- 1Department of Rehabilitation Medicine, New York University Langone Medical Center, New York, NY, United States
- 2Department of Neurology, New York University Langone Medical Center, New York, NY, United States
- 3Department of Psychology and Center for Neural Science, New York University, New York, NY, United States
- 4Department of Ophthalmology, New York University Langone Medical Center, New York, NY, United States
Acute and chronic disease processes that lead to cerebral injury can often be clinically challenging diagnostically, prognostically, and therapeutically. Neurodegenerative processes are one such elusive diagnostic group, given their often diffuse and indolent nature, creating difficulties in pinpointing specific structural abnormalities that relate to functional limitations. A number of studies in recent years have focused on eye–hand coordination (EHC) in the setting of acquired brain injury (ABI), highlighting the important set of interconnected functions of the eye and hand and their relevance in neurological conditions. These experiments, which have concentrated on focal lesion-based models, have significantly improved our understanding of neurophysiology and underscored the sensitivity of biomarkers in acute and chronic neurological disease processes, especially when such biomarkers are combined synergistically. To better understand EHC and its connection with ABI, there is a need to clarify its definition and to delineate its neuroanatomical and computational underpinnings. Successful EHC relies on the complex feedback- and prediction-mediated relationship between the visual, ocular motor, and manual motor systems and takes advantage of finely orchestrated synergies between these systems in both the spatial and temporal domains. Interactions of this type are representative of functional sensorimotor control, and their disruption constitutes one of the most frequent deficits secondary to brain injury. The present review describes the visually mediated planning and control of eye movements, hand movements, and their coordination, with a particular focus on deficits that occur following neurovascular, neurotraumatic, and neurodegenerative conditions. Following this review, we also discuss potential future research directions, highlighting objective EHC as a sensitive biomarker complement within acute and chronic neurological disease processes.
Introduction
Acute and chronic disease processes that lead to cerebral injury can often be clinically challenging diagnostically, prognostically, and therapeutically. Neurodegenerative processes are one such elusive diagnostic group, given their often diffuse and indolent nature, creating difficulties in pinpointing specific structural abnormalities that relate to functional limitations. Historically, experiments have concentrated on cerebral lesion-based approaches, significantly improving our understanding of the neurophysiology and underscoring the sensitivity of behavioral biomarkers to detect as well as predict the outcomes of cerebral injury. These focal lesion-based models and associated biomarkers can be combined synergistically and have significant potential in shedding light on acute and chronic neurological disease processes.
Eye–hand coordination (EHC) can be defined as the complex relationship between our visual system and our manual motor system. Visually guided reaching, grasping, and object manipulation depend on the ability to visually decipher environmental details and finely coordinate motor responses of the eye and hand to produce controlled, rapid and accurate movements. Independent deficits of either ocular or manual motor control have been studied extensively after acquired brain injury (ABI). More recently, the coordination between eye and hand movements in patients with central nervous system injury, as related to neurovascular, neurotraumatic, and neurodegenerative conditions, has been highlighted as a critical concept in understanding brain-behavior relationships.
Over the course of the past two decades, a number of studies have demonstrated that EHC deficits (i.e., eye–hand incoordination or dyssynergia) resulting from ABI are important thematic concepts within the field of rehabilitation following neurological injury (1–3). In response, a focused review was performed on the PubMed database using a series of key words that included the following phrases and/or words: eye–hand coordination, acquired brain injury, stroke, cerebrovascular accident (CVA), traumatic brain injury, and brain injury (including acute, subacute, and chronic time scales). The research included articles published over the past two decades. A total of 74 articles were surveyed, which varied significantly in scope and merit.
The aim of this narrative review on EHC was to clarify its conceptual importance in the setting of ABI, to improve understanding neuroanatomically, and to address implications therapeutically. The articles reviewed were focused on EHC or the integration of visual input secondary to ocular motor control and manual motor output and related pathology, including neurovascular, neurotraumatic, and neurodegenerative conditions. The overarching goal of this review is to engender dialogue between clinicians and scientists in a framework that will provide clarity, improve comprehension and precipitate translational, clinical research.
Literature Search Strategy
Our literature review was performed by J.R. and E.W. on publications available in the National Center for Biotechnology Information’s PubMed database using key words containing the phrase “eye hand coordination” and key words relevant to ABI (specific key words are listed in Table 1). The search of the literature included seminal and contemporary peer-reviewed articles on EHC in the setting of ABI, including injuries that were either secondary to trauma or CVAs. The research articles spanned publication dates between 1998 and 2015. The quality and the relevance of the resultant literature varied significantly in caliber and applicability. Articles were utilized based on their pertinence to ABI and its associated effects on EHC. Pertinence was determined by consensus between two authors based on whether there was a thematic focus on EHC, and also discussion of at least one of the patient populations of interest. A total of 74 articles were originally reviewed (surveyed); this compilation was ultimately distilled to 8 pertinent (utilized) references (see Figure 1 and Table 2).
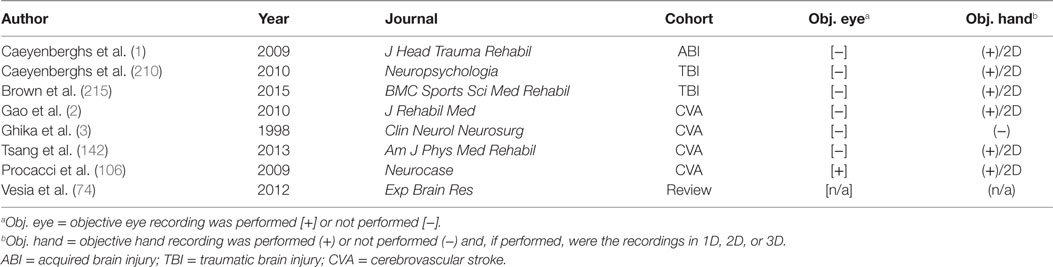
Table 2. Key comparisons of the articles utilized in the review following the literature search and selection process.
EHC Definition
Eye–hand coordination is the complex relationship between the visual and manual motor systems, at the intersection between vision and dexterity. EHC depends on vision to aid in directing goal-oriented hand movements, including pointing, reaching, grasping, object manipulation, and tool use, and encompasses many functionally relevant motor activities (4, 5). Optimal coordination relies on precise ocular motor control for high acuity visual perception and sound manual motor control, yielding robust effector coaction (6, 7). This visuomotor integration requires complex motor programs and near continuous, multimodal sensory feedback, and predictions thereof, to produce controlled and rapid task-specific movements (8).
EHC Neurophysiology and Neuroanatomy
The Visual System (Eye)
Primary visual cortex (V1), also known as striate cortex, is the first cortical region that processes visual input. V1 is located in the posterior pole of the occipital lobe. It mainly serves to process primitive visual features, such as bars of a specific orientation or edges and contours of solid objects within a specific portion of the retina’s visual field. From V1, visual processing continues through a sequence of adjacent cortical regions known as extrastriate cortex. A fundamental organizing principle of these visual areas is a topographic representation of the contralateral visual field. The spatial layout of a scene is represented in an orderly manner across a population of neurons that reflect input from the retina. This population of neurons constitutes a visual field map whereby adjacent neurons represent adjacent points in space (9), preserving the spatial layout of the retinal image in each of these cortical areas. This systematic organization is computationally and metabolically efficient as it shortens connection lengths between similarly tuned neurons. Interestingly, topographic organization extends beyond retinotopic coordinate space. Relevant for EHC, other areas represent space in head-centered coordinates (10–12), or a combination of coordinate systems (13, 14). The interactions between these areas likely facilitate sensorimotor transformations fundamental to EHC. Extrastriate regions (such as V2/V3), which emanate rostrally from V1, are believed to be responsible for processing features of progressively increasing complexity (15–17). This processing stream bifurcates into a ventral “what” pathway, processing object identity and visual features, and a dorsal “where” pathway, processing spatial attention and movement (15, 18). The dorsal pathway has also been implicated in processing visual input for predictive and anticipatory movements, including those coordinated between the eye and hand (17, 19, 20). The dorsal and ventral streams are thought to aid EHC (21, 22).
The Ocular Motor System
In order to examine our environment, we alternate between fixating a point of interest and making fast, darting eye movements (saccades) from one point of interest to another. For well over a century, scientists have measured saccades to investigate the link between brain and behavior (23, 24). Broadly, along with the subcortical superior colliculus (SC), four cortical areas contribute to the control of saccades: the frontal eye field (FEF), the supplementary eye field (SEF), the parietal eye field (PEF), and the cingulate eye field (CEF). Each region appears to play a distinct role in controlling eye movements. The FEF, SEF, and PEF directly project to the SC, while the CEF influences ocular motor control more indirectly through connections with the FEF, SEF, and PEF (25–29). Additionally, the FEF connects directly to the brainstem ocular motor nuclei, which house the ocular motor neurons that innervate the extraocular muscles (Figure 2).
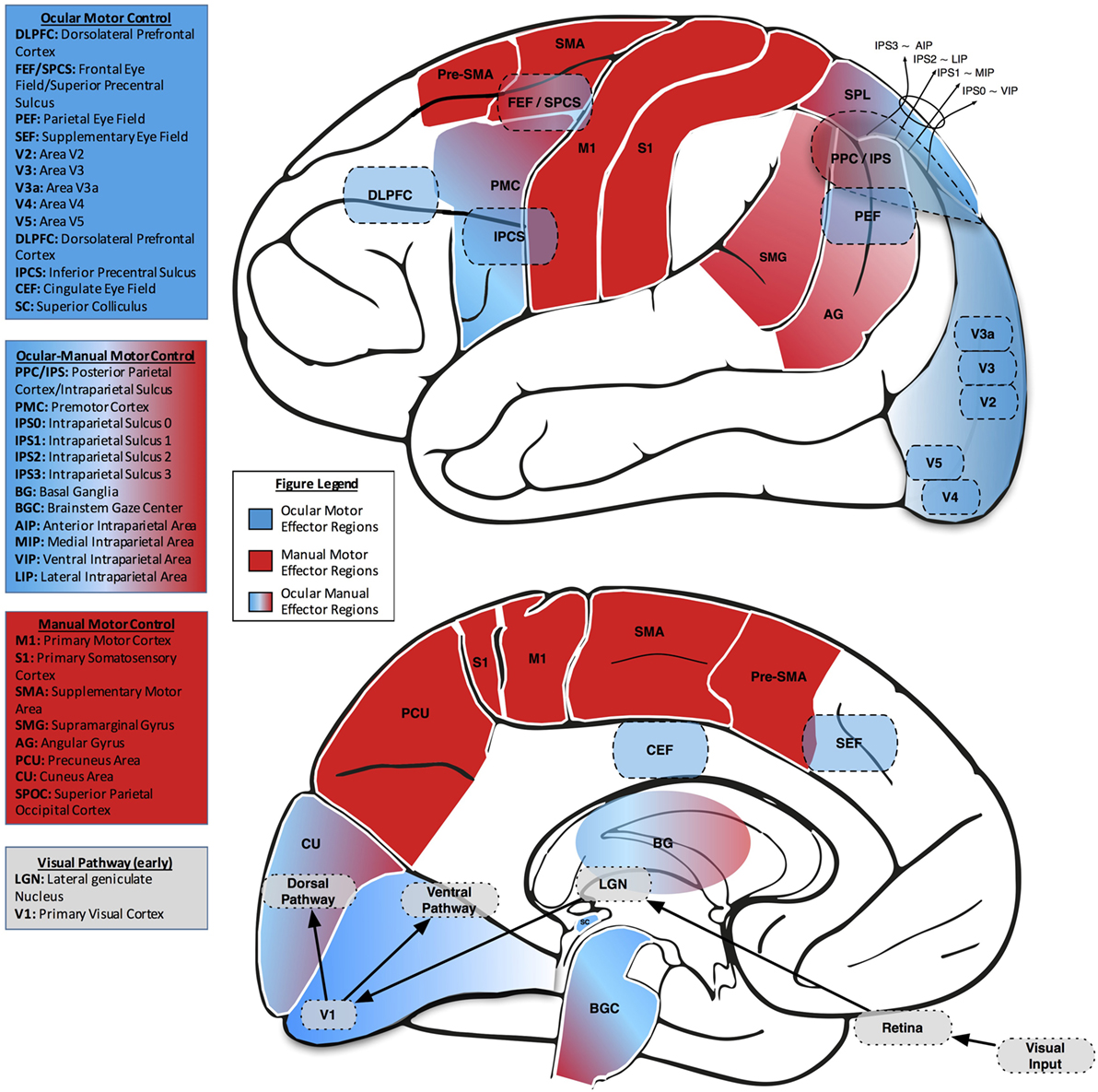
Figure 2. Lateral (upper) and midsaggital (lower) views of the human brain, labeled with neuroanatomical regions of interest related to eye–hand coordination. In both views, ocular motor areas are colored blue, manual motor areas red, and combined ocular-manual motor areas a blend of red and blue.
Frontal Eye Field
The FEF is crucial for the preparation and execution of voluntary saccades to either external (visually guided saccades) or internal targets (memory-guided saccades) (30–34). The majority of research characterizing the FEF has been with respect to the monkey ocular motor system, ever since Ferrier discovered that electrical stimulation of the FEF elicited eye movements (23). In the monkey, FEF is located in the anterior bank of the arcuate sulcus, just posterior to the principal sulcus (31). The FEF both projects to and receives connections from numerous cortical and subcortical brain regions (35, 36). It is retinotopically organized and primarily comprised of neurons that contribute to the holding or shifting of gaze (fixation neurons and saccade neurons, respectively) or neurons that generally respond to stimuli within their receptive field (visual neurons) (37, 38). Neurons within the FEF also have a preference for the contralateral visual field (31). In monkeys, there is also a rough topographic organization with regards to saccade amplitude. The superior portion of the FEF is responsible for generating larger amplitude saccades and shares connections with the dorsal visual stream, while the inferior portion of the FEF is responsible for generating smaller amplitude saccades and shares connections with the ventral visual stream (39). Interestingly, this topography and connectivity organized by dorsal and ventral streams has yet to be demonstrated in the human FEF. Instead, the putative human homolog of FEF, located in the superior precentral sulcus (SPCS), is organized into distinct visual field map clusters similar to early visual cortex (40).
Supplementary Eye Field
The SEF is involved in more indirect aspects of saccade control, such as monitoring the consequences or context of eye movements (41) and coordinating sequences of successive saccades (42, 43). Although believed to typically be found in the posteromedial part of the human superior frontal gyrus, there exists a great amount of variability between individuals in the exact location of the SEF, thus rendering it difficult to define by anatomy alone (44). The activity of neurons in the SEF is modulated by target position in multiple reference frames, aiding in maintaining eye position despite changes in body and head position (14, 45). This region receives both sensory and motor inputs and supplies outgoing connections to both the FEF and PEF (46). Although electrical stimulation of an FEF neuron elicits an eye movement of a fixed magnitude and direction, SEF stimulation elicits an eye movement to a fixed region of the visual field relative to the position of the head, irrespective of the starting position of the eye (47). Although much less is known about topographic organization in SEF, a recent human neuroimaging study suggests it also contains an orderly map of continuous space similar to other visual areas (48).
Parietal Eye Field
Visual input is received by the PEF and aids in triggering reflexive saccades toward visual stimuli found within the peripheral field of vision, as well as managing alterations in attention (49) and performing memory-guided saccades (40, 50). The PEF is located in the lateral intraparietal (LIP) area in monkeys and the intraparietal sulcus (IPS) in humans, and contains strong and reciprocal connections with the FEF. Similar to early visual areas, the IPS contains multiple visual field maps of contralateral space that have led to further parcelation (34, 51). These subregions are labeled numerically (IPS0, IPS1, IPS2, IPS3, etc.), starting from the most posterior area, IPS0, which borders V3A/V3B. Each of these subdivisions are activated during human neuroimaging studies involving eye movements (52). Therefore, which of these individual maps, if any, directly correspond to subdivisions of the monkey IPS (LIP, AIP, VIP, MIP) is still up for debate (52–55). It is also possible that some of the retinotopic IPS subdivisions are unique to humans.
Cingulate Eye Field
The CEF is involved in intentional but not reflexive saccade control (56), and projects to both the FEF and SEF (57). In non-human primates, the CEF is located on the medial wall in each hemisphere, ventral and partly anterior to the SEF. In humans, however, the CEF is located more posterior and ventral to the SEF (57). In humans, lesions of the CEF impair many types of saccades, including sequences of visually-guided saccades, memory-guided saccades, and antisaccades (56). Compared to the other ocular motor regions listed here, the CEF is the least studied and least understood.
Superior Colliculus
The SC plays a crucial role in saccade execution, as it projects directly to the brainstem ocular motor nuclei. It receives projections from a multitude of areas including FEF, SEF, and PEF. Like FEF, electrical stimulation of SC elicits saccades of a particular magnitude and direction. SC is also organized similarly to FEF, except in a rostral-caudal, rather than an inferior–superior, gradient of increasing saccade amplitude. Recent human neuroimaging studies have demonstrated that human SC contains a retinotopic map of the entire contralateral visual field (58, 59). In non-human primates, lesions of the SC alone impair but do not abolish eye movements, but lesions of SC and FEF together have catastrophic consequences for eye movements that do not recover with time (60).
Other Areas
The aforementioned areas are clearly not an exhaustive list of brain regions associated with ocular motor control, although they are the most studied. For example, dorsomedial frontal cortex, sometimes referred to as the presupplementary motor area, is critical for inhibition of reflexive saccades in humans (61). It has also been implicated in selecting among competing movements during action selection (62). Additionally, V1 also plays a role in ocular motor control and has projections directly to the SC. In the rhesus monkey, electrical stimulation of V1 can elicit saccades, but the required level of stimulation is much higher than what is necessary to elicit saccades via FEF or SC stimulation (63).
The role of human dorsolateral prefrontal cortex (dlPFC) in ocular motor control is still unclear. Electrophysiological and lesion studies in non-human primates show that the dlPFC contains spatially selective neurons that are critical for memory-guided saccades (64, 65). However, lesions to human dlPFC do not impair memory-guided saccades (34), and do not show spatial selectivity (34, 66). A handful of studies have examined the effects of transcranial magnetic stimulation (TMS) on dlPFC during a memory-guided saccade task (67–69). These results seem to parallel the results from non-human primate lesion studies, finding effects of TMS on memory-guided saccade performance. However, it is likely that the stimulation site in these studies overlapped with the FEF. All three papers used the identical method to localize and define dlPFC, first finding the motor hand area and then moving anteriorly a few centimeters; this method has also been described as an effective way to localize the human FEF (70). A more recent study using TMS to disrupt activity in human dlPFC found no impairment on memory-guided saccades (48).
The Manual Motor System (Hand)
Within EHC, the end goal is to place the hand/finger(s) or the manual effector in the position required for motor program execution or, in a dynamic sense, to work seamlessly and reciprocally with the eye to build and actualize complex motor programs. The neuroanatomical reach network most directly responsible for voluntary movements of the arm and hand includes motor cortical regions such as primary motor cortex (M1) and the supplementary and premotor cortices. The primary motor cortex begins on the anterior wall of the central sulcus and continues rostrally to comprise what is the anterior paracentral lobule. It is the cortical region responsible for the collective generation of action potentials that relay neural information to the descending corticospinal tract to produce hand movements (71). The premotor cortex (PMC) is located anterior to the primary motor cortex (M1) and in a lateral position from midline; this region is in close spatial proximity to the inferior precentral sulcus (70). PMC is the planning region for anticipatory movements, provides spatial guidance during hand movements, and processes the sensory input used to aid the guidance of hand movements. The supplementary motor cortex is closer to the midline and anterior to the primary motor cortex, and is used to plan sequential manual movements. These motor areas supply the bulk of the neurons whose axons compose the corticospinal tract (in conjunction with smaller inputs from somatosensory, posterior parietal, and cingulate cortex), which travels through the internal capsule and pons, decussates at the level of the medulla, and ultimately activates the alpha motorneurons in the spinal cord (primarily the lower cervical and first thoracic levels) either directly or via spinal interneurons.
This cortical reach network is supplemented by a larger network of cortical and subcortical regions, including the posterior parietal cortex (PPC), somatosensory cortex, basal ganglia, and cerebellum. The PPC is an associative region that translates visual information and input from the somatosensory cortex into motor commands (72, 73). Based on functional neuroimaging, TMS studies, and human case series with parietal injuries, a functional topography for reach, as it relates to the planning and control of visuomotor action, has been described within the human PPC (74). More specifically, midposterior intraparietal sulcus (mIPS), superior parietal occipital cortex (SPOC), and angular gyrus (AG) are reach-specific areas (Figure 2). Three main aspects in reach-dominant areas include effector specificity, hemispheric laterality and computational specificity. The area posteromedial to IPS contributes to the planning of reaching, while the area anterolateral to the IPS has a role in grasp-related information integration. Cortex anterior to the intraparietal area (AIP) is involved in object-directed hand grasping and hand preshaping. In hemispheric lateralization, bilateral activation due to reaching with more emphasis on contralateral movements has been identified (75).
The anterior portion of IPS monitors the compatibility of a planned reach/grasp with outgoing movement commands and incoming sensory inputs (74, 76). Eye movements frequently take place before a hand movement and may be spatially fixated on the object of interest until the end of reaching to improve accuracy (77–79). Decoupling of eye and hand movements requires reach and saccade goal separation (80–83). SPOC is more active in reaches toward peripheral (non-foveal) targets independent of gaze signals, while mIPS is more active in reach toward foveated targets with spatial congruence between gaze and reach goal (74).
In cortical reach-dominant regions, the anterior precuneus (aPCu) area, expanding into the medial IPS, is equally active in visual and non-visual reaching. Medial, anterior intraparietal and superior parietal cortices are also activated in both visual and non-visual reaching; areas located in the anterior distribution are more active during hand movements in comparison to those in the posterior distribution, which are more active during combined eye and hand movements. Another area, at the superior end of the parieto-occipital sulcus (sPOS), is more active during visual reaching. Taken together, aPCu may be a sensorimotor area with a prominent proprioceptive sensory input and sPOS, a visuomotor area that receives visual feedback during reaching (84).
In addition to these cortical contributions, the cerebellum plays a critical role in the timing and control aspects of manual dexterity, particularly multijoint movements, through both reciprocal connections with frontal motor areas, and through connections to the descending motor pathway through the red nucleus (85). The cerebellum receives inputs from a cortical network composed of motor, somatosensory, and posterior parietal areas via the pons. These inputs allow the cerebellum to compare the desired consequences of a movement (e.g., touching an elevator button), with the future progression of the hand through space as predicted from current motor commands. The cerebellum is often said to act as a “forward modeler” of the arm/hand for this reason (it can predict the consequences of the descending motor commands sent to the arm) (86, 87). The cerebellum is then able to modulate the ongoing stream of motor commands to correct anticipated errors, either through connections to SMA, or via a more direct modulation of the descending motor pathway via the red nucleus (86). Cerebellar damage results in motor incoordination, and a loss of the typical smoothness of manual motor trajectories through space (85, 88).
This highly interconnected reach network is further complicated by additional interconnections with the basal ganglia, the set of subcortical structures including the striatum (caudate nucleus and putamen), globus pallidus, subthalamic nucleus, and substantia nigra. Inputs to this functional grouping of nuclei from reach-related cortical areas are received by the striatum and processed by the remaining basal ganglia before being returned to the cortex (SMA) via the thalamus. The basal ganglia have a complex modulatory role in the reach motor network that appears to involve the choice of which movement to make, from among the possible alternatives, as well as the related function of assigning values to different possible movements (e.g., based on which are expected to be most rewarding) (89, 90).
Sensorimotor Control: Ocular (Eye) and Manual (Hand)
Overview
In humans, sensorimotor control strategies are essential for skilled somatic behavior; object manipulation performance aids in characterizing the interactions between the body and the article of interest (91). Before initiating a manual motor movement, the eyes very often fixate on the preferred object (92); however, a more invariant feature is that the eyes will spatially direct gaze on the target prior to the arrival of the hand (93), typically near the peak acceleration of the reach (94–96). The ocular motor system enables the needed visual information to direct the hand and successfully accomplish the task requirements; this is performed so fixations are “just in time,” providing information at the moment the additional foveal-based fine detail would be required for the task at hand (97). Change blindness and short-term memory limitations, features of normal visual function, support the notion that information acquired during prior fixations factors minimally into computation (98–100). The information that is integrated across the fixations when a visual scene, for example, is largely semantic in nature, i.e., the memory of an object’s identity but not specific features or the memory of a global scene but not particular details (101, 102). Therefore, eye movements are closely coupled to motor action in both time and space (103).
Sensorimotor coupling involves the fusion of visual perception and somatic motor control for action planning and behavioral execution; in fact, vision may best be understood through the “lens” of action production (104, 105). The line of sight is often directed at items of interest in an environment, upon which manual interactions may subsequently be focused. Based on the final goal of an intended manual interaction, grasping choices will be affected; this not only has relevance for motor control and planning requisite to finger position but also for eye fixation position, as gaze is paramount to precise manual action before execution (71). Eye fixations suggest a multitiered manual motor planning hierarchy. At the first level, it is determined where to grasp the object of interest, given the current descriptive content and the orientation of the object. If needed, at the second level the grasp is altered based on the type of secondary task to be accomplished with the grasped object, e.g., tool movement from location A to B. If needed, at the third level the movement plan incorporates a joint action component reflecting, e.g., the final resting place for the tool, handing it to a second person or placing it in a convenient location. Changes in the second and third levels of motor planning alter eye movement patterns and suggest a bidirectional sensorimotor coupling of eye to hand in coordinated activities (71).
The brain putatively plans visually guided action in the PPC, as suggested by neurophysiological studies in non-human primates, in imaging studies in healthy humans, and in human patients with cerebral injuries (74, 106–108). In non-human primate studies, electrophysiological results have revealed effector-specific regions in the PPC, with the parietal reach region relating to arm movements and the LIP area relating to saccadic activity. Given the relationship to effector preference but not dominance in these PPC subregions, functional imaging studies have sought to determine similar degrees of effector selectivity in human PPC, including area V7 and IPS areas 1 and 2 (IPS1 and IPS2) (109). Results indicate a limited degree of effector selectivity in the cortex and that transitions from the specificity surrounding one effector to another are gradual through the cerebral hierarchy in association with the frontal, parietal, and occipital cortices (109). In the visual cortex, there is a general preference noted for saccades, the PPC subregion, V7, has been specifically noted to activate relative to these fast eye movements. In the parietal cortex, IPS1 reflects a balance of saccade and reach activity, while IPS2 appears to be biased somewhat toward representing reach planning. In the frontal cortex, while regions near the central sulcus are more active for reach, FEF displays no effector preference (109), which may indirectly indicate a form of balance between eye and hand (Figure 2).
The PPC is of central importance given its strong feedforward connections to premotor and primary motor cortex (110). It has been suggested that the cytoarchitecture of networks between frontal and parietal cortices and their associative connections is ideal for integrating visual and somatic information (111). In fact, connections between the parietal and the dorsolateral (e.g., PMC) and medial (e.g., SMA) frontal motor areas may link vital neural information that assists in determining the visually deciphered target location and the somatic hand configuration required for execution (112). Expanding the integration network, the parieto-occipital junction shows activation when hand-motor goals are directed by a combination of gaze-oriented and proprioceptive body cues, suggesting some level of segregation within the reach-related regions of the PPC, while purely gaze-centered motor goals demonstrate activation in the anterior cuneus (113).
In visually guided reaching, studies in the macaque have shown that the ventral aspect of the parieto-occipital sulcus may act as a potential early node of the distributed eye–hand network, serving as a possible source of visual- and eye-position signals to parietal and frontal areas; this process has been described as re-entrant signaling, reciprocal associative connections leading to the interaction of eye and hand motor commands (110, 114). The ventral bank of the parieto-occipital sulcus, areas V6 and V6A, operates as an integrator of visual and somatic spatial information (115). There might be overlap between these two areas and the “parieto-occipital area” (PO) (116), but recent studies comparing the connections emphasize that V6Av (the ventral subregion of area V6A) is cytoarchitectonically and functionally distinct from the adjoining areas (V6 and V6Ad, the dorsal subregion) (117). More specifically, V6Av may serve as an integrator of visual and somatic/motor inputs (118). PPC is not only considered the sensorimotor interface for the planning and control of visually guided movements, but also conveys initial sensory-to-motor signals and online updates for the integration of sensory information from prior and current manual motor movement (119). The spatial position of the target is compared to the current spatial position of the hand which is thought to be represented in an eye-centered reference frame, mapping directly into motor error signals in a hand-centered reference frame; the superior parietal lobule (SPL) in the PPC is the primary location where these transformations are thought to occur with activation patterns mapped along a ventral–dorsal axis (119).
Coordinate Mapping Based on Visual Cues
Visual cues that translate into retinotopically coded information must be converted into meaningful output for effector-specific, goal-directed activity. The PPC may direct and plan movement by establishing a head/body-centered coordinate system, through both visual input and motor/proprioceptive cues, or, in contrast, utilize an eye-centered coordinate system (120, 121). An eye-centered frame of reference proves useful when considering the optimal dual-effector coordination, as eye movements are coded in eye-centered coordinates: extending this into PPC would ostensibly be strategic (122). In addition, the eye-centered reference frame used in PPC would help in accounting for online obstacles during visuomotor action and during error correction (123, 124). Evidence from macaque supports the concept of PPC operating under a common reference frame, where sensory targets are computationally processed for transformation from head-, body-, eye-, and limb-based coordinates into one eye-centered representation; this simplifies inter-effector motor planning (122).
The brain maintains a dynamic map of memory-based, geometric space in a gaze-centered coordinate system (125). On a cellular level, in the primate parietal cortex, the receptive fields of neurons have been shown to shift transiently in anticipation of an eye movement, predicting the sensory consequences of the intended eye movement (126). Given natural delays in sensory feedback and the anticipatory nature of this physiologic phenomenon, the mechanism is likely a forward model similar to what has been described for arm movements (87, 127, 128), which would combine sensory feedback with the predicted consequences of motor commands to facilitate online feedback control; additionally, this may impact the process by which the brain monitors and stores memories of previous movement execution and performance (125, 129, 130).
Impairment of the Visuomotor System
Pathology and Clinical Disease
Pathology and clinical disease provides neuroanatomical and neurophysiological “knockouts” that can be diagnosed and characterized behaviorally, shedding light on cerebral function. Connecting empirical data on clinical deficits with neuroimaging and anatomical correlates yields greater understanding behind the nature of specific visuomotor pathologies and more significantly on relevant connections, associations, pathways, and networks. Optic ataxia (OA), as a clinical entity, is an archetype; patients demonstrate difficulty in executing visually guided reaching without additional sensory cues, accompanied by deficits in prehension and hand orientation. As opposed to Balint’s syndrome or OA plus ocular apraxia and simultagnosia, an isolated optic ataxia often manifests with intact ocular motor function, full visual fields, normal depth perception, complete motor ability, and cerebellar function and no known cause of reaching ataxia. These clinical signs and disease patterns are attributed to lesions in the PPC or, more specifically, neurovascular injuries in the superior and inferior parietal lobule (SPL and IPL, respectively), around the IPS (131–134).
Optic ataxia, again defined as the inability to properly reach or grasp objects under visual control, particularly under peripheral vision, is associated neuroanatomically with dysfunction at the border of the SPL, near the IPS, but superior to the IPL, and behaviorally with poor motor performance when faced with moving targets that pose immediate motor programming challenges (135, 136). More precisely, the SPL receives afferent signals from the extrastriate areas of the occipital lobe and has reciprocal connections to and from the premotor and primary motor cortices of the frontal lobe, serving as a multisensory integration hub planning motor commands (137, 138). Optic ataxia has been interpreted as a combinatorial dysfunction in the ability of parietal neurons to integrate retinal, eye, and hand signals utilized for EHC (134). The neural mechanisms of hand movement corrections given rapid target changes shed light on the functional abilities of the eye and hand to maintain coupling and assist in further understanding the pathology of optic ataxia, highlighting clinical deficits that manifest as an inability to quickly adjust in-flight hand movement trajectories aimed at moving objects (132).
Sensorimotor Impairment
Cerebrovascular accident leads to sensorimotor impairments that result in a myriad of deficits in visually guided reaching and pointing movements, impairments that are noted in both the contralateral and ipsilateral hands (2, 139–145). The focus post-injury has been to examine the hand objectively during visually guided action without objective eye movement assessment, leaving one to question the abnormalities that may exist between effectors. In fact, the ocular motor system, when objectively assessed, has been shown to be a powerful tool in clinical neuroscience, serving as a marker of cerebral function (146–148). Recently, eye movements have been shown in stroke investigations to be a sensitive biomarker for cognitive and motor recovery (149, 150). Additionally, poststroke patients display unique pathophysiologic phenotypes that may include tactile deficits (151–153), proprioceptive losses (154, 155), hemiparesis and related motor synergies (156–158), and spasticity (159–161), which would suggest that these new sensory and motor “states” postinjury create new relationships between receptor and effector, requiring the need for re-integration (162–164).
In fact, poor visuomotor performance (EHC) has been associated with poorer accuracy and longer movement times in visually guided action poststroke, and these deficits have correlated significantly with impairments at the sensory and motor level; more specifically, poor chronometric and spatial performance in the more affected limbs of stroke subjects have correlated with tactile insensitivity, handgrip strength deficits and more severe motor impairment scores, as assessed by the Fugl-Meyer (2). It is well known that reaching depends on inputs from both vision and proprioception; tactile sensation is a component of proprioception, particularly when proprioceptive inputs may be impaired (165, 166). Evidence of this sensori-motor coupling in control physiology during multi-joint action tasks is well documented (167, 168). Optimality in functionally oriented somatic movements of the upper extremity is demonstrated through hand paths that are straight, smooth, and with bell-shaped velocity profiles that scale with distance, implying advance planning (169, 170). These control markers set comparative baselines for investigations into impairment and not surprisingly suggest impairments in motor control programming at the planning level and at the sensori-motor interface level (162, 171, 172).
Following stroke, sensorimotor uncoupling is a byproduct of new relationships between impaired sensory input and poor motor output (163). As these new relationships are learned, the execution of limb movements is altered, above and beyond what would be expected from the individual deficits themselves: take for example a velocity curve that has an earlier peak and a prolonged deceleration phase, allowing greater opportunity for feedback mechanisms to improve endpoint accuracy (141, 163, 173). An intriguing experimental paradigm is the double-step saccade task (174), in that goal-directed action can be tested while a spatial target is displaced between two locations during the primary saccade, a period in which there is no visual perception. This paradigm can be deployed as a part of a visually guided reach to point task and will decrease the performance of the arm movement without mechanical perturbation or cognitive understanding of the manipulation. It has been suggested that during visually guided rapid arm-movement control, in which saccadic double-stepped targets are implemented, that spatial corrections of the hand are driven by ocular motor corrections following spatial target shifting (175).
Vision is essential to the sensori-motor integration required for visuomotor action. Gaze position is a consequence of ocular motor control and supports hand movement planning. These spatial locations or fixations often mark key positions for fingertip placement and are a byproduct of the functional requirements of the task at hand (91, 176). Furthermore, vision-based hand feedback is vital to motor adjustments during online control, as saccadic behavior updates spatial understanding and improves goal localization (177); in fact, it has been suggested that there is parallel processing between effectors (77, 178). This could be particularly problematic in patients with ABI with eye movement deficits (179–185), in addition to somatic motor deficits (e.g., hemiparesis). While manual motor deficits are typically evident on clinical examination, ocular motor deficits frequently require objective recording techniques (186–194) for identification and prognostication (181, 183, 195). Nevertheless, even if eye movements are found to be sound post-ABI, clinically and subclinically, following objective recordings, an impaired limb with poor functional performance may lead to maladaptive ocular motor behavior to compensate for lost task ability.
An eye-hand dyssynergia, or a lack of coordination between effectors, may operate in suboptimal modes to re-establish premorbid skill level, impeding recovery. This sensorimotor impairment may by multifactorial and compromised secondary to not only ocular motor deficits but also visuospatial and visuoperceptual abnormalities (196–200), in addition to balance deficits; in fact, decreases in balance have been noted during EHC tasks with stroke patients (142). This may all be of significant interest given the increased sensitivity during poststroke periods to sensory reweighting (201).
Deficits of Predictive Control
Our visual world is ever changing and prediction is a necessary part of object manipulation and consequently an important aspect of eye–hand control. Catching a ball or grasping a pen being handed to you requires anticipating the motion and direction of the object, and planning a motor response that will intersect successfully with the predicted trajectory. If the afferent end of visual processing or perception was simply used to generate spatial cues for EHC, our hand would consistently miss the spatial target; rather, an integrated construct replete with anticipation and prediction is pivotal to successful outcomes, which translate into functional performance (202). Superior skill in sports demonstrates finely tuned ocular motor control that drives complex somatic motor control (203–207). For example, soccer goalkeepers at the expert level demonstrate more accurate soccer ball prediction during anticipation tasks, as compared to novice level players; differences also include efficient and more effective strategies during visual search, which consist in part of longer fixations that are less frequent and directed at disparate regions of interest (208, 209).
In ABI, including injuries secondary to neurovascular and neurotraumatic insults significant predictive control deficits have been demonstrated during dynamic EHC tasks in the absence of deficits during static visuomotor tasks, highlighting difficulties in rapidly processing sensory information rather than motor execution errors. Delaying or inefficiently managing sensory information may not only lead to problems with target anticipation during dynamic tasks (feedforward impairment), but also the use of sensory feedback for error correction (1, 210). In fact, studies have demonstrated ocular motor deficits in predictive control within ABI for moving targets with and without intermittent stimulus blanking, and these impairments have been correlated with cognitive performance (211–213). Moreover, increasing cognitive load during predictive ocular motor tasks degrades performance in ABI and may suggest an “overload” to the impaired neural network (214).
This opens several broader questions, as patients with ABI who suffer from impaired eye movements, or even decreases in exploratory eye movements, may have perceptual limitations that hampers the understanding of scenes and spatial relationships between objects. This, in combination with loss of sensory feedback systems typically in place during action production, may increase the cognitive complexity of the task at hand (3). This may be more problematic in tasks for which EHC needs to flexibly convert from coupled function to uncoupled or decoupled function. For example, consider reaching for your cell phone while reading a newspaper, thus executing a somatic motor movement toward one spatial target while simultaneously executing saccades during the reading task elsewhere (74). Even asymptomatic post-ABI patients have shown difficulty in visually guided action when there is a level of dissociation between the visual information used to guide the required motoric action, decoupling the eye and the hand and perhaps increasing the task complexity. Similarly, multidomain tasks that encompass cognitive and motoric skill are effective at “pushing” the brain during functionally relevant performance; these constructs must be viewed on a spectrum. A cognitive “load” in such dual tasks can be experimentally manipulated and made more or less challenging for more effective screening; at the mild end, this may be accomplished by increasing the cognitive difficulty (e.g., visually guided pointing coupled with a serial sevens countdown), or decreased for those on the severe end by decreasing the cognitive difficulty (e.g., adding an easily predictable element to a spatial sequence of visually guided pointing) (215).
These predictive control deficits are provocative when framed in neurovascular and neurotraumatic conditions, particularly when visually guided action is uncoupled and spatial targets are dynamic. However, in the setting of neurodegeneration, whether one considers vascular dementia following repeated multistepped strokes or chronic traumatic encephalopathy following repeated traumatic brain injuries, these constructs are even more compelling, given the cognitive impairments that may be superimposed on ocular motor and/or visual deficits (216–219).
Disorders in Visuomotor Planning
Predictive control is a central element of visuomotor planning; this is particularly relevant during dynamic motor tasks with spatial targets that are in motion and that require anticipation for successful interaction (202). However, at a more basic level, if one considers motor programming or feedforward control during tasks without dynamically moving targets, the planning of hand movements during reach is impaired after stroke or post-ABI (171, 172, 220, 221). Impaired planning results in an inability to program sequences of motor action in space and time (139, 222–225). As the environment undergoes incessant change, our body must adapt, a fundamental element to spatially accurate motoric action. During adaptation, previously observed errors in one’s own performance inform the correction of future motor plans. It has been suggested that sensory prediction errors are a primary input for motor programming revisions, during which planning is adjusted following a comparison between motor output and the predicted sensory outcomes of the original plan (226).
While planning is contingent on sensory information, e.g., vision and proprioception, laterality may also play a significant role. It has been even suggested that hemispheric specialization is paramount, producing dissociable differences in poststroke motor control. The left hemisphere is theorized to be motor-planning dominant for feedforward control while the right hemisphere is theorized to be feedback dominant for error correction during position control. Following this construct, a limb stabilized on a visual target may leverage right hemisphere resources, while a limb attempting to catch a moving ball may leverage left. In concert, optimizing ongoing action is undoubtedly the integration of feedforward and feedback control, and ABI has revealed deficits in initial trajectory profiles in left-brain injury and deficits in spatial accuracy in right-brain damage (227–230). Thorough assessment and targeted treatment of planning deficits may lead to improved motor relearning and functional recovery in ABI (221).
Clinical Implications and Outcome Measures
Acute and chronic disease processes that lead to cerebral injury are often challenging from a diagnostic and therapeutic standpoint; this is particularly true with neurodegenerative disorders secondary to their often diffuse and indolent nature, constraining our ability to isolate specific structural abnormalities with associations to functional limitations (231). To improve our understanding of neurophysiology and enhance our understanding of the clinical implications, experiments have historically concentrated on focal lesion-based approaches. These lesion-based models and associated biomarkers can be combined synergistically with the goal of detecting and characterizing the preclinical evolution of the neurobiological events that precede the cognitive impairments associated with neurodegeneration (232–235).
Objective eye movement recordings, when approached with methodological rigor, have already proven valuable as a research tool within ABI (236–238). In fact, ocular motor recordings have been used for their screening utility in a diagnostic capacity (211–214). As a response, rapid, vision-based performance measures that depend on time taken and errors made during visually presented number reading or object naming have been developed and extensively studied in the setting of ABI (239–244). More broadly, eye movements and visuomotor skill of the upper limb have been sensitive markers of cerebral injury (245). Taken further, eye and arm function following acute ABI has demonstrated good predictive capacity for outcomes in the subacute and chronic stages following injury with superior performance when compared to health status on self-report or based on neuropsychologic assessment (1, 246, 247). These prognostic capabilities have also enabled the identification of individuals who are poor responders or those who may require more aggressive intervention (248, 249). Ocular motor performance has even been demonstrated to be a biomarker of cognitive recovery beyond the times at which apparent full recovery had been deemed, as assessed by conventional metrics (150). While the literature is more extensive for neurovascular and neurotraumatic etiologies, the evidence base does extend into neurodegeneration (250, 251).
Given this framework, it is not difficult to see that there are extensive opportunities for translational ocular motor investigations that extend beyond the research setting and into the clinic. These opportunities are multiplied when ocular motor investigations are juxtaposed with manual motor investigations in ABI. While the clinical implications are significant, the literature has yet to see objective ocular motor and somatic motor control recordings enter the setting of ABI for unconstrained, coordinated eye and hand movements and frequently the motor output that is quantified during visually guided action is simply somatic in nature (Table 2). Though examples certainly exist where these two effectors have been objectively recorded, the movements have been constrained to one or two spatial dimensions, limiting the ecological validity; such constrained movements may require altered programmatic control between effectors, as a limb restricted to execute somatic motor output in an unnatural mode may have problematic effects on the ocular motor output, restricting comparisons. In the present narrative review, there was only one study that simultaneously recorded ocular and manual motor activity; the remaining manuscripts quantified movements of a single effector system (Table 2).
In fact, objective EHC tasks have already been designed for neurodegenerative disease processes, incorporating simultaneous ocular and manual motor recordings (252, 253). These investigations focused on integrated eye and hand assessments have yielded promising results and used simple tasks during which subjects are merely asked to perform a “look and reach,” revealing quantifiable deficits in visually guided action (254). Additionally, and perhaps more promising, are tasks that have combined more cognitively demanding elements, e.g., antisaccades and antitapping, as part of an effort to increase the diagnostic power of the measures (252, 253, 255). While it has been suggested that objectified visuomotor tasks and related deficits may assist in diagnosing prodromal neurodegenerative disease entities and monitoring their progression, similar tasks that further increase complexity with distractors and/or feedback perturbations may assist in preclinical detection.
Currently, a central focus of rehabilitative interventions for cerebral injury is to restore motor ability and increase function. However, the return of motor ability often does not ensure ecologically valid, meaningful gains in function (222, 256). A clearer characterization of ocular motor control and its relationship to manual motor control will improve our understanding of EHC in a functional context. The quantitative relationships and motor outputs from both effectors are likely to yield metrics that can be correlated and compared to existing assessments and outcome measures utilized in current care models. Positive relationships will yield significant opportunities on the diagnostic, prognostic and therapeutic fronts, driving toward the development of algorithmic approaches with tailored, patient-specific management plans.
Conclusion
During goal-directed movement, first-rate function often requires that visual perception, under precise ocular motor control, be translated optimally into somatic action. Leveraging focal lesion-based models and associated eye–hand biomarkers is a robust approach toward significantly improving our understanding of acute and chronic neurological disease processes. In recent years, a number of studies have focused on EHC in ABI. The present review describes a series of studies that directly or indirectly highlight EHC in ABI and the neuroanatomic, computational, and broader clinical implications. While there is ample evidence to suggest that coupling is essential to EHC and that it is a sensitive biomarker for cerebral injury, visually guided action in the experimental setting has typically been limited to quantifying one effector or two effectors in a limited or constrained fashion. As such, it is recommended that future studies addressing related behavior should concurrently objectify ocular and manual motor control in unconstrained and natural modes. These studies, while technically more challenging, are likely to further characterize coupling and potentially yield high-impact results along the care spectrum from diagnosis to neurorehabilitative treatment in the setting of neurovascular, neurotraumatic, and neurodegenerative pathology.
Author Contributions
Conception and design of the review: J-RR and EW. Substantial manuscript drafting: J-RR, MH, JF, WM, EA, PR, JR, ML, and TH.
Conflict of Interest Statement
The authors declare that the research was conducted in the absence of any commercial or financial relationships that could be construed as a potential conflict of interest.
Acknowledgments
We would like to thank Dr. David S. Zee and Dr. Tamara Bushnik for their thoughts, suggestions, and contributions.
Funding
This research was supported in part by National Institutes of Health Grants EY08266 (to MSL), HD071978 (to PR), TR000038 (to J-RR), R03 AG042379 (to J-RR, ML, and PR), and 5K12 HD001097 (to J-RR, ML, and PR).
References
1. Caeyenberghs K, van Roon D, van Aken K, De Cock P, Linden CV, Swinnen SP, et al. Static and dynamic visuomotor task performance in children with acquired brain injury: predictive control deficits under increased temporal pressure. J Head Trauma Rehabil (2009) 24:363–73. doi: 10.1097/HTR.0b013e3181af0810
2. Gao KL, Ng SS, Kwok JW, Chow RT, Tsang WW. Eye-hand coordination and its relationship with sensori-motor impairments in stroke survivors. J Rehabil Med (2010) 42:368–73. doi:10.2340/16501977-0520
3. Ghika J, Ghika-Schmid F, Bogousslasvky J. Parietal motor syndrome: a clinical description in 32 patients in the acute phase of pure parietal strokes studied prospectively. Clin Neurol Neurosurg (1998) 100:271–82. doi:10.1016/S0303-8467(98)00054-7
4. Bard C, Fleury M, Hay L. Development of Eye Hand Coordination across the Lifespan. Columbia, SC: University of South Carolina Press (1990).
5. Crawford JD, Medendorp WP, Marotta JJ. Spatial transformations for eye-hand coordination. J Neurophysiol (2004) 92:10–9. doi:10.1152/jn.00117.2004
8. Desrosiers J, Hebert R, Bravo G, Dutil E. Upper-extremity motor co-ordination of healthy elderly people. Age Ageing (1995) 24:108–12. doi:10.1093/ageing/24.2.108
9. Mountcastle VB. Modality and topographic properties of single neurons of cat’s somatic sensory cortex. J Neurophysiol (1957) 20:408–34.
10. Sereno MI, Huang RS. A human parietal face area contains aligned head-centered visual and tactile maps. Nat Neurosci (2006) 9:1337–43. doi:10.1038/nn1777
11. McKyton A, Zohary E. Beyond retinotopic mapping: the spatial representation of objects in the human lateral occipital complex. Cereb Cortex (2007) 17:1164–72. doi:10.1093/cercor/bhl027
12. Goossens HH, van Opstal AJ. Influence of head position on the spatial representation of acoustic targets. J Neurophysiol (1999) 81:2720–36.
13. Andersen RA, Zipser D. The role of the posterior parietal cortex in coordinate transformations for visual-motor integration. Can J Physiol Pharmacol (1988) 66:488–501. doi:10.1139/y88-078
14. Mushiake H, Fujii N, Tanji J. Visually guided saccade versus eye-hand reach: contrasting neuronal activity in the cortical supplementary and frontal eye fields. J Neurophysiol (1996) 75:2187–91.
15. Mishkin M, Ungerleider LG, Macko KA. Object vision and spatial vision: two cortical pathways. Trends Neurosci (1983) 6:414–7. doi:10.1016/0166-2236(83)90190-X
16. Goodale MA, Milner AD. Separate visual pathways for perception and action. Trends Neurosci (1992) 15:20–5. doi:10.1016/0166-2236(92)90344-8
17. Milner AD, Goodale MA. Two visual systems re-viewed. Neuropsychologia (2008) 46:774–85. doi:10.1016/j.neuropsychologia.2007.10.005
18. Ungerleider LG, Mishkin M. Two cortical visual systems. In:Goodale MA, Mansfield RJW, Ingle DJ, editors. Analysis of Visual Behavior. Cambridge, MA: MIT Press (1982). p. 549–86.
19. Sheth BR, Young R. Two visual pathways in primates based on sampling of space: exploitation and exploration of visual information. Front Integr Neurosci (2016) 10:37. doi:10.3389/fnint.2016.00037
20. Goodale MA, Westwood DA. An evolving view of duplex vision: separate but interacting cortical pathways for perception and action. Curr Opin Neurobiol (2004) 14:203–11. doi:10.1016/j.conb.2004.03.002
21. Sereno AB, Sereno ME, Lehky SR. Recovering stimulus locations using populations of eye-position modulated neurons in dorsal and ventral visual streams of non-human primates. Front Integr Neurosci (2014) 8:28. doi:10.3389/fnint.2014.00028
22. van Polanen V, Davare M. Interactions between dorsal and ventral streams for controlling skilled grasp. Neuropsychologia (2015) 79:186–91. doi:10.1016/j.neuropsychologia.2015.07.010
23. Ferrier D. The croonian lecture: experiments on the brain of monkeys (second series). Philos Trans R Soc Lond (1875) 165:433–88. doi:10.1098/rstl.1875.0016
24. Huey EB. The Psychology and Pedagogy of Reading: With a Review of the History of Reading and Writing and of Methods, Texts, and Hygiene in Reading. New York, NY: Macmillan Company (1908).
25. Parthasarathy HB, Schall JD, Graybiel AM. Distributed but convergent ordering of corticostriatal projections: analysis of the frontal eye field and the supplementary eye field in the macaque monkey. J Neurosci (1992) 12:4468–88.
26. Huerta MF, Kaas JH. Supplementary eye field as defined by intracortical microstimulation: connections in macaques. J Comp Neurol (1990) 293:299–330. doi:10.1002/cne.902930211
27. Luppino G, Rozzi S, Calzavara R, Matelli M. Prefrontal and agranular cingulate projections to the dorsal premotor areas F2 and F7 in the macaque monkey. Eur J Neurosci (2003) 17:559–78. doi:10.1046/j.1460-9568.2003.02476.x
28. Small DM, Gitelman DR, Gregory MD, Nobre AC, Parrish TB, Mesulam MM. The posterior cingulate and medial prefrontal cortex mediate the anticipatory allocation of spatial attention. Neuroimage (2003) 18:633–41. doi:10.1016/S1053-8119(02)00012-5
29. Wang SY, Wollin J. Falls among older people: identifying those at risk. Nurs Older People (2004) 15:14–6. doi:10.7748/nop2004.02.15.10.14.c2242
30. Keating EG. Frontal eye field lesions impair predictive and visually-guided pursuit eye movements. Exp Brain Res (1991) 86:311–23. doi:10.1007/BF00228954
31. Bruce CJ, Goldberg ME. Primate frontal eye fields. I. Single neurons discharging before saccades. J Neurophysiol (1985) 53:603–35.
32. Rivaud S, Muri RM, Gaymard B, Vermersch AI, Pierrot-Deseilligny C. Eye movement disorders after frontal eye field lesions in humans. Exp Brain Res (1994) 102:110–20. doi:10.1007/BF00232443
33. Dias EC, Segraves MA. Muscimol-induced inactivation of monkey frontal eye field: effects on visually and memory-guided saccades. J Neurophysiol (1999) 81:2191–214.
34. Mackey WE, Devinsky O, Doyle WK, Meager MR, Curtis CE. Human dorsolateral prefrontal cortex is not necessary for spatial working memory. J Neurosci (2016) 36:2847–56. doi:10.1523/jneurosci.3618-15.2016
35. Huerta MF, Krubitzer LA, Kaas JH. Frontal eye field as defined by intracortical microstimulation in squirrel monkeys, owl monkeys, and macaque monkeys. II. Cortical connections. J Comp Neurol (1987) 265:332–61. doi:10.1002/cne.902650304
36. Stanton GB, Bruce CJ, Goldberg ME. Topography of projections to posterior cortical areas from the macaque frontal eye fields. J Comp Neurol (1995) 353:291–305. doi:10.1002/cne.903530210
37. Bruce C, Friedman H, Kraus M, Stanton G. The primate frontal eye field. In: Chalupa LM, Werner JS, editors. The Visual Neurosciences (Vol. 1). Cambridge, MA: MIT Press (2004). p. 1428–48.
38. Schall JD, Stuphorn V, Brown JW. Monitoring and control of action by the frontal lobes. Neuron (2002) 36:309–22. doi:10.1016/S0896-6273(02)00964-9
39. Schall JD, Morel A, King DJ, Bullier J. Topography of visual-cortex connections with frontal eye field in macaque – convergence and segregation of processing streams. J Neurosci (1995) 15:4464–87.
40. Mackey WE, Devinsky O, Doyle WK, Golfinos JG, Curtis CE. Human parietal cortex lesions impact the precision of spatial working memory. J Neurophysiol (2016) 116:1049–54. doi:10.1152/jn.00380.2016
41. Stuphorn V, Bauswein E, Hoffmann KP. Neurons in the primate superior colliculus coding for arm movements in gaze-related coordinates. J Neurophysiol (2000) 83:1283–99.
42. Gaymard B, Pierrot-Deseilligny C, Rivaud S. Impairment of sequences of memory-guided saccades after supplementary motor area lesions. Ann Neurol (1990) 28:622–6. doi:10.1002/ana.410280504
43. Isoda M, Tanji J. Cellular activity in the supplementary eye field during sequential performance of multiple saccades. J Neurophysiol (2002) 88:3541–5. doi:10.1152/jn.00299.2002
44. Tehovnik EJ. The dorsomedial frontal cortex: eye and forelimb fields. Behav Brain Res (1995) 67:147–63. doi:10.1016/0166-4328(94)00151-5
45. Tehovnik EJ, Slocum WM, Tolias AS, Schiller PH. Saccades induced electrically from the dorsomedial frontal cortex: evidence for a head-centered representation. Brain Res (1998) 795:287–91. doi:10.1016/S0006-8993(98)00302-3
46. Pierrot-Deseilligny C, Muri RM, Nyffeler T, Milea D. The role of the human dorsolateral prefrontal cortex in ocular motor behavior. Ann N Y Acad Sci (2005) 1039:239–51. doi:10.1196/annals.1325.023
47. Tehovnik EJ, Lee K. The dorsomedial frontal cortex of the rhesus monkey: topographic representation of saccades evoked by electrical stimulation. Exp Brain Res (1993) 96:430–42. doi:10.1007/BF00234111
48. Mackey WE, Curtis CE. Distinct contributions by frontal and parietal cortices support working memory. bioRxiv (2017) (in press).
49. Pierrot-Deseilligny C, Milea D, Muri RM. Eye movement control by the cerebral cortex. Curr Opin Neurol (2004) 17:17–25. doi:10.1097/00019052-200402000-00005
50. Li CS, Mazzoni P, Andersen RA. Effect of reversible inactivation of macaque lateral intraparietal area on visual and memory saccades. J Neurophysiol (1999) 81:1827–38.
51. Silver MA, Ress D, Heeger DJ. Topographic maps of visual spatial attention in human parietal cortex. J Neurophysiol (2005) 94:1358–71. doi:10.1152/jn.01316.2004
52. Silver MA, Kastner S. Topographic maps in human frontal and parietal cortex. Trends Cogn Sci (2009) 13:488–95. doi:10.1016/j.tics.2009.08.005
53. Grefkes C, Fink GR. The functional organization of the intraparietal sulcus in humans and monkeys. J Anat (2005) 207:3–17. doi:10.1111/j.1469-7580.2005.00426.x
54. Shikata E, McNamara A, Sprenger A, Hamzei F, Glauche V, Buchel C, et al. Localization of human intraparietal areas AIP, CIP, and LIP using surface orientation and saccadic eye movement tasks. Hum Brain Mapp (2008) 29:411–21. doi:10.1002/hbm.20396
55. Bosley TM, Dann R, Silver FL, Alavi A, Kushner M, Chawluk JB, et al. Recovery of vision after ischemic lesions: positron emission tomography. Ann Neurol (1987) 21:444–50. doi:10.1002/ana.410210505
56. Gaymard B, Rivaud S, Cassarini JF, Dubard T, Rancurel G, Agid Y, et al. Effects of anterior cingulate cortex lesions on ocular saccades in humans. Exp Brain Res (1998) 120:173–83. doi:10.1007/s002210050391
57. Amiez C, Petrides M. Anatomical organization of the eye fields in the human and non-human primate frontal cortex. Prog Neurobiol (2009) 89:220–30. doi:10.1016/j.pneurobio.2009.07.010
58. Katyal S, Zughni S, Greene C, Ress D. Topography of covert visual attention in human superior colliculus. J Neurophysiol (2010) 104:3074–83. doi:10.1152/jn.00283.2010
59. DeSimone K, Viviano JD, Schneider KA. Population receptive field estimation reveals new retinotopic maps in human subcortex. J Neurosci (2015) 35:9836–47. doi:10.1523/jneurosci.3840-14.2015
60. Schiller PH, True SD, Conway JL. Deficits in eye movements following frontal eye-field and superior colliculus ablations. J Neurophysiol (1980) 44:1175–89.
61. Ploner CJ, Gaymard BM, Rivaud-Pechoux S, Pierrot-Deseilligny C. The prefrontal substrate of reflexive saccade inhibition in humans. Biol Psychiatry (2005) 57:1159–65. doi:10.1016/j.biopsych.2005.02.017
62. Nachev P, Wydell H, O’Neill K, Husain M, Kennard C. The role of the pre-supplementary motor area in the control of action. Neuroimage (2007) 36:T155–63. doi:10.1016/j.neuroimage.2007.03.034
63. Tehovnik EJ, Slocum WM, Schiller PH. Saccadic eye movements evoked by microstimulation of striate cortex. Eur J Neurosci (2003) 17:870–8. doi:10.1046/j.1460-9568.2003.02489.x
64. Funahashi S, Bruce CJ, Goldman-Rakic PS. Mnemonic coding of visual space in the monkey’s dorsolateral prefrontal cortex. J Neurophysiol (1989) 61:331–49.
65. Funahashi S, Bruce CJ, Goldman-Rakic PS. Dorsolateral prefrontal lesions and oculomotor delayed-response performance: evidence for mnemonic “scotomas”. J Neurosci (1993) 13:1479–97.
66. Kastner S, DeSimone K, Konen CS, Szczepanski SM, Weiner KS, Schneider KA. Topographic maps in human frontal cortex revealed in memory-guided saccade and spatial working-memory tasks. J Neurophysiol (2007) 97:3494–507. doi:10.1152/jn.00010.2007
67. Muri RM, Vermersch AI, Rivaud S, Gaymard B, Pierrot-Deseilligny C. Effects of single-pulse transcranial magnetic stimulation over the prefrontal and posterior parietal cortices during memory-guided saccades in humans. J Neurophysiol (1996) 76:2102–6.
68. Nyffeler T, Pierrot-Deseilligny C, Felblinger J, Mosimann UP, Hess CW, Muri RM. Time-dependent hierarchical organization of spatial working memory: a transcranial magnetic stimulation study. Eur J Neurosci (2002) 16:1823–7. doi:10.1046/j.1460-9568.2002.02252.x
69. Nyffeler T, Pierrot-Deseilligny C, Pflugshaupt T, von Wartburg R, Hess CW, Muri RM. Information processing in long delay memory-guided saccades: further insights from TMS. Exp Brain Res (2004) 154:109–12. doi:10.1007/s00221-003-1663-6
70. Ro T, Farne A, Chang E. Locating the human frontal eye fields with transcranial magnetic stimulation. J Clin Exp Neuropsychol (2002) 24:930–40. doi:10.1076/jcen.24.7.930.8385
71. Belardinelli A, Stepper MY, Butz MV. It’s in the eyes: planning precise manual actions before execution. J Vis (2016) 16:18. doi:10.1167/16.1.18
73. Rathelot JA, Strick PL. Muscle representation in the macaque motor cortex: an anatomical perspective. Proc Natl Acad Sci U S A (2006) 103:8257–62. doi:10.1073/pnas.0602933103
74. Vesia M, Crawford JD. Specialization of reach function in human posterior parietal cortex. Exp Brain Res (2012) 221:1–18. doi:10.1007/s00221-012-3158-9
75. Blangero A, Menz MM, McNamara A, Binkofski F. Parietal modules for reaching. Neuropsychologia (2009) 47:1500–7. doi:10.1016/j.neuropsychologia.2008.11.030
76. Tunik E, Frey SH, Grafton ST. Virtual lesions of the anterior intraparietal area disrupt goal-dependent on-line adjustments of grasp. Nat Neurosci (2005) 8:505–11. doi:10.1038/nn1430
77. Prablanc C, Echallier JF, Komilis E, Jeannerod M. Optimal response of eye and hand motor systems in pointing at a visual target. I. Spatio-temporal characteristics of eye and hand movements and their relationships when varying the amount of visual information. Biol Cybern (1979) 35:113–24. doi:10.1007/BF00337063
78. Vercher JL, Magenes G, Prablanc C, Gauthier GM. Eye-head-hand coordination in pointing at visual targets: spatial and temporal analysis. Exp Brain Res (1994) 99:507–23. doi:10.1007/BF00228987
79. Henriques DY, Klier EM, Smith MA, Lowy D, Crawford JD. Gaze-centered remapping of remembered visual space in an open-loop pointing task. J Neurosci (1998) 18:1583–94.
80. Grol MJ, Majdandzic J, Stephan KE, Verhagen L, Dijkerman HC, Bekkering H, et al. Parieto-frontal connectivity during visually guided grasping. J Neurosci (2007) 27:11877–87. doi:10.1523/jneurosci.3923-07.2007
81. Cavina-Pratesi C, Monaco S, Fattori P, Galletti C, McAdam TD, Quinlan DJ, et al. Functional magnetic resonance imaging reveals the neural substrates of arm transport and grip formation in reach-to-grasp actions in humans. J Neurosci (2010) 30:10306–23. doi:10.1523/jneurosci.2023-10.2010
82. Gorbet DJ, Staines WR, Sergio LE. Brain mechanisms for preparing increasingly complex sensory to motor transformations. Neuroimage (2004) 23:1100–11. doi:10.1016/j.neuroimage.2004.07.043
83. Prado J, Clavagnier S, Otzenberger H, Scheiber C, Kennedy H, Perenin MT. Two cortical systems for reaching in central and peripheral vision. Neuron (2005) 48:849–58. doi:10.1016/j.neuron.2005.10.010
84. Filimon F, Nelson JD, Huang RS, Sereno MI. Multiple parietal reach regions in humans: cortical representations for visual and proprioceptive feedback during on-line reaching. J Neurosci (2009) 29:2961–71. doi:10.1523/jneurosci.3211-08.2009
85. Gibbon J, Malapani C, Dale CL, Gallistel C. Toward a neurobiology of temporal cognition: advances and challenges. Curr Opin Neurobiol (1997) 7:170–84. doi:10.1016/S0959-4388(97)80005-0
86. Desmurget M, Grafton S. Forward modeling allows feedback control for fast reaching movements. Trends Cogn Sci (2000) 4:423–31. doi:10.1016/S1364-6613(00)01537-0
87. Miall RC, Weir DJ, Wolpert DM, Stein JF. Is the cerebellum a smith predictor? J Mot Behav (1993) 25:203–16. doi:10.1080/00222895.1993.9942050
88. Bienkiewicz MM, Craig CM. Parkinson’s is time on your side? Evidence for difficulties with sensorimotor synchronization. Front Neurol (2015) 6:249. doi:10.3389/fneur.2015.00249
89. Piray P, Toni I, Cools R. Human choice strategy varies with anatomical projections from ventromedial prefrontal cortex to medial striatum. J Neurosci (2016) 36:2857–67. doi:10.1523/jneurosci.2033-15.2016
90. Jocham G, Brodersen KH, Constantinescu AO, Kahn MC, Ianni AM, Walton ME, et al. Reward-guided learning with and without causal attribution. Neuron (2016) 90:177–90. doi:10.1016/j.neuron.2016.02.018
91. Flanagan JR, Bowman MC, Johansson RS. Control strategies in object manipulation tasks. Curr Opin Neurobiol (2006) 16:650–9. doi:10.1016/j.conb.2006.10.005
92. Carlton LG. Visual information: the control of aiming movements. Q J Exp Psychol (1981) 33:87–93. doi:10.1080/14640748108400771
93. Binsted G, Elliott D. Ocular perturbations and retinal/extraretinal information: the coordination of saccadic and manual movements. Exp Brain Res (1999) 127:193–206. doi:10.1007/s002210050789
94. Helsen WF, Elliott D, Starkes JL, Ricker KL. Temporal and spatial coupling of point of gaze and hand movements in aiming. J Mot Behav (1998) 30:249–59. doi:10.1080/00222899809601340
95. Helsen WF, Elliott D, Starkes JL, Ricker KL. Coupling of eye, finger, elbow, and shoulder movements during manual aiming. J Mot Behav (2000) 32:241–8. doi:10.1080/00222890009601375
96. Starkes J, Helsen W, Elliott D. A menage a trois: the eye, the hand and on-line processing. J Sports Sci (2002) 20:217–24. doi:10.1080/026404102317284772
97. Hayhoe MM, Shrivastava A, Mruczek R, Pelz JB. Visual memory and motor planning in a natural task. J Vis (2003) 3:49–63. doi:10.1167/3.1.6
98. Irwin DE. Information integration across saccadic eye movements. Cogn Psychol (1991) 23:420–56. doi:10.1016/0010-0285(91)90015-G
99. Irwin DE, Zacks JL, Brown JS. Visual memory and the perception of a stable visual environment. Percept Psychophys (1990) 47:35–46. doi:10.3758/BF03208162
100. Ballard DH, Hayhoe MM, Pelz JB. Memory representations in natural tasks. J Cogn Neurosci (1995) 7:66–80. doi:10.1162/jocn.1995.7.1.66
101. Irwin DE. Integrating information across saccadic eye movements. Curr Dir Psychol Sci (1996) 5:94–100. doi:10.1111/1467-8721.ep10772833
102. O’Regan JK. Solving the “real” mysteries of visual perception: the world as an outside memory. Can J Psychol (1992) 46:461–88. doi:10.1037/h0084327
103. Land M, Mennie N, Rusted J. The roles of vision and eye movements in the control of activities of daily living. Perception (1999) 28:1311–28. doi:10.1068/p2935
105. Cilo M, Politzer T, Ripley DL, Weintraub A. Vision examination of TBI patients in an acute rehabilitation hospital. NeuroRehabilitation (2010) 27:237–42. doi:10.3233/NRE-2010-0603
106. Procacci NM, Stanford TR, Wittenberg GF. The relationship between visual orienting and interlimb synchrony in a patient with a superior parietal infarction: a case study. Neurocase (2009) 15:73–88. doi:10.1080/13554790802620558
107. Jeannerod M, Arbib MA, Rizzolatti G, Sakata H. Grasping objects: the cortical mechanisms of visuomotor transformation. Trends Neurosci (1995) 18:314–20. doi:10.1016/0166-2236(95)93921-J
108. Snyder LH, Batista AP, Andersen RA. Coding of intention in the posterior parietal cortex. Nature (1997) 386:167–70. doi:10.1038/386167a0
109. Levy I, Schluppeck D, Heeger DJ, Glimcher PW. Specificity of human cortical areas for reaches and saccades. J Neurosci (2007) 27:4687–96. doi:10.1523/jneurosci.0459-07.2007
110. Caminiti R, Genovesio A, Marconi B, Mayer AB, Onorati P, Ferraina S, et al. Early coding of reaching: frontal and parietal association connections of parieto-occipital cortex. Eur J Neurosci (1999) 11:3339–45. doi:10.1046/j.1460-9568.1999.00801.x
111. Johnson PB, Ferraina S, Bianchi L, Caminiti R. Cortical networks for visual reaching: physiological and anatomical organization of frontal and parietal lobe arm regions. Cereb Cortex (1996) 6:102–19. doi:10.1093/cercor/6.2.102
112. Johnson PB, Ferraina S. Cortical networks for visual reaching: intrinsic frontal lobe connectivity. Eur J Neurosci (1996) 8:1358–62. doi:10.1111/j.1460-9568.1996.tb01598.x
113. Bernier PM, Grafton ST. Human posterior parietal cortex flexibly determines reference frames for reaching based on sensory context. Neuron (2010) 68:776–88. doi:10.1016/j.neuron.2010.11.002
114. Tanne J, Boussaoud D, Boyer-Zeller N, Rouiller EM. Direct visual pathways for reaching movements in the macaque monkey. Neuroreport (1995) 7:267–72. doi:10.1097/00001756-199512000-00064
115. Galletti C, Fattori P, Battaglini PP, Shipp S, Zeki S. Functional demarcation of a border between areas V6 and V6A in the superior parietal gyrus of the macaque monkey. Eur J Neurosci (1996) 8:30–52. doi:10.1111/j.1460-9568.1996.tb01165.x
116. Colby CL, Gattass R, Olson CR, Gross CG. Topographical organization of cortical afferents to extrastriate visual area PO in the macaque: a dual tracer study. J Comp Neurol (1988) 269:392–413. doi:10.1002/cne.902690307
117. Galletti C, Gamberini M, Kutz DF, Baldinotti I, Fattori P. The relationship between V6 and PO in macaque extrastriate cortex. Eur J Neurosci (2005) 21:959–70. doi:10.1111/j.1460-9568.2005.03911.x
118. Passarelli L, Rosa MG, Gamberini M, Bakola S, Burman KJ, Fattori P, et al. Cortical connections of area V6Av in the macaque: a visual-input node to the eye/hand coordination system. J Neurosci (2011) 31:1790–801. doi:10.1523/jneurosci.4784-10.2011
119. Buneo CA, Andersen RA. The posterior parietal cortex: sensorimotor interface for the planning and online control of visually guided movements. Neuropsychologia (2006) 44:2594–606. doi:10.1016/j.neuropsychologia.2005.10.011
120. Connolly JD, Vuong QC, Thiele A. Gaze-dependent topography in human posterior parietal cortex. Cereb Cortex (2015) 25:1519–26. doi:10.1093/cercor/bht344
121. Soechting JF, Flanders M. Moving in three-dimensional space: frames of reference, vectors, and coordinate systems. Annu Rev Neurosci (1992) 15:167–91. doi:10.1146/annurev.ne.15.030192.001123
122. Cohen YE, Andersen RA. A common reference frame for movement plans in the posterior parietal cortex. Nat Rev Neurosci (2002) 3:553–62. doi:10.1038/nrn873
123. Batista AP, Buneo CA, Snyder LH, Andersen RA. Reach plans in eye-centered coordinates. Science (1999) 285:257–60. doi:10.1126/science.285.5425.257
124. McGuire LM, Sabes PN. Sensory transformations and the use of multiple reference frames for reach planning. Nat Neurosci (2009) 12:1056–61. doi:10.1038/nn.2357
125. Medendorp WP, Beurze SM, Van Pelt S, Van Der Werf J. Behavioral and cortical mechanisms for spatial coding and action planning. Cortex (2008) 44:587–97. doi:10.1016/j.cortex.2007.06.001
126. Duhamel JR, Colby CL, Goldberg ME. The updating of the representation of visual space in parietal cortex by intended eye movements. Science (1992) 255:90–2. doi:10.1126/science.1553535
127. Sommer MA, Wurtz RH. A pathway in primate brain for internal monitoring of movements. Science (2002) 296:1480–2. doi:10.1126/science.1069590
128. Sommer MA, Wurtz RH. Brain circuits for the internal monitoring of movements. Annu Rev Neurosci (2008) 31:317–38. doi:10.1146/annurev.neuro.31.060407.125627
129. Vaziri S, Diedrichsen J, Shadmehr R. Why does the brain predict sensory consequences of oculomotor commands? Optimal integration of the predicted and the actual sensory feedback. J Neurosci (2006) 26:4188–97. doi:10.1523/jneurosci.4747-05.2006
130. Wolpert DM, Kawato M. Multiple paired forward and inverse models for motor control. Neural Netw (1998) 11:1317–29. doi:10.1016/S0893-6080(98)00066-5
131. Andersen RA, Andersen KN, Hwang EJ, Hauschild M. Optic ataxia: from Balint’s syndrome to the parietal reach region. Neuron (2014) 81:967–83. doi:10.1016/j.neuron.2014.02.025
132. Battaglia-Mayer A, Archambault PS, Caminiti R. The cortical network for eye-hand coordination and its relevance to understanding motor disorders of parietal patients. Neuropsychologia (2006) 44:2607–20. doi:10.1016/j.neuropsychologia.2005.11.021
133. Perenin MT, Vighetto A. Optic ataxia: a specific disruption in visuomotor mechanisms. I. Different aspects of the deficit in reaching for objects. Brain (1988) 111(Pt 3):643–74. doi:10.1093/brain/111.3.643
134. Battaglia-Mayer A, Caminiti R. Optic ataxia as a result of the breakdown of the global tuning fields of parietal neurones. Brain (2002) 125:225–37. doi:10.1093/brain/awf034
135. Rossetti Y, Pisella L, Vighetto A. Optic ataxia revisited: visually guided action versus immediate visuomotor control. Exp Brain Res (2003) 153:171–9. doi:10.1007/s00221-003-1590-6
136. Milner AD, Paulignan Y, Dijkerman HC, Michel F, Jeannerod M. A paradoxical improvement of misreaching in optic ataxia: new evidence for two separate neural systems for visual localization. Proc Biol Sci (1999) 266:2225–9. doi:10.1098/rspb.1999.0912
137. Andersen RA, Buneo CA. Intentional maps in posterior parietal cortex. Annu Rev Neurosci (2002) 25:189–220. doi:10.1146/annurev.neuro.25.112701.142922
138. Battaglia-Mayer A, Caminiti R, Lacquaniti F, Zago M. Multiple levels of representation of reaching in the parieto-frontal network. Cereb Cortex (2003) 13:1009–22. doi:10.1093/cercor/13.10.1009
139. Beer RF, Dewald JP, Rymer WZ. Deficits in the coordination of multijoint arm movements in patients with hemiparesis: evidence for disturbed control of limb dynamics. Exp Brain Res (2000) 131:305–19. doi:10.1007/s002219900275
140. Fisher BE, Winstein CJ, Velicki MR. Deficits in compensatory trajectory adjustments after unilateral sensorimotor stroke. Exp Brain Res (2000) 132:328–44. doi:10.1007/s002210000391
141. McCrea PH, Eng JJ. Consequences of increased neuromotor noise for reaching movements in persons with stroke. Exp Brain Res (2005) 162:70–7. doi:10.1007/s00221-004-2106-8
142. Tsang WW, Ng SS, Lee MW, Tse SP, Yip EW, Yuen JK. Does postural stability affect the performance of eye-hand coordination in stroke survivors? Am J Phys Med Rehabil (2013) 92:781–8. doi:10.1097/PHM.0b013e3182876adb
143. Velicki MR, Winstein CJ, Pohl PS. Impaired direction and extent specification of aimed arm movements in humans with stroke-related brain damage. Exp Brain Res (2000) 130:362–74. doi:10.1007/s002219900262
144. Wenzelburger R, Kopper F, Frenzel A, Stolze H, Klebe S, Brossmann A, et al. Hand coordination following capsular stroke. Brain (2005) 128:64–74. doi:10.1093/brain/awh317
145. Zackowski KM, Dromerick AW, Sahrmann SA, Thach WT, Bastian AJ. How do strength, sensation, spasticity and joint individuation relate to the reaching deficits of people with chronic hemiparesis? Brain (2004) 127:1035–46. doi:10.1093/brain/awh116
146. Leigh RJ, Kennard C. Using saccades as a research tool in the clinical neurosciences. Brain (2004) 127:460–77. doi:10.1093/brain/awh035
147. White OB, Fielding J. Cognition and eye movements: assessment of cerebral dysfunction. J Neuroophthalmol (2012) 32:266–73. doi:10.1097/WNO.0b013e3182688230
148. Ramat S, Leigh RJ, Zee DS, Optican LM. What clinical disorders tell us about the neural control of saccadic eye movements. Brain (2007) 130:10–35. doi:10.1093/brain/awl309
149. Anderson T. Could saccadic function be a useful marker of stroke recovery? J Neurol Neurosurg Psychiatry (2013) 84:242. doi:10.1136/jnnp-2012-304481
150. Dong W, Yan B, Johnson BP, Millist L, Davis S, Fielding J, et al. Ischaemic stroke: the ocular motor system as a sensitive marker for motor and cognitive recovery. J Neurol Neurosurg Psychiatry (2013) 84:337–41. doi:10.1136/jnnp-2012-303926
151. Lima NM, Menegatti KC, Yu E, Sacomoto NY, Scalha TB, Lima IN, et al. Sensory deficits in ipsilesional upper-extremity in chronic stroke patients. Arq Neuropsiquiatr (2015) 73:834–9. doi:10.1590/0004-282X20150128
152. Meyer S, Karttunen AH, Thijs V, Feys H, Verheyden G. How do somatosensory deficits in the arm and hand relate to upper limb impairment, activity, and participation problems after stroke? A systematic review. Phys Ther (2014) 94:1220–31. doi:10.2522/ptj.20130271
153. Scalha TB, Miyasaki E, Lima NM, Borges G. Correlations between motor and sensory functions in upper limb chronic hemiparetics after stroke. Arq Neuropsiquiatr (2011) 69:624–9. doi:10.1590/S0004-282X2011000500010
154. Findlater SE, Dukelow SP. Upper extremity proprioception after stroke: bridging the gap between neuroscience and rehabilitation. J Mot Behav (2017) 49:27–34. doi:10.1080/00222895.2016.1219303
155. Hughes CM, Tommasino P, Budhota A, Campolo D. Upper extremity proprioception in healthy aging and stroke populations, and the effects of therapist- and robot-based rehabilitation therapies on proprioceptive function. Front Hum Neurosci (2015) 9:120. doi:10.3389/fnhum.2015.00120
156. Ekstrand E, Lexell J, Brogardh C. Grip strength is a representative measure of muscle weakness in the upper extremity after stroke. Top Stroke Rehabil (2016) 23:400–5. doi:10.1080/10749357.2016.1168591
157. McCrea PH, Eng JJ, Hodgson AJ. Saturated muscle activation contributes to compensatory reaching strategies after stroke. J Neurophysiol (2005) 94:2999–3008. doi:10.1152/jn.00732.2004
158. Rohafza M, Fluet GG, Qiu Q, Adamovich S. Correlation of reaching and grasping kinematics and clinical measures of upper extremity function in persons with stroke related hemiplegia. Conf Proc IEEE Eng Med Biol Soc (2014) 2014:3610–3. doi:10.1109/EMBC.2014.6944404
159. Kong KH, Lee J, Chua KS. Occurrence and temporal evolution of upper limb spasticity in stroke patients admitted to a rehabilitation unit. Arch Phys Med Rehabil (2012) 93:143–8. doi:10.1016/j.apmr.2011.06.027
160. Opheim A, Danielsson A, Alt Murphy M, Persson HC, Sunnerhagen KS. Upper-limb spasticity during the first year after stroke: stroke arm longitudinal study at the University of Gothenburg. Am J Phys Med Rehabil (2014) 93:884–96. doi:10.1097/PHM.0000000000000157
161. Opheim A, Danielsson A, Alt Murphy M, Persson HC, Sunnerhagen KS. Early prediction of long-term upper limb spasticity after stroke: part of the SALGOT study. Neurology (2015) 85:873–80. doi:10.1212/WNL.0000000000001908
162. Levin MF. Interjoint coordination during pointing movements is disrupted in spastic hemiparesis. Brain (1996) 119(Pt 1):281–93. doi:10.1093/brain/119.1.281
163. Trombly CA. Deficits of reaching in subjects with left hemiparesis: a pilot study. Am J Occup Ther (1992) 46:887–97. doi:10.5014/ajot.46.10.887
164. Raghavan P. Upper limb motor impairment after stroke. Phys Med Rehabil Clin N Am (2015) 26:599–610. doi:10.1016/j.pmr.2015.06.008
165. Rao AK, Gordon AM. Contribution of tactile information to accuracy in pointing movements. Exp Brain Res (2001) 138:438–45. doi:10.1007/s002210100717
166. Van Beers RJ, Sittig AC, Denier Van Der Gon JJ. Localization of a seen finger is based exclusively on proprioception and on vision of the finger. Exp Brain Res (1999) 125:43–9. doi:10.1007/s002210050656
167. Flanagan JR, Ostry DJ, Feldman AG. Control of trajectory modifications in target-directed reaching. J Mot Behav (1993) 25:140–52. doi:10.1080/00222895.1993.9942045
168. Ghafouri M, Feldman AG. The timing of control signals underlying fast point-to-point arm movements. Exp Brain Res (2001) 137:411–23. doi:10.1007/s002210000643
169. Hogan N, Flash T. Moving gracefully: quantitative theories of motor coordination. Trends Neurosci (1987) 10:170–4. doi:10.1016/0166-2236(87)90043-9
170. Gordon J, Ghilardi MF, Ghez C. Accuracy of planar reaching movements. I. Independence of direction and extent variability. Exp Brain Res (1994) 99:97–111. doi:10.1007/BF00241415
171. Raghavan P, Krakauer JW, Gordon AM. Impaired anticipatory control of fingertip forces in patients with a pure motor or sensorimotor lacunar syndrome. Brain (2006) 129:1415–25. doi:10.1093/brain/awl070
172. Raghavan P, Santello M, Gordon AM, Krakauer JW. Compensatory motor control after stroke: an alternative joint strategy for object-dependent shaping of hand posture. J Neurophysiol (2010) 103:3034–43. doi:10.1152/jn.00936.2009
173. Nagasaki H. Asymmetric velocity and acceleration profiles of human arm movements. Exp Brain Res (1989) 74:319–26. doi:10.1007/BF00248865
174. Becker W, Jurgens R. An analysis of the saccadic system by means of double step stimuli. Vision Res (1979) 19:967–83. doi:10.1016/0042-6989(79)90222-0
175. Blouin J, Teasdale N, Bard C, Fleury M. Control of rapid arm movements when target position is altered during saccadic suppression. J Mot Behav (1995) 27:114–22. doi:10.1080/00222895.1995.9941704
176. Johansson RS, Westling G, Backstrom A, Flanagan JR. Eye-hand coordination in object manipulation. J Neurosci (2001) 21:6917–32.
177. Gaveau V, Pisella L, Priot AE, Fukui T, Rossetti Y, Pelisson D, et al. Automatic online control of motor adjustments in reaching and grasping. Neuropsychologia (2014) 55:25–40. doi:10.1016/j.neuropsychologia.2013.12.005
178. Prablanc C, Echallier JE, Jeannerod M, Komilis E. Optimal response of eye and hand motor systems in pointing at a visual target. II. Static and dynamic visual cues in the control of hand movement. Biol Cybern (1979) 35:183–7. doi:10.1007/BF00337436
179. Bogousslavsky J. Impairment of visually evoked eye movements with a unilateral parieto-occipital lesion. J Neurol (1987) 234:160–2. doi:10.1007/BF00314136
180. Bogousslavsky J, Meienberg O. Eye-movement disorders in brain-stem and cerebellar stroke. Arch Neurol (1987) 44:141–8. doi:10.1001/archneur.1987.00520140013011
181. Catz A, Ron S, Solzi P, Korczyn AD. Saccade characteristics in patients with hemispheric stroke. J Neurol Rehabil (1997) 11:175–80.
182. Colombo A, Gibertoni M, Sorgato P. [Clinical study of disorders of eye movements (voluntary and following) after acute vascular accidents involving the cerebral hemispheres (author’s transl)]. Riv Patol Nerv Ment (1981) 102:38–46.
183. Lekwuwa GU, Barnes GR. Cerebral control of eye movements. II. Timing of anticipatory eye movements, predictive pursuit and phase errors in focal cerebral lesions. Brain (1996) 119(Pt 2):491–505. doi:10.1093/brain/119.2.491
184. Pierrot-Deseilligny C, Ploner CJ, Muri RM, Gaymard B, Rivaud-Pechoux S. Effects of cortical lesions on saccadic: eye movements in humans. Ann N Y Acad Sci (2002) 956:216–29. doi:10.1111/j.1749-6632.2002.tb02821.x
185. Waespe W, Baumgartner R. Enduring dysmetria and impaired gain adaptivity of saccadic eye movements in Wallenberg’s lateral medullary syndrome. Brain (1992) 115(Pt 4):1123–46. doi:10.1093/brain/115.4.1125
186. Agrawal Y, Schubert MC, Migliaccio AA, Zee DS, Schneider E, Lehnen N, et al. Evaluation of quantitative head impulse testing using search coils versus video-oculography in older individuals. Otol Neurotol (2014) 35:283–8. doi:10.1097/MAO.0b013e3182995227
187. Eggert T. Eye movement recordings: methods. Dev Ophthalmol (2007) 40:15–34. doi:10.1159/000100347
188. Houben MM, Goumans J, van der Steen J. Recording three-dimensional eye movements: scleral search coils versus video oculography. Invest Ophthalmol Vis Sci (2006) 47:179–87. doi:10.1167/iovs.05-0234
189. Imai T, Sekine K, Hattori K, Takeda N, Koizuka I, Nakamae K, et al. Comparing the accuracy of video-oculography and the scleral search coil system in human eye movement analysis. Auris Nasus Larynx (2005) 32:3–9. doi:10.1016/j.anl.2004.11.009
190. Kimmel DL, Mammo D, Newsome WT. Tracking the eye non-invasively: simultaneous comparison of the scleral search coil and optical tracking techniques in the macaque monkey. Front Behav Neurosci (2012) 6:49. doi:10.3389/fnbeh.2012.00049
191. McCamy MB, Otero-Millan J, Leigh RJ, King SA, Schneider RM, Macknik SL, et al. Simultaneous recordings of human microsaccades and drifts with a contemporary video eye tracker and the search coil technique. PLoS One (2015) 10:e0128428. doi:10.1371/journal.pone.0128428
192. Stahl JS, van Alphen AM, De Zeeuw CI. A comparison of video and magnetic search coil recordings of mouse eye movements. J Neurosci Methods (2000) 99:101–10. doi:10.1016/S0165-0270(00)00218-1
193. van der Geest JN, Frens MA. Recording eye movements with video-oculography and scleral search coils: a direct comparison of two methods. J Neurosci Methods (2002) 114:185–95. doi:10.1016/S0165-0270(01)00527-1
194. Yee RD, Schiller VL, Lim V, Baloh FG, Baloh RW, Honrubia V. Velocities of vertical saccades with different eye movement recording methods. Invest Ophthalmol Vis Sci (1985) 26:938–44.
195. Machado L, Rafal RD. Control of fixation and saccades in humans with chronic lesions of oculomotor cortex. Neuropsychology (2004) 18:115–23. doi:10.1037/0894-4105.18.1.115
196. Kaplan J, Hier DB. Visuospatial deficits after right hemisphere stroke. Am J Occup Ther (1982) 36:314–21. doi:10.5014/ajot.36.5.314
197. Machner B, Sprenger A, Kompf D, Sander T, Heide W, Kimmig H, et al. Visual search disorders beyond pure sensory failure in patients with acute homonymous visual field defects. Neuropsychologia (2009) 47:2704–11. doi:10.1016/j.neuropsychologia.2009.05.016
198. Malhotra P, Coulthard E, Husain M. Hemispatial neglect, balance and eye-movement control. Curr Opin Neurol (2006) 19:14–20. doi:10.1097/01.wco.0000198101.87670.7e
199. Mennem TA, Warren M, Yuen HK. Preliminary validation of a vision-dependent activities of daily living instrument on adults with homonymous hemianopia. Am J Occup Ther (2012) 66:478–82. doi:10.5014/ajot.2012.004762
200. Rowe F. Visual perceptual consequences of stroke. Strabismus (2009) 17:24–8. doi:10.1080/09273970802678537
201. Bonan IV, Gaillard F, Ponche ST, Marquer A, Vidal PP, Yelnik AP. Early post-stroke period: a privileged time for sensory re-weighting? J Rehabil Med (2015) 47:516–22. doi:10.2340/16501977-1968
202. Hudson TE, Maloney LT, Landy MS. Movement planning with probabilistic target information. J Neurophysiol (2007) 98:3034–46. doi:10.1152/jn.00858.2007
203. Mann DT, Williams AM, Ward P, Janelle CM. Perceptual-cognitive expertise in sport: a meta-analysis. J Sport Exerc Psychol (2007) 29:457–78. doi:10.1123/jsep.29.4.457
204. McRobert AP, Williams AM, Ward P, Eccles DW. Tracing the process of expertise in a simulated anticipation task. Ergonomics (2009) 52:474–83. doi:10.1080/00140130802707824
205. Piras A, Lobietti R, Squatrito S. A study of saccadic eye movement dynamics in volleyball: comparison between athletes and non-athletes. J Sports Med Phys Fitness (2010) 50:99–108.
206. Willams AM, Hodges NJ, North JS, Barton G. Perceiving patterns of play in dynamic sport tasks: investigating the essential information underlying skilled performance. Perception (2006) 35:317–32. doi:10.1068/p5310
207. Williams AM. Perceptual skill in soccer: implications for talent identification and development. J Sports Sci (2000) 18:737–50. doi:10.1080/02640410050120113
208. Savelsbergh GJ, Williams AM, Van der Kamp J, Ward P. Visual search, anticipation and expertise in soccer goalkeepers. J Sports Sci (2002) 20:279–87. doi:10.1080/026404102317284826
209. Savelsbergh GJ, Van der Kamp J, Williams AM, Ward P. Anticipation and visual search behaviour in expert soccer goalkeepers. Ergonomics (2005) 48:1686–97. doi:10.1080/00140130500101346
210. Caeyenberghs K, Leemans A, Geurts M, Taymans T, Vander Linden C, Smits-Engelsman BC, et al. Brain-behavior relationships in young traumatic brain injury patients: fractional anisotropy measures are highly correlated with dynamic visuomotor tracking performance. Neuropsychologia (2010) 48:1472–82. doi:10.1016/j.neuropsychologia.2010.01.017
211. Suh M, Basu S, Kolster R, Sarkar R, McCandliss B, Ghajar J, et al. Increased oculomotor deficits during target blanking as an indicator of mild traumatic brain injury. Neurosci Lett (2006) 410:203–7. doi:10.1016/j.neulet.2006.10.001
212. Suh M, Kolster R, Sarkar R, McCandliss B, Ghajar J; Cognitive and Neurobiological Research Consortium. Deficits in predictive smooth pursuit after mild traumatic brain injury. Neurosci Lett (2006) 401:108–13. doi:10.1016/j.neulet.2006.02.074
213. Maruta J, Suh M, Niogi SN, Mukherjee P, Ghajar J. Visual tracking synchronization as a metric for concussion screening. J Head Trauma Rehabil (2010) 25:293–305. doi:10.1097/HTR.0b013e3181e67936
214. Contreras R, Ghajar J, Bahar S, Suh M. Effect of cognitive load on eye-target synchronization during smooth pursuit eye movement. Brain Res (2011) 1398:55–63. doi:10.1016/j.brainres.2011.05.004
215. Brown JA, Dalecki M, Hughes C, Macpherson AK, Sergio LE. Cognitive-motor integration deficits in young adult athletes following concussion. BMC Sports Sci Med Rehabil (2015) 7:25. doi:10.1186/s13102-015-0019-4
216. Armstrong R, Kergoat H. Oculo-visual changes and clinical considerations affecting older patients with dementia. Ophthalmic Physiol Opt (2015) 35:352–76. doi:10.1111/opo.12220
217. Rosler A, Billino J, Muller NG, Weidauer S, Steinmetz H, Kleinschmidt A. Visual search in patients with subcortical vascular dementia: short fixations but long reaction times. Dement Geriatr Cogn Disord (2005) 20:375–80. doi:10.1159/000089104
218. Schewe HJ, Uebelhack R, Vohs K. Abnormality in saccadic eye movement in dementia. Eur Psychiatry (1999) 14:52–3. doi:10.1016/S0924-9338(99)80716-0
219. Ling H, Kara E, Revesz T, Lees AJ, Plant GT, Martino D, et al. Concomitant progressive supranuclear palsy and chronic traumatic encephalopathy in a boxer. Acta Neuropathol Commun (2014) 2:24. doi:10.1186/2051-5960-2-24
220. Beer R, Dewald J, Rymer Z. Disturbances of voluntary movement coordination in stroke: problems of planning or execution? Prog Brain Res (1999) 123:455–60. doi:10.1016/S0079-6123(08)62881-2
221. Rizzo JR, Hudson TE, Abdou A, Rashbaum IG, George AE, Raghavan P, et al. Motor planning poststroke: impairment in vector-coded reach plans. Physiol Rep (2015) 3:e12650. doi:10.14814/phy2.12650
222. Cirstea MC, Levin MF. Compensatory strategies for reaching in stroke. Brain (2000) 123(Pt 5):940–53. doi:10.1093/brain/123.5.940
223. Ketcham CJ, Rodriguez TM, Zihlman KA. Targeted aiming movements are compromised in nonaffected limb of persons with stroke. Neurorehabil Neural Repair (2007) 21:388–97. doi:10.1177/1545968306297872
224. Kusoffsky A, Apel I, Hirschfeld H. Reaching-lifting-placing task during standing after stroke: coordination among ground forces, ankle muscle activity, and hand movement. Arch Phys Med Rehabil (2001) 82:650–60. doi:10.1053/apmr.2001.22611
225. Takahashi CD, Reinkensmeyer DJ. Hemiparetic stroke impairs anticipatory control of arm movement. Exp Brain Res (2003) 149:131–40. doi:10.1007/s00221-002-1340-1
226. Tseng YW, Diedrichsen J, Krakauer JW, Shadmehr R, Bastian AJ. Sensory prediction errors drive cerebellum-dependent adaptation of reaching. J Neurophysiol (2007) 98:54–62. doi:10.1152/jn.00266.2007
227. Sarlegna FR, Sainburg RL. The roles of vision and proprioception in the planning of reaching movements. Adv Exp Med Biol (2009) 629:317–35. doi:10.1007/978-0-387-77064-2_16
228. Schaefer SY, Mutha PK, Haaland KY, Sainburg RL. Hemispheric specialization for movement control produces dissociable differences in online corrections after stroke. Cereb Cortex (2012) 22:1407–19. doi:10.1093/cercor/bhr237
229. Schaefer SY, Haaland KY, Sainburg RL. Ipsilesional motor deficits following stroke reflect hemispheric specializations for movement control. Brain (2007) 130:2146–58. doi:10.1093/brain/awm145
230. Shabbott BA, Sainburg RL. Differentiating between two models of motor lateralization. J Neurophysiol (2008) 100:565–75. doi:10.1152/jn.90349.2008
231. Roy OW, Cohen NR, Nicoll JA. Pathophysiology of dementias and implications for therapy. Indian J Pathol Microbiol (2005) 48:289–99.
232. Masdeu JC. Future directions in imaging neurodegeneration. Curr Neurol Neurosci Rep (2017) 17:9. doi:10.1007/s11910-017-0718-1
233. Sperling RA, Aisen PS, Beckett LA, Bennett DA, Craft S, Fagan AM, et al. Toward defining the preclinical stages of Alzheimer’s disease: recommendations from the National Institute on aging-Alzheimer’s Association workgroups on diagnostic guidelines for Alzheimer’s disease. Alzheimers Dement (2011) 7:280–92. doi:10.1016/j.jalz.2011.03.003
234. Velazquez-Perez L, Rodriguez-Labrada R, Laffita-Mesa JM. Prodromal spinocerebellar ataxia type 2: prospects for early interventions and ethical challenges. Mov Disord (2017) 32:708–18. doi:10.1002/mds.26969
235. Meissner WG. When does Parkinson’s disease begin? From prodromal disease to motor signs. Rev Neurol (Paris) (2012) 168:809–14. doi:10.1016/j.neurol.2012.07.004
236. Rizzo JR, Hudson TE, Dai W, Birkemeier J, Pasculli RM, Selesnick I, et al. Rapid number naming in chronic concussion: eye movements in the King–Devick test. Ann Clin Transl Neurol (2016) 3:801–11. doi:10.1002/acn3.345
237. Rizzo JR, Hudson TE, Dai W, Desai N, Yousefi A, Palsana D, et al. Objectifying eye movements during rapid number naming: methodology for assessment of normative data for the King–Devick test. J Neurol Sci (2016) 362:232–9. doi:10.1016/j.jns.2016.01.045
238. Hutton SB. Cognitive control of saccadic eye movements. Brain Cogn (2008) 68:327–40. doi:10.1016/j.bandc.2008.08.021
239. Cobbs L, Hasanaj L, Amorapanth P, Rizzo JR, Nolan R, Serrano L, et al. Mobile universal lexicon evaluation system (MULES) test: a new measure of rapid picture naming for concussion. J Neurol Sci (2017) 372:393–8. doi:10.1016/j.jns.2016.10.044
240. Galetta KM, Barrett J, Allen M, Madda F, Delicata D, Tennant AT, et al. The King–Devick test as a determinant of head trauma and concussion in boxers and MMA fighters. Neurology (2011) 76:1456–62. doi:10.1212/WNL.0b013e31821184c9
241. Galetta KM, Brandes LE, Maki K, Dziemianowicz MS, Laudano E, Allen M, et al. The King–Devick test and sports-related concussion: study of a rapid visual screening tool in a collegiate cohort. J Neurol Sci (2011) 309:34–9. doi:10.1016/j.jns.2011.07.039
242. Galetta KM, Liu M, Leong DF, Ventura RE, Galetta SL, Balcer LJ. The King–Devick test of rapid number naming for concussion detection: meta analysis and systematic review of the literature. Concussion (2015) 15:1–15.
243. Leong DF, Balcer LJ, Galetta SL, Evans G, Gimre M, Watt D. The King–Devick test for sideline concussion screening in collegiate football. J Optom (2015) 8:131–9. doi:10.1016/j.optom.2014.12.005
244. Ventura RE, Balcer LJ, Galetta SL, Rucker JC. Ocular motor assessment in concussion: current status and future directions. J Neurol Sci (2016) 361:79–86. doi:10.1016/j.jns.2015.12.010
245. Heitger MH, Anderson TJ, Jones RD, Dalrymple-Alford JC, Frampton CM, Ardagh MW. Eye movement and visuomotor arm movement deficits following mild closed head injury. Brain (2004) 127:575–90. doi:10.1093/brain/awh066
246. Heitger MH, Jones RD, Dalrymple-Alford JC, Frampton CM, Ardagh MW, Anderson TJ. Mild head injury – a close relationship between motor function at 1 week post-injury and overall recovery at 3 and 6 months. J Neurol Sci (2007) 253:34–47. doi:10.1016/j.jns.2006.11.007
247. Heitger MH, Jones RD, Dalrymple-Alford JC, Frampton CM, Ardagh MW, Anderson TJ. Motor deficits and recovery during the first year following mild closed head injury. Brain Inj (2006) 20:807–24. doi:10.1080/02699050600676354
248. Heitger MH, Jones RD, Anderson TJ. A new approach to predicting postconcussion syndrome after mild traumatic brain injury based upon eye movement function. Conf Proc IEEE Eng Med Biol Soc (2008) 2008:3570–3. doi:10.1109/IEMBS.2008.4649977
249. Heitger MH, Jones RD, Macleod AD, Snell DL, Frampton CM, Anderson TJ. Impaired eye movements in post-concussion syndrome indicate suboptimal brain function beyond the influence of depression, malingering or intellectual ability. Brain (2009) 132:2850–70. doi:10.1093/brain/awp181
250. Galetta KM, Chapman KR, Essis MD, Alosco ML, Gillard D, Steinberg E, et al. Screening utility of the King–Devick test in mild cognitive impairment and Alzheimer disease dementia. Alzheimer Dis Assoc Disord (2016) 31:152–8. doi:10.1097/WAD.0000000000000157
251. van Stockum S, MacAskill M, Anderson T, Dalrymple-Alford J. Don’t look now or look away: two sources of saccadic disinhibition in Parkinson’s disease? Neuropsychologia (2008) 46:3108–15. doi:10.1016/j.neuropsychologia.2008.07.002
252. de Boer C, Pel JJ, van den Dorpel JJ, Boon AJ, van der Steen J. Behavioral inhibition errors in Parkinson’s disease tested using an antisaccade and antitapping task. J Parkinsons Dis (2014) 4:599–608. doi:10.3233/JPD-140401
253. Muilwijk D, Verheij S, Pel JJ, Boon AJ, van der Steen J. Changes in timing and kinematics of goal directed eye-hand movements in early-stage Parkinson’s disease. Transl Neurodegener (2013) 2:1. doi:10.1186/2047-9158-2-1
254. Verheij S, Muilwijk D, Pel JJ, van der Cammen TJ, Mattace-Raso FU, van der Steen J. Visuomotor impairment in early-stage Alzheimer’s disease: changes in relative timing of eye and hand movements. J Alzheimers Dis (2012) 30:131–43. doi:10.3233/JAD-2012-111883
255. Tippett WJ, Krajewski A, Sergio LE. Visuomotor integration is compromised in Alzheimer’s disease patients reaching for remembered targets. Eur Neurol (2007) 58:1–11. doi:10.1159/000102160
Keywords: coordination, eye, hand, stroke, brain injuries
Citation: Rizzo J-R, Hosseini M, Wong EA, Mackey WE, Fung JK, Ahdoot E, Rucker JC, Raghavan P, Landy MS and Hudson TE (2017) The Intersection between Ocular and Manual Motor Control: Eye–Hand Coordination in Acquired Brain Injury. Front. Neurol. 8:227. doi: 10.3389/fneur.2017.00227
Received: 10 March 2017; Accepted: 11 May 2017;
Published: 01 June 2017
Edited by:
Aasef G. Shaikh, Case Western Reserve University, United StatesReviewed by:
Nathan Evanson, Cincinnati Children’s Hospital Medical Center, United StatesRandel Lynn Swanson II, University of Pennsylvania, United States
Copyright: © 2017 Rizzo, Hosseini, Wong, Mackey, Fung, Ahdoot, Rucker, Raghavan, Landy and Hudson. This is an open-access article distributed under the terms of the Creative Commons Attribution License (CC BY). The use, distribution or reproduction in other forums is permitted, provided the original author(s) or licensor are credited and that the original publication in this journal is cited, in accordance with accepted academic practice. No use, distribution or reproduction is permitted which does not comply with these terms.
*Correspondence: John-Ross Rizzo, am9obnJvc3Mucml6em9Abnl1bWMub3Jn