by evaporative cooling like terrestrial mammals, they are instead able to rapidly redistribute their core body heat via increased peripheral blood flow to extremities (dorsal fin, flukes, and flippers) using counter-current heat exchange systems (Scholander and Schevill, 1955; Rommel et al., 1992, 1994; Favilla et al., 2022).
The thermal emissivity of marine mammal skin is similar to water, however the blowhole has been shown to provide a consistently detectable heat anomaly, likely due to the lack of insulating blubber in this region as well as sizeable venous plexuses associated with the pterygoid and accessory sinus system (Cuyler et al., 1992; Costidis and Rommel, 2012; Melero et al., 2015). Infrared thermography of the blowhole was found to be nearly identical to rectal temperatures collected in bottlenose dolphins (Tursiops truncatus) and approximately 1°C lower than rectal temperatures of beluga whales (Delphinapterus leucas) (Melero et al., 2015). Additionally, thermal imaging of the blowhole produced more accurate readings than imaging of the eye or oral mucosa, especially when taken at an angle perpendicular to the blowhole (Melero et al., 2015). The lacrimal caruncle of several species (including humans) is often used to estimate core temperature due to the blood irrigation and innervation of the eye, although in other species, infrared measurement of eye temperature is significantly lower than rectal temperature (Teunissen and Daanen, 2011; Katsoulos et al., 2016; Huggins and Rakobowchuk, 2019; Cugmas et al., 2020). Skin surface temperature is not a reliable indicator of core body temperature in cetaceans due to the insulating nature of skin and blubber, and has been shown to be within 0.90-1.00°C of ambient water temperature (Williams et al., 1999; Barbieri et al., 2010). In cetaceans, the blowhole may be more practical for temperature measurement than the eye simply because this part of the body is regularly exposed during respiration (Figure 1).
A reliable non-contact method of evaluating body temperature, such as infrared thermography, may not only have implications for individual animals receiving veterinary care, but may also serve as a useful data collection tool when performing health assessments of wild killer whale populations (McCafferty, 2007; Bossart, 2011; Barratclough et al., 2019; Rhodes et al., 2022). Studies using infrared thermography in marine mammals are limited, and, to the authors knowledge, no previous peer-reviewed literature exists validating the use of infrared thermography in killer whales. It is the aim of this study to prospectively compare infrared thermography of the blowhole to rectal temperature in healthy killer whales and report the observed ranges for each modality. It was hypothesized that temperatures collected using infrared thermography of the blowhole would be similar to those collected rectally. In addition, retrospective rectal temperature data was analyzed to establish healthy expected temperature ranges for this species.
2 Materials and methods
Methodology was approved by the SeaWorld Parks and Entertainment Animal Research Use Committee, as well as by the veterinary staff at each facility. Thirteen (n = 13) clinically healthy killer whales at SeaWorld Texas (SWT) and Sea World California (SWC) participated in two separate thermometer comparison studies. Health status was determined during regular veterinary clinical examinations, including monthly blood analysis (complete blood count [CBC], serum biochemistry, erythrocyte sedimentation rate [ESR], and fibrinogen), quarterly thoracic ultrasound, and annual dental radiographs. All animals were previously conditioned to accept a rectal probe thermometer and were already participating in daily rectal temperature measurements as part of routine husbandry practices.
At SWT, five killer whales (n = 5, two males ages 20 and 28 years, weighing 3,522 kg and 4,363 kg, respectively, and three females ages 6-28 years, weighing 1,172-2,068 kg) participated in the study. Trainers asked whales to rest at the surface of the water in sternal recumbency, parallel to the edge of the habitat, and breathe normally (i.e., without discriminative stimulus). It was previously reported that infrared imaging of the blowhole was more accurate during a natural breath cycle as compared to a trained exhalation or ‘chuff’ behavior (Melero et al., 2015). Infrared video was obtained from directly above the blowhole at a height of approximately 2 ft (0.61 m) using an infrared camera (FLIR E40 Thermal Imaging Camera, Teledyne FLIR, 27700 SW Parkway Ave., Wilsonville, OR 97070, USA; with variable 0.001 to 0.1 emissivity correction; minimum focus distance 0.4 m, spatial resolution of 2.72 milliradians, accurate up to a distance of 120 cm) (see Figure 2). The camera was in the ‘hot spot’ setting, which generated a dynamic crosshair that tracked the hottest area visible within the frame (see Figure 3). The animal was then immediately asked to roll over and rest in dorsal recumbency for rectal temperature measurement using a flexible probe thermometer (DataTherm II, 28351 Beck Rd, Wixom, MI, 48393, USA) (see Figure 4) the probe of which was measured and marked in 10 cm increments prior to study commencement. The flexibility of the DataTherm II probe impeded unidirectional passage into the colon. To mitigate this, a modified 18F/Ch red rubber catheter (Covidien, 15 Hampshire Street, Mansfield, MA 02048, USA) was used as a sheath-like speculum to facilitate passage of the probe, such that the thermocouple was still fully exposed to make direct contact with the subject (see Figure 5A). The probe was inserted to 50 cm depth, and a temperature was recorded. The probe was then removed slowly in 10 cm increments, with an additional reading taken, until the probe was completely removed (i.e., separate temperature measurements were collected at 50 cm, 40 cm, 30 cm, 20 cm, and 10 cm depth). At each depth, the probe was held in place for 5 seconds until the temperature reading stabilized (per manufacturer instructions). A total of 102 observations (i.e., 102 blowhole and rectal temperature pairs) were recorded. The highest observed rectal temperature overall for a given session was noted as the ‘maximum rectal temperature’ and the associated depth was recorded as the ‘depth at maximum rectal temperature.’
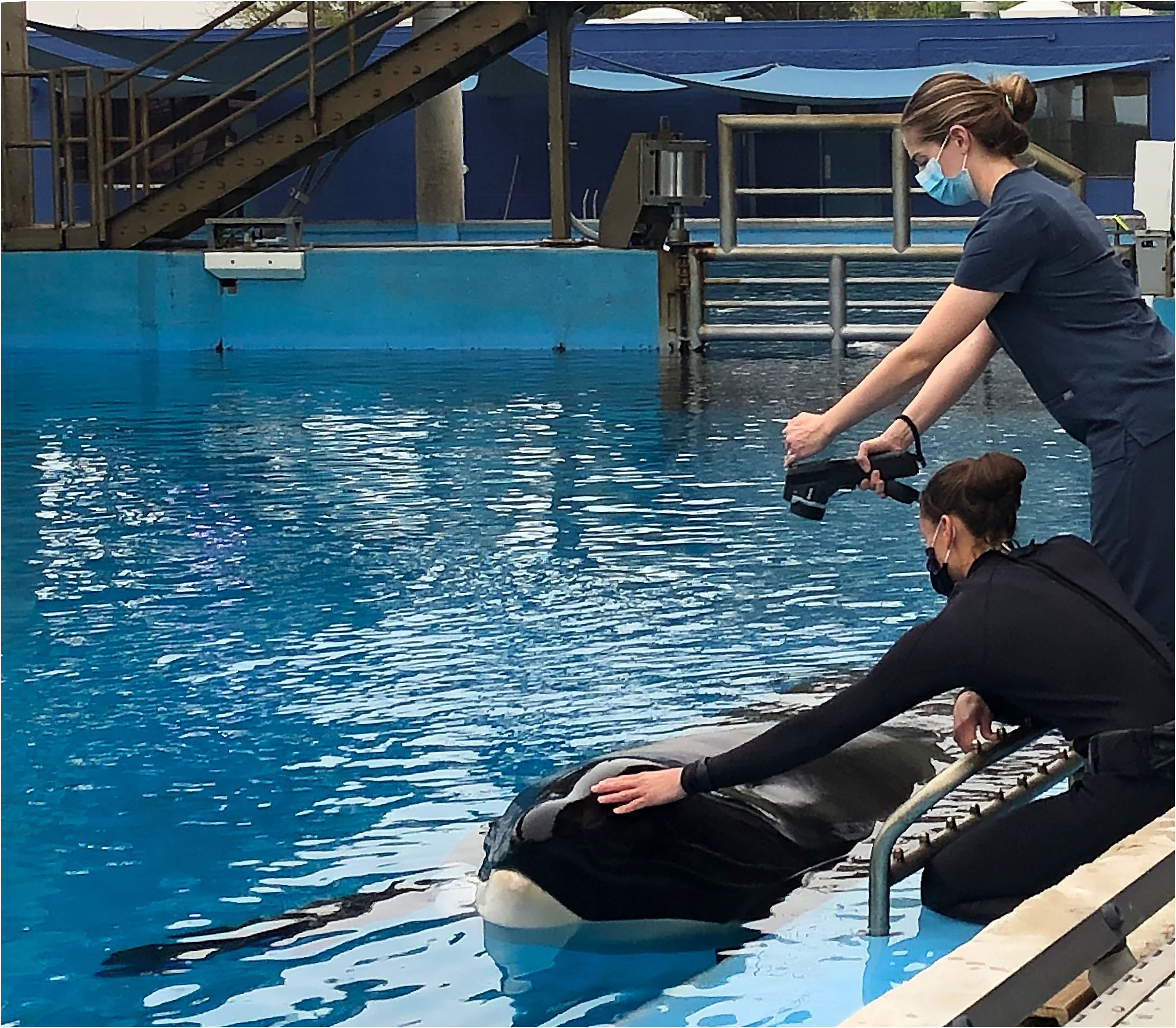
Figure 2 Blowhole temperature reading using a handheld infrared camera in a killer whale, Orcinus orca..
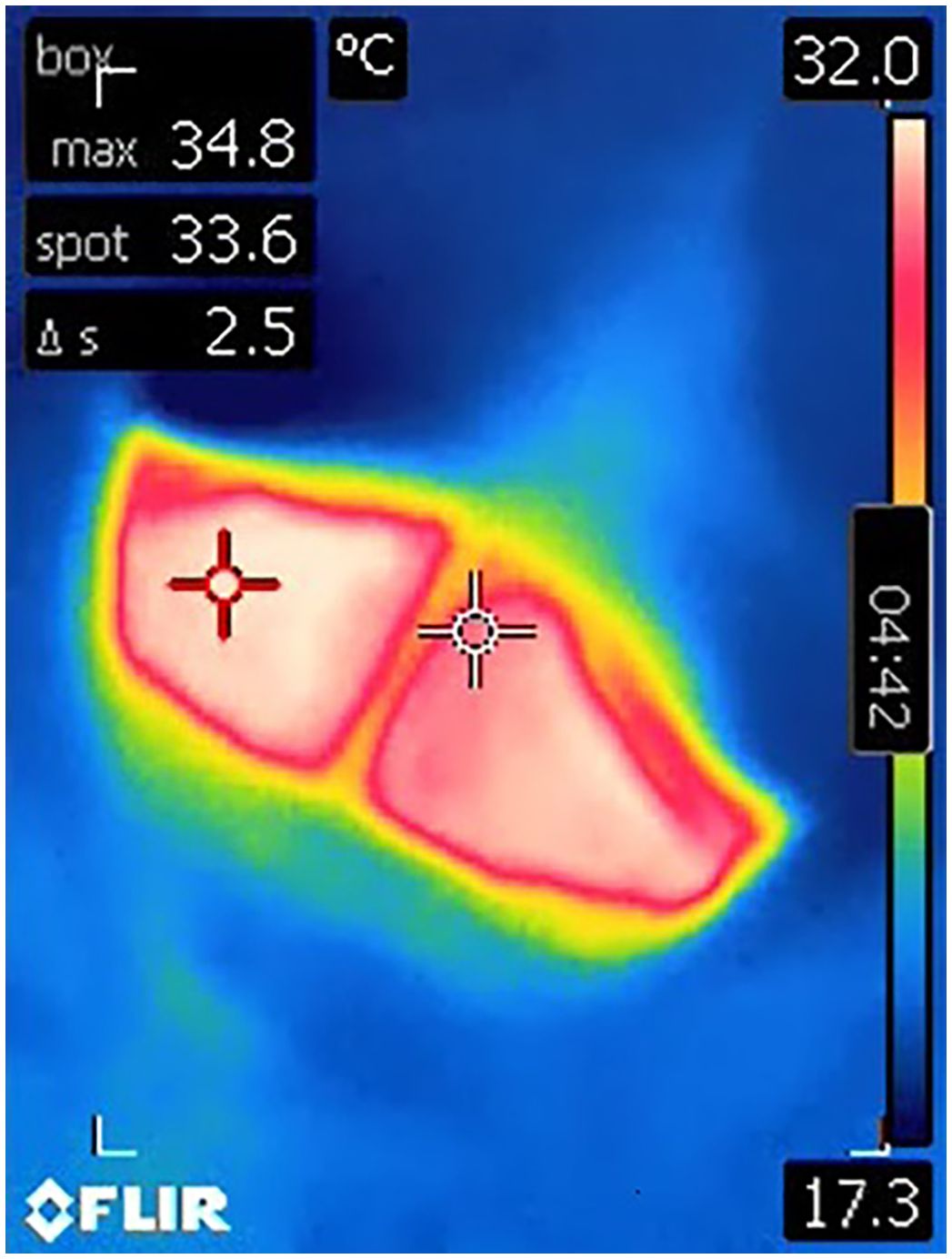
Figure 3 A still image from an infrared video clip of the blowhole of a male killer whale during the peak of breath using a handheld infrared camera. The red crosshair dynamically tracks the maximum temperature (‘max’) visible within the image frame, while the white crosshair is static and continuously reads the temperature at the center of the image (‘spot’). The bar to the right depicts the range of pixel colors assigned to temperature values. The maximum temperature in this image was recorded as 34.8°C.
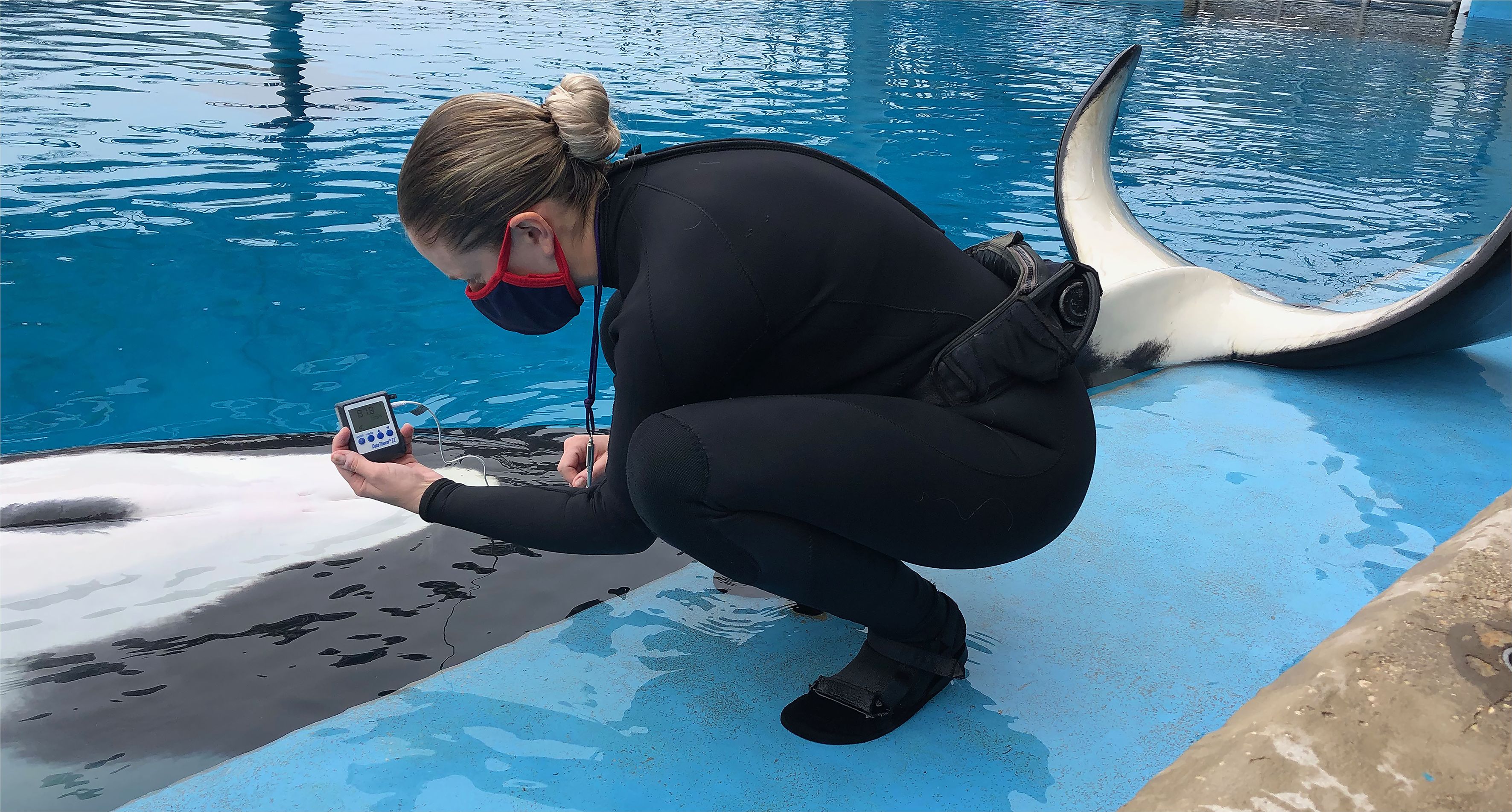
Figure 4 Rectal temperature measurement using a flexible probe thermometer in a killer whale, Orcinus orca.
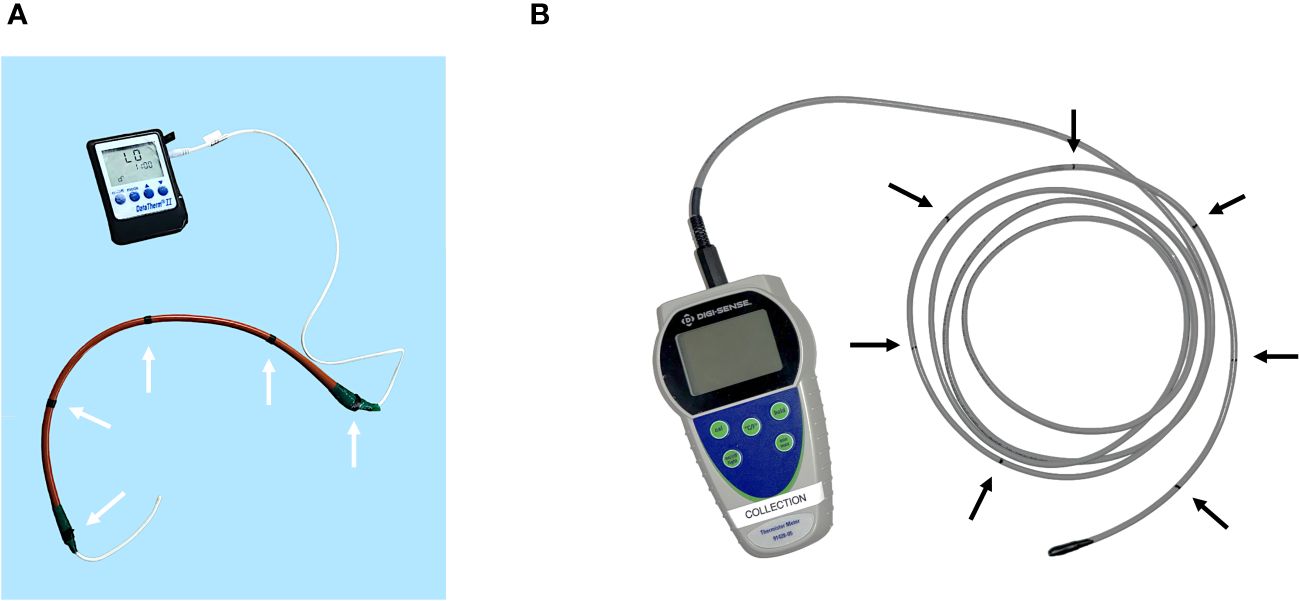
Figure 5 (A) The flexible rectal temperature probe used at SWT (DataTherm II). A red rubber catheter was utilized as a sheath-like speculum to facilitate passage of the probe into the colon. The catheter was measured and labelled with a black indicator every 10 cm (white arrows) to denote the depth for temperature recording. Note that the distal thermocouple was left exposed by 10 cm. (B) The flexible rectal temperature probe used at SWC (Digi-Sense). The probe was measured and labelled with a black indicator every 10 cm (black arrows) to denote the depth for temperature recording.
At SWC, eight killer whales (n = 8, four males ages 9 to 44 years, weighing 2,352 to 4,609 kg, and four females ages 17 to 57 years, weighing 2,281 to 4,113 kg) participated in the study. A second rectal thermometer (Digi-Sense Temp Series Thermocouple thermometer, Cole-Palmer, 625 East Bunker Court, Vernon Hills, LI 60061, USA) (see Figure 5B) was used for comparison to blowhole temperature. This probe was longer and more rigid than the DataTherm II probe and was inserted at 70 cm deep to the anus, and additional readings were taken at 10 cm increments as the probe was gradually removed in the same manner as described for SWT. A total of 60 observations (i.e., 60 blowhole and rectal temperature pairs) were recorded. Water temperature was controlled by each facility’s life support system team and ranged from 12.22 to 14.44°C at both facilities.
The same infrared camera was used for both studies, and the camera and both rectal thermometers were calibrated using melting ice at a temperature of 0.0°C. Rectal probes were calibrated once at the beginning of the study, and the infrared camera was calibrated using buckets of ice at the start of each poolside session. In all cases, blowhole temperature was recorded first, immediately followed by rectal temperature. The time of day was recorded, along with water surface temperature, air temperature, and pool location. Animals were sampled between the hours of 0800 and 1600 and assigned to a categorical variable based on time of temperature recording; AM (0800-1059), Midday (1100-1300), and PM (1301-1600). Each animal participated in repeated sessions in more than one time category, and up to three times per day. Recording was performed during the months of October and November at SWC, where the average air temperature was 19.1°C, and between the months of March to June at SWT, where the average air temperature was 23.7°C. Habitat location was recorded, as one of the pools in SWT was fully covered by a roof, while all other sampling locations in the study occurred in direct sunlight.
During preliminary trials using the infrared camera to collect thermography data, it was observed that the ‘hot spot’ had a slight time delay and was not always present directly inside the blowhole during peak breath (i.e., blowhole opening to its most eccentric point was occasionally faster than the camera could register the crosshair). While this was a very rare occurrence, it prompted the design of a processing algorithm that was coded using the programming language Python 3.9 (with libraries OpenCv v4.7, Numpy v1.24, Tesseract v0.3) to provide a secondary qualitative analysis of the thermography videos. For each video, a processing pipeline performed the following steps: 1) Collect all frames with the highest temperature pixels, 2) Rank each frame by the largest region of high temperature pixels, 3) Select for the largest collection of high temperature pixels, and 4) Draw a bounding box around the collection providing location and temperature for the frame. An image output of the highest-ranking processed frame was saved. The output results of the algorithm were notably consistent, showing a fully eccentric blowhole with the bounding box drawn around the inner-most point of the blowhole (Figure 6). Output results were reviewed manually to ensure the algorithm had selected frames appropriately. Maximum blowhole temperatures, from either the algorithm or the original camera output, were used for statistical analysis.
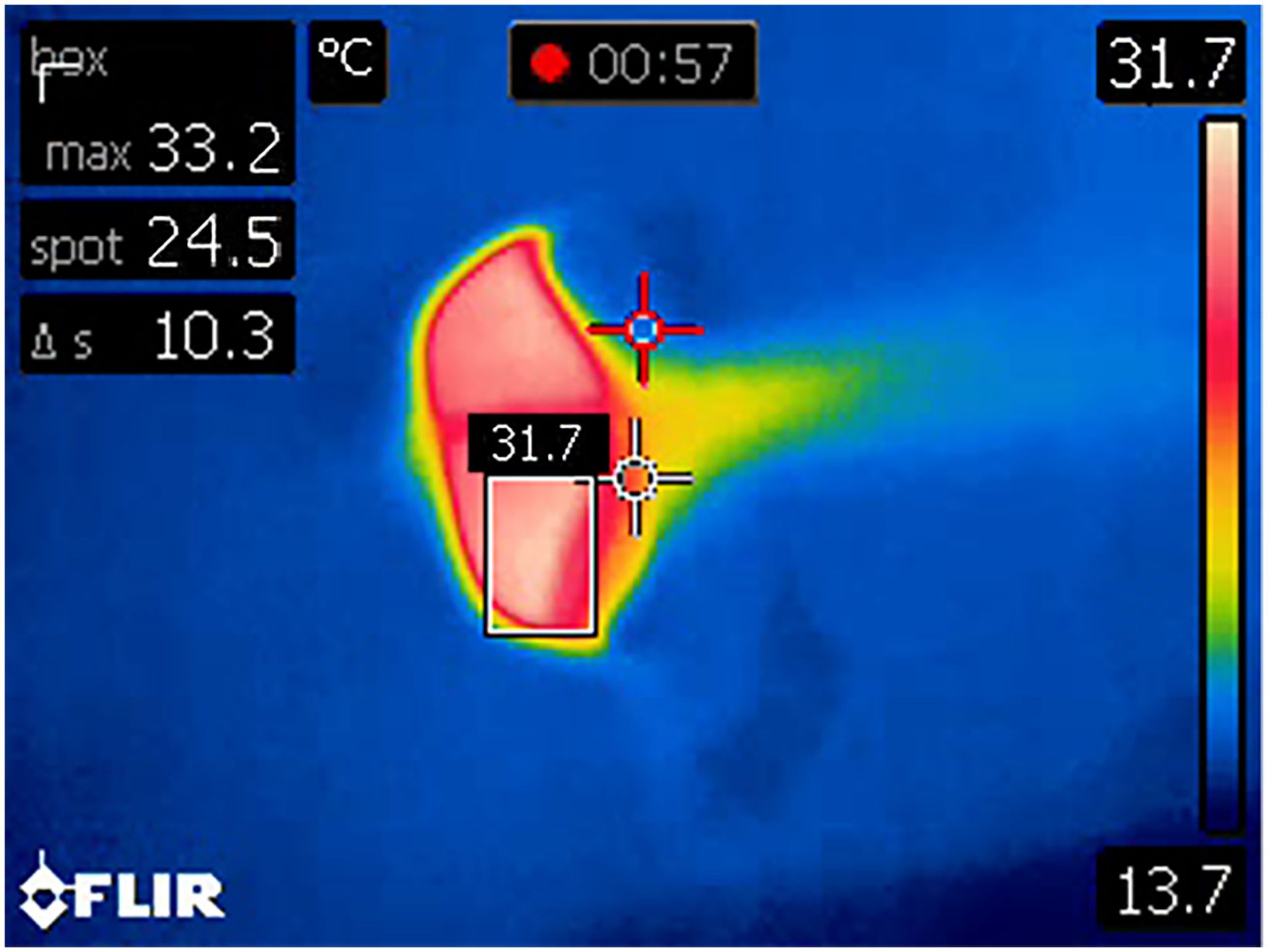
Figure 6 An example of an output result image from the custom-designed Python algorithm. Note that on rare occasions the dynamic crosshair (red) fell outside the margins of blowhole at peak breath (i.e., the speed of the blowhole opening and closing was faster than the speed of the crosshair). The algorithm generated an output from each video file as a single image frame with a bounding box around the hottest temperature pixels with the calculated temperature above the box.
Statistical analyses were performed using R software (R v 4.2.3, 2023 R Foundation for Statistical Computing, Vienna, Austria) or STATA (StataCorp, College Station, Texas 77845). Outliers were identified using the interquartile range method and removed, resulting in n=56 paired observations for SWC and n = 97 for SWT (i.e., four blowhole and rectal temperature pairs from SWC and five pairs from SWT were removed). A two or three level linear mixed effects (LMM) restricted maximum likelihood (REML) regression model was used to analyze the effects of independent variables on either dependent variable rectal (RT) or blowhole (BHT) temperature. For RT model development, the variance associated with the random portion of the model was first evaluated to determine if a two or three level model was appropriate. Repeated measurements from each animal would represent level 2 (Animal ID, random intercept), while each exam (Level 3, EXAM, random intercept) nested within each animal would account for the natural clustering of values around serial temperatures recorded at decreasing depths within one exam. Model estimates using a two or three-level model were compared using a Likelihood Ratio test (LR test) to determine if a three-level model would provide significantly improved variance explanation as compared to a two-level model. Once the random portion of the model was determined, the fixed portion of the model was added, which included the independent variables directly associated with physical characteristics of the animals or the environmental conditions. Variables generally associated with the animal included sex, mass (kg), diurnal rhythms (AM, Midday, PM), depth of temperature probe (depth: 20 to 70 cm in 10 cm increments), and all interactions between depth, mass, and sex, while environmental variables were considered to include the location/probe (SWT versus SWC, pool location), air temperature (air), and water temperature (water). For BHT analysis, the independent variables were the same as described for RT analysis except depth, which was not applicable. Non-significant variables (P > 0.05) were iteratively removed from the full RT model and the effects of its removal on model variance (ML estimates) were evaluated using the likelihood-ratio test (West et al., 2022). If removal of the variable had no significant effect on model variance estimation, the variable was removed. This reduced model (or new “full model”) was rerun and the process was repeated until only significant variables remained (final model). All final mixed effects models were checked for normality using quantile plots of the standard residuals. If quantile–quantile plots of standardized residuals exhibited non-normal distribution, then data was transformed as predicted by the Shapiro-Wilk test until residuals were normalized. The significance of the final model was determined using a Wald χ2 test. Marginal (predicted) means within each categorical variable were determined and if appropriate, multiple comparisons were performed using Šidák corrections at P < 0.05.
Comparisons between the BHT and maximum RT were conducted using repeated measures Bland Altman plots (Martin Bland and Altman, 1986; Bland and Altman, 2007; Myles and Cui, 2007). Post-hoc power calculations for repeated measures Bland Altman analysis with a sample size of 56 and an expected mean of difference at 1°C generated a power of 98.9%. A sample size of 97 generated a power of 99.2%. Normality of the data was assessed with Shapiro-Wilk tests. A value of P < 0.05 was considered statistically significant. It was hypothesized that blowhole temperatures measured using infrared thermography would be similar to temperatures measured rectally.
Given the sample size of 13 animals, actual observed ranges for blowhole and rectal temperatures, rather than reference intervals, are reported for the prospective study (Ozarda et al., 2018). To generate expected rectal temperature ranges (i.e., to approximate a reference interval for rectal temperature), retrospective temperature data collected from a population of 18 killer whales at three different locations (SWT, SWC, and SeaWorld Florida [SWF]) was analyzed nonparametrically using the ‘refineR’ package in R (Ammer et al., 2021), partitioning out the data for sex and body mass. There were n = 3591 total observations of rectal temperature between October 8, 2022, and December 6, 2023. The distributions were visually assessed with histograms, and box plots were evaluated prior to, and following, removal of outliers using the interquartile range method. Outliers (150) were identified and removed, leaving n = 3441 observations of rectal temperature for analysis. The upper (97.5th) and lower (2.5th) percentiles were computed using the estimated model parameters (lambda, mu, and sigma of a Box-Cox transformed normal distribution), and 1000 bootstrap iterations performed to provide a sampling distribution from which to calculate 95% confidence intervals.
3 Results
3.1 Rectal temperature characteristics
Three level (exams nested within animal ID) Linear Mixed Model (LMM) analysis of rectal temperature was significantly improved (χ2 = 285.1, P < 0.0001), over a two-level model using only ID. In addition, allowing the slopes to vary (random slopes) with the depth of measurement at both exam (χ2 = 13.2, P = 0.0003) and ID levels (χ2 = 69.7, P < 0.0001) was significant. Finally, residuals set to vary independently with depth also improved model predictions (χ2 = 53.1, P < 0.0001). Overall, the random effects portion of the mixed model was significant (χ2 = 673, P < 0.0001). For significant fixed animal variables, rectal temperature decreased -0.26°C (from a constant of 35.6 °C) with each 1000 kg increase in mass (χ2 = 19.1, P < 0.0001). Across any sex or size animal (mass) temperature increased with depth (χ2 = 44.6, P < 0.0001). Multiple comparison tests indicated that a temperature peaked at 40 cm (35.6 ± 0.064 °C) as compared to 20 and 30 cm while temperatures were similar thereafter (Supplementary Table 1). A significant (χ2 = 44.6, P < 0.0001) interaction between depth and mass was detected with all temperatures at each depth decreasing with mass, however, the rate of decrease varied with each depth (Supplementary Figure 1). Significant environmental effects were only detected with air temperature (χ2 = 56.5, P < 0.0001) whereby rectal temperatures increased 0.024 °C with each 1 °C increase in air temperature. Levene’s test showed homogeneity of variance within animals for probe depth at maximum RT, F(7) = 2.075, P = 0.054.
3.2 Blowhole temperature characteristics
A two level (animal ID as level two, random intercepts) Linear Mixed model analysis of BHT was significant with covariance set as unstructured (χ2 = 17.4., P = 0.0002). The use of random slopes for ID did not improve the model and was not included. Overall, the random effects portion of the mixed model (level 2, ID) was significant (χ2 = 12.9, P = 0.0002). No significant animal variables including sex, mass, or time of day were detected. The only environmental variables that significantly contributed to the model were air (χ2 = 6.25, P = 0.012) and water (χ2 = 10.0, P = 0.002) temperature. BHT increased by 0.03°C for every 1°C increase in air temperature, and 0.61°C for every 1°C increase water temperature. Average water temperature during the study was 13°C for both SWC and SWT.
3.3 Comparisons between rectal and blowhole temperatures
Location was not a significant variable for the LMM and so RT and BHT data from both parks was pooled for comparisons. Bland-Altman comparisons indicated that blowhole temperature had a bias of -1.28°C compared to rectal temperature (95% CI -1.77 to 080°C), with lower Limit of Agreement (LoA) -3.06°C (95% CI -3.86 to -2.58°C) and an upper LoA 0.49°C (95% CI 0.01 to 1.29°C) (see Figure 7). There was significant proportional bias; weak agreement between measurements at cooler mean temperatures (i.e., mean of the blowhole and rectal temperature) and good to near perfect agreement at warmer mean temperatures (i.e., agreement improved as mean temperature increased). Levene’s test showed that variances between blowhole and rectal temperatures were not equal, F(1) = 73.54, P < 0.001 Within animal variance for rectal temperatures was not equal, F(12) = 4.27, P < 0.001, but there wase homogeneity of variance within animals for blowhole temperature, F(12) = 1.12, P = 0.3576.
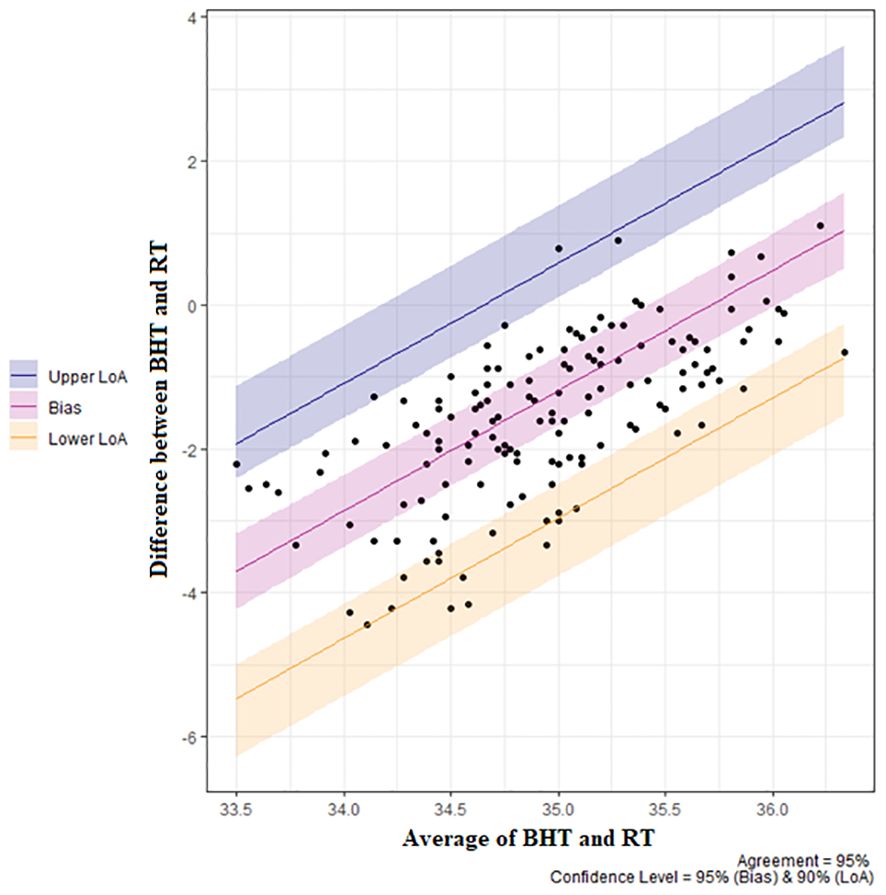
Figure 7 Repeated measures Bland Altman plot for blowhole (BHT) vs. rectal temperature (RT) for n=13 killer whales (Orcinus orca). Bias = -1.28°C (95% CI -1.77 to -0.80°C. Lower Limit of Agreement (LoA) -3.06°C (95% CI -3.86 to -2.58°C), upper LoA 0.49°C (95% CI 0.01 to 1.29°C).
3.4 Expected rectal temperature ranges from retrospective data
Observed rectal temperature ranges (OTR) for the populations (n = 13) during the study period are listed in Table 1.
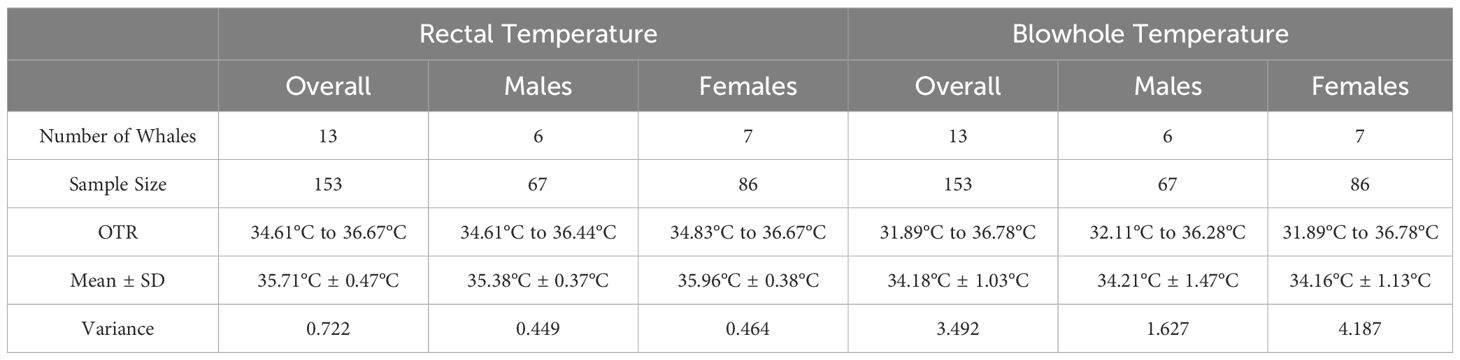
Table 1 Observed temperature ranges (OTR) for rectal and blowhole temperature for the thirteen killer whales included in the prospective study.
The expected rectal temperature range (ETR) generated from retrospective data for n = 18 whales (from SWT, SWC, and SeaWorld Florida [SWF]) was 34.90 to 36.10°C. For large males (> 4000 kg body mass) ETR is 35.10 to 35.70°C. Small males (2000 to 4000 kg) 35.10 to 35.50°C. Large females (> 2200 kg body mass) ETR is 35.40 to 36.00°C; small females (< 2200 kg) 35.90 to 36.50°C (See Table 2).
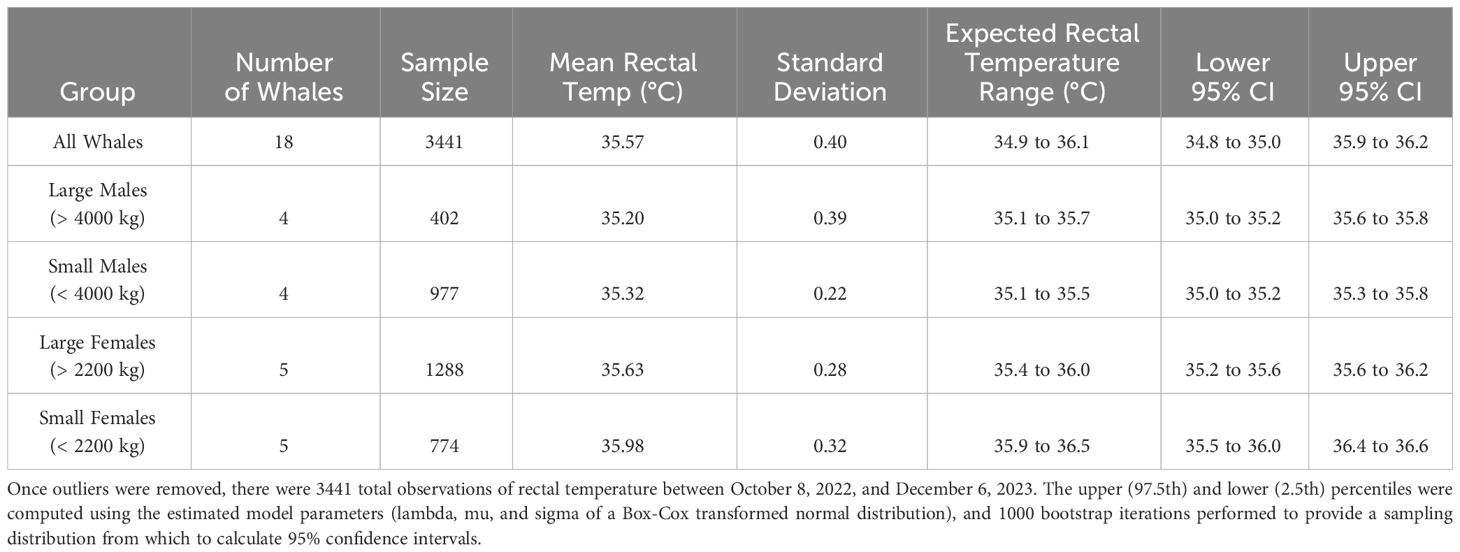
Table 2 Expected temperature ranges for rectal temperature in killer whales generated from retrospective data from eighteen whales from SWT, SWC, and SWF.
4 Discussion
BHT was generally colder than RT, with a bias of -1.28°C, which is slightly lower than reported for beluga whales (-1°C) and dolphins (near identical) (Melero et al., 2015). Proportional bias was observed (i.e., the rectal temperature probes had good to near perfect agreement with the infrared camera at higher mean temperature [mean BHT/RT pair], but weak agreement at lower mean temperature), and there was a wider range of observed BHT than RT. RT had an inverse relationship with body mass, where there was a -0.26°C decrease in temperature with every 1000 kg increase in mass. RT also increased with increasing probe depth up to 40 cm, after which further increases in depth did not necessarily provide higher or ‘more accurate’ temperatures. Probe depth and mass had a significant interaction; generally, all RT decreased with increasing body mass, however the rate of decrease varied with each depth. This is supportive of the concept that a countercurrent testicular vascular rete mirabile that reduces regional temperature in the retroperitoneal location may also lower colonic temperature (Rommel et al., 1994). This specialized vascular network is known as the counter current heat exchanger (CCHE), which flanks the colon and provides cooled blood to the testes in males, but also to the uterus in females, making RT variable depending on the size of the animal and depth of the probe (Rommel et al., 1993; Pabst and Rommel, 1999, 1999; Williams et al., 1999; Meagher et al., 2002; Rommel et al., 2007; Ponganis, 2015). Cetaceans have intra-abdominal testes, supplied by a spermatic arterial plexus. Cool blood from subcutaneous veins on peripheral surfaces of the extremities, particularly the dorsal fin and flukes, flows to a lumbo-caudal venous plexus, which runs counter to the spermatic arterial plexus (Rommel et al., 1992). This mechanism allows cetaceans to compensate for the potentially detrimental effects of core body temperature on sperm viability (Rommel et al., 1992, 1994). Females have a similar CCHE network that plays a role in regulating temperature of the uterus during gestation (Rommel et al., 2007).
Air temperature was a significant environmental factor for rectal temperature, and both air and water temperature were significant factors for blowhole temperature. Infrared thermography is not a direct measurement of temperature per se, but is a non-contact measurement of infrared radiation emitted by an object via a visible image (i.e., a thermogram) with colors arbitrarily assigned to each infrared energy level (Usamentiaga et al., 2014; Tattersall, 2016). Any object with a temperature above absolute zero emits infrared radiation, and radiation intensity increases with increasing temperature. In addition, radiation detected by an infrared camera is influenced by the absorptivity, transmissivity, and reflectivity of the object of interest (Usamentiaga et al., 2014).
Infrared thermography may be affected by ambient air temperature, wind speed, and relative humidity (Church et al., 2014; Almeida et al., 2022; Tran et al., 2023) and thus it seems intuitive that infrared thermography of the blowhole would be influenced by air temperature (although humidity and wind speed were not measured in the present study). It was, however, unexpected that it would be affected by the surrounding water temperature, and conversely that the rectal probe temperature would be affected by ambient air temperature. Animal position during the study may be a contributing factor. Rectal temperature was collected with animals in dorsal recumbency, with pectoral flippers and fluke blades partially or fully exposed to the air (see Figure 4). Similarly, flukes and flippers were in the water during blowhole temperature measurement. Given the influence of the extremities on the CCHE, this may account for the effects of air and water temperature on rectal and blowhole readings, respectively. Additionally, human studies have demonstrated that water on the skin (i.e., sweating) can act as a filter that can reduce skin temperature, resulting in erroneous estimations due to evaporation, and it is possible that a similar phenomenon may be involved with the effect of water on blowhole temperatures seen in the current study (Priego-Quesada et al., 2020).
The infrared camera used in this study did not store radiometric data, necessitating the use of the custom algorithm for secondary video analysis. In camera models with radiometric video capability, commercial software can be used. It is highly recommended that future projects use infrared cameras with radiometric video capability and store video files as.wav files to avoid image compression.
The use of infrared cameras for assessing temperature in animals relies on a low zenith angle (McCafferty et al., 2015; Horton et al., 2019). As killer whales take breaths very quickly, to maximize accuracy and eliminate inter-operator variability, a single camera operator was used to ensure consistency in height and camera angle relative to the blowhole. The camera was aimed as perpendicular to the blowhole as possible, however angle itself was not quantified. Intra-exam variability was minimal but not unavoidable, and slight variances may have occurred in height or angle relative to the blowhole due to operator or animal movement; appreciable shifts in angle from the animal or operator resulted in erroneous readings that were excluded from the data set. Additionally, variance due to differences in solar radiation, cloud cover, humidity, water vapor, or other factors not quantified during this study, may have contributed to some variability (Cilulko et al., 2013).
If a whale blew a plume of water into the camera the infrared signature would not register due to high density water vapor on the lens. Images were best obtained when breaths occurred a few seconds after the animal had settled into position, allowing pooled water to run off the blowhole. Future study examining application of infrared thermography via unoccupied aerial systems (i.e., drones) in moving animals will need to consider optimizing camera height relative to the blowhole (Burke et al., 2019). During this study the camera was held approximately 2 ft high (0.61 m) at each reading. It remains to be seen whether increasing the height of the camera will diminish or magnify sensitivity of the camera in terms of overall accuracy, position angle, and interference of water plumes, however aerial studies of free ranging baleen whales at higher altitudes did not report issues with blowhole vapor interference (Horton et al., 2019; Lonati et al., 2022).
Rectal temperature was chosen for the current study based on the animals’ comfortability with the procedure (animals were routinely participating in rectal temperature measurement prior to study commencement), ease of measurement, and to optimize human and animal safety during the study. However, rectal temperature is not always used for clinical diagnosis in cetaceans due to the influence of the counter current heat exchange mechanism (CCHE) on the measurement. A bottlenose dolphin study, using thermocouples positioned at five different points simultaneously within the colon, reported cooler temperatures at the anterior-most thermocouples adjacent to the CCHE (~1.30°C cooler), with the warmest temperatures recorded posterior to the CCHE at the shallowest thermocouple (Rommel et al., 1994). Given this principle, rectal temperature may not be the most sensitive proxy of core body temperature for cetaceans, and it is important to understand that while it is often used synonymously with core body temperature, any regional temperature reading is only a measurement of the metabolic heat production and blood flow of that specific anatomical region (McCafferty et al., 2015), thus future study may seek to investigate comparison of blowhole temperature with other body regions, for example with esophageal temperature probes such as previously described in manatees (Martony et al., 2020) or radio telemetry boluses, which have been used in many different species, including cetaceans and pinnipeds (Whittow et al., 1974; Kuhn and Costa, 2006; AlZahal et al., 2011; Heide-Jørgensen et al., 2014; McCafferty et al., 2015).
Previous studies using radio telemetry boluses found that, in one male false killer whale (Pseudorca crassidens), temperature recordings of the stomach ranged from approximately 32.00-37.20°C; temperatures increased with increased activity and with increasing water temperature. It was hypothesized that actual body temperature was between 36.00-37.20°C (excluding periods when food was in the stomach), while rectal temperature (not taken simultaneously) was 36.50°C. In one killer whale, the stomach temperature range was 37.50-38.50°C (Whittow et al., 1974). It was hypothesized that high water temperature influenced the higher temperature of this killer whale relative to the other two whales in the same study (of different species), however, this animal was also clinically unwell and died shortly afterward (Whittow et al., 1974). Based on current study data, this animal would be considered pyrexic, however, baseline stomach temperature data from healthy killer whales has not yet been reported.
Results from this study have applications for clinicians working with populations of killer whales under human care, as having reference data for RT and BHT from healthy animals in a controlled environment can assist clinicians in determining if a presenting animal is pyrexic, euthermic, or hypothermic. Data regarding probe depth is useful for maintaining consistency with data collection. The authors recommend inserting a flexible temperature probe to a depth of at least 40 cm for any killer whale. While the two different rectal thermometer probes did not yield statistically different readings, given the length, rigidity, and ease of use, the Digi-Sense probe is recommended for future study and for clinical use for RT measurement in killer whales.
Data presented here has implications for the study of free-ranging killer whale populations, particularly where disease, anthropogenic, or other environmental factors may be affecting health status or thermoregulation (Lacy et al., 2017). It may be helpful for study of reproductive status, as body temperature is a useful indicator of impending parturition in killer whales and other cetaceans (Tersawa et al., 1999; Katsumata et al., 2006).
The Southern Resident killer whales (SRKW) of the Salish Sea are a critically endangered population, and are threatened by reduced prey availability, vessel noise, polychlorinated biphenyls and other contaminants (Lacy et al., 2017). These factors likely have secondary immune system effects, putting them at risk of pathogens, including, but not limited to, ecto-, endo- and protozoal parasites, Erysipelothrix spp., Salmonella spp., Edwardsiella spp., poxviruses, herpesviruses, morbillivirus, marine Brucella spp, and mucoralean fungi (Gaydos et al., 2004, 2023; Huggins et al., 2020; Raverty et al., 2020). The ability to identify potentially pyrexic individuals could improve efforts to investigate disease prevalence and virulence within this population. Perhaps more importantly, doing so in a manner that is rapid and minimizes contact or population disturbance will maximize the increasingly limited opportunities available to perform health surveillance on these animals (Rhodes et al., 2022).
Further investigation into infrared blowhole thermography in managed-care killer whales is warranted and should aim to examine correlations between BHT and inflammatory changes in blood work, or for detection of peripartum temperature drops. Work in managed-care whales may also examine temperature variation with increasing camera altitude, as unoccupied aerial systems (drones) fitted with infrared cameras are a practical option for evaluating blowhole temperature in free-ranging animals. Recent studies have used drone infrared thermography to visualize blowholes of North Atlantic right whales, humpback whales, minke, blue, and sperm whales, which appeared as distinct heat anomalies compared to the water surface (Cuyler et al., 1992; Horton et al., 2019; Lonati et al., 2022). Blowholes were observed at an altitude of 9.84 ft (3 m) for the right whales and 65.62 ft (20 m) for the humpback whales (Horton et al., 2019; Lonati et al., 2022), whereas the present investigation used infrared thermography at a close range of ~ 2 ft (0.61 m). Studies performed only on free-ranging animals lack temperature reference data via direct contact as comparison, and the goal of the present study is to fill those data gaps.
Future study will need to determine whether infrared thermography of the blowhole can accurately detect clinically significant temperature elevation in killer whales. The current study reports observed BHT ranges from animals that were systemically well at the time of recording, and it cannot be assumed that a systemically unwell animal will have a detectable blowhole temperature above the reported range. Clinical case report data incorporating blowhole infrared thermography as part of the diagnostic toolkit will help to corroborate these findings.
Animals in this study were at rest during the time of data collection; it is unknown whether blowhole temperatures will vary beyond what is observed here during swimming or exercise. In studies examining the rectal temperature of bottlenose dolphins, it was found that rectal temperatures adjacent to the CCHE were 0.5°C cooler during exercise than temperatures at rest (Pabst et al., 1995).
BHT and RT are proxy measurements for core body temperature, and BHT tended to be lower than RT, with proportional bias, and thus cannot currently be recommended for measurement of absolute core body temperature in killer whales over rectal thermometry. The clinical use of blowhole infrared thermography will likely be best used as a population screening tool to discriminate against individuals who have a higher temperature than the rest of a group, rather than for determining absolute temperature values. The same holds true for ear, axillary, and temporal artery temperature measurement in humans; their use as screening tools is widespread in medical practice (Blahd et al., 2023) despite their lack of clinical agreement with rectal temperature (Fortuna et al., 2010; Edelu et al., 2011; Mogensen et al., 2018). For children, it is generally accepted that rectal temperature is most accurate proxy for core body temperature, with rectal temperature > oral > axillary and temporal temperature (Blahd et al., 2023). In addition, human axillary temperature and rectal temperature measurements have demonstrated proportional bias similar to that seen in the current study, and axillary temperature had better agreement at higher temperatures than at lower temperatures, and there was narrowing of the axillary-rectal temperature difference with increasing axillary temperature (Edelu et al., 2011). Human axillary temperature may be more representative of core body temperatures at higher temperatures (Edelu et al., 2011). It is therefore the authors’ opinion that blowhole infrared thermography remains a promising screening tool for wild populations to identify, and provide targeted health assessment for, individuals that may be systemically unwell. However, further investigation is needed to determine if this methodology can be used to consistently distinguish between euthermic and pyrexic individuals.
5 Conclusion
The mean RT of healthy killer whales in the study was 35.7°C ± 0.47°C (and overall the expected range using RT retrospective data was 34.9 to 36.1°C), and the mean observed BHT of healthy whales was 34.2°C ± 1.03°C. BHT had a bias of -1.28°C relative to RT; slightly lower than reported for beluga whales and dolphins (Melero et al., 2015). The authors recommend inserting a flexible temperature probe to a depth of at least 40 cm for any killer whale. While the two different rectal thermometer probes did not yield statistically different readings, given the length, rigidity, and ease of use, the Digi-Sense probe is recommended for future study and for clinical use for RT measurement in killer whales. Rectal temperatures had good to near perfect agreement with blowhole temperature at higher mean temperature, but weak agreement at lower mean temperature. Given the proportional bias observed with Bland Altman analysis, BHT cannot currently be recommended as a measurement for absolute core body temperature, however infrared thermography of the blowhole remains a promising tool for health assessment of free-ranging killer whale populations, as it may serve as a non-contact screening tool to detect pyrexic animals within a group.
Data availability statement
The original contributions presented in the study are included in the article/Supplementary Material. Further inquiries can be directed to the corresponding author.
Ethics statement
The animal study was approved by SeaWorld Parks and Entertainment animal welfare and research committee, and veterinary staff at each facility. The study was conducted in accordance with the local legislation and institutional requirements.
Author contributions
JR: Conceptualization, Data curation, Formal analysis, Investigation, Methodology, Project administration, Software, Supervision, Visualization, Writing – original draft, Writing – review & editing, Resources, Validation. MG: Formal analysis, Software, Writing – original draft, Writing – review & editing, Data curation. SO: Writing – review & editing. TS: Writing – review & editing. KH: Writing – review & editing. TR: Data curation, Formal analysis, Supervision, Validation, Writing – original draft, Writing – review & editing.
Funding
The author(s) declare that no financial support was received for the research, authorship, and/or publication of this article.
Acknowledgments
The authors thank Fran Rowley, Rebecca Rivera, Tara Klimek, Julie Sigman, Maria Love, Mary Nations, Amy Reese, Pete Peters, Jen Davenport, Amber Johnson-Reed, Caitlin Brush, Mike Felice, Laura Surovik, and the animal care and training staff at SeaWorld California, SeaWorld Texas, and SeaWorld Florida, as well as Gina Lonati of the University of New Brunswick. This is SeaWorld Parks & Entertainment Technical contribution 2023-16.
Conflict of interest
The authors declare that the research was conducted in the absence of any commercial or financial relationships that could be construed as a potential conflict of interest.
Publisher’s note
All claims expressed in this article are solely those of the authors and do not necessarily represent those of their affiliated organizations, or those of the publisher, the editors and the reviewers. Any product that may be evaluated in this article, or claim that may be made by its manufacturer, is not guaranteed or endorsed by the publisher.
Supplementary material
The Supplementary Material for this article can be found online at: https://www.frontiersin.org/articles/10.3389/fmars.2024.1369287/full#supplementary-material
References
Almeida R. M. S. F., Barreira E., Simões M. L., Sousa T. S. F. (2022). Infrared thermography to evaluate thermal comfort under controlled ambient conditions. Appl. Sci. 12, 12105. doi: 10.3390/app122312105
AlZahal O., AlZahal H., Steele M. A., Van Schaik M., Kyriazakis I., Duffield T. F., et al. (2011). The use of a radiotelemetric ruminal bolus to detect body temperature changes in lactating dairy cattle. J. Dairy Sci. 94, 3568–3574. doi: 10.3168/jds.2010-3944
Ammer T., Schützenmeister A., Prokosch H. U., Rauh M., Rank C. M., Zierk J. (2021). refineR: A novel algorithm for reference interval estimation from real-world data. Sci. Rep. 11, 16023. doi: 10.1038/s41598-021-95301-2
Andrade D. V. (2015). Thermal windows and heat exchange. Temperature: Multidiscip. Biomed. J. 2, 451. doi: 10.1080/23328940.2015.1040945
Andvik C., Jourdain E., Lyche J. L., Karoliussen R., Borgå K. (2021). High levels of legacy and emerging contaminants in killer whales (Orcinus orca) from Norway 2015 to 2017. Environ. Toxicol. Chem. 40, 1848–1858. doi: 10.1002/etc.5064
Barbieri M. M., McLellan W. A., Wells R. S., Blum J. E., Hofmann S., Gannon J., et al. (2010). Using infrared thermography to assess seasonal trends in dorsal fin surface temperatures of free-swimming bottlenose dolphins (Tursiops truncatus) in Sarasota Bay, Florida. Mar. Mammal Sci. 26, 53–66. doi: 10.1111/mms.2010.26.issue-1
Barratclough A., Wells R. S., Schwacke L. H., Rowles T. K., Gomez F. M., Fauquier D. A., et al. (2019). Health assessments of common bottlenose dolphins (Tursiops truncatus): past, present, and potential conservation applications. Front. Vet. Sci. 6. doi: 10.3389/fvets.2019.00444
Blahd W. H., et al. (2023) Fever Temperatures: Accuracy and Comparison, Kaiser Permanente. Available online at: https://healthy.kaiserpermanente.org/health-wellness/health-encyclopedia/he.fever-temperatures-accuracy-and-comparison.tw9223 (Accessed 3 May 2023).
Bland J. M., Altman D. G. (2007). Agreement between methods of measurement with multiple observations per individual. J. Biopharmaceutical Stat 17, 571–582. doi: 10.1080/10543400701329422
Bossart G. (2011). Marine mammals as sentinel species for oceans and human health. Vet. Pathol. 48, 676–690. doi: 10.1177/0300985810388525
Burke C., Rashman M., Wich S., Symons A., Theron C., Longmore S. (2019). Optimizing observing strategies for monitoring animals using drone-mounted thermal infrared cameras. Int. J. Remote Sens. 40, 439–467. doi: 10.1080/01431161.2018.1558372
Center for Whale Research Orca Population (CWR). Available online at: https://www.whaleresearch.com/orca-population (Accessed 9 January 2024).
Church J. S., Hegadoren P. R., Paetkau M. J., Miller C. C., Regev-Shoshani G., Schaefer A. L., et al. (2014). Influence of environmental factors on infrared eye temperature measurements in cattle. Res. Vet. Sci. 96, 220–226. doi: 10.1016/j.rvsc.2013.11.006
Cilulko J., Janiszewski P., Bogdaszewski M., Szczygielska E. (2013). Infrared thermal imaging in studies of wild animals. Eur. J. Wildlife Res. 59, 17–23. doi: 10.1007/s10344-012-0688-1
Costidis A., Rommel S. A. (2012). Vascularization of air sinuses and fat bodies in the head of the bottlenose dolphin (Tursiops truncatus): morphological implications on physiology. Front. Physiol. 3. doi: 10.3389/fphys.2012.00243
Cugmas B., Šušterič P., Gorenjec N. R., Plavec T. (2020). Comparison between rectal and body surface temperature in dogs by the calibrated infrared thermometer. Vet. Anim. Sci. 9, 100120. doi: 10.1016/j.vas.2020.100120
Cuyler L. C., Wiulsrød R., ØRitsland N. A. (1992). Thermal infrared radiation from free living whales. Mar. Mammal Sci. 8, 120–134. doi: 10.1111/j.1748-7692.1992.tb00371.x
Dold C., Ridgway S. (2014). “Cetaceans,” in Zoo Animal and Wildlife Immobilization and Anesthesia (Oxford: John Wiley & Sons, Ltd), 679–691. doi: 10.1002/9781118792919.ch49
Edelu B. O., Ojinnaka N. C., Ikefuna A. N. (2011). A comparison of axillary with rectal thermometry in under 5 children. Nigerian Med. Journal : J. Nigeria Med. Assoc. 52, 207–210. doi: 10.4103/0300-1652.93789
Favilla A. B., Horning M., Costa D. P. (2022). Advances in thermal physiology of diving marine mammals: The dual role of peripheral perfusion. Temperature: Multidiscip. Biomed. J. 9, 46–66. doi: 10.1080/23328940.2021.1988817
Fortuna E. L., Carney M. M., Macy M., Stanley R. M., Younger J. G., Bradin S. A. (2010). Accuracy of non-contact infrared thermometry versus rectal thermometry in young children evaluated in the emergency department for fever. J. Emergency Nurs. 36, 101–104. doi: 10.1016/j.jen.2009.07.017
Gaydos J. K., Balcomb K. C., Osborne R. W., Dierauf L. (2004). Evaluating potential infectious disease threats for southern resident killer whales, Orcinus orca: a model for endangered species. Biol. Conserv. 117, 253–262. doi: 10.1016/j.biocon.2003.07.004
Gaydos J. K., St. Leger J., Raverty S., Nollens H. H., Haulena M., Ward E. J., et al. (2023). Epidemiology of skin changes in endangered Southern Resident killer whales (Orcinus orca). PloS One 18, e0286551. doi: 10.1371/journal.pone.0286551
Hao Y. J., Zhao Q. Z., Wu H. P., Chen D. Q., Gong C., Li L., et al. (2009). Physiological responses to capture and handling of free-ranging male Yangtze finless porpoises (Neophocaena phocaenoides asiaeorientalis): Marine & Freshwater Behaviour & Physiology. Mar. Freshw. Behav. Physiol. 42, 315–327. doi: 10.1080/10236240903302161
Heide-Jørgensen M., Nielsen N. H., Hansen R. G., Blackwell S. B. (2014). Stomach temperature of narwhals (Monodon monoceros) during feeding events. Anim. Biotelemetry 2, 9. doi: 10.1186/2050-3385-2-9
Horton T. W., Hauser N., Cassel S., Klaus K. F., Fettermann T., Key N. (2019). Doctor drone: non-invasive measurement of humpback whale vital signs using unoccupied aerial system infrared thermography. Front. Mar. Sci. 6. doi: 10.3389/fmars.2019.00466
Huggins J. L., Garner M. M., Raverty S. A., Lambourn D. M., Norman S. A., Rhodes L. D. (2020). The emergence of mucormycosis in free-ranging marine mammals of the Pacific Northwest. Front. Mar. Sci. 7, 555. doi: 10.3389/fmars.2020.00555
Huggins J., Rakobowchuk M. (2019). Utility of lacrimal caruncle infrared thermography when monitoring alterations in autonomic activity in healthy humans. Eur. J. Appl. Physiol. 119, 531–538. doi: 10.1007/s00421-018-4041-6
Hunt K. E., Moore M. J., Rolland R. M., Kellar N. M., Hall A. J., Kershaw J., et al. (2013). Overcoming the challenges of studying conservation physiology in large whales: a review of available methods. Conserv. Physiol. 1, cot006. doi: 10.1093/conphys/cot006
Karaer M. C., Čebulj-Kadunc N., Snoj T. (2023). Stress in wildlife: comparison of the stress response among domestic, captive, and free-ranging animals. Front. Vet. Sci. 10. doi: 10.3389/fvets.2023.1167016
Katsoulos P. D., Athanasiou L. V., Karatzia M. A., Valasi I., Boscos C., Karatzias H. (2016). Comparison of a non-contact infrared thermometer with a rectal digital thermometer for use in ewes. Small Ruminant Res. 143, 84–88. doi: 10.1016/j.smallrumres.2016.09.004
Katsumata E., Jaroenporn S., Katsumata H., Konno S., Maeda Y., Watanabe G., et al. (2006). Body Temperature and Circulating Progesterone Levels before and after Parturition in Killer Whales (Orcinus orca). J. Reprod. Dev. 52, 65–71. doi: 10.1262/jrd.17063
Kuhn C. E., Costa D. P. (2006). Identifying and quantifying prey consumption using stomach temperature change in pinnipeds. J. Exp. Biol. 209, 4524–4532. doi: 10.1242/jeb.02530
Kusuda S., Kakizoe Y., Kanda K., Sengoku T., Fukumoto Y., Adachi I., et al. (2011). Ovarian cycle approach by rectal temperature and fecal progesterone in a female killer whale, Orcinus orca. Zoo Biol. 30, 285–295. doi: 10.1002/zoo.20336
Lacy R. C., Williams R., Ashe E., Balcomb K. C. III, Brent L. J. N., Clark C. W., et al. (2017). Evaluating anthropogenic threats to endangered killer whales to inform effective recovery plans. Sci. Rep. 7, 14119. doi: 10.1038/s41598-017-14471-0
Lonati G., Zitterbart D., Miller C., Corkeron P., Murphy C., Moore M. (2022). Investigating the thermal physiology of Critically Endangered North Atlantic right whales Eubalaena glacialis via aerial infrared thermography. Endangered Species Res. 48, 139–154. doi: 10.3354/esr01193
Martin Bland J., Altman D. G. (1986). Statistical methods for assessing agreement between two methods of clinical measurement. Lancet 1, 307–310. doi: 10.1016/S0140-6736(86)90837-8
Martony M., Isaza R., Erlacher-Reid C., Peterson J., Stacy N. I. (2020). Esophageal measurement of core body temperature in the Florida manatee (Trichechus manatus latirostris). J. Wildlife Dis. 56, 27–33. doi: 10.7589/2019-02-049
McCafferty D. J. (2007). The value of infrared thermography for research on mammals: previous applications and future directions. Mammal Rev. 37, 207–223. doi: 10.1111/j.1365-2907.2007.00111.x
McCafferty D. J., Gallon S., Nord A. (2015). Challenges of measuring body temperatures of free-ranging birds and mammals. Anim. Biotelemetry 3, 33. doi: 10.1186/s40317-015-0075-2
Meagher E. M., McLellan W. A., Westgate A. J., Wells R. S., Frierson D. Jr., Pabst D. A. (2002). The relationship between heat flow and vasculature in the dorsal fin of wild bottlenose dolphins Tursiops truncatus. J. Exp. Biol. 205, 3475–3486. doi: 10.1242/jeb.205.22.3475
Melero M., Rodríguez-Prieto V., Rubio-García A., García-Párraga D., Sánchez-Vizcaíno J. M. (2015). Thermal reference points as an index for monitoring body temperature in marine mammals. BMC Res. Notes 8, 411. doi: 10.1186/s13104-015-1383-6
Mogensen C. B., Wittenhoff L., Fruerhøj G., Hansen S. (2018). Forehead or ear temperature measurement cannot replace rectal measurements, except for screening purposes. BMC Pediatr. 18, 1–6. doi: 10.1186/s12887-018-0994-1
Morrison S. F., Nakamura K. (2011). Central neural pathways for thermoregulation. Front. Bioscience-Landmark 16, 74–104. doi: 10.2741/3677
Mota-Rojas D., Titto C. G., Orihuela A., Martínez-Burnes J., Gómez-Prado J., Torres-Bernal F., et al. (2021). Physiological and behavioral mechanisms of thermoregulation in mammals. Animals 11, 1733. doi: 10.3390/ani11061733
Mota-Rojas D., Pereira A. M. F., Martínez-Burnes J., Domínguez-Oliva A., Mora-Medina P., Casas-Alvarado A., et al. (2022). Thermal imaging to assess the health status in wildlife animals under human care: limitations and perspectives. Animals 12, 3558. doi: 10.3390/ani12243558
Myles P. S., Cui J. (2007). Using the Bland-Altman method to measure agreement with repeated measures. Br. J. Anesth. 99, 309–311. doi: 10.1093/bja/aem214
National Marine Fisheries Service (2005). Endangered and threatened wildlife and plants: endangered status for southern resident killer whales. 70th edn. Office of the Federal Register, National Archives and Records Administration, U.S. Government Publishing Office.
Ozarda Y., Higgins V., Adeli K. (2018). Verification of reference intervals in routine clinical laboratories: practical challenges and recommendations. Clin. Chem. Lab. Med. 57, 30–37. doi: 10.1515/cclm-2018-0059
Pabst D. A., Rommel S. A., McLellan W. A., Williams T. M., Rowles T. K. (1995). Thermoregulation of the intra-abdominal testes of the bottlenose dolphin (Tursiops truncatus) during exercise. J. Exp. Biol. 198, 221–226. doi: 10.1242/jeb.198.1.221
Pabst D. A., Rommel S. A. (1999) “Functional Anatomy of marine mammals,” in Biology of Marine Mammals. Eds. Reynolds ., Rommel S. (Smithsonian Institution, Washington, DC), 15–72.
Ponganis P. J. (Ed.) (2015). “Thermoregulation,” in Diving Physiology of Marine Mammals and Seabirds (Cambridge University Press, Cambridge), 145–161. doi: 10.1017/CBO9781139045490.009
Priego-Quesada J. I., Machado A. S., Gil-Calvo M., Jimenez-Perez I., Cibrian Ortiz de Anda R. M., Salvador Palmer R., et al. (2020). A methodology to assess the effect of sweat on infrared thermography data after running: Preliminary study. Infrared Phys. Technol. 109, 103382. doi: 10.1016/j.infrared.2020.103382
Raverty S., St. Leger J., Noren D. P., Burek Huntington K., Rotstein D. S., Gulland F. M. D., et al. (2020). Pathology findings and correlation with body condition index in stranded killer whales (Orcinus orca) in the northeastern Pacific and Hawaii from 2004 to 2013. PloS One 15, e0242505. doi: 10.1371/journal.pone.0242505
Rekant S. I., Lyons M. A., Pacheco J. M., Arzt J., Rodriguez L. L. (2016). Veterinary applications of infrared thermography. Am. J. Vet. Res. 77, 98–107. doi: 10.2460/ajvr.77.1.98
Rhodes L. D., Emmons C. K., Wisswaesser G. S., Wells A. H., Hanson M. B. (2022). Bacterial microbiomes from mucus and breath of southern resident killer whales (Orcinus orca). Conserv. Physiol. 10 (1), coac014. doi: 10.1093/conphys/coac014
Romanovsky A. A. (2014). Skin temperature: its role in thermoregulation. Acta Physiologica 210, 498–507. doi: 10.1111/apha.12231
Rommel S. A., Ann Pabst D., McLellan W. A. (1993). Functional morphology of the vascular plexuses associated with the cetacean uterus. Anatomical Rec. 237, 538–546. doi: 10.1002/ar.1092370414
Rommel S. A., Pabst D. A., McLellan W. A., Mead J. G., Potter C. W. (1992). Anatomical evidence for a countercurrent heat exchanger associated with dolphin testes. Anatomical Rec. 232, 150–156. doi: 10.1002/ar.1092320117
Rommel S. A., Pabst D. A., McLellan W. A., Williams T. M., Friedl W. A. (1994). Temperature regulation of the testes of the bottlenose dolphin (Tursiops truncatus): evidence from colonic temperatures. J. Comp. Physiol. B 164, 130–134. doi: 10.1007/BF00301654
Rommel S., Pabst D., McLellan W. (2007). “Functional Anatomy of the Cetacean Reproductive System, with Comparisons to the Domestic Dog,” in Reproductive Biology and Phylogeny of Cetacea. Ed. Miller D. (Science Publishers, Enfield, NH), 127–145. doi: 10.1201/b11001-5
Scholander P. F., Schevill W. E. (1955). Counter-current vascular heat exchange in the fins of whales. J. Appl. Physiol. 8, 279–282. doi: 10.1152/jappl.1955.8.3.279
Sweeney J. C., Ridgway S. H. (1975). Procedures for the clinical management of small cetaceans. J. Am. Vet. Med. Assoc. 167, 540–545.
Tattersall G. J. (2016). Infrared thermography: A non-invasive window into thermal physiology. Comp. Biochem. Physiol. Part A: Mol. Integr. Physiol. 202, 78–98. doi: 10.1016/j.cbpa.2016.02.022
Tersawa F., Yokoyama Y., Kitamura M. (1999). Rectal temperatures before and after parturition in bottlenose dolphins. Zoo Biol. 18, 153–156. doi: 10.1002/(SICI)1098-2361(1999)18:2%3C153::AID-ZOO7%3E3.0.CO;2-F
Teunissen L., Daanen H. (2011). Infrared thermal imaging of the inner canthus of the eye as an estimator of body core temperature. J. Med. Eng. Technol. 35, 134–138. doi: 10.3109/03091902.2011.554595
Tran Q. H., Dang Q. M., Pham X. T., Huh J. (2023). Impact of wind speed on the use of active infrared thermography. J. Nondestructive Eval. 42 (2), 35. doi: 10.1007/s10921-023-00948-8
Usamentiaga R., Venegas P., Guerediaga J., Vega L., Molleda J., Bulnes F. (2014). Infrared thermography for temperature measurement and non-destructive testing. Sensors 14, 12305–12348. doi: 10.3390/s140712305
Verdegaal E. L. J. M. M., Howarth G. S., McWhorter T. J., Delesalle C. J. G. (2024). Thermoregulation during field exercise in horses using skin temperature monitoring. Animals 14, 136. doi: 10.3390/ani14010136
Walsh M., Gaynor E. (2001). “Thermal imaging of marine mammals,” in CRC handbook of marine mammal medicine, 2nd edn. Eds. Dierauf L., Gulland F. M. D. (CRC Press, Florida), 643–652.
West B. T., Welch K. B., Galecki A. T. (2022). Linear mixed models: a practical guide using statistical software. 3rd edn (Boca Raton, FL: CRC Press). doi: 10.1201/9781003181064
Whittow G. C., Hampton I. F. G., Matsuura D. T., Ohata C. A., Smith R. M., Allen J. F. (1974). Body temperature of three species of whales. J. Mammalogy 55, 653–656. doi: 10.2307/1379555
Keywords: infrared thermography, blowhole, rectal temperature, killer whale, Orcinus orca
Citation: Russell JP, Germain MS, Osborn SD, Schmitt TL, Herrick KES and Robeck T (2024) Comparison of infrared thermography of the blowhole mucosa with rectal temperatures in killer whales (Orcinus orca). Front. Mar. Sci. 11:1369287. doi: 10.3389/fmars.2024.1369287
Received: 12 January 2024; Accepted: 09 May 2024;
Published: 24 May 2024.
Edited by:
Daniel Mota-Rojas, Metropolitan Autonomous University, MexicoReviewed by:
Shane B. Kanatous, Colorado State University, United StatesAdriana Domínguez-Oliva, Metropolitan Autonomous University, Mexico
Copyright © 2024 Russell, Germain, Osborn, Schmitt, Herrick and Robeck. This is an open-access article distributed under the terms of the Creative Commons Attribution License (CC BY). The use, distribution or reproduction in other forums is permitted, provided the original author(s) and the copyright owner(s) are credited and that the original publication in this journal is cited, in accordance with accepted academic practice. No use, distribution or reproduction is permitted which does not comply with these terms.
*Correspondence: Jennifer P. Russell, amVubmlmZXIucnVzc2VsbEBzZWF3b3JsZC5jb20=