- 1Department of Animal and Dairy Science, University of Georgia, Athens, GA, United States
- 2Regenerative Bioscience Center, University of Georgia, Athens, GA, United States
- 3Department of Kinesiology, University of Georgia, Athens, GA, United States
Reach-to-grasp is an evolutionarily conserved motor function that is adversely impacted following stroke and traumatic brain injury (TBI). Non-invasive brain stimulation (NIBS) methods, such as transcranial magnetic stimulation and transcranial direct current stimulation, are promising tools that could enhance functional recovery of reach-to-grasp post-brain injury. Though the rodent literature provides a causal understanding of post-injury recovery mechanisms, it has had a limited impact on NIBS protocols in human research. The high degree of homology in reach-to-grasp circuitry between humans and rodents further implies that the application of NIBS to brain injury could be better informed by findings from pre-clinical rodent models and neurorehabilitation research. Here, we provide an overview of the advantages and limitations of using rodent models to advance our current understanding of human reach-to-grasp function, cortical circuitry, and reorganization. We propose that a cross-species comparison of reach-to-grasp recovery could provide a mechanistic framework for clinically efficacious NIBS treatments that could elicit better functional outcomes for patients.
Introduction
Reach-to-grasp is an essential task that requires precise spatial and temporal integration of sensory and motor systems. Damage to these systems following stroke or traumatic brain injury (TBI), commonly leads to long-term deficits in reach-to-grasp function (1, 2). Given that reach-to-grasp movements are a fundamental skill for many daily activities, improving reach-to-grasp recovery after brain injury is a major goal of neurorehabilitation therapies (3, 4). Non-invasive brain stimulation (NIBS) is of interest as both a prognostic tool for predicting motor recovery after brain injury and as a novel option for rehabilitation treatment (5–8). When used as an intervention in clinical populations, NIBS techniques such as transcranial magnetic stimulation (TMS) and transcranial direct current stimulation (tDCS) have been shown to modulate localized regions of activity in the cortex and are administered either independently or in combination with task-specific training to promote functional recovery. Despite growing evidence of the benefits of NIBS in reducing motor impairment after brain injury, there are still large gaps in our understanding of the optimal treatment parameters, the underlying neural mechanisms, and factors that influence outcomes.
Rodent models have the potential to inform how neurostimulation can be useful in clinical applications, especially as it pertains to improving reach-to-grasp behavior after brain injury. The sensorimotor circuitry controlling volitional motor control and related reaching and grasping behavior is highly conserved in mammals, with significant cross-species similarities in posture and usage of forelimbs (9). Rodents exhibit a similar laminar organization of their M1 cortex compared to primates, and proximal and longitudinal connectivity patterns are also known to be mostly conserved (10). In addition to their comparable dexterity and homology to human cortical representation, rodent models offer several translational advantages—such as the availability of transgenic lines, feasibility of invasive function modulation, and imaging tools that can be exploited to study circuit function and behavioral outcomes under highly controlled environments (11, 12).
The purpose of this review is to compare and contrast current literature on reach-to-grasp deficits in pre-clinical rodent models and human studies, and to highlight how neurostimulation in pre-clinical rodent models of brain injury could provide a mechanistic basis for improving reach-to-grasp function in humans. We begin with an overview of the similarities and dissimilarities between the behavioral and neural mechanisms of reach-to-grasp movements in humans and rodents. We then compare how reach-to-grasp circuitry is affected post-injury in humans and rodents and describe the current efficacy of NIBS protocols in clinical applications and rodent models. In each section, we identify future directions and identify potential avenues for clinical translation.
Comparison of Reach-to-Grasp Function in Humans and Rodents
Characteristics of Reach-to-Grasp in Healthy Humans
Prehension is the act of reaching and grasping objects during activities of daily living. Picking up a coffee cup, lifting a pen to sign a check, or hammering a nail in the wall—all are movements that involve reaching and grasping an object to achieve a goal. Reaching movements are directed toward extrinsic properties of an object, such as the location and orientation, and involve recruitment of larger arm muscles to transport the hand to the object. Grasping movements are directed toward the intrinsic properties of the object, such as shape and size, and require synchronized recruitment of extrinsic and smaller intrinsic hand muscles to open and close the digits as the hand approaches the object (13). During a typical reach-to-grasp movement, there is first a gradual opening of the grip with straightening of the digits, followed by a progressive closing of the fingers to match the affordance of the object (14). The maximum grip aperture occurs at about 60–70% of the reach trajectory and is strongly correlated with the size of the object (15). Besides object size, the fragility of the object (16), texture, and weight (17) also influence digit pre-shaping during the reach to the object.
Humans typically grasp an object in either a precision or power grasp (18–20). Precision grasps (Figure 1) involve creation of an opposition space between the thumb and the remaining digits and the application of force on the object with digit tips (22). In contrast, power grasps are used when large forces need to be applied to the environment, e.g., while wielding a hammer, and involve the fingers to be flexed in opposition to the palm with the degree of flexion dictated by the dimensions of the object (15, 23).
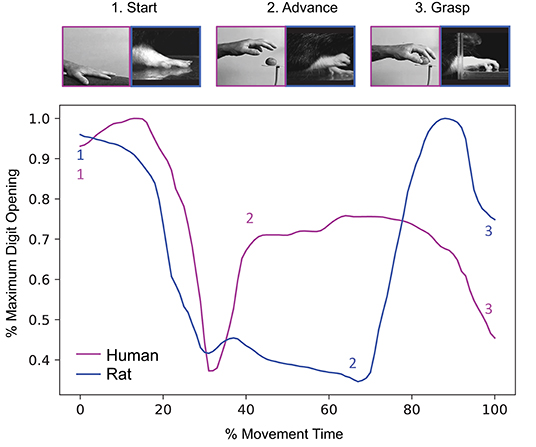
Figure 1. Rodents and humans present high similarity in kinematic stages and coordination. Top panel: Representative hand/paw positions for three stages (start, advance, and grasp). Adapted from (13). Bottom panel: Temporal evolution of maximum digit opening throughout reach-to-grasp, with corresponding timing of each movement stage as a function of overall movement time. While there are differences in kinematic strategies between the human hand and rodent paw, the gross dynamics, and morphology of reach-to-grasp remain the same. Adapted and republished with permission of Elsevier Science & Technology Journals from Sacrey et al. (21); permission conveyed through Copyright Clearance Center, Inc.
Reach-to-Grasp Assessments in Rodents
Much like humans, rodents demonstrate a high level of dexterity and fine usage of the limb while engaging in reach-to-grasp movements. In particular, direct kinematic comparison of reach-to-grasp between rodent and humans shows strong conserved motor and coordination strategies (21, 24) (Figure 1). Skilled reaching tasks in rodents provide multiple measurements that quantify dexterity and ability to perform the task via measures such as the number of hand changes, number of successful hits, efficiency, and response time. The assessment of forelimb function in rodents can be achieved through several modalities that distinguish power and precision grip, as in humans. Power grasp and strength can be simply measured using a Newton meter by allowing the rodent to grasp on a bar while being pulled to evaluate the strength at failure to grab. Assays such as the staircase assay (25), skilled reach task (26), the pasta matrix (27), and the pasta handling assays (28) were designed to assess the skilled use of the forelimb for precision reach and grasp or fine handling of food.
The scoring of hand shape and grasp movement has also been used to evaluate the extent of compensatory changes in movement strategy following injuries (26, 29, 30). More recently, feedback loop approaches have been developed to investigate motor adaptation and motor strength using lever pulling in static (31, 32) and dynamic conditions (33), which allows for a closer investigation of the neural correlates in motor planning and execution during power grasp experiments. Here, our focus is on precision grasp-related assays such as the skilled reach task or reach-and-grasp assay to draw a correspondence with similar assays described in human literature.
Scalability: Mapping Rodent Experimental Paradigms to Clinical Motor Outcomes
Despite many similarities, there are nonetheless important postural differences in reach-to-grasp movements between rodents and humans. Unlike humans, rodents also have to stabilize overall posture during reach-to-grasp, but this will not be reviewed here as they are exhaustively covered elsewhere (34–36).
A current limitation in using findings from rodent experiments to understand reach-to-grasp impairments in humans is the dichotomy in the scales and outcomes used to score task performance. The rodent literature has mostly focused on pure performance such as successful number of hits, and for the most part, has ignored compensatory mechanisms of task accomplishment, such as altered handshape, unconventional motor strategies, and two-limb assisted performance. In contrast, the human literature has emphasized kinematic and kinetic analyses that isolate specific features of movement that are impaired after injury (37–40). Specific parameters of reach-to-grasp movements are predictive of general upper extremity impairment and function (41). For example, maximum grip aperture is higher in patients following stroke, and is predictive of functional impairments (42). Thus, a closer examination of rodent aperture scaling in response to different haptic-based object cues may provide a window into behavioral measures that are clinically relevant (13).
Overall, pre-clinical animal models would benefit from the incorporation of higher sensitivity assessments in order to distinguish finer motor alterations and associated motor circuitry adaptations following brain injuries (43, 44). Recent advances in low-cost platforms for trajectory tracking (45) and open source software developed for markerless pose estimation (46, 47) makes measuring fine-grained changes in kinematics easily implementable in the lab setting and provides a relevant framework for cross-species comparisons.
Neural Mechanisms of Reach-to-Grasp
Neural Mechanisms of Reach-to-Grasp in Humans and Non-human Primates
Reach-to-grasp movements in primates are mediated by two cortical dorsal stream pathways involving cortical interactions between posterior parietal cortex (PPC), premotor cortex (PM), and primary motor cortex (M1) [reviewed in (48, 49)]. Reaching is enabled primarily by a dorsomedial pathway that projects through the superior parietal lobule (SPL) via the parietal reach region, including the superior parieto-occipital cortex, to the dorsal premotor cortex (PMd) and finally to M1 (50–52). The dorsomedial stream uses visual motion information to monitor spatial location of objects to allow for skilled reaching movements to occur (53). In contrast, the dorsolateral pathway is responsible for grasping and projects through the anterior intraparietal sulcus to ventral premotor cortex (PMv) and then to M1. This pathway primarily receives and processes visual information on object affordances (14, 54). The dorsolateral visual stream is also involved in recognition of object motion and self-motion (55). The basal ganglia and cerebellum each form reciprocal connections with the two cortical streams and play an important modulatory role in facilitating eye-hand coordination during corrective online responses and integrating reach and grasp components into a single motor program (56–58).
In addition to these neural substrates, the corticospinal tract also mediates integration of reach-to-grasp movements under visual control (59). Non-human primates and humans have direct connections between corticospinal neurons and the cervical motorneurons. The phylogenetic development of the corticospinal tract also correlates with dexterity, particularly in the ability to perform precision grips (60). Indeed, non-human primates with weaker corticospinal projections have no ability to form precision grips and possess limited manipulatory skills. In humans, TMS-induced motor evoked potentials, a measure of corticospinal excitability, are more suppressed during power grasps than precision grasps, suggesting a more pronounced role of the corticospinal tract in precision grasps (61, 62).
Reaching and grasping movements emerge independently in humans in the early stages of development, and foveal vision plays a critical role in facilitating the integration of these movements during development (13). Young infants primarily rely on proprioception to direct their hands toward objects and use somatosensory feedback to determine if they have contacted an object (63). Between 4 and 8 months of age, infants begin to use vision to organize sequential reach-then-grasp movements (64). By about 9 months of age, infants begin to exhibit feedforward visual control of functional reach movements by orienting the hand to match the orientation of the object. This suggests early ontogenetic maturation of the dorsomedial pathway compared to the dorsolateral pathway. The gradual maturation of the dorsal visual stream during the first decade of life (65) allows smooth integration of reach-to-grasp movements under feedforward visual control (66). In adults, simultaneous processing of visual inputs in these two streams allow the reach and grasp movements to be concurrently executed as a single smooth integrated act (67–70). When visual processing is limited, either during traumatic brain injuries or through experimental manipulation, prehension decomposes into its two elemental components, suggesting disruption in online processing and interactions in these two pathways.
Neural Mechanisms of Reach-to-Grasp in Rodents
Rodent models have facilitated the detailed investigation of motor circuits using techniques such as regional lesioning, electrical stimulation, and optogenetics. Much like humans, motor circuitry in rodents is also divided into 3 major groups: (1) higher order centers that include posterior parietal (PPC), primary (M1; also identified as the caudal forelimb area—CFA) and secondary/premotor (M2/PM; also identified as the rostral forelimb area—RFA) regions that are involved in sensorimotor integration, planning and volitional control, (2) modulatory and relay centers that include the thalamo-striatal-midbrain axis responsible for gating, selective sensory integration, and motor plasticity, and (3) motor coordination and execution centers such as the cerebello-cortico-spinal pathway that regulate the direct translation of efferent higher order signals into physical movements as well as providing feedback for fine movement tuning and learning. Primary motor regions modulate movement outcomes through both direct and indirect pathways involving corticospinal neurons and reticulo/rubro/tectospinal pathways, respectively (71, 72).
In contrast to the cerebellum and spinal control of posture and locomotion, skilled motor learning is thought to be orchestrated through the corticomotor and motor planning pathways in association with a descending pathway consisting of midbrain and spinal cord neurons (73, 74). In comparison to humans, the corticomotor and planning pathways in rodents are organized through a much reduced PPC mapping to CFA and RFA (75). Cholera toxin B (CTB) retrograde tracing coupled with optogenetic probing of CFA/RFA interconnectivity established asymmetric intra-hemispheric connections between the two regions, with CFA strongly projecting to L2/3 and L5a of RFA, and RFA strongly projecting to L1 and L5b of CFA (76).
With regards to reach-to-grasp, there is not a clear distinction of the CFA (primary) and RFA (secondary) function in rodents (Figure 2). Though there is some evidence for preferential reach-related activity in CFA and grasp-related activity in RFA (77, 78), it is believed that CFA and RFA might share the same motor planning and execution function and only differ in how internal state information is integrated with motor planning (71). Recent studies have demonstrated the sensitization of RFA to rewarding situations and internal adaptation to feedback stimuli, which is a key feature of neuroplastic changes and motor control flexibility (71).
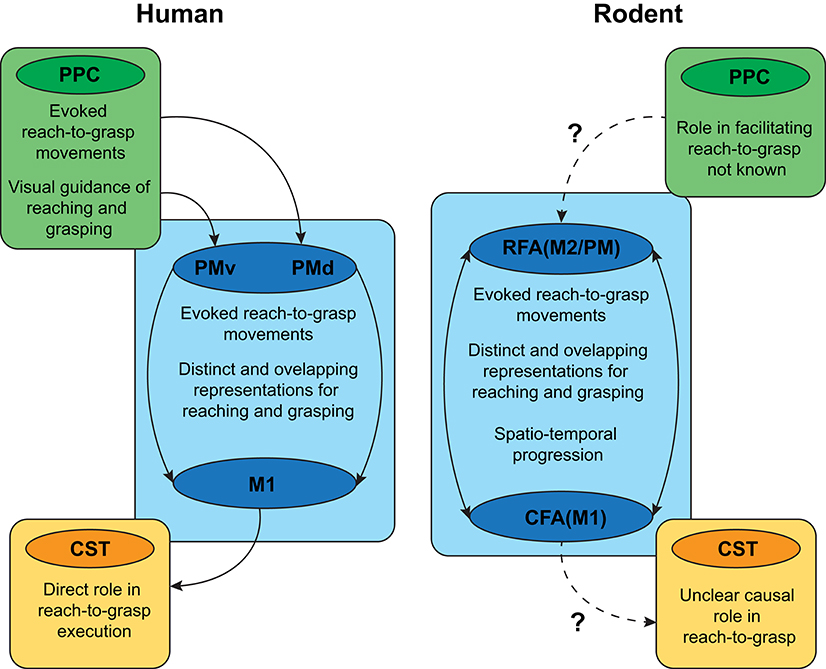
Figure 2. Overview of similarities and differences in the cortical circuitry supporting reach-to-grasp function in humans and rodents. PPC, posterior parietal cortex; CST, corticospinal tract; PMd, dorsal premotor cortex; PMv, ventral premotor cortex, M1, primary motor cortex; CFA/M1, Caudal forebrain area, primary motor cortex; RFA/M2/PM, rostral forebrain area/secondary motor cortex/pre-motor cortex. Solid arrows: known connectivity and circuitry; Dashed arrows: lack of knowledge on connectivity and circuitry.
The somatotopic organization of the motor cortex mapped using intracortical microstimulation (ICMS) has helped reveal part of the functional association between sub-divisions of the cortico-spinal layer (layer IV-V) of the RFA and CFA (79, 80). Short duration ICMS revealed that isolated body movements of the wrist, elbow, and neck had overlapping representations in RFA and CFA. Long duration stimulations, on the other hand, have been shown to elicit complex motor sequences such as reach, grasp, and retract (81), with distinct representations in RFA and CFA. These results suggest that sustained cortical activation might recruit corticospinal neurons into combined sequences of single movements and might also act as a basic template for the learning of skilled motor tasks. In summary, although rodents share homologous neural circuits with humans, the functional segregation of CFA and RFA in the rodent cortex is less distinct when compared to M1 and PM in the human cortex, suggesting that targeting anatomically analogous regions need not necessarily elicit similar functional outcomes.
Scalability: Defining the Overlap Between Rodent and Human Cortical Circuitry for Reach-to-Grasp
In addition to a shared somatotopic organization related to different parts of the body, motor cortical regions may contain spatially distinct representations of complex movements, such as reach-to-grasp, of the animals' natural motor repertoire (82, 83). The similarity between ethological “action maps” across species lends support to the view that a common organizational structure supports reach-to-grasp movements in humans, non-human primates, and rodents (81, 84, 85). The differential extent of cortical mappings devoted to a given movement category, between species (and across individuals within-species) depends on the behavioral relevance and specific experience with performing that movement type (86, 87). This suggests that rodent models of reach-to-grasp can focus on functional, rather than strictly anatomical, correspondence across interacting motor cortical areas to best inform mechanisms of human reach-to-grasp.
Rodent models can also improve our understanding of the functional specialization related to the neural substrates of reach-to-grasp action. In primates, though reaching and grasping components have been localized to dorsomedial and dorsolateral pathways, respectively, recent neurophysiological and neuroimaging evidence has revealed overlapping reach- and grasp-related neural activity in each pathway (88–91). Likewise, rodent models have associated CFA with the reaching/retracting motions associated with more proximal forelimb muscles and RFA with grasping movements associated with distal forelimb muscles (77, 78), whereas others have shown each area plays a role in both reaching and grasping (92, 93). This has led to the view that the posited functional segregation between reaching and grasping may simply be a byproduct of overlapping representations that are activated sequentially during naturalistic reach-to-grasp movements (94). Consequently, translating rodent models of reach-to-grasp to humans will depend on a stronger characterization of how the spatiotemporal progression of neural activity in rodent CFA and RFA maps onto a similar progression in human cortical pathways.
The main disadvantage of rodent models concerns evolutionary development of specialized neural circuits for more sophisticated control of prehension in primates (95). Though both rodents and primates have many descending motor cortex projections through the CST, lesions to the CST have a stronger effect on reach-to-grasp movements in primates than in rodents (96). The presence of direct monosynaptic connections between a phylogenetically newer portion of M1 and cervical motoneurons in primates make CST a prominent contributor to reach-to-grasp movements (59, 97, 98). The diverging function of CST in rodents and primates mirrors the behavioral advantage of primates for fractionated digit control and visual guidance for precision grip (13).
Relative to the motor cortex, fewer investigations of reach-to-grasp have targeted rodent PPC. Though PPC in rodents serves roles across a wide variety of sensory, cognitive, and motor domains (99, 100), whether rodent PPC contains complex movement representations similar to primates is unclear (101). Anatomically, the relative size of PPC in rodents suggests a much smaller role in coordination of reach-to-grasp movements. Functionally, a recent investigation by Soma et al. (102) suggests that PPC's contribution to the control of movement in rodents fundamentally differs from that of primate PPC: whereas neurons in primate PPC show a preference for movements of the contralateral limb (as with M1 and PM), neural activity in rat PPC showed a strong ipsilateral preference.
In summary, rodent models of neural organization show the greatest promise in elucidating the underlying spatial structure and temporal dynamics of premotor and motor areas throughout the course of a reach-to-grasp action. However, they have limited scalability in terms of understanding the role of associative regions and descending pathways in reaching and grasping function.
Loss Of Reach-to-Grasp Function Following Brain Injury
Reach-to-Grasp Dysfunction Following Brain Injury in Humans
Impaired reach-to-grasp after stroke is most commonly observed as a result of upper limb paresis following damage involving the CST or cortical motor areas (103–105). After stroke, paresis mainly affects the side of the body opposite the lesion, in addition to subtler deficits observed ipsilesionally (39). Stroke survivors with damage to the CST generally exhibit slower arm transport velocities, decreased movement smoothness, and more segmented hand opening during grasp (39, 42, 106), while other features, such as the temporal synchrony between the transport and grasp phases, are relatively preserved (42, 106, 107). In TBI, diffuse brain damage can lead to arm paresis and other motor impairments (108). Motor function is relatively preserved relative to neurocognitive effects, this may be partly because the standard clinical measurements do not capture the full extent of sensorimotor deficits after TBI (109, 110). Children with moderate or severe TBI showed slowing of both trajectory and grip formation in a reach-to-grasp task, and differences in performance persists months after TBI onset (111). Other observed deficits in movement preparation and execution are similar to those seen post-stroke, though recovery of function is likely to be different given the large disparities in post-injury plasticity (1, 108).
Behavioral changes following brain injury are coupled with substantial reorganization of cortical networks, and the extent of cortical reorganization beyond the focal tissue damage can have large facilitative or maladaptive effects on motor function and recovery (112, 113). Spontaneous reorganization mainly occurs within the first 3 months post-stroke in humans, a critical time period in which heightened plasticity can be exploited to facilitate recovery (114). The recent advances in brain connectivity analyses have led to a greater emphasis on understanding remote effects of the infarct on large-scale brain network structure (115–119). A meta-analysis found that across a wide variety of motor tasks and impairments, stroke patients exhibit higher activation in primary motor cortex opposite the lesioned hemisphere, as well as increased bilateral premotor activity relative to healthy controls (120). During performance of simple movements, TBI patients show altered brain activation patterns and connectivity within the motor network (121, 122). Though less is known about connectivity with parietal regions, recent neuroimaging studies suggest that weakened connections between the parietal, premotor, and motor areas underlie motor impairments after stroke (123, 124). In both TBI and stroke, changes in parietofrontal networks can persist even in patients who are well-recovered, suggesting a long-term reorganization of functional networks subserving compensatory motor planning and execution (108, 125).
Much of the focus in understanding cortical reorganization has concerned an altered balance of excitatory and inhibitory inputs (E/I balance). TMS has been used extensively as an assessment tool to probe the excitability of motor cortex and functional CST integrity via measurement of motor-evoked potentials. In early stroke, patients without upper-limb motor evoked potentials exhibited considerably worse functional recovery than patients with observable motor evoked potentials (126). When used in combination with standard clinical assessments of upper-limb impairment and MRI-based biomarkers of CST integrity, TMS-based assessments of corticospinal excitability strongly predicted potential (or lack of potential) for motor recovery after stroke (127, 128). More severe CST damage, especially damage to fibers originating in primary motor cortex, is associated with impaired hand function and poorer rehabilitation outcomes (129–131). TMS GABA receptor function and tonic GABA concentration, markers of inhibition, are generally reduced in the affected hemisphere post-stroke (132–134). In severe TBI, reduced corticospinal excitability is related to both greater diffuse axonal injury and greater motor impairment (135, 136).
Changes in relative excitation and inhibition and their relevance for motor recovery after ischemic stroke depend on the severity and location of the lesion. Di Pino et al. (137) proposed a “bimodal-balance recovery model,” in which the optimal interhemispheric interactions between motor cortices depends on the available neural pathways post-injury. According to the model, in patients with relatively low impairment (and preserved neural pathways), increased inhibition of hemisphere opposite of the lesion onto the lesioned hemisphere is maladaptive, whereas in patients with severe impairment (and more damaged neural pathways), an interhemispheric imbalance constitutes compensatory plasticity supportive of recovery, perhaps a consequence of shifting toward more ipsilateral control via the cortico-reticulo-propriospinal pathway (138). Likewise, the role of premotor cortex depends on impairment severity—whereas in less impaired patients, ipsilesional PM plays a larger role through increased connectivity with M1 and descending corticospinal tracts, in more impaired patients contralesional PM facilitates M1 to aid in movement execution (139, 140). However, recent evidence suggests that interhemispheric imbalance only emerges after the sensitive period when changes in motor behavior are minimal (141, 142). Thus, how targeting restoration of E/I balance may facilitate recovery post-injury remains an open question.
Motor Cortex Injury and Reach-to-Grasp Function Loss in Rodents
Stroke and TBI in rodent models cause sizeable lesions and significant cell death in brain tissue. Despite similar manifestations of hemorrhage and edema across both stroke and TBI, their pathophysiologies are quite different. Stroke can be ischemic (75% of all cases), hemorrhagic (15% of all cases) or both (143), leading to reduced blood perfusion of the adjacent brain tissue that causes an immediate loss of neuronal activity and functional impairment. The state of prolonged ischemia (oxygen depletion) triggers massive cell death that induces progressively larger lesions due to secondary insults mediated by excitotoxic agent release (neurotransmitters) and neuroimmune responses. Severe TBI results from a blunt force injury or severe penetrating lesions to brain tissue that leads to shearing of neuronal circuits and vasculature. The progressive damage of brain tissue in the days, months, and years thereafter are a result of the secondary injury cascades that are similar to stroke as described above. Following a severe TBI, the massive loss in tissue integrity in the lesion site and surrounding regions is due to the significant cell death and breach of the blood-brain barrier (BBB), which results in permanent loss of function.
The functional impairments observed in rats following TBI have been shown to be dependent on age (144), lesion size, and depth (145), as well as the presence of an edema (146). The extent of tissue loss dictates the initial functional impairment and the speed of recovery. Rodents subjected to small focal impact injuries (<3.0 diameter) might recover up to 90% of their initial pre-injury performance within 15 days (145). However, the same extent of recovery is not evidenced in the case of larger and deeper lesions, especially those spanning the pyramidal tract and white matter, with animals never recovering more than 80% of initial performance within 4 weeks post-TBI (146). The intervals between testing post-injury vary considerably across studies, which makes discriminating between causative factors of recovery quite challenging.
Severe TBI studies in rodents have demonstrated that the motor cortex undergoes reorganization post-TBI, involving either intra-cortical inhibition (147–150), inter-hemispheric crosstalk, axonal sprouting (151), or cholinergic/dopaminergic-dependent plasticity disruptions (152). Similar to humans (see Reach-to-Grasp Dysfunction Following Brain Injury in Humans section), an increase in activity in perilesional tissue and complementary motor regions such as PM, is indicative of the reorganization of motor function following injury (153–155). Motor reorganization and compensatory mechanisms observed in rodents are often associated with a return of function to near pre-lesion performance levels (146). It is now well-established that the extent of cortical reorganization and its associated re-functionalization, or lack thereof, is heavily dependent on the extent of exposure to stimulating environments and tasks prone to induce neuroplastic changes such as enriched environments and novel motor tasks (152, 156, 157), which also form the basis of some rehabilitative strategies used in humans (155, 158, 159).
Direct investigations of circuit refunctionalization after TBI using voltage-sensor dye imaging of the entire cortex showed a long-lasting, delayed, and decreased response of the ipsi- and contralateral cortex following forelimb stimulation (sensory). Whereas, direct optogenetic stimulation of the RFA cortical surface revealed a strong asymmetric contralateral response indicating the presence of a spontaneous inter-hemispheric rewiring at week 8 post-injury (160). These results supported the notion that motor learning of the intact limb (contralesional cortex) is further enhanced and might provide compensatory support for task completion (161). Indeed, these findings are further supported by observations indicating expansion of the contralesional somatotopic motor map following TBI, and studies demonstrating intact limb restraint leading to limited recovery of the impacted limb (162). In addition, restraining of the intact limb limited recovery potential of the impacted limb (146). Altogether, these results suggest that plasticity changes and motor reorganization of the hemisphere contralateral to the lesion might partially support functional recovery after TBI in rodents.
The convergence and overlap of peri-lesional, intra-cortical, inter-hemispheric, and systemic compensations promote a spontaneous reorganization and offloading of motor function following injury. All these plastic changes might ultimately be driven by trophic factor-dependent mechanisms such as those activated by brain-derived neurotrophic factor (BDNF) (163–166), which facilitates spontaneous rewiring-driven functional recovery observed in rodents after exercise rehabilitation, and potentially after drug and neurostimulation driven repair.
Scalability: Translating Rodent Injury Models to Post-injury Reorganization in Humans
Cortical reorganization of upper-limb function using ICMS protocols in rodents, and TMS/MRI in humans, have been instrumental in detecting enhanced perilesional and contralesional neural activity and excitatory/inhibitory (E/I) imbalance, both acutely and chronically post-injury. The E/I imbalance has been shown to drive acute cortical depression that further enhanced secondary excitotoxic cascades that accelerated intra- and perilesional neuronal death (167). It is therefore important to note that E/I imbalance might also be targeted to shape cortical reorganization post-injury through the promotion of long-term potentiation and depression (168–170). Dialysis and magnetic resonance spectroscopy studies in humans found E/I imbalance similar to that observed in rodents (171), however, the direct correspondence between rodent and human responses to a loss of E/I homeostasis remains to be explored.
Despite profound disparities in the anatomical correspondence in the CST between rodents and humans (see Scalability: Defining the Overlap Between Rodent and Human Cortical Circuitry for Reach-to-Grasp section), it is now evident that the extent of CST loss post-injury might proportionally correspond to the observed contralesional hemispheric activation (146). The induction of controlled cortical impact lesions of brain tissue and quantification of refunctionalizaton post-injury is quite feasible in rodent studies when compared to humans, where comorbidities and lesion variabilities might provide a very restricted spatial and mechanistic understanding of injury progression.
The reach-to-grasp task is considered a skilled learned task that involves the acquisition of specific and fine-tuned sequences of movements (172). As such, it has been demonstrated that skilled motor learning as opposed to other forms of repetitious motor learning tasks induces a reshaping of the motor cortical landscape (173) that is dependent on intra-cortical integration of sensory and planning information (174, 175), and on the cholinergic (152, 176) and the dopaminergic (177, 178) afferent projections to the motor cortex. Importantly, the morphology, laminar distribution and density of cholinergic basal forebrain innervation varies greatly between humans and non-human primates, let alone between humans and rodents (179, 180). Although the laminar distribution of cholinergic systems and control of plasticity by cholinergic inputs is highly conserved between rodent and humans (181, 182), the specific role of this system in motor learning and post-injury adaptation remains to be elucidated.
Despite several disparities, the shared principles of reorganization between rodent and human models such as anatomical and physiological changes, E/I imbalance, regional compensatory activity, and impact of environmental factors, point to the translational relevance of pre-clinical rodent studies.
Neuromodulation for Motor Recovery Post-Brain Injury
Neurostimulation to Promote Motor Recovery: Clinical Studies
The goal of non-invasive brain stimulation (NIBS) therapies is to augment existing rehabilitation protocols through shaping the cascade of reorganizational processes that occur post-injury. NIBS protocols aim to either upregulate preserved areas of cortex supporting effective recovery or downregulate areas that are thought to be maladaptive. Two of the most common methods in human clinical trials are repetitive transcranial magnetic stimulation (rTMS) and transcranial direct current stimulation (tDCS). In each of these methods, the after-effects of stimulation remain for minutes or hours beyond the stimulation itself, allowing for behavioral testing of effects or pairing stimulation with other rehabilitation interventions. rTMS involves a train of magnetic pulses for a set duration, intensity, interval, and frequency. Low-frequency (≤1 Hz) rTMS delivered continuously for 10–30 min induces inhibition of cortical excitability, whereas high-frequency (5–20 Hz) increases cortical excitability. A more recent form of rTMS is theta-burst stimulation (TBS), in which patterned pulses are delivered in triplets at 50 Hz either continuously (every 200 ms for 20 or 40 s) to increase cortical excitability (continuous TBS; cTBS) or intermittently in 10 bursts repeated every 10 s for 192 s to decrease cortical excitability (intermittent TBS, iTBS). TBS is appealing in clinical settings due to its shorter duration, lower intensity, and longer lasting after-effects relative to LF- or HF-rTMS (183).
In tDCS, application of low-intensity (1–2 mA) currents through two electrodes placed on the scalp for 5–30 min increases or reduces excitability in a polarity-specific manner. When compared to rTMS, tDCS has worse spatiotemporal resolution but generally has longer after-effects. Although more recently, high-definition tDCS has been used to improve spatial resolution (184, 185). Though TMS and tDCS have different mechanisms of action, they are both thought to activate mechanisms of neuroplasticity that are similar to long-term potentiation or long-term depression (186), both at the stimulation location and remotely (185). Typically, intervention studies investigating NIBS and motor recovery have designed stimulation paradigms based on principles of the interhemispheric inhibition model, either by exciting ipsilesional cortex (HF-rTMS, iTBS, anodal tDCS), inhibiting contralesional cortex (LF-rTMS, cTBS, cathodal tDCS), or both (187, 188).
There is currently a greater emphasis on implementing NIBS protocols for stroke than for TBI recovery. Of the currently registered clinical trials using rTMS or tDCS for treatment of stroke or TBI, 85% are focused on stroke recovery (as of Dec 2019—Figure 3). Research on the applicability of NIBS as an intervention for recovery of motor deficits is in the nascent stages in TBI as compared to stroke (6, 189, 190). Despite this rapidly increasing interest in clinical applications, there is little consensus on the efficacy of such treatment protocols [for systematic reviews and meta-analyses, see (191–198)]. The mixed effectiveness across studies is a confluence of many interrelated factors. In healthy humans, factors such as demographics, genetic polymorphisms, anatomical features, and current brain state all contribute to response variability (183). Compounding these differences, in patients, the level of initial functional impairment (mild to severe) and associated functional integrity of the CST (e.g., presence or absence of TMS-induced motor-evoked potentials) further influences which NIBS treatment protocol (excitation or inhibition) is most likely to be effective (199). Response to treatment also depends on the timing relative to the injury—whereas the goal of stimulation in the acute and subacute phases may be to augment motor learning during spontaneous recovery, in the chronic phase NIBS may instead be geared toward “re-opening” the sensitive period and/or focusing on compensation. From an experimental design perspective, the specific parameters selected, including intensity, duration, focality of stimulation, and number of stimulation sessions, can influence the directionality of changes to cortical excitability and treatment efficacy (200–202). Finally, how NIBS is paired with behavior modulates effectiveness, with treatment effects often most promising when stimulation is applied during motor training (185, 203, 204).
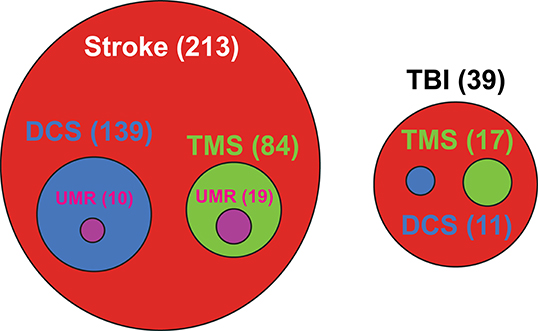
Figure 3. Current landscape for clinical trials investigating non-invasive brain stimulation treatments for stroke and traumatic brain injury. TBI, Traumatic brain injury; DCS, Direct current stimulation; TMS, Transcranial magnetic stimulation; UMR, Upper-limb motor rehabilitation. Number in parenthesis represents the number of matching clinical trials related to NIBS treatment. Source: https://www.globalclinicaltrialsdata.com/; data post-filtered for matching key words on conditions and treatments.
Few NIBS applications thus far have specifically pinpointed kinematic-based reach-to-grasp outcomes after brain injury, though many studies have used clinical assessments of dexterity (e.g., Jebsen-Taylor Hand Function Test, Perdue Pegboard Test) or tests with reaching and grasping subcomponents (e.g., Wolf Motor Function Test, Action Research Arm Test) (191, 205). Nowak et al. (206) examined the effects of LF-rTMS to the contralesional M1 in a cohort of patients with subcortical strokes with mild to moderate motor impairment. Consistent with the bimodal-balance recovery model, they found that reducing contralesional M1 excitability in this cohort resulted in improved peak wrist velocities and peak digit apertures of the affected hand, matching kinematic performance of their unaffected hand. Similarly, LF-rTMS to the contralesional hemisphere improved peak aperture and a composite measure of reach-to-grasp coordination, especially for grasps to smaller objects (207, 208). LF-rTMS and TBS have also been shown to augment effects of reach-to-grasp and precision grip training in chronic stroke patients (209, 210). Finally, Lefebvre et al. (211) found improved precision grip kinematics following bilateral tDCS, in which anodal stimulation over ipsilesional M1 and cathodal stimulation over contralesional M1 was applied simultaneously. Collectively, these studies provide evidence that the positive effects of NIBS on motor function can extend to more complex manual dexterity.
Despite obvious benefits, whether NIBS can aid in the recovery of complex motor functions like reach-to-grasp remains an open question. Clinical NIBS studies to date have almost exclusively targeted M1 for both practical (e.g., determining optimal stimulation coordinates) and theoretical (interhemispheric competition models) reasons. Compared to improvements in simple motor parameters, stimulating M1 sometimes have a muted effect on complex motor functions (212). Thus, given the wealth of evidence of widespread changes to cortico-cortical interactions governing motor control after brain injury, there is a need to investigate whether other nodes in the parietofrontal system are suitable stimulation targets for improving reach-to-grasp behavior. Supporting this notion, Lotze et al. (213) found that stimulating contralesional M1, PMd, and SPL each led to deficits in performance of a complex sequential motor task in well-recovered stroke patients, suggesting that all three areas play a role in compensatory reorganization. Though PMd has recently received more attention as a potential stimulation target for improving dexterity following brain injury (214, 215), similar investigations targeting PPC in reach-to-grasp function are lacking.
Neurostimulation to Promote Motor Recovery: Pre-clinical Models
Current clinical strategies to treat post brain injury trauma following a stroke or a TBI are mostly designed to alleviate immediate symptoms such as intracranial pressure, blood clots, overall blood perfusion and control of hemorrhage (216, 217). Despite the enormous physical, medical, and economic burden associated with post-brain injury, to this date, no clinical treatment is available for the repair and re-functionalization of the damaged brain (218–220).
NIBS techniques such as rTMS and tDCS/DCS allow for a high temporal and spatial resolution control of neuronal ensemble activity in humans as well as in rodents. The pre-clinical investigation of neurostimulation and reach-and-grasp has substantially improved our understanding of the underlying mechanisms of recovery post-injury, facilitated by combining NIBS with more invasive approaches. Mechanistically, in the rodents, TMS and DCS modulate excitability of cortical structures (221–223), BDNF-dependent motor learning (224–226), neurogenesis (227, 228), and can result in improved motor performance (229). Overall, the use of NIBS in rodents can be broadly classified into two distinct approaches:
Schedule Stimulation and Skilled Motor Recovery
It has been shown that the severity of the motor cortex injury, and particularly, the extent of damage to corticospinal neurons can seriously impair spontaneous recovery in rats (146). M1 electrical stimulation targeting the contralesional cortical column, including corticospinal neurons, promoted corticospinal axonal sprouting and re-functionalization at the contralesional spinal cord tracks, leading to improvements in reaching (230–232). While these previous studies used intensive and long stimulation protocols, more acute application of tDCS has also been shown to facilitate functional motor recovery (233, 234). Similarly, monopolar fast DCS could improve skilled reaching in rats over a 10 day period (235, 236). Such scheduled stimulation might cause transient responses in activated microglia (237, 238).
Pairing Neurostimulation and Behavior for Targeted Causal Recovery
Ramanathan et al. (153) have shown in rodents that the RFA (rostral forelimb area; M2/PM) produces short, slow oscillatory local field potentials for the duration of the reach-grasp-retract phase of a single pellet retrieval assay which disappear, intra- and peri-lesionally, following stroke along with a reduction in retrieval performance. Interestingly, the authors paralleled a similar loss in sensorimotor synchronization in patients with stroke performing a center-out reaching task. Timely triggering of DCS stimulations could re-ignite peri-lesional slow oscillations and reach-to-grasp performance. The cross-functionality of the RFA and CFA was demonstrated in another study where a CFA injury resulting in loss of reach-to-grasp performance could be recovered through closed-loop DCS re-pairing of the primary sensory cortex (S1) and RFA, resulting in a functional stream that bypasses the CFA and facilitates faster reach-and-grasp recovery (239). Pairing neurostimulation with behavior has a major advantage of creating associations with the stimulation in a reliable manner, leading to faster recovery (Figure 4). Ultimately, it is expected that neurostimulation paired with behavioral tasks would have a longer-lasting re-functionalizing impact than neurostimulation alone.
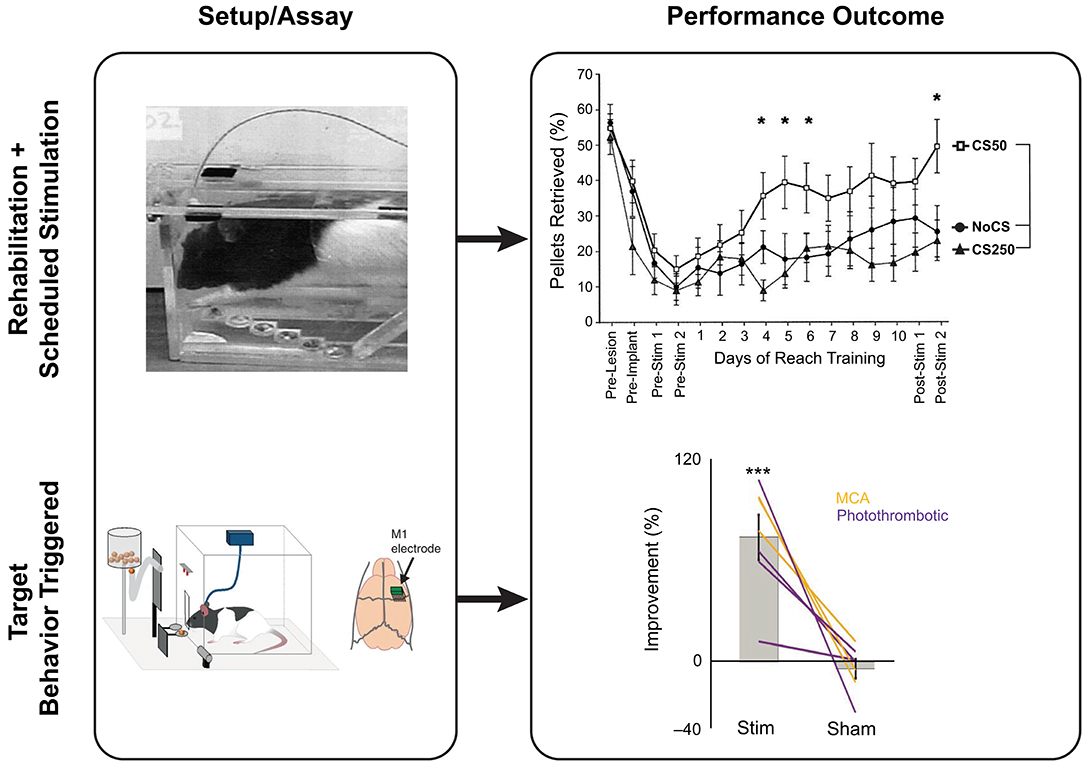
Figure 4. Neurostimulation strategies and reach-to-grasp recovery in rats post-injury showing two typical stimulation paradigms. Top panel: Scheduled rehabilitative stimulation using the staircase task on rodents post-controlled cortical impact injury and tDCS at various frequencies of stimulation. Bottom panel: Behavior-triggered stimulation. Using an automated skilled reach task box, the timing of stimulation can be performed relatively to the intended movement to reinforce cortical plasticity and recovery post-stroke in rats. Adapted from Ramanathan et al. (153), reprinted with permission from Springer Nature, Nature Medicine (advance online publication, 07/2020, doi: https://doi.org/10.1038/s41591-018-0058-y), and Adkins-Muir and Jones (233), reprinted by permission of Taylor & Francis (Taylor & Francis Ltd, http://www.tandfonline.com). *p < 0.05 and ***p < 0.001.
Altogether, these results show that rodent models allow for a wide exploration of parameters and combinatorial approaches, while providing mechanistic evidence for their efficacy.
Scalability: Using Pre-clinical Models to Refine Neurostimulation Parameters for Clinical Use
Pre-clinical use of neurostimulation has been essential for optimizing the wide parameter space that encompasses NIBS, as well as to define the essential mechanistic components that direct positive outcomes following stimulation such as, neurogenesis, neural stem cell proliferation/migration (240, 241), the triggering of plasticity enhancing pathways (224), the promotion of excitability (242, 243), dendritic spine and axonal sprouting (244) and the direct pairing between volition and reach-and-grasp control (153, 239). Yet, the role and translational relevance of these factors in mediating recovery in humans after stroke or TBI is not entirely clear and remains to be investigated.
Clinical studies remain very segregated from pre-clinical findings, and promising results from pre-clinical studies remain largely untested in humans (245). The disconnect between applied research and clinical practice suffers in part from medical conservatism and “guesswork” around known stimulation parameters. This divide could be bridged to a significant extent by evidence from pre-clinical research studies, which offer the exploration of stimulation parameters (246) and that could help reduce guesswork and improve chances of success in human testing (247). We propose that the exploratory data obtained from rodent studies could be integrated in human clinical trials by replacing current clinical protocols by relevant pre-clinically defined parameters within a safe and applicable framework (248). This information can be integrated into a decision-tree approach to select cross-cutting or distinct parameters, and behavioral pairing combinations, which can lead to favorable functional outcomes (Figure 5).
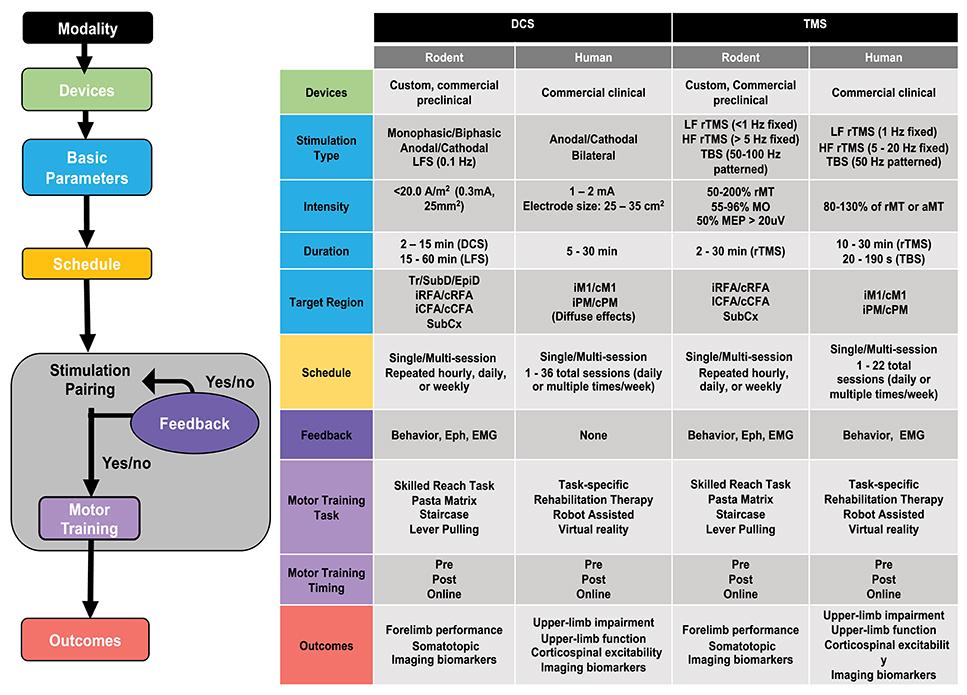
Figure 5. Decision tree for designing rehabilitative paradigm using non-invasive brain stimulation (NIBS). Left panel: Flow chart illustrating the various steps for designing a NIBS-based rehabilitative paradigm. Right panel: Table summarizing common, non-exhaustive ranges of parameters and protocols used for direct current stimulation (DCS) and transcranial magnetic stimulation (TMS) techniques in rodent models and human studies of motor recovery following acquired brain injury. LF, low frequency; HF, High frequency; TBS, theta burst stimulation; LFS, Low frequency stimulation; rTMS, repetitive TMS; rMT, resting motor threshold; aMT, active motor threshold; MO, maximum output (TMS device); MEP, motor-evoked potential; Tr, transcranial; SubD, subdural; EpiD, Epidural; SubCx, subcortical; i/c, ipsi/contralateral; RFA, rostral forelimb area; CFA, caudal forelimb area; M1, motor cortex; PM, premotor cortex; CST, corticospinal tract; Eph, Electrophysiology; EMG, electromyography. Representative parameters drawn from (153, 191, 194, 196, 198, 224, 233, 239, 249, 250).
Several studies have explored the effectiveness of tDCS stimulation in rats post-TBI. The comparison of tDCS parameters such as polarity (251) and train stimulation frequency (233), in combination with rehabilitation (252, 253) or intact limb-restriction (254), have demonstrated that the cathodic train stimulation of 100 Hz paired with motor rehabilitation could be used as an optimal tDCS-based rehabilitation scheme for enabling reach-to-grasp function recovery post-TBI—although this remains to be tested in humans. More recently, Ramanathan et al. (153) identified the initiation of slow oscillation pre-reach-to-grasp movement in rats and humans, and their causal relationship to upper-limb performance. Using a behavior-triggered stimulation regimen that reproduced the observed slow oscillations in the peri-lesional motor area, they demonstrated that paired behavior-stimulation could enhance reach-to-grasp performance. These examples illustrate stimulation parameters and timing in pre-clinical rodent studies, that have promising translational potential in humans. More importantly, these preclinical results suggest that NIBS parameters that target cortical plasticity, rather than E/I imbalance, have significant benefits, especially when performed in combination with upper-limb rehabilitation.
There are translational alternatives for when DCS parameters are not directly applicable to humans, particularly for closed-loop and repeated train stimulation parameters, which encompass rapid and abrupt changes of current. For example, transcranial alternative current (tACS) offers a less irritable and sudden change in stimulation than tDCS, which might be more suitable for human applications, as reflected in a growing body of literature showing effectiveness in both rodents and humans to treat brain injury (255, 256). In addition, online rTMS approaches that time stimulation onset to changes in movement, muscle activity, or brain state are novel avenues to approximate closed-loop stimulation in rodent models (257, 258).
Key challenges and questions need to be addressed to ease translation between rodent models and human studies of NIBS to improve motor recovery after brain injury (Table 1). For example, both rodent and human studies would benefit from incorporating a shared set of kinematic assessments in order to better quantify and translate motor outcomes (259). This can not only help resolve open questions about cortical organization for reach-to-grasp action (e.g., what is the functional correspondence between rodent CFA/RFA and human M1/PM?), but would provide a common framework for comparing stimulation parameters across species (e.g., does stimulation parameter A in rodents lead to a similar change in reach-to-grasp kinematics as stimulation parameter B in humans?). Rodent models could benefit from a more deliberate focus on stimulation devices and protocols that can be feasibly and safely implemented in humans, and to incorporate systematic heterogeneity in the study population as a way to address the high variability in individual responses to NIBS interventions in clinical trials (246, 260). Likewise, clinical studies can continue to optimize strategies for pairing NIBS with motor behavior to improve functional recovery (204). Finally, both studies in rodents and humans could explore novel potential targets beyond M1, both to inform our understanding of network plasticity mediating reach-to-grasp actions following brain injury and to potentially tailor interventions to individuals.
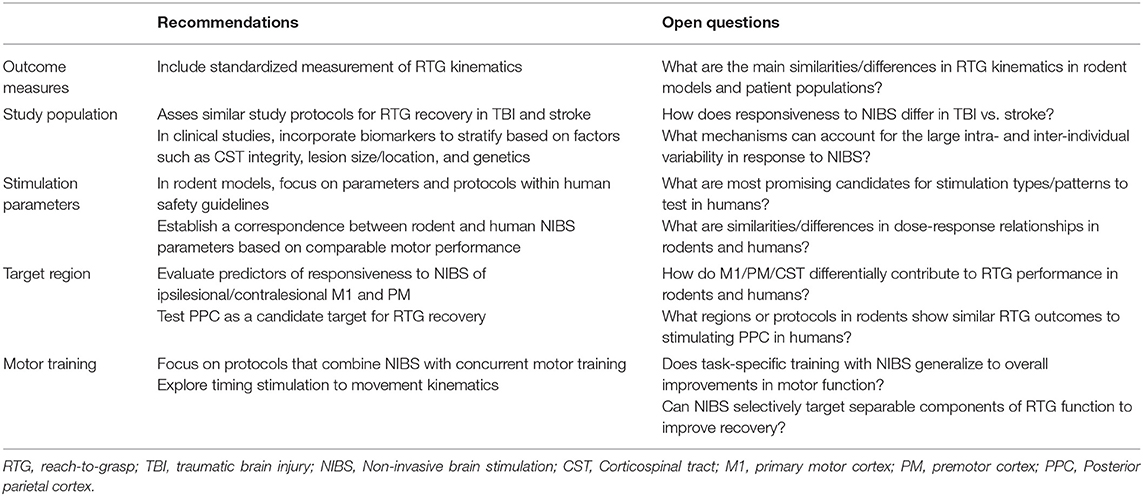
Table 1. Cross-cutting recommendations and open questions related to pre-clinical and clinical assessments of reach-to-grasp function.
Conclusion
In this review, we present the idea that the reach-to-grasp movement is an impaired motor function following acquired brain injury, such as traumatic brain injury or stroke. Though important dissimilarities in neural architecture and the behavioral response to injuries exist in humans and rodents, overall the reach-to-grasp movement presents a strongly conserved neural circuitry across the two species. Similar responses to brain injury and to treatment post-injury demonstrate that the rodent model could serve as a testbed for translational rehabilitation of brain injury using non-invasive brain stimulation (NIBS). We propose that pre-clinical parameters should be considered in current clinical trials to improve functional outcomes. Currently, clinical trials using NIBS are primarily focused on stroke, but TBI might deserve as much interest as stroke due to its prevalence, and in turn, because it is a major risk factor for stroke (261). More importantly, for both stroke and TBI, the rehabilitative applications and clinical trials targeting upper-limb recovery and reach-to-grasp are still largely lacking. The considerable knowledge associated with volitional motor control of reach-to-grasp should be used as a steppingstone to deepen our understanding of functional remapping post-injury as well as functional recovery post-NIBS.
Author Contributions
C-FL and DB edited the data and figures. C-FL and LK analyzed the data base entries. C-FL, DB, TS, and LK wrote and edited the manuscript. All authors contributed to the article and approved the submitted version.
Funding
DB received support from the American Heart Association (18POST34060183). A portion of this research was supported by a grant from the University of Georgia Research Foundation Inc. to TS. This work was supported in part by an NIH/NINDS R01 award (R01NS099596) to LK and Technology Development Funds from the Alliance for Regenerative Rehabilitation Research and Training, University of Pittsburgh to C-FL and LK.
Conflict of Interest
The authors declare that the research was conducted in the absence of any commercial or financial relationships that could be construed as a potential conflict of interest.
References
1. Walker WC, Pickett TC. Motor impairment after severe traumatic brain injury: a longitudinal multicenter study. J Rehabil Res Dev. (2007) 44:975–82. doi: 10.1682/JRRD.2006.12.0158
2. Langhorne P, Coupar F, Pollock A. Motor recovery after stroke: a systematic review. Lancet Neurol. (2009) 8:741–54. doi: 10.1016/S1474-4422(09)70150-4
3. Pelton T, van Vliet P, Hollands K. Interventions for improving coordination of reach to grasp following stroke: a systematic review. Int J Evid Based Healthc. (2012) 10:89–102. doi: 10.1111/j.1744-1609.2012.00261.x
4. Marshall S, Teasell R, Bayona N, Lippert C, Chundamala J, Villamere J, et al. Motor impairment rehabilitation post acquired brain injury. Brain Inj. (2007) 21:133–60. doi: 10.1080/02699050701201383
5. Morishita T, Hummel FC. Non-invasive Brain Stimulation (NIBS) in motor recovery after stroke: concepts to increase efficacy. Curr Behav Neurosci Rep. (2017) 4:280–9. doi: 10.1007/s40473-017-0121-x
6. Clayton E, Kinley-Cooper SK, Weber RA, Adkins DL. Brain stimulation: neuromodulation as a potential treatment for motor recovery following traumatic brain injury. Brain Res. (2016) 1640(Pt. A):130–8. doi: 10.1016/j.brainres.2016.01.056
7. Grefkes C, Fink GR. Noninvasive brain stimulation after stroke: it is time for large randomized controlled trials! Curr Opin Neurol. (2016) 29:714–20. doi: 10.1097/WCO.0000000000000395
8. Smith MC, Stinear CM. Transcranial magnetic stimulation (TMS) in stroke: ready for clinical practice? J Clin Neurosci. (2016) 31:10–4. doi: 10.1016/j.jocn.2016.01.034
9. Krubitzer LA, Seelke AM. Cortical evolution in mammals: the bane and beauty of phenotypic variability. Proc Natl Acad Sci USA. (2012) 109(Suppl. 1):10647–54. doi: 10.1073/pnas.1201891109
10. Papale AE, Hooks BM. Circuit changes in motor cortex during motor skill learning. Neuroscience. (2018) 368:283–97. doi: 10.1016/j.neuroscience.2017.09.010
11. Gerraty RT, Davidow JY, Foerde K, Galvan A, Bassett DS, Shohamy D. Dynamic flexibility in striatal-cortical circuits supports reinforcement learning. J Neurosci. (2018) 38:2442–3. doi: 10.1523/JNEUROSCI.2084-17.2018
12. Chung K, Deisseroth K. CLARITY for mapping the nervous system. Nat Methods. (2013) 10:508–13. doi: 10.1038/nmeth.2481
13. Karl JM, Whishaw IQ. Different evolutionary origins for the reach and the grasp: an explanation for dual visuomotor channels in primate parietofrontal cortex. Front Neurol. (2013) 4:208. doi: 10.3389/fneur.2013.00208
14. Gibson JJ. The Ecological Approach to Visual Perception: Classic Edition. New York, NY: Psychology Press (1979).
15. Castiello U. The neuroscience of grasping. Nat Rev Neurosci. (2005) 6:726–36. doi: 10.1038/nrn1744
16. Savelsbergh G, Steenbergen B, van der Kamp J. The role of fragility information in the guidance of the precision grip. Hum Move Sci. (1996) 15:115–27. doi: 10.1016/0167-9457(95)00039-9
17. Santello M, Soechting JF. Gradual molding of the hand to object contours. J Neurophysiol. (1998) 79:1307–20. doi: 10.1152/jn.1998.79.3.1307
18. Napier JR. The prehensile movements of the human hand. J Bone Joint Surg Br. (1956) 38-B:902–13. doi: 10.1302/0301-620X.38B4.902
19. Schlesinger G. Der mechanische aufbau der künstlichen glieder. In: Borchardt M editors. Ersatzglieder und Arbeitshilfen fur Kriegsbescliadigte und Unfaflverletzt. Berlin: Springer (1919). p. 321–661.
20. Landsmeer JM. Power grip and precision handling. Ann Rheum Dis. (1962) 21:164–70. doi: 10.1136/ard.21.2.164
21. Sacrey LA, Alaverdashvili M, Whishaw IQ. Similar hand shaping in reaching-for-food (skilled reaching) in rats and humans provides evidence of homology in release, collection, manipulation movements. Behav Brain Res. (2009) 204:153–61. doi: 10.1016/j.bbr.2009.05.035
22. Iberall T, Bingham G, Arbib MA. Opposition space as a structuring concept for the analysis of skilled hand movements. Exp Brain Res. (1986) 15:158–73. doi: 10.1007/978-3-642-71476-4_12
24. Whishaw IQ. Lateralization and reaching skill related: results and implications from a large sample of Long-Evans rats. Behav Brain Res. (1992) 52:45–8. doi: 10.1016/S0166-4328(05)80323-7
25. Baird AL, Meldrum A, Dunnett SB. The staircase test of skilled reaching in mice. Brain Res Bull. (2001) 54:243–50. doi: 10.1016/S0361-9230(00)00457-3
26. Whishaw IQ, Pellis SM, Gorny BP, Pellis VC. The impairments in reaching and the movements of compensation in rats with motor cortex lesions: an endpoint, videorecording, and movement notation analysis. Behav Brain Res. (1991) 42:77–91. doi: 10.1016/S0166-4328(05)80042-7
27. Ballermann M, McKenna J, Whishaw IQ. A grasp-related deficit in tactile discrimination following dorsal column lesion in the rat. Brain Res Bull. (2001) 54:237–42. doi: 10.1016/S0361-9230(01)00431-2
28. Allred RP, Adkins DL, Woodlee MT, Husbands LC, Maldonado MA, Kane JR, et al. The vermicelli handling test: a simple quantitative measure of dexterous forepaw function in rats. J Neurosci Methods. (2008) 170:229–44. doi: 10.1016/j.jneumeth.2008.01.015
29. Whishaw IQ, Gharbawie OA, Clark BJ, Lehmann H. The exploratory behavior of rats in an open environment optimizes security. Behav Brain Res. (2006) 171:230–9. doi: 10.1016/j.bbr.2006.03.037
30. Metz GA, Farr T, Ballermann M, Whishaw IQ. Chronic levodopa therapy does not improve skilled reach accuracy or reach range on a pasta matrix reaching task in 6-OHDA dopamine-depleted (hemi-Parkinson analogue) rats. Eur J Neurosci. (2001) 14:27–37. doi: 10.1046/j.0953-816x.2001.01615.x
31. Woodard CL, Bolanos F, Boyd JD, Silasi G, Murphy TH, Raymond LA. An automated home-cage system to assess learning and performance of a skilled motor task in a mouse model of huntington's disease. eNeuro. (2017) 4:ENEURO.0141-17.2017. doi: 10.1523/ENEURO.0141-17.2017
32. Hays SA, Khodaparast N, Sloan AM, Hulsey DR, Pantoja M, Ruiz AD, et al. The isometric pull task: a novel automated method for quantifying forelimb force generation in rats. J Neurosci Methods. (2013) 212:329–37. doi: 10.1016/j.jneumeth.2012.11.007
33. Mathis MW, Mathis A, Uchida N. Somatosensory cortex plays an essential role in forelimb motor adaptation in mice. Neuron. (2017) 93:1493–503.e6. doi: 10.1016/j.neuron.2017.02.049
34. Klein A. L, Sacrey AR, Whishaw IQ, Dunnett SB. The use of rodent skilled reaching as a translational model for investigating brain damage and disease. Neurosci Biobehav Rev. (2012) 36:1030–42. doi: 10.1016/j.neubiorev.2011.12.010
35. Whishaw IQ, Suchowersky O, Davis L, Sarna J, Metz GA, Pellis SM. Impairment of pronation, supination, and body co-ordination in reach-to-grasp tasks in human Parkinson's disease (PD) reveals homology to deficits in animal models. Behav Brain Res. (2002) 133:165–76. doi: 10.1016/S0166-4328(01)00479-X
36. Whishaw IQ, Pellis SM, Gorny BP. Skilled reaching in rats and humans: evidence for parallel development or homology. Behav Brain Res. (1992) 47:59–70. doi: 10.1016/S0166-4328(05)80252-9
37. Schaefer SY, DeJong SL, Cherry KM, Lang CE. Grip type and task goal modify reach-to-grasp performance in post-stroke hemiparesis. Motor Control. (2012) 16:245–64. doi: 10.1123/mcj.16.2.245
38. Álvarez AG, Roby-Brami A, Robertson J, Roche N. Functional classification of grasp strategies used by hemiplegic patients. PLoS ONE. (2017) 12:e0187608. doi: 10.1371/journal.pone.0187608
39. Nowak DA. The impact of stroke on the performance of grasping: usefulness of kinetic and kinematic motion analysis. Neurosci Biobehav Rev. (2008) 32:1439–50. doi: 10.1016/j.neubiorev.2008.05.021
40. Xu J, Ejaz N, Hertler B, Branscheidt M, Widmer M, Faria AV, et al. Separable systems for recovery of finger strength and control after stroke. J Neurophysiol. (2017) 118:1151–63. doi: 10.1152/jn.00123.2017
41. Michaelsen SM, Magdalon EC, Levin MF. Grip aperture scaling to object size in chronic stroke. Motor Control. (2009) 13:197–217. doi: 10.1123/mcj.13.2.197
42. Lang CE, Schieber MH. Stroke. In: Nowak DA, Hermsdörfer J, editors. Sensorimotor Control of Grasping: Physiology and Pathophysiology. Cambridge: University Press (2009). p. 296–310.
43. Lemke SM, Ramanathan DS, Guo L, Won SJ, Ganguly K. Emergent modular neural control drives coordinated motor actions. Nat Neurosci. (2019) 22:1122–31. doi: 10.1038/s41593-019-0407-2
44. Ryait H, Bermudez-Contreras E, Harvey M, Faraji J, Mirza Agha B, Gomez-Palacio Schjetnan A, et al. Data-driven analyses of motor impairments in animal models of neurological disorders. PLoS Biol. (2019) 17:e3000516. doi: 10.1371/journal.pbio.3000516
45. Belsey PP, Nicholas MA, Yttri EA. Open-source joystick manipulandum for decision-making, reaching, and motor control studies in mice. eNeuro. (2020) 7:ENEURO.0523-19.2020. doi: 10.1523/ENEURO.0523-19.2020
46. Nath T, Mathis A, Chen AC, Patel A, Bethge M, Mathis MW. Using DeepLabCut for 3D markerless pose estimation across species and behaviors. Nat Protoc. (2019) 14:2152–76. doi: 10.1038/s41596-019-0176-0
47. Mathis A, Mamidanna P, Cury KM, Abe T, Murthy VN, Mathis MW, et al. DeepLabCut: markerless pose estimation of user-defined body parts with deep learning. Nat Neurosci. (2018) 21:1281–9. doi: 10.1038/s41593-018-0209-y
48. Turella L, Lingnau A. Neural correlates of grasping. Front Hum Neurosci. (2014) 8:686. doi: 10.3389/fnhum.2014.00686
49. Caminiti R, Innocenti GM, Battaglia-Mayer A. Organization and evolution of parieto-frontal processing streams in macaque monkeys and humans. Neurosci Biobehav Rev. (2015) 56:73–96. doi: 10.1016/j.neubiorev.2015.06.014
50. Culham JC, Danckert SL, De Souza JF, Gati JS, Menon RS, Goodale MA. Visually guided grasping produces fMRI activation in dorsal but not ventral stream brain areas. Exp Brain Res. (2003) 153:180–9. doi: 10.1007/s00221-003-1591-5
51. Caminiti R, Ferraina S, Mayer AB. Visuomotor transformations: early cortical mechanisms of reaching. Curr Opin Neurobiol. (1998) 8:753–61. doi: 10.1016/S0959-4388(98)80118-9
52. Davare M, Kraskov A, Rothwell JC, Lemon RN. Interactions between areas of the cortical grasping network. Curr Opin Neurobiol. (2011) 21:565–70. doi: 10.1016/j.conb.2011.05.021
53. Vesia M, Crawford JD. Specialization of reach function in human posterior parietal cortex. Exp Brain Res. (2012) 221:1–18. doi: 10.1007/s00221-012-3158-9
54. Davare M, Rothwell JC, Lemon RN. Causal connectivity between the human anterior intraparietal area and premotor cortex during grasp. Curr Biol. (2010) 20:176–81. doi: 10.1016/j.cub.2009.11.063
55. Galletti C, Fattori P. The dorsal visual stream revisited: stable circuits or dynamic pathways? Cortex. (2018) 98:203–17. doi: 10.1016/j.cortex.2017.01.009
56. Zackowski KM, Thach WT Jr, Bastian AJ. Cerebellar subjects show impaired coupling of reach and grasp movements. Exp Brain Res. (2002) 146:511–22. doi: 10.1007/s00221-002-1191-9
57. Dudman JT, Krakauer JW. The basal ganglia: from motor commands to the control of vigor. Curr Opin Neurobiol. (2016) 37:158–66. doi: 10.1016/j.conb.2016.02.005
58. Cisek P, Kalaska JF. Neural mechanisms for interacting with a world full of action choices. Annu Rev Neurosci. (2010) 33:269–98. doi: 10.1146/annurev.neuro.051508.135409
59. Lemon RN. Descending pathways in motor control. Annu Rev Neurosci. (2008) 31:195–218. doi: 10.1146/annurev.neuro.31.060407.125547
60. Courtine G, Bunge MB, Fawcett JW, Grossman RG, Kaas JH, Lemon R, et al. Can experiments in nonhuman primates expedite the translation of treatments for spinal cord injury in humans? Nat Med. (2007) 13:561–6. doi: 10.1038/nm1595
61. Tazoe T, Perez MA. Cortical and reticular contributions to human precision and power grip. J Physiol. (2017) 595:2715–30. doi: 10.1113/JP273679
62. Federico P, Perez MA. Distinct corticocortical contributions to human precision and power grip. Cereb Cortex. (2017) 27:5070–82. doi: 10.1093/cercor/bhw291
63. Karl JM, Sacrey L, Whishaw IQ. Multiple motor channel theory the development of skilled hand movements in human infants. In: Corbetta D, Santello M, editors. Reach-to-Grasp Behavior. Brain, Behavior, and Modeling Across the Life Span. New York, NY: Routledge (2018). p. 42–68.
64. Karl JM, Whishaw IQ. Haptic grasping configurations in early infancy reveal different developmental profiles for visual guidance of the reach versus the grasp. Exp Brain Res. (2014) 232:3301–16. doi: 10.1007/s00221-014-4013-y
65. Loenneker T, Klaver P, Bucher K, Lichtensteiger J, Imfeld A, Martin E. Microstructural development: organizational differences of the fiber architecture between children and adults in dorsal and ventral visual streams. Hum Brain Mapp. (2011) 32:935–46. doi: 10.1002/hbm.21080
66. Kuhtz-Buschbeck JP, Stolze H, Johnk K, Boczek-Funcke A, Illert M. Development of prehension movements in children: a kinematic study. Exp Brain Res. (1998) 122:424–32. doi: 10.1007/s002210050530
67. Jeannerod M. The formation of finger grip during prehension. A cortically mediated visuomotor pattern. Behav Brain Res. (1986) 19:99–116. doi: 10.1016/0166-4328(86)90008-2
68. Lemon RN, Johansson RS, Westling G. Corticospinal control during reach, grasp, and precision lift in man. J Neurosci. (1995) 15:6145–56. doi: 10.1523/JNEUROSCI.15-09-06145.1995
69. James TW, Culham J, Humphrey GK, Milner AD, Goodale MA. Ventral occipital lesions impair object recognition but not object-directed grasping: an fMRI study. Brain. (2003) 126:2463–75. doi: 10.1093/brain/awg248
70. Davare M, Andres M, Cosnard G, Thonnard JL, Olivier E. Dissociating the role of ventral and dorsal premotor cortex in precision grasping. J Neurosci. (2006) 26:2260–8. doi: 10.1523/JNEUROSCI.3386-05.2006
71. Saiki A, Kimura R, Samura T, Fujiwara-Tsukamoto Y, Sakai Y, Isomura Y. Different modulation of common motor information in rat primary and secondary motor cortices. PLoS ONE. (2014) 9:e98662. doi: 10.1371/journal.pone.0098662
72. Harrison TC, Ayling OG, Murphy TH. Distinct cortical circuit mechanisms for complex forelimb movement and motor map topography. Neuron. (2012) 74:397–409. doi: 10.1016/j.neuron.2012.02.028
73. Alstermark B, Isa T. Circuits for skilled reaching and grasping. Annu Rev Neurosci. (2012) 35:559–78. doi: 10.1146/annurev-neuro-062111-150527
74. Klein A, Dunnett SB. Analysis of skilled forelimb movement in rats: the single pellet reaching test and staircase test. Curr Protoc Neurosci. (2012) 8:Unit8.28. doi: 10.1002/0471142301.ns0828s58
75. Whitlock JR. Navigating actions through the rodent parietal cortex. Front Hum Neurosci. (2014) 8:293. doi: 10.3389/fnhum.2014.00293
76. Hira R, Ohkubo F, Tanaka YR, Masamizu Y, Augustine GJ, Kasai H, et al. In vivo optogenetic tracing of functional corticocortical connections between motor forelimb areas. Front Neural Circuits. (2013) 7:55. doi: 10.3389/fncir.2013.00055
77. Wang X, Liu Y, Li X, Zhang Z, Yang H, Zhang Y, et al. Deconstruction of corticospinal circuits for goal-directed motor skills. Cell. (2017) 171:440–55.e14. doi: 10.1016/j.cell.2017.08.014
78. Brown AR, Teskey GC. Motor cortex is functionally organized as a set of spatially distinct representations for complex movements. J Neurosci. (2014) 34:13574–85. doi: 10.1523/JNEUROSCI.2500-14.2014
79. Kleim JA, Barbay S, Nudo RJ. Functional reorganization of the rat motor cortex following motor skill learning. J Neurophysiol. (1998) 80:3321–5. doi: 10.1152/jn.1998.80.6.3321
80. Tennant KA, Adkins DL, Donlan NA, Asay AL, Thomas N, Kleim JA, et al. The organization of the forelimb representation of the C57BL/6 mouse motor cortex as defined by intracortical microstimulation and cytoarchitecture. Cereb Cortex. (2011) 21:865–76. doi: 10.1093/cercor/bhq159
81. Ramanathan D, Conner JM, Tuszynski MH. A form of motor cortical plasticity that correlates with recovery of function after brain injury. Proc Natl Acad Sci USA. (2006) 103:11370–5. doi: 10.1073/pnas.0601065103
82. Graziano MS, Taylor CS, Moore T. Complex movements evoked by microstimulation of precentral cortex. Neuron. (2002) 34:841–51. doi: 10.1016/S0896-6273(02)00698-0
83. Gharbawie OA, Stepniewska I, Qi H, Kaas JH. Multiple parietal-frontal pathways mediate grasping in macaque monkeys. J Neurosci. (2011) 31:11660–77. doi: 10.1523/JNEUROSCI.1777-11.2011
84. Graziano MSA. Ethological action maps: a paradigm shift for the motor cortex. Trends Cogn Sci. (2016) 20:121–32. doi: 10.1016/j.tics.2015.10.008
85. Desmurget M, Richard N, Harquel S, Baraduc P, Szathmari A, Mottolese C, et al. Neural representations of ethologically relevant hand/mouth synergies in the human precentral gyrus. Proc Natl Acad Sci USA. (2014) 111:5718–22. doi: 10.1073/pnas.1321909111
86. Budri M, Lodi E, Franchi G. Sensorimotor restriction affects complex movement topography and reachable space in the rat motor cortex. Front Syst Neurosci. (2014) 8:231. doi: 10.3389/fnsys.2014.00231
87. Aflalo TN, Graziano MS. Possible origins of the complex topographic organization of motor cortex: reduction of a multidimensional space onto a two-dimensional array. J Neurosci. (2006) 26:6288–97. doi: 10.1523/JNEUROSCI.0768-06.2006
88. Stark E, Asher I, Abeles M. Encoding of reach and grasp by single neurons in premotor cortex is independent of recording site. J Neurophysiol. (2007) 97:3351–64. doi: 10.1152/jn.01328.2006
89. Fabbri S, Strnad L, Caramazza A, Lingnau A. Overlapping representations for grip type and reach direction. Neuroimage. (2014) 94:138–46. doi: 10.1016/j.neuroimage.2014.03.017
90. Fattori P, Raos V, Breveglieri R, Bosco A, Marzocchi N, Galletti C. The dorsomedial pathway is not just for reaching: grasping neurons in the medial parieto-occipital cortex of the macaque monkey. J Neurosci. (2010) 30:342–9. doi: 10.1523/JNEUROSCI.3800-09.2010
91. Monaco S, Cavina-Pratesi C, Sedda A, Fattori P, Galletti C, Culham JC. Functional magnetic resonance adaptation reveals the involvement of the dorsomedial stream in hand orientation for grasping. J Neurophysiol. (2011) 106:2248–63. doi: 10.1152/jn.01069.2010
92. Hyland B. Neural activity related to reaching and grasping in rostral and caudal regions of rat motor cortex. Behav Brain Res. (1998) 94:255–69. doi: 10.1016/S0166-4328(97)00157-5
93. Nishibe M, Urban ET, 3rd, Barbay S, Nudo RJ. Rehabilitative training promotes rapid motor recovery but delayed motor map reorganization in a rat cortical ischemic infarct model. Neurorehabil Neural Repair. (2015) 29:472–82. doi: 10.1177/1545968314543499
94. Rouse AG, Mazurek KA, Liu Z, Rivlis G, Schieber MH. How separate are reaching and grasping? In: Corbetta D, Santello M, editors. Reach-to-Grasp Behavior: Brain, Behavior, and Modelling Across the Life Span. New York, NY: Routledge (2018). p. 119–42.
95. Kaas JH. The evolution of neocortex in primates. Prog Brain Res. (2012) 195:91–102. doi: 10.1016/B978-0-444-53860-4.00005-2
96. Alstermark B, Pettersson LG. Skilled reaching and grasping in the rat: lacking effect of corticospinal lesion. Front Neurol. (2014) 5:103. doi: 10.3389/fneur.2014.00103
97. Rathelot JA, Strick PL. Subdivisions of primary motor cortex based on cortico-motoneuronal cells. Proc Natl Acad Sci USA. (2009) 106:918–23. doi: 10.1073/pnas.0808362106
98. Lawrence DG, Kuypers HG. The functional organization of the motor system in the monkey. II the effects of bilateral pyramidal lesions. Brain. (1968) 91:1–14. doi: 10.1093/brain/91.1.1
99. Whitlock JR. Posterior parietal cortex. Curr Biol. (2017) 27:R691–5. doi: 10.1016/j.cub.2017.06.007
100. Lyamzin D, Benucci A. The mouse posterior parietal cortex: anatomy and functions. Neurosci Res. (2019) 140:14–22. doi: 10.1016/j.neures.2018.10.008
101. Baldwin MK, Cooke DF, Krubitzer L. Intracortical microstimulation maps of motor, somatosensory, and posterior parietal cortex in tree shrews (Tupaia belangeri) reveal complex movement representations. Cereb Cortex. (2016) 27:bhv329. doi: 10.1093/cercor/bhv329
102. Soma S, Yoshida J, Kato S, Takahashi Y, Nonomura S, Sugimura YK, et al. Ipsilateral-dominant control of limb movements in rodent posterior parietal cortex. J Neurosci. (2019) 39:485–502. doi: 10.1523/JNEUROSCI.1584-18.2018
103. Winstein CJ, Stein J, Arena R, Bates B, Cherney LR, Cramer SC, et al. American heart association stroke council, C.O.stroke nursing CC, C. council on quality of, and outcomes R. Guidelines for adult stroke rehabilitation and recovery: a guideline for healthcare professionals from the american heart association/american stroke association. Stroke. (2016) 47:e98–169. doi: 10.1161/STR.0000000000000098
104. Nakayama H, Jorgensen HS, Raaschou HO, Olsen TS. Recovery of upper extremity function in stroke patients: the copenhagen stroke study. Arch Phys Med Rehabil. (1994) 75:394–8. doi: 10.1016/0003-9993(94)90161-9
105. Sathian K, Buxbaum LJ, Cohen LG, Krakauer JW, Lang CE, Corbetta M, et al. Neurological principles and rehabilitation of action disorders: common clinical deficits. Neurorehabil Neural Repair. (2011) 25:21S−32. doi: 10.1177/1545968311410941
106. van Vliet P, Pelton TA, Hollands KL, Carey L, Wing AM. Neuroscience findings on coordination of reaching to grasp an object: implications for research. Neurorehabil Neural Repair. (2013) 27:622–35. doi: 10.1177/1545968313483578
107. Wenzelburger R, Kopper F, Frenzel A, Stolze H, Klebe S, Brossmann A, et al. Hand coordination following capsular stroke. Brain. (2005) 128:64–74. doi: 10.1093/brain/awh317
108. Kozlowski DA, Leasure JL, Schallert T. The control of movement following traumatic brain injury. Compr Physiol. (2013) 3:121–39. doi: 10.1002/cphy.c110005
109. Debert CT, Herter TM, Scott SH, Dukelow S. Robotic assessment of sensorimotor deficits after traumatic brain injury. J Neurol Phys Ther. (2012) 36:58–67. doi: 10.1097/NPT.0b013e318254bd4f
110. Cantagallo A, Di Russo F, Favilla M, Zoccolotti P. Targeted isometric force impulses in patients with traumatic brain injury reveal delayed motor programming and change of strategy. J Neurotrauma. (2015) 32:563–70. doi: 10.1089/neu.2014.3571
111. Kuhtz-Buschbeck JP, Stolze H, Golge M, Ritz A. Analyses of gait, reaching, and grasping in children after traumatic brain injury. Arch Phys Med Rehabil. (2003) 84:424–30. doi: 10.1053/apmr.2003.50017
112. Rehme AK, Grefkes C. Cerebral network disorders after stroke: evidence from imaging-based connectivity analyses of active and resting brain states in humans. J Physiol. (2013) 591:17–31. doi: 10.1113/jphysiol.2012.243469
113. Grefkes C, Ward NS. Cortical reorganization after stroke: how much and how functional? Neuroscientist. (2014) 20:56–70. doi: 10.1177/1073858413491147
114. Zeiler SR, Krakauer JW. The interaction between training and plasticity in the poststroke brain. Curr Opin Neurol. (2013) 26:609–16. doi: 10.1097/WCO.0000000000000025
115. Carrera E, Tononi G. Diaschisis: past, present, future. Brain. (2014) 137:2408–22. doi: 10.1093/brain/awu101
116. Sharp DJ, Scott G, Leech R. Network dysfunction after traumatic brain injury. Nat Rev Neurol. (2014) 10:156–66. doi: 10.1038/nrneurol.2014.15
117. Ovadia-Caro S, Khalil AA, Sehm B, Villringer A, Nikulin VV, Nazarova M. Predicting the response to non-invasive brain stimulation in stroke. Front Neurol. (2019) 10:302. doi: 10.3389/fneur.2019.00302
118. Fagerholm ED, Hellyer PJ, Scott G, Leech R, Sharp DJ. Disconnection of network hubs and cognitive impairment after traumatic brain injury. Brain. (2015) 138:1696–709. doi: 10.1093/brain/awv075
119. Guggisberg AG, Koch PJ, Hummel FC, Buetefisch CM. Brain networks and their relevance for stroke rehabilitation. Clin Neurophysiol. (2019) 130:1098–124. doi: 10.1016/j.clinph.2019.04.004
120. Rehme AK, Eickhoff SB, Rottschy C, Fink GR, Grefkes C. Activation likelihood estimation meta-analysis of motor-related neural activity after stroke. Neuroimage. (2012) 59:2771–82. doi: 10.1016/j.neuroimage.2011.10.023
121. Wiese H, Tonnes C, de Greiff A, Nebel K, Diener HC, Stude P. Self-initiated movements in chronic prefrontal traumatic brain injury: an event-related functional MRI study. Neuroimage. (2006) 30:1292–301. doi: 10.1016/j.neuroimage.2005.11.012
122. Kasahara M, Menon DK, Salmond CH, Outtrim JG, Taylor Tavares JV, Carpenter TA, et al. Altered functional connectivity in the motor network after traumatic brain injury. Neurology. (2010) 75:168–76. doi: 10.1212/WNL.0b013e3181e7ca58
123. Inman CS, James GA, Hamann S, Rajendra JK, Pagnoni G, Butler AJ. Altered resting-state effective connectivity of fronto-parietal motor control systems on the primary motor network following stroke. Neuroimage. (2012) 59:227–37. doi: 10.1016/j.neuroimage.2011.07.083
124. Schulz R, Koch P, Zimerman M, Wessel M, Bonstrup M, Thomalla G, et al. Parietofrontal motor pathways and their association with motor function after stroke. Brain. (2015) 138:1949–60. doi: 10.1093/brain/awv100
125. Schulz R, Buchholz A, Frey BM, Bonstrup M, Cheng B, Thomalla G, et al. Enhanced effective connectivity between primary motor cortex and intraparietal sulcus in well-recovered stroke patients. Stroke. (2016) 47:482–9. doi: 10.1161/STROKEAHA.115.011641
126. Byblow WD, Stinear CM, Barber PA, Petoe MA, Ackerley SJ. Proportional recovery after stroke depends on corticomotor integrity. Ann Neurol. (2015) 78:848–59. doi: 10.1002/ana.24472
127. Stinear CM. Prediction of motor recovery after stroke: advances in biomarkers. Lancet Neurol. (2017) 16:826–36. doi: 10.1016/S1474-4422(17)30283-1
128. Stinear CM, Byblow WD, Ackerley SJ, Smith MC, Borges VM, Barber PA. PREP2: a biomarker-based algorithm for predicting upper limb function after stroke. Ann Clin Transl Neurol. (2017) 4:811–20. doi: 10.1002/acn3.488
129. Stinear CM, Barber PA, Smale PR, Coxon JP, Fleming MK, Byblow WD. Functional potential in chronic stroke patients depends on corticospinal tract integrity. Brain. (2007) 130:170–80. doi: 10.1093/brain/awl333
130. Peters DM, Fridriksson J, Stewart JC, Richardson JD, Rorden C, Bonilha L, et al. Cortical disconnection of the ipsilesional primary motor cortex is associated with gait speed and upper extremity motor impairment in chronic left hemispheric stroke. Hum Brain Mapp. (2018) 39:120–32. doi: 10.1002/hbm.23829
131. Sterr A, Dean PJ, Szameitat AJ, Conforto AB, Shen S. Corticospinal tract integrity and lesion volume play different roles in chronic hemiparesis and its improvement through motor practice. Neurorehabil Neural Repair. (2014) 28:335–43. doi: 10.1177/1545968313510972
132. Blicher JU, Near J, Naess-Schmidt E, Stagg CJ, Johansen-Berg H, Nielsen JF, et al. GABA levels are decreased after stroke and GABA changes during rehabilitation correlate with motor improvement. Neurorehabil Neural Repair. (2015) 29:278–86. doi: 10.1177/1545968314543652
133. Liepert J, Restemeyer C, Kucinski T, Zittel S, Weiller C. Motor strokes: the lesion location determines motor excitability changes. Stroke. (2005) 36:2648–53. doi: 10.1161/01.STR.0000189629.10603.02
134. Butefisch CM, Wessling M, Netz J, Seitz RJ, Homberg V. Relationship between interhemispheric inhibition and motor cortex excitability in subacute stroke patients. Neurorehabil Neural Repair. (2008) 22:4–21. doi: 10.1177/1545968307301769
135. Bernabeu M, Demirtas-Tatlidede A, Opisso E, Lopez R, Tormos JM, Pascual-Leone A. Abnormal corticospinal excitability in traumatic diffuse axonal brain injury. J Neurotrauma. (2009) 26:2185–93. doi: 10.1089/neu.2008.0859
136. Jang SH. Diffusion tensor imaging studies on corticospinal tract injury following traumatic brain injury: a review. NeuroRehabilitation. (2011) 29:339–45. doi: 10.3233/NRE-2011-0710
137. Di Pino G, Pellegrino G, Assenza G, Capone F, Ferreri F, Formica D, et al. Modulation of brain plasticity in stroke: a novel model for neurorehabilitation. Nat Rev Neurol. (2014) 10:597–608. doi: 10.1038/nrneurol.2014.162
138. Bradnam LV, Stinear CM, Byblow WD. Ipsilateral motor pathways after stroke: implications for non-invasive brain stimulation. Front Hum Neurosci. (2013) 7:184. doi: 10.3389/fnhum.2013.00184
139. Bestmann S, Swayne O, Blankenburg F, Ruff CC, Teo J, Weiskopf N, et al. The role of contralesional dorsal premotor cortex after stroke as studied with concurrent TMS-fMRI. J Neurosci. (2010) 30:11926–37. doi: 10.1523/JNEUROSCI.5642-09.2010
140. Kantak SS, Stinear JW, Buch ER, Cohen LG. Rewiring the brain: potential role of the premotor cortex in motor control, learning, and recovery of function following brain injury. Neurorehabil Neural Repair. (2012) 26:282–92. doi: 10.1177/1545968311420845
141. Schambra HM. Repetitive transcranial magnetic stimulation for upper extremity motor recovery: does it help? Curr Neurol Neurosci Rep. (2018) 18:97. doi: 10.1007/s11910-018-0913-8
142. Xu J, Branscheidt M, Schambra H, Steiner L, Widmer M, Diedrichsen J, et al. Rethinking interhemispheric imbalance as a target for stroke neurorehabilitation. Ann Neurol. (2019) 85:502–13. doi: 10.1002/ana.25452
143. Andersen KK, Olsen TS, Dehlendorff C, Kammersgaard LP. Hemorrhagic and ischemic strokes compared: stroke severity, mortality, risk factors. Stroke. (2009) 40:2068–72. doi: 10.1161/STROKEAHA.108.540112
144. Kolb B, Cioe J, Whishaw IQ. Is there an optimal age for recovery from motor cortex lesions? II, behavioural and anatomical consequences of unilateral motor cortex lesions in perinatal, infant, adult rats. Restor Neurol Neurosci. (2000) 17:61–70.
145. Nishibe M, Barbay S, Guggenmos D, Nudo RJ. Reorganization of motor cortex after controlled cortical impact in rats and implications for functional recovery. J Neurotrauma. (2010) 27:2221–32. doi: 10.1089/neu.2010.1456
146. Whishaw IQ. Loss of the innate cortical engram for action patterns used in skilled reaching and the development of behavioral compensation following motor cortex lesions in the rat. Neuropharmacology. (2000) 39:788–805. doi: 10.1016/S0028-3908(99)00259-2
147. Huntley G. Correlation between patterns of horizontal connectivity and the extend of short-term representational plasticity in rat motor cortex. CerebCortex. (1997) 7:143–56. doi: 10.1093/cercor/7.2.143
148. Farkas T, Perge J, Kis Z, Wolff JR, Toldi J. Facial nerve injury-induced disinhibition in the primary motor cortices of both hemispheres. Eur J Neurosci. (2000) 12:2190–4. doi: 10.1046/j.1460-9568.2000.00096.x
149. Toldi J, Farkas T, Perge J, Wolff JR. Facial nerve injury produces a latent somatosensory input through recruitment of the motor cortex in the rat. Neuroreport. (1999) 10:2143–7. doi: 10.1097/00001756-199907130-00027
150. Levy LM, Ziemann U, Chen R, Cohen LG. Rapid modulation of GABA in sensorimotor cortex induced by acute deafferentation. Ann Neurol. (2002) 52:755–61. doi: 10.1002/ana.10372
151. Castro-Alamancos MA, Garcia-Segura LM, Borrell J. Transfer of function to a specific area of the cortex after induced recovery from brain damage. Eur J Neurosci. (1992) 4:853–63. doi: 10.1111/j.1460-9568.1992.tb00195.x
152. Ramanathan D, Tuszynski MH, Conner JM. The basal forebrain cholinergic system is required specifically for behaviorally mediated cortical map plasticity. J Neurosci. (2009) 29:5992–6000. doi: 10.1523/JNEUROSCI.0230-09.2009
153. Ramanathan DS, Guo L, Gulati T, Davidson G, Hishinuma AK, Won SJ, et al. Low-frequency cortical activity is a neuromodulatory target that tracks recovery after stroke. Nat Med. (2018) 24:1257–67. doi: 10.1038/s41591-018-0058-y
154. Jones TA. Motor compensation and its effects on neural reorganization after stroke. Nat Rev Neurosci. (2017) 18:267–80. doi: 10.1038/nrn.2017.26
155. Shanina EV, Schallert T, Witte OW, Redecker C. Behavioral recovery from unilateral photothrombotic infarcts of the forelimb sensorimotor cortex in rats: role of the contralateral cortex. Neuroscience. (2006) 139:1495–506. doi: 10.1016/j.neuroscience.2006.01.016
156. Passineau MJ, Green EJ, Dietrich WD. Therapeutic effects of environmental enrichment on cognitive function and tissue integrity following severe traumatic brain injury in rats. Exp Neurol. (2001) 168:373–84. doi: 10.1006/exnr.2000.7623
157. Kleim JA, Jones TA, Schallert T. Motor enrichment and the induction of plasticity before or after brain injury. Neurochem Res. (2003) 28:1757–69. doi: 10.1023/A:1026025408742
158. Holden MK. Virtual environments for motor rehabilitation: review. Cyberpsychol Behav. (2005) 8:187–211. doi: 10.1089/cpb.2005.8.187
159. Dobkin BH. Motor rehabilitation after stroke, traumatic brain, and spinal cord injury: common denominators within recent clinical trials. Curr Opin Neurol. (2009) 22:563–9. doi: 10.1097/WCO.0b013e3283314b11
160. Lim DH, LeDue JM, Mohajerani MH, Murphy TH. Optogenetic mapping after stroke reveals network-wide scaling of functional connections and heterogeneous recovery of the peri-infarct. J Neurosci. (2014) 34:16455–66. doi: 10.1523/JNEUROSCI.3384-14.2014
161. Nudo RJ, Milliken GW. Reorganization of movement representations in primary motor cortex following focal ischemic infarcts in adult squirrel monkeys. J Neurophysiol. (1996) 75:2144–9. doi: 10.1152/jn.1996.75.5.2144
162. Axelson HW, Winkler T, Flygt J, Djupsjo A, Hanell A, Marklund N. Plasticity of the contralateral motor cortex following focal traumatic brain injury in the rat. Restor Neurol Neurosci. (2013) 31:73–85. doi: 10.3233/RNN-2012-120242
163. Griesbach GS, Hovda DA, Molteni R, Wu A, Gomez-Pinilla F. Voluntary exercise following traumatic brain injury: brain-derived neurotrophic factor upregulation and recovery of function. Neuroscience. (2004) 125:129–39. doi: 10.1016/j.neuroscience.2004.01.030
164. Wu H, Lu D, Jiang H, Xiong Y, Qu C, Li B, et al. Simvastatin-mediated upregulation of VEGF and BDNF, activation of the PI3K/Akt pathway, and increase of neurogenesis are associated with therapeutic improvement after traumatic brain injury. J Neurotrauma. (2008) 25:130–9. doi: 10.1089/neu.2007.0369
165. Griesbach GS, Hovda DA, Gomez-Pinilla F. Exercise-induced improvement in cognitive performance after traumatic brain injury in rats is dependent on BDNF activation. Brain Res. (2009) 1288:105–15. doi: 10.1016/j.brainres.2009.06.045
166. Chen A, Xiong LJ, Tong Y, Mao M. The neuroprotective roles of BDNF in hypoxic ischemic brain injury. Biomed Rep. (2013) 1:167–76. doi: 10.3892/br.2012.48
167. Ding MC, Wang Q, Lo EH, Stanley GB. Cortical excitation and inhibition following focal traumatic brain injury. J Neurosci. (2011) 31:14085–94. doi: 10.1523/JNEUROSCI.3572-11.2011
168. Froemke RC. Plasticity of cortical excitatory-inhibitory balance. Annu Rev Neurosci. (2015) 38:195–219. doi: 10.1146/annurev-neuro-071714-034002
169. Butler CR, Boychuk JA, Smith BN. Brain injury-induced synaptic reorganization in hilar inhibitory neurons is differentially suppressed by rapamycin. eNeuro. (2017) 4:ENEURO.0134-17.2017. doi: 10.1523/ENEURO.0134-17.2017
170. Benali A, Weiler E, Benali Y, Dinse HR, Eysel UT. Excitation and inhibition jointly regulate cortical reorganization in adult rats. J Neurosci. (2008) 28:12284–93. doi: 10.1523/JNEUROSCI.1952-08.2008
171. Guerriero RM, Giza CC, Rotenberg A. Glutamate and GABA imbalance following traumatic brain injury. Curr Neurol Neurosci Rep. (2015) 15:27. doi: 10.1007/s11910-015-0545-1
172. Buitrago MM, Ringer T, Schulz JB, Dichgans J, Luft AR. Characterization of motor skill and instrumental learning time scales in a skilled reaching task in rat. Behav Brain Res. (2004) 155:249–56. doi: 10.1016/j.bbr.2004.04.025
173. Nudo RJ. Adaptive plasticity in motor cortex: implications for rehabilitation after brain injury. J Rehabil Med. (2003) 41:7–10. doi: 10.1080/16501960310010070
174. Huber D, Gutnisky DA, Peron S, O'Connor DH, Wiegert JS, Tian L, et al. Multiple dynamic representations in the motor cortex during sensorimotor learning. Nature. (2012) 484:473–8. doi: 10.1038/nature11039
175. Heindorf M, Arber S, Keller GB. Mouse motor cortex coordinates the behavioral response to unpredicted sensory feedback. Neuron. (2018) 99:1040–54.e5. doi: 10.1016/j.neuron.2018.07.046
176. Conner JM, Kulczycki M, Tuszynski MH. Unique contributions of distinct cholinergic projections to motor cortical plasticity and learning. Cereb Cortex. (2010) 20:2739–48. doi: 10.1093/cercor/bhq022
177. Hosp JA, Molina-Luna K, Hertler B, Atiemo CO, Luft AR. Dopaminergic modulation of motor maps in rat motor cortex: an in vivo study. Neuroscience. (2009) 159:692–700. doi: 10.1016/j.neuroscience.2008.12.056
178. Hosp JA, Pekanovic A, Rioult-Pedotti MS, Luft AR. Dopaminergic projections from midbrain to primary motor cortex mediate motor skill learning. J Neurosci. (2011) 31:2481–7. doi: 10.1523/JNEUROSCI.5411-10.2011
179. Obermayer J, Verhoog MB, Luchicchi A, Mansvelder HD. Cholinergic modulation of cortical microcircuits is layer-specific: evidence from rodent, monkey and human brain. Front Neural Circuits. (2017) 11:100. doi: 10.3389/fncir.2017.00100
180. Coppola JJ, Disney AA. Is there a canonical cortical circuit for the cholinergic system? anatomical differences across common model systems. Front Neural Circuits. (2018) 12:8. doi: 10.3389/fncir.2018.00008
181. Verhoog MB, Obermayer J, Kortleven CA, Wilbers R, Wester J, Baayen JC, et al. Layer-specific cholinergic control of human and mouse cortical synaptic plasticity. Nat Commun. (2016) 7:12826. doi: 10.1038/ncomms12826
182. Ramanathan DS, Conner JM, Anilkumar AA, Tuszynski MH. Cholinergic systems are essential for late-stage maturation and refinement of motor cortical circuits. J Neurophysiol. (2015) 113:1585–97. doi: 10.1152/jn.00408.2014
183. Valero-Cabre A, Amengual JL, Stengel C, Pascual-Leone A, Coubard OA. Transcranial magnetic stimulation in basic and clinical neuroscience: a comprehensive review of fundamental principles and novel insights. Neurosci Biobehav Rev. (2017) 83:381–404. doi: 10.1016/j.neubiorev.2017.10.006
184. Gandiga PC, Hummel FC, Cohen LG. Transcranial DC stimulation (tDCS): a tool for double-blind sham-controlled clinical studies in brain stimulation. Clin Neurophysiol. (2006) 117:845–50. doi: 10.1016/j.clinph.2005.12.003
185. Liew SL, Santarnecchi E, Buch ER, Cohen LG. Non-invasive brain stimulation in neurorehabilitation: local and distant effects for motor recovery. Front Hum Neurosci. (2014) 8:378. doi: 10.3389/fnhum.2014.00378
186. Polania R, Nitsche MA, Ruff CC. Studying and modifying brain function with non-invasive brain stimulation. Nat Neurosci. (2018) 21:174–87. doi: 10.1038/s41593-017-0054-4
187. Auriat AM, Neva JL, Peters S, Ferris JK, Boyd LA. A review of transcranial magnetic stimulation and multimodal neuroimaging to characterize post-stroke neuroplasticity. Front Neurol. (2015) 8:226. doi: 10.3389/fneur.2015.00226
188. Pruski A, Cantarero G. Transcranial direct current stimulation for motor recovery following brain injury. Curr Phys Med Rehabil Rep. (2020). doi: 10.1007/s40141-020-00262-8. [Epub ahead of print].
189. Neville IS, Gomes-Osman J, Amorim RL, Hayashi CY, Galhardoni R, Zaninotto ALC. How can transcranial magnetic stimulation change the way we treat traumatic brain injury? Int J Clin Exp Med. (2018) 11:7643–50.
190. Kim WS, Lee K, Kim S, Cho S, Paik NJ. Transcranial direct current stimulation for the treatment of motor impairment following traumatic brain injury. J Neuroeng Rehabil. (2019) 16:14. doi: 10.1186/s12984-019-0489-9
191. Dionisio A, Duarte IC, Patricio M, Castelo-Branco M. The use of repetitive transcranial magnetic stimulation for stroke rehabilitation: a systematic review. J Stroke Cerebrovasc Dis. (2018) 27:1–31. doi: 10.1016/j.jstrokecerebrovasdis.2017.09.008
192. Butler AJ, Shuster M, O'Hara E, Hurley K, Middlebrooks D, Guilkey K. A meta-analysis of the efficacy of anodal transcranial direct current stimulation for upper limb motor recovery in stroke survivors. J Hand Ther. (2013) 26:162–70. doi: 10.1016/j.jht.2012.07.002
193. Klomjai W, Lackmy-Vallee A, Roche N, Pradat-Diehl P, Marchand-Pauvert V, Katz R. Repetitive transcranial magnetic stimulation and transcranial direct current stimulation in motor rehabilitation after stroke: an update. Ann Phys Rehabil Med. (2015) 58:220–4. doi: 10.1016/j.rehab.2015.05.006
194. Bornheim S, Thibaut A, Beaudart C, Maquet P, Croisier JL, Kaux JF. Evaluating the effects of tDCS in stroke patients using functional outcomes: a systematic review. Disabil Rehabil. (2020). doi: 10.1080/09638288.2020.1759703. [Epub ahead of print].
195. Graef P, Dadalt MLR, Rodrigués DAMDS, Stein C, Pagnussat AS. Transcranial magnetic stimulation combined with upper-limb training for improving function after stroke: a systematic review and meta-analysis. J Neurol Sci. (2016) 369:149–58. doi: 10.1016/j.jns.2016.08.016
196. Sebastianelli L, Versace V, Martignago S, Brigo F, Trinka E, Saltuari L, et al. Low-frequency rTMS of the unaffected hemisphere in stroke patients: a systematic review. Acta Neurol Scand. (2017) 136:585–605. doi: 10.1111/ane.12773
197. Adeyemo BO, Simis M, Macea D, Fregni F. Systematic review of parameters of stimulation, clinical trial design characteristics, and motor outcomes in non-invasive brain stimulation in stroke. Front Psychiatr. (2012) 3:88. doi: 10.3389/fpsyt.2012.00088
198. Peters HT, Edwards DJ, Wortman-Jutt S, Page SJ. Moving forward by stimulating the brain: transcranial direct current stimulation in post-stroke hemiparesis. Front Hum Neurosci. (2016) 10:394. doi: 10.3389/fnhum.2016.00394
199. Bolognini N, Pascual-Leone A, Fregni F. Using non-invasive brain stimulation to augment motor training-induced plasticity. J Neuroeng Rehabil. (2009) 6:8. doi: 10.1186/1743-0003-6-8
200. Kantak SS, Fisher BE, Sullivan KJ, Winstein CJ. Effects of different doses of low frequency rTMS on motor corticospinal excitability. J Neurol Neurophysiol. (2010) 1:102. doi: 10.4172/2155-9562.1000102
201. Chhatbar PY, Ramakrishnan V, Kautz S, George MS, Adams RJ, Feng W. Transcranial direct current stimulation post-stroke upper extremity motor recovery studies exhibit a dose-response relationship. Brain Stimul. (2016) 9:16–26. doi: 10.1016/j.brs.2015.09.002
202. Lefebvre S, Liew SL. Anatomical parameters of tDCS to modulate the motor system after stroke: a review. Fron Neurol. (2017) 8:29. doi: 10.3389/fneur.2017.00029
203. Hummel FC, Cohen LG. Non-invasive brain stimulation: a new strategy to improve neurorehabilitation after stroke? Lancet Neurol. (2006) 5:708–12. doi: 10.1016/S1474-4422(06)70525-7
204. Page SJ, Cunningham DA, Plow E, Blazak B. It takes two: noninvasive brain stimulation combined with neurorehabilitation. Arch Phys Med Rehabil. (2015) 96:S89-93. doi: 10.1016/j.apmr.2014.09.019
205. O'Brien AT, Bertolucci F, Torrealba-Acosta G, Huerta R, Fregni F, Thibaut A. Non-invasive brain stimulation for fine motor improvement after stroke: a meta-analysis. Eur J Neurol. (2018) 25:1017–26. doi: 10.1111/ene.13643
206. Nowak DA, Grefkes C, Dafotakis M, Eickhoff S, Kust J, Karbe H, et al. Effects of low-frequency repetitive transcranial magnetic stimulation of the contralesional primary motor cortex on movement kinematics and neural activity in subcortical stroke. Arch Neurol. (2008) 65:741–7. doi: 10.1001/archneur.65.6.741
207. Tretriluxana J, Kantak S, Tretriluxana S, Wu AD, Fisher BE. Low frequency repetitive transcranial magnetic stimulation to the non-lesioned hemisphere improves paretic arm reach-to-grasp performance after chronic stroke. Disabil Rehabil Assist Technol. (2013) 8:121–4. doi: 10.3109/17483107.2012.737136
208. Tretriluxana J, Kantak S, Tretriluxana S, Wu AD, Fisher BE. Improvement in paretic arm reach-to-grasp following low frequency repetitive transcranial magnetic stimulation depends on object size: a pilot study. Stroke Res Treat. (2015) 2015:498169. doi: 10.1155/2015/498169
209. Vongvaivanichakul P, Tretriluxana J, Bovonsunthonchai S, Pakaprot N, Laksanakorn W. Reach-to-grasp training in individuals with chronic stroke augmented by low-frequency repetitive transcranial magnetic stimulation. J Med Assoc Thai. (2014) 97(Suppl. 7):S45–9.
210. Ackerley SJ, Stinear CM, Barber PA, Byblow WD. Combining theta burst stimulation with training after subcortical stroke. Stroke. (2010) 41:1568–72. doi: 10.1161/STROKEAHA.110.583278
211. Lefebvre S, Thonnard JL, Laloux P, Peeters A, Jamart J, Vandermeeren Y. Single session of dual-tDCS transiently improves precision grip and dexterity of the paretic hand after stroke. Neurorehabil Neural Rep. (2014) 28:100–10. doi: 10.1177/1545968313478485
212. Volz LJ, Rehme AK, Michely J, Nettekoven C, Eickhoff SB, Fink GR, et al. Shaping early reorganization of neural networks promotes motor function after stroke. Cereb Cortex. (2016) 26:2882–94. doi: 10.1093/cercor/bhw034
213. Lotze M, Markert J, Sauseng P, Hoppe J, Plewnia C, Gerloff C. The role of multiple contralesional motor areas for complex hand movements after internal capsular lesion. J Neurosci. (2006) 26:6096–102. doi: 10.1523/JNEUROSCI.4564-05.2006
214. Cunningham DA, Varnerin N, Machado A, Bonnett C, Janini D, Roelle S, et al. Stimulation targeting higher motor areas in stroke rehabilitation: a proof-of-concept, randomized, double-blinded placebo-controlled study of effectiveness and underlying mechanisms. Restor Neurol Neurosci. (2015) 33:911–26. doi: 10.3233/RNN-150574
215. Lüdemann-Podubecká J, Bösl K, Nowak D. Inhibition of the contralesional dorsal premotor cortex improves motor function of the affected hand following stroke. Eur J Neurol. (2016) 23:823–30. doi: 10.1111/ene.12949
216. Marshall S, Bayley M, McCullagh S, Velikonja D, Berrigan L. Clinical practice guidelines for mild traumatic brain injury and persistent symptoms. Can Fam Phys. (2012) 58:257–67.
217. Maas AI, Stocchetti N, Bullock R. Moderate and severe traumatic brain injury in adults. Lancet Neurol. (2008) 7:728–41. doi: 10.1016/S1474-4422(08)70164-9
218. Peterson AB, Xu L, Daugherty J, Breiding MJ. Surveillance Report of Traumatic Brain Injury-Related Emergency Department Visits, Hospitalizations, and Deaths, United States. 2014. CDC (2019).
219. Finkelstein E, Corso PS, Miller TR. The Incidence and Economic Burden of Injuries in the United States. Oxford University Press (2006).
220. Demaerschalk BM, Hwang HM, Leung G. US cost burden of ischemic stroke: a systematic literature review. Am J Manag Care. (2010) 16:525–33.
221. Cambiaghi M, Velikova S, Gonzalez-Rosa JJ, Cursi M, Comi G, Leocani L. Brain transcranial direct current stimulation modulates motor excitability in mice. Eur J Neurosci. (2010) 31:704–9. doi: 10.1111/j.1460-9568.2010.07092.x
222. Maeda F, Keenan JP, Tormos JM, Topka H, Pascual-Leone A. Modulation of corticospinal excitability by repetitive transcranial magnetic stimulation. Clin Neurophysiol. (2000) 111:800–5. doi: 10.1016/S1388-2457(99)00323-5
223. Benali A, Trippe J, Weiler E, Mix A, Petrasch-Parwez E, Girzalsky W, et al. Theta-burst transcranial magnetic stimulation alters cortical inhibition. J Neurosci. (2011) 31:1193–203. doi: 10.1523/JNEUROSCI.1379-10.2011
224. Fritsch B, Reis J, Martinowich K, Schambra HM, Ji Y, Cohen LG, et al. Direct current stimulation promotes BDNF-dependent synaptic plasticity: potential implications for motor learning. Neuron. (2010) 66:198–204. doi: 10.1016/j.neuron.2010.03.035
225. Wang HY, Crupi D, Liu J, Stucky A, Cruciata G, Di Rocco A, et al. Repetitive transcranial magnetic stimulation enhances BDNF–TrkB signaling in both brain and lymphocyte. J Neurosci. (2011) 31:11044–54. doi: 10.1523/JNEUROSCI.2125-11.2011
226. Podda MV, Cocco S, Mastrodonato A, Fusco S, Leone L, Barbati SA, et al. Anodal transcranial direct current stimulation boosts synaptic plasticity and memory in mice via epigenetic regulation of Bdnf expression. Sci Rep. (2016) 6:22180. doi: 10.1038/srep22180
227. Ueyama E, Ukai S, Ogawa A, Yamamoto M, Kawaguchi S, Ishii R, et al. Chronic repetitive transcranial magnetic stimulation increases hippocampal neurogenesis in rats. Psychiatr Clin Neurosci. (2011) 65:77–81. doi: 10.1111/j.1440-1819.2010.02170.x
228. Pikhovych A, Stolberg NP, Jessica Flitsch L, Walter HL, Graf R, Fink GR, et al. Transcranial direct current stimulation modulates neurogenesis and microglia activation in the mouse brain. Stem Cells Int. (2016) 2016:2715196. doi: 10.1155/2016/2715196
229. Boychuk JA, Adkins DL, Kleim JA. Distributed versus focal cortical stimulation to enhance motor function and motor map plasticity in a rodent model of ischemia. Neurorehabil Neural Repair. (2011) 25:88–97. doi: 10.1177/1545968310385126
230. Carmel JB, Berrol LJ, Brus-Ramer M, Martin JH. Chronic electrical stimulation of the intact corticospinal system after unilateral injury restores skilled locomotor control and promotes spinal axon outgrowth. J Neurosci. (2010) 30:10918–26. doi: 10.1523/JNEUROSCI.1435-10.2010
231. Carmel JB, Kimura H, Berrol LJ, Martin JH. Motor cortex electrical stimulation promotes axon outgrowth to brain stem and spinal targets that control the forelimb impaired by unilateral corticospinal injury. Eur J Neurosci. (2013) 37:1090–102. doi: 10.1111/ejn.12119
232. Carmel JB, Kimura H, Martin JH. Electrical stimulation of motor cortex in the uninjured hemisphere after chronic unilateral injury promotes recovery of skilled locomotion through ipsilateral control. J Neurosci. (2014) 34:462–6. doi: 10.1523/JNEUROSCI.3315-13.2014
233. Adkins-Muir DL, Jones TA. Cortical electrical stimulation combined with rehabilitative training: enhanced functional recovery and dendritic plasticity following focal cortical ischemia in rats. Neurol Res. (2003) 25:780–8. doi: 10.1179/016164103771953853
234. Yoon KJ, Lee YT, Chae SW, Park CR, Kim DY. Effects of anodal transcranial direct current stimulation (tDCS) on behavioral and spatial memory during the early stage of traumatic brain injury in the rats. J Neurol Sci. (2016) 362:314–20. doi: 10.1016/j.jns.2016.02.005
235. Kleim JA, Bruneau R, VandenBerg P, MacDonald E, Mulrooney R, Pocock D. Motor cortex stimulation enhances motor recovery and reduces peri-infarct dysfunction following ischemic insult. Neurol Res. (2003) 25:789–93. doi: 10.1179/016164103771953862
236. Teskey GC, Young NA, van Rooyen F, Larson SE, Flynn C, Monfils MH, et al. Induction of neocortical long-term depression results in smaller movement representations, fewer excitatory perforated synapses, and more inhibitory synapses. Cereb Cortex. (2007) 17:434–42. doi: 10.1093/cercor/bhj160
237. Rueger MA, Keuters MH, Walberer M, Braun R, Klein R, Sparing R, et al. Multi-session transcranial direct current stimulation (tDCS) elicits inflammatory and regenerative processes in the rat brain. PLoS ONE. (2012) 7:e43776. doi: 10.1371/journal.pone.0043776
238. Keuters MH, Aswendt M, Tennstaedt A, Wiedermann D, Pikhovych A, Rotthues S, et al. Transcranial direct current stimulation promotes the mobility of engrafted NSCs in the rat brain. NMR Biomed. (2015) 28:231–9. doi: 10.1002/nbm.3244
239. Guggenmos DJ, Azin M, Barbay S, Mahnken JD, Dunham C, Mohseni P, et al. Restoration of function after brain damage using a neural prosthesis. Proc Natl Acad Sci USA. (2013) 110:21177–82. doi: 10.1073/pnas.1316885110
240. Liu J, Zhu B, Zhang G, Wang J, Tian W, Ju G, et al. Electric signals regulate directional migration of ventral midbrain derived dopaminergic neural progenitor cells via Wnt/GSK3beta signaling. Exp Neurol. (2015) 263:113–21. doi: 10.1016/j.expneurol.2014.09.014
241. Liu HH, Xiang Y, Yan TB, Tan ZM, Li SH, He XK. Functional electrical stimulation increases neural stem/progenitor cell proliferation and neurogenesis in the subventricular zone of rats with stroke. Chin Med J. (2013) 126:2361–7.
242. Latchoumane CV, Jackson L, Sendi MSE, Tehrani KF, Mortensen LJ, Stice SL, et al. Chronic electrical stimulation promotes the excitability and plasticity of ESC-derived neurons following glutamate-induced inhibition in vitro. Sci Rep. (2018) 8:10957. doi: 10.1038/s41598-018-29069-3
243. Ridding MC, Ziemann U. Determinants of the induction of cortical plasticity by non-invasive brain stimulation in healthy subjects. J Physiol. (2010) 588:2291–304. doi: 10.1113/jphysiol.2010.190314
244. English AW, Schwartz G, Meador W, Sabatier MJ, Mulligan A. Electrical stimulation promotes peripheral axon regeneration by enhanced neuronal neurotrophin signaling. Dev Neurobiol. (2007) 67:158–72. doi: 10.1002/dneu.20339
245. Regner GG, Pereira P, Leffa DT, de Oliveira C, Vercelino R, Fregni F, et al. Preclinical to clinical translation of studies of transcranial direct-current stimulation in the treatment of epilepsy: a systematic review. Front Neurosci. (2018) 12:189. doi: 10.3389/fnins.2018.00189
246. Rodger J, Sherrard RM. Optimising repetitive transcranial magnetic stimulation for neural circuit repair following traumatic brain injury. Neural Regen Res. (2015) 10:357–9. doi: 10.4103/1673-5374.153676
247. Brunoni AR, Fregni F. Clinical trial design in non-invasive brain stimulation psychiatric research. Int J Methods Psychiatr Res. (2011) 20:e19–30. doi: 10.1002/mpr.338
248. Cha YH, Urbano D, Pariseau N. Randomized single blind sham controlled trial of adjunctive home-based tDCS after rTMS for mal de debarquement syndrome: safety, efficacy, and participant satisfaction assessment. Brain Stimul. (2016) 9:537–44. doi: 10.1016/j.brs.2016.03.016
249. Bikson M, Grossman P, Thomas C, Zannou AL, Jiang J, Adnan T, et al. Safety of transcranial direct current stimulation: evidence based update 2016. Brain Stimul. (2016) 9:641–61. doi: 10.1016/j.brs.2016.06.004
250. Rossi S, Hallett M, Rossini PM, Pascual-Leone A, SOGroup TC. Safety, ethical considerations, and application guidelines for the use of transcranial magnetic stimulation in clinical practice and research. Clin Neurophysiol. (2009) 120:2008–39. doi: 10.1016/j.clinph.2009.08.016
251. Adkins DL, Boychuk J, Remple MS, Kleim JA. Motor training induces experience-specific patterns of plasticity across motor cortex and spinal cord. J Appl Physiol. (2006) 101:1776–82. doi: 10.1152/japplphysiol.00515.2006
252. Jefferson SC, Clayton ER, Donlan NA, Kozlowski DA, Jones TA, Adkins DL. Cortical stimulation concurrent with skilled motor training improves forelimb function and enhances motor cortical reorganization following controlled cortical impact. Neurorehabil Neural Rep. (2016) 30:155–8. doi: 10.1177/1545968315600274
253. O'Bryant AJ, Adkins DL, Sitko AA, Combs HL, Nordquist SK, Jones TA. Enduring poststroke motor functional improvements by a well–timed combination of motor rehabilitative training and cortical stimulation in rats. Neurorehabil Neural Repair. (2016) 30:143–54. doi: 10.1177/1545968314562112
254. Adkins DL, Ferguson L, Lance S, Pevtsov A, McDonough K, Stamschror J, et al. Combining multiple types of motor rehabilitation enhances skilled forelimb use following experimental traumatic brain injury in rats. Neurorehabil Neural Repair. (2015) 29:989–1000. doi: 10.1177/1545968315576577
255. Abd Hamid AI, Gall C, Speck O, Antal A, Sabel BA. Effects of alternating current stimulation on the healthy and diseased brain. Front Neurosci. (2015) 9:391. doi: 10.3389/fnins.2015.00391
256. Henrich-Noack P, Sergeeva EG, Sabel BA. Non-invasive electrical brain stimulation: from acute to late-stage treatment of central nervous system damage. Neural Regen Res. (2017) 12:1590. doi: 10.4103/1673-5374.217322
257. Zrenner C, Desideri D, Belardinelli P, Ziemann U. Real-time EEG-defined excitability states determine efficacy of TMS-induced plasticity in human motor cortex. Brain Stimul. (2018) 11:374–89. doi: 10.1016/j.brs.2017.11.016
258. Buetefisch C, Heger R, Schicks W, Seitz R, Netz J. Hebbian-type stimulation during robot-assisted training in patients with stroke. Neurorehabil Neural Repair. (2011) 25:645–55. doi: 10.1177/1545968311402507
259. Kwakkel G, Van Wegen E, Burridge J, Winstein C, Van Dokkum L, Alt Murphy M, et al. Standardized measurement of quality of upper limb movement after stroke: consensus-based core recommendations from the second stroke recovery and rehabilitation roundtable. Neurorehabil Neural Repair. (2019) 33:951–8. doi: 10.1177/1545968319886477
260. Voelkl B, Altman NS, Forsman A, Forstmeier W, Gurevitch J, Jaric I, et al. Reproducibility of animal research in light of biological variation. Nat Rev Neurosci. (2020) 21:384–93. doi: 10.1038/s41583-020-0313-3
Keywords: reach-and-grasp, stroke, traumatic brain injury, rodent, human, neuromodulation
Citation: Latchoumane C-FV, Barany DA, Karumbaiah L and Singh T (2020) Neurostimulation and Reach-to-Grasp Function Recovery Following Acquired Brain Injury: Insight From Pre-clinical Rodent Models and Human Applications. Front. Neurol. 11:835. doi: 10.3389/fneur.2020.00835
Received: 17 April 2020; Accepted: 06 July 2020;
Published: 21 July 2020.
Edited by:
Ina M. Tarkka, University of Jyväskylä, FinlandReviewed by:
Niamh C. Kennedy, Ulster University, United KingdomCristiano De Marchis, Roma Tre University, Italy
Copyright © 2020 Latchoumane, Barany, Karumbaiah and Singh. This is an open-access article distributed under the terms of the Creative Commons Attribution License (CC BY). The use, distribution or reproduction in other forums is permitted, provided the original author(s) and the copyright owner(s) are credited and that the original publication in this journal is cited, in accordance with accepted academic practice. No use, distribution or reproduction is permitted which does not comply with these terms.
*Correspondence: Tarkeshwar Singh, dHNpbmdoQHVnYS5lZHU=
†These authors have contributed equally to this work