- 1Dipartimento di Scienze Biomediche e Biotecnologiche (BIOMETEC), Università di Catania, Catania, Italy
- 2Center of Excellence for the Acceleration of Harm Reduction (CoEHAR), University of Catania, Catania, Italy
- 3CNR, Institute for Polymers, Composites and Biomaterials (IPCB), Catania, Italy
- 4Dipartimento di Scienze del Farmaco e della Salute, Università di Catania, Catania, Italy
Currently, the use of probiotic strains and their products represents a promising innovative approach as an antagonist treatment against many human diseases. Previous studies showed that a strain of Limosilactobacillus fermentum (LAC92), previously defined as Lactobacillus fermentum, exhibited a suitable amensalistic property. The present study aimed to purify the active components from LAC92 to evaluate the biological properties of soluble peptidoglycan fragments (SPFs). The cell-free supernatant (CFS) and bacterial cells were separated after 48 h of growth in MRS medium broth and treated for isolation of SPFs. Antimicrobial activity and proliferation analysis on the human cell line HTC116 were performed using technologies such as xCELLigence, count and viability, and clonogenic analysis. MALDI-MS investigation and docking analysis were performed to determine the molecular structure and hypothetical mode of action, respectively. Our results showed that the antimicrobial activity was mainly due to SPFs. Moreover, the results obtained when investigating the SPF effect on the cell line HCT116 showed substantial preliminary evidence, suggesting their significant cytostatic and quite antiproliferative properties. Although MALDI was unable to identify the molecular structure, it was subsequently revealed by analysis of the bacterial genome. The amino acid structure is called peptide 92. Furthermore, we confirmed by molecular docking studies the interaction of peptide 92 with MDM2 protein, the negative regulator of p53. This study showed that SPFs from the LAC92 strain exerted anticancer effects on the human colon cancer HCT116 cell line via antiproliferation and inducing apoptosis. These findings indicated that this probiotic strain might be a potential candidate for applications in functional products in the future. Further examination is needed to understand the specific advantages of this probiotic strain and improve its functional features to confirm these data. Moreover, deeper research on peptide 92 could increase our knowledge and help us understand if it will be possible to apply to specific diseases such as CRC.
1 Introduction
Currently, natural substances are considered a promising alternative to conventional therapies (Yuan et al., 2016; Kim et al., 2018; Kim et al., 2021; Lim et al., 2021; Kim et al., 2022). Among the macromolecules that play an essential role in cell growth and development, closely related to physiological functions are the bacterial cell wall peptidoglycan (PG) components (Wheeler et al., 2014; Semenov, 2021). The PG polymer is composed of N-acetylmuramic and N-acetylglucosamine sugar chains alternated with cross-linking peptide chains containing d- and l-amino acids. The polysaccharides and teichoic acids in the cell wall are covalently linked to PGs (Chapot-Chartier and Kulakauskas, 2014). As a whole, it is a dynamic and complex structure, with various chemical modifications and trimming mechanisms that produce disaccharide-containing elements (Bersch et al., 2021). In addition to playing an essential role in bacterial physiology and maintaining the cell’s shape and integrity, it acts as an interface between the bacterium and its environment. In fact, recently, attention toward it has been increasing due to its various pharmacological activities, such as antitumor effects (He et al., 2017; Wang et al., 2018).
Colorectal cancer (CRC) is the third most common cancer and the fourth most common cause of cancer-related death (McGuire, 2016). While environmental and genetic factors play a significant role in the pathogenesis of colon cancer, extensive research has suggested that nutrition and dysbiosis may play both a causal and protective role in the development of this cancer (Koliarakis et al., 2019; Thanikachalam and Khan, 2019). Indeed, Western dietary habits, obesity, and heavy alcohol consumption play significant roles in causing CRC (Cheng et al., 2020). Moreover, several bacterial species have been associated with CRC, such as Streptococcus bovis (Gupta et al., 2010), Bacteroides fragilis (Chung et al., 2018), Fusobacterium nucleatum (Bashir et al., 2015), and Peptostreptococcus anaerobius (Long et al., 2019; Cheng et al., 2020). On the contrary, several studies have demonstrated that probiotic bacteria can be effective for various medical conditions, including CRC (Ishikawa et al., 2005; Uccello et al., 2012; Chong, 2014; Fong et al., 2020).
In this context, many studies have shown that some Lactobacillus species, such as Lactobacillus casei, have remarkable antitumor activity both in vivo and in vitro (Kato et al., 1981; Yasutake et al., 1984; Shimizu et al., 1987; Kato et al., 1988; Matsuzaki et al., 1996). The antiproliferative activity appears due to mechanisms involving activated macrophages, modulation of the host’s immune response, and regulation of cellular apoptosis (Lidbeck et al., 1992; Saha and Saroj, 2022). Several lactobacilli are claimed to be health-benefiting maintaining intestinal microbial balance, protecting against the invasion of pathogenic microorganisms, and activating the innate immunity response of the host (Veiga et al., 2020). Therefore, some of these strains are currently used as probiotic supplements in technological additives and for therapeutic purposes (E.F.S.A., 2010; E.F.S.A., 2011a; E.F.S.A., 2011b; E.F.S.A., 2011c; E.F.S.A. and Panel, 2013; E.F.S.A. et al., 2021).
Extracts and fragments of peptidoglycan can induce apoptosis in several cell cancer lines (Troll et al., 2009; Vazquez-Sanchez et al., 2014; Wang et al., 2018; Patra et al., 2022). The principal tumor suppressor protein is p53, which induces cell death by apoptosis in response to various stress conditions (Aubrey et al., 2018). p53 binds explicitly to a 20-base pair (bp) consensus DNA sequence, acting as a transcription factor activator and leading to cell growth suppression and cell death (Kitayner et al., 2010). Inactivation of p53 is the most prevalent defect in human cancers.
p53 is negatively regulated by the oncoprotein MDM2 (murine double minute 2) (Zhao et al., 2014). MDM2 is an E3 ubiquitin ligase that targets the degradation of the p53 tumor suppressor and inhibits the expression of p53-related genes.
Since it has been discovered that MDM2 and p53 are part of an auto-regulatory feedback loop, a new approach for cancer treatment consists of stimulating p53 by inhibiting its interaction with MDM2 (Wu et al., 1993). It was already reported that compounds that activated the p53 pathway by inhibiting the mdm2 mRNA level execute anti-HCT116 cancer activity (Zhang et al., 2022).
Our previous studies showed that strains of Lactobacillus, isolated from clinical samples, exhibited suitable amensalistic properties, and their purified components have biological properties (Fuochi et al., 2018; Fuochi et al., 2019; Stivala et al., 2021). The present paper describes the production and isolation of the active fragments of peptidoglycan, belonging to a strain of Limosilactobacillus fermentum, responsible for the antimicrobial activity and antiproliferative events observed in tumor cells. Subsequently, an analysis of the bacterial genome was performed by searching for the mur genes to confirm the structure of the peptide fragment since MALDI investigations had failed to detect it. The amino acid structure is called peptide 92. Moreover, we performed docking analysis to confirm the hypothesis that the antiproliferative effects exerted by peptidoglycan fragments were due to the interaction of peptide 92 with MDM2, preventing the binding of the protein with p53. In fact, inhibitors of the Mdm2–p53 interaction, which restore the functional p53, constitute potential non-genotoxic anticancer agents with a novel mode of action. Taken together, our results provided a scientific reference for further investigations and applications in discovering natural antitumor drugs from lactic acid bacteria.
2 Materials and methods
2.1 Bacterial strain
For this purpose, the strain of Limosilactobacillus fermentum, LAC92 (GenBank ID:CP021790.1), earlier defined as Lactobacillus fermentum and isolated from the oral cavity, as previously described (collection of the Laboratory of Applied Microbiology, Department of Biomedical and Biotechnological Sciences, Università degli Studi di Catania, Italy), was used (Fuochi et al., 2017).
LAC92 was grown in de Man, Rogosa, and Sharpe (MRS) broth (Oxoid, Thermo Fisher Scientific Inc., Rodano (MI), Italy, CM0359) at 37°C for 48 h under microaerobic conditions before proceeding with subsequent investigations.
2.2 Composition of the culture media
The MRS broth used had the following components per liter: peptone, 10.0 g; yeast extract, 4.0 g; `Lab-Lemco’ powder, 8.0 g; glucose, 20.0 g; sodium acetate 3 H2O, 5.0 g; K2HPO4, 2.0 g; triammonium citrate, 2.0 g; MgSO4 7 H2O, 0.2 g; MnSO4 4 H2O, 0.05 g; sorbitan mono-oleate, 1 mL; pH 6.2 ± 0.2 at 25°C. The medium was sterilized by heating to 121°C for 20 min.
2.3 Isolation and storage of SPFs
For isolating soluble peptidoglycan fragments (SPFs), a modified method of Fichera et al., (2016) was performed. Briefly, bacterial cells were collected by centrifugation at 7000 g for 15 min at room temperature, resuspended in 500 mL sterile PBS containing 2.5 g of d-glucose and 5 mg of penicillin G, and incubated under agitation at 32°C for 30 min. The supernatant containing soluble peptidoglycan fragments was then harvested by centrifugation at 12,000 g for 30 min at 4°C, then heated at 65°C for 15 min, and finally concentrated at 4°C by flash evaporation.
CFS and the bacterial pellet discarded from the isolation process of SPFs were used for further investigation.
All products were immediately used for the assays described. Furthermore, SPFs were kept at +4°C and −30°C to carry out stability tests at 30 days and again at 90 and 180 days.
2.4 In vitro antimicrobial activity
The CFS, bacterial pellets, and SPFs obtained were tested against several pathogens. CFS was neutralized with NaOH 1 mol/L at pH 7.0 before use. The inhibitory activity was performed by the agar-well diffusion assay and microdilution broth method as described by CLSI (C.L.S.I., 2018). Antibacterial activity was evaluated against Escherichia coli ATCC 25922, Enterococcus faecalis ATCC 29212, Sarcina lutea ATCC 9341, Staphylococcus aureus ATCC 25923, Pseudomonas aeruginosa ATCC 27853, Klebsiella pneumoniae ATCC 70060, and Acinetobacter baumannii ATCC 19606.
Briefly, the substances (CFS, pellet, and SPF) were added to wells in pre-inoculated MH agar plates for an agar-well diffusion assay (Stivala et al., 2021). MH plates were incubated at 37°C overnight in aerobic conditions. Finally, the inhibition zones were measured in millimeter by a gauge. Results were expressed in mm ± SD.
Regarding the microdilution broth method, minimum inhibitory concentration (MIC) values were determined according to CLSI. The assay was performed in 96-well polystyrene plates (Corning® 96-well microplates) with CAMHB medium (Cationic Adjusted Muller Hinton Broth; Oxoid). Briefly, 100 µL of the medium was added to each well, and 100 µL of the test substance was added along the first column. The dilutions (50%–0.1%) were carried out by direct microdilution in the plate. No substance was added in the last two columns. Then, a microbial suspension was made for each strain under examination, and the broth dilutions were prepared to obtain the final concentration of 104–105 CFU/mL. The last column was filled with fresh medium (negative control), while in the penultimate column, the tested pathogen was inoculated (positive control). Finally, microplates were incubated at 37°C overnight, and the MIC value was defined as the lowest concentration that inhibited the pathogenic strains’ visible growth. Moreover, the minimum bactericidal concentration (MBC) was performed after the MIC assay had been completed. Briefly, the dilution representing the MIC and two more concentrated dilutions were plated on MHA and enumerated to determine viable colonies after incubation at 37°C overnight. The MBC is the lowest concentration that demonstrates a pre-determined reduction (99.9%) in CFU/mL compared to the MIC. Results were expressed as % v/v (Fuochi et al., 2021b).
2.5 In vitro antiproliferative evaluation of the HCT116 cancer cell line
2.5.1 Cell culture
Human colorectal cells HCT116 (ATCC® CCL-247™) were purchased from ATCC Company (Manassas, Virginia, United States). Cells were suspended in RPMI 1640 (Gibco, Cat. No. 21870076) culture medium containing 10% fetal bovine serum (FBS, Gibco, Cat. No. 10082147), 100 U/mL penicillin, and 100 U/mL streptomycin (Gibco, Cat. No. 15070063). At 80% confluency, cells were passaged using a trypsin–EDTA solution (0.05% trypsin and 0.02% EDTA, Gibco, Cat. No. 25300054).
2.5.2 Real-time monitoring of cell proliferation
xCELLigence experiments were performed using the RTCA (Real-Time Cell Analyzer) DP (Dual Plate) instrument according to the manufacturers’ instructions (Roche Applied Science, Mannheim, Germany and ACEA Biosciences, San Diego, CA). The RTCA DP instrument includes three main components.
RTCA DP Analyzer, which is placed inside a humidified incubator at 37°C with 5% CO2,
RTCA Control Unit with RTCA software, and
EPlate 16.
The optimal seeding number was determined by cell titration and growth experiments. After seeding the optimal cell number (3.0 × 103 cells per well), cells were automatically monitored every 20 min for 65 h. The optimal cell number was determined in a preliminary set of experiments to obtain a significant CI (Cell Index) value and constant cell growth during the entire duration of the experiment (Castruccio Castracani et al., 2020).
2.5.3 Count and cell viability
Cell viability measurements were performed by a Muse® Cell Analyzer after 24 and 48 h (Merck Millipore Corporation, Milan, Italy) as previously described by Fuochi et al. (2017). The measurement was based on the differential permeability of two DNA-binding dyes. The nuclear dye stains only nucleated cells, while the viability dye brightly stains dying and dead cells. A volume of 180 μL of Muse® Count & Viability Reagent was added to 20 μL of suspended cells. Cells were incubated at room temperature for 5 min, and then the measurements were performed.
2.5.4 Cell migration
Cell proliferation and migration were studied using the “wound healing” assay. Cells were seeded separately in 6-well dishes and cultured until confluence. Cells were scraped with a 200-μL micropipette tip and monitored at five time-points (0, 6, 24, 30, and 48 h). The uncovered wound area was measured and quantified at different intervals using ImageJ 1.37v.
2.5.5 Clonogenic assay
Colony assays were performed by seeding cells in 6-well plates at low density (2500 cells/well) and allowing growth for 9 days. Then, cells were washed once with PBS 1X and fixed with a solution of methanol/acetic acid (3:1) for 5 min. Furthermore, a solution of crystal violet was added to stain the colonies for 15 min. Finally, colony images were acquired using a Leica microscope.
2.6 MALDI-MS analysis
MALDI mass spectrometry analysis was performed on SPFs, while crude MRS broth and MALDI matrix preparations without the analyte were used as blanks.
Spectra were recorded using a 4800 Proteomics Analyzer MALDI-TOF/TOF mass spectrometer (Applied Biosystems, Framingham, MA) equipped with an Nd:YAG laser at a wavelength of 355 nm with a less than 500-psec pulse and 200-Hz firing rate. All measurements were performed in both negative and positive polarity reflector modes using CHCA and DHB matrices. External calibration was performed on a standard peptide mix (AB-SCIEX), and mass accuracy was 100 ppm in the MS mode and 0.25 Da in the MS/MS mode. Approximately 2000 laser shots were accumulated for each spectrum in the MS experiments; 4000 to 16,000 shots were summed for the MS/MS data acquisitions. The tandem mass spectra reported in this study were acquired with and without a collision gas (He, air at low pressure).
Peak lists were generated for every spectrum of different fractions, and peptide–signal candidates were selected (for MS/MS analysis) comparing SPF spectra with MRS crude broth (with no Lactobacillus strains) and with MALDI matrix blanks (DHB and CHCA 10 g/L in TFA 0.1%/ACN 3:2) so that the only signals observed exclusively in CFS fractions were fragmented.
Samples were analyzed before and after purification with C18 Zip Tips (following the manufacturer’s protocols) at different concentrations (dilutions 1:10 ÷ 1:500, solvent TFA 0.1%/ACN 3:2).
2.7 Determination of structural features by mur gene research
All mur genes in the LAC92 strain genome were searched. Indeed, the strain was previously sequenced by Illumina MiSeq and assembled by MIRA v 4.0.2. Moreover, the sequence was deposited in GenBank (ID:CP021790.1.) with the assembly identification code GCF_002192435.1.
2.8 Docking calculations
Molecular docking experiments of SPF (defined as peptide 92) with the selected protein were performed with AutoDock Vina provided in YASARA (v. 22.5.22, YASARA Biosciences GmbH, Vienna, Austria). The 2D chemical structure of peptide 92 was built using MarvinSketch (https://chemaxon.com/products/marvin) to obtain a 3D molecule from a 2D structure and saved in the pdb file format. The software supported the valence checking, atom and bond query, stereochemistry, and user-defined templates.
The protein crystal structure of MDM2-p53 (PDB ID: 1YCR) was collected from the Protein Data Bank (PDB, http://www.rcsb.org/pdb) and optimized using YASARA software (Krieger et al., 2002). A docking employing a cell with an extension encompassing all atoms spanning 5 Å from the exterior of the p53 structure was performed (Rescifina et al., 2014; Floresta et al., 2022). Screening uses YASARA structure software with the macro dock_runscreening.mcr set in the VINA method, runs = 100, and AMBER14 force field. All the parameters were inserted at their default settings, as previously reported (Varrica et al., 2018; Floresta et al., 2019; Crocetti et al., 2022). Docking results were analyzed using BIOVIA Discovery Studio.
2.9 Statistical analysis
All the experiments were carried out four times, each in triplicate. In addition, statistical analysis was performed using GraphPad Prism 8 software. Specifically, the unpaired Welch’s t-test was selected for two data groups. Likewise, the null hypothesis was tested by multiple comparison analysis of variance (ANOVA) to compare multiple treatment groups. Differences between experimental groups were determined considering the statistical significance p < 0.05. Data were reported as mean ± SD.
3 Results
3.1 Antimicrobial activity
The antagonistic activity of LAC92 components was assessed against the following target bacteria: E. coli ATCC 25922, E. faecalis ATCC 29212, S. lutea ATCC 9341, S. aureus ATCC 25923, P. aeruginosa ATCC 27853, K. pneumoniae ATCC 70060, and A. baumannii ATCC 19606. CFS and SPFs showed good activity both against Gram-positive and Gram-negative pathogens (Table 1). In particular, S. lutea showed the greatest sensitivity, followed by E. coli and A. baumannii. The strains that proved to be less sensitive, although still inhibited, were E. faecalis and S. aureus. No activity was shown by the LAC92 bacterial pellet. These results were also confirmed by the microdilution method (Table 2).

TABLE 1. Zones of inhibition caused by LAC92 components against human pathogens. Results are expressed in millimeter (diameter) ± SD.
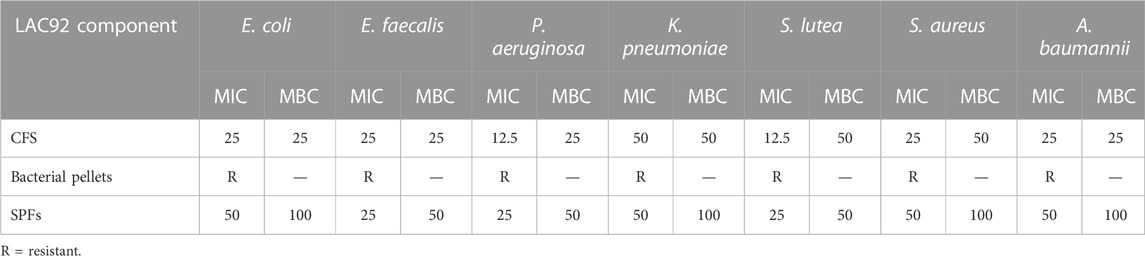
TABLE 2. Antibacterial activity of LAC92 components by the microdilution method. MIC and MBC values are expressed as % v/v.
3.2 Biological effects of SPFs on the HCT116 cancer cell line
3.2.1 xCELLigence real-time cell analysis
A preliminary investigation was performed by seeding cells at a defined concentration (3 × 103 cells per well) and monitoring the growth using a live cell analysis system xCELLigence for 65 h. Specifically, this analysis allowed evaluating the dynamic cell index in real-time upon exposure to different concentrations (% v/v) of SPFs (10%, 13%, 15%, 20%, and 25%) in the growth medium. Data shown in Figure 1 demonstrate the cytotoxicity of SPFs at concentrations above 15%. Conversely, the first non-lethal but cytostatic concentration whose cell index was maintained at lower levels compared to those of the control was the 13% SPFs. For this reason, to use a non-lethal but compromising concentration, the treatment SPFs at 13% v/v were selected to perform all the following in vitro experiments.
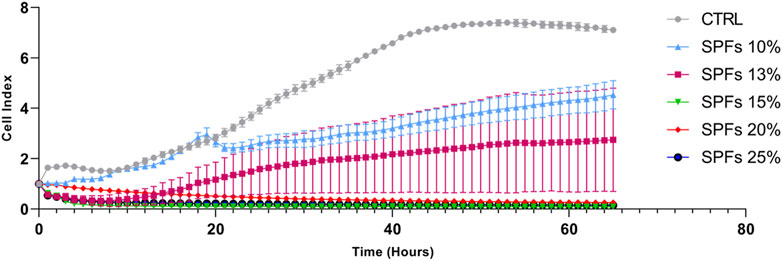
FIGURE 1. Real-time cell proliferation analysis at different concentrations (%v/v) of SPF (10%, 13%, 15%, 20%, and 25%) influencing cell index (standard cell index adhesion curve). From 15% onward SPF results are cytotoxic, while among the lower concentrations, the 13% deviates more from the cell index trend of the control. The curves represent the mean cell index value from 4 wells ±SD.
3.2.2 SPFs reduced cell count and viability
As shown in Figure 2, cell count and viability assay were performed on the HCT116 cell line both at 24 and 48 h (panel A and panel B, respectively). In particular, the treatment with SPFs induced a significant decrease in both parameters after 24 h compared to the control. This evidence suggested a remarkable antiproliferative and cytotoxic effect of SPFs on the tumor cell model.
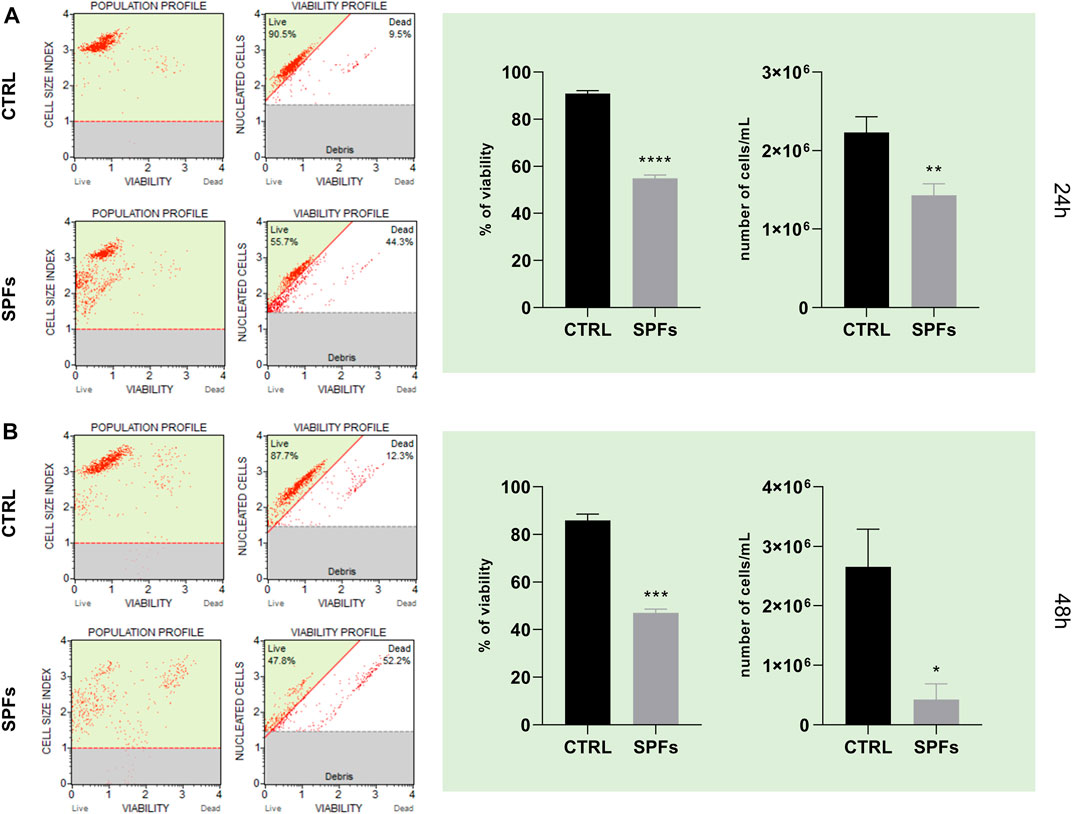
FIGURE 2. SPF affects cancer cell count and viability. (A) Cell population and viability profiles (from left to right) for CTRL and SPFs (from the top to bottom) after 24 h—histograms of percentage of viability and number of cells/mL of suspension after 24 h. (B) Cell population and viability profiles (from left to right) for CTRL and SPFs (from the top to bottom) after 48 h—histograms of percentage of viability and number of cells/mL of suspension after 48 h. Data are expressed as the means ± SD of three experiments performed in triplicate (*p < 0.05 versus CTRL).
3.2.3 SPFs affected cell migration and proliferation
Based on previous analysis, cell proliferation was further evaluated by performing the wound healing assay. The wound closure area was quantified at different time-points from T0 (6, 24, 30, and 48 h) and compared between SPF treatment and the untreated control (see Figure 3A). The results show a decrease in the wound closure rate of SPFs compared to the control, related to the SPF’s ability to inhibit the proliferation and migration rate in HCT116. The same evidence is more clearly represented in Figure.3B.
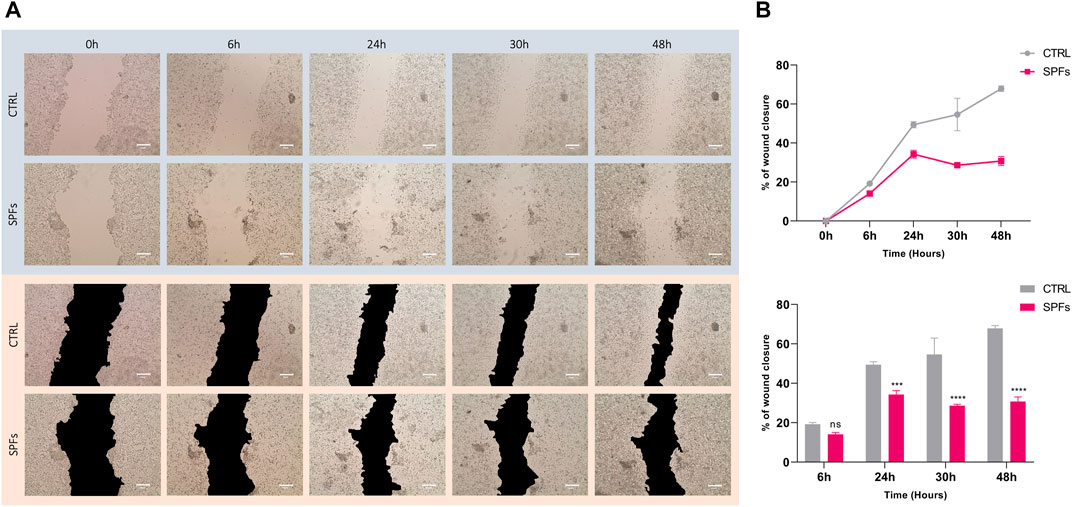
FIGURE 3. Inhibiting effects of SPFs on cancer cell migration. (A) Representative photos of wound healing assay with or without SPFs. Photos were taken from time 0–48 h (columns from left to right). All images were acquired using a Leica microscope with a magnification of ×20. The scale bar represents 200 µm. (B) Quantification of wound closure percentage expressed as a line graph (top) and bar chart (bottom). Data are expressed as the means ± SD of three experiments performed in triplicate (*p < 0.05 versus CTRL).
3.2.4 SPFs inhibited colony formation
In order to further evaluate the cytotoxic properties of SPFs in our cancer cell line, a clonogenic assay was performed. The data obtained showed a notable antiproliferative effect of SPFs compared to the control. Remarkably, the number of colonies was significantly reduced with SPFs, as reported in Figure 4.
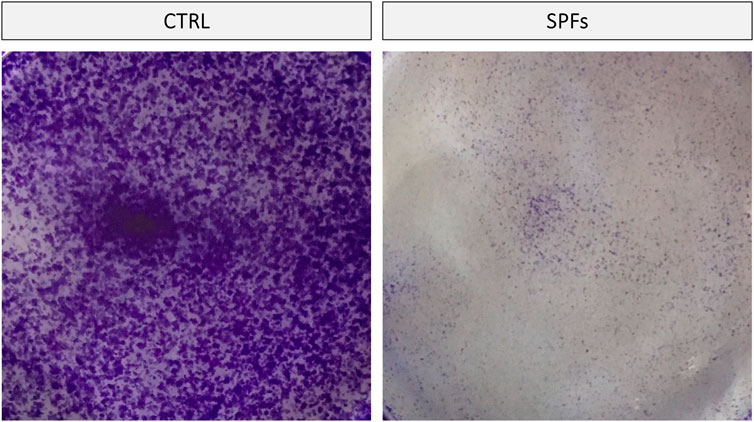
FIGURE 4. SPFs inhibited cancer cell capacity to form colonies. Representative photos of clonogenic assay performed using crystal violet staining. SPFs drastically reduced the number and size of cell colonies after 9 days of incubation compared to the untreated control.
3.3 MALDI-MS analysis
The most commonly employed MS-based strategy for identifying peptides employs low-energy collision-induced dissociation (CID) to produce characteristic fragments to determine peptide sequences. MALDI-MS/MS fragmentations generated by CID are typically dominated by cleavages along the peptide amide backbone, resulting in formation of a series of overlapping b- and y-type ions.
The first attempt to identify the possible peptide content of the SPF fraction consisted in searching protein databases (SWISS-PROT) of Gram-positive bacteria by setting the search criteria with no enzymatic cut, Firmicutes taxonomy, 150 ppm mass tolerance for precursors, and 0.25 Da mass tolerance for MS/MS fragments. However, this approach failed as databases containing information on the peptidoglycan composition of different bacterial strains have not yet been implemented. In addition, DB searches are based on homology, so unique species-specific sequences might not be identified, and this is particularly true for small and biologically active polypeptides (Medzihradszky and Chalkley, 2015).
For this reason, it was not possible to carry out a peptide mass fingerprinting search on the MALDI profiles of different soluble fractions of peptidoglycan nor was it possible to use methods such as an MS/MS ion search to search for peptide sequences by exploiting the fragmentation spectra of the signals present exclusively in the fraction CFS.
Bacterial PG hydrolases can cleave covalent bonds in peptidoglycan sacculi or their fragments (Vollmer et al., 2008). Bacterial PG hydrolases form a vast and highly diverse group of enzymes capable of cleaving bonds in polymeric PG and/or its soluble fragments. Each hydrolase class has cleavage site specificity, e.g., endopeptidases cleave peptide amide bonds, and N-acetyl-β-d-muramidases cleave the glycosidic bond between MurNAc and GlcNAc residues. Penicillin has the activity of PG hydrolases but not the specificity (of cleavage site).
The MS-based analysis of PG components is hampered by the diversity of penicillin treatment products; each can be originated from different cleavage sites of the cross-linked structure of PG. It is not possible to establish a priori the fragments produced, nor, through the knowledge of the amino acids involved in the peptidic part of the PG, to build a list of the expected products as is used in the classical proteomics approach.
However, CID can induce other fragmentation pathways that can potentially improve the confidence of peptide assignments, providing composition-specific information. One of these pathways results in the forming of internal immonium ions with the general structure RCH = NH2+ (where R is the amino acid side chain) and a mass of 27 Da less than the residue mass. These ions can be formed directly by fragmentation of the N-terminal residue of the peptide. Furthermore, they can also be formed by two cleavages surrounding a particular residue during CID, e.g., an a-type cleavage following either a b- or y-type cleavage.
In order to study the hypothetical amino acidic component of the SPFs, MS/MS spectra of putative peptides were analyzed by manual de novo sequencing; however, it was not possible to assign a unique sequence. The hypothesis of the existence in SPFs of a peptide/protein component, however, is corroborated by
1) The presence of (at least three) immonium ions (and related ions) in the low-mass region of spectra.
2) MS/MS spectra show a2 and b2 ion fragments as well as y2 fragments.
3) Loss of GlcNAc or MurNAc residues from the molecular ion.
4) Accurate delta masses of amino acid residues from the low-to high-mass region of the spectra.
5) The absence of these signals in MRS broth and DHB and CHCA blanks.
6) The presence of these signals in both DHB and CHCA preparations.
Significant results for SPF samples’ MS/MS spectra containing low-mass amino acidic immonium ions and delta mass between fragments related to amino acid residues are shown in Figure 5.
3.4 Biological activity of SPFs upon long-term storage
Biological analysis was performed to evaluate the stability of SPFs over 6-month storage at +4°C and −30°C. Unfortunately, when stored at +4°C, the products lose all biological activities after only 30 days. On the other hand, we found that SPFs stored at −30°C showed biological activity comparable to that of the fresh product at all times tested (Supplementary Figure S1). These data indicate that long-term storage at −30°C did not impair the SPFs that remained remarkably constant during the experiments.
3.5 Amino acid structure of peptide 92
Since it was unable to fully identify the peptides linked to the peptidoglycan by chromatography, as previously suggested by Fichera et al. (2016), we proceeded with another type of investigation. We have searched for the gene locus related to the synthesis of peptidoglycans (so called mur genes). The list of mur genes (CDS region) and their products involved in PG synthesis is described in Supplementary Table S1. From this analysis, the sequence of the pentapeptide bound to muramic acid was presumed. The pentapeptide, defined as peptide 92, linked to the peptidoglycan fragment has the following structure:
l-Ala-d-Glu-2,6DAP-d-Ala-d-Ala
3.6 Docking calculations
Molecular docking of peptide 92 revealed an interaction with the MDM2 protein, the negative regulator of p53 (Figure 6, left). By interacting with this protein, peptide 92 could aid the p53-driven induction of apoptosis. Indeed, docking studies performed with YASARA software show that peptide 92 allocates in the same groove as MDM2, which binds to p53 (Figure 6, right). Furthermore, the calculated Ki for p53 and peptide 92 of 185.5 µM and 62.7 µM, respectively, suggests that peptide 92 can displace p53 from the MDM2-binding site.
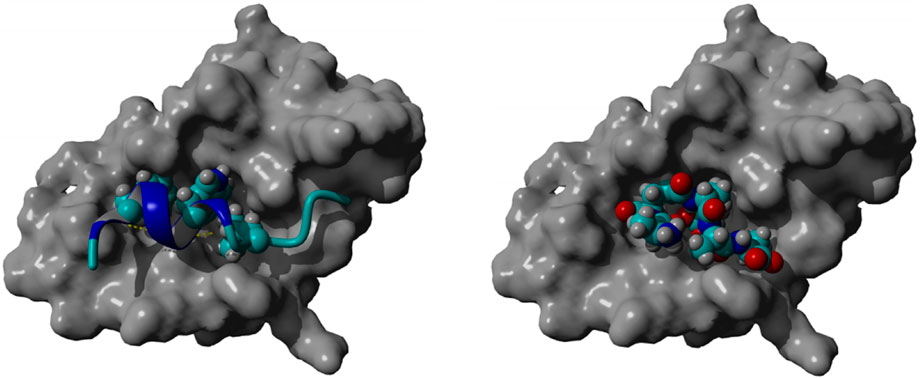
FIGURE 6. Crystallographic pose of p53 within the MDM2 groove (left); best-docked pose of peptide 92 within the MDM2 groove (right).
4 Discussion
Human microbiota has emerged as a key modulator of many disease treatment responses (Al-Qadami et al., 2022). In particular, it is already known that bacteria with probiotic properties, particularly Lactobacillus, can induce antitumor action by improving the apoptosis of tumor cells and protecting them from oxidative stress (Badgeley et al., 2021). In this regard, the gut microbiota has been involved in the efficacy of anti-PD1 and anti-PDL1 immunotherapies (Frankel et al., 2017; Matson et al., 2018; Peters et al., 2019), further confirming the crucial role of microbiota in cancer. Moreover, the antitumoral activity exerted by specific microbial species has been noticed in preclinical mouse models, suggesting that the specific presence of differential microorganisms due to co-housing or a fecal transplant was responsible for improvement in immune-checkpoint blockade agents (Sivan et al., 2015; Vetizou et al., 2015). Notably, the anticancer properties seem to be specifically related to peptidoglycan remodeling, which is involved in the dissemination of fragments into circulation, affecting immune response (Clarke et al., 2010) as well as the presence of biomarkers like NlpC/p60 hydrolases or NOD2-muropeptides. Based on this evidence, some papers suggest the importance of peptidoglycan remodeling enzymes in reprogramming probiotic bacteria to enhance the host’s immune response mechanisms (Griffin et al., 2021).
For a strain to be exploited in the therapeutic field, it is essential to define it as “probiotic” in terms of efficacy and safety. There are several criteria for recognizing bacteria as probiotics, such as their ability to survive in the GI-tract because of acidic conditions and bile salts, then being able to adhere to the intestinal cells, and, especially, have amensalistic properties (Fuochi et al., 2015; Stivala et al., 2021).
In this study, we aimed to investigate the antitumor effects of Limosilactobacillus fermentum strain against human colorectal cancer. In particular, the study targeted the effects of the peptidoglycan fragments released by the strain LAC92, for which the probiotic characteristics had already been proven (Fuochi et al., 2017). For this purpose, we screened the cytotoxicity and antiproliferative activity of LAC92 SPFs against human colorectal cancer HCT116 cells. Moreover, we analyzed the molecular structure of the peptidoglycan fragments to clarify the role of the peptide chains involved in the antitumor activity.
First of all, our observations confirmed amensalistic activity. Indeed, SPFs showed antibacterial activity against all pathogens tested. The best results were obtained against S. lutea, followed by A. baumannii, K. pneumoniae, and E. coli. Moreover, the iron-scavenging siderophore pyoverdine, a major virulence regulatory mechanism of P. aeruginosa, has also been inhibited. Nevertheless, the better activity shown by crude CFS than SPFs suggested the presence of other active molecules, which were probably lost during the peptidoglycan isolation process. Indeed, many LABs use narrow-spectrum toxins, such as bacteriocins, as a signature of ecological dominance (Fuochi et al., 2021a; Palmer and Foster, 2022). Moreover, Lactobacillus spp. synthesizes exopolysaccharides, which play an important role in preventing human diseases, thanks to their anticancer, immunomodulatory, and antimicrobial properties (Oleksy and Klewicka, 2018). Therefore, it is natural to hypothesize that molecules of different natures act synergistically by increasing the antibacterial effects demonstrated by CFS.
Based on the microbiological outcomes, we further investigated the role of SPFs in an in vitro model of a human colorectal cancer HCT116 cell line. In this regard, recent scientific literature widely focuses on the anticancer properties exerted by gut microbiota against colorectal cancer (Chen and Li, 2020; Sheikh et al., 2021). In particular, most of the clinical research between 2015 and 2017 shows the crucial role of specific gut microbiomes in postsurgical complications of colorectal cancer (Aisu et al., 2015; Consoli et al., 2016; Theodoropoulos et al., 2016; Flesch et al., 2017; Hibberd et al., 2017) acting on immune system functionality and inflammation.
The first preliminary analysis was based on selecting the proper SPF concentration of SPFs to perform all the biological investigations. In this regard, the data shown in Figure 1 showed the variable effects of SPFs on the cell index value in a concentration-dependent manner. The data obtained showed immediate cytotoxicity from 15%v/v upward. This evidence motivated us to select the first cytostatic but not cytotoxic concentration (13%v/v) to establish a proper model to evaluate further biological analyses. The subsequent investigations showed a substantial reduction in cell viability and the number of cells (Figure 2) after 24 (Figure 2A) and 48 h (Figure 2B).
Effectively, our data broadly coincided with scientific evidence showing the pro-apoptotic effect of peptidoglycan from Lacticaseibacillus paracasei in the cancer HT-29 cell line (Tian et al., 2015) through mechanisms involving endoplasmic reticulum damage. In addition, we obtained further encouraging results demonstrating the inhibitory properties of SPFs on cell migration, cell proliferation, and colony formation, as shown in Figure 3 and Figure 4.
Actually, PG derived from probiotics had encouraging antiproliferative activity on several cancer cell lines. Even though MS-MS could not assign a unique sequence to the peptide responsible for the excellent antiproliferative activity shown by SPFs deriving from LAC92, it was possible to confirm the amino acid structure involved in biological activity, thanks to the associated bacterial genomic sequence. These kinds of peptides are typically involved in the PG of L. fermentum (Barretto et al., 2016). So far, L. casei is the strain that has mostly been proven to have immunomodulatory and antitumor effects (Lee et al., 2004; Jacouton et al., 2018; Kim et al., 2019; Dadfarma et al., 2021; Spyridopoulou et al., 2021), but also live L. fermentum strain exerted the ability to inhibit cancer cells but not normal colon cells and protected them from the damaging effect of a carcinogen (Kahouli et al., 2017).
Although one critical point should be noted, which is that in vitro methods do not accurately represent in vivo conditions, taken together, these data demonstrated a crucial role of SPFs of L. fermentum LAC92 in the viability and proper functionality of cancer cells. These suggested its potential anticancer activity. This possibility was further investigated in silico by studying the interaction of peptide 92 with the MDM2 protein, the negative regulator of p53. By interacting with this protein, peptide 92 could induce apoptosis driven by p53.
The crystal structure of the amino-terminal domain of MDM2 bound to a 15-residue transactivation domain peptide of p53 disclosed that MDM2 has a deep hydrophobic cleft on which the p53 peptide binds as an amphipathic α-helix (Kussie et al., 1996). The contact between the two proteins is based on a triad of p53 amino acids, Phe19, Trp23, and Leu26, which fit perfectly with the groove of MDM2 (Figure 6, left).
The hypothesis is that MDM2 inactivates p53 by hiding its transactivation domain, disrupting its interaction with the general transcription machinery. Therefore, targeting the MDM2–p53 interaction by small molecules to reactivate p53 has emerged as a promising new cancer therapeutic strategy (Wiman, 2006; Vassilev, 2007).
Docking studies, performed using the YASARA software, of peptide 92 with only MDM2, employing the crystal structure in which it is bound to the transactivation domain of p53 (PDB: 1YCR), show that peptide 92 allocates in the same groove as MDM2, which binds to p53 (Figure 6, right). Moreover, the calculated Ki for p53 and peptide 92 of 185.5 μM and 62.7 μM, respectively, suggests that peptide 92 may displace p53 from the MDM2-binding site.
Furthermore, the biological evidences obtained, combined with the encouraging notions offered by scientific literature, lead us to speculate that LAC92 should represent an important resource in colorectal cancer treatment, in combination or not with the traditional therapies. The next steps to consider are the total purification of the bioactive molecule and its evaluation on ex vivo models, and subsequently, on in vivo cancer models to obtain results more congruous to its therapeutic potential.
Data availability statement
The original contributions presented in the study are included in the article/Supplementary Materials, further inquiries can be directed to the corresponding author.
Author contributions
VF and PMF designed the research. VF, MS, AD, CZ, and AP performed the research. CZ and AR performed molecular modeling and docking studies. VF, MS, AD, AP, DG, CZ, and AR were responsible for the analysis, interpretation of data, and graphing. VF, MS, AP, and CZ drafted the manuscript. All authors read and approved the final manuscript. VF, PMF, AR, and GLV supervised the whole analysis and provided guidance and instructions. All authors contributed to the article and approved the submitted version.
Funding
This work was partially supported by Piano di Incentivi per la ricerca di Ateneo 2020/2022 Linea di intervento 2 (GLV).
Conflict of interest
The authors declare that the research was conducted in the absence of any commercial or financial relationships that could be construed as a potential conflict of interest.
Publisher’s note
All claims expressed in this article are solely those of the authors and do not necessarily represent those of their affiliated organizations, or those of the publisher, the editors, and the reviewers. Any product that may be evaluated in this article, or claim that may be made by its manufacturer, is not guaranteed or endorsed by the publisher.
Supplementary material
The Supplementary Material for this article can be found online at: https://www.frontiersin.org/articles/10.3389/fmolb.2023.1082526/full#supplementary-material
References
Aisu, N., Tanimura, S., Yamashita, Y., Yamashita, K., Maki, K., Yoshida, Y., et al. (2015). Impact of perioperative probiotic treatment for surgical site infections in patients with colorectal cancer. Exp. Ther. Med. 10 (3), 966–972. doi:10.3892/etm.2015.2640
Al-Qadami, G., Van Sebille, Y., Bowen, J., and Wardill, H. (2022). Oral-gut microbiome Axis in the pathogenesis of cancer treatment-induced oral mucositis. Front. Oral Health 3, 881949. doi:10.3389/froh.2022.881949
Aubrey, B. J., Kelly, G. L., Janic, A., Herold, M. J., and Strasser, A. (2018). How does p53 induce apoptosis and how does this relate to p53-mediated tumour suppression? Cell Death Differ. 25 (1), 104–113. doi:10.1038/cdd.2017.169
Badgeley, A., Anwar, H., Modi, K., Murphy, P., and Lakshmikuttyamma, A. (2021). Effect of probiotics and gut microbiota on anti-cancer drugs: Mechanistic perspectives. Biochim. Biophys. Acta Rev. Cancer 1875 (1), 188494. doi:10.1016/j.bbcan.2020.188494
Barretto, C., Ngom-Bru, C., Genevaz, A., Fournier, C., Moine, D., Kassam, M., et al. (2016). Genome sequence of Lactobacillus fermentum strain NCC2970 (CNCM I-5068). Genome announc 4 (6), e01254–16. doi:10.1128/genomeA.01254-16
Bashir, A., Miskeen, A. Y., Bhat, A., Fazili, K. M., and Ganai, B. A. (2015). Fusobacterium nucleatum: An emerging bug in colorectal tumorigenesis. Eur. J. Cancer Prev. 24 (5), 373–385. doi:10.1097/CEJ.0000000000000116
Bersch, K. L., DeMeester, K. E., Zagani, R., Chen, S., Wodzanowski, K. A., Liu, S., et al. (2021). Bacterial peptidoglycan fragments differentially regulate innate immune signaling. ACS Cent. Sci. 7 (4), 688–696. doi:10.1021/acscentsci.1c00200
Castruccio Castracani, C., Longhitano, L., Distefano, A., Di Rosa, M., Pittala, V., Lupo, G., et al. (2020). Heme oxygenase-1 and carbon monoxide regulate growth and progression in glioblastoma cells. Mol. Neurobiol. 57 (5), 2436–2446. doi:10.1007/s12035-020-01869-7
Chapot-Chartier, M. P., and Kulakauskas, S. (2014). Cell wall structure and function in lactic acid bacteria. Microb. Cell Fact. 13, S9. doi:10.1186/1475-2859-13-S1-S9
Chen, C., and Li, H. (2020). The inhibitory effect of gut microbiota and its metabolites on colorectal cancer. J. Microbiol. Biotechnol. 30 (11), 1607–1613. doi:10.4014/jmb.2002.02032
Cheng, Y., Ling, Z., and Li, L. (2020). The intestinal microbiota and colorectal cancer. Front. Immunol. 11, 615056. doi:10.3389/fimmu.2020.615056
Chong, E. S. (2014). A potential role of probiotics in colorectal cancer prevention: Review of possible mechanisms of action. World J. Microbiol. Biotechnol. 30 (2), 351–374. doi:10.1007/s11274-013-1499-6
Chung, L., Orberg, E. T., Geis, A. L., Chan, J. L., Fu, K., DeStefano Shields, C. E., et al. (2018). Bacteroides fragilis toxin coordinates a pro-carcinogenic inflammatory cascade via targeting of colonic epithelial cells. Cell Host Microbe 23 (3), 421. doi:10.1016/j.chom.2018.02.004
Clarke, T. B., Davis, K. M., Lysenko, E. S., Zhou, A. Y., Yu, Y., and Weiser, J. N. (2010). Recognition of peptidoglycan from the microbiota by Nod1 enhances systemic innate immunity. Nat. Med. 16 (2), 228–231. doi:10.1038/nm.2087
C.L.S.I (2018). “Methods for dilution antimicrobial susceptibility tests for bacteria that grow aerobically,” in CLSI standard M07. (950 west valley road, suite 2500 (Wayne, Pennsylvania 19087 USA: Clinical and Laboratory Standards Institute).
Consoli, M. L., da Silva, R. S., Nicoli, J. R., Bruna-Romero, O., da Silva, R. G., de Vasconcelos Generoso, S., et al. (2016). Randomized clinical trial: Impact of oral administration of Saccharomyces boulardii on gene expression of intestinal cytokines in patients undergoing colon resection. JPEN J. Parenter. Enter. Nutr. 40 (8), 1114–1121. doi:10.1177/0148607115584387
Crocetti, L., Floresta, G., Zagni, C., Merugu, D., Mazzacuva, F., Silva, R. R. D., et al. (2022). Ligand growing experiments suggested 4-amino and 4-ureido pyridazin-3(2H)-one as novel scaffold for FABP4 inhibition. Pharmaceuticals 15 (11), 1335. doi:10.3390/ph15111335
Dadfarma, N., Nowroozi, J., Kazemi, B., and Bandehpour, M. (2021). Identification of the effects of acid-resistant Lactobacillus casei metallopeptidase gene under colon-specific promoter on the colorectal and breast cancer cell lines. Iran. J. Basic Med. Sci. 24 (4), 506–513. doi:10.22038/ijbms.2021.53015.11950
E.F.S.A. (2011a). Scientific Opinion on the safety and efficacy of Lactobacillus rhamnosus (NCIMB 30121) as a silage additive for all species. EFSA J. 9 (9). doi:10.2903/j.efsa.2011.2365
E.F.S.A. Bampidis, V., Azimonti, G., Bastos, M. d. L., Christensen, H., Dusemund, B., Durjava, M. F., et al. (2021). Safety and efficacy of a feed additive consisting of Lacticaseibacillus rhamnosus (formerly Lactobacillus rhamnosus) NCIMB 30121 for all animal species for the renewal of its authorisation (Lactosan GmbH & Co. KG). EFSA J. 19 (11), e06901. doi:10.2903/j.efsa.2021.6901
E.F.S.A. (2010). Scientific Opinion on the substantiation of health claims related to Lactobacillus gasseri CECT5714 and Lactobacillus coryniformis CECT5711 and “natural defence/immune system” (ID 930) pursuant to Article 13(1) of Regulation (EC) No 1924/2006. EFSA J. 8 (10), 1803. doi:10.2903/j.efsa.2010.1803
E.F.S.A. (2011b). Scientific Opinion on the substantiation of health claims related to Lactobacillus rhamnosus ATCC 53103 (LGG) and “gastro-intestinal health” (ID 906) and maintenance of tooth mineralisation (ID 3018) pursuant to Article 13(1) of Regulation (EC) No 1924/20. EFSA J. 9 (6), 2233. doi:10.2903/j.efsa.2011.2233
E.F.S.A. (2011c). Scientific Opinion on the substantiation of health claims related to Lactobacillus rhamnosus GR-1 (ATCC 55826) in combination with Lactobacillus reuteri RC-14 (ATCC 55845) and defence against vaginal pathogens by increasing the proportion of lactobacilli. EFSA J. 9 (6), 2232. doi:10.2903/j.efsa.2011.2232
E.F.S.A. Panel, N. D. A. (2013). Scientific Opinion on the substantiation of a health claim related to Lactobacillus rhamnosus GG and maintenance of normal defecation during antibiotic treatment pursuant to Article 13(5) of Regulation (EC) No 1924/2006. EFSA J. 11 (6), 3256. doi:10.2903/j.efsa.2013.3256
Fichera, G. A., Fichera, M., and Milone, G. (2016). Antitumoural activity of a cytotoxic peptide of Lactobacillus casei peptidoglycan and its interaction with mitochondrial-bound hexokinase. Anticancer Drugs 27 (7), 609–619. doi:10.1097/CAD.0000000000000367
Flesch, A. T., Tonial, S. T., Contu, P. C., and Damin, D. C. (2017). Perioperative synbiotics administration decreases postoperative infections in patients with colorectal cancer: A randomized, double-blind clinical trial. Rev. Col. Bras. Cir. 44 (6), 567–573. doi:10.1590/0100-69912017006004
Floresta, G., Cilibrizzi, A., Abbate, V., Spampinato, A., Zagni, C., and Rescifina, A. (2019). FABP4 inhibitors 3D-QSAR model and isosteric replacement of BMS309403 datasets. Data Brief. 22, 471–483. doi:10.1016/j.dib.2018.12.047
Floresta, G., Zagni, C., Gentile, D., Patamia, V., and Rescifina, A. (2022). Artificial intelligence technologies for COVID-19 de novo drug design. Int. J. Mol. Sci. 23 (6), 3261. doi:10.3390/ijms23063261
Fong, W., Li, Q., and Yu, J. (2020). Gut microbiota modulation: A novel strategy for prevention and treatment of colorectal cancer. Oncogene 39 (26), 4925–4943. doi:10.1038/s41388-020-1341-1
Frankel, A. E., Coughlin, L. A., Kim, J., Froehlich, T. W., Xie, Y., Frenkel, E. P., et al. (2017). Metagenomic shotgun sequencing and unbiased metabolomic profiling identify specific human gut microbiota and metabolites associated with immune checkpoint therapy efficacy in melanoma patients. Neoplasia 19 (10), 848–855. doi:10.1016/j.neo.2017.08.004
Fuochi, V., Cardile, V., Petronio Petronio, G., and Furneri, P. M. (2018). Biological properties and production of bacteriocins-like-inhibitory substances by Lactobacillus sp. strains from human vagina. J. Appl. Microbiol. 126, 1541–1550. doi:10.1111/jam.14164
Fuochi, V., Coniglio, M. A., Laghi, L., Rescifina, A., Caruso, M., Stivala, A., et al. (2019). Metabolic characterization of supernatants produced by Lactobacillus spp. with in vitro anti-Legionella activity. Front. Microbiol. 10, 1403. doi:10.3389/fmicb.2019.01403
Fuochi, V., Emma, R., and Furneri, P. M. (2021a). Bacteriocins, A natural weapon against bacterial contamination for greater safety and preservation of Food: A review. Curr. Pharm. Biotechnol. 22, 216–231. doi:10.2174/1389201021666200704145427
Fuochi, V., Li Volti, G., and Furneri, P. M. (2017). Probiotic properties of Lactobacillus fermentum strains isolated from human oral samples and description of their antibacterial activity. Curr. Pharm. Biotechnol. 18 (2), 138–149. doi:10.2174/1389201017666161229153530
Fuochi, V., Petronio, G. P., Lissandrello, E., and Furneri, P. M. (2015). Evaluation of resistance to low pH and bile salts of human Lactobacillus spp. isolates. Int. J. Immunopathol. Pharmacol. 28 (3), 426–433. doi:10.1177/0394632015590948
Fuochi, V., Rosato, A., Emma, R., and Furneri, P. M. (2021b). Colistin and kanamycin together in association with coridothymus capitatus to enhance their antimicrobial activity and fight multidrug-resistance pathogens. Biointerface Res. Appl. Chem. 11 (2), 8608–8625. doi:10.33263/Briac112.86088625
Griffin, M. E., Espinosa, J., Becker, J. L., Luo, J. D., Carroll, T. S., Jha, J. K., et al. (2021). Enterococcus peptidoglycan remodeling promotes checkpoint inhibitor cancer immunotherapy. Science 373 (6558), 1040, doi:10.1126/science.abc9113
Gupta, A., Madani, R., and Mukhtar, H. (2010). Streptococcus bovis endocarditis, a silent sign for colonic tumour. Colorectal Dis. 12 (3), 164–171. doi:10.1111/j.1463-1318.2009.01814.x
He, J., Wu, Z., Pan, D., Guo, Y., and Zeng, X. (2017). Effect of selenylation modification on antitumor activity of peptidoglycan from Lactobacillus acidophilus. Carbohydr. Polym. 165, 344–350. doi:10.1016/j.carbpol.2017.02.031
Hibberd, A. A., Lyra, A., Ouwehand, A. C., Rolny, P., Lindegren, H., Cedgard, L., et al. (2017). Intestinal microbiota is altered in patients with colon cancer and modified by probiotic intervention. Bmj Open Gastroenterol. 4 (1), e000145. doi:10.1136/bmjgast-2017-000145
Ishikawa, H., Akedo, I., Otani, T., Suzuki, T., Nakamura, T., Takeyama, I., et al. (2005). Randomized trial of dietary fiber and Lactobacillus casei administration for prevention of colorectal tumors. Int. J. Cancer 116 (5), 762–767. doi:10.1002/ijc.21115
Jacouton, E., Michel, M. L., Torres-Maravilla, E., Chain, F., Langella, P., and Bermudez-Humaran, L. G. (2018). Elucidating the immune-related mechanisms by which probiotic strain Lactobacillus casei BL23 displays anti-tumoral properties. Front. Microbiol. 9, 3281. doi:10.3389/fmicb.2018.03281
Kahouli, I., Malhotra, M., Westfall, S., Alaoui-Jamali, M. A., and Prakash, S. (2017). Design and validation of an orally administrated active L. fermentum-L. acidophilus probiotic formulation using colorectal cancer Apc (Min/+) mouse model. Appl. Microbiol. Biotechnol. 101 (5), 1999–2019. doi:10.1007/s00253-016-7885-x
Kato, I., Kobayashi, S., Yokokura, T., and Mutai, M. (1981). Antitumor activity of Lactobacillus casei in mice. Gan 72 (4), 517–523.
Kato, I., Yokokura, T., and Mutai, M. (1988). Correlation between increase in Ia-bearing macrophages and induction of T cell-dependent antitumor activity by Lactobacillus casei in mice. Cancer Immunol. Immunother. 26 (3), 215–221. doi:10.1007/BF00199932
Kim, A., Ha, J., Kim, J., Cho, Y., Ahn, J., Cheon, C., et al. (2021). Natural products for pancreatic cancer treatment: From traditional medicine to modern drug Discovery. Nutrients 13 (11), 3801. doi:10.3390/nu13113801
Kim, D. B., Lee, D. K., Cheon, C., Ribeiro, R., and Kim, B. (2022). Natural products for liver cancer treatment: From traditional medicine to modern drug Discovery. Nutrients 14 (20), 4252. doi:10.3390/nu14204252
Kim, E., Yang, J., Sung, M. H., and Poo, H. (2019). Oral administration of poly-gamma-glutamic acid significantly enhances the antitumor effect of HPV16 E7-expressing Lactobacillus casei in a TC-1 mouse model. J. Microbiol. Biotechnol. 29 (9), 1444–1452. doi:10.4014/jmb.1906.06021
Kim, J. H., Kismali, G., and Gupta, S. C. (2018). Natural products for the prevention and treatment of chronic inflammatory diseases: Integrating traditional medicine into modern chronic diseases care. Evid. Based Complement. Altern. Med. 2018, 9837863. doi:10.1155/2018/9837863
Kitayner, M., Rozenberg, H., Rohs, R., Suad, O., Rabinovich, D., Honig, B., et al. (2010). Diversity in DNA recognition by p53 revealed by crystal structures with Hoogsteen base pairs. Nat. Struct. Mol. Biol. 17 (4), 423–429. doi:10.1038/nsmb.1800
Koliarakis, I., Messaritakis, I., Nikolouzakis, T. K., Hamilos, G., Souglakos, J., and Tsiaoussis, J. (2019). Oral bacteria and intestinal dysbiosis in colorectal cancer. Int. J. Mol. Sci. 20 (17), 4146. doi:10.3390/ijms20174146
Krieger, E., Koraimann, G., and Vriend, G. (2002). Increasing the precision of comparative models with YASARA NOVA--a self-parameterizing force field. Proteins 47 (3), 393–402. doi:10.1002/prot.10104
Kussie, P. H., Gorina, S., Marechal, V., Elenbaas, B., Moreau, J., Levine, A. J., et al. (1996). Structure of the MDM2 oncoprotein bound to the p53 tumor suppressor transactivation domain. Science 274(5289), 948–953. doi: DOI doi:10.1126/science.274.5289.948
Lee, J. W., Shin, J. G., Kim, E. H., Kang, H. E., Yim, I. B., Kim, J. Y., et al. (2004). Immunomodulatory and antitumor effects in vivo by the cytoplasmic fraction of Lactobacillus casei and Bifidobacterium longum. J. Vet. Sci. 5 (1), 41–48. doi:10.4142/jvs.2004.5.1.41
Lidbeck, A., Nord, C. E., Gustafsson, J. A., and Rafter, J. (1992). Lactobacilli, anticarcinogenic activities and human intestinal microflora. Eur. J. Cancer Prev. 1 (5), 341–353. doi:10.1097/00008469-199208000-00002
Lim, S., Jeong, I., Cho, J., Shin, C., Kim, K. I., Shim, B. S., et al. (2021). The natural products targeting on allergic rhinitis: From traditional medicine to modern drug Discovery. Antioxidants (Basel) 10 (10), 1524. doi:10.3390/antiox10101524
Long, X., Wong, C. C., Tong, L., Chu, E. S. H., Ho Szeto, C., Go, M. Y. Y., et al. (2019). Peptostreptococcus anaerobius promotes colorectal carcinogenesis and modulates tumour immunity. Nat. Microbiol. 4 (12), 2319–2330. doi:10.1038/s41564-019-0541-3
Matson, V., Fessler, J., Bao, R., Chongsuwat, T., Zha, Y. Y., Alegre, M. L., et al. (2018). The commensal microbiome is associated with anti-PD-1 efficacy in metastatic melanoma patients. Science 359 (6371), 104, doi:10.1126/science.aao3290
Matsuzaki, T., Hashimoto, S., and Yokokura, T. (1996). Effects on antitumor activity and cytokine production in the thoracic cavity by intrapleural administration of Lactobacillus casei in tumor-bearing mice. Med. Microbiol. Immunol. 185 (3), 157–161. doi:10.1007/s004300050026
McGuire, S. (2016). World cancer report 2014. Geneva, Switzerland: World health organization, international agency for research on cancer, WHO press. Adv. Nutr. 7 (2), 418–419. doi:10.3945/an.116.012211
Medzihradszky, K. F., and Chalkley, R. J. (2015). Lessons in de novo peptide sequencing by tandem mass spectrometry. Mass Spectrom. Rev. 34 (1), 43–63. doi:10.1002/mas.21406
Oleksy, M., and Klewicka, E. (2018). Exopolysaccharides produced by Lactobacillus sp.: Biosynthesis and applications. Crit. Rev. Food Sci. Nutr. 58 (3), 450–462. doi:10.1080/10408398.2016.1187112
Palmer, J. D., and Foster, K. R. (2022). The evolution of spectrum in antibiotics and bacteriocins. Proc. Natl. Acad. Sci. U.S.A. 119 (38), e2205407119. doi:10.1073/pnas.2205407119
Patra, S., Sahu, N., Saxena, S., Pradhan, B., Nayak, S. K., and Roychowdhury, A. (2022). Effects of probiotics at the interface of metabolism and immunity to prevent colorectal cancer-associated gut inflammation: A systematic network and meta-analysis with molecular docking studies. Front. Microbiol. 13, 878297. doi:10.3389/fmicb.2022.878297
Peters, B. A., Wilson, M., Moran, U., Pavlick, A., Izsak, A., Wechter, T., et al. (2019). Relating the gut metagenome and metatranscriptome to immunotherapy responses in melanoma patients. Genome Med. 11 (1), 61. doi:10.1186/s13073-019-0672-4
Rescifina, A., Zagni, C., Mineo, P. G., Giofre, S. V., Chiacchio, U., Tommasone, S., et al. (2014). DNA recognition with polycyclic-aromatic-hydrocarbon-presenting calixarene conjugates. Eur. J. Org. Chem. 2014 (34), 7605–7613. doi:10.1002/ejoc.201403050
Saha, U. B., and Saroj, S. D. (2022). Lactic acid bacteria: Prominent player in the fight against human pathogens. Expert Rev. Anti Infect. Ther. 20, 1435–1453. doi:10.1080/14787210.2022.2128765
Semenov, A. V. (2021). Peptidoglycan of bacterial cell wall affects competitive properties of microorganisms. Bull. Exp. Biol. Med. 172 (2), 164–168. doi:10.1007/s10517-021-05356-4
Sheikh, A., Taube, J., and Greathouse, K. L. (2021). Contribution of the microbiota and their secretory products to inflammation and colorectal cancer pathogenesis: The role of toll-like receptors. Carcinogenesis 42 (9), 1133–1142. doi:10.1093/carcin/bgab060
Shimizu, T., Nomoto, K., Yokokura, T., and Mutai, M. (1987). Role of colony-stimulating activity in antitumor activity of Lactobacillus casei in mice. J. Leukoc. Biol. 42 (3), 204–212. doi:10.1002/jlb.42.3.204
Sivan, A., Corrales, L., Hubert, N., Williams, J. B., Aquino-Michaels, K., Earley, Z. M., et al. (2015). Commensal Bifidobacterium promotes antitumor immunity and facilitates anti-PD-L1 efficacy. Science 350 (6264), 1084–1089. doi:10.1126/science.aac4255
Spyridopoulou, K., Tryfonopoulou, E., Aindelis, G., Ypsilantis, P., Sarafidis, C., Kalogirou, O., et al. (2021). Biogenic selenium nanoparticles produced by Lactobacillus casei ATCC 393 inhibit colon cancer cell growth in vitro and in vivo. Nanoscale Adv. 3 (9), 2516–2528. doi:10.1039/d0na00984a
Stivala, A., Carota, G., Fuochi, V., and Furneri, P. M. (2021). Lactobacillus rhamnosus AD3 as a promising alternative for probiotic products. Biomolecules 11 (1), 94. doi:10.3390/biom11010094
Thanikachalam, K., and Khan, G. (2019). Colorectal cancer and nutrition. Nutrients 11 (1), 164. doi:10.3390/nu11010164
Theodoropoulos, G. E., Memos, N. A., Peitsidou, K., Karantanos, T., Spyropoulos, B. G., and Zografos, G. (2016). Synbiotics and gastrointestinal function-related quality of life after elective colorectal cancer resection. Ann. Gastroenterol. 29 (1), 56–62.
Tian, P. J., Li, B. L., Shan, Y. J., Zhang, J. N., Chen, J. Y., Yu, M., et al. (2015). Extraction of peptidoglycan from L. Paracasei subp. Paracasei X12 and its preliminary mechanisms of inducing immunogenic cell death in HT-29 cells. Int. J. Mol. Sci. 16 (8), 20033–20049. doi:10.3390/ijms160820033
Troll, J. V., Adin, D. M., Wier, A. M., Paquette, N., Silverman, N., Goldman, W. E., et al. (2009). Peptidoglycan induces loss of a nuclear peptidoglycan recognition protein during host tissue development in a beneficial animal-bacterial symbiosis. Cell. Microbiol. 11 (7), 1114–1127. doi:10.1111/j.1462-5822.2009.01315.x
Uccello, M., Malaguarnera, G., Basile, F., D'Agata, V., Malaguarnera, M., Bertino, G., et al. (2012). Potential role of probiotics on colorectal cancer prevention. BMC Surg. 12, S35. doi:10.1186/1471-2482-12-S1-S35
Varrica, M. G., Zagni, C., Mineo, P. G., Floresta, G., Monciino, G., Pistarà, V., et al. (2018). DNA intercalators based on (1,10-phenanthrolin-2-yl)isoxazolidin-5-yl core with better growth inhibition and selectivity than cisplatin upon head and neck squamous cells carcinoma. Eur. J. Med. Chem. 143, 583–590. doi:10.1016/j.ejmech.2017.11.067
Vassilev, L. T. (2007). MDM2 inhibitors for cancer therapy. Trends Mol. Med. 13 (1), 23–31. doi:10.1016/j.molmed.2006.11.002
Vazquez-Sanchez, E. A., Rodriguez-Romero, M., Sanchez-Torres, L. E., Rodriguez-Martinez, S., Cancino-Diaz, J. C., Rodriguez-Cortes, O., et al. (2014). Peptidoglycan from Staphylococcus aureus has an anti-apoptotic effect in HaCaT keratinocytes mediated by the production of the cellular inhibitor of apoptosis protein-2. Microbiol. Immunol. 58 (2), 87–95. doi:10.1111/1348-0421.12126
Veiga, P., Suez, J., Derrien, M., and Elinav, E. (2020). Moving from probiotics to precision probiotics. Nat. Microbiol. 5 (7), 878–880. doi:10.1038/s41564-020-0721-1
Vetizou, M., Pitt, J. M., Daillere, R., Lepage, P., Waldschmitt, N., Flament, C., et al. (2015). Anticancer immunotherapy by CTLA-4 blockade relies on the gut microbiota. Science 350 (6264), 1079–1084. doi:10.1126/science.aad1329
Vollmer, W., Joris, B., Charlier, P., and Foster, S. (2008). Bacterial peptidoglycan (murein) hydrolases. FEMS Microbiol. Rev. 32 (2), 259–286. doi:10.1111/j.1574-6976.2007.00099.x
Wang, S., Han, X., Zhang, L., Zhang, Y., Li, H., and Jiao, Y. (2018). Whole peptidoglycan extracts from the Lactobacillus paracasei subsp. paracasei M5 strain exert anticancer activity in vitro. Biomed. Res. Int. 2018, 2871710. doi:10.1155/2018/2871710
Wheeler, R., Chevalier, G., Eberl, G., and Boneca, I. G. (2014). The biology of bacterial peptidoglycans and their impact on host immunity and physiology. Cell. Microbiol. 16 (7), 1014–1023. doi:10.1111/cmi.12304
Wiman, K. G. (2006). Strategies for therapeutic targeting of the p53 pathway in cancer. Cell Death Differ. 13 (6), 921–926. doi:10.1038/sj.cdd.4401921
Wu, X. W., Bayle, J. H., Olson, D., and Levine, A. J. (1993). The P53 mdm-2 autoregulatory feedback loop. Genes & Dev. 7 (7), 1126–1132. doi:10.1101/gad.7.7a.1126
Yasutake, N., Kato, I., Ohwaki, M., Yokokura, T., and Mutai, M. (1984). Host-mediated antitumor activity of Lactobacillus casei in mice. Gan 75 (1), 72–80.
Yuan, H., Ma, Q., Ye, L., and Piao, G. (2016). The traditional medicine and modern medicine from natural products. Molecules 21 (5), 559. doi:10.3390/molecules21050559
Zhang, S. Y., Wang, Y. F., Sun, Y. J., Zhao, G. J., Wang, J., Liu, L., et al. (2022). Hinokiflavone, as a MDM2 inhibitor, activates p53 signaling pathway to induce apoptosis in human colon cancer HCT116 cells. Biochem. Biophysical Res. Commun. 594, 93–100. doi:10.1016/j.bbrc.2022.01.032
Keywords: Limosilactobacillus fermentum, soluble peptidoglycan fragments, antiproliferative activity, colorectal cancer, amino acids, antibacterial activity
Citation: Fuochi V, Spampinato M, Distefano A, Palmigiano A, Garozzo D, Zagni C, Rescifina A, Li Volti G and Furneri PM (2023) Soluble peptidoglycan fragments produced by Limosilactobacillus fermentum with antiproliferative activity are suitable for potential therapeutic development: A preliminary report. Front. Mol. Biosci. 10:1082526. doi: 10.3389/fmolb.2023.1082526
Received: 28 October 2022; Accepted: 31 January 2023;
Published: 15 February 2023.
Edited by:
Bart De Geest, KU Leuven, BelgiumReviewed by:
Teresa Semedo-Lemsaddek, Universidade de Lisboa, PortugalLucrecia C. Teran, National Scientific and Technical Research Council (CONICET), Argentina
Blaženka Kos, University of Zagreb, Croatia
Copyright © 2023 Fuochi, Spampinato, Distefano, Palmigiano, Garozzo, Zagni, Rescifina, Li Volti and Furneri. This is an open-access article distributed under the terms of the Creative Commons Attribution License (CC BY). The use, distribution or reproduction in other forums is permitted, provided the original author(s) and the copyright owner(s) are credited and that the original publication in this journal is cited, in accordance with accepted academic practice. No use, distribution or reproduction is permitted which does not comply with these terms.
*Correspondence: Virginia Fuochi, dmZ1b2NoaUB1bmljdC5pdA==