- 1Department of Psychiatry, University of Texas Southwestern Medical Center, Dallas, TX, United States
- 2Department of Neuroscience, University of Texas Southwestern Medical Center, Dallas, TX, United States
Contextual learning is a critical component of episodic memory and important for living in any environment. Context can be described as the attributes of a location that are not the location itself. This includes a variety of non-spatial information that can be derived from sensory systems (sounds, smells, lighting, etc.) and internal state. In this review, we first address the behavioral underpinnings of contextual memory and the development of context memory theory, with a particular focus on the contextual fear conditioning paradigm as a means of assessing contextual learning and the underlying processes contributing to it. We then present the various neural centers that play roles in contextual learning. We continue with a discussion of the current knowledge of the neural circuitry and physiological processes that underlie contextual representations in the Entorhinal cortex-Hippocampal (EC-HPC) circuit, as the most well studied contributor to contextual memory, focusing on the role of ensemble activity as a representation of context with a description of remapping, and pattern separation and completion in the processing of contextual information. We then discuss other critical regions involved in contextual memory formation and retrieval. We finally consider the engram assembly as an indicator of stored contextual memories and discuss its potential contribution to contextual memory.
Introduction
We have all experienced the recall of a specific memory when we are exposed to a similar situation; the feeling of a warm breeze on a beach might remind you of a summer day from your childhood when you enjoyed swimming with your family and a barbecue. It occurs because the target (the act of swimming with your family in this case), was memorized alongside multiple types of information that occurred at that moment (a warm breeze on the beach in this case). While we may focus on a specific event or person in the moment, many other pieces of information occurring simultaneously around the target become enmeshed in memory formation. Those other information streams can serve as a hint to facilitate recall of the target memory. Together, these are referred to as the “context” (Godden and Baddeley, 1975; Smith, 1979; Smith et al., 2004; Manns and Eichenbaum, 2009; McKenzie et al., 2014; Robin et al., 2018; Libby et al., 2019; de Voogd et al., 2020). Context can shape our decisions and our recall processes (Godden and Baddeley, 1975; Smith, 1979; Smith et al., 2004; Robin et al., 2018; de Voogd et al., 2020), and has been shown to be an important first step in processing and rebuilding of episodic memories, helping to streamline object representations (Manns and Eichenbaum, 2009; McKenzie et al., 2014; Libby et al., 2019), and has a role in the determination of motivation and valuation of actions and items (Zeithamova et al., 2018). Context includes external information and internal states (Spear, 1973; Bouton, 1993; Rudy et al., 2004; Maren et al., 2013). In laboratory observations of contextual learning, the external components collected by sensory systems such as visual information (e.g., a color of paint on the wall of a room, dark or brightness of light), odor, sound, and touch (e.g., texture of a floor) contribute to the formation of spatial-contextual information for animals. Thus, the external components prove easier to manipulate, and are often used as the primary means of modifying contexts. We can describe the context as the specifics of a place that are not the place itself. For example, it can be understood that the context changes if the color of paint on the walls in the room is changed, but the space of the room itself does not. The Internal elements can include emotions (e.g., happiness, fear, sadness, and anger etc.) and hormonal states such as hunger or stress experienced within the situation. These internal sorts of contextual stimuli are important; just as ones experience of a situation may vary with the context of an environment (dark vs. light) for example, ones experience of an event may differ depending on whether one is stressed or calm, or whether one is angry. The effects of these internal states can predispose a circuit to be more or less responsive to a given cue (Jezek et al., 2010; Maren et al., 2013; Tye, 2018). In animal behavioral models, there are various types of behavioral experiments in which an animal uses these “contextual” data to perform a task. Broadly, multiple tasks in which animals use place memory can be included as part of a context dependent memory paradigm such as contextual fear conditioning (CFC), passive avoidance, mazes, open field experiments etc. In this review, we focus primarily on CFC paradigms which are one of the most common behavioral tasks for assessing contextual memory, and highlight the brain regions that are involved in contextual memory processing. Among the many brain regions involved in contextual memory, the EC-hippocampal network has been widely studied across a number of behavioral paradigms, and is known to have a strong role in episodic memory formation. As a result, a great deal is known about the physiological processes that support learning and memory within this region (Scoville and Milner, 1957; Mahut et al., 1982; Kim and Fanselow, 1992; Phillips and LeDoux, 1992; Frankland et al., 1998; Eichenbaum, 2000; Tulving, 2002; Sutherland et al., 2008; Kitamura et al., 2012; Pilkiw et al., 2017). To that end, we begin with a main focus on the EC-HPC network as the most well studied region involved in contextual memory before shifting our focus to the large array of associated regions. We then discuss the contributions of engram assemblies both within and outside of the EC-HPC network to context-dependent memory.
Contextual Memory
Contextual Fear Conditioning
A common test used to assess contextual memory processes is the Pavlovian contextual fear conditioning (CFC) paradigm. An animal (a mouse or rat in most cases) is put into a conditioning chamber (conditioned stimuli, CS) which is designed to signal the delivery of an electrical foot-shock (unconditioned stimuli, US), and learns its specific context as paired with shock. The duration of freezing behavior is measured as an outcome when the animal is reintroduced into the same context vs. a novel context (Figures 1A,B). An animal shows freezing behavior in the conditioned context if an animal has formed a contextual fear memory following the association of a previous painful experience (Pavlov, 1927; Kim and Fanselow, 1992; Phillips and LeDoux, 1992; Frankland et al., 1998; Amaral and Lavenex, 2007; Sutherland et al., 2008; Maren et al., 2013; Kitamura et al., 2015, 2017). In order to learn the context, animals must form a representation of the context first.
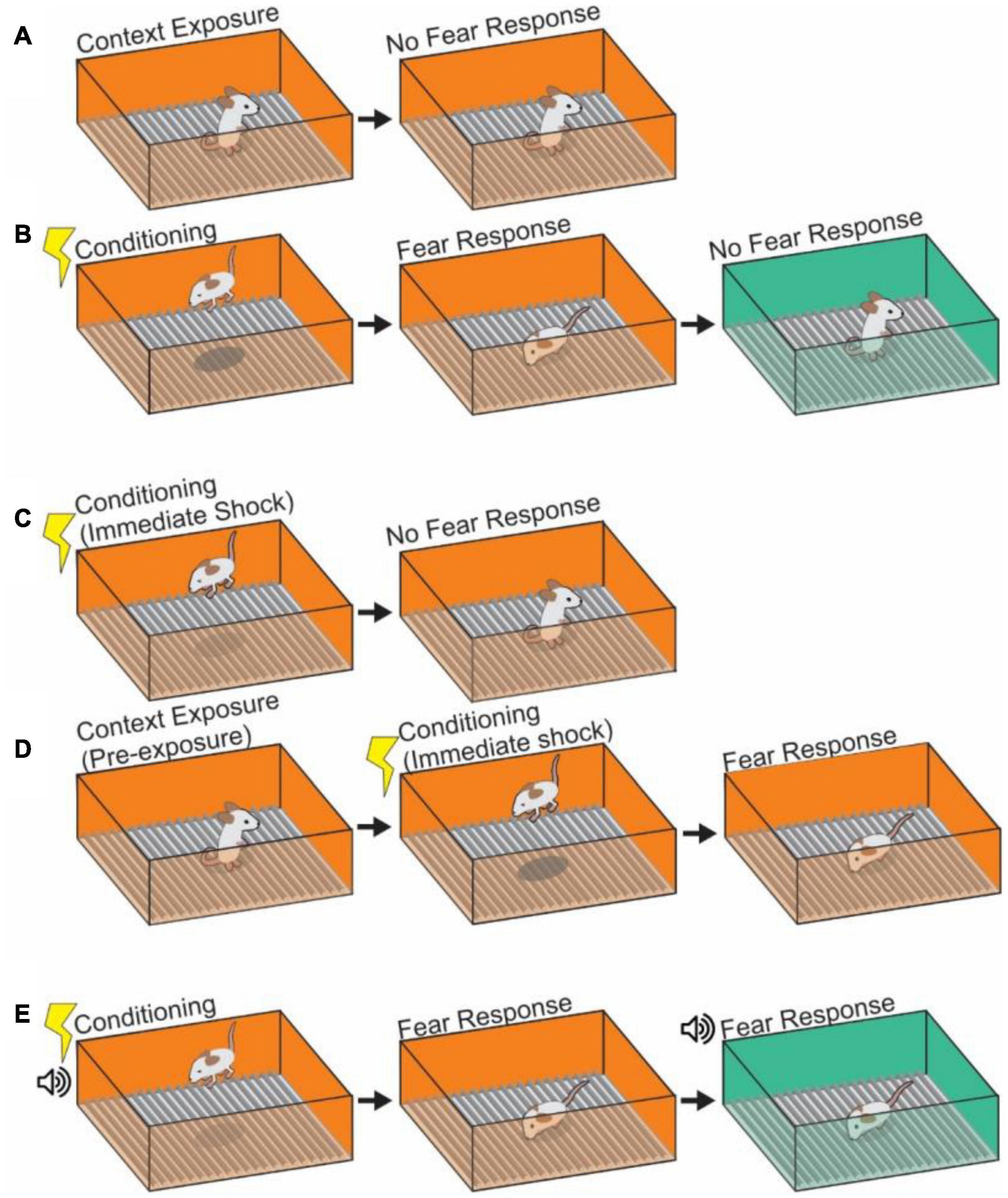
Figure 1. Depictions of contextual learning. (A) The exposure of an animal to a context results in learning of that context. However, in the absence of any frightening stimulus, no fear response will occur to the neutral context. (B) Pairing of a shock with a contextual stimulus will cause fear responses upon return to that conditioned context, indicating a pairing of shock memory with memory of the contextual cues. Subsequent placement in a second neutral context will not provoke a fear response. (C) Depiction of an immediate shock protocol. A mouse which is shocked immediately upon being placed in a context and removed after will not pair shock learning with context as insufficient encoding of the context itself has occurred. (D) Pre-exposure to a context will alleviate the failure to learn after immediate shock. Animals pre-exposed to the same or similar context 24 h prior to immediate shock protocol will associate the shock with the context. (E) Auditory fear conditioning can be added to create a more complex contextual pairing in which the fear response is tied both to the visual context and the auditory context (the tone). The tone can cause a fear response in a neutral context and generalization of fear to that context.
Immediate Shock and Context Pre-exposure
Previous studies demonstrated that the exposure time to the context chamber itself is crucial for context memory formation (Fanselow, 1986; Wiltgen et al., 2006). The experiments show that an animal does not learn the relationship of context and shock when a shock is presented immediately (less than 24 s) after animals are placed into a novel context (Figure 1C). This is referred to as the “immediate shock” effect. This phenomenon suggests that a certain amount of time processing the novel context, including texture, visual, auditory and sensory information in the environment, is necessary to form a contextual memory (Blanchard et al., 1976; Fanselow, 1986, 1990; Wiltgen et al., 2006), with longer durations spent in a context resulting in stronger unified representations of the context against which to form associations (Bae et al., 2015). Performing a pre-exposure to the context, in which an animal is habituated to the context in the absence of US (shocks) for 24 h before the US application works for the association between the context and shock even with immediate shock conditioning (Fanselow, 1990; Rudy and O’Reilly, 2001; Ohkawa et al., 2015). In this case an animal shows a significant level of freezing behavior in the context after the pre-exposure experience and following US conditioning (Figure 1D). This result indicates that pre-exposure to the shock chamber facilitates later association between the context and shock. Interestingly, several studies suggest that the degree of difference between the contexts may play a role in this context-shock association; when applying a pre-exposure and immediate shock across different, but closely related contexts it is possible to generate a false (generalized) fear memory (Rudy and O’Reilly, 1999; Bae et al., 2015; Lingawi et al., 2018; Libby et al., 2019), while an animal given pre-exposure in a vastly different context does not create false memories following US conditioning. The degree of similarity between two contexts (in this case the pre-exposure and immediate shock chambers) and the amount of time spent in a context both appear to be critical in determining how generalized or specific a fear memory can be. These interactions appear to be very sensitive due to the underlying complexities of the supportive processes (Lingawi et al., 2018; Sevenster et al., 2018), highlighting the need for increased study of the underlying mechanisms.
Cue Associated Contextual Learning
Beyond static contextual cues, discrete and salient sensory presentations of CS also become associated with the presentations of US through “cue associated” learning (Phillips and LeDoux, 1992; Goosens and Maren, 2001; Kim et al., 2013; Pellman and Kim, 2016). In auditory-cued fear learning (Figure 1E), for example, animals can learn through CS-US association that a neutral tone presentation (as CS) in a particular context (chamber A) is contingently paired with foot-shock (as US) that induces freezing behavior. After the conditioning, the animal shows freezing behavior when the tone is applied even in a different chamber (chamber B) (Curzon et al., 2009; Maren et al., 2013). A discrete CS, like this tone representation, that is separated from the context allows for the separation of which processes are related to fear memory itself, and which are due to the contextual element, as well as defining the linkage between the two processes (Anagnostaras et al., 2001; Rudy et al., 2004). The brain regions that contribute to cued fear conditioning can vary depending on the intensity of CS and US presentation, timing, and duration. Contextual fear memory (and fear memory in general) is known to be largely mediated by the amygdala (Phillips and LeDoux, 1992; Goosens and Maren, 2001; Kim et al., 2013; Pellman and Kim, 2016), and having a means to assess the contribution of amygdala to CFC by associating a tone has generated greater insight into the processes by which contextual and other cued information streams are combined in the HPC, for example, that fear association with a tone is sensitive to lesion in of the insular cortex, while fear association with a context is not, as well as the identification of the involvement of other brain regions in CFC including auditory cortex, Retrosplenial cortex (RSC) and thalamus (see detail in section “Other Brain Areas Involved in Contextual Memory”) (Phillips and LeDoux, 1992; Fanselow and LeDoux, 1999; Brunzell and Kim, 2001; Kochli et al., 2015; Kwapis et al., 2015; Bergstrom, 2016; Pollack et al., 2018; Chaaya et al., 2019). Depending on how the cued CS is presented, auditory-cued fear conditioning can be further subcategorized as delay fear conditioning or trace fear conditioning: Delay fear conditioning refers to a delay procedure in which the CS (Tone) is followed by US (foot-shock) and those presentations are temporally contiguous (Phillips and LeDoux, 1992; Fanselow and LeDoux, 1999). It is thought that the lateral amygdala receives tone information from the auditory cortex and auditory thalamus. Trace fear conditioning, by contrast, has a time interval introduced between the termination of the CS and the onset of the US (Clark and Squire, 1998; Buchel and Dolan, 2000; Misane et al., 2005; Kitamura et al., 2014; Kochli et al., 2015; Yokose et al., 2021). This paradigm requires an animal to track the structure of the temporal gap between CS and US presentations. This is controlled by specialized projections from MEC to HPC (McEchron et al., 1999; Quinn et al., 2002; Chowdhury et al., 2005; Gilmartin and McEchron, 2005; Suh et al., 2011; Kochli et al., 2015) (see section “Neural Circuits for the Contextual Memory in the Entorhinal Cortex-Hippocampal Formation”). As we described above, context information is composed of multiple elements in an animal’s environment. Here, we stop to ask if there is any systematic rule that allows for the individual contextual information components to be recorded in contextual memory. Researchers have been interested in this question for a long time, and have proposed mechanisms to explain the phenomenon.
The Hierarchical Nature of Contextual Information
Richard Hirsh hypothesized that contextual cues have a hierarchical property rather than a universal equivalence (Hirsh, 1974; Nadel and Willner, 1980). Hirsh’s hypothesis stated that context was i) hierarchical, operating in the background relative to other memories, and ii) acted to serve as an index system for other memories (Hirsh, 1974; Nadel and Willner, 1980), which contrasted with earlier ideas that treated context as an independent CS which was functionally equivalent to any other CS (Rescorla and Wagner, 1972; Wagner and Rescorla, 1972; Odling-Smee, 1975). In support of Hirsch’s theory, even in context-independent tasks, contingent context learning occurs; altering the presented context after the initial trial attenuates conditioned responses to the CS as well as latent inhibition (For example, animals conditioned to respond to air puffs delivered to the eyes (CS) in context A showed reduced responses to the same CS in context B), suggesting that animals are passively learning and integrating the contextual cues even though they are not directly relevant to the learned task (Penick and Solomon, 1991; Honey and Good, 1993), and indicating that context does not behave like a normal CS as previously thought. In a number of associative learning studies, contextual stimuli can be seen behaving as “occasion setters,” which, instead of becoming directly associated, modulate other associative linkages (Bouton and King, 1983; Bouton and Peck, 1989; Bouton and Brooks, 1993). For example; if establishing contextual fear conditioning in a given context (context A) paired with a tone, and performing tone-extinction relearning in a second (context B) with a tone, animals shows less freezing when re-exposed to context A (Bouton and King, 1983; Bouton and Peck, 1989; Bouton and Brooks, 1993), showing that the animal’s behavioral selection is dependent on the contextual presentation, that is, the suppression of responding that results from presentation of the extinction context works as a negative occasion setter whose role is to disambiguate the current meaning of the conditioned context. Notably, this disruption is affected by the context presentation itself, not the associative strength or conditioning efficiency (Bouton and King, 1983; Bouton and Peck, 1989; Hall and Honey, 1989; Kaye and Mackintosh, 1990). In this way, the associative strength of the CS is subject to selection by the occasion setting function of contextual stimuli; a secondary contextual association inhibits the original association once a novel association is formed in a secondary context, acting as a forced selector depending on the contextual presentation (McCloskey and Cohen, 1989; Swartzentruber, 1991), functionally setting the occasion, or in other words, selecting a behavioral state to operate in relative to and dependent on particular presentations of external stimuli. In light of these findings, it can be inferred that, due to the sensitivity of CS-US associations to contextual presentations, contextual stimuli must be accounted for before the association happens, supporting the idea that contextual activity is hierarchical and can be combined with other data streams (Harris et al., 2000; Chang and Liang, 2017). Thus, it appears that context is a collection of multiple background data that can operate as a map and modify other maps (Hirsh, 1974; Nadel and Willner, 1980).
The Role of the Entorhinal Cortex-Hippocampal Formation for Contextual Memory
Many different brain regions are involved in contextual fear memory acquisition, consolidation, and retrieval (Figure 2). In particular, there have been many reports on the necessity of EC-HPC formation in contextual memory processes. The HPC has been shown to be involved in the differentiation of contexts (Frankland et al., 1998), and integration of contextual information with learned behaviors and responses (Good and Honey, 1991; Honey and Good, 1993; Freeman et al., 1997; Smith et al., 2004; Kim et al., 2012). Lesion of the HPC disrupts CFC (McEchron et al., 1999) in a manner that is time sensitive, with recently acquired memories being destroyed by lesion, and longer term contextual memories being undamaged (Kim and Fanselow, 1992; Anagnostaras et al., 1999; Lee et al., 2017). Pharmacological inactivation of the dorsal HPC impairs context-dependent memory recall (Corcoran and Maren, 2001; Barrientos et al., 2002). On the other hand, there are also reports that contextual fear memories can be acquired even in the absence of the HPC. These findings raise the question of how critical the HPC is in the learning and recall of contextual fear memory. Several groups have performed contextual fear conditioning in HPC lesioned rodents to demonstrate that animals could indeed acquire contextual memory even in the absence of the HPC, however, this hippocampal independent conditioning required more shock events or more learning sessions to account for decreased efficiency of acquisition (Wiltgen et al., 2006; Lehmann et al., 2009). Depending on conditions of the experiment, these hippocampal independent memories can be less robust; disappearing more quickly that those generated with the hippocampus intact (Zelikowsky et al., 2012), while other studies demonstrate that memories generated without the hippocampus can be robust, lasting as long as thirty days (Lehmann et al., 2009; Gidyk et al., 2021). These results suggest that the acquisition of contextual fear may have redundant or compensatory systems, potentially explaining why hippocampus independent learning requires more shock events or more learning sessions (Wiltgen et al., 2006; Lehmann et al., 2009; Chaaya et al., 2018). The brain has a robust ability to compensate for damage, and these reports are presumed to be the result of the ability to acquire contextual fear memory by supplementing the absence of the HPC with other brain regions to counterbalance the disruption of normal operations. Entorhinal Cortex (EC), a major contributor to the functionality of the hippocampal circuit, is also known to play a key role in contextual memory processes, and it is reported that the lesion of EC results in decreased contextual learning, but not avoidance learning (Freeman et al., 1997). Chemical inactivation of the MEC also leads to alterations in contextual reconsolidation and extinction, supporting the regions’ role in processing contextual information (Baldi and Bucherelli, 2014). These two regions (EC-HPC) are part of a tightly interconnected network, which is collectively the most studied region involved in processing of contextual and episodic memories.
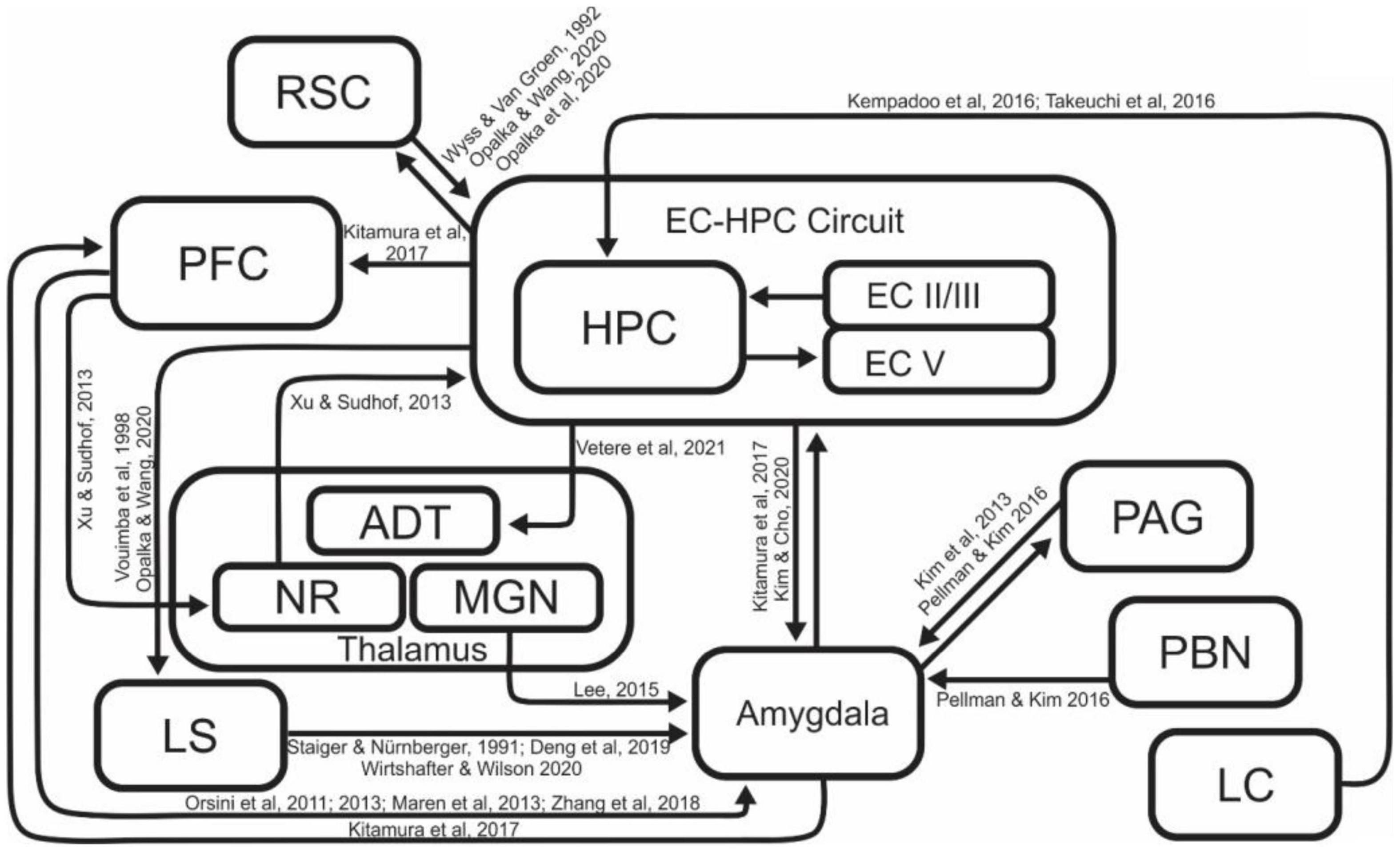
Figure 2. A schematic diagram of the brain regions and related connections involved in contextual fear learning. While several regions feature more prominently than others in this process, a number of regions are involved in generating and refining contextual representations and associations. ADT, Anterodorsal Thalamus; EC, Entorhinal Cortex; HPC, Hippocampus; LC, Locus Coeruleus; LS, Lateral Septum; MGN, Medial Geniculate Nucleus; NR, Nucleus Reuniens; PAG, Periaqueductal gray; PBN, Parabrachial Nucleus; PFC, Prefrontal Cortex; RSC, Retrosplenial Cortex.
Taken together, these reports highlight that the EC-HPC formation plays a core role in contextual memory processes with a broader network supporting the expression of contextual memories following their initial formation. In the following sections, we mainly focus on EC-HPC formation as one of best studied contributing brain regions in the processes of contextual memory, and discuss their neuronal circuit connectivity and physiological mechanisms in relation to contextual memory. We describe other relevant brain regions, and how the individual components are involved in CFC as well.
Neural Circuits for Contextual Memory in the Entorhinal Cortex-Hippocampal Formation
The EC-HPC formation have been considered to be crucial for learning and memory (Eichenbaum and Cohen(eds), 2004; Kitamura, 2017; Figure 3). In the main excitatory hippocampal network, there are parallel pathways referred to as the trisynaptic pathway (EC layer II→adentate gyrus (DG) →CA3 →CA1) and the monosynaptic pathway (EC layer III→CA1). The pathway From ECII to CA3 via the DG is understood to be critical in the creation of differential representations of contexts and spaces with high degrees of overlap, while the CA3-CA3/CA1 pathways are understood to be critical in the identification of contexts from partial cues (see section “Pattern Separation and Pattern Completion”).
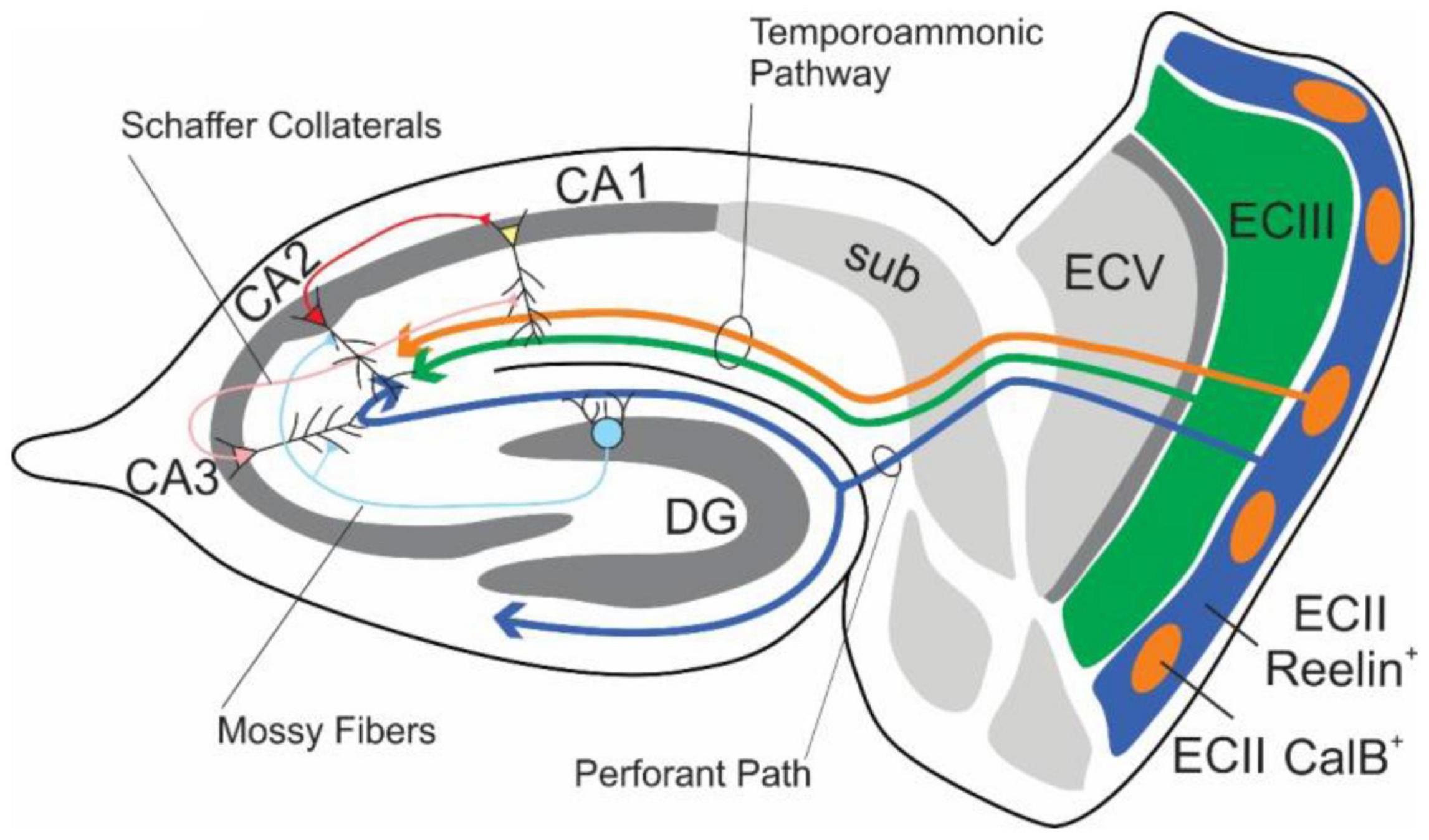
Figure 3. Schematic diagram of the major connections of the EC-HPC network. Axons from the EC layer III and CalB+ pyramidal cell clusters in EC layer II project along the temporoammonic pathway (ECIII/ECII CalB+ → CA1), which largely governs temporal features, The indirect pathway, which is involved in spatial and contextual learning, originates from Reelin+ stellate cells within EC layer II and projects to the classical tripartite synapse (ECII Reelin+ → DG → CA3 → CA1). Additional connections from DG to CA2, and CA2 to CA1 exist alongside the more heavily studied hippocampal pathways.
Most physiological studies of contextual processing were carried out in the HPC, however, it was more recently that studies of the role of the EC, upstream of HPC, in contextual memory were undertaken. A great deal of the contributing mechanism was unknown. For example, it was unknown whether contextual information was already processed in a subset of EC cells or not, and what the key driver for routing of contextual information to HPC was. Originally, grid cells, which are critical for various aspects of spatial navigation, were “thought to provide a context-independent metric representation of the local environment” (Giocomo et al., 2011). Recently, the question of upstream drivers of contextual learning has been gaining more attention.
To elucidate circuits upstream from HPC, previous studies demonstrated with electrolytic lesion or pharmacological inhibition that the EC is necessary for contextual fear conditioning (Maren et al., 1997; Lewis and Gould, 2007). However, due to the lack of cell-type specific manipulation, it remained unclear how the EC contributes to the contextual fear conditioning. One important study began to examine the role of specific cell types within individual layers of medial entorhinal cortex (MEC) in contextual memory processing. In the section above, we describe trace fear conditioning, which contains a temporal gap between the end of the CS and the onset of US called the “trace period” or “trace interval.” It was revealed that the synaptic plasticity of CA1 pyramidal neurons is necessary for the representation of the trace period (Tsien et al., 1996; Huerta et al., 2000; Fukaya et al., 2003). A monosynaptic pathway from MECIII to CA1 pyramidal neurons was shown to be crucial for trace fear conditioning, demonstrated using MECIII mutant mice that had tetanus toxin light chain expressed in dorsal MECIII (Suh et al., 2011), and optogenetic inhibition of MECIII input to CA1 neurons (Kitamura et al., 2014). Further study by Kitamura et al. (2014) revealed that there are two distinct subtypes of projection neurons found in MECII, CalbindinD-28K (CalB)+ pyramidal cells and Reelin+ stellate cells, with the former projecting to interneurons in stratum lacunosum (SL) of CA1 area and the latter monosynaptically projecting to DG neurons (Kitamura et al., 2014). The inputs from the CalB+ pyramidal cell clusters in MECII suppress the excitatory MECIII input into the CA1 pyramidal neurons via feedforward inhibition achieved by activation of SL interneurons to control the strength and duration of trace fear memory (Kitamura et al., 2014; Yokose et al., 2021).
On the other hand, optogenetic inhibition of Reelin+ cells in MECII, which project to DG neurons, during memory acquisition or retrieval disrupted both formation and recall of CFC memory (Kitamura et al., 2015). Kitamura et al. (2015) applied cell type-specific in vivo Ca2+ imaging in both Reelin+ stellate cells and CalB+ pyramidal cells individually in MEC layer II, and found that Reelin+ stellate cells, but not CalB+ pyramidal cells, have context specific neural activity and drive context-specific CA3 activation and CFC memory (Kitamura et al., 2015). The context-specific responses in Reelin+ stellate cells were still observed when dorsal CA1 activity was inhibited by muscimol, a gamma-aminobutyric acid subtype A (GABAA) receptor agonist (Kitamura et al., 2015). This finding suggests that ensembles of Reelin+ stellate cells in MEC layer II are essential, carry full contextual information, and transfer it to HPC. These results demonstrated that Reelin+ cells are essential for the formation and recall of CFC memory, while CalB+ cells were found to be crucial for temporal association learning, demonstrating that the two excitatory MEC layer II inputs to the HPC have different, but complementary roles in episodic memory (Kitamura et al., 2014, 2015; Yokose et al., 2021). Importantly, optogenetic inhibition of the MECIII inputs into hippocampal CA1 has no effect on CFC memory, indicating that the hippocampal trisynaptic pathway, but not the monosynaptic pathway, is crucial for the CFC memory (Suh et al., 2011; Kitamura et al., 2014). Since the optogenetic inhibition of DG granule cells does not impair the contextual fear response itself, but does increase the level of freezing in an unconditioned context (Kitamura et al., 2015), the direct MECII to CA3 pathway would be a main driver for contextual fear memory, while MECII-DG-CA3 pathway would be more specialized for contextual discrimination/pattern separation. These optogenetic experiments were temporally targeted during the conditioning phase. A recent work, however, has demonstrated the necessity of MEC inputs into HPC immediately after conditioning has concluded (Kang and Han, 2021). It was also revealed that the subpopulation in MEC which are not grid cells drive the context specific activity. Diehl et al. (2017) showed that approximately 95% of cells in the superficial layers of MEC were identified as spatially active cells spanning multiple functional categories including populations of grid cells, non-grid cells, and border cells. The non-grid spatial cells responded to contextual box manipulations with reorganized spatial firing, while the grid cell activity of the EC did not exhibit contextual sensitivity. Although context specific-activity and necessary circuits for contextual memory have been found in the projections from MEC II Reelin+ stellate cells, it still remains unclear exactly how the HPC processes and integrates contextual information.
These neurophysiological observations, discussed further in the sections “Remapping” and “Pattern Separation and Pattern Completion” below, open up further questions of differential and local processing modalities between these regions that can independently shape spatial and contextual representations as they form. Within the HPC, local networks also contribute to the control of CA subfield pyramidal neuron activity (Pelkey et al., 2017). HPC GABAergic interneurons have been shown to have a role in CFC expression (Gilmartin et al., 2012; Almada et al., 2013). Overactivation of GABA receptors has been shown to impair CFC without impairment of the ability to recall a shock in a separate, context independent paradigm (Chang and Liang, 2012; Misane et al., 2013). Additionally, specific GABA receptor subunits on pyramidal cells have been tied to different aspects and overall intensity of contextual learning (Lehner et al., 2010; Moore et al., 2010; Engin et al., 2016, 2020). These findings suggest that the local networks play a highly complex and input specific role in the formation of contextual memories. Moreover, outside of the EC-HPC network, there are multiple reports about the contributions of other brain regions involved in contextual memory formation which may directly modulate EC-HPC network activity (see section “Other Brain Areas Involved in Contextual Memory” below). Further study will be expected to understand neural circuit mechanisms for the formation and recall of CFC memory.
Physiological Representations in Contextual Memory
Remapping
The EC-HPC network is most known for its function in the processing and encoding of spatial information (O’Keefe and Nadel, 1978; Eichenbaum et al., 1999), with individual pyramidal cells able to act within an ensemble specific to a particular location and function as “place cells” which are generated in “place fields” (O’Keefe and Dostrovsky, 1971; O’Keefe et al., 1998). The functional activity of the place cells essentially allows for the mapping and representation of multiple discrete spaces by the HPC (O’Keefe and Conway, 1978; Kubie and Ranck, 1983; Skaggs and McNaughton, 1996; Louie and Wilson, 2001; Dragoi and Tonegawa, 2011; Kubie et al., 2020). Neuronal firing activity patterns within an ensemble change along with the changes in environment and reorganized cell ensembles represents the position of animal. This ensemble activity change is called “remapping” and is categorized differentially based on its associated representation.
The first report of remapping was observed by O’Keefe and Conway (1978), who analyzed the firing activity of place cells in rats on an elevated platform and on a T-maze. Across the two different environments, a population of place cells fired in the first environment but not second, while another set of cells fired in the second but not the first. This phenomenon was termed “global mapping” (O’Keefe and Conway, 1978), reflecting the discrete separations between environments as two discrete place cell ensembles.
By contrast, when spatial cues or other contextual modifiers are only partially altered, a subgroup of place cells change their firing patterns, creating a new ensemble consisting of some of the original place cells and some new place cells, termed “partial remapping” (Muller et al., 1987; Bostock et al., 1991; Kubie and Muller, 1991; Markus et al., 1995; Kubie et al., 2020). Anderson and Jeffery (2003) demonstrated this in the rat CA1 using compound contexts comprised of two differently colored boxes paired with two different odors. The majority of neurons changed their firing fields across varying combinations of both box and odor, but some neurons responded to the color or odor changes alone. This demonstrated that altering certain features of the environment can cause a partial shift in the population of active place cells.
Additionally, “rate remapping” can occur in which the firing field of individual neurons remains the same, but their firing frequency changes (Leutgeb et al., 2005; Kubie et al., 2020). Leutgeb et al. (2005) demonstrated this in ensemble activity recordings from dorsal CA1 and CA3 in freely moving rats. They examined the neuronal firing pattern and rate in two experimental conditions; either a variable cue in a constant place; or identical cues in varied places. Rate remapping was most evident in CA3, with particularly large firing rate changes and little change in firing field, particularly in the latter case. This is of particular interest, as CA3 is thought to be where contextual representations are built prior to their storage in CA1 (Mizumori et al., 1999; Wintzer et al., 2014; Zhao et al., 2020).
The different remapping modalities can be reflective of differential neuronal processing modalities. Global remapping in the HPC occurs almost immediately, consistent with the magnitude of the change between environments (Kentros et al., 1998; Hayman et al., 2003; Wills et al., 2005), and differential spatial ensembles stabilize quickly after animals are introduced to the novel environment (Wilson and McNaughton, 1993; Frank et al., 2004). However, ensembles stabilize more slowly in CA3 than in CA1 after exposure to a novel space (Leutgeb et al., 2004). This suggests that hippocampal representations are formed independently and utilizing unique local processing methods. The differential processes between CA1 and CA3 may be modulated by CA2 activity which has a critical role in reconciling differences between incoming contextual data (CA3) and stored contextual data (CA1) by facilitating remapping of the representations in CA3 and CA1 (Wintzer et al., 2014; Boehringer et al., 2017). The flexibility gained by remapping combined with the ability of the HPC to rapidly generate new ensembles (Ziv et al., 2013) allows for quick encoding of a context with ensemble activity (Dragoi and Tonegawa, 2011, 2013), and a high level of complexity in the encoding and integration of contextual information with other data streams (Chang and Liang, 2017; Gulli et al., 2020).
Pattern Separation and Pattern Completion
The prediction of place fields or generation of associational assemblies in CA1 are not directly correlated to CA3 or DG activity, meaning that somewhere along the information path through the hippocampus, divergent mechanisms for generating ensembles from contextual data, and reactivating previously generated ensembles from observed stimuli must exist; these are referred to as pattern separation and pattern completion, respectively (Leutgeb et al., 2007; Colgin et al., 2008; Sahay et al., 2011a; Rolls, 2013; Lee et al., 2020; Carrillo-Reid, 2021).
Pattern separation is the process of differentiating similar input patterns into distinct outputs to prevent incorrect associations. This process is believed to occur at the connection between DG and CA3. The idea that pattern separation takes place along this pathway is associated with the large number of DG granule cells and their relatively sparse activity, and the fact that DG-CA3 synaptic connections tend to have a low redundancy (Marr, 1971; O’Reilly and McClelland, 1994; Treves and Rolls, 1994; Leutgeb et al., 2004, 2007; McHugh et al., 2007; Bakker et al., 2008; Wintzer et al., 2014). The DG has a unique form of neural circuit plasticity, in which adult-born DG cells make novel and sparse connections to CA3. Studies of animals with either impaired adult neurogenesis (Altman and Das, 1965; Schlessinger et al., 1975; Seki and Arai, 1993; Eriksson et al., 1998) or blockade of DG-CA3 synapses demonstrated that contextual discrimination is not dependent on extant/stable mossy fiber inputs to CA3 (Nakashiba et al., 2012). Rather, this function is driven by newly generated granule cells and their novel connection with the network (Clelland et al., 2009; Kitamura et al., 2009; Scobie et al., 2009; Creer et al., 2010; Arruda-Carvalho et al., 2011; Sahay et al., 2011b; Nakashiba et al., 2012; Gage and Temple, 2013; Kitamura and Inokuchi, 2014; Alam et al., 2018; Terranova et al., 2019).
In animals with synaptic transmission-deficient CA3 pyramidal cells, contextual fear conditioning is impaired (Nakashiba et al., 2008), indicating that the CA3-CA1 and CA3-CA3 circuits have a central role in the processing of contextual information. In particular, the recurrent CA3-CA3 network is known to be crucial for pattern completion which is the process of reconstructing completed representations from parts of stored components (Guzman et al., 2016). Recurrent CA3-CA3 synapses appear to have a sparse interconnectivity, suggesting high specificity in their connections rather than a broad activation. This could account for much of the processing needed to complete a pattern from a partial cue; a small subset of recurrent connections activated by an even smaller subset within (Leutgeb et al., 2007; Rolls, 2013; Guzman et al., 2016). These connections are critical in fast object-place recall, as one may expect in a situation where an animal is exposed to a particular set of external stimuli and needs to determine which behavioral state is appropriate. As such, this necessitates rapid reconstruction from partial cues as a matter of efficiency (Nakazawa et al., 2002, 2003; Gold and Kesner, 2005; Leutgeb and Leutgeb, 2007). It is possible that this process, if not tightly controlled enough or presented with ambiguous cues might be responsible for the generation of generalized contextual fear memories discussed earlier (Rudy and O’Reilly, 1999; Bae et al., 2015; Lingawi et al., 2018). The pattern completion process can be understood as the mapping and remapping activity of the CA3/CA1 subfields; a novel field being formed, or a field being recalled in response to a (partial or complete) set of discrete contextual cues (Nakazawa et al., 2002, 2003; Gold and Kesner, 2005; Leutgeb and Leutgeb, 2007).
Other Brain Areas Involved in Contextual Memory
In addition to the EC-HPC network, a number of other brain regions were reported to contribute to contextual memory formation (Figure 2), either by contributing to the acquisition and retrieval of context memory directly, or regulating EC-HPC networks.
The basolateral amygdala (BLA) has been demonstrated to play a role in contextual fear conditioning, primarily by generating the emotional valence associated with the context-shock pairing. HPC interacts with the BLA via MECVa to encode contextual information during encoding of contextual fear memory. Not only the dorsal HPC (dHPC), but also the ventral CA1 (vHPC) projections to the Basal amygdala, paired with aversive stimuli, contribute to encoding conditioned fear memory (Kim and Cho, 2020). Hippocampal projections to the amygdala are involved in recent memory recall and association of contextual stimuli with pain. BLA receives foot-shock US information via the ascending pain pathways including the periaqueductal gray (PAG) and the parabrachial nucleus (PBN) and auditory CS information via thalamus (Kim et al., 2013; Lee, 2015; Pellman and Kim, 2016) and projects not only back to the HPC, but to the PFC (Kitamura et al., 2017).
Kitamura et al. (2017) demonstrated that in addition to receiving amygdalar input, PFC also receives contextual fear information from HPC via MECVa cells and that PFC engram cells are generated during the CFC encoding period. With optogenetic manipulation of both pathways, HPC-MECVa to PFC and BLA to PFC, it was revealed that both pathways are crucial for the generation and maturation of PFC engram cells following CFC, and are key for formation and recall of remote memory. Moreover, contextual activity in the PFC has been demonstrated to directly influence amygdalar activity (Orsini et al., 2011, 2013; Maren et al., 2013; Zhang et al., 2018).
Beyond the role of the Medial Geniculate Nucleus (MGN) in routing tonal information to the amygdala (Lee, 2015), the thalamic nuclei are also known to be involved in processing contextual information. Fear memories stored in PFC are either heightened or dampened in the nucleus reuniens (NR) of the thalamus prior to routing to HPC, resulting in a control of generalization of fear memory to differential contexts (Richter-Levin and Akirav, 2000; Xu and Sudhof, 2013; Rozeske et al., 2015). Additionally, a sparse inhibitory projection from CA3 to the anterodorsal thalamic nucleus is necessary for contextual fear memory retrieval at remote, but not recent time points (Vetere et al., 2021).
The Retrosplenial cortex (RSC) contributes to spatial navigation, head direction, as well as learning and memory for discrete cues such as auditory or visual stimuli. Lesion studies demonstrate that RSC processing contributes to configuration of contextual representation building (combining of elements). This is particularly interesting considering the previously demonstrated roles of RSC in hippocampal place field remapping (Wyss and Van Groen, 1992; Cooper and Mizumori, 2001; Mao et al., 2017; Todd et al., 2017; Alexander et al., 2020). Optogenetic inhibition of dHPC terminals in the RSC during memory acquisition causes impairment of subsequent memory performance (Opalka and Wang, 2020).
The Lateral septum (LS) is downstream from the HPC and has been implicated in various functions such as mood, motivation, anxiety, and spatial behavior, as reviewed in Wirtshafter and Wilson (2021). HPC terminal inhibition in LS during memory acquisition or retrieval tells us the dHPC-LS pathway plays a critical role in both contextual memory acquisition and retrieval (Opalka and Wang, 2020; Opalka et al., 2020). It thought that LS is important for matching context with behavior by taking contextually relevant information from the hippocampus and routing that information to the amygdala (Penick and Solomon, 1991; Staiger and Nurnberger, 1991; Deng et al., 2019; Wirtshafter and Wilson, 2021). Additionally, LS and full basal forebrain lesions can result in inappropriate pairing of context and movement response (Vouimba et al., 1998; Knox and Keller, 2016).
Recently the involvement of neuromodulatory inputs in contextual memory has begun to attract attention. It was reported that the Locus coeruleus (LC) tyrosine-hydroxylase-expressing (TH+) neurons project strongly to the HPC, and release dopamine. Optogenetic activation of LC TH+ neurons increased dopamine release into dHPC, promoting novelty associated spatial learning and memory (Kempadoo et al., 2016; Takeuchi et al., 2016). We expect further elucidation of relevant brain regions and understanding of the mechanism of contextual memory from the perspective of the entire brain.
Engrams and Contextual Memory
Neural representations of context, dependent on the repeated activation of neural ensembles, remain stable over a period of time to facilitate recall and integration. It is understood that repetitive exposures to the same context activates the same set of cells as assessed using the expression of immediate early genes (IEGs) such as c-Fos, Arc (activity-regulated cytoskeleton-associated protein) or Zif268 (Wilson and McNaughton, 1993; Radulovic et al., 1998; Guzowski et al., 1999; Guzowski, 2002). German evolutionary zoologist Richard Semon first posited the term “engram” to describe a hypothetical physical substrate for memory storage (Semon, 1921; Schacter et al., 1978). While Semon’s idea was impossible to prove in the early twentieth century, recent technologies have made it possible to revisit his idea and the definition was updated to fit our modern understanding of memory. The following three criteria must be met for an event to be considered as engram (Josselyn, 2010; Tonegawa et al., 2015, 2018; Denny et al., 2017; Josselyn and Tonegawa, 2020). An engram (i) is an enduring and significant physical or chemical alteration to a neural network (ii) due to activity in a subset of neurons caused by episodic stimuli (iii) that can be reactivated following presentation of all or part of the original stimulus set, leading to memory recall. Technological advances have allowed for the targeting of individual cell subtypes by gene expression, which can include constitutively active proteins or more transient expression caused by neuronal activity (Guzowski et al., 1999; Guzowski, 2002; Han et al., 2007, 2009; Reijmers et al., 2007; Guenthner et al., 2013; Roy et al., 2017a).
By utilizing IEG promoters to allow activity-dependent labeling of cells, and pairing this technique with optogenetically driven expression of opsins in previously activated cells, Liu et al., in 2012 made first observations of an “engram cell” under the modern definition (Reijmers et al., 2007; Liu et al., 2012). DG cells which had been active during CFC were targeted with ChR2, and stimulated with blue light at 20Hz were reactivated, and this reactivation artificially induced contextual memory recall without re-exposure to the original context information (Liu et al., 2012; Figure 4). Engram cells have since been found to have synapses with other engram cells resulting from the same memory event in the HPC (Choi et al., 2018). In this way, engrams can be further described as networks of individual engram cells which act together to store the components of memories, and reactivation of that network is both necessary and sufficient for recalling that encoded memory (Ryan et al., 2015; Tonegawa et al., 2015; Holtmaat and Caroni, 2016; Denny et al., 2017; Figure 4). Thus, we can understand an engram as the physical record of a representation, with the activated ensemble becoming reactivatable within a given set of stimuli (context) resulting in recall (Kelemen and Fenton, 2010; Jezek et al., 2011; Tayler et al., 2013). Importantly, engrams are distinctly encoded independently of other memory modalities (e.g., A spatial engram or a contextual engram) (Tanaka et al., 2018; Ghandour et al., 2019; Marks et al., 2021). Moreover, engram activation can even be sufficient to induce a false memory in the form of generalization of a fear response in one context onto a neutral context (Ramirez et al., 2013; Ohkawa et al., 2015; Oishi et al., 2019), or even to construct a representation of a context that has never been physically experienced (Garner et al., 2012). Kitamura et al. (2015) demonstrated how hippocampal engram cells are activated during memory recall. They found that the neural input from Reelin+ cells, but not CalB+ cells, in MEC drives contextual information into the HPC, is necessary for the formation and recall of CFC memory. They further showed that the neural input from Reelin+ cells in MEC is necessary for the reactivation of memory engram cells encoding CFC memory in the hippocampal CA3. These results indicate that the hippocampal trisynaptic pathway driven by Reelin+ cells in MEC layer II is crucial for CFC memory.
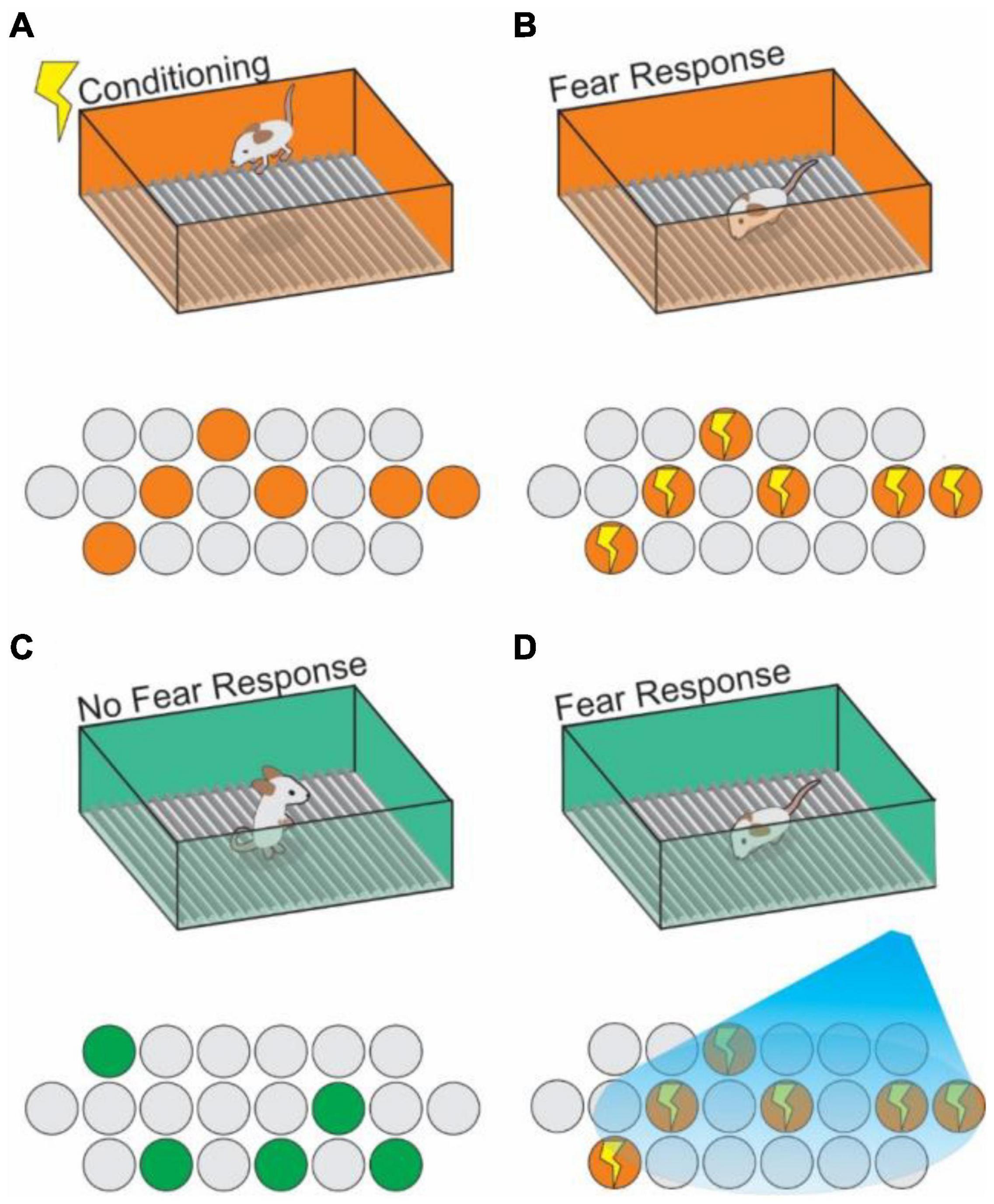
Figure 4. Context engram reactivation in the hippocampus can trigger fear memories. (A) An animal receives a shock in Context A (orange box) and generates a context specific ensemble (orange circles) into which the shock memory is tied (contextual fear conditioning). (B) Upon re-exposure to context A, the previously generated neural ensemble is activated (indicated by lightning bolts), and a fear response to the context is triggered. Using active cell tagging, the previous contextual ensemble is marked with an opsin for later reactivation. (C) The animal explores a novel context (Context B; green box), with no association to the shock training, learns the context and generates a new contextual ensemble (green circles) and exhibits no fear response. (D) Optogenetic reactivation of the Context A ensemble causes reactivation of the fear memory associated with Context A in the setting of Context B.
Recently, the question of when engram cells are formed during the process of memory acquisition and long-term storage has come into focus. Kitamura et al. (2017) found that engrams are actually formed simultaneously in the early stage of context memory acquisition in both the HPC and the medial prefrontal cortex (mPFC) where long-term memory is stored (Kitamura et al., 2017). This result went against the Standard Consolidation Model, which states that newly acquired episodic memories are initially stored in only HPC and then transferred to cortex as long-term memories, accompanied by “deletion” of HPC engrams with time. The mPFC engram cells which were generated in the early stage are not reactivated during the recent memory recall state but exist as “silent engrams” like those previously found by Ryan et al. (2015). These silent engrams become functionally mature with time, simultaneous with transition of the memory into the basolateral amygdala (BLA) which is necessary for fear memory, whereas HPC engram cells gradually became silent with time (Kitamura et al., 2017). This is an important finding, as it begins to explain how the long term storage of contextual memory remains stable, despite the observed shift in the active cell population makeup of HPC-stored representations over time (Mankin et al., 2012; Ziv et al., 2013); The hippocampal representations may need to be supported by representations constructed from engrams elsewhere in the brain, and that the maturation of the mPFC engrams over time may compensate for the decorrelation of hippocampal representations. Matos et al. (2019) demonstrated that activity of a small subset of mPFC neurons is sufficient and necessary for remote memory expression, and selective disruption of cAMP response element-binding protein (CREB) function in mPFC engram cells after CFC impairs remote memory formation (Matos et al., 2019), while another recent study suggests that the neural population in mPFC activated during memory recall changes over time (DeNardo et al., 2019). While further studies will be necessary for the understanding of mPFC engram cells for remote memory formation, these studies suggest that contextual information activates a set of neural ensembles and generates engrams in multiple brain regions during learning that are functionally connected (Kitamura et al., 2017; Roy et al., 2017b; Choi et al., 2018; DeNardo et al., 2019; Matos et al., 2019; Pignatelli et al., 2019). Recent advances in technology have the potential to generate an even deeper understanding of engram ensembles. Roy et al. (2019) applied a combination of genetic access to activated neurons labeled by the c-Fos promotor with a tamoxifen dependent Cre recombinase CreERT2 through the whole brain in a Fos-TRAP (targeted recombination in active populations) mouse line (Guenthner et al., 2013). All neurons activated during the CFC protocol within a narrow (< 12 h) time window were labeled with tdTomato fluorescence, then those brains were made transparent using tissue clearing techniques and imaged intact at the whole-brain scale. The mapping and subsequent optogenetic manipulations revealed new contextual engram populations in the anteromedial thalamus and the nucleus reuniens. They found that simultaneous chemogenetic reactivation of these multiple engram assemblies conferred a greater level of memory recall than reactivation of a single engram ensemble (Roy et al., 2019). Engram cells encoding CFC memory have also been identified in RSC (Cowansage et al., 2014), and optogenetic activation of those engram cells facilitates the systems consolidation of CFC memory (de Sousa et al., 2019). These results show that CFC engrams exist in brain regions other than the classically highlighted areas. Understanding the mechanisms of contextual memory at the systems level, such as ensemble activity or synchrony between brain regions will be of great interest for future studies.
Conclusion
In this review, we have discussed the behavioral neuroscience of contextual fear conditioning in the EC-HPC network. Contextual memory has been found to be a unique part of episodic memory, encoding information about the conditions within a space in such a way as to serve as a hierarchical basis for encoding and indexing memories. Contextual information is fed to the HPC largely by Reelin+ cells in MEC layer II via the trisynaptic pathway. This information is then used to form unique ensembles of activity that have the ability to nest within representations of locations. Questions still remain as to the nature of the local processing of contextual data within the HPC, but recent advances in technologies for cell type specific genetic manipulations and for in vivo recording of cellular activity can provide powerful opportunities for investigation of these local circuit mechanisms. For example, the in vivo visualization of calcium transients during behavior, or the stable recording of cellular arrays across multiple brain regions simultaneously that have recently become possible have the potential to yield critical new insights into contextual learning processes as context dependent memory is composed of multiple elements, and simultaneous measurement of physiological neuronal activity could tell us which sub-component is driving the changes in neuronal activities. At the same time, utilizing cell type specific techniques to visually identify neuronal activity in specific subsets of hippocampal neurons could be of great use in further developing our understanding of the local processes that shape contextual encoding.
Author Contributions
WM, TK, and SO conceived this project. WM, JY, TK, and SO wrote the manuscript and approved the final manuscript. WM and SO designed and created the figures. All authors contributed to the article and approved the submitted version.
Funding
This work was supported by the grants from Endowed Scholar Program at UTSW to TK and Faculty Science and Technology Acquisition and Retention Program in UT System to TK.
Conflict of Interest
The authors declare that the research was conducted in the absence of any commercial or financial relationships that could be construed as a potential conflict of interest.
Publisher’s Note
All claims expressed in this article are solely those of the authors and do not necessarily represent those of their affiliated organizations, or those of the publisher, the editors and the reviewers. Any product that may be evaluated in this article, or claim that may be made by its manufacturer, is not guaranteed or endorsed by the publisher.
References
Alam, M. J., Kitamura, T., Saitoh, Y., Ohkawa, N., Kondo, T., and Inokuchi, K. (2018). Adult neurogenesis conserves hippocampal memory capacity. J. Neurosci. 38, 6854–6863. doi: 10.1523/JNEUROSCI.2976-17.2018
Alexander, A. S., Robinson, J. C., Dannenberg, H., Kinsky, N. R., Levy, S. J., Mau, W., et al. (2020). Neurophysiological coding of space and time in the hippocampus, entorhinal cortex, and retrosplenial cortex. Brain Neurosci. Adv. 4:2398212820972871. doi: 10.1177/2398212820972871
Almada, R. C., Albrechet-Souza, L., and Brandao, M. L. (2013). Further evidence for involvement of the dorsal hippocampus serotonergic and gamma-aminobutyric acid (GABA)ergic pathways in the expression of contextual fear conditioning in rats. J. Psychopharmacol. 27, 1160–1168. doi: 10.1177/0269881113482840
Altman, J., and Das, G. D. (1965). Autoradiographic and histological evidence of postnatal hippocampal neurogenesis in rats. J. Comp. Neurol. 124, 319–335. doi: 10.1002/cne.901240303
Amaral, D., and Lavenex, P. (2007). “Hippocampal neuroanatomy,” in The Hippocampus Book, eds P. Anderson, R. Morris, D. Amaral, T. Bliss, and J. O’Keefe (New York, NY: Oxford University Press), 37–109.
Anagnostaras, S. G., Gale, G. D., and Fanselow, M. S. (2001). Hippocampus and contextual fear conditioning: recent controversies and advances. Hippocampus 11, 8–17. doi: 10.1002/1098-1063(2001)11:1<8::AID-HIPO1015>3.0.CO;2-7
Anagnostaras, S. G., Maren, S., and Fanselow, M. S. (1999). Temporally graded retrograde amnesia of contextual fear after hippocampal damage in rats: within-subjects examination. J. Neurosci. 19, 1106–1114. doi: 10.1523/JNEUROSCI.19-03-01106.1999
Anderson, M. I., and Jeffery, K. J. (2003). Heterogeneous modulation of place cell firing by changes in context. J. Neurosci. 23, 8827–8835. doi: 10.1523/JNEUROSCI.23-26-08827.2003
Arruda-Carvalho, M., Sakaguchi, M., Akers, K. G., Josselyn, S. A., and Frankland, P. W. (2011). Posttraining ablation of adult-generated neurons degrades previously acquired memories. J. Neurosci. 31, 15113–15127. doi: 10.1523/JNEUROSCI.3432-11.2011
Bae, S. E., Holmes, N. M., and Westbrook, R. F. (2015). False context fear memory in rats. Learn. Mem. 22, 519–525. doi: 10.1101/lm.039065.115
Bakker, A., Kirwan, C. B., Miller, M., and Stark, C. E. (2008). Pattern separation in the human hippocampal CA3 and dentate gyrus. Science 319, 1640–1642. doi: 10.1126/science.1152882
Baldi, E., and Bucherelli, C. (2014). Entorhinal cortex contribution to contextual fear conditioning extinction and reconsolidation in rats. Neurobiol. Learn. Mem. 110, 64–71. doi: 10.1016/j.nlm.2014.02.004
Barrientos, R. M., O’Reilly, R. C., and Rudy, J. W. (2002). Memory for context is impaired by injecting anisomycin into dorsal hippocampus following context exploration. Behav. Brain Res. 134, 299–306. doi: 10.1016/s0166-4328(02)00045-1
Bergstrom, H. C. (2016). The neurocircuitry of remote cued fear memory. Neurosci. Biobehav. Rev. 71, 409–417. doi: 10.1016/j.neubiorev.2016.09.028
Blanchard, R. J., Fukunaga, K. K., and Blanchard, D. C. (1976). Environmental-control of defensive reactions to footshock. Bull. Psychon. Soc. 8, 129–130.
Boehringer, R., Polygalov, D., Huang, A. J. Y., Middleton, S. J., Robert, V., Wintzer, M. E., et al. (2017). Chronic loss of CA2 transmission leads to hippocampal hyperexcitability. Neuron 94, 642.e9–655.e9. doi: 10.1016/j.neuron.2017.04.014
Bostock, E., Muller, R. U., and Kubie, J. L. (1991). Experience-dependent modifications of hippocampal place cell firing. Hippocampus 1, 193–205. doi: 10.1002/hipo.450010207
Bouton, M. E. (1993). Context, time, and memory retrieval in the interference paradigms of Pavlovian learning. Psychol. Bull. 114, 80–99. doi: 10.1037/0033-2909.114.1.80
Bouton, M. E., and Brooks, D. C. (1993). Time and context effects on performance in a pavlovian discrimination reversal. J. Exp. Psychol. Anim. Behav. Process. 19, 165–179.
Bouton, M. E., and King, D. A. (1983). Contextual control of the extinction of conditioned fear: tests for the associative value of the context. J. Exp. Psychol. Anim. Behav. Process. 9, 248–265.
Bouton, M. E., and Peck, C. A. (1989). Context effects on conditioning, extinction, and reinstatement in an appetitive conditioning preparation. Anim. Learn. Behav. 17, 188–198.
Brunzell, D. H., and Kim, J. J. (2001). Fear conditioning to tone, but not to context, is attenuated by lesions of the insular cortex and posterior extension of the intralaminar complex in rats. Behav. Neurosci. 115, 365–375.
Buchel, C., and Dolan, R. J. (2000). Classical fear conditioning in functional neuroimaging. Curr. Opin. Neurobiol. 10, 219–223.
Carrillo-Reid, L. (2021). Neuronal ensembles in memory processes. Semin. Cell Dev. Biol. [Epub ahead of print]. doi: 10.1016/j.semcdb.2021.04.004
Chaaya, N., Battle, A. R., and Johnson, L. R. (2018). An update on contextual fear memory mechanisms: transition between amygdala and hippocampus. Neurosci. Biobehav. Rev. 92, 43–54. doi: 10.1016/j.neubiorev.2018.05.013
Chaaya, N., Jacques, A., Belmer, A., Richard, D. J., Bartlett, S. E., Battle, A. R., et al. (2019). Localization of contextual and context removed auditory fear memory within the basolateral amygdala complex. Neuroscience 398, 231–251. doi: 10.1016/j.neuroscience.2018.12.004
Chang, S. D., and Liang, K. C. (2012). Roles of hippocampal GABA(A) and muscarinic receptors in consolidation of context memory and context-shock association in contextual fear conditioning: a double dissociation study. Neurobiol. Learn. Mem. 98, 17–24. doi: 10.1016/j.nlm.2012.04.004
Chang, S. D., and Liang, K. C. (2017). The hippocampus integrates context and shock into a configural memory in contextual fear conditioning. Hippocampus 27, 145–155. doi: 10.1002/hipo.22679
Choi, J. H., Sim, S. E., Kim, J. I., Choi, D. I., Oh, J., Ye, S., et al. (2018). Interregional synaptic maps among engram cells underlie memory formation. Science 360, 430–435. doi: 10.1126/science.aas9204
Chowdhury, N., Quinn, J. J., and Fanselow, M. S. (2005). Dorsal hippocampus involvement in trace fear conditioning with long, but not short, trace intervals in mice. Behav. Neurosci. 119, 1396–1402. doi: 10.1037/0735-7044.119.5.1396
Clark, R. E., and Squire, L. R. (1998). Classical conditioning and brain systems: the role of awareness. Science 280, 77–81. doi: 10.1126/science.280.5360.77
Clelland, C. D., Choi, M., Romberg, C., Clemenson, G. D. Jr., Fragniere, A., Tyers, P., et al. (2009). A functional role for adult hippocampal neurogenesis in spatial pattern separation. Science 325, 210–213. doi: 10.1126/science.1173215
Colgin, L. L., Moser, E. I., and Moser, M. B. (2008). Understanding memory through hippocampal remapping. Trends Neurosci. 31, 469–477. doi: 10.1016/j.tins.2008.06.008
Cooper, B. G., and Mizumori, S. J. (2001). Temporary inactivation of the retrosplenial cortex causes a transient reorganization of spatial coding in the hippocampus. J. Neurosci. 21, 3986–4001. doi: 10.1523/JNEUROSCI.21-11-03986.2001
Corcoran, K. A., and Maren, S. (2001). Hippocampal inactivation disrupts contextual retrieval of fear memory after extinction. J. Neurosci. 21, 1720–1726. doi: 10.1523/JNEUROSCI.21-05-01720.2001
Cowansage, K. K., Shuman, T., Dillingham, B. C., Chang, A., Golshani, P., and Mayford, M. (2014). Direct reactivation of a coherent neocortical memory of context. Neuron 84, 432–441. doi: 10.1016/j.neuron.2014.09.022
Creer, D. J., Romberg, C., Saksida, L. M., van Praag, H., and Bussey, T. J. (2010). Running enhances spatial pattern separation in mice. Proc. Natl. Acad Sci. U.S.A. 107, 2367–2372. doi: 10.1073/pnas.0911725107
Curzon, P., Rustay, N. R., and Browman, K. E. (2009). “Cued and contextual fear conditioning for rodents,” in Methods of Behavior Analysis in Neuroscience, ed. J. J. Buccafusco (Boca Raton, FL: CRC Press/Taylor & Francis).
de Sousa, A. F., Cowansage, K. K., Zutshi, I., Cardozo, L. M., Yoo, E. J., Leutgeb, S., et al. (2019). Optogenetic reactivation of memory ensembles in the retrosplenial cortex induces systems consolidation. Proc. Natl. Acad Sci. U.S.A. 116, 8576–8581. doi: 10.1073/pnas.1818432116
de Voogd, L. D., Murray, Y. P. J., Barte, R. M., van der Heide, A., Fernandez, G., Doeller, C. F., et al. (2020). The role of hippocampal spatial representations in contextualization and generalization of fear. Neuroimage 206:116308. doi: 10.1016/j.neuroimage.2019.116308
DeNardo, L. A., Liu, C. D., Allen, W. E., Adams, E. L., Friedmann, D., Fu, L., et al. (2019). Temporal evolution of cortical ensembles promoting remote memory retrieval. Nat. Neurosci. 22, 460–469. doi: 10.1038/s41593-018-0318-7
Deng, K., Yang, L., Xie, J., Tang, H., Wu, G. S., and Luo, H. R. (2019). Whole-brain mapping of projection from mouse lateral septal nucleus. Biol. Open 8:bio043554. doi: 10.1242/bio.043554
Denny, C. A., Lebois, E., and Ramirez, S. (2017). From engrams to pathologies of the brain. Front. Neural Circ. 11:23. doi: 10.3389/fncir.2017.00023
Diehl, G. W., Hon, O. J., Leutgeb, S., and Leutgeb, J. K. (2017). Grid and nongrid cells in medial entorhinal cortex represent spatial location and environmental features with complementary coding schemes. Neuron 94, 83.e6–92.e6. doi: 10.1016/j.neuron.2017.03.004
Dragoi, G., and Tonegawa, S. (2011). Preplay of future place cell sequences by hippocampal cellular assemblies. Nature 469, 397–401. doi: 10.1038/nature09633
Dragoi, G., and Tonegawa, S. (2013). Distinct preplay of multiple novel spatial experiences in the rat. Proc. Natl. Acad Sci. U.S.A. 110, 9100–9105. doi: 10.1073/pnas.1306031110
Eichenbaum, H. (2000). A cortical-hippocampal system for declarative memory. Nat. Rev. Neurosci. 1, 41–50. doi: 10.1038/35036213
Eichenbaum, H., and Cohen, N. J. (eds) (2004). “The hippocampal memory system,” in From Conditioning to Conscious Recollection, (Oxford: Oxford University Press), 305–343.
Eichenbaum, H., Dudchenko, P., Wood, E., Shapiro, M., and Tanila, H. (1999). The hippocampus, memory, and place cells: is it spatial memory or a memory space? Neuron 23, 209–226. doi: 10.1016/s0896-6273(00)80773-4
Engin, E., Sigal, M., Benke, D., Zeller, A., and Rudolph, U. (2020). Bidirectional regulation of distinct memory domains by alpha5-subunit-containing GABAA receptors in CA1 pyramidal neurons. Learn. Mem. 27, 423–428. doi: 10.1101/lm.052084.120
Engin, E., Smith, K. S., Gao, Y., Nagy, D., Foster, R. A., Tsvetkov, E., et al. (2016). Modulation of anxiety and fear via distinct intrahippocampal circuits. eLife 5:e14120. doi: 10.7554/eLife.14120
Eriksson, P. S., Perfilieva, E., Bjork-Eriksson, T., Alborn, A. M., Nordborg, C., Peterson, D. A., et al. (1998). Neurogenesis in the adult human hippocampus. Nat. Med. 4, 1313–1317.
Fanselow, M. S. (1986). Associative vs topographical accounts of the immediate shock freezing deficit in rats - implications for the response selection-rules governing species-specific defensive reactions. Learn. Motiv. 17, 16–39.
Fanselow, M. S. (1990). Factors governing one-trial contextual conditioning. Anim. Learn. Behav. 18, 264–270. doi: 10.3758/bf03205285
Fanselow, M. S., and LeDoux, J. E. (1999). Why we think plasticity underlying Pavlovian fear conditioning occurs in the basolateral amygdala. Neuron 23, 229–232. doi: 10.1016/s0896-6273(00)80775-8
Frank, L. M., Stanley, G. B., and Brown, E. N. (2004). Hippocampal plasticity across multiple days of exposure to novel environments. J. Neurosci. 24, 7681–7689. doi: 10.1523/JNEUROSCI.1958-04.2004
Frankland, P., Cestari, V., Filipkowski, R., McDonald, R., and Silva, A. (1998). The dorsal hippocampus is essential for context discrimination but not for contextual conditioning. Behav. Neurosci. 112, 863–874. doi: 10.1037//0735-7044.112.4.863
Freeman, J. H. Jr., Weible, A., Rossi, J., and Gabriel, M. (1997). Lesions of the entorhinal cortex disrupt behavioral and neuronal responses to context change during extinction of discriminative avoidance behavior. Exp. Brain Res. 115, 445–457. doi: 10.1007/pl00005714
Fukaya, M., Kato, A., Lovett, C., Tonegawa, S., and Watanabe, M. (2003). Retention of NMDA receptor NR2 subunits in the lumen of endoplasmic reticulum in targeted NR1 knockout mice. Proc. Natl. Acad. Sci. U.S.A. 100, 4855–4860. doi: 10.1073/pnas.0830996100
Gage, F. H., and Temple, S. (2013). Neural stem cells: generating and regenerating the brain. Neuron 80, 588–601. doi: 10.1016/j.neuron.2013.10.037
Garner, A. R., Rowland, D. C., Hwang, S. Y., Baumgaertel, K., Roth, B. L., Kentros, C., et al. (2012). Generation of a synthetic memory trace. Science 335, 1513–1516. doi: 10.1126/science.1214985
Ghandour, K., Ohkawa, N., Fung, C. C. A., Asai, H., Saitoh, Y., Takekawa, T., et al. (2019). Orchestrated ensemble activities constitute a hippocampal memory engram. Nat. Commun. 10:2637. doi: 10.1038/s41467-019-10683-2
Gidyk, D. C., McDonald, R. J., and Sutherland, R. J. (2021). Intact behavioral expression of contextual fear, context discrimination, and object discrimination memories acquired in the absence of the hippocampus. J. Neurosci. 41, 2437–2446. doi: 10.1523/JNEUROSCI.0546-20.2020
Gilmartin, M. R., Kwapis, J. L., and Helmstetter, F. J. (2012). Trace and contextual fear conditioning are impaired following unilateral microinjection of muscimol in the ventral hippocampus or amygdala, but not the medial prefrontal cortex. Neurobiol. Learn. Mem. 97, 452–464. doi: 10.1016/j.nlm.2012.03.009
Gilmartin, M. R., and McEchron, M. D. (2005). Single neurons in the medial prefrontal cortex of the rat exhibit tonic and phasic coding during trace fear conditioning. Behav. Neurosci. 119, 1496–1510. doi: 10.1037/0735-7044.119.6.1496
Giocomo, L. M., Moser, M. B., and Moser, E. I. (2011). Computational models of grid cells. Neuron 71, 589–603.
Godden, D. R., and Baddeley, A. D. (1975). Context-dependent memory in two natural environments: on land and underwater. Br. J. Psychol. 66, 325–331.
Gold, A. E., and Kesner, R. P. (2005). The role of the CA3 subregion of the dorsal hippocampus in spatial pattern completion in the rat. Hippocampus 15, 808–814. doi: 10.1002/hipo.20103
Good, M., and Honey, R. C. (1991). Conditioning and contextual retrieval in hippocampal rats. Behav. Neurosci. 105, 499–509. doi: 10.1037//0735-7044.105.4.499
Goosens, K. A., and Maren, S. (2001). Contextual and auditory fear conditioning are mediated by the lateral, basal, and central amygdaloid nuclei in rats. Learn. Mem. 8, 148–155. doi: 10.1101/lm.37601
Guenthner, C. J., Miyamichi, K., Yang, H. H., Heller, H. C., and Luo, L. (2013). Permanent genetic access to transiently active neurons via TRAP: targeted recombination in active populations. Neuron 78, 773–784. doi: 10.1016/j.neuron.2013.03.025
Gulli, R. A., Duong, L. R., Corrigan, B. W., Doucet, G., Williams, S., Fusi, S., et al. (2020). Context-dependent representations of objects and space in the primate hippocampus during virtual navigation. Nat. Neurosci. 23, 103–112. doi: 10.1038/s41593-019-0548-3
Guzman, S. J., Schlogl, A., Frotscher, M., and Jonas, P. (2016). Synaptic mechanisms of pattern completion in the hippocampal CA3 network. Science 353, 1117–1123. doi: 10.1126/science.aaf1836
Guzowski, J. F. (2002). Insights into immediate-early gene function in hippocampal memory consolidation using antisense oligonucleotide and fluorescent imaging approaches. Hippocampus 12, 86–104. doi: 10.1002/hipo.10010
Guzowski, J. F., McNaughton, B. L., Barnes, C. A., and Worley, P. F. (1999). Environment-specific expression of the immediate-early gene Arc in hippocampal neuronal ensembles. Nat. Neurosci. 2, 1120–1124. doi: 10.1038/16046
Hall, G., and Honey, R. C. (1989). Contextual effects in conditioning, latent inhibition, and habituation - associative and retrieval functions of contextual cues. J. Exp. Psychol. Anim. Behav. Process. 15, 232–241. doi: 10.1037/0097-7403.15.3.232
Han, J. H., Kushner, S. A., Yiu, A. P., Cole, C. J., Matynia, A., Brown, R. A., et al. (2007). Neuronal competition and selection during memory formation. Science 316, 457–460. doi: 10.1126/science.1139438
Han, J. H., Kushner, S. A., Yiu, A. P., Hsiang, H. L., Buch, T., Waisman, A., et al. (2009). Selective erasure of a fear memory. Science 323, 1492–1496. doi: 10.1126/science.1164139
Harris, J. A., Jones, M. L., Bailey, G. K., and Westbrook, R. F. (2000). Contextual control over conditioned responding in an extinction paradigm. J. Exp. Psychol. Anim. Behav. Process. 26, 174–185. doi: 10.1037//0097-7403.26.2.174
Hayman, R., Chakraborty, S., Anderson, M., and Jeffery, K. (2003). Context-specific acquisition of location discrimination by hippocampal place cells. Eur. J. Neurosci. 18, 2825–2834. doi: 10.1111/j.1460-9568.2003.03035.x
Hirsh, R. (1974). The hippocampus and contextual retrieval of information from memory: a theory. Behav. Biol. 12, 421–444. doi: 10.1016/s0091-6773(74)92231-7
Holtmaat, A., and Caroni, P. (2016). Functional and structural underpinnings of neuronal assembly formation in learning. Nat. Neurosci. 19, 1553–1562. doi: 10.1038/nn.4418
Honey, R. C., and Good, M. (1993). Selective hippocampal lesions abolish the contextual specificity of latent inhibition and conditioning. Behav. Neurosci. 107, 23–33. doi: 10.1037//0735-7044.107.1.23
Huerta, P. T., Sun, L. D., Wilson, M. A., and Tonegawa, S. (2000). Formation of temporal memory requires NMDA receptors within CA1 pyramidal neurons. Neuron 25, 473–480. doi: 10.1016/s0896-6273(00)80909-5
Jezek, K., Henriksen, E. J., Treves, A., Moser, E. I., and Moser, M. B. (2011). Theta-paced flickering between place-cell maps in the hippocampus. Nature 478, 246–249. doi: 10.1038/nature10439
Jezek, K., Lee, B. B., Kelemen, E., McCarthy, K. M., McEwen, B. S., and Fenton, A. A. (2010). Stress-induced out-of-context activation of memory. PLoS Biol. 8:e1000570. doi: 10.1371/journal.pbio.1000570
Josselyn, S. A. (2010). Continuing the search for the engram: examining the mechanism of fear memories. J. Psychiatry Neurosci. 35, 221–228. doi: 10.1503/jpn.100015
Josselyn, S. A., and Tonegawa, S. (2020). Memory engrams: recalling the past and imagining the future. Science 367:eaaw4325. doi: 10.1126/science.aaw4325
Kang, M. S., and Han, J. H. (2021). Optogenetic inhibition of medial entorhinal cortex inputs to the hippocampus during a short period of time right after learning disrupts contextual fear memory formation. Mol. Brain 14:2. doi: 10.1186/s13041-020-00719-w
Kaye, H., and Mackintosh, N. J. (1990). A change of context can enhance performance of an aversive but not of an appetitive conditioned response. Q. J. Exp. Psychol. B 42, 113–134.
Kelemen, E., and Fenton, A. A. (2010). Dynamic grouping of hippocampal neural activity during cognitive control of two spatial frames. PLoS Biol. 8:e1000403. doi: 10.1371/journal.pbio.1000403
Kempadoo, K. A., Mosharov, E. V., Choi, S. J., Sulzer, D., and Kandel, E. R. (2016). Dopamine release from the locus coeruleus to the dorsal hippocampus promotes spatial learning and memory. Proc. Natl. Acad. Sci. U.S.A. 113, 14835–14840. doi: 10.1073/pnas.1616515114
Kentros, C., Hargreaves, E., Hawkins, R. D., Kandel, E. R., Shapiro, M., and Muller, R. V. (1998). Abolition of long-term stability of new hippocampal place cell maps by NMDA receptor blockade. Science 280, 2121–2126. doi: 10.1126/science.280.5372.2121
Kim, E. J., Horovitz, O., Pellman, B. A., Tan, L. M., Li, Q., Richter-Levin, G., et al. (2013). Dorsal periaqueductal gray-amygdala pathway conveys both innate and learned fear responses in rats. Proc. Natl. Acad. Sci. U.S.A. 110, 14795–14800. doi: 10.1073/pnas.1310845110
Kim, J. J., and Fanselow, M. S. (1992). Modality-specific retrograde amnesia of fear. Science 256, 675–677. doi: 10.1126/science.1585183
Kim, S., Lee, J., and Lee, I. (2012). The hippocampus is required for visually cued contextual response selection, but not for visual discrimination of contexts. Front. Behav. Neurosci. 6:66. doi: 10.3389/fnbeh.2012.00066
Kim, W. B., and Cho, J. H. (2020). Encoding of contextual fear memory in hippocampal-amygdala circuit. Nat. Commun. 11:1382. doi: 10.1038/s41467-020-15121-2
Kitamura, T. (2017). Driving and regulating temporal association learning coordinated by entorhinal-hippocampal network. Neurosci. Res. 121, 1–6. doi: 10.1016/j.neures.2017.04.005
Kitamura, T., and Inokuchi, K. (2014). Role of adult neurogenesis in hippocampal-cortical memory consolidation. Mol. Brain 7:13. doi: 10.1186/1756-6606-7-13
Kitamura, T., Ogawa, S. K., Roy, D. S., Okuyama, T., Morrissey, M. D., Smith, L. M., et al. (2017). Engrams and circuits crucial for systems consolidation of a memory. Science 356, 73–78. doi: 10.1126/science.aam6808
Kitamura, T., Okubo-Suzuki, R., Takashima, N., Murayama, A., Hino, T., Nishizono, H., et al. (2012). Hippocampal function is not required for the precision of remote place memory. Mol. Brain 5:5. doi: 10.1186/1756-6606-5-5
Kitamura, T., Pignatelli, M., Suh, J., Kohara, K., Yoshiki, A., Abe, K., et al. (2014). Island cells control temporal association memory. Science 343, 896–901. doi: 10.1126/science.1244634
Kitamura, T., Saitoh, Y., Takashima, N., Murayama, A., Niibori, Y., Ageta, H., et al. (2009). Adult neurogenesis modulates the hippocampus-dependent period of associative fear memory. Cell 139, 814–827. doi: 10.1016/j.cell.2009.10.020
Kitamura, T., Sun, C., Martin, J., Kitch, L. J., Schnitzer, M. J., and Tonegawa, S. (2015). Entorhinal cortical ocean cells encode specific contexts and drive context-specific fear memory. Neuron 87, 1317–1331. doi: 10.1016/j.neuron.2015.08.036
Knox, D., and Keller, S. M. (2016). Cholinergic neuronal lesions in the medial septum and vertical limb of the diagonal bands of Broca induce contextual fear memory generalization and impair acquisition of fear extinction. Hippocampus 26, 718–726. doi: 10.1002/hipo.22553
Kochli, D. E., Thompson, E. C., Fricke, E. A., Postle, A. F., and Quinn, J. J. (2015). The amygdala is critical for trace, delay, and contextual fear conditioning. Learn. Mem. 22, 92–100. doi: 10.1101/lm.034918.114
Kubie, J. L., Levy, E. R. J., and Fenton, A. A. (2020). Is hippocampal remapping the physiological basis for context? Hippocampus 30, 851–864. doi: 10.1002/hipo.23160
Kubie, J. L., and Muller, R. U. (1991). Multiple representations in the hippocampus. Hippocampus 1, 240–242. doi: 10.1002/hipo.450010305
Kubie, J. L., and Ranck, J. B. Jr. (1983). “Sensory-behavioral correlates in individual hippocampal neurons in three situations: space and context,” in Neurobiology of the Hippocampus, ed. W. Seifert (New York, NY: Academic Press), 433–447.
Kwapis, J. L., Jarome, T. J., Lee, J. L., and Helmstetter, F. J. (2015). The retrosplenial cortex is involved in the formation of memory for context and trace fear conditioning. Neurobiol. Learn. Mem. 123, 110–116. doi: 10.1016/j.nlm.2015.06.007
Lee, C. C. (2015). Exploring functions for the non-lemniscal auditory thalamus. Front. Neural Circ. 9:69. doi: 10.3389/fncir.2015.00069
Lee, H., GoodSmith, D., and Knierim, J. J. (2020). Parallel processing streams in the hippocampus. Curr. Opin. Neurobiol. 64, 127–134. doi: 10.1016/j.conb.2020.03.004
Lee, J. Q., Sutherland, R. J., and McDonald, R. J. (2017). Hippocampal damage causes retrograde but not anterograde memory loss for context fear discrimination in rats. Hippocampus 27, 951–958. doi: 10.1002/hipo.22759
Lehmann, H., Sparks, F. T., Spanswick, S. C., Hadikin, C., McDonald, R. J., and Sutherland, R. J. (2009). Making context memories independent of the hippocampus. Learn. Mem. 16, 417–420. doi: 10.1101/lm.1385409
Lehner, M., Wislowska-Stanek, A., Skorzewska, A., Maciejak, P., Szyndler, J., Turzynska, D., et al. (2010). Differences in the density of GABA-A receptor alpha-2 subunits and gephyrin in brain structures of rats selected for low and high anxiety in basal and fear-stimulated conditions, in a model of contextual fear conditioning. Neurobiol. Learn. Mem. 94, 499–508. doi: 10.1016/j.nlm.2010.09.001
Leutgeb, J. K., Leutgeb, S., Moser, M. B., and Moser, E. I. (2007). Pattern separation in the dentate gyrus and CA3 of the hippocampus. Science 315, 961–966. doi: 10.1126/science.1135801
Leutgeb, S., and Leutgeb, J. K. (2007). Pattern separation, pattern completion, and new neuronal codes within a continuous CA3 map. Learn. Mem. 14, 745–757. doi: 10.1101/lm.703907
Leutgeb, S., Leutgeb, J. K., Barnes, C. A., Moser, E. I., McNaughton, B. L., and Moser, M. B. (2005). Independent codes for spatial and episodic memory in hippocampal neuronal ensembles. Science 309, 619–623.
Leutgeb, S., Leutgeb, J. K., Treves, A., Moser, M. B., and Moser, E. I. (2004). Distinct ensemble codes in hippocampal areas CA3 and CA1. Science 305, 1295–1298. doi: 10.1126/science.1100265
Lewis, M. C., and Gould, T. J. (2007). Reversible inactivation of the entorhinal cortex disrupts the establishment and expression of latent inhibition of cued fear conditioning in C57BL/6 mice. Hippocampus 17, 462–470. doi: 10.1002/hipo.20284
Libby, L. A., Reagh, Z. M., Bouffard, N. R., Ragland, J. D., and Ranganath, C. (2019). The hippocampus generalizes across memories that share item and context information. J. Cogn. Neurosci. 31, 24–35. doi: 10.1162/jocn_a_01345
Lingawi, N. W., Andrew, E., Laurent, V., Killcross, S., Westbrook, R. F., and Holmes, N. M. (2018). The conditions that regulate formation of a false fear memory in rats. Neurobiol. Learn. Mem. 156, 53–59. doi: 10.1016/j.nlm.2018.10.009
Liu, X., Ramirez, S., Pang, P. T., Puryear, C. B., Govindarajan, A., Deisseroth, K., et al. (2012). Optogenetic stimulation of a hippocampal engram activates fear memory recall. Nature 484, 381–385. doi: 10.1038/nature11028
Louie, K., and Wilson, M. A. (2001). Temporally structured replay of awake hippocampal ensemble activity during rapid eye movement sleep. Neuron 29, 145–156. doi: 10.1016/s0896-6273(01)00186-6
Mahut, H., Zola-Morgan, S., and Moss, M. (1982). Hippocampal resections impair associative learning and recognition memory in the monkey. J. Neurosci. 2, 1214–1220. doi: 10.1523/JNEUROSCI.02-09-01214.1982
Mankin, E. A., Sparks, F. T., Slayyeh, B., Sutherland, R. J., Leutgeb, S., and Leutgeb, J. K. (2012). Neuronal code for extended time in the hippocampus. Proc. Natl. Acad. Sci. U.S.A. 109, 19462–19467. doi: 10.1073/pnas.1214107109
Manns, J. R., and Eichenbaum, H. (2009). A cognitive map for object memory in the hippocampus. Learn. Mem. 16, 616–624. doi: 10.1101/lm.1484509
Mao, D., Kandler, S., McNaughton, B. L., and Bonin, V. (2017). Sparse orthogonal population representation of spatial context in the retrosplenial cortex. Nat. Commun. 8:243. doi: 10.1038/s41467-017-00180-9
Maren, S., Aharonov, G., and Fanselow, M. S. (1997). Neurotoxic lesions of the dorsal hippocampus and Pavlovian fear conditioning in rats. Behav. Brain Res. 88, 261–274. doi: 10.1016/s0166-4328(97)00088-0
Maren, S., Phan, K. L., and Liberzon, I. (2013). The contextual brain: implications for fear conditioning, extinction and psychopathology. Nat. Rev. Neurosci. 14, 417–428. doi: 10.1038/nrn3492
Marks, W. D., Yamamoto, N., and Kitamura, T. (2021). Complementary roles of differential medial entorhinal cortex inputs to the hippocampus for the formation and integration of temporal and contextual memory (systems neuroscience). Eur. J. Neurosci. 54, 6762–6779. doi: 10.1111/ejn.14737
Markus, E. J., Qin, Y. L., Leonard, B., Skaggs, W. E., McNaughton, B. L., and Barnes, C. A. (1995). Interactions between location and task affect the spatial and directional firing of hippocampal neurons. J. Neurosci. 15, 7079–7094. doi: 10.1523/JNEUROSCI.15-11-07079.1995
Marr, D. (1971). Simple memory: a theory for archicortex. Philos. Trans. R. Soc. Lond. B Biol. Sci. 262, 23–81.
Matos, M. R., Visser, E., Kramvis, I., van der Loo, R. J., Gebuis, T., Zalm, R., et al. (2019). Memory strength gates the involvement of a CREB-dependent cortical fear engram in remote memory. Nat. Commun. 10:2315. doi: 10.1038/s41467-019-10266-1
McCloskey, M., and Cohen, N. J. (1989). “Catastrophic interference in connectionist networks: the sequential learning problem,” in Psychology of Learning and Motivation, ed. G. H. Bower (Cambridge, MA: Academic Press), 109–165. doi: 10.1016/s0079-7421(08)60536-8
McEchron, M. D., Bouwmeester, H., Tseng, W., Weiss, C., and Disterhoft, J. F. (1999). Hippocampectomy disrupts auditory trace fear conditioning and contextual fear conditioning in the rat. Hippocampus 8, 638–646. doi: 10.1002/(SICI)1098-1063(1998)8:6<638::AID-HIPO6gt;3.0.CO;2-Q
McHugh, T. J., Jones, M. W., Quinn, J. J., Balthasar, N., Coppari, R., Elmquist, J. K., et al. (2007). Dentate gyrus NMDA receptors mediate rapid pattern separation in the hippocampal network. Science 317, 94–99. doi: 10.1126/science.1140263
McKenzie, S., Frank, A. J., Kinsky, N. R., Porter, B., Riviere, P. D., and Eichenbaum, H. (2014). Hippocampal representation of related and opposing memories develop within distinct, hierarchically organized neural schemas. Neuron 83, 202–215. doi: 10.1016/j.neuron.2014.05.019
Misane, I., Kruis, A., Pieneman, A. W., Ogren, S. O., and Stiedl, O. (2013). GABA(A) receptor activation in the CA1 area of the dorsal hippocampus impairs consolidation of conditioned contextual fear in C57BL/6J mice. Behav. Brain Res. 238, 160–169. doi: 10.1016/j.bbr.2012.10.027
Misane, I., Tovote, P., Meyer, M., Spiess, J., Ogren, S. O., and Stiedl, O. (2005). Time-dependent involvement of the dorsal hippocampus in trace fear conditioning in mice. Hippocampus 15, 418–426. doi: 10.1002/hipo.20067
Mizumori, S. J., Ragozzino, K. E., Cooper, B. G., and Leutgeb, S. (1999). Hippocampal representational organization and spatial context. Hippocampus 9, 444–451. doi: 10.1002/(SICI)1098-1063(1999)9:4<444::AID-HIPO10gt;3.0.CO;2-Z
Moore, M. D., Cushman, J., Chandra, D., Homanics, G. E., Olsen, R. W., and Fanselow, M. S. (2010). Trace and contextual fear conditioning is enhanced in mice lacking the alpha4 subunit of the GABA(A) receptor. Neurobiol. Learn. Mem. 93, 383–387. doi: 10.1016/j.nlm.2009.12.004
Muller, R. U., Kubie, J. L., and Ranck, J. B. Jr. (1987). Spatial firing patterns of hippocampal complex-spike cells in a fixed environment. J. Neurosci. 7, 1935–1950. doi: 10.1523/JNEUROSCI.07-07-01935.1987
Nadel, L., and Willner, J. (1980). Context and conditioning - a place for space. Physiol. Psychol. 8, 218–228. doi: 10.3758/bf03332853
Nakashiba, T., Cushman, J. D., Pelkey, K. A., Renaudineau, S., Buhl, D. L., McHugh, T. J., et al. (2012). Young dentate granule cells mediate pattern separation, whereas old granule cells facilitate pattern completion. Cell 149, 188–201. doi: 10.1016/j.cell.2012.01.046
Nakashiba, T., Young, J. Z., McHugh, T. J., Buhl, D. L., and Tonegawa, S. (2008). Transgenic inhibition of synaptic transmission reveals role of CA3 output in hippocampal learning. Science 319, 1260–1264. doi: 10.1126/science.1151120
Nakazawa, K., Quirk, M. C., Chitwood, R. A., Watanabe, M., Yeckel, M. F., Sun, L. D., et al. (2002). Requirement for hippocampal CA3 NMDA receptors in associative memory recall. Science 297, 211–218. doi: 10.1126/science.1071795
Nakazawa, K., Sun, L. D., Quirk, M. C., Rondi-Reig, L., Wilson, M. A., and Tonegawa, S. (2003). Hippocampal CA3 NMDA receptors are crucial for memory acquisition of one-time experience. Neuron 38, 305–315. doi: 10.1016/s0896-6273(03)00165-x
Odling-Smee, F. J. (1975). The role of background stimuli during Pavlovian conditioning. Q. J. Exp. Psychol. 27, 201–209. doi: 10.1080/14640747508400480
Ohkawa, N., Saitoh, Y., Suzuki, A., Tsujimura, S., Murayama, E., Kosugi, S., et al. (2015). Artificial association of pre-stored information to generate a qualitatively new memory. Cell Rep. 11, 261–269. doi: 10.1016/j.celrep.2015.03.017
Oishi, N., Nomoto, M., Ohkawa, N., Saitoh, Y., Sano, Y., Tsujimura, S., et al. (2019). Artificial association of memory events by optogenetic stimulation of hippocampal CA3 cell ensembles. Mol. Brain 12:2. doi: 10.1186/s13041-018-0424-1
O’Keefe, J., Burgess, N., Donnett, J. G., Jeffery, K. J., and Maguire, E. A. (1998). Place cells, navigational accuracy, and the human hippocampus. Philos. Trans. R. Soc. Lond. B Biol. Sci. 353, 1333–1340. doi: 10.1098/rstb.1998.0287
O’Keefe, J., and Conway, D. H. (1978). Hippocampal place units in the freely moving rat: why they fire where they fire. Exp. Brain Res. 31, 573–590. doi: 10.1007/BF00239813
O’Keefe, J., and Dostrovsky, J. (1971). The hippocampus as a spatial map. Preliminary evidence from unit activity in the freely-moving rat. Brain Res. 34, 171–175. doi: 10.1016/0006-8993(71)90358-1
Opalka, A. N., Huang, W. Q., Liu, J., Liang, H., and Wang, D. V. (2020). Hippocampal ripple coordinates retrosplenial inhibitory neurons during slow-wave sleep. Cell Rep. 30, 432.e3–441.e3. doi: 10.1016/j.celrep.2019.12.038
Opalka, A. N., and Wang, D. V. (2020). Hippocampal efferents to retrosplenial cortex and lateral septum are required for memory acquisition. Learn. Mem. 27, 310–318. doi: 10.1101/lm.051797.120
O’Reilly, R. C., and McClelland, J. L. (1994). Hippocampal conjunctive encoding, storage, and recall: avoiding a trade-off. Hippocampus 4, 661–682. doi: 10.1002/hipo.450040605
Orsini, C. A., Kim, J. H., Knapska, E., and Maren, S. (2011). Hippocampal and prefrontal projections to the basal amygdala mediate contextual regulation of fear after extinction. J. Neurosci. 31, 17269–17277. doi: 10.1523/JNEUROSCI.4095-11.2011
Orsini, C. A., Yan, C., and Maren, S. (2013). Ensemble coding of context-dependent fear memory in the amygdala. Front. Behav. Neurosci. 7:199. doi: 10.3389/fnbeh.2013.00199
Pavlov, I. P. (1927). Conditioned Reflexes: An Investigation of The Physiological Activity of the Cerebral Cortex. Oxford: Oxford Univ. Press.
Pelkey, K. A., Chittajallu, R., Craig, M. T., Tricoire, L., Wester, J. C., and McBain, C. J. (2017). Hippocampal GABAergic inhibitory interneurons. Physiol. Rev. 97, 1619–1747. doi: 10.1152/physrev.00007.2017
Pellman, B. A., and Kim, J. J. (2016). What can ethobehavioral studies tell us about the brain’s fear system? Trends Neurosci. 39, 420–431. doi: 10.1016/j.tins.2016.04.001
Penick, S., and Solomon, P. R. (1991). Hippocampus, context, and conditioning. Behav. Neurosci. 105, 611–617. doi: 10.1037//0735-7044.105.5.611
Phillips, R. G., and LeDoux, J. E. (1992). Differential contribution of amygdala and hippocampus to cued and contextual fear conditioning. Behav. Neurosci. 106, 274–285. doi: 10.1037//0735-7044.106.2.274
Pignatelli, M., Ryan, T. J., Roy, D. S., Lovett, C., Smith, L. M., Muralidhar, S., et al. (2019). Engram cell excitability state determines the efficacy of memory retrieval. Neuron 101, 274–284. doi: 10.1016/j.neuron.2018.11.029
Pilkiw, M., Insel, N., Cui, Y., Finney, C., Morrissey, M. D., and Takehara-Nishiuchi, K. (2017). Phasic and tonic neuron ensemble codes for stimulus-environment conjunctions in the lateral entorhinal cortex. eLife 6:e28611. doi: 10.7554/eLife.28611
Pollack, G. A., Bezek, J. L., Lee, S. H., Scarlata, M. J., Weingast, L. T., and Bergstrom, H. C. (2018). Cued fear memory generalization increases over time. Learn. Mem. 25, 298–308. doi: 10.1101/lm.047555.118
Quinn, J. J., Oommen, S. S., Morrison, G. E., and Fanselow, M. S. (2002). Post-training excitotoxic lesions of the dorsal hippocampus attenuate forward trace, backward trace, and delay fear conditioning in a temporally specific manner. Hippocampus 12, 495–504. doi: 10.1002/hipo.10029
Radulovic, J., Kammermeier, J., and Spiess, J. (1998). Relationship between Fos production and classical fear conditioning: effects of novelty, latent inhibition, and unconditioned stimulus preexposure. J. Neurosci. 18, 7452–7461. doi: 10.1523/JNEUROSCI.18-18-07452.1998
Ramirez, S., Liu, X., Lin, P. A., Suh, J., Pignatelli, M., Redondo, R. L., et al. (2013). Creating a false memory in the hippocampus. Science 341, 387–391. doi: 10.1126/science.1239073
Reijmers, L. G., Perkins, B. L., Matsuo, N., and Mayford, M. (2007). Localization of a stable neural correlate of associative memory. Science 317, 1230–1233. doi: 10.1126/science.1143839
Rescorla, R. A., and Wagner, A. R. (1972). “A theory of Pavlovian conditioning: variations in the effectiveness of reinforcement and nonreinforcement,” in Classical Conditioning II: Current Research and Theory, eds W. F. Black and A. H. Prokasy (New York, NY: Appleton-Century-Crofts), 64–99. doi: 10.1037/a0030892
Richter-Levin, G., and Akirav, I. (2000). Amygdala-hippocampus dynamic interaction in relation to memory. Mol. Neurobiol. 22, 11–20. doi: 10.1385/MN:22:1-3:011
Robin, J., Buchsbaum, B. R., and Moscovitch, M. (2018). The primacy of spatial context in the neural representation of events. J. Neurosci. 38, 2755–2765. doi: 10.1523/JNEUROSCI.1638-17.2018
Rolls, E. T. (2013). The mechanisms for pattern completion and pattern separation in the hippocampus. Front. Syst. Neurosci. 7:74. doi: 10.3389/fnsys.2013.00074
Roy, D. S., Okuyama, T., and Tonegawa, S. (2017a). Tagging activated neurons with light. Nat. Biotechnol. 35, 827–828. doi: 10.1038/nbt.3954
Roy, D. S., Muralidhar, S., Smith, L. M., and Tonegawa, S. (2017b). Silent memory engrams as the basis for retrograde amnesia. Proc. Natl. Acad. Sci. U.S.A. 114, E9972–E9979. doi: 10.1073/pnas.1714248114
Roy, D. S., Park, Y.-G., Ogawa, S. K., Cho, J. H., Choi, H., Kamensky, L., et al. (2019). Brain-wide mapping of contextual fear memory engram ensembles supports the dispersed engram complex hypothesis. bioRxiv [Preprint]. doi: 10.1101/668483
Rozeske, R. R., Valerio, S., Chaudun, F., and Herry, C. (2015). Prefrontal neuronal circuits of contextual fear conditioning. Genes Brain Behav. 14, 22–36. doi: 10.1111/gbb.12181
Rudy, J. W., Huff, N. C., and Matus-Amat, P. (2004). Understanding contextual fear conditioning: insights from a two-process model. Neurosci. Biobehav. Rev. 28, 675–685. doi: 10.1016/j.neubiorev.2004.09.004
Rudy, J. W., and O’Reilly, R. C. (1999). Contextual fear conditioning, conjunctive representations, pattern completion, and the hippocampus. Behav. Neurosci. 113, 867–880. doi: 10.1037//0735-7044.113.5.867
Rudy, J. W., and O’Reilly, R. C. (2001). Conjunctive representations, the hippocampus, and contextual fear conditioning. Cogn. Affect. Behav. Neurosci. 1, 66–82. doi: 10.3758/cabn.1.1.66
Ryan, T. J., Roy, D. S., Pignatelli, M., Arons, A., and Tonegawa, S. (2015). Memory. Engram cells retain memory under retrograde amnesia. Science 348, 1007–1013. doi: 10.1126/science.aaa5542
Sahay, A., Wilson, D. A., and Hen, R. (2011a). Pattern separation: a common function for new neurons in hippocampus and olfactory bulb. Neuron 70, 582–588. doi: 10.1016/j.neuron.2011.05.012
Sahay, A., Scobie, K. N., Hill, A. S., O’Carroll, C. M., Kheirbek, M. A., Burghardt, N. S., et al. (2011b). Increasing adult hippocampal neurogenesis is sufficient to improve pattern separation. Nature 472, 466–470. doi: 10.1038/nature09817
Schacter, D. L., Eich, J. E., and Tulving, E. (1978). Richard Semon’s theory of memory. J. Verbal Learn. Verbal Behav. 17, 721–743. doi: 10.3389/fnbeh.2020.632019
Schlessinger, A. R., Cowan, W. M., and Gottlieb, D. I. (1975). An autoradiographic study of the time of origin and the pattern of granule cell migration in the dentate gyrus of the rat. J. Comp. Neurol. 159, 149–175. doi: 10.1002/cne.901590202
Scobie, K. N., Hall, B. J., Wilke, S. A., Klemenhagen, K. C., Fujii-Kuriyama, Y., Ghosh, A., et al. (2009). Kruppel-like factor 9 is necessary for late-phase neuronal maturation in the developing dentate gyrus and during adult hippocampal neurogenesis. J. Neurosci. 29, 9875–9887. doi: 10.1523/JNEUROSCI.2260-09.2009
Scoville, W. B., and Milner, B. (1957). Loss of recent memory after bilateral hippocampal lesions. J. Neurol. Neurosurg. Psychiatry 20, 11–21. doi: 10.1136/jnnp.20.1.11
Seki, T., and Arai, Y. (1993). Highly polysialylated neural cell adhesion molecule (NCAM-H) is expressed by newly generated granule cells in the dentate gyrus of the adult rat. J. Neurosci. 13, 2351–2358. doi: 10.1523/JNEUROSCI.13-06-02351.1993
Sevenster, D., Alvares, L. D., and D’Hooge, R. (2018). Pre-exposure and retrieval effects on generalization of contextual fear. Learn. Motiv. 63, 20–26.
Skaggs, W. E., and McNaughton, B. L. (1996). Replay of neuronal firing sequences in rat hippocampus during sleep following spatial experience. Science 271, 1870–1873. doi: 10.1126/science.271.5257.1870
Smith, D. M., Wakeman, D., Patel, J., and Gabriel, M. (2004). Fornix lesions impair context-related cingulothalamic neuronal patterns and concurrent discrimination learning in rabbits (Oryctolagus cuniculus). Behav. Neurosci. 118, 1225–1239. doi: 10.1037/0735-7044.118.6.1225
Smith, S. M. (1979). Remembering in and out of context. J. Exp. Psychol. Hum. Learn. Mem. 5, 460–471.
Staiger, J. F., and Nurnberger, F. (1991). The efferent connections of the lateral septal nucleus in the guinea pig: intrinsic connectivity of the septum and projections to other telencephalic areas. Cell Tissue Res. 264, 415–426. doi: 10.1007/BF00319032
Suh, J., Rivest, A. J., Nakashiba, T., Tominaga, T., and Tonegawa, S. (2011). Entorhinal cortex layer III input to the hippocampus is crucial for temporal association memory. Science 334, 1415–1420. doi: 10.1126/science.1210125
Sutherland, R. J., O’Brien, J., and Lehmann, H. (2008). Absence of systems consolidation of fear memories after dorsal, ventral, or complete hippocampal damage. Hippocampus 18, 710–718. doi: 10.1002/hipo.20431
Swartzentruber, D. (1991). Blocking between occasion setters and contextual stimuli. J. Exp. Psychol. Anim. Behav. Process. 17, 163–173. doi: 10.1037//0097-7403.17.2.163
Takeuchi, T., Duszkiewicz, A. J., Sonneborn, A., Spooner, P. A., Yamasaki, M., Watanabe, M., et al. (2016). Locus coeruleus and dopaminergic consolidation of everyday memory. Nature 537, 357–362. doi: 10.1038/nature19325
Tanaka, K. Z., He, H., Tomar, A., Niisato, K., Huang, A. J. Y., and McHugh, T. J. (2018). The hippocampal engram maps experience but not place. Science 361, 392–397. doi: 10.1126/science.aat5397
Tayler, K. K., Tanaka, K. Z., Reijmers, L. G., and Wiltgen, B. J. (2013). Reactivation of neural ensembles during the retrieval of recent and remote memory. Curr. Biol. 23, 99–106. doi: 10.1016/j.cub.2012.11.019
Terranova, J. I., Ogawa, S. K., and Kitamura, T. (2019). Adult hippocampal neurogenesis for systems consolidation of memory. Behav. Brain Res. 372:112035. doi: 10.1016/j.bbr.2019.112035
Todd, T. P., DeAngeli, N. E., Jiang, M. Y., and Bucci, D. J. (2017). Retrograde amnesia of contextual fear conditioning: evidence for retrosplenial cortex involvement in configural processing. Behav. Neurosci. 131, 46–54.
Tonegawa, S., Morrissey, M. D., and Kitamura, T. (2018). The role of engram cells in the systems consolidation of memory. Nat. Rev. Neurosci. 19, 485–498. doi: 10.1038/s41583-018-0031-2
Tonegawa, S., Pignatelli, M., Roy, D. S., and Ryan, T. J. (2015). Memory engram storage and retrieval. Curr. Opin. Neurobiol. 35, 101–109. doi: 10.1016/j.conb.2015.07.009
Treves, A., and Rolls, E. T. (1994). Computational analysis of the role of the hippocampus in memory. Hippocampus 4, 374–391. doi: 10.1002/hipo.450040319
Tsien, J. Z., Huerta, P. T., and Tonegawa, S. (1996). The essential role of hippocampal CA1 NMDA receptor-dependent synaptic plasticity in spatial memory. Cell 87, 1327–1338. doi: 10.1016/s0092-8674(00)81827-9
Tye, K. M. (2018). Neural circuit motifs in valence processing. Neuron 100, 436–452. doi: 10.1016/j.neuron.2018.10.001
Vetere, G., Xia, F., Ramsaran, A. I., Tran, L. M., Josselyn, S. A., and Frankland, P. W. (2021). An inhibitory hippocampal-thalamic pathway modulates remote memory retrieval. Nat. Neurosci. 24, 685–693. doi: 10.1038/s41593-021-00819-3
Vouimba, R. M., Garcia, R., and Jaffard, R. (1998). Opposite effects of lateral septal LTP and lateral septal lesions on contextual fear conditioning in mice. Behav. Neurosci. 112, 875–884. doi: 10.1037//0735-7044.112.4.875
Wagner, A. R., and Rescorla, R. A. (1972). “Inhibition in Pavlovian conditioning: application of a theory,” in Inhibition and Learning, eds R. A. Boakes and M. S. Halliday (New York, NY: Academic).
Wills, T. J., Lever, C., Cacucci, F., Burgess, N., and O’Keefe, J. (2005). Attractor dynamics in the hippocampal representation of the local environment. Science 308, 873–876. doi: 10.1126/science.1108905
Wilson, M. A., and McNaughton, B. L. (1993). Dynamics of the hippocampal ensemble code for space. Science 261, 1055–1058.
Wiltgen, B. J., Sanders, M. J., Anagnostaras, S. G., Sage, J. R., and Fanselow, M. S. (2006). Context fear learning in the absence of the hippocampus. J. Neurosci. 26, 5484–5491. doi: 10.1523/JNEUROSCI.2685-05.2006
Wintzer, M. E., Boehringer, R., Polygalov, D., and McHugh, T. J. (2014). The hippocampal CA2 ensemble is sensitive to contextual change. J. Neurosci. 34, 3056–3066. doi: 10.1523/JNEUROSCI.2563-13.2014
Wirtshafter, H. S., and Wilson, M. A. (2021). Lateral septum as a nexus for mood, motivation, and movement. Neurosci. Biobehav. Rev. 126, 544–559. doi: 10.1016/j.neubiorev.2021.03.029
Wyss, J. M., and Van Groen, T. (1992). Connections between the retrosplenial cortex and the hippocampal formation in the rat: a review. Hippocampus 2, 1–11. doi: 10.1002/hipo.450020102
Xu, W., and Sudhof, T. C. (2013). A neural circuit for memory specificity and generalization. Science 339, 1290–1295. doi: 10.1126/science.1229534
Yokose, J., Marks, W. D., Yamamoto, N., Ogawa, S. K., and Kitamura, T. (2021). Entorhinal cortical Island cells regulate temporal association learning with long trace period. Learn. Mem. 28, 319–328. doi: 10.1101/lm.052589.120
Zeithamova, D., Gelman, B. D., Frank, L., and Preston, A. R. (2018). Abstract representation of prospective reward in the hippocampus. J. Neurosci. 38, 10093–10101. doi: 10.1523/JNEUROSCI.0719-18.2018
Zelikowsky, M., Bissiere, S., and Fanselow, M. S. (2012). Contextual fear memories formed in the absence of the dorsal hippocampus decay across time. J. Neurosci. 32, 3393–3397. doi: 10.1523/JNEUROSCI.4339-11.2012
Zhang, W., van Ast, V. A., Klumpers, F., Roelofs, K., and Hermans, E. J. (2018). Memory contextualization: the role of prefrontal cortex in functional integration across item and context representational regions. J. Cogn. Neurosci. 30, 579–593. doi: 10.1162/jocn_a_01218
Zhao, X., Wang, Y., Spruston, N., and Magee, J. C. (2020). Membrane potential dynamics underlying context-dependent sensory responses in the hippocampus. Nat. Neurosci. 23, 881–891. doi: 10.1038/s41593-020-0646-2
Keywords: hippocampus, contextual fear conditioning, entorhinal cortex, neural circuits, memory engram
Citation: Marks WD, Yokose J, Kitamura T and Ogawa SK (2022) Neuronal Ensembles Organize Activity to Generate Contextual Memory. Front. Behav. Neurosci. 16:805132. doi: 10.3389/fnbeh.2022.805132
Received: 29 October 2021; Accepted: 14 February 2022;
Published: 15 March 2022.
Edited by:
Liana Fattore, CNR Neuroscience Institute (IN), ItalyReviewed by:
Fraser Sparks, Regeneron Pharmaceuticals, Inc., United StatesRajan Dasgupta, Johns Hopkins University, United States
Copyright © 2022 Marks, Yokose, Kitamura and Ogawa. This is an open-access article distributed under the terms of the Creative Commons Attribution License (CC BY). The use, distribution or reproduction in other forums is permitted, provided the original author(s) and the copyright owner(s) are credited and that the original publication in this journal is cited, in accordance with accepted academic practice. No use, distribution or reproduction is permitted which does not comply with these terms.
*Correspondence: Takashi Kitamura, VGFrYXNoaS5LaXRhbXVyYUBVVFNvdXRod2VzdGVybi5lZHU=; Sachie K. Ogawa, U2FjaGllLk9nYXdhQFVUU291dGh3ZXN0ZXJuLmVkdQ==