- 1Key Laboratory of Environment Change and Resources Use in Beibu Gulf, Ministry of Education, Nanning Normal University, Nanning, China
- 2Alfred-Wegener-Institute Helmholtz Centre for Polar and Marine Research, Bremerhaven, Germany
- 3Institute of Oceanology–Bulgarian Academy of Sciences, Varna, Bulgaria
- 4Southern Marine Science and Engineering Guangdong Laboratory (Zhuhai), Zhuhai, China
- 5Ocean College, Zhejiang University, Zhoushan, China
- 6Beibu Gulf Marine Ecological Environment Field Observation and Research Station of Guangxi, Marine Environmental Monitoring Center of Guangxi, Beihai, China
The Beibu Gulf is located in the northwestern South China Sea. On August 2, 2023, a mass mortality event of cultured Trachinotus ovatus occurred in Lianzhou Bay and Tieshan Bay, suspected to be associated with a Karenia bloom. To identify the causative organism, investigate possible environmental drivers for bloom development, and determine the cause of fish mortality, in-situ bloom samples were collected for community characterization and toxicity analysis. Results showed that during the bloom, seawater quality remained within China’s highest classification, Class I, and RDA analyses indicated that protistan communities were primarily influenced by dissolved inorganic nitrogen (DIN) and pH. Based on a combination of microscopic observation, phylogenetic analyses of ITS and D1-D3 rDNA sequences obtained from clone libraries, and 18S rDNA V4 amplicon sequence variant (ASV) analysis, all approaches confirmed Karenia selliformis as the bloom-causing organism. Weak westward sea surface winds before and during the period facilitated the accumulation and probably the bloom formation of K. selliformis in Lianzhou and Tieshan coastal waters. A rabbit erythrocyte lysis assay detected hemolytic toxicity of 45.2–48.3% in tests with 5 × 107 rabbit erythrocyte cells exposed to 1.3–2.5 × 104 K. selliformis cells, suggesting it as a predominant factor in fish mortality. LC-MS/MS analysis did not detect neurotoxic shellfish toxins (BTX2, BTX3), diarrhetic shellfish toxins (DTX1-2, OA), or SPX1. However, gymnodimine-A (GYM-A), a “fast-acting” toxin known to be exclusively produced by K. selliformis, was detected at 2.2–2.5 pg GYM-A cell-1. To our knowledge, this study represents the first recorded fish-killing caused by a K. selliformis bloom in Chinese waters. The study provides the first biological and toxicity insights into K. selliformis bloom, crucial for the management and mitigation of fish-killing events associated to harmful algal blooms (HABs) in the Beibu Gulf and the South China Sea.
1 Introduction
The marine unarmored dinoflagellate Karenia is notorious for causing mass mortality of marine organisms and producing over 130 potentially toxic metabolites, including the potent neurotoxic brevetoxins and aerosols (Hort et al., 2021; Natsuike et al., 2023). Karenia is widely distributed across global marine waters, however, blooms predominantly occur in East Asia (China, Japan, and Russia), the Mediterranean Sea (Tunisia), the Arabian Sea (Kuwait and Iran), New Zealand, and Chile (Heil et al., 2001; Feki et al., 2013; Li et al., 2019; Iwataki et al., 2022; Orlova et al., 2022; Rolton et al., 2022; Dolatabadi and Attaran-Fariman, 2024). To date, a total of 11 species have been described (Cen et al., 2024; Guiry and Guiry, 2025).
In Chinese waters, eight Karenia species have been recorded, including K. bicuneiformis, K. hui, K. longicanalis, K. mikimotoi, K. papilionacea, K. brevis, K. brevisulcata, and K. selliformis (Cen et al., 2024). However, blooms have commonly been attributed to K. mikimotoi, which is ranked as the second most prominent harmful algal bloom (HAB)-causing species, only after Prorocentrum donghaiense (syn. P. obtusidens), with over 100 blooms reported between 2006 and 2018 in Chinese waters (Li et al., 2017; Lü et al., 2019). Notably, K. mikimotoi is recognized as the most deleterious HAB species in terms of economic losses due to fish and shellfish mortality along the Chinese coasts, particularly in the Pearl River Estuary, the East China Sea, and the Changjiang River Estuary (Gu et al., 2022). It accounts for 65.2% of fisheries damage associated with HAB species in China (Sakamoto et al., 2021).
The Beibu Gulf is a semi-enclosed bay in the northwestern South China Sea, characterized by an average annual seawater temperature of 24.5°C and a tropical monsoon climate, with prevailing southwest winds in summer and northeast winds in winter (Xu et al., 2019) (Figure 1). Unlike the South China Sea near Guangdong Province, the East China Sea, the Yellow Sea, and the Bohai Sea, where various red, brown, green, and golden HABs, including Karenia, have frequently occurred since the 1990s (Yu et al., 2023), the Beibu Gulf has long been regarded as the last “clean ocean” in Chinese coastal waters. Therefore, it played a vital role in supporting marine aquaculture in China. The situation changed in January 2015, when the haptophyte Phaeocystis globosa bloomed massively in the Beibu Gulf, and even blocked the cooling water supply for a nuclear power station in Fangcheng (Xu et al., 2019). Since then, Phaeocystis globosa has become the dominant HAB species in the region, and studies have since been conducted, focusing on its origin, outbreak mechanisms, and modified clay flocculation control strategies (He et al., 2021; Niu et al., 2022; Ren et al., 2022; Li et al., 2024). Dinoflagellate HABs have been rarely recorded over the past decades in the Beibu Gulf (Su et al., 2022).
However, on August 2, 2023, a Karenia sp. bloom was documented for the first time near Lianzhou Bay and Tieshan Bay, coinciding with mass mortality of cultured Trachinotus ovatus (common name golden pompanos) at the bloom sites, an event unprecedented in the Beibu Gulf in recent decades. This study aims to identify the causative organism through a combination of traditional microscopic observation, molecular marker cloning, and metabarcoding analysis, as well as to determine the potential environmental drivers of the bloom and investigate the association between Karenia cell toxicity and fish mortality.
2 Materials and methods
2.1 Study area and sample collection
On August 2, 2023, surface seawater samples were collected from the Beihai aquaculture area in the Beibu Gulf, China, following an incident of Trachinotus ovatus mortality (Figure 1). Zooplankton were initially removed using a 160 μm net. A 1000 mL aliquot was fixed with formaldehyde to a final concentration of 2% for microscopic quantification of phytoplankton. The 0.2–160 μm fraction was collected using 0.2 μm GF/C membranes (Cytiva, Massachusetts, USA) and subjected to the cloning of dominant microalgae species and high-throughput sequencing of the variable region 4 of the eukaryotic 18S rDNA (18S rDNA V4) (n=3). Additionally, a total of 2250 mL, 4000 mL, and 4000 mL of seawater from sites S1, S2, and S4, respectively, were filtered using 1.2 μm GF/C membranes (Cytiva, Massachusetts, USA) for hemolytic toxicity testing and high-performance liquid chromatography coupled with tandem mass spectrometry (LC-MS/MS) toxin analyses. Hemolytic toxicity and toxin LC-MS/MS analysis at site S3 was not assessed due to sample loss.
2.2 Environmental parameters
Seawater environmental parameters, including pH, dissolved oxygen (DO), dissolved inorganic nitrogen (DIN), phosphate (PO43-), chlorophyll-a (Chl-a), and heavy metals (Supplementary Table S1), were measured following the Specification for Marine Monitoring of China (GB17378.1-2007) (General Administration of Quality Supervision et al., 2008). Seawater quality was assessed based on Chinese seawater quality standards (National Environmental Protection Agency, 2004), which classify seawater into four categories (Class I–IV) according to marine functional uses and protection objectives. Class I represents the highest quality, suitable for marine fisheries, nature reserves, and habitats of rare and endangered marine species (National Environmental Protection Agency, 2004). Physical environmental elements, including sea surface temperature (SST), sea surface currents, wind speed, and Chl-a were obtained from Level 4 products of the EU Copernicus Marine Environment Monitoring Service (https://data.marine.copernicus.eu). SST had a spatial resolution of 0.05° × 0.05° and a daily temporal resolution. Sea surface currents (0.25° × 0.25°) were derived from geostrophic current fields based on sea level anomalies (SLA) observed by satellite altimeters. Wind speed (0.125° × 0.125°, hourly) was averaged daily for visualization. Chl-a (4 km × 4 km, daily) was used to represent primary productivity.
2.3 Phytoplankton microscopic counting and molecular marker analyses
Formaldehyde-preserved seawater samples were concentrated in phytoplankton sedimentation chambers for 24 hours and then counted under a microscope at 400× magnification (Ni-U, Nikon, Japan), revealing a high abundance of the Karenia cells in the seawater.
To identify the dominant Karenia at the species-level, DNA from the 0.2–160 μm fraction was extracted using the BioFastSpin DNA Extraction Kit (Bioer Technology, China) following the manufacturer’s protocol. The ITS sequences and D1-D3 LSU rDNA were amplified using the primer pairs ITSA/ITSB (5’-CCTCGTAACAAGGHTCCGTAGGT-3’/5’-CAGATGCTTAARTTCAGCRGG-3’) (Adachi et al., 1994) and 28S-D1R/D3B (5’-ACCCGCTGAATTTAAGCATA-3’/5’-TCGGAAGGAACCAGCTACTA-3’) (Scholin et al., 1994; Nunn et al., 1996), respectively. The PCR reaction system and conditions were identical to those described by Xu et al. (2024). The ITS (~600 bp) and D1-D3 LSU rDNA (~1000 bp) PCR products were extracted from agarose gels and purified using the Universal DNA Purification Kit (Tiangen, DP214, China), followed by cloning as described in Xu et al. (2021a). For both ITS and D1-D3 LSU rDNA, six clones from each site (S1–S4) were randomly selected for Sanger sequencing. Six ITS sequences (OR604560–OR604565) and two D1-D3 LSU rDNA sequences (OR604586–OR604587) have been deposited in the National Center for Biotechnology Information (NCBI).
2.4 Amplicon 18S rDNA sequencing and data analysis
The genomic DNA was extracted using the cetyltrimethylammonium bromide method (Allen et al., 2006). To target 18S rDNA V4, the DNA was PCR-amplified using the primer pairs AReuk454FWD1 5’-CCAGCASCYGCGGTAATTCC-3’/TAReukREV3 5’-ACTTTCGTTCTTGATYRA-3’ (Stoeck et al., 2010). The sequencing library was constructed using the TruSeq® DNA PCR-Free Sample Preparation Kit (Illumina, USA), and sequenced on the Illumina NovaSeq 6000 platform (Novogene, Beijing, China) with paired-end reads of 250 bp in length. Raw reads were processed using Cutadapt v2.8 to remove PCR primer matches and potential artificial leftover sequence segments (Martin, 2011). The DADA2 pipeline v1.18 of the software package (Callahan et al., 2016) implemented in R v4.0.4 (R Core Team, 2024) was applied to conduct 3’-end trimming, error filtering, sequence denoising, paired-end reads merging, chimera detection and removal, and the determination and annotation of Amplicon Sequence Variants (ASVs). The 18S sequencing reads were analyzed using the following parameters: maxEE = c (2.2, 2.1), minLen= 150, truncLen = c (220, 210), minBoot = 70, and minOverlap=10 bases, and the taxonomy was assigned using the reference database PR2 v5.0.0 (Guillou et al., 2012). ASVs annotated as macroalgae, fungi and metazoans were removed from the dataset. A summary of each filtering process on the sequences is shown in Supplementary Table S2. All raw data have been deposited in the NCBI (Bioproject PRJNA1205743). Twenty-one 18S rDNA V4 ASVs assigned to the genus Karenia were also deposited into NCBI (PQ822021-PQ822041).
The ASV table was further analyzed in RStudio v.2024.9.0.375 (Posit team, 2024). Protistan alpha diversity (α-diversity) was determined by calculating Hill numbers using iNEXT v3.0.1 package (bootstrap=100, confidence=0.95), for species richness (q=0), the exponential of Shannon’s entropy index (q=1), and the inverse of Simpson’s concentration index (q=2), respectively (Chao et al., 2014). To assess sequencing depth, rarefaction and extrapolation curves based on iNEXT function were plotted using the ggplot2 v3.5.1 package. ANOVA and pairwise t-tests with Bonferroni correction were conducted to assess α-diversity variations within and between sites S1-S4, and were visualized using ggplot2. For compositional analysis, singletons in the ASV table were removed and zero values were replaced using the Count Zero Multiplicative method via the cmultRepl function from the zCompositions v1.5.0-4 package. A Centered Log-Ratio (CLR) transformation was then applied to mitigate the compositional effect of microbiome ASV data. The top ten most abundant protistan taxa were plotted using ggplot2.
For protistan beta diversity (β-diversity) analysis, as the first axis length from the Detrended Correspondence Analysis (DCA) was<3, Redundancy Analysis (RDA) was applied to assess relationships between ASVs and environmental variables. Environmental metadata (pH, DO, DIN, PO43-) were z-standardized, multicollinearity was checked using vif.cca function, and highly collinear variables were removed. ANOVA with 999 permutations (p=0.05) was used to test the significance of the RDA analysis. Significant environmental factors were identified using the ordistep function, then the final RDA ordination plot was generated based on protistan CLR-transformed ASVs and these significant environmental factors (pH, DIN). Additionally, treemaps were generated using the treemapify v2.5.6 package to visualize phytoplankton categories and their relative compositions at both the phylum and species levels, based on traditional microscopy counts and 18S rDNA V4 ASVs.
2.5 rDNA phylogenetic analysis
The Karenia spp. sequences of ITS, D1-D3 LSU rDNA, 18S rDNA V4 ASVs obtained in this study along with reference sequences from the NCBI were aligned using MAFFT v7.526 (Katoh and Standley, 2013) and trimmed in MEGA v10.1.8 (Kumar et al., 2018). Maximum likelihood (ML) phylogenetic analysis was performed at CIPRES server (Stamatakis, 2014) (https://www.phylo.org/), with evolutionary models GTR+G+I, GTR+G, and GTR+G+I applied to ITS, D1-D3 LSU rDNA, and SSU sequences, respectively. Evolutionary models for Bayesian inference (BI) phylogenetic analysis were selected using jModelTest 2 v0.1.11 (Guindon and Gascuel, 2003; Darriba et al., 2012). The BI phylogenetic tree was conducted using MrBayes v3.2.7 (Ronquist et al., 2012), with the evolutionary models TIM3+G, TrN+G+I, and TIM1+G selected for the respective datasets. Bayesian analysis was performed in two independent runs, each consisting of four Markov chains and a temperature constant of 0.2. The analysis was run for 5 million generations, sampling every 100 generations, with convergence diagnostics calculated every 1000 generations. The first 25% of samples were discarded as burn-in. The phylogenetic trees were edited in FigTree v1.4.4 and Adobe Illustrator 2020 v24.1.1.
To infer the genealogical relationships among 21 ASVs annotated as K. selliformis, a phylogenetic haplotype network using the TCS network method was constructed in PopART v.1.7 (http://popart.otago.ac.nz) (Leigh et al., 2015).
2.6 Toxin analysis
2.6.1 Hemolytic toxicity
The assay followed the protocol of Song et al. (2023). Briefly, GF/C membranes containing phytoplankton cells were dissolved in 8 mL of rabbit erythrocyte lysis assay (ELA) buffer, and ultrasonically disrupted in an ice bath. The hemolytic toxin extract in the supernatant was collected by centrifugation. For hemolytic toxicity measurement, 1 mL of hemolysin extract was incubated with 1 mL of 5×107 cells mL-1 rabbit erythrocyte solution as the test sample (As). Three controls were included: 1 mL erythrocytes + 1 mL ELA buffer (background), 1 mL hemolysin extract + 1 mL ELA buffer (negative control), and 1 mL erythrocytes + 900 µL ELA buffer + 100 µL TritonX-100 (positive control). All mixtures were incubated at 20°C under a light intensity of 50 μmol m-2s-1 for 5 h, followed by centrifugation at 2000 g, 4°C for 5 min. A 200 µL aliquot of the supernatant was loaded into a 96-well microplate, and absorbance was measured at 414 nm using a microplate reader (Infinite M1000 Pro, Tecan, Switzerland). Here, the hemolytic toxicity was calculated according to Ling and Trick (2010):
Where As, Ab, An, and Ap represent the absorbance at 414 nm for phytoplankton cells from bloom seawater, background, negative and positive control, respectively. Therefore, the hemolytic toxicity here represented the percentage of hemolytic toxicity caused by the one-eighth (1/8) of total phytoplankton cells on the GF/C membrane to 5 × 107 rabbit erythrocyte cells.
2.6.2 LC-MS/MS
GF/C membranes containing phytoplankton cells were immersed in 5 mL of 50% methanol solution. The mixture was vortexed for 10 min, subjected to ultrasonic extraction for 15 min (JP-060S, Skymen, China), and centrifuged at 4000 g for 10 min. The supernatant was collected and filtered through a 0.22 μm organic nylon membrane (Tianjin Navigator Lab Instrument Co., Ltd, China) and further analyzed for toxin profiles using LC-MS/MS (Agilent 1260-6460, California, USA). The analyzed toxins included BTX-2, BTX-3, GYM-A, and SPX1 (ESI+ mode), as well as DTX1, DTX2, and OA (ESI- mode). Standards for DTX1, DTX2, GYM-A, OA, and SPX1 were purchased from the National Research Council, Canada. However, standards for BTX-2 and BTX-3 were unavailable. BTX-2 was characterized by m/z 895.5/877.5 and 895.5/859.6, and BTX-3 was identified by m/z 897.5/807.7 and 897.5/725.2, respectively.
The liquid chromatography was performed using an Agilent InfinityLab Poroshell 120 EC-C18 column (4.6 × 150 mm, 4 μm) with an injection volume of 10.0 μL. The mobile phase A consisted of 5 mmol/L ammonium acetate in 0.1% formic acid aqueous solution, while mobile phase B was acetonitrile. The column temperature and flow rate were set to 40.0°C and 0.8 mL min-1 for ESI+ mode, and 30.0°C and 0.6 mL min-1 for ESI- mode, respectively. Mass spectrometry was performed using electrospray ionization (ESI) as the ionization source, with a sheath gas temperature of 350°C and a flow rate of 11 L min-1. The nozzle voltage was set to 500 V, the capillary voltage to 4000 V, and analysis was conducted in Multiple Reaction Monitoring (MRM) mode. In ESI+ mode, the nebulizer pressure was 45 psi, and the dry gas flow rate was 5 L min-1. In ESI- mode, these values were 50 psi and 10 L min-1, respectively. The gradient elution program and MRM parameters for LC-MS/MS were listed in Supplementary Table S3.
3 Results
3.1 Environmental factors
Based on the Karenia cell concentration and fish mortality, the study period was divided into three phases: before the bloom (August 1, 2023), during the bloom (August 2, 2023), and after the bloom (August 3–4, 2023). Mortality of cultured Trachinotus ovatus was observed only on August 2, 2023, near Lianzhou Bay and Tieshan Bay in the Beibu Gulf, coinciding with the occurrence of a Karenia bloom (Figure 1). During the bloom, the water condition at sampling sites S1–S4 was good and classified as Class I according to Chinese seawater quality standards (National Environmental Protection Agency, 2004) (Supplementary Table S1). Therefore, fish mortality resulting from heavy metal contamination or general water pollution was ruled out.
Satellite data indicated that from August 1 to 4, 2023, sea surface temperature remained steady at 31.1–31.3°C (Figure 2; Supplementary Figure S1). Before and during the bloom (August 1–2), relatively weak westward sea surface winds facilitated the convergence of offshore and within the bay microalgae, including Karenia, toward the coast and small embayments via Ekman transport. After the bloom (August 3–4), sea surface winds intensified and shifted to a southwestern direction (Figure 2; Supplementary Figure S1). In response to these winds, sea surface currents flowed westward along the northern coast of the Beibu Gulf, potentially transporting phytoplankton from eastern to western part of Qinzhou and Fangcheng (Figure 2; Supplementary Figure S1). Additionally, sea level anomaly data revealed clear Kelvin wave propagation from east to west along the coast (Figure 2). Together, these physical environmental factors supported the occurrence of elevated Chl-a concentrations westward along the coast from August 1 to 4, 2023 (Figure 2; Supplementary Figure S1).
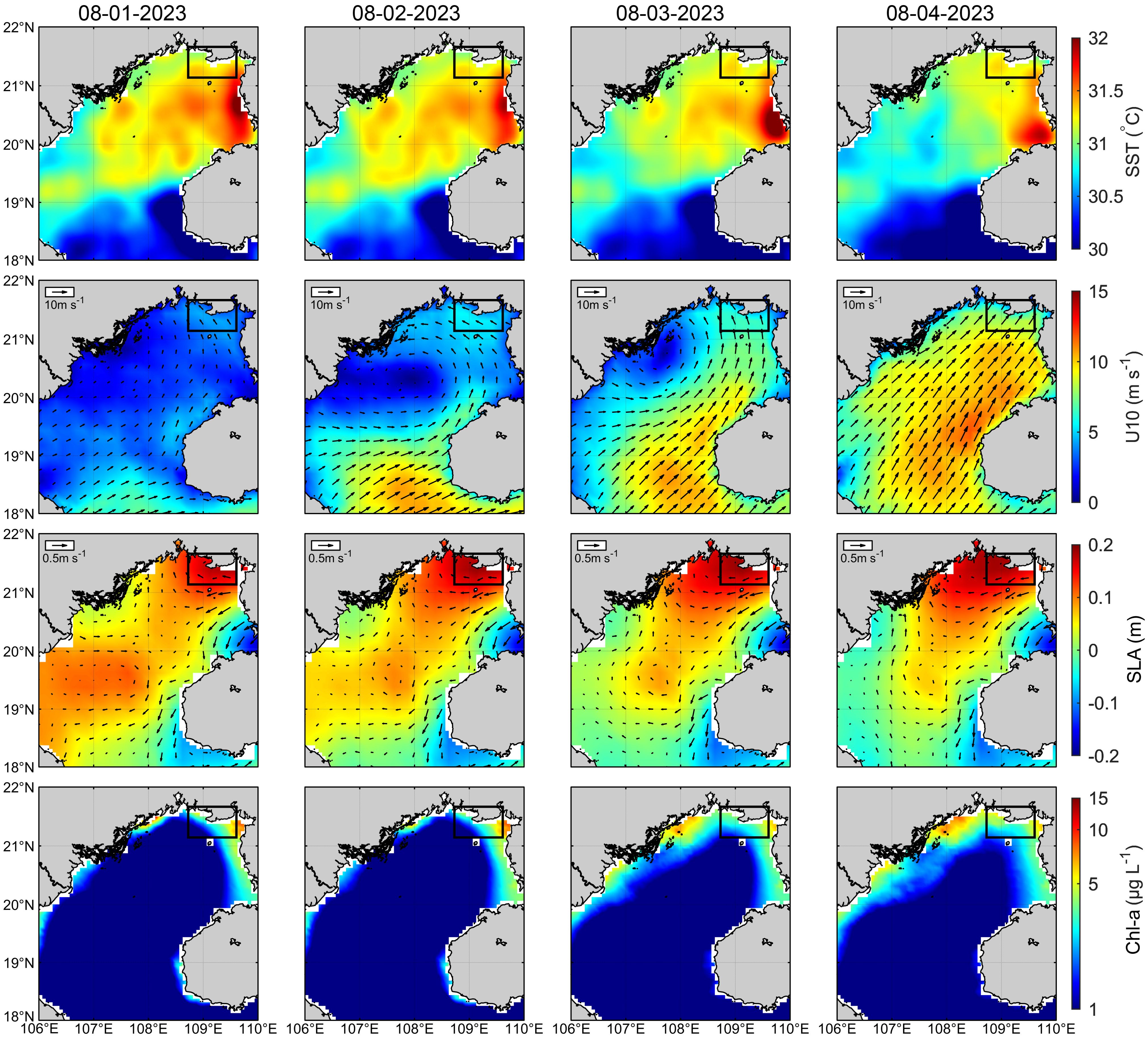
Figure 2. The distributions of sea surface temperature (SST), winds speed at 10m above the sea surface (U10), sea level anomaly (filled color) and geostrophic currents (vectors) (SLA), and chlorophyll-a concentration (Chl-a) from August 1 to 4, 2023, respectively. The rectangular area represents the study area.
3.2 Protistan community characteristics
The average raw read counts for 18S rDNA V4 were 140,528. After denoising, low-quality read removal, and chimera elimination, an average of 83.5% of the raw reads remained for further analysis (Supplementary Table S2). The rarefaction/extrapolation curves for 18S rDNA V4 (q = 0, q = 1, q = 2) plateaued, indicating that the sequencing depth was sufficient to reliably capture protistan community composition and diversity (Supplementary Figure S2). In the bloom seawater, the most abundant protists were Dinoflagellata (42.6%) and Gyrista (20.6%) at the division level; Dinophyceae (28%), Syndiniales (14.7%), and Mediophyceae (9.3%) at the class level; and Dino-Group-II (12.3%) and Gymnodiniales (8.6%) at the order level (Supplementary Figure S3). MOCH-5_XXX (2.9%) and Gyrodinium (2.7%), and MOCH-5_XXX_sp. (3.3%) and Dino-Group-II-Clade-1_X_sp. (2.3%) corresponded to the most abundant genera and species, respectively. The bloom causative organism Karenia generally occupied 1.2% and 1.4% at the genus and species levels, respectively.
Both protist richness and evenness varied significantly among the four sites (p<0.01), with richness at S2–S4 significantly higher than at S1, and evenness at S4 significantly greater than at S1–S3 (Supplementary Figure S4). The RDA analysis revealed that the RDA1 and RDA2 axes explained 32.3% and 26.0% of the variation, respectively. Among the environmental factors, pH and DIN were the primary drivers of community distribution, accounting for 58.3% of the total variation (Figure 3). The remaining RDA axes added another 1.8% to the constrained variance, while the remaining 39.9% was attributed to unconstrained factors. Site S1 was primarily influenced by pH, while S4 was driven by DIN. Sites S2 and S3 were less affected by pH and DIN, clustering closely and exhibiting more similar protist community composition compared to S1 and S4 (Figure 3). The genera Chlorodendrales_XX, Acanthometra, and Grammatodinium were positively correlated with pH and predominantly distributed at S1, while the genus Urgorri was positively correlated with DIN and mostly distributed at S4. The genus Peronosporomycetes_XXX ordinated between Site 1 and Site 4 (Figure 3).
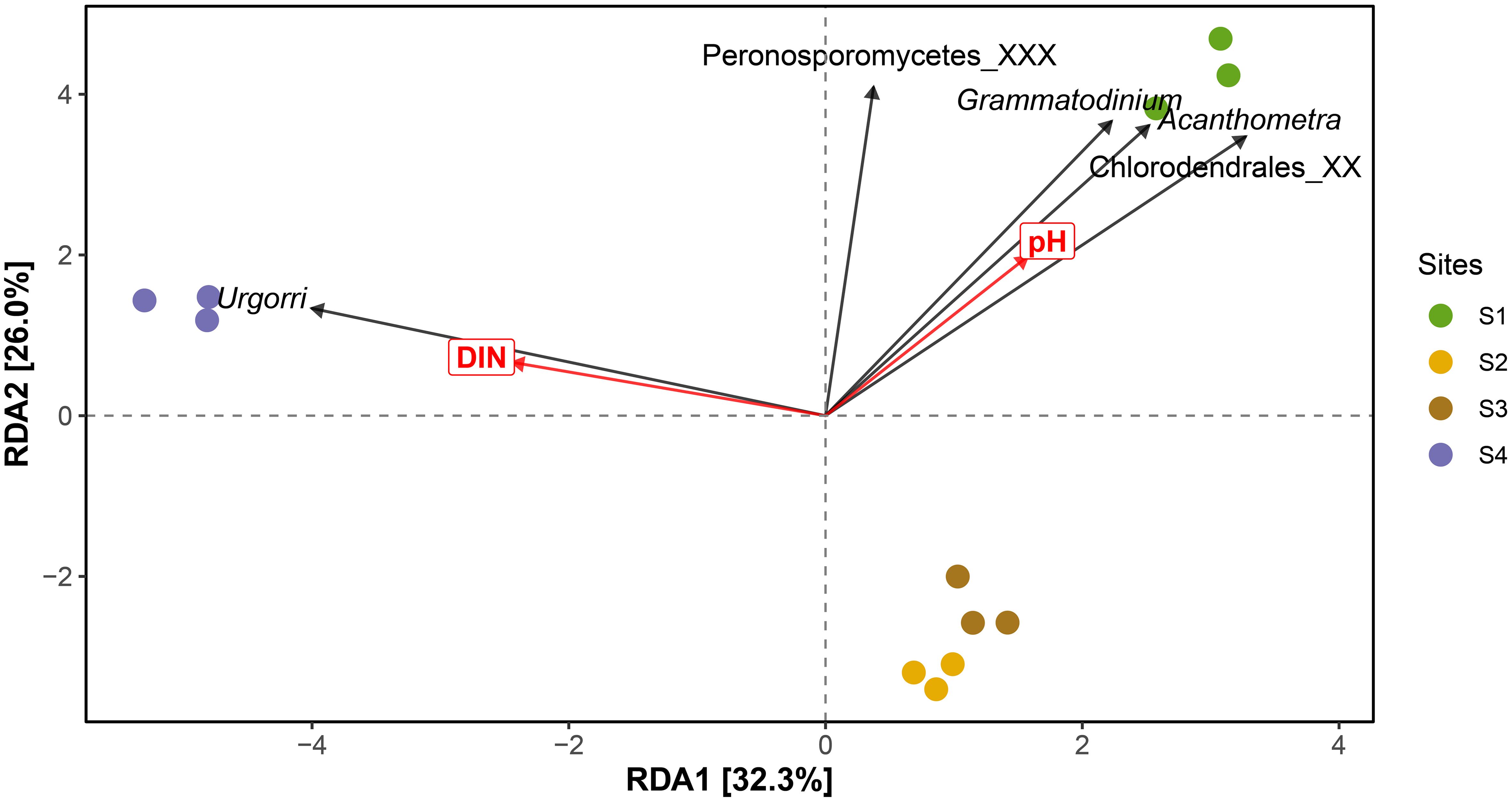
Figure 3. RDA analysis of the protistan community derived from the 18S rDNA V4 amplicon sequence variant (ASV) sequences in relation to environmental factors.
3.3 Phytoplankton community characteristics
The phytoplankton composition, determined by microscopic counting and 18S rDNA V4 ASVs showed substantial agreement, with both methods identifying Heterokontophyta and Dinoflagellata as the dominant phyla. These phyla accounted for 62.4% and 33.7% in microscopic counts, and 37.3% and 43.9% in ASV sequences, respectively (Figure 4; Supplementary Table S4). However, microscopic counting uniquely identified Cyanophyta, Euglenophyta, and Xanthophyta, whereas ASVs exclusively detected Haptophyta, Prasinodermophyta, and Rhodophyta (Figure 4). At the species level, microscopic counting identified 86 species, obviously fewer than the 171 species detected through ASVs. The bloom-forming organism, Karenia sp., accounted for an average of 32.6% of the total phytoplankton abundance in S1-S4 based on microscopic counts but only 3.1% in ASV-based estimates (Figure 4). Nevertheless, Karenia sp. was among the most abundant phytoplankton ASVs, alongside Spiniferites mirabilis (4.2%), Oodinium pouchetii (4.0%), and Dinophyceae_XXX_sp. (3.4%).
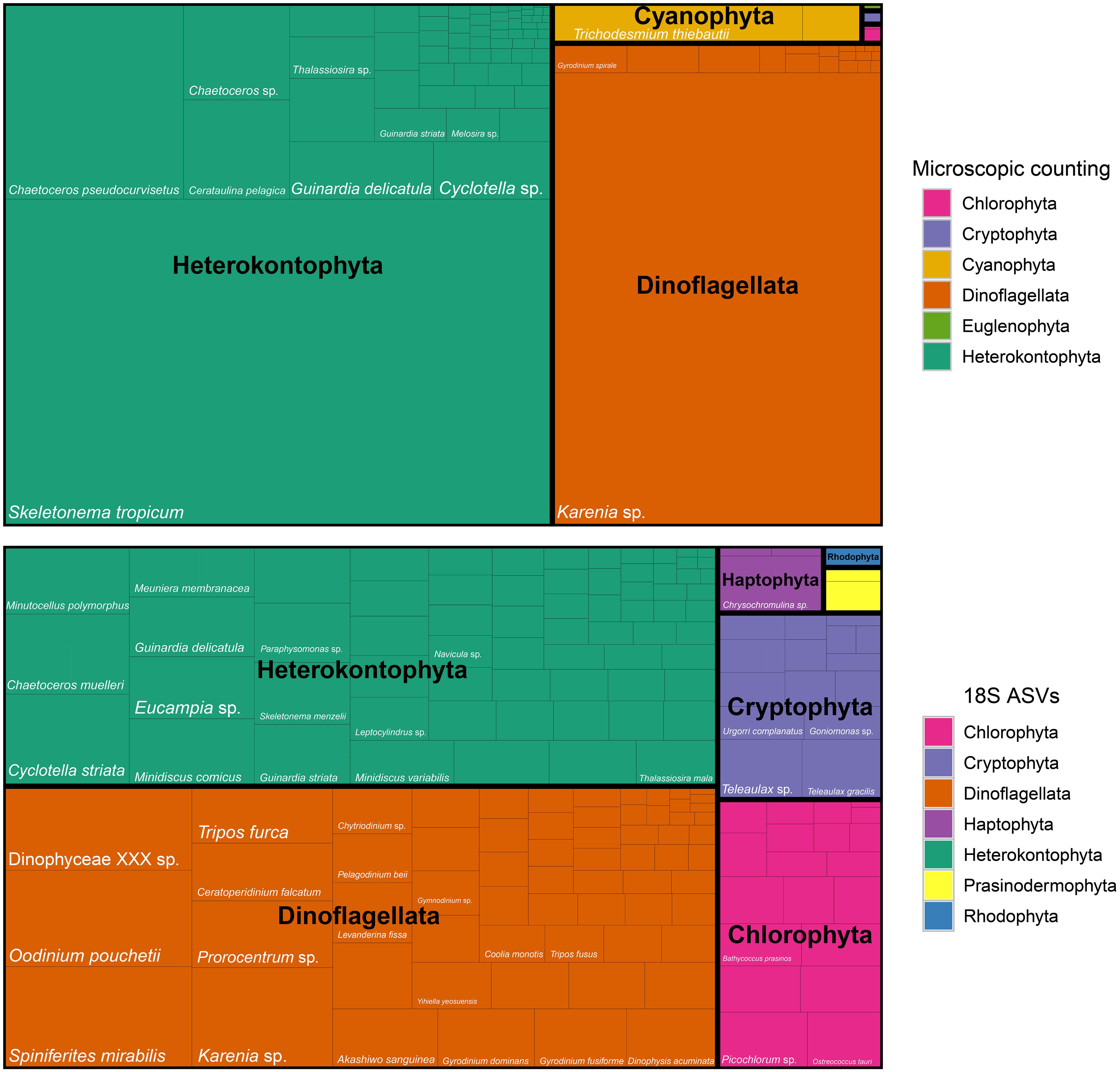
Figure 4. Treemap of phytoplankton composition at the phylum and species level derived from microscopic counting and 18S rDNA V4 sequences.
Karenia sp. was the most abundant species at S1, S2, and S3, contributing 91.3%, 70.5%, and 38.3% of the total abundance in microscopic counts, respectively. At S4, Skeletonema tropicum dominated, while the abundance of Karenia sp. ranked third, accounting for 7.5%. Despite these composition differences, the cell density of Karenia sp. remained within the same order of magnitude across S1–S4, with 8.9 × 10⁴, 4.9 × 10⁴, 1.4 × 10⁴, and 2.7 × 10⁴ cells L-1, respectively (Figure 5; Supplementary Table S4). The ASV abundance of Karenia sp. in S1–S4 remained relatively constant ranging from 13.9 (S1) to 19.6 (S3) in CLR-normalized values, accounting for 2.6% (S4) to 3.9% (S1) of the total ASV abundance (Figure 5).
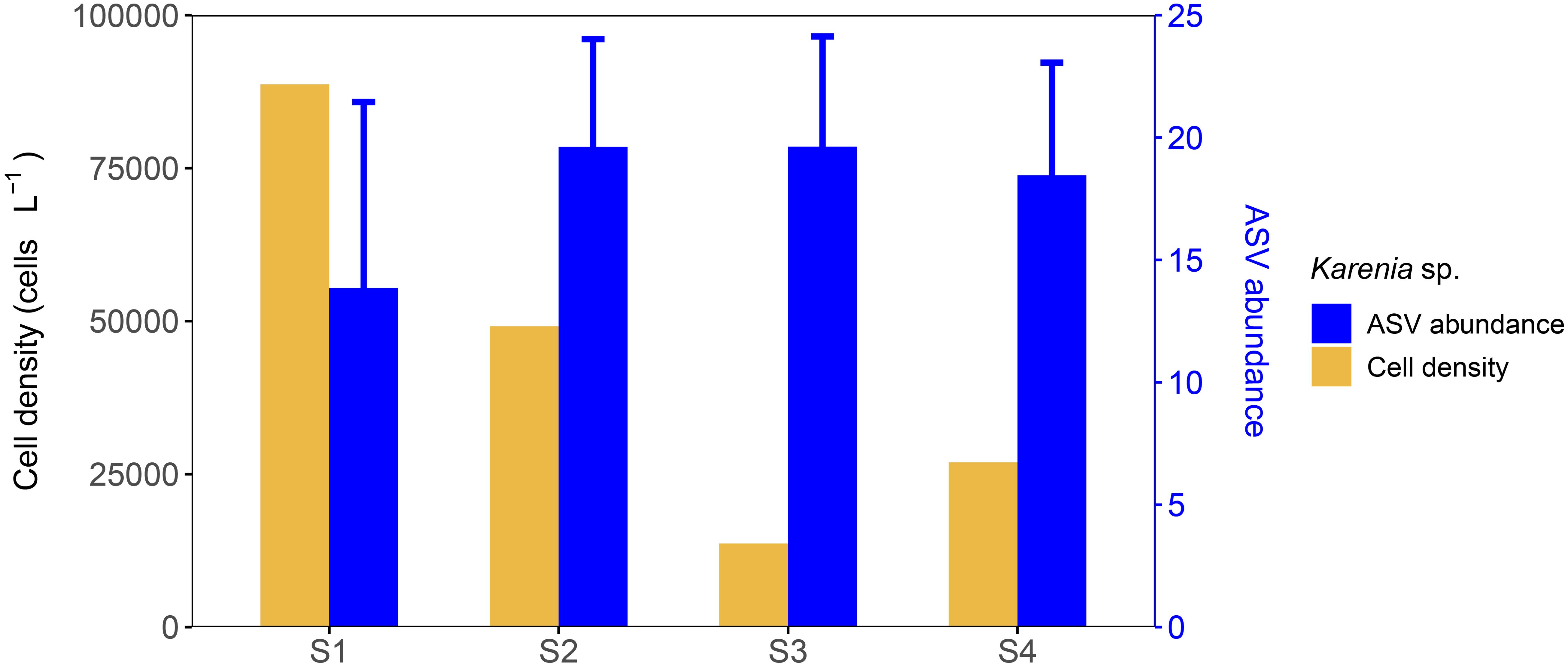
Figure 5. Karenia sp. cell density from microscopic counts and ASV relative abundance after CLR-normalized transformation.
3.4 Phylogenetic analysis of Karenia
The six ITS clone sequences and two D1-D3 LSU rDNA clone sequences were 676 bp and 1,042 bp in length, respectively, while the twenty-one 18S rDNA V4 ASV sequences ranged from 340 to 412 bp, with an average length of 378 bp. After trimming and alignment, the sequence lengths used for ITS, D1-D3 LSU rDNA, and SSU phylogenetic analyses were 658 bp, 897 bp, and 1,667 bp, respectively. The tree topologies generated by ML and BI analyses are mostly consistent, and the ML tree is presented here. The phylogenetic tree showed that both ITS clones BGERL260–BGERL265 and D1-D3 LSU rDNA clones BGERL266-267 clustered with strains of K. selliformis, supported by high bootstrap and posterior probability values of 88/1.0 and 98/1.0, respectively (Figure 6; Supplementary Figure S5). In the SSU phylogenetic tree, all 21 ASVs also clustered exclusively with K. selliformis strain CAWD79 (Figure 7).
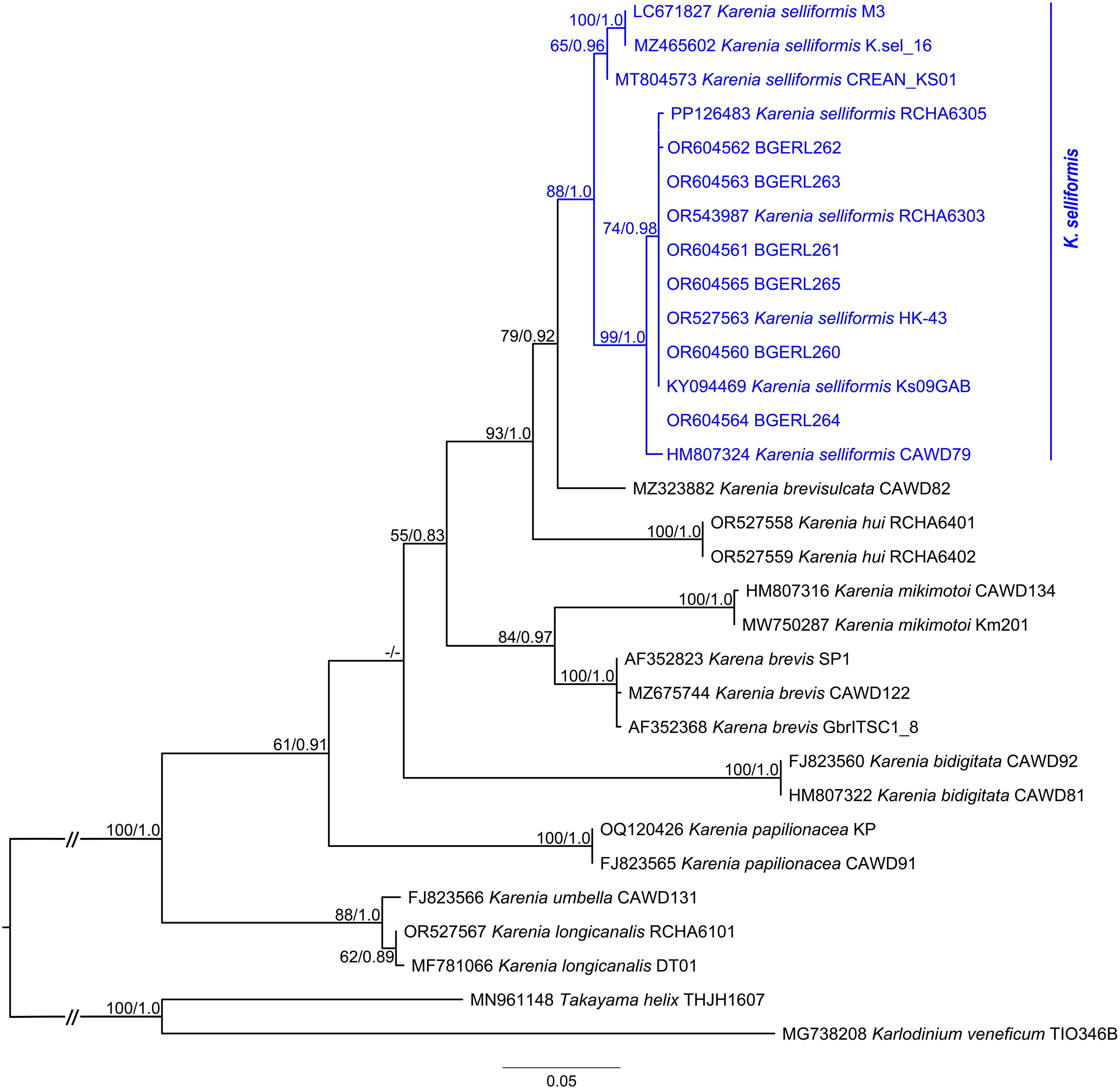
Figure 6. Phylogenetic tree based on ITS sequences. The bootstrap and posterior probability values below 50 and 0.75 are indicated by “-” in the tree.
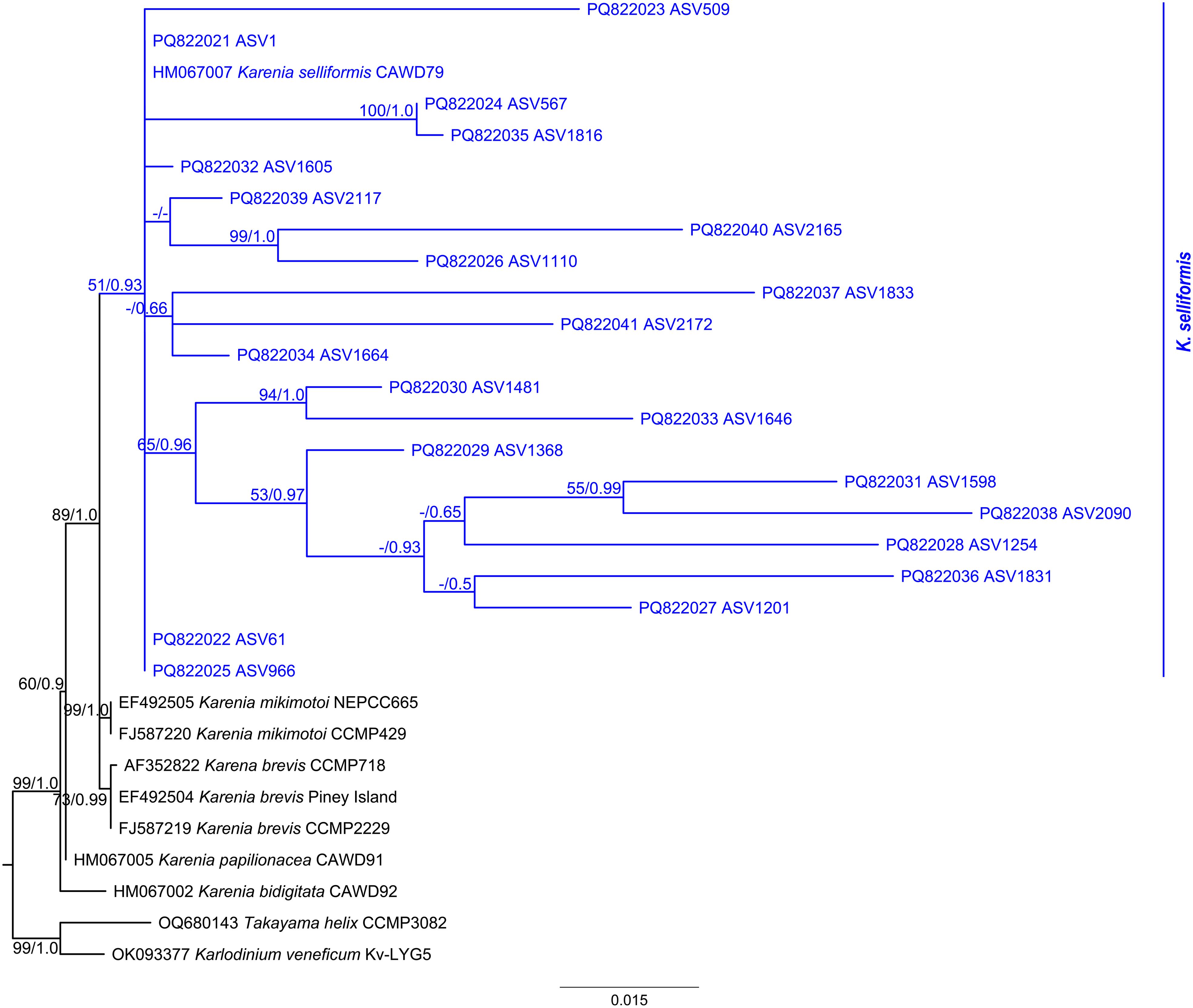
Figure 7. Amplicon placement phylogenetic tree based on SSU rDNA reference alignment and Karenia assigned 18S rDNA V4 amplicon sequence variant (ASV) sequences of this study. The bootstrap and posterior probability values below 50 and 0.50 are indicated by “-” in the tree.
Phylogenetic haplotype networks revealed notable genetic diversity among the 21 ASVs annotated as K. selliformis, with a nucleotide diversity of 0.342, 103 segregating sites, and 39 parsimony-informative sites. ASV1, ASV61, and ASV966 were grouped as the same haplotype and represented the most abundant ASVs, serving as the core vertex in the radiating network (Figure 8). Among these three ASVs, ASV1 was dominant (98.9%). Except for ASV1201, ASV1646, ASV1816, ASV1831, ASV2090, and ASV2165, all the remaining ASVs were connected to the core vertex by 1–19 base differences (Figure 8).
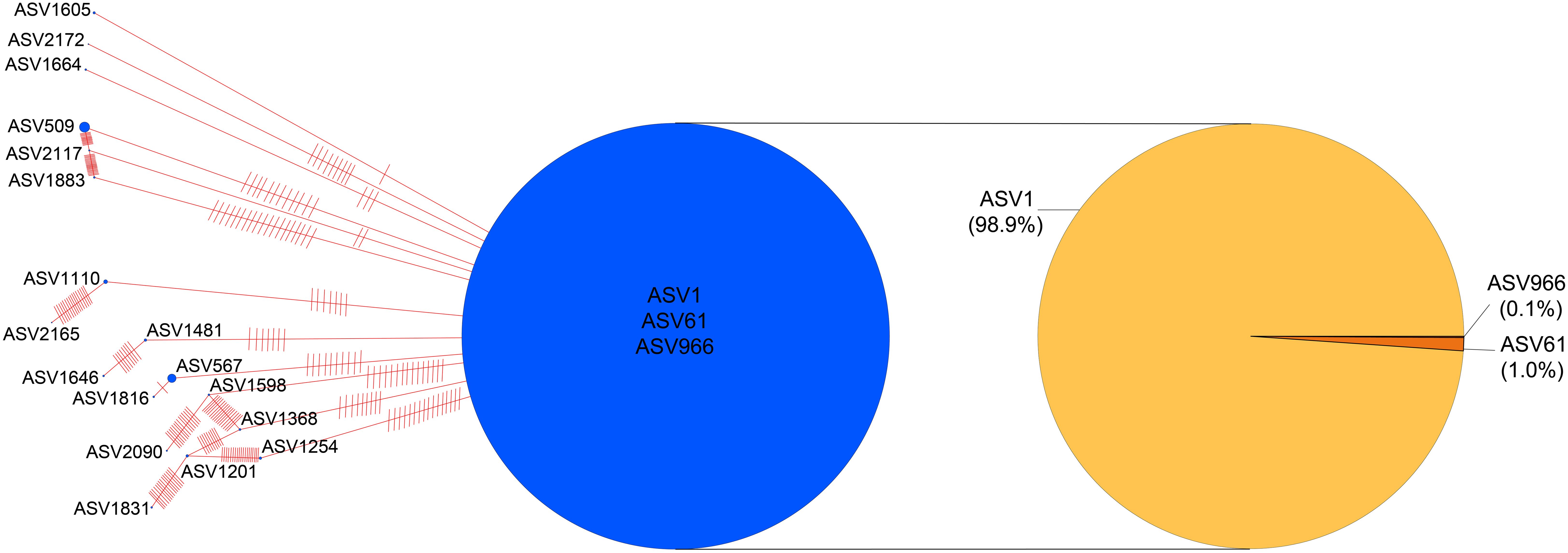
Figure 8. TCS network of ASVs annotated as Karenia selliformis. Vertex size corresponds to the relative abundance of ASVs, and red hatch marks on the edges indicate the number of base differences between ASVs.
3.5 Toxin analysis
Phytoplankton cells collected by filtration from 2250 mL, 4000 mL, and 4000 mL of seawater at sites S1, S2, and S4, respectively, were analyzed for hemolytic toxicity using rabbit erythrocytes. Combing the microscopic cell density for Karenia sp., 88,690, 49,143, and 26,931 cells L-1 and the molecular identification of bloom causative organism as unique K. selliformis (see 3.3 and 3.4), 1/8 of the total K. selliformis cell numbers were calculated as 2.5 × 104 from S1, 2.5 × 104 from S2, and 1.3 × 104 from S4, and exhibited hemolytic toxicities of 48.3%, 47.6%, and 45.2% to 5 × 107 rabbit erythrocyte cells, respectively.
In the LC-MS/MS analysis, BTX2, BTX3, DTX1-2, OA, and SPX1 were not detected. However, GYM-A was detected at all three sites (Figure 9). According to previous research, GYM-A is exclusively produced by K. selliformis (Hort et al., 2021; Wu et al., 2024). Therefore, K. selliformis from S1, S2, and S4 generated GYM-A contents of 2.3, 2.5, and 2.2 pg cell-1, respectively.
4 Discussion
4.1 Confirmation of K. selliformis as HAB causative organism
In this study, light microscopy identified Karenia sp. as the dominant species. However, the small cell size and the highly similar morphology within the genus made species-level identification challenging, especially given previous reports of the frequent coexistence of multiple Karenia species, along with other morphologically similar members of Kareniaceae, such as Karlodinium and Takayama, within the same bloom (Wolny et al., 2015; Orlova et al., 2022). The species from the in-situ bloom waters was successfully identified as K. selliformis based on the molecular cloning approach and sequencing of ITS (six) and D1-D3 LSU rDNA (two) clones. Phylogenetic trees supported the previous finding that this species can be divided into at least two phylotypes (Iwataki et al., 2022; Orlova et al., 2022; Cen et al., 2024), with ITS and D1-D3 rDNA sequences from this study grouped in the same phylotypes with those from South China Sea (Figures 6, 7; Supplementary Figure S5). The SSU rDNA tree topology further indicated that only K. selliformis has been found within all the twenty-one 18S rDNA V4 ASVs assigned to the genus Karenia. In addition, GYM-A was the only lipophilic toxin detected in the in-situ bloom water, which so far can only be produced by the dinoflagellate K. selliformis (Hort et al., 2021; Wu et al., 2024). The combination of the dominance of K. selliformis and GYM-A detection strongly suggest that K. selliformis is the causative organism for this harmful bloom event.
To the best of our knowledge, this is the first recorded K. selliformis bloom in Chinese waters. Although Karenia species are among the most detrimental HAB-causing organisms and frequently bloom across Chinese seawaters from north to south, their blooms have typically been attributed to K. mikimotoi (Li et al., 2019). Karenia selliformis was first identified in New Zealand and initially referred to as Gymnodinium sp. by Mackenzie et al. (1996). Later, Haywood et al. (2004) formally described the species K. selliformis using strain CAWD79 from Foveaux Strait, South Island, New Zealand. This species has caused blooms in Kuwait, Iran, New Zealand, Chile, Tunisia (Seki et al., 1995; Heil et al., 2001; Feki et al., 2013; Mardones et al., 2020; Baldrich et al., 2024; Dolatabadi and Attaran-Fariman, 2024), especially become serious in last years in East Asia waters (Russia, Japan), resulting in mass mortality of marine fauna in the region (Orlova et al., 2022; Takagi et al., 2022). In Japan, the economic loss caused by K. selliformis in Hokkaido in 2021 has exceeded the largest record set by the Chattonella bloom in the Seto Inland Sea in 1972 (Iwataki et al., 2022).
As a country heavily impacted by HABs since the 1990s, it is not surprising that China now records a K. selliformis bloom. In China, K. selliformis was firstly reported in 2016 during a K. mikimotoi dominant bloom in Tolor Harbor, Hong Kong, along with cells of K. longicanalis, K. papilionacea, and K. selliformis (Lin et al., 2020). Subsequently, more strains of K. selliformis were isolated from the South China Sea between 2021 and 2023, including the central Beibu Gulf in August 2023 (Cen et al., 2024). This occurred nearly two decades after the first large-scale K. mikimotoi bloom in the Zhujiang Estuary in 1998 (Li et al., 2019). However, recent studies on Karenia genetic diversity using DNA metabarcoding have consistently detected K. selliformis from environmental DNA in the East China Sea and South China Sea (Zhang et al., 2022, 2025). Combined with the recent confirmation of resting cysts in species within the genera Karenia and Karlodinium (Liu et al., 2020; Tang et al., 2021a), we speculate that K. selliformis should have persisted at low background densities in Chinese waters, including Beibu Gulf, for much longer than previously assumed.
To overcome the challenges of microscopic morphological observation in small and fragile cells, rDNA-based metabarcoding has been increasingly integrated with traditional microscopy in studies of phytoplankton diversity and abundance (Kezlya et al., 2023). This approach has led to the discovery of numerous new HAB species in marine environments (Stern et al., 2010; Cui et al., 2021; Smith et al., 2024). In this study, taxa such as Haptophyta, Prasinodermophyta, and Rhodophyta were uniquely detected through ASV analysis. However, it is noteworthy that results from metabarcoding and microscopy may differ substantially, especially for dinoflagellates. Dinoflagellates possess exceptionally large genome sizes among eukaryotes (~1–250 Gbp), with high and variable gene copy numbers both within and between species (Casabianca et al., 2017; Lin, 2024). These differences can be further amplified by PCR in metabarcoding approach. It likely accounts for the marked difference in Karenia abundance estimates in this study—32.6% by microscopy versus 3.1% by ASV analysis. To mitigate this issue in future work, in addition to 18S rDNA V4 primers, multiple longer or alternative primers targeting more variable regions such as ITS and LSU rDNA should be considered.
4.2 Potential drivers for K. selliformis outbreak
HABs in marine ecosystems are complex ecological disasters driven by internal and external factors. Biological characteristics of K. selliformis from the Beibu Gulf are currently limited. However, evidence from its bloom environmental conditions and cultures isolated from distinct regions suggests that K. selliformis has high physiological plasticity, enabling it to thrive across diverse marine environment (Medhioub et al., 2009; Vellojin et al., 2023). Blooms of K. selliformis have been documented across a range of temperatures, including 13.5–15°C in temperate waters of the Southern Pacific (42–53°S) (Clément et al., 2000; Uribe and Ruiz, 2001; Guillou et al., 2002; Mardones et al., 2020) and 7–23°C in temperate waters of the Northwestern Pacific (43–56°N) (Iwataki et al., 2022; Orlova et al., 2022). In subtropical Arabian waters (25°N), blooms have been reported at 25–28.6°C (Heil et al., 2001; Dolatabadi and Attaran-Fariman, 2024), while in this study, K. selliformis bloom was observed at 31°C in the tropical Beibu Gulf (21°N), pushing the upper limit of previously reported temperature regimes. The species has also bloomed across a wide salinity range of 20–50 in various water bodies (Medhioub et al., 2009; Feki-Sahnoun et al., 2017; Li et al., 2018; Guangxi Marine Bureau, 2023; Vellojin et al., 2023). In contrast, pH fluctuations during blooms tend to be narrower, mostly around 8.0 as the general seawater value. However, in laboratory experiment, K. selliformis can grow within a broad pH range of 7.0–9.0 (Vellojin et al., 2023). The increased pH values measured at field site S1 is in alignment with high Chl-a values and high photosynthetic rates reduce the CO2 and hence increases the pH in the water (Supplementary Table S1). In this study, nutrient levels including DIN and PO43- were low, with water quality classified as Class I. However, as the genus Karenia is mixotrophic, capable of utilizing a wide range of inorganic and organic nutrient sources (Li et al., 2019; Ahn and Glibert, 2024), nutrient availability is unlikely to have limited its outbreak in the Beibu Gulf, rather might be the mixotrophic an competitive advantage for the species in low nutrient environments. However, not all Karenia species are mixotrophic (Ok et al., 2023), and further study is needed to understand the nutritional acquisition strategy of K. selliformis in the Beibu Gulf.
In addition to the favorable marine environment supporting K. selliformis cell accumulation and maybe even growth in the Beibu Gulf, the bloom was also driven by physical factors, particularly sea surface wind. The weak westward onshore sea surface winds before and during the bloom on August 1-2, 2023 produced a stable water environment, which is favorable for unarmored dinoflagellates, such as Karenia, as they possess delicate cell structures due to the lack of cellulose thecae, highlighting their preference for low-disturbance environments (Brand et al., 2012; Stumpf et al., 2022). While onshore sea surface wind may drive the seawater towards shoreline and cause cell accumulation near coast. A similar phenomenon was found during the large-scale K. mikimotoi bloom along the Fujian coast in May–June 2012, when the northerly onshore winds accumulated water towards nearshore, and provide a stable hydrodynamic environment conducive to cell division and bloom formation (Deng et al., 2016). The unprecedented K. selliformis bloom in eastern Hokkaido, Japan, during autumn 2021, was also associated with southerly wind-driven current, which resulted in transportation of offshore water towards the shoreline, causing bloom outbreak and prolong due to algal accumulation in the semi-closed fishing port (Hasegawa et al., 2022). In this study, the strong southwest winds on August 3–4, following the bloom, contributed to the degradation of K. selliformis in Lianzhou Bay and Tieshan Bay, which is a common factor in HAB decline (Li et al., 2023).
The westward wind and currents most likely transported cells of K. selliformis from Lianzhou Bay and Tieshan Bay westward along the coastline, which explains the Karenia outbreak in Fangcheng coastal waters on August 7, 2023, with cell density ranging from 2.3-9.7×104 cells L-1, as well as scattered dead fish floating on the sea surface (Guangxi Marine Bureau, 2023). Similar to this study, seawater pH, DO, chemical oxygen demand, DIN, PO43-, Chl-a concentrations in Fangcheng waters were all within the typical range for the region, with most indices belonging to water quality class I (Guangxi Marine Bureau, 2023). The government reported Karenia cells as K. mikimotoi, possibly based on microscopic observation (Guangxi Marine Bureau, 2023). However, we speculate that the cells were actually K. selliformis, particularly given that K. mikimotoi has not been detected so far in the Beibu Gulf, even using DNA metabarcoding technology, neither through ASV analysis in this study, nor via the Operational Taxonomic Unit analysis in Li (2022).
4.3 Possible causes for cultured fish mortality
Fish mortality is the most common and devastating consequence of Karenia blooms, primarily attributed to gill damage caused by the hemolytic toxicity of Karenia cells (Li et al., 2019; Sakamoto et al., 2021). This toxin can lyse blood cells in the gills, trigger excessive mucus, entangle gill filaments, and ultimately lead to fish suffocation. Hemolytic toxins are not a single specific compound but a group of components, only some of which have been identified, such as all-cis-3,6,9,12,15-octadecapentaenoic acid, monogalactosyl diacylglycerol, and digalactosyl diacylglycerol (Brand et al., 2012; Li et al., 2019). Although in this study, some other potential fish-killing algal organisms, like Akashiwo sanguinea, Alexandrium leei, Margalefidinium fulvescens (Gu et al., 2022), were also detected, their abundance/reads is negligible comparing to K. selliformis (Supplementary Table S4). As all tested field samples exhibited strong hemolytic activity (45.2%–48.3%), it supports hemolytic toxicity produced by K. selliformis is responsible for the cultured Trachinotus ovatus mortality during the bloom in the Beibu Gulf.
Karenia selliformis generally has stronger hemolysis and cytotoxicity than other Karenia species. Among the four Karenia species from Hong Kong blooms in 2016, K. selliformis (strain CCHA-7) had lower hemolytic toxicity (42.3%) than K. longicanalis (46.4%), but higher than K. mikimotoi (37.8%) and K. papilionacea (37.0%) (Lin et al., 2020). Both cells and culture media of K. selliformis culture (CAWD79, New Zealand) consistently contained hemolytic activity, with mean hemolytic toxicity of 102 relative to K. brevis (Tatters et al., 2010). In gill cell-based assay, K. selliformis (CREAN-KS00, Chile) was highly cytotoxic to reduce gill cell viability down to 28.3–44.7% of controls by supernatant and lysed cell suspensions (Mardones et al., 2020). Similarly, K. bicuneiformis can be prey for common heterotrophic dinoflagellates, whereas K. selliformis (NIES 4541, Japan) lyse all predator (Park et al., 2024). The high potential of K. selliformis to exert toxic effects on marine organisms within the ecosystem has also been documented. The Hokkaido K. selliformis (Ks-01) could markedly decrease the survival and ingestion of copepod, no matter in direct contact, indirect interaction, or ingestion of K. selliformis (Ohnishi et al., 2024). During the interaction between calanoid copepod Acartia hongi and Karenia, Ok et al. (2024) concluded K. selliformis (NIES-4541, Japan) had almost the highest mortality to copepod at similar dinoflagellate concentrations, and the lowest ingestion rate among A. hongi feeding on harmful dinoflagellates. K. selliformis strain obtained from the South China Sea had much stronger acute toxicity to rotifer Brachionus plicatilis than K. umbella, with LD50 (lethal dose fifty) 2,750 mL-1 vs. 14,011 mL-1 (Li et al., 2018). Among the five Chinese Karenia species, K. hui, K. longicanalis, K. mikimotoi, K. papilionacea, and K. selliformis, K. selliformis (HK-43, China) showed the strongest acute toxicity on marine model organism of medaka Oryzias melastigma, resulting in 100% mortality within 4 h and the most shortest LD50 of 2h (Cen et al., 2024). The simulated food chain from K. selliformis (GM94GAB, Tunisia) to brine shrimp and further to marine medaka induced abnormal swimming behavior, pathological damage to the intestine and liver tissues, and energy metabolism disorders in medaka (Liu et al., 2023). Therefore, K. selliformis has been proved to be a highly toxic organism, which explains the mass mortality of aquaculture fish in the Beibu Gulf.
4.4 GYM-A produced by K. selliformis in the bloom
Besides hemolytic toxicity, GYM-A, a “fast-acting” lipophilic toxin was also detected in field samples in this study, whereas the other lipophilic toxins, i.e. neurotoxic shellfish toxins (BTX2, BTX3), diarrhetic shellfish toxins (DTX1-2, OA), and SPX1, were not detected. GYM-A belongs to cyclic imines toxins family, initially identified from oyster (Tiostrea chilensis) in New Zealand in 1994, and displays toxicity due to its potent antagonists of the nicotinic acetylcholine receptors (Seki et al., 1995; Molgó et al., 2017). GYM-A, along with its analogs GYM-B and GYM-C, is primarily produced by K. selliformis, though not all isolates of this species generate GYM-A (Miles et al., 2000, 2003; Mardones et al., 2020). In this study, K. selliformis from the bloom in the Beibu Gulf generated 2.2–2.5 pg GYM-A cell-1, which is comparable to a K. selliformis strain (GM94GAB) from Tunisia with a range of 1.2–22.4 pg cell-1 (Medhioub et al., 2009), but higher than 0.9 pg cell-1 produced by the strain (HK-43) from Tolo Harbor, South China Sea (Cen et al., 2024). Considering the extraction efficiency of GYM-A, the actual toxin quota collected by filtration in this study may represent only ~34% of the total GYM-A (Tang et al., 2021b), with the residual lost in the water during harvesting process.
The role of GYM-A in the marine species mortaility is unclear. Previous studies suggest that the neurotoxicity of K. selliformis, due to GYM-A and its analogues, can sensitize marine species to cytotoxins (Dragunow et al., 2005). However, Mardones et al. (2020) pointed out that the massive fauna mortality during K. selliformis bloom along the Chilean coast was likely induced by ichthyotoxic polyunsaturated fatty acids and/or uncharacterized toxic compounds, rather than GYMs. As a lipophilic toxin, GYM-A is well-known for its accumulation in shellfish and the potential risks to human health. Both GYM-A and its fatty acid conjugates have been widely detected in various shellfish worldwide (Finch et al., 2024). In China, shellfish with high GYM-A concentrations are primarily found in the South China Sea, particularly in the Beibu Gulf (Ji et al., 2020), which indirectly supports the widespread presence of K. selliformis in the region. In this area, GYM-A and SPX1 are the predominant lipophilic phycotoxins in shellfish, with a significant positive correlation observed between GYM-A levels in shellfish and seawater (Xu et al., 2021b; Ji et al., 2022). However, only recently have cultures and environmental DNA analyses confirmed the presence of K. selliformis for this toxin in the Beibu Gulf (Li, 2022; Cen et al., 2024). Further research is required to elucidate the role of GYM-A in K. selliformis blooms and its impact on aquaculture and other organisms within the ecosystem.
5 Conclusions
This study investigated the first mass fish mortality associated with a Karenia bloom in the Beibu Gulf, China. During the bloom, seawater maintained a good quality of Class I. Using a combination of traditional microscopic observation, rRNA gene cloning, and 18S rDNA ASV analysis of in-situ bloom samples, K. selliformis was identified as the bloom-causing organism. Weak westward sea surface winds before and during the bloom facilitated the accumulation and outbreak of K. selliformis in Lianzhou and Tieshan coastal waters, while hemolytic toxicity was the predominant cause of fish mortality. The “fast-acting” toxin of GYM-A was detected, but its role in K. selliformis bloom remains unclear. To our knowledge, this study represents the first documented fish-killing event caused by K. selliformis in Chinese waters. Further research on its physiology, toxicity mechanisms, and bloom formation under the background of climate change is essential for understanding its impact on marine aquaculture in the Beibu Gulf.
Data availability statement
The original contributions presented in the study are included in the article/Supplementary Material, further inquiries can be directed to the corresponding author/s.
Ethics statement
Ethical approval was not required for the studies on animals in accordance with the local legislation and institutional requirements because only commercially available established cell lines were used.
Author contributions
YX: Data curation, Formal analysis, Funding acquisition, Methodology, Visualization, Writing – original draft, Writing – review & editing. XW: Data curation, Visualization, Writing – original draft. CH: Data curation, Formal analysis, Methodology, Writing – review & editing. ND: Validation, Writing – review & editing, Methodology. XZ: Methodology, Writing – review & editing. SN: Data curation, Software, Writing – review & editing. MT: Writing – review & editing, Methodology. WL: Funding acquisition, Writing – review & editing, Investigation. UJ: Writing – review & editing, Conceptualization, Funding acquisition, Writing – original draft.
Funding
The author(s) declare that financial support was received for the research and/or publication of this article. This research was supported by the Natural Science Foundation of Guangxi Province (2020GXNSFDA297001, 2024GXNSFAA010136), the National Natural Science Foundation of China (41976155, 42376211), the Project of Southern Marine Science and Engineering Guangdong Laboratory (Zhuhai) (SML2024SP023), and the scientific research capacity building project for Beibu Gulf Marine Ecological Environment Field Observation and Research Station of Guangxi (Guike23-026-271).
Conflict of interest
The authors declare that the research was conducted in the absence of any commercial or financial relationships that could be construed as a potential conflict of interest.
Generative AI statement
The author(s) declare that no Generative AI was used in the creation of this manuscript.
Publisher’s note
All claims expressed in this article are solely those of the authors and do not necessarily represent those of their affiliated organizations, or those of the publisher, the editors and the reviewers. Any product that may be evaluated in this article, or claim that may be made by its manufacturer, is not guaranteed or endorsed by the publisher.
Supplementary material
The Supplementary Material for this article can be found online at: https://www.frontiersin.org/articles/10.3389/fmars.2025.1582234/full#supplementary-material
References
Adachi M., Sako Y., Ishida Y. (1994). Restriction fragment length polymorphism of ribosomal DNA internal transcribed spacer and 5.8s regions in Japanese Alexandrium species (Dinophyceae). J. Phycol. 30, 857–863. doi: 10.1111/j.0022-3646.1994.00857.x
Ahn S. H., Glibert P. M. (2024). Temperature-dependent mixotrophy in natural populations of the toxic dinoflagellate Karenia brevis. Water. 16, 1555. doi: 10.3390/w16111555
Allen G. C., Flores-Vergara M., Krasynanski S., Kumar S., Thompson W. (2006). A modified protocol for rapid DNA isolation from plant tissues using cetyltrimethylammonium bromide. Nat. Protoc. 1, 2320–2325. doi: 10.1038/nprot.2006.384
Baldrich Á.M., Díaz P. A., Rosales S. A., Rodríguez-Villegas C., Álvarez G., Pérez-Santos I., et al. (2024). An unprecedented bloom of oceanic Dinoflagellates (Karenia spp.) inside a fjord within a highly dynamic multifrontal ecosystem in Chilean Patagonia. Toxins. 16, 77. doi: 10.3390/toxins16020077
Brand L. E., Campbell L., Bresnan E. (2012). Karenia: The biology and ecology of a toxic genus. Harmful Algae. 14, 156–178. doi: 10.1016/j.hal.2011.10.020
Callahan B. J., McMurdie P. J., Rosen M. J., Han A. W., Johnson A. J. A., Holmes S. P. (2016). DADA2: High-resolution sample inference from Illumina amplicon data. Nat. Methods 13, 581–583. doi: 10.1038/nmeth.3869
Casabianca S., Cornetti L., Capellacci S., Vernesi C., Penna A. (2017). Genome complexity of harmful microalgae. Harmful algae. 63, 7–12. doi: 10.1016/j.hal.2017.01.003
Cen J. Y., Lu S. H., Moestrup Ø., Jiang T., Ho K. C., Li S., et al. (2024). Five Karenia species along the Chinese coast: With the description of a new species, Karenia hui sp. nov. (Kareniaceae, Dinophyta). Harmful Algae. 137, 102645. doi: 10.1016/j.hal.2024.102645
Chao A., Gotelli N. J., Hsieh T. C., Sander E. L., Ma K. H., Colwell R. K., et al. (2014). Rarefaction and extrapolation with Hill numbers: a framework for sampling and estimation in species diversity studies. Ecol. Monogr. 84, 45–67. doi: 10.1890/13-0133.1
Clément A., Seguel M., Arzul G., Guzman L., Alarcon C. (2000). “Widespread outbreak of a haemolytic, ichthyotoxic Gymnodinium sp. in southern Chile,” in Harmful algal blooms 2000. Eds. Hallegraeff G. M., Blackburn S. I., Bolch C. J., Lewis R. J. (Proceedings of the Ninth International Conference on Harmful Algal Blooms, Hobart, Australia), 66–69.
Cui Z., Xu Q., Gibson K., Liu S., Chen N. (2021). Metabarcoding analysis of harmful algal bloom species in the Changjiang Estuary, China. Sci. Total Environ. 782, 146823. doi: 10.1038/nmeth.2109
Darriba D., Taboada G. L., Doallo R., Posada D. (2012). jModelTest 2: more models, new heuristics and high-performance computing. Nat. Methods. 9, 772. doi: 10.1038/nmeth.2109
Deng H., Guan W., Cao Z., Bao M., Chen Q. (2016). Analysis of hydrological and meteorological factors causing Karenia mikimotoi bloom in 2012 along Fujian coast. J. Mar. Sci. 34, 28–38. doi: 10.3969/j.issn.1001-909X.2016.04.004
Dolatabadi F., Attaran-Fariman G. (2024). Bloom and phylogeny of the harmful dinoflagellate Karenia selliformis (Dinophyceae) isolated from Iran southeast coast (northern of Oman Sea). Nova Hedwigia. 119, 1–20. doi: 10.1127/nova_hedwigia/2024/0826
Dragunow M., Trzoss M., Brimble M. A., Cameron R., Beuzenberg V., Holland P., et al. (2005). Investigations into the cellular actions of the shellfish toxin gymnodimine and analogues. Environ. Toxicol. Pharmacol. 20, 305–312. doi: 10.1016/j.etap.2005.02.008
Feki W., Hamza A., Frossard V., Abdennadher M., Hannachi I., Jacquot M., et al. (2013). What are the potential drivers of blooms of the toxic dinoflagellate Karenia selliformis? A 10-year study in the Gulf of Gabes, Tunisia, southwestern Mediterranean Sea. Harmful Algae. 23, 8–18. doi: 10.1016/j.hal.2012.12.001
Feki-Sahnoun W., Hamza A., Njah H., Barraj N., Mahfoudi M., Rebai A., et al. (2017). A Bayesian network approach to determine environmental factors controlling Karenia selliformis occurrences and blooms in the Gulf of Gabès, Tunisia. Harmful Algae. 63, 119–132. doi: 10.1016/j.hal.2017.01.013
Finch S. C., Harwood D. T., Boundy M. J., Selwood A. I. (2024). A review of cyclic imines in shellfish: Worldwide occurrence, toxicity and assessment of the risk to consumers. Mar. Drugs 22, 129. doi: 10.3390/md22030129
General Administration of Quality Supervision, Inspection and Quarantine of China, National Standardization Administration (2008). National standard of the people’s republic of China (GB 17378.3-2007): the specification for marine monitoring (Beijing: The Chinese Standards Press).
Gu H., Wu Y., Lü S., Lu D., Tang Y. Z., Qi Y. (2022). Emerging harmful algal bloom species over the last four decades in China. Harmful Algae. 111, 102059. doi: 10.1016/j.hal.2021.102059
Guangxi Marine Bureau (2023). Toxic red tide monitoring information in Fangcheng sea area. Available online at: http://hyj.gxzf.gov.cn/hykp_66917/hyzh/t16954480.shtml (Accessed February 24, 2025).
Guillou L., Bachar D., Audic S., Bass D., Berney C., Bittner L., et al. (2012). The Protist Ribosomal Reference database (PR2): a catalog of unicellular eukaryote Small Sub-Unit rRNA sequences with curated taxonomy. Nucleic Acids Res. 41, D597–D604. doi: 10.1093/nar/gks1160
Guillou L., Nézan E., Cueff V., Erard-Le Denn E., Cambon-Bonavita M.-A., Gentien P., et al. (2002). Genetic diversity and molecular detection of three toxic dinoflagellate genera (Alexandrium, Dinophysis, and Karenia) from French coasts. Protist. 153, 223–238. doi: 10.1078/1434-4610-00100
Guindon S., Gascuel O. (2003). A simple, fast, and accurate algorithm to estimate large phylogenies by maximum likelihood. Syst. Biol. 52, 696–704. doi: 10.1080/10635150390235520
Guiry M. D., Guiry G. M. (2025). AlgaeBase. Available online at: https://www.algaebase.org (Accessed February 24, 2025).
Hasegawa N., Watanabe T., Unuma T., Yokota T., Izumida D., Nakagawa T., et al. (2022). Repeated reaching of the harmful algal bloom of Karenia spp. around the Pacific shoreline of Kushiro, eastern Hokkaido, Japan, during autumn 2021. Fish Sci. 88, 787–803. doi: 10.1007/s12562-022-01642-w
Haywood A. J., Steidinger K. A., Truby E. W., Bergquist P. R., Bergquist P. L., Adamson J., et al. (2004). Comparative morphology and molecular phylogenetic analysis of three new species of the genus Karenia (Dinophyceae) from New Zealand. J. Phycol. 40, 165–179. doi: 10.1046/j.1529-8817.2004.02149.x
He C., Xu S., Kang Z. J., Song S. Q., Li C. W. (2021). Prokaryotic community composition and structure during Phaeocystis globosa blooms in the Beibu Gulf, China. Aquat. Microb. Ecol. 86, 137–151. doi: 10.3354/ame01962
Heil C. A., Glibert P. M., Al-Sarawi M. A., Faraj M., Behbehani M., Husain M. (2001). First record of a fish-killing Gymnodinium sp. bloom in Kuwait Bay, Arabian Sea: chronology and potential causes. Mar. Ecol. Prog. Ser. 214, 15–23. doi: 10.3354/meps214015
Hort V., Abadie E., Arnich N., Dechraoui Bottein M.-Y., Amzil Z. (2021). Chemodiversity of brevetoxins and other potentially toxic metabolites produced by Karenia spp. and their metabolic products in marine organisms. Mar. Drugs 19, 656. doi: 10.3390/md19120656
Iwataki M., Lum W. M., Kuwata K., Takahashi K., Arima D., Kuribayashi T., et al. (2022). Morphological variation and phylogeny of Karenia selliformis (Gymnodiniales, Dinophyceae) in an intensive cold-water algal bloom in eastern Hokkaido, Japan. Harmful Algae. 114, 102204. doi: 10.1016/j.hal.2022.102204
Ji Y., Che Y. J., Wright E. J., McCarron P., Hess P., Li A. F. (2020). Fatty acid ester metabolites of gymnodimine in shellfish collected from China and in mussels (Mytilus galloprovincialis) exposed to Karenia selliformis. Harmful Algae. 92, 101774. doi: 10.1016/j.hal.2020.101774
Ji Y., Yan G. W., Wang G. X., Liu J. W., Tang Z. X., Yan Y. J., et al. (2022). Prevalence and distribution of domoic acid and cyclic imines in bivalve mollusks from Beibu Gulf, China. J. Hazard. Mater. 423, 127078. doi: 10.1016/j.jhazmat.2021.127078
Katoh K., Standley D. M. (2013). MAFFT multiple sequence alignment software version 7: improvements in performance and usability. Mol. Biol. Evol. 30, 772–780. doi: 10.1093/molbev/mst010
Kezlya E., Tseplik N., Kulikovskiy M. (2023). Genetic markers for metabarcoding of freshwater microalgae: Review. Biology. 12, 1038. doi: 10.3390/biology12071038
Kumar S., Stecher G., Li M., Knyaz C., Tamura K. (2018). MEGA X: molecular evolutionary genetics analysis across computing platforms. Mol. Biol. Evol. 35, 1547–1549. doi: 10.1093/molbev/msy096
Leigh J. W., Bryant D., Nakagawa S. (2015). POPART: full-feature software for haplotype network construction. Methods Ecol. Evol. 6, 1110–1116. doi: 10.1111/2041-210X.12410
Li W. (2022). Phytoplankton community structure and molecular diversity in Beibu Gulf. [dissertation/master’s thesis] (Gaungzhou (China: Jinan University).
Li S., Cen J., Wang J., Lv S. (2018). Study on acute toxicity of two Karenia (Dinophyceae) species to rotifer Brachionus plicatilis. Mar. Environ. Science. 37, 28–32. doi: 10.13634/j.cnki.mes20180105
Li J., Lai J. X., Xu G. L., Xu M. B., Wu M., Yan X. M., et al. (2024). Detecting the Phaeocystis globosa bloom and characterizing its bloom condition in the northern Beibu Gulf using MODIS measurements. Mar. pollut. Bull. 209, 117273. doi: 10.1016/j.marpolbul.2024.117273
Li X., Yan T., Lin J., Yu R., Zhou M. (2017). Detrimental impacts of the dinoflagellate Karenia mikimotoi in Fujian coastal waters on typical marine organisms. Harmful Algae. 61, 1–12. doi: 10.1016/j.hal.2016.11.011
Li X. D., Yan T., Yu R. C., Zhou M. J. (2019). A review of karenia mikimotoi: Bloom events, physiology, toxicity and toxic mechanism. Harmful Algae. 90, 101702. doi: 10.1016/j.hal.2019.101702
Li Y., Zhu S., Hang X., Sun L., Li X., Luo X., et al. (2023). Variation of local wind fields under the background of climate change and its impact on algal blooms in Lake Taihu, China. Water. 15, 4258. doi: 10.3390/w15244258
Lin S. (2024). A decade of dinoflagellate genomics illuminating an enigmatic eukaryote cell. BMC Genomics 25, 932. doi: 10.1186/s12864-024-10847-5
Lin Y., Cen J., Wang J., Liang Q., Lv S. (2020). Preliminary study on interspecific relationship and hemolytic activity of four Karenia species from South China Sea. Oceanologia Et Limnologia Sinica. 51, 1402–1411. doi: 10.11693/hyhz20200100002
Ling C., Trick C. G. (2010). Expression and standardized measurement of hemolytic activity in Heterosigma akashiwo. Harmful Algae. 9, 522–529. doi: 10.1016/j.hal.2010.04.004
Liu Q. Y., Chen Z. M., Li D. W., Li A. F., Ji Y., Li H. Y., et al. (2023). Toxicity and potential underlying mechanism of Karenia selliformis to the fish Oryzias melastigma. Aquat. Toxicol. 262, 106643. doi: 10.1016/j.aquatox.2023.106643
Liu Y., Hu Z., Deng Y., Tang Y. Z. (2020). Evidence for resting cyst production in the cosmopolitan toxic dinoflagellate Karlodinium veneficum and the cyst distribution in the China seas. Harmful Algae. 93, 101788. doi: 10.1016/j.hal.2020.101788
Lü S., Cen J., Wang J., Ou L. (2019). The research status quo, hazard, and ecological mechanisms of Karenia mikimotoi red tide in coastal waters of China. Oceanologia Et Limnologia Sinica. 50, 487–494. doi: 10.11693/hyhz20181000255
Mackenzie L., Haywood A., Adamson J., Truman P., Till D., Satake M., et al. (1996). “Gymnodimine contamination of shellfish in New Zealand,” in Harmful and toxic algal blooms proceeding of the seventh international conference on toxic phytoplankton. Eds. Yasumoto T., Oshima Y., Fukuyo Y. (Intergovernmental Oceanographic Commission of UNESCO, Sendai, Japan), 97–100.
Mardones J. I., Norambuena L., Paredes J., Fuenzalida G., Dorantes-Aranda J. J., Chang K. J. L., et al. (2020). Unraveling the Karenia selliformis complex with the description of a non-gymnodimine producing Patagonian phylotype. Harmful Algae. 98, 101892. doi: 10.1016/j.hal.2020.101892
Martin M. (2011). Cutadapt removes adapter sequences from high-throughput sequencing reads. EMBnet J. 17, 10–12. doi: 10.14806/ej.17.1.200
Medhioub A., Medhioub W., Amzil Z., Sibat M., Bardouil M., Ben Neila I., et al. (2009). Influence of environmental parameters on Karenia selliformis toxin content in culture. Cah. Biol. Mar. 50, 333–342.
Miles C. O., Wilkins A. L., Stirling D. J., MacKenzie A. L. (2000). New analogue of gymnodimine from a Gymnodinium species. J. Agric. Food Chem. 48, 1373–1376. doi: 10.1021/jf991031k
Miles C. O., Wilkins A. L., Stirling D. J., MacKenzie A. L. (2003). Gymnodimine C, an isomer of Gymnodimine B, from Karenia selliformis. J. Agric. Food Chem. 51, 4838–4840. doi: 10.1021/jf030101r
Molgó J., Marchot P., Aráoz R., Benoit E., Iorga B. I., Zakarian A., et al. (2017). Cyclic imine toxins from dinoflagellates: a growing family of potent antagonists of the nicotinic acetylcholine receptors. J. Neurochem. 142, 41–51. doi: 10.1111/jnc.13995
National Environmental Protection Agency (2004). GB 3097–1997, Marine water quality standard (Beijing: China Environmental Press).
Natsuike M., Kanamori M., Akino H., Sakamoto S., Iwataki M. (2023). Lethal effects of the harmful dinoflagellate Karenia selliformis (Gymnodiniales, Dinophyceae) on two juvenile kelp sporophytes Saccharina japonica and S. sculpera(Laminariales, Phaeophyceae). Reg. Stud. Mar. Sci. 65, 103094. doi: 10.1016/j.rsma.2023.103094
Niu Z., Guan W. B., Wang J. X., Yuan Y. Q., Kong F. Z., Liu C., et al. (2022). Dynamics of Phaeocystis globosa bloom and implications for its seed sources in the Beibu Gulf, China. J. Oceanol. Limnol. 40, 2385–2400. doi: 10.1007/s00343-022-1447-0
Nunn G., Theisen B., Christensen B., Arctander P. (1996). Simplicity-correlated size growth of the nuclear 28S ribosomal RNA D3 expansion segment in the crustacean order Isopoda. J. Mol. Evol. 42, 211–223. doi: 10.1007/BF02198847
Ohnishi T., Taniuchi Y., Watanabe T., Shikata T., Kasai H. (2024). Experimental assessment of copepod survival in response to the harmful dinofiagellate Karenia selliformis from the southeastern coast of Hokkaido, Japan. Plankton Benthos Res. 19, 88–97. doi: 10.3800/pbr.19.88
Ok J. H., Jeong H. J., Lim A. S., Kang H. C., You J. H., Park S. A., et al. (2023). Lack of mixotrophy in three Karenia species and the prey spectrum of Karenia mikimotoi (Gymnodiniales, Dinophyceae). Algae. 38, 39–55. doi: 10.4490/algae.2023.38.2.28
Ok J. H., Jeong H. J., You J. H., Park S. A., Kang H. C., Eom S. H., et al. (2024). Interactions between the calanoid copepod Acartia hongi and the bloom-forming dinoflagellates Karenia bicuneiformis and K. selliformis. Mar. Biol. 171, 112. doi: 10.1007/s00227-024-04427-0
Orlova T. Y., Aleksanin A. I., Lepskaya E. V., Efimova K. V., Selina M. S., Morozova T. V., et al. (2022). A massive bloom of Karenia species (Dinophyceae) off the Kamchatka coast, Russia, in the fall of 2020. Harmful Algae. 120, 102337. doi: 10.1016/j.hal.2022.102337
Park S. A., Ok J. H., Eom S. H., Kwon M. J., You J. H., Kang H. C., et al. (2024). Differential interactions between the bloom-forming dinoflagellates Karenia bicuneiformis and Karenia selliformis and heterotrophic dinoflagellates. Algae. 39, 255–275. doi: 10.4490/algae.2024.39.11.30
Posit team (2024). RStudio: Integrated development environment for R (Boston, MA: Posit Software, PBC). Available at: http://www.posit.co/ (Accessed April 18, 2025).
R Core Team (2024). R: A language and environment for statistical computing (Vienna, Austria: R Foundation for Statistical Computing). Available at: https://www.R-project.org/ (Accessed April 18, 2025).
Ren X., Yu Z., Song X., Zhu J., Wang W., Cao X. (2022). Effects of modified clay on the formation of Phaeocystis globosa colony revealed by physiological and transcriptomic analyses. Sci. Total Environ. 838, 155985. doi: 10.1016/j.scitotenv.2022.155985
Rolton A., Rhodes L., Hutson K. S., Biessy L., Bui T., MacKenzie L., et al. (2022). Effects of harmful algal blooms on fish and shellfish species: A case study of New Zealand in a changing environment. Toxins. 14, 341. doi: 10.3390/toxins14050341
Ronquist F., Teslenko M., van der Mark P., Ayres D. L., Darling A., Höhna S., et al. (2012). MrBayes 3.2: Efficient Bayesian phylogenetic inference and model choice across a large model space. Syst. Biol. 61, 539–542. doi: 10.1093/sysbio/sys029
Sakamoto S., Lim W. A., Lu D., Dai X., Orlova T., Iwataki M. (2021). Harmful algal blooms and associated fisheries damage in East Asia: Current status and trends in China, Japan, Korea and Russia. Harmful Algae. 102, 101787. doi: 10.1016/j.hal.2020.101787
Scholin C. A., Herzog M., Sogin M., Anderson D. M. (1994). Identification of group- and strain-specific genetic markers for globally distributed Alexandrium (Dinophyceae). II. sequence analysis of a fragment of the LSU rRNA gene. J. Phycology. 30, 999–1011. doi: 10.1111/j.0022-3646.1994.00999.x
Seki T., Satake M., Mackenzie L., Kaspar H. F., Yasumoto T. (1995). Gymnodimine, a new marine toxin of unprecedented structure isolated from New Zealand oysters and the dinoflagellate. Gymnodinium Tetrahedron Lett. sp, 36. doi: 10.1016/0040-4039(95)01434-J
Smith K. F., Stuart J., Rhodes L. L. (2024). Molecular approaches and challenges for monitoring marine harmful algal blooms in a changing world. Front. Protistol. 1. doi: 10.3389/frpro.2023.1305634
Song X., Xu Z., Zhang W., Tong M. (2023). Regulation of photosynthetic and hemolytic activity of Phaeocystis globosa under different light spectra. New Phytol. 239, 1852–1868. doi: 10.1111/nph.19056
Stamatakis A. (2014). RAxML version 8: a tool for phylogenetic analysis and post-analysis of large phylogenies. Bioinformatics. 30, 1312–1313. doi: 10.1093/bioinformatics/btu033
Stern R. F., Horak A., Andrew R. L., Coffroth M. A., Andersen R. A., Küpper F. C., et al. (2010). Environmental barcoding reveals massive dinoflagellate diversity in marine environments. PloS One 5, e13991. doi: 10.1371/journal.pone.0013991
Stoeck T., Bass D., Nebel M., Christen R., Jones M. D., Breiner H. W., et al. (2010). Multiple marker parallel tag environmental DNA sequencing reveals a highly complex eukaryotic community in marine anoxic water. Mol. Ecol. 19, 21–31. doi: 10.1111/j.1365-294X.2009.04480.x
Stumpf R. P., Li Y., Kirkpatrick B., Litaker R. W., Hubbard K. A., Currier R. D., et al. (2022). Quantifying Karenia brevis bloom severity and respiratory irritation impact along the shoreline of Southwest Florida. PloS One 17, e0260755. doi: 10.1371/journal.pone.0260755
Su Q., Lei X., Liu G., Sun Y., Lao Q., Sun T. (2022). Characteristics of red tide disaster in coastal waters of Beibu Gulf of Guangxi in recent 20 years. Guangxi Sci. 29, 552–557. doi: 10.13656/j.cnki.gxkx.20220720.018
Takagi S., Kuroda H., Hasegawa N., Watanabe T., Unuma T., Taniuchi Y., et al. (2022). Controlling factors of large-scale harmful algal blooms with Karenia selliformis after record-breaking marine heatwaves. Front. Mar. Sci. 9. doi: 10.3389/fmars.2022.939393
Tang Y. Z., Gu H., Wang Z., Liu D., Wang Y., Lu D., et al. (2021a). Exploration of resting cysts (stages) and their relevance for possibly HABs-causing species in China. Harmful Algae. 107, 102050. doi: 10.1016/j.hal.2021.102050
Tang Z. X., Qiu J. B., Wang G. X., Ji Y., Hess P., Li A. F. (2021b). Development of an efficient extraction method for harvesting Gymnodimine-A from large-scale cultures of Karenia selliformis. Toxins. 13, 793. doi: 10.3390/toxins13110793
Tatters A. O., Muhlstein H. I., Tomas C. R. (2010). The hemolytic activity of Karenia selliformis and two clones of Karenia brevis throughout a growth cycle. J. Appl. Phycol. 22, 435–442. doi: 10.1007/s10811-009-9476-z
Uribe J. C., Ruiz M. (2001). Gymnodinium brown tide in the Magellanic fjords, southern Chile. Rev. Biol. Mar. Oceanogr. 36, 155–164. doi: 10.4067/S0718-19572001000200004
Vellojin J. P., Mardones J. I., Vargas V., Leal P. P., Corredor-Acosta A., Iriarte J. L. (2023). Potential effects of climate change on the growth response of the toxic dinoflagellate Karenia selliformis from Patagonian waters of Chile. Prog. Oceanogr. 211, 102956. doi: 10.1016/j.pocean.2022.102956
Wolny J. L., Scott P. S., Tustison J., Brooks C. R. (2015). Monitoring the 2007 Florida east coast Karenia brevis (Dinophyceae) red tide and neurotoxic shellfish poisoning (NSP) event. Algae. 30, 49–58. doi: 10.4490/algae.2015.30.1.049
Wu X. Z., Wang G. X., Qiu J. B., Li A. F., Hess P. (2024). Effects of culture systems and nutrients on the growth and toxin production of Karenia selliformis. Toxins. 16, 518. doi: 10.3390/toxins16120518
Xu Y., Dzhembekova N., Smith K. F., Gu H., John U., Xie H., et al. (2024). Biodiversity and hemolytic toxicity of the genus Heterocapsa (Dinophyceae) in the Beibu Gulf, China. Mar. Drugs 22, 514. doi: 10.3390/md22110514
Xu Y., He X., Lee W. H., Chan L. L., Lu D., Wang P., et al. (2021a). Ciguatoxin-producing dinoflagellate Gambierdiscus in the beibu gulf: First report of toxic Gambierdiscus in Chinese waters. Toxins. 13, 643. doi: 10.3390/toxins13090643
Xu Y., Wei G., Wang Y., Jia Y., Gao H., Zhang T., et al. (2021b). Pollution of lipophilic shellfish toxins in Qinzhou Bay: Seawater and Crassostrea hongkongensis. Oceanologia Et Limnologia Sinica. 52, 144–152. doi: 10.11693/hyhz20200400126
Xu Y., Zhang T., Zhou J. (2019). Historical occurrence of algal blooms in the northern Beibu Gulf of China and implications for future trends. Front. Microbiol. 10. doi: 10.3389/fmicb.2019.00451
Yu Z., Tang Y., Gobler C. J. (2023). Harmful algal blooms in China: History, recent expansion, current status, and future prospects. Harmful Algae. 129, 102499. doi: 10.1016/j.hal.2023.102499
Zhang Q., Liu C., Qiu L., Zhang W., Sun L., Gu H., et al. (2025). Genetic diversity and distribution of Karenia in the eastern coastal seas of China and implications for the trends in Karenia blooms under global environmental changes. J. Environ. Manage. 373, 123465. doi: 10.1016/j.jenvman.2024.123465
Keywords: Karenia, phylogeny, hemolytic toxicity, gymnodimine, amplicon sequence variant, harmful algal blooms, Beibu Gulf
Citation: Xu Y, Wang X, Hörstmann C, Dzhembekova N, Zhu X, Neuhaus S, Tong M, Lan W and John U (2025) The first recorded fish-killing bloom in the Beibu Gulf, China: caused by dinoflagellate Karenia selliformis. Front. Mar. Sci. 12:1582234. doi: 10.3389/fmars.2025.1582234
Received: 24 February 2025; Accepted: 22 April 2025;
Published: 14 May 2025.
Edited by:
Antonella Leone, Istituto di Scienze delle Produzioni Alimentari, ItalyReviewed by:
Zhangxi Hu, Guangdong Ocean University, ChinaLaura Biessy, Cawthron Institute, New Zealand
Copyright © 2025 Xu, Wang, Hörstmann, Dzhembekova, Zhu, Neuhaus, Tong, Lan and John. This is an open-access article distributed under the terms of the Creative Commons Attribution License (CC BY). The use, distribution or reproduction in other forums is permitted, provided the original author(s) and the copyright owner(s) are credited and that the original publication in this journal is cited, in accordance with accepted academic practice. No use, distribution or reproduction is permitted which does not comply with these terms.
*Correspondence: Wenlu Lan, ZHIubGFuQDEzOS5jb20=; Uwe John, VXdlLkpvaG5AYXdpLmRl