- 1Division of Neurosurgery, Department of Clinical Neurosciences, University of Cambridge, Cambridge, United Kingdom
- 2Department of Clinical Neuroscience, Karolinska Institutet, Stockholm, Sweden
- 3Division of Anaesthesia, Department of Medicine, University of Cambridge, Cambridge, United Kingdom
- 4Rady Faculty of Health Sciences, Department of Surgery, University of Manitoba, Winnipeg, MB, Canada
- 5Clinician Investigator Program, Rady Faculty of Health Sciences, University of Manitoba, Winnipeg, MB, Canada
- 6Wolfson Brain Imaging Centre, Department of Clinical Neurosciences, University of Cambridge, Cambridge, United Kingdom
- 7Department of Epidemiology and Preventive Medicine, Monash University, Melbourne, VIC, Australia
- 8Department of Child Health, Barrow Neurological Institute at Phoenix Children’s Hospital, University of Arizona College of Medicine, Phoenix, Phoenix, AZ, United States
Traumatic brain injury (TBI) and subarachnoid hemorrhage (SAH) are major contributors to morbidity and mortality. Following the initial insult, patients may deteriorate due to secondary brain damage. The underlying molecular and cellular cascades incorporate components of the innate immune system. There are different approaches to assess and monitor cerebral inflammation in the neuro intensive care unit. The aim of this narrative review is to describe techniques to monitor inflammatory activity in patients with TBI and SAH in the acute setting. The analysis of pro- and anti-inflammatory cytokines in compartments of the central nervous system (CNS), including the cerebrospinal fluid and the extracellular fluid, represent the most common approaches to monitor surrogate markers of cerebral inflammatory activity. Each of these compartments has a distinct biology that reflects local processes and the cross-talk between systemic and CNS inflammation. Cytokines have been correlated to outcomes as well as ongoing, secondary injury progression. Alongside the dynamic, focal assay of humoral mediators, imaging, through positron emission tomography, can provide a global in vivo measurement of inflammatory cell activity, which reveals long-lasting processes following the initial injury. Compared to the innate immune system activated acutely after brain injury, the adaptive immune system is likely to play a greater role in the chronic phase as evidenced by T-cell-mediated autoreactivity toward brain-specific proteins. The most difficult aspect of assessing neuroinflammation is to determine whether the processes monitored are harmful or beneficial to the brain as accumulating data indicate a dual role for these inflammatory cascades following injury. In summary, the inflammatory component of the complex injury cascade following brain injury may be monitored using different modalities. Using a multimodal monitoring approach can potentially aid in the development of therapeutics targeting different aspects of the inflammatory cascade and improve the outcome following TBI and SAH.
Introduction
Pathophysiology of Brain Injury
Traumatic brain injury (TBI) and aneurysmal subarachnoid hemorrhage (SAH) are common neurological conditions (1, 2) associated with extensive morbidity and mortality (3, 4). While the two diseases are different entities, they share many common features in their secondary pathophysiology. Furthermore, they provide an experimental paradigm in which multimodality monitoring offers the opportunity to decipher common pathological processes. There are multiple potential mechanisms by which neuronal injury is inflicted following TBI and SAH. The traditional clinical paradigm for management of these conditions has focused on adequate delivery of oxygen and appropriate metabolic substrates to the injured brain, and prevention of secondary injuries caused by hypotension and hypoxia (5). These secondary processes lead to an increasingly inhospitable environment with ongoing excitotoxicity, oxidative stress, blood–brain barrier (BBB) disruption, cortical spreading depression, mitochondrial dysfunction, and subsequent cellular death in the tissue surrounding the initial damage (6, 7). There is an increasing recognition that inflammatory mediators are involved in the mechanistic link that underlies these injurious processes (8–10).
What Is Neuroinflammation?
Galen’s original description of inflammation as calor (heat), dolor (pain), rubor (redness), tumor (swelling), and subsequently functio laesa (loss of function) has been refined into a more complex phenomenon that represents the host response to any insult. This complex process involves the release of molecular mediators, the alteration of the cerebral vasculature, the activation and influx of immune cells to eliminate pathogens, damaged cells, and other perceived harmful stimuli. The brain was long thought to be immunoprivileged due to the presence of the BBB, which limits the cross-talk between the blood and brain-resident inflammatory cells, the lack of a classical lymphatic system, and the shielding of neural antigens from peripheral immune surveillance. More recently, all these assumptions have been questioned and revised (11). There is an increasing realization that cerebral inflammation or neuroinflammation occurs within the whole gamut of central nervous system (CNS) pathologies, whether it is an adaptive autoimmune response (e.g., multiple sclerosis) or a response to external stimuli (e.g., accumulation of red blood cells following brain hemorrhage). While microglia are considered the primary immune cells of the CNS, it is becoming increasingly clear that other glial cells (astrocytes and oligodendrocytes) as well as neurons display immune-competent functions. Activated resident cells of the CNS, in combination with migrating inflammatory cells from peripheral blood, form an intricate immune network (12, 13). Although this immune response may be initiated to protect the brain, it is becoming evident that it can result in harmful outcomes for the CNS (14). In fact, an ongoing neuroinflammatory response may not only contribute to increased edema, cellular death, and BBB disruption but also function as a potent scavenger of dead cells and support the regenerative processes in the injured CNS (15, 16). An outline of the pathophysiological mechanisms identified in the literature is illustrated in Figure 1, many of which are described in this review.
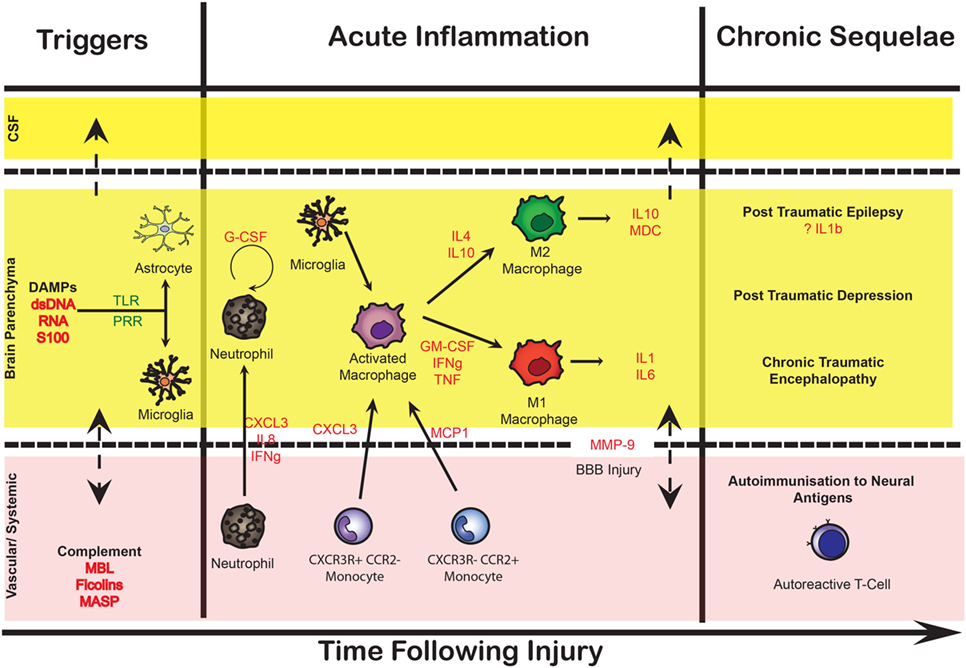
Figure 1. Conceptual summary of the neuroinflammatory response in acute brain injury. This illustration depicts some of the cellular and molecular sequelae and inflammatory cascades that occur following injury to the brain over time, divided into different compartments [cerebrospinal fluid (CSF), brain parenchyma, and vascular]. Initially, danger-associated molecular patterns (DAMPs) including double-stranded (ds)DNA, RNA, and brain-enriched proteins such as S100B provide early triggers to resident cells reflecting tissue injury and linking the initial insult to subsequent humoral and cellular cascades. These in turn lead to an increasingly cytotoxic environment promoting cellular responses, stimulating both immunocompetent cells in the central nervous system (resident microglia and astrocytes), as well as recruiting peripheral monocytes, through pattern recognition receptors (PRR) such as toll-like receptors (TLR). The secretion of cytokines, chemokines, and other potent chemotactic substances (including complement proteins) induces the recruitment of neutrophils and monocytes into the brain parenchyma through the increasingly permeable blood–brain barrier (BBB), as well as having a direct cytotoxic effect. Ongoing release of cytokines initiates a long-term inflammatory process, which allows the dynamic shift of macrophages and microglial canonical phenotype between M1 (classical activation) and M2 (alternative active presumably the result of antigen-presenting cells migrating from the periphery). These processes result in chronic inflammation as well as auto-immunization toward brain-enriched antigens. Key cytokines are highlighted in red. MBL, mannose-binding lectin; MASP, mannose-associated serine protease; MMP-9, matrix metalloproteinase 9.
Aspects of Neuroinflammation in SAH
Brain hemorrhage triggers a series of pathological processes resulting in neuronal damage and consequent neurological deficit (17–22). Early brain injury (EBI) refers to the damage ensuing in the first 72 h after the bleed caused by transient cerebral ischemia (CI), BBB disruption, edema, cell death, and brain tissue loss. Many of the patients who survive these phenomena deteriorate days later from delayed ischemic neurological deficit (DIND), which is responsible for poor outcomes or death in up to 30% of patients with SAH. DIND was traditionally attributed to narrowing of the large basal cerebral arteries, termed cerebral or angiographic vasospasm, causing a reduction in cerebral blood flow (CBF) and, if critically reduced, progressing to infarction in the relevant vascular territory. However, a growing number of reports shows that angiographic vasospasm does not always correlate with CI, cerebral infarction, or changes in CBF, indicating that DIND is a more complex (and complicated) phenomenon (23–33). The landmark CONSCIOUS-1 trial showed that an endothelin-1 antagonist ameliorates angiographic vasospasm, but fails to improve functional outcomes (34, 35), forever changing the concept of DIND, which is now suspected to arise from the combined effects of angiographic vasospasm, global reduction in blood flow, arteriolar constriction and thrombosis, cortical spreading depressions, and processes triggered by EBI (17–21, 36). With the complex interplay of the underlying pathological substrates, DIND is often (incorrectly) used as a blanket term for vascular spasm, CI, and neurological (clinical) deficit. It remains unclear to what extent these phenomena overlap or represent distinct processes (Figure 2).
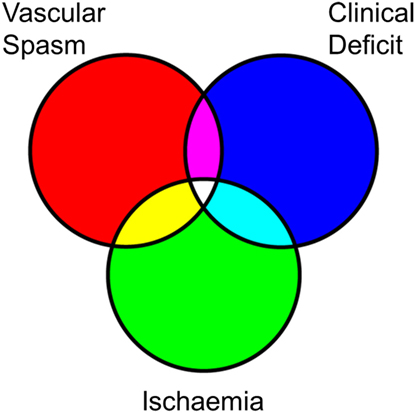
Figure 2. Overlapping characteristics of the pathophysiology in subarachnoid hemorrhage (SAH). Simplified Venn diagram of the different pathophysiological processes that follow SAH. Rather than a linear relationship between vascular spasm, ischemia, and clinical deficit, these may be overlapping and also occur as distinct phenomena. Vascular spasm is most closely linked with endothelin-1-mediated vasoconstriction but may also encompass swelling of the vascular endothelium leading to an angiographic appearance of reduced contrast flow. Ischemia may result from an absolute reduction in blood flow, which may be complicated by local microvascular collapse or thrombosis, or even hyperemia causing localized swelling and reducing the capillary perfusion in that locality. Neurological deficits may be caused by frank ischemia and also result from phenomena such as cortical spreading depression.
It is increasingly recognized that the neuroinflammatory cascade following SAH is the potential mechanistic link between the initial ictus and the progression of neuronal injury in EBI as well as the development of DIND (37–40). Both overlapping phenomena of ischemic injury and vasospasm lead to an unfavorable physiological environment that may inflict neuronal injury (Figure 2), possibly driven in part by neuroinflammatory mediators.
Monitoring Neuroinflammation in the Neuro-Critical Care Unit (NCCU)
Patients suffering from TBI and SAH are typically treated in specialized NCCUs where extensive intracranial monitoring is implemented in order to detect subsequent secondary insults, prevent further deterioration, and optimize conditions for brain recovery (41, 42). Monitoring neuroinflammatory processes is technically and logistically complex, and this has hampered the development of pharmaceutical compounds that act to modulate the neuroinflammatory cascade (43). As we acquire a greater insight into the interplay between neuroinflammation and secondary brain injury, monitoring the neuroinflammatory events may open an opportunity to both increase our understanding of the molecular basis of the underlying pathology as well as identify novel therapeutic targets.
The aim of this narrative review is to demonstrate how different aspects of the neuroinflammatory response contribute to the pathophysiology of secondary brain injury. In addition, we discuss the methods available to monitor cellular and humoral inflammation in TBI and SAH patients in the clinical setting.
Cytokine and Chemokine Monitoring in Acute Brain Injury
There is no accepted gold standard in how to assess the neuroinflammatory processes in TBI and SAH. In clinical studies, the most common approach is to measure the concentration of cytokines and chemokines as biomarkers of ongoing neuroinflammation. Cytokines are small proteins of approximately 20 kDa molecular weight that are synthesized primarily by immune cells to promote and regulate inflammatory responses in an autocrine and paracrine fashion.
There are currently a wide range of commercially available assays that can detect with high sensitivity (pg/ml range) the levels of cytokines in different compartments. Historically, enzyme-linked immunosorbant assay has most frequently been used, but these require relatively large volumes of fluid/tissue and can only measure one cytokine at the time, and are therefore not ideal to monitor a complex inflammatory network. In the last 10 years, multiplex bead arrays have allowed the use of very small sample sizes (~25 μl per sample) to simultaneously measure several cytokines, thus greatly increasing the technical efficiency and our understanding of the inflammatory response in the injured brain (44).
CNS Compartmentalization of Cytokine Measurements
The CNS has several inherent barriers, restricting and controlling the flow of substances. These include the BBB, the blood–cerebrospinal fluid (CSF) barrier, and the CSF–brain barrier. The brain compartment, or specifically the extracellular space of the brain, is accessible by using a microdialysis (MD) catheter. The MD catheter possesses a semipermeable membrane that is able to extract proteins like cytokines directly from the extracellular fluid (ECF) when inserted into the injured brain (13, 45).
The CSF is produced by cells of the choroid plexus located within the cerebral ventricles and provides a medium to embed the brain, maintain a homeostatic environment, and facilitate the transport of nutrients and waste products. Thus, while using the CSF for cytokine measurement may provide a global assessment of cerebral inflammation, it remains less specific in terms of regional monitoring. CSF is more easily accessible through, ideally, external ventricular drainage in sedated patients or via lumbar puncture in conscious patients where several milliliters can be drawn for analyses. While blood is biologically the most remote space from the organ of interest, it represents an easily accessible compartment for repeated sampling. To compare and monitor cytokine concentrations, collection of matched CSF and plasma samples over time allows a composite picture of ongoing immune responses, distinguishing central from systemic cytokine profiles and determining how immune activation in the brain may impact on mechanisms occurring in the periphery and vice versa.
Figure 1 illustrates the intricate relationship between different compartments in the CNS and the potential cross-talk between the different compartments.
Cytokines in Blood
Although controversial, some groups have correlated cytokine concentrations measured in blood/plasma in the acute and chronic setting of patients with TBI and SAH to functional outcome (46–48). This suggests that, while these mediators may represent non-brain-specific inflammatory activity, systemic inflammation is triggered by brain injury, potentially having long-term sequelae such as stimulating an acute-phase response in the liver (49). Importantly, the pattern of cytokine production is both qualitatively and quantitatively different in blood compared to CSF following injury (49–52), suggesting the existence of distinct biological processes at play.
Cytokines in CSF
Collection of CSF is the oldest and most prevalent method to test for inflammatory mediators following TBI and SAH and, and in contrast to blood sampling, it represents a global measure of cerebral immune activity. Access to ventricular CSF is achieved with surgical insertion of a drain in the ventricles to measure and monitor changes of intracranial pressure. Longitudinal measurement of cytokines in CSF of patients with severe TBI reveals a biphasic immune response being maximal in the first few days after injury followed by a second modest increase by 7–10 days as shown for IL-6 (49).
These data have been confirmed using principal component analysis (PCA) of CSF cytokines and chemokines, resulting in the identification of distinct clusters of cytokine response (53, 54). Interestingly, multivariate analysis has also demonstrated a significant difference in outcome between the two clusters. Thus, assessing inflammatory activity provides a potential window for appropriate pharmaceutical therapies targeting cytokine production (54). The difficulty is in disentangling the roles of individual mediators within these clusters to investigate rationalized treatment targets.
In SAH, CSF samples may not be a true measure of neuroinflammation. The volume of blood present within the subarachnoid space can affect the levels of cytokines present in CSF, and therefore, cytokine concentration in CSF is partly a consequence of the volume of SAH rather than a specific measure of the magnitude of the inflammatory response within the brain.
Microdialysate Monitoring of Brain ECF
Microdialysis is inevitably a spatially restricted approach that can be used to target pericontusional/infarcted tissue at risk for secondary injury and thus allow us to monitor ongoing or worsening focal brain damage. Notably, cytokine analysis revealed large variations in their concentrations when comparing CSF with brain-ECF (Table 1). However, the difference in cytokine levels between CSF and brain-ECF is smaller compared to the concentration gradients between these compartments and serum (55). Measurement of cytokines using MD have the additional complication of relative recovery. Relative recovery within the microdialysate fluid refers to the recovered fraction of the true concentration in the ECF, which is governed by a range of physicochemical factors including the molecular weight of the cytokine, its isoelectric point (which in turn determines the charge the protein carries at neutral pH and its solubility in water), and its native oligomerization characteristics (56). For some species, the relative recovery can be as low as 5% (exemplified in Table 1) making it technically difficult to reliably monitor.

Table 1. Comparison of selected cytokine concentrations in serum, CSF, and microdialysate in patients with traumatic brain injury.
There is an intricate interplay between the various cellular elements (both resident and recruited) within the brain, which are both the source and target of these mediators (13, 57, 58), further complicating the interpretation.
Using either a qualitative approach or an unbiased quantitative analysis (such as PCA), cytokine production appears as an intrinsic and ordered component among the multiple biochemical pathways contributing to delayed brain damage (57, 59). This fact would argue against the inflammatory response being a secondary form of injury per se, and rather a sequential cascade of molecular events [in the words of Julius Caesar: alea iacta est (the die is cast)], with a predetermined sequence following the initial injury.
Perfusate for Cytokine Recovery Using Microdialysis
MD is commonly used in the NCCU for metabolic monitoring of the injured brain, recovering small metabolites such as lactate, pyruvate, glucose and glycerol from the ECF (45). For these metabolites, the relative recovery is circa 70% from catheters inserted into the brain, using a flow rate of 0.3 µl/min (60). Importantly, the pore size of MD membranes used for metabolite analysis is significantly smaller (20 kDa) compared to the size used for larger proteins such as cytokines (100 kDa) (61, 62). By using 100 kDa cutoff catheters with the standard crystalloid solution, the relative recovery for cytokines has been shown to be typically 30%, but as low as 5% for cytokines that oligomerize (e.g., TNF) (56). In order to improve poor recovery rates, colloids such as human albumin solution (HAS) or dextran may be added to the perfusion fluid (63). The addition of colloids does not always improve the recovery of all cytokines; however, the relative recovery for TNF in 3.5% HAS has been shown to increase from 4.4 to 31.2%, a sevenfold increase (56). A 3% Dextran-500 solution shows equally encouraging results compared to crystalloid solutions (64), and has the capacity to improve extraction of even larger inflammatory mediators (65, 66) and has been empirically demonstrated to remain within a 100 kDa membrane catheter (67). In summary, the optimal recovery of cytokines within the MD samples is dependent upon perfusate supplemented with albumin or dextran, favoring an improved relative recovery and no net loss of fluid from the catheter. In our opinion, this is an essential adjunct for protein microdialysis in general and specifically in recovering inflammatory mediators.
Statistical Approaches to Cytokine Studies
While the neuroinflammatory cascade following TBI is complex and characterized by substantial collinearity and functional overlap among mediators, many studies have focused on single cytokines utilizing univariate comparative statistics without adequately assessing the interrelationships with other factors (68–70). A more appropriate statistical approach would be to incorporate several cytokines into multivariate analysis methods, such as PCA (71). By using PCA, it is possible to detect the greatest sources of variance in the dataset and thus highlight patterns in the cytokine response (59). A regression extension of PCA such as partial least square discriminant analysis (PLS-DA) can be used to identify variations in a particular domain such as time (58, 72). PLS-DA has the disadvantage of introducing bias, but can be used to test prespecified hypotheses.
Interpretations of Cytokine Studies in TBI
A systematic review has identified 32 studies describing the analysis of CSF cytokines in 1,363 severe TBI patients, of which 19 studies reported the analysis of cytokines via MD in 267 severe TBI patients (73). The majority of the MD studies have been published from a few centers of excellence in TBI research, known for their work in the field of cerebral MD. From these studies, it is evident that MD catheter positioning plays a crucial role in the amount of cytokines recovered, with peri-lesional or lesional locations displaying greatly different cytokine profiles compared to “healthy” non-lesioned tissue. There is also a stereotyped response to injury whereby the peaks of MD derived cytokines such as interleukin-1 beta (IL-1β), IL-6, and IL-8 occur within the first 48–72 h post-TBI, with IL-10 remaining elevated throughout the acute period up to 5 days of monitoring. Therapeutic modulation of the neuroinflammatory response to TBI has been demonstrated clinically in patients using subcutaneous administration of recombinant human IL-1 receptor antagonist, with the aim of attenuating the experimentally proven IL-1-mediated cellular damage in the injured brain (57, 58). Several studies have attempted to correlate CSF cytokine concentrations [IL-1β, interleukin-1 receptor antagonist (IL-1ra), IL-6, IL-8, IL-10 and TNF] with patient outcome, demonstrating a number of conflicting data [summarized in a review article (74)]. We would argue that this approach is flawed as it ascribes a unitary role for a given cytokine (as “good” or “bad”) as well as ignoring the high degree of collinearity between all these mediators (75). It is important to recognize the delicate interplay between mediators such that the ultimate effect of a given cytokine is determined by the specific cellular, chemical, and temporal milieu in which the cytokine is acting. Finally, the clinical complication profile with brain-ECF and CSF-based cytokine sampling appears to be quite low, attesting to the safety of both procedures in critically ill TBI patients. All these findings are necessarily limited by the small number of studies identified in the accompanying systematic review.
Interpretations of Cytokine Studies in SAH
Similar to the findings identified in the review on TBI, the studies reporting on the analysis of cytokines via MD and CSF in aneurysmal SAH cytokine can be found in the parent systematic review, included in this Research Topic in Frontiers in Neurology (Zeiler et al., 2017, II submitted). Overall, there are 9 studies describing the analysis of cytokines via MD in 246 aneurysmal SAH patients. Another 20 studies reported the analysis of CSF cytokines in 630 patients. As with the TBI cytokine literature, the MD and CSF studies identified within the review originated from a small number of centers of excellence in NCCU and SAH based research. This body of literature is smaller than that identified within the TBI population. Thus, the conclusions that can be made within the SAH systematic review are somewhat limited. However, as with the TBI literature, some interesting trends should be highlighted. Again, MD catheter location plays a role in the cytokine levels obtained in SAH patients, with lesioned tissue (i.e., ICH, ischemic brain) and peri-lesional tissue displaying higher cytokine levels than the “healthy” non-lesioned tissue. Second, SAH patients displayed peaks in MD IL-1β, IL-6, and IL-8 within the first 12 h’ post-hemorrhage, while (as in TBI) IL-10 appears to remain elevated within the acute period. Third, CSF-based cytokines in SAH appear to correlate with patient outcome, but as with the TBI data, our interpretation is that this type of solitary comparative analysis for each given cytokine may be misleading as there will be a potent collinearity between all these mediators. The association between cytokine profiles and the incidence of clinical vasospasm post-SAH has been documented in a previous systematic review and meta-analysis, with IL-6 and TNF linked to the development of vasospasm and delayed ischemic neurological deficit (DIND) (76). In addition, chronic hydrocephalus and shunt dependency may be associated with TGF-β levels within the CSF for SAH patients.
Tissue Sampling to Assess Neuroinflammation
A direct method to determine focal inflammatory activity is to biopsy samples adjacent to the injured tissue. Compared to indirect sampling of fluid, this provides a measure of tissue inflammation with a high degree of confidence; however, it remains a temporal snapshot that cannot readily reflect dynamic processes. Harish et al. found that surgically removed tissue demonstrates higher concentrations of several, predominantly pro-inflammatory, cytokines, and chemokines in contused brain vs pericontusional tissue (77). They also found a high degree of macrophage and microglia activity in contused regions, suggesting strong inflammatory activity. Similarly, Bellander et al. identified increased complement activation from pericontusional tissue (78), indicating a strong inflammatory response, especially in surrounding neurons, following TBI. Additionally, in postmortem tissue of TBI patients, it has been shown that pro-inflammatory cytokine mRNA and protein concentration are significantly elevated compared to cytokines with a more anti-inflammatory function (79). In the same study, regions with a profound cytokine production were associated with abundant inflammatory cell infiltration, astrogliosis, and axonal pathology. While in postmortem tissue, changes that occur at the time of death might influence results, this study directly demonstrates that cytokines are upregulated in the brain parenchyma as early as a few minutes from brain injury. In the future, it might be possible to directly assess the inflammatory profile of the brain by acquiring brain tissue samples.
Imaging Techniques to Measure the Neuroinflammatory Response
Advances in neuroimaging have allowed aspects of the neuroinflammatory cascade to be assessed with a high degree of spatial resolution. Ideally, these techniques can be combined with other tools such as MD, which are focal but provide temporal resolution, to get a more complete overview of inflammatory processes.
Positron Emission Tomography (PET)
Positron emission tomography relies on radioligands, which bind to a specific receptor or mimic a biological molecule of interest, and can be used in in vivo studies of neuroinflammation (80). Translocator protein (TSPO) is a 18 kDa mitochondrial membrane protein involved in steroid biosynthesis and is by far the most studied target to quantify microglial activation following TBI (81, 82). The first-generation TSPO ligand, [11C]-PK11195 is the most commonly employed; however, there are now several second-generation agents with improved signal to noise ratio and reduced non-specific binding. The second-generation agents all demonstrate variable binding between individuals relating to a specific genetic polymorphism resulting in different TSPO affinity for the radiolabeled ligands (83). PET studies have revealed that the binding of TSPO ligand PK-11195 remains elevated chronically, many years following TBI (81, 82), and that the degree of binding, and therefore chronic microglial activation, correlates with the degree of cognitive impairment (81). The second-generation TSPO ligand [11C]DPA-713 has been used to demonstrate cellular neuroinflammation in retired American football players (84, 85).
Translocator protein ligands are limited by their overexpression in reactive astrocytes, complicating the interpretation of the resulting binding (86, 87). Moreover, expense and the ability to generate radioligands on-site in the acute phase make the logistics of scanning complex. Nevertheless, currently, TSPO PET remains the only method for human in vivo imaging of microglial activation.
Other putative PET ligands to assess neuroinflammation include cannabinoid-2-receptor (88), cyclo-oxygenase (COX) (89), and matrix metalloproteinases (90) but these have not been adequately studied in acute brain injury. Furthermore, it is also possible that (18F)-FDG-PET may be applicable to imaging neuroinflammation, because of the apparent linkage between metabolic programing and inflammation (see below).
Magnetic Resonance Imaging (MRI)
The clinically used MRI sequences may be used to explore specific features of neuroinflammation (91), most commonly by assessing BBB integrity through the addition of the contrast agent gadolinium (92). In preclinical experimental settings, there are more advanced MRI applications, employing microparticles iron oxide, endothelial vascular cell adhesion molecule-1 (VCAM-1) (93), and macrophage-specific epitopes (94). However, in clinical studies in humans, MRI has not been employed to directly assess neuroinflammation, rather indirectly provide a measure of BBB dysfunction and endothelial cell activation.
Magnetic Resonance Spectroscopy (MRS)
The prevalent form of in vivo spectroscopy is 1H magnetic resonance spectroscopy (1H-MRS, also called proton MRS). This is employed to assess relative levels of cerebral metabolites. MRS is also known as NMR spectroscopy, but usually the term MRS refers to in vivo measurements and NMR to ex vivo or in vitro measurements. The most abundant signal in the brain 1H spectrum is usually N-acetylaspartate (NAA). The peptide N-acetylaspartylglutamate (NAAG) is a product of NAA and the two are interconvertible. NAAG is a small peak in the brain 1H-MRS that is difficult to distinguish from NAA. Often both are considered together as “total NAA” that is interpreted as a marker for neuronal health, viability, and/or number of neurons, particularly their mitochondria. Reduction in NAA is regarded as indicating dysfunction (permanent or temporary) of neuronal tissue. Other 1H signals include creatine (combined signal from creatine and phosphocreatine), glutamate and glutamine (often considered together as Glx as the two species’ signals are incompletely resolved), gamma-aminobutyric acid, and lactate. 1H MRS also allows detection of elevated levels of myo-inositol (osmolyte present in glial cells) and choline containing compounds. Commonly, MRS is used to measure metabolic activity in TBI (95), but these substances have also been shown to act as a surrogate of neuroinflammation (91, 96).
Another form of MRS less commonly used is 13C-MRS, which necessitates administration (usually intravenously, or sometimes orally) of substrates such as glucose, acetate, lactate etc. that have been manufactured to be artificially enriched in 13C (e.g., 99%), in contrast to the natural abundance of 13C that is only 1.1% of all carbons. 13C-MRS methodology is a powerful means of quantifying metabolic fluxes in vivo, e.g., the tricarboxylic acid cycle and glutamate–glutamine cycling (97). Also, in recent years, 13C hyperpolarization has evolved, a technique that boosts the 13C-MRS signal albeit for a very brief duration. Clinically, it utilizes hyperpolarized 1-13C pyruvate, given intravenously, to measure glycolysis vs TCA cycle, by means of signals for the respective products 1-13C lactate and 13C HCO3− (98). To date, it has mostly been used to image tumors (99), although results have emerged recently on 13C hyperpolarization in preclinical TBI (100, 101). 13C-MRS modalities may be useful in future in studies of neuroinflammation. A recent development (preclinically) is the use of hyperpolarized [6-13C] arginine to detect inflammatory cell function in cancer (102), and this could presumably be used in brain injury. A final MRS modality is 31P-MRS. No artificial enrichment is needed as 31P is virtually 100% of all naturally occurring phosphorus atoms. 31P-MRS is not commonly used, despite being able to measure energy-related phosphorus species non-invasively in vivo, including phosphocreatine, ATP, and inorganic phosphate. Notably, in a 31P-MRS study of TBI patients, Garnett et al. (103) suggested that the changes (vs healthy controls) might be due to reactive gliosis in the injured brain. The 31P-MRS modality is being actively investigated in acute brain injury.
The Adaptive Immune System
In comparison to the innate immune system, the adaptive immune response has not been as extensively studied. It is believed that the transition between the innate and adaptive immune response following acute brain injury is moderated by migrating antigen-presenting dendritic cells (104).
Cellular Components of the Adaptive Immune Response
The cellular components of the adaptive immune system can be measured in blood by identifying specific subpopulations of peripheral lymphocytes using flow cytometry. In severe TBI, this technology has revealed that the number of T-cells, specifically CD8+ cytotoxic cells, decrease significantly following TBI, while the number of B-cells remain constant (105).
Autoimmune Responses to Neural Antigens
The humoral adaptive immune system is also activated following TBI as a result of presentation of previously novel neural antigens to the peripheral immune system. Acute brain injury triggers the production of autoantibodies toward brain-specific proteins, including the brain-enriched proteins glial fibrillary acidic protein (106) and S100B (107). Moreover, Cox and coworkers showed that peripheral blood mononuclear cells isolated from patients with TBI have the capacity to proliferate in vitro when stimulated by myelin basic protein, the most abundant protein of the myelin sheath expressed in the brain by oligodendrocytes (108).
These adaptive responses are long lasting and are a candidate mechanism in the later stages of the pathophysiology of brain injury that may underlie chronic neurodegeneration (109); however, the mechanism by which empirically identified autoantibodies inflict neuronal injury is yet to be proven.
Failure of Clinical Trials Using “Anti-”Inflammatory Agents
There have been numerous failures to translate promising preclinical agents targeting the underlying conditions in brain injury studies into efficacious phase III human studies (110). Several reasons for this have been suggested, including small group sizes, inadequate dosing, inappropriate delivery route, inadequate therapy duration, and timing of drug delivery in relation to the occurrence of injury [several of the suggested harmful pro-inflammatory cytokines are elevated predominantly the first hours after injury (13)], the complexity of BBB and adequate drug penetration. In fact, large phase III studies, including corticosteroids (CRASH trial) (111), progesterone (112, 113), and erythropoietin (114) failed to detect the presence of the administered drug in the brain, something that could have been determined empirically using microdialysis (45). Moreover, due to poor penetration of pharmacological compounds across the BBB, lower concentrations of the drug may have reached the brain explaining the lack of expected neuroprotection (115). Before deciding to embark on large phase III ventures, which are costly and consume much time and resources, adequate phase II clinical studies with informative surrogate endpoints should be performed, including microdialysis, to ascertain the degree to which the drug can cross the BBB and exert changes on relevant biomarkers, including immune response-related molecules such as cytokines and chemokines.
Conceptual Understanding of Mechanism of Action
Within recent years, there have been several ongoing trials aimed at negating the neuroinflammatory response in brain injury (43). As we improve the monitoring of the inflammatory response, we can improve the accuracy of prediction of how new anti-inflammatory drugs impact on the innate inflammatory response, and when they should be delivered, in order to best modulate neuroinflammation following brain injury. However, improved monitoring techniques also lead to increased study complexity as more data become available. Helmy and coworkers treated 10 patients with an IL-1ra (Anakinra) and they demonstrated that the cytokine response in ECF in the treated group resembled that of the classical pro-inflammatory microglial activation (M1) (116), with increased IL-1β and low levels of IL-4 and IL-10. These findings were counterintutitive given the classification of IL1ra as an “anti-inflammatory cytokine” and therefore more in keeping with the M2 (anti-inflammatory, adaptive) response that would have led to a predominance of anti-inflammatory cytokines (57). Nevertheless, the more data we obtain from the complex cascades in the neuroinflammatory response, the greater the refinement in our understanding. There is an increasing recognition that microglia and macrophages have the capability to express both M1 and M2 specific phenotypes (117), and that the classification system does not accurately reflect the complexity of microglial function (118).
Over recent years, the idea of metabolic reprogramming of macrophages associated with inflammatory phenotype has gained ground. Very recently, a study by Ip et al., in the context of inflammatory bowel disease, has shown that IL-10 opposes the switch to the more glycolytic metabolic program induced by inflammatory stimuli in macrophages (119). Specifically, IL-10 inhibits lipopolysaccharide-induced glucose uptake and glycolysis and promotes oxidative phosphorylation. These findings may be relevant to harnessing metabolic reprogramming to treat inflammation in injured brain.
Chronic Sequelae of Neuroinflammation
Following brain injury, a chronic inflammatory state has been shown to correlate to several clinical conditions. One of the most common symptoms decreasing quality of life in patients that suffer from TBI is post-traumatic depression (PTD), which is present in up to 44% of patients (120). A study reported that higher levels of the acute CSF endothelial markers (sVCAM, sICAM-1, and sFAS) as well as IL-7 and IL-8, were associated with PTD as 6 and 12 months post-TBI, respectively (121).
Chronic traumatic encephalopathy (CTE) is a neurodegenerative condition leading to impairments in mood, behavior, cognition, and motor functioning and predominantly affecting patients with mild, repetitive TBI, common in contact sports (122). CTE has been defined as a tauopathy where phosphorylated tau accumulates within cells in the CNS (123). Recently, a cadaver study highlighted the presence of activated microglia (CD68-positive cells) close to tau deposits (124).
Another common complication following TBI is epilepsy (125), occurring in up to 20% of patients. Similarly, following SAH, a prevalence of between 7 and 25% has been reported (126, 127). Diamond et al. (128) found that a high CSF/plasma IL-1β ratio correlated with post-traumatic epilepsy (PTE) and that presence of a specific single-nucleotide peptide of the IL-1β gene (rs1143634) correlates with a higher risk of PTE following brain injury. Claassen and coworkers found an association between the inflammatory response following SAH (TNF-receptor 1 and high-sensitivity C-reactive protein) and the presence of seizures (129). Several reviews covering this field have been published, and all do suggest a strong link between neuroinflammatory cascades, increased excitotoxicity, and epileptogenesis in the aftermath of brain injury (130, 131).
It may therefore be possible to target inflammatory mediators after TBI and SAH, to alleviate the burden of these associated long-term morbidities.
Collinearity and Confounding Factors Between Inflammatory Mediators
Collinearity is a statistical term that describes how two (or more) variables correlate with each other to a high degree. As most inflammatory mediators are present at a low concentration in the uninjured brain, and the subsequent increase in concentration is a response to injury, they are likely to correlate with injury severity. It is therefore not surprising that many of the mediators can correlate with outcome and this may be confounded by the severity of injury, rather than a specific facet of the biology of the mediator of interest.
This is further complicated by the fact that most studies focus on a small panel of mediators such as the “pro”-inflammatory mediators such as IL-1β, IL-6, TNF, and IFN-γ as well as “anti”-inflammatory cytokines IL-4 and IL-10. However, we should not discount the importance of other unmeasured mediators in contributing to the detrimental inflammatory responses after TBI and SAH. Cytokines are known to act in complex cascades with some early potent mediators that subsequently trigger the synthesis of regulatory cytokines which act to temper the initial response. Although in some cases cytokines have partially overlapping functions, each cytokine has a unique role, pattern of expression, and cellular source. Moreover, cytokines and chemokines display effects that are both “damaging” and “reparative” that may occur simultaneously in the post-injury phase (57). This dual effect has been also acknowledged for microglia and macrophages as both play both a beneficial and detrimental role in the injured brain (9, 14).
Combination Therapy
Biological redundancy in complex interrelated systems means that there are multiple pathways to secondary neuronal injury (45). However, in most clinical trials, only a single therapy is used to target a single pathway limiting the potential efficacy. This is a potential reason why there is still no approved drug that has shown any clinical efficacy in mitigating neuronal injury in TBI and SAH. However, polytherapy using preclinically proven neuroprotective drugs (i.e., progesterone and Vitamin D hormone, creatinine, and choline) has also failed to show any treatment benefit (132), presumably as there are fundamental pathological differences between animal models and human brain injury (133) and also because we need a better understanding of the underlying pathophysiology and how the different pharmacological agents interact.
Nonetheless, a review of agents negating the neuroinflammatory cascades in brain injury indicates that there are many promising pharmaceutical agents currently being developed (43). Furthermore, with the tools currently available, it is increasingly feasible to monitor the neuroinflammatory response to therapy, thus allow researchers to rationalize specific combinations of therapy.
Challenges Ahead
There are several challenges and limitations in the field today. The major issues surrounding these techniques discussed in this manuscript are summarized in Table 2.
To measure and assess inflammatory activity, several current studies investigate a single marker in a single biological compartment. As discussed above, to adequately understand the neuroinflammatory response requires combinations of methodologies looking at multiple down-stream cytokines (59, 121), but also region specific focal inflammatory activity using PET-MRI (81, 82) and T-cell mediated autoreactivity toward brain-specific proteins (108).
Moreover, more sophisticated biostatistical methods analogous to the “-omic”-literature are required. Multivariate methods incorporating the entire cytokine profile using tools such as principal component analyses will presumably be necessary until we reach a greater understanding of these processes (57, 59).
In summary, we suggest a multimodal approach for the measurement of cytokines in various fluids, parallel to neuroimaging techniques to visualize changes in cellular/microglia activation with repeated measurement of both humoral and cellular immune activation to distinguish acute from chronic responses. Only this holistic approach will provide a deeper understanding of the longitudinal inflammatory processes in hope to develop accurately targeted and efficacious anti-inflammatory therapies.
Conclusion
The neuroinflammatory cascade following TBI and SAH comprises a wide array of both humoral and cellular players. An ever-growing literature has defined changes in cytokine production and cell activation in both the acute and chronic phases following brain injury. However, we are still confronted with the difficulty in placing the individual components into a coherent picture. The extended time course of inflammation following these conditions provides multiple opportunities for therapeutic intervention. However, there is a limit to what we can learn from observational clinical studies as to the role of individual mediators/cells and ultimately understand how they affect patient functional outcome. For this reason, it is pivotal to maintain a close dialog between clinical and experimental research, in order to identify the distinct role of cytokines and immune cells, acting in the large scheme of the complex inflammatory responses.
Author Contributions
ET, AH, TT, FZ, MM-K, DM, PH, and KC designed and planned the study. ET, AH, TT, FZ, MM-K, DM, PH, and KC drafted the manuscript, which all authors read and approved.
Conflict of Interest Statement
The authors declare that the research was conducted in the absence of any commercial or financial relationships that could be construed as a potential conflict of interest.
Funding
The following funding sources should be acknowledged: ET: Swedish Society of Medicine (Grant no. SLS-587221). FZ: this work was made possible through salary support through the Cambridge Commonwealth Trust Scholarship, the Royal College of Surgeons of Canada—Harry S. Morton Travelling Fellowship in Surgery, the University of Manitoba Clinician Investigator Program, R. Samuel McLaughlin Research and Education Award, the Manitoba Medical Service Foundation, and the University of Manitoba Faculty of Medicine Dean’s Fellowship Fund. DM: National Institute for Healthcare Research (NIHR, UK) through the Acute Brain Injury and Repair theme of the Cambridge NIHR Biomedical Research Centre, an NIHR Senior Investigator Award to DM. Authors were also supported by a European Union Framework Program 7 grant (CENTER-TBI; Grant Agreement No. 602150). PH: National Institute for Health Research Professorship, Academy of Medical Sciences/Health Foundation Senior Surgical Scientist Fellowship, and the National Institute for Health Research Biomedical Research Centre, Cambridge. KC: National Institute for Health Research Biomedical Research Centre, Cambridge. AH: Medical Research Council, Cambridge Biomedical Research Centre, Royal College of Surgeons of England. The funders had no role in study design, data collection and analysis, decision to publish, or preparation of the manuscript.
References
1. Roozenbeek B, Maas AI, Menon DK. Changing patterns in the epidemiology of traumatic brain injury. Nat Rev Neurol (2013) 9:231–6. doi:10.1038/nrneurol.2013.22
2. de Rooij NK, Linn FH, van der Plas JA, Algra A, Rinkel GJ. Incidence of subarachnoid haemorrhage: a systematic review with emphasis on region, age, gender and time trends. J Neurol Neurosurg Psychiatry (2007) 78:1365–72. doi:10.1136/jnnp.2007.117655
3. Hyder AA, Wunderlich CA, Puvanachandra P, Gururaj G, Kobusingye OC. The impact of traumatic brain injuries: a global perspective. NeuroRehabilitation (2007) 22:341–53.
4. Nieuwkamp DJ, Setz LE, Algra A, Linn FH, de Rooij NK, Rinkel GJ. Changes in case fatality of aneurysmal subarachnoid haemorrhage over time, according to age, sex, and region: a meta-analysis. Lancet Neurol (2009) 8:635–42. doi:10.1016/S1474-4422(09)70126-7
5. Maas AI, Stocchetti N, Bullock R. Moderate and severe traumatic brain injury in adults. Lancet Neurol (2008) 7:728–41. doi:10.1016/S1474-4422(08)70164-9
6. Werner C, Engelhard K. Pathophysiology of traumatic brain injury. Br J Anaesth (2007) 99:4–9. doi:10.1093/bja/aem131
7. Lozano D, Gonzales-Portillo GS, Acosta S, de la Pena I, Tajiri N, Kaneko Y, et al. Neuroinflammatory responses to traumatic brain injury: etiology, clinical consequences, and therapeutic opportunities. Neuropsychiatr Dis Treat (2015) 11:97–106. doi:10.2147/NDT.S65815
8. Schmidt OI, Heyde CE, Ertel W, Stahel PF. Closed head injury – an inflammatory disease? Brain Res Brain Res Rev (2005) 48:388–99. doi:10.1016/j.brainresrev.2004.12.028
9. Morganti-Kossmann MC, Satgunaseelan L, Bye N, Kossmann T. Modulation of immune response by head injury. Injury (2007) 38:1392–400. doi:10.1016/j.injury.2007.10.005
10. Dumont AS, Dumont RJ, Chow MM, Lin CL, Calisaneller T, Ley KF, et al. Cerebral vasospasm after subarachnoid hemorrhage: putative role of inflammation. Neurosurgery (2003) 53:123–33; discussion 133–5. doi:10.1227/01.NEU.0000068863.37133.9E
11. Louveau A, Smirnov I, Keyes TJ, Eccles JD, Rouhani SJ, Peske JD, et al. Structural and functional features of central nervous system lymphatic vessels. Nature (2015) 523:337–41. doi:10.1038/nature14432
12. Freidin M, Bennett MV, Kessler JA. Cultured sympathetic neurons synthesize and release the cytokine interleukin 1 beta. Proc Natl Acad Sci U S A (1992) 89:10440–3. doi:10.1073/pnas.89.21.10440
13. Helmy A, Carpenter KL, Menon DK, Pickard JD, Hutchinson PJ. The cytokine response to human traumatic brain injury: temporal profiles and evidence for cerebral parenchymal production. J Cereb Blood Flow Metab (2011) 31:658–70. doi:10.1038/jcbfm.2010.142
14. Morganti-Kossmann MC, Rancan M, Stahel PF, Kossmann T. Inflammatory response in acute traumatic brain injury: a double-edged sword. Curr Opin Crit Care (2002) 8:101–5. doi:10.1097/00075198-200204000-00002
15. Morganti-Kossmann MC, Rancan M, Otto VI, Stahel PF, Kossmann T. Role of cerebral inflammation after traumatic brain injury: a revisited concept. Shock (2001) 16:165–77. doi:10.1097/00024382-200116030-00001
16. Szalay G, Martinecz B, Lenart N, Kornyei Z, Orsolits B, Judak L, et al. Microglia protect against brain injury and their selective elimination dysregulates neuronal network activity after stroke. Nat Commun (2016) 7:11499. doi:10.1038/ncomms11499
17. Wan H, AlHarbi BM, Macdonald RL. Mechanisms, treatment and prevention of cellular injury and death from delayed events after aneurysmal subarachnoid hemorrhage. Expert Opin Pharmacother (2014) 15:231–43. doi:10.1517/14656566.2014.865724
18. Naraoka M, Matsuda N, Shimamura N, Asano K, Ohkuma H. The role of arterioles and the microcirculation in the development of vasospasm after aneurysmal SAH. Biomed Res Int (2014) 2014:253746. doi:10.1155/2014/253746
19. Chen S, Wu H, Tang J, Zhang J, Zhang JH. Neurovascular events after subarachnoid hemorrhage: focusing on subcellular organelles. Acta Neurochir Suppl (2015) 120:39–46. doi:10.1007/978-3-319-04981-6_7
20. Macdonald RL. Delayed neurological deterioration after subarachnoid haemorrhage. Nat Rev Neurol (2014) 10:44–58. doi:10.1038/nrneurol.2013.246
21. Brathwaite S, Macdonald RL. Current management of delayed cerebral ischemia: update from results of recent clinical trials. Transl Stroke Res (2014) 5:207–26. doi:10.1007/s12975-013-0316-8
22. Sanchez-Pena P, Pereira AR, Sourour NA, Biondi A, Lejean L, Colonne C, et al. S100B as an additional prognostic marker in subarachnoid aneurysmal hemorrhage. Crit Care Med (2008) 36:2267–73. doi:10.1097/CCM.0b013e3181809750
23. Graham DI, Macpherson P, Pitts LH. Correlation between angiographic vasospasm, hematoma, and ischemic brain damage following SAH. J Neurosurg (1983) 59:223–30. doi:10.3171/jns.1983.59.2.0223
24. Kawamura S, Sayama I, Yasui N, Uemura K. Sequential changes in cerebral blood flow and metabolism in patients with subarachnoid haemorrhage. Acta Neurochir (Wien) (1992) 114:12–5. doi:10.1007/BF01401107
25. Proust F, Debono B, Gerardin E, Hannequin D, Derrey S, Langlois O, et al. Angiographic cerebral vasospasm and delayed ischemic deficit on anterior part of the circle of Willis. Usefulness of transcranial Doppler. Neurochirurgie (2002) 48:489–99.
26. Geraud G, Tremoulet M, Guell A, Bes A. The prognostic value of noninvasive CBF measurement in subarachnoid hemorrhage. Stroke (1984) 15:301–5. doi:10.1161/01.STR.15.2.301
27. Powsner RA, O’Tuama LA, Jabre A, Melhem ER. SPECT imaging in cerebral vasospasm following subarachnoid hemorrhage. J Nucl Med (1998) 39:765–9.
28. Rabinstein AA, Friedman JA, Weigand SD, McClelland RL, Fulgham JR, Manno EM, et al. Predictors of cerebral infarction in aneurysmal subarachnoid hemorrhage. Stroke (2004) 35:1862–6. doi:10.1161/01.STR.0000133132.76983.8e
29. Weidauer S, Lanfermann H, Raabe A, Zanella F, Seifert V, Beck J. Impairment of cerebral perfusion and infarct patterns attributable to vasospasm after aneurysmal subarachnoid hemorrhage: a prospective MRI and DSA study. Stroke (2007) 38:1831–6. doi:10.1161/STROKEAHA.106.477976
30. Weidauer S, Vatter H, Beck J, Raabe A, Lanfermann H, Seifert V, et al. Focal laminar cortical infarcts following aneurysmal subarachnoid haemorrhage. Neuroradiology (2008) 50:1–8. doi:10.1007/s00234-007-0294-1
31. Dhar R, Scalfani MT, Blackburn S, Zazulia AR, Videen T, Diringer M. Relationship between angiographic vasospasm and regional hypoperfusion in aneurysmal subarachnoid hemorrhage. Stroke (2012) 43:1788–94. doi:10.1161/STROKEAHA.111.646836
32. Ibrahim GM, Weidauer S, Vatter H, Raabe A, Macdonald RL. Attributing hypodensities on CT to angiographic vasospasm is not sensitive and unreliable. Stroke (2012) 43:109–12. doi:10.1161/STROKEAHA.111.632745
33. Juvela S. Plasma endothelin concentrations after aneurysmal subarachnoid hemorrhage. J Neurosurg (2000) 92:390–400. doi:10.3171/jns.2000.92.3.0390
34. Macdonald RL, Higashida RT, Keller E, Mayer SA, Molyneux A, Raabe A, et al. Clazosentan, an endothelin receptor antagonist, in patients with aneurysmal subarachnoid haemorrhage undergoing surgical clipping: a randomised, double-blind, placebo-controlled phase 3 trial (CONSCIOUS-2). Lancet Neurol (2011) 10:618–25. doi:10.1016/S1474-4422(11)70108-9
35. Macdonald RL, Kassell NF, Mayer S, Ruefenacht D, Schmiedek P, Weidauer S, et al. Clazosentan to overcome neurological ischemia and infarction occurring after subarachnoid hemorrhage (CONSCIOUS-1): randomized, double-blind, placebo-controlled phase 2 dose-finding trial. Stroke (2008) 39:3015–21. doi:10.1161/STROKEAHA.108.519942
36. Udoetuk JD, Stiefel MF, Hurst RW, Weigele JB, LeRoux PD. Admission angiographic cerebral circulation time may predict subsequent angiographic vasospasm after aneurysmal subarachnoid hemorrhage. Neurosurgery (2007) 61:1152–9; discussion 1159–61. doi:10.1227/01.neu.0000306092.07647.6d
37. Provencio JJ. Inflammation in subarachnoid hemorrhage and delayed deterioration associated with vasospasm: a review. Acta Neurochir Suppl (2013) 115:233–8. doi:10.1007/978-3-7091-1192-5_42
38. Lucke-Wold BP, Logsdon AF, Manoranjan B, Turner RC, McConnell E, Vates GE, et al. Aneurysmal subarachnoid hemorrhage and neuroinflammation: a comprehensive review. Int J Mol Sci (2016) 17:497. doi:10.3390/ijms17040497
39. Muroi C, Hugelshofer M, Seule M, Tastan I, Fujioka M, Mishima K, et al. Correlation among systemic inflammatory parameter, occurrence of delayed neurological deficits, and outcome after aneurysmal subarachnoid hemorrhage. Neurosurgery (2013) 72:367–75; discussion 375. doi:10.1227/NEU.0b013e31828048ce
40. Schoch B, Regel JP, Wichert M, Gasser T, Volbracht L, Stolke D. Analysis of intrathecal interleukin-6 as a potential predictive factor for vasospasm in subarachnoid hemorrhage. Neurosurgery (2007) 60:828–36; discussion 828–36. doi:10.1227/01.NEU.0000255440.21495.80
41. Helmy A, Vizcaychipi M, Gupta AK. Traumatic brain injury: intensive care management. Br J Anaesth (2007) 99:32–42. doi:10.1093/bja/aem139
42. Connolly ES Jr, Rabinstein AA, Carhuapoma JR, Derdeyn CP, Dion J, Higashida RT, et al. Guidelines for the management of aneurysmal subarachnoid hemorrhage: a guideline for healthcare professionals from the American Heart Association/American Stroke Association. Stroke (2012) 43:1711–37. doi:10.1161/STR.0b013e3182587839
43. Hellewell S, Semple BD, Morganti-Kossmann MC. Therapies negating neuroinflammation after brain trauma. Brain Res (2015) 1640:36–56. doi:10.1016/j.brainres.2015.12.024
44. Buttram SD, Wisniewski SR, Jackson EK, Adelson PD, Feldman K, Bayir H, et al. Multiplex assessment of cytokine and chemokine levels in cerebrospinal fluid following severe pediatric traumatic brain injury: effects of moderate hypothermia. J Neurotrauma (2007) 24:1707–17. doi:10.1089/neu.2007.0349
45. Thelin EP, Carpenter KL, Hutchinson PJ, Helmy A. Microdialysis monitoring in clinical traumatic brain injury and its role in neuroprotective drug development. AAPS J (2017) 19:367–76. doi:10.1208/s12248-016-0027-7
46. Kumar RG, Boles JA, Wagner AK. Chronic inflammation after severe traumatic brain injury: characterization and associations with outcome at 6 and 12 months postinjury. J Head Trauma Rehabil (2015) 30:369–81. doi:10.1097/HTR.0000000000000067
47. Ferreira LC, Regner A, Miotto KD, Moura S, Ikuta N, Vargas AE, et al. Increased levels of interleukin-6, -8 and -10 are associated with fatal outcome following severe traumatic brain injury. Brain Inj (2014) 28:1311–6. doi:10.3109/02699052.2014.916818
48. Hollig A, Remmel D, Stoffel-Wagner B, Schubert GA, Coburn M, Clusmann H. Association of early inflammatory parameters after subarachnoid hemorrhage with functional outcome: a prospective cohort study. Clin Neurol Neurosurg (2015) 138:177–83. doi:10.1016/j.clineuro.2015.08.030
49. Kossmann T, Hans VH, Imhof HG, Stocker R, Grob P, Trentz O, et al. Intrathecal and serum interleukin-6 and the acute-phase response in patients with severe traumatic brain injuries. Shock (1995) 4:311–7. doi:10.1097/00024382-199511000-00001
50. Gruber A, Rossler K, Graninger W, Donner A, Illievich MU, Czech T. Ventricular cerebrospinal fluid and serum concentrations of sTNFR-I, IL-1ra, and IL-6 after aneurysmal subarachnoid hemorrhage. J Neurosurg Anesthesiol (2000) 12:297–306. doi:10.1097/00008506-200010000-00001
51. Stein DM, Lindell A, Murdock KR, Kufera JA, Menaker J, Keledjian K, et al. Relationship of serum and cerebrospinal fluid biomarkers with intracranial hypertension and cerebral hypoperfusion after severe traumatic brain injury. J Trauma (2011) 70:1096–103. doi:10.1097/TA.0b013e318216930d
52. Csuka E, Morganti-Kossmann MC, Lenzlinger PM, Joller H, Trentz O, Kossmann T. IL-10 levels in cerebrospinal fluid and serum of patients with severe traumatic brain injury: relationship to IL-6, TNF-alpha, TGF-beta1 and blood-brain barrier function. J Neuroimmunol (1999) 101:211–21. doi:10.1016/S0165-5728(99)00148-4
53. Kumar RG, Diamond ML, Boles JA, Berger RP, Tisherman SA, Kochanek PM, et al. Acute CSF interleukin-6 trajectories after TBI: associations with neuroinflammation, polytrauma, and outcome. Brain Behav Immun (2015) 45:253–62. doi:10.1016/j.bbi.2014.12.021
54. Kumar RG, Rubin JE, Berger RP, Kochanek PM, Wagner AK. Principal components derived from CSF inflammatory profiles predict outcome in survivors after severe traumatic brain injury. Brain Behav Immun (2016) 53:183–93. doi:10.1016/j.bbi.2015.12.008
55. Roberts DJ, Jenne CN, Leger C, Kramer AH, Gallagher CN, Todd S, et al. Association between the cerebral inflammatory and matrix metalloproteinase responses after severe traumatic brain injury in humans. J Neurotrauma (2013) 30:1727–36. doi:10.1089/neu.2012.2842
56. Helmy A, Carpenter KL, Skepper JN, Kirkpatrick PJ, Pickard JD, Hutchinson PJ. Microdialysis of cytokines: methodological considerations, scanning electron microscopy, and determination of relative recovery. J Neurotrauma (2009) 26:549–61. doi:10.1089/neu.2008.0719
57. Helmy A, Guilfoyle MR, Carpenter KL, Pickard JD, Menon DK, Hutchinson PJ. Recombinant human interleukin-1 receptor antagonist promotes M1 microglia biased cytokines and chemokines following human traumatic brain injury. J Cereb Blood Flow Metab (2016) 36:1434–48. doi:10.1177/0271678X15620204
58. Helmy A, Guilfoyle MR, Carpenter KL, Pickard JD, Menon DK, Hutchinson PJ. Recombinant human interleukin-1 receptor antagonist in severe traumatic brain injury: a phase II randomized control trial. J Cereb Blood Flow Metab (2014) 34:845–51. doi:10.1038/jcbfm.2014.23
59. Helmy A, Antoniades CA, Guilfoyle MR, Carpenter KL, Hutchinson PJ. Principal component analysis of the cytokine and chemokine response to human traumatic brain injury. PLoS One (2012) 7:e39677. doi:10.1371/journal.pone.0039677
60. Hutchinson PJ, O’Connell MT, Al-Rawi PG, Maskell LB, Kett-White R, Gupta AK, et al. Clinical cerebral microdialysis: a methodological study. J Neurosurg (2000) 93:37–43. doi:10.3171/jns.2000.93.1.0037
62. Ao X, Stenken JA. Microdialysis sampling of cytokines. Methods (2006) 38:331–41. doi:10.1016/j.ymeth.2005.11.012
63. Hillman J, Aneman O, Anderson C, Sjogren F, Saberg C, Mellergard P. A microdialysis technique for routine measurement of macromolecules in the injured human brain. Neurosurgery (2005) 56:1264–8; discussion 1268–70. doi:10.1227/01.NEU.0000159711.93592.8D
64. Chu J, Hjort K, Larsson A, Dahlin AP. Impact of static pressure on transmembrane fluid exchange in high molecular weight cut off microdialysis. Biomed Microdevices (2014) 16:301–10. doi:10.1007/s10544-013-9833-1
65. Dahlin AP, Purins K, Clausen F, Chu J, Sedigh A, Lorant T, et al. Refined microdialysis method for protein biomarker sampling in acute brain injury in the neurointensive care setting. Anal Chem (2014) 86:8671–9. doi:10.1021/ac501880u
66. Hillered L, Dahlin AP, Clausen F, Chu J, Bergquist J, Hjort K, et al. Cerebral microdialysis for protein biomarker monitoring in the neurointensive care setting – a technical approach. Front Neurol (2014) 5:245. doi:10.3389/fneur.2014.00245
67. Chu J, Koudriavtsev V, Hjort K, Dahlin AP. Fluorescence imaging of macromolecule transport in high molecular weight cut-off microdialysis. Anal Bioanal Chem (2014) 406:7601–9. doi:10.1007/s00216-014-8192-y
68. Kirchhoff C, Buhmann S, Bogner V, Stegmaier J, Leidel BA, Braunstein V, et al. Cerebrospinal IL-10 concentration is elevated in non-survivors as compared to survivors after severe traumatic brain injury. Eur J Med Res (2008) 13:464–8.
69. Hergenroeder GW, Moore AN, McCoy JP Jr, Samsel L, Ward NH III, Clifton GL, et al. Serum IL-6: a candidate biomarker for intracranial pressure elevation following isolated traumatic brain injury. J Neuroinflammation (2010) 7:19. doi:10.1186/1742-2094-7-19
70. Chiaretti A, Antonelli A, Mastrangelo A, Pezzotti P, Tortorolo L, Tosi F, et al. Interleukin-6 and nerve growth factor upregulation correlates with improved outcome in children with severe traumatic brain injury. J Neurotrauma (2008) 25:225–34. doi:10.1089/neu.2007.0405
71. Eriksson L, Antti H, Gottfries J, Holmes E, Johansson E, Lindgren F, et al. Using chemometrics for navigating in the large data sets of genomics, proteomics, and metabonomics (gpm). Anal Bioanal Chem (2004) 380:419–29. doi:10.1007/s00216-004-2783-y
72. Thelin EP, Just D, Frostell A, Haggmark-Manberg A, Risling M, Svensson M, et al. Protein profiling in serum after traumatic brain injury in rats reveals potential injury markers. Behav Brain Res (2016). doi:10.1016/j.bbr.2016.08.058
73. Zeiler FA, Thelin EP, Czosnyka M, Hutchinson PJ, Menon DK, Helmy A. Cerebrospinal fluid and microdialysis cytokines in severe traumatic brain injury: a scoping systematic review. Front Neurol (2017) 8:331. doi:10.3389/fneur.2017.00331
74. Woodcock T, Morganti-Kossmann MC. The role of markers of inflammation in traumatic brain injury. Front Neurol (2013) 4:18. doi:10.3389/fneur.2013.00018
75. Helmy A, De Simoni MG, Guilfoyle MR, Carpenter KL, Hutchinson PJ. Cytokines and innate inflammation in the pathogenesis of human traumatic brain injury. Prog Neurobiol (2011) 95:352–72. doi:10.1016/j.pneurobio.2011.09.003
76. Wu W, Guan Y, Zhao G, Fu XJ, Guo TZ, Liu YT, et al. Elevated IL-6 and TNF-alpha levels in cerebrospinal fluid of subarachnoid hemorrhage patients. Mol Neurobiol (2016) 53:3277–85. doi:10.1007/s12035-015-9268-1
77. Harish G, Mahadevan A, Pruthi N, Sreenivasamurthy SK, Puttamallesh VN, Keshava Prasad TS, et al. Characterization of traumatic brain injury in human brains reveals distinct cellular and molecular changes in contusion and pericontusion. J Neurochem (2015) 134:156–72. doi:10.1111/jnc.13082
78. Bellander BM, Singhrao SK, Ohlsson M, Mattsson P, Svensson M. Complement activation in the human brain after traumatic head injury. J Neurotrauma (2001) 18:1295–311. doi:10.1089/08977150152725605
79. Frugier T, Morganti-Kossmann MC, O’Reilly D, McLean CA. In situ detection of inflammatory mediators in post mortem human brain tissue after traumatic injury. J Neurotrauma (2010) 27:497–507. doi:10.1089/neu.2009.1120
80. Ory D, Celen S, Verbruggen A, Bormans G. PET radioligands for in vivo visualization of neuroinflammation. Curr Pharm Des (2014) 20:5897–913. doi:10.2174/1381612820666140613120212
81. Ramlackhansingh AF, Brooks DJ, Greenwood RJ, Bose SK, Turkheimer FE, Kinnunen KM, et al. Inflammation after trauma: microglial activation and traumatic brain injury. Ann Neurol (2011) 70:374–83. doi:10.1002/ana.22455
82. Folkersma H, Boellaard R, Yaqub M, Kloet RW, Windhorst AD, Lammertsma AA, et al. Widespread and prolonged increase in (R)-(11)C-PK11195 binding after traumatic brain injury. J Nucl Med (2011) 52:1235–9. doi:10.2967/jnumed.110.084061
83. Owen DR, Gunn RN, Rabiner EA, Bennacef I, Fujita M, Kreisl WC, et al. Mixed-affinity binding in humans with 18-kDa translocator protein ligands. J Nucl Med (2011) 52:24–32. doi:10.2967/jnumed.110.079459
84. Coughlin JM, Wang Y, Munro CA, Ma S, Yue C, Chen S, et al. Neuroinflammation and brain atrophy in former NFL players: an in vivo multimodal imaging pilot study. Neurobiol Dis (2015) 74:58–65. doi:10.1016/j.nbd.2014.10.019
85. Coughlin JM, Wang Y, Minn I, Bienko N, Ambinder EB, Xu X, et al. Imaging of glial cell activation and white matter integrity in brains of active and recently retired national football league players. JAMA Neurol (2017) 74:67–74. doi:10.1001/jamaneurol.2016.3764
86. Lavisse S, Guillermier M, Herard AS, Petit F, Delahaye M, Van Camp N, et al. Reactive astrocytes overexpress TSPO and are detected by TSPO positron emission tomography imaging. J Neurosci (2012) 32:10809–18. doi:10.1523/JNEUROSCI.1487-12.2012
87. Veiga S, Carrero P, Pernia O, Azcoitia I, Garcia-Segura LM. Translocator protein 18 kDa is involved in the regulation of reactive gliosis. Glia (2007) 55:1426–36. doi:10.1002/glia.20558
88. Evens N, Muccioli GG, Houbrechts N, Lambert DM, Verbruggen AM, Van Laere K, et al. Synthesis and biological evaluation of carbon-11- and fluorine-18-labeled 2-oxoquinoline derivatives for type 2 cannabinoid receptor positron emission tomography imaging. Nucl Med Biol (2009) 36:455–65. doi:10.1016/j.nucmedbio.2009.01.009
89. Takashima-Hirano M, Shukuri M, Takashima T, Goto M, Wada Y, Watanabe Y, et al. General method for the (11)C-labeling of 2-arylpropionic acids and their esters: construction of a PET tracer library for a study of biological events involved in COXs expression. Chemistry (2010) 16:4250–8. doi:10.1002/chem.200903044
90. Wagner S, Breyholz HJ, Faust A, Holtke C, Levkau B, Schober O, et al. Molecular imaging of matrix metalloproteinases in vivo using small molecule inhibitors for SPECT and PET. Curr Med Chem (2006) 13:2819–38. doi:10.2174/092986706778522002
91. Quarantelli M. MRI/MRS in neuroinflammation: methodology and applications. Clin Transl Imaging (2015) 3:475–89. doi:10.1007/s40336-015-0142-y
92. Rebeles F, Fink J, Anzai Y, Maravilla KR. Blood-brain barrier imaging and therapeutic potentials. Top Magn Reson Imaging (2006) 17:107–16. doi:10.1097/RMR.0b013e31802f5df9
93. McAteer MA, Sibson NR, von Zur Muhlen C, Schneider JE, Lowe AS, Warrick N, et al. In vivo magnetic resonance imaging of acute brain inflammation using microparticles of iron oxide. Nat Med (2007) 13:1253–8. doi:10.1038/nm1631
94. Pirko I, Johnson A, Ciric B, Gamez J, Macura SI, Pease LR, et al. In vivo magnetic resonance imaging of immune cells in the central nervous system with superparamagnetic antibodies. FASEB J (2004) 18:179–82. doi:10.1096/fj.02-1124fje
95. Croall I, Smith FE, Blamire AM. Magnetic resonance spectroscopy for traumatic brain injury. Top Magn Reson Imaging (2015) 24:267–74. doi:10.1097/RMR.0000000000000063
96. Chang L, Munsaka SM, Kraft-Terry S, Ernst T. Magnetic resonance spectroscopy to assess neuroinflammation and neuropathic pain. J Neuroimmune Pharmacol (2013) 8:576–93. doi:10.1007/s11481-013-9460-x
97. Rothman DL, De Feyter HM, de Graaf RA, Mason GF, Behar KL. 13C MRS studies of neuroenergetics and neurotransmitter cycling in humans. NMR Biomed (2011) 24:943–57. doi:10.1002/nbm.1772
98. Chaumeil MM, Najac C, Ronen SM. Studies of metabolism using (13)C MRS of hyperpolarized probes. Methods Enzymol (2015) 561:1–71. doi:10.1016/bs.mie.2015.04.001
99. Chaumeil MM, Lupo JM, Ronen SM. Magnetic resonance (MR) metabolic imaging in glioma. Brain Pathol (2015) 25:769–80. doi:10.1111/bpa.12310
100. Guglielmetti C, Najac C, Chou A, Van der Linden A, Ronen SM, Rosi S, et al. MR metabolic imaging of neuroinflammation using hyperpolarized 13C MR. 2017 HMTRC Workshop. San Francisco: Hyperpolarized MRI Technology Resource Center, Department of Radiology & Biomedical Imaging, University of California (2017).
101. DeVience SJ, Lu X, Proctor J, Rangghran P, Melhem ER, Gullapalli R, et al. Metabolic imaging of energy metabolism in traumatic brain injury using hyperpolarized [1-13C]pyruvate. Sci Rep (2017) 7:1907. doi:10.1038/s41598-017-01736-x
102. Najac C, Chaumeil MM, Kohanbash G, Guglielmetti C, Gordon JW, Okada H, et al. Detection of inflammatory cell function using (13)C magnetic resonance spectroscopy of hyperpolarized [6-(13)C]-arginine. Sci Rep (2016) 6:31397. doi:10.1038/srep31397
103. Garnett MR, Corkill RG, Blamire AM, Rajagopalan B, Manners DN, Young JD, et al. Altered cellular metabolism following traumatic brain injury: a magnetic resonance spectroscopy study. J Neurotrauma (2001) 18:231–40. doi:10.1089/08977150151070838
104. Yilmaz A, Fuchs T, Dietel B, Altendorf R, Cicha I, Stumpf C, et al. Transient decrease in circulating dendritic cell precursors after acute stroke: potential recruitment into the brain. Clin Sci (Lond) (2009) 118:147–57. doi:10.1042/CS20090154
105. Mrakovcic-Sutic I, Tokmadzic VS, Laskarin G, Mahmutefendic H, Lucin P, Zupan Z, et al. Early changes in frequency of peripheral blood lymphocyte subpopulations in severe traumatic brain-injured patients. Scand J Immunol (2010) 72:57–65. doi:10.1111/j.1365-3083.2010.02407.x
106. Wang KK, Yang Z, Yue JK, Zhang Z, Winkler EA, Puccio AM, et al. Plasma anti-glial fibrillary acidic protein autoantibody levels during the acute and chronic phases of traumatic brain injury: a transforming research and clinical knowledge in traumatic brain injury pilot study. J Neurotrauma (2016) 33:1270–7. doi:10.1089/neu.2015.3881
107. Marchi N, Bazarian JJ, Puvenna V, Janigro M, Ghosh C, Zhong J, et al. Consequences of repeated blood-brain barrier disruption in football players. PLoS One (2013) 8:e56805. doi:10.1371/journal.pone.0056805
108. Cox AL, Coles AJ, Nortje J, Bradley PG, Chatfield DA, Thompson SJ, et al. An investigation of auto-reactivity after head injury. J Neuroimmunol (2006) 174:180–6. doi:10.1016/j.jneuroim.2006.01.007
109. Kelso ML, Gendelman HE. Bridge between neuroimmunity and traumatic brain injury. Curr Pharm Des (2014) 20:4284–98.
110. Kabadi SV, Faden AI. Neuroprotective strategies for traumatic brain injury: improving clinical translation. Int J Mol Sci (2014) 15:1216–36. doi:10.3390/ijms15011216
111. Roberts I, Yates D, Sandercock P, Farrell B, Wasserberg J, Lomas G, et al. Effect of intravenous corticosteroids on death within 14 days in 10008 adults with clinically significant head injury (MRC CRASH trial): randomised placebo-controlled trial. Lancet (2004) 364:1321–8. doi:10.1016/S0140-6736(04)17188-2
112. Wright DW, Yeatts SD, Silbergleit R, Palesch YY, Hertzberg VS, Frankel M, et al. Very early administration of progesterone for acute traumatic brain injury. N Engl J Med (2014) 371:2457–66. doi:10.1056/NEJMoa1404304
113. Skolnick BE, Maas AI, Narayan RK, van der Hoop RG, MacAllister T, Ward JD, et al. A clinical trial of progesterone for severe traumatic brain injury. N Engl J Med (2014) 371:2467–76. doi:10.1056/NEJMoa1411090
114. Nichol A, French C, Little L, Haddad S, Presneill J, Arabi Y, et al. Erythropoietin in traumatic brain injury (EPO-TBI): a double-blind randomised controlled trial. Lancet (2015) 386:2499–506. doi:10.1016/S0140-6736(15)00386-4
115. Shannon RJ, Carpenter KL, Guilfoyle MR, Helmy A, Hutchinson PJ. Cerebral microdialysis in clinical studies of drugs: pharmacokinetic applications. J Pharmacokinet Pharmacodyn (2013) 40:343–58. doi:10.1007/s10928-013-9306-4
116. Mosser DM, Edwards JP. Exploring the full spectrum of macrophage activation. Nat Rev Immunol (2008) 8:958–69. doi:10.1038/nri2448
117. Kim CC, Nakamura MC, Hsieh CL. Brain trauma elicits non-canonical macrophage activation states. J Neuroinflammation (2016) 13:117. doi:10.1186/s12974-016-0581-z
118. Ransohoff RM. A polarizing question: do M1 and M2 microglia exist? Nat Neurosci (2016) 19:987–91. doi:10.1038/nn.4338
119. Ip WKE, Hoshi N, Shouval DS, Snapper S, Medzhitov R. Anti-inflammatory effect of IL-10 mediated by metabolic reprogramming of macrophages. Science (2017) 356:513–9. doi:10.1126/science.aal3535
120. Hibbard MR, Uysal S, Kepler K, Bogdany J, Silver J. Axis I psychopathology in individuals with traumatic brain injury. J Head Trauma Rehabil (1998) 13:24–39. doi:10.1097/00001199-199808000-00003
121. Juengst SB, Kumar RG, Failla MD, Goyal A, Wagner AK. Acute inflammatory biomarker profiles predict depression risk following moderate to severe traumatic brain injury. J Head Trauma Rehabil (2015) 30:207–18. doi:10.1097/HTR.0000000000000031
122. McKee AC, Daneshvar DH, Alvarez VE, Stein TD. The neuropathology of sport. Acta Neuropathol (2014) 127:29–51. doi:10.1007/s00401-013-1230-6
123. McKee AC, Cairns NJ, Dickson DW, Folkerth RD, Keene CD, Litvan I, et al. The first NINDS/NIBIB consensus meeting to define neuropathological criteria for the diagnosis of chronic traumatic encephalopathy. Acta Neuropathol (2016) 131:75–86. doi:10.1007/s00401-015-1515-z
124. Cherry JD, Tripodis Y, Alvarez VE, Huber B, Kiernan PT, Daneshvar DH, et al. Microglial neuroinflammation contributes to tau accumulation in chronic traumatic encephalopathy. Acta Neuropathol Commun (2016) 4:112. doi:10.1186/s40478-016-0382-8
125. Kolakowsky-Hayner SA, Wright J, Englander J, Duong T, Ladley-O’Brien S. Impact of late post-traumatic seizures on physical health and functioning for individuals with brain injury within the community. Brain Inj (2013) 27:578–86. doi:10.3109/02699052.2013.765595
126. Claassen J, Peery S, Kreiter KT, Hirsch LJ, Du EY, Connolly ES, et al. Predictors and clinical impact of epilepsy after subarachnoid hemorrhage. Neurology (2003) 60:208–14. doi:10.1212/01.WNL.0000038906.71394.DE
127. Olafsson E, Gudmundsson G, Hauser WA. Risk of epilepsy in long-term survivors of surgery for aneurysmal subarachnoid hemorrhage: a population-based study in Iceland. Epilepsia (2000) 41:1201–5. doi:10.1111/j.1528-1157.2000.tb00326.x
128. Diamond ML, Ritter AC, Failla MD, Boles JA, Conley YP, Kochanek PM, et al. IL-1beta associations with posttraumatic epilepsy development: a genetics and biomarker cohort study. Epilepsia (2015) 56:991–1001. doi:10.1111/epi.13100
129. Claassen J, Albers D, Schmidt JM, De Marchis GM, Pugin D, Falo CM, et al. Nonconvulsive seizures in subarachnoid hemorrhage link inflammation and outcome. Ann Neurol (2014) 75:771–81. doi:10.1002/ana.24166
130. Webster KM, Sun M, Crack P, O’Brien TJ, Shultz SR, Semple BD. Inflammation in epileptogenesis after traumatic brain injury. J Neuroinflammation (2017) 14:10. doi:10.1186/s12974-016-0786-1
131. Lucke-Wold BP, Nguyen L, Turner RC, Logsdon AF, Chen YW, Smith KE, et al. Traumatic brain injury and epilepsy: underlying mechanisms leading to seizure. Seizure (2015) 33:13–23. doi:10.1016/j.seizure.2015.10.002
132. Margulies S, Anderson G, Atif F, Badaut J, Clark R, Empey P, et al. Combination therapies for traumatic brain injury: retrospective considerations. J Neurotrauma (2016) 33:101–12. doi:10.1089/neu.2014.3855
Keywords: neuroinflammation, traumatic brain injury, subarachnoid hemorrhage, multimodal monitoring, secondary brain injury
Citation: Thelin EP, Tajsic T, Zeiler FA, Menon DK, Hutchinson PJA, Carpenter KLH, Morganti-Kossmann MC and Helmy A (2017) Monitoring the Neuroinflammatory Response Following Acute Brain Injury. Front. Neurol. 8:351. doi: 10.3389/fneur.2017.00351
Received: 15 May 2017; Accepted: 04 July 2017;
Published: 20 July 2017
Edited by:
Kenneth Curley, Iatrikos Research and Development Strategies LLC, United StatesReviewed by:
Marco Fidel Avila-Rodriguez, Universidad del Tolima, ColombiaDavid J. Loane, University of Maryland School of Medicine, United States
Copyright: © 2017 Thelin, Tajsic, Zeiler, Menon, Hutchinson, Carpenter, Morganti-Kossmann and Helmy. This is an open-access article distributed under the terms of the Creative Commons Attribution License (CC BY). The use, distribution or reproduction in other forums is permitted, provided the original author(s) or licensor are credited and that the original publication in this journal is cited, in accordance with accepted academic practice. No use, distribution or reproduction is permitted which does not comply with these terms.
*Correspondence: Eric Peter Thelin, ZXJpYy50aGVsaW5Aa2kuc2U=