- 1Department of Agricultural and Environmental Sciences, College of Agriculture, Tennessee State University, Nashville, TN, United States
- 2Department of Life and Physical Sciences, Fisk University, Nashville, TN, United States
Multidrug resistance (MDR) is a major concern in battling infectious bacterial diseases. The overuse of antibiotics contributes to the emergence of resistance by eradicating the drug-sensitive strains, leaving behind the resistant strains that multiply without any competition. Nanoparticles are becoming popular as novel antimicrobial agents that follow a different mode of action from standard antibiotics and are therefore desirable against MDR bacteria. In this study, we synthesized carbon dots from different precursors including glucosamine HCL (GlcNH2·HCl) and 4,7,10-trioxa-1,13-tridecanediamine (TTDDA, and studied their antimicrobial effects in a diverse list of Gram-negative bacteria including Escherichia coli, Pseudomonas syringae, Salmonella enterica subsp. enterica serovar Typhimurium, Pectobacterium carotovorum, Agrobacterium tumefaciens, and Agrobacterium rhizogenes. We demonstrated the antimicrobial properties of these carbon dots against these bacteria and provided the optimum concentration and incubation times for each bacterial species. Our findings indicated that not all carbon dots carry antimicrobial properties, and there is also a variation between different bacterial species in their resistance against these carbon dots.
Background
Microorganisms like bacteria and viruses are the major cause for many infectious diseases, resulting in millions of deaths each year (Jones et al., 2008). Lately, multidrug resistance (MDR) is on the rise in a wide range of pathogens. According to the Centers for Disease Control and Prevention (CDC) Annual Threat Report for 2019, there were more than 2.8 million antibiotic-resistant infections with the human death toll up to 35,000 in the United States. In addition, more than $4.6 billion annually is spent to treat infections caused by multidrug-resistant pathogens (Centers for Disease Control and Prevention, 2019). The surge in MDR is mainly due to the availability of a handful of antibiotics, which resulted in continuous exposure of pathogens to these antibiotics for a long period of time. It is predicted that by 2050, the rise of MDR could cause 300 million more deaths and cost up to US$100 trillion (Dong et al., 2020). Globally, antibiotic resistance is also one of the greatest health threats according to the World Health Organization (WHO) (Piddock, 2012). Hence, there is a significant urge in the scientific community for developing alternative antimicrobial strategies with superior properties. There are different approaches for eradicating these pathogens. One of the most common methods is the use of antiseptics and disinfectants. However, a downside of these methods includes extreme dermal irritation and toxicity, resulting in mild to severe health complications. Furthermore, these agents are becoming less effective owing to the increased bacterial resistance. The present well-tested strategy for combatting these pathogens is the use of antibiotics. The golden era of antibiotics began with the discovery of penicillin by Sir Alexander Fleming in 1928 (Piddock, 2012; Ventola, 2015; Sagbas and Sahiner, 2019). The use of antibiotics helped extend the life expectancy in the world (Piddock, 2012). For example, in the United States, the life expectancy has increased from 56.4 years in 1920 to 78.7 years (Xu et al., 2018). Due to popularity and overuse of antibiotics for the eradication of these pathogens, new resistant strains have emerged due to random mutation and evolution in these species. Epidemiological studies have shown a direct relationship between the use of antibiotics and emergence and dissemination of drug-resistant bacterial strains (Michael et al., 2014a). The overuse of antibiotics contributes to the emergence of resistance by eradicating the drug-sensitive strains leaving behind the resistant strains which multiply without any competition (Read and Woods, 2014).
One of the promising approaches for microbial inhibition is the use of disinfectants that carry photodynamic inhibition (PDI) properties, and they lead to non-specific oxidative damage to the vital biomolecules inside the cells (Dong et al., 2020). In PDT, the infected cells are killed locally by the release of free radicals such as reactive oxygen species (ROS) (Carrera et al., 2016; Qi et al., 2019) and reactive chlorine species (RCS) (Thangudu et al., 2020) produced by a photosensitizer (PS). It is reported that multi-antibiotic–resistant bacterial strains are easily killed by photodynamic therapy (PDT) and that bacteria do not readily develop resistance against PDT (Hamblin and Hasan, 2004). However, current applications of PDI compounds are often limited due to various drawbacks such as poor photostability, poor water dispersibility, and inability to be absorbed in the region (>700 nm) (Ge et al., 2014). Recently, carbon nanoparticles (carbon dots) are considered as novel PDI compounds having excellent photostability and autofluorescence with better antimicrobial properties including quick acting, minimal to zero cytotoxicity, and specificity towards pathogenic microorganisms (Gupta et al., 2019). Carbon dots (CDs) are zero-dimensional photoluminescent particles which are mostly less than 20 nm (Sun et al., 2006; Ponomarenko et al., 2008; Fernando et al., 2015; Sagbas and Sahiner, 2019). Carbon dots were accidentally produced and reported for the first time, while they were planning to purify single-walled carbon nanotubes (SWCNTs). Since then, scientists’ interest shifted toward carbon dots which carry functions similar the toxic metal-based nanomaterials but are less toxic to the mammalian cells and the environment (Li et al., 2010; Michael et al., 2014b). The inhibitory roles of CDs against bacteria are mostly associated with the production of reactive oxygen species (ROS). These mechanisms include adhesion of CDs to the bacterial surface, disruption of the bacterial cell wall, random oxidative damage to nucleic acids and proteins, altering the gene expression patterns and affecting other biomolecules (Meziani et al., 2016; AlAwak et al., 2017; Li et al., 2018). Li et al., 2018 developed carbon dots from vitamin C and reported their inhibitory effects against various bacteria like S. aureus, B. subtilis, and E. coli. Fabricated hydrophobic CDs and embedded them in polydimethylsiloxane and polyurethane. Similarly, Muktha et al. (2020) synthesized CDs from organic wastes of watermelons and pomegranates and observed that CDs synthesized from pomegranate were found to be an effective antimicrobial agent against various bacteria and fungi such as Staphylococcus aureus and Fusarium oxysporum (Muktha et al., 2020).
The objective of this study was to evaluate the antimicrobial properties of four different carbon dots which were reported for cell imaging (Balouiri et al., 2016; Cao et al., 2018; Wu et al., 2018) and screen their antimicrobial properties. Amine-coated carbon dots (NH2-FCDs), synthesized from glucosamine HCL (GlcNH2·HCl) and 4,7,10-trioxa-1,13-tridecanediamine (TTDDA), displayed better inhibitory effects on Escherichia coli (E. coli) cells, and they were used for further analysis in a diverse list of Gram-negative bacteria, such as Pseudomonas syringae pathovar tomato DC 3000 (PST) (P. syringae), Salmonella enterica pathovar typhimurium (13311) (S. enterica), Pectobacterium carotovorum (P. carotovorum), Agrobacterium tumefaciens (A. tumefaciens), and Agrobacterium rhizogenes (A. rhizogenes).
Materials and Methodology
Materials
The chemicals for synthesizing the carbon dots (glucosamine HCl (GlcNH2·HCl, CAS# 66–84–2), 4,7,10-trioxa-1,13-tridecanediamine (TTDDA, CAS#4246–51–9), β-alanine (C3H7NO2, CAS# 107–95–9), glucose (C6H12O6, CAS# 50–99–7), L-arginine (C6H14N4O2, CAS# 74–79–3), citric acid (C6H8O7, CAS# 5949–29–1), and polyethyleneimine (PEI) [(C37H24O6N2)n, CAS# 71–2353] were purchased from VWR. Components for the preparation of growth media for these bacteria such as bacteriological agar, yeast extract, tryptone, NaCl, proteose peptone, potassium phosphate dibasic, magnesium sulfate heptahydrate, glycerol, Bacto Peptone, and sterile water were also ordered from VWR or Fisher Scientific.
Synthesis of Amine-Coated Carbon Cots (NH2-FCD)s
The carbon dot was prepared according to a study conducted by Hill et al. (2016). Glucosamine hydrochloride (1.00 g, 4.63 mmol) was dissolved in distilled H2O (20 ml) and stirred to complete dissolution in a 250-ml conical flask. 4,7,10-trioxa-1,13-tridecanediamine (TTDDA) (1.11 ml, 5.09 mmol) was added to the sugar solution and agitated to ensure homogeneity. The conical flask was then placed in a domestic microwave 700 W, and the solution was heated for 3 min at 700 W (full power). A viscous brown residue was obtained and dissolved in distilled H2O (10 ml) and centrifuged for 3 min at 8500 rpm. The solution was purified by dialysis method for 4 days using Spectra/Por® seven dialysis membrane tubes of 11.5 mm diameter. The dialysis water was refreshed every 2 h for the first 8 h and then once a day for the remaining 4 days. Then the purified CDs were further sterilized by passing the solution through a filter of 0.45 µm pore size. Other carbon dots including COOH-FCDs (glucosamine + b-alanine), N-CDs (glucose + L-arginine), and PEI-CDs (citric acid + PEI) were also synthesized according to the studies conducted by Hill et al. (2016), Cao et al. (2018), and Wu et al. (2018), respectively (Supplementary Table S1).
Bacterial Culture
Six bacterial strains belonging to different taxonomic groups were used, namely, E. coli (DH5α), Pectobacterium carotovorum (Ecc7), Agrobacterium tumefaciens (EHA101), Agrobacterium rhizogenes (K599), Pseudomonas syringae, and Salmonella enterica subsp. enterica serovar Typhimurium 13311) (Figure 1). Agrobacterium and Pseudomonas were cultured in the YEP medium and King’s medium B, respectively. Pectobacterium, E. coli, and Salmonella strains were grown in the LB medium. Agrobacterium, Pectobacterium, and Pseudomonas were grown at 28°C, while E. coli and Salmonella were grown at 37°C. The OD (600 nm) was adjusted at 0.5 by subculturing the overnight grown cells and harvesting the cells at the logarithmic growth phase 5 ml of culture was centrifuged, and the cells were washed three times with sterile distilled water and eluted in 1 ml of 15% glycerol 50 µl of cells were further aliquoted to 0.65-ml tubes and stored at a −80°C freezer for future use.
Characterization of CDs and Confocal Imaging
FTIR analyses were performed to confirm the functional groups of CDs. The ATR–FTIR analysis of freeze-dried CDs was carried out using a Perkin Elmer Frontier Infrared spectrometer equipped with a liquid N2 cooled MCT-A (Mercury cadmium telluride) detector and an optic compartment purged with CO2- and H2O-free air delivered by a Balston–Parker air purger. The FTIR spectra of the CDs were recorded between 600 and 3800 cm−1. Furthermore, photoluminescence properties such as absorbance, emission, and excitation wavelength of the CDs were recorded using a Synergy H1 Hybrid Multi-Mode Microplate Reader (BioTek, Winooski, VT). A Zetasizer nano ZS (Malvern Panalytical Inc., Westborough MA) was used to measure the electrostatic charges carried by CDs. For the confocal microscopy, 5 µl of NH2-FCDs (15 µg/µl) and 50 µl of cells (OD600 = 0.5) were incubated for 15 min and 1 h, respectively. The cells were centrifuged and washed three times with sterile water to remove LB and carbon dots outside of the bacteria. Earlier reports indicated that NH2-FCDs synthesized through this approach are in 2–10 nm particle size range (Hill et al., 2016).
Antimicrobial Activity Assays
The agar plate well-diffusion method was utilized to examine the antimicrobial activity of CDs diluted at different concentrations on Gram-negative bacteria (Balouiri et al., 2016). The agar plates were inoculated by spreading 50 µl bacterial suspension (OD600 = 0.5) using sterile glass beads. The experiment consisted of three replicates for each treatment. A well of 0.6 mm diameter was cut on the inoculated plates using a cork borer (back of sterile 20–200 µl pipette tips can also be used instead). Then, 80 µl of CD solution was added into each well and allowed to diffuse overnight at 28°C or 37°C depending upon the organism. To determine the minimum incubation time for complete inhibition of bacterial cells, we mixed 50 µl of cells harvested at an OD600 of 0.5 with 5 µl of freshly prepared CDs and incubated the solution for different time intervals ranging from 1 to 24 h. The incubation time at which there is complete inhibition of cells was noted.
Statistical Analysis
The diameter of the inhibition zone for each bacterium was tabulated and analyzed using R software (V3.6.3). The mean of the treatments was calculated, and the post hoc analysis was carried out using the Tukey HSD test to compare mean difference between the treatments.
Result and Discussion
Synthesis and Characterization of CDs
Fourier-transform infrared (FT-IR) spectroscopy was carried out to characterize the chemical functional groups on the NH2-FCDs. Thus, the prepared CDs showed peaks at 870.2 cm-1(C-O) of double olefinic bonds in single vinyl (C=CH2), 1461 cm-1 (C≡N) of the nitrile group, 1616 cm-1 (C=O) of the ketone group, 2867 cm-1 (C=OH) of the aldehyde group, and 3255 cm-1 (-OH) of the hydroxyl (Nandiyanto et al., 2019) (Figure 2A). The photoluminescence properties showed an absorbance profile maximum of 280 nm which lies between t 200 and 450 nm range—a characteristic value for the CDs synthesized using a bottom-up approach (Figure 2). The defined peak of 280 nm could be attributed to π–π* transition of aromatic/alkenyl C=C bonds or C≡N bonds (Tan et al., 2016). The fluorescence emission profile showed an emission band at 450 nm when excited at 365 nm. This emission band at 450 nm is typical for carbon dots, which gives blue fluorescence (Carbonaro et al., 2018) (Figure 2B). The electrokinetic potential, commonly known as the zeta potential, of the NH2-FCDs was measured using a Malvern Zetasizer Nano-ZS ZEN 3600 (Figure 2C). The zeta potential value of NH2-FCDs was found to be (10.50 ± 0.3) mV. The positive charge is the result of primary amines derived from TTDA linker (Hill et al., 2016) (Figure 2C). The physical properties of other carbon dots were also measured as before and are presented in supplementary files (Supplementary Figures S1–S3).
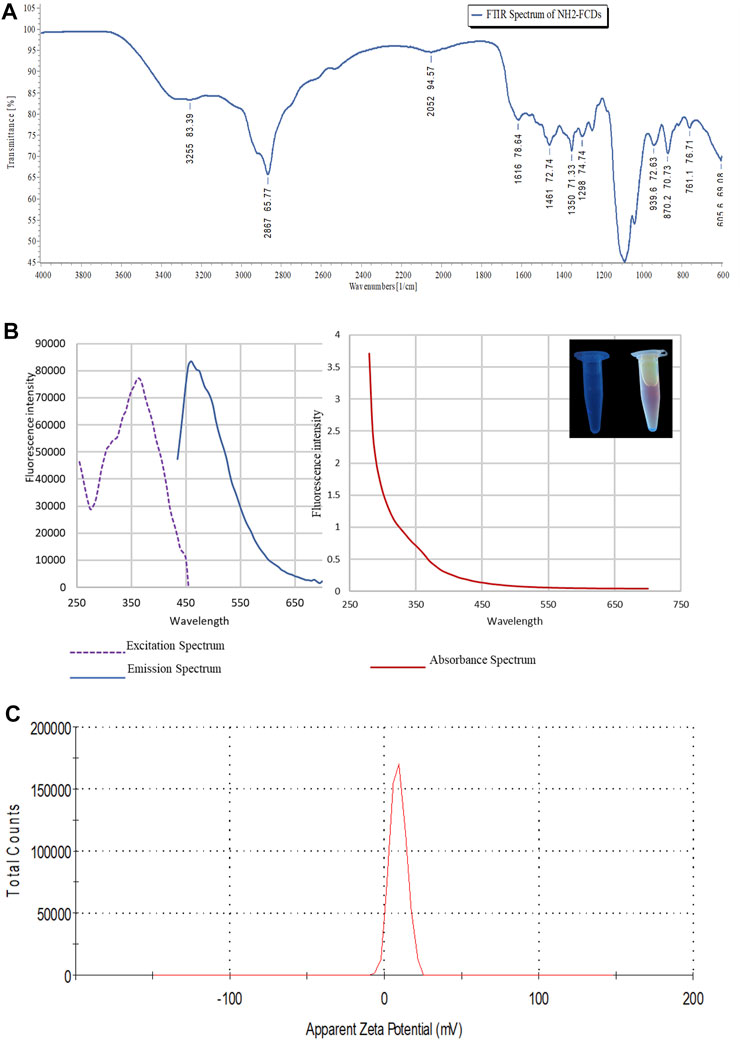
FIGURE 2. (A) Photoluminescence properties of NH2-FCDs. A) FTIR spectrum of NH2-FCDs. (B) Graphs representing excitation, emission, and absorbance wavelength for the CDs. (C) Zeta potential of NH2-FCDs [CDs were diluted with distilled water (pH 4)].
Confocal Microscopy
As indicated in Figure 3, the CDs are interacting with the bacterial membrane. For sensitive bacteria (e.g., Pseudomonas), no single colony was observed after 1 h of incubation, confirming complete dissolution of cell membranes in these bacteria. Under the microscope, CDs were found interacting all over the bacterial cell surfaces, indicating their adherence to the cell membranes even after multiple washing steps (Figure 3, Supplementary Figures S4, S5).
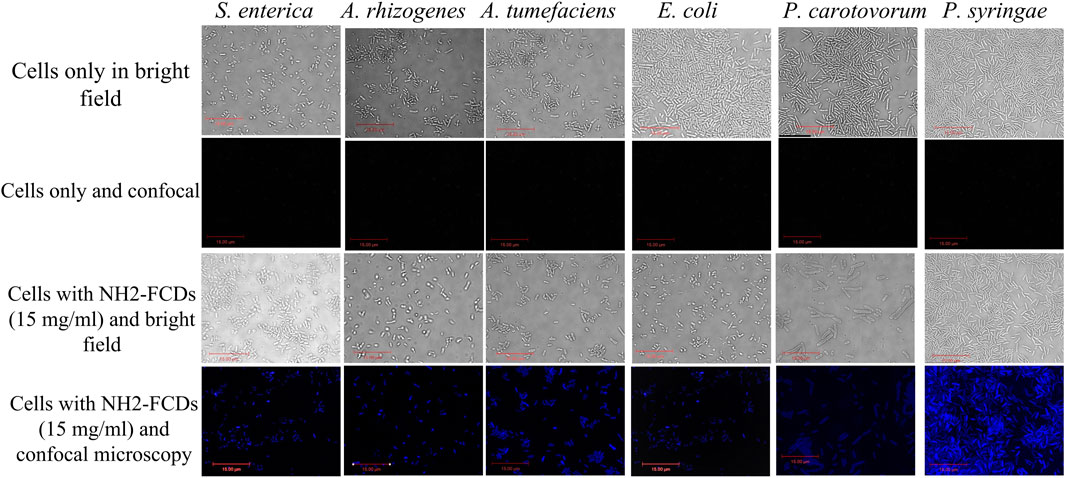
FIGURE 3. Bacterial strains under DIC and confocal fluorescence microscopy after 1 h incubation with NH2-FCDs (15 mg/ml) (Scale bar =15 um).
Antibacterial Activity
In an initial experiment, the antimicrobial properties of these four carbon dots were measured to identify the variation between these carbon dots in their toxicity to different bacterial strains. Except PEI-CDs that had no antimicrobial effects on these bacterial strains (Supplementary Figure S6), other carbon dots displayed some degree of bacterial growth inhibition at different concentrations (Figure 4, Supplementary Figures S7, S8). The agar well diffusion method was used to determine the minimum inhibitory concentration (MIC) of NH2-FCDs with different concentration of CDs from 0.5, 1, 5 to 10 mg/ml. The MIC was different depending on the species. Pseudomonas growth was inhibited at 0.5 mg/ml (Figures 4, 5), whereas for Agrobacterium, Salmonella, Pectobacterium, and E. coli at least 5 mg/ml was needed to observe growth inhibition. The growth inhibition is dependent on the concentration of carbon dots, and more growth inhibition was observed at higher concentration of CDs. These inhibitory concentrations are far more than that reported in the earlier reports for nanoparticles used as antimicrobial agents. For example, the MIC for AgNPs against different bacteria (E. coli, Pseudomonas syringae, and Staphylococcus aureus) was reported as low as 4, 2, and 4 μg/ml (Gondil et al., 2019). However, the MIC of CDs synthesized in our laboratory was as high as 0.5 mg/ml for Pseudomonas and 5 mg/ml for other bacteria. Heavy metal–based nanoparticles are known to be toxic to the bacterial and human cells. Thus, even small concentration of these nanomaterials was effective against bacterial growth. Other studies using carbon dots synthesized from 2,2′-(ethylenedioxy)bis(ethylamine) (EDA) also reported lower MIC (64 μg/ml) on E. coli cells (Dong et al., 2017). However, it should be noted that the number of E. coli cells that they have used (1 × 106) is 400x smaller than that used in our study (OD600 = 0.5 which equals to 4 × 108 number of cells).
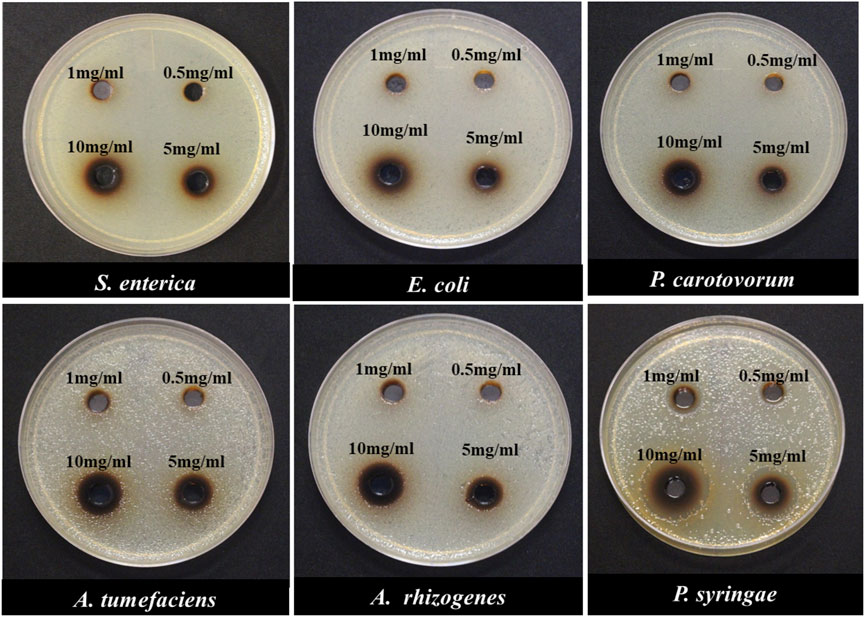
FIGURE 4. Minimum inhibitory concentration (MIC) of Gram-negative bacterial species in response to different concentrations of NH2-FCDs using the agar-plate well diffusion method.
In another experiment, both synthesized CDs and their precursors were tested against six bacterial species to determine if the antimicrobial effects are unique to the carbon dots or their precursors. The result indicated that the antimicrobial capacity was enhanced in NH2-FCDs compared to the precursor solution (Figures 6, 7). Similar results were also observed in COOH-CDs and N-CDs as well (Supplementary Figures S9, S10). NH2-FCDs were more effective in E. coli, Salmonella, Pectobacterium, and Agrobacterium. However, both solution and NH2-FCDs were found to be equally effective against Pseudomonas.
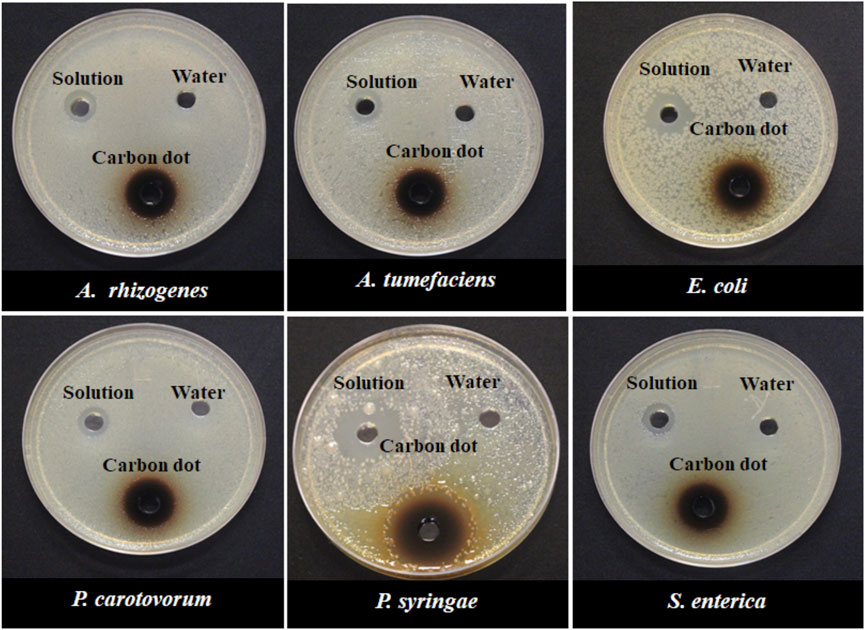
FIGURE 6. Growth inhibition of different Gram-negative bacteria in response to 15 mg ml−1 concentration of NH2-CDs. Water and unprepared reactants were used as control.
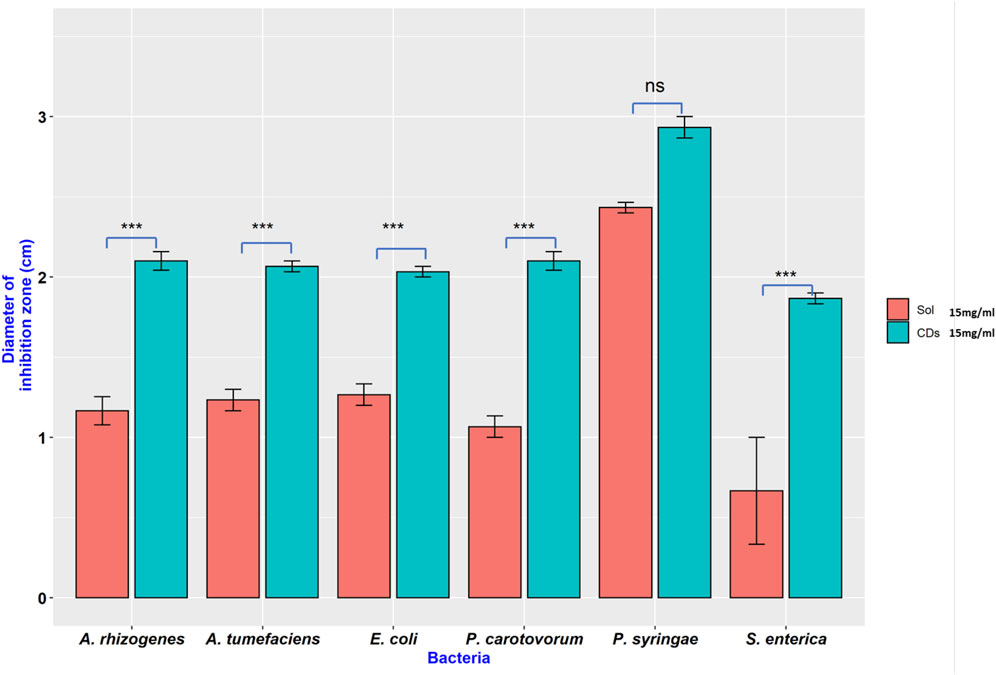
FIGURE 7. Inhibition on different Gram-negative bacteria in the presence of NH2-CDs and its precursors (Sol). Ns = no significant differences, *** = significant differences at p ≤ 0.001.
Incubation Time for Complete Inhibition of Bacterial Cells
An experiment was setup to determine the minimum incubation time required for complete inhibition of bacterial growth. 50 µl of bacterial culture harvested at the log phase was incubated at different time intervals with 5 µl (15.0 mg/ml) of freshly synthesized CDs. Depending on the species, different bacterial strains required different incubation times for complete inhibitions by NH2-FCDs (Figure 8). The complete inhibition was observed in E. coli after 16 h of incubation with CDs, and for Salmonella, 24 h was necessary for complete inhibition. While 2 h incubation time was required for Pectobacterium carotovorum, other bacteria, including A. tumefaciens, A. rhizogenes, and Pseudomonas syringae were more sensitive to the CDs and required as low as 1 h of incubation for complete inhibition. This experiment was also conducted for other CDs as well (Supplementary Figures S11, S12) in which E. coli and Salmonella displayed the highest resistance against these CDs.
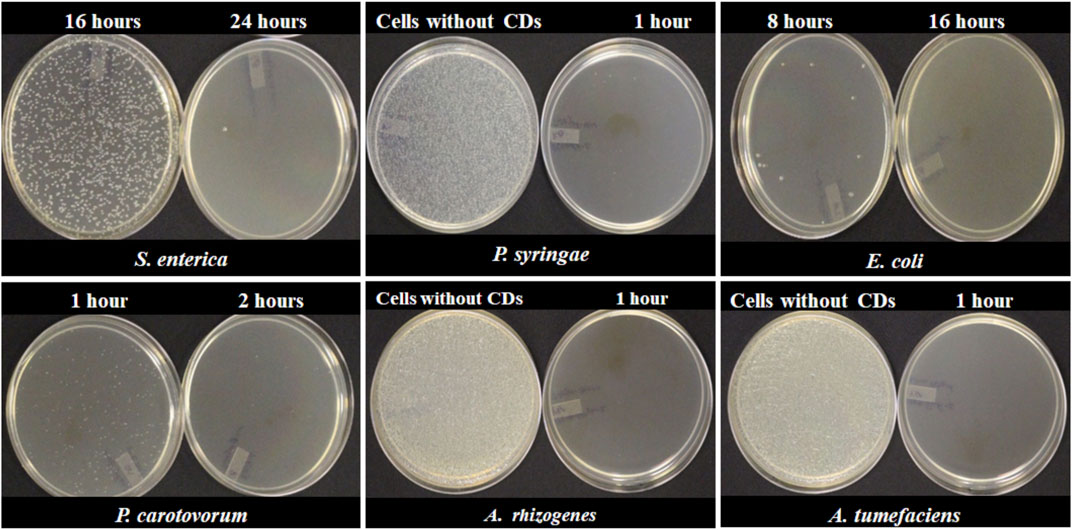
FIGURE 8. Minimum time required for complete inhibition of different bacterial cells using NH2-FCDs.
Conclusion
In conclusion, we synthesized four different carbon dots and evaluated their microbial activities against six different Gram-negative bacterial species including Escherichia coli (E. coli), Pseudomonas syringae pathovar tomato DC 3000 (P. syringae), Salmonella enterica pathovar typhimurium (S. enterica), Pectobacterium carotovorum (P. carotovorum), Agrobacterium tumefaciens (A. tumefaciens), and Agrobacterium rhizogenes (A. rhizogenes). Based on our findings, among the four different carbon dots, CDs prepared from citric acid and PEI did not have any antimicrobial effects against bacteria tested here, while the other carbon dots showed some degree of inhibition to the bacterial cells. It is an interesting observation that we do not know the exact mechanism. Interestingly, the PEI-CDs are also reported as a safe DNA delivery mechanism in other cells (plant cells), which is an indication that earlier reports also did not observe any inhibitory effects on using these CDs in their experiments. It is true that carbon dots synthesized from CA and PEI possessed positive charge. Despite their positive charge that could interfere with the negatively charged cell membrane, there are numerous other factors that could affect the cellular viability. Earlier reports indicate that the interaction between CDs and microorganisms and their inhibitory effects on bacterial growth depends on composition, size, shape, surface charge, and chemistry of the CDs, as well as the structure and surface chemistry of microorganisms (Lin et al., 2019).
In this report, we presented that the NH2-FCDs can be used as a novel antimicrobial agent for a wide range of Gram-negative bacteria in which some of them are pathogenic for human and animals. These positively charged carbon dots would interact strongly with a broad spectrum of bacteria via electrostatic interactions (Li et al., 2019). The carbon dots’ uptake by cells begins with the adhesion of these particles to the cells and interactions with biomolecules. Confocal microscopy showed that CDs were adhering all over the bacterial cell membrane. The process is potentially followed by an energy-dependent CD uptake mechanism and the internalization of carbon dots into the cells (Rejman et al., 2004; Kneipp et al., 2006; Dausend et al., 2008; Lesniak et al., 2013). The interactions of the CDs with the bacterial cell membrane trigger enzymatic inhibition, oxidative stress mechanisms (release of ROS species), and protein deactivation that eventually leads to cell death (Wang et al., 2017; Zaidi et al., 2017). In addition, the particle size also plays a role in the toxicity of CDs to the cells. Smaller particle sizes of NH2-FCDs (2.4 nm average size) as reported earlier (Hill et al., 2016) would easily penetrate the cell and interfere with the bacterial enzymatic processes. Furthermore, the surface of the NH2-FCDs consists of various alkyl and aromatic moieties, such as imidazole, pyridine, and pyrazine-type molecules, resulting from the desymmetrization of TTDDA (Hill et al., 2016). These N-heterocyclic complexes are described as antimicrobial agents in earlier reports (Bansal and Silakari, 2012).
We demonstrated that the extent of bacterial resistance against these NH2-FCDs varies depending on the bacterial species. The emerging issue of MDR can be addressed using these nanomaterials having antimicrobial capacity. However, further studies are required to understand the mechanisms involved in the antibiotic effect of these CDs and their efficacy against Gram-positive bacteria. Further studies are also required for measuring safety issues if they are going to be used for treating bacterial infections in human and livestock. Considering the antibiotic properties of these carbon dots against Agrobacterium which is a common tool in the delivery of foreign DNA into plants, this carbon dot can be used for the eradication of Agrobacterium from the cell and tissue culture media, instead of common antibiotics that are used for this purpose.
Data Availability Statement
The original contributions presented in the study are included in the article/Supplementary Material; further inquiries can be directed to the corresponding author.
Author Contributions
AD, AP, and AT all conducted the nanomaterials investigation; AD prepared the draft version; and AT, ZY, and KD prepared the resources, supervised the project progress, and edited the manuscript.
Funding
The authors wish to thank the USDA for an Evans Allen funding (1005722), Tennessee Soybean Promotion Board (16-123-P), and USDA NIFA EGP (2020-70410-32909).
Conflict of Interest
The authors declare that the research was conducted in the absence of any commercial or financial relationships that could be construed as a potential conflict of interest.
Publisher’s Note
All claims expressed in this article are solely those of the authors and do not necessarily represent those of their affiliated organizations, or those of the publisher, the editors, and the reviewers. Any product that may be evaluated in this article, or claim that may be made by its manufacturer, is not guaranteed or endorsed by the publisher.
Acknowledgments
We are also thankful to Dr Sudipta Rakshit and Dr Ying Wu for giving us access to their laboratories, training, and the use of FTIR, freeze dryer, and Zetasizer in their laboratories.
Supplementary Material
The Supplementary Material for this article can be found online at: https://www.frontiersin.org/articles/10.3389/fnano.2021.768487/full#supplementary-material
References
AlAwak, M. M., Wang, P., Wang, S., and Tang, Y. (2017). Correlation of Carbon Dots' Light-Activated Antimicrobial Activities and Fl Uorescence Quantum Yield. R. Soc. Chem. 7, 30177–30184. doi:10.1039/c7ra05397e
Balouiri, M., Sadiki, M., and Ibnsouda, S. K. (2016). Methods for In Vitro Evaluating Antimicrobial Activity: A Review. J. Pharm. Anal. 6, 71–79. doi:10.1016/j.jpha.2015.11.005
Bansal, Y., and Silakari, O. (2012). The Therapeutic Journey of Benzimidazoles: A Review. Bioorg. Med. Chem. 20, 6208–6236. doi:10.1016/j.bmc.2012.09.013
Cao, X., Wang, J., Deng, W., Chen, J., Wang, Y., Zhou, J., et al. (2018). Photoluminescent Cationic Carbon Dots as Efficient Non-Viral Delivery of Plasmid SOX9 and Chondrogenesis of Fibroblasts. Sci. Rep. 8, 7057. doi:10.1038/s41598-018-25330-x
Carbonaro, C. M., Chiriu, D., Stagi, L., Casula, M. F., Thakkar, S. V., Malfatti, L., et al. (2018). Carbon Dots in Water and Mesoporous Matrix: Chasing the Origin of Their Photoluminescence. J. Phys. Chem. C 122, 25638–25650. doi:10.1021/acs.jpcc.8b08012
Carrera, E. T., Dias, H. B., Corbi, S. C. T., Marcantonio, R. A. C., Bernardi, A. C. A., Bagnato, V. S., et al. (2016). The Application of Antimicrobial Photodynamic Therapy (aPDT) in Dentistry: A Critical Review. Laser Phys. 26, 1–23. doi:10.1088/1054-660X/26/12/123001
Centers for Disease Control and Prevention (2019). Antibiotic Resistance Threats in the United States, 2019. Atlanta, GA: Centers for Disease Control and Prevention; Available at: https://www.cdc.gov/drugresistance/pdf/threatsreport/2019-ar-threats-report-508.pdf.
Dausend, J., Musyanovych, A., Dass, M., Walther, P., Schrezenmeier, H., Landfester, K., et al. (2008). Uptake Mechanism of Oppositely Charged Fluorescent Nanoparticles in Hela Cells. Macromol. Biosci. 8, 1135–1143. doi:10.1002/mabi.200800123
Dong, X., Awak, M. A., Tomlinson, N., Tang, Y., Sun, Y. P., and Yang, L. (2017). Antibacterial Effects of Carbon Dots in Combination with Other Antimicrobial Reagents. PLoS One 12, e0185324–16. doi:10.1371/journal.pone.0185324
Dong, X., Liang, W., Meziani, M. J., Sun, Y. P., and Yang, L. (2020). Carbon Dots as Potent Antimicrobial Agents. Theranostics 10, 671–686. doi:10.7150/thno.39863
Fernando, K. A. S., Sahu, S., Liu, Y., Lewis, W. K., Guliants, E. A., Jafariyan, A., et al. (2015). Carbon Quantum Dots and Applications in Photocatalytic Energy Conversion. ACS Appl. Mater. Inter. 7, 8363–8376. doi:10.1021/acsami.5b00448
Ge, J., Lan, M., Zhou, B., Liu, W., Guo, L., Wang, H., et al. (2014). A Graphene Quantum Dot Photodynamic Therapy Agent with High Singlet Oxygen Generation. Nat. Commun. 5, 4596–4598. doi:10.1038/ncomms5596
Gondil, V. S., Kalaiyarasan, T., Bharti, V. K., and Chhibber, S. (2019). Antibiofilm Potential of Seabuckthorn Silver Nanoparticles (SBT@AgNPs) against Pseudomonas aeruginosa. 3 Biotech. 9, 1–13. doi:10.1007/s13205-019-1947-6
Gupta, A., Mumtaz, S., Li, C.-H., Hussain, I., and Rotello, V. M. (2019). Combatting Antibiotic-Resistant Bacteria Using Nanomaterials. Chem. Soc. Rev. 48, 415–427. doi:10.1039/c7cs00748e
Hamblin, M. R., and Hasan, T. (2004). Photodynamic Therapy: A New Antimicrobial Approach to Infectious Disease? Photochem. Photobiol. Sci. 3, 436–450. doi:10.1039/b311900a
Hill, S. A., Benito-Alifonso, D., Morgan, D. J., Davis, S. A., Berry, M., and Galan, M. C. (2016). Three-Minute Synthesis of Sp3nanocrystalline Carbon Dots as Non-Toxic Fluorescent Platforms for Intracellular Delivery. Nanoscale 8, 18630–18634. doi:10.1039/c6nr07336k
Jones, K. E., Patel, N. G., Levy, M. A., Storeygard, A., Balk, D., Gittleman, J. L., et al. (2008). Global Trends in Emerging Infectious Diseases. Nature 451, 990–993. doi:10.1038/nature06536
Kneipp, J., Kneipp, H., McLaughlin, M., Brown, D., and Kneipp, K. (2006). In Vivo molecular Probing of Cellular Compartments with Gold Nanoparticles and Nanoaggregates. Nano Lett. 6, 2225–2231. doi:10.1021/nl061517x
Lesniak, A., Salvati, A., Santos-Martinez, M. J., Radomski, M. W., Dawson, K. A., and Åberg, C. (2013). Nanoparticle Adhesion to the Cell Membrane and its Effect on Nanoparticle Uptake Efficiency. J. Am. Chem. Soc. 135, 1438–1444. doi:10.1021/ja309812z
Li, H., He, X., Kang, Z., Huang, H., Liu, Y., Liu, J., et al. (2010). Water-Soluble Fluorescent Carbon Quantum Dots and Photocatalyst Design. Angew. Chem. 122, 4532–4536. doi:10.1002/ange.200906154
Li, H., Huang, J., Song, Y., Zhang, M., Wang, H., Lu, F., et al. (2018). Degradable Carbon Dots with Broad-Spectrum Antibacterial Activity. ACS Appl. Mater. Inter. 10, 26936–26946. doi:10.1021/acsami.8b08832
Li, Z., Ma, J., Ruan, J., and Zhuang, X. (2019). Using Positively Charged Magnetic Nanoparticles to Capture Bacteria at Ultralow Concentration. Nanoscale Res. Lett. 14, 195. doi:10.1186/s11671-019-3005-z
Lin, F., Bao, Y.-W., and Wu, F.-G. (2019). Carbon Dots for Sensing and Killing Microorganisms. Carbon Res. 5, 33. doi:10.3390/c5020033
Meziani, M. J., Dong, X., Zhu, L., Jones, L. P., Lecroy, G. E., Yang, F., et al. (2016). Visible-Light-Activated Bactericidal Functions of Carbon "Quantum" Dots. ACS Appl. Mater. Inter. 8, 10761–10766. doi:10.1021/acsami.6b01765
Michael, C. A., Dominey-howes, D., Labbate, M., Maria, C., and Elisabeth, J. (2014). The Antimicrobial Resistance Crisis: Causes, Consequences, and Management. Front. Public Health 2, 145. doi:10.3389/fpubh.2014.00145
Michael, C. A., Dominey-Howes, D., and Labbate, M. (2014). The Antimicrobial Resistance Crisis: Causes, Consequences, and Management. Front. Public Health 2, 145. doi:10.3389/fpubh.2014.00145
Muktha, H., Sharath, R., Kottam, N., Smrithi, S. P., Samrat, K., and Ankitha, P. (2020). Green Synthesis of Carbon Dots and Evaluation of its Pharmacological Activities. BioNanoSci. 10, 731–744. doi:10.1007/s12668-020-00741-1
Nandiyanto, A. B. D., Oktiani, R., and Ragadhita, R. (2019). How to Read and Interpret Ftir Spectroscope of Organic Material. Indonesian J. Sci. Technol. 4, 97–118. doi:10.17509/ijost.v4i1.15806
Piddock, L. J. (2012). The Crisis of No New Antibiotics-What Is the Way Forward? Lancet Infect. Dis. 12, 249–253. doi:10.1016/s1473-3099(11)70316-4
Ponomarenko, L. A., Schedin, F., Katsnelson, M. I., Yang, R., Hill, E. W., Novoselov, K. S., et al. (2008). Chaotic Dirac Billiard in Graphene Quantum Dots. Science 320, 356–358. doi:10.1126/science.1154663
Qi, M., Chi, M., Sun, X., Xie, X., Weir, M. D., Oates, T. W., et al. (2019). Novel Nanomaterial-Based Antibacterial Photodynamic Therapies to Combat Oral Bacterial Biofilms and Infectious Diseases. Int. J. Nanomedicine 14, 6937–6956. doi:10.2147/ijn.s212807
Read, A. F., and Woods, R. J. (2014). Antibiotic Resistance Management. Evol. Med. Public Health 2014, 147. doi:10.1093/emph/eou024
Rejman, J., Oberle, V., Zuhorn, I. S., and Hoekstra, D. (2004). Size-Dependent Internalization of Particles via the Pathways of Clathrin- and Caveolae-Mediated Endocytosis. Biochem. J. 377, 159–169. doi:10.1042/bj20031253
Sagbas, S., and Sahiner, N. (2019). 22 - Carbon dots: preparation, properties, and application. In: Khan A, Jawaid M, Inamuddin, Asiri AMBT-N and its C, editors. Woodhead Publishing Series in Composites Science and Engineering. Woodhead Publishing. Nanocarbon and its Composites, 651–676. doi:10.1016/B978-0-08-102509-3.00022-5
Sun, Y.-P., Zhou, B., Lin, Y., Wang, W., Fernando, K. A. S., Pathak, P., et al. (2006). Quantum-Sized Carbon Dots for Bright and Colorful Photoluminescence. J. Am. Chem. Soc. 128, 7756–7757. doi:10.1021/ja062677d
Tan, J., Zou, R., Zhang, J., Li, W., Zhang, L., and Yue, D. (2016). Large-Scale Synthesis of N-Doped Carbon Quantum Dots and Their Phosphorescence Properties in a Polyurethane Matrix. Nanoscale 8, 4742–4747. doi:10.1039/c5nr08516k
Thangudu, S., Kulkarni, S. S., Vankayala, R., Chiang, C.-S., and Hwang, K. C. (2020). Photosensitized Reactive Chlorine Species-Mediated Therapeutic Destruction of Drug-Resistant Bacteria Using Plasmonic Core-Shell Ag@AgCl Nanocubes as an External nanomedicineAg@AgCl Nanocubes as an External Nanomedicine. Nanoscale 12, 12970–12984. doi:10.1039/d0nr01300e
Ventola, C. L. (2015). The Antibiotic Resistance Crisis: Part 1: Causes and Threats. P T 40, 277–283.
Wang, L., Hu, C., and Shao, L. (2017). The Antimicrobial Activity of Nanoparticles: Present Situation and Prospects for the Future. Int. J. Nanomedicine 12, 1227–1249. doi:10.2147/ijn.s121956
Wu, X., Wu, L., Cao, X., Li, Y., Liu, A., and Liu, S. (2018). Nitrogen-Doped Carbon Quantum Dots for Fluorescence Detection of Cu2+ and Electrochemical Monitoring of Bisphenol A. RSC Adv. 8, 20000–20006. doi:10.1039/c8ra03180k
Xu, J., Murphy, S. L., Kockanek, K. D., and Arias, E. (2018). Mortality in the United States. NCHS Data Brief 355, 1–8. PMID: 32487294.
Keywords: carbon dots, antimicrobial, MDR, gram-negative, amine coated
Citation: Devkota A, Pandey A, Yadegari Z, Dumenyo K and Taheri A (2021) Amine-Coated Carbon Dots (NH2-FCDs) as Novel Antimicrobial Agent for Gram-Negative Bacteria. Front. Nanotechnol. 3:768487. doi: 10.3389/fnano.2021.768487
Received: 31 August 2021; Accepted: 18 October 2021;
Published: 16 November 2021.
Edited by:
Raviraj Vankayala, Indian Institute of Technology Jodhpur, IndiaReviewed by:
Suresh Thangudu, Kaohsiung Chang Gung Memorial Hospital, TaiwanPoliraju Kalluru, University of Ulsan, South Korea
Copyright © 2021 Devkota, Pandey, Yadegari, Dumenyo and Taheri. This is an open-access article distributed under the terms of the Creative Commons Attribution License (CC BY). The use, distribution or reproduction in other forums is permitted, provided the original author(s) and the copyright owner(s) are credited and that the original publication in this journal is cited, in accordance with accepted academic practice. No use, distribution or reproduction is permitted which does not comply with these terms.
*Correspondence: Ali Taheri, YXRhaGVyaTFAdG5zdGF0ZS5lZHU=