Water-soluble trehalose glycolipids show superior Mincle binding and signaling but impaired phagocytosis and IL-1β production
- 1School of Chemical and Physical Sciences, Victoria University of Wellington, Wellington, New Zealand
- 2Centre for Biodiscovery, Victoria University of Wellington, Wellington, New Zealand
- 3Department of Molecular Immunology, Research Institute for Microbial Diseases, Osaka University, Osaka, Japan
- 4Laboratory of Molecular Immunology, Immunology Frontier Research Center, Osaka University, Osaka, Japan
- 5Division of Molecular Immunology, Medical Institute of Bioregulation, Kyushu University, Fukuoka, Japan
- 6Division of Molecular Immunology, Medical Mycology Research Center, Chiba University, Chiba, Japan
The tremendous potential of trehalose glycolipids as vaccine adjuvants has incentivized the study of how the structures of these ligands relate to their Mincle-mediated agonist activities. Despite this, structure-activity work in the field has been largely empirical, and less is known about how Mincle-independent pathways might be affected by different trehalose glycolipids, and whether Mincle binding by itself can serve as a proxy for adjuvanticity. There is also much demand for more water-soluble Mincle ligands. To address this need, we prepared polyethylene glycol modified trehalose glycolipids (PEG-TGLs) with enhanced water solubility and strong murine Mincle (mMincle) binding and signaling. However, only modest cytokine and chemokine responses were observed upon the treatment of GM-CSF treated bone-marrow cells with the PEG-TGLs. Notability, no IL-1β was observed. Using RNA-Seq analysis and a representative PEG-TGL, we determined that the more water-soluble adducts were less able to activate phagocytic pathways, and hence, failed to induce IL-1β production. Taken together, our data suggests that in addition to strong Mincle binding, which is a pre-requisite for Mincle-mediated cellular responses, the physical presentation of trehalose glycolipids in colloidal form is required for inflammasome activation, and hence, a strong inflammatory immune response.
1 Introduction
In recent years there has been much interest in the development of synthetic Macrophage Inducible C-type lectin (Mincle, Clec4e, or Clecsf9) agonists (Braganza et al., 2018; Williams, 2017; O’Hagan et al., 2020; Cramer, 2021). In particular, trehalose glycolipids have shown much promise as Mincle-mediated adjuvants (Foster et al., 2018; Ryter et al., 2020; Foster et al., 2020; Rasheed et al., 2020; Lynch et al., 2021; Ryter et al., 2021; Dangerfield et al., 2022). Trehalose dimycolate (TDM, 1, Figure 1A), a heterogenous pathogen associated molecular pattern (PAMP) isolated from the cell wall of Mycobacterium tuberculosis, and trehalose 6,6′-dibehenate (TDB, 2), a linear C22-synthetic derivative thereof, were the first non-proteinaceous Mincle ligands identified (Ishikawa et al., 2009; Schoenen et al., 2010). Following binding of TDM or TDB to Mincle, induction of the FcRγ-Syk-Card9-Bcl10-Malt1 signaling axis occurs, which leads to the NFκB-mediated expression of cytokines, chemokines, and small molecule mediators. (Ishikawa et al., 2009; Werninghaus et al., 2009; Schoenen et al., 2010; Kodar et al., 2017). Ultimately, these cellular mediators influence the adaptive immune response and T-helper cell differentiation. TDB has been formulated into a variety of dimethyldioctadecylammonium bromide (DDA)-containing liposomes, with or without other pathogen-associated molecular patterns (Pedersen et al., 2018). These liposomes have found wide application in several different vaccination models (Pedersen et al., 2018), with the DDA/TDB adjuvant system, CAF01, being used in clinical trials for HIV and TB vaccination (Kaufmann et al., 2010; Fomsgaard et al., 2011).
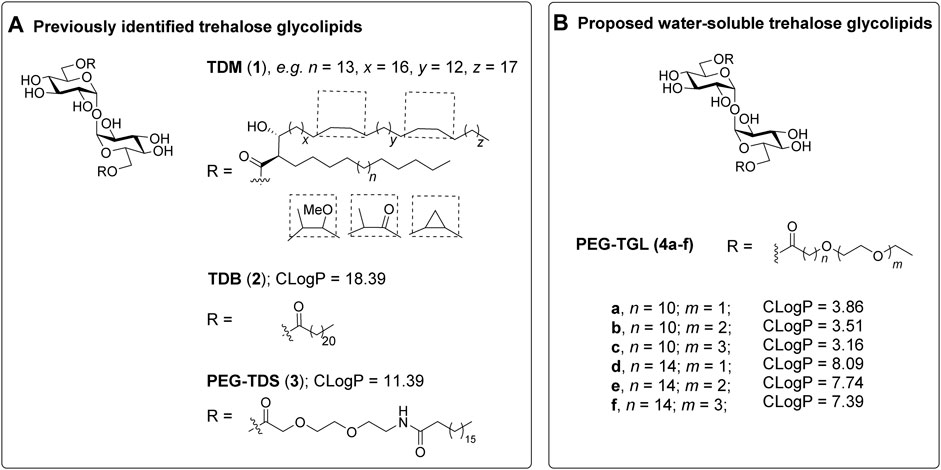
FIGURE 1. Trehalose glycolipids as Mincle-adjuvants. (A). The previously reported trehalose glycolipids TDM (1) (Ishikawa et al., 2009) and TDB (2) (Schoenen et al., 2010) exhibit good Mincle-mediated adjuvanticity, however water-soluble adduct, PEG-TDS (3) (Huber et al., 2016) exhibits only modest adjuvanticity, which was attributed to poor mMincle binding. (B) We proposed that PEGylated trehalose glycolipids 4a-f would retain good Mincle binding due to the positioning of the PEG groups at the terminus of the lipid. The calculated LogP values provide an indication of water solubility.
In addition to introducing new vaccine adjuvants, determining how changes to the structure of the trehalose glycolipid influences the ensuing immune response generates valuable mechanistic knowledge about those features of the trehalose glycolipid that are required for optimal agonist activity (Bird et al., 2018; Foster et al., 2018; Khan et al., 2018; Ryter et al., 2020; Dangerfield et al., 2022). As part of such studies, there has been interest in the development of TDB-analogues with improved water solubility to facilitate the formulation and delivery of this class of ligands (Jacobsen et al., 2015; Huber et al., 2016; O’Hagan et al., 2020). Lang and co-workers synthesized 6,6′-bis [8-(stearoylamido)-3,6-dioxaoctanoyl]-trehalose (PEG-TDS 3), which has a hydrophilic polyethylene glycol (PEG) group between the sugar moiety and the acyl chain (Huber et al., 2016). Unfortunately, PEG-TDS 3) exhibited modest adjuvanticity, both in vitro using bone marrow derived macrophage (BMDM) and bone marrow derived dendritic cell (BMDC) assays, and in vivo immunization assays using PEG-TDS/DDA liposomes with an antigen from Chlamydia trachomatis. The author’s attributed the limited immunostimulatory ability of PEG-TDS 3) to a lower binding affinity to Mincle (Huber et al., 2016).
Despite these observations, we were interested in determining whether a more water-soluble trehalose glycolipid with improved Mincle-binding, and hence, agonist-activity, could be developed. We reasoned that the placement of the water-soluble PEG spacer towards the end of an acyl chain would provide a portion of hydrophobic lipid that would be able to interact with the hydrophobic groove of Mincle (Feinberg et al., 2013; Furukawa et al., 2013; Feinberg et al., 2016), while the terminal PEG would improve the water solubility of the ligand. Molecular docking of PEG-modified TGLs showed that aliphatic chain lengths of around 12 carbons would be ideal to span Mincle’s lipophilic groove (Figure 2) (Maestro, 2021). This in turn allows for the incorporation of ethyleneglycol units at the lipid termini to provide for a more hydrophilic ligand. Thus, PEGylated trehalose glycolipids (PEG-TGLs, 4a-f) with differing lipophilic acyl chain lengths (n = 10 and 14) and number of PEG groups (m = 1, 2 or 3) were designed and the CLogP values calculated to provide an indication of lipophilicity (Leo et al., 1971), and hence, water solubility (Figure 1B).
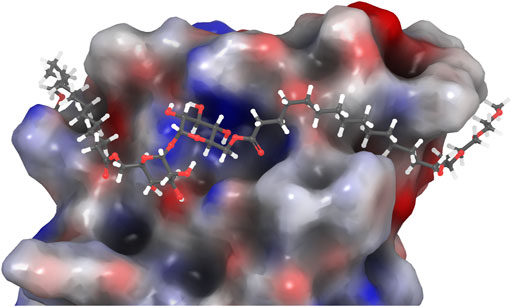
FIGURE 2. PEG-TGL 4f (m = 3, n = 14) docked in the binding site of hMincle (3WH2). The hydrophobic portion of the lipid binds to the hydrophobic groove of Mincle, while the hydrophilic PEG groups are found outside this binding groove and can increase the water solubility of the ligand (Maestro, 2021).
However, during our studies, we unexpectedly observed that Mincle binding affinity does not correlate to the immunomodulatory activity of the ligands, though does correlate to Mincle signaling. Moreover, our mechanistic studies suggest that this lack of functional immune response results from the inability of the water-soluble ligands to activate the inflammasome. Due to the importance of Mincle ligands as a burgeoning class of vaccine adjuvant (Williams, 2017; Braganza et al., 2018), our findings provide much needed guidance in the field of Mincle agonist design and shed light on the mechanisms involved in immune cell activation through Mincle- and non-Mincle-mediated pathways.
2 Materials and methods
2.1 Ethics
C57BL/6 wild-type and Mincle−/− mice were bred and housed in a conventional animal facility at the Malaghan Institute of Medical Research, Wellington, New Zealand. The experimental procedures used in this study were approved by the Victoria University Animal Ethics Committee (ethics approval number 26793) and by the Animal Care and Use Committee of the Research Institute for Microbial Diseases, Osaka University (Biken-AP-R03-17-0).
2.2 Chemical synthesis and analysis
PEG-TGLs 4a-f were synthesized from commercially available starting materials. TDB was prepared according to literature procedures (Khan et al., 2011). Full experimental procedures and compound characterization data are provided in the supporting information. Critical micelle concentrations were determined by dynamic light scattering (DSL) with a ZetasizerNano ZS (Malvern Instruments Ltd., Malvern, Worcestershire, United Kingdom) at 20°C. Data was analyzed using Malvern Dispersion Technology Software. All synthesized glycolipids were confirmed to be endotoxin free at a sensitivity level of ≤ 0.25 EU/mL by using the ToxinSensor™ Gel Clot Endotoxin Assay Kit (Gen Script) prior to biological evaluation. CLogP values were calculated using ChemDrawStd v 11.0.
2.3 Preparation of ligand-coated plates
Synthesized PEGylated glycolipids (4a-f) and TDB (2a) were dissolved in CHCl3:MeOH (2:1) to make stock solutions of 1 mM. The stock solutions were diluted to the appropriate concentration in isopropanol then added to 96-well plates (20 μL/well), and the solvent evaporated in a sterile hood.
2.4 NFAT-GFP Mincle reporter assays
2B4 T cells expressing NFAT-GFP along with mouse Mincle + FcRγ, human Mincle + FcRγ, or FcRγ only were maintained as previously described (Yamasaki et al., 2008). The 2B4 T cells transfected with NFAT-GFP were cultured in complete Roswell Park Memorial Institute medium [RPMI-1640 supplemented with 2 mM Glutamax (Gibco), 10% (v/v) fetal bovine serum (Gibco) and 1% (v/v) penicillin-streptomycin (Gibco)]. Cells (4 × 105 cells/mL, 100 μL/well) were then incubated with ligands coated on plates (0.1 or 1 nmol/well) for 18 h. The reporter cells were then harvested, stained with 4′,6-diamidino-2-phenylindole (DAPI), and NFAT-GFP expression monitored by flow cytometry (FACS Canto II). Expression of GFP by reporter cells were given as a percentage of total live, single cells.
2.5 GM-CSF treated bone-marrow cell assay with ligand-coated plates
Bone-marrow cells were collected from femurs and tibias of wild type (C57BL/6) or C57BL/6 Mincle−/− mice and the cells cultured as previously reported (50 ng/ml of GM-CSF) (Stocker et al., 2019). On day 8, all media together with non-adherent cells were removed by aspirating. Cells were harvested using Acutase (0.5 ml/well, 10–15 min) and resuspended at 1 × 106 cells/mL in cRPMI and were added (200 μL/well) to 96 well plates coated with TDB or trehalose glycolipids 4a-f (0.1 or 1 nmol/well). A positive control with 100 ng/ml LPS and a negative control with isopropanol were used. Stimulated cells were incubated for 24 h at 37°C before supernatants were collected and analyzed for cytokine or chemokine production. In an additional experimental set-up, bone-marrow cells from wild type (C57BL/6) mice were harvested and treated in the same manner as noted above except that 20 ng/ml of GM-CSF was added to the cell culture.
2.6 GM-CSF treated bone-marrow cell assay with solubilized ligands
Bone-marrow cells were collected from femurs and tibias of wild type (C57BL/6) or Mincle−/− mice and the cells cultured as previously reported (50 ng/ml of GM-CSF) (Stocker et al., 2019). On day 8, all media together with non-adherent cells were removed and 0.25 ml fresh media was added to each well (48 well plate). Stock solutions of TGLs 4a-f and TDB (1 mM, with 2% DMSO in sterile water) were prepared and kept sterile. From stock solutions, 1 or 10 μL were added to each well, to give a final ligand concentration of 4 or 40 μM in a total well volume of 250 μL. A positive control with 100 ng/ml LPS and a negative control with untreated cells were used. Stimulated cells were incubated for the indicated time (24 or 48 h) before supernatants were analyzed for cytokine production.
2.7 Cytokine and chemokine analysis
Levels of IL-1β (R&D systems), IL-6 (BD Biosciences), IL-10 (R&D systems), and MIP-2 (R&D systems) were determined using sandwich ELISA according to the manufacturer’s instructions.
2.8 mMincle binding assay
Trehalose glycolipids (4c and 4f) were coated on plates and incubated with mMincle-Ig or Ig alone [at a concentration of 3 μg/ml in binding buffer (1% BSA/TSM, BSA: bovine serum albumin; TSM buffer (pH7.0): 20 mM Tris-HCl, 1 mM CaCl2, 150 mM NaCl and 2 mM MgCl2)]. The preparation of Mincle fusion proteins was undertaken as described previously (Yamasaki et al., 2008). TDM (Sigma-Aldrich) and TDB were used as positive controls. Ligand bound protein mMincle-Ig was then detected using HRP-labelled anti-human Ig antibody via ELISA by optical density (OD) measurement at 450 nm [Multiskan JX and Ascent Software version 2.6 (Thermo Fisher Scientific)] and analyzed using Microsoft Excel (Microsoft).
2.9 mMincle competition assay
Glycolipids 4c or 4f (0, 0.01, 0.1 or 1 nmol/well) were co-plated with or without TDM or TDB (0.01 nmol/well) and incubated with NFAT-GFP reporter cells. GFP expression by the reporter cells, as determined using a flow cytometer (Attune NxT Flow Cytometer, Thermo Fisher), was used as a readout to measure the ability of the analogues to compete with TDM or TDB for recognition by Mincle.
2.10 RNA-Sequence protocol
Bone-marrow cells were collected from femurs and tibias of wild type (C57BL/6) mice and cells were cultured in RPMI-1640 supplemented with 10% (v/v) fetal bovine serum (NICHIREI), 2-mercaptoethanol (DS Pharma), penicillin G (Sigma-Aldrich), streptomycin (MP Biomedicals), and 20 ng/ml of mouse GM-CSF (BioLegend). On day 3, 10 ml of GM-CSF-containing media was added, and on day 9, cells were harvested and used for stimulation. 1 × 106 cells were stimulated with 0.6 nmol/well of indicated stimulants coated on a 24-well plate for 4 h. Cells were lysed with QIAsol (QIAGEN) to extract total RNA from the cells according to the manufacturer’s instructions. Library preparation was performed by TruSeq standard mRNA sample prep kit (Illumina) and whole transcriptome sequencing was applied to the RNA samples by using Illumina HiSeq 2,500 platform in 75-base single-end mode. The Illumina Casava ver.1.8.2 software was used for base calling. Sequenced reads were mapped to the mouse reference genome sequences (mm10) using TopHat ver.2.0.13 in combination with Bowtie2 ver.2.2.3 and SAMtools ver.0.1.19. The number of fragments per kilobase of exon per million mapped fragments was calculated using Cufflinks ver.2.2.1. Differential expression and pathway analysis of the RNA-Seq data was performed using iDEP (Ge et al., 2018), and KEGG pathway enrichment analysis and visualizations were generated using Pathview Web (Luo et al., 2017).
3 Results
3.1 Synthesis of PEGylated trehalose glycolipids
To synthesize the polyethyleneglycol modified trehalose glycolipids (PEG-TGLs) 4a-f, we first prepared a series of PEGylated carboxylic acids for subsequent coupling to trehalose. While 11-bromoundecanoic acid (8) is commercially available, 15-bromopentadecanoic acid (6) was synthesized from pentadecanolide (5) in a single step (78% yield), by treatment with HBr and H2SO4 (Davey and Hayman, 1998; Carter et al., 2012) (Figure 3A). Both bromides were then used in Williamson ether syntheses with mono-, di- and triethyleneglycol monomethyl ethers 7a-c to afford carboxylic acids 9a-f in 20–63% yield. For each ether, the formation of the linkage between bromo-carboxylic acids and ethylene glycol was confirmed by the observation of an HMBC between the terminal methylene protons of the alkyl chain (ca. δ 3.43 ppm) and the first carbon of the glycol moiety (ca. δ 70.1 ppm). During the ether syntheses, we observed the undesired β-elimination of the bromine atom in the starting carboxylic acid to give a terminal alkene in ca. 10% yield along with other unidentified by-products. Unfortunately, changes to the order and equivalents of reagents added, reaction temperatures, and solvent did not improve the yields. That said, it should be noted that similar yields have been reported for related compounds (Baker et al., 2013), illustrating the difficulties of undertaking Williamson ether syntheses using long lipophilic chains.
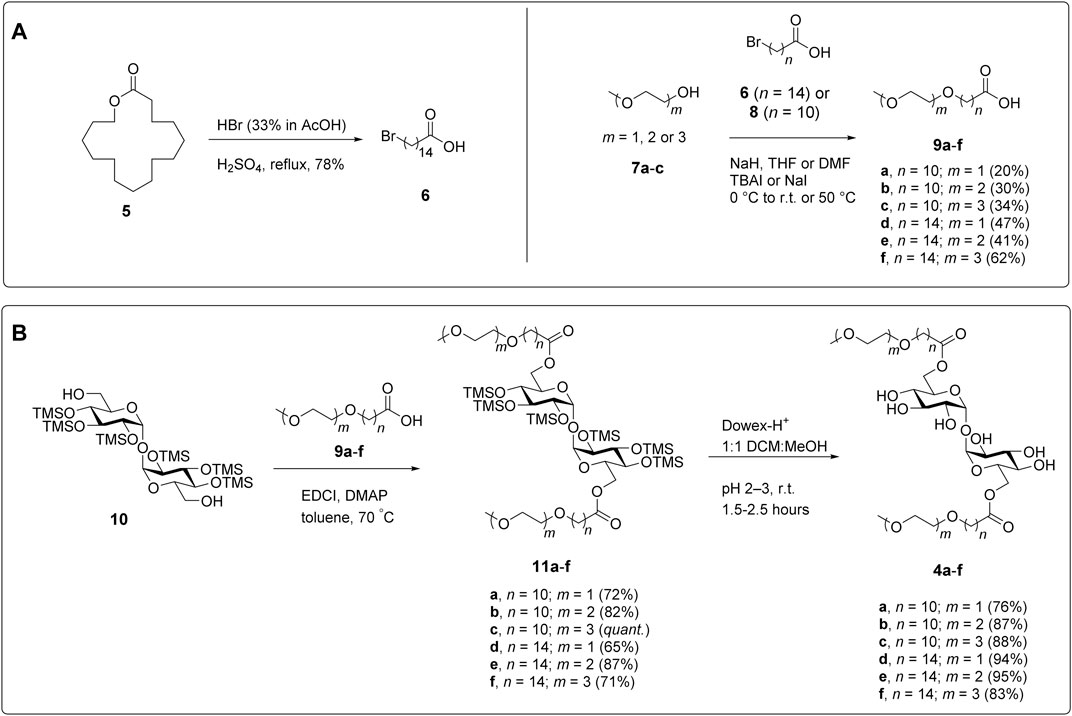
FIGURE 3. Synthesis of PEG-TGLs. (A) Preparation of the carboxylic acids required to install the PEGylated side chain. (B) Conjugation of the suitably functionalized trehalose scaffold (Khan et al., 2011) to the carboxylic acid side chains and deprotection to give the target PEG-TGLs (4a-f).
With the PEGylated side chains in hand, α,α′-D-trehalose was per-silylated under the agency of N,O-bis(trimethylsilyl)acetamide (BSA) and catalytic tetra-N-butylammonium fluoride (TBAF), followed by treatment with K2CO3 to afford TMS-protected trehalose 10 (Figure 3B) according to previously published procedures (Johnson, 1992; Khan et al., 2011). An EDCI-mediated esterification reaction between diol 10 and carboxylic acids 9a-f gave diesters 11a-f. Subsequent removal of the TMS groups under the agency of Dowex-H+ resin afforded the target PEG-TGLs 4a-f in good overall yield. When performing the final deprotection step it is important to ensure that the compounds are properly neutralized before storage otherwise they are prone to hydrolysis. We speculate that this might be due to the ability of the PEG-TGLs to co-ordinate to cations (e.g., H+, Na+) in much the same way that crown-ethers coordinate cations to augment base- or acid-catalyzed hydrolysis reactions.
3.2 PEGylated trehalose glycolipids exhibit robust mincle signaling but induce moderate chemokine and cytokine production by GM-CSF treated bone marrow cells
The PEGylated glycolipids 4a–f were tested for their ability to signal through Mincle using a NFAT-GFP reporter cell assay employing cells expressing mouse Mincle + FcRγ, or FcRγ only, at two different concentrations (0.1 and 1 nmol/well) of glycolipid. Analogues 4a (CLogP = 3.86) and 4b (CLogP = 3.51) with a shorter hydrophobic acyl length (n = 10) and with 1 or 2 glycol groups (m = 1 or 2, respectively), led to Mincle-mediated signaling in a dose dependent manner (Figure 4A). However, at the lower ligand concentration, the ability of 4a to signal through Mincle was slightly reduced compared to analogues 4d-f, which have longer lipid length (n = 14) and therefore greater CLogP values (i.e., CLogP = 8.09–7.39). The most water-soluble adduct, 4c (n = 10, m = 3, CLogP = 3.16) exhibited only a modest ability to signal through mMincle, and only at the highest ligand concentration tested (1 nmol/well). For the adducts containing a longer lipophilic portion (i.e., 4d-f, n = 14), there was little difference in the percentage of GFP-producing reporter cells at the two different ligand concentrations.
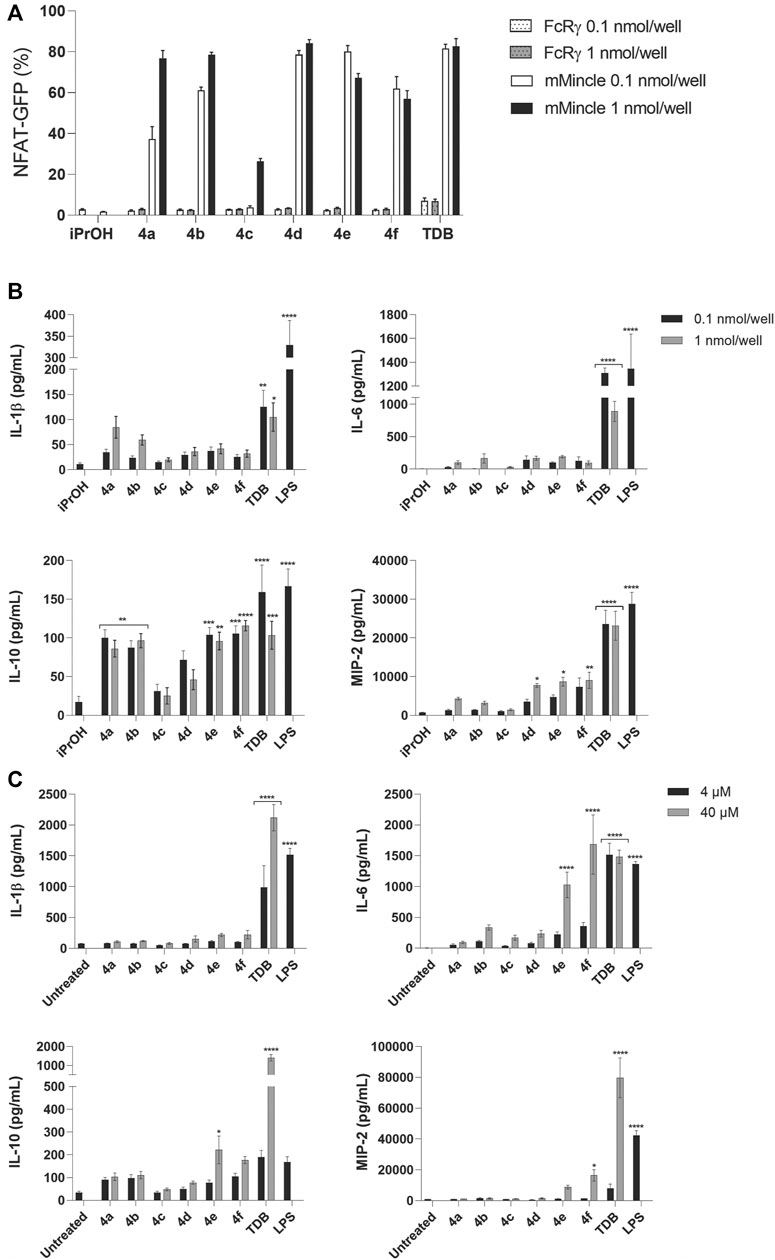
FIGURE 4. PEGylated TGLs (4a-f) led to strong mMincle signaling but modest cytokine production. (A) Reporter cells expressing mMincle + FcRγ or FcRγ only were stimulated with ligands coated on plates (0.1 and 1 nmol/well). NFAT-GFP expression by the harvested cells after 18 h was measured using flow cytometry. Data represents the mean of two independent experiments performed in duplicate (mean ± SEM). (B) WT C57 GM-CSF treated BMCs were stimulated with TDB or 4a-f (0.1 or 1 nmol/well) coated on plates, or LPS (100 ng/ml), and IL-1β, IL-6, IL-10 and MIP-2 were measured by ELISA from the supernatants collected after 24 h. Data represents the mean of three independent experiments performed in triplicate (mean ± SEM). Statistical significance was calculated in comparison to iPrOH control using two-way ANOVA (Dunnett’s multiple comparison test), *p ≤ 0.05; **p ≤ 0.01; ***p ≤ 0.001; ****p ≤ 0.0001. (C) GM-CSF treated BMCs were stimulated with TDB or trehalose glycolipids 4a-f (4 µM or 40 μM; solubilized in 2% DMSO in H2O), or LPS (100 ng/ml). IL-1β, IL-6, IL-10 and MIP-2 were measured by ELISA from the supernatants collected after 48 h. Data are representative of three independent experiments performed in triplicate (mean ± SEM). Statistical significance was calculated in comparison to untreated control using two-way ANOVA (Dunnett’s multiple comparison test), *p ≤ 0.05; **p ≤ 0.01; ***p ≤ 0.001; ****p ≤ 0.0001.
The PEGylated glycolipids 4a-f were then evaluated for their ability to generate a functional immune response, as determined by the production of the cytokines IL-1β, IL-6, and IL-10, and chemokine MIP-2, by GM-CSF-treated bone marrow cells (BMCs) in response to the ligands (Figure 4B). The GM-CSF promoted differentiation of cultured BMCs produces a heterogenous mixture of monocytes/macrophages, granulocytes, and dendritic cells (DCs) (Na et al., 2016; Sun et al., 2018). Using a high concentration of GM-CSF (50 ng/ml) favors a high proportion of monocytes/macrophages (Sun et al., 2018). Plates were coated with the ligands at concentrations of 0.1 and 1 nmol/well and cytokine and chemokine production measured at 24 h. As anticipated, TDB led to significant levels of IL-1β, IL-6, IL-10, and MIP-2, and the most water-soluble adduct 4c, which led to poor Mincle signaling, did not elicit a functional immune response. In contrast, the PEGylated glycolipids 4e and 4f led to statistically significant levels of IL-10 and MIP-2 but did not lead to the production of IL-6 or IL-1β, while 4a and 4b led to only the production of IL-10. All responses were Mincle-dependent, as demonstrated using Mincle−/− GM-CSF-treated BMCs (Supplementary Figure S1). In addition, we measured the response of BMCs treated with 20 ng/ml of GM-CSF, followed by stimulation with the glycolipids. This concentration of GM-CSF should also lead to a high proportion of monocytes/macrophages (Sun et al., 2018). With lower levels of GM-CSF added to the culture media, TDB, but not the other PEGylated adducts, led to significant levels of IL-1β, IL-10 and MIP-2 (Supplementary Figure S2). The small differences that were observed between the assay setups may be due to minor differences in the heterogeneity of the cell culture, or it could be due to the effect of GM-CSF itself (Bergamini et al., 2000).
To determine whether the activity of the PEGylated adducts could be improved by altering their physical presentation (Stocker et al., 2019), we added 4a-f to PBS (Khan et al., 2011; Stocker et al., 2014; Stocker et al., 2019) rather than using the ligand-coated format and measured the production of IL-1β, IL-6, IL-10 and MIP-2 by BMCs at 24 h (Supplementary Figure S3) and 48 h (Figure 4C). As anticipated, greater cytokine production was observed at 48 h (Khan et al., 2011), with levels of IL-6 being significantly enhanced by 4f and being comparable to those induced by TDB. However, no IL-1β was observed in response to any of the PEGylated glycolipids. To confirm the solubility of compound 4f, we analyzed both 4f and TDB using dynamic light scattering (DLS), and found that while TDB formed micelles at concentrations above 1.0 μM, no aggregates were observed for 4f.
3.3 PEGylated TGL 4f binds more strongly to mMincle than TDB but is not a competitive inhibitor
To investigate whether cytokine production was related to Mincle binding affinity, we determined the mMincle binding affinity of two representative compounds with the same number of PEG groups (m = 3) but with acyl chain portions of n = 10 (4c) or n = 14 (4f). The compounds were assessed for their binding affinity to mMincle-Ig at 0.01, 0.1, and 1.0 nmol/well, with ligand bound mMincle-Ig being detected through HRP-labelled anti-human Ig antibody using ELISA. Analogue 4c with the shorter hydrophobic portion (n = 10, CLogP = 3.16) showed no mMincle-Ig binding affinity, while 4f, with the longer hydrophobic portion (n = 14, CLogP = 7.39), bound mMincle with a higher affinity than TDB (2, CLogP = 18.39) at concentrations of 0.1 and 1 nmol/well (Figure 5A). Moreover, the mMincle binding affinity of 4f was slightly greater than that of TDM (1) at 1 mol/well, and much greater than that of TDB (2). Molecular docking of TDB and PEG-TGLs 4c and 4f into the hMincle binding site supported this observation with PEG-TGL 4f possessing the ideal number of CH2 units to span the Mincle hydrophobic groove (cf. Figure 2). The strong mMincle binding affinity of 4f prompted us to explore whether 4f might be a competitive inhibitor of TDB, and hence perhaps able to reduce the strong inflammatory response induced by TDM. However, 4f was not able to inhibit reporter cell activation by TDB or TDM at any of the concentrations tested (Figure 5B).
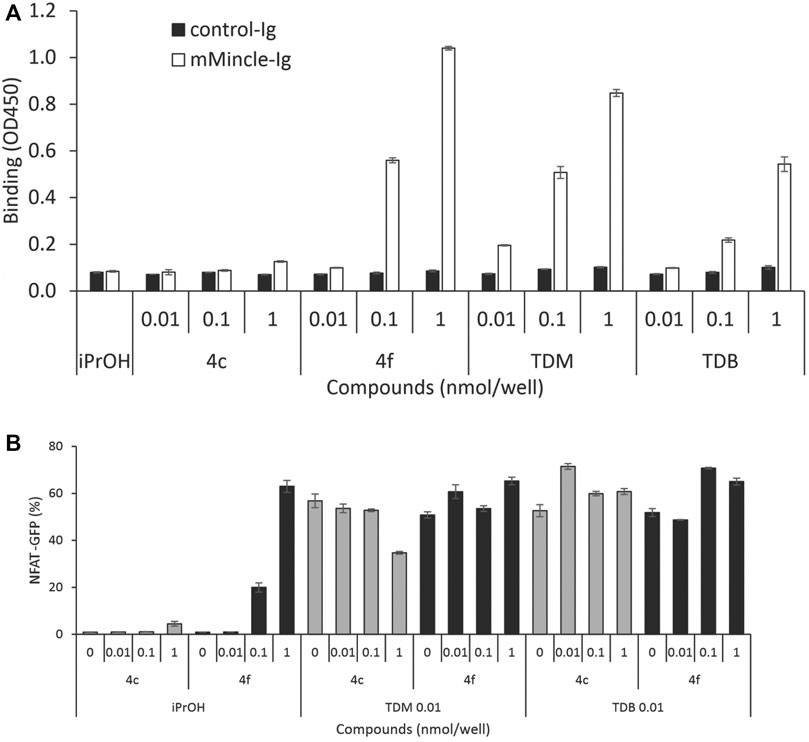
FIGURE 5. Water-soluble analogue 4f is a better ligand for mMincle compared to TDB and TDM but does not competitively inhibit the binding of TDB or TDM to mMincle. (A) Ligand coated plates (0.01, 0.1 or 1 nmol/well) were incubated with mMincle-Ig or Ig alone (3 μg/ml) for 14 h, and ligand bound protein determined using ELISA. Data obtained in a representative experiment performed in triplicate. (B) 2B4-NFAT-GFP reporter cells expressing mMincle were stimulated with 4c or 4f coated on plates (0, 0.01, 0.1 or 1 nmol/well) with TDM or TDB (0.01 nmol/well). GFP expression by the reporter cells was measured using flow cytometry after 18 h. Data obtained in a representative experiment performed in triplicate.
3.4 TDB and 4f activate similar immune cell signalling pathways although differences include pathways related to phagocytosis
We used RNA sequencing to analyze the transcriptome control of immune cell activation pathways upon the stimulation of GM-CSF treated BMCs with TDM or 4f (Figure 6). A total of 13,797 genes were detected, of which 947 and 656 were differentially upregulated with statistical significance (FDR >0.05, and fold change [FC] > 2) in the TDB and 4f-treated cells, respectively. Further hierarchical clustering analysis of the differentially expressed genes (DEGs) revealed four clusters of expression patterns (A–D) (Figures 6A,B) (Ge et al., 2018). KEGG pathway enrichment of cluster A and B revealed 15 significantly enriched pathway terms in each cluster. The majority of the pathway terms were linked to inflammation and immunity, including cytokine-cytokine receptor interaction, TNF signaling pathway, NF-κB signaling pathway, IL-17 signaling pathway, MAPK signaling pathway and C-type lectin receptor signaling pathway (Figure 6B). Further analysis of DEGs between the 4f and TDB treated groups revealed the relative down-regulation of pathways associated with phagocytosis (Figure 6C), including ribosome, phagosome (including FcRγ-mediated phagocytosis) and lysosome pathways, along with regulation of the actin cytoskeleton and focal adhesion (Luo et al., 2017).
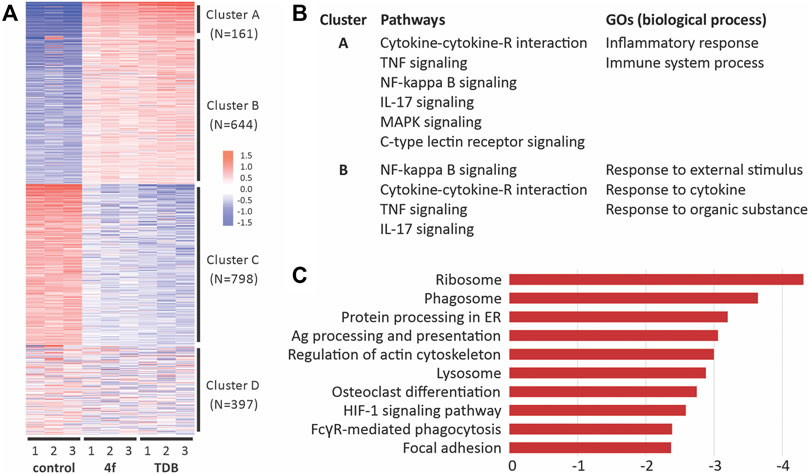
FIGURE 6. RNA-Seq analysis of gene pathway following the activation of GM-CSF treated BMCs with 4f and TDB reveals similarities in immune cell signaling but also differences in phagocytic pathways. (A). Hierarchical clustering of differentially expressed genes. (B). Functional enrichment of differentially expressed genes (DEGs) in response to 4f and TDB. (C). KEGG pathway enrichment analysis showing the top 10 down-regulated pathways between 4f vs TDB control samples.
4 Discussion
The desire to understand how the structure of trehalose glycolipids influences their immunomodulatory activity has been a topic of much interest in recent years (Foster et al., 2018; Ryter et al., 2020; Dangerfield et al., 2022). In particular, the poor solubility of long chain trehalose diesters potentially decreases their attractiveness as adjuvant candidates and has led to interest in more water-soluble adducts (Jacobsen et al., 2015; Huber et al., 2016; O’Hagan et al., 2020). One way to increase the water-solubility of trehalose glycolipids is to include polyethylene glycol (PEG) groups along the backbone of the lipid, with Lang and co-workers adopting this approach to produce 6,6′-bis [8-(stearoylamido)-3,6-dioxaoctanoyl]-trehalose (PEG-TDS 3) (Huber et al., 2016). Unfortunately, this adduct exhibited only modest Mincle-binding, and was not a promising Mincle adjuvant.
We reasoned that by incorporating PEG groups at the terminus of the trehalose glycolipid acyl chains, we could develop Mincle ligands (PEG-TGLs) that would not only be more water-soluble but would also retain good Mincle binding since the lipophilic acyl portion of the PEG-TGL would be sufficiently apolar to interact with the hydrophobic groove of Mincle (Feinberg et al., 2013; Furukawa et al., 2013; Feinberg et al., 2016). To this end, we successfully synthesized six PEGylated trehalose glycolipids 4a-f with improved water-solubility, as illustrated by the calculated LogP values of 3.16–8.09 (compared to TDB with a CLogP = 18.39), and these adducts were then tested for their ability to signal through mMincle using GFP reporter cells. All PEG-TGLs, except the most water-soluble adduct 4c, exhibited good mMincle signaling, thus confirming our hypothesis that through the judicious placement of the PEG groups on the acyl chain, more water-soluble trehalose glycolipids that maintain strong Mincle binding could be prepared. Both the hydrophilicity and length of the lipophilic portion engaging with Mincle affected the ensuing immune response with a longer lipophilic portion in the 6- and 6′-acyl chains (i.e., n = 14 vs. n = 10) tending to enhance Mincle signaling, while an increase in hydrophilicity (i.e., an increase in the number of ethylene glycol units) led to a slight decrease in Mincle signaling where n = 14.
In contrast, the poor ability of the PEG-TGLs to lead to the production of cytokines by GM-CSF treated BMCs was unexpected. While we anticipated that 4c, which exhibited poor mMincle signaling, would elicit a poor functional response, as previously reported in other studies (Huber et al., 2016; Foster et al., 2018), we were surprised to observe that PEG-TGL 4f, which had a stronger affinity for mMincle than TDB (2), also led to modest cytokine production. Knowing that assay set-up could affect the ensuing immune response (Stocker et al., 2019), we performed both ligand-coated and ligand solubilized assays. However, on the whole, the response to the PEG-TGLs was modest compared to TDB and less pro-inflammatory in nature. Notably, no IL-1β, a cytokine indicative of promising adjuvanticity (Nakae et al., 2001), was observed. Given that macrophage, rather than DC, are responsible for inflammatory activity the GM-CSF model (Erlich et al., 2019), we can assume that it is macrophage, and not DCs, that effect IL-1β production in our experimental set-up.
The distinct lack of IL-1β production in response to 4f and the other PEG-TGLs prompted us to consider the mechanism by which 4f and TDB lead to IL-1β production. Although we, and others, had previously determined that glycolipid presentation can influence the immune response to trehalose glycolipids (Kallerup et al., 2017; Stocker et al., 2019), the molecular mechanisms for this were not investigated. Moreover, we had previously observed that short-chain trehalose glycolipids led to a less inflammatory immune response (Khan et al., 2011; Khan et al., 2018), though had assumed that this might be related to poor Mincle binding. However, our data in this study indicated that lipid binding was not the only consideration for IL-1β production.
Two signals are required for inflammasome activation and IL-1β production. Mincle binding is a prerequisite for induction of the FcRγ-Syk-Card9 signaling axis which mediates the TDB- or TDM-induced signaling for NF-κB activation and the production of pro-inflammatory cytokines (Ishikawa et al., 2009; Werninghaus et al., 2009; Schoenen et al., 2010). Insomuch, this constitutes signal-1 for inflammasome activation. Signal-2 of NLPR3 inflammasome activation by TDB has been reported to require phagocytosis, lysosomal acidification, and cathepsin activity, with the particulate nature of TDB believed to be key in this process (Schweneker et al., 2013). While this may indicate that the induction of phagocytic pathways plays an essential role in the ability of trehalose glycolipids to generate an inflammatory immune response, there are many ways by which the inflammasome can be activated (Swanson et al., 2019; Zheng et al., 2020).
To understand the mechanistic rationale behind the ability of TDB, and to a lesser extent, PEG-TGL 4f, to activate GM-CSF treated BMCs, we used RNA sequencing to analyze the transcriptome control of immune cell activation pathways. Lang and co-workers had explored the transcriptome responses by wildtype and Mincle−/− bone marrow derived macrophages (BMDMs) in response to TDM and TDB and revealed a variety of Mincle-dependent and Mincle-independent pathways that were associated with the innate immune responses and cell cycle regulation, respectively (Hansen et al., 2019). The Mincle-dependent pathways included strong enrichment for endo/lysosomal and transport proteins, a finding that we also observed upon the treatment of GM-CSF treated BMCs with TDB. In contrast, compared to TDB, PEG-TGL 4f did not lead to similar upregulation of Fcγ-R-mediated phagocytosis or associated pathways such as phagosome, lysosome, and actin-cytoskeleton regulation. Taken together, this suggests that the water-soluble nature of PEG-TGL 4f fails to initiate the phagocytosis-mediated signal-2 of inflammasome activation, which in turn, prevents the cleavage of pro-IL-1β into IL-1β (Figure 7).
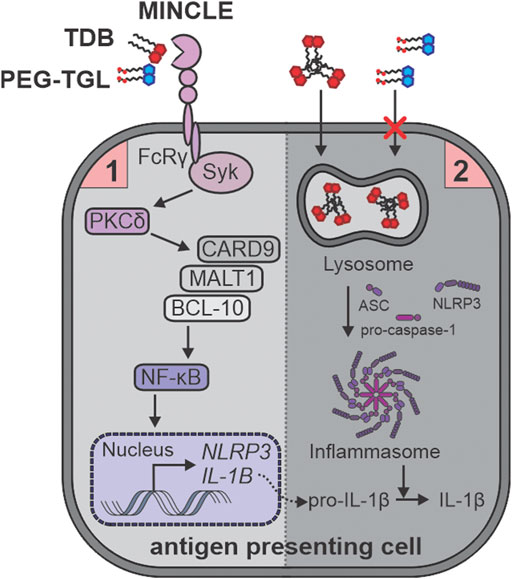
FIGURE 7. TDB (2), but not PEG-TGL (4f), leads to lysosome-mediated activation of the inflammasome and IL-1b production. TDB (2) and PEG-TGL (4f) are both bind to and signal via Mincle, thus providing the priming signal-1 for inflammasome activation. Only TDB (2) can sufficiently induce phagocytosis, and thus, signal-2 for inflammasome activation. This leads to TDB (2), but not PEG-TGL (4f), mediated IL-1b production.
With these findings in mind, it begs the question whether PEG-TGL 4f, or indeed, any water-soluble trehalose glycolipid, would make a good adjuvant? Strong Mincle binding is clearly a pre-requisite for good Mincle-mediated cellular responses, and ligands need to be designed to ensure that the Mincle binding affinity is retained. Following this, understanding how Mincle ligands activate the inflammasome, and hence, the production of IL-1β, is more complex. It has been proposed that TDM and TDB lead to the multimerization of Mincle and other related CLRs, such as the macrophage C-type lectin (MCL), with this multimerization enhancing inflammatory gene responses and phagocytosis (Lobato-Pascual et al., 2013; Yamasaki, 2013). Mincle has also recently been shown to mediate self-lipid endocytosis (Kostarnoy et al., 2022). However, our data demonstrated that any such Mincle multimerization does not sufficiently engage the Fcγ-R-mediated phagocytotic pathway that is needed for IL-1β production in response to trehalose glycolipids, and that colloids, rather than the coordinated properties of discrete compounds, are required for a good pro-inflammatory response to Mincle ligands.
It is difficult to say whether water-solubility can be used as a proxy for inflammasome activation by trehalose glycolipids, however, this is something to be considered when designing new Mincle agonists. The lack of IL-1β production in response to short-chain trehalose glycolipids (CLogP = 8.61, for iso-C12 + 1) supports the notion that water-solubility is related to inflammasome activation (Khan et al., 2011; Khan et al., 2018), although the monoester of TDB (CLogP = 7.56) has been reported to lead to IL-1β by macrophages (Stocker et al., 2014). Notwithstanding, the immune responses towards the mono-esters are generally poorer than those elicited by their diester counterparts (Huber et al., 2016; Bird et al., 2018). Whether the delivery of water-soluble ligands with particulate adjuvants, such as alum (Eisenbarth et al., 2008), or in liposomal formulations (Kallerup et al., 2017), can be used to develop effective water-soluble Mincle ligands also remains to be seen.
With the tremendous amount of synthetic effort that is put into the design and synthesis of Mincle agonists (Williams, 2017; Braganza et al., 2018), it is of value to have more guidance as it relates to optimizing Mincle ligands for their potential adjuvanticity. To this end, we have demonstrated that both Mincle binding, and the ability of the ligands to activate the inflammasome, through mechanisms that are presumably related to the particulate nature of the substrates, are important. Indeed, it may well be that the amphiphilic nature of trehalose glycolipids, which is what makes them somewhat water insoluble and which can complicate the interpretation of experiments, plays a critical role in their effectiveness as adjuvants. There also appears to be a fine balance between improved water solubility and retention of the pro-inflammatory activity of this class of compound. Accordingly, when screening for Mincle agonists, it is suggested that Mincle ligand binding and signaling, along with the use of the appropriate functional assays, are undertaken to better allow for the identification of lead compounds.
Data availability statement
The datasets presented in this study can be found in online repositories. The names of the repository/repositories and accession number(s) can be found below: https://www.ncbi.nlm.nih.gov/, GSE210869.
Ethics statement
The animal study was reviewed and approved by Victoria University Animal Ethics Committee (ethics approval number 26793) Animal Care and Use Committee of the Research Institute for Microbial Diseases, Osaka University (Biken-AP-R03-17-0).
Author contributions
BS and MT conceived the presented ideas. TM and AW synthesized the compounds. TM, ED, SI, and BL performed the in vitro experiments, with support from BS, MT, and SY. MT contributed to statistical analyses and undertook the computational studies. BS wrote the manuscript with support from MT. All authors provided critical feedback and helped shape the research, analysis, and manuscript.
Funding
The research in this publication was supported by the Royal Society of New Zealand Marsden Fund (VUW1401), the Health Research Council of New Zealand (VUW20/928), and AMED (JP223fa727001) for financial support.
Conflict of interest
The authors declare that the research was conducted in the absence of any commercial or financial relationships that could be construed as a potential conflict of interest.
Publisher’s note
All claims expressed in this article are solely those of the authors and do not necessarily represent those of their affiliated organizations, or those of the publisher, the editors and the reviewers. Any product that may be evaluated in this article, or claim that may be made by its manufacturer, is not guaranteed or endorsed by the publisher.
Supplementary material
The Supplementary Material for this article can be found online at: https://www.frontiersin.org/articles/10.3389/fmolb.2022.1015210/full#supplementary-material
References
Baker, Y. R., Galloway, W. R. J. D., Hodgkinson, J. T., and Spring, D. R. (2013). Design and synthesis of a biotinylated chemical probe for detecting the molecular targets of an inhibitor of the production of the Pseudomonas aeruginosa virulence factor pyocyanin. Molecules 18 (10), 11783–11796. doi:10.3390/molecules181011783
Bergamini, A., Bolacchi, F., Bongiovanni, B., Cepparulo, M., Ventura, L., Capozzi, M., et al. (2000). Granulocyte–macrophage colony-stimulating factor regulates cytokine production in cultured macrophages through CD14-dependent and -independent mechanisms. Immunology 101 (2), 254–261. doi:10.1046/j.1365-2567.2000.00117.x
Bird, J. H., Khan, A. A., Nishimura, N., Yamasaki, S., Timmer, M. S. M., and Stocker, B. L. (2018). Synthesis of branched trehalose glycolipids and their Mincle agonist Activity. J. Org. Chem. 83 (15), 7593–7605. doi:10.1021/acs.joc.7b03269
Braganza, C. D., Teunissen, T., Timmer, M. S. M., and Stocker, B. L. (2018). Identification and biological activity of synthetic macrophage inducible C-type lectin ligands. Front. Immunol. 8, 1940. doi:10.3389/fimmu.2017.01940
Carter, B. M., Wiesenauer, B. R., Hatakeyama, E. S., Barton, J. L., Noble, R. D., and Gin, D. L. (2012). Glycerol-based bicontinuous cubic lyotropic liquid crystal monomer system for the fabrication of thin-film membranes with uniform nanopores. Chem. Mater. 24 (21), 4005–4007. doi:10.1021/cm302027s
Cramer, J. (2021). Medicinal chemistry of the myeloid C-type lectin receptors Mincle, Langerin, and DC-SIGN. RSC Med. Chem. 12, 1985–2000. doi:10.1039/d1md00238d
Dangerfield, E. M., Lynch, A. T., Kodar, K., Stocker, B. L., and Timmer, M. S. M. (2022). Amide-linked brartemicin glycolipids exhibit Mincle-mediated agonist activity in vitro. Carbohydr. Res. 511, 108461. doi:10.1016/j.carres.2021.108461
Davey, T. W., and Hayman, A. R. (1998). Synthesis of ω-hydroxy quaternary ammonium bolaform surfactants. Aust. J. Chem. 51 (7), 581–586. doi:10.1071/C98001
Eisenbarth, S. C., Colegio, O. R., O’Connor, W., Sutterwala, F. S., and Flavell, R. A. (2008). Crucial role for the Nalp3 inflammasome in the immunostimulatory properties of aluminium adjuvants. Nature 453, 1122–1126. doi:10.1038/nature06939
Erlich, Z., Shlomovitz, I., Edry-Botzer, L., Cohen, H., Frank, D., Wang, H., et al. (2019). Macrophages, rather than DCs, are responsible for inflammasome activity in the GM-CSF BMDC model. Nat. Immunol. 20 (4), 397–406. doi:10.1038/s41590-019-0313-5
Feinberg, H., Rambaruth, N. D. S., Jégouzo, S. A. F., Jacobsen, K. M., Djurhuus, R., Poulsen, T. B., et al. (2016). Binding sites for acylated trehalose analogs of glycolipid ligands on an extended carbohydrate recognition domain of the macrophage receptor Mincle. J. Biol. Chem. 291 (40), 21222–21233. doi:10.1074/jbc.M116.749515
Feinberg, H., Thomas, S. A. F., Rowntree, T. J. W., Guan, Y., Brash, M. A., Brash, M. A., et al. (2013). Mechanism for recognition of an unusual mycobacterial glycolipid by the macrophage receptor mincle. J. Biol. Chem. 288 (40), 28457–28465. doi:10.1074/jbc.M113.497149
Fomsgaard, A., Karlsson, I., Gram, G., Schou, C., Tang, S., Bang, P., et al. (2011). Development and preclinical safety evaluation of a new therapeutic HIV-1 vaccine based on 18 T-cell minimal epitope peptides applying a novel cationic adjuvant CAF01. Vaccine 29 (40), 7067–7074. doi:10.1016/j.vaccine.2011.07.025
Foster, A. J., Kodar, K., Timmer, M. S. M., and Stocker, B. L. (2020). ortho-Substituted lipidated Brartemicin derivative shows promising Mincle-mediated adjuvant activity. Org. Biomol. Chem. 18, 1095–1103. doi:10.1039/C9OB02397F
Foster, A. J., Nagata, M., Lu, X., Lynch, A. T., Omahdi, Z., Ishikawa, E., et al. (2018). Lipidated brartemicin analogues are potent Th1-stimulating vaccine adjuvants. J. Med. Chem. 61 (3), 1045–1060. doi:10.1021/acs.jmedchem.7b01468
Furukawa, A., Kamishikiryo, J., Mori, D., Toyonaga, K., Okabe, Y., Toji, A., et al. (2013). Structural analysis for glycolipid recognition by the C-type lectins Mincle and MCL. Proc. Natl. Acad. Sci. U. S. A. 110 (43), 17438–17443. doi:10.1073/pnas.1312649110
Ge, S. X., Son, E. W., and Yao, R. (2018). iDEP: an integrated web application for differential expression and pathway analysis of RNA-Seq data. BMC Bioinformatics 19, 534. doi:10.1186/s12859-018-2486-6
Hansen, M., Peltier, J., Killy, B., Amin, B., Bodendorfer, B., Härtlova, A., et al. (2019). Macrophage phosphoproteome analysis reveals MINCLE-dependent and -independent mycobacterial cord factor signaling. Mol. Cell. Proteomics 18 (4), 669–685. doi:10.1074/mcp.RA118.000929
Huber, A., Kallerup, R. S., Korsholm, K. S., Franzyk, H., Lepenies, B., Christensen, D., et al. (2016). Trehalose diester glycolipids are superior to the monoesters in binding to Mincle, activation of macrophages in vitro and adjuvant activity in vivo. Innate Immun. 22 (6), 405–418. doi:10.1177/1753425916651132
Ishikawa, E., Ishikawa, T., Morita, Y. S., Toyonaga, K., Yamada, H., Takeuchi, O., et al. (2009). Direct recognition of the mycobacterial glycolipid, trehalose dimycolate, by C-type lectin Mincle. J. Exp. Med. 206 (13), 2879–2888. doi:10.1084/jem.20091750
Jacobsen, K. M., Keiding, U. B., Clement, L. L., Schaffert, E. S., Ramaruth, N. D. S., Johannsen, M., et al. (2015). The natural product brartemicin is a high affinity ligand for the carbohydrate-recognition domain of the macrophage receptor mincle. Med. Chem. Commun. 6 (4), 647–652. doi:10.1039/c4md00512k
Johnson, D. A. (1992). Simple procedure for the preparation of trimethylsilyl ethers of carbohydrates and alcohols. Carbohydr. Res. 237, 313–318. doi:10.1016/S0008-6215(92)84254-P
Kallerup, R. S., Franzyk, H., Schiøth, M. L., Martin-Bertelsen, B., Rose, F., Madsen, C. M., et al. (2017). Adjuvants based on synthetic mycobacterial cord factor analogues: Biophysical properties of neat glycolipids and nanoself-assemblies with DDA. Mol. Pharm. 14 (7), 2294–2306. doi:10.1021/acs.molpharmaceut.7b00170
Kaufmann, S. H. E., Hussey, G., and Lambert, P.-H. (2010). New vaccines for tuberculosis. Lancet 375 (9731), 2110–2119. doi:10.1016/S0140-6736(10)60393-5
Khan, A. A., Chee, S. H., McLaughlin, R. J., Harper, J. L., Kamena, F., Timmer, M. S. M., et al. (2011). Long-chain lipids are required for the innate immune recognition of trehalose diesters by macrophages. ChemBioChem 12 (17), 2572–2576. doi:10.1002/cbic.201100451
Khan, A., Kodar, K., Timmer, M. S. M., and Stocker, B. L. (2018). Lipid length and iso-branching of trehalose diesters influences Mincle agonist activity. Tetrahedron 74 (12), 1269–1277. doi:10.1016/j.tet.2017.11.076
Kodar, K., Harper, J. L., McConnell, M. J., Timmer, M. S. M., and Stocker, B. L. (2017). The Mincle ligand trehalose dibehenate differentially modulates M1-like and M2-like macrophage phenotype and function via Syk signaling. Immun. Inflamm. Dis. 5 (4), 503–514. doi:10.1002/iid3.186
Kostarnoy, A. V., Gancheva, P. G., Kireev, I. I., Soloviev, A. I., Lepenies, B., Kulibin, A. Y., et al. (2022). A mechanism of self-lipid endocytosis mediated by the receptor Mincle. Proc. Natl. Acad. Sci. U. S. A. 119 (30), e2120489119. doi:10.1073/pnas.2120489119
Leo, A., Hansch, C., and Elkins, D. (1971). Partition coefficients and their uses. Chem. Rev. 71 (6), 525–616. doi:10.1021/cr60274a001
Lobato-Pascual, A., Saether, P. C., Fossum, S., Dissen, E., and Daws, M. R. (2013). Mincle, the receptor for mycobacterial cord factor, forms a functional receptor complex with MCL and Fc εRI-γ. Eur. J. Immunol. 43 (12), 3167–3174. doi:10.1002/eji.201343752
Luo, W., Pant, G., Bhavnasi, Y. K., Blanchard, S. G., and Brouwer, C. (2017). Pathview web: User friendly pathway visualization and data integration. Nucleic Acids Res. 45, W501–W508. doi:10.1093/nar/gkx372
Lynch, A. T., Motozono, C., Foster, A. J., Kodar, K., Dangerfield, E. M., Yamasaki, S., et al. (2021). Trehalose diamide glycolipids augment antigen-specific antibody responses in a Mincle-dependent manner. Bioorg. Chem. 110, 104747. doi:10.1016/j.bioorg.2021.104747
Maestro, S. (2021). Molecular docking was performed using the Schrödinger software package, using the crystal structure of hMincle 3wh2 from the Protein Data Bank (PDB). LLC, New York, NY: Release.
Na, Y. R., Jung, D., Gu, G. J., and Seok, S. H. (2016). GM-CSF grown bone marrow derived cells are composed of phenotypically different dendritic cells and macrophages. Mol. Cells 39 (10), 734–741. doi:10.14348/molcells.2016.0160
Nakae, S., Asano, M., Horai, R., and Iwakura, Y. (2001). Interleukin-1 beta, but not interleukin-1 alpha, is required for T-cell-dependent antibody production. Immunology 104 (4), 402–409. doi:10.1046/j.1365-2567.2001.01337.x
O’Hagan, D. T., Lodaya, R. N., and Lofano, G. (2020). The continued advance of vaccine adjuvants – ‘we can work it out. Semin. Immunol. 50, 101426. doi:10.1016/j.smim.2020.101426
Pedersen, G. K., Andersen, P., and Christensen, D. (2018). Immunocorrelates of CAF family adjuvants. Semin. Immunol. 39, 4–13. doi:10.1016/j.smim.2018.10.003
Rasheed, O. K., Ettenger, G., Buhl, C., Child, R., Miller, S. M., Evans, J. T., et al. (2020). 6, 6´-Aryl trehalose analogs as potential Mincle ligands. Bioorg. Med. Chem. 28 (14), 115564. doi:10.1016/j.bmc.2020.115564
Ryter, K. T., Ettenger, G., Rasheed, O. K., Buhl, C., Child, R., Miller, S. M., et al. (2020). Aryl trehalose derivatives as vaccine adjuvants for Mycobacterium tuberculosis. J. Med. Chem. 63 (1), 309–320. doi:10.1021/acs.jmedchem.9b01598
Ryter, K. T., Rasheed, O. K., Buhl, C., and Evans, J. T. (2021). Design of trehalose-based amide/sulfonamide C-type lectin receptor signaling compounds. ChemMedChem 16 (8), 1246–1251. doi:10.1002/cmdc.202000775
Schoenen, H., Bodendorfer, B., Hitchens, K., Manzanero, S., Werninghaus, K., Nimmerjahn, F., et al. (2010). Cutting edge: Mincle is essential for recognition and adjuvanticity of the mycobacterial cord factor and its synthetic analog trehalose-dibehenate. J. Immunol. 184 (6), 2756–2760. doi:10.4049/jimmunol.0904013
Schweneker, K., Gorka, O., Schweneker, M., Poeck, H., Tschopp, J., Peschel, C., et al. (2013). The mycobacterial cord factor adjuvant analogue trehalose-6, 6′-dibehenate (TDB) activates the Nlrp3 inflammasome. Immunobiology 218 (4), 664–673. doi:10.1016/j.imbio.2012.07.029
Stocker, B. L., Khan, A. A., Chee, S. H., Kamena, F., and Timmer, M. S. M. (2014). On one leg: Trehalose mono-esters activate macrophages in a Mincle-dependant Manner. ChemBioChem 15 (3), 382–388. doi:10.1002/cbic.201300674
Stocker, B. L., Kodar, K., Wahi, K., Foster, A. J., Harper, A. J., Mori, D., et al. (2019). The effects of trehalose glycolipid presentation on cytokine production by GM-CSF macrophages. Glycoconj. J. 36 (1), 69–78. doi:10.1007/s10719-018-09857-9
Sun, L., Rautela, J., Delconte, R. B., Souza-Fonseca-Guimaraes, F., Carrington, E. M., Schenk, R. L., et al. (2018). GM-CSF quantity has a selective effect on granulocytic vs. monocytic myeloid development and function. Front. Immunol. 9, 1922. doi:10.3389/fimmu.2018.01922
Swanson, K. V., Deng, M., and Ting, J. P.-Y. (2019). The NLRP3 inflammasome: molecular activation and regulation to therapeutics. Nat. Rev. Immunol. 19, 477–489. doi:10.1038/s41577-019-0165-0
Werninghaus, K., Babiak, A., Gross, O., Hölscher, C., Dietrich, H., Agger, E. M., et al. (2009). Adjuvanticity of a synthetic cord factor analogue for subunit Mycobacterium tuberculosis vaccination requires FcRgamma-Syk-Card9-dependent innate immune activation. J. Exp. Med. 206 (1), 89–97. doi:10.1084/jem.20081445
Williams, S. J. (2017). Sensing lipids with mincle: Structure and function. Front. Immunol. 8, 1662. doi:10.3389/fimmu.2017.01662
Yamasaki, S., Ishikawa, E., Sakuma, M., Hara, H., Ogata, K., and Saito, T. (2008). Mincle is an ITAM-coupled activating receptor that senses damaged cells. Nat. Immunol. 9 (10), 1179–1188. doi:10.1038/ni.1651
Yamasaki, S. (2013). Signaling while eating: MCL is coupled with Mincle. Eur. J. Immunol. 43 (12), 3156–3158. doi:10.1002/eji.201344131
Keywords: Mincle, glycolipid, chemical immunology, synthesis, trehalose glycolipid, C-type lectin, adjuvant, inflammasome
Citation: Manthrirathna MATP, Dangerfield EM, Ishizuka S, Woods A, Luong BS, Yamasaki S, Timmer MSM and Stocker BL (2022) Water-soluble trehalose glycolipids show superior Mincle binding and signaling but impaired phagocytosis and IL-1β production. Front. Mol. Biosci. 9:1015210. doi: 10.3389/fmolb.2022.1015210
Received: 09 August 2022; Accepted: 25 October 2022;
Published: 24 November 2022.
Edited by:
M. Florencia Haurat, United States Food and Drug Administration, United StatesReviewed by:
Roland Lang, University Hospital Erlangen, GermanyKendal T. Ryter, University of Montana, United States
Copyright © 2022 Manthrirathna, Dangerfield, Ishizuka, Woods, Luong, Yamasaki, Timmer and Stocker. This is an open-access article distributed under the terms of the Creative Commons Attribution License (CC BY). The use, distribution or reproduction in other forums is permitted, provided the original author(s) and the copyright owner(s) are credited and that the original publication in this journal is cited, in accordance with accepted academic practice. No use, distribution or reproduction is permitted which does not comply with these terms.
*Correspondence: Bridget L. Stocker, bridget.stocker@vuw.ac.nz; Mattie S. M. Timmer, mattie.timmer@vuw.ac.nz