Human Sulfotransferase Assays With PAPS Production in situ
- 1Pharmaceutical and Medicinal Chemistry (Pharmaceutical Analyses), Institute of Pharmacy, Freie Universitaet Berlin, Berlin, Germany
- 2School of Pharmaceutical Science and Technology, Health Sciences Platform, Tianjin University, Tianjin, China
For in vitro investigations on human sulfotransferase (SULT) catalyzed phase II metabolism, the costly cofactor 3′-phosphoadenosine-5′-phosphosulfate (PAPS) is generally needed. In the present study, we developed and optimized a new approach that combines SULT-dependent biotransformation using recombinant and permeabilized fission yeast cells (enzyme bags) with PAPS production in situ applying quality by design principles. In the initial application of the procedure, yeast cells expressing human SULT1A3 were used for the production of 4′-hydroxypropranolol-4-O-sulfate from 4-hydroxypropranolol. The optimized protocol was then successfully transferred to other sulfonation reactions catalyzed by SULT2A1, SULT1E1, or SULT1B1. The concomitant degradation of some sulfoconjugates was investigated, and further optimization of the reaction conditions was performed in order to reduce product loss. Also, the production of stable isotope labelled sulfoconjugates was demonstrated utilizing isotopically labelled substrates or 34S-sulfate. Overall, this new approach results in higher space-time yields while at the same time reducing experimental cost.
Introduction
The study of metabolic pathways of drug substances in humans relies both on in vivo and in vitro experiments. After administration drugs are either directly excreted unchanged or metabolized first. The main specimen for excretion of most drugs and metabolites is urine. The parent drugs often show shorter detection windows due to extensive metabolism. Therefore, in toxicological, forensic or doping control analysis metabolites are often used as target analytes in urine samples (Balcells et al., 2017; Esquivel et al., 2019). Even though in vivo techniques are widely applied in anti-doping research, the high expenditure and unpredicted toxicological effect of many prohibited drugs are considerable drawbacks. In the last two decades, modern in vitro techniques became a viable alternative and furthermore a great extension to in vivo studies (Ekins et al., 2000). Moreover, they allow for precise reaction phenotyping. In in vitro studies, tissues or fractions of tissues like liver microsomes or homogenized liver fractions are commonly applied. In recent years, biosynthesis of sulfoconjugates using genetically modified microorganisms has been developed as well (Taskinen et al., 2003; Nishikawa et al., 2018). Metabolism of drug substances occurs in the complementary phases I and II. While enzymes of phase I metabolism transform parent compounds by hydroxylation, oxidation, or reduction, phase II metabolism consists of the attachment of small moieties to the target molecules, thus allowing them to be excreted from the body rapidly and efficiently. The majority of phase II metabolites are glucuronide- or sulfoconjugates. The formation of the latter species is catalyzed by sulfotransferases (SULTs), which for their activity depend on the cofactor 3′-phosphoadenosine-5′-phosphosulfate (PAPS).
For laboratory use PAPS is highly expensive (approx. 286 US$/mg). This fact might contribute to the limited number of sulfonation studies as compared with research on glucuronidation. In biological sulfonation, conversion of inorganic sulfate into the high-energy cofactor PAPS is a prerequisite. In this pathway, adenosine triphosphate (ATP) sulfonation is initially catalyzed by ATP-sulfurylase to generate adenosine-5′-phosphosulfate (APS), which is subsequently phosphorylated by APS kinase to yield PAPS (Burkart et al., 2000). Afterwards, the sulfo-group is transferred from PAPS to the parent drug or its phase I metabolite in a reaction catalyzed by a SULT enzyme. The released 3′‐phosphoadenosine‐5′‐phosphate (PAP) is subsequently dephosphorylated and re‐phosphorylated in several enzymatically catalyzed steps to regenerate ATP (Robbins and Lipmann, 1958). In animal cells, ATP sulfurylase and APS kinase are expressed as a bifunctional enzyme named PAPS synthase (PAPSS), whereas in bacteria, yeasts, fungi, and plants, the two enzymes are generally encoded by separate genes (Besset et al., 2000).
In the budding yeast Saccharomyces cerevisiae ATP sulfurylase and APS kinase are encoded by the genes MET3 and MET14, respectively (Masselot and Surdin-Kerjan, 1977; Cherest et al., 1985; Cherest et al., 1987; Mountain and Korch, 1991). In the fission yeast Schizosaccharomyces pombe there is a MET14 homologue which is predicted to encode a APS kinase (Lock et al., 2019). While this has yet to be demonstrated experimentally, PAPS synthesis in S. pombe as such has already been reported (Song and Roe, 2008). Previously, all 14 human SULTs have been functionally expressed in S. pombe and, moreover, using this microbial host the functionality of SULT4A1 and SULT6B1 was demonstrated for the first time (Sun et al., 2020). In comparison to whole-cell biotransformation, sulfonation of drugs with permeabilized recombinant fission yeast cells (enzyme bags) provided higher sensitivity and shorter reaction times. However, the required PAPS addition makes this approach expensive for extensive substrate screening or for up-scaling of biosynthetic metabolite production. In this study, we investigated the possibility of generating PAPS by endogenous fission yeast enzymes in the presence of (comparatively cheaper) ATP and ammonium sulfate. For this purpose, the experimental conditions were first optimized using SULT1A3 and 4-hydroxypropranolol (4HP) as model compound. Further verification was then performed with several other SULTs and substrates.
Material and Methods
Chemicals and Reagents
Na2HPO4 and CaCl2 • 2 H2O were purchased from Riedel de Haen (Seelze, Germany). KH2PO4, CuSO4 • 5 H2O, H3BO3, potassium hydrogen phthalate, Na2SO4, nicotinic acid, MnSO4 • H2O, and KI were from Merck (Darmstadt, Germany). ZnSO4 • 7 H2O was purchased from Acros (Geel, Belgium). Tris, agar, NH4HCO3, NH4Cl, FeCl3 • 6 H2O, MgCl2 • 6 H2O, glucose, Triton-X100, and biotin were from Roth (Karlsruhe, Germany). Inositol was from Th.Geyer (Berlin, Germany), and MoO4 • 2 H2O was purchased from Alfa Aesar (Kandel, Germany). Dehydroepiandrosterone (DHEA) was obtained from Steraloids (Newport, RI, United States). 4HP, ATP, and citric acid were purchased from Sigma Aldrich (Steinheim, Germany). 7-Hydroxycoumarin (7HC) and formic acid were purchased from TCI (Zwijindrecht, Belgium). 34S labelled (NH4)2SO4 and D9-Salbutamol (D9-SA) were purchased from Sigma-Aldrich (Taufkirchen, Germany), D6-DHEA was obtained from Sigma-Aldrich (Saint Louis, MO, United States). Acetonitrile was from Fischer Scientific (Geel, Belgium), and HCOONH4 was from VWR Chemicals (Damstadt, Germany). Ultrapure water was prepared with a Milli-Q water purification system LaboStar 2-DI/UV from SG Wasseraufbereitung und Regenerierstation GmbH (Barsbüttel, Germany). All other chemicals and reagents used were also of the highest grade available.
Fission Yeast Strains, Media and General Techniques
The recombined fission yeast strains YN3, YN4, YN20, and YN25 used in this project were described before (Sun et al., 2020). The preparation of media and basic manipulation methods of S. pombe were carried out as described (Alfa et al., 1993). Briefly, strains were generally cultivated at 30°C in Edinburgh Minimal Medium (EMM). EMM was prepared with NH4Cl (93.5 mM), glucose (2% w/v), Na2HPO4 (15.5 mM), potassium hydrogen phthalate (14.7 mM) and standard amounts of salt, vitamin and mineral stock solutions. Liquid cultures were kept shaking at 230 rpm.
Biotransformation With Enzyme Bags
This was essentially done as described before (Sun et al., 2020) with slight modifications. Briefly, fission yeast strains were grown in 10 ml liquid culture of EMM at 30°C and 230 rpm for 24 h. Incubation of main cultures in 250 ml Erlenmeyer flasks was performed subsequently. For each assay a certain number of cells were transferred to micro centrifuge tubes or falcons, pelleted and incubated in 0.3% Triton-X100 in Tris-KCl buffer (200 mM KCl, 100 mM Tris-Cl pH 7.8) at 30°C for 60 min at 230 rpm to allow for permeabilization. Cells were then washed thrice with NH4HCO3 buffer (50 mM, pH 7.8) and directly used for SULT-dependent reactions. Enzyme bags were resuspended in 200 µL of aqueous NH4HCO3 buffer (50 mM, pH 7.8) or phosphate buffer (50 mM, pH 7.8) containing PAPS or ATP, ammonium sulfate, magnesium chloride and substrate as indicated. Biotransformations were carried out at 37°C in a shaking incubator (300 rpm). Enzymatic reactions were stopped by short sharp centrifugation at 14,100 rcf for 2 min and 200 µL of sample in 1.5 ml micro centrifuge tubes were directly frozen at –20°C. After defrosting, samples were centrifuged again (14,100 rcf, 2 min). Supernatants were directly analyzed by ultra-high performance liquid chromatography tandem mass spectrometry (UHPLC-MS/MS) or diluted with a mixture of acetonitrile and ultrapure water (50/50, v/v) prior to analysis. Negative control samples were incubated without cofactors (ATP, (NH4)2SO4, and MgCl2) or without cells, respectively.
Multifactorial Optimization
The optimization process was performed applying quality-by-design (QbD) principles. Design of experiments (DoE) was used for multivariate statistical analysis aiming to ensure robust protocol conditions. It started with a broad systematic screening of the influence of several factors on the incubation of 4HP with YN20 (SULT1A3): ATP concentration (1–50 mM, while (NH4)2SO4 concentration was always kept to the half of ATP concentration), incubation time (3–72 h), cell number per incubation, pre-incubation time, and magnesium chloride concentration (1–100 mM) were altered. Results of the pre-screening disclosed some limits and trends of the factors. For further optimization a Box-Behnken design was used to investigate the effect of the five dependent variables in biotransformation and to optimize the experimental conditions to achieve the highest yield. The variables in this design involved ATP concentration (11–20 mM), magnesium chloride concentration (10–100 mM), pre-incubation time (i.e. incubation without substrate for either 0, 3, or 5 h), incubation time (3–24 h), and cell number (either 5
Biosynthesis of Isotope-Labelled Metabolites and Evaluation of in situ PAPS Generation
D6-DHEA (100 µM) and D9-SA (100 µM) were used as substrates in enzyme bags experiments. Enzyme bags experiments were also carried out with DHEA (100 µM) and SA (100 µM) utilizing (NH4)234SO4 (5.5 mM) and ATP (11 mM) as educts for the cofactor PAPS. Production of labelled sulfoconjugates was monitored by UHPLC-MS/MS.
Degradation Experiments
Degradation of an already sulfonated metabolite in enzyme bags experiment was tested by incubating 7-hydroxycoumarin sulfate (7HCSU) with either YN3 or YN4 for 5 h at 37°C. All experiments were carried out in duplicates.
Optimized Enzyme Bags Biosynthesis
Based on the optimization experiments the final enzyme bag method used 2.5 × 108 precultured and pelleted fission yeast cells that are permeabilized using 200 µL of Triton-X100 [0.3% in Tris-KCl buffer (200 mM KCl, 100 mM Tris-Cl pH 7.8)] at 30°C for 60 min at 230 rpm. After washing with NH4HCO3 buffer (50 mM, pH 7.8, three times) enzyme bags were resuspended in 200 µL of aqueous NH4HCO3 buffer (50 mM, pH 7.8) and supplied with ATP at 11 mM, ammonium sulfate at 22 mM, and magnesium chloride at 20 mM. Following substrate addition (final concentration of 100 µM in incubation solution) mixtures are incubated at 37°C at 300 rpm. Sharp centrifugation at 14,100 rcf for 2 min followed by a freeze-thaw cycle at −20°C and a second centrifugation at 14,100 rcf for 2 min yielded the sulfoconjugates in the supernatant.
UHPLC-MS/MS Instrumentation and Analytical Methods
Separation was conducted on a 1290 Infinity UHPLC System (Agilent Technologies, Waldbronn, Germany) with an Agilent InfinityLab Poroshell 120 Phenyl Hexyl (100
Statistical Analysis
All data are presented as mean ± SD. Statistical analysis was done using Origin 2021 (Originlab Corporation, Northampton, MA. United States).
Results
Optimization of Reaction Conditions for Sulfoconjugate Production With Enzyme Bags
Initially, a sulfonation assay of 4HP (for reaction schemes see Supplementary Figure S1) by SULT1A3 (strain YN20) with external PAPS (100 µM) was carried out as described (Sun et al., 2020). For substitution of the cofactor PAPS by ATP and SO42- and optimization of the product yields multifactorial screening supported by Minitab software was started with five factors: ATP concentration, MgCl2 concentration, number of cells, reaction incubation time, and time of preincubation without substrate. Initial results indicated that a reduction of yield was correlated with longer preincubation time (Figure 1). Therefore, later rounds were performed without any preincubation. Two optimization rounds were conducted within a more targeted range of each factor using Box-Behnken design. More specifically, ATP concentrations of 11 mM or 15 mM, cell numbers of 1.25
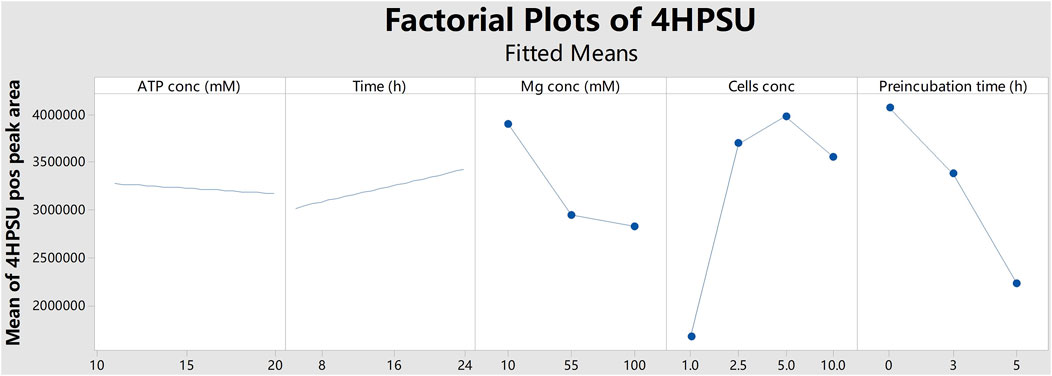
FIGURE 1. Factorial plots of 4HPSU production with different ATP concentrations (ATP conc), reaction times (Time), MgCl2 concentrations (Mg conc), cells concentrations (Cells conc), and preincubation times (Preincubation time). The peak areas of 4HPSU were obtained in positive ion mode and mean values were calculated by Minitab.
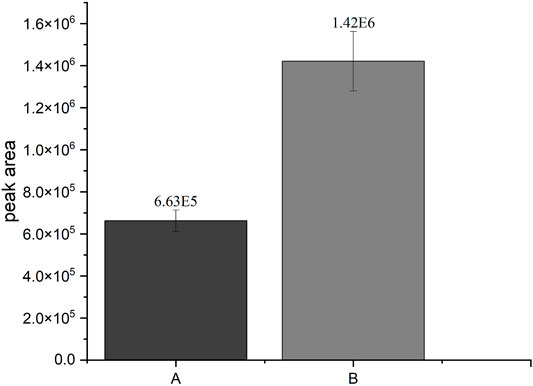
FIGURE 2. Comparison of the SULT1A3-dependend production of 4HPSU from 4HP under different conditions. A: Peak area of 4HPSU produced with 100 µM PAPS after 3 h incubation; B: Peak area of 4HPSU produced under optimized conditions (11 mM ATP, 20 mM MgCl2, 2.5 × 108 cells per sample, and after 5 h incubation time). Each experiment was done in triplicates. Error bars show the standard deviations.
Evaluation of Optimal Conditions for Additional Substrates and Enzyme Isoforms
As proof of concept the above-mentioned standard protocol developed with the SULT1A3 (YN20) and 4HP was then applied to 7HC, DHEA, salbutamol (SA) and 4HP using various SULTs (Figure 3). Experimental conditions were as follows: 11 mM ATP, 5.5 mM (NH4)2SO4, 100 µM substrate, 20 mM MgCl2, and 2.5
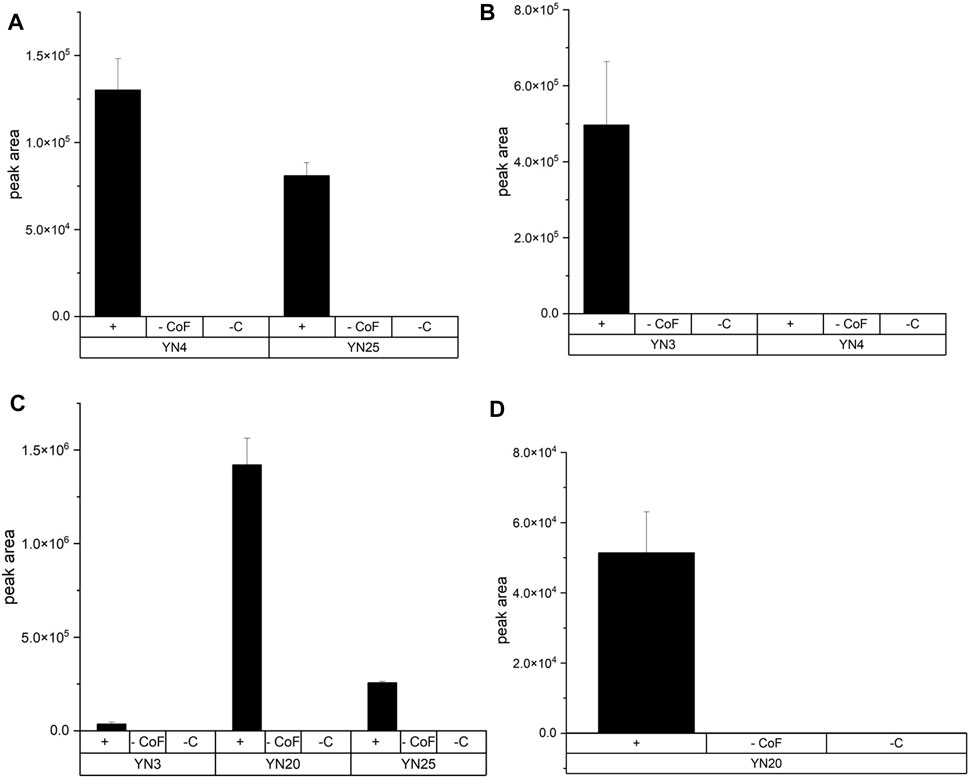
FIGURE 3. Production of three different sulfoconjugated metabolites by various human SULTs. Experimental conditions are described in the text. Names of fission yeast strain are given in brackets. +: incubation with cells and cofactors—CoF: incubation without cofactors but with cells—C: incubation without cells but with cofactors. (A) Production of DHEASU from DHEA. (B) Production of 7HCSU from 7HC. (C) Production of 4HPSU from 4HP. Each experiment was done in triplicates. (D) Production of SASU from SA.
In the past, recombinant fission yeast strains that express human UDP glucuronosyltransferases were successfully used for the production of stable isotope-labelled glucuronides (Dragan et al., 2010; Buchheit et al., 2011). In order to demonstrate the usefulness of our new protocol for the production of stable isotope-labelled sulfometabolites, biotransformations with D6-DHEA, D9-salbutamol (D9-SA), and (NH4)234SO4 were conducted in the present study (Figure 4 and Figure 5). Non-labelled DHEA or D6-DHEA were subjected to SULT2A1-dependend enzyme bag biotransformations either with (NH4)2SO4 or (NH4)234SO4. Results of UHPLC-MS/MS analysis proved same retention time of DHEASU, D6-DHEASU, and DHEA-34S-SU (Figures 4A,C,E) the respective pattern of mass transitions (Figures 4B,D,F) showed the successful production of non-labelled and isotope-labelled sulfometabolites. The same strategy was performed using non-labelled SA and D9-SA with SULT1A3 (YN20) as well (Figure 5).
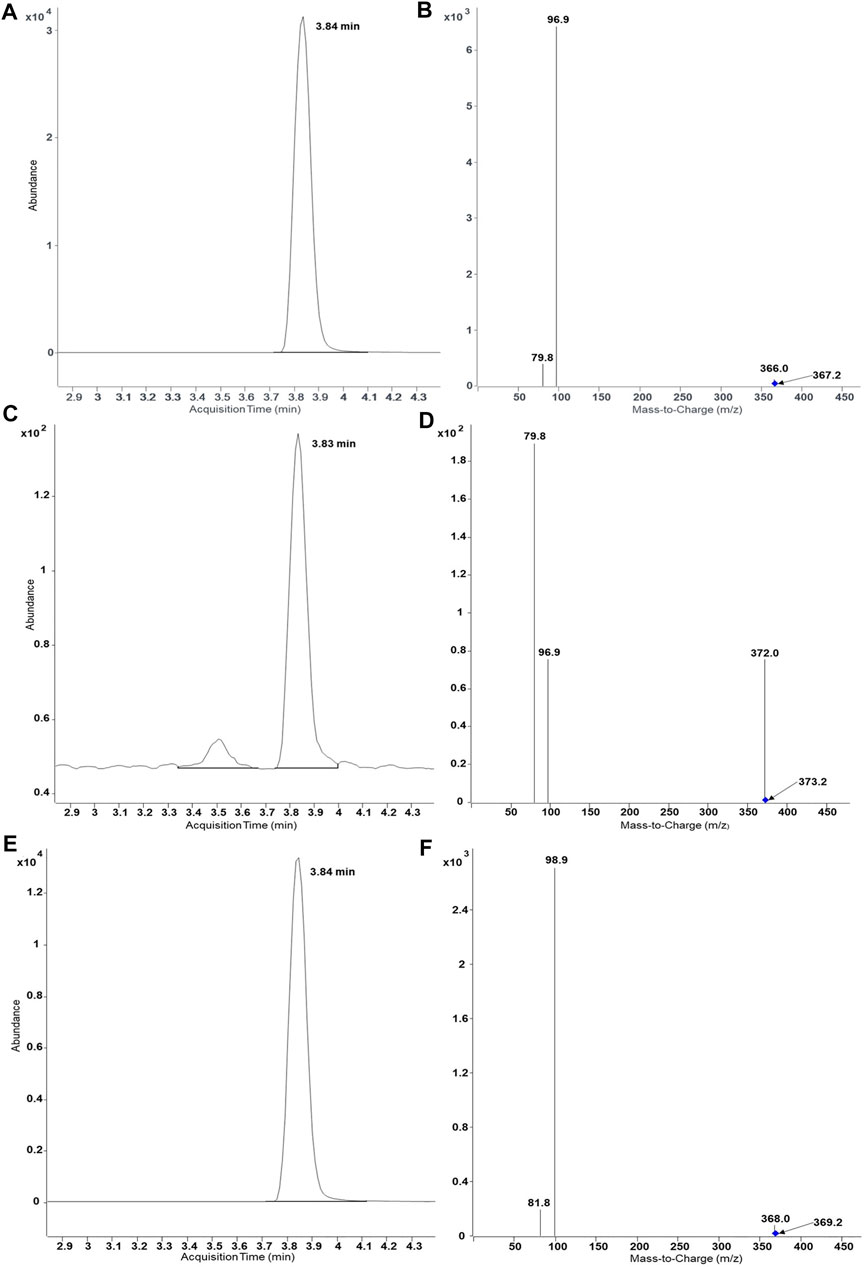
FIGURE 4. UHPLC-MS/MS results of biosynthesis of DHEASU, D6-DHEASU, and DHEA-34S-SU by SULT2A1 (YN4). Product ion spectra generated in ESI− are dominated by the fragment HSO4− (96.9) or H34SO4− (98.9). (A) Chromatogram of DHEASU displaying qualifier transition m/z 367.2 → 96.9; (B) MRM transitions in DHEASU assay (m/z 367.2→366, 367.2→96.9 and 367.2→79.8); (C) Chromatogram of D6-DHEASU displaying qualifier transition m/z 373.2 → 96.9 (D) MRM transitions in D6-DHEASU assay (m/z 373.2→372, 373.2→96.9 and 373.2→79.8); (E) Chromatogram of DHEA-34S-SU displaying qualifier transition m/z 369.2 → 98.9; (F) MRM transitions in DHEA34SU assay (m/z 369.2→368, 369.2→98.9 and 369.2→81.8).
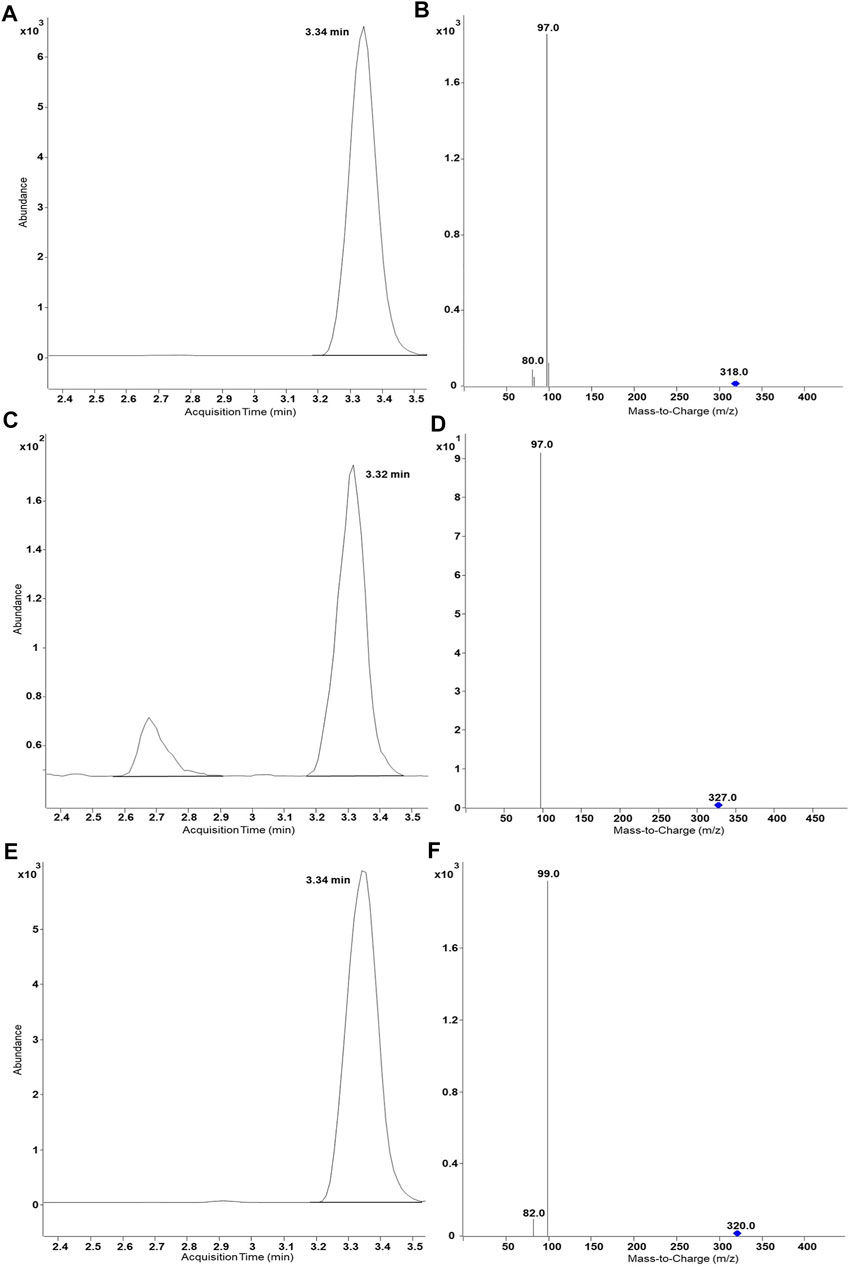
FIGURE 5. UHPLC-MS/MS results of biosynthesis of SASU, D9-SASU, and SA-34S-SU by SULT1A3 (YN20). Product ion spectra generated in ESI− are dominated by the fragment HSO4− (96.9) or H34SO4− (98.9). (A) Chromatogram of SASU shows ion transition m/z 318.0 → 97; (B) MRM transitions in SASU assay (m/z 318.0→97.0 and 318.0→80.0); (C) Chromatogram of D9-SASU shows m/z 327.0 → 97.0; (D) MRM transitions in D9-SASU assay (m/z 327.0→97.0 and 327.0→79.8); (E) Chromatogram of SA-34S-SU shows m/z 320.0 → 99.0; (F) MRM transitions in SASU assay (m/z 320.0→99.0 and 320.0→82.0).
Further Optimization of Buffer, (NH4)2SO4 and Substrate Concentrations
Unexpectedly, the sulfonation of 7HC by SULT2A1 (YN4) could not be shown using above mentioned conditions, even though the enzyme is known to metabolize this substrate (Nishikawa et al., 2018; Sun et al., 2020). It was suspected that product lability might be a reason. In order to confirm this suspicion, degradation assays were performed. Indeed, the incubation of 7HCSU with enzyme bags generated using YN3 at pH 7.8 led to more than 99% loss of the compound within 5 hours. By contrast, degradation tests in buffer without cells proved good stability of 7HCSU (Supplementary Figure S2). Apparently, there are endogenous fission yeast enzymes which can catalyze a cleavage of this sulfated metabolite.
Further investigations of the degradation of 7HCSU were performed by incubating permeabilized YN3 cells either in NH4HCO3 buffer (at pH 7.8 or 7.4) or in phosphate buffer (at pH 7.8, 7.4, or 6.5). The results showed that in pH 7.8 phosphate buffer 7HCSU displays the smallest amount of degradation (Supplementary Figure S3). With the intention of avoiding total degradation of 7HCSU and increasing sulfonation yield of 7HC by SULT2A1 (YN4), and also with the purpose for exploring the possibility of further optimization of the general protocol, enzyme bag assays were subsequently conducted at higher substrate concentrations (7HC at 100 µM or 1 mM), higher (NH4)2SO4 concentrations (5.5, 22, 33, or 44 mM), and also in phosphate buffer (pH 7.8). The results demonstrated that the peak area of 7HCSU reached the highest levels at 1 mM substrate concentration, while the influence of the (NH4)2SO4 concentration on the yield was minor (Figure 6). The same optimization with higher substrate and ammonium sulfate concentrations was performed for the biotransformation of 4HP with SULT1A3 (YN20) as well. In this case, no particularly obvious differences were found among the different conditions (Supplementary Figure S4).
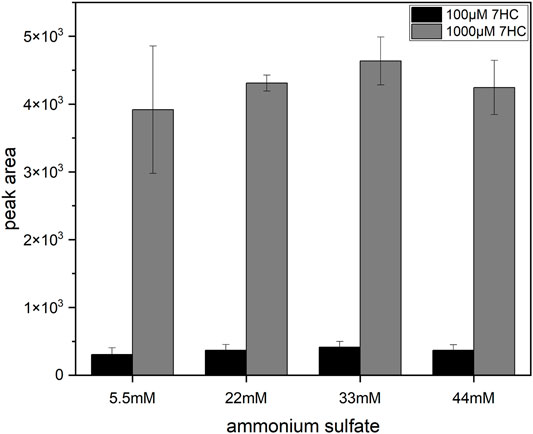
FIGURE 6. Influence of (NH4)2SO4 and substrate concentrations on 7HCSU formation from 7HC by SULT2A1. Each experiment was done in triplicates. Error bars show the standard deviations.
Final Protocol
The final protocol (Figure 7) for this sulfonation assay uses 20 mM Mg2+, 11 mM ATP, 5.5 mM SO42-, and 2.5 × 108 cells per incubation in ammonium bicarbonate buffer at pH 7.8 for 5 h at 37°C. In the case of 4HP, the most efficient substrate concentration was 100 µm. Sulfonation of compounds with a low affinity to a SULT can be achieved by enhancing substrate concentration to 1 mM.
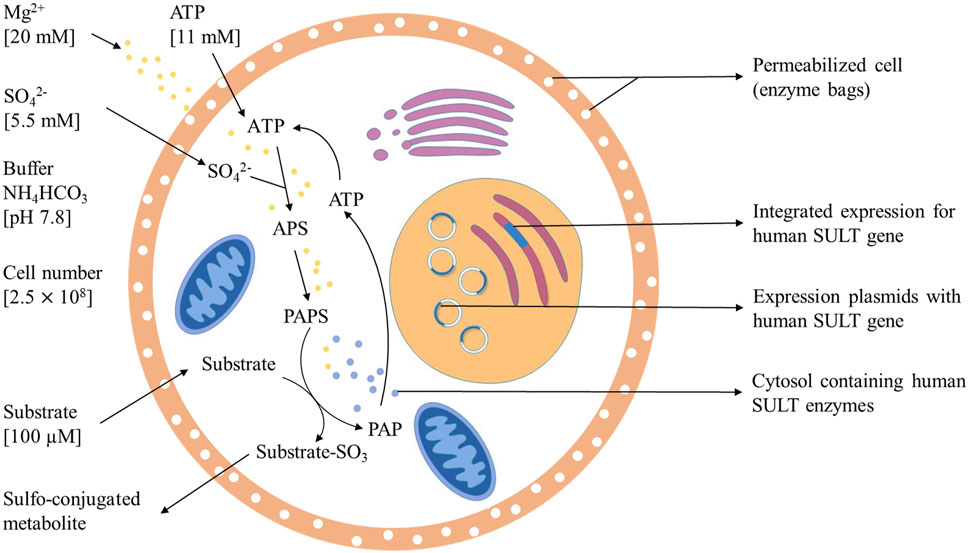
FIGURE 7. Optimized method for sulfotansferase catalyzed metabolite generation including PAPS replacement protocol.
Discussion
In this study, the successful replacement of the SULT cofactor PAPS by ATP and (NH4)2SO4 in a recombinant sulfoconjugate biosynthesis system is reported. While production and regeneration of PAPS were previously described in a chemoenzymatic approach (An et al., 2017), in an enzymatic approach (Burkart et al., 2000), and in liver S9 fraction-based biosynthesis (Weththasinghe et al., 2018), this assay successfully combines both, PAPS (re-)generation and sulfonation of xenobiotics. Multifactorial optimization was performed applying DoE principles using the model substrate 4HP and human SULT1A3, which is expressed by recombinant fission yeast strain YN20 (Figure 1). Compared with the biosynthesis where PAPS was used as cofactor, the optimized method with ATP and (NH4)2SO4 resulted in a more than doubled yield of product (Figure 2). At the same time, the cost per experiment was reduced by a factor of 60. Further optimization was performed using 4HP with SULT1A3 and 7HC with SULT2A1. Permeabilized fission yeast (enzyme bags) assays with PAPS regeneration combine the advantage of high sensitivity and low cost. Small molecules like substrates, cofactors, and products can move in and out of the cells freely. Meanwhile, the enzymes needed for sulfonation remain trapped within the enzyme bags and can therefore be employed to catalyze the reactions of interest.
The concentration of magnesium ions was observed to be one of the most crucial parameters in the optimization process. Magnesium is an essential electrolyte in the human body. As a cofactor, magnesium participates in more than 300 enzyme systems that modulate multiple biochemical reactions in the body (Gröber et al., 2015a). In biological systems that generate PAPS or sulfonated metabolites, magnesium functions as an assistant inorganic ion (Burkart et al., 2000; Weththasinghe et al., 2018). In this study, the concentration of MgCl2 was tested in a range from 1 to 100 mM. Ultimately, 20 mM was found to be the optimal concentration. The results demonstrated that within certain limits, the MgCl2 concentration had an evident impact on the yield of 4HPSU. Although the mechanism of magnesium in biological sulfonation is not yet completely understood, magnesium is reported to be essential for the pathway of PAPS synthesis in S. cerevisiae (Thomas and Surdin-Kerjan, 1997). It is reasonable to assume that magnesium either functions as an enzyme activator or is involved in ATP production within the sulfonation system (Swaminathan, 2003; Gröber et al., 2015b).
By screening several ATP concentrations, it was observed that higher ATP concentrations (≥50 mM) led to a significant decline of yield in sulfonated product formation. As a structural analogue of PAPS, ATP has been reported to competitively inhibit the sulfonation of human M and P phenol sulfotransferase (SULT1A3, SULT1A1) (Rens-Domiano and Roth, 1987; Dooley et al., 1994). This property might be responsible for the effects observed in here as well.
Afterwards, the standard protocol was applied to additional substrates and SULTs (Figure 3). Furthermore, production of stable isotope-labelled sulfometabolites, with D6-DHEA, D9-salbutamol (SA), and (NH4)234SO4 were achieved applying the established protocol as well (Figures 4, 5). The competence of SULT2A1 to sulfonate 7HC was reported by Nishikawa (Nishikawa et al., 2018) and Sun (Sun et al., 2020). However, using our PAPS replacing protocol, the enzymatic activity of SULT2A1 towards 7HC could not be demonstrated under initial standard protocol conditions. A possible reason is a rapid degradation of the product 7HCSU, presumably by cleavage of the sulfate group. Therefore, the degradation of 7HCSU was subsequently investigated in reaction mixtures with and without cells (Supplementary Figure S1). Degradation was only found in assays with cells, which indicated that the degradation of 7HCSU is a result of enzymatical catalysis rather than of chemical instability. Less degradation was observed when phosphate buffer (pH 7.8) was used instead of hydrogen carbonate buffer, which is in line with earlier reports of sulfatase inhibition by phosphate (Lee and Van Etten, 1975; Bostick et al., 1978; Metcalfe et al., 1979). Furthermore, using phosphate buffer higher product yields were obtained with both SULT2A1 and SULT1B1. Therefore, for 7HC sulfonation experiments with enzyme bags, the usage of phosphate buffer (pH 7.8) is superior to that of NH4HCO3 buffer (pH 7.8).
In a previous study by Burkart et al. (2000) the generation of PAPS from ATP and inorganic sulfate was also achieved using genetically modified E. coli. Highest yields were obtained when the sulfate concentration dramatically exceeded that of ATP. Consequently, we increased the (NH4)2SO4 concentration to 44 mM. However, the yield of 4HPSU did not show a significant rise with increasing (NH4)2SO4 concentrations. This might indicate that the concentration of PAPS is not the main limiting factor of 4HPSU yield in this case.
Being a known hydroxysteroid converting SULT, SULT2A1 shows low affinity to phenolic compounds like 7HC (Tibbs et al., 2015), which might explain the lack of sulfoconjugated metabolite in initial experiments. Therefore, the concentration of 7HC was increased to 1 mM to facilitate the enzymatic reaction. A remarkable increase of 7HCSU production (Figure 6) was observed. In this manner, the standard protocol was modified for biotransformation of low affinity substrates in enzyme bags.
The developed assay allows to determine whether a substrate is sulfonated by any one of the 14 human SULTs and also permits a comparison of their sulfonation activity. Due to the fact that the biosynthesized sulfoconjugates are not available as references, a quantitation of the results by UHPLC-MS/MS is not possible. Therefore, metabolite formation rates cannot be given in absolute values in this study.
The successful development of an optimized PAPS replacement protocol (details in Figure 7) provides an economic and efficient way for further research of SULT-depended phase II metabolism pathways of drugs. Bigger scale screening experiments will be performed to demonstrate broad applicability and to further evaluate the possibilities of this great sulfonation technique. The biotechnological generation of sulfonated compounds and metabolites may be achieved on reasonable costs applying this assay.
Data Availability Statement
The original contributions presented in the study are included in the article/Supplementary Material, further inquiries can be directed to the corresponding authors.
Author Contributions
Conceptualization, MP and MB; methodology, YS, LH, MB and MP; software, YS and LH; investigation, YS and LH, resources, MP, and MB; data curation, MP and MB; writing—original draft preparation, YS and LH; writing—review and editing, MP, and MB; visualization, YS and LH; supervision, MP, and MB; project administration, MP and MB; funding acquisition, MP and MB. All authors have read and agreed to the published version of the manuscript.
Funding
This research was funded by the World Anti-Doping Agency (WADA grant 19A10MP). The APC was funded by the Open Access Publication Fund of the Freie Universität Berlin. We would like to acknowledge the assistance of the Core Facility BioSupraMol supported by the DFG.
Conflict of Interest
The authors declare that the research was conducted in the absence of any commercial or financial relationships that could be construed as a potential conflict of interest.
Publisher’s Note
All claims expressed in this article are solely those of the authors and do not necessarily represent those of their affiliated organizations, or those of the publisher, the editors and the reviewers. Any product that may be evaluated in this article, or claim that may be made by its manufacturer, is not guaranteed or endorsed by the publisher.
Supplementary Material
The Supplementary Material for this article can be found online at: https://www.frontiersin.org/articles/10.3389/fmolb.2022.827638/full#supplementary-material
References
Alfa, C., Fantes, P., Hyams, J., McLeod, M., and Warbrick, E. (1993). Experiments with Fission yeastA Laboratory Course Manual. NY: Cold Spring Harbor Press, Cold Spring Harbor.
An, C., Zhao, L., Wei, Z., and Zhou, X. (2017). Chemoenzymatic Synthesis of 3′-Phosphoadenosine-5′-Phosphosulfate Coupling with an ATP Regeneration System. Appl. Microbiol. Biotechnol. 101, 7535–7544. doi:10.1007/s00253-017-8511-2
Balcells, G., Gómez, C., Garrostas, L., Pozo, Ó. J., and Ventura, R. (2017). Sulfate Metabolites as Alternative Markers for the Detection of 4-chlorometandienone Misuse in Doping Control. Drug Test. Anal. 9, 983–993. doi:10.1002/dta.2101
Besset, S., Vincourt, J.-B., Amalric, F., and Girard, J.-P. (2000). Nuclear Localization of PAPS Synthetase 1: a Sulfate Activation Pathway in the Nucleus of Eukaryotic Cells. FASEB j. 14, 345–354. doi:10.1096/fasebj.14.2.345
Bostick, W. D., Dinsmore, S. R., Mrochek, J. E., and Waalkes, T. P. (1978). Separation and Analysis of Arylsulfatase Isoenzymes in Body Fluids of Man. Clin. Chem. 24, 1305–1316. doi:10.1093/clinchem/24.8.1305
Buchheit, D., Drăgan, C.-A., Schmitt, E. I., and Bureik, M. (2011). Production of Ibuprofen Acyl Glucosides by Human UGT2B7. Drug Metab. Dispos 39, 2174–2181. doi:10.1124/dmd.111.041640
Burkart, M. D., Izumi, M., Chapman, E., Lin, C.-H., and Wong, C.-H. (2000). Regeneration of PAPS for the Enzymatic Synthesis of Sulfated Oligosaccharides. J. Org. Chem. 65, 5565–5574. doi:10.1021/jo000266o
Cherest, H., Nguyen, N. T., and Surdin-Kerjan, Y. (1985). Transcriptional Regulation of the MET3 Gene of Saccharomyces cerevisiae. Gene 34, 269–281. doi:10.1016/0378-1119(85)90136-2
Cherest, H., Kerjan, P., and Surdin-Kerjan, Y. (1987). The Saccharomyces cerevisiae MET3 Gene: Nucleotide Sequence and Relationship of the 5′ Non-coding Region to that of MET25. Mol. Gen Genet 210, 307–313. doi:10.1007/bf00325699
Dooley, T. P., Mitchison, H. M., Munroe, P. B., Probst, P., Neal, M., Siciliano, M. J., et al. (1994). Mapping of Two Phenol Sulfotransferase Genes, STP and STM, to 16p: Candidate Genes for Batten Disease. Biochem. Biophysical Res. Commun. 205, 482–489. doi:10.1006/bbrc.1994.2691
Dragan, C. A., Buchheit, D., Bischoff, D., Ebner, T., and Bureik, M. (2010). Glucuronide Production by Whole-Cell Biotransformation Using Genetically Engineered Fission Yeast Schizosaccharomyces pombe. Drug Metab. Dispos 38, 509–515. doi:10.1124/dmd.109.030965
Ekins, S., Ring, B. J., Grace, J., McRobie-Belle, D. J., and Wrighton, S. A. (2000). Present and Future In Vitro Approaches for Drug Metabolism. J. Pharmacol. Toxicol. Methods 44, 313–324. doi:10.1016/s1056-8719(00)00110-6
Esquivel, A., Alechaga, É., Monfort, N., Yang, S., Xing, Y., Moutian, W., et al. (2019). Evaluation of Sulfate Metabolites as Markers of Intramuscular Testosterone Administration in Caucasian and Asian Populations. Drug Test. Anal. 11, 1218–1230. doi:10.1002/dta.2598
Gamage, N., Barnett, A., Hempel, N., Duggleby, R. G., Windmill, K. F., Martin, J. L., et al. (2006). Human Sulfotransferases and Their Role in Chemical Metabolism. Toxicol. Sci. 90, 5–22. doi:10.1093/toxsci/kfj061
Gröber, U., Schmidt, J., and Kisters, K. (2015). Magnesium in Prevention and Therapy. Nutrients 7, 8199–8226. doi:10.3390/nu7095388
Gröber, U., Schmidt, J., and Kisters, K. (2015). Magnesium in Prevention and Therapy. Nutrients 7, 8199–8226. doi:10.3390/nu7095388
Ko, K., Kurogi, K., Davidson, G., Liu, M.-Y., Sakakibara, Y., Suiko, M., et al. (2012). Sulfation of Ractopamine and Salbutamol by the Human Cytosolic Sulfotransferases. J. Biochem. 152, 275–283. doi:10.1093/jb/mvs073
Lee, G. D., and Van Etten, R. L. (1975). Purification and Properties of a Homogeneous Aryl Sulfatase A from Rabbit Liver. Arch. Biochem. Biophys. 166, 280–294. doi:10.1016/0003-9861(75)90389-6
Lock, A., Rutherford, K., Harris, M. A., Hayles, J., Oliver, S. G., Bähler, J., et al. (2019). PomBase 2018: User-Driven Reimplementation of the Fission Yeast Database Provides Rapid and Intuitive Access to Diverse, Interconnected Information. Nucleic Acids Res. 47, D821–D827. doi:10.1093/nar/gky961
Masselot, M., and Surdin-Kerjan, Y. (1977). Methionine Biosynthesis in Saccharomyces cerevisiae. Mol. Gen. Genet. 154, 23–30. doi:10.1007/bf00265572
Metcalfe, D. D., Corash, L. M., and Kaliner, M. (1979). Human Platelet Arylsulphatases: Identification and Capacity to Destroy SRS-A. Immunology 37, 723–729.
Miyano, J., Yamamoto, S., Hanioka, N., Narimatsu, S., Ishikawa, T., Ogura, K., et al. (2005). Involvement of SULT1A3 in Elevated Sulfation of 4-hydroxypropranolol in Hep G2 Cells Pretreated with β-naphthoflavone. Biochem. Pharmacol. 69, 941–950. doi:10.1016/j.bcp.2004.12.012
Mountain, H. A., and Korch, C. (1991). TDH2 Is Linked toMET3 on Chromosome X ofSaccharomyces Cerevisiae. Yeast 7, 873–880. doi:10.1002/yea.320070814
Nishikawa, M., Masuyama, Y., Nunome, M., Yasuda, K., Sakaki, T., and Ikushiro, S. (2018). Whole-cell-dependent Biosynthesis of Sulfo-Conjugate Using Human Sulfotransferase Expressing Budding Yeast. Appl. Microbiol. Biotechnol. 102, 723–732. doi:10.1007/s00253-017-8621-x
Rens-Domiano, S. S., and Roth, J. A. (1987). Inhibition of M and P Phenol Sulfotransferase by Analogues of 3'-Phosphoadenosine-5'-Phosphosulfate. J. Neurochem. 48, 1411–1415. doi:10.1111/j.1471-4159.1987.tb05679.x
Robbins, P. W., and Lipmann, F. (1958). Enzymatic Synthesis of Adenosine-5′-Phosphosulfate. J. Biol. Chem. 233, 686–690. doi:10.1016/s0021-9258(18)64728-3
Song, J.-Y., and Roe, J.-H. (2008). The Role and Regulation of Trxl, a Cytosolic Thioredoxin in Schizosaccharomyces pombe. J. Microbiol. 46, 408–414. doi:10.1007/s12275-008-0076-4
Sun, Y., Machalz, D., Wolber, G., Parr, M. K., and Bureik, M. (2020). Functional Expression of All Human Sulfotransferases in Fission Yeast, Assay Development, and Structural Models for Isoforms SULT4A1 and SULT6B1. Biomolecules 10, 1517. doi:10.3390/biom10111517
Taskinen, J., Ethell, B. T., Pihlavisto, P., Hood, A. M., Burchell, B., and Coughtrie, M. W. H. (2003). Conjugation of Catechols by Recombinant Human Sulfotransferases, UDP-Glucuronosyltransferases, and Soluble Catechol O-Methyltransferase: Structure-Conjugation Relationships and Predictive Models. Drug Metab. Dispos 31, 1187–1197. doi:10.1124/dmd.31.9.1187
Thomas, D., and Surdin-Kerjan, Y. (1997). Metabolism of Sulfur Amino Acids in Saccharomyces cerevisiae. Microbiol. Mol. Biol. Rev. 61, 503–532. doi:10.1128/mmbr.61.4.503-532.1997
Tibbs, Z. E., Rohn-Glowacki, K. J., Crittenden, F., Guidry, A. L., and Falany, C. N. (2015). Structural Plasticity in the Human Cytosolic Sulfotransferase Dimer and its Role in Substrate Selectivity and Catalysis. Drug Metab. Pharmacokinet. 30, 3–20. doi:10.1016/j.dmpk.2014.10.004
Keywords: fission yeast, in vitro metabolism, method optimization, PAPS, sulfonation, SULT, quality by design, isotopic labelling
Citation: Sun Y, Harps LC, Bureik M and Parr MK (2022) Human Sulfotransferase Assays With PAPS Production in situ. Front. Mol. Biosci. 9:827638. doi: 10.3389/fmolb.2022.827638
Received: 02 December 2021; Accepted: 24 January 2022;
Published: 28 February 2022.
Edited by:
Jon Wolf Mueller, University of Birmingham, United KingdomReviewed by:
Yang Xie, Brigham and Women’s Hospital and Harvard Medical School, United StatesChristoph Müller, Ludwig Maximilian University of Munich, Germany
Copyright © 2022 Sun, Harps, Bureik and Parr. This is an open-access article distributed under the terms of the Creative Commons Attribution License (CC BY). The use, distribution or reproduction in other forums is permitted, provided the original author(s) and the copyright owner(s) are credited and that the original publication in this journal is cited, in accordance with accepted academic practice. No use, distribution or reproduction is permitted which does not comply with these terms.
*Correspondence: Matthias Bureik, matthias@tju.edu.cn; Maria Kristina Parr, maria.parr@fu-berlin.de
†These authors have contributed equally to this work and share first authorship