- 1Department of Chemistry and Biotechnology, Graduate School of Engineering Tottori University Koyama-Minami 4-101, Tottori, Japan
- 2Centre for Research on Green Sustainable Chemistry Tottori University Koyama-Minami 4-101, Tottori, Japan
Cytoskeletons such as microtubules and actin filaments are natural protein assemblies, which dynamically control cellular morphology by reversible polymerization/depolymerization. Recently, the control of polymerization/depolymerization of fibrous protein/peptide assemblies by external stimuli has attracted significant attention. However, as far as we know, the creation of an “artificial cytoskeleton” that reversibly controls the polymerization/depolymerization of peptide nanofiber in giant unilamellar vesicles (GUVs) has not been reported. Here, we developed peptide nanofiber self-assembled from spiropyran (SP)-modified β-sheet-forming peptides, which can be reversibly polymerized/depolymerized by light. The reversible photoisomerization of the SP-modified peptide (FKFECSPKFE) to the merocyanine-peptide (FKFECMCKFE) by ultraviolet (UV) and visible light irradiation was confirmed by UV–visible spectroscopy. Confocal laser scanning microscopy with thioflavin T staining and transmission electron microscopy of the peptides showed that the SP-peptide formed β-sheet nanofibers, whereas the photoisomerization to the merocyanine-peptide almost completely dissociated the nanofibers. The merocyanine peptide was encapsulated in spherical GUVs comprising of phospholipids as artificial cell models. Interestingly, the morphology of GUV encapsulating the merocyanine-peptide dramatically changed into worm-like vesicles by the photoisomerization to the SP-modified peptide, and then reversibly changed into spherical GUV by the photoisomerization to the MC-modified peptide. These dynamic morphological changes in GUVs by light can be applied as components of a molecular robot with artificially controlled cellular functions.
Introduction
Cytoskeletons in eukaryotic cells such as microtubules, actin filaments, and intermediate filaments are fibrous protein assemblies, which spatiotemporally polymerize and depolymerize to control cell morphology and intracellular/extracellular movements (Pederson and Aebi, 2002; Wade, 2009; Eldirany et al., 2021). The dynamic polymerization/depolymerization of the cytoskeleton plays important roles in the deformation and movement of various membrane systems and the arrangement of cell organelles, cell division, muscle contraction, and ciliary movement (Luna and Hitt, 1992; Ramaekers et al., 2004; Hall, 2009; Fletcher and Mullins, 2010). The polymerization and depolymerization of microtubules and actin filaments are dynamically controlled by the hydrolysis of guanosine triphosphate (GTP) and adenosine triphosphate (ATP), respectively. It is challenging to control the dynamics of the polymerization and depolymerization of the cytoskeletons using artificial materials.
Giant unilamellar vesicles (GUVs) with diameters near cell size are widely utilized in research as cellular models for the physicochemical understanding of processes involving biological lipid membranes (Lasic and Papahadjopoulos, 1995). Recently, it was reported that the polymerization/depolymerization of cytoskeletons and physical perturbation to GUVs induced deformation in GUVs, as in natural cells (Sekine et al., 2012; Shi and Baumgart, 2015; Sato et al., 2017; Steinkühler et al., 2020; Ganar et al., 2021). For example, Tanaka et al. (2018) reported that the adjustment of osmotic pressure and cleavage of actin filaments with gelsolin induced reversible deformation in GUVs between the spindle and spherical morphologies. Koseki and Suzuki (2020) successfully constructed neuron-like vesicles by osmotic shrinking in GUVs. Li et al. (2021) developed self-driven artificial cells encapsulating active mitochondria that can generate ATP and demonstrated reversible morphological changes in GUVs with the growth of actin filaments by the generated ATP. However, there were problems such as the irreversibility of GUV deformation (Loiseau et al., 2016), long response time (Fanalista et al., 2018), and small morphology change (Liu et al., 2021).
Recently, the polymerization/depolymerization of various peptide/protein fibers has been controlled by external stimuli, such as enzymatic reactions (Haines, et al., 2005; Tanaka, et al., 2015; Chen, et al., 2016; West, et al., 2018), light (Bosques and Imperiali, 2003; Doran, et al., 2014; Nakamura, et al., 2021), and redox (D’Souza, et al., 2022; Yao, et al., 2021; Bowerman and Nilsson, 2010). For example, Pires et al. (2015) successfully formed peptide nanofibers on the cell surface by enzymatic dephosphorylation to induce cell death. Sendai et al. (2013) reported the light-induced reversible polymerization/depolymerization of protein nanotubes comprising GroEL (a natural molecular chaperone) modified with photochromic spiropyran (SP). Bashirzadeh et al. (2020) recently reported the light-controlled reversible formation/dissociation of hydrogels self-assembled from SP-modified short peptide, Fmoc-KKSPKF-NH2, by the photoisomerization of SP to protonated merocyanine (MCH+). However, to the best of our knowledge, there is no example of the dynamic control of GUV deformation by reversibly controlled polymerization/depolymerization of self-assembling peptide nanofibers mimicking natural cytoskeletons. Major challenge of this study is to develop a self-assembling material that can reversibly and dramatically deform GUVs by polymerization/depolymerization upon external stimuli.
Previously, we pioneered a photoinduced peptide nanofiber growth system by conjugating a β-sheet-forming peptide (FKFEFKFE) with DNA (dA20), an assembly inhibitory site, using a photodissociative amino acid (Furutani et al., 2015). The equipping of this system with the GUV and nucleosphere (DNA microassembly) promotes motility driven by light-induced nanofiber formation (Inaba, et al., 2018; Inaba, et al., 2021). Considering that the light-induced spatiotemporal control of nanofiber growth is irreversible, the reversible control of the polymerization/depolymerization mimicking of natural cytoskeletons has not been realized.
Here, to create artificial cytoskeletons that dynamically control the deformation of GUVs by the light-induced reversible polymerization/depolymerization of peptide nanofibers, we designed a β-sheet-forming peptide modified with a photochromic dye, SP (Figure 1A). Uncharged SP with a dipole moment of 4.3 D was isomerized by ultraviolet (UV) light irradiation to zwitterionic merocyanine (MC) with a dipole moment of 17.7 D (Klajn, 2014). The photoisomerization of SP/MC with changes in the structure and dipole moment can be harnessed for the reversible control of the polymerization/depolymerization of peptide/protein assemblies (Sendai et al., 2013; Bashirzadeh et al., 2020). We demonstrated the reversible control of the polymerization/depolymerization of peptide nanofibers self-assembled from the SP/MC-modified β-sheet-forming peptide (Figure 1A) and dramatic morphological changes in the GUVs encapsulating the peptide by light irradiation (Figure 1B). By creating a photo-responsive artificial cytoskeleton that mimics the dynamic control of the eukaryotic cell cytoskeleton, the dramatic deformation of the GUV through the polymerization and depolymerization of peptide nanofibers by light irradiation was achieved. From the perspective of molecular robotics reconstructed from artificial molecules, it is proposed here that polymerization and depolymerization of peptide nanofibers is another mechanism that enables the dynamic properties of living cells.
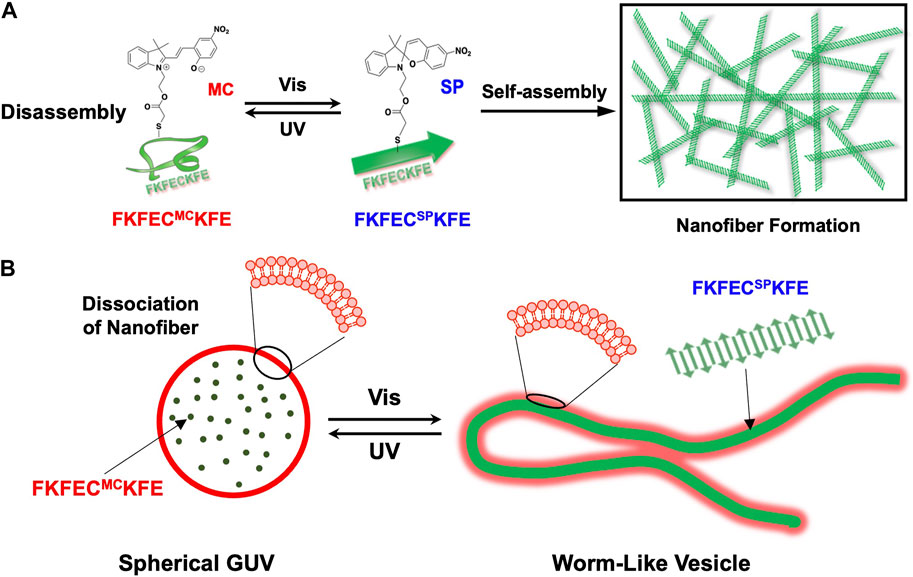
FIGURE 1. (A) Schematic of reversible nanofiber formation and dissociation of FKFECSP/MCKFE peptides by photoisomerization. (B) Schematic of the reversible and dramatic morphological changes that occurred after the photoisomerization of FKFECSP/MCKFE in the giant unilamellar vesicle (GUV).
Materials and methods
General
Ultrapure water with high resistivity (>18 MΩ cm) purified using the Millipore Purification System (Milli-Q water) was used as a solvent for the peptides. Reagents were obtained from a commercial source and used without further purification. Reversed-phase high-performance liquid chromatography (RP-HPLC) was performed at ambient temperature using a Shimadzu LC-6AD system equipped with a UV–visible detector [220 and 280 nm, Shimadzu SPD-10 A(V) vp] and Inertsil ODS-3 (GL Science) columns (250 × 4.6 or 250 × 20 mm). Matrix-assisted laser desorption/ionization time-of-flight mass spectrometry (MALDI-TOF MS) was conducted using an UltrafleXtreme instrument (Bruker Daltonics) in the linear/positive mode with α-cyano-4-hydroxy cinnamic acid (α-CHCA) as the matrix. Circular dichroism (CD) spectra were recorded at 25°C in a 1.0 cm quartz cell using a Jasco J-820 spectrophotometer equipped with a Peltier-type thermostatic cell holder.
Synthesis of β-sheet-forming peptide, FKFECKFE
β-Sheet-forming peptide bearing Cys (FKFECKFE) was synthesized on Fmoc-Glu (OtBu)-Alko resin (543 mg, 0.125 mmol/g; Watanabe Chemical Ind. Ltd.,) by fluorenylmethyloxycarbonyl (Fmoc)-based coupling reactions (4 equiv. of Fmoc amino acids). An N-methyl pyrrolidone (NMP) solution containing (1-cyano-2-ethoxy-oxoethylidenaminooxy) dimethyl amino-morpholino-carbenium hexafluorophosphate (COMU, 4 equiv.) and N, N-diisopropylethylamine (DIPEA, 8 equiv.) was used as the coupling reagent. Fmoc deprotection was achieved by adding 2 mL of a solution of piperidine/N, N-dimethylformamide (DMF) = 40/60 (vol) to the column and stirring for 3 min, then removing the solution. Next, 2 mL of a solution of piperidine/DMF = 20/80 (vol) was added and stirred for 10 min. After removing the solution, the resin was washed five times with NMP. The progression of the coupling reaction and Fmoc deprotection was confirmed using a 2,4,6-trinitrobenzene sulfonic acid (TNBS) test kit (TCI Co. Ltd.,). The peptidyl resins were washed with NMP and dried under a vacuum. The peptide was deprotected and cleaved from the resin through treatment with a cocktail of trifluoroacetic acid (TFA)/1,2-ethanedithiol/triisopropylsilane/thioanisole/water at a ratio of 8.25/0.25/0.1/0.5/0.5 (mL) at room temperature for 4 h. The reaction mixture was filtered to remove the resins, and the filtrates were concentrated under vacuum conditions. The peptide was precipitated by adding methyl tert-butyl ether (MTBE) to the residue, and the supernatant was decanted. After washing thrice with MTBE, the crude product was purified by RP-HPLC, eluting with a linear gradient of CH3CN/water containing 0.1% TFA (5/95 to 50 min). The fraction containing the desired peptide was lyophilized to afford 2.34 mg of a flocculent solid (45.1% yield), confirmed by MALDI-TOF MS (matrix, α-CHCA; m/z = 1077 [M]+).
Synthesis of bromoacetyl spiropyran
Here, 1-(2-hydroxyethyl)-3,3-dimethylindolino-6′-nitrobenzopyrylospiran (251.0 mg, 0.71 mmol) and 4-dimethylaminopyridine (137.5 mg, 1.12 mmol) were placed in an eggplant flask and purged with N2 gas. The mixture was dissolved in dry toluene (6 mL), and bromoacetyl bromide (100 μL, 1.07 mmol) was added dropwise at 25°C with stirring. The reaction mixture was stirred for 2 h at 25°C under a nitrogen atmosphere and quenched by adding water (10 mL). The mixture was extracted with dichloromethane (30 mL) and washed thrice with water. The organic layer was dried over anhydrous MgSO4 overnight, and the MgSO4 was removed by filtration. The filtrate was concentrated under reduced pressure to obtain a reddish-brown solid. Subsequently, the product was purified by silica-gel column chromatography using dichloromethane as the eluent. After the evaporation of dichloromethane under reduced pressure, 269 mg (80% yield) of bromoacetyl spiropyran was obtained as a yellow-brown powder. MALDI-TOF MS: m/z found: 472.3 ([SP-79Br]+), calcd. 472.1, m/z found: 474.3 ([SP-81Br]+), calcd. 474.1.1H NMR (500 MHz, CDCl3, Supplementary Figure S8): δ/ppm; 8.01–8.03 (2H, m); 7.22 (1H, t); 7.10 (1H, d); 6.90–6.94 (2H, m); 6.75 (1H, d); 6.67 (1H, d); 5.93 (1H, d); 4.32 (2H, s); 3.77 (2H, t); 3.50 (2H, q); 1.16–1.2 (6H, s).
Synthesis of spiropyran-modified peptide FKFECSPKFE
FKFECKFE peptide (3.53 mg, 3.28 μmol) was added to a solution of bromoacetyl spiropyran (2.2 mg, 4.59 μmol) in DMF (700 μL) and DIPEA (100 μL), and the mixture was stirred for 4 h at 4°C. The product was purified by RP-HPLC, eluting with a linear gradient of CH3CN/water containing 0.1% TFA (5/95 to 50 min). The fraction containing the FKFECSPKFE peptide was lyophilized to afford 1.33 mg of flocculent solid (27.6% yield), confirmed by MALDI-TOF MS (matrix, α-CHCA; m/z = 1469 [M]+).
Photoisomerization of FKFECSP/MCKFE
FKFECSPKFE peptide (100 μM) was dissolved in a 10-mM phosphate buffer (pH, 7.4) and incubated for 30 min at 60°C, followed by incubation for 10 min at 25°C. The peptide solution was irradiated with UV light (365 nm, 5 mW, and 5 cm) using a Jasco FP-8200 spectrofluorometer for 10–180 min at 25°C, and UV-visible spectra were recorded using a Jasco V-630 spectrophotometer. Afterward, the peptide solution was irradiated with visible light (580 nm, 5 mW, and 5 cm) for 10–180 min at 25°C, and UV-visible spectra were recorded.
Circular dichroism spectra of FKFECSP/MCKFE
A solution of FKFECSPKFE peptide (100 μM) in a 10-mM phosphate buffer (pH, 7.4) was incubated at 60°C for 30 min and left in the dark at 25°C for 24 h. The CD spectrum of the peptide solution was measured at 25°C in a 1.0-cm quartz cell using a Jasco J-820 spectrophotometer. After UV light irradiation of the solution (365 nm, 5 mW, and 5 cm) for 80 min using the Jasco FP-8200 spectrofluorometer, followed by incubation in the dark at 25°C for 24 h, the CD spectrum of the FKFECMCKFE peptide solution was recorded at 25°C.
Fluorescence spectra of thioflavin T
A solution of thioflavin T (100 μM) and FKFECSPKFE peptide (100 μM) in a 10-mM phosphate buffer (pH, 7.4) was incubated at 60 °C for 30 min and left in the dark at 25°C for 24 h. The fluorescence spectrum of the solution was recorded using a Jasco FP-8200 spectrofluorometer at 25°C at an excitation wavelength of 450 nm. After the UV light irradiation of the solution (365 nm, 5 mW, and 5 cm) for 80 min using a Jasco FP-8200 spectrofluorometer, followed by incubation for 24 h in the dark at 25°C, the fluorescence spectrum of the solution was recorded. The fluorescence spectra of the FKFECSPKFE peptide (100 μM) alone and thioflavin T (100 μM) alone in a 10-mM phosphate buffer were recorded separately under the same condition.
Transmission electron microscopy (TEM)
Carbon-coated Cu grids (thin carbon film TEM grids; Alliance Biosystems) were hydrophilized by plasma treatment (25°C, 60 Hz, 500 VA, 40 s, JEOL HD Treatment). Aliquots (5 μL) of the aqueous sample solutions were applied to the hydrophilized carbon-coated Cu grids for 1 min and removed using filter paper. Subsequently, the TEM grids were instilled in a staining solution, 2% phosphotungstic acid (Na3(PW12O40) (H2O)n) (5 µL), for 2 min and removed using filter paper. After the sample-loaded Cu grids were dried in vacuo, they were observed by TEM (JEOL JEM 1400 Plus) at an accelerating voltage of 80 kV.
Preparation of giant unilamellar vesicle-encapsulating peptide
Here, 1-palmitoyl-2-oleoyl phosphatidylcholine (POPC, Funakoshi Co., Ltd.) and Atto 550-labeled 1,2-dioleoyl-sn-glycero-3-phosphoethanolamine (Atto 550-DOPE, Funakoshi Co., Ltd.) with a molar ratio of 200:1 were mixed with D-glucose (1 equiv. of the lipids) in chloroform/methanol (2/1, v/v). The solution was poured into a glass test tube and dried under reduced pressure overnight. A 10 μM FKFECSPKFE peptide solution in a 10-mM phosphate buffer (pH, 7.4) containing 100 μM thioflavin T was incubated at 60°C for 30 min, followed by 10 min at 25°C. Thereafter, the peptide solution was irradiated with UV light (365 nm, 5 mW, and 5 cm) using the Jasco FP-8200 spectrofluorometer for 80 min and incubated for 24 h at 25°C in the dark. Thereafter, the dried lipid film was hydrated with the peptide solution (100 μL) in a 10-mM phosphate buffer (pH, 7.4) containing 100 μM thioflavin T at 25°C and incubated for 1 h in the dark at 25°C. The final concentration was [POPC] = 1 mM [Atto 550 DOPE] = 5 μM [FKFECSP/MCKFE] = 10 μM [thioflavin T] = 100 μM.
Confocal laser scanning microscopy (CLSM)
The thioflavin T-stained FKFECSPKFE, FKFECMCKFE peptides, and GUVs encapsulating peptides were observed by CLSM using a FluoView FV10i (Olympus). The FKFECSPKFE peptide solution (100 μM) in a 10-mM phosphate buffer (pH, 7.4) containing 100 μM thioflavin T was incubated at 60 °C for 30 min, followed by 10 min at 25°C. CLSM was performed by placing the prepared peptide solution of FKFECSPKFE in a glass bottom dish. Thereafter, the peptide solution was irradiated with UV light (5 mW, 5 cm) for 80 min using the Jasco FP-8200 spectrofluorometer at 365 nm and incubated for 24 h at 25°C in the dark. Afterward, the CLSM of the peptide solution of FKFECMCKFE (100 μM) in a 10-mM phosphate buffer (pH, 7.4) containing 100 μM thioflavin T was performed.
The dispersion of GUV encapsulating FKFECMCKFE peptide (2 μL) was dropped onto a glass slide and covered with a cover glass, and CLSM was performed. The dispersions of GUV encapsulating FKFECMCKFE peptide on the glass slide were irradiated with visible light at 505 nm (CL-1503, Asahi Spectra, 52 mW, 10 cm) for 3 min, and images were obtained every 10 s in the CLSM time-lapse mode, followed by 1 h of continuous video recording. The dispersions of GUV encapsulating FKFECSPKFE peptide on the glass slide were irradiated with UV light at 365 nm (CL-1503, Asahi Spectra, 30 mW, 10 cm) for 3 min, and images were obtained every 10 s in the CLSM time-lapse mode, followed by 1 h of continuous video recording.
The final concentration was [POPC] = 1 mM [Atto 550 DOPE] = 5 μM [FKFECSP/MCKFE] = 10 μM [thioflavin T] = 100 μM. Thioflavin T was excited at 491 nm and observed through a 508-nm emission band-pass filter (green). Atto 550 and merocyanine was excited at 553 nm and observed through a 577-nm emission band-pass filter (red).
Results
Synthesis of spiropyran-modified β-sheet-forming peptide
To create nanofibers that reversibly polymerize and depolymerize upon photoisomerization, we designed a β-sheet-forming peptide with SP (Figure 1). The central Phe residue of the β-sheet-forming peptide, FKFEFKFE (Marini et al., 2002), which forms nanofibers based on antiparallel β-sheets, was substituted with Cys to modify SP. The FKFECKFE peptide was synthesized using the standard Fmoc solid-phase method, then purified by RP-HPLC (Supplementary Figure S1A), the molecular weight was confirmed by MALDI-TOF MS (Supplementary Figure S1B). The CD spectrum (Supplementary Figure S1C) and TEM image (Supplementary Figure S1D) showed that the formation of peptide nanofibers with β-sheet structure. Subsequently, 1-(2-hydroxyethyl)-3,3-dimethylindolino-6′-nitrobenzopyrylospiran was reacted with bromoacetyl bromide to obtain bromoacetyl spiropyran (Supplementary Figure S2A). The nucleophilic substitution of the thiol group of the FKFECKFE peptide to bromoacetyl spiropyran in the presence of DIPEA afforded the SP-modified peptide, FKFECSPKFE (Supplementary Figure S2B), which was purified by RP-HPLC (Supplementary Figure S3A) and confirmed by MALDI-TOF MS (Supplementary Figure S3B).
Photoisomerization of FKFECSP/MCKFE peptides
The photoisomerization of FKFECSPKFE peptides in a 10-mM phosphate buffer (pH, 7.4) was confirmed by UV–visible spectroscopy (Figure 2). An aqueous solution of 100 μM FKFECSPKFE in a 10-mM phosphate buffer (pH, 7.4) was incubated at 60°C for 30 min, followed by UV light irradiation at 365 nm (80 min, 5 mW, 5 cm). The absorbance of SP (around 350 nm) in the peptide decreased, whereas that of MC (around 580 nm) increased upon UV irradiation (Figure 2B). Contrarily, after UV light irradiation, the peptide solution was irradiated with visible light at 580 nm (80 min, 5 mW, 5 cm), which increased the absorbance of SP and decreased the absorption of MC (Figure 2C). The time course of absorbance of the FKFECSP/MCKFE peptides due to photoisomerization reached a steady state at 80 min for both UV- and visible light irradiation (Figures 2D, E). These results indicated that the FKFECSP/MCKFE peptides were reversibly photoisomerized at approximately 80 min. RP-HPLC confirmed that the peptides were 91.4% FKFECMCKFE and 8.6% FKFECSPKFE after 80 min of UV light irradiation at 365 nm (Supplementary Figure S4; Supplementary Table S1).
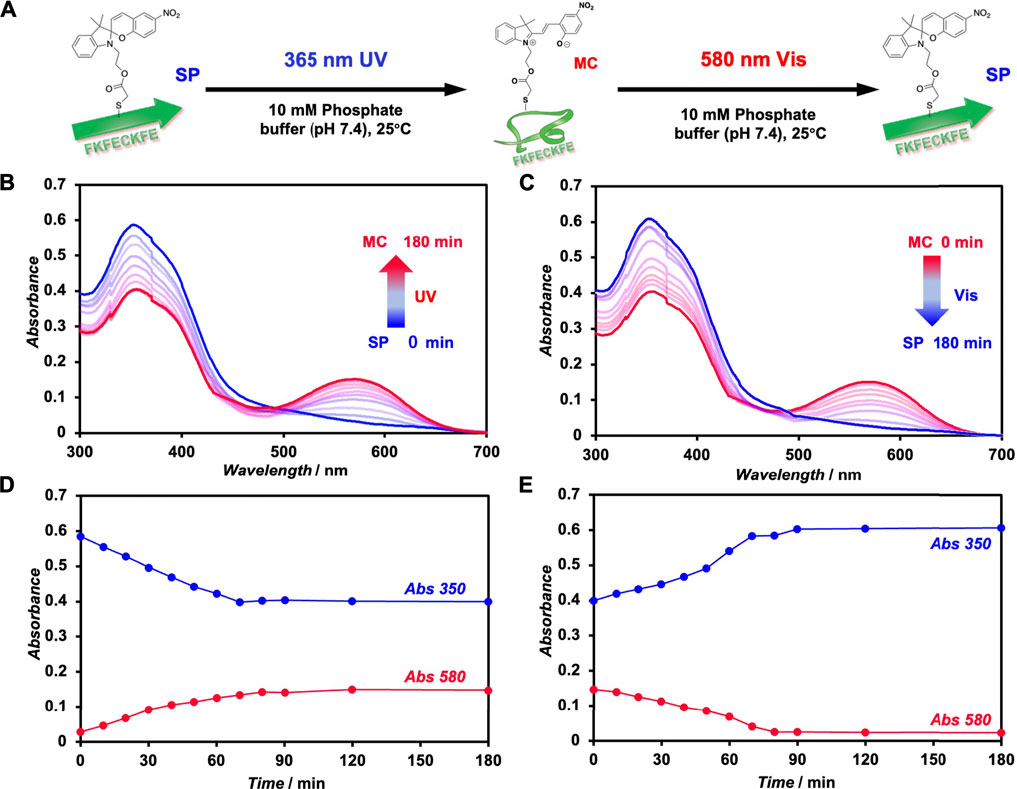
FIGURE 2. (A) Schematic of the photoisomerization of FKFECSP/MCKFE peptides. UV–visible spectra of (B) spiropyran (SP)-modified peptide ([FKFECSPKFE] = 100 μM, blue) during UV light (365 nm, 5 mW, 5 cm) irradiation and (C) merocyanine (MC)-modified peptide ([FKFECMCKFE] = 100 μM, red) in a 10-mM phosphate buffer (pH, 7.4; 25°C) during visible light (580 nm, 5 mW, 5 cm) irradiation. Time course of absorbance of the SP (350 nm) and MC (580 nm) peptides during (D) UV- and (E) visible light irradiation.
Secondary structure of FKFECSP/MCKFE peptides
The CD spectrum of FKFECSPKFE peptides in a 10-mM phosphate buffer (pH 7.4), which was incubated at 60°C for 30 min and left in the dark for 24 h at 25°C, exhibited positive and negative peaks at 197 and 214 nm, respectively, indicating the formation of a typical β-sheet structure (Figure 3A). Contrarily, the CD spectrum of the photoisomerized FKFECMCKFE peptides in the same buffer exhibited a negative peak at 198 nm, indicating a random coil structure. After irradiation of visible light at 580 nm (80 min, 5 mW, 5 cm) to the FKFECMCKFE peptide and subsequent incubation in the dark for 24 h at 25°C, the CD spectrum indicated the reformation of β-sheet structure. As most of the peptides reverted to the β-sheet conformation, the reversibility of the photoisomerization process of this peptide was confirmed. However, the secondary structure of the peptide was slightly different from that before photoisomerization, which is probably due to the generation of small amount of other structures such as α-helix after photoisomerization.
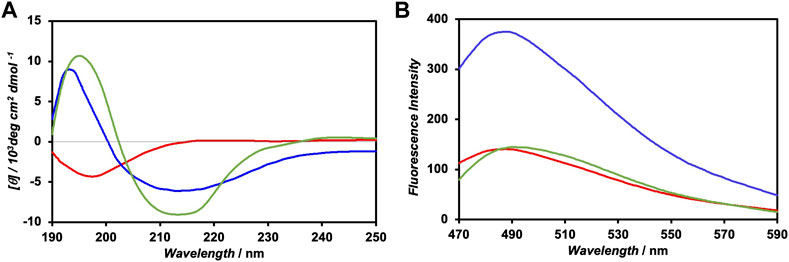
FIGURE 3. (A) CD spectra of 100-μM FKFECSPKFE (blue), 100-μM FKFECMCKFE (red), and FKFECSPKFE resulted by 580 nm visible light irradiation to 100-μM FKFECMCKFE and subsequent incubation in the dark for 24 h (green) in a 10-mM phosphate buffer (pH, 7.4) at 25°C. (B) Fluorescence spectra of thioflavin T (100 μM) in the presence of 100-μM FKFECSPKFE (blue), 100-μM FKFECMCKFE (red), and thioflavin T alone (green) in a 10-mM phosphate buffer (pH 7.4) at 25°C.
The secondary structure of the FKFECSP/MCKFE peptides was also confirmed by the fluorescence spectra of thioflavin T; the fluorescence intensity increased when bound to nanofibers comprising β-sheet structures. The fluorescence intensity of thioflavin T in the presence of FKFECSPKFE increased approximately three-fold, compared with that of thioflavin T alone, whereas FKFECMCKFE minimally affected the intensity of thioflavin T (Figure 3B). These results suggested that FKFECSPKFE peptides formed nanofibers with a β-sheet structure, whereas FKFECMCKFE peptides exhibited a random coil structure.
Polymerization and depolymerization of nanofibers controlled by the photoisomerization of FKFECSP/MCKFE peptides
The change in self-assembling behavior driven by the photoisomerization of FKFECSP/MCKFE peptides was evaluated by CLSM with thioflavin T staining and TEM with phosphotungstic acid staining (Figure 4A). No nanofiber images were observed immediately after the FKFECSPKFE peptide solution was incubated at 60°C for 30 min and left in the dark at 25°C. After the incubation of the solution for 24 h, the formation of peptide nanofibers with lengths in the range of 1–7 μm was observed by CLSM and TEM, as shown in Figures 4B, C, respectively. After the FKFECSPKFE peptide nanofibers were irradiated with UV light at 365 nm (80 min, 5 mW, 5 cm), followed by incubation in the dark for 24 h, the nanofibers completely disappeared (Figures 4B, C). This indicated that the structural change from β-sheets to random coils caused by the isomerization of SP to MC was responsible for the dissociation of the nanofibers. The reformation of nanofibers with lengths in the range of 0.6–3.5 μm was achieved when the FKFECMCKFE solution was irradiated with visible light at 580 nm (80 min, 5 mW, 5 cm), followed by incubation in the dark for 24 h (Figures 4B, C). These results showed that the photoisomerization of FKFECSP/MCKFE can reversibly control the formation and dissociation of nanofibers. It is possible that the initial heating step at 60°C to promote rapid dissolution of the powdered peptide in phosphate buffer provided the necessary energy to form longer fibers more rapidly. The shorter fibers observed in the second round of photoisomerization may be formed because there is no energy boost. In addition, the secondary structural change due to photoisomerization was not completely reversible (Figure 3A), which may be one of the factors contributing to the shorter length of the peptide nanofibers.
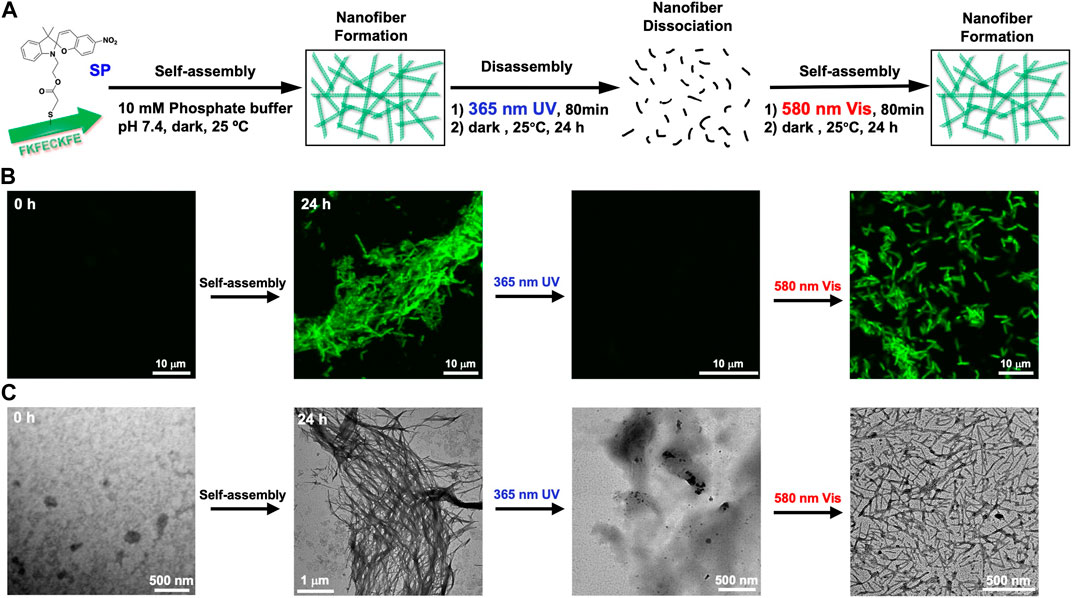
FIGURE 4. (A) Schematic of the formation and dissociation of the peptide nanofiber by photoisomerization. CLSM (B) and TEM images (C) of 100-μM FKFECSP/MCKFE and 100 μM thioflavin T during photoisomerization to form and dissociate peptide nanofibers in a 10-mM phosphate buffer (pH, 7.4) at 25°C.
Morphological changes in the GUV by the photoisomerization of FKFECSP/MCKFE peptides
Furthermore, we evaluated if the photoisomerization of FKFECSP/MCKFE can transform the morphology of spherical GUVs. A mixed lipid membrane of POPC and Atto 550-labeled DOPE was hydrated to form spherical GUVs (Figure 5A). Also, the mixed lipid membrane was hydrated with an aqueous solution of random coil FKFECMCKFE peptide to encapsulate the peptide with GUVs. The TEM image of the complex of FKFECMCKFE peptides and POPC shows the formation of normal spherical GUVs (Figure 5B). After visible light irradiation at 580 nm (80 min, 5 mW, 5 cm), the spherical GUV was significantly deformed to a rod-like structure. When this sample solution was irradiated with UV light at 365 nm (80 min, 5 mW, 5 cm), spherical GUVs were again observed (Figure 5B).
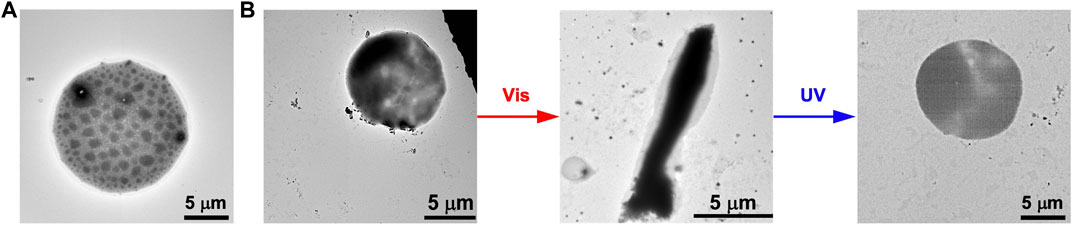
FIGURE 5. TEM images of (A) the GUVs alone and (B) the morphological change in the GUVs ([POPC] = 1 mM) by the photoisomerization of FKFECSP/MCKFE peptides in a 10-mM phosphate buffer (pH, 7.4) at 25°C.
While dry samples were observed by TEM, CLSM was used to observe in aqueous solution the morphological changes in the GUVs due to the photoisomerization of FKFECMCKFE peptide by thioflavin T staining (Figure 6A). The GUV composed of POPC/Atto-550 POPE encapsulating FKFECMCKFE peptide exhibited a normal spherical morphology in the aqueous solution. Interestingly, visible light irradiation at 580 nm (80 min, 5 mW, 5 cm) induced a dramatic morphological change into a worm-like vesicle (Figure 6B; after 505 nm, 52 mW, 10 cm, 3 min irradiated, Supplementary Video S1). This change was probably caused by the formation of peptide nanofibers inside the GUV by photoisomerization from MC to SP. Visible light irradiation of the GUV encapsulating FKFECMCKFE peptide induced a variety of morphologies of worm-like vesicles, and an intermediate state of worms extending from inside the spherical GUVs was also observed (Figure 7). The worm-like vesicle completely reverted to the original spherical GUV after UV light irradiation at 365 nm (after 80 min, 5 mW, 5 cm, Figure 6B; and after 30 mW, 10 cm, 3 min irradiated, Supplementary Video S2). Although the photoisomerization of the peptide at 10 μM does not cause bursting, the peptide could not be encapsulated by GUV when higher peptide concentrations (50 and 100 μM) were mixed with GUV. Furthermore, the photoisomerization of the peptide at 5 μM induced small morphological changes in the GUV, and at 1 μM did not induced morphological changes (Supplementary Figure S9).
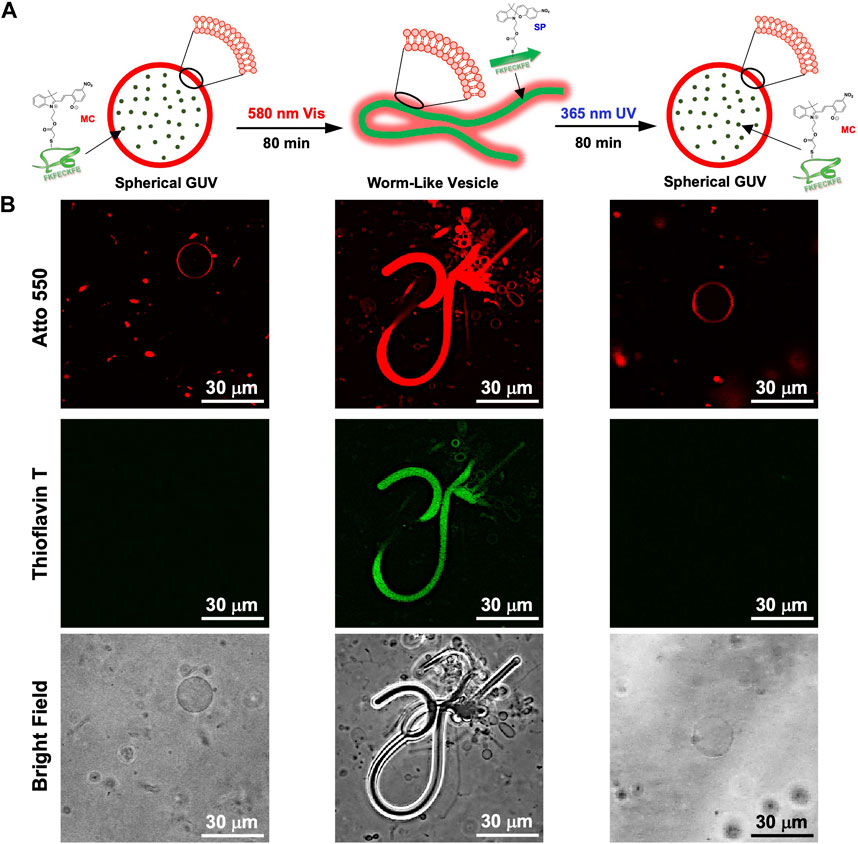
FIGURE 6. (A) Schematic of the morphological change in the GUV by the photoisomerization of peptides. (B) CLSM images of the morphological change in the GUV ([Atto 550-DOPE] = 5 μM [POPC] = 1 mM) encapsulated using 10-μM FKFECMCKFE and 100 μM thioflavin T by photoisomerization in a 10-mM phosphate buffer (pH, 7.4) at 25°C. Channel for Atto 550 (top), thioflavin T (middle), bright field (bottom).
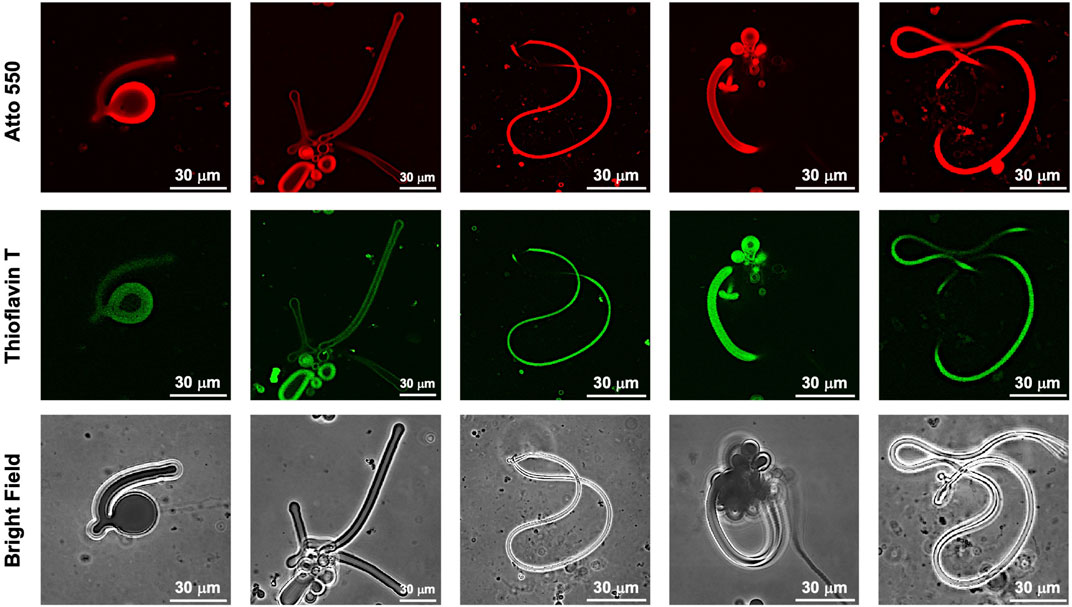
FIGURE 7. CLSM images of various morphologies of worm-like vesicles ([Atto 550-DOPE)] = 5 μM [POPC] = 1 mM) encapsulated using 10-μM FKFECSPKFE and 100 μM Thioflavin T in a 10-mM phosphate buffer (pH, 7.4) at 25°C. Channels for Atto 550 (top), thioflavin T (middle), and bright field (bottom).
The presence of the FKFECSPKFE peptide inside the worm-like vesicle was confirmed by the fluorescence profile in the CLSM image (Supplementary Figure S5A). The fluorescence profile of thioflavin T can be observed inside that of Atto 550 on the white line in the merged image, suggesting that the FKFECSPKFE peptide was internalized within the GUV and partially adsorbed in the membrane (Supplementary Figure S5B). Since merocyanine dye itself fluoresces at 577 nm when excited at 553 nm, the CLSM of the unstained FKFECMCKFE peptide-GUV mixture revealed MC-derived red fluorescence within the GUV (Supplementary Figure S6A). The fluorescence profiles revealed that the FKFECMCKFE peptide was localized within the GUV and partially adsorbed on the membrane (Supplementary Figure S6B).
Discussion
Dynamics of cytoskeleton are important for deformation and movement of various membrane systems in the cell, arrangement of cell organelles, cell division, muscle contraction, and ciliary motility. Mimicking the cytoskeleton dynamics, we developed a short peptide FKFECSP/MCKFE which can be reversibly polymerized and depolymerized by the photoisomerization. In addition, dramatic and reversible morphological change of cell model GUV encapsulating FKFECMCKFE was induced by the photoisomerization.
Although several studies have been reported on changing the morphology of GUVs in various ways, there were some problems such as irreversibility (Loiseau et al., 2016), long response time (Fanalista et al., 2018), and small morphology change (Li et al., 2021). In this study, dramatic and reversible morphology change of GUVs was achieved by brief photoisomerization of FKFECSP/MCKFE peptide inside the GUV using a strong light source. This morphology change is attributed to the formation of the β-sheet peptide nanofibers inside GUV due to the large structural change from MC to SP upon visible light irradiation. The polymerization of the numerous peptides accompanied by the lipid bilayer-peptide interaction inside GUV could enhance extrusion of the membrane outward to form worm-like vesicles (Figure 6B; Supplementary Video S1). The worm-like vesicles were reverted to the spherical morphology by UV light irradiation (Figure 6B; Supplementary Video S2). The relaxation of deformation stress could be caused by the depolymerization of peptide nanofibers inside vesicle due to photoisomerization from SP to MC.
In this study, we succeeded in reversibly changing the morphology of GUVs by photoisomerization of nanofiber-forming peptides. The molecular design mimicking the polymerization and depolymerization of the cytoskeleton provides a guideline for a photo-controlled artificial cytoskeleton and can be used as a component of molecular robots and artificial cell systems (Hagiya, et al., 2014; Murata, et al., 2022). However, the present study has shown that construction of artificial cytoskeletons is hindered by the relatively slow photoisomerization process compared to the fast time scale of the natural cytoskeleton. To overcome this limitation, the design of nanofiber-forming peptide could be optimized to allow faster assembly and disassembly kinetics. Alternatively, the use of alternative approaches, such as light-activated proteins or other molecular switches, could allow more precise and rapid control of cytoskeletal deformation. In the future, we are planning to actually introduce the photo-controlled artificial cytoskeleton into cells to control cell deformation and migration by photoisomerization. We will introduce the peptide fibers into cells to evaluate their effects on light-induced migration, thereby providing of the possibility of light-driven artificial cytoskeletons for manipulation of cellular structures.
Conclusion
We synthesized a FKFECSP/MCKFE peptide in which the central Phe residue of the β-sheet structure-forming peptide FKFEFKFE was replaced by a Cys residue modified with a photoisomerizable SP/MC. The photoisomerization ability and the secondary structure were confirmed by UV-Vis spectra and CD spectra. Reversible control of polymerization and depolymerization of nanofibers was achieved by photoisomerization of the FKFECSP/MCKFE peptide. Interestingly, the visible light irradiation of the spherical GUV-encapsulating FKFECMCKFE peptide induced a dramatic morphological change that the spherical vesicles transformed from spherical to worm-like vesicles. Polymerization and depolymerization of peptide nanofibers by photoisomerization in the vicinity of the lipid bilayer seems to promote extrusion and relaxation of the membrane. This molecular design provides guidelines for light-controlled artificial cytoskeletons that mimic the polymerization/depolymerization of the cytoskeleton. We envisage that light-controlled artificial cytoskeletons can be introduced into cells, which will be an innovative molecular technology to control cellular deformation and migration by light.
Data availability statement
The original contributions presented in the study are included in the article/; Supplementary Material, further inquiries can be directed to the corresponding author.
Author contributions
KM conceived the experiments, YL performed the experiment. The synthesis and purification of bromoacetyl spiropyran were performed by SO, HI has made a lot of useful suggestions for the experiment. YL wrote the first draft of the paper, KM and HI made suggestions and revisions to it. All authors agree to be accountable for the content of the work.
Funding
This research was supported by a Grant-in-Aid for Challenging Research (Exploratory) (JSPS KAKENHI Grant No. JP21K19008), Grant-in-Aid for Transformative Research Areas (A) “Molecular Cybernetics” (JSPS KAKENHI Grant number: JP20H05970), and ACT-X (Grant No. JPMJAX 2012) from the Japan Science and Technology Agency (JST).
Acknowledgments
The authors would like to thank Enago (www.enago.jp) for the English language review.
Conflict of interest
The authors declare that the research was conducted in the absence of any commercial or financial relationships that could be construed as a potential conflict of interest.
Publisher’s note
All claims expressed in this article are solely those of the authors and do not necessarily represent those of their affiliated organizations, or those of the publisher, the editors and the reviewers. Any product that may be evaluated in this article, or claim that may be made by its manufacturer, is not guaranteed or endorsed by the publisher.
Supplementary material
The Supplementary Material for this article can be found online at: https://www.frontiersin.org/articles/10.3389/fmolb.2023.1137885/full#supplementary-material
References
Bashirzadeh, Y., Wubshet, N., and Liu, A. (2020). Confinement geometry tunes fascin-actin bundle structures and consequently the shape of a lipid bilayer vesicle. Front. Mol. Biosci. 7, 610277. doi:10.3389/fmolb.2020.610277
Bosques, C., and Imperiali, B. (2003). Photolytic control of peptide self-assembly. J. Am. Chem. Soc. 125 (25), 7530–7531. doi:10.1021/ja035360b
Bowerman, C., and Nilsson, B. (2010). A reductive trigger for peptide self-assembly and hydrogelation. J. Am. Chem. Soc. 132 (28), 9526–9527. doi:10.1021/ja1025535
Chen, C., Zhang, Y., Fei, R., Cao, C., Wang, M., Wang, J., et al. (2016). Hydrogelation of the short self-assembling peptide I3QGK regulated by transglutaminase and use for rapid hemostasis. ACS Appl. Mat. Interfaces 8 (28), 17833–17841. doi:10.1021/acsami.6b04939
Doran, T., Ryan, D., and Nilsson, B. (2014). Reversible photocontrol of self-assembled peptide hydrogel viscoelasticity. Polym. Chem. 5, 241–248. doi:10.1039/C3PY00903C
D’Souza, A., Marshall, L., Yoon, J., Kulesha, A., Edirisinghe, D., Chandrasekaran, S., et al. (2022). Peptide hydrogel with self-healing and redox-responsive properties. Nano Converg. 9, 18. doi:10.1186/s40580-022-00309-7
Eldirany, S., Lomakin, I., Ho, M., and Bunick, C. (2021). Recent insight into intermediate filament structure. Curr. Opin. Cell Biol. 68, 132–143. doi:10.1016/j.ceb.2020.10.001
Fanalista, F., Deshpande, S., Lau, A., Pawlik, G., and Dekker, C. (2018). FtsZ-induced shape transformation of coacervates. Adv. Biosyst. 2 (9), 1800136. doi:10.1002/adbi.201800136
Fletcher, D., and Mullins, R. (2010). Cell mechanics and the cytoskeleton. Nature 463, 485–492. doi:10.1038/nature08908
Furutani, M., Uemura, A., Shigenaga, A., Komiya, C., Matsuura, K., and Otaka, A. (2015). A photoinduced growth system of peptide nanofibres addressed by DNA hybridization. Chem. Commun. 51, 8020–8022. doi:10.1039/C5CC01452B
Ganar, K., Honaker, L., and Deshpande, S. (2021). Shaping synthetic cells through cytoskeleton-condensate-membrane interactions. Curr. Opin. Colloid Interface Sci. 54, 101459. doi:10.1016/j.cocis.2021.101459
Hagiya, M., Konagaya, A., Kobayashi, S., Saito, H., and Satoshi Murata, S. (2014). Molecular robots with sensors and intelligence. Acc. Chem. Res. 47 (6), 1681–1690. doi:10.1021/ar400318d
Haines, L., Rajagopal, K., Ozbas, B., Salick, D., Pochan, D., and Schneider, J. (2005). Light-activated hydrogel formation via the triggered folding and self-assembly of a designed peptide. J. Am. Chem. Soc. 127 (48), 17025–17029. doi:10.1021/ja054719o
Hall, A. (2009). The cytoskeleton and cancer. Cancer Metastasis Rev. 28, 5–14. doi:10.1007/s10555-008-9166-3
Inaba, H., Hatta, K., and Matsuura, K. (2021). Directional propulsion of DNA microspheres based on light-induced asymmetric growth of peptide nanofibers. ACS Appl. Bio Mater. 4 (7), 5425–5434. doi:10.1021/acsabm.1c00146
Inaba, H., Uemura, A., Morishita, K., Kohiki, T., Shigenaga, A., Matsuura, K., et al. (2018). Light-induced propulsion of a giant liposome driven by peptide nanofibre growth. Sci. Rep. 8, 6243. doi:10.1038/s41598-018-24675-7
Klajn, R. (2014). Spiropyran-based dynamic materials. Chem. Soc. Rev. 43, 148–184. doi:10.1039/C3CS60181A
Koseki, K., and Suzuki, H. (2020). Deformation dynamics of giant unilamellar vesicles in the large surface-to-volume ratio regime: The emergence of neuron-like morphology. Langmuir 36 (22), 6238–6244. doi:10.1021/acs.langmuir.0c00872
Lasic, D., and Papahadjopoulos, D. (1995). Liposomes revisited. Science 267 (5202), 1275–1276. doi:10.1126/science.7871422
Li, C., Zhang, X., Yang, B., Wei, F., Han, X., Mu, W., et al. (2021). Reversable deformation of artificial cell colony for muscle behavior mimicry triggered by actin polymerization. BioRxiv. doi:10.1101/2021.12.18.473289
Liu, M., Creemer, C., Reardona, T., and Parquette, J. (2021). Light-driven dissipative self-assembly of a peptide hydrogel. Chem. Commun. 57, 13776–13779. doi:10.1039/D1CC04971B
Loiseau, E., Schneider, J., Keber, F., Pelzl, C., Massiera, G., Salbreux, G., et al. (2016). Shape remodeling and blebbing of active cytoskeletal vesicles. Sci. Adv. 2 (4), e1500465. doi:10.1126/sciadv.1500465
Luna, E. J., and Hitt, A. L. (1992). Cytoskeleton—plasma membrane interactions. Science 258 (5084), 955–964. doi:10.1126/science.1439807
Marini, D., Hwang, W., Lauffenburger, D., Zhang, S., and Kamm, R. (2002). Left-handed helical ribbon intermediates in the self-assembly of a β-sheet peptide. Nano Lett. 2 (4), 295–299. doi:10.1021/nl015697g
Murata, S., Toyota, T., Nomura, S., Nakakuki, T., and Kuzuya, A. (2022). Molecular Cybernetics: Challenges toward cellular chemical artificial intelligence. Adv. Funct. Mater. 32 (37), 2201866. doi:10.1002/adfm.202201866
Nakamura, K., Tanaka, W., Sada, K., Kubota, R., Aoyama, T., Urayama, K., et al. (2021). Phototriggered spatially controlled out-of-equilibrium patterns of peptide nanofibers in a self-sorting double network hydrogel. J. Am. Chem. Soc. 143 (46), 19532–19541. doi:10.1021/jacs.1c09172
Pederson, T., and Aebi, U. (2002). Actin in the nucleus: What form and what for? J. Struct. Biol. 140 (1-3), 3–9. doi:10.1016/S1047-8477(02)00528-2
Pires, R., Abul-Haija, Y., Costa, D., Novoa-Carballal, R., Reis, R., Ulijn, R. V., et al. (2015). Controlling cancer cell fate using localized biocatalytic self-assembly of an aromatic carbohydrate amphiphile. J. Am. Chem. Soc. 137 (2), 576–579. doi:10.1021/ja5111893
Ramaekers, F., Bosman, F., and Bosman, T. (2004). The cytoskeleton and disease. J. Pathol. 204 (4), 351–354. doi:10.1002/path.1665
Sato, Y., Hiratsuka, Y., Kawamata, I., Murata, S., and Nomura, S. (2017). Micrometer-sized molecular robot changes its shape in response to signal molecules. Sci. Robot. 2 (4), eaal3735. doi:10.1126/scirobotics.aal3735
Sekine, Y., Shimizu, A., Sasaki, Y., Sawadad, S., and Akiyoshi, K. (2012). Shear flow-induced nanotubulation of surface-immobilized liposomes. RSC Adv. 2, 2682–2684. doi:10.1039/C2RA00629D
Sendai, T., Biswas, S., and Aida, T. (2013). Photoreconfigurable supramolecular nanotube. J. Am. Chem. Soc. 135 (31), 11509–11512. doi:10.1021/ja4060146
Shi, Z., and Baumgart, T. (2015). Membrane tension and peripheral protein density mediate membrane shape transitions. Nat. Commun. 6, 5974. doi:10.1038/ncomms6974
Steinkühler, J., Knorr, R., Bartelt, S., Wegner, S., Dimova, R., Lipowsky, R., et al. (2020). Controlled division of cell-sized vesicles by low densities of membrane-bound proteins. Nat. Commun. 11, 905. doi:10.1038/s41467-020-14696-0
Tanaka, A., Fukuoka, Y., Morimoto, Y., Honjo, T., Maruyama, T., Goto, M., et al. (2015). Cancer cell death induced by the intracellular self-assembly of an enzyme-responsive supramolecular gelator. J. Am. Chem. Soc. 137 (2), 770–775. doi:10.1021/ja510156v
Tanaka, S., Takiguchi, K., and Hayashi, M. (2018). Repetitive stretching of giant liposomes utilizing the nematic alignment of confined actin. Commun. Phys. 1, 18. doi:10.1038/s42005-018-0019-2
Wade, R. (2009). On and around microtubules: An overview. Mol. Biotechnol. 43, 177–191. doi:10.1007/s12033-009-9193-5
West, H., Csizmar, C., and Wagner, C. (2018). Tunable supramolecular assemblies from amphiphilic nucleoside phosphoramidate nanofibers by enzyme activation. Biomacromolecules 19 (7), 2650–2656. doi:10.1021/acs.biomac.8b00254
Keywords: peptide nanofiber, liposome, photoisomerization, self-assembly, polymerization/depolymerization, spiropyran, merocyanine, artificial cytoskeleton
Citation: Liang Y, Ogawa S, Inaba H and Matsuura K (2023) Dramatic morphological changes in liposomes induced by peptide nanofibers reversibly polymerized and depolymerized by the photoisomerization of spiropyran. Front. Mol. Biosci. 10:1137885. doi: 10.3389/fmolb.2023.1137885
Received: 04 January 2023; Accepted: 15 March 2023;
Published: 30 March 2023.
Edited by:
Marcelo Kogan, University of Chile, ChileReviewed by:
Md. Rizwanullah, Jamia Hamdard University, IndiaManzar Abbas, Khalifa University, United Arab Emirates
Sílvia Pujals, Institute of Advanced Chemistry of Catalonia (CSIC), Spain
Copyright © 2023 Liang, Ogawa, Inaba and Matsuura. This is an open-access article distributed under the terms of the Creative Commons Attribution License (CC BY). The use, distribution or reproduction in other forums is permitted, provided the original author(s) and the copyright owner(s) are credited and that the original publication in this journal is cited, in accordance with accepted academic practice. No use, distribution or reproduction is permitted which does not comply with these terms.
*Correspondence: Kazunori Matsuura, bWEycmEta0B0b3R0b3JpLXUuYWMuanA=