- 1Institute of Medical Sciences, University of Aberdeen, Aberdeen, United Kingdom
- 2School of Microbiology, University College Cork, Cork, Ireland
- 3Departamento de Bioquímica, Microbiología, Biología Celular y Genética, Universidad de La Laguna, Tenerife, Spain
- 4Institute for Research in Biomedicine (IRB Barcelona), The Barcelona Institute of Science and Technology, Barcelona, Spain
- 5Centro de Investigaciones Biológicas Margarita Salas, Consejo Superior de Investigaciones Científicas, Madrid, Spain
Promoter recognition by RNA polymerase is a key step in the regulation of gene expression. The bacterial RNA polymerase core enzyme is a complex of five subunits that interacts transitory with one of a set of sigma factors forming the RNA polymerase holoenzyme. The sigma factor confers promoter specificity to the RNA polymerase. In the Gram-positive pathogenic bacterium Streptococcus pneumoniae, most promoters are likely recognized by SigA, a poorly studied housekeeping sigma factor. Here we present a sequence conservation analysis and show that SigA has similar protein architecture to Escherichia coli and Bacillus subtilis homologs, namely the poorly conserved N-terminal 100 residues and well-conserved rest of the protein (domains 2, 3, and 4). Further, we have purified the native (untagged) SigA protein encoded by the pneumococcal R6 strain and reconstituted an RNA polymerase holoenzyme composed of the E. coli core enzyme and the sigma factor SigA (RNAP-SigA). By in vitro transcription, we have found that RNAP-SigA was able to recognize particular promoters, not only from the pneumococcal chromosome but also from the S. agalactiae promiscuous antibiotic-resistance plasmid pMV158. Specifically, SigA was able to direct the RNA polymerase to transcribe genes involved in replication and conjugative mobilization of plasmid pMV158. Our results point to the versatility of SigA in promoter recognition and its contribution to the promiscuity of plasmid pMV158.
Introduction
Bacteria in their natural habitats are often subjected to rapid changes in the provision of nutrients (the so-called feast-famine situation). These environmental fluctuations may lead from moderate to drastic changes in the bacterial lifestyle, affecting many transcription regulatory pathways of the bacterial cells (Yokoyama et al., 2006). Transcription patterns can also be severely affected when bacteria colonize new niches where the availability of nutrients can be far different (Bravo et al., 2018). This is true in the case of the Gram-positive bacterium Streptococcus pneumoniae (the pneumococcus), which colonizes the human nasopharynx of about 70% of healthy individuals (Gamez and Hammerschmidt, 2012). However, under hospital conditions or in immunocompromised individuals, pneumococci can invade, among other niches, the lower respiratory tract, thus leading to pneumococcal pneumonia. This deadly infection causes up to 1.5 million deaths per year (Dockrell et al., 2012). Pneumococcal pneumonia is the single largest infectious cause of death in children worldwide, being responsible for the death of nearly 1 million children under the age of five in 2017 (https://www.who.int/en/news-room/fact-sheets/detail/pneumonia). Also, pneumococcal pneumonia is a heavy economic burden on society (O’Brien et al., 2009). Changes in the pneumococcal transcriptome under various infection-relevant conditions have been reported, revealing profound changes in the relative amount of the RNAs synthesized by this microorganism (Aprianto et al., 2018).
Transcription of bacterial genes requires the assembly of five protein subunits (α2ββ´ω) that constitute the RNA polymerase core enzyme. Subsequently, the core enzyme associates temporarily with one of a set of sigma factors forming the RNA polymerase holoenzyme. The holoenzyme, but not the core enzyme, can recognize promoters and initiate transcription. Promoter recognition by RNA polymerase is a crucial step in the regulation of gene expression. The promoter specificity of the RNA polymerase depends on its sigma factor, which can contact several sequence motifs, including the −35 element, the extended −10 element, the −10 element and the discriminator region (Browning and Busby, 2016).
Nearly all bacteria have various sigma factors that direct the RNA polymerase to transcribe particular genes depending on the bacterial needs. Nevertheless, it is the housekeeping sigma factor the one responsible for recognizing the majority of promoters and, consequently, for cell proliferation and wellbeing. Most of the transcription processes taking place in exponentially growing bacteria are initiated by the RNA polymerase that contains the housekeeping sigma factor (Saecker et al., 2011). Leading investigations on Escherichia coli identified the consensus sequences of the −35 (5′-TTGACA-3′) and −10 (5′-TATAAT-3′) elements recognized by its housekeeping sigma factor (σ70; also termed RpoD). The optimal spacer length between the two promoter elements was reported to be 17 nucleotides (nt). Compared to the housekeeping sigma factor, alternative sigma factors usually recognize a smaller set of promoters, have more stringent sequence specificities, and associate with fewer transcription factors (Browning and Busby, 2016). Moreover, numerous alternative sigma factors play key roles when bacteria enter into the stationary phase and/or they are confronted with stressful environmental conditions (Saecker et al., 2011). The housekeeping sigma factors have four main regions: 1.1, 2 (consisting of 1.2, 2.1–2.4), 3 (3.0–3.2) and 4 (4.1–4.2) that group into four structured helical domains (1.1, 2, 3, and 4). The poorly conserved domain 1.1 is found only in Group 1 of housekeeping sigma factors and is involved in restraining the sigma factor apo-form from DNA binding, thereby inhibiting its non-productive interaction with promoter DNA in the absence of the RNA polymerase core complex. In the RNA polymerase holoenzyme, the negatively charged domain 1.1 (a mimicry of DNA negative charge) needs to be displaced from its initial position for the DNA-RNA polymerase complex to become transcriptionally competent. The displacement process takes place more easily at some promoters while behaving more inhibitory or difficult to move out at others, therefore it assists in the promoter selection process (Paget, 2015; Fang et al., 2020; Shin et al., 2021).
In S. pneumoniae, most housekeeping genes are transcribed by RNA polymerase complexes that contain the sigma factor SigA, also known as RpoD and σ43. However, it is the alternative sigma factor SigX the best characterized so far (Luo et al., 2003; Inniss et al., 2019). SigA (369 amino acids) belongs to the family of σ70 factors, despite its small size, and is thought to be responsible for the transcription of most pneumococcal genes. SigX, the only known alternative sigma factor in pneumococcus (Inniss and Morrison, 2020), is involved in the transcription of a set of genes that participate in the development of genetic competence for transformation and is encoded by the comX gene (Tovpeko and Morrison, 2014). The pneumococcal promoters recognized by SigA share many features with those recognized by the σ70 factor of E. coli in terms of the consensus sequences defined for the −35 and −10 regions (Puyet and Espinosa, 1993; Sabelnikov et al., 1995; Ruiz-Cruz et al., 2010).
Although bioinformatics can predict promoter sequences in genomic DNAs, their definitive identification requires the use of different experimental approaches, both in vivo and in vitro (Ross and Gourse, 2009). This is particularly necessary when dealing with genomic DNAs that have a high A + T content, as it is the case of the pneumococcal R6 chromosome (60% A + T) (Hoskins et al., 2001) and the S. agalactiae plasmid pMV158 (63% A + T), which can replicate in a broad variety of bacterial species, including S pneumoniae and E. coli (Lacks et al., 1986; del Solar et al., 1987). In such DNAs, stretches resembling the consensus −10 element (5′-TATAAT-3′) of the promoters recognized by housekeeping sigma factors are frequent.
Bacterial RNA polymerase holoenzymes share a conserved architecture of the core enzyme (Griesenbeck et al., 2017). In the present work, we report the purification of the native (untagged version) pneumococcal SigA protein encoded by the strain R6, the reconstitution of RNA polymerase complexes constituted by the E. coli RNA polymerase core enzyme and the pneumococcal SigA factor (RNAP-SigA), and the ability of the RNAP-SigA complexes to recognize particular streptococcal promoters and initiate transcription. Specifically, we used DNA templates that contain promoters identified previously by in vivo approaches: i) the promoter region of the pneumococcal mgaSpn gene, which encodes a transcriptional regulator, and ii) the promoter regions of the copG (replication control) and mobM (conjugative mobilization) genes from the streptococcal plasmid pMV158. Our results support the contribution of SigA to the promiscuous behaviour of pMV158.
Materials and Methods
Bacterial Strains, Plasmids, and Oligonucleotides
E. coli BL21 (DE3) (a gift of F. W. Studier) was used for the overproduction of SigA. The strain is based on the DE3 lambda lysogen developed in Studier’s laboratory (Studier and Moffatt, 1986; Studier et al., 1990) in which the gene that encodes the RNA polymerase of bacteriophage T7 is under the control of the lacUV5 promoter, inducible by isopropyl β-D-1-thiogalactopyranoside (IPTG). This bacterial strain is also deficient in the Lon protease. The pneumococcal sigA gene was cloned into the E. coli expression vector pET24b (Novagen), under the control of the ϕ10-promoter of phage T7, generating its derivative pET24b-sigA (see below). Both plasmid pET24b and the BL21 (DE3) chromosome carry the lacI repressor gene to ensure tight repression of the lacUV5 promoter. For small-scale preparations of plasmid DNA, a High Pure Plasmid Isolation Kit (Roche Applied Science) was used. Chromosomal DNA was isolated from S. pneumoniae R6 (Hoskins et al., 2001) as previously described (Lacks, 1966). Plasmid pMV158 (Lacks et al., 1986; Priebe and Lacks, 1989) was purified from S. pneumoniae 708 (trt-1 hex-4 end-1 exo-2 malM594) (Lacks and Greenberg, 1977) by two consecutive CsCl gradients as previously described (del Solar et al., 1987). The oligonucleotides used in this work are listed in Table 1.
Growth and Transformation of Bacteria
E. coli BL21 (DE3) cells harbouring a pET24b derivative were grown in tryptone-yeast extract (TY) medium (Pronadisa) supplemented with kanamycin (Km; 30 μg/ml), at 37°C under aerobic conditions. Bacterial growth was measured by determination of the optical density of the cultures at 600 nm (OD600). The protocol used to transform E. coli by electroporation has been described (Dower et al., 1988). S. pneumoniae cells were grown in AGCH medium (Lacks, 1968; Ruiz-Cruz et al., 2010) supplemented with 0.3% sucrose and 0.2% yeast extract, at 37°C in a static water bath. Bacterial growth was measured at OD650. For pneumococcal cells harbouring plasmid pMV158, the medium was supplemented with tetracycline (1 µg/ml).
Polymerase Chain Reaction
The Phusion High-Fidelity DNA polymerase (Thermo Scientific) and the Phusion HF buffer were used. Reaction mixtures (50 µl) contained 5–30 ng of template DNA, 20 pmol of each primer, 200 µM each dNTP, and one unit of DNA polymerase. PCR conditions have been described (Ruiz-Cruz et al., 2010). PCR products were purified with the QIAquick PCR purification kit (QIAGEN).
Construction of Plasmid pET24b-sigA
The complete genome sequence of S. pneumoniae R6 has been published (Hoskins et al., 2001) (NCBI RefSeq NC_003098.1). The sigA gene (locus_tag SPR_RS04905; coordinates 965,281–966,390) encodes the sigma factor SigA (also known as RpoD). To overproduce SigA, a 1,166-bp DNA region of the pneumococcal R6 chromosome, which contains the promoter-less sigA gene was amplified by PCR using the sigA-F and sigA-R oligonucleotides (Table 1). These primers have a single restriction site for NdeI and XhoI, respectively. The amplified product was digested with both enzymes, and the 1,142-bp restriction fragment was ligated to pET24b digested with the same enzymes. E. coli BL21 (DE3) transformants harbouring the recombinant plasmid (pET24b-sigA) were selected, and the inserted fragment and the regions of pET24b flanking the inserted fragment were sequenced. Dye-terminator sequencing was carried out at Secugen (CIB Margarita Salas, Madrid, Spain).
Overproduction and Purification of SigA
E. coli BL21 (DE3) cells carrying plasmid pET24b-sigA were grown at 37°C with rotary shaking in TY medium containing Km (30 µg/ml) to an OD600 of 0.45. Then, IPTG (1 mM) was added to induce the expression of the T7 RNA polymerase-encoding gene and, therefore, the expression of sigA. After 25 min, rifampicin (200 µg/ml) was added to inhibit specifically the activity of the E. coli RNA polymerase. After 60 min, cells were harvested by centrifugation (9,000 rpm in an SLA-3000 rotor for 20 min at 4°C) and washed twice with buffer A (50 mM Tris-HCl, pH 7.6, 5% glycerol, 1 mM DTT, 1 mM EDTA) containing 400 mM NaCl. The cell pellet was concentrated (40x) in buffer A containing 400 mM NaCl and a protease inhibitor cocktail (Roche). Cells were disrupted by two passages through a pre-chilled French pressure cell, and the whole-cell extract was centrifuged (10,000 rpm in an Eppendorf F-34-6-38 rotor for 40 min at 4°C). The cleared lysate was mixed with 0.2% polyethyleneimine (PEI), kept on ice for 30 min, and centrifuged (9,000 rpm in an Eppendorf F-34-6-38 rotor for 20 min at 4°C). Under these conditions, SigA was recovered in the PEI pellet, which was then washed with buffer A containing 100 mM NaCl. SigA was eluted from the PEI pellet with buffer A containing 700 mM NaCl. Proteins recovered in the supernatant were precipitated with 70% saturated ammonium sulphate. After centrifugation (9,000 rpm in an Eppendorf F-34-6-38 rotor for 20 min at 4°C), the precipitate was dissolved in buffer A containing 100 mM NaCl and dialyzed against the same buffer at 4°C. The protein preparation was applied to a heparin affinity column (Affi-gel BioRad Heparin Gel, Econo-column BioRad) equilibrated with buffer A containing 100 mM NaCl. About 10% of SigA was in the flow-through, and about 80% of SigA was recovered when the column was washed with buffer A containing 100 mM NaCl. The latter protein fraction was concentrated by filtering through a 10-kDa-cutoff membrane (Macrosep, Pall), and dialyzed against buffer A containing 100 mM NaCl. Then, gel filtration chromatography was carried out in an AKTA HPLC system (Amersham Biosciences) using a HiLoad Superdex 200 gel filtration column (Amersham Biosciences), equilibrated with buffer A containing 100 mM NaCl. Fractions were analysed by Coomassie-stained SDS polyacrylamide (10%) gels, pooled, concentrated as described above, and stored at −80°C.
DNA Regions Amplified by Polymerase Chain Reaction
The following DNA regions were amplified by PCR: a) a 224-bp region of the R6 chromosome (coordinates 1,598,452–1,598,229) using the 1622C and 1622D oligonucleotides, b) a 265-bp region of the R6 chromosome (coordinates 1,598,452–1,598,188) using the 1622C and 1622F oligonucleotides, c) a 246-bp region of the pMV158 plasmid (NCBI RefSeq NC_010096.1; coordinates 489–734) using the copG-F and copG-R oligonucleotides, d) a 288-bp region of the pMV158 plasmid (coordinates 3,461–3,748) using the mobM-F and mobM-R1 oligonucleotides, and e) a 309-bp region of the pMV158 plasmid (coordinates 3,461–3,769) using the mobM-F and mobM-R2 oligonucleotides. The oligonucleotides are listed in Table 1.
In vitro Transcription Assays
The interaction of purified RNA polymerase with a particular promoter is affected greatly by several parameters, including temperature (Ross and Gourse, 2009). Although the optimum conditions for RNA polymerase complex formation with a particular promoter must be determined empirically, typical multiple-round in vitro transcription reactions are incubated at 25, 30 or 37°C (Ross and Gourse, 2009). Linear DNA fragments amplified by PCR were used as templates for in vitro transcription assays under multiple-round conditions. The procedure involved three steps. First, the reaction mixture (41.5 µl) contained 0.5 µl DTT (100 mM), 0.5 µl BSA (10 mg/ml), 10 µl transcription buffer (200 mM Tris-HCl, pH 7.5, 750 mM KCl, 50 mM MgCl2, 0.05% Triton X-100), 1 µl (700 ng; 1 unit) E. coli RNA polymerase core (Epicentre), 0.5 µl (250 ng) SigA and 29 µl H2O. The reaction mixture was incubated at 30°C for 15 min (Reconstitution Step). Then, 2.5 µl of DNA template (200 nM) was added and the reaction mixture was incubated at 30°C for 15 min (Binding Step). Finally, 0.5 µl (20 units) SUPERase-In (RNase inhibitor, Ambion), 5 µl rNTPs (2.5 mM of each rNTP) and 0.5 µl (α-32P)-UTP (10 µCi/µl; 3,000 Ci/mmol; GE Healthcare) were added to the reaction mixture, which was then incubated at 30°C for 15 min (Transcription Step). As a control, transcription reactions using E. coli RNA polymerase holoenzyme (500 ng/µl; 1 unit/µl; Epicentre) (here named RNAP-σ70) were performed. Reactions were stopped with 50 µl of STOP buffer (2% SDS, 100 mM EDTA). Non-incorporated nucleotides were removed using MicroSpinTM G-25 columns (GE Healthcare). Then, RNA was ethanol precipitated using Pellet Paint Co-Precipitant (Millipore), a visible dye-labelled carrier. The pellet was dissolved in 10 µl of loading buffer (80% formamide, 10 mM EDTA, pH 8.0, 0.1% xylene cyanol, 0.1% bromophenol blue), heated at 95°C for 5 min, and subjected to electrophoresis in 8 M urea-6% polyacrylamide gels. To estimate the size of the runoff transcripts, dideoxy-mediated chain termination sequencing reactions of a particular DNA were run in the same gel. Labelled products were visualized using a Fujifilm Image Analyzer FLA-3000. Single-stranded DNA molecules have been previously used to estimate chain lengths of RNA molecules by comparing their electrophoretic mobilities on polyacrylamide gels that contain a high concentration of urea (8 M) (del Solar et al., 1990; del Solar and Espinosa, 1992).
Bioinformatics Analyses
The DNA sequence of gene sigA (rpoD) (1,110-nt) was extracted by selecting coordinates 965,281–966,390 from the S. pneumoniae R6 genome (NC_003098.1). A standard nucleotide Megablast (Altschul et al., 1990) was performed with the default parameters. Only representative strains of those Streptococcus species related to humans (pathogenic, commensal or probiotic) were selected from retrieved sequences. Sequences were then aligned in MEGA-X software (Kumar et al., 2018) using the ClustalW algorithm configured for the highest accuracy (with default settings). The phylogenetic tree was inferred by using the Maximum Likelihood method and the Tamura-Nei model (Tamura and Nei, 1993). The sequence of the SigA from S. pneumoniae R6 (identity entry: P0A4J0) was used for performing a search for homologous proteins in the Uniprot database (The UniProt Consortium, 2019). Proteins with similar lengths related to SigA from S. pneumoniae R6 were then aligned with Clustal Omega (Sievers and Higgins, 2014) as implemented in the UniprotKB tools, highlighting the sequence motifs and other regions of interest. The comparative analysis and three-dimensional modelling of the SigA were constructed with the SWISS-MODEL server (Waterhouse et al., 2018), by using the crystal structure of the E. coli holoenzyme as reference (https://swissmodel.expasy.org/templates/4lk1.1, chain F). The second-best scoring model, which used as the template Bacillus subtilis SigA structure from residue 100 to residue 371 (https://swissmodel.expasy.org/templates/7ckq.1, chain F), was also taken for the comparison to highlight structural conservation between SigA (RpoD) sigma factors core region (domains 2, 3, and 4). Additionally, a deep learning transform-restrained Rosetta (trRosetta) protein structure prediction server was used to validate the SWISS-MODEL results and compare the models obtained by both methods (Yang et al., 2020). trRosetta uses both homologous templates and de novo modelling through deep learning-based prediction to inter-residue orientations and distances coupled with Rosetta-based optimization method that combines the predicted restraints with components of the Rosetta energy minimization function. This approach let trRosetta outperform all previously described structure-prediction methods in the 13th Community-Wide Experiment on the Critical Assessment of Techniques for Protein Structure Prediction (CASP13)- and Continuous Automated Model Evaluation (CAMEO)-derived sets benchmark. The trRosetta-made model of SigA was built with restraints from both deep learning and homologous templates from E. coli (PDBs 6pst and 4yfk) and Mycobacterium tuberculosis (PDBs 6c05 and 5uh5).
Results and Discussion
The sigA Gene of S. pneumoniae R6
In the genome of S. pneumoniae strain R6 (NC_003098.1) (Hoskins et al., 2001), the ATG codon at coordinate 965,281 is the translation start site of the sigA gene. Translation from this ATG codon generates a protein of 369 residues (P0A4J0). Using the sigA gene sequence of S. pneumoniae R6 as query (SPR_RS04905), we performed a Megablast analysis and selected 36 representative Streptococcus strains (Supplementary Table S1). The Phylogenetic tree of the sigA gene from such representative strains (Figure 1) showed two main clades. The sigA gene of S. pneumoniae was included in a subclade and found to be closely related to its homologs in the genomes of S. mitis, S. gwangjuense and S. pseudopneumoniae (mean base substitutions per site of 0.064).
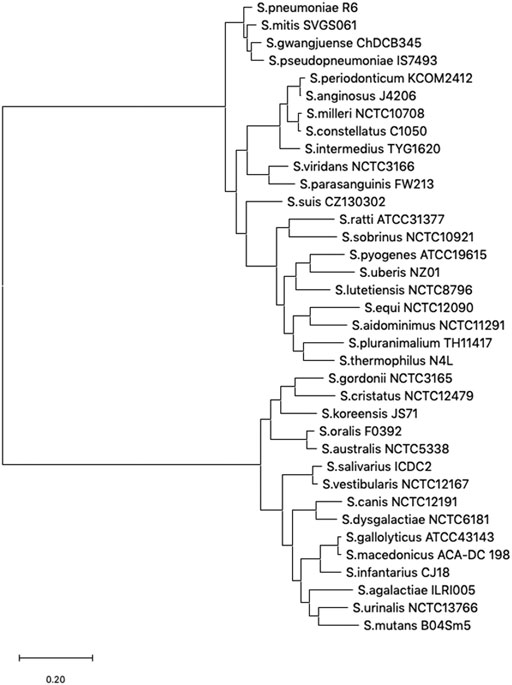
FIGURE 1. Phylogenetic tree of the sigA gene. Evolutionary analyses were conducted in MEGA X by comparing sigA gene sequences from the 36 representative Streptococcus species listed in Supplementary Table S1 (Supplementary Material). The tree with the highest log likelihood (−19,316.02) is shown. Initial tree(s) for the heuristic search were obtained automatically by applying Neighbor-Join and BioNJ algorithms to a matrix of pairwise distances estimated using the Tamura-Nei model, and then selecting the topology with a superior log-likelihood value. The tree is drawn to scale, with branch lengths measured in the number of substitutions per site.
We run BLAST to analyse the sequence conservation between the pneumococcal SigA factor and the housekeeping sigma factors σ70 from E. coli (613 residues) and SigA from Bacillus subtilis (371 residues). The analysis revealed that the essential core region (domains 2, 3, and 4) has a very high level of conservation between the pneumococcal SigA factor and the two other proteins, reaching 66% identity and 88% similarity for σ70 of E. coli and 80% identity and 92% similarity for SigA of B. subtilis (Supplementary Table S2 and Figure S1). We also used SWISS-MODEL and trRosetta (transform-restrained Rosetta) servers to obtain theoretical 3D models of the pneumococcal SigA factor (Waterhouse et al., 2018; Yang et al., 2020). The top model from SWISS-MODEL covered almost the entire sequence of the SigA protein, from residue D12 to residue E368 using the E. coli σ70 structure (PDB 4lk1) as the template (Figure 2A). As illustrated in Figure 2B, the main difference between both proteins is that the region from residue N128 to residue A372 of the σ70 factor is absent in SigA. Such a region is important for promoter escape and hinders early elongation pausing in σ70-like sigma factors (Shin et al., 2021). The second-best model from SWISS-MODEL used the B. subtilis SigA structure (PDB 7ckq), which due to flexibility of the domain 1.1 misses that region in the determined structure. Although 60% similarity (22% identity) for this N-terminal less conserved region (residues 1–96 of S. pneumoniae SigA) between the E. coli σ70 and the pneumococcal SigA sequences seems to provide sufficient similarity for homology modelling, we also used trRosetta server for complementary prediction, relying on its top scores at CASP13 experiment. The trRosetta model was obtained with restraints from both deep learning and homologous templates and supported the ones generated with SWISS-MODEL (superposition of models is shown in Supplementary Figure S2). The RMSD between both models equals 2.7 Å (Z score = 21.6) for the highly conserved core region (residues 97–369), and 4.0 Å (Z score = 4.3) for the N-terminal less conserved and mobile region, when comparing separated fragments at the DALI server (Holm, 2020). Submission of the complete models results in RMSD of 3.2 Å (Z score = 20.4) calculated over a region covering residues 80–369 of both models. Additionally, a superposition of SigA models on holoenzymes structures is shown in Supplementary Figure S3.
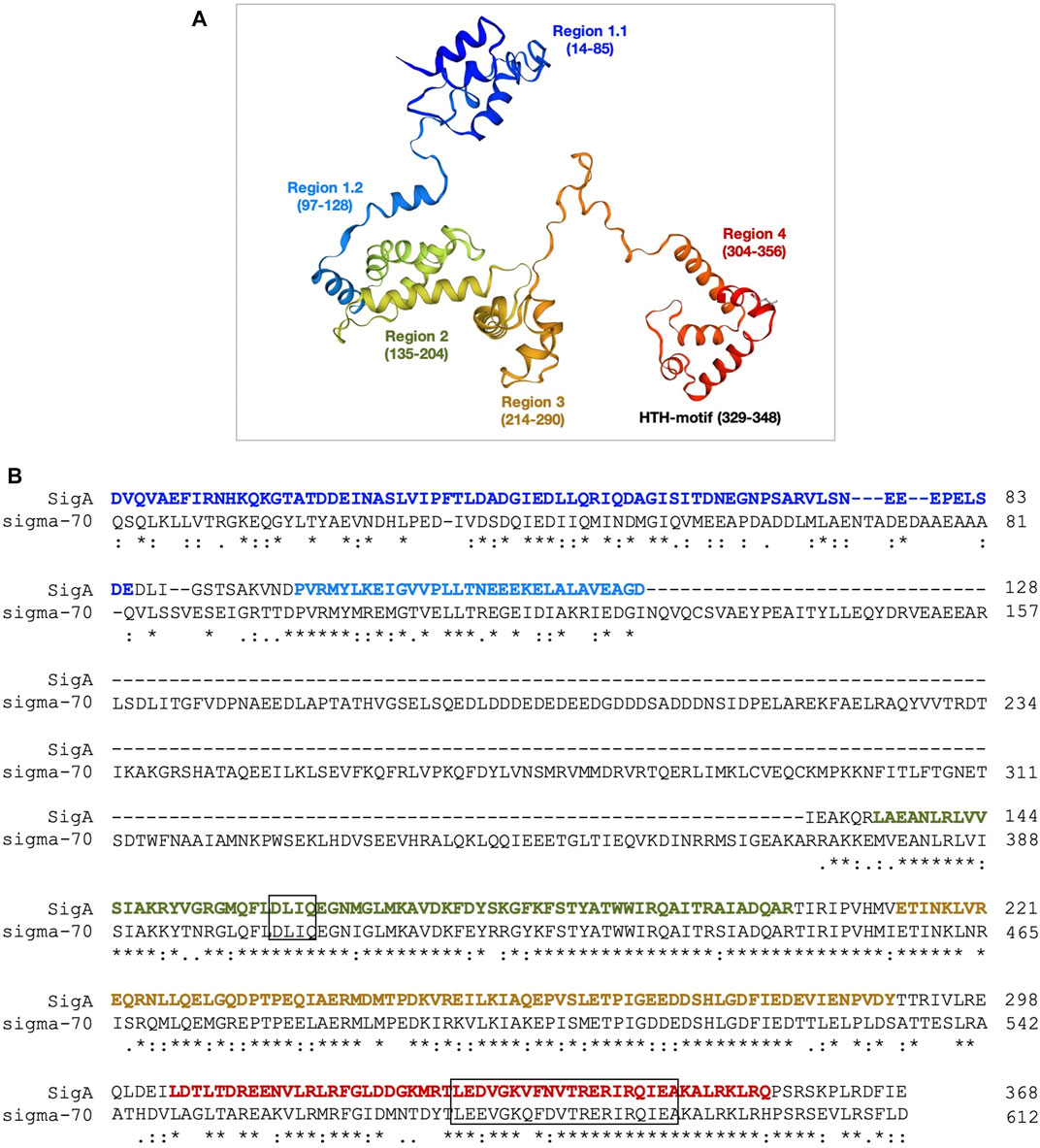
FIGURE 2. 3D structure model of the SigA protein. (A) The homology model of SigA (P0A4J0) from S. pneumoniae strain R6 was constructed in the SWISS-MODEL server using the structure of the E. coli holoenzyme (RNAP-σ70) as the template. The model includes residue D12 to residue E368. Different regions are indicated. (B) Sequence alignment of the SigA and E. coli σ70 proteins. The DLIQ and HTH motifs are highlighted in boxes. The sequence colours correspond to the colours assigned to the regions shown in panel A. The region N128-A372 of E. coli σ70 is not present in SigA. The asterisks (*), colons (:) and dots (.) indicate identical amino acid residues, conserved substitutions, and semi-conserved substitutions, respectively.
The 3D structure model of SigA let us visualize the protein functional regions assigned by sequence alignment at Pfam (Mistry et al., 2021) (Supplementary Figure S4, Figure 2): i) region 1.1 (residues 14–85) involved in the promoter selection; ii) region 2 (residues 135–204) that contains both the −10 promoter recognition helix and the primary core RNA polymerase binding determinant; iii) region 3 (residues 214–290) involved in binding to the core RNA polymerase; with a role in the recognition of some specific promoters containing an extended −10 element; and iv) region 4 (residues 304–356) involved in the binding to the −35 element. Out of the four regions, the most important domains are represented by region 2, in which the highly conserved DLIQ motif involved in the interaction with the β′ subunit (RpoC) is found; and region 4 containing a helix-turn-helix (HTH) motif that binds to the −35 element (Figure 2B). To study the degree of conservation of these motifs, 12 SigA proteins belonging to the σ70 factor SigA subfamily were selected from a total of 75 manually annotated (curated) protein sequences available in the Uniprot database. After performing a multiple sequence alignment, the results showed a high degree of diversity in region 1, whereas regions 2–4 were highly conserved among several Gram-positive bacteria, including B. subtilis, Enterococcus faecalis, Staphylococcus aureus, S. mutans, and S. pneumoniae (Supplementary Figure S5 and Table S3).
Purification of the Pneumococcal SigA Protein
To overproduce the pneumococcal SigA protein (untagged version), we used an inducible expression system based on the pET24b plasmid, which carries the ϕ10-promoter recognized by the bacteriophage T7 RNA polymerase, and the E. coli BL21 (DE3) strain, which carries the T7 RNA polymerase-encoding gene fused to the lacUV5 promoter (Studier and Moffatt, 1986) (see Materials and Methods). To purify SigA (369 amino acids), we set up a procedure that included the following steps: i) precipitation of nucleic acids with 0.2% PEI in the presence of 400 mM NaCl. SigA was recovered in the PEI pellet; ii) elution of SigA from the PEI pellet using a higher ionic strength buffer (700 mM NaCl); iii) after elution, proteins recovered in the supernatant (including SigA) were precipitated with 70% saturated ammonium sulphate; iv) chromatography on a heparin column equilibrated with a low ionic strength buffer (100 mM NaCl). About 80% of SigA was recovered when the column was washed with the same buffer, and v) chromatography on a gel filtration column. Both the equilibration buffer and the running buffer contained 100 mM NaCl. Purified SigA was analysed by SDS-polyacrylamide (10%) gel electrophoresis (Figure 3). The SigA preparation obtained after gel filtration chromatography was ∼95% pure. SigA (42.04 kDa, calculated from the predicted amino acid sequence) migrated slightly above the band corresponding to a 45 kDa standard protein.
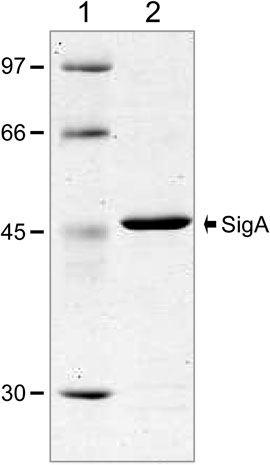
FIGURE 3. Purification of the pneumococcal SigA protein. Purified SigA was analysed by SDS-polyacrylamide (10%) gel electrophoresis. The gel was stained with Coomassie Blue. The molecular weight (in kDa) of proteins used as markers (GE Healthcare) is indicated on the left of the gel.
Recognition of the Pneumococcal Pmga Promoter by RNAP-SigA
The pneumococcal mgaSpn gene encodes a transcriptional regulator (MgaSpn) (Solano-Collado et al., 2012; Solano-Collado et al., 2013). In the pneumococcal R6 genome (NCBI RefSeq NC_003098.1) (Hoskins et al., 2001), the ATG codon at coordinate 1,598,270 is likely the translation initiation codon of mgaSpn, as it is preceded by a putative Shine-Dalgarno sequence (5′-AAAGAGAGAAAG-3′) (coordinates 1,598,288–1,598,277) (Figure 4A). Previous in vivo experiments showed that i) the mgaSpn gene is transcribed in exponentially growing pneumococcal cells, ii) the transcription start site (position +1) of mgaSpn is located at coordinate 1,598,309, and iii) the promoter of mgaSpn (known as Pmga) has the features of a promoter recognized by a housekeeping sigma factor: a consensus −10 element (5′-TATAAT-3′), a consensus −10 extension element (5′-TGTG-3′), and shows a 3/6 match at the −35 element (5′-aTGctA-3′) (Solano-Collado et al., 2012) (see Figure 4A).
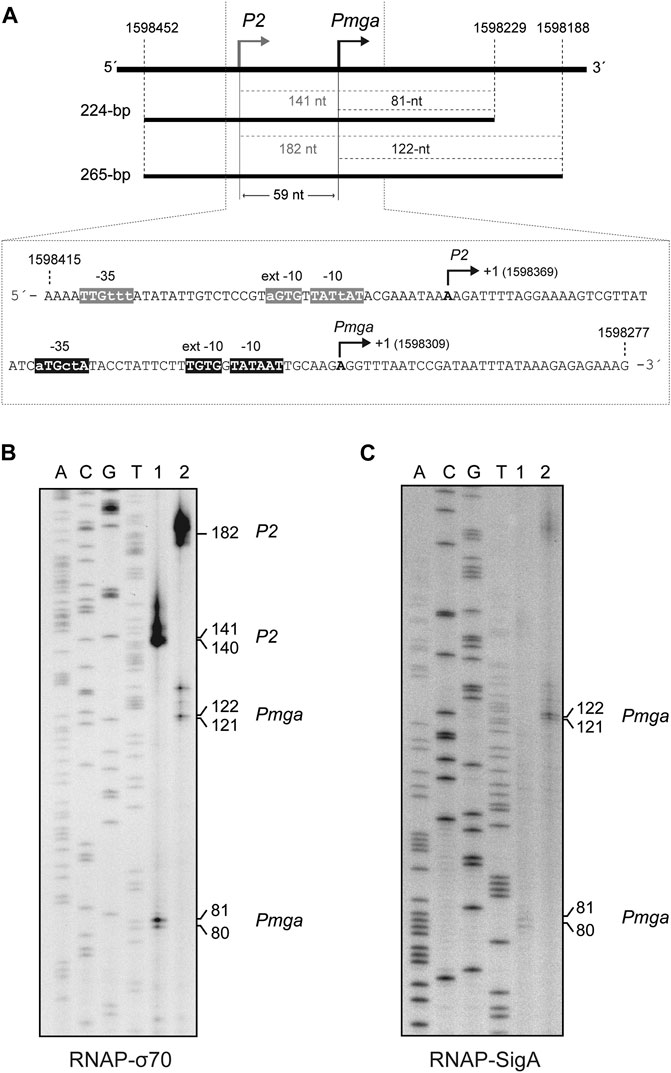
FIGURE 4. SigA recognizes the Pmga promoter. (A) The pneumococcal Pmga promoter. Upper part: Region of the pneumococcal R6 genome (Hoskins et al., 2001) that contains the P2 promoter (this work) and the Pmga promoter (Solano-Collado et al., 2012). The coordinates of the 224-bp and 265-bp DNA fragments are indicated. Both DNA fragments were used for in vitro transcription assays (see Figures 4B,C). The size (in nucleotides) of the expected run-off transcripts is indicated. Lower part: Nucleotide sequence of the region spanning coordinates 1,598,415 and 1,598,277. The main sequence elements of the P2 and Pmga promoters are indicated. Their transcription start site (position +1) is shown. (B)In vitro transcription experiments using RNAP-σ70. (C)In vitro transcription experiments using RNAP-SigA. In (B) and (C), two DNA fragments of 224-bp (lanes 1) and 265-bp (lanes 2) were used as DNA templates (see Figure 4A). Denaturing gels (8 M urea-6% polyacrylamide) were used for resolving transcripts. Dideoxy-mediated chain termination sequencing reactions were run in the same gel (lanes A, C, G, T). In (B), the sequencing reactions were prepared using a PCR-amplified fragment from the pneumococcal R6 genome (1,221-bp; coordinates 1,597,232–1,598,452) and the 5′-radiolabeled 1622D oligonucleotide (Table 1). Such a 1,221-bp DNA fragment was obtained by PCR using R6 chromosomal DNA as a template and the 1622A and 1622C oligonucleotides (Table 1). In (C), the sequencing reactions were prepared using a PCR-amplified fragment from the R6 genome (421-bp; coordinates 1,598,229–1,598,649) and the 5′-radiolabeled 1622C oligonucleotide (Table 1). Such a 421-bp DNA fragment was obtained by PCR using R6 chromosomal DNA as a template and the 1622D and 1622B oligonucleotides (Table 1). The size (in nucleotides) of the transcription products is indicated on the right of the gels.
By electrophoretic mobility shift assays (Supplementary Figure S6), we determined that the holoenzyme constituted by the E. coli RNA polymerase core and the pneumococcal SigA factor (RNAP-SigA) was able to bind to linear DNA. We used a radioactively labelled 222-bp DNA fragment (coordinates 1,598,519–1,598,298 of the R6 genome), which contains the P1623B and Pmga divergent promoters (Solano-Collado et al., 2013). To further analyse whether RNAP-SigA was able to recognize specifically the Pmga promoter, we performed in vitro transcription experiments under multiple-round conditions using two linear DNA fragments of 224-bp (coordinates 1,598,452–1,598,229) and 265-bp (coordinates 1,598,452–1,598,188) as DNA templates (Figure 4A). Such fragments contain the Pmga promoter but not the P1623B promoter (Solano-Collado et al., 2013). Transcription from the Pmga promoter was expected to generate run-off transcripts of 81-nt (224-bp template) and 122-nt (265-bp template), respectively. The transcription products were resolved on denaturing gels (6% PAA, 8M urea) (Figure 4B,C), and their sizes were estimated taking into account that an RNA molecule migrates slightly slower than a DNA molecule of the same size (Sambrook et al., 1989). Using RNAP-SigA, transcripts of the expected size were detected with both the 224-bp template and the 265-bp template (Figure 4C, lanes 1 and 2). However, in addition to such products, transcripts of 141-nt (224-bp template) and 182-nt (265-bp template) were detected using the E. coli RNA polymerase holoenzyme that contains the housekeeping σ70 factor (RNAP-σ70) (Figure 4B, lanes 1 and 2). This result indicated that RNAP-σ70 was able to recognize, in addition to the Pmga promoter, a sequence (here named P2) located upstream of Pmga and to initiate transcription at coordinate 1,598,369 (see Figure 4A). The P2 promoter sequence has a near-consensus −10 element (5′-TATtAT-3′), a near-consensus −10 extension element (5′-aGTG-3′), and a 3/6 match at the −35 element (5′-TTGttt-3′). The −10 and −35 regions are separated by 20-nt. Therefore, we conclude that the pneumococcal SigA factor recognizes mainly the Pmga promoter. Upstream of this promoter, there are sequence motifs that can be recognized by RNAP-σ70.
Recognition of the Pcr Promoter of pMV158 by RNAP-SigA
Plasmid pMV158 (5,540 bp) replicates by the rolling circle mechanism and was isolated from S. agalactiae. However, it replicates in many bacterial species, including S. pneumoniae and E. coli, although at different copy numbers, around 30 in the pneumococcus and seven in the Gram-negative host (Lacks et al., 1986; del Solar et al., 1997; Lorenzo-Díaz and Espinosa, 2009; Lorenzo-Díaz et al., 2017). The Pcr promoter directs the synthesis of a bicistronic mRNA, which encodes a transcriptional repressor involved in plasmid copy-number control, CopG, and a replication initiator protein, RepB. Promoter Pcr has been characterized by in vivo and in vitro assays. Specifically, using total RNA from S. pneumoniae cells harbouring a pMV158-derivative, the copG-repB transcription start site was identified at coordinate 633 of pMV158 (del Solar et al., 1990). This conclusion was further confirmed by in vitro transcription experiments using the E. coli RNA polymerase holoenzyme that contains σ70 (del Solar et al., 1990; del Solar and Espinosa, 1992). Compared to the consensus sequence of the promoters recognized by the E. coli σ70 factor, the Pcr promoter has a 4/6 match at the −10 element (5′-TActAT-3′), a consensus −10 extension element (5′-TATG-3′), and a 3/6 match at the −35 element (5′-TTGcat-3′) (see Figure 5A).
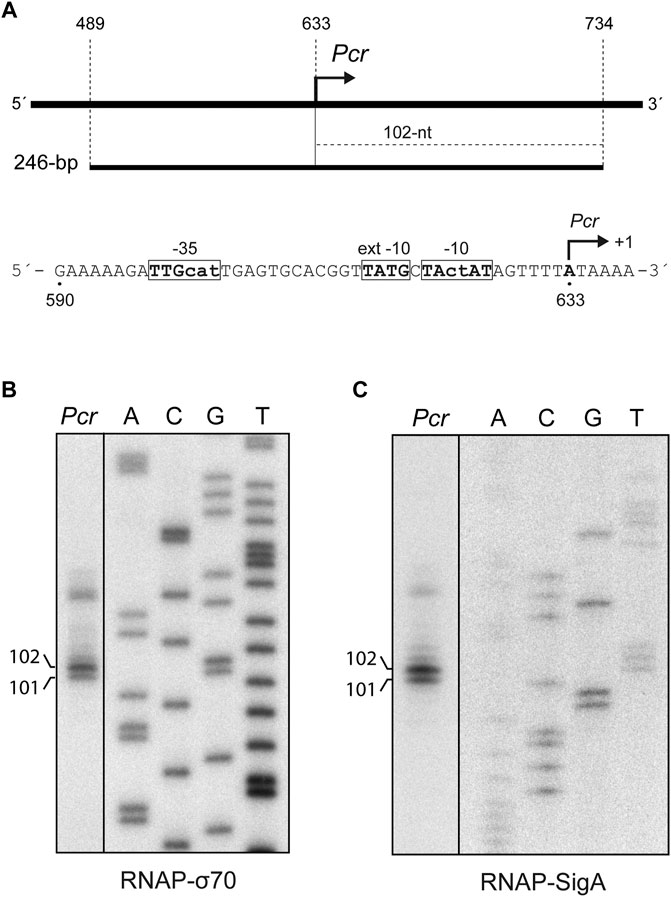
FIGURE 5. SigA recognizes the Pcr promoter. (A) Region of the pMV158 plasmid that contains the Pcr promoter (del Solar et al., 1990; del Solar and Espinosa, 1992). The coordinates of the 246-bp DNA fragment are indicated. This fragment was used for in vitro transcription assays (see Figures 5B,C). The size of the expected run-off transcript is indicated. The main sequence elements of the Pcr promoter, including the transcription start site (position +1), are shown. (B)In vitro transcription assays using RNAP-σ70. (C)In vitro transcription assays using RNAP-SigA. In (B) and (C), the 246-bp DNA fragment was used as the DNA template. Denaturing gels (8 M urea-6% polyacrylamide) were used for resolving transcripts. Dideoxy-mediated chain termination sequencing reactions were run in the same gel (lanes A, C, G, T). All the lanes displayed came from the same gel (delineation with dividing lines). Sequencing reactions were prepared (B) using M13mp18 DNA and the 5′-radiolabeled-40 M13 oligonucleotide (Table 1; Yanisch-Perron et al., 1985) or (C) using a Sau3AI DNA fragment from plasmid pKN1562 and the 5′-radiolabeled oligo-2 oligonucleotide (Table 1; Monti et al., 2007). The size (in nucleotides) of the transcription products is indicated on the left of the gels.
To know whether the reconstituted RNAP-SigA enzyme was able to initiate transcription from the Pcr promoter, we performed in vitro transcription assays. A 246-bp DNA fragment (coordinates 489–734 of plasmid pMV158) was used as the DNA template (Figure 5A). As shown in Figure 5C, two main RNAs of about 101-nt and 102-nt were synthesized. Both products were also detected in assays performed with RNAP-σ70 (Figure 5B). The synthesized RNAs have the size expected for run-off transcripts initiated at coordinates 633 and 634, respectively (see Figure 5A). Thus, we conclude that the pneumococcal SigA factor can recognize the Pcr promoter of pMV158.
Recognition of the Pmob1 and Pmob2 Promoters of pMV158 by RNAP-SigA
Although the streptococcal broad host range plasmid pMV158 is not conjugative, it can be mobilized among a wide number of bacterial species by the activity of the plasmid-encoded MobM protein, which is the prototype of the MOBV1 family of relaxases (Garcillán-Barcia et al., 2009; Fernández-López et al., 2014; Lorenzo-Díaz et al., 2014). Plasmids of the Inc18 family, such as pIP501 or pAMβ1, are considered auxiliary plasmids that provide the machinery (coupling protein and the T4 secretion system; reviewed by Grohmann et al., 2018) for the conjugative mobilization of pMV158 (Priebe and Lacks, 1989). The mobilization module of pMV158 includes the mobM gene and the origin of transfer, oriT. To initiate the conjugative mobilization, MobM binds to oriT on supercoiled plasmid DNA and performs a histidine-mediated nucleophilic attack at the phosphate group of the specific dinucleotide 5′-GpT-3´ (coordinates 3,595–3,596; nick site) (Guzmán and Espinosa, 1997; Pluta et al., 2017). By in vitro studies, the minimal oriT sequence was delimited to a stretch of 26 nucleotides (coordinates 3,570–3,595) that is located just upstream of the nick site (Lorenzo-Díaz et al., 2011) (see Figure 6A). In addition to its role as initiator of transfer, MobM self-regulates its synthesis at the transcriptional level (Lorenzo-Díaz et al., 2012) and participates in the regulation of the plasmid copy number (Lorenzo-Díaz et al., 2017), contributing to the promiscuity of pMV158.
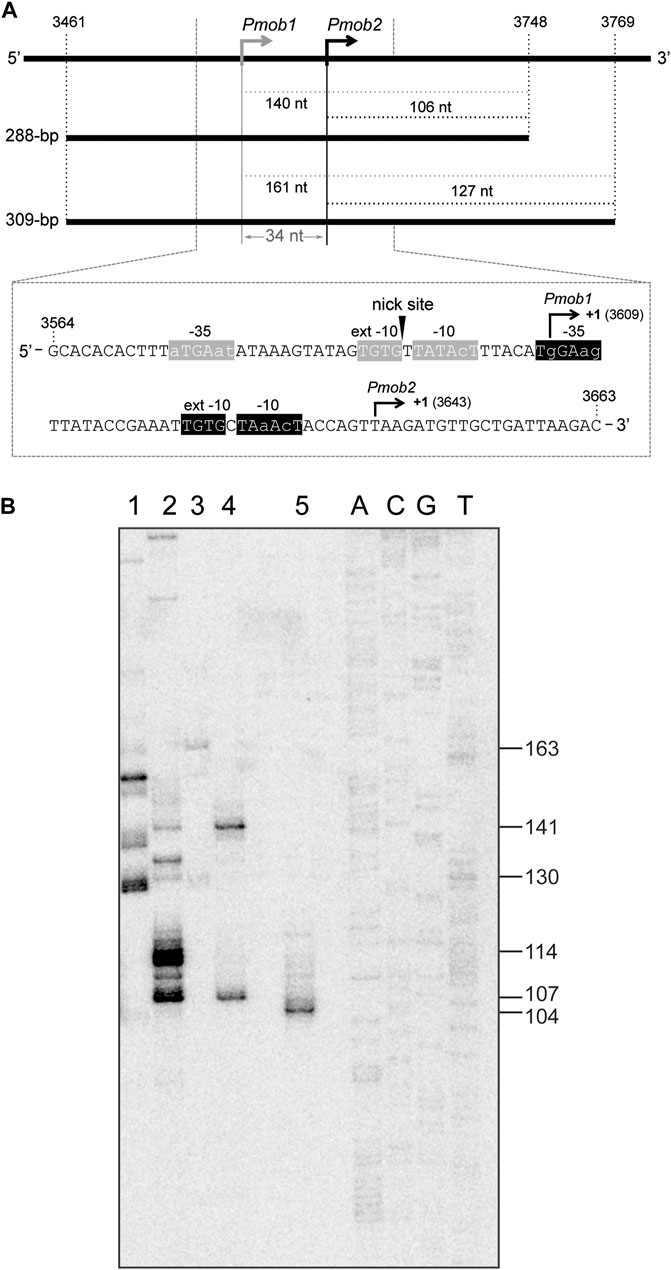
FIGURE 6. SigA recognizes the Pmob1 and Pmob2 promoters. (A) Region of the pMV158 plasmid that contains the Pmob1 and Pmob2 promoters (Farías et al., 1999; Lorenzo-Díaz et al., 2012). Upper part: the coordinates of the 288-bp and 309-bp DNA fragments are indicated. Both DNA fragments were used for in vitro transcription assays (see Figure 6B). The size (in nucleotides) of the expected run-off transcripts is indicated. Lower part: the nucleotide sequence of the region spanning coordinates 3,564 and 3,663 of pMV158 is shown. The arrowhead indicates the nick site. The main sequence elements of the Pmob1 and Pmob2 promoters are indicated. Their transcription start site (position +1) is shown. (B)In vitro transcription assays using RNAP-σ70 and RNAP-SigA. The 288-bp and 309-bp DNA fragments shown in Figure 6A were used as DNA templates. A denaturing gel (8 M urea-6% polyacrylamide) was used for resolving transcripts. Lane 1: 309-bp DNA template and RNAP-σ70. Lane 2: 288-bp DNA template and RNAP-σ70. Lane 3: 309-bp DNA template and RNAP-SigA. Lane 4: 288-bp DNA template and RNAP-SigA. Lane 5: transcript of 102-nt that comigrates with the 104-nt DNA marker. Dideoxy-mediated chain termination sequencing reactions were run in the same gel (lanes A, C, G, T). Sequencing reactions were prepared using a PCR-amplified fragment from the pneumococcal ST556 genome (1,009-bp IR1.2 fragment; oligonucleotides pr14686/pr15159) and the 5′-radiolabeled pr14687 oligonucleotide (Table 1) (see Li et al., 2020). The size (in nucleotides) of the DNA molecules that comigrate with the runoff transcripts is indicated on the right of the gel.
Studies on the transcription of the mobM gene led to the identification of two promoters, Pmob1 and Pmob2 (Figure 6A). Specifically, in Lactococcus lactis, the transcription start site of the mobM gene was located at coordinate 3,609 (promoter Pmob1) (Farías et al., 1999), whereas the E. coli RNA polymerase was shown to initiate transcription of mobM preferentially at coordinate 3,643 (promoter Pmob2) (Lorenzo-Díaz et al., 2012). Both promoters are subjected to self-regulation by MobM protein (Lorenzo-Díaz et al., 2012). As shown in Figure 6A, the Pmob1 promoter has a near-consensus −10 element (5′-TATAcT-3′), a consensus −10 extension element (5′-TGTG-3′) and shows a 3/6 match at the −35 element (5′-aTGAat-3′). Additionally, this promoter is located within the oriT sequence, being its −10 element adjacent to the nick site. The Pmob2 promoter, which is placed just downstream of the Pmob1 promoter, shows a 4/6 match at the −10 element (5′-TAaAcT-3′), has a consensus −10 extension (5′-TGTG-3′) and shows a 3/6 match at the −35 element (5′-TgGAag-3′). The organization of the mobM promoter region has been considered a strategy of the promiscuous plasmid pMV158 to cope with the different types of transcription machinery of Gram-positive and Gram-negative bacteria (Lorenzo-Díaz et al., 2012).
In this study, we investigated whether the pneumococcal SigA factor was able to recognize the Pmob1 and Pmob2 promoters. Specifically, we performed in vitro transcription assays under multiple-round conditions using RNAP-SigA and two linear DNA fragments of 288-bp (coordinates 3,461–3,748) and 309-bp (coordinates 3,461–3,769) (Figure 6A). Depending on the promoter recognized by RNAP-SigA, runoff transcripts of 140-nt (Pmob1) or 106-nt (Pmob2) should be synthesized using the 288-bp DNA template, whereas runoff transcripts of 161-nt (Pmob1) or 127-nt (Pmob2) should be synthesized using the 309-bp DNA template. The in vitro transcription products were resolved on a denaturing gel (Figure 6B). As an internal control, a transcript of 102-nt was run in the same gel (Figure 6B, lane 5). This transcript comigrated with the 104-nt DNA marker, confirming that an RNA molecule migrates slightly more slowly than a DNA molecule of the same length on a denaturing polyacrylamide gel (Sambrook et al., 1989). Regarding RNAP-SigA, with the 288-bp DNA template (lane 4), two RNA products that comigrated with the 141-nt and 107-nt DNA markers were detected, indicating that RNAP-SigA was able to initiate transcription from both promoters, Pmob1 and Pmob2. This conclusion was confirmed with the 309-bp DNA template (lane 3). In this case, two RNA products that comigrated with the 163-nt and 130-nt DNA markers were detected. Concerning RNAP-σ70, the RNA product that corresponds to transcription initiated at the Pmob2 promoter was detected using the 288-bp DNA template (lane 2), as well as the 309-bp DNA template (lane 1). Moreover, RNA products that do not correspond to Pmob1-initiated events were detected. Taken together, we conclude that the pneumococcal SigA factor can recognize the Pmob1 and Pmob2 promoters, which ensures the conjugative mobilization of pMV158 from S. pneumoniae to other bacterial species.
Conclusion
Definitive identification of promoters involves the use of various experimental approaches, including in vitro transcription (Ross and Gourse, 2009). In bacteria, four different subunits (β, β′, two copies of α and ω) form the structurally conserved RNA polymerase core, which transiently binds to a member of the sigma factor family forming the holoenzyme. As pointed out (Griesenbeck et al., 2017), even though there is only a low sequence identity among the core subunits across the domains of life, there is a high degree of structural conservation. To date, the pneumococcal sigma factor SigA has been poorly studied. Recently, it has been reported that a His-tagged SigA factor bound to the E. coli RNA polymerase core was able to recognize the promoter region of the pneumococcal amiA gene, a housekeeping gene required for log-phase growth (Inniss and Morrison, 2020). Here we have set up for the first time a procedure to purify an untagged version of SigA and analysed its functionality using the E. coli core enzyme and particular streptococcal promoters. We have shown that SigA recognizes in vitro the pneumococcal Pmga promoter, which had been defined previously using promoter-reporter fusions and primer extension assays (Solano-Collado et al., 2012). Furthermore, we have shown that SigA provides the RNA polymerase with the ability to transcribe genes involved in replication (copG-repB) and conjugative mobilization (mobM) of the S. agalactiae plasmid pMV158. Thus, SigA contributes to the broad-host-range of this plasmid, and consequently to the spread of antibiotic-resistance genes among bacteria. Finally, our bioinformatics analyses have revealed that essential SigA domains (binding to the core enzyme and binding to DNA) are highly conserved among relevant Gram-positive bacteria, including staphylococcal and enterococcal pathogens.
Data Availability Statement
The original contributions presented in the study are included in the article/Supplementary Material, further inquiries can be directed to the corresponding authors.
Author Contributions
VS-C and SR-C performed laboratory work. FL-D and RP performed bioinformatics analyses. ME and AB designed and supervised the study. All authors performed data analysis and discussed the results. ME and AB wrote the first draft of the manuscript. All authors reviewed different versions of the manuscript and approved the final version.
Funding
This study was financially supported by grants BIO2016-76412-C2-2-R (AEI/FEDER, UE) to AB from the Spanish Ministry of Economy and Competitiveness, and PID2019-104553RB-C21 to AB from the Spanish Ministry of Science and Innovation.
Conflict of Interest
The authors declare that the research was conducted in the absence of any commercial or financial relationships that could be construed as a potential conflict of interest.
Acknowledgments
Thanks are due to F. W. Studier for his gift of the E. coli BL21 (DE3) strain and to L. Rodríguez for her technical help in protein purification.
Supplementary Material
The Supplementary Material for this article can be found online at: https://www.frontiersin.org/articles/10.3389/fmolb.2021.666504/full#supplementary-material
Abbreviations
HTH, helix-turn-helix; IPTG, isopropyl β-D-1-thiogalactopyranoside; Km, kanamycin; nt, nucleotides; OD600, optical density at 600 nm; PAA, polyacrylamide; PEI, polyethyleneimine; RNAP-σ70, E. coli RNA polymerase holoenzyme; RNAP-SigA, E. coli RNA polymerase core and pneumococcal SigA factor; TY, tryptone-yeast extract.
References
Altschul, S. F., Gish, W., Miller, W., Myers, E. W., and Lipman, D. J. (1990). Basic Local Alignment Search Tool. J. Mol. Biol. 215, 403–410. doi:10.1016/S0022-2836(05)80360-2
Aprianto, R., Slager, J., Holsappel, S., and Veening, J.-W. (2018). High-resolution Analysis of the Pneumococcal Transcriptome under a Wide Range of Infection-Relevant Conditions. Nucleic Acids Res. 46, 9990–10006. doi:10.1093/nar/gky750
Bravo, A., Ruiz-Cruz, S., Alkorta, I., and Espinosa, M. (2018). When Humans Met Superbugs: Strategies to Tackle Bacterial Resistances to Antibiotics. Biomol. Concepts 9, 216–226. doi:10.1515/bmc-2018-0021
Browning, D. F., and Busby, S. J. W. (2016). Local and Global Regulation of Transcription Initiation in Bacteria. Nat. Rev. Microbiol. 14, 638–650. doi:10.1038/nrmicro.2016.103
del Solar, G., Acebo, P., and Espinosa, M. (1997). Replication Control of Plasmid pLS1: the Antisense RNA II and the Compact rnaII Region Are Involved in Translational Regulation of the Initiator RepB Synthesis. Mol. Microbiol. 23, 95–108. doi:10.1046/j.1365-2958.1997.1981561.x
del Solar, G., and Espinosa, M. (1992). The Copy Number of Plasmid pLS1 Is Regulated by Two Trans-acting Plasmid Products: the Antisense RNA II and the Repressor Protein, RepA. Mol. Microbiol. 6, 83–94. doi:10.1111/j.1365-2958.1992.tb00840.x
del Solar, G., Díaz, R., and Espinosa, M. (1987). Replication of the Streptococcal Plasmid pMV158 and Derivatives in Cell-free Extracts of Escherichia coli. Mol. Gen. Genet. 206, 428–435. doi:10.1007/BF00428882
del Solar, G. H., Pérez-Martín, J., and Espinosa, M. (1990). Plasmid pLS1-Encoded RepA Protein Regulates Transcription from repAB Promoter by Binding to a DNA Sequence Containing a 13-base Pair Symmetric Element. J. Biol. Chem. 265, 12569–12575. doi:10.1016/s0021-9258(19)38382-6
Dockrell, D. H., Whyte, M. K. B., and Mitchell, T. J. (2012). Pneumococcal Pneumonia. Chest 142, 482–491. doi:10.1378/chest.12-0210
Dower, W. J., Miller, J. F., and Ragsdale, C. W. (1988). High Efficiency Transformation of E. Coli by High Voltage Electroporation. Nucleic Acids Res. 16, 6127–6145. doi:10.1093/nar/16.13.6127
Fang, C., Li, L., Zhao, Y., Wu, X., Philips, S. J., You, L., et al. (2020). The Bacterial Multidrug Resistance Regulator BmrR Distorts Promoter DNA to Activate Transcription. Nat. Commun. 11, 6284. doi:10.1038/s41467-020-20134-y
Farías, M., Grohmann, E., and Espinosa, M. (1999). Expression of the mobM Gene of the Streptococcal Plasmid pMV158 in Lactococcus Lactis Subsp. Lactis. FEMS Microbiol. Lett. 176, 403–410. doi:10.1111/j.1574-6968.1999.tb13690.x10.1016/s0378-1097(99)00265-7
Fernández-López, C., Bravo, A., Ruiz-Cruz, S., Solano-Collado, V., Garsin, D. A., Lorenzo-Díaz, F., et al. (2014). Mobilizable Rolling-circle Replicating Plasmids from Gram-Positive Bacteria: a Low-Cost Conjugative Transfer. Microbiol. Spectr. 2 (5). doi:10.1128/microbiolspec.PLAS-0008-2013
Gamez, G., and Hammerschmidt, S. (2012). Combat Pneumococcal Infections: Adhesins as Candidates for Protein- Based Vaccine Development. Curr. Drug. Targets. 13, 323–337. doi:10.2174/138945012799424697
Garcillán-Barcia, M. P., Francia, M. V., and de la Cruz, F. (2009). The Diversity of Conjugative Relaxases and its Application in Plasmid Classification. FEMS Microbiol. Rev. 33, 657–687. doi:10.1111/j.1574-6976.2009.00168.x
Griesenbeck, J., Tschochner, H., and Grohmann, D. (2017). “Structure and Function of RNA Polymerases and the Transcription Machineries,” in Macromolecular Protein Complexes Subcellular Biochemistry. Editors J. Harris, and J. Marles-Wright (Cham: Springer), Vol. 83, 225–270. doi:10.1007/978-3-319-46503-6_9
Grohmann, E., Christie, P. J., Waksman, G., and Backert, S. (2018). Type IV Secretion in Gram-Negative and Gram-Positive Bacteria. Mol. Microbiol. 107, 455–471. doi:10.1111/mmi.13896
Guzmán, L. M., and Espinosa, M. (1997). The Mobilization Protein, MobM, of the Streptococcal Plasmid pMV158 Specifically Cleaves Supercoiled DNA at the Plasmid oriT. J. Mol. Biol. 266, 688–702. doi:10.1006/jmbi.1996.0824
Holm, L. (2020). DALI and the Persistence of Protein Shape. Protein Sci. 29, 128–140. doi:10.1002/pro.3749
Hoskins, J., Alborn, W. E., Arnold, J., Blaszczak, L. C., Burgett, S., DeHoff, B. S., et al. (2001). Genome of the Bacterium Streptococcus pneumoniae Strain R6. J. Bacteriol. 183, 5709–5717. doi:10.1128/JB.183.19.5709-5717.2001
Inniss, N. L., and Morrison, D. A. (2020). ComWΔ6 Stimulates Transcription of Pneumococcal Competence Genes In Vitro. Front. Mol. Biosci. 7, 61. doi:10.3389/fmolb.2020.00061
Inniss, N. L., Prehna, G., and Morrison, D. A. (2019). The Pneumococcal σX Activator, ComW, Is a DNA-Binding Protein Critical for Natural Transformation. J. Biol. Chem. 294, 11101–11118. doi:10.1074/jbc.RA119.007571
Kumar, S., Stecher, G., Li, M., Knyaz, C., and Tamura, K. (2018). MEGA X: Molecular Evolutionary Genetics Analysis across Computing Platforms. Mol. Biol. Evol. 35, 1547–1549. doi:10.1093/molbev/msy096
Lacks, S. A., López, P., Greenberg, B., and Espinosa, M. (1986). Identification and Analysis of Genes for Tetracycline Resistance and Replication Functions in the Broad-Host-Range Plasmid pLS1. J. Mol. Biol. 192, 753–765. doi:10.1016/0022-2836(86)90026-4
Lacks, S. (1968). Genetic Regulation of Maltosaccharide Utilization in Pneumococcus. Genetics 60, 685–706. doi:10.1093/genetics/60.4.685
Lacks, S., and Greenberg, B. (1977). Complementary Specificity of Restriction Endonucleases of Diplococcus pneumoniae with Respect to DNA Methylation. J. Mol. Biol. 114, 153–168. doi:10.1016/0022-2836(77)90289-3
Lacks, S. (1966). Integration Efficiency and Genetic Recombination in Pneumococcal Transformation. Genetics 53, 207–235. doi:10.1093/genetics/53.1.207
Li, J., Wang, J., Ruiz-Cruz, S., Espinosa, M., Zhang, J.-R., and Bravo, A. (2020). In Vitro DNA Inversions Mediated by the PsrA Site-specific Tyrosine Recombinase of Streptococcus pneumoniae. Front. Mol. Biosci. 7, 43. doi:10.3389/fmolb.2020.00043
Lorenzo-Díaz, F., Dostál, L., Coll, M., Schildbach, J. F., Menéndez, M., and Espinosa, M. (2011). The MobM Relaxase Domain of Plasmid pMV158: thermal Stability and Activity upon Mn2+ and Specific DNA Binding. Nucleic Acids Res. 39, 4315–4329. doi:10.1093/nar/gkr049
Lorenzo-Díaz, F., and Espinosa, M. (2009). Lagging Strand DNA Replication Origins Are Required for Conjugal Transfer of the Promiscuous Plasmid pMV158. J. Bacteriol. 191, 720–727. doi:10.1128/JB.01257-08
Lorenzo-Díaz, F., Fernández-López, C., Garcillán-Barcia, M. P., and Espinosa, M. (2014). Bringing Them Together: Plasmid pMV158 Rolling circle Replication and Conjugation under an Evolutionary Perspective. Plasmid 74, 15–31. doi:10.1016/j.plasmid.2014.05.004
Lorenzo-Díaz, F., Fernández-López, C., Lurz, R., Bravo, A., and Espinosa, M. (2017). Crosstalk between Vertical and Horizontal Gene Transfer: Plasmid Replication Control by a Conjugative Relaxase. Nucleic Acids Res. 45, 7774–7785. doi:10.1093/nar/gkx450
Lorenzo-Díaz, F., Solano-Collado, V., Lurz, R., Bravo, A., and Espinosa, M. (2012). Autoregulation of the Synthesis of the MobM Relaxase Encoded by the Promiscuous Plasmid pMV158. J. Bacteriol. 194, 1789–1799. doi:10.1128/JB.06827-11
Luo, P., Li, H., and Morrison, D. A. (2003). ComX Is a Unique Link between Multiple Quorum Sensing Outputs and Competence in Streptococcus pneumoniae. Mol. Microbiol. 50, 623–633. doi:10.1046/j.1365-2958.2003.03714.x
Mistry, J., Chuguransky, S., Williams, L., Qureshi, M., Salazar, G. A., Sonnhammer, E. L. L., et al. (2021). Pfam: The Protein Families Database in 2021. Nucleic Acids Res. 49, D412–D419. doi:10.1093/nar/gkaa913
Monti, M. C., Hernández-Arriaga, A. M., Kamphuis, M. B., López-Villarejo, J., Heck, A. J. R., Boelens, R., et al. (2007). Interactions of Kid-Kis Toxin-Antitoxin Complexes with the parD Operator-Promoter Region of Plasmid R1 Are Piloted by the Kis Antitoxin and Tuned by the Stoichiometry of Kid-Kis Oligomers. Nucleic Acids Res. 35, 1737–1749. doi:10.1093/nar/gkm073
O'Brien, K. L., Wolfson, L. J., Watt, J. P., Henkle, E., Deloria-Knoll, M., McCall, N., et al. (2009). Burden of Disease Caused by Streptococcus pneumoniae in Children Younger Than 5 years: Global Estimates. The Lancet 374, 893–902. doi:10.1016/S0140-6736(09)61204-6
Paget, M. (2015). Bacterial Sigma Factors and Anti-sigma Factors: Structure, Function and Distribution. Biomolecules 5, 1245–1265. doi:10.3390/biom5031245
Pluta, R., Boer, D. R., Lorenzo-Díaz, F., Russi, S., Gómez, H., Fernández-López, C., et al. (2017). Structural Basis of a Histidine-DNA Nicking/joining Mechanism for Gene Transfer and Promiscuous Spread of Antibiotic Resistance. Proc. Natl. Acad. Sci. U.S.A. 114, E6526–E6535. doi:10.1073/pnas.1702971114
Priebe, S. D., and Lacks, S. A. (1989). Region of the Streptococcal Plasmid pMV158 Required for Conjugative Mobilization. J. Bacteriol. 171, 4778–4784. doi:10.1128/jb.171.9.4778-4784.1989
Puyet, A., and Espinosa, M. (1993). Structure of the Maltodextrin-Uptake Locus of Streptococcus pneumoniae. J. Mol. Biol. 230, 800–811. doi:10.1006/jmbi.1993.1202
Ross, W., and Gourse, R. L. (2009). Analysis of RNA Polymerase-Promoter Complex Formation. Methods 47, 13–24. doi:10.1016/j.ymeth.2008.10.018
Ruiz-Cruz, S., Solano-Collado, V., Espinosa, M., and Bravo, A. (2010). Novel Plasmid-Based Genetic Tools for the Study of Promoters and Terminators in Streptococcus pneumoniae and Enterococcus faecalis. J. Microbiol. Methods 83, 156–163. doi:10.1016/j.mimet.2010.08.004
Sabelnikov, A. G., Greenberg, B., and Lacks, S. A. (1995). An Extended −10 Promoter Alone Directs Transcription of the DpnII Operon of Streptococcus pneumoniae. J. Mol. Biol. 250, 144–155. doi:10.1006/jmbi.1995.0366
Saecker, R. M., Record, M. T., and deHaseth, P. L. (2011). Mechanism of Bacterial Transcription Initiation: RNA Polymerase - Promoter Binding, Isomerization to Initiation-Competent Open Complexes, and Initiation of RNA Synthesis. J. Mol. Biol. 412, 754–771. doi:10.1016/j.jmb.2011.01.018
Sambrook, J., Fritsch, E. F., and Maniatis, T. (1989). Molecular Cloning: A Laboratory Manual. Cold Spring Harbor; Cold Spring Harbor Laboratory Press.
Shin, Y., Qayyum, M. Z., Pupov, D., Esyunina, D., Kulbachinskiy, A., and Murakami, K. S. (2021). Structural Basis of Ribosomal RNA Transcription Regulation. Nat. Commun. 12, 528. doi:10.1038/s41467-020-20776-y
Sievers, F., and Higgins, D. G. (2014). “Clustal Omega, Accurate Alignment of Very Large Numbers of Sequences,” in Multiple Sequence Alignment Methods. Methods in Molecular Biology (Methods and Protocols). Editor D. Russell (Totowa, N. J.: Humana Press), 1079, 105–116. doi:10.1007/978-1-62703-646-7_6
Solano-Collado, V., Espinosa, M., and Bravo, A. (2012). Activator Role of the Pneumococcal Mga-like Virulence Transcriptional Regulator. J. Bacteriol. 194, 4197–4207. doi:10.1128/jb.00536-12
Solano-Collado, V., Lurz, R., Espinosa, M., and Bravo, A. (2013). The Pneumococcal MgaSpn Virulence Transcriptional Regulator Generates Multimeric Complexes on Linear Double-Stranded DNA. Nucleic Acids Res. 41, 6975–6991. doi:10.1093/nar/gkt445
Studier, F. W., and Moffatt, B. A. (1986). Use of Bacteriophage T7 RNA Polymerase to Direct Selective High-Level Expression of Cloned Genes. J. Mol. Biol. 189, 113–130. doi:10.1016/0022-2836(86)90385-2
Studier, F. W., Rosenberg, A. H., Dunn, J. J., and Dubendorff, J. W. (1990). Use of T7 RNA Polymerase to Direct Expression of Cloned Genes. Meth. Enzymol. 185, 60–89. doi:10.1016/0076-6879(90)85008-c
Tamura, K., and Nei, M. (1993). Estimation of the Number of Nucleotide Substitutions in the Control Region of Mitochondrial DNA in Humans and Chimpanzees. Mol. Biol. Evol. 10, 512–526. doi:10.1093/oxfordjournals.molbev.a040023
The UniProt Consortium (2019). UniProt: a Worldwide Hub of Protein Knowledge. Nucleic Acids Res. 47, D506–D515. doi:10.1093/nar/gky1049
Tovpeko, Y., and Morrison, D. A. (2014). Competence for Genetic Transformation in Streptococcus pneumoniae: Mutations in σA Bypass the ComW Requirement. J. Bacteriol. 196, 3724–3734. doi:10.1128/jb.01933-14
Waterhouse, A., Bertoni, M., Bienert, S., Studer, G., Tauriello, G., Gumienny, R., et al. (2018). SWISS-MODEL: Homology Modelling of Protein Structures and Complexes. Nucleic Acids Res. 46, W296–W303. doi:10.1093/nar/gky427
Yang, J., Anishchenko, I., Park, H., Peng, Z., Ovchinnikov, S., and Baker, D. (2020). Improved Protein Structure Prediction Using Predicted Interresidue Orientations. Proc. Natl. Acad. Sci. U.S.A. 117, 1496–1503. doi:10.1073/pnas.1914677117
Yanisch-Perron, C., Vieira, J., and Messing, J. (1985). Improved M13 Phage Cloning Vectors and Host Strains: Nucleotide Sequences of the M13mpl8 and pUC19 Vectors. Gene 33, 103–119. doi:10.1016/0378-1119(85)90120-9
Keywords: plasmid pMV158, RNA polymerase, SigA protein, sigma factor, streptococcal promoters, Streptococcus pneumoniae
Citation: Solano-Collado V, Ruiz-Cruz S, Lorenzo-Díaz F, Pluta R, Espinosa M and Bravo A (2021) Recognition of Streptococcal Promoters by the Pneumococcal SigA Protein. Front. Mol. Biosci. 8:666504. doi: 10.3389/fmolb.2021.666504
Received: 10 February 2021; Accepted: 14 June 2021;
Published: 24 June 2021.
Edited by:
Emil Alexov, Clemson University, United StatesReviewed by:
Lin Li, The University of Texas at El Paso, United StatesYunhui Peng, National Institutes of Health (NIH), United States
Copyright © 2021 Solano-Collado, Ruiz-Cruz, Lorenzo-Díaz, Pluta, Espinosa and Bravo. This is an open-access article distributed under the terms of the Creative Commons Attribution License (CC BY). The use, distribution or reproduction in other forums is permitted, provided the original author(s) and the copyright owner(s) are credited and that the original publication in this journal is cited, in accordance with accepted academic practice. No use, distribution or reproduction is permitted which does not comply with these terms.
*Correspondence: Manuel Espinosa, bWVzcGlub3NhQGNpYi5jc2ljLmVz; Alicia Bravo, YWJyYXZvQGNpYi5jc2ljLmVz