- 1Marine Immunobiology Laboratory, Department of Earth and Marine Sciences, University of Palermo, Palermo, Italy
- 2NBFC, National Biodiversity Future Center, Palermo, Italy
- 3Marine Botany Laboratory, Department of Earth and Marine Sciences, University of Palermo, Palermo, Italy
- 4Cell Biology and Genetics Laboratory, Department of Biological, Chemical and Pharmaceutical Sciences and Technologies, University of Palermo, Palermo, Italy
- 5Marine Conservation Laboratory, Department of Earth and Marine Sciences, University of Palermo, Palermo, Italy
Stress memory is a key ecological and evolutionary response for sessile organisms under changing environmental conditions, but the ubiquity of this phenomenon among coral species and habitat is unknown. We exposed colonies of the Mediterranean coral Cladocora caespitosa to two short-term thermal profiles (constant high and pulse) and quantified the genome-wide DNA methylation level before stress testing 75 days later. Here we show that a protective effect could be induced using short-term thermal profiles, which significantly improved immune tolerance and bleaching resistance. We found a significant relationship between genomic methylation levels and accumulated thermal stress by corals, preliminarily suggesting an epigenetic regulation dynamic of temperate coral tolerance in response to climate change. Our results represent new mechanistic insights into the stress memory of Mediterranean corals, supporting a role for DNA methylation in crucial cryptic complexity of plasticity avenues.
1 Introduction
Global warming represents a challenge for marine organisms, which can respond to fast environmental changes through non-genetic mechanisms involving changes in phenotype, without changes to the underlying DNA sequences (Hackerott et al., 2021; Drury et al., 2022). Stress memory, a transient “priming” stimulus that leads to a modified response to a subsequent “triggering” stress exposure (also called pre-exposure or preconditioning; Putnam and Gates, 2015; Hawkins and Warner, 2017; NASEM, 2019; Martell, 2023), becomes particularly important in the context of rapid climate change because it occurs within the time of a generation (Hilker et al., 2016; Putnam et al., 2017; Hackerott et al., 2021). This short-term response to rapid environmental shift may bridge the gap between reproductive events, during which genetic adaptation occurs (Munday et al., 2013; Hackerott et al., 2021), potentially allowing organisms to cope with rapid climate change. Stress memory is well-documented in marine plants (Kishimoto et al., 2019; Nguyen et al., 2020), but has also been observed in planktonic and benthic invertebrates (Klein et al., 2017; Li et al., 2020), and may be particularly important for long-lived, sessile organisms living near their thermal limits such as corals (Coles and Jokiel, 1978; Putnam et al., 2017).
Coral health is declining worldwide due to a complex network of local and global environmental drivers (Pandolfi et al., 2003), with the impacts of climate change (e.g., warming seawater and ocean acidification) representing a serious threat to their long-term survival (Hoegh-Guldberg et al., 2007). Thermal stress has been identified as the main factor affecting coral health-state (Lough, 2011), disrupting the symbiotic partnership with the dinoflagellate algae of the Symbiodiniaceae family (i.e., coral bleaching) (Glynn, 1993; LaJeunesse et al., 2018). Since most carbon requirements of corals are obtained from photosynthetic products translocated from their symbionts (Muscatine et al., 1981; Goldberg, 2018), this breakdown leads to an overall reduction in metabolic resources, loss of reserves and decrease in growth rate (Thurber et al., 2009; Grottoli et al., 2014). Meanwhile, coral innate immunity is also affected by thermal stress (Traylor-Knowles and Connelly, 2017; Palmer, 2018a; Bisanti et al., 2024a); for example, immune-related enzymatic pathways have been widely shown to be critical for coral response under elevated temperature conditions (such us antioxidant enzymes and melanin pathways) (Palmer, 2018b; Bisanti et al., 2024b). This emerging complex scenario, combined with well-known thermal-induced shifts in microbiomes (Vezzulli et al., 2013; Vompe et al., 2024), results in increased susceptibility of corals to disease and mortality during the warmer periods of the years. Indeed, the frequency and severity of coral bleaching and mortality events have increased in the last few decades around the world (Hughes et al., 2018; Garrabou et al., 2022) and massive bleaching is expected to occur annually by mid-century, under current emissions scenarios (Van Hooidonk et al., 2016).
Stress memory will play a crucial role as a mechanism by which sessile organisms can respond to rapid climate change (Palumbi et al., 2014; Hackerott et al., 2021). Coral priming to thermal stress can result from changes in the symbiotic algal community (Silverstein et al., 2015), microbiome composition (Ziegler et al., 2019), and gene expression patterns (Rivera et al., 2021; Drury et al., 2022), allowing for differential responses in growth, morphology, skeletal characteristics and bleaching tolerance (e.g., Todd, 2008; Drury et al., 2017; Drury and Lirman, 2021; Martell, 2023). Interestingly, colonies exposed to environmental stress display a variety of epigenetic marks that regulate gene expression and affect the phenotype (Putnam et al., 2016; Dixon et al., 2018; Eirin-Lopez and Putnam, 2019), and are influenced by the community dynamics of coral symbionts (Hackerott et al., 2021; Rodriguez-Casariego et al., 2022).
Epigenetic mechanisms act as a link between environmental conditions and genetic processes, dynamically altering and in some cases perpetuating gene activation (Cavalli and Heard, 2019; Hackerott et al., 2021). As carriers of environmental information, epigenetic regulation underlies the creation and maintenance of stress memory, within the limits inherent to the organism’s genome (Adrian-Kalchhauser et al., 2020). Epigenetic modifications include post-translational modifications of histones (Lämke and Bäurle, 2017; Fabrizio et al., 2019), reduced nucleosome occupancy (Brzezinka et al., 2016), DNA methylation (Wibowo et al., 2016), as well as genetic regulation by small RNAs (Stief et al., 2014). In particular, the regulation of DNA methylation appears to be involved in the reduction of spurious transcription in corals (Putnam et al., 2016; Liew et al., 2018; Dixon et al., 2018) and other cnidarians (Li et al., 2018), which could be fundamental in the activation of stress tolerance in primed colonies. Seasonal patterns of DNA methylation have also been reported in Acropora cervicornis (Rodríguez-Casariego et al., 2020), suggesting a role for epigenetic modifications in mediating seasonal acclimation in corals (Jurriaans and Hoogenboom, 2020).
Stress memory, priming, or stress-hardening have all been identified as promising research avenues in corals conservation (van Oppen et al., 2015; NASEM, 2019). A key question is how the stress dose (i.e., temporal and magnitude variability) affects coral functional traits, which is complicated given the intricate network of interactions between the host animal, symbionts, and the microbiome. In fact, previous work has documented both negative and positive feedbacks between these components (Kellett et al., 2005; Calosi et al., 2008; Gerken et al., 2015), which reflects the presence of complex “hormetic” barriers (Putnam et al., 2017) where a small dose of stress may benefit an organism, but higher doses will cause deleterious effects (Calabrese et al., 2007; Mattson, 2008; Carelli and Iavicoli, 2016). Our understanding of priming duration and its ability to support thermal tolerance has been limited by experimental approaches, that so far have assessed only short-term phenotype responses (Bellantuono et al., 2012; Bay and Palumbi, 2015). Nevertheless, some recent experiments have used longer recovery periods (up to 4 months), and found that persistent changes in gene expression accounted for improved physiological performance, mainly in apoptosis and cell-death pathways (Ainsworth et al., 2016; Majerova et al., 2021; Drury et al., 2022). The importance of relative temperature variability is also object of debate, as regimes with greater variations may give corals greater thermal tolerance than constant exposures (Oliver and Palumbi, 2011; DeMerlis et al., 2022), but this effect is not universal (Bay and Palumbi, 2015; Klepac and Barshis, 2020; Drury et al., 2022).
Here, we make use of artificial thermal profiles in a laboratory setting to induce warming tolerance associated with stress memory in the zooxanthellate Cladocora caespitosa (Linnaeus, 1767), the only Mediterranean coral forming localized bioconstructions comparable to tropical reefs. The sensitivity of this species to elevated seawater temperatures has been assessed across the Mediterranean during field studies, and some laboratory experiments have investigated its thermal tolerance with different results among populations, geographical areas and depth range (Supplementary Table S1). Using this approach, (1) we investigate the effectiveness of two temperature profiles in inducing performance benefits in C. caespitosa, assessed by changes in innate immune responses and symbiont photosynthetic efficiency, and (2) we run a genome-wide analysis to investigate the role of DNA methylation as an epigenetic control mechanism underlying stress memory. We considered as proxy of immune response the activity of several immune-related enzymes: the non-specific lysozyme, which act in the primary and rapid defense against pathogens and some viruses, exerting an anti-inflammatory action (Li et al., 2008; Leśnierowski and Yang, 2021; La Corte et al., 2023); the phenoloxidase, responsible for cytotoxic defense and melanin depositions with photoprotective effects on the algal endosymbionts and bleaching mitigation (Nappi and Ottaviani, 2000; Palmer et al., 2010); the glutathione peroxidase, a scavenger antioxidant enzyme that counteracts the effects of reactive oxygen species (ROS) under thermal stress conditions (Lesser, 2006; Hawkins et al., 2014; Parisi et al., 2020). This work provides new insights into the use of artificially induced stress memory to increase thermal tolerance in threatened coral species, and sets a baseline for relevant priming dose that could induce beneficial responses by organisms.
2 Materials and methods
2.1 Experimental design and temperature profiles
Ten large colonies of C. caespitosa corals were collected at ~ 5m depth from Capo Zafferano Bay (38° 06′ 25″ N; 13° 32′ 10″ E; NW coast of Sicily, Italy) during June 2023. Fragments (n = 130) were returned to the Marine Immunobiology Laboratory of the University of Palermo, mounted on labeled plugs, and transferred to indoor mesocosms (Figure 1A). Only colonies from a single sampling area were used to limit potential genetic background effects. After 2 weeks of indoor acclimation, corals were exposed to either a control or one of two short-term thermal profiles - constant high and pulse - between 28 and 31°C for 5 days (Figure 1B) in replicate tanks (n = 2). In order to determine the appropriate maximum temperature of thermal profiles, C. caespitosa fragments of opportunity were exposed to increasing peak temperatures over the course of 12 hours, then kept at each temperature for six days. Based on analyses of seawater surface temperatures over the last decade experienced by these coral species at the sampling site (Supplementary Figure S1), fragments were exposed to maximum temperatures of 30, 30.5, 31, 31.5, and 32°C, and monitored daily to detect bleaching responses. After the six-day trial, corals in the 31.5°C and higher temperature treatments had visibly bleached. Thus, 31°C was chosen as the maximum temperature for the thermal profiles in order to ensure coral fragments in all profiles, including constant high temperature conditions, would be exposed to sub-lethal thermal stress. The pulse profile varied from the lowest temperature point of 28 to 31°C daily. The constant profile remained at 31°C throughout the exposure period, while the control tanks were kept at ~ 28°C, close to the environmental temperature. Temperatures in the tanks were programmed and controlled using an Haquoss thermo-control system (Aquarialand s.a.s) and recorded throughout the experiment using HOBO Pendant temperature loggers (Onset Computer Corporation). Thermal profiles have accumulated 0.03 - 2.14 degree heating weeks (DHWs; Figure 1C) had a ~ 12-fold range in rate of change (ROC; Figure 1D). Light conditions were maintained with a 15:9 h photoperiod of daily light/dark cycles to match the natural photoperiod at the collection sites (metal halide lights, with a maximum irradiance of 200 µmol photons m−2 s−1; Martin and Gattuso, 2009; Carbonne et al., 2024). After the priming, a subset of corals (n = 35 fragments per thermal profile, N = 105) were returned to the collection site on July 25th and mounted on a platform at ~ 5m depth for recovery prior to subsequent stress testing 75 days later. In situ temperatures were recorded during the recovery period using 3 HOBO loggers.
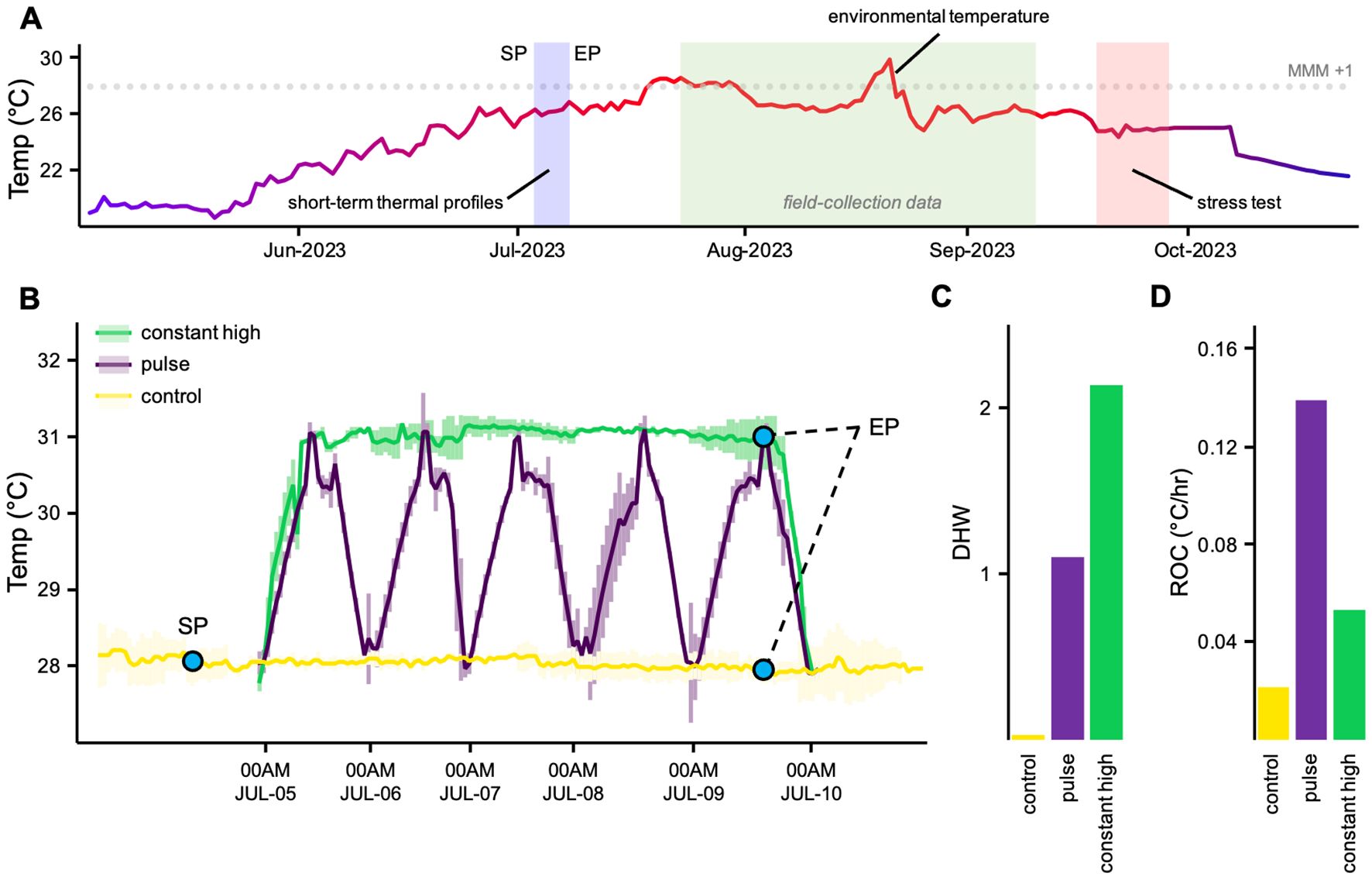
Figure 1. Experimental Design (A) experimental timeline showing short-term thermal profiles, field deployment and stress testing, overlaid on ambient temperatures (B) five-day short-term thermal profiles including a control at environmental temperature and two high temperature profiles with different characteristics (C) experimental degree heating weeks (DHWs) during short-term thermal profiles (D) rate of change during short-term thermal profiles, calculated as °C/hr, averaged across replicate tanks. Thermal profiles colours are maintained across figures. SP, start of exposures to short-term thermal profiles; EP, end of exposures to short-term thermal profiles.
2.2 Epigenomic assessment of DNA methylation
At noon on the day before the start of exposure to thermal profiles, we collected a subset of 10 randomly chosen fragments as pre-exposure controls to thermal profiles, flash-frozen and stored them at -80°C until processing. We repeated this sampling at noon on day 5 of the thermal profiles (Figure 1B) when all profiles (excluding control) had reached 31°C. We collected 5 coral samples per thermal profile (n = 3), for a total of 15 samples. We used the nuclei isolation method to extract DNA, minimizing contamination with symbiont DNA (Voolstra et al., 2017). Cells of C. caespitosa were harvested from a fragment of about 5 cm in 50 ml of 0.2 M EDTA solution refrigerated at 4°C using an air-gun. Extracts were passed through a 100-µm and a 40- µm cell strainer (Falcon) to eliminate as many algal symbionts as possible. Extracts were then centrifuged at 2000g for 10 min at 4°C. The supernatant was discarded, and the resulting pellets were homogenized with a lysis buffer (G2) included in the Qiagen Genomic DNA Isolation Kit (Qiagen). The DNA was extracted following the manufacturer’s instructions using Genomic-tip 100/G (Qiagen) with few changes (Volpes et al., 2023). DNA concentration was determined at the NanoDrop® ND-1000.
Dot Spot Hybridization Analysis was performed to assess genome-wide changes in coral DNA methylation (Jia et al., 2017; Bellavia et al., 2022). Briefly, 500 μg of genomic DNA were spotted on the Hybond N+ membrane and fixed with U.V. treatment for 2 min. The membranes were soaked in 5% BSA in TBS-T (NaCl 150 mM, Tris-HCl 10 mM, 1% Tween-20, pH 7.4) for 1 h, to block non-specific antibody binding sites. The membranes were rinsed in TBS-T for 5 min three times and incubated with a rabbit anti-5-methylcytosine (5-mC) (InvitrogenTM, MA5-24694; 1:1000 dilution) monoclonal antibody in TBS-T at 4°C overnight. After three more washes, in TBS-T, the membranes were incubated with a secondary anti-rabbit IgG HRP-linked antibody (1:2000, #7074-Cell Signaling) in TBS-T for 1 h at room temperature. The membranes were washed again for 5 min three times in TBS-T and the enzyme substrate was added. The secondary antibody signals were visualized using a chemiluminescence kit (NovexTM ECL Chemiluminescent Substrate Reagent Kit, InvitrogenTM), through a ChemiDoc apparatus (Bio-Rad Laboratories, Hercules, CA, USA), and we performed densitometric analyses of images using the open-source software Image J (Gallo-Oller et al., 2018; Schneider et al., 2012; Naselli et al., 2024).
2.3 Stress testing and coral response variables
Fragments of C. caespitosa were collected from the field in September 2023, 75 days after the conclusion of thermal profile exposures, and randomly allocated into two tanks for stress testing. Each tank was heated from 28 to 32°C over 5 days and then maintained at 32°C for the 10-day stress test (Supplementary Figure S2). We collected data from randomly chosen coral samples at noon on the day before the temperature ramp (n = 5 for each thermal profile), on day 1 and every 2 days during stress testing, totaling 5 samples per profile (n = 3) per timepoint (n = 6), for a grand total of 105 samples. Coral performances were assessed throughout the stress test duration using 4 response variables, of which 3 enzymatic activities related to coral immune activities, namely (1) lysozyme, (2) glutathione peroxidase and (3) phenoloxidase, and (4) the maximum yield of photosystem II (fv/fm) of dark adapted in hospite dinoflagellate endosymbionts (Pulse Amplitude-Modulated fluorescence; PAM). Details for each are below.
2.3.1 Extract preparation and protein concentration
Tissue samples of C. caespitosa were excised under ice using an air-gun in TBS-buffer containing an EDTA-free cocktail of protease inhibitors (Sigma-Aldrich); the resultant tissue slurry was then centrifuged (36,200 x g for 20 min at 4°C). The supernatant was collected and protein concentration was measured with a Bradford assay (Bradford, 1976). The sample absorbance was read at 595 nm (RAYTO RT-2100C) using TBS as a blank, and a calibration curve defined through bovine serum albumin was used to obtain the protein concentration (mg/ml). Extracts were adjusted to 0.5 mg/ml before performing enzymatic assays.
2.3.2 Lysozyme activity
LYS activity was evaluated following Parry et al. (1965). Briefly, 30 µl of each sample were placed in a 96-well flat-bottomed plate and incubated with 270 µl of bacterial suspension (Micrococcus lysodeikticus ATCC 4698, Sigma-Aldrich, USA) in triplicate. The reaction was started by incubation at 25°C, and absorbance (450 nm; microplate reader, RAYTO RT-2100C) was measured every 30 s for 10 min. A unit of LYS was defined as the amount of sample causing a decrease in absorbance of 0.001/min (U min-1), and U/ml was calculated according to the formula: U/ml = (Δ abs/min-1 * dilution factor * 1000)/enzyme volume buffer.
2.3.3 Glutathione peroxidase activity
GPx activity was measured according to Ross et al. (2000). In 96-well flat-bottomed plates, 50 µl of sample at standard concentration (0.5 mg/ml) were incubated with 100 µl TMB (3,3′ 5,5′-tetramethylbenzidine; Sigma-Aldrich, USA). The reaction was stopped after 30 min of incubation in the dark with sulfuric acid (H2SO4) 2 M. The absorbance was read spectrophotometrically at 450 nm in a microplate reader (RAYTO RT-2100C), and the GPx produced was quantified in U/mg of protein according to the equation: U/mg = Abs * Vf/CP (Vf, final volume of the well; CP, protein concentration of the sample).
2.3.4 Phenoloxidase activity
PO activity was measured spectrophotometrically according to Winder and Harris (1991), by using L-Dopa (3,4 dihydroxy-L-phenylalanine; Sigma-Aldrich, USA) as a substrate and MBTH (3-methyl-2 benzothiazolinone hydrazone hydrochloride; Sigma-Aldrich, USA) as a specific reagent. 50 μl of coral tissue sample and 50 μl of trypsin from bovine pancreas (1 mg/ml; Sigma-Aldrich, USA) were incubated for 20 min at 20°C in a 50 μl reaction mixture (5 mM L-DOPA and 20.7 mM MBTH in distilled water). The absorbance was read for 60 min at 5 min intervals by spectrophotometry at 505 nm (microplate reader, RAYTO RT-2100C). PO activity was calculated as units (U) per min, where 1 U = 0.001 ΔA540 min-1 mg-1 protein.
2.3.5 Chlorophyll-a fluorescence
A diving-PAM (Walz, Germany) fluorescence was used to measure the maximum quantum yield (fv/fm) of photosystem II, which is commonly used as a measure of damage to the photosynthetic apparatus of the algal symbiont following thermal stress and bleaching (Warner et al., 1999; Jones et al., 2000). Corals were dark-acclimated for 45 min and fv/fm measurements were recorded by applying a saturation pulse of actinic light (9000 μmol photon m-2 s-1, 800 ms). The maximum quantum yield was calculated using the formula fv/fm = (fm - fo)/fm (Murchie and Lawson, 2013), where fv is variable fluorescence, fm is the maximum fluorescence and fo is the basal fluorescence. Triplicate measurements were recorded for each coral fragment, before the temperature was ramped to assess latent impacts of thermal profiles and confirm that photochemical data had a consistent baseline, in addition to measurements at 6 key timepoints throughout the experiment. During each PAM measurement session, one area of interest per coral was selected (avoiding fragment edges) and fo was kept at values between 200 and 400 prior to applying the saturation pulse. Data were downloaded from the diving-PAM and filtered. fv/fm values less than 0.52 were removed from the data set, as lower densities of light-absorbing Symbiodiniaceae in thermally stressed coral tissues can cause problems with PAM fluorometry measurements, due to back-scattering of light by the coral skeleton (Wangpraseurt et al., 2019). fv/fm values above 0.84 were also removed from the data set, as they are higher than theoretical maximum values of fv/fm using PAM fluorometry (Walz, 2020).
2.4 Data analysis
All data analyses were conducted in XLSTAT-R software (Lumivero 2023.3.0). We compared raw values of response variables before stress testing across thermal profile using a one-way ANOVA to ensure recovery from thermal profiles. Normalized data were analyzed using a linear mixed-effects model with thermal profile (three levels) and timepoint (six levels) as fixed effects, and fragment as random intercepts. A post-hoc interaction analysis was used to detect differences between factor levels.
In order to determine which characteristics of temperature profiles may be important for inducing stress memory, we explored the relative importance of total thermal stress accumulated by corals during the temperature profiles and physiological responses to stress testing. We performed polynomial regressions to examine the relationship between the response variables during the stress test and the accumulated DHWs. DHWs were approximated as the time spent above the 28°C (average summer-monthly maximum +1°C at sampling site), a measure of the total accumulation of coral thermal stress above the typical warmest conditions for a location, which is integrative of temperature and duration (Dilworth et al., 2021). We used this value to calculate the experimental DHW (Leggat et al., 2022) for each experimental thermal profile. Seawater temperature data series at the sampling site were obtained from the Copernicus Marine Environment Monitoring Service (CMEMS) products (Buongiorno Nardelli et al., 2013).
We run in vegan (Dixon, 2003) a permutational multivariate analysis of variance (PERMANOVA) considering response variables together and visualized them by principal coordinate analysis (PCoA). The design included two fixed factors, thermal profile (three levels) and timepoint (six levels). PERMANOVA was based on Euclidean distance matrix after square root transformation of the data (Anderson, 2001). When significant differences were found, a pairwise comparison was done to explore differences among all pairs of the factors. Pearson correlations were also calculated between the multivariate distribution of samples and the coral response variables, superimposing them as vectors on the PCoA plot.
Changes in the DNA methylation occurrence was analyzed using a linear mixed-effects model with fixed effects of thermal profile, and random intercepts of fragment. A post-hoc interaction analysis was used to detect differences between factor levels. We also compared coral methylation patterns between profiles using a linear regression to compare levels of DNA methylation with accumulated DHWs and ROCs during thermal profiles.
3 Results
3.1 Stress testing
We exposed fragments of C. caespitosa to two different high-temperature profiles for 5 days before returning them to their sampling site (Figure 1). We stress tested corals 75 days after the conclusion of thermal profiles, at which point there was no difference in baseline of response variables between profiles (LYS: one-way ANOVA F = 3.543, p = 0.051; GPx: one-way ANOVA F = 0.974, p = 0.510; PO: one-way ANOVA F = 1.790, p = 0.218; fv/fm: one-way ANOVA F = 0.445, p = 0.902). For the whole duration of the stress test, no direct necrosis occurred in corals and an overall retraction of polyps were observed over time. There was a significant effect only for timepoint (p < 0.001) on LYS activities. Instead, there was a significant effect of thermal profiles (p < 0.001, GPx; p < 0.05, PO; p < 0.001, fv/fm) and timepoint (p < 0.001) on GPx, PO and fv/fm variables, as well as an interactive effect of thermal profile and timepoint (p < 0.05) on GPx and PO. Mean LYS activities in pulse and constant high corals were also lower than control, but there were no significant differences among profiles (Figure 2). Corals that were exposed to the pulse and constant high thermal profiles had lower mean GPx and PO activities than those in control corals, even though there were significant differences only for the constant high fragments (Figure 2). Both constant high and pulse corals had higher mean fv/fm than the controls, but only the constant high thermal profile significantly differed from control corals (Figure 2).
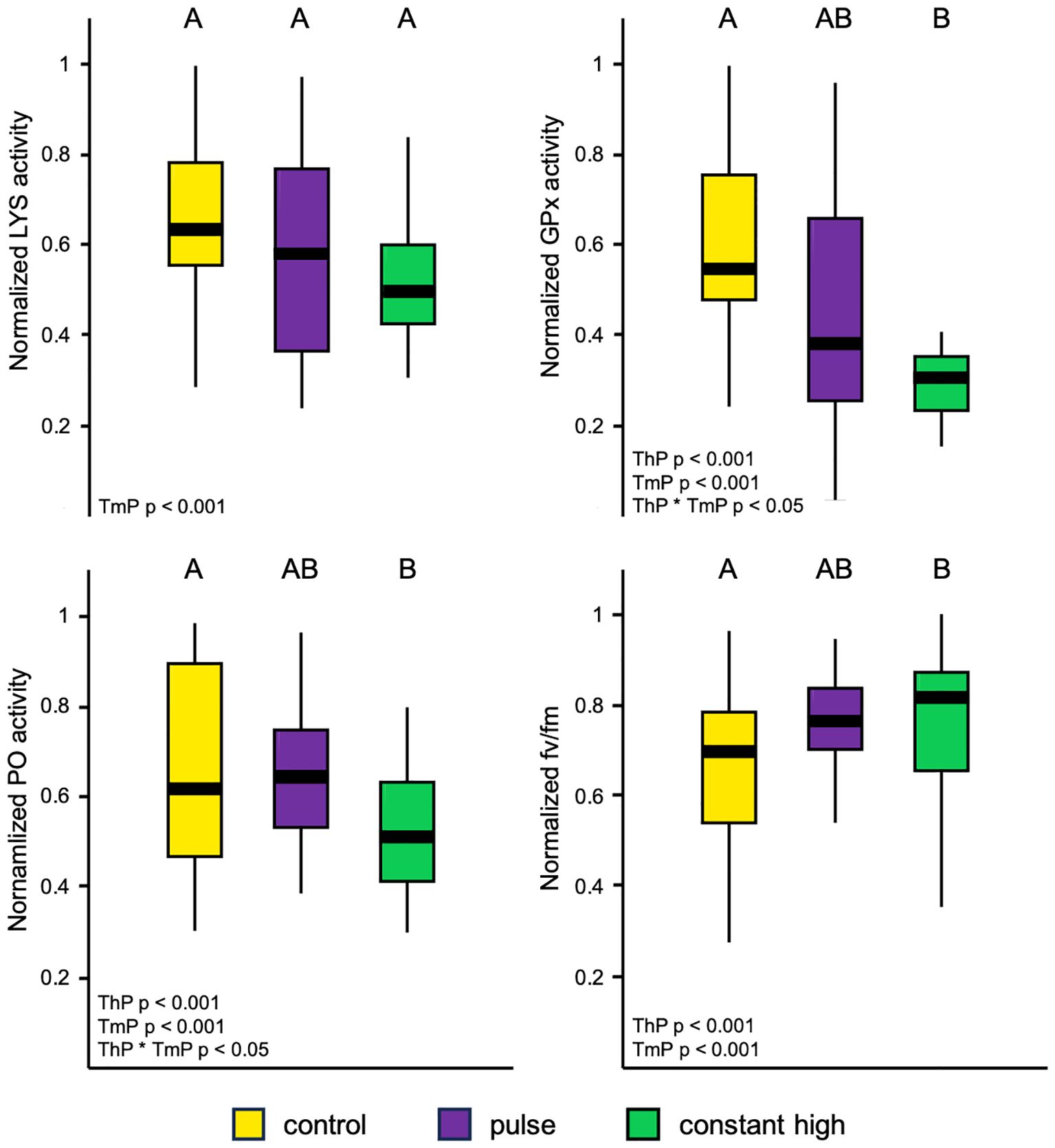
Figure 2. Boxplots of normalized response variables (lysozyme, LYS; glutathione peroxidase, GPx; Phenoloxidase, PO; maximum quantum yield of photosystem II, fv/fm) by thermal profiles averaged over time in fragments of C. caespitosa, with the middle horizontal line showing mean normalized values. Letters represent the differences between profiles found during post-hoc interaction analysis (Tukey test, p < 0.05). ThP, thermal profiles; TmP, timepoint.
Corals from the control profile showed significant relationships in enzymatic variables as accumulated thermal stress increased (LYS: R-square = 0.27, p < 0.05; GPx: R-square = 0.64, p < 0.05; PO: R-square = 0.37, p < 0.05), decreasing steeply after reaching a peak with the highest values found among thermal profiles (Figure 3). Pulsed corals showed similar significant patterns (LYS: R-square = 0.25, p < 0.05; GPx: R-square = 0.50, p < 0.05; PO: R-square = 0.36, p < 0.05) with an initial rapid increase in enzymatic activity followed by a collapse, with lower peaks compared to control corals (Figure 3). Different significant relationships emerged from constant high corals in enzymatic pattern (LYS: R-square = 0.20, p < 0.05; GPx: R-square = 0.44, p < 0.05; PO: R-square = 0.41, p < 0.05), which experienced lower and almost constant activities as thermal stress increased, compared to other thermal profiles (Figure 3). There was also a significant relationship between photo-chemical efficiency and accumulated thermal stress by corals (control: R-square = 0.88, p < 0.05; pulse: R-square = 0.73, p < 0.05; constant high: R-square = 0.59, p < 0.05), with less decline in bleaching tendency of corals at constant high compared to control and pulse profiles (Figure 3).
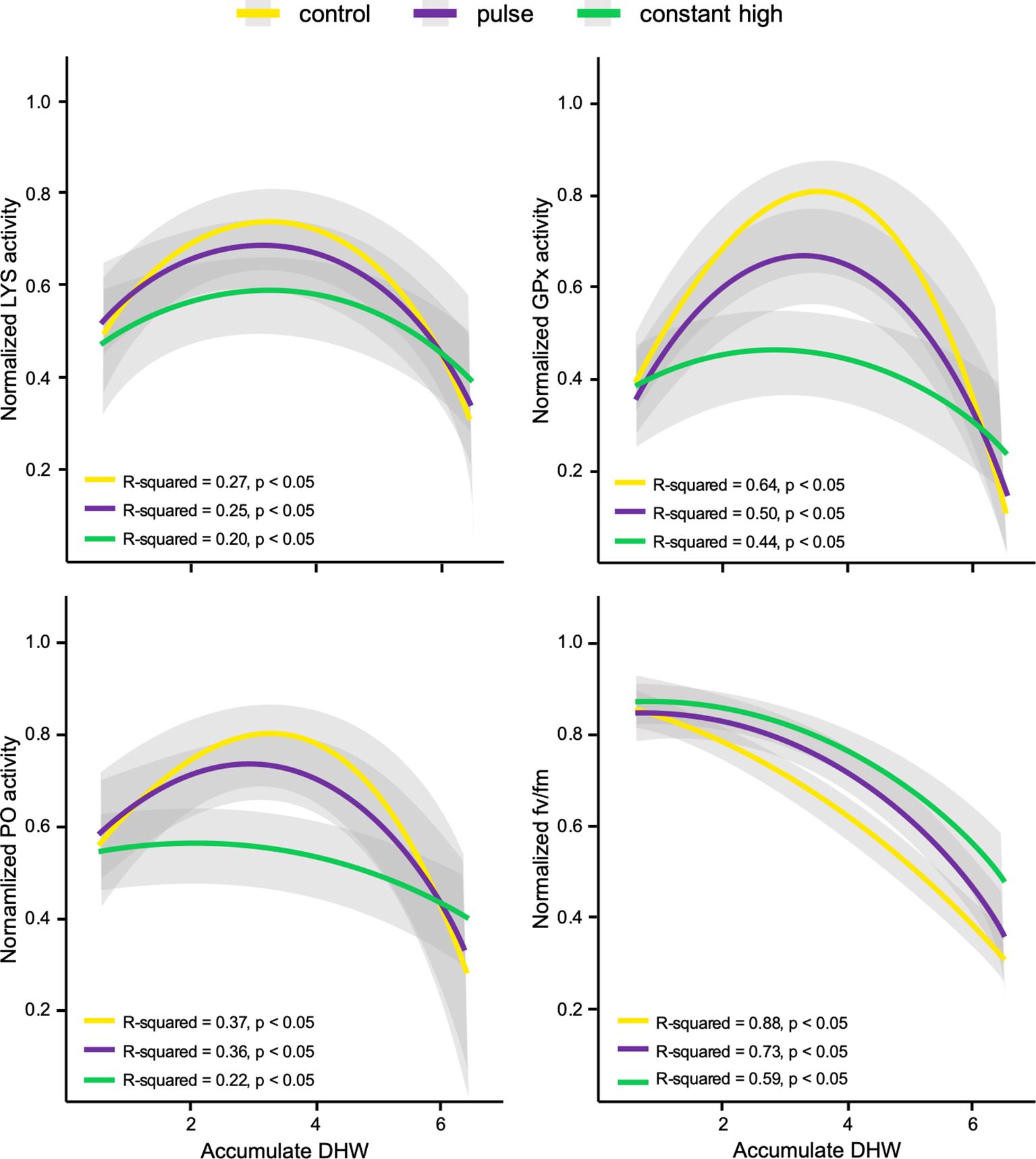
Figure 3. Polynomial regression plots between normalized response variables (lysozyme, LYS; glutathione peroxidase, GPx; Phenoloxidase, PO; maximum quantum yield of photosystem II, fv/fm) by thermal profiles in fragments of C. caespitosa and the accumulated degree heating weeks (DHWs) during the stress test. All regression fits include shaded error intervals which represent the 95% CI.
There were significant differences in overall coral responses for thermal profile (PERMANOVA, p < 0.001), timepoint (PERMANOVA, p < 0.001), and their interaction (PERMANOVA, p < 0.001). PCoA accounted for 77.9% of the total variation, with the first axis (PCo1) explaining 56.1% and the second axes (PCo2) explaining 21.7% of multivariate distribution (Figure 4). There was significant variation in each direction of the PCoA plot, along both the first and second axes (ANOVA PCo1, p < 0.001; PCo2, p < 0.05), with a grouped distribution by thermal profile (Figure 4A). This was particularly evident for timepoints T3, T5 and T6 with a clear and separate clustering for the control and constant high corals (Figure 4B). The variable contributing most to multivariate distribution of coral samples was GPx (+ 0.914) for the first PCoA axis, while fv/fm was the variable most related to the second axis (− 0.982) (Figure 4C).
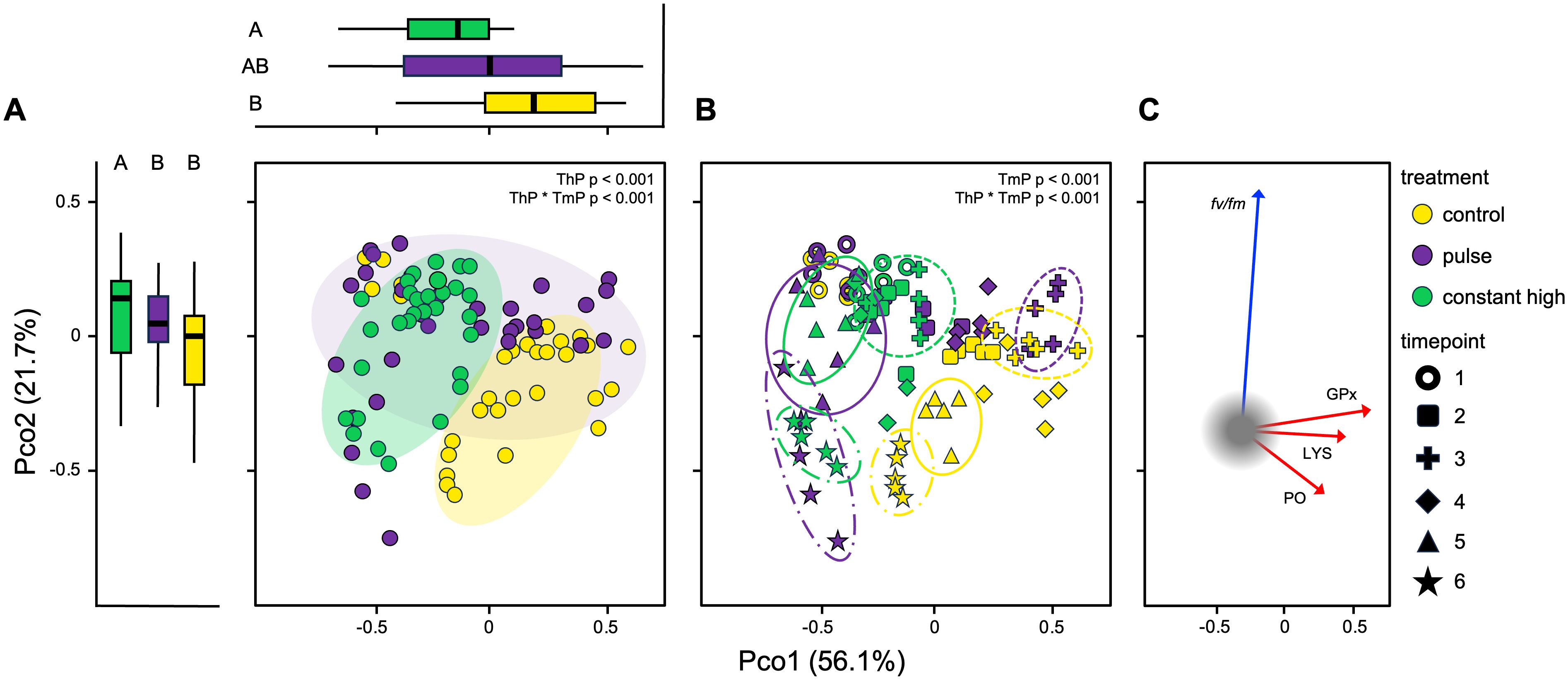
Figure 4. Principal Coordinates Analysis (PCoA) visualizing ordination constructed using distances generated from response variables (lysozyme, LYS; glutathione peroxidase, GPx; Phenoloxidase, PO; maximum quantum yield of photosystem II, fv/fm) between (A) thermal profiles and (B) timepoints in fragments of C. caespitosa during the stress test. Thermal profiles (ThP) and timepoints (TmP) were compared using PERMANOVA. Boxplots on the top and far left indicate the distribution of coral samples by thermal profiles along the first and second PCoA axes and letters represent the differences between groups found during the post-hoc interaction analysis (Tukey test, p < 0.05). Line shapes of clusters indicate T3 (dashed), T5 (solid), T6 (dash-dot) timepoints. (C) Relationships between the multivariate distribution of samples and coral response variables along the first two principal component axes (immune activities: red arrows; photosynthetic efficiency: blue arrow).
3.2 DNA methylation response to thermal profiles
Starting from the hypothesis that stress memory acts at the level of the regulation of DNA methylation, a spot hybridization of DNA extracted by fragments of C. caespitosa was carried out to assess modifications in the methylation status after the thermal profiles, using antibody anti-methyl-cytosine. The pulse and constant high thermal profiles induced a general hyper-methylation of coral genomic DNA, with mean values increasing from 1.6 to 3.1-fold compared to control fragments (Figure 5A). There was a significant effect of thermal profiles on methylation levels (p < 0.001), although a post-hoc interaction analysis revealed significantly higher values only for constant high corals (p < 0.001; Figure 5A). A strong and significant relationship was also found between the accumulated DHWs due to thermal profiles and the methylation levels of corals in the corresponding groups (R-squared = 0.63, p < 0.001; Figure 5B). However, there was no significant relationship between the ROC and the methylation levels of each coral profiles (R-squared = 0.0005, p = 0.926; Figure 5C).
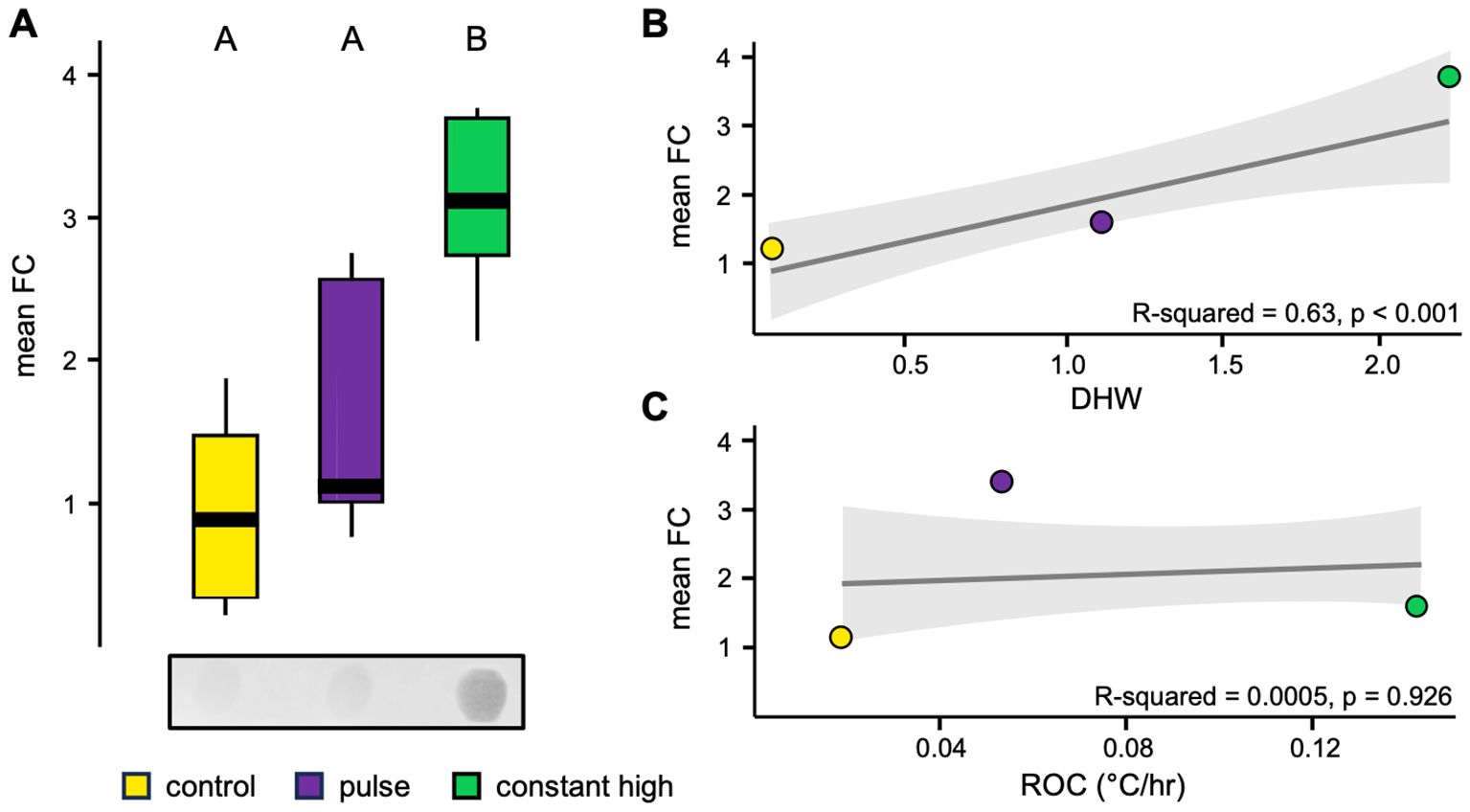
Figure 5. (A) Representative image and boxplot of densitometric analysis (expressed as fold change compared to pre-thermal profile controls, FC) of spot hybridization of genomic DNA from fragments of C. caespitosa by thermal profiles using antibody through methyl-cytosine, with the middle line showing the mean values (see Supplementary Materials for full-length spot images). Letters represent the differences between profiles found during post hoc interaction analysis (Tukey test, p < 0.05). Linear regression plot between fold change values by thermal profiles and (B) the accumulated degree heating weeks (DHWs) or (C) the rate of change during short-term thermal profiles (°C/hr). The gray line represents overall relationship and shaded area is 95% CI of linear regression fit.
4 Discussion
The resistance of marine ecosystems to ocean warming requires both short- and long-term stress responses. For long-lived sessile organisms that have a limited ability to move towards better environmental conditions, stress memory could play a crucial role, especially in view of the rapid climate changes expected in the near future. Here we show that changes in genome-wide DNA methylation levels during short-term thermal profiles could lead to a latent, long-lasting improvement of thermal tolerance during a subsequent stress test in a reef-forming Mediterranean coral species. This acclimatization phenomenon suggests the presence of plastic potential even in temperate organisms in response to climate change.
Our results confirm a potential long duration of tolerance effects by stress memory in corals (Middlebrook et al., 2012; Hackerott et al., 2021; Drury et al., 2022), using an acute thermal profile that still elicits ecologically relevant changes after 75 days. We did not re-collect DNA samples after the recovery period, and there is little information available on the persistence of acquired methylation patterns over time; however, corals that undergo environmental activation of DNA methylation have the potential to fix epigenetic marks over time and transfer them to the offspring (Putnam et al., 2016; Liew et al., 2020). The persistence of the response observed here suggests that a moderate exposition to thermal stress outside the warmest period of the year could influence subsequent tolerance levels of corals, which has critical ecological implications for the seasonal dynamics on marine ecosystems.
Significant changes in immune-related patterns after the thermal profiles were found in one of our profiles (constant high temperature) but were absent in the other (pulse temperature). Immune functions sustain organism health and survival, contributing to stress tolerance (Sheldon and Verhulst, 1996; Mydlarz et al., 2006; Palmer, 2018a). Indeed, it has been shown before that temperature can affect coral immune activities and trigger immune-modulations in response to detrimental environmental cues (Lee, 2006; Palmer, 2018a). Ramping of constitutive enzymatic activities promoting homeostasis under warmer conditions has been documented for both tropical and Mediterranean coral species (Palmer, 2018b; Bisanti et al., 2024b). Here, stress test conditions induced a general increase in LYS activities of C. caespitosa fragments, with no significant differences among thermal profiles. Sustained constitutive activities of coral LYS could represent a viable strategy under elevated temperature conditions, since both disease and bleaching pose higher threats during summer months (Lee, 2006; Mydlarz et al., 2010; Palmer, 2018b; Bisanti et al., 2024b). As accumulated thermal stress increased, control and pulse corals improved also their PO production probably in order to mitigate bleaching. PO modulations play a crucial role in thermal bleaching susceptibility of zooxanthellate species (Mydlarz et al., 2010; Palmer, 2018b); deposition of melanin (a redox-active brown/black pigment that absorbs light) by coral mobile cells provide photoprotection to Symbiodiniaceae (Palmer et al., 2010). In contrast, constant high corals did not experience elevated PO activities, suggesting a lower photoprotection demand to maintain algal endosymbiosis. Meanwhile, an increase in GPx values was observed in control and pulse profiles as accumulated thermal stress increased, which was missing in constant high corals. Increased antioxidant activities are indicative of ROS production by Symbiodiniaceae under thermal stress (Mydlarz and Jacobs, 2004; Palmer et al., 2011) and as a result of invertebrate immune activities (e.g., activation of the melanin synthesis pathway) (Nappi and Ottaviani, 2000; Sadd and Siva-Jothy, 2006; Palmer and Traylor-Knowles, 2018). Thus, fragments in control and pulse profiles may have experienced more ROS damage compared to constant high corals. This is consistent with previous observations in Acropora pruinosa, where lower values of superoxide dismutase and catalase (two antioxidant enzymes) in primed corals compared to naïve colonies have already been correlated with lower oxidative stress (Yu et al., 2020). Based on these evidences, we have postulated that fragments showing lower immune activation would be less impacted by temperatures and, therefore, would show improved thermal tolerance (Mydlarz et al., 2010; Palmer, 2018a; Yu et al., 2020). Here, the lower and constant immune-related patterns of constant high profile may suggest a boosted immune-tolerance acquired via stress memory.
Thermal stress is also known to induce saturation of photosynthetic electron transport leading to degradation of the photosystem II and promoting the generation of reactive oxygen species, triggering coral bleaching by losing symbiont or by photopigment degradation (Warner et al., 1999; Oakley and Davy, 2018). C. caespitosa has been reported to respond differently across geographical source areas, depth range of sampled organisms, and experimental temperature thresholds (e.g., Rodolfo-Metalpa et al., 2006, 2010; Jurriaans et al., 2021; Carbonne et al., 2024), although a general declining effects in photosynthetic efficiency occurred as a result of warming temperatures. Here, the stress test caused an efficiency reduction for all thermal profiles, although the constant high profile would appear to confer a relative protective effect on coral bleaching, given the significantly higher efficiency values compared to controls. The ability to improve photo-chemical efficiency has been associated with fine-scale differences in temperature regime, host genotype and relatively stable symbiont community composition (Dilworth et al., 2021). Temperature variability may confer major bleaching tolerance to corals compared to constant exposures (Oliver and Palumbi, 2011; DeMerlis et al., 2022), but these observations are not universal (Bay and Palumbi, 2015; Klepac and Barshis, 2020), and our results suggest that constant high profiles create more beneficial responses. We have not investigated coral genotype and composition of harbored symbiont communities in the natural population of C. caespitosa considered in this experiment. However, recent findings indicate that both host genotype and Symbiodiniaceae (both tolerant and non-tolerant species) do not intrinsically limit the acquisition of thermal tolerance in Montipora capitata colonies (Drury et al., 2022).
Our results support a strong and positive correlation between accumulated total thermal stress and global changes in DNA methylation levels. Thermal profile exposures have elicited changes in addition of methyl groups (-CH3) to DNA nucleotides, probably related to the lack of cooler and darker night-time conditions, during which corals can respond to DNA alterations (Bertucci et al., 2015). In corals, stress memory activation via DNA methylation may be driven by temperature-related signals, reflective of the surrounding environment, (e.g., Dixon et al., 2018; Dimond and Roberts, 2020; Rodriguez-Casariego et al., 2022). A recent cross-transplantation experiment revealed that colonies showing closer gene methylation-patterns match to local corals reached higher fitness, via balancing between expressions of housekeeping and environmentally responsive genes (Dixon et al., 2018). Additionally, DNA methylation also underpins changes in symbiont communities, favoring thermal tolerant associations in coral hosts (Rodriguez-Casariego et al., 2022).
We documented a 2-fold difference in genomic DNA methylation levels between thermal profiles, with an hypermethylation of DNA in corals at constant high temperatures. These methylation patterns of genomic DNA are consistent with recent studies showing hypermethylation on genome-wide levels involved in stress responses of bleaching tolerant corals (Liew et al., 2018, 2020). These variations in DNA methylation levels of C. caespitosa may have provided the required homeostatic control through alternative transcriptomic responses under dynamic acclimatization mechanisms, such as increased constitutive expression of a variety of genes (Barshis et al., 2013; Putnam et al., 2016). However, a genomic hypermethylation does not directly indicate changes in coral adaptive responses to thermal stress. Adaptative epigenetic changes can also act at the gene level without global differences in methylation levels; for example, increased methylation levels may occur in single gene-body, intergenic regions, and transposable elements (Rodriguez-Casariego et al., 2022), potentially generating new genetic combinations by selective inhibition of mobile elements (Venkataraman et al., 2020; Choi et al., 2020). Pairing DNA bisulfite sequencing with RNASeq to identify methylation-associated expression patterns will likely provide further insights to correlate with coral performances, and allow to identify mechanistic links to stress memory.
The results presented here suggest that an adaptive effect can be induced by artificial short-term thermal profiles in temperate corals. Thermal tolerance during stress testing resulted in boosted immune tolerance and, accordingly, greater coral bleaching resistance. We also document substantial variation in DNA methylation levels in relation to accumulated thermal stress, warranting further investigation on the extent, longevity, and heritability of these epigenetic tags in primed corals. Identification of genomic locations and resulting transcriptional control in a subset of nursery-propagated C. caespitosa genotypes is the next step to assess the relevance of epigenetics in survivorship via stress memory. These efforts may prove crucial to averting large-scale losses of corals in the Mediterranean basin, and potentially worldwide, due to future climate change scenarios.
Data availability statement
The raw data supporting the conclusions of this article will be made available by the authors, without undue reservation.
Ethics statement
This study considers marine invertebrates not subject to regulation or ethical approval. The study was conducted in accordance with the local legislation and institutional requirements.
Author contributions
LB: Conceptualization, Data curation, Formal Analysis, Validation, Writing – original draft, Writing – review & editing. CLC: Formal Analysis, Validation, Writing – review & editing. MD: Formal Analysis, Validation, Writing – review & editing. FB: Formal Analysis, Validation, Writing – review & editing. GR: Data curation, Formal Analysis, Validation, Writing – review & editing. RV: Data curation, Formal Analysis, Validation, Writing – review & editing. FN: Writing – review & editing. DP: Writing – review & editing. MP: Writing – review & editing. AT: Writing – review & editing. FC: Writing – review & editing. RC: Conceptualization, Supervision, Writing – review & editing. MC: Conceptualization, Supervision, Validation, Writing – review & editing.
Funding
The author(s) declare that financial support was received for the research and/or publication of this article. This work was supported by the Robotics and AI for Socio-Economic Empowerment Project (RAISE), Spoke 3: Sustainable technologies for monitoring in Marine Protected Areas of the Lower Tyrrhenian Sea (TEC-SOS), CUP B33C22000700006 (identification code: ECS00000035).
Conflict of interest
The authors declare that the research was conducted in the absence of any commercial or financial relationships that could be construed as a potential conflict of interest.
Generative AI statement
The author(s) declare that no Generative AI was used in the creation of this manuscript.
Publisher’s note
All claims expressed in this article are solely those of the authors and do not necessarily represent those of their affiliated organizations, or those of the publisher, the editors and the reviewers. Any product that may be evaluated in this article, or claim that may be made by its manufacturer, is not guaranteed or endorsed by the publisher.
Supplementary material
The Supplementary Material for this article can be found online at: https://www.frontiersin.org/articles/10.3389/fmars.2025.1579913/full#supplementary-material
References
Adrian-Kalchhauser I., Sultan S. E., Shama L. N., Spence-Jones H., Tiso S., Valsecchi C. I. K., et al. (2020). Understanding “non-genetic” inheritance: insights from molecular-evolutionary crosstalk. Trends Ecol. Evol. 35, 1078–1089. doi: 10.1016/j.tree.2020.08.011
Ainsworth T. D., Heron S. F., Ortiz J. C., Mumby P. J., Grech A., Ogawa D., et al. (2016). Climate change disables coral bleaching protection on the Great Barrier Reef. Science 352, 338–342. doi: 10.1126/science.aac7125
Anderson M. J. (2001). A new method for non-parametric multivariate analysis of variance. Austral Ecol. 26, 32–46. doi: 10.1111/j.1442-9993.2001.01070.pp.x
Barshis D. J., Ladner J. T., Oliver T. A., Seneca F. O., Traylor-Knowles N., Palumbi S. R., et al. (2013). Genomic basis for coral resilience to climate change. Proc. Natl. Acad. Sci. 110, 1387–1392. doi: 10.1073/pnas.1210224110
Bay R. A., Palumbi S. R. (2015). Rapid acclimation ability mediated by transcriptome changes in reef-building corals. Genome Biol. Evol. 7, 1602–1612. doi: 10.1093/gbe/evv085
Bellantuono A. J., Granados-Cifuentes C., Miller D. J., Hoegh-Guldberg O., Rodriguez-Lanetty M. (2012). Coral thermal tolerance: Tuning gene expression to resist thermal stress. PloS One 7, e50685. doi: 10.1371/journal.pone.0050685
Bellavia D., Costa V., De Luca A., Cordaro A., Fini M., Giavaresi G., et al. (2022). The binomial “inflammation-epigenetics” in breast cancer progression and bone metastasis: IL-1β actions are influenced by TET inhibitor in MCF-7 cell line. Int. J. Mol. Sci. 23, 15422. doi: 10.3390/ijms232315422
Bertucci A., Foret S., Ball E. E., Miller D. J. (2015). Transcriptomic differences between day and night in Acropora millepora provide new insights into metabolite exchange and light-enhanced calcification in corals. Mol. Ecol. 24, 4489–4504. doi: 10.1111/mec.13328
Bisanti L., La Corte C., Dara M., Bertini F., Parisi M. G., Chemello R., et al. (2024a). Global warming-related response after bacterial challenge in Astroides calycularis, a Mediterranean thermophilic coral. Sci. Rep. 14, 8495. doi: 10.1038/s41598-024-58652-0
Bisanti L., La Corte C., Dara M., Bertini F., Parrinello D., Chemello R., et al. (2024b). How does warmer sea water change the sensitivity of a Mediterranean thermophilic coral after immune-stimulation? Coral Reefs 43, 137–150. doi: 10.1007/s00338-023-02454-9
Bradford M. M. (1976). A rapid and sensitive method for the quantitation of microgram quantities of protein utilizing the principle of protein-dye binding. Anal. Biochem. 72, 248–254. doi: 10.1016/0003-2697(76)90527-3
Brzezinka K., Altmann S., Czesnick H., Nicolas P., Gorka M., Benke E., et al. (2016). Arabidopsis FORGETTER1 mediates stress-induced chromatin memory through nucleosome remodeling. elife 5, e17061. doi: 10.7554/eLife.17061
Buongiorno Nardelli B., Tronconi C., Pisano A., Santoleri R. (2013). High and Ultra-High resolution processing of satellite Sea Surface Temperature data over Southern European Seas in the framework of MyOcean project. Remote Sens. Environ. 129, 1–16. doi: 10.1016/j.rse.2012.10.012
Calabrese E. J., Bachmann K. A., Bailer A. J., Bolger P. M., Borak J., Cai L., et al. (2007). Biological stress response terminology: Integrating the concepts of adaptive response and preconditioning stress within a hormetic dose-response framework. Toxicol. Appl. Pharmacol. 222, 122–128. doi: 10.1016/j.taap.2007.02.015
Calosi P., Bilton D. T., Spicer J. I. (2008). Thermal tolerance, acclimatory capacity and vulnerability to global climate change. Biol. Lett. 4, 99–102. doi: 10.1098/rsbl.2007.0408
Carbonne C., Comeau S., Plichon K., Schaub S., Gattuso J. P., Teixidó N. (2024). Response of two temperate scleractinian corals to projected ocean warming and marine heatwaves. R. Soc Open Sci. 11, 231683. doi: 10.1098/rsos.231683
Carelli G., Iavicoli I. (2016). Defining hormesis: the necessary tool to clarify experimentally the low dose-response relationship. Hum. Exp. Toxicol. 21, 103–104. doi: 10.1191/0960327102ht219oa
Cavalli G., Heard E. (2019). Advances in epigenetics link genetics to the environment and disease. Nature 571, 489–499. doi: 10.1038/s41586-019-1411-0
Choi J., Lyons D. B., Kim M. Y., Moore J. D., Zilberman D. (2020). DNA methylation and histone H1 jointly repress transposable elements and aberrant intragenic transcripts. Mol. Cell 77, 310–323. doi: 10.1016/j.molcel.2019.10.011
Coles S. L., Jokiel P. L. (1978). Synergistic effects of temperature, salinity and light on the hermatypic coral Montipora verrucosa. Mar. Biol. 49, 187–195. doi: 10.1007/BF00391130
DeMerlis A., Kirkland A., Kaufman M. L., Mayfield A. B., Formel N., Kolodziej G., et al. (2022). Pre-exposure to a variable temperature treatment improves the response of Acropora cervicornis to acute thermal stress. Coral Reefs 41, 435–445. doi: 10.1007/s00338-022-02232-z
Dilworth J., Caruso C., Kahkejian V. A., Baker A. C., Drury C. (2021). Host genotype and stable differences in algal symbiont communities explain patterns of thermal stress response of Montipora capitata following thermal pre-exposure and across multiple bleaching events. Coral Reefs 40, 151–163. doi: 10.1007/s00338-020-02024-3
Dimond J. L., Roberts S. B. (2020). Convergence of DNA methylation profiles of the reef coral Porites astreoides in a novel environment. Front. Mar. Sci. 6. doi: 10.3389/fmars.2019.00792
Dixon P. (2003). VEGAN, a package of R functions for community ecology. J. Veg. Sci. 14, 927–930. doi: 10.1111/j.1654-1103.2003.tb02228.x
Dixon G., Liao Y., Bay L. K., Matz M. V. (2018). Role of gene body methylation in acclimatization and adaptation in a basal metazoan. Proc. Natl. Acad. Sci. 115, 13342–13346. doi: 10.1073/pnas.1813749115
Drury C., Dilworth J., Majerová E., Caruso C., Greer J. B. (2022). Expression plasticity regulates intraspecific variation in the acclimatization potential of a reef-building coral. Nat. Commun. 13, 4790. doi: 10.1038/s41467-022-32452-4
Drury C., Lirman D. (2021). Genotype by environment interactions in coral bleaching. Proc. R. Soc B 288, 20210177. doi: 10.1098/rspb.2021.0177
Drury C., Manzello D., Lirman D. (2017). Genotype and local environment dynamically influence growth, disturbance response and survivorship in the threatened coral, Acropora cervicornis. PloS One 12, e0174000. doi: 10.1371/journal.pone.0174000
Eirin-Lopez J. M., Putnam H. M. (2019). Marine environmental epigenetics. Ann. Rev. Mar. Sci. 11, 335–368. doi: 10.1146/annurev-marine-010318-095114
Fabrizio P., Garvis S., Palladino F. (2019). Histone methylation and memory of environmental stress. Cells 8, 339. doi: 10.3390/cells8040339
Gallo-Oller G., Ordonez R., Dotor J. (2018). A new background subtraction method for Western blot densitometry band quantification through image analysis software. J. Immunol. Methods 457, 1–5. doi: 10.1016/j.jim.2018.03.004
Garrabou J., Gómez-Gras D., Medrano A., Cerrano C., Ponti M., Schlegel R., et al. (2022). Marine heatwaves drive recurrent mass mortalities in the Mediterranean Sea. Glob. Change Biol. 28, 5708–5725. doi: 10.1111/gcb.16301
Gerken A. R., Eller O. C., Hahn D. A., Morgan T. J. (2015). Constraints, independence, and evolution of thermal plasticity: probing genetic architecture of long-and short-term thermal acclimation. Proc. Natl. Acad. Sci. 112, 4399–4404. doi: 10.1073/pnas.1503456112
Glynn P. W. (1993). Coral reef bleaching: ecological perspectives. Coral reefs 12, 1–17. doi: 10.1007/BF00303779
Goldberg W. M. (2018). “Coral food, feeding, nutrition, and secretion: a review,” in Marine organisms as model systems in biology and medicine. Eds. Kloc M., Kubiak J. (Springer, Cham, Switzerland), 377–421. doi: 10.1007/978-3-319-92486-1_18
Grottoli A. G., Warner M. E., Levas S. J., Aschaffenburg M. D., Schoepf V., McGinley M., et al. (2014). The cumulative impact of annual coral bleaching can turn some coral species winners into losers. Glob. Change Biol. 20, 3823–3833. doi: 10.1111/gcb.12658
Hackerott S., Martell H. A., Eirin-Lopez J. M. (2021). Coral environmental memory: causes, mechanisms, and consequences for future reefs. Trends Ecol. Evol. 36, 1011–1023. doi: 10.1016/j.tree.2021.06.014
Hawkins T. D., Krueger T., Becker S., Fisher P. L., Davy S. K. (2014). Differential nitric oxide synthesis and host apoptotic events correlate with bleaching susceptibility in reef corals. Coral Reefs 33, 141–153. doi: 10.1007/s00338-013-1103-4
Hawkins T. D., Warner M. E. (2017). Warm preconditioning protects against acute heat-induced respiratory dysfunction and delays bleaching in a symbiotic sea anemone. J. Exp. Biol. 220, 969–983. doi: 10.1242/jeb.150391
Hilker M., Schwachtje J., Baier M., Balazadeh S., Bäurle I., Geiselhardt S., et al. (2016). Priming and memory of stress responses in organisms lacking a nervous system. Biol. Rev. Camb. Philos. Soc. 91, 1118–1133. doi: 10.1111/brv.12215
Hoegh-Guldberg O., Mumby P. J., Hooten A. J., Steneck R. S., Greenfield P., Gomez E., et al. (2007). Coral reefs under rapid climate change and ocean acidification. Science 318, 1737–1742. doi: 10.1126/science.1152509
Hughes T. P., Kerry J. T., Baird A. H., Connolly S. R., Dietzel A., Eakin C. M., et al. (2018). Global warming transforms coral reef assemblages. Nature 556, 492–496. doi: 10.1038/s41586-018-0041-2
Jia Z., Liang Y., Ma B., Xu X., Xiong J., Duan L., et al. (2017). A 5-mC dot blot assay quantifying the DNA methylation level of chondrocyte dedifferentiation in vitro. JoVE 123), e55565. doi: 10.3791/55565
Jones R. J., Ward S., Amri A. Y., Hoegh-Guldberg O. (2000). Changes in quantum efficiency of Photosystem II of symbiotic dinoflagellates of corals after heat stress, and of bleached corals sampled after the 1998 Great Barrier Reef mass bleaching event. Mar. Freshw. Res. 51, 63–71. doi: 10.1071/MF99100
Jurriaans S., Hoogenboom M. O. (2020). Seasonal acclimation of thermal performance in two species of reef-building corals. Mar. Ecol. Prog. Ser. 635, 55–70. doi: 10.3354/meps13203
Jurriaans S., Hoogenboom M. O., Ferrier-Pages C. (2021). Similar thermal breadth of two temperate coral species from the Mediterranean Sea and two tropical coral species from the Great Barrier Reef. Coral Reefs 40, 1281–1295. doi: 10.1007/s00338-021-02139-1
Kellett M., Hoffmann A. A., Mckechnie S. W. (2005). Hardening capacity in the Drosophila melanogaster species group is constrained by basal thermotolerance. Funct. Ecol. 19 (5), 853–858. Available at: http://www.jstor.org/stable/3599347.
Kishimoto I., Ariga I., Itabashi Y., Mikami K. (2019). Heat-stress memory is responsible for acquired thermotolerance in bangia fuscopurpurea. J. Phycol. 55, 971–975. doi: 10.1111/jpy.12895
Klein S. G., Pitt K. A., Carroll A. R. (2017). Pre-exposure to simultaneous, but not individual, climate change stressors limits acclimation capacity of Irukandji jellyfish polyps to predicted climate scenarios. Coral Reefs 36, 987–1000. doi: 10.1007/s00338-017-1590-9
Klepac C. N., Barshis D. J. (2020). Reduced thermal tolerance of massive coral species in a highly variable environment. Proc. R. Soc B 287, 20201379. doi: 10.1098/rspb.2020.1379
La Corte C., Dara M., Bertini F., Parrinello D., Piazzese D., Parisi M. G. (2023). Response of Sabella spallanzanii to multiple stressors. The combined effect of infection and copper sulphate. Comp. Biochem. Physiol. C Toxicol. Pharmacol. 263, 109475. doi: 10.1016/j.cbpc.2022.109475
LaJeunesse T. C., Parkinson J. E., Gabrielson P. W., Jeong H. J., Reimer J. D., Voolstra C. R., et al. (2018). Systematic revision of Symbiodiniaceae highlights the antiquity and diversity of coral endosymbionts. Curr. Biol. 28, 2570–2580. doi: 10.1016/j.cub.2018.07.008
Lämke J., Bäurle I. (2017). Epigenetic and chromatin-based mechanisms in environmental stress adaptation and stress memory in plants. Genome Biol. 18, 1–11. doi: 10.1186/s13059-017-1263-6
Leśnierowski G., Yang T. (2021). Lysozyme and its modified forms: A critical appraisal of selected properties and potential. Trends Food. Sci. Technol. 107, 333–342. doi: 10.1016/j.tifs.2020.11.004
Lee K. A. (2006). Linking immune defenses and life history at the levels of the individual and the species. Integr. Comp. Biol. 46, 1000–1015. doi: 10.1093/icb/icl049
Leggat W., Heron S. F., Fordyce A., Suggett D. J., Ainsworth T. D. (2022). Experiment Degree Heating Week (eDHW) as a novel metric to reconcile and validate past and future global coral bleaching studies. J. Environ. Manag. 301, 113919. doi: 10.1016/j.jenvman.2021.113919
Lesser M. P. (2006). Oxidative stress in marine environments: biochemistry and physiological ecology. Annu. Rev. Physiol. 68, 253–278. doi: 10.1146/annurev.physiol.68.040104.110001
Li Y., Liew Y. J., Cui G., Cziesielski M. J., Zahran N., Michell C. T., et al. (2018). DNA methylation regulates transcriptional homeostasis of algal endosymbiosis in the coral model Aiptasia. Sci. Adv. 4, eaat2142. doi: 10.1126/sciadv.aat2142
Li H., Huang X., Zhan A. (2020). Stress memory of recurrent environmental challenges in marine invasive species: Ciona robusta as a case study. Front. Physiol. 11. doi: 10.3389/fphys.2020.00094
Li H., Parisi M. G., Toubiana M., Cammarata M., Roch P. (2008). Lysozyme gene expression and hemocyte behaviour in the Mediterranean mussel, Mytilus galloprovincialis, after injection of various bacteria or temperature stresses. Fish Shellfish Immunol. 25, 143–152. doi: 10.1016/j.fsi.2008.04.001
Liew Y. J., Zoccola D., Li Y., Tambutté E., Venn A. A., Michell C. T., et al. (2018). Epigenome-associated phenotypic acclimatization to ocean acidification in a reef-building coral. Sci. Adv. 4, eaar8028. doi: 10.1126/sciadv.aar8028
Liew Y. J., Howells E. J., Wang X., Michell C. T., Burt J. A., Idaghdour Y., et al. (2020). Intergenerational epigenetic inheritance in reef-building corals. Nat. Clim. Change 10, 254–259. doi: 10.1038/s41558-019-0687-2
Lough J. M. (2011). Climate change and coral reefs in Encyclopedia of modern coral reefs: Structure, form and process. Ed. Hopley D. (Dordrecht: Springer), 198–210. doi: 10.1007/978-90-481-2639-2
Majerova E., Carey F. C., Drury C., Gates R. D. (2021). Preconditioning improves bleaching tolerance in the reef-building coral Pocillopora acuta through modulations in the programmed cell death pathways. Mol. Ecol. 30 (14), 3560–3574. doi: 10.1111/mec.15988
Martell H. A. (2023). Thermal priming and bleaching hormesis in the staghorn coral, Acropora cervicornis (Lamarck 1816). J. Exp. Mar. Bio. Ecol. 560, 151820. doi: 10.1016/j.jembe.2022.151820
Martin S., Gattuso J. P. (2009). Response of Mediterranean coralline algae to ocean acidification and elevated temperature. Glob. Change Biol. 15, 2089–2100. doi: 10.1111/j.1365-2486.2009.01874.x
Middlebrook R., Anthony K. R., Hoegh-Guldberg O., Dove S. (2012). Thermal priming affects symbiont photosynthesis but does not alter bleaching susceptibility in Acropora millepora. J. Exp. Mar. Bio. Ecol. 432, 64–72. doi: 10.1016/j.jembe.2012.07.005
Munday P. L., Warner R. R., Monro K., Pandolfi J. M., Marshall D. J. (2013). Predicting evolutionary responses to climate change in the sea. Ecol. Lett. 16, 1488–1500. doi: 10.1111/ele.12185
Murchie E. H., Lawson T. (2013). Chlorophyll fluorescence analysis: a guide to good practice and understanding some new applications. J. Exp. Bot. 64, 3983–3998. doi: 10.1093/jxb/ert208
Muscatine L., McCloskey L. R., Marian R. E. (1981). Estimating the daily contribution of carbon from zooxanthellae to coral animal respiration 1. Limnol. Oceanogr. 26, 601–611. doi: 10.4319/lo.1981.26.4.0601
Mydlarz L. D., Jacobs R. S. (2004). Comparison of an inducible oxidative burst in free-living and symbiotic dinoflagellates reveals properties of the pseudopterosins. Phytochem 65, 3231–3241. doi: 10.1016/j.phytochem.2004.09.014
Mydlarz L. D., Jones L. E., Harvell C. D. (2006). Innate immunity, environmental drivers, and disease ecology of marine and freshwater invertebrates. Annu. Rev. Ecol. Evol. Syst. 37, 251–288. doi: 10.1146/annurev.ecolsys.37.091305.110103
Mydlarz L. D., McGinty E. S., Harvell C. D. (2010). What are the physiological and immunological responses of coral to climate warming and disease? J. Exp. Biol. 213, 934–945. doi: 10.1242/jeb.037580
Nappi A. J., Ottaviani E. (2000). Cytotoxicity and cytotoxic molecules in invertebrates. Bioessays 22, 469–480. doi: 10.1002/(SICI)1521-1878(200005)22:5<469::AID-BIES9>3.0.CO;2-4
Naselli F., Cardinale P. S., Volpes S., Martino C., Cruciata I., Valenti R., et al. (2024). An alternative approach of TUNEL assay to specifically characterize DNA fragmentation in cell model systems. Histochem. Cell Biol. 162, 429–442. doi: 10.1007/s00418-024-02306-9
National Academies of Sciences, Division on Earth, Life Studies, Board on Life Sciences, Ocean Studies Board, Committee on Interventions to Increase the Resilience of Coral Reefs (2019). A research review of interventions to increase the persistence and resilience of coral reefs (Washington, DC: The National Academies Press). doi: 10.17226/25279
Nguyen H. M., Kim M., Ralph P. J., Marín-Guirao L., Pernice M., Procaccini G. (2020). Stress memory in seagrasses: first insight into the effects of thermal priming and the role of epigenetic modifications. Front. Plant Sci. 11. doi: 10.3389/fpls.2020.00494
Oakley C. A., Davy S. K. (2018). Cell biology of coral bleaching in Coral bleaching: patterns, processes, causes and consequences. Eds. van O. M., L J. M. (Cham: Springer), 189–211. doi: 10.1007/978-3-319-75393-5_8
Oliver T. A., Palumbi S. R. (2011). Do fluctuating temperature environments elevate coral thermal tolerance? Coral reefs 30, 429–440. doi: 10.1007/s00338-011-0721-y
Palmer C. V. (2018a). Immunity and the coral crisis. Commun. Biol. 1, 91. doi: 10.1038/s42003-018-0097-4
Palmer C. V. (2018b). Warmer water affects immunity of a tolerant reef coral. Front. Mar. Sci. 5. doi: 10.3389/fmars.2018.00253
Palmer C. V., Bythell J. C., Willis B. L. (2010). Levels of immunity parameters underpin bleaching and disease susceptibility of reef corals. FASEB J. 24, 1935–1946. doi: 10.1096/fj.09-152447
Palmer C. V., McGinty E. S., Cummings D. J., Smith S. M., Bartels E., Mydlarz L. D. (2011). Patterns of coral ecological immunology: variation in the responses of Caribbean corals to elevated temperature and a pathogen elicitor. J. Exp. Biol. 214, 4240–4249. doi: 10.1242/jeb.061267
Palmer C. V., Traylor-Knowles N. G. (2018). “Cnidaria: anthozoans in the hot seat,” in Advances in Comparative Immunology. Ed. Cooper E. (Springer, Cham). doi: 10.1007/978-3-319-76768-0_3
Palumbi S. R., Barshis D. J., Traylor-Knowles N., Bay R. A. (2014). Mechanisms of reef coral resistance to future climate change. Science 344, 895–898. doi: 10.1126/science.1251336
Pandolfi J. M., Bradbury R. H., Sala E., Hughes T. P., Bjorndal K. A., Cooke R. G., et al. (2003). Global trajectories of the long-term decline of coral reef ecosystems. Science 301, 955–958. doi: 10.1126/science.1085706
Parisi M. G., Parrinello D., Stabili L., Cammarata M. (2020). Cnidarian immunity and the repertoire of defense mechanisms in anthozoans. Biology 9, 283. doi: 10.3390/biology9090283
Parry R. M., Chandan R. C., Shahani K. M. (1965). A rapid and sensitive assay of muramidase. Exp. Biol. Med. 119, 384–386. doi: 10.3181/00379727-119-30188
Putnam H. M., Barott K. L., Ainsworth T. D., Gates R. D. (2017). The vulnerability and resilience of reef-building corals. Curr. Biol. 27, R528–R540. doi: 10.1016/j.cub.2017.04.047
Putnam H. M., Davidson J. M., Gates R. D. (2016). Ocean acidification influences host DNA methylation and phenotypic plasticity in environmentally susceptible corals. Evol. Appl. 9, 1165–1178. doi: 10.1111/eva.12408
Putnam H. M., Gates R. D. (2015). Preconditioning in the reef-building coral Pocillopora damicornis and the potential for trans-generational acclimatization in coral larvae under future climate change conditions. J. Exp. Biol. 218, 2365–2372. doi: 10.1242/jeb.123018
Rivera H. E., Aichelman H. E., Fifer J. E., Kriefall N. G., Wuitchik D. M., Smith S. J., et al. (2021). A framework for understanding gene expression plasticity and its influence on stress tolerance. Mol. Ecol. 30, 1381–1397. doi: 10.1111/mec.15820
Rodolfo-Metalpa R., Martin S., Ferrier-Pagès C., Gattuso J. P. (2010). Response of the temperate coral Cladocora caespitosa to mid-and long-term exposure to pCO2 and temperature levels projected for the year 2100 AD. Biogeosciences 7, 289–300. doi: 10.5194/bg-7-289-2010
Rodolfo-Metalpa R., Richard C., Allemand D., Ferrier-Pagès C. (2006). Growth and photosynthesis of two Mediterranean corals, Cladocora caespitosa and Oculina patagonica, under normal and elevated temperatures. J. Exp. Biol. 209, 4546–4556. doi: 10.1242/jeb.02550
Rodriguez-Casariego J. A., Cunning R., Baker A. C., Eirin-Lopez J. M. (2022). Symbiont shuffling induces differential DNA methylation responses to thermal stress in the coral Montastraea cavernosa. Mol. Ecol. 31, 588–602. doi: 10.1111/mec.16246
Rodríguez-Casariego J. A., Mercado-Molina A. E., Garcia-Souto D., Ortiz-Rivera I. M., Lopes C., Baums I. B. (2020). Genome-wide DNA methylation analysis reveals a conserved epigenetic response to seasonal environmental variation in the staghorn coral Acropora cervicornis. Front. Mar. Sci. 7. doi: 10.3389/fmars.2020.560424
Ross N. W., Firth K. J., Wang A., Burka J. F., Johnson S. C. (2000). Changes in hydrolytic enzyme activities of naive Atlantic salmon Salmo salar skin mucus due to infection with the salmon louse Lepeophtheirus salmonis and cortisol implantation. Dis. Aquat. Org. 41, 43–51. doi: 10.3354/dao041043
Sadd B. M., Siva-Jothy M. T. (2006). Self-harm caused by an insect’s innate immunity. Proc. Biol. Sci. 273, 2571–2574. doi: 10.1098/rspb.2006.3574
Schneider C. A., Rasband W. S., Eliceiri K. W. (2012). NIH Image to ImageJ: 25 years of image analysis. Nat. Methods 9, 671–675. doi: 10.1038/nmeth.2089
Sheldon B. C., Verhulst S. (1996). Ecological immunology: costly parasite defences and trade-offs in evolutionary ecology. Trends Ecol. Evol. 11, 317–321. doi: 10.1016/0169-5347(96)10039-2
Silverstein R. N., Cunning R., Baker A. C. (2015). Change in algal symbiont communities after bleaching, not prior heat exposure, increases heat tolerance of reef corals. Glob. Change Biol. 21, 236–249. doi: 10.1111/gcb.12706
Stief A., Altmann S., Hoffmann K., Pant B. D., Scheible W. R., Bäurle I. (2014). Arabidopsis miR156 regulates tolerance to recurring environmental stress through SPL transcription factors. Plant Cell 26, 1792–1807. doi: 10.1105/tpc.114.123851
Thurber R. V., Willner-Hall D., Rodriguez-Mueller B., Desnues C., Edwards R. A., Angly F., et al. (2009). Metagenomic analysis of stressed coral holobionts. Environ. Microbiol. 11, 2148–2163. doi: 10.1111/j.1462-2920.2009.01935.x
Todd P. A. (2008). Morphological plasticity in scleractinian corals. Biol. Rev. 83, 315–337. doi: 10.1111/j.1469-185X.2008.00045.x
Traylor-Knowles N., Connelly M. T. (2017). What is currently known about the effects of climate change on the coral immune response. Curr. Clim. Change Rep. 3, 252–260. doi: 10.1007/s40641-017-0077-7
Van Hooidonk R., Maynard J., Tamelander J., Gove J., Ahmadia G., Raymundo L., et al. (2016). Local-scale projections of coral reef futures and implications of the Paris Agreement. Sci. Rep. 6, 1–8. doi: 10.1038/srep39666
van Oppen M. J., Oliver J. K., Putnam H. M., Gates R. D. (2015). Building coral reef resilience through assisted evolution. Proc. Natl. Acad. Sci. 112, 2307–2313. doi: 10.1073/pnas.1422301112
Venkataraman Y. R., Downey-Wall A. M., Ries J., Westfield I., White S. J., Roberts S. B., et al. (2020). General DNA methylation patterns and environmentally-induced differential methylation in the eastern oyster (Crassostrea virginica). Front. Mar. Sci. 7. doi: 10.3389/fmars.2020.00225
Vezzulli L., Colwell R. R., Pruzzo C. (2013). Ocean warming and spread of pathogenic vibrios in the aquatic environment. Microb. Ecol. 65, 817–825. doi: 10.1007/s00248-012-0163-2
Volpes S., Cruciata I., Ceraulo F., Schimmenti C., Naselli F., Pinna C., et al. (2023). Nutritional epigenomic and DNA-damage modulation effect of natural stilbenoids. Sci. Rep. 12, 658. doi: 10.1038/s41598-022-27260-1
Vompe A. D., Epstein H. E., Speare K. E., Schmeltzer E. R., Adam T. C., Burkepile D. E., et al. (2024). Microbiome ecological memory and responses to repeated marine heatwaves clarify variation in coral bleaching and mortality. Glob. Change Biol. 30, e17088. doi: 10.1111/gcb.17088
Voolstra C. R., Li Y., Liew Y. J., Baumgarten S., Zoccola D., Flot J. F., et al. (2017). Comparative analysis of the genomes of Stylophora pistillata and Acropora digitifera provides evidence for extensive differences between species of corals. Sci. Rep. 7, 17583. doi: 10.1038/s41598-017-17484-x
Wangpraseurt D., Lichtenberg M., Jacques S. L., Larkum A. W., Kühl M. (2019). Optical properties of corals distort variable chlorophyll fluorescence measurements. Plant Physiol. 179, 1608–1619. doi: 10.1104/pp.18.01275
Warner M. E., Fitt W. K., Schmidt G. W. (1999). Damage to photosystem II in symbiotic dinoflagellates: a determinant of coral bleaching. Proc. Natl. Acad. Sci. 96, 8007–8012. doi: 10.1073/pnas.96.14.8007
Wibowo A., Becker C., Marconi G., Durr J., Price J., Hagmann J., et al. (2016). Hyperosmotic stress memory in Arabidopsis is mediated by distinct epigenetically labile sites in the genome and is restricted in the male germline by DNA glycosylase activity. elife 5, e13546. doi: 10.7554/eLife.13546
Winder A. J., Harris H. (1991). New assays for the tyrosine hydroxylase and dopa oxidase activities of tyrosinase. Eur. J. Mol. Biol. Biochem. 198, 317–326. doi: 10.1111/j.1432-1033.1991.tb16018.x
Yu X., Yu K., Huang W., Liang J., Qin Z., Chen B., et al. (2020). Thermal acclimation increases heat tolerance of the scleractinian coral Acropora pruinosa. Sci. Total Environ. 733, 139319. doi: 10.1016/j.scitotenv.2020.139319
Keywords: climate change, priming, coral immunity, epigenetics, DNA-methylation
Citation: Bisanti L, La Corte C, Dara M, Bertini F, Rizzuto G, Valenti R, Naselli F, Parrinello D, Parisi MG, Tomasello A, Caradonna F, Chemello R and Cammarata M (2025) Stress memory and epigenome variations: insights into the thermal tolerance potential of Cladocora caespitosa (Linnaeus, 1767), a Mediterranean hermatypic coral. Front. Mar. Sci. 12:1579913. doi: 10.3389/fmars.2025.1579913
Received: 19 February 2025; Accepted: 27 March 2025;
Published: 09 May 2025.
Edited by:
Thanos Dailianis, Hellenic Centre for Marine Research (HCMR), GreeceReviewed by:
Elisavet Kaitetzidou, Aristotle University of Thessaloniki, GreeceAdam Barno, King Abdullah University of Science and Technology, Saudi Arabia
Copyright © 2025 Bisanti, La Corte, Dara, Bertini, Rizzuto, Valenti, Naselli, Parrinello, Parisi, Tomasello, Caradonna, Chemello and Cammarata. This is an open-access article distributed under the terms of the Creative Commons Attribution License (CC BY). The use, distribution or reproduction in other forums is permitted, provided the original author(s) and the copyright owner(s) are credited and that the original publication in this journal is cited, in accordance with accepted academic practice. No use, distribution or reproduction is permitted which does not comply with these terms.
*Correspondence: Matteo Cammarata, bWF0dGVvLmNhbW1hcmF0YUB1bmlwYS5pdA==