- 1Inserm U894, Université Paris Descartes, Paris, France
- 2Plateforme de Recherche Clinique en Gériatrie, Hôpitaux universitaires Pitié-Salpêtrière-Charles Foix, APHP, Ivry-sur-Seine, France
- 3Service de Gériatrie à orientation Cardiologique et Neurologique, Sorbonne Université, Hôpitaux Universitaires Pitié-Salpêtrière-Charles Foix, APHP, Ivry-sur-Seine, France
- 4Département de soins ambulatoires, Hôpitaux universitaires Pitié-Salpêtrière-Charles Foix, APHP, Ivry-sur-Seine, France
- 5FR3636 CNRS, Université Paris Descartes, Paris, France
- 6Department of Life Sciences, Université Paris Diderot, Paris, France
Manual dexterity measures can be useful for early detection of age-related functional decline and for prediction of cognitive decline. However, what aspects of sensorimotor function to assess remains unclear. Manual dexterity markers should be able to separate impairments related to cognitive decline from those related to healthy aging. In this pilot study, we aimed to compare manual dexterity components in patients diagnosed with cognitive decline (mean age: 84 years, N = 11) and in age comparable cognitively intact elderly subjects (mean age: 78 years, N = 11). In order to separate impairments due to healthy aging from deficits due to cognitive decline we also included two groups of healthy young adults (mean age: 26 years, N = 10) and middle-aged adults (mean age: 41 years, N = 8). A comprehensive quantitative evaluation of manual dexterity was performed using three tasks: (i) visuomotor force tracking, (ii) isochronous single finger tapping with auditory cues, and (iii) visuomotor multi-finger tapping. Results showed a highly significant increase in force tracking error with increasing age. Subjects with cognitive decline had increased finger tapping variability and reduced ability to select the correct tapping fingers in the multi-finger tapping task compared to cognitively intact elderly subjects. Cognitively intact elderly subjects and those with cognitive decline had prolonged force release and reduced independence of finger movements compared to young adults and middle-aged adults. The findings suggest two different patterns of impaired manual dexterity: one related to cognitive decline and another related to healthy aging. Manual dexterity tasks requiring updating of performance, in accordance with (temporal or spatial) task rules maintained in short-term memory, are particularly affected in cognitive decline. Conversely, tasks requiring online matching of motor output to sensory cues were affected by age, not by cognitive status. Remarkably, no motor impairments were detected in patients with cognitive decline using clinical scales of hand function. The findings may have consequences for the development of manual dexterity markers of cognitive decline.
Introduction
Cognitive aging represents the reduction of mental abilities with age, such as attention, memory function, and information processing speed (1). The prevalence of Mild Cognitive Impairment (MCI) and Alzheimer's disease (AD) increases strongly with age. These conditions are common in approximately 10% of the population over 65 years of age. And in about 50% of those over 85 years, who develop AD (2). Due to high prevalence of dementia in age, early detection and prediction of cognitive decline remains a key challenge in public health. Previous studies suggest a relationship between cognitive decline and impairments in hand motor function (3, 4). There is an increasing number of studies on sensorimotor markers of cognitive decline in AD and MCI. Sensorimotor performances have been investigated by several methods available in clinical settings like gait, postural equilibrium (5–7) or neuropsychological tests (8, 9). Markers of impaired manual dexterity have also been used (3), and a recent longitudinal 4-year cohort study found that prolonged time taken in two simple manual dexterity tasks (including putting on and buttoning a shirt) was related to higher risk of developing cognitive decline [according to MMSE; (10)]. Sensorimotor markers are considered independent of educational level (11), which is an advantage for clinical use. However, despite these promising results some issues remain unresolved. First, as a potential marker, what type of manual dexterity task and which type of performance variable is optimal? Performance measures previously used were most often global task-based measures, i.e., time taken to complete task (5, 6, 10). Thus, it remained unclear what aspect of sensorimotor control was being measured, making the rationale for detecting cognitive decline uncertain. A second issue, also relevant for comparing different sensorimotor markers, concerns the role of cognition in a given sensorimotor task. Most motor tasks also involve cognitive control such as attention, planning, prediction (12), and cognitive factors are increasingly being recognized as important for motor control (13, 14). Cognitive assessments, probing executive functions, can also be used to predict cognitive decline (8, 15). Improved detection of sensorimotor impairments in MCI patients has been found when assessed in a dual-task condition, with enhanced effect in counting tasks compared to verbal fluency tasks (5). Therefore, it is likely that sensorimotor performance measures incorporating cognitive control would enhance discrimination and improve detection of cognitive decline.
Manual dexterity is complex and can be defined as the ability to accurately and rapidly control finger movements in a coordinated and adaptive manner, such as fine control in grasping and manipulation of small objects. Manual dexterity is highly specialized in humans (16) allowing a rich repertoire of goal- and object-oriented manual control. Manual dexterity deteriorates with aging and can negatively impact activities of daily living and independence (17). Studies have reported age-related impairments in maximal grip force (18) sensory functioning (19, 20) and in grasping and manipulation of objects [Box and Block test (21, 22), NHPT (23, 24)]. Regarding specific manual dexterity components, accuracy in force control tasks is reduced in age (25, 26) and independence of finger movements may deteriorate (27). Increased variability of finger movements (28) and motor slowing (29) have also been documented. These studies suggest a complex multi-component decline in manual dexterity in older people, especially in the very old (30). However, it is less clear how age-related sensorimotor impairments relate to cognitive decline, and how those two compare. In particular, whether different measures of manual dexterity reflect sensorimotor or rather cognitive control has not been investigated so far.
The aim in this study was to use the Finger Force Manipulandum, developed for the measurement of multiple components of manual dexterity (31), to disentangle manual dexterity impairments due to cognitive decline from those related to age-related sensorimotor impairment (25). We hypothesized that manual dexterity tasks strongly dependent on executive functions (attention, working memory) would be differently affected by cognitive decline compared to tasks involving fewer cognitive constraints.
Methods
Participants
This cross-sectional observational study included four groups of participants recruited from Hôpital Pitié-Salpêtrière-Charles Foix, Paris and the Centre de Psychiatrie et Neurosciences, Paris. We studied three groups of healthy participants: young adults [YA, N = 10, 6F/4M, mean age ± SD = 26 ± 3 y, range (21–30 y)], middle-aged adults [MA, N = 8, 3F/5M, mean age = 41 ± 9y, (32–55 y)], cognitively intact elderly subjects [ES, N = 11, 7F/4M, mean age = 78 ± 8y, (68–93 y)] and one group of elderly subjects with cognitive decline [CD, N = 11, 8F/3M, mean age = 84 ± 7 y, (73–96 y)], consisting of either MCI or early Alzheimer's disease (AD). All participants reported being right-handed with a laterality quotient above than 0 according to the Edinburgh Handedness Inventory (32). Patients in the CD group had been previously diagnosed of MCI or early AD by an experienced geriatrician, accordingly to the National Institute on Aging—Alzheimer's Association criteria (33).
Exclusion criteria were any neurological, orthopedic, or age-related disorders that could affect their manual dexterity. A brief interview preceded all testing, to determine whether subjects met the inclusion criteria. Elderly subjects with cognitive decline also underwent additional clinical neuropsychological evaluation (see below).
Elderly subjects were participants of a larger study on health and functional recovery in a geriatric population post-transaortic valve implantation. Young and middle-aged adults were volunteers who underwent dexterity assessment for the purpose of another study. Ethical approval was obtained from local ethical committee (CPP, Ile de France). Informed consent was obtained from all participants and the study was conducted in accordance to the Declaration of Helsinki.
Clinical Measures
Upper extremity sensorimotor function was assessed in all elderly subjects (i.e., in healthy elderly and in subjects with cognitive decline) using the following tests. The Nine-Hole Peg Test [NHPT, (22)] was used to qualitatively evaluate precision grip and object manipulation. Both the dominant and non-dominant hands were tested twice, and the average time taken to place and remove all pegs of each hand was calculated. The Box and Blocks Test [BBT, (34)] was used to measure gross manual dexterity. The Jebsen Taylor hand function test [JTHFT, (24)] was used to evaluate fine and gross motor hand function. The pinch gauge [Patterson Medical Inc. (35)] was used to measure maximal strength in precision, key (lateral), palmar (three-jaw chuck) and pinch grips (best of three attempts recorded). Performance in right and left hands was measured in the participants. The Instrumental Activities of Daily Living Scale [IADL, (36)] as used to assess independent living skills. Patients were scored according to their highest level of functioning using a summary score that ranges from 0 (low function, dependent) to 14 (high function, independent) (37). Sensory function was tested through light touch-test (Semmes-Weinstein Monofilaments). This provided an evaluation of cutaneous sensitivity of finger tips (38).
Neuropsychological assessments were performed by a neuropsychologist. It included the Mini-Mental State Examination (MMSE) and a more detailed neuropsychological assessment to document the presence or absence of cognitive decline. Neuropsychological testing included in most cases the Dubois test of verbal episodic memory (39), the French version of the Free and cued selective reminding test [RL/RI-16; (40)], the French version of the Listening Span Test (EMPANS), the French version of the Frontal Assessment Battery [Batterie rapide d'efficience mentale, BREF; (41)], the Verbal Fluency Test, which assesses semantic memory (42), as well as the figure of Rey test.
Finger Force Manipulandum Tasks
Manual dexterity components were measured using the Finger Force Manipulandum (FFM; http://www.sensix.fr1), a device with force sensitive pistons linked to various visuo-motor tasks [Figure 1, (31)]. Individual force data for each finger (index, middle, ring and little finger) were sampled at 10 KHz using a CED1401® (www.ced.co.uk) connected to a computer running Spike2V6®, which provided real-time visual display of finger forces together with target instructions. Three FFM tasks, as described previously (43) were used (Figure 2A):
(i) The finger force tracking task was used to measure precision of index fingertip force modulation. Subjects were instructed to accurately match the applied index finger force to the target force. The applied force was displayed in real-time as a cursor moving vertically as a function of force. The target force was displayed by a moving line. Each trial was composed of a ramp (linearly increasing force), a hold (static maintenance of force), and a release phase (instantaneous drop in target force back to baseline level, 0N). Trials were separated by 3 s rest. A total of 48 trials were performed in eight blocks (four with 1N and four with 2N target hold force) in alternating order.
(ii) The single finger tapping task was used to measure performance of rhythmic tapping at 1, 2, and 3 Hz. For each finger, subjects were instructed first to follow auditory rhythmic cues by tapping on the piston. After 15 auditory-cued trials, subjects had to continue tapping 15 trials at the same rate without auditory cues (total number of trials per frequency for each finger: 30).
(iii) The multi-finger tapping task was used to measure the independence of finger movements. Subjects were instructed to reproduce different finger tap combinations according to displayed target instructions within a 2 s time window. Trials consisted of single finger taps (separate tap of index, middle, ring or little finger; each performed 8 times for a total of 32 single finger trials) or two-finger tap combinations (simultaneous taps of index-middle, index-ring, index-little, middle-ring, middle-little, or ring-little fingers; each performed 5 times for a total of 30 two-finger trials). The sequence of trials was pseudo-randomized.
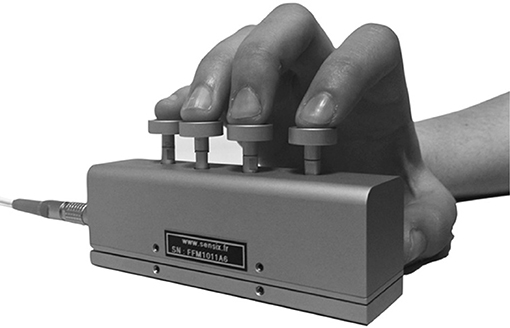
Figure 1. The Finger Force Manipulandum (FFM). Index, middle, ring, and little finger each apply forces on separate spring-loaded pistons. In the force tracking task, graduated force was exerted on one piston (index finger). In single and multi-finger tapping tasks the subject was instructed to tap on the corresponding piston(s) in response to auditory or visual cues without trying to match a particular force level (no force constraint).
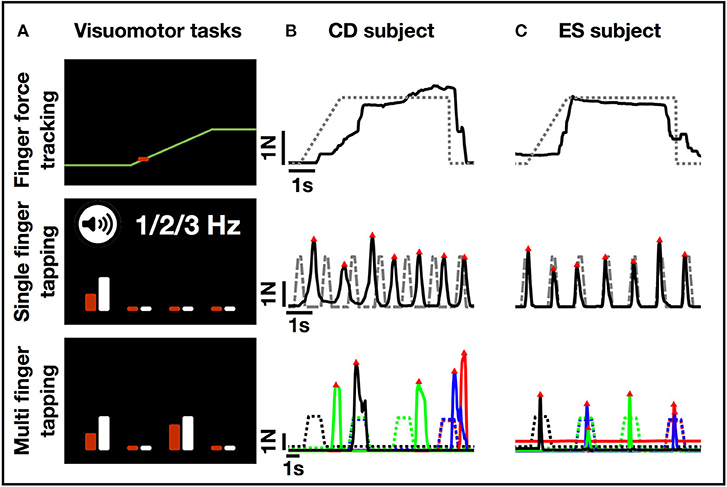
Figure 2. FFM task display and single trial performance examples. (A) Examples from the visual display of the three FFM tasks. In the finger force tracking task, the subject matched the force applied on the piston (represented as a red cursor that moves vertically as a function of force) to the target force trajectory (yellow right-to-left moving line) displayed on computer screen. In the single finger tapping task, the subject performed repeated tapping with a single finger following auditory cues at a given rate. The white bar on the screen indicated which finger had to perform the tapping task (here the index finger), while the red bar gave a visual feedback of which finger is being selected by the subject. The length (height) of each bar was a function of force. In the multi-finger tapping task, the subject was instructed to perform a one or two-finger tap with fingers matching the visual cues on the screen (here the instruction indicates a two-finger tap using the index and ring finger). (B) Single trial recordings from a subject in the cognitive decline (CD) group. (C) Single trial recordings from a subject in the elderly subjects (ES) group. Note: greater variability in single finger tapping and difficulty selecting correct finger to tap with in multi-finger tapping task (the performed taps do not match the cues indicated by the stippled line). Color code: blue, index; red, middle; green, ring; black, little finger. The four trials from left to right represent: a single (little) finger tap, followed by a two-finger (index, little) tap, a single (ring) finger tap, and another two-finger (index, middle) tap.
Data Analysis
Visuomotor performance was analyzed using MatlabV9.1 (The MathWorks, Inc., Natick, MA, USA). Raw data of the four finger force signals was first down-sampled to 100 Hz (and then smoothed using a 20 ms sliding window). The following measures were first extracted trial-by-trial and then averaged across trials for each task and condition (e.g., for a single subject in CD and ES groups in Figures 2B,C).
(i) Finger force tracking:
• Tracking error (N) was calculated as the absolute summed error between the ideal target force and the user applied force. The tracking error was extracted separately in the ramp and hold phase for each trial (total of 48 trials).
• Release duration (ms) was calculated as the time taken to instantaneously reduce the user-applied force from 75 to 25% of the target force (total of 48 trials).
The data of the two tapping tasks were analyzed with a peak detection algorithm allowing identification of finger taps of a minimal force amplitude (>0.5N). All detected taps were then categorized as correct (detected tap = target instruction) or incorrect (detected tap ≠ target instruction). Incorrect taps included “overflow taps” (presence of unwanted extra finger tap while correctly matching the target finger) and “error taps” (presence of unwanted extra finger tap in absence of a correct finger tap). The following task-specific measures were calculated:
(ii) Single finger tapping:
• Tap frequency: mean tapping frequency (Hz) performed during 1, 2, or 3 Hz conditions during auditory cues (15 taps) or without auditory cues (equivalent time).
• Standard deviation (SD) Tap interval: tap interval (ms) variability between two successive finger taps during 1, 2, or 3 Hz condition.
(iii) Multi-finger tapping:
• Selectivity index: rate (%) of correct finger taps matching the target (non-target taps were not considered).
• Individuation index: rate (%) of correct finger taps matching the target in absence of incorrect taps.
Statistical Analysis
Statistical analyses of clinical and behavioral measures were performed using Statistica10 (StatSoft, Inc., USA). Student's t-test or Mann-Whitney U-test were used to test for group differences in demographic and clinical outcomes. Group differences of FFM measures were analyzed using a general linear model repeated measures ANOVA with one GROUP factor (YA/A/ES/CD) and task-related within-group factors:
(i) Finger force tracking: FORCE (1N and 2N) and PHASE (RAMP and HOLD)
(ii) Single finger tapping: FREQUENCY (1, 2, and 3 Hz), FINGER (index, middle, ring, little finger), PHASE (auditory-cued, without feedback)
(iii) Multi-finger tapping: FINGER (index, middle, ring, little finger), COMBINATION (single and two-finger taps)
Fisher LSD post-hoc test was used to investigate differences revealed by ANOVA.
Results
Demographic and clinical details of elderly subjects and patients with cognitive decline are shown in Table 1.
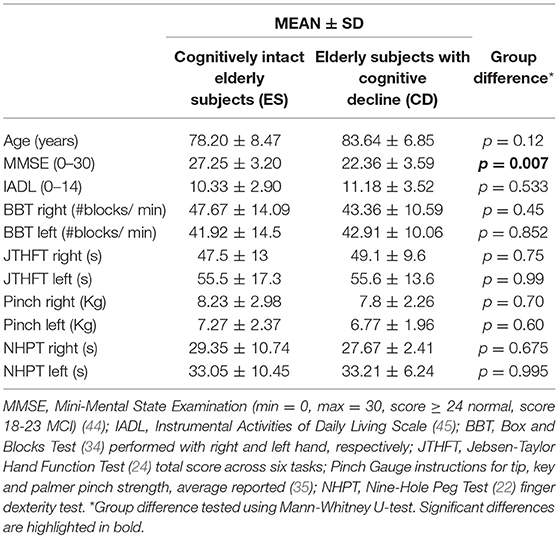
Table 1. Clinical data for the two groups: elderly subjects (ES) and patients with cognitive decline (CD).
FFM Task Feasibility
All subjects successfully performed the finger force tracking task. Two participants in the elderly subjects group were not able to complete the single and multi-finger tapping tasks due to limited time or unwillingness to complete the full protocol. In the cognitive decline group, four participants were not able to complete the multi-finger tapping task due to an inability to use the visual feedback in the allotted time window [task-related issue similar to (31)].
Group Comparisons for Dexterity Components
Finger Force Tracking
Qualitatively, this task revealed striking differences in the ability to precisely control forces with increasing age. Single subject/single trial examples are shown in Figure 2A. The ANOVA of tracking error showed significant group differences [F(3, 36) = 18.60, p < 0.001, Figure 3A]. Post-hoc testing revealed that young adults (YA) had smaller errors compared to other groups (Table 2, p < 0.05). Young adults also had decreased error compared to elderly subjects (p = 0.03) and to subjects with cognitive decline (p = 0.001). However, error did not differ between elderly subjects and those with cognitive decline (p = 0.16). Release duration also changed as a function of age [GROUP F(3, 36) = 3.18, p = 0.02). Post-hoc testing showed that both elderly subjects and those with cognitive decline had increased release duration compared to young adults (Table 2, p < 0.05).
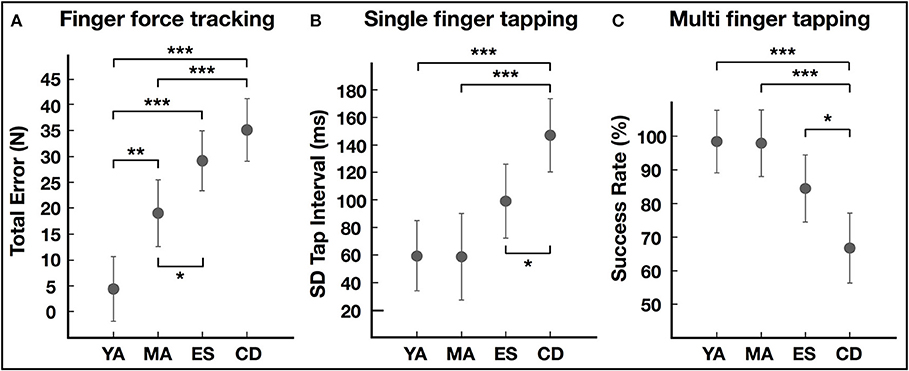
Figure 3. Group comparisons of performance in FFM tasks. (A) Error during finger force tracking task. (B) Variability of intertap interval in single finger tapping task. (C) Success rate (also termed the selectivity index) in the multi-finger tapping task. YA, young adults; MA, middle-aged adults; ES, elderly subjects; CD, subjects with cognitive decline. Group differences in LSD post-hoc tests: *p < 0.05, **p < 0.01, ***p < 0.001.
Single Finger Tapping
The ANOVA of tap frequency showed GROUP differences [F(3, 34) = 9.94, p < 0.001] and significant interaction with frequency conditions [GROUP*FREQ, F(6, 68) = 11.07, p < 0.001]. Post-hoc testing revealed that all groups performed similarly at 1 Hz (YA: 1.06 Hz ± 0.04; MA: 1.02 Hz ± 0.03; ES: 1.09 Hz ± 0.14; CD: 1.02 Hz ± 0.14) and 2 Hz (YA: 2.07 Hz ± 0.12; MA: 2.01 Hz ± 0.08; ES: 1.80 Hz ± 0.28; CD: 1.74 Hz ± 0.19). However, at 3 Hz elderly subjects as well as subjects with cognitive decline had reduced tap frequency compared to young adults and middle-aged adults (p < 0.001), detailed in Table 2. No difference was found between elderly subjects and those with cognitive decline.
The variability of tapping at 3 Hz also differed significantly between groups [ANOVA, GROUP, F(3, 34) = 8.44, p < 0.001, Figure 3B]. Post-hoc tests showed no effect of age and only the subjects with cognitive decline had significantly increased tap interval variability compared to the other groups (Table 2, p < 0.05).
Multi Finger Tapping
The ANOVA of the selectivity index showed significant GROUP differences [F(3, 28) = 6.91, p = 0.001, Figure 3C]. Only the cognitive decline group had greater difficulty to tap with the correctly selected finger compared to the other groups (Table 2, p < 0.05). Furthermore, the ANOVA showed a significant interaction between GROUP and COMBINATION [F(3, 28) = 4.90, p = 0.007]. Post-hoc testing revealed that the performance of elderly subjects did not differ from those of young adults (p = 0.16) or middle-aged adults (0.19) when tapping with one finger. The selectivity of taps was reduced in elderly subjects when tapping with two fingers (ES*YA, p = 0.02; ES*MA, p = 0.02). In contrast, the cognitive decline group had reduced selectivity compared to all groups in both one and two-finger taps.
The individuation index also varied between groups [GROUP F(3, 28) = 10.30, p < 0.001] but there was no interaction between GROUP × COMBINATION [F(3, 28) = 1.50, p = 0.24]. Thus, elderly subjects and those with cognitive impairment showed a significantly decreased group performance compared to young adults and middle-aged adults (Table 2, p < 0.05). Furthermore, this index showed no significant differences between elderly subjects and those with cognitive impairment.
Clinical Measures of Hand Sensory and Motor Impairment and ADL
Clinical measures were obtained for all elderly subjects, i.e., for healthy elderly subjects and those with cognitive impairment. Sensory function (light touch) was normal in all subjects. The MMSE score was, as expected, significantly lower in the subjects with cognitive impairment compared to elderly subjects. However, the mean MMSE score of 22.36 in the cognitive impairment group indicated the absence of a major cognitive disorder. For the clinical manual dexterity tests (pinch grip strength, gross and fine manual dexterity; see Table 1) elderly subjects and those with cognitive impairment showed comparable performance (no significant group differences). In both of these groups no dependency in activity of daily living was observed.
Discussion
This study provides a first multi-component characterization of changes in manual dexterity related to aging and to cognitive decline. The behavioral data suggest two different patterns of deterioration in manual dexterity. (i) The first pattern of impaired performance was found in patients with a medical history of cognitive decline. These patients had increased variability of finger tapping and reduced ability to correctly select a finger in response to a visual target in the multi-finger tapping task. Remarkably, impaired performance was only related to cognitive status and not to increasing age. The second pattern of decline in performance was related to increasing age and was present in tasks that required fine-graded sensorimotor processing, such as visuo-motor precision during force tracking, finger tapping rate at 3 Hz and individuation of finger movements. Independent finger movements are considered a hallmark of manual dexterity (46), and we found impaired independence of finger movements in elderly subjects. These pilot findings need to be confirmed in larger samples. Nonetheless, the two patterns of impaired performance suggest a dissociation between manual tasks involving mainly sensorimotor processing (sensorimotor integration, speed of execution and motor inhibition) and those involving a greater cognitive contribution (attention and working memory).
Cognitive Effects
Two specific impairments were only detected in subjects with cognitive decline. First, tap interval variability, in the audio-motor single finger tapping task, was higher (Figure 3B), replicating previous findings of increased intra-individual variability in finger tapping in MCI (47–49). Increased tapping variability in MCI is considered to arise from impaired working memory and attentional processing (48, 49), not from impaired motor control. Furthermore, increased tapping variability has also been shown in patients with attention deficit hyperactivity disorder (50). Impaired working memory and attention may compromise matching of internal task expectancies with external temporal demands (51) or affect task planning (52) and prediction (53). Second, subjects with cognitive decline showed reduced ability to select the correct finger according to the visual cue during the multi-finger tapping task (Figure 3C). This was not the case in healthy middle-aged and young adults, suggesting absence of an age effect. This task resembles the serial reaction time task (SRTT), which revealed prolonged processing times in MCI (9, 54, 55). Although older healthy subjects showed prolonged reaction times in SRTT, they had similar error rates compared to young subjects (56), coherent with our findings of similar selectivity index in healthy subjects of different age. Selecting the correct finger (effector), in response to the visual cue, requires spatial mapping between cue and effector according to rules maintained in short-term memory (57). This stimulus-response relation and selection process is likely affected in MCI, consistent with impaired associative memory and decision making (58–60).
Aging Effects
A strong age effect was found in the ability to precisely match finger force to a visual target in the force tracking task. Error increased linearly with age across groups, with elderly subjects and subjects with cognitive decline having the highest error values (Figure 3A). Even the group of adults had increased error compared to the group of young adults, providing evidence of an early age-related decline in the precision of sensorimotor control. This shows that the capacity to adapt motor performance in accordance to visual feedback deteriorates with age. Previous studies showed a two-fold increase in force-tracking error in subjects 60–70 years old compared to young subjects (age ~20) (61, 62). Our results extend these findings, showing an even greater decline (~6-fold) in force tracking precision in elderly subjects and subjects with cognitive decline (age >70). Importantly, subjects with cognitive decline did not perform worse than elderly subjects of comparable age, suggesting that MCI does not impact visuomotor force tracking, which relies primarily on on-line sensorimotor integration, less on cognitive resources. This is consistent with absence of visuo-motor upper limb deficits in MCI, as long as cognitive demands (mapping, memory, learning) are minor (63).
Our findings also suggest an age-related decline in motor inhibition: longer release duration (during tracking) and reduced finger individuation, both considered to involve processes of motor inhibition (31, 64, 65), were found in elderly subjects and those with cognitive decline. Age-related increase in release duration has been reported previously in healthy subjects (62), whereas altered finger individuation has not been shown consistently (27, 66), probably due to task-related differences. Our tapping task, resembling keyboard typing or piano playing, revealed evidence for a similar reduction of independent finger movements in elderly subjects and, in subjects with cognitive decline. This suggests that MCI does not influence these measures linked to motor inhibition, in line with previous reports using Go-Nogo paradigms or Stroop (67).
Our data also point to age-related motor slowing. Both elderly subjects [as those in (68)] and those with cognitive decline were unable to maintain single finger tapping at 3 Hz, but showed no speed deficit at slower tapping rates (1 or 2 Hz). Tapping speed did not differentiate between elderly subjects and those with cognitive decline, similar to previous reports (69–71).
Differential Cognitive Involvement in Manual Dexterity Tasks
We propose a qualitative, explanatory model that accounts for the observed differences in manual dexterity as a function of cognitive decline vs. healthy aging. The model incorporates (i) the cognitive and sensorimotor constraints of each task, and (ii) the presumably involved brain structures and processes (Figure 4).
(i) Task constraints: the single and multi-finger tapping tasks both require a mapping between motor performance and stimulus-based rules. In the single-finger tapping task the rule involves tapping (with one finger at a time) in synchrony with the auditory cue and then continuing without cue. Single finger tapping is thus the one task among the three that contains an explicit memory condition (other than task instructions) on the timing of repetitive motor action, which determines the degree of performance. In the multi-finger tapping task the rule requires mapping the visually displayed target tap to the effector configuration. Thus, finger selection based on this trial-by-trial mapping determines here successful task performance. In these two tasks, specific rule-based (temporal or spatial) information is maintained in short-term memory. Attention and working memory processes are therefore key to good performance in these two tasks, that do not require high-level sensorimotor integration. In contrast, the force-tracking task requires constant on-line modulation of finger force based on real-time visual feedback (sensorimotor integration), but depends, most likely, less on working memory (since the feedback is available at all times and the task rules are simple and invariant).
(ii) Neural correlates: we presume that implementation of (temporal or spatial) stimulus-response rules, requiring attention and working memory, depend on prefrontal cortical areas and hippocampus (72). This concerns primarily the two tapping tasks. In contrast, we assume that force tracking, requiring a high degree of visuo-motor integration, depends predominantly on sensorimotor (parieto-motor) cortical networks (73, 74).
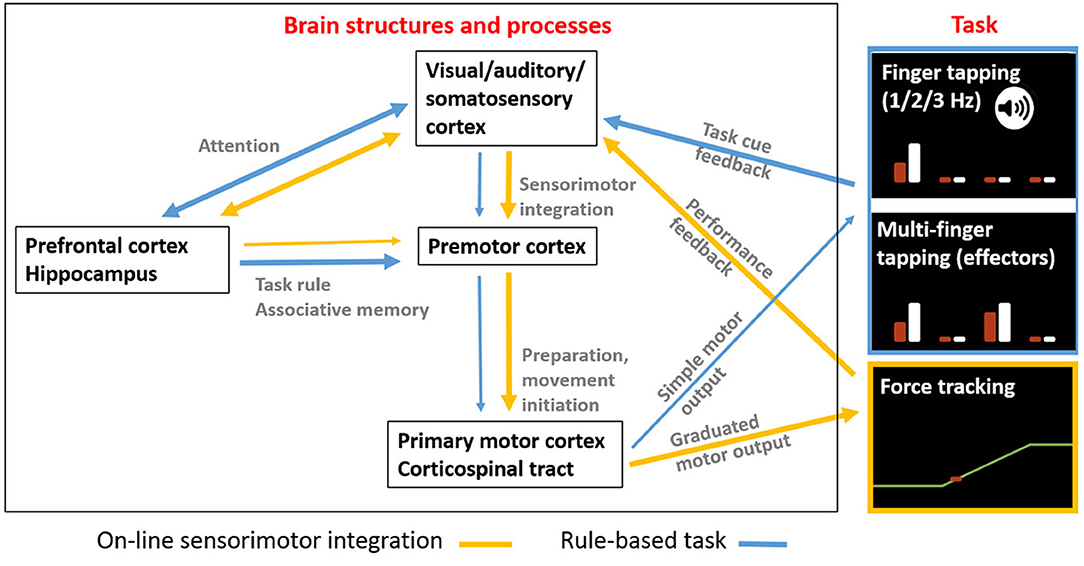
Figure 4. A hypothetical overview of how different brain areas are more or less involved depending on manual dexterity tasks involving more cognitive rule-making or more sensorimotor integration requirements. Arrow thickness reflects degree of task-related involvement processes. The hypothesis is that brain areas and processing involved in manual dexterity tasks depend on whether the task requires (i) on-line sensorimotor integration (yellow arrows) to adapt performance according to feedback or (ii) rule-based associations (blue arrows) that result in performance predictions that are adapted during performance. The force tracking task requires a greater level of on-line matching of motor output to sensory feedback with engagement of sensory-premotor-motor networks. In contrast, tasks requiring more cognitive processing including stimulus-based decisions (temporal or spatial) according to task rules (maintained in working memory) involve a greater contribution from prefrontal cortex and hippocampus. The basal ganglia and the cerebellum, also involved in manual dexterity processing, are not shown for simplicity.
The proposed model is compatible with studies suggesting a disassociation of neural mechanisms related to age-related sensorimotor deterioration or cognitive decline. Neuroimaging studies provide compelling evidence of reduced structural and functional integrity of prefrontal cortex and hippocampus in patients with MCI (72, 75), which has been correlated with gait slowing and cognitive dysfunction in elderly subjects (76). Decision making and response selection are closely linked to the prefrontal cortex (77–79), which is dysregulated in MCI subjects (80). In contrast, age-related decline in motor function has been mainly related to loss of structural and functional integrity in descending motor and ascending sensory pathways (20, 25, 81, 82). Furthermore, healthy aging has been related with increased recruitment of prefrontal and sensorimotor networks to successfully accomplish more cognitively demanding motor tasks (83). Our results suggest that elderly subjects affected by MCI cannot use compensatory cognitive reserves, consistent with decreased performance in more cognitively demanding tasks (81, 84, 85).
Limitations
The cognitive decline group consisted of a heterogeneous sample of patients with clinical MCI or early stage AD diagnosis. Group size was small and sub-group characterization was not feasible. Nonetheless, this study provides a first multi-component description of dexterity in patients with cognitive decline. Future studies with a larger sample would be needed to (i) assess the presence of different manual dexterity profiles in various types of MCI and AD, (ii) replicate the absence of an age effect in particular key dexterity scores, and (iii) include a finger motor sequence (memorization) task (43) to potentially evaluate differences between amnestic and non-amnestic types of MCI (86).
Conclusions
Although conventional clinical testing of hand function (BBT, 9-HPT, JTHFT) did not reveal any differences between elderly subjects with cognitive decline and those without, the quantitative assessment of manual dexterity showed clearly distinct task performance. Subjects with cognitive impairment showed decreased single-finger tapping regularity and reduced finger selectivity (compared to healthy elderly, age-matched subjects). In contrast, accuracy of force control was significantly reduced with age (even between young adults and adults), but not more so in subjects with cognitive decline. This dissociation suggests that rule-based dexterity tasks are useful for the detection of MCI and that on-line sensorimotor integration tasks are sensitive for determining age-related decline in manual dexterity in healthy subjects. Furthermore, our findings imply that these performance measures, suitable for rapid quantification in the clinical setting, could provide valuable clinical markers for early sensitive detection of age-related cognitive decline. Further studies in longitudinal cohorts are warranted to investigate whether these measures could be useful for predicting the development of MCI (87).
Author Contributions
PL, JB, and MM conceptualization, LC, AA, and PL formal analysis, LC and AA investigation, PL, JB, MM, and CL-L methodology, SP and JB resources, PL and JB supervision, LC, AA, and PL writing—original draft, LC, AA, PL, JB, MM, CL-L, and SP writing—review and editing.
Funding
This work was supported by the Pitié-Salpêtrière-Charles Foix Hospital and was in part funded by the Jacques and Gloria Gossweiler Foundation.
Conflict of Interest Statement
PL owns shares in Aggero MedTech AB, a company commercializing a measurement instrument for spasticity. PL and MM have patented a method for measurement of manual dexterity (EP2659835A1), but do not own commercialization rights. JB reports fees or invitations unrelated to this work from Boehringer Ingelheim, GlaxoSmithKline, MSD, Amgen, Novartis, Sanofi Aventis, Pfizer, and Santor Edition.
The remaining authors declare that the research was conducted in the absence of any commercial or financial relationships that could be construed as a potential conflict of interest.
Acknowledgments
LC reports Ph.D. grant from Université Pierre et Marie Curie (UPMC), Paris VI.
Footnotes
1. ^SENSIX FORCE-TORQUE SENSOR FOR BIOMECHANICS Available online at: http://www.sensix.fr/ (Accessed June 3, 2018).
References
1. Harada CN, Natelson Love MC, Triebel K. Normal cognitive aging. Clin Geriatr Med. (2013) 29:737–52. doi: 10.1016/j.cger.2013.07.002
2. Hebert LE, Scherr PA, Bienias JL, Bennett DA, Evans DA. Alzheimer's disease in the US population: prevalence estimates using the 2000 census. Arch Neurol. (2003) 60:1119–22. doi: 10.1001/archneur.60.8.1119
3. Kluger A, Gianutsos JG, Golomb J, Ferris SH, George AE, Franssen E, et al. Patterns of motor impairement in normal aging, mild cognitive decline, and early Alzheimer's disease. J Gerontol B Psychol Sci Soc Sci. (1997) 52B:P28–39.
4. Scherder E, Dekker W, Eggermont L. Higher-level hand motor function in aging and (preclinical) dementia: its relationship with (instrumental) activities of daily life—A mini-review. Gerontology (2008) 54:333–41. doi: 10.1159/000168203
5. Bahureksa L, Najafi B, Saleh A, Sabbagh M, Coon D, Mohler MJ, et al. The impact of mild cognitive impairment on gait and balance: a systematic review and meta-analysis of studies using instrumented assessment. Gerontology (2017) 63:67–83. doi: 10.1159/000445831
6. Franssen EH, Souren LE, Torossian CL, Reisberg B. Equilibrium and limb coordination in mild cognitive impairment and mild Alzheimer's disease. J Am Geriatr Soc. (1999) 47:463–9.
7. Kikkert LHJ, Vuillerme N, van Campen JP, Hortobágyi T, Lamoth CJ. Walking ability to predict future cognitive decline in old adults: a scoping review. Ageing Res Rev. (2016) 27:1–14. doi: 10.1016/j.arr.2016.02.001
8. Brandt J, Aretouli E, Neijstrom E, Samek J, Manning K, Albert MS, et al. Selectivity of executive function deficits in mild cognitive impairment. Neuropsychology (2009) 23:607–18. doi: 10.1037/a0015851
9. Darby D, Maruff P, Collie A, McStephen M. Mild cognitive impairment can be detected by multiple assessments in a single day. Neurology (2002) 59:1042–6. doi: 10.1212/wnl.59.7.1042
10. Curreri C, Trevisan C, Carrer P, Facchini S, Giantin V, Maggi S, et al. Difficulties with fine motor skills and cognitive impairment in an elderly population: the progetto veneto anziani. J Am Geriatr Soc. (2018) 66:350–6. doi: 10.1111/jgs.15209
11. Kluger A, Gianutsos JG, Golomb J, Wagner A, Wagner D, Scheurich S. Clinical features of MCI: motor changes. Int Psychogeriatr. (2008) 20:32–9. doi: 10.1017/S1041610207006461
12. Kobayashi-Cuya KE, Sakurai R, Suzuki H, Ogawa S, Takebayashi T, Fujiwara Y. Observational evidence of the association between handgrip strength, hand dexterity, and cognitive performance in community-dwelling older adults: a systematic review. J Epidemiol. (2018) 28:373–81. doi: 10.2188/jea.JE20170041
13. Mullick AA, Subramanian SK, Levin MF. Emerging evidence of the association between cognitive deficits and arm motor recovery after stroke: a meta-analysis. Restor Neurol Neurosci. (2015) 33:389–403. doi: 10.3233/RNN-150510
14. Rinne P, Hassan M, Fernandes C, Han E, Hennessy E, Waldman A, et al. Motor dexterity and strength depend upon integrity of the attention-control system. Proc Natl Acad Sci USA. (2018) 115:E536–45. doi: 10.1073/pnas.1715617115
15. Haworth J, Phillips M, Newson M, Rogers PJ, Torrens-Burton A, Tales A. Measuring information processing speed in mild cognitive impairment: clinical vs. Research Dichotomy. J Alzheimers Dis. (2016) 51:263–75. doi: 10.3233/JAD-150791
16. Krampe RT. Aging, expertise and fine motor movement. Neurosci Biobehav Rev. (2002) 26:769–76. doi: 10.1016/s0149-7634(02)00064-7
17. Ranganathan VK, Siemionow V, Sahgal V, Liu JZ, Yue GH. Skilled finger movement exercise improves hand function. J Gerontol A Biol Sci Med Sci. (2001) 56:M518–22. doi: 10.1093/gerona/56.8.M518
18. Vieluf S, Godde B, Reuter E-M, Voelcker-Rehage C. Age-related differences in finger force control are characterized by reduced force production. Exp Brain Res. (2013) 224:107–17. doi: 10.1007/s00221-012-3292-4
19. Cole KJ, Rotella DL, Harper JG. Mechanisms for age-related changes of fingertip forces during precision gripping and lifting in adults. J Neurosci. (1999) 19:3238–47. doi: 10.1523/JNEUROSCI.19-08-03238.1999
20. Goble DJ, Coxon JP, Wenderoth N, Van Impe A, Swinnen SP. Proprioceptive sensibility in the elderly: degeneration, functional consequences and plastic-adaptive processes. Neurosci Biobehav Rev. (2009) 33:271–8. doi: 10.1016/j.neubiorev.2008.08.012
21. Friedman N, Chan V, Zondervan D, Bachman M, Reinkensmeyer DJ. MusicGlove: motivating and quantifying hand movement rehabilitation by using functional grips to play music. Conf Proc IEEE Eng Med Biol Soc. (2011) 2011:2359–63. doi: 10.1109/IEMBS.2011.6090659
22. Goodkin DE, Hertsgaard D, Seminary J. Upper extremity function in multiple sclerosis: improving assessment sensitivity with box-and-block and nine-hole peg tests. Arch Phys Med Rehabil. (1988) 69:850–4.
23. Canfield JS, Hackel ME, Wolfe GA, Bang SM, Other G, Hackel ME, et al. Changes in hand function in the aging adult as determined by the Jebsen test of hand function. Phys Ther. (1992) 72:373–7.
24. Sears ED, Chung KC. Validity and Responsiveness of the Jebsen-Taylor hand function Test. J Hand Surg Am. (2010) 35:30–7. doi: 10.1016/j.jhsa.2009.09.008
25. Lindberg PG, Feydy A, Maier MA. White matter organization in cervical spinal cord relates differently to age and control of grip force in healthy subjects. J Neurosci. (2010) 30:4102–9. doi: 10.1523/JNEUROSCI.5529-09.2010
26. Santisteban L, Térémetz M, Bleton J-P, Baron J-C, Maier MA, Lindberg PG. Upper limb outcome measures used in stroke rehabilitation studies: a systematic literature review. PLoS ONE (2016) 11:e0154792. doi: 10.1371/journal.pone.0154792
27. Mirakhorlo M, Maas H, Veeger HEJ. Increased enslaving in elderly is associated with changes in neural control of the extrinsic finger muscles. Exp Brain Res. (2018) 236:1583–92. doi: 10.1007/s00221-018-5219-1
28. Sommervoll Y, Ettema G, Vereijken B. Effects of age, task, and frequency on variability of finger tapping. Percept Mot Skills (2011) 113:647–61. doi: 10.2466/10.25.PMS.113.5.647-661
29. Bosman EA. Age-related differences in the motoric aspects of transcription typing skill. Psychol Aging (1993) 8:87–102.
30. Ostwald SK, Snowdon DA, Rysavy DM, Keenan NL, Kane RL. Manual dexterity as a correlate of dependency in the elderly. J Am Geriatr Soc. (1989) 37:963–9.
31. Térémetz M, Colle F, Hamdoun S, Maier MA, Lindberg PG. A novel method for the quantification of key components of manual dexterity after stroke. J Neuroeng Rehabil. (2015) 12:64. doi: 10.1186/s12984-015-0054-0
32. Oldfield RC. The assessment and analysis of handedness: the Edinburgh inventory. Neuropsychologia (1971) 9:97–113. doi: 10.1016/0028-3932(71)90067-4
33. Jack CR, Albert MS, Knopman DS, McKhann GM, Sperling RA, Carrillo MC, et al. Introduction to the recommendations from the National Institute on Aging-Alzheimer's Association workgroups on diagnostic guidelines for Alzheimer's disease. Alzheimer's Dement. (2011) 7:257–62. doi: 10.1016/j.jalz.2011.03.004
34. Desrosiers J, Bravo G, Hébert R, Dutil E, Mercier L. Validation of the box and Block test as a measure of dexterity of elderly people: reliability, validity, and norms studies. Arch Phys Med Rehabil. (1994) 75:751–5.
35. Mathiowetz V, Kashman N, Volland G, Weber K, Dowe M, Rogers S. Grip and pinch strength: normative data for adults. Arch Phys Med Rehabil. (1985) 66:69–74.
36. Lawton MP, Brody EM. Assessment of older people: self-maintaining and instrumental activities of daily living. Gerontologist (1969) 9:179–86.
37. Meinerding M, DeFeis B, Sunderaraman P, Azar M, Lawless S, Perez-Vivaldo C, et al. Assessing dependency in a multiethnic community cohort of individuals with Alzheimer's disease. Innov Aging (2018) 2:igy011. doi: 10.1093/geroni/igy011
38. Silva PG, Jones A, Araujo PMP, Natour J. Assessment of light touch sensation in the hands of systemic sclerosis patients. Clinics (Sao Paulo) (2014) 69:585–8. doi: 10.6061/clinics/2014(09)02
39. Ojea Ortega T, González Álvarez de Sotomayor MM, Pérez González O, Fernández Fernández O. [A new assessment for episodic memory. Episodic memory test and caregiver's episodic memory test]. Neurologia (2013) 28:488–96. doi: 10.1016/j.nrl.2012.10.004
40. Lemos R, Simões MR, Santiago B, Santana I. The free and cued selective reminding test: validation for mild cognitive impairment and Alzheimer's disease. J Neuropsychol. (2015) 9:242–57. doi: 10.1111/jnp.12048
41. Kopp B, Rösser N, Tabeling S, Stürenburg HJ, de Haan B, Karnath H-O, et al. Performance on the frontal assessment battery is sensitive to frontal lobe damage in stroke patients. BMC Neurol. (2013) 13:179. doi: 10.1186/1471-2377-13-179
42. Brucki SMD, Rocha MSG. Category fluency test: effects of age, gender and education on total scores, clustering and switching in Brazilian Portuguese-speaking subjects. Braz J Med Biol Res. (2004) 37:1771–7. doi: 10.1590/S0100-879X2004001200002
43. Térémetz M, Carment L, Brénugat-Herne L, Croca M, Bleton J-P, Krebs M-O, et al. Manual dexterity in schizophrenia—A neglected clinical marker? Front Psychiatr. (2017) 8:120. doi: 10.3389/fpsyt.2017.00120
44. Kim R, Kim H-J, Kim A, Jang M-H, Kim HJ, Jeon B. Validation of the Conversion between the mini-mental state examination and montreal cognitive assessment in Korean patients with Parkinson's disease. J Mov Disord. (2018) 11:30–4. doi: 10.14802/jmd.17038
45. Aydin G, Mucuk S. The evaluation of daily living activities, pressure sores and risk factors. Rehabil Nurs. (2015) 40:84–91. doi: 10.1002/rnj.145
46. Lemon RN. Descending pathways in motor control. Annu Rev Neurosci. (2008) 31:195–218. doi: 10.1146/annurev.neuro.31.060407.125547
47. Henley SMD, Downey LE, Nicholas JM, Kinnunen KM, Golden HL, Buckley A, et al. Degradation of cognitive timing mechanisms in behavioural variant frontotemporal dementia. Neuropsychologia (2014) 65:88–101. doi: 10.1016/j.neuropsychologia.2014.10.009
48. Kay CD, Seidenberg M, Durgerian S, Nielson KA, Smith JC, Woodard JL, et al. Motor timing intraindividual variability in amnestic mild cognitive impairment and cognitively intact elders at genetic risk for Alzheimer's disease. J Clin Exp Neuropsychol. (2017) 39:866–75. doi: 10.1080/13803395.2016.1273321
49. Rabinowitz I, Lavner Y. Association between finger tapping, attention, memory, and cognitive diagnosis in elderly patients. Percept Mot Skills (2014) 119:259–78. doi: 10.2466/10.22.PMS.119c12z3
50. Toplak ME, Dockstader C, Tannock R. Temporal information processing in ADHD: findings to date and new methods. J Neurosci Methods (2006) 151:15–29. doi: 10.1016/j.jneumeth.2005.09.018
51. Bangert AS, Balota DA. Keep up the pace: declines in simple repetitive timing differentiate healthy aging from the earliest stages of Alzheimer's disease. J Int Neuropsychol Soc. (2012) 18:1052–63. doi: 10.1017/S1355617712000860
52. Ameli M, Kemper F, Sarfeld A-S, Kessler J, Fink GR, Nowak DA. Arbitrary visuo-motor mapping during object manipulation in mild cognitive impairment and Alzheimer's disease: a pilot study. Clin Neurol Neurosurg. (2011) 113:453–8. doi: 10.1016/j.clineuro.2011.01.011
53. Delevoye-Turrell Y, Wilquin H, Giersch A. A ticking clock for the production of sequential actions: where does the problem lie in schizophrenia? Schizophr Res. (2012) 135:51–4. doi: 10.1016/j.schres.2011.12.020
54. Anstey KJ, Mack HA, Christensen H, Li S-C, Reglade-Meslin C, Maller J, et al. Corpus callosum size, reaction time speed and variability in mild cognitive disorders and in a normative sample. Neuropsychologia (2007) 45:1911–20. doi: 10.1016/j.neuropsychologia.2006.11.020
55. Jackson JD, Balota DA, Duchek JM, Head D. White matter integrity and reaction time intraindividual variability in healthy aging and early-stage Alzheimer's disease. Neuropsychologia (2012) 50:357–66. doi: 10.1016/j.neuropsychologia.2011.11.024
56. Meissner SN, Keitel A, Südmeyer M, Pollok B. Implicit motor sequence learning and working memory performance changes across the adult life span. Front Aging Neurosci. (2016) 8:89. doi: 10.3389/fnagi.2016.00089
57. Proctor RW, Vu K-PL, Pick DF. Aging and response selection in spatial choice tasks. Hum Factors (2005) 47:250–70. doi: 10.1518/0018720054679425
58. Boyle PA, Yu L, Wilson RS, Gamble K, Buchman AS, Bennett DA. Poor decision making is a consequence of cognitive decline among older persons without Alzheimer's disease or mild cognitive impairment. PLoS ONE (2012) 7:e43647. doi: 10.1371/journal.pone.0043647
59. Hamdy RC, Lewis JV, Kinser A, Depelteau A, Copeland R, Kendall-Wilson T, et al. Too many choices confuse patients with dementia. Gerontol Geriatr Med. (2017) 3. doi: 10.1177/2333721417720585
60. Oedekoven CSH, Jansen A, Keidel JL, Kircher T, Leube D. The influence of age and mild cognitive impairment on associative memory performance and underlying brain networks. Brain Imaging Behav. (2015) 9:776–89. doi: 10.1007/s11682-014-9335-7
61. Lindberg P, Ody C, Feydy A, Maier MA. Precision in isometric precision grip force is reduced in middle-aged adults. Exp Brain Res. (2009) 193:213–24. doi: 10.1007/s00221-008-1613-4
62. Voelcker-Rehage C, Alberts JL. Age-related changes in grasping force modulation. Exp Brain Res. (2005) 166:61–70. doi: 10.1007/s00221-005-2342-6
63. Salek Y, Anderson ND, Sergio L. Mild cognitive impairment is associated with impaired visual-motor planning when visual stimuli and actions are incongruent. Eur Neurol. (2011) 66:283–93. doi: 10.1159/000331049
64. Lindberg PG, Roche N, Robertson J, Roby-Brami A, Bussel B, Maier MA. Affected and unaffected quantitative aspects of grip force control in hemiparetic patients after stroke. Brain Res. (2012) 1452:96–107. doi: 10.1016/j.brainres.2012.03.007
65. Teremetz M, Amado I, Bendjemaa N, Krebs M-O, Lindberg PG, Maier MA. Deficient grip force control in schizophrenia: behavioral and modeling evidence for altered motor inhibition and motor noise. PLoS ONE (2014) 9:e111853. doi: 10.1371/journal.pone.0111853
66. Oliveira MA, Hsu J, Park J, Clark JE, Shim JK. Age-related changes in multi-finger interactions in adults during maximum voluntary finger force production tasks. Hum Mov Sci. (2008) 27:714–27. doi: 10.1016/j.humov.2008.04.005
67. Zhang Y, Han B, Verhaeghen P, Nilsson L-G. Executive functioning in older adults with mild cognitive impairment: MCI has effects on planning, but not on inhibition. Neuropsychol Dev Cogn B Aging Neuropsychol Cogn. (2007) 14:557–70. doi: 10.1080/13825580600788118
68. Turgeon M, Wing AM, Taylor LW. Timing and aging: slowing of fastest regular tapping rate with preserved timing error detection and correction. Psychol Aging (2011) 26:150–61. doi: 10.1037/a0020606
69. Bailon O, Roussel M, Boucart M, Krystkowiak P, Godefroy O. Psychomotor slowing in mild cognitive impairment, Alzheimer's disease and lewy body dementia: mechanisms and diagnostic value. Dement Geriatr Cogn Disord. (2010) 29:388–96. doi: 10.1159/000305095
70. Goldman WP, Baty JD, Buckles VD, Sahrmann S, Morris JC. Motor dysfunction in mildly demented AD individuals without extrapyramidal signs. Neurology (1999) 53:956–62.
71. Hall JR, Harvey MB. Behavioral regulation: factor analysis and application of the behavioral dyscontrol scale in dementia and mild cognitive impairment. Int J Geriatr Psychiatr. (2008) 23:314–8. doi: 10.1002/gps.1881
72. Yu J, Lam CLM, Lee TMC. White matter microstructural abnormalities in amnestic mild cognitive impairment: a meta-analysis of whole-brain and ROI-based studies. Neurosci Biobehav Rev. (2017) 83:405–16. doi: 10.1016/j.neubiorev.2017.10.026
73. Castiello U. The neuroscience of grasping. Nat Rev Neurosci. (2005) 6:726–36. doi: 10.1038/nrn1744
74. Janssen P, Scherberger H. Visual guidance in control of grasping. Annu Rev Neurosci. (2015) 38:69–86. doi: 10.1146/annurev-neuro-071714-034028
75. Rémy F, Vayssière N, Saint-Aubert L, Barbeau E, Pariente J. White matter disruption at the prodromal stage of Alzheimer's disease: relationships with hippocampal atrophy and episodic memory performance. Neuroimage Clin. (2015) 7:482–92. doi: 10.1016/j.nicl.2015.01.014
76. Rosso AL, Verghese J, Metti AL, Boudreau RM, Aizenstein HJ, Kritchevsky S, et al. Slowing gait and risk for cognitive impairment: the hippocampus as a shared neural substrate. Neurology (2017) 89:336–42. doi: 10.1212/WNL.0000000000004153
77. Ridderinkhof KR, Ullsperger M, Crone EA, Nieuwenhuis S. The role of the medial frontal cortex in cognitive control. Science (2004) 306:443–7. doi: 10.1126/science.1100301
78. Rowe JB, Hughes L, Nimmo-Smith I. Action selection: a race model for selected and non-selected actions distinguishes the contribution of premotor and prefrontal areas. Neuroimage (2010) 51:888–96. doi: 10.1016/j.neuroimage.2010.02.045
79. Alameda-Bailen JR, Salguero-Alcaniz MP, Merchan-Clavellino A, Paino-Quesada S. Cognitive mechanisms in decision-making in patients with mild Alzheimer's disease. Curr Alzheimer's Res. (2017) 14:1248–55. doi: 10.2174/1567205014666170417113834
80. Yap KH, Ung WC, Ebenezer EGM, Nordin N, Chin PS, Sugathan S, et al. Visualizing hyperactivation in neurodegeneration based on prefrontal oxygenation: a comparative study of mild Alzheimer's disease, mild cognitive impairment, and healthy controls. Front Aging Neurosci. (2017) 9:00287. doi: 10.3389/fnagi.2017.00287
81. Seidler RD, Bernard JA, Burutolu TB, Fling BW, Gordon MT, Gwin JT, et al. Motor control and aging: links to age-related brain structural, functional, and biochemical effects. Neurosci Biobehav Rev. (2010) 34:721–33. doi: 10.1016/j.neubiorev.2009.10.005
82. Vien C, Boré A, Lungu O, Benali H, Carrier J, Fogel S, et al. Age-related white-matter correlates of motor sequence learning and consolidation. Neurobiol Aging (2016) 48:13–22. doi: 10.1016/j.neurobiolaging.2016.08.006
83. Heuninckx S, Wenderoth N, Debaere F, Peeters R, Swinnen SP. Neural basis of aging: the penetration of cognition into action control. J Neurosci. (2005) 25:6787–96. doi: 10.1523/JNEUROSCI.1263-05.2005
84. Aggarwal NT, Wilson RS, Beck TL, Bienias JL, Bennett DA. Motor dysfunction in mild cognitive impairment and the risk of incident Alzheimer's disease. Arch Neurol. (2006) 63:1763–9. doi: 10.1001/archneur.63.12.1763
85. de Paula JJ, Albuquerque MR, Lage GM, Bicalho MA, Romano-Silva MA, Malloy-Diniz LF. Impairment of fine motor dexterity in mild cognitive impairment and Alzheimer's disease dementia: association with activities of daily living. Rev Bras Psiquiatr. (2016) 38:235–8. doi: 10.1590/1516-4446-2015-1874
86. Csukly G, Sirály E, Fodor Z, Horváth A, Salacz P, Hidasi Z, et al. The differentiation of amnestic type MCI from the non-amnestic types by structural MRI. Front Aging Neurosci. (2016) 8:52. doi: 10.3389/fnagi.2016.00052
Keywords: manual dexterity, sensorimotor integration, aging, cognitive decline, Alzheimer disease
Citation: Carment L, Abdellatif A, Lafuente-Lafuente C, Pariel S, Maier MA, Belmin J and Lindberg PG (2018) Manual Dexterity and Aging: A Pilot Study Disentangling Sensorimotor From Cognitive Decline. Front. Neurol. 9:910. doi: 10.3389/fneur.2018.00910
Received: 26 July 2018; Accepted: 09 October 2018;
Published: 29 October 2018.
Edited by:
Antonio Oliviero, Fundación del Hospital Nacional de Parapléjicos, SpainReviewed by:
Rebecca Jane Rylett, University of Western Ontario, CanadaFederico Ranieri, Università Campus Bio-Medico, Italy
Copyright © 2018 Carment, Abdellatif, Lafuente-Lafuente, Pariel, Maier, Belmin and Lindberg. This is an open-access article distributed under the terms of the Creative Commons Attribution License (CC BY). The use, distribution or reproduction in other forums is permitted, provided the original author(s) and the copyright owner(s) are credited and that the original publication in this journal is cited, in accordance with accepted academic practice. No use, distribution or reproduction is permitted which does not comply with these terms.
*Correspondence: Påvel G. Lindberg, pavel.lindberg@inserm.fr
†These authors have contributed equally to this work