- Department of Aquaculture, Zhejiang Ocean University, Zhoushan, China
Continuing global warming intensifies the thermal stress suffered by fish, urgently necessitating effective mitigating techniques. This study aims to investigate the mechanisms by which astaxanthin alleviates oxidative stress and inflammatory damage induced by thermal stress. Under thermal stress, an increase in oxidative stress was observed in the myocytes of Micropterus salmoides, however, intervention of astaxanthin exerted a notable alleviating effect on oxidative stress. Evidence of thermal stress experiment on primary myocytes indicates that astaxanthin resists thermal stress by enhancing the activity of antioxidant enzymes, heat shock proteins and activating the antioxidant gene Nrf2. Further integrated multi-omics analysis revealed a significant upregulation of several antioxidant biomarkers, such as Glutathione (GSH), glutathione peroxidase (GPX) and glutathione S-transferase (GST). This study proposes the hypothesis that astaxanthin may enhance the GSH-dependent endogenous antioxidant enzyme system by activating the Keap1-Nrf2 signaling pathway. Notably, the supplementation of astaxanthin, compared to thermal stress alone, inhibited the expression of inflammatory factors and apoptosis-related genes, including TLR2, IL8, EIF4E, IL2RB, CASP3, and CASP9. These results, combined with the observed inhibition of the Toll-like receptor signaling pathway and the NF-κB signaling pathway, indicate that the TLR2/4-NF-κB signaling pathway plays a crucial role in mediating the alleviation of oxidative stress-induced inflammatory damage by astaxanthin. Furthermore, astaxanthin remodels amino acid and lipid metabolism under thermal stress, establishing an adaptive anti-thermal stress metabolic mechanism that encompasses both phase I and phase II metabolism. These findings offer novel insights into the mechanisms underlying astaxanthin-induced protection against oxidative stress and inflammatory damage.
1 Introduction
Global warming has led to elevated temperatures in inland water bodies, particularly during summer, exacerbating thermal stress on fish populations. This leads to metabolic alterations and increased energy consumption in fish, and when substrate oxidation cannot adequately compensate for these heightened demands, oxidative stress ensues. This causes metabolic imbalances, endoplasmic reticulum stress, inflammation, and the activation of growth-inhibiting signaling pathways (Beemelmanns et al., 2021; Cheng et al., 2018a). As oxidative stress intensifies, substantial amounts of reactive oxygen species (ROS) accumulate within cells, disrupting cellular redox homeostasis, impairing the antioxidant system, and leading to inflammation and apoptosis (Gallagher, 2013). Carotenoids, such as astaxanthin (AX), which is particularly effective due to its strong capacity to neutralize oxygen free radicals, are widely utilized as aquaculture additives to mitigate environmental stress. Astaxanthin interacts directly with cell membranes to scavenge intracellular oxygen free radicals (Ambati et al., 2014; Nakagawa et al., 2011), enhancing antioxidant capacity by modulating the antioxidant enzyme system and suppressing inflammatory responses (Li et al., 2014; Yi et al., 2014). Thus, investigating the regulatory mechanisms of astaxanthin on oxidative stress induced by high-temperature conditions in fish is imperative.
The primary antioxidant defense in cells is mediated through enzymatic and non-enzymatic systems, including factors such as glutathione (GSH), glutathione peroxidase (GPX), catalase (CAT), and superoxide dismutase (SOD) (Jiang et al., 2016), with the enzymatic system being the principal protective mechanism in fish cells (Mourente et al., 2002). Astaxanthin has been shown to enhance the activity of antioxidant enzymes SOD, CAT, and GPX, thereby improving the total antioxidant capacity (T-AOC) of serum, reducing malondialdehyde (MDA) levels indicative of oxidative damage, preventing infectious diseases in aquaculture, and mitigating environmental stress (Jiang et al., 2020; Nakano et al., 2018). Moreover, astaxanthin upregulates the expression of heat shock proteins (HSPs) in various animal tissues and cells to counteract environmental stress (Fleischmann et al., 2020; Tan et al., 2020). Therefore, astaxanthin may alleviate oxidative stress induced by high temperatures by activating the antioxidant enzyme system, warranting further investigation into the specific mechanisms involved.
The Keap1-Nrf2 signaling pathway plays a crucial role in mediating the antioxidant system. During oxidative stress, Nrf2 translocates into the nucleus to regulate the transcription and expression of antioxidant response elements (ARE), activating the intracellular antioxidant enzyme system to eliminate excess ROS and bolster the cell’s antioxidant capacity (Lu et al., 2016). Research has confirmed that astaxanthin enhances the activity of antioxidant enzymes by activating the Keap1-Nrf2 signaling pathway (Jaiswal, 2004), upregulating Nrf2 expression to combat environmental stress from peroxides. GSH, a critical component of the antioxidant system, is regulated by Nrf2, which influences the expression of GSH synthesis-dependent genes, affecting GSH synthesis, redox processes, and homeostasis (Nguyen et al., 2009; Suzuki and Yamamoto, 2015). Thus, activating the Keap1-Nrf2 pathway is vital for promoting GSH synthesis and activating the endogenous antioxidant system.
The inflammatory response is intricately linked to redox balance, with ROS serving as key pro-inflammatory mediators. These mediators directly participate in pathogen clearance and activate the expression of pro-inflammatory factors, creating a positive feedback loop with pro-inflammatory cytokines (Fierro and Serhan, 2001; Liu et al., 2001). Therefore, timely interruption of this feedback loop is essential; otherwise, excessive oxidative stress can lead to apoptosis and tissue damage. Astaxanthin, due to its potent antioxidant properties, helps maintain cellular redox balance, inhibits tissue inflammation (Hwang et al., 2017; Ohgami et al., 2003), and reduces the expression of pro-inflammatory factors such as IL-15, IL-10, TNF-α, CASP3, and CASP9 (Song et al., 2017), thereby alleviating cell damage from excessive inflammation. Further studies indicate that astaxanthin may exert anti-inflammatory effects by regulating Toll-like receptor signaling pathways (TLR2/4) and the NF-κB signaling pathway (Li et al., 2019; Liu et al., 2015; Yang et al., 2019). Consequently, astaxanthin may inhibit apoptosis induced by excessive inflammatory responses by enhancing the cellular antioxidant enzyme system, though the specific mechanisms remain unclear and necessitate further research.
Aquaculture is increasingly becoming a vital source of high-quality protein. Largemouth bass (Micropterus salmoides), a freshwater fish with substantial commercial value, is widely cultivated in China. However, high summer temperatures induce oxidative stress, metabolic imbalances, and inflammatory damage, leading to a decline in immune function, reduced food intake, and lower hatching rates, severely hindering its industrialization (Shehata et al., 2020). Moreover, increased temperatures, resulting in insufficient dissolved oxygen and deteriorating water quality, are more likely to induce disease outbreaks, causing severe economic losses (Roushdy et al., 2018). Studies have demonstrated that supplementing feed with astaxanthin can enhance fish growth performance under high temperatures (Cheng et al., 2018b) and play a significant role in mitigating high-temperature stress in IPEC-J2 cells (Mo et al., 2022). Therefore, understanding the regulatory mechanisms of astaxanthin against high-temperature stress is crucial. There is an urgent need to devise effective strategies to mitigate the adverse effects of high-temperature stress. Given astaxanthin’s potent antioxidant properties (Li et al., 2014), this study aims to explore the mechanisms by which astaxanthin alleviates oxidative stress and inflammatory damage in largemouth bass subjected to high-temperature stress. This will be achieved through supplementation experiments at both cellular and individual levels, complemented by comprehensive multi-omics analyses. Additionally, the study seeks to elucidate, for the first time, the reconstruction processes of amino acid and lipid metabolism facilitated by astaxanthin, thereby providing a theoretical basis for developing nutritional regulation strategies to enhance fish stress resistance.
2 Materials and methods
2.1 Fish maintenance and ethics statement
The largemouth bass (8–10g) were purchased from Chia Tai Aquatic Products Co. Ltd. (Huzhou, Zhejiang, China), and maintained in aquarium tanks with continuous aeration at 28°C. Prior to the experiment, fish were acclimated for at least two weeks and given basal pellets daily. All the study designs and animal experiments were conducted following the guidelines of the Animal Care and Use Committee of the Zhejiang Ocean University.
2.2 Primary culture of myocytes and thermal stress trial
2.2.1 Primary culture of myocytes
Juvenile fish, each weighing approximately 5 g, were dissected under sterile conditions to obtain muscle tissue, which was then cut into pieces and transferred to a 24-well cell culture plate. Antibiotic medium (MEM, 2% PS, 12.5 μg/mL amphotericin B, 0.5 mg/mL gentamicin sulfate) was added and the cells were cultured in an incubator (28°C + 5% CO2) for 2 hours, with a medium change every 30 minutes. Subsequently, the tissue fragments were inoculated into T75 culture flasks and, once the tissue adhered, the medium was replaced with complete medium (MEM, 20% FBS, 1% PS, 2 μg/mL epidermal growth factor EGF, 25 ng/mL fibroblast growth factor FGF) to facilitate cell migration. Following significant cell migration, purification was performed. The first-generation cells were digested with trypsin (0.25%) and cultured in fresh medium. After adherence, the cells were passaged twice to obtain pure primary myocytes.
2.2.2 Thermal stress of myocytes
Myocytes were seeded at a density of 1×106 per well in a 24-well plate, and subjected to high-temperature culture at 37°C for 2, 4, 6, 12, and 24 hours. After thermal stress, the supernatant was collected for lactate dehydrogenase (LDH) measurement using a commercial detection kit. After removing the supernatant, cell activity and intracellular MDA were measured. For cell activity, the CCK-8 solution and corresponding medium were added to the wells, incubated at 28°C for 2 hours, and absorbance was measured at 450 nm. To determine intracellular MDA content, 0.2 mL of MDA extraction solution from the kit was added to the wells to fully lyse the Myocytes and form a cell suspension.
2.2.3 Thermal stress of myocytes treated with astaxanthin
AX at a concentration of 12 mg/mL was diluted with a complete cell culture medium to prepare working solutions with concentrations of 0 μM, 2 μM, 5 μM, 10 μM, and 20 μM. Myocytes were seeded at a density of 1×106 per well in a 24-well plate and pre-cultured with varying concentrations of AX at 28°C for 6 hours. Subsequently, the Myocytes were cultured at either 28°C or 37°C for an additional 6 hours, serving as the negative control group (28°C + 0 μM AX, CON) and the thermal stress group, respectively. Each group had six replicates.
2.2.4 Biochemical and gene expression analysis
Cell activity was measured using the previously described method. Antioxidant indices were determined using commercial kits (Nanjing Jiancheng Bioengineering Inc., Nanjing, China). Available kit information includes: MDA detection kit (A003-4-1), LDH detection kit (A020-2-1), SOD detection kit (A001-3-2), CAT detection kit (A007-1-1), and T-AOC detection kit (A015-2-1). All experimental procedures were conducted on ice, and parameters were measured using a microplate reader (iMark Bio-Rad, Hercules, USA). ROS levels were quantified by observing cells with an inverted fluorescence microscope and measuring the average fluorescence intensity within Myocytes using Image J software after pre-culturing with various concentrations of AX for 6 hours, followed by 2 hours of thermal stress at 37°C, with 6 replicates per group.
Myocytes were divided into two groups: thermal stress and thermal stress combined with AX, with 10 μM chosen as the optimal concentration for AX addition. Following thermal stress treatment, total RNA was extracted at 20, 40, 60, 120, and 240 minutes. The RNA was then reverse-transcribed into cDNA using the PrimeScript™ RT reagent kit (Takara, Dalian, China) (Chomczynski and Sacchi, 2006). Quantitative analysis was conducted with a Real-Time PCR system (QuantStudio™ 6 Flex, Life Technologies, Carlsbad, USA), and target gene expression levels were determined using the 2-△△CT method. Primer sequences are provided in Data sheet 1: Supplementary Table S1 (synthesized by Sangon Biotech Co., Ltd., Shanghai).
2.3 Animal feeding procedure and thermal stress trial
Juvenile largemouth bass were acquired from Chia Tai Aquatic Products Co., Ltd. in Huzhou, Zhejiang, China. Following a two-week acclimation period to the experimental conditions, 180 healthy fish of similar size (mean weight: 6.82 ± 0.09 g) were randomly distributed among 9 tanks (water volume, 150 L). The water conditions, with a temperature of 26 ± 2°C and dissolved oxygen levels of 6–7 mg/L, were maintained at optimal levels for the growth of largemouth bass during the experimental period. The experimental diets incorporated fish meal as the protein source, corn starch as the carbohydrate source, and a combination of fish oil and soybean oil as the fat sources. These diets were formulated with AX concentrations of 0 mg/kg and 150 mg/kg, maintaining isonitrogenous and isolipidic conditions, and stored at -20°C for future use. The 150 mg/kg AX concentration of diets has been validated as optimal for growth (Zhang et al., 2024).
Fish were fed to apparent satiation twice daily at 8:00 and 17:00 for 8 weeks, with triplicate groups assigned to each diet. After the 8-week feeding trial, largemouth bass were fasted for 24 h prior to undergoing a thermal stress test at 35°C. Each of the 9 tanks was equipped with a 300 W heater, and the temperature was gradually increased to 35°C at a rate of 1°C per hour, with 3 groups and 3 replicates per group. Three groups were established with varying concentrations of astaxanthin (AX) in the diet and different temperature conditions: the NC group (0 mg/kg, 26 ± 2°C), the HTC group (0 mg/kg, 35 ± 2°C), and the HTV group (150 mg/kg, 35 ± 2°C). After 12 hours of thermal stress, 6 fish were randomly sampled from each tank, anesthetized with MS-222 (0.125 g/L), and dissected to collect liver tissues, which were then frozen in liquid nitrogen for subsequent analysis. No fish mortality was observed during the thermal stress process.
2.4 Transcriptome and untargeted metabolomics analysis
9 liver transcriptome samples, comprising 3 each from NC, HTC, and HTV groups, and 18 metabolome samples (6 per group), were submitted to Biomarker Technology Co. Ltd. (Qingdao, China) for omics sequencing. Transcriptomic analysis was conducted using established protocols with high-quality RNA sequenced via the Illumina NovaSeq 6000 platform (Sun et al., 2022). Bioinformatics analysis encompassed Kyoto Encyclopedia of Genes and Genomes (KEGG) enrichment and differential expression gene (DEGs) analysis, utilizing OmicShare tools(https://www.omicshare.com/).
For metabolome data, quality evaluation, annotation analysis, and metabolite functional enrichment were conducted. Annotated metabolites were uploaded to BMKCloud (www.biocloud.net) for further investigation. In brief, metabolites were identified based on the Progenesis QI online METLIN database, public databases, and Biomarker Technology’s self-built database. The Variable Importance in Projection (VIP) was ranked in the OPLS-DA (orthogonal projections to latent structures discriminant analysis) based on each variable’s total effect on the OPLS-DA model, with variables having a VIP ≥1 and p <0.05 considered significant. Metaboanalyst software was used for metabolite annotation through KEGG pathway analysis. Bioinformatics analysis of different metabolites (DEMs) was performed on the OmicShare platform (https://www.omicshare.com/).
2.5 Integration analysis of transcriptome and metabolome
OmicShare platform were employed to conduct an integrated analysis of the metabolome and transcriptome. To investigate the metabolome and transcriptome within KEGG pathways, a graph was produced illustrating the number and p-values of DEGs and DEMs within each pathway. A gene-metabolite association heatmap was created based on the abundances of DEGs and DEMs. A Sankey diagram depicting the correlation between DEGs, DEMs, and pathways was constructed using the data from the gene-metabolite association heatmap. Additionally, KEGG mapping results for DEGs and DEMs were generated using the Pathview tool (https://pathview.uncc.edu/) (Figure 1).
2.6 Statistical analysis
All data, excluding those from RNA-seq and untargeted metabolomics, were analyzed using SPSS 22.0. Results are presented as mean ± standard deviation (SD). Levene’s test assessed the normality and homogeneity of variances. One-way ANOVA and Duncan’s multiple range test were conducted to evaluate significant differences, with statistical significance set at p < 0.05.
3 Results
3.1 Biochemical results of myocytes following astaxanthin treatment under thermal stress
This study initially evaluated the biochemical responses of myocytes subjected to varying durations of thermal stress, focusing on cell viability, MDA content, and the activity of LDH in the culture medium. Cell viability significantly declined after 6 hours of thermal stress, with the lowest relative cell viability observed after 24 hours (Figures 2A-C). MDA content differed significantly from the 0-hour group after 2 hours of thermal stress (P < 0.05), and levels progressively increased with the duration of stress. LDH content also exhibited a significant rise at 2 hours (P < 0.05) and subsequently maintained a stable trend. Following a 6-hour pre-treatment with culture media containing varying concentrations of astaxanthin, myocytes were exposed to thermal stress at 37°C. After 6 hours of thermal stress, cell viability was significantly higher in groups treated with 5 μM, 10 μM, and 20 μM astaxanthin compared to the 0 μM group (Figure 2D). Furthermore, after 2 hours of thermal stress, the introduction of 10 μM and 20 μM astaxanthin significantly reduced MDA content compared to the 0 μM group (Figure 2E). The 10 μM astaxanthin group exhibited the highest levels of CAT activity and T-AOC, while the 20 μM group showed the highest SOD activity, both significantly exceeding the group without astaxanthin (Figures 2F-H). The LDH content in the culture medium of all astaxanthin-treated groups significantly decreased, with further reductions at higher concentrations (Figure 2I). Measurements of intracellular reactive oxygen species showed that the addition of 5 μM, 10 μM, and 20 μM astaxanthin significantly reduced their levels (Figure 2J).
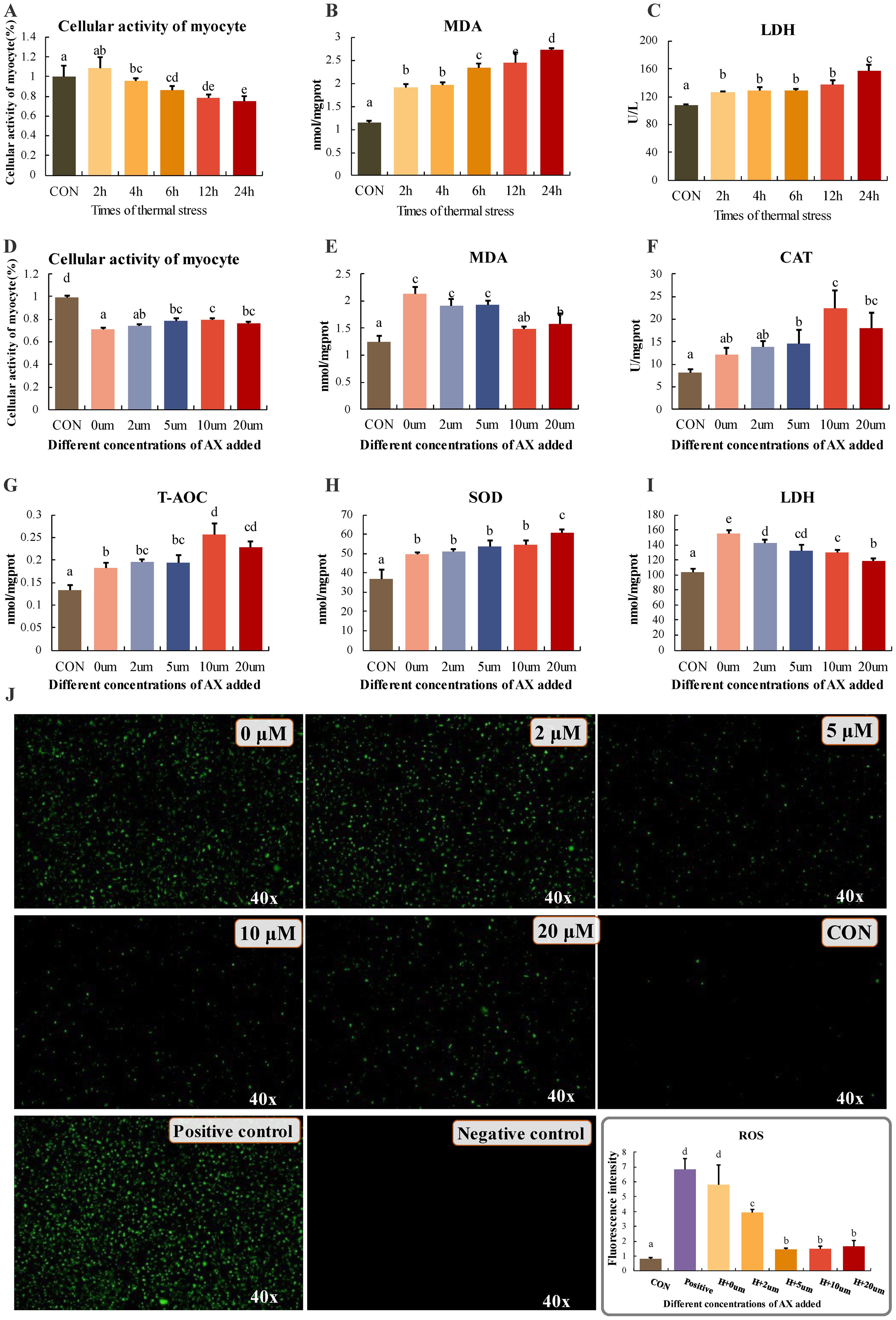
Figure 2. (A-C) Changes in myocyte activity, MDA and LDH content at different times of thermal stress. (D-F) Changes in cellular activity and enzyme activity in groups under stress after addition of different concentrations of astaxanthin. (J) Determination of cellular reactive oxygen content. CON: reactive oxygen content of cells incubated at 28°C; negative control: cells without probe; positive control: addition of reactive oxygen donors, magnified 40x. Differences between groups with different letters (mean ± SD, n = 3) were statistically significant (P < 0.05) using one-way ANOVA.
Myocytes pre-treated with 0 μM and 10 μM astaxanthin for 6 hours were subjected to thermal stress, with samples collected at 20, 40, 60, 120, and 240 minutes to evaluate the expression of antioxidant and immune-related genes. The results demonstrated that at 40 and 60 minutes, the expression levels of HSP70 and HSP90 genes were significantly higher in the 10 μM astaxanthin group compared to the 0 μM group (Figures 3A, B), with a subsequent decrease observed at 120 minutes. Additionally, following astaxanthin treatment, CASP3 expression was significantly lower at 60 and 240 minutes compared to the control group (Figure 3C). Similarly, CASP9 gene expression was significantly reduced at 120 minutes post-treatment compared to the 0 μM group (Figure 3D). Moreover, at 120 and 240 minutes post-treatment, Nrf2 gene expression was significantly elevated compared to the group without astaxanthin (Figure 3E). After normalizing the qRT-PCR results, a heat map was employed to illustrate the expression patterns of immune and antioxidant genes under thermal stress following astaxanthin treatment. This revealed that HSP70 and HSP90 exhibited a clear trend of initial increase followed by a decrease, while CASP3 and CASP9 expression levels significantly decreased (Figure 3F). A molecular interaction network of key genes under thermal stress was constructed using Cytoscape software, further validating the impact of astaxanthin addition on the antioxidant capacity and immune function of largemouth bass under thermal stress, with HSP70 at the initial position of the interaction network, indicating it’s more important regulatory role and clarifying other key genes related to antioxidation.
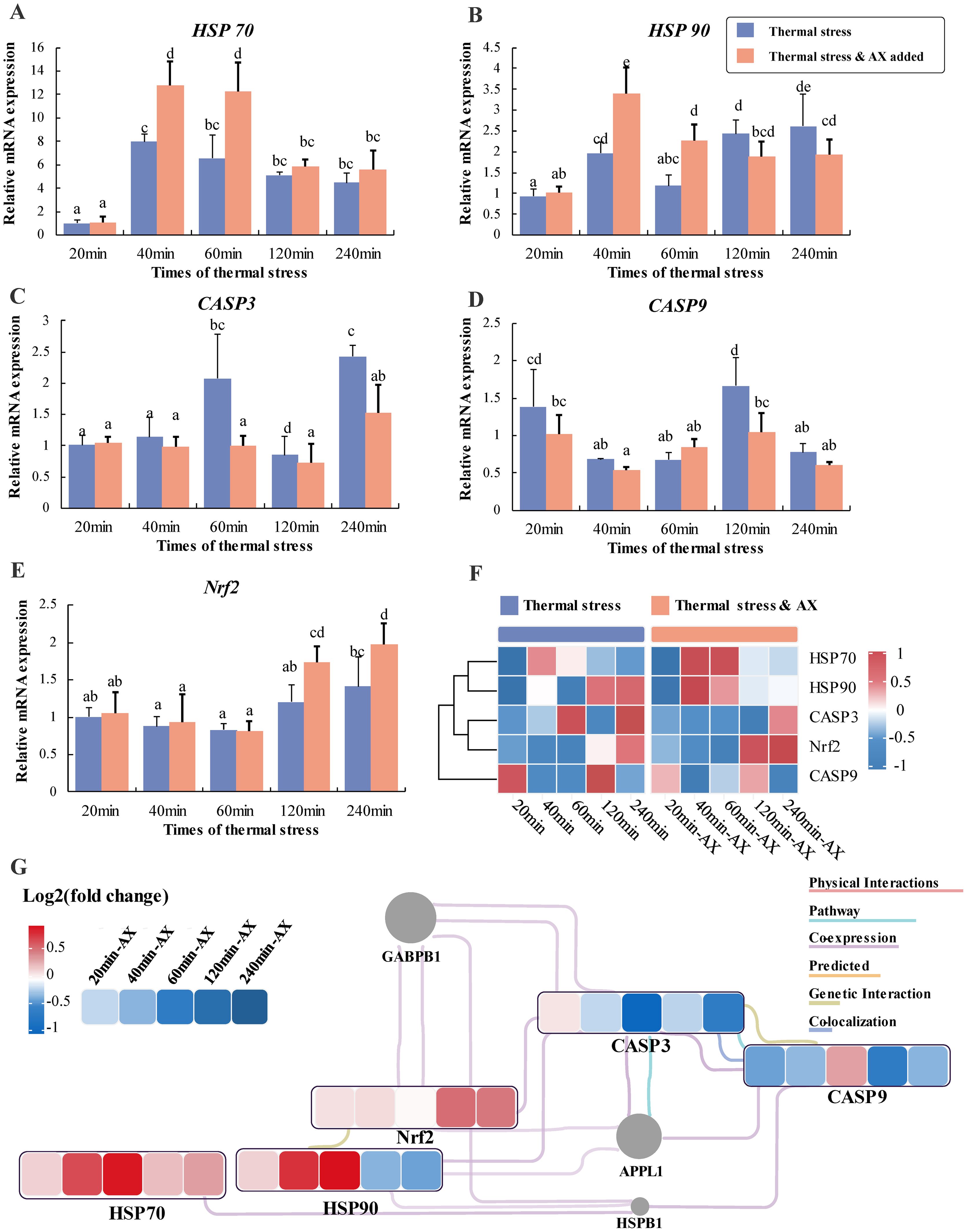
Figure 3. Relative expression of genes under different thermal stress durations after addition of 0 μM and 10 μM astaxanthin. (A) HSP70, heat shock protein 70; (B) HSP90, heat shock protein 90; (C) Caspase-3, cysteine protease-3; (D) Caspase 9, cysteine protease-9; (E) nrf2, nuclear factor erythroid 2-related factor 2. Differences between groups with different letters (mean ± SD, n = 3) were statistically significant (P < 0.05) using one-way ANOVA. (F) Heatmap analysis of key immunity and antioxidant genes in myocytes under different thermal stress durations after addition of 10 μM astaxanthin. The color scale at the far right of the heatmap represents expression, whereby red, blue, and white indicate up-regulated, down-regulated and unaltered expression, respectively. (G) The interaction networks of key immunity and antioxidant genes, representing the expression level of change as effects of thermal stress durations after addition of 0 μM and 10 μM astaxanthin based on qPCR results. The color represents the relative expression value (Log2 fold change), where red, blue, and white colors indicate up-regulation, down-regulation, and unaltered expression, respectively. The genes not subject to research are marked in gray.
3.2 Transcriptome profiles in the liver indicate astaxanthin reduces inflammatory damage under thermal stress
The preceding results preliminarily reveal the regulatory roles of astaxanthin supplementation on the immune and antioxidant functions of largemouth bass subjected to thermal stress. To investigate the mechanisms by which astaxanthin influences immune and metabolic functions, transcriptome analysis was conducted on liver samples. In comparing the HTC and HTV groups, a total of 2,264 differentially expressed genes (DEGs) were identified, comprising 1,104 downregulated genes and 1,160 upregulated genes (Data sheet 1: Supplementary Figure S1A). To further explore the key pathways associated with DEGs under thermal stress following astaxanthin supplementation, all DEGs underwent KEGG database enrichment analysis using OmicShare tools, allowing for mapping to corresponding pathways to assess potential functional implications. KEGG enrichment results indicated that 221 DEGs were enriched in the Immune System, 239 in Global and Overview Maps, 66 in Lipid Metabolism, and 63 in Amino Acid Metabolism (Data sheet 1: Supplementary Figure S1B). Further pathway enrichment analysis revealed that DEGs were predominantly enriched in immune-related pathways as well as those related to amino acid and lipid metabolism. Immune-related pathways included the Toll-like receptor signaling pathway, NF-κB signaling pathway, and HIF-1 signaling pathway, whereas pathways associated with amino acid and lipid metabolism included Glutathione metabolism, Arginine and proline metabolism, and Arachidonic acid metabolism, among others (Data sheet 1: Supplementary Figure S1C). Based on the expression patterns of DEGs in significantly enriched pathways, a bubble plot of these signaling pathways was constructed to elucidate the overall regulatory trends of key signaling pathways involved in immune and metabolic regulation (Data sheet 1: Supplementary Figure S1D).
To elucidate the expression patterns of immune and metabolic regulatory functions in the liver of largemouth bass under thermal stress alone, a comparative analysis was conducted between the NC and HTC groups. A total of 3,811 DEGs were identified, with 1,666 downregulated and 2,145 upregulated (Data sheet 1: Supplementary Figure S2A). KEGG enrichment analysis revealed that 331 DEGs were associated with the Immune System, 117 with Lipid Metabolism, and 92 with Amino Acid Metabolism (Data sheet 1: Supplementary Figure S2B). Pathway enrichment analysis indicated significant involvement in immune-related pathways, including apoptosis, Toll-like receptor signaling, NF-κB signaling, and HIF-1 signaling, as well as metabolic pathways such as Glutathione metabolism, Arginine and proline metabolism, Glycolysis/gluconeogenesis, and Arachidonic acid metabolism (Data sheet 1: Supplementary Figures S2C, D). Compared to the dietary astaxanthin supplementation group (HTC vs. HTV), thermal stress (NC vs. HTC) resulted in more DEGs and a more pronounced regulation of immune-related genes.
Based on the enrichment results of the aforementioned immune-related pathways, it is indicated that dietary astaxanthin regulates the expression of these pathways in largemouth bass under thermal stress. The findings revealed that 3,200 genes exhibited an expression trend characterized by initial increase followed by decrease (Figure 4A). Functional analysis of these genes uncovered significant enrichment in several key immune-related pathways, including Apoptosis, the Toll-like receptor signaling pathway, the NF-κB signaling pathway, the p53 signaling pathway, and the TNF signaling pathway (Figure 4A). Gene Set Enrichment Analysis (GSEA) results indicated that immune pathways were highly enriched during the regulation of immune responses by astaxanthin (HTC vs. HTV). Specifically, the Toll-like receptor signaling pathway, NF-κB signaling pathway, PI3K-AKT signaling pathway, and HIF-1 signaling pathway exhibited statistically significant differences, generally presenting a downward expression trend (Figure 4B). The enrichment of inflammation-related pathways elucidates the alleviating function of astaxanthin on inflammatory damage. However, the mTOR signaling pathway did not exhibit significant expression pattern regulation, possibly due to its involvement in various biological functions, including protein synthesis and genetic material synthesis (Figure 4B). Interaction analysis of immune-related key genes indicated that BCL2, MYC, TLR2, MAP2K1, EGFR, PTEN, TSC2, and EIF4E were at the core of the regulatory network, potentially playing a central role in immune regulation (Figure 4C). Subsequent expression analysis of these key DEGs showed that TLR2, EGFR, IL8, EIF4E, PTEN, CASP8, pckA, TSC2, IL2RB, HSP70, and HSP90 exhibited a consistent trend of initial increase followed by decrease (Figure 4D), aligning with gene trend analysis (Figure 4A) and GSEA results (Figure 4B). The construction of a molecular interaction network of key DEGs revealed an overall decrease in expression relative to the HTC group, except for TLR5, indicating that astaxanthin alleviates inflammatory damage caused by thermal stress and enhances the organism’s ability to resist environmental stress (Figure 4E).
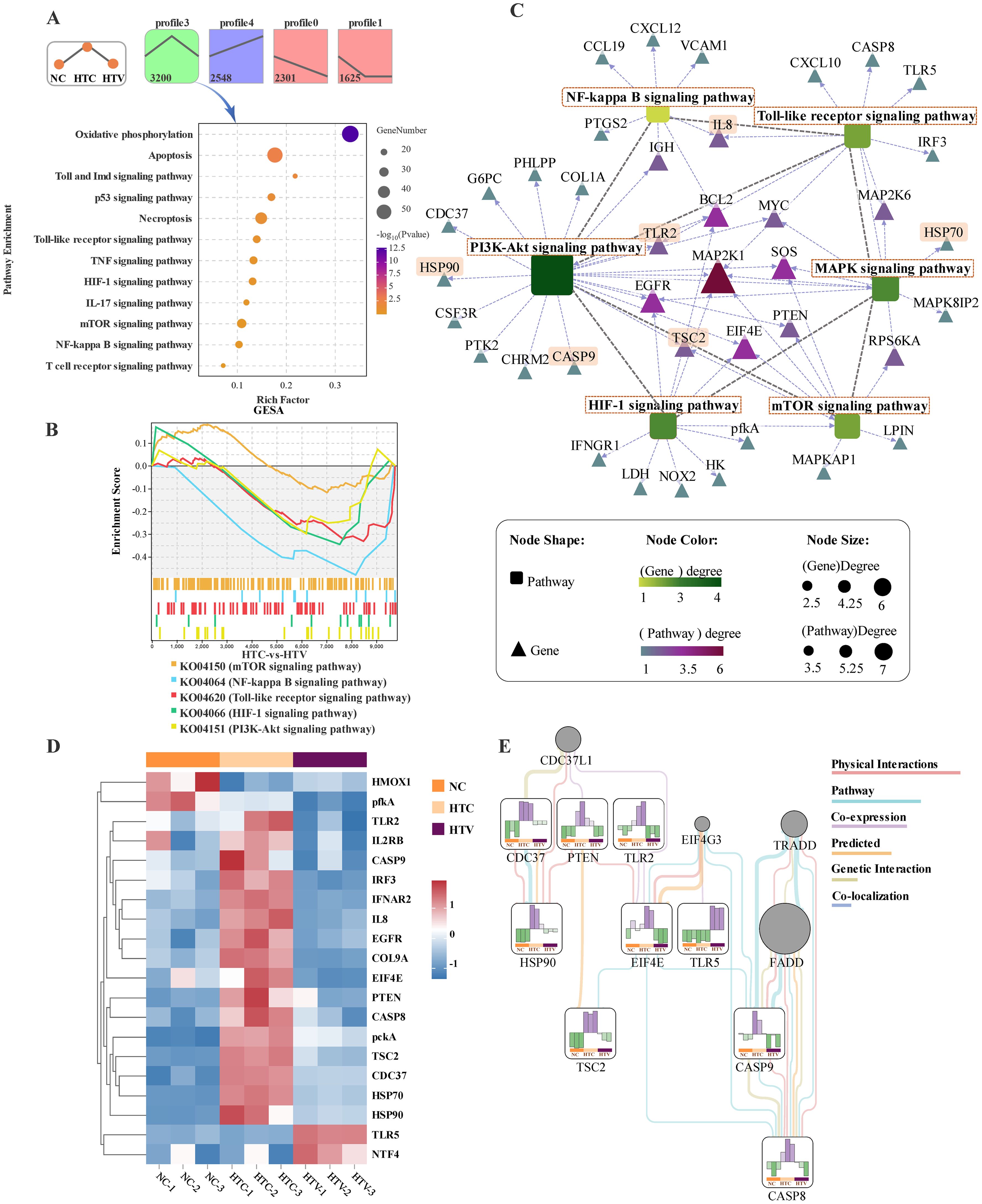
Figure 4. Transcriptomic analysis of expression pattern of immune-related pathways and DEGs in the liver of largemouth bass (HTC vs HTV; n = 3). (A) Analysis of gene expression trend in NC, HTC, and HTV groups; kegg enrichment bubble plot of key immune-related pathways of profile3. (B) GSEA for the KEGG terms related to immune-related pathways. (C) Regulatory network between key immune-related pathways and genes in the liver. Round rectangle represents pathways and triangles represent genes, the closer the genes color is to red, the stronger its importance in the regulatory network. The length of the dotted arrows reflects the correlation between immune-related pathways and genes, and the length of the lines represents the correlation between immune-related. (D) Heat map of key genes associated with immune capacity in NC, HTC and HTV. (E) The interaction networks of key immunity genes, representing the expression level of change as effects of thermal stress after addition of astaxanthin. The color represents the relative expression value, where red and green colors indicate up-regulation and down-regulation, respectively. The genes not subject to research are marked in gray.
Based on the aforementioned results, several key immune pathways appear to be regulated by astaxanthin. However, environmental stress is intricately associated not only with immune function but also with metabolic remodeling processes. Consequently, the subsequent step involves analyzing the regulatory mechanisms of metabolic pathways in response to astaxanthin (HTC vs. HTV). KEGG enrichment analysis revealed that DEGs were predominantly enriched in amino acid metabolism pathways, including Glutathione metabolism, Arginine and proline metabolism, Glycine, serine, and threonine metabolism, and Cysteine and methionine metabolism. Lipid metabolism pathways included Arachidonic acid metabolism, Fatty acid metabolism, and Glycerophospholipid metabolism. Carbohydrate metabolism was represented by the citrate cycle (TCA cycle) (Figure 5A). The GESA results further illustrate that astaxanthin intervention induces a complex regulatory pattern in these metabolic pathways (Figure 5B). Given that the enriched pathways encompass amino acid, lipid, and carbohydrate metabolism, a comprehensive regulatory network was constructed to integrate energy metabolism pathways and DEGs. Analysis of this regulatory network identified key metabolic pathways and DEGs involved in the response to astaxanthin. Amino acid metabolic pathways, specifically Glycine, serine, and Threonine metabolism and Glutathione metabolism, occupy central positions within the regulatory network and connect with other metabolic pathways through core DEGs, including pckA, LDH, SDS, BHMT, GPX, ALDH, and PLA2G (Figure 5C). Notably, Cysteine and methionine metabolism was linked to Glycolysis/Gluconeogenesis through shared LDH, while Glutathione metabolism was associated with Arachidonic acid metabolism via shared GPX (Figure 5C).
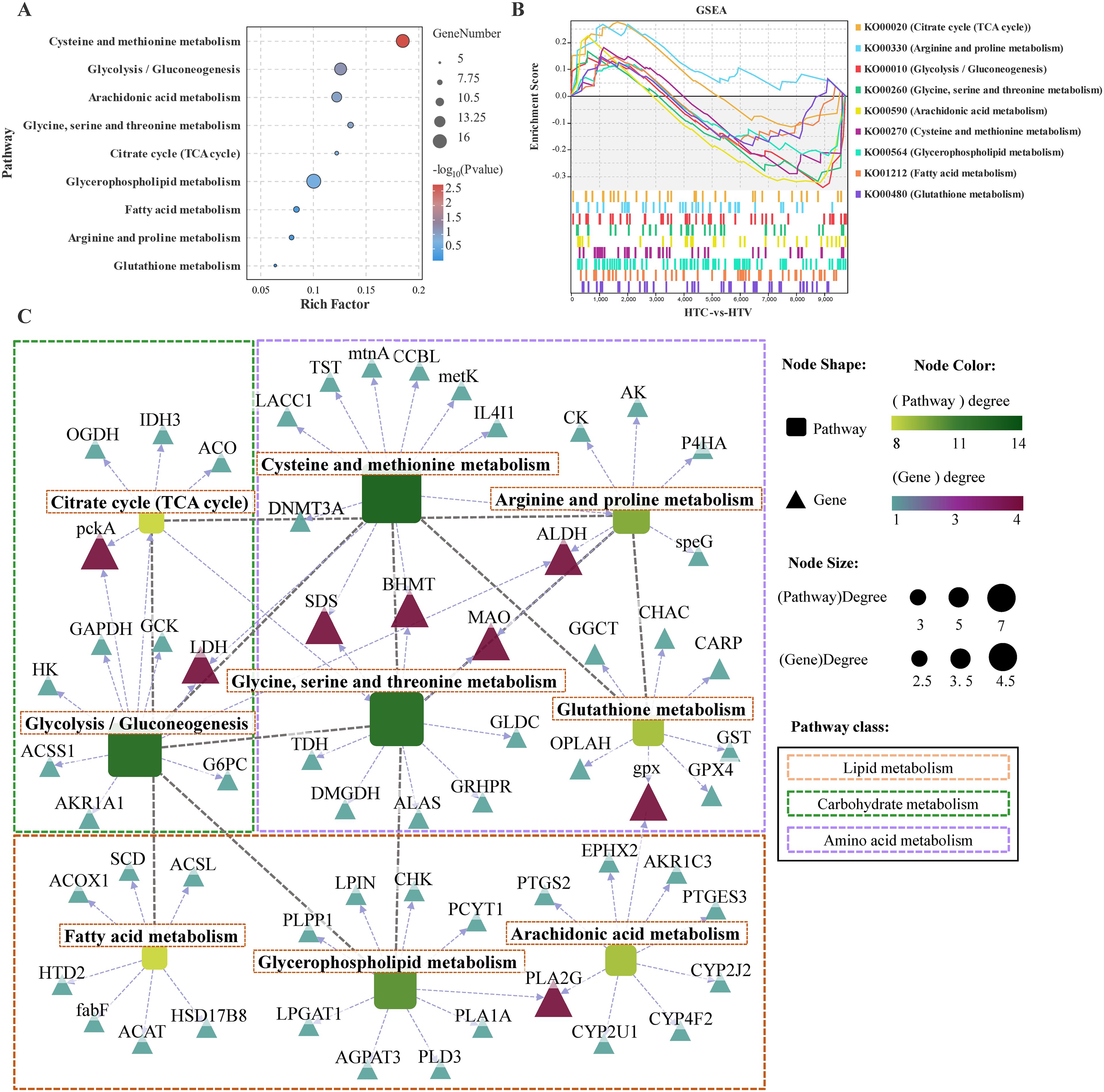
Figure 5. Transcriptomic analysis of expression pattern of metabolism-related pathways and DEGs in the liver of largemouth bass (HTC vs HTV; n = 3). (A) Kegg enrichment bubble plot of key metabolism-related pathways. (B) GSEA for the KEGG terms related to metabolism-related pathways. (C) Regulatory network between key metabolism-related pathways and genes in the liver. Round rectangle represents pathways and triangles represent genes, the closer the genes color is to red, the stronger its importance in the metabolism regulatory network.
3.3 Metabolome profile in response to astaxanthin supplementation in the liver
Transcriptomic analysis reveals that dietary astaxanthin significantly affects amino acid, lipid, and carbohydrate metabolism. Consequently, a further analysis of the metabolic profile in the liver was conducted using metabolomics. To enhance differentiation between the two groups, we employed multivariate statistical analysis using the OPLS-DA model to identify differential metabolites (DEMs). The score plot from the OPLS-DA analysis demonstrated a clear separation between the NC vs. HTC and HT vs. HTV comparison groups. The calculated classification parameters for the two groups were R2Y = 0.995 and R2Y = 0.993, respectively, indicating that the model was stable and effective for both fit and prediction (Data sheet 1: Supplementary Figures S3A, B). Furthermore, variability among the NC, HTC, and HTV samples was assessed, revealing a significant trend of separation within each group (Figure 6A), suggesting notable changes in metabolite profiles following thermal stress and the addition of astaxanthin. A total of 1,614 DEMs were identified in the HTC vs. HTV comparison (VIP ≥ 1, p < 0.05), comprising 857 downregulated and 759 upregulated metabolites. In the NC vs. HTC comparison, 1,692 DEMs were identified, including 798 downregulated and 894 upregulated genes (Data sheet 1: Supplementary Figure S3C, D; Figure 6B). Based on the HMDB database, the identified DEMs were primarily concentrated in Carboxylic acids and derivatives, Fatty Acyls, and Prenol lipids (Figure 6C). There were 1,113 co-enriched differential DEMs (co-DEMs) shared between the NC vs. HTC and HTC vs. HTV comparison groups (Figure 6D). KEGG enrichment analysis indicated that both groups were mainly co-enriched in amino acid and lipid metabolic pathways, including Glutathione metabolism, Glycine, serine and threonine metabolism, Cysteine and methionine metabolism, Arachidonic acid metabolism, and Glycerophospholipid metabolism. Additionally, the HIF-1 signaling pathway was significantly enriched (Figure 6E).
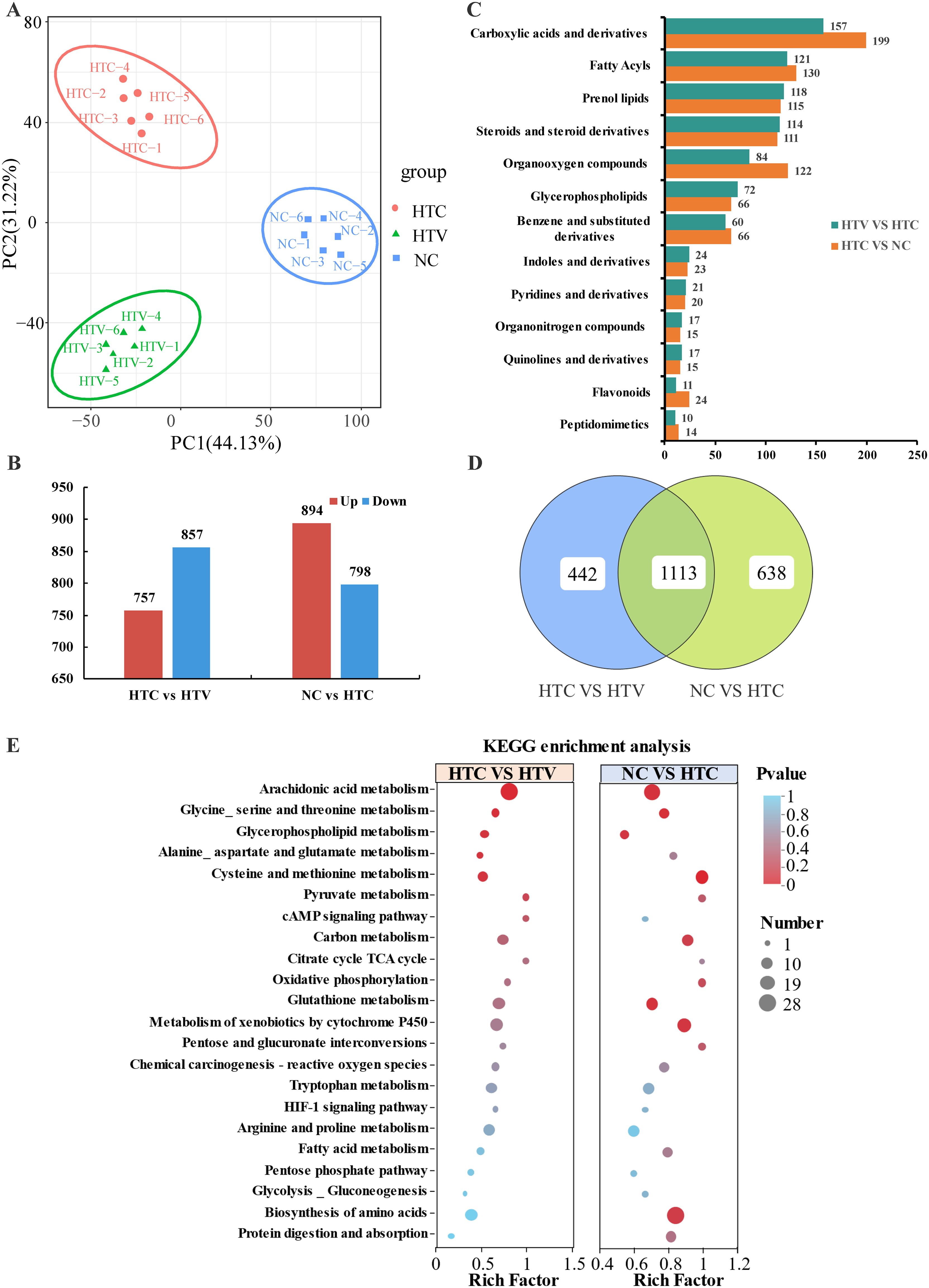
Figure 6. Metabolomic analysis of the DEMs in liver of largemouth bass under thermal stress after addition of astaxanthin (HTC vs HTV; NC vs HTC n = 6). (A) PCA analysis of the different samples. (B) Statistics on the number of up-regulated and down-regulated DMs. (C) HMDB classification map of DMs in different comparison groups (HTC vs HTV; NC vs HTC). (D) Vene plot of the intersection of DEMs among each comparison group. (E) KEGG co-enrichment pathway of DMs in each comparison group.
As metabolic pathways responding to both thermal stress and astaxanthin addition, these pathways will be the focus of subsequent research. Screening the metabolites within these pathways identified 75 DEMs and 72 DEMs in the NC vs HTC and HTC vs HTV comparison groups, respectively (Figure 7A). An analysis of the shared DEMs between the two comparison groups using an UPSET plot identified 51 co-DEMs that can respond to both thermal stress and astaxanthin addition (Figure 7A). These key biomarkers may directly trigger or participate in the regulatory mechanisms by which astaxanthin resists environmental stress in largemouth bass(Data sheet 1; Supplementary Figure S3E; Supplementary Table S2; Figure 7B). KEGG enrichment results for the co-DEMs revealed significant co-enrichment of Glutathione metabolism and Arachidonic acid metabolism in both groups (Figure 7C), highlighting their critical roles in reconstructing the liver metabolic profile under astaxanthin influence. Furthermore, a regulatory network was constructed linking co-DEMs to metabolic pathways, which showed that the amino acid metabolic pathways, including Cysteine and methionine metabolism and Glutathione metabolism, occupy central positions in the regulatory network. These pathways are interconnected through co-DEMs such as GSH, S-Adenosylmethioninamine, S-Adenosylmethionine, L-Cystathionine and Choline. Glutathione metabolism connects to Cysteine and methionine metabolism through shared GSH and further links to Arginine and proline metabolism via S-Adenosylmethioninamine and S-Adenosylmethionine. Glycine, serine and threonine metabolism connects with Cysteine and methionine metabolism through shared L-Cystathionine and Choline. Biomarkers (co-DEMs) located at the core of the regulatory network will play a crucial role in the regulatory process of astaxanthin response (Figure 7D).
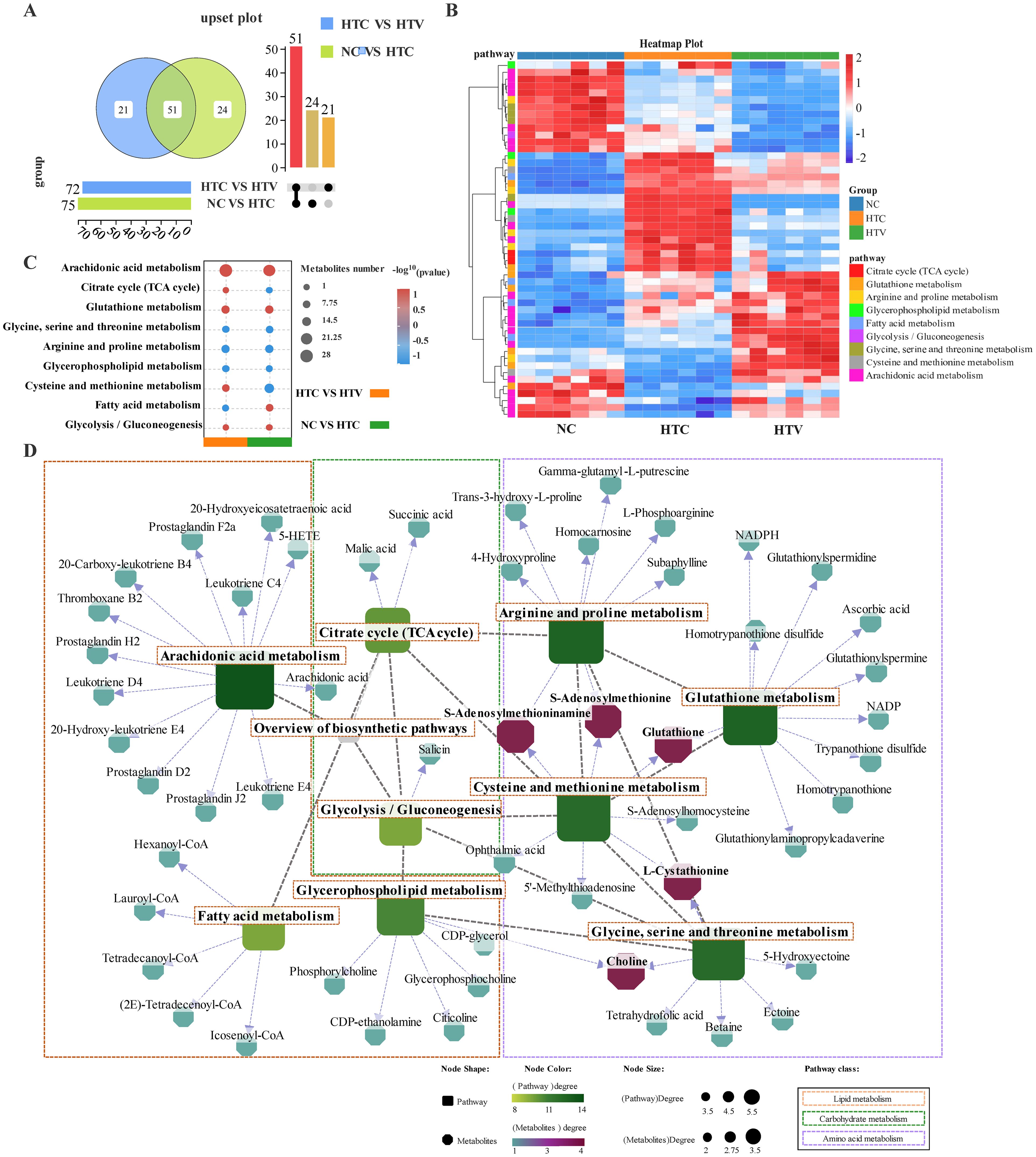
Figure 7. Metabolomic analysis of regulation pattern of key metabolism pathways and DEMs in the liver of largemouth bass after addition of astaxanthin (HTC vs HTV; NC vs HTC n = 6). (A) Upset plot of the intersection of key DEMs among each comparison group. (B) Heat map of key DMs profile in the liver of largemouth bass in NC, HTC and HTV group. (C) KEGG co-enrichment of key metabolism pathways in each comparison group. (D) Regulatory network between key metabolism pathways and DEMs. Round rectangle represents pathways and octagon represent metabolites, the closer the metabolites color is to red, the stronger its importance in the metabolism regulatory network.
3.4 Integrated analysis of DEGs and DEMs demonstrates that astaxanthin enhances antioxidative capacity by remodeling glutathione metabolism
Transcriptomic and metabolomic data indicate that dietary astaxanthin significantly influences amino acid, lipid metabolism, and immune-related pathways. To better elucidate the DEMs and their relationship with DEGs, a comprehensive analysis of the transcriptomic and metabolomic data was performed. Key immune-related and metabolic pathways were identified based on co-enriched DEGs and DEMs. These pathways include Glutathione metabolism, Arachidonic acid metabolism, Cysteine and methionine metabolism, and the HIF-1 signaling pathway, among others (Figure 8A).
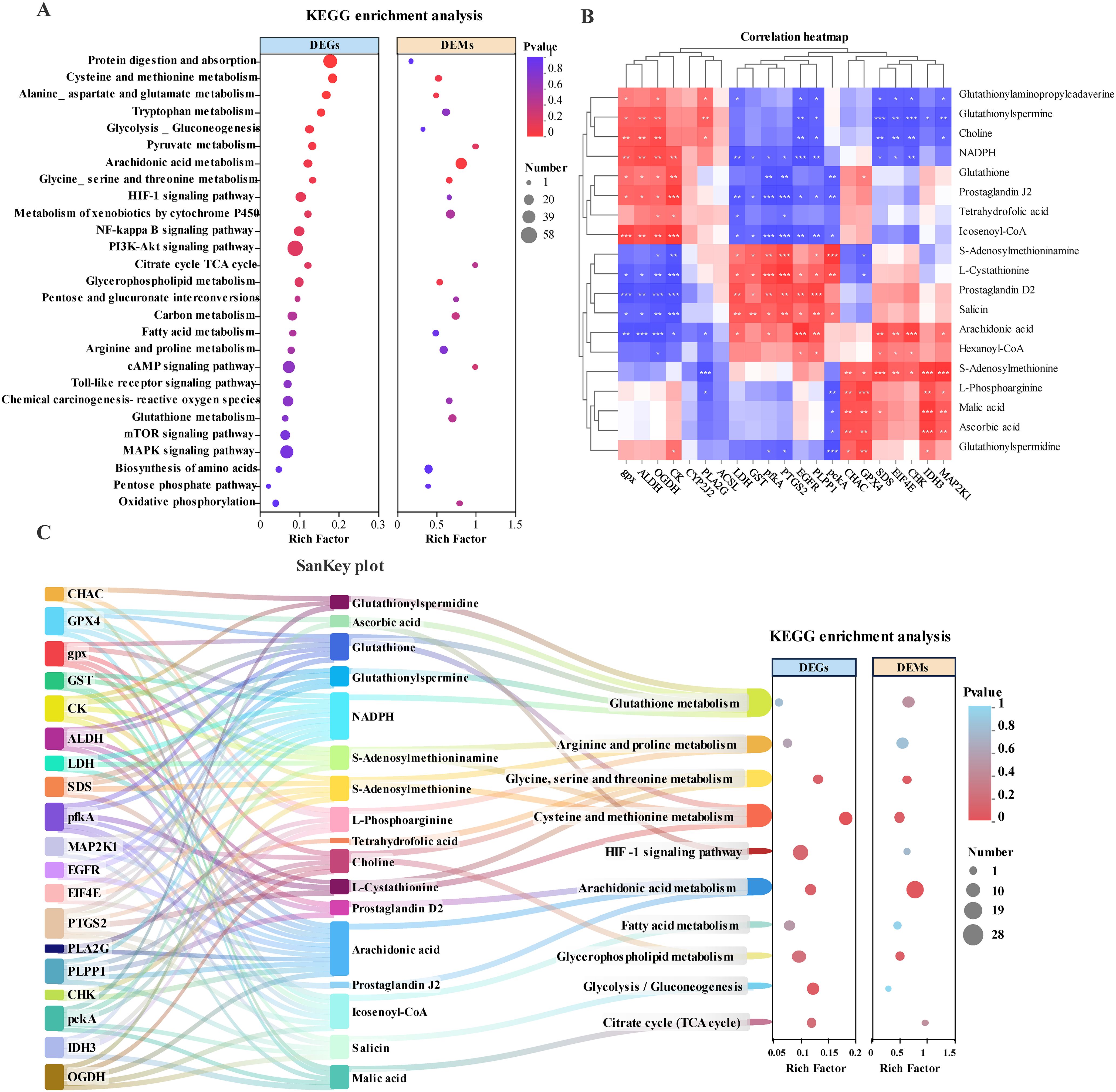
Figure 8. Integrated analysis of DEGs and DEMs in the liver of largemouth bass under thermal stress after addition of astaxanthin (HTC vs HTV). (A) KEGG co-enrichment of key metabolism and immune-related pathways for DEGs and DEMs (HTC vs HTV). (B) DEGs-DEMs association heatmap. The red color represents the positive correlation between DEGs and DEMs, and the blue color represents the negative correlation. * represents a significant correlation between DEGs and DEMs, asterisks indicate significance levels: * for 0.01 < p < 0.05, ** for 0.001 < p < 0.01, and *** for p ≤ 0.001. (C) DEGs-DEMs-pathway correlation Sankey plot in the liver of largemouth bass. The left box is highly representative of the importance of genes involved in the network; the center represents metabolites, and the right represents the key metabolism pathways involved.
To further investigate the interaction between DEGs and DEMs within co-enriched pathways, the study examined the correlation between DEGs and DEMs within these pathways. Correlation heatmaps and a Sankey diagram were constructed to illustrate the associations among DEGs, DEMs, and pathways (Figure 8B). The clustering analysis in the correlation heatmap grouped genes and metabolites with similar correlation coefficients, partially reflecting the interactions between genes and metabolites (Figure 8B). In the Sankey diagram, significant correlations between genes, metabolites, and pathways were connected, ultimately forming a complete regulatory network that illustrates potential biological information flow channels. This significant relationship suggests that the regulatory patterns of biomarkers may exhibit similar or opposing changes in response to dietary astaxanthin (Figure 8C). Further analysis of the flow of biological information from genes to metabolites to pathways revealed that genes and metabolites primarily enriched in Glycine, serine and threonine metabolism, Arginine and proline metabolism, Arachidonic acid metabolism, Fatty acid metabolism, and Glycolysis/Gluconeogenesis constitute a metabolic regulatory network involving amino acids, carbohydrates, and lipids (Figure 8C). Importantly, this network also includes glutathione metabolism and the HIF-1 signaling pathway, which are related to oxidative stress and immune regulation (Figure 8C). This indicates that dietary astaxanthin significantly regulates energy metabolism under thermal stress, thereby enhancing the antioxidant capacity and immune response processes of M. salmoides under environmental stress.
Based on the correlation analysis between DEGs and DEMs in amino acid metabolism, an association network consisting of 11 key genes and 11 differential metabolic markers was identified, divided into two distinct co-expression modules: one exhibiting positive correlation and the other exhibiting negative correlation (Figure 9A). Further examination of the most significantly correlated DEGs and DEMs facilitated the construction of a metabolic regulatory network. The core components of this network are primarily situated at the intersections of multiple pathway regulations, prominently influencing Glutathione metabolism, Arginine and proline metabolism, as well as Glycine, serine, and Threonine metabolism (Figure 9B). In-depth investigation into Glutathione metabolism revealed the enrichment of several metabolites, including GSH, Glutathionylspermidine, Ascorbic acid, Glutathionylspermine, NADPH, and NADP, along with key metabolic genes such as CHAC, GST, GPX4, GGCT, and GPX, which collectively demonstrate an active regulatory pattern (Figure 9C) in HTV. Notably, GST plays a pivotal role in the antioxidant enzyme system, significantly contributing to the mitigation of oxidative stress damage following environmental stress. The observed increase in GSH and GPX levels in HTV indicating that astaxanthin may enhance antioxidant capacity by promoting glutathione metabolism(Figure 9C).
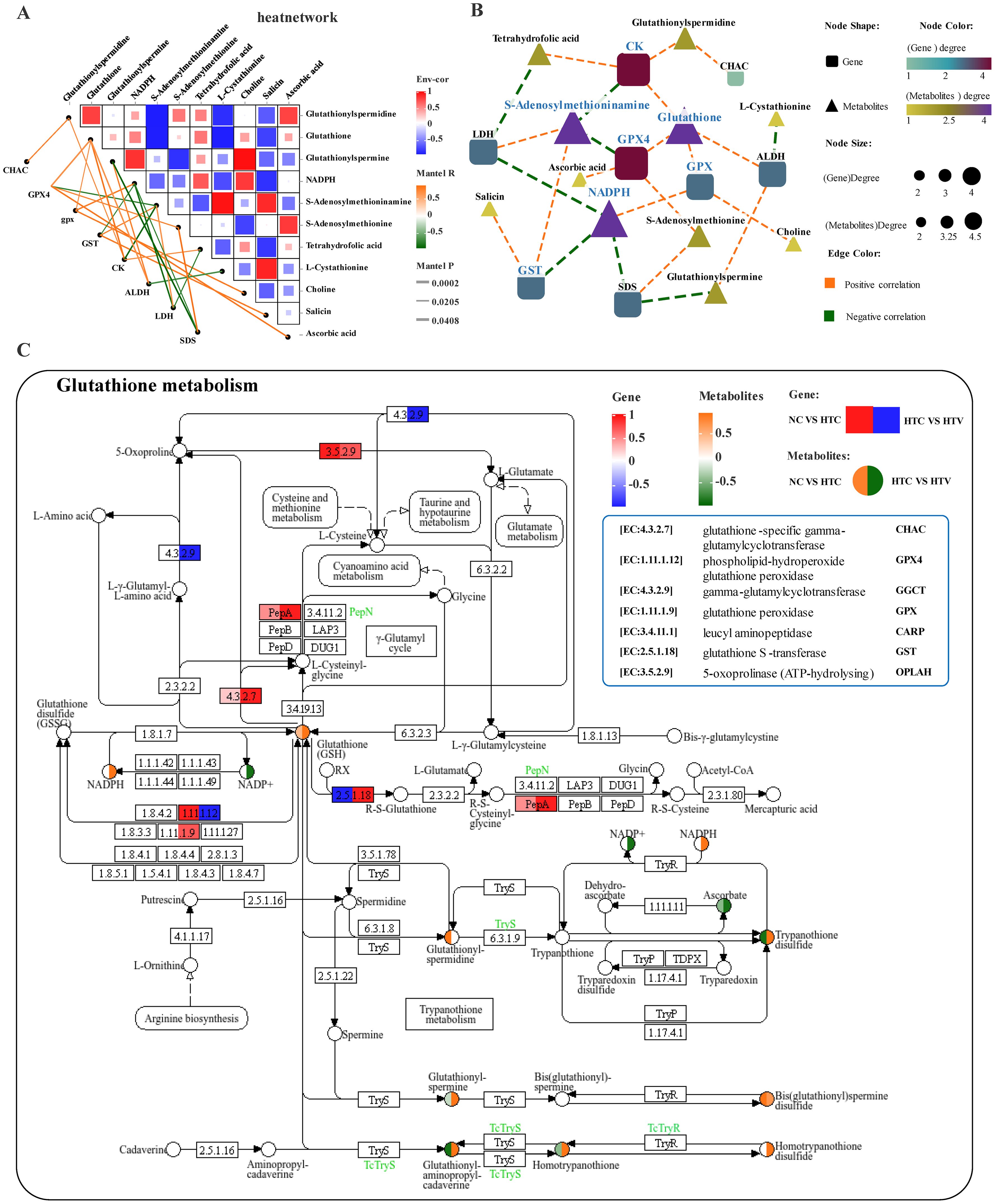
Figure 9. Interaction analysis of amino acid metabolism-related genes and metabolites under thermal stress after addition of astaxanthin (HTC vs HTV). (A) Association network heatmap of DEGs and DEMs at the core of amino acid metabolism. (B) Interaction network of DEGs - DEMs related to amino acid metabolism; (C) KEGG mapping results of DEGs and DEM in the glutathione metabolism pathway. The green or red box illustrated significant downregulation or upregulation of the gene, respectively. The blue or yellow circle reflected significant downregulation or upregulation of the metabolite, respectively.
Correlation analysis of key biomarkers in lipid metabolism revealed a complex association pattern between DEGs and DEMs (Figure 10A). Further investigation of the most significantly correlated DEGs and DEMs identified highly interconnected core genes such as CYP2J2, PLA2G, PLPP1, and CHK, along with metabolites including arachidonic acid, Prostaglandin D2, Prostaglandin J2, Icosenoyl-CoA, and Choline. These biomarkers occupy critical positions within the lipid metabolism interaction network and may serve as core factors linking various lipid metabolic pathways, particularly dominating Arachidonic acid metabolism, Fatty acid metabolism, and Glycerophospholipid metabolism (Figure 10B). The Arachidonic acid metabolism pathway was enriched with various metabolites, including Arachidonic acid, Prostaglandin D2, and Prostaglandin J2, as well as several key metabolic genes such as PLA2G, CYP2J2, and GPX (Figure 10C). Notably, secretory phospholipase A2 (PLA2G) plays a crucial role in synthesizing arachidonic acid by hydrolyzing phospholipids to provide precursors for the biosynthesis of linoleic acid and arachidonic acid (Arora and Damodaran, 2011). The cytochrome P450 family 2 subfamily J enzyme (CYP2J) is an important phase I metabolic enzyme that is not only enriched in Arachidonic acid metabolism but also plays a significant regulatory role in the Tryptophan metabolic. The enrichment of genes encoding P450 enzymes in this pathway indicates a close association between arachidonic acid and phase I metabolism in the liver of M. salmoides. Levels of PLA2G and CYP2J significantly increased following dietary astaxanthin supplementation (Figure 10C), indicating that astaxanthin markedly alters lipid metabolism processes in M. salmoides, enhancing lipid metabolic capacity. Furthermore, the HIF-1 signaling pathway is linked to Glutathione metabolism through the metabolic marker Ascorbic acid (Figures 9C, 11). The co-enrichment results of DEGs and DEMs demonstrate that multiple immune and inflammatory regulatory genes, including pfkA, HK, ARNT (HIF-1β), MAP2K1, IFNGR1, LDH, EGFR, and EIF4E, are enriched (Figure 11). Following astaxanthin supplementation, the overall trend of this pathway exhibited a significant downward trajectory (HTC vs HTV), suggesting that astaxanthin may alleviate immune responses induced by thermal stress, thereby enhancing the organism’s ability to cope with environmental stress and providing protection against inflammatory damage, regulated by glutathione metabolism.
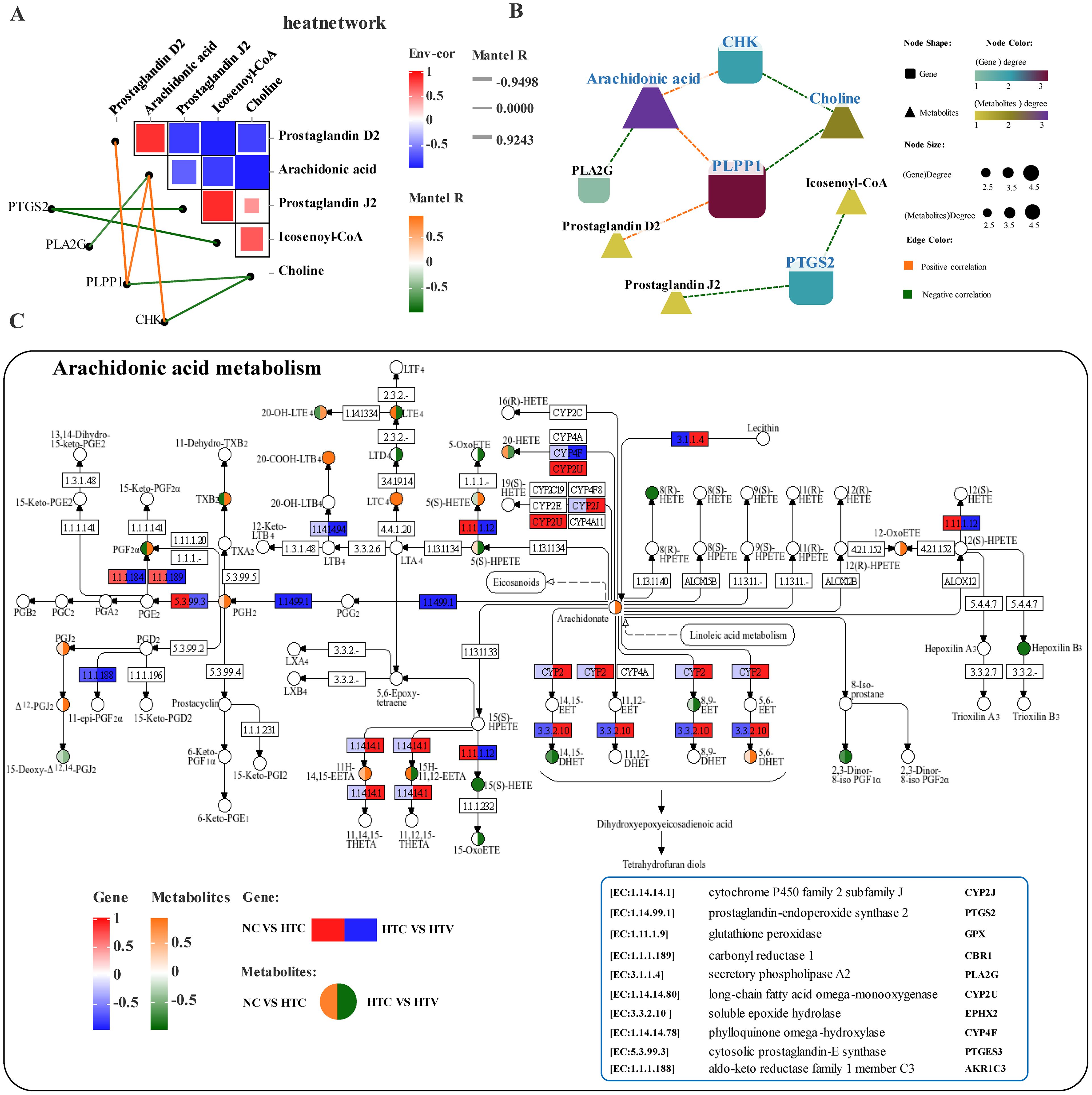
Figure 10. Interaction analysis of lipid metabolism-related genes and metabolites under thermal stress after addition of astaxanthin (HTC vs HTV). (A) Association network heatmap of DEGs and DEMs at the core of lipid metabolism. (B) Interaction network of DEGs - DEMs related to lipid metabolism; (C) KEGG mapping results of DEGs and DEMs in the arachidonic acid metabolism pathway. The green or red box illustrated significant downregulation or upregulation of the gene, respectively. The blue or yellow circle reflected significant downregulation or upregulation of the metabolite, respectively.
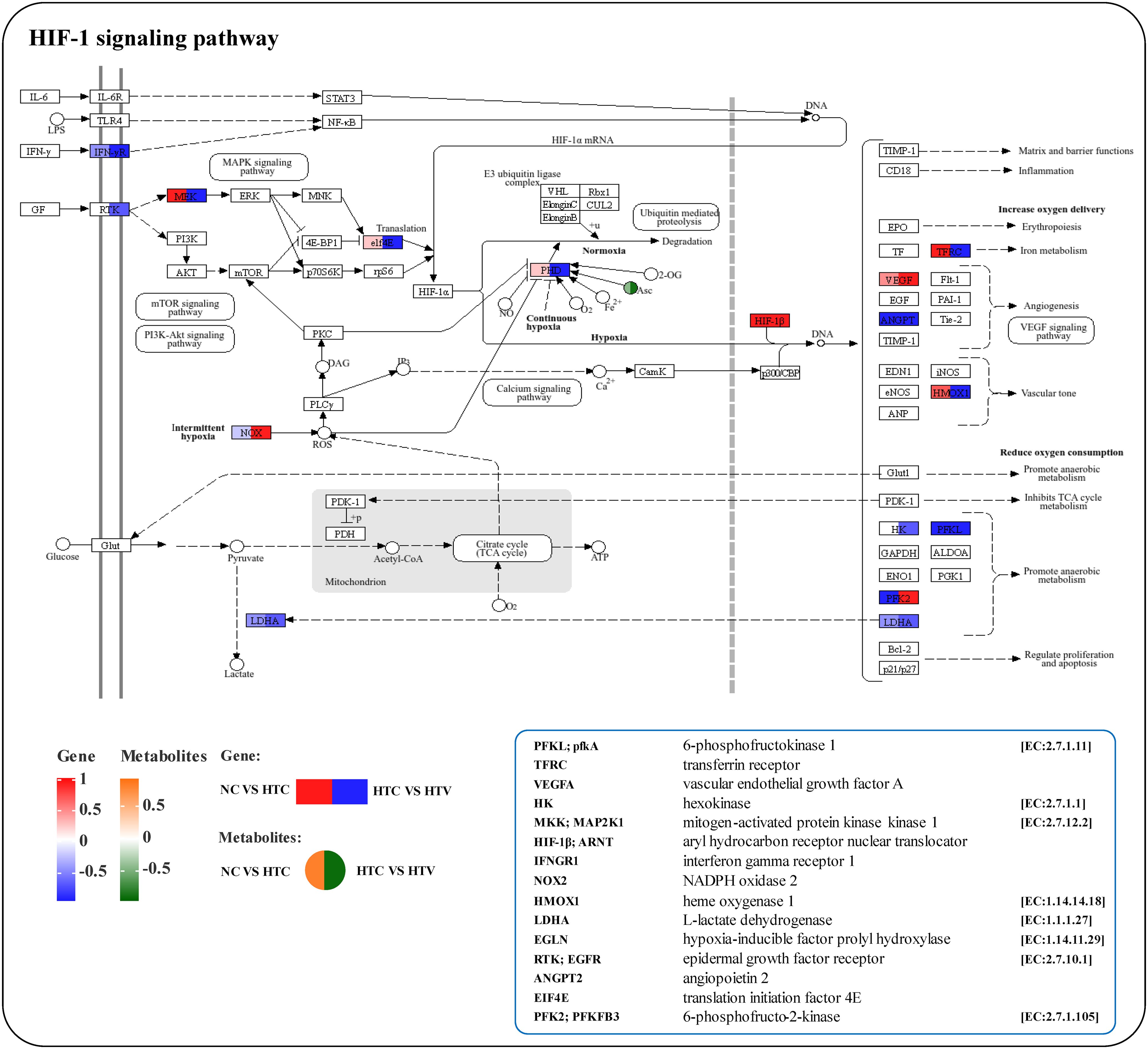
Figure 11. KEGG mapping results of DEGs and DEMs in the HIF-1 signaling pathway. The green or red box illustrated significant downregulation or upregulation of the gene, respectively. The blue or yellow circle reflected significant downregulation or upregulation of the metabolite, respectively.
4 Discussion
Fish health is intrinsically linked to environmental conditions, which regulate physiological, metabolic, and developmental processes (Beemelmanns et al., 2021; Cheng et al., 2018a). Fish are frequently exposed to stressors such as temperature fluctuations, disease outbreaks, and water quality deterioration, necessitating effective mitigation strategies. To counter these challenges, researchers have explored various interventions, including herbal compounds (Tan et al., 2017) and vitamins (El-Sayed and Izquierdo, 2022), aimed at enhancing fish resilience. Studies on Trachinotus ovatus have shown that dietary supplementation with hawthorn extract can enhance growth performance, improve immune capacity, and increase resistance to Vibrio harveyi (Tan et al., 2017). Similarly, research on Oreochromis niloticus has demonstrated that vitamin E can alleviate oxidative damage induced by microcystins and enhance the activities of CAT, SOD, and GPX (El-Sayed and Izquierdo, 2022). These findings provide new insights into addressing environmental stressors.
The ongoing global climate warming exacerbates thermal stress in fish. Astaxanthin demonstrates remarkable antioxidant activity, which significantly enhances the growth, metabolism, immunity, and antioxidant capacity of aquatic animals (Li et al., 2014). By integrating cellular and individual experiments with multi-omics analysis, we systematically investigated the effects of astaxanthin on the antioxidant system, inflammatory response, and metabolic reprogramming in largemouth bass under thermal stress. Furthermore, we elucidated the potential mechanisms by which astaxanthin alleviates stress-induced damage and remodels amino acid and lipid metabolism.
4.1 Astaxanthin enhances the antioxidant capacity of myocytes by promoting antioxidant enzyme activity and HSPs
Thermal stress induces excessive production of ROS in animals (Cheng et al., 2001), resulting in mitochondrial oxidative stress, cellular damage and apoptosis. This further results in the release of extracellular enzymes and an increase in intracellular peroxides, such as LDH and MDA, which are typically referred to as cell damage-related enzymes (Amani et al., 2013; Chen et al., 2013). In this research, after 2 hours of thermal stress, the levels of MDA and LDH in cultured myocytes significantly increased compared to before stress, and enzyme activity showed a clear upward trend with prolonged exposure. Cell viability only began to decline after 6 hours of thermal stress, indicating that the myocytes of the largemouth bass possess strong resistant, allowing them to withstand temperature fluctuations for a period.
In aquatic animals studied, cells primarily exert antioxidant functions through enzyme and non-enzyme systems, including antioxidant factors such as GPX, CAT, SOD and GSH (Jiang et al., 2016). The antioxidant enzyme system is the main protective mechanism for fish cells against oxidative stress (Mourente et al., 2002). When exposed to environmental stress, cells require urgent mechanisms, such as enhanced antioxidant enzyme activity. SOD and CAT, as key components of the antioxidant defense system, directly participate in the removal of ROS, protecting the organism from oxidative damage (Lortz et al., 2000; Zhang et al., 2013b). Numerous studies have shown that adding astaxanthin to feed can reduce oxidative stress levels and enhance antioxidant capacity, with increased astaxanthin content in feed significantly boosting the T-AOC and SOD in liver and serum, while MDA levels, reflecting ROS-induced cellular damage, tend to decrease (Eldessouki et al., 2024; Zhang et al., 2013a). In this study, after 2 hours of thermal stress, groups supplemented with 10μM and 20μM astaxanthin showed increased intracellular SOD and CAT activity and reduced MDA and extracellular LDH levels. Moreover, astaxanthin supplementation (5μM, 10μM, 20μM) significantly improved cell survival rates, indicating that astaxanthin enhances the ability to resist thermal stress by promoting antioxidant enzyme activity.
Astaxanthin also alleviates symptoms such as cell apoptosis and hepatopancreas tissue damage (Wang et al., 2018). Cells exposed to thermal stress activate molecularly mediated apoptotic signaling pathways, while HSPs provide protection by enhancing thermotolerance, promoting anti-apoptotic pathways, and regulating redox balance (Kabakov et al., 2003). Previous studies have shown that HSP70 and HSP90 are key members of the HSPs family, with HSP70 binding to nascent, unfolded, misfolded, or aggregated proteins, reducing the risks posed by aberrant protein expression, which is crucial for maintaining protein solubility and resistance to apoptosis (Kabakov et al., 2003; Voellmy and Boellmann, 2007). HSP90 mainly maintains protein stability and enhances cellular stress tolerance (Hartl and Hayer-Hartl, 2002). Therefore, under thermal stress, HSP70 may play a more significant role than HSP90. Research has demonstrated that astaxanthin can increase HSPs gene expression in lymphocytes induced by lipopolysaccharides (LPS) (Li et al., 2020), and similar findings were observed in rats under thermal stress (Fleischmann et al., 2020). Our results indicate that, as compared to the unsupplemented group, HSP70 and HSP90 expression levels in myocytes supplemental with astaxanthin increased significantly in the early stages of thermal stress, while MDA, CASP3, and CASP9 levels decreased significantly. These findings suggest that astaxanthin may have a pronounced anti-thermal stress effect on myocytes and mitigate apoptosis induced by thermal stress. In summary, astaxanthin can enhance heat tolerance and inhibit apoptosis in myocytes, thereby increasing the threshold for thermal damage. The induction of sufficient HSPs is a key factor in its anti-thermal stress mechanism.
4.2 Astaxanthin regulates the Keap1-Nrf2 signaling pathway to activate the glutathione-dependent endogenous antioxidant enzyme system
Vertebrate cells primarily rely on the Keap1-Nrf2 signaling pathway to achieve antioxidant stress protection, functioning through the regulation of expression and activity of antioxidant enzyme-dependent genes. For example, research by Wang et al. on Ctenopharyngodon idella demonstrated that the Keap1-Nrf2 pathway alleviates oxidative stress damage in gill cells induced under environmental stress by upregulating the expression and translation of antioxidant elements (SOD, CAT, GPX and GST) (Wang et al., 2015b), and increases the content of antioxidants in the intestine and muscles (Wang et al., 2015a). When cells suffer oxidative stress, the degradation of Nrf2 decreases, causing Nrf2 proteins to dissociate from Keap1. Nrf2 then translocates into the nucleus, binding with specific sites on the ARE cis-acting elements to regulate the transcription and expression of numerous antioxidant elements (Turley et al., 2015), activating phase II detoxifying enzymes and antioxidant systems to eliminate excessive ROS and enhance cellular antioxidant capacity (Lu et al., 2016). Studies have confirmed that astaxanthin enhances the activity of antioxidant enzymes by activating the Keap1-Nrf2 signaling pathway (Jaiswal, 2004). Similarly, our previous study on the long-term effects of dietary astaxanthin demonstrated that astaxanthin may enhance the activity of antioxidant enzymes (SOD, CAT) in the largemouth bass liver and serum by regulating the Nrf2 signaling pathway, potentially leading to decreased cortisol and MDA levels in these tissues (Zhang et al., 2024). In this study, during 120 min and 240 min of thermal stress, the expression of the Nrf2 gene in the group supplemented with 10 μM astaxanthin was significantly higher than in the unsupplemented group. Consistent with these findings, Brasil et al. revealed that astaxanthin supplementation enhances Nrf2 expression in SH-SY5Y cells to counteract environmental stress from peroxide exposure.
On the other hand, GSH, as an endogenous antioxidant, plays an essential role in preventing oxidative stress, scavenging free radicals, and reducing biological damage (Matthaiou et al., 2014). The synthesis of GSH is determined by glutamate-cysteine ligase (GCL) and GSH synthase (GS) (Lu, 2013). GST is an important factor in the antioxidant enzyme system, playing a significant role in scavenging free radicals and reducing biological damage. Nrf2 can regulate the expression of GSH synthesis-dependent genes (GS, GCL, GST and GPX) through transcription, affecting GSH de novo synthesis and redox processes, and regulating the mechanism of GSH homeostasis (Nguyen et al., 2009; Suzuki and Yamamoto, 2015). Additionally, Nrf2 can induce the transcription and translation of antioxidant element-dependent genes, such as SOD, CAT, and NQO1, hence controlling cellular oxidative stress defense mechanisms (Saw et al., 2013; Tabei et al., 2017; Wang et al., 2017). Therefore, activating the Keap1-Nrf2 pathway is crucial for upregulating GSH synthesis to perform endogenous antioxidant responses. Unsurprisingly, in this study, the largemouth bass given dietary astaxanthin exhibited a glutathione metabolism process dominated by GST, GPX4, GPX, and GGCT, with an overall more active regulatory pattern. Key DEMs, such as GSH, showed a significantly upregulated pattern, suggesting that astaxanthin enhances glutathione metabolism, participating in the liver’s antioxidant stress process, thereby reducing cell damage from environmental stress. Based on the observed upregulation of Nrf2 expression and its critical role in maintaining GSH homeostasis, this study hypothesizes that astaxanthin may enhance the activity of the Keap1-Nrf2 signaling pathway, thereby activating the GSH-dependent endogenous cellular antioxidant system and improving the antioxidant capacity of largemouth bass. However, further targeted metabolic validation and mechanistic studies are required to confirm this hypothesis.
4.3 Astaxanthin inhibits the TLR2/4-NF-κB signaling pathway to alleviate inflammatory damage under thermal stress
Previous results of this research have already demonstrated the crucial role of astaxanthin in enhancing the antioxidant capacity of largemouth bass and in alleviating cellular damage induced by thermal stress. Since oxidative stress can cause inflammatory damage, and excessive inflammation can lead to tissue damage, investigating the key role of astaxanthin in alleviating inflammatory damage under oxidative stress will be the focus of subsequent research. The inflammatory response, as a critical process in humoral immunity, essentially serves as a self-protective defense reaction of the organism against various injurious stimuli (White, 1999). The pro-inflammatory mediators primarily include ROS, transforming growth factor-beta (TGF-β), interferons, and others, playing a major role during the occurrence of inflammatory responses (Fierro and Serhan, 2001). ROS is an important means by which the inflammatory response exerts its immune function. On one hand, ROS can kill pathogens or eliminate damaged tissues as terminal effector molecules; on the other hand, it upregulates the expression of pro-inflammatory factors, acting as a trigger for inflammation and forming a positive feedback loop with pro-inflammatory cytokines. Antioxidants and antioxidant enzymes can inhibit the gene expression of inflammatory factors and block this positive feedback loop (Liu et al., 2001). Therefore, during the exercise of immune functions in the inflammatory response, the organism must quickly achieve a balance between damage and recovery; otherwise, excessive oxidative stress damage can lead to tissue injury (Ivanenkov et al., 2011).
In the innate immune system, astaxanthin can directly or indirectly enhance the organism’s antioxidant capacity and promote cell-mediated humoral immune responses by affecting lysosomal release, complement components, cationic peptides, and the synthesis of reactive oxygen species (Wu et al., 2023), thereby enhancing the resistance of aquatic animals to pathogenic microorganisms (Smith et al., 2013). Due to its unique chemical structure, astaxanthin possesses strong antioxidant properties, which can significantly inhibit the inflammatory response within tissues (Hwang et al., 2017; Ohgami et al., 2003). Further studies have shown that astaxanthin can exert anti-inflammatory effects by modulating the Toll-like receptor signaling pathway and the NF-κB signaling pathway (Li et al., 2019; Liu et al., 2015; Yang et al., 2019). TLR2/4 can recognize pathogen-associated molecular patterns (PAMPs) on different pathogen surfaces and transmit them to MyD88 (Myeloid Differentiation Factor 88), which then activates the NF-κB signaling pathway, leading to the release of pro-inflammatory factors such as TNF-α, IL-1, IL-2, and IL-8, thereby activating the inflammatory response through these cytokines (He et al., 2013; Lee et al., 2010; Tan et al., 2010). Inhibiting the TLR2/4-NF-κB signaling pathway can mitigate the damage caused by inflammation to tissues (Miyachi et al., 2015). For instance, in mammals, astaxanthin can inhibit the secretion and expression of pro-inflammatory cytokines (TNFα, IL-1β, IL-6, IL-8) within tissues (Kim et al., 2018; Santos et al., 2012; Zhang et al., 2018), thereby exerting an anti-inflammatory function and significantly alleviating the inflammatory response induced by LPS both in vivo and in vitro (Ohgami et al., 2003). In aquatic animals, astaxanthin has an inhibitory effect on the inflammatory response induced by LPS, reducing the upregulation of pro-inflammatory cytokines TNF-α, IL-6, and IL-1β, and decreasing the expression of the TLR4 gene, thereby inhibiting the activation of the NF-κB signaling pathway. This suggests that the anti-inflammatory effect of astaxanthin may be related to the regulation of the activation process of the TLR2/4-NF-κB signaling pathway (Li et al., 2019). The results of this study provide evidence for this hypothesis, showing that under thermal stress, the group supplemented with astaxanthin exhibited an overall downregulation in the expression of the Toll-like receptor signaling pathway and the NF-κB signaling pathway compared to the unsupplemented group. Moreover, in the NC, HTC, and HTV groups, TLR2, IL8, EIF4E, PTEN, CASP8, TSC2, and IL2RB showed a significant trend of first increasing and then decreasing. Based on these findings, we speculate that thermal stress alone can trigger an inflammatory response in largemouth bass, while the addition of astaxanthin alleviated the inflammatory damage caused by environmental stress by inhibiting the activation of the TLR2/4-NF-κB signaling pathway, enhancing the body’s ability to cope with adverse environments. In combination with the results of cell experiments and multi-omics analyses in this study, as well as the regulatory mechanisms of astaxanthin in antioxidant and anti-inflammatory processes, we propose the following hypothesis: astaxanthin may enhance antioxidant capacity by activating glutathione metabolism, thereby mitigating inflammatory damage mediated by the TLR2/4-NF-κB signaling pathway under thermal stress (Figure 12). However, further validation using pathway inhibitors or gene knockdown approaches is required to confirm the involvement of the Keap1-Nrf2 regulatory mechanism and refine this conclusion.
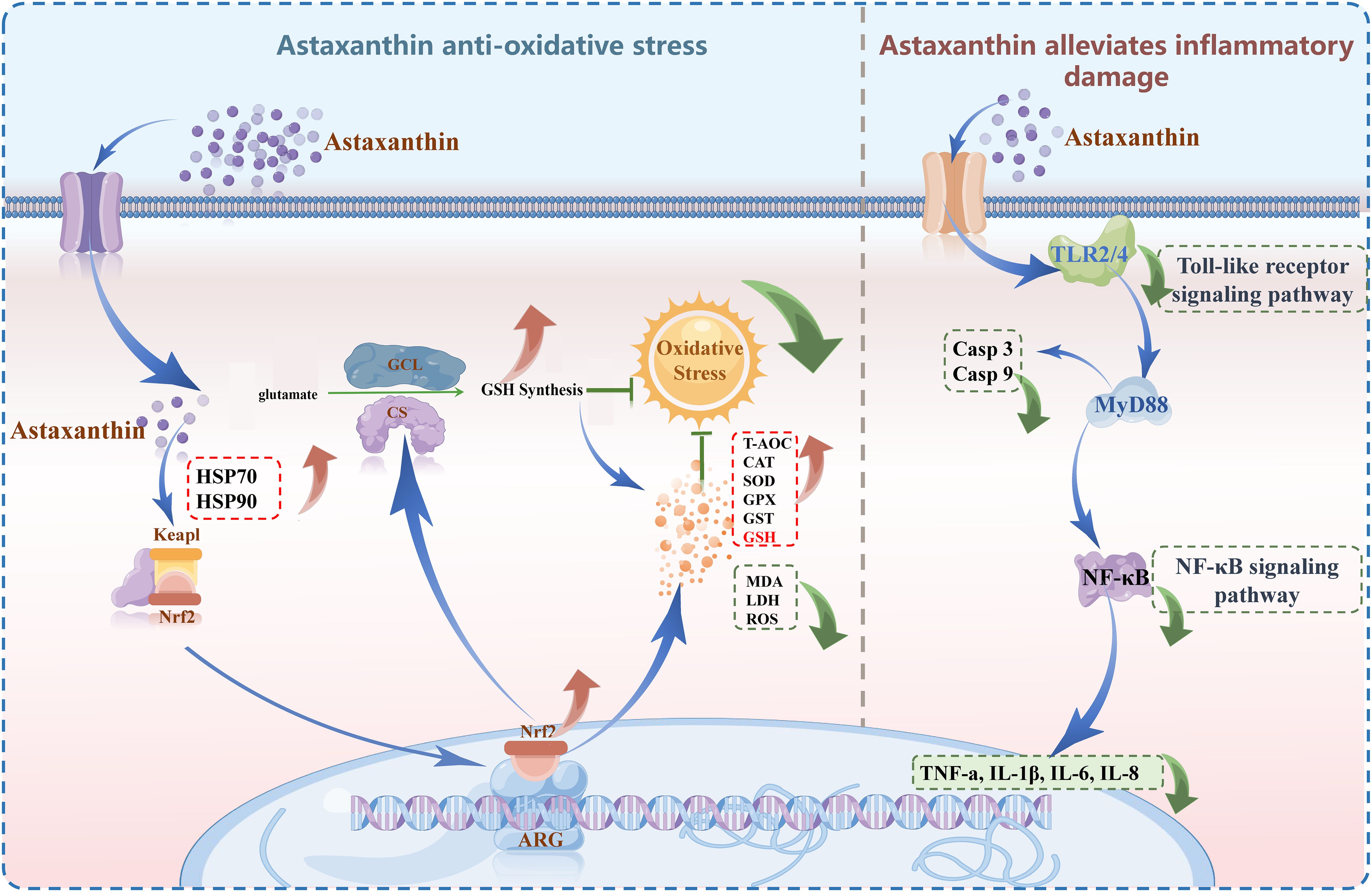
Figure 12. Graphical abstract: molecular regulation mechanism of astaxanthin enhances antioxidant capacity, reducing TLR2/4-NF-κB pathway-mediated liver inflammation under thermal stress.
4.4 Astaxanthin establishes adaptive stress-resistant metabolic mechanisms in largemouth bass by remodeling amino acid and lipid metabolism
During environmental stress, energy and material metabolism are integral components. A combined analysis of DEGs and DEMs identified pathways related to amino acid and lipid metabolism that respond to astaxanthin regulation, constructing a comprehensive regulatory network comprising various energy substance metabolisms. This indicates that astaxanthin induces remodeling of amino acid and lipid metabolism in largemouth bass. The immune-related HIF-1 signaling pathway was also significantly enriched, showing that astaxanthin notably regulates both energy metabolism and innate immune regulatory processes. In vivo amino acid metabolism not only provides energy and intermediate metabolites for cellular metabolism but also plays a crucial role in signal transduction, gene expression regulation, and antioxidant activity (Wendisch, 2014; Wu, 2009). In this study, DEMs (such as GSH, Glutathionylspermidine, Ascorbic acid, Glutathionylspermine, NADPH, NADP, etc.) and DEGs (such as GST, GPX, GPX4, GGCT, etc.) were jointly enriched in the amino acid metabolism pathway, constructing a regulatory network from genes to metabolites, and overall exhibiting a more active regulatory pattern. This suggests that under environmental stress, amino acid-related metabolism is undergoing significant metabolic remodelling. Simultaneously, research indicates that excessively high environmental temperatures can induce the metabolic shift of energy substances from carbohydrates and fats to proteins (Bayne, 1973), consistent with the active amino acid metabolism observed in this study. Notably, multiple key biomarkers in glutathione metabolism, such as GSH and GST, are significantly enriched. Considering the crucial role of glutathione metabolism in the antioxidant system, it suggests that under high temperature stress, astaxanthin enhances the ability of largemouth bass to cope with thermal stress by upregulating amino acid metabolism and remodelling the energy metabolism process, especially glutathione metabolism.
On the other hand, astaxanthin also affects lipid metabolism in fish, particularly regulating hepatic lipid metabolism, including the biosynthesis of terpenoids and steroids. In this study, several biomarkers (Arachidonic acid, Prostaglandin D2, CYP2J2, GPX, PLA2G) were collectively enriched in lipid metabolism pathways, especially in Arachidonic acid metabolism, which exhibited a complex regulatory pattern in response to astaxanthin induction. Arachidonic acid, as a precursor of eicosanoids, plays a crucial role in immune regulation throughout the body by generating a series of prostaglandins (PGs) (Gómez-Abellán and Sepulcre, 2016). Studies have shown that dietary supplementation with arachidonic acid can increase the activity of the alternative complement pathway (ACP) and SOD in Lateolabrax japonicus (Xu et al., 2010). Additionally, PGs play a significant role in the progress of the immune response at multiple levels, often in concert with microbial ligands, cytokines, and various other factors (Simmons et al., 2004). Additionally, CYP2J2, an important member of the cytochrome P450 enzyme system, along with various DEMs such as Arachidonic acid, were enriched in the Arachidonic acid metabolism pathway. As a significant phase I metabolic enzyme (Sisignano et al., 2020), CYP2J2 plays an essential role in the redox metabolism of xenobiotics (Lewis et al., 1999). This indicates that lipid metabolic processes, represented by arachidonic acid metabolism, respond to astaxanthin induction and participate in immune regulation and antioxidant processes under thermal stress. The results from simple transcriptomics are limited, as there is a lengthy biochemical process from genes to the execution of antioxidant or metabolic regulatory biological functions (Ytrestøyl et al., 2021). This study, for the first time, employed a combined analysis of metabolomics and transcriptomics to investigate the remodelling process of stress-resistant metabolism in largemouth bass induced by astaxanthin, and mapped the energy metabolism regulatory network of metabolites and genes. In summary, the arachidonic acid metabolism pathway is involved in the phase I metabolic regulation process induced by astaxanthin, while glutathione metabolism, as a critical component of the antioxidant system, represents an important phase II metabolic pathway (Yang et al., 2006). This suggests that the phase I to phase II metabolic adaptive mechanisms in largemouth bass under thermal stress may respond to astaxanthin induction.
5 Conclusion
This study investigates the role of astaxanthin in countering oxidative stress, inhibiting inflammatory responses, and remodeling metabolism by combining thermal stress experiments with cellular and individual-level supplementation. Under thermal stress, oxidative damage is closely linked to inflammatory responses. astaxanthin enhances antioxidant enzymes (CAT and SOD) and HSPs to resist thermal stress. Comprehensive multi-omics analysis identified significant upregulation of key biomarkers such as GSH, GPX, and GST. Further analysis suggests that astaxanthin may activate the glutathione-dependent cellular endogenous antioxidant enzyme system by regulating the Keap1-Nrf2 signaling pathway. This activation enhances the organism’s overall antioxidant capacity, which subsequently alleviates inflammatory damage mediated by the TLR2/4-NF-κB signaling pathway. Investigations into innate immune-related pathways revealed downregulation of several key immune pathways, suggesting that astaxanthin mitigates inflammatory damage by inhibiting these pathways. Additionally, the enrichment results of DEGs and DEMs suggest that astaxanthin could regulate adaptive mechanisms from phase I to phase II metabolism in the liver by remodeling amino acid and lipid metabolic processes under thermal stress. In summary, this study proposes the following hypothesis: astaxanthin may enhance antioxidant capacity by remodeling glutathione metabolism, thereby potentially alleviating TLR2/4-NF-κB pathway-mediated inflammatory damage in largemouth bass under thermal stress. However, further pathway mechanistic validation is required to confirm these findings and refine this conclusion.
6 Study Limitations
Cellular and in vivo studies suggest that astaxanthin supplementation activates the endogenous antioxidant system by upregulating antioxidant enzymes such as CAT and SOD. Multi-omics analyses further indicate that astaxanthin may exert protective effects through modulation of the Nrf2-Keap1 signaling pathway, which activates the glutathione-dependent antioxidant system. However, functional validation of the Nrf2-Keap1 signaling pathway, using pathway inhibitors or gene knockdown techniques, remains to be performed. Similarly, pharmacological inhibitors could be utilized to confirm astaxanthin’s inhibitory effects on the TLR2/4-NF-κB signaling pathway. Incorporating specific GSH activity enhancers as positive controls would provide additional evidence for astaxanthin’s efficacy. Moreover, further immunostaining of myogenic markers or related gene expression analysis could strengthen myocyte identity verification. Hence, while this study provides preliminary insights into the relevant mechanisms, further long-term research is warranted, particularly in vitro experiments incorporating RNA knockdown and specific enhancers, and improved validation techniques.
Data availability statement
The original contributions presented in the study are included in the article/Supplementary Material. Further inquiries can be directed to the corresponding author/s.
Ethics statement
All experiments involving fish in this study were meticulously conducted in accordance with the Management Rule of Laboratory Animals (Chinese Order No. 676 of the State Council, revised on March 1, 2017). These experiments were executed under the vigilant supervision and inspection of the Experimental Animal Welfare Ethics Committee of Zhejiang Ocean University. The study was conducted in accordance with the local legislation and institutional requirements.
Author contributions
YS: Investigation, Formal analysis, Writing – original draft, Methodology, Writing – review & editing. WZ: Data curation, Methodology, Investigation, Writing – original draft, Formal analysis. JW: Writing – review & editing, Supervision, Funding acquisition, Conceptualization. JH: Writing – review & editing, Methodology, Data curation. JZ: Formal analysis, Writing – original draft, Methodology, Investigation. TH: Funding acquisition, Writing – review & editing.
Funding
The author(s) declare that financial support was received for the research and/or publication of this article. This work was supported by Major Scientific and Technological Research Projects of Zhoushan (2023C13017), Zhejiang Province “three agricultural six-party” Science and Technology Collaboration Program (2024SNJF059), The Key Research and Development Program of Zhejiang Province (2021C04016), National Natural Science Foundation of China (32273151), The Research Fund of Zhejiang Ocean University, China (No. JX6311032423).
Conflict of interest
The authors declare that the research was conducted in the absence of any commercial or financial relationships that could be construed as a potential conflict of interest.
Generative AI statement
The author(s) declare that no Generative AI was used in the creation of this manuscript.
Publisher’s note
All claims expressed in this article are solely those of the authors and do not necessarily represent those of their affiliated organizations, or those of the publisher, the editors and the reviewers. Any product that may be evaluated in this article, or claim that may be made by its manufacturer, is not guaranteed or endorsed by the publisher.
Supplementary material
The Supplementary Material for this article can be found online at: https://www.frontiersin.org/articles/10.3389/fmars.2025.1595039/full#supplementary-material
References
Amani M., Ahmadiasl N., Usefzade N., and Zaman J. (2013). Effect of HEMADO on level of CK-MB and LDH enzymes after ischemia/reperfusion injury in isolated rat heart. BioImpacts: BI. 3, 101. doi: doi: 10.5681/bi.2013.003
Ambati R. R., Phang S.-M., Ravi S., and Aswathanarayana R. G. (2014). Astaxanthin: Sources, extraction, stability, biological activities and its commercial applications—A review. Mar. Drugs 12, 128–152. doi: 10.3390/md12010128
Arora A. and Damodaran S. (2011). Removal of soy protein-bound phospholipids by a combination of sonication, β-cyclodextrin, and phospholipase A2 treatments. Food Chem. 127, 1007–1013. doi: 10.1016/j.foodchem.2011.01.073
Bayne B. (1973). Physiological changes in Mytilus edulis L. induced by temperature and nutritive stress. J. Mar. Biol. Assoc. United Kingdom. 53, 39–58. doi: 10.1017/S0025315400056629
Beemelmanns A., Zanuzzo F. S., Xue X., Sandrelli R. M., Rise M. L., and Gamperl A. K. (2021). The transcriptomic responses of Atlantic salmon (Salmo salar) to high temperature stress alone, and in combination with moderate hypoxia. BMC Genomics 22, 1–33. doi: 10.1186/s12864-021-07464-x
Chen T.-H., Yang Y.-C., Wang J.-C., and Wang J.-J. (2013). “Curcumin treatment protects against renal ischemia and reperfusion injury–induced cardiac dysfunction and myocardial injury,” in Transplantation proceedings (Elsevier) 45, 3546–3549. doi: 10.1016/j.transproceed.2013.09.006
Cheng C.-H., Guo Z.-X., Luo S.-W., and Wang A.-L. (2018a). Effects of high temperature on biochemical parameters, oxidative stress, DNA damage and apoptosis of pufferfish (Takifugu obscurus). Ecotoxicology Environ. safety. 150, 190–198. doi: 10.1016/j.ecoenv.2017.12.045
Cheng C.-H., Guo Z.-X., Ye C.-X., and Wang A.-L. (2018b). Effect of dietary astaxanthin on the growth performance, non-specific immunity, and antioxidant capacity of pufferfish (Takifugu obscurus) under high temperature stress. Fish Physiol. Biochem. 44, 209–218. doi: 10.1007/s10695-017-0425-5
Cheng T.-Y., Zhu Z., Masuda S., and Morcos N. (2001). Effects of multinutrient supplementation on antioxidant defense systems in healthy human beings. J. Nutr. Biochem. 12, 388–395. doi: 10.1016/S0955-2863(01)00153-X
Chomczynski P. and Sacchi N. (2006). The single-step method of RNA isolation by acid guanidinium thiocyanate–phenol–chloroform extraction: twenty-something years on. Nat. Protoc. 1, 581–585. doi: 10.1038/nprot.2006.83
Eldessouki E. A., Elshopakey G. E., Elbahnaswy S., Shakweer M. S., Abdelwarith A. A., Younis E. M., et al. (2024). Influence of astaxanthin-enriched Haematococcus pluvialis microalgae on the growth efficacy, immune response, antioxidant capacity, proinflammatory cytokines, and tissue histomorphology of hybrid red tilapia. Aquaculture Int. 32, 7447–7468. doi: 10.1007/s10499-024-01524-1
El-Sayed A. F. M. and Izquierdo M. (2022). The importance of vitamin E for farmed fish—A review. Rev. Aquaculture. 14, 688–703. doi: 10.1111/raq.12619
Fierro I. and Serhan C. (2001). Mechanisms in anti-inflammation and resolution: the role of lipoxins and aspirin-triggered lipoxins. Braz. J. Med. Biol. Res. 34, 555–566. doi: 10.1590/S0100-879X2001000500002
Fleischmann C., Bar-Ilan N., Horowitz M., Bruchim Y., Deuster P., and Heled Y. (2020). Astaxanthin supplementation impacts the cellular HSP expression profile during passive heating. Cell Stress Chaperones. 25, 549–558. doi: 10.1007/s12192-019-01061-4
Gallagher P. M. (2013). Stress sensors of skeletal muscle: heat shock-induced cytokine expression. Focus on “Skeletal muscle interleukin-6 regulation in hyperthermia. Am. J. Physiology-Cell Physiol. 305, C375–C376. doi: 10.1152/ajpcell.00184.2013
Gómez-Abellán V. and Sepulcre M. P. (2016). The role of prostaglandins in the regulation of fish immunity. Mol. Immunol. 69, 139–145. doi: 10.1016/j.molimm.2015.09.022
Hartl F. U. and Hayer-Hartl M. (2002). Molecular chaperones in the cytosol: from nascent chain to folded protein. Science 295, 1852–1858. doi: 10.1126/science.1068408
He W., Qu T., Yu Q., Wang Z., Lv H., Zhang J., et al. (2013). LPS induces IL-8 expression through TLR 4, M y D 88, NF-kappa B and MAPK pathways in human dental pulp stem cells. Int. endodontic J. 46, 128–136. doi: 10.1111/j.1365-2591.2012.02096.x
Hwang Y.-H., Hong S.-G., Mun S.-K., Kim S.-J., Lee S.-J., Kim J.-J., et al. (2017). The protective effects of astaxanthin on the OVA-induced asthma mice model. Molecules 22, 2019. doi: 10.3390/molecules22112019
Ivanenkov Y. A., Balakin K. V., and Lavrovsky Y. (2011). Small molecule inhibitors of NF-κB and JAK/STAT signal transduction pathways as promising anti-inflammatory therapeutics. Mini Rev. medicinal Chem. 11, 55–78.
Jaiswal A. K. (2004). Nrf2 signaling in coordinated activation of antioxidant gene expression. Free Radical Biol. Med. 36, 1199–1207. doi: 10.1016/j.freeradbiomed.2004.02.074
Jiang W.-D., Wen H.-L., Liu Y., Jiang J., Wu P., Zhao J., et al. (2016). Enhanced muscle nutrient content and flesh quality, resulting from tryptophan, is associated with anti-oxidative damage referred to the Nrf2 and TOR signalling factors in young grass carp (Ctenopharyngodon idella): Avoid tryptophan deficiency or excess. Food Chem. 199, 210–219. doi: 10.1016/j.foodchem.2015.12.003
Jiang X., Zu L., Wang Z., Cheng Y., Yang Y., and Wu X. (2020). Micro-algal astaxanthin could improve the antioxidant capability, immunity and ammonia resistance of juvenile Chinese mitten crab, Eriocheir sinensis. Fish shellfish Immunol. 102, 499–510. doi: 10.1016/j.fsi.2020.05.021
Kabakov A. E., Budagova K. R., Bryantsev A. L., and Latchman D. S. (2003). Heat shock protein 70 or heat shock protein 27 overexpressed in human endothelial cells during posthypoxic reoxygenation can protect from delayed apoptosis. Cell Stress chaperones. 8, 335. doi: 10.1379/1466-1268(2003)008<0335:HSPOHS>2.0.CO;2
Kim S. H., Lim J. W., and Kim H. (2018). Astaxanthin inhibits mitochondrial dysfunction and interleukin-8 expression in Helicobacter pylori-infected gastric epithelial cells. Nutrients 10, 1320. doi: 10.3390/nu10091320
Lee B. C., Kim M. S., Choi S. H., and Kim T. S. (2010). Involvement of capsular polysaccharide via a TLR2/NF-κB pathway in Vibrio vulnificus-induced IL-8 secretion of human intestinal epithelial cells. Int. J. Mol. Med. 25, 581–591. doi: 10.3892/ijmm_00000380
Lewis D. F., Dickins M., Eddershaw P. J., Tarbit M., and Goldfarb P. S. (1999). Cytochrome P450 substrate specificities, substrate structural templates and enzyme active site geometries. Drug Metab. Drug interactions. 15, 1–50. doi: 10.1515/DMDI.1999.15.1.1
Li M. Y., Gao C. S., Du X. Y., Zhao L., Niu X. T., Wang G. Q., et al. (2020). Amelioration of LPS-induced inflammatory response and oxidative stress by astaxanthin in Channa argus lymphocyte via activating glucocorticoid receptor signalling pathways. Aquaculture Res. 51, 2687–2697. doi: 10.1111/are.14608
Li M.-Y., Sun L., Niu X.-T., Chen X.-M., Tian J.-X., Kong Y.-D., et al. (2019). Astaxanthin protects lipopolysaccharide-induced inflammatory response in Channa argus through inhibiting NF-κB and MAPKs signaling pathways. Fish shellfish Immunol. 86, 280–286. doi: 10.1016/j.fsi.2018.11.011
Li M., Wu W., Zhou P., Xie F., Zhou Q., and Mai K. (2014). Comparison effect of dietary astaxanthin and Haematococcus pluvialis on growth performance, antioxidant status and immune response of large yellow croaker Pseudosciaena crocea. Aquaculture 434, 227–232. doi: 10.1016/j.aquaculture.2014.08.022
Liu Q.-S., Cheng Z.-W., Xiong J.-G., Cheng S., He X.-F., and Li X.-C. (2015). “Erythropoietin pretreatment exerts anti-inflammatory effects in hepatic ischemia/reperfusion-injured rats via suppression of the TLR2/NF-κB pathway,” in Transplantation Proceedings (Elsevier) 47, 283–289. doi: 10.1016/j.transproceed.2014.10.045
Liu T.-Z., Lee K.-T., Chern C.-L., Cheng J.-T., Stern A., and Tsai L.-Y. (2001). Free radical-triggered hepatic injury of experimental obstructive jaundice of rats involves overproduction of proinflammatory cytokines and enhanced activation of nuclear factor κB. Ann. Clin. Lab. Science. 31, 383–390.
Lortz S., Tiedge M., Nachtwey T., Karlsen A. E., Nerup J., and Lenzen S. (2000). Protection of insulin-producing RINm5F cells against cytokine-mediated toxicity through overexpression of antioxidant enzymes. Diabetes 49, 1123–1130. doi: 10.2337/diabetes.49.7.1123
Lu S. C. (2013). “Glutathione synthesis,” in Biochimica et Biophysica Acta (BBA)-General Subjects, Elsevier 1830, 3143–3153.
Lu M. C., Ji J. A., Jiang Z. Y., and You Q. D. (2016). The Keap1–Nrf2–ARE pathway as a potential preventive and therapeutic target: an update. Medicinal Res. Rev. 36, 924–963. doi: 10.1002/med.2016.36.issue-5
Matthaiou C. M., Goutzourelas N., Stagos D., Sarafoglou E., Jamurtas A., Koulocheri S. D., et al. (2014). Pomegranate juice consumption increases GSH levels and reduces lipid and protein oxidation in human blood. Food Chem. toxicology. 73, 1–6. doi: 10.1016/j.fct.2014.07.027
Miyachi M., Matsuno T., Asano K., and Mataga I. (2015). Anti-inflammatory effects of astaxanthin in the human gingival keratinocyte line NDUSD-1. J. Clin. Biochem. Nutr. 56, 171–178. doi: 10.3164/jcbn.14-109
Mo F., Zhou X., Yang M., Chen L., Tang Z., Wang C., et al. (2022). Trehalose attenuates oxidative stress and endoplasmic reticulum stress-mediated apoptosis in IPEC-J2 cells subjected to heat stress. Animals 12, 2093. doi: 10.3390/ani12162093
Mourente G., Dıaz-Salvago E., Bell J. G., and Tocher D. R. (2002). Increased activities of hepatic antioxidant defence enzymes in juvenile gilthead sea bream (Sparus aurata L.) fed dietary oxidised oil: attenuation by dietary vitamin E. Aquaculture 214, 343–361. doi: 10.1016/S0044-8486(02)00064-9
Nakagawa K., Kiko T., Miyazawa T., Burdeos G. C., Kimura F., Satoh A., et al. (2011). Antioxidant effect of astaxanthin on phospholipid peroxidation in human erythrocytes. Br. J. Nutr. 105, 1563–1571. doi: 10.1017/S0007114510005398
Nakano T., Hayashi S., and Nagamine N. (2018). Effect of excessive doses of oxytetracycline on stress-related biomarker expression in coho salmon. Environ. Sci. pollut. Res. 25, 7121–7128. doi: 10.1007/s11356-015-4898-4
Nguyen T., Nioi P., and Pickett C. B. (2009). The Nrf2-antioxidant response element signaling pathway and its activation by oxidative stress. J. Biol. Chem. 284, 13291–13295. doi: 10.1074/jbc.R900010200
Ohgami K., Shiratori K., Kotake S., Nishida T., Mizuki N., Yazawa K., et al. (2003). Effects of astaxanthin on lipopolysaccharide-induced inflammation in vitro and in vivo. Invest. Ophthalmol. Visual Sci. 44, 2694–2701. doi: 10.1167/iovs.02-0822
Roushdy E. M., Zaglool A. W., and El-Tarabany M. S. (2018). Effects of chronic thermal stress on growth performance, carcass traits, antioxidant indices and the expression of HSP70, growth hormone and superoxide dismutase genes in two broiler strains. J. Thermal Biol. 74, 337–343. doi: 10.1016/j.jtherbio.2018.04.009
Santos S. D., Cahú T. B., Firmino G. O., de Castro C. C., Carvalho J. L. B., Bezerra R. S., et al. (2012). Shrimp waste extract and astaxanthin: rat alveolar macrophage, oxidative stress and inflammation. J. Food Sci. 77, H141–H146. doi: 10.1111/j.1750-3841.2012.02762.x
Saw C. L. L., Yang A. Y., Guo Y., and Kong A.-N. T. (2013). Astaxanthin and omega-3 fatty acids individually and in combination protect against oxidative stress via the Nrf2–ARE pathway. Food Chem. toxicology. 62, 869–875. doi: 10.1016/j.fct.2013.10.023
Shehata A. M., Saadeldin I. M., Tukur H. A., and Habashy W. S. (2020). Modulation of heat-shock proteins mediates chicken cell survival against thermal stress. Animals 10, 2407. doi: 10.3390/ani10122407
Simmons D. L., Botting R. M., and Hla T. (2004). Cyclooxygenase isozymes: the biology of prostaglandin synthesis and inhibition. Pharmacol. Rev. 56, 387–437. doi: 10.1124/pr.56.3.3
Sisignano M., Steinhilber D., Parnham M. J., and Geisslinger G. (2020). Exploring CYP2J2: lipid mediators, inhibitors and therapeutic implications. Drug Discovery Today 25, 1744–1753. doi: 10.1016/j.drudis.2020.07.002
Smith C., Cortes R., and Gomez L. (2013). Astaxanthin effect on reactive oxygen species and leukocytes counts in rainbow trout (Oncorhynchus mykiss). Glob Virtual Conf., 8e12.
Song X., Wang L., Li X., Chen Z., Liang G., and Leng X. (2017). Dietary astaxanthin improved the body pigmentation and antioxidant function, but not the growth of discus fish (Symphysodon spp.). Aquaculture Res. 48, 1359–1367. doi: 10.1111/are.13200
Sun Y., Yao C., Zhu Y., Wang Y., and Zhang Z. (2022). Metabolism response of fasting in Octopus sinensis paralarvae revealed by RNA-seq. Aquaculture 550, 737859. doi: 10.1016/j.aquaculture.2021.737859
Suzuki T. and Yamamoto M. (2015). Molecular basis of the Keap1–Nrf2 system. Free Radical Biol. Med. 88, 93–100. doi: 10.1016/j.freeradbiomed.2015.06.006
Tabei Y., Murotomi K., Umeno A., Horie M., Tsujino Y., Masutani B., et al. (2017). Antioxidant properties of 5-hydroxy-4-phenyl-butenolide via activation of Nrf2/ARE signaling pathway. Food Chem. Toxicology. 107, 129–137. doi: 10.1016/j.fct.2017.06.039
Tan X., Sun Z., Huang Z., Zhou C., Lin H., Tan L., et al. (2017). Effects of dietary hawthorn extract on growth performance, immune responses, growth-and immune-related genes expression of juvenile golden pompano (Trachinotus ovatus) and its susceptibility to Vibrio harveyi infection. Fish shellfish Immunol. 70, 656–664. doi: 10.1016/j.fsi.2017.09.041
Tan B., Yin Y., Kong X., Li P., Li X., Gao H., et al. (2010). L-Arginine stimulates proliferation and prevents endotoxin-induced death of intestinal cells. Amino Acids 38, 1227–1235. doi: 10.1007/s00726-009-0334-8
Tan K., Zhang H., Lim L.-S., Ma H., Li S., and Zheng H. (2020). Roles of carotenoids in invertebrate immunology. Front. Immunol. 10, 3041. doi: 10.3389/fimmu.2019.03041
Turley A. E., Zagorski J. W., and Rockwell C. E. (2015). The Nrf2 activator tBHQ inhibits T cell activation of primary human CD4 T cells. Cytokine 71, 289–295. doi: 10.1016/j.cyto.2014.11.006
Voellmy R. and Boellmann F. (2007). “Chaperone regulation of the heat shock protein response,” in Molecular aspects of the stress response: chaperones, membranes and networks New York: Springer 89–99.
Wang B., Feng L., Jiang W.-D., Wu P., Kuang S.-Y., Jiang J., et al. (2015b). Copper-induced tight junction mRNA expression changes, apoptosis and antioxidant responses via NF-κB, TOR and Nrf2 signaling molecules in the gills of fish: preventive role of arginine. Aquat. toxicology. 158, 125–137. doi: 10.1016/j.aquatox.2014.10.025
Wang P., Gao Y.-M., Sun X., Guo N., Li J., Wang W., et al. (2017). Hepatoprotective effect of 2′-O-galloylhyperin against oxidative stress-induced liver damage through induction of Nrf2/ARE-mediated antioxidant pathway. Food Chem. Toxicology. 102, 129–142. doi: 10.1016/j.fct.2017.02.016
Wang W., Ishikawa M., Koshio S., Yokoyama S., Hossain M. S., and Moss A. S. (2018). Effects of dietary astaxanthin supplementation on juvenile kuruma shrimp, Marsupenaeus japonicus. Aquaculture 491, 197–204. doi: 10.1016/j.aquaculture.2018.03.025
Wang B., Liu Y., Feng L., Jiang W.-D., Kuang S.-Y., Jiang J., et al. (2015a). Effects of dietary arginine supplementation on growth performance, flesh quality, muscle antioxidant capacity and antioxidant-related signalling molecule expression in young grass carp (Ctenopharyngodon idella). Food Chem. 167, 91–99. doi: 10.1016/j.foodchem.2014.06.091
Wendisch V. F. (2014). Microbial production of amino acids and derived chemicals: synthetic biology approaches to strain development. Curr. Opin. Biotechnol. 30, 51–58. doi: 10.1016/j.copbio.2014.05.004
White M. (1999). Mediators of inflammation and the inflammatory process. J. Allergy Clin. Immunol. 103, S378–S381. doi: 10.1016/S0091-6749(99)70215-0
Wu G. (2009). Amino acids: metabolism, functions, and nutrition. Amino Acids 37, 1–17. doi: 10.1007/s00726-009-0269-0
Wu Y., Xiao Y., Li W., Yang C., Ma W., Pang Z., et al. (2023). Tea polyphenols, astaxanthin, and melittin can significantly enhance the immune response of juvenile spotted knifejaw (Oplegnathus punctatus). Fish Shellfish Immunol. 138, 108817. doi: 10.1016/j.fsi.2023.108817
Xu H., Ai Q., Mai K., Xu W., Wang J., Ma H., et al. (2010). Effects of dietary arachidonic acid on growth performance, survival, immune response and tissue fatty acid composition of juvenile Japanese seabass, Lateolabrax japonicus. Aquaculture 307, 75–82. doi: 10.1016/j.aquaculture.2010.07.001
Yang P., Ebbert J. O., Sun Z., and Weinshilboum R. M. (2006). Role of the glutathione metabolic pathway in lung cancer treatment and prognosis: a review. J. Clin. Oncol. 24, 1761–1769. doi: 10.1200/JCO.2005.02.7110
Yang C., Hassan Y. I., Liu R., Zhang H., Chen Y., Zhang L., et al. (2019). Anti-inflammatory effects of different astaxanthin isomers and the roles of lipid transporters in the cellular transport of astaxanthin isomers in Caco-2 cell monolayers. J. Agric. Food Chem. 67, 6222–6231. doi: 10.1021/acs.jafc.9b02102
Yi X., Xu W., Zhou H., Zhang Y., Luo Y., Zhang W., et al. (2014). Effects of dietary astaxanthin and xanthophylls on the growth and skin pigmentation of large yellow croaker Larimichthys croceus. Aquaculture 433, 377–383. doi: 10.1016/j.aquaculture.2014.06.038
Ytrestøyl T., Afanasyev S., Ruyter B., Hatlen B., Østbye T.-K., and Krasnov A. (2021). Transcriptome and functional responses to absence of astaxanthin in Atlantic salmon fed low marine diets. Comp. Biochem. Physiol. Part D: Genomics Proteomics. 39, 100841.
Zhang J., Li X., Leng X., Zhang C., Han Z., and Zhang F. (2013a). Effects of dietary astaxanthins on pigmentation of flesh and tissue antioxidation of rainbow trout (Oncorhynchus mykiss). Aquaculture Int. 21, 579–589. doi: 10.1007/s10499-012-9590-9
Zhang J., Liu Y. J., Tian L. X., Yang H. J., Liang G. Y., Yue Y. R., et al. (2013b). Effects of dietary astaxanthin on growth, antioxidant capacity and gene expression in Pacific white shrimp L itopenaeus vannamei. Aquaculture Nutr. 19, 917–927. doi: 10.1111/anu.2013.19.issue-6
Zhang H., Yang W., Li Y., Hu L., Dai Y., Chen J., et al. (2018). Astaxanthin ameliorates cerulein-induced acute pancreatitis in mice. Int. Immunopharmacology. 56, 18–28. doi: 10.1016/j.intimp.2018.01.011
Keywords: astaxanthin, thermal stress, anti-oxidative, Micropterus salmoides, glutathione metabolism, Keap1-Nrf2 signaling pathways
Citation: Sun Y, Zong W, Wang J, He J, Zhang J and Han T (2025) Astaxanthin enhances antioxidant capacity to alleviate thermal stress-induced liver inflammation in largemouth bass (Micropterus salmoides): a multi-omics insight into glutathione metabolism remodeling. Front. Mar. Sci. 12:1595039. doi: 10.3389/fmars.2025.1595039
Received: 17 March 2025; Accepted: 07 May 2025;
Published: 27 May 2025.
Edited by:
Lei Wang, Anhui Normal University, ChinaReviewed by:
Amit Ranjan, Tamil Nadu Fisheries University, IndiaSena Ardicli, Bursa Uludağ University, Türkiye
Copyright © 2025 Sun, Zong, Wang, He, Zhang and Han. This is an open-access article distributed under the terms of the Creative Commons Attribution License (CC BY). The use, distribution or reproduction in other forums is permitted, provided the original author(s) and the copyright owner(s) are credited and that the original publication in this journal is cited, in accordance with accepted academic practice. No use, distribution or reproduction is permitted which does not comply with these terms.
*Correspondence: Jiteng Wang, d2FuZ2ppdGVuZzE5NzFAMTYzLmNvbQ==