- 1Department of Nephrology, The First Affiliated Hospital, Hengyang Medical School, University of South China, Hengyang, Hunan, China
- 2Department of Pathology, Yueyang People's Hospital, Yueyang, Hunan, China
- 3Hengyang Medical School, The First Affiliated Hospital, Institute of Clinical Medicine, University of South China, Hengyang, Hunan, China
- 4Department of Nephrology, Xiangya Hospital, Central South University, Changsha, Hunan, China
Atherosclerosis (AS) is characterized by the accumulation of lipids, fibrous elements, and calcification in the innermost layers of arteries. Vascular calcification (VC), the deposition of calcium and phosphate within the arterial wall, is an important characteristic of AS natural history. However, medial arterial calcification (MAC) differs from intimal calcification and cannot simply be explained as the consequence of AS. Endothelial cells (ECs) and vascular smooth muscle cells (VSMCs) are directly involved in AS and VC processes. Understanding the communication between ECs and VSMCs is critical in revealing mechanisms underlying AS and VC. Extracellular vesicles (EVs) are found as intercellular messengers in kinds of physiological processes and pathological progression. Non-coding RNAs (ncRNAs) encapsulated in EVs are involved in AS and VC, including microRNAs (miRNAs), long non-coding RNAs (lncRNAs), and circular RNAs (circRNAs). The effects of ncRNAs have not been comprehensively understood, especially encapsulated in EVs. Some ncRNAs have demonstrated significant roles in AS and VC, but it remains unclear the functions of the majority ncRNAs detected in EVs. In this review, we summarize ncRNAs encapsulated in EC-EVs and VSMC-EVs, and the signaling pathways that are involved in AS and VC.
1. Introduction
Atherosclerosis (AS) is associated with systemic risk factors, including hypertension, hyperlipidemia, and diabetes mellitus, and is characterized by the accumulation of lipids, fibrous elements, and calcification in the innermost layers of arteries (1, 2). Several cell types are directly involved in the pathological progression of AS, such as endothelial cells (ECs), vascular smooth muscle cells (VSMCs), platelets, and foam cells (3). As a cellular monolayer lining the blood vessel wall, ECs first contact endogenous metabolite-related signals in the bloodstream, in which ECs function as danger signal sensors (4). ECs play an important role in inhibiting AS by regulating vascular tension and regulating inflammation (5). However, ECs can respond to oxidized low-density lipoprotein (ox-LDL) through different mechanisms, including EC dysfunction (6). Endothelial dysfunction is essential in the pathogenesis of AS (7–10), which releases inflammatory cytokines to VSMCs contributing to AS, including interleukin-1β (IL-1β), tumor necrosis factor-a (TNF-α), and transforming growth factor-β (TGF-β) (11, 12). VSMCs are the sole component of the center layer of the vessel wall, the tunica media (13). VSMCs are hypothesized whose principal function is contraction, so the contribution of VSMCs to AS has been greatly underestimated (14, 15). VSMCs have remarkable plasticity and reprogramming capacity during the complex AS process, including contractile phenotype, macrophage-like, foam cell-like, osteochondrogenic-like, myofibroblast-like, and mesenchymal stem cell-like (11, 16). The hypothesis that VSMCs possess a “transitional stage between smooth muscle and foam cells” has been demonstrated, which highlights the importance of VSMC phenotypic modulation in AS (17–19). The switching of VSMCs to macrophage-like cells may be driven by lipids accumulation which was Krüppel-like factor 4 (KLF4) dependent (20, 21).
Vascular calcification (VC), the deposition of calcium and phosphate within the arterial wall, is an important part of AS natural history and an independent predictor of cardiovascular morbidity and mortality (22–25). VC occurs in both the intimal and medial layers of the arteries, and intimal arterial calcification (IAC) is mainly involved in the calcification of atherosclerotic lesions (26, 27). Calcifying vascular cells are derived from local VSMCs, especially in IAC (28). On the contrary, medial arterial calcification (MAC) is a chronic systemic vascular disorder distinct from AS, in which its hallmark is the dissemination and progressive precipitation of calcium phosphate within the medial layer (29). MAC differs in several ways from the IAC seen in atherosclerotic lesions, including cellular aging linked to mechanical stress cooperated and buffered by ECs (27, 30, 31). Initially, VC was regarded as a passive degenerative process, and now VC is elucidated to be a multifactorial process through the phenotypic modulation of VSMCs (26, 32–36). It means that ECs have a potential role in MAC. Correspondingly, endothelial dysfunction under aging or injured condition promoted VC (37–39). More importantly, it has been demonstrated that endothelial dysfunction aggravated MAC in vivo (40, 41). Moreover, extracellular vesicles (EVs) found a novel messenger in the cellular crosstalk between ECs and VSMCs in VC (42, 43) (Figure 1).
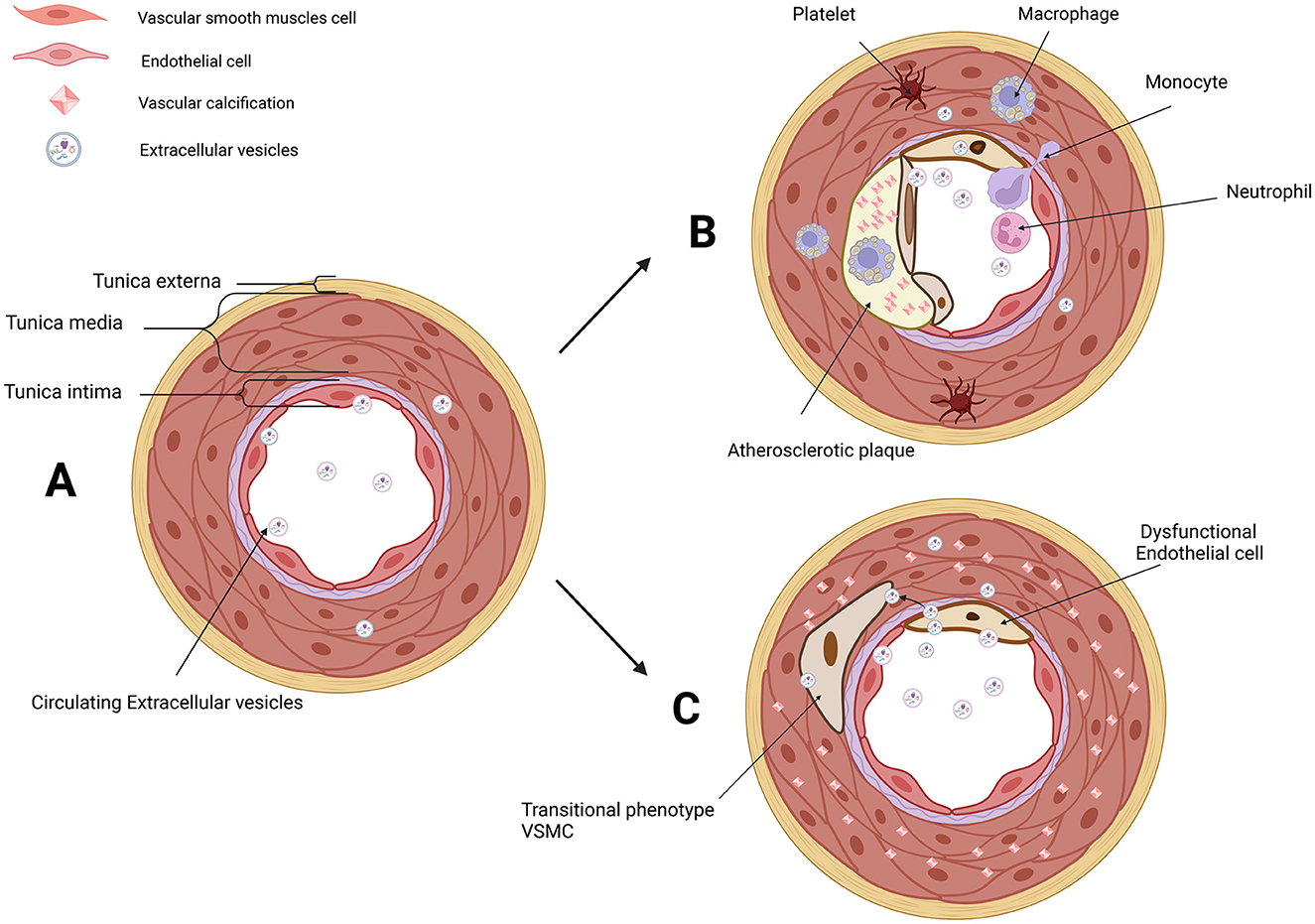
Figure 1. EVs in the microenvironment of IAC in AS and MAC. (A) Three layers of arteries: tunica externa, tunica media (mainly composed of VSMCs), and tunica intima (composed of ECs and subendothelial layer). VSMCs and ECs are both the donor cells and recipient cells in the microenvironment of vascular. Circulating EVs in serum and EVs derived from VSMCs and ECs have been involved in cellular communication. (B) Platelet, monocyte, macrophage, neutrophil, transitional VSMCs, and dysfunctional ECs are involved in AS and IAC. EVs derived from VSMCs and ECs are novel messengers in the microenvironment of AS. (C) Dysfunctional ECs and transitional VSMCs are involved in MAC. EVs derived from VSMCs and ECs are novel messengers in the microenvironment of MAC. EVs, extracellular vesicles; IAC, intimal arterial calcification; AS, atherosclerosis; MAC, medial arterial calcification; VSMCs, vascular smooth muscle cells; ECs, endothelial cells. Created with BioRender.com.
Extracellular vesicles, as the generic term for particles, are derived from cells and are delimited by a lipid bilayer (44). Non-coding RNAs (ncRNAs), messenger RNAs (mRNAs), and lipids encapsulated in EVs can involve in intracellular function regulation and intercellular communication. It has been reported that there were a growing number of studies about microRNAs (miRNAs) encapsulated in EVs related to VC (45–50). Nevertheless, there were few special studies aimed to summarize the miRNAs encapsulated in EVs during intercellular communication. Therefore, considering the prospect of cellular communication through EVs, first, we propose to summarize that the miRNAs have appeared in EVs derived from ECs (EC-EVs) and EVs derived from VSMCs (VSMC-EVs) in the AS and VC processes. Subsequently, we will discuss the potential prospect of long non-coding RNAs (lncRNAs) and circular RNAs (circRNAs) in EC-EVs and VSMC-EVs during AS and VC formation.
2. The flexibility and versatility of miRNAs encapsulated in EC-EVs in AS and VC
The emerging role of EVs and intercellular communication has gained momentum in the past few years. In the process of biological message transferring from ECs to other cells, the presence of EVs has been verified in numerous physiological and pathological pathways, including AS and VC.
2.1. miRNAs encapsulated in EC-EVs regulate the process of AS
It has been shown that miRNAs encapsulated in EC-EVs exerted atheroprotective or proatherogenic function in several studies. The differential expression of miRNAs encapsulated in EC-EVs during AS was analyzed by miRNA-Sequence (miRNA-seq) (51, 52), providing a reasonable prospect to illuminate the detailed mechanisms miRNAs are involved in. Here, miRNAs encapsulated in EC-EVs including miR-92a-3p, miR-155-5p, miR-505-3p, miR-19b-3p, and miR-4306 promoted AS, while miR-126-5p and miR-145-5p inhibited AS process (53–61).
Xiang et al. (51) performed miRNA-Seq analysis and found that EVs derived from lipopolysaccharide (LPS)-stimulated human umbilical vein endothelial cells (HUVECs) promoted human aortic smooth muscle cell (HASMC) proliferation and migration, in which four upregulated miRNAs (including miR-27a-3p, miR-126-5p, miR-155-5p, and miR-365b-3p) and nine downregulated miRNAs (including let-7b-5p, miR-10a-5p, miR-10b-5p, miR-21-3p, miR-30a-5p, miR-92a-3p, miR-125a-3p, miR-143-3p, and miR-181a-2-3p) participated in various aspects of atherosclerotic lesion formation. In another RNA-seq research, a significant upregulation of 49 miRNAs (including miR-19b-3p and miR-126-5p) and downregulation of 19 miRNAs in extra-virgin olive-induced HUVECs-EVs compared to sunflower oil-induced HUVECs-EVs were observed, which closely linked to the development of AS (52). The finding of higher expression of miR-126-5p was detected in both studies (51, 52). It is no coincidence that miR-126-5p was found to have a linear relationship with coronary calcium in outpatients with AS (53). However, the upregulation or transfection of miR-126-5p promoted ECs proliferation by the suppression of delta-like 1 homolog (Dlk1) and modulation of nuclear factor-κB (NF-κB)-mediated serine/threonine kinase (AKT) signaling pathway (62, 63). On the contrary, the upregulation or transfection of miR-126-5p limited ox-LDL-induced VSMC (ox-LDL-VSMC) proliferation and migration as an atheroprotective miRNA targeting high-mobility group box 1 (HMGB1) (64). In ECs and VSMCs, miR-126-5p showed opposite regulation: miR-126-5p promoted ECs proliferation and inhibited VSMCs proliferation and migration. From above, we suppose that miR-126-5p encapsulated in EC-EVs has a double atheroprotective regulation mechanism when recipient cells assimilate it: it promoted ECs proliferation and inhibited VSMCs proliferation and migration.
MiR-155, named miR-155-5p now according to miRNA online database miRBase (https://www.mirbase.org/), was upregulated according to the profile of miRNAs in EC-EVs (51) and was also enriched in ox-LDL-HUVEC-EVs (54). By enhancing monocyte activation toward proinflammatory M1 macrophages, miR-155 showed a proatherogenic function and was negatively regulated by Krüppel-like factor 2 (KLF2) in an experimental mouse model (54). MiR-155-5p has emerged as a significant component in AS (65). In senescent HUVECs, upregulated miR-155-5p mediated endothelial dysfunction through NF-κB/endothelial nitric oxide synthase (eNOS) axis and inhibited the proliferation of ECs by targeting Ras homolog enriched in brain (RHEB) expression (66–68). In VSMCs, miR-155-5p inhibited the viability of VSMCs and induced VSMCs phenotypic switching by inhibiting cyclic guanosine monophosphate (cGMP)-dependent protein kinase 1 (PKG1) (69, 70). In both ECs and VSMCs, miR-155-5p inhibited the proliferation and migration in AS by inhibiting AKT1 (71). MiR-155-5p encapsulated in EC-EVs, as distinct from miR-126-5p, was demonstrated as an element of inflammatory signal transduction in AS.
Additionally, miR-505, called miR-505-3p at present, is also highly expressed in exosomes from ox-LDL-HUVEC, which was finally proved to be a proatherogenic factor by inducing neutrophil extracellular trap (NET) formation in vitro (55). MiR-505-3p markedly inhibited the proliferation, migration, and tube formation capacity of HUVECs by targeting vascular endothelial growth factor A (VEGFA) (72, 73). Therefore, circulating miR-505-3p was a prognostic biomarker of endothelial dysfunction and inflammation in hypertension patients (74, 75). However, the biological function of miR-505-3p in VSMCs has not been reported, leaving to further investigation.
MiR-19b encapsulated in HUVECs-EVs mentioned above (52), the previous name of miR-19b-3p, reduced HUVECs migration and damaged lymphatic system function by negatively regulating TGF-βRII expression (56, 76). MiR-19b-3p in endothelial microparticles (MPs) exaggerated AS by targeting the suppressor of cytokine signaling 3 (SOCS3) (77). The upregulation of miR-19b-3p in ECs contributed to endothelial dysfunction by inhibiting syndecan-1 mRNA and protein expression (78–80). MiR-19b-3p exerted an inflammatory effect on the vascular microenvironment and promoted human retinal microvascular ECs apoptosis through SOCS6-mediated Janus kinase 2 (JAK2)/signal transducer and activator of transcription 3 (STAT3) axis (81, 82). Similarly, miR-19b-3p reduced hypoxia-induced HUVECs apoptosis, autophagy, and inflammation by the inhibition of hypoxia-induced factor-1 (HIF-1α) (83). MiR-19b-3p was increased by more than 3-fold in LPS-ECs (84). Inhibition of miR-19b-3p reduced TNF-α and IL-1β expression levels in ox-LDL-HUVEC and AS mice (85) and mitigated endothelial dysfunction (86). Overexpression of miR-19b-3p in human aortic endothelial cells (HAECs) caused intracellular reactive oxygen species accumulation, promoting apoptosis (87). However, Li et al. adopted a different perspective toward the role of miR-19b-3p in AS (88, 89). MiR-19b-3p inhibited HUVECs proliferation, migration, and angiogenesis targeting STAT3, which may, however, delay unstable plaque progression in patients with unstable angina (88, 89). This opinion was contrary to the mentioned studies (85–87). This was not the only case, Tang et al. (90) demonstrated that miR-19b-3p attenuated TNF-α-induced ECs apoptosis. We speculate that this biological difference might be due to the different stimuli (LPS, TNF-α, and so on), the different types of ECs (HAECs and HUVECs), and different periods of AS.
Furthermore, miR-4306 encapsulated in EVs derived from ox-LDL condition (ox-LDL-EVs) from human coronary artery vascular endothelial cells (HCAECs) upregulated the AKT/NF-κB signaling pathway enhancing the lipid formation of human monocyte-derived macrophages (HMDMs), while miR-4306 encapsulated in ox-LDL-EVs from HMDMs inhibited capillary tubule formation of HCAECs in vitro (57). Wang et al. (91) found that miR-4306 plays a key role in urban particulate matter-induced endothelial damage. As a result, miR-4306 manifested promotion in AS.
The previously reported MiR-92a-3p, named miR-92 or miR-92a before, performed a different function with its recipient cells during AS (51). MiR-92a-3p encapsulated in EVs from HUVEC treated with TNF-α or subjected to shear flows could be transported to macrophages to suppress KLF4, leading to the atheroprone phenotypes of macrophage in AS (58). The progression of AS was associated with a maladaptive form of angiogenesis which contributed to plaque disruption and intraplaque hemorrhage (92). Independent atherosclerotic stimuli (ox-LDL and IL-6) increased miR-92a-3p expression in donor HCAEC-EVs, promoting an angiogenic phenotype in the recipient ECs through the downregulation of thrombospondin 1 (THBS1) (59). Meaningfully, miR-92a-3p encapsulated in HCAEC-EVs increased in a balloon injury rat model and inhibited the atheroprotective expression of KLF2 (60). Uremic toxins accumulation upregulated the expression level of miR-92a-3p in ECs, contributing to endothelial dysfunction (93). MiR-92a-3p overexpression inhibited VSMCs proliferation and migration, while miR-92a-3p inhibition promoted the proliferation and migration of VSMCs via the KLF4 pathway (94, 95). MiR-92a-3p encapsulation in EC-EVs demonstrated a double effect on AS when recipient cells assimilate it: it promoted ECs phenotype switching while it inhibited VSMCs proliferation and migration.
MiR-143 (miR-143-3p) and miR-145 (miR-145-5p) encapsulated in KLF2-transduced or shear-stress-stimulated HUVEC-EVs induced an atheroprotective HASMC phenotype and reduced AS in the aorta of Apolipoprotein E Knock-Out (ApoE(−/−)) mice (61), supporting the bioinformatic prediction of Xiang et al. (51). MiR-143-3p and miR-145-5p have been reported frequently, their roles in AS and VC are discussed later.
In brief, numerous studies show plenty of potential EC-EV-miRNAs that are involved in the progress of AS and illustrate the multiple signaling pathways. Particularly, miR-126-5p was upregulated in EC-EVs in atherogenic condition, while previous studies showed its atheroprotective effect, addressing the complicated role of miR-126-5p in the AS process. Further studies are essential to explore the distribution of miR-126-5p between EVs and cells, and how biological function diversities are feasible. In addition, miR-155-5p promoted AS and KLF2 downregulated the expression of miR-155-5p showing its atheroprotective role. Correspondingly, the suppression of KLF2 by miR-92a-3p and the suppression of KLF4 by miR-92a-3p lead to AS. Additionally, the upregulation of miR-143-3p and miR-145-5p encapsulated in EC-EVs was transduced by KLF2 and showed an atheroprotective effect. KLF2, a shear-responsive transcription factor, was critical because it played a major role in exerting atheroprotective effects in ECs, which promised a novel target for AS prevention and treatment (96, 97). Similarly, KLF4, as a therapeutic target of atherosclerosis, regulates ECs inflammation, VSMC phenotypic transformation, and foam cell formation during the process of AS (98). Several miRNAs encapsulated in EC-EVs that regulate the process of AS are summarized in Table 1 and Supplementary Table 1.
2.2. miRNAs encapsulated in EC-EVs regulate the process of VC
We have demonstrated for the first time that high phosphate (HP)-induced EVs from HUVECs directly promoted and accelerated VC through astrocyte elevated gene-1 (AEG-1) (99). Furthermore, miRNAs encapsulated in EC-EVs demonstrated significant messengers in the biogenesis of VC. Lin et al. (100) found that miR-670-3p encapsulated in EC-EVs stimulated by HP could target insulin-like growth factor-1 (IGF-1) resulting in potentiating the VC process in vitro and in vivo. Freise et al. applied EC-EVs with a miR-221 (miR-221-3p) inhibitor which provoked a distinct reduction of VSMC calcification, and miR-143 or miR-145 mimics also provoked a distinct reduction of VSMC calcification (101). MiR-29b (miR-29b-3p) and miR-126-5p in EC-EVs also negatively regulated VC (102, 103). Additionally, we detected the profile of miRNAs in HP-EVs from HUVECs by RNA-seq, finding that miR-3182 increased while 13 known miRNAs decreased in HP-EVs from HUVECs (104), indicating the potential function of miRNAs encapsulated in EC-EVs during VC.
MiR-29b-3p was named miR-29b previously according to miRBase. Wang et al. confirmed miR-29b-3p encapsulated in endothelial exosomes mediated anti-calcification and was associated with C-X-C motif chemokine receptor 6 (CXCR6) (102). Similarly, miR-29b-3p downregulated VSMC calcification by targeting matrix metalloproteinase 2 (MMP2) and Wnt family member 7B (Wnt7b)/β-catenin signal (105–107). The expression of miR-29b in the coronary artery calcification group was significantly lower than that in the control group, indicating that decreased miR-29b-3b level may be a coronary artery calcification risk factor (108). However, it is a paradox that Fang et al. found that miR-29b was increased in calcific aortic tissue and the inhibition of miR-29b mitigated aortic calcification in tissues of calcific aortic valve diseased rats via directly targeting TGF-β3 (109). Additionally, inorganic phosphorus (Pi) stimulation increased miR-29b-3p expression and overexpression of miR-29b-3p facilitated Pi-induced VSMC calcification (110). Intriguingly, the level of miR-29b shows no statistical significance in VSMCs during the exposure of 3 mM Pi, compared with normal VSMCs (111). Taken together, miR-29b-3p encapsulated in EC-EVs inhibited VSMC calcification, while the role of miR-29b-3p in VSMC calcification is still ambiguous. Considering specific distribution, further studies explaining the relationship between miR-29b-3p and VC are required in the future.
Freise et al. (101) revealed that three miRNAs (miR-221, miR-222, and miR-126) were significantly enhanced in uremic toxins-induced-EVs from human ECs. Subsequently, VSMC calcification was inhibited by a miR-221 inhibitor and a stronger reduction with a miR-222 inhibitor. Both miR-221 and miR-222 contribute to the atherogenic calcification of VSMCs (112). Correspondingly, increased calcium deposition was observed in the combined treatment of miR-221 and miR-222 that mimics synergistically but not in individual treatments, with significant changes in ectonucleotide phosphodiesterase 1 (Enpp1) and Pit-1 expression (113).
By contrast, Freise et al. (101) confirmed that the levels of miR-143 and miR-145 were significantly decreased during VSMC calcification. MiR-143 and miR-145 can both inhibit AS process as mentioned above (61). Similarly, an individual transfection with miR-143 or miR-145 mimics provoked a distinct reduction of VSMC calcification and a stronger reduction in a concomitant transfection (101). Massy et al. (114) suggested that decreasing levels of miR-143, miR-145, and miR-126, and increasing levels of miR-223 were potential biomarkers of vascular calcification associated with chronic kidney disease (CKD). Elevated Pi significantly downregulated miR-143 and miR-145 and upregulated miR-223 during VSMCs calcification in vitro and in ApoE(−/−) mice (111, 115).
In conclusion, miR-143-3p and miR-145-5p inhibited VC, whereas miR-221-3p and miR-222-3p potentiated VC.
Based on Freise et al. (101) and Massy et al. (114) studies, the expression of miR-126 was found to be adverse, which probably was attributed to the different distribution of miR-126 among EC-EVs, VSMCs, and serum of CKD mice. Massy et al. (114) detected that the expression of miRNAs (including miR-126, miR-143, miR-145, and miR-223) in the endothelium or media of the thoracic and abdominal aorta is different. For example, miR-126 expression of VSMCs in the upper abdominal aorta is equal to ECs, lower than ECs in the low thoracic and low abdominal aorta, and higher than ECs in the upper thoracic (114). However, it must be remembered that miR-126 is known as miR-126-3p, and miR-126* is referred to as miR-126-5p. Due to some reason, the specific sequence of miR-126 was not shown in all studies. As a result, miR-126 refers to miR-126-3p as default. It suggested that miR-126 can be considered a potential cardiovascular risk and VC biomarker in cardiovascular disease (CVD) and cerebral troubles associated with CKD (53, 114, 116, 117). Additionally, miR-126 expression is increased in CKD and ApoE(−/−) mice aorta (118). Furthermore, miR-126 markedly enhanced the regeneration of vascular smooth muscle (119). In the progress of Pi-induced HASMCs calcification, miR-126 was involved in the Wnt/β-catenin signaling pathway (120). However, miR-126-5p encapsulated in HUVEC-EVs stimulated with advanced glycation end-products led to the reduction of calcium deposition by blocking the smad1/5/9 signaling pathway both in vitro and in a mouse model (103). In short, the biological effects are different between miR-126-3p and miR-126-5p. The upregulation of miR-126-3p encapsulated in EC-EVs probably enhances VC, while the upregulation of miR-126-5p encapsulated in EC-EVs prohibits VC. However, the versatility of miR-126-5p is still worth exploring because of its atherogenic function. Thus, it is necessary to validate these results in further study.
To determine whether the HP condition altered the profile of miRNAs, we (104) detected the upregulation expression of has-miR-3182, while the downregulation expression of 13 known hsa-miRs (including miR-10a-5p, miR-10b-5p, miR-30a-3p, miR-30a-5p, miR-30c-2-3p, miR-99b-5p, miR-143-3p, miR-193b-5p, miR-365a-5p, miR-486-5p, miR-941, and miR-7706) in the HP-EC-EVs group compared to the PBS-EC-EVs group by miRNA-seq bioinformatics analysis. Target mRNAs for hsa-miR-3182 included acyloxyacyl hydrolase (AOAH), nucleotide-binding oligomerization domain containing 2 (NOD2), and RNA-binding motif protein 47 (RBM47), implying that miR-3182 might be a novel miRNA in an HP-induced VC. In addition, accumulating evidence showed that AOAH, NOD2, and RBM47 were also related to AS, which has a close connection with VC (121–124). Interestingly, miR-10a-5p, miR-10b-5p, miR-30a-5p, and miR-143-3p were also downregulated and discovered in accordance with the mentioned research (50), indicating the protective roles of these miRNAs in the cardiovascular system. Correspondingly, miR-30a-5p, known as miR-30a, attenuated osteoblast maturation by suppressing runt-related transcription factor 2 (Runx2) and smad1/5 (125). Nevertheless, miR-30a was increased during osteogenic processes and the progression of VC (126–129). MiR-143-3p, which was referred to as miR-143, has been reported as a protective factor in AS and VC. MiR-486-5p, called miR-486 previously, increased in the calcification of aortic valve, promoting osteoblastic biomarkers Runx2, osterix (Osx), and calcium deposition of human aortic valve interstitial cells (AVICs) (130, 131). A further novel finding was that we discovered another 34 novel hsa-miRNAs, which included 31 downregulated miRNAs and 3 upregulated miRNAs. In the future, it is essential to confirm our data through in vitro and in vivo experiments to take a step forward.
We have discussed the ambiguous role of miR-29b-3p, anti-calcification roles of miR-143-3p, miR-145-5p, and miR-126-5p, and pro-calcification roles of miR-221-3p, miR-222-3p, and miR-126-3p. Overall, the results above show a novel perspective in intercellular communication during VC: miRNAs encapsulated in EC-EVs potentiate an anti-calcification or pro-calcification effect when transmitted to VSMCs. Several miRNAs encapsulated in EC-EVs that regulate the process of VC are summarized in Table 2 and Supplementary Table 2.
3. The flexibility and versatility of miRNAs encapsulated in VSMC-EVs in AS and VC
The phenotype change from the contractible type to the synthetic type of VSMCs plays a crucial role in the biogenesis of AS, VC, and vascular dysfunction. VSMCs are one of the main cells implicated in AS, retaining remarkable plasticity (132). VSMCs can adopt alternative phenotypes resembling foam cells contributing to AS (133). VSMCs are also key players in VC, which transform into calcifying VSMCs and secrete mineralizing EVs that form microcalcifications, subsequently increasing plaque instability and consequential plaque rupture (134). In this review, we summarized miRNAs encapsulated in VSMC-EVs in the process of AS and VC.
3.1. miRNAs encapsulated in VSMC-EVs regulate the process of AS
The function and number of VSMCs in AS plaques according to lineage-tracing studies have been greatly underestimated (135). The majority of research on EVs that mediate cellular communication between VSMCs and other cell types has focused on foam cells, especially EVs from foam cells. As far as miRNAs are concerned, a couple of studies have illuminated the atherogenic function of miRNAs encapsulation in EVs from foam cells to VSMCs, including miR-19b-3p, miR-21-3p, miR-106a-3p, and miR-186-5p (136–139). Here, we aimed to review miRNAs encapsulated in VSMC-EVs in the progression of AS.
Gonzalo et al. (140) demonstrated first that MPs derived from human coronary artery smooth muscle cells (HCASMCs) contain miRNAs. Moreover, the packaging of both miR-143-3p and miR-222-3p was decreased, which is considered a response to hypercholesterolemia stress in HCASMC and conditioned medium of AS (140). Thus, it is conceivable that miR-143-3p and miR-222-3p in HCASMC-MPs would be a source of cardiovascular biomarkers. In 2009, Boettger et al. defined the miR-143/145 gene cluster as a major regulator of the contractile phenotype of VSMC (141). In 2012, Hergenreider et al. (61) elucidated a novel pathway linking endothelial KLF2 expression to VSMC phenotype regulating miR-143/145 and provided an intercellular communication mediated by miR-143/145 encapsulated in EC-EVs. In 2020, Chung et al. (142) demonstrated that miR-143 and miR-145 downregulated Ras and Ras homolog family member A (RhoA) expressions, which potentially prevented AS. Dégano et al. (143) identified that miR-143-3p was independently associated with time-to-coronary events and that a higher expression in healthy individuals was related to less risk of an incident coronary artery disease (CAD) event at 10 years. By contrast, González et al. (144) suggested that miR-143-3p may be implicated in carotid plaque instability by the modulation of IGF-IIR, contributing to AS.
The characteristics of miR-222-3p in the process of AS were subtle, which were different from the ones in the process of VC, as we summarized in EC-EVs. In 2010, Dentelli et al. demonstrated that miR-222 acted as an anti-angiogenic miRNA that negatively regulated STAT5A, providing a promising perspective to prevent the biogenesis of AS (145). Atherosclerotic plaque rupture exhibited a decrease in miR-221/222 (146). Both miR-221-3p and miR-222-3p decreased significantly in patients with AS, indicating that the downregulation of these two miRNAs may be connected with AS (147). In addition, miR-222 was involved in VSMCs proliferation and upregulated by Ang II in promoting ECs migration and inflammation (148). In 2015, Xue et al. (87) unveiled miR-221/222-induced EC dysfunction by the suppression of peroxisome proliferator-activated receptor-γ coactivator-1α (PGC-1α) in AS. Taraldsen et al. (149) observed that circulating levels of miR-92a-3p, miR-221-3p, and miR-222-3p were associated with baseline coronary necrotic core volume. We speculate that the duality of miR-221-3p and miR-222-3p is due to the different distribution of VC and that miR-221-3p and miR-222-3p probably play key roles in MAC.
Efforts would be essential to understand the conflicting role of miR-222-3p in AS. As a result, increasing number of evidence is required to address its time-based and spatial location in ECs, VSMCs, and EVs. The protective function of miR-143-3p seemed more specific than miR-222-3p in AS. Nevertheless, further studies are required to elucidate the relationship between the functional and distributional diversity of miR-143-3p and miR-222-3p encapsulated in EV.
Zheng et al. (150) found miR-155 encapsulated in KLF5-induced exosomes from VSMCs to ECs, which destroyed the integrity of endothelial barriers, resulting in AS. This result is consistent with the research mentioned above that elevated miR-155 enhanced AS progress (54, 151–154). González et al. (144) suggested that miR-155-5p might be implicated in plaque instability by targeting AKT, contributing to AS. MiR-155 activated the NLRP3 by regulating NF-κB in the AS progression of ApoE(−/−) mice (155). However, evidence showed that miR-155 exerted inhibition on AS in ApoE(−/−) mice (156). Park et al. (157) suggested that miR-155 was a novel negative regulator in the soluble guanylyl cyclase (sGC)/cGMP pathway, providing a novel therapeutic target for AS. MiR-155 attenuated ox-LDL-induced apoptosis in various cells by targeting p85α, revealing its therapeutic effect in AS (158). miR-155 was implicated in several signaling pathways in AS mouse models, as shown by the evidence. In the future, the distinction and differential expression of miR-155 in different conditions still need to be elucidated, and potential signaling pathways associated with AS also need to be uncovered.
Gonzalo et al. (159) discovered that atherogenic lipoproteins significantly reduced miR-15b-5p, −24-3p, −29b-3p, −130a-3p, −143-3p, −146a-3p, −222-3p, and −663a in microvesicles (MVs) released by HCASMC. MiR-143-3p, miR-222, and miR-29b have been discussed above. MiR-24-3p and miR-130a-3p were also reduced in circulating MVs from familial hypercholesterolemia (FH) patients. In addition, the expression of miR-130a-3p was reduced in uremia toxin indoxyl sulfate-induced HCAEC-EVs (160). Downregulation of miR-130a-3p expression leads to endothelial barrier dysfunction in the development of AS (161). Paradoxically, serum miR-130a-3p was elevated in cerebral AS patients and provided a possible method to predict cerebrovascular events (162). The different expressions of miR-130a-3p in AS period indicated the potential ability in AS diagnosis. However, we suppose that the signaling pathways of miR-130a-3p that implicate AS need to be discovered.
We have discussed the protective effect of miR-143-3p and the versatility of miR-222-3p, miR-155-5p, and miR-130a-3p in the process of AS. On the whole, versatile distributions between EVs and intracellular location probably perform profound effects on cellular metabolism, which implicated a variety of signaling pathways (Figure 2). Table 3 and Supplementary Table 3 indicate several miRNA-encapsulated VSMC-EVs that regulate the AS process.
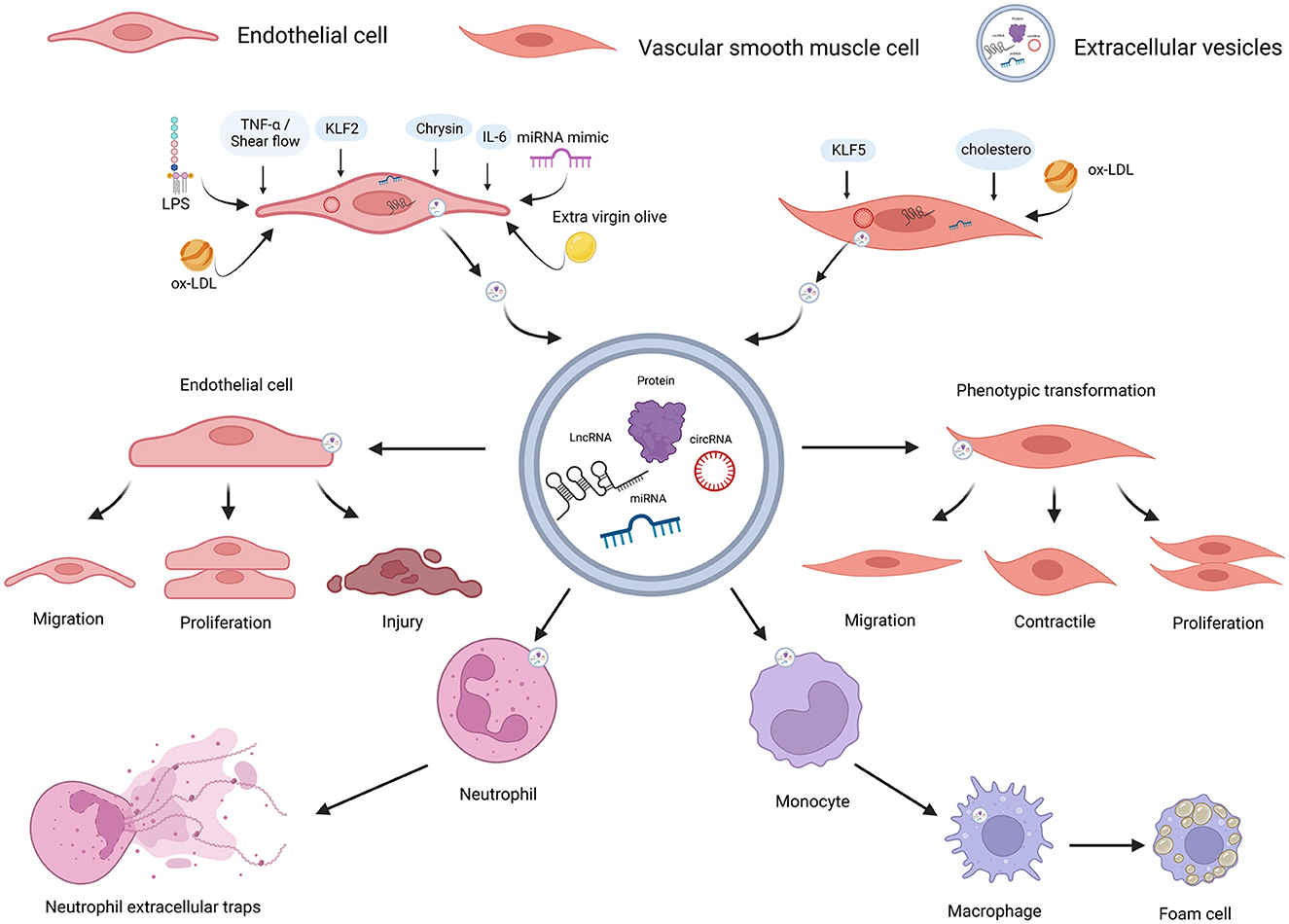
Figure 2. ncRNAs encapsulated in EC-EVs and VSMC-EVs regulate the AS process. Various stimuli stimulate the release of EVs and ncRNAs encapsulated in EC-EVs, and VSMC-EVs are assimilated by different recipient cells, resulting in AS inhibition or promotion. ncRNAs (miR-155-5p, miR-505-3p, miR-19b-3p, miR-4306, miR-92a-3p, MALAT1, ZEB1-AS1, CLDN10-AS1, LINC01005, and hsa_circ_0086296) encapsulated in EVs derived from ECs induced by different stimuli (LPS, extra-virgin-olive, ox-LDL, IL-6, TNF-α, shear flows, and chrysin) were linked to AS via enhancing ECs and VSMCs phenotype switching, promoting M1 macrophages activation, M2 macrophage polarization, NETs formation, lipid formation of macrophages, and endothelial injuries; miR-143-3p and miR-145-5p encapsulated in EVs derived from ECs stimulated by KLF2-transduced and shear-stress induced an atheroprotective VSMC phenotype; ncRNAs (miR-143-3p, miR-222-3p, miR-155-5p, and hsa_circ_0001445) encapsulated in EVs derived from VSMCs induced by stimuli (including hypercholesterolemia, KLF5, and ox-LD) were linked to AS by reducing expressions and enhancing endothelial injuries. ncRNAs, non-coding RNAs; VSMCs, vascular smooth muscle cells; ECs, endothelial cells; EVs, extracellular vesicles; AS, atherosclerosis; LPS, lipopolysaccharide; ox-LDL, oxidized low-density lipoprotein; IL-6, interleukin-6; TNF-α, tumor necrosis factor-a; NETs, neutrophil extracellular traps; KLF2, Krüppel-like factor 2; KLF5, Krüppel-like factor 5. Created with BioRender.com.
3.2. miRNAs encapsulated in VSMC-EVs regulate the process of VC
In VC, increased miR-92b-3p expression in both VSMCs and exosomes downregulated KLF4 and Runx2 expression in rat VSMCs (163). Similarly, Wang et al. (164) found that miR-92b-3p inhibited hypoxia-induced proliferation, migration, and phenotype switch of VSMCs.
MiR-204/miR-211 encapsulated in exosomes from melatonin-treated VSMCs attenuated VC by targeting bone morphogenetic protein-2 (BMP2) in vitro and in a 5/6 nephrectomy in C57BL/6 mice model (165). Indeed, miR-204 deficiency elevated valvular calcification activity and was independently associated with coronary artery calcification (CAC) and CVD in patients with type 2 diabetes mellitus (T2DM) (130, 131, 166–171). Correspondingly, the overexpression of miR-204 alleviated the osteoblastic differentiation of VSMCs in vitro and in female mice by regulating Runx2 (172, 173). In uremic rats, the expression of miR-29b increased, and the expressions of miR-133b and miR-211 decreased in calcifying conditions (107). Moreover, the expressions of miR-31, miR-106a, miR-148a, miR-204, miR-211, and miR-424 were lower in the aortic stenosis group than in the controls, whereas the levels of miR-30c were higher than in the controls (129). In conclusion, miR-92b-3p encapsulated in VSMC-EVs accelerated VC, while miR-204/miR-211 encapsulated in VSMC-EVs exhibited an anti-calcification function.
Pan et al. found that 987 miRNAs were significantly upregulated, while 92 miRNAs were downregulated in exosomes from MOVAS-1 cells stimulated with β-glycerophosphate (β-GP) and pyruvic acid (174). Chaturvedi et al. (175) concluded that 14 miRNAs increased and 19 miRNAs decreased in MVs isolated from calcifying VSMCs obtained from descending thoracic aorta in three CKD rats. It is no coincidence that both research studies shared several common miRNAs: 7 miRNAs were up-regulated (miR-92b-5p, miR-204-3p, miR-296-3p, miR-494-3p, miR-667-5p, miR-702-5p, and miR-770-3p) while 6 miRNAs were down-regulated (let-7b-5p, miR-19b-3p, miR-24-3p, miR-30e-5p, miR-99b, and miR-199a-5p). Especially, miR-99b and miR-24-3p were decreased in VC condition in previous studies (104, 159). It also suggested that Runx2 was controlled by miR-30e, and the downregulation of miR-30e indicated osteoblast differentiation regulation (175). These findings facilitate the understanding of the effect of upregulation and downregulation of miRNAs in VSMC-EVs and VC, providing a bright prospect in the investigation of VC (Figure 3). Table 4 and Supplementary Table 4 describe several miRNAs that encapsulated VSMC-EVs that were regulated during the process of VC.
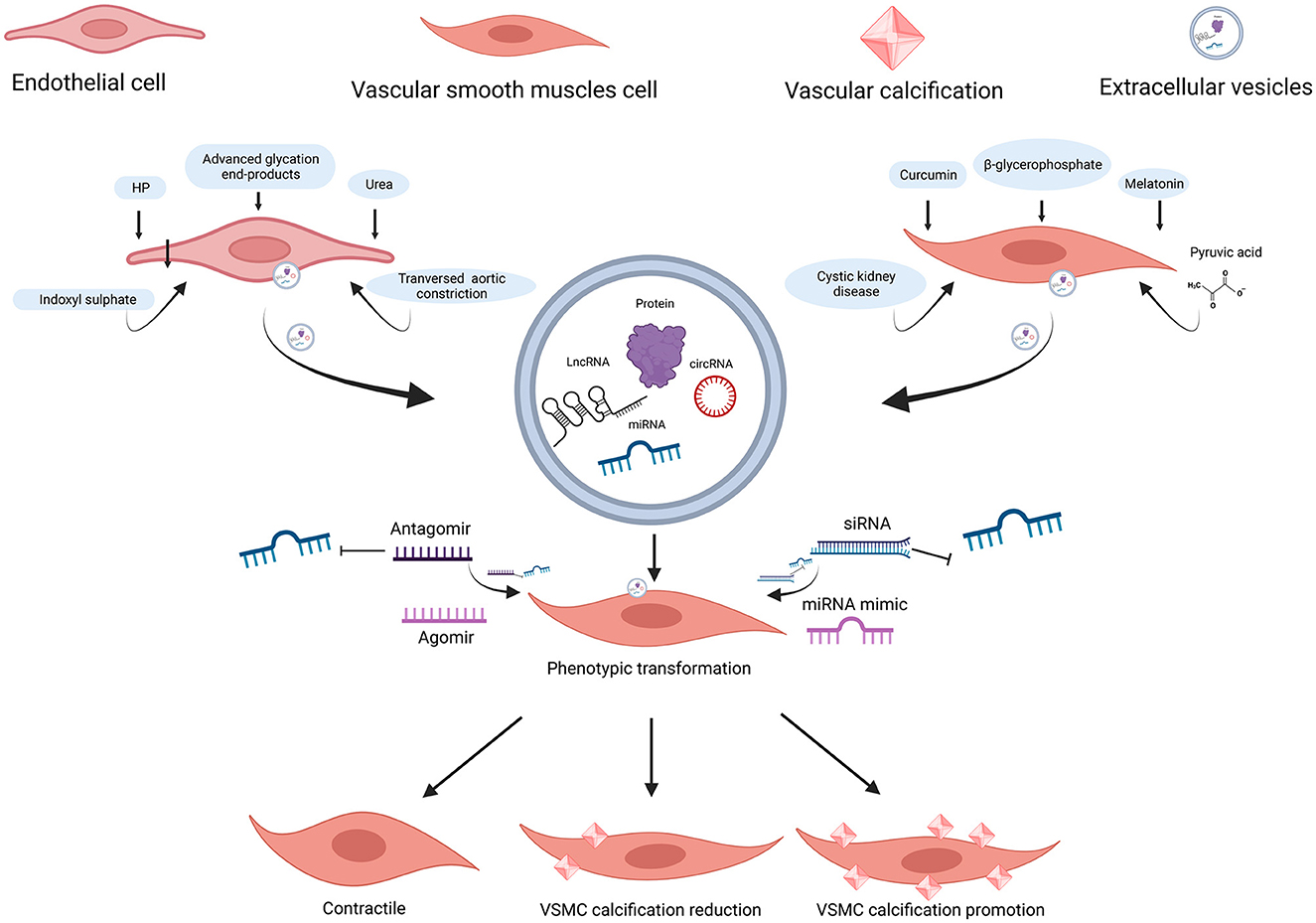
Figure 3. ncRNAs encapsulated in EC-EVs and VSMC-EVs regulate the VC process. Various stimuli stimulate the release of EVs and ncRNAs encapsulated in EC-EVs, and VSMC-EVs are assimilated by VSMCs, resulting in VC inhibition or promotion. miR-670-3p, miR-221-3p, and miR-222-3p encapsulated in EVs derived from ECs induced by stimuli (HP, uremic toxins, transverse aortic constriction, and advanced glycation end-products) promoted VC, while miR-143-3p, miR-145-5p, miR-29b-3p, and miR-126-5p inhibited VC; miR-92b-3p, miR-204-5p, and miR-211-5p encapsulated in EVs derived from VSMCs induced by stimuli (curcumin and melatonin), inhibited VC; the profiles of miRNAs encapsulated in EVs derived from VSMCs (β-GP and pyruvic acid-induced MOVAS-1 and cystic kidney disease rats VSMCs) have been detected. ncRNAs, non-coding RNAs; EVs, extracellular vesicles; VSMCs, vascular smooth muscle cells; ECs, endothelial cells; VC, vascular calcification; HP, high phosphate; β-GP, β-glycerophosphate. Created with BioRender.com.
4. The emerging role of lncRNAs encapsulated in EVs in AS and VC processes
LncRNAs, another type of non-coding RNA larger than 200 base pairs, have significant enrichment in the nucleus compared with mRNAs, emerging as crucial regulators of tissue physiology and pathology mechanism (176–179). Many lncRNAs modulated gene expression in neuronal disorders and cancer (180). In turn, therapeutic targeting of lncRNAs provided a promising approach to the treatment of multiple diseases (181). Furthermore, lncRNA encapsulated in EVs represented an approach to utilizing lncRNAs in disease diagnostics and therapy (182). Here, we aimed to review studies related to lncRNAs encapsulated in EV in the process of AS and VC.
4.1. lncRNAs encapsulated in EVs involved in the process of AS
Indeed, different lncRNAs involved in AS showed opposite effects, such as lncRNA RNCR3 has an atheroprotective effect while lncRNA MIAT has a proinflammatory effect on VSMCs (183, 184). Moreover, lncRNAs encapsulated in EVs were also demonstrated to be tightly associated with AS (185). In mesenchymal stem cells (MSCs)-EVs, lncRNA FENDRR induced by ox-LDL alleviated HUVECs injury and AS by binding with miR-28 (186); knockdown of LOC100129516 alleviated the progression of AS (187). Encapsulated in ox-LDL-EVs from THP-1 cells, lncRNA GAS5 enhanced the apoptosis of HUVECs and HAECs and lncRNA LIPCAR-enhanced AS (188–190).
In ox-LDL-HUVEC-EVs, lncRNA MALAT1 promoted AS by inducing M2 macrophage polarization and NETs (191, 192); LncRNA ZEB1-AS1 promoted endothelial injuries in AS by competitively binding to miR-590-5p (193); LINC01005 promoted VSMC phenotype switch through miR-128-3p/KLF4 axis in the development of AS (194); lncRNA CLDN10-AS1 promoted endothelial injuries by sponging miR-186 (195).
In conclusion, lncRNAs encapsulated in EVs provided a new perspective on the intercellular communication of AS. The potential role of lncRNAs in the prevention and progress of AS still needs to be illuminated. Several lncRNAs encapsulated in EVs involved in the process of AS are summarized in Table 5.
4.2. lncRNAs involved in the process of VC
It has also been shown that the lncRNAs were new players regulating VC, providing therapeutic targets (196–198).
Bao et al. (199) revealed 728 different lncRNA expressions in HVSMCs under HP condition compared to the control group, in which 8 lncRNAs were the most potential lncRNAs in VC. Jeong et al. (200) discovered 100's of lncRNAs differentially expressed in rat VSMCs under HP condition, and Lrrc75a-as1 acted as an inhibitor of VC. LncRNA GAS5, as mentioned above (188, 189), promoted VC by modulating miR-26-5p (201). LncRNA SNHG1 alleviated high glucose-induced VSMC calcification by regulating basic helix–loop–helix family member e40 (Bhlhe40) (202). Similarly, lncRNA EPS inhibited VC in diabetic mice through the TGF-β/Wnt/β-catenin pathway (203). On the contrary, lncRNA LEF1-AS1 promoted VC (204). LncRNA-ES3 promoted VC by the modulation of miR-34c-5p and Bhlhe40 (205, 206).
Treated with HP, lncRNA H19/Runx2 axis promoted VC by the modulation of miR-103-3p (207–209). The modulation between LncRNA H19 and miR-138 was also discovered in the HP environment (210). Nevertheless, lncRNA H19 attenuated VC under ox-LDL and HP conditions in the development of AS (211).
However, there are no studies aimed to investigate the profile of lncRNAs encapsulated in EVs in the intercellular communication between ECs and VSMCs. This might be the reason that lncRNA expressions are relatively low in EVs so their transcripts cannot be analyzed in RNA-seq. More importantly, the lncRNA sequence–function relationship needs to be explored in the future (212). LncRNAs for the in-depth study often follow a specific physiologic or pathological state (213). The relationship between EVs and lncRNAs has the potential to advance our understanding of AS, VC, and other diseases.
5. The emerging role of circRNAs encapsulated in EVs in AS and VC processes
CircRNAs, generated from intronic lariats during colinear splicing and characterized by their covalently closed circular structure, showed tissue-specific and development stage-specific expression (214–216). Hansen et al. (217) first revealed that circRNAs were regarded as miRNAs sponges generally in gene regulation. Some endogenous circRNAs were highly abundant and evolutionarily conserved, providing potential implications for research and treatment applications (218). We have discussed miRNA encapsulated in EVs in AS and VC so far and subsequently aimed to summarize circRNA encapsulated in EVs in AS and VC.
5.1. circRNAs encapsulated in EVs involved in the process of AS
CircRNAs have been identified in the process of AS, such as circ_USP36, circ_0007478, circCHFR, and circ_0001879 (219–223). CircRNAs encapsulated in EVs were also involved in the process of AS (224). CircNPHP4 in monocyte-EVs regulated heterogeneous adhesion in coronary heart atherosclerotic disease by the modulation of miR-1231 (225). Hsa_circ_0001445 acted as a biomarker in CAD patients and was decreased in VSMCs-EVs on AS condition (226). Hsa_circ_0005699 was downregulated in the blood exosomes of patients with coronary heart disease and upregulated in ox-LDL-treated macrophage, providing candidate targets for the diagnosis of AS (227). Encapsulation in serum EVs of patients with unstable/vulnerable plaque AS, upregulated circRNA_0006896 plays a crucial role in carotid plaque destabilization by modulation of miR-1264 (228); circ_0043837 and circ_0001801 were independent predictive factors for artery atherosclerotic stroke (229). Hsa_circ_0086296 was also observed to be higher in AS patient serum EVs and ox-LDL-treated HUVECs, which promoted ECs injury and AS by the modulation of miR-576-3p (230). Although many circRNAs in AS have been revealed, circRNAs encapsulated in EVs during AS between intercellular communication will be focused, discussing issues and trends in studies in the future. Several circRNAs encapsulated in EVs involved in the process of AS are summarized in Table 6.
5.2. circRNAs involved in the process of VC
Ryu et al. discovered that circSamd4a reduced VC by modulating miR-125a-3p and miR-483-5p; circSmoc1-2 reduced VC by the modulation of miR-874-3p (231, 232). Moreover, CircSamd4a could act as a biomarker for the diagnosis of VC (233). In contrast, circRNA CDR1 promoted VC by sponging miR-7-5p (234). Similarly, hsa_circRNA_0008028-modulated miR-182-5p and promoted high glucose-induced VC (235). Notably, there were few articles concentrated on circRNAs participating in VC. Further studies are essential to elucidate the emerging role of circRNAs in the process of VC.
6. Discussion
In this review, we have summarized almost all miRNAs encapsulated in EVs secreted by ECs and VSMCs in the process of AS and VC. We concluded on the function of different miRNAs encapsulated in EVs which are effective to inhibit or aggravate AS and VC, depending on the stimuli and distributions. In addition, miRNAs encapsulated in EVs were expected to be indicators in the diagnosis and prognosis of cardiovascular diseases. There is still a long way to go. Different methods (direct electroporation, cell transfection, and chemical transfection) were developed to vehicle therapeutic molecules through EVs (236). Nevertheless, limitations, such as EV disruption and aggregation, still exist. The application of the atheroprotective miRNAs encapsulated in EVs in the treatment is still needed to be further investigated. In addition to elucidating the versatility of miRNAs in different signaling pathways, the complicated and subtle effects in the animal model should be illustrated.
Moreover, lncRNAs and circRNAs are expressed differentially during AS and VC. We have discussed the current understanding of lncRNAs and circRNAs in AS and VC. We have noticed the potential prospect of lncRNAs and circRNAs in the prevention, diagnosis, and treatment of AS/VC.
In conclusion, the versatile non-coding RNAs encapsulated in EVs provide a novel perspective on the biogenesis mechanisms of AS and VC.
Author contributions
ZH and XX: conceptualization and supervision. FY and YD: writing—original draft preparation. HH, CL, and ZH: writing—review and editing. CL, HH, ZH, and XX: visualization. FY, CL, and ZH: reversion of the manuscript. ZH: project administration and funding acquisition. All authors have read and agreed to the published version of the manuscript.
Funding
This study was supported by the National Natural Science Foundation of China (grant number 81900678), the Natural Science Foundation of Hunan Province (grant number 2021JJ40491), the Hunan Innovation Guidance Project (grant number 2021SK51811), and the Scientific Research Project of Hunan Provincial Health Commission (grant numbers 202103051523 and B202303109098).
Conflict of interest
The authors declare that the research was conducted in the absence of any commercial or financial relationships that could be construed as a potential conflict of interest.
Publisher's note
All claims expressed in this article are solely those of the authors and do not necessarily represent those of their affiliated organizations, or those of the publisher, the editors and the reviewers. Any product that may be evaluated in this article, or claim that may be made by its manufacturer, is not guaranteed or endorsed by the publisher.
Supplementary material
The Supplementary Material for this article can be found online at: https://www.frontiersin.org/articles/10.3389/fmed.2023.1193660/full#supplementary-material
Abbreviations
AS, Atherosclerosis; VC, Vascular calcification; MAC, Medial arterial calcification; ECs, Endothelial cells; VSMCs, Vascular smooth muscle cells; EVs, Extracellular vesicles; ncRNAs, Non-coding RNAs; miRNAs, microRNAs; lncRNAs, Long non-coding RNAs; circRNAs, Circular RNAs; EC-EVs, EVs derived from ECs; HUVECs-EVs, EVs derived from HUVECs; VSMC-EVs, EVs derived from VSMCs; HCASMC-MPs, MPs derived from HCASMC; ox-LDL, Oxidized low-density lipoprotein; IL-1β, Interleukin-1β; TNF-α, Tumor necrosis factor-a; TGF-β, Transforming growth factor-β; KLF4, Krüppel-like factor 4; IAC, Intimal arterial calcification; mRNAs, Messenger RNAs; miRNA-seq, miRNA-sequence; RNA-seq, RNA-seq; LPS, Lipopolysaccharide; HUVECs, Human umbilical vein endothelial cells; HASMCs, Human aortic smooth muscle cells; Dlk1, Delta-like 1 homolog; NF-κB, Nuclear factor-κB; AKT, Serine/threonine kinase; ox-LDL-VSMC, ox-LDL-induced VSMC; ox-LDL-HUVEC, ox-LDL-induced HUVEC; HMGB1, High-mobility group box 1; KLF2, Krüppel-like factor 2; eNOS, Endothelial nitric oxide synthase; RHEB, Ras homolog enriched in brain; cGMP, Cyclic guanosine monophosphate; PKG1, Protein kinase 1; NETs, Neutrophil extracellular traps; VEGFA, Vascular endothelial growth factor A; MPs, Microparticles; SOCS3, Suppressor of cytokine signaling 3; JAK2, Janus kinase 2; STAT3, Signal transducer and activator of transcription 3; HIF-1α, Hypoxia-induced factor-1; HAECs, Human aortic endothelial cells; ox-LDL-EVs, EVs derived from ox-LDL condition; HP-EVs, EVs derived from high phosphate condition; HCAECs, Human coronary artery vascular endothelial cells; THBS1, Thrombospondin 1; ApoE(-/-), Apolipoprotein E knockout; HP, High phosphate; AEG-1, Astrocyte elevated gene-1; CXCR6, C-X-C motif chemokine receptor 6; MMP2, Matrix metalloproteinase 2; Wnt7b, Wnt family member 7B; Pi, Inorganic phosphorus; Enpp1, Ectonucleotide phosphodiesterase 1; CKD, Chronic kidney disease; CVD, Cardiovascular disease; PBS, Phosphate buffer saline; AOAH, Acyloxyacyl hydrolase; NOD2, Nucleotide-binding oligomerization domain containing 2; RBM47, RNA-binding motif protein 47; Runx2, Runt-related transcription factor 2; Osx, Osterix; AVICs, Aortic valve interstitial cells; HCASMC, Human coronary artery smooth muscle cells; RhoA, Ras homolog family member A; CAD, Coronary artery disease; IGF-1, Insulin-like growth factor-1; PGC-1α, Peroxisome proliferator-activated receptor-γ coactivator-1α; sGC, Soluble guanylyl cyclase; FH, Familial hypercholesterolemia; BMP2, Bone morphogenetic protein-2; CAC, Coronary artery calcification; T2DM, Diabetes mellitus type 2; β-GP, β-glycerophosphate; MVs, Microvesicles; MSCs, Mesenchymal stem cells.
References
1. Malek AM, Alper SL, Izumo S. Hemodynamic shear stress and its role in atherosclerosis. JAMA. (1999) 282:2035–42. doi: 10.1001/jama.282.21.2035
2. Kwak BR, Bäck M, Bochaton-Piallat M-L, Caligiuri G, Daemen MJAP, Davies PF, et al. Biomechanical factors in atherosclerosis: mechanisms and clinical implications. Eur Heart J. (2014) 35:3013–20. doi: 10.1093/eurheartj/ehu353
3. Gardin C, Ferroni L, Leo S, Tremoli E, Zavan B. Platelet-derived exosomes in atherosclerosis. Int J Mol Sci. (2022) 23:12546. doi: 10.3390/ijms232012546
4. Mai J, Virtue A, Shen J, Wang H, Yang XF. An evolving new paradigm: endothelial cells—conditional innate immune cells. J Hematol Oncol. (2013) 6:61. doi: 10.1186/1756-8722-6-61
5. Duan H, Zhang Q, Liu J, Li R, Wang D, Peng W, et al. Suppression of apoptosis in vascular endothelial cell, the promising way for natural medicines to treat atherosclerosis. Pharmacol Res. (2021) 168:105599. doi: 10.1016/j.phrs.2021.105599
6. Kianmehr A, Qujeq D, Bagheri A, Mahrooz A. Oxidized LDL-regulated microRNAs for evaluating vascular endothelial function: molecular mechanisms and potential biomarker roles in atherosclerosis. Crit Rev Clin Lab Sci. (2022) 59:40–53. doi: 10.1080/10408363.2021.1974334
7. Henein MY, Vanch eri S, Longo G, et al. The role of inflammation in cardiovascular disease. Int J Mol Sci. (2022) 23:12906. doi: 10.3390/ijms232112906
8. Mućka S, Miodońska M, Jakubiak GK, Starzak M, Cieślar G, Stanek A. Endothelial function assessment by flow-mediated dilation method: a valuable tool in the evaluation of the cardiovascular system. Int J Environ Res Public Health. (2022) 19:11242. doi: 10.3390/ijerph191811242
9. Maruhashi T, Higashi Y. Pathophysiological association between diabetes mellitus and endothelial dysfunction. Antioxidants. (2021) 10:1306. doi: 10.3390/antiox10081306
10. Pi X, Xie L, Patterson C. Emerging roles of vascular endothelium in metabolic homeostasis. Circ Res. (2018) 123:477–94. doi: 10.1161/CIRCRESAHA.118.313237
11. Sorokin V, Vickneson K, Kofidis T, Woo CC, Lin XY, Foo R, et al. Role of vascular smooth muscle cell plasticity and interactions in vessel wall inflammation. Front Immunol. (2020) 11:599415. doi: 10.3389/fimmu.2020.599415
12. Hu D, Yin C, Luo S, Habenicht AJR, Mohanta SK. Vascular smooth muscle cells contribute to atherosclerosis immunity. Front Immunol. (2019) 10:1101. doi: 10.3389/fimmu.2019.01101
13. Lacolley P, Regnault V, Nicoletti A, Li Z, Michel JB. The vascular smooth muscle cell in arterial pathology: a cell that can take on multiple roles. Cardiovasc Res. (2012) 95:194–204. doi: 10.1093/cvr/cvs135
14. Lacolley P, Regnault V, Segers P, Laurent S. Vascular smooth muscle cells and arterial stiffening: relevance in development, aging, and disease. Physiol Rev. (2017) 97:1555–617. doi: 10.1152/physrev.00003.2017
15. Owens GK. Regulation of differentiation of vascular smooth muscle cells. Physiol Rev. (1995) (3):487–517. doi: 10.1152/physrev.1995.75.3.487
16. Grootaert MOJ, Bennett MR. Vascular smooth muscle cells in atherosclerosis: time for a re-assessment. Cardiovasc Res. (2021) 117:2326–39. doi: 10.1093/cvr/cvab046
17. Alencar GF, Owsiany KM, Karnewar S, Sukhavasi K, Mocci G, Nguyen AT, et al. Stem cell pluripotency genes klf4 and oct4 regulate complex smc phenotypic changes critical in late-stage atherosclerotic lesion pathogenesis. Circulation. (2020) 142:2045–59. doi: 10.1161/CIRCULATIONAHA.120.046672
18. Pan H, Xue C, Auerbach BJ, et al. Single-cell genomics reveals a novel cell state during smooth muscle cell phenotypic switching and potential therapeutic targets for atherosclerosis in mouse and human. Circulation. (2020) 142:2060–75. doi: 10.1161/CIRCULATIONAHA.120.048378
19. Miano JM, Fisher EA, Majesky MW. Fate and state of vascular smooth muscle cells in atherosclerosis. Circulation. (2021) 143:2110–6. doi: 10.1161/CIRCULATIONAHA.120.049922
20. Bennett MR, Sinha S, Owens GK. Vascular smooth muscle cells in atherosclerosis. Circ Res. (2016) 118:692–702. doi: 10.1161/CIRCRESAHA.115.306361
21. Shankman LS, Gomez D, Cherepanova OA, et al. KLF4-dependent phenotypic modulation of smooth muscle cells has a key role in atherosclerotic plaque pathogenesis. Nat Med. (2015) 21:628–37. doi: 10.1038/nm.3866
22. Pugliese G, Iacobini C, Fantauzzi CB, Menini S. The dark and bright side of atherosclerotic calcification. Atherosclerosis. (2015) 238:220–30. doi: 10.1016/j.atherosclerosis.2014.12.011
23. Waring OJ, Skenteris NT, Biessen EAL, et al. Two-faced Janus: the dual role of macrophages in atherosclerotic calcification. Cardiovasc Res. (2022) 118:2768–77. doi: 10.1093/cvr/cvab301
24. Bäck M, Michel JB. From organic and inorganic phosphates to valvular and vascular calcifications. Cardiovasc Res. (2021) 117:2016–29. doi: 10.1093/cvr/cvab038
25. Hou YC, Lu CL, Zheng CM, Liu WC, Yen TH, Chen RM, et al. The role of vitamin D in modulating mesenchymal stem cells and endothelial progenitor cells for vascular calcification. Int J Mol Sci. (2020) 21:2466. doi: 10.3390/ijms21072466
26. Li M, Wang ZW, Fang LJ, Cheng SQ, Wang X, Liu NF. Programmed cell death in atherosclerosis and vascular calcification. Cell Death Dis. (2022) 13:467. doi: 10.1038/s41419-022-04923-5
27. Durham AL, Speer MY, Scatena M, Giachelli CM, Shanahan CM. Role of smooth muscle cells in vascular calcification: implications in atherosclerosis and arterial stiffness. Cardiovasc Res. (2018) 114:590–600. doi: 10.1093/cvr/cvy010
28. Nakahara T, Dweck MR, Narula N, Pisapia D, Narula J, Strauss HW. Coronary artery calcification: from mechanism to molecular imaging. JACC Cardiovasc Imaging. (2017) 10:582–93. doi: 10.1016/j.jcmg.2017.03.005
29. Lanzer P, Hannan FM, Lanzer JD, Janzen J, Raggi P, Furniss D, et al. Medial arterial calcification: JACC state-of-the-art review. J Am Coll Cardiol. (2021) 78:1145–65. doi: 10.1016/j.jacc.2021.06.049
30. He L, Zhang C-L, Chen Q, Wang L, Huang Y. Endothelial shear stress signal transduction and atherogenesis: from mechanisms to therapeutics. Pharmacol Ther. (2022) 235:108152. doi: 10.1016/j.pharmthera.2022.108152
31. Dessalles CA, Leclech C, Castagnino A, Barakat AI. Integration of substrate- and flow-derived stresses in endothelial cell mechanobiology. Commun Biol. (2021) 4:764. doi: 10.1038/s42003-021-02285-w
32. Johnson RC, Leopold JA, Loscalzo J. Vascular calcification: pathobiological mechanisms and clinical implications. Circ Res. (2006) 99:1044–59. doi: 10.1161/01.RES.0000249379.55535.21
33. Yao Y, Bennett BJ, Wang X, Rosenfeld ME, Giachelli C, Lusis AJ, et al. Inhibition of bone morphogenetic proteins protects against atherosclerosis and vascular calcification. Circ Res. (2010) 107:485–94. doi: 10.1161/CIRCRESAHA.110.219071
34. Andrews J, Psaltis PJ, Di Bartolo BA, Nicholls SJ, Puri R. Coronary arterial calcification: A review of mechanisms, promoters and imaging. Trends Cardiovasc Med. (2018) 28:491–501. doi: 10.1016/j.tcm.2018.04.007
35. Düsing P, Zietzer A, Goody PR, Hosen MR, Kurts C, Nickenig G, et al. Vascular pathologies in chronic kidney disease: pathophysiological mechanisms and novel therapeutic approaches. J Mol Med. (2021) 99:335–48. doi: 10.1007/s00109-021-02037-7
36. Lee SJ, Lee IK, Jeon JH. Vascular calcification-new insights into its mechanism. Int J Mol Sci. (2020) 21:2685. doi: 10.3390/ijms21082685
37. Tesauro M, Mauriello A, Rovella V, Annicchiarico-Petruzzelli M, Cardillo C, Melino G, et al. Arterial ageing: from endothelial dysfunction to vascular calcification. J Intern Med. (2017) 281:471–82. doi: 10.1111/joim.12605
38. Zhang Y-X, Tang R-N, Wang L-T, Liu B-C. Role of crosstalk between endothelial cells and smooth muscle cells in vascular calcification in chronic kidney disease. Cell Prolif. (2021) 54:e12980. doi: 10.1111/cpr.12980
39. Bouabdallah J, Zibara K, Issa H, Lenglet G, Kchour G, Caus T, et al. Endothelial cells exposed to phosphate and indoxyl sulphate promote vascular calcification through interleukin-8 secretion. Nephrol Dial Transplant. (2019) 34:1125–34. doi: 10.1093/ndt/gfy325
40. Van den Bergh G, Van den Branden A, Opdebeeck B, Fransen P, Neven E, De Meyer GRY, et al. Endothelial dysfunction aggravates arterial media calcification in warfarin administered rats. FASEB J. (2022) 36:e22315. doi: 10.1096/fj.202101919R
41. Van den Bergh G, De Moudt S, Van den Branden A, Neven E, Leysen H, Maudsley S, et al. Endothelial contribution to warfarin-induced arterial media calcification in mice. Int J Mol Sci. (2021) 22:11615. doi: 10.3390/ijms222111615
42. Alique M, Bodega G, Corchete E, García-Menéndez E, de Sequera P, Luque R, et al. Microvesicles from indoxyl sulfate-treated endothelial cells induce vascular calcification in vitro. Comput Struct Biotechnol J. (2020) 18:953–66. doi: 10.1016/j.csbj.2020.04.006
43. Lin X, Li S, Wang YJ, Wang Y, Zhong JY, He JY, et al. Exosomal Notch3 from high glucose-stimulated endothelial cells regulates vascular smooth muscle cells calcification/aging. Life Sci. (2019) 232:116582. doi: 10.1016/j.lfs.2019.116582
44. Théry C, Witwer KW, Aikawa E. Minimal information for studies of extracellular vesicles 2018 (MISEV2018): a position statement of the international society for extracellular vesicles and update of the MISEV2014 guidelines. J Extracell Vesicles. (2018) 7:1535750. doi: 10.1080/20013078.2018.1535750
45. Goettsch C, Hutcheson JD, Aikawa E. MicroRNA in cardiovascular calcification: focus on targets and extracellular vesicle delivery mechanisms. Circ Res. (2013) 112:1073–84. doi: 10.1161/CIRCRESAHA.113.300937
46. Ciceri P, Falleni M, Tosi D. High-phosphate induced vascular calcification is reduced by iron citrate through inhibition of extracellular matrix osteo-chondrogenic shift in VSMCs. Int J Cardiol. (2019) 297:94–103. doi: 10.1016/j.ijcard.2019.09.068
47. Luo F, Guo W, Liu W. Exosomes derived from bone marrow mesenchymal stem cells inhibit human aortic vascular smooth muscle cells calcification via the miR-15a/15b/16/NFATc3/OCN axis. Biochem Biophys Res Commun. (2022) 635:65–76. doi: 10.1016/j.bbrc.2022.09.076
48. Peng M, Sun R, Hong Y, Wang J, Xie Y, Zhang X, et al. Extracellular vesicles carrying proinflammatory factors may spread atherosclerosis to remote locations. Cell Mol Life Sci. (2022) 79:430. doi: 10.1007/s00018-022-04464-2
49. Liu Y, Sun Y, Lin X, Zhang D, Hu C, Liu J, et al. Perivascular adipose-derived exosomes reduce macrophage foam cell formation through miR-382-5p and the BMP4-PPARγ-ABCA1/ABCG1 pathways. Vascul Pharmacol. (2022) 143:106968. doi: 10.1016/j.vph.2022.106968
50. Niu M, Li H, Li X, Yan X, Ma A, Pan X, et al. Circulating exosomal miRNAs as novel biomarkers perform superior diagnostic efficiency compared with plasma miRNAs for large-artery atherosclerosis stroke. Front Pharmacol. (2021) 12:791644. doi: 10.3389/fphar.2021.791644
51. Xiang D, Li Y, Cao Y, Huang Y, Zhou L, Lin X, et al. Different effects of endothelial extracellular vesicles and LPS-induced endothelial extracellular vesicles on vascular smooth muscle cells: role of curcumin and its derivatives. Front Cardiovasc Med. (2021) 8:649352. doi: 10.3389/fcvm.2021.649352
52. Santiago-Fernandez C, Rodríguez-Díaz C, Ho-Plagaro A, Gutierrez-Repiso C, Oliva-Olivera W, Martin-Reyes F, et al. EVOO promotes a less atherogenic profile than sunflower oil in smooth muscle cells through the extracellular vesicles secreted by endothelial cells. Front Nutr. (2022) 9:867745. doi: 10.3389/fnut.2022.867745
53. Rozhkov AN, Shchekochikhin DY, Ashikhmin YI, Mitina YO, Evgrafova VV, Zhelankin AV, et al. The profile of circulating blood microRNAs in outpatients with vulnerable and stable atherosclerotic plaques: associations with cardiovascular risks. Noncoding RNA. (2022) 8:47. doi: 10.3390/ncrna8040047
54. He S, Wu C, Xiao J, Li D, Sun Z, Li M. Endothelial extracellular vesicles modulate the macrophage phenotype: Potential implications in atherosclerosis. Scand J Immunol. (2018) 87:e12648. doi: 10.1111/sji.12648
55. Chen L, Hu L, Li Q. Exosome-encapsulated miR-505 from ox-LDL-treated vascular endothelial cells aggravates atherosclerosis by inducing NET formation. Acta Biochim Biophys Sin. (2019) 51:1233–41. doi: 10.1093/abbs/gmz123
56. Yu SR, Cui YX, Song Z-Q, Li SF, Zhang CY, Song JX, et al. Endothelial microparticle-mediated transfer of microRNA-19b inhibits the function and distribution of lymphatic vessels in atherosclerotic mice. Front Physiol. (2022) 13:850298. doi: 10.3389/fphys.2022.850298
57. Yang Y, Luo H, Zhou C, Zhang R, Liu S, Zhu X, et al. Regulation of capillary tubules and lipid formation in vascular endothelial cells and macrophages via extracellular vesicle-mediated microRNA-4306 transfer. J Int Med Res. (2019) 47:453–69. doi: 10.1177/0300060518809255
58. Chang YJ, Li YS, Wu CC, Wang KC, Huang TC, Chen Z, et al. Extracellular microRNA-92a mediates endothelial cell-macrophage communication. Arterioscler Thromb Vasc Biol. (2019) 39:2492–504. doi: 10.1161/ATVBAHA.119.312707
59. Liu Y, Li Q, Hosen MR, Zietzer A, Flender A, Levermann P, et al. Atherosclerotic conditions promote the packaging of functional microRNA-92a-3p into endothelial microvesicles. Circ Res. (2019) 124:575–87. doi: 10.1161/CIRCRESAHA.118.314010
60. Lin CM, Wang BW, Pan CM, Fang WJ, Chua SK, Cheng WP, et al. Chrysin boosts KLF2 expression through suppression of endothelial cell-derived exosomal microRNA-92a in the model of atheroprotection. Eur J Nutr. (2021) 60:4345–55. doi: 10.1007/s00394-021-02593-1
61. Hergenreider E, Heydt S, Tréguer K, Boettger T, Horrevoets AJG, Zeiher AM, et al. Atheroprotective communication between endothelial cells and smooth muscle cells through miRNAs. Nat Cell Biol. (2012) 14:249–56. doi: 10.1038/ncb2441
62. Schober A, Nazari-Jahantigh M, Wei Y. MicroRNA-126-5p promotes endothelial proliferation and limits atherosclerosis by suppressing Dlk1. Nat Med. (2014) 20:368–76. doi: 10.1038/nm.3487
63. Jia W, Liu J, Tian X. MircoRNA-126-5p inhibits apoptosis of endothelial cell in vascular arterial walls via NF-κB/PI3K/AKT/mTOR signaling pathway in atherosclerosis. J Mol Histol. (2022) 53:51–62. doi: 10.1007/s10735-022-10091-9
64. Chen Z, Pan X, Sheng Z, Yan G, Chen L, Ma G. Baicalin Suppresses the Proliferation and Migration of Ox-LDL-VSMCs in atherosclerosis through upregulating miR-126-5p. Biol Pharm Bull. (2019) 42:1517–23. doi: 10.1248/bpb.b19-00196
65. Ma X, Ma C, Zheng X. MicroRNA-155 in the pathogenesis of atherosclerosis: a conflicting role? Heart Lung Circ. (2013) 22:811–8. doi: 10.1016/j.hlc.2013.05.651
66. Kim TH, Kim JY, Bae J, Kim YM, Won MH, Ha KS, et al. Korean red ginseng prevents endothelial senescence by downregulating the HO-1/NF-κB/miRNA-155-5p/eNOS pathway. J Ginseng Res. (2021) 45:344–53. doi: 10.1016/j.jgr.2020.08.002
67. Wang X, Han W, Zhang Y, Zong Y, Tan N, Zhang Y, et al. Soluble epoxide hydrolase inhibitor t-AUCB ameliorates vascular endothelial dysfunction by influencing the NF-κB/miR-155-5p/eNOS/NO/IκB cycle in hypertensive rats. Antioxidants. (2022) 11:1372. doi: 10.3390/antiox11071372
68. Xu L, Zhang L, Zhang X, Li G, Wang Y, Dong J, et al. HDAC6 negatively regulates miR-155-5p expression to elicit proliferation by targeting RHEB in microvascular endothelial cells under mechanical unloading. Int J Mol Sci. (2021) 22:10527. doi: 10.3390/ijms221910527
69. Zhao L, Ouyang Y, Bai Y. miR-155-5p inhibits the viability of vascular smooth muscle cell via targeting FOS and ZIC3 to promote aneurysm formation. Eur J Pharmacol. (2019) 853:145–52. doi: 10.1016/j.ejphar.2019.03.030
70. Choi S, Park M, Kim J. TNF-α elicits phenotypic and functional alterations of vascular smooth muscle cells by miR-155-5p-dependent down-regulation of cGMP-dependent kinase 1. J Biol Chem. (2018) 293:14812–22. doi: 10.1074/jbc.RA118.004220
71. Chen L, Zheng SY, Yang CQ. MiR-155-5p inhibits the proliferation and migration of VSMCs and HUVECs in atherosclerosis by targeting AKT1. Eur Rev Med Pharmacol Sci. (2019) 23:2223–33.
72. Liu S, Gao J, Chen J. Knockdown of lncRNA TUG1 suppresses corneal angiogenesis through regulating miR-505-3p/VEGFA. Microvasc Res. (2021) 138:104233. doi: 10.1016/j.mvr.2021.104233
73. Zhao S, Lu L, Liu Q. MiR-505 promotes M2 polarization in choroidal neovascularization model mice by targeting transmembrane protein 229B. Scand J Immunol. (2019) 90:e12832. doi: 10.1111/sji.12832
74. Yang Q, Jia C, Wang P. MicroRNA-505 identified from patients with essential hypertension impairs endothelial cell migration and tube formation. Int J Cardiol. (2014) 177:925–34. doi: 10.1016/j.ijcard.2014.09.204
75. Yang Q, Wang P, Cai Y, Cui Y, Cui J, Du X, et al. Circulating microRNA-505 may serve as a prognostic biomarker for hypertension-associated endothelial dysfunction and inflammation. Front Cardiovasc Med. (2022) 9:834121. doi: 10.3389/fcvm.2022.834121
76. Liang HZ, Li SF, Zhang F, Wu MY, Li CL, Song JX, et al. Effect of endothelial microparticles induced by hypoxia on migration and angiogenesis of human umbilical vein endothelial cells by delivering microRNA-19b. Chin Med J. (2018) 131:2726–33. doi: 10.4103/0366-6999.245271
77. Li C, Li S, Zhang F, Wu M, Liang H, Song J, et al. Endothelial microparticles-mediated transfer of microRNA-19b promotes atherosclerosis via activating perivascular adipose tissue inflammation in apoE-/- mice. Biochem Biophys Res Commun. (2018) 495:1922–9. doi: 10.1016/j.bbrc.2017.11.195
78. Wu F, Wang JY, Chao W. miR-19b targets pulmonary endothelial syndecan-1 following hemorrhagic shock. Sci Rep. (2020) 10:15811. doi: 10.1038/s41598-020-73021-3
79. Wu F, Wang JY, Dorman B. c-Jun-mediated miR-19b expression induces endothelial barrier dysfunction in an in vitro model of hemorrhagic shock. Mol Med. (2022) 28:123. doi: 10.1186/s10020-022-00550-0
80. Zeineddin A, Wu F, Chao W, Zou L, Vesselinov R, Chipman AM, et al. Biomarkers of endothelial cell dysfunction persist beyond resuscitation in patients with hemorrhagic shock. J Trauma Acute Care Surg. (2022) 93:572–8. doi: 10.1097/TA.0000000000003758
81. Song T, Zhou M, Li W, Lv M, Zheng L, Zhao M. The anti-inflammatory effect of vasoactive peptides from soybean protein hydrolysates by mediating serum extracellular vesicles-derived miRNA-19b/CYLD/TRAF6 axis in the vascular microenvironment of SHRs. Food Res Int. (2022) 160:111742. doi: 10.1016/j.foodres.2022.111742
82. Xiao F, Li L, Fu JS, Hu Y-X, Luo R. Regulation of the miR-19b-mediated SOCS6-JAK2/STAT3 pathway by lncRNA MEG3 is involved in high glucose-induced apoptosis in hRMECs. Biosci Rep. (2020) 40:BSR20194370. doi: 10.1042/BSR20194370
83. Liu H, Shi C, Deng Y. MALAT1 affects hypoxia-induced vascular endothelial cell injury and autophagy by regulating miR-19b-3p/HIF-1α axis. Mol Cell Biochem. (2020) 466:25–34. doi: 10.1007/s11010-020-03684-z
84. Katta S, Karnewar S, Panuganti D. Mitochondria-targeted esculetin inhibits PAI-1 levels by modulating STAT3 activation and miR-19b via SIRT3: Role in acute coronary artery syndrome J Cell Physiol. (2018) 233:214–25. doi: 10.1002/jcp.25865
85. Wang J, Xu X, Li P, Zhang B, Zhang J. HDAC3 protects against atherosclerosis through inhibition of inflammation via the microRNA-19b/PPARγ/NF-κB axis. Atherosclerosis. (2021) 323:1–12. doi: 10.1016/j.atherosclerosis.2021.02.013
86. Chipman AM, Wu F, Kozar RA. Fibrinogen inhibits microRNA-19b, a novel mechanism for repair of haemorrhagic shock-induced endothelial cell dysfunction. Blood Transfus. (2021) 19:420–7. doi: 10.2450/2021.0361-20. [Epub ahead of print].
87. Xue Y, Wei Z, Ding H. MicroRNA-19b/221/222 induces endothelial cell dysfunction via suppression of PGC-1α in the progression of atherosclerosis. Atherosclerosis. (2015) 241:671–81. doi: 10.1016/j.atherosclerosis.2015.06.031
88. Li S, Geng Q, Chen H. The potential inhibitory effects of miR-19b on vulnerable plaque formation via the suppression of STAT3 transcriptional activity. Int J Mol Med. (2018) 41:859–67. doi: 10.3892/ijmm.2017.3263
89. Li S, Ren J, Xu N. MicroRNA-19b functions as potential anti-thrombotic protector in patients with unstable angina by targeting tissue factor. J Mol Cell Cardiol. (2014) 75:49–57. doi: 10.1016/j.yjmcc.2014.06.017
90. Tang Y, Zhang YC, Chen Y, Xiang Y, Shen CX, Li YG. The role of miR-19b in the inhibition of endothelial cell apoptosis and its relationship with coronary artery disease. Sci Rep. (2015) 5:15132. doi: 10.1038/srep15132
91. Wang Y, Tang M. Integrative analysis of mRNAs, miRNAs and lncRNAs in urban particulate matter SRM 1648a-treated EA.hy926 human endothelial cells. Chemosphere. (2019) 233:711–23. doi: 10.1016/j.chemosphere.2019.05.294
92. Aplin AC, Nicosia RF. Tissue oxygenation stabilizes neovessels and mitigates hemorrhages in human atherosclerosis-induced angiogenesis. Angiogenesis. (2022) 2:10. doi: 10.1007/s10456-022-09851-8
93. Shang F, Wang SC, Hsu CY, et al. MicroRNA-92a mediates endothelial dysfunction in CKD. J Am Soc Nephrol. (2017) 28:3251–61. doi: 10.1681/ASN.2016111215
94. Iaconetti C, Polimeni A, Sorrentino S, Sabatino J, Pironti G, Esposito G, et al. Inhibition of miR-92a increases endothelial proliferation and migration in vitro as well as reduces neointimal proliferation in vivo after vascular injury. Basic Res Cardiol. (2012) 107:296. doi: 10.1007/s00395-012-0296-y
95. Jiang F, Zhang B, Zhang X. miRNA-92a inhibits vascular smooth muscle cell phenotypic modulation and may help prevent in-stent restenosis. Mol Med Rep. (2023) 27:40. doi: 10.3892/mmr.2023.12927
96. Dabravolski SA, Sukhorukov VN, Kalmykov VA, Grechko AV, Shakhpazyan NK, Orekhov AN. The role of KLF2 in the regulation of atherosclerosis development and potential use of KLF2-targeted therapy. Biomedicines. (2022) 10:254. doi: 10.3390/biomedicines10020254
97. Niu N, Xu S, Xu Y, Little PJ, Jin ZG. Targeting mechanosensitive transcription factors in atherosclerosis. Trends Pharmacol Sci. (2019) 40:253–66. doi: 10.1016/j.tips.2019.02.004
98. Yang C, Xiao X, Huang L, Zhou F, Chen LH, Zhao YY, et al. Role of Kruppel-like factor 4 in atherosclerosis. Clin Chim Acta. (2021) 512:135–41. doi: 10.1016/j.cca.2020.11.002
99. Xiang Y, Duan Y, Peng Z, Huang H, Ding W, Chen E, et al. Microparticles from hyperphosphatemia-stimulated endothelial cells promote vascular calcification through astrocyte-elevated gene-1. Calcif Tissue Int. (2022) 111:73–86. doi: 10.1007/s00223-022-00960-6
100. Lin X, Shan SK, Xu F, Zhong JY, Wu F, Duan JY, et al. The crosstalk between endothelial cells and vascular smooth muscle cells aggravates high phosphorus-induced arterial calcification. Cell Death Dis. (2022) 13:650. doi: 10.1038/s41419-022-05064-5
101. Freise C, Querfeld U, Ludwig A, Hamm B, Schnorr J, Taupitz M. Uraemic extracellular vesicles augment osteogenic transdifferentiation of vascular smooth muscle cells via enhanced AKT signalling and PiT-1 expression. J Cell Mol Med. (2021) 5:5602–14. doi: 10.1111/jcmm.16572
102. Wang S, Wu J, Li X, Tan R, Chen L, Yang L, et al. CXCR6 mediates pressure overload-induced aortic stiffness by increasing macrophage recruitment and reducing exosome-miRNA29b. J Cardiovasc Transl Res. (2022). doi: 10.1007/s12265-022-10304-2
103. Guo B, Shan SK, Xu F, Lin X, Li FXZ, Wang Y, et al. Protective role of small extracellular vesicles derived from HUVECs treated with AGEs in diabetic vascular calcification. J Nanobiotechnology. (2022) 20:334. doi: 10.1186/s12951-022-01529-z
104. Peng Z, Duan Y, Zhong S. RNA-seq analysis of extracellular vesicles from hyperphosphatemia-stimulated endothelial cells provides insight into the mechanism underlying vascular calcification. BMC Nephrol. (2022) 23:192. doi: 10.1186/s12882-022-02823-6
105. Jiang W, Zhang Z, Yang H, Lin Q, Qin X. The miR-29b/matrix metalloproteinase 2 axis regulates transdifferentiation and calcification of vascular smooth muscle cells in a calcified environment. Blood Purif. (2020) 49:524–34. doi: 10.1159/000505571
106. Zhang H, Chen J, Shen Z, Gu Y, Xu L, Hu J, et al. Indoxyl sulfate accelerates vascular smooth muscle cell calcification via dependent regulation of Wnt/β-catenin signaling. Toxicol Lett. (2018) 284:29–36. doi: 10.1016/j.toxlet.2017.11.033
107. Panizo S, Naves-Díaz M, Carrillo-López N, Martínez-Arias L, Fernández-Martín JL, Ruiz-Torres MP, et al. MicroRNAs 29b, 133b, and 211 regulate vascular smooth muscle calcification mediated by high phosphorus. J Am Soc Nephrol. (2016) 27:824–34. doi: 10.1681/ASN.2014050520
108. Yang N, Song Y, Dong B, Yang J, Li Y, Qin Q, et al. Analysis of miRNA associated with coronary artery calcification. Comput Math Methods Med. (2022) 2022:3708547. doi: 10.1155/2022/3708547
109. Fang M, Liu K, Li X. AntagomiR-29b inhibits vascular and valvular calcification and improves heart function in rats. J Cell Mol Med. (2020) 24:11546–57. doi: 10.1111/jcmm.15770
110. Sudo R, Sato F, Azechi T. MiR-29-mediated elastin down-regulation contributes to inorganic phosphorus-induced osteoblastic differentiation in vascular smooth muscle cells. Genes Cells. (2015) 20:1077–87. doi: 10.1111/gtc.12311
111. Louvet L, Metzinger L, Büchel J, Steppan S, Massy ZA. Magnesium attenuates phosphate-induced deregulation of a MicroRNA signature and prevents modulation of smad1 and osterix during the course of vascular calcification. Biomed Res Int. (2016) 2016:7419524. doi: 10.1155/2016/7419524
112. Chistiakov DA, Sobenin IA, Orekhov AN, Bobryshev YV. Human miR-221/222 in physiological and atherosclerotic vascular remodeling. Biomed Res Int. (2015) 2015:354517. doi: 10.1155/2015/354517
113. Mackenzie NC, Staines KA, Zhu D. miRNA-221 and miRNA-222 synergistically function to promote vascular calcification. Cell Biochem Funct. (2014) 32:209–16. doi: 10.1002/cbf.3005
114. Massy ZA, Metzinger-Le Meuth V, Metzinger L. MicroRNAs Are associated with uremic toxicity, cardiovascular calcification, and disease. Contrib Nephrol. (2017) 189:160–8. doi: 10.1159/000450774
115. Rangrez AY. M'Baya-Moutoula E, Metzinger-Le Meuth V. Inorganic phosphate accelerates the migration of vascular smooth muscle cells: evidence for the involvement of miR-223. PLoS ONE. (2012) 7:e47807. doi: 10.1371/journal.pone.0047807
116. Chen D, Liang M, Jin C, Sun Y, Xu D, Lin Y. Expression of inflammatory factors and oxidative stress markers in serum of patients with coronary heart disease and correlation with coronary artery calcium score. Exp Ther Med. (2020) 20:2127–33. doi: 10.3892/etm.2020.8958
117. Metzinger-Le Meuth V, Andrianome S, Chillon JM, et al. microRNAs are dysregulated in the cerebral microvasculature of CKD mice. Front Biosci. (2014) 6:80–8. doi: 10.2741/E693
118. Taïbi F, Metzinger-Le Meuth V. M'Baya-Moutoula E. Possible involvement of microRNAs in vascular damage in experimental chronic kidney disease. Biochim Biophys Acta. (2014) 1842:88–98. doi: 10.1016/j.bbadis.2013.10.005
119. Wei Y, Wu Y, Zhao R. MSC-derived sEVs enhance patency and inhibit calcification of synthetic vascular grafts by immunomodulation in a rat model of hyperlipidemia. Biomaterials. (2019) 204:13–24. doi: 10.1016/j.biomaterials.2019.01.049
120. Chen Y, Huang C, Zhu SY, Zou HC, Xu CY, Chen YX. Overexpression of HOTAIR attenuates Pi-induced vascular calcification by inhibiting Wnt/β-catenin through regulating miR-126/Klotho/SIRT1 axis. Mol Cell Biochem. (2021) 476:3551–61. doi: 10.1007/s11010-021-04164-8
121. Wang L, Beecham A, Zhuo D, Dong C, Blanton SH, Rundek T, et al. Fine mapping study reveals novel candidate genes for carotid intima-media thickness in Dominican Republican families. Circ Cardiovasc Genet. (2012) 5:234–41. doi: 10.1161/CIRCGENETICS.111.961763
122. Yuan H, Zelkha S, Burkatovskaya M, Gupte R, Leeman SE, Amar S. Pivotal role of NOD2 in inflammatory processes affecting atherosclerosis and periodontal bone loss. Proc Natl Acad Sci U S A. (2013) 110:E5059–68. doi: 10.1073/pnas.1320862110
123. Vlacil A-K, Schuett J, Ruppert V, Soufi M, Oberoi R, Shahin K, et al. Deficiency of Nucleotide-binding oligomerization domain-containing proteins (NOD) 1 and 2 reduces atherosclerosis. Basic Res Cardiol. (2020) 115:47. doi: 10.1007/s00395-020-0806-2
124. Liu C, Zhang H, Chen Y, Wang S, Chen Z, Liu Z, et al. Identifying RBM47, HCK, CD53, TYROBP, and HAVCR2 as Hub genes in advanced atherosclerotic plaques by network-based analysis and validation. Front Genet. (2021) 11:602908. doi: 10.3389/fgene.2020.602908
125. Kim DK, Bandara G, Cho YE, et al. Mastocytosis-derived extracellular vesicles deliver miR-23a and miR-30a into pre-osteoblasts and prevent osteoblastogenesis and bone formation. Nat Commun. (2021) 12:2527. doi: 10.1038/s41467-021-22754-4
126. Liu J, Xiao X, Shen Y. MicroRNA-32 promotes calcification in vascular smooth muscle cells: Implications as a novel marker for coronary artery calcification. PLoS One. (2017) 12:e0174138. doi: 10.1371/journal.pone.0174138
127. Eguchi T, Watanabe K, Hara ES. OstemiR: a novel panel of microRNA biomarkers in osteoblastic and osteocytic differentiation from mesencymal stem cells. PLoS ONE. (2013) 8:e58796. doi: 10.1371/journal.pone.0058796
128. Vasuri F, Ciavarella C, Fittipaldi S, Pini R, Vacirca A, Gargiulo M, et al. Different histological types of active intraplaque calcification underlie alternative miRNA-mRNA axes in carotid atherosclerotic disease. Virchows Arch. (2020) 476:307–16. doi: 10.1007/s00428-019-02659-w
129. Takahashi K, Satoh M, Takahashi Y, Osaki T, Nasu T, Tamada M, et al. Dysregulation of ossification-related miRNAs in circulating osteogenic progenitor cells obtained from patients with aortic stenosis. Clin Sci. (2016) 130:1115–24. doi: 10.1042/CS20160094
130. Song R, Fullerton DA, Ao L, Zhao KS, Meng X. An epigenetic regulatory loop controls pro-osteogenic activation by TGF-β1 or bone morphogenetic protein 2 in human aortic valve interstitial cells. J Biol Chem. (2017) 292:8657–66. doi: 10.1074/jbc.M117.783308
131. Song R, Fullerton DA, Ao L, Zhao K-S, Reece TB, Jr JCC, et al. Altered microRNA expression is responsible for the pro-osteogenic phenotype of interstitial cells in calcified human aortic valves. J Am Heart Assoc. (2017) 6:e005364. doi: 10.1161/JAHA.116.005364
132. Bonetti J, Corti A, Lerouge L, Pompella A, Gaucher C. Phenotypic modulation of macrophages and vascular smooth muscle cells in atherosclerosis-nitro-redox interconnections. Antioxidants. (2021) 10:516. doi: 10.3390/antiox10040516
133. Bennett MR, Sinha S, Owens GK. Vascular smooth muscle cells in atherosclerosis. Nat Rev Cardiol. (2019) 16:727–44. doi: 10.1038/s41569-019-0227-9
134. Atkins SK, Sonawane AR, Brouwhuis R, Barrientos J, Ha A, Rogers M, et al. Induced pluripotent stem cell-derived smooth muscle cells to study cardiovascular calcification. Front Cardiovasc Med. (2022) 9:925777. doi: 10.3389/fcvm.2022.925777
135. Liu YX, Yuan PZ, Wu JH, Hu B. Lipid accumulation and novel insight into vascular smooth muscle cells in atherosclerosis. J Mol Med. (2021) 99:1511–26. doi: 10.1007/s00109-021-02109-8
136. Wang Q, Dong Y, Wang H. microRNA-19b-3p-containing extracellular vesicles derived from macrophages promote the development of atherosclerosis by targeting JAZF1. J Cell Mol Med. (2022) 26:48–59. doi: 10.1111/jcmm.16938
137. Zhu J, Liu B, Wang Z, Wang D, Ni H, Zhang L, et al. Exosomes from nicotine-stimulated macrophages accelerate atherosclerosis through miR-21-3p/PTEN-mediated VSMC migration and proliferation. Theranostics. (2019) 9:6901–19. doi: 10.7150/thno.37357
138. Liu Y, Zhang WL, Gu JJ. Exosome-mediated miR-106a-3p derived from ox-LDL exposed macrophages accelerated cell proliferation and repressed cell apoptosis of human vascular smooth muscle cells. Eur Rev Med Pharmacol Sci. (2020) 24:7039–50.
139. Ren L, Chen S, Yao D. OxLDL-stimulated macrophage exosomes promote proatherogenic vascular smooth muscle cell viability and invasion via delivering miR-186-5p then inactivating SHIP2 mediated PI3K/AKT/mTOR pathway. Mol Immunol. (2022) 146:27–37. doi: 10.1016/j.molimm.2022.02.018
140. de Gonzalo-Calvo D, Cenarro A, Civeira F. microRNA expression profile in human coronary smooth muscle cell-derived microparticles is a source of biomarkers. Clin Investig Arterioscler. (2016) 28:167–77. doi: 10.1016/j.arteri.2016.05.005
141. Boettger T, Beetz N, Kostin S, Schneider J, Krüger M, Hein L, et al. Acquisition of the contractile phenotype by murine arterial smooth muscle cells depends on the Mir143/145 gene cluster. J Clin Invest. (2009) 119:2634–47. doi: 10.1172/JCI38864
142. Chung DJ, Wu YL, Yang MY, Chan KC, Lee HJ, Wang CJ. Nelumbo nucifera leaf polyphenol extract and gallic acid inhibit TNF-α-induced vascular smooth muscle cell proliferation and migration involving the regulation of miR-21, miR-143 and miR-145. Food Funct. (2020) 11:8602–11. doi: 10.1039/D0FO02135K
143. Dégano IR, Camps-Vilaró A, Subirana I, García-Mateo N, Cidad P, Muñoz-Aguayo D, et al. Association of circulating microRNAs with coronary artery disease and usefulness for reclassification of healthy individuals: the REGICOR study. J Clin Med. (2020) 9:1402. doi: 10.3390/jcm9051402
144. González-López P, Ares-Carral C, López-Pastor AR, Infante-Menéndez J, Illaness TG, de Ceniga MV, et al. Implication of miR-155-5p and miR-143-3p in the vascular insulin resistance and instability of human and experimental atherosclerotic plaque. Int J Mol Sci. (2022) 23:10253. doi: 10.3390/ijms231810253
145. Dentelli P, Rosso A, Orso F. microRNA-222 controls neovascularization by regulating signal transducer and activator of transcription 5A expression. Arterioscler Thromb Vasc Biol. (2010) 30:1562–8. doi: 10.1161/ATVBAHA.110.206201
146. Bazan HA, Hatfield SA, O'Malley CB, Brooks AJ, Jr DL, Woods TC. Acute loss of miR-221 and miR-222 in the atherosclerotic plaque shoulder accompanies plaque rupture. Stroke. (2015) 46:3285–7. doi: 10.1161/STROKEAHA.115.010567
147. Zhang X, Shao S, Geng H, Yu Y, Wang C, Liu Z, et al. Expression profiles of six circulating microRNAs critical to atherosclerosis in patients with subclinical hypothyroidism: a clinical study. J Clin Endocrinol Metab. (2014) 99:E766–74. doi: 10.1210/jc.2013-1629
148. Leung A, Trac C, Jin W, Lanting L, Akbany A, Sætrom P, et al. Novel long noncoding RNAs are regulated by angiotensin II in vascular smooth muscle cells. Circ Res. (2013) 113:266–78. doi: 10.1161/CIRCRESAHA.112.300849
149. Taraldsen MD, Wiseth R, Videm V, Bye A, Madssen E. Associations between circulating microRNAs and coronary plaque characteristics: potential impact from physical exercise. Physiol Genomics. (2022) 54:129–40. doi: 10.1152/physiolgenomics.00071.2021
150. Zheng B, Yin WN, Suzuki T. Exosome-mediated miR-155 transfer from smooth muscle cells to endothelial cells induces endothelial injury and promotes atherosclerosis. Mol Ther. (2017) 25:1279–94. doi: 10.1016/j.ymthe.2017.03.031
151. Gomez I, Ward B, Souilhol C, Recarti C, Ariaans M, Johnston J, et al. Neutrophil microvesicles drive atherosclerosis by delivering miR-155 to atheroprone endothelium. Nat Commun. (2020) 11:214. doi: 10.1038/s41467-019-14043-y
152. Fitzsimons S, Oggero S, Bruen R. microRNA-155 is decreased during atherosclerosis regression and is increased in urinary extracellular vesicles during atherosclerosis progression. Front Immunol. (2020) 11:576516. doi: 10.3389/fimmu.2020.576516
153. Wang G, Chen JJ, Deng WY, Ren K, Yin SH, Yu XH. CTRP12 ameliorates atherosclerosis by promoting cholesterol efflux and inhibiting inflammatory response via the miR-155-5p/LXRα pathway. Cell Death Dis. (2021) 12:254. doi: 10.1038/s41419-021-03544-8
154. Nguyen DDN, Zain SM, Kamarulzaman MH, Low TY, Chilian WM, Pan Y, et al. Intracellular and exosomal microRNAome profiling of human vascular smooth muscle cells during replicative senescence. Am J Physiol Heart Circ Physiol. (2021) 321:H770–83. doi: 10.1152/ajpheart.00058.2021
155. Peng Q, Yin R, Zhu X. miR-155 activates the NLRP3 inflammasome by regulating the MEK/ERK/NF-κB pathway in carotid atherosclerotic plaques in ApoE-/- mice. J Physiol Biochem. (2022) 78:365–75. doi: 10.1007/s13105-022-00871-y
156. Tang Y, Song H, Shen Y. MiR-155 acts as an inhibitory factor in atherosclerosis-associated arterial pathogenesis by down-regulating NoxA1 related signaling pathway in ApoE-/- mouse. Cardiovasc Diagn Ther. (2021) 11:1–13. doi: 10.21037/cdt-20-518
157. Park M, Choi S, Kim S. NF-κB-responsive miR-155 induces functional impairment of vascular smooth muscle cells by downregulating soluble guanylyl cyclase. Exp Mol Med. (2019) 51:1–12. doi: 10.1038/s12276-019-0212-8
158. Ruan Z, Chu T, Wu L. miR-155 inhibits oxidized low-density lipoprotein-induced apoptosis in different cell models by targeting the p85α/AKT pathway. J Physiol Biochem. (2020) 76:329–43. doi: 10.1007/s13105-020-00738-0
159. de Gonzalo-Calvo D, Cenarro A, Garlaschelli K, Pellegatta F, Vilades D, Nasarre L, et al. Translating the microRNA signature of microvesicles derived from human coronary artery smooth muscle cells in patients with familial hypercholesterolemia and coronary artery disease. J Mol Cell Cardiol. (2017) 106:55–67. doi: 10.1016/j.yjmcc.2017.03.005
160. Zietzer A, Steffen E, Niepmann S. MicroRNA-mediated vascular intercellular communication is altered in chronic kidney disease. Cardiovasc Res. (2022) 118:316–33. doi: 10.1093/cvr/cvaa322
161. Fan A, Wang Q, Yuan Y. Liver X receptor-α and miR-130a-3p regulate expression of sphingosine 1-phosphate receptor 2 in human umbilical vein endothelial cells. Am J Physiol Cell Physiol. (2016) 310:C216–26. doi: 10.1152/ajpcell.00102.2015
162. Xu J, Gao F. Circulating miR-130a-3p is elevated in patients with cerebral atherosclerosis and predicts 2-year risk of cerebrovascular events. BMC Neurol. (2022) 22:308. doi: 10.1186/s12883-022-02829-5
163. Chen C, Li Y, Lu H, Liu K, Jiang W, Zhang Z, et al. Curcumin attenuates vascular calcification via the exosomal miR-92b-3p/KLF4 axis. Exp Biol Med. (2022) 247:1420–32. doi: 10.1177/15353702221095456
164. Wang J, Hu L, Huang H. CAR (CARSKNKDC) peptide modified rencell-derived extracellular vesicles as a novel therapeutic agent for targeted pulmonary hypertension therapy. Hypertension. (2020) 76:1147–60. doi: 10.1161/HYPERTENSIONAHA.120.15554
165. Xu F, Zhong JY, Lin X, Shan SK, Guo B, Zheng MH, et al. Melatonin alleviates vascular calcification and ageing through exosomal miR-204/miR-211 cluster in a paracrine manner. J Pineal Res. (2020) 68:e12631. doi: 10.1111/jpi.12631
166. Ding YD, Pei YQ. Rui-Wang. Association of plasma MiRNA-204 and the presence and severity of coronary artery calcification in patients with type 2 diabetes. Angiology. (2021) 72:451–8. doi: 10.1177/0003319720984592
167. Wang R, Ding YD, Gao W, Pei YQ, Yang JX, Zhao YX, et al. Serum microRNA-204 levels are associated with long-term cardiovascular disease risk based on the Framingham risk score in patients with type 2 diabetes: results from an observational study. J Geriatr Cardiol. (2020) 17:330–7. doi: 10.21203/rs.3.rs-24135/v1
168. Song R, Zhai Y, Ao L. MicroRNA-204 deficiency in human aortic valves elevates valvular osteogenic activity. Int J Mol Sci. (2019) 21:76. doi: 10.3390/ijms21010076
169. Toshima T, Watanabe T, Narumi T, Otaki Y, Shishido T, Aono T, et al. Therapeutic inhibition of microRNA-34a ameliorates aortic valve calcification via modulation of Notch1-Runx2 signalling. Cardiovasc Res. (2020) 116:983–94. doi: 10.1093/cvr/cvz210
170. Yu C, Li L, Xie F, Guo S, Liu F, Dong N, et al. LncRNA TUG1 sponges miR-204-5p to promote osteoblast differentiation through upregulating Runx2 in aortic valve calcification. Cardiovasc Res. (2018) 114:168–79. doi: 10.1093/cvr/cvx180
171. Wang Y, Han D, Zhou T, Zhang J, Liu C, Cao F, et al. Melatonin ameliorates aortic valve calcification via the regulation of circular RNA CircRIC3/miR-204-5p/DPP4 signaling in valvular interstitial cells. J Pineal Res. (2020) 69:e12666. doi: 10.1111/jpi.12666
172. Lin X, Xu F, Cui RR, Xiong D, Zhong JY, Zhu T, et al. Arterial calcification is regulated via an miR-204/DNMT3a regulatory circuit both in vitro and in female mice. Endocrinology. (2018) 159:2905–16. doi: 10.1210/en.2018-00320
173. Cui RR Li SJ, Liu LJ. MicroRNA-204 regulates vascular smooth muscle cell calcification in vitro and in vivo. Cardiovasc Res. (2012) 96:320–9. doi: 10.1093/cvr/cvs258
174. Pan W, Liang J, Tang H, Fang X, Wang F, Ding Y, et al. Differentially expressed microRNA profiles in exosomes from vascular smooth muscle cells associated with coronary artery calcification. Int J Biochem Cell Biol. (2020) 118:105645. doi: 10.1016/j.biocel.2019.105645
175. Chaturvedi P, Chen NX, O'Neill K, McClintick JN, Moe SM, Janga SC. Differential miRNA expression in cells and matrix vesicles in vascular smooth muscle cells from rats with kidney disease. PLoS One. (2015) 10:e0131589. doi: 10.1371/journal.pone.0131589
176. Rinn JL, Chang HY. Genome regulation by long noncoding RNAs. Annu Rev Biochem. (2012) 81:145–66. doi: 10.1146/annurev-biochem-051410-092902
177. Derrien T, Johnson R, Bussotti G, Tanzer A, Djebali S, Tilgner H, et al. The GENCODE v7 catalog of human long noncoding RNAs: analysis of their gene structure, evolution, and expression. Genome Res. (2012) 22:1775–89. doi: 10.1101/gr.132159.111
178. St Laurent G, Wahlestedt C, Kapranov P. The Landscape of long noncoding RNA classification. Trends Genet. (2015) 31:239–51. doi: 10.1016/j.tig.2015.03.007
179. Iyer MK, Niknafs YS, Malik R, Singhal U, Sahu A, Hosono Y, et al. The landscape of long noncoding RNAs in the human transcriptome. Nat Genet. (2015) 47:199–208. doi: 10.1038/ng.3192
180. Statello L, Guo CJ, Chen LL, Huarte M. Gene regulation by long non-coding RNAs and its biological functions. Nat Rev Mol Cell Biol. (2021) 22:96–118. doi: 10.1038/s41580-020-00315-9
181. Winkle M, El-Daly SM, Fabbri M, Calin GA. Noncoding RNA therapeutics - challenges and potential solutions. Nat Rev Drug Discov. (2021) 20:629–51. doi: 10.1038/s41573-021-00219-z
182. O'Brien K, Breyne K, Ughetto S, Laurent LC, Breakefield XO. RNA delivery by extracellular vesicles in mammalian cells and its applications. Nat Rev Mol Cell Biol. (2020) 21:585–606. doi: 10.1038/s41580-020-0251-y
183. Shan K, Jiang Q, Wang XQ, Wang YNZ, Yang H, Yao MD, et al. Role of long non-coding RNA-RNCR3 in atherosclerosis-related vascular dysfunction. Cell Death Dis. (2016) 7:e2248. doi: 10.1038/cddis.2016.145
184. Fasolo F, Jin H, Winski G, Chernogubova E, Pauli J, Winter H, et al. Long non-coding RNA MIAT controls advanced atherosclerotic lesion formation and plaque destabilization. Circulation. (2021) 144:1567–83. doi: 10.1161/CIRCULATIONAHA.120.052023
185. Xu H, Ni YQ, Liu YS. Mechanisms of action of MiRNAs and LncRNAs in extracellular vesicle in atherosclerosis. Front Cardiovasc Med. (2021) 8:733985. doi: 10.3389/fcvm.2021.733985
186. Zhang N, Luo Y, Zhang H, Zhang F, Gao X, Shao J. Exosomes Derived from mesenchymal stem cells ameliorate the progression of atherosclerosis in ApoE-/- Mice via FENDRR. Cardiovasc Toxicol. (2022) 22:528–44. doi: 10.1007/s12012-022-09736-8
187. Sun L, He X, Zhang T. Knockdown of mesenchymal stem cell-derived exosomal LOC100129516 suppresses the symptoms of atherosclerosis via upregulation of the PPARγ/LXRα/ABCA1 signaling pathway. Int J Mol Med. (2021) 48:208. doi: 10.3892/ijmm.2021.5041
188. Chen L, Yang W, Guo Y, Chen W, Zheng P, Zeng J, et al. Exosomal lncRNA GAS5 regulates the apoptosis of macrophages and vascular endothelial cells in atherosclerosis. PLoS ONE. (2017) 12:e0185406. doi: 10.1371/journal.pone.0185406
189. Liang W, Fan T, Liu L, Zhang L. Knockdown of growth-arrest specific transcript 5 restores oxidized low-density lipoprotein-induced impaired autophagy flux via upregulating miR-26a in human endothelial cells. Eur J Pharmacol. (2019) 843:154–61. doi: 10.1016/j.ejphar.2018.11.005
190. Hu N, Zeng X, Tang F, Xiong S. Exosomal long non-coding RNA LIPCAR derived from oxLDL-treated THP-1 cells regulates the proliferation of human umbilical vein endothelial cells and human vascular smooth muscle cells. Biochem Biophys Res Commun. (2021) 575:65–72. doi: 10.1016/j.bbrc.2021.08.053
191. Huang C, Han J, Wu Y, Li S, Wang Q, Lin W, et al. Exosomal MALAT1 derived from oxidized low-density lipoprotein-treated endothelial cells promotes M2 macrophage polarization. Mol Med Rep. (2018) 18:509–15. doi: 10.3892/mmr.2018.8982
192. Gao H, Wang X, Lin C, An Z, Yu J, Cao H, et al. Exosomal MALAT1 derived from ox-LDL-treated endothelial cells induce neutrophil extracellular traps to aggravate atherosclerosis. Biol Chem. (2020) 401:367–76. doi: 10.1515/hsz-2019-0219
193. Chen D, Wang K, Zheng Y, et al. Exosomes-Mediated LncRNA ZEB1-AS1 facilitates cell injuries by miR-590-5p/ETS1 axis through the TGF-β/Smad pathway in oxidized low-density lipoprotein-induced human umbilical vein endothelial cells. J Cardiovasc Pharmacol. (2021) 77:480–90. doi: 10.1097/FJC.0000000000000974
194. Zhang Z, Yi D, Zhou J, Zheng Y, Gao Z, Hu X, et al. Exosomal LINC01005 derived from oxidized low-density lipoprotein-treated endothelial cells regulates vascular smooth muscle cell phenotypic switch. Biofactors. (2020) 46:743–53. doi: 10.1002/biof.1665
195. Fu X, Liu H, Fan Y, Yuan J. Extracellular vesicles-mediated transfer of lncRNA CLDN10-AS1 aggravates low-density lipoprotein-induced vascular endothelial injury. Physiol Genomics. (2022).
196. Kim YK, Kook H. Diverse roles of noncoding RNAs in vascular calcification. Arch Pharm Res. (2019) 42:244–51. doi: 10.1007/s12272-019-01118-z
197. Demer LL, Tintut Y. Interactive and multifactorial mechanisms of calcific vascular and valvular disease. Trends Endocrinol Metab. (2019) 30:646–57. doi: 10.1016/j.tem.2019.06.001
198. Ryu J, Ahn Y, Kook H, Kim YK. The roles of non-coding RNAs in vascular calcification and opportunities as therapeutic targets. Pharmacol Ther. (2021) 218:107675. doi: 10.1016/j.pharmthera.2020.107675
199. Bao S, Guo Y, Diao Z. Genome-wide identification of lncRNAs and mRNAs differentially expressed in human vascular smooth muscle cells stimulated by high phosphorus. Ren Fail. (2020) 42:437–46. doi: 10.1080/0886022X.2020.1758722
200. Jeong G, Kwon D-H, Shin S, Choe N, Ryu J, Lim Y-H, et al. Long noncoding RNAs in vascular smooth muscle cells regulate vascular calcification. Sci Rep. (2019) 9:5848. doi: 10.1038/s41598-019-42283-x
201. Chang Z, Yan G, Zheng J, Liu Z. The lncRNA GAS5 inhibits the osteogenic differentiation and calcification of human vascular smooth muscle cells. Calcif Tissue Int. (2020) 107:86–95. doi: 10.1007/s00223-020-00696-1
202. Li S, Ni Y, Li C, Xiang Q, Zhao Y, Xu H, et al. Long noncoding RNA SNHG1 alleviates high glucose-induced vascular smooth muscle cells calcification/senescence by post-transcriptionally regulating Bhlhe40 and autophagy via Atg10. J Physiol Biochem. (2022) 3:25.
203. Li Y, Xi Z, Yu Z, et al. LincRNA-EPS increases TGF-β expression to inhibit the Wnt/β-catenin pathway, VSMC osteoblastic differentiation and vascular calcification in diabetic mice. Exp Ther Med. (2022) 23:425. doi: 10.3892/etm.2022.11352
204. Ma X, Wang Y, Liu Q, Han B, Wang G, Zhang R, et al. Vaspin alleviates the lncRNA LEF1-AS1-induced osteogenic differentiation of vascular smooth muscle cells via the Hippo/YAP signaling pathway. Exp Cell Res. (2022) 421:113407. doi: 10.1016/j.yexcr.2022.113407
205. Lin X, Zhan JK, Zhong JY. lncRNA-ES3/miR-34c-5p/BMF axis is involved in regulating high-glucose-induced calcification/senescence of VSMCs. Aging. (2019) 11:523–35. doi: 10.18632/aging.101758
206. Zhong JY, Cui XJ, Zhan JK. LncRNA-ES3 inhibition by Bhlhe40 is involved in high glucose-induced calcification/senescence of vascular smooth muscle cells. Ann N Y Acad Sci. (2020) 1474:61–72. doi: 10.1111/nyas.14381
207. Zhou W, Feng Q, Cheng M, Zhang D, Jin J, Zhang S, et al. LncRNA H19 sponges miR-103-3p to promote the high phosphorus-induced osteoblast phenotypic transition of vascular smooth muscle cells by upregulating Runx2 cell. Signal. (2022) 91:110220. doi: 10.1016/j.cellsig.2021.110220
208. Liu F, Yang XC, Chen ML, Zhuang Z-W, Jiang Y, Wang J, et al. LncRNA H19/Runx2 axis promotes VSMCs transition via MAPK pathway. Am J Transl Res. (2020) 12:1338–47.
209. Dai X, Liu S, Cheng L, Huang T, Guo H, Wang D, et al. Epigenetic upregulation of H19 and AMPK inhibition concurrently contribute to S-adenosylhomocysteine hydrolase deficiency-promoted atherosclerotic calcification. Circ Res. (2022) 130:1565–82. doi: 10.1161/CIRCRESAHA.121.320251
210. Liu Q, Qi H, Yao L. A long non-coding RNA H19/microRNA-138/TLR3 network is involved in high phosphorus-mediated vascular calcification and chronic kidney disease. Cell Cycle. (2022) 21:1667–83. doi: 10.1080/15384101.2022.2064957
211. Song Z, Wei D, Chen Y, Chen L, Bian Y, Shen Y, et al. Association of astragaloside IV-inhibited autophagy and mineralization in vascular smooth muscle cells with lncRNA H19 and DUSP5-mediated ERK signaling. Toxicol Appl Pharmacol. (2019) 364:45–54. doi: 10.1016/j.taap.2018.12.002
212. Uszczynska-Ratajczak B, Lagarde J, Frankish A. Towards a complete map of the human long non-coding RNA transcriptome. Nat Rev Genet. (2018) 19:535–48. doi: 10.1038/s41576-018-0017-y
213. Kopp F, Mendell JT. Functional classification and experimental dissection of long non-coding RNAs. Cell. (2018) 172:393–407. doi: 10.1016/j.cell.2018.01.011
214. Misir S, Wu N, Yang BB. Specific expression and functions of circular RNAs. Cell Death Differ. (2022) 29:481–91. doi: 10.1038/s41418-022-00948-7
215. Memczak S, Jens M, Elefsinioti A, Torti F, Krueger J, Rybak A, et al. Circular RNAs are a large class of animal RNAs with regulatory potency. Nature. (2013) 495:333–8. doi: 10.1038/nature11928
216. Holdt LM, Kohlmaier A, Teupser D. Molecular roles and function of circular RNAs in eukaryotic cells. Cell Mol Life Sci. (2018) 75:1071–98. doi: 10.1007/s00018-017-2688-5
217. Hansen TB, Jensen TI, Clausen BH, Bramsen JB, Finsen B, Damgaard CK, et al. Natural RNA circles function as efficient microRNA sponges. Nature. (2013) 495:384–8. doi: 10.1038/nature11993
218. Jeck WR, Sharpless NE. Detecting and characterizing circular RNAs. Nat Biotechnol. (2014) 32:453–61. doi: 10.1038/nbt.2890
219. Peng K, Jiang P, Du Y, Zeng D, Zhao J, Li M, et al. Oxidized low-density lipoprotein accelerates the injury of endothelial cells via circ-USP36/miR-98-5p/VCAM1 axis. IUBMB Life. (2021) 73:177–87. doi: 10.1002/iub.2419
220. Ye B, Liang X, Zhao Y, Cai X, Wang Z, Lin S, et al. Hsa_circ_0007478 aggravates NLRP3 inflammasome activation and lipid metabolism imbalance in ox-LDL-stimulated macrophage via miR-765/EFNA3 axis. Chem Biol Interact. (2022) 368:110195. doi: 10.1016/j.cbi.2022.110195
221. Li Y, Wang B. Circular RNA circCHFR downregulation protects against oxidized low-density lipoprotein-induced endothelial injury via regulation of microRNA-15b-5p/growth arrest and DNA damage inducible gamma. Bioengineered. (2022) 13:4481–92. doi: 10.1080/21655979.2022.2032967
222. Li F, Chen Y, He Z, Wang C, Wang X, Pan G, et al. Hsa_circ_0001879 promotes the progression of atherosclerosis by regulating the proliferation and migration of oxidation of low density lipoprotein (ox-LDL)-induced vascular endothelial cells via the miR-6873-5p-HDAC9 axis. Bioengineered. (2021) 12:10420–9. doi: 10.1080/21655979.2021.1997224
223. Huang X, Zhao Y, Zhou H, Li Y. Circular RNAs in atherosclerosis. Clin Chim Acta. (2022) 531:71–80. doi: 10.1016/j.cca.2022.03.016
224. Wen C, Li B, Nie L, Mao L, Xia Y. Emerging Roles of extracellular vesicle-delivered circular RNAs in atherosclerosis. Front Cell Dev Biol. (2022) 10:804247. doi: 10.3389/fcell.2022.804247
225. Xiong F, Mao R, Zhang L, Zhao R, Tan K, Liu C, et al. CircNPHP4 in monocyte-derived small extracellular vesicles controls heterogeneous adhesion in coronary heart atherosclerotic disease. Cell Death Dis. (2021) 12:948. doi: 10.1038/s41419-021-04253-y
226. Vilades D, Martínez-Camblor P, Ferrero-Gregori A, Bär C, Lu D, Xiao K, et al. Plasma circular RNA hsa_circ_0001445 and coronary artery disease: performance as a biomarker. FASEB J. (2020) 34:4403–14. doi: 10.1096/fj.201902507R
227. Gu Y, Ma X, Li J, Ma Y, Zhang Y. Identification of candidate targets for the diagnosis and treatment of atherosclerosis by bioinformatics analysis. Am J Transl Res. (2021) 13:4137–51.
228. Wen Y, Chun Y, Lian ZQ, et al. circRNA-0006896-miR1264-DNMT1 axis plays an important role in carotid plaque destabilization by regulating the behavior of endothelial cells in atherosclerosis. Mol Med Rep. (2021) 23:311. doi: 10.3892/mmr.2021.11950
229. Xiao Q, Hou R, Li H, Zhang S, Zhang F, Zhu X, et al. Circulating exosomal circRNAs contribute to potential diagnostic value of large artery atherosclerotic stroke. Front Immunol. (2022) 12:830018. doi: 10.3389/fimmu.2021.830018
230. Zhang M, Zhu Y, Zhu J, Xie Y, Wu R, Zhong J, et al. Circ_0086296 induced atherosclerotic lesions via the IFIT1/STAT1 feedback loop by sponging miR-576-3p. Cell Mol Biol Lett. (2022) 27:80. doi: 10.1186/s11658-022-00372-2
231. Ryu J, Kwon D-H, Choe N, Shin S, Jeong G, Lim Y-H, et al. Characterization of circular rnas in vascular smooth muscle cells with vascular calcification. Mol Ther Nucleic Acids. (2020) 19:31–41. doi: 10.1016/j.omtn.2019.11.001
232. Ryu J, Choe N, Kwon D-H, Shin S, Lim Y-H, Yoon G, et al. Circular RNA circSmoc1-2 regulates vascular calcification by acting as a miR-874-3p sponge in vascular smooth muscle cells. Mol Ther Nucleic Acids. (2021) 27:645–55. doi: 10.1016/j.omtn.2021.12.031
233. Zhou Y, Liu Y, Xuan S. CircSamd4: a novel biomarker for predicting vascular calcification. J Clin Lab Anal. (2022) 36:e24156. doi: 10.1002/jcla.24156
234. Ma C, Gu R, Wang X. circRNA CDR1as promotes pulmonary artery smooth muscle cell calcification by upregulating CAMK2D and CNN3 via sponging miR-7-5p. Mol Ther Nucleic Acids. (2020) 22:530–41. doi: 10.1016/j.omtn.2020.09.018
235. Shi L, Li Y, Shi M, Li X, Li G, Cen J, et al. Hsa_circRNA_0008028 deficiency ameliorates high glucose-induced proliferation, calcification, and autophagy of vascular smooth muscle cells via miR-182-5p/TRIB3 axis. Oxid Med Cell Longev. (2022) 2022:5142381. doi: 10.1155/2022/5142381
Keywords: atherosclerosis (AS), vascular calcification (VC), extracellular vesicles (EVs), non-coding RNAs (ncRNAs), uremia
Citation: Yu F, Duan Y, Liu C, Huang H, Xiao X and He Z (2023) Extracellular vesicles in atherosclerosis and vascular calcification: the versatile non-coding RNAs from endothelial cells and vascular smooth muscle cells. Front. Med. 10:1193660. doi: 10.3389/fmed.2023.1193660
Received: 25 March 2023; Accepted: 12 June 2023;
Published: 04 July 2023.
Edited by:
Shan Mou, Shanghai Jiao Tong University, ChinaReviewed by:
Bisheng Zhou, University of Illinois Chicago, United StatesWan Nazatul Shima Shahidan, Universiti Sains Malaysia Health Campus, Malaysia
Copyright © 2023 Yu, Duan, Liu, Huang, Xiao and He. This is an open-access article distributed under the terms of the Creative Commons Attribution License (CC BY). The use, distribution or reproduction in other forums is permitted, provided the original author(s) and the copyright owner(s) are credited and that the original publication in this journal is cited, in accordance with accepted academic practice. No use, distribution or reproduction is permitted which does not comply with these terms.
*Correspondence: Zhangxiu He, aGV6aGFuZ3hpdTIwMTUwNzI0QDEyNi5jb20=; Xiangcheng Xiao, eGlhb3hjQGNzdS5lZHUuY24=