Role of Calpain-1 in Neurogenesis
- 1Graduate College of Biomedical Sciences, Western University of Health Sciences, Pomona, CA, United States
- 2College of Osteopathic Medicine of the Pacific, Western University of Health Sciences, Pomona, CA, United States
While calpains have been implicated in neurogenesis for a long time, there is still little information regarding the specific contributions of various isoforms in this process. We took advantage of the availability of mutant mice with complete deletion of calpain-1 to analyze its contribution to neurogenesis. We first used the incorporation of BrdU in newly-generated cells in the subgranular zone of the dentate gyrus to determine the role of calpain-1 deletion in neuronal proliferation. Our results showed that the lack of calpain-1 decreased the rate of cell proliferation in adult hippocampus. As previously shown, it also decreased the long-term survival of newly-generated neurons. We also used data from previously reported RNA and miRNA sequencing analyses to identify differentially expressed genes in brain of calpain-1 knock-out mice related to cell division, cell migration, cell proliferation and cell survival. A number of differentially expressed genes were identified, which could play a significant role in the changes in neurogenesis in calpain-1 knock out mice. The results provide new information regarding the role of calpain-1 in neurogenesis and have implications for better understanding the pathologies associated with calpain-1 mutations in humans.
Introduction
Mammalian adult neurogenesis was first observed in 1965, but the last two decades have seen considerable expansion of interest and effort to understand the biology of this phenomenon in mammals (Altman and Das, 1965; Hamburger, 1980; Spalding et al., 2013; Christian et al., 2014; Ernst et al., 2014). Adult neurogenesis is the process by which new neurons and glial cells are generated in the adult brain (Gage, 2000). It is both temporally and spatially regulated over the continuum of an organism‘s lifespan (Suh et al., 2009; Ming and Song, 2005; Christian et al., 2014). It was originally assumed that neurogenesis did not occur appreciably in mammals after the early postnatal period (Hamburger, 1980; Spalding et al., 2013). However, new evidence indicates that neurogenesis occurs throughout the lifetime (Ibrayeva and Bonaguidi, 2015). In adult humans, it is estimated that seven hundred new neurons are generated every week (Spalding et al., 2013; Ernst et al., 2014). The brain experiences the highest rate of neurogenesis in the immediate days following birth. As mammals transition to adulthood, the endogenous rate of proliferation begins to decline and the ability of newly generated neurons to survive and become incorporated into established neural networks decreases (Doetsch et al., 1999; Merkle et al., 2004; Ming and Song, 2005; Lepousez et al., 2013; Christian et al., 2014).
There are two discrete regions in the adult mammalian brain where neurogenesis is readily observable, the subventricular zone (SVZ) and the subgranular zone (SGZ) of the dentate gyrus (Altman and Das, 1965; Altman, 1969). In these two regions, stem cells undergo asymmetrical cell division wherein the stem cell self-renews, retaining its genetic and phenotypic identity, while the “daughter-cell” becomes a more differentiated neural progenitor cell (NPC) relative to its “mother” (Santos et al., 2012; Lepousez et al., 2013; Christian et al., 2014). The daughter NPCs then proliferate, differentiate, and migrate (Suh et al., 2009; Santos et al., 2012; Lepousez et al., 2013; Christian et al., 2014). NPCs generated in the SGZ and the SVZ can become neurons, glia, and other cell types, which migrate and integrate into neural networks in hippocampus and olfactory bulbs (Lepousez et al., 2013; Vadodaria and Gage, 2014).
Several studies have shown that calpains are actively involved in mammalian adult neurogenesis (Santos et al., 2012; Persson et al., 2013; Chung et al., 2015; Machado et al., 2015; Wang and Zhang, 2014). Furthermore, many studies provide additional support for a critical role for calpains in cell proliferation, survival, migration, and differentiation (Huttenlocher et al., 1997; Yajima and Kawashima, 2002; Moyen et al., 2004; Raynaud et al., 2008; Jessberger et al., 2009; Moudilou et al., 2010; Storr et al., 2011; Lade et al., 2012; Wang and Zhang, 2014; Chung et al., 2015; Liang et al., 2015). Calpains have been shown to be critical for cell proliferation and differentiation through their interactions with and cleavage of proteins involved in cell cycle regulation (Joy et al., 2006; Jessberger et al., 2009; Storr et al., 2011). In particular, the cyclin-dependent kinase 5 (cdk5) plays important roles in neural development (Kawauchi, 2014), and its activation requires the binding of its regulatory subunit, p35. Calpain cleaves p35 into p25, which increases cdk5 activity and changes its substrate selectivity and subcellular localization. Calpains also play an important role for cell survival during postnatal development, as demonstrated by the loss of many cells during development in Capn1-KO mice and the embryological lethality in Capns1−/− and Capn2−/− mice (Arthur et al., 2000; Zimmerman et al., 2000; Dutt et al., 2006; Takano et al., 2011; Amini et al., 2013; Wang et al., 2016). In addition, calpains could regulate distinct steps in neuronal differentiation by regulation of epigenetic DNA modifications, cell cycle progression, migration, and response to extracellular trophic signals (Moors et al., 2007; Suh et al., 2009; Santos et al., 2012; Wang and Zhang, 2014). Calpains have been shown to cleave several proteins involved in the differentiation of several cell types, including Ten-eleven translocation (Tet) proteins, ß-catenin and CCAAT/enhancer-binding protein (C/EBP) β (Moyen et al., 2004; Abe and Takeichi, 2007; Zhang et al., 2012; Wang and Zhang, 2014).
It has been difficult to evaluate the specific roles of the two major calpain isoforms in the brain, calpain-1 and calpain-2, in neurogenesis, due to the lack of selective inhibitor and the lack of mutant mice with selective down-regulation of calpain-1 or calpain-2. During neuronal differentiation, calpain-1 is highly expressed in neural stem cells (NSCs) and immature neural progenitor cells (NPCs), while the expression of calpain-2 increases with continuing neuronal differentiation (Wu et al., 2010; Santos et al., 2012; Machado et al., 2015). Differential expression of calpain isoforms has also been observed in many differentiating cell types, including myocytes, hepatocytes, osteoblasts, and adipocytes (Yajima and Kawashima, 2002; Moyen et al., 2004; Lade et al., 2012). Similar to what is observed in neuronal differentiation, high levels of calpain-1 are observed in immature progenitors, while calpain-2 levels increase with continuing states of differentiation (Yajima and Kawashima, 2002; Dedieu et al., 2003; Honda et al., 2008; Wu et al., 2010; Lade et al., 2012; Wamstad et al., 2012). The present studies took advantage of the availability of calpain-1 knock-out (C1KO) mice to more precisely analyze the role of calpain-1 in neurogenesis and the potential mechanisms by which calpain-1 could regulate neuronal division, differentiation, proliferation, migration and survival.
Materials and Methods
Animals
Animal use in all experiments followed the National Institutes of Health (NIH) guidelines and all protocols were approved by the Institution Animal Care and Use Committee of Western University of Health Sciences.
5-Bromo-2-Deoxyuridine Labeling
Three-month-old C57/Bl6 (wild-type (WT) and Capn1-KO on a C57/Bl6 background) male mice were kept under standard laboratory conditions with a 12:12 h light/dark cycle. Mice were injected intraperitoneally (i.p.) with 5-bromo-2-deoxyuridine (BrdU) (50 mg/kg) every 8 h for 3 days, a total of nine injections. Brains were collected following transcardial perfusion after 3 or 30 days from the first BrdU injection. They were immersed in 4% PFA for 24–48 h before transfer to a 30% sucrose solution for 48 h. Preparation of 30 μm-thick tissue sections was performed by embedding brains in 2% agarose and sectioning them with a Leica VT000 S vibratome. Sections were rinsed (3 × 5 min) in 0.01 M phosphate buffer saline containing 0.3% Triton X-100 (PBS-TX). For BrdU immunostaining, sections were pre-treated with 2 M hydrochloride and Triton-X 100 at 37°C for 45 min followed by 10 min washing in borate buffer (pH 8.5) in order to denaturate DNA. Sections were incubated in anti-NeuN (abcam ab104224, ab177487), anti–BrdU (SCBT sc-32323), or anti–Ki67 (abcam ab16667) antibodies for 48 h at 4°C followed by incubation in secondary antibodies (Life technologies Alexa-fluor) for 2 h at room temperature. One tenth of the collected sections were stained and imaged with a Nikon Te-2000 confocal microscope at ×20 magnification. Images were then analyzed using Macro enabled Image-J open source software. All BrdU- or Ki67- positive cells in a 500 × 500 µm area were counted using ImageJ. Five sections from each mouse at various levels of the hippocampus or the olfactory bulbs were analyzed and the data averaged for this mouse. Quantitative data were normalized to percent of control.
P35-P25 Conversion
Mice were deeply anesthetized using gaseous isoflurane and decapitated. The whole brain was removed, and hippocampus dissected. Tissue was homogenized in radioimmunoprecipitation assay (RIPA) buffer (10 mM Tris, pH 8, 140 mM NaCl, 1 mM EDTA, 0.5 mM EGTA, 1% NP-40, 0.5% sodium deoxycholate, and 0.1% SDS). Protein concentrations were determined with a BCA protein assay kit (Pierce). Western blots were performed according to published protocols (Sun et al., 2015). Briefly, proteins were separated by SDS-PAGE and transferred onto a PVDF membrane (Millipore). After blocking with 3% BSA for 1 h, membranes were incubated with p35 antibodies (1:500; catalog #sc-820; Santa Cruz Biotechnology) overnight at 4°C followed by incubation with IRDye secondary antibodies for 2 h at room temperature. Antibody binding was detected with the Odyssey® imaging system (LI-COR Biosciences), and Western blots were analyzed with the Image Studio Software.
miRNA Target Prediction
To explore neurogenesis-related DE miRNAs found in the miRNA-seq results, the targets of DE miRNAs were provided from the miRTarBase database via the MIENTURNET (MicroRNA Enrichment TURend NETwork) tool (Licursi et al., 2019), and then filter on the basis of a p-value <0.05.
Gene Ontology Analysis
DAVID (https://david.ncifcrf.gov/) and GENEONTOLOGY (http://geneontology.org/) were used for extraction of the genes belonging to the “neurogenesis” (GO:0022008) GO terms. These programs may utilize different dataset for assessing GO (Gene Ontology) enrichment and therefore may complement each other. We used a combined list as candidate genes related to neurogenesis.
Statistical Analyses
Data are generally presented as means ± SD or means ± SEM. In all experiments, only two groups were compared, thus, a two-tail t-test was used for determining statistical significance with no correction of variability of standard deviation. p values less than 0.05 were considered statistically significant. Due to the small sample size, no attempt was made to test the normality of data.
Results
Role of Calpain-1 in Neurogenesis in the Dentate Gyrus
Evidence suggests that calpain-1 is important for cell proliferation and self-renewal of NSCs (Santos et al., 2012; Wang and Zhang, 2014). We investigated the rate of proliferation in the dentate gyrus of C1KO mice using the canonical proliferation expression marker Ki67, and BrdU-incorporation. We found a >25% decrease for both BrdU+ and Ki67+ cells (n = 5–6 per group, *p < 0.05 WT vs. C1KO) in the DG of C1KO mice (Figures 1, 2). The similarities between the changes in BrdU+ and Ki67+ cells suggest that most BrdU+ cells are neurons. This result suggests that calpain-1 activity is important for the proliferation of newly-born cells in the dentate gyrus. We also analyzed the migration/survival of newly-born cells by determining the number of BrdU+ cells in the olfactory bulbs 30 days after injection (Figure 3). The number of BrdU+ cells was significantly decreased in C1KO mice, as compared to WT mice.
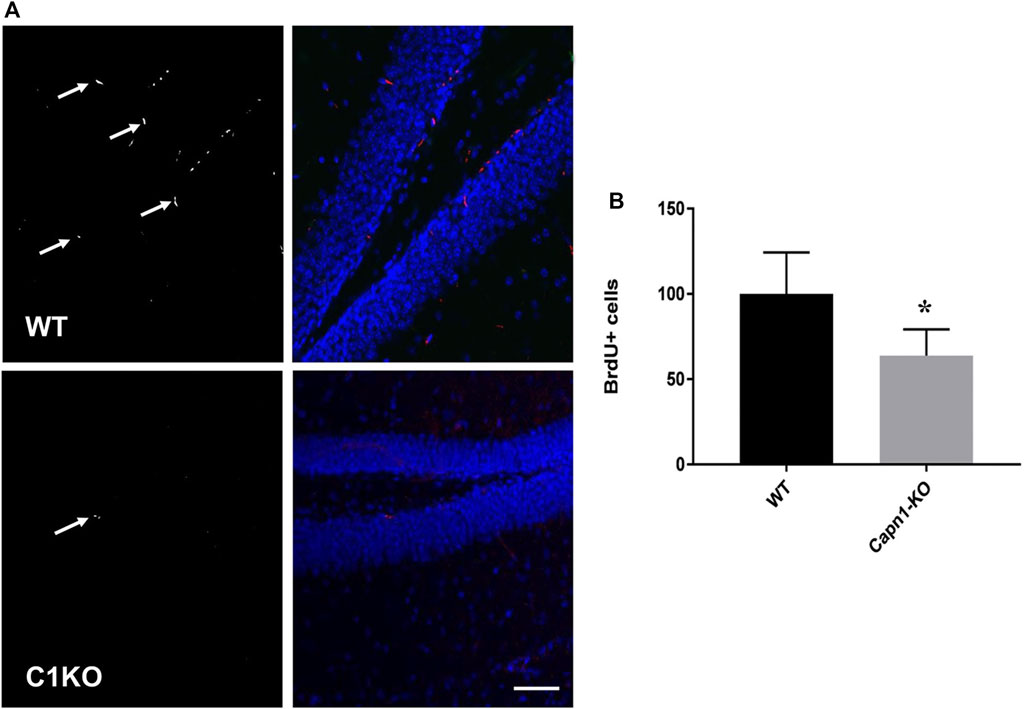
FIGURE 1. BrdU+ cells in the dentate gyrus of WT and C1KO mice. WT and C1KO mice were injected i.p. with 5-bromo-2-deoxyuridine (BrdU) (50 mg/kg) every 8 h for 3 days, for a total of nine injections. Brains were collected following transcardial perfusion 3 days after the first BrdU injection. Brains were sectioned and sections were processed for immunolabeling with antibodies against BrdU (A), left panels, arrows) and NeuN (A), right panels, blue). Numbers of BrdU+ cells were quantified as indicated in the Materials and Methods section (B). Sections were counterstained with DAPI (blue). Five sections per mouse were quantified and 5 WT and C1KO mice were analyzed. Results are expressed as percent of the average number of cells in the WT mice and are Means ± SEM of 5 mice per group. *p < 0.01, Student’s t-test. Scale bar: 50 µm.
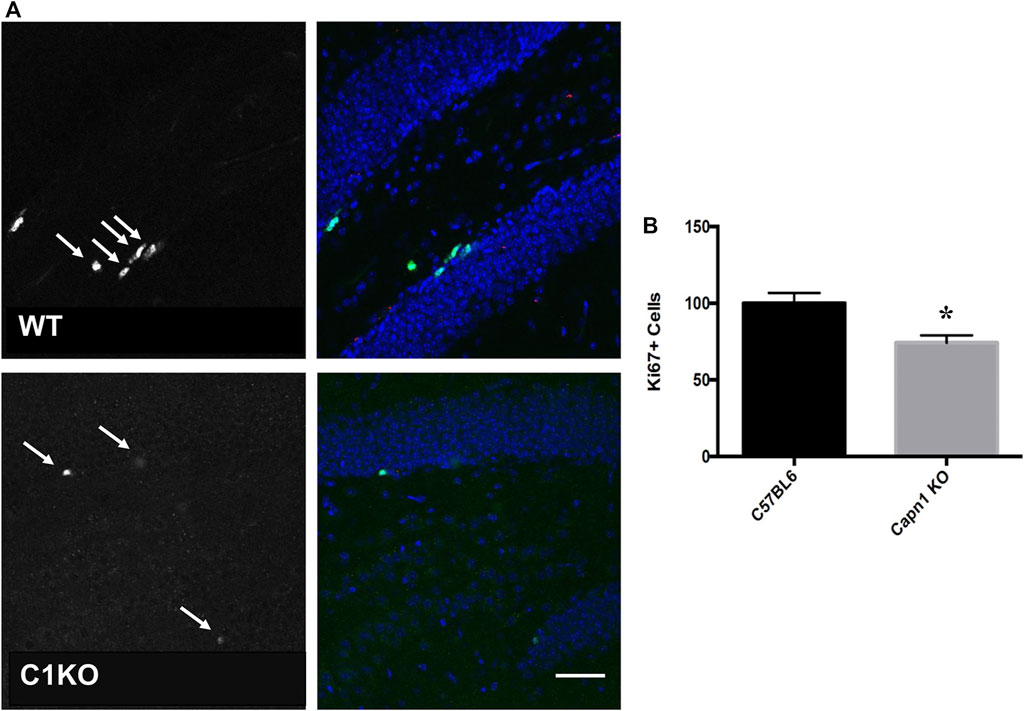
FIGURE 2. Ki67+ cells in the dentate gyrus of WT and C1KO mice. Adjacent sections from the ones used for the BrdU analysis were used for immunolabeling with antibodies against Ki67 (A), left panels, arrows) and NeuN (A), right panels, green). Numbers of Ki67+ cells were quantified as indicated in the Materials and Methods section (B). Sections were counterstained with DAPI (blue). Five sections per mouse were quantified and 5 WT and C1KO mice were analyzed. Results are expressed as percent of the average number of cells in the WT mice and are Means ± SEM of 5 mice per group. *p < 0.01, Student’s t-test. Scale bar: 50 µm.
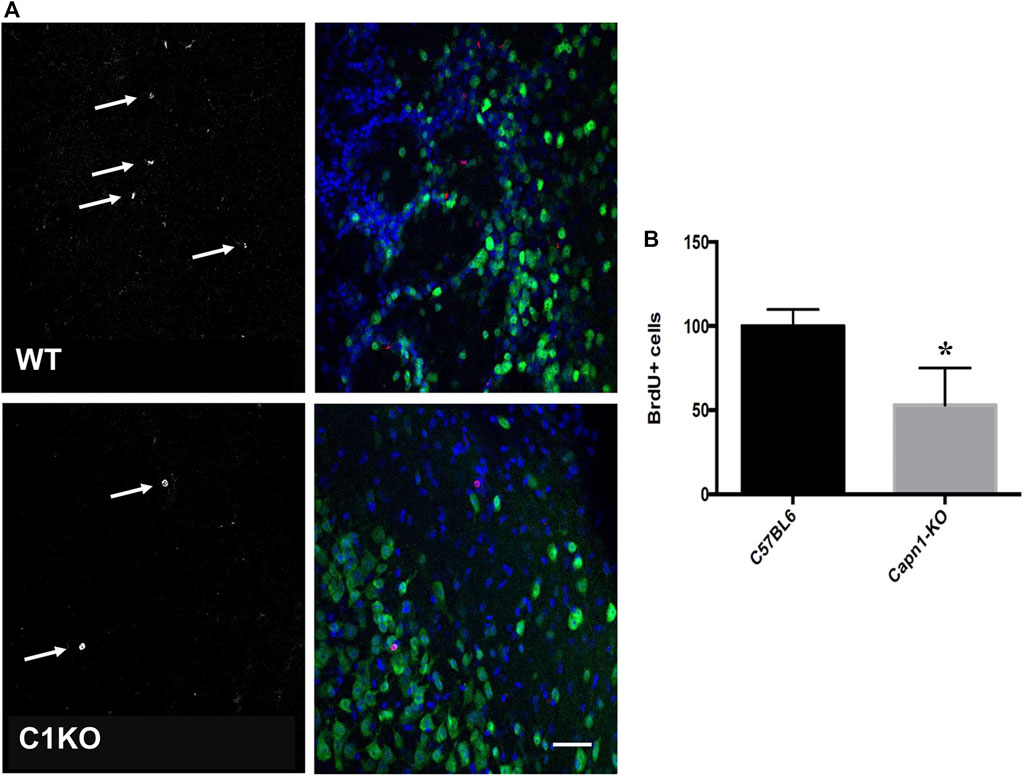
FIGURE 3. BrdU+ cells in the olfactory bulbs of WT and C1KO mice. WT and C1KO mice were injected with 5-bromo-2-deoxyuridine (BrdU) (50 mg/kg) every 8 h for 3 days, a total of nine injections. Brains were collected following transcardial perfusion 30 days after the first BrdU injection. Brains were sectioned and sections were processed for immunolabeling with antibodies against BrdU (A), left panels arrows, red in left panels) and NeuN (A), right panels, green). Sections were counterstained with DAPI (blue). Numbers of BrdU+ cells were quantified as indicated in the Materials and Methods section (B). Five sections per mouse were quantified and 5 WT and C1KO mice were analyzed. Results are expressed as percent of the average number of cells in the WT mice and are Means ± SEM of 5 mice per group. *p < 0.01, Student’s t-test. Scale bar: 50 µm.
Cdk5 plays important roles in neuronal development and survival (Kawauchi, 2014), and it has been shown that calpain-mediated truncation of p35–p25 promotes neural development and differentiation (Jessberger et al., 2009; Shu et al., 2018). We therefore evaluated the levels of p35 and p25 in hippocampus of WT and C1KO mice (Figure 4). While p35 levels were not significantly different between WT and C1KO mice, the ratio p25/p35 was significantly decreased in hippocampus from C1KO mice as compared to WT mice. These results suggest that p35 is tonically cleaved to p25 by calpain-1 and that the lack of calpain-1 results in decreased cleavage, which would be associated with reduced cdk5 activity in the C1KO mice.
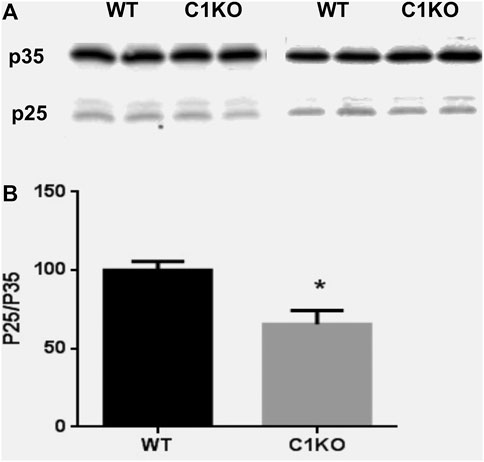
FIGURE 4. Levels of p35 and p25 in the hippocampus of WT and C1KO mice. WT and C1KO mice were sacrificed, and their hippocampi collected. They were homogenized and aliquots of the homogenates processed for immunoblotting with p35 antibodies (A). (B) Quantification analysis. Ratios of p25/p35 were calculated and results were expressed as percent of the averaged value in WT mice and represent Means ± SEM of 4 animals. *p < 0.01, Student’s t-test.
Changes in Neurogenesis Genes and Gene Networks in Calpain-1 Knock-Out Mice
We recently performed RNA-Seq analysis to compare gene expression in brains of WT and C1KO mice (Su et al., 2020). We reanalyzed the data to identify which genes related to neurogenesis were differentially expressed in brains of these mice (Supplementary Table S1). We identified 60 genes that were differentially expressed (DE), with nine genes upregulated and 51 downregulated. We categorized these genes into four clusters related to cell cycle, cell migration, cell division and cell death (Table 1). Interestingly, one of the genes significantly down-regulated in the brains of C1KO mice is Nr4a1, which we previously validated in our RNA-Seq analysis by RT-qPCR and showed that the protein was also down-regulated in hippocampus. Nr4a1 is involved in many functions including apoptosis, and we found that it colocalized with doublecortin in the dentate gyrus and was present in newly-born neurons (Su et al., 2020). Nr4a3 has also been previously identified as playing a role in hippocampal neurogenesis (Ashbrook et al., 2014) and is significantly downregulated in brains of C1KO mice.

TABLE 1. GO analysis of differentially expressed neurogenesis-related genes in brains of C1KO, as compared to WT mice.
We also recently analyzed changes in miRNAs in brains of C1KO mice, as compared to WT mice (Su et al., 2021). Again, we reanalyzed the data to identify those miRNAs related to neurogenesis (Table 2). We identified 24 miRNAs that were differentially expressed in brains of C1KO mice as compared to WT mice, with three upregulated and 21 downregulated mi-RNAs. We then analyzed the target genes for the DE miRNAs and identified those involved in cell cycle, cell migration, cell division and cell death (Table 3). Surprisingly, there was no overlap between the DE RNAs and the genes targeted by the DE miRNAs. To further analyze the important target genes from the DE miRNAs, we selected those for which the miRNAs exhibited the largest changes in expression between WT and C1KO mice (Table 4). Five genes appeared to be regulated by multiple miRNAs, including Map2 (microtubule-associated protein 2), Mtor (mechanistic target of rapamycin kinase), Qk (quaking, KH domain containing RNA binding), Dcx (doublecortin) and Zeb1 (zinc finger E-box binding homeobox1). Overall, there were 50 genes regulated by calpain-1 involved in cell cycle, cell migration, cell division and cell death (Figure 5). We used the GeneMANIA tool to build a gene interaction network using all the genes identified in the cell cycle, cell migration, cell division and cell death GO terms (Figure 6). A number of nodes were clearly revealed by this analysis, and the genes in these nodes are indeed related to cell death, growth cone and brain development.
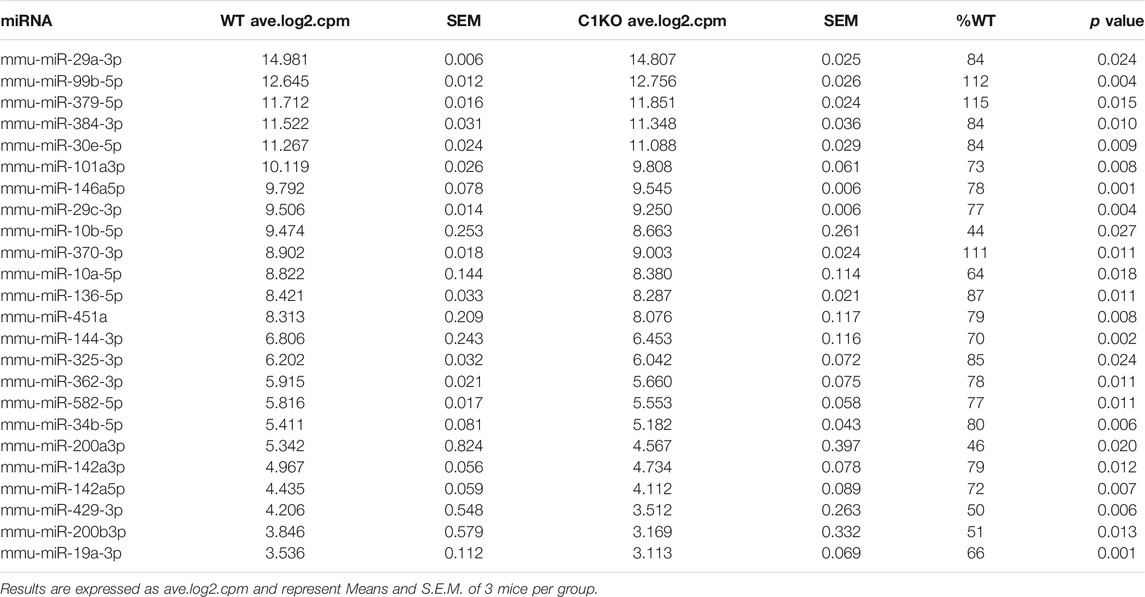
TABLE 2. Differentially expressed miRNAs related to neurogenesis in brains of C1KO, as compared to WT mice.
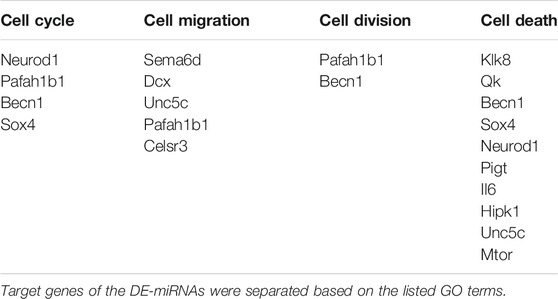
TABLE 3. Target genes of the DE miRNAs in C1KO mice, as compared to WT mice, and corresponding GO terms.
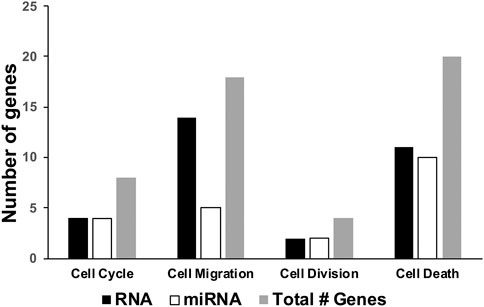
FIGURE 5. Number of differentially expressed genes related to neurogenesis in brains of C1KO mice as compared to WT mice. The number of differentially expressed genes, based on RNA sequencing or miRNA-targeted or both, were compiled for cell cycle, cell migration, cell division or cell death.
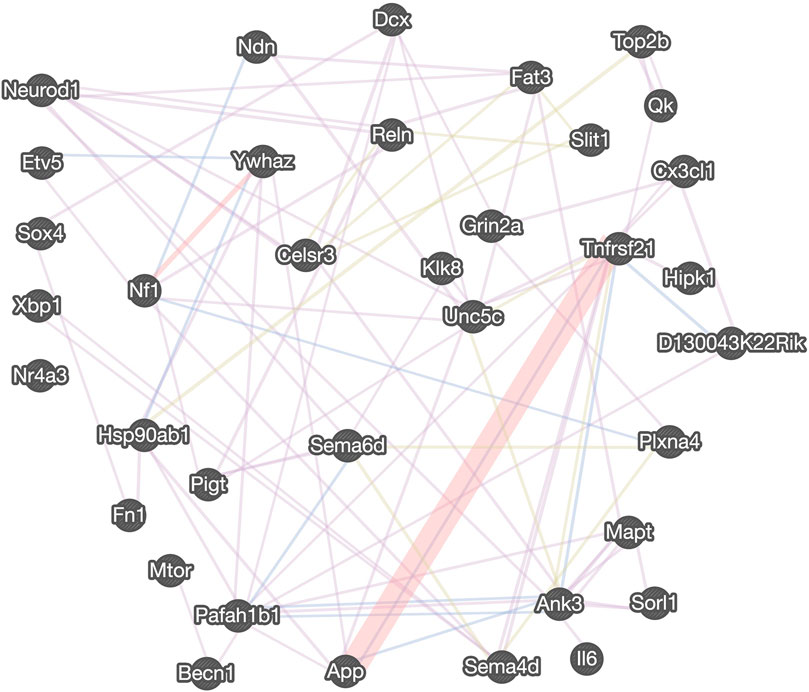
FIGURE 6. Gene interaction network regulated by calpain-1 for cell cycle, cell division and cell survival. The gene interaction network was derived from the GeneMANIA tool using the neurogenesis-related DE genes and targets of DE miRNAs, assigned to “cell cycle,” “cell migration,” “cell division” and “cell death” GO terms. The distinct colors of the network line indicate the dataset applied: co-expression (purple), physical interactions (red), co-localization (blue), shared protein domains (light green). Five transcription factors are listed on the left.
Discussion
Our study sought to investigate the role of the calpain specific isoform, calpain-1, in proliferation, survival and migration of adult neural stem cells in vivo. We found that the survival of newborn cells in the OB of adult C1KO mice labeled with BrdU was significantly reduced, as compared to that in WT mice. No significant difference was found in the amount of BrdU+ cells migrating in the rostral migratory stream (RMS) (data not shown). The similarities on the changes in BrdU+ and Ki67+ cells suggest that these cells are newly-born neurons. This result suggests that survival of newly-born neurons is dependent on calpain-1 activity, which is in good agreement with the notion that calpain-1 is neuroprotective (Wang et al., 2013; Wang et al., 2016).
We also observed that proliferation of newly-born cells in the dentate gyrus was decreased in C1KO mice. The decrease in proliferation we observed was comparable to that found in a previous study on neural stem cells (NSC) proliferation in the dentate gyrus in the Capns1-KO mice (Santos et al., 2012; Amini et al., 2013), in which the activities of both calpain-1 and -2 were disrupted. To our knowledge, our study is the first to investigate the effect of the loss of function of a specific calpain isoform in adult neurogenesis in vivo. It remains to be investigated whether deletion of calpain-2 would have a similar effect on proliferation of NSCs in the dentate gyrus. Studies investigating proliferation in other cell systems have provided ample evidence for a role for calpain-1 (Moudilou et al., 2010; Storr et al., 2011; Santos et al., 2012).
We found no significant difference in the number of BrdU+ cells in the RMS between C1KO and WT mice. This result suggests that the migration of differentiating NSCs from the SVZ is not critically dependent on calpain-1. Previous reports examining the differential role of calpain-1 and calpain-2 in adult neurogenesis in vitro have suggested that calpain-1 activity is important for NSC proliferation and self-renewal, while calpain-2 activity has been shown in many different cell types to be important for cell migration (Moyen et al., 2004; Santos et al., 2012). Investigation of the molecular mechanisms regulating differentiation of several cell types (embryonic stem cells, induced pluripotent cells, adipose derived stromal/cells, myocytes) has demonstrated that calpain-2 is critically important for migration of differentiating cells (Dedieu et al., 2003; Raynaud et al., 2008). Our evidence that calpain-1 deletion does not negatively affect the migration of NSCs from the SVZ, is consistent with these previous studies.
Analysis of genes and miRNAs differentially expressed in C1KO mice offers several potential mechanisms responsible for the changes in cell proliferation and survival we observed between WT and C1KO mice. The expression of a significant number of genes involved in cell cycle, cell division, cell differentiation and cell survival is altered in brain of C1KO mice. Likewise, the expression of many miRNAs targeting genes involved in these processes are altered. At least 50 genes involved in these cellular processes are differentially expressed in brain of C1KO mice. The gene network analysis provided a number of critical nodes regulating cell survival and cell proliferation, which could be responsible for the various effects we observed in C1KO mice. This is in particular the case for Grin2a, which encodes the NR2A subunit of the NMDA receptors and has been shown to be upstream of calpain-1 and involved in neuroprotection (Wang et al., 2013). Several DE genes are also involved in growth cone motility, including Sema6d, Unc5c, and Ank3. In addition, calpain-1 has been shown to regulate the differentiation of mouse embryonic stem cells to neuronal cells through degradation of ten-eleven translocation (TET) proteins, which regulate DNA demethylation (Wang and Zhang, 2014). Calpain-1 has also been shown to regulate developmental organogenesis via N-terminal truncation and activation of β-catenin, a family member of the Wnt signaling pathway (Lade et al., 2012) and through the STAT3/HIF-1α/VEGF pathway (Wu et al., 2018). Loss of regulation of distinct TFs and epigenetic modifications of DNA are possible molecular mechanisms underlying the reduced proliferation observed in the dentate gyrus of C1KO mice. As we used the whole brain and not the dentate gyrus for the mRNA and miRNA analysis, it is possible that we missed some genes and miRNAs with low levels of expression. We also found that ratios of p25/p35 are decreased in hippocampus of C1KO mice as compared to WT mice, suggesting that cdk5 is less active in the C1KO mice, which could participate in impaired development in these mice. This could also play a role in the learning and memory impairment we previously reported in these mice (Zhu et al., 2015), as cdk5 also plays a crucial role in spatial learning (Mishiba et al., 2014).
In conclusion, our results clearly demonstrate that calpain-1 plays an important role in neurogenesis in the mouse brain, which could account for several of the phenotypic alterations previously reported, including cerebellar ataxia, impaired synaptic plasticity and learning, and increased susceptibility to brain injury. These results underscore the needs to further evaluate human subjects with calpain-1 mutations for possible defects in neurogenesis.
Data Availability Statement
The original contributions presented in the study are included in the article/Supplementary Material, further inquiries can be directed to the corresponding author.
Ethics Statement
This animal study was reviewed and approved by Western University of Health Sciences Institutional Animal Care and Use Committee.
Author Contributions
MB designed the study, supervised the work and wrote the manuscript. WS generated some of the data, analyzed them, prepared tables and figures and edited the manuscript. JS collected some of the data, prepared figures and wrote a draft of the manuscript. JDS collected some of the data and prepared figures. XB organized and interpreted the data and worked with MB to finalize the manuscript.
Funding
This work was supported by the National Institutes of Health Grant R01N S104078 to MB. XB is supported in part by funds from the Daljit and Elaine Sarkaria Chair.
Conflict of Interest
The authors declare that the research was conducted in the absence of any commercial or financial relationships that could be construed as a potential conflict of interest.
Supplementary Material
The Supplementary Material for this article can be found online at: https://www.frontiersin.org/articles/10.3389/fmolb.2021.685938/full#supplementary-material
References
Abe, K., and Takeichi, M. (2007). NMDA-Receptor Activation Induces Calpain-Mediated β-Catenin Cleavages for Triggering Gene Expression. Neuron 53 (3), 387–397. doi:10.1016/j.neuron.2007.01.016
Altman, J. (1969). Autoradiographic and Histological Studies of Postnatal Neurogenesis. IV. Cell Proliferation and Migration in the Anterior Forebrain, With Special Reference to Persisting Neurogenesis in the Olfactory Bulb. J. Comp. Neurol. 137 (4), 433–457. doi:10.1002/cne.901370404
Altman, J., and Das, G. D. (1965). Autoradiographic and Histological Evidence of Postnatal Hippocampal Neurogenesis in Rats. J. Comp. Neurol. 124 (3), 319–335. doi:10.1002/cne.901240303
Amini, M., Ma, C.-l., Farazifard, R., Zhu, G., Zhang, Y., Vanderluit, J., et al. (2013). Conditional Disruption of Calpain in the CNS Alters Dendrite Morphology, Impairs LTP, and Promotes Neuronal Survival Following Injury. J. Neurosci. 33 (13), 5773–5784. doi:10.1523/jneurosci.4247-12.2013
Arthur, J. S. C., Elce, J. S., Hegadorn, C., Williams, K., and Greer, P. A. (2000). Disruption of the Murine Calpain Small Subunit Gene, Capn4: Calpain is Essential for Embryonic Development But Not for Cell Growth and Division. Mol. Cell Biol. 20 (12), 4474–4481. doi:10.1128/mcb.20.12.4474-4481.2000
Ashbrook, D. G., Delprato, A., Grellmann, C., Klein, M., Wetzel, R., Overall, R. W., et al. (2014). Transcript Co-Variance With Nestin in Two Mouse Genetic Reference Populations Identifies Lef1 as a Novel Candidate Regulator of Neural Precursor Cell Proliferation in the Adult Hippocampus. Front. Neurosci. 8, 418. doi:10.3389/fnins.2014.00418
Christian, K. M., Song, H., and Ming, G.-l. (2014). Functions and Dysfunctions of Adult Hippocampal Neurogenesis. Annu. Rev. Neurosci. 37, 243–262. doi:10.1146/annurev-neuro-071013-014134
Chung, K. M., Park, H., Jung, S., Ha, S., Yoo, S.-J., Woo, H., et al. (2015). Calpain Determines the Propensity of Adult Hippocampal Neural Stem Cells to Autophagic Cell Death Following Insulin Withdrawal. Stem Cells 33 (10), 3052–3064. doi:10.1002/stem.2082
Dedieu, S., Mazères, G., Poussard, S., Brustis, J.-J., and Cottin, P. (2003). Myoblast Migration is Prevented by a Calpain-Dependent Accumulation of MARCKS. Biol. Cell 95 (9), 615–623. doi:10.1016/j.biolcel.2003.09.005
Doetsch, F., García-Verdugo, J. M., and Alvarez-Buylla, A. (1999). Regeneration of a Germinal Layer in the Adult Mammalian Brain. Proc. Natl. Acad. Sci. 96 (20), 11619–11624. doi:10.1073/pnas.96.20.11619
Dutt, P., Croall, D. E., Arthur, J. S. C., De Veyra, T., Williams, K., Elce, J. S., et al. (2006). m-Calpain is Required for Preimplantation Embryonic Development in Mice. BMC Dev. Biol. 6, 3. doi:10.1186/1471-213x-6-3
Ernst, A., Alkass, K., Bernard, S., Salehpour, M., Perl, S., Tisdale, J., et al. (2014). Neurogenesis in the Striatum of the Adult Human Brain. Cell 156 (5), 1072–1083. doi:10.1016/j.cell.2014.01.044
Gage, F. H. (2000). Mammalian Neural Stem Cells. Science 287 (5457), 1433–1438. doi:10.1126/science.287.5457.1433
Hamburger, V. (1980). Trophic Interactions in Neurogenesis: A Personal Historical Account. Annu. Rev. Neurosci. 3 (1), 269–278. doi:10.1146/annurev.ne.03.030180.001413
Honda, M., Masui, F., Kanzawa, N., Tsuchiya, T., and Toyo-oka, T. (2008). Specific Knockdown of m-Calpain Blocks Myogenesis with cDNA Deduced From the Corresponding RNAi. Am. J. Physiol. Cell Physiol. 294 (4), C957–C965. doi:10.1152/ajpcell.00505.2007
Huttenlocher, A., Palecek, S. P., Lu, Q., Zhang, W., Mellgren, R. L., Lauffenburger, D. A., et al. (1997). Regulation of Cell Migration by the Calcium-Dependent Protease Calpain. J. Biol. Chem. 272 (52), 32719–32722. doi:10.1074/jbc.272.52.32719
Ibrayeva, A., and Bonaguidi, M. A. (2015). Pushing and Pulling on Adult Neural Stem Cells. Cell Stem Cell 16 (5), 451–452. doi:10.1016/j.stem.2015.04.011
Jessberger, S., Gage, F. H., Eisch, A. J., and Lagace, D. C. (2009). Making a Neuron: Cdk5 in Embryonic and Adult Neurogenesis. Trends Neurosci. 32 (11), 575–582. doi:10.1016/j.tins.2009.07.002
Joy, J., Nalabothula, N., Ghosh, M., Popp, O., Jochum, M., Machleidt, W., et al. (2006). Identification of Calpain Cleavage Sites in the G1 Cyclin-Dependent Kinase Inhibitor p19INK4d. Biol. Chem. 387 (3), 329–335. doi:10.1515/bc.2006.044
Kawauchi, T. (2014). Cdk5 Regulates Multiple Cellular Events in Neural Development, Function and Disease. Develop. Growth Differ. 56, 335–348. doi:10.1111/dgd.12138
Lade, A., Ranganathan, S., Luo, J., and Monga, S. P. S. (2012). Calpain Induces N-Terminal Truncation of β-Catenin in Normal Murine Liver Development. J. Biol. Chem. 287 (27), 22789–22798. doi:10.1074/jbc.m112.378224
Lepousez, G., Valley, M. T., and Lledo, P.-M. (2013). The Impact of Adult Neurogenesis on Olfactory Bulb Circuits and Computations. Annu. Rev. Physiol. 75, 339–363. doi:10.1146/annurev-physiol-030212-183731
Liang, Z., Brown, R. C., Fletcher, J. C., and Opsahl-Sorteberg, H.-G. (2015). Calpain-Mediated Positional Information Directs Cell Wall Orientation to Sustain Plant Stem Cell Activity, Growth and Development. Plant Cell Physiol. 56 (9), 1855–1866. doi:10.1093/pcp/pcv110
Licursi, V., Conte, F., Fiscon, G., and Paci, P. (2019). MIENTURNET: An Interactive Web Tool for microRNA-Target Enrichment and Network-Based Analysis. BMC Bioinformatics 20 (1), 1–10. doi:10.1186/s12859-019-3105-x
Machado, V. M., Morte, M. I., Carreira, B. P., Azevedo, M. M., Takano, J., Iwata, N., et al. (2015). Involvement of Calpains in Adult Neurogenesis: Implications for Stroke. Front. Cell Neurosci. 9, 22. doi:10.3389/fncel.2015.00022
Merkle, F. T., Tramontin, A. D., García-Verdugo, J. M., and Alvarez-Buylla, A. (2004). Radial Glia Give Rise to Adult Neural Stem Cells in the Subventricular Zone. Proc. Natl. Acad. Sci. 101 (50), 17528–17532. doi:10.1073/pnas.0407893101
Ming, G.-l., and Song, H. (2005). Adult Neurogenesis in the Mammalian Central Nervous System. Annu. Rev. Neurosci. 28, 223–250. doi:10.1146/annurev.neuro.28.051804.101459
Mishiba, T., Tanaka, M., Mita, N., He, X., Sasamoto, K., Itohara, S., et al. (2014). Cdk5/p35 Functions as a Crucial Regulator of Spatial Learning and Memory. Mol. Brain 7, 82. doi:10.1186/s13041-014-0082-x
Moors, M., Cline, J., Abel, J., and Fritsche, E. (2007). ERK-Dependent and -Independent Pathways Trigger Human Neural Progenitor Cell Migration. Toxicol. Appl. Pharmacol. 221 (1), 57–67. doi:10.1016/j.taap.2007.02.018
Moudilou, E. N., Mouterfi, N., Exbrayat, J.-M., and Brun, C. (2010). Calpains Expression During Xenopus laevis Development. Tissue Cell 42 (5), 275–281. doi:10.1016/j.tice.2010.07.001
Moyen, C., Goudenege, S., Poussard, S., Sassi, A. H., Brustis, J.-J., and Cottin, P. (2004). Involvement of Micro-Calpain (CAPN 1) in Muscle Cell Differentiation. Int. J. Biochem. Cell Biol. 36 (4), 728–743. doi:10.1016/s1357-2725(03)00265-6
Persson, A., Lindberg, O. R., and Kuhn, H. G. (2013). Radixin Inhibition Decreases Adult Neural Progenitor Cell Migration and Proliferation In Vitro and In Vivo. Front. Cell Neurosci. 7, 161. doi:10.3389/fncel.2013.00161
Raynaud, F., Marcilhac, A., Chebli, K., Benyamin, Y., and Rossel, M. (2008). Calpain 2 Expression Pattern and Sub-Cellular Localization During Mouse Embryogenesis. Int. J. Dev. Biol. 52 (4), 383–388. doi:10.1387/ijdb.072448fr
Santos, D. M., Xavier, J. M., Morgado, A. L., Sola, S., and Rodrigues, C. M. (2012). Distinct Regulatory Functions of Calpain 1 and 2 During Neural Stem Cell Self-Renewal and Differentiation. PLoS One 7 (3), e33468. doi:10.1371/journal.pone.0033468
Shu, Y., Xiang, M., Zhang, P., Qi, G., He, F., and Zhang, Q. (2018). Wnt-5a Promotes Neural Development and Differentiation by Regulating CDK5 via Ca2+/Calpain Pathway. Cell Physiol. Biochem. 51 (6), 2604–2615. doi:10.1159/000495932
Spalding, K. L., Bergmann, O., Alkass, K., Bernard, S., Salehpour, M., Huttner, H. B., et al. (2013). Dynamics of Hippocampal Neurogenesis in Adult Humans. Cell 153 (6), 1219–1227. doi:10.1016/j.cell.2013.05.002
Storr, S. J., Carragher, N. O., Frame, M. C., Parr, T., and Martin, S. G. (2011). The Calpain System and Cancer. Nat. Rev. Cancer 11 (5), 364–374. doi:10.1038/nrc3050
Su, W., Bi, X., Wang, Y., and Baudry, M. (2021). Changes in Neurodegeneration-Related miRNAs in Brains From CAPN1−/− Mice. BBA Adv. 1, 100004. doi:10.1016/j.bbadva.2021.100004
Su, W., Zhou, Q., Wang, Y., Chishti, A., Li, Q. Q., Dayal, S., et al. (2020). Deletion of the Capn1 Gene Results in Alterations in Signaling Pathways Related to Alzheimer’s Disease, Protein Quality Control and Synaptic Plasticity in Mouse Brain. Front. Genet. 11, 334. doi:10.3389/fgene.2020.00334
Suh, H., Deng, W., and Gage, F. H. (2009). Signaling in Adult Neurogenesis. Annu. Rev. Cell Dev. Biol. 25, 253–275. doi:10.1146/annurev.cellbio.042308.113256
Sun, J., Liu, Y., Moreno, S., Baudry, M., and Bi, X. (2015). Imbalanced Mechanistic Target of Rapamycin C1 and C2 Activity in the Cerebellum of Angelman Syndrome Mice Impairs Motor Function. J. Neurosci. 35, 4706–4718. doi:10.1523/jneurosci.4276-14.2015
Takano, J., Mihira, N., Fujioka, R., Hosoki, E., Chishti, A. H., and Saido, T. C. (2011). Vital Role of the Calpain-Calpastatin System for Placental Integrity-Dependent Embryonic Survival. Mol. Cell. Biol. 31, 4097. doi:10.1128/MCB.05189-11
Vadodaria, K. C., and Gage, F. H. (2014). SnapShot: Adult Hippocampal Neurogenesis. Cell 156 (5), 1114. doi:10.1016/j.cell.2014.02.029
Wamstad, J. A., Alexander, J. M., Truty, R. M., Shrikumar, A., Li, F., Eilertson, K. E., et al. (2012). Dynamic and Coordinated Epigenetic Regulation of Developmental Transitions in the Cardiac Lineage. Cell 151 (1), 206–220. doi:10.1016/j.cell.2012.07.035
Wang, Y., Briz, V., Chishti, A., Bi, X., and Baudry, M. (2013). Distinct Roles for -Calpain and m-Calpain in Synaptic NMDAR-Mediated Neuroprotection and Extrasynaptic NMDAR-Mediated Neurodegeneration. J. Neurosci. 33 (48), 18880–18892. doi:10.1523/jneurosci.3293-13.2013
Wang, Y., Hersheson, J., Lopez, D., Hammer, M., Liu, Y., Lee, K.-H., et al. (2016). Defects in the CAPN1 Gene Result in Alterations in Cerebellar Development and Cerebellar Ataxia in Mice and Humans. Cell Rep. 16 (1), 79–91. doi:10.1016/j.celrep.2016.05.044
Wang, Y., and Zhang, Y. (2014). Regulation of TET Protein Stability by Calpains. Cell Rep. 6 (2), 278–284. doi:10.1016/j.celrep.2013.12.031
Wu, J. Q., Habegger, L., Noisa, P., Szekely, A., Qiu, C., Hutchison, S., et al. (2010). Dynamic Transcriptomes During Neural Differentiation of Human Embryonic Stem Cells Revealed by Short, Long, and Paired-End Sequencing. Proc. Natl. Acad. Sci. 107 (11), 5254–5259. doi:10.1073/pnas.0914114107
Wu, X., Liu, S., Hu, Z., Zhu, G., Zheng, G., and Wang, G. (2018). Enriched Housing Promotes Post-Stroke Neurogenesis Through Calpain 1-STAT3/HIF-1α/VEGF Signaling. Brain Res. Bull. 139, 133–143. doi:10.1016/j.brainresbull.2018.02.018
Yajima, Y., and Kawashima, S. (2002). Calpain Function in the Differentiation of Mesenchymal Stem Cells. Biol. Chem. 383 (5), 757–764. doi:10.1515/bc.2002.079
Zhang, Y.-y., Li, S.-F., Qian, S.-W., Zhang, Y.-Y., LiuTang, Y. Q.-Q., Tang, Q.-Q., et al. (2012). Phosphorylation Prevents C/EBPβ From the Calpain-Dependent Degradation. Biochem. Biophys. Res. Commun. 419, 550–555. doi:10.1016/j.bbrc.2012.02.058
Zhu, G., Liu, Y., Wang, Y., Bi, X., and Baudry, M. (2015). Different Patterns of Electrical Activity Lead to Long-Term Potentiation by Activating Different Intracellular Pathways. J. Neurosci. 35, 621–633. doi:10.1523/jneurosci.2193-14.2015
Keywords: calpain, neurogenesis, dentate gyrus, gene expression, miRNA
Citation: Baudry M, Su W, Seinfeld J, Sun J and Bi X (2021) Role of Calpain-1 in Neurogenesis. Front. Mol. Biosci. 8:685938. doi: 10.3389/fmolb.2021.685938
Received: 26 March 2021; Accepted: 01 June 2021;
Published: 15 June 2021.
Edited by:
Marianna Crispino, University of Naples Federico II, ItalyReviewed by:
Carla Perrone-Capano, University of Naples Federico II, ItalyGuy Massicotte, Université du Québec à Trois-Rivières, Canada
Copyright © 2021 Baudry, Su, Seinfeld, Sun and Bi. This is an open-access article distributed under the terms of the Creative Commons Attribution License (CC BY). The use, distribution or reproduction in other forums is permitted, provided the original author(s) and the copyright owner(s) are credited and that the original publication in this journal is cited, in accordance with accepted academic practice. No use, distribution or reproduction is permitted which does not comply with these terms.
*Correspondence: Michel Baudry, mbaudry@westernu.edu