- 1Laboratory of Brain Metabolic Diseases, Institute of Metabolic Disease Research and Drug Development, China Medical University, Shenyang, China
- 2Magnetic Resonance Research Center, Diagnostic Radiology and Biomedical Engineering, Yale University, New Haven, CT, USA
It is firmly believed that the mechanism of action of SSRIs in major depression is to inhibit the serotonin transporter, SERT, and increase extracellular concentration of serotonin. However, this undisputed observation does not prove that SERT inhibition is the mechanism, let alone the only mechanism, by which SSRI’s exert their therapeutic effects. It has recently been demonstrated that 5-HT2B receptor stimulation is needed for the antidepressant effect of fluoxetine in vivo. The ability of all five currently used SSRIs to stimulate the 5-HT2B receptor equipotentially in cultured astrocytes has been known for several years, and increasing evidence has shown the importance of astrocytes and astrocyte-neuronal interactions for neuroplasticity and complex brain activity. This paper reviews acute and chronic effects of 5-HT2B receptor stimulation in cultured astrocytes and in astrocytes freshly isolated from brains of mice treated with fluoxetine for 14 days together with effects of anti-depressant therapy on turnover of glutamate and GABA and metabolism of glucose and glycogen. It is suggested that these events are causally related to the mechanism of action of SSRIs and of interest for development of newer antidepressant drugs.
Introduction: SERT, the 5-HT2B Receptor and SSRI
SSRI Targets
It is generally thought that the molecular mechanism of SSRIs is the long-known blockage of serotonin (5-HT) reuptake by its transporter SERT (Fuller and Wong, 1977; Wong and Bymaster, 1995). Binding of SSRIs to this transporter (Langer et al., 1986; Launay et al., 2006; Diaz et al., 2012) leads to elevated extracellular 5-HT levels, assumed to cause their antidepressant effects. SSRIs do exert a direct inhibitory effect on the SERT protein on the neuronal presynaptic plasma membrane (Zhou et al., 2009). Recently the molecular basis for high-affinity recognition of fluoxetine in the SERT molecule was unraveled (Andersen et al., 2014). However, these findings do not prove that SERT inhibition is the mechanism, let alone the only mechanism, by which SSRI’s exert their therapeutic effects in major depression.
Recently Diaz et al. (2012) demonstrated in intact brain a raphe nuclei 5-HT2B receptor with relatively high affinity for SSRIs, which was indispensable for the antidepressant effect of fluoxetine. However, SSRI effects exerted via the 5-HT2B receptor had previously been described not only in cultured neurons (Launay et al., 2006) but also in cultured astrocytes (Kong et al., 2002), where they have been thoroughly studied (Li et al., 2008a, 2009; Zhang et al., 2010). The resultant induction of signaling pathways in glia and neurons has been further characterized in astrocytic and neuronal fractions from mice treated for 2 weeks with fluoxetine. They may play a key role in the antidepressant mechanism of SSRIs. Given the dominant role ascribed to neurons in the mechanisms of SSRIs and other psychiatric drugs it may appear surprising that studies found the major chronic impact of SSRIs in the fluoxetine-treated mice to be on astrocytes. However they are consistent with the growing evidence for a major role of these cells in major depression and its therapy studied in patients (Abdallah et al., 2014a,b), postmortem brain (Rajkowska and Stockmeier, 2013) or in models of this disease (Gosselin et al., 2009; Banasr et al., 2010).
Astrocytes account for ~25% of brain cortical volume and are responsible for at least a corresponding fraction of oxidative energy metabolism, mainly required for maintaining extracellular glutamate, GABA, and K+ homeostasis, and synthesis of glutamate and GABA via the glutamine-glutamate (GABA) cycle (reviewed in Hertz, 2011). This cycle is well established in the brain in vivo, where it represents the quantitatively most important interaction between neurons and astrocytes. It will be described in more detail below. Astrocytes synthesize all transmitter glutamate and GABA and accumulate most after neuronal release. According to recent research (Duarte and Gruetter, 2013), astrocytes account for an even larger fraction of oxidative brain metabolism, when their role in subsequent metabolism of released GABA before its partial return via astrocytes to neurons also is taken into account. These major roles of astrocytes are likely to be relevant for antidepressant effects on glutamate homeostasis, excitatory and inhibitory signaling, and glucose metabolism. Involvement of the glutamine-glutamate (GABA) cycle remains to be studied after SSRI administration, but it has been investigated in patients suffering from major depression (Abdallah et al., 2014a). Moreover, studies of the rapidly acting anti-depressant drugs ketamine or riluzole (which have no known effect on 5-HT2B receptors) have shown that increases in flux in this cycle parallel recovery from experimental and clinical depression (Chowdhury et al., 2008, 2012; Brennan et al., 2010).
Besides discussing the 5-HT2B receptor as an SSRI target, this review will deal with 5-HT2B receptors’ cellular locations; the signaling pathways activated; short term effect on cell signaling; and long-term-effects in cultured astrocytes and in fluoxetine-treated animals. Consequences of long term (14 days) SSRI treatment on gene up-regulation and editing in primary cultures of astrocytes and in neurons and astrocytes freshly isolated from the brains of mice treated with fluoxetine and/or in whole brains from such animals are described in detail. Some of these effects are exerted on genes mediating glutamate/glutamine transport and interconversion and on glutamate and GABA receptor genes. Other effects are exercised on genes which are not directly related to glutamate signaling, but are important for the well-established correlation between recovery from major depression and increase in glucose metabolism in brain (Buchsbaum et al., 1997; Mayberg et al., 2000; Kennedy et al., 2001). Finally, the reviewed studies pinpoint acute and chronic effects on pathways for glycogen turnover. This is important because glycogenolysis is known to impact glutamate formation, learning and longer term neuroplasticity (Gibbs et al., 2007, 2008; Duran et al., 2013). Together, these changes may be the link between the molecular and cellular changes due to 5-HT2B receptor binding and the longer-term impact on depressive symptoms. Selective activation of this receptor or intermediates of its downstream pathways may accordingly constitute potential targets for pharmaceutical development. Such development would be important, since (i) a considerable fraction of patients suffering from major depression do not respond adequately to current antidepressant therapy; (ii) the response is slow except for a few recently tested drugs (O’Leary et al., 2014); and (iii) even the relatively safe SSRIs can have severe side effects if used in pregnant women (Ellfolk and Malm, 2010) or after acute coronary occlusion (Rieckmann et al., 2013).
The Critical Importance of the 5-HT2B Receptor for SSRI Effects
The 5-HT2B receptor was identified in 1987 (Cohen and Fludzinski, 1987) and was thus unknown when SSRIs were introduced and believed to lack relevant receptor effects. Like other 5-HT2 receptors, the 5-HT2B receptor is Gq/11 protein-coupled and stimulates phospholipase C (PLC) to generate diacylglycerol (DAG) and inositol 1,4,5-trisphosphate (IP3) by hydrolysis of phosphatidyl-inositol 4,5-bisphosphate (PIP2). This triggers IP3 receptor-mediated increase of free cytosolic calcium concentration ([Ca2+]i) (Deecher et al., 1993; Roth et al., 1998; Porter et al., 1999) and additional second messenger effects. During chronic exposure to fluoxetine these second messenger effects may be responsible for the many reported changes in gene expression, as well as alterations in metabolism and the glutamine-glutamate (GABA) cycle that will be described later.
The 5-HT2B receptor is expressed in mouse, rat, and human brain (Kursar et al., 1994; Baez et al., 1995; Bonhaus et al., 1995; Choi and Maroteaux, 1996). In astrocytes obtained from mouse brain (Zhang et al., 2010) its mRNA expression is ~2 times higher than in neurons (Li et al., 2012, see Figure 1). It is also a major 5-HT2 receptor in astrocyte cultures (Kong et al., 2002). Neuronal expression has been reported in Purkinje cells (Choi and Maroteaux, 1996) and raphe nuclei (Diaz et al., 2012). Diaz et al. (2012) showed in an in vivo rat model that 5-HT2B receptors are needed for long-term behavioral effects of fluoxetine. These effects were abolished in mice or after pharmacologic inactivation of 5-HT2B receptors, whereas stimulation by a selective 5-HT2B receptor agonist induced similar responses as fluoxetine in behavioral assays. Fluoxetine-mediated neurogenesis (Manev et al., 2003) was eliminated in 5-HT2B knock-out animals, and acute fluoxetine administration to mice induced a much smaller increase in hippocampal 5-HT levels than in wild type mice.
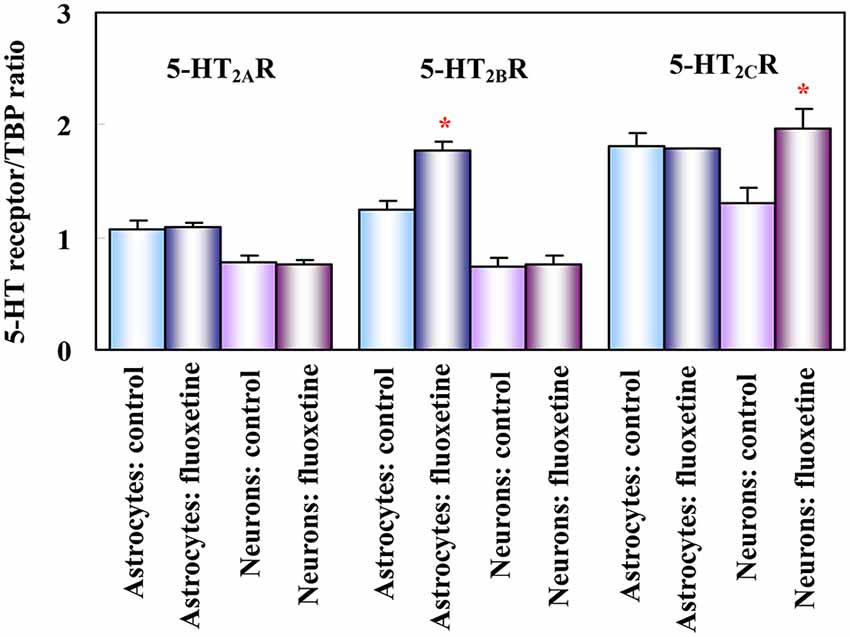
Figure 1. Effect of fluoxetine on 5-HT2 receptor mRNA in astrocytes and neurons obtained separately from both control mice (injected with saline) and mice treated for 14 days with fluoxetine. mRNA expression was measured by reverse transcription polymerase chain reaction (RT-PCR) of 5-HT2A, 5-HT2B and 5-HT2C receptors in astrocytes and neurons isolated by fluorescence-activated cell sorting (FACS) from cerebral hemispheres of two different adult mice strains (FVB/NTg(GFAP-GFP)14Mes/J, providing astrocyte-specific fluorescence, and B6.Cg-Tg(Thy1-YFPH)2Jrs/J, providing neuron-specific fluorescence, although mainly of large glutamatergic neurons). Results are shown for mice chronically treated with fluoxetine (10 mg/kg per day) and for untreated controls. They are means ± SEM of ratios between scanned 5-HT2A, 5-HT2B or 5-HT2C receptor expression and scanned expression of TATA-binding protein (TBP), used as housekeeping gene. n = 3 (neurons) or 4 (astrocytes). *P > 0.05 vs. control group in astrocytes (5-HT2B receptor) or in neurons (5-HT2C receptor). (Li et al., 2012).
Acute Effects of SSRIs on 5-HT2B Receptors and Their Targets
Acute Effects on 5-HT2B Receptors on Neurons
5-HT2B receptor effects on SERT or inhibition of 5-HT release has been shown in very young cultures of serotonergic neurons and in neuronal-enriched cultures from the raphe nuclei (Azmitia et al., 1990; Launay et al., 2006). However, these nuclei in addition contain glia cells (Azmitia and Whitaker-Azmitia, 1991), which may also be present in the cultures. Unfortunately our own cultured neurons tolerate fluoxetine poorly. Moreover, they are also young and the immature nervous system is different from the mature brain (e.g., Hertz, 2013). However, future studies to establish potential direct neuronal responses to fluoxetine would be of high importance.
Glial 5-HT2B Binding is Critical for in Vivo Effects of SSRIs
Support for the concept that direct binding to 5-HT2B receptors is important for SSRI’s mechanisms of action is that all presently used SSRIs have virtually identical affinity for the 5-HT2B receptor in cultured astrocytes (Zhang et al., 2010). This contrasts the huge differences in their potency as inhibitors of 5-HT uptake (Wong and Bymaster, 1995; Popik, 1999). Also inconsistent with SSRIs owing their therapeutic effects solely to an action on SERT are findings that substantial SERT occupancy (about 80%) occurs at sub-therapeutic SSRI doses, and that increasing doses to clinically effective levels causes only very minor increases in SERT binding (Meyer et al., 2004). SERT-unrelated acute and chronic effects of fluoxetine, including those by 5-HT2B receptor stimulation of astrocytes may therefore be important for their mechanisms of action.
Acute Effect of Fluoxetine on 5-HT2B Receptors and Their Targets in Cultured Astrocytes
Cultured astrocytes are well suited to acute and chronic studies of SSRIs as they can survive undamaged in culture for a long time. Effects on gene expression and editing can be compared with those exerted in the brain in vivo after separation of an astrocytic and a neuronal cell fraction as described by Lovatt et al. (2007).
Fluoxetine displaces serotonin binding to cultured astrocytes (Hertz et al., 1979). Kong et al. (2002) found expression of mRNA expression of 5-HT2B and 5-HT2A receptors, but not of 5-HT2C receptors in the cultures (Zhang et al., 1993). Lack of inhibition by ketanserin showed that this was not a 5-HT2A receptor effect. They also obtained quantitative data for displacement of the universal 5-HT2 receptor ligand mesulergine by fluoxetine. Based on these data the Ki value for fluoxetine’s displacement of 5-HT was calculated to be 70 nM (Hertz et al., 2012). This affinity is 4 times higher than that originally determined for binding of fluoxetine in brain tissue to the 5-HT2C receptor, for which fluoxetine initially was found to have the highest affinity (Wong and Bymaster, 1995).
Studies on post receptor signaling in these glia cells have shown effects on signaling targets such as growth factors and glycogen synthesis. Both of these are known to influence neuroplasticity, which is believed to be key factor in the efficacy of SSRIs as antidepressants (Eom and Jope, 2009; Freitas et al., 2013). Acute administration of fluoxetine increases [Ca2+]i and stimulates glycogenolysis (a [Ca2+]i-dependent process) in cultured astrocytes (Zhang et al., 1993; Chen et al., 1995). These effects are not limited to cultured cells, since both fluoxetine and paroxetine support learning in day-old chickens. This effect is inhibited by an antagonist of the 5-HT2B,C receptor and by a glycogenolytic inhibitor (Gibbs and Hertz, 2014). Further evidence that the effects were specific to 5-HT2B binding as opposed to SERT was that the same effects were obtained with almost identical doses of SSRIs (Zhang et al., 2010) that affect 5-HT uptake with widely different potency (Wong and Bymaster, 1995).
The signaling pathway activated by fluoxetine in cultured astrocytes (Li et al., 2008a) is complex (Figure 2). Abolishment of 5-HT2B receptor activity by its siRNA or administration of the 5-HT2B receptor inhibitor SB204741 prevented any response, further validating the concept that the 5-HT2B receptor is directly stimulated. Phosphorylation of extracellular kinases 1 and 2 (ERK1/2) was established as an end point without examining further downstream effects, except for a rapid increase in the expression of the immediate early genes cfos and fosB. The increase in [Ca2+]i (Chen et al., 1995) leads to activation of metalloproteinases (MMPs) and shedding of growth factor(s), perhaps mainly heparin-binding epidermal growth factor (HB-EGF). This EGF receptor agonist is known to be present in adult brain and to be required for synaptic plasticity (Oyagi et al., 2011).
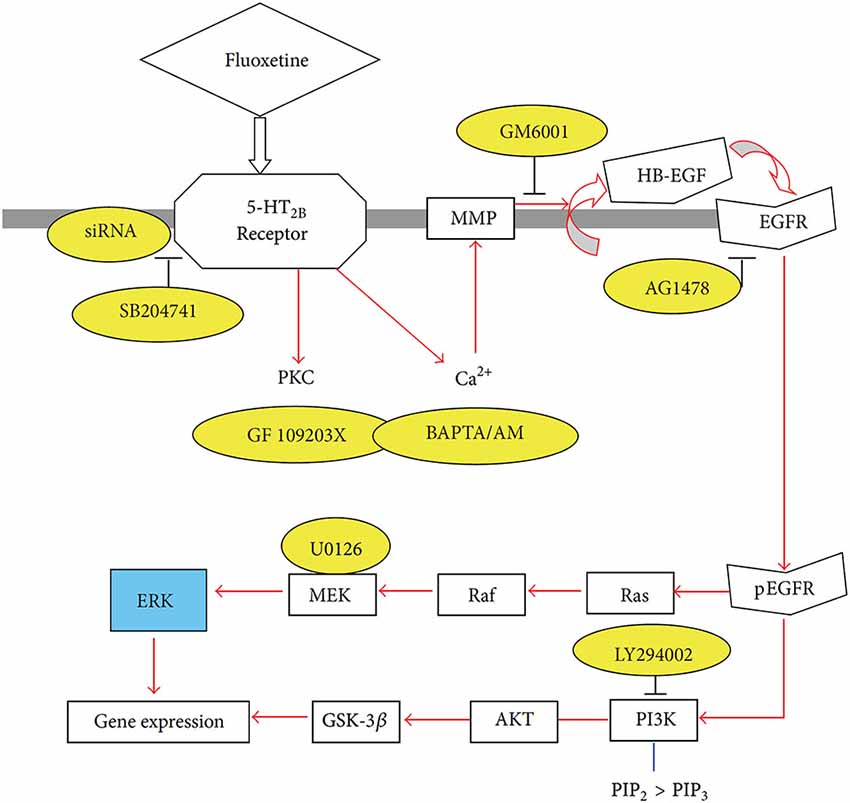
Figure 2. Schematic illustration of pathways leading to stimulation of extracellular-regulated kinase (ERK) and AKT phosphorylation by fluoxetine in astrocytes. Fluoxetine binds to 5-HT2B receptors. The activation of the receptors induces protein kinase C (PKC) activity and increase of intracellular Ca2+ concentration ([Ca2+]i) by Ca2+ release from intracellular stores. The latter activates Zn-dependent metalloproteinases (MMPs) and leads to shedding of growth factor(s). The released epidermal growth factor receptor (EGFR) ligand stimulates phosphorylation of the EGFR. The downstream target of EGFR, ERK (shown in blue) is phosphorylated via the Ras/Raf/MEK pathway, and AKT is phosphorylated via PI3K pathway. PIK3 is also known to catalyze the formation of PIP3 from PIP2. During fluoxetine administration, phosphorylation of ERK and AKT was prevented after siRNA administration against the 5-HT2B receptor or after administration of inhibitors (shown in yellow) of this receptor (SB204741), of PKC (GF 109293X), of intracellular Ca2+ homeostasis (BAPTA/AM, an intracellular Ca2+ chelator), of Zn-dependent MMPs (GM6001), of the receptor-tyrosine kinase of the EGFR (AG1478), of ERK phosphorylation (U0126, a mitogen-activated kinase (MEK) inhibitor) or of the AKT pathway (LY294002, a PI3K inhibitor). This inhibition is an indication of participation of all the inhibited factors in the normal signaling pathway. (Hertz et al., 2012).
Growth factor release may link glial 5-HT2B binding to the longer-term cellular and behavioral changes induced by SSRI stimulation of receptor tyrosine kinases of the epidermal growth factor (EGF) receptor (EGFR). Such an effect of G protein-coupled receptors represents a transactivation process, a common mechanism in astrocytes (Daub et al., 1997; Peavy et al., 2001; Peng, 2004; Du et al., 2009). mRNA expression of the EGFR is approximately 4 times higher in freshly isolated mouse brain astrocytes than in corresponding neurons (Peng et al., 2014). EGFR phosphorylation leads to activation of ERK1/2 and of PI3K, with the latter causing AKT phosphorylation (Hertz et al., 2012; Peng et al., 2014). Moreover, released growth factor also acts on neurons (Li et al., 2008b) and may be at least one of the stimuli for the well known fluoxetine-stimulated neurogenesis (Manev et al., 2003) and effects on synaptic activity (Oyagi et al., 2011). Within 1 h the phosphorylation of ERK1/2 induces gene expression of cfos and fosB in astrocytes (Li et al., 2008a), shown in Figure 3 to be abolished by inhibitors of the pathway indicated in Figure 2. Fluoxetine also rapidly induces ERK1/2-dependent enhancement of gene expression of glial-derived nerve factor (GDNF) in the cultured astrocytes used by Mercier et al. (2004). These cultures differ in several respects from ours, e.g., by being prepared from rats instead of mice and without exposing the cells to the differentiating agent dibutyryl cyclic AMP. It is re-assuring that several aspects of fluoxetine effects are the same. However, some differences were also found, which is not unexpected when cultured cells are used.
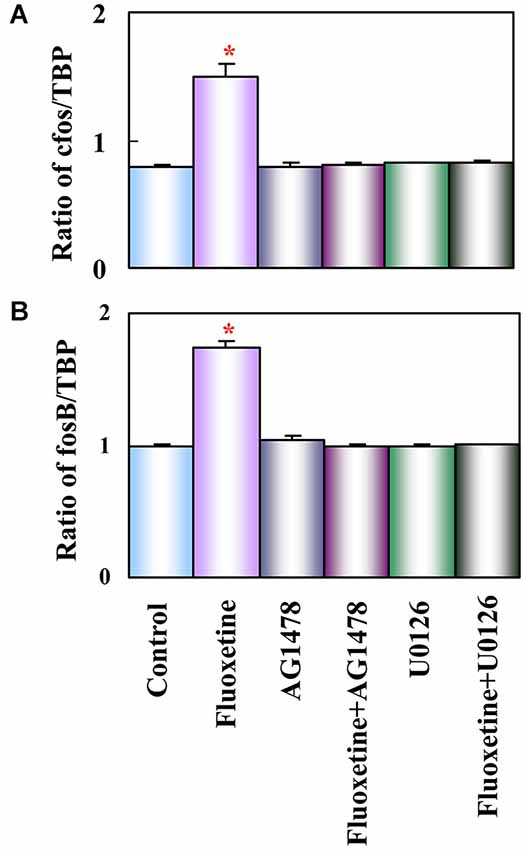
Figure 3. Activation of EGF receptors and of ERK is required for up-regulation of mRNA expression of c-fos and fosB by fluoxetine in astrocytes. Cells were incubated for 60 min in serum-free medium in the absence of any drug (Control) or in the presence of 10 μM fluoxetine alone or together with 1 μM AG 1478 or 10 μM U0126. Average mRNA expression (n = 3) was quantitated as ratios between c-fos and TBP, used as a house-keeping gene (A) and between fosB and TBP (B). SEM values are indicated by vertical bars. *Indicates statistically significant (P < 0.05) difference from Control, AG 1478, fluoxetine plus AG 1478, U0126, and fluoxetine plus U0126 groups for c-fos and fosB. (Li et al., 2008a).
A further important step where glial 5-HT2B receptor binding may influence the longer-term cellular and behavioral effects of SSRIs is the impact on glycogen synthesis. Glycogen turnover, i.e., interspersed glycogen synthesis and glycogenolysis, is indispensable during learning (Gibbs and Hutchinson, 2012; Hertz et al., 2013a). The acute memory-enhancing, glycogenolysis-dependent effect of both fluoxetine and paroxetine has been mentioned (Gibbs and Hertz, 2014). Knock-out of brain glycogen synthase abolishes learning of new motor and cognitive skills (Duran et al., 2013). It is likely that fluoxetine also affects glycogen synthesis, since the AKT pathway (Figure 2) is stimulated, as shown by AKT phosphorylation in the cultured cells (Hertz et al., 2012; Peng et al., 2014). Phosphorylated AKT in turn inhibits glycogen synthase kinase-3β (GSK-3β) by phosphorylation (Fang et al., 2005). This probably stimulates glycogen synthesis, since activation of glycogen synthase by GSK-3 decreases its activity (Embi et al., 1980; De Sarno et al., 2002).
Consistent with these findings in cultured astrocytes Plenge (1976) found an acute increase in brain glycogen after administration of Li+ (“lithium”), a known inhibitor of GSK3. As shown in Figure 4, this reflected increased glycogen synthesis (Plenge, 1982). Effects of fluoxetine (and of electroconvulsive therapy) by inhibitory phosphorylation of GSK-3 have also been summarized by Gould et al. (2006), and the role of GSK-3 in synaptic plasticity, including memory, discussed by Bradley et al. (2012).
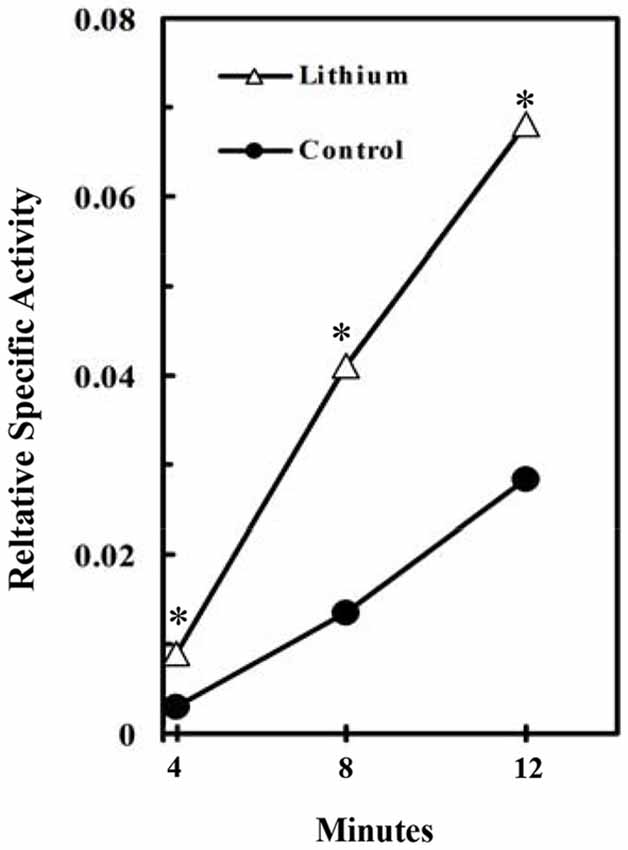
Figure 4. Stimulation of glycogen synthesis by inhibition of GSK3. Specific activity of brain glycogen was measured relatively to the specific activity of blood glucose in lithium-treated rats. At 4–12 min after injection of radioactive glucose, 15 μmol LiCl was intracisternally injected into the rats. The brains were frozen 2 h after the injection and incorporation of radioactivity into glycogen determined. *P < 0.05 (4–8 rats at each point). (Plenge, 1976).
The Jope group found in whole brain that 5-HT2B stimulation or acute fluoxetine administration decreases the levels of phosphorylated GSK3 (Li et al., 2004; Polter et al., 2012). This response was blunted or absent in young mice (Beurel et al., 2012), consistent with astrocytic localization since astrocytes are mainly generated postnatally (Schousboe, 1971; Ge et al., 2012). Other papers from this group showed that deficiency in inhibitory phosphorylation of GSK-3 increases sensitivity to mood disturbances (Polter et al., 2010) and that GSK-3 is required for the antidepressant effect of ketamine (Beurel et al., 2011).
Further Interactions Between Astrocytes and Neurons Required for Normal Function in Adult Brain
Astrocytes Receive Transmitter Signals from Neurons and Release “Gliotransmitters” and Other Neuroactive Compounds
The response of astrocytes to the SSRI 5-HT2B agonists is only one example of how astrocyte neurotransmitter receptors may modulate synaptic strength and plasticity. An increasing number of studies have demonstrated that astrocytes express receptors for most neurotransmitters and release neuroactive substances that modulate neuronal activity (Ben Achour and Pascual, 2010). Besides the already mentioned growth factor release in response to stimulation by 5-HT2B receptors (and many other neurotransmitter receptors) they release compounds like adenosine triphosphate (ATP) and glutamate as gliotransmitters. The amount of glutamate released is much smaller than that released from glutamatergic neurons, but it is of special importance because it acts on glutamate receptors which are not located postsynaptically. In this manner astrocytes contribute to regulation of long-term potentiation (LTP), long-term depression (LTD) and neuroplasticity (Ben Achour and Pascual, 2010).
Astrocytes Synthesize All Transmitter Glutamate and GABA and Accumulate Most After Neuronal Release
Neurons cannot carry out glutamate synthesis from glucose because they lack an enzyme (pyruvate carboxylase), which is critical for glutamate synthesis in vivo (Shank et al., 1985) and in culture (Yu et al., 1983). The majority of glutamate released as a neurotransmitter is taken up by glial cells. Subsequently glutamate is transferred to neurons in the glutamine-glutamate (GABA) cycle via astrocytic glutamine formation, release of glutamine, and its uptake in neurons, where it is deamidated to glutamate (Hertz and Zielke, 2004; Schousboe et al., 2013). In glutamatergic neurons glutamate is used as a transmitter, and in GABAergic neurons it is converted to GABA (Figure 5). In gray matter (Lebon et al., 2002) of the awake human brain the rate of this process equals about 75% of the rate of total glucose consumption, and in the deeply anesthetized, iso-electric brain it is abolished (Sibson et al., 1998; Hyder and Rothman, 2012; Duarte and Gruetter, 2013). The correlation between cycle flux and brain glucose utilization is linear (Sibson et al., 1998; Hyder and Rothman, 2012). Key processes in this massive astrocyte-neuronal interaction are strictly regulated and/or complex. This applies to astrocytic release of glutamine (Nissen-Meyer and Chaudhry, 2013) and neuronal formation of glutamate from glutamine (Palaiologos et al., 1989), which requires concomitant stimulation of glycolysis (Chowdhury et al., 2014; Verkhratsky et al., 2014). This requirement explains part of the linear correlation between glucose utilization and the glutamine-glutamate (GABA) cycle. The cycle also brings previously released transmitter amino acids back to neurons after an initial uptake in astrocytes (Figure 5). This is associated with a small amount of energy expenditure (for glutamate uptake and glutamine formation). Return to astrocytes of previously released transmitter and its transport back to neurons represents the major part of the flux in the glutamine-glutamate (GABA) cycle. Only 15–25% of the flux serves to transfer newly synthesized glutamate from astrocytes to neurons (an anaplerotic process) and return glutamate to astrocytes for oxidative degradation (a cataplerotic process). The anaplerotic de novo synthesis requires glucose utilization in astrocytes (one glucose molecule for each molecule of glutamate). Cycle flux is also significantly correlated with glutamate content in human brain (Abdallah et al., 2014a). Since the glutamate content in astrocytes is very low (Lebon et al., 2002), this mainly represents neuronal glutamate.
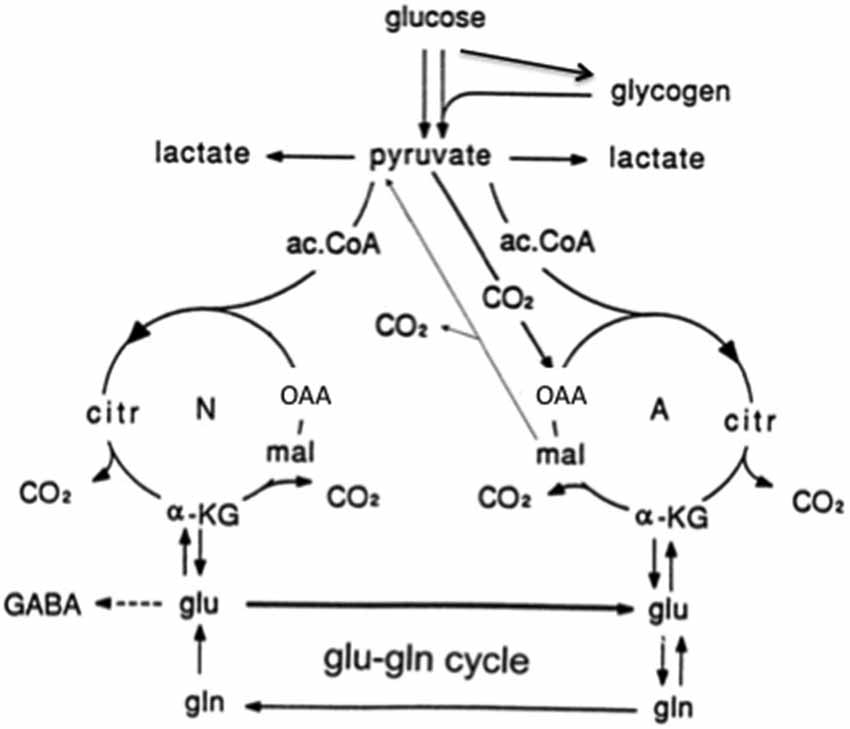
Figure 5. Cartoon of glucose metabolism via pyruvate in neurons (left—N) and astrocytes (right—A) and of glutamine–glutamate (GABA) cycling. In both cell types 2 molecules pyruvate are formed from one molecule glucose by glycolysis. Their metabolism via acetyl Coenzyme A (ac.CoA) leads to formation of citrate by condensation with pre-existing oxaloacetate (OAA) in the tricarboxylic acid (TCA), an end-result of the previous turn of the cycle. Citrate oxidation in the TCA cycle includes two decarboxylations, leading to re-formation of OAA, ready for another turn of the cycle, and to production of large amounts of energy (ATP). Pyruvate carboxylation occurs only in astrocytes. It creates a new molecule of OAA, which after condensation with ac.CoA, derived from a second molecule of pyruvate, forms a new molecule of citrate. This process can be used for replacement of worn TCA cycle intermediates. More important in the present context is that α-ketoglutarate (α-KG), one of the intermediates of the TCA cycle can leave the cycle to form glutamate (glu), catalyzed by aspartate aminotransferase and/or glutamate dehydrogenase. In turn, glutamate is amidated to glutamine (gln), catalyzed by the cytosolic and astrocyte-specific enzyme glutamine synthetase. The arrows between neuronal α-KG and glu do not indicate net synthesis but only isotope exchange. After release from astrocytes glutamine is accumulated in glutamatergic and GABAergic neurons (lower line of the glutamine–glutamate(GABA) cycle [Glu–gln cycle]), converted to glutamate (and in GABAergic cells onward to GABA) and released as transmitter. Released glutamate is almost quantitatively re-accumulated in the astrocytic cytosol. Part of the released GABA is also accumulated in astrocytes [upper line of the glutamine–glutamate (GABA) cycle], but its conversion to glutamate requires oxidative metabolism in astrocytes as described in the text. Here, 75–85% of accumulated glutamate is converted to glutamine and re-enters the glutamine–glutamate (GABA) cycle. The remaining 15–25% is oxidatively degraded after re-conversion via α-ketoglutarate to malate, exit of malate to the cytosol, decarboxylation to pyruvate by cytosolic malic enzyme and further pyruvate oxidation in the TCA cycle via ac.CoA. This part must in the long term be replaced by a quantitatively similar de novo production of glutamate from glucose as described above. Alternatively, malate may not exit the mitochondria but after formation of OAA and condensation with ac.CoA be used for re-synthesis of another molecule of glutamate. Under some conditions de novo synthesis of glutamate exceeds its oxidation, leading to an increase in tissue glutamate (e.g., Gibbs et al., 2007; Mangia et al., 2012). Moreover the 15–25% of total flux in the glutamine-glutamate (GABA) cycle, which is resynthesized and oxidized might change, if the equilibrium is disturbed between the activities of enzymes catalyzing the interconversion between glutamate and α-ketoglutarate (aspartate aminotransferase and/or glutamate dehydrogenase) and that catalyzing glutamate conversion to glutamine (glutamine synthetase). This could potentially also happen if the concentrations of the reactants are altered, e.g., as a result of a change in pyruvate carboxylase activity. There is no proof of such effects, but they can also not be excluded. Neuronal re-supply of GABA may be less dependent on the glutamate-glutamine (GABA) cycle than their supply of glutamate, as discussed in the text. (Hertz et al., 2013b).
The GABA component of the glutamine-glutamate (GABA) cycle accounts for ~20% of cycle flux from neurons to astrocytes (Patel et al., 2005; Duarte and Gruetter, 2013). GABA synthesis and metabolism to glutamine shows similarities and differences from production and degradation of glutamate. In contrast to direct astrocytic uptake of most neuronally released glutamate, astrocytically accumulated GABA must first be converted to glutamate in the TCA cycle as described below. This requires condensation of oxaloacetate (OAA) with acetyl Coenzyme A (ac.CoA; Figure 5) but no pyruvate carboxylation (Patel et al., 2005; Duarte and Gruetter, 2013; Lanz et al., 2013). The generated glutamate might be directly converted to GABA. Cultured GABAergic neurons show also a substantial uptake and re-utilization of released GABA (Schousboe et al., 2013). Both of these mechanisms may make GABA synthesis less dependent upon the classical glutamate-glutamine (GABA) cycle. However a recent in vivo study in which neuronal reuptake was inhibited showed minimal impact on the fraction of GABA synthesis from glutamine suggesting that direct reuptake may be significantly lower at least in the cerebral cortex (Patel et al., 2015). Further studies will be needed to fully understand the regional and activity dependance of direct neuronal reuptake vs. astrocyte uptake.
The conversion of GABA to glutamate is a complex process, in which GABA initially enters the astrocytic mitochondria, possibly in exchange with glutamate allowing exit of newly synthesized glutamate to the cytosol. It is then transaminated to succinic semialdehyde and oxidized to succinate, which enters the TCA cycle and via oxaloacetate (OAA) and citrate is converted to α-ketoglutarate, from which glutamate is generated by the same transamination that catalyzes the conversion of GABA to succinic semialdehyde. The participation of TCA cycle activity means that complete oxidation also could have occurred after exit of malate (Figure 5). However, Duarte and Gruetter (2013) found no increase in pyruvate carboxylation from previously reported values, indicating no major increase in anaplerosis/cataplerosis, compared to cycling related to glutamatergic signaling.
In the long term, rates of anaplerosis and cataplerosis must be identical (Lebon et al., 2002; Sonnewald, 2014), but this is not necessarly so in the short term, since brain glutamate content can transiently increase. This happens during specific phases of learning (Gibbs et al., 2007), although children with reading difficulties (Pugh et al., 2014) or with ADHD (Carrey et al., 2007) have an increased brain glutamate content. The latter findings might be related to hyperexcitability. In cases of epileptic seizure and even physiological visual stimulation there is an acute increase in brain glutamate (Peca et al., 2010; Mangia et al., 2012). The larger involvement of astrocytic metabolism during anaplerosis/cataplerosis provides increased possibilities for astrocytic regulation of the cycle. Nevertheless even simple astrocytic involvement in return of previously released transmitter provides a possibility for astrocytic regulation, because glutamine can traverse the astrocytic syncytium (Cruz et al., 2007). It cannot even be excluded that incoming glutamate from neuronal release might be re-directed to GABA-ergic neurons and vice-versa.
K+ Homeostasis
There is increasing evidence that increased extracellular K+ resulting from neuronal excitation is initially accumulated in astrocytes by the astrocytic Na+, K+-ATPase, which in contrast to the neuronal enzyme is stimulated by above-normal extracellular K+ concentrations (reviewed by Hertz et al., 2014b). It is subsequently released via Kir4.1 channels, probably after transport through the astrocytic syncytium, distributing the amount released over a larger area and thus preventing excessive local extracellular K+ increase. This allows secondary uptake by the neuronal Na+, K+-ATPase, preventing neuronal K+ depletion. This process is strikingly reminiscent of how astrocytes handle released neuronal glutamate. However in neither case is it known which advantages the energetically costly double uptake provides for the brain.
Chronic Effects of Fluoxetine
Objectives and Methodologies
The several weeks delay between SSRI administration and improvement in depressive symptoms show that chronic effects are the most clinically relevant (Nierenberg et al., 2000). The long life span of astrocyte cultures allows chronic studies of both functional properties and gene expression and editing. In the studies described below all effects on gene expression were confirmed in isolated neuronal and astrocytic cell fractions (Lovatt et al., 2007) prepared from chronically fluoxetine-treated animals as described in the legend of Figure 1. Our own observations using these methodologies will be supplemented with information from studies by other authors, which provide no information about cellular location(s). While the absence of SERT in cultured astrocytes excludes that the gene effects could be secondary to SERT inhibition, those shown in neurons in intact animals could be SERT-related.
The effects of SSRIs on gene expression and editing in both neurons and astrocytes from fluoxetine-treated animals (10 mg fluoxetine hydrochloride/kg per day for 14 days).are summarized in Table 1. The expression and editing changes in both neurons and astrocytes are present in whole brain as also shown in the Table. Many of the genes studied are relevant for major depression. This includes a key role for glutamate in major depression and its treatment (Barbon et al., 2006; Sanacora et al., 2007, 2012; Chowdhury et al., 2008, 2012; Banasr et al., 2010; Hertz et al., 2012; Li et al., 2012; Sanacora and Banasr, 2013; Niciu et al., 2014). Furthemore some oppositely-directed gene changes have been shown in an animal model of depression, indicating their therapeutic relevance (Li et al., 2012; Peng et al., 2014).
Effects on Glutamate, GABA and Energy Metabolism
Patients suffering from major depression show evidence of increased glutamatergic activity (Mitani et al., 2006; Hashimoto et al., 2007; Kanner, 2014) and decreased GABA-ergic activity (Bajbouj et al., 2006; Kanner, 2014) as well as of cortical GABA levels (Sanacora et al., 2004). Successful therapy of major depression lowers cortical glutamate in brain (Abdallah et al., 2014a) and raises GABA (Sanacora et al., 2002; Bhagwagar et al., 2004).
Glutamine synthetase is down-regulated in brains from depressed patients (Rajkowska and Stockmeier, 2013). This is consistent with the increased amino acid neurotransmitter (glutamate and GABA) cycling between astrocytes and neurons after antidepressant treatment with ketamine or riluzole (Chowdhury et al., 2008, 2012), Moreover, inhibition of glutamine synthetase or of the transporter mediating glutamine uptake into neurons causes depression-like symptoms in animal models (Lee et al., 2013).
In a recent 13C MRS study no significant difference in cycling flux, determined from glutamate and glutamine 13C labeling from [1-13C]glucose, (0.19 ± 0.05 (SD) vs. 0.18 ± 0.04 (SD) μmol/g per min) was found between healthy and depressed individuals (Abdallah et al., 2014a). The rate of GABA synthesis was also unaltered. However, inhibition of glutamine synthase or glutamate uptake does not necessarily decrease glutamate flux to glutamine. The flux can still be maintained if glutamate release is not inhibited but at the cost of higher extracellular and glial glutamate levels, as has been seen when glial glutamate uptake or glutamine synthase is inhibited in animal models (Rothstein et al., 1996; Eid et al., 2012). The elevated glutamate would be expected to alter synaptic function. Furthermore it could lead to a change in the ratio of released glutamate that is oxidized in astrocytes vs. glutamate that is directly converted to glutamine.
In patients suffering from depression, brain glucose metabolism is reduced (Little et al., 1996, 2005; Videbech, 2000; Rasgon et al., 2008; Abdallah et al., 2014a) in parallel with the severity of the illness (Kimbrell et al., 2002). Normalization occurs following SSRI treatment (Buchsbaum et al., 1997; Mayberg et al., 2000; Kennedy et al., 2001). Anti-depressant doses of ketamine increase oxidative metabolism in neuronal and glial cells in the brain in vivo (Chowdhury et al., 2012). Consistent with this therapeutic effect Abdallah et al. (2014a) found a large (one quarter) decrease in the rate of glucose oxidation by glutamatergic neurons in depressed patients.
On account of the linear relationship between neuronal activity and neuronal oxidative demand beyond isoelectricity, the metabolic decrease in glutamatergic neurons suggests a drastic reduction of neuronal activity. An increased cycling after treatment with riluzole or ketamine might remedy this deficiency regardless whether or not decreased glutamate cycling is a key component of the pathophysiology of major depression. Increased energy metabolism in glutamatergic neurons is required not only for release of neurotransmitter but probably even more for effects exerted via their glutamate receptors.
In conclusion, in spite of convincing evidence for alterations in glutamine-glutamate (GABA) fluxes and associated energetics in animal models of major depression and its treatment there are still unanswered questions in human depression. This is perhaps partly because the cycle is of key importance for both glutamatergic and GABAergic signaling, and partly because there are many different glutamate receptors. Effects of chronic fluoxetine treatment of mice on glutamate and GABA receptors in whole brain, astrocytes and or neurons might provide further clues.
Glutamate and GABA Receptor and Transporter Genes
Fluoxetine effects on up-regulation and/or editing of genes of several glutamate receptors are shown in Table 1. Receptor up-regulation might potentially correct the abnormal ratio between energetics and the glutamine-glutamate (GABA) cycle observed by Abdallah et al. (2014a), whereas the effects of editing may vary between receptors and editing sites. Editing requires ADARs, a family of adenosine deaminases, which catalyze deamination of adenosine to inosine in mRNAs. This changes the amino acids in the translated protein sequence, since inosine is read as guanosine (Bass, 2002). ADAR 2 is upregulated in astrocytes but not in neurons in mice treated with fluoxetine (Table 1). GluK2 is up-regulated and edited in astrocytes at all its 3 editing sites (Li et al., 2011a, 2012), and GluK4 is upregulated in neurons (Li et al., 2012). The Gluk2 editing may be the reason why a normal increase in [Ca2+]i in cultured astrocytes in response to 100 μM glutamate is abolished by fluoxetine treatment (Li et al., 2011a). The human GluK2c splice variant in brain is mainly expressed in non-neuronal cells (Barbon et al., 2008). Mice with GluK2 receptor knock-out, exhibit less anxious or more risk-taking type behavior and less manifestation of despair (Shaltiel et al., 2008).
Obsessive-compulsive disorder (OCD) is genetically linked to abnormalities in the GluK2 gene, Grik2 (Delorme et al., 2004; Sampaio et al., 2011). The neuronal up-regulation of GluK4 by fluoxetine may appear paradoxical since genetic ablation of this receptor subunit causes anxiolytic and antidepressant-like behavior in mice (Catches et al., 2012). However, there is a risk of elevated suicidality during initiation of antidepressant therapy (Fava and Rosenbaum, 1991; Kraus et al., 2010; Trivedi et al., 2011; Singh et al., 2013), which might be related to this up-regulation.
The GluA2 gene is expressed in both neurons and astrocytes (Cahoy et al., 2008), and it is up-regulated in whole brain by fluoxetine treatment (Ampuero et al., 2010; Vialou et al., 2010; Barbon et al., 2011). Up-regulation in neuronal dendrites leads to structural plasticity (Rubio et al., 2013). Mice susceptible to chronic social defeat show a significant decrease in GluA2 levels, while resilient mice showed increased GluA2 levels (Vialou et al., 2010). GluA1 (Barbon et al., 2006; Ampuero et al., 2010) and GluA4 (Barbon et al., 2011) are also upregulated in brains of fluoxetine-treated animals, but GluA3 is less affected (Barbon et al., 2006). In contrast to the lack of fluoxetine effect on mGlu5 in both astrocytes and neurons (Table 1) and of an unaltered mGluR2 and mGluR3 expression in whole brain in major depression (Muguruza et al., 2014), mGluR7 is up-regulated in whole brain after fluoxetine treatment (Ampuero et al., 2010; O’Connor et al., 2013). Very little information is available about possible fluoxetine effect on NMDA receptors. The GABA-synthesizing enzyme glutamic acid decarboxylase (GAD) is upregulated in hippocampus but not in prefontal cortex by 2 weeks of fluoxetine treatment (Guirado et al., 2012). A hippocampal up-regulation of the GABAB receptor has also been reported (Sands et al., 2004). A decrease in cerebrospinal fluid of patients with depression of the neurosteroid 3α-hydroxy 5α-pregnan-20-one (allopregnanolone), which enhances GABA action at GABAA receptors, is corrected by fluoxetine treatment (Pinna et al., 2009). The likely reason for this is that fluoxetine, sertraline, and paroxetine acutely cause a large decrease of the Km for conversion of 5α-dihydroprogesterone to allopregnanolone by human 3α-HSD type III (Griffin and Mellon, 1999). In turn, 5α-dihydroprogesterone is formed from progesterone (and some other substrates) by the rate-limiting enzyme 5α-reductase. In cerebellum this enzyme is mainly expressed in astrocytes and oligodendrocytes (Kiyokage et al., 2014). However, in frontal cortex, where allopregnanolone is down-regulated in depressed patients, it was reported to be synthesized in glutamatergic neurons (Agis-Balboa et al., 2014). A decrease of corticolimbic allopregnanolone content induced in mice by social isolation as well as the induced behavioral changes are sterospecifically normalized by fluoxetine by a mechanism independent from 5-HT reuptake inhibition (Pinna et al., 2009). Thus, fluoxetine facilitates GABAA receptor neurotransmission and effectively ameliorates depression by stimulating brain steroidogenesis in a SERT-independent manner.
Phospholipase Genes
A polymorphism in the calcium-dependent phospholipase 2 (cPLA2) gene is connected with increased risk for major depression (Pae et al., 2004). This enzyme is strongly expressed in astrocytes (Lautens et al., 1998; Balboa and Balsinde, 2002; Sun et al., 2004). In cultured astrocytes cPLA2 causes transactivation of the EGF receptor and ERK1/2 phosphorylation (Xia and Zhu, 2011). Its activation releases the unsaturated fatty acid arachidonic acid from membrane-bound phospholipids (Felder et al., 1990; Qu et al., 2003; Rapoport, 2008). In agreement with an up-regulation reported in whole brain (Rapoport, 2008), Li et al. (2009) showed a slow and selective up-regulation of mRNA and protein expression of cPLA2a, the major cPLA2 isoform, in cultured mouse astrocytes during chronic incubation with fluoxetine (Table 1). The up-regulation was abrogated by the 5-HT2B antagonist SB 204741, and by inhibitors of the fluoxetine signaling pathway shown in Figure 2. Up-regulation, specifically of cPLA2a was confirmed in freshly dissociated mouse astrocytes after 2 weeks fluoxetine treatment, whereas no effect was found in neurons (Li et al., 2012).
sPLA2 is upregulated by fluoxetine in neurons and whole brain, but not in astrocytes (Table 1). Some subtypes of sPLA2 also stimulate arachidonic acid release (Murakami et al., 1998). Exogenously applied sPLA2 causes an increase in neurotransmitter release from cultured hippocampal neurons (Wei et al., 2003).
One of the many effects of arachidonic acid is to stimulate glucose metabolism in cultured astrocytes (Yu et al., 1993). So does treatment with 10 μM fluoxetine for 24 h (Allaman et al., 2011), which might have sufficed to induce an increase in cPLA2, whereas acute exposure to fluoxetine has no corresponding effect (L. Peng and L. Hertz, unpublished experiments). Stimulation of glucose metabolism by arachidonic acid in vivo (Sublette et al., 2009) may be important in the treatment of depressive illness, as shown in Figure 6. Arachidonic acid in addition stimulates glycogenolysis (Sorg et al., 1995; Hertz et al., 2015) and seems to enhance signaling via glutamatergic receptors of the AMPA and mGluR subtypes (Schaeffer and Gattaz, 2008).
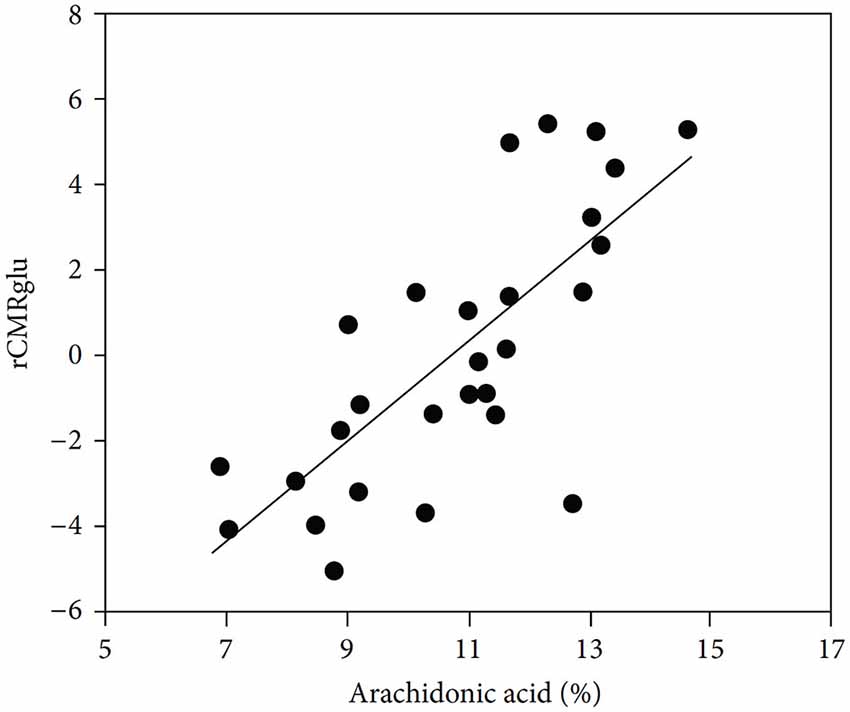
Figure 6. Correlation between cerebral metabolic rate of glucose metabolism and plasma arachidonic acid levels. Correlation between arachidonic acid as a percentage of total phospholipid polyunsaturated essential fatty acids and glucose utilization (rCMRglu) measured by fluoro-deoxyglucose PET scan in an area of right temporoparietal cortex that included precentral gyrus, superior temporal gyrus, and inferior parietal lobule in medication-free patients. (Sublette et al., 2009).
Brain glycogenolysis is activated by a multitude of transmitters (Xu et al., 2014; Hertz et al., 2015), Increased glycogenolysis has been found in paroxetine-treated mice (Webhofer et al., 2013) and in astrocyte cultures treated with 10 μM fluoxetine for more than 1 week, whereas shorter treatment led to a decrease (Kong et al., 2002). Astrocytic glutamate production depends on glycogenolysis (Gibbs et al., 2007). However, glycogenolysis has additional wide-reaching effects on neuroplastic changes, which are likely to be associated with chronic actions of SSRIs. Thus, Duran et al. (2013) found an absence of appropriate postsynaptic LTP responses to high-frequency stimulation in alert, behaving mice with a specific knock-out of brain glycogen synthase.
The acute effect of fluoxetine on GSK-3 via PI3K/AKT and the demonstration that the GSK-3 inhibitor lithium stimulates glycogen synthesis (Plenge, 1982) suggest that potential chronic effects of fluoxetine on glycogen formation might be very important for its mechanism of action. This conclusion is consistent with the suggestion by Li and Jope (2010) that GSK-3 is a central modulator of mood regulation. Recent studies by Gu and Peng showed a tripling of astrocytic glycogen content after 14 days of treatment with 1 μM fluoxetine, but a caveat is that with this concentration of fluoxetine the transient decrease in glycogenolysis described above (Kong et al., 2002) may not have subsided after 2 weeks. However, the findings by Plenge (1982) of acute effects of lithium strongly suggest that the stimulation of the PI3K/AKT pathway by chronic treatment with 10 μM fluoxetine does stimulate glycogen synthesis, and treatment of cultured astrocytes with 10 μM fluoxetine treatment for 14 days increased fluoxetine-induced AKT phosphorylation (Hertz et al., 2012). In contrast, chronic lithium treatment abolishes the increased incorporation of glucose into glycogen seen after acute exposure to lithium (Plenge, 1982). The importance of SSRI-induced effects on glycogen is consistent with its crucial involvement in astrocytic signaling (Gibbs et al., 2007; Obel et al., 2012; Hertz et al., 2013a, 2014b; Mangia et al., 2013; Xu et al., 2013; Webhofer et al., 2013).
The Calcium Channel Gene Cav1.2
[Ca2+]i is low in resting cells (~100 nM, compared to 1–2 mM in the extracellular fluid). Increases in [Ca2+]i are an essential component of virtually all astrocytic activities (e.g., formation of glutamate, glycogenolysis, and effects of many transmitters) (Gibbs et al., 2007; Hertz et al., 2015). [Ca2+]i increase is also required for transmitter-induced stimulation of several TCA-cycle enzymes and glutaminase (Halestrap, 1989; Denton and McCormack, 1990; Rutter et al., 1996) and for a direct stimulation of oxidative phosphorylation (Gaspers and Thomas, 2008) in many cell types. Such enzyme stimulations have been demonstrated in cultured astrocytes (Subbarao and Hertz, 1991; Huang and Hertz, 1995; Huang et al., 2000; Hertz et al., 2010). Ca2+ transport across the cell membrane is therefore of great importance for astrocytic functions. One route of entry is via L-channels opened by membrane depolarization (Li et al., 2011b; Du et al., 2014). The evoked [Ca2+]i rise is increased after chronic treatment with fluoxetine, because of up-regulation of the L-channel gene Cav1.2 (Du et al., 2014), shown both in cultured cells and in astrocytes freshly obtained from fluoxetine-treated mice. It is not up-regulated in neurons (Table 1). The fluoxetine-induced up-regulation counteracts a down-regulation of capacitative Ca2+ uptake via store-operated channels (Socs), found in astrocytes after treatment with fluoxetine (Li et al., 2011b). The antibipolar drug carbamazepine has a similar effect on Socs in both astrocytes (Yan et al., 2013) and neurons (unpublished), but it has no effect on Cav1.2. A similar neuronal down-regulation of Socs by fluoxetine would be advantageous by reducing the ability of stress-released neuropeptide corticotropin-releasing hormone (CRH) to decrease dendritic spine density in an NMDA- and Ca2+-dependent process (Andres et al., 2013). SSRI effects on [Ca2+]i is not limited to cultured cells, since citalopram or fluoxetine acutely induces astrocytic [Ca2+]i increase in mouse brain slices (Schipke et al., 2011).
5-HT Receptors Genes and SERT
Fluoxetine interactions with 5-HT receptors are age-dependent (Sarkar et al., 2014) and only effects on adult individuals will be discussed. In contrast to the 5-HT2B receptor’s up-regulation by 14 days of in vivo treatment with fluoxetine, the astrocytic 5-HT2A and 5-HT2C receptors are unaltered (Table 1). After 2 weeks fluoxetine treatment all editing sites in the 5-HT2B receptor become edited in astrocytes (Li et al., 2012). In cultured astrocytes up-regulation of the 5-HT2B receptor occurs slowly (Hertz et al., 2014a). Editing of the receptor is faster and abolishes the 5-HT-induced increase in IP3 turnover. This together with a similar up-regulation and editing of 5-HT2C receptors in neurons (Table 1) are consistent with an early suggestion that chronic SSRI treatment reduces 5-HT2C/5-HT2B receptor responsivity in rats (Lightowler et al., 1994).
In whole brain fluoxetine-induced editing of the HT2C receptor has repeatedly been described (Niswender et al., 2001; Gurevich et al., 2002; Englander et al., 2005). It requires PKC and ADAR activity (Schmauss et al., 2010). The fluoxetine-induced changes in editing are opposite to those seen in suicide victims (Gurevich et al., 2002). These findings might suggest that a reduced agonist activity after editing (see above) neutralizes a reported anxiogenic effect of the un-edited 5-HT2C receptor (Kennett et al., 1997; Vicente and Zangrossi, 2014). It is consistent with this conclusion that Vicente and Zangrossi (2014) found acute injection of the 5-HT2C receptor agonist MK-212 into the amygdala to have anxiogenic effect, and that chronic treatment with fluoxetine abolished the anxiety. However, there is also strong evidence that acute administration of specific agonists of 5-HT2C receptor are therapeutically effective in depression, obsessive compulsive disorder and anxiety (Jenck et al., 1998; Martin et al., 1998; Leysen, 1999; Cryan and Lucki, 2000; Rosenzweig-Lipson et al., 2007).
The expression of the inhibitory 5-HT1A autoreceptor, suggested to contribute to the slow manifestation of the therapeutic effect of SSRIs (Blier and De Montigny, 1983), was unaltered after fluoxetine treatment in both neurons and astrocytes (Peng et al., 2014) and whole brain (Le Poul et al., 2000; Johnson et al., 2009). This does not mean that its activity could not have decreased, since a fluoxetine-induced internalization of the receptor was shown by Descarries and Riad (2012) and a reduced 5-HT1A receptor expression was found in the raphe nuclei (Le Poul et al., 2000).
The cell culture finding that SERT is absent in astrocytes (Kong et al., 2002) was confirmed in freshly isolated astrocytes from the cerebral hemispheres, excluding raphe nuclei (Peng et al., 2014). This also applies to neurons, although a minor presence cannot be excluded (Table 1). Expression of the EGF receptor, involved in 5-HT2B receptor signaling (Figure 2), is not altered by chronic fluoxetine treatment in either astrocytes or neurons (Peng et al., 2014). However mRNA of the equilibrative nucleoside transporter ENT2 is increased in neurons and even more in astrocytes after fluoxetine treatment (Table 1), probably a reflection of the fluoxetine-induced increase in cell generation (Manev et al., 2003).
The 5-HT2B receptor is downregulated in mice becoming anhedonic after chronic stress (Li et al., 2012). No similar downregulation was found of the 5-HT2C receptor, but anhedonia is only one component of depressive symptomatology, and effects on the 5-HT2C receptor may mainly influence anxiety.
Conclusions
The importance of the 5-HT2B receptor in the mechanism of action of SSRIs has been demonstrated both in vivo (Diaz et al., 2012) and in cultured astrocytes (Li et al., 2008a). This paper elucidates its pathway, emphasizes astrocytic-neuronal interactions in brain function and shows identical effects of fluoxetine on astrocytic gene expression in our cultured astrocytes, expressing no SERT, and in drug treated animals. The latter study also found neuronal effects. The signaling pathway, studies by other authors and the observed gene effects point towards the importance in SSRI’s mechanism of action of glucose and glycogen metabolism, glutamate and GABA turnover and signaling, cPLA2 and sPLA2 activities, arachidonic acid, cellular Ca2+ regulation, and alterations in 5-HT2 receptor expression and editing. Many of the observed effects might be useful targets for drug development.
Contribution Statement
Professors Hertz and Rothman wrote and revised the manuscript. Professor Rothman is also an author of many of the studies referred to. Professor Peng approved and corrected the initially submitted manuscript, had designed the many experiments from her own group (partly with some input from Professor Hertz) and supported all research from her group. Dr. Li provided essential help to Professor Hertz by preparing the reference list and and quite complex Table 1. She also performed the majority of the experiments in Professor Peng’s group related to this review.
Conflict of Interest Statement
The authors declare that the research was conducted in the absence of any commercial or financial relationships that could be construed as a potential conflict of interest.
Acknowledgments
Drs. Ting Du and Li Gu are cordially thanked for their important studies which have been essential for the present review. This study was supported by Grant No. 31000479 from the National Natural Science Foundation of China.
References
Abdallah, C. G., Jiang, L., De Feyter, H. M., Fasula, M., Krystal, J. H., Rothman, D. L., et al. (2014a). Glutamate metabolism in major depressive disorder. Am. J. Psychiatry doi: 10.1176/appi.ajp.2014.14010067. [Epub ahead of print].
Pubmed Abstract | Pubmed Full Text | CrossRef Full Text | Google Scholar
Abdallah, C. G., Niciu, M. J., Fenton, L. R., Fasula, M. K., Jiang, L., Black, A., et al. (2014b). Decreased occipital cortical glutamate levels in response to successful cognitive-behavioral therapy and pharmacotherapy for major depressive disorder. Psychother. Psychosom. 83, 298–307. doi: 10.1159/000361078
Pubmed Abstract | Pubmed Full Text | CrossRef Full Text | Google Scholar
Agis-Balboa, R. C., Guidotti, A., and Pinna, G. (2014). 5α-reductase type I expression is downregulated in the prefrontal cortex/Brodmann’s area 9 (BA9) of depressed patients. Psychopharmacology (Berl) 231, 3569–3580. doi: 10.1007/s00213-014-3567-5
Pubmed Abstract | Pubmed Full Text | CrossRef Full Text | Google Scholar
Allaman, I., Fiumelli, H., Magistretti, P. J., and Martin, J. L. (2011). Fluoxetine regulates the expression of neurotrophic/growth factors and glucose metabolism in astrocytes. Psychopharmacology (Berl) 216, 75–84. doi: 10.1007/s00213-011-2190-y
Pubmed Abstract | Pubmed Full Text | CrossRef Full Text | Google Scholar
Ampuero, E., Rubio, F. J., Falcon, R., Sandoval, M., Diaz-Veliz, G., Gonzalez, R. E., et al. (2010). Chronic fluoxetine treatment induces structural plasticity and selective changes in glutamate receptor subunits in the rat cerebral cortex. Neuroscience 169, 98–108. doi: 10.1016/j.neuroscience.2010.04.035
Pubmed Abstract | Pubmed Full Text | CrossRef Full Text | Google Scholar
Andersen, J., Stuhr-Hansen, N., Zachariassen, L. G., Koldsø, H., Schiøtt, B., Strømgaard, K., et al. (2014). Molecular basis for selective serotonin reuptake inhibition by the antidepressant agent fluoxetine (Prozac). Mol. Pharmacol. 85, 703–714. doi: 10.1124/mol.113.091249
Pubmed Abstract | Pubmed Full Text | CrossRef Full Text | Google Scholar
Andres, A. L., Regev, L., Phi, L., Seese, R. R., Chen, Y., Gall, C. M., et al. (2013). NMDA receptor activation and calpain contribute to disruption of dendritic spines by the stress neuropeptide CRH. J. Neurosci. 33, 16945–16960. doi: 10.1523/JNEUROSCI.1445-13.2013
Pubmed Abstract | Pubmed Full Text | CrossRef Full Text | Google Scholar
Anthony, J. P., Sexton, T. J., and Neumaier, J. F. (2000). Antidepressant-induced regulation of 5-HT(1b) mRNA in rat dorsal raphe nucleus reverses rapidly after drug discontinuation. J. Neurosci. Res. 61, 82–87. doi: 10.1002/1097-4547(20000701)61:1<82::aid-jnr10>3.0.co;2-e
Pubmed Abstract | Pubmed Full Text | CrossRef Full Text | Google Scholar
Azmitia, E. C., Murphy, R. B., and Whitaker-Azmitia, P. M. (1990). MDMA (ecstasy) effects on cultured serotonergic neurons: evidence for Ca2(+)-dependent toxicity linked to release. Brain Res. 510, 97–103. doi: 10.1016/0006-8993(90)90732-q
Pubmed Abstract | Pubmed Full Text | CrossRef Full Text | Google Scholar
Azmitia, E. C., and Whitaker-Azmitia, P. M. (1991). Awakening the sleeping giant: anatomy and plasticity of the brain serotonergic system. J. Clin. Psychiatry 52(Suppl.), 4–16.
Baez, M., Kursar, J. D., Helton, L. A., Wainscott, D. B., and Nelson, D. L. (1995). Molecular biology of serotonin receptors. Obes. Res. 3, 441S–447S. doi: 10.1002/j.1550-8528.1995.tb00211.x
Bajbouj, M., Lisanby, S. H., Lang, U. E., Danker-Hopfe, H., Heuser, I., and Neu, P. (2006). Evidence for impaired cortical inhibition in patients with unipolar major depression. Biol. Psychiatry 59, 395–400. doi: 10.1016/j.biopsych.2005.07.036
Pubmed Abstract | Pubmed Full Text | CrossRef Full Text | Google Scholar
Balboa, M. A., and Balsinde, J. (2002). Involvement of calcium-independent phospholipase A2 in hydrogen peroxide-induced accumulation of free fatty acids in human U937 cells. J. Biol. Chem. 277, 40384–40389. doi: 10.1074/jbc.m206155200
Pubmed Abstract | Pubmed Full Text | CrossRef Full Text | Google Scholar
Banasr, M., Chowdhury, G. M., Terwilliger, R., Newton, S. S., Duman, R. S., Behar, K. L., et al. (2010). Glial pathology in an animal model of depression: reversal of stress-induced cellular, metabolic and behavioral deficits by the glutamate-modulating drug riluzole. Mol. Psychiatry 15, 501–511. doi: 10.1038/mp.2008.106
Pubmed Abstract | Pubmed Full Text | CrossRef Full Text | Google Scholar
Barbon, A., Caracciolo, L., Orlandi, C., Musazzi, L., Mallei, A., La Via, L., et al. (2011). Chronic antidepressant treatments induce a time-dependent up-regulation of AMPA receptor subunit protein levels. Neurochem. Int. 59, 896–905. doi: 10.1016/j.neuint.2011.07.013
Pubmed Abstract | Pubmed Full Text | CrossRef Full Text | Google Scholar
Barbon, A., Gervasoni, A., LaVia, L., Orlandi, C., Jaskolski, F., Perrais, D., et al. (2008). Human GluR6c, a functional splicing variants of GluR6, is mainly expressed in non-nervous cells. Neurosci. Lett. 434, 77–82. doi: 10.1016/j.neulet.2008.01.049
Pubmed Abstract | Pubmed Full Text | CrossRef Full Text | Google Scholar
Barbon, A., Popoli, M., La Via, L., Moraschi, S., Vallini, I., Tardito, D., et al. (2006). Regulation of editing and expression of glutamate alpha-amino-propionic-acid (AMPA)/kainate receptors by antidepressant drugs. Biol. Psychiatry 59, 713–720. doi: 10.1016/j.biopsych.2005.10.018
Pubmed Abstract | Pubmed Full Text | CrossRef Full Text | Google Scholar
Bass, B. L. (2002). RNA editing by adenosine deaminases that act on RNA. Annu. Rev. Biochem. 71, 817–846. doi: 10.1146/annurev.biochem.71.110601.135501
Pubmed Abstract | Pubmed Full Text | CrossRef Full Text | Google Scholar
Ben Achour, S., and Pascual, O. (2010). Glia: the many ways to modulate synaptic plasticity. Neurochem. Int. 57, 440–445. doi: 10.1016/j.neuint.2010.02.013
Pubmed Abstract | Pubmed Full Text | CrossRef Full Text | Google Scholar
Beurel, E., Mines, M. A., Song, L., and Jope, R. S. (2012). Glycogen synthase kinase-3 levels and phosphorylation undergo large fluctuations in mouse brain during development. Bipolar Disord. 14, 822–830. doi: 10.1111/bdi.12023
Pubmed Abstract | Pubmed Full Text | CrossRef Full Text | Google Scholar
Beurel, E., Song, L., and Jope, R. S. (2011). Inhibition of glycogen synthase kinase-3 is necessary for the rapid antidepressant effect of ketamine in mice. Mol. Psychiatry 16, 1068–1070. doi: 10.1038/mp.2011.47
Pubmed Abstract | Pubmed Full Text | CrossRef Full Text | Google Scholar
Bhagwagar, Z., Wylezinska, M., Taylor, M., Jezzard, P., Matthews, P. M., and Cowen, P. J. (2004). Increased brain GABA concentrations following acute administration of selective serotinonin reuptake inhibitor. Am. J. Psychiatry 161, 368–370. doi: 10.1176/appi.ajp.161.2.368
Pubmed Abstract | Pubmed Full Text | CrossRef Full Text | Google Scholar
Blier, P., and De Montigny, C. (1983). Electrophysiological investigations on the effect of repeated zimelidine administration on serotonergic neurotransmission in the rat. J. Neurosci. 3, 1270–1278.
Bonhaus, D. W., Bach, C., DeSouza, A., Salazar, F. H., Matsuoka, B. D., Zuppan, P., et al. (1995). The pharmacology and distribution of human 5-hydroxytryptamine2B (5-HT2B) receptor gene products: comparison with 5-HT2A and 5-HT2C receptors. Br. J. Pharmacol. 115, 622–628. doi: 10.1111/j.1476-5381.1995.tb14977.x
Pubmed Abstract | Pubmed Full Text | CrossRef Full Text | Google Scholar
Bradley, C. A., Peineau, S., Taghibiglou, C., Nicolas, C. S., Whitcomb, D. J., Bortolotto, Z. A., et al. (2012). A pivotal role of GSK-3 in synaptic plasticity. Front. Mol. Neurosci. 5:13. doi: 10.3389/fnmol.2012.00013
Pubmed Abstract | Pubmed Full Text | CrossRef Full Text | Google Scholar
Brennan, B. P., Hudson, J. I., Jensen, J. E., McCarthy, J., Roberts, J. L., Prescot, A. P., et al. (2010). Rapid enhancement of glutamatergic neurotransmission in bipolar depression following treatment with riluzole. Neuropsychopharmacology 35, 834–846. doi: 10.1038/npp.2009.191
Pubmed Abstract | Pubmed Full Text | CrossRef Full Text | Google Scholar
Buchsbaum, M. S., Wu, J., Siegel, B. V., Hackett, E., Trenary, M., Abel, L., et al. (1997). Effect of sertraline on regional metabolic rate in patients with affective disorder. Biol. Psychiatry 41, 15–22. doi: 10.1016/s0006-3223(96)00097-2
Pubmed Abstract | Pubmed Full Text | CrossRef Full Text | Google Scholar
Cahoy, J. D., Emery, B., Kaushal, A., Foo, L. C., Zamanian, J. L., Christopherson, K. S., et al. (2008). A transcriptome database for astrocytes, neurons and oligodendrocytes: a new resource for understanding brain development and function. J. Neurosci. 28, 264–278. doi: 10.1523/JNEUROSCI.4178-07.2008
Pubmed Abstract | Pubmed Full Text | CrossRef Full Text | Google Scholar
Carrey, N. J., MacMaster, F. P., Gaudet, L., and Schmidt, M. H. (2007). Striatal creatine and glutamate/glutamine in attention-deficit/hyperactivity disorder. J. Child Adolesc. Psychopharmacol. 17, 11–17. doi: 10.1089/cap.2006.0008
Pubmed Abstract | Pubmed Full Text | CrossRef Full Text | Google Scholar
Catches, J. S., Xu, J., and Contractor, A. (2012). Genetic ablation of the GluK4 kainate receptor subunit causes anxiolytic and antidepressant-like behavior in mice. Behav. Brain Res. 228, 406–414. doi: 10.1016/j.bbr.2011.12.026
Pubmed Abstract | Pubmed Full Text | CrossRef Full Text | Google Scholar
Chen, Y., Peng, L., Zhang, X., Stolzenburg, J. U., and Hertz, L. (1995). Further evidence that fluoxetine interacts with a 5-HT2C receptor in glial cells. Brain Res. Bull. 38, 153–159. doi: 10.1016/0361-9230(95)00082-p
Pubmed Abstract | Pubmed Full Text | CrossRef Full Text | Google Scholar
Choi, D. S., and Maroteaux, L. (1996). Immunohistochemical localisation of the serotonin 5-HT2B receptor in mouse gut, cardiovascular system and brain. FEBS. Lett. 391, 45–51. doi: 10.1016/0014-5793(96)00695-3
Pubmed Abstract | Pubmed Full Text | CrossRef Full Text | Google Scholar
Chowdhury, G. M., Banasr, M., de Graaf, R. A., Rothman, D. L., Behar, K. L., and Sanacora, G. (2008). Chronic riluzole treatment increases glucose metabolism in rat prefrontal cortex and hippocampus. J. Cereb. Blood Flow Metab. 28, 1892–1897. doi: 10.1038/jcbfm.2008.78
Pubmed Abstract | Pubmed Full Text | CrossRef Full Text | Google Scholar
Chowdhury, G. M., Behar, K. L., Cho, W., Thomas, M. A., Rothman, D. L., and Sanacora, G. (2012). 1H-[13C]-nuclear magnetic resonance spectroscopy measures of ketamine’s effect on amino acid neurotransmitter metabolism. Biol. Psychiatry 71, 1022–1025. doi: 10.1016/j.biopsych.2011.11.006
Pubmed Abstract | Pubmed Full Text | CrossRef Full Text | Google Scholar
Chowdhury, G. M., Jiang, L., Rothman, D. L., and Behar, K. L. (2014). The contribution of ketone bodies to basal and activity-dependent neuronal oxidation in vivo. J. Cereb. Blood Flow Metab. 34, 1233–1242. doi: 10.1038/jcbfm.2014.77
Pubmed Abstract | Pubmed Full Text | CrossRef Full Text | Google Scholar
Cohen, M. L., and Fludzinski, L. A. (1987). Contractile serotonergic receptor in rat stomach fundus. J. Pharmacol. Exp. Ther. 243, 264–269.
Cruz, N. F., Ball, K. K., and Dienel, G. A. (2007). Functional imaging of focal brain activation in conscious rats: impact of [(14)C]glucose metabolite spreading and release. J. Neurosci. Res. 85, 3254–3266. doi: 10.1002/jnr.22077
Pubmed Abstract | Pubmed Full Text | CrossRef Full Text | Google Scholar
Cryan, J. F., and Lucki, I. J. (2000). Antidepressant-like behavioral effects mediated by 5-Hydroxytryptamine(2C) receptors. Pharmacol. Exp. Ther. 295, 1120–1126.
Daub, H., Wallasch, C., Lankenau, A., Herrlich, A., and Ullrich, A. (1997). Signal characteristics of G protein-transactivated EGF receptor. EMBO J. 16, 7032–7044. doi: 10.1093/emboj/16.23.7032
Pubmed Abstract | Pubmed Full Text | CrossRef Full Text | Google Scholar
Deecher, D. C., Wilcox, B. D., Dave, V., Rossman, P. A., and Kimelberg, H. K. (1993). Detection of 5-hydroxytryptamine2 receptors by radioligand binding, northern blot analysis and Ca2+ responses in rat primary astrocyte cultures. J. Neurosci. Res. 35, 246–256. doi: 10.1002/jnr.490350304
Pubmed Abstract | Pubmed Full Text | CrossRef Full Text | Google Scholar
Delorme, R., Krebs, M. O., Chabane, N., Roy, I., Millet, B., Mouren-Simeoni, M. C., et al. (2004). Frequency and transmission of glutamate receptors GRIK2 and GRIK3 polymorphisms in patients with obsessive compulsive disorder. Neuroreport 15, 699–702. doi: 10.1097/00001756-200403220-00025
Pubmed Abstract | Pubmed Full Text | CrossRef Full Text | Google Scholar
Denton, R. M., and McCormack, J. G. (1990). Ca2+ as a second messenger within mitochondria of the heart and other tissues. Annu. Rev. Physiol. 52, 451–466. doi: 10.1146/annurev.physiol.52.1.451
Pubmed Abstract | Pubmed Full Text | CrossRef Full Text | Google Scholar
De Sarno, P., Li, X., and Jope, R. S. (2002). Regulation of Akt and glycogen synthase kinase-3 beta phosphorylation by sodium valproate and lithium. Neuropharmacology 43, 1158–1164. doi: 10.1016/s0028-3908(02)00215-0
Pubmed Abstract | Pubmed Full Text | CrossRef Full Text | Google Scholar
Descarries, L., and Riad, M. (2012). Effects of the antidepressant fluoxetine on the subcellular localization of 5-HT1A receptors and SERT. Philos. Trans. R. Soc. Lond. B Biol. Sci. 367, 2416–2425. doi: 10.1098/rstb.2011.0361
Pubmed Abstract | Pubmed Full Text | CrossRef Full Text | Google Scholar
Diaz, S. L., Doly, S., Narboux-Nême, N., Fernández, S., Mazot, P., Banas, S. M., et al. (2012). 5-HT(2B) receptors are required for serotonin-selective antidepressant actions. Mol. Psychiatry 17, 154–163. doi: 10.1038/mp.2011.159
Pubmed Abstract | Pubmed Full Text | CrossRef Full Text | Google Scholar
Du, T., Li, B., Liu, S., Zang, P., Prevot, V., Hertz, L., et al. (2009). ERK phosphorylation in intact, adult brain by alpha(2)-adrenergic transactivation of EGF receptors. Neurochem. Int. 55, 593–600. doi: 10.1016/j.neuint.2009.05.016
Pubmed Abstract | Pubmed Full Text | CrossRef Full Text | Google Scholar
Du, T., Liang, C., Li, B., Hertz, L., and Peng, L. (2014). Chronic fluoxetine administration increases expression of the L-channel gene Cav1.2 in astrocytes from the brain of treated mice and in culture and augments K(+)-induced increase in [Ca(2+)]i. Cell Calcium 55, 166–174. doi: 10.1016/j.ceca.2014.01.002
Pubmed Abstract | Pubmed Full Text | CrossRef Full Text | Google Scholar
Duarte, J. M., and Gruetter, R. (2013). Glutamatergic and GABAergic energy metabolism measured in the rat brain by (13) C NMR spectroscopy at 14.1 T. J. Neurochem. 126, 579–590. doi: 10.1111/jnc.12333
Pubmed Abstract | Pubmed Full Text | CrossRef Full Text | Google Scholar
Duran, J., Saez, I., Gruart, A., Guinovart, J. J., and Delgado-García, J. M. (2013). Impairment in long-term memory formation and learning-dependent synaptic plasticity in mice lacking glycogen synthase in the brain. J. Cereb. Blood Flow Metab. 33, 550–556. doi: 10.1038/jcbfm.2012.200
Pubmed Abstract | Pubmed Full Text | CrossRef Full Text | Google Scholar
Eid, T., Behar, K., Dhaher, R., Bumanglag, A. V., and Lee, T.-S. (2012). Roles of glutamine synthetase inhibition in epilepsy. Neurochem. Res. 37, 2339–2350. doi: 10.1007/s11064-012-0766-5
Pubmed Abstract | Pubmed Full Text | CrossRef Full Text | Google Scholar
Ellfolk, M., and Malm, H. (2010). Risks associated with in utero and lactation exposure to selective serotonin reuptake inhibitors (SSRIs). Reprod. Toxicol. 30, 249–260. doi: 10.1016/j.reprotox.2010.04.015
Pubmed Abstract | Pubmed Full Text | CrossRef Full Text | Google Scholar
Embi, N., Rylatt, D. B., and Cohen, P. (1980). Glycogen synthase kinase-3 from rabbit skeletal muscle. Separation from cyclic-AMP-dependent protein kinase and phosphorylase kinase. Eur. J. Biochem. 107, 519–527. doi: 10.1111/j.1432-1033.1980.tb06059.x
Pubmed Abstract | Pubmed Full Text | CrossRef Full Text | Google Scholar
Englander, M. T., Dulawa, S. C., Bhansali, P., and Schmauss, C. (2005). How stress and fluoxetine modulate serotonin 2C receptor pre-mRNA editing. J. Neurosci. 25, 648–651. doi: 10.1523/jneurosci.3895-04.2005
Pubmed Abstract | Pubmed Full Text | CrossRef Full Text | Google Scholar
Eom, T. Y., and Jope, R. S. (2009). Blocked inhibitory serine-phosphorylation of glycogen synthase kinase-3alpha/beta impairs in vivo neural precursor cell proliferation. Biol. Psychiatry 66, 494–502. doi: 10.1016/j.biopsych.2009.04.015
Pubmed Abstract | Pubmed Full Text | CrossRef Full Text | Google Scholar
Fang, C. H., Li, B. G., James, J. H., King, J. K., Evenson, A. R., Warden, G. D., et al. (2005). Protein breakdown in muscle from burned rats is blocked by insulin-like growth factor i and glycogen synthase kinase-3beta inhibitors. Endocrinology 146, 3141–3149. doi: 10.1210/en.2004-0869
Pubmed Abstract | Pubmed Full Text | CrossRef Full Text | Google Scholar
Fava, M., and Rosenbaum, J. F. (1991). Suicidality and fluoxetine: is there a relationship? J. Clin. Psychiatry 52, 108–111.
Felder, C. C., Kanterman, R. Y., Ma, A. L., and Axelrod, J. (1990). Serotonin stimulates phospholipase A2 and the release of arachidonic acid in hippocampal neurons by a type 2 serotonin receptor that is independent of inositolphospholipid hydrolysis. Proc. Natl. Acad. Sci. U S A 87, 2187–2191. doi: 10.1073/pnas.87.6.2187
Pubmed Abstract | Pubmed Full Text | CrossRef Full Text | Google Scholar
Freitas, A. E., Machado, D. G., Budni, J., Neis, V. B., Balen, G. O., Lopes, M. W., et al. (2013). Fluoxetine modulates hippocampal cell signaling pathways implicated in neuroplasticity in olfactory bulbectomized mice. Behav. Brain Res. 237, 176–184. doi: 10.1016/j.bbr.2012.09.035
Pubmed Abstract | Pubmed Full Text | CrossRef Full Text | Google Scholar
Gaspers, L. D., and Thomas, A. P. (2008). Calcium-dependent activation of mitochondrial metabolism in mammalian cells. Methods 46, 224–232. doi: 10.1016/j.ymeth.2008.09.012
Pubmed Abstract | Pubmed Full Text | CrossRef Full Text | Google Scholar
Ge, W. P., Miyawaki, A., Gage, F. H., Jan, Y. N., and Jan, L. Y. (2012). Local generation of glia is a major astrocyte source in postnatal cortex. Nature 484, 376–380. doi: 10.1038/nature10959
Pubmed Abstract | Pubmed Full Text | CrossRef Full Text | Google Scholar
Gibbs, M. E., and Hertz, L. (2014). Serotonin mediation of early memory formation via 5-HT2B receptor-induced glycogenolysis in the day-old chick. Front. Pharmacol. 5:54. doi: 10.3389/fphar.2014.00054
Pubmed Abstract | Pubmed Full Text | CrossRef Full Text | Google Scholar
Gibbs, M. E., and Hutchinson, D. S. (2012). Rapid turnover of glycogen in memory formation. Neurochem. Res. 37, 2456–2463. doi: 10.1007/s11064-012-0805-2
Pubmed Abstract | Pubmed Full Text | CrossRef Full Text | Google Scholar
Gibbs, M. E., Hutchinson, D., and Hertz, L. (2008). Astrocytic involvement in learning and memory consolidation. Neurosci. Biobehav. Rev. 32, 927–944. doi: 10.1016/j.neubiorev.2008.02.001
Pubmed Abstract | Pubmed Full Text | CrossRef Full Text | Google Scholar
Gibbs, M. E., Lloyd, H. G., Santa, T., and Hertz, L. (2007). Glycogen is a preferred glutamate precursor during learning in 1-day-old chick: biochemical and behavioral evidence. J. Neurosci. Res. 85, 3326–3333. doi: 10.1002/jnr.21307
Pubmed Abstract | Pubmed Full Text | CrossRef Full Text | Google Scholar
Gosselin, R. D., Gibney, S., O’Malley, D., Dinan, T. G., and Cryan, J. F. (2009). Region specific decrease in glial fibrillary acidic protein immunoreactivity in the brain of a rat model of depression. Neuroscience 159, 915–925. doi: 10.1016/j.neuroscience.2008.10.018
Pubmed Abstract | Pubmed Full Text | CrossRef Full Text | Google Scholar
Gould, T. D., Picchini, A. M., Einat, H., and Manji, H. K. (2006). Targeting glycogen synthase kinase-3 in the CNS: implications for the development of new treatments for mood disorders. Curr. Drug Targets 7, 1399–1409. doi: 10.2174/1389450110607011399
Pubmed Abstract | Pubmed Full Text | CrossRef Full Text | Google Scholar
Griffin, L. D., and Mellon, S. H. (1999). Selective serotonin reuptake inhibitors directly alter activity of neurosteroidogenic enzymes. Proc. Natl. Acad. Sci. U S A 96, 13512–13517. doi: 10.1073/pnas.96.23.13512
Pubmed Abstract | Pubmed Full Text | CrossRef Full Text | Google Scholar
Guirado, R., Sanchez-Matarredona, D., Varea, E., Crespo, C., Blasco-Ibáñez, J. M., and Nacher, J. (2012). Chronic fluoxetine treatment in middle-aged rats induces changes in the expression of plasticity-related molecules and in neurogenesis. BMC Neurosci. 13:5. doi: 10.1186/1471-2202-13-5
Pubmed Abstract | Pubmed Full Text | CrossRef Full Text | Google Scholar
Gurevich, I., Tamir, H., Arango, V., Dwork, A. J., Mann, J. J., and Schmauss, C. (2002). Altered editing of serotonin 2C receptor pre-mRNA in the prefrontal cortex of depressed suicide victims. Neuron 34, 349–356. doi: 10.1016/s0896-6273(02)00660-8
Pubmed Abstract | Pubmed Full Text | CrossRef Full Text | Google Scholar
Halestrap, A. P. (1989). The regulation of the matrix volume of mammalian mitochondria in vivo and in vitro and its role in the control of mitochondrial metabolism. Biochim. Biophys. Acta. 973, 355–382. doi: 10.1016/s0005-2728(89)80378-0
Pubmed Abstract | Pubmed Full Text | CrossRef Full Text | Google Scholar
Hashimoto, K., Sawa, A., and Iyo, M. (2007). Increased levels of glutamate in brains of patients with mood disorders. Biol. Psychiatry 25, 1310–1316. doi: 10.1016/j.biopsych.2007.03.017
Pubmed Abstract | Pubmed Full Text | CrossRef Full Text | Google Scholar
Hertz, L. (2011). Astrocytic energy metabolism and glutamate formation–relevance for 13C-NMR spectroscopy and importance of cytosolic/mitochondrial trafficking. Magn. Reson. Imaging 29, 1319–1329. doi: 10.1016/j.mri.2011.04.013
Pubmed Abstract | Pubmed Full Text | CrossRef Full Text | Google Scholar
Hertz, L. (2013). The Glutamate-Glutamine (GABA) cycle: importance of late postnatal development and potential reciprocal interactions between biosynthesis and degradation. Front. Endocrinol. (Lausanne) 4:59. doi: 10.3389/fendo.2013.00059
Pubmed Abstract | Pubmed Full Text | CrossRef Full Text | Google Scholar
Hertz, L., Baldwin, F., and Schousboe, A. (1979). Serotonin receptors on astrocytes in primary cultures: effects of methysergide and fluoxetine. Can. J. Physiol. Pharmacol. 57, 223–226. doi: 10.1139/y79-034
Pubmed Abstract | Pubmed Full Text | CrossRef Full Text | Google Scholar
Hertz, L., Gerkau, N. J., Xu, J., Durry, S., Song, D., Rose, C., et al. (2014b). Roles of astrocytic Na+,K+-ATPase and glycogenolysis for K+ homeostasis in mammalian brain. J. Neurosci. Res. doi: 10.1002/jnr.23499. [Epub ahead of print].
Hertz, L., Li, B., Song, D., Ren, J., Dong, L., Chen, Y., et al. (2012). Astrocytes as a 5-HT2B-mediated, SERT-independent SSRI target, slowly altering depression-associated genes and functions. Curr. Signal Transduct. Ther. 7, 65–80. doi: 10.2174/1574362799278154
Hertz, L., Lovatt, D., Goldman, S. A., and Nedergaard, M. (2010). Adrenoceptors in brain: cellular gene expression and effects on astrocytic metabolism and [Ca(2+)]i. Neurochem. Int. 57, 411–420. doi: 10.1016/j.neuint.2010.03.019
Pubmed Abstract | Pubmed Full Text | CrossRef Full Text | Google Scholar
Hertz, L., Song, D., Li, B., Du, T., Xu, J., Gu, L., et al. (2014a). Signal transduction in astrocytes during chronic or acute treatment with drugs (SSRIs; anti-bipolar drugs; GABA-ergic drugs; benzodiazepines) ameliorating mood disorders. J. Signal Transduct. 2014:593934. doi: 10.1155/2014/593934
Hertz, L., Xu, J., Song, D., Du, T., Li, B., Yan, E., et al. (2015). Astrocytic glycogenolysis: mechanisms and functions. Metab. Brain Dis. 30, 317–333. doi: 10.1007/s11011-014-9536-1
Pubmed Abstract | Pubmed Full Text | CrossRef Full Text | Google Scholar
Hertz, L., Xu, J., Song, D., Du, T., Yan, E., and Peng, L. (2013a). Brain glycogenolysis, adrenoceptors, pyruvate carboxylase, Na(+),K(+)-ATPase and Marie E. Gibbs’ pioneering learning studies. Front. Integr. Neurosci. 7:20. doi: 10.3389/fnint.2013.00020
Pubmed Abstract | Pubmed Full Text | CrossRef Full Text | Google Scholar
Hertz, L., Xu, J., Song, D., Yan, E., Gu, L., and Peng, L. (2013b). Astrocytic and neuronal accumulation of elevated extracellular K(+) with a 2/3 K(+)/Na(+) flux ratio-consequences for energy metabolism, osmolarity and higher brain function. Front. Comput. Neurosci. 7:114. doi: 10.3389/fncom.2013.00114
Pubmed Abstract | Pubmed Full Text | CrossRef Full Text | Google Scholar
Hertz, L., and Zielke, H. R. (2004). Astrocytic control of glutamatergic activity: astrocytes as stars of the show. Trends Neurosci. 27, 735–743. doi: 10.1016/j.tins.2004.10.008
Pubmed Abstract | Pubmed Full Text | CrossRef Full Text | Google Scholar
Huang, R., Chen, Y., Yu, A. C., and Hertz, L. (2000). Dexmedetomidine-induced stimulation of glutamine oxidation in astrocytes: a possible mechanism for its neuroprotective activity. J. Cereb. Blood Flow Metab. 20, 895–898. doi: 10.1097/00004647-200006000-00001
Pubmed Abstract | Pubmed Full Text | CrossRef Full Text | Google Scholar
Huang, R., and Hertz, L. (1995). Noradrenaline-induced stimulation of glutamine metabolism in primary cultures of astrocytes. J. Neurosci. Res. 41, 677–683. doi: 10.1002/jnr.490410514
Pubmed Abstract | Pubmed Full Text | CrossRef Full Text | Google Scholar
Hyder, F., and Rothman, D. L. (2012). Quantitative fMRI and oxidative neuroenergetics. Neuroimage 62, 985–994. doi: 10.1016/j.neuroimage.2012.04.027
Pubmed Abstract | Pubmed Full Text | CrossRef Full Text | Google Scholar
Jenck, F., Bös, M., Wichmann, J., Stadler, H., Martin, J. R., and Moreau, J. L. (1998). The role of 5-HT2C receptors in affective disorders. Expert Opin. Investig. Drugs 7, 1587–1599. doi: 10.1517/13543784.7.10.1587
Pubmed Abstract | Pubmed Full Text | CrossRef Full Text | Google Scholar
Johnson, D. A., Ingram, C. D., Grant, E. J., Craighead, M., and Gartside, S. E. (2009). Glucocorticoid receptor antagonism augments fluoxetine-induced downregulation of the 5-HT transporter. Neuropsychopharmacology 34, 399–409. doi: 10.1038/npp.2008.70
Pubmed Abstract | Pubmed Full Text | CrossRef Full Text | Google Scholar
Kanner, A. M. (2014). Is depression associated with an increased risk of treatment-resistant epilepsy? Research strategies to investigate this question. Epilepsy Behav. 38, 3–7. doi: 10.1016/j.yebeh.2014.06.027
Pubmed Abstract | Pubmed Full Text | CrossRef Full Text | Google Scholar
Kennedy, S. H., Evans, K. R., Krüger, S., Mayberg, H. S., Meyer, J. H., McCann, S., et al. (2001). Changes in regional brain glucose metabolism measured with positron emission tomography after paroxetine treatment of major depression. Am. J. Psychiatry 158, 899–905. doi: 10.1176/appi.ajp.158.987.899
Pubmed Abstract | Pubmed Full Text | CrossRef Full Text | Google Scholar
Kennett, G. A., Wood, M. D., Bright, F., Trail, B., Riley, G., Holland, V., et al. (1997). SB 242084, a selective and brain penetrant 5-HT2C receptor antagonist. Neuropharmacology 36, 609–620. doi: 10.1016/s0028-3908(97)00038-5
Pubmed Abstract | Pubmed Full Text | CrossRef Full Text | Google Scholar
Kimbrell, T. A., Ketter, T. A., George, M. S., Little, J. T., Benson, B. E., Willis, M. W., et al. (2002). Regional cerebral glucose utilization in patients with a range of severities of unipolar depression. Biol. Psychiatry 51, 237–252. doi: 10.1016/S0006-3223(01)01216-1
Pubmed Abstract | Pubmed Full Text | CrossRef Full Text | Google Scholar
Kiyokage, E., Toida, K., Suzuki-Yamamoto, T., and Ishimura, K. (2014). Cellular localization of 5α-reductase in the rat cerebellum. J. Chem. Neuroanat. 59–60, 8–16. doi: 10.1016/j.jchemneu.2014.04.002
Kong, E. K., Peng, L., Chen, Y., Yu, A. C. H., and Hertz, L. (2002). Up-regulation of 5-HT2B receptor density and receptor-mediated glycogenolysis in mouse astrocytes by long-term fluoxetine administration. Neurochem. Res. 27, 113–120. doi: 10.1023/A:1014862808126
Pubmed Abstract | Pubmed Full Text | CrossRef Full Text | Google Scholar
Kraus, J. E., Horrigan, J. P., Carpenter, D. J., Fong, R., Barrett, P. S., and Davies, J. T. (2010). Clinical features of patients with treatment-emergent suicidal behavior following initiation of paroxetine therapy. J. Affect. Disord. 120, 40–47. doi: 10.1016/j.jad.2009.04.004.
Pubmed Abstract | Pubmed Full Text | CrossRef Full Text | Google Scholar
Kursar, J. D., Nelson, D. L., Wainscott, D. B., and Baez, M. (1994). Molecular cloning, functional expression and mRNA tissue distribution of the human 5-hydroxytryptamine2B receptor. Mol. Pharmacol. 46, 227–234.
Langer, S. Z., Galzin, A. M., Lee, C. R., and Schoemaker, H. (1986). Antidepressant-binding sites in brain and platelets. Ciba Found. Symp. 123, 3–29.
Lanz, B., Gruetter, R., and Duarte, J. M. (2013). Metabolic flux and compartmentation analysis in the brain in vivo. Front. Endocrinol. (Lausanne) 4:156. doi: 10.3389/fendo.2013.00156
Pubmed Abstract | Pubmed Full Text | CrossRef Full Text | Google Scholar
Launay, J. M., Schneider, B., Loric, S., Da Prada, M., and Kellermann, O. (2006). Serotonin transport and serotonin transporter-mediated antidepressant recognition are controlled by 5-HT2B receptor signaling in serotonergic neuronal cells. FASEB J. 20, 1843–1854. doi: 10.1096/fj.06-5724com
Pubmed Abstract | Pubmed Full Text | CrossRef Full Text | Google Scholar
Lautens, L. L., Chiou, X. G., Sharp, J. D., Young, W. S. 3rd, Sprague, D. L., Ross, L. S., et al. (1998). Cytosolic phospholipase A2 (cPLA2) distribution in murine brain and functional studies indicate that cPLA2 does not participate in muscarinic receptor-mediated signaling in neurons. Brain Res. 809, 18–30. doi: 10.1016/s0006-8993(98)00806-3
Pubmed Abstract | Pubmed Full Text | CrossRef Full Text | Google Scholar
Lebon, V., Petersen, K. F., Cline, G. W., Shen, J., Mason, G. F., Dufour, S., et al. (2002). Astroglial contribution to brain energy metabolism in humans revealed by 13C nuclear magnetic resonance spectroscopy: elucidation of the dominant pathway for neurotransmitter glutamate repletion and measurement of astrocytic oxidative metabolism. J. Neurosci. 22, 1523–1531.
Lee, Y., Son, H., Kim, G., Kim, S., Lee, D. H., Roh, G. S., et al. (2013). Glutamine deficiency in the prefrontal cortex increases depressive-like behaviours in male mice. J. Psychiatry Neurosci. 38, 183–191. doi: 10.1503/jpn.120024
Pubmed Abstract | Pubmed Full Text | CrossRef Full Text | Google Scholar
Le Poul, E., Boni, C., Hanoun, N., Laporte, A. M., Laaris, N., Chauveau, J., et al. (2000). Differential adaptation of brain 5-HT1A and 5-HT1B receptors and 5-HT transporter in rats treated chronically with fluoxetine. Neuropharmacology 39, 110–122. doi: 10.1016/s0028-3908(99)00088-x
Pubmed Abstract | Pubmed Full Text | CrossRef Full Text | Google Scholar
Lesch, K. P., Aulakh, C. S., Wolozin, B. L., Tolliver, T. J., Hill, J. L., and Murphy, D. L. (1993). Regional brain expression of serotonin transporter mRNA and its regulation by reuptake inhibiting antidepressants. Brain Res. Mol. Brain Res. 17, 31–35. doi: 10.1016/0169-328x(93)90069-2
Pubmed Abstract | Pubmed Full Text | CrossRef Full Text | Google Scholar
Li, B., Dong, L., Fu, H., Wang, B., Hertz, L., and Peng, L. (2011b). Effects of chronic treatment with fluoxetine on receptor-stimulated increase of [Ca2+]i in astrocytes mimic those of acute inhibition of TRPC1 channel activity. Cell Calcium 50, 42–53. doi: 10.1016/j.ceca.2011.05.001
Pubmed Abstract | Pubmed Full Text | CrossRef Full Text | Google Scholar
Li, B., Dong, L., Wang, B., Cai, L., Jiang, N., and Peng, L. (2012). Cell type-specific gene expression and editing responses to chronic fluoxetine treatment in the in vivo mouse brain and their relevance for stress-induced anhedonia. Neurochem. Res. 37, 2480–2495. doi: 10.1007/s11064-012-0814-1
Pubmed Abstract | Pubmed Full Text | CrossRef Full Text | Google Scholar
Li, B., Du, T., Li, H., Gu, L., Zhang, H., Huang, J., et al. (2008b). Signalling pathways for transactivation by dexmedetomidine of epidermal growth factor receptors in astrocytes and its paracrine effect on neurons. Br. J. Pharmacol. 154, 191–203. doi: 10.1038/bjp.2008.58
Pubmed Abstract | Pubmed Full Text | CrossRef Full Text | Google Scholar
Li, B., Gu, L., Hertz, L., and Peng, L. (2013). Expression of nucleoside transporter in freshly isolated neurons and astrocytes from mouse brain. Neurochem. Res. 38, 2351–2358. doi: 10.1007/s11064-013-1146-5
Pubmed Abstract | Pubmed Full Text | CrossRef Full Text | Google Scholar
Li, X., and Jope, R. S. (2010). Is glycogen synthase kinase-3 α central modulator in mood regulation? Neuropsychopharmacology 35, 2143–2154. doi: 10.1038/npp.2010.105
Pubmed Abstract | Pubmed Full Text | CrossRef Full Text | Google Scholar
Li, B., Zhang, S., Li, M., Hertz, L., and Peng, L. (2009). Chronic treatment of astrocytes with therapeutically relevant fluoxetine concentrations enhances cPLA2 expression secondary to 5-HT2B-induced, transactivation-mediated ERK1/2 phosphorylation. Psychopharmacology (Berl) 207, 1–12. doi: 10.1007/s00213-009-1631-3
Pubmed Abstract | Pubmed Full Text | CrossRef Full Text | Google Scholar
Li, B., Zhang, S., Zhang, H., Hertz, L., and Peng, L. (2011a). Fluoxetine affects GluK2 editing, glutamate-evoked Ca(2+) influx and extracellular signal-regulated kinase phosphorylation in mouse astrocytes. J. Psychiatry Neurosci. 36, 322–338. doi: 10.1503/jpn.100094
Pubmed Abstract | Pubmed Full Text | CrossRef Full Text | Google Scholar
Li, B., Zhang, S., Zhang, H., Nu, W., Cai, L., Hertz, L., et al. (2008a). Fluoxetine-mediated 5-HT2B receptor stimulation in astrocytes causes EGF receptor transactivation and ERK phosphorylation. Psychopharmacology (Berl) 201, 443–458. doi: 10.1007/s00213-008-1306-5
Pubmed Abstract | Pubmed Full Text | CrossRef Full Text | Google Scholar
Li, X., Zhu, W., Roh, M. S., Friedman, A. B., Rosborough, K., and Jope, R. S. (2004). In vivo regulation of glycogen synthase kinase-3beta (GSK3beta) by serotonergic activity in mouse brain. Neuropsychopharmacology 29, 1426–1431. doi: 10.1038/sj.npp.1300439
Pubmed Abstract | Pubmed Full Text | CrossRef Full Text | Google Scholar
Lightowler, S., Kennett, G. A., Williamson, I. J., Blackburn, T. P., and Tulloch, I. F. (1994). Anxiolytic-like effect of paroxetine in a rat social interaction test. Pharmacol. Biochem. Behav. 49, 281–285. doi: 10.1016/0091-3057(94)90422-7
Pubmed Abstract | Pubmed Full Text | CrossRef Full Text | Google Scholar
Little, J. T., Ketter, T. A., Kimbrell, T. A., Danielson, A., Benson, B. E., Willis, M. W., et al. (1996). Venlafaxine or bupropion responders but not nonresponders show baseline prefrontal and paralimbic hypometabolism compared with controls. Psychopharmacol. Bull. 32, 629–635.
Little, J. T., Ketter, T. A., Kimbrell, T. A., Dunn, R. T., Benson, B. E., Willis, M. W., et al. (2005). Bupropion and venlafaxine responders differ in pretreatment regional cerebral metabolism in unipolar depression. Biol. Psychiatry 57, 220–228. doi: 10.1016/j.biopsych.2004.10.033
Pubmed Abstract | Pubmed Full Text | CrossRef Full Text | Google Scholar
Lovatt, D., Sonnewald, U., Waagepetersen, H. S., Schousboe, A., He, W., Lin, J. H., et al. (2007). The transcriptome and metabolic gene signature of protoplasmic astrocytes in the adult murine cortex. J. Neurosci. 27, 12255–12266. doi: 10.1523/jneurosci.3404-07.2007
Pubmed Abstract | Pubmed Full Text | CrossRef Full Text | Google Scholar
Manev, H., Uz, T., and Manev, R. (2003). Glia as a putative target for antidepressant treatments. J. Affect. Disord. 75, 59–64. doi: 10.1016/s0165-0327(02)00044-7
Pubmed Abstract | Pubmed Full Text | CrossRef Full Text | Google Scholar
Mangia, S., Giove, F., and Dinuzzo, M. (2012). Metabolic pathways and activity-dependent modulation of glutamate concentration in the human brain. Neurochem. Res. 37, 2554–2561. doi: 10.1007/s11064-012-0848-4
Pubmed Abstract | Pubmed Full Text | CrossRef Full Text | Google Scholar
Mangia, S., Giove, F., and Dinuzzo, M. (2013). K+ homeostasis in the brain: a new role for glycogenolysis. Neurochem. Res. 38, 470–471. doi: 10.1007/s11064-012-0962-3
Pubmed Abstract | Pubmed Full Text | CrossRef Full Text | Google Scholar
Martin, J. R., Bös, M., Jenck, F., Moreau, J., Mutel, V., Sleight, A. J., et al. (1998). 5-HT2C receptor agonists: pharmacological characteristics and therapeutic potential. J. Pharmacol. Exp. Ther. 286, 913–924.
Mayberg, H. S., Brannan, S. K., Tekell, J. L., Silva, J. A., Mahurin, R. K., McGinnis, S., et al. (2000). Regional metabolic effects of fluoxetine in major depression: serial changes and relationship to clinical response. Biol. Psychiatry 48, 830–843. doi: 10.1016/s0006-3223(00)01036-2
Pubmed Abstract | Pubmed Full Text | CrossRef Full Text | Google Scholar
Mercier, G., Lennon, A. M., Renouf, B., Dessouroux, A., Ramaugé, M., Courtin, F., et al. (2004). MAP kinase activation by fluoxetine and its relation to gene expression in cultured rat astrocytes. J. Mol. Neurosci. 24, 207–216. doi: 10.1385/jmn:24:2:207
Pubmed Abstract | Pubmed Full Text | CrossRef Full Text | Google Scholar
Meyer, J. H., Wilson, A. A., Sagrati, S., Hussey, D., Carella, A., Potter, W. Z., et al. (2004). Serotonin transporter occupancy of five selective serotonin reuptake inhibitors at different doses: an [11C]DASB positron emission tomography study. Am. J. Psychiatry 161, 826–835. doi: 10.1176/appi.ajp.161.5.826
Pubmed Abstract | Pubmed Full Text | CrossRef Full Text | Google Scholar
Mitani, H., Shirayama, Y., Yamada, T., Maeda, K., Ashby, C. R. Jr., and Kawahara, R. (2006). Correlation between plasma levels of glutamate, alanine and serine with severity of depression. Prog. Neuropsychopharmacol. Biol. Psychiatry 30, 1155–1158. doi: 10.1016/j.pnpbp.2006.03.036
Pubmed Abstract | Pubmed Full Text | CrossRef Full Text | Google Scholar
Muguruza, C., Miranda-Azpiazu, P., Díez-Alarcia, R., Morentin, B., González-Maeso, J., Callado, L. F., et al. (2014). Evaluation of 5-HT2A and mGlu2/3 receptors in postmortem prefrontal cortex of subjects with major depressive disorder: effect of antidepressant treatment. Neuropharmacology 86, 311–318. doi: 10.1016/j.neuropharm.2014.08.009
Pubmed Abstract | Pubmed Full Text | CrossRef Full Text | Google Scholar
Murakami, M., Shimbara, S., Kambe, T., Kuwata, H., Winstead, M. V., Tischfield, J. A., et al. (1998). The functions of five distinct mammalian phospholipase A2S in regulating arachidonic acid release. Type IIa and type V secretory phospholipase A2S are functionally redundant and act in concert with cytosolic phospholipase A2. J. Biol. Chem. 273, 14411–14423. doi: 10.1074/jbc.273.23.14411
Pubmed Abstract | Pubmed Full Text | CrossRef Full Text | Google Scholar
Niciu, M. J., Henter, I. D., Luckenbaugh, D. A., Zarate, C. A. Jr., and Charney, D. S. (2014). Glutamate receptor antagonists as fast-acting therapeutic alternatives for the treatment of depression: ketamine and other compounds. Annu. Rev. Pharmacol. Toxicol. 54, 119–139. doi: 10.1146/annurev-pharmtox-011613-135950
Pubmed Abstract | Pubmed Full Text | CrossRef Full Text | Google Scholar
Nierenberg, A. A., Farabaugh, A. H., Alpert, J. E., Gordon, J., Worthington, J. J., Rosenbaum, J. F., et al. (2000). Timing of onset of antidepressant response with fluoxetine treatment. Am. J. Psychiatry 157, 1423–1428. doi: 10.1176/appi.ajp.157.9.1423
Pubmed Abstract | Pubmed Full Text | CrossRef Full Text | Google Scholar
Nissen-Meyer, L. S., and Chaudhry, F. A. (2013). Protein Kinase C Phosphorylates the System N Glutamine Transporter SN1 (Slc38a3) and regulates its membrane trafficking and degradation. Front. Endocrinol. (Lausanne) 4:138. doi: 10.3389/fendo.2013.00138
Pubmed Abstract | Pubmed Full Text | CrossRef Full Text | Google Scholar
Niswender, C. M., Herrick-Davis, K., Dilley, G. E., Meltzer, H. Y., Overholser, J. C., Stockmeier, C. A., et al. (2001). RNA editing of the human serotonin 5-HT2C receptor. Alterations in suicide and implications for serotonergic pharmacotherapy. Neuropsychopharmacology 24, 478–491. doi: 10.1016/s0893-133x(00)00223-2
Pubmed Abstract | Pubmed Full Text | CrossRef Full Text | Google Scholar
Obel, L. F., Müller, M. S., Walls, A. B., Sickmann, H. M., Bak, L. K., Waagepetersen, H. S., et al. (2012). Brain glycogen-new perspectives on its metabolic function and regulation at the subcellular level. Front. Neuroenergetics 4:3. doi: 10.3389/fnene.2012.00003
Pubmed Abstract | Pubmed Full Text | CrossRef Full Text | Google Scholar
O’Connor, R. M., Pusceddu, M. M., Dinan, T. G., and Cryan, J. F. (2013). Impact of early-life stress, on group III mGlu receptor levels in the rat hippocampus: effects of ketamine, electroconvulsive shock therapy and fluoxetine treatment. Neuropharmacology 66, 236–241. doi: 10.1016/j.neuropharm.2012.05.006
Pubmed Abstract | Pubmed Full Text | CrossRef Full Text | Google Scholar
O’Leary, O. F., Dinan, T. G., and Cryan, J. F. (2014). Faster, better, stronger: towards new antidepressant therapeutic strategies. Eur. J. Pharmacol. doi: 10.1016/j.ejphar.2014.07.046. [Epub ahead of print].
Pubmed Abstract | Pubmed Full Text | CrossRef Full Text | Google Scholar
Oyagi, A., Moriguchi, S., Nitta, A., Murata, K., Oida, Y., Tsuruma, K., et al. (2011). Heparin-binding EGF-like growth factor is required for synaptic plasticity and memory formation. Brain Res. 1419, 97–104. doi: 10.1016/j.brainres.2011.09.003
Pubmed Abstract | Pubmed Full Text | CrossRef Full Text | Google Scholar
Pae, C. U., Yu, H. S., Kim, J. J., Lee, C. U., Lee, S. J., Lee, K. U., et al. (2004). BanI polymorphism of the cytosolic phospholipase A2 gene and mood disorders in the Korean population. Neuropsychobiology 49, 185–188. doi: 10.1159/000077364
Pubmed Abstract | Pubmed Full Text | CrossRef Full Text | Google Scholar
Palaiologos, G., Hertz, L., and Schousboe, A. (1989). Role of aspartate aminotransferase and mitochondrial dicarboxylate transport for release of endogenously and exogenously supplied neurotransmitter in glutamatergic neurons. Neurochem. Res. 14, 359–366. doi: 10.1007/bf01000039
Pubmed Abstract | Pubmed Full Text | CrossRef Full Text | Google Scholar
Patel, A. B., de Graaf, R. A., Mason, G. F., Rothman, D. L., Shulman, R. G., and Behar, K. L. (2005). The contribution of GABA to glutamate/glutamine cycling and energy metabolism in the rat cortex in vivo. Proc. Natl. Acad. Sci. U S A 102, 5588–5593. doi: 10.1073/pnas.0501703102
Pubmed Abstract | Pubmed Full Text | CrossRef Full Text | Google Scholar
Patel, A. B., de Graaf, R. A., Rothman, D. L., and Behar, K. L. (2015). Effects of GAT1 inhibtion by tiagabine on brain glutamate and GABA metabolism in the anesthetized rat in vivo. J. Neurosci. Res.
Peavy, R. D., Chang, M. S., Sanders-Bush, E., and Conn, P. J. (2001). Metabotropic glutamate receptor 5-induced phosphorylation of extracellular signal-regulated kinase in astrocytes depends on transactivation of the epidermal growth factor receptor. J. Neurosci. 21, 9619–9628.
Peca, S., Carnì, M., Di Bonaventura, C., Aprile, T., Hagberg, G. E., Giallonardo, A. T., et al. (2010). Metabolic correlatives of brain activity in a FOS epilepsy patient. NMR Biomed. 23, 170–178. doi: 10.1002/nbm.1439
Pubmed Abstract | Pubmed Full Text | CrossRef Full Text | Google Scholar
Peng, L. (2004). “Transactivation in astrocytes as a novel mechanism of neuroprotection,” in Non-neuronal Cells of the Nervous System: Function and Dysfunction, ed L. Hertz (Amsterdam: Elsevier), 503–518.
Peng, L., Gu, L., Li, B., and Hertz, L. (2014). Fluoxetine and all other SSRIs are 5-HT2B Agonists–Importance for their therapeutic effects. Curr. Neuropharmacol. 12, 365–379. doi: 10.2174/1570159x12666140828221720
Pubmed Abstract | Pubmed Full Text | CrossRef Full Text | Google Scholar
Pinna, G., Costa, E., and Guidotti, A. (2009). SSRIs act as selective brain steroidogenic stimulants (SBSSs) at low doses that are inactive on 5-HT reuptake. Curr. Opin. Pharmacol. 9, 24–30. doi: 10.1016/j.coph.2008.12.006
Pubmed Abstract | Pubmed Full Text | CrossRef Full Text | Google Scholar
Plenge, P. (1976). Acute lithium effects on rat brain glucose metabolism-in vivo. Int. Pharmacopsychiatry 11, 84–92.
Plenge, P. (1982). Lithium effects on rat brain glucose metabolism in vivo. Effects after administration of lithium by various routes. Psychopharmacology (Berl) 77, 348–355. doi: 10.1007/bf00432769
Pubmed Abstract | Pubmed Full Text | CrossRef Full Text | Google Scholar
Polter, A., Beurel, E., Yang, S., Garner, R., Song, L., Miller, C. A., et al. (2010). Deficiency in the inhibitory serine-phosphorylation of glycogen synthase kinase-3 increases sensitivity to mood disturbances. Neuropsychopharmacology 35, 1761–1774. doi: 10.1038/npp.2010.43
Pubmed Abstract | Pubmed Full Text | CrossRef Full Text | Google Scholar
Polter, A. M., Yang, S., Jope, R. S., and Li, X. (2012). Functional significance of glycogen synthase kinase-3 regulation by serotonin. Cell Signal. 24, 265–271. doi: 10.1016/j.cellsig.2011.09.009
Pubmed Abstract | Pubmed Full Text | CrossRef Full Text | Google Scholar
Popik, P. (1999). Preclinical pharmacology of citalopram. J. Clin. Psychopharmacol. 19, 4S–22S. doi: 10.1097/00004714-199910001-00002
Pubmed Abstract | Pubmed Full Text | CrossRef Full Text | Google Scholar
Porter, R. H., Benwell, K. R., Lamb, H., Malcolm, C. S., Allen, N. H., Revell, D. F., et al. (1999). Functional characterization of agonists at recombinant human 5-HT2A, 5-HT2B and 5-HT2C receptors in CHO-K1 cells. Br. J. Pharmacol. 128, 13–20. doi: 10.1038/sj.bjp.0702751
Pubmed Abstract | Pubmed Full Text | CrossRef Full Text | Google Scholar
Pugh, K. R., Frost, S. J., Rothman, D. L., Hoeft, F., Del Tufo, S. N., Mason, G. F., et al. (2014). Glutamate and choline levels predict individual differences in reading ability in emergent readers. J. Neurosci. 34, 4082–4089. doi: 10.1523/JNEUROSCI.3907-13.2014
Pubmed Abstract | Pubmed Full Text | CrossRef Full Text | Google Scholar
Qu, Y., Chang, L., Klaff, J., Seemann, R., and Rapoport, S. I. (2003). Imaging brain phospholipase A2-mediated signal transduction in response to acute fluoxetine administration in unanesthetized rats. Neuropsychopharmacology 28, 1219–1226. doi: 10.1038/sj.npp.1300177
Pubmed Abstract | Pubmed Full Text | CrossRef Full Text | Google Scholar
Rajkowska, G., and Stockmeier, C. A. (2013). Astrocyte pathology in major depressive disorder: insights from human postmortem brain tissue. Curr. Drug Targets 14, 1225–1236. doi: 10.2174/13894501113149990156
Pubmed Abstract | Pubmed Full Text | CrossRef Full Text | Google Scholar
Rao, J. S., Ertley, R. N., Lee, H. J., Rapoport, S. I., and Bazinet, R. P. (2006). Chronic fluoxetine upregulates activity, protein and mRNA levels of cytosolic phospholipase A2 in rat frontal cortex. Pharmacogenomics J. 6, 413–420. doi: 10.1038/sj.tpj.6500391
Pubmed Abstract | Pubmed Full Text | CrossRef Full Text | Google Scholar
Rapoport, S. I. (2008). Brain arachidonic and docosahexaenoic acid cascades are selectively altered by drugs, diet and disease. Prostaglandins Leukot. Essent. Fatty Acids 79, 153–156. doi: 10.1016/j.plefa.2008.09.010
Pubmed Abstract | Pubmed Full Text | CrossRef Full Text | Google Scholar
Rasgon, N. L., Kenna, H. A., Geist, C., Small, G., and Silverman, D. (2008). Cerebral metabolic patterns in untreated postmenopausal women with major depressive disorder. Psychiatry Res. 164, 77–80. doi: 10.1016/j.pscychresns.2007.12.006
Pubmed Abstract | Pubmed Full Text | CrossRef Full Text | Google Scholar
Rieckmann, N., Kronish, I. M., Shapiro, P. A., Whang, W., and Davidson, K. W. (2013). Serotonin reuptake inhibitor use, depression and long-term outcomes after an acute coronary syndrome: a prospective cohort study. JAMA Intern. Med. 173, 1150–1151. doi: 10.1001/jamainternmed.2013.910
Pubmed Abstract | Pubmed Full Text | CrossRef Full Text | Google Scholar
Rosenzweig-Lipson, S., Sabb, A., Stack, G., Mitchell, P., Lucki, I., Malberg, J. E., et al. (2007). Antidepressant-like effects of the novel, selective, 5-HT2C receptor agonist WAY-163909 in rodents. Psychopharmacology (Berl) 192, 159–170. doi: 10.1007/s00213-007-0710-6
Pubmed Abstract | Pubmed Full Text | CrossRef Full Text | Google Scholar
Roth, B. L., Willins, D. L., Kristiansen, K., and Kroeze, W. K. (1998). 5-Hydroxytryptamine2-family receptors (5-hydroxytryptamine2A, 5-hydroxytryptamine2B, 5-hydroxytryptamine2C): where structure meets function. Pharmacol. Ther. 79, 231–257. doi: 10.1016/s0163-7258(98)00019-9
Pubmed Abstract | Pubmed Full Text | CrossRef Full Text | Google Scholar
Rothstein, J. D., Dykes-Hoberg, M., Pardo, C. A., Bristol, L. A., Jin, L., Kunci, R. W., et al. (1996). Knockout of glutamate transporters reveals a major role for astroglial transport in excitotoxicity and clearance of glutamate. Neuron 16, 675–686. doi: 10.1016/s0896-6273(00)80086-0
Pubmed Abstract | Pubmed Full Text | CrossRef Full Text | Google Scholar
Rubio, F. J., Ampuero, E., Sandoval, R., Toledo, J., Pancetti, F., and Wyneken, U. (2013). Long-term fluoxetine treatment induces input-specific LTP and LTD impairment and structural plasticity in the CA1 hippocampal subfield. Front. Cell. Neurosci. 7:66. doi: 10.3389/fncel.2013.00066
Pubmed Abstract | Pubmed Full Text | CrossRef Full Text | Google Scholar
Rutter, G. A., Burnett, P., Rizzuto, R., Brini, M., Murgia, M., Pozzan, T., et al. (1996). Subcellular imaging of intramitochondrial Ca2+ with recombinant targeted aequorin: significance for the regulation of pyruvate dehydrogenase activity. Proc. Natl. Acad. Sci. U S A 93, 5489–5494. doi: 10.1073/pnas.93.11.5489
Pubmed Abstract | Pubmed Full Text | CrossRef Full Text | Google Scholar
Sampaio, A. S., Fagerness, J., Crane, J., Leboyer, M., Delorme, R., Pauls, D. L., et al. (2011). Association between polymorphisms in GRIK2 gene and obsessive-compulsive disorder: a family-based study. CNS Neurosci. Ther. 17, 141–147. doi: 10.1111/j.1755-5949.2009.00130.x
Pubmed Abstract | Pubmed Full Text | CrossRef Full Text | Google Scholar
Sanacora, G., and Banasr, M. (2013). From pathophysiology to novel antidepressant drugs: glial contributions to the pathology and treatment of mood disorders. Biol. Psychiatry 73, 1172–1179. doi: 10.1016/j.biopsych.2013.03.032
Pubmed Abstract | Pubmed Full Text | CrossRef Full Text | Google Scholar
Sanacora, G., Gueorguieva, R., Epperson, C. N., Wu, Y. T., Appel, M., Rothman, D. L., et al. (2004). Subtype-specific alterations of gamma-aminobutyric acid and glutamate in patients with major depression. Arch. Gen. Psychiatry 61, 705–713. doi: 10.1001/archpsyc.61.7.705
Pubmed Abstract | Pubmed Full Text | CrossRef Full Text | Google Scholar
Sanacora, G., Kendell, S. F., Levin, Y., Simen, A. A., Fenton, L. R., Coric, V., et al. (2007). Preliminary evidence of riluzole efficacy in antidepressant-treated patients with residual depressive symptoms. Biol. Psychiatry 61, 822–825. doi: 10.1016/j.biopsych.2006.08.037
Pubmed Abstract | Pubmed Full Text | CrossRef Full Text | Google Scholar
Sanacora, G., Mason, G. F., Rothman, D. L., and Krystal, J. H. (2002). Increased occipital cortex GABA concentrations in depressed patients after therapy with selective Serotonin Reuptake inhibitors. Am. J. Psychiatry 159, 663–665. doi: 10.1176/appi.ajp.159.4.663
Pubmed Abstract | Pubmed Full Text | CrossRef Full Text | Google Scholar
Sanacora, G., Treccani, G., and Popoli, M. (2012). Towards a glutamate hypothesis of depression an emerging frontier of neuropsychopharmacology for mood disorders. Neuropharmacology 62, 63–77. doi: 10.1016/j.neuropharm.2011.07.036
Pubmed Abstract | Pubmed Full Text | CrossRef Full Text | Google Scholar
Sands, S. A., Reisman, S. A., and Enna, S. J. (2004). Effect of antidepressants on GABA(B) receptor function and subunit expression in rat hippocampus. Biochem. Pharmacol. 68, 1489–1495. doi: 10.1016/j.bcp.2004.07.027
Pubmed Abstract | Pubmed Full Text | CrossRef Full Text | Google Scholar
Sarkar, A., Chachra, P., and Vaidya, V. A. (2014). Postnatal fluoxetine-evoked anxiety is prevented by Concomitant 5-HT(2A/C) receptor blockade and mimicked by postnatal 5-HT(2A/C) receptor stimulation. Biol. Psychiatry 76, 858–868. doi: 10.1016/j.biopsych.2013.11.005
Pubmed Abstract | Pubmed Full Text | CrossRef Full Text | Google Scholar
Schaeffer, E. L., and Gattaz, W. F. (2008). Cholinergic and glutamatergic alterations beginning at the early stages of Alzheimer disease: participation of the phospholipase A2 enzyme. Psychopharmacology (Berl) 198, 1–27. doi: 10.1007/s00213-008-1092-0
Pubmed Abstract | Pubmed Full Text | CrossRef Full Text | Google Scholar
Schipke, C. G., Heuser, I., and Peters, O. (2011). Antidepressants act on glial cells: SSRIs and serotonin elicit astrocyte calcium signaling in the mouse prefrontal cortex. J. Psychiatr. Res. 45, 242–248. doi: 10.1016/j.jpsychires.2010.06.005
Pubmed Abstract | Pubmed Full Text | CrossRef Full Text | Google Scholar
Schmauss, C., Zimnisky, R., Mehta, M., and Shapiro, L. P. (2010). The roles of phospholipase C activation and alternative ADAR1 and ADAR2 pre-mRNA splicing in modulating serotonin 2C-receptor editing in vivo. RNA 16, 1779–1785. doi: 10.1261/rna.2188110
Pubmed Abstract | Pubmed Full Text | CrossRef Full Text | Google Scholar
Schousboe, A. (1971). Development of potassium effects on ion concentrations and indicator spaces in rat brain-cortex slices during postnatal ontogenesis. Exp. Brain Res. 15, 521–531. doi: 10.1007/bf00236406
Pubmed Abstract | Pubmed Full Text | CrossRef Full Text | Google Scholar
Schousboe, A., Bak, L. K., and Waagepetersen, H. S. (2013). Astrocytic control of Biosynthesis and turnover of the Neurotransmitters Glutamate and GABA. Front. Endocrinol. (Lausanne) 4:102. doi: 10.3389/fendo.2013.00102
Pubmed Abstract | Pubmed Full Text | CrossRef Full Text | Google Scholar
Shaltiel, G., Maeng, S., Malkesman, O., Pearson, B., Schloesser, R. J., Tragon, T., et al. (2008). Evidence for the involvement of the kainate receptor subunit GluR6 (GRIK2) in mediating behavioral displays related to behavioral symptoms of mania. Mol. Psychiatry 13, 858–872. doi: 10.1038/mp.2008.20
Pubmed Abstract | Pubmed Full Text | CrossRef Full Text | Google Scholar
Shank, R. P., Bennett, G. S., Freytag, S. O., and Campbell, G. L. (1985). Pyruvate carboxylase: an astrocyte-specific enzyme implicated in the replenishment of amino acid neurotransmitter pools. Brain Res. 329, 364–367. doi: 10.1016/0006-8993(85)90552-9
Pubmed Abstract | Pubmed Full Text | CrossRef Full Text | Google Scholar
Sibson, N. R., Dhankhar, A., Mason, G. F., Rothman, D. L., Behar, K. L., and Shulman, R. G. (1998). Stoichiometric coupling of brain glucose metabolism and glutamatergic neuronal activity. Proc. Natl. Acad. Sci. U S A 95, 316–321. doi: 10.1073/pnas.95.1.316
Pubmed Abstract | Pubmed Full Text | CrossRef Full Text | Google Scholar
Singh, A. B., Bousman, C. A., Ng, C. H., and Berk, M. (2013). High impact child abuse may predict risk of elevated suicidality during antidepressant initiation. Aust. N Z J. Psychiatry 47, 1191–1195. doi: 10.1177/0004867413510212
Pubmed Abstract | Pubmed Full Text | CrossRef Full Text | Google Scholar
Sonnewald, U. (2014). Glutamate synthesis has to be matched by its degradation- where do all the carbons go? J. Neurochem. 131, 399–406. doi: 10.1111/jnc.12812
Pubmed Abstract | Pubmed Full Text | CrossRef Full Text | Google Scholar
Sorg, O., Pellerin, L., Stolz, M., Beggah, S., and Magistretti, P. J. (1995). Adenosine triphosphate and arachidonic acid stimulate glycogenolysis in primary cultures of mouse cerebral cortical astrocytes. Neurosci. Lett. 188, 109–112. doi: 10.1016/0304-3940(95)11410-x
Pubmed Abstract | Pubmed Full Text | CrossRef Full Text | Google Scholar
Subbarao, K. V., and Hertz, L. (1991). Stimulation of energy metabolism by alpha-adrenergic agonists in primary cultures of astrocytes. J. Neurosci. Res. 28, 399–405. doi: 10.1002/jnr.490280312
Pubmed Abstract | Pubmed Full Text | CrossRef Full Text | Google Scholar
Sublette, M. E., Milak, M. S., Hibbeln, J. R., Freed, P. J., Oquendo, M. A., Malone, K. M., et al. (2009). Plasma polyunsaturated fatty acids and regional cerebral glucose metabolism in major depression. Prostaglandins Leukot. Essent. Fatty Acids 80, 57–64. doi: 10.1016/j.plefa.2008.11.004
Pubmed Abstract | Pubmed Full Text | CrossRef Full Text | Google Scholar
Sun, G. Y., Xu, J., Jensen, M. D., and Simonyi, A. (2004). Phospholipase A2 in the central nervous system: implications for neurodegenerative diseases. J. Lipid. Res. 45, 205–213. doi: 10.1194/jlr.r300016-jlr200
Pubmed Abstract | Pubmed Full Text | CrossRef Full Text | Google Scholar
Trivedi, M. H., Wisniewski, S. R., Morris, D. W., Fava, M., Kurian, B. T., Gollan, J. K., et al. (2011). Concise associated symptoms tracking scale: a brief self-report and clinician rating of symptoms associated with suicidality. J. Clin. Psychiatry 72, 765–774. doi: 10.4088/jcp.11m06840
Pubmed Abstract | Pubmed Full Text | CrossRef Full Text | Google Scholar
Verkhratsky, A., Nedergaard, M., and Hertz, L. (2014). Why are astrocytes important? Neurochem. Res. doi: 10.1007/s11064-014-1403-2. [Epub ahead of print].
Pubmed Abstract | Pubmed Full Text | CrossRef Full Text | Google Scholar
Vialou, V., Robison, A. J., Laplant, Q. C., Covington, H. E. 3rd, Dietz, D. M., Ohnishi, Y. N., et al. (2010). DeltaFosB in brain reward circuits mediates resilience to stress and antidepressant responses. Nat. Neurosci. 13, 745–752. doi: 10.1038/nn.2551
Pubmed Abstract | Pubmed Full Text | CrossRef Full Text | Google Scholar
Vicente, M. A., and Zangrossi, H. Jr. (2014). Involvement of 5-HT2C and 5-HT1A receptors of the basolateral nucleus of the amygdala in the anxiolytic effect of chronic antidepressant treatment. Neuropharmacology 79, 127–135. doi: 10.1016/j.neuropharm.2013.11.007
Pubmed Abstract | Pubmed Full Text | CrossRef Full Text | Google Scholar
Videbech, P. (2000). PET measurements of brain glucose metabolism and blood flow in major depressive disorder: a critical review. Acta Psychiatr. Scand. 101, 11–20. doi: 10.1034/j.1600-0447.2000.101001011.x
Pubmed Abstract | Pubmed Full Text | CrossRef Full Text | Google Scholar
Webhofer, C., Gormanns, P., Reckow, S., Lebar, M., Maccarrone, G., Ludwig, T., et al. (2013). Proteomic and metabolomic profiling reveals time-dependent changes in hippocampal metabolism upon paroxetine treatment and biomarker candidates. J. Psychiatr. Res. 47, 289–298. doi: 10.1016/j.jpsychires.2012.11.003
Pubmed Abstract | Pubmed Full Text | CrossRef Full Text | Google Scholar
Wei, S., Ong, W. Y., Thwin, M. M., Fong, C. W., Farooqui, A. A., Gopalakrishnakone, P., et al. (2003). Group IIA secretory phospholipase A2 stimulates exocytosis and neurotransmitter release in pheochromocytoma-12 cells and cultured rat hippocampal neurons. Neuroscience 121, 891–898. doi: 10.1016/s0306-4522(03)00525-6
Pubmed Abstract | Pubmed Full Text | CrossRef Full Text | Google Scholar
Wong, D. T., and Bymaster, F. P. (1995). Development of antidepressant drugs. Fluoxetine (Prozac) and other selective serotonin uptake inhibitors. Adv. Exp. Med. Biol. 363, 77–95. doi: 10.1007/978-1-4615-1857-0_11
Pubmed Abstract | Pubmed Full Text | CrossRef Full Text | Google Scholar
Xia, M., and Zhu, Y. (2011). Signaling pathways of ATP-induced PGE2 release in spinal cord astrocytes are EGFR transactivation-dependent. Glia 59, 664–674. doi: 10.1002/glia.21138
Pubmed Abstract | Pubmed Full Text | CrossRef Full Text | Google Scholar
Xu, J., Song, D., Bai, Q., Cai, L., Hertz, L., and Peng, L. (2014). Basic mechanism leading to stimulation of glycogenolysis by isoproterenol, EGF, elevated extracellular K+ concentrations, or GABA. Neurochem. Res. 39, 661–667. doi: 10.1007/s11064-014-1244-z
Xu, J., Song, D., Xue, Z., Gu, L., Hertz, L., and Peng, L. (2013). Requirement of glycogenolysis for uptake of increased extracellular K+ in astrocytes: potential implications for K+ homeostasis and glycogen usage in brain. Neurochem. Res. 38, 472–485. doi: 10.1007/s11064-012-0938-3
Pubmed Abstract | Pubmed Full Text | CrossRef Full Text | Google Scholar
Yan, E., Li, B., Gu, L., Hertz, L., and Peng, L. (2013). Mechanisms for L-channel-mediated increase in [Ca(2+)](i) and its reduction by anti-bipolar drugs in cultured astrocytes combined with its mRNA expression in freshly isolated cells support the importance of astrocytic L-channels. Cell Calcium 54, 335–342. doi: 10.1016/j.ceca.2013.08.002
Pubmed Abstract | Pubmed Full Text | CrossRef Full Text | Google Scholar
Yu, A. C., Drejer, J., Hertz, L., and Schousboe, A. (1983). Pyruvate carboxylase activity in primary cultures of astrocytes and neurons. J. Neurochem. 41, 1484–1487. doi: 10.1111/j.1471-4159.1983.tb00849.x
Pubmed Abstract | Pubmed Full Text | CrossRef Full Text | Google Scholar
Yu, N., Martin, J. L., Stella, N., and Magistretti, P. J. (1993). Arachidonic acid stimulates glucose uptake in cerebral cortical astrocytes. Proc. Natl. Acad. Sci. U S A 90, 4042–4046. doi: 10.1073/pnas.90.9.4042
Pubmed Abstract | Pubmed Full Text | CrossRef Full Text | Google Scholar
Zhang, S., Li, B., Lovatt, D., Xu, J., Song, D., Goldman, S. A., et al. (2010). 5-HT2B receptors are expressed on astrocytes from brain and in culture and are a chronic target for all five conventional ‘serotonin-specific reuptake inhibitors’. Neuron Glia Biol. 6, 113–125. doi: 10.1017/s1740925x10000141
Pubmed Abstract | Pubmed Full Text | CrossRef Full Text | Google Scholar
Zhang, X., Peng, L., Chen, Y., and Hertz, L. (1993). Stimulation of glycogenolysis in astrocytes by fluoxetine, an antidepressant acting like 5-HT. Neuroreport 4, 1235–1238. doi: 10.1097/00001756-199309000-00006
Pubmed Abstract | Pubmed Full Text | CrossRef Full Text | Google Scholar
Zhou, Z., Zhen, J., Karpowich, N. K., Law, C. J., Reith, M. E., and Wang, D. N. (2009). Antidepressant specificity of serotonin transporter suggested by three LeuT-SSRI structures. Nat. Struct. Mol. Biol. 16, 652–657. doi: 10.1038/nsmb.1602
Pubmed Abstract | Pubmed Full Text | CrossRef Full Text | Google Scholar
Keywords: calcium homeostasis, fluoxetine signaling, 5-HT2 receptors, glucose metabolism, glutamate/GABA, glycogen, major depression, PLA2
Citation: Hertz L, Rothman DL, Li B and Peng L (2015) Chronic SSRI stimulation of astrocytic 5-HT2B receptors change multiple gene expressions/editings and metabolism of glutamate, glucose and glycogen: a potential paradigm shift. Front. Behav. Neurosci. 9:25. doi: 10.3389/fnbeh.2015.00025
Received: 28 October 2014; Accepted: 23 January 2015;
Published online: 20 February 2015.
Edited by:
Allan V. Kalueff, International Stress and Behavior Society (ISBS), USAReviewed by:
Douglas Fields, National Institute of Health, USACharles Patrick Gilman, Nazarbayev University, Kazakhstan
Copyright © 2015 Hertz, Rothman, Li and Peng. This is an open-access article distributed under the terms of the Creative Commons Attribution License (CC BY). The use, distribution and reproduction in other forums is permitted, provided the original author(s) or licensor are credited and that the original publication in this journal is cited, in accordance with accepted academic practice. No use, distribution or reproduction is permitted which does not comply with these terms.
*Correspondence: Liang Peng, Laboratory of Brain Metabolic Diseases, Institute of Metabolic Disease Research and Drug Development, China Medical University, No.77 Puhe Road, Shenyang North New Area, Shenyang, Liaoning Province 110122, China e-mail:aGtraWQwOEB5YWhvby5jb20=