- 1Marine Science Institute, University of the Philippines Diliman, Quezon City, Philippines
- 2Research Center for Environmental Changes, Academia Sinica, Taipei, Taiwan
- 3Institute for Oceanography, National Taiwan University, Taipei, Taiwan
Trichodesmium is a unique cyanobacterium that concurrently performs both oxygen-generating photosynthesis and oxygen-sensitive nitrogen fixation in the same cells during the photoperiod. We have previously shown that nickel availability positively influences growth and nitrogen fixation rates of Trichodesmium under intense light conditions. The activity of the nickel-containing superoxide dismutase (Ni-SOD) is a critical component of the cellular mechanism exploited by Trichodesmium to attain the sensitive balance between photosynthesis and nitrogen fixation. Here, we studied the interactive effects of Ni and Fe availability on growth and nitrogen fixation rates of Trichodesmium because these act as metal centers of essential enzymes critical in various biochemical processes. Our results show that Ni availability enhances production of hydrogen by Trichodesmium but intracellular levels may be influenced by activity of a NiFe-uptake hydrogenase. Further, we used immunocytochemistry to demonstrate that Ni-SOD was significantly expressed in Trichodesmium, and that it co-occurred with both the Fe-component of nitrogenase enzyme and the D1 protein of PSII. The concurrence of these enzymes indicates that Ni-SOD aids in maintaining low intracellular levels of reactive oxygen species (ROS) by rapidly converting superoxide released by photosynthetic systems into hydrogen peroxide. Our results showing that Ni-SOD coexists with a photosynthetic protein and the nitrogenase enzyme supports the role of Ni-SOD in the protective mechanism employed by Trichodesmium.
Introduction
Cyanobacteria are prokaryotic organisms that serve as important primary producers, some of which are capable of nitrogen (N2) fixation and are critical sources of bioavailable nitrogen in specific ecological niches (Capone et al., 1997; Karl et al., 1997). Nitrogen fixation is an energy intensive process that is anchored on the activity of the nitrogenase enzyme, which is prone to damage caused by oxygen and other ROS occurring intracellularly (Gallon, 1981; Ohki et al., 1992; Latifi et al., 2009). Diazotrophic cyanobacteria have evolved distinct mechanisms to perform photosynthesis and N2 fixation through spatial or temporal separation enabling protection of the nitrogenase enzyme activity. Spatial separation is attained through formation of differentiated cells called heterocysts specifically intended for N2 fixation, while temporal separation is accomplished by carrying out N2 fixation at night and photosynthesis during the day (Gallon, 1981; Ohki et al., 1992; Latifi et al., 2009). Trichodesmium is unique among cyanobacteria because it performs these incompatible processes at the same time during the day in the absence of heterocysts (Carpenter and Capone, 1992). The mechanisms exploited by the organism to allow for carbon fixation while maintaining low intracellular ROS levels to permit N2 fixation has continually been debated, especially with differing observations provided by techniques probing intracellular processes. There are several proposed mechanisms on how Trichodesmium performs the two incongruous processes including the presence of diazocytes, cells that act similar to heterocysts but are not fully differentiated, and the rapid switching of photosynthesis and N2-fixing activities throughout the photoperiod. It has been demonstrated that the cyanobacterium employs an intricate mechanism resulting in tightly controlled cellular machinery facilitating temporal and spatial separation between the two processes (Berman-Frank et al., 2001a). The intracellular interactions between N2 fixation, photosynthesis, respiration, and oxygen-consuming reactions in Trichodesmium confer a strategy that is critical for its growth. A review of the various mechanisms on how the cyanobacterium performs both processes have been covered comprehensively by Bergman et al. (2012) and revisited by Hania et al. (2023) with the latter review providing information from recent techniques in cellular imaging.
The genus Trichodesmium is one of the major contributors of bioavailable nitrogen in oligotrophic tropical and subtropical oceanic regions. It is estimated that blooms of the cyanobacterium produce about 65 Tg of fixed N2 every year (Carpenter et al., 2004; Capone et al., 2005). The ecological niche preferred by Trichodesmium is characterized by high light intensities that reach about 2000 µmol photons m-2 s-1 of incident radiation (Carpenter et al., 1993; Breitbarth et al., 2008). High light intensities provide the energy required by photosynthesis but also lead to photoinhibition due to production of reactive oxygen radicals that deactivate light harvesting complexes and protein components of photosynthetic reaction systems (Carpenter et al., 1993; Breitbarth et al., 2008). These entail that the cyanobacterium must allocate cellular resources for continuous production and repair of its photosynthetic systems. In addition to photoinhibitory effects, Trichodesmium also contends with ROS damage to nitrogenase, the requisite enzyme for N2 fixation. In previous reports, we have demonstrated that higher Ni availability promotes better growth and supports higher N2 fixation activities of Trichodesmium in high light conditions (Ho, 2013; Rodriguez and Ho, 2014; Chen et al., 2022). Further, the work discussed by Chen et al. (2022) demonstrated that Ni-SOD expression closely corresponded to N2 fixation rates in high light conditions, which provided strong evidence for the role of Ni-SOD in maintaining low intracellular superoxide levels. In addition to Ni-SOD, it was also revealed that the expression of PsbA and PsbC, two important photosynthetic proteins of PS1, were positively correlated with Ni-SOD expression under high light intensity (Chen et al., 2022). This supports the proposed role of Ni-SOD as a lynchpin in processes critical for rapid transformation of ROS radicals to protect both the nitrogenase enzyme and the components of photosynthetic systems.
In this work, we probed for Ni-SOD, Fe-nitrogenase, and D1 proteins in cells of representative Trichodesmium trichomes using immunocytochemistry. The cells were grown in culture media with different bioavailable concentrations of iron and nickel. Iron (Fe) is a critical trace element for the cyanobacterium because of inherent Fe requirement for photosynthesis, nitrogen fixation, and other biochemical processes. Previous studies have revealed that Fe availability strongly influences both the photosynthetic and N2-fixing activities of the cyanobacterium (Berman-Frank et al., 2001b; Mills et al., 2004; Whittaker et al., 2011). The use of immunocytochemistry was applied to visualize the localization of different enzymes within the cell and investigate whether Ni-SOD, nitrogenase, and photosynthetic proteins are expressed at the same time within the same cells. The interactive effect of Fe and Ni availability on the expression of D1, Fe-nitrogenase, and Ni-SOD shall provide a distinct view on their intracellular distribution and infer on specific biochemical processes occurring within the cells during the photoperiod.
There are few reported biological functions for Ni. Aside from use in SOD, Ni is required in urease and uptake hydrogenases. Ureases are important for organisms that rely on urea as a nitrogen source and uptake hydrogenases are employed in regulation of proton levels in the cells (Palenik et al., 2003). Trichodesmium has the genetic platform to produce uptake hydrogenases, which contain both Ni and Fe as metal centers. There are two types of hydrogenases, uptake or bidirectional hydrogenases, which are characterized depending on their function (Tamagnini et al., 2007). Uptake hydrogenases take up H2 and facilitates its conversion to H+. Bidirectional hydrogenases mediate the conversion of H2 to H+ and vice versa depending on their cellular concentrations (Tamagnini et al., 2007). Hydrogen is produced as a byproduct of nitrogen fixation and because we have repeatedly observed that higher Ni availability supports higher N2 fixation, the influence of Ni supply on H2 production thus warrants to be studied. Here, we endeavored to investigate the effect of Ni and Fe availability on H2 production by Trichodesmium and its correlation with its N2-fixing activity.
Results
Trichodesmium growth and metal quotas
We subjected Trichodesmium to four treatment conditions with different concentrations of Fe (high and low Fe from hereon) and Ni (high and low Ni from hereon). As expected, the results showed that different combinations of Fe and Ni conditions supported high biomass of the diazotroph reaching about 0.5 to 1.0 x 107 µm3 ml-1 cellular volumes (Figure 1). Evaluation of growth rates revealed that the influence of Ni availability was only significantly different at low Fe concentration (Figure 2a; F3,11 = 7.29, p = 0.011). Rates were estimated during the exponential growth phase of the diazotroph, which typically covered the period from day 3 to 15, and reached about 0.4 day-1. On day 15, aliquots of the cultures were subjected to parallel acetylene reduction assay, to assess N2 fixation activity, and H2 production assay. Aliquots were also collected for POC/PON content determination, which resulted in values with insignificant differences (Figure 2b; F3,11 = 2.82, p = 0.107). Aliquots for intracellular metal quota determination were also collected in parallel with POC/PON analysis. The determined Fe quotas, or intracellular Fe normalized against phosphorus content, reflected Fe availability in the growth medium with about two-fold difference between low and high Fe treatments, and were consistent with previous quotas observed in bottle incubations of Trichodesmium (Figure 2c; F3,22 = 26.76, p < 0.00001). Evaluation of Ni quotas showed that the high Fe-high Ni treatment had significantly lower intracellular Ni content compared to others (Figure 2d; F3,20 = 18.2, p < 0.00001).
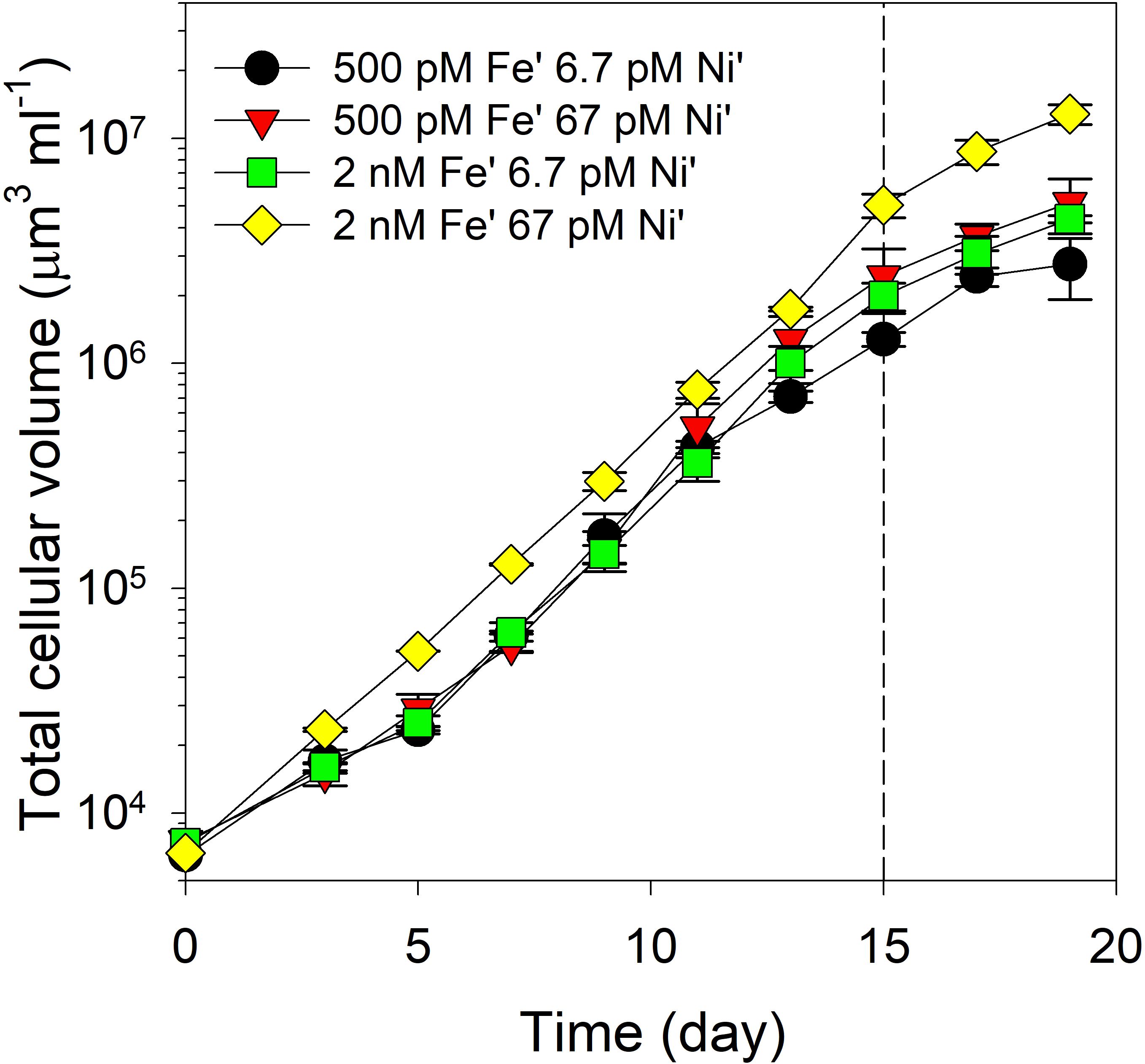
Figure 1. Growth profiles of Trichodesmium erythraeum subjected to different Fe and Ni availability in the growth medium. The values represent mean and error bars represent standard deviation of biological triplicates per treatment. Aliquots of cultures were collected for different analyses and assays on day 15. Fe was added at total dissolved concentrations of 100 and 400 nM resulting in expected inorganic Fe concentrations of 0.5 and 2.0 nM Fe′, respectively. Ni was added at total dissolved concentrations of 10 and 100 nM resulting in expected inorganic concentrations of 6.7 and 67 pM Ni′, respectively.
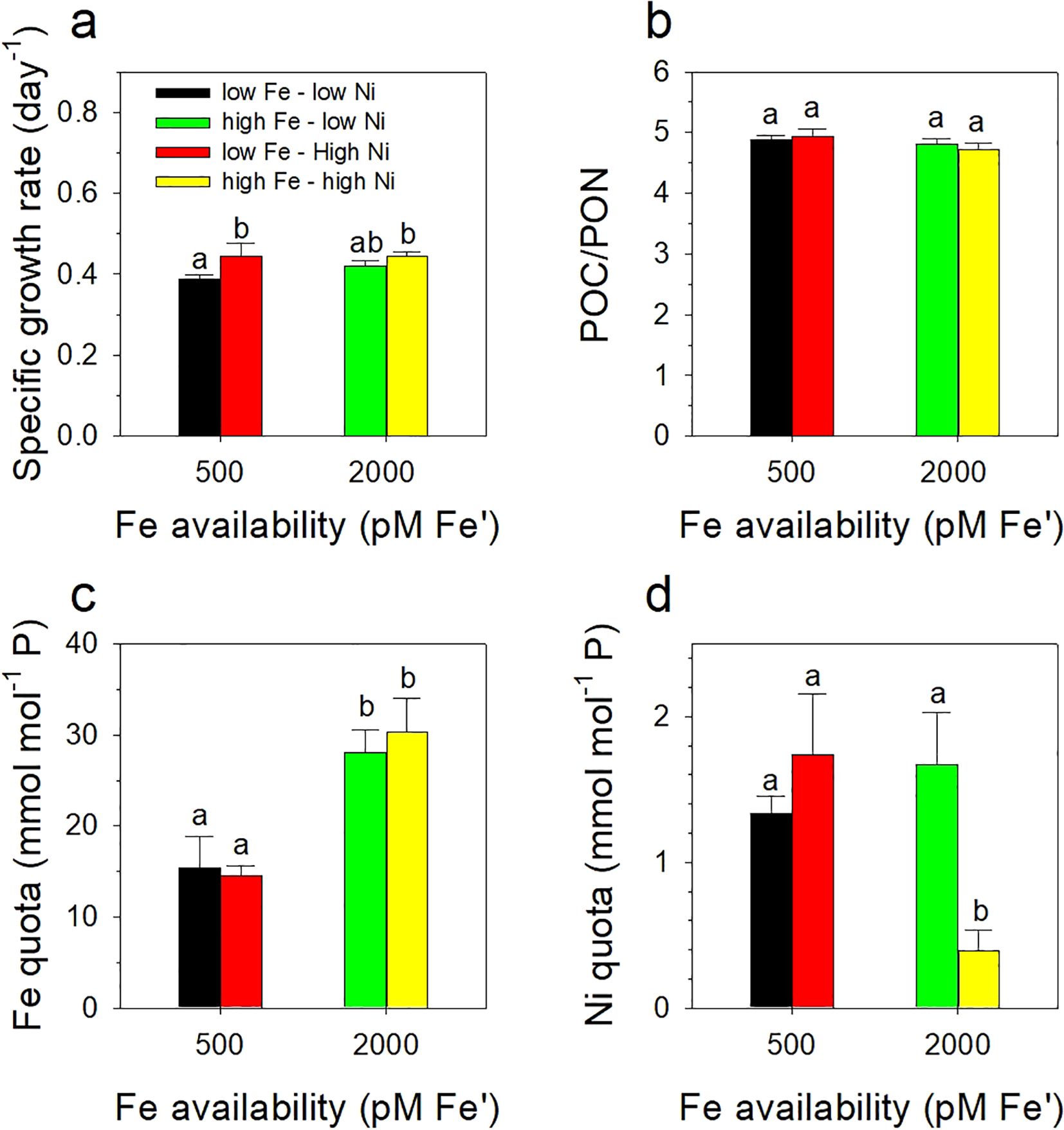
Figure 2. Growth rates (a), POC/PON content (b), and intracellular Fe (c) and Ni (d) quotas of Trichodesmium erythraeum subjected to different Fe and Ni availability in the growth medium. The values represent means ± SD (N = 3, P < 0.05, ANOVA, post hoc Tukey HSD). Different letters indicate significant differences between treatments. Fe was added at total dissolved concentrations of 100 and 400 nM resulting in expected inorganic Fe concentrations of 0.5 and 2.0 nM Fe', respectively. Ni was added at total dissolved concentrations of 10 and 100 nM resulting in expected inorganic concentrations of 6.7 and 67 pM Ni', respectively.
Interactive effects of Ni and Fe on N2 fixation and H2 production by Trichodesmium
The levels of N2 fixed by Trichodesmium displayed that the low Fe-high Ni treatment processed the highest amounts of N2 while the low Fe-low Ni treatment performed the least (Figure 3a). Between high Fe treatments, high Ni availability favored higher levels of N2 fixed. The levels of H2 produced by Trichodesmium in different growth conditions revealed that higher Fe availability favored higher H2 release (Figure 3b). The treatments supplied with high Ni also demonstrated high H2 production levels. A strong correlation between H2 production and N2 fixation is evinced when values for H2 produced and N2 fixed were plotted together (Figure 3c). Except for the high Fe-low Ni treatment, which had a correlation value of 0.74 (p < 0.95), all treatments exhibited strong correlation with r values equal to 0.9 (p < 0.95). Both treatments with high Fe concentrations reflected higher levels of H2 produced even though levels of N2 fixed were comparable with that in low Fe treatments. The cells subjected to hydrogen production assay were collected after each time point during the 12-hr experiment to probe the cells for the different proteins of interest by immunocytochemistry.
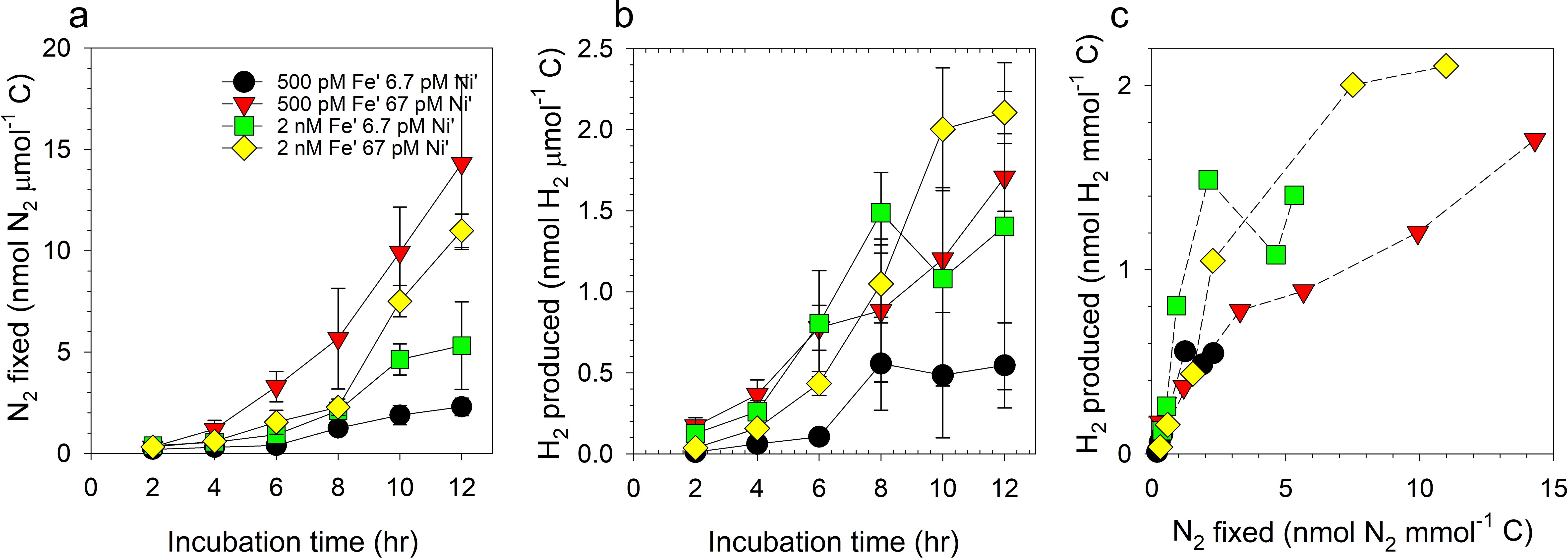
Figure 3. Levels of nitrogen fixed (a), hydrogen produced (b), and correlation of N2 fixed and H2 produced (c) by Trichodesmium erythraeum subjected to different Fe and Ni availability in the growth medium. The values represent mean and error bars represent standard deviation of biological triplicates per treatment. Fe was added at total dissolved concentrations of 100 and 400 nM resulting in expected inorganic Fe concentrations of 0.5 and 2.0 nM Fe′, respectively. Ni was added at total dissolved concentrations of 10 and 100 nM resulting in expected inorganic concentrations of 6.7 and 67 pM Ni′, respectively.
Immunolocalization of Ni-SOD, Fe-nitrogenase, and D1
One of the major objectives for this work was to investigate whether Ni-SOD is produced by Trichodesmium and if its expression co-occurs with nitrogenase or a photosynthetic protein. We used D1 as a representative photosynthetic protein because of its crucial role in the PSI system. The trichomes were probed simultaneously for nitrogenase and D1 or nitrogenase and Ni-SOD (representative images presented in Figures 4, 5). For each panel, the images with overlaid signals were presented on top of trichomes probed for nitrogenase and D1 or Ni-SOD. Our results showed that Ni-SOD was produced by the diazotroph and that it occurred in the same cells expressing the nitrogenase enzyme. In another set of trichomes where we probed for nitrogenase and D1, both proteins were also expressed on the same cells providing evidence that Ni-SOD co-occurred with both nitrogenase and D1 in the same cells.
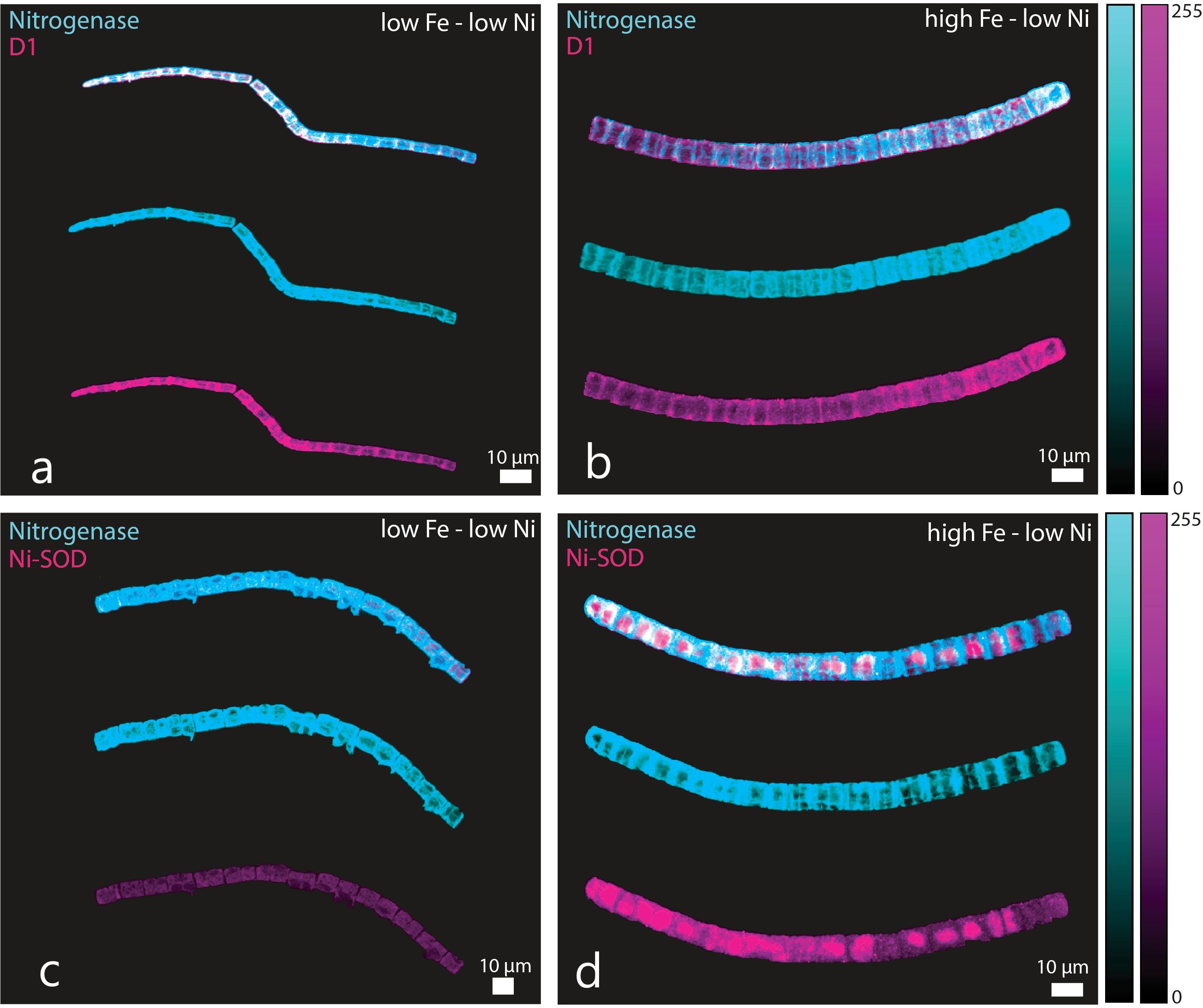
Figure 4. Immunocytochemistry images showing location of nitrogenase (cyan), D1 (purple), and Ni-SOD (purple) in Trichodesmium erythraeum cells subjected to different Fe and low Ni availability in the growth medium: (a) nitrogenase and D1 in low Fe – low Ni, (b) nitrogenase and D1 in high Fe – low Ni, (c) nitrogenase and Ni-SOD in low Fe – low Ni, and (d) nitrogenase and Ni-SOD in high Fe – low Ni treatments. The trichomes were probed simultaneously for nitrogenase and D1 or nitrogenase and Ni-SOD. For each panel, the image of the trichome with overlaid signals are presented on top of images for trichomes probed for nitrogenase and D1 or Ni-SOD. Fe was added at total dissolved concentrations of 100 and 400 nM resulting in expected inorganic Fe concentrations of 0.5 and 2.0 nM Fe′, respectively. Ni was added at total dissolved concentrations of 10 and 100 nM resulting in expected inorganic concentrations of 6.7 and 67 pM Ni′, respectively.
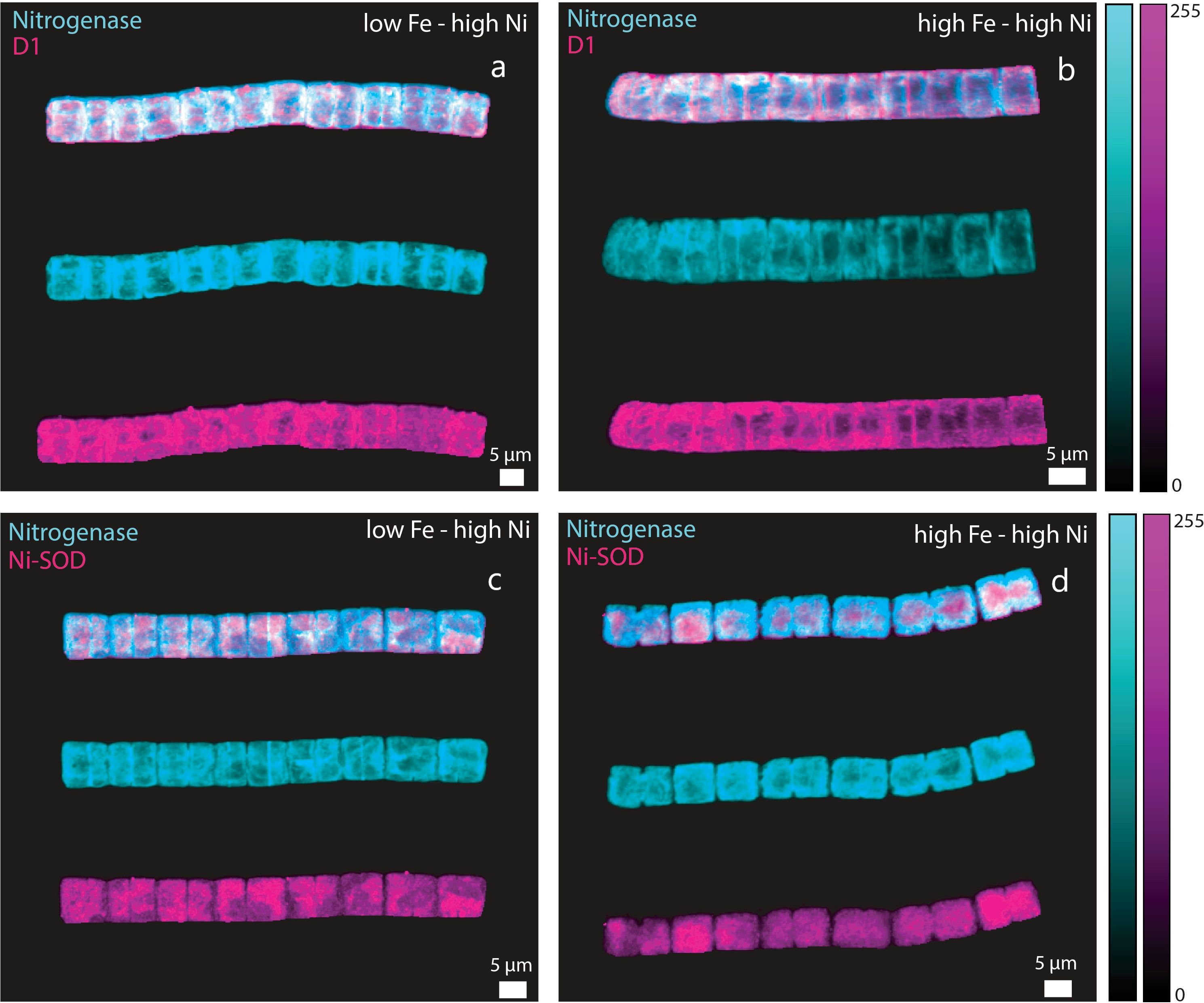
Figure 5. Immunocytochemistry images showing location of nitrogenase (cyan), D1 (purple), and Ni-SOD (purple) in Trichodesmium erythraeum cells subjected to different Fe and high Ni availability in the growth medium: (a) nitrogenase and D1 in low Fe – high Ni, (b) nitrogenase and D1 in high Fe – high Ni, (c) nitrogenase and Ni-SOD in low Fe – high Ni, and (d) nitrogenase and Ni-SOD in high Fe – high Ni treatments. The trichomes were probed simultaneously for nitrogenase and D1 or nitrogenase and Ni-SOD. For each panel, the image of the trichome with overlaid signals are presented on top of images for trichomes probed for nitrogenase and D1 or Ni-SOD. Fe was added at total dissolved concentrations of 100 and 400 nM resulting in expected inorganic Fe concentrations of 0.5 and 2.0 nM Fe′, respectively. Ni was added at total dissolved concentrations of 10 and 100 nM resulting in expected inorganic concentrations of 6.7 and 67 pM Ni′, respectively.
Discussion
Our results validated previous observations that the availability of Ni and Fe influenced the growth and N2 fixation of Trichodesmium. The Fe and Ni conditions we used were based on previous studies and represented sufficient concentrations to support growth of Trichodesmium in the light intensity employed (Ho, 2013; Rodriguez and Ho, 2014; Chen et al., 2022). The growth rates and metal quotas were comparable with reported values in earlier studies (Rodriguez and Ho, 2014, 2017) and support our hypothesis that Ni availability enhances growth of Trichodesmium under intense light. The POC: PON ratios in different treatments exhibited comparable values highlighting that Fe and Ni combinations used favored growth of the diazotroph (Figure 2b). The C:N content observed was lower than the canonical Redfield ratio of 6.6:1 but equivalent values in all treatments indicate that the cyanobacteria was able to fix the required nitrogen for its growth, which was also reflected in the results of the N2-fixing assay. The Fe quotas determined in this work reflected the availability of the essential metal in the growth medium while Ni quotas presented a trend that was previously observed (Rodriguez and Ho, 2014). The treatment with highest bioavailable concentrations of both Fe and Ni had significantly lower Ni content compared with others (Figure 2d). It is tempting to ascribe this to a vastly different requirement for Ni at the high Fe – high Ni conditions but understanding the cellular mechanisms resulting in this observation clearly warrants further studies.
This work further strengthens the evidence that Ni is critical to support higher N2 fixation rates, which we posited to be attributed to the activity of Ni-SOD in protecting the nitrogenase enzyme (Ho, 2013). The genome of Trichodesmium reveals that it contains genes required for production of both Ni- and Mn-containing SOD (Dupont et al., 2008). Superoxide dismutases are considered the first line of defense against oxidative stress because SODs neutralize the highly reactive superoxide preventing it from inflicting damage on cellular components (Palenik et al., 2003; Dupont et al., 2008). This current work builds on previous research done and was focused on the importance of Ni-SOD in Trichodesmium to provide insights on how Ni availability influences biochemical processes in the cyanobacterium (Ho, 2013; Ho et al., 2013; Rodriguez and Ho, 2014, 2017). Our results validate previous observation about the importance of Ni in Trichodesmium and we show through immunocytochemistry that it expresses Ni-SOD, which has been established to be positively influenced by Ni bioavailability (Chen et al., 2022). The previous work presented by Chen et al. (2022) revealed that Ni-SOD expression by Trichodesmium was linearly correlated with Ni availability. Our current results employing immunocytochemistry demonstrate that Ni-SOD is produced by Trichodesmium and that it co-occurred with both the nitrogenase enzyme and PsbA (D1) protein in the cells (Figures 4, 5). Immunocytochemical images show that nitrogenase (shown in cyan color) co-occur in the same cells as the D1 protein (in purple color) in the same trichome. Also, the trichomes probed for nitrogenase (shown in cyan color) and Ni-SOD (shown in purple color) demonstrated the co-occurrence of these two enzymes. It is worth noting that this is the first report of intracellular localization of Ni-SOD in Trichodesmium. This co-occurrence strengthens our supposition that Ni-SOD is important in the regulation of intracellular ROS levels via the Mehler reaction when Trichodesmium is carrying out both photosynthesis and N2 fixation.
Nitrogen fixation is an energy intensive process and H2 production is an inadvertent by-product of this N2-fixing activity. Theoretically, a mole of H2 is liberated for every mole of N2 fixed. Previous investigations evinced that most cyanobacteria release H2 at much lower molar equivalents than the levels of N2 fixed (Tuo et al., 2020; Li et al., 2022). Compared with both Cyanothece sp. and C. watsonii, T. erythraeum releases a higher ratio of H2 to N2 fixed (Wilson et al., 2010, 2012). We have repeatedly observed that higher Ni availability supports higher N2 fixation, thus we also investigated the influence of Ni supply on H2 production by Trichodesmium. Our results show that H2 production is proportional to N2 fixed (Figure 3), which was expected because H2 is liberated during N2-fixing activities. The treatments that exhibited higher N2-fixing activities, especially the high Fe treatments, also presented higher levels of H2 produced. Except for the high Fe – low Ni treatment, the correlation between H2 produced to N2 fixed in the treatments was 0.9. This observation of lower correlation at the high Fe – low Ni treatment indicates either a lower H2 production from N2 fixation or elevated consumption of H2 because of the activity of a Ni/Fe-uptake hydrogenase. The availability of protons and hydrogen in the cells is critical for various biochemical processes, one of which is the dismutation of superoxide producing hydrogen peroxide, a less reactive ROS, catalyzed by Ni-SOD via the Mehler reaction (Asada, 1999; Berman-Frank et al., 2001a; Chen et al., 2022). This dismutation requires protons that may be supplied through the activity of a Ni/Fe-uptake hydrogenase, the production of which may be limited by Ni availability in the growth medium. Aside from ensuring the supply of protons for the dismutation reaction, the activity of uptake hydrogenase also liberates electrons that may be used for other biochemical processes (Berman-Frank et al., 2001b; Tamagnini et al., 2007). Additionally, the conversion of H2 to protons results in elevated N2-fixing activity because H2 can inhibit nitrogenase activity (Tamagnini et al., 2007). This cascade of reactions highlights the importance of Ni-containing enzymes in the anti-oxidative defense mechanism employed by Trichodesmium. The cellular mechanism controlling hydrogen homeostasis and the influence of Ni and Fe availability on this process certainly merits further studies.
The results of this work underscore the need to further explore the role of Ni because this has consequences for naturally occurring blooms of Trichodesmium. Nickel concentration in the surface ocean is about 2 nM and its availability for phytoplankton utilization is largely unknown (Mackey et al., 2002). This has implications in biogeochemical cycling of nutrients because Trichodesmium blooms trigger succession of other species through introduction of new bioavailable nitrogen especially in oligotrophic waters (Karl et al., 2002; Mulholland et al., 2006). Previous studies have demonstrated that Trichodesmium benefits from micronutrients derived from dust and other particles through various strategies including release of metal-sequestration compounds or concerted activities of associated organisms (Rubin et al., 2011; Basu and Shaked, 2018). Further, a recent work by Held et al. (2021) through single colony metaproteomics showed that Ni-SOD was significantly more abundant in mineral-associated colonies, which was attributed to the need for regulation of ROS. The results presented by Held et al. (2021) combined with our results from immunocytochemical imaging of Trichodesmium filaments point to the importance of Ni-SOD in the cyanobacterium. The contribution of other nitrogen fixers including Crocosphaera watsonii and other unidentified unicellular cyanobacteria is widely regarded to be significant (Wilson et al., 2010, 2012). However, the contribution of Trichodesmium especially during blooms is still considered as critical to dramatically impact nutrient cycling and biogeochemical cycles.
Conclusion
Our results indicate that Ni availability increases H2 production, potentially through enhanced N2 fixation activities. The effect of Ni is highly linked to the activity of Ni-SOD in conjunction with a Ni/Fe-uptake hydrogenase resulting in the regulation of intracellular proton levels in Trichodesmium. The proton gradient in the cell is critical in various biochemical processes including photosynthesis, nitrogen fixation, ATP generation, photorespiration, and others. The role of Ni-containing enzymes in Trichodesmium merits further research to unravel cellular mechanisms that dictate growth and blooms of this cyanobacterium. The results show that availability of trace metal cofactors influences growth of phytoplankton because of the inherent requirement for these metals in critical biochemical functions. In Trichodesmium, Ni availability influences growth through production of Ni-SOD and possibly of NiFe-uptake hydrogenase. The production of these enzymes control N2 fixation activities as well as H2 evolution by the cyanobacteria through a tightly controlled series of biochemical processes involving photosynthesis, nitrogen fixation, respiration, and anti-oxidative defenses. In this work, we present results employing immunocytochemistry that reveal co-occurrence of Ni-SOD with the nitrogenase enzyme and D1 protein, enzymes needed for nitrogen fixation and photosynthetic activities respectively. Our results underpin the role of Ni-SOD in these critical biological processes in the nonheterocystous diazotroph Trichodesmium and point to an additional role of Ni-containing enzymes in photosynthetic organisms.
Methods
Culture conditions
Trichodesmium erythraeum IMS101 (Trichodesmium from here onwards) was obtained from National Center for Marine Algae and Microbiota (East Boothbay, ME, USA). Cultures were kept in 1 L acid washed polycarbonate (PC) bottles with trace metal-defined culture medium (Ho, 2013) modified from the original YBC-II recipe (Chen et al., 1996). Artificial seawater was filtered using Whatman® Polycap filters, passed through a column packed with Chelex-100® resin to remove trace metal contents, and filter-sterilized using 0.22 µm pore size filters prior to use. Trichodesmium was grown in two different Fe and Ni concentrations resulting in a total of four treatments with differing Fe and Ni bioavailability. The bioavailable metal concentration, which was defined as the expected inorganic concentration, was controlled by addition of 20 µM ethylenediaminetetraacetic acid (EDTA) and calculated using MineQL version 4.0 (Westall et al., 1976). Fe was added at total dissolved concentrations of 100 and 400 nM resulting in expected inorganic Fe concentrations of 0.5 and 2.0 nM Fe′, respectively. Ni was added at total dissolved concentrations of 10 and 100 nM resulting in expected inorganic concentrations of 6.7 and 67 pM Ni′, respectively.
All treatments were carried out in triplicates and were inoculated from a source that was acclimatized to respective growth conditions for at least two passages. The inoculation volume was set at 1/1000 to achieve a starting cell density of about 6000 µm3 ml-1 while ensuring minimum carry-over of trace metals. Trichodesmium cultures were kept free of fixed nitrogen whereas phosphate was added at a total initial concentration of 50 µM. A mixture of B-vitamins, composed of thiamine, biotin and cyanocobalamin, was added to have final concentrations in the medium equivalent to 300, 2.0, and 4.0 nM, respectively. Stock solutions of phosphate, thiamine, and biotin were all passed through columns packed with Chelex-100® to remove metal contaminants. The stock cyanocobalamin solution was not passed through the column to avoid loss of cyanocobalamin.
Cultures were kept in a temperature-controlled growth chamber set at 26°C. Light was supplied at a photon flux density of 680 µmol photons m-2 s-1 using full-spectrum white light fluorescent lamps (Philips). Light intensity was validated by measuring photon flux density using a submersible radiometer (Biospherical Instruments Inc. QSL 2100). The light and dark periods were programmed under a square-wave 12:12 h light:dark regime. Bottles and other plastic materials used for culturing and other related work were carefully washed with 2% Micro-90® solution, rinsed, soaked with 10% hydrochloric acid solution, and rinsed thoroughly with ultrapure water prepared using a Milli-Q system. All necessary procedures were performed in a class 100 trace-metal clean laboratory.
Growth rates, particulate organic carbon and particulate organic nitrogen content, and intracellular metal quotas
Trichodesmium growth was monitored by observing changes in cell density, expressed as total cellular volume per ml, using a Beckman Coulter Counter Multisizer 3 fitted with a 100 µm aperture tube, for a period up to 20 days. Other more robust methods are available for growth monitoring of filamentous phytoplankton. However, we find the Coulter counter to be a reliable method offering a precision within 5% and suitability for cellular volumes down to 5000 µm3 ml-1. This cellular volume corresponds to presence of 1–2 trichomes per ml of culture and assuming each trichome consists of 10–20 cells. The use of a Coulter Counter for monitoring changes in cellular volume of Trichodesmium has been explained in detail in a previous report (Ho, 2013).
Growth rates, POC/POC content, and intracellular metal quotas were determined while cultures were in the exponential growth stage, typically between days 3 to 15. Rates were calculated by taking the natural logarithmic values of cellular volume during the initial and final days over the change in time. Samples for determination of intracellular quotas were collected during the middle of the photoperiod by filtering cells onto acid-washed polycarbonate filters (25 mm diameter, 5 µm pore size, Millipore). Filtered cells were washed with ultrapure water and acid-digested prior to elemental analysis using HR-ICPMS (Element XR, Thermo Scientific). Metal quotas or intracellular metal content were normalized against phosphorus content as the biomass indicator. Filter blanks were subjected to the same procedures including digestion, dilution, and analysis. The blank values were subtracted from sample measurements. Parallel samples for POC/PON content determination were processed together with the assays and intracellular metal quota determination. Aliquots were filtered using GFF filters (25 mm diameter, 0.75 µm pore size, Whatman), subsequently processed and analyzed using a CHNS elemental analyzer (Thermo Scientific).
Nitrogen fixation by Trichodesmium
The amount of N2 fixed by Trichodesmium cultures was estimated using the acetylene reduction method following steps outlined elsewhere (Ho, 2013; Rodriguez and Ho, 2014). The assay was carried out while cells were in the exponential phase of growth and when biomass was higher than 1 x 106 µm3 ml-1. Previous experiments in the laboratory showed that this cellular volume was the minimum cell that may be subjected to the acetylene reduction assay (Rodriguez and Ho, 2014). Briefly, 10 ml aliquots from each replicate culture were transferred to 20 ml vials (Agilent). The vials were sealed using Teflon-coated caps and 2 ml headspace was drawn using a syringe, which was then replaced by adding 2 ml of commercially available acetylene to initiate the experiment. The vials were incubated in the same culture conditions for 12 hours with time points for sample collection set every two hours during the day. After incubation for the desired period, 2 ml of headspace was drawn, and the gaseous sample was subsequently analyzed for ethylene using an Agilent 7890A gas chromatograph equipped with a Poropak N column and a flame ionization detector. Estimation of N2 fixation was taken from acetylene reduction using a conversion ratio of 4:1, and assumption that the Bunsen coefficient for ethylene is 0.084. Procedural blanks prepared by using vials filled with filtered YBC-II were also subjected to the same acetylene reduction assay. The blank values were subtracted from sample measurements.
Hydrogen production by Trichodesmium
The amount of H2 produced by Trichodesmium was estimated during the same time as the acetylene reduction assay using a parallel set of 10 ml aliquots of the respective cultures. Time points for collection were also set at the same time and frequency. The levels of H2 produced were determined by subjecting 1 ml of headspace into a reduced gas analyzer that incorporated a mercuric bed oxide coupled to a reducing compound photometer (Peak Laboratories, Mountain View, CA, USA). Headspace samples from procedural blanks using filtered YBC-II that were incubated in similar conditions were also subjected to the same analysis. The blank values were subtracted from sample measurements.
Immunocytochemistry
Trichodesmium cultures (about 10 ml, the same set of aliquots from three biological replicates used for the H2 production assay) were filtered using a PC membrane filter (5 µm pore size, Millipore). The membrane containing trichomes was transferred to a 50-ml falcon tube containing 10 ml chilled ethanol, stored at -20°C for at least 24 hours, then filtered again using the same membrane filter. The membrane was washed 3 times with 1 ml PBS (phosphate buffered saline, Bio-Rad Laboratories) then transferred into a 2 ml Eppendorf tube with 1.5 ml 10% dimethyl sulfoxide (DMSO) in PBS for 4 hours at 4°C for permeabilization. The solution containing permeabilized trichomes was transferred to a PLL (Sigma-Aldrich, P4707) coated 8 well substrate (Mattek, CCS-8), then washed with PBS before incubating with the blocking buffer solution, 4% BSA (Sigma-Aldrich, A9205), 1% goat serum (Thermo Fisher Scientific, 50197Z) in PBS, for 24 hours at 4°C. The nitrogenase primary antibody (Agrisera, AS01 021A), and Ni-SOD (Yao-Hong Biotechnology) or PsBA primary antibodies (Agrisera, AS05 084), diluted 200 times in blocking buffer, were simultaneously incubated for 24 hours followed by washing with PBS. The corresponding secondary antibodies (Abcam, Goat anti-chicken, 405 nm: ab175674, Goat anti-rabbit, 488 nm: ab150077, diluted 200 times with blocking buffer) were incubated simultaneously for 1 hour at room temperature. The trichomes were washed again with PBS then subjected to confocal microscopy imaging. At least 5 trichomes from each time point for each treatment was subjected to imaging resulting in 30 images of trichomes per treatment for each of Ni-SOD, nitrogenase, or D1 protein. Determining the trichomes to be subjected for analysis was based on filaments or fragments of filaments that were intact in terms of cellular structure (i.e., not visibly leaching cellular contents) after the immunolabeling procedure, which resulted in various lengths. Thus, the filament sizes are a result of the immunolabeling procedure and not by the differing Fe and Ni treatments in the growth medium.
Image acquisition and analysis
The image stacks were taken using 40X water lens objective attached to a Zeiss LSM 780 confocal microscope with confocal scanning module Zeiss LSM780 with 32 GaAsP photomultiplier tube as the detector. Excitation was performed using a 405 nm diode laser for detecting autofluorescence and for exciting Alexa Fluor 405, and a 488 nm argon laser for exciting Alexa Fluor 488. Emission was detected within 410–500 nm for the 405 nm excitation and 500–550 nm for the 488 nm excitation. These settings were chosen to optimize signal detection while minimizing spectral overlap. To remove the background noise, we subtracted the spectral profile of the auto-fluorescence measured using the linear unmixing feature of the Zeiss software. Briefly, to enable the use of negative controls and the distinction between autofluorescence and antibody signal, we performed a negative control experiment where trichomes underwent the same sample preparation and imaging protocol but without the primary antibody. This allowed the confirmation of the presence of autofluorescence in unstained samples. To accurately separate autofluorescence from specific antibody signals, we employed spectral imaging with linear unmixing in Zeiss ZEN software. A lambda scan of unstained trichomes was acquired to record their autofluorescence spectrum, this is done by exciting the sample by 405 nm or 488 nm and detecting fluorescence at different spectral bins from 400 nm to 700 nm, which was then used as a reference to mathematically subtract autofluorescence from the antibody-stained sample. Microscope images of both unstained (negative control) and stained samples from the same batch, presented at the same scale of pixel intensity values for direct comparison are presented in the Supplementary Figure. The images were further processed by extracting the intensities of the trichome by applying a threshold segmentation using ImageJ. Concerning post-acquisition image processing, we projected the Z-stack into a 2D maximum intensity projection to create a single image that captures the brightest fluorescence signals from all focal planes. To enhance visual clarity, we applied automatic brightness adjustment, ensuring that fluorescence signals were clearly distinguishable for qualitative comparisons. This brightness normalization was performed uniformly across all images by mapping pixel intensity values from 0 to 255, without altering the actual fluorescence intensity values stored in the raw data. Since our analysis focused on signal localization rather than fluorescence quantification, this method ensured that the spatial distribution of fluorescence was clearly visible without misrepresenting the data. To further refine the images, we segmented the trichomes using thresholding or manual selection to isolate the regions of interest. Any background noise was removed by setting background pixel values to 0, ensuring that only relevant fluorescence signals were displayed. This preprocessing step allowed for a clearer distinction between autofluorescence and antibody-specific signals while maintaining consistency across all images. No additional enhancements, such as gamma correction or manual contrast adjustments, were applied.
Statistical analyses
One-way analysis of variance (ANOVA) was conducted to determine statistical differences between treatments (post hoc Tukey HSD, P < 0.05; SPSS).
Data availability statement
The original contributions presented in the study are included in the article/Supplementary Material. Further inquiries can be directed to the corresponding author/s.
Author contributions
IR: Conceptualization, Data curation, Formal Analysis, Investigation, Methodology, Writing – original draft, Writing – review & editing. IL: Data curation, Formal Analysis, Investigation, Methodology, Visualization, Writing – review & editing. T-YH: Conceptualization, Formal Analysis, Funding acquisition, Project administration, Resources, Supervision, Writing – review & editing, Methodology.
Funding
The author(s) declare that financial support was received for the research and/or publication of this article. The financial support partially came from the National Science and Technology Council under grants 108–2611-M-001–006-MY3 and 111–2611-M-001–006-MY3, as well as the Investigator Award AS-IA-110-M03 from Academia Sinica. The work was also partially funded by grants from The Marine Science Institute, UP Diliman.
Conflict of interest
The authors declare that the research was conducted in the absence of any commercial or financial relationships that could be construed as a potential conflict of interest.
Generative AI statement
The author(s) declare that no Generative AI was used in the creation of this manuscript.
Publisher’s note
All claims expressed in this article are solely those of the authors and do not necessarily represent those of their affiliated organizations, or those of the publisher, the editors and the reviewers. Any product that may be evaluated in this article, or claim that may be made by its manufacturer, is not guaranteed or endorsed by the publisher.
Supplementary material
The Supplementary Material for this article can be found online at: https://www.frontiersin.org/articles/10.3389/fmars.2025.1525315/full#supplementary-material
Supplementary Figure 1 | Control experiment for immunostaining of trichome. (A) The upper panel shows an image of a trichome without the primary antibody, acquired using 405 nm excitation with an emission detection range of 410–500 nm. The lower panel presents a trichome from the same batch of immunostaining, but stained with a primary antibody targeting Nitrogenase. (B) Similar to (A), but using 488 nm excitation with an emission detection range of 500–550 nm. The lower panel displays a trichome stained with a primary antibody against Ni-SOD.
References
Asada K. (1999). The water–water cycle in chloroplasts: Scavenging of active oxygens and dissipation of excess photons. Annu. Rev. Plant Biol. 50, 601–639. doi: 10.1146/annurev.arplant.50.1.601
Basu S. and Shaked Y. (2018). Mineral iron utilization by natural and cultured Trichodesmium and associated bacteria. Limnol Oceanogr. 63, 2307–2320. doi: 10.1002/lno.10939
Bergman B., Sandh G., Lin S., Larsson J., and Carpenter E. J. (2012). Trichodesmium – a widespread marine cyanobacterium with unusual nitrogen fixation properties. FEMS Microbiol. Rev. 37, 286–302. doi: 10.1111/j.1574-6976.2012.00352.x
Berman-Frank I., Cullen J. T., Shaked Y., Sherrell R. M., and Falkowski P. G. (2001a). Iron availability, cellular iron quotas, and nitrogen fixation in Trichodesmium. Limnol. Oceanogr. 46, 1249–1260. doi: 10.4319/lo.2001.46.6.1249
Berman-Frank I., Lundgren P., Chen Y. B., Küpper H., Kolber Z., Bergman B, et al. (2001b). Segregation of nitrogen fixation and oxygenic photosynthesis in the marine cyanobacterium Trichodesmium. Science 294, 1534–1537. doi: 10.1126/science.1064082
Breitbarth E., Wohlers J., Kläs J., LaRoche J., and Peeken I. (2008). Nitrogen fixation and growth rates of Trichodesmium IMS-101 as a function of light intensity. Mar. Ecol. Prog. Ser. 359, 25–36. doi: 10.3354/meps07241
Capone D. G., Burns J. A., Montoya J. P., Subramaniam A., Mahaffey C., Gunderson T., et al. (2005). Nitrogen fixation by Trichodesmium spp.: An important source of new nitrogen to the tropical and subtropical North Atlantic Ocean. Global Biogeochem. Cycle 19, GB2024. doi: 10.1029/2004GB002331
Capone D. G., Zehr J. P., Paerl H. W., Bergman B., and Carpenter E. J. (1997). Trichodesmium, a globally significant marine cyanobacterium. Science 276, 1221–1229. doi: 10.1126/science.276.5316.1221
Carpenter E. J. and Capone D. G. (1992). “Nitrogen fixation in Trichodesmium blooms,” in Marine pelagic cyanobacteria: Trichodesmium and other diazotrophs, vol. 362. (Springer, Dordrecht), 211–217. doi: 10.1007/978-94-015-7977-3_13
Carpenter E. J., O’Neil J. M., Dawson R., Capone D. G., Siddiqui P. J. A., Roenneberg T., et al. (1993). The tropical diazotrophic phytoplankter Trichodesmium: biological characteristics of two common species. Mar. Ecol. Prog. Ser. 95, 295–304. doi: 10.3354/meps095295
Carpenter E. J., Subramaniam A., and Capone D. G. (2004). Biomass and primary productivity of the cyanobacterium, Trichodesmium spp, in the southwestern tropical N Atlantic Ocean. Deep-Sea Res. 51, 173–203. doi: 10.1016/j.dsr.2003.10.006
Chen C.-C., Rodriguez I. B., Y.-l. L., Zehr J. P., Chen Y.-R., Hsu S.-T. D., et al. (2022). Nickel superoxide dismutase protects nitrogen fixation in Trichodesmium. Limnol. Oceanogr. Lett. 7, 363–371. doi: 10.1002/lol2.10263
Chen Y. B., Zehr J. P., and Mellon M. (1996). Growth and nitrogen fixation of the diazotrophic filamentous nonheterocystous cyanobacterium Trichodesmium sp. IMS 101 in defined media: evidence for a circadian rhythm. J. Phycol. 32, 916–923. doi: 10.1111/j.0022-3646.1996.00916.x
Dupont C. L., Barbeau K., and Palenik B. B. (2008). Ni uptake and limitation in marine Synechococcus strains. Appl. Environ. Microbiol. 74, 23–31. doi: 10.1128/AEM.01007-07
Gallon J. R. (1981). The oxygen sensitivity of nitrogenase: a problem for biochemists and micro-organisms. Trends Biochem. Sci. 6, 19–23. doi: 10.1016/0968-0004(81)90008-6
Hania A., López-Adams R., PrášIl O., and Eichner M. (2023). Protection of nitrogenase from photosynthetic O2 evolution in Trichodesmium: methodological pitfalls and advances over 30 years of research. Photosynthetica 61, 58–72. doi: 10.32615/ps.2023.007
Held N. A., Sutherland K. M., Webb E. A., McIlvin M. R., Cohen N. R., Devaux A. J., et al. (2021). Mechanisms and heterogeneity of in situ mineral processing by the marine nitrogen fixer Trichodesmium revealed by single-colony metaproteomics. ISME Commun. 1, 35. doi: 10.1038/s43705-021-00034-y
Ho T. Y. (2013). Nickel limitation of nitrogen fixation by Trichodesmium. Limnol. Oceanogr. 58, 112–120. doi: 10.4319/lo.2013.58.1.0112
Ho T. Y., Chu T. H., and Hu C. L. (2013). Interrelated influence of light and Ni on Trichodesmium growth. Front. Microbiol. 4. doi: 10.3389/fmicb.2013.00139
Karl D., Michaels A., Bergman B., Capone D., Carpenter E., Letelier R., et al. (2002). Dinitrogen fixation in the world’s oceans. Biogeochem 57, 47–98. doi: 10.1023/A:1015798105851
Karl D., Letelier R., Tupas L., Dore J., Christian J., and Hebel D. (1997). The role of nitrogen fixation in biogeochemical cycling in the subtropical North Pacific Ocean. Nature 388, 533–538. doi: 10.1038/41474
Latifi A., Ruiz M., and Zhang C. C. (2009). Oxidative stress in cyanobacteria. FEMS Microbiol. Rev. 33, 258–278. doi: 10.1111/j.1574-6976.2008.00134.x
Li S., Li F., Zhu X., Liao Q., Chang J., and Ho S. (2022). Biohydrogen production from microalgae for environmental sustainability. Chemosphere. 291. doi: 10.1016/j.chemosphere.2021.132717
Mackey D. J., O’Sullivan J. E., Watson R. J., and Dal Pont G. (2002). Trace metals in the Western Pacific: Temporal and spatial variability in the concentrations of Cd, Cu, Mn and Ni. Deep-Sea Res. Part I Oceanogr. Res. Pap. 49, 2241–2259. doi: 10.1016/S0967-0637(02)00124-3
Mills M. M., Ridame C., Davey M., La Roche J., and Geider R. J. (2004). Iron and phosphorus co-limit nitrogen fixation in the eastern tropical North Atlantic. Nature 429, 292–294. doi: 10.1038/nature02550
Mulholland M. R., Bernhardt P. W., Heil C. A., Bronk D. A., and O`Neil J. M. (2006). Nitrogen fixation and release of fixed nitrogen by Trichodesmium spp. in the Gulf of Mexico. Limnol. Oceanogr. 51, 1762–1776. doi: 10.4319/lo.2006.51.4.1762
Ohki K., Zehr J. P., and Fujita Y. (1992). Regulation of nitrogenase activity in relation to the light-dark regime in the filamentous non-heterocystous cyanobacterium Trichodesmium sp. NIBB 1067. J. Gen. Microbiol. 138, 2679–2685. doi: 10.1099/00221287-138-12-2679
Palenik B., Brahamsha B., Larimer F. W., Land M., Hauser L., Chain P., et al. (2003). The genome of a motile marine Synechococcus. Nature 424, 1037–1042. doi: 10.1038/nature01943
Rodriguez I. B. and Ho T. Y. (2014). Diel nitrogen fixation pattern of Trichodesmium: the interactive control of light and Ni. Sci. Rep. 4, 4445. doi: 10.1038/srep04445
Rodriguez I. B. and Ho T.-Y. (2017). Interactive effects of spectral quality and trace metal availability on the growth of Trichodesmium and Symbiodinium. PloS One 12, e0188777. doi: 10.1371/journal.pone.0188777
Rubin M., Berman-Frank I., and Shaked Y. (2011). Dust-and mineral-iron utilization by the marine dinitrogen-fixer Trichodesmium. Nat. Geosci. 4, 529–534. doi: 10.1038/ngeo1181
Tamagnini P., Leitao E., Oliviera P., Ferreira D., Pinto F., Harris D. J., et al. (2007). Cyanobacterial hydrogenases: diversity, regulation and applications. FEMS Microbiol. Rev. 31, 692–720. doi: 10.1111/j.1574-6976.2007.00085.x
Tuo S., Rodriguez I. B., and Ho T.-Y. (2020). H2 accumulation and N2 fixation variation by Ni limitation in Cyanothece. Limnol. Oceanogr. 65, 377–386. doi: 10.1002/lno.113
Westall J. C., Zachary J. L., and Morel F. M. M. (1976). MINEQL: A computer program for the calculation of chemical equilibrium composition of aqueous systems, civil eng. Tech. Note no. 18. Cambridge: massachusetts institute of technology.
Whittaker S., Bidle K. D., Kustka A. B., and Falkowski P. G. (2011). Quantification of nitrogenase in Trichodesmium IMS101: implications for iron limitation of nitrogen fixation in the ocean. Environ. Microbiol. Rep. 3, 54–58. doi: 10.1111/j.1758-2229.2010.00187.x
Wilson S. T., Foster R. A., Zehr J. P., and Karl D. M. (2010). Hydrogen production by Trichodesmium erythraeum, Cyanothece sp. and Crocosphaera watsonii. Aquat. Microb. Ecol. 59, 197–206. doi: 10.3354/ame01407
Keywords: nitrogen fixation, nitrogen cycling, diazotoph, nickel availability, nickel enzymes
Citation: Rodriguez IB, Lazarte IAP and Ho T-Y (2025) Immunocytochemical evidence of Ni-SOD expression in Trichodesmium. Front. Mar. Sci. 12:1525315. doi: 10.3389/fmars.2025.1525315
Received: 09 November 2024; Accepted: 02 May 2025;
Published: 23 May 2025.
Edited by:
Eric ‘Pieter Achterberg, Association of German Research Centres (HZ), GermanyReviewed by:
Meri J Eichner, Academy of Sciences of the Czech Republic (ASZR), CzechiaAnxhela Hania, Institute of Microbiology, Academy of Sciences of the Czech Republic (ASCR), in collaboration with reviewer ME
Asma Sakka Hlaili, University of Carthage, Tunisia
Copyright © 2025 Rodriguez, Lazarte and Ho. This is an open-access article distributed under the terms of the Creative Commons Attribution License (CC BY). The use, distribution or reproduction in other forums is permitted, provided the original author(s) and the copyright owner(s) are credited and that the original publication in this journal is cited, in accordance with accepted academic practice. No use, distribution or reproduction is permitted which does not comply with these terms.
*Correspondence: Irene B. Rodriguez, aWJyb2RyaWd1ZXpAbXNpLnVwZC5lZHUucGg=