Infant Trauma Alters Social Buffering of Threat Learning: Emerging Role of Prefrontal Cortex in Preadolescence
- 1Emotional Brain Institute, Nathan Kline Institute for Psychiatric Research, Orangeburg, NY, United States
- 2Department of Child and Adolescent Psychiatry, Child Study Center at NYU Langone Medical Center, NYU School of Medicine, New York, NY, United States
- 3Department of Psychology, Stockholm University, Stockholm, Sweden
- 4Department of Neural Science, New York University, New York, NY, United States
- 5Department of Psychology, Florida Atlantic University, Boca Raton, FL, United States
- 6Department of Biology, Yeshiva University, New York, NY, United States
- 7Instituto de Investigaciones Médicas A Lanari, IDIM-CONICET, Universidad de Buenos Aires, Combatientes de Malvinas 3150 (CP 1427), Buenos Aires, Argentina
Within the infant-caregiver attachment system, the primary caregiver holds potent reward value to the infant, exhibited by infants’ strong preference for approach responses and proximity-seeking towards the mother. A less well-understood feature of the attachment figure is the caregiver’s ability to reduce fear via social buffering, commonly associated with the notion of a “safe haven” in the developmental literature. Evidence suggests this infant system overlaps with the neural network supporting social buffering (attenuation) of fear in the adults of many species, a network known to involve the prefrontal cortex (PFC). Here, using odor-shock conditioning in young developing rats, we assessed when the infant system transitions to the adult-like PFC-dependent social buffering of threat system. Rat pups were odor-shock conditioned (0.55 mA–0.6 mA) at either postnatal day (PN18; dependent on mother) or 28 (newly independent, weaned at PN23). Within each age group, the mother was present or absent during conditioning, with PFC assessment following acquisition using 14C 2-DG autoradiography and cue testing the following day. Since the human literature suggests poor attachment attenuates the mother’s ability to socially buffer the infants, half of the pups at each age were reared with an abusive mother from PN8–12. The results showed that for typical control rearing, the mother attenuated fear in both PN18 and PN28 pups, although the PFC [infralimbic (IL) and ventral prelimbic (vPL) cortices] was only engaged at PN28. Abuse rearing completely disrupted social buffering of pups by the mother at PN18. The results from PN28 pups showed that while the mother modulated learning in both control and abuse-reared pups, the behavioral and PFC effects were attenuated after maltreatment. Our data suggest that pups transition to the adult-like PFC social support circuit after independence from the mother (PN28), and this circuit remains functional after early-life trauma, although its effectiveness appears reduced. This is in sharp contrast to the effects of early life trauma during infancy, where social buffering of the infant is more robustly impacted. We suggest that the infant social buffering circuit is disengaged by early-life trauma, while the adolescent PFC-dependent social buffering circuit may use a safety signal with unreliable safety value.
Introduction
For infants, the mother and other significant caregivers serve as potent reward stimuli and induce robust proximity-seeking in the infant, regardless of the quality of care received. This infant attachment to the caregiver is learned during a sensitive period and rodent work suggests there is a unique neural network that robustly supports learning proximity-seeking (Moriceau et al., 2010; Raineki et al., 2010; Bisaz and Sullivan, 2012; Perry et al., 2016; Opendak et al., 2017). This open attachment system permits the infant to attach to multiple caregivers, including non-biological caregivers, within the context of diverse rearing conditions. Strikingly, this proximity-seeking characteristic of the attachment system is maintained even when the caregiver is the source of the threat, as occurs in maltreatment in a wide variety of species, including humans (Bowlby, 1982; Tottenham and Sheridan, 2009; Sanchez et al., 2015; Drury et al., 2016; Howell et al., 2017; Zajac et al., 2019).
A less well-known feature of the attachment figure is his or her ability to suppress or block fear/threat responding during early life, also referred to as social buffering (Hostinar et al., 2014; Gunnar et al., 2015; Hostinar and Gunnar, 2015; Callaghan et al., 2019). This fear reduction system was first characterized within Bowlby’s Attachment Theory (Bowlby, 1969, 1978) and is critical for the infant to approach the caregiver (safe base) for protection when threatened, rather than showing adult-like threat response behaviors (e.g., freezing, attacking or hiding; Coss, 2016). This phenomenon of social buffering of threat by the parent was first demonstrated in infant rats when the presence of the mother reduced the young infants’ responses to shock and blocked stress hormone release. This system is strongly phylogenetically represented and has been shown in rodents (Stanton and Levine, 1985; Levine et al., 1988; Suchecki et al., 1993; Hennessy et al., 2006, 2009, 2015; Gunnar et al., 2015; Sullivan and Perry, 2015; Al Aïn et al., 2017; Opendak et al., 2019), nonhuman primates and children (Coe et al., 1978; Wiener et al., 1987; Nachmias et al., 1996; Hennessy et al., 2009; Tottenham et al., 2012, accepted; Gee et al., 2013a; Sanchez et al., 2015; Howell et al., 2017). This social buffering supports the role of the attachment figure as a regulator of the immature infant (Bowlby, 1982; Hofer, 1994; Sroufe, 2005; Blair and Raver, 2015; Chambers, 2017; Feldman, 2017; Perry et al., 2017).
We have some understanding of the neural network supporting infant social buffering. This system involves caregiver suppression of the paraventricular nucleus (PVN) of the hypothalamus to block engagement of the stress axis (Shionoya et al., 2007) and attenuation of the amygdala and ventral tegmental response to threat (Hennessy et al., 2006, 2009; Moriceau and Sullivan, 2006; Moriceau et al., 2006, 2009; Opendak et al., 2019). This network analysis has, in part, been replicated in children (Gee et al., 2014; Tottenham et al., accepted), and nonhuman primates (Gunnar et al., 2015; Sanchez et al., 2015; Howell et al., 2017). Importantly, the literature across these species suggests that social buffering by maternal presence is disrupted in mother-infant dyads with poor quality attachment (Nachmias et al., 1996; Gunnar and Quevedo, 2007; Hostinar et al., 2014; Gunnar et al., 2015; Sanchez et al., 2015; Gunnar and Sullivan, 2017; Opendak et al., 2019). Yet, the neurobiology of this compromised social buffering system has received little attention.
Social buffering wanes with maturation, although this effect can still be seen in adults of many species. While there appears to be some overlap in the neural mechanisms across development, the late-developing prefrontal cortex (PFC) appears critical in adult social buffering (Hennessy et al., 2006, 2015, 2018; Kiyokawa et al., 2007, 2012; Taylor et al., 2008; Upton and Sullivan, 2010; Inagaki and Eisenberger, 2012; Tottenham et al., 2012; Hostinar et al., 2015; Hornstein et al., 2016; Harrison et al., 2017; Hornstein and Eisenberger, 2017). Here, we focus on the PFC and its evolving role in social buffering of the threat response, targeting a developmental transition from dependence on the mother (postnatal day [PN] 18) to independence in preadolescent rats (PN28) weaned from the mother. To further probe the dynamics of this developing circuit, we perturbed the system by exposing half of the animals to maternal maltreatment in early infancy. Overall, our results suggest that the neurobehavioral substrates of maternal social buffering and its perturbation are distinct during sensitive periods in development.
Materials and Methods
Subjects
A total of 322 Long Evans rats (178 PN18 ±1 day, 144 PN28 ±1 day), with approximately equal males and females, were bred and reared in our animal facility with ad libitum food and water. Animals were reared with an abusive mother or control mother from PN8-PN12–an age range documented to induce neurobehavioral deficits. Animals were tested at PN18 while still living with the mother or PN28 when pups live independently of the mother (all animal only tested once). Animals were always housed in an enclosure with solid floors, with both breeding and rearing occurring in a private animal room within the lab. Two weeks before giving birth, pregnant females were moved from large breeding cages to standard cages for birth and pup rearing (34 long × 29 wide × 17 high cm). General health and births were checked twice daily with the day of birth designated PN0. Litters were culled to 12 pups (approximately equal males and females) at PN1. Cages were cleaned twice a week except for the nest, which was saved and placed back with the mother and pups. All procedures were approved by the Institutional Animal Care and Use Committee in accordance with guidelines from the National Institutes of Health.
Scarcity-Adversity Model of Low Bedding (LB; PN8–12)
Early-life trauma was modeled in rats using a well-established Scarcity-Adversity Model previously utilized by our lab and others (Sullivan et al., 2000; Raineki et al., 2010; Opendak and Sullivan, 2016; Opendak et al., 2017; Walker et al., 2017; Yan et al., 2017). As illustrated in Figure 1, the low bedding (LB) rearing takes place from PN8–12 and included the following manipulations: nest hutch removal, bedding material reduced from 4,000 mL to 100 mL and solid floor cage cleaned daily with bedding replaced to reduce odor and maintain a clean cage environment. As illustrated in Table 1, this procedure increases instances of maternal maltreatment of the pups (e.g., reduced time with pups, rough handling pups) and results in neurobehavioral dysfunction, including depressive-like behavior, disrupted social behavior and dysregulation of fear expression in pups, although major neurobehavioral effects show significant emergence at weaning age (Perry and Sullivan, 2014; Al Aïn et al., 2017; Opendak et al., 2017). Age-matched control litters were reared concurrently but with abundant bedding and nest-building materials. Pups were videotaped three times a week and data analyzed using Ethovision (Noldus Information Technologies Inc., Leesburg, VA, USA). Maternal behavior and infant-mother interactions were hand-scored using BORIS (Life Sciences and Systems Biology) behavioral coding software to validate abusive and non-abusive care.
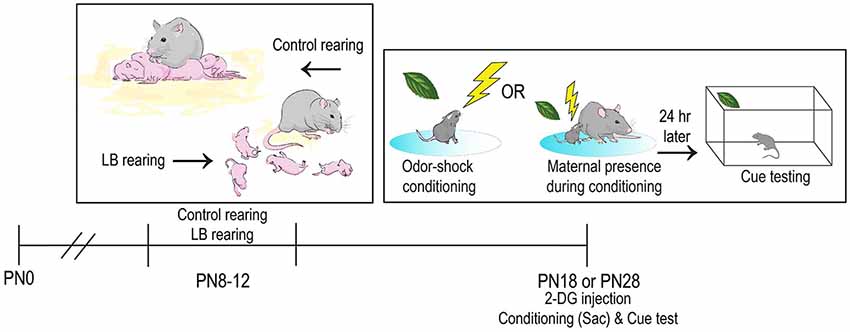
Figure 1. Schematic of methodology and experimental timeline. In infancy, pups received either Scarcity-Adversity Model of Low Bedding (LB) rearing or control rearing from the mother (ages PN8–12). LB rearing involved providing the mother with insufficient bedding for nest building, which produces maltreatment of pups but growth indistinguishable from controls. Pups are odor-shock conditioned in the mother’s presence or absence at one of two ages, with the goal of better understanding the neural mechanisms involved in social suppression of threat. A portion of the pups had the brain removed immediately after conditioning, while the other half were tested the next day (Cue test involving odor only presentations). The younger subjects were PN18, an age when pups are still with the mother but only for about 5 days before weaning. The other age tested was PN28, when pups have been independent for about 5 days.
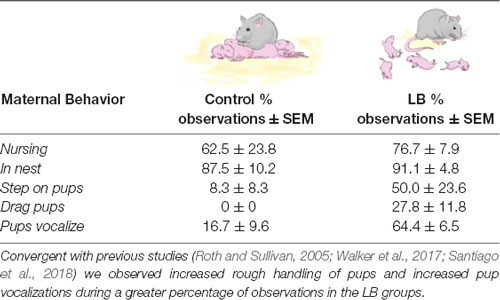
Table 1. The Scarcity-Adversity Model of Low Bedding (LB) is a validated procedure of inducing abuse by providing the mother with insufficient nest building material.
Odor-Shock Conditioning (Dependent on Mother PN18 or Independent PN28)
Conditioning took place in standard mouse fear conditioning (Coulbourn Instruments) apparatus within a sound attenuation chamber (Med Associates) with Coulbourn FreezeFrame software controlling stimuli delivery and video recording. Animals received a 20 min habituation session in the conditioning chambers a day prior to conditioning. On conditioning day, animals were given a 10 min adaptation period to the conditioning chamber before the start of conditioning. The conditioned stimulus (CS) was a 30 s peppermint odor (McCormick Pure Peppermint; 2 L/min; 1:10 peppermint vapor to air) controlled with a solenoid valve that minimized pressure changes by diverting airflow from the clean air to the peppermint air stream. To ventilate the chamber and ensure removal of odor CS, a standard attenuating chamber fan provided a constant stream of deodorized air flow through the chambers (2 L/min). The unconditioned stimulus (US) was a 1 s 0.6 mA foot shock delivered through a grid floor. The Paired experimental animals received a total of seven CS-US presentations administered at a 4 min inter-trial interval (ITI) and co-terminated with the 1 s footshock during the last second of the odor. Unpaired (behavioral control) animals received the same number of odor and shock presentations, however, the stimuli were separated by a 2 min inter-stimulus interval (ISI). Animals in the Odor-only condition also received the seven odor presentations but no shocks. Half of the experimental animals were conditioned in the presence of a urethane-anesthetized dam placed directly adjacent to the conditioning chamber where her odor was perceptible but she was not visible. Following conditioning, animals were either sacrificed and brains assessed for regional activity or retained for behavioral cue testing the next day to assess learning. PN18 and PN28 animals were only used at one age. These procedures were done according to published laboratory protocols (Boulanger Bertolus et al., 2014; Debiec and Sullivan, 2014; Tallot et al., 2016).
Neural Assessment
Animals used for neural assessment were injected with 14C-labeled 2-deoxyglucose (2-DG; 20 μCi/100 g, i.p.) just prior to being placed in the conditioning chamber and brains removed after conditioning (45 min after injection). Brains were stored in a −80°C freezer before being sectioned in a cryostat (20 μm) at −20°C. Through the region of interest (ROI), every third slice was collected onto a coverslip and slices along with 14C standards (10 × 0.02 mCi, American Radiolabeled Chemicals Inc., St. Louis, MO, USA) were exposed to X-ray film (Kodak) for 5 days. The autoradiograph was then digitally scanned and prepared for analysis. All procedures occurred according to published lab protocols (Perry et al., 2016; Opendak et al., 2019).
PFC Analysis
Autoradiographs were analyzed using ImageJ software (National Institutes of Health) for quantitative optical densitometry with an increase in autoradiographic density indicating increased 2-DG metabolism. Using Paxinos and Watson (2013) as a guide, two medial prefrontal regions were identified and analyzed for regional activity: Prelimbic (PL) and Infralimbic (IL), each of which was subdivided into additional subregions. At least three sections from the rostro-caudal extent were analyzed for each brain area.
Regional engagement levels were expressed as 2-DG uptake relative to that observed in white matter tracts (e.g., the anterior commissure or forceps minor) to control for differences in exposure levels or section thickness (Sullivan et al., 2000). Autoradiographic density was measured in both hemispheres of the brain for each region of interest and then averaged across both hemispheres, as no statistical difference was found between hemispheres.
Cue Test
Twenty-four hours following conditioning, learning was assessed using a cue test in a new context: novel room, placed in a 5,000 mL glass beaker inside a sound attenuating chamber (Coulbourn) with the fan placed outside the attenuating box. Context was further changed by cleaning the attenuating chamber with Windex (SC Johnson) 5 min before animals were placed within the beaker. For cue testing, animals were placed in the beaker and given a 5 min acclimation period prior to the first odor onset. Five 30 s presentations of the peppermint odor were presented using a 4-min ITI, as described for conditioning. Learning was measured by total time (in seconds) freezing during the odor with freezing defined as the cessation of all body movements with the exception of that minimally required for breathing. Freezing was scored automatically by FreezeFrame, although all freezing was checked by a blind scorer to determine freezing vs. inactivity. All animals were videotaped using two cameras, a side view and a top view to ensure accurate behavioral scoring.
Statistical Analysis
All behavior data were separated by age and rearing condition and analyzed using a two-way analysis of variance (ANOVA) with repeated measures [maternal presence (alone vs. with mom) × cue presentation (cue #1–5)] for training day data and two-way ANOVA [learning condition (paired, unpaired, odor only) × maternal presence (alone vs. with mom)] for cue test data, followed by Bonferroni-corrected pairwise tests. Planned comparisons were used when justified by a priori hypotheses (see Results section below). No sex effects or interactions were found in freezing behavior at either PN18 or PN28 and therefore data were collapsed across sex for analysis of maternal presence effects on behavior and 2-DG uptake. 2-DG uptake data were analyzed separately for each age using two-way ANOVA (rearing × maternal presence), followed by Bonferroni-corrected pairwise tests. All differences were considered significant when p < 0.05. All data analysis was performed by an experimenter blind to the experimental conditions.
Results
Mother-Infant Response to Scarcity-Adversity Model
Offline, blinded observations of videos of mother-infant interactions during control and LB Adversity-Rearing (PN8–12) indicated that the LB pups received more rough handling by the mother than controls (see Table 1 for further details).
Odor-Shock Conditioning Acquisition Curves
Assessment of paired animals with and without maternal presence during conditioning revealed significantly higher freezing in animals conditioned with the mom relative to animals conditioned alone during later trials except in PN28 control animals (Figure 2). For PN18 controls (Figure 2A), there was no main effect of maternal presence (F(1,14) = 2.601, p = 0.129) but there was a main effect of cue presentation (F(6,84) = 37.90, p < 0.001) and a cue presentation by maternal presence interaction (F(6,84) = 4.567, p = 0.0005). Post hoc tests showed that during the sixth (p = 0.016) and seventh (p = 0.001) odor presentations animals conditioned with the mother showed higher freezing relative to animals conditioned alone. For the PN18 LB group (Figure 2B), there also was no main effect of maternal presence (F(1,14) = 2.885, p = 0.112) but there was a main effect of cue presentation (F(6,84) = 50.66, p < 0.001) and a cue presentation by maternal presence interaction (F(6,84) = 5.563, p < 0.001). Similar to the control animals, during later cue presentations [fifth (p = 0.040), sixth (p = 0.019) and seventh (p < 0.001)] animals conditioned with the mother showed higher freezing relative to animals conditioned alone.
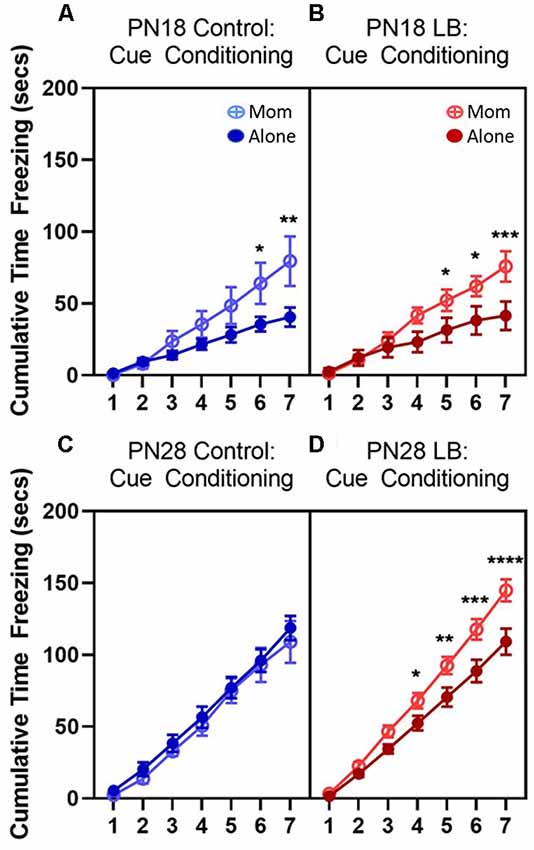
Figure 2. Odor-shock cue conditioning increases freezing to odor during training. Total cumulative (seconds) freezing (±SEM) during paired odor-shock cue conditioning at (A,B) PN18 (Control: Alone n = 8; Mom n = 8; LB: Alone n = 8; Mom n = 8) and (C,D) PN28 (Control: Alone n = 9; Mom n = 8; LB: Alone n = 14; Mom n = 15). Cue conditioning increased freezing at both ages in both control and low bedding (LB) rearing conditions however increased freezing was observed in animals conditioned with the mom (except in PN28 Control group) relative to animals conditioned alone. Open circle = conditioned with the mom; filled circles = conditioned alone; blue = controls; red = LB. *p < 0.05, **p < 0.01, ***p < 0.001, ****p < 0.0001.
At PN28, similar effects were observed in the LB group although there were fewer differences observed in the control animals (Figure 2C). For controls, there was no main effect of maternal presence (F(1,15) = 0.331, p = 0.574) or cue presentation by maternal presence interaction (F(6,90) = 0.177, p = 0.983), but there was a main effect of cue presentation (F(6,90) = 167.0, p < 0.001). The increase in freezing over time did not differ between animals conditioned alone or with the mom. In contrast, in the PN28 LB group (Figure 2D) there was a main effect of maternal presence (F(1,27) = 6.810, p = 0.015), cue presentation (F(6,162) = 410.9, p < 0.001) and a cue presentation by maternal presence interaction (F(6,162) = 7.722, p < 0.001). During the fourth (p = 0.046), fifth (p = 0.006), sixth (p < 0.001) and seventh (p = <0.001) odor presentations, animals conditioned with the mother showed higher freezing relative to animals conditioned alone.
Cue Test
Overall, all paired animals at both ages and in both rearing conditions showed increased freezing to the CS relative to controls, indicating retention of the learned association between the odor and the shock (Figure 3; Johansen et al., 2011). For PN18 Controls (Figure 3A), there was a main effect of learning condition (F(2,66) = 49.07, p < 0.001), maternal presence (F(1,66) = 7.27 p = 0.009) and a trending interaction (F(2,66) = 2.784, p = 0.069). Post hoc tests revealed that freezing in Paired groups with and without mom was significantly higher than control groups (all p’s < 0.05) and maternal presence increased paired group freezing relative to paired animals conditioned alone (p < 0.001). For the PN18 LB group (Figure 3B), there was a main effect of learning condition (F(2,65) = 60.00, p < 0.001), no effect of maternal presence (F(1,65) = 1.27, p = 0.264) nor an interaction effect (F(2,65) = 0.22, p = 0.80). Post hoc tests revealed that paired group freezing with and without mom was significantly higher than control groups (all p’s < 0.05) and no significant difference between the two paired groups with and without the mother (p = 0.359) suggesting the mother did not suppress learning.
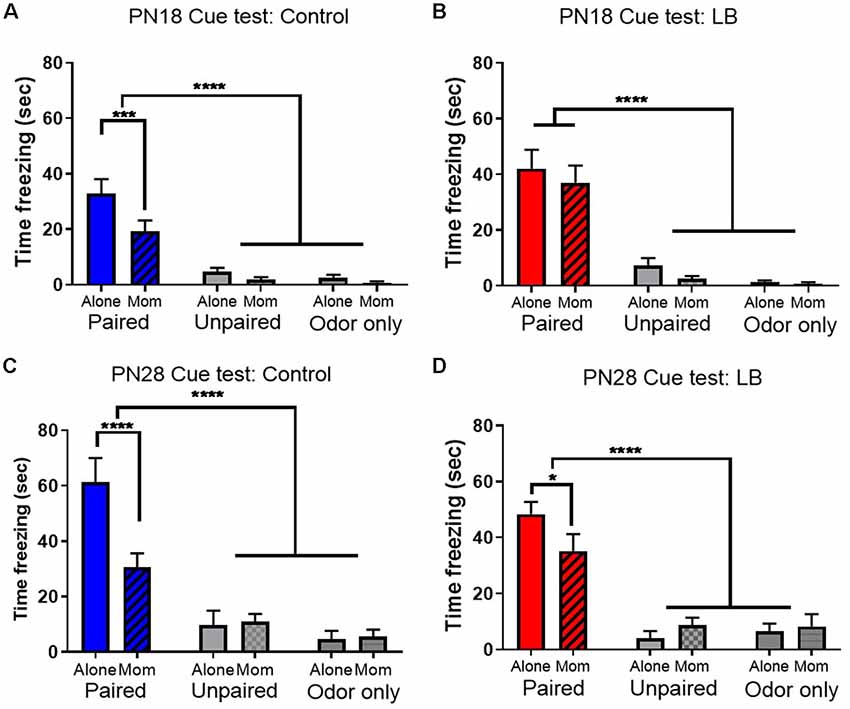
Figure 3. Early abuse modulates maternal buffering of odor-shock conditioning. Total (seconds) freezing (±SEM) to a conditioned stimulus (CS) was higher in Paired odor-shock conditions than Unpaired and Odor only conditions. Maternal presence during conditioning attenuated learning at both (A,B) PN18 Control (Paired: Alone n = 12; Mom n = 12; Unpaired: Alone n = 12; Mom n = 12; odor Only: Alone n = 12; Mom n = 12) and LB (Paired: Alone n = 11; Mom n = 12; Unpaired: Alone n = 12; Mom n = 12; odor Only: Alone n = 12; Mom n = 12) and (C,D) PN28 Control (Paired: Alone n = 9; Mom n = 10; Unpaired: Alone n = 8; Mom n = 8; odor Only: Alone n = 8; Mom n = 8) and LB (Paired: Alone n = 14; Mom n = 14; Unpaired: Alone n = 8; Mom n = 8; odor Only: Alone n = 8; Mom n = 8), although this maternal presence effect was not present following early life PN18 LB maltreatment and present but attenuated following early life PN28 LB maltreatment. *p < 0.05, ****p < 0.0001.
At PN28, similar effects were found for both controls and LB: only paired animals learned, although both rearing conditions showed attenuated learning with maternal presence. Specifically, for controls (Figure 3C) there were significant main effects of learning (F(2,45) = 36.93, p < 0.001), maternal presence (F(1,45) = 4.872, p = 0.032) and an interaction (F(2,45) = 6.363, p = 0.004). Post hoc tests revealed that both paired freezing with and without the mom was significantly higher than all control groups (p’s < 0.05) and there was a significant difference between the two paired groups (with mother freezing increased relative to without the mother, p < 0.01). A similar behavioral pattern was found in LB-reared animals (Figure 3D); there was a main effect of learning condition (F(2,54) = 39.81, p < 0.001), no effect for maternal presence (F(1,54) = 0.311, p = 0.579) nor was there an interaction (F(2,54) = 2.215, p = 0.119). Post hoc tests revealed that the paired groups with and without mom were significantly higher than all control groups (p’s < 0.001) and maternal presence increased paired group freezing (with mother vs. without the mother, p = 0.026).
Neural Analysis of Prefrontal Cortex (PFC)
Overall, we found significant evidence that PFC activation in several subregions at PN28 varied as a function of rearing condition (Control and LB) and whether the mother was present during conditioning. In contrast, no such PFC activation patterns at age PN18 were observed.
Infralimbic Prefrontal Cortex (IL)
The PFC showed significant differences across the dorsal-ventral axis at PN28, but not at PN18. Specifically for PN28 animals (Figure 4B), IL 2-DG uptake was higher when pups received paired CS-US conditioning with the mother vs. conditioned alone, while abused pups failed to show this effect [two-way ANOVA (rearing × maternal presence): main effect of rearing (F(1,117) = 30.77, p < 0.0001), main effect of maternal presence (F(1,117) = 6.754, p = 0.011), and a trending interaction (F(1,117) = 2.965, p = 0.088)]. Post hoc tests showed that maternal presence during paired odor-shock conditioning was associated with increased 2-DG uptake in IL in controls, but not LB-reared PN28 pups (control alone vs. control with mom, t(117) = 3.041, p = 0.003; LB alone vs. LB with mom, t(117) = 0.623, p = 0.535).
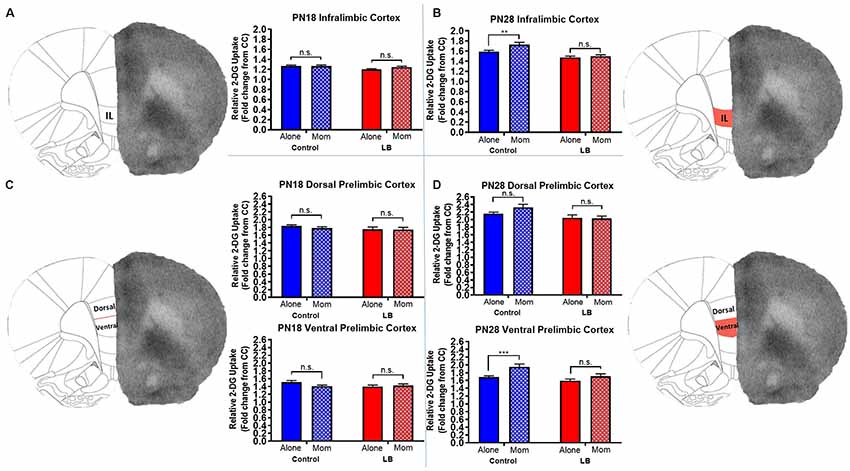
Figure 4. Previous abuse impairs maternal regulation of vmPFC regions during threat in older pups. Average (±SEM) fold change in activity for (A,C) PN18 Control infralimbic (IL: Alone n = 40; Mom n = 44; dorsal PL: Alone n = 41; Mom n = 33; ventral PL: Alone n = 30; Mom n = 33) and LB (IL: Alone n = 28; Mom n = 28; dorsal PL: Alone n = 28; Mom n = 21; ventral PL: Alone n = 21; Mom n = 21) or (B,D) PN28 Control (IL: Alone n = 32; Mom n = 28; dorsal PL: Alone n = 24; Mom n = 24; ventral PL: Alone n = 32; Mom n = 32) and LB (IL: Alone n = 32; Mom n = 298; dorsal PL: Alone n = 24; Mom n = 27; ventral PL: Alone n = 32; Mom n = 36). Solid bars represent pups conditioned alone while hashed bars represent pups conditioned with the mother. Right, schematics of brain regions; red, subregions with statistically significant effect of abuse on 2-DG uptake. n.s., indicates a non-significant effect (p > 0.05); **p < 0.01; ***p < 0.001.
At PN18 (Figure 4A), we failed to observe an effect of maternal presence on 2-DG uptake, though a main effect of rearing was observed [two-way ANOVA (rearing × maternal presence), main effect of rearing (F(1,136) = 4.705, p = 0.032); no main effect of maternal presence (F(1,136) = 1.127, p = 0.293); no interaction (F(1,136) = 1.557, p = 0.214)]. Post hoc tests showed that LS-reared pups exhibited lower 2-DG uptake levels compared to controls (LS with mom vs. control with mom, t(136) = 2.44, p = 0.016; LB with mom vs. control alone, t(136) = 2.26, p = 0.025).
Prelimbic Prefrontal Cortex (PL)
We observed that in PN28 pups (Figure 4D), the ventral region of the PL showed significant changes in 2-DG metabolism depending on rearing condition (Control vs. LS) as well as maternal presence [two-way ANOVA (rearing × maternal presence), main effect of rearing (F(1,128) = 9.127, p = 0.003), main effect of maternal presence (F(1,128) = 12.13, p = 0.001), no interaction (F(1,128) = 1.902, p = 0.170)]. Post hoc tests showed that maternal presence increased activity in Control pups conditioned with the mom but not LB (control alone vs. control with mom, t(128) = 3.39, p = 0.001; LB alone vs. LB with mom (t(128) = 1.51, p = 0.134). In the dorsal PL, only a main effect of rearing was observed (F(1,95) = 9.045, p = 0.003) with no main effect of maternal presence (F(1,95) = 1.288, p = 0.259) or interaction (F(1,95) = 1.869, p = 0.175). 2-DG uptake was decreased in all LS-reared groups compared to controls (LS with mom vs. control with mom, t(95) = 3.138, p = 0.002; LB alone vs. control mom, t(95) = 2.888, p = 0.005). At PN18 (Figure 4C), dorsal PL had no effects of maternal presence, rearing, or an interaction observed in the ventral (F(1,101) = 1.066, p = 0.304, F(1,101) = 1.764, p = 0.1871, and F(1,101) = 3.199, p = 0.076, respectively) or dorsal (F(1,119) = 0.480, p = 0.490, F(1,119) = 1.831, p = 0.1785, F(1,119) = 0.243, p = 0.623, respectively).
Discussion
Here, we assessed the neurobiology of social buffering of threat learning in typical and perturbed development. We focused on a developmental transition from dependence on the mother (PN18) to independence in preadolescent rats (PN28) weaned from the mother. Overall, our results show that social buffering of threat occurs across the lifespan, although the underlying neural circuit diverges, with the present results suggesting a late emerging role for the PFC after weaning from the mother. We summarize these results and integrate them into the existing social buffering literature in Figure 5: maternal presence blocks fear learning in early development, but switches to attenuation of threat responding, which behaviorally appears similar from PN16 into adulthood. This system is disrupted by early life trauma: PN18 maltreated pups were not socially buffered by the mother, but social buffering of threat emerged again by PN28. Most surprisingly, expression of social buffering in maltreated preadolescents did not require PFC engagement. Taken together, these results suggest that social buffering is a dynamic process that is sensitive to developmental events in an age-dependent manner.

Figure 5. Developmental transitions in amygdala inputs regulating social suppression of threat. The neural circuit supporting infant fear learning and its maternal presence blockade (≤PN15) or attenuation (≥PN16) undergoes developmental changes. Social buffering in early infancy is supported by VTA-amygdala connectivity (≤PN15), while in older pups (PN28) and adults social attenuation of fear is supported by vmPFC-amygdala connectivity. This system is disrupted following early life abusive rearing. At PN18, the ability of the mother to block fear learning is abolished and VTA showed compromised suppression of the amygdala. Early life maltreatment leaves social suppression of fear learning intact at PN28, although it is effectiveness is reduced and social modulation of vmPFC engagement is significantly reduced.
Using an age range when the PFC and its connectivity with the amygdala are maturing (Bouwmeester et al., 2002; Cressman et al., 2010; Willing and Juraska, 2015; Arruda-Carvalho et al., 2017), we asked if the PFC is involved in maternal suppression of fear learning in infant rats during a developmentally significant transitional period. In humans, the late-developing PFC shows a switch from positive to negative connectivity with the amygdala as children develop into adolescents and amygdala-prefrontal circuitry is associated with increased behavioral modulation of children by their mothers (Gee et al., 2013b, 2014). Furthermore, early life trauma is associated with dysregulated cortico-limbic network connectivity through adolescence, impaired stress responding, and cortico-limbic hyperactivity in response to negative social cues (Andersen and Teicher, 2008; Suzuki et al., 2014; Teicher et al., 2014, 2016; Kaiser et al., 2018). Together with the current results, these reports suggest that prefrontal modulation of interacting fear and social systems contributes to the developmental profile of maternal fear regulation and this system can be disrupted following early life trauma. However, further investigation is needed to confirm this hypothesis.
Typical Rearing: Social Buffering of Threat Occurs at Both PN18 and PN28, but the PFC Is Only Engaged in Newly Independent PN28 Pups
The similar social buffering effects on the behavioral level at PN18 and PN28 appear to be supported by different neural networks; the ventromedial (vm)PFC IL and PL subregions were only modulated by the mother in the PN28 animals. The PFC is a late-developing structure (Gee et al., 2013b; Schubert et al., 2015; Hennessy et al., 2018) and the older infant/child and adult literature validates the important role of the amygdala and vmPFC for social buffering in humans (Lungwitz et al., 2014; Hornstein et al., 2016; Hornstein and Eisenberger, 2017; van Rooij et al., 2017), nonhuman primates (Winslow et al., 2003; Suomi et al., 2008; Sanchez et al., 2015; Howell et al., 2017) and rodents (Hennessy et al., 2015; Penha Farias et al., 2019). The absence of a PFC effect in the youngest pups is consistent with the literature as well. These reports suggest that the rodent vmPFC is not engaged by simple maternal presence, simple innate threat presentation, or learning about threat until around weaning age (~PN23; Kim et al., 2009; Chan et al., 2011; Ball and Slane, 2012; Li et al., 2012; Shechner et al., 2014; Takahashi, 2014; Almada et al., 2015; Perry et al., 2016; Heroux et al., 2017; Robinson-Drummer et al., 2018). It should be noted that the PFC appears to be involved in the appetitive system in PN18 pups (Lilliquist et al., 1999; Nair et al., 2001a,b), suggesting a staggered developmental functional onset for various PFC functions.
The newly emerging role of the vmPFC by PN28 to support social buffering of threat is consistent with vmPFC importance in adult fear conditioning social presence literature in humans and rodents. For example, in adult rats, the presence of a cage mate significantly attenuates fear learning, compared to those conditioned alone and engages the vmPFC (Kiyokawa et al., 2014, 2007; Penha Farias et al., 2019). This effect also occurs in humans and involves the vmPFC; in adults, the presence of an important social partner (i.e., mother, romantic partner, cage mate) or a stimulus that provokes the memory of an individual (i.e., odor, photo) dampens fear through amygdala-vmPFC to block adult fear learning across species (Guzmán et al., 2009; Fuzzo et al., 2015; Hornstein et al., 2016; Hornstein and Eisenberger, 2017; van Rooij et al., 2017; Toumbelekis et al., 2018). Our results also overlap with the literature involving non-social cues predicting safety within a threatening situation: conditioned inhibitors/safety signals use a similar network of PFC input suppressing the amygdala (Rogan et al., 2005; Pollak et al., 2008; Christianson et al., 2012; Harrison et al., 2017; Levin et al., 2017). The specific connection between the vmPFC and amygdala has not been documented within the social buffering of threat literature, although our general understanding of vmPFC-amygdala functional connectivity suggests the PFC is required to modulate the amygdala’s output response to threat (Phelps et al., 2004; Corcoran and Quirk, 2007; Marek et al., 2013). In general, the IL appears to reduce fear (Quirk et al., 2000; Sotres-Bayon et al., 2004; Do-Monte et al., 2015), and this is consistent with our findings; the largest maternal response in the PFC was found in the IL. In contrast, the PL is generally associated with enhanced amygdala responding and enhanced amygdala-dependent response to threat (Sharpe and Killcross, 2015a,b, 2018; Ye et al., 2017) although prelimbic-infralimbic projections have been shown to contribute to reductions in fear expression (Marek et al., 2018). The dorso-ventral gradient of activity observed at PN28 support a role for a subset of PL contributing to fear reduction with ventral regions sharing function with the IL cortex; a finding not surprising due to their close anatomical proximity.
As we consider the functional significance of late PFC engagement by social buffering of threat during early life, we suggest that as pups leave the nest they encounter a far more complicated environment where higher order brain areas (such as the vmPFC) are required for processing complex threat and safety cues. Indeed, outside the nest an animal must use changing, context- and time-dependent safety/threat cues to choose appropriate approach/avoidance responses in environments with complex social hierarchy (Cunningham et al., 2002; Holland and Gallagher, 2004; Taylor et al., 2008; Maren et al., 2013; Opendak et al., 2017). Development of functional connectivity between the vmPFC, threat and social circuits would allow necessary integration of these cues thereby facilitating proper social interactions and threat evaluation.
Maltreatment Rearing Blocked Social Buffering of Threat at PN18, but Returns at PN28 Without PFC Engagement
One of the more intriguing aspects of the present data is the effect of rearing on social buffering at across development; early life maltreatment transiently suppressed social buffering at PN18 (replicating effects observed in Opendak et al., 2019) and social buffering returned at PN28. Our experiments do not suggest a mechanism for this transition, although the evidence points to the slow decline of the infant VTA social buffering system and the protracted emergence of the adult-like, PFC-dependent, social buffering system (see Figure 5). Specifically, our previous work suggests this PN18 maltreatment effect is due to disruption of the infant VTA dopaminergic input to the basolateral amygdala, the mechanism supporting social blockade and suppression of fear learning in younger pups (Barr et al., 2009; Opendak et al., 2019). In further support of this framework, in typically-reared PN28 pups, social buffering was associated with PFC engagement, which was not observed at PN18.
Another striking feature of these data is the dissociation between PFC and social buffering following maltreatment in preadolescents. Specifically, buffering was still observed at PN28 following maltreatment, though we failed to observe the engagement of the PFC documented in control-reared pups. We should note that early maltreatment seemed to reduce the effect of maternal presence on fear learning; LB pups showed a smaller difference in freezing between paired conditioning alone and with mom groups although this result requires replication and direct comparison in a future study. However this complements existing literature on the impact of early life stress on the infant PFC and infant learning (Callaghan and Richardson, 2012; Pattwell et al., 2012; Fareri et al., 2017; Peña et al., 2017; Bath, 2018; Callaghan et al., 2019; Junod et al., provisionally accepted) and extends these results to include reduced social reduction of fear.
While it is abundantly clear that early life stress disrupts pups’ neurobehavioral development (Barbosa Neto et al., 2012; Tang et al., 2014; Doherty and Roth, 2016; Pattwell and Bath, 2017; Walker et al., 2017), including PFC development (Braun and Bock, 2011; Kunzler et al., 2015; Schubert et al., 2015; Hanson et al., 2018; VanTieghem and Tottenham, 2018), we speculate that a critical feature of this effect is that the ability of the mother to impact pups’ brains has failed to acquire the strength or value it has in typically reared pups. Indeed, our previous assessment of the value of maternal odor in control-reared vs. maltreatment-reared pups shows a slight yet significant decrease in approach to the maternal odor and decreased activation of amygdala and PFC in response to a maternal odor presentation without threat (Perry et al., 2016). It should be noted that the maltreatment-associated maternal odor increases in value across development. Indeed, adults reared with maltreatment have greater reduction of threat by maternal odor compared to controls, as evidenced by suppression of amygdala, attenuated fear conditioning and normalization of depressive-like behaviors (Sevelinges et al., 2011; Rincón-Cortés et al., 2015). This phenomenon may contribute to the transient effect of LB on behavior between PN18 and 28; as weaned animals approach adulthood, the weakened maternal cue naturally regains value and is able to reduce fear behavior. This would suggest that modulation of LB fear behavior is redirected through other circuit nodes (e.g., the VTA) when maternal presence fails to modulate vmPFC activity at this age. Thus, in addition to the social buffering network changing during development, the social signal processing within a larger social brain network may contribute to maltreatment-associated effects on social buffering.
Conclusion
As we consider the implications of these results for infant neurobehavioral development and integration with the broader human development work, this work may inform our understanding of attachment. Within Attachment Theory, the mother is considered a “safe haven” or a source of safety, wherein the infant approaches the caregiver for safety and the caregiver reduces fear (Kerns et al., 2015; Hornstein et al., 2016). Here, using a fear conditioning paradigm, we show that maltreatment diminishes the mother’s ability to serve as a “safe haven” and social buffering of threat takes on a nonlinear effect across development.
Data Availability
All datasets generated for this study are included in the manuscript.
Ethics Statement
This study was carried out in accordance with the recommendations of National Institutes of Health. The protocol was approved by the Institutional Animal Care and Use Committees.
Author Contributions
RS, MO and PR-D designed the experiments. PR-D, KW, MO and RS conducted the research/analyzed behavior. MO and AB made illustrations. ST, AB, PR-D, MO, SC, EF, CS, AS, DC, CD and ST analyzed the IA data. KW, LJ and GK performed histology and autoradiography. RS, PR-D, MO and AB wrote the manuscript. PR-D, MO, AB and RS performed and were consulted on data analysis and statistics.
Funding
This research was funded by Foundation for the National Institutes of Health (NIH) F32MH112232, BBRF NARSAD Young Investigator Award T32MH019524 (MO), Gålö-stiftelsen (AB), NYU undergraduate DURF award (SC, ST, CD), Fulbright Fellowship (GK), and R37HD083217 (RS).
Conflict of Interest Statement
The authors declare that the research was conducted in the absence of any commercial or financial relationships that could be construed as a potential conflict of interest.
Acknowledgments
We thank Joyce Woo for the contribution of her drawings to use as illustrations in Figure 1 and Table 1.
References
Al Aïn, S., Perry, R. E., Nuñez, B., Kayser, K., Hochman, C., Brehman, E., et al. (2017). Neurobehavioral assessment of maternal odor in developing rat pups: implications for social buffering. Soc. Neurosci. 12, 32–49. doi: 10.1080/17470919.2016.1159605
Almada, R. C., Coimbra, N. C., and Brandão, M. L. (2015). Medial prefrontal cortex serotonergic and GABAergic mechanisms modulate the expression of contextual fear: intratelencephalic pathways and differential involvement of cortical subregions. Neuroscience 284, 988–997. doi: 10.1016/j.neuroscience.2014.11.001
Andersen, S. L., and Teicher, M. H. (2008). Stress, sensitive periods and maturational events in adolescent depression. Trends Neurosci. 31, 183–191. doi: 10.1016/j.tins.2008.01.004
Arruda-Carvalho, M., Wu, W. C., Cummings, K. A., and Clem, R. L. (2017). Optogenetic examination of prefrontal-amygdala synaptic development. J. Neurosci. 37, 2976–2985. doi: 10.1523/JNEUROSCI.3097-16.2017
Ball, K. T., and Slane, M. (2012). Differential involvement of prelimbic and infralimbic medial prefrontal cortex in discrete cue-induced reinstatement of 3,4-methylenedioxymethamphetamine (MDMA; ecstasy) seeking in rats. Psychopharmacology 224, 377–385. doi: 10.1007/s00213-012-2762-5
Barbosa Neto, J. B., Tiba, P. A., Faturi, C. B., de Castro-Neto, E. F., da Graca Naffah-Mazacoratti, M., de Jesus Mari, J., et al. (2012). Stress during development alters anxiety-like behavior and hippocampal neurotransmission in male and female rats. Neuropharmacology 62, 518–526. doi: 10.1016/j.neuropharm.2011.09.011
Barr, G. A., Moriceau, S., Shionoya, K., Muzny, K., Gao, P., Wang, S., et al. (2009). Transitions in infant learning are modulated by dopamine in the amygdala. Nat. Neurosci. 12, 1367–1369. doi: 10.1038/nn.2403
Bath, K. G. (2018). “Early life stress is associated with precocious amygdala development and an unexpected dip in threat-associated freezing,” in Paper Presented at the International Society for Developmental Psychobiology, San Diego, CA.
Bisaz, R., and Sullivan, R. M. (2012). Developmental neurobiology of the rat attachment system and its modulation by stress. Behav. Sci. 2, 79–102. doi: 10.3390/bs2020079
Blair, C., and Raver, C. C. (2015). School readiness and self-regulation: a developmental psychobiological approach. Annu. Rev. Psychol. 66, 711–731. doi: 10.1146/annurev-psych-010814-015221
Boulanger Bertolus, J., Hegoburu, C., Ahers, J. L., Londen, E., Rousselot, J., Szyba, K., et al. (2014). Infant rats can learn time intervals before the maturation of the striatum: evidence from odor fear conditioning. Front. Behav. Neurosci. 8:176. doi: 10.3389/fnbeh.2014.00176
Bouwmeester, H., Smits, K., and Van Ree, J. M. (2002). Neonatal development of projections to the basolateral amygdala from prefrontal and thalamic structures in rat. J. Comp. Neurol. 450, 241–255. doi: 10.1002/cne.10321
Bowlby, J. (1982). Attachment and loss: retrospect and prospect. Am. J. Orthopsychiatry 52, 664–678. doi: 10.1111/j.1939-0025.1982.tb01456.x
Braun, K., and Bock, J. (2011). The experience-dependent maturation of prefronto-limbic circuits and the origin of developmental psychopathology: implications for the pathogenesis and therapy of behavioural disorders. Dev. Med. Child Neurol. 53, 14–18. doi: 10.1111/j.1469-8749.2011.04056.x
Callaghan, B. L., and Richardson, R. (2012). Early-life stress affects extinction during critical periods of development: an analysis of the effects of maternal separation on extinction in adolescent rats. Stress 15, 671–679. doi: 10.3109/10253890.2012.667463
Callaghan, B. L., Meyer, H., Opendak, M., Van Tieghem, M. R., Li, A., Harmon, C., et al. (2019). The developmental ecology of fear neurobiology: scaffolding by caregiving. Annu. Rev. Clin. Psychol. 15, 345–369. doi: 10.1146/annurev-clinpsy-050718-095727
Chambers, J. (2017). The neurobiology of attachment: from infancy to clinical outcomes. Psychodyn. Psychiatry 45, 542–563. doi: 10.1521/pdps.2017.45.4.542
Chan, T., Kyere, K., Davis, B. R., Shemyakin, A., Kabitzke, P. A., Shair, H. N., et al. (2011). The role of the medial prefrontal cortex in innate fear regulation in infants, juveniles and adolescents. J. Neurosci. 31, 4991–4999. doi: 10.1523/JNEUROSCI.5216-10.2011
Christianson, J. P., Fernando, A. B., Kazama, A. M., Jovanovic, T., Ostroff, L. E., and Sangha, S. (2012). Inhibition of fear by learned safety signals: a mini-symposium review. J. Neurosci. 32, 14118–14124. doi: 10.1523/JNEUROSCI.3340-12.2012
Coe, C. L., Mendoza, S. P., Smotherman, W. P., and Levine, S. (1978). Mother-infant attachment in the squirrel monkey: adrenal response to separation. Behav. Biol. 22, 256–263. doi: 10.1016/s0091-6773(78)92305-2
Corcoran, K. A., and Quirk, G. J. (2007). Recalling safety: cooperative functions of the ventromedial prefrontal cortex and the hippocampus in extinction. CNS Spectr. 12, 200–206. doi: 10.1017/s1092852900020915
Coss, R. G. (2016). Sex difference in choice of concealed or exposed refuge sites by preschool children viewing a model leopard in a playground simulation of antipredator behavior. Int. J. Psychol. Res. 9, 8–19. doi: 10.21500/20112084.2325
Cressman, V. L., Balaban, J., Steinfeld, S., Shemyakin, A., Graham, P., Parisot, N., et al. (2010). Prefrontal cortical inputs to the basal amygdala undergo pruning during late adolescence in the rat. J. Comp. Neurol. 518, 2693–2709. doi: 10.1002/cne.22359
Cunningham, M. G., Bhattacharyya, S., and Benes, F. M. (2002). Amygdalo-cortical sprouting continues into early adulthood: implications for the development of normal and abnormal function during adolescence. J. Comp. Neurol. 453, 116–130. doi: 10.1002/cne.10376
Debiec, J., and Sullivan, R. M. (2014). Intergenerational transmission of emotional trauma through amygdala-dependent mother-to-infant transfer of specific fear. Proc. Natl. Acad. Sci. U S A 111, 12222–12227. doi: 10.1073/pnas.1316740111
Doherty, T. S., and Roth, T. L. (2016). Insight from animal models of environmentally driven epigenetic changes in the developing and adult brain. Dev. Psychopathol. 28, 1229–1243. doi: 10.1017/s095457941600081x
Do-Monte, F. H., Manzano-Nieves, G., Quiñones-Laracuente, K., Ramos-Medina, L., and Quirk, G. J. (2015). Revisiting the role of infralimbic cortex in fear extinction with optogenetics. J. Neurosci. 35, 3607–3615. doi: 10.1523/JNEUROSCI.3137-14.2015
Drury, S. S., Sánchez, M. M., and Gonzalez, A. (2016). When mothering goes awry: challenges and opportunities for utilizing evidence across rodent, nonhuman primate and human studies to better define the biological consequences of negative early caregiving. Horm. Behav. 77, 182–192. doi: 10.1016/j.yhbeh.2015.10.007
Fareri, D. S., Gabard-Durnam, L., Goff, B., Flannery, J., Gee, D. G., Lumian, D. S., et al. (2017). Altered ventral striatal-medial prefrontal cortex resting-state connectivity mediates adolescent social problems after early institutional care. Dev. Psychopathol. 29, 1865–1876. doi: 10.1017/s0954579417001456
Feldman, R. (2017). The neurobiology of human attachments. Trends Cogn. Sci. 21, 80–99. doi: 10.1016/j.tics.2016.11.007
Fuzzo, F., Matsumoto, J., Kiyokawa, Y., Takeuchi, Y., Ono, T., and Nishijo, H. (2015). Social buffering suppresses fear-associated activation of the lateral amygdala in male rats: behavioral and neurophysiological evidence. Front. Neurosci. 9:99. doi: 10.3389/fnins.2015.00099
Gee, D. G., Gabard-Durnam, L. J., Flannery, J., Goff, B., Humphreys, K. L., Telzer, E. H., et al. (2013a). Early developmental emergence of human amygdala-prefrontal connectivity after maternal deprivation. Proc. Natl. Acad. Sci. U S A 110, 15638–15643. doi: 10.1073/pnas.1307893110
Gee, D. G., Humphreys, K. L., Flannery, J., Goff, B., Telzer, E. H., Shapiro, M., et al. (2013b). A developmental shift from positive to negative connectivity in human amygdala-prefrontal circuitry. J. Neurosci. 33, 4584–4593. doi: 10.1523/JNEUROSCI.3446-12.2013
Gee, D. G., Gabard-Durnam, L., Telzer, E. H., Humphreys, K. L., Goff, B., Shapiro, M., et al. (2014). Maternal buffering of human amygdala-prefrontal circuitry during childhood but not during adolescence. Psychol. Sci. 25, 2067–2078. doi: 10.1177/0956797614550878
Gunnar, M. R., Hostinar, C. E., Sanchez, M. M., Tottenham, N., and Sullivan, R. M. (2015). Parental buffering of fear and stress neurobiology: reviewing parallels across rodent, monkey, and human models. Soc. Neurosci. 10, 474–478. doi: 10.1080/17470919.2015.1070198
Gunnar, M. R., and Sullivan, R. M. (2017). The neurodevelopment of social buffering and fear learning: integration and crosstalk. Soc. Neurosci. 12, 1–7. doi: 10.1080/17470919.2016.1151824
Gunnar, M. R., and Quevedo, K. M. (2007). Early care experiences and HPA axis regulation in children: a mechanism for later trauma vulnerability. Prog. Brain Res. 167, 137–149. doi: 10.1016/s0079-6123(07)67010-1
Guzmán, Y. F., Tronson, N. C., Guedea, A., Huh, K. H., Gao, C., and Radulovic, J. (2009). Social modeling of conditioned fear in mice by non-fearful conspecifics. Behav. Brain Res. 201, 173–178. doi: 10.1016/j.bbr.2009.02.024
Hanson, J. L., Knodt, A. R., Brigidi, B. D., and Hariri, A. R. (2018). Heightened connectivity between the ventral striatum and medial prefrontal cortex as a biomarker for stress-related psychopathology: understanding interactive effects of early and more recent stress. Psychol. Med. 48, 1835–1843. doi: 10.1017/s0033291717003348
Harrison, B. J., Fullana, M. A., Via, E., Soriano-Mas, C., Vervliet, B., Martinez-Zalacain, I., et al. (2017). Human ventromedial prefrontal cortex and the positive affective processing of safety signals. Neuroimage 152, 12–18. doi: 10.1016/j.neuroimage.2017.02.080
Hennessy, M. B., Hornschuh, G., Kaiser, S., and Sachser, N. (2006). Cortisol responses and social buffering: a study throughout the life span. Horm. Behav. 49, 383–390. doi: 10.1016/j.yhbeh.2005.08.006
Hennessy, M. B., Kaiser, S., and Sachser, N. (2009). Social buffering of the stress response: diversity, mechanisms, and functions. Front. Neuroendocrinol. 30, 470–482. doi: 10.1016/j.yfrne.2009.06.001
Hennessy, M. B., Schiml, P. A., Willen, R., Watanasriyakul, W., Johnson, J., and Garrett, T. (2015). Selective social buffering of behavioral and endocrine responses and Fos induction in the prelimbic cortex of infants exposed to a novel environment. Dev. Psychobiol. 57, 50–62. doi: 10.1002/dev.21256
Hennessy, M. B., Watanasriyakul, W. T., Price, B. C., Bertke, A. S., and Schiml, P. A. (2018). Adult males buffer the cortisol response of young guinea pigs: changes with age, mediation by behavior, and comparison with prefrontal activity. Horm. Behav. 98, 165–172. doi: 10.1016/j.yhbeh.2017.12.017
Heroux, N. A., Robinson-Drummer, P. A., Sanders, H. R., Rosen, J. B., and Stanton, M. E. (2017). Differential involvement of the medial prefrontal cortex across variants of contextual fear conditioning. Learn. Mem. 24, 322–330. doi: 10.1101/lm.045286.117
Hofer, M. A. (1994). Hidden regulators in attachment, separation, and loss. Monogr. Soc. Res. Child Dev. 59, 192–207. doi: 10.1111/j.1540-5834.1994.tb01285.x
Holland, P. C., and Gallagher, M. (2004). Amygdala-frontal interactions and reward expectancy. Curr. Opin. Neurobiol. 14, 148–155. doi: 10.1016/j.conb.2004.03.007
Hornstein, E. A., and Eisenberger, N. I. (2017). Unpacking the buffering effect of social support figures: social support attenuates fear acquisition. PLoS One 12:e0175891. doi: 10.1371/journal.pone.0175891
Hornstein, E. A., Fanselow, M. S., and Eisenberger, N. I. (2016). A safe haven: investigating social-support figures as prepared safety stimuli. Psychol. Sci. 27, 1051–1060. doi: 10.1177/0956797616646580
Hostinar, C. E., and Gunnar, M. R. (2015). Social support can buffer against stress and shape brain activity. AJOB Neurosci. 6, 34–42. doi: 10.1080/21507740.2015.1047054
Hostinar, C. E., Johnson, A. E., and Gunnar, M. R. (2015). Parent support is less effective in buffering cortisol stress reactivity for adolescents compared to children. Dev. Sci. 18, 281–297. doi: 10.1111/desc.12195
Hostinar, C. E., Sullivan, R. M., and Gunnar, M. R. (2014). Psychobiological mechanisms underlying the social buffering of the hypothalamic-pituitary-adrenocortical axis: a review of animal models and human studies across development. Psychol. Bull. 140, 256–282. doi: 10.1037/a0032671
Howell, B. R., McMurray, M. S., Guzman, D. B., Nair, G., Shi, Y., McCormack, K. M., et al. (2017). Maternal buffering beyond glucocorticoids: impact of early life stress on corticolimbic circuits that control infant responses to novelty. Soc. Neurosci. 12, 50–64. doi: 10.1080/17470919.2016.1200481
Inagaki, T. K., and Eisenberger, N. I. (2012). Neural correlates of giving support to a loved one. Psychosom. Med. 74, 3–7. doi: 10.1097/psy.0b013e3182359335
Johansen, J. P., Cain, C. K., Ostroff, L. E., and LeDoux, J. E. (2011). Molecular mechanisms of fear learning and memory. Cell 147, 509–524. doi: 10.1016/j.cell.2011.10.009
Junod, A., Opendak, M., LeDoux, J., and Sullivan, R. M. (provisionally accepted). Development of threat expression following infant maltreatment: infant and adult enhancement but adolescent attenuation. Front. Behav. Neurosci. doi: 10.3389/fnbeh.2019.00130
Kaiser, R. H., Clegg, R., Goer, F., Pechtel, P., Beltzer, M., Vitaliano, G., et al. (2018). Childhood stress, grown-up brain networks: corticolimbic correlates of threat-related early life stress and adult stress response. Psychol. Med. 48, 1157–1166. doi: 10.1017/s0033291717002628
Kerns, K. A., Mathews, B. L., Koehn, A. J., Williams, C. T., and Siener-Ciesla, S. (2015). Assessing both safe haven and secure base support in parent-child relationships. Attach. Hum. Dev. 17, 337–353. doi: 10.1080/14616734.2015.1042487
Kim, J. H., Hamlin, A. S., and Richardson, R. (2009). Fear extinction across development: the involvement of the medial prefrontal cortex as assessed by temporary inactivation and immunohistochemistry. J. Neurosci. 29, 10802–10808. doi: 10.1523/JNEUROSCI.0596-09.2009
Kiyokawa, Y., Hiroshima, S., Takeuchi, Y., and Mori, Y. (2014). Social buffering reduces male rats’ behavioral and corticosterone responses to a conditioned stimulus. Horm. Behav. 65, 114–118. doi: 10.1016/j.yhbeh.2013.12.005
Kiyokawa, Y., Takeuchi, Y., and Mori, Y. (2007). Two types of social buffering differentially mitigate conditioned fear responses. Eur. J. Neurosci. 26, 3606–3613. doi: 10.1111/j.1460-9568.2007.05969.x
Kiyokawa, Y., Wakabayashi, Y., Takeuchi, Y., and Mori, Y. (2012). The neural pathway underlying social buffering of conditioned fear responses in male rats. Eur. J. Neurosci. 36, 3429–3437. doi: 10.1111/j.1460-9568.2012.08257.x
Kunzler, J., Braun, K., and Bock, J. (2015). Early life stress and sex-specific sensitivity of the catecholaminergic systems in prefrontal and limbic regions of Octodon degus. Brain Struct. Funct. 220, 861–868. doi: 10.1007/s00429-013-0688-2
Levin, N., Kritman, M., Maroun, M., and Akirav, I. (2017). Differential roles of the infralimbic and prelimbic areas of the prefrontal cortex in reconsolidation of a traumatic memory. Eur. Neuropsychopharmacol. 27, 900–912. doi: 10.1016/j.euroneuro.2017.06.007
Levine, S., Stanton, M. E., and Gutierrez, Y. R. (1988). Maternal modulation of pituitary-adrenal activity during ontogeny. Adv. Exp. Med. Biol. 245, 295–310. doi: 10.1007/978-1-4899-2064-5_24
Li, S., Kim, J. H., and Richardson, R. (2012). Differential involvement of the medial prefrontal cortex in the expression of learned fear across development. Behav. Neurosci. 126, 217–225. doi: 10.1037/a0027151
Lilliquist, M. W., Nair, H. P., Gonzalez-Lima, F., and Amsel, A. (1999). Extinction after regular and irregular reward schedules in the infant rat: influence of age and training duration. Dev. Psychobiol. 34, 57–70. doi: 10.1002/(sici)1098-2302(199901)34:1<57::aid-dev7>3.0.co;2-r
Lungwitz, E. A., Stuber, G. D., Johnson, P. L., Dietrich, A. D., Schartz, N., Hanrahan, B., et al. (2014). The role of the medial prefrontal cortex in regulating social familiarity-induced anxiolysis. Neuropsychopharmacology 39, 1009–1019. doi: 10.1038/npp.2013.302
Marek, R., Strobel, C., Bredy, T. W., and Sah, P. (2013). The amygdala and medial prefrontal cortex: partners in the fear circuit. J. Physiol. 591, 2381–2391. doi: 10.1113/jphysiol.2012.248575
Marek, R., Xu, L., Sullivan, R. K. P., and Sah, P. (2018). Excitatory connections between the prelimbic and infralimbic medial prefrontal cortex show a role for the prelimbic cortex in fear extinction. Nat. Neurosci. 21, 654–658. doi: 10.1038/s41593-018-0137-x
Maren, S., Phan, K. L., and Liberzon, I. (2013). The contextual brain: implications for fear conditioning, extinction and psychopathology. Nat. Rev. Neurosci. 14, 417–428. doi: 10.1038/nrn3492
Moriceau, S., and Sullivan, R. M. (2006). Maternal presence serves as a switch between learning fear and attraction in infancy. Nat. Neurosci. 9, 1004–1006. doi: 10.1038/nn1733
Moriceau, S., Roth, T. L., and Sullivan, R. M. (2010). Rodent model of infant attachment learning and stress. Dev. Psychobiol. 52, 651–660. doi: 10.1002/dev.20482
Moriceau, S., Shionoya, K., Jakubs, K., and Sullivan, R. M. (2009). Early-life stress disrupts attachment learning: the role of amygdala corticosterone, locus ceruleus corticotropin releasing hormone, and olfactory bulb norepinephrine. J. Neurosci. 29, 15745–15755. doi: 10.1523/JNEUROSCI.4106-09.2009
Moriceau, S., Wilson, D. A., Levine, S., and Sullivan, R. M. (2006). Dual circuitry for odor-shock conditioning during infancy: corticosterone switches between fear and attraction via amygdala. J. Neurosci. 26, 6737–6748. doi: 10.1523/JNEUROSCI.0499-06.2006
Nachmias, M., Gunnar, M., Mangelsdorf, S., Parritz, R. H., and Buss, K. (1996). Behavioral inhibition and stress reactivity: the moderating role of attachment security. Child Dev. 67, 508–522. doi: 10.2307/1131829
Nair, H. P., Berndt, J. D., Barrett, D., and Gonzalez-Lima, F. (2001a). Maturation of extinction behavior in infant rats: large-scale regional interactions with medial prefrontal cortex, orbitofrontal cortex, and anterior cingulate cortex. J. Neurosci. 21, 4400–4407. doi: 10.1523/JNEUROSCI.21-12-04400.2001
Nair, H. P., Berndt, J. D., Barrett, D., and Gonzalez-Lima, F. (2001b). Metabolic mapping of brain regions associated with behavioral extinction in preweanling rats. Brain Res. 903, 141–153. doi: 10.1016/s0006-8993(01)02469-6
Opendak, M., Gould, E., and Sullivan, R. (2017). Early life adversity during the infant sensitive period for attachment: programming of behavioral neurobiology of threat processing and social behavior. Dev. Cogn. Neurosci. 25, 145–159. doi: 10.1016/j.dcn.2017.02.002
Opendak, M., Robinson-Drummer, P., Blomkvist, A., Zanca, R. M., Wood, K., Jacobs, L., et al. (2019). Neurobiology of maternal regulation of infant fear: the role of mesolimbic dopamine and its disruption by maltreatment. Neuropsychopharmacology 44, 1247–1257. doi: 10.1038/s41386-019-0340-9
Opendak, M., and Sullivan, R. M. (2016). Unique neurobiology during the sensitive period for attachment produces distinctive infant trauma processing. Eur. J. Psychotraumatol. 7:31276. doi: 10.3402/ejpt.v7.31276
Pattwell, S. S., and Bath, K. G. (2017). Emotional learning, stress, and development: an ever-changing landscape shaped by early-life experience. Neurobiol. Learn. Mem. 143, 36–48. doi: 10.1016/j.nlm.2017.04.014
Pattwell, S. S., Duhoux, S., Hartley, C. A., Johnson, D. C., Jing, D., Elliott, M. D., et al. (2012). Altered fear learning across development in both mouse and human. Proc. Natl. Acad. Sci. U S A 109, 16318–16323. doi: 10.1073/pnas.1206834109
Paxinos, G., and Watson, C. (2013). The Rat Brain in Stereotaxic Coordinates. 7th Edn. Boston, MA: Academic Press.
Peña, C. J., Kronman, H. G., Walker, D. M., Cates, H. M., Bagot, R. C., Purushothaman, I., et al. (2017). Early life stress confers lifelong stress susceptibility in mice via ventral tegmental area OTX2. Science 356, 1185–1188. doi: 10.1126/science.aan4491
Penha Farias, C., Guerino Furini, C. R., Godfried Nachtigall, E., Kielbovicz Behling, J. A., Silva de Assis Brasil, E., Bühler, L., et al. (2019). Extinction learning with social support depends on protein synthesis in prefrontal cortex but not hippocampus. Proc. Natl. Acad. Sci. U S A 116, 1765–1769. doi: 10.1073/pnas.1815893116
Perry, R. E., Al Aïn, S., Raineki, C., Sullivan, R. M., and Wilson, D. A. (2016). Development of odor hedonics: experience-dependent ontogeny of circuits supporting maternal and predator odor responses in rats. J. Neurosci. 36, 6634–6650. doi: 10.1523/JNEUROSCI.0632-16.2016
Perry, R. E., Blair, C., and Sullivan, R. M. (2017). Neurobiology of infant attachment: attachment despite adversity and parental programming of emotionality. Curr. Opin. Psychol. 17, 1–6. doi: 10.1016/j.copsyc.2017.04.022
Perry, R. E., and Sullivan, R. M. (2014). Neurobiology of attachment to an abusive caregiver: short-term benefits and long-term costs. Dev. Psychobiol. 56, 1626–1634. doi: 10.1002/dev.21219
Phelps, E. A., Delgado, M. R., Nearing, K. I., and LeDoux, J. E. (2004). Extinction learning in humans: role of the amygdala and vmPFC. Neuron 43, 897–905. doi: 10.1016/j.neuron.2004.08.042
Pollak, D. D., Monje, F. J., Zuckerman, L., Denny, C. A., Drew, M. R., and Kandel, E. R. (2008). An animal model of a behavioral intervention for depression. Neuron 60, 149–161. doi: 10.1016/j.neuron.2008.07.041
Quirk, G. J., Russo, G. K., Barron, J. L., and Lebron, K. (2000). The role of ventromedial prefrontal cortex in the recovery of extinguished fear. J. Neurosci. 20, 6225–6231. doi: 10.1523/JNEUROSCI.20-16-06225.2000
Raineki, C., Moriceau, S., and Sullivan, R. M. (2010). Developing a neurobehavioral animal model of infant attachment to an abusive caregiver. Biol. Psychiatry 67, 1137–1145. doi: 10.1016/j.biopsych.2009.12.019
Rincón-Cortés, M., Barr, G. A., Mouly, A. M., Shionoya, K., Nuñez, B. S., and Sullivan, R. M. (2015). Enduring good memories of infant trauma: rescue of adult neurobehavioral deficits via amygdala serotonin and corticosterone interaction. Proc. Natl. Acad. Sci. U S A 112, 881–886. doi: 10.1073/pnas.1416065112
Robinson-Drummer, P. A., Chakraborty, T., Heroux, N. A., Rosen, J. B., and Stanton, M. E. (2018). Age and experience dependent changes in Egr-1 expression during the ontogeny of the context preexposure facilitation effect (CPFE). Neurobiol. Learn. Mem. 150, 1–12. doi: 10.1016/j.nlm.2018.02.008
Rogan, M. T., Leon, K. S., Perez, D. L., and Kandel, E. R. (2005). Distinct neural signatures for safety and danger in the amygdala and striatum of the mouse. Neuron 46, 309–320. doi: 10.1016/j.neuron.2005.02.017
Roth, T., and Sullivan, R. (2005). Memory of early maltreatment: neonatal behavioral and neural correlates of maternal maltreatment within the context of classical conditioning. Biol. Psychiatry 57, 823–831. doi: 10.1016/j.biopsych.2005.01.032
Sanchez, M. M., McCormack, K. M., and Howell, B. R. (2015). Social buffering of stress responses in nonhuman primates: maternal regulation of the development of emotional regulatory brain circuits. Soc. Neurosci. 10, 512–526. doi: 10.1080/17470919.2015.1087426
Santiago, A. N., Lim, K. Y., Opendak, M., Sullivan, R. M., and Aoki, C. (2018). Early life trauma increases threat response of peri-weaning rats, reduction of axo-somatic synapses formed by parvalbumin cells and perineuronal net in the basolateral nucleus of amygdala. J. Comp. Neurol. 526, 2647–2664. doi: 10.1002/cne.24522
Schubert, D., Martens, G. J., and Kolk, S. M. (2015). Molecular underpinnings of prefrontal cortex development in rodents provide insights into the etiology of neurodevelopmental disorders. Mol. Psychiatry 20, 795–809. doi: 10.1038/mp.2014.147
Sevelinges, Y., Mouly, A. M., Raineki, C., Moriceau, S., Forest, C., and Sullivan, R. M. (2011). Adult depression-like behavior, amygdala and olfactory cortex functions are restored by odor previously paired with shock during infant’s sensitive period attachment learning. Dev. Cogn. Neurosci. 1, 77–87. doi: 10.1016/j.dcn.2010.07.005
Sharpe, M. J., and Killcross, S. (2015a). The prelimbic cortex uses higher-order cues to modulate both the acquisition and expression of conditioned fear. Front. Syst. Neurosci. 8:235. doi: 10.3389/fnsys.2014.00235
Sharpe, M. J., and Killcross, S. (2015b). The prelimbic cortex directs attention toward predictive cues during fear learning. Learn. Mem. 22, 289–293. doi: 10.1101/lm.038273.115
Sharpe, M. J., and Killcross, S. (2018). Modulation of attention and action in the medial prefrontal cortex of rats. Psychol. Rev. 125, 822–843. doi: 10.1037/rev0000118
Shechner, T., Hong, M., Britton, J. C., Pine, D. S., and Fox, N. A. (2014). Fear conditioning and extinction across development: evidence from human studies and animal models. Biol. Psychol. 100, 1–12. doi: 10.1016/j.biopsycho.2014.04.001
Shionoya, K., Moriceau, S., Bradstock, P., and Sullivan, R. M. (2007). Maternal attenuation of hypothalamic paraventricular nucleus norepinephrine switches avoidance learning to preference learning in preweanling rat pups. Horm. Behav. 52, 391–400. doi: 10.1016/j.yhbeh.2007.06.004
Sotres-Bayon, F., Bush, D. E., and LeDoux, J. E. (2004). Emotional perseveration: an update on prefrontal-amygdala interactions in fear extinction. Learn. Mem. 11, 525–535. doi: 10.1101/lm.79504
Sroufe, L. A. (2005). Attachment and development: a prospective, longitudinal study from birth to adulthood. Attach. Hum. Dev. 7, 349–367. doi: 10.1080/14616730500365928
Stanton, M. E., and Levine, S. (1985). Brief separation elevates cortisol in mother and infant squirrel monkeys. Physiol. Behav. 34, 1007–1008. doi: 10.1016/0031-9384(85)90029-0
Suchecki, D., Rosenfeld, P., and Levine, S. (1993). Maternal regulation of the hypothalamic-pituitary-adrenal axis in the infant rat: the roles of feeding and stroking. Dev. Brain Res. 75, 185–192. doi: 10.1016/0165-3806(93)90022-3
Sullivan, R. M., Landers, M., Yeaman, B., and Wilson, D. A. (2000). Good memories of bad events in infancy. Nature 407, 38–39. doi: 10.1038/35024156
Sullivan, R. M., and Perry, R. E. (2015). Mechanisms and functional implications of social buffering in infants: lessons from animal models. Soc. Neurosci. 10, 500–511. doi: 10.1080/17470919.2015.1087425
Suomi, S. J., van der Horst, F. C., and van der Veer, R. (2008). Rigorous experiments on monkey love: an account of Harry F. Harlow’s role in the history of attachment theory. Integr. Psychol. Behav. Sci. 42, 354–369. doi: 10.1007/s12124-008-9072-9
Suzuki, H., Luby, J. L., Botteron, K. N., Dietrich, R., McAvoy, M. P., and Barch, D. M. (2014). Early life stress and trauma and enhanced limbic activation to emotionally valenced faces in depressed and healthy children. J. Am. Acad. Child Adolesc. Psychiatry 53, 800.e10–813.e10. doi: 10.1016/j.jaac.2014.04.013
Takahashi, L. K. (2014). Olfactory systems and neural circuits that modulate predator odor fear. Front. Behav. Neurosci. 8:72. doi: 10.3389/fnbeh.2014.00072
Tallot, L., Doyère, V., and Sullivan, R. M. (2016). Developmental emergence of fear/threat learning: neurobiology, associations and timing. Genes Brain Behav. 15, 144–154. doi: 10.1111/gbb.12261
Tang, A. C., Reeb-Sutherland, B. C., Romeo, R. D., and McEwen, B. S. (2014). On the causes of early life experience effects: evaluating the role of mom. Front. Neuroendocrinol. 35, 245–251. doi: 10.1016/j.yfrne.2013.11.002
Taylor, S. E., Burklund, L. J., Eisenberger, N. I., Lehman, B. J., Hilmert, C. J., and Lieberman, M. D. (2008). Neural bases of moderation of cortisol stress responses by psychosocial resources. J. Pers. Soc. Psychol. 95, 197–211. doi: 10.1037/0022-3514.95.1.197
Teicher, M. H., Anderson, C. M., Ohashi, K., and Polcari, A. (2014). Childhood maltreatment: altered network centrality of cingulate, precuneus, temporal pole and insula. Biol. Psychiatry 76, 297–305. doi: 10.1016/j.biopsych.2013.09.016
Teicher, M. H., Samson, J. A., Anderson, C. M., and Ohashi, K. (2016). The effects of childhood maltreatment on brain structure, function and connectivity. Nat. Rev. Neurosci. 17, 652–666. doi: 10.1038/nrn.2016.111
Tottenham, N., and Sheridan, M. A. (2009). A review of adversity, the amygdala and the hippocampus: a consideration of developmental timing. Front. Hum. Neurosci. 3:68. doi: 10.3389/neuro.09.068.2009
Tottenham, N., Shapiro, M., Flannery, J., Caldera, C., and Sullivan, R. (accepted). Parental presence switches avoidance to attraction learning in children. Nature Hum. Beh.
Tottenham, N., Shapiro, M., Telzer, E. H., and Humphreys, K. L. (2012). Amygdala response to mother. Dev. Sci. 15, 307–319. doi: 10.1111/j.1467-7687.2011.01128.x
Toumbelekis, M., Liddell, B. J., and Bryant, R. A. (2018). Thinking of attachment figures blocks differential fear conditioning. Soc. Cogn. Affect. Neurosci. 13, 989–994. doi: 10.1093/scan/nsy065
Upton, K. J., and Sullivan, R. M. (2010). Defining age limits of the sensitive period for attachment learning in rat pups. Dev. Psychobiol. 52, 453–464. doi: 10.1002/dev.20448
van Rooij, S. J., Cross, D., Stevens, J. S., Vance, L. A., Kim, Y. J., Bradley, B., et al. (2017). Maternal buffering of fear-potentiated startle in children and adolescents with trauma exposure. Soc. Neurosci. 12, 22–31. doi: 10.1080/17470919.2016.1164244
VanTieghem, M. R., and Tottenham, N. (2018). Neurobiological programming of early life stress: functional development of amygdala-prefrontal circuitry and vulnerability for stress-related psychopathology. Curr. Top. Behav. Neurosci. 38, 117–136. doi: 10.1007/7854_2016_42
Walker, C. D., Bath, K. G., Joels, M., Korosi, A., Larauche, M., Lucassen, P. J., et al. (2017). Chronic early life stress induced by limited bedding and nesting (LBN) material in rodents: critical considerations of methodology, outcomes and translational potential. Stress 20, 421–448. doi: 10.1080/10253890.2017.1343296
Wiener, S. G., Johnson, D. F., and Levine, S. (1987). Influence of postnatal rearing conditions on the response of squirrel monkey infants to brief perturbations in mother-infant relationships. Physiol. Behav. 39, 21–26. doi: 10.1016/0031-9384(87)90339-8
Willing, J., and Juraska, J. M. (2015). The timing of neuronal loss across adolescence in the medial prefrontal cortex of male and female rats. Neuroscience 301, 268–275. doi: 10.1016/j.neuroscience.2015.05.073
Winslow, J. T., Noble, P. L., Lyons, C. K., Sterk, S. M., and Insel, T. R. (2003). Rearing effects on cerebrospinal fluid oxytocin concentration and social buffering in rhesus monkeys. Neuropsychopharmacology 28, 910–918. doi: 10.1038/sj.npp.1300128
Yan, C. G., Rincón-Cortés, M., Raineki, C., Sarro, E., Colcombe, S., Guilfoyle, D. N., et al. (2017). Aberrant development of intrinsic brain activity in a rat model of caregiver maltreatment of offspring. Transl. Psychiatry 7:e1005. doi: 10.1038/tp.2016.276
Ye, X., Kapeller-Libermann, D., Travaglia, A., Inda, M. C., and Alberini, C. M. (2017). Direct dorsal hippocampal-prelimbic cortex connections strengthen fear memories. Nat. Neurosci. 20, 52–61. doi: 10.1038/nn.4443
Zajac, L., Raby, K. L., and Dozier, M. (2019). Attachment state of mind and childhood experiences of maltreatment as predictors of sensitive care from infancy through middle childhood: results from a longitudinal study of parents involved with Child Protective Services. Dev. Psychopathol. 31, 113–125. doi: 10.1017/s0954579418001554
Keywords: early-life trauma, social buffering, social support, threat, fear, prefrontal cortex, infralimbic, prelimbic
Citation: Robinson-Drummer PA, Opendak M, Blomkvist A, Chan S, Tan S, Delmer C, Wood K, Sloan A, Jacobs L, Fine E, Chopra D, Sandler C, Kamenetzky G and Sullivan RM (2019) Infant Trauma Alters Social Buffering of Threat Learning: Emerging Role of Prefrontal Cortex in Preadolescence. Front. Behav. Neurosci. 13:132. doi: 10.3389/fnbeh.2019.00132
Received: 21 February 2019; Accepted: 04 June 2019;
Published: 21 June 2019.
Edited by:
Jee Hyun Kim, Florey Institute of Neuroscience and Mental Health, AustraliaReviewed by:
Brittany R. Howell, University of Minnesota Twin Cities, United StatesTimothy J. Jarome, Virginia Tech, United States
Copyright © 2019 Robinson-Drummer, Opendak, Blomkvist, Chan, Tan, Delmer, Wood, Sloan, Jacobs, Fine, Chopra, Sandler, Kamenetzky and Sullivan. This is an open-access article distributed under the terms of the Creative Commons Attribution License (CC BY). The use, distribution or reproduction in other forums is permitted, provided the original author(s) and the copyright owner(s) are credited and that the original publication in this journal is cited, in accordance with accepted academic practice. No use, distribution or reproduction is permitted which does not comply with these terms.
*Correspondence: Regina M. Sullivan, regina.sullivan@nyumc.org
† These authors have contributed equally to this work and are joint first authors