- Department of Psychological and Brain Sciences, Texas A&M Institute for Neuroscience, Texas A&M University, College Station, TX, United States
The locus coeruleus norepinephrine (LC-NE) system plays a broad role in learning and memory. Here we begin with an overview of the LC-NE system. We then consider how both direct and indirect manipulations of the LC-NE system affect cued and contextual aversive learning and memory. We propose that NE dynamically modulates Pavlovian conditioning and extinction, either promoting or impairing learning aversive processes under different levels of behavioral arousal. We suggest that under high levels of stress (e.g., during/soon after fear conditioning) the locus coeruleus (LC) promotes cued fear learning by enhancing amygdala function while simultaneously blunting prefrontal function. Under low levels of arousal, the LC promotes PFC function to promote downstream inhibition of the amygdala and foster the extinction of cued fear. Thus, LC-NE action on the medial prefrontal cortex (mPFC) might be described by an inverted-U function such that it can either enhance or hinder learning depending on arousal states. In addition, LC-NE seems to be particularly important for the acquisition, consolidation and extinction of contextual fear memories. This may be due to dense adrenoceptor expression in the hippocampus (HPC) which encodes contextual information, and the ability of NE to regulate long-term potentiation (LTP). Moreover, recent work reveals that the diversity of LC-NE functions in aversive learning and memory are mediated by functionally heterogeneous populations of LC neurons that are defined by their projection targets. Hence, LC-NE function in learning and memory is determined by projection-specific neuromodulation that accompanies various states of behavioral arousal.
Introduction
The locus coeruleus norepinephrine (LC-NE) system has numerous functions including regulating the sleep-wake cycle, arousal, respiration, motivation, cognition, and learning and memory. In particular, NE plays a broad role in the formation and retrieval of emotional memories. As such, NE is a candidate molecule for the treatment of trauma- and stressor-related disorders and a number of studies suggest that the NE system may be dysregulated in posttraumatic stress disorder (PTSD; Yehuda et al., 1992; Bremner et al., 1996; Southwick et al., 1997, 1999a,b,c; Morilak et al., 2005; Arnsten, 2009, 2015; Rodrigues et al., 2009; Arnsten et al., 2015; Raio and Phelps, 2015; Giustino et al., 2016b; Kroes et al., 2016a).
Trauma- and stressor-related disorders, including PTSD, are pervasive in our society, making improved treatment options an important issue for researchers and clinicians alike. Although most individuals will experience or witness a traumatic event during their lifetime, only of portion of those will go on to develop such disorders. PTSD has a life time prevalence of approximately 10% in the general population (Kessler et al., 1995, 2005) and this number only increases for members of the military (Koenen et al., 2008; Pitman et al., 2012). Research has attempted to understand the underlying pathophysiology of these disorders with an aim toward empirically driven therapeutic approaches. However, most approaches, whether it is cognitive behavioral therapies (such as exposure therapy), pharmaceutical agents, or a combination of the two, fall short in treating these debilitating disorders (Tawa and Murphy, 2013). Despite the extensive research on this topic, the use of NE-altering drugs for PTSD treatment remains controversial.
In the laboratory, Pavlovian fear conditioning procedures are used to understand the neurobiology of aversive learning and memory. In a typical paradigm, a neutral cue (conditioned stimulus, CS, such as an auditory tone) is paired with an aversive unconditioned stimulus (US, such as a mild electric footshock). Rodents quickly and reliably learn the predictive nature of the CS and US; that is, the CS predicts the US and the CS-alone comes to elicit conditioned behavioral and autonomic fear responses (CRs) such as freezing behavior, increased heart rate, and respiration. Importantly, these CRs can be reduced by presentation of the CS in the absence of the US, a process termed extinction which results in a new CS-no US memory. In this review, we aim to dissect the existing literature on the role for LC-NE in mediating the effects of acute stress on Pavlovian fear conditioning and extinction in animals. We posit that aversive stimuli (“stressors”, such as footshock) increase arousal by driving LC-NE release in the forebrain. In the present review, we operationally define “arousal” as the heightened noradrenergic tone that follows an aversive event. We suggest that NE dynamically modulates Pavlovian conditioning and extinction, either promoting or impairing learning aversive processes under different levels of behavioral arousal.
The LC-NE System Anatomy and Physiology
The LC is a bilateral brainstem nucleus located adjacent to the fourth ventricle. While the LC is small in terms of cell count (~1500 in rats and ~15,000 in humans) it has been implicated in a range of behavioral phenomenon (Aston-Jones and Cohen, 2005; Arnsten, 2009, 2015; Robbins and Arnsten, 2009; Sara, 2009, 2015; Aston-Jones and Waterhouse, 2016). The LC was first described in detail by Dahlstrom and Fuxe in 1964 (Dahlström and Fuxe, 1964). This discovery led to a surge of interest into this small nucleus in the subsequent decades. Below we review the broad afferent and efferent connectivity of the LC and briefly describe the different receptor subtypes of the NE system.
Efferents
The LC projects broadly throughout the brain and is largely thought to be the sole source of cortical NE. Due to its vast projections, it is not surprising that NE has an important role in many aspects of behavior and cognition. LC neurons fire at low basal rates (~1–3 Hz) and can fire in two modes: phasic states of firing (i.e., bursts of activity) occur in response to relevant environmental stimuli whereas the LC fires tonically during periods of stress. Increased tonic firing rates are associated with less phasic activity (Aston-Jones et al., 1999; Aston-Jones and Cohen, 2005). How these distinct firing modes affect aversive learning and memory is not well characterized. The LC consists of at least two cell types with the smaller fusiform cells being found in more dorsal portions of the nucleus whereas larger multipolar cells tend to be located more ventrally (Swanson, 1976; Grzanna and Molliver, 1980). Because this review is largely focused on fear conditioning and extinction, we restrict our focus to projections to the medial prefrontal cortex (mPFC), the basolateral amygdala (BLA, encompassing all nuclei), the central amygdala (CeA) and the hippocampus (HPC) all of which are heavily innervated by the LC (Descarries and Lapierre, 1973; Lapierre et al., 1973; Pickel et al., 1974; Segal and Landis, 1974; Swanson and Hartman, 1975; Swanson, 1976; Amaral and Sinnamon, 1977; Descarries et al., 1977; Jones and Moore, 1977; Fallon et al., 1978; Gerfen and Clavier, 1979; Moore and Bloom, 1979; Morrison et al., 1979; Loughlin et al., 1982; Foote et al., 1983). Recent work has led to an increasingly complex view of LC function based on the discovery of target-specific subpopulations within this small nucleus. We will discuss the contribution of distinct LC efferents in more detail throughout the review as it relates to aversive learning and memory.
Homogeneous or Heterogeneous Output?
Upon the initial discovery of the LC it was determined that all neurons within this nucleus were noradrenergic (Dahlström and Fuxe, 1964). Further anatomical work on the extensive projections of the LC promoted the idea that this nucleus was largely homogenous, serving to distribute NE throughout the forebrain to coordinate global brain states. For example, several tracing studies describe collateralization of LC projections (Swanson and Hartman, 1975; Nagai et al., 1981; Room et al., 1981; Steindler, 1981; Jones and Yang, 1985). In addition, physiological evidence supported this idea–LC firing properties were found to be topographically homogeneous, phasic activity was synchronized amongst neurons, and local field potentials also displayed high synchronization (Aston-Jones and Bloom, 1981a; Ishimatsu and Williams, 1996). How could the LC-NE system dynamically modulate so many different aspects of behavior and cognition which depend on distinct brain regions/systems if its effects are global, rather than task and target specific?
Indeed, others have argued that the LC is comprised of distinct target-specific subpopulations. Several tracing studies have shown the LC consists of largely non-overlapping populations of neurons that can be defined based on their downstream target (Loughlin et al., 1982, 1986; Waterhouse et al., 1983; Chandler and Waterhouse, 2012; Agster et al., 2013; Chandler et al., 2013, 2014; Uematsu et al., 2015, 2017; Waterhouse and Chandler, 2016; Hirschberg et al., 2017). Waterhouse and colleagues have provided extensive anatomical and physiological evidence to suggest that the LC does not simply distribute NE equally to its many targets. For example, in a series of studies using retrograde tracers infused into different cortical regions, they have shown strong evidence for separate populations of cells within the LC (Waterhouse et al., 1983; Chandler and Waterhouse, 2012; Agster et al., 2013; Chandler et al., 2013, 2014; Waterhouse and Chandler, 2016). Moreover, they have recorded from these distinct LC populations and demonstrated that LC neurons projecting to the mPFC (compared to motor cortex) show different molecular properties that promote increased excitability. The cellular properties of target-specific LC populations may be related to the functional needs of their unique downstream targets (Chandler et al., 2014; Waterhouse and Chandler, 2016). A recent study has further confirmed the LC has highly divergent projections (i.e., it is not completely homogeneous) and suggests that small subpopulations may be selective for target regions, but propose that the LC may still serve to dictate brain-wide states (Schwarz and Luo, 2015; Schwarz et al., 2015). Moving forward, it will be important to examine the target specificity of the LC as well as how phasic vs. tonic firing in these discrete populations affect their downstream target to influence learning and memory.
Synaptic or Volume Transmission?
A second issue regarding how the LC influences both brain-wide states, as well as distinct target regions, revolves around the potential mechanism of NE transmission. How the LC releases NE has been an area of debate with two possible mechanisms receiving attention. Traditional synaptic release of NE being one possibility and the other being volume transmission, or nonsynaptic release. Some evidence suggests that LC terminals release NE at traditional synapses (Papadopoulos et al., 1987, 1989; Papadopoulos and Parnavelas, 1990). In contrast, others have proposed that LC-NE functions primarily via volume transmission (i.e., nonsynaptic or extrasynaptic release; Descarries and Lapierre, 1973; Lapierre et al., 1973; Descarries et al., 1977; Agster et al., 2013). It is likely that the LC-NE system supports both synaptic and nonsynaptic release and this may be area specific (Olschowka et al., 1981; Farb et al., 2010). It is possible that volume transmission preferentially influences brain-wide states/NE-tone whereas synaptic release is dependent upon local needs of specific target regions, though these ideas remain to be tested.
Afferents
Complementing its widespread projections throughout the forebrain, the LC receives dense reciprocal feedback from many of it’s targets. Indeed the LC is highly interconnected with the mPFC, BLA, CeA and HPC (Cedarbaum and Aghajanian, 1978; Arnsten and Goldman-Rakic, 1984; Aston-Jones et al., 1986; Sara and Hervé-Minvielle, 1995; Jodo et al., 1998; Van Bockstaele et al., 1998; Valentino and Van Bockstaele, 2008; Schwarz and Luo, 2015; Schwarz et al., 2015). The LC expresses several peptides including, but not limited to, vasopressin, somatostatin, neuropeptide y, enkephalin, neurotensin, corticotropin releasing hormone, galanin, glutamate, acetycholine and serotonin (Berridge and Waterhouse, 2003; Aston-Jones et al., 2004; Schwarz and Luo, 2015; Schwarz et al., 2015). These observations suggest that the LC is highly responsive to numerous transmitter and peptide systems and likely integrates information from several incoming sources. A recent study has shown that the LC receives direct projections from 111 brain regions (Schwarz et al., 2015). How the LC integrates this information is a subject of great interest. The LC has extensive dendritic arborization extending into the periocoerulear region which receives widespread non-NE synaptic contact (Shipley et al., 1996) and nearby GABAergic cells within this dendritic zone likely serve to regulate LC function (Aston-Jones et al., 2004). Understanding how the LC reciprocal network affects both LC signaling and target regions remains an important question.
Receptor Subtypes
NE exerts its function via action at three G-protein coupled receptor subtypes with the α2-adrenoceptors (ARs; A, B and C subtypes) having the highest affinity, followed by α1-ARs (A, B and D), and the lowest affinity β-ARs (1, 2 and 3; Berridge and Waterhouse, 2003; Ramos and Arnsten, 2007). The heterogeneous distribution, distinct subtypes, and differing affinities of each class of receptor provide yet another mechanism by which NE may exert target-specific effects. The α2-ARs are Gi-coupled leading to the inhibition of cAMP and thereby reducing neuronal excitability and primarily serve as presynaptic autoreceptors, although they are also expressed postsynaptically (MacDonald et al., 1997; Ramos et al., 2006; Ramos and Arnsten, 2007). Several studies have demonstrated strong expression patterns in the mPFC, HPC and amygdala using in situ hybridization (Zeng and Lynch, 1991; McCune et al., 1993; Nicholas et al., 1993b; Scheinin et al., 1994; Wang et al., 1996), radioligand binding (Unnerstall et al., 1984; Boyajian et al., 1987), and immunohistochemical techniques (Aoki et al., 1994; Rosin et al., 1996; Talley et al., 1996).
The α1-ARs are generally thought to be excitatory in nature and are Gq-coupled. Activation of these receptors acts via phospholipase C and phosphatidyl inositol intracellular signaling mechanisms, activating protein kinase C and subsequent release of intracellular calcium (Johnson and Minneman, 1985; Marshall et al., 1999; Birnbaum et al., 2004; Ramos and Arnsten, 2007). This class of ARs can also be found throughout the cortex, HPC, and amygdala (Young and Kuhar, 1980; Rainbow and Biegon, 1983; Jones et al., 1985; Palacios et al., 1987; McCune et al., 1993; Pieribone et al., 1994; Day et al., 1997; Domyancic and Morilak, 1997); however, α2-ARs tend to be more widespread than α1-ARs (McCune et al., 1993). This may serve as a mechanism for target regions to regulate NE action to reduce signaling by having densely expressed, high-affinity autoreceptors.
Lastly, the lowest-affinity β-ARs are Gs-coupled to adenylyl cyclase resulting in increased cAMP and enhanced cellular excitability (Ordway et al., 1987; Ferry et al., 1999a,b; Zhang H.-T. et al., 2005). β-ARs show high expression levels throughout the brain, particularly in the HPC, mPFC, and amygdala (Rainbow et al., 1984; Booze et al., 1993; Nicholas et al., 1993a; Summers et al., 1995; Milner et al., 2000). Interestingly, β-ARs are also expressed on astrocytes which may indirectly influence neural signaling (Milner et al., 2000). Signaling via α1- and β-ARs has been proposed to have opposing effects on the mPFC and BLA. High levels of NE may bias instinctive and reflexive responses mediated by NE action at α1- and β-ARs in the BLA and whereas activation of these receptors may impair mPFC function. This has important implications for aversive learning and memory (Arnsten, 2009, 2015; Arnsten et al., 2015).
Stress, the LC-NE System and the Fear Circuit
The LC responds to both appetitive and aversive stimuli (Aston-Jones and Bloom, 1981b; Sara and Segal, 1991; Aston-Jones et al., 1999; Bouret and Sara, 2004; Aston-Jones and Cohen, 2005; Ventura et al., 2008; Aston-Jones and Waterhouse, 2016), however the focus of this section will be to examine how NE affects key nodes in the fear circuit. Footshock serves as the US in the majority of Pavlovian fear conditioning experiments, and it is well document that footshock and other acute stressors increase LC activity (Thierry et al., 1968; Sara and Segal, 1991; Smith et al., 1992; Pezzone et al., 1993; Passerin et al., 2000; Sved et al., 2002; Chen and Sara, 2007; George et al., 2013; Uematsu et al., 2017). Below we discuss how LC activity and NE affects the fear circuit.
NE and the Amygdala
The BLA plays a crucial role in the formation and retrieval of fear conditioning and extinction memories (LeDoux, 2000; Maren, 2001, 2011; Maren and Quirk, 2004; Myers and Davis, 2007; Johansen et al., 2011; Herry and Johansen, 2014; Dejean et al., 2015). NE signaling in the amygdala appears to be critical for most aspects of Pavlovian fear conditioning and extinction (see below). Increased LC activity in response to acute stressors (including footshock) produces robust increases in amygdalar NE content (Galvez et al., 1996; Quirarte et al., 1998; McGaugh, 2000, 2004; Morilak et al., 2005; Ramos and Arnsten, 2007; Arnsten, 2009, 2015). How increased NE affects BLA signaling is therefore a fundamental question when studying emotional learning and memory. It has been proposed that heightened NE levels in the amygdala promote instinctive and reflexive responses to environmental stimuli (which would presumably bias responses for emotional events and memories); (Southwick et al., 1999a; Ramos and Arnsten, 2007; Arnsten, 2009, 2015; Arnsten et al., 2015). For example, one study found that footshock-induced increases in LC and BLA Fos were significantly reduced by LC inhibition prior to footshock (using the GABAA antagonist muscimol). Moreover, drugs that increase NE efflux (such as the α2 autoreceptor antagonist yohimbine) produce robust increases in BLA Fos expression (Singewald et al., 2003). This suggests that heightened noradrenergic activity in the BLA promotes excitability which would likely strengthen fear memories. However, a pair of studies has demonstrated that footshock, LC stimulation, or iontophoresis of NE (or NE-increasing drugs) into the BLA produced heterogeneous responses in BLA single-unit activity, although the BLA was generally suppressed in response to increased NE (Buffalari and Grace, 2007; Chen and Sara, 2007). This is perhaps counterintuitive if increased amygdalar excitability is associated with fear memory formation and recall, but NE did increase the spontaneous firing rate of a smaller subpopulation of BLA neurons (Buffalari and Grace, 2007). Interestingly, the suppression of BLA firing is dependent upon α2-AR signaling insofar as iontophoresis of clonidine mimicked the effects of NE and these inhibitory effects are potentiated with the systemic administration of propranolol (Buffalari and Grace, 2007). It is possible that the smaller population of BLA cells that showed excitation is sufficient for memory formation or that higher levels of NE (that engage the lower affinity receptors) would result in more excitation.
The central amygdala (CeA) is viewed as the output region of the amygdala that drives fear expression, although mounting data indicate it too plays a role in the acquisition of fear conditioning (Goosens and Maren, 2003; Yu et al., 2017). That said, CeA microcircuits and projections to downstream targets such as the periaqueductal gray are particularly important for generating freezing behavior (Ciocchi et al., 2010; Haubensak et al., 2010; Fadok et al., 2017). Importantly, the CeA is reciprocally connected with the LC (Van Bockstaele et al., 1998; Valentino and Van Bockstaele, 2008). Under stress, the CeA activates the LC via corticotropin-releasing hormone (Van Bockstaele et al., 1998; McCall et al., 2015; Prouty et al., 2017) which may act as a positive feedforward mechanism to maintain high levels of LC activity and NE transmission, particularly in the amygdala. This circuit could provide a way to generate sustained fear responses, particularly in the aftermath of conditioning.
NE and the Hippocampus
The HPC is critical for integrating and processing spatial information which is important in context fear conditioning among other types of learning and memory (Bouton et al., 2006; Maren et al., 2013; Jin and Maren, 2015; Chen et al., 2017; Hansen, 2017) and LC input to the HPC has been shown to impact learning about a novel context (Wagatsuma et al., 2018). Indeed, NE has a major influence on hippocampal function and LC stimulation, footshock, and other acute stressors increase hippocampal NE levels (Abercrombie et al., 1988; Hajós-Korcsok et al., 2003; Yavich et al., 2005). In addition, drugs that increase NE levels, such as yohimbine, amplify this effect whereas drugs that reduce NE levels, such as clonidine, blunt stress-induced hippocampal NE release (Abercrombie et al., 1988). Moreover, a number of studies suggest that NE enhances hippocampal long-term potentiation (LTP), particularly in the dentate gyrus and CA1, which is dependent upon both α1 and β-AR mechanisms (Bliss et al., 1983; Neuman and Harley, 1983; Lacaille and Harley, 1985; Stanton and Sarvey, 1985a,b, 1987; Hopkins and Johnston, 1988; Dahl and Sarvey, 1989; Segal et al., 1991; Dunwiddie et al., 1992; Katsuki et al., 1997; Chaulk and Harley, 1998; Izumi and Zorumski, 1999; Yang et al., 2002; Harley, 2007). For example, NE applied either directly to the dentate gyrus or applied to the perforant pathway increases excitatory postsynaptic potentials, decreases spike onset latency, and increases the population spike amplitude; these effects promote LTP induction and may be important for memory formation (Neuman and Harley, 1983; Lacaille and Harley, 1985; Harley, 2007). However, NE effects on LTP may depend on stimulation parameters and the areas being stimulated which suggests that NE can dynamically modulate HPC function (Dahl and Sarvey, 1989; Harley, 2007). In line with this idea, restraint stress and tail shock (which would presumably increase hippocampal NE) have been shown to impair hippocampal LTP (Foy et al., 1987). It may be that stress-induced increases in HPC-NE are beyond optimal levels and exceed the levels used in many of the recording studies showing that NE enhances HPC-LTP. Despite these possibilities, it appears NE generally enhances hippocampal synaptic efficacy which may function to enhance emotional learning and memory. Of course, this may be sensitive to the prevailing level of NE and the subregions being examined, allowing NE to bidirectionally modulate HPC function.
NE and the mPFC
The prelimbic (PL) and infralimbic (IL) subdivisions of the mPFC are thought to regulate the expression and suppression of fear, respectively (Quirk and Mueller, 2008; Knapska and Maren, 2009; Herry et al., 2010; Milad and Quirk, 2012; Dejean et al., 2015; Giustino and Maren, 2015). Several studies have examined the effects of NE and stress on prefrontal function. Footshock and other acute stressors increase NE levels in the mPFC (Korf et al., 1973; Gresch et al., 1994; Finlay et al., 1995; Hatfield et al., 1999; Ishizuka et al., 2000; Morilak et al., 2005; Girotti et al., 2017). Similar to other brain regions, NE effects on PFC function are highly dependent upon the prevailing level of NE and the task requirements. Lower levels of NE (engaging postsynaptic α2-ARs) appear to promote cortical function such as cognitive flexibility and working memory, whereas high levels impair prefrontal signaling via α1- and β-AR dependent mechanisms (Arnsten and Li, 2005; Ramos et al., 2006; Ramos and Arnsten, 2007; Arnsten, 2009, 2015). Interestingly, the PFC has more dopamine-β hydroxylase (DβH) varicosities relative to sensory cortical areas (Agster et al., 2013). This raises the possibility that prefrontal regions might be subjected to greater release of NE (via volume transmission in addition to synaptic transmission), which may help explain why the PFC is highly sensitive to stress. An important topic for future research will be to address differences in LC projections to PL and IL and how these affect fear expression.
NE and Fear Conditioning
As mentioned above the mPFC, HPC and BLA are a triad of interconnected brain regions with important roles in Pavlovian fear conditioning. Here we will review direct and indirect manipulations of LC-NE on both cued and context fear conditioning and the neural circuit mechanisms underlying these effects.
Cued Fear Conditioning
Several studies reveal that LC-NE plays an essential role in the acquisition of cued fear conditioning and this may be dependent upon projections to the BLA (Oei and King, 1980; Goldstein et al., 1996; Pitman and Delahanty, 2005; Liu et al., 2007; Tully et al., 2007; Rodrigues et al., 2009; Tully and Bolshakov, 2010; Johnson et al., 2011; Krugers et al., 2011; Pitman et al., 2012; Uematsu et al., 2017). This is likely due to stress-induced LC activation in response to the footshock US. However, a trained CS can also elicit increased LC activity. For example, early investigations demonstrated that c-fos mRNA is increased in the LC after presentation of a CS and that 6-hydroxydopamine (6-OHDA) lesions of NE (particularly the dorsal noradrenergic bundle, DNAB) impair fear conditioning to explicit cues (Cole and Robbins, 1987; Selden et al., 1990; Smith et al., 1992). However, some studies found no effects of 6-OHDA lesions (Mason and Fibiger, 1979) while others saw enhanced aversion with DNAB lesions (Selden et al., 1991). These differences may relate to the extent of damage.
Indirect manipulations of LC-NE (i.e., genetic and/or pharmacological approaches targeting NE transmission) lend support to the idea that NE is a critical factor for the acquisition of cued fear conditioning. For example, there is extensive evidence to suggest that β-ARs are important for fear acquisition insofar as systemic or intra-BLA propranolol (non-selective β1,2 antagonist) prior to training impairs cued fear conditioning (Cole and Koob, 1988; Bush et al., 2010; Díaz-Mataix et al., 2017; Schiff et al., 2017). Interestingly, methylphenidate (increases NE/DA levels by blocking NET and DAT) enhanced cued fear memory formation at lower levels, but had an impairing effect at higher doses (Carmack et al., 2014). In addition, blunting NE transmission with the α2-AR agonist dexmedetomidine resulted in weakened cued conditioning; this was associated with decreased Fos expression in both the BLA and CeA (Davies et al., 2004). In line with this, intra-BLA infusions of clonidine also impaired fear acquisition (Schulz et al., 2002). Curiously, α1 blockade (via terazosin), either systemically or intra-BLA, actually enhanced fear memory formation (Lazzaro et al., 2010). The authors found that terazosin facilitated LTP in the lateral amygdala which would support fear learning (Lazzaro et al., 2010). These findings suggest that NE can have opposing and dynamic effects on the acquisition of fear learning depending on the circuits being investigated and the subtype of AR being manipulated.
Genetic manipulations also suggest that NE signaling primarily promotes fear learning. Mutations that result in reduced NE transmission blunt, whereas those that increase NE signaling enhance conditioning (Kim et al., 1997; Kobayashi and Kobayashi, 2001; Davies et al., 2003). However, mice completely lacking NE or epinephrine appear to acquire cued fear normally (Murchison et al., 2004, 2011; Ouyang and Thomas, 2005; Toth et al., 2013). Several of these studies were done with DBH knockout (KO) mice using a single conditioning trial. While the interpretation of these studies would suggest that NE is not necessary for most aspects of fear conditioning (both cued and contextual) a recent study may help explain these paradoxical results. NE enhances fear learning for multi-trial, but not single-trial, conditioning protocols (Díaz-Mataix et al., 2017). It may be that weaker conditioning protocols, such as single-trial conditioning paradigms, do not engage the LC-NE system to the same extent as more typical, multi-trial training protocols. This will be noted where necessary throughout the review because the results from several DBH KO studies have led to a view that NE may play little to no role in many aspects of cued and context fear that were examined in a series of studies using weak conditioning protocols that may not rely on NE transmission (Murchison et al., 2004; Ouyang and Thomas, 2005; Zhang W.-P. et al., 2005; Schutsky et al., 2011a,b). It would be of great interest to know if these mice show deficits in most aspects of aversive learning and memory when trained with multiple trials.
Fanselow and colleagues have developed a stress-enhanced fear learning paradigm in which they have shown that pre exposure to unsignaled shocks enhances fear conditioning to both contexts and cues (Rau et al., 2005; Rau and Fanselow, 2009). It would be interesting to examine the role of the LC and NE in modulating these effects. We have recently shown that the mPFC is particularly sensitive to footshock stress. Both the PL and IL showed rapid and in some cases sustained alterations in spontaneous firing rate. In particular, IL activity was suppressed for nearly an hour following cued fear conditioning (which corresponded with high levels of freezing) and these effects were mitigated by systemic propranolol (Fitzgerald et al., 2015). A recent study has shown that LC projections to the BLA, but not the mPFC, underlie the acquisition of cued fear memories (Uematsu et al., 2017). Overall, the existing data suggest that NE strongly affects the acquisition of cued fear conditioning and these effects may be due to enhanced BLA activity as well as impaired mPFC function under high levels of stress associated with conditioning (see Figure 1).
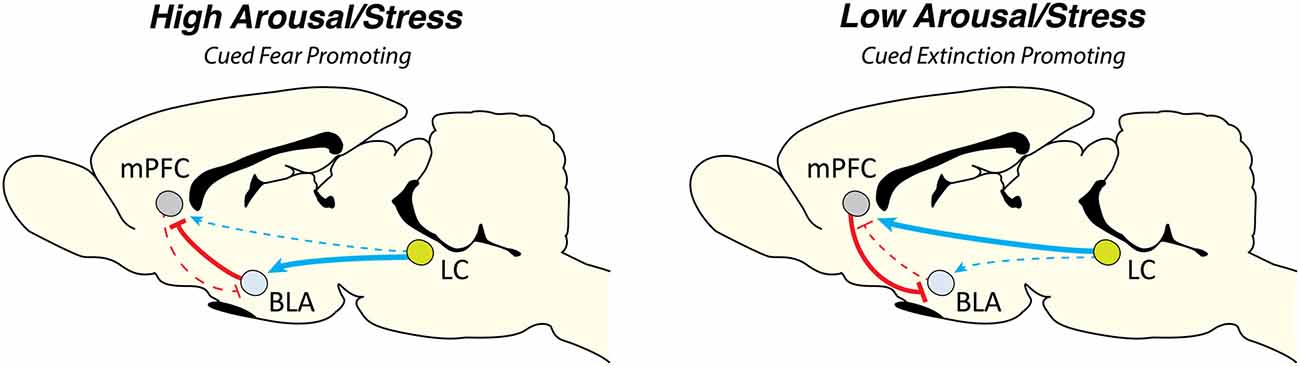
Figure 1. Locus coeruleus norepinephrine (LC-NE) dynamically regulates cued aversive learning and memory. We propose a model by which the LC-NE system can dynamically regulate cued Pavlovian fear conditioning and extinction based on the prevailing level of stress at the onset of learning. Under high levels of arousal the LC-NE system acts via α1 and β-ARs, a state that would favor elevated levels of fear and hinder new learning (for example, in the case of immediate extinction). High levels of stress may act to increase BLA function while simultaneously impairing mPFC output which is necessary for extinction learning. In contrast, under low levels of arousal, LC-NE acts via α2-ARs in both the BLA and mPFC. This scenario would likely promote new learning (particularly extinction learning) by leaving mPFC function intact. Arrow width indicates projection strength. Abbreviations: mPFC, medial prefrontal cortex; BLA, basolateral complex of the amygdala; LC, locus coeruleus.
Contextual Fear Conditioning
Norepinephrine also influences the acquisition of contextual fear conditioning, although these effects may be due to the aforementioned effects on hippocampal LTP in addition to effects on the BLA. Evidence suggests LC-NE may be a critical mediator of contextual fear learning insofar as LC neurons (and GABAergic neurons near/within the LC) show increased Fos expression following context fear conditioning (Ishida et al., 2002). In addition, 6-OHDA lesions of the LC result in impaired contextual fear conditioning (Neophytou et al., 2001). These data suggest that footshock stress strongly activate the LC-NE system to promote contextual fear learning. However, others have reported that either 6-OHDA or DNAB (but not VNAB) lesions actually enhance context fear (Selden et al., 1990, 1991). It is possible the conflicting results stem from differences in the extent of LC-NE damage.
Genetic and pharmacological manipulations of NE largely support the idea that NE regulates context fear learning. For instance, genetic or pharmacological depletion of NE results in impaired contextual fear (Onaka et al., 1996; Murchison et al., 2004, 2011; Ouyang and Thomas, 2005; Zhang W.-P. et al., 2005; Hott et al., 2012) and this corresponded with a reduction in CA1 activation (Zhang W.-P. et al., 2005). Moreover, increasing levels of NE or epinephrine promotes context fear learning (Kim et al., 1997; Frankland et al., 2004; Hu et al., 2007; Carmack et al., 2014). Somewhat paradoxically, increasing NE levels with dexmedetomidine or via α2-AR KO seemingly had no effects on context fear (Davies et al., 2003, 2004), although a recent study has demonstrated that intra-CeA clonidine blocks the acquisition of context fear (Holmes et al., 2017). These discrepancies may relate to differing levels of NE with each manipulation (i.e., differences in the conditioning protocols), species differences, and/or compensatory mechanisms in mutant mice.
The HPC richly expresses low affinity β-ARs and their activation has a demonstrated role in enhanced HPC-LTP (Harley, 2007). It seems likely that HPC-LTP is highly sensitive to the level of NE efflux which may explain how NE can bidirectionally modulate context fear acquisition, by either enhancing or impairing LTP. Connectivity between the HPC and mPFC may importantly mediate effects on context learning, which has been shown to be affected by NE in the BLA. Recent work suggests that NE in the BLA bidirectionally affects HPC-mPFC plasticity. NE enhanced HPC-mPFC LTP in an α2-AR dependent fashion and β-AR stimulation decreased LTP in this pathway (Lim et al., 2017). These data provide compelling evidence that the BLA may gate HPC-mPFC plasticity which may promote high and low fear states in a receptor-dependent manner. How this affects contextual fear learning remains an open question.
NE and Fear Expression
Cued Fear Expression
In line with the idea that NE is important for the acquisition of both cued and contextual fear conditioning, it appears that NE also modulates fear expression. As mentioned above, a trained CS has been demonstrated to engage the LC-NE system and CS-exposure produces a conditional fear response associated with noradrenergic arousal. In terms of cued fear expression, systemic administration of propranolol prior to extinction training reduced within session freezing which corresponded with a reduction in PL activity (Rodriguez-Romaguera et al., 2009). Similarly, decreasing NE transmission with clonidine produced dose-dependent reductions in fear expression whereas elevating NE (piperoxane or yohimbine) increased fear expression as measured by potentiated startle in the presence of a formerly trained CS (Davis et al., 1979). This finding was replicated with intra-LA infusions of clonidine (Schulz et al., 2002). These effects may depend upon interactions between the BLA and mPFC insofar as post-conditioning lesions of the BLA blocked CS-induced increases in mPFC NE levels which correlated with lower fear expression (Goldstein et al., 1996). Moreover, slice recordings have shown that while NE did not disrupt glutamatergic BLA signaling, inhibitory neurotransmission was decreased in the BLA following recall of a cued fear memory. These data suggest that NE and heightened fear expression is associated with BLA excitability (Skelly et al., 2017). Interestingly, a handful of reports suggest NE manipulations had no effect on cued fear expression (Murchison et al., 2004, 2011; Ouyang and Thomas, 2005; Schutsky et al., 2011b; Laitman et al., 2014). As discussed above, these findings come from studies with DBH KO mice. Importantly, the training protocols used in these studies may help explain the observed effects (or lack thereof). These studies used a single shock conditioning trial and a recent study has demonstrated that NE blockade has no effects on single-trial conditioning, but is necessary for multi-trial enhancement of learning and subsequent expression of that memory (Díaz-Mataix et al., 2017). Weaker conditioning protocols are apparently less likely to recruit LC-NE given that they appear insensitive to NE manipulations.
Contextual Fear Expression
Similar to the effects reported on the acquisition of context fear, NE manipulations impact context fear expression. For instance, genetic depletion of NE produces deficits in context fear retrieval and this effect is dependent upon β-AR signaling insofar as these deficits are recapitulated by propranolol (Murchison et al., 2004, 2011; Ouyang and Thomas, 2005; Zhang W.-P. et al., 2005). Interestingly, xamoterol (β1 partial agonist) actually impairs context freezing in a dose-dependent fashion; however, the authors argue that these effects are mediated by Gi-coupled β2 signaling which acts to oppose Gs-coupled β1 signaling which is necessary for hippocampal-dependent memory retrieval (Schutsky et al., 2011a,b). Moreover, pharmacologically driven increases in NE (acute treatment with reobxetine, a norepinephrine reuptake inhibitor) enhanced context fear expression (Inoue et al., 2006). It would be interesting to know if DBH KO mice show similar deficits using a multi-trial conditioning protocol which would more likely to result in stress-induced LC-NE signaling.
NE and Memory Consolidation
After initial training occurs short-term memory undergoes consolidation processes to form a long-term memory. Post-training manipulations have been used to examined the involvement of NE in both cued and context fear memory consolidation. An interesting dichotomy emerges here, where it seems that NE is not necessarily needed for the consolidation of cued fear learning, but is for context.
Cued Fear Consolidation
While NE (particularly in the BLA) is critical for the formation of cued fear memories it may not be essential for the consolidation of such memories. Mice lacking NE showed no deficits in cued fear memory consolidation using single-trial conditioning procedures (Murchison et al., 2004; Ouyang and Thomas, 2005). Further supporting this idea, post training systemic administration of either epinephrine or amphetamine had no effects on consolidation (Lee et al., 2001). Moreover, systemic, intra-mPFC, or intra-BLA propranolol has no impact on fear memory consolidation (Bush et al., 2010; Fitzgerald et al., 2015; Giustino et al., 2017; Schiff et al., 2017). and post training propranolol had no effects on LA synaptic strengthening (Clem and Huganir, 2013). In line with this, intra-BLA atenolol only impaired corticosterone enhanced cued fear memories, but had no effect in rats that just received auditory fear conditioning and post-training BLA infusions of atenolol (Roozendaal et al., 2006). Interestingly, mice heterozygous for a mutation in the gene encoding tyrosine hydroxylase (a precursor enzyme in the biosynthetic pathway for NE) show impaired cued fear memory and this could be restored by drug-induced NE stimulation after training (Kobayashi et al., 2000; Kobayashi and Kobayashi, 2001). However, these mutant mice only show a modest reduction in NE accumulation and release; they may also have differences in the distribution and density of AR expression as a compensatory mechanism complicating the interpretation of the results. The existing literature primarily suggests that NE manipulations have minimal consequences for the consolidation of cued fear conditioning memories.
Contextual Fear Consolidation
As noted above, an intriguing difference emerges when comparing the role of NE in cued vs. contextual fear memory consolidation. This may relate to the extensive and long-lasting effects of NE manipulations on hippocampal LTP, which would favor the strengthening of contextual memories. Increasing NE with yohimbine administration has been shown to enhance context fear memory consolidation. This enhancement was blocked with propranolol administration, suggesting a role for β-ARs. In addition, dampening NE-transmission with clonidine reduced contextual fear memory consolidation (Gazarini et al., 2013, 2014). These effects seem to be dependent on both the BLA and HPC insofar as post training NE into the BLA enhances consolidation (McGaugh and Roozendaal, 2002; LaLumiere et al., 2003) and blockade of β-ARs in CA1 has impairing effects (Ji et al., 2003). These findings lend support to the idea that NE importantly regulates the consolidation of HPC-dependent contextual fear memories in a β-ARs dependent manner. The involvement of NE in the consolidation of contextual, but not cued, fear conditioning suggests that NE is operating on the consolidation of context representations, rather than context-US or CS-US associations. Moreover, signaled and unsignaled shocks produced marked differences in neuronal activity in the mPFC (Fitzgerald et al., 2015; Giustino et al., 2016a), which may play a role in contextual memory (Zelikowsky et al., 2013, 2014; Sharpe and Killcross, 2014, 2015; Heroux et al., 2017; Pennington et al., 2017).
NE and Reconsolidation
When a memory is reactivated it enters a labile state and undergoes protein synthesis dependent reconsolidation (Nader et al., 2000; Duvarci et al., 2008). This provides a window of opportunity to disrupt long-term memories. We and others have previously reviewed the effects of NE (mainly examining the effects of propranolol) on reconsolidation and will therefore only briefly touch on this area in this review (Steckler and Risbrough, 2012; Giustino et al., 2016b; Kroes et al., 2016a). Disrupting the reconsolidation of a fear memory can potentially have far reaching clinical implications for trauma- and stressor-related disorders. Many labs have provided compelling evidence that propranolol delivered after brief memory reactivation disrupts the reconsolidation of both cued and context fear memories in rodents (Przybyslawski et al., 1999; Dębiec and Ledoux, 2004; Abrari et al., 2008; Muravieva and Alberini, 2010; Dębiec et al., 2011; Pitman et al., 2011; Schneider et al., 2014; Taherian et al., 2014). In addition, systemic clonidine has also mirrored these effects (Gamache et al., 2012). An important consideration regarding these findings is that reconsolidation effects may be subject to certain boundary conditions such as the age and strength of a memory, thus making interference with fear memories more complicated (Duvarci et al., 2006; Wang et al., 2009). Moreover, many of these conditioning protocols use a single-trial, producing a relatively weak fear memory that is less dependent upon NE transmission (Díaz-Mataix et al., 2017), which may be more susceptible to blockade.
NE and Fear Extinction
Behavioral and pharmacological approaches aimed at improving extinction learning in rodents have received much attention due to the clinical importance of extinction based cognitive behavioral therapies (Myers and Davis, 2007; Quirk and Mueller, 2008; Holmes and Quirk, 2010; Mueller and Cahill, 2010; Vervliet et al., 2013; Fitzgerald et al., 2014; Giustino and Maren, 2015; Giustino et al., 2016b). It is generally thought that stress impairs extinction learning and this may relate to alterations in NE transmission (Mason and Fibiger, 1979; Mason et al., 1979; Holmes and Quirk, 2010; Steckler and Risbrough, 2012; Fitzgerald et al., 2015; Giustino et al., 2016b, 2017; Maren and Holmes, 2016).
Cued Fear Extinction
While NE primarily strengthens the acquisition of fear learning, it has bidirectional effects on extinction learning that may depend on the extant level of noradrenergic arousal. For example, reducing or depleting NE levels impairs delayed extinction (occurring at least 24 h following fear conditioning; Mason and Fibiger, 1979; Tsaltas et al., 1984; Cole and Robbins, 1987; Cain et al., 2004; Mueller et al., 2008; Fitzgerald et al., 2015) and this may be due to effects on IL activity (Mueller et al., 2008). In line with this, recent work has shown that LC projections to the mPFC promote extinction learning (Uematsu et al., 2017). Indeed increasing NE with yohimbine can facilitate delayed extinction learning (Cain et al., 2004; Morris and Bouton, 2007; Hefner et al., 2008; Mueller et al., 2009). These findings suggest that NE is important for the formation of a new and competing CS-no US extinction memory.
Our lab and others have demonstrated that the timing of extinction training relative to conditioning is an important factor regulating the long-term success of extinction in rodent models and humans (Maren and Chang, 2006; Chang et al., 2010; Kim et al., 2010; MacPherson et al., 2013; Stafford et al., 2013; Maren, 2014; Fitzgerald et al., 2015; Hollis et al., 2016; Merz et al., 2016; Giustino et al., 2017). These effects may be due to high levels of stress (and NE) soon after conditioning that interferes with new learning. In line with this idea, we have shown that systemic or intra-BLA propranolol enables learning where it normally fails (Fitzgerald et al., 2015; Giustino et al., 2016b, 2017). In addition, systemic propranolol administered prior to delayed extinction, when stress levels are presumably lower, impaired learning when tested in a drug free state the following day (Fitzgerald et al., 2015). These data are supported by the idea that stress generally impairs extinction learning which may be due to alterations in NE signaling (Lin et al., 2016; Maren and Holmes, 2016). Importantly, the fact that reducing NE signaling prior to delayed extinction has impairing effects suggests that CS-evoked NE actually facilitates learning under lower levels of arousal. In the case of immediate extinction (a state of higher arousal at the onset of learning), it is likely that propranolol is reducing NE signaling to more optimal levels, thus facilitating learning. Our data and others suggest that heightened NE in the BLA strengthens fear memories perhaps at the expense of forming a new extinction memory. Overall, NE can bidirectionally modulate extinction learning and this seems to depend on the prevailing level of stress at the onset of learning. Low levels of NE can enhance prefrontal function which may act to blunt downstream signaling in the BLA to promote extinction learning (Figure 1). These findings have important clinical implications and warrant careful consideration when interpreting effects of NE-altering drugs and their effects on the treatment of trauma- and stressor-related disorders (discussed further below).
Contextual Fear Extinction
As with context fear, the extinction of contextual fear may rely heavily on hippocampal function. It seems that NE is critical to context fear extinction (and its consolidation). Methylphenidate (a NET and DAT blocker) delivered either before or after context extinction enhanced learning in a dose-dependent manner (Abraham et al., 2012). These effects may be due to increased synaptic efficacy in the HPC and BLA insofar as NE infusion into CA1 or the BLA immediately after context extinction mirror these enhancing effects (Berlau and McGaugh, 2006; Chai et al., 2014). In addition, NE in the vmPFC has been implicated in enhanced context extinction learning such that β-AR agonism (via isoproterenol) facilitates learning whereas systemic propranolol or prazosin hinder learning (Cain et al., 2004; Bernardi and Lattal, 2010; Do-Monte et al., 2010). These data support the notion that NE is a critical factor for the extinction of contextual fear. It is likely that these effects are at least in part mediated by HPC plasticity, which can be regulated by NE transmission. As discussed above, recent work has shown that NE in the BLA can alter HPC-mPFC plasticity (Lim et al., 2017). Given the important role of the mPFC in extinction learning and evidence that NE levels that engage α2-AR signaling enhance HPC-mPFC plasticity, it remains possible that this may be a neural mechanism underlying contextual fear extinction.
NE and PTSD
Noradrenergic modulating drugs are used to treat an array of neuropsychiatric disorders, though the only two FDA approved drugs for PTSD are selective serotonin reuptake inhibitors (Steckler and Risbrough, 2012; Tawa and Murphy, 2013; Fitzgerald et al., 2014; Arnsten et al., 2015). Drugs that either elevate or reduce NE transmission have been studied and used off-label for the treatment of PTSD and its symptoms with varying success (Southwick et al., 1997, 1999a,b,c; Holmes and Quirk, 2010; Bukalo et al., 2014; Giustino et al., 2016b). It has recently been shown that threat is associated with increased LC activity in healthy human volunteers and this acts to strengthen prioritized memory representations (Clewett et al., 2018). This suggests that heightened states of arousal may promote fear memory formation and maintenance. In addition, a number of studies have demonstrated that elevated NE plays a major role in the pathophysiology of PTSD (Kosten et al., 1987; Yehuda et al., 1992; Bremner et al., 1996; Southwick et al., 1997, 1999a,b,c; Geracioti et al., 2001; Strawn and Geracioti, 2008; Naegeli et al., 2018). Despite this link, success with pharmacological erasure of fear memories and/or enhancement of extinction based cognitive behavioral therapies has been limited (Bos et al., 2014).
It has been proposed that individuals with PTSD may “hypercondition” to fearful stimuli and this is coupled with impaired extinction, ultimately resulting in a heavy bias towards fearful responses in inappropriate situations (Orr et al., 2000; Lissek et al., 2005; Guthrie and Bryant, 2006; Blechert et al., 2007; Wessa and Flor, 2007; Milad et al., 2008, 2009; Pitman et al., 2012; VanElzakker et al., 2014; Norrholm et al., 2015). While the LC-NE system plays an important role in learning and memory, including extinction learning (Sterpenich et al., 2006; Ramos and Arnsten, 2007; Arnsten, 2009, 2015; Sara, 2009, 2015; Arnsten et al., 2015), stress (and elevated NE beyond optimal levels) may only exacerbate these effects by impairing extinction learning and/or increasing generalization (Hartley et al., 2014; Raio et al., 2014, 2017; Raio and Phelps, 2015; Maren and Holmes, 2016; Dunsmoor et al., 2017). Indeed, yohimbine has been used in healthy human subjects to enhance fear learning and it also has been shown to hinder extinction learning (van Stegeren et al., 2010; Soeter and Kindt, 2011b, 2012; Visser et al., 2015). Despite this, there has been some interest in yohimbine as a pharmaceutical agent to augment the treatment of PTSD, which has yielded mixed results (Powers et al., 2009; Holmes and Quirk, 2010; Wangelin et al., 2013).
Clonidine and guanfacine are two α2-AR agonists that are used to treat a number of conditions. While the evidence for the efficacy of either drug is somewhat limited, these drugs may have some use in the treatment of PTSD and related disorders (Arnsten et al., 2015; Belkin and Schwartz, 2015). These NE-reducing agents have been shown to reduce symptoms of hyperarousal associated with PTSD as well as sleep disturbances (Kinzie and Leung, 1989; Horrigan and Barnhill, 1996; Porter and Bell, 1999; Boehnlein and Kinzie, 2007; Detweiler et al., 2016). Importantly, both compounds have also shown safety and promise for treating PTSD in children (Harmon and Riggs, 1996; Connor et al., 2013). Unfortunately, some studies have found little evidence for the efficacy of α2-AR agonists in the treatment of PTSD (Neylan et al., 2006; Davis et al., 2008). It remains possible that these, or related drugs, may only be effective for individuals who have dysregulated/elevated NE signaling which may explain discrepant findings. Further research is warranted on the efficacy of these compounds.
Results with noradrenergic receptor antagonists, such as propranolol, have yielded mixed results in both healthy human volunteers and individuals with PTSD. Propranolol is already used safely in humans for other conditions and has been shown to reduce long-term memory for an emotionally arousing story (Cahill et al., 1994) and reduced the strength of context conditioning in healthy human subjects (Grillon et al., 2004). These data suggest that β-AR activation underlies memories for emotional events. However, some have suggested that propranolol treatment soon after trauma has no effects, although this was done in the absence of any extinction based therapy (Stein et al., 2007; McGhee et al., 2009; Nugent et al., 2010). In addition, one report found that propranolol has no effects on the acquisition or retention of extinction learning (Orr et al., 2006). Others have shown that propranolol impairs extinction learning in healthy subjects (Bos et al., 2012). At first glance, these conflicting reports would imply that researchers and clinicians alike should look elsewhere for a pharmaceutical adjunct for exposure therapy. However, we have argued that the timing of propranolol administration coupled with behavioral therapy is an often overlooked, and highly important, factor regulating the long-term outcome of extinction learning (Giustino et al., 2016b). This may be due to differences in the prevailing level of noradrenergic arousal at the time of administration. There is some empirical evidence to support this idea in humans though more research coupling propranolol and extinction soon after trauma is needed (Pitman et al., 2002; Vaiva et al., 2003).
One area that has shown promise centers on blocking the reconsolidation of a fearful memory. Several studies in both healthy human volunteers and individuals with PTSD have suggested that propranolol can be used to disrupt reconsolidation (Brunet et al., 2008, 2011, 2014; Kindt et al., 2009; Soeter and Kindt, 2011a, 2012; Poundja et al., 2012; Schwabe et al., 2012; Lonergan et al., 2013). As discussed previously, effects on reconsolidation may be subject to certain boundary conditions and memories do not necessarily even undergo reconsolidation unless new learning occurs (Sevenster et al., 2012). Moreover, many of these reconsolidation effects have not been replicated which further complicates approaches focusing on reconsolidation blockade as an effective treatment strategy for PTSD (Tollenaar et al., 2009; Bos et al., 2014; Spring et al., 2015; Wood et al., 2015). It seems unlikely that acute administration of propranolol, or any drug, would effectively eradicate a long-standing fear memory, such as those observed in individuals suffering from PTSD. However, this does not preclude the idea that propranolol may reduce fear under some circumstances and thus has utility moving forward (Giustino et al., 2016b; Kroes et al., 2016a,b).
Another noradrenergic receptor antagonist that has been used primarily to combat disordered sleep in individuals with PTSD is the α1-AR antagonist prazosin. Prazosin has shown promise in ameliorating nightmares and sleep disturbances associated with PTSD (Raskind et al., 2003; Taylor et al., 2008; Koola et al., 2014; Writer et al., 2014; de Dassel et al., 2017; Keeshin et al., 2017; Miller et al., 2017; Short et al., 2018). However, a recent clinical trial demonstrated that prazosin did not ameliorate sleep-related disturbances in military veterans with PTSD (Raskind et al., 2018). Less is known about how prazosin affects other aspects of PTSD symptomatology. A recent study in healthy human subjects suggests that prazosin delivered prior to fear conditioning enhanced future discrimination between fearful and safe stimuli during extinction (Homan et al., 2017). Further work is needed to examine the effects of prazosin as well as other NE-altering drugs in both healthy human volunteers and those with PTSD.
Conclusion
Overall, the LC-NE system critically regulates most aspects of emotional learning and memory in rodent models, healthy human subjects, and individuals suffering from trauma- and stressor-related disorders. Recent advances in technology for basic science research will be crucial to further our understanding of how stress and the LC-NE system regulate these effects in rodent models. Target-specific approaches have led to a new appreciation of LC function and while the precise effects of distinct LC subpopulations are not well characterized several recent articles have pinpointed unique contributions to learning and memory as well as anxiety in rodents (Schwarz and Luo, 2015; Schwarz et al., 2015; Uematsu et al., 2015, 2017; Li et al., 2016; Hirschberg et al., 2017; McCall et al., 2017).
An important area of research moving forward may center around individualized medicine based on differences in the LC-NE system and stress responsivity. Human imaging protocols have improved to better isolate the LC (Keren et al., 2009; Murphy et al., 2014; Betts et al., 2017; Brooks et al., 2017; Krebs et al., 2017; Langley et al., 2017; Priovoulos et al., 2017; Song et al., 2017; Tona et al., 2017). Understanding if and how LC-NE is contributing to an individual’s symptomatology will likely improve therapeutic outcomes. Patients often undergo several “rounds” of drug treatment as they (and their doctor) search for either a single or combination of agents that ameliorate their condition. An improved appreciation of how the LC-NE system contributes to aversive learning and memory and its subsequent extinction may help improve empirically driven treatment options.
We propose that the LC-NE system dynamically regulates the acquisition and extinction of both cued and contextual fear and these effects are dependent upon the prevailing level of stress (and NE) at the onset of learning (Figure 1). LC-NE likely influences learning and memory processes in a manner described by an inverted-U function, albeit acting in different brain regions/circuits to regulate cued and contextual learning. Regarding contextual fear conditioning, we suggest that the LC-NE system acts to regulate hippocampal LTP, which is involved in the acquisition, consolidation, and extinction of contextual representations (as opposed to context-US associations). In terms of cued fear conditioning, low levels of NE release prior to delayed extinction would enhance extinction learning by promoting mPFC function, which would, in turn, inhibit BLA output to enable extinction learning. In contrast, high levels of NE released under stress (such as that accompanying footshock) promotes fear expression while inhibiting new learning (as is observed with immediate extinction procedures) by strengthening BLA function and simultaneously impairing mPFC function via target-specific LC subpopulations.
Author Contributions
TFG and SM wrote and edited the manuscript.
Funding
This work was supported by grants from the National Institutes of Health (R01MH065961 to SM and F31MH112208 to TFG). SM was also supported by a McKnight Foundation Memory and Cognitive Disorders Award.
Conflict of Interest Statement
The authors declare that the research was conducted in the absence of any commercial or financial relationships that could be construed as a potential conflict of interest.
References
Abercrombie, E. D., Keller, R. W. Jr., and Zigmond, M. J. (1988). Characterization of hippocampal norepinephrine release as measured by microdialysis perfusion: pharmacological and behavioral studies. Neuroscience 27, 897–904. doi: 10.1016/0306-4522(88)90192-3
Abraham, A. D., Cunningham, C. L., and Lattal, K. M. (2012). Methylphenidate enhances extinction of contextual fear. Learn. Mem. 19, 67–72. doi: 10.1101/lm.024752.111
Abrari, K., Rashidy-Pour, A., Semnanian, S., and Fathollahi, Y. (2008). Administration of corticosterone after memory reactivation disrupts subsequent retrieval of a contextual conditioned fear memory: dependence upon training intensity. Neurobiol. Learn. Mem. 89, 178–184. doi: 10.1016/j.nlm.2007.07.005
Agster, K. L., Mejias-Aponte, C. A., Clark, B. D., and Waterhouse, B. D. (2013). Evidence for a regional specificity in the density and distribution of noradrenergic varicosities in rat cortex. J. Comp. Neurol. 521, 2195–2207. doi: 10.1002/cne.23270
Amaral, D. G., and Sinnamon, H. M. (1977). The locus coeruleus: neurobiology of a central noradrenergic nucleus. Prog. Neurobiol. 9, 147–196. doi: 10.1016/0301-0082(77)90016-8
Aoki, C., Go, C.-G., Venkatesan, C., and Kurose, H. (1994). Perikaryal and synaptic localization ofα2A-adrenergic receptor-like immunoreactivity. Brain Res. 650, 181–204. doi: 10.1016/0006-8993(94)91782-5
Arnsten, A. F. T. (2009). Stress signalling pathways that impair prefrontal cortex structure and function. Nat. Rev. Neurosci. 10, 410–422. doi: 10.1038/nrn2648
Arnsten, A. F. T. (2015). Stress weakens prefrontal networks: molecular insults to higher cognition. Nat. Neurosci. 18, 1376–1385. doi: 10.1038/nn.4087
Arnsten, A. F., and Goldman-Rakic, P. S. (1984). Selective prefrontal cortical projections to the region of the locus coeruleus and raphe nuclei in the rhesus monkey. Brain Res. 306, 9–18. doi: 10.1016/0006-8993(84)90351-2
Arnsten, A. F. T., and Li, B.-M. (2005). Neurobiology of executive functions: catecholamine influences on prefrontal cortical functions. Biol. Psychiatry 57, 1377–1384. doi: 10.1016/j.biopsych.2004.08.019
Arnsten, A. F. T., Raskind, M. A., Taylor, F. B., and Connor, D. F. (2015). The effects of stress exposure on prefrontal cortex: translating basic research into successful treatments for post-traumatic stress disorder. Neurobiol. Stress 1, 89–99. doi: 10.1016/j.ynstr.2014.10.002
Aston-Jones, G., and Bloom, F. E. (1981a). Activity of norepinephrine-containing locus coeruleus neurons in behaving rats anticipates fluctuations in the sleep-waking cycle. J. Neurosci. 1, 876–886.
Aston-Jones, G., and Bloom, F. E. (1981b). Norepinephrine-containing locus coeruleus neurons in behaving rats exhibit pronounced responses to non-noxious environmental stimuli. J. Neurosci. 1, 887–900.
Aston-Jones, G., and Cohen, J. D. (2005). An integrative theory of locus coeruleus-norepinephrine function: adaptive gain and optimal performance. Annu. Rev. Neurosci. 28, 403–450. doi: 10.1146/annurev.neuro.28.061604.135709
Aston-Jones, G., Ennis, M., Pieribone, V. A., Nickell, W. T., and Shipley, M. T. (1986). The brain nucleus locus coeruleus: restricted afferent control of a broad efferent network. Science 234, 734–737. doi: 10.1126/science.3775363
Aston-Jones, G., Rajkowski, J., and Cohen, J. (1999). Role of locus coeruleus in attention and behavioral flexibility. Biol. Psychiatry 46, 1309–1320. doi: 10.1016/s0006-3223(99)00140-7
Aston-Jones, G., and Waterhouse, B. (2016). Locus coeruleus: from global projection system to adaptive regulation of behavior. Brain Res. 1645, 75–78. doi: 10.1016/j.brainres.2016.03.001
Aston-Jones, G., Zhu, Y., and Card, J. P. (2004). Numerous GABAergic afferents to locus ceruleus in the pericerulear dendritic zone: possible interneuronal pool. J. Neurosci. 24, 2313–2321. doi: 10.1523/JNEUROSCI.5339-03.2004
Belkin, M. R., and Schwartz, T. L. (2015). α-2 receptor agonists for the treatment of posttraumatic stress disorder. Drugs Context 4:212286. doi: 10.7573/dic.212286
Berlau, D. J., and McGaugh, J. L. (2006). Enhancement of extinction memory consolidation: the role of the noradrenergic and GABAergic systems within the basolateral amygdala. Neurobiol. Learn. Mem. 86, 123–132. doi: 10.1016/j.nlm.2005.12.008
Bernardi, R. E., and Lattal, K. M. (2010). A role for α-adrenergic receptors in extinction of conditioned fear and cocaine conditioned place preference. Behav. Neurosci. 124, 204–210. doi: 10.1037/a0018909
Berridge, C. W., and Waterhouse, B. D. (2003). The locus coeruleus-noradrenergic system: modulation of behavioral state and state-dependent cognitive processes. Brain Res. Rev. 42, 33–84. doi: 10.1016/s0165-0173(03)00143-7
Betts, M. J., Cardenas-Blanco, A., Kanowski, M., Jessen, F., and Düzel, E. (2017). In vivo MRI assessment of the human locus coeruleus along its rostrocaudal extent in young and older adults. Neuroimage 163, 150–159. doi: 10.1016/j.neuroimage.2017.09.042
Birnbaum, S. G., Yuan, P. X., Wang, M., Vijayraghavan, S., Bloom, A. K., Davis, D. J., et al. (2004). Protein kinase C overactivity impairs prefrontal cortical regulation of working memory. Science 306, 882–884. doi: 10.1126/science.1100021
Blechert, J., Michael, T., Vriends, N., Margraf, J., and Wilhelm, F. H. (2007). Fear conditioning in posttraumatic stress disorder: evidence for delayed extinction of autonomic, experiential, and behavioural responses. Behav. Res. Ther. 45, 2019–2033. doi: 10.1016/j.brat.2007.02.012
Bliss, T. V., Goddard, G. V., and Riives, M. (1983). Reduction of long-term potentiation in the dentate gyrus of the rat following selective depletion of monoamines. J. Physiol. 334, 475–491. doi: 10.1113/jphysiol.1983.sp014507
Boehnlein, J. K., and Kinzie, J. D. (2007). Pharmacologic reduction of CNS noradrenergic activity in PTSD: the case for clonidine and prazosin. J. Psychiatr. Pract. 13, 72–78. doi: 10.1097/01.pra.0000265763.79753.c1
Booze, R. M., Crisostomo, E. A., and Davis, J. N. (1993). β-adrenergic receptors in the hippocampal and retrohippocampal regions of rats and guinea pigs: autoradiographic and immunohistochemical studies. Synapse 13, 206–214. doi: 10.1002/syn.890130303
Bos, M. G. N., Beckers, T., and Kindt, M. (2012). The effects of noradrenergic blockade on extinction in humans. Biol. Psychol. 89, 598–605. doi: 10.1016/j.biopsycho.2012.01.007
Bos, M. G. N., Beckers, T., and Kindt, M. (2014). Noradrenergic blockade of memory reconsolidation: a failure to reduce conditioned fear responding. Front. Behav. Neurosci. 8:412. doi: 10.3389/fnbeh.2014.00412
Bouret, S., and Sara, S. J. (2004). Reward expectation, orientation of attention and locus coeruleus-medial frontal cortex interplay during learning. Eur. J. Neurosci. 20, 791–802. doi: 10.1111/j.1460-9568.2004.03526.x
Bouton, M. E., Westbrook, R. F., Corcoran, K. A., and Maren, S. (2006). Contextual and temporal modulation of extinction: behavioral and biological mechanisms. Biol. Psychiatry 60, 352–360. doi: 10.1016/j.biopsych.2005.12.015
Boyajian, C. L., Loughlin, S. E., and Leslie, F. M. (1987). Anatomical evidence for α-2 adrenoceptor heterogeneity: differential autoradiographic distributions of [3H]rauwolscine and [3H]idazoxan in rat brain. J. Pharmacol. Exp. Ther. 241, 1079–1091.
Bremner, J. D., Krystal, J. H., Southwick, S. M., and Charney, D. S. (1996). Noradrenergic mechanisms in stress and anxiety: I. Preclinical studies. Synapse 23, 28–38. doi: 10.1002/(sici)1098-2396(199605)23:1<28::aid-syn4>3.3.co;2-4
Brooks, J. C. W., Davies, W.-E., and Pickering, A. E. (2017). Resolving the brainstem contributions to attentional analgesia. J. Neurosci. 37, 2279–2291. doi: 10.1523/JNEUROSCI.2193-16.2016
Brunet, A., Orr, S. P., Tremblay, J., Robertson, K., Nader, K., and Pitman, R. K. (2008). Effect of post-retrieval propranolol on psychophysiologic responding during subsequent script-driven traumatic imagery in post-traumatic stress disorder. J. Psychiatr. Res. 42, 503–506. doi: 10.1016/j.jpsychires.2007.05.006
Brunet, A., Poundja, J., Tremblay, J., Bui, E., Thomas, E., Orr, S. P., et al. (2011). Trauma reactivation under the influence of propranolol decreases posttraumatic stress symptoms and disorder: 3 open-label trials. J. Clin. Psychopharmacol. 31, 547–550. doi: 10.1097/JCP.0b013e318222f360
Brunet, A., Thomas, É., Saumier, D., Ashbaugh, A. R., Azzoug, A., Pitman, R. K., et al. (2014). Trauma reactivation plus propranolol is associated with durably low physiological responding during subsequent script-driven traumatic imagery. Can. J. Psychiatry 59, 228–232. doi: 10.1177/070674371405900408
Buffalari, D. M., and Grace, A. A. (2007). Noradrenergic modulation of basolateral amygdala neuronal activity: opposing influences of α-2 and β receptor activation. J. Neurosci. 27, 12358–12366. doi: 10.1523/JNEUROSCI.2007-07.2007
Bukalo, O., Pinard, C. R., and Holmes, A. (2014). Mechanisms to medicines: elucidating neural and molecular substrates of fear extinction to identify novel treatments for anxiety disorders. Br. J. Pharmacol. 171, 4690–4718. doi: 10.1111/bph.12779
Bush, D. E. A., Caparosa, E. M., Gekker, A., and Ledoux, J. (2010). β-adrenergic receptors in the lateral nucleus of the amygdala contribute to the acquisition but not the consolidation of auditory fear conditioning. Front. Behav. Neurosci. 4:154. doi: 10.3389/fnbeh.2010.00154
Cahill, L., Prins, B., Weber, M., and McGaugh, J. L. (1994). β-adrenergic activation and memory for emotional events. Nature 371, 702–704. doi: 10.1038/371702a0
Cain, C. K., Blouin, A. M., and Barad, M. (2004). Adrenergic transmission facilitates extinction of conditional fear in mice. Learn. Mem. 11, 179–187. doi: 10.1101/lm.71504
Carmack, S. A., Howell, K. K., Rasaei, K., Reas, E. T., and Anagnostaras, S. G. (2014). Animal model of methylphenidate’s long-term memory-enhancing effects. Learn. Mem. 21, 82–89. doi: 10.1101/lm.033613.113
Cedarbaum, J. M., and Aghajanian, G. K. (1978). Afferent projections to the rat locus coeruleus as determined by a retrograde tracing technique. J. Comp. Neurol. 178, 1–15. doi: 10.1002/cne.901780102
Chai, N., Liu, J.-F., Xue, Y.-X., Yang, C., Yan, W., Wang, H.-M., et al. (2014). Delayed noradrenergic activation in the dorsal hippocampus promotes the long-term persistence of extinguished fear. Neuropsychopharmacology 39, 1933–1945. doi: 10.1038/npp.2014.42
Chandler, D. J., Gao, W.-J., and Waterhouse, B. D. (2014). Heterogeneous organization of the locus coeruleus projections to prefrontal and motor cortices. Proc. Natl. Acad. Sci. U S A 111, 6816–6821. doi: 10.1073/pnas.1320827111
Chandler, D. J., Lamperski, C. S., and Waterhouse, B. D. (2013). Identification and distribution of projections from monoaminergic and cholinergic nuclei to functionally differentiated subregions of prefrontal cortex. Brain Res. 1522, 38–58. doi: 10.1016/j.brainres.2013.04.057
Chandler, D., and Waterhouse, B. D. (2012). Evidence for broad versus segregated projections from cholinergic and noradrenergic nuclei to functionally and anatomically discrete subregions of prefrontal cortex. Front. Behav. Neurosci. 6:20. doi: 10.3389/fnbeh.2012.00020
Chang, C., Berke, J. D., and Maren, S. (2010). Single-unit activity in the medial prefrontal cortex during immediate and delayed extinction of fear in rats. PLoS One 5:e11971. doi: 10.1371/journal.pone.0011971
Chaulk, P. C., and Harley, C. W. (1998). Intracerebroventricular norepinephrine potentiation of the perforant path-evoked potential in dentate gyrus of anesthetized and awake rats: a role for both α- and β-adrenoceptor activation. Brain Res. 787, 59–70. doi: 10.1016/s0006-8993(97)01460-1
Chen, F.-J., and Sara, S. J. (2007). Locus coeruleus activation by foot shock or electrical stimulation inhibits amygdala neurons. Neuroscience 144, 472–481. doi: 10.1016/j.neuroscience.2006.09.037
Chen, W., Wang, Y., Wang, X., and Li, H. (2017). Neural circuits involved in the renewal of extinguished fear. IUBMB Life 69, 470–478. doi: 10.1002/iub.1636
Ciocchi, S., Herry, C., Grenier, F., Wolff, S. B. E., Letzkus, J. J., Vlachos, I., et al. (2010). Encoding of conditioned fear in central amygdala inhibitory circuits. Nature 468, 277–282. doi: 10.1038/nature09559
Clem, R. L., and Huganir, R. L. (2013). Norepinephrine enhances a discrete form of long-term depression during fear memory storage. J. Neurosci. 33, 11825–11832. doi: 10.1523/JNEUROSCI.3317-12.2013
Clewett, D., Huang, R., Velasco, R., Lee, T.-H., and Mather, M. (2018). Locus coeruleus activity strengthens prioritized memories under arousal. J. Neurosci. 38, 1558–1574. doi: 10.1523/JNEUROSCI.2097-17.2017
Cole, B. J., and Koob, G. F. (1988). Propranolol antagonizes the enhanced conditioned fear produced by corticotropin releasing factor. J. Pharmacol. Exp. Ther. 247, 902–910.
Cole, B. J., and Robbins, T. W. (1987). Dissociable effects of lesions to the dorsal or ventral noradrenergic bundle on the acquisition, performance, and extinction of aversive conditioning. Behav. Neurosci. 101, 476–488. doi: 10.1037/0735-7044.101.4.476
Connor, D. F., Grasso, D. J., Slivinsky, M. D., Pearson, G. S., and Banga, A. (2013). An open-label study of guanfacine extended release for traumatic stress related symptoms in children and adolescents. J. Child Adolesc. Psychopharmacol. 23, 244–251. doi: 10.1089/cap.2012.0119
Dahl, D., and Sarvey, J. M. (1989). Norepinephrine induces pathway-specific long-lasting potentiation and depression in the hippocampal dentate gyrus. Proc. Natl. Acad. Sci. U S A 86, 4776–4780. doi: 10.1073/pnas.86.12.4776
Dahlström, A., and Fuxe, K. (1964). Localization of monoamines in the lower brain stem. Experientia 20, 398–399. doi: 10.1007/bf02147990
Davies, M. F., Tsui, J. Y., Flannery, J. A., Li, X., DeLorey, T. M., and Hoffman, B. B. (2003). Augmentation of the noradrenergic system in α-2 adrenergic receptor deficient mice: anatomical changes associated with enhanced fear memory. Brain Res. 986, 157–165. doi: 10.1016/s0006-8993(03)03248-7
Davies, M. F., Tsui, J. Y., Flannery, J. A., Li, X., DeLorey, T. M., and Hoffman, B. B. (2004). Activation of α2 adrenergic receptors suppresses fear conditioning: expression of c-Fos and phosphorylated CREB in mouse amygdala. Neuropsychopharmacology 29, 229–239. doi: 10.1038/sj.npp.1300324
Davis, M., Redmond, D. E., and Baraban, J. M. (1979). Noradrenergic agonists and antagonists: effects on conditioned fear as measured by the potentiated startle paradigm. Psychopharmacology 65, 111–118. doi: 10.1007/bf00433036
Davis, L. L., Ward, C., Rasmusson, A., Newell, J. M., Frazier, E., and Southwick, S. M. (2008). A placebo-controlled trial of guanfacine for the treatment of posttraumatic stress disorder in veterans. Psychopharmacol. Bull. 41, 8–18.
Day, H. E., Campeau, S., Watson, S. J., and Akil, H. (1997). Distribution of α1a-, α1b- and α1d-adrenergic receptor mRNA in the rat brain and spinal cord. J. Chem. Neuroanat. 13, 115–139. doi: 10.1016/S0891-0618(97)00042-2
de Dassel, T., Wittmann, L., Protic, S., Höllmer, H., and Gorzka, R. J. (2017). Association of posttraumatic nightmares and psychopathology in a military sample. Psychol. Trauma doi: 10.1037/tra0000319 [Epub ahead of print].
Dębiec, J., Bush, D. E. A., and LeDoux, J. E. (2011). Noradrenergic enhancement of reconsolidation in the amygdala impairs extinction of conditioned fear in rats–a possible mechanism for the persistence of traumatic memories in PTSD. Depress. Anxiety 28, 186–193. doi: 10.1002/da.20803
Dębiec, J., and Ledoux, J. E. (2004). Disruption of reconsolidation but not consolidation of auditory fear conditioning by noradrenergic blockade in the amygdala. Neuroscience 129, 267–272. doi: 10.1016/j.neuroscience.2004.08.018
Dejean, C., Courtin, J., Rozeske, R. R., Bonnet, M. C., Dousset, V., Michelet, T., et al. (2015). Neuronal circuits for fear expression and recovery: recent advances and potential therapeutic strategies. Biol. Psychiatry 78, 298–306. doi: 10.1016/j.biopsych.2015.03.017
Descarries, L., and Lapierre, Y. (1973). Noradrenergic axon terminals in the cerebral cortex of rat. I. Radioautographic visualization after topical application of dl-[3H]norepinephrine. Brain Res. 51, 141–160. doi: 10.1016/0006-8993(73)90369-7
Descarries, L., Watkins, K. C., and Lapierre, Y. (1977). Noradrenergic axon terminals in the cerebral cortex of rat: III. Topometric ultrastructural analysis. Brain Res. 133, 197–222. doi: 10.1016/0006-8993(77)90759-4
Detweiler, M. B., Pagadala, B., Candelario, J., Boyle, J. S., Detweiler, J. G., and Lutgens, B. W. (2016). Treatment of post-traumatic stress disorder nightmares at a veterans affairs medical center. J. Clin. Med. 5:E117. doi: 10.3390/jcm5120117
Díaz-Mataix, L., Piper, W. T., Schiff, H. C., Roberts, C. H., Campese, V. D., Sears, R. M., et al. (2017). Characterization of the amplificatory effect of norepinephrine in the acquisition of Pavlovian threat associations. Learn. Mem. 24, 432–439. doi: 10.1101/lm.044412.116
Do-Monte, F. H. M., Kincheski, G. C., Pavesi, E., Sordi, R., Assreuy, J., and Carobrez, A. P. (2010). Role of β-adrenergic receptors in the ventromedial prefrontal cortex during contextual fear extinction in rats. Neurobiol. Learn. Mem. 94, 318–328. doi: 10.1016/j.nlm.2010.07.004
Domyancic, A. V., and Morilak, D. A. (1997). Distribution of α1A adrenergic receptor mRNA in the rat brain visualized by in situ hybridization. J. Comp. Neurol. 386, 358–378. doi: 10.1002/(sici)1096-9861(19970929)386:3<358::aid-cne3>3.0.co;2-0
Dunsmoor, J. E., Otto, A. R., and Phelps, E. A. (2017). Stress promotes generalization of older but not recent threat memories. Proc. Natl. Acad. Sci. U S A 114, 9218–9223. doi: 10.1073/pnas.1704428114
Dunwiddie, T. V., Taylor, M., Heginbotham, L. R., and Proctor, W. R. (1992). Long-term increases in excitability in the CA1 region of rat hippocampus induced by β-adrenergic stimulation: possible mediation by cAMP. J. Neurosci. 12, 506–517.
Duvarci, S., Mamou, C. B., and Nader, K. (2006). Extinction is not a sufficient condition to prevent fear memories from undergoing reconsolidation in the basolateral amygdala. Eur. J. Neurosci. 24, 249–260. doi: 10.1111/j.1460-9568.2006.04907.x
Duvarci, S., Nader, K., and LeDoux, J. E. (2008). De novo mRNA synthesis is required for both consolidation and reconsolidation of fear memories in the amygdala. Learn. Mem. 15, 747–755. doi: 10.1101/lm.1027208
Fadok, J. P., Krabbe, S., Markovic, M., Courtin, J., Xu, C., Massi, L., et al. (2017). A competitive inhibitory circuit for selection of active and passive fear responses. Nature 542, 96–100. doi: 10.1038/nature21047
Fallon, J. H., Koziell, D. A., and Moore, R. Y. (1978). Catecholamine innervation of the basal forebrain. II. Amygdala, suprarhinal cortex and entorhinal cortex. J. Comp. Neurol. 180, 509–532. doi: 10.1002/cne.901800308
Farb, C. R., Chang, W., and Ledoux, J. E. (2010). Ultrastructural characterization of noradrenergic axons and β-adrenergic receptors in the lateral nucleus of the amygdala. Front. Behav. Neurosci. 4:162. doi: 10.3389/fnbeh.2010.00162
Ferry, B., Roozendaal, B., and McGaugh, J. L. (1999a). Basolateral amygdala noradrenergic influences on memory storage are mediated by an interaction between β- and α1-adrenoceptors. J. Neurosci. 19, 5119–5123.
Ferry, B., Roozendaal, B., and McGaugh, J. L. (1999b). Role of norepinephrine in mediating stress hormone regulation of long-term memory storage: a critical involvement of the amygdala. Biol. Psychiatry 46, 1140–1152. doi: 10.1016/s0006-3223(99)00157-2
Finlay, J. M., Zigmond, M. J., and Abercrombie, E. D. (1995). Increased dopamine and norepinephrine release in medial prefrontal cortex induced by acute and chronic stress: effects of diazepam. Neuroscience 64, 619–628. doi: 10.1016/0306-4522(94)00331-x
Fitzgerald, P. J., Giustino, T. F., Seemann, J. R., and Maren, S. (2015). Noradrenergic blockade stabilizes prefrontal activity and enables fear extinction under stress. Proc. Natl. Acad. Sci. U S A 112, E3729–E3737. doi: 10.1073/pnas.1500682112
Fitzgerald, P. J., Seemann, J. R., and Maren, S. (2014). Can fear extinction be enhanced? A review of pharmacological and behavioral findings. Brain Res. Bull. 105, 46–60. doi: 10.1016/j.brainresbull.2013.12.007
Foote, S. L., Bloom, F. E., and Aston-Jones, G. (1983). Nucleus locus ceruleus: new evidence of anatomical and physiological specificity. Physiol. Rev. 63, 844–914. doi: 10.1152/physrev.1983.63.3.844
Foy, M. R., Stanton, M. E., Levine, S., and Thompson, R. F. (1987). Behavioral stress impairs long-term potentiation in rodent hippocampus. Behav. Neural Biol. 48, 138–149. doi: 10.1016/s0163-1047(87)90664-9
Frankland, P. W., Josselyn, S. A., Anagnostaras, S. G., Kogan, J. H., Takahashi, E., and Silva, A. J. (2004). Consolidation of CS and US representations in associative fear conditioning. Hippocampus 14, 557–569. doi: 10.1002/hipo.10208
Galvez, R., Mesches, M. H., and McGaugh, J. L. (1996). Norepinephrine release in the amygdala in response to footshock stimulation. Neurobiol. Learn. Mem. 66, 253–257. doi: 10.1006/nlme.1996.0067
Gamache, K., Pitman, R. K., and Nader, K. (2012). Preclinical evaluation of reconsolidation blockade by clonidine as a potential novel treatment for posttraumatic stress disorder. Neuropsychopharmacology 37, 2789–2796. doi: 10.1038/npp.2012.145
Gazarini, L., Stern, C. A. J., Carobrez, A. P., and Bertoglio, L. J. (2013). Enhanced noradrenergic activity potentiates fear memory consolidation and reconsolidation by differentially recruiting α1- and β-adrenergic receptors. Learn. Mem. 20, 210–219. doi: 10.1101/lm.030007.112
Gazarini, L., Stern, C. A. J., Piornedo, R. R., Takahashi, R. N., and Bertoglio, L. J. (2014). PTSD-like memory generated through enhanced noradrenergic activity is mitigated by a dual step pharmacological intervention targeting its reconsolidation. Int. J. Neuropsychopharmacol. 18:pyu026. doi: 10.1093/ijnp/pyu026
George, S. A., Knox, D., Curtis, A. L., Aldridge, J. W., Valentino, R. J., and Liberzon, I. (2013). Altered locus coeruleus-norepinephrine function following single prolonged stress. Eur. J. Neurosci. 37, 901–909. doi: 10.1111/ejn.12095
Geracioti, T. D., Baker, D. G., Ekhator, N. N., West, S. A., Hill, K. K., Bruce, A. B., et al. (2001). CSF norepinephrine concentrations in posttraumatic stress disorder. Am. J. Psychiatry 158, 1227–1230. doi: 10.1176/appi.ajp.158.8.1227
Gerfen, C. R., and Clavier, R. M. (1979). Neural inputs to the prefrontal agranular insular cortex in the rat: horseradish peroxidase study. Brain Res. Bull. 4, 347–353. doi: 10.1016/s0361-9230(79)80012-x
Girotti, M., Adler, S. M., Bulin, S. E., Fucich, E. A., Paredes, D., and Morilak, D. A. (2017). Prefrontal cortex executive processes affected by stress in health and disease. Prog. Neuropsychopharmacol. Biol. Psychiatry doi: 10.1016/j.pnpbp.2017.07.004 [Epub ahead of print].
Giustino, T. F., Fitzgerald, P. J., and Maren, S. (2016a). Fear expression suppresses medial prefrontal cortical firing in rats. PLoS One 11:e0165256. doi: 10.1371/journal.pone.0165256
Giustino, T. F., Fitzgerald, P. J., and Maren, S. (2016b). Revisiting propranolol and PTSD: memory erasure or extinction enhancement? Neurobiol. Learn. Mem. 130, 26–33. doi: 10.1016/j.nlm.2016.01.009
Giustino, T. F., and Maren, S. (2015). The role of the medial prefrontal cortex in the conditioning and extinction of fear. Front. Behav. Neurosci. 9:298. doi: 10.3389/fnbeh.2015.00298
Giustino, T. F., Seemann, J. R., Acca, G. M., Goode, T. D., Fitzgerald, P. J., and Maren, S. (2017). β-adrenoceptor blockade in the basolateral amygdala, but not the medial prefrontal cortex, rescues the immediate extinction deficit. Neuropsychopharmacology 42, 2537–2544. doi: 10.1038/npp.2017.89
Goldstein, L. E., Rasmusson, A. M., Bunney, B. S., and Roth, R. H. (1996). Role of the amygdala in the coordination of behavioral, neuroendocrine, and prefrontal cortical monoamine responses to psychological stress in the rat. J. Neurosci. 16, 4787–4798.
Goosens, K. A., and Maren, S. (2003). Pretraining NMDA receptor blockade in the basolateral complex, but not the central nucleus, of the amygdala prevents savings of conditional fear. Behav. Neurosci. 117, 738–750. doi: 10.1037/0735-7044.117.4.738
Gresch, P. J., Sved, A. F., Zigmond, M. J., and Finlay, J. M. (1994). Stress-induced sensitization of dopamine and norepinephrine efflux in medial prefrontal cortex of the rat. J. Neurochem. 63, 575–583. doi: 10.1046/j.1471-4159.1994.63020575.x
Grillon, C., Cordova, J., Morgan, C. A., Charney, D. S., and Davis, M. (2004). Effects of the β-blocker propranolol on cued and contextual fear conditioning in humans. Psychopharmacology 175, 342–352. doi: 10.1007/s00213-004-1819-5
Grzanna, R., and Molliver, M. E. (1980). The locus coeruleus in the rat: an immunohistochemical delineation. Neuroscience 5, 21–40. doi: 10.1016/0306-4522(80)90068-8
Guthrie, R. M., and Bryant, R. A. (2006). Extinction learning before trauma and subsequent posttraumatic stress. Psychosom. Med. 68, 307–311. doi: 10.1097/01.psy.0000208629.67653.cc
Hajós-Korcsok, E., Robinson, D. D., Yu, J. H., Fitch, C. S., Walker, E., and Merchant, K. M. (2003). Rapid habituation of hippocampal serotonin and norepinephrine release and anxiety-related behaviors, but not plasma corticosterone levels, to repeated footshock stress in rats. Pharmacol. Biochem. Behav. 74, 609–616. doi: 10.1016/s0091-3057(02)01047-x
Hansen, N. (2017). The longevity of hippocampus-dependent memory is orchestrated by the locus coeruleus-noradrenergic system. Neural Plast. 2017:2727602. doi: 10.1155/2017/2727602
Harley, C. W. (2007). Norepinephrine and the dentate gyrus. Prog. Brain Res. 163, 299–318. doi: 10.1016/s0079-6123(07)63018-0
Harmon, R. J., and Riggs, P. D. (1996). Clonidine for posttraumatic stress disorder in preschool children. J. Am. Acad. Child Adolesc. Psychiatry 35, 1247–1249. doi: 10.1097/00004583-199609000-00022
Hartley, C. A., Gorun, A., Reddan, M. C., Ramirez, F., and Phelps, E. A. (2014). Stressor controllability modulates fear extinction in humans. Neurobiol. Learn. Mem. 113, 149–156. doi: 10.1016/j.nlm.2013.12.003
Hatfield, T., Spanis, C., and McGaugh, J. L. (1999). Response of amygdalar norepinephrine to footshock and GABAergic drugs using in vivo microdialysis and HPLC. Brain Res. 835, 340–345. doi: 10.1016/s0006-8993(99)01566-8
Haubensak, W., Kunwar, P. S., Cai, H., Ciocchi, S., Wall, N. R., Ponnusamy, R., et al. (2010). Genetic dissection of an amygdala microcircuit that gates conditioned fear. Nature 468, 270–276. doi: 10.1038/nature09553
Hefner, K., Whittle, N., Juhasz, J., Norcross, M., Karlsson, R.-M., Saksida, L. M., et al. (2008). Impaired fear extinction learning and cortico-amygdala circuit abnormalities in a common genetic mouse strain. J. Neurosci. 28, 8074–8085. doi: 10.1523/JNEUROSCI.4904-07.2008
Heroux, N. A., Robinson-Drummer, P. A., Sanders, H. R., Rosen, J. B., and Stanton, M. E. (2017). Differential involvement of the medial prefrontal cortex across variants of contextual fear conditioning. Learn. Mem. 24, 322–330. doi: 10.1101/lm.045286.117
Herry, C., Ferraguti, F., Singewald, N., Letzkus, J. J., Ehrlich, I., and Lüthi, A. (2010). Neuronal circuits of fear extinction. Eur. J. Neurosci. 31, 599–612. doi: 10.1111/j.1460-9568.2010.07101.x
Herry, C., and Johansen, J. P. (2014). Encoding of fear learning and memory in distributed neuronal circuits. Nat. Neurosci. 17, 1644–1654. doi: 10.1038/nn.3869
Hirschberg, S., Li, Y., Randall, A., Kremer, E. J., and Pickering, A. E. (2017). Functional dichotomy in spinal- vs. prefrontal-projecting locus coeruleus modules splits descending noradrenergic analgesia from ascending aversion and anxiety in rats. Elife 6:e29808. doi: 10.7554/elife.29808
Hollis, F., Sevelinges, Y., Grosse, J., Zanoletti, O., and Sandi, C. (2016). Involvement of CRFR1 in the basolateral amygdala in the immediate fear extinction deficit. eNeuro 3:ENEURO.0084-16.2016. doi: 10.1523/ENEURO.0084-16.2016
Holmes, A., and Quirk, G. J. (2010). Pharmacological facilitation of fear extinction and the search for adjunct treatments for anxiety disorders—the case of yohimbine. Trends Pharmacol. Sci. 31, 2–7. doi: 10.1016/j.tips.2009.10.003
Holmes, N. M., Crane, J. W., Tang, M., Fam, J., Westbrook, R. F., and Delaney, A. J. (2017). α2-adrenoceptor-mediated inhibition in the central amygdala blocks fear-conditioning. Sci. Rep. 7:11712. doi: 10.1038/s41598-017-12115-x
Homan, P., Lin, Q., Murrough, J. W., Soleimani, L., Bach, D. R., Clem, R. L., et al. (2017). Prazosin during threat discrimination boosts memory of the safe stimulus. Learn. Mem. 24, 597–601. doi: 10.1101/lm.045898.117
Hopkins, W. F., and Johnston, D. (1988). Noradrenergic enhancement of long-term potentiation at mossy fiber synapses in the hippocampus. J. Neurophysiol. 59, 667–687. doi: 10.1152/jn.1988.59.2.667
Horrigan, J. P., and Barnhill, L. J. (1996). The suppression of nightmares with guanfacine. J. Clin. Psychiatry 57:371.
Hott, S. C., Gomes, F. V., Fabri, D. R. S., Reis, D. G., Crestani, C. C., Côrrea, F. M. A., et al. (2012). Both α1- and β1-adrenoceptors in the bed nucleus of the stria terminalis are involved in the expression of conditioned contextual fear. Br. J. Pharmacol. 167, 207–221. doi: 10.1111/j.1476-5381.2012.01985.x
Hu, H., Real, E., Takamiya, K., Kang, M.-G., Ledoux, J., Huganir, R. L., et al. (2007). Emotion enhances learning via norepinephrine regulation of AMPA-receptor trafficking. Cell 131, 160–173. doi: 10.1016/j.cell.2007.09.017
Inoue, T., Nakagawa, S., Izumi, T., Kitaichi, Y., and Koyama, T. (2006). Effect of combined treatment with noradrenaline and serotonin reuptake inhibitors on conditioned freezing. Eur. J. Pharmacol. 540, 91–95. doi: 10.1016/j.ejphar.2006.08.020
Ishida, Y., Hashiguchi, H., Takeda, R., Ishizuka, Y., Mitsuyama, Y., Kannan, H., et al. (2002). Conditioned-fear stress increases Fos expression in monoaminergic and GABAergic neurons of the locus coeruleus and dorsal raphe nuclei. Synapse 45, 46–51. doi: 10.1002/syn.10086
Ishimatsu, M., and Williams, J. T. (1996). Synchronous activity in locus coeruleus results from dendritic interactions in pericoerulear regions. J. Neurosci. 16, 5196–5204.
Ishizuka, Y., Ishida, Y., Jin, Q., Kato, K., Kunitake, T., Mitsuyama, Y., et al. (2000). Differential profiles of nitric oxide and norepinephrine releases in the paraventricular nucleus region in response to mild footshock in rats. Brain Res. 862, 17–25. doi: 10.1016/s0006-8993(00)02061-8
Izumi, Y., and Zorumski, C. F. (1999). Norepinephrine promotes long-term potentiation in the adult rat hippocampus in vitro. Synapse 31, 196–202. doi: 10.1002/(sici)1098-2396(19990301)31:3<196::aid-syn4>3.0.co;2-k
Ji, J.-Z., Wang, X.-M., and Li, B.-M. (2003). Deficit in long-term contextual fear memory induced by blockade of β-adrenoceptors in hippocampal CA1 region. Eur. J. Neurosci. 17, 1947–1952. doi: 10.1046/j.1460-9568.2003.02620.x
Jin, J., and Maren, S. (2015). Prefrontal-hippocampal interactions in memory and emotion. Front. Syst. Neurosci. 9:170. doi: 10.3389/fnsys.2015.00170
Jodo, E., Chiang, C., and Aston-Jones, G. (1998). Potent excitatory influence of prefrontal cortex activity on noradrenergic locus coeruleus neurons. Neuroscience 83, 63–79. doi: 10.1016/s0306-4522(97)00372-2
Johansen, J. P., Cain, C. K., Ostroff, L. E., and LeDoux, J. E. (2011). Molecular mechanisms of fear learning and memory. Cell 147, 509–524. doi: 10.1016/j.cell.2011.10.009
Johnson, L. R., Hou, M., Prager, E. M., and Ledoux, J. E. (2011). Regulation of the fear network by mediators of stress: norepinephrine alters the balance between cortical and subcortical afferent excitation of the lateral amygdala. Front. Behav. Neurosci. 5:23. doi: 10.3389/fnbeh.2011.00023
Johnson, R. D., and Minneman, K. P. (1985). α1-adrenergic receptors and stimulation of [3H]inositol metabolism in rat brain: regional distribution and parallel inactivation. Brain Res. 341, 7–15. doi: 10.1016/0006-8993(85)91466-0
Jones, L. S., Gauger, L. L., and Davis, J. N. (1985). Anatomy of brain α 1-adrenergic receptors: in vitro autoradiography with [125I]-heat. J. Comp. Neurol. 231, 190–208. doi: 10.1002/cne.902310207
Jones, B. E., and Moore, R. Y. (1977). Ascending projections of the locus coeruleus in the rat. II. Autoradiographic study. Brain Res. 127, 25–53. doi: 10.1016/0006-8993(77)90378-x
Jones, B. E., and Yang, T. Z. (1985). The efferent projections from the reticular formation and the locus coeruleus studied by anterograde and retrograde axonal transport in the rat. J. Comp. Neurol. 242, 56–92. doi: 10.1002/cne.902420105
Katsuki, H., Izumi, Y., and Zorumski, C. F. (1997). Noradrenergic regulation of synaptic plasticity in the hippocampal CA1 region. J. Neurophysiol. 77, 3013–3020. doi: 10.1152/jn.1997.77.6.3013
Keeshin, B. R., Ding, Q., Presson, A. P., Berkowitz, S. J., and Strawn, J. R. (2017). Use of prazosin for pediatric PTSD-associated nightmares and sleep disturbances: a retrospective chart review. Neurol. Ther. 6, 247–257. doi: 10.1007/s40120-017-0078-4
Keren, N. I., Lozar, C. T., Harris, K. C., Morgan, P. S., and Eckert, M. A. (2009). In vivo mapping of the human locus coeruleus. Neuroimage 47, 1261–1267. doi: 10.1016/j.neuroimage.2009.06.012
Kessler, R. C., Berglund, P., Demler, O., Jin, R., Merikangas, K. R., and Walters, E. E. (2005). Lifetime prevalence and age-of-onset distributions of DSM-IV disorders in the National Comorbidity Survey Replication. Arch. Gen. Psychiatry 62, 593–602. doi: 10.1001/archpsyc.62.6.593
Kessler, R. C., Sonnega, A., Bromet, E., Hughes, M., and Nelson, C. B. (1995). Posttraumatic stress disorder in the National Comorbidity Survey. Arch. Gen. Psychiatry 52, 1048–1060. doi: 10.1001/archpsyc.1995.03950240066012
Kim, S. C., Jo, Y. S., Kim, I. H., Kim, H., and Choi, J.-S. (2010). Lack of medial prefrontal cortex activation underlies the immediate extinction deficit. J. Neurosci. 30, 832–837. doi: 10.1523/JNEUROSCI.4145-09.2010
Kim, J. J., Shih, J. C., Chen, K., Chen, L., Bao, S., Maren, S., et al. (1997). Selective enhancement of emotional, but not motor, learning in monoamine oxidase A-deficient mice. Proc. Natl. Acad. Sci. U S A 94, 5929–5933. doi: 10.1073/pnas.94.11.5929
Kindt, M., Soeter, M., and Vervliet, B. (2009). Beyond extinction: erasing human fear responses and preventing the return of fear. Nat. Neurosci. 12, 256–258. doi: 10.1038/nn.2271
Kinzie, J. D., and Leung, P. (1989). Clonidine in Cambodian patients with posttraumatic stress disorder. J. Nerv. Ment. Dis. 177, 546–550. doi: 10.1097/00005053-198909000-00005
Knapska, E., and Maren, S. (2009). Reciprocal patterns of c-Fos expression in the medial prefrontal cortex and amygdala after extinction and renewal of conditioned fear. Learn. Mem. 16, 486–493. doi: 10.1101/lm.1463909
Kobayashi, K., and Kobayashi, T. (2001). Genetic evidence for noradrenergic control of long-term memory consolidation. Brain Dev. 23, S16–S23. doi: 10.1016/s0387-7604(01)00329-1
Kobayashi, K., Noda, Y., Matsushita, N., Nishii, K., Sawada, H., Nagatsu, T., et al. (2000). Modest neuropsychological deficits caused by reduced noradrenaline metabolism in mice heterozygous for a mutated tyrosine hydroxylase gene. J. Neurosci. 20, 2418–2426.
Koenen, K. C., Stellman, S. D., Sommer, J. F. Jr., and Stellman, J. M. (2008). Persisting posttraumatic stress disorder symptoms and their relationship to functioning in Vietnam veterans: a 14-year follow-up. J. Trauma. Stress 21, 49–57. doi: 10.1002/jts.20304
Koola, M. M., Varghese, S. P., and Fawcett, J. A. (2014). High-dose prazosin for the treatment of post-traumatic stress disorder. Ther. Adv. Psychopharmacol. 4, 43–47. doi: 10.1177/2045125313500982
Korf, J., Aghajanian, G. K., and Roth, R. H. (1973). Increased turnover of norepinephrine in the rat cerebral cortex during stress: role of the locus coeruleus. Neuropharmacology 12, 933–938. doi: 10.1016/0028-3908(73)90024-5
Kosten, T. R., Mason, J. W., Giller, E. L., Ostroff, R. B., and Harkness, L. (1987). Sustained urinary norepinephrine and epinephrine elevation in post-traumatic stress disorder. Psychoneuroendocrinology 12, 13–20. doi: 10.1016/0306-4530(87)90017-5
Krebs, R. M., Park, H. R. P., Bombeke, K., and Boehler, C. N. (2017). Modulation of locus coeruleus activity by novel oddball stimuli. Brain Imaging Behav. doi: 10.1007/s11682-017-9700-4 [Epub ahead of print].
Kroes, M. C. W., Schiller, D., LeDoux, J. E., and Phelps, E. A. (2016a). Translational approaches targeting reconsolidation. Curr. Top. Behav. Neurosci. 28, 197–230. doi: 10.1007/7854_2015_5008
Kroes, M. C. W., Tona, K.-D., den Ouden, H. E. M., Vogel, S., van Wingen, G. A., and Fernández, G. (2016b). How administration of the β-blocker propranolol before extinction can prevent the return of fear. Neuropsychopharmacology 41, 1569–1578. doi: 10.1038/npp.2015.315
Krugers, H. J., Zhou, M., Joëls, M., and Kindt, M. (2011). Regulation of excitatory synapses and fearful memories by stress hormones. Front. Behav. Neurosci. 5:62. doi: 10.3389/fnbeh.2011.00062
Lacaille, J. C., and Harley, C. W. (1985). The action of norepinephrine in the dentate gyrus: β-mediated facilitation of evoked potentials in vitro. Brain Res. 358, 210–220. doi: 10.1016/0006-8993(85)90965-5
Laitman, B. M., Gajewski, N. D., Mann, G. L., Kubin, L., Morrison, A. R., and Ross, R. J. (2014). The α1 adrenoceptor antagonist prazosin enhances sleep continuity in fear-conditioned Wistar-Kyoto rats. Prog. Neuropsychopharmacol. Biol. Psychiatry 49, 7–15. doi: 10.1016/j.pnpbp.2013.11.004
LaLumiere, R. T., Buen, T.-V., and McGaugh, J. L. (2003). Post-training intra-basolateral amygdala infusions of norepinephrine enhance consolidation of memory for contextual fear conditioning. J. Neurosci. 23, 6754–6758.
Langley, J., Huddleston, D. E., Liu, C. J., and Hu, X. (2017). Reproducibility of locus coeruleus and substantia nigra imaging with neuromelanin sensitive MRI. MAGMA 30, 121–125. doi: 10.1007/s10334-016-0590-z
Lapierre, Y., Beaudet, A., Demianczuk, N., and Descarries, L. (1973). Noradrenergic axon terminals in the cerebral cortex of rat. II. Quantitative data revealed by light and electron microscope radioautography of the frontal cortex. Brain Res. 63, 175–182. doi: 10.1016/0006-8993(73)90086-3
Lazzaro, S. C., Hou, M., Cunha, C., LeDoux, J. E., and Cain, C. K. (2010). Antagonism of lateral amygdala α1-adrenergic receptors facilitates fear conditioning and long-term potentiation. Learn. Mem. 17, 489–493. doi: 10.1101/lm.1918210
LeDoux, J. E. (2000). Emotion circuits in the brain. Annu. Rev. Neurosci. 23, 155–184. doi: 10.1146/annurev.neuro.23.1.155
Lee, H. J., Berger, S. Y., Stiedl, O., Spiess, J., and Kim, J. J. (2001). Post-training injections of catecholaminergic drugs do not modulate fear conditioning in rats and mice. Neurosci. Lett. 303, 123–126. doi: 10.1016/s0304-3940(01)01733-5
Li, Y., Hickey, L., Perrins, R., Werlen, E., Patel, A. A., Hirschberg, S., et al. (2016). Retrograde optogenetic characterization of the pontospinal module of the locus coeruleus with a canine adenoviral vector. Brain Res. 1641, 274–290. doi: 10.1016/j.brainres.2016.02.023
Lim, E. P., Dawe, G. S., and Jay, T. M. (2017). Activation of β- and α-2-adrenoceptors in the basolateral amygdala has opposing effects on hippocampal-prefrontal long-term potentiation. Neurobiol. Learn. Mem. 137, 163–170. doi: 10.1016/j.nlm.2016.11.020
Lin, C.-C., Tung, C.-S., Lin, P.-H., Huang, C.-L., and Liu, Y.-P. (2016). Traumatic stress causes distinctive effects on fear circuit catecholamines and the fear extinction profile in a rodent model of posttraumatic stress disorder. Eur. Neuropsychopharmacol. 26, 1484–1495. doi: 10.1016/j.euroneuro.2016.06.004
Lissek, S., Powers, A. S., McClure, E. B., Phelps, E. A., Woldehawariat, G., Grillon, C., et al. (2005). Classical fear conditioning in the anxiety disorders: a meta-analysis. Behav. Res. Ther. 43, 1391–1424. doi: 10.1016/j.brat.2004.10.007
Liu, X., Lonart, G., and Sanford, L. D. (2007). Transient fear-induced alterations in evoked release of norepinephrine and GABA in amygdala slices. Brain Res. 1142, 46–53. doi: 10.1016/j.brainres.2007.01.038
Lonergan, M. H., Olivera-Figueroa, L. A., Pitman, R. K., and Brunet, A. (2013). Propranolol’s effects on the consolidation and reconsolidation of long-term emotional memory in healthy participants: a meta-analysis. J. Psychiatry Neurosci. 38, 222–231. doi: 10.1503/jpn.120111
Loughlin, S. E., Foote, S. L., and Bloom, F. E. (1986). Efferent projections of nucleus locus coeruleus: topographic organization of cells of origin demonstrated by three-dimensional reconstruction. Neuroscience 18, 291–306. doi: 10.1016/0306-4522(86)90155-7
Loughlin, S. E., Foote, S. L., and Fallon, J. H. (1982). Locus coeruleus projections to cortex: topography, morphology and collateralization. Brain Res. Bull. 9, 287–294. doi: 10.1016/0361-9230(82)90142-3
MacDonald, E., Kobilka, B. K., and Scheinin, M. (1997). Gene targeting—homing in on α2-adrenoceptor-subtype function. Trends Pharmacol. Sci. 18, 211–219. doi: 10.1016/s0165-6147(97)01063-8
MacPherson, K., Whittle, N., Camp, M., Gunduz-Cinar, O., Singewald, N., and Holmes, A. (2013). Temporal factors in the extinction of fear in inbred mouse strains differing in extinction efficacy. Biol. Mood Anxiety Disord. 3:13. doi: 10.1186/2045-5380-3-13
Maren, S. (2001). Neurobiology of Pavlovian fear conditioning. Annu. Rev. Neurosci. 24, 897–931. doi: 10.1146/annurev.neuro.24.1.897
Maren, S. (2011). Seeking a spotless mind: extinction, deconsolidation, and erasure of fear memory. Neuron 70, 830–845. doi: 10.1016/j.neuron.2011.04.023
Maren, S. (2014). Nature and causes of the immediate extinction deficit: a brief review. Neurobiol. Learn. Mem. 113, 19–24. doi: 10.1016/j.nlm.2013.10.012
Maren, S., and Chang, C. (2006). Recent fear is resistant to extinction. Proc. Natl. Acad. Sci. U S A 103, 18020–18025. doi: 10.1073/pnas.0608398103
Maren, S., and Holmes, A. (2016). Stress and fear extinction. Neuropsychopharmacology 41, 58–79. doi: 10.1038/npp.2015.180
Maren, S., Phan, K. L., and Liberzon, I. (2013). The contextual brain: implications for fear conditioning, extinction and psychopathology. Nat. Rev. Neurosci. 14, 417–428. doi: 10.1038/nrn3492
Maren, S., and Quirk, G. J. (2004). Neuronal signalling of fear memory. Nat. Rev. Neurosci. 5, 844–852. doi: 10.1038/nrn1535
Marshall, I., Burt, R. P., and Chapple, C. R. (1999). Signal transduction pathways associated with α1-adrenoceptor subtypes in cells and tissues including human prostate. Eur. Urol. 36, 42–47; discussion 65. doi: 10.1159/000052317
Mason, S. T., and Fibiger, H. (1979). Noradrenaline, fear and extinction. Brain Res. 165, 47–56. doi: 10.1016/0006-8993(79)90043-x
Mason, S. T., Roberts, D. C., and Fibiger, H. C. (1979). Interaction of brain noradrenaline and the pituitary-adrenal axis in learning and extinction. Pharmacol. Biochem. Behav. 10, 11–16. doi: 10.1016/0091-3057(79)90161-8
McCall, J. G., Al-Hasani, R., Siuda, E. R., Hong, D. Y., Norris, A. J., Ford, C. P., et al. (2015). CRH engagement of the locus coeruleus noradrenergic system mediates stress-induced anxiety. Neuron 87, 605–620. doi: 10.1016/j.neuron.2015.07.002
McCall, J. G., Siuda, E. R., Bhatti, D. L., Lawson, L. A., McElligott, Z. A., Stuber, G. D., et al. (2017). Locus coeruleus to basolateral amygdala noradrenergic projections promote anxiety-like behavior. Elife 6:e18247. doi: 10.7554/eLife.18247
McCune, S. K., Voigt, M. M., and Hill, J. M. (1993). Expression of multiple α adrenergic receptor subtype messenger RNAs in the adult rat brain. Neuroscience 57, 143–151. doi: 10.1016/0306-4522(93)90116-w
McGaugh, J. L. (2000). Memory—a century of consolidation. Science 287, 248–251. doi: 10.1126/science.287.5451.248
McGaugh, J. L. (2004). The amygdala modulates the consolidation of memories of emotionally arousing experiences. Annu. Rev. Neurosci. 27, 1–28. doi: 10.1146/annurev.neuro.27.070203.144157
McGaugh, J. L., and Roozendaal, B. (2002). Role of adrenal stress hormones in forming lasting memories in the brain. Curr. Opin. Neurobiol. 12, 205–210. doi: 10.1016/s0959-4388(02)00306-9
McGhee, L. L., Maani, C. V., Garza, T. H., Desocio, P. A., Gaylord, K. M., and Black, I. H. (2009). The effect of propranolol on posttraumatic stress disorder in burned service members. J. Burn Care Res. 30, 92–97. doi: 10.1097/BCR.0b013e3181921f51
Merz, C. J., Hamacher-Dang, T. C., and Wolf, O. T. (2016). Immediate extinction promotes the return of fear. Neurobiol. Learn. Mem. 131, 109–116. doi: 10.1016/j.nlm.2016.03.013
Milad, M. R., Orr, S. P., Lasko, N. B., Chang, Y., Rauch, S. L., and Pitman, R. K. (2008). Presence and acquired origin of reduced recall for fear extinction in PTSD: results of a twin study. J. Psychiatr. Res. 42, 515–520. doi: 10.1016/j.jpsychires.2008.01.017
Milad, M. R., and Quirk, G. J. (2012). Fear extinction as a model for translational neuroscience: ten years of progress. Annu. Rev. Psychol. 63, 129–151. doi: 10.1146/annurev.psych.121208.131631
Milad, M. R., Pitman, R. K., Ellis, C. B., Gold, A. L., Shin, L. M., Lasko, N. B., et al. (2009). Neurobiological basis of failure to recall extinction memory in posttraumatic stress disorder. Biol. Psychiatry 66, 1075–1082. doi: 10.1016/j.biopsych.2009.06.026
Miller, K. E., Brownlow, J. A., Woodward, S., and Gehrman, P. R. (2017). Sleep and dreaming in posttraumatic stress disorder. Curr. Psychiatry Rep. 19:71. doi: 10.1007/s11920-017-0827-1
Milner, T. A., Shah, P., and Pierce, J. P. (2000). β-Adrenergic receptors primarily are located on the dendrites of granule cells and interneurons but also are found on astrocytes and a few presynaptic profiles in the rat dentate gyrus. Synapse 36, 178–193. doi: 10.1002/(sici)1098-2396(20000601)36:3<178::aid-syn3>3.0.co;2-6
Moore, R. Y., and Bloom, F. E. (1979). Central catecholamine neuron systems: anatomy and physiology of the norepinephrine and epinephrine systems. Annu. Rev. Neurosci. 2, 113–168. doi: 10.1146/annurev.ne.02.030179.000553
Morilak, D. A., Barrera, G., Echevarria, D. J., Garcia, A. S., Hernandez, A., Ma, S., et al. (2005). Role of brain norepinephrine in the behavioral response to stress. Prog. Neuropsychopharmacol. Biol. Psychiatry 29, 1214–1224. doi: 10.1016/j.pnpbp.2005.08.007
Morris, R. W., and Bouton, M. E. (2007). The effect of yohimbine on the extinction of conditioned fear: a role for context. Behav. Neurosci. 121, 501–514. doi: 10.1037/0735-7044.121.3.501
Morrison, J. H., Molliver, M. E., and Grzanna, R. (1979). Noradrenergic innervation of cerebral cortex: widespread effects of local cortical lesions. Science 205, 313–316. doi: 10.1126/science.451605
Mueller, D., and Cahill, S. P. (2010). Noradrenergic modulation of extinction learning and exposure therapy. Behav. Brain Res. 208, 1–11. doi: 10.1016/j.bbr.2009.11.025
Mueller, D., Olivera-Figueroa, L. A., Pine, D. S., and Quirk, G. J. (2009). The effects of yohimbine and amphetamine on fear expression and extinction in rats. Psychopharmacology (Berl) 204, 599–606. doi: 10.1007/s00213-009-1491-x
Mueller, D., Porter, J. T., and Quirk, G. J. (2008). Noradrenergic signaling in infralimbic cortex increases cell excitability and strengthens memory for fear extinction. J. Neurosci. 28, 369–375. doi: 10.1523/JNEUROSCI.3248-07.2008
Muravieva, E. V., and Alberini, C. M. (2010). Limited efficacy of propranolol on the reconsolidation of fear memories. Learn. Mem. 17, 306–313. doi: 10.1101/lm.1794710
Murchison, C. F., Schutsky, K., Jin, S.-H., and Thomas, S. A. (2011). Norepinephrine and ß1-adrenergic signaling facilitate activation of hippocampal CA1 pyramidal neurons during contextual memory retrieval. Neuroscience 181, 109–116. doi: 10.1016/j.neuroscience.2011.02.049
Murchison, C. F., Zhang, X.-Y., Zhang, W.-P., Ouyang, M., Lee, A., and Thomas, S. A. (2004). A distinct role for norepinephrine in memory retrieval. Cell 117, 131–143. doi: 10.1016/s0092-8674(04)00259-4
Murphy, P. R., O’Connell, R. G., O’Sullivan, M., Robertson, I. H., and Balsters, J. H. (2014). Pupil diameter covaries with BOLD activity in human locus coeruleus. Hum. Brain Mapp. 35, 4140–4154. doi: 10.1002/hbm.22466
Myers, K. M., and Davis, M. (2007). Mechanisms of fear extinction. Mol. Psychiatry 12, 120–150. doi: 10.1038/sj.mp.4001939
Nader, K., Schafe, G. E., and Le Doux, J. E. (2000). Fear memories require protein synthesis in the amygdala for reconsolidation after retrieval. Nature 406, 722–726. doi: 10.1038/35021052
Naegeli, C., Zeffiro, T., Piccirelli, M., Jaillard, A., Weilenmann, A., Hassanpour, K., et al. (2018). Locus coeruleus activity mediates hyper-responsiveness in posttraumatic stress disorder. Biol. Psychiatry 83, 254–262. doi: 10.1016/j.biopsych.2017.08.021
Nagai, T., Satoh, K., Imamoto, K., and Maeda, T. (1981). Divergent projections of catecholamine neurons of the locus coeruleus as revealed by fluorescent retrograde double labeling technique. Neurosci. Lett. 23, 117–123. doi: 10.1016/0304-3940(81)90027-6
Neophytou, S. I., Aspley, S., Butler, S., Beckett, S., and Marsden, C. A. (2001). Effects of lesioning noradrenergic neurones in the locus coeruleus on conditioned and unconditioned aversive behaviour in the rat. Prog. Neuropsychopharmacol. Biol. Psychiatry 25, 1307–1321. doi: 10.1016/s0278-5846(01)00181-6
Neuman, R. S., and Harley, C. W. (1983). Long-lasting potentiation of the dentate gyrus population spike by norepinephrine. Brain Res. 273, 162–165. doi: 10.1016/0006-8993(83)91106-x
Neylan, T. C., Lenoci, M., Samuelson, K. W., Metzler, T. J., Henn-Haase, C., Hierholzer, R. W., et al. (2006). No improvement of posttraumatic stress disorder symptoms with guanfacine treatment. Am. J. Psychiatry 163, 2186–2188. doi: 10.1176/appi.ajp.163.12.2186
Nicholas, A. P., Pieribone, V. A., and Hökfelt, T. (1993a). Cellular localization of messenger RNA for β-1 and β-2 adrenergic receptors in rat brain: an in situ hybridization study. Neuroscience 56, 1023–1039. doi: 10.1016/0306-4522(93)90148-9
Nicholas, A. P., Pieribone, V., and Hökfelt, T. (1993b). Distributions of mRNAs for α-2 adrenergic receptor subtypes in rat brain: an in situ hybridization study. J. Comp. Neurol. 328, 575–594. doi: 10.1002/cne.903280409
Norrholm, S. D., Glover, E. M., Stevens, J. S., Fani, N., Galatzer-Levy, I. R., Bradley, B., et al. (2015). Fear load: the psychophysiological over-expression of fear as an intermediate phenotype associated with trauma reactions. Int. J. Psychophysiol. 98, 270–275. doi: 10.1016/j.ijpsycho.2014.11.005
Nugent, N. R., Christopher, N. C., Crow, J. P., Browne, L., Ostrowski, S., and Delahanty, D. L. (2010). The efficacy of early propranolol administration at reducing PTSD symptoms in pediatric injury patients: a pilot study. J. Trauma. Stress 23, 282–287. doi: 10.1002/jts.20517
Oei, T. P., and King, M. G. (1980). Catecholamines and aversive learning: a review. Neurosci. Biobehav. Rev. 4, 161–173. doi: 10.1016/0149-7634(80)90014-7
Olschowka, J. A., Molliver, M. E., Grzanna, R., Rice, F. L., and Coyle, J. T. (1981). Ultrastructural demonstration of noradrenergic synapses in the rat central nervous system by dopamine-β-hydroxylase immunocytochemistry. J. Histochem. Cytochem. 29, 271–280. doi: 10.1177/29.2.7019303
Onaka, T., Palmer, J. R., and Yagi, K. (1996). Norepinephrine depletion impairs neuroendocrine responses to fear but not novel environmental stimuli in the rat. Brain Res. 713, 261–268. doi: 10.1016/0006-8993(95)01552-3
Ordway, G. A., O’Donnell, J. M., and Frazer, A. (1987). Effects of clenbuterol on central β-1 and β-2 adrenergic receptors of the rat. J. Pharmacol. Exp. Ther. 241, 187–195.
Orr, S. P., Metzger, L. J., Lasko, N. B., Macklin, M. L., Peri, T., and Pitman, R. K. (2000). De novo conditioning in trauma-exposed individuals with and without posttraumatic stress disorder. J. Abnorm. Psychol. 109, 290–298. doi: 10.1037/0021-843x.109.2.290
Orr, S. P., Milad, M. R., Metzger, L. J., Lasko, N. B., Gilbertson, M. W., and Pitman, R. K. (2006). Effects of β blockade, PTSD diagnosis, and explicit threat on the extinction and retention of an aversively conditioned response. Biol. Psychol. 73, 262–271. doi: 10.1016/j.biopsycho.2006.05.001
Ouyang, M., and Thomas, S. A. (2005). A requirement for memory retrieval during and after long-term extinction learning. Proc. Natl. Acad. Sci. U S A 102, 9347–9352. doi: 10.1073/pnas.0502315102
Palacios, J. M., Hoyer, D., and Cortés, R. (1987). α1-adrenoceptors in the mammalian brain: similar pharmacology but different distribution in rodents and primates. Brain Res. 419, 65–75. doi: 10.1016/0006-8993(87)90569-5
Papadopoulos, G. C., and Parnavelas, J. G. (1990). Distribution and synaptic organization of serotoninergic and noradrenergic axons in the lateral geniculate nucleus of the rat. J. Comp. Neurol. 294, 345–355. doi: 10.1002/cne.902940304
Papadopoulos, G. C., Parnavelas, J. G., and Buijs, R. (1987). Monoaminergic fibers form conventional synapses in the cerebral cortex. Neurosci. Lett. 76, 275–279. doi: 10.1016/0304-3940(87)90414-9
Papadopoulos, G. C., Parnavelas, J. G., and Buijs, R. M. (1989). Light and electron microscopic immunocytochemical analysis of the noradrenaline innervation of the rat visual cortex. J. Neurocytol. 18, 1–10. doi: 10.1007/bf01188418
Passerin, A. M., Cano, G., Rabin, B. S., Delano, B. A., Napier, J. L., and Sved, A. F. (2000). Role of locus coeruleus in foot shock-evoked Fos expression in rat brain. Neuroscience 101, 1071–1082. doi: 10.1016/s0306-4522(00)00372-9
Pennington, Z. T., Anderson, A. S., and Fanselow, M. S. (2017). The ventromedial prefrontal cortex in a model of traumatic stress: fear inhibition or contextual processing? Learn. Mem. 24, 400–406. doi: 10.1101/lm.046110.117
Pezzone, M. A., Lee, W. S., Hoffman, G. E., Pezzone, K. M., and Rabin, B. S. (1993). Activation of brainstem catecholaminergic neurons by conditioned and unconditioned aversive stimuli as revealed by c-Fos immunoreactivity. Brain Res. 608, 310–318. doi: 10.1016/0006-8993(93)91472-5
Pickel, V. M., Segal, M., and Bloom, F. E. (1974). A radioautographic study of the efferent pathways of the nucleus locus coeruleus. J. Comp. Neurol. 155, 15–42. doi: 10.1002/cne.901550103
Pieribone, V. A., Nicholas, A. P., Dagerlind, A., and Hökfelt, T. (1994). Distribution of α 1 adrenoceptors in rat brain revealed by in situ hybridization experiments utilizing subtype-specific probes. J. Neurosci. 14, 4252–4268.
Pitman, R. K., and Delahanty, D. L. (2005). Conceptually driven pharmacologic approaches to acute trauma. CNS Spectr. 10, 99–106. doi: 10.1017/s109285290001943x
Pitman, R. K., Milad, M. R., Igoe, S. A., Vangel, M. G., Orr, S. P., Tsareva, A., et al. (2011). Systemic mifepristone blocks reconsolidation of cue-conditioned fear; propranolol prevents this effect. Behav. Neurosci. 125, 632–638. doi: 10.1037/a0024364
Pitman, R. K., Rasmusson, A. M., Koenen, K. C., Shin, L. M., Orr, S. P., Gilbertson, M. W., et al. (2012). Biological studies of post-traumatic stress disorder. Nat. Rev. Neurosci. 13, 769–787. doi: 10.1038/nrn3339
Pitman, R. K., Sanders, K. M., Zusman, R. M., Healy, A. R., Cheema, F., Lasko, N. B., et al. (2002). Pilot study of secondary prevention of posttraumatic stress disorder with propranolol. Biol. Psychiatry 51, 189–192. doi: 10.1016/s0006-3223(01)01279-3
Porter, D. M., and Bell, C. C. (1999). The use of clonidine in post-traumatic stress disorder. J. Natl. Med. Assoc. 91, 475–477.
Poundja, J., Sanche, S., Tremblay, J., and Brunet, A. (2012). Trauma reactivation under the influence of propranolol: an examination of clinical predictors. Eur. J. Psychotraumatol. 3:15470. doi: 10.3402/ejpt.v3i0.15470
Powers, M. B., Smits, J. A. J., Otto, M. W., Sanders, C., and Emmelkamp, P. M. G. (2009). Facilitation of fear extinction in phobic participants with a novel cognitive enhancer: a randomized placebo controlled trial of yohimbine augmentation. J. Anxiety Disord. 23, 350–356. doi: 10.1016/j.janxdis.2009.01.001
Priovoulos, N., Jacobs, H. I. L., Ivanov, D., Uludag, K., Verhey, F. R. J., and Poser, B. A. (2017). High-resolution in vivo imaging of human locus coeruleus by magnetization transfer MRI at 3T and 7T. Neuroimage doi: 10.1016/j.neuroimage.2017.07.045 [Epub ahead of print].
Prouty, E. W., Waterhouse, B. D., and Chandler, D. J. (2017). Corticotropin releasing factor dose-dependently modulates excitatory synaptic transmission in the noradrenergic nucleus locus coeruleus. Eur. J. Neurosci. 45, 712–722. doi: 10.1111/ejn.13501
Przybyslawski, J., Roullet, P., and Sara, S. J. (1999). Attenuation of emotional and nonemotional memories after their reactivation: role of β adrenergic receptors. J. Neurosci. 19, 6623–6628.
Quirarte, G. L., Galvez, R., Roozendaal, B., and McGaugh, J. L. (1998). Norepinephrine release in the amygdala in response to footshock and opioid peptidergic drugs. Brain Res. 808, 134–140. doi: 10.1016/s0006-8993(98)00795-1
Quirk, G. J., and Mueller, D. (2008). Neural mechanisms of extinction learning and retrieval. Neuropsychopharmacology 33, 56–72. doi: 10.1038/sj.npp.1301555
Rainbow, T. C., and Biegon, A. (1983). Quantitative autoradiography of [3H]prazosin binding sites in rat forebrain. Neurosci. Lett. 40, 221–226. doi: 10.1016/0304-3940(83)90042-3
Rainbow, T. C., Parsons, B., and Wolfe, B. B. (1984). Quantitative autoradiography of β 1- and β 2-adrenergic receptors in rat brain. Proc. Natl. Acad. Sci. U S A 81, 1585–1589. doi: 10.1073/pnas.81.5.1585
Raio, C. M., Brignoni-Perez, E., Goldman, R., and Phelps, E. A. (2014). Acute stress impairs the retrieval of extinction memory in humans. Neurobiol. Learn. Mem. 112, 212–221. doi: 10.1016/j.nlm.2014.01.015
Raio, C. M., Hartley, C. A., Orederu, T. A., Li, J., and Phelps, E. A. (2017). Stress attenuates the flexible updating of aversive value. Proc. Natl. Acad. Sci. U S A 114, 11241–11246. doi: 10.1073/pnas.1702565114
Raio, C. M., and Phelps, E. A. (2015). The influence of acute stress on the regulation of conditioned fear. Neurobiol. Stress 1, 134–146. doi: 10.1016/j.ynstr.2014.11.004
Ramos, B. P., and Arnsten, A. F. T. (2007). Adrenergic pharmacology and cognition: focus on the prefrontal cortex. Pharmacol. Ther. 113, 523–536. doi: 10.1016/j.pharmthera.2006.11.006
Ramos, B. P., Stark, D., Verduzco, L., van Dyck, C. H., and Arnsten, A. F. T. (2006). α2A-adrenoceptor stimulation improves prefrontal cortical regulation of behavior through inhibition of cAMP signaling in aging animals. Learn. Mem. 13, 770–776. doi: 10.1101/lm.298006
Raskind, M. A., Peskind, E. R., Chow, B., Harris, C., Davis-Karim, A., Holmes, H. A., et al. (2018). Trial of prazosin for post-traumatic stress disorder in military veterans. N. Engl. J. Med. 378, 507–517. doi: 10.1056/NEJMoa1507598
Raskind, M. A., Peskind, E. R., Kanter, E. D., Petrie, E. C., Radant, A., Thompson, C. E., et al. (2003). Reduction of nightmares and other PTSD symptoms in combat veterans by prazosin: a placebo-controlled study. Am. J. Psychiatry 160, 371–373. doi: 10.1176/appi.ajp.160.2.371
Rau, V., DeCola, J. P., and Fanselow, M. S. (2005). Stress-induced enhancement of fear learning: an animal model of posttraumatic stress disorder. Neurosci. Biobehav. Rev. 29, 1207–1223. doi: 10.1016/j.neubiorev.2005.04.010
Rau, V., and Fanselow, M. S. (2009). Exposure to a stressor produces a long lasting enhancement of fear learning in rats. Stress 12, 125–133. doi: 10.1080/10253890802137320
Robbins, T. W., and Arnsten, A. F. T. (2009). The neuropsychopharmacology of fronto-executive function: monoaminergic modulation. Annu. Rev. Neurosci. 32, 267–287. doi: 10.1146/annurev.neuro.051508.135535
Rodrigues, S. M., LeDoux, J. E., and Sapolsky, R. M. (2009). The influence of stress hormones on fear circuitry. Annu. Rev. Neurosci. 32, 289–313. doi: 10.1146/annurev.neuro.051508.135620
Rodriguez-Romaguera, J., Sotres-Bayon, F., Mueller, D., and Quirk, G. J. (2009). Systemic propranolol acts centrally to reduce conditioned fear in rats without impairing extinction. Biol. Psychiatry 65, 887–892. doi: 10.1016/j.biopsych.2009.01.009
Room, P., Postema, F., and Korf, J. (1981). Divergent axon collaterals of rat locu Demonstration by a fluorescent double labeling technique. Brain Res. 221, 219–230. doi: 10.1016/0006-8993(81)90773-3
Roozendaal, B., Hui, G. K., Hui, I. R., Berlau, D. J., McGaugh, J. L., and Weinberger, N. M. (2006). Basolateral amygdala noradrenergic activity mediates corticosterone-induced enhancement of auditory fear conditioning. Neurobiol. Learn. Mem. 86, 249–255. doi: 10.1016/j.nlm.2006.03.003
Rosin, D. L., Talley, E. M., Lee, A., Stornetta, R. L., Gaylinn, B. D., Guyenet, P. G., et al. (1996). Distribution of α2C-adrenergic receptor-like immunoreactivity in the rat central nervous system. J. Comp. Neurol. 372, 135–165. doi: 10.1002/(sici)1096-9861(19960812)372:1<135::aid-cne9>3.3.co;2-b
Sara, S. J. (2009). The locus coeruleus and noradrenergic modulation of cognition. Nat. Rev. Neurosci. 10, 211–223. doi: 10.1038/nrn2573
Sara, S. J. (2015). Locus Coeruleus in time with the making of memories. Curr. Opin. Neurobiol. 35, 87–94. doi: 10.1016/j.conb.2015.07.004
Sara, S. J., and Hervé-Minvielle, A. (1995). Inhibitory influence of frontal cortex on locus coeruleus neurons. Proc. Natl. Acad. Sci. U S A 92, 6032–6036. doi: 10.1073/pnas.92.13.6032
Sara, S. J., and Segal, M. (1991). “Plasticity of sensory responses of locus coeruleus neurons in the behaving rat: implications for cognition,” in Neurobiology of the Locus Coeruleus, Progress in Brain Research, eds C. D. Barnes and O. Pompeiano (Amsterdam: Elsevier), 571–585.
Scheinin, M., Lomasney, J. W., Hayden-Hixson, D. M., Schambra, U. B., Caron, M. G., Lefkowitz, R. J., et al. (1994). Distribution of α2-adrenergic receptor subtype gene expression in rat brain. Brain Res. Mol. Brain Res. 21, 133–149. doi: 10.1016/0169-328x(94)90386-7
Schiff, H. C., Johansen, J. P., Hou, M., Bush, D. E. A., Smith, E. K., Klein, J. E., et al. (2017). β-adrenergic receptors regulate the acquisition and consolidation phases of aversive memory formation through distinct, temporally regulated signaling pathways. Neuropsychopharmacology 42, 895–903. doi: 10.1038/npp.2016.238
Schneider, A. M., Simson, P. E., Daimon, C. M., Mrozewski, J., Vogt, N. M., Keefe, J., et al. (2014). Stress-dependent opioid and adrenergic modulation of newly retrieved fear memory. Neurobiol. Learn. Mem. 109, 1–6. doi: 10.1016/j.nlm.2013.11.013
Schulz, B., Fendt, M., and Schnitzler, H.-U. (2002). Clonidine injections into the lateral nucleus of the amygdala block acquisition and expression of fear-potentiated startle. Eur. J. Neurosci. 15, 151–157. doi: 10.1046/j.0953-816x.2001.01831.x
Schutsky, K., Ouyang, M., Castelino, C. B., Zhang, L., and Thomas, S. A. (2011a). Stress and glucocorticoids impair memory retrieval via β2-adrenergic, Gi/o-coupled suppression of cAMP signaling. J. Neurosci. 31, 14172–14181. doi: 10.1523/JNEUROSCI.2122-11.2011
Schutsky, K., Ouyang, M., and Thomas, S. A. (2011b). Xamoterol impairs hippocampus-dependent emotional memory retrieval via Gi/o-coupled β2-adrenergic signaling. Learn. Mem. 18, 598–604. doi: 10.1101/lm.2302811
Schwabe, L., Nader, K., Wolf, O. T., Beaudry, T., and Pruessner, J. C. (2012). Neural signature of reconsolidation impairments by propranolol in humans. Biol. Psychiatry 71, 380–386. doi: 10.1016/j.biopsych.2011.10.028
Schwarz, L. A., and Luo, L. (2015). Organization of the locus coeruleus-norepinephrine system. Curr. Biol. 25, R1051–R1056. doi: 10.1016/j.cub.2015.09.039
Schwarz, L. A., Miyamichi, K., Gao, X. J., Beier, K. T., Weissbourd, B., DeLoach, K. E., et al. (2015). Viral-genetic tracing of the input-output organization of a central noradrenaline circuit. Nature 524, 88–92. doi: 10.1038/nature14600
Segal, M., and Landis, S. (1974). Afferents to the hippocampus of the rat studied with the method of retrograde transport of horseradish peroxidase. Brain Res. 78, 1–15. doi: 10.1016/0006-8993(74)90349-7
Segal, M., Markram, H., and Richter-Levin, G. (1991). “Actions of norepinephrine in the rat hippocampus,” in Progress in Brain Research, eds C. D. Barnes and O. Pompeiano (Amsterdam: Elsevier), 323–330.
Selden, N. R., Everitt, B. J., and Robbins, T. W. (1991). Telencephalic but not diencephalic noradrenaline depletion enhances behavioural but not endocrine measures of fear conditioning to contextual stimuli. Behav. Brain Res. 43, 139–154. doi: 10.1016/s0166-4328(05)80064-6
Selden, N. R., Robbins, T. W., and Everitt, B. J. (1990). Enhanced behavioral conditioning to context and impaired behavioral and neuroendocrine responses to conditioned stimuli following ceruleocortical noradrenergic lesions: support for an attentional hypothesis of central noradrenergic function. J. Neurosci. 10, 531–539.
Sevenster, D., Beckers, T., and Kindt, M. (2012). Retrieval per se is not sufficient to trigger reconsolidation of human fear memory. Neurobiol. Learn. Mem. 97, 338–345. doi: 10.1016/j.nlm.2012.01.009
Sharpe, M. J., and Killcross, S. (2014). The prelimbic cortex uses higher-order cues to modulate both the acquisition and expression of conditioned fear. Front. Syst. Neurosci. 8:235. doi: 10.3389/fnsys.2014.00235
Sharpe, M., and Killcross, S. (2015). The prelimbic cortex uses contextual cues to modulate responding towards predictive stimuli during fear renewal. Neurobiol. Learn. Mem. 118, 20–29. doi: 10.1016/j.nlm.2014.11.005
Shipley, M. T., Fu, L., Ennis, M., Liu, W. L., and Aston-Jones, G. (1996). Dendrites of locus coeruleus neurons extend preferentially into two pericoerulear zones. J. Comp. Neurol. 365, 56–68. doi: 10.1002/(sici)1096-9861(19960129)365:1<56::aid-cne5>3.0.co;2-i
Short, N. A., Allan, N. P., Stentz, L., Portero, A. K., and Schmidt, N. B. (2018). Predictors of insomnia symptoms and nightmares among individuals with post-traumatic stress disorder: an ecological momentary assessment study. J. Sleep Res. 27, 64–72. doi: 10.1111/jsr.12589
Singewald, N., Salchner, P., and Sharp, T. (2003). Induction of c-Fos expression in specific areas of the fear circuitry in rat forebrain by anxiogenic drugs. Biol. Psychiatry 53, 275–283. doi: 10.1016/s0006-3223(02)01574-3
Skelly, M. J., Ariwodola, O. J., and Weiner, J. L. (2017). Fear conditioning selectively disrupts noradrenergic facilitation of GABAergic inhibition in the basolateral amygdala. Neuropharmacology 113, 231–240. doi: 10.1016/j.neuropharm.2016.10.003
Smith, M. A., Banerjee, S., Gold, P. W., and Glowa, J. (1992). Induction of c-fos mRNA in rat brain by conditioned and unconditioned stressors. Brain Res. 578, 135–141. doi: 10.1016/0006-8993(92)90240-a
Soeter, M., and Kindt, M. (2011a). Disrupting reconsolidation: pharmacological and behavioral manipulations. Learn. Mem. 18, 357–366. doi: 10.1101/lm.2148511
Soeter, M., and Kindt, M. (2011b). Noradrenergic enhancement of associative fear memory in humans. Neurobiol. Learn. Mem. 96, 263–271. doi: 10.1016/j.nlm.2011.05.003
Soeter, M., and Kindt, M. (2012). Stimulation of the noradrenergic system during memory formation impairs extinction learning but not the disruption of reconsolidation. Neuropsychopharmacology 37, 1204–1215. doi: 10.1038/npp.2011.307
Song, A. H., Kucyi, A., Napadow, V., Brown, E. N., Loggia, M. L., and Akeju, O. (2017). Pharmacological modulation of noradrenergic arousal circuitry disrupts functional connectivity of the locus ceruleus in humans. J. Neurosci. 37, 6938–6945. doi: 10.1523/JNEUROSCI.0446-17.2017
Southwick, S. M., Bremner, J. D., Rasmusson, A., Morgan, C. A. III., Arnsten, A., and Charney, D. S. (1999a). Role of norepinephrine in the pathophysiology and treatment of posttraumatic stress disorder. Biol. Psychiatry 46, 1192–1204. doi: 10.1016/s0006-3223(99)00219-x
Southwick, S. M., Morgan, C. A. III., Charney, D. S., and High, J. R. (1999b). Yohimbine use in a natural setting: effects on posttraumatic stress disorder. Biol. Psychiatry 46, 442–444. doi: 10.1016/s0006-3223(99)00107-9
Southwick, S. M., Paige, S., Morgan, C. A. III., Bremner, J. D., Krystal, J. H., and Charney, D. S. (1999c). Neurotransmitter alterations in PTSD: catecholamines and serotonin. Semin. Clin. Neuropsychiatry 4, 242–248.
Southwick, S. M., Krystal, J. H., Bremner, J. D., Morgan, C. A. III., Nicolaou, A. L., Nagy, L. M., et al. (1997). Noradrenergic and serotonergic function in posttraumatic stress disorder. Arch. Gen. Psychiatry 54, 749–758. doi: 10.1001/archpsyc.1997.01830200083012
Spring, J. D., Wood, N. E., Mueller-Pfeiffer, C., Milad, M. R., Pitman, R. K., and Orr, S. P. (2015). Prereactivation propranolol fails to reduce skin conductance reactivity to prepared fear-conditioned stimuli. Psychophysiology 52, 407–415. doi: 10.1111/psyp.12326
Stafford, J. M., Maughan, D. K., Ilioi, E. C., and Lattal, K. M. (2013). Exposure to a fearful context during periods of memory plasticity impairs extinction via hyperactivation of frontal-amygdalar circuits. Learn. Mem. 20, 156–163. doi: 10.1101/lm.029801.112
Stanton, P. K., and Sarvey, J. M. (1985a). Blockade of norepinephrine-induced long-lasting potentiation in the hippocampal dentate gyrus by an inhibitor of protein synthesis. Brain Res. 361, 276–283. doi: 10.1016/0006-8993(85)91299-5
Stanton, P. K., and Sarvey, J. M. (1985b). Depletion of norepinephrine, but not serotonin, reduces long-term potentiation in the dentate gyrus of rat hippocampal slices. J. Neurosci. 5, 2169–2176.
Stanton, P. K., and Sarvey, J. M. (1987). Norepinephrine regulates long-term potentiation of both the population spike and dendritic EPSP in hippocampal dentate gyrus. Brain Res. Bull. 18, 115–119. doi: 10.1016/0361-9230(87)90039-6
Steckler, T., and Risbrough, V. (2012). Pharmacological treatment of PTSD—established and new approaches. Neuropharmacology 62, 617–627. doi: 10.1016/j.neuropharm.2011.06.012
Stein, M. B., Kerridge, C., Dimsdale, J. E., and Hoyt, D. B. (2007). Pharmacotherapy to prevent PTSD: results from a randomized controlled proof-of-concept trial in physically injured patients. J. Trauma. Stress 20, 923–932. doi: 10.1002/jts.20270
Steindler, D. A. (1981). Locus coerules neurons have axons that branch to the forebrain and cerebellum. Brain Res. 223, 367–373. doi: 10.1016/0006-8993(81)91149-5
Sterpenich, V., D’Argembeau, A., Desseilles, M., Balteau, E., Albouy, G., Vandewalle, G., et al. (2006). The locus ceruleus is involved in the successful retrieval of emotional memories in humans. J. Neurosci. 26, 7416–7423. doi: 10.1523/JNEUROSCI.1001-06.2006
Strawn, J. R., and Geracioti, T. D. Jr. (2008). Noradrenergic dysfunction and the psychopharmacology of posttraumatic stress disorder. Depress. Anxiety 25, 260–271. doi: 10.1002/da.20292
Summers, R. J., Papaioannou, M., Harris, S., and Evans, B. A. (1995). Expression of β 3-adrenoceptor mRNA in rat brain. Br. J. Pharmacol. 116, 2547–2548. doi: 10.1111/j.1476-5381.1995.tb17205.x
Sved, A. F., Cano, G., Passerin, A. M., and Rabin, B. S. (2002). The locus coeruleus, Barrington’s nucleus, and neural circuits of stress. Physiol. Behav. 77, 737–742. doi: 10.1016/s0031-9384(02)00927-7
Swanson, L. W. (1976). The locus coeruleus: a cytoarchitectonic, Golgi and immunohistochemical study in the albino rat. Brain Res. 110, 39–56. doi: 10.1016/0006-8993(76)90207-9
Swanson, L. W., and Hartman, B. K. (1975). The central adrenergic system. An immunofluorescence study of the location of cell bodies and their efferent connections in the rat utilizing dopamine-β-hydroxylase as a marker. J. Comp. Neurol. 163, 467–505. doi: 10.1002/cne.901630406
Taherian, F., Vafaei, A. A., Vaezi, G. H., Eskandarian, S., Kashef, A., and Rashidy-Pour, A. (2014). Propranolol-induced impairment of contextual fear memory reconsolidation in rats: a similar effect on weak and strong recent and remote memories. Basic Clin. Neurosci. 5, 231–239.
Talley, E. M., Rosin, D. L., Lee, A., Guyenet, P. G., and Lynch, K. R. (1996). Distribution of α2A-adrenergic receptor-like immunoreactivity in the rat central nervous system. J. Comp. Neurol. 372, 111–134. doi: 10.1002/(sici)1096-9861(19960812)372:1<111::aid-cne8>3.0.co;2-6
Tawa, J., and Murphy, S. (2013). Psychopharmacological treatment for military posttraumatic stress disorder: an integrative review. J. Am. Assoc. Nurse Pract. 25, 419–423. doi: 10.1111/1745-7599.12016
Taylor, H. R., Freeman, M. K., and Cates, M. E. (2008). Prazosin for treatment of nightmares related to posttraumatic stress disorder. Am. J. Health Syst. Pharm. 65, 716–722. doi: 10.2146/ajhp070124
Thierry, A. M., Javoy, F., Glowinski, J., and Kety, S. S. (1968). Effects of stress on the metabolism of norepinephrine, dopamine and serotonin in the central nervous system of the rat. I. Modifications of norepinephrine turnover. J. Pharmacol. Exp. Ther. 163, 163–171.
Tollenaar, M. S., Elzinga, B. M., Spinhoven, P., and Everaerd, W. (2009). Psychophysiological responding to emotional memories in healthy young men after cortisol and propranolol administration. Psychopharmacology 203, 793–803. doi: 10.1007/s00213-008-1427-x
Tona, K.-D., Keuken, M. C., de Rover, M., Lakke, E., Forstmann, B. U., Nieuwenhuis, S., et al. (2017). In vivo visualization of the locus coeruleus in humans: quantifying the test-retest reliability. Brain Struct. Funct. 222, 4203–4217. doi: 10.1007/s00429-017-1464-5
Toth, M., Ziegler, M., Sun, P., Gresack, J., and Risbrough, V. (2013). Impaired conditioned fear response and startle reactivity in epinephrine-deficient mice. Behav. Pharmacol. 24, 1–9. doi: 10.1097/FBP.0b013e32835cf408
Tsaltas, E., Gray, J. A., and Fillenz, M. (1984). Alleviation of response suppression to conditioned aversive stimuli by lesions of the dorsal noradrenergic bundle. Behav. Brain Res. 13, 115–127. doi: 10.1016/0166-4328(84)90142-6
Tully, K., and Bolshakov, V. Y. (2010). Emotional enhancement of memory: how norepinephrine enables synaptic plasticity. Mol. Brain 3:15. doi: 10.1186/1756-6606-3-15
Tully, K., Li, Y., Tsvetkov, E., and Bolshakov, V. Y. (2007). Norepinephrine enables the induction of associative long-term potentiation at thalamo-amygdala synapses. Proc. Natl. Acad. Sci. U S A 104, 14146–14150. doi: 10.1073/pnas.0704621104
Uematsu, A., Tan, B. Z., and Johansen, J. P. (2015). Projection specificity in heterogeneous locus coeruleus cell populations: implications for learning and memory. Learn. Mem. 22, 444–451. doi: 10.1101/lm.037283.114
Uematsu, A., Tan, B. Z., Ycu, E. A., Cuevas, J. S., Koivumaa, J., Junyent, F., et al. (2017). Modular organization of the brainstem noradrenaline system coordinates opposing learning states. Nat. Neurosci. 20, 1602–1611. doi: 10.1038/nn.4642
Unnerstall, J. R., Kopajtic, T. A., and Kuhar, M. J. (1984). Distribution of α2 agonist binding sites in the rat and human central nervous system: analysis of some functional, anatomic correlates of the pharmacologic effects of clonidine and related adrenergic agents. Brain Res. Rev. 7, 69–101. doi: 10.1016/0165-0173(84)90030-4
Vaiva, G., Ducrocq, F., Jezequel, K., Averland, B., Lestavel, P., Brunet, A., et al. (2003). Immediate treatment with propranolol decreases posttraumatic stress disorder two months after trauma. Biol. Psychiatry 54, 947–949. doi: 10.1016/s0006-3223(03)00412-8
Valentino, R. J., and Van Bockstaele, E. (2008). Convergent regulation of locus coeruleus activity as an adaptive response to stress. Eur. J. Pharmacol. 583, 194–203. doi: 10.1016/j.ejphar.2007.11.062
Van Bockstaele, E. J., Colago, E. E., and Valentino, R. J. (1998). Amygdaloid corticotropin-releasing factor targets locus coeruleus dendrites: substrate for the co-ordination of emotional and cognitive limbs of the stress response. J. Neuroendocrinol. 10, 743–758. doi: 10.1046/j.1365-2826.1998.00254.x
van Stegeren, A. H., Roozendaal, B., Kindt, M., Wolf, O. T., and Joëls, M. (2010). Interacting noradrenergic and corticosteroid systems shift human brain activation patterns during encoding. Neurobiol. Learn. Mem. 93, 56–65. doi: 10.1016/j.nlm.2009.08.004
VanElzakker, M. B., Dahlgren, M. K., Davis, F. C., Dubois, S., and Shin, L. M. (2014). From Pavlov to PTSD: the extinction of conditioned fear in rodents, humans, and anxiety disorders. Neurobiol. Learn. Mem. 113, 3–18. doi: 10.1016/j.nlm.2013.11.014
Ventura, R., Latagliata, E. C., Morrone, C., La Mela, I., and Puglisi-Allegra, S. (2008). Prefrontal norepinephrine determines attribution of “high” motivational salience. PLoS One 3:e3044. doi: 10.1371/journal.pone.0003044
Vervliet, B., Craske, M. G., and Hermans, D. (2013). Fear extinction and relapse: state of the art. Annu. Rev. Clin. Psychol. 9, 215–248. doi: 10.1146/annurev-clinpsy-050212-185542
Visser, R. M., Kunze, A. E., Westhoff, B., Scholte, H. S., and Kindt, M. (2015). Representational similarity analysis offers a preview of the noradrenergic modulation of long-term fear memory at the time of encoding. Psychoneuroendocrinology 55, 8–20. doi: 10.1016/j.psyneuen.2015.01.021
Wagatsuma, A., Okuyama, T., Sun, C., Smith, L. M., Abe, K., and Tonegawa, S. (2018). Locus coeruleus input to hippocampal CA3 drives single-trial learning of a novel context. Proc. Natl. Acad. Sci. U S A 115, E310–E316. doi: 10.1073/pnas.1714082115
Wang, S.-H., de Oliveira Alvares, L., and Nader, K. (2009). Cellular and systems mechanisms of memory strength as a constraint on auditory fear reconsolidation. Nat. Neurosci. 12, 905–912. doi: 10.1038/nn.2350
Wang, R., Macmillan, L. B., Fremeau, R. T. Jr., Magnuson, M. A., Lindner, J., and Limbird, L. E. (1996). Expression of α 2-adrenergic receptor subtypes in the mouse brain: evaluation of spatial and temporal information imparted by 3 kb of 5’ regulatory sequence for the α 2A AR-receptor gene in transgenic animals. Neuroscience 74, 199–218. doi: 10.1016/0306-4522(96)00116-9
Wangelin, B. C., Powers, M. B., Smits, J. A. J., and Tuerk, P. W. (2013). Enhancing exposure therapy for PTSD with yohimbine HCL: protocol for a double-blind, randomized controlled study implementing subjective and objective measures of treatment outcome. Contemp. Clin. Trials 36, 319–326. doi: 10.1016/j.cct.2013.08.003
Waterhouse, B. D., and Chandler, D. J. (2016). Heterogeneous organization and function of the central noradrenergic system. Brain Res. 1641, v–x. doi: 10.1016/j.brainres.2015.12.050
Waterhouse, B. D., Lin, C. S., Burne, R. A., and Woodward, D. J. (1983). The distribution of neocortical projection neurons in the locus coeruleus. J. Comp. Neurol. 217, 418–431. doi: 10.1002/cne.902170406
Wessa, M., and Flor, H. (2007). Failure of extinction of fear responses in posttraumatic stress disorder: evidence from second-order conditioning. Am. J. Psychiatry 164, 1684–1692. doi: 10.1176/appi.ajp.2007.07030525
Wood, N. E., Rosasco, M. L., Suris, A. M., Spring, J. D., Marin, M.-F., Lasko, N. B., et al. (2015). Pharmacological blockade of memory reconsolidation in posttraumatic stress disorder: three negative psychophysiological studies. Psychiatry Res. 225, 31–39. doi: 10.1016/j.psychres.2014.09.005
Writer, B. W., Meyer, E. G., and Schillerstrom, J. E. (2014). Prazosin for military combat-related PTSD nightmares: a critical review. J. Neuropsychiatry Clin. Neurosci. 26, 24–33. doi: 10.1176/appi.neuropsych.13010006
Yang, H.-W., Lin, Y.-W., Yen, C.-D., and Min, M.-Y. (2002). Change in bi-directional plasticity at CA1 synapses in hippocampal slices taken from 6-hydroxydopamine-treated rats: the role of endogenous norepinephrine. Eur. J. Neurosci. 16, 1117–1128. doi: 10.1046/j.1460-9568.2002.02165.x
Yavich, L., Jäkälä, P., and Tanila, H. (2005). Noradrenaline overflow in mouse dentate gyrus following locus coeruleus and natural stimulation: real-time monitoring by in vivo voltammetry. J. Neurochem. 95, 641–650. doi: 10.1111/j.1471-4159.2005.03390.x
Yehuda, R., Southwick, S., Giller, E. L., Ma, X., and Mason, J. W. (1992). Urinary catecholamine excretion and severity of PTSD symptoms in Vietnam combat veterans. J. Nerv. Ment. Dis. 180, 321–325. doi: 10.1097/00005053-199205000-00006
Young, W. S., and Kuhar, M. J. (1980). Noradrenergic α 1 and α 2 receptors: light microscopic autoradiographic localization. Proc. Natl. Acad. Sci. U S A 77, 1696–1700. doi: 10.1073/pnas.77.3.1696
Yu, K., Ahrens, S., Zhang, X., Schiff, H., Ramakrishnan, C., Fenno, L., et al. (2017). The central amygdala controls learning in the lateral amygdala. Nat. Neurosci. 20, 1680–1685. doi: 10.1038/s41593-017-0009-9
Zelikowsky, M., Bissiere, S., Hast, T. A., Bennett, R. Z., Abdipranoto, A., Vissel, B., et al. (2013). Prefrontal microcircuit underlies contextual learning after hippocampal loss. Proc. Natl. Acad. Sci. U S A 110, 9938–9943. doi: 10.1073/pnas.1301691110
Zelikowsky, M., Hersman, S., Chawla, M. K., Barnes, C. A., and Fanselow, M. S. (2014). Neuronal ensembles in amygdala, hippocampus, and prefrontal cortex track differential components of contextual fear. J. Neurosci. 34, 8462–8466. doi: 10.1523/jneurosci.3624-13.2014
Zeng, D., and Lynch, K. R. (1991). Distribution of α2-adrenergic receptor mRNAs in the rat CNS. Mol. Brain Res. 10, 219–225. doi: 10.1016/0169-328X(91)90064-5
Zhang, W.-P., Guzowski, J. F., and Thomas, S. A. (2005). Mapping neuronal activation and the influence of adrenergic signaling during contextual memory retrieval. Learn. Mem. 12, 239–247. doi: 10.1101/lm.90005
Keywords: fear, extinction, consolidation, locus coeruleus, norepinephrine
Citation: Giustino TF and Maren S (2018) Noradrenergic Modulation of Fear Conditioning and Extinction. Front. Behav. Neurosci. 12:43. doi: 10.3389/fnbeh.2018.00043
Received: 01 December 2017; Accepted: 26 February 2018;
Published: 13 March 2018.
Edited by:
Nuno Sousa, Instituto de Pesquisa em Ciências da Vida e da Saúde (ICVS), PortugalReviewed by:
Seth Davin Norrholm, Emory University School of Medicine, United StatesFrauke Nees, Zentralinstitut für Seelische Gesundheit, Germany
Copyright © 2018 Giustino and Maren. This is an open-access article distributed under the terms of the Creative Commons Attribution License (CC BY). The use, distribution or reproduction in other forums is permitted, provided the original author(s) and the copyright owner are credited and that the original publication in this journal is cited, in accordance with accepted academic practice. No use, distribution or reproduction is permitted which does not comply with these terms.
*Correspondence: Stephen Maren, bWFyZW5AdGFtdS5lZHU=